- 1Centro de Investigación en Ecosistemas de la Patagonia (CIEP), Coyhaique, Chile
- 2Department of Earth, Environmental and Life Sciences (DISTAV), University of Genoa, Genoa, Italy
- 3Centro Tecnológico para la Acuicultura (CTPA) Putemún, Instituto de Fomento Pesquero, Castro, Chile
- 4Departamento de Ingeniería en Obras Civiles, Facultad de Ingeniería y Ciencias, Universidad de La Frontera, Temuco, Chile
- 5Centro de Investigación: Dinámica de Ecosistemas Marinos de Altas Latitudes (FONDAP-IDEAL), Punta Arenas, Chile
Global estimates of the supply of dissolved and suspended materials to the ocean, in order to be relevant at either political or ecological scales, belie a finer-scale analysis necessary for understanding specific terrestrial-marine interactions. This is especially true for continental runoff to the marine critical zone of inland fjords and channels, where mechanisms, drivers, and predictions need to be elaborated in the context of changing land use and shifting climate forcing. In fjords in south-western Patagonia, runoff from small coastal and large continental basins (~310 x103 km2), sourced from a diverse geography and wide climatic gradient (<150 – 6,000+ mm/year), correspond with a very low density of hydrological and water quality observations. Based on the recently developed regional runoff model (FLOW), we estimated the coastal freshwater discharges and characterized flow-weighted sourcing (land use-cover type, climate, glaciers/geology, and soil province) for Pacific drainages from 41° to 56° south latitude. An estimated 692 km3/year (mean across 1979-2018), or 2% of worldwide total, is more than 85% of previous estimates for the much larger Pacific South American input. Based on limited water quality observations and inference from runoff sourcing, we predict general patterns of export for four groups of continental resources important for marine productivity, including: significant regional variation in flow seasonality, a N-S gradient in declining input of silicic acid and increased glacial input of sediment and iron, and potential shift in dissolved organic matter input sources from rainforest (potentially labile) to peatlands (refractory). Finally, we emphasize the temporal and spatial consequences of near-reference condition river runoff for marine ecosystem productivity and function in the Patagonian fjords, with specific recommendations for water quality standards and sustained monitoring for coupled river and marine ecosystems.
1 Introduction
Rivers are an essential connection between terrestrial and marine ecosystems, with annual global coastal discharges estimated between 36 to 45 x 103 km3 of freshwater together with an estimated 20 billion tons of particulate and dissolved solids (Oki and Kanae, 2006; Milliman and Farnsworth, 2011). The significant riverine export affecting channels and fjords creates a highly variable freshwater-brackish lens overlying more uniform near-seawater salinity (Calvete and Sobarzo, 2011; Schneider et al., 2014). This vertical structure in water column salinity (Calvete and Sobarzo, 2011; Schneider et al., 2014), also corresponds with temperature and physical stability (Schneider et al., 2014), biogeochemistry (Vandekerkhove et al., 2016; Torres et al., 2020), particulate load (Vandekerkhove et al., 2020; Amann et al., 2022) and ultimately light availability (Marín et al., 2013).
All of these effects have continental origins: the quality and chemical composition of continental waters, the array of dissolved and suspended constituents that flow from land to sea, begins with the evolution of dilute precipitation inputs in contact with the land, initially through weathering, leaching and erosion processes. Land forms, vegetation, soils, and corresponding physical conditions and alterations from land use may generate distinct water quality signatures at the source. Subsequently, the transformation of these initial sources may occur through physical, chemical and biological reactions, degradation and complexation, along variable hydrologic networks and flow paths, and over short to long residence times. From these complex origins, continental runoff may generate a wide range of elements, complexes and diversity of dissolved organic compounds, subsequently exported by rivers to coastal areas. In simpler terms, we recognize four principal classes of riverine export relevant to marine ecosystems: (1) macronutrients (e.g. Nitrogen-N and Phosphorous-P); (2) resources (dissolved silica and carbon); (3) limiting trace elements (esp. bioavailable iron), and; (4) suspended sediments.
Inorganic macronutrients (NO3-, NO2-, NH4+; and PO4+3 being the most readily available for assimilation) play a crucial role in limiting primary productivity within ecosystems, while excessive loading may drive tipping points in marine ecosystems at large scales (Voss et al., 2011; Heinze et al., 2021). Dissolved silica (dSi) is an important resource essential for marine diatoms, while a regional dSi deficit (Si*; Torres et al., 2014) may constitute a critical factor in determining the proportion of diatom production vs potentially harmful algal taxa (Torres et al., 2014). Organic carbon exported from rivers provides energy, via Particulate Organic Matter (POM) inputs to benthic communities (Quiroga et al., 2013, 2016), or Dissolved Organic Carbon (DOC) contribution to microbial production. Dissolved iron (dFe) could modulate the ability of diatom proliferations to use organic macronutrients during early spring (Torres et al., 2020, 2023), and like dSi, is largely sourced from river inputs. Finally, suspended sediment inputs, sourced from glacial meltwater or soil erosion, may simultaneously supplement or inhibit marine productivity, via light regime (Marín et al., 2013) or substrate, in the latter example often co-occurring with POM inputs (Quiroga et al., 2016).
There has been a noteworthy proliferation of global estimates of resource export to marine systems: particulate C and N (Beusen et al., 2005), dissolved N (Bouwman et al., 2005; Dumont et al., 2005), P (Seitzinger et al., 2005), dissolved silica (dSi; Beusen et al., 2009), and total alkalinity (AT; Fry et al., 2015). Nevertheless, the most comprehensive global synthesis of riverine export, Milliman and Farnsworth (2011), highlights a data deficit at regional scales, for example, estimating basic suspended sediment export (<5 rivers with reliable or comprehensive data are available for the western Andes). According to these authors, potentially over 200 x106 t/year of suspended sediments and 20 x106 t/year of dissolved solids are exported from rivers in southern Chile, approximately the same order of magnitude as any regional basin in South America except for the Amazon, and among the highest globally in terms of specific yield. The southern cone of South America (including Patagonia) is the largest land mass in the southern oceans (> 30°S, excluding Antarctica). Almost none of this area is included in the aforementioned global estimates of riverine export to marine systems, while continental scale estimates of yield lack validation from this region (van der Struijk and Kroeze, 2010).
The consequences of the land-sea interaction in south-western Patagonian fjords is illustrated by observations during an extreme drought in 2016, where a relation between reduced freshwater input and water column stability to harmful algal blooms (HAB) has been suggested (León-Muñoz et al., 2018). In general, however, interpretations of cause and effect between continental and marine systems in Patagonia have taken into account water quality only to a very limited extent (Torres et al., 2014), or causation suggested with limited evidence from the terrestrial or freshwater perspective (response to Vargas et al., 2018; see Torres et al., 2021). The often-highlighted importance of freshwater inputs on Patagonian marine fjords (Aracena et al., 2011; Torres et al., 2014; Cuevas et al., 2019; León-Muñoz et al., 2021), together with the corresponding limited insight into the magnitude, spatial distribution, seasonality and especially quality of raw freshwater coastal discharge, suggest the need for a general evaluation “state-of the science” and conceptual model for continental runoff at the regional and finer scale for Patagonia. The characterization of runoff sources in Patagonia, in terms dissolved and suspended materials, or even a basic comprehension of how runoff quality is associated with specific terrestrial characteristics such as soils, geology, land use/cover, and climate, for example, has not been considered. This is further underscored by data poor region in terms of water quality and time series hydrologic observations, especially with respect to near-coastal observations.
Our objective is to synthesize and advance a framework for dynamic runoff inputs to Patagonian fjords. To achieve this, we present and interpret the modeled runoff simulated by the hydrological FLOW model (Reche et al., 2021) developed by the Fisheries Development Institute (IFOP). The model provides a spatially segregated estimate of continental runoff to the fjords, along with an analysis of regional patterns in seasonality and spatially distributed estimates of runoff sourcing (land use, climate, geologic and soil origin). Together with a synthesis of publicly available water quality monitoring observations, we provide a conceptual model of latitudinal trends of runoff quantity and quality, and corresponding patterns of inland marine (fjord) productivity. Finally, we identify and discuss the patterns of export of key terrestrially derived nutrients, energy, and materials to the marine critical zone of south-western Patagonia.
2 Methods
2.1 Study site description
We define Pacific south-western Patagonia based on the combination of continental drainage basins, within the generalized Patagonia region, that are directly or indirectly linked to inland marine fjords, canals, and coastal waters, in turn associated with the Pacific Ocean (Figure 1A). The study area is similar to the one by Astorga et al. (2018) from a terrestrial perspective, and Torres et al. (2014) from a marine focus. The inland drainage area starts with the Río Petrohué basin (discharged at 41.5° S into the estuary of Reloncaví), continuing southward with several major trans-Andean catchments, including the Puelo, Yelcho, Palena, Cisnes, Aysén, Baker, Pascua and Serrano basins. The insular area starts in the north with the Inner Sea of Chiloé (41° S), bounded to the south by Cape Horn, Darwin Passage, and Magellan Straits (55° S). The site includes the political jurisdictions of the Los Lagos Region (Llanquihue, Palena, and Chiloé provinces), Aysén, and Magallanes Regions in Chile, and the Provinces of Chubut, Santa Cruz, and Tierra del Fuego in Argentina.
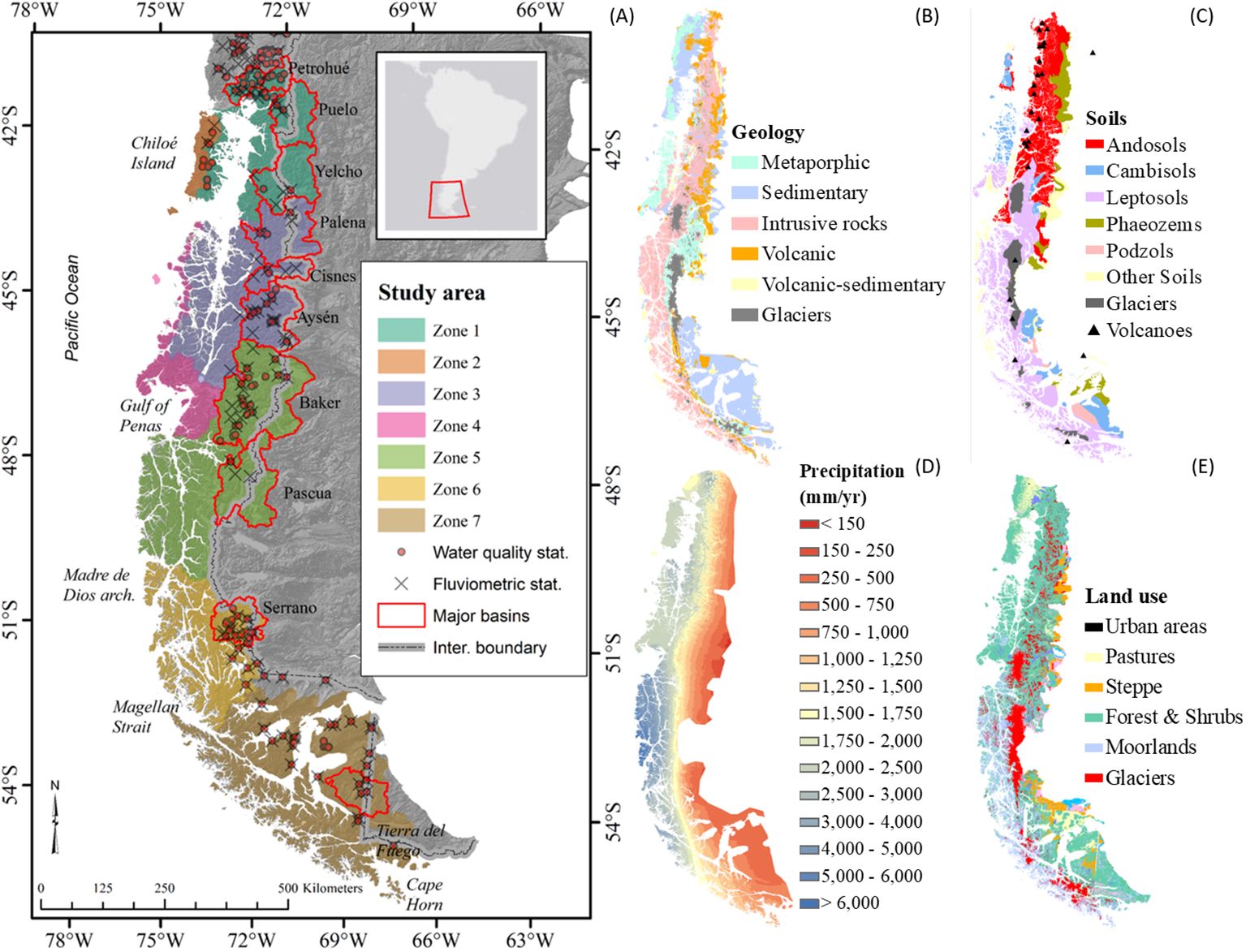
Figure 1. South-western (A) Patagonia, showing coastal watershed Zones 1-7, internal basin boundaries and major continental basins, based on the FLOW hydrologic modeling domain. (B) Geology classification (SERNAGEOMIN, 2003; SegemAR, 2021). (C) Soils classification (Dijkshoorn et al., 2014). (D) Precipitation classification (Fick and Hijmans, 2017). (E) Land use/cover classification (IGN, 2013; CONAF, 2016).
The geomorphology of this region is characterized by lower elevations of both the Cordilleran and coastal ranges of the southern Andes, along with relatively short fluvial courses from source to the sea. The central valley, effectively flooded, translates into an extensive network of fjords, channels, and islands and an extended coastline, one the most significant worldwide (Kelso and Patterson, 2010; Schneider et al., 2014). The region includes many of the major rivers in the western Andes, some of the largest lakes on the continent and deepest in the world, together with the largest temperate icefields globally (Reid et al., 2021).
With the exception of the northwest of Chiloe Island, the region was entirely covered by glaciers in the late Holocene (Davies et al., 2020). Bedrock geology is diverse (Figure 1B), ranging from sedimentary and metamorphic complexes in the coastal range extending from Chiloé island to the Taitao Peninsula (the principal hydrographic and biogeographic barrier, north of the Gulf of Penas), the Patagonia Batholith which dominates all latitudes (cordilleran systems in the north to the coastal range in the south), and metamorphic, sedimentary and volcanic formations in the cordillera and pre-cordillera for latitudes south of 45° S (see Torres et al., 2014 for further description). The region is also characterized by highly active volcanism, with Holocene volcanic formations frequently between 41-47° S (Figure 1C; SERNAGEOMIN, 2003) and associated with tephra-based soil series (Vandekerkhove et al., 2016). Elsewhere, pedogenesis is commonly based on regolith or unconsolidated quaternary material. Chonos Formation soils are mainly cambisols (coastal basins; 41-47° S), with clay minerals and iron oxides. The archipelago soils south of 47° S are thin, mainly leptosols with different origins due to parent material or vegetation presence. In the steppe, the predominant soils are podzols, cambisols, and phaeozems (Dijkshoorn et al., 2014).
Climate is strongly oceanic, precipitation driven by southern Westerlies and orography of the Andes Mountain ranges. The hyper-humid climate on the west slopes (5,000 to 6,000 mm/year on average, but extremes up to 10,000 mm/year) grades to cold steppe in the lee-slope rain shadow (< 300 mm/year), the two extremes often less than 50-100 km apart, considered among the most pronounced precipitation gradients on the planet (Figure 1D; Garreaud et al., 2013). The Southern Annular Mode (SAM) and El Niño/Southern Oscillation (ENSO), influence intra-seasonal and decadal scale precipitation variability (Aceituno et al., 2021). An extreme dry event in 2016 was produced by one of the strongest warm-phase ENSO records, combined with negative SAM (Blunden and Arndt, 2017), resulted in the lowest stream flows on record (Garreaud, 2018). Precipitation trends show a decrease of 20 mm per decade for stations in the Aysén region, while a positive trend above 50 mm-decade in the Magallanes region (Castro and Gironás, 2021), generally corresponding with regional predictions from global climate models (Garreaud et al., 2013). Mean temperature ranges from 10°C (41° S) to 5°C (55° S) with significant local variation across elevational gradients (Castro and Gironás, 2021). Vegetation in south-western Patagonia is highly correlated with the east-west climate gradient (Figure 1E; Luebert and Pliscoff, 2006). The hyper-humid climate is associated with a mixed broadleaf evergreen forest with some conifers (Donoso, 1993). More extreme environments exposed to oceanic influence are dominated by moorland, shrubs, Sphagnum bogs and other wetlands. Colder, drier and/or higher altitudes feature deciduous southern beech (Nothofagus spp.) forests. Eastward, the vegetation shifts to cold-steppe dominated by shrub and graminoid vegetation (Luebert and Pliscoff, 2006).
Land use alterations in Patagonia, resulting from very recent colonization in the last century, are generally limited: Astorga et al. (2021) identified 66,000 km2 of intact forested watersheds between 41-56° S. However, where impacts have occurred, the extreme effects, such as wildfires (Veblen et al., 1996) and the subsequent transformation of forests to rangeland, and more recent signs of forest recovery (Hernández-Moreno et al., 2023) are still plainly evident. Industrial forest management/plantations, mining, urbanization, roads, and associated interventions on the hydrologic cycle (dams, withdrawals) are all very limited in the region. Further information on land cover and land use impacts can be found in Astorga et al. (2018).
2.2 Land/sea zoning
Runoff zoning is based on a marine perspective (Pickard and Stanton, 1980), including differences between inner seas, channel zones, and offshore coasts, combined with a continental perspective based on the distribution/clusters of major basins (Figure 1A; Table 1). Zone 1 corresponds with the Inner Sea of Chiloé, including Reloncaví Fjord, with watersheds from inland and Chiloé island, and major basins Petrohué, Puelo, and Yelcho. Zone 2 comprises offshore Chiloé runoff, with Pudeto, Chepu, and Huillinco basins. Zone 3 corresponds with the Aysén Sea and associated fjords and channels, and includes the Palena, Cisnes, Aysén, and Exploradores basins (the latter originating from the Northern Patagonia Icefield, NPI). Offshore runoff from Guaitecas island group until the Taitao Peninsula and northern Gulf of Penas corresponds to Zone 4. Zone 5 comprises the inland catchments that discharge south of the Gulf of Penas until the north of the Madre de Dios archipelago (MDD): Rios Baker and Pascua are the most extensive basins and two of largest rivers in Chile, with runoff from the Northern and southern Patagonian Icefields (NPI and SPI). Zone 6 corresponds with the catchments from the Madre de Dios Archipelago to the outer Magellan Strait, with the Serrano basin as southernmost large cordilleran basin. Finally, Zone 7 starts with the Strait of Magellan until Cape Horn. The total area of the seven zones is 310 x103 km2 over 15° of latitude range (Table 1). A proposal for marine zoning for the northern fjords based on salinity (Leon-Muñoz, pers. com.), is comparable, albeit more finely segmented.
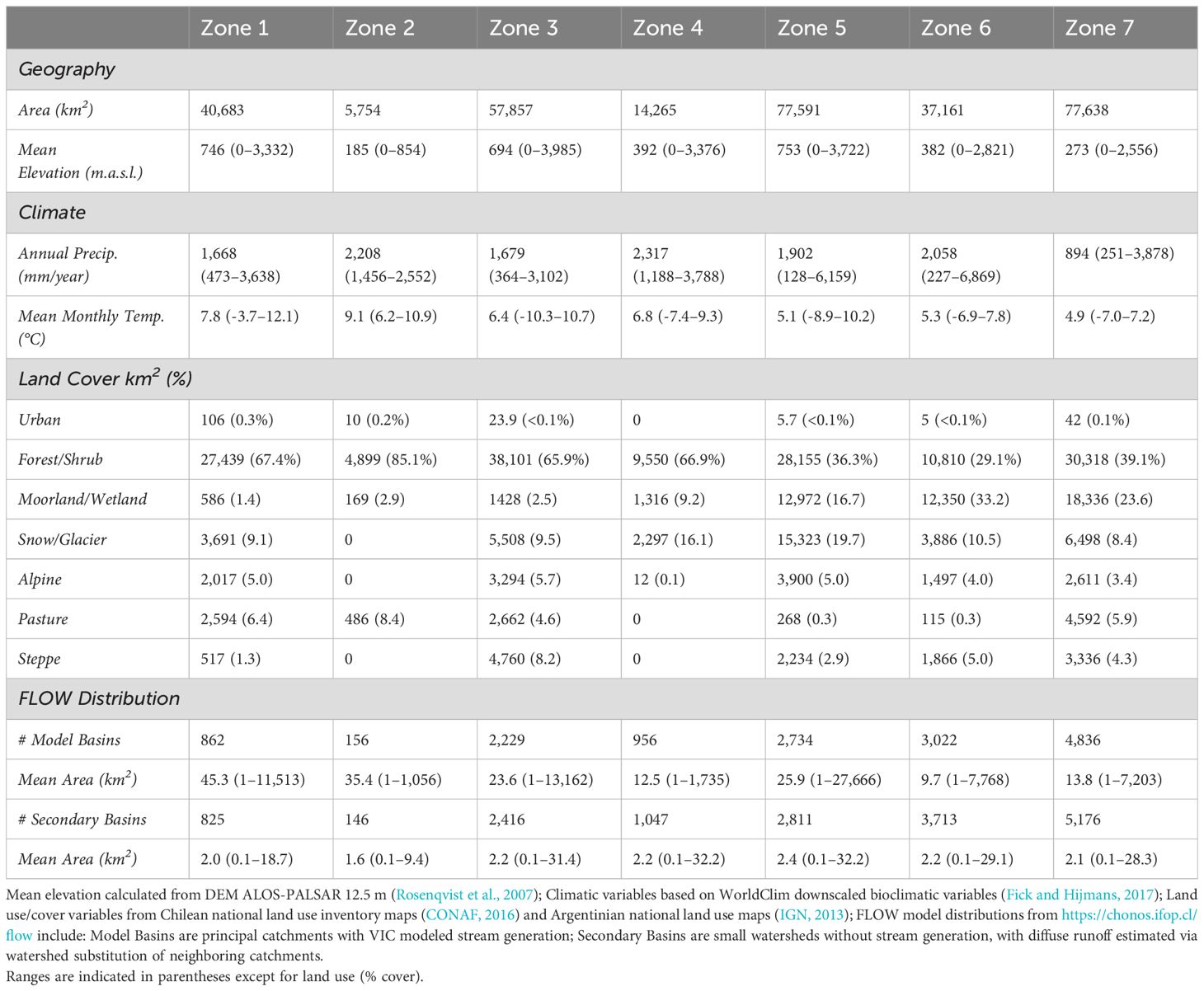
Table 1. Characterization of runoff zones based on geography, climate, land use/cover and FLOW model distribution (number, area and type of sub-basins).
2.3 Freshwater runoff estimation: FLOW platform
FLOW is a web tool that allows exploring and visualizing hydrological runoff simulation for southern Chilean fjords (https://chonos.ifop.cl/flow). The FLOW platform provides daily time series estimates of point runoff for major basins, including diffuse inputs (>1 km2; 31,220 coastal discharge points; 315,740 km2), over nearly 40 years (1979-2018). The platform also offers fractional estimates of glacial meltwater. Hydrologic simulations were performed with the widely used Variable Infiltration Capacity (VIC) model (Liang et al., 1994; Nijssen et al., 2001; Hamman et al., 2018), which solves energy and water balance equations driven by atmospheric forcing (examples from Chile include Demaria et al., 2013; Bozkurt et al., 2018; Fustos et al., 2022). VIC is currently used for the recently updated national water balance of the Chilean General Water Directorate (DGA, 2017; https://www.balancehidrico.uchile.cl/). A more detailed description of the FLOW platform and the parameterization, calibration and validation of the VIC model is presented in Supplementary Material.
2.4 Flow-weighted sourcing of freshwater runoff
FLOW discharges were assigned to respective runoff zones (see Figure 1A), and weighted with landscape attributes that influence biogeochemical characteristics, such as soils, geology, climate, and land use/cover following methodology from Marcus et al. (2024). Discharges weighted by geologic attributes were calculated from Chilean and Argentinian digital geologic maps (SERNAGEOMIN, 2003; SegemAR, 2021). For soils, flows were assigned to three general categories based on origin: Andosols/tephra, weathered regolith, and unconsolidated quaternary material (sources include the Soil and Terrain database for Latin America and the Caribbean - SOTERLAC; version 2.0, scale of 1:5 million; Dijkshoorn et al., 2014). Flow sourced by land use/cover was based on land use inventory maps (Argentina - IGN, 2013; Chile -CONAF, 2016) supplemented by moorland/wetland extent in the outer fjords based on Luebert and Pliscoff (2006). Flow sourced by climatic zones was determined from WorldClim downscaled annual precipitation (Fick and Hijmans, 2017) assigning humid, intermediate, and dry classes ranging from >1200 mm, 1200-600 mm, and <600 mm, respectively. Differences in the annual amount of runoff by zone were identified by analyses of variance, with the Holm method for p-values adjustment for multiple comparisons. All statistical analyses were performed in R software (Core-Team, 2017).
2.5 Surface water quality of rivers in Patagonia
Water quality observations are sparse for the region, either generally or specific to the four previously defined export functional categories (macronutrients, resources, trace nutrients and suspended load). We generated a synthesis based on the only existing time series of surface water quality observations for representative rivers in south-western Patagonia (establishing general boundaries and latitudinal/climate variation of resource/nutrient export) including all near-coastal discharges (DGA; https://snia.mop.gob.cl/BNAConsultas/reportes). Unless otherwise noted, parameter averages and variance were based on a minimum ten years of observations (2006 – 2015), often limited to 2-4 observations per year. We also compiled from the published scientific literature all relevant short-term water quality observations for coastal river discharges and corresponding near-shore marine surface water observations representing the seven zones (simultaneous observations wherever possible, but also including independent research efforts; sources noted in Supplementary Table 2).
2.6 Identification of nutrient/resource export for the region
A qualitative assessment of latitudinal patterns in runnoff quality was developed for substrate, resources, nutrients, and trace resources using the flow-weighted sourcing of freshwater runoff and an index for relative export magnitude, based on a weighting matrix (Supplementary Table 3). For each land unit class, we assigned a value of 0, 1 or 2, representing neutral, intermediate, and strong effects on the potential concentration of the respective export parameter. For example, for dSi, each catchment has its exportation index such as EIdSi= A1(CLAS1:COV1) +…+ Az(CLASx: COVy) where Az is the % cover for respective watershed coastal discharge z, as a function of x Cover Class (e.g. Soils, Climate, Vegetation) and y Cover type. Specific justification of the weighting matrix is detailed in the Supplementary Material.
Finally, by combining the latitudinal index of freshwater runoff quality, and examining existing water quality observations for corresponding zones (Table 2 and Suplementary Table 2), we were able to construct a conceptual model, illustrating the potential link between regional flow magnitude from continental runoff, latitudinal patterns in runoff quality, and corresponding patterns in marine productivity.
3 Results
3.1 Total and seasonal coastal freshwater runoff
The coastal runoff discharge in south-western Patagonia was estimated at 692 km3/year averaged over 40 years (95% CI [679.8,711.8]). Discharge magnitude varied by zone, a function of catchment size and precipitation (Figure 2). Zone 5 presented the highest runoff volume, with an average close to 190 km3/year (95% CI [186.1,196.0]), followed by Zone 3, with ~150 km3/year (95% CI [144.9,154.9]). Together with Zone 1, these inland basins also had a higher inter-annual dispersion in discharges (Figure 2). In contrast, those zones associated with coastal islands, Zones 2, 4, 6, and 7 showed relatively low variation between years. However, when we look at normalized discharges by area, Zone 7 is the lowest in terms of inter-annual variation (Supplementary Figure 1B). A summary of runoff for each watershed/zone/season are shown in the Supplementary Material. In terms of seasonal discharges, Zone 5 decreases in relative importance in winter, and is surpassed by Zone 3 (Figure 3). More generally, fall/winter runoff is highest and summer runoff lowest for zones 1 and 2 in the north, while the pattern is reversed in the south, where spring and summer discharges are highest (Figure 3B). The reduced inter-annual variation of zones associated with offshore discharge and coastal/island catchments is conserved at the seasonal time scale (sharp peak-shaped distributions for Zones 2 and 4; Figure 3). Slightly greater seasonal variance is evident from southernmost runoff in zones 6 and 7, although still much reduced compared to zones 3 and 5.
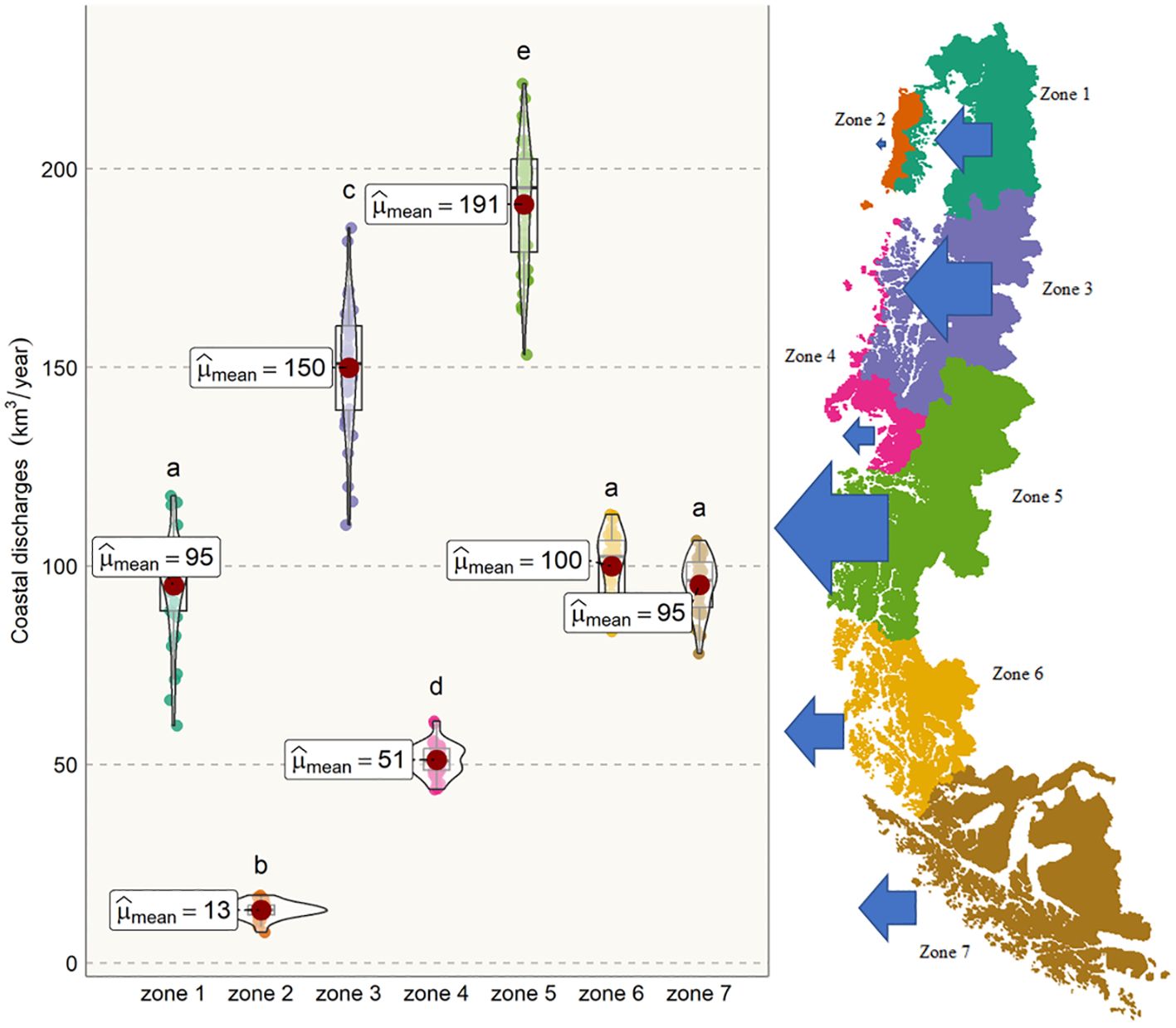
Figure 2. Box-violin plots of estimated mean annual freshwater runoff discharge distribution by zone, based on FLOW model outputs (1979 - 2018). Means and multiple pair-comparisons are indicated by letters, arrows in panel right represent relative magnitude of annual coastal discharges.
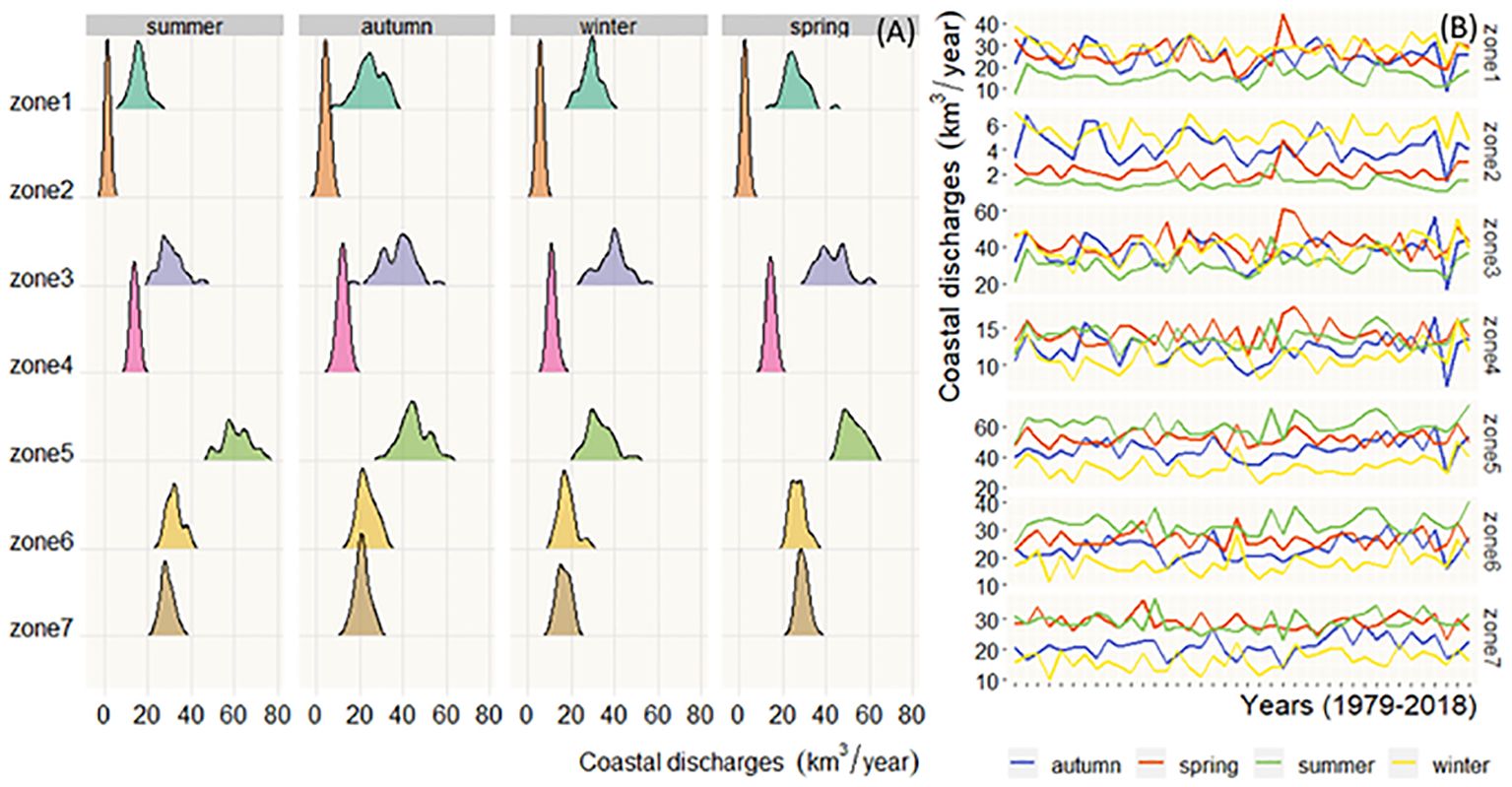
Figure 3. Seasonal discharges by Zone based on FLOW model outputs (1979 - 2018). (A) Distribution of coastal discharges. (B) Interannual variability of seasonal trends.
3.2 Source characterization of freshwater runoff
Source characterization of freshwater discharges based on climatic origin showed high variability across runoff zones. As expected, the most significant contributions to runoff originate from wet climates (i.e., precipitation >1200 mm; Figure 4). However, Zone 5 had a noteworthy influence from dry climate (rainfall <600 mm). The most austral Zone 7 was dominated by flows from intermediate and dry climate regions. Runoff from the more coastal Zones 2 and 4 were sourced exclusively from hyper-humid climates.
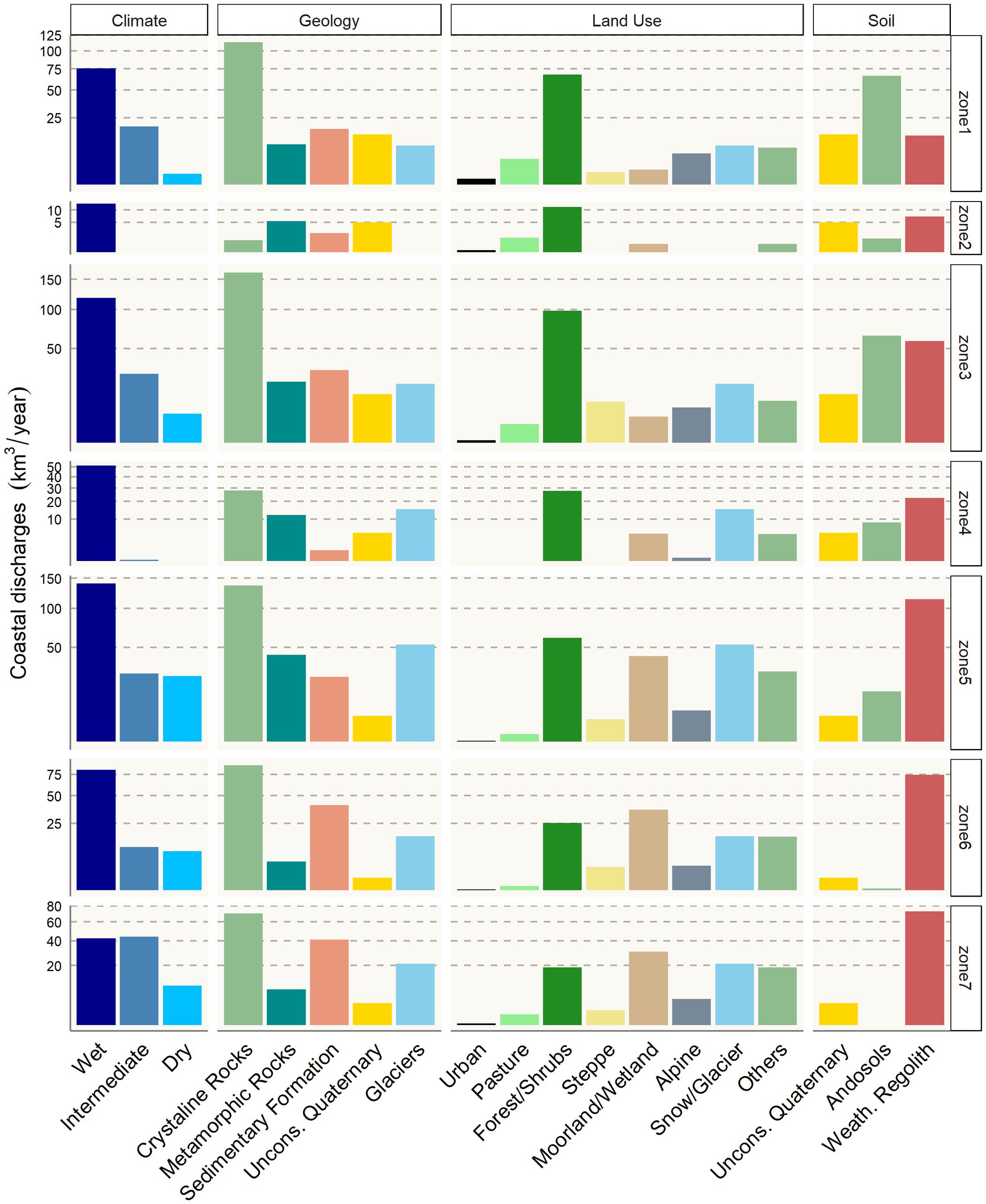
Figure 4. Flow-weighted runoff by climatic zones, geology, land use/cover, and soil attributes. Climate categories based on annual precipitation include: humid (>1200 mm), intermediate (600-1200 mm), and arid (<600 mm). Soil origin categorized by unconsolidated Quaternary/Holocene (alluvium, till, moraine etc.), Weathered Regolith (limited tephra deposition) and Andosol/tephra-based soil series. Note square root scale for the y axis.
In the case of lithologic origin of flow-weighted runoff sources, all zones are strongly influenced by crystalline rocks, mainly granitoids. However, distinct geologic characteristics of runoff source are present in Zone 2, where the most significant flows originate from metamorphic and unconsolidated quaternary sediments. Runoff from sedimentary formations is noteworthy for all except Zone 4, but with a general increase in proportional contribution to the south (Zone 5-7), areas that are also associated with relatively flat topography, dry climate and cold-steppe vegetation. Glacial sourced runoff, while most pronounced for Zone 5 (corresponding with the northern and southern Patagonian Icefields), is significant for all zones except Zone 2. Meanwhile, the contribution from unconsolidated quaternary formations shows a general decline in importance from north to south.
Forest is the primary land use/cover associated with runoff from Zone 1 to 5, and forested source areas are associated with an order of magnitude greater runoff from northern Zones 1-3. Runoff from moorlands, bogs and other wetlands begin to dominate overall runoff from the runoff from Zone 5 and southward. Alpine areas above tree line are present in most zones, but with noteworthy absence of alpine generated runoff from coastal Zones 2 and 4. In terms of runoff sources from land uses associated with more intensive human activities, pastures and urban areas contribute a very minor proportion throughout, although slightly elevated in northern Zones 1-3.
Runoff sourcing segregated by soils, indicate that Andosols (Figure 1C) are the most common soil class in the study area, dominating Zones 1 and 3. Soil origin also shows perhaps the strongest latitudinal trends compared to other runoff classifications, with northern zones dominated by volcanic tephra and unconsolidated material as origin for soil formation, while southern zone are dominated by weathered regolith and a near-complete absence of volcanic soils. Weathered regolith is also dominant in the coastal runoff zones (Zones 2 and 4) upwind of major volcanos (Figure 1C).
3.3 Runoff characterization: review of water quality observations for continental waters
Observations for representative rivers based on the Chilean national water quality monitoring program (Table 2; Supplementary Figure 2) demonstrate that Patagonian rivers are moderately to highly dilute (indicated by e.g. Specific conductivity < 100 μS/cm for most volcanic lithology, < 50 μS/cm for granitic catchments, and 10-20 μS/cm for coastal catchments). Specific conductivity may surpass 150 to 200 μS/cm in areas transitioning to cold steppe, which forms the headwaters of major rivers, or from more arid coastal climates with less precipitation (e.g. Zone 7; Table 2). Mildly basic systems (pH 7.5-8.5) are not unusual in arid pampas transitions, circum-neutral pH of 7 to 7.5 is common in the cordilleran range, while mildly acidic conditions pH 6-7 are typical of coastal catchments with high runoff (Table 2). Mean stream temperature is low for all systems, with minimum temperatures around 5°C in the north, near zero °C to the south, and maximum temperatures around 15°C across the latitudinal range.
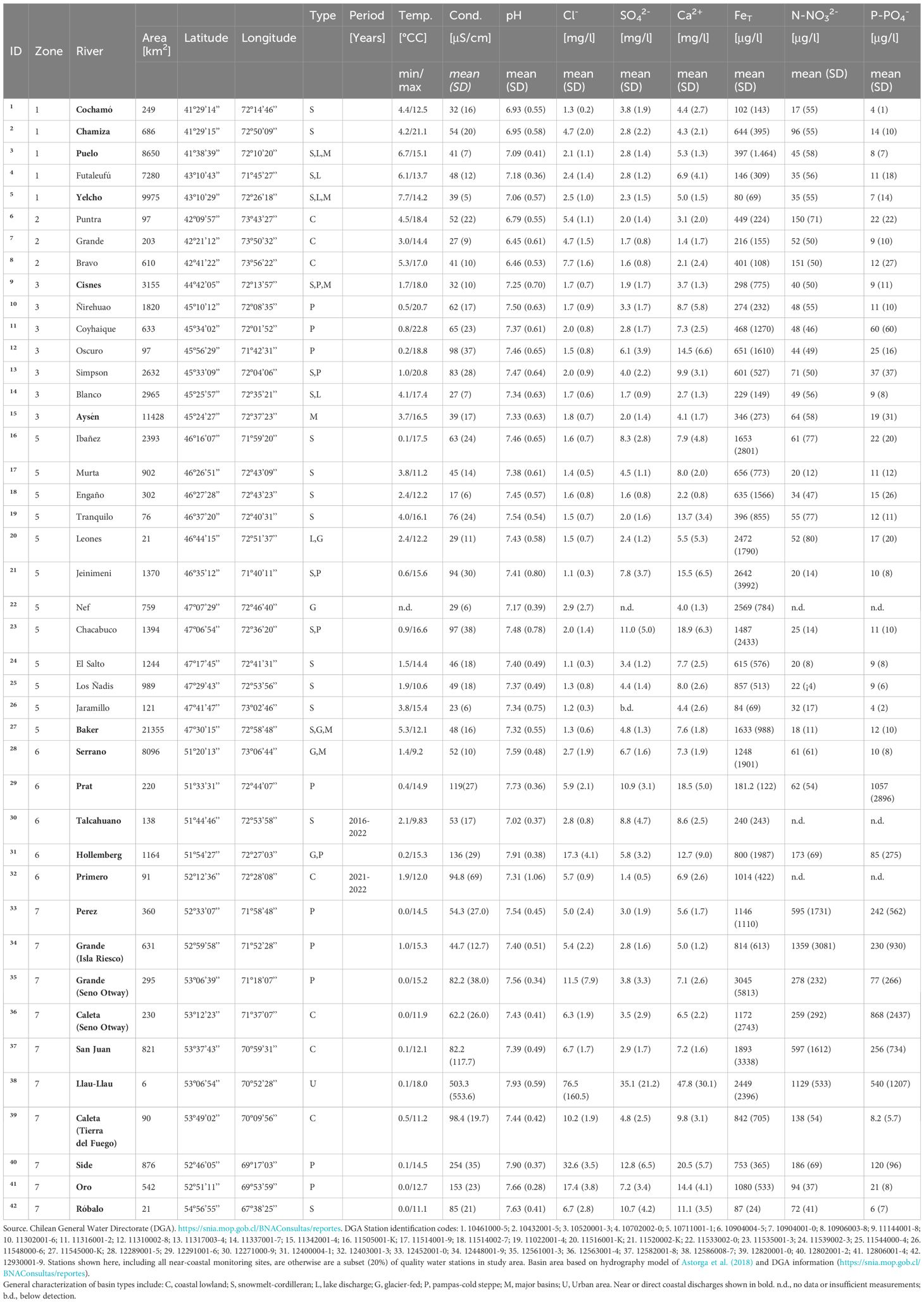
Table 2. Biogeochemical characterization of rivers in Patagonia by Zone, based on minimum 10 years of observations (approximately 2-4 observations per year between 2006 a 2015; exceptions noted in text and column period).
For macronutrients, concentrations of inorganic phosphorous of 2-10 ug/L P-PO4 are typical, while values 5-6 ug/L are common especially in granitic lithology or glacial or snowmelt origin in the cordillera. Phosphate values may be elevated by an order of magnitude for streams of arid origin (Coyhaique River in the Aysen River headwaters, and the coastal Side River; Table 2). Concentrations of inorganic nitrate of 30-60 ug/L N-NOx are evident for a wide range of rivers, being somewhat lower (20-40 ug/L) for coastal rivers, but otherwise limited trend in term of river origin, latitude, and also with high within-site variance (Table 2). Exceptions based on single locations indicate very low NOx for the glacially influenced Baker River (18 ± 11 ug/L; Zone 5), and high values for Puntra and Bravo Rivers (≈150 ug/L; Zone 2) and Side River (120 ± 96 ug/L; Zone 7). General north to south increases in concentration for some parameters are evident, including sulfate and calcium ions, and total iron (Table 2).
Note that these observations, the most comprehensive available for the region, include only 22 near-coastal observation stations, of which 15 are small coastal basins in the southern Zones 6 and 7. Six stations are major basins, contributing water and resources sourced across a widely varying geographic, climatic and hydrologic regime (detailed in the previous section). Many parameters important for understanding land-sea coupling are not available (e.g. dSi, DOC, dFe, NH4 and non-inorganic sources of macronutrients). Moreover, inorganic macronutrient observations were seldom reported after 2010-2011, and discontinued after 2015. This principal data source may be complemented by the summary of coastal river and fjord surface water quality observations based on the academic literature (Supplementary Table 2). These observations are much more intermittent, relating to synoptic or seasonal observations, only some of which consider both river runoff and marine end members simultaneously. Despite these limitations, it is evident that dSi, DOC and dFe are typically 1-2 orders of magnitude lower in marine zones compared to riverine sources, while macronutrient concentrations are more moderately elevated (generally no more than 2-4x) in marine waters. Observations on suspended sediments (in fjords) and NH4+ (in both systems) are almost non-existent. Finally, it is noted that only seven studies considered simultaneously both freshwater and marine observation (References 2, 4-6, 9, 13, 15 in Supplementary Table 2), only one of which represented at least 4 seasons, none with monthly or more frequent observations, and none of which represented more than half of the parameters of interest for coupled freshwater-marine systems.
Latitudinal patterns of nutrient and resource export for the region, including indices for TSS, dSi, DOC, inorganic nitrogen (N inorg.), total nitrogen (N total), inorganic phosphorus (P inorg.), total phosphorus (P total), and dFe, developed as a first iteration (Supplementary Figure 3) imply a potential similarity in large-scale behavior for export of TSS and dFe, with higher levels associated with continental basins. The dSi indices also implies a strong positive correlation with Zones 1 and 3. It is important to note that macronutrient inputs (N, P) from runoff are very low and are presumed negligible compared to internal marine cycling.
4 Discussion
The estimated mean annual discharge of 692 km3/year corresponds with 1.5 to 2% of world freshwater runoff depending on the source (Oki and Kanae, 2006; Milliman and Farnsworth, 2011). Milliman and Farnsworth (2011) estimate a 79.3 km3/year for south-western Patagonia (base on five major rivers) and 700 km3/year for the western Andes (Colombia to Chile). The significantly increased estimate is clearly a result of including simulated runoff from smaller coastal drainages (e.g. FLOW model). The results agree generally with Dávila et al. (2002), who found somewhat higher runoff of ~876 km3/year but with a larger area (35-55°S, including additional major rivers). A recent national assessment on climate change trajectory (Álvarez Garretón et al., 2023) suggests a somewhat lower runoff volume (e.g. the FLOW estimate for Patagonia is within the range of the national estimate of 680 +/- 16 km3/year). Direct comparison is hindered by scale and geographic extent (e.g. political vs. watershed boundaries for reporting precipitation inputs), together with different data sources, assumptions and modeling methodology. If anything, this indicates the level of uncertainty and range in error, notably unquantified (i.e. Winter, 1981) and the need for better quantitative estimates of coastal groundwater exchange and also land use effects on evapotranspiration.
Beyond this simple comparison in total volume, we address the more relevant regional factors of concentrated runoff to more confined inland marine fjords, extensive and geographically complex coastline, and high variability of runoff sources. South-western Patagonia coastline, estimated at 27.2 x 103 km, represents 34% of the South American continent (79.7 x 103 km; Natural-Earth, 2022). The “coast/area ratio” of 87.5 m/km2 for the study area, compared to 59.7 m/km2 for Chile and 0.004 m/km2 in South America (Natural-Earth, 2022), underscores the importance of regional characterization of terrestrial-freshwater-marine coupling, and site-specific relevance for near-shore or littoral marine ecosystems. In summary, terrestrial export, among the most significant of the Southern Ocean in terms of volume, are concentrated regionally in the fjord system, yet locally diffuse over a vast network of coastline. This combination is highlighted among the principal findings in the ensuing discussion: the high spatial variability and asynchronization of runoff (Sec. 4.1), together with the potential major spatial variation in runoff sourcing, contrasts with the severely limited level of knowledge on runoff quality (Sec. 4.2). The latter, essential for understanding regional scale patterns of fjord productivity (Sec. 4.3), is illustrated here as a conceptual model of land-sea forcing of continental runoff on the Patagonia fjords. Finally, we summarize and comment (Sec. 4.4) on the implications for policy, management and future investigations on the land-sea continuum of southern Patagonia fjords.
4.1 Spatial and temporal runoff variability
Runoff timing and seasonality will result in varied continental forcing of marine systems depending on the zone. For example, interannual hydrologic variability is much more evident in Zones 1, 3 and 5 corresponding with extensive continental basins such as Puelo, Yelcho, Palena, Aysén, Baker and Pascua basins. As a consequence, corresponding inland marine areas linked to continental systems are potentially more susceptible to drought influences on both physical (density) stratification, and also where water quality of inputs may be linked to flow (often the case for suspended sediments), potentially sensitive to drought-induced reduction in resource/nutrient loading (see Figure 2). Meanwhile, coastal systems (Zones 2, 4) have the least flow variation across years: heightened resilience to drought conditions might be expected here.
Similar latitudinal differences are evident in the seasonal patterns, coastal compared to continental zones. Coastal systems experienced significantly less variability throughout the seasons (see Figure 3). In the northern zones 1 and 2, summer discharges are lower due to less precipitation in rain-fed basins observed in small to medium-sized headwater streams (Sosnovsky et al., 2022; Garcia et al., 2023) and also large continental basins (León-Muñoz et al., 2023) in the same latitudes. Specifically, the oceanic watersheds of Chiloé (Zone 2) experience a prolonged period of low discharges between spring and summer. However, this behavior changes to the south, where certain homogeneity between seasons exists in the central zones 3 and 4. Summer discharge is the most important for the zones 5-7, noteworthy for an opposite seasonality compared to northern zones. In southern zones encompassing Zone 4 to 7, lower runoff levels characterize the winter season. Notably, Zone 5, housing the Baker/Pascua Rivers, exhibits elevated coastal freshwater discharges throughout most seasons, except for winter, whereas Zone 3 has the maximum winter runoff observed.
Precipitation interannual variability marked mainly by ENSO in its warm phase, generates dry anomalies in Patagonia with a reduction of runoff in summer and autumn (Garreaud et al., 2013). This effect is pronounced from Zone 1 to 4, ENSO response in southern zones, especially associated with archipelagos, is less pronounced, or otherwise poorly characterized in terms of magnitude and extent. Precipitation, and hence runoff, in Zones 5 to 7 is also influenced by SAM. The FLOW model estimated a record (across 40 years) of minimal coastal discharges in the autumn of 2016 (most pronounced in zones 1-4), corresponding with the extreme combined ENSO/SAM event of that year (Blunden and Arndt, 2017; León-Muñoz et al., 2018).
Although climate change effects on runoff patterns are expected, initial trends and rates are still unclear (Falvey and Garreaud, 2009). Global models predict a drying climate northward, more humid trends toward the south (Garreaud et al., 2013) although the rate and precise location of this transition, in the complex mountain geography of Patagonia, has not been evaluated adequately with regional climate modeling. Other studies report changes in precipitation (Boisier et al., 2018; León-Muñoz et al., 2018; Aguayo et al., 2021), reduced runoff of some rivers (León-Muñoz et al., 2018, 2021), reduced snowpack (Schaefer et al., 2015; Pérez et al., 2018; Aguayo et al., 2021), etc. A shift in runoff dominance from fall to spring, a potential consequence of declining annual runoff, seasonally declining fall rains together with only a somewhat less significant declining snowpack, has been observed for the Puelo basin (Zone 1; 41°S; León-Muñoz et al., 2021).
4.2 Freshwater runoff sourcing and potential consequences for export
The results and discussion so far have revealed considerable variability in the south-western Patagonian region’s flows and chemical characteristics. Although previous extrapolations of export to Patagonia fjords have been based on average concentrations and/or flows (van der Struijk and Kroeze, 2010; Marshall et al., 2021; Tornquist et al., 2024), a comprehensive analysis of this area cannot rely on averages, as the wide range of flows and sources significantly influences the concentration and load of continental subsidies. Additionally, the marine systems exhibit distinct features between small offshore (coastal) and inland (continental) basins, inner seas, channels, over a latitude range of 15 degrees. This variability is expected to amplify in future scenarios of global change, particularly in the climate and land use/cover changes specific to Patagonia.
The results showing 1-2 orders of magnitude greater resource concentration in rivers (e.g. dSi, DOC) and similarly elevated trace nutrients such as dFe, and suspended particulates (TSS) in runoff compared to zones, are perhaps the most prominent indication of strong potential land-sea coupling from runoff in Patagonia. Meanwhile, macronutrient concentrations are of the same order across riverine and fjord systems, albeit usually greater by several factors in marine waters. Based on these general end members, zonal water quality observations (Table 2; Supplementary Table 2), and a spatial distribution analysis of sources (Figure 4), together with the trends in exportation indices (Supplementary Figure 3) we propose a conceptual model illustrating the potential link between regional flow magnitude from continental runoff, latitudinal patterns in runoff quality, and corresponding patterns in marine productivity (see Figure 5).
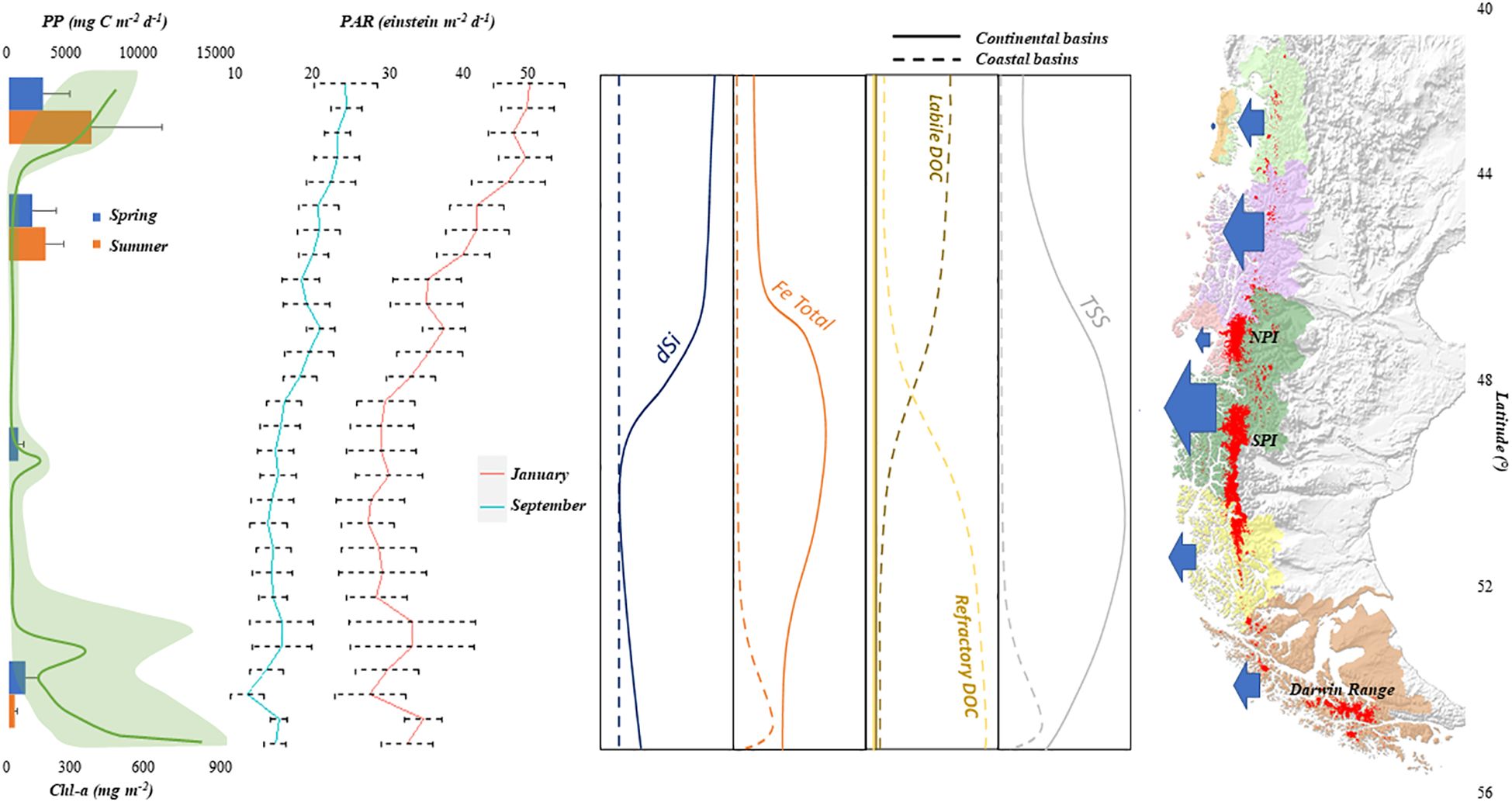
Figure 5. Conceptual model of the potential link between regional flow magnitude from continental runoff, latitudinal patterns in runoff quality, and corresponding latitudinal patterns in marine productivity. Left panel shows estimate of primary productivity (data from Aracena et al., 2011) and Chlorophyl A (redrawn from Cuevas et al., 2019). Middle-left panel shown the MODIS monthly (Jan and Sep) mean photosynthetically available radiation (PAR) at the sea surface (res. 4km). PAR is defined as the quantum energy flux from the Sun in the 400-700nm range from 2019-2023 (https://oceancolor.gsfc.nasa.gov/l3/order/). Middle-right panel shown general latitudinal trends in concentration for significant subsidies/drivers of marine systems for both coastal and continental sources (e.g. Table 2). Note that macronutrient inputs (N, P) from runoff are not shown, and are presumed negligible compared to internal cycling. Right panel shows regional runoff inputs, with size of arrows proportional to annual flow magnitude (Figure 2). Glaciers/Ice Fields shown in red. Note also that latitudinal subsidies showing concentration may diverge from seasonal or annual load based on sign of exponent of the Q:C function (see text).
In term of export of suspended material (TSS), glacial sources are the most widely observed riverine sources (Supplementary Table 2). Figure 5 shows a maximum input for mid latitudes (Zone 5), corresponding with continental meltwater from NPI and SPI discharges in continental basins and the Darwin Mountain ranges in coastal basins (Zone 7). These flows, characterized by higher TSS concentrations, are predominantly observed in spring and summer meltwater peaks for snow and glaciers, respectively. Despite the increased radiation levels during these seasons (see Figure 5), the elevated concentration of TSS will diminish light availability (Vandekerkhove et al., 2020; Amann et al., 2022), resulting in lower levels of primary productivity. Overall, spatial patterns and finer-scale dynamics of light vs. nutrients in the river-estuarine mixing zone are highly complex and poorly understood. Nevertheless, Modenutti et al. (2013) suggest the possibility of runoff turbidity (or the TSS) having both inhibitory and promoting effects, depending on the local context. A similar pattern is expected for trace elements such as dFe, coinciding with high TSS load and/or glacial input (Serrano and Nef Rivers; Table 2). Consequently, dFe is predicted to show a pattern similar to TSS on a macro scale (Figure 5) although with local deviations from this pattern which may be variably and unpredictably sourced by local geology, groundwater flow paths, and reduced conditions associated with wetland soils.
For materials such as dissolved silica (dSi), concentrations in Patagonia are substantially higher than global means, higher levels sourced from the weathering of volcanic glass compared to weathering of regolith or glacial material (Pedrozo and Chillrud, 1998). Additionally, dSi levels may be substantially greater as a function of depth of tephra soil horizons (Vandekerkhove et al., 2016), potentially significant in the northern half of the region dominated by active volcanos (Figure 1C) and volcanic andosols (Figure 4). Figure 5 therefore predicts higher levels of dSi in river export (Zones 1, 3, 5), but with exceptions on the leeward side of the volcanic sources (Zones 2, 4) and dilution by glacial meltwater (Zone 4). This pattern corresponds with the latitudinal range of zero-salinity end members shown in Torres et al. (2014), and the same general trend in marine dSi (Beusen et al., 2009). In terms of the dominant form of carbon (DOC) as both material and energy export, limited local observations (Supplementary Table 2) suggest elevated DOC for coastal basins characterized by humid climate and evergreen forest (Curra-Sánchez et al., 2022), and also for peatland, grassland and forest dominated in Zone 7 (Pérez-Rodríguez and Biester, 2022). The results of Pérez-Rodríguez and Biester (2022) also suggest that smaller catchments might have higher DOC concentrations than larger ones due to shorter residence times and less DOC degradation (which, we add, may also be related to the high density of mid-catchment lakes in the region). Humid forest and peatlands are dominant cover types throughout the seven zones (Figure 4), with the former somewhat more characteristic of the northern zones (2, 4) and the latter of the southern zones (5-7). Hence, we expect a relatively uniform latitudinal distribution of DOC inputs (Figure 5). However, we predict that lability will shift along the north-south transition: moderate to high DOC inputs of relatively labile quality (leaching of forests litter, Kalbitz et al., 2007), meanwhile, colder humid regions dominated by peatlands may have variable to high DOC loads of a more refractory quality (e.g., humic substances sourced from Sphagnum bogs, Mastný et al., 2018).
Finally, in terms of macronutrients, riverine levels of inorganic N and P are expected to be naturally very low throughout (Table 2; Supplementary Table 2), significantly lower than global averages (Pedrozo et al., 1993; Diaz et al., 2007). Nitrate in pacific (Chilean) forested headwater streams are among the lowest observed worldwide (Perakis and Hedin, 2002), and represent a global outlier in terms of fractionation favoring organic forms (e.g., DON>>N-NH4+>N-NO3-). Natural sources of phosphate may be constrained by the high sorption capacity of volcanic soils which dominate the region (Besoain et al., 1995). Anomalously high P-PO43- documented locally for some glacial rivers may be due to local geochemical processes (Chillrud et al., 1994). The possibility of significantly high episodic runoff inputs of inorganic P in coastal discharges should not be discarded, given the very sparse observations (Table 2; Supplementary Table 2) together with the significant and widespread glacial runoff sources (Figure 4).
Spatial variation in macronutrient inputs is presumed to lack regional patterns, nevertheless are significantly lower in fresh water compared to marine fjords (Supplementary Table 2), hence are not shown in Figure 5. Locally, however, this presumed baseline may be affected by urban and agricultural development, cropland cover being proportional to N export and increased urbanization is generally related to N/P export (Kalff, 2002). In Patagonia, only in Zone 3 might this be a (minor) factor, with two of the three largest urban centers (Figure 4) and moderate agricultural activity concentrated in the Aysén basin (not shown). Specific human activities, such as aquaculture (DOC composition and N/P nutrients; Ryan et al., 2022) or mining (Marquet et al., 2024), may generate higher levels of contamination. However, their effects are localized and currently low in density within the study area. More subtle spatial patterns of N and P export might be estimated for the region based on potentially comparable coefficients and land use classes for New Zealand developed by Snelder et al. (2018). However, a similar analysis would be inconclusive, as most (if not all) of the available data for Patagonia would not likely meet the screening criteria established by these authors.
We have so far focused on characterizing riverine export in terms of composition (i.e. relative concentration in Figure 5) however it is also important to understand dynamics of load, the product of concentration and discharge. Resource concentration is relevant for understanding assimilation of nutrients and resources at the organismal level. Meanwhile, load will determine the geographic area of influence of riverine export. High concentration of macronutrients typical during low flows may have an important local effect on marine ecosystems, while low concentrations during high flow periods or flow pulses will have a broader but more subtle geographic effect. Implicit in this example is the relationship between discharge (Q) and concentration (C), or Q:C relationship (Speir et al., 2024). Concentration may have a strong nonlinear response to flow, and is often modeled as a power function (Petts and Calow, 1996), may be is often site-specific with some ambiguous results (Fazekas et al., 2021). For the sake of advancing a simple first iteration model for runoff effects on Patagonian fjords, we discuss only the Q:C tendency, subject to local and regional validation. These include: (1) a positive Q:C, or strong non-linear increase in concentration with discharge, for DOC and TSS (Raymond et al., 2016); (2) corresponding positive Q:C for dFe, following the potential link with TSS described above; (3) negative Q:C or dilution effect for macronutrients (Mosquera et al., 2022); (4) unknown or neutral Q:C tendency for dSi (the authors are unaware of relevant observations); (5) the previous distinction made between runoff concentration and runoff load, as a function of the above variation in Q:C relationship, which in turn may vary spatially (across zones, or other geographic patterns linked to the distribution of cover types: Figure 1). The conceptual model proposed by Mosquera et al. (2022), comparing Q:C relationships (and also resource ratios) for forest vs. mire catchments, suggests a contrast across the two systems, of inverse patterns for DOC, or varying strength of the Q:C relationship for nutrients. This pattern may be broadly relevant to respective Patagonian systems, based on regional DOC sourcing from peatlands discussed in preceding paragraphs. Moreover, it is probable that some variation of peatland vs. forest Q:C relationship may apply to the hyper-humid coastal evergreen forests of Patagonia.
4.3 Consequences in marine ecosystems based on current patterns and expected change in runoff
Freshening of subantarctic surface water constitutes the principal driver of the physical, chemical and biological characteristics (i.e. photosynthesis) in the euphotic layer at the Pacific eastern boundary of Patagonia. While the physical effects of freshening (e.g. stratification and hydrodynamics) are largely independent of runoff quality, coastal productivity most likely depends more on runoff quality (Torres et al., 2020). The assumption of uniform runoff quality or flow-independence of water quality to infer potential effects of runoff variability may be equivocal. Moreover, the general effect of mixing between marine and continental water is both a supplement (esp. dSi, dFe) relative surrounding waters, and also may stimulate entrainment of nutrients from deeper water into the surface water. Significant local variability in marine productivity probably relies on the interaction of many physical and chemical factors, including the quantity of nutrients introduced and/or entrained, the settling out of river/glacial silt which allows light to penetrate deeper, the depth of the mixed layer, and a highly variable intensity of top-down processes such as grazing, which also is not independent of freshening effect (e.g. salinity tolerance of grazer community). The potential role of spatio-temporal runoff variability on coastal productivity, is especially well exemplified in Zone 5, where higher amounts of runoff of glacial origin corresponds with lower levels of primary productivity (Aracena et al., 2011) and Chl-a (Cuevas et al., 2019) (left panel Figure 5). Conversely, the northern and southern areas demonstrate higher levels of productivity.
A detailed examination of latitudinal concentration trends of continental and coastal runoff (previous section) is therefore an essential antecedent for proposing and testing hypotheses on the role of continental subsidies as drivers to marine fjord ecosystems. Specifically, from Figure 5 we can hypothesize that: (1) a spring to summer increase of primary productivity in northern Patagonia is supported by allochthonous dSi, while low dSi continental subsides in southern Patagonia could explain the low summer primary productivity, since diatom communities could be severely limited by dSi rather than the lower solar radiation at higher latitudes (PAR, or Fe-PAR; see Figure 5); (2) low springtime PAR levels in southern regions are consistent with low primary productivity and high Chl-a levels, since low light regimes may induce the production of chlorophyll to overcome light limitation, as well as increase the requirement of bioavailable forms of Fe (light-Fe colimitation; Torres et al., 2023); (3) high summer TSS in Zone 5 may inhibit light penetration and reduce local primary production in a variable zone of influence relative to discharge points; (4) low dSi in Zone 5 may have reduce the persistence of diatom blooms (outside the area of TSS influence) and corresponding diatom productivity; (5) changes in DOM quality along latitudinal gradients may correspond with decomposition and/or production of re-mineralized nutrients, and also could be linked with capacity to form bioactive trace nutrients complexes (thereby affecting its trace nutrient residence time and area of influence).
Note that specific water quality parameters described here may not be the precise species or phases bioavailable to marine biota: the speciation of bioactive terrestrial solutes (such iron and silica), and colloidal fractions specifically contributed from glacial systems (Pryer et al., 2020), or speciation of DOM (Fellman et al., 2014; Yates et al., 2023), for example. Proportions of organic versus inorganic forms of macronutrients, exemplified by the sequence of DON>>N-NH4+>N-NO3- (Perakis and Hedin, 2002), demonstrated for pristine headwater catchments in Patagonia, lack wider observations that might establish generalizations for larger fluvial basins, land use and climate change. Meanwhile, macronutrient speciation also lacks corresponding observations from the marine systems in Patagonia, suggested by the emphasis on NOx observations relative to NH4+ (Supplementary Table 2). Also, there is clearly a need to explore the role of short-term estuarine processes associated with short-term mixing (e.g. flocculation, aggregation, sedimentation, etc.) in providing bioavailable continental nutrients to the inner fjord. Overall, noting that these are general predictions, local basin-specific exceptions are expected, while closer examination at finer scales or specific parameters and their evolution, will help strengthen our understanding of mechanisms in the terrestrial-freshwater-marine linkage.
All of these external runoff inputs depend on physical factors that enhance water column stratification in marine zones, confining the turbulent layer closer to the surface, and improving the phytoplankton light regimen. The latter is in turn dependent on the seasonality of the light regime, across latitudinal gradients. Hence, more generally, higher productivity levels optimizing the land-sea connection may be associated with increased radiation exposure, together with elevated dSi, labile DOC concentrations, lower TSS levels, and high dFe, the latter from non-physical/glacial weathering (e.g. not offset by high TSS). Specific local evidence of this seasonality from Patagonian systems is limited to a few generalizations. Montero et al. (2017b) in Zone 3 observed strong northern winds correlated with a water column mixing and decreasing salinity/temperature profiles, provoking high rates of primary production in winter. This would correlate with minimum runoff flows and minimum loads throughout the region, although intermittent storm flows are common in late fall and occasionally in winter. The availability of more light and the enrichment of silica and iron have been identified as critical factors promoting diatom blooms during the beginning of the productive season in late spring/early summer (Torres et al., 2023). The persistence of these diatom proliferation depends on silica availability, among other factors (Montero et al., 2017a). This period corresponds with the rising limb of seasonal snowmelt for continental runoff zones 1, 3 and 5 (Figure 3), which implies increasing resource loads, macronutrient dilution, and increasing TSS. The positive (>dSi) and negative light limitation via TSS effects of runoff may therefore be complex, both spatially and temporally. Meanwhile spring runoff subsidy in coastal runoff zones (1, 2) or mixed regimes (glacial/coastal in zones 6, 7) may be less affected by inhibiting effects of TSS during winter. In general, more observations from the side of marine effects (e.g. Montero et al., 2017a, b; Montero et al., 2021; Torres et al., 2023) need to be validated in the context of respective local (zonal) runoff regimes, representing the wider spatial and temporal context within the vast Patagonian fjord system. These potential generalizations should be contextualized, based on geographic area (i.e. zones proposed here), season, and also inter-annual variability (climate change tendencies and climate cycles, which also vary geographically). Hence, noting that these observations represent only a fraction of the possible combinations of geography, latitude, climate regime, and seasonality, we conclude that greater effort is needed towards representation (e.g. Vargas et al., 2018; León-Muñoz et al., 2021) of the freshwater-marine continuum, or based on salinity as proxy (Torres et al., 2014).
Climate and land use are anticipated to change with many uncertainties on the impact the freshwater discharge quality into marine ecosystems. In this context, the potential decline in DOM input in the Reloncavi Fjord (Zone 1) from reduced flows (León-Muñoz et al., 2021), may be counteracted by changes in organic matter quality (currently unknown), nutrient inputs, and weathering rates (also unknown). Noting that both the reduction in flows and also weathering processes may respond to both land use and climate drivers, the two processes may have additive or negating effects (the latter shown by León-Muñoz et al., 2021, in their example reduced flows driven by climate are somewhat negated by estimated evapotranspiration shifts resulting from wildfires). Specifically, the implications of extreme low flows from reduced summer precipitation on the Q:C relationships remain unclear. How the flip side possibility of increased extreme high-flow events, and the water chemistry pulse-shunt events (in the context of DOM sensu Raymond et al. (2016)) will have consequences for other resources (e.g. across the four categories presented here) remains unexplored. The Inner Sea of Chiloé (Zone 1) and the Inner Sea of Aysén (Zone 3) are expected to experience an elevated rate of land use change linked to increased human population density (Nahuelhual et al., 2014). This may produce distinct changes in concentration, loadings and bioavailability in the future. Links between harmful algal blooms (HABs) due to reduced freshwater flows have been proposed (León-Muñoz et al., 2018; Mardones et al., 2023). It is important to note that these potential causal relationships remain speculative, and have not adequately considered the combined effects of hydrologic regime alteration and corresponding resource load change from altered runoff.
In summary, the discharge patterns in south-western Patagonia are influenced by a confluence of climatic variables (annual and seasonal), soil characteristics (predominantly observed in the northern region due to volcanic activity), geological attributes (particularly pronounced in the southern area due to weathering), and land use/cover dynamics (both existing conditions and rates of change). This complex interplay modulates a part of the dynamics of marine ecosystems, such as alterations in the thickness and spatial extent of surface brackish water, as documented by various studies (Calvete and Sobarzo, 2011; Schneider et al., 2014; Castillo et al., 2016; León-Muñoz et al., 2018). Additionally, these environmental factors contribute to the occurrence and persistence of intense harmful algal blooms (HABs) (León-Muñoz et al., 2018; Mardones et al., 2023), fluctuations in water temperature (Calvete and Sobarzo, 2011; Mardones et al., 2023), impacts on aquaculture production (Lara et al., 2016; Mardones et al., 2023), sediment composition (Quiroga et al., 2016; Amann et al., 2022), nutrient levels (Jacob et al., 2014; Torres et al., 2014; Quiroga et al., 2016), chlorophyll concentrations (Jacob et al., 2014; Lara et al., 2016; Cuevas et al., 2019; Torres et al., 2020), salinity variations (Dávila et al., 2002), and primary production rates (Aracena et al., 2011; Montero et al., 2011; Torres et al., 2014), among other effects.
4.4 Recommendations for policy and management in south-western Patagonia
The present analysis, focused on the state of knowledge on interconnected terrestrial, freshwater and marine ecosystems in south-western Patagonia, is simultaneously a determination of a rather limited “state-of-the art.” However, in the context of a region with relatively limited intervention, there is a clear opportunity for integrative management, incorporating development and planning models that anticipate increased extractive and industrial activities, rural land use pressures and urban development. While the proposed concept of “half earth” as a conservation target (Wilson, 2016) has already been surpassed in this region (at least for terrestrial systems), how the other half is managed is just as important, as the same author later articulates. This vision would depend on strategic monitoring and management targets (indicators), whose development and future implementation is perhaps the real sense of “frontier” for Patagonia in the global temperate zone context.
First, a watershed perspective is critical towards advancing an integrative vision that consider both conservation and development. That “freshwater runoff lost to sea” expressed as an opportunity missed, an adage in the political sectors of Chile, deserves sustained rebuttal and a more nuanced explanation from the scientific community. Alterations and variations in the productivity of terrestrial ecosystems, whether natural or anthropogenic, may ultimately have consequences for the productivity and functioning of marine ecosystems (Priya et al., 2023). These teleconnections are mediated through streams and rivers, their connectivity via the watersheds, while the semi-closed nature of interior seas, fjords, and canals of south-western Patagonia may likely amplify the signal (and impact). The “Consejo de Cuencas” (regional watershed panels), a recent initiative in Chile (https://agua.mma.gob.cl/consejos-de-cuenca-piloto/) presents an opportunity for advancing integrative watershed management, with proposed pilots in the Aysén basin (one of the major rivers in zone 3) and some coastal watersheds in zone 7. Argentina, which contributes to significant Pacific watershed drainages in Zones 1, 3, 5 and 7, has implemented the “Comités de Cuencas” a system of watershed administrative units for decades (https://www.argentina.gob.ar/obras-publicas/hidricas/comites-de-cuencas). Without dwelling on the efficacy of these initiatives, we highlight three challenges specific to the region of interest: (1) how to ensure that the watershed-to-sea perspective is considered in the integrated watershed management approach? – the boundary should extend beyond the estuary; (2) how to integrate this watershed-to-sea concept in a binational context? and; (3) how the integrated watershed management approach is carried into practice?. Initiatives based on coastal systems that work their way inland (e.g., the Chesapeake Bay and Buzzards Bay (Tango and Batiuk, 2016; Jakuba et al., 2021)) may provide a valuable model for more developed zones, while Hudson Bay in Canada (Deschepper et al., 2023) might be a more appropriate reference point for larger-scale, wilder areas with limited human populations, and also the binational perspective.
In turn, watershed-scale planning could potentially provide the platform for initiatives nested within, measures that would enhance the terrestrial-marine basis for territorial planning and management in south-western Patagonia. Vega et al. (2018) presented a framework that resumes the previous themes for a science-based water quality approach, that is entirely appropriate for watershed-based management. Mainly, the framework incorporates conceptual models, quantitative models, and a monitoring plan focused on water quality, creating crucial information for stakeholders, sustainable development goals, and policies for governance. In addition, developing secondary water quality standards for some specific and essential basins in Patagonia must be the initial stage for a policy and management of water quality in south-western Patagonia. It is especially noteworthy, that there is significant potential for developing secondary water quality standards in some basins with a low human footprint, as a baseline for other more contaminated watersheds.
In the context of this overarching framework, we propose targeted recommendations to refine the interconnected dynamics of flow and water quality, with a particular focus on the terrestrial-marine interface. Firstly, there is a crucial need to expand and sustain time series water-quality observations for coastal discharges. This expansion should encompass a representation of major basins within each zone, accompanied by a reasonable subset of minor catchments aligned with the land-use/cover analysis outlined in Figure 4. In response to the general necessity for watershed observation points covering the full extent of watershed influence (Milliman and Farnsworth, 2011), a reconsideration and potential relocation of existing sampling points may be imperative. Moreover, we advocate for increasing manual water quality sampling frequency, preferably every month, and strategically correlated with flow monitoring stations.
In addressing the logistical challenges of monitoring in remote regions, a comprehensive approach is proposed. This new effort involves a combination of citizen-based sampling, analytical processing by local institutions (considering the substantial distances involved in shipping samples to the central zone of Chile), and the incorporation of sensor suites. Recent pilots of citizen water quality monitoring completed in the Puelo, Yelcho, Palena, Cisnes, and Aysén basins, indicate the potential for high quality and high frequency (weekly) observations over annual time scales (Reid, unpubl.). Supplemented by high frequency (sub-hourly) time series observation using low-cost sensor technology (ej. Temperature, dissolved oxygen, electrical conductivity, turbidity, and, where feasible, macronutrients and DOM (Rode et al., 2016; Bieroza et al., 2023) represent an especially potent combination for remote regions such as southwest Patagonia. It is important that the parameters measured align with those relevant to terrestrial-marine connectivity (Vega et al., 2018), considering elements such as total suspended solids, various organic and inorganic forms of macronutrients, iron species, and dissolved silica, as proposed in the introduction.
The FLOW model is a pioneering open-access platform providing information on stream flows in the Chilean Patagonia. This platform delivers crucial data for decision-makers, researchers, and the general public, facilitating informed decision-making and academic research. The FLOW model was assessed and calibrated with DGA stations in its implementation. Although the preliminary iteration indicates that ongoing calibration and validation efforts are required for further enhancement, the model is currently available for use. More information on model performance and respective recommendations can be found in Supplementary Material.
Any hydrologic model is a work in progress, and specific validation efforts are recommended for small to medium-sized coastal drainages in mid latitudes (e.g. between Chiloé and mid-Magellan Region - Figure 1). Model parameters presented in Supplementary Materials should undergo routine re-evaluation, incorporating cover types from Figure 4 and accounting for variations over latitude and elevational ranges. It is important to address the acceleration of snowmelt processes and the potential underestimation of observational discharges, as highlighted by Olivares (2022). In mountainous regions like southern Chile, scaling down climate observations is a challenging task (Falvey and Garreaud, 2009). However, a climate model based on the region could significantly improve model forcing (Damseaux et al., 2020; Pessacg et al., 2020). By supplementing the existing meteorological observation network with fine-scale reanalysis of climate data series, e.g. Patagonia MET (Aguayo et al., 2024), closing glacier mass balance (Dussaillant et al., 2019), and recent observations on icefield discharge (calving; Minowa et al., 2021), we can better estimate peak water.
Finally, there is a need for the development of models that can compare flow with corresponding water quality, known as “Q:C” models (Speir et al., 2024), for representative watersheds. It is important to have coordinated efforts between intensive monitoring of terrestrial runoff quality, and less frequent marine campaigns or transects. It is also essential to include forecasting models that are based on future climate and land use change scenarios. All these efforts should be consolidated within an open visualization platform that can be linked to applications or tools like CHONOS (https://chonos.ifop.cl/), which will help in informed decision-making within the policy and management sector.
5 Conclusions
Our study demonstrates a substantial runoff contribution in southwestern Patagonia, whereby including diffuse sources experiencing substantial rainfall, surpasses previous estimations from global discharge studies from 0.2 to 2% of world freshwater runoff. The diverse spatial variability in flow characteristics/seasonality, origins, and potential resource compositions within this region suggests correspondingly diverse influences on fjord ecosystems. For example, we found high hydrologic variability across zones, with interannual variability higher in continental basins, together with a marked seasonal shift along latitudinal gradients, ranging from higher winter discharges in the north to higher summer runoff in the austral zones.
Our proposed conceptual model, derived from limited water quality observations and inference from runoff sources, provide the first regional framework for linking resource variability from runoff to potential patterns of marine primary productivity in the region. These predictions encompass notable regional disparities in flow seasonality, a discernible north-south gradient characterized by diminishing silicic acid input and augmented glacial contributions of sediment and iron. Additionally, there is a conceivable transition in the sources of dissolved organic matter, transitioning from more northern rain forests to peatlands, the latter characterized by refractory properties.
Validating and refining this first regional conceptual model demands a more comprehensive understanding of land-rivers-sea processes, and greater focus the runoff supply vs. marine system demand for resources and nutrients. Expansion of efforts on the spatial and parameter-specific representation of terrestrial runoff monitoring, together with a more efficient coordination with (logistically challenging) marine studies, is essential. From a policy perspective, basin-specific recommendations for water quality standards and sustained monitoring of interconnected river and marine ecosystems are needed for Pacific Patagonian systems. In this context, despite the limited and/or sporadic observational data, the remarkably limited intervention of this area offers a unique prospect for holistic conservation management strategies, bridging terrestrial, freshwater, and marine ecosystems.
Data availability statement
The original contributions presented in the study are included in the article/Supplementary Material. Further inquiries can be directed to the corresponding author.
Author contributions
PM-M: Conceptualization, Data curation, Formal analysis, Investigation, Methodology, Writing – original draft, Writing – review & editing. OA: Data curation, Software, Writing – original draft, Writing – review & editing. RT: Writing – original draft, Writing – review & editing. BR: Conceptualization, Data curation, Investigation, Methodology, Supervision, Validation, Writing – original draft, Writing – review & editing.
Funding
The author(s) declare financial support was received for the research, authorship, and/or publication of this article. Development of the FLOW platform was funded through the Subsecretary of Fisheries and Aquaculture and the Ministry of Economy, Development, and Tourism of the Chilean Government. This study was supported by ANID R20F0002: “Long Term Socio-Ecological Research in Patagonia” (PATSER) and ANID FSEQ210030: “Risk analysis as a tool for the prioritization of secondary environmental water quality standards in the main tributary rivers to the fjord system of northwestern Patagonia, under hydrological drought scenarios”.
Acknowledgments
The authors would like to thank Marcelo Somos and Jorge León-Muñoz for their valuable observations and recommendations on the manuscript. We thank the entire group of IFOP in Putemún- Castro and Meteodata for its contribution in the development and web support of FLOW.
Conflict of interest
The authors declare that the research was conducted in the absence of any commercial or financial relationships that could be construed as a potential conflict of interest.
Publisher’s note
All claims expressed in this article are solely those of the authors and do not necessarily represent those of their affiliated organizations, or those of the publisher, the editors and the reviewers. Any product that may be evaluated in this article, or claim that may be made by its manufacturer, is not guaranteed or endorsed by the publisher.
Supplementary material
The Supplementary Material for this article can be found online at: https://www.frontiersin.org/articles/10.3389/fmars.2024.1396570/full#supplementary-material
References
Aceituno P., Boisier J. P., Garreaud R., Rondanelli R., Rutllant J. A. (2021). “Climate and Weather in Chile,” in Water Resources of CHILE. Eds. Fernández B., Gironás J. (Springer International Publishing, Cham), 7–29.
Aguayo R., León-Muñoz J., Aguayo M., Baez-Villanueva O., Fernandez A., Zambrano-Bigiarini M., et al. (2024). PatagoniaMet: A multi-source hydrometeorological dataset for Western Patagonia. Sci. Data. 11, 6. doi: 10.1038/s41597-023-02828-2
Aguayo R., León-Muñoz J., Garreaud R., Montecinos A. (2021). Hydrological droughts in the southern Andes (40–45°S) from an ensemble experiment using CMIP5 and CMIP6 models. Sci. Rep. 11, 5530. doi: 10.1038/s41598-021-84807-4
Álvarez Garretón C., Boisier J. P., Blanco G., Billi M., Nicolas-Artero C., Maillet A., et al. (2023). Seguridad Hídrica en Chile: Caracterización y Perspectivas de Futuro (Santiago-Chile: Centro de Ciencia del Clima y la Resiliencia, CR2).
Amann B., Bertrand S., Alvarez-Garreton C., Reid B. (2022). Seasonal variations in fjord sediment grain size: A pre-requisite for hydrological and climate reconstructions in partially glacierized watersheds (Baker river, Patagonia). J. Geophys. Res. Earth Surf. 127, e2021JF006391. doi: 10.1029/2021JF006391
Aracena C., Lange C. B., Luis Iriarte J., Rebolledo L., Pantoja S. (2011). Latitudinal patterns of export production recorded in surface sediments of the Chilean Patagonian fjords (41–55°S) as a response to water column productivity. Cont. Shelf Res. 31, 340–355. doi: 10.1016/j.csr.2010.08.008
Astorga A., Moreno P., Reid B. (2018). Watersheds and trees fall together: an analysis of intact forested watersheds in southern Patagonia (41–56° S). Forests 9, 385. doi: 10.3390/f9070385
Astorga A., Reid B., Moreno P., Rojas P. (2021). “Donde nacen los ríos: cuencas de bosques prístinos en la Patagonia occidental austral,” in Conservación en la Patagonia Chilena: evaluación del conocimiento, oportunidades y desafíos. Eds. Castilla J. C., Armesto J. J., Martínez-Harms M. J. (Ediciones Universidad Católica, Santiago, Chile), 167–198.
Besoain E., Ruiz R., Hepp C. (1995). La erupción del Volcán Hudson, XI Región, y sus consecuencias para la agricultura. Agricult. Técnica 55, 204–219.
Beusen A., Bouwman A., Dürr H., Dekkers A., Hartmann J. (2009). Global patterns of dissolved silica export to the coastal zone: Results from a spatially explicit global model. Glob. Biogeochem. Cycles 23. doi: 10.1029/2008GB003281
Beusen A., Dekkers A., Bouwman A., Ludwig W., Harrison J. (2005). Estimation of global river transport of sediments and associated particulate C, N and P. Glob. Biogeochem. Cycles 19. doi: 10.1029/2005GB002453
Bieroza M., Acharya S., Benisch J., ter Borg R. N., Hallberg L., Negri C., et al. (2023). Advances in catchment science, hydrochemistry, and aquatic ecology enabled by high-frequency water quality measurements. Environ. Sci. Tech. 57, 4701–4719. doi: 10.1021/acs.est.2c07798
Blunden J., Arndt D. S. (2017). State of the climate in 2016. Bull. Am. Meterol. Soc 98, Si–S280. doi: 10.1175/2017BAMSStateoftheClimate.1
Boisier J. P., Alvarez-Garreton C., Cordero R. R., Damiani A., Gallardo L., Garreaud R. D., et al. (2018). Anthropogenic drying in central-southern Chile evidenced by long-term observations and climate model simulations. Elem. Sci. Anth. 6, 74. doi: 10.1525/elementa.328
Bouwman A. F., Van Drecht G., Knoop J. M., Beusen A. H. W., Meinardi C. R. (2005). Exploring changes in river nitrogen export to the world’s oceans. Glob. Biogeochem. Cycles 19. doi: 10.1029/2004gb002314
Bozkurt D., Rojas M., Boisier J. P., Valdivieso J. (2018). Projected hydroclimate changes over Andean basins in central Chile from downscaled CMIP5 models under the low and high emission scenarios. Clim. Change 150, 131–147. doi: 10.1007/s10584-018-2246-7
Calvete C., Sobarzo M. (2011). Quantification of the surface brackish water layer and frontal zones in southern Chilean fjords between Boca del Guafo (43°30′S) and Estero Elefantes (46°30′S). Cont. Shelf Res. 31, 162–171. doi: 10.1016/j.csr.2010.09.013
Castillo M. I., Cifuentes U., Pizarro O., Djurfeldt L., Caceres M. (2016). Seasonal hydrography and surface outflow in a fjord with a deep sill: the Reloncaví fjord, Chile. Ocean Sci. 12, 533–544. doi: 10.5194/os-12-533-2016
Castro L., Gironás J. (2021). “Precipitation, Temperature and Evaporation,” in Water Resources of Chile. Eds. Fernández B., Gironás J. (Springer International Publishing, Cham), 31–60.
Chillrud S., Pedrozo F., Temporetti P., Planas H., Froelich P. (1994). Chemical weathering of phosphate and germanium in glacial meltwater streams: effects of subglacial pyrite oxidation. Limnol. Oceanogr. 39, 1130–1140. doi: 10.4319/lo.1994.39.5.1130
CONAF. (2016). Catastro de uso de suelo y vegetación. Available online at: http://www.ide.cl/descarga/capas/item/catastros-de-uso-de-suelo-y-vegetacion.html (Accessed 02 october 2017).
Core-Team. (2017). R: A language and environment for statistical computing (Vienna, Austria: R Foundation for Statistical Computing).
Cuevas L. A., Tapia F. J., Iriarte J. L., González H. E., Silva N., Vargas C. A. (2019). Interplay between freshwater discharge and oceanic waters modulates phytoplankton size-structure in fjords and channel systems of the Chilean Patagonia. Prog. Oceanogr. 173, 103–113. doi: 10.1016/j.pocean.2019.02.012
Curra-Sánchez E. D., Lara C., Cornejo-D’Ottone M., Nimptsch J., Aguayo M., Broitman B. R., et al. (2022). Contrasting land-uses in two small river basins impact the colored dissolved organic matter concentration and carbonate system along a river-coastal ocean continuum. Sci. Total Environ. 806, 150435. doi: 10.1016/j.scitotenv.2021.150435
Damseaux A., Fettweis X., Lambert M., Cornet Y. (2020). Representation of the rain shadow effect in Patagonia using an orographic-derived regional climate model. Int. J. Climatol. 40, 1769–1783. doi: 10.1002/joc.6300
Davies B. J., Darvill C. M., Lovell H., Bendle J. M., Dowdeswell J. A., Fabel D., et al. (2020). The evolution of the Patagonian Ice Sheet from 35 ka to the present day (PATICE). Earth Sci. Res. 204, 103152. doi: 10.1016/j.earscirev.2020.103152
Dávila P. M., Figueroa D., Müller E. (2002). Freshwater input into the coastal ocean and its relation with the salinity distribution off austral Chile (35–55°S). Cont. Shelf Res. 22, 521–534. doi: 10.1016/S0278-4343(01)00072-3
Demaria E., Maurer E. P., Thrasher B., Vicuña S., Meza F. J. (2013). Climate change impacts on an alpine watershed in Chile: Do new model projections change the story? J. Hydrol. 502, 128–138. doi: 10.1016/j.jhydrol.2013.08.027
Deschepper I., Myers P. G., Lavoie D., Papakyriakou T., Maps F. (2023). Understanding the physical forcings behind the biogeochemical productivity of the Hudson bay complex. J. Geophys. Res. Biogeosci. 128, e2022JG007294. doi: 10.1029/2022JG007294
DGA. (2017). “Metodología para la Actualización del Balance Hídrico Nacional,”. Ed. Planificación D. (Ministerio de Obras Públicas, Santiago, Chile).
Diaz M., Pedrozo F., Reynolds C., Temporetti P. (2007). Chemical composition and the nitrogen-regulated trophic state of Patagonian lakes. Limnologica 37, 17–27. doi: 10.1016/j.limno.2006.08.006
Dijkshoorn J., Huting J., Tempel P. (2014). Soil and Terrain Database for Latin America and the Caribbean (version 2.0)-scale 1: 5 million (SOTERLAC). Available online at: https://data.isric.org/geonetwork/srv/api/records/436bd4b0-7ffc-4272-be57-686b7d7eea7d (Accessed 02 october 2017).
Donoso C. (1993). Bosques templados de Chile y Argentina: variación, estructura y dinámica (Santiago de Chile: Editorial Universitaria).
Dumont E., Harrison J. A., Kroeze C., Bakker E. J., Seitzinger S. P. (2005). Global distribution and sources of dissolved inorganic nitrogen export to the coastal zone: Results from a spatially explicit, global model. Glob. Biogeochem. Cycles 19. doi: 10.1029/2005GB002488
Dussaillant I., Berthier E., Brun F., Masiokas M., Hugonnet R., Favier V., et al. (2019). Two decades of glacier mass loss along the Andes. Nat. Geosci. 12, 802–808. doi: 10.1038/s41561-019-0432-5
Falvey M., Garreaud R. D. (2009). Regional cooling in a warming world: Recent temperature trends in the southeast Pacific and along the west coast of subtropical South America, (1979–2006). J. Geophys. Res. Atmos. 114. doi: 10.1029/2008JD010519
Fazekas H. M., McDowell W. H., Shanley J. B., Wymore A. S. (2021). Climate variability drives watersheds along a transporter-transformer continuum. Geophys. Res. Lett. 48, e2021GL094050. doi: 10.1029/2021GL094050
Fellman J. B., Hood E., Spencer R. G. M., Stubbins A., Raymond P. A. (2014). Watershed glacier coverage influences dissolved organic matter biogeochemistry in coastal watersheds of southeast Alaska. Ecosyst 17, 1014–1025. doi: 10.1007/s10021-014-9777-1
Fick S. E., Hijmans R. J. (2017). WorldClim 2: new 1-km spatial resolution climate surfaces for global land areas. Int. J. Climatol. 37, 4302–4315. doi: 10.1002/joc.5086
Fry C. H., Tyrrell T., Hain M. P., Bates N. R., Achterberg E. P. (2015). Analysis of global surface ocean alkalinity to determine controlling processes. Mar. Chem. 174, 46–57. doi: 10.1016/j.marchem.2015.05.003
Fustos I., Abarca-del-Río R., Artal O., Alvial F., Sepúlveda H. (2022). Impact on discharge modelling using different spatial and temporal resolution scenarios in South of Chile. J. South. Am. Earth Sci. 115, 103727. doi: 10.1016/j.jsames.2022.103727
Garcia R. D., Diéguez M. C., Garcia P. E., Reissig M. (2023). Spatial and temporal patterns in the chemistry of temperate low order Andean streams: effects of landscape gradients and hydrology. Aquat. Sci. 85, 102. doi: 10.1007/s00027-023-01001-6
Garreaud R. D. (2018). A plausible atmospheric trigger for the 2017 coastal El Niño. Int. J. Climatol. 38, e1296–e1302. doi: 10.1002/joc.5426
Garreaud R., Lopez P., Minvielle M., Rojas M. (2013). Large-scale control on the patagonian climate. J. Clim. 26, 215–230. doi: 10.1175/JCLI-D-12-00001.1
Hamman J. J., Nijssen B., Bohn T. J., Gergel D. R., Mao Y. (2018). The Variable Infiltration Capacity model version 5 (VIC-5): Infrastructure improvements for new applications and reproducibility. Geosci. Model. Dev. 11, 3481–3496. doi: 10.5194/gmd-11-3481-2018
Heinze C., Blenckner T., Martins H., Rusiecka D., Döscher R., Gehlen M., et al. (2021). The quiet crossing of ocean tipping points. Proc. Natl. Acad. Sci. U. S. A. 118, e2008478118. doi: 10.1073/pnas.2008478118
Hernández-Moreno Á., Soto D. P., Miranda A., Holz A., Armenteras-Pascual D. (2023). Forest landscape dynamics after large-scale fires in western Patagonia: evidencing surprising temperate forest recovery trends. Landsc. Ecol. 38, 2207–2225. doi: 10.1007/s10980-023-01687-x
IGN. (2013). Cobertura del Suelo. Available online at: http://www.ign.gob.ar/NuestrasActividades/InformacionGeoespacial/CapasSIG (Accessed 2 october 2017).
Jacob B. G., Tapia F. J., Daneri G., Iriarte J. L., Montero P., Sobarzo M., et al. (2014). Springtime size-fractionated primary production across hydrographic and PAR-light gradients in Chilean Patagonia (41–50°S). Prog. Oceanogr. 129, 75–84. doi: 10.1016/j.pocean.2014.08.003
Jakuba R. W., Williams T., Neill C., Costa J. E., McHorney R., Scott L., et al. (2021). Water quality measurements in Buzzards Bay by the Buzzards Bay Coalition Baywatchers Program from 1992 to 2018. Sci. Data 8, 76. doi: 10.1038/s41597-021-00856-4
Kalbitz K., Meyer A., Yang R., Gerstberger P. (2007). Response of dissolved organic matter in the forest floor to long-term manipulation of litter and throughfall inputs. Biogeochemistry 86, 301–318. doi: 10.1007/s10533-007-9161-8
Kalff J. (2002). Limnology, Inland Water Ecosystems. 1st ed (Upper Saddle River, New Yersey: Prentice Hall).
Kelso N. V., Patterson T. (2010). Introducing natural earth data-naturalearthdata.com. Geogr. Tech. 5, 25.
Lara C., Saldías G. S., Tapia F. J., Iriarte J. L., Broitman B. R. (2016). Interannual variability in temporal patterns of Chlorophyll–a and their potential influence on the supply of mussel larvae to inner waters in northern Patagonia (41–44°S). J. Mar. Syst. 155, 11–18. doi: 10.1016/j.jmarsys.2015.10.010
León-Muñoz J., Aguayo R., Marcé R., Catalán N., Woelfl S., Nimptsch J., et al. (2021). Climate and land cover trends affecting freshwater inputs to a fjord in Northwestern patagonia. Front. Mar. Sci. 8. doi: 10.3389/fmars.2021.628454
León-Muñoz J., Urbina M. A., Garreaud R., Iriarte J. L. (2018). Hydroclimatic conditions trigger record harmful algal bloom in western Patagonia (summer 2016). Sci. Rep. 8, 1330. doi: 10.1038/s41598-018-19461-4
León-Muñoz J., Aguayo R., Soto D., Avendaño-Herrera R., Nimptsch J., Wolfl S., et al. (2023). Landscape dependency of land-based salmon farming under climate change. Clim. Risk Manag. 40, 100504. doi: 10.1016/j.crm.2023.100504
Liang X., Lettenmaier D. P., Wood E. F., Burges S. J. (1994). A simple hydrologically based model of land surface water and energy fluxes for general circulation models. J. Geophys. Res. D: Atmos. 99, 14415–14428. doi: 10.1029/94JD00483
Luebert F., Pliscoff P. (2006). Sinopsis bioclimática y vegetacional de Chile (Santiago, Chile: Editorial Universitaria).
Marcus L., Mardones J. I., Rioseco J. T., Pinochet J., Montes C., Corredor-Acosta A., et al. (2024). Evidence of plastic pollution from offshore oceanic sources in southern Chilean Patagonian fjords. Sci. Total Environ. 911, 168706. doi: 10.1016/j.scitotenv.2023.168706
Mardones J. I., Paredes-Mella J., Flores-Leñero A., Yarimizu K., Godoy M., Artal O., et al. (2023). Extreme harmful algal blooms, climate change, and potential risk of eutrophication in Patagonian fjords: Insights from an exceptional Heterosigma akashiwo fish-killing event. Prog. Oceanogr. 210, 102921. doi: 10.1016/j.pocean.2022.102921
Marín V. H., Tironi A., Paredes M. A., Contreras M. (2013). Modeling suspended solids in a Northern Chilean Patagonia glacier-fed fjord: GLOF scenarios under climate change conditions. Ecol. Model. 264, 7–16. doi: 10.1016/j.ecolmodel.2012.06.017
Marquet P. A., Buschmann A. H., Corcoran D., Díaz P. A., Fuentes-Castillo T., Garreaud R., et al. (2024). “Global change and acceleration of anthropic pressures on Patagonian ecosystems,” in Conservation in Chilean Patagonia: Assessing the State of Knowledge, Opportunities, and Challenges, vol. 33-65 . Eds. Castilla J. C., Armesto Zamudio J. J., Martínez-Harms M. J., Tecklin D. (Springer International Publishing, Cham).
Marshall M. G., Kellerman A. M., Wadham J. L., Hawkings J. R., Daneri G., Torres R., et al. (2021). Seasonal changes in dissolved organic matter composition in a patagonian fjord affected by glacier melt inputs. Front. Mar. Sci. 8. doi: 10.3389/fmars.2021.612386
Mastný J., Kaštovská E., Bárta J., Chroňáková A., Borovec J., Šantrůčková H., et al. (2018). Quality of DOC produced during litter decomposition of peatland plant dominants. Soil Biol. Biochem. 121, 221–230. doi: 10.1016/j.soilbio.2018.03.018
Milliman J. D., Farnsworth K. (2011). River Discharge to the Coastal Ocean – A Global Synthesis (Cambridge, United Kingdom: Cambridge University Press).
Minowa M., Schaefer M., Sugiyama S., Sakakibara D., Skvarca P. (2021). Frontal ablation and mass loss of the Patagonian icefields. Earth Planet. Sci. Lett. 561, 116811. doi: 10.1016/j.epsl.2021.116811
Modenutti B. E., Balseiro E. G., Elser J. J., Navarro M. B., Cuassolo F., Laspoumaderes C., et al. (2013). Effect of volcanic eruption on nutrients, light, and phytoplankton in oligotrophic lakes. Limnol. Oceanogr. 58, 1165–1175. doi: 10.1016/j.csr.2010.09.003
Montero P., Daneri G., Tapia F., Iriarte J. L., Crawford D. (2017a). Diatom blooms and primary production in a channel ecosystem of central Patagonia. Lat. Am. J. Aquat. Res. 45, 999–1016. doi: 10.3856/vol45-issue5-fulltext-16
Montero P., Pérez-Santos I., Daneri G., Gutiérrez M. H., Igor G., Seguel R., et al. (2017b). A winter dinoflagellate bloom drives high rates of primary production in a Patagonian fjord ecosystem. Estuar. Coast. Shelf Sci. 199, 105–116. doi: 10.1016/j.ecss.2017.09.027
Montero P., Coppari M., Betti F., Bavestrello G., Daneri G. (2021). Feeding of Aulacomya atra Under Different Organic Matter Sources (Autochthonous and Allochthonous) in a Chilean Patagonia Fjord Ecosystem. Front. Mar. Sci. 8. doi: 10.3389/fmars.2021.612406
Montero P., Daneri G., González H. E., Iriarte J. L., Tapia F. J., Lizárraga L., et al. (2011). Seasonal variability of primary production in a fjord ecosystem of the Chilean Patagonia: Implications for the transfer of carbon within pelagic food webs. Cont. Shelf Res. 31(3), 202–215. doi: 10.1016/j.csr.2010.09.003
Mosquera V., Hasselquist E. M., Sponseller R. A., Laudon H. (2022). Co-occurrence of browning and oligotrophication in a boreal stream network. Limnol. Oceanogr. 67, 2325–2339. doi: 10.1002/lno.12205
Nahuelhual L., Carmona A., Aguayo M., Echeverria C. (2014). Land use change and ecosystem services provision: a case study of recreation and ecotourism opportunities in southern Chile. Landsc. Ecol. 29, 329–344. doi: 10.1007/s10980-013-9958-x
Natural-Earth. (2022). Ocean Coastline, including major islands v. 4.1.0. Available online at: https://www.naturalearthdata.com/downloads/10m-physical-vectors/10m-coastline/ (Accessed 4 november 2022).
Nijssen B., Schnur R., Lettenmaier D. P. (2001). Global retrospective estimation of soil moisture using the variable infiltration capacity land surface model 1980–93. J. Clim. 14, 1790–1808. doi: 10.1175/1520-0442(2001)014<1790:GREOSM>2.0.CO;2
Oki T., Kanae S. (2006). Global hydrological cycles and world water resources. Science 313, 1068–1072. doi: 10.1126/science.1128845
Olivares J. (2022). Variabilidad e incerteza de la herramienta FLOW (IFOP): Ríos de la Patagonia modelados con VIC. Concepción, Chile: Universidad de Concepción.
Pedrozo F., Chillrud S. (1998). Relative water fluxes and silicate weathering from the tributaries of a small glaciated watershed in the Southern Patagonian Andes (Upper manso watershed, Argentina). Verh. Int. Ver. Theor. Angew. Limnol. 26, 935–939. doi: 10.1080/03680770.1995.11900856
Pedrozo F., Chillrud S., Temporetti P., Diaz M. (1993). Chemical composition and nutrient limitation in rivers and lakes of northern Patagonian Andes (39.5°-42° S; 71° W) (Rep. Argentina). Verh. Int. Ver. Theor. Angew. Limnol. 25, 207–214. doi: 10.1080/03680770.1992.11900093
Perakis S. S., Hedin L. O. (2002). Nitrogen loss from unpolluted South American forests mainly via dissolved organic compounds. Nature 415, 416. doi: 10.1038/415416a
Pérez T., Mattar C., Fuster R. (2018). Decrease in Snow Cover over the Aysén River Catchment in Patagonia, Chile. Water 10(5), 619. doi: 10.3390/w10050619
Pérez-Rodríguez M., Biester H. (2022). Sensitivity of river catchments to discharge-controlled dissolved carbon export: a study of eight catchments in southern Patagonia. Biogeochemistry 160, 177–197. doi: 10.1007/s10533-022-00947-3
Pessacg N., Flaherty S., Solman S., Pascual M. (2020). Climate change in northern Patagonia: critical decrease in water resources. Theor. Appl. Climatol. 140, 807–822. doi: 10.1007/s00704-020-03104-8
Petts G. E., Calow P. (1996). River restoration: Selected extracts from the rivers handbook (QC, Canada: Blackwell Science).
Pickard G. L., Stanton B. R. (1980). “Pacific Fjords - A Review of Their Water Characteristics,” in Fjord Oceanography. Eds. Freeland H. J., Farmer D. M., Levings C. D. (Springer US, Boston, MA), 1–51.
Priya A. K., Muruganandam M., Rajamanickam S., Sivarethinamohan S., Gaddam M. K. R., Velusamy P., et al. (2023). Impact of climate change and anthropogenic activities on aquatic ecosystem – A review. Environ. Res. J. 238, 117233. doi: 10.1016/j.envres.2023.117233
Pryer H. V., Hawkings J. R., Wadham J. L., Robinson L. F., Hendry K. R., Hatton J. E., et al. (2020). The influence of glacial cover on riverine silicon and iron exports in Chilean Patagonia. Global Biogeochem. Cycles 34, e2020GB006611. doi: 10.1029/2020GB006611
Quiroga E., Ortiz P., González-Saldías R., Reid B., Tapia F. J., Pérez-Santos I., et al. (2016). Seasonal benthic patterns in a glacial Patagonian fjord: the role of suspended sediment and terrestrial organic matter. Mar. Ecol. Prog. Ser. 561, 31–50. doi: 10.3354/meps11903
Quiroga E., Ortiz P., Reid B., Gerdes D. (2013). Classification of the ecological quality of the Aysen and Baker Fjords (Patagonia, Chile) using biotic indices. Mar. pollut. Bull. 68, 117–126. doi: 10.1016/j.marpolbul.2012.11.041
Raymond P. A., Saiers J. E., Sobczak W. V. (2016). Hydrological and biogeochemical controls on watershed dissolved organic matter transport: pulse-shunt concept. Ecology 97, 5–16. doi: 10.1890/14-1684.1
Reche P., Artal O., Pinilla E., Ruiz C., Venegas O., Arriagada A., et al. (2021). CHONOS: Oceanographic information website for Chilean Patagonia. Ocean Coast. Manage. 208, 105634. doi: 10.1016/j.ocecoaman.2021.105634
Reid B., Astorga A., Madriz I., Correa C. (2021). “Estado del conocimiento y conservación de los ecosistemas dulceacuícolas de la Patagonia occidental austral,” in Conservación en la Patagonia Chilena: evaluación del conocimiento, oportunidades y desafíos. Eds. Castilla J. C., Armesto J. J., Martínez-Harms M. J. (Ediciones Universidad Católica, Santiago, Chile).
Rode M., Wade A. J., Cohen M. J., Hensley R. T., Bowes M. J., Kirchner J. W., et al. (2016). Sensors in the stream: the high-frequency wave of the present. Environ. Sci. Tech. 50, 10297–10307. doi: 10.1021/acs.est.6b02155
Rosenqvist A., Shimada M., Ito N., Watanabe M. (2007). ALOS PALSAR: A pathfinder mission for global-scale monitoring of the environment. IEEE Trans. Geosci. Remote Sens. 45, 3307–3316. doi: 10.1109/TGRS.2007.901027
Ryan K. A., Palacios L. C., Encina F., Graeber D., Osorio S., Stubbins A., et al. (2022). Assessing inputs of aquaculture-derived nutrients to streams using dissolved organic matter fluorescence. Sci. Total Environ. 807, 150785. doi: 10.1016/j.scitotenv.2021.150785
Schaefer M., Machguth H., Falvey M., Casassa G., Rignot E. (2015). Quantifying mass balance processes on the Southern Patagonia Icefield. Cryosphere 9, 25–35. doi: 10.5194/tc-9-25-2015
Schneider W., Pérez-Santos I., Ross L., Bravo L., Seguel R., Hernández F. (2014). On the hydrography of Puyuhuapi Channel, Chilean Patagonia. Prog. Oceanogr. 129, 8–18. doi: 10.1016/j.pocean.2014.03.007
SegemAR. (2021). Mapa Geológico de Bicontinental de la República Argentina, escala 1:5.000.000. Available online at: https://repositorio.segemar.gov.ar/handle/308849217/2778;jsessionid=8F9E78B31821B980246BB282E6211D53 (Accessed 9 of november 2022).
Seitzinger S., Harrison J., Dumont E., Beusen A. H., Bouwman A. (2005). Sources and delivery of carbon, nitrogen, and phosphorus to the coastal zone: An overview of Global Nutrient Export from Watersheds (NEWS) models and their application. Global Biogeochem. Cycles 19. doi: 10.1029/2005GB002606
SERNAGEOMIN (2003). Mapa geologico de Chile version digital, scale 1/1.000.000. Available online at: https://www.geoportal.cl/geoportal/catalog/34864/Mapa%20Geol%C3%B3gico%20de%20Chile%20escala%201:1.000.000 (Accessed 8 of november 2022).
Snelder T. H., Larned S. T., McDowell R. W. (2018). Anthropogenic increases of catchment nitrogen and phosphorus loads in New Zealand. N. Z. J. Mar. Freshw. Res. 52, 336–361. doi: 10.1080/00288330.2017.1393758
Sosnovsky A., Lallement M. E., Rechencq M., Zattara E. E., Fernández M. V., Leiva S., et al. (2022). The influence of topography and land use on hydrological and nutrient dynamics in two Andean streams from Northern Patagonia. New Z. J. Mar. Freshw. Res. 56, 78–97. doi: 10.1080/00288330.2020.1853575
Speir S. L., Rose L. A., Blaszczak J. R., Kincaid D. W., Fazekas H. M., Webster A. J., et al. (2024). Catchment concentration–discharge relationships across temporal scales: A review. WIREs Water 11, e1702. doi: 10.1002/wat2.1702
Tango P. J., Batiuk R. A. (2016). Chesapeake Bay recovery and factors affecting trends: Long-term monitoring, indicators, and insights. Reg. Stud. Mar. Sci. 4, 12–20. doi: 10.1016/j.rsma.2015.11.010
Tornquist F., Bigg G. R., Bryant R. G. (2024). Physical mechanisms affecting phytoplankton variability along the Chilean coast. J. Mar. Syst. 242, 103934. doi: 10.1016/j.jmarsys.2023.103934
Torres R., Alarcón E., Reid B. (2021). Simultaneous CO 2 and O 2 Supersaturation in Waters of Southern Patagonia? The Importance of Evaluating Overall Carbonate System Parameters Uncertainty and External Consistency. A Comment to Vargas et al. 2018. J. Geophys. Res. G: Biogeosci. 126. doi: 10.1029/2019JG005523
Torres R., Reid B., Frangópulos M., Alarcón E., Márquez M., Häussermann V., et al. (2020). Freshwater runoff effects on the production of biogenic silicate and chlorophyll-a in western Patagonia archipelago (50–51°S). Estuar. Coast. Shelf Sci. 241, 106597. doi: 10.1016/j.ecss.2020.106597
Torres R., Reid B., Pizarro G., Frangópulos M., Alarcón E., Márquez M., et al. (2023). Iron and silicic acid addition effects on early Spring macronutrient drawdown and biogenic silica production of Patagonia estuarine waters. Prog. Oceanogr. 214, 102982. doi: 10.1016/j.pocean.2023.102982
Torres R., Silva N., Reid B., Frangopulos M. (2014). Silicic acid enrichment of subantarctic surface water from continental inputs along the Patagonian archipelago interior sea (41–56°S). Prog. Oceanogr. 129, 50–61. doi: 10.1016/j.pocean.2014.09.008
Vandekerkhove E., Bertrand S., Crescenzi Lanna E., Reid B., Pantoja S. (2020). Modern sedimentary processes at the heads of Martínez Channel and Steffen Fjord, Chilean Patagonia. Mar. Geol. 419, 106076. doi: 10.1016/j.margeo.2019.106076
Vandekerkhove E., Bertrand S., Reid B., Bartels A., Charlier B. (2016). Sources of dissolved silica to the fjords of northern Patagonia (44–48 S): the importance of volcanic ash soil distribution and weathering. Earth Surf. Process. Landf. 41, 499–512. doi: 10.1002/esp.3840
van der Struijk L. F., Kroeze C. (2010). Future trends in nutrient export to the coastal waters of South America: Implications for occurrence of eutrophication. Global Biogeochem. Cycles 24. doi: 10.1029/2009GB003572
Vargas C. A., Cuevas L. A., Silva N., González H. E., De Pol-Holz R., Narváez D. A. (2018). Influence of glacier melting and river discharges on the nutrient distribution and DIC recycling in the southern Chilean Patagonia. J. Geophys. Res. G: Biogeosci. 123, 256–270. doi: 10.1002/2017JG003907
Veblen T., Donoso C., Kitzberger T., Rebertus A. (1996). “Ecology of southern Chilean and Argentinean Nothofagus forests,” in The ecology and biogeography of nothofagus forests. Eds. Veblen T., Hill R., Read J. (Yale University Press, New Haven), 293–353.
Vega A. S., Lizama K., Pastén P. A. (2018). “Water quality: trends and challenges,” in Water Policy in Chile. Global Issues in Water Policy. Ed. Donoso G. (Springer, Cham), 25–51.
Voss M., Baker A., Bange H. W., Conley D., Deutsch B., Engel A., et al. (2011). “Nitrogen processes in coastal and marine ecosystems,” in The European Nitrogen Assessment. Sources, effects and perspectives. Eds. MA Sutton C. H., Erisman J. W., Billen G., Bleeker A., Grennfelt P., van Grinsven H., Grizzetti B. (Cambridge University Press, Cambridge, UK), 147–176.
Winter T. C. (1981). Uncertainties in estimating the water balance of lakes 1. J. Am. Water Resour. Assoc. 17, 82–115. doi: 10.1111/j.1752-1688.1981.tb02593.x
Keywords: coastal discharges, freshwater, inner seas, fjords, water quality, nutrients, suspended sediments
Citation: Moreno-Meynard P, Artal O, Torres R and Reid B (2024) Flow-weighted sourcing of freshwater runoff from Pacific-draining continental and coastal basins in south-western Patagonia (41-56° S): characterizing regional inputs to Chilean fjords. Front. Mar. Sci. 11:1396570. doi: 10.3389/fmars.2024.1396570
Received: 05 March 2024; Accepted: 14 August 2024;
Published: 19 September 2024.
Edited by:
Margarita Fernández Tejedor, Institute of Agrifood Research and Technology (IRTA), SpainReviewed by:
Patricia Elizabeth Garcia, National Scientific and Technical Research Council (CONICET), ArgentinaManel Grifoll, Universitat Politecnica de Catalunya, Spain
Copyright © 2024 Moreno-Meynard, Artal, Torres and Reid. This is an open-access article distributed under the terms of the Creative Commons Attribution License (CC BY). The use, distribution or reproduction in other forums is permitted, provided the original author(s) and the copyright owner(s) are credited and that the original publication in this journal is cited, in accordance with accepted academic practice. No use, distribution or reproduction is permitted which does not comply with these terms.
*Correspondence: Paulo Moreno-Meynard, cGF1bG8ubW9yZW5vQGNpZXAuY2w=