- Faculty of Economics, University of Gdansk, Sopot, Poland
Introduction: Like in many parts of the world, the Baltic Sea experiences a multitude of processes and stressors that influence fish stock dynamics. This paper compartmentalizes 250 publications that examine the cumulative effects and trade-offs of some of the most significant environmental drivers (temperature change, hypoxia, nutrient enrichment, acidification, low salinity, and food-web dynamics) on the ecology of top commercial fish species in the Baltic Sea (cod, sprat, whiting, herring, flounder, and plaice).
Methods: A systematic review method was applied to ensure rigorous coverage of existing literature and to provide a comprehensive synthesis of the current knowledge on the subject.
Results: The results illustrate the extent of scientific research applicable to commercial fisheries knowledge in the Baltic Sea and identify which pressures have the greatest negative impacts on which stocks. Additionally, the findings demonstrate how well top commercial fish species have adapted to the changing environmental conditions of the Baltic Sea. In doing so, the review illustrates the upcoming challenges and underscores which stocks are likely to dominate in the future and which will face difficulties.
Discussion: By considering ecosystem-based fisheries management, this paper emphasizes the need to account for complex ecosystem interactions beyond single-stock monitoring. With increased natural hazards, top commercial fish species have reacted differently, depending on the region and their adaptive capabilities. In most cases, Clupeidae species have adapted the best to their new surroundings, Pleuronectidae resilience is varied, while Gadidae species are finding the Baltic Sea increasingly challenging.
1 Introduction
The Baltic Sea is the largest body of brackish water in the world, with a surface area of 420,000 km², surrounded by 85 million inhabitants from 9 European states. Consequently, this semi-enclosed, moderately shallow sea, with a mean depth of 54 m, is one of the most exploited marine habitats. Human activities, coupled with the increasing symptoms of climate change, have imposed intense pressures on the health of the Baltic’s ecosystem and its capacity to produce goods and services (Meier et al., 2011a; Voss et al., 2019; Scharsack et al., 2021). This negatively affects the economic contribution of marine-related activities. This is most felt in the fisheries sector, which has drastically changed in the last 40 years (Kijewska et al., 2016; Cederqvist et al., 2020; HELCOM, 2020). Despite its decline, in 2015, the Baltic Sea had 6,192 active fishing vessels, employing 4,704 full-time and 4,336 part-time fishermen (HELCOM, 2020). Their landing value totalled EUR 217 million, with a gross profit of EUR 116 million (HELCOM, 2020).
Traditionally, cod (Gadus morhua), herring (Clupea harengus) and sprat (Sprattus sprattus) stocks had the highest commercial value in the Baltic Sea (ICES, 2019; HELCOM, 2020; ICES, 2022a–h). These stocks are managed by the EU Common Fisheries Policy (CFP) through the implementation of total allowable catch (TAC) allowances for the International Council for the Exploration of the Sea (ICES) Baltic Sea sub-divisions (SD): Eastern cod—SD 25-32, Western cod—SD 22-24, Bothnian herring—SD 30-31, Western herring—SD 22-24, Central herring—SD 25-27, 28.2, 29, 32, Riga herring—SD 28.1 and Sprat—SD 22-32 (European Commission, 2020, 2022). These stocks make up 90% of the total fish catch in the Baltic Sea (HELCOM, 2020). In addition, Pleuronectidae species including flounder (Platichthys flesus) and plaice (Pleuronectes platessa) are important for small-scale fisheries. Plaice—SD 22-32 is monitored as a single stock, while flounder has no TAC quotas (ICES, 2019; HELCOM, 2020; European Commission, 2022; ICES, 2022a-h).
ICES is responsible for gathering statistics for Baltic fish stocks, including information on spawning stock biomass (SSB), fishing mortality, recruitment rates and other ecological parameters such as age and size structures, distribution and genetic diversity. Their findings guide the decision-making process of the CFP (ICES, 2019, a–h). Other areas ICES and the CFP are concerned with incude wider environmental drivers such as temperature rise, hypoxia, nutrient enrichment, ocean acidification, low salinity, food-web dynamics, invasive species and parasitic infections (Almqvist et al., 2010). This paper explores those drivers by analysing 250 peer-reviewed, English-language papers that highlight the intricate interplay between stock dynamics and environmental change.
A comprehensive global framework that manages fishery stocks sustainably is vital for maintaining the health of marine ecosystems and fishing communities. Ecosystem-based fisheries management (EBFM) is steadily being adopted as a core concept in achieving sustainability. EBFM emphasises the need to consider complex interactions within marine ecosystems, including food-web dynamics and environmental pressures (Townsend et al., 2019). Fisheries impact marine organisms and ecosystems directly as well as indirectly, with cascading effects on the region’s biodiversity, habitats and food-web structures (Ojeda-Martı́nez et al., 2009; Rodriguez-Perez et al., 2023). EBFM also addresses the necessity of managing fisheries within the context of environmental pressures that contribute to a changing environment (Martins et al., 2012). The framework expands fisheries management beyond traditional single-species fisheries monitoring and is crucial for the long-term sustainability of marine resources (Rodriguez-Perez et al., 2023). Numerous global policies require the implementation of EBFM. For instance, in the EU, CFP explicitly states that an “ecosystem-based approach to fisheries management needs to be implemented” (CINEA, 2022). Managing these complex interactions helps towards Goal 14 of the United Nations Sustainable Development Goals (SDGs), which aims to “conserve and sustainably use the oceans, seas and marine resources for sustainable development” (United Nations, 2016).
2 Methods
2.1 Definitions
To enhance transparency and promote better comprehension of the review, it is essential to establish clear definitions for key terms.
1. “Environmental drivers” studies have been divided into the following categories: (1) temperature rise, (2) hypoxia, (3) nutrient enrichment, (4) ocean acidification, (5) low salinity and (6) food-web dynamics. In this paper, “environmental drivers” refer to two main aspects:
2. “Top commercial fish stocks” studies have been divided into the following categories: (1) Gadidae, (2) Clupeidae and (3) Pleuronectidae (Figure 1). By examining species individually, the review builds on studies highlighted in the ‘environmental drivers’ category, clarifying specific impacts, and facilitating comparisons between the stocks. This approach ensures a thorough analysis, supporting targeted management strategies, and identifying gaps in current knowledge for each stock.
• Human-Induced Factors: This includes pollutants, carbon dioxide (CO2) emissions, and other greenhouse gases that have resulted in a large-scale shift in climatic, oceanographic, and biogeochemical parameters in the Baltic Sea region (Eilola et al., 2011; Viitasalo and Bonsdorff, 2022).
• Food-Web Dynamics: This refers to the interactions within the marine ecosystem, where fish stock health is influenced by their ability to adapt to changing conditions. Adaptation involves factors such as reproductive capacity, habitat use, metabolic functions, growth, and resilience to parasites and invasive species. Food resource availability, competition with other wildlife, and natural predation pressures are also key environmental drivers.
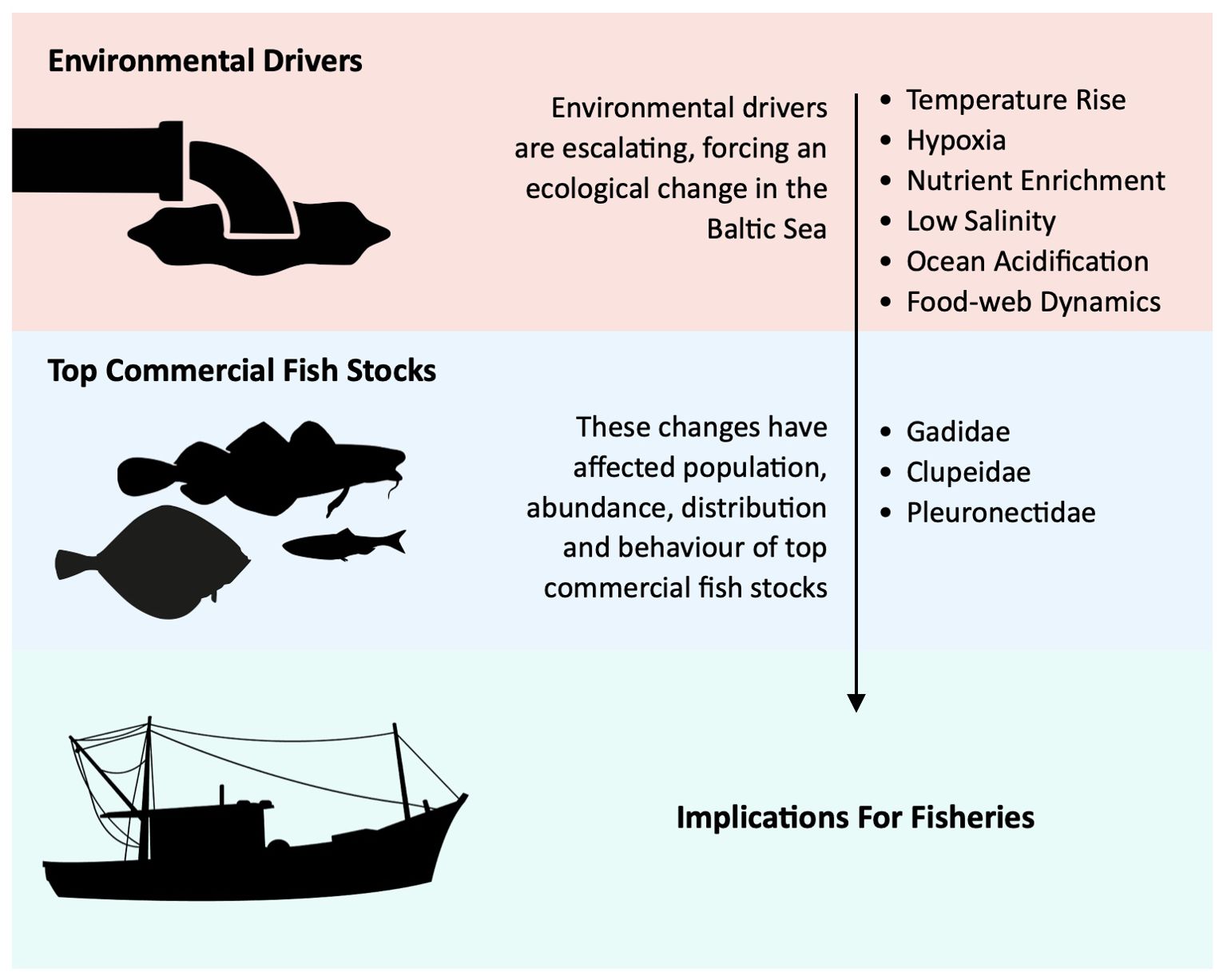
Figure 1. Factors influencing the connections between environmental drivers, top commercial fish stocks and their implications for fisheries.
2.2 Systematic review
To ensure the systematic review was rigorous and reproducible, a meticulous approach was utilised when sorting and selecting papers (Salvador-Oliván et al., 2021). Five search filters were applied during the systematic review.
• Temporal focus—The study filtered out articles published before 2010. This cutoff date was chosen due to the dynamic nature of stocks and environmental conditions in the Baltic Sea region. By focusing on more recent research from 2010 onwards, the review captures the more up-to-date understanding of these dynamics and their implications for fisheries management.
• Database selection—Papers were sourced using various databases: Google Scholar, Scopus, Science Direct, Sage, Directory of Open Access Journals, and Google. The search process was not only confined to these databases but also embraced a dynamic snowball sampling approach. This process involved reading the reference section of each relevant paper to generate other viable articles (Naderifar et al., 2017).
• Language criteria—Paper considered for review had to be written in English to focus on academia accessible globally.
• Fish stock relevancy—Articles included in the review had to meet the predefined criteria of documenting “top” commercial fish species in the Baltic Sea. By “top” commercial fish species, the review refers to stocks that have the greatest economic contribution to the fishing industry in the Baltic Sea; these include cod, whiting, herring, sprat, flounder, and plaice (ICES, 2019, a–h). Species with lower commercial value, such as salmon (Salmo salar), sea trout (Salmo trutta) and the European perch (Perca fluviatilis), were excluded from the study. Subsequently, search terms always included “Baltic Sea” and the fish species “cod, sprat, whiting, herring, flounder or plaice.”
• Environmental drivers relevancy—To establish a connection between a fish species and environmental drivers, one of the following search terms was added: climate change, temperature rise, hypoxia, eutrophication, salinity, ocean acidification, nutrient enrichment, food-web dynamics, parasites, competition, status, health, predators, zooplankton, Cyanobacteria, phytoplankton, invasive species, diet composition, larvae, benthic habitat, nursery habitat, stock, resilience, recovery, survival, changes, biodiversity, biomass and environment, ecosystem.
This systematic sorting continued until no new papers appeared, indicating comprehensive coverage of the relevant literature. Publications were selected using strategic and critical reading methods (Matarese, 2012). Over the 12-month data collection phase from March 2022 to March 2023, more than 12,150 articles were identified. Ultimately, 250 publications fit the inclusion criteria within the nine key topic areas (Figure 2).

Figure 2. Article selection results after applying the search filter for the systematic literature review.
2.3 Analysis for key topics
Within each key topic, articles have been analysed against the following categories:
• Number of papers—This review method numerically illustrated the amount of research conducted in the Baltic Sea region. In cases where articles addressed more than one research area, they were added to the statistics of multiple topics by their relevance.
• Country of publication—This category refers to the nationality and university affiliation of the authors of the journal article. In many cases, an article had multiple authors from different countries; in those instances, each country was marked as having a journal contributing to the research.
• Baltic Sea sub-divisions—ICES, a regional fishery advisory body, divides the Baltic Sea region into 11 sub-divisions, each unique for its environment, bordering country, and fish stocks. If the study did not specify a sub-division, this review categorised the paper as having findings relevant to the entire Baltic Sea region.
• Principal results—Once the final papers were selected, significant time was spent reading and summarising the most crucial findings for each topic within Section 3.
3 Results and discussion
3.1 Key findings
Each article that met the inclusion criteria within the key topic areas was categorised and compiled into a table available in the Supplementary Material. Among environmental drivers, nutrient enrichment studies are the most numerous (67 articles). This is followed by food-web dynamics (44), ocean acidification (34), temperature rise (31), and hypoxia (29). Conversely, articles that focus on the adverse consequences of low salinity are the hardest to find, with 20 identified in this paper (Figure 3—top left). Within the topic of top commercial fish stocks, Gadidae species garnered the highest scientific interest (41 articles), while articles discussing Pleuronectidae generated the fewest results (19); Figure 3—bottom left).
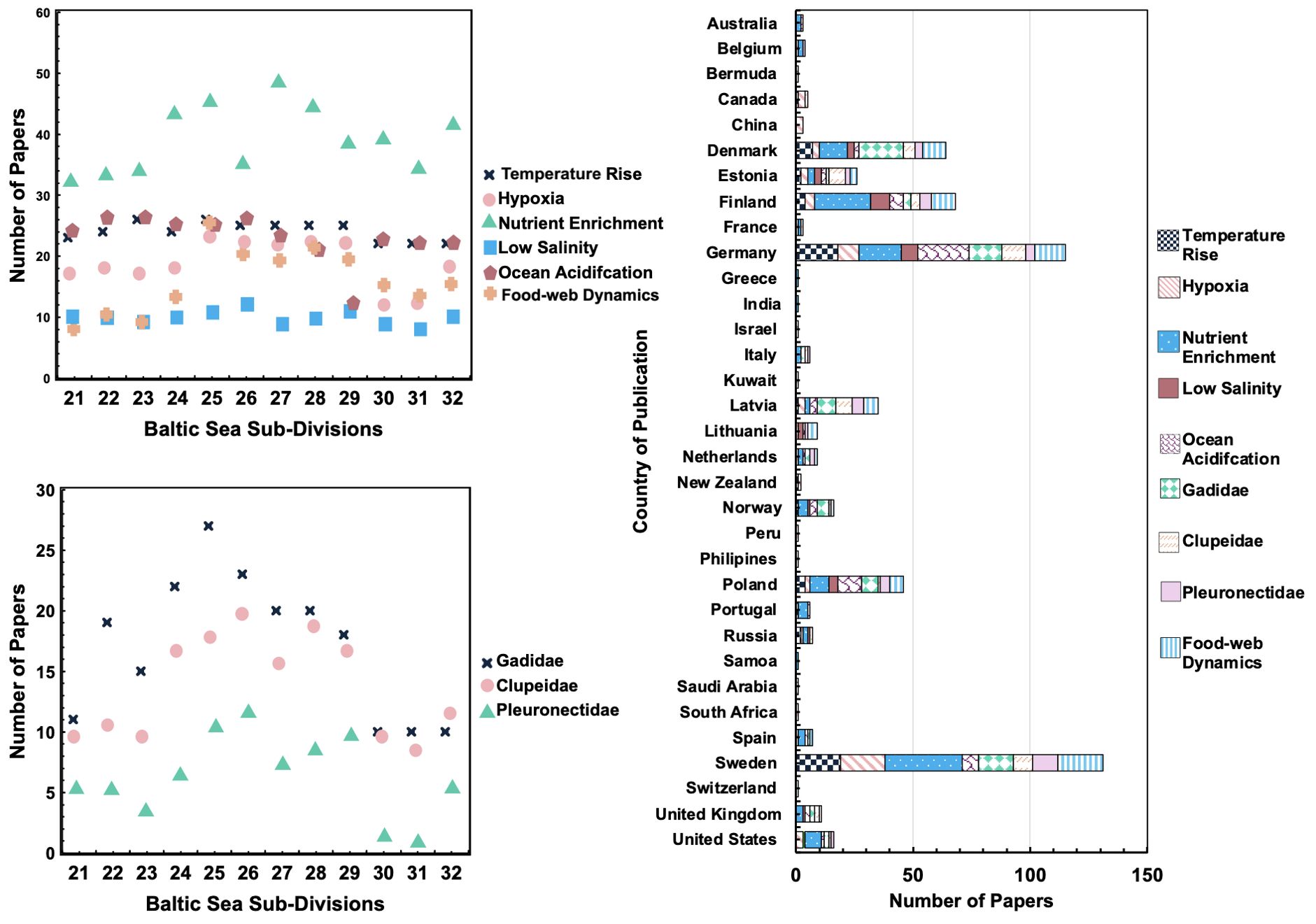
Figure 3. Summary of research distribution in the Baltic Sea: (top left) scatter plot chart showing the number of papers written on environmental drivers, categorised by topic; (bottom left) scatter plot chart illustrating the number of papers written on top commercial fish stocks, categorised by topic; (right) bar chart depicting the number of papers published per country and topic.
Thirty-three countries are credited with publications related to the 9 topics areas, with all nations bordering the Baltic Sea making appearances. Countries with the greatest number of entries are Sweden (131), Germany (115), Finland (68), Denmark (64) and Poland (46) (Figure 3—right). These countries achieved the highest overall research output and dominated the discussion in Sections 3.2. to 3.4. Most research focuses on SD 25, known as the Bornholm Basin, with over 225 papers discussing fish stocks within its borders. Other sub-divisions in the southwest also garnered significant attention, with SD 26, 27 and 28 having 207, 204 and 206 publications, respectively. Sub-divisions 30 and 31, also known as the Bothnian Sea and Bay, have the lowest scientific interest with 150 and 139 papers, respectively (Figure 4). Despite this, the difference between the most and least researched sub-divisions did not exceed 38%, indicating a relatively balanced study effort.
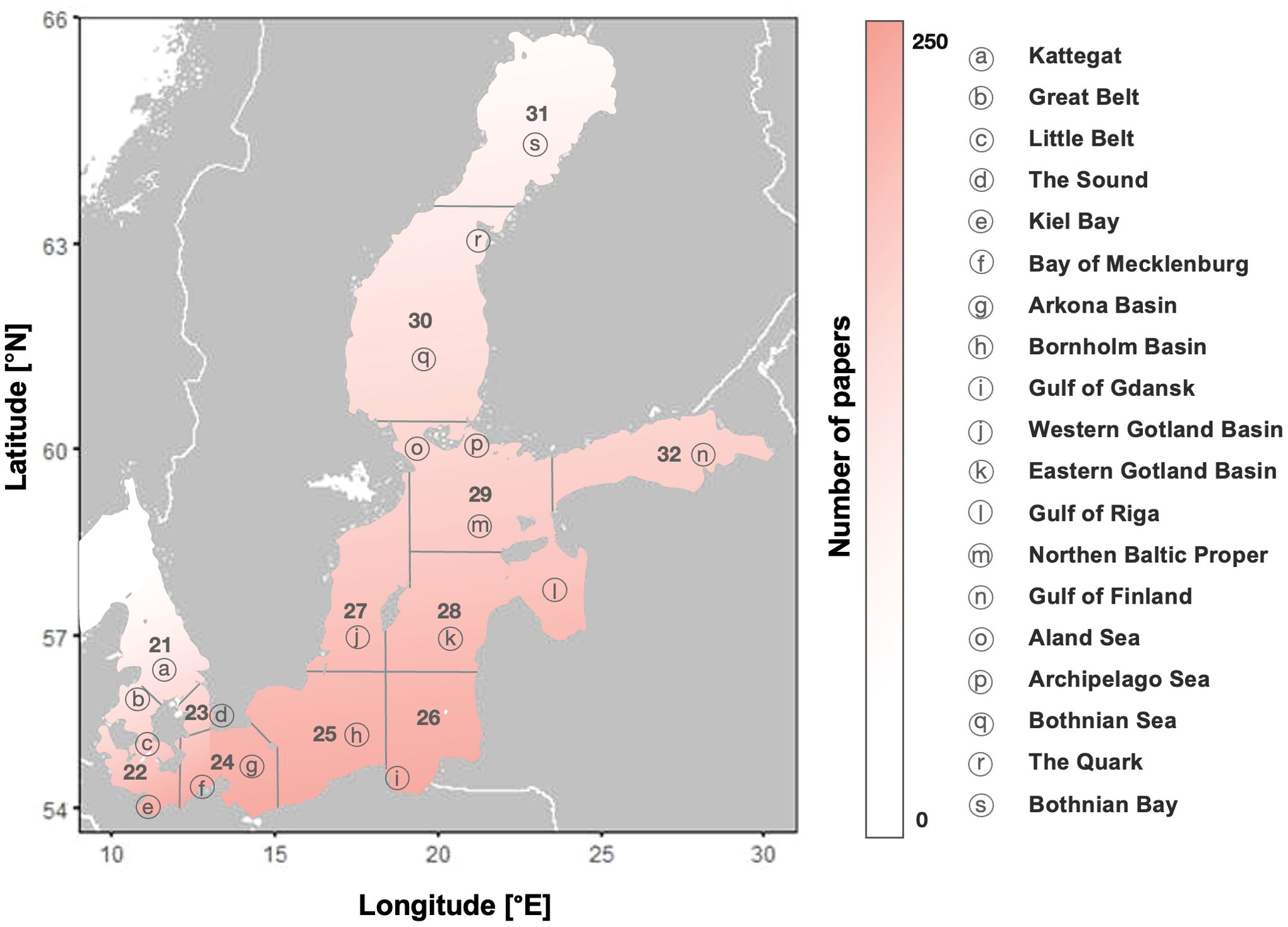
Figure 4. Map of the Baltic Sea, showing its sub-divisions and their respective names. The map includes a density gradient indicating the number of papers per region, ranging from highest to lowest.
The study reviews a range of scientific papers, collectively demonstrating the impact of environmental drivers on commercially important fish stocks. The findings highlight the increasing prevalence of hazards in the Baltic Sea and reveal diverse responses per species. The analysis of the papers uncover clear patterns of success and decline among these stocks (Figure 5). The review emphasises the vulnerability of Western and Eastern cod stocks to temperature rises, low salinity, and nutrient enrichment, which negatively affect their growth, reproduction and survival. Hypoxia has caused a shift in cod behaviour, leading to habitat loss and heightened competition with other species. Knowledge of whiting’s responses to environmental drivers are limited due to the scarcity of scientific studies. However, shared taxonomic affinity, behaviour and diet with cod suggest that whiting may face similar challenges. Herring stocks have varying statuses across regions, with Riga herring performing well and Western herring experiencing significant declines. Algal blooms and eutrophication benefit herring populations by providing extra food and reducing predation pressures from declining cod populations. However, sprat outcompete them. Flounder’s status varies regionally, with different areas reporting population increases and declines, due to low salinity, deoxygenation, and increased parasite infections. Plaice populations have increased in response to reduced cod numbers, but there is limited academia on this stock. Finally, Sprat benefit from changing environmental conditions in the Baltic Sea, with abundant food resources and reduced predation. This is reflected in their improved survival rates, distribution, feeding efficiency, and low susceptibility to parasites. Consequently, sprat have emerged as one of the most adaptive and thriving commercial fish stocks, although regional variations exist with greater population abundances in the north.
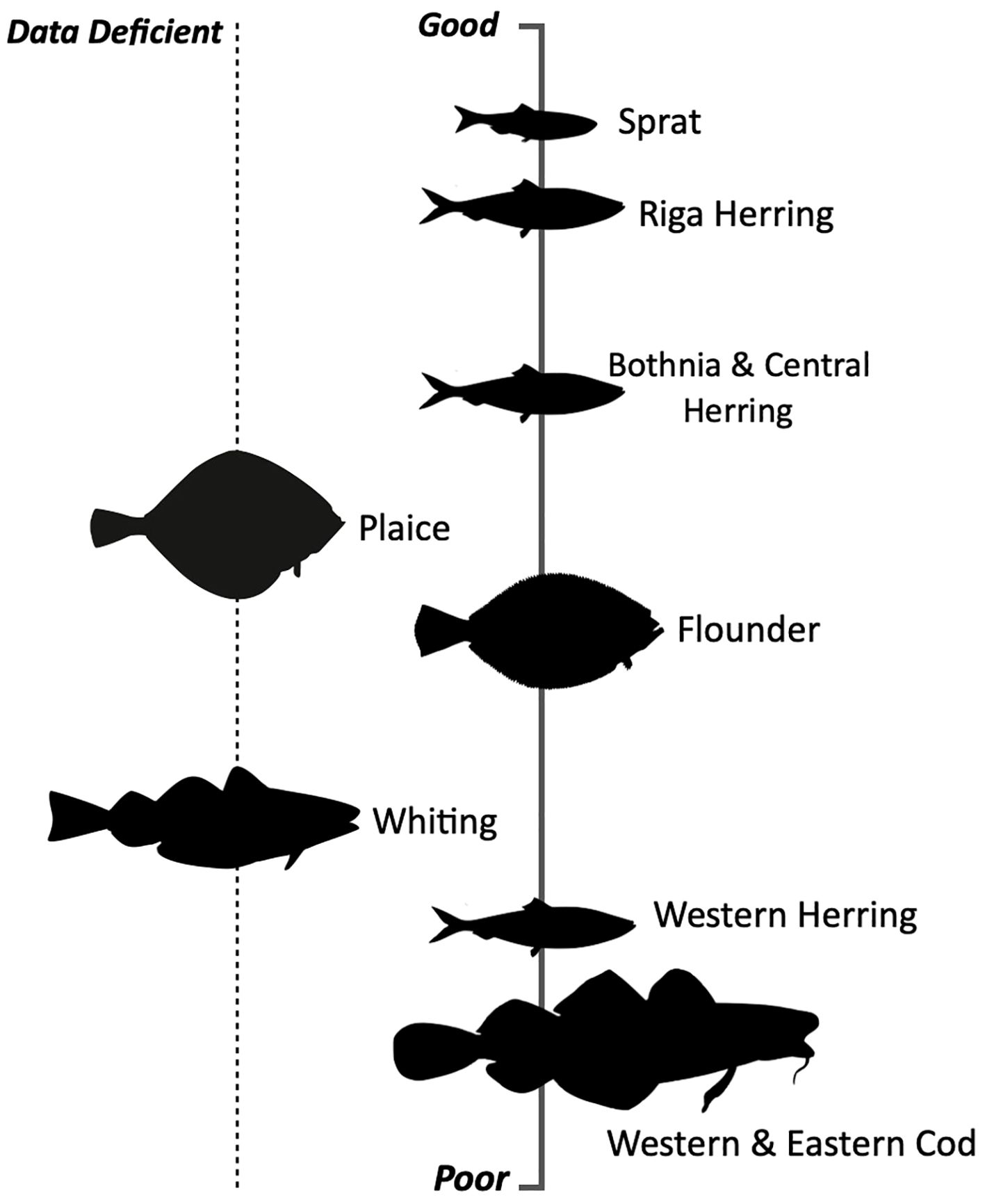
Figure 5. The scale of adaptability success ranges from good to poor in relation to the presented findings.
3.2 Environmental drivers
3.2.1 Temperature rise
Studies identifying the impacts of long-term shifts in temperatures and weather patterns affecting the Baltic Sea ecosystem are prominent in the analysis. The consensus among the 31 identified papers is that the Baltic Sea and its ecosystem have already exhibited clear evidence of change in response to rising temperatures. For example, Voss et al. (2019) demonstrates how human-induced ocean warming of +1.7°C drastically decreases cod fishing opportunities in the region, whilst a +2°C eliminates cod’s recovery chances due larvae preferring colder temperatures. Lindegren et al. (2010); Margonski et al. (2010) and Voss et al. (2012) further demonstrates how temperature change negatively influences cod and herring stock-recruitment abilities.
Thøgersen et al. (2015) forecasts the management difficulties and economic impacts of climate change on Baltic Sea fisheries using management scenarios based on age-structured models. Hinrichsen et al. (2011) underlines that reducing fish catch mortality is insufficient, emphasising how climate-driven long-term temperature trends in oxygen concentrations will be key contributors to the downfall of Western and Eastern cod populations. Hiddink and Coleby (2012) reveals that the effect of temperature rise on marine fish biodiversity is most pronounced in areas with low population connectivity, such as the Baltic Sea, because species in these areas have limited opportunities to migrate to more favourable conditions, leading to a higher risk of local extinctions.
Neumann (2010) calculates that the most serious impact of temperature change will be alterations in the physical environment, leading to changes in habitat conditions and distribution patterns of species. This includes shifts in temperature, salinity, and oxygen levels, which in turn affect the biological parameters of species such as growth rates, reproductive success, and survival. Furthermore, Neumann (2010) emphasises how increased warming will favour cyanobacteria growth, leading to longer lasting and more widespread blooms. Studies conducted a decade later by Cederqvist et al. (2020) and Meier et al. (2021) reinforce those earlier findings.
Brander (2010) discusses the implications of climate cycles on fish stocks, suggesting that temperature increases could exacerbate existing pressures on marine life. Meier et al. (2011b, 2011c, 2011d) and Nowicki et al. (2016) uses simulation models to predict future temperature scenarios, highlighting potential shifts in species distributions. Andersson et al. (2015a) explores future ecosystem management strategies against warming trends. Meanwhile, Hordoir and Meier (2012) investigates the effects of thermal stratification on the Baltic Sea, predicting significant changes in water column stability and nutrient cycling. Hägg et al. (2014) and Meier et al. (2012a, 2012c) study future nutrient loading scenarios, projecting that warming temperatures would likely increase the frequency and intensity of algal blooms.
Other notable research includes Barbosa and Donner (2016), who examine the interplay between temperature rise and marine ecosystem health; Philippart et al. (2011) on the long-term ecological impacts of temperature rise; Lehmann et al. (2011) on species adaptation mechanisms; Friedland et al. (2012) on fisheries management challenges; Niiranen et al. (2013) on ecosystem resilience; Rutgersson et al. (2014) on climate modelling; and Viitasalo and Bonsdorff (2022) on future biodiversity projections. Overall, rising sea temperatures have been identified as a major driver of ecological change in the Baltic Sea, influencing deoxygenation, eutrophication and saltwater inflow events.
3.2.2 Hypoxia
The phenomenon of deoxygenation in the Baltic Sea and the creation of hypoxia or dead zones is the focus of intense research, with 29 articles highlighted in this review. The collective findings underscore the expansion of dead zones in the Baltic Sea. For example, Conley et al. (2011) identifies 115 hypoxic sites between 1955 and 2009, underscoring how the Baltic Sea contains over 20% of all known hypoxic sites worldwide. Modelling studies by Hansson and Gustafsson (2011); Gustafsson (2012) and Savchuk (2013) consistently reveal a worsening trend in the long-term development, volume and dynamics of hypoxic areas in various Baltic sub-regions. Meier et al. (Meier et al., 2011a, 2017) reinforce this, demonstrating how accelerated mean sea level rise will exacerbate the spread of dead zones. Bendtsen and Hansen (2013) find that a 3°C temperature rise on the seafloor will reduce oxygen production by 30%. Other studies indicating a significant expansion of hypoxic areas include Almroth-Rosell et al. (2011, 2021); Bange et al. (2010); Gilbert et al. (2010); Rabalais et al. (2010); Hansson and Gustafsson (2011); Reed et al. (2011); Vaquer-Sunyer and Duarte (2011); Lehmann et al. (2014); Breitburg et al. (2018) and Zilí and Conley (2010) suggest that a worst-case scenario for the extent of hypoxic areas in the Baltic Sea has been reached.
Academics also research the effects of increasing hypoxia ranges and durations on the local ecosystem of the Baltic Sea (Meier et al., 2019c). Díaz and Rosenberg (2011) discusses the potential environmental and economic consequences of deoxygenation, highlighting how hypoxia increases fish vulnerability to diseases and reduces mobility, survivability, growth and reproductive success. Similar impacts are discussed by Vaquer-Sunyer and Duarte (2010); Hansson et al. (2011); Carstensen et al. (2014) and Villnäs et al. (2012), emphasising how increasing bottom-water hypoxia is the main stressor impacting benthic communities, including cod, whiting, flounder and plaice, creating barren wastelands on the seafloor.
Casini et al (Casini et al., 2016 and Casini et al., 2021) detail the adverse consequences of hypoxia on cod, in relation to their size, physiology, behaviour, and trophic interactions. Statistical evidence indicates hypoxia-induced restrictions for cod, forcing them to crowd and compete for fewer resources. Moreover, Norkko et al. (2012) emphasises how increased abundances of polychaetes Marenzelleria spp. in the Baltic Sea have helped mitigate the spread of hypoxia. Dietze and Löptien (2016); Neumann et al. (2017) and Stigebrandt and Kalén (2013) highlight the potential of major Baltic inflows that could oxygenate the central Baltic Sea. This oxygenated water could settle in oxygen-deprived areas, potentially revitalising them (Stigebrandt and Kalén, 2013). However, the absence of a major saltwater inflow since 2015 has created a prolonged period without new oxygenated water mixing in the Baltic Sea, causing further hypoxic expansion.
3.2.3 Nutrient enrichment
Nutrient enrichment, often resulting in eutrophication, is a primary driver of harmful algal and bacterial blooms in the Baltic Sea. This phenomenon is mainly fuelled by excessive nitrogen and phosphorus inputs, as highlighted by 67 studies emphasising its trends and ecological risks. One of the most visible signs of eutrophication is increased algal growth, which reduces water clarity and blocks sunlight from penetrating as deeply as before (Zilí and Conley, 2010).
Bergström et al. (2019) find that species exhibit varied responses to cyanobacteria blooms; for example, carnivorous fish are more negatively affected, while herbivores benefit from the increased algae. This adversely impacts cod, whiting, plaice and flounder, which prey on other fish. Benthic habitats housing Gadidae and Pleuronectidae species are expected to be under the biggest pressure, with cyanobacteria blooms contributing to the spread of hypoxia on the seafloor (Dzierzbicka-Głowacka et al., 2011a; Legrand et al., 2015). Additionally, Suikkanen et al. (2010, 2013) show how plankton communities in the Baltic Sea have shifted the food-web structure of smaller-sized organisms, leading to decreased energy available for zooplankton and consequently for herring, which rely on zooplankton as a food source (Adam et al., 2016). The decomposition of cyanobacteria releases nitrogen and depletes oxygen, creating uninhabitable environments for many fish species (Ferreira et al., 2011; Karlson et al., 2015; Kahru et al., 2020, Meier et al., 2012b, Walve and Larsson, 2010; Viitasalo and Bonsdorff, 2022).
Phytoplankton composition and growth trends indicate that large-scale climatic shifts will likely increase harmful algal blooms (Klais et al., 2011; Mazur-Marzec et al., 2013; Kahru and Elmgren, 2014; Kaiser et al., 2020, Meier et al., 2018b, Olenina et al., 2010; Ploug et al., 2010; Wasmund et al., 2011; Varjopuro et al., 2014; Munkes et al., 2021). Cyanobacteria life cycle models by Hense et al. (2013) demonstrate that cyanobacteria thrive in higher water temperatures, enabling them to benefit from climate change. Neumann et al. (2012) predict that cyanobacteria blooms contribute up to 50% of the total nitrogen load in the Baltic Sea. Studies by El-Shehawy et al. (2012), Wasmund (2017), Meier et al. (2018a, 2018b, 2019a, 2019b), Murray et al. (2019), Voss et al. (2011a) and Śliwińska-Wilczewska et al. (2019) anticipate record-breaking cyanobacteria bloom events in the region. Long-term temporal and spatial trend studies by Andersen J. H. et al. (2015), Fleming-Lehtinen et al. (2015) and Gustafsson et al. (2012) reveal that the Baltic Sea eutrophication problem is expanding.
The Baltic Sea has long been polluted by excessive nutrients from agricultural runoff, with over 250 rivers discharging into the sea. Nutrient enrichment detrimentally impacts the health of commercially valuable stocks, which frequently spawn in coastal lagoons and estuaries (Dodson et al., 2018, Kuss et al., 2020). Excess nutrients promote earlier and more intense cyanobacteria blooms, creating longer and more damaging cycles (Andersson et al., 2015b, Mort et al., 2010; Ploug et al., 2010; Raateoja et al., 2011; Viktorsson et al., 2012; Stigebrandt et al., 2014; Olofsson et al., 2016; Yli-Hemminki et al., 2016; Gustafsson et al., 2017). Projections by Pihlainen et al. (2020) indicate that if current conditions persist, by 2100 the overall nutrient loading in the Baltic Sea will increase from 52% to 115%. Räike et al. (2020) note that despite water protection measures, nutrient exports from Finnish rivers into the Baltic Sea have not decreased.
Contrarily, Kuss et al. (2020) observe a gradual 30% decrease in anthropogenic nitrogen input in the Western Baltic Sea since 1995, with phosphorus input declining by 25% between 1995 and 2000. Hägg et al. (Hägg et al., 2010, 2014) suggest that improved agricultural waste management could lead to better conditions for the Baltic Sea, despite warming trends. Simultaneous reductions in nitrogen and phosphorus loads could significantly improve oxygen conditions on the seafloor, combating hypoxic areas and allowing marine life to return to benthic regions (Gustafsson et al., 2012; Riemann et al., 2016). However, other studies underscore that climate change will only exacerbate the eutrophic dangers associated with riverine nutrient enrichment and limit the recovery success for many commercially valuable fish species, notably Gadidae and Pleuronectidae species (Carstensen et al., 2011; Arheimer et al., 2012; Meier et al., 2012a; Bring et al., 2015). While direct studies linking nutrient enrichment effects on top commercial fish species are lacking, research by Hongisto (2011; Hong et al. (2012)), Korpinen et al. (2012); Wikner and Andersson (2012); Savchuk (2018) and Wulff et al. (2014) offer insights into the increased pressures of nutrient cycling dynamics, interactions with algal blooms, and nutrient transport pathways in the Baltic Sea, which disrupt the food-web and habitat of commercial fish stocks.
3.2.4 Low salinity
Salinity levels and their impacts on marine life are highly researched in the Baltic Sea, with 20 articles highlighted in this review. The Baltic Sea has the lowest salinity level of any sea in the world. Key studies include Kniebusch et al. (2019); Maar et al. (2011) and MohHrholz et al. (2015) on the changing salinity gradients in the Baltic Sea. They highlight how unfavourable weather patterns for saltwater inflows from the North Sea, coupled with freshwater inflow from rivers, have resulted in significantly reduced salinity (Fu et al. 2010; Kniebuschet al., 2019). If significant inflow events do not occur in this century, the Baltic Sea could potentially become a lake (Mohrholz et al., 2015, Reusch et al., 2018). Lehmann et al. (2022) underscores how ecosystems adapted to the current salinity level may face ecological stress from sudden changes. Consequently, salt-tolerant species may struggle to adapt to shifting salinity levels, leading to reduced distribution and challenges in larval survival in oxygen-poor depths (Lehmann et al., 2022).
Miethe et al. (2014) suggests that the distribution pattern of herring is influenced by saltwater inflow events into the Baltic Sea. Despite herring’s tolerance to varying salinities and temperatures, central herring stocks are observed to avoid water layers with the lowest oxygen saturation and salinity levels. Nissling and Dahlman (2010) and Vuorinen et al. (2015) highlight how declining salinity levels in the Baltic Sea affect crucial marine benthic foundation species such as Eelgrass (Zostera marina) and Wrack (Fucus radicans). These species, integral in providing food and shelter for other marine organisms, see their distributions altered, potentially impacting commercially important species like cod and flounder.
Kijewska et al. (2016) examine the stress response of Eastern and Western Baltic cod populations to varying salinities. Their results show how cod accustomed to higher salinity conditions in the Kiel Bight are unlikely to migrate to less saline regions like Gdansk Bay, maintaining both physical and genetic separations among cod stocks. Berg et al. (2015) demonstrates how cod expanded into the Central Baltic Sea when the surface salinity is higher. Consequently, the presence of low-saline waters significantly impedes the spawning success of cod in the Baltic Sea (Berg et al., 2015). Additionally, low salinity promotes harmful bacterial blooms, creating a favourable environment for Dolichospermum spp (Brutemark et al., 2015). Similar experiments are conducted by Engström-Öst et al. (2011) and Rakko and Seppälä (2014), with their results showing the positive effect that low salinity has on the growth rates of various cyanobacteria species contributing to eutrophication and hypoxia.
3.2.5 Ocean acidification
The marine CO2 system is a key focus in marine research aimed at understanding ocean acidification and the consumption or production of CO2 in the Baltic Sea. This region is especially vulnerable to ocean acidification due to its considerably lower alkalinity levels compared to the ocean (Omstedt et al., 2014). Thirty-three studies focus on the distribution and spread of CO2 within this review. Beldowski et al. (2010) mapped the distribution pattern of CO2 and alkalinity in the region. They calculate that an annual increase of CO2 by 1.2–1.5 ppm will decrease pH by 0.002 per year. Other studies measure the unfavourable impacts of organic carbon, nitrogen and phosphorus cycling the Baltic Sea, including Brutemark et al. (2011); Dzierzbicka-Glowacka et al. (2011b); Skoog et al. (2011); Omstedt et al. (2012); Szczepańska et al. (2012); Gustafsson et al. (2014); Müller et al. (2016); and Hammer et al. (2017). These papers discuss how the accumulation of CO2 is influenced by organic matter from rivers, saltwater renewal, and atmospheric warming. Consequently, Kuliński and Pempkowiak (2011) and Kuliński et al. (2014) notes how the Baltic Sea has 3–5 times higher organic carbon concentrations than the North Sea.
Melzner et al. (2020) underscores how ocean acidification amplifies coastal hypoxia. Higher concentrations of methane also exacerbate warming and acidification in the Baltic Sea (Gülzow et al., 2011, Reindl and Bolałek 2013). This has serious consequences for marine life that are unable to tolerate the more acidic environment (Hammer et al., 2014, 2017). Moreover, Melzner et al. (2011) emphasise that calcifying organisms, such as blue mussel (Mytilus edulis), will be particularly vulnerable to pH alterations, leading to decreases in abundance and size. This observation is confirmed by Fitzer et al. (2012); Thomsen et al. (2017); Sanders et al. (2018), and Wahl et al. (2018). Graiff et al. (2017) notes how ocean acidification and warming could negatively impact the reproduction of rocky seaweeds, such as Fucus vesiculosus, which are pivotal in forming expansive underwater forests and providing habitat and nurseries for many fish in the Baltic Sea. They find that elevated CO2 and warming accelerated the spring maturation of Fucus vesiculosus (Graiff et al., 2015, 2017). However, Takolander et al. (2019) observe that CO2 increases did not affect the growth rates of F. vesiculosus, except for seasonal variations. Additionally, Wahl et al. (2020) reports that ocean warming has a more significant impact than acidification, causing structural and functional shifts in coastal communities of F. vesiculosus.
This destruction of blue mussels and seaweed beds could cascade up the food chain and negatively affect cod, plaice, and flounder who rely on these species as nursery grounds (Seitz et al., 2013). There is also evidence to show that ocean acidification negatively affects cod and herring larvae survival (Frommel et al., 2012, 2014; Stiasny et al., 2016).
3.3 Food-web dynamics
3.3.1 Prey-predator relationships
Fishing and environmental changes significantly alter the food-web of the Baltic Sea, affecting species composition, population dynamics and inter-species interactions (Tomczak et al., 2012, 2013). Compared with other regions, the brackish Baltic Sea exhibits the distinctive characteristic of low biodiversity, resulting in simpler predator-prey relationships and a higher level of interdependence among the existing species (Ojaveer et al., 2010). There are 44 articles under the food-web dynamics grouping, with 35 articles highlighting the impact of other marine predators and prey on the status of commercial fish.
Bivalves such as clams, oysters and mussels are among the most important taxonomic groups in benthic habitats (Zaiko et al., 2010). Studies by Koivisto and Westerbom (2010); Koivisto and Westerbom (2012); Darr et al. (2014) and Löfstrand et al. (2010) highlight the impact of blue mussels on wider population structures, namely Pleuronectidae that depend on mussels as a food source. Blue mussels also help mitigate eutrophication by filtering the water (Koivisto and Westerbom, 2010, 2012). Westerbom et al. (2019) note how blue mussel abundance is related to salinity, winter severity, wave exposure and depth, with peak densities in high saline areas. Their study highlights how blue mussel biomass has fallen by 80% in the Gulf of Finland since 1998, raising concerns for benthic stocks as the Baltic Sea continues to become less saline.
Studies on the community structures and dynamics of zooplankton and copepods are also crucial in determining the health of herring and sprat, which feed on these organisms (Vehmaa et al., 2013; Otto et al., 2014; Gorokhova et al., 2016; Labuce et al., 2021). Otto et al. (2014) investigates the long-term dynamics of three dominant zooplankton in the central Baltic Sea: Acartia spp., Temora longicornis and Pseudocalanus acuspes. They highlight these species’ behavioural and physiological adaptations over time, including preferred water depths, feeding habits and diel vertical migration patterns. Notably, Otto et al. (2014) highlights how Acartia spp. and Temora longicornis exhibits the highest rates of egg production as temperatures increased up to 18°C. Furthermore, bloom-forming cyanobacteria promote higher copepod reproduction, providing a greater food source for adult herring and sprat (Hogfors et al., 2014; Otto et al., 2014).
Moreover, Ojaveer and Kalejs (2010) and Ojaveer et al. (2018) analyses the stomach contents of herring and sprat, revealing a positive correlation between predators and prey, with the stomach fullness of Clupeidae fish increasing in areas with higher zooplankton. Considering the dietary dynamics of predators targeting commercially valuable fish is also critical. Lundström et al. (2010) observes how grey seals (Halichoerus grypus) predominately prey on herring, followed by sprat in the South, and whitefish in the North, with juveniles consuming small non-commercial species. However, MacKenzie et al. (2011) emphasise that seal predation has a lower impact on cod recovery compared to the effects of environmental drivers and fisheries. Roos et al. (2012) underscores that, despite the increasing number of grey seals, they do not impact stock dynamics. Similarly, Edrén et al. (2010) underscore that harbour porpoises (Phocoena Phocoena) prey on herring, cod and sprat, but their small population does not negatively affect stock dynamics. Other predators, such as the Northern pike (Esox Lucius), prey on smaller fish, but their interactions with sprat and herring are poorly understood (Engstedt et al., 2010; Nilsson et al., 2014; Larsson et al., 2015; Olsson, 2019).
Additionally, Järv et al. (2011) illustrate how the recent introduction of round gobies (Neogobius melanostomusin) in Mugga Bay may negatively affect flounder. They highlight a significant overlap in diet composition between these species, with gobies shown to have a larger diet variability and fuller stomach contents, potentially outcompeting flounder in the area (Järv et al., 2011). Likewise, three-spined stickleback (Gasterosteus aculeatus) populations have increased in many coastal areas of the Baltic Sea due to reduced predation and nutrient enrichment (Olin et al., 2022). Their presence can alter the food-web dynamics as they prey on their larvae (Lefébure et al., 2014; Jakubavičiūtė et al., 2017).
Furthermore, great cormorants (Phalacrocorax carbo) in the Eastern and Northern Baltic Sea are increasing in number, putting pressure on food-web dynamics (Veneranta et al., 2020; Van Eerden et al., 2022). So far, studies on cormorants primarily focus on their consumption of perch (Perca fluviatilis), with Veneranta et al. (2020) highlighting how high cormorant densities can locally reduce perch catches. Van Eerden et al. (2022) note how interactions between cormorants and fisheries are unlikely in the Gulf of Finland, although local effects on perch may exist. Also, moon jellyfish (Aurelia aurita) and lion’s mane jellyfish (Cyanea capillata) serve as competitors and predators for commercially valuable species, as both consume cod larvae and compete with sprat and herring for zooplankton resources (Janßen et al., 2013). Stoltenberg et al. (2021) notes large outbreaks of moon jellyfish overlapping in time with late spawning cod in the Bornholm basin. They conclude that both species heavily prey on Eastern cod eggs, highlighting that cod eggs are found in 49.3% of lion’s mane jellyfish and in 5.5% of moon jellyfish guts (Stoltenberg et al., 2021).
Despite this, Stoltenberg et al. (2021) mentions that adult whiting and herring prey on moon jellyfish, although no studies in the Baltic Sea have recorded this behaviour. In the Western Baltic, the invasive warty comb jelly (Mnemiopsis leidyi) was first recorded in 2006 (Schaber et al., 2011). Although the species cannot sustain itself due to lowering salinity, the comb jelly travels with currents and is often found preying in the spawning grounds of top fish stocks in the Baltic Sea (Jaspers et al., 2011; Schaber et al., 2011). Brulińska et al. (2016) highlights that increased jellyfish outbreaks are caused by climate change and nutrient enrichment and are likely to become more frequent in the Baltic Sea. Therefore, understanding the population dynamics and feeding preferences of these predators could inform broader fisheries management strategies.
3.3.2 Parasites of fish
Parasitic presence in the alimentary tract of fish pose a universal problem, leading to organ, physiological, behavioural and reproductive damage in many species. This review highlights nine papers on parasitic infections in top commercial fish species. Ryberg et al. (2021) reveal that in the Eastern Baltic, the probability of cod being in a critical condition increased when the parasitic nematode (Contracaecum osculatum) is detected. Horbowy et al. (2016); Podolska et al. (2016) and Polak-Juszczak (2017) shows how infected individuals in the Eastern Baltic cod stock has a 20% lower body condition compared to those free of Contracaecum parasites. This is further reinforced by Mohamed et al. (2020), who illustrates that parasitic presence slows down the growth regulation in cod. Furthermore, adult cod measuring 70-80 cm exhibited the highest prevalence and intensity of infection.
Unger et al. (2014) observe differences in parasite distribution among Central, Western and Gulf of Finland herring stocks, with a decreasing presence towards the East Baltic. Skrzypczak and Rolbiecki (2015) reported a low overall prevalence (3.2%) of parasites in sprat. This is reinforced by Kleinertz et al. (2011) who highlight that the Baltic Sea contains sprat with a lower number of parasite infections compared to the North Sea. Additionally, Kuciński et al. (2023) notes a significant decline in the fitness and catch volume of Pleuronectidae species, particularly on Slupsk Bank, attributing it to prevalent parasitic presence, including Glugea stephani, affecting 42% of investigated flounder. Despite these findings, the impact of parasitic fauna on commercial fish species in the Baltic Sea remains unclear.
3.4 Top commercial fish stocks
3.4.1 Gadidae: cod and whiting
The Gadidae family, including whiting (M. merlangus) and the Atlantic cod (G. morhua), is extensively researched in the Baltic Sea. Cod, divided into Eastern and Western stocks, have seen a steep population decline in the last four decades, leading to the subsequent closure of targeted cod fisheries in 2019 (ICES, 2019). Scientists have been intensely studying the species to understand the reasons for this decline, with 41 papers highlighted in this review. Stiasny et al. (2016) estimates Western cod larvae mortality under ocean acidification, showing a recruitment reduction by 8%. Frommel et al. (2012) demonstrates how exposure to increased CO2 can lead to lethal tissue damage in many internal organs of cod larvae, contributing to early-life mortality. Hüssy (2011) emphasises how salinity and oxygen play a key role in cod egg buoyancy, influencing survival success in oxygen-poor depths. Petereit et al. (2014) observes that cod larvae have the highest chances of survival in April and May, but no cohorts that drifted into lower saline and oxygen levels in the Central Baltic Sea survived. Similar results are published by Ljunggren et al. (2010); Margonski et al. (2010); Ojaveer et al. (2011); Hinrichsen et al. (2012); Maneja et al. (2013), and Voss et al. (2012).
Mion et al. (2018) notes how the disappearance of larger female individuals also reduced the spawning capacity for cod because larger and older individuals produce a higher number of eggs. Consequently, the remaining smaller females are producing fewer eggs, limiting their ability to repopulate. ICES (2022a) cite reduced spawning capacity as one of the key reasons that led to a population decline in cod stocks, prompting a targeted ban on cod fisheries in 2019.
Research on population distribution, feeding and growth of commercially valuable fish stocks has also been extensive. Neuenfeldt et al. (2020) identifies factors behind the reduction in cod size over the last two decades, with decreased food availability leading to higher densities of smaller cod competing for scarce resources, stunting their growth. Pachur and Horbowy (2013) reinforced this by studying Eastern cod catches in the Polish Exclusive Economic Zone and noting shifts in their diet composition since the 1980s, with sprat replacing herring as their primary food source. Gårdmark et al. (2015) also underscores the changes in cod’s diet, emphasising the consequential impacts on the spatial-temporal dynamics of other structured populations. Casini et al. (2016) investigates the body conditions of cod, revealing how alterations are linked with food limitations. Furthermore, Casini et al. (Casini et al., 2012, 2016) demonstrates how hypoxic areas further deteriorate cod’s health through mechanisms related to physiology, behaviour and trophic interactions.
Moreover, Schaber et al. (2012) study cod distribution patterns in the Bornholm Basin and observe that both salinity and oxygen concentration are key parameters affecting cod distribution. Consequently, increased hypoxia and eutrophication forced cod out of their previously favoured habitats. Similarly, Nielsen et al. (2013) observe changes in cod abundance linked with variations in the environment, noting differences in juvenile cod behaviour compared to their North Sea counterparts, as Baltic Sea cod did not form dense schooling patterns. Other notable cod-related studies that look at the age structure and spatial-temporal dynamics correspondingly suggest that there are fewer large adults (Hüssy et al., 2010a, 2010b; Mackenzie and Gislason, 2011; Hüssy et al., 2012; Kristensen et al., 2014, Nielsen et al., 2014, Orio et al., 2017a, 2019). As cod biomass decreased, sprat and herring emerged as key species in the Baltic Sea. Tomczak et al. (Tomczak et al., 2012, 2013) proposed that the decline of cod resulted in trophic cascades affecting Clupeidae fish, who lost their primary predator.
Whiting, although commercially fished in the Western Baltic Sea, holds lesser economic value compared to cod. Reflecting its less prominent status, there is a scarcity of published research on whiting, with two papers highlighted in this review from the same leading author. These papers examine the diet of whiting in the Western Baltic Sea, finding that clupeids making up 90% of adult diets, while gobies, brown shrimps and polychaetes as primary prey for juvenile whiting (Ross and Hüssy, 2013; Ross et al., 2016). The authors emphasise whiting’s role as a top predator in the Western Baltic Sea, stressing the importance of investigating its ecology and population dynamics. Comprehensive population assessments of whiting have only been conducted in the North Sea, and not much is known about the impact of environmental divers on their population.
3.4.2 Clupeidae: herring and sprat
Herring and sprat are key marine fish species with the highest commercial value in the Baltic Sea. ICES began managing Baltic herring in the 1970s, assessing them in four stocks: Bothnian, Western, Central and Riga herring. Their status varies, with Bothnian and Central herring SSB stabilising over the last six years, while Riga herring populations continue to grow. In contrast, Western herring faces collapse, having experienced substantial SSB declines over the last decade (ICES, 2022b-e). Extensive research is carried out to closely monitor herring, with 22 papers highlighted in this review.
Raid et al. (2010) examine the recruitment dynamics of herring populations in the Gulf of Riga, revealing extensive geographical variability, with all 12 local populations adapting to the highly variable environmental conditions in the region, allowing stocks to grow. Atmore et al. (2022) shows varied spawning success across different stocks, with autumn spawners making up 90% of Riga herring landings. However, MacKenzie and Ojaveer (2018) underscores how, despite high reproductive rates and rapid growth, herring can still undergo drastic population declines over time. Dodson et al. (2018) find that the optimal temperatures for healthy yolk-sac herring are between 9°C and 13°C, with rising temperatures resulting in increasing larval deformities. Polte et al. (2021) notes how Western Baltic herring has reduced reproductive success during warmer winters, reducing the herring reproduction index by 3% per day. Frommel et al. (2014) also shows that exposure to increased CO2 can cause lethal tissue damage and increased mortality in herring larvae.
Herring stocks in SD 25-32 are more abundant, but this does not necessarily indicate a healthy population. Dziaduch (2011) finds that out of the 1,615 analysed herring, no stomach was full of food. On the contrary, in the Bornholm Basin, 90% of all herring had empty stomachs due to changes in prey composition (zooplankton) and increased competition with sprat (Dziaduch, 2011). Livdane et al. (2016) reinforces this point by highlighting zooplankton availability as a major factor affecting herring fitness. Additionally, Casini et al. (2010) demonstrates that herring growth is positively influenced by the abundance of sprat, resulting in lower growth in areas with high sprat density. Dippner et al. (2019) observe a decreasing trend of 3-year-old central Baltic herring, with their mean weight dropping from 50-70 g in the 1970s to 25-30 g today, attributing this to increased precipitation, habitat reduction and changes in the prey composition.
Atmore et al. (2022) highlight the vulnerability of herring stocks to overfishing, nutrient enrichment, climate change, low salinity and competition from expanding sprat populations. Soerensen et al. (2022) observe a decline in selenium levels in Baltic herring, linking this to external source loads and changing atmospheric emissions. Miethe et al. (2014) notes that environmental conditions, specifically marine inflow events, strongly influence the distribution patterns of Western Baltic spring-spawning herring during autumn migrations. Rajasilta et al. (2019) attributes declining lipid content of Baltic herring and stock size to decreasing salinity. These studies underscore the significance of environmental factors in shaping herring fitness, abundance and distribution in the Baltic Sea.
Sprat is the smallest fish species in the Clupeidae family. There is less research published on sprat compared to herring, with 10 studies highlighted in this review. Sprat is monitored as a single stock covering SD 22-32, currently the largest stock in the Baltic Sea with the largest TAC quotas (ICES, 2022h). Voss et al. (2011b) observe significant population fluctuations in sprat due to rising temperatures, enhancing recruitment success during their early life stages. In addition, higher temperatures lead to faster larval development, resulting in decreased egg mortality as their most vulnerable life stage passes more quickly. MacKenzie et al. (2012) identifies the greatest positive impact of rising temperatures on sprat. Using a climate-ocean-fish population model, they projected that sprat spawning biomass could increase to 1.5 million tonnes with a 2-5°C sea temperature rise (MacKenzie et al., 2012). Therefore, sprat is among the few commercial fish species that could benefit from the effects of ocean warming.
Sprat experiences varying levels of success depending on the region, with 75% of the total sprat stock caught in the Northern Baltic and the Gulf of Finland (Casini et al., 2011). Kulke et al. (2018) find higher mean stomach contents in sprat from the Bornholm Basin compared with the Aroka and Gotland Basin, with a preferred feeding depth of 26 m, where light intensity is higher and plankton is visible. Ojaveer et al. (2010) highlights how the most stable environment for sprat is in the southwestern region, attributing freshwater discharge variations as the main factor affecting their abundance. During periods of high river runoff, sprat habitat expands and their stocks increase (Ojaveer et al., 2010). Voss et al. (2012) observe that the survival time of sprat larvae increased by an additional four days (~45%), indicating a reproductive advantage under higher rainfall.
Correspondingly, Eero (2012) notes how sprat has positively reacted to the changing environment as well as reduced cod populations and is outperforming herring in many categories. For example, Ojaveer et al. (2018) find that sprat have fewer empty stomachs compared to herring, indicating that they are more successful at finding and consuming zooplankton. Similar conclusions are made by Margonski et al. (2010); Casini et al. (2011); Heikinheimo (2011) and Tomczak et al. (2012), who study the relationships between cod, herring and sprat, noting how sprat shows the best adaptation in more hostile environments.
3.4.3 Pleuronectidae: flounder and plaice
Since the decline of cod, Pleuronectidae have become one of the most economically important species for small-scale fisheries in the Central Baltic Sea (Dabrowska et al., 2017). Among these, the European flounder (P. flesus) is the focus of most scientific studies. The European plaice (P. platessa) is the only Pleuronectidae species with imposed TAC limits, which have increased annually due to high recruitment. ICES (ICES, 2022f, g) monitors plaice as two stocks, in SD 21-23 and 24–32. Meanwhile, the CFP monitors plaice as a single unit (European Commission, 2022). Flounder has no catch restrictions due to its perceived healthy status with 17 studies highlighted in this review.
Haase et al. (2020) observe that flounder populations have increased in the past two decades, hypothesising that they have deprived cod of benthic food resources. Orio et al. (2017a) illustrates how both flounder and cod have migrated to shallower depths because of expanded hypoxia in deep waters, leading to increased competition. This has resulted in a general decline in size and weight for both species, with smaller individuals progressively dominating the demersal ecosystem (Orio et al., 2017a). Flounders in the Baltic Sea exhibit two reproductive behaviours: pelagic and demersal. Orio et al. (2017b) notes that pelagic spawning flounder negatively react to rising temperatures, leading to a reduction in their spawning areas in the Bornholm Basin despite favourable salinity levels. Further studies by Orios et al. (Orio et al., 2019, 2020) show additional spatial contraction of cod and flounder populations in the last 40 years due to significant environmental and hydrological changes.
Momigliano et al. (2018) attributes poor genetic diversity as a major threat to flounder adaptability, using DNA samples, they show how reduced genetic diversity in the Åland Sea and the Gulf of Finland made flounder more vulnerable to changing environmental conditions (Momigliano et al., 2019). Jokinen et al. (2019) supports this, revealing that in 1983, the fishery unknowingly targeted a single gene pool of flounder, depleting the genetic diversity of those stocks. Jokinen et al. (2016) emphasises that shallow habitats for juvenile Pleuronectidae in the North Baltic Sea are most vulnerable to coastal eutrophication, showing a negative correlation between juvenile flounder abundance and vegetation density. They also reported a 40-time decline in juvenile flounder densities since the 1980s.
Furthermore, Nissling and Dahlman (2010) notes how low salinity negatively affects the ability of flounder to reproduce in SD 25, 27, 28 and 29. Ustups et al. (2013) reinforced this by showing that flounder’s recruitment success depends on producing eggs that float in low-salinity waters. Borg et al. (2014) highlights significant year-to-year variations in flounder recruitment and distribution, with differences in diet and habitat preferences between males and females. Florin and Lavados (2010) and Momigliano et al. (2018) notes how flounder in the Baltic Sea display contrasting reproductive behaviours and diet preferences, which affect their resilience in different locations.
Despite the TAC allowance and its regional significance, plaice studies in the Baltic Sea are limited, with two highlighted in this review. Ulrich et al. (2013) examine the variability and connectivity of plaice populations from the Eastern North Sea and Western Baltic Sea. They find that plaice in Skagerrak, Kattegat, the Belts and the Sound Baltic Sea regions are closely linked with plaice in the North Sea (Ulrich et al., 2013). Spawning in the Kattegat occurs between February and March at depths of 30-40 m, with temperatures around 4°C (Ulrich et al., 2013). Many eggs and larvae settling in the shallow waters of Skagerrak are believed to originate from the North Sea. This drift is reinforced in winter when stronger winds prevail. Free-floating eggs are also found in the deeper basins of the South Baltic Sea, with spermatozoa and eggs well adapted to low salinity conditions, allowing plaice to thrive there (Ulrich et al., 2013).
Ulrich et al. (2013) find that plaice stocks have strong genetic diversity, indicating a healthy population. However, tagging data reveals limited movements for both adults and juveniles in the Belts, Western Baltic, Kattegat and the North Sea, with up to 90% of plaice recaptured in the same area, indicating non-migratory behaviours that make them more susceptible to habitat loss. Cardinale et al. (2010) reports a 60% reduction in adult plaice biomass and a 10 cm decrease in average maximum length in the Kattegat region since the 1960s. Their study linked these declines to increased fishing mortality. However, a recent ICES (2022f) report shows a positive shift, with relative fishing mortality declining, suggesting improving trends for plaice stocks.
4 Conclusion
In summary, this systematic review of 250 scientific papers provides comprehensive insights into the complex interplay of environmental drivers affecting top commercial fish stocks in the Baltic Sea. The findings highlight diverse responses among species, with cod proving most vulnerable to temperature changes, nutrient enrichment and hypoxia, impacting larval survival, habitat availability and feeding conditions. Parasitic infections and invasive species further exacerbate these challenges, with potential implications for whiting stocks as well. Pleuronectidae species exhibit resilience to various environmental pressures but remain susceptible to parasitic infections and habitat destruction. Nutrient enrichment benefits Clupeidae species by enhancing food availability, while reduced cod populations alleviate predation pressure. However, herring stocks vary, with Riga herring increasing while Western herring face decline due to intensified competition. Sprat populations thrive amidst favourable environmental conditions, demonstrating robustness in survival, distribution, body condition and predator avoidance, solidifying their position as the predominant commercial stock in the Baltic Sea (Rosciszewski-Dodgson and Cirella, 2023).
However, gaps persist in understanding stock dynamics amidst growing environmental pressures. Substantial research focuses on SD 25-28, and discusses nutrient enrichment or the status of cod populations, reflecting uneven scientific interest. Concerns mount over rising threats from invasive species, parasitic infections, eutrophication, and projected temperature increases. While this systematic review provides a rigorous overview of current drivers, ongoing and future research efforts are crucial. We recommend further studies on Baltic Sea commercial fish stocks aligned with EBFM principles and SDG 14, which aims to ensure sustainable use of marine resources. Additionally, we suggest that readers interested in this topic refer to the cited papers for additional context.
Data availability statement
The datasets used in this study are available in the Figshare online repository. The repository name and accession numbers can be accessed at: https://doi.org/10.6084/m9.figshare.22885913.
Author contributions
MR-D: Formal analysis, Investigation, Writing – review & editing, Conceptualization, Data curation, Methodology, Resources, Validation, Writing – original draft. GC: Writing – review & editing, Formal analysis, Investigation, Project administration, Supervision, Visualization.
Funding
The author(s) declare financial support was received for the research, authorship, and/or publication of this article. This research was funded by the Narodowe Centrum Nauki under the project titled "Socio-economic Resilience of Small-Scale Fishermen in the South Baltic Sea: Shaping Governance for Sustainable Fisheries," Grant Number 2023/49/N/HS4/01136.
Acknowledgments
The authors are grateful to the Faculty of Economics, University of Gdansk, for supporting this work. We are also thankful to several faculty members who have given us advice in piecing together the manuscript and conceptual development. This article was submitted as a preprint as Rosciszewski-Dodgson and Cirella, 2023.
Conflict of interest
The authors declare that the research was conducted in the absence of any commercial or financial relationships that could be construed as a potential conflict of interest.
Publisher’s note
All claims expressed in this article are solely those of the authors and do not necessarily represent those of their affiliated organizations, or those of the publisher, the editors and the reviewers. Any product that may be evaluated in this article, or claim that may be made by its manufacturer, is not guaranteed or endorsed by the publisher.
Supplementary material
The Supplementary Material for this article can be found online at: https://www.frontiersin.org/articles/10.3389/fmars.2024.1399707/full#supplementary-material
References
Adam B., Klawonn I., Svedén J. B., Bergkvist J., Nahar N., Walve J., et al. (2016). N 2 -fixation, ammonium release and N-transfer to the microbial and classical food web within a plankton community. ISME J. 10, 450–459. doi: 10.1038/ismej.2015.126
Almqvist G., Strandmark A. K., Appelberg M. (2010). Has the invasive round goby caused new links in Baltic food webs? Environ. Biol. Fish. 89, 79–93. doi: 10.1007/s10641-010-9692-z
Almroth-Rosell E., Eilola K., Hordoir R., Meier H. E. M., Hall P. O. J. (2011). Transport of fresh and resuspended particulate organic material in the Baltic Sea - a model study. J. Mar. Syst. 87, 1–12. doi: 10.1016/j.jmarsys.2011.02.005
Almroth-Rosell E., Wåhlström I., Hansson M., Väli G., Eilola K., Andersson P., et al. (2021). A regime shift toward a more anoxic environment in a eutrophic sea in Northern Europe. Front. Mar. Sci. 8. doi: 10.3389/fmars.2021.799936
Andersen J. H., Carstensen J., Conley D. J., Dromph K., Fleming-Lehtinen V., Gustafsson B. G., et al. (2015). Long-term temporal and spatial trends in eutrophication status of the Baltic Sea. Biol. Rev. 92, 135–149. doi: 10.1111/brv.12221
Andersson A., Höglander H., Karlsson C., Huseby S. (2015a). Key role of phosphorus and nitrogen in regulating cyanobacterial community composition in the northern Baltic Sea. Estuarine Coast. Shelf Sci. 164, 161–171. doi: 10.1016/j.ecss.2015.07.013
Andersson A., Meier H. E. M., Ripszam M., Rowe O., Wikner J., Haglund P., et al. (2015b). Projected future climate change and Baltic Sea ecosystem management. Ambio 44, 345–356. doi: 10.1007/s13280-015-0654-8
Arheimer B., Dahné J., Donnelly C. (2012). Climate change impact on riverine nutrient load and land-based remedial measures of the Baltic Sea action plan. Ambio 41, 600–612. doi: 10.1007/s13280-012-0323-0
Atmore L. M., Martinez-Garcia L., Makowiecki D., Andre C., Lougas L., Barrett J. H., et al. (2022). Population dynamics of Baltic herring since the Viking Age revealed by ancient DNA and genomics. Proc. Natl. Acad. Sci. United States America 119, e2208703119. doi: 10.1073/pnas.2208703119
Bange H. W., Bergmann K., Hansen H. P., Kock A., Koppe R., Malien F., et al. (2010). Dissolved methane during hypoxic events at the Boknis Eck time series station (Eckernförde Bay, SW Baltic Sea). Biogeosciences 7, 1279–1284. doi: 10.5194/bg-7-1279-2010
Barbosa S. M., Donner R. V. (2016). Long-term changes in the seasonality of Baltic Sea level. Tellus Ser. A: Dynam. Meteorol. Oceanogr. 68, e30540. doi: 10.3402/tellusa.v68.30540
Beldowski J., Löffler A., Schneider B., Joensuu L. (2010). Distribution and biogeochemical control of total CO2 and total alkalinity in the Baltic Sea. J. Mar. Syst. 81, 252–259. doi: 10.1016/j.jmarsys.2009.12.020
Bendtsen J., Hansen J. L. S. (2013). Effects of global warming on hypoxia in the Baltic Sea-North Sea transition zone. Ecol. Model. 264, 17–26. doi: 10.1016/j.ecolmodel.2012.06.018
Berg P. R., Jentoft S., Star B., Ring K. H., Knutsen H., Lien S., et al. (2015). Adaptation to low salinity promotes genomic divergence in Atlantic Cod (Gadus morhua L.). Genome Biol. Evol. 7, 1644–1663. doi: 10.1093/gbe/evv093
Bergström L., Karlsson M., Bergström U., Pihl L., Kraufvelin P. (2019). Relative impacts of fishing and eutrophication on coastal fish assessed by comparing a no-take area with an environmental gradient. Ambio 48, 565–579. doi: 10.1007/s13280-018-1133-9
Borg J. P. G., Westerbom M., Lehtonen H. (2014). Sex-specific distribution and diet of Platichthys flesus at the end of spawning in the northern Baltic Sea. J. Fish Biol. 84, 937–951. doi: 10.1111/jfb.12326
Brander K. (2010). Impacts of climate change on fisheries. J. Mar. Syst. 79, 389–402. doi: 10.1016/j.jmarsys.2008.12.015
Breitburg D., Levin L. A., Oschlies A., Grégoire M., Chavez F. P., Conley D. J., et al. (2018). Declining oxygen in the global ocean and coastal waters. Science 359, e7240. doi: 10.1126/science.aam7240
Bring A., Rogberg P., Destouni G. (2015). Variability in climate change simulations affects needed long-term riverine nutrient reductions for the Baltic Sea. Ambio 44, 381–391. doi: 10.1007/s13280-015-0657-5
Brulińska D., Olenycz M., Ziółkowska M., Mudrak-Cegiołka S., Wołowicz M. (2016). Moon jellyfish, Aurelia aurita, in the Gulf of Gdansk: Threatening predator or not? Boreal Environ. Res. 21, 528–540. Available at: http://hdl.handle.net/10138/225818.
Brutemark A., Engström-Öst J., Vehmaa A. (2011). Long-term monitoring data reveal pH dynamics, trends and variability in the western Gulf of Finland. Oceanol. Hydrobiol. Stud. 40, 91–94. doi: 10.2478/s13545-011-0034-3
Brutemark A., Vandelannoote A., Engström-Öst J., Suikkanen S. (2015). A less saline Baltic Sea promotes cyanobacterial growth, hampers intracellular microcystin production, and leads to strain-specific differences in allelopathy. PloS One 10, e0128904. doi: 10.1371/journal.pone.0128904
Cardinale M., Hagberg J., Svedäng H., Bartolino V., Gedamke T., Hjelm J., et al. (2010). Fishing through time: Population dynamics of plaice (Pleuronectes platessa) in the Kattegat-Skagerrak over a century. Popul. Ecol. 52, 251–262. doi: 10.1007/s10144-009-0177-x
Carstensen J., Conley D. J., Bonsdorff E., Gustafsson B. G., Hietanen S., Janas U., et al. (2014). Hypoxia in the Baltic Sea: Biogeochemical cycles, benthic fauna, and management. Ambio 43, 26–36. doi: 10.1007/s13280-013-0474-7
Carstensen J., Sánchez-Camacho M., Duarte C. M., Krause-Jensen D., Marbà N. (2011). Connecting the dots: Responses of coastal ecosystems to changing nutrient concentrations. Environ. Sci. Technol. 45, 9122–9132. doi: 10.1021/es202351y
Casini M., Bartolino V., Molinero J. C., Kornilovs G. (2010). Linking fisheries, trophic interactions and climate: Threshold dynamics drive herring clupea harengus growth in the central Baltic Sea. Mar. Ecol. Prog. Ser. 413, 241–252. doi: 10.3354/meps08592
Casini M., Blenckner T., Möllmann C., Gårdmark A., Lindegren M., Llope M., et al. (2012). Predator transitory spillover induces trophic cascades in ecological sinks. Proc. Natl. Acad. Sci. United States America 109, 8185–8189. doi: 10.1073/pnas.1113286109
Casini M., Hansson M., Orio A., Limburg K. (2021). Changes in population depth distribution and oxygen stratification are involved in the current low condition of the eastern Baltic Sea cod (Gadus morhua). Biogeosciences 18, 1321–1331. doi: 10.5194/bg-18-1321-2021
Casini M., Käll F., Hansson M., Plikshs M., Baranova T., Karlsson O., et al. (2016). Hypoxic areas, density-dependence and food limitation drive the body condition of a heavily exploited marine fish predator. R. Soc. Open Sci. 3, e160416. doi: 10.1098/rsos.160416
Casini M., Kornilovs G., Cardinale M., Möllmann C., Grygiel W., Jonsson P., et al. (2011). Spatial and temporal density dependence regulates the condition of central Baltic Sea clupeids: Compelling evidence using an extensive international acoustic survey. Popul. Ecol. 53, 511–523. doi: 10.1007/s10144-011-0269-2
Cederqvist J., Lidström S., Sörlin S., Svedäng H. (2020). Swedish environmental history of the Baltic Sea: A review of Current Knowledge and Perspectives for the Future. Scandinavian J. History 45, 663–688. doi: 10.1080/03468755.2019.1692067
CINEA (2022). The implementation of ecosystem-based approaches applied to fisheries management under the CFP (European Climate, Infrastructure and Environment Executive Agency: Publications Office of the European Union) (Accessed 12 June 2024).
Conley D. J., Carstensen J., Aigars J., Axe P., Bonsdorff E., Eremina T., et al. (2011). Hypoxia is increasing in the coastal zone of the Baltic Sea. Environ. Sci. Technol. 45, 6777–6783. doi: 10.1021/es201212r
Dabrowska H., Kopko O., Lehtonen K. K., Lang T., Waszak I., Balode M., et al. (2017). An integrated assessment of pollution and biological effects in flounder, mussels and sediment in the southern Baltic Sea coastal area. Environ. Sci. pollut. Res. 24, 3626–3639. doi: 10.1007/s11356-016-8117-8
Darr A., Gogina M., Zettler M. L. (2014). Detecting hot-spots of bivalve biomass in the south-western Baltic Sea. J. Mar. Syst. 134, 69–80. doi: 10.1016/j.jmarsys.2014.03.003
Díaz R. J., Rosenberg R. (2011). Introduction to environmental and economic consequences of hypoxia. Int. J. Water Resour. Dev. 27, 71–82. doi: 10.1080/07900627.2010.531379
Dietze H., Löptien U. (2016). Effects of surface current-wind interaction in an eddy-rich general ocean circulation simulation of the Baltic Sea. Ocean Sci. 12, 977–986. doi: 10.5194/os-12-977-2016
Dippner J. W., Fründt B., Hammer C. (2019). Lake or Sea? The unknown future of central Baltic Sea herring. Front. Ecol. Evol. 7, e143. doi: 10.3389/fevo.2019.00143
Dodson J. J., Daigle G., Hammer C., Polte P., Kotterba P., Winkler G., et al. (2018). Environmental determinants of larval herring (Clupea harengus) abundance and distribution in the western Baltic Sea. Limnol. Oceanogr. 64, 317–329. doi: 10.1002/lno.11042
Dziaduch D. (2011). Diet composition of herring (Clupea harengus L.) and cod (Gadus morhua L.) in the southern Baltic Sea in 2007 and 2008. Oceanol. Hydrobiol. Stud. 40, 96–109. doi: 10.2478/s13545-011-0046-z
Dzierzbicka-Głowacka L., Jakacki J., Janecki M., Nowicki A., Dzierzbicka-Głowacka L., Jakacki J., et al. (2011a). Variability in the distribution of phytoplankton as affected by changes to the main physical parameters in the Baltic Sea. Oceanologia 53, 449–470. doi: 10.5697/oc.53-1-TI.449
Dzierzbicka-Glowacka L., Kulí K., Maciejewska A., Jakacki J., Pempkowiak J. (2011b). Numerical modelling of POC Numerical modelling of POC yearly dynamics in the southern Baltic under variable scenarios of nutrients, light and temperature. Ocean Sci. Discuss 8, 675–700. doi: 10.5194/osd-8-675-2011
Edrén S. M. C., Wisz M. S., Teilmann J., Dietz R., Söderkvist J. (2010). Modelling spatial patterns in harbour porpoise satellite telemetry data using maximum entropy. Ecography 33, 698–7080. doi: 10.1111/j.1600-0587.2009.05901.x
Eero M. (2012). Reconstructing the population dynamics of sprat (Sprattus sprattus balticus) in the Baltic Sea in the 20th century. ICES J. Mar. Sci. 69, 1010–1018. doi: 10.1093/icesjms/fss051
Eilola K., Gustafsson B. G., Kuznetsov I., Meier H. E. M., Neumann T., Savchuk O. P. (2011). Evaluation of biogeochemical cycles in an ensemble of three state-of-the-art numerical models of the Baltic Sea. J. Mar. Syst. 88, 267–284. doi: 10.1016/j.jmarsys.2011.05.004
El-Shehawy R., Gorokhova E., Fernández-Piñas F., del Campo F. F. (2012). Global warming and hepatotoxin production by cyanobacteria: What can we learn from experiments? Water Res. 46, 1420–1429. doi: 10.1016/j.watres.2011.11.021
Engstedt O., Stenroth P., Larsson P., Ljunggren L., Elfman M. (2010). Assessment of natal origin of pike (Esox lucius) in the Baltic Sea using Sr: Ca in otoliths. Environ. Biol. Fish. 89, 547–555. doi: 10.1007/s10641-010-9686-x
Engström-Öst J., Repka S., Mikkonen M. (2011). Interactions between plankton and cyanobacterium Anabaena with focus on salinity, growth and toxin production. Harmful Algae 10, 530–535. doi: 10.1016/j.hal.2011.04.002
European Commission (2020). Council agreement on 2022 catch limits in the Baltic Sea (Press European Commission Press Release). Available online at: https://www.consilium.europa.eu/media/52388/baltic-fish-table-2022_final.pdf (Accessed 12 June 2024).
European Commission (2022). Commission proposes fishing opportunities for 2023 in the Baltic Sea in an effort to recover species, European Commission. Available online at: https://ec.europa.eu/commission/presscorner/detail/en/IP_22_5064 (Accessed 11 June 2024).
Ferreira J. G., Andersen J. H., Borja A., Bricker S. B., Camp J., Cardoso da Silva M., et al. (2011). Overview of eutrophication indicators to assess environmental status within the European Marine Strategy Framework Directive. Estuarine Coast. Shelf Sci. 93, 117–131. doi: 10.1016/j.ecss.2011.03.014
Fitzer S. C., Caldwell G. S., Close A. J., Clare A. S., Upstill-Goddard R. C., Bentley M. G. (2012). Ocean acidification induces multi-generational decline in copepod naupliar production with possible conflict for reproductive resource allocation. J. Exp. Mar. Biol. Ecol. 418–419, 30–36. doi: 10.1016/j.jembe.2012.03.009
Fleming-Lehtinen V., Andersen J. H., Carstensen J., Łysiak-Pastuszak E., Murray C., Pyhälä M., et al. (2015). Recent developments in assessment methodology reveal that the Baltic Sea eutrophication problem is expanding. Ecol. Indic. 48, 380–388. doi: 10.1016/j.ecolind.2014.08.022
Florin A. B., Lavados G. (2010). Feeding habits of juvenile flatfish in relation to habitat characteristics in the Baltic Sea. Estuarine Coast. Shelf Sci. 86, 607–612. doi: 10.1016/j.ecss.2009.11.028
Friedland R., Neumann T., Schernewski G. (2012). Climate change and the Baltic Sea action plan: Model simulations on the future of the western Baltic Sea. J. Mar. Syst. 105–108, 175–186. doi: 10.1016/j.jmarsys.2012.08.002
Frommel A. Y., Maneja R., Lowe D., Malzahn A. M., Geffen A. J., Folkvord A., et al. (2012). Severe tissue damage in Atlantic cod larvae under increasing ocean acidification. Nat. Climate Change 2, 42–46. doi: 10.1038/nclimate1324
Frommel A. Y., Maneja R., Lowe D., Pascoe C. K., Geffen A. J., Folkvord A., et al. (2014). Organ damage in Atlantic herring larvae as a result of ocean acidification. Ecol. Appl. 24, 1131–1143. doi: 10.1890/13-0297.1
Fu W., Høyer J. L., She J. (2010). Assessment of the 3-D temperature and salinity observational networks in the Baltic Sea and North Sea. Ocean Sci. Discuss 7, 1627–1668. doi: 10.5194/osd-7-1627-2010
Gårdmark A., Casini M., Huss M., van Leeuwen A., Hjelm J., Persson L., et al. (2015). Regime shifts in exploited marine food webs: Detecting mechanisms underlying alternative stable states using size structured community dynamics theory. Philos. Trans. R. Soc. B: Biol. Sci. 370, e262. doi: 10.1098/rstb.2013.0262
Gilbert D., Rabalais N. N., Díaz R. J., Zhang J. (2010). Evidence for greater oxygen decline rates in the coastal ocean than in the open ocean. Biogeosciences 7, 2283–2296. doi: 10.5194/bg-7-2283-2010
Gorokhova E., Lehtiniemi M., Postel L., Rubene G., Amid C., Lesutiene J., et al. (2016). Indicator properties of Baltic zooplankton for classification of environmental status within marine strategy framework directive. PloS One 11, e158326. doi: 10.1371/journal.pone.0158326
Graiff A., Bartsch I., Ruth W., Wahl M., Karsten U. (2015). Season exerts differential effects of ocean acidification and warming on growth and carbon, metabolism of the seaweed Fucus vesiculosus in the Western Baltic Sea. Front. Mar. Sci. 2, e112. doi: 10.3389/fmars.2015.00112
Graiff A., Dankworth M., Wahl M., Karsten U., Bartsch I. (2017). Seasonal variations of Fucus vesiculosus fertility under ocean acidification and warming in the western Baltic Sea. Botanica Marina 60, 239–255. doi: 10.1515/bot-2016-0081
Gülzow W., Rehder G., Schneider B., Schneider J., Sadkowiak B. (2011). A new method for continuous measurement of methane and carbon dioxide in surface waters using off-axis integrated cavity output spectroscopy (ICOS): An example from the Baltic Sea. Limnol. Oceanogr.: Methods 9, 176–184. doi: 10.4319/lom.2011.9.176
Gustafsson E. (2012). Modelled long-term development of hypoxic area and nutrient pools in the Baltic Proper. J. Mar. Syst. 94, 120–134. doi: 10.1016/j.jmarsys.2011.11.012
Gustafsson E., Deutsch B., Gustafsson B. G., Humborg C., Mörth C. M. (2014). Carbon cycling in the Baltic Sea—The fate of allochthonous organic carbon and its impact on air-sea CO2 exchange. J. Mar. Syst. 129, 289–302. doi: 10.1016/j.jmarsys.2013.07.005
Gustafsson E., Savchuk O. P., Gustafsson B. G., Müller-Karulis B. (2017). Key processes in the coupled carbon, nitrogen, and phosphorus cycling of the Baltic Sea. Biogeochemistry 134, 301–317. doi: 10.1007/s10533-017-0361-6
Gustafsson B. G., Schenk F., Blenckner T., Eilola K., Meier H. E. M., Müller-Karulis B., et al. (2012). Reconstructing the development of Baltic Sea eutrophication 1850-2006. Ambio 41, 534–548. doi: 10.1007/s13280-012-0318-x
Haase K., Orio A., Pawlak J., Pachur M., Casini M. (2020). Diet of dominant demersal fish species in the Baltic Sea: Is flounder stealing benthic food from cod? Mar. Ecol. Prog. Ser. 645, 159–170. doi: 10.3354/meps13360
Hägg H. E., Humbohg C., Morth C. M., Medina M. R., Wulff F. (2010). Scenario analysis on protein consumption and climate change effects on riverine N export to the Baltic Sea. Environ. Sci. Technol. 44, 2379–2385. doi: 10.1021/es902632p
Hägg H. E., Lyon S. W., Wällstedt T., Mörth C. M., Claremar B., Humborg C. (2014). Future nutrient load scenarios for the Baltic Sea due to climate and lifestyle changes. Ambio 43, 337–351. doi: 10.1007/s13280-013-0416-4
Hammer K., Schneider B., Kuliński K., Schulz-Bull D. E. (2014). Precision and accuracy of spectrophotometric pH measurements at environmental conditions in the Baltic Sea. Estuarine Coast. Shelf Sci. 146, 24–32. doi: 10.1016/j.ecss.2014.05.003
Hammer K., Schneider B., Kuliński K., Schulz-Bull D. E. (2017). Acid-base properties of Baltic Sea dissolved organic matter. J. Mar. Syst. 173, 114–121. doi: 10.1016/j.jmarsys.2017.04.007
Hansson M., Andersson L., Axe P. (2011). Areal Extent and Volume of Anoxia and Hypoxia in the Baltic Sea 1960-2011 (Norrköping: SMHI Report Oceanography). Available online at: https://www.smhi.se/polopoly_fs/1.19219!Oxygen_timeseries_1960_2010_20111219.pdf (Accessed 12 June 2024).
Hansson D., Gustafsson E. (2011). Salinity and hypoxia in the Baltic Sea since A.D. 1500. J. Geophys. Res.: Oceans 116, e6676. doi: 10.1029/2010JC006676
Heikinheimo O. (2011). Interactions between cod, herring and sprat in the changing environment of the Baltic Sea: A dynamic model analysis. Ecol. Model. 222, 1731–1742. doi: 10.1016/j.ecolmodel.2011.03.005
HELCOM (2020). Commercial Fisheries. Available online at: https://helcom.fi/action-areas/fisheries/commercial-fisheries/ (Accessed 12 June 2024).
Hense I., Meier H. E. M., Sonntag S. (2013). Projected climate change impact on Baltic Sea cyanobacteria: Climate change impact on cyanobacteria. Clim. Change 119, 391–406. doi: 10.1007/s10584-013-0702-y
Hiddink J. G., Coleby C. (2012). What is the effect of climate change on marine fish biodiversity in an area of low connectivity, the Baltic Sea? Global Ecol. Biogeogr. 21, 637–646. doi: 10.1111/j.1466-8238.2011.00696.x
Hinrichsen H. H., Hüssy K., Huwer B. (2012). Spatio-temporal variability in western Baltic cod early life stage survival mediated by egg buoyancy, hydrography and hydrodynamics. ICES J. Mar. Sci. 69, 1744–1752. doi: 10.1093/icesjms/fss137
Hinrichsen H. H., Huwer B., Makarchouk A., Petereit C., Schaber M., Voss R. (2011). Climate-driven long-term trends in Baltic Sea oxygen concentrations and the potential consequences for eastern Baltic cod (Gadus morhua). ICES J. Mar. Sci. 68, 2019–2028. doi: 10.1093/icesjms/fsr145
Hogfors H., Motwani N. H., Hajdu S., El-Shehawy R., Holmborn T., Vehmaa A., et al. (2014). Bloom-forming cyanobacteria support copepod reproduction and development in the Baltic Sea. PloS One 9, e112692. doi: 10.1371/journal.pone.0112692
Hong B., Swaney D. P., Mörth C. M., Smedberg E., Eriksson Hägg H., Humborg C., et al. (2012). Evaluating regional variation of net anthropogenic nitrogen and phosphorus inputs (NANI/NAPI), major drivers, nutrient retention pattern and management implications in the multinational areas of Baltic Sea basin. Ecol. Model. 227, 117–135. doi: 10.1016/j.ecolmodel.2011.12.002
Hongisto M. (2011). Variability of the marine boundary layer parameters over Baltic Sea sub-basins and their impact on nitrogen deposition. Oceanologia 53, 391–413. doi: 10.5697/oc.53-1-TI.391
Horbowy J., Podolska M., Nadolna-Ałtyn K. (2016). Increasing occurrence of Anisakid nematodes in the liver of cod (Gadus Morhua) from the Baltic Sea: Does infection affect the condition and mortality of fish? Fish. Res. 179, 98–103. doi: 10.1016/j.fishres.2016.02.011
Hordoir R., Meier H. E. M. (2012). Effect of climate change on the thermal stratification of the Baltic Sea: A sensitivity experiment. Climate Dynam. 38, 1703–1713. doi: 10.1007/s00382-011-1036-y
Hüssy K. (2011). Review of western Baltic cod (Gadus morhua) recruitment dynamics. ICES J. Mar. Sci. 68, 1459–1471). doi: 10.1093/icesjms/fsr088
Hüssy K., Hinrichsen H. H., Fey D. P., Walther Y., Velasco A. (2010a). The use of otolith microstructure to estimate age in adult Atlantic cod Gadus morhua. J. Fish Biol. 76, 1640–1654. doi: 10.1111/j.1095-8649.2010.02602.x
Hüssy K., Hinrichsen H. H., Huwer B. (2012). Hydrographic influence on the spawning habitat suitability of western Baltic cod (Gadus morhua). ICES J. Mar. Sci. 69, 1736–1743. doi: 10.1093/icesjms/fss136
Hüssy K., Hüssy K. (2010b). Why is age determination of Baltic cod (Gadus morhua) so difficult? ICES J. Mar. Sci. 67, 1198–1205. doi: 10.1093/icesjms/fsq023
ICES (2019). “Cod (Gadus morhua) in sub-divisions 24-32, eastern Baltic stock (eastern Baltic Sea),” in Report of the ICES Advisory Committe. (Copenhagen: International Council for the Exploration of the Sea). cod.27.24-32. doi: 10.17895/ices.advice.4747
ICES (2022a). “Cod (Gadus morhua) in sub-divisions 22–24, western Baltic stock (western Baltic Sea),” in Report of the ICES Advisory Committe. (Copenhagen: International Council for the Exploration of the Sea). ICES Advice 2022, cod.27.22–24. doi: 10.17895/ices.advice.19447868
ICES (2022b). “Herring (Clupea harengus) in sub-divisions 30 and 31 (Gulf of Bothnia),” in Report of the ICES Advisory Committe. (Copenhagen: International Council for the Exploration of the Sea). ICES Advice 2022, her.27.3031. doi: 10.17895/ices.advice.19447979
ICES (2022c). “Herring (Clupea harengus) in sub-divisions 20-24, spring spawners (Skagerrak, Kattegat, and western Baltic),” in Report of the ICES Advisory Committe. (Copenhagen: International Council for the Exploration of the Sea). ICES Advice 2022, her.27.20-24. doi: 10.17895/ices.advice.19447964
ICES (2022d). “Herring (Clupea harengus) in sub-division 28.1 (Gulf of Riga),” in Report of the ICES Advisory Committe. (Copenhagen: International Council for the Exploration of the Sea). ICES Advice 2022, her.27.28. doi: 10.17895/ices.advice.19447976
ICES (2022e). “Herring (Clupea harengus) in sub-divisions 25–29 and 32, excluding the Gulf of Riga (central Baltic Sea),” in Report of the ICES Advisory Committe. (Copenhagen: International Council for the Exploration of the Sea). ICES Advice 2021, her.27.25–2932. doi: 10.17895/ices.advice.19447970
ICES (2022f). “Plaice (Pleuronectes platessa) in sub-divisions 21–23 (Kattegat, Belt Seas, and the Sound),” in Report of the ICES Advisory Committe. (Copenhagen: International Council for the Exploration of the Sea). ICES Advice 2022, ple.27.21–23. doi: 10.17895/ices.advice.19453550
ICES (2022g). “Plaice (Pleuronectes platessa) in sub-divisions 24-32 (Baltic Sea, excluding the Sound and Belt Seas),” in Report of the ICES Advisory Committe. (Copenhagen: International Council for the Exploration of the Sea). ICES Advice 2022, ple.27.24-32. doi: 10.17895/ices.advice.19453583
ICES (2022h). “Sprat (Sprattus sprattus) in sub-divisions 22-32 (Baltic Sea),” in Report of the ICES Advisory Committe. (Copenhagen: International Council for the Exploration of the Sea). ICES Advice 2022, spr.27.22-32. doi: 10.17895/ices.advice.19453856
Jakubavičiūtė E., Ulf Bergström U., Eklöf J. S., Haenel Q., Bourlat S. J. (2017). DNA metabarcoding reveals diverse diet of the Three-spined stickleback in a coastal ecosystem. PloS One 12, e186929. doi: 10.1371/journal.pone.0186929
Janßen H., Augustin C. B., Hinrichsen H. H., Kube S. (2013). Impact of secondary hard substrate on the distribution and abundance of Aurelia aurita in the western Baltic Sea. Mar. pollut. Bull. 75, 224–234. doi: 10.1016/j.marpolbul.2013.07.027
Järv L., Kotta J., Kotta I., Raid T. (2011). Linking the structure of benthic invertebrate communities and the diet of native and invasive fish species in a brackish water ecosystem. Annales Zool. Fennici 48, 129–141. doi: 10.5735/086.048.0301
Jaspers C., Møller L. F., Kiørboe T. (2011). Salinity gradient of the Baltic Sea limits the reproduction and population expansion of the newly invaded Comb Jelly Mnemiopsis leidyi. PloS One 6, e0024065. doi: 10.1371/journal.pone.0024065
Jokinen H., Momigliano P., Merilä J., Grant W. S. (2019). From ecology to genetics and back: The tale of two flounder species in the Baltic Sea. ICES J. Mar. Sci. 76, 2267–2275. doi: 10.1093/icesjms/fsz151
Jokinen H., Wennhage H., Ollus V., Aro E., Norkko A. (2016). Juvenile flatfish in the northern Baltic Sea - long-term decline and potential links to habitat characteristics. J. Sea Res. 107, 67–75. doi: 10.1016/j.seares.2015.06.002
Kahru M., Elmgren R. (2014). Multidecadal time series of satellite-detected accumulations of cyanobacteria in the Baltic Sea. Biogeosciences 11, 3619–3633. doi: 10.5194/bg-11-3619-2014
Kahru M., Elmgren R., Kaiser J., Wasmund N., Savchuk O. (2020). Cyanobacterial blooms in the Baltic Sea: Correlations with environmental factors. Harmful Algae 92, e101739. doi: 10.1016/j.hal.2019.101739
Kaiser J., Wasmund N., Kahru M., Wittenborn A. K., Hansen R., Häusler K., et al. (2020). Reconstructing N2—fixing cyanobacterial blooms in the Baltic Sea beyond observations using 6- and 7-methylheptadecane in sediments as specific biomarkers. Biogeosciences 17, 2579–2591. doi: 10.5194/bg-17-2579-2020
Karlson A. M. L., Duberg J., Motwani N. H., Hogfors H., Klawonn I., Ploug H., et al. (2015). Nitrogen fixation by cyanobacteria stimulates production in Baltic food webs. Ambio 44, 413–426. doi: 10.1007/s13280-015-0660-x
Kijewska A., Kalamarz-Kubiak H., Arciszewski B., Guellard T., Petereit C., Wenne R. (2016). Adaptation to salinity in Atlantic cod from different regions of the Baltic Sea. J. Exp. Mar. Biol. Ecol. 478, 62–67. doi: 10.1016/j.jembe.2016.02.003
Klais R., Tamminen T., Kremp A., Spilling K., Olli K. (2011). Decadal-scale changes of Dinoflagellates and Diatoms in the Anomalous Baltic Sea spring bloom. PloS One 6, e21567. doi: 10.1371/journal.pone.0021567
Kleinertz S., Klimpel S., Palm H. W. (2011). Parasite communities and feeding ecology of the European Sprat (Sprattus Sprattus L.) over its range of distribution. Parasitol. Res. 110, 1147–1157. doi: 10.1007/s00436-011-2605-z
Kniebusch M., Meier H. E. M., Neumann T., Börgel F. (2019). Temperature variability of the baltic sea since 1850 and attribution to atmospheric forcing variables. J. Geophysical Research: Oceans 124 (6), 4168–4187. doi: 10.1029/2018JC013948
Koivisto M. E., Westerbom M. (2010). Habitat structure and complexity as determinants of biodiversity in blue mussel beds on sublittoral rocky shores. Mar. Biol. 157, 1463–1474. doi: 10.1007/s00227-010-1421-9
Koivisto M., Westerbom M. (2012). Invertebrate communities associated with blue mussel beds in a patchy environment: A landscape ecology approach. Mar. Ecol. Prog. Ser. 471, 101–110. doi: 10.3354/meps10010
Korpinen S., Meski L., Andersen J. H., Laamanen M. (2012). Human pressures and their potential impact on the Baltic Sea ecosystem. Ecol. Indic. 15, 105–114. doi: 10.1016/j.ecolind.2011.09.023
Kristensen K., Thygesen U. H., Andersen K. H., Beyer J. E. (2014). Estimating spatio-temporal dynamics of size-structured populations. Can. J. Fish. Aquat. Sci. 71, 326–336. doi: 10.1139/cjfas-2013-0151
Kuciński M., Złoch l., Trzeciak P., Kycko A., Nadolna-Ałtyn K., Mierzejewska M. (2023). Infection of the European flounder (Platichthys Flesus) with Glugea Stephani, a possible new indicator of the weakening of the Baltic population. Fish. Res. 260, 106590. doi: 10.1016/j.fishres.2022.106590
Kuliński K., Pempkowiak J. (2011). The carbon budget of the Baltic Sea. Biogeosciences 8, 3219–3230. doi: 10.5194/bg-8-3219-2011
Kuliński K., Schneider B., Hammer K., Machulik U., Schulz-Bull D. (2014). The influence of dissolved organic matter on the acid-base system of the Baltic Sea. J. Mar. Syst. 132, 106–115. doi: 10.1016/j.jmarsys.2014.01.011
Kulke R., Bödewadt V., Hänselmann K., Herrmann J. P., Temming A. (2018). Contribution to the symposium: “sustainable use of Baltic Sea resources” original article ignoring the vertical dimension: Biased view on feeding dynamics of vertically migrating sprat (Sprattus sprattus). ICES J. Mar. Sci. 75, 2450–2462. doi: 10.1093/icesjms/fsy136
Kuss J., Nausch G., Engelke C., von Weber M., Lutterbeck H., Naumann M., et al. (2020). Changes of nutrient concentrations in the western Baltic sea in the transition between inner coastal waters and the central basins: time series from 1995 to 2016 with source analysis. Front. Earth Sci. 8, e106. doi: 10.3389/feart.2020.00106
Labuce A., Ikauniece A., Jurgensone I., Aigars J. (2021). Environmental impacts on zooplankton functional diversity in brackish semi-enclosed gulf. Water 13, e13141881. doi: 10.3390/w13141881
Larsson P., Tibblin P., Koch-Schmidt P., Engstedt O., Nilsson J., Nordahl O., et al. (2015). Ecology, evolution, and management strategies of northern pike populations in the Baltic Sea. Ambio 44, 451–461. doi: 10.1007/s13280-015-0664-6
Lefébure R., Larsson S., Byström P. (2014). Temperature and size-dependent attack rates of the three-spined stickleback (Gasterosteus aculeatus): Are sticklebacks in the Baltic Sea Resource-limited? J. Exp. Mar. Biol. Ecol. 451, 82–90. doi: 10.1016/j.jembe.2013.11.008
Legrand C., Fridolfsson E., Bertos-Fortis M., Lindehoff E., Larsson P., Pinhassi J., et al. (2015). Interannual variability of phyto-bacterioplankton biomass and production in coastal and offshore waters of the Baltic Sea. Ambio 44, 427–438. doi: 10.1007/s13280-015-0662-8
Lehmann A., Getzlaff K., Harlaß J. (2011). Detailed assessment of climate variability in the Baltic Sea area for the period 1958 to 2009. Climate Res. 46, 185–196. doi: 10.3354/cr00876
Lehmann A., Hinrichsen H. H., Getzlaff K., Myrberg K. (2014). Quantifying the heterogeneity of hypoxic and anoxic areas in the Baltic Sea by a simplified coupled hydrodynamic-oxygen consumption model approach. J. Mar. Syst. 134, 20–28. doi: 10.1016/j.jmarsys.2014.02.012
Lehmann A., Myrberg K., Post P., Chubarenko I., Dailidiene I., Hinrichsen H.-H., et al. (2022). Salinity dynamics of the baltic sea. Earth System Dynamics 13 (1), 373–392. doi: 10.5194/esd-13-373-2022
Lindegren M., Möllmann C., Nielsen A., Brander K., MacKenzie B. R., Stenseth N. C. (2010). Ecological forecasting under climate change: The case of Baltic cod. Proc. R. Soc. B: Biol. Sci. 277, 2121–2130. doi: 10.1098/rspb.2010.0353
Livdane L., Putnis I., Rubene G., Elferts D., Ikauniece A. (2016). Baltic herring prey selectively on older copepodites of Eurytemora affinis and Limnocalanus macrurus in the Gulf of Riga. Oceanologia 58, 46–53. doi: 10.1016/j.oceano.2015.09.001
Ljunggren L., Sandström A., Bergström U., Mattila J., Lapalainen A., Johansson G., et al. (2010). Recruitment failure of coastal predatory fish in the Baltic Sea coincident with an offshore ecosystem regime shift. ICES J. Mar. Sci. 67, 1587–1595. doi: 10.1093/icesjms/fsq109
Löfstrand K., Malmvärn A., Haglund P., Bignert A., Bergman Å., Asplund L. (2010). Brominated phenols, anisoles, and dioxins present in blue mussels from the Swedish coastline. Environ. Sci. pollut. Res. 17, 1460–1468. doi: 10.1007/s11356-010-0331-1
Lundström K., Hjerne O., Lunneryd S.-G., Karlsson Lundström O., Lundström K., Lunneryd S. (2010). Understanding the diet composition of marine mammals: Grey seals (Halichoerus grypus) in the Baltic Sea. ICES J. Mar. Sci. 67, 1230–1239. Available at: http://icesjms.oxfordjournals.org/.
Maar M., Møller E. F., Larsen J., Madsen K. S., Wan Z., She J., et al. (2011). Ecosystem modelling across a salinity gradient from the North Sea to the Baltic Sea. Ecol. Model. 222, 1696–1711. doi: 10.1016/j.ecolmodel.2011.03.006
MacKenzie B. R., Eero M., Ojaveer H. (2011). Could seals prevent cod recovery in the Baltic Sea? PloS One 6, 2476–2487. doi: 10.1371/journal.pone.0018998
Mackenzie B. R., Gislason H. (2011). Multi-decadal responses of a cod (Gadus morhua) population to human-induced trophic changes, fishing, and climate. Ecol. Soc. America 21, 214–226. doi: 10.2307/29779648
MacKenzie B. R., Meier H. E. M., Lindegren M., Neuenfeldt S., Eero M., Blenckner T., et al. (2012). Impact of climate change on fish population dynamics in the Baltic Sea: A dynamical downscaling investigation. Ambio 41, 626–636. doi: 10.1007/s13280-012-0325-y
MacKenzie B. R., Ojaveer H. (2018). Evidence from the past: Exploitation as cause of commercial extinction of autumn-spawning herring in the Gulf of Riga, Baltic Sea. ICES J. Mar. Sci. 75, 2476–2487. doi: 10.1093/icesjms/fsy028
Maneja R. H., Frommel A. Y., Browman H. I., Clemmesen C., Geffen A. J., Folkvord A., et al. (2013). The swimming kinematics of larval Atlantic cod, Gadus morhua L., are resilient to elevated seawater pCO2. Mar. Biol. 160, 1963–1972. doi: 10.1007/s00227-012-2054-y
Margonski P., Hansson S., Tomczak M. T., Grzebielec R. (2010). Climate influence on Baltic cod, sprat, and herring stock-recruitment relationships. Prog. Oceanogr. 87, 277–288. doi: 10.1016/j.pocean.2010.08.003
Martins J. H., Camanho A. S., Gaspar M. B. (2012). A review of the application of driving forces – Pressure – State – Impact – Response framework to fisheries management. Ocean Coast. Manage. 69, 273–281. doi: 10.1016/j.ocecoaman.2012.07.029
Matarese V. (2012). Using strategic, critical reading of research papers to teach scientific writing: The reading-research-writing continuum. Support. Res. Writing: Roles Challenges Multilingual Settings 5, 73–89. doi: 10.1016/B978-1-84334-666-1.50005-9
Mazur-Marzec H., Sutryk K., Kobos J., Hebel A., Hohlfeld N., Błaszczyk A., et al. (2013). Occurrence of cyanobacteria and cyanotoxin in the Southern Baltic Proper. Filamentous cyanobacteria versus single-celled picocyanobacteria. Hydrobiologia 701, 235–252. doi: 10.1007/s10750-012-1278-7
Meier H. E. M., Andersson H. C., Arheimer B., Blenckner T., Chubarenko B., Donnelly C., et al. (2012a). Comparing reconstructed past variations and future projections of the Baltic Sea ecosystem - First results from multi-model ensemble simulations. Environ. Res. Lett. 7, e034005. doi: 10.1088/1748-9326/7/3/034005
Meier M., Andersson H., Dieterich C., Eilola K., Gustafsson B., Höglund A., et al. (2011a). Transient scenario simulations for the Baltic Sea region during the 21st century (Norrköping). Available online at: https://www.smhi.se/polopoly_fs/1.166692!/Oceanografi_108%20Transient%20scenario%20simulations%20for%20the%20Baltic%20Sea%20Region%20during%20the%2021st%20century.pdf (Accessed 12 June 2024). SMHI Report Oceanography No. 108.
Meier H. E. M., Andersson H. C., Eilola K., Gustafsson B. G., Kuznetsov I., Miller-Karulis B., et al. (2011b). Hypoxia in future climates: A model ensemble study for the Baltic Sea. Geophys. Res. Lett. 38. doi: 10.1029/2011GL049929
Meier H. E. M., Dieterich C., Eilola K., Gröger M., Höglund A., Radtke H., et al. (2019a). Future projections of record- breaking sea surface temperature and cyanobacteria bloom events in the Baltic Sea. Ambio 48, 1362–1376. doi: 10.1007/s13280-019-01235-5
Meier H. E., Dieterich C., Gröger M., Dutheil C., Safonova K., Christensen O. B., et al. (2021). Oceanographic regional climate projections for the Baltic Sea 1 until 2100. Earth Sys. Dynam. 68, 1–66. doi: 10.5194/esd-2021-68
Meier H. E. M., Edman M., Eilola K., Placke M., Neumann T., Andersson H. C., et al. (2019b). Assessment of uncertainties in scenario simulations of biogeochemical cycles in the Baltic Sea. Front. Mar. Sci. 6, e46. doi: 10.3389/fmars.2019.00046
Meier H. E. M., Edman M. K., Eilola K. J., Placke M., Neumann T., Andersson H. C., et al. (2018a). Assessment of eutrophication abatement scenarios for the Baltic Sea by multi-model ensemble simulations. Front. Mar. Sci. 5. doi: 10.3389/fmars.2018.00440
Meier M. H.E., Eilola K., Almroth E. (2011c). Climate-related changes in marine ecosystems simulated with a 3-dimensional coupled physical- biogeochemical model of the Baltic Sea. Climate Res. 48, 31–55. doi: 10.3354/cr00968
Meier H. E. M., Eilola K., Almroth-Rosell E., Schimanke S., Kniebusch M., Höglund A., et al. (2019c). Disentangling the impact of nutrient load and climate changes on Baltic Sea hypoxia and eutrophication since 1850. Climate Dynam. 53, 1145–1166. doi: 10.1007/s00382-018-4296-y
Meier H. E. M., Höglund A., Döscher R., Andersson H., Löptien U., Kjellström E. (2011d). Quality assessment of atmospheric surface fields over the Baltic Sea from an ensemble of regional climate model simulations with respect to ocean dynamics. Oceanologia 53, e193. doi: 10.5697/oc.53-1-TI.193
Meier H. E. M., Höglund A., Eilola K., Almroth-Rosell E. (2017). Impact of accelerated future global mean sea level rise on hypoxia in the Baltic Sea. Climate Dynam. 49, 163–172. doi: 10.1007/s00382-016-3333-y
Meier H. E. M., Hordoir R., Andersson H. C., Dieterich C., Eilola K., Gustafsson B. G., et al. (2012b). Modeling the combined impact of changing climate and changing nutrient loads on the Baltic Sea environment in an ensemble of transient simulations for 1961-2099. Climate Dynam. 39, 2421–2441. doi: 10.1007/s00382-012-1339-7
Meier H. E. M., Müller-Karulis B., Andersson H. C., Dieterich C., Eilola K., Gustafsson B. G., et al. (2012c). Impact of climate change on ecological quality indicators and biogeochemical fluxes in the Baltic Sea: A multi-model ensemble study. Ambio 41, 558–573. doi: 10.1007/s13280-012-0320-3
Meier H. E. M., Väli G., Naumann M., Eilola K., Frauen C. (2018b). Recently accelerated oxygen consumption rates amplify deoxygenation in the Baltic Sea. J. Geophys. Res.: Oceans 123, 3227–3240. doi: 10.1029/2017JC013686
Melzner F., Stange P., Trübenbach K., Thomsen J., Casties I., Panknin U., et al. (2011). Food Supply and Seawater pCO2 Impact Calcification and Internal Shell Dissolution in the Blue Mussel Mytilus edulis. PloS One 6, e24223. doi: 10.1371/journal.pone.0024223
Melzner F., Mark F. C., Seibel B. A., Tomanek L. (2020). Ocean acidification and coastal marine invertebrates: Tracking CO2 effects from seawater to the cell. Annu. Rev. Mar. Sci. 12, 499–523. doi: 10.1146/annurev-marine-010419-010658
Miethe T., Gröhsler T., Böttcher U., von Dorrien C. (2014). The effects of periodic marine inflow into the Baltic Sea on the migration patterns of Western Baltic spring-spawning herring. ICES J. Mar. Sci. 71, 519–527. doi: 10.1093/icesjms/fst166
Mion M., Thorsen A., Vitale F., Dierking J., Herrmann J. P., Huwer B., et al. (2018). Effect of fish length and nutritional condition on the fecundity of distressed Atlantic cod Gadus morhua from the Baltic Sea. J. Fish Biol. 92, 1016–1034. doi: 10.1111/jfb.13563
Mohamed A., Zuo S., Karami A. M., Marnis H., Setyawan A., Mehrdana F., et al. (2020). Contracaecum osculatum (sensu lato) infection of Gadus Morhua in the Baltic Sea: Inter- and intraspecific interactions. Int. J. Parasitol. 50, 891–898. doi: 10.1016/j.ijpara.2020.06.003
Mohrholz V., Naumann M., Nausch G., Krüger S., Gräwe U. (2015). Fresh oxygen for the baltic sea — an exceptional saline inflow after a decade of stagnation. J. Mar. Syst. 148, 152–166. doi: 10.1016/j.jmarsys.2015.03.005
Momigliano P., Denys G. P. J., Jokinen H., Merilä J. (2018). Platichthys solemdali sp. nov. (Actinopterygii, Pleuronectiformes): A new flounder species from the Baltic Sea. Front. Mar. Sci. 5, e225. doi: 10.3389/fmars.2018.00225
Momigliano P., Jokinen H., Calboli F., Aro E., Merilä J. (2019). Cryptic temporal changes in stock composition explain the decline of a flounder (Platichthys spp.) assemblage. Evolution. Appl. 12, 549–559. doi: 10.1111/eva.12738
Mort H. P., Slomp C. P., Gustafsson B. G., Andersen T. J. (2010). Phosphorus recycling and burial in Baltic Sea sediments with contrasting redox conditions. Geochim. Cosmochimica Acta 74, 1350–1362. doi: 10.1016/j.gca.2009.11.016
Müller J. D., Schneider B., Rehder G. (2016). Long-term alkalinity trends in the Baltic Sea and their implications for CO2-induced acidification. Limnol. Oceanogr. 61, 1984–2002. doi: 10.1002/lno.10349
Munkes B., Löptien U., Dietze H. (2021). Cyanobacteria blooms in the Baltic Sea: A review of models and facts. Biogeosciences 18, 2347–2378. doi: 10.5194/bg-18-2347-2021
Murray C. J., Müller-Karulis B., Carstensen J., Conley D. J., Gustafsson B. G., Andersen J. H. (2019). Past, present and future eutrophication status of the Baltic Sea. Front. Mar. Sci. 6, e00002. doi: 10.3389/fmars.2019.00002
Naderifar M., Goli H., Ghaljaie F. (2017). Snowball sampling: A purposeful method of sampling in qualitative research. Strides Dev. Med. Educ. 14, e67670. doi: 10.5812/sdme.67670
Neuenfeldt S., Bartolino V., Orio A., Andersen K. H., Andersen N. G., Niiranen S., et al. (2020). Feeding and growth of Atlantic cod (Gadus morhua L.) in the eastern Baltic Sea under environmental change. ICES J. Mar. Sci. 77, 624–632. doi: 10.1093/icesjms/fsz224
Neumann T. (2010). Climate-change effects on the Baltic Sea ecosystem: A model study. J. Mar. Syst. 81, 213–224. doi: 10.1016/j.jmarsys.2009.12.001
Neumann T., Eilola K., Gustafsson B., Müller-Karulis B., Kuznetsov I., Meier H. E. M., et al. (2012). Extremes of temperature, oxygen and blooms in the Baltic Sea in a changing climate. Ambio 41, 574–585. doi: 10.1007/s13280-012-0321-2
Neumann T., Radtke H., Seifert T. (2017). On the importance of Major Baltic Inflows for oxygenation of the central Baltic Sea. J. Geophys. Res.: Oceans 122, 1090–1101. doi: 10.1002/2016JC012525
Nielsen J. R., Kristensen K., Lewy P., Bastardie F. (2014). A statistical model for estimation of fish density including correlation in size, space, time and between species from research survey data. PloS One 9, e0099151. doi: 10.1371/journal.pone.0099151
Nielsen J. R., Lundgren B., Kristensen K., Bastardie F. (2013). Localisation of nursery areas based on comparative analyses of the horizontal and vertical distribution patterns of juvenile Baltic cod (Gadus morhua). PloS One 8, e0070668. doi: 10.1371/journal.pone.0070668
Niiranen S., Yletyinen J., Tomczak M. T., Blenckner T., Hjerne O., Mackenzie B. R., et al. (2013). Combined effects of global climate change and regional ecosystem drivers on an exploited marine food web. Global Change Biol. 19, 3327–3342. doi: 10.1111/gcb.12309
Nilsson J., Engstedt O., Larsson P. (2014). Wetlands for northern pike (Esox lucius L.) recruitment in the Baltic Sea. Hydrobiologia 721, 145–154. doi: 10.1007/s10750-013-1656-9
Nissling A., Dahlman G. (2010). Fecundity of flounder, Pleuronectes flesus, in the Baltic Sea—Reproductive strategies in two sympatric populations. J. Sea Res. 64, 190–198. doi: 10.1016/j.seares.2010.02.001
Norkko J., Reed D. C., Timmermann K., Norkko A., Gustafsson B. G., Bonsdorff E., et al. (2012). A welcome can of worms? Hypoxia mitigation by an invasive species. Global Change Biol. 18, 422–434. doi: 10.1111/j.1365-2486.2011.02513.x
Nowicki A., Rak D., Janecki M., Dzierzbicka-Glowacka L. (2016). Accuracy assessment of temperature and salinity computed by the 3D coupled ecosystem model of the Baltic Sea (3D CEMBS) in the southern Baltic. J. Operation. Oceanogr. 9, 67–73. doi: 10.1080/1755876X.2016.1209368
Ojaveer E., Arula T., Lankov A., Shpilev H. (2011). Impact of environmental deviations on the larval and year-class abundances in the spring spawning herring (Clupea harengus membras L.) of the Gulf of Riga (Baltic Sea) in 1947-2004. Fish. Res. 107, 159–168. doi: 10.1016/j.fishres.2010.11.001
Ojaveer H., Jaanus A., Mackenzie B. R., Martin G. (2010). Status of biodiversity in the Baltic sea. PloS One 5, e12467. doi: 10.1371/journal.pone.0012467
Ojaveer E., Kalejs M. (2010). Ecology and long-term forecasting of sprat (Sprattus sprattus balticus) stock in the Baltic Sea: A review. Rev. Fish Biol. Fish. 20, 203–217. doi: 10.1007/s11160-009-9130-5
Ojaveer H., Lankov A., Raid T., Põllumäe A., Klais R. (2018). Selecting for three copepods—feeding of sprat and herring in the Baltic Sea. ICES J. Mar. Sci. 75, 2439–2449. doi: 10.1093/icesjms/fsx249
Ojeda-Martínez C., Giménez Casalduero F., Bayle-Sempere J. T., Barbera Cebrián C., Valle C., Luis Sanchez-Lizaso J., et al. (2009). A conceptual framework for the integral management of marine protected areas. Ocean Coast. Manage. 52, 89–101. doi: 10.1016/j.ocecoaman.2008.10.004
Olenina I., Wasmund N., Hajdu S., Jurgensone I., Gromisz S., Kownacka J., et al. (2010). Assessing impacts of invasive phytoplankton: The Baltic Sea case. Mar. pollut. Bull. 60, 1691–1700. doi: 10.1016/j.marpolbul.2010.06.046
Olin A. B., Olsson J., Eklöf J. S., Eriksson B. K., Kaljuste O., Briekmane L., et al. (2022). Increases of opportunistic species in response to ecosystem change: The case of the Baltic Sea three-spined stickleback. ICES J. Mar. Sci. 79, 1419–1434. doi: 10.1093/icesjms/fsac073
Olofsson M., Egardt J., Singh A., Ploug H. (2016). Inorganic phosphorus enrichments in Baltic Sea water have large effects on growth, carbon fixation, and N2 fixation by Nodularia spumigena. Aquat. Microbial. Ecol. 77, 111–123. doi: 10.3354/ame01795
Olsson J. (2019). Past and current trends of coastal predatory fish in the Baltic Sea with a focus on perch, pike, and pikeperch. Fishes 4, e10007. doi: 10.3390/fishes4010007
Omstedt A., Edman M., Claremar B., Frodin P., Gustafsson E., Humborg C., et al. (2012). Future changes in the Baltic Sea acid–base (pH) and oxygen balances. Tellus B: Chem. Phys. Meteorol. 64, e19586. doi: 10.3402/tellusb.v64i0.19586
Omstedt A., Humborg C., Pempkowiak J., Pertillä M., Rutgersson A., Schneider B., et al. (2014). Biogeochemical control of the coupled CO2-O2 system of the Baltic Sea: A review of the results of Baltic-C. Ambio 43, 49–59. doi: 10.1007/s13280-013-0485-4
Orio A., Bergström U., Casini M., Erlandsson M., Eschbaum R., Hüssy K., et al. (2017b). Characterizing and predicting the distribution of Baltic Sea flounder (Platichthys flesus) during the spawning season. J. Sea Res. 126, 46–55. doi: 10.1016/j.seares.2017.07.002
Orio A., Bergström U., Florin A. B., Lehmann A., Šics I., Casini M. (2019). Spatial contraction of demersal fish populations in a large marine ecosystem. J. Biogeogr. 46, 633–645. doi: 10.1111/jbi.13510
Orio A., Bergström U., Florin A. B., Šics I., Casini M. (2020). Long-term changes in spatial overlap between interacting cod and flounder in the Baltic Sea. Hydrobiologia 847, 2541–2553. doi: 10.1007/s10750-020-04272-4
Orio A., Florin A. B., Bergström U., Šics I., Baranova T., Casini M. (2017a). Modelling indices of abundance and size-based indicators of cod and flounder stocks in the Baltic Sea using newly standardized trawl survey data. ICES J. Mar. Sci. 74, 1322–1333. doi: 10.1093/icesjms/fsx005
Otto S. A., Diekmann R., Flinkman J., Kornilovs G., Möllmann C. (2014). Habitat heterogeneity determines climate impact on zooplankton community structure and dynamics. PloS One 9, e18998. doi: 10.1371/journal.pone.0090875.0018998
Pachur M. E., Horbowy J. (2013). Food composition and prey selection of cod, Gadus morhua (Actinopterygii: Gadiformes: Gadidae), in the southern Baltic Sea. Acta Ichthyol. Piscatoria 43, 109–118. doi: 10.3750/AIP2013.43.2.03
Petereit C., Hinrichsen H. H., Franke A., Köster F. W. (2014). Floating along buoyancy levels: Dispersal and survival of western Baltic fish eggs. Prog. Oceanogr. 122, 131–152. doi: 10.1016/j.pocean.2014.01.001
Philippart C. J. M., Anadón R., Danovaro R., Dippner J. W., Drinkwater K. F., Hawkins S. J., et al. (2011). Impacts of climate change on European marine ecosystems: Observations, expectations and indicators. J. Exp. Mar. Biol. Ecol. 400, 52–69. doi: 10.1016/j.jembe.2011.02.023
Pihlainen S., Zandersen M., Hyytiäinen K., Andersen H. E., Bartosova A., Gustafsson B., et al. (2020). Impacts of changing society and climate on nutrient loading to the Baltic Sea. Sci. Total Environ. 731, e138935. doi: 10.1016/j.scitotenv.2020.138935
Ploug H., Musat N., Adam B., Moraru C. L., Lavik G., Vagner T., et al. (2010). Carbon and nitrogen fluxes associated with the cyanobacterium Aphanizomenon sp. in the Baltic Sea. ISME J. 4, 1215–1223. doi: 10.1038/ismej.2010.53
Podolska M., Polak-Juszczak L., Nadolna-Ałtyn K. (2016). Host condition and accumulation of metals by acanthocephalan parasite Echinorhynchus Gadi in cod Gadus morhua from the southern Baltic Sea. Mar. pollut. Bull. 113, 287–292. doi: 10.1016/j.marpolbul.2016.09.049
Polak-Juszczak L. (2017). Toxic metals (Cd, Pb) in flatfish, mollusc macoma balthica, water and sediments from the Southern Baltic Sea. J. Elementol. 22, 487–496. doi: 10.5601/jelem.2016.21.3.1279
Polte P., Gröhsler T., Kotterba P., von Nordheim L., Moll D., Santos J., et al. (2021). Reduced reproductive success of western Baltic herring (Clupea harengus) as a response to warming winters. Front. Mar. Sci. 8, e589242. doi: 10.3389/fmars.2021.589242
Raateoja M., Kuosa H., Hällfors S. (2011). Fate of excess phosphorus in the Baltic Sea: A real driving force for cyanobacterial blooms? J. Sea Res. 65, 315–321. doi: 10.1016/j.seares.2011.01.004
Rabalais N. N., Díaz R. J., Levin L. A., Turner R. E., Gilbert D., Zhang J. (2010). Dynamics and distribution of natural and human-caused hypoxia. Biogeosciences 7, 585–619. doi: 10.5194/bg-7-585-2010
Raid T., Kornilovs G., Lankov A., Nisumaa A.-M., Shpilev H., Järvik A., et al. (2010). Recruitment dynamics of the Gulf of Riga herring stock: density-dependent and environmental effects. ICES J. Mar. Sci. 67, 1914–1920. doi: 10.1093/icesjms/fsq128
Räike A., Taskinen A., Knuuttila S. (2020). Nutrient export from Finnish rivers into the Baltic Sea has not decreased despite water protection measures. Ambio 49, 460–474. doi: 10.1007/s13280-019-01217-7
Rajasilta M., Hänninen J., Laaksonen L., Laine P., Suomela J. P., Vuorinen I., et al. (2019). Influence of environmental conditions, population density, and prey type on the lipid content in Baltic herring (Clupea harengus membras) from the northern Baltic Sea. Can. J. Fish. Aquat. Sci. 76, 576–585. doi: 10.1139/cjfas-2017-0504
Rakko A., Seppälä J. (2014). Effect of salinity on the growth rate and nutrient stoichiometry of two Baltic Sea filamentous cyanobacterial species. Estonian J. Ecol. 63, 55–70. doi: 10.3176/eco.2014.2.01
Reed D. C., Slomp C. P., Gustafsson B. G. (2011). Sedimentary phosphorus dynamics and the evolution of bottom-water hypoxia: A coupled benthic-pelagic model of a coastal system. Limnol. Oceanogr. 56, 1075–1092. doi: 10.4319/lo.2011.56.3.1075
Reindl A. R., Bolałek J. (2013). Methane flux from sediment into near-bottom water and its variability along the Hel Peninsula-Southern Baltic Sea. Continent. Shelf Res. 74, 88–93. doi: 10.1016/j.csr.2013.12.006
Reusch T. B. H., Dierking J., Andersson H. C., Bonsdorff E., Carstensen J., Casini M., et al. (2018). The Baltic Sea as a time machine for the future coastal ocean. Sci. Adv. 4, e8195. doi: 10.1126/sciadv.aar8195
Riemann B., Carstensen J., Dahl K., Fossing H., Hansen J. W., Jakobsen H. H., et al. (2016). Recovery of Danish coastal ecosystems after reductions in nutrient loading: A holistic ecosystem approach. Estuaries Coasts 39, 82–97. doi: 10.1007/s12237-015-9980-0
Rodriguez-Perez A., Tsikliras A. C., Gal G., Steenbeek J., Falk-Andersson J., Heymans J. (2023). Using ecosystem models to inform ecosystem-based fisheries management in Europe: A review of the policy landscape and related stakeholder needs. Front. Mar. Sci. 10, e1196329. doi: 10.3389/fmars.2023.1196329
Roos A. M., Bäcklin B. M. V. M., Helander B. O., Rigét F. F., Eriksson U. C. (2012). Improved reproductive success in otters (Lutra lutra), grey seals (Halichoerus grypus) and sea eagles (Haliaeetus albicilla) from Sweden in relation to concentrations of organochlorine contaminants. Environ. pollut. 170, 268–275. doi: 10.1016/j.envpol.2012.07.017
Rosciszewski-Dodgson M. J., Cirella G. T. (2023). Anthropogenic and ecology research indicators of top commercial fish species in the Baltic Sea: Review. Syst. Sci. Data Discuss. [Preprint]. doi: 10.5194/essd-2023-185
Ross S. D., Gislason H., Andersen N. G., Lewy P., Nielsen J. R. (2016). The diet of whiting Merlangius merlangus in the western Baltic Sea. J. Fish Biol. 88, 1965–1988. doi: 10.1111/jfb.12959
Ross S. D., Hüssy K. (2013). A reliable method for ageing of whiting (Merlangius merlangus) for use in stock assessment and management. J. Appl. Ichthyol. 29, 825–832. doi: 10.1111/jai.12204
Rutgersson A., Jaagus J., Schenk F., Stendel M. (2014). Observed changes and variability of atmospheric parameters in the Baltic Sea region during the last 200 years. Climate Res. 61, 177–190. doi: 10.3354/cr01244
Ryberg M. P., Huwer B., Nielsen A., Dierking J., Buchmann K., Sokolova M., et al. (2021). Parasite load of Atlantic cod gadus morhua in the Baltic Sea assessed by the liver category method, and associations with infection density and critical condition. Fish. Manage. Ecol. 29, 88–99. doi: 10.1111/fme.12516
Śliwińska-Wilczewska S., Cieszyńska A., Konik M., Maculewicz J., Latała A. (2019). Environmental drivers of bloom-forming cyanobacteria in the Baltic Sea: Effects of salinity, temperature, and irradiance. Estuarine Coast. Shelf Sci. 219, 139–150. doi: 10.1016/j.ecss.2019.01.016
Salvador-Oliván J. A., Marco-Cuenca G., Arquero-Avilés R. (2021). Development of an efficient search filter to retrieve systematic reviews from PubMed. J. Med. Library Assoc. 109, e1223. doi: 10.5195/jmla.2021.1223
Sanders T., Schmittmann L., Nascimento-Schulze J., Melzner F. (2018). High calcification costs limit mussel growth at low salinity. Front. Mar. Sci. 5, e352. doi: 10.3389/fmars.2018.00352
Savchuk O. P. (2013). Large-scale dynamics of hypoxia in the Baltic Sea. Handb. Environ. Chem. 22, 137–160. doi: 10.1007/698_2010_53
Savchuk O. P. (2018). Large-scale nutrient dynamics in the Baltic Sea 1970-2016. Front. Mar. Sci. 5, e00095. doi: 10.3389/fmars.2018.00095
Schaber M., Haslob H., Huwer B., Harjes A., Hinrichsen H. H., Köster F. K., et al. (2011). The invasive ctenophore mnemiopsis leidyi in the central Baltic Sea: Seasonal phenology and hydrographic influence on spatio-temporal distribution patterns. J. Plankton Res. 33, 1053–1065. doi: 10.1093/plankt/fbq167
Schaber M., Hinrichsen H. H., Gröger J. (2012). Seasonal changes in vertical distribution patterns of cod (Gadus morhua) in the Bornholm Basin, central Baltic Sea. Fish. Oceanogr. 21, 33–43. doi: 10.1111/j.1365-2419.2011.00607.x
Scharsack J. P., Koske D., Straumer K., Kammann U. (2021). Effects of climate change on marine dumped munitions and possible consequence for inhabiting biota. Environ. Sci. Europe 33, e5374. doi: 10.1186/s12302-021-00537-4
Seitz R. D., Wennhage H., Bergström U., Lipcius R. N., Ysebaert T. (2013). Ecological value of coastal habitats for commercially and ecologically important species. ICES J. Mar. Sci. 71, 648–665. doi: 10.1093/icesjms/fst152
Skoog A., Wedborg M., Fogelqvist E. (2011). Decoupling of total organic carbon concentrations and humic substance fluorescence in an extended temperate estuary. Mar. Chem. 124, 68–77. doi: 10.1016/j.marchem.2010.12.003
Skrzypczak M., Rolbiecki L. (2015). Endoparasitic helminths of the European sprat, Sprattus Sprattus (linnaeus 1758) from the Gulf of Gdańsk (the southern Baltic Sea) with a checklist of its parasites. Russian J. Mar. Biol. 41, 167–175. doi: 10.1134/s1063074015030104
Soerensen A. L., Feinberg A., Schartup A. T. (2022). Selenium concentration in herring from the Baltic Sea tracks decadal and spatial trends in external sources. Environ. Sci.: Processes Impacts 24, 1319–1329. doi: 10.1039/d1em00418b
Stiasny M. H., Mittermayer F. H., Sswat M., Voss R., Jutfelt F., Chierici M., et al. (2016). Ocean acidification effects on Atlantic cod larval survival and recruitment to the fished population. PloS One 11, e0155448. doi: 10.1371/journal.pone.0155448
Stigebrandt A., Kalén O. (2013). Improving oxygen conditions in the deeper parts of Bornholm Sea by pumped injection of winter water. Ambio 42, 587–595. doi: 10.1007/s13280-012-0356-4
Stigebrandt A., Rahm L., Viktorsson L., Ödalen M., Hall P. O. J., Liljebladh B. (2014). A new phosphorus paradigm for the Baltic proper. Ambio 43, 634–643. doi: 10.1007/s13280-013-0441-3
Stoltenberg I., Dierking J., Müller-Navarra D. C., Javidpour J. (2021). Review of jellyfish trophic interactions in the Baltic Sea. Mar. Biol. Res. 17, 311–326. doi: 10.1080/17451000.2021.1964532
Suikkanen S., Pulina S., Engström-Öst J., Lehtiniemi M., Lehtinen S., Brutemark A. (2013). Climate change and eutrophication induced shifts in northern summer plankton communities. PloS One 8, e66475. doi: 10.1371/journal.pone.0066475
Suikkanen S., Kaartokallio H., Hällfors S., Huttunen M., Laamanen M. (2010). Life cycle strategies of bloom-forming, filamentous cyanobacteria in the baltic sea. Deep Sea Res. Part II: Topical Stud. Oceanography Phytoplankton Life-Cycles Their Impacts Ecol. Harmful Algal Bloom 57 (3), 199–209. doi: 10.1016/j.dsr2.2009.09.014
Szczepańska A., Zaborska A., Maciejewska A., Kuliński K., Pempkowiak J. (2012). Distribution and origin of organic matter in the Baltic Sea sediments dated with 210pb and 137cs. Geochronometria 39, 1–9. doi: 10.2478/s13386-011-0058-x
Takolander A., Cabeza M., Leskinen E. (2019). Seasonal interactive effects of pCO2 and irradiance on the ecophysiology of brown macroalga Fucus vesiculosus L. Eur. J. Phycol. 54, 380–392. doi: 10.1080/09670262.2019.1572226
Thøgersen T., Hoff A., Frost H. S. (2015). Fisheries management responses to climate change in the Baltic Sea. Climate Risk Manage. 10, 51–62. doi: 10.1016/j.crm.2015.09.001
Thomsen J., Stapp L. S., Haynert K., SChade H., Danelli M., Lannig G., et al. (2017). Naturally acidified habitat selects for ocean acidification-tolerant mussels. Sci. Adv. 3, e1602411. doi: 10.1126/sciadv.1602411
Tomczak M. T., Heymans J. J., Yletyinen J., Niiranen S., Otto S. A., Blenckner T. (2013). Ecological network indicators of ecosystem status and change in the Baltic sea. PloS One 8, e75439. doi: 10.1371/journal.pone.0075439
Tomczak M. T., Niiranen S., Hjerne O., Blenckner T. (2012). Ecosystem flow dynamics in the Baltic Proper-Using a multi-trophic dataset as a basis for food-web modelling. Ecol. Model. 230, 123–147. doi: 10.1016/j.ecolmodel.2011.12.014
Townsend H., Harvey C. J., deReynier Y., Davis D., Zador S. G., Gaichas S., et al. (2019). Progress on implementing ecosystem-based fisheries management in the United States through the use of ecosystem models and analysis. Front. Mar. Sci. 6, e641. doi: 10.3389/fmars.2019.00641
Ulrich C., Boje J., Cardinale M., Gatti P., LeBras Q., Andersen M., et al. (2013). Variability and connectivity of plaice populations from the Eastern North Sea to the Western Baltic Sea, and implications for assessment and management. J. Sea Res. 84, 40–48. doi: 10.1016/j.seares.2013.04.007
Unger P., Klimpel S., Lang T., Palm H. (2014). Metazoan parasites from Herring (Clupea harengus L.) as biological indicators in the Baltic Sea. Acta Parasitol. 59, e2765. doi: 10.2478/s11686-014-0276-5
United Nations (2016). Goal 14: Conserve and sustainably use the oceans, seas and marine resources. Available online at: https://www.un.org/sustainabledevelopment/oceans/ (Accessed 12 June 2024).
Ustups D., Müller-Karulis B., Bergstrom U., Makarchouk A., Sics I. (2013). The influence of environmental conditions on early life stages of flounder (Platichthys flesus) in the central Baltic Sea. J. Sea Res. 75, 77–84. doi: 10.1016/j.seares.2012.05.001
Van Eerden M. R., Rijn S., Kilpi M., Lehikoinen A., Lilleleht V., Millers K., et al. (2022). Expanding east: Great cormorants phalacrocorax carbo thriving in the eastern Baltic and Gulf of Finland. Ardea 109, e1095. doi: 10.5253/arde.v109i2.a5
Vaquer-Sunyer R., Duarte C. M. (2010). Sulfide exposure accelerates hypoxia-driven mortality. Limnol. Oceanogr. 55, 1075–1082. doi: 10.4319/lo.2010.55.3.1075
Vaquer-Sunyer R., Duarte C. M. (2011). Temperature effects on oxygen thresholds for hypoxia in marine benthic organisms. Global Change Biol. 17, 1788–1797. doi: 10.1111/j.1365-2486.2010.02343.x
Varjopuro R., Andrulewicz E., Blenckner T., Dolch T., Heiskanen A. S., Pihlajamäki M., et al. (2014). Coping with persistent environmental problems: Systemic delays in reducing eutrophication of the Baltic Sea. Ecol. Soc. 19, e190448. doi: 10.5751/ES-06938-190448
Vehmaa A., Hogfors H., Gorokhova E., Brutemark A., Holmborn T., Engström-Öst J. (2013). Projected marine climate change: Effects on copepod oxidative status and reproduction. Ecol. Evol. 3, 4548–4557. doi: 10.1002/ece3.839
Veneranta L., Heikinheimo O., Marjomäki T. J. (2020). Cormorant (phalacrocorax carbo) predation on a coastal perch (perca fluviatilis) population: Estimated effects based on pit tag mark-recapture experiment. ICES J. Mar. Sci. 77, 2611–2622. doi: 10.1093/icesjms/fsaa124
Viitasalo M., Bonsdorff E. (2022). Global climate change and the Baltic Sea ecosystem: Direct and indirect effects on species, communities and ecosystem functioning. Earth Sys. Dynam. 13, 711–747. doi: 10.5194/esd-13-711-2022
Viktorsson L., Almroth-Rosell E., Tengberg A., Vankevich R., Neelov I., Isaev A., et al. (2012). Benthic phosphorus dynamics in the Gulf of Finland, Baltic sea. Aquat. Geochem. 18, 543–564. doi: 10.1007/s10498-011-9155-y
Villnäs A., Norkko J., Lukkari K., Hewitt J., Norkko A. (2012). Consequences of increasing hypoxic disturbance on benthic communities and ecosystem functioning. PloS One 7, e44920. doi: 10.1371/journal.pone.0044920
Voss M., Dippner J. W., Humborg C., Hürdler J., Korth F., Neumann T., et al. (2011a). History and scenarios of future development of Baltic Sea eutrophication. Estuarine Coast. Shelf Sci. 92, 307–322. doi: 10.1016/j.ecss.2010.12.037
Voss R., Hinrichsen H. H., Quaas M. F., Schmidt J. O., Tahvonen O. (2011b). Temperature change and Baltic sprat: From observations to ecological economic modelling. ICES J. Mar. Sci. 68, 1244–1256. doi: 10.1093/icesjms/fsr063
Voss R., Petereit C., Schmidt J. O., Lehmann A., Makarchouk A., Hinrichsen H. H. (2012). The spatial dimension of climate-driven temperature change in the Baltic Sea and its implication for cod and sprat early life stage survival. J. Mar. Syst. 100–101, 1–8. doi: 10.1016/j.jmarsys.2012.03.009
Voss R., Quaas M. F., Stiasny M. H., Hänsel M., Stecher Justiniano Pinto G. A., Lehmann A., et al. (2019). Ecological economic sustainability of the Baltic cod fisheries under ocean warming and acidification. J. Environ. Manage. 238, 110–118. doi: 10.1016/j.jenvman.2019.02.105
Vuorinen I., Hänninen J., Rajasilta M., Laine P., Eklund J., Montesino-Pouzols F., et al. (2015). Scenario simulations of future salinity and ecological consequences in the baltic sea and adjacent north sea areas–implications for environmental monitoring. Ecol. Indic. 50, 196–205. doi: 10.1016/j.ecolind.2014.10.019
Wahl M., Schneider Covachã S., Saderne V., Hiebenthal C., Müller J. D., Pansch C., et al. (2018). Macroalgae may mitigate ocean acidification effects on mussel calcification by increasing pH and its fluctuations. Limnol. Oceanogr. 63, 3–21. doi: 10.1002/lno.10608
Wahl M., Werner F. J., Buchholz B., Raddatz S., Graiff A., Matthiessen B., et al. (2020). Season affects strength and direction of the interactive impacts of ocean warming and biotic stress in a coastal seaweed ecosystem. Limnol. Oceanogr. 65, 807–827. doi: 10.1002/lno.11350
Walve J., Larsson U. (2010). Seasonal changes in Baltic Sea seston stoichiometry: The influence of diazotrophic cyanobacteria. Mar. Ecol. Prog. Ser. 407, 13–25. doi: 10.3354/meps08551
Wasmund N. (2017). Recruitment of bloom-forming cyanobacteria from winter/spring populations in the Baltic Sea verified by a mesocosm approach. Boreal Environ. Res. 22, 445–455. doi: 10.1594/PANGAEA.873874
Wasmund N., Tuimala J., Suikkanen S., Vandepitte L., Kraberg A. (2011). Long-term trends in phytoplankton composition in the western and central Baltic Sea. J. Mar. Syst. 87, 145–159. doi: 10.1016/j.jmarsys.2011.03.010
Westerbom M., Mustonen O., Jaatinen K., Kilpi M., Norkko A. (2019). Population dynamics at the range margin: Implications of climate change on sublittoral blue mussels (Mytilus trossulus). Front. Mar. Sci. 6, e292. doi: 10.3389/fmars.2019.00292
Wikner J., Andersson A. (2012). Increased freshwater discharge shifts the trophic balance in the coastal zone of the northern Baltic Sea. Global Change Biol. 18, 2509–2519. doi: 10.1111/j.1365-2486.2012.02718.x
Wulff F., Humborg C., Andersen H. E., Blicher-Mathiesen G., Czajkowski M., Elofsson K., et al. (2014). Reduction of Baltic Sea nutrient inputs and allocation of abatement costs within the Baltic Sea catchment. Ambio 43, 11–25. doi: 10.1007/s13280-013-0484-5
Yli-Hemminki P., Sara-Aho T., Jørgensen K. S., Lehtoranta J. (2016). Iron–manganese concretions contribute to benthic release of phosphorus and arsenic in anoxic conditions in the Baltic Sea. J. Soils Sediments 16, 2138–2152. doi: 10.1007/s11368-016-1426-1
Zaiko A., Păskauskas R., Krevš A. (2010). Biogeochemical alteration of the benthic environment by the zebra mussel Dreissena polymorpha (Pallas). Oceanologia 52, 649–667. doi: 10.5697/oc.52-4.649
Keywords: cod, flounder, herring, plaice, sprat, whiting, food-web interactions, ecosystem-based management
Citation: Rosciszewski-Dodgson MJ and Cirella GT (2024) Environmental drivers affecting the status of top commercial fish stocks in the Baltic Sea: review. Front. Mar. Sci. 11:1399707. doi: 10.3389/fmars.2024.1399707
Received: 12 March 2024; Accepted: 02 August 2024;
Published: 03 September 2024.
Edited by:
Fabio Fiorentino, National Research Council (CNR), ItalyReviewed by:
Filipe Martinho, University of Coimbra, PortugalRoberto Carlucci, University of Bari Aldo Moro, Italy
Copyright © 2024 Rosciszewski-Dodgson and Cirella. This is an open-access article distributed under the terms of the Creative Commons Attribution License (CC BY). The use, distribution or reproduction in other forums is permitted, provided the original author(s) and the copyright owner(s) are credited and that the original publication in this journal is cited, in accordance with accepted academic practice. No use, distribution or reproduction is permitted which does not comply with these terms.
*Correspondence: Michael J. Rosciszewski-Dodgson, bWljaGFlbC5yb3NjaXN6ZXdza2ktZG9kZ3NvbkBwaGRzdHVkLnVnLmVkdS5wbA==
†ORCID: Michael J. Rosciszewski-Dodgson, orcid.org/0000-0001-9648-2240
Giuseppe T. Cirella, orcid.org/0000-0002-0810-0589