- 1College of Earth, Ocean and Atmospheric Sciences, Oregon State University, Corvallis, OR, United States
- 2Antarctic Ecosystem Research Division, Southwest Fisheries Science Center, National Oceanic and Atmospheric Administration, La Jolla, CA, United States
- 3School of Aquatic and Fishery Sciences, College of the Environment, University of Washington, Seattle, WA, United States
- 4Institute of Arctic and Alpine Research, University of Colorado, Boulder, CO, United States
The northwestern Antarctic Peninsula is an important spawning, recruitment, and overwintering ground for Antarctic krill. The region is warming rapidly, and the current impacts of climate and environmental variability on the reproductive cycle of krill remain unclear. Here, we examine the reproductive stage of female krill in the austral winter from 2012 to 2016 in relation to climate and environmental data to assess what factors influence the timing of reproductive development. We observed significant interannual variability in the degree of maturation in female krill, ranging from 48% of female krill measured at a station in 2016 to a maximum of 94% of female krill measured at a station in 2014. On average, across all five years, three-quarters of the female krill sampled were in the stage known as previtellogenesis, the point at which the onset of sexual maturity begins. The preceding spring, summer, and autumn Southern Annular Mode and the Multivariate El Niño Index explained most of the variance in the data and indicated a strong, preconditioning storm-related effect on environmental conditions leading up to winter, affecting krill maturation status at the end of the winter season. Results from our study can be used to improve krill population models that are necessary for the management of the krill fishery and for conservation at the northwestern Antarctic Peninsula.
1 Introduction
The northwestern Antarctic Peninsula (nAP) is a year-round habitat for Antarctic krill, Euphausia superba (hereafter, krill), serving as an important spawning and recruitment ground in the spring and summer and as an overwintering hotspot (Siegel and Watkins, 2016; Reiss et al., 2017). The bathymetry of the region and the high levels of productivity that are common during the spring and summer make it an ideal place for krill to grow and reproduce (Murphy et al., 2017). However, climate-induced changes in water temperature, circulation patterns and seasonal sea ice extent and duration have altered the ecosystem in a way that may threaten the successful reproduction and growth of krill at the nAP in the future (Flores et al., 2012). Warming atmospheric and ocean temperatures and increasing winds have driven both the decline in seasonal sea ice extent and duration (Stammerjohn et al., 2008a, 2012), causing decreases in ice algal biomass during the autumn and winter (Lizotte, 2001). Increased wind speeds and temperatures have also limited spring and summer phytoplankton blooms in response to a weakly stratified surface layer (Schofield et al., 2018). A warming climate has also been linked to a size shift in the dominant phytoplankton community from diatoms (the preferred phytoplankton prey of krill) to smaller phytoplankton, such as cryptophytes (not generally consumed by krill) (Haberman et al., 2003; Montes-Hugo et al., 2008), as well as a regional shift, with greater phytoplankton biomass observed in more southerly regions of the Antarctic Peninsula (Montes-Hugo et al., 2009). Regional warming has been correlated with a southward contraction of the krill population toward higher latitudes of the Antarctic Peninsula over the last century (Atkinson et al., 2019). Further, the mean length of krill at the nAP has increased, suggesting a decline in krill recruitment in the region (Atkinson et al., 2019).
Successful krill recruitment events are determined by krill fecundity and the winter survival of krill larvae (Quetin and Ross, 2003). The physiological condition of female krill during the reproductive season (spring and summer) is positively correlated with recruitment success the following spring, emphasizing the importance of female fecundity to krill recruitment (Steinke et al., 2021). Krill have a variable spawning season ranging from 1 to 3 months (Ross and Quetin, 2000) and undergo sexual regression in the autumn to conserve energy through the winter, followed by a re-maturation the following spring (Ross and Quetin, 2000; Kawaguchi et al., 2007; Kawaguchi, 2016). Re-maturation is cued by environmental light intensity and an internal clock mechanism (Teschke et al., 2011; Höring et al., 2018), but previtellogenesis, the sexual development stage in female krill that directly precedes spawning, can only be initiated when food concentrations are sufficiently high (Ross and Quetin, 2000). Indeed, early spring phytoplankton blooms have been shown to induce superfluous feeding in krill and are correlated with enhanced sexual maturity and early-season mating, both of which may lead to an early initiation of spawning (Schmidt et al., 2012). Thus, the timing of the spring bloom could influence the start date of the spawning season. Further, at lower latitude locations of the Antarctic, such as the nAP, higher light levels persist throughout the autumn and winter, compared to higher latitude environments, and baseline food concentrations may be sufficient for krill to either (i) reduce their degree of sexual regression and remain in a more sexually advanced stage throughout the winter (Siegel, 1989; Kawaguchi, 2016), or (ii) encourage early maturation by krill at the end of winter (Steinke et al., 2022). A shorter re-maturation period is likely to lead to greater reproductive output and subsequent recruitment success in krill populations at the nAP via an earlier start to, and a lengthening of, the spawning season.
An understanding of the variability in the maturation state of female krill toward the end of winter and its relationship to environmental and climate conditions would provide valuable information on variability in the timing of the spawning season. This is particularly important at the nAP because of the rapid warming and concomitant physical and ecological changes occurring there (Siegert et al., 2019). A recent study by Reiss et al. (2020) found that most of the female krill at the nAP were in their resting reproductive stage (stage F2, identified from external morphology using the key of Makarov and Denys, 1980) at the end of the austral winter from 2012 to 2016. Although the study was conducted during a period when sea ice coverage and environmental conditions were highly variable between years, driven largely by alternating phases of the Southern Annular Mode (SAM) and El-Niño Southern Oscillation (ENSO), the authors found no significant interannual variability in female reproductive stage (Reiss et al., 2020). The reason for this may, in part, be due to the external morphology approach used by those authors. Female krill are at the F2 stage when the thelycum, the external organ used to distinguish females and which starts to form during oogenesis, is present. However, the distinction between stages F2 (oogenesis) and F3A (previtellogenesis) is that in the latter stage the thelycum is fully developed, red in color and apparent through the gills (Makarov and Denys, 1980). Sexual staging based on external morphology requires the individual researcher staging the krill to decide whether the thelycum is sufficiently red and developed to warrant the leap from F2 to F3, making it relatively subjective. An alternative approach is the “squash” method developed by Cuzin-Roudy and Amsler (1991), in which ovarian cells are gently squashed under a cover slip and examined under a compound microscope. The squash technique is useful in objectively detecting subtle changes in sexual maturation at the cellular level that could not otherwise be determined using external morphology alone (Cuzin-Roudy and Amsler, 1991). Indeed, in a laboratory study in which sub-adult krill were maintained in tanks and provided supplemental food through the winter months (April to September), the squash method was used to show that 27% of female krill were in previtellogenesis in August, despite not having fully developed, red thelycums (Steinke et al., 2022).
Although no significant differences were found in the sexual development stages of female krill at the nAP between 2012 and 2016 by Reiss et al. (2020), the high degree of environmental variability between the years studied and the limitations of external morphological characteristics to represent cellular processes warrant further investigation. Consequently, we examined the reproductive stages of female krill at the nAP collected in late winter during the same set of cruises (2012 to 2016) presented in Reiss et al. (2020). We hypothesized that the late winter sexual maturity of female krill varied between years and that at least a proportion of female krill would be in previtellogenesis in late winter (either because of reduced sexual regression or earlier initiation of re-maturation) when climate and environmental conditions were such that they encouraged sufficiently high food availability leading up to and during the winter sampling period. An understanding of the variability in the sexual maturity stage of female krill in late winter will elucidate previously unknown relationships between climate and environmental conditions and reproductive development in female krill. Results from this study can improve krill population models that are used to better manage the krill fishery thereby ensuring the sustainability of this species.
2 Materials and methods
2.1 Study site and krill collection
Antarctic krill were collected during the austral winter (August and early September) at the northwestern Antarctic Peninsula (nAP) from 2012 to 2016 aboard the RVIB Nathaniel B. Palmer as part of a multi-year winter study conducted by the U.S. Antarctic Marine Living Resources (AMLR) program (Table 1). The survey area was divided into the West Area (Figure 1; 5 stations), Elephant Island (Figure 1; 3 stations), and the South Area (Figure 1; 5 stations). Krill were collected using an Isaacs-Kidd Midwater Trawl (IKMT) towed obliquely to an average depth of 170 meters or 10m off the bottom in shallow water. Upon collection, the krill used in this study were preserved in a 10% buffered formalin solution and stored for subsequent analyses (Reiss et al., 2017).
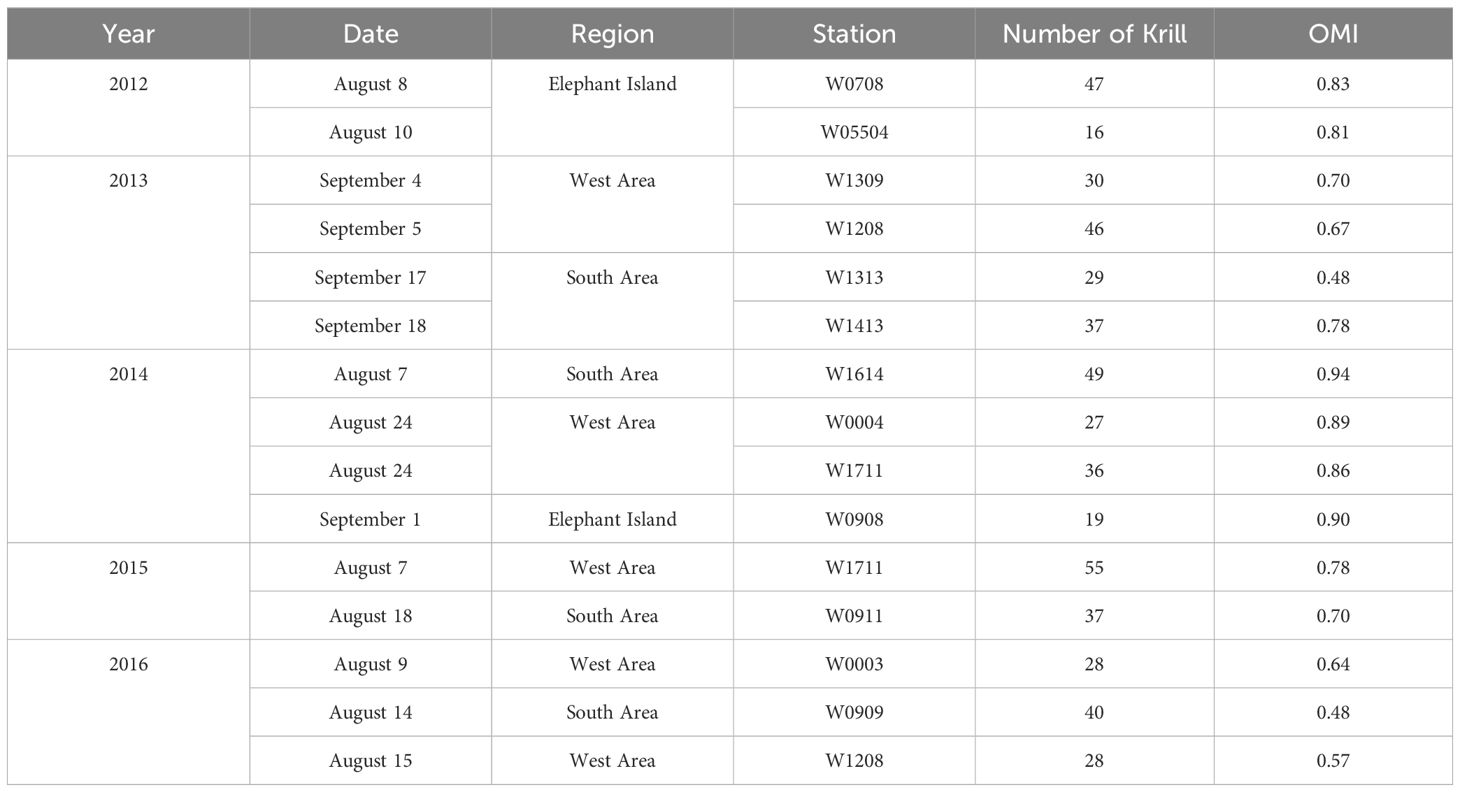
Table 1 Sampling years, date, regions, stations, number of krill sampled per station, and the average Onset of Maturity Index (OMI) at each sampling station in the northwestern Antarctic Peninsula from 2012 to 2016.
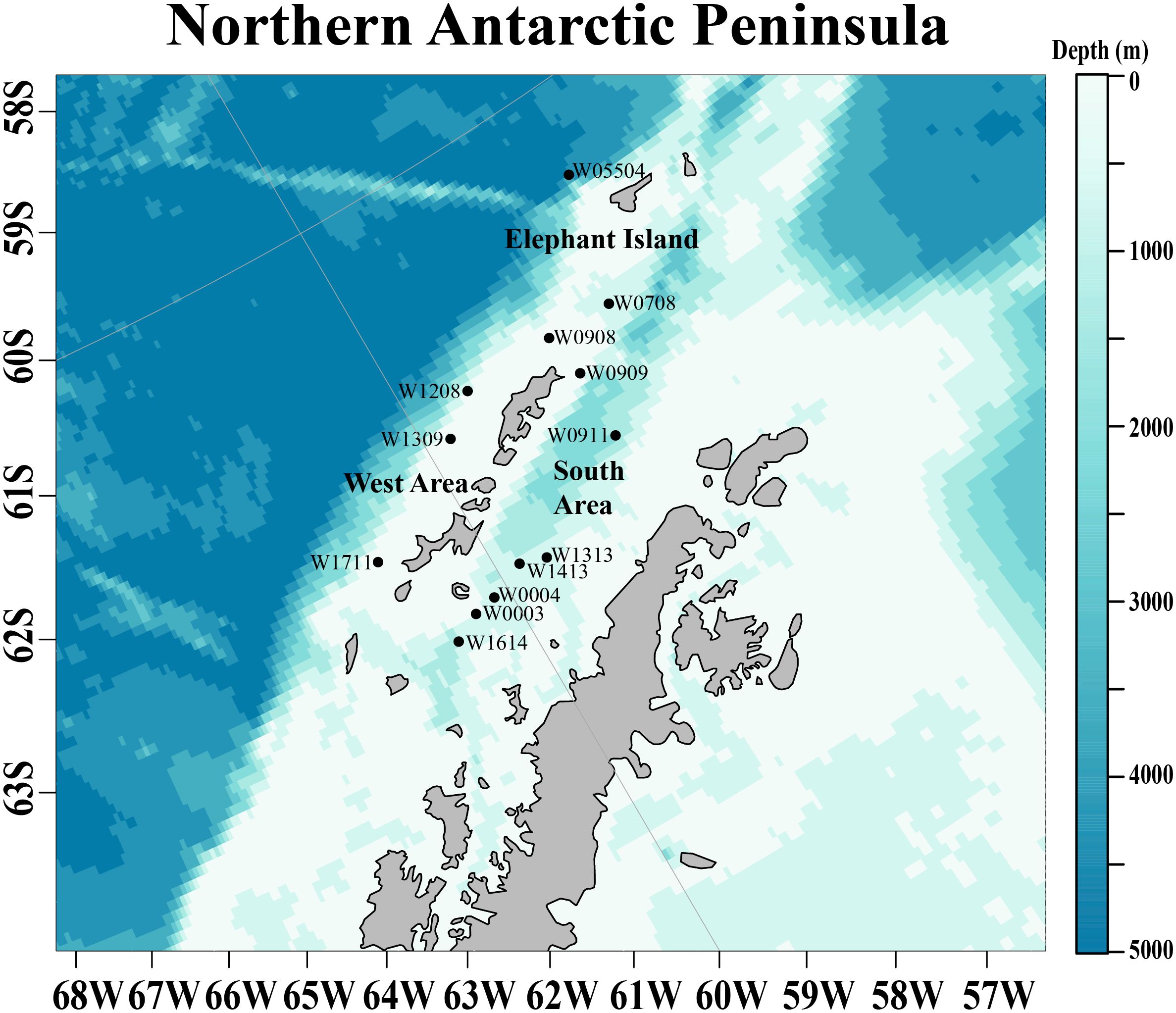
Figure 1 The study area at the northwestern Antarctic Peninsula (nAP) where krill were collected in one of three regions: Elephant Island, the West, or the South Area from 2012 to 2016. Sampling stations are represented by points with their corresponding station names shown.
2.2 Ovarian cell quantification and staging
Sexual development of female Antarctic krill is divided into ten stages that are referred to here as sexual development stages (SDS). For a more detailed description of the SDS, the reader is referred to Cuzin-Roudy and Amsler (1991) and Steinke et al. (2022). Briefly, the most immature stage (SDS 1) is gametogenesis where primary and secondary oogonia are formed. Oogenesis (SDS 2) occurs after females begin developing their external sexual organ, the thelycum, and when oogonia grow into primary oocytes. This stage is defined as F2 by Makarov and Denys (1980) and is considered the sub-adult stage. Previtellogenesis (SDS 3–4) is reached when krill develop primary oocytes and begin to accumulate glycoproteic yolk, becoming type 1 oocytes. Previtellogenesis is defined as stages F3A by Makarov and Denys (1980) and is considered the first adult stage. Vitellogenesis (SDS 5–7), correlative to stages F3B-E [Makarov and Denys (1980)], is achieved when there is sufficient yolk in the oocytes to carry out a spawning event and occurs cyclically, often with repeat spawning events. The oviposition stage (SDS 8) occurs after females finish a spawning event, and once all oocytes have been used or females have exhausted their energy reserves to the point that they are unable to spawn again, they enter the reproductive resting stages (SDS 9–10). Once all residual oocytes from the spawning season are resorbed by the female, they are considered to be back in gametogenesis (SDS 1–2), and the maturity cycle starts over (Cuzin-Roudy and Amsler, 1991). Krill begin to sexually mature in response to increasing food concentrations that usually coincide with the spring bloom (Kawaguchi et al., 2007). The rate of maturation fluctuates annually in response to environmental variability (Quetin and Ross, 2001; Kawaguchi et al., 2007).
In this study, we identified the SDS of each female krill using the squash method described by Cuzin-Roudy and Amsler (1991) and adapted by Steinke et al. (2022). We used the relationship between the diameter and area of the largest ovarian cell observed in each individual krill to determine the SDS of each female. Prior to dissection, each female krill was measured for total length (Standard Length 1 (mm); Mauchline, 1981). For each female, after a dissection, a single lobe of the ovary was removed and placed in phosphate-buffered saline (PBS) overnight to remove trace formalin. The ovary lobe was then stained with Biotium© DAPI, 10 mg/mL, for 24 hours. The lobe was subsequently rinsed in fresh PBS, placed onto a microscope slide, covered with a glass coverslip, and gently squashed. This squash technique separates the germ cells into a single layer, which were then examined using a Nikon H600L fluorescence microscope at 100x magnification. Cells were photographed, and the images were converted to 8-bit, 256-level grayscale for subsequent quantification using image analysis software (Figure 2).
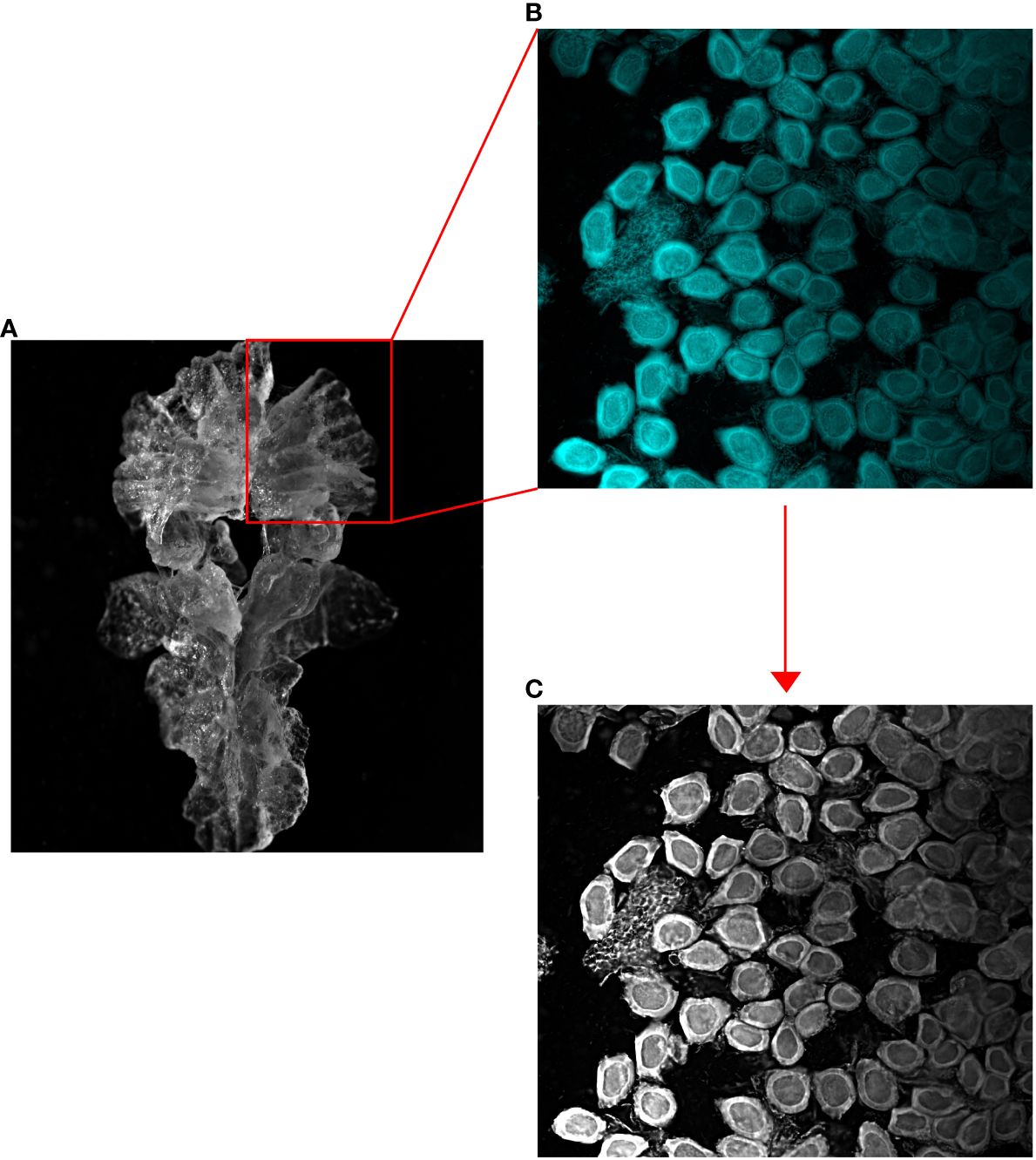
Figure 2 Microscope images of (A) an entire female krill ovary, (B) a lobe of the ovary stained with DAPI and subsequently squashed, and (C) a greyscale image of the fluorescently dyed ovarian section.
Like the study carried out by Steinke et al. (2022), the machine learning software programs ilastik and CellProfiler were used to quantify the ovarian cell areas (McQuin et al., 2018; Berg et al., 2019). Ilastik segmented the images into two categories: cells and background (Berg et al., 2019). Cell and background pixels in each image were annotated and a Random Forest classifier was applied to segment the images based on the annotations. Training was carried out in ilastik on a subset of the images (referred to here as the training set), until the program could accurately predict the cell objects in the images that were not trained (referred to here as the validation set).
CellProfiler was used to classify and quantify the objects within the images (McQuin et al., 2018). A pipeline in CellProfiler was developed to (i) remove the background from each image, (ii) threshold the objects within each image, and (iii) quantify the respective cell areas of each cell found in each image. Batch processing was applied in CellProfiler to quantify the objects quickly and accurately (i.e., cell areas) from all images in the image set. For a more detailed explanation of the ilastik and CellProfiler pipelines used in this study, the reader is referred to Steinke et al. (2022) Supplementary Materials.
2.3 Estimation of the onset of maturity index
The onset of maturity index (OMI) is a measure of the proportion of the female population that are in previtellogenesis (SDS 3–4), as previtellogenesis is the point at which female krill begin to undergo sexual maturation and accumulate glycoproteic yolk that they use to fuel the growth of oocytes in preparation for future spawning (Kawaguchi et al., 2007). We calculated the OMI for each sampling station where krill were caught. OMI was calculated using the same formula as in Steinke et al. (2022):
Where n is the number of females at a particular SDS at a sampling station. The largest cell area (µm2) in each image was used to estimate SDS, because the largest cell area represents the most advanced oocytes, and thus the most advanced SDS of the female ovary. A high OMI suggests that more of the female population were in previtellogenesis than in either gametogenesis/oogenesis (nSDS1-2) or resting stages (nSDS9-10).
2.4 Environmental data collection
2.4.1 Climate indices
Data on the Southern Annular Mode (SAM) Index and the Multivariate El Niño Southern Oscillation Index (MEI) were obtained from online data repositories. As the two dominant modes of climate variability in the Southern Hemisphere, SAM and the El Niño Southern Oscillation (ENSO) alter atmospheric circulation patterns (e.g., winds) and can impact ecosystem functionality by changing the physical conditions that structure Antarctic food webs and ecosystems (Thompson and Wallace, 2000; Massom et al., 2006; Stammerjohn et al., 2008b).
Monthly SAM data were acquired from the online database established by the National Environmental Research Council (NERC) British Antarctic Survey (BAS) (https://www.bas.ac.uk/data/our-data/publication/the-southern-annular-mode-variability-trends-and-climate-impacts-across/) and monthly MEI data were taken from the National Oceanic and Atmospheric Administration (NOAA), Earth System Research Laboratory (ESRL), Physical Sciences Division (https://psl.noaa.gov/enso/mei/) (Zhang et al., 2019; Fogt and Marshall, 2020). Seasonal SAM indices and the MEI were calculated based on the average values observed during the preceding austral spring (September-November), summer (December-February) and autumn (March-May) leading up to the winter sampling season, and during the winter (June-August) sampling season. We note that in 2013 and 2014 some of the sampling took place in early-to-mid September, rather than in August (Table 1). Winter values of the SAM index and the MEI during these years were calculated in the same way as the other sampling years for comparison purposes, averaging the monthly values from June to August to obtain climate values for the SAM index and for MEI during the sampling season. September sampling took place during the first few weeks of the spring season, thus the maturation state of females collected during September of 2013 and 2014 was likely impacted more by the seasonal climate and environmental variability leading up to the time of sampling, rather than the spring climate phases at the time of sampling. The seasonal SAM and ENSO anomalies for 2012 to 2016 were calculated by subtracting the seasonal values for a given year (e.g., 2012) from the average seasonal values of the full dataset (1957 to 2021 for the SAM Index and 1979 to 2021 for the MEI). The seasonal grouping of SAM and ENSO indices was done for two primary reasons (i) krill reproductive cycles happen on seasonal timescales (Ross and Quetin, 2000), and (ii) previous studies (e.g., Saba et al., 2014) have found that seasonal SAM and ENSO can impact ecological and food web dynamics at the Antarctic Peninsula.
2.4.2 Environmental variables
A Seabird 911 CTD was used to measure salinity (PSU), temperature (°C) and chlorophyll-a (chl-a; µg L-1; determined from fluorescence) in the water column at the sampling stations that corresponded with krill collected in each year. Salinity and temperature values used in our study were calculated as the average values over the top 100 meters of the water column at each station and are subsequently referred to in the text as AvgTemp and AvgSal. Integrated chl-a (IntChl, mg m-2) was calculated using trapezoidal integration of chl-a over the top 100 meters of the water column.
Sea ice data were downloaded from the National Snow and Ice Data Center (https://nsidc.org/data/nsidc-0079/versions/3) and were derived from the Nimbus-7 SMMR and DMSP SSMI/S-SSMIS satellite temperature brightness data, which were binned into 25 by 25 km grid cells. Daily sea ice concentration was assessed as percent sea ice cover (Comiso, 2017; Stammerjohn and Maksym, 2017). Average sea ice coverage at the sampling station two weeks prior to each sampling event (SIC2Wks) and the number of days between the date of sea ice advance and the sampling date each year (DaysAdv) were determined. Sea ice advance was determined to occur when the sea ice in a study region was greater than 15% for at least 5 consecutive days (Stammerjohn and Maksym, 2017). After running linear models assessing the relationships between multiple sea ice variables and OMI, we chose to include SIC2Wks and DaysAdv as the sea ice variables in our models because they had stronger univariate relationships (i.e., lowest AIC scores) with female krill OMI relative to the other sea ice variables we tested (i.e., maximum sea ice coverage and the concentration of sea ice at the time of sampling).
2.5 Statistical analyses
Our first hypothesis was that the maturity stage of female krill (represented here as the OMI) in late winter would vary between years. Although we collected samples over a timespan of five years, during two of those years (2012 and 2015), we only had samples from two stations, which is insufficient for inclusion in comparative statistics. Therefore, to address our first hypothesis, we have only used data from 2013, 2014 and 2016. Because the average size of krill in the population generally increases each year with growth of the dominant cohort, mean total length of the female krill was a potential covariate with OMI. Therefore, we chose to use an analysis of covariance (ANCOVA) testing for significant differences in OMI by year accounting for length as a possible covariate. After running the ANCOVA, we verified that the model residuals were normally distributed and had equal variance and ran a post-hoc Tukey’s honestly significant difference (HSD) test to look at pairwise comparisons between the years.
Our second hypothesis was that OMI would be positively correlated with climate and environmental conditions that encourage high food availability leading up to and during the winter. To test this, we used principal component regression (PCR) to reduce the climate (seasonal SAM and MEI) and measured environmental (IntChl, SIC2Wks, DaysAdv, AvgTemp, and AvgSal) variables, characterize the conditions at the nAP in each year, and test for significant relationships between OMI and the first and second principal components (PC1 and PC2). PCR is a statistically robust regression technique that is based on principal component analysis (PCA) and is used to estimate the relationship between principal components in a PCA and a dependent variable using a standard linear regression model (Agarwal et al., 2019). For this analysis, we used data collected during all five years of our study (2012 to 2016). Seasonal indices of SAM and MEI were included in the PCR because their phases can impact krill condition, reproduction and recruitment (Saba et al., 2014; Steinke et al., 2021). SIC2Wks and DaysAdv were included in the PCR because sea ice concentration and the timing of sea ice advance can impact chl-a content and krill dynamics at the Antarctic Peninsula (Nicol, 2006; Veytia et al., 2021). IntChl is a proxy for phytoplankton biomass (Garibotti et al., 2003) and was included in the PCR because of its established correlation with female krill reproductive development (Quetin and Ross, 2001). AvgTemp was included in the PCR because it can impact the growth and development of Antarctic krill with higher temperatures often resulting in enhanced growth rates (Brown et al., 2010). AvgSal was included in the PCR because of its ability to reflect changes in sea ice and climate patterns (Hellmer et al., 2011; Reiss et al., 2020). We included seasonal climate phases for SAM and MEI, sea ice dynamics leading up to the time of sampling, and measurements of local environmental conditions at the time of sampling in the PCR. Local environmental variables measured at the time of sampling provide only a snapshot of what krill are exposed to, while climate and environmental conditions that occur over longer timescales leading up to and throughout the winter may help us infer what krill are seasonally exposed to. Due to the varying scales of the variables of interest, data included in the PCR were normalized prior to running the analyses. All statistical tests were performed in RStudio 2022.07.01 (RStudio Team, 2022).
In addition, we ran PCR tests using seasonal mean values of satellite-derived chl-a and SST data in lieu of the seasonal climate and in situ winter measurements of IntChl and AvgTemp. However, there were no significant relationships between OMI and either principal component for the satellite-derived seasonal chl-a and the SST PCR, therefore these parameters will be shown from purely a qualitative standpoint in the Supplementary Material (see Supplementary Figures 1 and 2).
3 Results
3.1 The onset of maturity in female krill
The OMI of female krill at the northwestern Antarctic Peninsula (nAP) during this study ranged from 0.48 – 0.94 (Table 1), with an average of 0.74 (s.d. = ± 0.15) across all five years. Of all the females staged (n = 524), 73.9% were in previtellogenesis, 25.8% were in oogenesis, and 0.3% were in gametogenesis. Using the area of the largest ovarian cell for each female sampled, we determined that 95.8% of the krill that were in previtellogenesis were in SDS 3 and 4.2% were in SDS 4 (Table 2). Female krill OMI differed significantly between the three sampling years included in our analyses (i.e., 2013, 2014 and 2016) (Table 3, F = 4.45, d.f. = 2, p = 0.05). A larger proportion of female krill were found to be in previtellogenesis (i.e., higher OMI) in 2014 than in either 2013 and 2016 (Figure 3). OMI was unrelated to krill body length (Table 3, F = 0.88, d.f. = 1, p = 0.38), indicating that OMI and krill body length varied independently of each other. While the total length of females ranged from 34.01 to 38.82 mm, there were no interannual differences in female total length between 2013, 2014, and 2016 (F = 4.01, d.f. = 2, p > 0.05).
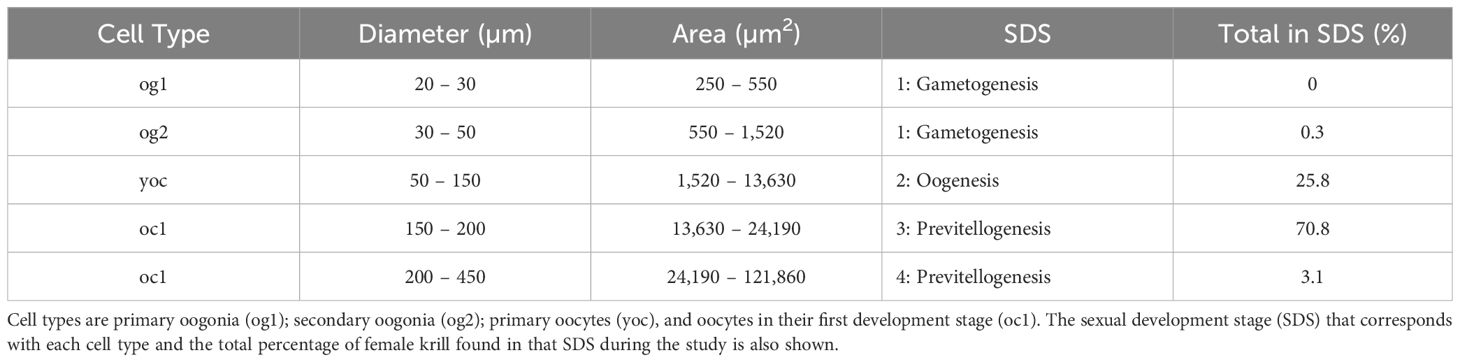
Table 2 Ovarian cell types with corresponding cell diameters (µm) [as determined by Cuzin-Roudy and Amsler (1991)] and cell areas (µm2) measured in this study.
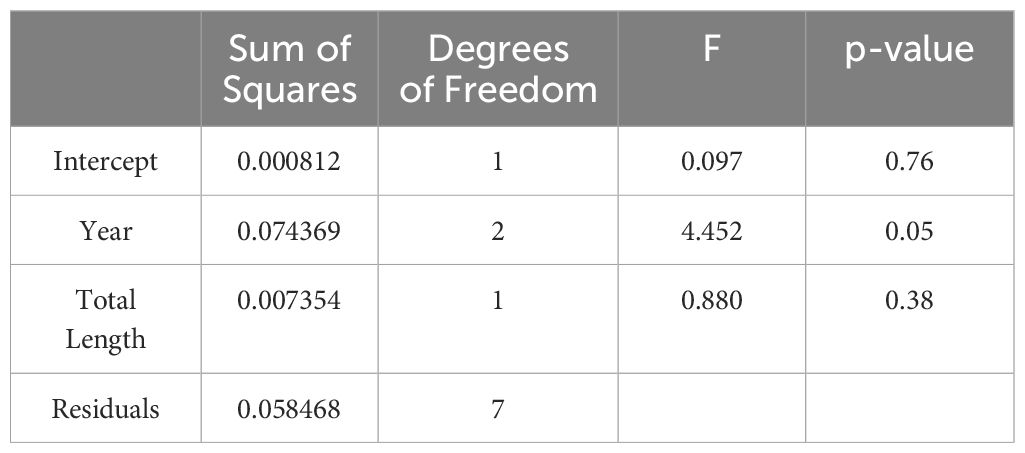
Table 3 Results of the Analysis of Covariance (ANCOVA) showing the response of the dependent variable, the Onset of Maturity Index (OMI), to year (2013, 2014 and 2016) with total body length (mm) as the covariate.
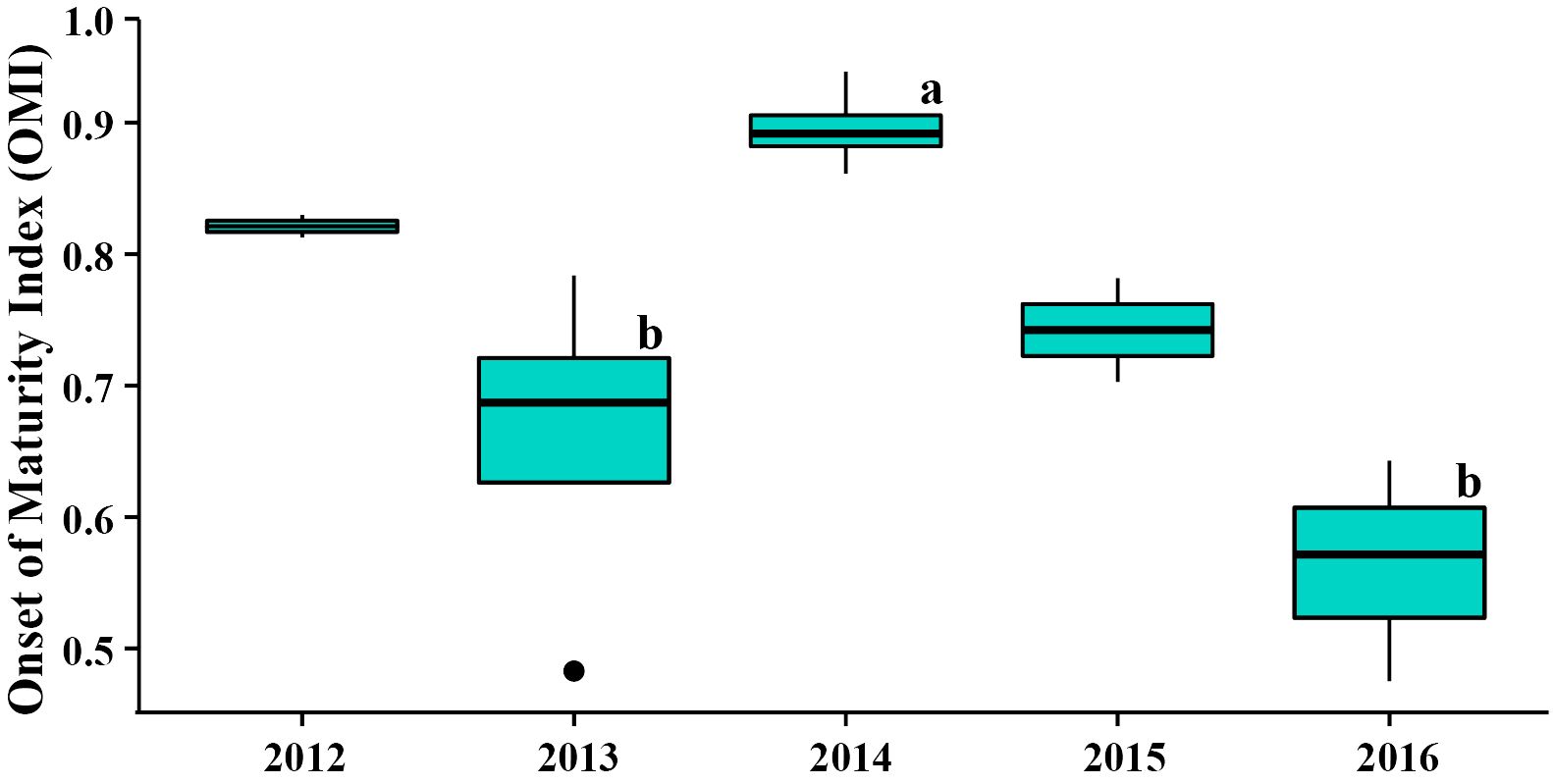
Figure 3 The Onset of Maturity Index (OMI) of female krill during the austral winter from 2012 to 2016 at the northwestern Antarctic Peninsula (nAP). Letters above 2013, 2014, and 2016 indicate significant differences between the years, as determined by the ANCOVA and post-hoc Tukey’s honestly significant difference (HSD) test. The OMI in 2012 and 2015 are also shown, however they were not included in the ANCOVA and cannot be considered when assessing interannual differences.
3.2 Climate, environmental conditions, and OMI
Climate and environmental conditions varied among the five years investigated in this study, with different conditions during each year (Figure 4; Table 4). In 2012, sea ice was present for more days leading up to sampling than in other years, salinity was low during the survey, while the spring and summer MEI and spring SAM prior to winter sampling, were negative (Figure 4; Table 4). In 2013, autumn SAM was strongly positive (Figure 4; Table 4). In 2014, high chl-a concentrations and low salinity were observed during the winter along with a strongly negative spring SAM and a negative spring, summer and autumn MEI (Figure 4; Table 4). SAM and MEI were positive prior to the 2015 winter survey, while SAM the previous year was slightly negative (Figure 4; Table 4). High salinity was also observed during the winter of 2015 (Figure 4; Table 4). In 2016, sea ice advance occurred less than a month, on average, before sampling, and only 5.76% of the survey area, on average, was covered in sea ice at the time of sampling (Figure 4; Table 4). Chl-a concentration was low, and salinity was high in the winter of 2016 (Figure 4; Table 4). Seasonal SAM and MEI were positive leading up to and throughout the winter of 2016, except for winter MEI; which was slightly negative throughout 2016 (Figure 4; Table 4).
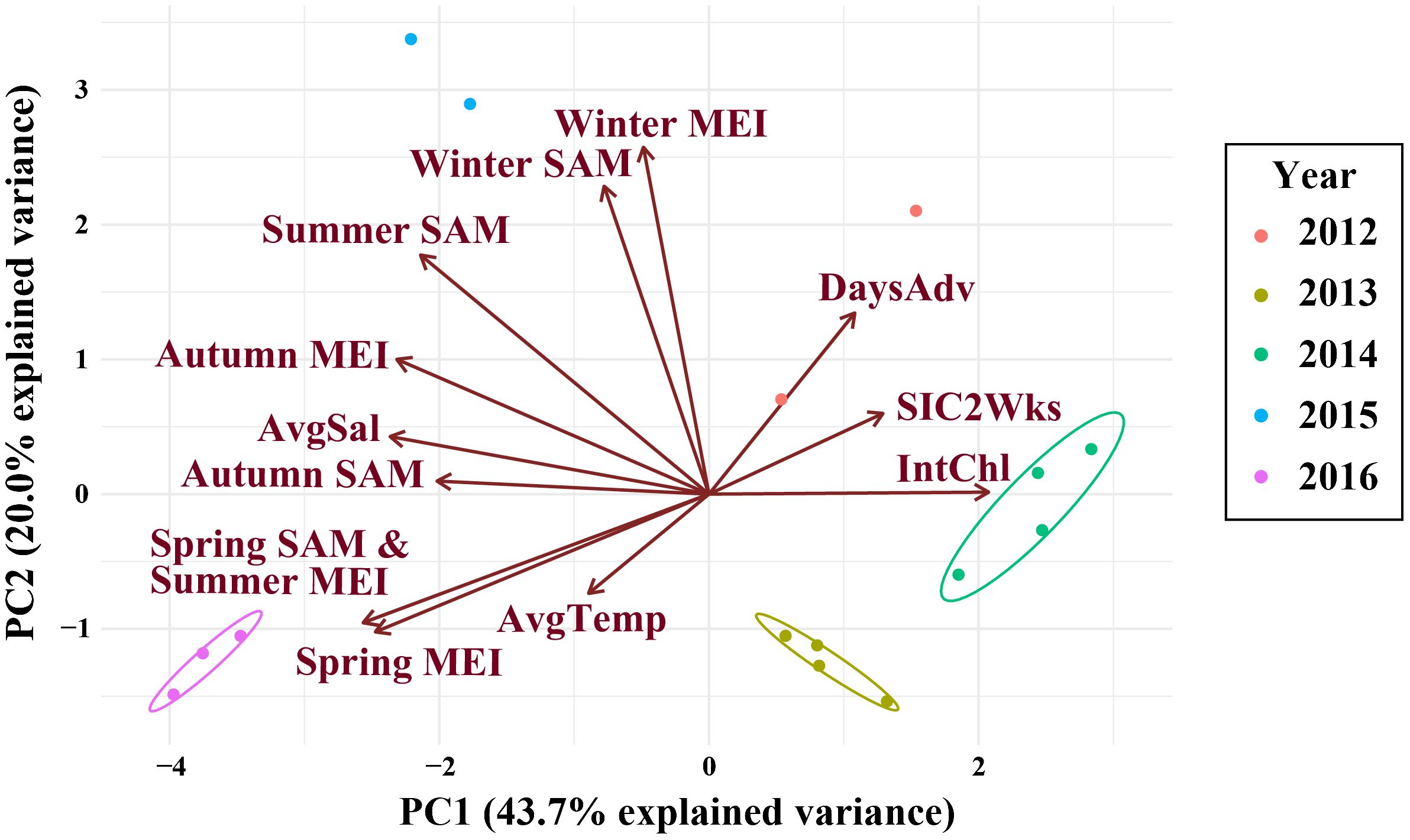
Figure 4 The PCR for the climate and environmental variables of interest at the northwestern Antarctic Peninsula (nAP) from 2012 to 2016. Variables included: the spring, summer, autumn, and winter Southern Annular Mode (SAM) and Multivariate El Niño Index (MEI), average salinity (AvgSal; PSU), integrated chl-a (IntChl; mg m-2), the average sea ice coverage at the sampling station two weeks prior to each sampling event (SIC2Wks), and the number of days between the date of sea ice advance and the sampling date each year (DaysAdv). Years are color-coded and the ellipses are drawn around data that are more similar. No ellipses were drawn around data from 2012 and 2015 because there were two few data points in each year to accurately group the data.
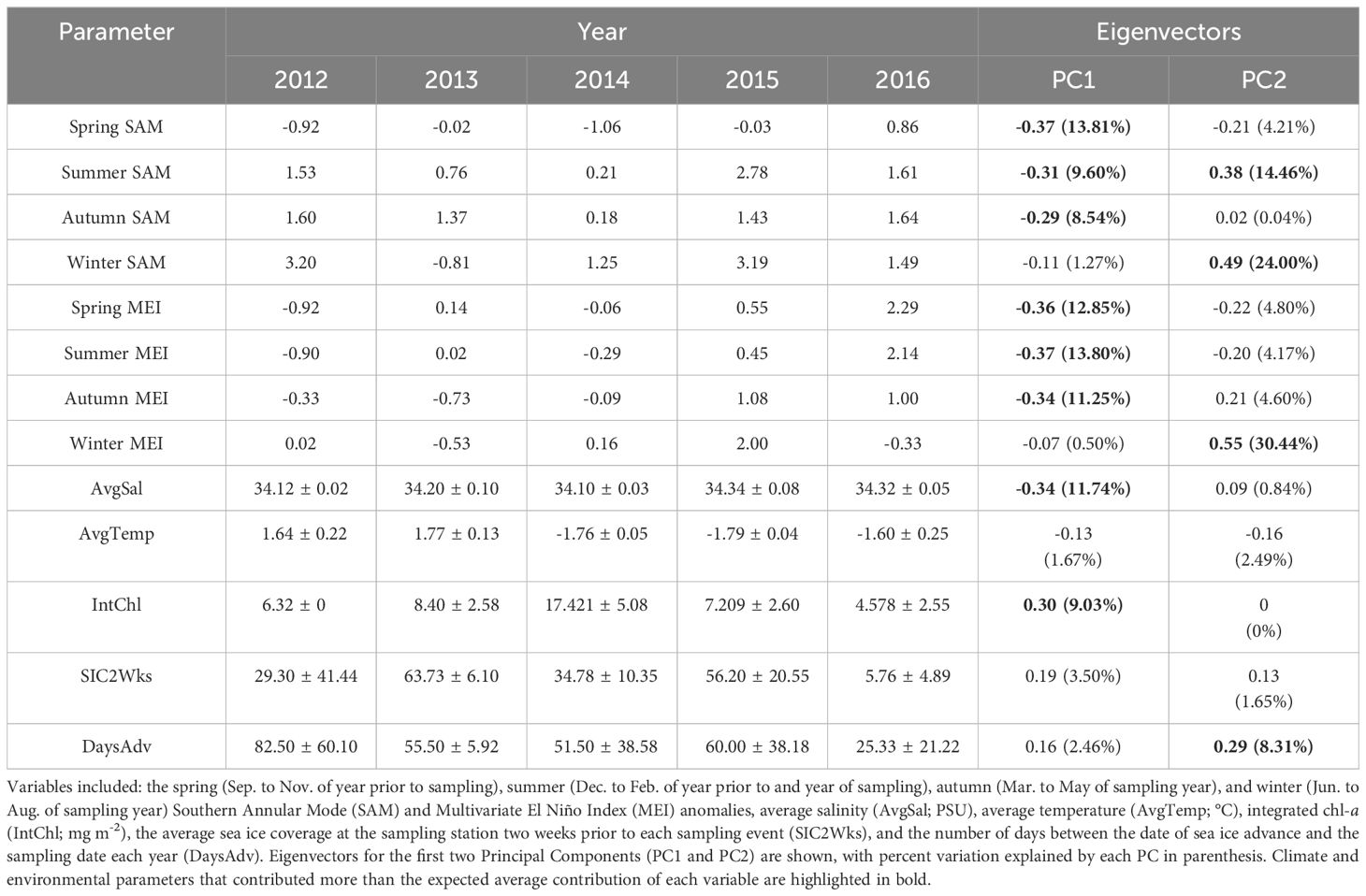
Table 4 The climate (mean) and environmental (mean ± s.d.) parameters for each year of the study (2012 to 2016).
OMI was positively correlated with PC1 (Figure 5A; R2 = 0.44), but not correlated with PC2 (Figure 5B; R2 = 0.06). Principal component 1 (PC1) explained 43.7% of the variance in the climate and environmental data, and principal component 2 (PC2) explained 20.0%, with a combined total of 63.7% (Figure 4). The spring, summer and autumn Southern Annular Mode (SAM) and Multivariate El Niño Index (MEI) anomalies, average salinity in the top 100m (AvgSal), and integrated chl-a within the top 100m (IntChl) contributed more than the estimated average variable contribution to PC1 (Table 4). All variables, except for IntChl, the average sea ice coverage at the sampling station two weeks prior to each sampling event (SIC2Wks), and the number of days between the date of sea ice advance and the sampling date each year (DaysAdv), decreased with increasing values of PC1. The winter SAM and MEI anomalies, summer SAM anomaly, and DaysAdv contributed more than the estimated average variable contribution to PC2 (Table 4). All variables increased with increasing values of PC2, except for summer SAM and MEI anomalies, spring MEI anomaly, and AvgTemp. Thus, the spring, summer and autumn SAM and MEI, IntChl and AvgSal were most strongly correlated with changes in OMI.
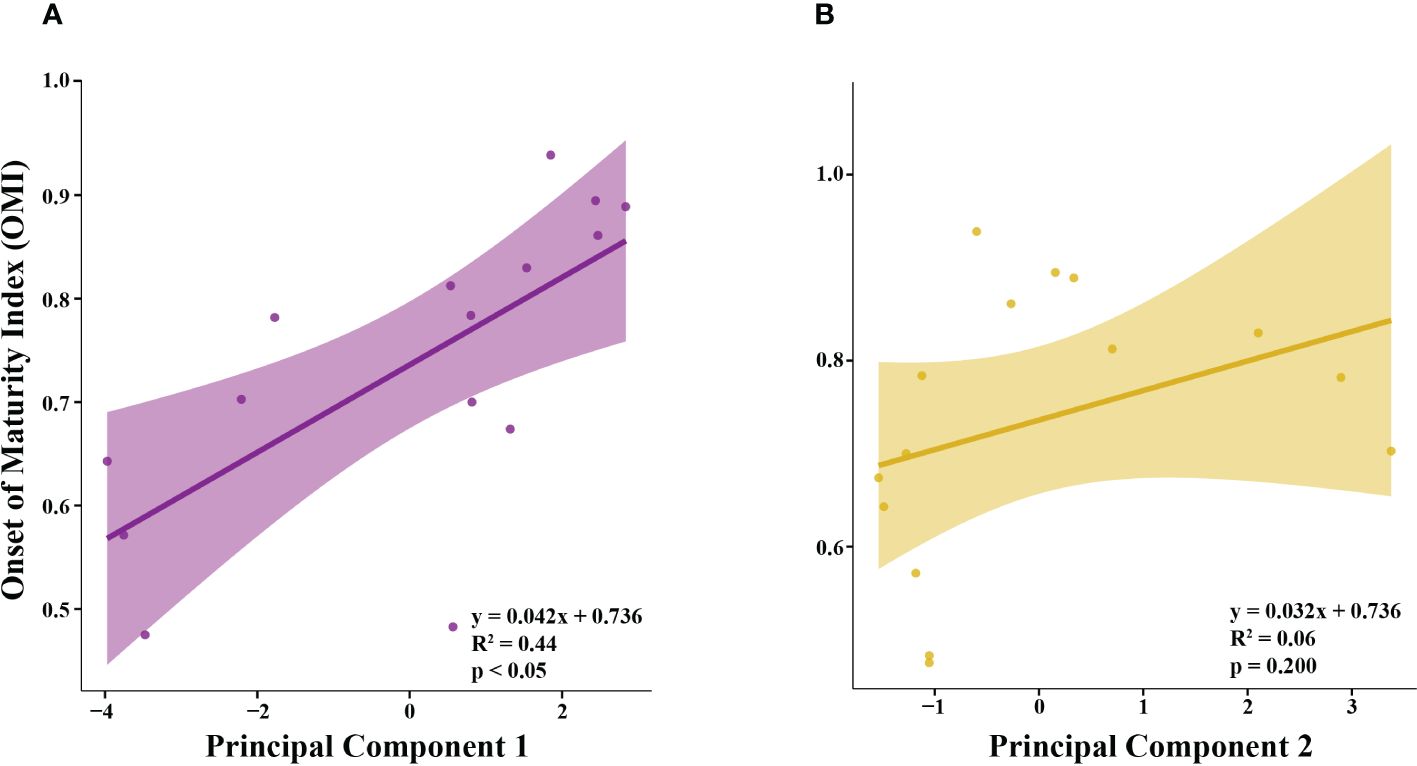
Figure 5 The relationship between the Onset of Maturity Index (OMI) of female krill and (A) Principal Component 1 (PC1) and (B) Principal Component 2 (PC2).
4 Discussion
For female Antarctic krill, being in previtellogenesis in late winter (either because of reduced sexual regression at the onset of winter or early initiation of re-maturation toward the end of winter) may increase fecundity, as it would allow for an earlier start to the spawning season. Understanding interannual variability in the late winter sexual maturity state of female krill and the relationships between that and climate and environmental conditions will improve our ability to predict changes to krill populations in the future. By examining female krill ovarian cells, we identified the sexual development stages (SDS) of female krill during the winter at the northwestern Antarctic Peninsula (nAP) and derived an Onset of Maturity Index (OMI) as an indication of the proportion of all female krill in previtellogenesis. We found that more than 70% of the female krill sampled during the late winter from 2012 to 2016 were in early previtellogenesis. As we hypothesized, there were interannual differences in OMI, and we found that extreme OMIs (i.e., high and low) were correlated with large-scale climate variability, particularly in the preceding spring, summer, and autumn months. We also found that the localized chl-a and salinity concentration at the time of sampling were correlated with female krill OMI.
4.1 The onset of maturity in female krill
OMI varied among the years studied, from a low of 0.56 in 2016 to a high of 0.9 in 2014. Despite this, the annual average was always > 0.5, indicating that at least 50% of the female krill in each year of our study were in previtellogenesis. Female krill body length was not significantly related to OMI indicating that the interannual variability we observed in OMI was caused by something other than simply body size. Across all five years, an average of almost 75% of the females staged were in previtellogenesis, categorized as sexual development stages 3 and 4 (SDS 3–4). Of those females, only 4% were in late-stage previtellogenesis (SDS 4), while the rest were in early previtellogenesis (SDS 3). When females move from SDS 3 to SDS 4, their ovaries grow and accumulate glycoproteic yolk, while their thelycum turns red and matures from partially developed to fully developed so that females are able to accept spermatophores during the upcoming mating season (Cuzin-Roudy and Amsler, 1991). Some females in SDS 4 are also observed with spermatophores attached to their thelycum (Cuzin-Roudy and Amsler, 1991). The length of time it takes for a female to transition from SDS 3 to SDS 4 varies in response to environmental conditions and energetic input, however previous studies suggest that it takes, on average, ~1 month to move from early to late-stage previtellogenesis (Cuzin-Roudy, 2000; Ross and Quetin, 2000).
Progression from SDS 3 to SDS 4 requires a substantial amount of energy suggesting that, either (i) despite the varied environmental conditions at the nAP during the winters studied, conditions were never sufficient to promote continued reproductive development past SDS 3 or (ii), not enough time passed after the maturation to SDS 3 at the end of winter to realistically acquire enough energy to transition to SDS 4. This finding is supported by a recent laboratory study of the reproductive development of female krill under various experimental food environment scenarios during the austral winter at the Western Antarctic Peninsula, which found that females were unable to mature past SDS 3 in late winter (Steinke et al., 2022). Interestingly, while Reiss et al. (2020) found that most of the females sampled from 2012 to 2016 were in their immature stages according to external morphology, they did observe a few females that were in the more sexually advanced stages F3A and F3B in 2014 and 2015; both years where the present study found more than 70% of the females in early-stage previtellogenesis.
Across all five years, an average of only 25.8% of the female krill staged were in oogenesis (SDS 2), and less than 1% of the females were found to be in the most immature stage, gametogenesis (SDS 1). This suggests that the majority (~75%) of the female krill population sampled at the nAP during our study either did not sexually regress to an earlier SDS than previtellogenesis or initiated re-maturation in winter. Being in previtellogenesis before winter ends would likely result in earlier spawning events, prolonging the spawning season and increasing krill reproductive success through higher reproductive output and a greater probability of larval survival and recruitment the following year (Siegel and Loeb, 1995). However, it is important to note that a trade-off exists for female krill during the process of sexual maturation. Steinke et al. (2022) found that female krill that were more reproductively advanced during the winter were also more likely to have a reduced body condition, defined by an empirical relationship between an individual’s wet weight and total length. Whether this trade-off is unique to small females (female krill in that study were, on average, 28.74 ± 2.92 mm in length, while females in the present study were, on average, 36.15 ± 1.90 mm in length) and/or is regionally specific (the krill in that study were collected further south than the present study, in the Gerlache Strait) is unclear. However, Walsh and Reiss (2023) found that post-larval Euphausia superba collected during the same surveys as ours had significantly higher total lipid content in 2013 and 2016 compared to 2014, indicating better body condition in years where we found lowest OMI. While interannual variability in total body condition is almost certainly caused by numerous factors, a trade-off between sexual maturation and body condition could have important implications on an individual’s health and population fecundity.
4.2 Climate, environmental conditions, and OMI
The principal component regression (PCR) of climate and environmental conditions at the nAP during our study period highlighted a unique suite of conditions each year. The first principal component (PC1) was positively correlated with OMI, and was primarily driven by spring, summer, and autumn anomalies of the Southern Annular Mode (SAM) Index and the Multivariate El Niño Index (MEI), as well as integrated chl-a and average salinity during the winter of observation. Thus, OMI in late winter was likely to be higher when the preceding spring, summer and autumn anomalies of SAM and MEI were in their negative phases (-SAM/La Niña), when winter chl-a was high, and when winter salinity was low. Below, we discuss each relationship and potential driving mechanism in further detail.
4.2.1 Climate and environmental conditions preceding and during a winter with high OMI
When SAM and MEI are in the same phase (i.e., -SAM/La Niña and +SAM/El Niño), they offset each other with regard to their impact on storms, creating milder conditions at the nAP (Clem and Fogt, 2013; Stammerjohn et al., 2008a). Although decreased storm activity occurs during both -SAM/La Niña and +SAM/El Niño paradigms, they impact the ocean environment differently. Unlike +SAM, -SAM encourages the growth of larger phytoplankton, notably diatoms, at the Antarctic Peninsula creating a favorable feeding environment for diatom grazers, like krill (Montes-Hugo et al., 2008). And, in contrast to El Niño, La Niña, which typically corresponds with reduced sea ice extent and duration in the winter and spring around the nAP, is associated with an earlier sea ice retreat that could initiate an earlier onset of seasonal primary productivity at the nAP, potentially encouraging earlier maturation in krill (Reiss et al., 2020). Thus, a combination of -SAM/La Niña is more likely to prompt early diatom blooms, while +SAM/El Niño is more likely to result in milder conditions with no associated diatom bloom.
As already noted, we observed the highest OMI during the winter of 2014. Here, we will explore the climate and environmental conditions leading up to, and during that survey. In the spring preceding our 2014 survey (September to November 2013), the nAP was forced by a -SAM/La Niña. As a result, storm activity would have been minimal, with SAM and ENSO offsetting each other. La Niña conditions would have resulted in greater inflow of warmer, mCDW into the study region resulting in a shallower seasonal mixed layer (Walsh and Reiss, 2023). A shallower mixed layer would promote early spring phytoplankton growth, primarily constituted of diatoms (Schofield et al., 2017), which are the preferred phytoplankton prey source of krill (Haberman et al., 2003). Indeed, seasonally averaged satellite-derived ocean color data indicate a robust phytoplankton biomass in our study area that spring (Supplementary Figure 2). That summer (December 2013 to February 2014), SAM switched to its positive phase and La Niña remained in effect through autumn (March to May 2014). This combination would have created warmer conditions, but because both indices were relatively neutral in the summer and autumn, storm activity was likely minimal. Although seasonally averaged satellite-derived ocean color data indicate phytoplankton biomass was relatively high through the summer and autumn of 2014 (Supplementary Figure 2), Mendes et al. (2018) found that cryptophytes were the dominant phytoplankton in the neighboring Gerlache Strait during the summer that same year, and thus, were likely the dominant phytoplankton in our study region too. Cryptophytes are not typically consumed by krill due to their small cell size (Haberman et al., 2003).
Copepods, on the other hand, are more efficient foragers of small phytoplankton (Deppeler and Davidson, 2017), and could have provided an alternative food source to krill during the summer and autumn of 2014. When krill feed carnivorously, they tend to accumulate storage lipids, primarily in the form of triacylglycerols (TAGs) more readily than when feeding on phytoplankton (Hagen et al., 2007). Thus, the summer and autumn conditions preceding winter 2014 may have promoted a more carnivorous diet in the local krill, resulting in an elevation of their total body lipid contents and improving body condition leading into the winter. With higher body condition at the onset of winter, krill would likely be in better condition toward the end of winter, even if food had been scarce. Bernard et al. (2022) found that krill given a carnivorous diet in the autumn and then starved in the winter had higher total body lipid contents by the end of winter than those krill fed diatoms the entire time. Because early reproductive development requires metabolic resources, krill with lipid reserves toward the end of winter would be more likely to initiate early reproductive development if they encountered sufficient phytoplankton due to their ability to fuel the process. In the winter of 2014, phytoplankton concentrations were significantly higher than those measured in any of the other years of our study. Despite this, analysis of fatty acid biomarkers on krill collected during the same cruise showed that krill had a low diatom index (the ratio of 16:1n-7 and 18:4n-3) (Walsh et al., 2020). Walsh et al. (2020) observed significantly lower total lipid contents in krill collected in 2014 compared to those collected in 2016, suggesting that, with cryptophytes dominating the phytoplankton community the previous summer (Mendes et al., 2018), krill would have likely been feeding largely carnivorously in the summer and autumn, which would have allowed them to build up their lipid reserves prior to the onset of winter (Bernard et al., 2022). This may have enabled female krill to limit sexual regression (Kawaguchi, 2016), allowing early and quick maturation toward the end of winter and early spring when blooms were initiated. In both scenarios, OMI would have been high at the end of winter.
4.2.2 Climate and environmental conditions preceding and during a winter with low OMI
In addition to the impacts that SAM and ENSO have on ocean circulation patterns and sea ice dynamics, when SAM and ENSO are in opposing phases, they amplify the climate conditions at the nAP (i.e., +SAM/La Niña = amplified warming, -SAM/El Niño = amplified cooling) (Stammerjohn et al., 2008b). In the spring preceding 2013, the nAP was dominated by an El Niño and a negative SAM. This resulted in amplified stormy conditions that created an unfavorable environment for phytoplankton growth (Supplementary Figure 2), with a late spring phytoplankton bloom causing a potential delay in krill physiological processes, such as growth, reproductive development, and spawning that year (Schmidt et al., 2012; Saba et al., 2014). However, in the summer of 2013, SAM shifted toward its positive phase and El Niño remained constant, resulting in neutral conditions, reduced winds, and favorable conditions for diatom blooms (Supplementary Figure 2). These conditions continued through the autumn of 2013, with high chl-a observed throughout the study region (Supplementary Figure 2). With plentiful phytoplankton in the water during the summer and autumn, female krill may have fed more herbivorously in the seasons preceding the winter sampling period, which may have limited their ability to accumulate lipid reserves prior to that winter.
Following the La Niña of 2014, ENSO shifted into its positive phase, El Niño, gaining strength through 2015 and reached a maximum in the spring (September to November 2015) prior to our winter survey in 2016. This was accompanied by a strongly positive SAM. With the nAP being dominated by a +SAM/El Niño from spring to autumn the region experienced little-to-no storm activity, cooler weather (primarily in response to the strong El Niño), and, based on seasonally averaged satellite-derived ocean color data, an intensive spring phytoplankton bloom at the retreating edge of the marginal ice zone (Supplementary Figure 2). Although a -SAM is more highly associated with diatom presence than a + SAM, the strong El Niño prevailing over the system in 2016 resulted in phytoplankton blooms from spring to autumn (Supplementary Figure 2) with the most intense blooms dominated by the large centric diatom, Odontella weissflogii (Costa et al., 2020). Interestingly, the large size (>70 µm) of O. weissflogii probably made it hard for krill to feed on, reducing their foraging efficiency during these blooms (Opaliński et al., 1997). Indeed, Costa et al. (2020) observed low grazing indices and a healthy, rapidly sinking diatom community. Therefore, although phytoplankton biomass was high, krill and indeed other smaller grazers like copepods, could not consume it. Thus, it is likely that copepods were less abundant given the paucity of edible phytoplankton resources and krill may have fed preferentially on protozoans, including dinoflagellates, which were a dominant part of the nanoplankton community and the second-most representative taxonomic group in the region (Costa et al., 2020). Through a process known as trophic upgrading, dinoflagellates can improve the biochemical composition of low-quality nano- and picophytoplankton, providing important polyunsaturated fatty acids that krill need for growth, eicosapentanoic acid (EPA) and docosahexaenoic acid (DHA) (Tang and Taal, 2005; Schmidt et al., 2006). However, krill obtain significantly less storage lipids when feeding solely on dinoflagellates compared to feeding on zooplankton, such as copepods, that are rich in TAGs (Dalsgaard et al., 2003). Thus, these krill may have entered the winter with relatively lower lipid reserves than those in 2014.
In the winter of 2016, ENSO shifted to a La Niña, which combined with the +SAM to result in warmer conditions, delayed sea ice formation, and reduced overall sea ice cover in the study region. Winter phytoplankton biomass was the lowest observed across all five years of our study (Table 4). Interestingly, fatty acid profile analysis on krill collected in the same survey indicated that post-larval individuals had been feeding primarily on diatoms in the weeks-to-months leading up to collection in August 2016 because they had a high diatom index (the ratio of 16:1n-7 and 18:4n-3) (Walsh et al., 2020). It is possible that high grazing rates by krill could have led to a dramatic decrease in phytoplankton biomass, but adult krill do not tend to increase their grazing rates in the winter, even if food is plentiful (Atkinson et al., 2002). Bernard et al. (2019) measured winter grazing rates of adult krill using the gut fluorescence technique and found that grazing rates in August 2016 were negligible. Thus, an alternate explanation is that the fatty acid biomarkers measured were more strongly representative of the autumn diet of krill rather than the winter diet as the turnover rates of these biomarkers would likely have been reduced by the low winter feeding rates of adult krill (Atkinson et al., 2002) and diminished food availability (Graeve et al., 2020). This notion supports our theory that, like the krill measured in 2013, the krill measured in 2016 were likely feeding herbivorously in the months leading up to winter. Without the resources necessary to initiate early reproductive development, female krill may have delayed reproductive maturation in favor of conserving their limited lipid reserves. It is possible that in doing so, their total lipid content would have been higher at the end of winter than those female krill that underwent early maturation in 2014, which was observed by Walsh and Reiss (2023).
4.2.3 The influence of large-scale climate forcing on environmental conditions at the nAP
Although the SAM and MEI anomalies are large-scale phenomena, they have been previously used to effectively forecast environmental conditions at the Antarctic Peninsula (e.g., Loeb, 2007; Stammerjohn et al., 2008b; Clem et al., 2016). For organisms that are highly mobile, and for which predicting their precise location nine months prior to sampling is impossible, the use of these indices is an appropriate way to broadly describe the environmental conditions they would have been faced with preceding the period of sampling. When SAM is in its negative phase, the westerly wind belt is geographically farther from Antarctica, maintaining colder and calmer conditions at the nAP (Clem et al., 2016). In its positive phase, westerly winds shift closer to the Peninsula, bringing more storms, warmer air and precipitation to the nAP (Clem et al., 2016). Positive SAM conditions are also associated with less sea ice, a deeper mixed layer, a shift in the phytoplankton community toward smaller cryptophytes, and a reduction in krill recruitment at the Antarctic Peninsula (Montes-Hugo et al., 2009; Atkinson et al., 2019).
During La Niña (negative MEI) conditions, the Amundsen Sea Low (ASL) is stronger than average, bringing warmer northerly winds to the nAP, resulting in less sea ice. During La Niña, there is also an enhanced delivery of warm, modified Circumpolar Deep Water (mCDW) into the Bransfield Strait (Ruiz Barlett et al., 2018). During El Niño (positive MEI) conditions, the ASL is weaker than average and Weddell Sea Shelf Water (WSSW) outflow into the Bransfield Strait is enhanced, resulting in cooler conditions, more extensive sea ice coverage, and potentially more sea-ice-associated food sources, in the region (Yuan, 2004; Clem et al., 2016; Nie et al., 2022; Walsh and Reiss, 2023). Further, WSSW has a higher salinity content and the PCR results showing the effects of salinity on OMI in this study may indicate the presence or absence of WSSW in the nAP region. A higher salinity content in the winter may also be observed when there is minimal sea ice and stratification in the upper water column (Venables et al., 2013; Saba et al., 2014). Despite being a year dominated by El Niño and cooler surface temperatures (Supplementary Figure 1), there was anomalously low sea ice at the nAP in 2016 (Stuecker et al., 2017; Wang et al., 2019), which could have contributed to high salinity and low stratification during the winter of 2016 (Walsh and Reiss, 2023). A lack of stratification due to extremely low sea ice coverage makes potential prey items for krill, including pelagic phytoplankton, zooplankton, and organic debris, more widely dispersed through the water column, rather than being restricted to the surface ocean by a strong thermocline where they would constitute a concentrated food source for krill (Montes-Hugo et al., 2009; Kirillin et al., 2012).
Sexual maturation in female krill requires substantial nutritional input from their diet (Quetin and Ross, 2001). While diatoms are a critical food source for krill, krill are omnivorous and also feed on organisms such as copepods and dinoflagellates (Ericson et al., 2018). Feeding carnivorously provides krill with key lipids and fatty acids that they use to survive the winter and to aid in the progress of certain physiological processes, such as growth and reproduction (Hagen et al., 2007). Notably, Yoshida et al. (2011) found that the hatching success of embryos increased when the female krill that released them had fed omnivorously, transferring favorable ratios of key fatty acids that promote survival and growth to their embryos. Variability in seasonal climate and environmental conditions may impact the quality and quantity of food available for female krill to feed on throughout the year, encouraging them to be flexible in their foraging behavior (Walsh et al., 2020).
While our study reveals important seasonal climate and environmental relationships with krill reproductive development, it is important to be aware of the assumptions and limitations of this study when using these results to forecast how krill populations may change in the future. Due to the logistical limitations of winter research in Antarctica, we had a relatively small sample size for our study, with an uneven spread of samples throughout the study region and over time. Only three of our five survey years yielded sufficient sample sizes to allow for statistical comparisons between years. In these years, we observed strikingly different environmental and climate conditions, resulting in different OMIs of female krill. However, the number of potential unique combinations of seasonal SAM and ENSO as well as other environmental parameters is large, and our study has only just scraped the surface of these. Thus, while we can discuss implications of climate and environmental variability within the timeframe of our study, further work is needed to allow for the prediction of krill reproductive development in the future. In addition, while we have explored the seasonal impacts of climate and environmental variability on krill reproduction, we fully acknowledge that there are likely many more impacts that climate has on environmental conditions and food availability than we were able to discuss within the scope of this paper.
5 Conclusions
Our study describes, for the first time, the interannual variability in female krill maturation at the cellular level at the end of winter and its correlation with both climate and environmental factors. A high OMI at the end of the winter season suggests that female krill either initiated re-maturation early in response to sufficient food availability throughout the fall and winter (Steinke et al., 2022), or that they did not fully regress to their more immature stages of gametogenesis or oogenesis at the onset of winter due to plentiful food availability, and thus a build-up of energetic reserves, in the seasons leading up to winter (Kawaguchi, 2016). In this study, we saw that the seasonal SAM and ENSO phases leading up to winter were more important in structuring the OMI of female krill at the end of winter than the winter phases of SAM and ENSO, nor the winter environmental conditions including chl-a and salinity. This implies that favorable feeding conditions in the spring, summer and autumn may be more critical than those in the winter and suggests that the elevated OMI observed in female krill in 2014 was likely due to a reduced reproductive regression rather than an early initiation of re-maturation. Large-scale climate indices were found to be important factors influencing the late-winter reproductive stage of female krill at the nAP. Spring, summer, and autumn SAM and ENSO anomalies showed a strong, negative correlation with the OMI that we used to represent female krill reproductive stage, with -SAM and La Niña phases encouraging a higher OMI. This was likely due to the indirect effects climate has on food availability throughout the year by altering patterns in storm activity, the preceding spring sea-ice retreat, summer ocean conditions, and the subsequent autumn sea-ice advance.
The nAP is warming rapidly, primarily in response to climate change and a SAM that has been moving toward a predominantly positive phase since the 1950s (Vaughan et al., 2003; Swart and Fyfe, 2012). In addition to a positively trending SAM, ENSO is predicted to increase in strength, with extreme El Niños being more likely to occur in the future (Cai et al., 2014). A future at the nAP where both SAM and ENSO are positive (El Niño) may result in less females in previtellogenesis at the end of the winter season. To fully understand how the timing of sexual maturation is likely to change in response to climate and environmental variability, and its impacts on krill reproductive success, more studies, especially during the spring, autumn and winter, are needed. Gaining a better understanding of the seasonal reproductive dynamics of krill is a top priority for krill fishery management and will allow for better predictions of how krill and their dependent predator populations may change at the Antarctic Peninsula in the future.
Data availability statement
The raw data supporting the conclusions of this article will be made available by the authors, without undue reservation. Data will be made available upon request.
Ethics statement
The manuscript presents research on animals that do not require ethical approval for their study.
Author contributions
KS: Conceptualization, Data curation, Formal analysis, Investigation, Methodology, Validation, Visualization, Writing – original draft, Writing – review & editing. KB: Funding acquisition, Project administration, Resources, Supervision, Validation, Writing – review & editing. CR: Data curation, Project administration, Resources, Supervision, Writing – review & editing. JW: Data curation, Project administration, Resources, Supervision, Writing – review & editing. GC: Formal analysis, Methodology, Writing – review & editing. SS: Data curation, Writing – review & editing.
Funding
The author(s) declare financial support was received for the research, authorship, and/or publication of this article. Funding for this study was provided by the United States National Science Foundation (NSF) Office for Polar Programs (OPP) as an Early Career Award. Grant number 2038145.
Acknowledgments
This work could not have been completed without the cooperation of the U.S. Antarctic Marine Living Resources (AMLR) Program. We would also like to thank L. Ciannelli and M. Kavanaugh who provided valuable insight on statistical analyses and model selection used in this study.
Conflict of interest
The authors declare that the research was conducted in the absence of any commercial or financial relationships that could be construed as a potential conflict of interest.
Publisher’s note
All claims expressed in this article are solely those of the authors and do not necessarily represent those of their affiliated organizations, or those of the publisher, the editors and the reviewers. Any product that may be evaluated in this article, or claim that may be made by its manufacturer, is not guaranteed or endorsed by the publisher.
Supplementary material
The Supplementary Material for this article can be found online at: https://www.frontiersin.org/articles/10.3389/fmars.2024.1383175/full#supplementary-material
References
Agarwal A., Shah D., Shen D., Song D. (2019). On robustness of principal component regression. Adv. Neural Inf. Process. Syst. (Curran Associates Inc.) 32. doi: 10.48550/arXiv.1902.10920
Atkinson A., Hill S. L., Pakhomov E. A., Siegel V., Reiss C. S., Loeb V. J., et al. (2019). Krill ( Euphausia superba ) distribution contracts southward during rapid regional warming. Nat. Climate Change 9, 142–147. doi: 10.1038/s41558–018-0370-z
Atkinson A., Meyer B., Stuϋbing D., Hagen W., Schmidt K., Bathmann U. V. (2002). Feeding and energy budgets of Antarctic krill Euphausia superba at the onset of winter-II. Juveniles and adults. Limnology Oceanography 47, 953–966. doi: 10.4319/lo.2002.47.4.0953
Berg S., Kutra D., Kroeger T. (2019). ilastik: interactive machine learning for (bio)image analysis. Nat. Methods 16, 1226–1232. doi: 10.1038/s41592-019-0582-9
Bernard K. S., Gunther L. A., Mahaffey S. H., Qualls K. M., Sugla M., Saenz B. T., et al. (2019). The contribution of ice algae to the winter energy budget of juvenile Antarctic krill in years with contrasting sea ice conditions. ICES J. Mar. Sci. 76, 206–216. doi: 10.1093/icesjms/fsy145
Bernard K. S., Steinke K. B., Fontana J. M. (2022). Winter condition, physiology, and growth potential of juvenile Antarctic krill. Front. Mar. Sci. 9. doi: 10.3389/fmars.2022.990853
Brown M., Kawaguchi S., Candy S., Virtue P. (2010). Temperature effects on the growth and maturation of Antarctic krill (Euphausia superba). Deep Sea Res. Part II: Topical Stud. Oceanography 57, 672–682. doi: 10.1016/j.dsr2.2009.10.016
Cai W., Borlace S., Lengaigne M., van Rensch P., Collins M., Vecchi G., et al. (2014). Increasing frequency of extreme El Niño events due to greenhouse warming. Nat. Clim Change 4, 111–116. doi: 10.1038/nclimate2100
Clem K. R., Renwick J. A., McGregor J., Fogt R. L. (2016). The relative influence of ENSO and SAM on Antarctic Peninsula climate. J. Geophysical Research: Atmospheres 121, 9324–9341. doi: 10.1002/2016JD025305
Comiso J. C. (2017). Bootstrap sea ice concentrations from nimbus-7 SMMR and DMSP SSM/I-SSMIS, version 3 [Data set] (Boulder, Colorado USA: NASA National Snow and Ice Data Center Distributed Active Archive Center). doi: 10.5067/7Q8HCCWS4I0R
Costa R. R., Mendes C. R. B., Tavano V. M., Dotto T. S., Kerr R., Monteiro T., et al. (2020). Dynamics of an intense diatom bloom in the Northern Antarctic Peninsula, February 2016. Limnology Oceanography 65, 2056–2075. doi: 10.1002/lno.11437
Cuzin-Roudy J. (2000). Seasonal reproduction, multiple spawning, and fecundity in northern krill, Meganyctiphanes norvegica, and Antarctic krill, Euphausia superba. Can. J. Fish. Aquat. Sci. 57, 6–15. doi: 10.1139/f00–165
Cuzin-Roudy J., Amsler M. O. (1991). Ovarian development and sexual maturity staging in antarctic krill, euphausia superba dana (Euphausiacea). J. Crustacean Biol. 11, 236. doi: 10.2307/1548361
Dalsgaard J., St. John M., Kattner G., Müller-Navarra D., Hagen W. (2003). “Fatty acid trophic markers in the pelagic marine environment,” in Advances in marine biology (Amsterdam, Netherlands: Elsevier), 225–340. doi: 10.1016/S0065–2881(03)46005–7
Deppeler S. L., Davidson A. T. (2017). Southern ocean phytoplankton in a changing climate. Front. Mar. Sci. 4. doi: 10.3389/fmars.2017.00040
Ericson J. A., Hellessey N., Nichols P. D., Kawaguchi S., Nicol S., Hoem N., et al. (2018). Seasonal and interannual variations in the fatty acid composition of adult Euphausia superba Dana 1850 (Euphausiacea) samples derived from the Scotia Sea krill fishery. J. Crustacean Biol. 38, 662–672. doi: 10.1093/jcbiol/ruy032
Flores H., Atkinson A., Kawaguchi S., Krafft B. A., Milinevsky G., Nicol S., et al. (2012). Impact of climate change on Antarctic krill. Mar. Ecol. Prog. Ser. 458, 1–19. doi: 10.3354/meps09831
Fogt R. L., Marshall G. J. (2020). The Southern Annular Mode: Variability, trends, and climate impacts across the Southern Hemisphere. WIREs Climate Change 11, e652. doi: 10.1002/wcc.652
Garibotti I. A., Vernet M., Kozlowski W. A., Ferrario M. E. (2003). Composition and biomass of phytoplankton assemblages in coastal Antarctic waters: a comparison of chemotaxonomic and microscopic analyses. Mar. Ecol. Prog. Ser. 247, 27–42. doi: 10.3354/meps247027
Graeve M., Boissonnot L., Niehoff B., Hagen W., Kattner G. (2020). Assimilation and turnover rates of lipid compounds in dominant Antarctic copepods fed with 13C-enriched diatoms. Phil. Trans. R. Soc B 375. doi: 10.1098/rstb.2019.0647
Haberman K. L., Ross R. M., Quetin L. B. (2003). Diet of the Antarctic krill (Euphausia superba Dana): II. Selective grazing in mixed phytoplankton assemblages. J. Exp. Mar. Biol. Ecol. 283, 97–113. doi: 10.1016/S0022–0981(02)00467–7
Hagen W., Yoshida T., Virtue P., Kawaguchi S., Swadling K. M., Nicol S., et al. (2007). Effect of a carnivorous diet on the lipids, fatty acids and condition of Antarctic krill, Euphausia superba. Antarctic. Sci. 19, 183–188. doi: 10.1017/S0954102007000259
Hellmer H. H., Huhn O., Gomis D., Timmermann R. (2011). On the freshening of the northwestern Weddell Sea continental shelf. Ocean Sci. 7, 305–316. doi: 10.5194/os-7–305-2011
Höring F., Teschke M., Suberg L., Kawaguchi S., Meyer B. (2018). Light regime affects the seasonal cycle of Antarctic krill ( Euphausia superba ): impacts on growth, feeding, lipid metabolism, and maturity. Can. J. Zool. 96, 1203–1213. doi: 10.1139/cjz-2017–0353
Kawaguchi S. (2016). “Reproduction and larval development in antarctic krill (Euphausia superba),” in Biology and ecology of antarctic krill advances in polar ecology. Ed. Siegel V. (Springer International Publishing, Cham), 225–246. doi: 10.1007/978–3-319–29279-3_6
Kawaguchi S., Yoshida T., Finley L., Cramp P., Nicol S. (2007). The krill maturity cycle: a conceptual model of the seasonal cycle in Antarctic krill. Polar Biol. 30, 689–698. doi: 10.1007/s00300–006-0226–2
Kirillin G., Grossart H.-P., Tang K. W. (2012). Modeling sinking rate of zooplankton carcasses: Effects of stratification and mixing. Limnology Oceanography 57, 881–894. doi: 10.4319/lo.2012.57.3.0881
Lizotte M. P. (2001). The contributions of sea ice algae to antarctic marine primary production. Integr. Comp. Biol. 41, 57–73. doi: 10.1093/icb/41.1.57
Loeb V. (2007). “Environmental variability and the antarctic marine ecosystem,” in The impact of environmental variability on ecological systems the peter yodzis fundamental ecology series. Eds. Vasseur D. A., McCann K. S. (Springer Netherlands, Dordrecht), 197–225. doi: 10.1007/978–1-4020–5851-6_10
Makarov R., Denys C. J. (1980). Stages of sexual maturity of euphausia supera dana. Biomass Handb. 11, 1–11.
Massom R. A., Stammerjohn S. E., Smith R. C., Pook M. J., Iannuzzi R. A., Adams N., et al. (2006). Extreme anomalous atmospheric circulation in the west antarctic peninsula region in austral spring and summer 2001/02, and its profound impact on sea ice and biota. J. Climate 19, 3544–3571. doi: 10.1175/JCLI3805.1
Mauchline J. (1981). “Measurement of body length of Euphausia superba Dana,” in BIOMASS handbook, vol. 4. , 1–9.
McQuin C., Goodman A., Chernyshev V., Kamentsky L., Cimini B. A., Karhohs K. W., et al. (2018). CellProfiler 3.0: Next-generation image processing for biology. PloS Biol. 16, 1–17. doi: 10.1371/journal.pbio.2005970
Mendes C. R. B., Tavano V. M., Kerr R., Dotto T. S., Maximiano T., Secchi E. R. (2018). Impact of sea ice on the structure of phytoplankton communities in the northern Antarctic Peninsula. Deep Sea Res. Part II: Topical Stud. Oceanography 149, 111–123. doi: 10.1016/j.dsr2.2017.12.003
Montes-Hugo M., Doney S. C., Ducklow H. W., Fraser W. R., Martinson D. G., Stammerjohn S. E., et al. (2009). Recent changes in phytoplankton communities associated with rapid regional climate change along the western antarctic peninsula. Science 323, 1470–1473. doi: 10.1126/science.1164533
Montes-Hugo M., Vernet M., Martinson D. G., Smith R. C., Iannuzzi R. A. (2008). Variability on phytoplankton size structure in the western Antarctic Peninsula, (1997–2006). Deep Sea Res. Part II: Topical Stud. Oceanography 55, 2106–2117. doi: 10.1016/j.dsr2.2008.04.036
Murphy E. J., Thorpe S. E., Tarling G. A., Watkins J. L., Fielding S., Underwood P. (2017). Restricted regions of enhanced growth of Antarctic krill in the circumpolar Southern Ocean. Sci. Rep. 7, 6963. doi: 10.1038/s41598–017-07205–9
Nicol S. (2006). Krill, currents, and sea ice: euphausia superba and its changing environment. BioScience 56, 111–120. doi: 10.1641/0006–3568(2006)056[0111:KCASIE]2.0.CO;2
Nie S., Xiao W., Wang R. (2022). Mid-Late Holocene climate variabilities in the Bransfield Strait, Antarctic Peninsula driven by insolation and ENSO activities. Palaeogeograph Palaeoclimatolog Palaeoecol. 601, 111140. doi: 10.1016/j.palaeo.2022.111140
Opaliński K. W., Maciejewska K., Georgieva L. V. (1997). Notes of food selection in the Antarctic krill, Euphausia superba. Polar Biol. 17, 350–357. doi: 10.1007/PL00013376
Quetin L. B., Ross R. M. (2001). Environmental variability and its impact on the reproductive cycle of antarctic krill. Am. Zoologist 41, 74–89. doi: 10.1093/icb/41.1.74
Quetin L. B., Ross R. M. (2003). Episodic recruitment in Antarctic krill Euphausia superba in the Palmer LTER study region. Mar. Ecol. Prog. Ser. 259, 185–200. doi: 10.3354/meps259185
Reiss C., Cossio A., Santora J., Dietrich K., Murray A., Mitchell B., et al. (2017). Overwinter habitat selection by Antarctic krill under varying sea-ice conditions: implications for top predators and fishery management. Mar. Ecol. Prog. Ser. 568, 1–16. doi: 10.3354/meps12099
Reiss C. S., Hinke J. T., Watters G. M. (2020). Demographic and maturity patterns of Antarctic krill (Euphausia superba) in an overwintering hotspot. Polar Biol. 43, 1233–1245. doi: 10.1007/s00300–020-02704–4
Ross R. M., Quetin L. B. (2000). “Reproduction in euphausiacea,” in Krill. Ed. Everson I. (Blackwell Science Ltd, Oxford, UK), 150–181. doi: 10.1002/9780470999493.ch6
RStudio Team. (2022). RStudio: integrated development environment for R. Available online at: http://www.rstudio.com/.
Ruiz Barlett E. M., Tosonotto G. V., Piola A. R., Sierra M. E., Mata M. M. (2018). On the temporal variability of intermediate and deep waters in the Western Basin of the Bransfield Strait. Deep Sea Res. Part II: Topical Stud. Oceanography 149, 31–46. doi: 10.1016/j.dsr2.2017.12.010
Saba G. K., Fraser W. R., Saba V. S., Iannuzzi R. A., Coleman K. E., Doney S. C., et al. (2014). Winter and spring controls on the summer food web of the coastal West Antarctic Peninsula. Nat. Commun. 5, 4318. doi: 10.1038/ncomms5318
Schmidt K., Atkinson A., Venables H. J., Pond D. W. (2012). Early spawning of Antarctic krill in the Scotia Sea is fuelled by “superfluous” feeding on non-ice associated phytoplankton blooms. Deep Sea Res. Part II: Topical Stud. Oceanography 59–60, 159–172. doi: 10.1016/j.dsr2.2011.05.002
Schofield O., Brown M., Kohut J., Nardelli S., Saba G., Waite N., et al. (2018). Changes in the upper ocean mixed layer and phytoplankton productivity along the West Antarctic Peninsula. Philos. Trans. R. Soc. A: Mathematical Phys. Eng. Sci. 376, 20170173. doi: 10.1098/rsta.2017.0173
Schofield O. M., Saba G. K., Coleman K., Carvalho F., Couto N., Ducklow H., et al. (2017). Decadal variability in coastal phytoplankton community composition in a changing West Antarctic Peninsula. Deep Sea Res. Part I: Oceanographic Res. Papers 124, 42–54. doi: 10.1016/j.dsr.2017.04.014
Siegel V. (1989). Winter and spring distribution and status of the krill stock in Antarctic Peninsula waters. Arch. Fisch 39, 45–72.
Siegel V., Loeb V. J. (1995). Recruitment of Antarctic krill Euphausia superba and possible causes for its variability. Mar. Ecol. Prog. Ser. 123, 45–56. doi: 10.3354/meps123045
Siegel V., Watkins J. L. (2016). “Distribution, biomass and demography of antarctic krill, euphausia superba,” in Biology and ecology of antarctic krill advances in polar ecology. Ed. Siegel V. (Springer International Publishing, Cham), 21–100. doi: 10.1007/978–3-319–29279-3_2
Siegert M., Atkinson A., Banwell A., Brandon M., Convey P., Davies B., et al. (2019). The antarctic peninsula under a 1.5°C global warming scenario. Front. Environ. Sci. 7. doi: 10.3389/fenvs.2019.00102
Stammerjohn S. E., Maksym T. (2017). Gaining (and losing) Antarctic sea ice: variability, trends and mechanisms. (Hoboken, New Jersey: John Wiley & Sons).
Stammerjohn S. E., Martinson D. G., Smith R. C., Iannuzzi R. A. (2008a). Sea ice in the western Antarctic Peninsula region: Spatio-temporal variability from ecological and climate change perspectives. Deep Sea Res. Part II: Topical Stud. Oceanography 55, 2041–2058. doi: 10.1016/j.dsr2.2008.04.026
Stammerjohn S. E., Martinson D. G., Smith R. C., Yuan X., Rind D. (2008b). Trends in Antarctic annual sea ice retreat and advance and their relation to El Niño–Southern Oscillation and Southern Annular Mode variability. J. Geophysical Res. 113, C03S90. doi: 10.1029/2007JC004269
Stammerjohn S., Massom R., Rind D., Martinson D. (2012). Regions of rapid sea ice change: An inter-hemispheric seasonal comparison. Geophysical Res. Lett. 39, L06501. doi: 10.1029/2012GL050874
Steinke K. B., Bernard K. S., Fontana J. M., Copeman L. A., Garcia L. M. (2022). The energetic cost of early reproductive development in juvenile Antarctic krill at the Western Antarctic Peninsula. Front. Mar. Sci. 9. doi: 10.3389/fmars.2022.1009385
Steinke K., Bernard K., Ross R., Quetin L. (2021). Environmental drivers of the physiological condition of mature female Antarctic krill during the spawning season: implications for krill recruitment. Mar. Ecol. Prog. Ser. 669, 65–82. doi: 10.3354/meps13720
Stuecker M., Bitz C., Armour K. (2017). Conditions leading to the unprecedented low Antarctic sea ice extent during the 2016 austral spring season - Stuecker - 2017 - Geophysical Research Letters - Wiley Online Library. Geophys. Res. Lett. 44, 9008–9019. doi: 10.1002/2017GL074691
Swart N. C., Fyfe J. C. (2012). Observed and simulated changes in the Southern Hemisphere surface westerly wind-stress. Geophysical Res. Lett. 39, L16711. doi: 10.1029/2012GL052810
Tang K., Taal M. (2005). Trophic modification of food quality by heterotrophic protists: Species-specific effects on copepod egg production and egg hatching. J. Exp. Mar. Biol. Ecol. 318, 85–98. doi: 10.1016/j.jembe.2004.12.004
Teschke M., Wendt S., Kawaguchi S., Kramer A., Meyer B. (2011). A circadian clock in antarctic krill: an endogenous timing system governs metabolic output rhythms in the euphausid species euphausia superba. PloS One 6, e26090. doi: 10.1371/journal.pone.0026090
Thompson D. W. J., Wallace J. M. (2000). Annular modes in the extratropical circulation. Part I: month-to-month variability*. J. Climate 13, 1000–1016. doi: 10.1175/1520–0442(2000)013<1000:AMITEC>2.0.CO;2
Vaughan D. G., Marshall G. J., Connolley W. M., Parkinson C., Mulvaney R., Hodgson D. A., et al. (2003). Recent rapid regional climate warming on the antarctic peninsula. Clim Change 60, 243–274. doi: 10.1023/A:1026021217991
Venables H. J., Clarke A., Meredith M. P. (2013). Wintertime controls on summer stratification and productivity at the western Antarctic Peninsula. Limnology Oceanography 58, 1035–1047. doi: 10.4319/lo.2013.58.3.1035
Veytia D., Bestley S., Kawaguchi S., Meiners K. M., Murphy E. J., Fraser A. D., et al. (2021). Overwinter sea-ice characteristics important for Antarctic krill recruitment in the southwest Atlantic. Ecol. Indic. 129, 107934. doi: 10.1016/j.ecolind.2021.107934
Walsh J., Reiss C. (2023). Extreme El Niño southern oscillation conditions have contrasting effects on the body condition of five euphausiid species around the northern Antarctic Peninsula during winter. Polar Biol 46, 319–338. doi: 10.1007/s00300–023-03129–5
Walsh J., Reiss C., Watters G. (2020). Flexibility in Antarctic krill Euphausia superba decouples diet and recruitment from overwinter sea-ice conditions in the northern Antarctic Peninsula. Mar. Ecol. Prog. Ser. 642, 1–19. doi: 10.3354/meps13325
Wang Z., Turner J., Wu Y., Liu C. (2019). Rapid Decline of Total Antarctic Sea Ice Extent during 2014–16 Controlled by Wind-Driven Sea Ice Drift in: Journal of Climate Volume 32 Issue 17 (2019). JCLI 32, 5381–5395. doi: 10.1175/JCLI-D-18–0635.1
Yoshida T., Virtue P., Kawaguchi S., Nichols P. (2011). Factors determining the hatching success of Antarctic krill Euphausia superba embryo: lipid and fatty acid composition. Mar. Biol. 158, 2313–2325. doi: 10.1007/s00227-011-1735-2
Yuan X. (2004). ENSO-related impacts on Antarctic sea ice: a synthesis of phenomenon and mechanisms. Antartic Sci. 16, 415–425. doi: 10.1017/S0954102004002238
Keywords: Antarctic krill, reproduction, environmental variability, climate, ecology
Citation: Steinke KB, Bernard KS, Reiss CS, Walsh J, Correa GM and Stammerjohn SE (2024) Factors impacting the timing of reproductive development in female Antarctic krill at the northwestern Antarctic Peninsula. Front. Mar. Sci. 11:1383175. doi: 10.3389/fmars.2024.1383175
Received: 07 February 2024; Accepted: 27 May 2024;
Published: 01 July 2024.
Edited by:
Doreen Kohlbach, UiT The Arctic University of Norway, NorwayReviewed by:
David Ainley, H.T. Harvey & Associates, United StatesFokje Schaafsma, Wageningen University and Research, Netherlands
Copyright © 2024 Steinke, Bernard, Reiss, Walsh, Correa and Stammerjohn. This is an open-access article distributed under the terms of the Creative Commons Attribution License (CC BY). The use, distribution or reproduction in other forums is permitted, provided the original author(s) and the copyright owner(s) are credited and that the original publication in this journal is cited, in accordance with accepted academic practice. No use, distribution or reproduction is permitted which does not comply with these terms.
*Correspondence: Kirsten B. Steinke, c3RlaW5ra2lAb3JlZ29uc3RhdGUuZWR1