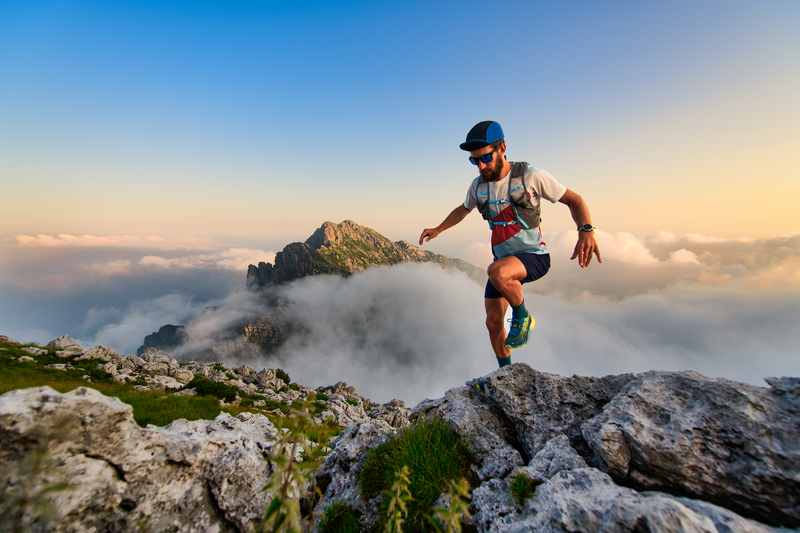
95% of researchers rate our articles as excellent or good
Learn more about the work of our research integrity team to safeguard the quality of each article we publish.
Find out more
ORIGINAL RESEARCH article
Front. Mar. Sci. , 11 June 2024
Sec. Marine Biotechnology and Bioproducts
Volume 11 - 2024 | https://doi.org/10.3389/fmars.2024.1376768
This article is part of the Research Topic Current Genetic Diversity in the Red Sea and Related Aquatic Environments. View all 5 articles
Crabs of the genus Charybdis are some of the world’s most aggressive and voracious marine invasive crustaceans. They are found in a wide variety of ecosystems in the Red Sea. Their near ubiquity in diverse marine habitats, however, makes them a possible resource for novel marine-derived bioactive products. To provide an important insight into the potential for C. natator as a beneficial product, its shell methanolic extract was chromatographically analyzed for identification of potential bioactive ingredients. Additionally, two different doses, i.e. a low and a high dose, of this extract were tested for their ability to protect against copper-induced oxidative stress and proinflammatory response in adult zebrafish Danio rerio. Gene expression levels of transcripts of proinflammatory mediators, i.e. nf-κβ and tnf-α; antioxidant enzymes, i.e. sod, cat, and gpx; and lipid-metabolizing enzymes, i.e., acox1 and fasn, were determined. The results showed that C. natator shell is rich in potential bioactive metabolites, including diverse unsaturated fatty acids, alkanes, flavonoids, and phenolic acids. Most antioxidant and proinflammatory transcripts in the protected groups were restored to levels that were lower than those in the CuSO4-stressed group. The low dose showed special success in inducing these effects. Also, the low-dose-protected group showed significantly elevated acox1 and decreased fasn, suggesting the capability of crab shell extract at a low dose to assist lipolysis and inhibit lipogenesis. The abundant presence of saturated fatty acids in the shell extract can be the reason for the inadequacy of the high dose to promote the antioxidant and anti-inflammatory activities. These results suggest that there is a potential to develop therapeutic industrial uses for C. natator shell, instead of considering it as a discard. Its wealth of bioactive metabolites may contribute to the market for natural products to combat inflammatory and oxidative stress, the origin of many diseases in the modern world.
Fierce predators, very successful invasive species, and globally expanding decapods, crabs belonging to the genus Charybdis are well-known as ecological disruptors by ecology and conservation biology researchers around the world. However, also because of their global distribution, some promising bioactivities of crabs are becoming a focus of another category of researchers, those interested in the diversity and exploration of marine bioactive metabolites and their potential as pharmaceutical products.
As a group, crabs are ranked the third group of invertebrates, after shrimps and lobsters, that offer major health benefits due to the richness of their bodies with vitamins, proteins, and unsaturated essential fatty acids, as well as peptides that may help treat different diseases (Narayanasamy et al., 2020). In addition, crab shell contains a variety of bioactive compounds that play roles as anti-inflammation and antioxidant agents (Devi et al., 2015; Zhou et al., 2021; Galal-Khallaf et al., 2022a). Charybdis crabs appear to have diverse antimicrobial, antioxidant, and anti-lipopolysaccharide activities (Rameshkumar et al., 2009; Soundarapandian et al., 2014; Sruthy & Philip, 2021). There is little information available about potential bioactive compounds in Charybdis natator (Herbst, 1789), which is widely distributed throughout the Indo-West Pacific from the Red Sea to China and Australia. Leg musculature extract of C. natator was found to have anti-inflammatory properties (Narayanasamy et al., 2020).
Oxidative stress occurs when reactive oxygen species (ROS) production and accumulation in cells and tissues exceeds the ability of biological systems to remove or protect the body against them (Pizzino et al., 2017). Reactive oxygen species represent a normal by-product of biological processes, such as phosphorylation, differentiation, activation of transcription factors, and apoptosis (Pizzino et al., 2017). They are highly reactive ions, which contain at least one oxygen atom (superoxide (O2.-), singlet oxygen) and oxygen-containing radical (hydroxyl radical (·OH)) (Auten and Davis, 2009). Normally, they are formed in low to moderate quantities during cell processes, for example during inflammation or aerobic respiration, and they are important for signal transduction regulation, receptor activation, and gene expression (Hajam et al., 2022; Zheng et al., 2024). When modulated appropriately, ROS contribute to cancer treatment, in chemotherapeutic agents that induce apoptosis (Jakubczyk et al., 2020). Moreover, ROS production can be stimulated by environmental stresses such as ionizing radiation or toxic compounds (Helston and Amaya, 2021). Overproduction of ROS leads to harmful impacts on cellular structures, including oxidative damage to cellular macromolecules such as DNA, proteins, and lipids (Zheng et al., 2024). Oxidative damage contributes to cancer, atherosclerosis, diabetes, neurodegenerative diseases, cardiovascular disease, and chronic inflammation (Vladkova et al., 2022).
Aerobic organisms possess antioxidant systems that protect cells against oxidative stress, including non-enzymatic antioxidants (e.g., vitamin E, serum albumin, glutathione, ascorbate), and enzymatic ones (e.g. catalase, glutathione peroxidase, superoxide dismutases, etc.) (Cai et al., 2022; Suo et al., 2022). All these molecules are collectively known as endogenous antioxidants (Pizzino et al., 2017). Some of the major endogenous antioxidant systems are superoxide dismutase (SOD), which mainly catalyzes O2- transformation into H2O2; as well as catalase (CAT) and glutathione peroxidase (GPX) that detoxify hydrogen peroxide to water (Collin, 2019). The biological systems depend on these mechanisms to maintain a convenient “oxidative balance”. If there is excessive generation of ROS and/or an imbalance of antioxidant mechanisms, oxidative stress begins. Many marine organisms produce or store non-enzymatic antioxidants, which can be used as dietary or nutritional supplements intended to reduce oxidative damage (Galal-Khallaf et al., 2022a).
Inflammation is a protective response of the innate immune system to protect the organism from necrotic cell signals or pathogenic stimuli (Fernando et al., 2016). Chronic inflammatory diseases can be triggered, as well, by high levels of ROS, and mediated by the activation of mitogen-activated protein kinase (MAPK) signaling pathways, activating the transcription factor nuclear factor kappa beta (NF-κβ). This, in turn, elevates the expression of pro-inflammatory mediators, e.g. interleukin (IL)6, tumor necrosis factor-alpha (TNF-α), and the immune cells recruiting chemokines like IL-8 (Sahu et al., 2014; Ma et al., 2015; Malik et al., 2015). Despite the initial benefits of inflammatory responses, prolonged inflammation leads to many diseases, for example, atherosclerosis, rheumatoid arthritis, and other hazardous manifestations (Luo et al., 2024).
The use of antioxidant and anti-inflammatory compounds is a growing trend in biological sciences due to their elucidated, very important roles in treating these perturbances (Vieira et al., 2020). Currently, the available therapy for inflammation includes the use of non-steroidal anti-inflammatory drugs (e.g., acetylsalicylic acid, ibuprofen), corticosteroids (e.g., dexamethasone), as well as conventional disease-modifying anti-rheumatic drugs and biological agents (e.g., anti-TNF-α). However, these therapeutic agents can have serious side effects (Shivaji et al., 2019). So, the discovery of new, safe, and effective antioxidants and anti-inflammatory drugs is an ongoing need for treating a variety of inflammatory diseases.
To the best of the authors’ knowledge, the bioactivity of C. natator shell extract is very scarcely studied. The current study aimed first to provide a glance at the major bioactive ingredients present in the shell of C. natator. Second, it aimed to test whether the shell extract of this crab has any anti-inflammatory or antioxidant activities. Zebrafish Danio rerio was chosen as an animal model in the current work owing to its extensive application in drug discovery. Its low maintenance costs, ease of care, and transcriptomic similarity to higher vertebrates make it a widely used model of choice for such investigations (He et al., 2023; Paramakrishnan et al., 2023).
Phosphate buffer (10% w/v) was prepared as 17.85 g of Na2HPO4.7H2O+4.61 g NaH2PO4.H2O, and completed to 1 L with sterile double distilled water (sddw) after adjusting the pH to 7.2. Tris-acetate EDTA (TAE 1X) buffer was prepared as 4.84 gm Tris-base+ 2 mL of 0.5 M EDTA.2Ka+ 1.142 mL Glacial acetic acid and completed to 1 L with Diethyl pyrocarbonate (DEPC) treated waters.
Two kilograms of male ridged swimming crab C. natator were collected from commercial fish catches from the Red Sea to Abo Zenima city market, South Sinai Governorate, Egypt (Figure 1). The species was morphologically identified according to Abbas et al. (2016). The specimens were transferred in ice boxes to the Molecular Biology and Biotechnology laboratory of the Zoology Department in the Faculty of Science, Menoufia University, Shebin El-Kom, Egypt. Samples were stored at – 20 °C until further processing (Figure 2).
Figure 1 Map of Charybdis natator collection site in Abo Zenima area, South Sinai Governorate, Egypt (coordinates: 29.2129034792315, 33.10344650708862). Photo credits GoogleMaps™ (details below the map).
Figure 2 Dorsal (A) and ventral (B) views for Charybdis natator collected from the Red Sea in South Sinai Governorate, Egypt. Ruler grading: cm.
Charybdis natator shells were collected and thoroughly cleansed of soft tissue residues. Then, they were chopped into small pieces of about 3 mm in length and extracted by soaking at room temperature in 750 ml aqueous methanol (80%) for 48 h. while covered by aluminum foil. The solvent was distilled off by a rotary evaporator at 50°C to obtain the dry residues, which were reconstituted in 10 mL of absolute ethanol and then subjected to gas chromatography-mass spectrometry (GC-MS) and high-performance liquid chromatography (HPLC) to identify the crab shell’s bioactive metabolites.
Different bioactive metabolites in shell methanolic extract were resolved using GC–MS, following the procedures mentioned by Hewavitharana et al. (2020) and Galal-Khallaf et al. (2022a). GC-MS results were obtained using Trace GC-ISQ mass spectrometer (ThermoFisher Scientific, USA) supplied with an A3000 autosampler and TG-5MS Capillary column of (length: 30 m, i.d.: 0.25 mm, film thickness: 0.25 μm). Temperature was programmed from 50˚C - 280˚C at a rate of 10˚C min-1. The mass spectrometer was set in EI mode at 70 eV, with a source temperature of 200˚C; interface temperature of 220˚C, and injector temperature of 220˚C. A diluted sample of 1 μL from the crab extract was injected in splitless mode and mass scan, 50 - 600 amu. Helium was used as a carrier gas with a 1 mL min-1 flow rate. Bioactive ingredients identification was carried out tentatively by comparing their relative retention times and mass spectra against the WILEY Registry of Mass Spectral Data (9th Edition, Version 1.02) and NIST 05 database (NIST/EPA/NIH mass spectral library version 2.0d).
Phenolic acids and flavonoids in C. natator assayed in the current study were identified following the procedures described in Hassan et al. (2022), Mosa et al. (2021), and Galal-Khallaf et al. (2022a), using an Agilent 1100 HPLC System (Agilent, CA, USA) equipped with a quaternary pump, UV/Vis detector and C18 column (125 mm × 4.60 mm, 5 μm particle size). All standards (HPLC grade) for commonly found phenolic acids and flavonoids were purchased from Sigma-Aldrich (St. Louis, MO, USA). The resulting chromatograms were analyzed using the Agilent ChemStation chromatography data system. Phenolic acids were separated by employing a gradient mobile phase of two solvents, i.e. Solvent A (methanol) and Solvent B [acetic acid in water (1:25)]. The gradient program started with 100% B and held at this concentration for the first 3 min. This was followed by 50% eluent A for the next 5 min, then the concentration of A was increased to 80% for the next 2 min and finally reduced to 50% again for the following 5 min detection wavelength at 250 nm. Therefore, the order of phenolic acids was according to authenticating standard acids by using this mobile phase.
The identification of flavonoid compounds in the extract was performed using HPLC (Agilent 1100), composed of two LC pumps, a UV/Vis detector, and a C18 column (125 mm × 4.60 mm, 5 μm). The mobile phase was acetonitrile (A) and 0.2% (v/v) aqueous formic acid (B) with an isocratic elution (70:30) program. The detection wavelength was set at 360 nm.
Adult zebrafish (Danio rerio) were purchased from a local pet supply store in Shebin El-Kom City, Menoufia, Egypt. The mean body weight and body length of zebrafish were 1.6 ± 0.2 gm and 1.3 ± 0.3 cm, respectively. Mixed-sex fishes were acclimated to dechlorinated tap water at ambient temperature (22 ± 2 °C) for 2 weeks, with a 14h:10h light: dark cycle. They were fed twice daily with commercial tropical fish food, as 1% of total fish weight. The dead zebrafish, if any, and wastes were removed daily. During acclimation, 20% of aquarium water was renewed daily. Zebrafish were transferred to plastic aquaria containing dechlorinated tap water and fasted 24 hours before and during the experiments. All experimentation procedures were approved by the ethical committee for the use of animals in scientific trials, with code: MUFSFGE623.
Copper sulfate (CuSO4) was employed to induce inflammation, through the induction of oxidative stress. Both processes are mainly mediated by the downregulation of the antioxidant enzyme systems and upregulation of the NF-κβ inflammatory pathway (Jian et al., 2020; Zhang et al., 2023). A preliminary trial for identifying the dose of CuSO4 that induces oxidative stress in zebrafish was conducted with concentrations of 45, 30, and 15 µg L-1. These concentrations were obtained by diluting a CuSO4 stock of 45 mg L-1 with dechlorinated tap water used for filling the 3 L aquaria. Then, fish (n=5 in each aquarium) were stocked in the aquaria and kept there for 48 hours. As a negative control, a group of 5 fish was placed in a CuSO4-free, 3 L aquarium. Fish mortalities were checked daily. After 48 h, the remaining zebrafish were euthanized in ice-cold dechlorinated tap water. They were dissected and livers were immediately used for the measurement of total antioxidant activity.
Liver tissue from each fish was homogenized in 10% (w/v) ice-cold phosphate buffer (pH 7.2) and centrifuged at 10,000g at 4°C for 10 min. The total antioxidant capacities of these homogenates were directly measured according to Khalil et al. (2018) and Galal-Khallaf et al. (2022a), using a commercial kit (Biodiagnostic, Egypt, Cat. No. TA2513). The absorbance of light by the reaction end-products was spectrophotometrically measured using a 96-well plate reader (TECAN, infinite F50, Switzerland) at 500 nm.
The treatment doses to be utilized in the main experiment were determined according to Zhang et al., 2019, with slight modifications. To identify the toxic dose of C. natator shell extract, different doses were prepared from an extract stock whose concentration was 94 mg dissolved in 1 ml 1% DMSO. Fish (n=6 per treatment, weight: 1.8 ± 0.4 gm) were intraperitoneally (i.p.) injected with 20 μL of the extract using a 1 mL sterile disposable syringe. Each fish received one of the following doses: 1500, 1000, and 500 µg. Control fish received 20 μL of 1% DMSO. Fish mortalities were recorded for 24 hours. This experiment was repeated twice to confirm the shell extract dose that produced 100% mortality. A sublethal dose of 50 µg was selected as the high dose of C. natator and 1/10 of the selected high dose (i.e., 5 µg) was chosen as the low dose in the main experiment.
Following acclimation for 2 weeks to lab conditions, zebrafish were transferred into four, 3-L capacity plastic aquaria containing aerated dechlorinated water, as 10 fish/aquarium. Two groups of them received a single injection with 20 µL of 1% DMSO, being destined to be the negative (naïve) and positive control groups. The third group was injected with 20 µL of crab shell extract with a dose of 2.5 µg µL-1,i.e., 50 µg/fish, and this was considered the high-dose group. The fourth group was injected with 20 µL of crab shell extract with a dose of 0.25 of µg µL-1, i.e., 5 µg/fish, and this was considered as the low-dose group. All groups were left in their corresponding aquaria for 2 h. Subsequently, oxidative stress was induced in all of them, except the negative control group, by placing them into new 3-L aquaria containing 30 µg L-1 CuSO4. The negative control group was also transferred to a new 3-L aquarium containing only aerated dechlorinated water. Oxidative stress induction persisted by incubating fish in these new aquaria for 48 h.
After 48 h exposure, ten fish from each group were quickly euthanized by placing them in melting ice and then were dissected. In each group, liver tissues of 5 fish were removed and immediately stored in 10 (w/v) of RNAlater (Invitrogen™ AM7021) at 4 °C overnight, then transferred to - 20 °C until subsequent gene expression analysis. The other 5 fish were rapidly dissected. Their livers were immediately removed into sterile 1.5 mL tubes, flash flash-frozen in liquid Nitrogen until the end of sampling time. Next, they were subjected to the same procedures as in section 2.4.3 herein for measuring the total antioxidant capacity in each sample.
Total RNA from liver tissues (preserved in RNA later) was isolated using Gene JET RNA Purification Kit (ThermoFisher Scientific, USA, Cat. No. K0731) according to the manufacturer’s instructions. UV spectrophotometry (Biometra, Germany) at 260 nm and RNase-free 1% agarose gel electrophoresis were employed to check total RNA quantity and quality, respectively. RNA purity (1.8 - 2) was verified by the spectrophotometer at a 260/280 nm ratio in each sample. Subsequently, cDNA was synthesized from high-quality and quantity RNA (300 ng) by reverse transcriptase enzyme using TOPscript™ cDNA Synthesis kit (Cat. No. EZ005s, Enzynomics, South Korea) according to the manufacturer’s instructions.
Expression of mRNAs for glutathione peroxidase (gpx), catalase (cat), copper/zinc superoxide dismutase (cu/zn sod), acyl-CoA oxidase (acox1), fatty acid synthase (fasn), tumor necrosis factor (tnf-α), nuclear factor kappa B (nf-κb) was evaluated in the cDNAs using semi-quantitative real-time PCR (abb. herein QPCR). Additionally, the expression of beta-actin (actb) was measured in a separate reaction for each sample as the reference gene. The reaction mixes were placed in UltraFlux®flat 0.2 mL flat cap PCR strips (Cat. No. 3135–00, SSIbio, USA) using gene-specific primers (Table 1). All QPCR reactions were run in triplicate for each sample in an ABI-7300 real-time PCR cycler (ThermoFisher Scientific, USA). The QPCR was operated in a 20 μL reaction system including 10 μL 2X ABT SYBR Mix (Applied biotechnology, Egypt), 0.5 μM primer, and 100 ng cDNA. The reaction program started with an initial denaturation step at 95 °C for 10 min, then followed by 35 cycles of 95 °C for 30 sec., and a combined annealing/extension step at 60 °C for 1 min. All reactions were run in triplicate. A melting curve was added to assure the existence of a single amplicon for each transcript as well as the primer’s specificity. Serial dilutions of liver cDNA were prepared to create standard curves for each target gene. Values of target gene expression were assessed relative to the expression of β-actin as a housekeeping gene, using the 2−ΔΔCt method (Livak and Schmittgen, 2001).
Table 1 Genes’ name, GenBank locus, primer sequence, and amplicon length of the QPCR primers used in this study.
STATGRPHICS Centurion XVI software was used to compare the expression of targeted genes and total antioxidant capacities, using the One-Way analysis of variance (ANOVA). As fundamental prerequisites for ANOVA, normal distribution and homogeneity of variance in all tested groups were checked using Shapiro-Wilk and Leven’s tests, respectively. The least significant difference (LSD) was applied as a post-hoc test. Values were considered statistically significant at P < 0.01.
A wide variety of potentially bioactive constituents were revealed in the Red Sea crab C. natator shell methanolic extract according to GC/MS and HPLC analyses (Supplementary Figure A). The most abundant category was fatty acids (FAs), occupying an area of > 44% of all potentially bioactive ingredients (Figure 3). Most of this percentage consisted of unsaturated fatty acids (61%), with saturated fatty acids comprising 39%. The unsaturated fatty acids and their derivatives identified were: i) 11-octadecenoic acid (ω-7 fatty acid), occupying an area of 13.26% among all GC-MS-resolved metabolites; ii) dielaidin (2-Hydroxy-3-[(9e)-9-Octadecenoyloxy]propyl (9e)-9-octadecenoate), a diacylglycerol that contains elaidic acid (ω-9 monounsaturated FA, (4.14%); iii) arachidonic acid (5,8,11,14-eicosatetraenoic acid, ω-6 polyunsaturated FA) (3.65%); iv) methyl 9-cis,11-trans-octadecadienoate (conjugated linoleic acid, ω-6 polyunsaturated FA) (2.59%); v) 9-hexadecenoic acid (palmitoleic acid, ω-7 monounsaturated FA) (1.76%); and vi) 5,8,11,14,17-Eicosapentaenoic acid (ω-3 polyunsaturated FA (1.64%). The saturated fatty acids included: palmitic acid (10.03%), stearic acid (5.89%), and docosanoic acid (saturated FA, 1.08%).
Figure 3 Different bioactive ingredients identified in the methanolic extract of the ridged swimming crab C. natator. Small pie chart: Fatty acids. Large pie chart: alkanes, sesqueterpenooids, and bufadienolids.
Several alkanes were also identified. These included dotriacontane (paraffin, lipid alkane, 8.04%), dodecane (7.61%), octadecane (straight-chain alkane, 5.45%), heptacosane (straight-chain alkane, 2.19%), and tetratriacontane (long-chain alkane, 1.86%). Two steroid derivatives were found, i.e., cholest-5-en-3-ol (6.11%) and β-Sitosterol (2.34%). Moreover, a single representative of sesquiterpenoids and bufadienolides were identified in the extract, i.e., isochiapin b (sesquiterpene lactone, 2.99%) and bufa-20,22-dienolide, 14, 15-epoxy-3,5,16-trihydrox y-, (7.9%), respectively. Structures and areas of the detected bioactive metabolites are shown in Supplementary Figure A; Figure 3.
Numerous phenolic compounds were detected in the crab shell extract. The most abundant was pyrogallol (9.58 μg mL−1) followed by p-coumaric (6.89 μg mL−1), caffeic (4.12 μg mL−1), ferulic (2.14 μg mL−1) and finally the lowest concentration was para-hydroxy benzoic acid, as shown in Figure 4A. Furthermore, diverse flavonoids compounds were found in the crab shell extract; the most prevalent one was catechin (15.79 μg mL−1), followed by 7-OH flavone (11.29 μg mL−1), quercetin (6.43 μg mL−1), rutin (5.22 μg mL−1), naringinin (4.13 μg mL−1), and kampferol (3.89 μg mL−1) as shown in Figure 4B.
Figure 4 Chromatograms for Red Sea crab Charybdis natator shell extract phenolic acids (A) and Flavonoids (B). Compounds of both classes were ordered ascendingly according to the retention times (RT). Phenolic acids identified were 1: pyrogallol, 2: p-coumaric, 3: Caffeic, 4: Ferulic, 5: P-OH benzoic. Flavonoids were 1: Catechin, 2: 7-OH flavone, 3: Querestin, 4: Rutin, 5: Naringinin, 6: Kampferol.
CuSO4 concentration of 30 μg L-1 in waters was identified in the preliminary experiment as the maximum amount that induced significant elevation of H2O2 scavenging in adult zebrafish without any mortality. Exposure to 30 μg L-1 of CuSO4 resulted in a significant increase in nf-kβ expression in the positive control group that did not receive a prior C. natator extract injection. However, fish exposed to the same CuSO4 concentration after receiving prior injections with either extract high dose (50 μg), or low dose (5 μg) showed no increase in nf-kβ expression relative to the negative control group (Figure 5A). Meanwhile, tnf-α didn’t change in all experimental groups except the low-dose one. Unexpectedly, fish receiving the low dose of crab shell extract showed a significant, 5-fold higher tnf-α level than in all other groups (Figure 5B).
Figure 5 mRNA levels of the proinflammatory mediators and antioxidant enzymes’ transcripts in response to protective doses of crab shell extract against oxidative stress induced by CuSO4 in zebrafish (n=4/group) (A): Nuclear Factor Kappa Beta (nf-κb) and (B): Tumor Necrosis Factor α (tnf-α). Mean ± Standard error of the means (SEM) was used to represent data. The different letters (a, b) denoted statistically significant variations among experimental groups (one-way ANOVA, LSD as test a posteriori, P<0.01). Groups: negative control: zebrafish group was injected with DMSO only, positive control: the group immersed in 30 μg L-1 CuSO4, High dose: zebrafish injected with 50 μg of crab extract then immersed in 30 μg L-1 of CuSO4 for 48 hours, and Low dose: zebrafish injected with 5 μg of crab extract then immersed in 30 μg L-1 of CuSO4 for 48 hours.
For the expression of antioxidant enzyme genes, i.e. sod, cat, and gpx, two features were shared. First, the negative control group (no CuSO4) showed the lowest expression levels among all experimental groups. Second, positive control and high dose (50 µg) groups showed significantly higher expression levels than both negative control and low dose (5 µg) groups (P <0.01). Interestingly, sod expression in the high-dose groups was the highest among all experimental groups (Figure 6A). For cat, mRNA levels in both high and low crab extract treatments were significantly lower than those in the positive control group but higher than the negative control one (Figure 6B). For the gpx, the low dose-injected group showed levels that are the same as the negative control group (Figure 6C).
Figure 6 mRNA levels of the antioxidant enzymes’ transcripts in response to protective doses of crab shell extract against oxidative stress induced by CuSO4. In zebrafish (n=4/group) (A): Cu-Zn Superoxide Dismutase (sod), (B): Catalase (cat), (C): Glutathione Peroxidase (gpx). Mean ± Standard error of the means (SEM) was used to represent data. The different letters (a-d) denoted statistically significant variations among experimental groups (one-way ANOVA, LSD as test a posteriori, P<0.01). Groups: negative control: zebrafish group was injected with DMSO only, positive control: the group immersed in 30 μg L-1 CuSO4, High dose: zebrafish injected with 50 μg of crab extract then immersed in 30 μg L-1 of CuSO4 for 48 hours, and Low dose: zebrafish injected with 5 μg of crab extract then immersed in 30 μg L-1 of CuSO4 for 48 hours.
Finally, lipid metabolizing genes showed completely inverse patterns. The transcripts of the lipolytic acox1 were significantly lower in the positive control and high dose-injected groups than both the negative control and low dose-injected zebrafish individuals (Figure 7A). Inversely, mRNA levels of the lipogenic enzyme, i.e., fasn, were 7–8 fold higher in the positive control and high dose-injected groups than both the negative control and low dose-injected individuals (Figure 7B).
Figure 7 mRNA levels of the lipid-metabolizing genes transcripts in response to protective doses of crab shell extract against oxidative stress induced by CuSO4. In zebrafish (n=4/group) (A): Acyl-CoA Oxidase (acox1), (B): Fatty Acid Synthase (fasn). Mean ± Standard error of the means (SEM) was used to represent data. The different letters (a-c) denoted statistically significant variations among experimental groups (one-way ANOVA, LSD as test a posteriori, P<0.01). Groups: negative control: zebrafish group was injected with DMSO only, positive control: the group immersed in 30 μg L-1 CuSO4, High dose: zebrafish injected with 50 μg of crab extract then immersed in 30 μg L-1 of CuSO4 for 48 hours, and Low dose: zebrafish injected with 5 μg of crab extract then immersed in 30 μg L-1 of CuSO4 for 48 hours.
The current study is an early report for the characterization of potentially bioactive components in the shell of the ridged swimming crab C. natator. Though it has the potential to be a major fishery and a rich source of seafood proteins (Sallam & Gab-Alla, 2009), the species appears to be the least studied within the genus Charybdis. Many studies have assessed the invasive capabilities and biological activities of other Charybdis species, mainly C. japonica and C. hellerii (Hilliam and Tuck, 2023; Izar et al., 2023). The current work positions C. natator to be a focus of future investigations for bioactive activities, and therapeutics from the Red Sea.
Excess copper elicits ROS stress in many organisms, and causes oxidative damage to DNA, lipids, and proteins (Valko et al., 2006; Olivari et al., 2008). There are also strong associations between copper, ROS overproduction, and inflammation (Kouadri et al., 2021). Severe ROS imbalance typically leads to activation of NF-κβ and activator protein-1 (AP-1), which increases the production of proinflammatory cytokines and chemokines (Kunsch and Medford, 1999). Antioxidant enzymes counteract ROS-induced stress and inflammation by neutralization of superoxide anion and other free radicals. Superoxide dismutase activity transforms O2- to H2O2, which is converted to H2O by the actions of glutathione (GSH) and CAT (Wang et al., 2021). The ability of crab tissue extracts to reduce oxidative stress and inflammatory markers has been reported previously. Atlantic blue crab (Callinectes sapidus) hepatopancreas and hemolymph extracts protected against H2O2-induced lipid peroxidation, and increased intracellular antioxidant activities (Zakzok et al., 2021). Mud crab (Scylla olivacea) tissue extract quenched free radicals and stimulated the activities of the antioxidant enzymes (Wan Yusof et al., 2019). The observation that C. natator shell extract modulates the expression of zebrafish proinflammatory and antioxidant enzyme transcripts agrees therefore with the known ameliorative potentials of the bioactive molecules in crab extracts.
The results of the current studies showed that C. natator shell extract has a very rich mixture of saturated and unsaturated fatty acids, phenolics, and flavonoids. In general, each of these categories has ameliorative effects against oxidative stress and inflammation, with saturated FAs being pro-inflammatory, while unsaturated FAs and polyphenols have antioxidant and anti-inflammatory properties (Zhou et al., 2020; Coniglio et al., 2023). Fatty acids comprised almost half of the C. natator methanolic shell extract ingredients identified in the current study. This is consistent with the knowledge about the prime role of these nutrients in the formation of crustacean cellular and organellar membranes and as their main energy stores (Azra et al., 2020). Poly- and mono-unsaturated fatty acids were the majority of FA in C. natator shell extract, in agreement with the FA profile of other portunid crab shells, e.g. C. cruciate and P. pelagicus (Sachindra et al., 2005; Fahmi et al., 2023). Moreover, C. natator shell extract contained a variety of flavonoids and polyphenols that were also found in other portunids, such as P. segnis and C. lucifera (Soundarapandian et al., 2014; Hamdi et al., 2020). Unsaturated FAs, flavonoids, and polyphenols exert their antioxidant actions either alone or in synergy with other compounds in biologically active extracts. For instance, potent antioxidant activities were found for extracts that are rich in 11-octadecenoic acid (Marrez et al., 2021), dotriacontane and isochiapin B (Qanash et al., 2022). However, the presence of palmitic acid (PA) as the most abundant unsaturated FA in C. natator shell extract can underlie the perturbances identified in the expression of different lipid-metabolizing and antioxidant enzyme genes noted in the current study. This was especially clear in the high-dose-protected group which showed more elevated levels of sod, cat, gpx and fasn than the low-dose-protected group, relative to the negative control one. This may be due to the role of PA excess in inducing ROS overproduction, apoptosis, oxidative stress, and inflammation (Harada et al., 2002).
Phenolic compound detected in C. natator shell extract included ferulic acid (FrA). Numerous studies show that foods rich in FrA may help to reduce hypertension. It exhibited a dual role by activating antioxidant enzymes that scavenge free radicals, and also by scavenging them directly (Alam, 2019). Other studies reported that FrA in association with other polyphenols, like p-coumaric acid, increased SOD, GPX, and CAT mRNA expression and activities (Yeh et al., 2009; Liu et al., 2020). P-coumaric acid was also found in C. natator extract in the current study. For the flavonoids, the most abundant was one was catechin, whose antioxidant properties are well-known from studies of tea extracts for example (Higdon and Frei, 2003). Recently, Zhao et al. (2022) reported on the ability of catechin to abrogate oxidative damage in pesticide-exposed zebrafish. Therefore, it can be expected that the diverse antioxidant biomolecules in C. natator shell extracts have the potential as healthy food-based supplements against oxidative stress and related health problems.
This study documented the complex effects of C. natator shell extracts on the expression of genes in oxidative and inflammatory pathways in zebrafish. In agreement with vertebrate studies, CuSO4 induced ROS overproduction and strongly triggered nf-κβ and other inflammatory mediators upregulation (Nguyen et al., 2020; Zhang et al., 2023). Pretreatment with both high and low doses of the extract dampened this expression of nf-κβ, which is an indicator of the presence of anti-inflammatory activity. This process was also reported before for crab shell extracts. For example, the shell extract of the Nile crab Potamonautes niloticus was found to counteract the inflammatory impacts of CuSO4 in zebrafish by down-regulating nf-κβ (Galal-Khallaf et al., 2022a). Among the potentially anti-inflammatory constituents of C. natator shell are compounds that were reported to have these activities in other systems, such as dotriacontane and isochiapin B from marine algal extracts (Orabi et al., 2020; Teleb et al., 2022). Additionally, most of the identified flavonoids and polyphenols were reported to interfere with NF-κβ classical pro-inflammatory and inflammatory mediators and cytokines (Alnawajha et al., 2023; Truong et al., 2024). Hence, the role of C. natator shell extract in precluding nf-κβ in response to CuSO4 can be understood in light of its bioactive components.
Interestingly, in the current study, tnf-α was unexpectedly upregulated in the group that received crab shell extract low dose only, not in any other experimental group. We would explain this with the capability of TNF-α to perform pleiotropic biological effects in various tissues. This usually aim to protect body cells and subcellular structures from the deleterious effects of xenobiotics (Metryka et al., 2018). CuSO4 is well known to induce hepatotoxicity through several mechanisms, including induction of fatty degeneration, accumulation of lipids, mitochondrial damage, reduction of mitochondrial biogenesis, and several other injuries (Wang et al., 2021, 2024). Meanwhile, TNF-α is well known to stimulate hepatocyte regeneration by binding its type 1 receptor, the pathway whose failure leads to NF-κβ activation, DNA synthesis failure, and increased cell mortality (Yamada et al., 1997; James et al., 2005). Some studies point to the role of TNF-α receptor 2 binding in stimulating tissue regeneration rather than binding of receptor 1 (Yang et al., 2018). A surge in TNF-α production in response to microinjuries was noted to be related to cellular regeneration and wound healing (Jin et al., 2023). Hence, a possible explanation for this increased tnf-α levels in the group that was protected with low crab shell extract dose in the current study is a TNF-α mediated stimulation of hepatic recovery from the deleterious effects of CuSO4. The absence of nf-κβ stimulation in response to both high and low doses of crab extract may suggest that this pattern of regulation is independent of NF-κβ pro-inflammatory pathway activation. Future works are being planned by our research group to test these hypotheses about the interaction between xenobiotics and the TNF-α system, and how marine natural products can modulate the multiple functionalities of this system in response to those chemicals.
Regarding the assessed lipid-regulatory transcripts, ACOX1 is known to play a key role in maintaining healthy lipid and free radical homeostasis (Kim et al., 2017). This is for being the primary enzyme involved in peroxisomal fatty acids β oxidation. Aging and several pathologies are related to the downregulation of lipolytic genes, like acox1, and upregulation of lipogenic genes, such as fasn (Kim et al., 2017). The current study observed coordinated downregulation of acox1 and upregulation of fasn in response to CuSO4 exposure. The low dose of C. natator extract reversed these responses, yet curiously, the high dose did not. A key explanation for that pattern is the richness of C. natator shell extract with diverse types of straight-chain fatty acids, the most abundant of which was 11-octadecenoic acid. Esters of these fatty acids are known as the key substrates for ACOX1 (Szrok-Jurga et al., 2023). Therefore, the significant upregulation of acox1 in response to low-extract-dose aligns well with the abundant presence of its substrates. It is also possible to assume that the elevated levels of H2O2 produced because of FA β oxidation were the trigger for the significant upregulation of catalase mRNA in comparison to the negative control group. The presence of natural antioxidant metabolites in the shell extract, such as flavonoids and polyphenolics, can further contribute to ROS scavenging, hence reducing CuSO4 oxidative impacts. In contrast, the downregulation of acox1 in response to the high dose of crab shell extract can be attributed to another different pattern of regulation. In general, a high-fat diet can reduce the expression of proteins related to fatty acids β oxidation, including the peroxisome proliferator-activated receptor α (PPAR α) and its subsequent targets, mainly ACOX1 (Nie et al., 2024). Considering the richness of crab shell extract with fatty acids, especially PA, an inhibition of FA β oxidation in the high-dose-treated group can be also expected. Likewise, the abundance of PUFA in C. natator extract can be the reason for fasn downregulation, which indicated a balanced function of de novo lipid synthesis inhibition in response to this PUFA abundance (Jensen-Urstad and Semenkovich, 2012). Conversely, fasn upregulation in response to the high dose of crab extract can refer to a deleterious effect of this dose in the liver, being increased de novo lipogenesis a key feature for hepatic steatosis (Dorn et al., 2010).
In conclusion, C. natator demonstrated highly intriguing potential as a source of various biologically active metabolites and as a formidable defense against oxidative stress and inflammatory processes. Therefore, many more studies can be conducted in the future to clarify the crab’s additional capabilities based on these factors. Its unique composition, which includes pheromone-like compounds, neuroprotectors, antidiabetic, antibacterial, and antifungal properties, as well as other bioactive chemicals, can certainly find numerous uses in the pharmaceutical industry.
The original contributions presented in the study are included in the supplementary Figure A, for bioactive chemical substances identified, and supplementary Table A, for gene expression and antioxidant capacity data. Further inquiries can be directed to the corresponding author/s.
The animal study was approved by Committee for Ethics of Use of Experimental Animals in Laboratory Experimentation at the Faculty of Science, Menoufia University, Egypt with the code: MUFSFGE623. The study was conducted in accordance with the local legislation and institutional requirements.
AG-K: Visualization, Data curation, Validation, Investigation, Supervision, Writing – original draft, Writing – review & editing. ESA: Investigation, Visualization, Data curation, Validation, Writing – review & editing, Writing – original draft. SE-S: Writing – review & editing, Supervision. AE-T: Writing – original draft, Writing – review & editing. EJS: Formal analysis, Visualization, Validation, Investigation, Writing – review & editing, Writing – original draft. KM-G: Writing – original draft, Writing – review & editing.
The author(s) declare that no financial support was received for the research, authorship, and/or publication of this article.
The authors would like to acknowledge the role of Ms. Reham Salah Elshaarawy for her valuable help and aid during the development of this work.
The authors declare that the research was conducted in the absence of any commercial or financial relationships that could be construed as a potential conflict of interest.
All claims expressed in this article are solely those of the authors and do not necessarily represent those of their affiliated organizations, or those of the publisher, the editors and the reviewers. Any product that may be evaluated in this article, or claim that may be made by its manufacturer, is not guaranteed or endorsed by the publisher.
The Supplementary Material for this article can be found online at: https://www.frontiersin.org/articles/10.3389/fmars.2024.1376768/full#supplementary-material
acox1, Acyl coenzyme A; cat, catalase; cDNA, complementary DNA; CuSo4, Cupric Sulphate; FA, fatty acid; fasn, fatty acid synthase; FrA, ferulic acid; GC-MS, gas chromatography-mass spectrometry; gpx, glutathione peroxidase; HPLC, high-performance liquid chromatography; nf-κβ, nuclear factor Kappa Beta; ROS, reactive oxygen species; sod, superoxide dismutase; tnf-α, tumor necrosis factor Alpha; ΔΔCt, delta delta threshold cycle; ω, omega.
Abbas E. M., Abdelsalam K. M., Mohammed-Geba K., Ahmed H. O., Kato M. (2016). Genetic and morphological identification of some crabs from the Gulf of Suez, Northern Red Sea, Egypt. Egyptian. J. Aquat. Res. 42, 319–329. doi: 10.1016/j.ejar.2016.08.003
Alam M. A. (2019). Anti-hypertensive effect of cereal antioxidant ferulic acid and its mechanism of action. Front. Nutr. 6, 121. doi: 10.3389/fnut.2019.00121
Alnawajha A., Endharti A. T., Santoso S., Santosaningsih D., Satriotomo I. (2023). A comparison study of the influence of caffeic acid phenethyl ester and mitoxantrone in experimental autoimmune encephalomyelitis balb/C mice model: comparison effect cape and mitoxantrone. J. Trop. Life Sci. 13, 445–452. doi: 10.11594/jtls.13.03.03
Auten R. L., Davis J. M. (2009). Oxygen toxicity and reactive oxygen species: the devil is in the details. Pediatr. Res. 66, 121–127. doi: 10.1203/PDR.0b013e3181a9eafb
Azra M. N., Tavares C. P. D. S., Abol-Munafi A. B., Ikhwanuddin M. (2020). Growth rate and fatty acid composition of orange mud crab instars, Scylla olivacea, reared at different temperatures. Egyptian. J. Aquat. Res. 46, 97–102. doi: 10.1016/j.ejar.2019.11.006
Cai W. W., Hu X. M., Wang Y. M., Chi C. F., Wang B. (2022). Bioactive peptides from Skipjack tuna cardiac arterial bulbs: Preparation, identification, antioxidant activity, and stability against thermal, pH, and simulated gastrointestinal digestion treatments. Mar. Drugs 20, 626. doi: 10.3390/md20100626
Collin F. (2019). Chemical basis of reactive oxygen species reactivity and involvement in neurodegenerative diseases. Int. J. Mol. Sci. 20, 2407. doi: 10.3390/ijms20102407
Coniglio S., Shumskaya M., Vassiliou E. (2023). Unsaturated fatty acids and their immunomodulatory properties. Biology 12, 279. doi: 10.3390/biology12020279
Devi A. R., Smija M. K., Latha N. P. (2015). Fatty acid composition of the freshwater crab travancoriana schirnerae. Fishery. Technol. 52, 246–251.
Dorn C., Riener M. O., Kirovski G., Saugspier M., Steib K., Weiss T. S., et al. (2010). Expression of fatty acid synthase in nonalcoholic fatty liver disease. Int. J. Clin. Exp. Pathol. 3, 505.
Fahmi A. S., Agustini T. W., Jayanto B. B., Prihantoko K. E. (2023). “Sensory value, proximate analysis, amino acid and fatty acid profile of steamed and boiled blue swimming crab (Portunus pelagicus),” in IOP Conference Series: Earth and Environmental Science (EES), Vol. 1224. 012034 (Bristol, UK: IOP Publishing).
Fernando I. S., Nah J. W., Jeon Y. J. (2016). Potential anti-inflammatory natural products from marine algae. Environ. Toxicol. Pharmacol. 48, 22–30. doi: 10.1016/j.etap.2016.09.023
Galal-Khallaf A., Al-Awthan Y. S., Al-Duais M. A., Mohammed-Geba K. (2022a). Nile crab Potamonautes niloticus shell extract: Chromatographic and molecular elucidation of potent antioxidant and anti-inflammatory capabilities. Bioorg. Chem. 127, 106023. doi: 10.1016/j.bioorg.2022.106023
Galal-Khallaf A., Shetaia A., Khalifa S. A., Zou X., Sakr H. H., Chen L., et al. (2022b). Pilot molecular assessment for components and capability of Northern Nile crab Potamonautes niloticus soft tissue extract for reduction of early obesity markers. Res. Square. doi: 10.21203/rs.3.rs-2143204/v1
Hajam Y. A., Rani R., Ganie S. Y., Sheikh T. A., Javaid D., Qadri S. S., et al. (2022). Oxidative stress in human pathology and aging: molecular mechanisms and perspectives. Cells 11, 552. doi: 10.3390/cells11030552
Hamdi M., Nasri R., Dridi N., Li S., Nasri M. (2020). Development of novel high-selective extraction approach of carotenoproteins from blue crab (Portunus segnis) shells, contribution to the qualitative analysis of bioactive compounds by HR-ESI-MS. Food Chem. 302, 125334. doi: 10.1016/j.foodchem.2019.125334
Harada H., Yamashita U., Kurihara H., Fukushi E., Kawabata J., Kamei Y. (2002). Antitumor activity of palmitic acid found as a selective cytotoxic substance in a marine red alga. Anticancer Res. 22, 2587–2590.
Hassan H. S., EL-Hefny M., Ghoneim I. M., El-Lahot M. S. A., Akrami M., Al-Huqail A. A., et al. (2022). Assessing the use of aloe vera gel alone and in combination with lemongrass essential oil as a coating material for strawberry fruits: HPLC and EDX analyses. Coatings 12 (4), 489.
Helston O., Amaya E. (2021). Reactive oxygen species during heart regeneration in zebrafish: Lessons for future clinical therapies. Wound Repair Regeneration. 29, 211–224. doi: 10.1111/wrr.12892
He B. L., Hu T. G., Wu H. (2023). Phenotypic screening of novel probiotics with potential anti-neuroinflammation activity based on cell and zebrafish models. Food Bioscience 55, 102949.
Hewavitharana G. G., Perera D. N., Navaratne S. B., Wickramasinghe I. (2020). Extraction methods of fat from food samples and preparation of fatty acid methyl esters for gas chromatography: A review. Arabian. J. Chem. 13, 6865–6875. doi: 10.1016/j.arabjc.2020.06.039
Higdon J. V., Frei B. (2003). Tea catechins and polyphenols: health effects, metabolism, and antioxidant functions. Crit. Rev. Food Sci. Nutr. 43, 89-143. doi: 10.1080/10408690390826464
Hilliam K., Tuck I. D. (2023). Range expansion of the invasive Portunid crab Charybdis japonica in New Zealand. New Z. J. Mar. Freshw. Res. 57, 518–534. doi: 10.1080/00288330.2022.2071301
Izar G. M., de Oliveira D. N., Laurino I. R. A., Zara F. J., Christofoletti R. A. (2023). Is the population increase of an invasive portunid crab (Charbydis hellerii) a concern? The effect on native prey. Estuarine. Coast. Shelf. Sci. 282, 108249. doi: 10.1016/j.ecss.2023.108249
Jakubczyk K., Dec K., Kałduńska J., Kawczuga D., Kochman J., Janda K. (2020). Reactive oxygen species-sources, functions, oxidative damage. Polski. Merkuriusz. Lekarski.: Organ Polskiego. Towarzystwa. Lekarskiego. 48, 124–127.
James L. P., Kurten R. C., Lamps L. W., McCullough S., Hinson J. A. (2005). Tumour necrosis factor receptor 1 and hepatocyte regeneration in acetaminophen toxicity: a kinetic study of proliferating cell nuclear antigen and cytokine expression. Basic. Clin. Pharmacol. Toxicol. 97, 8–14. doi: 10.1111/j.1742-7843.2005.pto_97102.x
Jensen-Urstad A. P., Semenkovich C. F. (2012). Fatty acid synthase and liver triglyceride metabolism: housekeeper or messenger? Biochim. Biophys. Acta (BBA)-Mol. Cell Biol. Lipids 1821, 747–753. doi: 10.1016/j.bbalip.2011.09.017
Jian Z., Guo H., Liu H., Cui H., Fang J., Zuo Z., et al. (2020). Oxidative stress, apoptosis and inflammatory responses involved in copper-induced pulmonary toxicity in mice. Aging (Albany. NY). 12, 16867. doi: 10.18632/aging.v12i17
Jin G. R., Hwang S. B., Park H. J., Lee B. H., Boisvert W. A. (2023). Microinjury-Induced Tumor Necrosis Factor-α Surge Stimulates Hair Regeneration in Mice. Skin Pharmacol. Physiol. 36 (1), 27–37.
Khalil S. R., Elhady W. M., Elewa Y. H., Abd El-Hameed N. E., Ali S. A. (2018). Possible role of Arthrospira platensis in reversing oxidative stress-mediated liver damage in rats exposed to lead. Biomed. Pharmacother. 97, 1259–1268. doi: 10.1016/j.biopha.2017.11.045
Kim S. M., Lee B., An H. J., Kim D. H., Park K. C., Noh S. G., et al. (2017). Novel PPARα agonist MHY553 alleviates hepatic steatosis by increasing fatty acid oxidation and decreasing inflammation during aging. Oncotarget 8, 46273. doi: 10.18632/oncotarget.v8i28
Kouadri A., Cormenier J., Gemy K., Macari L., Charbonnier P., Richaud P., et al. (2021). Copper-associated oxidative stress contributes to cellular inflammatory responses in cystic fibrosis. Biomedicines 9, 329. doi: 10.3390/biomedicines9040329
Kunsch C., Medford R. M. (1999). Oxidative stress as a regulator of gene expression in the vasculature. Circ. Res. 85, 753–766. doi: 10.1161/01.RES.85.8.753
Liu X., Ji D., Cui X., Zhang Z., Li B., Xu Y., et al. (2020). p-Coumaric acid induces antioxidant capacity and defense responses of sweet cherry fruit to fungal pathogens. Postharvest. Biol. Technol. 169, 111297. doi: 10.1016/j.postharvbio.2020.111297
Livak K. J., Schmittgen T. D. (2001). Analysis of relative gene expression data using real-time quantitative PCR and the 2– ΔΔCT method. Methods 25, 402–408. doi: 10.1006/meth.2001.1262
Luo M., Zhao F., Cheng H., Su M., Wang Y. (2024). Macrophage polarization: an important role in inflammatory diseases. Front. Immunol. 15, 1352946. doi: 10.3389/fimmu.2024.1352946
Ma X., Dang C., Kang H., Dai Z., Lin S., Guan H., et al. (2015). Saikosaponin-D reduces cisplatin-induced nephrotoxicity by repressing ROS-mediated activation of MAPK and NF-κB signalling pathways. Int. Immunopharmacol. 28, 399–408. doi: 10.1016/j.intimp.2015.06.020
Malik S., Suchal K., Gamad N., Dinda A. K., Arya D. S., Bhatia J. (2015). Telmisartan ameliorates cisplatin-induced nephrotoxicity by inhibiting MAPK mediated inflammation and apoptosis. Eur. J. Pharmacol. 748, 54–60. doi: 10.1016/j.ejphar.2014.12.008
Marrez D. A., Sultan Y. Y., Naguib M. M., Higazy A. M. (2021). Antimicrobial activity, cytotoxicity and chemical constituents of the freshwater microalga oscillatoria princeps. Biointerface. Res. App. Chem. 12, 961. doi: 10.33263/BRIAC
Metryka E., Chibowska K., Gutowska I., Falkowska A., Kupnicka P., Barczak K., et al. (2018). Lead (Pb) exposure enhances expression of factors associated with inflammation. Int. J. Of. Mol. Sci. 19, 1813. doi: 10.3390/ijms19061813
Mosa W. F., El-Shehawi A. M., Mackled M. I., Salem M. Z., Ghareeb R. Y., Hafez E. E., et al. (2021). Productivity performance of peach trees, insecticidal and antibacterial bioactivities of leaf extracts as affected by nanofertilizers foliar application. Sci. Rep. 11 (1), 10205.
Narayanasamy A., Balde A., Raghavender P., Shashanth D., Abraham J., Joshi I., et al. (2020). Isolation of marine crab (Charybdis natator) leg muscle peptide and its anti-inflammatory effects on macrophage cells. Biocatalysis. Agric. Biotechnol. 25, 101577. doi: 10.1016/j.bcab.2020.101577
Nguyen T. H., Le H. D., Nguyen Thi Kim T., Pham The H., Nguyen T. M., Cornet V., et al. (2020). Anti-inflammatory and antioxidant properties of the ethanol extract of clerodendrum cyrtophyllum turcz in copper sulfate-induced inflammation in zebrafish. Antioxidants 9, 192. doi: 10.3390/antiox9030192
Nie T., Wang X., Li A., Shan A., Ma J. (2024). The promotion of fatty acid β-oxidation by hesperidin via activating SIRT1/PGC1α to improve NAFLD induced by a high-fat diet. Food Funct. 15, 372–386. doi: 10.1039/D3FO04348G
Olivari F. A., Hernández P. P., Allende M. L. (2008). Acute copper exposure induces oxidative stress and cell death in lateral line hair cells of zebrafish larvae. Brain Res. 1244, 1–12. doi: 10.1016/j.brainres.2008.09.050
Orabi S. H., Al-Sabbagh E. S., Khalifa H. K., Mohamed M. A. E. G., Elhamouly M., Gad-Allah S. M., et al. (2020). Commiphora myrrha resin alcoholic extract ameliorates high fat diet induced obesity via regulation of UCP1 and adiponectin proteins expression in rats. Nutrients 12, 803. doi: 10.3390/nu12030803
Paramakrishnan N., Chavan L., Lim K. G., Paramaswaran Y., Muthuraman A. (2023). Reversal of neuralgia effect of beta carotene in streptozotocin-associated diabetic neuropathic pain in female zebrafish via matrix metalloprotease-13 inhibition. Pharmaceuticals 16, 157. doi: 10.3390/ph16020157
Pizzino G., Irrera N., Cucinotta M., Pallio G., Mannino F., Arcoraci V., et al. (2017). Oxidative stress: harms and benefits for human health. Oxid. Med. Cell. Longevity 2017. doi: 10.1155/2017/8416763
Qanash H., Yahya R., Bakri M. M., Bazaid A. S., Qanash S., Shater A. F., et al. (2022). Anticancer, antioxidant, antiviral and antimicrobial activities of Kei Apple (Dovyalis caffra) fruit. Sci. Rep. 12, 5914. doi: 10.1038/s41598-022-09993-1
Rameshkumar G., Aravindhan T., Ravichandran S. (2009). Antimicrobial proteins from the crab Charybdis lucifera (Fabricius 1798). Middle-East. J. Sci. Res. 4, 40–43.
Sachindra N. M., Bhaskar N., Mahendrakar N. S. (2005). Carotenoids in crabs from marine and fresh waters of India. LWT-Food. Sci. Technol. 38, 221–225. doi: 10.1016/j.lwt.2004.06.003
Sahu B. D., Kalvala A. K., Koneru M., Mahesh Kumar J., Kuncha M., Rachamalla S. S., et al. (2014). Ameliorative effect of fisetin on cisplatin-induced nephrotoxicity in rats via modulation of NF-κB activation and antioxidant defence. PloS One 9, e105070. doi: 10.1371/journal.pone.0105070
Sallam W., Gab-Alla A. (2009). Edibility assessment of the swimming crab Charybdis natator (Brachyura: Portunidae) from the Gulf of Suez, Red Sea, Egypt. Egyptian. J. Aquat. Biol. Fisheries. 13, 43–54. doi: 10.21608/ejabf.2009.2022
Shivaji U. N., Sharratt C. L., Thomas T., Smith S. C., Iacucci M., Moran G. W., et al. (2019). Managing the adverse events caused by anti-TNF therapy in inflammatory bowel disease. Alimentary. Pharmacol. Ther. 49, 664–680. doi: 10.1111/apt.15097
Soundarapandian P., Roy S., Varadharajan D. (2014). Antioxidant activity in hard and soft shell crabs of Charybdis lucifera (Fabricius 1798). J. Aquacult. Res. Dev. 5.
Sruthy K. S., Philip R. (2021). Anti-lipopolysaccharide factor from crucifix crab Charybdis feriatus, Cf-ALF2: molecular cloning and functional characterization of the recombinant peptide. Probiotics. Antimicrobial. Proteins 13, 885–898. doi: 10.1007/s12602-020-09716-w
Suo S. K., Zheng S. L., Chi C. F., Luo H. Y., Wang B. (2022). Novel angiotensin-converting enzyme inhibitory peptides from tuna byproducts—milts: Preparation, characterization, molecular docking study, and antioxidant function on H2O2-damaged human umbilical vein endothelial cells. Front. Nutr. 9, 957778. doi: 10.3389/fnut.2022.957778
Szrok-Jurga S., Czumaj A., Turyn J., Hebanowska A., Swierczynski J., Sledzinski T., et al. (2023). The physiological and pathological role of acyl-coA oxidation. Int. J. Mol. Sci. 24, 14857. doi: 10.3390/ijms241914857
Teleb W. K., Tantawy M. A., Osman N. A., Abdel-Rahman M. A., Hussein A. A. (2022). Structural and cytotoxic characterization of the marine red algae Sarconema filiforme and Laurencia obtusa. Egyptian. J. Aquat. Biol. Fisheries. 26, 549-573. doi: 10.21608/ejabf.2022.252760
Truong T. M. T., Jang H. J., Ghosh M., Son Y. O., Kang I. (2024). p-Coumaric acid alleviates skeletal muscle atrophy by improving muscular inflammation and mitochondrial dysfunction in high-fat and high-sucrose diet-fed C57BL/6 male mice. J. Funct. Foods. 112, 105979. doi: 10.1016/j.jff.2023.105979
Valko M., Rhodes C. J. B., Moncol J., Izakovic M. M., Mazur M. (2006). Free radicals, metals and antioxidants in oxidative stress-induced cancer. Chemico-Biological. Interact. 160, 1–40. doi: 10.1016/j.cbi.2005.12.009
Vieira S. F., Ferreira H., Neves N. M. (2020). Antioxidant and anti-inflammatory activities of cytocompatible Salvia officinalis extracts: A comparison between traditional and Soxhlet extraction. Antioxidants 9, 1157. doi: 10.3390/antiox9111157
Vladkova T., Georgieva N., Staneva A., Gospodinova D. (2022). Recent progress in antioxidant active substances from marine biota. Antioxidants 11, 439. doi: 10.3390/antiox11030439
Wang Y., Tian J., Shi F., Li X., Hu Z., Chu J. (2021). Protective effect of surfactin on copper sulfate-induced inflammation, oxidative stress, and hepatic injury in zebrafish. Microbiol. Immunol. 65, 410–421. doi: 10.1111/1348-0421.12924
Wang Y., Zhu Y., Cui H., Deng H., Zuo Z., Fang J., et al. (2024). Effects of CuSO4 on hepatic mitochondrial function, biogenesis and dynamics in mice. Environ. Toxicol. 39, 2208–2217. doi: 10.1002/tox.24085
Wan Yusof W. R., Badruddin Ahmad F., Ahmad N. M., Husaini A. S. A., Swamy M. (2019). Proximate composition and antioxidant properties of orange mud crab, Scylla olivacea. J. Aquat. Food Product. Technol. 28, 365–374. doi: 10.1080/10498850.2019.1594482
Wu S., Ji G., Liu J., Zhang S., Gong Y., Shi L. (2016). TBBPA induces developmental toxicity, oxidative stress, and apoptosis in embryos and zebrafish larvae (Danio rerio). Environ. Toxicol. 31, 1241–1249. doi: 10.1002/tox.v31.10
Yamada Y., Kirillova I., Peschon J. J., Fausto N. (1997). Initiation of liver growth by tumor necrosis factor: deficient liver regeneration in mice lacking type I tumor necrosis factor receptor. Proc. Natl. Acad. Sci. 94, 1441–1446. doi: 10.1073/pnas.94.4.1441
Yang S., Wang J., Brand D. D., Zheng S. G. (2018). Role of TNF–TNF receptor 2 signal in regulatory T cells and its therapeutic implications. Front. Immunol. 9, 360533. doi: 10.3389/fimmu.2018.00784
Yeh C. T., Ching L. C., Yen G. C. (2009). Inducing gene expression of cardiac antioxidant enzymes by dietary phenolic acids in rats. J. Nutr. Biochem. 20, 163–171. doi: 10.1016/j.jnutbio.2008.01.005
Zakzok M. S., Alkaradawe R. M., Mohammad S. H., Tawfik M. M. (2021). Antiproliferative and antioxidant activities of the edible crab Callinectes sapidus hepatopancreas and hemolymph extracts. Egyptian. J. Aquat. Biol. Fisheries. 25, 531–550. doi: 10.21608/ejabf.2021.179659
Zhang D., Tang J., Zhang J., Hu C. X. (2019). Responses of pro-and anti-inflammatory cytokines in zebrafish liver exposed to sublethal doses of Aphanizomenon flosaquae DC-1 aphantoxins. Aquat. Toxicol. 215, 105269.
Zhang P., Liu N., Xue M., Zhang M., Liu W., Xu C., et al. (2023). Anti-inflammatory and antioxidant properties of β-sitosterol in copper sulfate-induced inflammation in zebrafish (Danio rerio). Antioxidants 12, 391. doi: 10.3390/antiox12020391
Zhao Y., Fang C., Jin C., Bao Z., Yang G., Jin Y. (2022). Catechin from green tea had the potential to decrease the chlorpyrifos induced oxidative stress in larval zebrafish (Danio rerio). Pesticide. Biochem. Physiol. 182, 105028. doi: 10.1016/j.pestbp.2021.105028
Zheng S. L., Wang Y. M., Chi C. F., Wang B. (2024). Chemical characterization of honeysuckle polyphenols and their alleviating function on ultraviolet B-damaged haCaT cells by modulating the Nrf2/NF-κB signaling pathways. Antioxidants 13, 294. doi: 10.3390/antiox13030294
Zhou H., Urso C. J., Jadeja V. (2020). Saturated fatty acids in obesity-associated inflammation. J. Inflammation Res. 13, 1–14. doi: 10.2147/JIR
Keywords: anti-inflammatory, antioxidant, bioactive metabolites, Charybdis natator, methanolic extract, Red Sea
Citation: Galal-Khallaf A, Samir Aboali E, El-Sayed Hassab El-Nabi S, El-Tantawy AI, Schott EJ and Mohammed-Geba K (2024) As healthy as invasive: Charybdis natator shell extract reveals beneficial metabolites with promising antioxidant and anti-inflammatory potentials. Front. Mar. Sci. 11:1376768. doi: 10.3389/fmars.2024.1376768
Received: 04 March 2024; Accepted: 20 May 2024;
Published: 11 June 2024.
Edited by:
Santhiyagu Prakash, Tamil Nadu Fisheries University, IndiaReviewed by:
Michael Habte-Tsion, University of Maine, United StatesCopyright © 2024 Galal-Khallaf, Samir Aboali, El-Sayed Hassab El-Nabi, El-Tantawy, Schott and Mohammed-Geba. This is an open-access article distributed under the terms of the Creative Commons Attribution License (CC BY). The use, distribution or reproduction in other forums is permitted, provided the original author(s) and the copyright owner(s) are credited and that the original publication in this journal is cited, in accordance with accepted academic practice. No use, distribution or reproduction is permitted which does not comply with these terms.
*Correspondence: Khaled Mohammed-Geba, a2dlYmFAdW1jZXMuZWR1; a2hhbGVkLW1vaGFtZWQuZ2ViYUBmdWxicmlnaHRtYWlsLm9yZw==
Disclaimer: All claims expressed in this article are solely those of the authors and do not necessarily represent those of their affiliated organizations, or those of the publisher, the editors and the reviewers. Any product that may be evaluated in this article or claim that may be made by its manufacturer is not guaranteed or endorsed by the publisher.
Research integrity at Frontiers
Learn more about the work of our research integrity team to safeguard the quality of each article we publish.