- 1School of Natural Sciences, Macquarie University, Sydney, NSW, Australia
- 2Centre for Marine Science and Innovation, School of Biological, Earth and Environmental Science, University of New South Wales, Sydney, NSW, Australia
- 3School of Life and Environmental Sciences, The University of Sydney, Sydney, NSW, Australia
- 4NSW Department of Primary Industries, Port Stephens Fisheries Institute, Taylors Beach, NSW, Australia
- 5The Australian Institute of Marine Science, Townsville, QLD, Australia
Current rates of ocean warming are predicted to exacerbate ongoing declines in seagrass populations. Above-ground responses of seagrass to increasing temperatures have been studied from a direct physiological perspective while indirect effects, including changes to microbially-mediated below-ground processes, remain poorly understood. To test potential effects of increased temperature on seagrass growth and associated microbial communities, we sampled seagrass beds experiencing ambient and elevated water temperatures at Lake Macquarie, Australia. Sites with warmer water were associated with a plume from a power station discharge channel with temperatures analogous to conditions predicted by 2100 under current rates of ocean warming (+3°C). The microbial community composition in both sediments and leaf tissues varied significantly between warm and ambient water temperatures with higher relative abundances of putative sulphate-reducing bacteria such as Desulfocapsaceae, Desulfobulbaceae and Desulfosarcinaceae in sedimentary communities in warm water. Above-ground biomass and seagrass growth rates were greater at warm sites while below-ground biomass and detrital decomposition rates showed no difference suggesting potential buffering of temperature effects below-ground. These findings suggest a 3°C rise in temperate regions is unlikely to induce mortality in seagrass however, it may shift microbial communities towards more homogenous structure and composition.
1 Introduction
The world’s oceans have been warming by approximately 0.13°C decade-1 over the past 100 years, with mean global ocean temperatures predicted to rise 1-4°C by 2100 (Howes et al., 2015) Temperatures in shallow estuaries that have restricted water exchange with the ocean appear to be rising at a greater rate than oceanic waters, e.g. 2.16°C over the last 12 years in New South Wales, Australia (Scanes et al., 2020). This rise in temperature is having negative effects on marine and estuarine habitats including macrophytes such as seagrasses (Chefaoui et al., 2018; Babcock et al., 2019). Although slight temperature increases can in some cases enhance seagrass growth rates, once a species’ thermal threshold is reached, there can be negative impacts (York et al., 2013; Anton et al., 2020; Nguyen et al., 2021). For example, prolonged marine heatwaves up to 3°C above ambient have caused seagrass mortality in temperate and subtropical regions (Marba and Duarte, 2010; Thomson et al., 2015; Serrano et al., 2021; Jung et al., 2023). While responses of some seagrasses to temperature changes have been well studied, the response of microbes and the role they play in ameliorating these environmental stressors has rarely been investigated (Garcias-Bonet et al., 2016; Fuggle et al., 2023).
Seagrasses host a wide variety of microorganisms on their leaves, rhizomes, roots, and in surrounding sediments (Weidner et al., 2000; Uku et al., 2007; Garcias-Bonet et al., 2016). Below-ground microbial processes in the plant rhizosphere govern much of the above-ground attributes of plants (Gribben et al., 2017; Wardle et al., 2004; Lugtenberg and Kamilova, 2009). Microbes can also influence growth, health, and productivity of seagrasses, and thus underpin ecosystem services such as nursery habitat, carbon sequestration, and coastal protection provided by seagrasses (Ugarelli et al., 2017; Brodersen et al., 2018). Microbes can have negative effects on plant performance through parasitism, resource competition, and pathogenesis, however, many microbes in the same physical niche can benefit host plant performance through nutrient acquisition and hormone production (Hansen et al., 2000; Mendes et al., 2013; Tarquinio et al., 2019; Tarquinio et al., 2019; Fuggle et al., 2023). Environmental stressors such as warming temperatures may disturb microbes above and below-ground which could in turn have serious implications for seagrass function (Seymour et al., 2018). Therefore, understanding how below-ground processes and microbial communities interact within seagrass meadows may be critical to our understanding of sediment function and important for the resilience and conservation of seagrasses under multiple environmental stressors.
While microbes associated with seagrass leaves, rhizosphere (i.e. root structure and sediment particles in-between and in close contact with the roots), and surrounding sediments all play important roles in the health and functioning of seagrasses, the microbes which occupy the rhizosphere and sediments in seagrass meadows are considered to be the most vital as they can influence below-ground processes in seagrass meadows (Gribben et al., 2017; Brodersen et al., 2018; Gribben et al., 2018; Martin et al., 2020). Seagrasses, commonly thrive in anoxic sediments because they can transport oxygen from their leaves to their rhizosphere (Borum et al., 2007) and their root-associated microbes can oxidise toxic sulphides (Martin et al., 2020). Further, microbes, both above and below-ground, in seagrass meadows can alter biogeochemical processes and maximise the positive metabolic responses required for seagrass to survive under stress (Lehnen et al., 2016; Crump et al., 2018). For example, bacteria provide a mechanism for sulphide oxidation, ammonium production, nitrogen fixation, and absorption of nutrients within seagrasses (Hemminga et al., 1991; Hansen et al., 2000; Cifuentes et al., 2003). In this example, seagrasses provide habitat and energy sources to hosted microbial assemblages through the exudation of nutrients while the microbes are benefiting from protection from environmental stressors (Kirchman et al., 1984; Holmer et al., 2006; Ugarelli et al., 2017). Changes in microbial communities can result in seagrasses becoming more susceptible to disease, environmental change, and competition with invasive species (Gribben et al., 2018; Tarquinio et al., 2019; Fuggle et al., 2023).
Interactions between seagrasses and their associated microbes have garnered increasing attention but little is known about the effects of temperature on seagrass microbes and the consequences for ecosystem functions (Seymour et al., 2018). It is possible that increased water temperature will have the greatest impact on above-ground components (e.g., leaf tissue production) and processes (e.g., photosynthesis and nutrient cycling) because of direct exposure in the water column, while below-ground components may be buffered by the limited water exchange between water column and sediment bed (York et al., 2013; Sawall et al., 2021; Marbà et al., 2022).
Generally, increases in temperature lead to increases in microbial metabolism and growth, until temperatures exceed the optimal thermal range of the microbes (Pendall et al., 2004; von Lützow and Kögel-Knabner, 2009). Different species of microbes have different functions and different thermal tolerances. For example, in plant-microbial interactions, when temperatures exceed the optimal range, above-ground microbes have fewer beneficial effects on plants and can have detrimental effects, such as increased physiological costs (i.e., respiration rates) (O’Brien et al., 2020). Seagrasses can release organic compounds and exudates into the surrounding sediment, which can attract and support different microbes (Mohapatra et al., 2022). This can occur through a variety of mechanisms, including competition for resources, adaptation to environmental conditions, and interactions with other members of the microbial community (Cúcio et al., 2016; Lin et al., 2021). When certain microbial species are favoured or selected over others in a particular environment this is known as selection. As some proportion of microbial composition is selected by environmental conditions (Zhang et al., 2020), we predict that elevated temperatures may reduce variation in diversity of above and below-ground microbial communities because some thermal tolerances will be exceeded, leading to selection of a more consistent group of microbes with similar environmental functions.
The aim of this study was to investigate changes in above and below-ground microbial communities, and seagrass productivity in meadows of the seagrass Zostera muelleri subsp. capricorni (Asch.) (Hereafter referred to as Z. muelleri) in response to consistently elevated water temperatures (+ 3°C above ambient; ambient: 18°C in winter to 26°C in summer; warm: maximum 30°C in summer) created by a coal-fired power station. Specifically, this study determined differences in above- (seagrass leaf biomass and growth rates) and below-ground processes (root and rhizome biomass and detrital decomposition rates) and microbial communities between ambient water temperatures and elevated water temperatures. Z. muelleri was selected as the target species of the study because it has a wide geographical range across temperate and tropical coastal regions in the southern hemisphere (Short et al., 2007) and is susceptible to stressors associated with climate change, particularly temperature (Valle et al., 2014; Lefcheck et al., 2017). Finally, this study also examined which above and below-ground abiotic variables, independent of temperature (e.g., sediment properties and water quality), accounted for differences in seagrass productivity, detrital decomposition, and above and below-ground bacterial communities.
We hypothesised that the warm conditions would enhance seagrass productivity given the thermal optimum for Z. muelleri is ~27°C with negative effects not apparent until 32°C (York et al., 2013). We also predicted that detrital decomposition would be greater at warm sites because decomposition in typical anoxic sediments occurs via microbial mediation which can increase with warming temperatures (Trevathan-Tackett et al., 2017). Due to the temperature sensitive nature of many bacteria, we also predicted differences in composition of both leaf tissue and sedimentary bacterial communities between warm and ambient sites. Specifically, we predicted a reduction in variation between bacterial community assemblages in warm sites both above and below-ground in accordance with the stress gradient hypothesis which suggests that communities may tend towards higher homogeneity in stressful environmental conditions (Bertness and Callaway, 1994).
2 Methods
2.1 Survey location and sampling design
We used a thermal plume in Lake Macquarie (NSW, Australia) as analogue to future estuarine conditions under current rates of warming. Lake Macquarie is one of the largest saltwater coastal lakes in Australia, which has predominantly urbanised catchments. Lake Macquarie is a wave dominated estuary with a restricted permanent opening to the Pacific Ocean to the east, which results in limited water exchange and < 0.1 m tidal variation (Ingleton and McMinn, 2012) and freshwater inputs from Wyee Creek, Dora Creek, and Cockle Creek to the south, west and north respectively. The plume enters Myuna Bay on the western shoreline of Lake Macquarie where estuarine water used to cool Australia’s largest power station has been discharged into the lake since 1980’s (Figure 1).
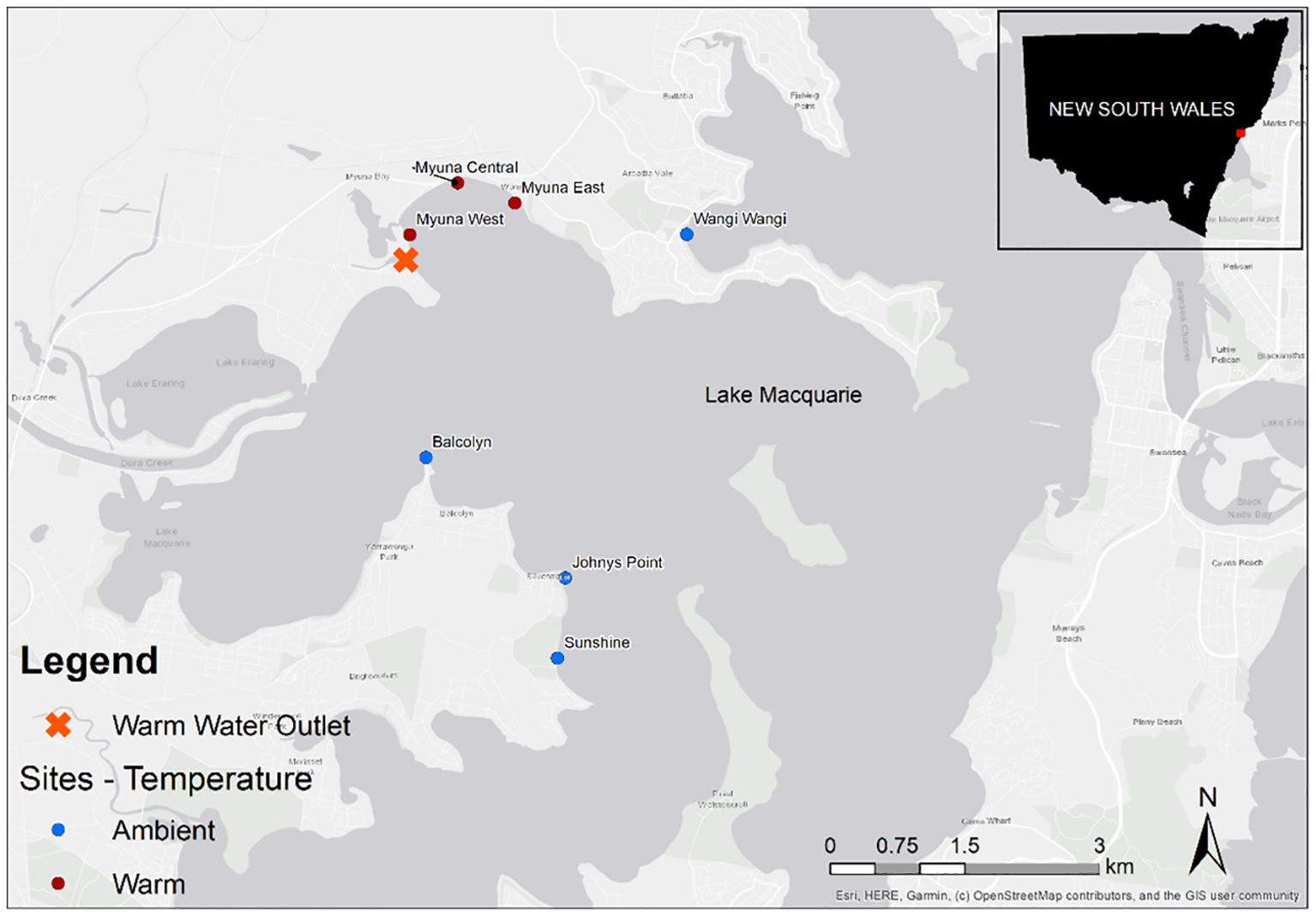
Figure 1 Location of study sites within Lake Macquarie, Australia. Orange cross represents the warm water outlet. Blue points represent ambient sites and brown points represent warm sites.
Field surveys of Z. muelleri seagrass beds were conducted across seven sites (three warm and four ambient) in Lake Macquarie (Figure 1). The study sites located within this warm plume experienced an increase in water temperature of 2- 4°C above ambient (Ingleton and McMinn, 2012). Temperatures in this warm plume are analogous to IPCC predictions for global ocean temperature increases by the end of the century (Howes et al., 2015), thus it is an ideal system for investigating seagrass response to elevated water temperatures under current warming scenarios. All study sites were located on the western side of the lake at a minimum distance of 750 m apart (straight line) and were selected based on temperature profiles from previous studies (Glasby unpublished data). All sites were between 0.5-1 m water depth with large beds (extending >100 m from the shore) of Z. muelleri.
All sampling was conducted in the Austral summer during September 2020 and January 2021 (Supplementary Table 1). September sampling included: surveys of water and sediment quality, seagrass biomass, and the bacterial communities associated with seagrass sediments and seagrass leaf tissues while January sampling included seagrass growth rates and detrital decomposition rates. Each of these variables were collected from eight randomly selected plots (30 cm x 30 cm) at each site (total n = 56). Plots (30 cm x 30 cm) were ~2 m apart to maintain independent sampling. It is important to note the difference between warm and ambient temperatures did not change between sampling periods.
2.2 Environmental variables
2.2.1 Water and sediment variables
Temperature, dissolved oxygen (DO), pH and conductivity of the water were measured in situ at each site in September 2020 and January 2021 using a Multiparameter Water Quality Sonde (YSI, USA) deployed 0.5 m below the water surface and ~0.25 m above each replicate plot (n= 56).
Sediment pH and oxidation-reduction potential (ORP) were measured using an Orion Redox combination electrode (Thermo Fisher Scientific, USA) in each replicate plot. The probe was inserted into the sediments to a depth of 5-10 cm to sample around the seagrass rhizosphere and pH and ORP values recorded immediately (Marba et al., 2010).
Within each replicate plot, a 50 ml sediment core was collected from the top 10 cm of the seagrass bed for particle size analysis and to determine organic matter. Any visible organic matter (seagrass matter, shells, leaves, sticks etc.) and excess water was removed from the sample before storage on ice for transport to the laboratory where samples were frozen at -20°C.
2.2.2 Sediment analyses
To determine the particle size distribution at each site and to test for relationships with seagrass productivity and bacterial community measures, particle size analysis by laser diffractometry was conducted on all sediment samples following manufacturer’s protocols with minor alterations (Malvern Instruments 2007). Firstly, a 30 g (wet weight) sediment sub-sample from each replicate plot was treated with hydrogen peroxide (30% H2O2) for at least 12 h to remove all organics. Sub-samples were stirred once for 30 s to ensure complete reaction with the hydrogen peroxide. Sub-samples were then centrifuged at 2,000 rpm for 5 min. Excess hydrogen peroxide was then removed by pouring off the top layer without losing any fine sediment particles. The remaining sediment was transferred into a new beaker. Sub-samples were then wet sieved with a 2 mm aperture sieve, transferred to a new beaker and mixed using a mechanical stirrer to ensure complete dispersion before a subsample was extracted using a pipette. Each subsample was measured in the Mastersizer 2000 laser diffraction particle size analyser (Malvern Instruments, UK) with particle refractive index of 1.54 and water refractive index of 1.33. Each sub-sample was analysed three times using a measurement time of 30 s. The average particle size distribution of the three runs was used as this is considered most representative of the true particle size distribution (Šinkovičová et al., 2017). Grain sizes were classified into two different categories: fines (0.01-62 μm) and sand (62.5-2000 μm).
Loss-on-ignition (LOI) was analysed with a Lindberg Blue box furnace to measure the organic matter (OM) of sediment sub-samples at each site and to observe relationships with seagrass productivity and bacterial community measures. Firstly, the sample was homogenised and ~3 g (wet weight) sediment was sub-sampled into a pre-weighed crucible. Sediments were then dried at 105°C for at least 12 h and weighed again to obtain dry weight. The crucible was then placed in the muffle furnace at 560°C for 12 h. The sample was then weighed immediately after being taken out of the furnace to obtain ash dry weight. The weight before entering the furnace minus final weight equals the percentage of organic carbon loss on ignition (Wang and Wang, 2011).
2.3 Bacterial assemblage analysis
2.3.1 Above and below-ground bacterial assemblages
For assessing the above-ground microbial assemblages, seagrass leaf samples were collected aseptically from each replicate plot (same plots used for measuring seagrass biomass and environmental variables) by cutting 5-10 shoots at their base and placing them in a sterile 50 ml centrifuge tube. To assess below-ground bacterial assemblages, sediment samples were collected aseptically from each replicate plot by inserting a 50 ml centrifuge tube into the top 10 cm of sediment (the same depth as for sediment pH and ORP), ensuring minimal seagrass leaf fragments and infauna were collected. Samples were immediately put on ice and frozen at -80°C within 5 h of collection.
2.3.2 DNA extraction
For the leaf and sediment samples, genomic DNA was extracted from 0.25 g of plant tissue or sediment, using bead beating and chemical lysis with the DNeasy PowerSoil Pro Kit (QIAGEN, Germany) according to the manufacturer’s standard instructions. DNA concentrations and potential contamination were evaluated using a Nanodrop-1000 spectrophotometer (Thermo Fisher Scientific, USA).
2.3.3 Bacterial community characterisation
To examine bacterial community composition, the 16S rRNA gene was amplified with the universal forward primer 27F (V1) (5′-AGAGTTTGATCMTGGCTCAG-3′) and the universal reverse primer 519R (V3) (5′-CGGTTACCTTGTTACGACTT-3′) (Weisburg et al., 1991). The V1-V3 region was polymerase chain reaction (PCR) amplified using 35 cycles at the temperatures of 94°C for 30 s, 55°C for 10 s and 72°C for 45 s. The resultant amplicons were visualised on 1% agarose gel with GelRed (1:10000). We included a positive and no template control in 60 PCR reactions.
Genomic DNA was used to prepare DNA libraries with the Illumina TruSeq DNA library preparation protocol. Sequencing was performed on the Illumina MiSeq platform at Ramaciotti Centre for Genomics (UNSW Sydney, Australia). All raw sequences and meta-data have been uploaded to NCBI Sequence Read Archive (SRA) under BioProject ID: PRJNA925291.
2.4 Seagrass productivity metrics and detrital decomposition rates
2.4.1 Above and below-ground biomass
Within each plot, a seagrass biomass sample was collected by inserting a PVC core (10 cm diameter) 15 cm into the sediments (Jacobs, 1979). The material collected above and below the sediment surface was rinsed with seawater in the field to remove excess sediment and placed in a Ziplock sample bag. All samples were placed on ice for transportation and stored at -20°C until processing.
All biomass samples were washed with tap water to remove all remaining mud, sand, shell grit and gravel. Above-ground seagrass biomass samples (all shoots and flowering shoots) were cut at the point where they intersected the rhizome (Jacobs, 1979). The separated above and below-ground materials were oven-dried at 60°C for at least 72 h to constant weight and then weighed to obtain total above and below-ground biomasses (dry weights). Total biomass was then calculated using the combined weight of the above and below-ground biomass values.
2.4.2 Growth rates
At each site, all Z. muelleri plants within three 5 x 5 cm plots (~2 m apart, depth 0.5-1 m) were marked using a pin prick method to assess growth rates. Specifically, two small holes were made in the sheath region of each shoot using a sharp probe to create two corresponding reference scars (Short and Duarte, 2001). One scar was on the sheath which does not grow, and the other on the leaf blades inside the sheath which continues to grow. All plants within each plot were resampled after 10-11 d using a trowel for collection and were placed on ice for transport and stored at 4°C until analysis.
Plants were rinsed in the laboratory to remove all sediment and the below-ground components of the plants were discarded leaving only the marked shoots. The entirety of each marked shoot was then cut at the bottom of each shoot’s lowest corresponding reference scar and above the highest reference scar (Short and Duarte, 2001). The remaining plant tissue between the reference scars is the new growth which developed between the time of marking and time of sampling. The combined new leaf tissue for the five shoots was oven dried at 60°C for at least 72 h and weighed to obtain an average value of new growth per shoot per day between shoot marking and collection (Short and Duarte, 2001).
2.4.3 Detrital decomposition rates
A detrital decomposition experiment was conducted to examine the rate at which seagrass detritus decomposed within seagrass sediments. Three sample bags (~2 L each) of wet Z. muelleri detritus were collected from each site to prepare litter bags. All detritus was mixed and dried for at least 72 h at 60°C.
For the decomposition experiment, green, senescent Z. muelleri leaf detritus was added to 1.0 mm nylon mesh litter bags (whole leaf detritus ~10 g dry weight in 15 cm × 15 cm bag: nylon mesh from Allied Filter Fabrics Pty Ltd., NSW, Australia). Litter bags enable tracking of seagrass biomass loss and have been used previously in decomposition studies (Harrison, 1989; Mateo and Romero, 1996; Trevathan-Tackett et al., 2020b). To replicate natural decomposition conditions, litter bags were secured to the sediments using metal stakes. Three litter bags per site were secured 10-20 m from shore at a depth of 0.5-1 m, central to the meadow and left 13 - 14 d before being collected. Each bag was then washed thoroughly to remove any sediment from the bags taking care to ensure that no detrital matter was lost. Litter bags were then dried in an oven at 60°C for at least 72 h and then the detritus was weighed. Dry weight after decomposition was then compared to dry weight before decomposition to determine the percentage of detritus that had decomposed.
2.5 Statistical analyses
2.5.1 Bioinformatics
FASTQ files were quality trimmed [maximum truncation lengths of 290 bps (forward) and 260 bps (reverse; maximum expected error of 2 (forward) and 6 (reverse)]. Sample inference was made using the core DADA2 denoising algorithm to reconstruct exact Amplicon Sequence Variants (ASVs) and filter rare ASVs and chimera sequences. After denoising, paired reads were merged to unique sequences and ASV taxonomic classifications were obtained using the SILVA reference database (v. 138). Quality of sampling and processing was also assessed through the calculation of Good’s coverage and rarefaction curves. From this table, chimera removal was done through a consensus method and with a conservative approach (Callahan et al., 2016). Chloroplast, archaea and mitochondria were removed from the ASV table prior to statistical analysis. All steps of this pipeline were done using the dada2 package (Callahan et al., 2016) in R Studio (V.3.6 R Core Team 2017).
2.5.2 Bacterial community
ASVs from sedimentary and leaf tissue bacterial datasets were removed if their total abundance was <0.01% of the total dataset. Each dataset was then standardised for sequencing depth by dividing each cell by total reads per sample.
Sedimentary and leaf surface tissue bacterial community data were compared between temperature category (fixed, two levels: warm versus ambient) and sites (random, 3 levels for warm and 4 levels for ambient) using PERMANOVA (Anderson, 2005) in the PERMANOVA+ add‐on for primer V7 (PRIMER‐E, UK) (Clarke and Gorley, 2006). Bray–Curtis similarity matrices were generated using square root‐transformed relative abundance (community structure) of ASVs or ASV presence/absence (community composition), using 999 permutations of residuals under a reduced model. Bacterial diversity within temperature categories (alpha diversity) was estimated using, Shannon and Chao1 diversity indices on rarefied ASV data.
PERMANOVA was also used to generate estimates of components of variation for fixed and random effects to compare the relative importance of each factor in explaining total variation in the model (Underwood and Chapman, 1996; Anderson, 2007). Multivariate patterns in bacterial assemblages were visualised using non‐metric multidimensional scaling (nMDS) derived from Bray–Curtis similarity matrices (PRIMER-E).
Relationships between environmental variables and bacterial community structure and composition were identified using multivariate multiple regression (DISTLM) within the PERMANOVA+ add‐on (Clarke and Gorley, 2006). Where two or more environmental variables were strongly correlated (R2 > 0.9), just one variable was retained in the model to represent the correlated variables while the others were excluded from the model. Analyses of these relationships used Akaike Information Criterion (AIC) and a stepwise selection procedure to select the best explanatory model.
Differences in the abundances of ASVs between warm and ambient temperatures were identified using the ANCOMBC package (Lin and Peddada, 2020) in R Studio using default parameters (version 3.5.4; R Core Team 2017).
2.5.3 Seagrass and environmental variables
All analyses were performed on untransformed data after first checking data for homogeneity of variance using Shapiro-Wilk test and Levene’s test. All statistical analyses were conducted using R Studio (version 3.5.4; R Core Team 2017) with the lme4 (Bates et al., 2014), and vegan packages (version 2.5–2) (Oksanen et al., 2013).
To determine differences in seagrass response variables (above and below-ground biomass, growth rates and detrital decomposition) between temperature categories (fixed factor with two levels: warm, ambient), a linear mixed-effects models (LMM) was fitted, including site as a random factor nested in temperature.
To examine the relationship between each seagrass response variable and all water and sediment variables, a stepwise model selection procedure and the AIC was used to select the best model. Before fitting models, each abiotic covariate was plotted against each seagrass response variable to check for linearity. No transformations were required as all relationships were linear.
Pearson correlation coefficient was used to determine relationships between highly correlated variables (P>0.8). ORP and sediment pH were the only covariates with a Pearson’s correlation coefficient (R > 0.8) (Supplementary Table 2). Model selection was performed for each seagrass response variable with both ORP, and sediment pH included, and also with one excluding the other. As the model with only ORP included had the lowest AIC value, sediment pH was removed from each stepwise model selection. All remaining covariates were normalised using the formula, X-mean/SD, before the model selection was run.
3 Results
3.1 Water and sediment variables
Water temperatures were consistently higher (~3°C) at warm sites than ambient sites (F1,5 = 33.1, p < 0.05) at both sampling times (F1,103 = 0.01, p (interaction) = 0.93). Temperatures were higher in January (warm mean 29.4°C) compared to September (warm mean 21.5°C) (F1,103 = 7800, p < 0.05; Figure 2). The maximum water temperature recorded in summer was 29.96°C.
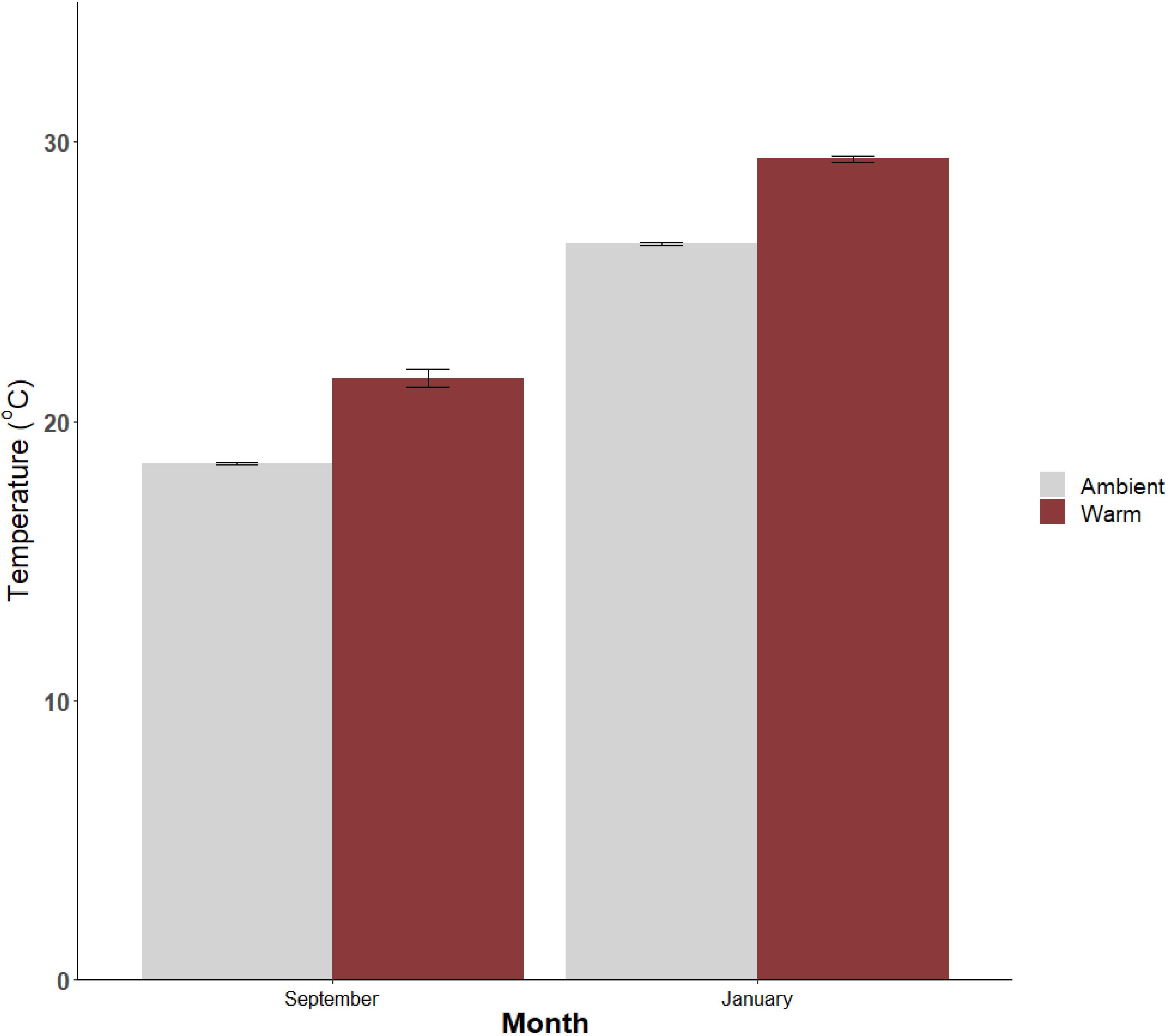
Figure 2 Mean (± SE) water temperature recorded at warm and ambient seagrass sites in Lake Macquarie, Australia during each sampling period (September 2020 and January 2021; N=56).
The above-ground abiotic variables (dissolved oxygen (DO), conductivity, and pH of the water column) did not differ significantly between warm and ambient sites (Supplementary Table 3). However, the below-ground variables organic matter (OM) and sediment pH were greater, and sediments were finer (smaller grain size) at warmer sites, and ORP was greater in ambient sites (Supplementary Table 3).
3.2 Bacterial communities and alpha diversity
3.2.1 Bacterial community structure and composition
Overall, 1135 ASVs were obtained from leaf tissues and 1907 ASVs from sediments. Bacterial community structure (relative abundance of individual ASVs) in leaf tissues and sediments differed between warm and ambient sites (leaf: F1,34 = 3.29, p = 0.02, sediments: F1,34 = 2.42, p = 0.04; Figures 3A, B; Supplementary Table 4). A similar trend was observed for leaf tissue and sedimentary bacterial community composition (presence/absence of ASVs) between warm and ambient sites, although this was not significant (leaf: F1,34 = 2.54, p = 0.05, sediments: F1,34 = 1.98, p = 0.085; Figures 3C, D; Supplementary Table 4).
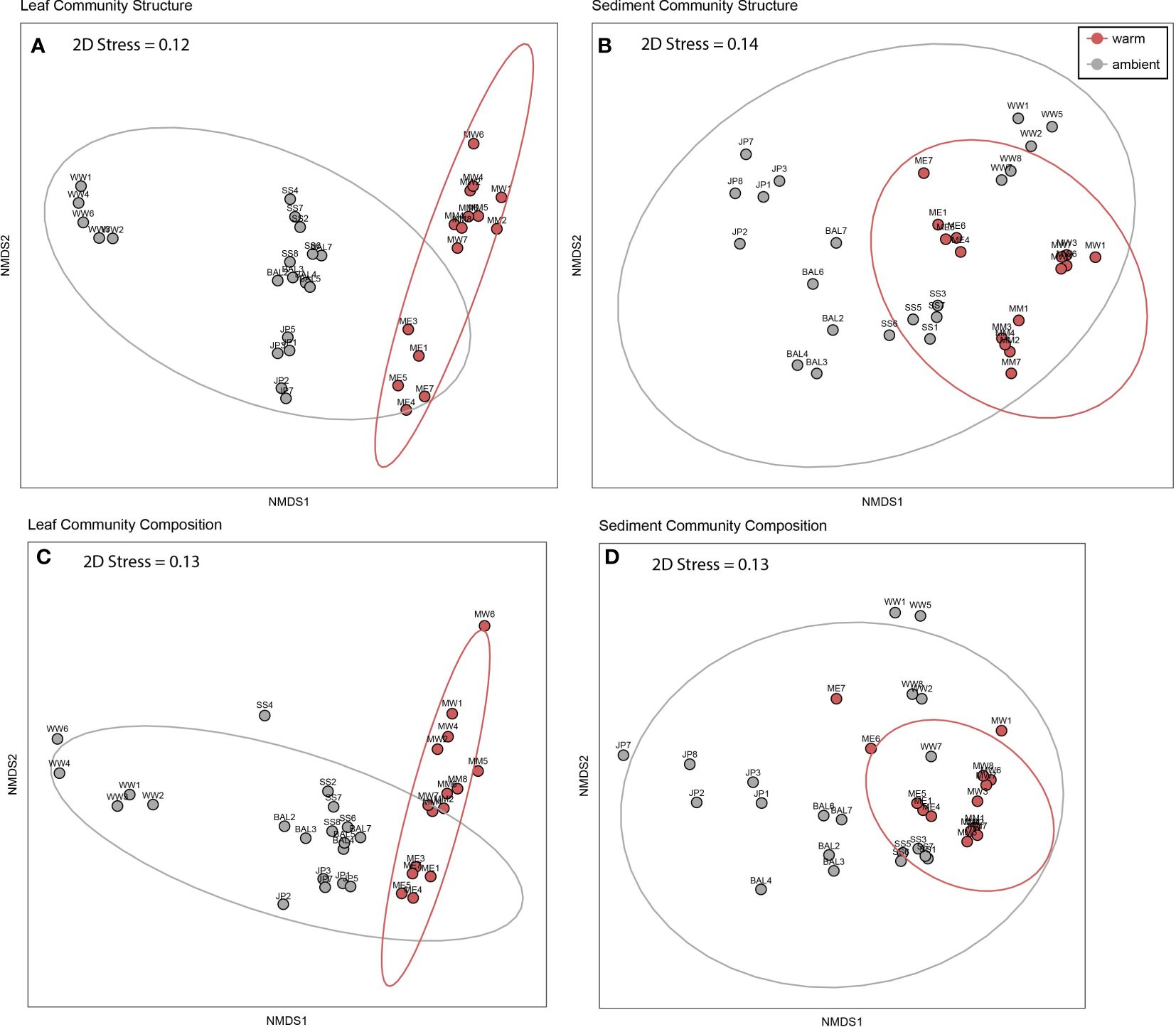
Figure 3 Non-metric multidimensional scaling (nMDS) of leaf tissue and sedimentary bacterial assemblages in warm and ambient sites sampled from seagrass meadows in Lake Macquarie (N=35). (A) Structure of leaf tissue communities (square root transformed) (B) Structure of sedimentary communities (C) Composition of leaf tissue communities (D) Composition of sedimentary communities. Data was standardised by total ASV abundances and then nMDS plots were constructed with Bray-Curtis dissimilarity matrices.
Leaf tissue and sedimentary bacterial community structure had less dispersion among replicate samples in warm sites compared to ambient sites (leaf: F1,34 = 18.11, p = 0.0001, sediments: F1,34 = 6.6, p= 0.0001, Figures 3A, B). This was similar to community composition (leaf: F1,34 = 16.71, p = 0.0001, sediments: F1,34 = 6.6, p = 0.0001, Figures 3C, D).
3.2.2 Bacterial diversity
Neither of the alpha diversity measures for leaf bacterial communities differed between warm and ambient sites (Shannon diversity: p = 0.2154; Figure 4A, Chao1 diversity: p = 0.7718; Figure 4B).
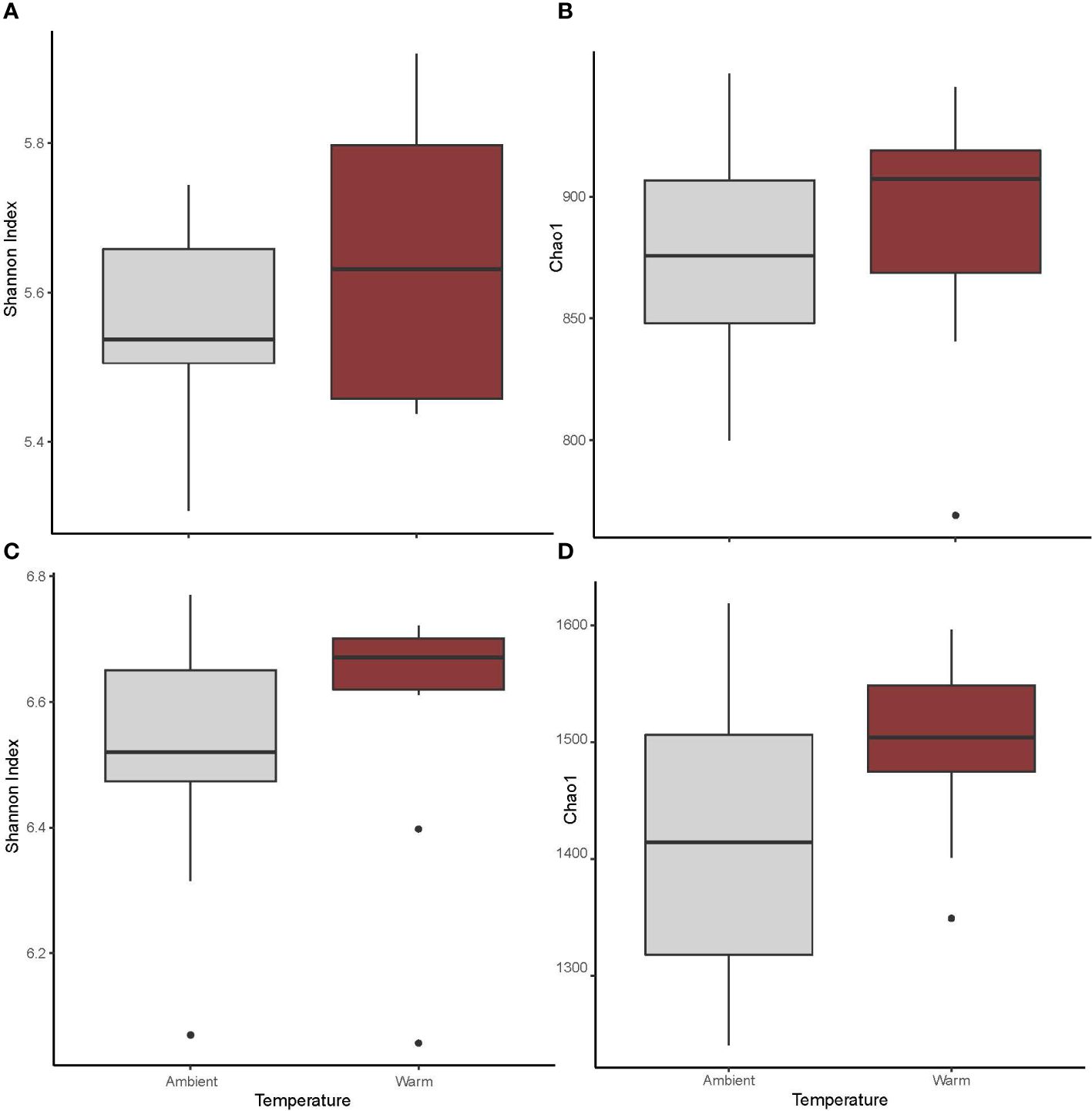
Figure 4 Alpha diversity of microbial communities associated with Z. muelleri leaves and sediments at warm and ambient sites within Lake Macquarie. Mean species richness estimated as (A) Leaf-Shannon index, and (B) leaf-Chao1, and as (C) sediment-Shannon index, and (D) sediment-Chao1. Mean species richness estimated, n = 25.
Of the two alpha diversity analyses for sedimentary communities, one showed no differences between warm and ambient sites (Shannon diversity: p > 0.05; Figure 4C), while the other (Chao1, p < 0.05; Figure 4D) showed warm sites had significantly higher alpha diversity than ambient sites.
3.2.3 Environmental influences
The stepwise model selection for leaf tissue bacterial community structure found that pH (10.4%), DO (5.4%), conductivity (15.9%), ORP (3%), and OM (17.7%) were significant explanatory variables accounting for 52.4% of the variance among replicates (Figure 5A; Supplementary Table 5). Bacterial community composition variation on leaves between warm and ambient communities was partitioned along decreasing OM (18.2%), pH (6.8%), and DO (4.7%) and increasing conductivity (15.7%) and ORP (3.5%) (Figure 5B).
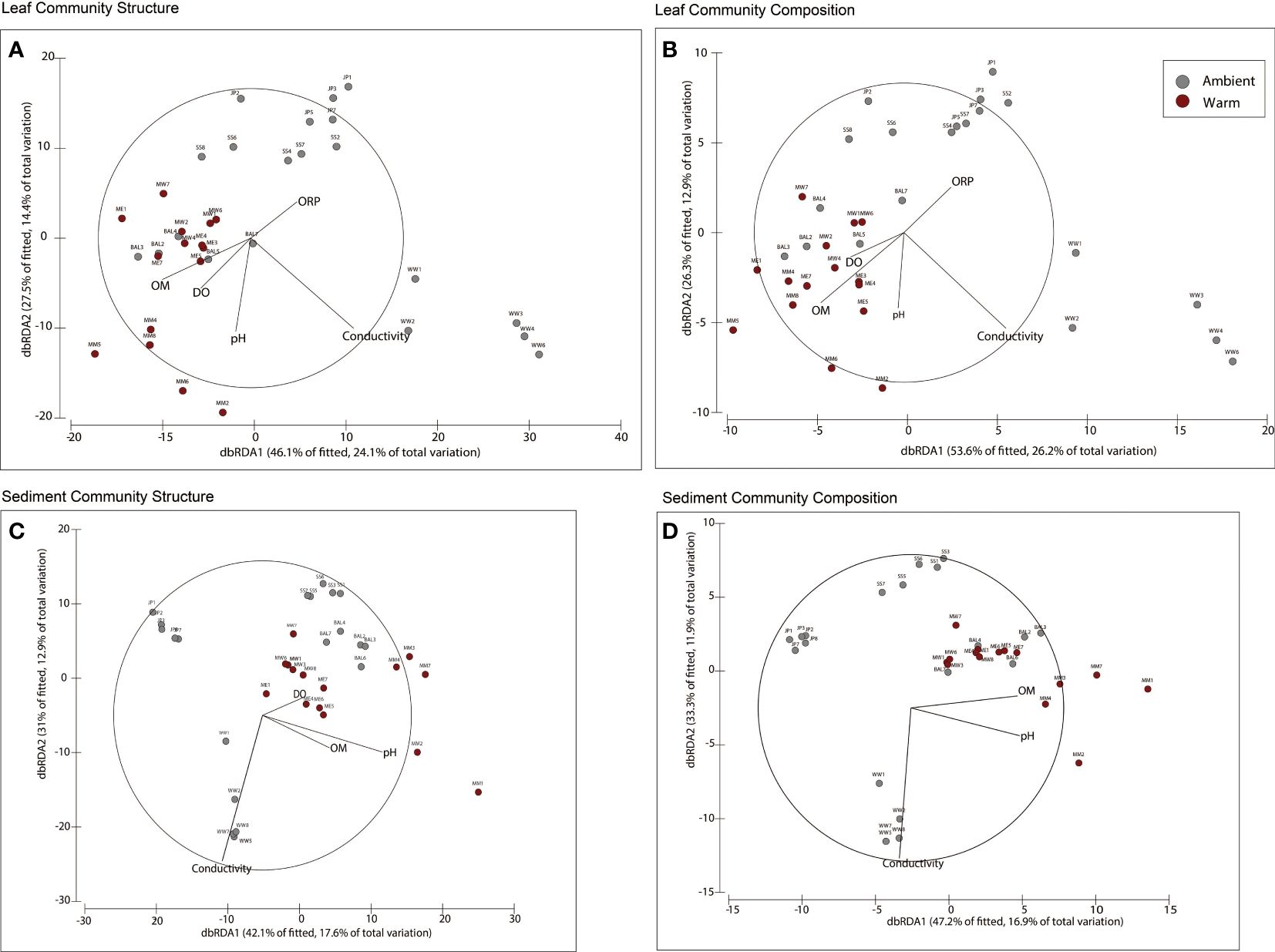
Figure 5 Distance-based redundancy analyses with vectors overlayed for variables selected by model selection for (A) Leaf tissue bacterial community structure, and (B) Leaf tissue bacterial community composition. (C) Sedimentary bacterial community structure, and (D) Sedimentary bacterial community composition. OM, Organic matter; DO, Dissolved oxygen; ORP, Oxidation-Reduction Potential.
The stepwise model selection for sedimentary bacterial community structure explained 42% of variance (R2 = 0.42) and included water quality variables – pH (16.5%), conductivity (12%), and DO (4%), and a sediment variable - OM (8.5%) (Figure 5C; Supplementary Table 5). Variation in sedimentary bacterial community composition was explained by the same variables except DO (R2 = 0.36). The dissimilarity of warm and ambient community composition was also along increasing pH (15%), and OM (9%) and decreasing conductivity (12%) (Figure 5D).
3.2.4 Bacterial assemblage changes
A total of 353 bacterial ASVs (31%) on leaf tissue differed significantly in abundance between warm and ambient sites. The following taxa were relatively more abundant at warm sites Aliikangiella (g), Aquimarina (g), Bdellovibrionaceae OM27 clade (g), Candidatus Amoebophilus (g), Coxiella (g), Flavobacteriaceae (f), Folkiniaceae MD3-55 (g), Lacinutrix (g), Microtrichaceae (f), Nannocystaceae (f), Planctomycetota OM190 (cl), Planktomarina (g), Rhodobacteraceae (f), Sandaracinaceae (f), Ulvibacter (g), Woeseia (g), and Yoonia-Loktanella (g). In contrast, Robiginitalea (g), Schizothrix LEGE 07164 (g), and Sagittlula (g) were relatively more abundant at ambient temperatures (Supplementary Figure 1).
Most bacterial ASVs within the top 25 ASVs that were differentially abundant in leaf tissue between warm and ambient sites were from aerobic bacteria [Flavobacteriaceae (f), Coxiella (g), Planktomarina (g)] (Supplementary Figure 1). The top 25 differentially abundant ASVs also included a mix of heterotrophs [Ulvibacter (g)] and chemotrophs [Sandaracinaceae (f), Robiginitalea (g)] related to various functions including nutrient cycling, and toxin defence [Aquimarina (g)] (Supplementary Figure 1).
In sedimentary bacterial communities the relative abundance of 337 ASVs (18%) differed significantly between warm and ambient temperatures. ASVs with the highest increase in relative abundance in sediments at warm sites belonged to the following taxa: Bacteroidales SB-5 (f), BD2-2 (f), Desulfocapsaceae (f), Desulfobulbaceae (f), Desulfosarcinaceae (f), Sva0081 sediment group (g), Woeseia (g), Gammaproteobacteria Incertae Sedis (o), Robiginitalea (g), Sva0485 (p), Thiogranum (g), and Thiotrichaceae (f). At ambient sites, ASVs with the highest increase relative abundance belonged to the following taxa: Bacteroidales (o), BD2-2 (f), Candidatus Amoebophilus (g), Desulfobulbaceae (f), Desulfosarcinaceae (f), LCP-80 (g), SEEP-SRB1 (g), Gammaproteobacteria (cl), Woeseia (g), Halioglobus (g), and Gammaproteobacteria HOC36 (o) (Supplementary Figure 3).
Among the 25 bacterial ASVs most significantly different in sedimentary communities between warm and ambient sites, there was a mix of aerobes [Halioglobus (g), Thiogranum (g)] and anaerobes [Desulfocapsaceae, Desulfosarcinaceae (f)] (Supplementary Figure 3) which are related to various functions such as nitrogen fixing, sulfur oxidation, and pathogen defence that were more abundant in warm sites. ASVs which were more abundant in ambient sites were chemotrophs associated with sulfur cycling [Desulfocapsaceae (f)] (Supplementary Figure 3).
3.3 Seagrass biomass, growth rates & decomposition rates
Above-ground seagrass biomass (F1,55 = 22.2, p < 0.001; sampled in September; Figure 6A) and growth rates (F1,55 = 15.9, p < 0.001; sampled in January; Figure 6B; Supplementary Table 6) were both over 1.5 times greater at warm sites compared to ambient sites.
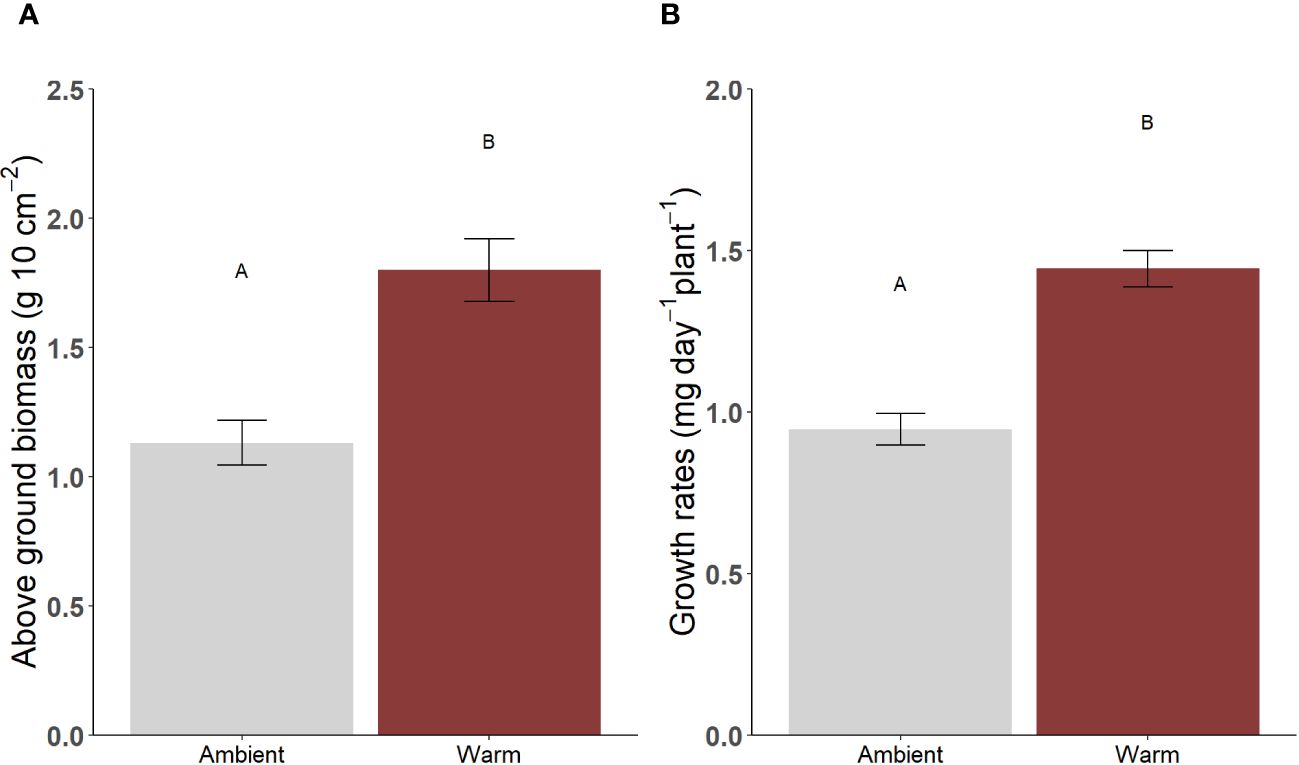
Figure 6 Mean (± SE) (A) Above-ground biomass, (B) Seagrass leaf tissue growth rates, at ambient and warm sites in Lake Macquarie. Sampling was conducted in September for (A) and January for (B). AB used to indicate statistical significance between temperature categories (AB used to indicate statistical significance between temperature categories; N=56).
Stepwise model selection indicated that above-ground seagrass tissue biomass was best predicted by sediment organic matter (31%), with the two being positively associated (AIC = 95.09; Supplementary Figure 2A; Supplementary Table 7).
Seagrass leaf growth rates were positively correlated with increased pH (11.2%), increased water conductivity (7.2%), and increased water temperature (27.1%) and negatively correlated with increasing sediment ORP (10%) (AIC = 4.67; Supplementary Tables 4, 7; Supplementary Figures 2B–E).
Considering conductivity ranged from 49.6-50.9 S/m across all sites, we believe this proportion of variation to be biologically insignificant.
Below-ground biomass of seagrass (sampled in September) and detrital decomposition rates (sampled in January) did not differ between warm and ambient sites but tended to be greater at warm compared to ambient sites (F1,34 = 1.08, p > 0.05 and F1,20 = 4.0, p > 0.05, respectively; Supplementary Table 6; Figure 7).
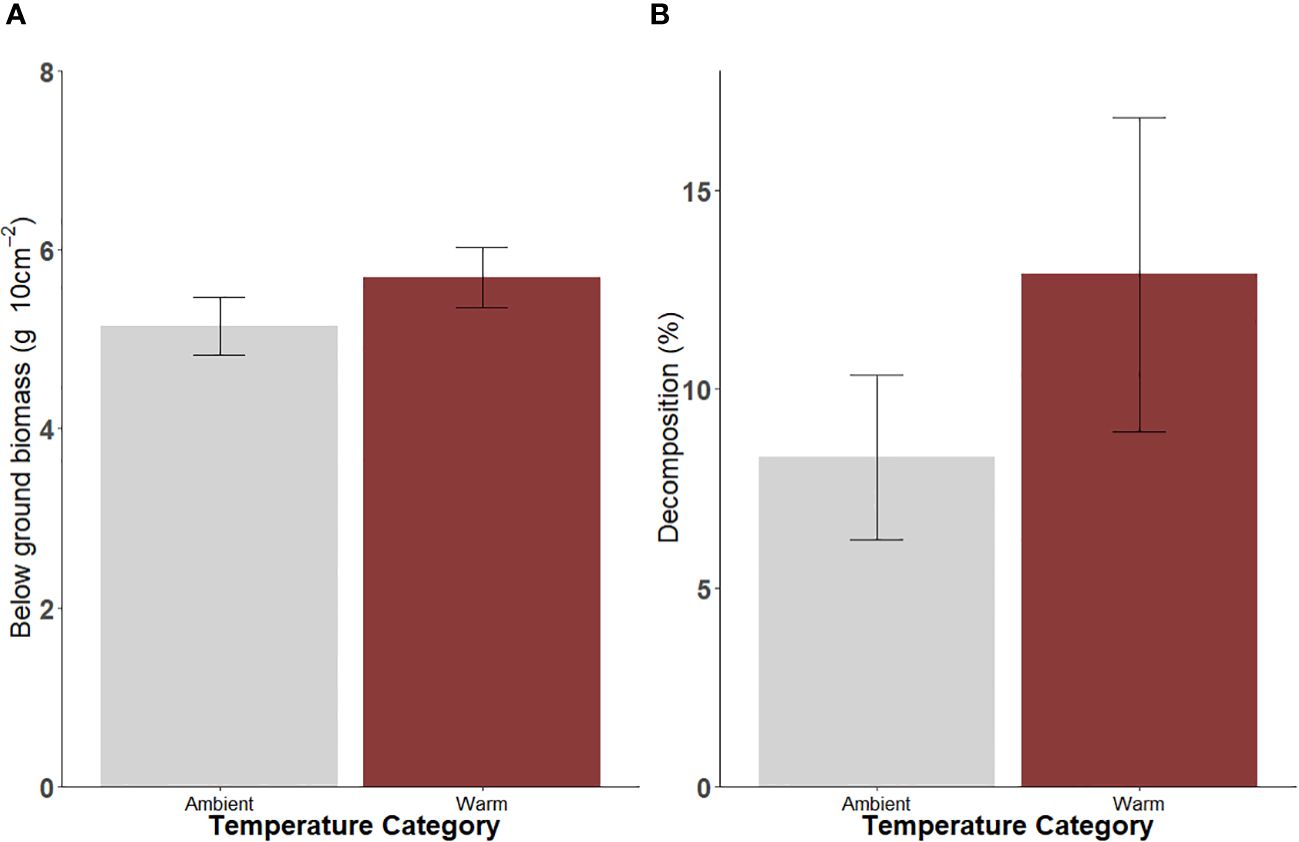
Figure 7 Mean (± SE) (A) below-ground biomass (B) detrital decomposition at ambient and warm sites in Lake Macquarie. Sampling was conducted in September for (A) and January for (B). AB used to indicate statistical significance between temperature categories (n=56).
Variation in below-ground biomass was best explained by sediment organic matter (35%) and temperature (5%), where below-ground biomass increased with increasing sediment organic matter and slightly increased with increasing temperature (AIC = 218.24; Supplementary Figures 4A, B; Supplementary Table 7).
Rates of detrital decomposition decreased as sediment ORP (0.57) increased but were not correlated to any other environmental variable (AIC = 163.64; Supplementary Figure 4C; Supplementary Table 7).
4 Discussion
In this study, a warm plume from a power station discharge channel in Lake Macquarie, Australia provided a thermal plume ~3°C above ambient lake temperatures which was used to investigate in situ differences in above and below-ground microbial communities, and seagrass productivity, in response to long term exposure to elevated water temperatures. Overall, we observed stronger changes in above-ground than below-ground bacterial communities and productivity with increased temperatures which support our hypothesis that the effects of water temperature are stronger above-ground and processes occurring below-ground may be buffered from increases in water temperature.
4.1 Microbial community response to temperature
Bacterial community composition in both sediment and leaf tissue was more homogeneous (i.e. less variable) in warm sites compared to ambient temperature sites. This result aligns with the Stress Gradient Hypothesis (SGH) which posits that environments under stress will have lower community richness where the remaining species occupy niches that directly affect the function of plants (Bertness and Callaway, 1994; Callaway, 2007). In this case under increased temperatures, facilitative interactions between bacteria and seagrass should be stronger compared with ambient/benign conditions where facilitative interactions are weaker (Bertness and Callaway, 1994). Interestingly, above-ground bacterial communities were more homogeneous than below-ground communities in both warm and ambient sites. This result suggests above-ground communities may have greater responses to elevated temperatures than the adjacent below-ground communities potentially due to thermal buffering provided by the top layer of sediments in seagrass meadows. The lower variation in microbial communities under elevated temperatures might indicate a stronger relationship between seagrass and their microbes under stressful conditions. A lower variation in microbial community structure signifies a consistent diversity across samples which suggests little environmental fluctuation which ensures consistent uniform nutrient availability, disease suppression, and overall plant performance through beneficial relationships between microbes and plants (Waymouth et al., 2020). The increase in community homogeneity may also be due to a more consistent assemblage of bacterial groups which can tolerate higher temperature (ASV’s pertaining to thermally tolerant bacteria are discussed later in this section).
An alternative cause of the differences in microbial communities to environmental selection is the selection of preferential bacterial species by plants to aid plant performance under less favourable conditions may be facilitated through the exudation of organic compounds such as sugars, amino acids, and volatile organic compounds into the surrounding water (Naylor et al., 2017; Sasse et al., 2018; Jones et al., 2019). These compounds can function as chemoattractants, attracting certain bacterial species to the area around the plant’s roots. As these exudates occur in response to external environmental forcing on the plants, the change in bacterial communities recorded in this study in warm and ambient temperatures may be related to seagrass nutrient exudation, but this was beyond the scope of testing in the current study.
We also cannot discount the possibility that the increased variability among ambient sites is due to the larger spatial separation across all four ambient sites relative to the three warm sites. The environmental conditions and terrestrial inputs may be more similar between the warm sites which leads to more similar microbial communities (Stadler and del Giorgio, 2022). However, between all sites a spatial separation of at least 750m was maintained, and all sites had a similar neighbouring land use (vegetated parkland) to control for these variables. A key knowledge gap to be determined, is whether differences in microbial communities under environmental stressors are linked to direct environmental selection of microbes or a case of plants selecting the microbes for their own benefits. Three of the four alpha diversity results indicate no changes in diversity between ambient and warm sites however, all show a non-significant trend towards increased diversity in warm sites. This indicates warmer water may select for a slightly wider range of microbes without removing any taxa which may already be present at ambient sites, but overall, warm communities were more homogenous than ambient sites.
Leaf tissue bacterial communities at warm sites contained greater relative abundances of bacteria related to nutrient cycling functions are known to be thermally tolerant [e.g., Woeseia (g) (Hoffmann et al., 2020)]. Of the ASVs which varied most significantly between warm and ambient temperatures, ASV3170 (belonging to family Rhodobacteraceae) was the most abundant in warm temperatures. This result of Rhodobacteraceae tolerating increased temperatures has been shown in multiple studies before (Elifantz et al., 2013; Pootakham et al., 2019; Miyamoto et al., 2023). Rhodobacteraceae spp. can have mutualistic relationships with eukaryotes such as micro- and macroalgae (Bischoff et al., 2021). Additionally, some Rhodobacteraceae spp. are capable of harvesting light to increase biomass yields under stressed environmental conditions, which may explain its presence in warm sites. It can also breakdown antagonistic bacterial pathogens which may protect the seagrass host in this case (Zhang et al., 2016). This pattern has also been shown in other marine systems by Pootakham et al. (2019), which found that various Rhodobacteraceae spp. in coral species Porites lutea are indicator species of thermal stress. Stratil et al. (2013) and Qiu et al. (2019) found that warming caused a shift in epiphytic bacterial communities on brown macroalgae Fucus vesiculosus and Ecklonia radiata. In both cases diversity decreased under warmer temperature treatments where bacteria such as Rhodobacteraceae, Thalassomonas and Alteromonadaceae were more abundant in warmer temperatures. The results of this present study found no differences in bacteria belonging to Thalassomonas or Alteromonadaceae; however, bacteria belonging to Rhodobacteraceae were relatively more abundant in warm sites. As previously mentioned, some Rhodobacteraceae species are regarded as extremophiles due to the stressed marine environments they can survive in which may account for their higher relative abundance in warm conditions (Pujalte et al., 2014). As such, there is potential that this bacterial family may have the same applicability as an indicator species of thermal stress in seagrass meadows.
The leaf tissue ASVs with higher relative abundances in ambient sites were mostly aerobes with functions associated with nutrient and carbon cycling [Robiginitalea, Sagittula (Egan et al., 2013; Tarquinio et al., 2021)]. Interestingly, Sagittula spp. can mineralise aromatic compounds from marine vascular plants, which results in the sequestration of inorganic carbon (Gulvik and Buchan, 2013). In the context of seagrass meadows, Sagittula spp. could influence carbon storage potential. As this bacterium has an optimum temperature of 25°C and was relatively less abundant in warm temperatures, further temperature increases could have consequences for the storage of inorganic carbon in seagrass sediments (Lee et al., 2013).
Sedimentary bacterial communities within seagrass meadows can provide specific functions within typically anoxic and organic enriched sediments (Lugtenberg and Kamilova, 2009; Trevathan-Tackett et al., 2017; Tarquinio et al., 2019; Martin et al., 2020). The interactions between seagrasses and their associated bacterial communities are key to survival in the anoxic marine sediments (Fraser et al., 2018). Although few studies have focussed on the effects of warming on bacterial communities in seagrass sediments, Hicks et al. (2018) found bacterial communities from intertidal mud flats were significantly affected by increases in temperature with bacteria belonging to Proteobacteria phylum increasing significantly between temperatures. This phylum contains a diverse range of functional groups ranging from sulphide-oxidisers to nitrogen cyclers (Seymour et al., 2018; Zhang et al., 2020; Tarquinio et al., 2021). In the present study, nine of the 25 ASVs most differentially abundant in sedimentary communities between warm and ambient sites belonged to the Proteobacteria phylum. In general, sedimentary bacterial communities in warm sites had higher relative abundances of sulphide reducing bacteria [e.g., Bacteroidetes BD2-2 (f), Desulfocapsaceae (f), Desulfosarcinaceae (f)] commonly found in sulphide rich environments (Holmer et al., 2006; Trevathan-Tackett et al., 2017; Martin et al., 2020). This suggests warm sites are richer in sulphide than ambient sites, which is supported by the results of lower ORP (commonly associated with sulphide rich conditions) in warm sites and high organic matter in warm sites (Martin et al., 2020). Sedimentary bacteria with higher relative abundances in the ambient sites included Halioglobus and other chemotrophs with a broad range of associated functions including nitrogen cycling, ferric iron reduction, and other nutrient cycling capabilities (Park et al., 2012; Lehnen et al., 2016; Szitenberg et al., 2022). More oxygenated conditions likely support more diverse and variable microbial communities and decrease the reliance of seagrass on facilitative interactions with sediment bacterial communities.
Variation in leaf tissue bacterial community structure and composition between warm and ambient sites was best explained by increasing pH and increasing organic matter (variables not colinear to temperature). While precise effects of elevated water temperature on epiphytic bacterial communities in seagrasses is still unclear, this interaction has been examined in habitat-forming macroalgae (Stratil et al., 2013). Increasing pH and organic matter also explained the most variation in sedimentary bacterial community structure and composition between warm and ambient sites. The greater separation of warm and ambient bacterial communities with increased organic matter is supported by the higher abundances of bacteria which are found in more anoxic, sulphide rich sediments commonly associated with high organic matter such as Desulfocapsaceae and Sva0081 sediment group (Waite et al., 2020; Jantharadej et al., 2021). The separation of bacterial communities due to variability in pH has important implications similar to temperature, as climate change is predicted to enhance the effects of ocean acidification in many marine ecosystems. Su et al. (2021) found changes in pH levels significantly altered sedimentary bacterial community composition which supports the findings of this study. None of the genera among the 20 most significantly different between warm and ambient sites in this study were identified as pH-sensitive or pH-tolerant by Su et al. (2021). However, five pH-sensitive genera did belong to Proteobacteria phylum which was commonly found in both warm and ambient sites.
4.2 Seagrass response to elevated temperatures
The above-ground biomass production of Z. muelleri was elevated by 11% (± 0.18) on average at warm sites with water temperatures ~3°C above ambient, suggesting a potential increase in seagrass metabolism and photosynthesis under warmer conditions. The same patterns between temperature, seagrass metabolism, and photosynthesis have been previously observed at Lake Macquarie (Cams Wharf; York et al., 2013) and elsewhere [Great Barrier Reef; Collier et al. (2017); Red Sea, Anton et al. (2020)]. York et al. (2013) found 27°C led to the highest seagrass biomass while 32°C resulted in 100% mortality of seagrass within 42 days. Collier et al. (2017) found 31°C led to the highest seagrass biomass while temperatures exceeding 35°C negatively affected growth of Z. muelleri. It is important to note that the Collier et al. (2017) study was conducted in tropical waters with much warmer ambient temperatures compared to Lake Macquarie. Differences in optimal temperatures found in these previous studies and the higher above-ground biomass at warm sites (long-term thermal exposure) recorded in the present study may therefore indicate that this species is able to adapt to local conditions over time. Additionally, an increase in the ratio of above to below-ground partitioning of resources has previously been recorded as a physiological response to environmental stress and may indicate poor survival outlook (Nixon et al., 2001).
Contrary to our predictions, below-ground biomass production in this study was not significantly related to warmer temperatures. This result is similar to those of Kaldy and Dunton (2000) who found no seasonal differences in seagrass below-ground biomass, while identifying a peak in above-ground biomass and biomass production in warmer months. Similarly, detrital decomposition rates did not differ between ambient and warm temperatures, despite an increase in anoxic sediment conditions, and potentially sulphide production (as evidenced through changes in microbial composition). However, previous studies found that increased temperatures increase detrital decomposition rates (Trevathan-Tackett et al., 2017; Trevathan-Tackett et al., 2020a). The non-significant trend in this study may be a result of different deployment intervals, daily temperature pulses, or differences in anoxic vs aerobic decay. The aforementioned studies were conducted under lab conditions for 30 and 80 d while this decomposition component was conducted over 12 d in the field with temperatures fluctuating based on time of day, hence greater deployment times may be required in future experiments.
5 Conclusions
Under current rates of warming, seagrass ecosystems are likely to experience a shift in bacterial communities both above-ground in plant tissue and below-ground in surrounding sediments, as well as an increase in above-ground plant productivity. Our results suggest that sedimentary processes may receive some physical thermal buffering from the rising temperatures, however, these increased temperatures may cause decoupling of processes that contribute to nutrient cycling above and below-ground. This study provided further evidence that increased temperature of ~3°C may increase above-ground biomass production in seagrasses in temperate regions (York et al., 2013; Collier et al., 2017), with changes observed in above-ground productivity of plants, potentially as a response to environmental conditions. This study also advances our understanding of seagrass-temperature interactions and explores associated changes in above and below-ground bacterial communities that might lead to changes in ecosystem functions of seagrass meadows. Future research direction on this topic should involve examining the effects of seasonal variation on seagrass-associated microbial communities and should also integrate manipulative experiments to examine the extent to which temperature, among other environmental changes in estuaries, impacts the interactions between seagrass and associated above and below-ground bacterial communities to determine the importance of these interactions to the resilience of seagrass in the face of warming temperatures.
Data availability statement
The original contributions presented in the study are publicly available. This data can be found here: https://www.ncbi.nlm.nih.gov/bioproject/PRJNA925291/.
Author contributions
LW: Conceptualization, Data curation, Formal analysis, Investigation, Methodology, Project administration, Writing – original draft, Writing – review & editing. PG: Conceptualization, Formal analysis, Funding acquisition, Supervision, Writing – review & editing. TG: Conceptualization, Methodology, Project administration, Supervision, Validation, Writing – review & editing. EM: Formal analysis, Methodology, Software, Supervision, Writing – review & editing. DV: Formal analysis, Supervision, Writing – review & editing. KD: Conceptualization, Funding acquisition, Investigation, Methodology, Project administration, Supervision, Validation, Writing – review & editing.
Funding
The author(s) declare financial support was received for the research, authorship, and/or publication of this article. This research was supported by funding from the Australian Research Council (LP200200220 awarded to PG) and Macquarie University HDR funding (awarded to LW).
Acknowledgments
We acknowledge the provision of laboratory space and equipment from Sydney institute of Marine Science and Macquarie Geoanalytical. And support in DNA sequencing from Ramaccioti centre for Genomics. The authors would like to thank all students, volunteers, and colleagues for their significant contribution to the various stages of this study, in particular Amy MacIntosh and Sebastian Vadillo. We also thank the reviewers who have improved this manuscript through their insightful comments.
Conflict of interest
The authors declare that the research was conducted in the absence of any commercial or financial relationships that could be construed as a potential conflict of interest.
The author(s) declared that they were an editorial board member of Frontiers, at the time of submission. This had no impact on the peer review process and the final decision.
Publisher’s note
All claims expressed in this article are solely those of the authors and do not necessarily represent those of their affiliated organizations, or those of the publisher, the editors and the reviewers. Any product that may be evaluated in this article, or claim that may be made by its manufacturer, is not guaranteed or endorsed by the publisher.
Supplementary material
The Supplementary Material for this article can be found online at: https://www.frontiersin.org/articles/10.3389/fmars.2024.1374946/full#supplementary-material
References
Anderson M. J. (2005). Permutational multivariate analysis of variance Vol. 26 (Auckland: Department of Statistics, University of Auckland), 32–46.
Anderson D. R. (2007). Model based inference in the life sciences: a primer on evidence (Springer Science & Business Media).
Anton A., Baldry K., Coker D. J., Duarte C. M. (2020). Drivers of the low metabolic rates of seagrass meadows in the Red Sea. Front. Mar. Sci. 7, 69. doi: 10.3389/fmars.2020.00069
Babcock R. C., Bustamante R. H., Fulton E. A., Fulton D. J., Haywood M. D. E., Hobday A. J., et al. (2019). Severe continental-scale impacts of climate change are happening now: extreme climate events impact marine habitat forming communities along 45% of Australia’s coast. Front. Mar. Sci. 6. doi: 10.3389/fmars.2019.00411
Bates D., Mächler M., Bolker B., Walker S. (2014). Fitting linear mixed-effects models using lme4. J. Stat. Softw. 67 (1), 1–48. doi: 10.18637/jss.v067.i01
Bertness M. D., Callaway R. (1994). Positive interactions in communities. Trends Ecol. Evol. 9, 191–193. doi: 10.1016/0169-5347(94)90088-4
Bischoff V., Zucker F., Moraru C. (2021). “Marine bacteriophages,” in Encyclopedia of Virology, 4th ed. Eds. Bamford D. H., Zuckerman M. (Academic Press, Oxford), 322–341. doi: 10.1016/B978-0-12-809633-8.20988-6
Borum J., Sand-Jensen K., Binzer T., Pedersen O., Greve T. (2007). Oxygen movement in seagrasses, seagrasses: biology, ecology and conservation (Netherlands: Springer), 255–270.
Brodersen K. E., Siboni N., Nielsen D. A., Pernice M., Ralph P. J., Seymour J., et al. (2018). Seagrass rhizosphere microenvironment alters plant-associated microbial community composition. Environ. Microbiol. 20 (8), 2854–2864. doi: 10.1111/1462-2920.14245
Callahan B. J., McMurdie P. J., Rosen M. J., Han A. W., Johnson A. J. A., Holmes S. P. (2016). DADA2: high-resolution sample inference from Illumina amplicon data. Nat. Methods 13, 581–583. doi: 10.1038/nmeth.3869
Callaway R. M. (2007). Positive interactions and interdependence in plant communities (Springer Science & Business Media).
Chefaoui R. M., Duarte C. M., Serrão E. A. (2018). Dramatic loss of seagrass habitat under projected climate change in the Mediterranean Sea. Global Change Biol. 24, 4919–4928. doi: 10.1111/gcb.14401
Cifuentes A., Antón J., De Wit R., Rodríguez-Valera F. (2003). Diversity of bacteria and Archaea in sulphate-reducing enrichment cultures inoculated from serial dilution of Zostera noltii rhizosphere samples. Environ. Microbiol. 5, 754–764. doi: 10.1046/j.1470-2920.2003.00470.x
Collier C. J., Ow Y. X., Langlois L., Uthicke S., Johansson C. L., O'Brien K. R., et al. (2017). Optimum temperatures for net primary productivity of three tropical seagrass species. Front. Plant Sci. 8, 1446.
Crump B. C., Wojahn J. M., Tomas F., Mueller R. S. (2018). Metatranscriptomics and amplicon sequencing reveal mutualisms in seagrass microbiomes. Front. Microbiol. 9, 388. doi: 10.3389/fmicb.2018.00388
Cúcio C., Engelen A. H., Costa R., Muyzer G. (2016). Rhizosphere microbiomes of European seagrasses are selected by the plant, but are not species specific. Front. Microbiol. 7. doi: 10.3389/fmicb.2016.00440
Egan S., Harder T., Burke C., Steinberg P., Kjelleberg S., Thomas T. (2013). The seaweed holobiont: understanding seaweed–bacteria interactions. FEMS Microbiol. Rev. 37, 462–476. doi: 10.1111/1574-6976.12011
Elifantz H., Horn G., Ayon M., Cohen Y., Minz D. (2013). Rhodobacteraceae are the key members of the microbial community of the initial biofilm formed in Eastern Mediterranean coastal seawater. FEMS Microbiol. Ecol. 85 (2), 348–357. doi: 10.1111/1574-6941.12122
Fraser M. W., Gleeson D. B., Grierson P. F., Laverock B., Kendrick G. A. (2018). Metagenomic evidence of microbial community responsiveness to phosphorus and salinity gradients in seagrass sediments. Front. Microbiol. 9, 1703. doi: 10.3389/fmicb.2018.01703
Fuggle R. E., Gribben P. E., Marzinelli E. M. (2023). Experimental evidence root-associated microbes mediate seagrass response to environmental stress. J. Ecol. 111, 1079–1093. doi: 10.1111/1365-2745.14081
Garcias-Bonet N., Arrieta J. M., Duarte C. M., Marba N. (2016). Nitrogen-fixing bacteria in Mediterranean seagrass (Posidonia oceanica) roots. Aquat. Bot. 131, 57–60. doi: 10.1016/j.aquabot.2016.03.002
Gribben P. E., Thomas T., Pusceddu A., Bonechi L., Bianchelli S., Buschi E., et al. (2018). Below-ground processes control the success of an invasive seaweed. J. Ecol. 106, 2082–2095. doi: 10.1111/1365-2745.12966
Gribben P. E., Nielsen S., Seymour J. R., Bradley D. J., West M. N., Thomas T. (2017). Microbial communities in marine sediments modify success of an invasive macrophyte. Sci. Rep. 7, 9845. doi: 10.1038/s41598-017-10231-2
Gulvik C. A., Buchan A. (2013). Simultaneous catabolism of plant-derived aromatic compounds results in enhanced growth for members of the roseobacter lineage. Appl. Environ. Microbiol. 79, 3716–3723. doi: 10.1128/AEM.00405-13
Hansen J. W., Udy J. W., Perry C. J., Dennison W. C., Lomstein B. A. (2000). Effect of the seagrass Zostera capricorni on sediment microbial processes. Mar. Ecol. Prog. Ser. 199, 83–96. doi: 10.3354/meps199083
Harrison P. G. (1989). Detrital processing in seagrass systems: a review of factors affecting decay rates, remineralization and detritivory. Aquat. Bot. 35, 263–288. doi: 10.1016/0304-3770(89)90002-8
Hemminga M. A., Harrison P. G., Van Lent F. (1991). The balance of nutrient losses and gains in seagrass meadows. Mar. Ecol. Prog. Ser., 85–96. doi: 10.3354/meps071085
Hicks N., Liu X., Gregory R., Kenny J., Lucaci A., Lenzi L., et al. (2018). Temperature driven changes in benthic bacterial diversity influences biogeochemical cycling in coastal sediments. Front. Microbiol. 9, 1730.
Hoffmann K., Bienhold C., Buttigieg P. L., Knittel K., Laso-Pérez R., Rapp J. Z., et al. (2020). Diversity and metabolism of Woeseiales bacteria, global members of marine sediment communities. ISME J. 14, 1042–1056. doi: 10.1038/s41396-020-0588-4
Holmer M., Pedersen O., Ikejima K. (2006). Sulfur cycling and sulfide intrusion in mixed Southeast Asian tropical seagrass meadows. Botanica Marina 49, 91–102. doi: 10.1515/BOT.2006.013
Howes E. L., Joos F., Eakin M., Gattuso J.-P. (2015). An updated synthesis of the observed and projected impacts of climate change on the chemical, physical and biological processes in the oceans. Front. Mar. Sci. 2, 36. doi: 10.3389/fmars.2015.00036
Ingleton T., McMinn A. (2012). Thermal plume effects: A multi-disciplinary approach for assessing effects of thermal pollution on estuaries using benthic diatoms and satellite imagery. Estuar. Coast. Shelf Sci. 99, 132–144. doi: 10.1016/j.ecss.2011.12.024
Jacobs R. (1979). Distribution and aspects of the production and biomass of eelgrass, Zostera marina L., at Roscoff, France. Aquat. Bot. 7, 151–172. doi: 10.1016/0304-3770(79)90019-6
Jantharadej K., Limpiyakorn T., Kongprajug A., Mongkolsuk S., Sirikanchana K., Suwannasilp B. B. (2021). Microbial community compositions and sulfate-reducing bacterial profiles in malodorous urban canal sediments. Arch. Microbiol. 203, 1981–1993. doi: 10.1007/s00203-020-02157-7
Jones P., Garcia B. J., Furches A., Tuskan G. A., Jacobson D. (2019). Plant host-associated mechanisms for microbial selection. Front. Plant Sci. 10, 862. doi: 10.3389/fpls.2019.00862
Jung E. M. U., Abdul Majeed N. A. B., Booth M. W., Austin R., Sinclair E. A., Fraser M. W., et al. (2023). Marine heatwave and reduced light scenarios cause species-specific metabolomic changes in seagrasses under ocean warming. New Phytol. 239 (5), 1692–1706. doi: 10.1111/nph.19092
Kaldy J., Dunton K. (2000). Above and below-ground production, biomass and reproductive ecology of Thalassia testudinum (turtle grass) in a subtropical coastal lagoon. Mar. Ecol. Prog. Ser. 193, 271–283. doi: 10.3354/meps193271
Kirchman D. L., Mazzella L., Alberte R. S., Mitchell R. (1984). Epiphytic bacterial production on Zostera marina. Marine ecology progress series. Oldendorf 15, 117–123. doi: 10.3354/meps015117
Lee D.-H., Cho S. J., Kim S. M., Lee S. B. (2013). Sagittula marina sp. nov., isolated from seawater and emended description of the genus Sagittula. Int. J. Sys. Evolutionary Microbiol. 63, 2101–2107. doi: 10.1099/ijs.0.040766-0
Lefcheck J. S., Wilcox D. J., Murphy R. R., Marion S. R., Orth R. J. (2017). Multiple stressors threaten the imperilled coastal foundation species eelgrass (Zostera marina) in Chesapeake Bay, USA. Global Change Biol. 23, 3474–3483. doi: 10.1111/gcb.13623
Lehnen N., Marchant H. K., Schwedt A., Milucka J., Lott C., Weber M., et al. (2016). High rates of microbial dinitrogen fixation and sulfate reduction associated with the Mediterranean seagrass Posidonia oceanica. Sys. Appl. Microbiol. 39, 476–483. doi: 10.1016/j.syapm.2016.08.004
Lin H., Peddada S. D. (2020). Analysis of compositions of microbiomes with bias correction. Nat. Commun. 11, 3514. doi: 10.1038/s41467-020-17041-7
Lin X., Wang S., Zhang X., Hsieh T.-H., Sun Z., Xu T. (2021). Near-field route optimization-supported polar ice navigation via maritime radar videos. J. Advanced Transportation 2021, 2798351. doi: 10.1155/2021/2798351
Lugtenberg B., Kamilova F. (2009). Plant-growth-promoting rhizobacteria. Annu. Rev. Microbiol. 63, 541–556. doi: 10.1146/annurev.micro.62.081307.162918
Marba N., Duarte C. M. (2010). Mediterranean warming triggers seagrass (Posidonia oceanica) shoot mortality. Global Change Biol. 16, 2366–2375. doi: 10.1111/j.1365-2486.2009.02130.x
Marba N., Duarte C., Terrados J., Halun Z., Gacia E., Fortes M. (2010). Effects of seagrass rhizospheres on sediment redox conditions in SE Asian coastal ecosystems. Estuaries Coasts 33, 107–117. doi: 10.1007/s12237-009-9250-0
Marbà N., Jordà G., Bennett S., Duarte C. M. (2022). Seagrass thermal limits and vulnerability to future warming. Front. Mar. Sci. 9. doi: 10.3389/fmars.2022.860826
Martin B. C., Alarcon M. S., Gleeson D., Middleton J. A., Fraser M. W., Ryan M. H., et al. (2020). Root microbiomes as indicators of seagrass health. FEMS Microbiol. Ecol. 96, fiz201. doi: 10.1093/femsec/fiz201
Mateo M., Romero J. (1996). Evaluating seagrass leaf litter decomposition: an experimental comparison between litter-bag and oxygen-uptake methods. J. Exp. Mar. Biol. Ecol. 202, 97–106. doi: 10.1016/0022-0981(96)00019-6
Mendes R., Garbeva P., Raaijmakers J. M. (2013). The rhizosphere microbiome: significance of plant beneficial, plant pathogenic, and human pathogenic microorganisms. FEMS Microbiol. Rev. 37 (5), 634–663.
Miyamoto H., Kawachi N., Kurotani A., Moriya S., Suda W., Suzuki K., et al. (2023). Computational estimation of sediment symbiotic bacterial structures of seagrasses overgrowing downstream of onshore aquaculture. Environ. Res. 219, 115130. doi: 10.1016/j.envres.2022.115130
Mohapatra M., Manu S., Dash S. P., Rastogi G. (2022). Seagrasses and local environment control the bacterial community structure and carbon substrate utilization in brackish sediments. J. Environ. Manage. 314, 115013. doi: 10.1016/j.jenvman.2022.115013
Naylor D., DeGraaf S., Purdom E., Coleman-Derr D. (2017). Drought and host selection influence bacterial community dynamics in the grass root microbiome. ISME J. 11, 2691–2704. doi: 10.1038/ismej.2017.118
Nguyen H. M., Ralph P. J., Marín-Guirao L., Pernice M., Procaccini G. (2021). Seagrasses in an era of ocean warming: a review. Biol. Rev. 96, 2009–2030. doi: 10.1111/brv.12736
Nixon S., Buckley B., Granger S., Bintz J. (2001). Responses of very shallow marine ecosystems to nutrient enrichment. Hum. Ecol. Risk Assess.: Int. J. 7 (5), 1457–1481.
O’Brien A. L., Dafforn K., Chariton A., Airoldi L., Schäfer R. B., Mayer-Pinto M. (2023). Multiple stressors. In Marine pollution–monitoring, management and mitigation (Cham: Springer Nature Switzerland) pp. 305–315. doi: 10.1101/2020.05.19.105098
Oksanen J., Blanchet F. G., Kindt R., Legendre P., Minchin P. R., O’hara R., et al. (2013). Package ‘vegan’ Community ecology package, version. 2 (9), 1–295.
Park S., Yoshizawa S., Inomata K., Kogure K., Yokota A. (2012). Halioglobus japonicus gen. nov., sp. nov. and Halioglobus pacificus sp. nov., members of the class Gammaproteobacteria isolated from seawater. Int. J. Syst. Evol. Microbiol. 62, 1784–1789. doi: 10.1099/ijs.0.031443-0
Pendall E., Bridgham S., Hanson P. J., Hungate B., Kicklighter D. W., Johnson D. W., et al. (2004). Below-ground process responses to elevated CO2 and temperature: a discussion of observations, measurement methods, and models. New Phytol. 162, 311–322. doi: 10.1111/j.1469-8137.2004.01053.x
Pootakham W., Mhuantong W., Yoocha T., Putchim L., Jomchai N., Sonthirod C., et al. (2019). Heat-induced shift in coral microbiome reveals several members of the Rhodobacteraceae family as indicator species for thermal stress in Porites lutea. MicrobiologyOpen 8, e935. doi: 10.1002/mbo3.935
Pujalte M. J., Lucena T., Ruvira M. A., Arahal D. R., Macián M. C. (2014). “The family rhodobacteraceae,” in The Prokaryotes: Alphaproteobacteria and Betaproteobacteria. Eds. Rosenberg E., DeLong E. F., Lory S., Stackebrandt E., Thompson F. (Springer Berlin Heidelberg, Berlin, Heidelberg), 439–512. doi: 10.1007/978-3-642-30197-1_377
Qiu Z., Coleman M. A., Provost E., Campbell A. H., Kelaher B. P., Dalton S. J., et al. (2019). Future climate change is predicted to affect the microbiome and condition of habitat-forming kelp. Proc. R. Soc. B: Biol. Sci. 286, 20181887. doi: 10.1098/rspb.2018.1887
R Core Team (2017). R: A language and environment for statistical computing (Vienna: R Foundation for Statistical Computing). Available at: https://www.R-project.org/.
Sasse J., Martinoia E., Northen T. (2018). Feed your friends: do plant exudates shape the root microbiome? Trends Plant Sci. 23, 25–41. doi: 10.1016/j.tplants.2017.09.003
Sawall Y., Ito M., Pansch C. (2021). Chronically elevated sea surface temperatures revealed high susceptibility of the eelgrass Zostera marina to winter and spring warming. Limnol. Oceanogr. 66, 4112–4124. doi: 10.1002/lno.11947
Scanes E., Scanes P., Ross P. M. (2020). Climate change rapidly warms and acidifies Australian estuaries. Nat. Commun. 11, 1803. doi: 10.1038/s41467-020-15550-z
Serrano O., Arias-Ortiz A., Duarte C. M., Kendrick G. A., Lavery P. S. (2021). “Impact of marine heatwaves on seagrass ecosystems,” in Ecosystem Collapse and Climate Change. Eds. Canadell J. G., Jackson R. B. (Springer International Publishing, Cham), 345–364. doi: 10.1007/978-3-030-71330-0_13
Seymour J. R., Laverock B., Nielsen D. A., Trevathan-Tackett S. M., Macreadie P. I. (2018). “The microbiology of seagrasses,” in Seagrasses of Australia: Structure, Ecology and Conservation. Eds. Larkum A. W. D., Kendrick G. A., Ralph P. J. (Springer International Publishing, Cham), 343–392. doi: 10.1007/978-3-319-71354-0_12
Short F. T., Duarte C. M. (2001). Methods for the measurement of seagrass growth and production. Global Seagrass Res. Methods 2001, 155–198. doi: 10.1016/B978-044450891-1/50009-8
Short F., Carruthers T., Dennison W., Waycott M. (2007). Global seagrass distribution and diversity: a bioregional model. J. Exp. Mar. Biol. Ecol. 350 (1–2), 3–20.
Šinkovičová M., Igaz D., Aydın E., Jarošová M. (2017). Soil particle size analysis by laser diffractometry: result comparison with pipette method. IOP Conf. Series: Mater. Sci. Eng. 245, 072025. doi: 10.1088/1757-899X/245/7/072025
Stadler M., del Giorgio P. A. (2022). Terrestrial connectivity, upstream aquatic history and seasonality shape bacterial community assembly within a large boreal aquatic network. ISME J. 16, 937–947. doi: 10.1038/s41396-021-01146-y
Stratil S. B., Neulinger S. C., Knecht H., Friedrichs A. K., Wahl M. (2013). Temperature-driven shifts in the epibiotic bacterial community composition of the brown macroalga Fucus vesiculosus. MicrobiologyOpen 2, 338–349. doi: 10.1002/mbo3.79
Su X., Yang X., Li H., Wang H., Wang Y., Xu J., et al. (2021). Bacterial communities are more sensitive to ocean acidification than fungal communities in estuarine sediments. FEMS Microbiol. Ecol. 97 (5), fiab058. doi: 10.1093/femsec/fiab058
Szitenberg A., Beca-Carretero P., Azcárate-García T., Yergaliyev T., Alexander-Shani R., Winters G. (2022). Teasing apart the host-related, nutrient-related and temperature-related effects shaping the phenology and microbiome of the tropical seagrass Halophila stipulacea. Environ. Microbiome 17, 1–17. doi: 10.1186/s40793-022-00412-6
Tarquinio F., Attlan O., Vanderklift M. A., Berry O., Bissett A. (2021). Distinct endophytic bacterial communities inhabiting seagrass seeds. Front. Microbiol. 12. doi: 10.3389/fmicb.2021.703014
Tarquinio F., Hyndes G. A., Laverock B., Koenders A., Säwström C. (2019). The seagrass holobiont: understanding seagrass-bacteria interactions and their role in seagrass ecosystem functioning. FEMS Microbiol. Lett. 366 (6), fnz057. doi: 10.1093/femsle/fnz057
Thomson J. A., Burkholder D. A., Heithaus M. R., Fourqurean J. W., Fraser M. W., Statton J., et al. (2015). Extreme temperatures, foundation species, and abrupt ecosystem change: an example from an iconic seagrass ecosystem. Global Change Biol. 21, 1463–1474. doi: 10.1111/gcb.12694
Trevathan-Tackett S. M., Brodersen K. E., Macreadie P. I. (2020a). Effects of elevated temperature on microbial breakdown of seagrass leaf and tea litter biomass. Biogeochemistry 151, 171–185. doi: 10.1007/s10533-020-00715-1
Trevathan-Tackett S. M., Jeffries T. C., Macreadie P. I., Manojlovic B., Ralph P. (2020b). Long-term decomposition captures key steps in microbial breakdown of seagrass litter. Sci. Total Environ. 705, 135806. doi: 10.1016/j.scitotenv.2019.135806
Trevathan-Tackett S. M., Seymour J. R., Nielsen D. A., Macreadie P. I., Jeffries T. C., Sanderman J., et al. (2017). Sediment anoxia limits microbial-driven seagrass carbon remineralization under warming conditions. FEMS Microbiol. Ecol. 93 (6), fix033. doi: 10.1093/femsec/fix033
Ugarelli K., Chakrabarti S., Laas P., Stingl U. (2017). The seagrass holobiont and its microbiome. Microorganisms 5, 81. doi: 10.3390/microorganisms5040081
Uku J., Björk M., Bergman B., Díez B. (2007). Characterization and comparison of prokaryotic epiphytes associated with three East African seagrasses. J. Phycol. 43 (4), 768–779. doi: 10.1111/j.1529-8817.2007.00371.x
Underwood A. J., Chapman M. G. (1996). Scales of spatial patterns of distribution of intertidal invertebrates. Oecologia 107, 212–224. doi: 10.1007/BF00327905
Valle M., Chust G., del Campo A., Wisz M. S., Olsen S. M., Garmendia J. M., et al. (2014). Projecting future distribution of the seagrass Zostera noltii under global warming and sea level rise. Biol. Conserv. 170, 74–85. doi: 10.1016/j.biocon.2013.12.017
von Lützow M., Kögel-Knabner I. (2009). Temperature sensitivity of soil organic matter decomposition—what do we know? Biol. Fertil. Soils 46, 1–15. doi: 10.1007/s00374-009-0413-8
Waite D. W., ChuvoChina M., Pelikan C., Parks D. H., Yilmaz P., Wagner M., et al. (2020). Proposal to reclassify the proteobacterial classes Deltaproteobacteria and Oligoflexia, and the phylum Thermodesulfobacteria into four phyla reflecting major functional capabilities. Int. J. Sys. Evolutionary Microbiol. 70, 5972–6016. doi: 10.1099/ijsem.0.004213
Wang Q., Wang Y. (2011). Optimizing the weight loss-on-ignition methodology to quantify organic and carbonate carbon of sediments from diverse sources. Environ. Monit. Assess. 174, 241–257. doi: 10.1007/s10661-010-1454-z
Wardle D., Bardgett R., Klironomos J., Setälä H., Putten W., Wall D. (2004). Ecological linkages between aboveground and belowground biota. Sci. (New York N.Y.) 304, 1629–1633. doi: 10.1126/science.1094875
Waymouth V., Miller R. E., Ede F., Bissett A., Aponte C. (2020). Variation in soil microbial communities: elucidating relationships with vegetation and soil properties, and testing sampling effectiveness. Plant Ecol. 221 (9), 837–851. doi: 10.1007/s11258-020-01029-w
Weidner S., Arnold W., Stackebrandt E., Pühler A. (2000). Phylogenetic analysis of bacterial communities associated with leaves of the seagrass Halophila stipulacea by a culture-independent small-subunit rRNA gene approach. Microbial Ecol. 39, 22–31. doi: 10.1007/s002489900194
Weisburg W. G., Barns S. M., Pelletier D. A., Lane D. J. (1991). 16S ribosomal DNA amplification for phylogenetic study. J. Bacteriol. 173 (2), 697–703.
York P. H., Gruber R. K., Hill R., Ralph P. J., Booth D. J., Macreadie P. I. (2013). Physiological and morphological responses of the temperate seagrass Zostera muelleri to multiple stressors: investigating the interactive effects of light and temperature. PloS One 8 (10), e76377. doi: 10.1371/journal.pone.0076377
Zhang Y., Sun Y., Jiao N., Stepanauskas R., Luo H. (2016). Ecological genomics of the uncultivated marine roseobacter lineage CHAB-I-5. Appl. Environ. Microbiol. 82, 2100–2111. doi: 10.1128/AEM.03678-15
Keywords: microbial community, foundation species, sediment, climate change, bacteria, microbiome, Zostera muelleri
Citation: Walker LD, Gribben PE, Glasby TM, Marzinelli EM, Varkey DR and Dafforn KA (2024) Above and below-ground bacterial communities shift in seagrass beds with warmer temperatures. Front. Mar. Sci. 11:1374946. doi: 10.3389/fmars.2024.1374946
Received: 23 January 2024; Accepted: 28 March 2024;
Published: 08 May 2024.
Edited by:
Michael Rappe, University of Hawaii at Manoa, United StatesReviewed by:
Myrsini Chronopoulou, Conidia Bioscience Ltd, United KingdomXia Zhang, Chinese Academy of Sciences (CAS), China
Copyright © 2024 Walker, Gribben, Glasby, Marzinelli, Varkey and Dafforn. This is an open-access article distributed under the terms of the Creative Commons Attribution License (CC BY). The use, distribution or reproduction in other forums is permitted, provided the original author(s) and the copyright owner(s) are credited and that the original publication in this journal is cited, in accordance with accepted academic practice. No use, distribution or reproduction is permitted which does not comply with these terms.
*Correspondence: Luke DA. Walker, bHVrZS53YWxrZXIzQGhkci5tcS5lZHUuYXU=