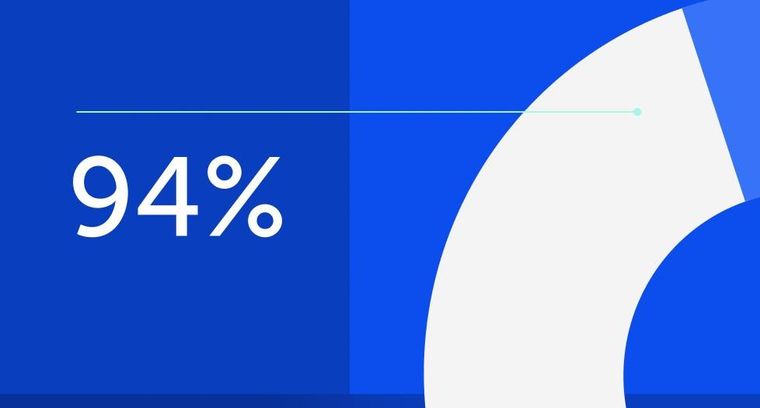
94% of researchers rate our articles as excellent or good
Learn more about the work of our research integrity team to safeguard the quality of each article we publish.
Find out more
ORIGINAL RESEARCH article
Front. Mar. Sci., 26 June 2024
Sec. Marine Fisheries, Aquaculture and Living Resources
Volume 11 - 2024 | https://doi.org/10.3389/fmars.2024.1397379
This article is part of the Research TopicTowards an Expansion of Sustainable Global Marine AquacultureView all 13 articles
Seaweed functional traits provide insights on natural populations, their adaptations to the local environment, which can be utilized for commercial exploitation. Here, we analyzed the functional traits of Gracilaria dura from two intertidal populations in Veraval and Adri, from the coast of Gujarat, India, over a period of three months. Functional traits were measured by analyzing growth rates, respiration, primary production, antioxidant activity and the content of plant growth hormones. The weight-to-length allometric exponent ≈3 indicated that G. dura grew almost isometrically. Furthermore, frond shape was not significantly different. Fronds in Veraval, resting submerged, grew faster than the fronds in Adri, which, lay exposed in the flat bare rock during low tide. Accordingly, the simultaneous increase in antioxidant activity, O2 production and chlorophyll content in fronds from Adri suggests that stress from desiccation and UV led to the detrimental accumulation of Reactive Oxygen Species, leading to decreased growth and decreased production of growth hormones. The increased Chl-a may indicate enhanced non-photochemical quenching (NPQ) for the dissipation of excess absorbed light. These results aid in establishing the best practices for maximizing biomass yield or the yield of specific molecules. For maximized biomass yield, fronds should not be subject to emersion nor cultivated on the sea-surface. On the other hand, fronds grown subject to emersion or at the sea-surface yield less biomass but more content on molecules such as antioxidants (flavonoids, phenolics, enzymes), that fight stress from desiccation high temperatures and UV. In this case, stress should still be avoided during the initial growth, at the onset of the growth season, in order to not disrupt the production of growth hormones. The increased O2 production at Adri was initially mistakenly perceived as enhanced Net Primary Production. Only a posterior holistic perspective over the whole data allowed to conclude that it was likely the stress-induced detrimental accumulation of Reactive Oxygen Species. More robust experiments are required to establish if the differences observed between locations have led to the evolution of genetic strains specific to each habitat that may show different performances and yields when cultivated in similar environments.
Seaweeds are the most efficient primary producers on the planet, surpassing plants in their ability to concentrate biomass per unit area (Creed et al., 2019). Therefore, seaweed farming has great potential to contribute to the ever-increasing human demand for food and raw materials. Seaweed applications are no novelty, and society has long cultivated seaweeds using traditional methods (McHugh, 2003; Valero et al., 2017; Cai et al., 2021). Red algae are the most harvested seaweeds, both from natural and farmed populations, for uses other than as a food source (McHugh, 2003; Cai et al., 2021). Their major uses are the extraction of agar and carrageenans, mostly from Gelidiales and Gracilariales. Harvesting of wild Gelidiales severely declined in Europe during the latter decades, mainly due to over exploitation. Gracilariales, yielding better-quality agarose, became its predominant source (McHugh, 2003). Most of the Gracilariales production is harvested in Asia, although there is also a fair amount of harvesting in South America (McHugh, 2003; Alemañ et al., 2019). For the extraction of hydrocolloids, there is no natural alternative to the cultivation of red seaweeds yielding agarose and carrageenans, given that the cultivation of brown seaweeds is too expensive and inefficient for the industrial production of alginate (McHugh, 2003). Hence, worldwide, Gracilariales play a pivotal role in supplying feedstock to local agarose production units. Time is due to optimize cultivation practices for Gracilariales as well as adapt them according to local needs and constraints.
Domestic agar (including agarose) demand in India is 400 ton·year-1, of which only 10–20% has been supplied through the indigenous supply chain. Gracilaria dura from India has been found to produce commercial-grade agarose. The technique employed for this process was a specially-developed surfactant-induced coagulation technology (Meena et al., 2014). Further commercially lucrative products from G. dura such as pigments, lipids, agar, agriculturally important nutrient-rich liquids, and energy-dense cellulose have been produced through a green processing route (Reddy et al., 2018). The farming of this species has thus been initiated at two coastal villages, namely Simar and Rajpara, along the north-west coast of India (Shah et al., 2022). About 10–12 tons of fresh biomass have been produced in pre-commercial trials at these locations.
Gracilaria dura (C. Agardh) J. Agardh is an intertidal red alga present in the Mediterranean, Arabian Sea and Indian Ocean. Along the north-west coast of India, the growth season goes from January to March. During this period (slightly extending from December to April) both gametophytic and sporophytic thalli coexist. Beyond this season, we never observed G. dura thalli, leading us to assume that bare holdfasts and dormant spores are most likely the only remnants, lying on the rocky surface until the next growth season arrives. This strong seasonality and scarcity of biomass in the wild (Mantri et al., 2009) drove the research and development of farming methods, namely, the floating bamboo raft, polypropylene net, net bag, hanging rope, monoline, net pouch and tube net (Veeragurunathan et al., 2015; Mantri et al., 2020; Shah et al., 2021). Among these, the floating bamboo raft, monoline and tube net methods have been used by commercial seaweed farmers (Kavale et al., 2021).
The seasonal occurrence of G. dura and the limited availability of natural biomass, even during the favorable season, were initially perceived as impediments to large-scale commercial aquaculture. However, new knowledge and improved practices enabled a year-round seedling production (see Saminathan et al., 2015; Vignesh et al., 2020; Shah et al., 2022). Commercial exploitation for aquaculture purposes has only been undertaken with the germplasm collected from Veraval, in the province of Gujarat, India. A tight control of the germplasm is fundamental for selecting and preserving the best strains for specific purposes or environments (Valero et al., 2017). However, for the G. dura in Gujarat, continuous and repeated farming from the same germplasm has resulted in declining growth rates due to aging or senescence. For most domesticated algal species, the best practice is to select and preserve strains through asexual cultivation (Valero et al., 2017). Whether from spores or through vegetative propagation, G. dura cultivation in Gujarat still requires determining which strains are the best to cultivate. This leads to the urgent need for a greater and more in-depth understanding of the variation in functional traits such as morphology, growth, pigments, antioxidant activity, plant growth regulators and net productivity. These traits have been demonstrated essential in the determination of growth, survival and production of molecules of commercial relevance (Gupta et al., 2015; Sambhwani et al., 2020, 2022; Dawange et al., 2023a, b).
Farmed species have evolved specific functional traits related to reproduction, growth and survival. Consequently, highly specific local aquaculture practices have led to the involuntary selection of traits with economic importance (Valero et al., 2017; Usandizaga et al., 2019; Sambhwani et al., 2020, 2022). In natural populations of G. dura, such traits also showed considerable seasonal variation (Sambhwani et al., 2020, 2022). Here, we analyzed functional traits, namely morphology, growth, pigments, antioxidant activity, plant growth regulators, productivity and respiration, for G. dura sampled during the growth season from two locations with distinct environmental conditions. The present study provides practical and novel information for the optimization of the commercial cultivation of G. dura selecting specimens retrieved from natural populations. To the best of our knowledge, this is the first study investigating spatial and temporal patterns of variation in six different functional traits crucial for the growth and survival of G. dura.
Gracilaria dura is an intertidal red alga with the isomorphic byphasic life cycle typical of rhodophytes - also known as the haploid-diploid life cycle - alternating isomorphic free living tetrasporophytes (diploid) and gametophytes (haploid) (Guillemin et al., 2014; Vieira et al., 2018a, b, 2021, 2022). Individuals are fixed to the rocky bottom by a holdfast. Along the north-west coast of India, G. dura has only been reported in Adri, Okha, and Veraval (Jha et al., 2009), where it is found occasionally in a few restricted locations (Mantri et al., 2009). In Veraval, fronds can be found permanently submerged within intertidal rockpools, whereas in Adri, fronds lie on the flat bare rock exposed to desiccation during low tide. The environmental conditions observed at Adri and Veraval during the growth season are presented in Table 1.
Healthy vegetative thalli of Gracilaria dura were collected from the coasts at Veraval (20.910404°N, 70.351273°E) and Adri (20.961213°N, 70.277051°E). Because reproductive fronds were not collected and biochemical tracers of the ploidy phase were not tested, it is not known whether each thallus was a male or female gametophyte (haploid) or a tetrasporophyte (diploid). Ecological differences have been reported between phases and sexes in various Gracilaria species, e.g., G. gracilis (Engel et al., 2001), G. tenuistipitata (Skriptsova and Nabivailo, 2009), Gracilaria caudata (Faria et al., 2017) and G. chilense [former Agarophyton chilensis] (Vieira et al., 2022). Here, we assumed that whatever the proportions among life-cycle phases, these were not significantly different among treatments of the experimental design (i.e., the Adri and Veraval populations and the three months) and thus did not bias the analysis. In this case, the different proportions of life-cycle phases would be accounted for by the homoscedastic within-group variances and would not affect the results relative to sites and seasons. Three sampling episodes took place, namely in January, February and March of 2021. This is the natural growth season for G. dura in India. Samples were collected during low tide at distances of 50, 100, 150, 200, and 250 m from the high tide limit. Based on these distances, samples were named V50, V100, V150, V200, and V250, and A50, A100, A150, A200, and A250, respectively, for Veraval and Adri. Each sample consisted of 5 G. dura thalli (having 5 – 10 fronds, but no discs) with lengths of 5–18 cm. Samples were placed in seawater and cool conditions, and immediately transported to the CSIR-CSMCRI laboratory, where they were cleaned with filtered seawater to remove calcareous and extraneous adhering detritus materials. To estimate daily growth rate (DGR), respiration and productivity, 5 fronds (one from each thallus) were selected from each sample and acclimatized in the laboratory for 5 days in filtered seawater at 12:12 h photoperiod, 25°C, 35 ppt salinity and 40 µmol photons m -2 s-1 light. To estimate the fresh weight (FW) to dry weight (DW) calibration, 15 frond fragments of various sizes were randomly collected. Dry weights were determined after 48 h at 60°C. For analyzing pigments, antioxidant capacity and plant growth hormones, the remaining thalli were surface dried with blotting paper to remove extra seawater, frozen in liquid N2 and stored at -80°C. Later, 5 fragments were taken from each site × month × distance combination and subjected to the procedures described below for the estimation of each variable. The final reading was the average of the five fragments. Sampling for the morphological analysis followed a different protocol described below.
The morphological characterization of G. dura relied on total length (L), diameter (D), fresh weight (W) and count of primary branches (B) measured on 50 single fronds collected each month (January, February and March of 2021) from the coasts of Veraval and Adri. The weight-to-length allometric exponent was estimated. In isometric growth, individuals grow proportionally in all 3 spatial dimensions, thus keeping their shape. Consequently, biomass = a×L3.
Five fronds (= 5 replicates) were selected from each site at each census. For each replicate, 10 fragments approx. 2 cm in length were excised and placed in a 500-ml flask containing Erdschreiber seawater (ESS) culture medium (Suto, 1959) with 35 ppt salinity, at 25°C, 7.8 pH, 40 µmol photons m -2 s-1 light and a 12:12 h photoperiod. Media was replenished every 5 days and fragments were cleaned with a soft brush to reduce fouling. After 15 days, all fragments were weighed and the daily growth rate (DGR) was estimated using the formula DGR (day -1) = ln [(W2/W1)/t], where W1 is the initial weight, W2 is the final weight and t is the number of days (see Dawes et al., 1993).
To prepare the extract, 100 mg of frozen algal material was ground to powder in the pre-chilled mortar and pestle using liquid nitrogen. Sequential extraction was performed in two steps: first, to the crushed sample was added 1 ml of distilled water, incubated overnight at 4°C and thereafter centrifuged at 11384g (Eppendorf Centrifuge – 5424-R, Germany) for 15 min at 4°C. Then, the supernatant was collected and stored to be mixed later with the second extract, 1 ml of 70% methanol was added to the pellet, and the mix was incubated overnight at 4°C followed by centrifugation at 11384g for 15 min at 4°C. Both supernatants were pooled and the extract was used for the analysis of total antioxidant capacity (TAC) and CUPric Reducing Antioxidant Capacity (CUPRAC).
A TAC assay was implemented according to the method of Prieto et al. (1999). Briefly, 100 µl of the above extract were mixed with 1 ml of mixed reagent (28 mM sodium phosphate, 4 mM ammonium molybdate and 0.6 M sulfuric acid) and incubated for 1 h at 100°C. After cooling to room temperature, absorbance was recorded at 695 nm (detection limits 200–999nm) using a UV-Vis spectrophotometer (EPOCH/2 Biotek). Using a calibration curve, TAC (mg·g-1) was standardized to the ascorbic acid equivalent antioxidant capacity (AAEAC) (Gonzales et al., 2021).
The CUPRAC spectrophotometric method was used for determining antioxidant capacity (Apak et al., 2004). To 100 µl of the extract mentioned above were added 50 µl of 10 mM copper chloride, 50 µl of ammonium acetate buffer (pH 7) and 50 ml of 7.5 mM neocuproine, followed by 1 h incubation at room temperature. Absorbance was recorded at 450 nm (detection limits 200–999nm). Using a calibration curve, CUPRAC (mg·g-1) was standardized to the ascorbic acid equivalent antioxidant capacity (AAEAC) (Gonzales et al., 2021).
A 100-mg sample of each of the 5 fragments was taken and crushed to powder, and homogenized in a mortar and pestle using liquid nitrogen. To determine R-Phycoerythrin (PE) and R-Phycocyanin (PC) content, 0.8 ml of 0.1 M phosphate buffer (6.8 pH) was added to the homogenate and incubated overnight at 4°C. Samples were then vortexed and centrifuged at 15900g for 10 min at 4°C (Eppendorf Centrifuge – 5424-R, Germany). The supernatant was collected and 0.2 ml of phosphate buffer was added to the pellet for re-extraction. The chemicals used in the experiments were all analytical grade (Sigma Aldrich Pvt. Ltd.). The absorbances at 564 nm (A564), 618 nm (A618) and 730 nm (A730) were recorded on a dual beam UV-Vis spectrophotometer (EPOCH BIOTEK) with detection limits 200–999nm (Sampath-Wiley and Neefus, 2007). A similar process was implemented for the extraction of Chlorophyll-a (Chl-a) using 90%acetone as a solvent and absorbance was recorded at 664 nm (A664) and 647 nm (A647) (Jeffrey and Humphrey, 1975). The R-PE (µg·g-1) and R-PC (µg·g-1) content was estimated from Equations 1, 2, respectively:
Chl-a (µg·g-1) content was estimated following Equation 3:
Net primary productivity (NPP) (mg O2·gDW-1·h-1) and respiration (R) (mg O2·gDW-1·h-1) were measured using the light and dark bottle method (Guillemin et al., 2014). Five frond fragments of approximately 0.3 g were placed in 100-ml glass bottles filled with autoclaved filtered seawater (salinity 35) and kept at 25°C, 40 µmole photons m -2 s-1 light for 12 h (the “Light” bottles). Another 5 frond fragments of approximately 0.3 g were placed in 100-ml glass bottles filled with autoclaved filtered seawater (salinity 35), kept at 25°C and covered with black plastic sheets (the “Dark” bottles). The 12-h time intervals mimicked the diel pattern as well as the 5-day acclimatization photoperiod. Initial and final dissolved oxygen (O2) concentrations were recorded using a HACH HQ30D DO probe, USA. As established in previous studies, there was no O2 saturation after 12 h (Sambhwani et al., 2022). Respiration (R), net primary productivity (NPP), and gross primary productivity (GPP) were calculated by using Equations 4–6 respectively.
The extractions were implemented separately for auxin (µg·g-1), cytokinin (µg·g-1) and gibberellic acid (µg·g-1). Each frond fragment (1 g) was homogenized in a mortar and pestle and collected in a 50-ml tube. Distilled water (5 ml) was added and stirred overnight on the tabletop stirrer. The supernatant was collected upon centrifugation at 7500g for 15 min at 4°C (Eppendorf Centrifuge – 5430-R, Germany). Auxin, cytokinin and gibberellic acid were extracted using diethyl ether (DEE), n-butanol and ethyl acetate (EA), respectively (Prasad et al., 2010; Sambhwani et al., 2022).
For estimating auxin (µg·g-1), the pH of the above extract was adjusted to 3 with 1N hydrochloric acid (HCl). Extraction was implemented with equal volumes of di-ethyl ether (DEE) and repeated thrice. Equal volumes of 5% sodium bicarbonate were added to the DEE layer. The bicarbonate layer was then collected in another fresh tube and its pH was adjusted to 3.0 by dropwise addition of 1N HCl. This was extracted with equal volumes of DEE and repeated thrice for maximum extraction. Both the DEE layers were pooled and washed with distilled water. The solvents were then evaporated and the residue was dissolved in 1 ml of HPLC-grade methanol.
To estimate cytokinin (µg·g-1), the pH of the extract from Section 2.8 was adjusted to pH 3 by dropwise addition of 1N HCl. Equal volumes of dichloromethane (DCM) were used thrice for extraction and collected in a separate tube. The pH of the aqueous layer was adjusted to 8 with 1N NaOH. For the second extraction, equal volumes of n-butanol were used thrice. Both DCM and n-butanol layers were pooled and then evaporated. The residue was dissolved in 1 ml of HPLC-grade methanol.
To estimate gibberellic acid (µg g-1), the pH of the extract from Section 2.8 was adjusted to 2.5 by 3.2 N HCl, followed by extraction with equal volumes of ethyl acetate (EA) three times. The pH of the aqueous layer was adjusted to 11.0 with 3.75 M NaOH. This was hydrolyzed at 60°C for 1 h in a water bath. The pH was then readjusted to 2.5, followed by re-extraction with EA. Both the EA layers were combined and an equal volume of NaHCO3 was added. The combined EA layer was transferred to a fresh tube. The pH of the NaHCO3 layer was adjusted to 2.5. For maximum extraction, the EA extraction was repeated and all EA samples were pooled. The solvent was evaporated and the residue was dissolved in 1 ml of HPLC-grade methanol.
The growth hormone content was quantified by High Performance Liquid Chromatography (HPLC). First, the extracted residue (see explanation above) was dissolved in methanol and passed through a 0.22-µm syringe filter. Standards of 25 ppm were prepared for all three growth hormones as follows: for auxin — indole-3-acetic acid Batch No: 2283040 of Sisco Research Laboratories; for cytokinin — zeatin Batch:0000008065 of Sigma Aldrich, and for gibberellins — gibberellic acid (GA3) Batch No:8962367 of Sisco Research Laboratories. Samples were analyzed in HPLC for the estimation of concentration with respect to the standards. The retention times were 14.7, 12.8 and 3.6 min for the standard IAA, GA3 and trans-zeatin, respectively. Prominence model of M/s. Shimadzu, Japan, including a Rheodyne injector, was used for HPLC analysis. The C18 stainless steel column of M/s. Thermo Scientific with specifications of 250 mm x 4.6 mm (i.d.) Nucleosil (5 μm particle size, 300 A° pore size) was used at a constant temperature of 37°C. The binary elution system of separation was carried out using mobile phases A (water with 0.1% formic acid) and B (methanol with 0.1% formic acid). A constant flow rate of 1 ml min-1 was maintained. Further, UV detection was attempted at 254 nm. The detector was an SPD M20A with wavelength range from 190 nm to 900 nm and operated at 25°C. The elution was achieved through a linear gradient as follows: 70% A and 30% B at 0 min, 70% A and 30% B (2 min), 0% A and 100% B (20 min), 0% A and 100% B (22 min), 70% A and 30% B (25 min) and 70% A and 30% B (30 min). The detection of the eluted plant growth regulators was performed at 254 nm and the identification of different PGRs measured against standards was based on HPLC peak areas.
The morphological growth dynamic (isometric vs. allometric) of G. dura fronds was inferred from the log10DW-to-log10L fit. Several model I and model II regression methods were tested, including Ordinary Least Squares (OLS), Reduced Major Axis (RMA) Principal Components Analysis (PCA), Quantile Regression (QR), among others. Differences in morphological growth between fronds from Adri or Veraval were tested by ANCOVA. The significance of these differences was estimated by permutation tests (Vieira and Creed, 2013a, b).
The morphometry data set, comprising 300 sampling units (2 sites × 3 months × 50 thalli), was subject to a Principal Components Analysis (PCA) to search for consistent associations between variables. The PCA was performed on the correlation matrix. The significances of the associations (described by the principal components – pc) and of the variables contributing to them (described by the respective loadings) were estimated by permutation tests following the protocol and software by Vieira (2012). Meaningful pcs replaced the original variables significantly contributing to them. Hence, each sampling unit had the original measurements replaced by the z-score estimated from the corresponding pc. To test for significant differences among sites (fixed effects) and/or months (fixed effects), 2-way permutation tests (also known as Permanova) were implemented using either the z-scores or uncorrelated original variables as the dependent variable.
The hormones, pigments, respiration and primary production data set comprising 30 sampling units (2 sites × 3 months × 5 distances from shore) were subject to a Principal Components Analysis (PCA) performed on the correlation matrix, as above. To test for significant differences among sites (fixed effects), months (fixed effects) or distances from shore (random effects), 3-way permutation tests were implemented using either the z-scores or uncorrelated original variables as the dependent variable.
The best log10DW fit to log10L (Figure 1) was obtained by Reduced Major Axis (RMA, a model II linear regression), leading to the allometric relation DW=0.0003·L2.87. The allometric exponent close to 3 indicates that G. dura growth is almost isometric. This almost isometric growth was not significantly different between G. dura fronds from Veraval and from Adri (p=0.08), as determined by an ANCOVA procedure.
Figure 1 Scatter plot of length vs weight of fronds of Gracilaria dura from Adri and Veraval. The morphometric relation between frond length and dry weight was tested by Reduced Major Axis (RMA) for G. dura collected from Adri and Veraval coasts. An ANCOVA showed that this relation was isometric and not significantly different between fronds from the two sites.
The PCA upon all the morphometric variables was meaningful (p<0.0001). Only the first (largest) principal component (pc1) extracted was meaningful (p<0.0001). This component was a weighted average of all morphometric variables under the form zi=0.54·log10Li+0.57·log10DWi+0.42·Di+0.44·Bi, where the subscript i stands for the ith frond. When selecting the variables significantly contributing to this component, the IL metric (see Vieira, 2012) selected all variables, in which case the pc represents 55.9% of the total normalized variation. On the other hand, the Correlation Index (see Vieira, 2012) selected only log10L and log10DW, in which case pc1 represents 34,8% of the total normalized variation. We chose the latter pc1 version with only the L and DW association as the subsequent analysis provided a clearer picture of the morphometric growth dynamics (Figure 2). Frond size, as represented by pc1, was significantly different among sites (p<0.0001) as well as among months (p<0.0001). At the start of the monitoring experiment, G. dura fronds were already larger at Veraval than at Adri and, as the growth season progressed, this pattern enhanced, leading G. dura fronds to end the growth season on average 2.3 times larger in Veraval than in Adri (Figure 2A). The larger divergence in the morphological growth dynamics occurred from February to March, when fronds enhanced growth in Veraval but stopped growing in Adri. During the monitoring experiment, G. dura fronds did not increase their diameter (Figure 2B), as shown by the non-significant differences among months (p=0.129). Nevertheless, G. dura branches were consistently thicker at Veraval than at Adri (p<0.0001) (Figure 2B). During the monitoring experiment, G. dura fronds slightly increased their number of primary branches (Figure 2C), as shown by the significant differences among months (p<0.0005). Furthermore, G. dura fronds were bushier at Veraval than at Adri (p=0.0114) (Figure 2C).
Figure 2 Gracilaria dura morphometry (length, dry weight, diameter and number of primary branches) during the growth season. (A) Principal component 1 (z in the left vertical axis) combining Length (L in cm, in the right vertical axis) and Dry Weight (DW in g, in the right vertical axis) into z=0.54·log10L+0.57·log10DW. PCA was performed using the correlation matrix, which implied that log10L and log10DW were normalized to zero mean and unit variance. (B) Diameter (D in mm) of the primary branches. (C) Number of primary branches in each frond. This data was log transformed for statistical inference. Error bars are standard deviations (n=50).
The PCA of the molecular, respiration and primary production data set generated a significant result (p<0.0001). However, each of the variables respiration, NPP, GPP, cytokinin and chlorophyll was uncorrelated with any other variable. Hence, a new PCA was performed with only the remaining correlated variables, namely DGR, TAC, CUPRAC, PE, PC, auxin and gibberellins. The two largest principal components extracted were significant (p<0.0001 for pc1 and p<0.0001 for pc2). Pc1 was a weighted contrast between growth and antioxidant activity given by z1i=0.53·DGRi-0.56·TACi-0.53·CUPRACi (Supplementary Figure S1), and explaining 31.9% of the total normalized variation. The 3-way Permanova was applied using z1 as the response (dependent) variable (Figure 3). Differences between months (fixed factor) were significant (p=0.0037), but only January and March were significantly different from each other (p<0.0137). Differences between sites (fixed factor) were significant (p=0.0008). Differences between distances from shore (random factor) were not significant (p=0.9241). There were no interactions (always p>0.7994). Pc2 was a weighted average of PE and growth hormones, given by z2i=0.42·PEi+0.44·PCi+0.5·Auxini+0.52·Gibberelinsi (Supplementary Figure S2), and explaining 27.8% of the total normalized variation. The 3-way Permanova was applied using z2 as the response (dependent) variable (Figure 3). Differences between months (fixed factor) were not significant (p=0.1895), as were differences between sites (fixed factor; p=0.2448), and differences between distances from shore (random factor; p=0.8775). There were interactions between month and site (p<0.0408), with z2 being significantly larger at Veraval during January.
Figure 3 Principal Components Analysis (PCA) of the growth, molecular composition, respiration and primary production data set of Gracilaria dura. The largest principal component (z1 in the left panel vertical axis) corresponds to z1i=0.53·Growthi-0.56·TACi-0.53·CUPRACi. The second largest principal component (z2 in the right panel vertical axis) corresponds to z2i=0.42·PEi+0.0.44·PCi+0.5·Auxini+0.52·Gibberelinsi. Both z1 and z2 were tested for their relation with month, site, and distance from shoreline. Months were January (Jan), February (Feb) and March (Mar). Sites were Adri (A) and Veraval (V). Distances from shoreline were 50, 100, 150, 200 and 250m. Markers identify sampling locations (A50) Adri, 50m, (A100) Adri, 100m, (A150) Adri, 150m, (A200) Adri, 200m, (A250) Adri, 250m, (V50) Veraval, 50m, (V100) Veraval, 100m, (V150) Veraval, 150m, (V200) Veraval, 200m and (V250) Veraval, 250m. PCA calculated using correlation matrix in which original variables were normalized to zero mean and unit variance.
Since the remaining variables Chlorophyll-a (Chl-a), Cytokinin, Respiration, Gross Primary Production (GPP) and Net Primary Production (NPP) were not significantly correlated among themselves or with any other variable, they were analyzed separately (Figure 4). The 3-way Permanova using Chl-a as response variable yielded significant differences between sites (p<0.0001). Fronds from Adri had significantly more Chl-a than fronds from Veraval. Differences between months and between distances from shore were not significant (p=0.643 and 0.622, respectively), and there were no interactions (always p>0.508). In a similar manner, the 3-way Permanova using NPP as response variable yield significant differences between sites (p=0.0019). Because NPP was measured by the O2 method, this meant that fronds from Adri produced significantly more O2 than fronds from Veraval. Differences between months were significant (p=0.037), with February showing significantly less NPP than January (p=0.044). Differences between distances from shore were not significant (p=0.5439). There were no interactions (always p>0.455). This pattern is close to the pattern above described by pc1. In fact, the PCA results yield Chl-a and NPP almost significantly associated with (or contributing to) pc1. The reason they were not so well correlated with growth, TAC and CUPRAC is because they do not match the time series evolution. While the association between growth, TAC and CUPRAC evolves consistently with time (Figure 3), Chl-a and NPP do not evolve consistently with time (Figure 4). Nevertheless, the key finding is the differentiation between sites shared by all these variables. The 3-way Permanova using Cytokinin as a response variable yields non-significant differences between sites (p=0.718). Differences between months were not significant (p=0.108). However, differences between distances from shore were significant (p=0.0008), with fronds collected 100m away having significantly more Cytokinin than the fronds collected anywhere else. We question whether this was simply a coincidence. There were no interactions (always p>0.242). The 3-way Permanova using respiration (R) as response variable yield non-significant differences between sites (p=0.643). Differences between months were significant (p=00025). Respiration was significantly less during January than during February (p=0.0003) or during March (p=0.0025). Differences between distances from shore were not significant (p=0.654). There were no interactions (always p>0.187). The 3-way Permanova using Gross Primary Production (GPP) as response variable yield significant differences between sites (p=0.001). Fronds from Adri had significantly more GPP than fronds from Veraval. Differences between months were not significant (p=0.217). Differences between distances from shore were not significant (p=0.729). There were no interactions (always p>0.24).
Figure 4 Chlorophyll-a (Chl a), cytokinin, respiration, gross primary production (GPP) and net primary production (NPP) of Gracilaria dura in different months, sites (A, Adri; V, Veraval), and distances from the shoreline (m). Distances from shoreline were 50, 100, 150, 200 and 250m. Markers identify sampling locations (A50) Adri, 50m, (A100) Adri, 100m, (A150) Adri, 150m, (A200) Adri, 200m, (A250) Adri, 250m, (V50) Veraval, 50m, (V100) Veraval, 100m, (V150) Veraval, 150m, (V200) Veraval, 200m and (V250) Veraval, 250m.
The weight-to-length exponent indicates whether or not individuals preserve their shape while growing. Fronds that do preserve their shape (i.e., isometric growth) have exponents ≈ 3. Fronds that do not preserve their shape (i.e., allometric growth) have exponents smaller than 3. Exponents larger than 3 indicate that something more happened besides fronds elongating along the three spatial dimensions; which could be increased branching or branch thickness. Scrosati et al. (2020) present examples of seaweeds corresponding to all these cases. The G. dura weight-to-length allometric exponent of 2.87 indicates that it grows almost isometrically (i.e., equally along the three spatial dimensions) (Figure 1). Thus, the fronds grew preserving their shape. However, while growing, G. dura barely increased the number of its primary branches (Figure 2C) or thickened them (Figure 2B), which goes in accordance with the isometric exponent ≈ 3. In conclusion, G. dura growth is basically a process of frond elongation along the three spatial dimensions. The same happens with the Kelp Saccharina latissima cultivated in longlines in Norway, showing an isometric exponent = 3.03 (r2 = 0.941) (Overrein et al., 2024). Despite G. dura growth being basically a process of frond elongation along the three spatial dimensions, fronds where bushier and branches were thicker at Veraval than at Adri, which also contributed to the larger biomass yield in Veraval. This differentiation was present and constant since the beginning of the growing season, and not something that evolved with time; raising the question about whether this was strictly forced by the environment or is there a genetic differentiation between Veraval and Adri strains. Supporting a strictly environmental justification, the environment should have been particularly stressful at Adri, leading to the reduced production of growth hormones at the onset of the growing season (Figure 3.right panel – z1) and the reduced growth coupled with the enhanced antioxidant activity during the entire growth season (Figure 3.left panel – z2). Notice that the major differentiation between Veraval and Adri for frond branching was during January, at the onset of the growing season (Figure 2C), which exactly matched the Veraval and Adri differentiation in growth hormones (Figure 3.right panel).
Gracilaria dura grew much larger at Veraval than at Adri. This was demonstrated both by the morphometric data (Figures 1, 2) and by the growth rate data (pc1\z1 in Figure 3). The principal component contrasting growth with antioxidant activity (pc1) suggested the reason for this differentiation between Veraval and Adri was growth inhibition by stress. The cause of stress was desiccation during low tide, which is debated in detail in a paragraph below. Presently, we highlight that stress from UV, excessive temperature and excessive light leads photoautotrophs (plants and seaweeds) to the detrimental uncontrolled accumulation of reactive oxygen species (ROS) that negatively impact metabolism, deter growth, oxidize tissue, and ultimately lead to cell death (Cruces et al., 2018; Savchenko and Tikhonov, 2021). To avoid such effects, photoautotrophs evolved countermeasures including increased antioxidative activity (Kusvuran et al., 2016; Cruces et al., 2018; Khaleghi et al., 2019; Kowalczewski et al., 2020; Savchenko and Tikhonov, 2021), here demonstrated by increased TAC and CUPRAC. Corroborating the hypothesis that ROS stress played an important part in the decreased frond growth and size observed in Adri, were the concomitant increases in O2 production and Chl-a content. Oxidative stress in photoautotrophs commonly results from the saturation of the photosynthetic electron transport chain, leading to the increased production of ROS, including O2 (Tietz et al., 2017; Savchenko and Tikhonov, 2021). This may explain the increased O2 production by fronds from Adri, which was initially mistakenly perceived as an increase in NPP. The saturation of the photosynthetic electron transport chain is promoted by excessive illumination or a reduced rate of CO2 fixation (Savchenko and Tikhonov, 2021). The increased Chl-a content may represent an adaptation to the saturating light environment by enhancing the Non-photochemical quenching (NPQ). This is a process for the harmless dissipation of excess absorbed light energy, most often as heat, although other forms of energy dissipation are possible (Demmig-Adams et al., 2014; Ruban, 2016; Tietz et al., 2017). NPQ prevents the harmful build-up of excess energy that could damage the antenna, the pigments and ultimately lead to cell death. This process (and molecular adaptation), taking place in the antenna and requiring photosynthetic pigments for energy transfers, is considered the most efficient and fastest response of the photosynthetic membrane to excess light (Demmig-Adams et al., 2014; Ruban, 2016; Tietz et al., 2017). Chl-a has a fundamental role in NPQ (Papageorgiou and Govindjee, 2014).
Fighting stress - from desiccation as well as other sources - demands allocating significant amounts of resources (nutrients and energy) that become unavailable for growth. In the case of antioxidant activity, this is generally performed by phenolic compounds (Cruces et al., 2018; Marinho et al., 2019; Kowalczewski et al., 2020), flavonoid compounds (Marinho et al., 2019) and enzymes (Kusvuran et al., 2016; Khaleghi et al., 2019). Furthermore, seaweeds also produce other costly metabolites to protect themselves directly from UV radiation (Gómez et al., 2005; Jiang et al., 2008; Kumar et al., 2011; Cruces et al., 2018). For fronds in Adri, the direct detrimental effects of desiccation and ROS stress may have been added to the cost of allocating resources to fight them, with these resources having become unavailable for growth. The strongest evidence suggesting that such was the case came from the content of costly metabolites (namely, R-phycoerythrin, R-phycocyanin, auxin and gibberellic acid) being lower in Adri than in Veraval (pc2\z2 in Figure 3). Auxins and gibberellins in particular have been found responsible for the induction of frond elongation, apical dominance, and tissue differentiation (Tarakhovskaya et al., 2007; Sambhwani et al., 2022). These hormones were found in much higher quantities in the fronds from Veraval than in the fronds from Adri, as demonstrated by pc2. However, this only occurred during the early growth season (i.e., during January, when growth hormones peaked at Veraval) and for the remaining monitorization, these hormones remained similarly lower at both sites. This suggests that the environment was so stressful at Adri at the onset of the growth season, demanding so much resource allocation for antioxidant activity, that the fronds in there lacked resources to produce growth hormones and photosynthetic pigments in larger quantities. Later on, during February and March, either the environment became more favorable and this bias tended to dissipate, or the posterior reduction in the content of growth hormones is a natural process in the biology of G. dura. Either way, growth had already been triggered in January with the discrepancies between Veraval and Adri.
The different stress levels experienced by G. dura fronds growing at Veraval or Adri likely resulted from the type of substrate. In both cases, the locations were intertidal. However, while fronds in Veraval occupied rockpools enabling them to stay submerged during low tide, fronds in Adri laid in the flat bare rocks, thus desiccating during low tide. This was exactly the same that happened to Gracilaria chilensis (also named Agarophyton chilensis) fronds in the Valdivia River estuary. In this case, the fronds occupying Niebla intertidal rock pools outperformed the fronds laying (and desiccating) in the Corral intertidal flat bare rocks. This outperformance was evident in adult survival (Vieira et al., 2018a), growth (Vieira et al., 2021), fertility and sporeling survival (Vieira et al., 2018b), ultimately leading to more vigorous and productive sub-populations that occupied the niche more efficiently (Vieira et al., 2022). Intertidal seaweed stands subject to desiccation and excessive light due to emersion during low tide suffer from decreased photosynthesis and growth, bleaching and mortality (Figueroa and Gómez, 2001; Hays, 2007; Zheng and Gao, 2009; Miller et al., 2011; Mueller et al., 2015). For algae occupying these habitats, forming denser turfs mitigates these stresses and their consequences, while also decreasing the energetic costs of copping with them (Hay, 1981; Scrosati and Dewreede, 1998; Kamiya et al., 2021).
The first conclusion that can be drawn is that G. dura fronds cultivated permanently submerged could grow faster and larger. Thus, this should be the protocol to maximize biomass production. However, for the specific production of antioxidative molecules of commercial interest, it is better to cultivate fronds with some degree of stress from emersion, provided that this stress is initially avoided (i.e., avoided for G. dura germlings and young fronds). Hence, the optimization of G. dura production on floating structures (as described in the methods) requires that these are always submerged, and thus never placed in intertidal locations. Furthermore, the fronds placed on top (i.e., closer to, or even on, the sea-surface) will be richer in antioxidative compounds. On the other hand, the optimized exploration of natural G. dura stands in Gujarat should harvest in Veraval for maximum biomass yield and in Adri for antioxidative compounds. Furthermore, for the specific objective of producing antioxidative compounds, after the onset of the growth season, some G. dura fronds in rockpools may be relocated to more exposed substrates. These relocated fronds shall not lack for biomass production as, according to the sell-thinning theory (see Creed et al., 2019 and references therein), competition in crowded rockpools would induce mortality in some of these fronds, anyway (Vieira et al., 2018a, 2022). Then, it becomes a matter of determining how crowded the rockpools get and how many fronds may be relocated without impacting biomass yield.
Gracilaria spp. from the Indo-Pacific are commonly adapted to high temperatures and irradiances (Raikar et al., 2001), and such is also the case for G. dura. A previous study suggests that marked phenotypical differentiation should not be expected in G. dura in Gujarat (Dawange et al., 2023b). Still, the differentiation between Veraval and Adri in the light environment, temperature and desiccation led G. dura individuals from either location to show marked differences in ecophysiology, metabolism and growth. Subject to more stress, G. dura in Adri clearly underperformed. This is common in rhodophytes (see, as examples, Duarte and Ferreira, 1995; Mueller et al., 2015; Vieira et al., 2018a, b, 2021, 2022). The question arose about whether the differences between environmental conditions observed in Veraval and Adri led to the evolution of genetic strains specific to either habitat that may show different performances and yields when cultivated in similar environments. A marked phenotypical differentiation, whether of a genetic origin or acquired during development (photo-acclimatation), in response to these stressors can be observed at spatial resolution as thin as among individuals occupying different bands (i.e., heights) of the same intertidal population or among individuals occupying the same habitat during different seasons (Smith and Berry, 1986; Matta and Chapman, 1991; Britting and Chapman, 1993; Figueroa and Gómez, 2001; Wright et al., 2004; Williams and Dethier, 2005; Hays, 2007; Miller et al., 2011). For these differences to be driven from genetics, they need to be observed among individuals cultivated for a long time in similar conditions. However, in our study some variables were measured from fronds subject to only 5-day acclimatization while other variables were measured from fronds subject to no acclimatization at all. Therefore, our work does not allow us to determine whether the observed phenotypic differences resulted from genetic differentiation or acquired memory of recent ecological history (i.e., preserved adaptations to recent ecological conditions).
A word of caution about the estimation of NPP and GPP for intertidal photoautotrophs. We think that the results of enhanced NPP were false and that the likeliest explanation is the application of the O2 method in situations where stress from desiccation, excess temperature and excess light lead photoautotrophs to an uncontrolled accumulation of reactive oxygen species (ROS), including O2. Because the estimation of GPP depends on the NPP input (GPP=R–NPP), we also have no confidence in the GPP readings. Respiration seems not to have been affected by the challenging environmental conditions at Veraval.
The differences between the two locations studied, namely Veraval and Adri, were preserved even when the frond fragments were acclimatized and grown under the same conditions. Therefore, we infer that either there is a genetic differentiation or G. dura fronds preserve a phenotypic memory of their recent ecological past. Veraval is better for biomass production, although it is uncertain whether this is exclusively due to the environmental conditions of the site or a site-specific strain has also evolved. On the other hand, the intertidal fronds of G. dura desiccating in Adri’s flat bare rock during low tide, despite growing less (possibly due to the stress from the detrimental uncontrolled accumulation of Reactive Oxygen Species), still have commercial value as they show increased accumulation of antioxidants.
The raw data supporting the conclusions of this article will be made available by the authors, without undue reservation.
VV: Data curation, Formal analysis, Investigation, Methodology, Software, Validation, Visualization, Writing – original draft, Writing – review & editing. PD: Data curation, Formal analysis, Writing – original draft. SJ: Data curation, Formal analysis, Funding acquisition, Project administration, Writing – original draft, Writing – review & editing. JS: Funding acquisition, Project administration, Writing – review & editing. VM: Conceptualization, Funding acquisition, Methodology, Project administration, Supervision, Writing – review & editing.
The author(s) declare financial support was received for the research, authorship, and/or publication of this article. PD and SJ were funded by the Science and Engineering Research Board (SERB), New Delhi (India) under the project EEQ/2018/000562. VM received funding from the Council of Scientific and Industrial Research, New Delhi (India) under the project MLP 0051. JS received funding from the Mar2020 program (Portugal) under project PhycosPT (MAR02.01.01-FEAMP-0039). PD received funding through a research fellowship from MAR2020 program (Portugal). VV was supported by FCT/MCTES (PIDDAC) through project LARSyS - FCT Pluriannual funding 2020-2023 (UIDB/EEA/50009/2020).
The authors would like to thank the Director, CSIR-Central Salt and Marine Chemicals Research Institute, Bhavnagar for providing facilities. This communication has CSMCRI PRIS approval number 38/2023.
The authors declare that the research was conducted in the absence of any commercial or financial relationships that could be construed as a potential conflict of interest.
All claims expressed in this article are solely those of the authors and do not necessarily represent those of their affiliated organizations, or those of the publisher, the editors and the reviewers. Any product that may be evaluated in this article, or claim that may be made by its manufacturer, is not guaranteed or endorsed by the publisher.
The Supplementary Material for this article can be found online at: https://www.frontiersin.org/articles/10.3389/fmars.2024.1397379/full#supplementary-material
Supplementary Figure 1 | Association between z1 and its contributing variables Daily Growth Rates (DGR), CUPRAC and TAC of Gracilaria dura.
Supplementary Figure 2 | Association between z2 and its contributing variables R-Phycoerythrin (PE) and R-Phycocyanin (PC), Auxin and Gibberellic acid of Gracilaria dura.
Alemañ A. E., Robledo D., Hayashi L. (2019). Development of seaweed cultivation in Latin America: current trends and future prospects. Phycologia 58, 462–471. doi: 10.1080/00318884.2019.1640996
Apak R., Güçlü K., Özyürek M., Karademir S. E. (2004). Novel total antioxidant capacity index for dietary polyphenols and vitamins C and E, using their cupric ion reducing capability in the presence of neocuproine: CUPRAC method. J. Agric. Food. Chem. 52, 7970–7981. doi: 10.1021/jf048741x
Britting S., Chapman D. (1993). Physiological comparison of the isomorphic life history phases of the high intertidal alga Endocladia muricata (Rhodophyta). J. Phycol. - J. Phycol. 29, 739–745. doi: 10.1111/j.0022-3646.1993.00739.x
Cai J., Lovatelli A., Aguilar-Manjarrez J., Cornish L., Dabbadie L., Desrochers A., et al. (2021). Seaweeds and microalgae: an overview for unlocking their potential in global aquaculture development. FAO Fisheries and Aquaculture Circular No. 1229. (Rome: FAO). doi: 10.4060/cb5670en
Creed J., Vieira V.M.N.C.S., Norton T. A., Caetano D. (2019). A meta-analysis shows that seaweeds surpass plants, setting life-on-Earth’s limit for biomass packing. BMC Ecol. 19, 1–11. doi: 10.1186/s12898-019-0218-z
Cruces E., Flores-Molina M. R., Dıaz M. J., Huovinen P., Gómez I. (2018). Phenolics as photoprotective mechanism against combined action of UV radiation and temperature in the red alga Gracilaria Chilensis? J. Appl. Phycol. 30, 1247–1257. doi: 10.1007/s10811-017-1304-2
Dawange P., Mantri V. A., Jaiswar S. (2023a). Selection and development of superior strains through functional trait- based approach in agarophytic red alga Gracilaria dura (Rhodophyta). J. Envin. Biol. 44, 795–803. doi: 10.22438/jeb/
Dawange P. S., Vieira V.M.N.C.S., Sardinha J. P., Jaiswar S., Mantri V. A. (2023b). Resource-limited Gracilaria dura partitions resources between growth and survival: Knowledge base for optimizing production. J. Appl. Phycol. 35, 2985–2994. doi: 10.1007/s10811-023-03101-0
Dawes C. J., Trono G. C., Lluisma A. O. (1993). Clonal propagation of Eucheuma denticulatum and Kappaphycus alvarezii for Philippine seaweed farms. Hydrobiologia 260, 379–383. doi: 10.1007/BF00049044
Demmig-Adams B., Garab G., Adams W. III, Govindgee (2014). Non-photochemical quenching and energy dissipation in plants, algae and cyanobacteria, Advances in Photosynthesis and Respiration 40 (Dordrecht: Springer). doi: 10.1007/978-94-017-9032-1
Duarte P., Ferreira J. G. (1995). Seasonal adaptation and short-term metabolic responses of Gelidium sesquipedale to varying light and temperature. Mar. Ecol. Prog. Ser. 121, 289–300. doi: 10.3354/meps121289
Engel C., Åberg P., Gaggiotti O. E., Destombe C., Valero M. (2001). Population dynamics and stage structure in a haploid-diploid red seaweed, Gracilaria gracilis. J. Ecol. 89, 436–450. doi: 10.1046/j.1365-2745.2001.00567.x
Faria A. V. F., Bonomi-Barufi J., Plastino E. M. (2017). Ecotypes of Gracilaria caudata (Gracilariales, Rhodophyta): physiological and morphological approaches considering life history phases. J. Appl. Phycol. 29, 707–719. doi: 10.1007/s10811-016-1018-x
Figueroa F. L., Gómez I. (2001). Photosynthetic acclimation to solar UV radiation of marine red algae from the warm-temperate coast of southern Spain: A review. J. Appl. Phycol. 13, 233–245. doi: 10.1023/A:1011126007656
Gómez I., Figueroa F. L., Huovinen P., Ulloa N., Morales V. (2005). Photosynthesis of the red alga Gracilaria Chilensis under natural solar radiation in an estuary in southern Chile. Aquaculture 244, 369–382. doi: 10.1016/j.aquaculture.2004.11.037
Gonzales M., Villena G. K., Kitazono A. A. (2021). Evaluation of the antioxidant activities of aqueous extracts from seven wild plants from the Andes using an in vivo yeast assay. Results. Chem. 3, 100098. doi: 10.1016/j.rechem.2021.100098
Guillemin M. L., Valenzuela P., Gaitán-Espitia J. D., Destombe C. (2014). Evidence of reproductive cost in the triphasic life history of the red alga Gracilaria Chilensis (Gracilariales, Rhodophyta). J. Appl. Phycol. 26, 569–575. doi: 10.1007/s10811-013-0072-x
Gupta V., Kumari P., Reddy C. (2015). Development and Characterization of Somatic Hybrids of Ulva reticulata Forsskål (×) Monostroma oxyspermum (Kutz.)Doty. Front. Plant Sci. 6. doi: 10.3389/fpls.2015.00003
Hay M. E. (1981). The functional morphology of turf-forming seaweeds: persistence in stressful marine habitats. Ecology 62, 739–750. doi: 10.2307/1937742
Hays C. G. (2007). Adaptive phenotypic differentiation across the intertidal gradient in the alga Silvetia compressa. Ecology 88, 149–157. doi: 10.1890/0012-9658(2007)88[149:APDATI]2.0.CO;2
Jeffrey S. T., Humphrey G. F. (1975). New spectrophotometric equations for determining chlorophylls a, b, c1 and c2 in higher plants, algae and natural phytoplankton. Biochem. Physiol. Pflanz. 167, 191–194. doi: 10.1016/S0015-3796(17)30778-3
Jha B., Reddy C. R. K., Thakur M. C., Rao M. U. (2009). Seaweeds of India: the diversity and distribution of seaweeds of Gujarat coast (Dordrecht, The Netherland: Springer), 215 pp.
Jiang H., Gao K., Helbling E. W. (2008). UV-absorbing compounds in Porphyra haitanensis (Rhodophyta) with special reference to effects of desiccation. J. Appl. Phycol. 20, 387–395. doi: 10.1007/s10811-007-9268-2
Kamiya M., Inoue N., Suzuki C., Abe S. (2021). Ecological, physiological, and biomechanical differences between gametophytes and sporophytes of Chondrus ocellatus (Gigartinales, Rhodophyta). J. Phycol. 57, 1590–1603. doi: 10.1111/jpy.13193
Kavale M. G., Yadav A., Mantri V. A. (2021). Initial comparison between monoline and tube net method of farming in red agarophyte Gracilaria dura: Evidence from hydrodynamic CFD simulations. Aquaculture 536, 736485. doi: 10.1016/j.aquaculture.2021.736485
Khaleghi A., Naderi R., Brunetti C., Maserti B. E., Salami S. A., Babalar M. (2019). Morphological, physiochemical and antioxidant responses of Maclura pomifera to drought stress. Sci. Rep. 9, 19250. doi: 10.1038/s41598-019-55889-y
Kowalczewski P.Ł., Radzikowska D., Ivanišová E., Szwengiel A., Kačániová M., Sawinska Z. (2020). Influence of abiotic stress factors on the antioxidant properties and polyphenols profile composition of green barley (Hordeum vulgare L.). Int. J. Mol. Sci. 21, 397. doi: 10.3390/ijms21020397
Kumar M., Gupta V., Trivedi N., Kumari P., Bijo A. J., Reddy C. R. K., et al. (2011). Desiccation induced oxidative stress and its biochemical responses in intertidal red alga Gracilaria corticata (Gracilariales, Rhodophyta). Environ. Exp. Bot. 72, 194–201. doi: 10.1016/j.envexpbot.2011.03.007
Kusvuran S., Kiran S., Ellialtioglu S. S. (2016). “Antioxidant Enzyme Activities and Abiotic Stress Tolerance Relationship in Vegetable Crops,” in Abiotic and Biotic Stress in Plants. Eds. Shanker A. K., Shanker C. (IntechOpen). doi: 10.5772/60477
Mantri V. A., Shah Y., Thiruppathi S. (2020). Feasibility of farming the agarose-yielding red alga Gracilaria dura using tube-net cultivation in the open sea along the Gujarat coast of NW India. Appl. Phycol. 1, 12–19. doi: 10.1080/26388081.2019.1648181
Mantri V. A., Thakur M. C., Kumar M., Reddy C. R. K., Jha B. (2009). The carpospore culture of industrially important red alga Gracilaria dura (Gracilariales, Rhodophyta). Aquaculture 297, 85–90. doi: 10.1016/j.aquaculture.2009.09.004
Marinho G. S., Sørensen A. D. M., Safafar H., Pedersen A. H., Holdt S. L. (2019). Antioxidant content and activity of the seaweed Saccharina latissima: A seasonal perspective. J. Appl. Phycol. 31, 1343–1354. doi: 10.1007/s10811-018-1650-8
Matta J., Chapman D. (1991). Photosynthetic responses and daily carbon balance ofColpomenia peregrina: Seasonal variations and differences between intertidal and subtidal populations. Mar. Biol. 108, 303–313. doi: 10.1007/BF01344345
McHugh D. J. (2003). A guide to the seaweed industry. FAO Fisheries Technical Paper. No. 441 (Rome: FAO), 105p.
Meena R., Chaudhary J. P., Agarwal P. K., Maiti P., Chatterjee S., Raval H. D., et al. (2014). Surfactant-induced coagulation of agarose from aqueous extract of Gracilaria dura seaweed as an energy-efficient alternative to the conventional freeze–thaw process. RSC. Adv. 4, 28093–28098. doi: 10.1039/C4RA04476B
Miller S. M., Hurd C. L., Wing S. R. (2011). Variations in growth, erosion, productivity, and morphology of Ecklonia radiata (Alariaceae; Laminariales) along a fjord in southern New Zealand. J. Phycol. 47, 505–516. doi: 10.1111/jpy.2011.47.issue-3
Mueller R., Fischer A. M., Bolch C. J., Wright J. T. (2015). Environmental correlates of phenotypic variation: do variable tidal regimes influence morphology in intertidal seaweeds? J. Phycol. 51, 859–871. doi: 10.1111/jpy.12329
Overrein M. M., Tinn P., Aldridge D., Johnsen G., Fragoso G. M. (2024). Biomass estimations of cultivated kelp using underwater RGB images from a mini-ROV and computer vision approaches. Front. Mar. Sci. 11. doi: 10.3389/fmars.2024.1324075
Papageorgiou G. C., Govindjee (2014). “The Non-Photochemical Quenching of the Electronically Excited State of Chlorophyll a in Plants: Definitions, Timelines, Viewpoints, Open Questions,” in Non-Photochemical Quenching and Energy Dissipation in Plants, Algae and Cyanobacteria. Advances in Photosynthesis and Respiration, vol. 40 . Eds. Demmig-Adams B., Garab G., Adams W. III, Govindjee (Dordrecht, Springer). doi: 10.1007/978-94-017-9032-1_1
Prasad K., Das A. K., Oza M. D., Brahmbhatt H., Siddhanta A. K., Meena R., et al. (2010). Detection and quantification of some plant growth regulators in a seaweed-based foliar spray employing a mass spectrometric technique sans chromatographic separation. J. Agric. Food Che. 58, 4594–4601. doi: 10.1021/jf904500e
Prieto P., Pineda M., Aguilar M. (1999). Spectrophotometric quantitation of antioxidant capacity through the formation of a phosphomolybdenum complex: specific application to the determination of vitamin E. Anal. Biochem. 269, 337–341. doi: 10.1006/abio.1999.4019
Raikar S. V., Iima M., Fujita Y. (2001). Effect of temperature, salinity and light intensity on the growth of Gracilaria spp. (Gracilariales, Rhodophyta) from Japan, Malaysia and India. Indian. J. Mar. Sci. 30, 98–104.
Reddy C. R. K., Baghel R. S., Trivedi N., Kumari P., Gupta V., Prasad K., et al. (2018). U.S. Patent No. 10,000,579 (Washington, DC: U.S. Patent and Trademark Office).
Ruban A. V. (2016). Nonphotochemical Chlorophyll fluorescence quenching: mechanism and effectiveness in protecting plants from photodamage. Plant Physiol. 170, 1903–1916. doi: 10.1104/pp.15.01935
Sambhwani K., Mathukiya G., Dawange P., Sequeira R. A., Prasad K., Mantri V. A. (2022). Analysis of functional traits in Gracilaria dura (Rhodophyta: Gracilariacae) reveals variation in wild and farmed populations. J. Appl. Phycol. 34, 1017–1031. doi: 10.1007/s10811-022-02697-z
Sambhwani K., Modi J., Singhala A., Bramhabatt H., Mishra A., Mantri V. A. (2020). Analysis of functional traits in female gametophytic and tetrasporophytic life phases of industrially important red alga Gracilaria dura (Rhodophyta: Gracilariacae). J. Appl. Phycol. 32, 1961–1969. doi: 10.1007/s10811-020-02116-1
Saminathan K. R., Ashok K. S., Veeragurunathan V., Mantri V. A. (2015). Seedling production in the industrially important agarophyte Gracilaria dura (Gracilariales, Rhodophyta). J. Appl. Phycol. 27, 1541–1548. doi: 10.1007/s10811-014-0450-z
Sampath-Wiley P., Neefus C. D. (2007). An improved method for estimating R-phycoerythrin and R-phycocyanin contents from crude aqueous extracts of Porphyra (Bangiales, Rhodophyta). J. Appl. Phycol. 19, 123–129. doi: 10.1007/s10811-006-9118-7
Savchenko T., Tikhonov K. (2021). Oxidative stress-induced alteration of plant central metabolism. Life (Basel). 11, 304. doi: 10.3390/life11040304
Scrosati R., Dewreede R. E. (1998). The impact of frond crowding on frond bleaching in the clonal intertidal alga Mazzaella cornucopiae (Rhodophyta, Gigartinaceae) from British Columbia, Canada. J. Phycol. 34, 228–232. doi: 10.1046/j.1529-8817.1998.340228.x
Scrosati R. A., MacDonald H. L., Córdova C. A., Casas G. N. (2020). Length and biomass data for Atlantic and Pacific seaweeds from both hemispheres. Front. Mar. Sci. 7, 592675. doi: 10.3389/fmars.2020.592675
Shah Y., Rathod M., Kavale M. G., Jaiswar S., Mantri V. A. (2022). Socio-demographic profiling and asset indicators of Gracilaria dura farmers from northern west coast of India useful for longitudinal analysis. Aquacult. Int. 30, 273–287. doi: 10.1007/s10499-021-00797-0
Shah Y., Yadav A., Kumar M. A., Kavale M. G., Prasad K., Mantri V. A. (2021). ‘Proof of concept’ of how tube-net diameter affects growth and agar content in industrially important farmed red seaweed Gracilaria dura. J. Appl. Phycol. 33, 2349–2358. doi: 10.1007/s10811-021-02443-x
Skriptsova A. V., Nabivailo Y. V. (2009). Comparison of three gracilarioids: growth rate, agar content and quality. J. Appl. Phycol. 21, 443–450. doi: 10.1007/s10811-008-9389-2
Smith C., Berry J. (1986). Recovery of photosynthesis after exposure of intertidal algae to osmotic and temperature stresses: comparative studies of species with differing distributional limits. Oecologia 70, 6–12. doi: 10.1007/BF00377105
Tarakhovskaya E. R., Maslov Y. I., Shishova M. F. (2007). Phytohormones in algae. Russ. J. Plant Physiol. 54, 163–170. doi: 10.1134/S1021443707020021
Tietz S., Hall C. C., Cruz J. A., Kramer D. M. (2017). NPQ(T): a chlorophyll fluorescence parameter for rapid estimation and imaging of non-photochemical quenching of excitons in photosystem-II-associated antenna complexes. Plant Cell Environ. 40, 1243–1255. doi: 10.1111/pce.12924
Usandizaga S., Camus C., Kappes J. L., Guillemin M. L., Buschmann A. H. (2019). Effect of temperature variation in Agarophyton Chilensis: Contrasting the response of natural and farmed populations. J. Appl. Phycol. 31, 2709–2717. doi: 10.1007/s10811-019-1757-6
Valero M., Guillemin M. L., Destombe C., Jacquemin B., Gachon C. M., Badis Y., et al. (2017). Perspectives on domestication research for sustainable seaweed aquaculture. Perspect. Phycol. 4, 33–46. doi: 10.1127/pip/2017/0066
Veeragurunathan V., Eswaran K., Saminathan K. R., Mantri V. A., Ajay G., Jha B. (2015). Feasibility of Gracilaria dura cultivation in the open sea on the South-eastern coast of India. Aquaculture 438, 68–74. doi: 10.1016/j.aquaculture.2015.01.009
Vieira V.M.N.C.S. (2012). Permutation tests to estimate significances on Principal Components Analysis. Comput. Ecol. Software. 2, 103–123.
Vieira V.M.N.C.S., Creed J. (2013a). Estimating significances of differences between slopes: A new methodology and software. Comput. Ecol. Software. 3, 44–52.
Vieira V.M.N.C.S., Creed J. (2013b). Significances of differences between slopes: An upgrade for replicated time series. Comput. Ecol. Software. 3, 102–109.
Vieira V.M.N.C.S., Engelen A. H., Huanel O. R., Guillemin M. L. (2018a). Haploid females in the isomorphic biphasic life-cycle of Gracilaria Chilensis excel in survival. BMC Evol. Biol. 18, 174. doi: 10.1186/s12862-018-1285-z
Vieira V.M.N.C.S., Engelen A. H., Huanel O. R., Guillemin M. L. (2018b). Differentiation of haploid and diploid fertilities in Gracilaria Chilensis affect ploidy ratio. BMC Evol. Biol. 18, 183. doi: 10.1186/s12862-018-1287-x
Vieira V.M.N.C.S., Engelen A. H., Huanel O. R., Guillemin M.-L. (2021). Differential frond growth in the isomorphic haploid-diploid red seaweed Agarophyton Chilense by long-term in situ monitoring. J. Phycol. 57, 592–605. doi: 10.1111/jpy.13110
Vieira V.M.N.C.S., Engelen A. H., Huanel O. R., Guillemin M.-L. (2022). An individual-based model of the red alga agarophyton Chilense unravels the complex demography of its intertidal stands. Front. Ecol. Evol. 10, 797350. doi: 10.3389/fevo.2022.797350
Vignesh M., Kazi M. A., Rathore M. S., Kavale M. G., Dineshkumar R., Mantri V. A. (2020). Artificial neural network modelling for seedling regeneration in Gracilaria dura (Rhodophyta) under different physiochemical conditions. Plant Cell Tissue Organ Cult. (PCTOC). 143, 583–591.
Williams S., Dethier M. (2005). High and dry: Variation in net photosynthesis of the intertidal seaweed Fucus gardneri. Ecology 86, 2373–2379. doi: 10.1890/04-1569
Wright J., Williams S., Dethier M. (2004). No zone is always greener: Variation in the performance of Fucus gardneri embryos, juveniles and adults across tidal zone and season. Mar. Biol. 145, 1061–1073. doi: 10.1007/s00227-004-1399-2
Keywords: aquaculture, functional traits, plant growth hormones, morphology, Rhodophyta
Citation: Vieira VMNCS, Dawange PS, Jaiswar S, Sardinha JP and Mantri VA (2024) Adaptation of functional traits in Gracilaria dura with the local environment: implications for resource management and exploitation. Front. Mar. Sci. 11:1397379. doi: 10.3389/fmars.2024.1397379
Received: 07 March 2024; Accepted: 04 June 2024;
Published: 26 June 2024.
Edited by:
Xinxin Wang, Fisheries and Aquaculture Research (Nofima), NorwayReviewed by:
Sasi Nayar, South Australian Research and Development Institute, AustraliaCopyright © 2024 Vieira, Dawange, Jaiswar, Sardinha and Mantri. This is an open-access article distributed under the terms of the Creative Commons Attribution License (CC BY). The use, distribution or reproduction in other forums is permitted, provided the original author(s) and the copyright owner(s) are credited and that the original publication in this journal is cited, in accordance with accepted academic practice. No use, distribution or reproduction is permitted which does not comply with these terms.
*Correspondence: Vaibhav A. Mantri, dmFpYmhhdkBjc21jcmkucmVzLmlu; Vasco M. N. C. S. Vieira, dmFzY28udmllaXJhQHRlY25pY28udWxpc2JvYS5wdA==; Santlal Jaiswar, c2FudGxhbEBjc21jcmkucmVzLmlu
Disclaimer: All claims expressed in this article are solely those of the authors and do not necessarily represent those of their affiliated organizations, or those of the publisher, the editors and the reviewers. Any product that may be evaluated in this article or claim that may be made by its manufacturer is not guaranteed or endorsed by the publisher.
Research integrity at Frontiers
Learn more about the work of our research integrity team to safeguard the quality of each article we publish.