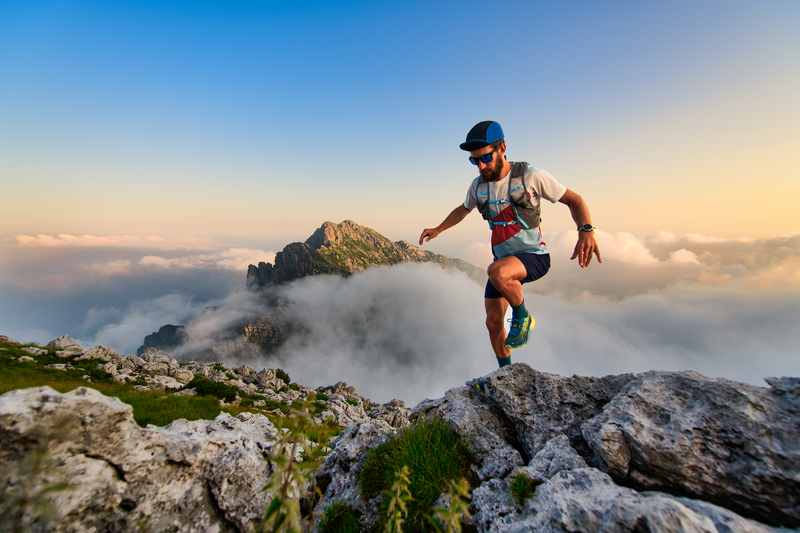
95% of researchers rate our articles as excellent or good
Learn more about the work of our research integrity team to safeguard the quality of each article we publish.
Find out more
ORIGINAL RESEARCH article
Front. Mar. Sci. , 25 March 2024
Sec. Coastal Ocean Processes
Volume 11 - 2024 | https://doi.org/10.3389/fmars.2024.1378724
Pollen and spore records in prodeltaic sediments hold significant potential for reconstructing paleoecologic and paleoclimatic evolution. However, uncertainties in these reconstructions arise from millennial-scale prodelta evolution, which dominates stratigraphic development and consequently influences sedimentary processes and/or pollen provenance. Here we explore the intricate relationship between pollen/spore records and prodelta stratigraphic evolution, using established seismic stratigraphy and ten sediment cores (five new, five from literature) within both the proximal and distal (mud belt) parts of the Huanghe (Yellow River) prodelta. In the proximal region, dominant lobate stratigraphic development, accompanied by shifts in river mouth and depocenter, leads to variations in pollen assemblages and contents within individual cores and differences in vertical pollen distribution across core sites. Transport distance appears to be a key factor, with arboreal pollens, particularly saccate ones (e.g., Pinus), positively correlating with the distance from the river mouth in their percentages within a single delta lobe, while non-arboreal and non-saccate arboreal pollens show higher percentages within shorter transport distances, despite longer distances leading to decreased total pollen concentrations. Likely due to the total pollen concentration after extended long-distance transport, this pattern is not observable in the distal mud belt. Subsurface stratigraphy in this mud belt reveals a complex pollen provenance characterized by Artemisia-Ulmus-Chenopodiaceae-Pinus, with non-arboreal pollens in dominance. Therein, non-arboreal pollens are not consistent with deposition from long-distance transport, and Ulmus pollens are uncommon in the western Bohai Sea. Interestingly, surface sediments in the mud belt display a different assemblage, characterized by Pinus-Artemisia-Quercus, consistent with the nearby Luanhe River prodelta, suggesting recent pollen supply from nearby sources, possibly due to the recent abandonment of the mud belt. Additionally, an energetic longshore transport/erosional regime reduces pollen content at the mud-belt margins and create pollen sinks (with the highest concentration) in the mud patch (accumulation area) within the erosion-dominated region adjacent to the mud belt. Our findings confirm that stratigraphic evolution, alongside hydrodynamic conditions and pollen provenance, governs pollen assemblages in deltaic/prodeltaic sediments. They can provide insights for palynological and pollen-based paleoclimatic and paleoecologic studies in other deltas.
Pollen and spore are crucial environmental proxies widely utilized in the realm of paleoecology and paleoclimatology (Faegri and Iversen, 1989; Nakagawa et al., 2003; Di Rita et al., 2015). They have been extensively employed for paleoenvironmental and paleoclimatic reconstructions on river deltas, particularly on prodeltas (the subaqueous domain below fair-weather wave base, encompassing the mud belt and mud-dominated longshore dispersal system if present; Anthony et al., 2010; Korus and Fielding, 2015), which have long been recognized as significant sinks for terrestrial materials, holding great potential as sedimentary archives. Such reconstructions have been conducted worldwide on deltas, including those on the Pacific Ocean Coast (Yi et al., 2003, 2006; Li et al., 2006; Song et al., 2017; Hao et al., 2021; Ye et al., 2024), Atlantic Ocean Coast (Smith et al., 2020; Yao et al., 2020, 2022; Adojoh et al., 2023), Indian Ocean Coast (Hait and Behling, 2009; Mohapatra et al., 2019, 2021), and Mediterranean Coast (Pantaléon-Cano et al., 2003; Di Rita et al., 2015; Zhao et al., 2020).
However, within prodeltaic sediments, the pollen and spore records also bear to uncertainties to some extent, potentially influencing the aforementioned reconstructions. These uncertainties are predominantly attributed to the prodeltas’ sensitivity to diverse sediment-transport and dispersal regimes, coupled with variations in riverine input fluxes and provenance during their development. Some scholars even propose that shifts in pollen assemblages in prodeltaic sediments are more likely linked to changes in depositional environments/processes rather than climate-related factors (Xu et al., 1996; DeBusk, 1997; Tian et al., 2008; Xu et al., 2016). This argument is partly supported by studies of pollen assemblages in modern seafloor sediments, which reveals influences from factors dominating sediment accumulation at such underwater environment, including oceanographic currents (e.g., tidal) and circulation (Heusser and Balsam, 1985), sediment transport (Sun et al., 1999; van der Kaars, 2001; Beaudouin et al., 2007), sediment density and grain size (Yang et al., 2019; Ouyang et al., 2021), and water depth and offshore distance (Hooghiemstra et al., 2006; Beaudouin et al., 2007; Luo et al., 2013; Yang et al., 2019).
Two aspects related to the evolution of prodelta can further complicate the aforementioned uncertainties and influence the pollen-based paleoenvironmental and paleoclimatic reconstruction: (1) the evolution of the prodelta may undergo complex cycles of deposition and erosion, as observed in deltas like the Huanghe (Yellow River) and Po River Deltas, characterized by frequent lobe switching (Correggiari et al., 2005; He et al., 2019), which can cause discontinuities in sedimentary archives; (2) the delta progradation is often accompanied by shoreline advancement, resulting in changes in the distance from the source, water depth, and marine dynamic processes over different intervals in one sediment core (Xue et al., 2018). Despite insights from modern prodelta sediments (seafloor samples; Chmura et al., 1999; Hooghiemstra et al., 2006; Yang et al., 2019; Ouyang et al., 2021), understanding how pollen and spore records within subsurface stratigraphy correlate with the sedimentary evolution of prodeltas, especially the influences from hydrodynamic conditions, transport regimes, and provenance during the evolution, remains less clear.
To address this issue, we focus on the Huanghe delta, particularly its prodelta in the western and northwestern Bohai Sea, in this study, which, renowned as one of the largest deltas on Earth, has been extensively studied for its sedimentary and stratigraphic evolution in recent years (He et al., 2019; Liu et al., 2019, 2020, 2022). Building on the well-established seismic stratigraphy (Liu et al., 2019; 2022), we utilized nine borehole cores and one gravity core (five newly acquired, five referenced from literature) (Table 1; Figure 1B) to establish seismic-to-core correlation and further examine the relationship between pollen and spore records and evolution of the prodelta stratigraphy. Our primary focus is to analyze variations in pollen and spore records within the same depositional interval in different prodelta locations, as well as to examine differences in pollen and spore assemblages between various depositional intervals, to identify the driving factors. We anticipate our findings will provide insights for pollen-based paleoenvironmental and paleoclimatic reconstructions in other deltaic regions.
Table 1 Basic information of the cores examined in this study. Except for BH264, which is a gravity core, the others are borehole cores.
Figure 1 (A, B) Physiographic representation of the Bohai Sea, detailing bathymetry, present shoreline, and the delta plain formed since the mid-Holocene (yellow fill). In Panel (A), historical shorelines (grey, black, and orange dashed lines with age labels) and the winter coastal current system (representative of long-term transport patterns) are superimposed (after Xue et al., 2018). Panel (B) illustrates subaqueous mud (blue fill) formed since the mid-Holocene highstand period, also known as the subaqueous highstand systems tract (HST), primarily composed of prodeltaic sediments (Liu et al., 2022). Isopachs of HST in the western and northwestern Bohai Sea, along with the positions of seismic profiles (bold black lines) and sediment cores from this study (yellow circles) and previously published data (blue circles) detailing in Figures 2–4, are indicated. Red dashed arrows indicate the directions of prodelta deflection in the Huanghe-dominated system. In the northwestern Bohai Sea, the Huanghe-dominated prodelta is separated from the Luanhe River prodelta by an elongated erosion-dominated region, delineated by heavy orange dashed lines. Modified after Liu et al. (2016a); Liu et al. (2019); Liu et al. (2022). Abbreviations: MHD=Modern Huanghe Delta; LRD=Luanhe River Delta; R.=river. (C) Overview of Bohai’s location and full extension of the Huanghe and Changjiang Rivers.
The Bohai Sea covers ~77,000 km2 and is characterized as an inland, shallow gulf (Liu et al., 2016a; He et al., 2019). The three major bays within the Bohai Sea (Liaodong, Bohai, and Laizhou Bays) have a water depth of less than 20 m, except for the central Bohai Sea, where depths can reach up to 40 m (Qin et al., 1990). It is connected to the North Yellow Sea through a 104.3 km-wide passage, known as Bohai Strait, where the water depth can reach ~60 m (Figure 1A).
The Huanghe originates from the Tibetan Plateau, and flows across the Chinese Loess Plateau and the North China Plain. It is renowned for its substantial sediment load, and annually carries approximately 1.1×109 tons of sediment into the Bohai Sea over the past 2000 years (Milliman et al., 1987; Wang et al., 2007). The total sediment discharge from other rivers around the Bohai Sea is minimal, constituting only 3.6% of the Huanghe’s sediment discharge (Xue et al., 2018).
Tidal patterns in the Bohai Sea are semidiurnal, with average and spring tidal ranges of 1.5-2 m and 3-4 m, respectively, in the western Bohai Sea (Xiong, 2012). Flood tidal currents can reach velocities of up to 5 knots (~2.5 m/s) around the north Bohai Strait (Liu et al., 2016a). The coastal current and oceanographic circulation within the Bohai Sea is predominantly influenced by high-salinity water from the Yellow Sea Warm Current, a branch of the Kuroshio Current. This circulation is counterclockwise in Liaodong Bay and clockwise in Bohai Bay (Liu et al., 2016a). The winter coastal current system, crucial for long-term sediment transport and prodelta evolution, is dominant because the Yellow Sea Warm Current is less intrusive in the summer; as a result, the circulation system predominantly transport river-borne sediments in winter, while storing them in summer (Su and Yuan, 2005; Xue et al., 2018). A bifurcation of the current occurs off the Luanhe River delta in the northwestern Bohai Sea (Figure 1A) (Su and Yuan, 2005; Liu et al., 2016a; 2016b).
The Bohai Sea experienced transgression since the deglaciation period, and as an isolated gulf, seawater has entered Bohai Sea from the Bohai Strait since ~12 kyr BP. During the maximum flooding of the transgression ~7 kyr BP, the shoreline extended 50-90 km west of its present position (Xue, 1993; Xue et al., 2018). From the mid-Holocene onward, the shoreline advanced along the northern, western, and southern coasts of Bohai Bay, associated with the deltaic/alluvial progradation of the ancient Huanghe and other smaller river (e.g., Luanhe and Haihe Rivers) (Tian, 2010; Liu et al., 2019) (Figure 1A). These rivers, particularly the Huanghe, contribute the most sediment loads to the western and northwestern Bohai Sea (He et al., 2019).
The substantial sediment discharge originating from the Huanghe has played a crucial role in shaping the Huanghe delta both onshore (Xue, 1993; Saito et al., 2000; He et al., 2019) and offshore (Liu et al., 2009; Liu et al., 2016b, 2019) in the western Bohai Sea. The Huanghe River has experienced frequent avulsion and lobe-switching events (Cheng and Xue, 1997; Wang et al., 2007), leading to the formation of a deltaic plain comprised of distinct deltaic lobe (referred to as superlobes). Tranditionally, the delta plain was divided into 10 superlobes (Xue and Cheng, 1989; Xue, 2009), but a recent analysis of borehole cores along the western Bohai coast (He et al., 2019) has suggested a revised division into eight superlobes, accompanied by refined dating spans (Supplementary Figure 1A). Notably, except for the period from 1128 to 1855 AD when the Huanghe altered its course to the South Yellow Sea, forming a separate superlobe, all other superlobes are located along the western Bohai coast (Supplementary Figure 1A). North of the Huanghe delta plain, the northernmost regions of the western Bohai coast and the northwestern Bohai coast are dominated by the Haihe and Luanhe River delta plains, respectively (Figure 1; Supplementary Figure 1A). Similar to the Huanghe delta plain, these areas have undergone evolution since the mid-Holocene, driven by the progradation of the two deltaic systems (Tian, 2010; Liu et al., 2019).
Offshore, there is a pervasive Huanghe-dominated mud region across the western, southern, and northwestern Bohai Sea (Figure 1B). The subaqueous delta clinoform of the Huanghe, as the subaqueous extension of the Huanghe delta plain, is situated predominantly in the western Bohai Sea with a thickness of up to 20 m (Figure 1B) (Liu et al., 2019). Dominated by eastward/southeastward and northeastward coastal currents, the Huanghe prodelta experiences deflection along these two directions, resulting in the formation of a unique dual-mud belt system (Figure 1B) (Liu et al., 2022). The eastward/southeastward deflected prodelta (mud belt) eventually reaches east of the Bohai Strait, entering the Yellow Sea and forming a mud wedge (up to ~40 m thick) offshore of the Shandong Peninsula (Yang and Liu, 2007). The northeastward deflected prodelta extends to the offshore regions of the Luanhe delta and even farther northeast, forming a depocenter (~10 m thick) near the furthest end of this mud belt (Figure 2C). This mud belt is separated from the adjacent Luanhe River prodelta (attached to the Luanhe River delta plain) by a shore-parallel erosion-dominated sandy region, which is ~10-20 km in width (Figure 1B) (Liu et al., 2022).
Figure 2 Interpreted seismic profiles covering approximately two-thirds of the offshore region around the MHD (A), extending east-west north of MHD (B), and along shore-parallel (C) and shore-normal (D) directions in the northwestern Bohai Sea, along with their correlation with sediment cores. The seismic interpretation in (A, B) follow Liu et al. (2019), with SUs 4-6 redefined as subaqueous lobes (SL) 1-3 in this study (the seismic profile in Panel (A) is a new addition from this study). (C, D) are modified from Liu et al. (2022), outlining boundaries of the Luanhe River prodelta, erosion-dominated area, and Huanghe-dominated prodelta with dashed orange lines. Solid-line columns represent sediment cores precisely aligned with seismic profiles, while dashed-line columns offer an approximate correspondence, emphasizing their relationship with seismic stratigraphy (especially for core H1 in Panel D). Refer to Figure 1B for profile and core locations, and see Supplementary Figure 2 and Table 1 for uninterpreted seismic profiles and core information, respectively. Abbreviations: LGM, Last Glacial Maximum; wRs, transgressive (wave) ravinement surface; MFS, Maximum flooding surface; SF, Seafloor.
The seismic stratigraphy in the western and northwestern Bohai Sea has been extensively examined recently (Tian et al., 2017; Liu et al., 2019; 2020; 2022), providing a critical foundation for this research (Figure 2). Postglacial stratigraphy in these regions comprises three sets of stratigraphic units (Liu et al., 2020): (1) terrestrial sediments formed during Late Pleistocene when the study area was subaerially exposed and devoid of seawater influence, primarily representing the Lowstand Systems Tract; (2) transgressive lags formed during the transgression through winnowing processes, especially wave action and ocean currents, often exhibiting as sand sheets and corresponding to the Transgressive Systems Tract; and (3) a unit of prodelta sediments formed since the mid-Holocene due to the progradation of neighboring river deltas, representing the Highstand Systems Tract (HST). These units are bounded by a transgressive (wave) ravinement surface (wRs) and a Maximum Flooding Surface (MFS).
The focus of this study is on the HST, and its internal configuration reveals notable disparities between the western and northwestern Bohai Sea. In the former, the unit exhibits a lobate configuration, consisting of four subunits associated with the Huanghe-dominated prodelta (Liu et al., 2019; corresponding to seismic units 4-6 and 8 in that study) (Figure 2B). Therein, the most recent subunit represents the accumulation of the Modern Huanghe Delta (MHD) formed since 1855 CE. Its basal boundary truncates internal dipping reflections of the underlying subunits on their tops (Figure 2B), representing a regional erosional unconformity; this aligns with the shift of the Huanghe course to the South Yellow Sea between 1128 and 1855 CE, resulting in a lack of sediment supply and degradation of the prodelta in our study area (Liu et al., 2013; 2019). In the northwestern Bohai Sea, the HST is internally uniform, characterized by stratified reflections, with no lobate structure observed (Figures 2C, D). Due to energetic longshore transport regimes, transgressive sand sheets have transformed into a series of alongshore-arranged erosional ridges, a phenomenon particularly pronounced in the erosion-dominated region (Figures 2C, D). Consequently, HST sediments have been partitioned by this erosional region along the shore-normal direction, with (1) a wedge attached to the Luanhe River delta plain corresponding to the Luanhe River-dominated prodelta, (2) isolated mud patches filling the troughs among the ridges in the erosion-dominated area, and (3) the Huanghe-dominated prodelta, extending from coast to offshore (Figures 2C, D) (Liu et al., 2022).
The Bohai Sea and its surrounding area are characterized by a warm and wet climate during summers and a cold and dry climate in winters, influenced by the East Asian Monsoon, with southerly and northerly winds prevailing in the summer and winter, respectively (Zang, 1996). The lowest temperatures (-1 to 4°C) typically occur in January, while the highest temperatures (24 to 27°C) are recorded in July and August. Precipitation is particularly concentrated in the summer, with an annual average of ~613.6 mm (IOCAS, 1985; Wang, 2013).
The vegetation surrounding the Bohai Sea (Supplementary Figure 1B) is predominantly characterized by deciduous broadleaved forests associated with warm temperate climates and shrub grasslands (Wang, 1993). Natural vegetation is predominantly observed in mountainous areas due to intensive agricultural and human activities on the plains (Meng and Wang, 1987). In the hills, deciduous broadleaved forests are dominated by Quercus, co-dominated with Pinus. Some deciduous broadleaved trees are present in the plain area, while others, e.g., Betula, Tilia, and Carpinus, are mainly found in the hills and lowlands. Coastal salt marshes are characterized by herb dominance, particularly Chenopodiaceae and Artemisia (Wang, 1993). Within the Huanghe delta plain, the vegetation is predominantly composed of herbs and shrubs, with trees dominated by Pinus densiflora, Pinus tabuliformis, and some other deciduous broadleaved forests. In the Luanhe delta plain, dominance is observed in P. tabuliformis, Quercus liaotungensis, and Quercus dentate (Supplementary Figure 1B).
In the Bohai Sea, the distribution of pollens and spores in seafloor sediments has been investigated by Yang et al. (2019), suggesting that Huanghe serves as the primary source. They also proposed that (1) pollen concentration is related to grain size, and (2) water depth is proportional to the percentages of Pinus pollen and Pteridophyte spores, while inversely related to other arboreal (excluding Pinus) and herbaceous pollen.
Previous palynological studies on sediment cores in this region primarily focused on paleoclimatic reconstruction (Yi et al., 2003; Chen et al., 2012; Lu et al., 2023), a scope does not align with the objective of this study. Nevertheless, these studies offer a valuable dataset for us to reevaluate the distribution of pollen and spore records in relation to the mid- and late-Holocene prodelta evolution.
As mentioned earlier, the seismic stratigraphy in the western and northwestern Bohai Sea has been well characterized through recent investigations. To place our pollen records in the context of stratigraphic evolution via a seismic-to-core correlation, we employed three seismic profiles from these studies: one from the western Bohai Sea, extending east-west north of MHD (Liu et al., 2019), and two from the northwestern Bohai Sea along shore-parallel and shore-normal directions (Liu et al., 2022) (Figures 1B; 2B-D). The acquisition of these profiles utilized the Applied Acoustic Engineering CSP2200 subbottom system (United Kingdom). Additionally, we presented a new seismic profile covering approximately two-thirds of the offshore region around the MHD (Figures 1B; 2A). The eastern quarter of this profile employed the same subbottom system and firing parameters as the boomer profiles reported by Liu et al. (2022), while the western three-quarters utilized chirp data collected with an Edgetech 512i towfish featuring a 0.5-7.2 kHz, 30 ms pulse.
Seismic interpretation in the western and northwestern Bohai Sea followed Liu et al. (2019) and (2022), respectively. The interpretation of early Holocene sand and the overlying MFS at the location east of MHD was not addressed in Liu et al. (2019), as this sand body did not develop within their study area. These interpretations, instead, is based on the findings of Li et al. (2023) (detailed in section 4.1). Depth estimation used two-way travel time (TWTT) with an average acoustic velocity of 1650 m/s, following Liu et al. (2016a); Liu et al. (2019).
Five new boreholes (BHB1, YRD1, H1, H3, and H4) were drilled in the western and northwestern Bohai Sea along with the seismic profiles (Figure 1B). The recovery rates for these cores exceed 90% (considering total length), except for the top 1 m of BHB1 and top 1.2 m of YRD1, which were unrecovered. Core positions were determined using DGPS. Given the primary focus on pollen records within prodeltaic sediments in this study, specific segments of these cores were selected for analysis: the top ~6 m of core BHB1 and the top 5 m of cores YRD1, H1, H3-H4. The selection of these segments was based on their correlation with the seismic stratigraphy. In the laboratory, these segments were longitudinally split, described, photographed, and subsampled for subsequent analysis. Additionally, five sediment cores (H9601, H9602, BH264, L1, H2) were sourced from existing literature, selected based on established chronological constraints and palynological data availability.
Cores BHB1, YRD1, H9601, H9602, and BH264 are situated in the western Bohai Sea, both onshore and offshore of the Huanghe delta plain. The remaining five boreholes (L1 and H1−4) are located in the northwestern Bohai Sea, both onshore and offshore of the Luanhe River Delta (Figure 1B). Basic information for these cores is summarized in Table 1.
Ten samples (including bulk sediments, peat, plant and benthic foraminifera) from the newly acquired cores were selected for 14C dating at Beta Analytic Inc. (Miami, United States) and Pilot National Laboratory for Marine science and Technology (Qingdao, China) using accelerator mass spectrometry (AMS). Past research in and around the Bohai Sea has suggested that conventional ages of marine samples should be calibrated using the Marine curve when their δ13C values exceed -10‰, while the IntCal curve is more appropriate for values typically around -25‰ (e.g., He et al., 2018; Liu et al., 2023). Given this, our bulk sediment, peat, and plant samples, characterized by δ13C values nearing −25‰, were calibrated using the latest IntCal 20 curve, while shell and benthic foraminifera samples were calibrated using the most recent Marine 20 model (Reimer et al., 2020) with the ΔR=-334 ± 50. All calibrations were conducted using Calib Rev. 8.2 software with a two standard deviation (2σ) of uncertainty (Stuiver et al., 2022).
Thirty-nine AMS 14C ages have been documented along with the other five cores (from literature sources), but they were not calibrated with the same standards (i.e., the Marine20 and IntCal20 models were not used). In this regard, a recalibration was conducted using these latest models. The dating (and calibration/recalibration) results newly reported in this study and those from earlier research are summarized in Tables 2, 3, respectively.
Table 2 Accelerator mass spectrometry (AMS) 14C ages obtained from borehole cores newly reported in this study.
A total of 178 samples were collected at 5-10 cm intervals (for cores BHB1 and YRD1) and 15-20 cm (for cores H1, H3 and H4) intervals for grain size analysis. The grain size data were determined using a Malvern Mastersizer-2000 laser particle size analyzer (United Kingdom) after pretreating the samples with 10% H2O2 and 0.1 N HCl to remove organic matter and biogenic carbonate. The grain size parameters (e.g., mean diameter) were calculated following the procedure of Folk and Ward (1957). The classification of sediment types was determined using the classification scheme established by Shepard (1954).
Fifty-two samples were taken at 5-30 cm (for cores YRD1 and BHB1) or 50-100 cm (for cores H1, H3 and H4) intervals for pollen and spore analysis. Each sample, weighing 5.0 ± 0.3 g (cores YRD1 and BHB1) or 10.0 ± 0.3 g (cores H1, H3 and H4) in dry weight, were processed following the presentative palynological treatment procedures (Moore et al., 1991). This involved the use of 10% HCl to dissolve calcareous minerals, 10% KOH treatment for the removal of organic matter, and 45% HF to eliminate siliceous materials. The residues were sieved over a 7-mm mesh screen in an ultrasonic water bath to remove tiny impurities and facilitate pollen identification. To facilitate identification, exotic Lycopodium marker spores (10,315 ± 281 grains/tablet) were added before the initial sample treatment. The prepared specimens were subsequently mounted in glycerin jelly for examination and identification.
Pollen identification and counting were conducted using a Nikon Eclipse Ni stereomicroscope at 400× or 1000× magnification. Palynomorphs were identified in accordance with the Quaternary Pollen Atlas (Wang et al., 1995; Tang et al., 2020). For each sample, a minimum of 200 pollen grains (excluding spores) were counted. A pollen diagram was created using TILIA 2.0.29 (Grimm, 1991 and the subsequent updated version). The pollen and spore percentages, indicating relative concentrations, were calculated based on the terrigenous pollens and spores.
All ten sediment cores examined in this study have been integrated into the pre-existing seismic stratigraphic framework (section 2.2), utilizing seismic-to-core correlation (Figures 2A-D) and incorporating AMS 14C dating results (Tables 2, 3). Stratigraphic transects of the core columns, integrated with the seismic stratigraphic framework, from the western and northwestern Bohai Sea are depicted in Figures 3, 4, respectively. The variations in mean grain size for the five newly acquired cores are illustrated in Figures 5–9.
Figure 3 Stratigraphic transect of borehole cores L1 and H1-4 in the northwestern Bohai Sea. The stratigraphic pattern in the transect aligns with the seismic stratigraphic interpretation in Figures 2C, D and incorporates evidence from lithology and chronology from the cores. Basic information and radiocarbon ages along these cores are summarized in Tables 1–3, respectively. Note that there is a mismatch in the proportions of cores L1 and cores H1-H4. Please refer to legend in Figure 3 for additional details.
Figure 4 Stratigraphic transect of cores H9601, H9602, BHB1, BH264, and YRD1 in the vicinity of the MHD. The stratigraphic pattern in the transect corresponds to the seismic stratigraphic interpretation in Figures 2A, B and incorporates the documented stratigraphic divisions of the cores, along with evidence from lithology, chronology, and proxy indicators. The location of the transect is highlighted in the inset map with a bold orange line. Basic information and radiocarbon ages along these cores are summarized in Tables 1–3, respectively. The interpretation of delta formation phases aligns with the evolutionary history of super lobes in the delta plain (per He et al., 2019), detailed in Supplementary Figure 1.
Figure 5 Downcore distribution of palynomorphs (percentage and concentration) and mean grain size recorded from borehole BHB1. Supplementary Figure 3 depicts the downcore distribution of grain size component of this core, as well as cores YRD1, L1, and H1-H4.
Figure 6 Downcore distribution of palynomorphs (percentage and concentration) and mean grain size recorded from borehole YRD1.
Figure 7 Downcore distribution of palynomorphs (percentage and concentration) and mean grain size recorded from borehole H1.
Figure 8 Downcore distribution of palynomorphs (percentage and concentration) and mean grain size recorded from borehole H3.
Figure 9 Downcore distribution of palynomorphs (percentage and concentration) and mean grain size recorded from borehole H4. Additional distributions of palynomorphs in cores L1 and H2 are presented in Supplementary Figures 4, 5, respectively, as they were solely published in a Chinese dissertation, potentially limiting accessibility.
To simplify the description of seismic stratigraphy, we redefined the lobate subunits within the Huanghe prodelta, which corresponds to the HST, as subaqueous lobes (SLs), with SLs 1-3 and the lobe associated with MHD corresponding to seismic units 4-6, and 8 in Liu et al. (2019), respectively (Figures 2A, B). In core BHB1, HST sediments locate in the top 5.4 m and cover SL2, SL3, and the MHD lobe, from bottom to top. The sediments below 5.4 m is dominated by transgressive coastal/estuarine muds, with a peat layer observable inside dating to 10.93 cal. kyr BP (Figure 4). In core YRD1, HST sediments correspond to SL3 in the top 3.5 m; a similar corresponding relation is also identifiable in core BH264. Below the HST sediments in both cores YRD1 and BH264 lies a sand unit formed during the early Holocene (~8-9.2 kyr) (Figure 4), extending thicker seaward but thinning and displaying apparent truncation landward (Figure 2A). This sand body appears to align with the early Holocene depocenter in the southern Bohai Sea documented by Li et al. (2023), corresponding to the Transgressive Systems Tract.
Based on the ages of SLs 2 and 3 (Figure 4), despite some deviation, the two lobes likely correspond to superlobes I (~7-5.5 kyr BP) and II (~5.5-3.6 kyr BP) in the Huanghe delta plain (Supplementary Figure 1A). As for onshore boreholes H9601 and H9602, although seismic-to-core correlation is not achievable, we establish correlation between sediment intervals in these cores and delta lobes based on abundant previously reported chronological and sedimentological (especially lithological changes) data (Figure 4). SLs 2-3 have been identified within both cores, situated at relatively deeper portions of the cores (~15 to 19.3 m in core H9601 and ~20.3-21.5 in core H9602). Several younger lobes formed ~3-1 kyr BP overlie these two lobes, where over half sediments originate from the MHD (Figure 4).
The four offshore boreholes (H1-H4) do not penetrate on the ridge-shaped transgressive sand sheets (Figures 2C, D). Instead, ~2-3 m thick HST sediments (SU2) at their uppermost sections (lacking dating for confirming the formation age) overlie directly late Pleistocene terrestrial sand bodies, which dated older than ~20 kyr BP, reaching to 41.5 kyr BP (Figure 3). HST sediments in the four cores are situated in different zones that have been introduced in section 2.2: the unit in core H1 and those in cores H3 and H4 are positioned in the Luanhe River prodelta and Huanghe-dominated prodelta, respectively; the unit in core H2 is within the erosion-dominated area but corresponds to isolated mud patch, an area of accumulation/erosional remnants (Figure 3). Notably, HST sediments in cores H1 and H4 represent the marginal deposition of prodeltas because the two cores are located at the boundary between the prodeltas and the erosion-dominated area (Figure 1B). The onshore borehole L1 is characterized by HST sediments exceeding 10 m in thickness, which encompasses a unit of Luanhe River prodelta in the lower part (~6-10.2 m) and a unit of Luanhe River delta plain in the upper part (0-6 m) (Figure 3).
In the five newly acquired boreholes, over 80 sporopollen types were identified, predominantly originating from deciduous trees and herbs (Figures 5–9). Therein, the arboreal pollens (AP) were dominated by gymnosperms, particularly Pinus and Picea, while angiosperm pollens included mainly deciduous Quercus, Betula, Ulmus, Ephedra, Morus, and Carpinus. Non-arboreal pollen (NAP) grains were mainly composed of Artemisia, Gramineae, Chenopodiaceae, Typha, and Cyperaceae. Fern spores included Selaginella, Triletes, and unidentified Monoletes. Pollen concentrations ranged from 1 to 8723 grains/g, with varying percentages and concentrations of AP and NAP. Pinus was notably abundant in several sections (Figures 5-9).
The two cores in the western Bohai Sea (BHB1 and YRD1) reveal diverse palynomorph assemblages; a total of 58 palynoflora, including 47 pollen, 5 spores, and 6 algae, were identified (Figures 5, 6). The average concentration and percentage of AP are greater than those of NAP. For the AP pollen, the gymnosperm pollen is dominated by Pinus, while the angiosperm pollen predominantly consists of deciduous Quercus, Betula, Ephedra, and Moraceae. NAP grains were primarily composed of Artemisia, Gramineae, Chenopodiaceae, and Typha. Fern spores and algae are dominated by Triletes, and Spiniferitaceae and Zygnemataceae, respectively.
As mentioned earlier, BHB1 predominantly encompasses two lobes, i.e., SLs 2 and 3 (Figure 4). Pollen records associated with SL2 were dominated by AP, primarily consisting of Pinus (~52%), and NAP, mainly comprising Chenopodiaceae (~13%). The average pollen concentration is ~3500 grains/g. Notably, Gramineae (~8.8%), Typha (~4.9%), Quercus (~4.4%), and Artemisia (~3.3%) exhibited relatively high concentration (Figure 5). The frequency and concentration of AP remained high throughout this segment, but gradually increase toward the upper boundary of the segment, while that of NAP decreased upwards (Figure 5). Saccate pollen (SP) was abundant and exhibited a significant trend of upward increasing, which is contrasting with the distribution of non-saccate arboreal pollen (NSP). Fern spores were scarce, but their frequency and concentration increased upwards in this zone (Figure 5).
In SL3, the average pollen concentration exceeded 4500 grains/g, which surpasses SL2 (Figure 5). The percentage and concentration of NAP were slightly higher than those of AP. Prominent AP elements (i.e., that with high percentage) included Pinus (~30%), and prominent NAP elements comprised Chenopodiaceae (~15%). Other accessory elements in this segment featured pollen grains of Gramineae (~13.8%), Artemisia (~7.3%), Typha (~4.2%), Quercus (~2.2%), Betulaceae (~1.6%), and ferns (~1.3%). The NSP, in contrast to that within SL2, exhibits a trend of decrease upward (Figure 5).
SL3 in core YRD1, the exclusive lobe covered in the core (Figure 4), shows a relatively lower average pollen concentration (~720 grains/g) compared to core BHB1 (Figure 5). The AP were much greater than NAP in frequency and concentration. Therein, AP constitutes ~82% of the total concentration, primarily comprising Pinus (~77%) and Betulaceae (~0.7%), while NAP account for ~10.3%, dominated by Gramineae (~4.3%), Chenopodiaceae (~2%), and Artemisia (~1%). The prevalent fern spore taxa include Triletes (~7.2%) and Monoletes (~1.2%). The SP (~83%) was significantly greater than NSP (~12%) in terms of the percentage, and shows a slight trend of increase upward (Figure 6).
In the northwestern Bohai Sea, a total of 41 palynoflora, comprising 33 pollens and 8 spores, were identified in cores H1, H3, and H4. These cores exhibit low pollen concentrations ranging from 21 to 85 grains/g, with NAP percentages generally surpassing those of AP. Specific pollen records reveal that gymnosperm pollen is predominantly Pinus and Picea, angiosperm pollen is characterized by deciduous Ulmus and Juglans, and NAP grains consisted mainly of Artemisia, Chenopodiaceae, and Gramineae. Fern spores and algae were rare (Figures 7-9).
The prodeltaic sediments (SU2; HST) in core H1 exhibit poorly preservation state of pollen characterized by low concentrations ranging from 21 to 33 grains/g (Figure 7). Within this segment, NAP prevails as the dominant pollen element, constituting ~61.8% of the total concentration, and it is primarily composed of Chenopodiaceae (~30.3%), Gramineae (~18.8%), and Artemisia (~5.8%). AP is also present but with a low concentration, mainly attributed to Pinus (~18%) and Ulmus (~1.9%). The percentage frequency of NAP and NSP exhibits a gentle decrease upwards. Conversely, the percentages of AP and SP show slight fluctuations (Figure 7).
The HST sediments within this core exhibit a low pollen concentration ranging between 22 and 57 grains/g. The AP and NAP contribute almost equally to the total pollen content within this section. Specifically, Artemisia and Chenopodiaceae dominate the AP component, constituting ~32.2% and 13.3%, respectively, while Ulmus and Pinus are the predominant contributors to NAP, accounting for ~30.3% and ~11.3%, respectively (Figure 8). The percentage and frequency of NAP and NSP show a trend of upward increase, while that of AP and SP show an opposite trend of decrease slightly upward (Figure 8).
The pollen assemblage from HST sediments in core H4 displays consistently low concentrations, ranging from 21 to 85 grains/g (Figure 9). It shows a pattern aligns with the observations from core H3, where both NAP and AP were equally abundant and t dominant elements within these two components are consistent with those in core H3. Specifically, NAP is characterized by Artemisia and Chenopodiaceae, contributing ~38.8% and ~12%, respectively, while AP is dominated by Ulmus and Pinus, comprising ~26% and ~11.6%, respectively. A notable feature in core H4 is the significant predominance of SP, accounting for approximately ~85%, compared to SP, which represents only ~15%. The percentage and frequency trends of both NAP and AP, as well as NSP and SP, exhibit fluctuations. Additionally, the presence of fern spores is rare, and algae are entirely absent within this core (Figure 9).
Examining core transects in both proximity and distal to the sediment source (i.e., the contemporaneous Huanghe mouth) (Figure 1B), allow us to investigate how pollen and spore records respond to a range of environmental factors associated with prodelta evolution. In the western Bohai Sea (proximal location), the stratigraphic evolution is primarily influenced by the spatial-temporal dynamics of Huanghe’s delta lobes (He et al., 2019; Liu et al., 2019). The cores (Figure 4) offer insights into how pollen records respond to the lobe-switching-dominated evolution and sediment dispersal. In the northwestern Bohai Sea (distal position), the stratigraphy is characterized by a longshore erosion-dominated area, leading to the separation of the Huanghe-dominated mud belt from the Luanhe River prodelta (Figure 3). Situated in different zones, these cores provide understanding of pollen records in response to the distal accumulation associated with complex provenance and hydrodynamic conditions.
In this section, we organize our discuss around individual influence of these factors. We acknowledge that some pollen records may be affected by the interaction of two or more factors, which will be addressed in the relevant text. To facilitate this analysis, we present the concentration and percentage of representative pollen elements from each core along with stratigraphic transects (Figures 10, 11 and Supplementary Figures 6, 7). The selection of pollen elements is based on their significant percentages and distinct distribution patterns identified in earlier studies on pollen assemblages within the surficial sediments (Yang et al., 2019), as well as in sediment cores analyzed in this study.
Figure 10 Concentration (grains/g; red curves) and percentage (navy bars) of representative pollen assemblages along the subsurface core transect of the Huanghe delta plain and subaqueous delta; see Figure 4 for the stratigraphic interpretation of the transect. The gray numbers on the right side of the diagrams indicate the depth of the section presented from each core. In this figure and Figure 11, only a subset of representative pollen elements is shown for the two core transects; additional elements along the two transects are detailed in Supplementary Figures 6, 7. AP, arboreal pollen; NAP, non-arboreal pollen.
Figure 11 Concentration (grains/g; red curves) and percentage (navy bars) of representative pollen assemblages along the core transect in the northwestern Bohai Sea; See Figure 3 for the stratigraphic interpretation of the transect. Note that there is a mismatch in the proportions of cores L1 and cores H1-H4, and the Pleistocene sediments underlie the color-filled sections in each core. SP, saccate pollen; NSP, non-arboreal pollen.
In the proximal core transect, core BHB1 shows the highest percentages of SP and AP for SL2, while core YRD1 exhibits the highest percentages for SL3 (Figure 10). Considering the alignment of SLs 2 and 3 with superlobes I and II on the Huanghe delta plain, along with the locations of these superlobes and contemporaneous paleo-shorelines, core YRD1 is the farthest core site from the contemporaneous Huanghe mouth along the direction of the offshore sediment dispersal regime during the evolution of SL3/superlobe II, and core BHB1, although not the farthest, is situated far away from the Huanghe mouth and within the upcurrent location of the sediment dispersal regime during the formation of SL2/superlobe I (Figure 1A; Supplementary Figure 1A). From a source-to-sink perspective, the upcurrent location can also be considered a considerably far distance for sediment transport, as it is not the typical destination for sediment deposition. In this context, our observation suggests that SP and AP can disperse to more distant locations in the proximal region, aligning with previous studies indicating that pollen with air sacs can travel greater distances compared to NSP (Wodehouse, 1935; Schwendemann et al., 2007; Leslie, 2010). The consistent dispersal pattern between SP and AP is likely due to a significant portion of AP consisting of airborne pollen species (Nilsson et al., 1977; Rahman et al., 2020). These findings also extend to individual pollen species; Pinus, a representative species of SP due to its lightweight nature allowing it to float in both water and air for an extended period with two air sacs (Schwendemann et al., 2007), shows a consistent increase in percentage within both SLs 2 and 3 as the transport distance increases (Figure 10).
Contrastingly, core BHB1 exhibits the lowest percentages for NSP and NAP in SL2, while core YRD1 shows the lowest percentages for SL3 (Figure 10). This suggests that the dispersal of NSP and NAP differs from that of SP and AP and is not dominant in long-distance transport. Despite NAP grains being generally smaller than AP and presumably dominated by water transport over longer distances, both NAP and Artemisia (a representative NAP species) show decreasing percentages with dispersal distance in SL2 and SL3 (Figure 10). This implies that NAP tends to accumulate earlier in the dispersal process, reflecting short-distance transport, which is consistent with previous studies indicating NAP has concentrated in the nearshore areas of the Bohai Sea (Xu and Sun, 1988; Yang et al., 2019). In summary, in the proximal accumulations of the Huanghe prodelta, the percentage of SP and AP correlates positively with offshore dispersal, while that of NSP and NAP shows a negative correlation. Notably, this process is also influenced by the direction and strength of dispersal and the hydrodynamic regime, discussed further in Section 5.4.
The HST sediments in core H3 represent the Huanghe-dominated distal accumulation (Figure 1). Although its formation age is unclear, it provides an opportunity to compare pollen records between proximal and distal accumulations. The five proximal cores cover most of the evolutionary stages of the Huanghe delta, potentially including the timing for distal accumulation. Therefore, differences in pollen assemblages between core H3 and these cores can be attributed to disparities between proximal and distal accumulations. In core H3, the percentage of AP is slightly lower than NAP, and Pinus percentage is insignificant (Figure 11). This indicates that, while AP, particularly Pinus pollen, can travel longer distances than NAP, both AP and NAP show low concentrations after extended long-distance transport, and the difference in their percentage observed in proximal sediments is no longer significant. Previous studies on seafloor sediments in the Bohai Sea suggest that wind-driven dispersal (in air) becomes the primary driver of pollen dispersal when water depth exceeds 20 m (Yang et al., 2019). However, this is not observed in core H3 (core top over 20 m water depth; Table 1), with lower SP and an insignificant percentage of Pinus (Figure 8). This could be due to complex pollen provenance and preservation processes in this distal mud, as further discussed in Section 5.3.
Pollen records in deltaic-prodeltaic sediments were often used for paleoclimatic reconstruction (Hao et al., 2021; Ye et al., 2024). In the Huanghe delta, Yi et al. (2003) used variations in Pinus and NAP percentages in cores H9601 and H9602 to indicate climatic warmth and cooling. They associated a decrease in Pinus in Pollen Zone I/1 and Zone IIb/2 with a warm climate, while an increase in NAP and Pinus in Pollen Zone II/1 and Zone III/2 suggested cooling.
Our stratigraphic analysis (Figure 4) reveals that in core H9601, Zones I/1 and II/1 roughly correspond to SL2 and SL3, respectively, while in core H9602, Zone III/2 spans SL2, SL3, and superlobe V. In contrast to cores H9601 and H9602, where Pinus decreases in SL2 and increases in SL3, our core BHB1 shows the highest Pinus percentage in SL2 but a sharp decline in SL3, accompanied by an increase in NAP. Core YRD1 also does not exhibit an increase in NAP and Pinus in SL3; instead, both decrease slightly (Figure 10). This comparison highlights a noticeable variation in the vertical (downcore) distribution of pollen assemblages in prodeltaic sediments across different locations within the Huanghe delta. Given that significant changes occurred among different delta lobes, this variation is likely attributed to lobe switching, which involves alterations in offshore distance, sediment/pollen transport distance, and different time spans of accumulation (even for a single lobe, it may accumulate diachronously) across various sites.
In the northwestern Bohai Sea, core site L1 underwent a transition from a prodelta to a delta-plain environment due to recent lobe switching of the Luanhe River, which resulted in resulting in the shoreline protrusion and creation of deltaic land at this location (Yu, 2019; Liu et al., 2022) (Figures 1, 3). In prodeltaic sediments, the pollen assemblages are dominated by AP, particularly Pinus and Quercus, with AP significantly higher than NAP in percentages and concentrations (Figure 11, Supplementary Figure 4). In delta-plain sediments, NAP becomes the dominant taxa, and the percentage of Selaginella is significantly higher than in prodeltaic sediments (Figure 11). Although the increased NAP and Selaginella percentages in delta-plain sediments most likely associated with the transition in sedimentary facies related to lobe-switching, these changes might be alternatively interpreted as indicators of increased precipitation and more frequent river floods in pollen-based paleoclimatic studies. In earlier studies, these elements are typically used for as climatic indictors because Selaginella spores, being large in grains, settle and sediment primarily through river flooding (Xu et al., 1995), and NAP content is generally higher than AP during flooding periods (Xu et al., 2004). In addition, the decline in AP from prodeltaic to delta-plain sediments is also probably influenced more by lobe-switching-related changes in the sedimentary environment than by climate. Previous studies (e.g., Xu et al., 1995) have indicated a gradual decline in AP content with greater transport distance on the Luanhe River alluvial plain-delta plain. This pattern likely plays a significant role in the decline of AP during the prodelta to delta plain transition at core site L1, associated with increased onshore transport distance for this core site.
The northwestern Bohai Sea, receiving sediment from both the Huanghe and Luanhe River (Liu et al., 2022), is expected to show varied pollen assemblages in their prodeltas due to diverse vegetation in their drainage basins (Supplementary Figure S1B) (Yang et al., 2019). Along the core transect (Figure 3), HST sediments in core L1, representing Luanhe River input, displays a Pinus-Artemisia-Quercus-dominated pollen assemblage (Supplementary Figure S4), with the dominance of AP, which is consistent with offshore seafloor sediments (Xu and Sun, 1988). However, subsurface sediments within this offshore region, identified as the Huanghe-dominated mud belt distinct from the Luanhe River prodelta (Liu et al., 2022), exhibit variations from surface sediments and among themselves, showing Artemisia-Ulmus-Chenopodiaceae-Pinus (Figures 8, 9) with NAP dominance in cores H3 and H4 (Figure 11). Such a discrepancy in pollen assemblages between surface sediments and subsurface records, aligning otherwise with those of the separated Luanhe River delta, likely results from the abandonment of the Huanghe-dominated mud belt. We hypothesize that the mud belt has not received Huanghe supply for at least over 1 kyr; historical evidence indicates that the Huanghe flowed into the southern Yellow Sea between 1128 and 1855 CE (Liu et al., 2013), and there is no evidence of sediment supply from the Huanghe to the mud belt since 1855 CE (instead prone to disperse southeastward from MHD) when it re-entered the Bohai Sea (Liu et al., 2023). Thus, while subsurface pollen records may reflect the influence of the Huanghe, surface sediments appear to receive pollen sedimentation sourced from the surrounding region. This discovery underscores the complex nature of pollen provenance in seafloor sediments, especially during periods of sediment hiatus.
In cores H3 and H4, Ulmus dominates the AP, constituting ~41.2% of the total pollen assemblage (Figure 11). Notably, no such high content of Ulmus has been observed in sediment cores from the Luanhe River and Huanghe drainage basins, delta plains, or the Bohai Sea seafloor (Yi et al., 2003; Chen et al., 2012; Yang et al., 2019), where the representatives of AP are Pinus, Quercus, Tilia, and Corylus (Xu et al., 2007). Instead, Ulmus and other broad-leaved trees were dominant in the forests of the eastern Northeast China region during the early to mid-Holocene (Ren, 1999). Particularly, in the pollen assemblage of core DYD in the eastern Liaodong Peninsula, Ulmus pollen accounted for as much as 37.65% in Holocene sediments (Liu, 2022). As Ulmus is an airborne pollen primarily flowering from April to June, coinciding with prevailing southerly winds in the Bohai Sea area (Yang et al., 2019), we hypothesize that the Ulmus pollen in cores H3 and H4 originated from airborne and/or seawater dispersion from the Northeast China region rather than being transported with sediment from the Huanghe or Luanhe River. This hypothesis can be supported by the water depths of cores H3 and H4, both ~20 m (Table 1), and the Bohai Sea circulation system (Figure 1A); both facts favor westward transport from Northeast China, facilitating dispersion with water flow (cf., Yang et al., 2019). The abundant Ulmus pollen in cores H3 and H4 provides insights into how prodeltaic sediments preserve pollen from other regions through airborne and seawater dispersion during prodeltaic sedimentation, adding greater uncertainty and complexity to the pollen assemblages of prodeltaic sediments.
In the northwestern Bohai Sea, HST sediments in core H2 exhibit higher pollen concentrations (excluding Ulmus) than adjacent offshore cores H1, H3 and H4 (Figure 11 and Supplementary Figure 5), even surpassing delta-plain sediments in the onshore core L1 (Supplementary Figure 4), indicating that H2 is a pollen sink. Our stratigraphic analysis places core H2 in the erosion-dominated area with energetic longshore tidal currents (Xue et al., 2009), strong enough to remold the transgressive sand sheets into erosional sand ridges, but the core site is on an isolated mud patch filling a trough between the ridges, representing an area of accumulation/erosional relict (Liu et al., 2022) (Figures 2, 3). Despite the prevailing belief that quiescent conditions favor pollen deposition (Chmura and Eisma, 1995), the concentration of pollen in core H2 suggests that accumulations in energetic hydrodynamic conditions might provide an ideal setting for pollen deposition, potentially more so than in prodeltaic sediments (compared to cores H1, H3, and H4).
Fern spores, predominantly Selaginella, also exhibit the highest percentage and concentration in core H2 along the transect of the five cores (Figure 11), which can further support the afore mentioned hypothesis. Specifically, Selaginella grains, as previously noted, being large and heavy, rely on strong hydrodynamic regimes for transport (Xu et al., 1995). Additionally, the high percentage of fern spores aligns with their buoyant nature, which allows them to be transported over longer distances (Dai and Weng, 2011; Dai et al., 2014; Yang et al., 2019). This nature may contribute to their high percentage in regions with strong transport regimes, particularly in seafloor sediments with increasing offshore distance and abundant precipitation, where fern spores far exceed pollen in percentage (Wang et al., 1987; Sun et al., 1999; van der Kaars and de Deckker, 2003).
Energetic longshore currents in the erosion-dominated area likely also influence pollen deposition in neighboring prodeltas, especially at their marginal parts. Cores H1 and H4, located at the boundary between the prodelta and the erosion-dominated area (Figures 1B; 3), represent such marginal sediments and exhibit lower pollen content compared to non-marginal core H3 (Figure 11). Additionally, we hypothesize that these currents control the sedimentation of Ulmus pollen along different zones. As Ulmus is likely transported from the east, possibly via seawater dispersal, strong longshore currents may act as a barrier, hindering Ulmus pollen from reaching more landward (northwestward) locations. This hypothesis explains the observed high Ulmus content in core sites (H3 and H4) southeast of the erosion-dominated area, while the content is much lower in the remaining cores (Figure 11).
Regionally, transport regimes can significantly impact pollen records, creating pollen sinks, due to their flow directions. This phenomenon is primarily observable in the western Bohai Sea, providing three lines of evidence. Firstly, an anomalous pattern in SL2 shows higher percentages of fern spores and Quercus near the river mouth in core H9601 but higher concentrations in core BHB1 farther away (Figure 10; Supplementary Figure 6). Despite typical nearshore concentration for Quercus (showing high percentages) in the western Bohai Sea (Yang et al., 2019) and an expected increase in fern percentage after long-distance transport, opposite trends for both elements were observed, potentially influenced by the local coastal current direction promoting nearshore pollen accumulation. Secondly, ferns exhibit nearshore concentration with percentages increasing offshore, a normal trend. However, the highest Quercus percentage and concentration appear in core H9602, not in core BHB1 closer to the contemporaneous river mouth (Figure 10; Supplementary Figure 1A), possibly due to core BHB1 being more offshore with deeper water depth, and the southeastward longshore transport regime at that time influencing a shallower region than core BHB1. The third evidence comes from total pollen concentration in core BHB1, which is the highest in both SL2 and SL3 (Figure 5). Given the core site BHB1 is neither the farthest nor closest to the contemporaneous river mouth for both SLs, the highest pollen concentration is most likely associated with a pollen sink likely induced by the local transport regime. Similar pollen sinks associated with the transport regime direction are observable in Bohai Sea surface sediments, particularly in Laizhou Bay (displaying the highest pollen concentration; Yang et al., 2019), a down-current region for the coastal current, indicating a sink (Figure 1A).
Considering that clinoform-scale construction of prodelta typically leads to specific arrangements of sediment grain size distribution (Patruno and Helland-Hansen, 2018), it is crucial to examine how this distribution affects the pollen records. We observed a positive correlation between grain size/mud content and pollen concentrations in various core segments (Figure 12). Specifically, in core BHB1, SL3 and SL2 exhibit the highest pollen concentrations (>4000 n/g; Figure 5) with the smallest grain size (clay or silt clay; Figure 4), while SL2 in core H9602 shows the lowest concentration (<50;Yi et al., 2003) with a significant increase in grain size (fine sand; Figure 4). In core L1, lower pollen concentrations are associated with coarser delta-plain sediments compared to the higher concentrations in prodeltaic sediments (Figures 3, 11).
Figure 12 Correlation between total pollen concentration and mud percentage in sediment cores from the western Bohai Sea (A) and northwestern Bohai Sea (B).
This positive correlation, unsurprisingly, is also evident in seafloor sediments across various continental shelf regions, e.g., the northern South China Sea (Tong et al., 2012) and the Bohai Sea (Yang et al., 2019), likely attributed to the susceptibility of both pollen and fine-grained sediments to suspension-related dispersion in underwater conditions (Heusser, 1988). Nevertheless, in Huanghe prodelta sediments, this positive correlation is valid only when the mud content exceeds ~8-12%, and lower mud content may impede pollen accumulation (Figure 12).
In this study, we investigated the relationship between pollen and spore records and the stratigraphic evolution of the Huanghe-dominated prodelta, employing ten sediment cores (five new, five from literature) from both proximal and distal regions of the prodelta.
Our findings reveal that, in the proximal location of the Huanghe prodelta, variations in pollen assemblages, percentages, and concentrations among cores, as well as within different intervals within a single core, are dominated by avulsions and lobe switching of Huanghe, which were typically misattributed to climate change in earlier studies. Within a single delta lobe, pollen records are influenced by transport distance; typically, NAP and NSP percentages display an inverse relationship with distance, while AP and SP (particularly Pinus) percentages exhibit a positive correlation.
These patterns of pollen distribution in the proximal prodelta are absent in the distal accumulation of the prodelta (distal mud belt), suggesting a weakened connection with transport distance, following an extended period of transport. Instead, this Huanghe-dominated distal mud belt is characterized by a complex pollen provenance, sourced from both the surrounding regions and even more distant locations (e.g., the Liaodong Peninsula), rather than being dominated solely by Huanghe supply. The energetic longshore transport regime appears to favor deposition of pollens (especially Quercus) and fern spore in accumulation/erosional-relict areas within a condition of pervasive erosion. This regime also affects neighboring prodeltas, reducing pollen content at their marginal parts. Additionally, pollen concentration is controlled by sediment grain size distribution associated with prodelta evolution, with higher concentrations associated with more fine-grained sediments.
These findings underscore the constraints on pollen records in prodeltaic sediments, shaped by prodelta evolution, regional pollen provenance, and hydrodynamic conditions, which potentially surpass the impact of climate change. These complexities and uncertainties should be considered in pollen-based paleoenvironmental and paleoclimatic reconstructions in the delta/prodelta of Huanghe and other deltas worldwide. It is recommended to incorporate comprehensive stratigraphic, sedimentological and provenance analyses in conjunction with these studies.
The raw data supporting the conclusions of this article will be made available by the authors, without undue reservation.
WH: Data curation, Formal analysis, Methodology, Visualization, Writing – original draft. SL: Conceptualization, Funding acquisition, Project administration, Supervision, Visualization, Writing – review & editing. YL: Data curation, Methodology, Supervision, Writing – review & editing. AF: Writing – review & editing. WF: Writing – review & editing. XW: Writing – review & editing. SC: Conceptualization, Funding acquisition, Project administration, Supervision, Writing – review & editing.
The author(s) declare financial support was received for the research, authorship, and/or publication of this article. This research received funding from the National Natural Science Foundation of China (grants 42076067, and 42276175).
This research received funding from the National Natural Science Foundation of China (grants 42076067, and 42276175). We extend our gratitude to the crews of the Research Center for Islands and Coastal Zone, First Institute of Oceanography, MNR, for their valuable contributions to data collection in the northwestern Bohai Sea. We appreciate three reviewers for their valuable inputs.
The authors declare that the research was conducted in the absence of any commercial or financial relationships that could be construed as a potential conflict of interest.
All claims expressed in this article are solely those of the authors and do not necessarily represent those of their affiliated organizations, or those of the publisher, the editors and the reviewers. Any product that may be evaluated in this article, or claim that may be made by its manufacturer, is not guaranteed or endorsed by the publisher.
The Supplementary Material for this article can be found online at: https://www.frontiersin.org/articles/10.3389/fmars.2024.1378724/full#supplementary-material
Adojoh O. C., Marret F., Duller R., Osterloff P. L., Oboh-Ikuenobe F. E., Saylor B. Z. (2023). Stages of palaeoenvironmental evolution, climate and sea level change of the Niger Delta, east Equatorial Atlantic: Novelty from elemental tracers, sedimentary facies and pollen records. Holocene. 33 (7), 781–790. doi: 10.1177/09596836231163506
Anthony E. J., Gardel A., Gratiot N., Proisy C., Allison M. A., Dolique F., et al. (2010). The Amazon-influenced muddy coast of South America: A review of mud-bank–shoreline interactions. Earth-Science Rev. 103, 99–121. doi: 10.1016/j.earscirev.2010.09.008
Beaudouin C., Suc J. P., Escarguel G., Arnaud M., Charmasson S. (2007). The significance of pollen signal in present-day marine terrigenous sediments: the example of the Gulf of Lions (western Mediterranean Sea). Geobios 40, 159–172. doi: 10.1016/j.geobios.2006.04.003
Chen J. X., Shi X. F., Qiao S. Q. (2012). Holocene palynological sequences and palaeoenvironmental changes in the Bohai Sea area. Acta Oceanologica Sin. 3, 99–105.
Cheng G., Xue C. (1997). Sedimentology of the Yellow River Delta (Beijing: Geological Publishing House), 147.
Chmura G. L., Eisma D. (1995). A palynological study of surface and suspended sediments on a tidal flat: implications for pollen transport and deposition in coastal waters. Mar. Geology 128, 183–200. doi: 10.1016/0025-3227(95)00096-H
Chmura G. L., Smirnov A., Campbell I. D. (1999). Pollen transport through distributaries and depositional patterns in coastal waters. Palaeogeography Palaeoclimatology Palaeoecol. 149, 257–270. doi: 10.1016/S0031-0182(98)00205-3
Correggiari A., Cattaneo A., Trincardi F. (2005). The modern Po Delta system: lobe switching and asymmetric prodelta growth. Mar. Geology 222, 49–74. doi: 10.1016/j.margeo.2005.06.039
Dai L., Weng C. (2011). A survey on pollen dispersal in the western Pacific Ocean and its paleoclimatological significance as a proxy for variation of the Asian winter monsoon. Sci. China Earth Sci. 54, 249–258. doi: 10.1007/s11430-010-4027-7
Dai L., Weng C., Lu J., Mao L. (2014). Pollen quantitative distribution in marine and fluvial surface sediments from the northern South China Sea: new insights into pollen transportation and deposition mechanisms. Quaternary Int. 325, 136–149. doi: 10.1016/j.quaint.2013.09.031
DeBusk J. G.H. (1997). The distribution of pollen in the surface sediments of Lake Malawi, Africa, and the transport of pollen in large lakes. Rev. Palaeobotany Palynology 97, 123–153. doi: 10.1016/S0034-6667(96)00066-8
Di Rita F., Celant A., Milli S., Magri D. (2015). Lateglacial–early Holocene vegetation history of the Tiber delta (Rome, Italy) under the influence of climate change and sea level rise. Rev. Palaeobotany Palynology 218, 204–216. doi: 10.1016/j.revpalbo.2014.12.005
Faegri K., Kaland P. E., Krzywinski K. (1989). Textbook of pollen analysis. 4 (Chichester, UK: John Wiley & Sons Ltd).
Folk R. L., Ward W. C. (1957). Branos river bar: a study in the significance of grain size parameter. J. sedimentary Petrology 27, 3–26. doi: 10.1306/74D70646-2B21-11D7-8648000102C1865D
Grimm E. C. (1991). TILIA v2.0.4 (Computer Software) (Springfield, IL: Illinois State Museum, Research and Collections Centre).
Hait A. K., Behling H. (2009). Holocene mangrove and coastal environmental changes in the western Ganga-Brahmaputra Delta, India. Vegetation History Archaeobotany 18, 159–169. doi: 10.1007/s00334-008-0203-5
Hao X., Li L., Ouyang X., Culligan N., Hu B., Zhao X., et al. (2021). Coastal morphodynamics and Holocene environmental changes in the Pearl River Delta, southern China: New evidence from palynological records. Geomorphology 389, 107846. doi: 10.1016/j.geomorph.2021.107846
He L., Xue C., Ye S., Amorosi A., Yuan H., Yang S., et al. (2019). New evidence on the spatial-temporal distribution of superlobes in the Yellow River Delta Complex. Quaternary Sci. Rev. 214, 117–138. doi: 10.1016/j.quascirev.2019.05.003
He L., Xue C., Ye S., Laws E. A., Yuan H., Yang S., et al. (2018). Holocene evolution of the Liaohe Delta, a tide-dominated delta formed by multiple rivers in Northeast China. J. Asian Earth Sci. 152, 52–68. doi: 10.1016/j.jseaes.2017.11.035
Heusser L. E. (1988). Pollen distribution in marine sediments on the continental margin off northern California. Mar. Geology 80, 131–147. doi: 10.1016/0025-3227(88)90076-X
Heusser L. E., Balsam W. L. (1985). Pollen sedimentation in the northwest Atlantic: effects of the Western Boundary Undercurrent. Mar. Geology 69, 149–153. doi: 10.1016/0025-3227(85)90138-0
Hooghiemstra H., Lézine A. M., Leroy S. A., Dupont L., Marret F. (2006). Late Quaternary palynology in marine sediments: a synthesis of the understanding of pollen distribution patterns in the NW African setting. Quaternary Int. 148, 29–44. doi: 10.1016/j.quaint.2005.11.005
IOCAS (Institute of Oceanography, Chinese Academy of Sciences) (1985). Geology of the Bohai Sea (Beijing: Science Press).
Korus J. T., Fielding C. R. (2015). Asymmetry in Holocene river deltas: patterns, controls, and stratigraphic effects. Earth-Science Rev. 150, 219–242. doi: 10.1016/j.earscirev.2015.07.013
Leslie A. B. (2010). Flotation preferentially selects saccate pollen during conifer pollination. New Phytol. 188, 273–279. doi: 10.1111/j.1469-8137.2010.03356.x
Li X., Zhao Y., Yang Z., Qiao S., Liu B., Xie Q., et al. (2023). Holocene marine deposits in the Bohai Sea: Depocenters, sediment sources, and oceanic and tectonic influences. Geomorphology 442, 108913. doi: 10.1016/j.geomorph.2023.108913
Li Z., Saito Y., Matsumoto E., Wang Y., Tanabe S., Vu Q. L. (2006). Climate change and human impact on the Song Hong (Red River) Delta, Vietnam, during the Holocene. Quaternary Int. 144, 4–28. doi: 10.1016/j.quaint.2005.05.008
Liu C. (2022). Analysis of sedimentary environment characteristics of boreholes in the eastern plain of the Dayang River area of eastern Liaoning. [M.S thesis]. Dalian, China: Liaoning Normal University.
Liu S., Feng A., Gao S., Wang Y. P., Jia J., Du J., et al. (2022). Evidence for a second deflected prodelta of the Yellow River: Insights into a complex pattern of delta asymmetry. Mar. Petroleum Geology 143, 105815. doi: 10.1016/j.marpetgeo.2022.105815
Liu S., Feng A., Liu Y. (2023). Buried channels under the northern Bohai Sea: Evidence for a great Bohai Paleolake during the late MIS3. Mar. Pet. Geol. 155, 106413. doi: 10.1016/j.marpetgeo.2023.106413
Liu S., Feng A., Liu C., Zheng Y., Li P., Zhang Z. (2019). Seismic stratigraphy and morphology of the Holocene progradational system beneath Bohai Bay, Bohai Sea: Lobate evolution of a multi-sourced subaqueous fluviodeltaic complex. Mar. Geology 409, 31–47. doi: 10.1016/j.margeo.2018.12.009
Liu S., Feng A., Yang L., Du J., Yu Y., Feng W., et al. (2020). Stratigraphic and three-dimensional morphological evolution of the late Quaternary sequences in the western Bohai Sea, China: Controls related to eustasy, high sediment supplies and neotectonics. Mar. Geology 427, 106246. doi: 10.1016/j.margeo.2020.106246
Liu J., Kong X., Saito Y., Liu J. P., Yang Z., Wen C. (2013). Subaqueous deltaic formation of the Old Yellow River (AD 1128–1855) on the western South Yellow Sea. Mar. Geology 344, 19–33. doi: 10.1016/j.margeo.2013.07.003
Liu S., Li P., Feng A., Du J., Gao W., Xu Y., et al. (2016b). Seismic and core investigation on the modern Yellow River Delta reveals the development of the uppermost fluvial deposits and the subsequent transgression system since the postglacial period. J. Asian Earth Sci. 128, 158–180. doi: 10.1016/j.jseaes.2016.07.009
Liu J., Saito Y., Wang H., Zhou L., Yang Z. (2009). Stratigraphic development during the Late Pleistocene and Holocene offshore of the Yellow River delta, Bohai Sea. J. Asian Earth Sci. 36, 318–331. doi: 10.1016/j.jseaes.2009.06.007
Liu J., Wang H., Wang F., Qiu J., Saito Y., Lu J., et al. (2016a). Sedimentary evolution during the last~ 1.9 Ma near the western margin of the modern Bohai Sea. Palaeogeography Palaeoclimatology Palaeoecol. 451, 84–96. doi: 10.1016/j.palaeo.2016.03.012
Lu J., Huang W., Sun J., Wang F., Gao Y., Fan X., et al. (2023). Quaternary pollen record and paleoenvironmental evolution of the Bohai Sea strait. Regional Stud. Mar. Sci. 68, 103278. doi: 10.1016/j.rsma.2023.103278
Luo C., Chen M., Xiang R., Liu J., Zhang L., Lu J., et al. (2013). Characteristics of modern pollen distribution in surface sediment samples for the northern South China Sea from three transects. Quaternary Int. 286, 148–158. doi: 10.1016/j.quaint.2012.11.001
Meng G., Wang S. (1987). Studies on the quaternary sporo-pollen assemblage from the Bohai Sea drilling core BC-1 and its paleoclimate. Oceanologia Limnologia Sin. 18, 253–264.
Milliman J. D., Yun-Shan Q., Mei-e R., Saito Y. (1987). Man's influence on the erosion and transport of sediment by Asian rivers: the Yellow River (Huanghe) example. J. Geology 95, 751–762. doi: 10.1086/629175
Mohapatra P. P., Stephen A., Prasad S., Singh P., Anupama K. (2019). Late Pleistocene and Holocene vegetation changes and anthropogenic impacts in the Cauvery delta plains, southern India. Quaternary Int. 507, 249–261. doi: 10.1016/j.quaint.2018.12.008
Mohapatra P. P., Stephen A., Singh P., Prasad S., Anupama K. (2021). Pollen based inference of Holocene sea level changes, depositional environment and climatic history of Cauvery delta, Southern India. Catena 199, 105029. doi: 10.1016/j.catena.2020.105029
Moore P. D., Webb J. A., Collison M. E. (1991). Pollen analysis (Oxford: Blackwell scientific publications).
Nakagawa T., Kitagawa H., Yasuda Y., Tarasov P. E., Nishida K., Gotanda K., et al. (2003). Asynchronous climate changes in the North Atlantic and Japan during the last termination. Science 299, 688–691. doi: 10.1126/science.1078235
Nilsson S., Praglowski J., Nilsson L. (1977). Atlas of airborne pollen grains and spores in northern Europe. Verlag Natur och Kultur. Stockholm. 160. doi: 10.1163/9789004612211
Ouyang X., Hao X., Culligan N., Dai L., Cheng Z., Li S. (2021). Distribution of suspended sediments and pollen in the northern south China sea: Implications for pollen source, transport, and deposition in surface ocean waters. Continental Shelf Res. 231, 104600. doi: 10.1016/j.csr.2021.104600
Pantaléon-Cano J., Yll E. I., Pérez-Obiol R., Roure J. M. (2003). Palynological evidence for vegetational history in semi-arid areas of the western Mediterranean (Almería, Spain). Holocene 13, 109–119. doi: 10.1191/0959683603hl598rp
Patruno S., Helland-Hansen W. (2018). Clinoforms and clinoform systems: Review and dynamic classification scheme for shorelines, subaqueous deltas, shelf edges and continental margins. Earth-Science Rev. 185, 202–233. doi: 10.1016/j.earscirev.2018.05.016
Qin Y., Zhao Y., Chen L., Zhao S. (1990). Geology of Bohai Sea. China Ocean Press Beijing pp, 1–354.
Rahman A., Luo C., Chen B., Haberle S., Khan M. H. R., Jiang W., et al. (2020). Regional and seasonal variation of airborne pollen and spores among the cities of South China. Acta Ecologica Sin. 40, 283–295. doi: 10.1016/j.chnaes.2019.05.012
Reimer P. J., Austin W. E., Bard E., Bayliss A., Blackwell P. G., Ramsey C. B., et al. (2020). The IntCal20 Northern Hemisphere radiocarbon age calibration curve (0–55 cal kBP). Radiocarbon 62, 725–757. doi: 10.1017/RDC.2020.41
Ren G. Y. (1999). Pollen distribution map and analysis of Holocene in northeast China. Acta Palaeontologica Sin. 38, 365–385.
Saito Y., Wei H., Zhou Y., Nishimura A., Sato Y., Yokota S. (2000). Delta progradation and chenier formation in the Huanghe (Yellow River) delta, China. J. Asian Earth Sci. 18, 489–497. doi: 10.1016/S1367-9120(99)00080-2
Schwendemann A. B., Wang G., Mertz M. L., McWilliams R. T., Thatcher S. L., Osborn J. M. (2007). Aerodynamics of saccate pollen and its implications for wind pollination. Am. J. Bot. 94, 1371–1381. doi: 10.3732/ajb.94.8.1371
Shepard F. P. (1954). Nomenclature based on sand-silt-clay ratios. J. Sedimentary Res. 24, 151–158. doi: 10.1306/D4269774-2B26-11D7-8648000102C1865D
Smith V., Warny S., Jarzen D. M., Demchuk T., Vajda V., Gulick S. P. (2020). Paleocene-Eocene palynomorphs from the Chicxulub impact crater, Mexico. Part 2: angiosperm pollen. Palynology 44, 489–519. doi: 10.1080/01916122.2019.1705417
Song B., Li Z., Lu H., Mao L., Saito Y., Yi S., et al. (2017). Pollen record of the centennial climate changes during 9-7 cal ka BP in the Changjiang (Yangtze) River Delta plain, China. Quaternary Res. 87, 275–287. doi: 10.1017/qua.2017.1
Stuiver M., Reimer P. J., Reimer R. W. (2022) Calib 8.2. Available online at: http://calib.org.
Sun X., Li X., Beug H. J. (1999). Pollen distribution in hemipelagic surface sediments of the South China Sea and its relation to modern vegetation distribution. Mar. Geology 156, 211–226. doi: 10.1016/S0025-3227(98)00180-7
Tang L., Mao L., Shu J., Li C., Shen C., Zhou Z. (2020). Atlas of Quaternary pollen and spores in China (Singapore: Springer Nature). doi: 10.1007/978-981-13-7103-5
Tian L. (2010). Deglacial Sequence and Sedimentary Evolution in the West Bohai Bay, China (Beijing: Phd Thesis of China University of Geosciences (Beijing).
Tian L., Chen Y., Jiang X., Wang F., Pei Y., Chen Y., et al. (2017). Post-glacial sequence and sedimentation in the western Bohai Sea, China, and its linkage to global sea-level changes. Mar. Geology 388, 12–24. doi: 10.1016/j.margeo.2017.04.006
Tian F., Xu Q., Li Y., Cao X., Wang X., Zhang L. (2008). Pollen assemblage characteristics of lakes in the monsoon fringe area of China. Chin. Sci. Bull. 53, 3354–3363. doi: 10.1007/s11434-008-0408-0
Tong G., Chen L., Long J., Li T., Xiao X., Tong S. (2012). Surface pollen distribution patterns in Beibu Gulf and corresponding sediment dynamics environment. Chin. Sci. Bull. 57, 902–911. doi: 10.1007/s11434-011-4912-2
van der Kaars S. (2001). Pollen distribution in marine sediments from the south-eastern Indonesian waters. Palaeogeography Palaeoclimatology Palaeoecol. 171, 341–361. doi: 10.1016/S0031-0182(01)00253-X
van der Kaars S., de Deckker P. (2003). Pollen distribution in marine surface sediments offshore Western Australia. Rev. Palaeobotany Palynology 124, 113–129. doi: 10.1016/S0034-6667(02)00250-6
Wang K. (1993). Spore-pollen and algal assemblages in the sediments of the Bohai Sea and palaeoenvironments (Beijing: Geological Publishing House).
Wang H. (2013). Climate Change around Bohai Region during 1956 to 2011 and its Relationship with ENSO. M.D (Thesis of Northwest Normal University).
Wang K., Sun Y., Zhang Y., Jiang H., Zhang Y. (1987). The Spore-Pollen and Algal Assemblage in the East China Sea Sediments (Beijing: China Ocean Press), 10–80.
Wang H., Yang Z., Saito Y., Liu J. P., Sun X., Wang Y. (2007). Stepwise decreases of the Huanghe (Yellow River) sediment load (1950–2005): Impacts of climate change and human activities. Global Planetary Change 57, 331–354. doi: 10.1016/j.gloplacha.2007.01.003
Wodehouse R. P. (1935). Pollen grains. Their structure, identification and significance in science and medicine (London: Mc Graw-Hill Publishing Co. Ltd.).
Xiong X. (2012). Chinese Offshore Oceanography – Physical Oceanography and Marine Meteorology (Beijing: Ocean Press).
Xu Q., Li Y., Yang X., Zheng Z. (2007). Quantitative relationship between pollen and vegetation in northern China. Sci. China Ser. D: Earth Sci. 50, 582–599. doi: 10.1007/s11430-007-2044-y
Xu Q., Yang X., Wu C., Meng L., Wang Z. (1996). Alluvial pollen on the North China plain. Quat. Res. 46 (3), 270–280. doi: 10.1006/qres.1996.0066
Xu J., Sun T. (1988). The assemblage feature of the spore-pollen in the surface sediments of the western Bohai Sea. Bull. Mar. Sci. 7, 49–54.
Xu Q., Yang X. L., Wang Z., Wu C., Meng L., Yao Z. (1995). Study on pollen transportation by rivers. Acta Botanica Boreali-Occidentalia Sin. 37, 829–832.
Xu Q., Yang X., Yang Z. (2004). Relationship between pollen assemblages and vegetation in alluvial sediments of Luanhe River Basin. J. Palaeogeogr. 6, 69–77s.
Xu Q., Zhang S., Gaillard M. J., Li M., Cao X., Tian F., et al. (2016). Studies of modern pollen assemblages for pollen dispersal-deposition-preservation process understanding and for pollen-based reconstructions of past vegetation, climate, and human impact: a review based on case studies in China. Quaternary Sci. Rev. 149, 151–166. doi: 10.1016/j.quascirev.2016.07.017
Xue C. (1993). Historical changes in the Yellow River delta, China. Mar. geology 113, 321–330. doi: 10.1016/0025-3227(93)90025-Q
Xue C. T. (2009). Historical changes of coastlines on west and south coasts of Bohai Sea since 7000 a BP. Scientia Geographica Sin. 29, 217–222. doi: 10.13249/j.cnki.sgs.2009.02.217
Xue C., Cheng G. (1989). “Shelly ridges in west coast of Bohai Sea and Holocene Yellow River delta system,” in Quaternary Processes and Events in China Offshore and Onshore Areas. Eds. Yang Z., Lin H. (China Ocean Press, Beijing), 117–125.
Xue Z., Feng A., Yin P., Xia D. (2009). Coastal erosion induced by human activities: A northwest Bohai Sea case study. J. Coast. Res. 25, 723–733. doi: 10.2112/07-0959.1
Xue C., Qin Y., Ye S., Laws E. A., Wang Z. (2018). Evolution of Holocene ebb-tidal clinoform off the Shandong Peninsula on East China Sea shelf. Earth-Science Rev. 177, 478–496. doi: 10.1016/j.earscirev.2017.12.012
Yang Z., Liu J. P. (2007). A unique Yellow River-derived distal subaqueous delta in the Yellow Sea. Mar. Geology 240, 169–176. doi: 10.1016/j.margeo.2007.02.008
Yang S., Song B., Ye S., Laws E. A., He L., Li J., et al. (2019). Large-scale pollen distribution in marine surface sediments from the Bohai Sea, China: Insights into pollen provenance, transport, deposition, and coastal-shelf paleoenvironment. Prog. Oceanography 178, 102183. doi: 10.1016/j.pocean.2019.102183
Yao Q., Liu K. B., Aragón-Moreno A. A., Rodrigues E., Xu Y. J., Lam N. S. (2020). A 5200-year paleoecological and geochemical record of coastal environmental changes and shoreline fluctuations in southwestern Louisiana: implications for coastal sustainability. Geomorphology 365, 107284. doi: 10.1016/j.geomorph.2020.107284
Yao Q., Rodrigues E., Liu K. B., Snyder C., Culligan N. (2022). A Late-Holocene palynological record of coastal ecological change and climate variability from Apalachicola, Florida, USA. Climate Change Ecol. 3, 100056. doi: 10.1016/j.ecochg.2022.100056
Ye L., Gao L., Han M., Li Y., Xiao X., Long H. (2024). The late Quaternary palynological record of the northern Yangtze Delta: Implication for palaeoclimate change in East Asia. Catena 234, 107630. doi: 10.1016/j.catena.2023.107630
Yi S., Saito Y., Oshima H., Zhou Y., Wei H. (2003). Holocene environmental history inferred from pollen assemblages in the Huanghe (Yellow River) delta, China: climatic change and human impact. Quaternary Sci. Rev. 22, 609–628. doi: 10.1016/S0277-3791(02)00086-0
Yi S., Saito Y., Yang D. (2006). Palynological evidence for Holocene environmental change in the Changjiang (Yangtze River) delta, China. Palaeogeography Palaeoclimatology Palaeoecol. 241, 103–117. doi: 10.1016/j.palaeo.2006.06.016
Yu X. (2019). Sedimentary characteristics and evolutions of the Luan River Delta during the Holocene. [Phd thesis]. (Beijing: Institute of Oceanlogy, Chinese Academy of Sciences).
Keywords: pollen transport, pollen preservation, prodelta, source-to-sink, Huanghe
Citation: Hu W, Liu S, Liu Y, Feng A, Feng W, Wang X and Chen S (2024) Pollen and spore records constrained by millennial prodelta evolution: a case study of the Huanghe (Yellow River) delta. Front. Mar. Sci. 11:1378724. doi: 10.3389/fmars.2024.1378724
Received: 30 January 2024; Accepted: 11 March 2024;
Published: 25 March 2024.
Edited by:
Yang Yang, Nanjing Normal University, ChinaReviewed by:
Bing Song, Chinese Academy of Sciences (CAS), ChinaCopyright © 2024 Hu, Liu, Liu, Feng, Feng, Wang and Chen. This is an open-access article distributed under the terms of the Creative Commons Attribution License (CC BY). The use, distribution or reproduction in other forums is permitted, provided the original author(s) and the copyright owner(s) are credited and that the original publication in this journal is cited, in accordance with accepted academic practice. No use, distribution or reproduction is permitted which does not comply with these terms.
*Correspondence: Shihao Liu, c2hsaXVAc2tsZWMuZWNudS5lZHUuY24=; Shenliang Chen, c2xjaGVuQHNrbGVjLmVjbnUuZWR1LmNu
Disclaimer: All claims expressed in this article are solely those of the authors and do not necessarily represent those of their affiliated organizations, or those of the publisher, the editors and the reviewers. Any product that may be evaluated in this article or claim that may be made by its manufacturer is not guaranteed or endorsed by the publisher.
Research integrity at Frontiers
Learn more about the work of our research integrity team to safeguard the quality of each article we publish.