- 1Department of Biology, Norwegian University of Science and Technology, Trondheim, Norway
- 2Nutrimar AS, Frøya, Norway
- 3Norwegian Institute of Bioeconomy Research, Ås, Norway
- 4Norwegian College of Fishery Science, Faculty of Bioscience, Fisheries, and Economics, UiT the Arctic University of Norway, Tromsø, Norway
The feed legislation allows the use of fish protein hydrolysates in feed for the same species in which it came from, since enzymatic hydrolysis degrades the proteins and eliminates potential prions, which have caused disease in mammals, but not in fish. In this trial, we investigated the effects of partially replacing dietary fishmeal (FM) with salmon protein hydrolysate (FPH) on the intestinal gene expression and microbiota. Atlantic salmon post smolts were either fed a control diet containing 30% fishmeal (FM), a 20% FM diet with 9% salmon hydrolysate (FPH-09) or a 10% FM diet with 18% salmon hydrolysate (FPH-18), until doubling of weight. Gene expression analysis by RNA sequencing of pyloric caeca (PC), midgut (MG) and hindgut (HG) revealed a downregulation of immunological genes involved in inflammation in the intestine of FPH-18 fed salmon compared to salmon fed the FM control. The gene expression of paralogous peptide transporters (PepT) was analyzed by real time quantitative PCR in PC, anterior midgut (AMG), posterior midgut (PMG) and HG of salmon fed all the three diets. The PepT1b paralog had highest relative expression levels in PC and AMG, suggesting that PepT1b is most important for peptide uptake in the anterior intestine. PepT1a was also mainly expressed in the PC and AMG, but at lower levels than PepT1b and PepT2b in the AMG. The PepT2b paralog had high levels of expression in AMG, PMG and HG indicating that it contributed significantly to peptide uptake in the posterior part of the gastrointestinal tract. The gut microbiota in the mucosa and digesta of the MG and HG, were dominated by the phyla Cyanobacteria and Proteobacteria, but also Firmicutes were present. The only dietary effect on the microbiota was the higher prevalence of the phyla Spirochaetes in the mucosa of FPH-18 fed salmon compared to the FM fed salmon. In conclusion, replacing FM with salmon hydrolysate reduced the expression of inflammatory markers in the Atlantic salmon intestine suggesting improved health benefits. The reduced inflammation may be related to the reduced FM content, potentially bioactive peptides in the hydrolysate and/or the altered gut microbial composition.
1 Introduction
The need for novel and sustainable feed ingredients for cultivated fish and land animals is increasing with the growing human population. By-products from marine food production are sustainable alternatives for production of feed ingredients (Rustad et al., 2011; Almås et al., 2020). In Norway a significant biomass of by-products is produced from farmed Atlantic salmon amounting to > 0.5 million tons in 2021 (Myhre et al., 2022). However, the feed legislation prohibits the reuse of proteins from Atlantic salmon into feed of the same species, unless it is hydrolyzed. Complete processing by enzymatic hydrolysis will degrade potential prions and is therefore an exception from the transmissible spongiform encephalopathy (TSE)-legislation (Regulation (EC) No 999/2001; Commission Regulation (EU) No 142/2011; Sandbakken et al., 2023).
Novel feed ingredients should be nutritious, palatable, digestible, and not have any negative impact on the immune response and health of the animal (Glencross, 2020). Protein hydrolysates are mixtures of peptides and free amino acids, which are nutritious, highly digestible and known to be palatable (Kristinsson and Rasco, 2000; Kousoulaki et al., 2013; Khosravi et al., 2015). Hydrolysates may also contain bioactive peptides (Zamora-Sillero et al., 2018; Gao et al., 2021; Siddik et al., 2021). Marine peptides in fish protein hydrolysates (FPH) have shown beneficial effects including antimicrobial, anti-inflammatory and immunomodulatory activities when consumed in vivo (Kang et al., 2019). Dietary inclusion of FPH in aquaculture has induced growth performance, digestibility and altered the immune response (Zheng et al., 2014; Siddik et al., 2018).
Optimized diets can improve the general health and immune functions of fish, thereby reduce losses due to diseases, as well as improving recovery after diseases in aquaculture (Waagbø, 1994; Dawood, 2020). Therefore, nutritional status has a major impact on the ability of fish to resist pathogens and cope with stress (Martin et al., 2016). Some feed additives have immunomodulatory properties, which either activates the immune system or downregulates immunological genes. Activating the innate immune system can be beneficial when the fish experiences pathogenic threats, but a general activation can be energy consuming and have negative impact on other processes like growth (Tacchi et al., 2011). A downregulation of genes related to inflammation saves energy for the organism to grow and thrive.
Intestinal protein absorption is performed by different transporters in the brush border membrane of absorptive epithelial cells (Evans and Claiborne, 2006; Kiela and Ghishan, 2016). Peptide transporters (PepT) provide a more efficient uptake mechanism for protein building blocks than amino acid transporters since they transport di- and tripeptides instead of single amino acids (Wang et al., 2017). Due to the whole genome duplication event, Atlantic salmon has at least 4 genes encoding paralogous PepT which are part of the solute carrier 15a (Slc15a) family of transporter molecules. These 4 transporters are slc15a1a, slc15a1b, slc15a2a and slc15a2b, also known as PepT1a, PepT1b, PepT2a and PepT2b, respectively. PepT1 paralogs are low affinity/high-capacity transporters that serve a key role in peptide absorption, mainly in the proximal part of the intestine (Verri et al., 2003; Rønnestad et al., 2007; Ostaszewska et al., 2010; Wang et al., 2017). Most research available is on the PepT1b paralog, since PepT1a was identified and characterized in Atlantic salmon (Salmo salar) quite recently by Gomes et al. (2020). PepT2 paralogs are high affinity/low-capacity transporters which recently were characterized in Atlantic salmon by Vacca et al. (2022). Whereas PepT2b mainly is expressed in the kidney and midgut (MG) to hindgut (HG) (Del Vecchio et al., 2021; Vacca et al., 2022), PepT2a is more abundant in brain and gills, and appears to have a minor role in peptide uptake along the gastrointestinal tract (Del Vecchio et al., 2021). There is however little information on how the expression of peptide transporters is regulated by dietary proteins, and especially available peptides and free amino acids in Atlantic salmon.
Intestinal microbiota composition depends on the diet, environmental factors, and host selection. The microbiota affects the nutrient absorption, immune system, and disease resistance of the host (Luan et al., 2023). Mucosal microbiota is colonizing the mucus layer on the intestinal epithelial cells and is more stable and less affected by diet than the microbiota found in the digesta (feces) (Gajardo et al., 2016). This was clearly shown in Atlantic salmon fed diets with and without insect meal (Li et al., 2021), where the digesta-associated microbiota also showed the highest microbial richness and diversity. Dietary protein can affect the intestinal microbiota composition and function, and interactions between proteins and microbiota can impact the host health (Bartlett and Kleiner, 2022). Furthermore, if the protein fraction is absorbed much faster than in normal diets, it is possible that remaining bacteria in midgut and hindgut sections will rely more on utilization of feed components reaching there in larger amounts such as fiber and carbohydrates. Such changes in available nutrients for the microbiota can have profound effect on fermentation patterns altering profiles of short chain fatty acids and other products of fermentation (Bartlett and Kleiner, 2022), and on production of inflammatory/anti-inflammatory components.
The current study aimed at assessing the effects of replacing 2/3 of the fishmeal (FM) in a standard diet, with salmon protein hydrolysate (FPH) on intestinal microbiota and health parameters by RNA sequencing analysis. Furthermore, we also report on the response of intestinal peptide transporter expression when the salmon were fed diets with 1/3 and 2/3 of the FM protein replaced with salmon hydrolysate.
2 Materials and methods
2.1 Feed and fish husbandry
A detailed description of the feed composition, experimental design and fish facilities have been reported in Sandbakken et al. (2023). In brief, Atlantic salmon (Salmo salar) post smolts were fed one of three diets with spray dried salmon hydrolysate (Nutrimar AS) replacing parts of the fish meal: a control diet with 30% fish meal (FM), a 9% hydrolysate diet with 20% fish meal (FPH-09) and a 18% hydrolysate diet with 10% fish meal (FPH-18) (Supplementary Table 1). The diets were designed to be iso-energetic, iso-nitrogenous, iso-lipidic as well as having the same amount of EPA, DHA and phospholipids. Salmon (mean start weight 141.5 ± 23.6 g (standard deviation)) were PIT-tagged and randomly distributed into 9 tanks (24 fish/tank, ~ 300 L, 10-14 L min-1 water flow, > 80% O2 saturation in the outlet water, 8.2-8.8°C seawater, continuous light) and fed for 2 h twice a day until satiety. After 9 weeks of feeding, the mean salmon weight was 313.8 ± 50 g at sampling. The feeding trial was approved by the Norwegian Food Safety Authority (FOTS ID:23021) and was conducted at NTNU Sealab (Department of Biology, Trondheim, Norway).
2.2 Sampling procedures
Final samples were taken 60 and 62 days after the experimental start, giving RNA samples and microbiota samples, respectively. Before the first sampling day, the fish were not fed for 48 hours to ensure empty intestines for RNA analysis. Five fish from each tank were randomly sampled and euthanized in seawater with Finquel, MS-222 (800 mg/l). Weight, length and PIT-ID was registered before the 5 fish were put on ice and processed immediately. The sampling was performed one tank at a time.
The fish abdomen was opened, and fish were sexed by looking at the gonads. For each fish, 5 intestinal samples were collected for RNA analysis, by cutting ~2 mm of pyloric caeca, 3 segments of the midgut (anterior, mid, and posterior) and a middle segment of the hindgut (HG). Tissue samples for RNA analysis were put in 1 ml cold RNA later (RNA stabilization solution, Ambion Inc.), and stored for 24 h at 4°C before the samples were transferred to -20°C.
The remaining fish (19 per tank) were fed five 2-hour meals over the next 42 hours, before the next sampling two days later. Five random fish from the tanks fed FM control and FPH-18 were euthanized (MS-222) to collect microbiota from midgut (MG) and hindgut (HG) digesta and mucosa. The fish were sexed followed by aseptic removal of the intestine from the abdominal cavity. Sampling equipment was sterilized between each sample by burning 96% ethanol from the metal. The intestine was opened longitudinally with scissors, followed by digesta collection in cryotubes that were snap-frozen on dry ice. The remaining intestine was washed 3 times in cold sterile Phosphate Buffered Saline (PBS, P3813, Sigma-Aldrich) to remove traces of remaining digesta. Some of the intestinal mucosa was scratched off with the backside of a scalpel and snap frozen on dry ice. The same procedure was performed aseptically on both the MG and HG, resulting in four microbiota samples from each fish that were stored at -80°C until analysis.
An overview of analysis performed on the different intestinal segments from fish fed different diets is shown in Table 1.
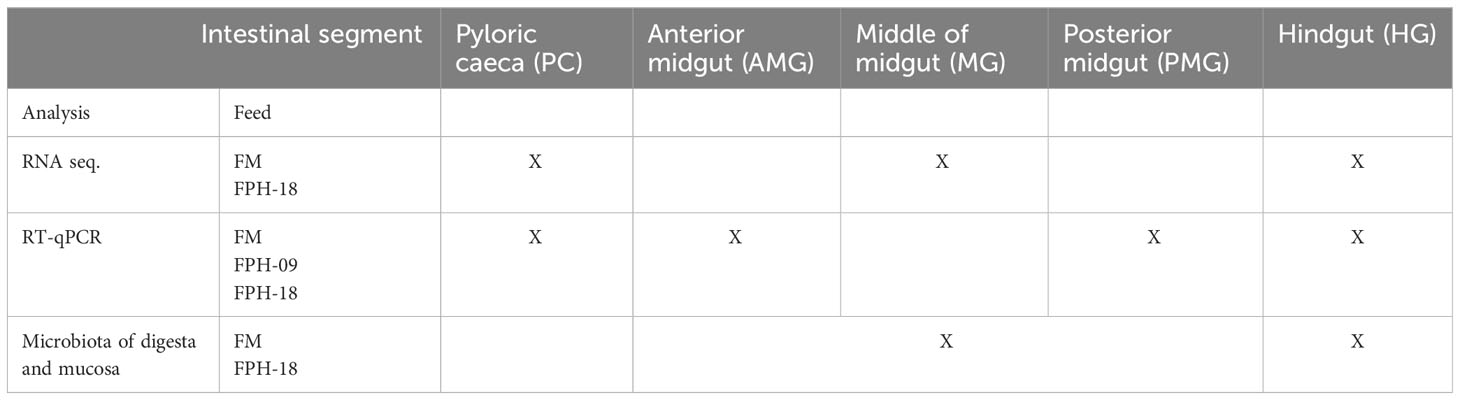
Table 1 Overview of analysis performed on the different intestinal segments in fish fed different diets: control diet with 30% fish meal (FM), 9% salmon hydrolysate diet with 20% fish meal (FPH-09) and 18% salmon hydrolysate with 10% fish meal (FPH-18).
2.3 RNA extraction and quantification
Intestinal samples were thawed on ice, dried off on a clean piece of paper and weighed (averagely 32 mg) in an Eppendorf tube (1.5 ml). Samples were added 1 ml of an RLT Lysis buffer (RNeasy Mini kit) mixed with β-mercaptoethanol (1% in RLT) before homogenization using a rotor-stator homogenizator (Polytron Kinematica PT3000) for up to 10 seconds at max speed (28 100 rpm). All samples were kept on ice between homogenization, and frozen at -80°C before further processing. RNA was isolated as described in the RNeasy Mini kit (Qiagen) including a DNase treatment step with RNase-Free DNase set (ID:79256, Qiagen). RNA concentration was measured with Nanodrop 2000c Spectrophotometer (Thermo Scientific) and RNA quality was evaluated by 2100 Bioanalyzer using RNA 6000 Nano Kit (both from Agilent Technologies) following the manufacturer’s protocol. All samples with RNA integrity number (RIN) of ≥ 7 were accepted for further use. RNA was kept at -80°C between analysis.
2.4 RNA sequencing and assembly
Extracted RNA samples (6 from each intestinal segment and diet, 36 in total) from pyloric caeca (PC), MG and HG from fish fed FM and FPH-18 diets were sent to The Beijing Genomics Institute (BGI, Beijing, China) for sequencing. RNA quality control, library construction and sequencing were done by BGI, including (i) mRNA enrichment and purification by using Oligo dT beads to enrich mRNA with a polyA tail, (ii) RNA fragment and reverse transcription by random N6-primed reverse transcription, followed by the second-strand cDNA synthesis, (iii) end repair of cDNA fragments, 3’ adenylation and adaptor ligation, (iv) PCR amplification to enrich the purified cDNA template, (v) cyclization by heating PCR product, oligos and DNA ligase, (vi) DNA nanoball synthesis, and (vii) sequencing on DNBSeq Technology platform.
Firstly, reads mapped to rRNAs and low-quality reads (where > 20% of the bases had qualities < 10 in base quality score) were removed. Secondly, reads with adaptors and/or with unknown bases (N bases more than 5%) were filtered. Clean reads were mapped onto the reference genome assembly Ssal_v3.1 (GCF_905237065.1), followed by novel gene prediction. After novel transcript detection, coding transcripts were merged with reference transcripts to get complete reference, then clean reads were mapped to it using STAR (Dobin et al., 2013), then gene expression level was calculated for each sample with RSEM (Li and Dewey, 2011). RSEM is a software package for estimating gene and isoform expression levels from RNA-Seq data. Pearson correlation between all samples was calculated using cor. Hierarchical clustering between all samples was performed using hclust. PCA analysis with all samples was performed using princomp, and the diagrams were drawn with ggplot2 in R. Circos software package (version 1.2.12.) was used to visualize data (Krzywinski et al., 2009). The clustering results were displayed with javaTreeview (Saldanha, 2004) using cluster (Eisen et al., 1998; De Hoon et al., 2004) software to analyze the expression genes and sample scheme at the same time by using the Euclidean distance matrix as the matrix formula.
In order to reflect the gene expression correlation between samples, all the samples were hierarchical clustered by the expression level of all genes, which can directly reflect the relationship between each two samples. Sample variation was visualized by a principal component analysis (PCA) plot (Supplementary Figure 1A), which shows that all samples were divided into three parts according to intestinal segments, indicating the reliability of the sequenced samples. Differentially expression genes (DEGs) were detected between groups based on gene expression levels using DEseq2 algorithms. Summary of DEGs is shown in Supplementary Figure 1B. DEGs were detected including an average of 105 up-regulated genes and on average 61 down-regulated DEGs in the three intestinal segments.
Differentially expressed genes (DEGs) between the FM and FPH-18 group (log fold change ≥ 1 and adjusted p-value ≤ 0.05), were detected based on gene expression levels using DEseq2 algorithms. Clustering analysis and functional annotations including Gene Ontology (GO) (http://www.geneontology.org) and Kyoto Encyclopedia of Genes and Genomes (KEGG) (http://www.genome.jp/kegg/) enrichment were performed. The raw data has been uploaded to NCBI SRA database with BioProject No. PRJNA1072556.
2.5 Reverse transcription and real-time quantitative PCR
Reverse transcription was performed using LunaScript RT SuperMix Kit (New England BioLabs) with 1 µg RNA per 20 µl reaction volume, following the manufacturers protocol. A control without reverse transcriptase (no-RT) was added to each plate (Thermo Scientific, cat. No. AB0600) to evaluate the presence of genomic DNA in a sample. The plate was centrifuged (1 min, 900 rpm, Sigma 2-6 Compact Centrifuge) before incubation in a thermal cycler (Bio-Rad T100) for 2 min at 25°C for primer annealing, followed by cDNA synthesis at 55°C for 10 min and finally heat inactivation at 95°C for 1 min. The cDNA plates were stored at -20°C.
Primers were screened for specificity in silico by using BLAST (Ye et al., 2012) and this tool was also used to create new primers when the BLAST results of published primers were insufficient. The primers used for the analysis are listed in Table 2 (Sigma Aldrich). The primer efficiencies were tested by preparing dilution curves of the pooled samples and running a qPCR analysis as described below.
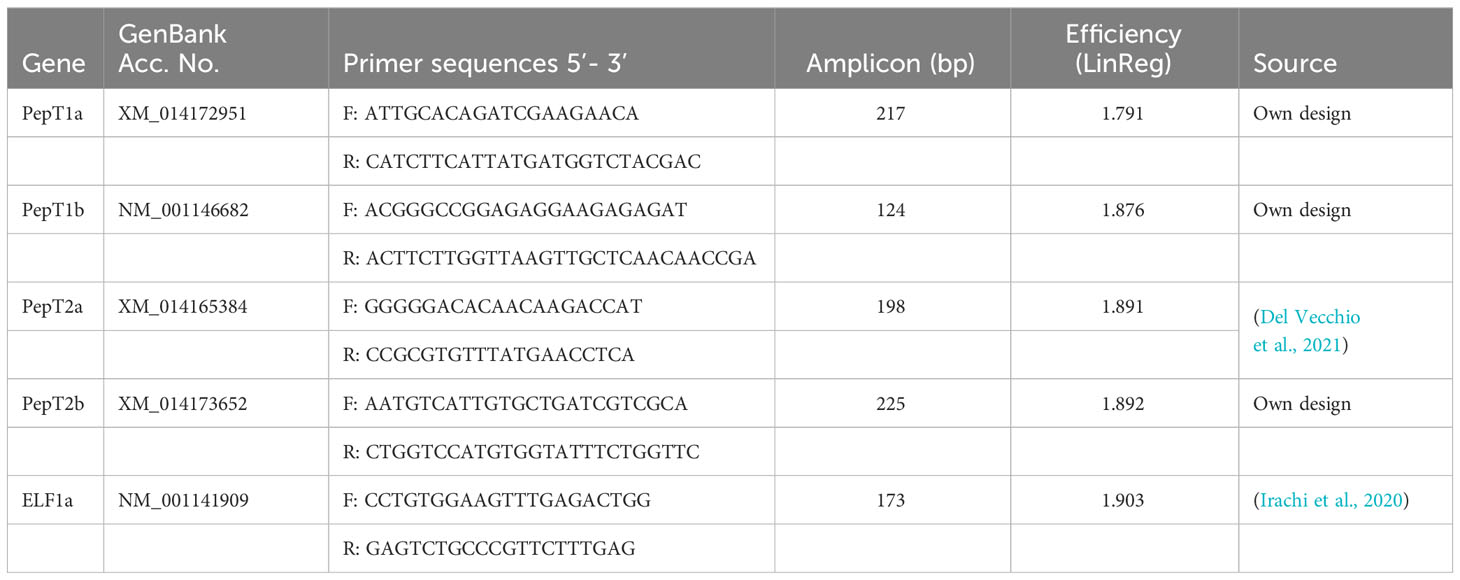
Table 2 Primers for RT-qPCR analysis of paralogous peptide transporters (PepT1a, -1b, -2a and -2b) and elongation factor 1 alpha (ELF1a).
Quantitative real-time PCR (qPCR) was performed by using the LightCycler 480 SYBR Green I Master Kit (Life Science Roche, Cat. No. 04887352001) containing FastStart Taq DNA Polymerase. Primer specific master mixes were made by mixing SYBR Green (10 µl), primers (10 µM, 1 µl each) and nuclease free water (3 µl), multiplying the volumes with sample number and distributing 15 µl per well into 96 well qPCR plates (VWR, Cat. No. 732-1463). Diluted cDNA (1:6, 5 µl) from each sample, no-RT control from the cDNA reaction, no template control (NTC) and pooled cDNA samples of PC, MG and HG (all with the same dilution 1:6) were manually transferred into single wells in a plate and mixed by pipetting. The pooled samples were used as internal calibrators for the reference gene and gene of interest on each plate to facilitate comparisons between different plates. The plate was run in the Lightcycler 480 qPCR instrument (Roche) with these cycling parameters: 1) Preincubation at 95°C for 10 min (1 cycle), 2a) denaturation at 95°C for 15 s, 2b) annealing at 60°C for 15 s, 2c) elongation at 72°C for 15 s (40 cycles of 2a-2c), followed by melt curve 3a) 95°C for 1 min, 3b) 65°C for 30 s, 3c) 97°C for 1 s and finally cooling at 37°C for 30 s.
The ΔCt (Ctgene – Ctref.) values per sample were plotted by diet and intestinal segment in R (version 4.3.1) to reveal outliers, which were removed if they were deviating more than 1.5 interquartile ranges (IQR) above the 75% quartile or below the 25% quartile. After outlier removal, we had 8-15 (averagely 13.6) biological replicates per diet and intestinal segment. The NTC and no-RT controls showed Ct values ranges between 32.03-36.87 and 31.67-35.07, respectively. The choice of elongation factor 1α (ELF1a) as reference gene was based on little variation in Ct values when testing effects of diets and different intestinal segments (Olsvik et al., 2005).
2.6 Bacterial microbiota analysis
DNA of mucosa and feces from MG and HG were extracted using QIAamp Fast DNA Stool Mini Kit (Qiagen, Switzerland). For bacterial microbiota analysis, DNA samples were sequenced at BGI (China), using 16S rRNA gene amplicon sequencing.
DNA template (30 ng) and the 16S rRNA fusion primers (V3-V4 region) were added for PCR. All PCR products were purified by Agencourt AMPure XP beads, dissolved in elution buffer and eventually labeled to finish library construction. Library size and concentration were detected by Agilent 2100 Bioanalyzer. Qualified libraries were sequenced on HiSeq platform according to their insert size.
Raw data were filtered to obtain high-quality clean data, and overlapping clean reads were merged to tags and further clustered to operational taxonomic units (OTU). Taxonomic classifications were assigned to OTU representative sequence using Ribosomal Project Database. Analysis like alpha diversity, beta diversity, differential species analysis, network and model prediction were carried out on the OTU profile table and taxonomic annotation results. Taxonomic analysis of OTU representative sequences was carried out by RDP classifier Bayesian algorithm to identify the composition of microbial structure. Abundances of species on seven levels (Phylum, Class, Order, Family, Genus, Species) was calculated after annotation (Cole et al., 2014). In the present study, only phylum level is presented.
2.7 Statistical analysis
Statistical analysis of RT-qPCR results was performed in R Studio (version R-4.3.1) (R Core Team, 2023). Homogeneity of variance in the residuals after removing outliers was tested using Levene’s test. Normal distribution of the residuals was tested by using Q-Q plot and Shapiro-Wilk’s normality test. Dietary effects were tested by One-way ANOVA of ΔCt (Ctgene – Ctref.) values for each gene and intestinal segment separately. In cases where ANOVA revealed significant difference of diet, Tukey’s Honest Significant Difference (HSD) post hoc test was used to find where the differences were. The significance level was set to p < 0.05.
3 Results
3.1 Fish performance
The specific growth rate, digestibility and intestinal histology results from this specific trial have been presented elsewhere (Sandbakken et al., 2023). In summary, salmon fed the hydrolysate diets showed significantly higher specific growth rate over the first 25 feeding days, which may indicate feed stimulatory properties of the hydrolysate. The FPH-18 diet had significantly higher protein and amino acid digestibility than the other diets, and the ash digestibility increased linearly with the FPH content, indicating increased mineral uptake from the hydrolysate diets. Histological analysis of midgut (MG) and hindgut (HG) of the FM and FPH-18 diets did not reveal any significant differences, and the intestines appeared normal and healthy.
3.2 RNA sequencing
Gene expression analysis by RNA sequencing in pyloric caeca (PC), midgut (MG) and hindgut (HG) generally revealed few differentially expressed genes (DEGs) between the salmon fed FM and FPH-18 diets (Supplementary Figure 1B). Gene ontology (GO) enrichments and KEGG (Kyoto Encyclopedia of Genes and Genomes) enriched pathways mostly grouped the downregulated genes, even though more upregulated DEGs (69-129), than downregulated DEGs (44-78) were detected in all intestinal segments of salmon fed FPH-18 compared to FM (Supplementary Figure 1B).
GO biological process enrichment revealed a downregulation of antimicrobial and antiviral immunity in PC, MG and HG of salmon fed the FPH-18 diet compared to the FM diet (Figure 1). In the PC, a downregulation of several immune-related GO biological processes was seen (Figure 1A), like interferon stimulated gene (ISG)15- protein conjugation (GO:0032020), cellular defense response (GO:0006968), positive regulation of T cell cytokine production (GO:0002726) and positive regulation of cytokine production involved in immune response (GO:0002720), which are part of cellular responses to viral and bacterial infections. In the MG, few downregulated GO biological processes were found, and they were linked to triglyceride biosynthetic process (GO:0019432) and superoxide metabolic process (GO:0006801), which are related to lipid metabolism and prevention of oxidative damage (Figure 1B).
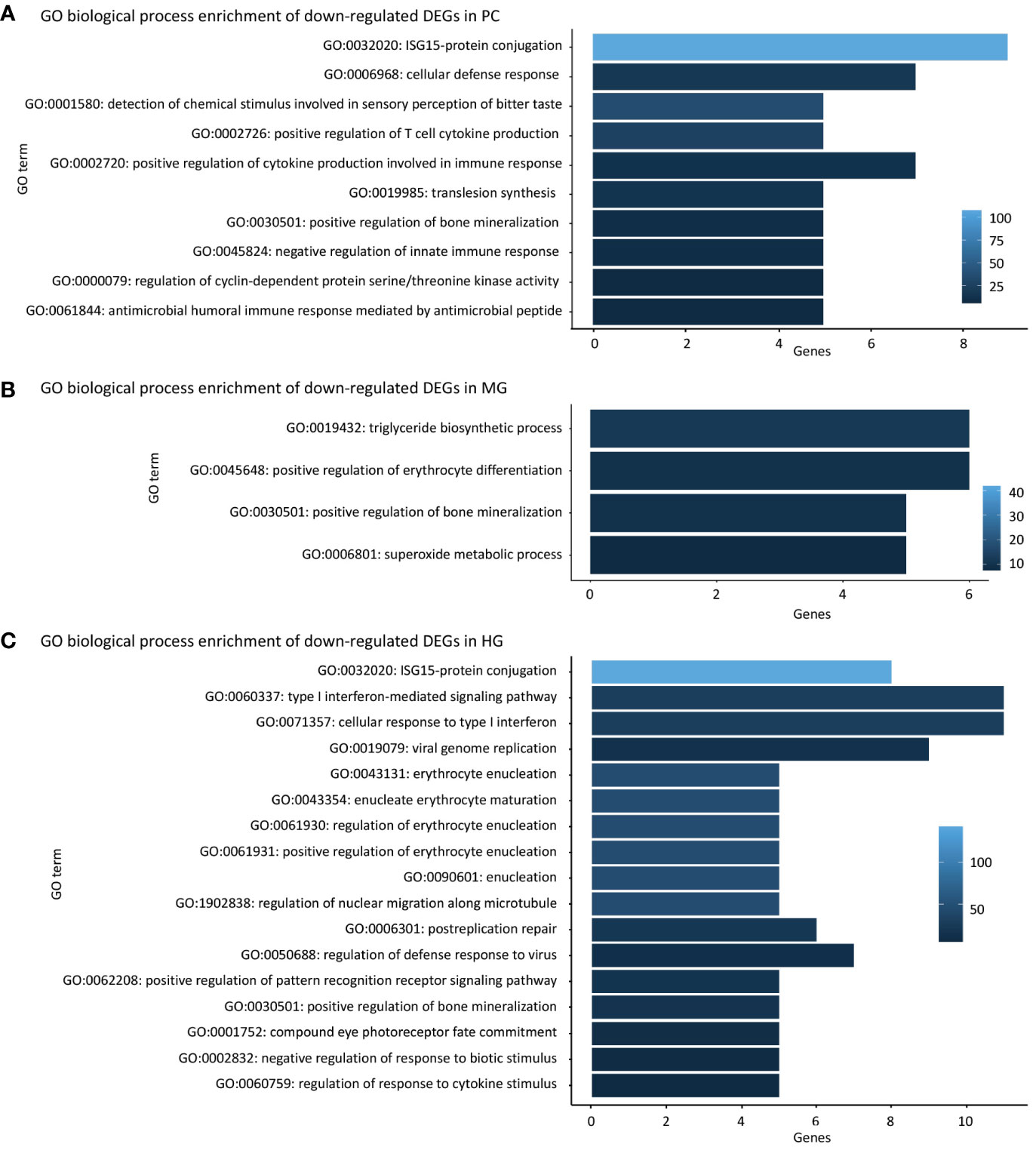
Figure 1 FPH-18 reduces inflammatory responses in salmon intestinal segments. Gene ontology (GO) enrichments of differentially expressed genes (DEGs) in 18% salmon protein hydrolysate diet (FPH-18) compared to the fishmeal control diet (FM). GO biological process enrichment of down-regulated DEGs in pyloric caeca (PC), midgut (MG) and hindgut (HG) are shown in (A–C) respectively. Only DEGs ≥ 5 genes are displayed, and the color intensity represents fold enrichment.
In the HG, a downregulation of several GO biological processes related to viral immune response was prominent in salmon fed FPH-18 compared to FM (Figure 1C). The processes ISG15-protein conjugation (GO:0032020), type I interferon-mediated signaling pathway (GO:0060337), cellular response to type I interferon (GO:0071357), viral genome replication (GO:0019079), regulation of defense response to virus (GO:0050688), positive regulation of pattern recognition receptor signaling pathway (GO:0062208), negative regulation of response to biotic stimulus (GO:0002832) and regulation of response to cytokine stimulus (GO:0060759) are related to pathogen recognition and antimicrobial immunity. This indicates that the immune system did not identify any threats and could therefore downregulate expression of immune-response genes to save energy.
GO molecular function enrichment showed that some downregulated DEGs were related to molecular binding, such as protein tag activity (GO:0031386) in the PC and HG, dynein heavy chain binding (GO:0045504), nuclear androgen receptor binding (GO:0050681) and protein self-association (GO:0043621) in HG (Figure 2). This indicates that the salmon hydrolysate diet (FPH-18) may reduce molecular interactions and protein binding compared to the FM diet.
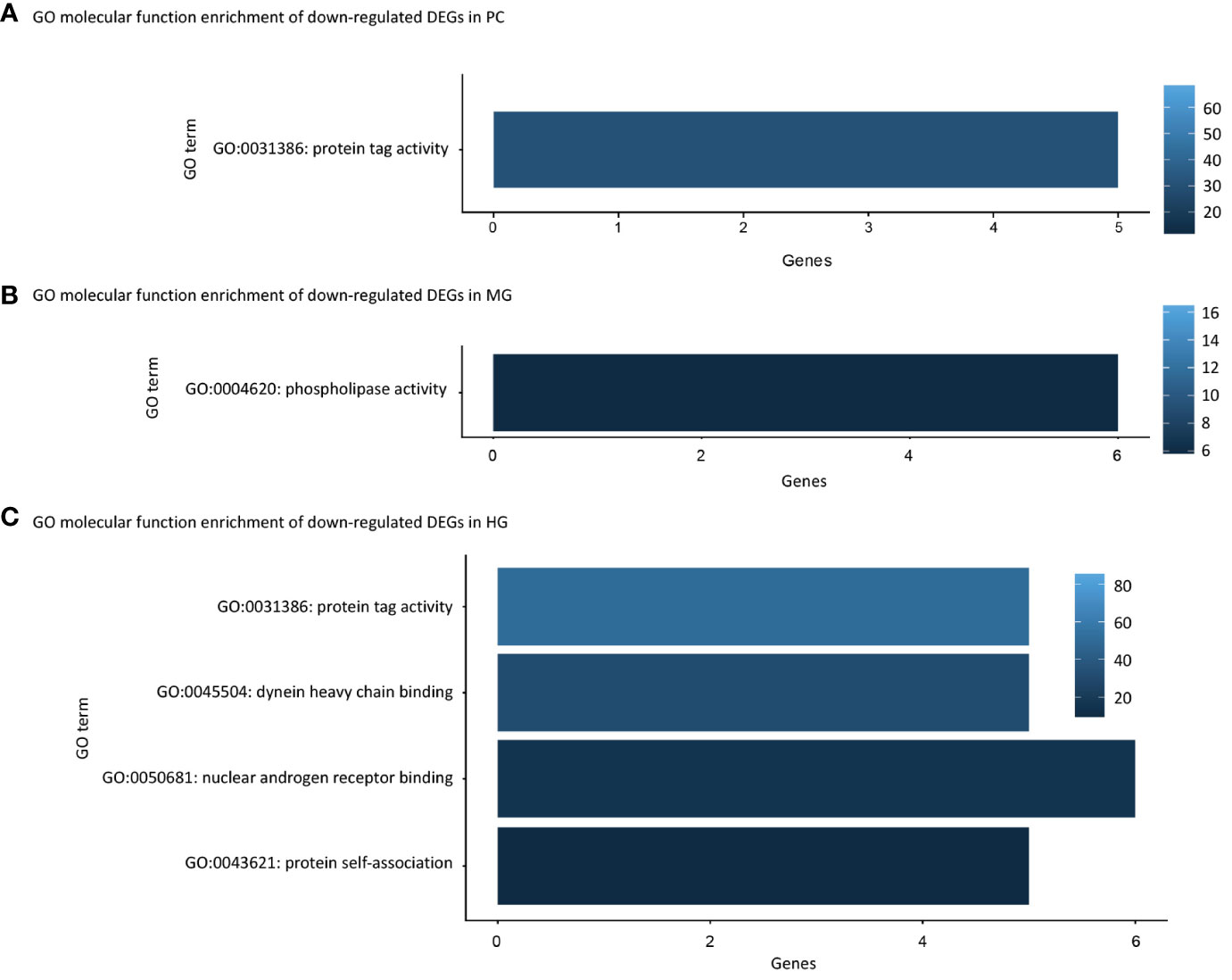
Figure 2 Gene ontology (GO) molecular function enrichments of downregulated differentially expressed genes (DEGs) in pyloric caeca (PC), midgut (MG) and hindgut (HG), shown in (A-C) respectively. Only DEGs ≥ 5 genes are displayed, and the color intensity represents fold enrichment.
The comparison between FPH-18 and FM fed salmon had 5 KEGG enriched pathways, where 4 were downregulated and 1 upregulated (Table 3). The downregulated pathways in the PC are related to viral, microbial and pathogen sensing and include the RIG-I-like receptor, NOD-like receptor and toll-like receptor signaling pathways as well as the cytosolic DNA sensing pathway.
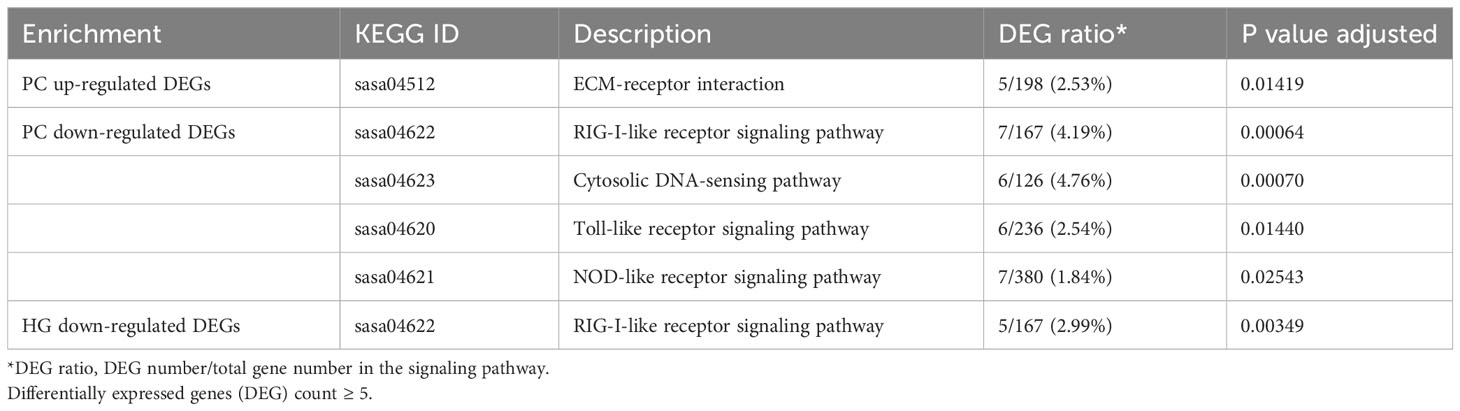
Table 3 Results from KEGG enrichment of RNA sequencing data of intestinal samples from salmon fed FPH-18 compared to FM fed salmon.
3.3 Expression of specifically interesting genes
Some specifically interesting genes were picked for in depth analysis of immune response and ion transport. Heatmap of gene expression in the three intestinal segments related to immune response (Figure 3A) and ion transporters (Figure 3B) shows the differences between the diets by FPKM (Fragments Per Kilobase of transcript per Million mapped reads). Molecules related to immune responses, such as TLR3 (toll-like receptor 3) and Mx (myxovirus resistance protein), seems to have higher expression in the hydrolysate diet (FPH-18), but the variation among the samples were large (Figure 3A). Guanylin is involved in regulating ion transport, and showed highest expression in MG, while the solute carrier 15 (Slc15) family of transporter molecules showed highest expression in PC (Figure 3B). Slc15 is a group of nutrient transporters that mediate the transport of nutrients across the cellular membrane. Peptide transporters (PepT) are in this group (Slc15a), and we looked specifically at 4 paralogous peptide transporters with RT-qPCR.
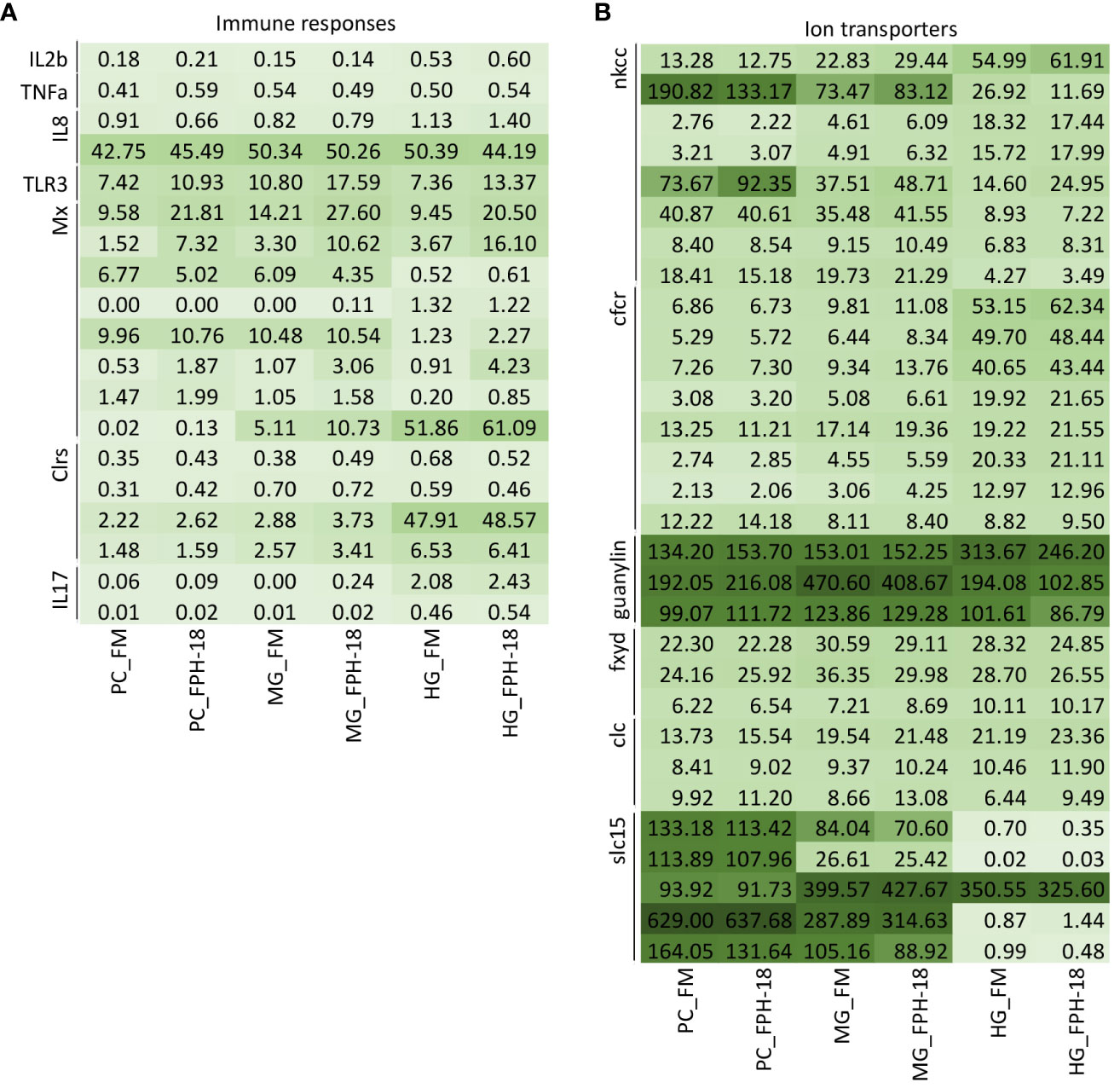
Figure 3 Heatmap of gene expression (FPKM) of interesting genes in different intestinal segments: pyloric caeca (PC), midgut (MG) and hindgut (HG) of salmon fed FM or FPH-18 diets. Genes related to immune responses (A) (without genes with average FPKM<0.5 in all groups) and ion transporters (without genes with average FPKM<10 in all groups) (B). Darker color shows higher FPKM.
3.4 Intestinal gene expression by RT-qPCR
Transcriptomic analysis by RT-qPCR was performed on paralogous peptide transporters (PepT, Slc15a) to evaluate the dietary effects in different intestinal segments. Relative expression of the four paralogous peptide transporters PepT1a, PepT1b, PepT2a and PepT2b differed among each other and between intestinal segments. Delta cycle threshold (ΔCt) between the gene of interest and reference gene was plotted for each diet in different intestinal segments to visualize which peptide transporter that mainly contributed to peptide uptake in that specific segment, and how the relative differences change along the gastrointestinal tract (Figure 4).
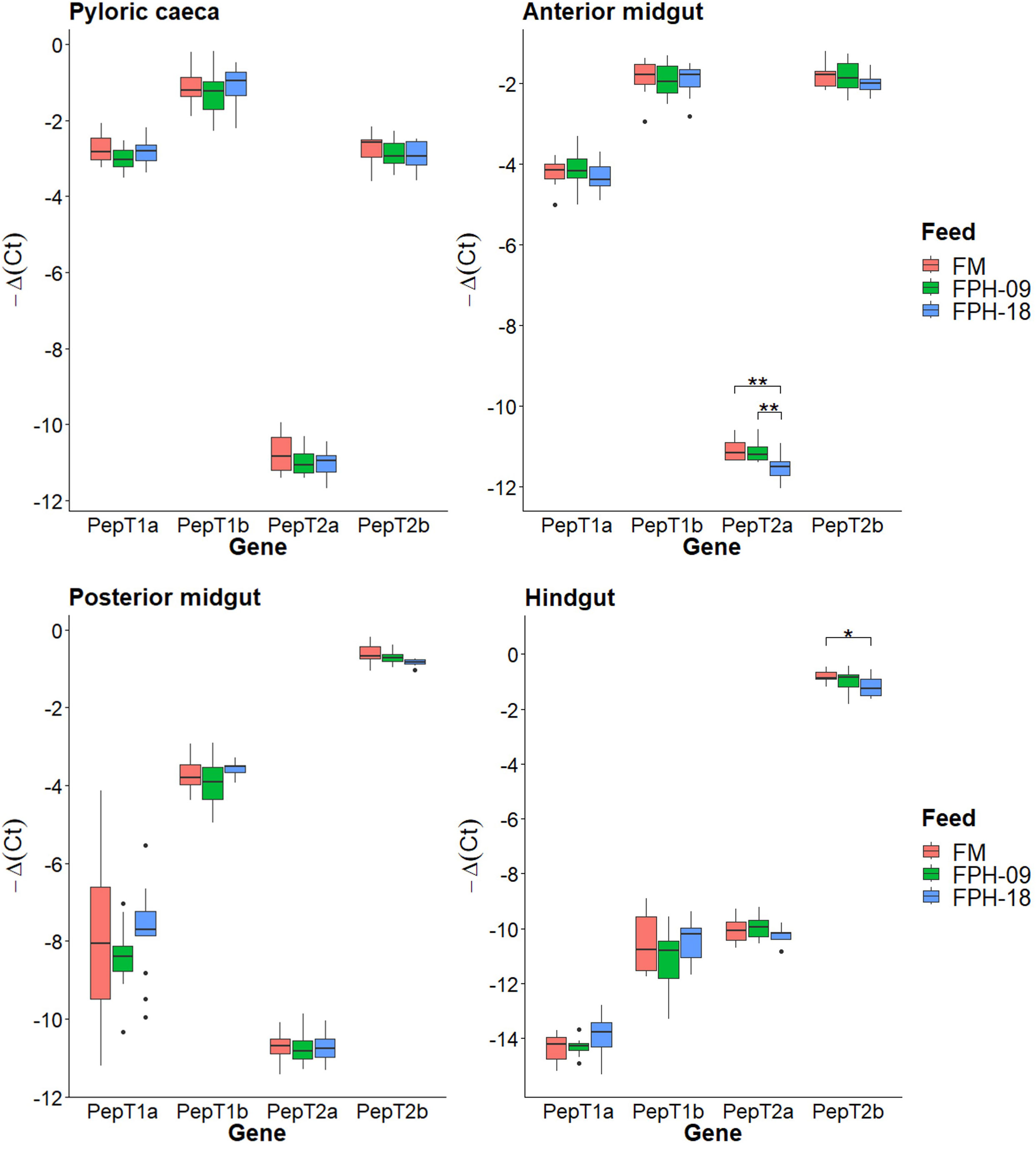
Figure 4 Boxplots of relative gene expression ratio as – Δ(Ctgene – Ctref) for each peptide transporter gene in the different intestinal segments of fish fed fishmeal control diet (FM), 9% and 18% salmon hydrolysate (FPH-09 and FPH-18, respectively). This shows the relative expression levels in the different intestinal segments, comparing which peptide transporters contribute the most to peptide uptake. Significantly different relative expression between diets are marked with *(p < 0.05) and **(p < 0.01).
Generally, there were few dietary effects on PepT expression. The most notable finding was that the PepTs appeared to be differently expressed along the intestinal tract. In PC, PepT1b had the highest mRNA level, followed by PepT1a and PepT2b, which both had about 4 times (2-2) lower mRNA levels. Pept2a generally showed very low expression levels throughout the intestine, with significantly lower expression in fish fed the FPH-18 diet compared to the FM and FPH-09 diets in the anterior midgut (AMG). In the AMG, PepT1b and PepT2b seem to contribute equally to peptide uptake, due to similar relative expressions to the reference gene.
The relative amount of PepT1b mRNA tended to decrease along the intestine, while the opposite was seen for PepT2b mRNA, which contributed the most to peptide uptake from posterior midgut (PMG) to HG. Significantly less PepT2b mRNA was found in the FPH-18 diet compared to FM control in the HG. The relative expression of PepT2b in the HG was 500 times (29) higher than any of the other paralogous PepT in this intestinal segment.
3.5 Bacterial microbiota
In the present study, numerous bacterial phyla were identified. Figure 5 shows the relative abundance of the predominant gut phyla levels in mucosa (A) and digesta (B) from MG and HG of salmon fed a FM or FPH-18 diet. At the phylum level, Cyanobacteria, Proteobacteria, Firmicutes, Spirochaetes and Actinobacteria were predominant in mucosa from MG and HG of salmon fed the two diets, but some dietary effects were noticed (Figure 5A; Supplementary Table 2). The relative abundance of Cyanobacteria, Proteobacteria and Firmicutes in HG mucosa decreased by FPH-18 feeding, in contrast to the relative abundance of Spirochaetes which increased. In the MG, the relative abundance of Cyanobacteria and Spirochaetes increased in the mucosa of salmon fed FPH-18. The relative abundance of Actinobacteria decreased while Firmicutes were unaffected by dietary treatment. The only dietary effect seen in mucosa was the increase in Spirochaetes in the MG and especially the HG of salmon fed FPH-18. Preliminary results on genus level, revealed a high abundance of Brevinema, and especially Brevinema andersonii on a species level, in mucosa of fed fish FPH-18 in contrast to the FM fed fish.
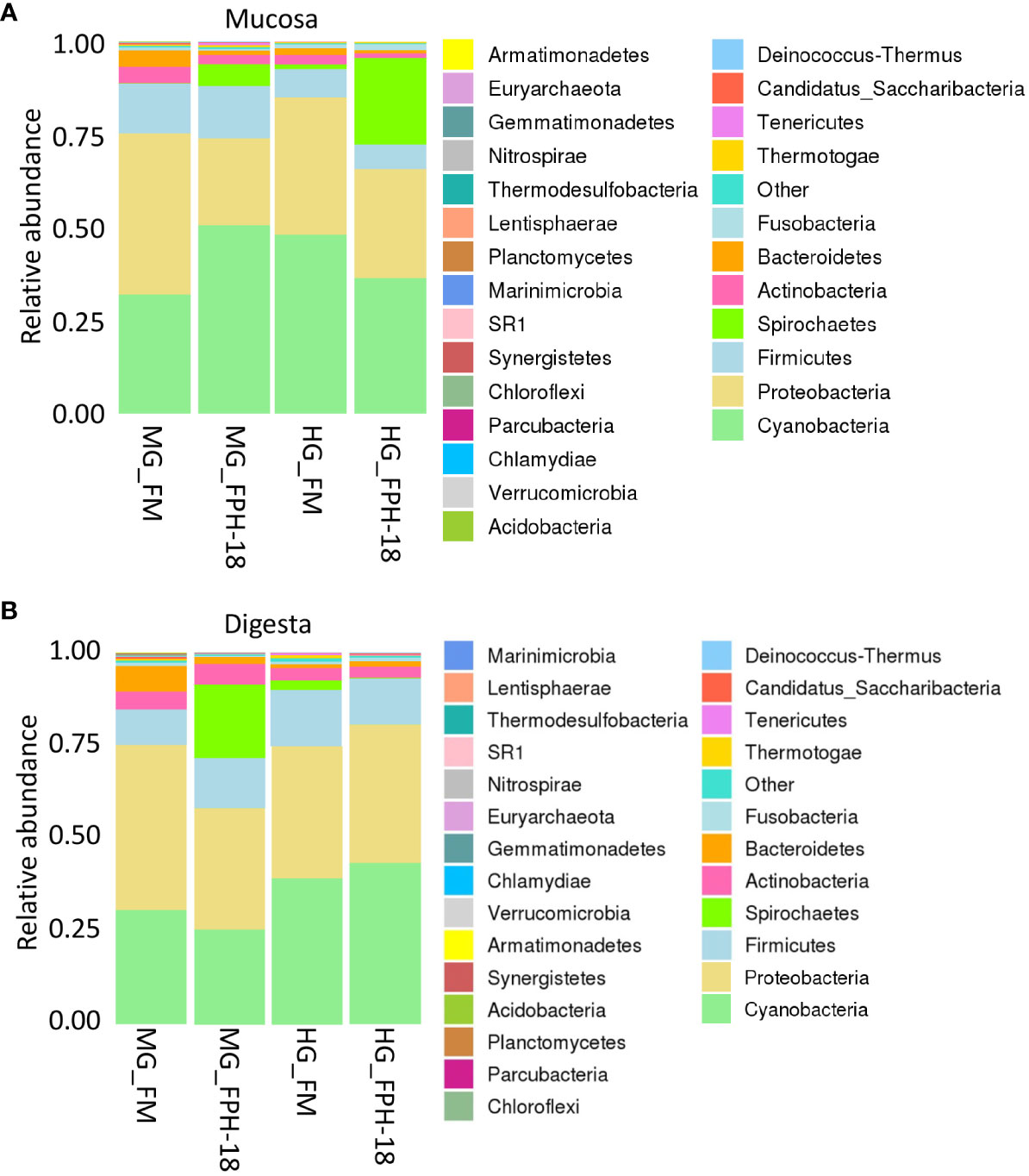
Figure 5 The composition of bacterial gut microbiota at phylum level in mucosa (A) and digesta (B) from midgut (MG) and hindgut (HG) of salmon fed the fishmeal (FM) diets or 18% salmon hydrolysate (FPH-18) diet (n = 6).
In the digesta, the relative abundance of phyla was predominated by Cyanobacteria, Proteobacteria, Firmicutes, Spirochaetes, Actinobacteria and Bacteroides (Figure 5B; Supplementary Table 3). The highest relative abundance of Cyanobacteria (43.4%) was noticed in HG digesta from fish fed the FPH-18 diet, and Proteobacteria (44.4%) in MG digesta from fish fed FM. Firmicutes abundance was relatively stable (values between 9.7-15.3%) in digesta samples, while the abundance of Spirochaetes (19.6%) was highest in the digesta from fish fed the FPH-18 diet. The only clear dietary effect in digesta composition was the higher abundance of Spirochaetes in MG of FPH-18 fed salmon.
4 Discussion
4.1 Gene expression by RNA sequencing
The results from GO and KEGG clearly shows that replacing fishmeal with salmon hydrolysate reduced the inflammatory load as viewed by the reduction of pro-inflammatory markers in the intestinal tissue, particularly towards pathogens like viruses. Along with the downregulation of RIG-I-like receptors, NOD-like receptors and toll-like receptors, which are intracellular pattern recognition receptors involved in the recognition of pathogen-associated molecular patterns (PAMPs) by the innate immune system (Barton and Medzhitov, 2003; Kanneganti et al., 2007; Loo and Gale, 2011). It therefore appears likely that the immune system does not sense immediate threats when salmon hydrolysate is partially replaced by FM in the diet. This could suggest that there are components in FM that cause a general upregulation of inflammatory and immune pathways, and that reduction of these would reduce the need to mount a response. Downregulation of genes associated with the immune system has also been seen in the liver of Atlantic salmon that were starved for 28 days. It was also interesting to note that when these fish were challenged with a pathogen, they showed a higher upregulation of immune genes than the non-starved fish (Martin et al., 2010), thus having a potentially better response to a real threat. Downregulation of the immune-related genes is beneficial for saving energy and may still be activated as necessary upon pathogenic threats. It can be speculated that the improved growth response during early phases of the feeding trial these fishes were analyzed from (Sandbakken et al., 2023) can be related to reduced need for energy to mount a continuous low-level inflammatory response.
Another possibility for the downregulation of immune parameters, may lie in a direct effect of protein hydrolysates containing potentially bioactive peptides. Some hydrolysates have been shown to have immunomodulatory functions, which may activate or downregulate the immune system, depending on the hydrolysate source and composition (Kiewiet et al., 2018). Bioactive peptides have been found in salmon protein hydrolysates made by enzymatic hydrolysis of salmon rest raw materials (Opheim et al., 2015). A peptide fraction from salmon hydrolysate showed anti-inflammatory activity in vitro by reducing the expression level of pro-inflammatory interleukins in macrophage cells upon lipopolysaccharide (LPS) stimulation (Ahn et al., 2012). Feeding 5% bioactive peptides from farmed Atlantic salmon to European sea bass also downregulated inflammatory responses in the intestine, but the anti-inflammatory effect was not seen at a 10% dietary inclusion level (Parma et al., 2023). Protein hydrolysates from shrimp and tilapia fed to European sea bass also showed a down-regulation of interferon-related genes (Leduc et al., 2018) as seen in this study with salmon hydrolysate. Interferons are signaling molecules produced by immune cells during viral infections, and a down-regulation of these indicate a reduction in viral threats (Ellis, 2001). Furthermore, Leduc et al. (2018) detected positive effects on immune responses and gut health by including 5% hydrolysate from shrimp, tilapia, or a mix of those to a 5% FM diet, and the European sea bass showed similar growth performance as the positive control fed a 20% FM diet. This indicates that also low inclusion levels of hydrolysates can have beneficial effects in restoring immune function and gut health of fish fed low FM diets, without compromising the growth. In this trial, we only compared the FPH-18 diet with the FM control which had positive effects on growth, digestibility, and gut health (Sandbakken et al., 2023). However, it would be interesting to explore a possible dose-response relationship of salmon hydrolysate to find the optimal inclusion level for maintaining a functional immune system and a good general gut health.
Peptides can also exert immunomodulatory effects by directly stimulating receptors, or by interfering with inflammatory signaling pathways after cellular uptake via a peptide transporter or via endocytosis (Kiewiet et al., 2018). There is also an interesting connection to PepT1 where studies have shown that PepT1 is upregulated in the colon during intestinal inflammation in mammals, and that specific soy peptides transported through PepT1 reduce the inflammatory response in vitro. After transport of peptides through PepT1 to the cytosol, some bioactive peptides resist degradation by peptidases and may inhibit the main inflammatory signaling pathways in human intestinal Caco2 cells (Dalmasso et al., 2008; Kovacs-Nolan et al., 2012; Kiewiet et al., 2018).
Some marine peptides have also shown antimicrobial effects (Kang et al., 2019), which may affect the mucosal microbiota. Thus, the immunomodulatory effects of hydrolysates may be due to a direct effect by bioactive peptides, an indirect effect through modulation of the bacterial microbiota or a combination of these.
4.2 Intestinal expression of peptide transporter genes
Based on previous literature from vertebrates (Chen et al., 2005; Gilbert et al., 2008; Bakke et al., 2010), our initial hypothesis was that high dietary peptide content would increase expression of intestinal peptide transporter (PepT) in the anterior part of the intestine, and perhaps reduce the expression in posterior regions since these components are readily absorbed in the anterior part. However, the only significant differences were a slight reduction of PepT2a in the anterior midgut and PepT2b in the hindgut (HG) of FPH-18 fed fish. The difference in the HG could be explained by rapid absorption of the hydrolysate peptides and free amino acids in the proximal part of the intestine, while the FM proteins had to be enzymatically degraded before absorption. The small differences observed may be due to the same protein content in all experimental diets and efficient protein degradation by endogenous enzymes in the digestive tract of Atlantic salmon.
In this trial, the fish were not fed for 48 hours prior to collection of RNA samples. Luminal content can affect PepT expression, but 4 days of fasting had minimal effect on expression of PepT paralogs in intestinal segments of Atlantic salmon (Del Vecchio et al., 2021).
The relative expression of PepT1b was highest in the anterior part of the intestine, followed by a decreasing expression level further down the gastrointestinal tract as previously observed in zebrafish (Verri et al., 2003), Atlantic cod (Rønnestad et al., 2007), European sea bass (Terova et al., 2009) and Atlantic salmon (Gomes et al., 2020). PepT1b had about 4 times (2-2) higher expression levels than PepT1a in PC and AMG, which is in line with what was reported by (Gomes et al., 2020). The relative expression of PepT1b and PepT2b was similar in the anterior midgut (AMG), while PepT2b had the highest mRNA concentration of all paralogs in posterior midgut (PMG) and HG. These results are comparable to previous data on Atlantic salmon (Del Vecchio et al., 2021). Expression of PepT2a was generally negligibly low in all intestinal segments, and 500-1000x lower than PepT2b in PMG and HG, as previously reported (Del Vecchio et al., 2021; Vacca et al., 2022).
In this trial, intestinal expression of PepT paralogs were comparable to previous data in Atlantic salmon, and minimal effects of increased level of dietary peptide were observed. PepT1b showed highest expression levels in the anterior part of the intestine, while PepT2b was most highly expressed in the posterior part of the intestine, thus they both contribute to intestinal peptide absorption in different parts of the gastrointestinal (GI) tract.
4.3 Bacterial microbiota
The present study revealed dietary effect on phyla level of the gut microbiota, an effect well known in fish (Cahill, 1990; Ringø et al., 1995; Egerton et al., 2018; 2020; Kokou et al., 2022; Luan et al., 2023). In addition, the bacterial microbiota in the digesta differed to some extent from the mucosal microbiota (Ringø et al., 2016; Li et al., 2021; Nyholm et al., 2022). The present study investigated the mucosal gut microbiota, but most fish studies have focused only on the digesta microbiota. Since the mucosal microbiota might influence fish health, more focus should be directed towards the mucosal community and furthermore on the importance of the gut microbiota on lipid-, carbohydrate-, protein- and amino acid metabolism of aquatic animals (Ringø et al., 2022).
Different segments of the teleost GI tract must be investigated separately, as the colonization patterns between beneficial bacteria and pathogens may vary along the intestine (Ringø et al., 2003). The difference in colonization of mucosal phyla between MG and HG of Atlantic salmon fed the two diets in the present study was revealed, and the findings are in accordance with the results described in previous studies evaluating the gut microbiota (Ringø et al., 1995; Gajardo et al., 2016). As evaluation of gut microbiota is relatively new, it is important to analyze the different GI tract segments to fully see the relationship between these communities. Furthermore, outcompeting beneficial intestinal microorganisms may lead to dysbiosis and increased gut permeability (Zhang et al., 2020).
The gut microbiota of marine fish of economic interest for aquaculture are being dominated by the phyla Proteobacteria (Egerton et al., 2018) and the results of the present study agrees with the above mentioned studies. Regarding Cyanobacteria, the phyla is seldomly reported as a part of the fish gut microbiota and not at the relative abundance level as revealed in the present study. The reason for this observation is not known, and merits further investigations.
Spirochaetes, Gram negative bacteria with long corkscrew or spiraled morphology are reported to be a part of the intestinal microbiota in several fish species (Egerton et al., 2020; Iwatsuki et al., 2021; Li et al., 2021). A dietary effect was noticed on the relative high abundance of Spirochaetes in mucosa of MG (6%) and HG (23%) of FPH-18 fed Atlantic salmon, while Egerton et al. (2020) revealed significantly lower abundance of intestinal Spirochaetes in Atlantic salmon fed 80% plant protein. The abundance of Spirochaetes in the mucosa of FPH-18 fed salmon were however considerably lower than the 50 and 70% Spirochaetes found in the hindgut mucosa of Atlantic salmon fed a commercial and insect meal diet, respectively (Li et al., 2021). The Spirochaetes detected in this trial were dominated by the genera Brevinema, more specifically the species Brevinema andersonii, but the prevalence varied between the individual fish. Among the Phyla Spirochaetes, the species B. andersonii has been associated with altered expression of pro- and anti-inflammatory genes, and Spirochaetaceae with genes related to intestinal barrier function, when found in the hindgut mucosa (Li et al., 2021). High abundance of Spirochaetes including B. andersonii has also been detected in the hindgut mucosa of Atlantic salmon fed a prebiotic alginate oligosaccharide derived from brown macroalgae (Gupta et al., 2019). The Spirochaete B. andersonii has genes associated with butyrate production, which is favorable for the gut health, since butyrate is one of the main short chain fatty acids (SCFAs) that provide energy for enterocytes and maintain mucosal integrity and immune homeostasis (O’Keefe, 2016). Based on our results, the downregulation of immune responses seen in the present study could be linked to the mucosa associated bacteria, especially Spirochaetes, and their fermentation products. However, as the interactions between immune system and intestinal bacteria are more investigated in mammals than in fish (Maynard et al., 2012; Daniel et al., 2021; Schlechte et al., 2022), so this topic merits further research. It is important to increase the knowledge on how dietary composition influences the immunity and health of farmed aquaculture species.
5 Conclusion
The first paper from this trial (Sandbakken et al., 2023) clearly showed that salmon protein hydrolysate can partially replace fishmeal in diets for Atlantic salmon giving good growth, increased digestibility of protein and ash and good intestinal health as viewed by histology. Intestinal gene expression analysis of the current manuscript showed a downregulation of several inflammatory pathways particularly towards virus and to some extent bacteria when salmon post-smolts were fed 18% salmon hydrolysate compared to a FM control diet. This could reduce energy expenditure by the immune system and free energy available for improved growth. The cause for this downregulation is not known at present but is being studied further. On one hand, it is possible that FM contain molecules that activates the immune system. On the other hand, the salmon hydrolysate with its high load of potentially bioactive peptides may have modulated the immune response through several possible pathways. It is also possible that bacteria selected by salmon hydrolysate could have contributed to reduce the presence of potential pathogens. The gut microbiota in the digesta and mucosa of the MG and HG, were dominated by the phyla Cyanobacteria and Proteobacteria in both diets. However, the phyla Spirochaetes were more prevalent in the mucosa of FPH-18 fed salmon, compared to the FM fed salmon. This dietary difference on mucosal microbiota and the microbial fermentation products may have influenced the inflammatory responses as seen in the gene expression analysis. Further research is needed to fully understand the relationship between fish nutrition, intestinal microbiome, and the immune system. Furthermore, it is well known that dietary manipulation modulates the intestinal microbiota which may improve the resistance towards bacterial infection, this topic must be investigated in future studies when evaluating the effect of salmon protein hydrolysate.
Peptide transporter gene expression was minimally affected by different dietary peptide content, and mostly varied between the intestinal segments. PepT1b contributed most to peptide uptake in the PC, while in AMG PepT1b and PepT2b showed similar relative expression levels. From PMG to HG, PepT2b showed the highest relative expression levels, indicating that PepT2b contributed the most to peptide uptake in the posterior part of the gastrointestinal tract.
Data availability statement
The raw data has been uploaded to NCBI SRA database with BioProject No. PRJNA1072556.
Ethics statement
The animal study was approved by Norwegian Food Safety Authority (FOTS ID:23021). The study was conducted in accordance with the local legislation and institutional requirements.
Author contributions
IS: Writing – original draft, Visualization, Validation, Supervision, Project administration, Investigation, Formal analysis, Data curation, Conceptualization. HS: Writing – original draft, Visualization, Investigation, Formal analysis, Data curation. LJ: Writing – review & editing, Data curation. YZ: Writing – review & editing, Visualization, Formal analysis, Data curation. ER: Writing – original draft. RR: Writing – review & editing, Supervision, Data curation. IY: Writing – review & editing, Formal analysis, Data curation. KF: Writing – review & editing, Data curation. RO: Writing – original draft, Supervision, Conceptualization.
Funding
The author(s) declare financial support was received for the research, authorship, and/or publication of this article. Nutrimar AS, the Norwegian research council [project no: 298839] and project Feed2Food [Grant 319693] funded this research. The funding sources had no role in study design or data interpretation from this experiment.
Acknowledgments
The authors would like to thank Jihong Liu Clarke (NIBIO) for providing funding for the RNA sequencing and microbiota analysis (Feed2Food project) and for the supervision of HS. We also thank Ralph Kissen (Norwegian University of Science and Technology) for supervision and assistance with RNA testing and RT-qPCR data management.
Conflict of interest
Authors IS and KF were employed by the company Nutrimar AS.
The remaining authors declare that the research was conducted in the absence of any commercial or financial relationships that could be construed as a potential conflict of interest.
The authors declare that this study received funding from Nutrimar AS. The funder was not involved in the study design, collection, analysis, interpretation of data, the writing of this article, or the decision to submit it for publication.
The author(s) declared that they were an editorial board member of Frontiers, at the time of submission. This had no impact on the peer review process and the final decision.
Publisher’s note
All claims expressed in this article are solely those of the authors and do not necessarily represent those of their affiliated organizations, or those of the publisher, the editors and the reviewers. Any product that may be evaluated in this article, or claim that may be made by its manufacturer, is not guaranteed or endorsed by the publisher.
Supplementary material
The Supplementary Material for this article can be found online at: https://www.frontiersin.org/articles/10.3389/fmars.2024.1376516/full#supplementary-material
References
Ahn C. B., Jae Y. J., Young S. C. (2012). Antioxidant and anti-inflammatory peptide fraction from salmon byproduct protein hydrolysates by peptic hydrolysis. Food Res. Int. 49, 92–985. doi: 10.1016/j.foodres.2012.08.002
Almås K. A., Josefsen K. D., Gjøsund S. H., Skjermo J., Forbord S., Jafarzadeh S., et al. (2020) Bærekraftig Fôr Til Norsk Laks. Available online at: https://hdl.handle.net/11250/2758913.
Bakke S., Jordal A. E. O., Gómez-Requeni P., Verri T., Kousoulaki K., Aksnes A., et al. (2010). Dietary protein hydrolysates and free amino acids affect the spatial expression of peptide transporter pepT1 in the digestive tract of atlantic cod (Gadus morhua). Comp. Biochem. Physiol. - B Biochem. Mol. Biol. 156, 48–555. doi: 10.1016/j.cbpb.2010.02.002
Bartlett A., Kleiner M. (2022). Dietary protein and the intestinal microbiota: an understudied relationship. IScience. 25, 105313. doi: 10.1016/j.isci.2022.105313
Barton G. M., Medzhitov R. (2003). Toll-like receptor signaling pathways. Science 300, 1524–1525. doi: 10.1126/science.1085536
Cahill M. M. (1990). Bacterial flora of fishes: A review. Microbial. Ecol. 19, 21–41. doi: 10.1007/BF02015051/METRICS
Chen H., Pan Y., Wong E. A., Webb K. E. (2005). Nutrient-gene interactions dietary protein level and stage of development affect expression of an intestinal peptide transporter (CPepT1) in chickens 1. J. Nutr. 135 (2), 193–198. doi: 10.1016/j.clnu.2016.02.005
Cole J. R., Wang Q., Fish J. A., Chai B., McGarrell D. M., Sun Y., et al. (2014). Ribosomal database project: data and tools for high throughput RRNA analysis. Nucleic Acids Res. 42, D633–D642. doi: 10.1093/nar/gkt1244
Commission Regulation (EU) No 142/2011 (2011). Implementing Regulation (EC) No. 1069/2009 of the European Parliament and of the Council Laying down Health Rules as Regards Animal by-Products and Derived Products Not Intended for Human Consumption and Implementing Council Directive 97/78/EC as Regards Certain Samples and Items Exempt from Veterinary Checks at the Border under That Directive. Available online at: https://www.fao.org/faolex/results/details/en/c/LEX-FAOC109216/#:~:text=This%20Regulation%20lays%20down%20implementing,by%2Dproducts%20and%20derived%20products.
Dalmasso G., Charrier-Hisamuddin L., Thi H., Nguyen T., Yan Y., Sitaraman S., et al. (2008). PepT1-mediated tripeptide KPV uptake reduces intestinal inflammation. Gastroenterology 134, 166–178. doi: 10.1053/j.gastro.2007.10.026
Daniel N., Lécuyer E., Chassaing B. (2021). Host/microbiota interactions in health and diseases-time for mucosal microbiology! Mucosal Immunol. 14, 1006–1016. doi: 10.1038/s41385-021-00383-w
Dawood M. A. O. (2020). Nutritional immunity of fish intestines: important insights for sustainable aquaculture. Rev. Aquac. 13, 642–663. doi: 10.1111/raq.12492
De Hoon M. J. L., Imoto S., Nolan J., Miyano S. (2004). Open source clustering software. Bioinf. Appl. NOTE 20, 1453–1545. doi: 10.1093/bioinformatics/bth078
Del Vecchio G., Lai F., Gomes A. S., Verri T., Kalananthan T., Barca A., et al. (2021). Effects of short-term fasting on MRNA expression of ghrelin and the peptide transporters pepT1 and 2 in Atlantic salmon (Salmo salar). Front. Physiol. 12. doi: 10.3389/fphys.2021.666670
Dobin A., Davis C. A., Schlesinger F., Drenkow J., Zaleski C., Jha S., et al. (2013). STAR: ultrafast universal RNA-seq aligner. Bioinformatics 29, 15–215. doi: 10.1093/bioinformatics/bts635
Egerton S., Culloty S., Whooley J., Stanton C., Ross R.P. (2018). The gut microbiota of marine fish. Front. Microbiol. 9. doi: 10.3389/fmicb.2018.00873
Egerton S., Wan A., Murphy K., Collins F., Ahern G., Sugrue I., et al. (2020). Replacing fishmeal with plant protein in Atlantic salmon (Salmo salar) diets by supplementation with fish protein hydrolysate. Sci. Rep. 10, 1–16. doi: 10.1038/s41598-020-60325-7
Eisen M. B., Spellman P. T., Brown P. O., Botstein D. (1998). Cluster analysis and display of genome-wide expression patterns. Proc. Natl. Acad. Sci. United States America 95, 14863–14685. doi: 10.1073/pnas.95.25.14863
Ellis A. E. (2001). Innate Host Defense Mechanisms of Fish against Viruses and Bacteria. Available online at: www.elsevier.com/locate/devcompimm. doi: 10.1016/S0145-305X(01)00038-6
Evans D. H., Claiborne J. B. (2006). The physiology of fishes. 3rd ed (Boca Raton: CRC Press). doi: 10.1201/9781420058093
Gajardo K., Rodiles A., Kortner T. M., Krogdahl Å., Bakke A. M., Merrifield D. L., et al. (2016). A high-resolution map of the gut microbiota in Atlantic salmon (Salmo salar): A basis for comparative gut microbial research. Sci. Rep. 6, 30893. doi: 10.1038/srep30893
Gao R., Yu Q., Shen Y., Chu Q., Chen G., Fen S., et al. (2021). Production, bioactive properties, and potential applications of fish protein hydrolysates: developments and challenges. Trends Food Sci. Technol. 110, 687–699. doi: 10.1016/j.tifs.2021.02.031
Gilbert E. R., Wong E. A., Webb K. E. (2008). Peptide absorption and utilization: implications for animal nutrition and health. J. Anim. Sci. 86, 2135–2155. doi: 10.2527/jas.2007-0826
Glencross B. D. (2020). A feed is still only as good as its ingredients: an update on the nutritional research strategies for the optimal evaluation of ingredients for aquaculture feeds. Aquacult. Nutr. 26, 1871–1883. doi: 10.1111/anu.13138
Gomes A. S., Vacca F., Cinquetti R., Murashita K., Barca A., Bossi E., et al. (2020). Identification and characterization of the Atlantic salmon peptide transporter 1a. Am. J. Physiol. - Cell Physiol. 318, C191–C204. doi: 10.1152/ajpcell.00360.2019
Gupta S., Lokesh J., Abdelhafiz Y., Siriyappagouder P., Pierre R., Sørensen M., et al. (2019). Macroalga-derived alginate oligosaccharide alters intestinal bacteria of Atlantic salmon. Front. Microbiol. 10. doi: 10.3389/fmicb.2019.02037
Irachi S., Hall D. J., Fleming M. S., Maugars G., Björnsson B. T., Dufour S., et al. (2020). Photoperiodic regulation of pituitary thyroid-stimulating hormone and brain deiodinase in Atlantic salmon. Mol. Cell. Endocrinol. 519, 111056. doi: 10.1016/j.mce.2020.111056
Iwatsuki T., Kanazawa T., Ogasawara T., Hosotani K., Tsuchiya K., Watanabe S., et al. (2021). 16S RRNA gene amplicon sequencing of gut microbiota in three species of deep-sea fish in suruga bay, Japan. Microbiol. Resource Announce. 10, 10:10.1128/mra.01260-20. doi: 10.1128/mra.01260-20
Kang H. K., Lee H. H., Seo C. H., Park Y. (2019). Antimicrobial and immunomodulatory properties and applications of marine-derived proteins and peptides. Mar. Drugs. 17, 350. doi: 10.3390/md17060350
Kanneganti T. D., Lamkanfi M., Núñez G. (2007). Intracellular NOD-like receptors in host defense and disease. Immunity. 27, 549–559. doi: 10.1016/j.immuni.2007.10.002
Khosravi S., Bui H. T. D., Rahimnejad S., Herault M., Fournier V., Kim S. S., et al. (2015). Dietary supplementation of marine protein hydrolysates in fish-meal based diets for red sea bream (Pagrus major) and olive flounder (Paralichthys olivaceus). Aquaculture 435, 371–376. doi: 10.1016/j.aquaculture.2014.10.019
Kiela P. R., Ghishan F. K. (2016). Physiology of intestinal absorption and secretion. Best Pract. Res. Clin. Gastroenterol. 30, 145–595. doi: 10.1016/J.BPG.2016.02.007
Kiewiet M. B. G., Faas M. M., de Vos P. (2018). Immunomodulatory protein hydrolysates and their application. Nutrients 10, 9045. doi: 10.3390/nu10070904
Kokou F., Gupta S., Kumar V. (2022). Editorial: understanding the interplay between diet, feed ingredients and gut microbiota for sustainable aquaculture. Front. Mar. Sci. 9. doi: 10.3389/fmars.2022.853548
Kousoulaki K., Rønnestad I., Olsen H. J., Rathore R., Campbell P., Nordrum S., et al. (2013). Krill hydrolysate free amino acids responsible for feed intake stimulation in Atlantic salmon (Salmo salar). Aquacult. Nutr. 19, 47–61. doi: 10.1111/anu.2013.19.issue-s1
Kovacs-Nolan J., Zhang H., Ibuki M., Nakamori T., Yoshiura K., Turner P. V., et al. (2012). The pepT1-transportable soy tripeptide VPY reduces intestinal inflammation. Biochimica et Biophysica Acta – General Subjects 1820, 1753–1763. doi: 10.1016/j.bbagen.2012.07.007
Kristinsson H. G., Rasco B. A. (2000). Fish protein hydrolysates: production, biochemical, and functional properties. Crit. Rev. Food Sci. Nutr. 40, 43–81. doi: 10.1080/10408690091189266
Krzywinski M., Schein J., Birol I., Connors J., Gascoyne R., Horsman D., et al. (2009). Circos: an information aesthetic for comparative genomics. Genome Res. 19, 1639–1455. doi: 10.1101/gr.092759.109
Leduc A., Zatylny-Gaudin C., Robert M., Corre E., Corguille G. L., Castel H., et al. (2018). Dietary aquaculture by-product hydrolysates: impact on the transcriptomic response of the intestinal mucosa of European seabass (Dicentrarchus labrax) fed low fish meal diets. BMC Genomics 19, 396. doi: 10.1186/s12864-018-4780-0
Li B., Dewey C. N. (2011). RSEM: accurate transcript quantification from RNA-seq data with or without a reference genome. BMC Bioinf. 12, 1–16. doi: 10.1186/1471-2105-12-323/TABLES/6
Li Y., Bruni L., Jaramillo-Torres A., Gajardo K., Kortner T. M., Krogdahl Å. (2021). Differential response of digesta- and mucosa-associated intestinal microbiota to dietary insect meal during the seawater phase of Atlantic salmon. Anim. Microb. 3, 8. doi: 10.1186/s42523-020-00071-3
Loo Y.-M., Gale M. (2011). Immune Signaling by RIG-I-like Receptors. Immunity 34, 680–692. doi: 10.1016/j.immuni.2011.05.003
Luan Y., Li M., Zhou W., Yao Y., Yang Y., Zhang Z., et al. (2023). The fish microbiota: research progress and potential applications. Engineering. 29, 137–146. doi: 10.1016/j.eng.2022.12.011
Martin S. A.M., Dehler C. E., Król E. (2016). Transcriptomic responses in the fish intestine. Dev. Comp. Immunol. 64, 103–117. doi: 10.1016/j.dci.2016.03.014
Martin S. A. M., Douglas A., Houlihan D. F., Secombes. C. J. (2010) Starvation alters the liver transcriptome of the innate immune response in Atlantic salmon (Salmo salar). Available online at: http://www.biomedcentral.com/1471-2164/11/418. doi: 10.1186/1471-2164-11-418
Maynard C. L., Elson C. O., Hatton R. D., Weaver C. T. (2012). Reciprocal interactions of the intestinal microbiota and immune system. Nature. 489, 231–241. doi: 10.1038/nature11551
Myhre M., Richardsen R., Nystøyl R., Strandheim G. (2022) Analyse marint restråstoff 2021. Available online at: https://hdl.handle.net/11250/3013196.
Nyholm L., Odriozola I., Bideguren G. M., Aizpurua O., Alberdi A. (2022). Gut microbiota differences between paired intestinal wall and digesta samples in three small species of fish. PeerJ 10, e12992. doi: 10.7717/peerj.12992
O’Keefe S. J.D. (2016). Diet, microorganisms and their metabolites, and colon cancer. Nat. Reviews Gastroenterol. Hepatol. 13, 697–706. doi: 10.1038/nrgastro.2016.165
Olsvik P. A., Lie K. K., Jordal A. E. O., Nilsen T. O., Hordvik I. (2005). Evaluation of potential reference genes in real-time RT-PCR studies of Atlantic salmon. BMC Mol. Biol. 6, 21. doi: 10.1186/1471-2199-6-21
Opheim M., Šližytė R., Sterten H., Provan F., Larssen E., Kjos N. P. (2015). Hydrolysis of Atlantic salmon (Salmo salar) rest raw materials - effect of raw material and processing on composition, nutritional value, and potential bioactive peptides in the hydrolysates. Process Biochem. 50, 1247–1575. doi: 10.1016/j.procbio.2015.04.017
Ostaszewska T., Kamaszewski M., Grochowski P., Dabrowski K., Verri T., Aksakal E., et al. (2010). The effect of peptide absorption on pepT1 gene expression and digestive system hormones in rainbow trout (Oncorhynchus mykiss). Comp. Biochem. Physiol. - A Mol. Integr. Physiol. 155, 107–145. doi: 10.1016/j.cbpa.2009.10.017
Parma L., Busti S., Ciulli S., Volpe E., Errani F., Oterhals Å., et al. (2023). Growth, plasma biochemistry and immune-related gene expression of European sea bass (Dicentrarchus labrax) fed bioactive peptides from farmed salmon by-products. Aquaculture 563, 738982. doi: 10.1016/j.aquaculture.2022.738982
R Core Team (2023). R: A language and environment for statistical computing (Vienna, Austria: R Foundation for Statistical Computing). Available at: https://www.R-project.org/.
Regulation (EC) No 999/2001 (2001). Laying down Rules for the Prevention, Control and Eradication of Certain Transmissible Spongiform Encephalopathies [2001] OJ L147. Available online at: https://eur-lex.europa.eu/legal-content/EN/TXT/?uri=celex%3A32001R0999.
Ringø E., Harikrishnan R., Soltani M., Ghosh K. (2022). The effect of gut microbiota and probiotics on metabolism in fish and shrimp. Animals 12, 30165. doi: 10.3390/ani12213016
Ringø E., Olsen R. E., Mayhew T. M., Myklebust R. (2003). Electron microscopy of the intestinal microflora of fish. Aquaculture. 227, 395–415. doi: 10.1016/j.aquaculture.2003.05.00
Ringø E., Strøm E., Tabachek J.-A. (1995). Intestinal microflora of salmonids: A review. Aquacult. Res. 26, 773–895. doi: 10.1111/j.1365-2109.1995.tb00870.x
Ringø E., Zhou Z., Vecino J. L. G., Wadsworth S., Romero J., Krogdahl Å., et al. (2016). Effect of dietary components on the gut microbiota of aquatic animals. A never-ending story? Aquacult. Nutr. 22, 219–282. doi: 10.1111/ANU.12346
Rønnestad I., Gavaia P. J., Viegas C. S. B., Verri T., Romano A., Nilsen T. O., et al. (2007). Oligopeptide transporter pepT1 in Atlantic cod (Gadus morhuaL.): cloning, tissue expression and comparative aspects. J. Exp. Biol. 210, 3883–3965. doi: 10.1242/JEB.007898
Rustad T., Storrø I., Slizyte R. (2011). Possibilities for the utilisation of marine by-products. Int. J. Food Sci. Technol. 46, 2001–2145. doi: 10.1111/j.1365-2621.2011.02736.x
Saldanha A. J. (2004). Java treeview—Extensible visualization of microarray data. Bioinformatics 20, 3246–3248. doi: 10.1093/bioinformatics/bth349
Sandbakken I. S., Five K. K., Bardal T., Knapp J. L., Olsen R. E. (2023). Salmon hydrolysate as a protein source for Atlantic salmon; prion content and effects on growth, digestibility and gut health. Aquaculture. 576, 739863. doi: 10.1016/j.aquaculture.2023.739863
Schlechte J., Skalosky I., Geuking M. B., Mcdonald B. (2022). Long-distance relationships-regulation of systemic host defense against infections by the gut microbiota. Mucosal Immunol. 15, 809–818. doi: 10.1038/s41385-022-00539-2
Siddik M. A. B., Howieson J., Fotedar R., Partridge G. J. (2021). Enzymatic fish protein hydrolysates in finfish aquaculture: A review. Rev. Aquacult. 13, 406–305. doi: 10.1111/raq.12481
Siddik M. A.B., Howieson J., Partridge G. J., Fotedar R., Gholipourkanani H. (2018). Dietary tuna hydrolysate modulates growth performance, immune response, intestinal morphology and resistance to Streprococcus iniae in juvenile barramundi, Lates calcarifer OPEN. Nat. - Sci. Rep. 8, 15942. doi: 10.1038/s41598-018-34182-4
Tacchi L., Bickerdike R., Douglas A., Secombes C. J., Martin S. A. M. (2011). Transcriptomic responses to functional feeds in Atlantic salmon (Salmo salar). Fish Shellf. Immunol. 31, 704–155. doi: 10.1016/j.fsi.2011.02.023
Terova G., Corà S., Verri T., Rimoldi S., Bernardini G., Saroglia M. (2009). Impact of feed availability on pepT1 MRNA expression levels in sea bass (Dicentrarchus labrax). Aquaculture 294, 288–299. doi: 10.1016/j.aquaculture.2009.06.014
Vacca F., Gomes A. S., Murashita K., Cinquetti R., Roseti C., Barca A., et al. (2022). Functional characterization of Atlantic salmon (Salmo salar L.) pepT2 transporters. J. Physiol. 600, 2377–24005. doi: 10.1113/JP282781
Verri T., Kottra G., Romano A., Tiso N., Peric M., Maffia M., et al. (2003). Molecular and functional characterisation of the zebrafish (Danio rerio) PEPT1-type peptide transporter. FEBS Lett. 549, 115–122. doi: 10.1016/S0014-5793(03)00759-2
Waagbø R. (1994). The impact of nutritional factors on the immune system in Atlantic salmon, Salmo salar L.: A review. Aquacult. Res. 25, 175–197. doi: 10.1111/j.1365-2109.1994.tb00573.x
Wang J., Yan X., Lu R., Meng X., Nie G. (2017). Peptide transporter 1 (PepT1) in fish: A review. Aquacult. Fish. 2, 193–206. doi: 10.1016/j.aaf.2017.06.007
Ye J., Coulouris G., Zaretskaya I., Cutcutache I., Rozen S., Madden T. L. (2012). Primer-BLAST: A tool to design target-specific primers for polymerase chain reaction. Available online at: http://www.biomedcentral.com/1471-2105/13/134. doi: 10.1186/1471-2105-13-134
Zamora-Sillero J., Gharsallaoui A., Prentice C. (2018). Peptides from fish by-product protein hydrolysates and its functional properties: an overview. Mar. Biotechnol. 20, 118–130. doi: 10.1007/s10126-018-9799-3
Zhang H., Ran C., Teame T., Ding Q., Hoseinifar S. H., Xie M., et al. (2020). Research progress on gut health of farmers teleost fish: A viewpoint concerning the intestinal mucosal barrier and the impact of its damage. Rev. Fish Biol. Fish. 30, 569–586. doi: 10.1007/s11160-020-09614-y
Keywords: Atlantic salmon (Salmo salar), feed, hydrolysate, gene expression, RNAseq, microbiota, peptide transporters
Citation: Sandbakken IS, Su H, Johansen L, Zhang Y, Ringø E, Røsbak R, Yakovlev I, Five KK and Olsen RE (2024) Replacing fishmeal with salmon hydrolysate reduces the expression of intestinal inflammatory markers and modulates the gut microbiota in Atlantic salmon (Salmo salar). Front. Mar. Sci. 11:1376516. doi: 10.3389/fmars.2024.1376516
Received: 25 January 2024; Accepted: 01 March 2024;
Published: 22 March 2024.
Edited by:
Ida Grong Aursand, SINTEF Ocean, NorwayReviewed by:
Carlos Alfonso Alvarez-González, Universidad Juárez Autónoma de Tabasco, MexicoErick Perera, Spanish National Research Council (CSIC), Spain
Copyright © 2024 Sandbakken, Su, Johansen, Zhang, Ringø, Røsbak, Yakovlev, Five and Olsen. This is an open-access article distributed under the terms of the Creative Commons Attribution License (CC BY). The use, distribution or reproduction in other forums is permitted, provided the original author(s) and the copyright owner(s) are credited and that the original publication in this journal is cited, in accordance with accepted academic practice. No use, distribution or reproduction is permitted which does not comply with these terms.
*Correspondence: Ingrid Schafroth Sandbakken, SW5ncmlkLnNhbmRiYWtrZW5AbnV0cmltYXIubm8=