- 1National Engineering Research Center for Marine Aquaculture, Zhejiang Ocean University, Zhoushan, China
- 2Ocean College, Zhejiang University, Hangzhou, China
- 3Marine Science and Technology College, Zhejiang Ocean University, Zhoushan, China
- 4Information and Intelligent Engineering, Zhejiang Wanli University, Ningbo, China
In the process of ascending and descending in deep-sea submerging and lifting cage nets, accompanied by changes in water pressure, pressure is one of the crucial environmental factors affecting the physiology and growth of fish. It directly relates to the comfort of farmed fish in the cage, thereby influencing the quality of aquaculture, especially for high-demanding species on the culture environment like the Larimichthys crocea. Investigating the stress level changes exhibited by L.crocea under environmental pressure variations, understanding the limit tolerance pressure, and its changes, can provide a theoretical basis for the design, application, and increased production of deep-sea submersible cage nets and L.crocea aquaculture. This study explores the effects of varying submersion depths (0–20 m) and speeds (0.6, 1, 1.3, 2, 4 m/min) on the behavioral responses of L.crocea in deep-sea cage environments. Key findings demonstrate that increased submersion depths and speeds significantly influence the physical behaviors and stress responses of the species. At submersion depths of 15 meters and 20 meters, the average swimming speed of the L.crocea exceeds 0.05 meters per second, the tail movement frequency is 1.42 times that of normal pressure, and the gasping frequency exceeds 20%., and at the highest speed of 4 m/min, the swimming speed reaches 0.0902 m/s—3.76 times greater than under normal pressure. The study determines that a submersion depth of 10 meters and a submersion speed of 0.6 meters per minute can minimize stress responses, providing critical insights for optimizing deep-sea aquaculture operations of L.crocea. These results offer valuable guidelines for the design and management of submersible cage systems.
1 Introduction
The global demand for aquaculture is continuously increasing, yet traditional nearshore aquaculture practices are no longer sufficient to meet this growing need (Bostock et al., 2010). Deep-sea aquaculture, as an emerging mode of aquaculture, has attracted widespread attention. Particularly, submersible cage technology, which can adapt to extreme deep-sea conditions and ensure the safety of aquaculture equipment and species, is seen as an effective mode to expand aquaculture space and reduce ecological pressure on coastal areas (Zhang, 2023). However, current research primarily focuses on the physical design and structural optimization of submersible cages, there is a lack of research on the influence of the depth and speed of cage diving on the behavior of L.crocea.This gap in research underscores the need for further investigation into these parameters to optimize deep-sea farming practices.
The L.crocea, a significant economic marine fish, is renowned for its tender and tasty meat, rich nutrition, and high economic value. It has long been favored by people along the southeastern coast of China (Liu et al., 2021). However, the current culture of L.crocea is still mainly dependent on the traditional open surface cage culture mode and purse Seine culture mode, and the quality and survival rate of L.crocea are difficult to guarantee. By adjusting the depth, the submersible cage culture mode can effectively reduce the burden of parasites such as sea lice, improve the survival rate and meat quality of L.crocea, and provide a more stable and controlled breeding environment. Especially in the face of extreme weather such as typhoon, the risk of fish escape can be significantly reduced. Currently, the feasibility of underwater or semi-submersible cage aquaculture has been successfully demonstrated for several fish species, including Pacific bluefin tuna (Engelhaupt, 2007), greater amberjack (Benetti et al., 2008), European sea bass, black cod (Chambers and Howell, 2006), and Atlantic salmon (Dempster et al., 2009). Due to overfishing and scarcity of L.crocea, most research on this species has simply focused on breeding and seedling cultivation (Fernandez-Jover et al., 2008). However, the characteristics of L.crocea cultivation, quality requirements, and limitations of traditional farming methods determine that the adoption of deep-sea submersible cage aquaculture is imperative. Therefore, this study investigates the behaviors of L.crocea in the submersible cage aquaculture model, filling the gap on behavioral safety and health quality during the L.crocea cultivation process in submersible cages.
The submersion process in submersible cages significantly influences the aquaculture environment by altering water pressure, which affects fish behavior and physiology. Notably, research has shown that increasing depth impacts species differently based on their tolerance to static water pressure (Carney, 2005; Lemaire et al., 2016). Furthermore, adjustments in cage height can regulate behavioral responses such as buoyancy in cod, linked to changes in water pressure (Korsoen et al., 2010). These adjustments are critical as they can lead to severe behavioral stress, potentially resulting in injury or death, particularly in species with slow rates of swim bladder adaptation (Fänge, 1966). This study aims to systematically explore how controlled variations in submersion depth and speed impact these responses, emphasizing the optimization of aquaculture practices to enhance fish welfare and productivity. And the Revealer Motion Analysis software, applied in engineering survey, is applied for object tracking, thereby enhancing result accuracy and feasibility. Additionally, computer vision technology provides an automated, non-invasive, and cost-effective method for recording behavioral parameters quantified and analyzed changes in fish behavior by monitoring fish behavior recorded and quantified behavioral parameters of one or two fish in a container, such as position coordinates, speed, swimming distance, trajectory, and turning direction, using computer vision technology to study various fish behaviors (Nogita et al., 1988; Chuang et al., 2016; Petrellis, 2021).
Based on the considerations above, this study aims to investigate the impact of pressure changes during the submersion process of the submersible cage on fish behavior. It explores the optimal submersion speed of the cage and the tolerance limit of L.crocea to sudden pressure changes. The goal is to ensure that the submersion depth and speed of the cage do not induce strong stress responses in L.crocea. The research focuses on the behaviors of L.crocea under different submersion depths (0–20 m) and various submersion speeds (0.6, 1, 1.3, 2, 4 m/min). In this experimental process, we conducted pressurized simulation tests under two conditions: different depths at the same speed and different speeds at the same depth. These tests simulated the variations in submersion pressure under realistic sea conditions and observed the behavioral indicators of fish during the experiment. The aim is to minimize stress responses in L.crocea during the submersion process, ultimately enhancing the survival rate of deep-sea aquaculture for L.crocea. This research will bring about significant theoretical and engineering value for enhancing the quality of L.crocea aquaculture.
2 Materials and methods
2.1 Experimental setup
The experimental setup for simulating pressure changes is illustrated in Figure 1, primarily consisting of four components: an acrylic pressure-resistant chamber, a gas pathway, a control system, and a recording system. The acrylic pressure-resistant chamber is designed to simulate the pressure changes experienced by the L.crocea in the submersible cage and possesses sufficient pressure-bearing capacity. The gas passage connects the pressurization equipment to ensure stable and unobstructed pressurization. The control system is responsible for adjusting the pressurization speed to meet the requirements of the experiment. The recording system is employed for observing and documenting the activities of the experimental fish within the acrylic pressure-resistant chamber.
2.1.1 Acrylic pressure-resistant chamber
The acrylic pressure-resistant chamber is divided into two parts: the inner cylinder and the outer one. The inner cylinder is a cylindrical structure with an internal height of 80 cm and an internal diameter of 30 cm. It is equipped with an inlet valve, an outlet valve, and a pressure sensor at the top. The outer one is a rectangular structure with an internal height of 50 cm and internal dimensions of 35 cm by 35 cm, the experimental space can ensure the normal activities of experimental fish. The chamber is constructed from transparent acrylic sheets, providing excellent pressure resistance and visibility. To avoid refraction of the water surface during observation, an equal height of seawater is added to both the outer and inner cylinders, allowing for clear and detailed observation of the behavioral indicators of the experimental fish to pressure changes within the chamber.
2.1.2 The gas passage
The gas passage is composed of an air compressor, air hose, air filter pressure regulator valve, and proportional valve. The air compressor (Dongcheng DQE800–24 L) can reach a maximum pressure of 7 bar and has a gas capacity of 24 L, ensuring the stability of pressurization during the experiment. The air hose (Dongcheng EVA5*8) connects the experimental equipment in the gas passage. The air filter pressure regulator valve (AW20–02BCG) maintains the pressure in the gas pipeline within a safe and stable range through pressure settings, achieving the regulation of gas settings, ensuring stable pressure, and providing a safe pressurization environment during the experiment. The proportional valve (SMC voltage type ITV1030–312CL) continuously and proportionally controls the airflow, pressure, and direction of the air based on the input analog signal. By opening the air compressor for pressurization, the air flows through the air hose, undergoes filtration and stabilization through the air filter pressure regulator valve, and then enters the acrylic pressure-resistant chamber by controlling the pressurization through the proportional valve based on changes in the valve opening.
2.1.3 The control system
The control system consists of a DC power supply, pressure transmitter, PLC controller, and control panel. The DC power supply (Delta CKDU-S150 W/24 V/6.5 A) provides stable regulated DC output for the entire system. The pressure transmitter (CYYZ11A) monitors real-time changes in pressure inside the acrylic pressure-resistant chamber. The differential voltage signal generated by the pressure resistance reaction is amplified through a dedicated amplifier, converting the signal corresponding to the full scale into a standard analog signal, which is then sent back to the PLC controller. The PLC controller (SK2N PLC), based on the input signals and the program written in Gx Works 2 software, executes instructions and outputs controls. The control panel (FLEXEM control panel), programmed with FStudio 2 software on the host computer, allows the setting of pressure and speed for pressurization and depressurization. It accurately displays the internal pressure of the acrylic pressure-resistant chamber and provides manual or automatic control of the proportional valve’s opening. The control panel visually presents all the equipment of the control system in both modes.
2.1.4 The recording system
The recording system consists of two cameras and a computer, providing real-time monitoring of the activity of experimental fish inside the acrylic pressure-resistant chamber. Cameras are installed on the front and side of the water tank, connected to the computer via a switch. By adjusting the eight vertices of the water tank to the internal video image, the selected camera (Hanbang High-tech HB772S) with a resolution of 1960×1280 pixels and a frame rate of 25 frames/s captures the activity of the fish.
Before the experiment, set the pressurization pressure and pressurization speed through the control panel, then open the intake valve and the air compressor to start pressurization. During the experiment, monitor the real-time pressure on the pressure transmitter and control panel. Once the internal pressure reaches the preset value, close the intake valve to stabilize the pressure inside the chamber. A high-definition camera synchronously records the entire experimental process, capturing the behavioral states of the experimental fish in the chamber. The recorded videos are then imported for image processing and analysis.
2.2 Experimental design
2.2.1 Experimental fish
The L.crocea used in this experiment were selected from the East China Sea area of Zhejiang Province, China, with a length of 90–110 mm and width of 4–8 mm. The fish were in good health with intact scales. Based on the actual effective avoidance depth of typhoon submersion in the submerged cage, the pressure range of the experiment was set at 0–200 kPa, corresponding to the submerged depth of the cage from 0 m (standard atmospheric pressure) to -20 m water depth pressure. Half of the acrylic pressure-resistant chamber’s inner cylinder was filled with seawater (depth of 40 cm), and the upper half was filled with air. The pressure inside the chamber was adjusted by the control system to simulate various pressure changes during the descent process of fish in a real submerged cage under sea conditions.
2.2.2 Working condition settings
This experiment investigated the behaviors of L.crocea under different pressurization speeds (corresponding to the respective test for the descent speed of the cage) and different pressure peaks (corresponding to the respective test for the descent depth of the cage). The experimental conditions are shown in Table 1. The control group represents L.crocea under normal atmospheric pressure. In the experiment, ΔP refers to the actual value of the pressure in the box minus one atmosphere. H-number represents different pressure peaks, where H1, H2, H3, and H4 correspond to pressures of 50, 100, 150, and 200 kPa, respectively, corresponding to descent depths of 5, 10, 15, and 20 m in the cage, the descent speeds adopted was uniform at 1 m/min. K-number represents different pressurization speeds, where K1, K2, K3, K4, and K5 represent pressurization speeds and cage descent speeds of 0.6,1.0, 1.3,2.0 and 4.0, m/min, respectively. Thirty L.crocea were used in this experiment, and Each set of conditions was repeated with three L.crocea.
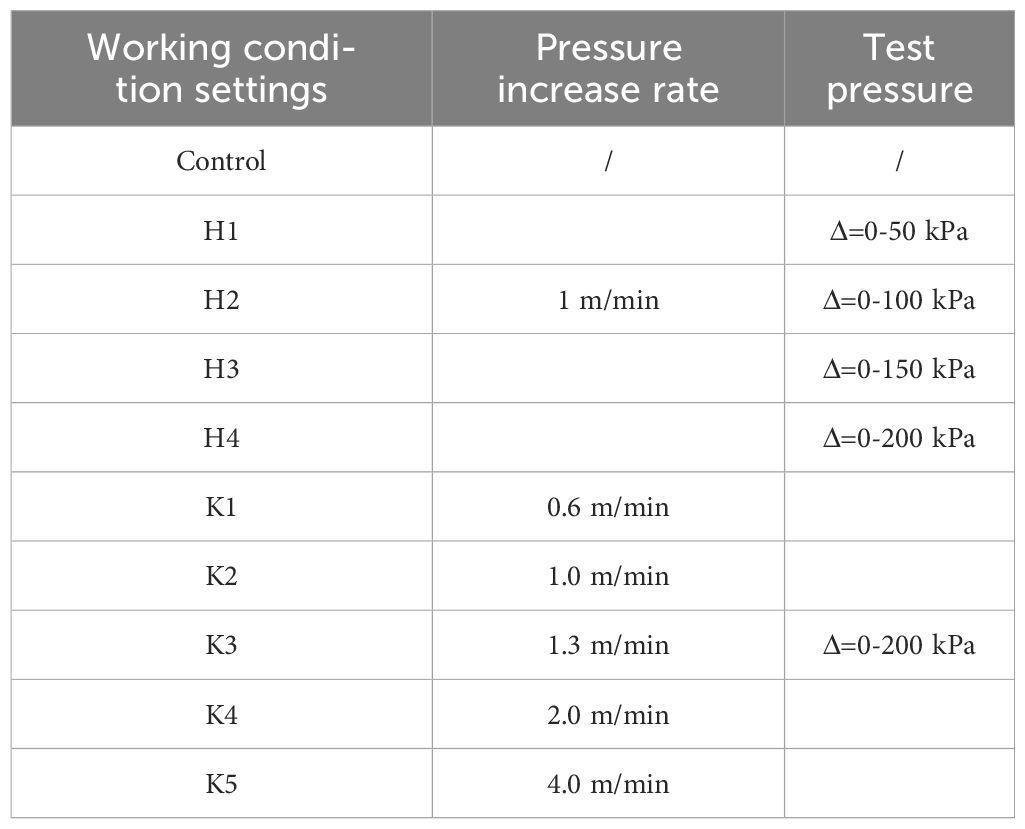
Table 1 Setting of test conditions for different pressurization speeds and different pressure peaks.
2.3 Behavioral indices and image-processing methods
This study investigated the impact of pressure changes during the descent of the cage on the cultured L.crocea. The stress responses in fish refer to the behavior where fish bodies suddenly experience intense harmful stimuli, leading to slower growth and development, reduced reproductive capacity, and even death. A systematic examination was conducted on stress responses, including changes in behaviors of the L.crocea before and after the experiment. Physical behavior analysis included a quantitative assessment of tailbeat frequency, swimming tilt angle, swimming speed, and gasping frequency in L.crocea. The entire pressurization experiment process was recorded by a video system. After completion of observation and recording, the video data were imported into a computer for further video analysis.
Swimming speed (m/s) was calculated based on the displacement of the L.crocea per second. The experimental videos captured by the video system were imported into the Revealer Motion Analysis software and converted into image data processing procedure is depicted in Figure 2.
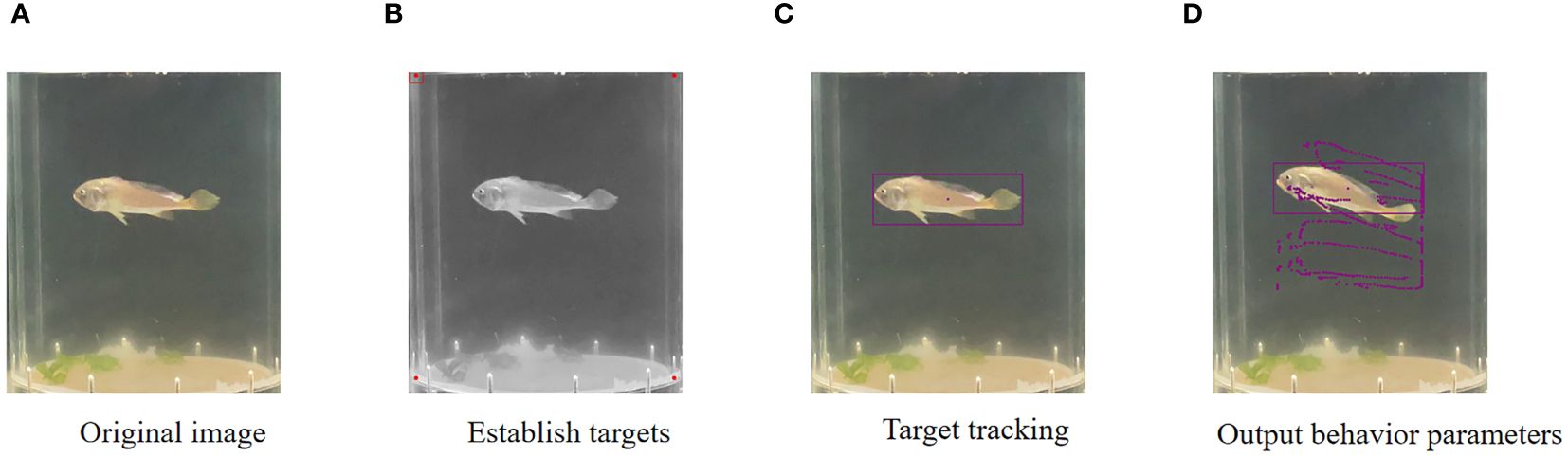
Figure 2 Process of determining swimming speed: (A) Original image; (B) Establish targets; gamma correction, establishing four targets in the image, and setting corresponding coordinates. (C) Target tracking; defines the L.crocea in the image for automatic tracking of the target. (D) Output behavior parameters. tracks the target in image, combined with motion trajectory parameters, to output the swimming speed of the fish.
Tilt angle (°) was defined as the angle between the L.crocea’s swimming position and the horizontal plane. MATLAB was used for image processing, and the specific data processing procedure is depicted in Figure 3.
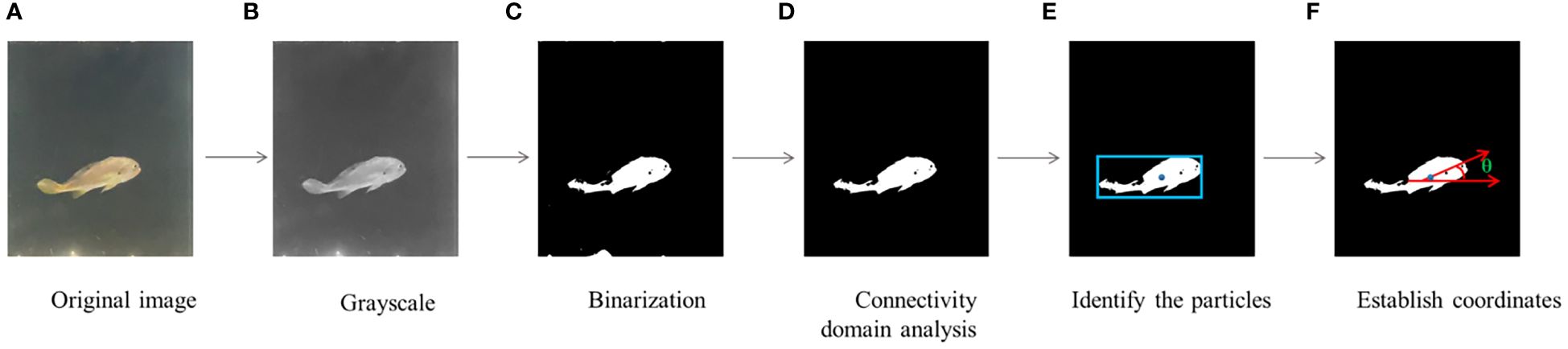
Figure 3 Process of determining tilt angle. (A) Original image; (B) Grayscale; Grayscale processing to generate image. (C) Binarization; the conversion of the image to black and white by a single threshold method. (D) Connectivity domain analysis; (E) Identify the particles; obtain the average coordinates of each vertex as the centroid of the final fish area (F) Establish coordinates. establishes the coordinates based on the obtained centroid, and the angle with the horizontal is the tilt angle.
Gasping frequency (Hz): The gasping frequency is the number of times a L.crocea emerges from the water per minute (gasping refers to a stress behavior where the fish jumps out of the water due to insufficient oxygen). It is obtained by recording the experimental video through the video system and manually counting the gasping frequency.
Tailbeat frequency (Hz): The tailbeat frequency is the number of tailbeats per second of the L.crocea (each tail beat is the process of the fish going from relaxed to bent state and then back to relaxed situation). It is obtained by recording the experimental video through the video system and manually counting the tail beat frequency. The final results for each experiment are the average of three sets of repeated experiments.
2.4 Animal ethics statement
Institutional Review Board Statement: All experimental and sampling procedures were conducted according to the Guidelines of the Animal Care of the Zhejiang Ocean University and were approved by the Ethics Committee of the university (Approval code: 2023088).
3 Results
3.1 The impact of submergence depth on the behavior of L.crocea in submergible cages
3.1.1 Swimming speed
Figure 4 illustrates the impact of different pressure values resulting from the submersion depth on the swimming speed of L.crocea. As shown in Figure 4, at a submersion depth of 0 m (Control group H0—no pressure applied), the average swimming speed of L.crocea is approximately 0.024 ± 0.003 m/s. At submersion depths of 5 m (H1—50 kPa), 10 m (H2—100 kPa), 15 m (H3—150 kPa), and 20 m (H4—200 kPa), the average swimming speeds of L.crocea are 0.029 ± 0.006, 0.039 ± 0.009, 0.055 ± 0.015, and 0.058 ± 0.016m/s, respectively, coefficient of variation <0.3. With increasing pressure, the swimming speed of L.crocea significantly increases. Clearly, the variation in submersion depth of the net cage, causing changes in environmental pressure, holds a certain impact on the physical behavior of L.crocea—swimming speed. Particularly, at submersion depths of 15 m and 20 m, corresponding to environmental pressures of 150 kPa and 200 kPa, the average swimming speed of L.crocea increases significantly, exceeding 0.05 m/s. This represents an increase of over 50% in comparison to L.crocea freely swimming in a comfortable environment (Control group H0—no pressure applied).
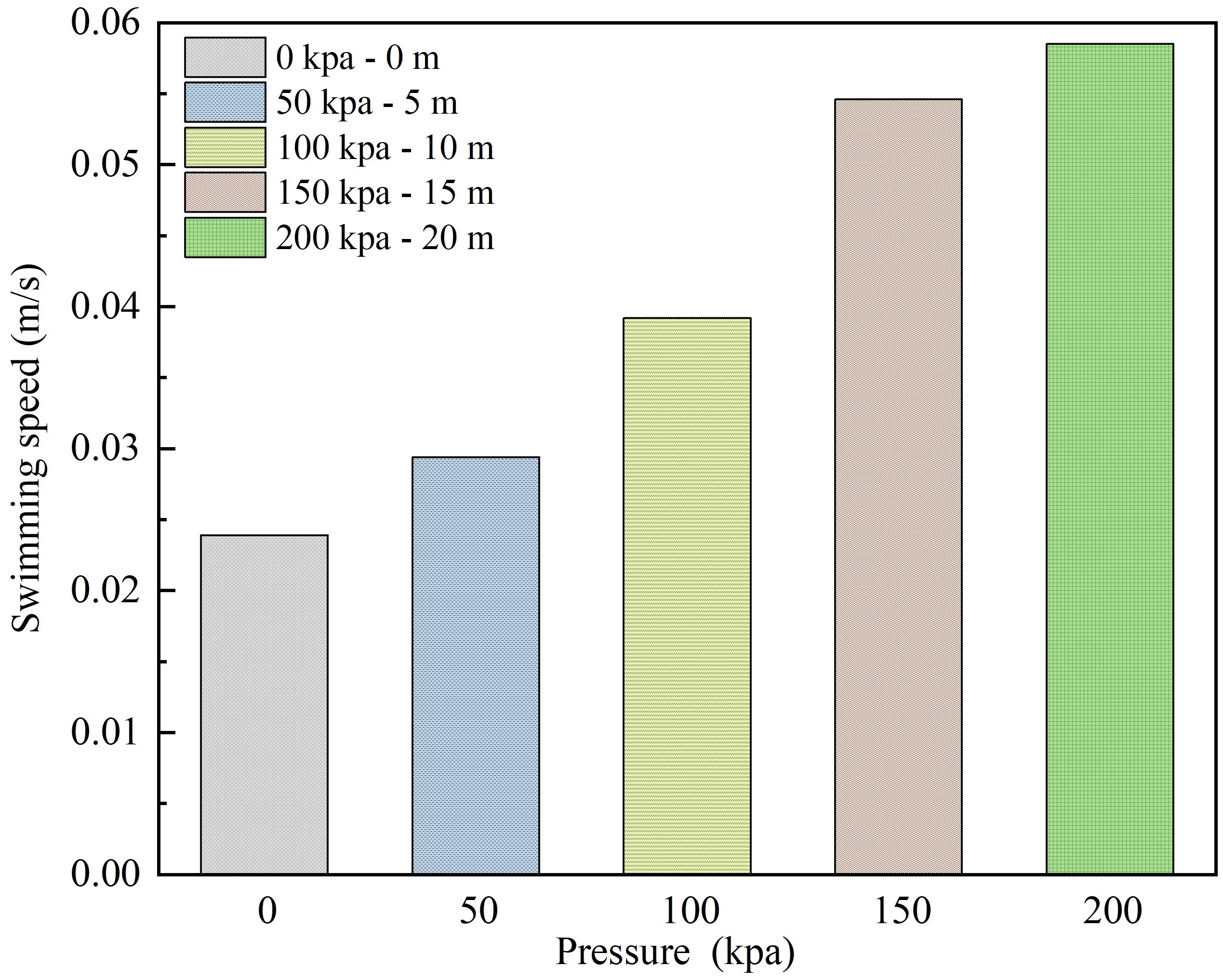
Figure 4 The swimming speed of L.crocea under different pressures corresponding to different diving depths of the net cage.
3.1.2 Tilt angle
Figure 5 presents the impact of different pressure values resulting from the submersion depth on the tilt angle of L.crocea. A tilt angle of 0° indicates that L.crocea inside the net cage maintains a horizontal swimming state. Tilt angles of ±90° indicate that L.crocea inside the net cage is in a state of vertical ascent or descent. As shown in Figure 5, in the control group H1, where the net cage has no submersion, and thus, L.crocea experiences no additional external pressure, L.crocea is predominantly distributed in a ‘clustered’ pattern, with tilt angles mostly falling within the range of 0°- ± 15°. As the submersion pressure increases, the tilt angles of L.crocea show a ‘forked’ pattern, and with increasing pressure, the range of forked distribution gradually widens, and tilt angles increase with pressure. When the pressure transitions from 100 kPa to approximately the midpoint value of 125 kPa, L.crocea in a horizontal steady state, i.e., 0° tilt angle, is almost non-existent. When the submersion depth reaches 20 m, corresponding to a pressure of 200 kPa, it is evident that the tilt angles of L.crocea approach 90° (vertical state). This indicates that if the pressure is above 125 kPa, L.crocea exhibits an unstable state of either floating or sinking, and at 200 kPa, the discomfort in L.crocea, as reflected by the tilt angle indicator, is most pronounced.
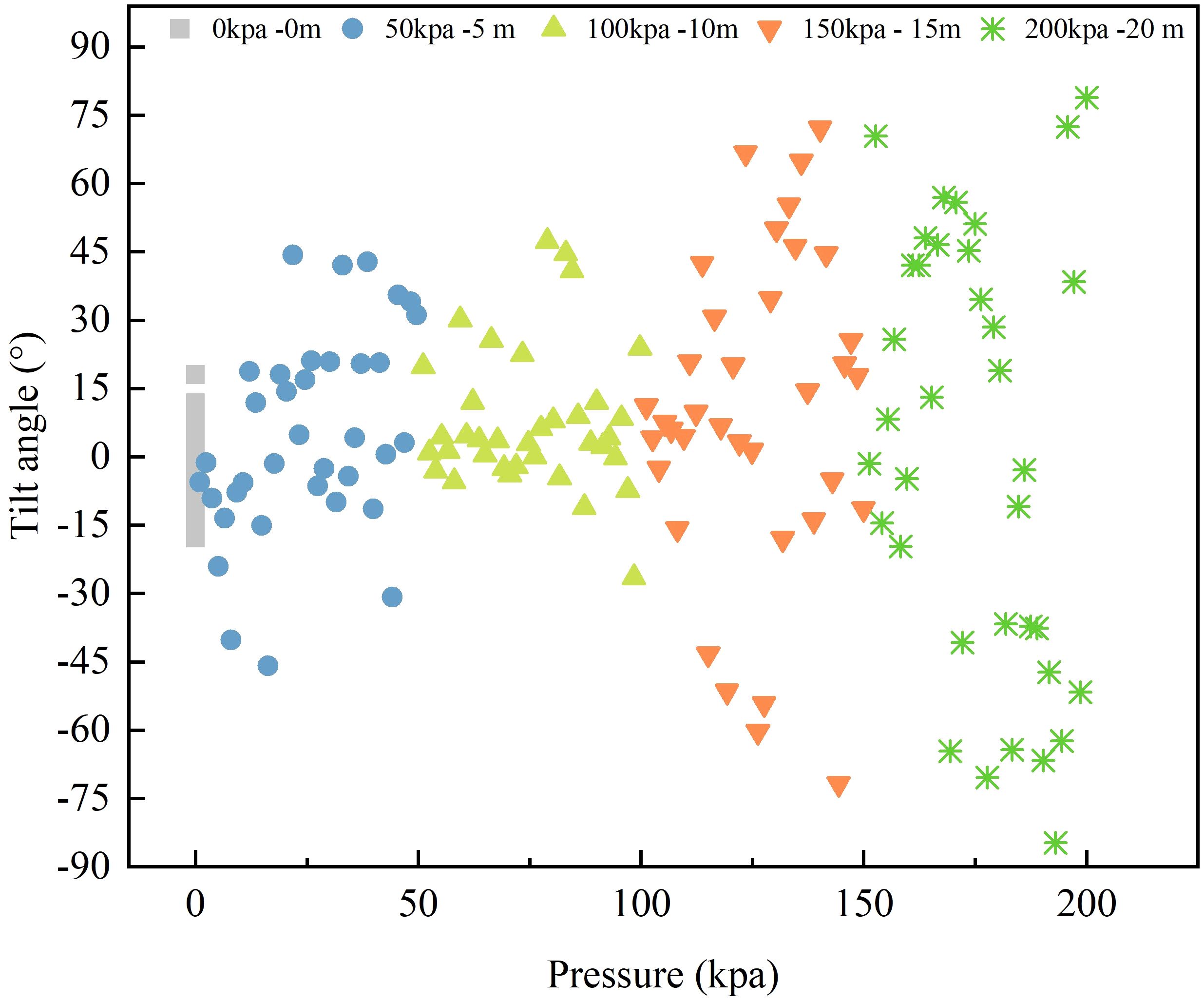
Figure 5 The tilt angle and distribution of L.crocea under different pressures corresponding to different diving depths of the net cage.
3.1.3 Gasping frequency
Figure 6 depicts the impact of different net cage submersion depths on the gasping frequency of L.crocea under corresponding pressure values. As shown in Figure 6, at submersion depths of 0 m (free state), 5 m (50 kPa), and 10 m (100 kPa), the gasping frequency is 0, indicating no occurrence of gasping behavior in L.crocea. However, as the pressure increases to 150 kPa at a depth of 15 m and 200 kPa at 20 m, frequent gasping occurrences are observed, with frequencies of 11 ± 3.21 times/min and 21 ± 6.57 times/min, respectively. The gasping frequency is positively correlated with submersion depth. The research results suggest that L.crocea can adapt to the pressure inside the net cage without the need for gasping behavior to replenish oxygen at submersion depths of 0–10 m. As the submersion depth continues to increase, excessive environmental pressure prevents the exchange of gases within the bodies of fish, necessitating gasping behavior to maintain pressure. Additionally, there is a close correlation between the gasping and tilt angle, especially when a 90° tilt angle is often accompanied by gasping behavior. The trends in tilt angle from Section 3.1.2 and gasping from Section 3.1.3 are consistent.
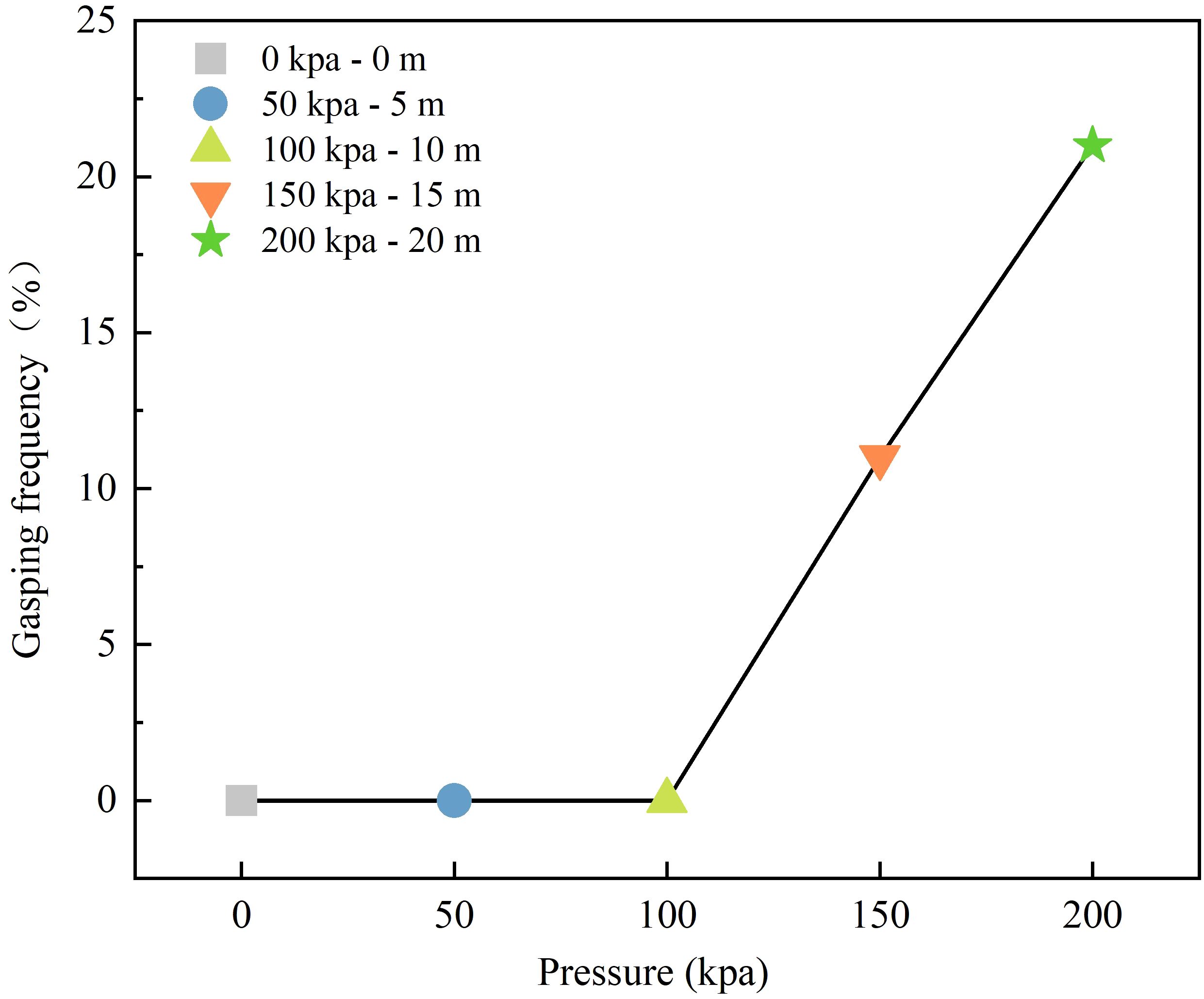
Figure 6 The gasping frequency of L.crocea under different pressures corresponding to different diving depths of the net cage.
3.1.4 Tailbeat frequency
Figure 7 illustrates the impact of different net cage submersion depths on the tail-beat frequency of L.crocea under corresponding pressure values. Tailbeat frequency is closely related to the behavioral stress response of L.crocea. As shown in Figure 7, the pressure generated by the submersion depth of the net cage significantly influences the tail-beat frequency of L.crocea. In the control group, where the aquaculture net cage is not equipped with lifting and submersion (resulting in no additional environmental pressure), the tail-beat frequency of L.crocea remains approximately 1 time/s. With increasing pressure, corresponding to the submersion of the net cage, the tailbeat frequency of L.crocea increases. The trend in tailbeat frequency aligns with the results of swimming speed, tilt angle, and gasping frequency with pressure, demonstrating consistent outcomes across the experimental results.
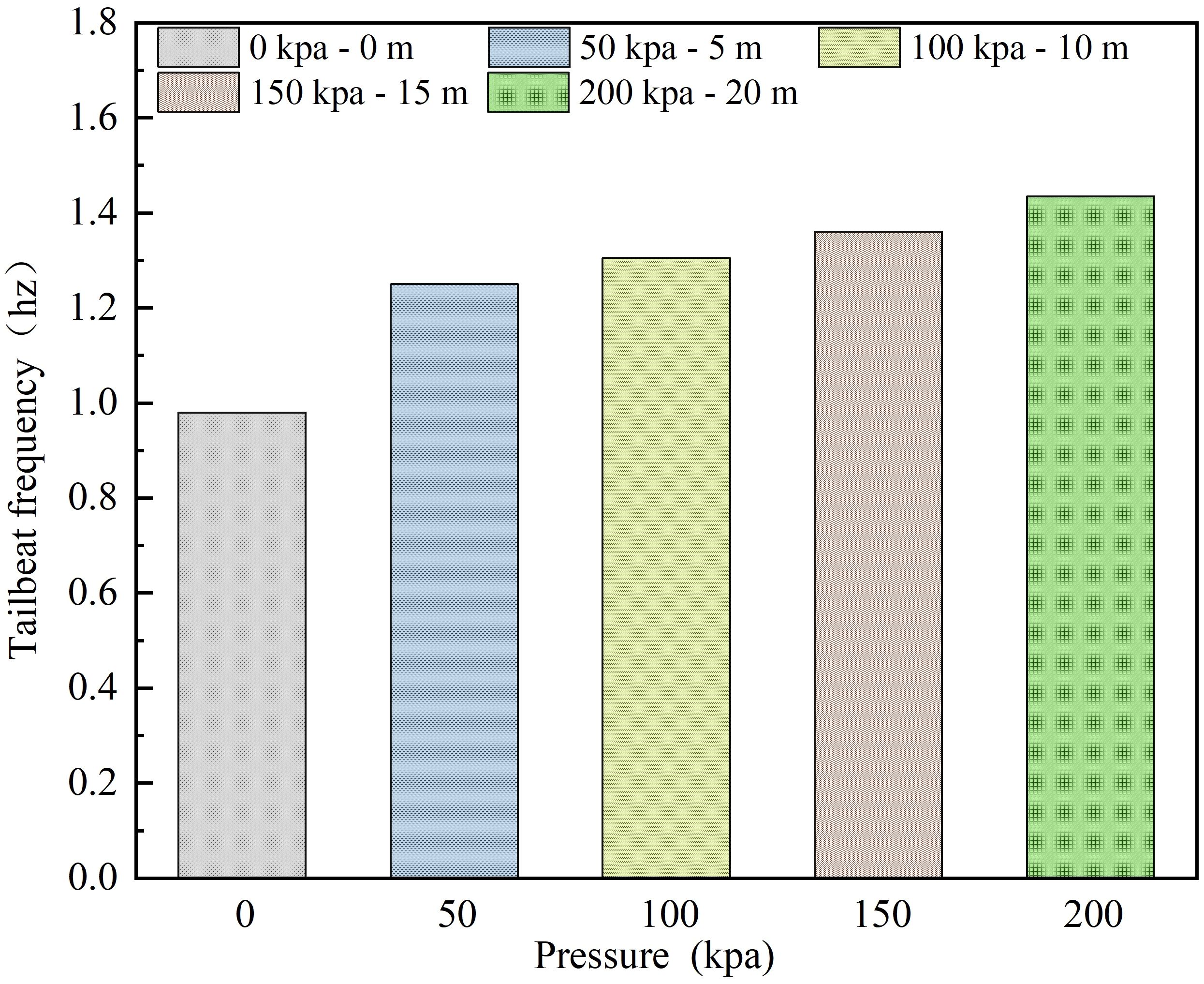
Figure 7 The tailbeat frequency of L.crocea under different pressures corresponding to different diving depths of the net cage.
3.2 The effect of net cage descent speed on the behavior of L.crocea
3.2.1 Swimming speed
Figure 8 depicts the impact of different net cage submersion speeds on the instantaneous swimming speed of L.crocea under varying pressure change speeds. In Figure 8, the solid line represents the instantaneous swimming speed of L.crocea, while the dashed lines represent the average swimming speed of L.crocea under different pressure change speeds corresponding to different net cage submersion speeds. In the control group, the instantaneous swimming speed of L.crocea fluctuates relatively smoothly within the range of 0.05 m/s, and the average swimming speed is maintained at 0.0239 m/s. When the submersion speeds, corresponding to pressure change speeds, are set to 0.6, 1.0, 1.3, 2.0, and 4.0 m/min, the instantaneous swimming speed exhibits significant fluctuations. Specifically, at pressure change speeds of 0.6 and 1.0 m/min, the average swimming speed increases to 0.0570 and 0.0590 m/s, respectively. When the pressure change speeds are 1.3, 2.0, and 4.0 m/min, the average swimming speed of L.crocea increases from that of the control group 0.0239 m/s to 0.0742, 0.0801, and 0.0902 m/s, representing a respective increase of 210.5%, 235.1%, and 277.4%. The instantaneous swimming speed of L.crocea is significantly influenced by the net cage submersion speed. As the submersion speed increases (corresponding to an increase in pressure change speed), the swimming speed of L.crocea increases accordingly, especially when the submersion speed reaches 1.3 m/min and beyond.
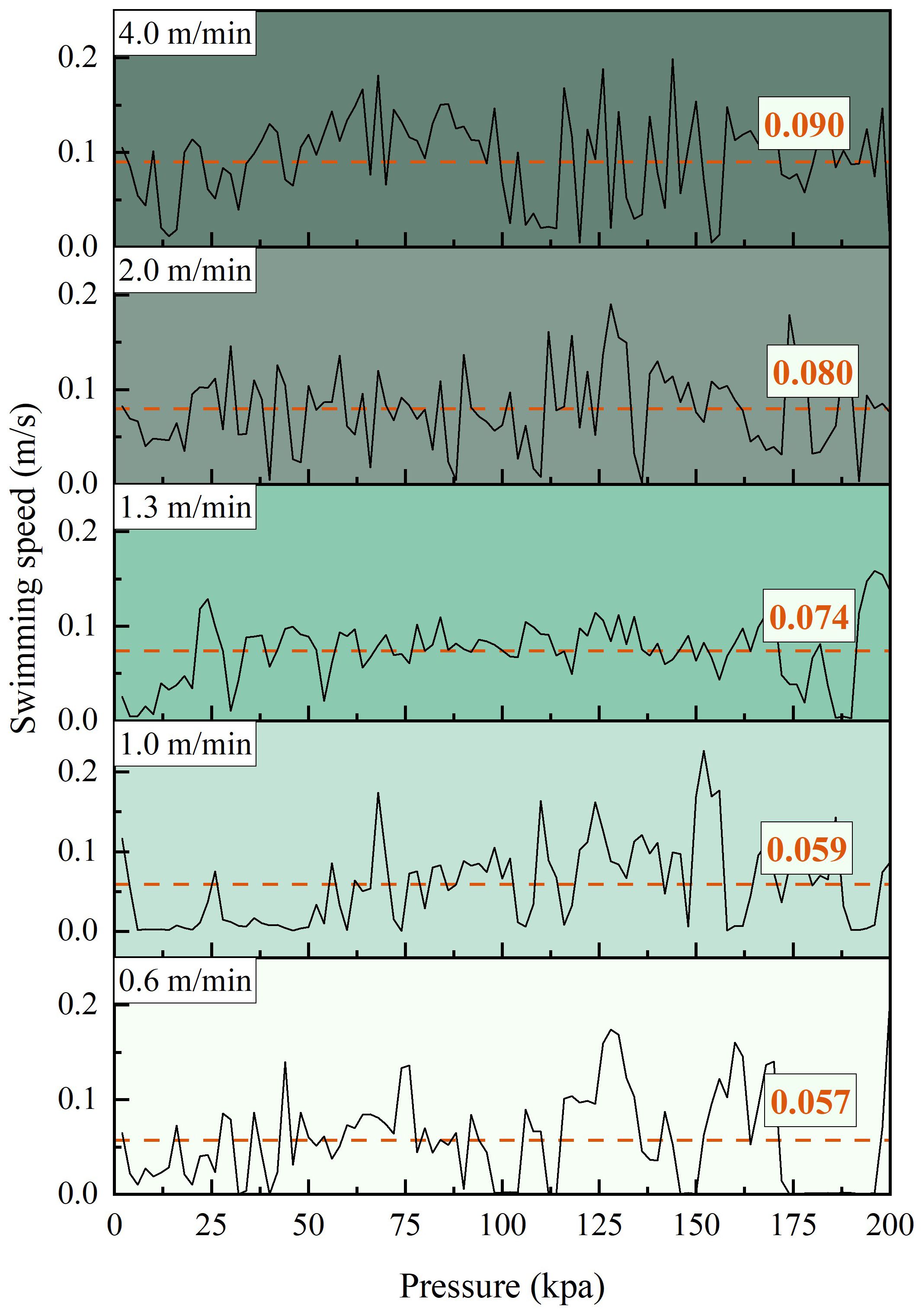
Figure 8 The swimming speed of L.crocea under different pressure change rates corresponding to different diving speeds of net cages.
3.2.2 Tilt angle
The excessive submersion speed prevents aquaculture fish from rapidly adjusting their buoyancy by introducing air into their swim bladders. This results in negative buoyancy, and fish often compensate for or adjust this negative buoyancy by continuously changing their swimming direction and angle. Figure 9 presents the distribution of the tilt angles of L.crocea under different pressure change speeds at various net cage submersion speeds. As shown in Figure 9, firstly, with an increase in pressure change speed, the distribution of tilt angles of L.crocea becomes more scattered. The tilt angles of normal swimming L.crocea are relatively concentrated, showing stable and slight variations within the range of 0 ± 10° (as seen in Figure 5). As the pressure change speed increases, the tilt angles of L.crocea vary within the range of 90°, and with a further increase in pressure change speed, the tilt angles of L.crocea significantly increase. Secondly, as the pressure change speed increases, the distribution of tilt angles of L.crocea begins to disperse at smaller pressure values. This indicates that as the pressure change speed increases, the tilt angles of L.crocea become larger at the same pressure, showing that at faster net cage submersion speeds, L.crocea feel more discomfort at the same net cage depth, adjusting their bodies to cope with the rapid changes in pressure.
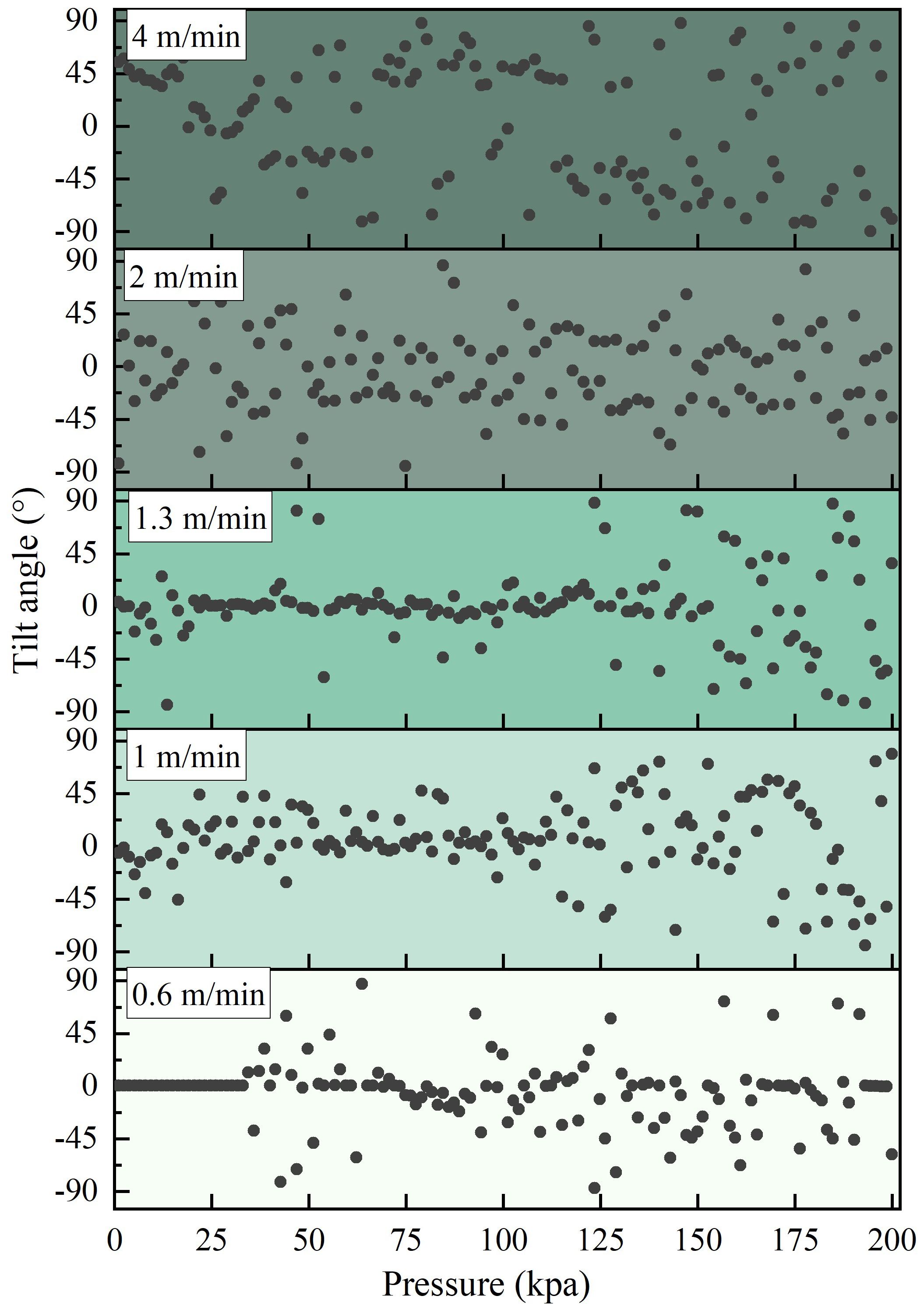
Figure 9 The tilt angle of L.crocea under different pressure change rates corresponding to different diving speeds of net cages.
Precise quantitative analysis of the distribution of tilt angles under different net cage submersion speeds can provide accurate insights into the impact of pressure changes on fish behavior during the submersion process. Figure 10 illustrates the probability distribution of tilt angles for L.crocea under different net cage submersion speeds. Observations from Figure 10 include: (1) With decreasing submersion speed, the frequency of tilt angles within range of 0°- ± 15° gradually increases. (2) At the maximum submersion speed of 4.0 m/min, tilt angles are primarily distributed within the range of ±30°- ± 45°, with less frequent occurrences in the range of 0°- ± 15°.
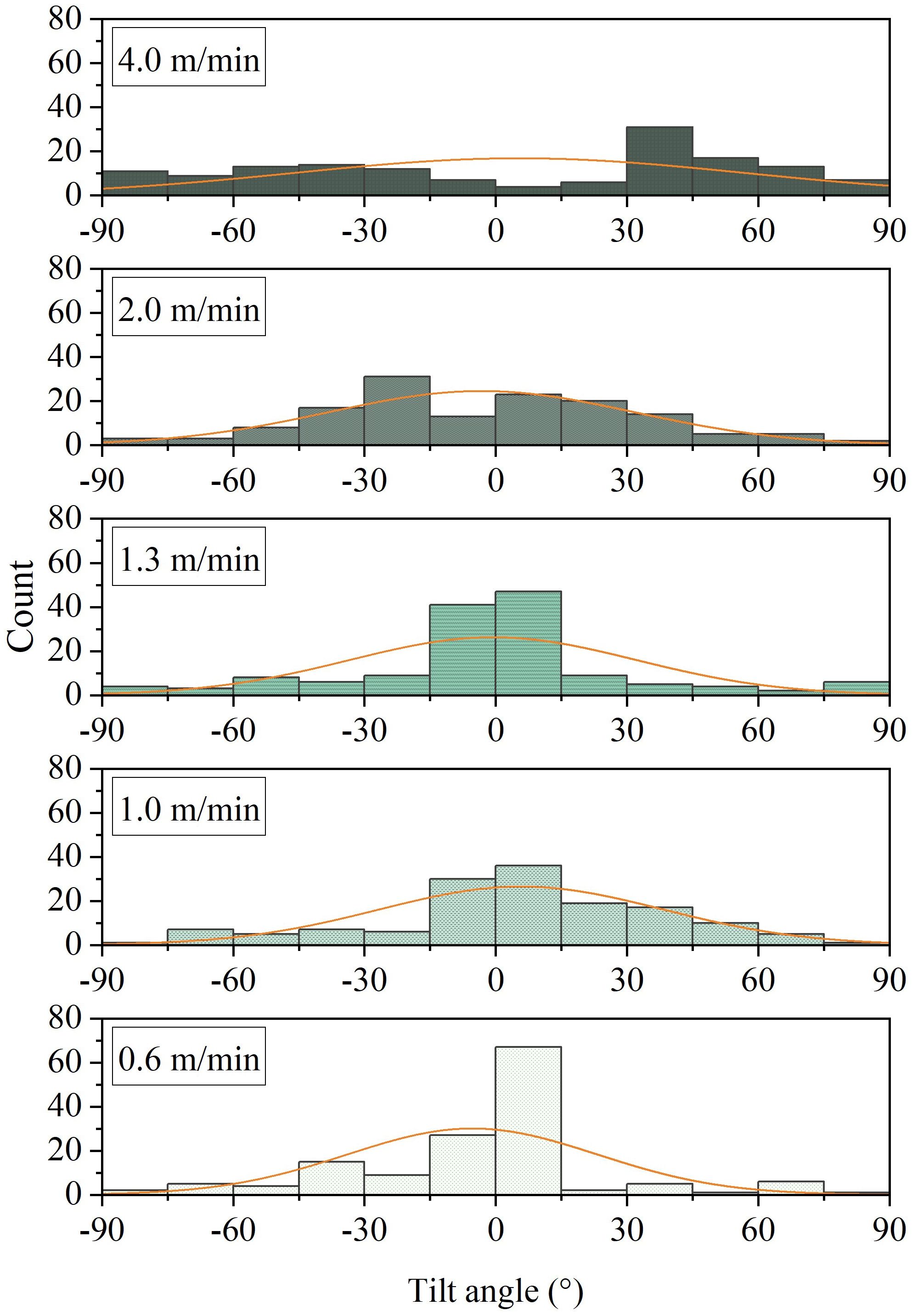
Figure 10 The tilt angle distribution of L.crocea under different pressure change rates corresponding to different diving speeds of net cages.
3.2.3 Gasping frequency
Due to the varying rates of pressure changes caused by different submersion speeds, the rapid decline in dissolved oxygen content in the net cage due to environmental pressure and pressure changes leads L.crocea to float at the water surface to gulp air due to oxygen deficiency. Mild floating may impact the growth rate of farmed fish, while severe floating can result in mass fish mortality. Figure 11 illustrates the frequency of air gulping by L.crocea under different net cage submersion speeds corresponding to various pressure change rates. As shown in Figure 11: First, the frequency of air gulping generally increases with the rise in submersion speed. Second, at submersion speeds of 0.6, 1.0, and 1.3 m/min, the air gulping frequency changes are not significant, fluctuating around 8 times/min. When the submersion speed increases to 2.0 m/min, the air gulping frequency significantly increases by a magnitude. At the maximum submersion speed of 4.0 m/min, the air gulping frequency of L.crocea approaches 30 times/min, due to the rapid increase in pressure inside the net cage leading to a sharp decrease in oxygen levels, prompting L.crocea to gulp air frequently to maintain possibly oxygen supply.
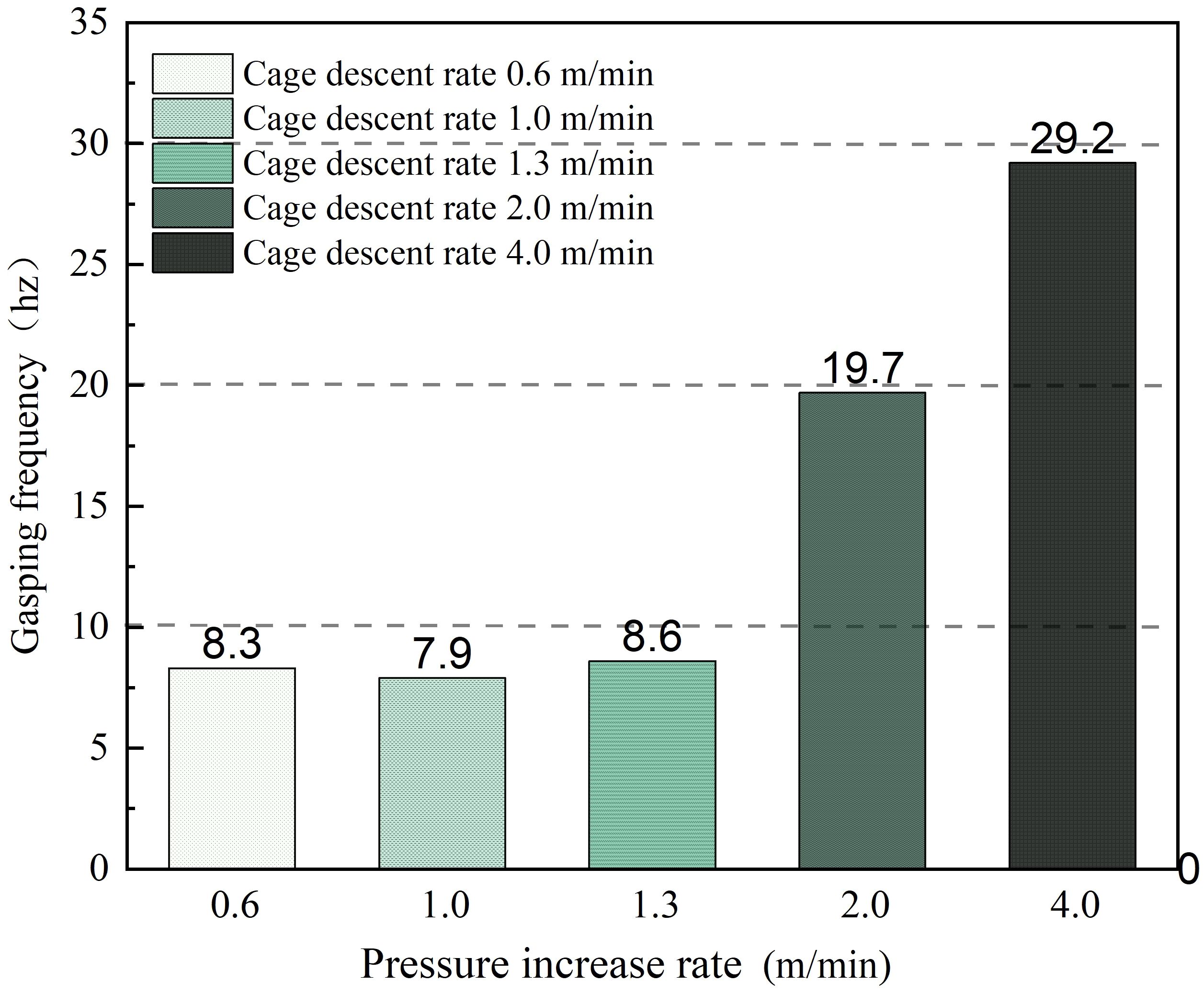
Figure 11 The gasping frequency of L.crocea under different pressure change rates corresponding to different diving speeds of net cages.
3.2.4 Tailbeat frequency
Based on the experiment videos recorded by the video system and manually counted, the tail-beating frequency of L.crocea under different pressure change rates is shown in Figure 12. As depicted in the figure, the tail-beating frequency generally increases with the rise in the speed of environmental pressure. The trend of tail-beating frequency under different net cage submersion speeds corresponding to various pressure change rates maintains a good correlation with the trend of swimming speed changes.
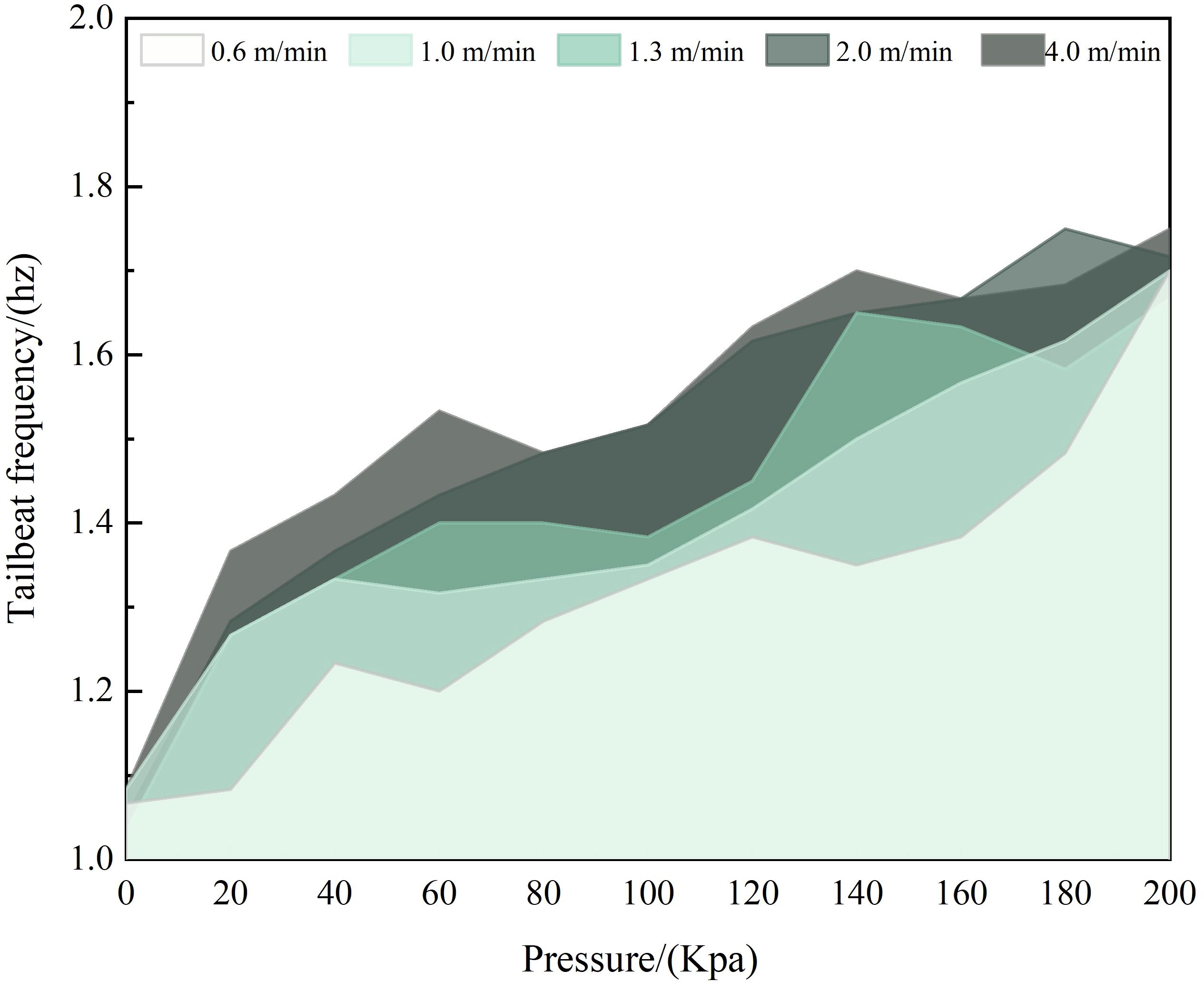
Figure 12 The tailbeat frequency of L.crocea under different pressure change rates corresponding to different diving speeds of net cages.
4 Discussion
4.1 Impact of different descent depths on pressure changes
This study delves into the effects of submersion depth on the swimming speed and gasping frequency of L.crocea, revealing significant increases in these parameters as water pressure rises from 10m to 15m: swimming speed increased from 0.039m/s to 0.055m/s and gasping frequency increased from 0 times to 11 times. This indicates that further increases in descent depth markedly affect the behavior of L.crocea, with the notable increases in swimming speed and gasping frequency suggesting discomfort, typically considered a stress response. These changes clearly demonstrate the direct impact of environmental pressure on the behavior of L.crocea, aligning with findings by Barton (2002), who reported that stress behaviors have long-term impacts on aquaculture quality. If the stress from pressure is too severe or prolonged, preventing fish from restoring internal balance, the response itself may become maladaptive and threaten the health and welfare of the fish. The stress responses observed in fish, such as increased swimming speeds and respiratory rates under adverse environmental conditions, are evident when comparing different pressures. For instance, the swimming speed of large yellow croaker increases by 141.6% at a depth of 20 meters compared to surface pressure, and its respiratory rate rises from 0 to 21 breaths per minute, likely represent adjustment to adverse environmental conditions (Korsoen et al., 2010). Moreover, Wendelaar Bonga (1997) emphasized the importance of adapting to environmental pressure, noting its critical role in physiological functions such as growth and gonadal development. This is further supported by Ginneken et al. (2010), who highlighted that maintaining necessary energy distribution under pressure is vital for survival and reproduction. Therefore, choosing an appropriate descent depth to mitigate the adverse effects of environmental pressure is crucial for maintaining high-quality aquaculture environments and ensuring the welfare of L.crocea. Based on our findings, we recommend setting the optimal cage descent depth at 10 meters (100 kPa) as a balance point to ensure economic efficiency in L.crocea aquaculture while minimizing behavioral issues due to stress responses. Additionally, long-term stress responses may lead to inhibited growth, decreased disease resistance, and reduced reproductive success (Wedemeyer, 1981; Vaughan et al., 1984), which are key factors for sustainable aquaculture development.
The experimental results for various parameters, including swimming speed, tilt angle, gasping frequency, and tail movement frequency, under different descent depths in cage culture exhibit consistent trends. Similar patterns were observed in the study conducted by Korsoen et al. (2010), which investigated the ascent of Atlantic cod in aquaculture cages (Naylor et al., 2000; Mangel et al., 1999). It was found that as the cage height increased, both swimming speed and tail movement frequency followed specific patterns of variation (Syme and Shadwick, 2002; Hayden, 2009). The ascent and descent processes of cages impose pressure on the farmed fish, leading to behavioral responses when the expansion of the swimming bladder exceeds the fish’s capacity to cope by absorbing gases. This study proposes behavioral stress patterns in L.crocea associated with pressure changes during cage descent, along with recommendations for the optimal descent depth. These findings provide insights into the behavioral response mechanisms of farmed fish, aid in the design of aquaculture equipment, and contribute to enhancing the efficiency, quantity, and product quality of aquaculture.
4.2 The impact of different submersion speeds on pressure changes
Beyond considering the depth of submersion, setting the appropriate speed of cage descent is critical for managing extreme weather conditions such as typhoons. Rapidly lowering cages to designated underwater areas is advantageous for avoiding typhoons; however, too swift a descent can lead to rapid increases in environmental pressure, compressing the air in the swim bladders of L.crocea, potentially causing them to rupture. This can drastically reduce buoyancy and even result in death. As reported by Liem (1988), rapid changes in pressure can directly damage the swim bladders of aquatic organisms.
Our study analyzes the impact of various descent speeds (ranging from 0.6 to 4.0 m/min) under consistent pressure conditions on the behavioral indicators of L.crocea. Observations demonstrate that at the slowest descent speed of 0.6 m/min, the swimming speed of L.crocea was 0.057 m/s, with a gasping frequency of approximately eight times per minute. At a descent speed of 2.0 m/min, there was a significant increase in swimming speed, up by 40.4% compared to the 0.6 m/min speed, and the gasping frequency significantly increased by 137%. When the descent speed reached 4.0 m/min, the swimming speed of L.crocea further escalated to 0.09 m/s, with the head floating frequency approaching 30 times per minute. These findings indicate that increased descent speeds provoke substantial changes in respiratory and locomotor behaviors, signaling heightened stress responses in L.crocea. Such stress states indicate that the fish’s tolerance to environmental stimuli is nearing its physiological limit, a condition also highlighted by Schreck and Tort (2016), who noted that continuous environmental pressure can trigger significant behavioral changes, thereby affecting health.
Moreover, this research also explores mechanisms other than the direct impact of pressure changes from cage lifting and lowering on the swim bladder’s pressure tolerance in L.crocea. Studies have shown that rapid pressure increases can significantly enhance dissolved oxygen levels in the water, accelerating the metabolism of L.crocea, thereby leading to a rise in various physical behavioral indicators (Petersen and Gamperl, 2010). Research by Videler and Wardle (1991); Wannamaker and Rice (2000), has also demonstrated that short-term changes in oxygen content can slightly increase fish swimming activity, aligning with our experimental outcomes.
Future studies will delve deeper into the long-term effects of pressure on L.crocea. This is particularly pertinent to the ongoing ascent and descent processes of cages under prolonged adverse weather conditions and their impact on L.crocea aquaculture. Additionally, research will be expanded to examine the impact of pressure on different aquaculture species to elucidate how pressure affects various fish populations, providing more concrete scientific bases and management recommendations for the sustainable development of the aquaculture industry.
5 Conclusion
This study assessed the impacts of different submersion depths and speeds on the behavioral indicators of L.crocea. Results indicate that under pressure ranges of 0–200 kPa and pressure speed changes of 0–4.0 m/min, increases in swimming speed, tilt angle, gasping frequency, and tail movement frequency are observed as both pressure and the rate of pressure change increase. These effects are especially pronounced when the pressure reaches 150 kPa or the submersion speed hits 2.0 m/min, where stress responses are significantly heightened. Therefore, to minimize stress and optimize the aquaculture environment for L.crocea, a submersion depth of 10 meters and a speed of 1.0 m/min are recommended. These findings provide a theoretical foundation for the design of deep-sea cage systems and offer new insights into the production of L.crocea in deep-sea conditions, enhancing our understanding of the impact of pressure changes in submersion cage systems on aquaculture species.
Data availability statement
The original contributions presented in the study are included in the article/supplementary material. Further inquiries can be directed to the corresponding authors.
Ethics statement
The animal studies were approved by Ethics Committee of Zhejiang Ocean University (approval code: 2023088). The studies were conducted in accordance with the local legislation and institutional requirements. Written informed consent was obtained from the owners for the participation of their animals in this study.
Author contributions
TT: Data curation, Formal analysis, Writing – original draft. XY: Conceptualization, Funding acquisition, Resources, Writing – review & editing. FG: Conceptualization, Funding acquisition, Resources, Writing – review & editing. JH: Data curation, Formal analysis, Writing – original draft. SN: Investigation, Methodology, Writing – original draft. LT: Investigation, Methodology, Writing – original draft. HH: Methodology, Software, Writing – original draft. YJ: Methodology, Software, Writing – original draft.
Funding
The author(s) declare financial support was received for the research, authorship, and/or publication of this article. This research was funded by the National Key Research and Development Project of China under grant number 2020YFE0200100, and the National Natural Science Foundation of China under grant number 42306229.
Acknowledgments
The authors would like to thank all the reviewers who participated in the review.
Conflict of interest
The authors declare that the research was conducted in the absence of any commercial or financial relationships that could be construed as a potential conflict of interest.
Publisher’s note
All claims expressed in this article are solely those of the authors and do not necessarily represent those of their affiliated organizations, or those of the publisher, the editors and the reviewers. Any product that may be evaluated in this article, or claim that may be made by its manufacturer, is not guaranteed or endorsed by the publisher.
References
Barton A. B. (2002). Stress in fishes: A diversity of responses with particular reference to changes in circulating corticosteroids. Integr. Comp. Biol. 42, 517–525. doi: 10.1093/icb/42.3.517
Benetti D. D., Orhun M. R., Sardenberg B., O’Hanlon B., Welch A., Hoenig R., et al. (2008). Advances in hatchery and grow-out technology of cobia Rachycentron canadum (Linnaeus). Aquacult. Res. 39, 701–711. doi: 10.1111/j.1365-2109.2008.01922.x
Bostock J., Mcandrew B., Richards R., Jauncey K., Telfer T., Lorenzen K., et al. (2010). Aquaculture: global status and trends. Philos. Trans. R. Soc. B: Biol. Sci. 365, 2897–2912. doi: 10.1098/rstb.2010.0170
Carney R. S. (2005). Zonation of deep biota on continental margins. Oceanogr. Mar. Biol. 43, 211–278. doi: 10.1201/9781420037449-8
Chambers M. D., Howell W. H. (2006). Preliminary information on cod and haddock production in submerged cages off the coast of New Hampshire, USA. Ices J. Mar. Sci. 63, 385–392. doi: 10.1016/j.icesjms.2005.10.008
Chuang M. C., Hwang J. N., Williams K. (2016). A feature learning and object recognition framework for underwater fish images. IEEE Trans. Image Process. 25, 1. doi: 10.1109/TIP.2016.2535342
Dempster T., Korsøen Ø., Folkedal O., Juell J.-E., Oppedal F. (2009). Submergence of Atlantic salmon (Salmo salar L.) in commercial scale sea-cages: A potential short-term solution to poor surface conditions. AQUACULTURE 288, 254–263. doi: 10.1016/j.aquaculture.2008.12.003
Engelhaupt E. (2007). Farming the deep blue sea. Environ. Sci. Technol. 41, 4188–4191. doi: 10.1021/es0725596
Fänge R. (1966). Physiology of the swimbladder. Physiol. Rev. 46, 299–322. doi: 10.1152/physrev.1966.46.2.299
Fernandez-Jover D., Sanchez-Jerez P., Bayle-Sempere J. T., Valle C., Dempster T. (2008). Seasonal patterns and diets of wild fish assemblages associated with Mediterranean coastal fish farms. Ices J. Mar. Sci. 65, 1153–1160. doi: 10.1093/icesjms/fsn091
Ginneken V. J. T., Van Eersel R., Balm P., Nieveen M., Van Den Thillart G. (2010). Tilapia are able to withstand long-term exposure to low environmental pH, judged by their energy status, ionic balance and plasma cortisol. J. Fish Biol. 51, 795–806. doi: 10.1111/j.1095-8649.1997.tb02000.x
Hayden T. A. (2009). Analyzing life history characteristics of lake erie fishes: migration and philopatry. Diss. Bowling Green State University.
Korsoen O. J., Dempster T., Fosseidengen J. E., Ferno A., Heegaard E., Kristiansen T. S. (2010). Behavioural responses to pressure changes in cultured Atlantic cod (Gadus morhua):Defining practical limits for submerging and lifting sea-cages. AQUACULTURE 308, 106–115. doi: 10.1016/j.aquaculture.2010.08.011
Lemaire B., Mignolet E., Debier C., Calderon P. B., Thomé J. P., Rees J. F. (2016). High hydrostatic pressure influences the in vitro response to xenobiotics in Dicentrarchus labrax liver. Aquat. Toxicol. 173, 43–52. doi: 10.1016/j.aquatox.2016.01.004
Liem K. F. (1988). Form and function of lungs: the evolution of air breathing mechanisms. Am. Zool. 28, 739–759. doi: 10.1093/icb/28.2.739
Liu Y., Tveteras S., Xie J. (2021). Efficiency in Chinese large yellow croaker aquaculture: implication for sustainable aquaculture in China. Sustainability 13 (24), 13952. doi: 10.3390/su132413952
Naylor R. L., Goldburg R. J., Primavera J. H., Kautsky N., Beveridge M. C. M., Clay J., et al. (2000). Effect of aquaculture on wild fish supplies. Nature. 405 (6790), 1017–1024. doi: 10.1038/35016500
Nogita S., Baba K., Yahagi H., Watanabe S., Mori S. (1988). Acute toxicant warning system based on a fish movement analysis by use of AI concept. IEEE. 273–276. doi: 10.1109/AIIA.1988.13305
Petersen L. H., Gamperl A. K. (2010). Effect of acute and chronic hypoxia on the swimming performance, metabolic capacity and cardiac function of Atlantic cod (Gadus morhua). J. Exp. Biol. 213, 808–819. doi: 10.1242/jeb.033746
Petrellis N. (2021). Measurement of fish morphological features through image processing and deep learning techniques. Appl. Sci. 11 (10), 4416. doi: 10.3390/app11104416
Schreck C. B., Tort L. (2016). “The concept of stress in fish,” in Fish physiology. (Academic Press). 35, 1–34.
Syme D. A., Shadwick R. E. (2002). Effects of longitudinal body position and swimming speed on mechanical power of deep red muscle from skipjack tuna (Katsuwonus pelamis). J. Exp. Biol. 205, 189–200. doi: 10.1242/jeb.205.2.189
Vaughan D. S., Yoshiyama R. M., Break J. E., Deangelis D. L. (1984). Modeling approaches for assessing the effects of stress on fish populations. Contam. Effects Fish. 259–278.
Videler J. J., Wardle C. S. (1991). Fish swimming stride by stride: speed limits and endurance. Rev. Fish Biol. Fish. 1, 23–40. doi: 10.1007/BF00042660
Wannamaker C. M., Rice J. A. (2000). Effects of hypoxia on movements and behavior of selected estuarine organisms from the southeastern United States. J. Exp. Mar. Biol. Ecol. 249, 145–163. doi: 10.1016/S0022-0981(00)00160-X
Wedemeyer G. (1981). Methods for determining the tolerance of fishes to environmental stressors. Stress Fish. 247–276. doi: 10.1046/j.1444-2906.2002.00491.x
Wendelaar Bonga S. E. (1997). The stress response in fish. Physiol. Rev. 77, 591–625. doi: 10.1152/physrev.1997.77.3.591
Keywords: cage descent rate, gasping frequency, depth of descent, tailbeat frequency, swimming speed, tilt angle
Citation: Tong T, Yang X, Gui F, Hu J, Niu S, Tang L, Huang H and Jiang Y (2024) The influence of simulated pressure changes on the behavior of Larimichthys crocea during the deep sea submarine descent of net cages. Front. Mar. Sci. 11:1402762. doi: 10.3389/fmars.2024.1402762
Received: 18 March 2024; Accepted: 06 June 2024;
Published: 04 July 2024.
Edited by:
Stephen J. Newman, Western Australian Fisheries and Marine Research Laboratories, AustraliaCopyright © 2024 Tong, Yang, Gui, Hu, Niu, Tang, Huang and Jiang. This is an open-access article distributed under the terms of the Creative Commons Attribution License (CC BY). The use, distribution or reproduction in other forums is permitted, provided the original author(s) and the copyright owner(s) are credited and that the original publication in this journal is cited, in accordance with accepted academic practice. No use, distribution or reproduction is permitted which does not comply with these terms.
*Correspondence: Xu Yang, eWFuZ3h1QHpqb3UuZWR1LmNu; Fukun Gui, R3VpMjIzN0AxNjMuY29t