- 1Marine Department, Coral Ecophysiology Team, Centre Scientifique de Monaco, Monaco, Monaco
- 2Sorbonne Université, Collège doctoral, Paris, France
- 3UPF, ILM, Ifremer, IRD, UMR 241 SECOPOL, Punaauia, Tahiti, French Polynesia
Seawater temperature and the availability of dissolved inorganic nutrients (DINut) have a major influence on the stability of the symbiosis between corals and Symbiodiniaceae. In particular, seawater warming or DINut depletion can lead to coral bleaching, the loss of Symbiodiniaceae from coral tissue. However, the combined effects of heat stress and DINut deficiency on the coral energy metabolism are still understudied. Here, we investigated the physiological and energetic responses of the octocoral Heteroxenia fuscescens and the hexacoral Stylophora pistillata exposed to two levels of inorganic nutrients in seawater (control, depleted) and two temperatures, 25°C (control) and 30°C (high temperature), in a crossed factorial design. Our results show that thermal and DINut stress both decreased the photosynthesis to respiration ratio of the two species and induced bleaching in H. fuscescens. While nutrient deprivation had little effect on the corals’ energy metabolism, heat stress led to higher concentrations of macromolecules such as carbohydrates and lipids, as well as anaerobic metabolism, and decreased ATP production in H. fuscescens. Given that the intensity and frequency of marine heatwaves will significantly increase in the future, there is an urgent need to investigate the processes by which corals can overcome starvation.
1 Introduction
Tropical shallow-water reefs harbor a remarkable diversity and abundance of marine life, although they are characterized by low nutrient concentrations in seawater (Hoegh-Guldberg and Dove, 2008; Knowlton et al., 2010). To cope with the nutrient-poor conditions, most corals live in mutualistic symbiosis with dinoflagellates from the Symbiodiniaceae family (LaJeunesse et al., 2018). These symbionts cover most of the host’s energy requirements by efficiently taking up dissolved inorganic nutrients (DINut) from seawater and translocating photosynthates to their host (Muscatine et al., 1984; Tremblay et al., 2014; Schubert et al., 2017). This autotrophic nutrient source can be supplemented by heterotrophy, the capture by the host of particulate (detritus and particles of different sizes ranging from bacteria to microzooplankton) and dissolved organic matter (reviewed in Houlbrèque and Ferrier-Pagès, 2009 and Goldberg, 2018).
Autotrophic and heterotrophic nutrients are mainly converted into three major macromolecules- proteins, lipids and carbohydrates- which are used for biomass and structural material building as well as for energy production and storage (Rodrigues and Grottoli, 2007; Kochman et al., 2021). While most carbohydrates are rapidly respired to meet the basic energy requirements for the maintenance and productivity of coral and symbiont cells (Davies, 1991; Kochman et al., 2021), they can also be incorporated into biomass or transformed into lipid via lipogenesis (Chen et al., 2017). Lipids are either structural (such as phospholipids in membranes) or used as the primary energy reserve. Such reserve can be consumed during periods of high energy demand (Grottoli et al., 2004; Rodrigues and Grottoli, 2007; Anthony et al., 2009) because they have energy-rich bonds that generate via oxidation ATP substrate, which is the primary energy currency in cells (Hardie et al., 2003). Finally, proteins are mainly used to build coral biomass, but can also be respired when carbohydrates and lipids are depleted (Lesser, 2013). Lipid and protein stores in coral tissue can be greatly increased when corals have access to a mixotrophic diet (Grottoli et al., 2006; Treignier et al., 2008). Under optimal living conditions, energetic homeostasis is maintained by a functioning symbiosis between corals and Symbiodiniaceae. However, when energy requirements are too high, animals can switch to anaerobic respiration, which takes place in the cytoplasm of the cell, yields far fewer ATP molecules than aerobic respiration and also produces lactate (Berg et al., 2002).
In recent decades, reef ecosystems have been impacted by a variety of environmental stressors ranging from local disturbances to global threats, including ocean warming, acidification, overfishing and pollution (Zaneveld et al., 2016; Hughes et al., 2017; Guan et al., 2020). Seawater warming is one of the greatest threats and the first cause of coral bleaching, the loss of Symbiodiniaceae and/or their photosynthetic pigments (Heron et al., 2016; Hoegh-Guldberg et al., 2019). At the organism level, bleaching leads to coral starvation, because they are deprived from their main source of autotrophic nutrient acquisition. Therefore, corals may undergo metabolic depression during heat stress (i.e., decreased cellular respiration and calcification) to reduce their energy costs (Guppy, 2004; Jacobson et al., 2016). They are also able to increase their reliance on energetic reserves (Rodrigues and Grottoli, 2007; Grottoli et al., 2017; Kochman et al., 2021), and/or predation on zooplankton depending on the heterotrophic capabilities of each coral species (Grottoli et al., 2006; Houlbrèque and Ferrier-Pagès, 2009). It has therefore been demonstrated that corals with high lipid reserves (Rodrigues and Grottoli, 2007; Grottoli et al., 2017; Kochman et al., 2021), or benefiting from increased heterotrophy (Towle et al., 2015; Levas et al., 2016; Grottoli et al., 2006; Palardy et al., 2008) can be more resistant and resilient to stress. In particular, heterotrophy helps in restoring the translocation of photosynthates from the Symbiodiniaceae to the coral host (Tremblay et al., 2016). However, heterotrophy is species-specific, some corals being more heterotrophic than others (Conti-Jerpe et al., 2020), and depends on plankton availability (Anthony et al., 2009). Seawater warming notably reduces the availability of plankton and DINut in shallow waters. This reduction occurs because of water column stratification, which limits the mixing of deeper waters with the surface layer (Coma et al., 2009). Additionally, increased productivity in the warmer, stratified surface waters exacerbates DINut depletion, further contributing to coral starvation (Ezzat et al., 2019). A minimum level of DINut such as nitrogen (N) and phosphorus (P) in seawater is also necessary for an optimal coral health (Rosset et al., 2017; Godinot et al., 2011; Béraud et al., 2013). Béraud et al. (2013) have demonstrated that corals supplied with 2 µM ammonium presented a limited bleaching state and were able to maintain photosynthesis and chlorophyll pigments during heat stress. In addition, a complete lack of P or an imbalanced N:P ratio can enhance bleaching (Rosset et al., 2017; Ezzat et al., 2019; Blanckaert et al., 2023; Crehan et al., 2024).
The use of energy reserves during heat-stress has mainly been studied in fed scleractinian (hexacoral) species (e.g. Rodrigues and Grottoli, 2007; Grottoli et al., 2017; Cziesielski et al., 2019; Kochman et al., 2021; Kochman-Gino and Fine, 2023). To date, only two studies have investigated the energetic response of corals to the combined stressors of elevated temperature and zooplankton depletion (Rivera et al., 2023), or DINut depletion (Ezzat et al., 2019). In addition, the energetic response to thermal stress of coral classes other than Hexacorallia, such as Octocorallia, has also hardly been investigated (Imbs and Yakovleva, 2012; Lõhelaid et al., 2015; Farag et al., 2018; Sikorskaya et al., 2020; Travesso et al., 2023), although corals with different trophic strategies can use their energy reserves differently. For example, most octocorals have lower rates of DINut acquisition by symbionts, either because they are unable to fully exploit this nutrient source or because their nutrient requirements are met through heterotrophy (Pupier et al., 2019a, b, Pupier et al., 2021a, b; Ferrier-Pagès et al., 2022; Hill et al., 2023). In the latter case, a depletion of DINut in seawater should not affect them to the same extent as hexacorals with high DINut uptake rates (Pupier et al., 2019b, 2021b). Therefore, how hexacorals and octocorals can react to nutrient deficiencies caused either by bleaching due to heat stress or by DINut deficiency in seawater still remains unclear.
In this experiment, the physiological and energetic response of the scleractinian coral Stylophora pistillata and the malacalcyonacean coral Heteroxenia fuscescens (respectively referred to as hexacoral and octocoral hereafter) to stress induced by high temperatures and DINut starvation was compared. Zooplankton starvation was not tested as these species are mainly autotrophic in shallow waters (Bednarz et al., 2012; Levas et al., 2016; Tremblay et al., 2016; Vollstedt et al., 2020). Corals from the Red Sea are known for their extreme thermal resistance (Fine et al., 2013), though several episodes of bleaching have been observed in the Southern part of the Red Sea (Osman et al., 2017; Fine et al., 2019). Although Red Sea corals can sustain relatively high temperature conditions when they are fed, they can be more susceptible when facing DINut depletion (Ezzat et al., 2019). It is therefore of major interest to investigate how they use their energetic reserves under multi-stress conditions. Our study also aims to determine whether the two species respond similarly to thermal stress and DINut deficiency, at the physiological and metabolic levels. We hypothesized that the combination of these two stressors would be more detrimental than either stressor alone. The results help to better understand the ability of corals to survive periods of nutrient deficiency.
2 Material and methods
2.1 Experimental design
Twelve mother colonies of the octocoral Heteroxenia fuscescens (Xeniidae family) and six mother colonies of the hexacoral Stylophora pistillata (Pocilloporidae family), originally sampled in the Gulf of Aqaba (Northern Red Sea) under CITES permit no. DCI/89/32 and then cultured at the Monaco Scientific Centre, were used to prepare a total of 72 nubbins per species (6 and 12 nubbins per colony respectively). H. fuscescens and S. pistillata were, respectively, in symbiosis with Durusdinium glynnii and Symbiodinium microadriaticum, which was determined by Ezzat et al. (2016) and Levy et al. (2016) following the protocol of Santos et al. (2002). The assignment (formerly Symbiodinium clade D1 and A1, respectively) corresponds to the updated nomenclature for Symbiodiniaceae (LaJeunesse et al., 2018). Nubbins (of 5-6 big polyps) of Heteroxenia fuscescens were attached to individual plates while nubbins of S. pistillata (ca. 17 cm2) were individually hung on nylon threads. Nubbins were randomly distributed in twelve experimental 20 L tanks (i.e., n = 6 per species per tank) and were left to recover without being fed (i.e., no supply of particulate organic matter) for one month prior to starting the experiment (Figure 1A). The tanks received a continuous supply of oligotrophic Mediterranean seawater, which was filtered through sand filters before being delivered to the aquaria. Therefore, very little particulate organic matter reached the corals. DINut levels were regularly measured (once a week) using a AA3 Seal autoanalyzer according to Aminot et al. (2009). Control DINut concentrations were PO4 = 0.22 ± 0.03 µM, NO3 = 0.84 ± 0.12 µM, NH4 = 0.17 ± 0.05 µM. These concentrations were similar to natural oligotrophic reef conditions, which are generally <1 µM for dissolved inorganic nitrogen sources and <0.3 µM for phosphate concentrations (Silbiger et al., 2018; Kim et al., 2022). Seawater was continuously renewed at a flow rate of 20 L h-1. In the tanks, seawater was mixed using mini-pumps (404 L h-1, Newa, Loreggia, Italy), and kept at a temperature of 25°C with submersible resistance heaters (300 W Titanium, Schego, Offenbach, Germany). Light level was set up to 200 µmol photons m−2 s−1 (12:12) using metal halide lamps (Philips, HPIT 400 W, Distrilamp, Bossée, France). After the recovery, half of the tanks were supplied with water depleted in DINut until the end of the experiment (Figure 1B). For this purpose, each depleted tank was connected to a 100 L re-circulating water reservoir at a flow rate of 20 L h-1. To deplete the water in DINut, water was passed through a biological filter (Fluidized bed filter BF-700, Otto, Australia). It was entirely renewed once a day to keep the salinity (37‰, salinity probe, Ponsel-mesure, France) and pH (8.1, pH probe, Ponsel-mesure, France) constant in the tanks. DINut concentrations were decreased by 30% in these depleted conditions, with PO4 = 0.17 ± 0.01 µM, NO3 = 0.51 ± 0.04 µM, NH4 = 0.10 ± 0.01 µM. After exposing the nubbins to depleted or control water treatment for one month, water temperature was gradually increased (+0.5°C every two days) in half of the tanks per treatment until reaching 30°C. Nubbins were then maintained in these conditions for three additional weeks (Figure 1C). This resulted in four different treatments, with triplicate tanks per treatment: control conditions at 25°C (C25) and 30°C (C30), and DINut depleted conditions at 25°C (D25) and 30°C (D30). At the end of the experiment, 4 to 6 nubbins of each species per treatment were sampled in the different tanks for the measurements of the physiological parameters while the remaining nubbins were flash-frozen and kept at -80°C for the determination of the energetic parameters.
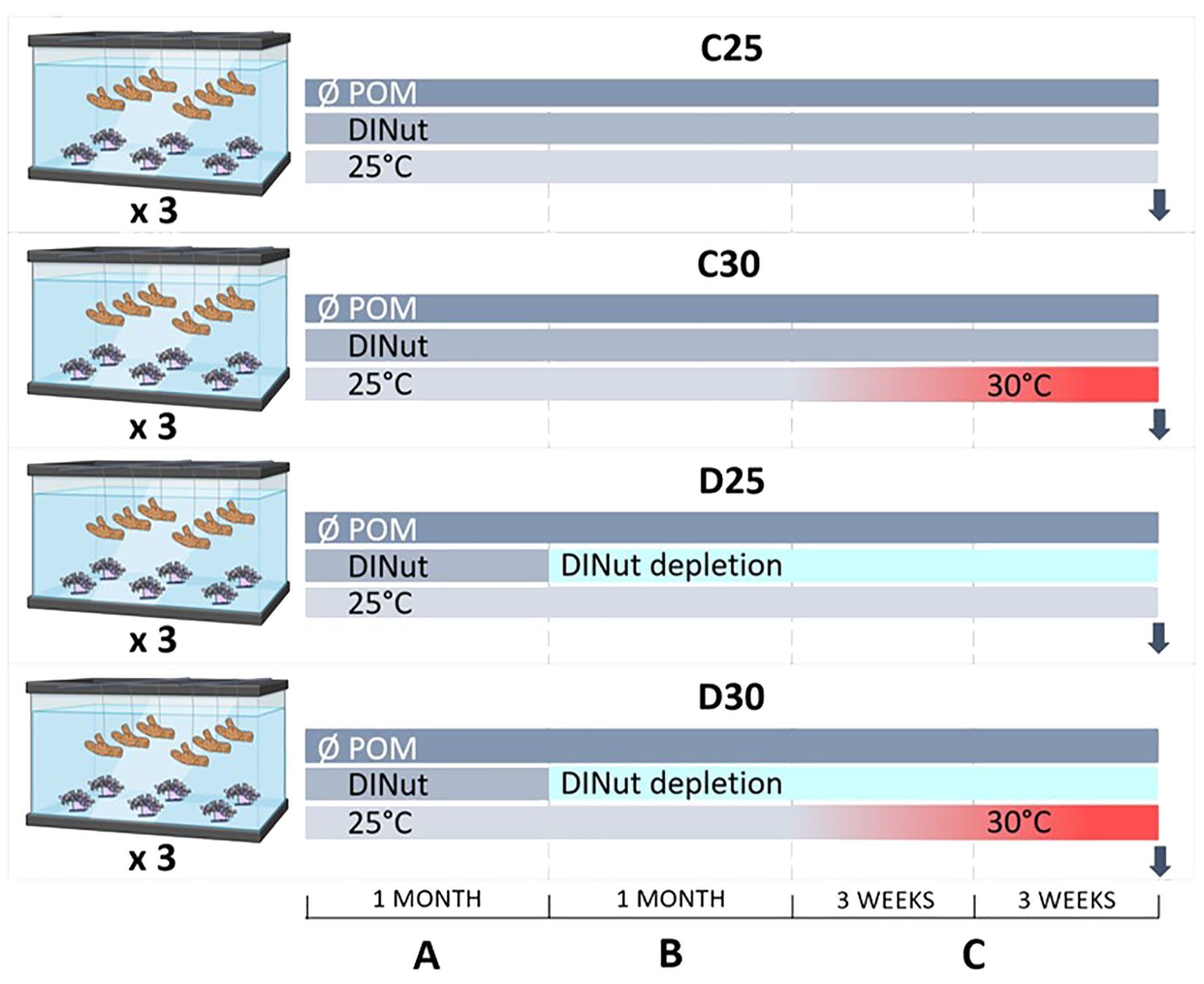
Figure 1. Overview of the experimental design applied to the coral species Heteroxenia fuscescens and Stylophora pistillata. Corals were not supplied with particulate organic matter (POM) for one month (A) prior to starting the dissolved inorganic nutrient (DINut) depletion in half of the tanks for another month (B). Temperature was then gradually increased in the high-temperature tanks until reaching 30°C. Corals were maintained under these conditions for three additional weeks (C). Arrows indicate the sampling time. C25 = DINut control water, 25°C. C30 = DINut control water, 30°C. D25 = DINut depleted water, 25°C. D30 = DINut depleted water, 30°C.
2.2 Physiological and tissue parameters
2.2.1 Physiological measurements
Oxygen fluxes were measured in the dark (respiration) and at 200 µmol photons m-2 s-1 (net photosynthesis) for 20 min following a 15 min acclimation period. Nubbins (n = 6 per condition for S. pistillata and n = 4 per condition for H. fuscescens) were individually placed in closed chambers, continuously mixed with a stirrer, filled with a known volume of 0.45 µm-filtered seawater corresponding to the right DINut and temperature conditions. Chambers were equipped with a Unisense optode oxygen-sensitive minisensor connected to the Oxy-4 software (Channel fiber-optic oxygen meter, PreSens, Regensburg, Germany). Optodes were calibrated prior to each incubation with air-saturated (100% oxygen) and nitrogen-saturated (0% oxygen) seawater. Gross photosynthesis (Pg) was calculated by adding the oxygen production to the absolute value of oxygen consumption. Photosynthesis to respiration ratios (P:R) were calculated by dividing the gross photosynthesis over 12h by the respiration over 24h. A subsample of these nubbins (n = 3 per treatment and species) were also used (after a 20 min-dark acclimation) to determine the maximum quantum yield of photosystem II of the symbionts (Fv/Fm) using Pulse Amplitude Modulation fluorometer (dual-PAM/F, Walz, Germany). These measurements being not destructive, the nubbins were then frozen at -80°C for the subsequent determination of ash-free dry weight (AFDW) and protein concentration, with a subsample (n = 3 per condition and species) also used for the measurement of Symbiodiniaceae density and chlorophyll concentration.
2.2.2 Tissue descriptors
Soft coral samples were freeze-dried, weighed to obtain their dry weight (DW) and crushed into powder. For hard coral samples, the tissue was first removed from the skeleton using an air brush and directly homogenized in distilled water with a potter tissue grinder prior to the freeze-drying step. For both coral groups, a first subsample of the powder was dissolved into distilled water and homogenized with a potter tissue grinder. To determine protein concentration, 500 µL were sampled from the homogenate and assessed using the method described by Smith et al. (1985) with a BCAssay Protein Quantification Kit (Uptima, Interchim) and a Xenius spectrofluorometer (SAFAS, Monaco).
The Symbiodiniaceae density was quantified by sampling another 500 µL from the homogenate and using a Coulter Counter (Beckman Coulter, France).
The remaining homogenate was used for the determination of the total chlorophyll concentration, which was measured as described in Pupier et al. (2018). Briefly, the homogenate was centrifuged for 10 min (at 8000 g at 4°C). The symbiont pellet was kept and treated with 5 mL of acetone (100%) amended with magnesium chloride (Sigma-Aldrich, Germany) to extract the chlorophyll over 24 h in the dark (at 4°C). After centrifugation at 11 000 g for 15 min at 4°C, absorbances were measured at 750, 663 and 630 nm using a Xenius spectrophotometer (SAFAS, Monaco) and chlorophyll concentrations calculated according to Jeffrey and Humphrey (1975).
For the determination of the AFDW, the remaining of the powder was first weighed, and then combusted at 450°C for 4 h in a muffle furnace (Thermolyne 62700, Thermo Fischer Scientific, the United States). AFDW was determined as the difference between the dry weight and ash weight of the subsample and extrapolated to the total weight of the nubbin. All data are expressed per g-1 AFDW. For S. pistillata, skeletal surface area was measured using the wax dipping technique (Veal et al., 2010), allowing the data to also be expressed per cm-2 of skeletal surface area.
Finally, a correlation was established between protein content and AFDW to extrapolate the AFDW of carbohydrate, lactate and ATP samples from their protein content, as these measurements were destructive. Similarly, this approach was also used to determine the surface area of S. pistillata skeletons for these specific samples.
2.3 Energetic parameters
2.3.1 Lipids
Total lipids were extracted twice from three freeze-dried nubbins (n = 3 per species and treatment) in a 2:1 dichloromethane:methanol solution followed by stirring and ultrasonication for 10 min, according to Bligh and Dyer (1959). The lipid extracts were collected after centrifugation (2000g; 10 min), filtered and washed with dichloromethane and water (1:1). The liquid was evaporated and the two aliquots from both extractions were pooled for gravimetric quantification of the dried lipid extracts. Data are expressed as mg lipids g-1 AFDW, with the AFDW of the nubbins being quantified from a subsample of the freeze-dried powder, as described in the previous section. For S. pistillata, data are also expressed as mg lipids cm-2, determined as described in the previous section.
2.3.2 Sample preparation for the biochemical assays
Prior to the determination of carbohydrate, lactate and ATP concentrations, small coral pieces (n = 3 per condition and species) were cut (ca. 0.5 cm²) and homogenized using 250-300 µL of the buffer corresponding to the measured parameter (see below) in ice by ultrasound (frequency 70 kHz, Vibra-CellTM 75, 185, Bioblock Scientific, France). After sonication, the homogenized solution was centrifuged and used for analysis. Total protein concentration of each sample homogenates was determined using Coomassie Brilliant Blue Assay (23,200, Thermo Scientific, USA) according to Bradford (1976). This step is essential to ensure that the same amount of material is used for all samples when measuring carbohydrate, lactate and ATP levels.
2.3.3 Carbohydrates
Carbohydrates, which are major energy reserves in corals, were quantified according to the pheno-sulfuric acid method (Dubois et al., 1956) by using a Total Carbohydrate Assay Kit (MAK104, Sigma-Aldrich, USA) with glucose as standard. Data are expressed as mg carbohydrates g-1 AFDW and also as mg carbohydrates cm-2 for S. pistillata.
2.3.4 Lactate
L(+)-Lactate, which is a byproduct of anaerobic metabolism and indicates that corals are experiencing hypoxic conditions, was quantified using the Lactate Assay kit (MAK064, Sigma-Aldrich, USA), which results in a fluorimetric (λex = 535, λem = 587 nm) product proportional to the lactate present in the sample homogenates. Following the manufacturer instructions, samples were first deproteinized with a 10 kDa Molecular Weight Cut-off (MWCO) spin filter, to avoid the degradation of lactate by the lactate dehydrogenase. Data are expressed as pg lactate g-1 AFDW and also as pg lactate cm-2 for S. pistillata.
2.3.5 ATP
ATP concentration, which is the primary energy currency in cells, and directly reflects the cell’s metabolic activity, was determined by phosphorylating glycerol using the ATP Assay kit (MAK190, Sigma-Aldrich, USA), which results in a fluorimetric (λex = 535, λem = 587 nm) product proportional to the amount of ATP present in the sample homogenates. Following the manufacturer instructions, samples were first deproteinized with a 10 kDa Molecular Weight Cut-off (MWCO) spin filter, to avoid the degradation of ATP by enzymatic activities. Data are expressed as µg ATP g-1 AFDW and also as µg ATP cm-2 for S. pistillata.
2.3.6 Energy storage
Total stored energy values were calculated by multiplying the mean of each macromolecule per condition by its combustion enthalpy (Gnaiger and Bitterlich, 1984) and summing them (Grottoli et al., 2017).
2.4 Statistical analysis
Analyses were performed using R version 4.3.1 software. respectively, generalized linear models (family = Gaussian) were performed on each species separately (with temperature and nutrient level (DINut) as fixed factors) on all of the measured parameters. Compliance with the assumptions of a normal distribution of the model residuals and homoscedasticity was verified using, respectively, Shapiro-Wilk’s and Levene’s tests, as implemented in the R-package car (Fox and Weisberg, 2018). Data were log-transformed to meet these assumptions when necessary. When a factor or the interaction of both was significant (p-value < 0.05), Tukey’s HSD tests were performed as a posteriori testing using the R-package multcomp (Hothorn et al., 2008). Statistical tests were not performed on the total energy storage since lipids and carbohydrates were measured on different samples. Student’s t-tests were used to test for differences between species under the control condition (C25).
3 Results
3.1 Comparison of the physiology of H. fuscescens and S. pistillata under the control condition
Under the control condition (C25), there was no significant difference between the two species regarding Symbiodiniaceae density (Figures 2A, 3A and Supplementary Table S1), chlorophyll concentration (Figures 2B, 3B and Supplementary Table S1) and the P:R (Figures 2F, 3F and Supplementary Table S1). S. pistillata, however, exhibited ca. five-fold higher gross photosynthesis (Figures 2D, 3D and Supplementary Table S1) and respiration rates (Figures 2E, 3E and Supplementary Table S1). The Fv/Fm was slightly lower in S. pistillata (-16%, Figures 2C, 3C and Supplementary Table S1). There was no difference between species for the concentrations of lactate (Figures 4E, 5E and Supplementary Table S1) and ATP (Figures 4F, 5F and Supplementary Table S1).
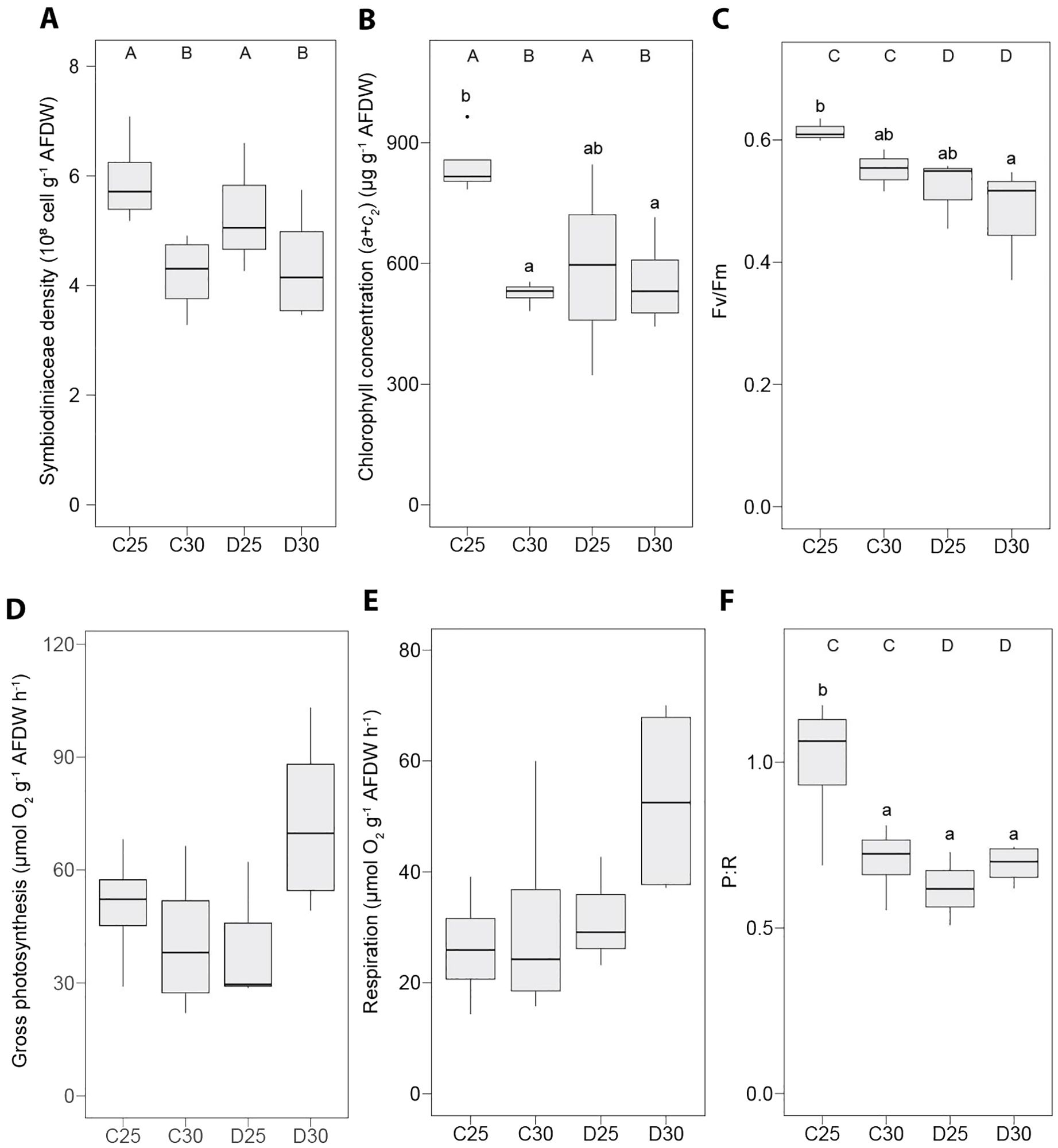
Figure 2. Physiological parameters measured in the soft coral Heteroxenia fuscescens exposed to different nutrient conditions and temperature treatments. (A) Symbiodiniaceae density, (B) chlorophyll concentration, (C) photochemical efficiency of the photosystem II (Fv/Fm), (D, E) oxygen fluxes, (F) gross photosynthesis to respiration ratio (P:R). AFDW = ash-free dry weight. C25 = DINut control water, 25°C. C30 = DINut control water, 30°C. D25 = DINut depleted water, 25°C. D30 = DINut depleted water, 30°C. Upper-case letters indicate significant main effects of temperature (A, B) and DINut (C, D) as determined by generalized linear models. Pairwise significant differences resulting from Tukey’s HDS testing are displayed by lower-case letters (P < 0.05).
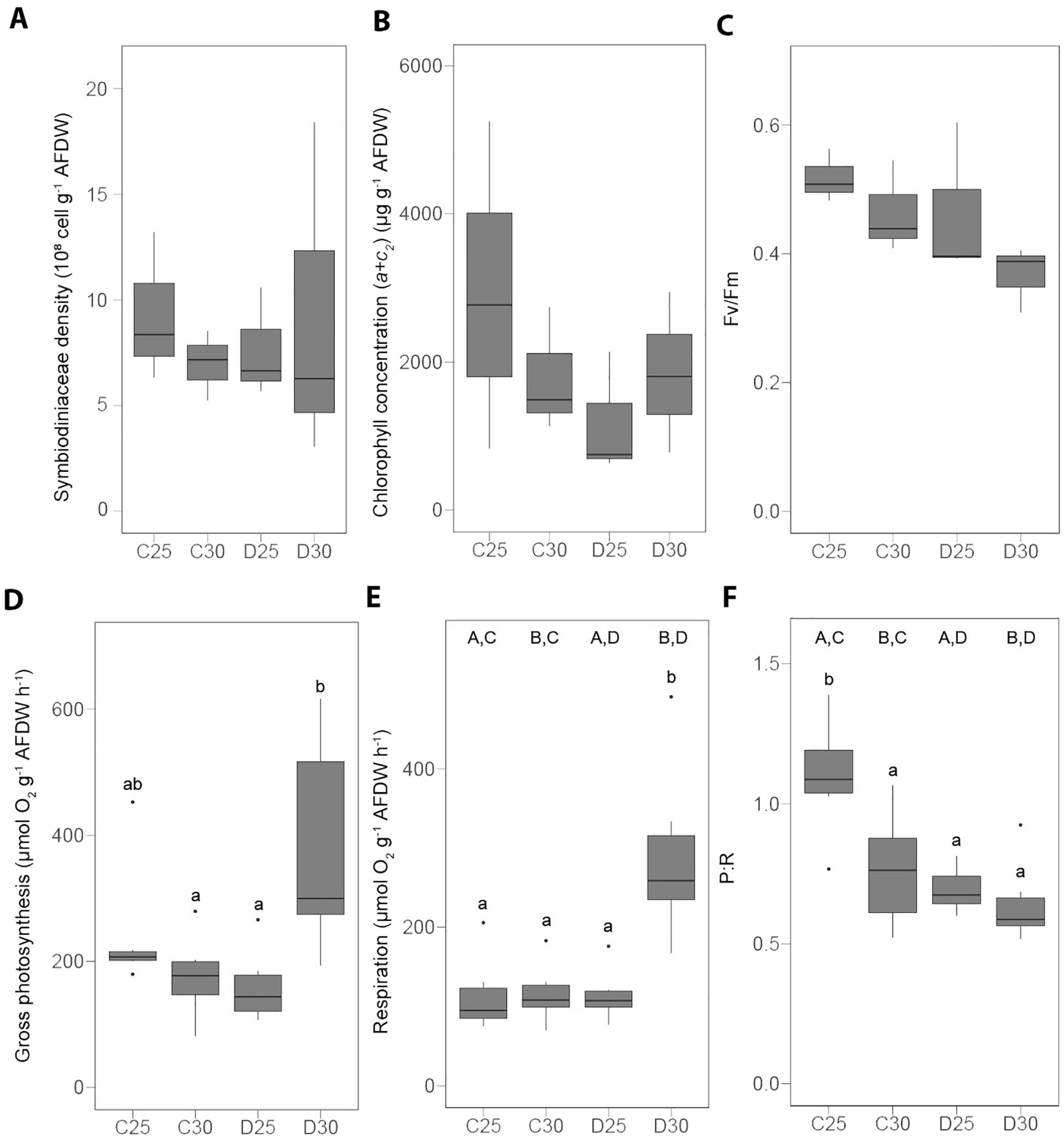
Figure 3. Physiological parameters measured in the hard coral Stylophora pistillata exposed to different nutrient conditions and temperature treatments. (A) Symbiodiniaceae density, (B) chlorophyll concentration, (C) photochemical efficiency of the photosystem II (Fv/Fm), (D, E) oxygen fluxes, (F) gross photosynthesis to respiration ratio (P:R). AFDW = ash-free dry weight. C25 = DINut control water, 25°C. C30 = DINut control water, 30°C. D25 = DINut depleted water, 25°C. D30 = DINut depleted water, 30°C. Upper-case letters indicate significant main effects of temperature (A, B) and DINut (C, D) as determined by generalized linear models. Pairwise significant differences resulting from Tukey’s HDS testing are displayed by lower-case letters (P < 0.05).
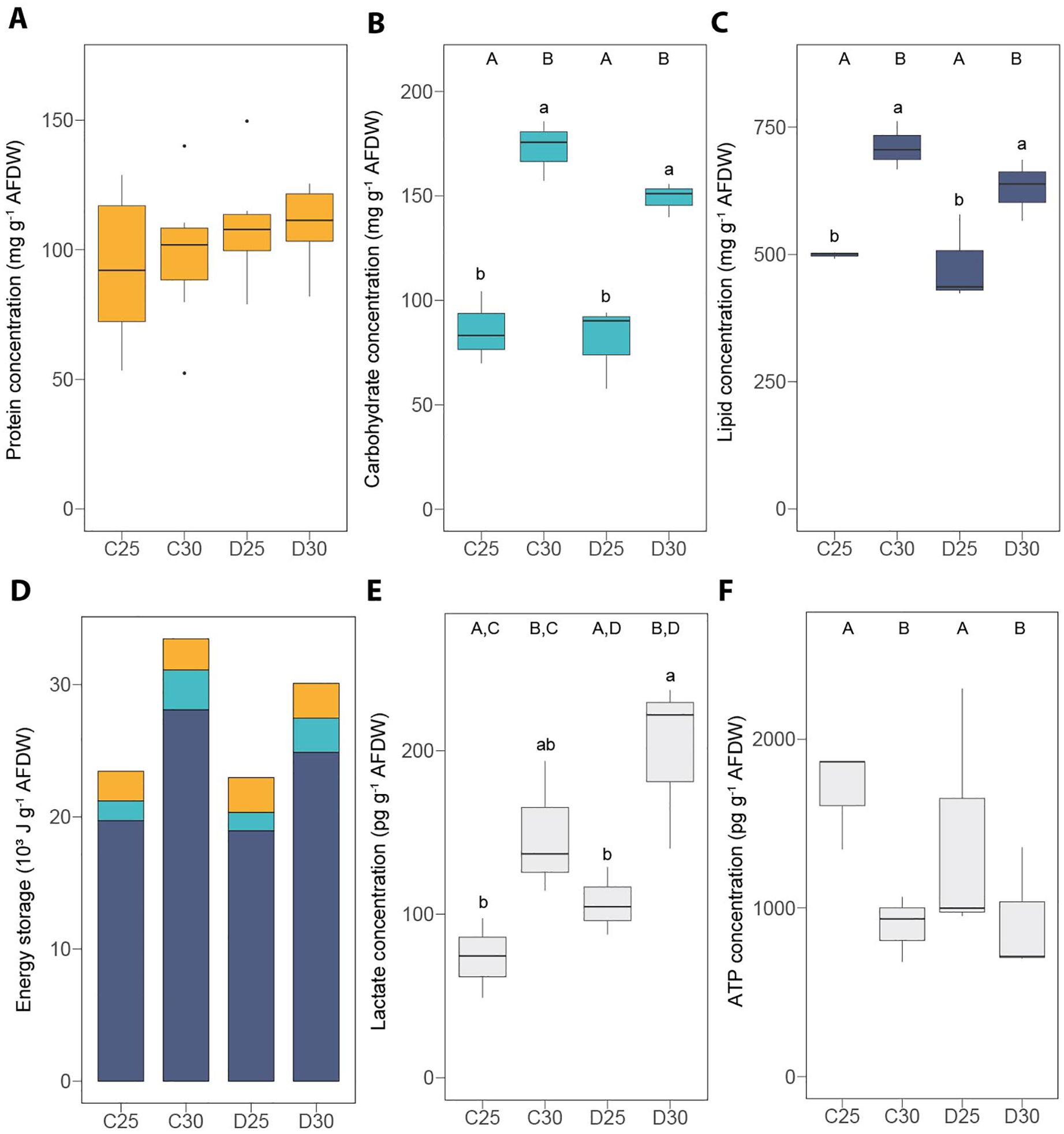
Figure 4. Energy reserves and metabolic compounds measured in the soft coral Heteroxenia fuscescens exposed to different nutrient conditions and temperature treatments. (A) protein concentration, (B) carbohydrate concentration, (C) lipid concentration, (D) total energy storage, (E) lactate concentration and (F) ATP concentration. AFDW = ash-free dry weight. ATP = adenosine triphosphate. C25 = DINut control water, 25°C. C30 = DINut control water, 30°C. D25 = DINut depleted water, 25°C. D30 = DINut depleted water, 30°C. Upper-case letters indicate significant main effects of temperature (A, B) and DINut (C, D) as determined by generalized linear models. Pairwise significant differences resulting from Tukey’s HDS testing are displayed by lower-case letters (P < 0.05). Statistical tests were not performed on the total energy storage since lipids and carbohydrates were measured on different samples.
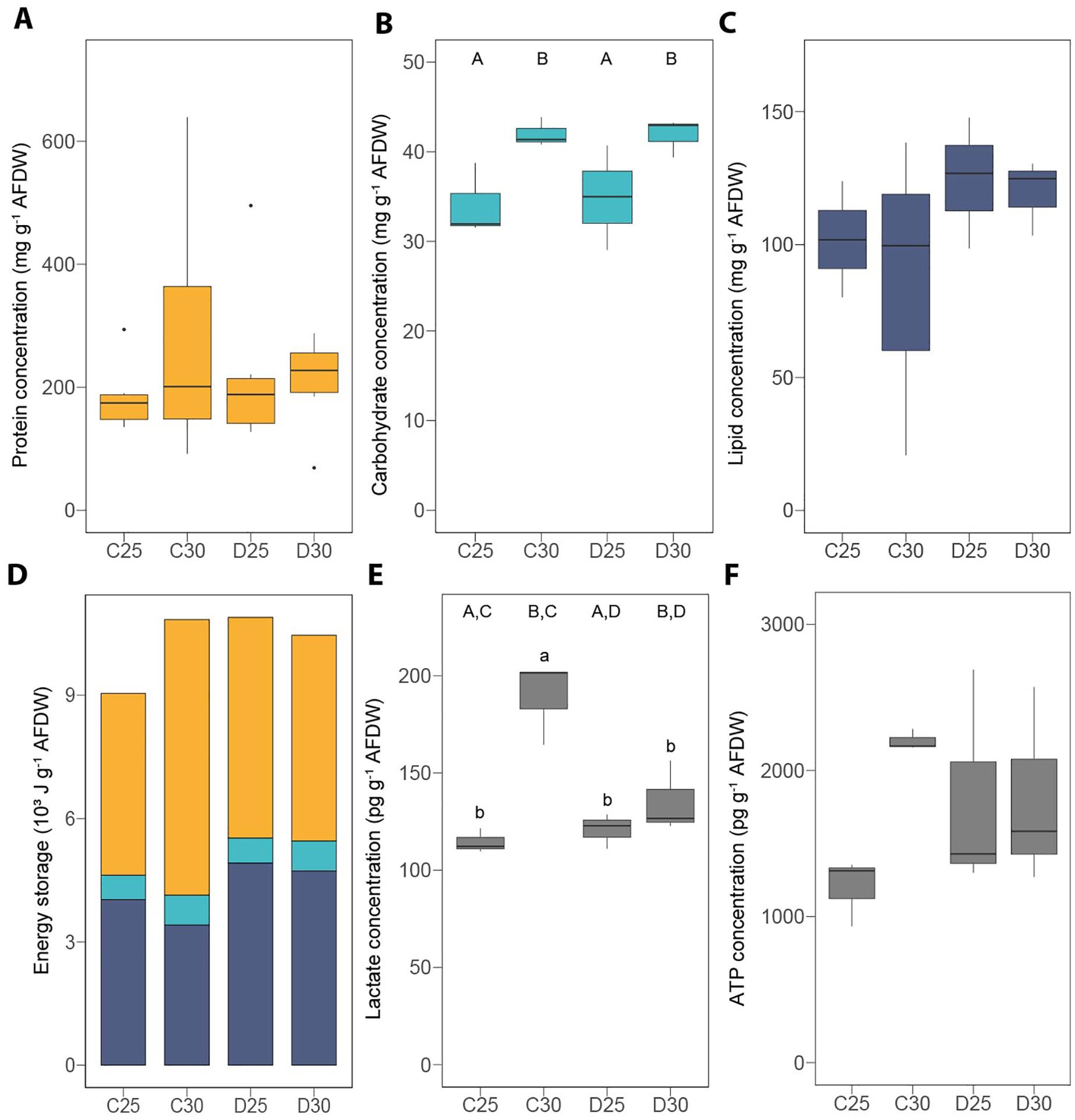
Figure 5. Energy reserves and metabolic compounds measured in the hard coral Stylophora pistillata exposed to different nutrient conditions and temperature treatments. (A) protein concentration, (B) lipid concentration, (C) carbohydrate concentration, (D) total energy storage, (E) lactate concentration and (F) ATP concentration. AFDW = ash-free dry weight. ATP = adenosine triphosphate. C25 = DINut control water, 25°C. C30 = DINut control water, 30°C. D25 = DINut depleted water, 25°C. D30 = DINut depleted water, 30°C. Upper-case letters indicate significant main effects of temperature (A, B) and DINut (C, D) as determined by generalized linear models. Pairwise significant differences resulting from Tukey’s HDS testing are displayed by lower-case letters (P < 0.05). Statistical tests were not performed on the total energy storage since lipids and carbohydrates were measured on different samples.
A very different composition in macromolecules was observed between the soft and hard coral species since S. pistillata had ca. two-fold higher protein concentrations than H. fuscescens (Figures 4A, 5A and Supplementary Table S1), while this latter had 2.5-fold more carbohydrates (Figures 4B, 5C and Supplementary Table S1) and ca. five-fold more lipids (Figures 4C, 5B and Supplementary Table S1) than S. pistillata. This translated into 2.6-fold higher energy stored in H. fuscescens than in S. pistillata (Figures 4D, 5D). The above trends remained similar in the other conditions of temperature and DINut depletion.
3.2 Effect of high temperature and DINut depletion on H. fuscescens
High temperature significantly affected the Symbiodiniaceae density and chlorophyll concentration of H. fuscescens, resulting in respective decreases of -23 ± 3% (Figure 2A and Supplementary Table S2) and -22 ± 4% (Figure 2B and Supplementary Table S2) in average as compared with 25°C. DINut depletion alone led to lower Fv/Fm (-14 ± 3%, Figure 2C and Supplementary Table S2) and P:R (-21 ± 8%, Figure 2F and Supplementary Table S2). While there was no significant effect of high temperature or DINut depletion on the rates of gross photosynthesis (Figure 2D and Supplementary Table S2) and respiration (Figure 2E and Supplementary Table S2), the interaction of these two factors significantly affected the chlorophyll concentration (Figure 2B and Supplementary Table S2), gross photosynthesis (Figure 2D and Supplementary Table S2) and P:R (Figure 2F and Supplementary Table S2). Particularly for the latter, the highest value was observed in the control (C25) treatment compared to all others Figure 2F and Supplementary Table S2).
The different treatments had no impact on the protein concentration of H. fuscescens (Figure 4A and Supplementary Table S2). However, carbohydrate (+94 ± 9%) and lipid (+37 ± 5%) concentrations were significantly higher under high temperature, regardless the DINut condition (Figures 4B, C and Supplementary Table S2). This resulted in a steep increase in total energy storage under high temperature (+37 ± 4%, Figure 4D). On the contrary, the ATP concentration declined of -41 ± 3% under the high-temperature treatments (Figure 4F and Supplementary Table S2). DINut depletion had no significant impact on macromolecule or ATP concentrations (Figures 4A–C, F and Supplementary Table S2). However, lactate concentration was affected by both temperature and DINut conditions (Figure 4E and Supplementary Table S2), leading to an increase of +129 ± 42% under D30 compared with C25 and D25.
3.3 Effect of high temperature and DINut depletion on S. pistillata
Temperature and DINut depletion had no significant effect on the Symbiodiniaceae density (Figure 3A and Supplementary Table S3), chlorophyll concentration (Figure 3B and Supplementary Table S3) or on the Fv/Fm (Figure 3C and Supplementary Table S3) of S. pistillata. However, they both affected respiration rates and P:R (Figures 3E, F and Supplementary Table S3). Their interaction led to increased rates of gross photosynthesis (+101 ± 24%, Figure 3D and Supplementary Table S3) and respiration at D30 (+152 ± 2%, Figure 3E and Supplementary Table S3). This resulted in significant differences between P:R, with the higher ratio measured in the control (C25) condition (Figure 3F and Supplementary Table S3).
While there was no difference between treatments regarding the protein (Figure 5A and Supplementary Table S2) and lipid (Figure 5C and Supplementary Table S3) concentrations of S. pistillata, the concentrations of carbohydrates were higher under high temperature (+22 ± 1%, Figure 5B and Supplementary Table S3). Overall, this resulted in a total energy storage of +20% at C30 and D25, and +16% at D30, as compared to C25 (Figure 5D). Finally, the concentration of lactate was significantly affected by temperature and DINut conditions, and their interaction led to higher values at C30 as compared with the other conditions (+54 ± 7%, Figure 5E and Supplementary Table S3). The concentration of ATP remained statistically unchanged (Figure 5F and Supplementary Table S3).
Similar trends were observed for the physiological (Supplementary Figures S1A–D and Supplementary Table S4) and energy (Supplementary Figures S1E–I and Supplementary Table S4) parameters normalized to the skeletal surface area of S. pistillata.
4 Discussion
Although Red Sea corals have unusual high thermal tolerance and typically bleach only at much higher temperatures than elsewhere (e.g. Bellworthy and Fine, 2017; Grottoli et al., 2017; Voolstra et al., 2021; Evensen et al., 2022), this study shows that both temperature stress and DINut depletion reduced the autotrophic capacity of the two coral species investigated. There was indeed a significant decrease in the P:R ratio at elevated temperature and under DINut deficiency. In terms of energy metabolism, the two coral species responded primarily to seawater warming. Both species engaged into anaerobic metabolism under elevated temperature, with a decrease in ATP production in H. fuscescens, but none of them catabolized their energy reserves. Overall, the combination of the two stressors in our experiment did not intensify their individual effects, except for 1) the oxygen fluxes in S. pistillata, and 2) the lactate concentration in H. fuscescens, both of which showed an increase under the combined stress of high temperature and DINut depletion.
4.1 Differences in basal maintenance between H. fuscescens and S. pistillata under similar culture conditions (C25)
Under similar control culture conditions (DINut control and 25°C), without external particulate organic matter supply, the average P:R was above the compensation threshold (P:R = 1.0) in both species, indicating that the energy produced by photosynthesis compensated for the energy consumed by respiration over the course of a day. However, photosynthetic rates were up to 5-fold higher in S. pistillata than in H. fuscescens, despite similar concentrations in total chlorophyll and symbionts. This difference can be due to a different pattern of symbiont distribution within the host tissue, with a homogeneous versus a heterogeneous distribution of symbionts in S. pistillata (Loussert-Fonta et al., 2020) and H. fuscescens (Benayahu et al., 1989; Yoffe et al., 2012), respectively. In addition, while the tissue layer surrounding the skeleton of S. pistillata is relatively thin, soft corals exhibit a low surface area to volume ratio due to their thick tissue, which does not favor light exposure or gas and nutrient exchange throughout the colony (Wangpraseurt et al., 2014). Such morphological features could thus partly explain the observed differences in oxygen production, along with the different Symbiodiniaceae species hosted by the corals (Starzak et al., 2014; Ros et al., 2020) or the presence of a skeleton in the hard coral species, that enables multiple light scattering towards the symbionts (Enríquez et al., 2017).
H. fuscescens and S. pistillata also exhibited completely different tissue composition under the same culture conditions. Despite lower photosynthetic rates, H. fuscescens had two-fold more carbohydrates and five-fold more lipids per tissue biomass than S. pistillata, indicating that the soft coral could allocate more energy to build up energy reserves. Furthermore, hard corals have additional maintenance costs due to the calcification process. The energy stored by H. fuscescens is also of better quality, as lipids, which have a higher energy value than carbohydrates and proteins (Gnaiger and Bitterlich, 1984), accounted for most of the energy storage (80%), compared to 45% for S. pistillata. The tissue composition of H. fuscescens agrees with Schlichter et al. (1983), who emphasized that the highest proportion of photosynthetically derived carbon in this species is contained in lipids. In S. pistillata, the tissue composition is consistent with what is usually found in hard coral species, with lipids accounting for 10-40% of the total biomass (Imbs and Dembitsky, 2023). Moreover, when normalized to skeletal surface area, the physiological and energy parameters of S. pistillata were in the same range than samples collected in the Gulf of Aqaba (Kochman et al., 2021; Kochman-Gino and Fine, 2023).
4.2 Metabolic responses of H. fuscescens and S. pistillata to heat stress and DINut depletion
The symbionts of the two species exhibited different responses to stress. In H. fuscescens symbionts, DINut depletion and elevated temperatures impaired the photosynthetic machinery by reducing the efficiency of the photosystem II (PSII) and lowering chlorophyll concentration (i.e., bleaching). These factors are crucial for capturing and converting light energy. The lack of dissolved inorganic nitrogen and phosphorus in seawater hinders the production of pigments and proteins essential for photosynthesis (Wiedenmann et al., 2012). Additionally, elevated temperatures can disrupt the function of photosynthetic components, such as photosystems (Warner et al., 1999) and thylakoid membranes (Tchernov et al., 2004), and inhibit the repair of photodamaged PSII (Takahashi et al., 2004), further reducing the overall capacity for photosynthesis. Despite these stressors, the photosynthetic apparatus in H. fuscescens symbionts remained efficient in converting absorbed light into chemical energy, as photosynthesis rates were not affected and even tended to increase at D30. In contrast, the photosynthetic efficiency of PSII in S. pistillata symbionts remained unaffected and comparable to that of corals from the Gulf of Aqaba under C30 (Voolstra et al., 2021). The differing responses between S. pistillata and H. fuscescens could be related to the genotype of the symbionts harbored by each coral species, despite both symbiont species being thermally tolerant (Krämer et al., 2012; Silverstein et al., 2017).
When exposed to DINut deficiency, high temperatures, or their combined effect, both species exhibited a common pattern where photosynthesis could not meet respiratory demand over a day. This resulted in a significant decrease in P:R ratio (P:R < 1.0), indicating stress, since autotrophy alone could not sustain the elevated coral metabolism in the long-term.
Surprisingly, our study found that heat-stressed corals had more energy reserves (carbohydrates across the two coral species and lipids in H. fuscescens) per ash-free dry weight tissue compared to those at lower temperatures (25°C). This is contrary to the general observation that corals under stress, and in absence of external particulate organic matter supply, are forced to rely on their stored energy reserves (e.g. Grottoli and Rodrigues, 2011; Imbs and Yakovleva, 2012; Tignat-Perrier et al., 2022; Rivera et al., 2023). However, some studies have already found a significant increase in energy reserves in various host-symbiont associations exposed to thermal stress (Hoadley et al., 2015, 2019). Such discrepancies in the coral energetics can be due to a reduced loss of energy in the form of mucus (Ferrier-Pagès et al., 1998; de Goeij and van Duyl, 2007; Bythell and Wild, 2011), an increased reliance on dissolved organic matter (Lange et al., 2023), or even a carbon-nitrogen negative feedback loop (Cui et al., 2023). Symbiodiniaceae and coral gastrodermal cells can also accumulate lipids as a survival mechanism under stress, likely due to limitations in ATP production (Hillyer et al., 2017; Petrou et al., 2018; Al-Hammady et al., 2022). This mechanism is probably at play in our study, as ATP concentrations in H. fuscescens significantly decreased under thermal stress, and the concentrations of lactate, the major end-product of anaerobic metabolism (Sokolova, 2013), increased in both species. We hypothesize that the reduced ATP production was likely due to the induction of anaerobic glycolysis. This process consumes carbohydrates instead of lipids and is less efficient than aerobic metabolism, producing significantly fewer ATP molecules (Sebens, 1987; Shick et al., 1988; Lesser, 2013) and generating lactate as a byproduct (Berg et al., 2002).
The activation of anaerobic pathways for energy production in response to thermal stress has been poorly studied in cnidarians, although few studies have documented this phenomenon (e.g. Marangoni et al., 2020; Nevarez-Lopez et al., 2020; Linsmayer et al., 2024). In our study, we did not detect the oxidation of carbohydrates, which typically leads to energy production (Sokolova et al., 2012). However, an increased lactate concentration may indirectly indicate destructive processes in the mitochondrial membranes (Marangoni et al., 2020). Thus, we propose using lactate concentration as an indicator of energetic stress in corals. Despite compensatory ATP production through both aerobic and anaerobic respiration, ATP depletion was not prevented in H. fuscescens. In contrast, S. pistillata maintained cellular homeostasis. S. pistillata may utilize phosphagens other than ATP to produce energy during stress (Fonseca et al., 2017), possibly through stimulating the pentose phosphate pathway (Lauer et al., 2012), which was not measured in this study.
5 Conclusions
Long-term tolerance to environmental stress depends on the sustained provision of nutrients and energy, both of which support essential physiological processes (Pan et al., 2015). Corals are, however, subject to nutrient restriction during heat stress, when rising sea surface temperatures impair coral photosynthetic capacity and increase stratification in shallow waters, limiting the supply of essential nutrients from deeper waters (Moore et al., 2013). The metabolic response of corals to nutrient limitation and thermal stress may be species dependent, due to different feeding strategies (e.g. Derviche et al., 2022), different associations with Symbiodiniaceae (e.g. Ziegler et al., 2019) or different utilization of energy reserves (e.g. Grottoli et al., 2017). All together, these differences underline the need to find proxies for coral health that are also the same for all corals. In this study, we observed that H. fuscescens and S. pistillata exhibited distinct physiological responses to heat stress and DINut depletion, yet both species experienced a significant decline in P:R ratio. Additionally, both corals showed a similar metabolic response, with a notable increase in lactate concentration under heat stress. These findings suggest that these two parameters warrant further investigation as potential indicators of stress in corals. Our study thus contributes to improving knowledge of the factors that may be important for the survival and resilience of corals during heat stress and DINut deficiency. It may therefore be interesting to further investigate the conditions that trigger the accumulation of reserves (e.g. Nielsen and Petrou, 2023) and the involvement of anaerobic metabolism in corals, to better understand how corals cope with stress.
Data availability statement
The raw data supporting the conclusions of this article will be made available by the authors, without undue reservation.
Ethics statement
The manuscript presents research on animals that do not require ethical approval for their study.
Author contributions
CP: Writing – review & editing, Writing – original draft, Validation, Methodology, Data curation, Conceptualization. RG: Writing – review & editing, Validation, Conceptualization. CR: Writing – review & editing, Validation, Methodology. CF: Writing – review & editing, Validation, Supervision, Funding acquisition, Conceptualization.
Funding
The author(s) declare financial support was received for the research, authorship, and/or publication of this article. This study was funded by the Centre Scientifique de Monaco.
Acknowledgments
The authors would like to thank L. Marangoni for laboratory assistance and fruitful discussions. We also would like to thank the two reviewers for their constructive comments on the manuscript.
Conflict of interest
The authors declare that the research was conducted in the absence of any commercial or financial relationships that could be construed as a potential conflict of interest.
Publisher’s note
All claims expressed in this article are solely those of the authors and do not necessarily represent those of their affiliated organizations, or those of the publisher, the editors and the reviewers. Any product that may be evaluated in this article, or claim that may be made by its manufacturer, is not guaranteed or endorsed by the publisher.
Supplementary material
The Supplementary Material for this article can be found online at: https://www.frontiersin.org/articles/10.3389/fmars.2024.1423034/full#supplementary-material
References
Al-Hammady M. A. M. M., Silva T. F., Hussein H. N. M., Saxena G., Modolo L. V., Belasy M. B. I., et al. (2022). How do algae endosymbionts mediate for their coral host fitness under heat stress? A comprehensive mechanistic overview. Algal Res. 67, 102850. doi: 10.1016/j.algal.2022.102850
Aminot A., Kérouel R., Coverly S. (2009). “Nutrients in seawater using segmented flow analysis,” in Practical guidelines for the analysis of seawater, ed Wurl O. (Boca Raton: CRC Press), 143–178.
Anthony K. R. N., Hoogenboom M. O., Maynard J. A., Grottoli A. G., Middlebrook R. (2009). Energetics approach to predicting mortality risk from environmental stress: A case study of coral bleaching. Funct. Ecol. 23, 539–550. doi: 10.1111/j.1365-2435.2008.01531.x
Bednarz V. N., Naumann M. S., Niggl W., Wild C. (2012). Inorganic nutrient availability affects organic matter fluxes and metabolic activity in the soft coral genus Xenia. J. Exp. Biol. 215, 3672–3679. doi: 10.1242/jeb.072884
Bellworthy J., Fine M. (2017). Beyond peak summer temperatures, branching corals in the Gulf of Aqaba are resilient to thermal stress but sensitive to high light. Coral Reefs 36, 1071–1082. doi: 10.1007/s00338-017-1598-1
Benayahu Y., Achituv Y., Berner T. (1989). Metamorphosis of an octocoral primary polyp and its infection by algal symbiosis. Symbiosis 7, 159–169.
Béraud E., Gevaert F., Rottier C., Ferrier-Pagès C. (2013). The response of the scleractinian coral Turbinaria reniformis to thermal stress depends on the nitrogen status of the coral holobiont. J. Exp. Biol. 216, 2665–2674. doi: 10.1242/jeb.085183
Berg J., Tymoczko J., Stryer L. (2002). “The regulation of cellular respiration is governed primarily by the need for ATP,” in Biochemistry (Freeman, New York).
Blanckaert A. C. A., Grover R., Marcus M. I., Ferrier-Pagès C. (2023). Nutrient starvation and nitrate pollution impairs the assimilation of dissolved organic phosphorus in coral-Symbiodiniaceae symbiosis. Sci. Total Environ. 858, 159944. doi: 10.1016/j.scitotenv.2022.159944
Bligh E. G., Dyer W. J. (1959). A rapid method of total lipid extraction and purification. Can. J. Biochem. Physiol. 37, 911–917. doi: 10.1139/y59-099
Bradford M. M. (1976). A rapid and sensitive method for the quantitation of microgram quantities of protein utilizing the principle of protein-dye binding. Anal. Biochem. 72, 248–254. doi: 10.1016/0003-2697(76)90527-3
Bythell J. C., Wild C. (2011). Biology and ecology of coral mucus release. J. Exp. Mar. Biol. Ecol. 408, 88–93. doi: 10.1016/j.jembe.2011.07.028
Chen H. K., Wang L. H., Chen W. N. U., Mayfield A. B., Levy O., Lin C. S., et al. (2017). Coral lipid bodies as the relay center interconnecting diel-dependent lipidomic changes in different cellular compartments. Sci. Rep. 7, 3244. doi: 10.1038/s41598-017-02722-z
Coma R., Ribes M., Serrano E., Jiménez E., Salat J., Pascual J. (2009). Global warming-enhanced stratification and mass mortality events in the mediterranean. Proc. Natl. Acad. Sci. U.S.A. 106, 6176–6181. doi: 10.1073/pnas.0805801106
Conti-Jerpe I. E., Thompson P. D., Wong C. W. M., Oliveira N. L., Duprey N. N., Moynihan M. A., et al. (2020). Trophic strategy and bleaching resistance in reef-building corals. Sci. Adv. 6, eaaz5443. doi: 10.1126/sciadv.aaz5443
Crehan O., Davy S. K., Grover R., Ferrier-Pagès C. (2024). Nutrient depletion and heat stress impair the assimilation of nitrogen compounds in a scleractinian coral. J. Exp. Biol. 227, jeb246466. doi: 10.1242/jeb.246466
Cui G., Mi J., Moret A., Menzies J., Zhong H., Li A., et al. (2023). A carbon-nitrogen negative feedback loop underlies the repeated evolution of cnidarian–Symbiodiniaceae symbioses. Nat. Commun. 14, 6949. doi: 10.1038/s41467-023-42582-y
Cziesielski M. J., Schmidt-Roach S., Aranda M. (2019). The past, present, and future of coral heat stress studies. Ecol. Evol. 9, 10055–10066. doi: 10.1002/ece3.5576
Davies P. S. (1991). Effect of daylight variations on the energy budgets of shallow-water corals. Mar. Biol. 108, 137–144. doi: 10.1007/BF01313481
de Goeij J. M., van Duyl F. C. (2007). Coral cavities are sinks of dissolved organic carbon (DOC). Limnol Oceanogr 52, 2608–2617. doi: 10.4319/lo.2007.52.6.2608
Derviche P., Menegotto A., Lana P. (2022). Carbon budget trends in octocorals: a literature review with data reassessment and a conceptual framework to understand their resilience to environmental changes. Mar. Biol. 169, 159. doi: 10.1007/s00227-022-04146-4
Dubois M., Gilles K. A., Hamilton J. K., Rebers P. A., Smith F. (1956). Colorimetric method for determination of sugars and related substances. Anal. Chem. 28, 350–356. doi: 10.1021/ac60111a017
Enríquez S., Méndez E. R., Hoegh-Guldberg O., Iglesias-Prieto R. (2017). Key functional role of the optical properties of coral skeletons in coral ecology and evolution. Proc. R. Soc. London Ser. B 284, 20161667. doi: 10.1098/rspb.2016.1667
Evensen N. R., Voolstra C. R., Fine M., Perna G., Buitrago-López C., Cárdenas A., et al. (2022). Empirically derived thermal thresholds of four coral species along the Red Sea using a portable and standardized experimental approach. Coral Reefs 41, 239–252. doi: 10.1007/s00338-022-02233-y
Ezzat L., Maguer J. F., Grover R., Ferrier-Pagès C. (2016). Limited phosphorus availability is the Achilles heel of tropical reef corals in a warming ocean. Sci. Rep. 6, 1–11. doi: 10.1038/srep31768
Ezzat L., Maguer J. F., Grover R., Rottier C., Tremblay P., Ferrier-Pagès C. (2019). Nutrient starvation impairs the trophic plasticity of reef-building corals under ocean warming. Funct. Ecol. 33, 643–653. doi: 10.1111/1365-2435.13285
Farag M. A., Meyer A., Ali S. E., Salem M. A., Giavalisco P., Westphal H., et al. (2018). Comparative metabolomics approach detects stress-specific responses during coral bleaching in soft corals. J. Proteome Res. 17, 2060–2071. doi: 10.1021/acs.jproteome.7b00929
Ferrier-Pagès C., Bednarz V., Grover R., Benayahu Y., Maguer J. F., Rottier C., et al. (2022). Symbiotic stony and soft corals: Is their host-algae relationship really mutualistic at lower mesophotic reefs? Limnol Oceanogr 67, 261–271. doi: 10.1002/lno.11990
Ferrier-Pagès C., Gattuso J.-P., Cauwet G., Jaubert J., Allemand D. (1998). Release of dissolved organic carbon and nitrogen by the zooxanthellate coral Galaxea fascicularis. Mar. Ecol. Prog. Ser. 172, 265–274. doi: 10.3354/meps172265
Fine M., Cinar M., Voolstra C. R., Safa A., Rinkevich B., Laffoley D., et al. (2019). Coral reefs of the Red Sea — Challenges and potential solutions. Reg. Stud. Mar. Sci. 25, 100498. doi: 10.1016/j.rsma.2018.100498
Fine M., Gildor H., Genin A. (2013). A coral reef refuge in the Red Sea. Glob Chang Biol. 19, 3640–3647. doi: 10.1111/gcb.2013.19.issue-12
Fonseca J. S., Marangoni L. F. B., Marques J. A., Bianchini A. (2017). Effects of increasing temperature alone and combined with copper exposure on biochemical and physiological parameters in the zooxanthellate scleractinian coral. Mussismilia harttii. Aquat. Toxicol. 190, 121–132. doi: 10.1016/j.aquatox.2017.07.002
Fox J., Weisberg S. (2018). An R companion to applied regression. (Thousand Oaks, CA: Sage publications).
Gnaiger E., Bitterlich G. (1984). Proximate biochemical composition and caloric content calculated from elemental CHN analysis: a stoichiometric concept. Oecologia 62, 289–298. doi: 10.1007/BF00384259
Godinot C., Houlbrèque F., Grover R., Ferrier-Pagès C. (2011). Coral uptake of inorganic phosphorus and nitrogen negatively affected by simultaneous changes in temperature and pH. PloS One 6, 1–10. doi: 10.1371/journal.pone.0025024
Goldberg W. M. (2018). “Coral food, feeding, nutrition, and secretion: a review” in Marine organisms as model systems in biology and medicine, eds Kloc M., Kubiak J. Z. (Cham: Springer), 377–421.
Grottoli A. G., Rodrigues L. J. (2011). Bleached Porites compressa and Montipora capitata corals catabolize δ13C-enriched lipids. Coral Reefs 30, 687–692. doi: 10.1007/s00338-011-0756-0
Grottoli A. G., Rodrigues L. J., Juarez C. (2004). Lipids and stable carbon isotopes in two species of Hawaiian corals, Porites compressa and Montipora verrucosa, following a bleaching event. Mar. Biol. 145, 621–631. doi: 10.1007/s00227-004-1337-3
Grottoli A. G., Rodrigues L. J., Palardy J. E. (2006). Heterotrophic plasticity and resilience in bleached corals. Nature 440, 1186–1189. doi: 10.1038/nature04565
Grottoli A. G., Tchernov D., Winters G. (2017). Physiological and biogeochemical responses of super-corals to thermal stress from the Northern Gulf of Aqaba, Red Sea. Front. Mar. Sci. 4, 215. doi: 10.3389/fmars.2017.00215
Guan Y., Hohn S., Wild C., Merico A. (2020). Vulnerability of global coral reef habitat suitability to ocean warming, acidification and eutrophication. Glob Chang Biol. 26, 5646–5660. doi: 10.1111/gcb.15293
Guppy M. (2004). The biochemistry of metabolic depression: A history of perceptions. Comp. Biochem. Physiol. - B Biochem. Mol. Biol. 139, 435–442. doi: 10.1016/j.cbpc.2004.02.019
Hardie D. G., Scott J. W., Pan D. A., Hudson E. R. (2003). Management of cellular energy by the AMP-activated protein kinase system. FEBS Lett. 546, 113–120. doi: 10.1016/S0014-5793(03)00560-X
Heron S. F., Maynard J. A., Van Hooidonk R., Eakin C. M. (2016). Warming trends and bleaching stress of the world’s coral reefs 1985-2012. Sci. Rep. 6, 38402. doi: 10.1038/srep38402
Hill C. E. L., Abbass S. G., Caporale G., El-Khaled Y. C., Kuhn L., Schlenzig T., et al. (2023). Physiology of the widespread pulsating soft coral Xenia umbellata is affected by food sources, but not by water flow. Ecol. Evol. 13, e10483. doi: 10.1002/ece3.10483
Hillyer K. E., Dias D. A., Lutz A., Wilkinson S. P., Roessner U., Davy S. K. (2017). Metabolite profiling of symbiont and host during thermal stress and bleaching in the coral Acropora aspera. Coral Reefs 36, 105–118. doi: 10.1007/s00338-016-1508-y
Hoadley K. D., Lewis A. M., Wham D. C., Pettay D. T., Grasso C., Smith R., et al. (2019). Host–symbiont combinations dictate the photo-physiological response of reef-building corals to thermal stress. Sci. Rep. 9, 9985. doi: 10.1038/s41598-019-46412-4
Hoadley K. D., Pettay D. T., Grottoli A. G., Cai W. J., Melman T. F., Schoepf V., et al. (2015). Physiological response to elevated temperature and pCO2 varies across four Pacific coral species: Understanding the unique host+symbiont response. Sci. Rep. 5, 18371. doi: 10.1038/srep18371
Hoegh-Guldberg O., Dove S. G. (2008). “Primary production, nutrient recycling and energy flow through coral reef ecosystems,” in The Great Barrier Reef: Biology, Environment and Management, eds Hutchings P., Kingsford M., Hoegh-Guldberg O. (Clayton: CSIRO publishing), 59–73.
Hoegh-Guldberg O., Jacob D., Taylor M., Guillén Bolaños T., Bindi M., Brown S., et al. (2019). The human imperative of stabilizing global climate change at 1.5°C. Science 365, eaaw6974. doi: 10.1126/science.aaw6974
Hothorn T., Bretz F., Westfall P. (2008). Simultaneous inference in general parametric models. Biometrical J. 50, 346–363. doi: 10.1002/bimj.200810425
Houlbrèque F., Ferrier-Pagès C. (2009). Heterotrophy in tropical scleractinian corals. Biol. Rev. 84, 1–17. doi: 10.1111/j.1469-185X.2008.00058.x
Hughes T. P., Kerry J. T., Álvarez-Noriega M., Álvarez-Romero J. G., Anderson K. D., Baird A. H., et al. (2017). Global warming and recurrent mass bleaching of corals. Nature 543, 373–377. doi: 10.1038/nature21707
Imbs A. B., Yakovleva I. M. (2012). Dynamics of lipid and fatty acid composition of shallow-water corals under thermal stress: An experimental approach. Coral Reefs 31, 41–53. doi: 10.1007/s00338-011-0817-4
Jacobson L. M., Edmunds P. J., Muller E. B., Nisbet R. M. (2016). The implications of reduced metabolic rate in resource-limited corals. J. Exp. Biol. 219, 870–877. doi: 10.1242/jeb.136044
Jeffrey S. W., Humphrey G. F. (1975). New spectrophotometric equations for determining chlorophylls a, b, c1 and c2 in higher plants, algae and natural phytoplankton. Biochemie und Physiologie der Pflanzen 167, 191–194. doi: 10.1016/s0015-3796(17)30778-3
Kim C. J. S., Roelfsema C., Dove S., Hoegh-Guldberg O. (2022). The Condition of Four Coral Reefs in Timor-Leste before and after the 2016–2017 Marine Heatwave. Oceans 3, 147–171. doi: 10.3390/oceans3020012
Knowlton N., Brainard R. E., Fisher R., Moews M., Plaisance L., Caley M. J. (2010). “Coral reef biodiversity,” in Life in the World’s Oceans. Ed. McIntyre A. D. (Oxford: Blackwell Publishing Ltd), 65–77.
Kochman N. R., Grover R., Rottier C., Ferrier-Pages C., Fine M. (2021). The reef building coral Stylophora pistillata uses stored carbohydrates to maintain ATP levels under thermal stress. Coral Reefs 40, 1473–1485. doi: 10.1007/s00338-021-02174-y
Kochman-Gino N. R., Fine M. (2023). Reef building corals show resilience to the hottest marine heatwave on record in the Gulf of Aqaba. Front. Mar. Sci. 10. doi: 10.3389/fmars.2023.1215567
Krämer W. E., Caamaño-Ricken I., Richter C., Bischof K. (2012). Dynamic regulation of photoprotection determines thermal tolerance of two phylotypes of Symbiodinium clade A at two photon fluence rates. Photochem. Photobiol. 88, 398–413. doi: 10.1111/j.1751-1097.2011.01048.x
LaJeunesse T. C., Parkinson J. E., Gabrielson P. W., Jeong H. J., Reimer J. D., Voolstra C. R., et al. (2018). Systematic revision of Symbiodiniaceae highlights the antiquity and diversity of coral endosymbionts. Curr. Biol. 28, 2570–2580. doi: 10.1016/j.cub.2018.07.008
Lange K., Maguer J. F., Reynaud S., Ferrier-Pagès C. (2023). Nutritional ecology of temperate octocorals in a warming ocean. Front. Mar. Sci. 10. doi: 10.3389/fmars.2023.1236164
Lauer M. M. H., De Oliveira C. B., Yano N. L. I., Bianchini A. (2012). Copper effects on key metabolic enzymes and mitochondrial membrane potential in gills of the estuarine crab Neohelice granulata at different salinities. Comp. Biochem. Physiol. - C Toxicol. Pharmacol. 156, 140–147. doi: 10.1016/j.cbpc.2012.08.001
Lesser M. P. (2013). Using energetic budgets to assess the effects of environmental stress on corals: Are we measuring the right things? Coral Reefs 32, 25–33. doi: 10.1007/s00338-012-0993-x
Levas S., Grottoli A. G., Schoepf V., Aschaffenburg M., Baumann J., Bauer J. E., et al. (2016). Can heterotrophic uptake of dissolved organic carbon and zooplankton mitigate carbon budget deficits in annually bleached corals? Coral Reefs 35, 495–506. doi: 10.1007/s00338-015-1390-z
Levy O., Karako-Lampert S., Ben-Asher H. W., Zoccola D., Pagès G., Ferrier-Pagès C. (2016). Molecular assessment of the effect of light and heterotrophy in the scleractinian coral Stylophora Pistillata. Proc. R. Soc. B: Biol. Sci. 283, 20153025. doi: 10.1098/rspb.2015.3025
Linsmayer L. B., Noel S. K., Leray M., Wangpraseurt D., Hassibi C., Kline D. I., et al. (2024). Effects of bleaching on oxygen dynamics and energy metabolism of two Caribbean coral species. Sci. Total Environ. 919, 170753. doi: 10.1016/j.scitotenv.2024.170753
Lõhelaid H., Teder T., Samel N. (2015). Lipoxygenase-allene oxide synthase pathway in octocoral thermal stress response. Coral Reefs 34, 143–154. doi: 10.1007/s00338-014-1238-y
Loussert-Fonta C., Toullec G., Paraecattil A. A., Jeangros Q., Krueger T., Escrig S., et al. (2020). Correlation of fluorescence microscopy, electron microscopy, and NanoSIMS stable isotope imaging on a single tissue section. Commun. Biol. 3, 362. doi: 10.1038/s42003-020-1095-x
Marangoni L. F. B., Ferrier-Pagès C., Rottier C., Bianchini A., Grover R. (2020). Unravelling the different causes of nitrate and ammonium effects on coral bleaching. Sci. Rep. 10, 11975. doi: 10.1038/s41598-020-68916-0
Moore C. M., Mills M. M., Arrigo K. R., Berman-Frank I., Bopp L., Boyd P. W., et al. (2013). Processes and patterns of oceanic nutrient limitation. Nat. Geosci 6, 701–710. doi: 10.1038/ngeo1765
Muscatine L., Falkowski P. G., Porter J. W., Dubinsky Z. (1984). Fate of photosynthetic fixed carbon in light- and shade-adapted colonies of the symbiotic coral Stylophora pistillata. Proc. R Soc. Lond B Biol. Sci. 222, 181–202. doi: 10.1098/rspb.1984.0058
Nevarez-Lopez C. A., Sanchez-Paz A., Lopez-Martinez J., Llera-Herrera R., Muhlia-Almazan A. (2020). Metabolic response of the cannonball jellyfish Stomolophus meleagris upon short-term exposure to thermal stress. J. Sea Res. 166, 101959. doi: 10.1016/j.seares.2020.101959
Nielsen D. A., Petrou K. (2023). Lipid stores reveal the state of the coral-algae symbiosis at the single-cell level. ISME Commun. 3, 29. doi: 10.1038/s43705-023-00234-8
Osman E. O., Smith D. J., Ziegler M., Kürten B., Conrad C., El-Haddad K. M., et al. (2017). Thermal refugia against coral bleaching throughout the northern Red Sea. Glob Chang Biol. 24, e474–e484. doi: 10.1111/gcb.13895
Palardy J. E., Rodrigues L. J., Grottoli A. G. (2008). The importance of zooplankton to the daily metabolic carbon requirements of healthy and bleached corals at two depths. J. Exp. Mar. Biol. Ecol. 367, 180–188. doi: 10.1016/j.jembe.2008.09.015
Pan T. C. F., Applebaum S. L., Manahan D. T. (2015). Experimental ocean acidification alters the allocation of metabolic energy. Proc. Natl. Acad. Sci. U.S.A. 112, 4696–4701. doi: 10.1073/pnas.1416967112
Petrou K., Nielsen D. A., Heraud P. (2018). Single-cell biomolecular analysis of coral algal symbionts reveals opposing metabolic responses to heat stress and expulsion. Front. Mar. Sci. 5. doi: 10.3389/fmars.2018.00110
Pupier C. A., Bednarz V. N., Ferrier-Pagès C. (2018). Studies with soft corals - recommendations on sample processing and normalization metrics. Front. Mar. Sci. 5. doi: 10.3389/fmars.2018.00348
Pupier C. A., Bednarz V. N., Grover R., Fine M., Maguer J.-F., Ferrier-Pagès C. (2019a). Divergent capacity of scleractinian and soft corals to assimilate and transfer diazotrophically derived nitrogen to the reef environment. Front. Microbiol. 10. doi: 10.3389/fmicb.2019.01860
Pupier C. A., Fine M., Bednarz V. N., Rottier C., Grover R., Ferrier-Pagès C. (2019b). Productivity and carbon fluxes depend on species and symbiont density in soft coral symbioses. Sci. Rep. 9, 17819. doi: 10.1038/s41598-019-54209-8
Pupier C. A., Grover R., Fine M., Rottier C., van de Water J. A. J. M., Ferrier-Pagès C. (2021a). Dissolved nitrogen acquisition in the symbioses of soft and hard corals with symbiodiniaceae: A key to understanding their different nutritional strategies? Front. Microbiol. 12. doi: 10.3389/fmicb.2021.657759
Pupier C. A., Mies M., Fine M., Bastos Francini-Filho R., Pereira Brandini F., Zambotti-Villela L., et al. (2021b). Lipid biomarkers reveal the trophic plasticity of octocorals along a depth gradient. Limnol Oceanogr 66, 2078–2087. doi: 10.1002/lno.11746
Rivera H. E., Tramonte C. A., Samaroo J., Dickerson H., Davies S. W. (2023). Heat challenge elicits stronger physiological and gene expression responses than starvation in symbiotic Oculina arbuscula. J. Heredity 114, 312–325. doi: 10.1093/jhered/esac068
Rodrigues L. J., Grottoli A. G. (2007). Energy reserves and metabolism as indicators of coral recovery from bleaching. Limnol Oceanogr 52, 1874–1882. doi: 10.4319/lo.2007.52.5.1874
Ros M., Camp E. F., Hughes D. J., Crosswell J. R., Warner M. E., Leggat W. P., et al. (2020). Unlocking the black-box of inorganic carbon-uptake and utilization strategies among coral endosymbionts (Symbiodiniaceae). Limnol Oceanogr 65, 1747–1763. doi: 10.1002/lno.11416
Rosset S., Wiedenmann J., Reed A. J., D’Angelo C. (2017). Phosphate deficiency promotes coral bleaching and is reflected by the ultrastructure of symbiotic dinoflagellates. Mar. pollut. Bull. 118, 180–187. doi: 10.1016/j.marpolbul.2017.02.044
Santos S. R., Taylor D. J., Kinzie R. A., Hidaka M., Sakai K., Coffroth M. A. (2002). Molecular phylogeny of symbiotic dinoflagellates inferred from partial chloroplast large subunit (23S)-rDNA sequences. Mol. Phylogenet Evol. 23, 87–11. doi: 10.1016/s1055-7903(02)00010-6
Schlichter D., Svoboda A., Kremer B. P. (1983). Functional autotrophy of Heteroxenia fuscescens (Anthozoa: Alcyonaria): carbon assimilation and translocation of photosynthates from symbionts to host. Mar. Biol. 78, 29–38. doi: 10.1007/BF00392968
Schubert N., Brown D., Rossi S. (2017). “Symbiotic versus non-symbiotic octocorals: physiological and ecological implications,” in Marine Animal Forests: the Ecology of Benthic Biodiversity Hotspots, eds Bramanti L., Gori A., Rossi S. (Cham: Springer), 887–918. doi: 10.1007/978-3-319-17001-5
Sebens K. P. (1987). “Coelenterata,” in Animal energetics. Eds. Pandian T., Vernberg F. (Academic Press, New York), 55–120.
Shick J. M., Widdows J., Gnaiger E. (1988). Calorimetric studies of behavior, metabolism and energetics of sessile intertidal animals. Am. Zool 28, 161–181. doi: 10.1093/icb/28.1.161
Sikorskaya T. V., Ermolenko E. V., Imbs A. B. (2020). Effect of experimental thermal stress on lipidomes of the soft coral Sinularia sp. and its symbiotic dinoflagellates. J. Exp. Mar. Biol. Ecol. 524, 151295. doi: 10.1016/j.jembe.2019.151295
Silbiger N. J., Nelson C. E., Remple K., Sevilla J. K., Quinlan Z. A., Putnam H. M., et al. (2018). Nutrient pollution disrupts key ecosystem functions on coral reefs. Proc. R. Soc. B: Biol. Sci. 285, 20172718. doi: 10.1098/rspb.2017.2718
Silverstein R. N., Cunning R., Baker A. C. (2017). Tenacious D: Symbiodinium in clade D remain in reef corals at both high and low temperature extremes despite impairment. J. Exp. Biol. 220, 1192–1196. doi: 10.1242/jeb.148239
Smith P. K., Krohn R. I., Hermanson G. T., Mallia A. K., Gartner F. H., Provenzano M. D., et al. (1985). Measurement of protein using bicinchoninic acid. Anal. Biochem. 150, 76–85. doi: 10.1016/0003-2697(85)90442-7
Sokolova I. M. (2013). Energy-limited tolerance to stress as a conceptual framework to integrate the effects of multiple stressors. Integr. Comp. Biol. 53, 597–608. doi: 10.1093/icb/ict028
Sokolova I. M., Frederich M., Bagwe R., Lannig G., Sukhotin A. A. (2012). Energy homeostasis as an integrative tool for assessing limits of environmental stress tolerance in aquatic invertebrates. Mar. Environ. Res. 79, 1–15. doi: 10.1016/j.marenvres.2012.04.003
Starzak D. E., Quinnell R. G., Nitschke M. R., Davy S. K. (2014). The influence of symbiont type on photosynthetic carbon flux in a model cnidarian-dinoflagellate symbiosis. Mar. Biol. 161, 711–724. doi: 10.1007/s00227-013-2372-8
Takahashi S., Nakamura T., Sakamizu M., Van Woesik R., Yamasaki H. (2004). Repair machinery of symbiotic photosynthesis as the primary target of heat stress for reef-building corals. Plant. Cell Physiol. 45, 251–255. doi: 10.1093/pcp/pch028
Tchernov D., Gorbunov M. Y., De Vargas C., Yadav S. N., Milligan A. J., Häggblom M., et al. (2004). Membrane lipids of symbiotic algae are diagnostic of sensitivity to thermal bleaching in corals. Proc. Natl. Acad. Sci. 101, 13531–13535. doi: 10.1073/pnas.0402907101
Tignat-Perrier R., van de Water J. A. J. M., Guillemain D., Aurelle D., Allemand D., Ferrier-Pagès C. (2022). The effect of thermal stress on the physiology and bacterial communities of two key mediterranean gorgonians. AEM 88, e02340–e02321. doi: 10.1128/aem.02340-21
Towle E. K., Enochs I. C., Langdon C. (2015). Threatened Caribbean coral is able to mitigate the adverse effects of ocean acidification on calcification by increasing feeding rate. PloS One 10, e0123394. doi: 10.1371/journal.pone.0123394
Travesso M., Missionário M., Cruz S., Calado R., Madeira D. (2023). Combined effect of marine heatwaves and light intensity on the cellular stress response and photophysiology of the leather coral Sarcophyton cf. glaucum. Sci. Total Environ. 861, 160460. doi: 10.1016/j.scitotenv.2022.160460
Treignier C., Grover R., Ferrier-Pagès C., Tolosa I. (2008). Effect of light and feeding on the fatty acid and sterol composition of zooxanthellae and host tissue isolated from the scleractinian coral Turbinaria reniformis. Limnol Oceanogr 53, 2702–2710. doi: 10.4319/lo.2008.53.6.2702
Tremblay P., Gori A., Maguer J.-F., Hoogenboom M., Ferrier-Pagès C. (2016). Heterotrophy promotes the re-establishment of photosynthate translocation in a symbiotic coral after heat stress. Sci. Rep. 6, 38112. doi: 10.1038/srep38112
Tremblay P., Grover R., Maguer J.-F., Hoogenboom M., Ferrier-Pagès C. (2014). Carbon translocation from symbiont to host depends on irradiance and food availability in the tropical coral. Stylophora pistillata. 33, 1–13. doi: 10.1007/s00338-013-1100-7
Veal C. J., Carmi M., Fine M., Hoegh-Guldberg O. (2010). Increasing the accuracy of surface area estimation using single wax dipping of coral fragments. Coral Reefs 29, 893–897. doi: 10.1007/s00338-010-0647-9
Vollstedt S., Xiang N., Simancas-Giraldo S. M., Wild C. (2020). Organic eutrophication increases resistance of the pulsating soft coral Xenia umbellata to warming. PeerJ 8, e9182. doi: 10.7717/peerj.9182
Voolstra C. R., Valenzuela J. J., Turkarslan S., Cárdenas A., Hume B. C. C., Perna G., et al. (2021). Contrasting heat stress response patterns of coral holobionts across the Red Sea suggest distinct mechanisms of thermal tolerance. Mol. Ecol. 30, 4466–4480. doi: 10.1111/mec.16064
Wangpraseurt D., Polerecky L., Larkum A. W. D., Ralph P. J., Nielsen D. A., Pernice M., et al. (2014). The in situ light microenvironment of corals. Limnol Oceanogr 59, 917–926. doi: 10.4319/lo.2014.59.3.0917
Warner M. E., Fitt W. K., Schmidt G. W. (1999). Damage to photosystem II in symbiotic dinoflagellates: A determinant of coral bleaching. Proc. Natl. Acad. Sci. 96, 8007–8012. doi: 10.1073/pnas.96.14.8007
Wiedenmann J., D’Angelo C., Smith E. G., Hunt A. N., Legiret F.-E., Postle A. D., et al. (2012). Nutrient enrichment can increase the susceptibility of reef corals to bleaching. Nat. Clim Chang 3, 160–164. doi: 10.1038/nclimate1661
Yoffe C., Lotan T., Benayhau Y. (2012). A modified view on octocorals: Heteroxenia fuscescens nematocysts are diverse, featuring both an ancestral and a novel type. PloS One 7, 1–5. doi: 10.1371/journal.pone.0031902
Zaneveld J. R., Burkepile D. E., Shantz A. A., Pritchard C. E., McMinds R., Payet J. P., et al. (2016). Overfishing and nutrient pollution interact with temperature to disrupt coral reefs down to microbial scales. Nat. Commun. 7, 1–12. doi: 10.1038/ncomms11833
Keywords: ocean warming, nutrient limitation, energetics, stress, biomarker
Citation: Pupier CA, Grover R, Rottier C and Ferrier-Pagès C (2024) Impact of seawater warming and nutrient deprivation on the physiology and energy metabolism of corals. Front. Mar. Sci. 11:1423034. doi: 10.3389/fmars.2024.1423034
Received: 25 April 2024; Accepted: 13 September 2024;
Published: 01 October 2024.
Edited by:
Stefano Goffredo, University of Bologna, ItalyReviewed by:
Marleen Stuhr, Leibniz Centre for Tropical Marine Research (LG), GermanyDavide Seveso, University of Milano-Bicocca, Italy
Copyright © 2024 Pupier, Grover, Rottier and Ferrier-Pagès. This is an open-access article distributed under the terms of the Creative Commons Attribution License (CC BY). The use, distribution or reproduction in other forums is permitted, provided the original author(s) and the copyright owner(s) are credited and that the original publication in this journal is cited, in accordance with accepted academic practice. No use, distribution or reproduction is permitted which does not comply with these terms.
*Correspondence: Chloé A. Pupier, Y2hsb2UucHVwaWVyQHVwZi5wZg==; Christine Ferrier-Pagès, ZmVycmllckBjZW50cmVzY2llbnRpZmlxdWUubWM=