- 1Department of Applied Ecology, North Carolina State University, Morehead City, NC, United States
- 2U.S. Geological Survey, North Carolina Cooperative Fish and Wildlife Research Unit, Department of Applied Ecology, North Carolina State University, Raleigh, NC, United States
- 3National Centers for Coastal Ocean Science, National Ocean Service, National Oceanic and Atmospheric Administration, Beaufort, NC, United States
Artificial reefs can play an important role in marine fisheries management by supplementing or enhancing natural habitats. Despite their increased use in recent years, the choice of structures used at artificial reefs remains largely haphazard due to the lack of information on reef structure performance. Few studies have examined the use of different artificial reef structures by individual fish. From 2021-2022, we acoustically tagged 72 black sea bass (Centropristis striata), 34 gag (Mycteroperca mircrolepis), 27 greater amberjack (Seriola dumerili), nine almaco jack (S. rivoliana), and eight red snapper (Lutjanus campechanus) on four artificial reef complexes near Cape Lookout, North Carolina, U.S. Available artificial reef structures consisted of materials of various sizes and heights made of concrete and metal. We tracked tagged fish using a fine-scale positioning system for ~100 days. Black sea bass exhibited high site fidelity to the artificial structure where we caught them, rarely moving away from that structure. The limited movement resulted in low transition probabilities; we conclude that black sea bass do not select for particular artificial structures. Gag and red snapper moved greater distances away from artificial structures and routinely moved between them. Greater amberjack and almaco jack moved the most within the complexes displaying circling behavior around individual structures and were the only species that regularly moved off the artificial reef complexes. Greater amberjack movements away from artificial sites were most commonly directed to surrounding shipwrecks. Whereas gag, red snapper, almaco jack, and greater amberjack used all available structures, they consistently selected for high relief structures, such as vessels, more than other structures. These results will be useful to managers charged with decisions on what types of structures to place at artificial reef complexes to supplement or enhance habitat for economically important fishes.
1 Introduction
Artificial reefs can play an important role in marine fisheries management by supplementing or enhancing natural habitats (Becker et al., 2018). Globally, they can function similarly to natural reefs as they can host equivalent fish community metrics such as biomass, density, diversity, and species richness (Paxton et al., 2020b). On newly deployed artificial reefs, fish community metrics can increase quickly and can equal or exceed those on natural reefs in as little as five months (Paxton et al., 2018). In addition to ecological benefits, artificial reefs can offer significant financial yields to fishers and may reduce fishing pressure on natural reefs (Leitão et al., 2009).
Since the 1950’s, the popularity of artificial reefs has increased among reef management groups and recreational anglers in the United States, with many coastal states deploying them in nearshore and offshore waters (Paxton et al., 2020b). A large reason for the popularity of these reefs is their ability to attract and/or produce fish at locations devoid of structured habitat. One explanation for the rapid increase in fish numbers after artificial reef deployment (Roa-Ureta et al., 2019; Folpp et al., 2020) comes from the structural complexity that artificial reefs can provide over a relatively small footprint. A recent study found that <0.01% of the total southeast United States Atlantic coast (SEUS) seafloor area has artificial reef coverage whereas 2.6% of the seafloor is covered by natural reefs (Steward et al., 2022). Thus, while artificial reefs cover only a small proportion of the SEUS seafloor, a growing trend in increasing cover of artificial reefs (Paxton et al., 2024) could lead them to become an increasingly vital habitat for fish communities and stakeholder utilization. Indeed, increased use of marine resources by recreational fishers in the SEUS is a growing trend with an increase in average annual trips from 38 million in the 1980’s to 50 million in recent years (MRIP 2023). Moreover, as small isolated areas whose locations are often well known, artificial reefs are likely to feel the effects of increased recreational fishing power (Shertzer et al., 2019). For reef fishes, such as those in the snapper-grouper complex in the SEUS, the recreational sector now accounts for 68% of the landings and is the dominant source of fishing mortality (Shertzer et al., 2019).
Despite increasing use of artificial reefs in recent years, choices of structures and deployment remains largely haphazard due to the lack of ecological information on artificial structure performance (Becker et al., 2018). Managers have access to a variety of artificial structures from reef modules or pipes, to intentionally sunk ships and unintentional shipwrecks (Paxton et al., 2020a). Each structure can vary in size, relief, and complexity (Komyakova et al., 2019; Paxton et al., 2020b), and productivity of artificial reefs varies with structure choice, design, and location (Strelcheck et al., 2005). Such variation can provide different opportunities for foraging, shelter, and spawning in reef species, but can also make optimizing reef structures and their placement for management goals difficult (Becker et al., 2018). For example, secondary-use concrete structures (e.g., rubble and bridge pieces) that are repurposed from their original use to be reefs are readily available and composed of materials compatible with the marine environment, but they tend to have a high cost for deployment and require waterfront land for staging (Broughton, 2012). Steel vessels, on the other hand, can generate economic gains as popular recreational diving sites, host more diverse communities of aquatic life, and provide surface for epibenthic life to colonize, but their stability during strong storms is variable and they are expensive to prepare for marine deployment (Broughton, 2012). Given the diversity of available artificial reef structures and potential pros and cons for each, it is necessary to examine artificial structures in more detail to determine if fish select for certain structures and how selection may vary across fish species.
One effective way to determine how fishes use different artificial structures is to track fish movements (we use the term movement as a general term to describe the movement of an individual from point A to point B, independent of scale). Studying individual movements has been used to demonstrate that particular fish species exhibit varying degrees of residency on reefs, as well as movement between or among reefs. For example, 72% of acoustically tagged and tracked individual red snapper (Lutjanus campechanus) spent over one year on specific artificial reefs in the Gulf of Mexico (Topping and Szedlmayer, 2011). Examinations of movement between artificial and natural reefs reveal that certain species, such as acoustically tagged white sea bream (Diplodus sargus) in Portugal, occupy both artificial and nearby natural reefs, taking excursions from one another, likely related to feeding dynamics (Abecasis et al., 2013). Other species, such as acoustically tagged Atlantic cod (Gadus morhua) around European wind farms, exhibit more transient or seasonal residency on artificial structures (Reubens et al., 2013). Advances in acoustic telemetry now allow examination of fish behavior at a greater detail, providing more information about fine-scale habitat use, movement, and behavioral states (we use the terms “fine-scale movement” or “fine-scale” to describe the movement of an individual from point A to B where their positions are determined with meter-level accuracy). For example, fine-scale acoustic telemetry studies have led to increased understanding of green sturgeon (Acipenser medirostris) habitat preferences on spawning grounds (Klimley et al., 2020), great barracuda (Sphyraena barracuda) home ranges over a tropical reef (Becker et al., 2020), optimal habitat use areas for flatfishes at an artificial site compared to a nearby natural site (Burns et al., 2019), and habitat use and partitioning in red drum (Fodrie et al., 2015). In the SEUS, fine-scale telemetry studies determined that gray triggerfish (Balistes capriscus) and red snapper responded to baited camera traps by altering their fine-scale movement and behavior (Bacheler et al., 2018, 2019, 2021; Bacheler et al., 2022b).
Among the most targeted and valued species in SEUS commercial and recreational fisheries are black sea bass (Centroprisits striata), gag (Mycteroperca microlepis), greater amberjack (Seriola dumerili), almaco jack (S. rivoliana), and red snapper (Coleman et al., 1999, 2000; SEDAR 59, 2020; SEDAR 71, 2021; SEDAR 73, 2021; SEDAR 76, 2023). Given the value of these species among fishers, they have been the subjects of intensive management and research. Several studies have used broad-scale acoustic telemetry arrays to study black sea bass movements at natural (Fabrizio et al., 2013, 2014) and artificial sites (Secor et al., 2019, 2021; Wiernicki et al., 2020); gag at natural sites (Ellis et al., 2019; Keller et al., 2020); red snapper at artificial sites (Topping and Szedlmayer, 2011; Curtis et al., 2015); greater amberjack at artificial sites (Jackson et al., 2018; Boyle et al., 2022a, b); and almaco jack at natural sites (Fontes et al., 2014; Gandra et al., 2020). However, despite intensive research and management, gag (SEDAR 71, 2021), red snapper (SEDAR 73, 2021), and black sea bass are currently overfished and experiencing overfishing (SEDAR 76, 2023). As reef associated species, choice and placement of artificial structure could be an important consideration to the management of these species as increased artificial reef coverage could increase available habitat. However, increased coverage could also lead to increased access and exploitation by fishers as fishes could be attracted to or produce new communities around artificial sites (Roa-Ureta et al., 2019). Thus, as the cover of artificial structures on the seascape increases, there is a growing need to understand the fine-scale movements of these fishes on and among artificial structures, and among artificial and natural reefs.
In this paper, we use structure to refer to a particular structure type and complex as an area where either one or more structure types are provided. The goals of our study were to determine fine-scale artificial structure selection within artificial complexes and connectivity between artificial and natural reef complexes for black sea bass, gag, red snapper, greater amberjack, and almaco jack using acoustic telemetry. To achieve this, we addressed the following two questions: (1) is there selection for certain reef structures over others and does selection differ by species and (2) do fishes use other artificial and natural reefs surrounding a single artificial reef complex and does this differ by species? Knowledge of how these species use different artificial structures, the amount of reef area each species uses, and the amount of time each species spends on reefs, can help guide decisions on how to target future habitat enhancement and to best support these species.
2 Methods
2.1 Study areas
Our fine-scale telemetry studies took place near Cape Lookout, North Carolina, U.S. at four offshore artificial reef complexes: artificial reefs 285 (AR285), 300 (AR300), 330 (AR330), and 345 (AR345; Figure 1). Artificial reef 285 has an average depth of 20 meters and five artificial structure types; AR300 has an average depth of 27 meters and two artificial structure types; AR330 has an average depth of 18 meters and six artificial structure types; and AR345 has an average depth of 18 meters and five artificial structure types. These sites were chosen because both demersal and water-column associated fish species of recreational importance are known to frequent these reefs and because these reefs offer a diversity of reef size, layout, and artificial structures present. Additionally, they all have natural reef bottom within close proximity.
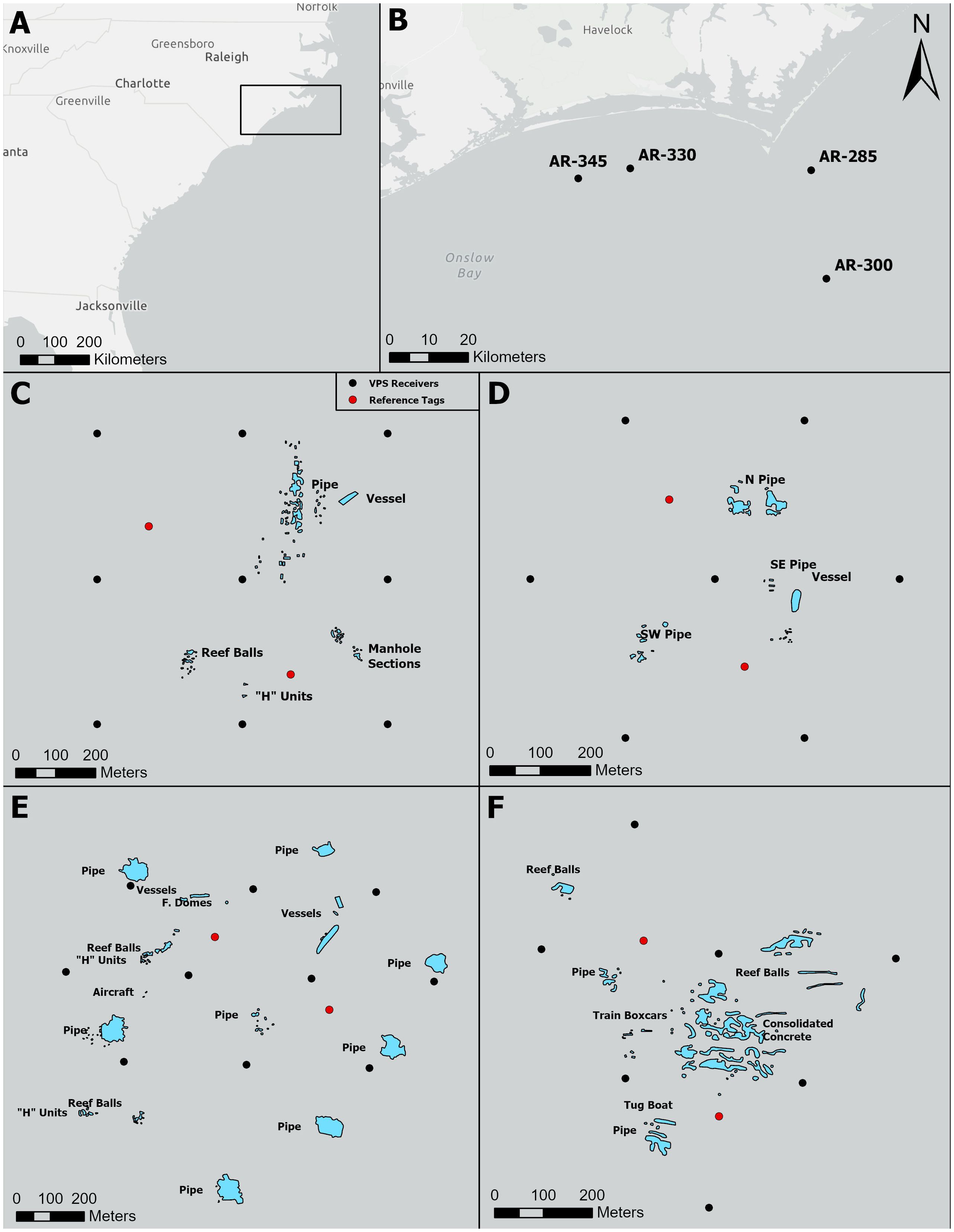
Figure 1 Locations of four monitored artificial reefs, the structures present at each reef, and the respective Vemco Positioning Arrays deployed at each reef. (A) Larger geographic context of study where the black square highlights the study region. (B) Locations of the four monitored artificial reefs. Layouts of artificial structures at AR285 (C), AR300 (D), AR330 (E), and AR345 (F). Black dots represent the locations of Vemco Positioning System receivers and red dots represent the locations of reference tags.
2.2 Tagging procedure
We caught all fishes using hook-and-line tackle. Black sea bass, red snapper, and small gag were externally tagged via a spaghetti loop method with high power V9-1x-BLU-3 transmitters (Innovasea Inc.) programmed with an average delay of 180 seconds and a battery life of 278 days. We chose this tagging method because it has a high tag retention rate and causes minimal trauma (Runde et al., 2022), is faster than internal tagging, and since external tagging tends to have improved detection range over internal tagging (Dance et al., 2016). We internally tagged greater amberjack, almaco jack, and larger gag with high power V16-4x-BLU-1 transmitters (Innovasea Inc.) programmed with an average delay of 180 seconds and a battery life of 1,460 days. We chose to internally tag these particular species because we wanted longer-term data beyond the timeframe of this project. We distributed fishing effort and acoustic tags of demersal species as evenly as possible across the various structures at each reef so as to not introduce bias for the habitat selection portion of the project. We tagged water-column associated species opportunistically, concentrating our fishing effort wherever schools were observed on the surface or Garmin fishfinder.
2.3 Receiver array set-up
We used four Vemco Positioning Systems (VPS) to monitor fine-scale positions of reef fishes at and among artificial reef structures at each of the four artificial reef complexes. A VPS is a fine-scale positioning system that uses coded transmitters and a strategically placed array of receivers to detect and position individuals with high resolution and spatial accuracy, often within one meter (Espinoza et al., 2011). Our VPS arrays also contained two reference tags each to calculate the horizontal position error of animal tags and receivers, and detection efficiency of an array. Prior to receiver deployment, we conducted a range test at AR285 to determine ideal receiver spacing. We deployed five receivers at distances of 100 m, 200 m, 250 m, 300 m, and 350 m from the range test tag for a duration of two weeks. We found that ~90% of transmissions were detected at a distance of 300 m and this distance was selected for our study; although Innovasea Inc. recommends using a distance where ~ 60% of transmissions are detected, we went with a shorter distance and higher detection rate to account for higher boat and biological noise that were expected later in the summer.
We deployed our VPS arrays during the summers of 2021 and 2022 (Figure 1). The VPS array at AR285 consisted of nine VR2AR receivers and was deployed for 103 days. The VPS array at AR300 consisted of seven VR2AR receivers and was deployed for 129 days. The array at AR330 consisted of ten receivers and the array at AR345 consisted of seven receivers. Both arrays were deployed for 138 days. In the summer of 2021, VR2AR receivers were deployed at natural bottom areas near AR285 (n=4) and AR300 (n=2; Figure 2). In the summer of 2022, seven VR2AR receivers were placed at natural bottom (n=3), artificial reef complexes (n=2), or shipwrecks (n=2; Figure 2) near AR330 and AR345. These offsite receivers were used to monitor for any movements of tagged fishes off the artificial reef complexes that they were tagged on during 2021 and 2022 experimental periods. Additionally, detection data of our tagged fishes from sites we did not monitor were shared with us from colleagues at the North Carolina Aquarium at Pine Knoll Shores and North Carolina State University; these sites included the shipwrecks Atlas, USS Tarpon, and Portland, and the natural bottom site Big Rock.
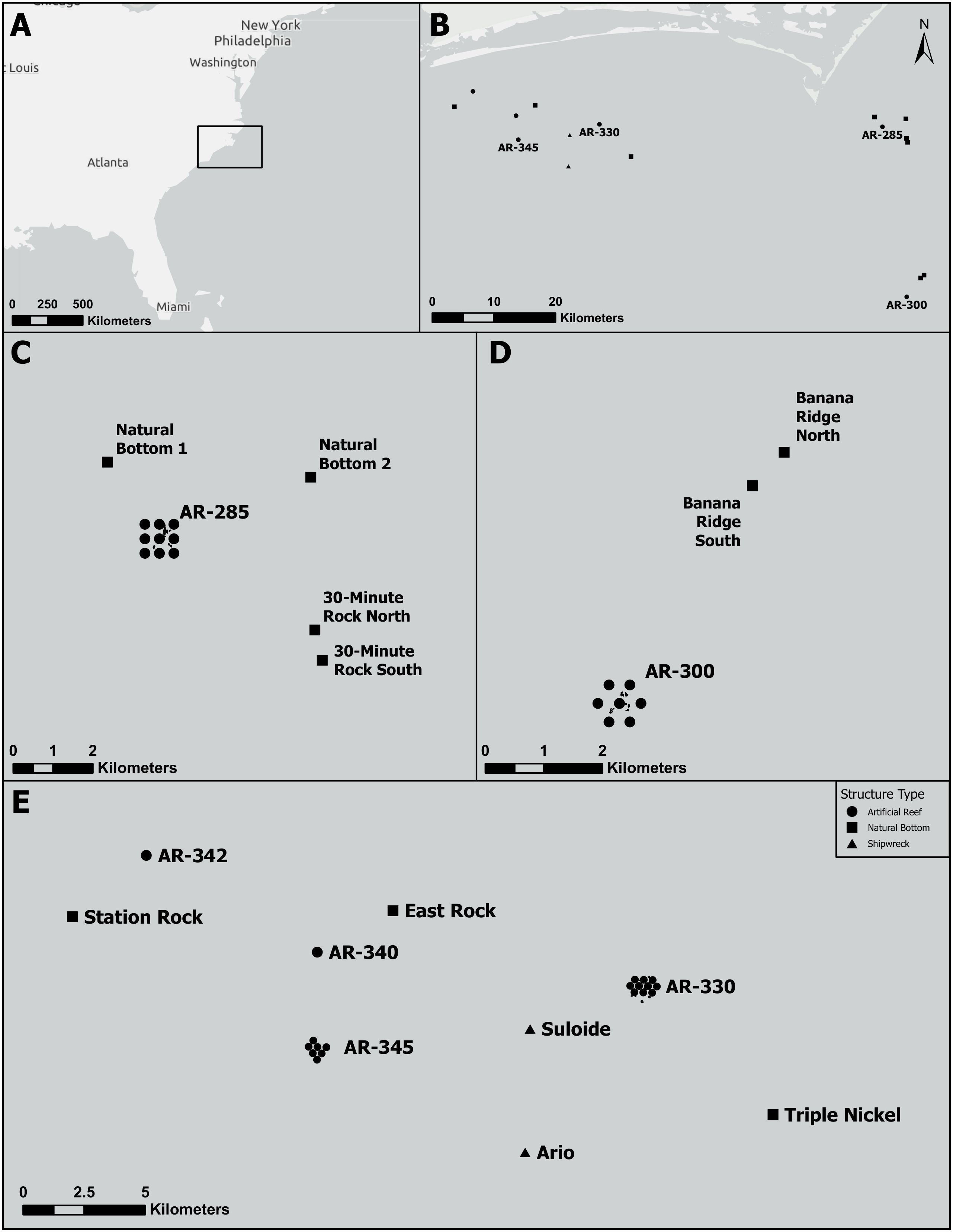
Figure 2 Locations of offsite receivers relative to their nearby Vemco Positioning System sites. (A) Larger geographic context of study where the black square highlights the study region. (B) Locations of the four monitored artificial reefs (AR) and surrounding offsite receivers. (C) Receivers at natural bottom near AR285 deployed in 2021. (D) Receivers at natural bottom near AR300 deployed in 2021. (E) Receivers at natural bottom, shipwrecks, and other artificial reefs near AR330 and AR345 deployed in 2022. Black circles represent artificial reefs, squares represent natural bottom, and triangles represent shipwrecks.
2.4 Array performance and fish inclusion
We downloaded the detection data from the receivers using the Vemco VUE software and sent them to Innovasea for initial processing. With the resulting data, we calculated a daily mean horizontal positional error (HPE) for each reference tag by comparing their known positions to the Innovasea estimated positions. We then compared reference tag performance to environmental data such as water temperature, wind speed, wind direction, wave height, and atmospheric pressure to see if any declines in detection efficiency were tied to environmental conditions. We also compared the deployed, retrieved, and Innovasea-derived receiver locations to see if there were any receivers that were moved or in the incorrect location. After assessing and confirming that the array performance was robust, we plotted the fine-scale movement tracks and visually assessed them for any individuals who lacked data or had non-useful data (e.g., the greater amberjack tagged at AR285 had five positions total and was therefore not used in analysis). We then created a series of abacus plots to view the days when VPS positions were estimated for each tag. There were days when no VPS positions were estimated; however, fishes were still detected by individual receivers, so days when individuals were detected but not positioned within the arrays were added to the abacus plots. Hereafter, we use detections to describe a detection of a telemetry tag at a single receiver, and use positions to describe a calculated VPS position of a telemetry tag. Lastly, detections at offsite receivers were added to the abacus plots for those individuals that left the VPS arrays and were detected elsewhere.
2.5 Fate assignments
To estimate habitat selection of our tagged species, we had to ensure that the tags represented tagged individuals and not predators, tags lying on the bottom, or deceased individuals. To assign black sea bass fates, we created a decision tree on how to use data based on fine-scale movement tracks and movement speeds of “positive controls” (Figure 3; Capizzano et al., 2019; Runde et al., 2020; Zemeckis et al., 2020). We define the positive control data as observed sea bass movements and movement speeds that occur from the time of the initial tagging event to the time of a reported recapture. Several black sea bass were recaptured and reported by anglers at AR285, AR330, and AR345 and served as positive controls for movement speeds for each tagging year using the maximum observed speed of each individual. For individuals who were re-released with their telemetry tags still attached, only movements prior to the recapture event were used as positive controls. We calculated movement speeds using VPS positions for each individual black sea bass as distance over time using the VPS data, and we then plotted speeds against time to examine movement speeds throughout the study. We then calculated the mean of the observed maximum movement speeds, and added two standard deviations to the value to create an upper threshold of speed. We calculated this value separately for 2021 and 2022 using positive controls from AR285 and ARs 330 and 345, respectively, and examined any black sea bass movement speed value above these thresholds in greater detail. We then compared resulting movement speeds to movement speeds of known black sea bass positive controls to assign fates. Any individual that was determined to have undergone a predation event or was determined to be a release mortality following a reported recapture event had their data filtered to include only positions before the time when the event was determined to have occurred.
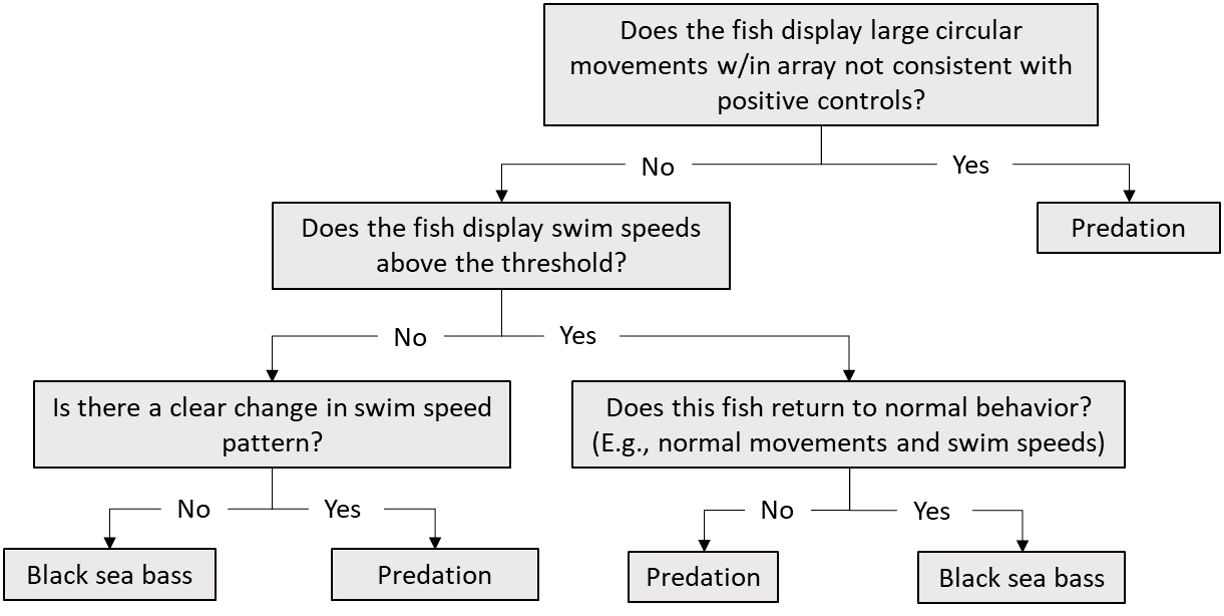
Figure 3 Black sea bass fate decision tree based on known black sea bass behaviors (i.e. positive controls).
We did not calculate movement speeds for other species due to a lack of positive controls; only one tagged red snapper and only two tagged greater amberjack were recaptured. To assign fates of other species, we visually assessed fine-scale movement tracks for signs of tag loss (e.g., non-moving tag), tagging mortality (e.g., erratic behavior followed by lack of movement or immediate emigration following tagging event), release mortality (e.g., erratic behavior followed by lack of movement or immediate emigration following release event), or predation (e.g., clear change in behavior). For fishes tagged with V16 transmitters, we also used release conditions to help assign fates. However, we note here that examples of these fates (e.g. tag loss, predation) were rare for species other than black sea bass. We used the assigned fates to filter out positions that were assumed to not belong to the tagged individual. The resulting filtered data for all species were used to calculate days at liberty as the number of days where the individual is still known to be alive.
2.6 Interpolating locations
To position a tag within the VPS system, it must be detected by three or more receivers simultaneously. Doing so can be difficult in marine habitats because artificial structures can block acoustic transmissions, biological (e.g., snapping shrimp) and anthropogenic (e.g., boat propellers) noise can disrupt transmissions, and increased wave activity from inclement weather can drown out tag transmissions (Kessel et al., 2014). For black sea bass, gag, and red snapper, there were long periods of time where an individual was not positioned because it was likely in or very near a structure and it could not be detected by three or more receivers. There were also a few days during the study where zero tags, or very few, were positioned at a reef. However, this did not mean that the individual had left the reef as we observed no movement tracks suggesting the individual emigrated and because the individual was still being detected during the time. Rather, we assumed the fish had not emigrated and that the individual was unable to be positioned, preventing VPS locations during those periods.
Once the fate of each individual was determined, we used the ‘crawl’ package (Johnson et al., 2022) in R (R Core Team, 2023), to fit a continuous-time correlated random walk model to each individual. We then predicted locations at fixed times to account for periods of time where individuals were unable to be positioned. We evaluated predictions at every one hour, six hours, 12 hours, and 24 hours; no noticeable changes in the overall results (see below) were observed; thus, we elected to use the one-hour estimated positions for further analysis. Long time periods without VPS-derived positions were less of an issue with greater amberjack and almaco jack, but for consistency we also used the crawl package to determine their hourly positions. To help standardize the number, frequency, and timing of positions per individual, we used crawl-predicted positions for subsequent analyses.
2.7 Habitat selection
To determine artificial structure selection for each species, we used a habitat selection function (HSF), also referred to as a resource selection function (RSF). We used the HSF framework presented by Fieberg et al. (2021) where the model is performed as a simple weighted logistic regression combining observed positions (i.e., “used” positions) with randomly generated available positions (i.e., “unused” positions). We chose to weight our available positions at 5,000 and observed positions at 1 as it is recommended that the available positions be assigned large weight values to ensure that results are consistent (Fithian and Hastie, 2013; Fieberg et al., 2021). Weighting values of 500, 1,000, and 10,000 were also evaluated, and indicated that results stabilized at weights ≥5,000. We used the North Carolina Division of Marine Fisheries (NCDMF) artificial reef boundaries to delineate available habitat in our model; however, our VPS array at AR330 did not have complete coverage of the NCDMF reef complex. Thus, we also performed the habitat selection model using available habitat where the boundaries were the VPS array and compared results between the two boundary types. We then used weighted logistic regression to evaluate selection via the ‘glm’ function in R (R Core Team 2023), where position type was the response (observed = 1, available = 0) and habitat associated with each position was the predictor (specific artificial reef structure or sand).
We used the model-generated estimates to determine selection for different artificial reef structures. Selection strength of one structure relative to another (relative selection strength) was determined by exponentiating the model-estimated coefficients of two structures and taking their ratio (Fieberg et al., 2021). It is important to note the calculated selection for one structure is relative to another structure, and that the calculated selection is dependent on the baseline structure. For example, if the ratio between vessel and Reef Balls equals 2.5 that means that the vessel is 2.5 times more likely to be selected compared to the Reef Balls, assuming equal availability. For our study, we used sand as a baseline comparison at all sites. We performed this process for each species individually at each artificial reef complex.
2.8 Transition matrices
To measure residency at artificial structures and transitions among them, we calculated transition matrices for each species at each artificial reef complex. We calculated transition matrices using a non-homogenous discrete time Markov chain process to estimate transition probabilities between consecutive observations. We performed our calculations using the ‘markovchain’ package (Spedicato 2017) in R. The calculated transition matrices represented the probability of an individual being positioned at a structure at time t+1 given that its current position was at a structure at time t with a time step of one hour. For example, if a black sea bass was positioned on a Reef Ball at time t and the transition probability from Reef Ball to pipe was 0.05, then there is a 0.05 probability of that black sea bass moving to the pipe at time t+1.
3 Results
3.1 Tagging
We tagged 65 fishes during the summer of 2021, 40 at AR285 and 25 at AR300 and tagged 85 fishes during the summer of 2022, 47 at AR330 and 38 at AR345 (Table 1). As part of a separate study occurring simultaneous to this one, an additional seven greater amberjack were tagged at AR330 and one at the Suloide shipwreck, increasing the total number of tagged fish to 158. For each year and reef combination, VPS positions were obtained for all tagged individuals unless otherwise stated below and all sizes presented are total length (mm) unless otherwise specified. Across all sites and species, the average time to attach an external tag was one minute 34 seconds for V9 telemetry tags, and average surgery time was six minutes 52 seconds for V16 telemetry.
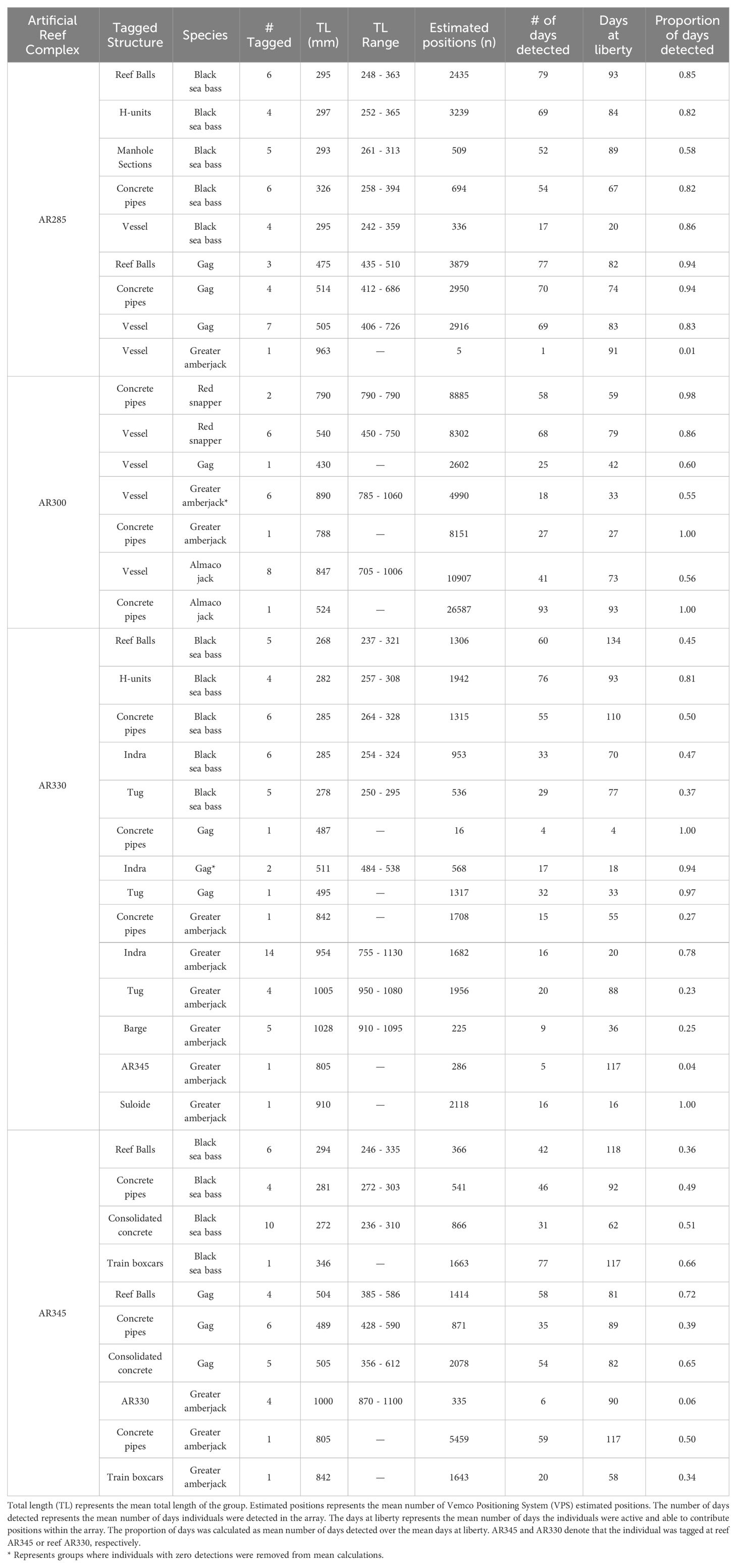
Table 1 Tagging information on all fishes used to examine artificial structure selection by economically important reef fishes at North Carolina artificial reefs. Tagging information is averaged across tagging structure.
Of the 150 fishes tagged specifically for this study, there were 72 black sea bass (2021: n=25; 2022: n=47), 34 gag (2021: n=15; 2022: n=19), 27 greater amberjack (2021: n=8; 2022: n=19), nine almaco jack (2021: n=9; 2022: n=0), and eight red snapper (2021: n=8; 2022: n=0). Two black sea bass and one greater amberjack were caught by anglers and had their tags returned; we redeployed those telemetry tags on the same species within the same reef complex and those are included in the above count. The eight fish from a companion study (8 greater amberjack) had a sufficient number of estimated positions within arrays to be included in our analyses. Sizes, number of positions, and days detected varied across tagged individuals (Table 1). Tags that were not positioned within the array, or lacked sufficient data, were removed from all further analysis (Table 2).
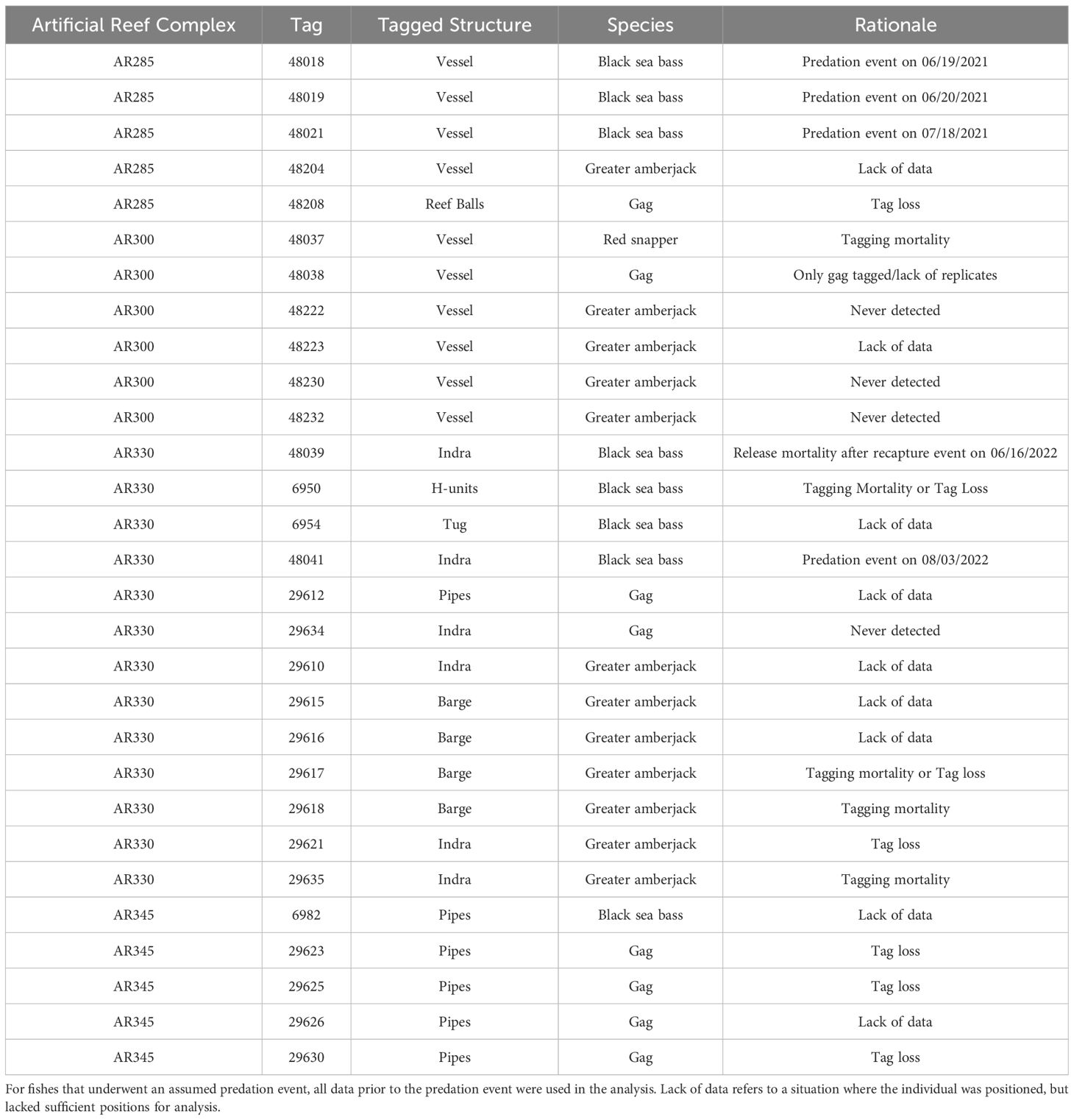
Table 2 List of tagged fishes removed from analyses of artificial structure selection by economically important reef fishes at North Carolina artificial reefs and the rationale for the removals.
3.2 Array performance
Our receiver arrays performed as expected with minimal positional error. Deployed, retrieved, and derived locations for all receivers at AR285 and AR330, and most receivers at AR345 were within ~10 m of each other. At AR300, the retrieved locations of receivers were more than 10 m away from deployed and derived locations. We assume this discrepancy is due to strong currents on the day of retrieval at AR300 causing the receivers to drift in the current during their ascent to the surface. Across all sites, mean daily HPE for V9 reference tags was 1.40 m and for V16 reference tags was 1.35 m. Temperature ranges monitored by the receiver arrays were homogenous within artificial reef complexes throughout the study.
3.3 Fate assignments
Eight black sea bass were recaptured and reported at AR285; the mean maximum movement speed was 0.214 m/s ± 0.0928 standard deviation (s.d.), resulting in an upper threshold speed of 0.399 m/s (mean + 2 * s.d.). Three black sea bass at AR330 and three at AR345 were recaptured and reported; the mean maximum movement speed was 0.133 m/s ± 0.104, resulting in an upper threshold speed of 0.341 m/s. The calculated movement thresholds and decision support tree (Figure 3) were then used to assign black sea bass fates; due to a lack of positive controls, we assigned fates for gag, red snapper, greater amberjack, and almaco jack by visually assessing their movement patterns. All individuals assumed to have undergone tag loss events, tagging mortalities, or whose fates were unknown were removed from further analyses. Individuals who were determined to have experienced a predation event had their data filtered to include only positions prior to the predation event. For a complete list of excluded individuals and rationale, see Table 2. For ease of interpretation, we have pooled counts of each species across reefs.
Of the 72 tagged black sea bass, we assumed that 50 individuals were alive and in their respective arrays until the conclusion of the study (Figure 4A); for the other 22 black sea bass, nine individuals emigrated, five individuals were recaptured and their telemetry tags returned, four were assumed to have undergone predation events, two were assumed to be release mortalities, and two had unknown fates due to a lack of position data. Of the original 34 tagged gag, we assumed 25 were alive and in their respective arrays at the conclusion of the study (Figure 5A), four were assumed tag loss events, four were assumed to have emigrated, and one was unknown due to a lack of positions. Of the 35 tagged greater amberjack, we assumed that all emigrated from their respective tagging site at some point (Figure 6A). Seven greater amberjack were assumed alive and in their respective array at the conclusion of the study, several individuals emigrated and returned to their release sites, four were assumed to be tagging mortalities, and three are unknown due to a lack of positions. Of the nine tagged almaco jack at AR300, four were assumed alive and in the array (Figure 7A) and five individuals emigrated and did not return. Of the eight tagged red snapper at AR300, five individuals were assumed alive and in the array (Figure 8A), one individual was recaptured and had its telemetry tag returned, one emigrated, and one was assumed to be a tagging or release mortality.
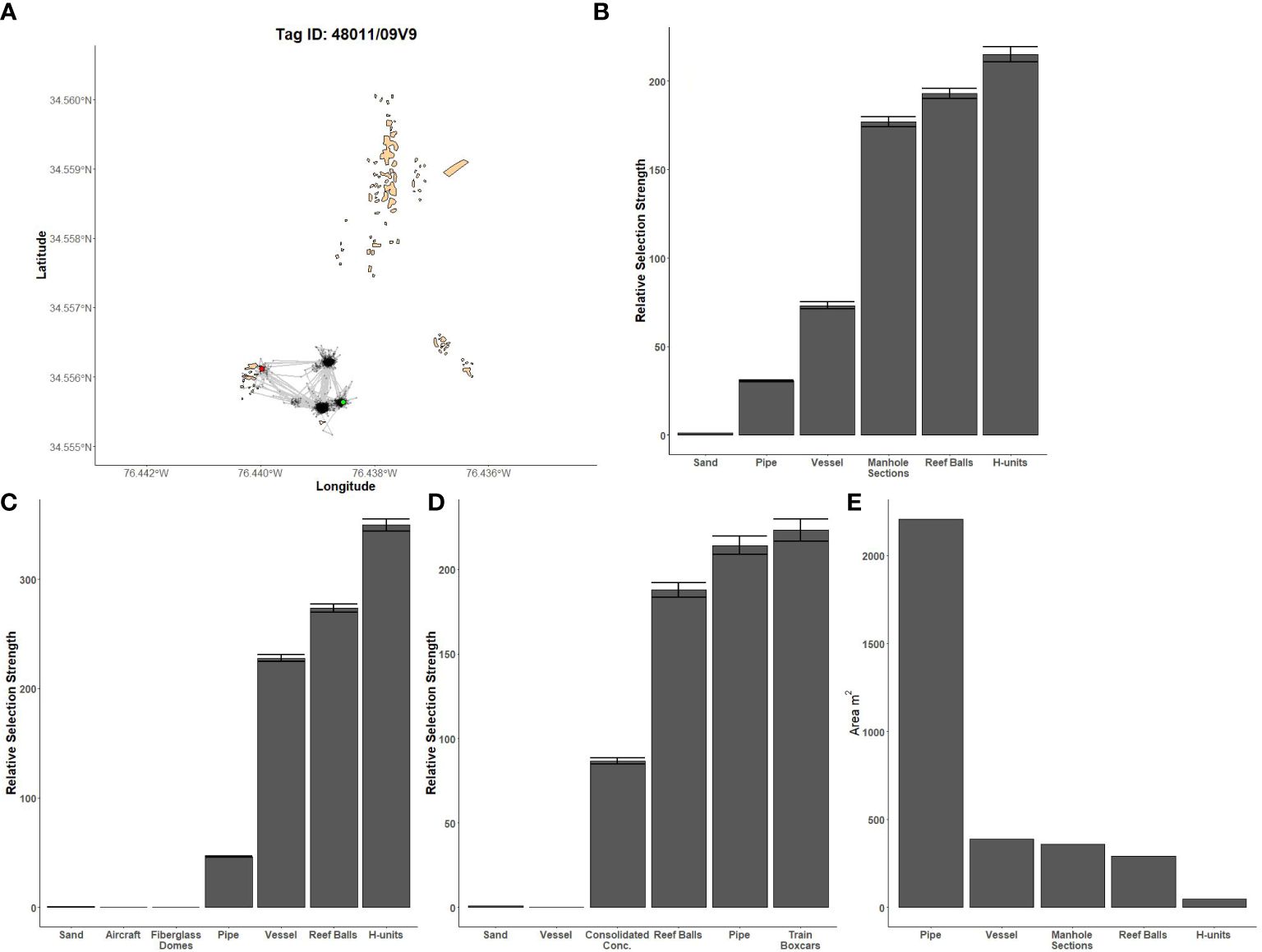
Figure 4 Fine-scale movements of an example black sea bass and habitat selection results for all black sea bass. (A) Example movement tracks based on estimated positions from a Vemco Positioning System for a black sea bass tagged at AR285, where green dots represent the first position, red dots represent the last position, and beige shapes represent artificial structures. Relative selection strengths for black sea bass at AR285 (B), AR330 (C), and AR345 (D) using sand as the baseline comparison. Error bars represent standard error estimates for their respective selection strengths. (E) Available area of each artificial structure at AR285. Refer to Figure 1 for location information.
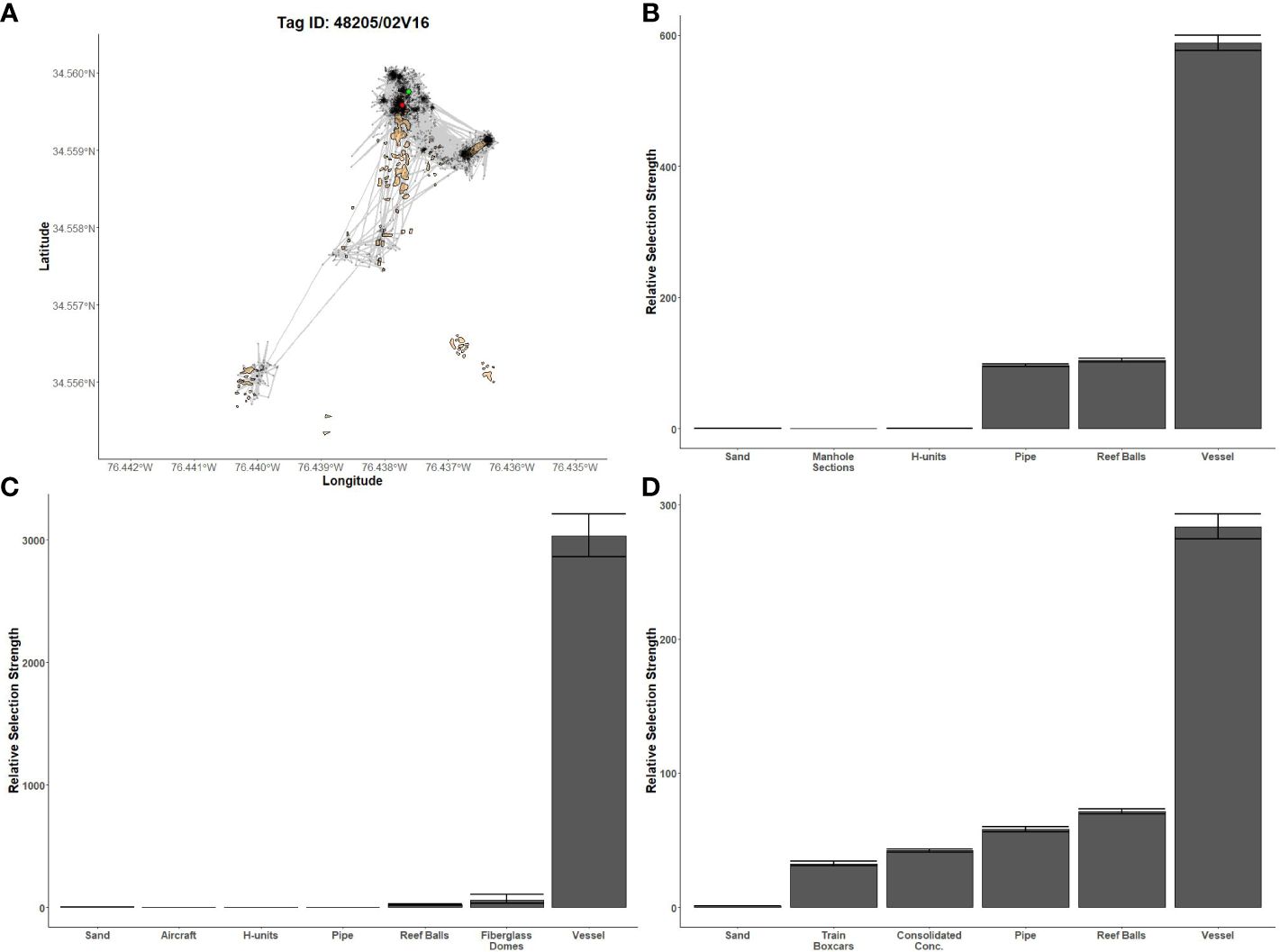
Figure 5 Fine-scale movements of an example gag and habitat selection results for all gag. (A) Example movement tracks based on estimated positions from a Vemco Positioning System for a gag tagged at AR285, where green dots represent the first position, red dots represent the last position, and beige shapes represent artificial structures. Relative selection strengths for gag at AR285 (B), AR330 (C), and AR345 (D) using sand as the baseline comparison. Error bars represent standard error estimates for their respective selection strengths. Refer to Figure 1 for location information.
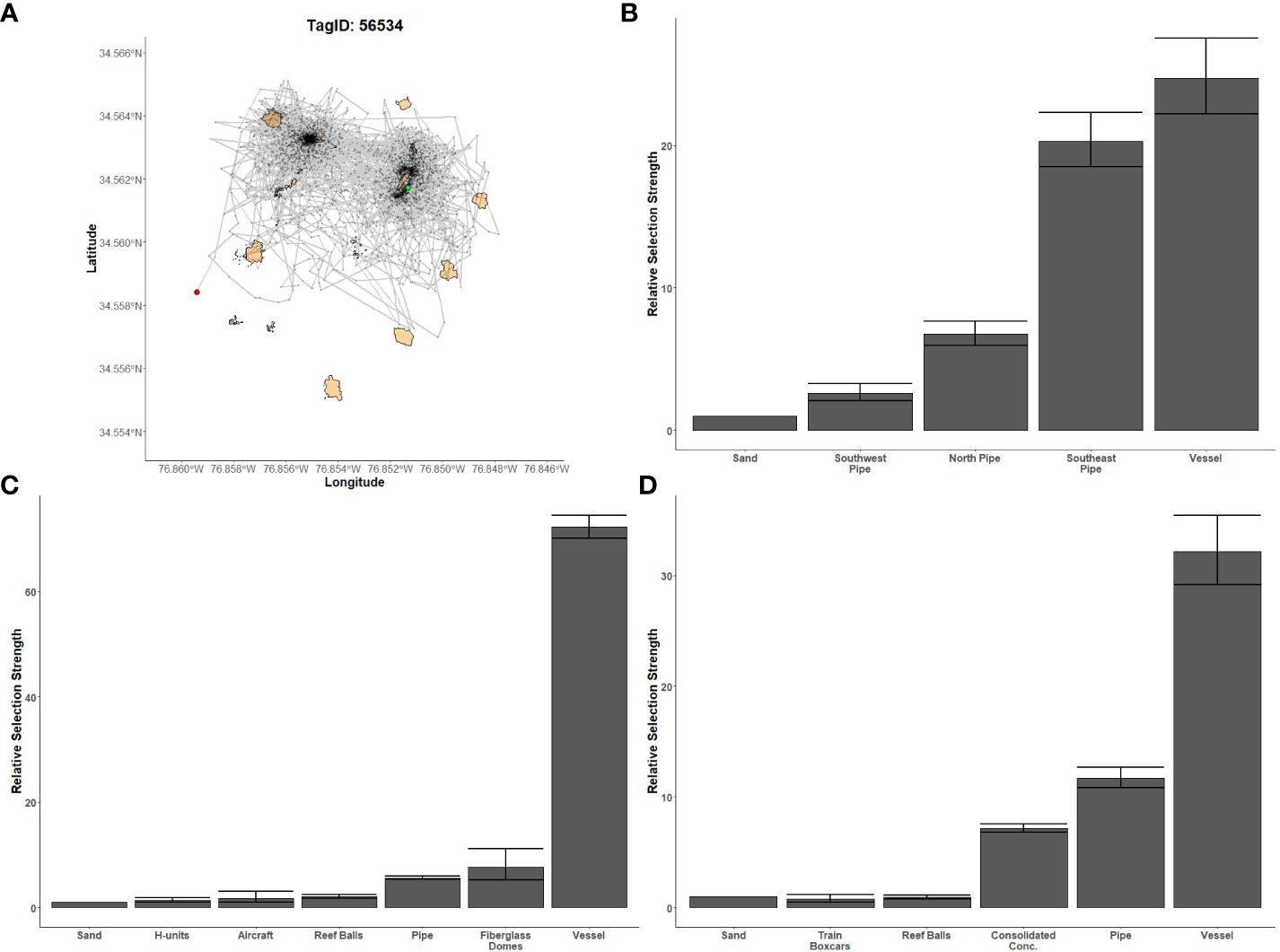
Figure 6 Fine-scale movements of an example greater amberjack and habitat selection results for all greater amberjack. (A) Example movement tracks based on estimated positions from a Vemco Positioning System for a greater amberjack tagged at AR330, where green dots represent the first position, red dots represent the last position, and beige shapes represent artificial structures. Relative selection strengths for greater amberjack at AR285 (B), AR330 (C), and AR345 (D) using sand as the baseline comparison. Error bars represent standard error estimates for their respective selection strengths. Refer to Figure 1 for location information.
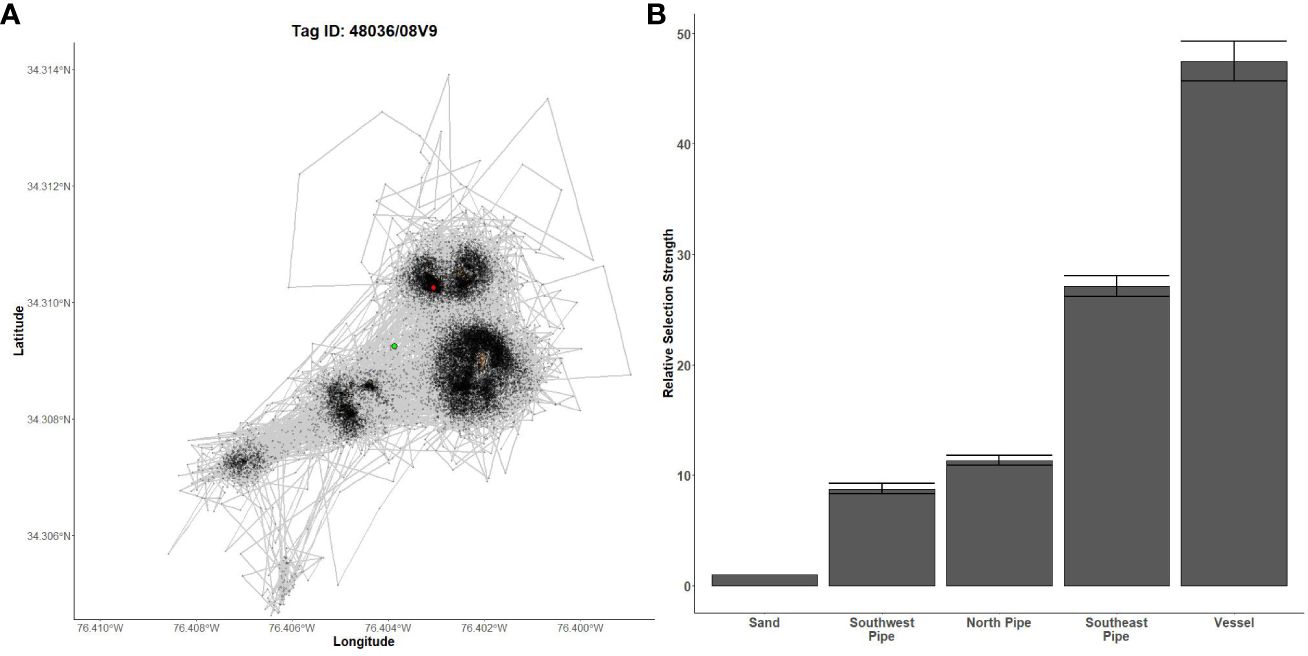
Figure 7 Fine-scale movements of an example almaco jack and habitat selection results for all almaco jack. (A) Example movement tracks based on estimated positions from a Vemco Positioning System for an almaco jack tagged at AR300, where green dots represent the first position, red dots represent the last position, and beige shapes represent artificial structures. (B) Relative selection strengths for almaco jack at AR300 using sand as the baseline comparison. Error bars represent standard error estimates for their respective selection strengths. Refer to Figure 1 for location information.
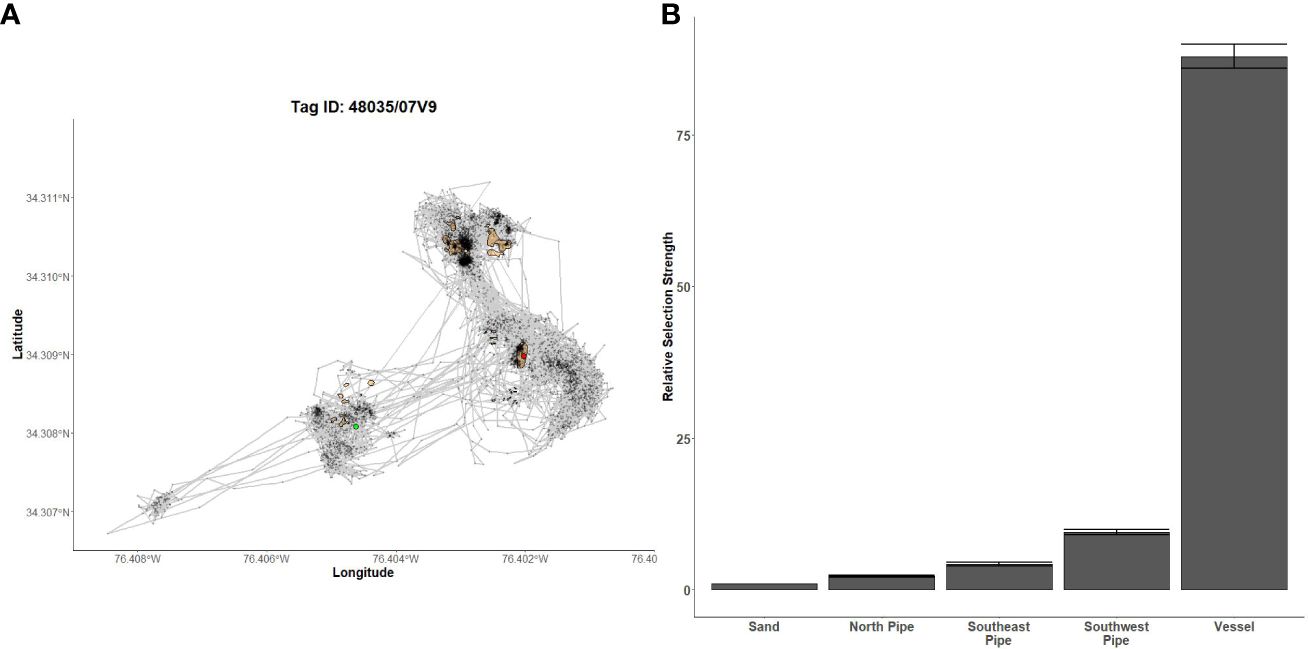
Figure 8 Fine-scale movements of an example red snapper and habitat selection results for all red snapper. (A) Example movement tracks based on estimated positions from a Vemco Positioning System for a red snapper tagged at AR300, where green dots represent the first position, red dots represent the last position, and beige shapes represent artificial structures. (B) Relative selection strengths for red snapper at AR300 using sand as the baseline comparison. Error bars represent standard error estimates for their respective selection strengths. Refer to Figure 1 for location information.
3.4 Habitat selection
Model results were qualitatively similar between the models using the NCDMF boundaries and those using the VPS boundaries. Moreover, on days with favorable acoustic conditions, some individuals were positioned slightly outside the VPS boundary but within the NCDMF boundary; we therefore present results from the model using the NCDMF boundaries as these boundaries cover more artificial reef area and include more data. Estimates of artificial structure selection from the HSF model were different for black sea bass compared to gag, red snapper, or the two jack species; the latter four species had similar structure selection estimates. Black sea bass had the highest selection for H-units at AR285 and AR330 and train boxcars at AR330 while the least selected structures were concrete pipe at AR285, aircraft and fiberglass domes at AR330, and the vessel at AR345 (Figures 4B–D). However, black sea bass selection was found to be the inverse of the available area of each artificial structure (Figure 4E) and is likely an artifact of the observed lack of movement between structures.
Gag had the highest selection for the vessels at AR285, AR300, and AR345 while the least selected structures were manhole sections at AR285, aircraft, H-units, and pipe at AR330, and the train boxcars at AR345 (Figures 5B–D). Greater amberjack had the highest selection for the vessels at AR300, AR330, and AR345 while least selected structures were the southwest concrete pipes at AR300, H-units at AR330, and train boxcars at AR345 (Figures 6B–D). Almaco jack had the highest selection for the vessel at AR300 with the southwest pipe field being the least selected (Figure 7B). Red snapper had the highest selection for the vessel at AR300 with the north pipe field being the least selected (Figure 8B).
3.5 Transition matrix
We found that transition probabilities between structures varied with species and reef (Table 3). Black sea bass at AR285 regularly remained on their initial structures as displayed by a transition probability of nearly 1 at all structures (Table 3 diagonal), suggesting they were unlikely to move to nearby structures. Indeed, black sea bass had the lowest probabilities of transitioning to other structures across 1-hr periods; the highest transition probabilities between structures were 0.003 ± 0.001 standard error (s.e.) at AR285 (vessel to the concrete pipe), 0.013 ± 0.001 at AR330 (H-units to the Reef Balls), and 0.001 ± 0.001 at AR345 (Reef Balls to the consolidated concrete; Table 3). Gag had higher probabilities of transitioning to other structures with probabilities of 1.00 ± 1.00 at AR285 (H-units to the Reef Balls), 1.000 ± 0.577 at AR330 (fiberglass domes to the vessel), and 0.111 ± 0.017 at AR345 (train boxcars to the consolidated concrete). Gag at AR285 had high probabilities of remaining at the vessel, pipe, and Reef Balls, but always left the H-units in less than 1-hr (Table 3), supporting the selection results. The highest red snapper transition probability between structures was 0.374 ± 0.0444 from southeast concrete pipes to the vessel at AR300. This high transition probability, coupled with the high probability of remaining at the vessel (0.961; Table 3), supports the selection results. At AR300, the highest almaco jack transition probabilities between structures were 0.285 ± 0.019 from the vessel to the southeast concrete pipes and 0.259 ± 0.017 from the southeast pipes back to the vessel, again supporting the selection results described above. The highest greater amberjack transition probabilities between structures were 0.418 ± 0.068 at AR300 (vessel to the southeast concrete pipes), 0.857 ± 0.35 at AR330 (fiberglass domes to the vessel), and 0.600 ± 0.346 at AR345 (train boxcars to the concrete pipes). Greater amberjack at AR330 regularly transitioned to the vessel from nearly all structures, and had high probabilities of remaining at the vessel (0.90; Table 3), which supports results of high selection for the vessel at AR330 (Figure 6C). Conversely, greater amberjack at AR330 would occasionally transition to the aircraft or fiberglass domes but always left in less than 1-hr (Table 3) suggesting that these structures served as short-term use structures as opposed to long-term use of the vessel.
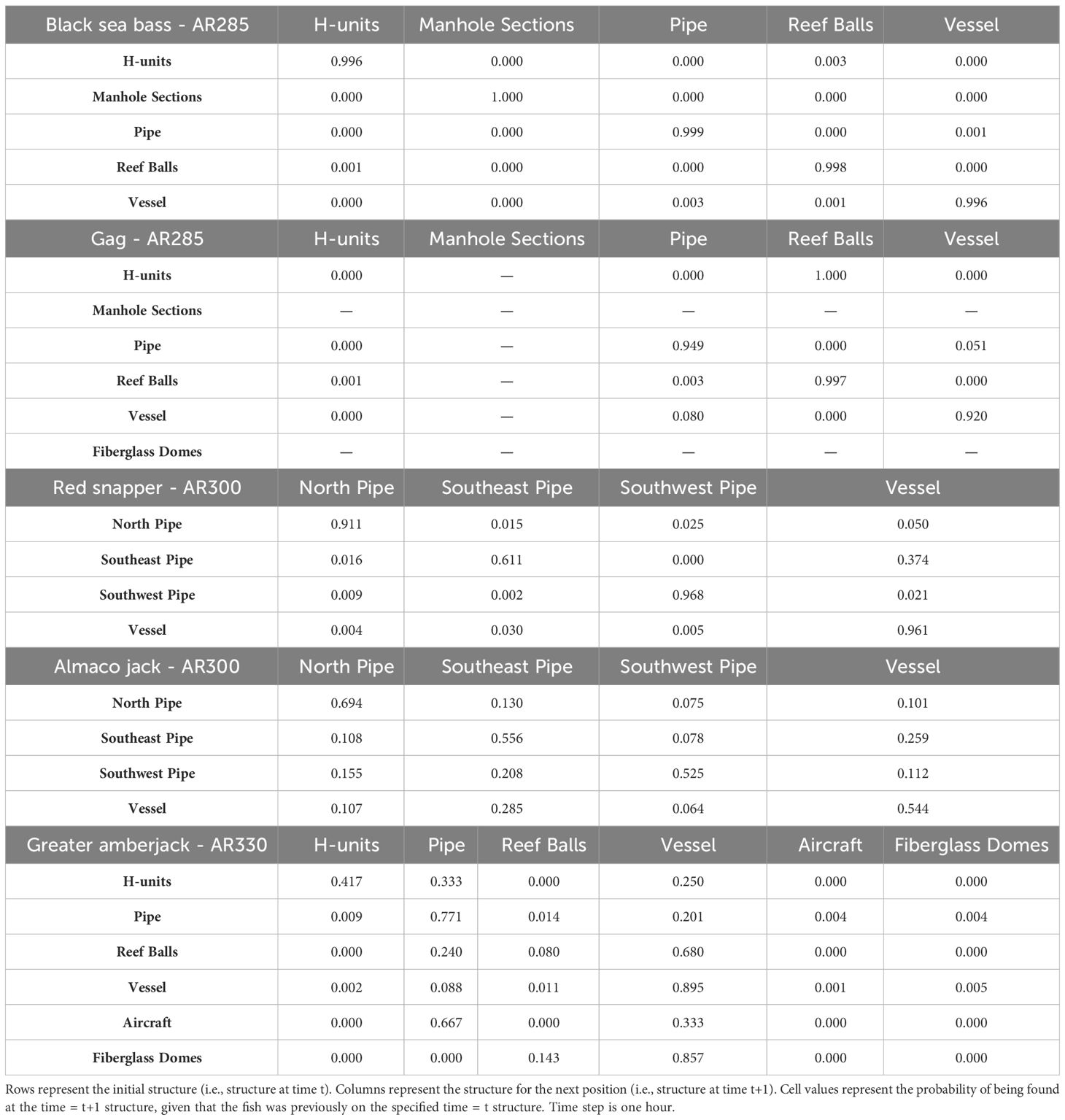
Table 3 Transition matrices for black sea bass and gag tagged at AR285, red snapper and almaco jack at AR300, and greater amber jack at AR330.
3.6 Offsite movements
Offsite movements varied by species and year, with greater amberjack and almaco jack moving offsite more than the other tagged species, and the most detected movements occurring in the summer of 2022. In 2021, three black sea bass were detected offsite of AR285: two at the northeast natural bottom site for one day and one at the northwest natural bottom site for one day. One gag and the lone greater amberjack from AR285 were detected offsite at a natural bottom reef called 30-Minute Rock, each for one day. Two almaco jack and two greater amberjack were detected at a natural reef called Banana Ridge northwest of AR300 (Table 4). Time spent at Banana Ridge ranged from a single day, to several consecutive days; additionally, two of these four fish moved between AR300 and Banana Ridge on multiple occasions. An individual greater amberjack was detected at the Portland wreck near Cape Lookout for several days and a separate greater amberjack was detected at a natural reef called Big Rock for a single day (Table 4); these detections were provided to us by colleagues that had receivers deployed as part of separate studies. The Portland shipwreck had the highest proportion of offsite detections (0.751) in 2021, with the two Banana Ridge receivers having the next most (0.238), and Big Rock the least (0.011; Table 4). In 2022, no black sea bass or gag were detected offsite while greater amberjack visited every offsite receiver that we had deployed with the exception of the natural reef Station Rock. Greater amberjack were also detected by our colleagues’ receivers at the Atlas and USS Tarpon shipwrecks. Eight of the 24 greater amberjack tagged at AR330 (including the seven tagged for a separate study), both greater amberjack tagged at AR345, and the individual amberjack tagged at the Suloide were detected at reef complexes other than their tagging reef complex. There were more frequent movements of greater amberjack from the artificial reef arrays to other artificial sites and fewer movements from the arrays to natural bottom sites. Artificial sites, consisting of the Suloide, Ario, and Atlas shipwrecks and AR340, had a very high proportion of offsite detections (0.97) relative to detections on receivers at natural bottom sites (Table 4).
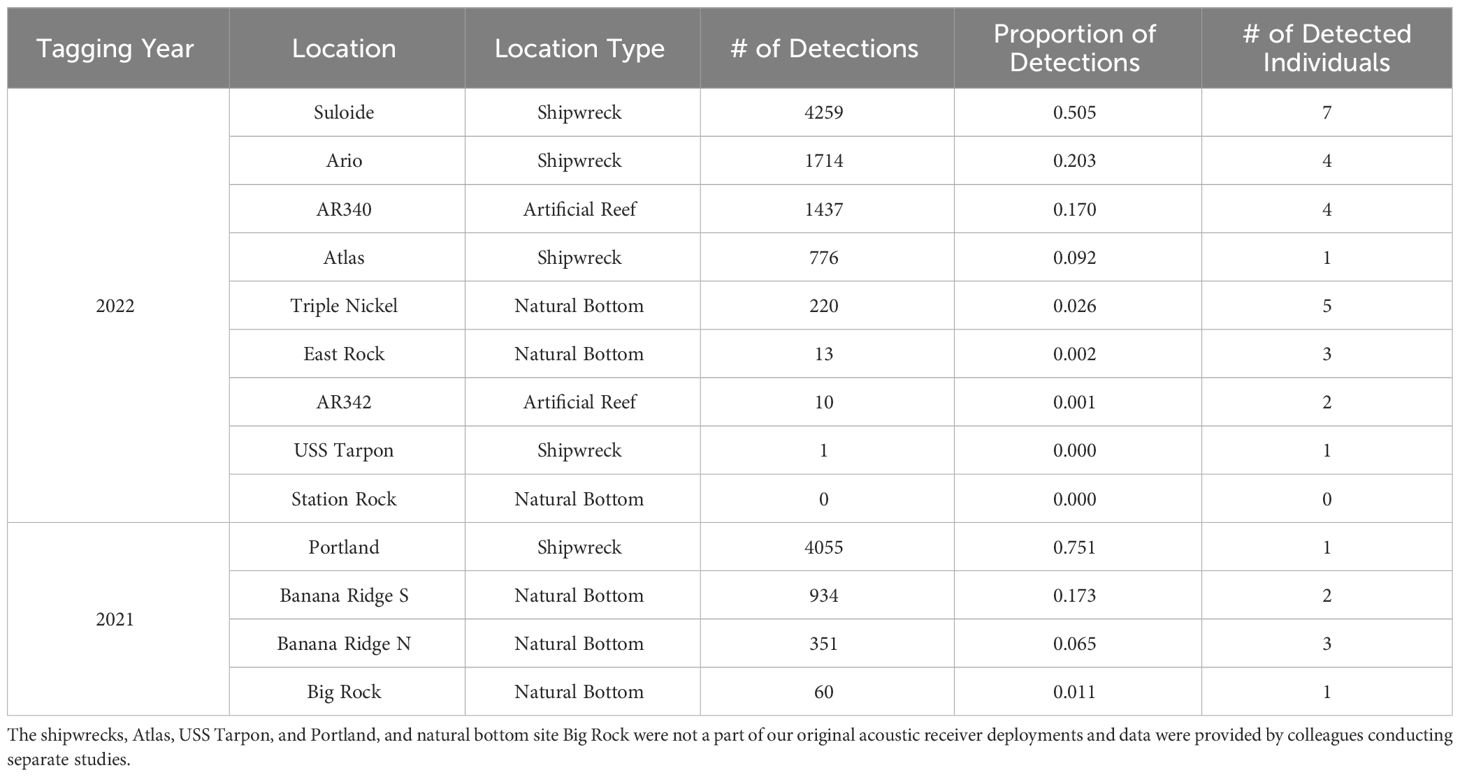
Table 4 Number and proportion of offsite (non VPS receiver locations) detections of greater amberjack at locations with single receivers.
4 Discussion
We found significant selection for certain artificial structures and that this selection differed by species. Gag, red snapper, greater amberjack, and almaco jack displayed a high selection for vessels while black sea bass selected for lower relief structures, such as Reef Balls. However, the selection estimates for black sea bass are biased because transition matrices showed that they moved very little from the structure where they were caught, tagged, and released. Black sea bass had a much lower likelihood of moving from structure to structure while gag and red snapper did so regularly. Greater amberjack and almaco jack had the highest probabilities of moving between structures. Offsite movements out of artificial reef complexes were rare for black sea bass, gag, and red snapper, but were commonly observed with greater amberjack and almaco jack.
4.1 Habitat use
Knowing if different species of management concern are selecting for some artificial structures over others can be valuable information for the deployment of new and modification of existing structures within artificial reef complexes. Our habitat selection results for gag, red snapper, almaco jack, and greater amberjack showed the highest selection for vessels. Our observed high selection for vessels in four out of five species could be due to a variety of reasons.
First, vessels tend to hold a higher abundance and biomass of marine life compared to other artificial structures (Paxton et al., 2017; Lemoine et al., 2019) and could improve foraging opportunities. Food abundance is known to be a key driver of fish movements (Cooke et al., 2022), and it follows that fishes are more likely to use habitats that increase their chances of finding food. In this region, common prey groups, such as Carangidae, Clupeidae, Haemulidae, and Sparidae can occur in high abundances on vessels (Stephan and Lindquist, 1989; Lemoine et al., 2019) and could lead predatory species, such as those we studied, to select for, move to, and spend more time at vessels over other structures.
Second, vessels provide more vertical structure as they often extend further off the seafloor than other structures. The increased vertical surface that vessels provide allows more surface over which epibenthic life can grow. Structures such as Reef Balls and pipes have comparably more horizontal surface, which tends to host less epibenthic life than vertical surfaces (Walker et al., 2007). An increase in epibenthic life could lead to increases in smaller fishes that would increase foraging opportunities for predatory fishes. Higher vertical structure could be advantageous for greater amberjack (Tone et al., 2022) and red snapper (Williams-Grove and Szedlmayer, 2017; Bacheler et al., 2021) as both species have been observed making daily vertical movements, thought in part to do with increased forging opportunities. However, our observed selection in red snapper could also be an artifact of where they were tagged; seven of our nine snapper were tagged at the vessel where the probability of remaining at the vessel during 1-hour intervals was 0.96 despite some individuals displaying a clear ability to move among structures. Red snapper monitored with a fine-scale positioning system at an artificial site in the Gulf of Mexico were also determined to select vessels over concrete structures like culverts and pyramids, but they too were observed spending the greatest amount of time on their tagging structure compared to other structures (Gibson Banks et al., 2021).
Third, vessels tend to have higher structural complexity than other artificial structures (Paxton et al., 2017; Lemoine et al., 2019). Increased complexity often leads to more area or volume, and an increase in holes, which can be beneficial to many reef fishes, particularly gag (Hackradt et al., 2011). Gag on experimental artificial reef complexes in Florida selected for structures that contain openings as opposed to solid-faced structures (Lindberg et al., 2006). Brotto and Araujo (2001) observed the same behavior in several serranid species by deploying structures made of clay tiles in varying degrees of complexity and observing the highest abundances on the most complex structures. Primary uses of such openings in structures could be for shelter from larger fishes or to aid in ambush feeding. When monitoring shelter use by reef fishes, Khan et al. (2017) rarely observed individuals more than 2 m from a point of shelter. Using structure to support ambush behavior is well known in many grouper species (Harmelin-Vivien and Harmelin, 2022) and has even been observed in the pelagic species, bluefin trevally (Caranx melampygus), at a coral reef in the central Pacific (Sancho, 2000). However, it is important to note that artificial structures can change over time and the current structure condition may not be completely representative of the condition of the structure when it was deployed. For example, lower relief structures such as reef balls and concrete pipes can be buried in sand and higher profile structures such as vessels could collapse or break down, changing the physical characteristics of the structure such as area, perimeter, or relief (Tassetti et al., 2015). We did not examine these quantitative metrics in this study, but they should be considered in future work.
We found conflicting results with regards to structure selection in black sea bass. The results from the habitat selection model suggested that black sea bass select for low relief structures, such as H-units and Reef Balls. However, the transition matrices suggest that black sea bass have very high site fidelity to the structure they are caught and released over and are unlikely to move. Indeed, the fine-scale positions of our bass were highly concentrated around the structure that they were caught, tagged, and returned to. This lack of movement displayed by black sea bass has been observed in other systems. For example, researchers in South Carolina conventionally-tagged black sea bass on and off an artificial reef complex and found that most recaptured black sea bass were at or very near their initial tagging structure; only 10% of sea bass were recaptured on different structures while the other 90% were recaptured at their tagging structure (Low and Waltz, 1991). Another conventional tag study at an artificial reef complex in New Jersey found that 78% percent of recaptures occurred at the exact site they were tagged (Figley, 2003). We conclude that black sea bass may not actively select for one structure type and that HSF results should be interpreted with caution when fish do not move from one habitat to another (see below); rather, black sea bass appear to be habitat generalists in regards to structure types with high site fidelity.
It is worth noting that the lack of movements of black sea bass could be impacted by reproductive and social behaviors. Black sea bass are protogynous hermaphrodites, beginning life and undergoing initial spawning seasons as females, later transitioning to males at larger sizes (Watanabe 2011). Such life history patterns in reef fishes typically lead to territorial behavior in males as they develop and protect harems (Mumby and Wabnitz, 2002; Kline et al., 2011). The resulting behavior could be that males are remaining where they are to protect their territory or harem, potentially explaining the lack of movement. However, we do not think this had a strong role in our results for two reasons. The first is that black sea bass have a 50% probability of transitioning to males at 355mm total length (Provost et al., 2017). Of our tagged sea bass, 66 of the 71 individuals were below this size and were likely females. The second is that while haremic and territorial behaviors with conspecifics are common for other reef fish, such behaviors have not been documented for black sea bass in the wild (Fabrizio et al., 2013), only in laboratory settings during spawning seasons (Nelson et al., 2003; Stuart and Smith, 2003). Importantly, spawning for the mid-Atlantic stock occurs in the spring from mainly March to May (Watanabe 2011); our study occurred after spawning season during the summer from June to September. Therefore, it is difficult to say if density had an impact on the observed movements. Future studies would benefit from additional work on this topic.
We also observed differences in the use of the surrounding, non-structured sandy bottom by species at our monitored artificial reef complexes. Fine-scale positioning data show that black sea bass rarely ventured away from hard structure, spending much of their time either over or directly next to structure. A similar pattern in habitat use has been observed in the northern stock off New Jersey where telemetry-tagged black sea bass were more likely to be detected at sites with varying bathymetry and placed structures as opposed to open bottom (Fabrizio et al., 2013). While no studies could be found regarding area use around individual structures, it has been shown that reef fish abundance declines with distance from a reef (dos Santos et al., 2010; Lemoine et al., 2019). Gag and red snapper on the other hand more frequently moved through the sandy bottom, but their movements were highly directed between structures; any observed off structure movements were straight-line movements suggesting that they are only displaying transient behavior over sand. Similar behavior has been observed in red snapper at an artificial reef in Texas where individuals displayed significant differences in habitat use between structures and open sand, using the structures more (Gibson Banks et al., 2021). Lastly, greater amberjack and almaco jack displayed large, circular movements over sandy bottom and frequently moved off structures. As water-column associated species, this behavior is not unexpected; greater amberjack and almaco jack typically do not shelter in holes or under ledges like demersal species. As these species spend more time in the water column than the demersal species in this study, they are more likely to follow and feed on smaller pelagic fishes that aggregate near or above structures (Paxton et al., 2017; Lemoine et al., 2019).
4.2 Limitations of habitat selection functions
Black sea bass display high site fidelity to the structure location of capture. Extreme site fidelity (i.e., lack of movement among structures) causes challenges to the interpretation of HSF parameters as it is not clear if selection arises from preference or an inability to access other features (e.g., distance between features). Habitat selection functions rely on selection to occur (i.e., an individual moving between available habitats), so when selection does not occur (i.e., no movement between available habitats), HSF model results can be biased. In the case of black sea bass, we identified two sources of bias.
The first source of bias is that HSFs use the available area (i.e., footprint) of each structure when calculating selection strengths; they assume equal area for each structure and account for differences in available area by treating smaller structures as more important. Under random fish movement, the number of positions should be proportional to the area of the structure. Thus, if it were observed that the number of positions was not proportional to the area of the structures, some form of selection would be occurring. However, if no movement between structures occurs, and the number of positions at each structure is roughly equal, the HSF model would estimate a higher selection for the lower area structures even though there was no fish selection of structures. We anticipated that black sea bass might have infrequent or short movements, so we dispersed their telemetry tags as equally as possible over each structure type within each reef complex. In doing so, we expected that if the black sea bass did not move, the selection for each structure would be roughly equal. In reality, the observed selection was the inverse of the available area; the most selected structure was the least available structure type and the least selected structure was the most available. Selection strongly biased towards available structure area was not an issue with other tagged species because they frequently moved between structures.
The second source of bias is that, when no movement is occurring, HSFs are unable to account for unequal mortality rates across structures. In the model framework, selection strength is dependent on the total number of positions present at a structure. Thus, when a structure has a high number of positions, it is thought to be highly selected whereas when a structure has a small number of positions, it is thought to be minimally selected. Ideally, tagged individuals would move between structures and contribute positions at different structures dependent on their movement choices in addition to the amount of time spent at a structure. If one of those individuals was removed, the other individuals that were still moving within the system would mask the loss of positions that the removed individual would have contributed. However, in the absence of movement, loss of an individual at a structure would result in a decrease in the number of positions and an underestimate of selection strength. Using AR285 as an example, black sea bass tagged at the vessel were in the array for an average 19.75 days while those tagged at the remaining structures ranged from an average of 64.8 to 92.3 days. The result of this was an underestimated selection of the vessel because the bass were not leaving the structures they were tagged on and the vessel was receiving fewer positions. Therefore, given that the habitat selection function at the spatial scale of an entire site was dependent on movement between structures, we conclude here that HSFs should be interpreted with caution for species, like black sea bass, that display very high site fidelity. Future research to tease apart selection and distances among features would further resolve uncertainties in how black sea bass use artificial structures and their placement.
4.3 Offsite movements
When managing artificial reefs, it is important to consider how target species are using surrounding habitat. We observed frequent offsite movements for our tagged water-column associated species, but offsite movements were rare for demersal species. Three black sea bass tags were detected at a natural bottom site nearby AR285, but it was not possible to confirm whether they were bass or predators as they never returned to AR285, nor had they been recaptured and reported.
Despite larger within-artificial reef complex movements for gag and red snapper, relative to black sea bass, no red snapper and only one gag were ever detected offsite, suggesting that these two species have high site fidelity to the artificial reef complex where they were found. Similar results have been found along the west coast of Florida; gag tracked over two broad acoustic arrays were most detected at the section of reef where they were tagged and they rarely left their tagging reef (Ellis et al., 2019). However, Ellis et al. (2019) do not specify whether the tagging reefs were natural or artificial. Afonso et al. (2016) tracked acoustically tagged dusky grouper (Epinephelus marginatus) at a natural site in the Azores and also found that individuals leaving the tagging area was rare. Red snapper tagged at an artificial reef complex in Texas were also rarely observed moving to nearby natural bottom (Froehlich et al., 2019). However, one factor that could bias the offsite movement results is the study duration. We tracked our fish for ~100 days during the summer and fall, which could be too short to consider offsite movements. Ellis et al. (2019) and Froehlich et al.’s (Froehlich et al., 2019) studies were over a similar time frame, roughly 100 to 120 days, and observed similar results. Importantly, Afonso et al. (2016) tracked dusky grouper over a much longer time frame, a median of 1,620 days, and also determined that offsite movements were rare. Given that the longer term results of Afonso et al. (2016) are similar to the shorter terms results of Ellis et al. (2019), Froehlich et al. (2019), and our own study, it is possible that the observed lack of offsite movement for gag and red snapper are not a result of the study duration.
Greater amberjack and almaco jack displayed very large and frequent offsite movements to nearby natural bottom, artificial reef complexes, and shipwrecks that had acoustic receivers. At AR300, almaco jack were observed spending longer periods of time at the reef complex that they were tagged compared to greater amberjack. Between AR300, AR330, and AR345, greater amberjack spent similar numbers of days at each reef with individuals tagged at AR300 being detected offsite less frequently than those tagged at AR330 or AR345. Fewer offsite detections for greater amberjack tagged at AR300 could be due to a lack of receiver coverage at surrounding sites. In summer 2021, only one nearby site had deployed receivers, so it is possible that tagged greater amberjack left AR300 and visited unmonitored sites. This is supported by the presence of one of our tagged greater amberjacks being detected by a colleague’s receiver at the Portland shipwreck north of AR300. By comparison, we monitored several natural and artificial sites in the summer of 2022 and had far more offsite detections. The greater amberjack tagged at AR300, however, were observed returning to AR300 far less than those tagged AR330 or AR345. That is to say that greater amberjack that left AR300 were unlikely to revisit the site while those that left AR330 and AR345 frequently returned to their tagging reef complex during our study. This could be because AR300 is a more isolated artificial reef complex than either AR330 or AR345. The three nearest known locations to AR300 are the natural reef Banana Ridge 4.5 km to the northeast, the Portland shipwreck 25 km to the north, and AR305 27 km to the west. In contrast, there were multiple natural and artificial reef complexes near the VPS array sites in 2022; eight sites were within 24 km of AR330 with three of them within 10 km and the same eight sites were within 20 km of AR345 with four of the within 10 km. With the number and close proximity of other reefs and structures, it is possible the greater amberjack tagged at AR330 and AR345 were more likely to frequently move between reef complexes than what we observed for AR300. It is also possible that greater amberjack were less likely to revisit AR300 after leaving because of the distance traveled to move between neighboring sites. Acoustically tagged greater amberjack in the Gulf of Mexico displayed similar behavior; 14% of tagged individuals left their tagging reef and the distance they traveled to a second site ranged from 4.5 to 18.6 km (Boyle et al., 2022b). Another study showed that over a 35 year time-span, nearly half of conventionally tagged greater amberjack in the SEUS that were recaptured had zero net movement while one third moved within ~46 km of their tagging location; most individuals were recaptured within a year of tagging (McClellan and Cummings, 1997).
Greater amberjack frequented off-site locations with high relief structure, like vessels, more often than they frequented locations without vessels, suggesting that they select for areas or reef complexes where high relief structures are present. Four of the five most visited sites by greater amberjack were the shipwrecks Portland in 2021, and Suloide, Atlas, and Ario in 2022. Thus, these between reef complex movements provide data that support our within-complex results showing selection for vessel structures. This high selection for vessels was also observed by Arena et al. (2007); they used visual SCUBA censuses to monitor fish communities around vessels and surrounding natural reefs in Florida and only observed greater amberjack at the vessels. Arena et al. (2007) even go so far as to mention that greater amberjacks have never been observed in natural reef surveys in Broward County, FL. This high selection could also be due to the high vertical relief provided by vessels compared to natural sites as Seriola spp. have also been observed in high numbers on other high relief structures like FADs (Stephan and Lindquist, 1989). Indeed, greater amberjack tagged in 2021 were detected over three-times as much at the Portland shipwreck compared to Banana Ridge; the Portland is 25 km from AR300 while Banana Ridge is only 4.5 km. This same pattern was observed in greater amberjacks in 2022 as they were detected nearly four-times as much at the Atlas shipwreck compared to Triple Nickel, the most frequented natural bottom site, when the distance to travel to those sites from AR330 was 56 km and 6.5 km, respectively.
The importance of high relief structure to greater amberjack can be seen further when removing the shipwrecks from consideration in our results. Greater amberjack frequented higher-relief artificial sites, like AR340, more often than lower-relief natural sites, like Triple Nickel. Becker et al. (2017) observed similar behavior in yellowtail amberjack (S. lalandi) at a high relief artificial reef complex, absent a vessel, compared to nearby coral reefs in Australia; yellowtail were more common on the artificial reef. The importance of high relief habitat has also been observed when considering only natural sites. Using extensive stationary video data, Bacheler et al. (2022a) show that, among SEUS natural reef habitat, all four Seriola species were observed more frequently at high relief sites than low relief. Thus, it appears that patterns of among-reef movement are emerging. However, there are two important notes to be made. Of the most visited sites without vessels, AR340 was the nearest site to AR345 and Triple Nickel was the nearest site to AR330, where most of our greater amberjacks were tagged, and the proportion of detections decreased at farther sites. Moreover, natural sites are extensive and often larger than artificial sites (Steward et al., 2022), so one receiver is not likely to cover the entire area like a single receiver could cover an entire artificial reef complex or vessel. As such, in the absence of a high relief vessel, greater amberjack could be using sites based on proximity in addition to artificial or natural composition and it is possible greater amberjacks visited our monitored natural sites in locations that were outside our receivers’ detection ranges. We did not control for the confounding effects of distance or receiver coverage when identifying these offsite patterns. Examining these effects is beyond the scope of this paper, but should be considered for any future analyses.
5 Conclusions
We provide the following conclusions based on our findings as considerations for future artificial reef planning. During the time period of our study, black sea bass displayed very limited movement among structures and a very high site fidelity to their tagging locations that represented a diversity of structures, rarely venturing off structure. Thus, black sea bass did not display selection for any particular artificial reef structure type and they can be considered habitat generalists. When planning artificial reefs, any available artificial structure (based on those we monitored) appears to meet their needs. An additional implication of high site fidelity is the potential for a put-and-take fishery at artificial reefs if the costs of black sea bass propagation (Watanabe et al., 2021) could be offset by angler payments for that type of fishery, assuming the ecological impact of stocked fish was minimal. Gag and red snapper had moderate movements between structures, using most of the available structures, but showed the highest selection for vessels. Therefore, when planning artificial reefs with gag and red snapper in mind, it appears best to use a variety of structures, including vessels or similar types of structures, greater amberjack and almaco jack had the largest movements and used the entire reef area, but they showed the highest selection for the vessels. They also frequently left the artificial reefs and most frequently visited other artificial locations, especially those with vessels. Thus, when planning artificial reefs with greater amberjack or almaco in mind, it appears that they will use all available structures, but a vessel with relatively higher relief appears to be the most selected structure when occupying artificial sites.
Data availability statement
The raw data supporting the conclusions of this article will be made available by the authors, without undue reservation.
Ethics statement
The animal study was approved by North Carolina State University Institutional Animal Care and Use Committee. The study was conducted in accordance with the local legislation and institutional requirements.
Author contributions
RT: Conceptualization, Data curation, Formal analysis, Investigation, Methodology, Visualization, Writing – original draft, Writing – review & editing. NH: Methodology, Validation, Writing – review & editing. AP: Conceptualization, Funding acquisition, Methodology, Project administration, Writing – review & editing. JT: Conceptualization, Funding acquisition, Methodology, Project administration, Writing – review & editing. JB: Conceptualization, Funding acquisition, Project administration, Methodology, Supervision, Writing – review & editing.
Funding
The author(s) declare financial support was received for the research, authorship, and/or publication of this article. This study was funded by a North Carolina Coastal Recreational Fishing License Grant 8072. Supplemental acoustic tags were provided by NOAA - MS-AL Sea Grant - University of South Alabama grant number NA20OAR4170494.
Acknowledgments
We would like to thank C. Mahnke, R. Dorroh, R. Mahoney, J. Brooks, O. Mulvey-McFerron, S. Birath, A. Rocco, M. Damiano, B. Runde, A. Matthews, P. Rudershausen and Mount Maker Charters for their help during field tagging trips. We acknowledge the tag detection data from acoustic receivers that were part of separate projects either in our laboratory (greater amberjack abundance estimation) or the Pine Knoll Shores Aquarium (sand tiger shark ecology). We thank N. Bacheler for lending us the acoustic receivers necessary to conduct this work. We also thank K. Shertzer, A. Combatt and T. Grothues for providing comments that improved earlier versions of this manuscript. This study was funded by a North Carolina Coastal Recreational Fishing License Grant. The scientific results and conclusions, as well as any views or opinions expressed herein, are those of the author(s) and do not necessarily reflect those of the National Oceanic and Atmospheric Administration or the Department of Commerce. Any use of trade, firm, or product names is for descriptive purposes only and does not imply endorsement by the U.S. Government.
Conflict of interest
The authors declare that the research was conducted in the absence of any commercial or financial relationships that could be construed as a potential conflict of interest.
Publisher’s note
All claims expressed in this article are solely those of the authors and do not necessarily represent those of their affiliated organizations, or those of the publisher, the editors and the reviewers. Any product that may be evaluated in this article, or claim that may be made by its manufacturer, is not guaranteed or endorsed by the publisher.
Supplementary material
The Supplementary Material for this article can be found online at: https://www.frontiersin.org/articles/10.3389/fmars.2024.1373494/full#supplementary-material
References
Abecasis D., Bentes L., Lino P. G., Santos M. N., Erzini K. (2013). Residency, movements and habitat use of adult white seabream (Diplodus sargus) between natural and artificial reefs. Estuarine Coast. Shelf Sci. 118, 80–85. doi: 10.1016/j.ecss.2012.12.014
Afonso P., Abecasis D., Santos R. S., Fontes J. (2016). Contrasting movements and residency of two serranids in a small Macaronesian MPA. Fish. Res. 177, 59–70. doi: 10.1016/j.fishres.2015.12.014
Arena P. T., Jordan L. K. B., Spieler R. E. (2007). Fish assemblages on sunken vessels and natural reefs in southeast Florida, USA. Hydrobiologia 580, 157–171. doi: 10.1007/s10750-006-0456-x
Bacheler N., Shertzer K., Buckel J., Rudershausen P., Runde B. (2018). Behavior of gray triggerfish Balistes capriscus around baited fish traps determined from fine-scale acoustic tracking. Mar. Ecol. Prog. Ser. 606, 133–150. doi: 10.3354/meps12780
Bacheler N. M., Michelot T., Cheshire R. T., Shertzer K. W. (2019). Fine-scale movement patterns and behavioral states of gray triggerfish Balistes capriscus determined from acoustic telemetry and hidden Markov models. Fish. Res. 215, 76–89. doi: 10.1016/j.fishres.2019.02.014
Bacheler N. M., Shertzer K. W., Runde B. J., Rudershausen P. J., Buckel J. A. (2021). Environmental conditions, diel period, and fish size influence the horizontal and vertical movements of red snapper. Sci. Rep. 11, 9580. doi: 10.1038/s41598-021-88806-3
Bacheler N. M., Gregalis K. C., Gillum Z. D., Pickett E. P., Schobernd C. M., Schobernd Z. H., et al. (2022a). Using stationary video data to infer relative abundance and distribution of four Seriola species along the southeast United States Atlantic coast. Fish. Res. 249, 106238. doi: 10.1016/j.fishres.2022.106238
Bacheler N. M., Runde B. J., Shertzer K. W., Buckel J. A., Rudershausen P. J. (2022b). Fine-scale behavior of red snapper (Lutjanus campechanus) around bait: approach distances, bait plume dynamics, and effective fishing area. Can. J. Fish. Aquat. Sci. 79, 458–471. doi: 10.1139/cjfas-2021-0044
Becker S. L., Finn J. T., Novak A. J., Danylchuk A. J., Pollock C. G., Hillis-Starr Z., et al. (2020). Coarse- and fine-scale acoustic telemetry elucidates movement patterns and temporal variability in individual territories for a key coastal mesopredator. Environ. Biol. Fish 103, 13–29. doi: 10.1007/s10641-019-00930-2
Becker A., Taylor M. D., Folpp H., Lowry M. B. (2018). Managing the development of artificial reef systems: The need for quantitative goals. Fish Fish 19, 740–752. doi: 10.1111/faf.12288
Becker A., Taylor M. D., Lowry M. B. (2017). Monitoring of reef associated and pelagic fish communities on Australia’s first purpose built offshore artificial reef. ICES J. Mar. Sci. 74, 277–285. doi: 10.1093/icesjms/fsw133
Boyle K. S., Hightower C. L., Powers S. P. (2022a). Evaluation of factors contributing to postrelease mortality of greater amberjack (Seriola dumerili) in the northern Gulf of Mexico with depth and acceleration data from acoustic tags. FB 120, 218–233. doi: 10.7755/FB.120.3-4.3
Boyle K. S., Hightower C. L., Powers S. P. (2022b). Diel patterns of depth use and swimming activity of post-release greater amberjack (Seriola dumerili) in the northern Gulf of Mexico. Environ. Biol. Fish. 106, 491–518. doi: 10.1007/s10641-022-01385-8
Brotto D. S., Araujo F. G. (2001). Habitat selection by fish in an artificial reef in Ilha Grande Bay, Brazil. Braz. Arch. Biol. Technol. 44, 319–324. doi: 10.1590/S1516-89132001000300015
Broughton K. (2012). Office of National Marine Sanctuaries science review of artificial reefs (Silver Spring, Maryland: National Oceanic and Atmospheric Administration, Office of National Marine Sanctuaries).
Burns E. S., Armstrong J., Tang D., Sakamoto K., Lowe C. G. (2019). The residency, movement patterns and habitat association of several demersal fish species to the Orange County Sanitation District wastewater outfall. Mar. pollut. Bull. 149, 110638. doi: 10.1016/j.marpolbul.2019.110638
Capizzano C. W., Zemeckis D. R., Hoffman W. S., Benoît H. P., Jones E., Dean M. J., et al. (2019). Fishery-scale discard mortality rate estimate for haddock in the gulf of maine recreational fishery. North Am. J. Fish Manage 39, 964–979. doi: 10.1002/nafm.10328
Coleman F. C., Koenig C. C., Eklund A. M., Grimes C. B. (1999). Management and conservation of temperate reef fishes in the grouper-snapper complex of the southeastern United States. Am. Fish. Soc. Symposium 23, 233–242.
Coleman F. C., Koenig C. C., Huntsman G. R., Musick J. A., Eklund A. M., McGovern J. C., et al. (2000). Long-lived reef fishes: the grouper-snapper complex. Fisheries 25, 14–21. doi: 10.1577/1548-8446(2000)025<0014:LRF>2.0.CO;2
Cooke S. J., Bergman J. N., Twardek W. M., Piczak M. L., Casselberry G. A., Lutek K., et al. (2022). The movement ecology of fishes. J. Fish Biol. 101, 756–779. doi: 10.1111/jfb.15153
Curtis J. M., Johnson M. W., Diamond S. L., Stunz G. W. (2015). Quantifying delayed mortality from barotrauma impairment in discarded red snapper using acoustic telemetry. Mar. Coast. Fish. 7, 434–449. doi: 10.1080/19425120.2015.1074968
Dance M. A., Moulton D. L., Furey N. B., Rooker J. R. (2016). Does transmitter placement or species affect detection efficiency of tagged animals in biotelemetry research? Fish. Res. 183, 80–85. doi: 10.1016/j.fishres.2016.05.009
dos Santos L. N., Brotto D. S., Zalmon I. R. (2010). Fish responses to increasing distance from artificial reefs on the Southeastern Brazilian Coast. J. Exp. Mar. Biol. Ecol. 386, 54–60. doi: 10.1016/j.jembe.2010.01.018
Ellis R. D., Flaherty-Walia K. E., Collins A. B., Bickford J. W., Boucek R., Walters Burnsed S. L., et al. (2019). Acoustic telemetry array evolution: From species- and project-specific designs to large-scale, multispecies, cooperative networks. Fish. Res. 209, 186–195. doi: 10.1016/j.fishres.2018.09.015
Espinoza M., Farrugia T. J., Webber D. M., Smith F., Lowe C. G. (2011). Testing a new acoustic telemetry technique to quantify long-term, fine-scale movements of aquatic animals. Fish. Res. 108, 364–371. doi: 10.1016/j.fishres.2011.01.011
Fabrizio M. C., Manderson J. P., Pessuti J. P. (2014). Home range and seasonal movements of Black Sea Bass (Centropristis striata) during their inshore residency at a reef in the mid-Atlantic Bight. FB 112, 71–81. doi: 10.7755/FB.112.1.5
Fabrizio M., Manderson J., Pessutti J. (2013). Habitat associations and dispersal of black sea bass from a mid-Atlantic Bight reef. Mar. Ecol. Prog. Ser. 482, 241–253. doi: 10.3354/meps10302
Fieberg J., Signer J., Smith B., Avgar T. (2021). A ‘How to’ guide for interpreting parameters in habitat-selection analyses. J. Anim. Ecol. 90, 1027–1043. doi: 10.1111/1365-2656.13441
Figley B. (2003). Micro-Movements of Black Sea Bass on the Atlantic city Reef site. NJ Division of Fish and Wildlife. Federal Aid to Fisheries Project. 17 pp.
Fithian W., Hastie T. (2013). Finite-sample equivalence in statistical models for presence-only data. Ann. Appl. Stat. 7, 1917–1939. doi: 10.1214/13-AOAS667
Fodrie F. J., Yeager L. A., Grabowski J. H., Layman C. A., Sherwood G. D., Kenworthy M. D. (2015). Measuring individuality in habitat use across complex landscapes: approaches, constraints, and implications for assessing resource specialization. Oecologia 178, 75–87. doi: 10.1007/s00442-014-3212-3
Folpp H. R., Schilling H. T., Clark G. F., Lowry M. B., Maslen B., Gregson M., et al. (2020). Artificial reefs increase fish abundance in habitat-limited estuaries. J. Appl. Ecol. 57, 1752–1761. doi: 10.1111/1365-2664.13666
Fontes J., Schmiing M., Afonso P. (2014). Permanent aggregations of a pelagic predator at shallow seamounts. Mar. Biol. 161, 1349–1360. doi: 10.1007/s00227-014-2423-9
Froehlich C. Y. M., Garcia A., Kline R. J. (2019). Daily movement patterns of red snapper (Lutjanus campechanus) on a large artificial reef. Fish. Res. 209, 49–57. doi: 10.1016/j.fishres.2018.09.006
Gandra M., Afonso P., Fontes J. (2020). Intra- and interspecific associations in two predatory reef fishes at a shallow seamount. Mar. Ecol. Prog. Ser. 654, 93–107. doi: 10.3354/meps13501
Gibson Banks K., Curtis J. M., Williams J. A., Wetz J. J., Stunz G. W. (2021). Designing cost-effective artificial reefs: fine-scale movement and habitat use of red snapper around a nearshore artificial reef complex. N Am. J. Fish Manag 41, 1850–1862. doi: 10.1002/nafm.10698
Hackradt C. W., Félix-Hackradt F. C., García-Charton J. A. (2011). Influence of habitat structure on fish assemblage of an artificial reef in southern Brazil. Mar. Environ. Res. 72, 235–247. doi: 10.1016/j.marenvres.2011.09.006
Harmelin-Vivien M., Harmelin J. G. (2022). “Feeding Biology of Groupers,” in Biology and Ecology of Groupers (CRC Press, Taylor & Francis Group, Boca Raton).
Jackson L. S., Drymon J. M., Nelson T. R., Powers S. P. (2018). Biotelemetry based estimates of greater amberjack (Seriola dumerili) post-release mortality in the northern Gulf of Mexico. Fish. Res. 208, 239–246. doi: 10.1016/j.fishres.2018.07.017
Johnson D., London J. M., McClintock B. (2022). NMML/crawl: Last CRAN release (v2.3.0-1). Zenodo. doi: 10.5281/zenodo.7154089
Keller J. A., Herbig J. L., Morley D., Wile A., Barbera P., Acosta A. (2020). Grouper tales: use of acoustic telemetry to evaluate grouper movements at western dry rocks in the Florida keys. Mar. Coast. Fish 12, 290–307. doi: 10.1002/mcf2.10109
Kessel S. T., Cooke S. J., Heupel M. R., Hussey N. E., Simpfendorfer C. A., Vagle S., et al. (2014). A review of detection range testing in aquatic passive acoustic telemetry studies. Rev. Fish Biol. Fish. 24, 199–218. doi: 10.1007/s11160-013-9328-4
Khan J. A., Goatley C. H. R., Brandl S. J., Tebbett S. B., Bellwood D. R. (2017). Shelter use by large reef fishes: long-term occupancy and the impacts of disturbance. Coral Reefs 36, 1123–1132. doi: 10.1007/s00338-017-1604-7
Klimley A. P., McDonald R., Thomas M. J., Chapman E., Hearn A. (2020). Green sturgeon habitat suitability varies in response to drought related flow regimes. Environ. Biol. Fish 103, 425–435. doi: 10.1007/s10641-020-00946-z
Kline R. J., Khan I. A., Holt G. J. (2011). Behavior, color change and time for sexual inversion in the protogynous grouper (Epinephelus adscensionis). PloS One 6, e19576. doi: 10.1371/journal.pone.0019576
Komyakova V., Chamberlain D., Jones G. P., Swearer S. E. (2019). Assessing the performance of artificial reefs as substitute habitat for temperate reef fishes: Implications for reef design and placement. Sci. Total Environ. 668, 139–152. doi: 10.1016/j.scitotenv.2019.02.357
Leitão F., Santos M. N., Erzini K., Monteiro C. C. (2009). Diplodus spp. assemblages on artificial reefs: importance for near shore fisheries. Fish. Manage. Ecol. 16, 88–99. doi: 10.1111/j.1365-2400.2008.00646.x
Lemoine H. R., Paxton A. B., Anisfeld S. C., Rosemond R. C., Peterson C. H. (2019). Selecting the optimal artificial reefs to achieve fish habitat enhancement goals. Biol. Conserv. 238, 108200. doi: 10.1016/j.biocon.2019.108200
Lindberg W. J., Frazer T. K., Portier K. M., Vose F., Loftin J., Murie D. J., et al. (2006). Density-dependent habitat selection and performance by A large mobile reef fish. Ecol. Appl. 16, 731–746. doi: 10.1890/1051-0761(2006)016[0731:DHSAPB]2.0.CO;2
Low R. A., Waltz C. W. (1991). Seasonal utilization and movement of black sea bass on a South Carolina artificial reef. North Am. J. Fish. Manage. 11, 131–138. doi: 10.1577/1548-8675(1991)011<0131:SUAMOB>2.3.CO;2
Marine Recreational Information Program MRIP (2023) About the Marine Recreational Information Program (NOAA Fisheries). Available online at: https://www.fisheries.noaa.gov/recreational-fishing-data/about-marine-recreational-information-program (Accessed November 15, 2023).
McClellan D. B., Cummings N. J. (1997). Preliminary analysis of tag and recapture data of the greater amberjack, Seriola dumerili, in the southeastern United States. Proc. Gulf Caribbean Fish. Instit. 49, 25–45.
Mumby P. J., Wabnitz C. C. C. (2002). Spatial patterns of aggression, territory size, and harem size in five sympatric Caribbean parrotfish species. Environ. Biol. Fish. 63, 265–279. doi: 10.1023/A:1014359403167
Nelson D. A., Perry D., Baker E. (2003). Natural spawning of black sea bass, Centropristis striata, at the NMFS Milford Laboratory and the UMASS Dartmouth laboratory with observations on spawning behavior. J. Shellf. Res. 22, 297–298.
Paxton A. B., Newton E. A., Adler A. M., Van Hoeck R. V., Iversen E. S., Taylor J. C., et al. (2020a). Artificial habitats host elevated densities of large reef-associated predators. PloS One 15, e0237374. doi: 10.1371/journal.pone.0237374
Paxton A. B., Pickering E. A., Adler A. M., Taylor J. C., Peterson C. H. (2017). Flat and complex temperate reefs provide similar support for fish: Evidence for a unimodal species-habitat relationship. PloS One 12, e0183906. doi: 10.1371/journal.pone.0183906
Paxton A. B., Revels L. W., Rosemond R. C., Van Hoeck R. V., Lemoine H. R., Taylor J. C., et al. (2018). Convergence of fish community structure between a newly deployed and an established artificial reef along a five-month trajectory. Ecol. Eng. 123, 185–192. doi: 10.1016/j.ecoleng.2018.09.012
Paxton A. B., Shertzer K. W., Bacheler N. M., Kellison G. T., Riley K. L., Taylor J. C. (2020b). Meta-analysis reveals artificial reefs can be effective tools for fish community enhancement but are not one-size-fits-all. Front. Mar. Sci. 7. doi: 10.3389/fmars.2020.00282
Paxton A. B., Steward D., Mille K. J., Renchen J., Harrison Z. H., Byrum J. S., et al. (2024). Artificial reef footprint in the U.S. ocean. Nat. Sustainabil. 7, 140–147. doi: 10.1038/s41893-023-01258-7
Provost M. M., Jensen O. P., Berlinsky D. L. (2017). Influence of size, age, and spawning season on sex change in black sea bass. Mar. Coast. Fish 9, 126–138. doi: 10.1080/19425120.2016.1274696
R Core Team (2023). _R: A Language and Environment for Statistical Computing_ (Vienna, Austria: R Foundation for Statistical Computing). Available at: https://www.R-project.org/.
Reubens J. T., Pasotti F., Degraer S., Vincx M. (2013). Residency, site fidelity and habitat use of Atlantic cod (Gadus morhua) at an offshore wind farm using acoustic telemetry. Mar. Environ. Res. 90, 128–135. doi: 10.1016/j.marenvres.2013.07.001
Roa-Ureta R. H., Santos M. N., Leitão F. (2019). Modelling long-term fisheries data to resolve the attraction versus production dilemma of artificial reefs. Ecol. Model. 407, 108727. doi: 10.1016/j.ecolmodel.2019.108727
Runde B. J., Buckel J. A., Bacheler N. M., Tharp R. M., Rudershausen P. J., Harms C. A., et al. (2022). Evaluation of six methods for external attachment of electronic tags to fish: assessment of tag retention, growth and fish welfare. J. Fish Biol. 101, 419–430. doi: 10.1111/jfb.14989
Runde B. J., Michelot T., Bacheler N. M., Shertzer K. W., Buckel J. A. (2020). Assigning fates in telemetry studies using hidden markov models: an application to deepwater groupers released with descender devices. N Am. J. Fish Manag 40, 1417–1434. doi: 10.1002/nafm.10504
Sancho G. (2000). PREDATORY BEHAVIORS OF CARANX MELAMPYGUS (CARANGIDAE) FEEDING ON SPAWNING REEF FISHES: A NOVEL AMBUSHING STRATEGY. Bull. OF Mar. Sci. 66, 487–496.
Secor D. H., Bailey H., Carroll A., Lyubchich V., O’Brien M. H. P., Wiernicki C. J. (2021). Diurnal vertical movements in black sea bass (Centropristis striata): Endogenous, facultative, or something else? Ecosphere 12, e03616. doi: 10.1002/ecs2.3616
Secor D. H., Zhang F., O’Brien M. H. P., Li M. (2019). Ocean destratification and fish evacuation caused by a Mid-Atlantic tropical storm. ICES J. Mar. Sci. 76, 573–584. doi: 10.1093/icesjms/fsx241
SEDAR 59 (2020). SEDAR – South Atlantic Greater Amberjack Stock Assessment Report (North Charleston SC: SEDAR). Available at: http://sedarweb.org/sedar-59. 142 pp.
SEDAR 71 (2021). SEDAR 71 South Atlantic Gag Stock Assessment Report (North Charleston SC: SEDAR). Available at: http://sedarweb.org/sedar–71. 164 pp.
SEDAR 73 (2021). SEDAR 73 South Atlantic Red Snapper Stock Assessment Report (North Charleston SC: SEDAR). Available at: http://sedarweb.org/sedar-73. 194 pp.
SEDAR 76 (2023). SEDAR 76 South Atlantic Black Sea Bass Stock Assessment Report (North Charleston SC: SEDAR). Available at: https://sedarweb.org/assessments/sedar–76/. 182 pp.
Shertzer K. W., Williams E. H., Craig J. K., Fitzpatrick E. E., Klibansky N., Siegfried K. I. (2019). Recreational sector is the dominant source of fishing mortality for oceanic fishes in the Southeast United States Atlantic Ocean. Fish Manag Ecol. 26, 621–629. doi: 10.1111/fme.12371
Spedicato G. A. (2017). Discrete Time Markov Chains with R. The R Journal 9, 84–104. doi: 10.32614/RJ-2017-036
Stephan D. C., Lindquist D. G. (1989). A comparative analysis of the fish assemblages associated with old and new shipwrecks and fish aggregating devices in Onslow bay, North Carolina. Bull. OF Mar. Sci. 44, 20.
Steward D. N., Paxton A. B., Bacheler N. M., Schobernd C. M., Mille K., Renchen J., et al. (2022). Quantifying spatial extents of artificial versus natural reefs in the seascape. Front. Mar. Sci. 9. doi: 10.3389/fmars.2022.980384
Strelcheck A. J., Cowan J. H., Shah A. (2005). Influence of reef location on artificial-reef fish assemblages in the northcentral Gulf of Mexico. Bull. OF Mar. Sci. 77, 425–440.
Stuart K. R., Smith T. I. J. (2003). Development of nursery systems for black sea bass Centropristis striata. J. World Aquacult. Soc. 34, 359–367. doi: 10.1111/j.1749-7345.2003.tb00073.x
Tassetti A. N., Malaspina S., Fabi G. (2015). USING A MULTIBEAM ECHOSOUNDER TO MONITOR AN ARTIFICIAL REEF. Int. Arch. Photogramm. Remote Sens. Spatial Inf. Sci. XL-5/W5, 207–213. doi: 10.5194/isprsarchives-XL-5-W5-207-2015
Tone K., Chiang W., Yeh H., Hsiao S., Li C., Komeyama K., et al. (2022). Two-way habitat use between reefs and open ocean in adult greater amberjack: evidence from biologging data. Mar. Ecol. Prog. Ser. 699, 135–151. doi: 10.3354/meps14169
Topping D., Szedlmayer S. (2011). Site fidelity, residence time and movements of red snapper Lutjanus campechanus estimated with long-term acoustic monitoring. Mar. Ecol. Prog. Ser. 437, 183–200. doi: 10.3354/meps09293
Walker S. J., Schlacher T. A., Schlacher-Hoenlinger M. A. (2007). Spatial heterogeneity of epibenthos on artificial reefs: fouling communities in the early stages of colonization on an East Australian shipwreck. Mar. Ecol. 28, 435–445. doi: 10.1111/j.1439-0485.2007.00193.x
Watanabe W. O., Carroll P. M., Alam M. S., Dumas C. F., Gabel J. E., Davis T. M., et al. (2021). The status of black sea bass, Centropristis striata, as a commercially ready species for U.S. marine aquaculture. J. World Aquacult. Soc. 52, 541–565. doi: 10.1111/jwas.12803
Wiernicki C. J., O’Brien M. H. P., Zhang F., Lyubchich V., Li M., Secor D. H., et al (2020). The recurring impact of storm disturbance on black sea bass (Centropristis striata) movement behaviors in the Mid-Atlantic Bight. PLoS ONE 15, e0239919. doi: 10.1371/journal.pone.0239919
Williams-Grove L. J., Szedlmayer S. T. (2017). Depth preferences and three-dimensional movements of red snapper, Lutjanus campechanus, on an artificial reef in the northern Gulf of Mexico. Fish. Res. 190, 61–70. doi: 10.1016/j.fishres.2017.01.003
Zemeckis D. R., Kneebone J., Capizzano C. W., Bochenek E. A., Hoffman W. S., Grothues T. M., et al. (2020). Discard mortality of black sea bass (Centropristis striata) in a deepwater recreational fishery off New Jersey: role of swim bladder venting in reducing mortality. FB 118, 105–119. doi: 10.7755/FB.118.2.1
Keywords: artificial reef, habitat selection, telemetry, fish tagging, fine-scale habitat use
Citation: Tharp RM, Hostetter NJ, Paxton AB, Taylor JC and Buckel JA (2024) Artificial structure selection by economically important reef fishes at North Carolina artificial reefs. Front. Mar. Sci. 11:1373494. doi: 10.3389/fmars.2024.1373494
Received: 19 January 2024; Accepted: 19 March 2024;
Published: 11 April 2024.
Edited by:
Jorge Paramo, University of Magdalena, ColombiaReviewed by:
Anthony Combatt, Independent researcher, Santa Marta, ColombiaThomas Marcellin Grothues, The State University of New Jersey, United States
Copyright © 2024 Tharp, Hostetter, Paxton, Taylor and Buckel. This is an open-access article distributed under the terms of the Creative Commons Attribution License (CC BY). The use, distribution or reproduction in other forums is permitted, provided the original author(s) and the copyright owner(s) are credited and that the original publication in this journal is cited, in accordance with accepted academic practice. No use, distribution or reproduction is permitted which does not comply with these terms.
*Correspondence: Ryan M. Tharp, cm10aGFycEBuY3N1LmVkdQ==
†ORCID: Ryan M. Tharp, orcid.org/0000-0003-1977-5650
Nathan J. Hostetter, orcid.org/0000-0001-6075-2157
Avery B. Paxton, orcid.org/0000-0002-4871-9167