- 1Royal Netherlands Institute for Sea Research (NIOZ), Ocean Systems, Texel, Netherlands
- 2Department of Geosciences, Utrecht University, Utrecht, Netherlands
The isotopic and elemental composition of the fossil shells of foraminifera are often used for reconstructing past environments and climates. These so-called proxy relations are based on the effect of environmental conditions (e.g. seawater temperature, pH) on the isotopic ratio (e.g. δ11B or δ18O) or partitioning of elements (commonly expressed as El/Ca or DEl) during calcification. Whereas many studies focused on proxy-calibrations of divalent cations, incorporation of monovalent cations are less well constrained. Here we calibrate shell potassium content (K/Cacc) as a function of 1) seawater K+ concentration, 2) the ratio of potassium and calcium in seawater (K/Casw) and 3) temperature. Moreover, we analyze Na+ incorporation into the calcite as a function of seawater K+ and Ca2+ concentrations. First, we cultured specimens of the larger benthic foraminifer Amphistegina lessonii at four different seawater [Ca2+] and constant [K+], resulting in a range of K/Casw. Secondly, we cultured specimens of the same species at four different [Ca2+]sw and [K+]sw while keeping the ratio between these two ions constant. Finally, we tested the effect of temperature (from 18 to 28°C) on K-incorporation in this species. Measured K/Cacc values are not notably affected by [Ca2+]sw, while seawater [K+] positively influences potassium incorporation, resulting in a positive correlation between seawater K/Ca values and K/Cacc. Although the [Na+] in the culture media was constant throughout both experiments, incorporated Na responded positively to decreasing [Ca2+]sw, resulting in a positive correlation between sea water Na/Ca and Na/Cacc. The difference in the controls on K- and Na-incorporation suggests that the (biological) control on these ions differs. Part of the observed variability in element partitioning may be explained by differences in chemical speciation and crystallographic coordination in the calcite lattice.
1 Introduction
Our climate is rapidly changing as a consequence of anthropogenic greenhouse gas emissions to the atmosphere. Predicting the effects of increases in atmospheric CO2 levels are greatly helped by reconstructions of past climate change. Such reconstructions, in turn, can be derived from the chemical composition of the shells of foraminifera: the boron isotopic composition of their calcite reflects primarily seawater pH (Sanyal et al., 1995), while for example the Mg/Ca can be used to reconstruct past seawater temperature (Nürnberget al., 1996; Holland et al., 2020). Incorporation of elements is usually also affected by biological control during calcification. This is apparent from the large deviation in El/Ca between species (Bentov and Erez, 2006; De Nooijer et al., 2023), within species (De Nooijer et al., 2014; Sadekov et al., 2016) and even within single chamber walls (Kunioka et al., 2006; Fehrenbacher et al., 2017; Levi et al., 2019). These observations have prompted investigating the physiological controls acting on ion uptake by foraminifera during calcification (Erez, 2003; De Nooijer et al., 2014; Schmidt et al., 2016; Evans et al., 2018; Tyszka et al., 2019). The active proton pumping (Bentov et al., 2009; De Nooijer et al., 2009) and calcium uptake (Toyofuku et al., 2017) may be the key in understanding divalent cation uptake and incorporation and at the same time may affect reconstruction of such elements’ concentrations.
Concentrations of the major ions in seawater (Na+, Mg2+, Ca2+, K+) are not constant on geological time scales with residence times of 1.1 to 100 Myrs (Horibe et al., 1974; Hardie, 1996; Lécuyer, 2016; Zeebe and Tyrrell, 2018; Hauzer et al., 2021). The changes of [Ca2+]sw result in changes in the incorporation of other elements into the calcite of foraminifera, likely as a result of competition of these ions with Ca2+ for a place in the crystal lattice (Dueñas-Bohórquez et al., 2011; Mewes et al., 2015a; Hauzer et al., 2018). In theory, past concentrations of calcium in seawater may be estimated from foraminiferal El/Ca ratios of elements that have a higher residence time than calcium (Na; (Hauzer et al., 2018). This, however, requires calibration of El/Ca as a function of [Ca2+]. For Mg2+ incorporation, a non-linear response to [Ca2+] is described (Segev and Erez, 2006; Hauzer et al., 2018), It has been described linear correlations for Li/Cacc versus Li/Casw, Sr/Cacc versus Sr/Casw (Mewes et al., 2015b; Hauzer et al., 2018) and Na/Cacc versus Na/Casw (Hauzer et al., 2018).
One of the major elements in seawater is K+ with an average concentration of 380 mg/L (Wang et al., 2020) and a residence time of ~12 Myrs (Culkin and Cox, 1966). The 2 main sources for potassium in seawater are continental runoff and hydrothermal fluxes, while K is removed from seawater by pore-water entrapment or adsorption into clay minerals (Kronberg, 1985; Sun et al., 2016). However, K+ incorporation into foraminiferal calcite and its potential dependency on environmental parameters are at the first steps with only a few publications. Culturing experiments with benthic foraminifera suggested both foraminiferal K/Cacc and Na/Cacc as a potential proxy for seawater [Ca2+] (Hauzer et al., 2018; Nambiar et al., 2023). Such reconstructions are important as they can reveal long-term changes in the oceans’ major ion composition and thereby allow studying changes in the rates of geological processes including weathering, seafloor spreading and authigenic mineral formation (Horita et al., 2002; Fantle and DePaolo, 2005).
Compared to other major elements in seawater, K+ has a large ionic radius and can interfere with the structure of proteins, requiring cells to regulate the concentration of this ion. Studies looking at the impact of temperature showed no apparent effect on K/Ca in a variety of organisms (Li et al., 2021) and suggest that K-incorporation is correlated only to the concentration of K+ in the medium during inorganic precipitation experiments (Ishikawa and Ichikuni, 1984). Another very well-known monovalent ion in seawater is Na+, with an average concentration of 10.6 g/L (Duxbury et al., 2024). Na/Cacc has being studied as a proxy before for changes in salinity in the past (Wit et al., 2013; Geerken et al., 2018), although this was debated to be the case by Hauzer et al. (2021). In addition, there is no effect of temperature on Na-incorporation (Allen et al., 2016).
Incorporation of monovalent cations and whether these elements are affected by the so-called vital effects of foraminifera remain largely unknown. Incorporation of K+ by skeleton of corals has been shown to be related to incorporation of Na+ and explains the similarity in the way they are incorporated (Mitsuguchi and Kawakami, 2012). Moreover, using the incorporation of divalent cations such as Mg2+ and Sr2+ as proxies also requires known sea water element to calcium ratios, which is on geological time scales can rely on changes in seawater [Ca2+]. It is also vital to understand the inter-species’ effect of environmental parameters on element incorporation. Finally, it is necessary to quantify the interaction between incorporated ions, for example Na+ and K+, to correct fossil El/Ca for changes in the composition of the seawater’s major ion inventory.
In this study, we report the results of a set of experiments to calibrate K+ incorporation in the benthic foraminifer Amphistegina lessonii as a function of [Ca2+], [K+]and [Ca2+] and temperature. We set up controlled growth experiments to decouple the effect of these variables on calcitic K/Ca, which are inherently correlated in nature to deconvolve the underlying processes involved in K+ and Na+ uptake. Hence, grown specimens were also used to analyze their Na/Ca to test potential impacts of [Ca2+], [K+] and temperature on sodium incorporation. Accordingly, we aim to evaluate the potential of foraminiferal K/Ca and Na/Ca values as a proxy for [Ca2+]sw and seawater chemistry in general.
2 Materials and methods
Three sets of controlled growth experiments were performed (Table 1). In the first set only the seawater’s calcium concentration (i.e. [Ca2+]sw) was varied. In the second set of experiments, we varied both [K+]sw and [Ca2+]sw, while keeping K/Casw constant. These two sets of experiments were done under identical, constant temperature, salinity, and inorganic carbon chemistry. Finally, a set of experiments was conducted with varying temperatures, while keeping element concentrations, salinity and inorganic carbon chemistry constant.
2.1 Culture set-up: [Ca2+]sw, and [K+]sw and [Ca2+]sw
For the two experiments in which Ca- and/or K-concentrations were varied, specimens of A. lessonii were isolated from coral rubble retrieved from the tropical aquarium of Burgers’ Zoo [Arnhem, the Netherlands; (Ernst et al., 2011)]. Approximately 100 living specimens were selected and transferred to Petri dishes and placed at 26°C with a light/dark cycle of 12h/12h to stimulate asexual reproduction. Within 2 weeks, between 80 and 90 juveniles were released from several adults that were incubated in pre-made culture media.
For these two sets of experiments, the amounts of salts were added following the recipes of (Kester, 1967) and (Wit et al., 2013), however changing the concentrations of CaCl2 and KCl (Supplementary Tables S4, S5). We mixed the artificial seawater with filtered (< 2 μm) North Sea Water, using a ratio of 1 to 5 (20% North Sea Water and 80% artificial seawater) and we measured and controlled the salinity (within +/-0.1 salinity units) at the start and at the end of the experiment using a conductometer.
The prepared media were divided over culture flasks (De Goeyse et al., 2021) that were placed in a cabinet with a controlled atmospheric pCO2 of 600 ppm and a light/dark cycle of 12h/12h. Evaporation of the culture media was minimized by saturating the atmosphere inside the cabinet with water vapor. The culture media were subsampled for DIC and TA by filling replicate 5mL vials, pre-poisoned with HgCl2, and storage at 4°C until analyses using a Continuous Segmented Flow Analyzer (QuAAtro). For DIC the method was the same as the one used in the temperature experiment (2.1.1) but for TA an adapted method from (Sarazin et al., 1999) was used, changing the organic acid from formic acid to Phthalic acid, keeping the same indicator Bromo Phenol Blue which is blue at a pH of 3.7 and giving a green color at more alkaline phases having an absorbance at 590nm (Supplementary Tables S1, S2). A. lessonii was grown under these pre-set conditions for six weeks. Almost all replicates had sufficient material to have one or two analysis per replicate for K/Cacc using ICP-MS (Supplementary Tables S1, S2). Every batch of foraminifera measured contained between 10-25 individuals per condition. The specimens that were selected for analysis were fully grown (i.e. consisted of more than 15 chambers) to minimize the contribution to the chemical signal by the first 1 or 2 chambers that were built before the experimental incubation a correlation with weight of Ca against K/Cacc was plotted to ensure there was no correlation and hence no effect of growth rate when K and Na incorporated into the calcite (Supplementary Table S12, Supplementary Figure S2) The mortality rate during the experiments was derived from the temperature experiment and equaled 22% (i.e. 78% of the incubated specimens were alive at the end of the experiment).
2.2 Culture set-up: temperature experiment
The procedure for selecting specimens, feeding, and subsampling of the culture media were identical to that in the first two sets of experiments. Juveniles derived from asexual reproduction events were divided into 4 equal groups, with at least 20 juveniles per group, which were transferred to Petri dishes with filtered (< 2 μm) North Sea water. At this stage, the juveniles had not more than seven chambers. Three or four replicates (depending on the number of juveniles available per condition) were subsequently incubated at each of the four different temperatures: 18°C, 22°C, 26°C, and 28°C (Table 1). All incubations used a light/dark cycle of 12h/12h and a light intensity of approximately 180 μmol photons m-2 s-1 for a total of eight weeks. During the experiment, the foraminifera were observed weekly to monitor how many of the incubated specimens were alive. Every week, culture media were replaced and the foraminifera were fed freeze-dried algae Dunialiella salina (Van Dijk et al., 2017a) suspended in precleaned water (cleaned by rinsing the Falcon tubes with Milli-Q water three times).
To monitor potential changes in inorganic carbon chemistry, culture media were subsampled for dissolved inorganic carbon (DIC) and total alkalinity (TA) (Supplementary Table S3). Vials for these sub-samples were pre-poisoned with HgCl2 and stored at 4°C until they were analyzed. DIC samples were acidified, and the CO2 was dialyzed to reduce the phenolphthalein indicator and spectrophotometrically measured at 550nm using Continuous Segmented Flow Analyzer (QuAAtro); TA was measured by using an acid buffered solution of formic acid and was measured spectrophotometrically (Sarazin et al., 1999) using a QuAAtro. For the temperature experiments salinity of the culture media was monitored using a refractometer and was always 36 +/- 0.5. Because we used the same culture water for the different temperature experiments, we do not expect offsets between these experiments. Measured values for DIC and TA together with the temperature and salinity were used to calculate pH, pCO2, [HCO3-], [CO32-] and the saturation state with respect to calcium (ΩCa) using PyCO2SYS (Humphreys et al., 2022) (Supplementary Table S3).
2.3 Element/Ca ratios
2.3.1 Element/Ca in seawater
To analyze the El/Ca values of the culture media, we sub-sampled the culture media from every replicate of the two experiments (2.1.1). Samples were diluted 900 times in 2 steps using a 30x dilution with 1M HNO3 sub boiled distilled quality acid, with a standard element mix containing Sc, Rh and In added to it. Rhodium was used as an internal standard to correct instrumental drift during the analysis of the mass spectrometer. Samples were measured on a sector field SF-ICPMS (ThermoFisher Scientific, Element-2) at the Royal NIOZ. Resolutions for the measurements were based on 3 slits, measuring at high resolution: 10000; medium resolution: 4000; low resolution: 300. To fully separate the 39K-signal from 38Ar1H, potassium was measured in high resolution. This strategy was based on the ratio of the mass over the mass difference: 39K has an atomic mass (M) of 38.96371 and 38Ar1H has an M of: 38.97056. Dividing the M of potassium over the difference in M, gives 5688 > 4000, which requires the high-resolution settings of the detector (Supplementary Figure S1). Similarly, measuring in medium resolution is also necessary for 32S due to interference from 16O16O, dividing the M of sulfur over the difference in M gives 479 > 300. 11B, 23Na, 25Mg, 43Ca, 88Sr and 103Rh were all measured in low resolution (Tables 2, 3) and values El/Casw (Tables 4, 5).
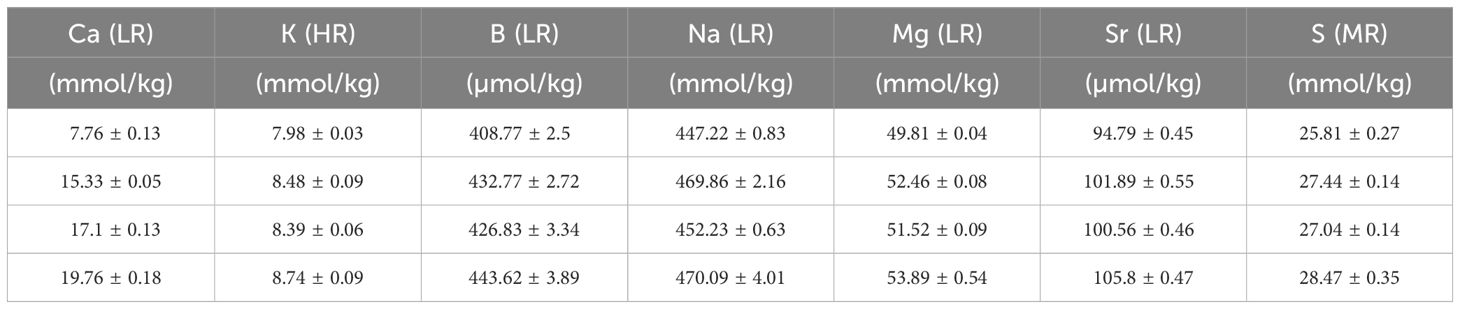
Table 2 Elements in the seawater (mean +/- SD) after mixing with North Sea water and measured with SF-ICPMS in the [Ca2+]sw experiment. Low resolution analysis (LR), Medium resolution analysis (MR), High resolution analysis (HR).
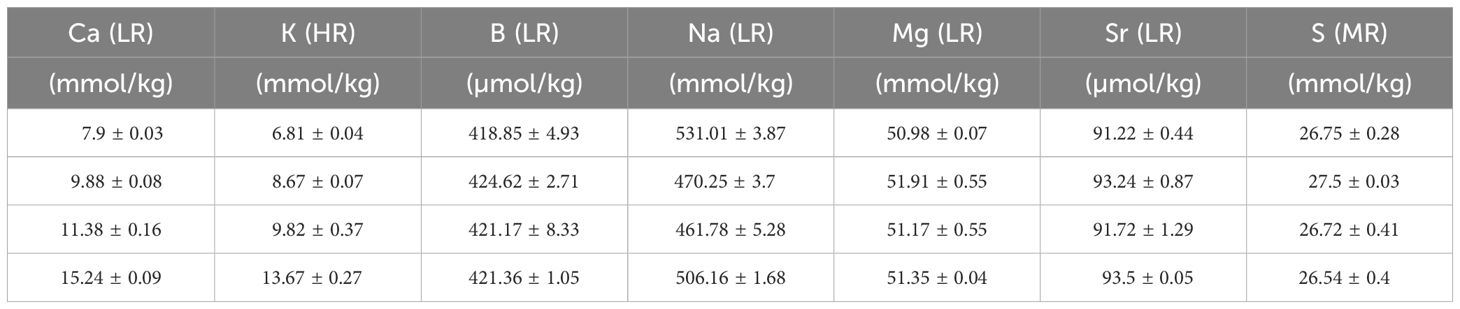
Table 3 Elements in the seawater (mean +/- SD) after mixing with North Sea water and measured with SF-ICPMS in the [Ca2+]sw and [K+]sw experiment. Low resolution analysis (LR), Medium resolution analysis (MR), High resolution analysis (HR).
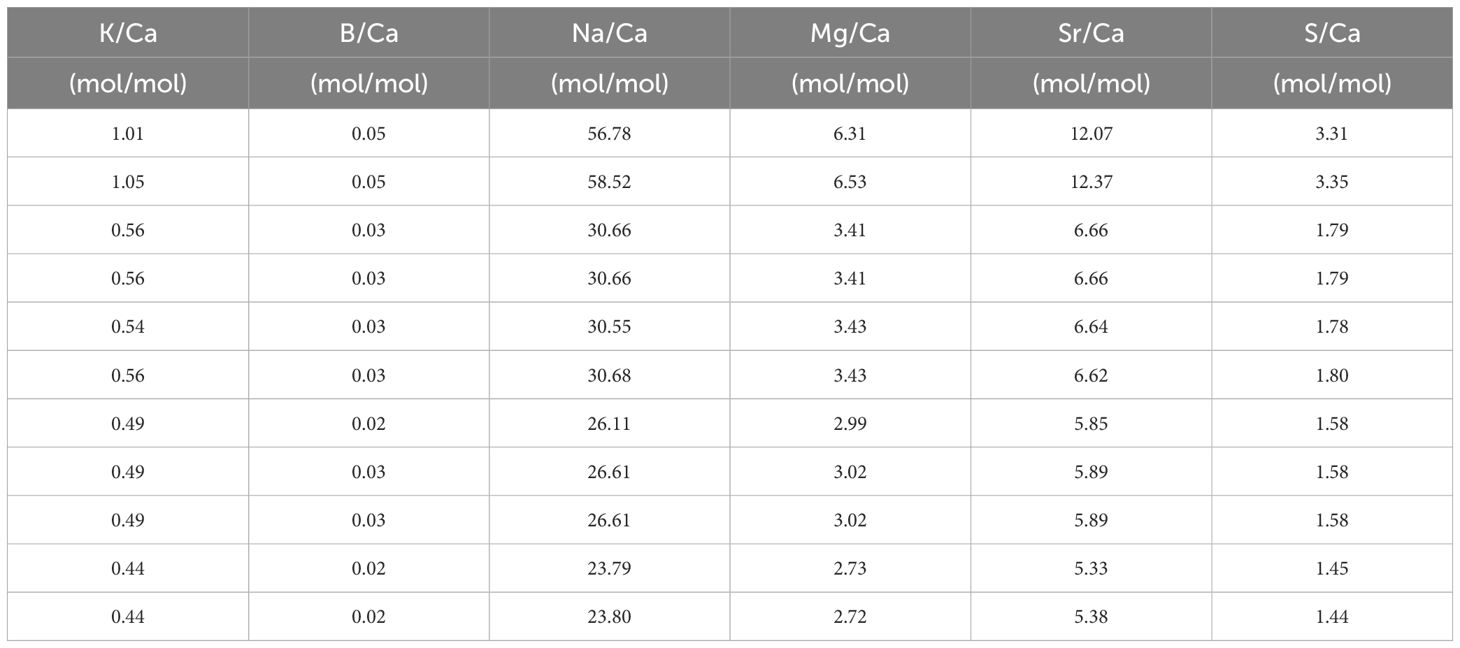
Table 4 El/Ca in the seawater after mixing with North Sea water and measured with SF-ICPMS in the [Ca2+]sw experiment.
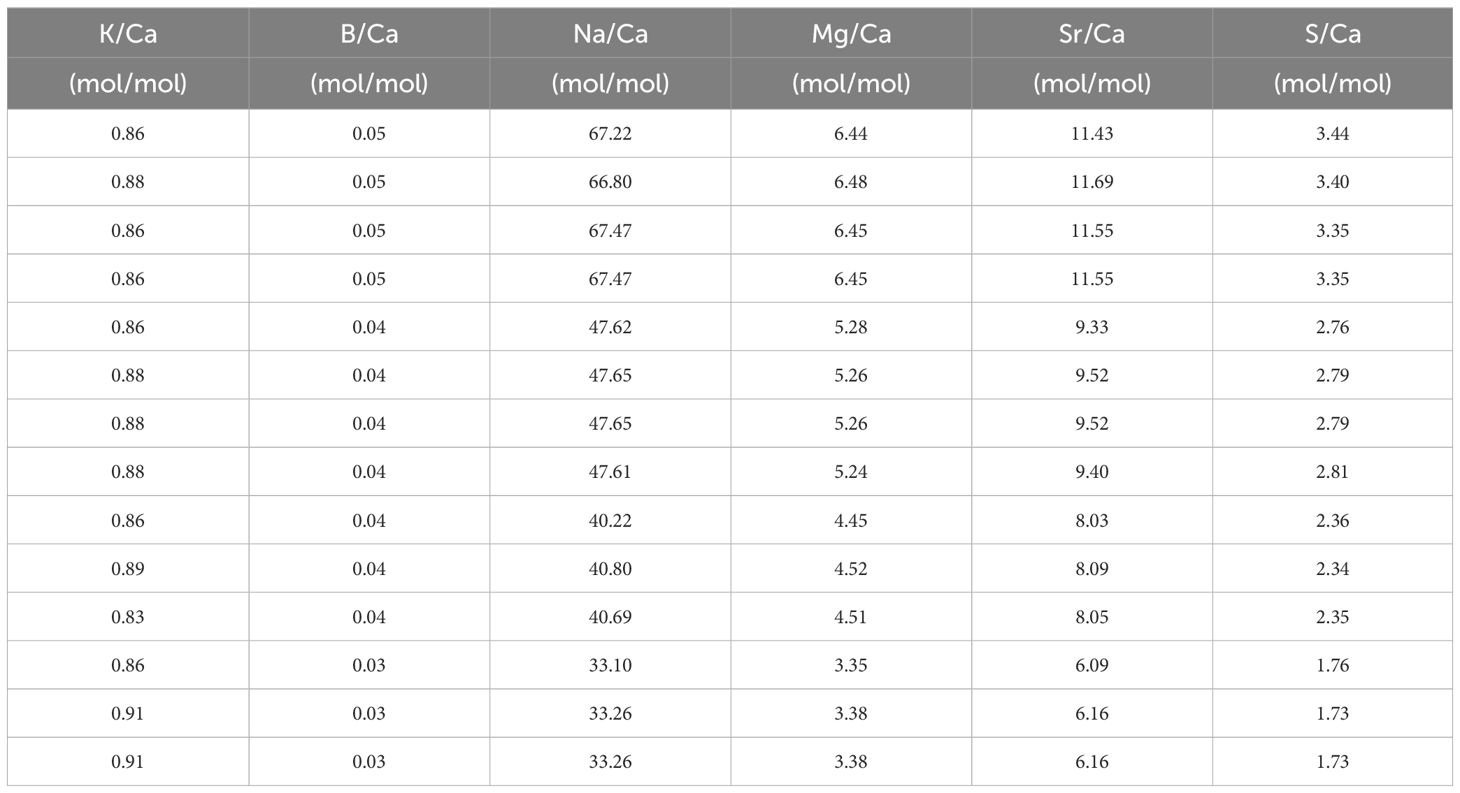
Table 5 El/Ca in the seawater (mean +/- SD) after mixing with North Sea water and measured with SF-ICPMS in the [Ca2+]sw and [K+]sw experiment.
2.3.2 Foraminiferal element uptake
2.3.2.1 Cleaning procedure for shells from the culture experiments
Even though the foraminifera were all derived from culture experiments in which no sediment was present, all samples were carefully cleaned prior to element analyses. We followed a cleaning procedure based on the protocol from Barker et al. (2003). The foraminifera were not crushed, because the specimens do not contain sedimentary infillings. Crushing or over-crushing can lead to losses of carbonate material (Barker et al., 2003). First organic matter was removed using a 1% H2O2 buffered with a NH4OH solution, adding 250 µL to each 500 μL Eppendorf, which was then transferred to a pre-warmed water-bath at 95°C for 10 minutes and ultrasonicated. Afterwards, the solution was removed with a 0.1 ml pipette. This procedure was repeated twice and the uncrushed foraminifera and buffered H2O2 solution probably gave a neglectable loss of carbonate during this organic removal step. After this step, the foraminifera were rinsed three times with ultrapure water (Milli-Q, >18.2 MΩ) by filling the tube fully with ultrapure water and removing after fully settling of the foraminifera. Possible remaining contaminants at the surface of the foraminiferal shells were finally removed by a leaching step with diluted HNO3 (250µL 0.001 M) and concurrent gentle ultrasonication for 30 seconds. The HNO3 was removed, and samples were cleaned with ultrapure water. The cleaned foraminifera were dissolved in 0.5 ml 0.1M ultra-pure HNO3 (two times sub-boiled analytical grade acid in the NIOZ clean lab). To enhance the dissolution, vials were placed in an ultrasonic bath for 15 min and visually inspected to ensure complete dissolution.
2.3.2.2 SF-ICPMS analyses
For all solutions with dissolved calcium carbonate, the [Ca2+] were pre-determined in medium resolution with the SF-ICPMS against a 5-point external calibration line using 45Sc as an internal standard. Based on this data, samples were diluted to obtain a 100 ppm Ca matrix for each sample, minimizing mass bias effects between samples and standards. To determine foraminiferal element to calcium ratios with the SF-ICPMS, a ratio calibration method (de Villiers et al., 2002) was employed using standards with similar matrices as the samples. Samples (between 20-40 individuals per condition) were divided to obtain a minimum weight of 300µg. Samples were measured in triplicate using a low sample flow of 50μl/min (ESI, microFAST). To monitor accuracy, JCp-1 (Geological Survey of Japan, coral; Porites sp (Okai et al., 2004), JCt-1 (giant clam), and NFHS-2NP (Boer et al., 2022) were included in the analyses. NFHS-1(NIOZ Foraminifera House Standard; (Mezger et al., 2016) was used as a drift standard. Accuracies are listed in (Supplementary Table S6).
2.4 Statistical analysis
To analyze (potential) correlations between seawater temperature/chemistry and foraminiferal calcite composition, a bootstrap method was applied. This allows calculating confidence intervals for potential correlations through iterative resampling of the dataset generating distinct simulated sub-sets of samples (in this case 1000 iterations were used). The results of these regressions are only shown when p-values< 0.05. Confidence intervals displayed in the figures were taken from the means and standard deviations obtained from the bootstrap analysis. Analyses were performed using a standard package available for Python: scipy.stats (Virtanen et al., 2020).
2.5 Element partitioning
The incorporation of elements is often presented as the El/Ca (where El is the element analyzed) or DEL, known as the partition coefficient:
When D > 1 the element is more concentrated in the shell than in the seawater and when D< 1 the element is excluded from the shell (Lea, 2003). As it is explained by (Lea, 2003) this relation is not strictly following thermodynamic properties, but also affected by biology. Nevertheless, expressing element incorporation into the calcite in terms of D allows better comparison between different ocean chemistries and also between experiments.
3 Results
3.1 Experiment [Ca2+]sw
In the first experiment, both [K+]sw and [Na+]sw were kept constant and therefore variability in foraminiferal calcite composition must be related to changes in [Ca2+]sw (Supplementary Table S7). The K/Cacc values of the different conditions used are statistically the same. The K/Cacc at the lowest K/Casw appear higher than at higher K/Casw, but this is likely caused by the contribution of pre-existing calcite (see also discussion, 4.1). Na/Cacc, however, increases with increasing Na/Casw values (and hence decreasing [Ca2+]sw) with a significant offset between the lower and the higher Na/Cacc conditions used in the experiments (Figure 1B; bootstrap analysis, p<0.05). Also clear from these figures is that the partitioning coefficients does not remain constant with changing [Ca2+]sw, for both K and Na incorporation.
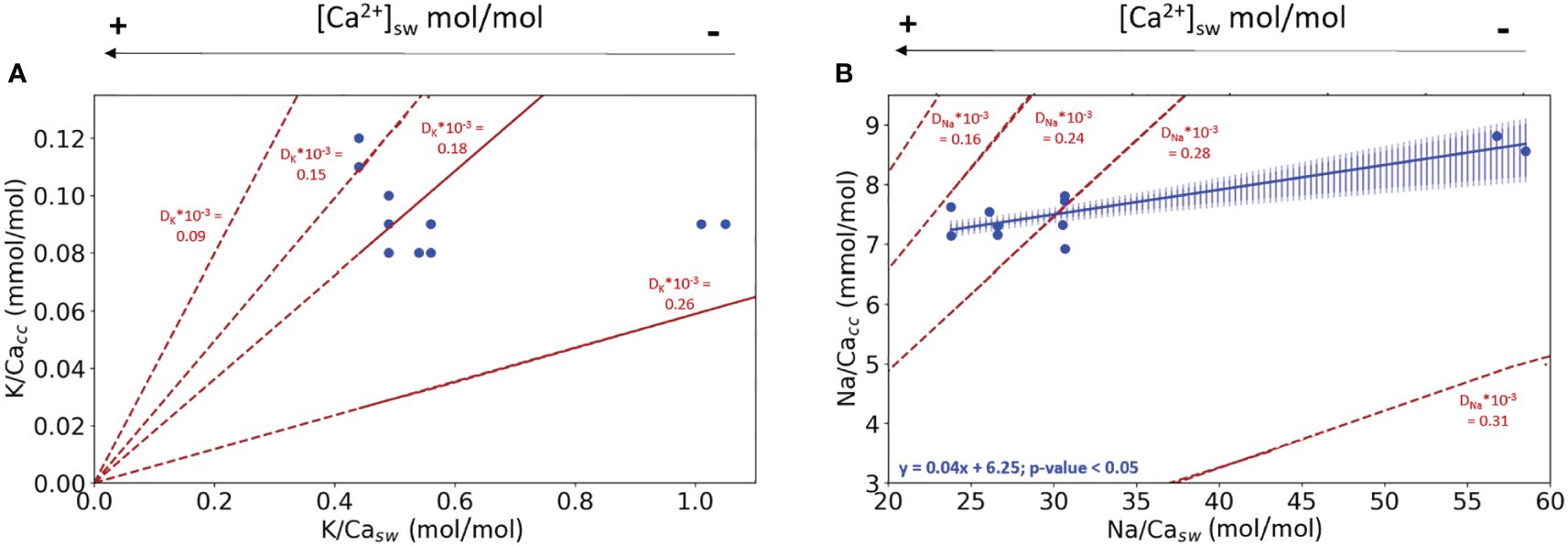
Figure 1 (A) K/Cacc in cultured A. lessonii versus K/Casw from the experiment with varying [Ca2+], keeping [K+] constant; (B) Na/Cacc from the same specimens versus Na/Casw, keeping [Na+]sw constant. Blue dots are the measured El/Cacc, the blue line in (B) indicates the linear correlation between Na/Ca in the culture media and the foraminiferal calcite. The shading surrounding this correlation represents the confidence interval (0.99 darker blue and 0.95 lighter shade of blue) based on a bootstrap analysis (1000 iterations). In both plots, lines with constant partition coefficients (DK and DNa) are plotted (dashed red lines) to facilitate interpretation of the observed changes in the relative incorporation of elements. The standard deviation for every replicate using the internal error from the SF-ICPMS was very small making it imperceptible in the plotted data (Supplementary Table S10).
3.2 Experiment [Ca2+]sw and [K+]sw
When varying both [K+]sw and [Ca2+]sw and keeping K/Casw constant, K/Cacc increases linearly (Figure 2A). In the same cultured foraminifera, the Na/Cacc also increases significantly with higher seawater Na/Ca ratios (Figure 2B). When directly comparing these results with those from the first experimental set, it is clear that K-incorporation depends not on [Ca2+] but does respond when both [K+] and [Ca2+] are varied (Figure 2C). Na-incorporation, on the other hand, does react to changes in [Ca2+]sw, irrespective of changes in [K+] (Figure 2, Supplementary Table S8).
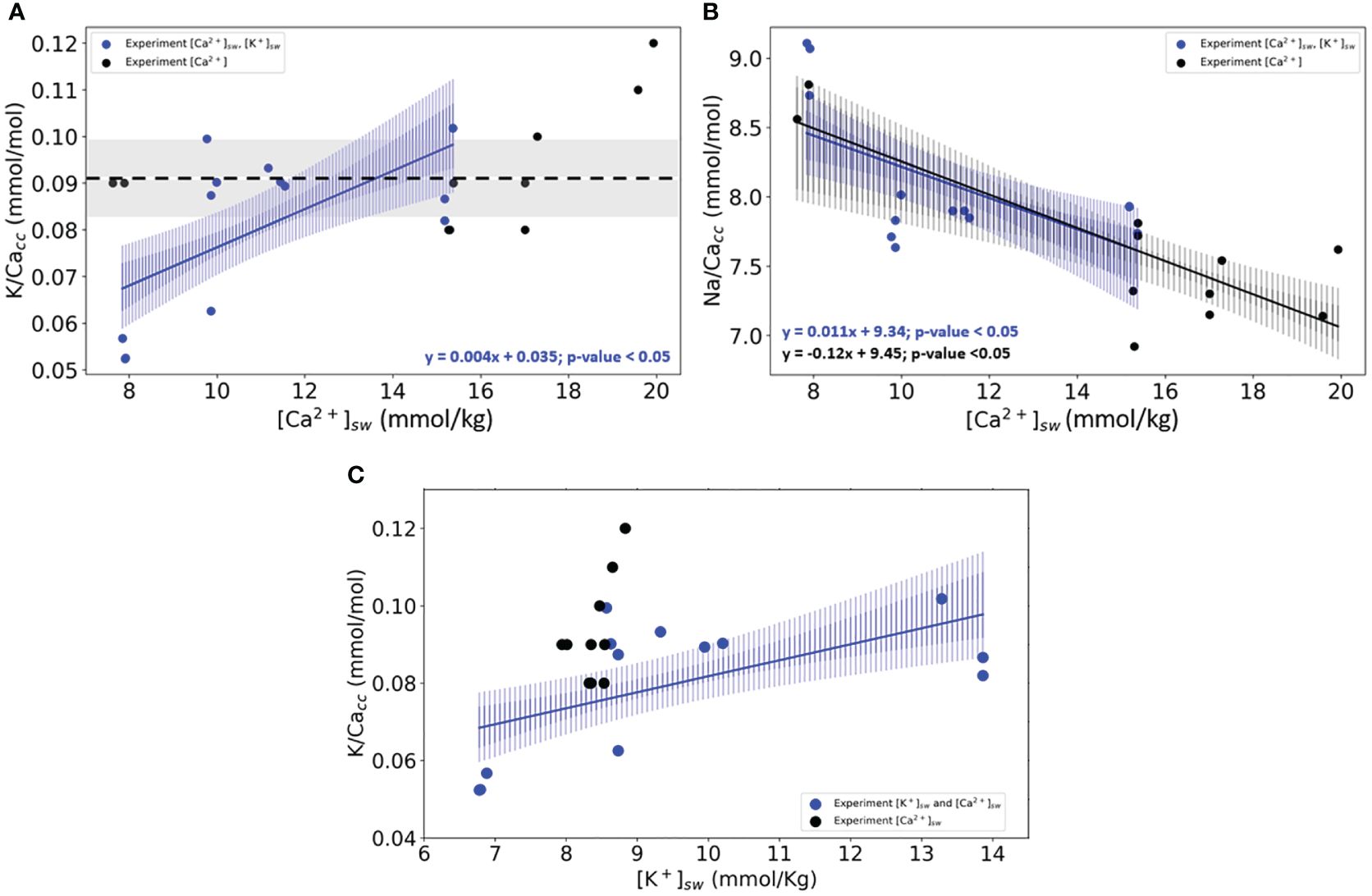
Figure 2 (A) K/Cacc in cultured specimens of (A) lessonii as a function of the culture’s media [Ca2+]sw. (B) Na/Cacc of the same specimens versus [Ca2+]sw. (C) K/Cacc versus [K+]sw. In panels (A, B), blue dots and lines are the results from the experiment in which both [Ca2+] and [K+] were varied. In black are the results from the experiments (Figure 1) where only [Ca2+] was varied. Lines indicate the linear correlations (both with p-values<0.05) based on the bootstrap analysis, for which the confidence intervals are added as envelopes (0.99 darker blue and 0.95 lighter shade of blue). The standard deviation for every replicate using the internal error from the SF-ICPMS was very small making it imperceptible in the plotted data (Supplementary Table S10). Panel (C) shows the same statistical analysis for both experiments axys X changes, and instead of [Ca2+]sw, the results are plotted against [K+]sw.
3.3 Temperature experiment
Both K/Cacc and Na/Cacc of the cultured A. lessonii do not show consistent changes with varying temperature (Figures 3A, B), although the average K/Cacc was slightly higher at the lowest temperature (0.21 +/- 0.06 mmol/mol) (Supplementary Table S9). Variability observed for K/Cacc was higher than that of Na/Cacc but does not change consistently between the four temperature conditions using a regression analysis giving for both plots (Figure 3) p-values > 0.05.
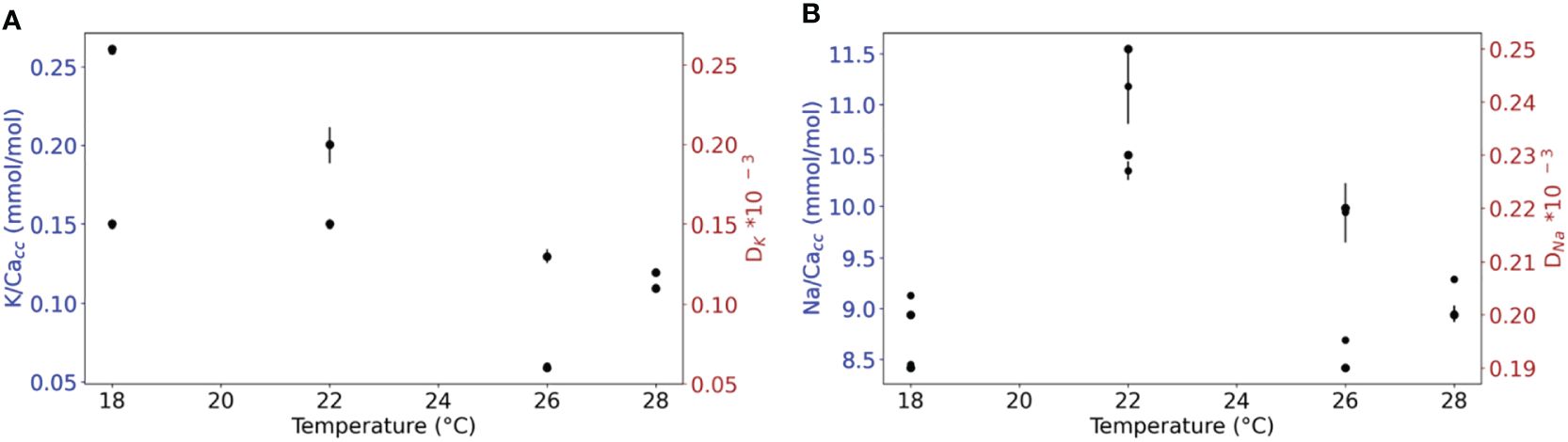
Figure 3 (A) K/Cacc and DK versus temperature. (B) Na/Cacc and DNa versus temperature. The RSD for every replicate using the internal error from the SF-ICPMS are plotted with black lines (Supplementary Table S11). For either of the elements, a regression analysis shows no significant correlation between El/Cacc and temperature (p< 0.05).
3.4 K/Cacc versus Na/Cacc
Combining all data, Na/Cacc and K/Cacc of the cultured A. lessonii do not correlate with each other (Figure 4) when varying temperature or changing only [Ca2+]. However, when considering the experiments in which both [K+] and [Ca2+] varied with a constant K/Casw K- and Na-incorporation were negatively correlated, this correlation is due to the difference in [Ca2+]sw that changes the Na incorporated, and not because of a Na+ and K+ compete for the same spot in the calcite crystal lattice during incorporation.
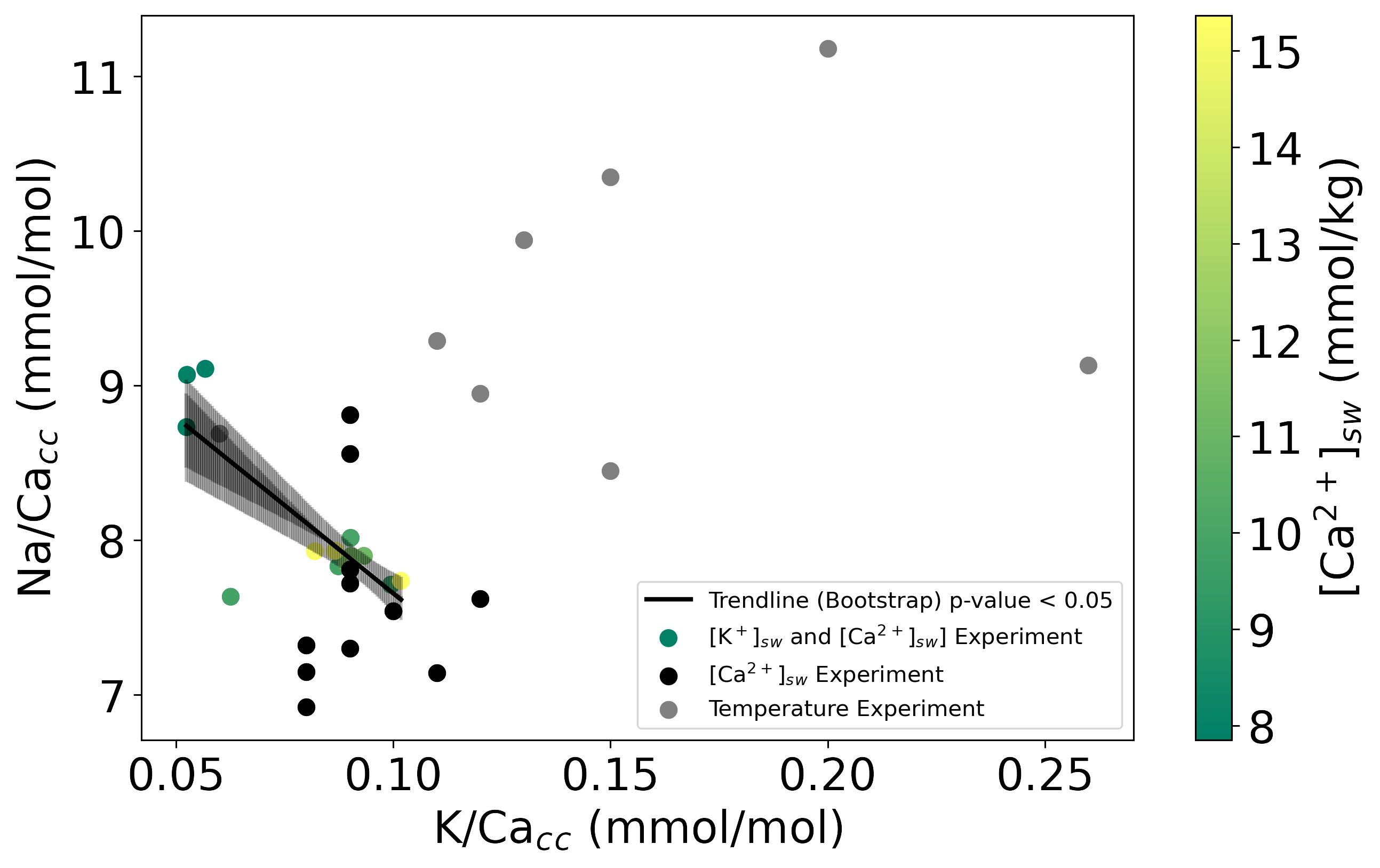
Figure 4 Na/Cacc versus K/Cacc for the three experiments. Solid lines indicate the linear correlations (both with p-values<0.05) based on the bootstrap analysis, for which the confidence intervals (0.99 darker black and 0.95 lighter black) are added as shading. The color-scale in experiment [K+]sw and [Ca2+]sw shows the [Ca2+]sw from green (higher [Ca2+]sw to yellow (lower [Ca2+]sw).
4 Discussion
4.1 Consistency of the data, the effect of temperature and the proxy value of K/Ca and Na/Ca
The K/Cacc values obtained for A. lessonii in all experiments ranged between 0.05 and 0.26 mmol/mol (Supplementary Table S9), without a noticeable effect of temperature (Figure 3A). The total amount of calcite added per treatment, either when changing [Ca2+]sw or temperature did not correlate to K/Cacc (Supplementary Figures S2 and S3, respectively). This means that the average K/Cacc does not depend on growth rate and changes therein reflect directly the effects of temperature or seawater chemistry. The relatively high variability observed for K/Cacc at the lowest temperature (Figure 3A) may be explained by uneven growth of foraminiferal specimens across temperatures. At the lowest temperature (18°C) on average fewer chambers were added per specimen than at higher temperatures (Supplementary Tables S13, S14). As this increases the relative contribution of pre-existing carbonate (i.e. from before the actual experiment) this would add to the variability in the data. The contribution of chambers formed before start of the incubation may have also biased average K/Ca and Na/Ca values, with a larger impact for specimens that added less chambers during the experiment.
The potassium-to-calcium ratio in A. lessonii (0.10 – 0.26; Figure 1A) overlap with those established for inorganically precipitated calcites (Ishikawa and Ichikuni, 1984); K/Cacc: 0.01-0.1 and (Okumura and Kitano, 1986). Still, it is challenging to compare these coefficients directly as the overall composition of the culture media (artificial seawater) in our experiment differed considerably from that used in the inorganic precipitation experiments, which only contained few elements.
The only other report on foraminiferal K/Cacc indicated similar ratios for the benthic O. ammonoides (0.12-0.30; Nambiar et al., 2023), as those reported here (Supplementary Tables S10, S11). The small offset in the average ratios could be caused by differences in culturing conditions or by inter-species differences in El/Ca values as observed for many elements (Bentov and Erez, 2006). Although we used a slightly different analytical approach (higher mass resolution) this is unlikely to have resulted in appreciable offsets between studies (methods and Supplementary Figure S1).
The average and range in Na/Cacc of cultured specimens (Supplementary Tables S9-S11) are similar to ratios reported previously for this genus (Geerken et al., 2019; Levi et al., 2019). The Na/Cacc in specimens cultured from 18 to 28°C vary between 8.8 and 11 mmol/mol (Supplementary Table S9) and are not correlated to temperature, which is in line with previous reports (Allen et al., 2016; Geerken et al., 2019). In the experiment with temperatures ranging from 18 to 28°C (Supplementary Table S9, Figure 3), K/Cacc values show no significant differences between different temperature conditions, agreeing with the field data from (Nambiar et al., 2023). The absence of an effect of temperature on either K/Cacc or Na/Cacc underscores the potential for applying these ratios in reconstructing (long-term) changes in seawater composition.
Element/Ca ratios vary greatly between genera and species and generally show elevated ratios for multiple elements in the same taxon (Evans et al., 2015; Mewes et al., 2015a; Hauzer et al., 2018; Van Dijk et al., 2019; Dämmer et al., 2021). The magnitude by which the different elements vary among species, however, is not constant. For K/Cacc, ratios are on average slightly higher in O. ammonoides (Nambiar et al., 2023) than in A. lessonii. (Figures 1–3), which is in line with the observed difference in Mg/Cacc between these species. Mg/Cacc is approximately 140 mmol/mol for O. ammonoides (Evans et al., 2015) and varies between 25 and 30 mmol/mol for Amphistegina sp (De Nooijer et al., 2017). Similarly, Na/Cacc in A. lessonii varies between 7 and 11 mmol/mol (Figures 1B, 2B and 3B; (Van Dijk et al., 2017c) and around 20 - 25 mmol/mol in O. ammonoides (Hauzer et al., 2018). In contrast, interspecific differences in Sr/Ca are relatively small [~1.7 versus ~2.6 mmol/mol for Amphistegina and Operculina, respectively; (Geerken et al., 2019; Hauzer et al., 2021)], but still, as for the other elements, they are all higher in Operculina than in Amphistegina. The consistent offsets, albeit with different magnitudes, suggest that differences in biomineralization pathways, e.g. Ca2+ pumping rate, between genera and species are reflected in element incorporation (De Nooijer et al., 2023). These inter-specific differences in element uptake are consistent with the reported relatively low K/Cacc values for planktonic foraminiferal species [0.06-0.12 mmol/mol; (Li et al., 2021)], as these are also known to have low values for Mg/Ca (1-5 mmol/mol; (Nürnberg et al., 1996; Anand et al., 2003) compared to Amphistegina and O. ammonoides (Figure 5). More recently (Nambiar et al., 2023), however, showed ratios (about 0.25 mmol/mol) for G. ruber comparable to those reported here and for A. lessonii (Nambiar et al., 2023).
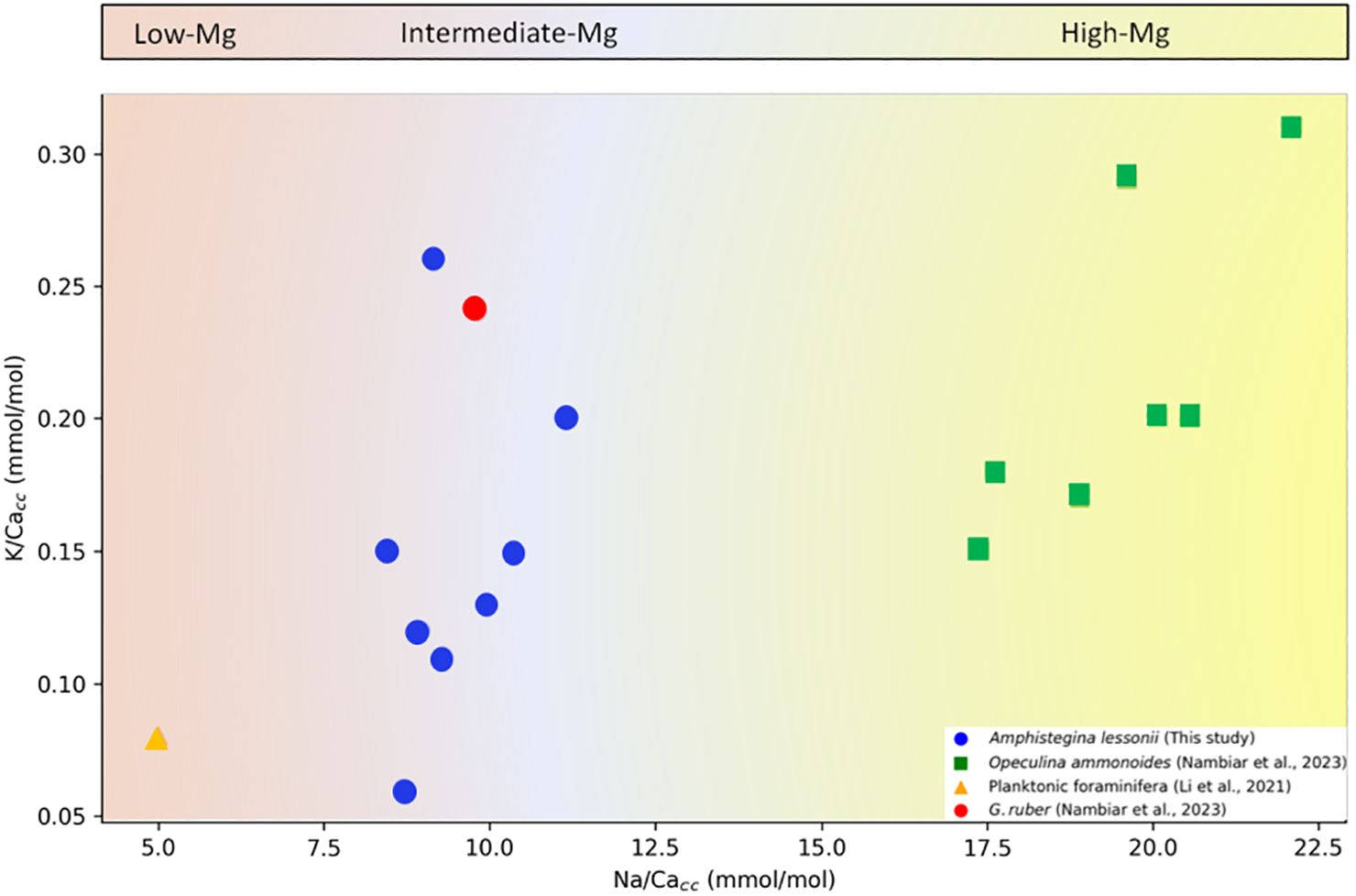
Figure 5 K/Cacc versus Na/Cacc for groups with different average Mg/Cacc. Low-Mg/Ca (<40 mmol/mol), Intermediate-Mg/Ca (40 – 100 mmol/mol) and High-Mg/Ca (>100 mmol/mol) (Blackmon and Todd, 1959).
Recently, foraminiferal Na/Cacc was suggested to act as a proxy for seawater [Ca2+] (Hauzer et al., 2018) in addition to its potential to reconstruct salinity (Wit et al., 2013; Mezger et al., 2019). Our results confirm that foraminiferal Na/Cacc is responsive to changes in [Ca2+] (Figures 1, 2) and the salinity range in Tables 2 and 4 in this case, show no significant effect in the Na or K incorporation, due to [Ca2+]sw differences act as the main driver where they are incorporated. Here we show that foraminiferal K/Cacc may be used to reconstruct past seawater [K+], adding to our understanding of the long-term cycling of the oceans’ major ions as was suggested by Nambiar et al., 2023). For seawater potassium concentrations, such a reconstruction may indicate rates of continental weathering (Kronberg, 1985; Sun et al., 2016) and indirectly, may reflect long-term changes in total alkalinity. Alternatively, seawater potassium concentrations may reflect rates of oceanic crust formation due to the difference between seawater and the mantle (Bloch and Bischoff, 1979).
4.2 Incorporation of potassium in foraminiferal calcite
K/Cacc in A. lessonii does not vary consistently with [Ca2+]sw (and hence K/Casw; Figure 1A). Conversely, K/Cacc increases when [Ca2+] and [K+] both increase (and K/Casw remains similar; Figure 2A). The absence of an effect of seawater Ca2+ and a positive effect of the combined K+ and Ca2+ elevation suggests that seawater [K+] is the main driver of K-incorporation into foraminiferal calcite (Figure 2C), whereas it is not affected by [Ca2+]. This resembles results from inorganic experiments in which K-incorporation was found to depend on solution [K+] (Ishikawa and Ichikuni, 1984; Okumura and Kitano, 1986). Such a dependency is hence fundamentally different from that observed for many divalent cations like Ba2+, Mn2+, Mg2+, or Sr2+, for which the concentration in seawater relative to [Ca2+]sw directly translates into a calcitic El/Ca ratio (Okumura and Kitano, 1986; Alkhatib et al., 2022). Such an incorporation pattern is caused by the competition of these ions with Ca2+ for a place in the calcite crystal lattice: for the monovalent cation incorporation is not governed by competition with Ca2+. However, incorporation of monovalent ions (Li+, Na+, K+, etc.) may be affected by different processes such as crystal surface sorption, speciation, and crystal growth rate, which complicates a straightforward interpretation of the environmental controls on element partitioning during calcification. Most likely a combination of all these effects impacts the uptake of monovalent cations.
More precisely, incorporation of an element into foraminiferal calcite is at least a two-step process (Figure 6; Bentov and Erez, 2006). There is the biological activity that configures the size and shape of the calcifying space and sets the composition of the fluid from which CaCO3 precipitates (Erez, 2003; Bentov and Erez, 2006; Bentov et al., 2009; De Nooijer et al., 2014). The exact controls are only partly characterized and may vary between species, but include selective ion transport (Nehrke et al., 2013; Toyofuku et al., 2017), pH manipulation (Bentov et al., 2009; De Nooijer et al., 2009; Glas et al., 2012) and production of organic templates (Branson et al., 2016; Tyszka et al., 2019). Secondly, there are various (physico-) chemical processes that operate within the calcifying space that determine how much of each ion in the fluid is incorporated into the shell. Relevant processes include Rayleigh fractionation (Elderfield et al., 1996; Evans et al., 2018), kinetics (Uchikawa and Zeebe, 2012; Devriendt et al., 2021), CaCO3 phase-transformations (Gray and Evans, 2019), chemical speciation (Van Dijk et al., 2017b) and configuration of ions within the crystal lattice (Branson et al., 2015) (Figure 6). For K one would expect a different trend as K uptake does not depend on Ca concentration (Figures 1, 2), but should reflect K concentration at the site of calcification (SOC). Nambiar et al. (2023), however, suggested an effect of [Ca2+] on K-incorporation, as was observed in corals (Ram and Erez, 2021). The within chamber wall K profiles of Geerken et al. (2019) actually show a gradual increase towards the end of a low concentration band (i.e. towards the outer surface of the shell wall). Other studies, however, have shown a tight correlation between the alternating high- and low-K bands with those of other elements (e.g. Mg) in Amphistegina lobifera and A. lessonii (Levi et al., 2019), which hints at a similarity in their incorporation dynamics. In addition, phosphorus is present in relatively narrow bands, similar to those of potassium, which hints to a coupling in their incorporation mode.
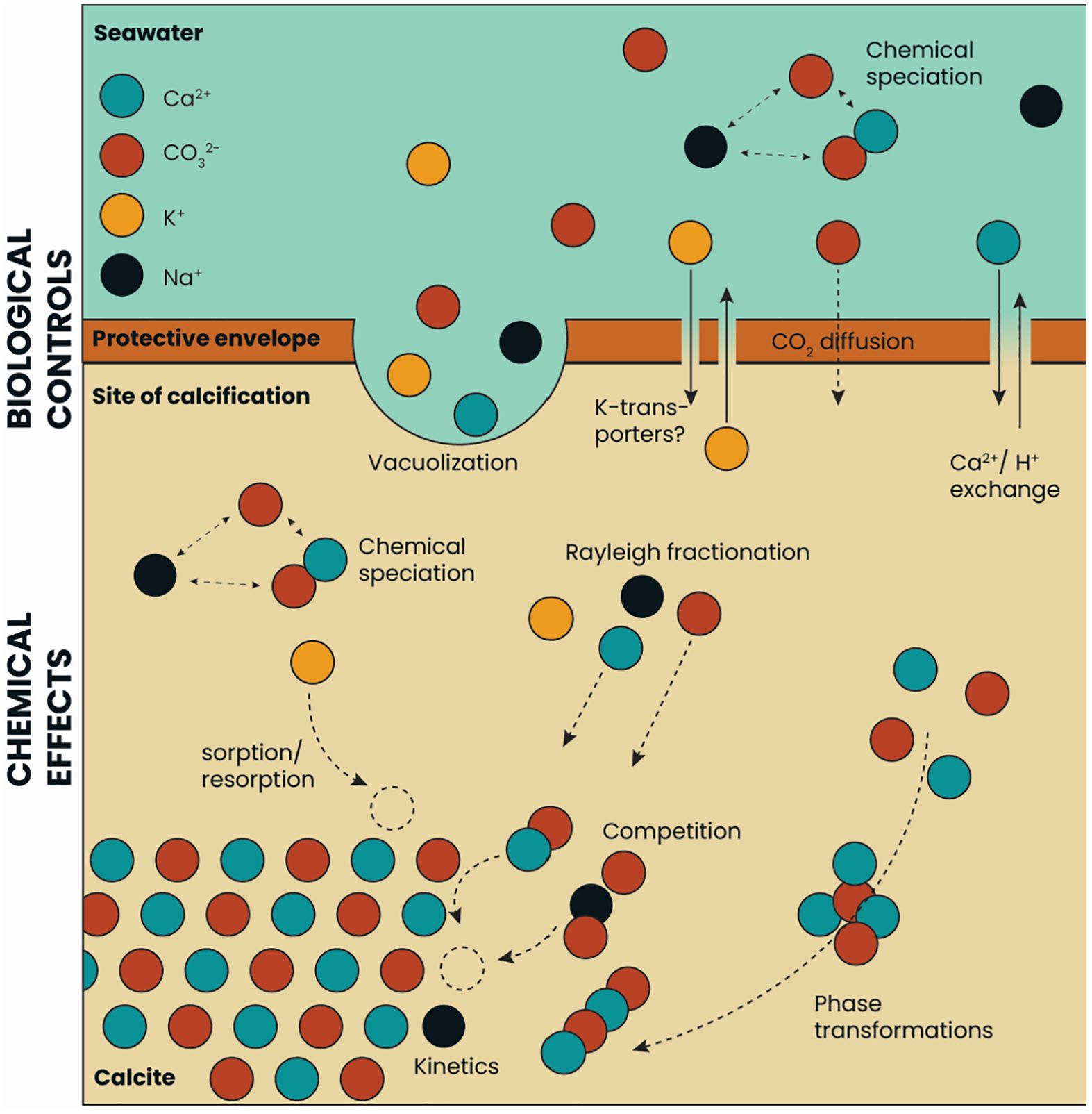
Figure 6 Simplified calcification scheme for rotaliid foraminifera. Biological and chemical processes at the site of calcification are presented, with a focus on the differences between Na+ and K+ incorporation into the calcite.
The observed relation between a solution’s El/Ca and the calcitic El/Ca is likely affected by both biological and ‘chemical’ steps during foraminiferal biomineralization. Transport of K-ions over a cell membrane is not likely facilitated by Ca-transporters, while an occasional Mg-ion may well pass a Ca-pump (e.g. Dudev and Lim, 2013). For the precipitation dynamics, the monovalent K ion may occupy the interstitial sites in the calcite lattice (Ishikawa and Ichikuni, 1984), in the organic phases, or be incorporated in amorphous K2CO3 (Li et al., 2021). It is likely that all or most of these processes together determine the eventual K/Cacc and Na/Cacc of the foraminiferal calcite (Figure 6).
Our results show that the seawater’s [K+], rather than [Ca2+] is the main driver for K partitioning. This is in line with free K+ being the main species present in seawater (Johnson and Pytkowicz, 1979), with K sorption at the crystal lattice being the primary way K is incorporated. It should be noted however, that the chemical speciation of potassium in the calcifying fluid may differ from that in seawater due to the foraminfer’s biological control on that fluid’s composition (Erez, 2003; Bentov and Erez, 2006; De Nooijer et al., 2014) In contrast, for Na-incorporation, our results, and the dependence of Na/Cacc on the solution’s Na/Ca (Figure 2) suggest that sodium and calcium compete for the same place in the crystal lattice. This would imply that sodium incorporation substitutes by replacement of two Na ions for one Ca ion (Devriendt et al., 2021) in the calcite and form a quadratic relationship. Since we do not observe this, alternatives including lattice vacancies can be filled by other divalent elements such as Mg2+ or Sr2+ may also play a role in the calcite’s El/Ca.
Although our results cannot exclude that K-incorporation at interstitial sites is responsible for a (minor) part of the foraminiferal K/Cacc, (Nambiar et al., 2023) showed an increasing K/Cacc with K/Casw for O. ammonoides with similar conditions as the one show in the [Ca2+]sw experiment from this study. This may indicate that the crystallographic orientation of potassium differs between foraminiferal species but may also indicate that biological uptake of potassium ions varies among species. The presence of specialized K-transporters in addition to Ca-transporters (Toyofuku et al., 2017) promoting K-incorporation into the shell would imply an advantage of higher K/Ca, which is not immediately apparent. Alternatively, inward K-transport into the site of calcification may result from the organismal need to accompany inward transport of a negatively charged ions and/or to counter the outward transport of another positively charged ion in the calcifying fluid. Major anions are SO42-, Cl-, but both do not seem to be enriched in foraminiferal calcite compared to seawater and inorganically precipitated calcite (Kontrec et al., 2004; Van Dijk et al., 2017b; Roepert et al., 2020). Cations that are pumped out during calcification include H+ (Glas et al., 2012) and are hypothesized to be coupled to an inward Ca2+ pumping (De Nooijer et al., 2017). The outward pumping of H+ may sometimes also affect Na+- and/or K+-transport. With it is considerably larger ionic radius K+ (152 pm) is less likely to be ‘accidentally’ transported through a transporter designed for H+ (ionic radius of 66 pm) compared to Na+ (116 pm).
When pumping of K+ by the foraminifer is somehow affecting the K/Ca at the site of calcification incorporation of potassium in foraminiferal calcite may be impacted by an intracellular role of [K+]. Potassium is often used as an agent to regulate osmosis and is found in cells at [K+]/[Na+] ratios in the 0.1–1.0 range (Dibrova et al., 2015). Sodium ions form relatively strong hydrogen bonds, therefore attract water, and disrupt intracellular processes. For this reason, many (marine) cells have [K+]/[Na+] antiporters (Nakamura et al., 1992; Shabala et al., 2009). Such a transport mechanism may (partly) explain the observed difference in Na/Ca and K/Ca in foraminifera when compared to inorganically precipitated calcite. The partition coefficients for potassium are relatively similar between inorganic and foraminifera calcite, while the partition coefficients for sodium are much lower in inorganically precipitated calcite (compared to foraminiferal shells). Overall, this makes K uptake in foraminiferal calcite a much more reliable proxy for reconstructing past sea water major ion composition.
5 Conclusions
Potassium in the shells of the benthic foraminifera A. lessonii was found at concentrations of 0.05-0.26 mmol/mol Ca. The incorporation of potassium was found not to depend on temperature, nor on seawater [Ca2+]. This also implies a fundamental difference in uptake compared to that of Na, the other major monovalent cation in sea water. Experiments in which [K+] and [Ca2+] varied simultaneously, resulted in a change in the foraminifer’s K/Ca, indicating the direct and unique effect of seawater [K+] on potassium partitioning during calcification. This implies that fossil foraminiferal K/Ca may directly reflect changes in seawater [K+], without being affected by changes in [Ca2+]. We did find an effect of [Ca2+]sw on Na- incorporation, highlighting the potential to use these two elements combined to reconstruct both seawater potassium and calcium concentrations.
Data availability statement
The original contributions presented in the study are included in the article/Supplementary Material. Further inquiries can be directed to the corresponding authors.
Ethics statement
The manuscript presents research on animals that do not require ethical approval for their study.
Author contributions
LP: Conceptualization, Data curation, Formal Analysis, Investigation, Writing – original draft, Writing – review & editing. LD: Conceptualization, Formal Analysis, Methodology, Project administration, Supervision, Writing – original draft, Writing – review & editing. WB: Data curation, Methodology, Writing – original draft, Writing – review & editing. G-JR: Conceptualization, Formal Analysis, Funding acquisition, Investigation, Methodology, Project administration, Supervision, Writing – original draft, Writing – review & editing.
Funding
The author(s) declare financial support was received for the research, authorship, and/or publication of this article. This work was carried out under the program of the Netherlands Earth System Science Centre (NESSC), financially supported by the Ministry of Education Culture and Science (OCW).
Acknowledgments
To Patrick Laan for the SF-ICP-MS analyses for the seawater, and to Karel Baker for the DIC and TA analyses. To Joji Uchikawa for the helpful discussion during the experimental work.
Conflict of interest
The authors declare that the research was conducted in the absence of any commercial or financial relationships that could be construed as a potential conflict of interest.
Publisher’s note
All claims expressed in this article are solely those of the authors and do not necessarily represent those of their affiliated organizations, or those of the publisher, the editors and the reviewers. Any product that may be evaluated in this article, or claim that may be made by its manufacturer, is not guaranteed or endorsed by the publisher.
Supplementary material
The Supplementary Material for this article can be found online at: https://www.frontiersin.org/articles/10.3389/fmars.2024.1385347/full#supplementary-material
References
Alkhatib M., Qutob M., Alkhatib S., Eisenhauer A. (2022). Influence of precipitation rate and temperature on the partitioning of magnesium and strontium in calcite overgrowths. Chem. Geol. 599, 120841. doi: 10.1016/j.chemgeo.2022.120841
Allen K. A., Eggins S. M., Haynes L. L., Rosenthal Y., Yu J. (2016). Trace element proxies for surface ocean conditions: A synthesis of culture calibrations with planktic foraminifera. Geochim. Cosmochim. Acta 193, 197–221. doi: 10.1016/j.gca.2016.08.015
Anand P., Elderfield H., Conte M. H. (2003). Calibration of Mg/Ca thermometry in planktonic foraminifera from a sediment trap time series. Paleoceanography 18, 1050. doi: 10.1029/2002pa000846
Barker S., Greaves M., Elderfield H. (2003). A study of cleaning procedures used for foraminiferal Mg/Ca paleothermometry. Geochem. Geophys. Geosyst. 4, 1–20. doi: 10.1016/j.quascirev.2004.07.016
Bentov S., Erez J. (2006). Impact of biomineralization processes on the Mg content of foraminiferal shells: A biological perspective. Geochem. Geophys. Geosyst. 7, Q01P08. doi: 10.1029/2005GC001015
Bentov S., Brownlee C., Erez J. (2009). The role of seawater endocytosis in the biomineralization process in calcareous foraminifera. Proc. Nat. Acad. Sci. 106 (51), 21500–21504. doi: 10.1073/pnas.0906636106
Blackmon P. D., Todd R. (1959). Mineralogy of some foraminifera as related to their classification and ecology. J. Paleontol. 33, 1–15. Available at: http://www.jstor.org/stable/1300802
Bloch S., Bischoff J. L. (1979). The effect of low-temperature alteration of basalt on the oceanic budget of potassium. Geology 7, 193–196. https://doi.org/10.1130/0091-7613(1979)7<193:TEOLAO>2.0.CO;2
Boer W., Weber M., Mertz-Kraus R., Hönisch B., Bijma J., Raitzsch M., et al. (2022). New calcium carbonate nano-particulate pressed powder pellet (NFHS-2-NP) for LA-ICP-OES, LA-(MC)-ICP-MS and µXRF. Geostandards. Geoanalytical. Res. 46, 411–432. doi: 10.1111/ggr.12425
Branson O., Bonnin E. A., Perea D. E., Gagnon A. C. (2016). Nanometer-scale chemistry of a calcite biomineralization template: implications for skeletal composition and nucleation. Proc. Natl. Acad. Sci. United. States America 113, 12934–12939. doi: 10.1073/pnas.1522864113
Branson O., Kaczmarek K., Redfern S. A. T., Misra S., Langer G., Tyliszczak T., et al. (2015). The coordination and distribution of B in foraminiferal calcite. Earth Planet. Sci. Lett. 416, 67–72. doi: 10.1016/j.epsl.2015.02.006
Culkin F., Cox R. A. (1966). Sodium, potassium, magnesium, calcium and strontium in sea water. Deep. Sea. Res. Oceanographic. Abstracts. 13, 789–804. doi: 10.1016/0011-7471(76)90905-0
Dämmer L. K., Van Dijk I., Nooijer L. J., van der Wagt B., Wilckens F. W., Zoetemelk B., et al. (2021). Temperature impact on magnesium isotope fractionation in cultured foraminifera. Front. Earth Sci. 9. doi: 10.3389/feart.2021.642256
De Goeyse S., Webb A. E., Reichart G.-J., De Nooijer L. J. (2021). Carbonic anhydrase is involved in calcification by the benthic foraminifer Amphistegina lessonii. Biogeosciences 18, 393–401. doi: 10.5194/bg-18-393-2021
De Nooijer L. J., Hathorne E. C., Reichart G. J., Langer G., Bijma J. (2014). Biomineralization in perforate foraminifera. Earth-Sci. Rev. 135, 48–58. doi: 10.1016/j.earscirev.2014.03.013
De Nooijer L. J., Pacho Sampedro L., Jorissen F. J., Pawlowski J., Rosenthal Y., Dissard D., et al. (2023). 500 million years of foraminiferal calcification. Earth-Sci. Rev. 243, 104484. doi: 10.1016/j.earscirev.2023.104484
De Nooijer L. J., Toyofuku T., Kitazato H. (2009). Foraminifera promote calcification by elevating their intracellular pH. Proc. Natl. Acad. Sci. United. States America 106, 15374–15378. doi: 10.1073/pnas.0904306106
De Nooijer L. J., Van Dijk I., Toyofuku T., Reichart G. J. (2017). The impacts of seawater mg/ca and temperature on element incorporation in benthic foraminiferal calcite. Geochem. Geophys. Geosyst. 18, 3617–3630. doi: 10.1002/2017GC007183
de Villiers S., Greaves M., Elderfield H. (2002). ‘An intensity ratio calibration method for the accurate determination of Mg/Ca and Sr/Ca of marine carbonates by ICP-AES’. Geochem. Geophys. Geosyst. 3, 1001. doi: 10.1029/2001gc000169
Devriendt L. S., Mezguer E. M., Olsen E. K., Watkins J. M., Kaczmarek K., Nehrke G., et al. (2021). Sodium incorporation into inorganic CaCO3 and implications for biogenic carbonates. Geochim. Cosmochim. Acta 314, 294–312. doi: 10.1016/j.gca.2021.07.024
Dibrova D. V., Galperin M. Y., Koonin E. V., Mulkidjanian A. Y. (2015). Ancient systems of sodium/potassium homeostasis as predecessors of membrane bioenergetics. Biochem. (Moscow). 80, 495–516. doi: 10.1134/S0006297915050016
Dudev T., Lim C. (2013). Importance of metal hydration on the selectivity of Mg2+ versus Ca2+ in magnesium ion channels. J. Am. Chem. Soc. 135, 17200–17208. doi: 10.1021/ja4087769
Dueñas-Bohórquez A., Raitzsch M., De Nooijer L. J., Reichart G. J. (2011). Independent impacts of calcium and carbonate ion concentration on Mg and Sr incorporation in cultured benthic foraminifera. Mar. Micropaleontol. 81, 122–130. doi: 10.1016/j.marmicro.2011.08.002
Duxbury A. C., Mackenzie F. T., Byrne R. H. (2024). “seawater”. Encyclopedia britannica. Available online at: https://www.britannica.com/science/seawater (Accessed 9 February 2024).
Elderfield H., Bertram C. J., Erez J. (1996). A biomineralization model for the incorporation of trace elements into foraminiferal calcium carbonate. Earth Planet. Sci. Lett. 142, 409–423. doi: 10.1016/0012-821x(96)00105-7
Erez J. (2003). The source of ions for biomineralization in foraminifera and their implications for paleoceanographic proxies. Rev. Mineral. Geochem. 54, 115–149. https://doi.org/10.2113/0540115
Ernst S., Janse M., Renema W., Kouwenhoven T., Goudeau M.-L., Reichart G. J. (2011). Benthic foraminifera in a large Indo-Pacific coral reef aquarium. J. Foraminiferal. Res. 41, 101–113. doi: 10.2113/gsjfr.41.2.101
Evans D., Erez J., Oron S., Müller W. (2015). Mg/Ca-temperature and seawater-test chemistry relationships in the shallow-dwelling large benthic foraminifera Operculina ammonoides. Geochim. Cosmochim. Acta 148, 325–342. doi: 10.1016/j.gca.2014.09.039
Evans D., Müller W., Erez J. (2018). Assessing foraminifera biomineralisation models through trace element data of cultures under variable seawater chemistry. Geochim. Cosmochim. Acta 236, 198–217. doi: 10.1016/j.gca.2018.02.048
Fantle M. S., DePaolo D. J. (2005). Variations in the marine Ca cycle over the past 20 million years. Earth Planet. Sci. Lett. 237, 102–117. doi: 10.1016/j.epsl.2005.06.024
Fehrenbacher J. S., Russell A. D., Davis C. V., Gagnon A. C., Spero H., Cliff J. B., et al. (2017). Link between light-triggered Mg-banding and chamber formation in the planktic foraminifera Neogloboquadrina dutertrei. Nat. Commun. 8, 15441. doi: 10.1038/ncomms15441
Geerken E., De Nooijer L. J., Roepert A., Polerecky L., King H. E., Reichart G. J. (2019). Element banding and organic linings within chamber walls of two benthic foraminifera. Sci. Rep. 9, 3598. doi: 10.1038/s41598-019-40298-y
Geerken E., De Nooijer L. J., Van Dijk I., Reichart G. J. (2018). Impact of salinity on element incorporation in two benthic foraminiferal species with contrasting magnesium contents. Biogeosciences 15, 2205–2218. doi: 10.5194/bg-15-2205-2018
Glas M. S., Langer G., Keul N. (2012). Calcification acidifies the microenvironment of a benthic foraminifer (Ammonia sp.). J. Exp. Mar. Biol. Ecol., 424-425, 424–425. doi: 10.1016/j.jembe.2012.05.006
Gray W. R., Evans D. (2019). Nonthermal influences on mg/ca in planktonic foraminifera: A review of culture studies and application to the last glacial maximum. Paleoceanogr. Paleoclimatol. 34, 306–315. doi: 10.1029/2018PA003517
Hardie L. A. (1996). Secular variation in seawater chemistry: An explanation for the coupled secular variation in the mineralogies of marine limestones and potash evaporites over the past 600 m.y. Available online at: http://pubs.geoscienceworld.org/gsa/geology/article-pdf/24/3/279/3516554/i0091-7613-24-3-279.pdf.
Hauzer H., Evans D., Müller W., Rosenthal Y., Erez J. (2018). Calibration of Na partitioning in the calcitic foraminifer Operculina ammonoides under variable Ca concentration: Toward reconstructing past seawater composition. Earth Planet. Sci. Lett. 497, 80–91. doi: 10.1016/j.epsl.2018.06.004
Hauzer H., Evans D., Müller W., Rosenthal Y., Erez J. (2021). Salinity effect on trace element incorporation in cultured shells of the large benthic foraminifer operculina ammonoides. Paleoceanogr. Paleoclimatol. 36, e2021PA004218. doi: 10.1029/2021PA004218
Holland K., Branson O., Haynes L. L., Hönisch B., Allen K. A., Russell A. D., et al. (2020). Constraining multiple controls on planktic foraminifera Mg/Ca. Geochim. Cosmochim. Acta 273, 116–136. doi: 10.1016/j.gca.2020.01.015
Horibe Y., Endo K., Tsubota H. (1974). Calcium in the South Pacific, and its correlation with carbonate alkalinity. Earth Planet. Sci. Lett. 23, 136–140. doi: 10.1016/0012-821X(74)90040-5
Horita J., Zimmermann H., Holland H. D. (2002). Chemical evolution of seawater during the Phanerozoic: Implications from the record of marine evaporites. Geochim. Cosmochim. Acta 66, 3733–3756. doi: 10.1016/S0016-7037(01)00884-5
Humphreys M. P., Lewis E. R., Sharp J. D., Pierrot D. (2022). PyCO2SYS v1.8: Marine carbonate system calculations in Python. Geosci. Model. Dev. 15, 15–43. doi: 10.5194/gmd-15-15-2022
Ishikawa M., Ichikuni M. (1984). Uptake of sodium and potassium by calcite. Chem. Geol. 42, 137–146. doi: 10.1016/0009-2541(84)90010-X
Johnson K. S., Pytkowicz R. M. (1979). Ion association of chloride and sulphate with sodium, potassium, magnesium and calcium in seawater at 25°C. Mar. Chem. 8, 87–93. doi: 10.1016/0304-4203(79)90034-3
Kester D. R. (1967). Preparation of artificial seawater 1. Limnol. Oceanogr. 12, 176–179. doi: 10.4319/lo.1967.12.1.0176
Kontrec J., Kralj D., Brecevic L., Falini G., Fermani S., Noethig-Laslo V., et al. (2004). Incorporation of inorganic anions in calcite. Eur. J. Inorganic. Chem. 23, 4579–4585. doi: 10.1002/ejic.200400268
Kronberg B. I. (1985). Weathering dynamics and geosphere mixing with reference to the potassium cycle. Phys. Earth Planet. Interiors. 41, 125–132. doi: 10.1016/0031-9201(85)90027-5
Kunioka D., Toyofuku T., Ujiie Y. (2006). Microdistribution of Mg/Ca, Sr/Ca, and Ba/Ca ratios in Pulleniatina obliquiloculata test by using a NanoSIMS: Implication for the vital effect mechanism. Geochem. Geophys. Geosyst. 7, Q12P20. doi: 10.1029/2006GC001280
Lea D. W. (2003). “Trace elements in foraminiferal calcite,” in Modern foraminifera. Ed. Sen Gupta B. K. (Springer Netherlands, Dordrecht), 259–277. doi: 10.1007/0-306-48104-9_15
Lécuyer C. (2016). Seawater Residence times of some elements of geochemical interest and the salinity of the oceans. Bull. la Soc. Geol. France. 187, 245–260. doi: 10.2113/gssgfbull.187.6.245
Levi A., Müller W., Erez J. (2019). Intrashell variability of trace elements in benthic foraminifera grown under high CO2 levels. Front. Earth Sci. 7. doi: 10.3389/feart.2019.00247
Li W., Liu X. M., Wang K., Fodrie F. J., Yoshimura T., Hu Y. F. (2021). Potassium phases and isotopic composition in modern marine biogenic carbonates. Geochim. Cosmochim. Acta 304, 364–380. doi: 10.1016/j.gca.2021.04.018
Mewes A., Langer G., Thoms S., Nehrke G., Reichart G. J., De Nooijer L. J., Bijm J.. (2015a). ‘Impact of seawater [Ca2+] on the calcification and calcite Mg/Ca of Amphistegina lessonii’ Biogeosciences, 12(7), 2153–2162. doi: 10.5194/bg-12-2153-2015
Mewes A., Langer G., Reichart G. J., De Nooijer L. G., Nehrke G., Bijma J.. (2015b). ‘The impact of Mg contents on Sr partitioning in benthic foraminifers’ Chemical Geology, 412, pp. 92–98. doi: 10.1016/j.chemgeo.2015.06.026
Mezger E. M., De Nooijer L. J., Bertlich J., Bijma J., Nürnberg D., Reichart G. J. (2019). Planktonic foraminiferal spine versus shell carbonate Na incorporation in relation to salinity. Biogeosciences 16, 1147–1165. doi: 10.5194/bg-16-1147-2019
Mezger E. M., De Nooijer L. J., Boer W., Brummer G. J. A., Reichart G. J. (2016). Salinity controls on Na incorporation in Red Sea planktonic foraminifera. Paleoceanography 31, 1562–1582. doi: 10.1002/2016PA003052
Mitsuguchi T., Kawakami T. (2012). ‘Potassium and other minor elements in Porites corals: Implications for skeletal geochemistry and paleoenvironmental reconstruction’. Coral. Reefs. 31, 671–681. doi: 10.1007/s00338-012-0902-3
Nakamura T., Kawasaki S., Unemoto T. (1992). Roles of K+ and Na+ in pH homeostasis and growth of the marine bacterium Vibrio alginolyticus. J. Gen. Microbiol. 138, 1271–1276. doi: 10.1099/00221287-138-6-1271
Nambiar R., Hauzer H., Gray W. R., Henehan M. J., Cotton L., Erez J., et al. (2023). Controls on potassium incorporation in foraminifera and other marine calcifying organisms. Geochim. Cosmochim. Acta 351, 125–138. doi: 10.1016/j.gca.2023.04.020
Nehrke G., Keul N., Langer G., De Nooijer L. J., Bijma J., Meibom A. (2013). A new model for biomineralization and trace-element signatures of Foraminifera tests. Biogeosciences 10, 6759–6767. doi: 10.5194/bg-10-6759-2013
Nürnberg D., Bijma J., Hemleben C. (1996). Assessing the reliability of magnesium in foraminiferal calcite as a proxy for water mass temperatures. Geochim. Cosmochim. Acta 60, 803–814. doi: 10.1016/0016-7037(95)00446-7
Okai T., Suzuki A., Terashima S., Mayuri I., Nohara M., Kawahata H., et al. (2004). Collaborative analysis of GSJ/AIST geochemical reference materials JCp-1 (Coral) and JCt-1 (Giant Clam). Chikyukagaku. (Geochemistry). 38, 281–286.
Okumura M., Kitano Y. (1986). Coprecipitation of alkali metal ions with calcium carbonate. Geochim. Cosmochim. Acta 50, 49–58. doi: 10.1016/0016-7037(86)90047-5
Ram S., Erez J. (2021). The distribution coefficients of major and minor elements in coral skeletons under variable calcium seawater concentrations. Front. Earth Sci. 9. doi: 10.3389/feart.2021.657176
Roepert A., Polerecky L., Geerken E., Reichart G. J., Middelburg J. J. (2020). ‘Distribution of chlorine and fluorine in benthic foraminifera’. Biogeosciences 17, 4727–4743. doi: 10.5194/bg-17-4727-2020
Sadekov A. Y., Darling K. F., Ishimura T., Wade C. M., Kimoto K., Singh A. D., et al. (2016). ‘Geochemical imprints of genotypic variants of Globigerina bulloides in the Arabian Sea’. Paleoceanography 31, 1440–1452. doi: 10.1002/2016PA002947
Sanyal A., Hemming N. G., Hanson G. N., Broecker W. S. (1995). ‘Evidence for a higher pH in the glacial ocean from boron isotopes in foraminifera’. Nature 373, 234–236. doi: 10.1038/373234a0
Sarazin G., Michard G., Prevot F. (1999). ‘A rapid and accurate spectroscopic method for alkalinity measurements in sea water samples’. Water Res. 33, 290–294. doi: 10.1016/S0043-1354(98)00168-7
Schmidt C., Titelboim D., Brandt J., Herut B., Abramovich S., Almogi-Labin A., et al. (2016). ‘Extremely heat tolerant photo-symbiosis in a shallow marine benthic foraminifera’. Sci. Rep. 6, 1–9. doi: 10.1038/srep30930
Segev E., Erez J. (2006). ‘Effect of Mg/Ca ratio in seawater on shell composition in shallow benthic foraminifera’. Geochem. Geophys. Geosyst. 7, 1–8. doi: 10.1029/2005GC000969
Shabala L., McMeekin T., Shabala S. (2009). ‘Osmotic adjustment and requirement for sodium in marine protist thraustochytrid’. Environ. Microbiol. 11, 1835–1843. doi: 10.1111/j.1462-2920.2009.01908.x
Sun X., Higgins J., Turchyn A. V. (2016). ‘Diffusive cation fluxes in deep-sea sediments and insight into the global geochemical cycles of calcium, magnesium, sodium and potassium’. Mar. Geol. 373, 64–77. doi: 10.1016/j.margeo.2015.12.011
Toyofuku T., Matsuo M. Y., De Nooijer L. J., Nagai Y., Kawada S., Fujita K., et al. (2017). ‘Proton pumping accompanies calcification in foraminifera’. Nat. Commun. 8, 1–6. doi: 10.1038/ncomms14145
Tyszka J., Bickmeyer U., Raitzsch M., Bijma J., Kaczmarek K., Mewes A., et al. (2019). ‘Form and function of F-actin during biomineralization revealed from live experiments on foraminifera’. Proc. Natl. Acad. Sci. United. States America 116, 4111–4116. doi: 10.1073/pnas.1810394116
Uchikawa J., Zeebe R. E. (2012). ‘The effect of carbonic anhydrase on the kinetics and equilibrium of the oxygen isotope exchange in the CO2-H2O system: Implications for δ18O vital effects in biogenic carbonates’. Geochim. Cosmochim. Acta 95, 15–34. doi: 10.1016/j.gca.2012.07.022
Van Dijk I., De Nooijer L. J., Boer W., Reichart G. J. (2017b). Sulfur in foraminiferal calcite as a potential proxy for seawater carbonate ion concentration. Earth Planet. Sci. Lett. 470, 64–72. doi: 10.1016/j.epsl.2017.04.031
Van Dijk I., De Nooijer L. J., Reichart G. J. (2017c). Trends in element incorporation in hyaline and porcelaneous foraminifera as a function of pCO2. Biogeosciences 14, 497–510. doi: 10.5194/bg-14-497-2017
Van Dijk I., De Nooijer L. J., Wolthers M., Reichart G. J. (2017a). Impacts of pH and [CO32–] on the incorporation of Zn in foraminiferal calcite. Geochim. Cosmochim. Acta 197, 263–277. doi: 10.1016/j.gca.2016.10.031
Van Dijk I., Mouret A., Cotte M., Le Houedec S., Oron S., Reichart G. J., et al. (2019). Chemical heterogeneity of mg, mn, na, S, and sr in benthic foraminiferal calcite. Front. Earth Sci. 7. doi: 10.3389/feart.2019.00281
Virtanen P., Gommers R., Oliphant T.-E., Reddy T., Cournapeau D., Burovski E., et al. (2020). ‘{SciPy} 1.0: fundamental algorithms for scientific computing in python’. Nat. Methods 17, 261–272. doi: 10.1038/s41592-019-0686-2
Wang K., Close H.-G., Tuller-Ross B., Chen H. (2020). ‘Global average potassium isotope composition of modern seawater’. ACS Earth Space. Chem. 4, 1010–1017. doi: 10.1021/acsearthspacechem.0c00047
Wit J. C., De Nooijer L. J., Wolthers M., Reichart G. J. (2013). ‘A novel salinity proxy based on Na incorporation into foraminiferal calcite’. Biogeosciences 10, 6375–6387. doi: 10.5194/bg-10-6375-2013
Zeebe R. E., Tyrrell T. (2018). ‘Comment on “The Effects of Secular Calcium and Magnesium Concentration Changes on the Thermodynamics of Seawater Acid/Base Chemistry: Implications for Eocene and Cretaceous Ocean Carbon Chemistry and Buffering” by Hain et al., (2015)’. Global Biogeochem. Cycles. 32, 895–897. doi: 10.1002/2017GB005786
Keywords: foraminifera, biomineralization, K/Ca, Na/Ca, proxy, culture experiment
Citation: Pacho L, De Nooijer LJ, Boer W and Reichart G-J (2024) Differences between potassium and sodium incorporation in foraminiferal shell carbonate. Front. Mar. Sci. 11:1385347. doi: 10.3389/fmars.2024.1385347
Received: 12 February 2024; Accepted: 16 July 2024;
Published: 01 August 2024.
Edited by:
Ed Hathorne, Helmholtz Association of German Research Centres (HZ), GermanyReviewed by:
Sambuddha Misra, Indian Institute of Science (IISc), IndiaToshihiro Yoshimura, Japan Agency for Marine-Earth Science and Technology (JAMSTEC), Japan
Copyright © 2024 Pacho, De Nooijer, Boer and Reichart. This is an open-access article distributed under the terms of the Creative Commons Attribution License (CC BY). The use, distribution or reproduction in other forums is permitted, provided the original author(s) and the copyright owner(s) are credited and that the original publication in this journal is cited, in accordance with accepted academic practice. No use, distribution or reproduction is permitted which does not comply with these terms.
*Correspondence: Laura Pacho, bGF1cmEucGFjaG8uc2FtcGVkcm9Abmlvei5ubA==; Lennart Jan De Nooijer, bGVubmFydC5kZS5ub29pamVyQG5pb3oubmw=