- 1Ocean College, Hebei Agricultural University, Qinhuangdao, China
- 2Ocean Survey Department, Qinhuangdao Marine Center of the Ministry of Natural Resources, Qinhuangdao, China
Thraustochytrids, as a distinct group of heterotrophic protists, have garnered considerable attention owing to their remarkable adaptability in extreme marine environments, pronounced capacity for metabolic regulation and prolific production of high-value metabolites. The taxonomic classification of these microorganisms presents a substantial challenge due to the variability in morphological characteristics under different culture conditions. And this undermines the efficacy of traditional classification systems on physiological and biochemical traits. The establishment of a polyphasic taxonomic system integrating genomic characteristics in the future will provide new avenues for more accurate classification and identification. Thraustochytrids can effectively accumulate bioactive substances such as docosahexaenoic acid (DHA), eicosapentaenoic acid (EPA), squalene and carotenoids. Through fermentation optimization and genetic modification, scientists have significantly enhanced the production of these metabolites. Moreover, the application of thraustochytrids in aquaculture, poultry and livestock feed has significantly improved animal growth and physiological indicators meanwhile increasing their DHA content. Natural bioactive substances in thraustochytrids, such as terpenoid compounds with antioxidant properties, have been proposed for application in the cosmetics industry. In the field of pharmacology, thraustochytrids have shown certain anti-inflammatory and anti-cancer activities and provide potential for the development of new oral vaccines. Additionally, they can degrade various industrial and agricultural wastes for growth and fatty acid production, demonstrating their potential in environmental bioremediation. Therefore, thraustochytrids not only exhibit tremendous application potential in the field of biotechnology, but also hold significant value in environmental protection and commercialization.
1 Introduction
Thraustochytrids, belonging to the phylum Labyrinthulomycetes, were ubiquitous marine heterotrophic protists and exert of significance roles in coastal and oceanic ecosystems (Liu et al., 2017; Xie et al., 2022). They were widely considered as significant decomposers of organic matters in humus-rich environment or phytoplankton bloom areas by secreting various extracellular enzymes, e.g. cellulase, amylases and proteases (Liu et al., 2014b). Thraustochytrids participated in the carbon cycle and particle sink from the surface to the deep sea by facilitating the formation of marine aggregates (Damare and Raghukumar, 2012). These were contributed largely to the physiological and morphological features of thraustochytrids such as the attachment of biflagellated zoospores and ectoplasmic net to the substrates, hydrophobic effect of the cell wall as well as the production of extracellular polysaccharides (Damare et al., 2020). The diverse habitats of thraustochytrids have led to the production of complex metabolites, positioning them alongside marine bacteria, fungi and microalgae as crucial sources for the development of natural bioactive substances. Due to containing abundant ω-3 polyunsaturated fatty acids (PUFAs), squalene and carotenoids, thraustochytrids are excellent nutrient providers for marine animals through marine food web. And they exhibit great biotechnological application potentials in food, feed and healthy industries.
Ω-3 PUFAs of thraustochytrids were mainly composed of docosahexaenoic acid (DHA) which was significant for modulating the cardiovascular system and promoting cell growth and development of nervous system for human infants (Guesnet and Alessandri, 2011; Graham et al., 2021). Recently, most of the concerns about thraustochytrids were put on the biosynthesis process optimization of PUFAs using fermentation strategies combined with genetic modification and omics techniques as well as wastes screening for fermentation substrates (Heggeset et al., 2019; Yu et al., 2020; Wang et al., 2021a, b; Watanabe et al., 2022; Li et al., 2023). Although former studies had testified the feasibility of utilizing varied agricultural and industrial wastes for DHA production by thraustochytrids, and exhibited great industrial application potential in sustainable DHA production (Chi et al., 2007; Lee Chang et al., 2013; Ryu et al., 2013; Song et al., 2017; Villarroel Hipp and Silva Rodriguez, 2018). High-value utilization of novel wastes such as tofu whey wastewater (Wang et al., 2020), soybean curd wastewater (Lee et al., 2020), volatile fatty acids (Patel et al., 2020), waste acid oil (Laddha et al., 2021), sugarcane bagasse (Watanabe et al., 2022), dairy and brewery wastes (Russo et al., 2021), digestate from methanization (de la Broise et al., 2022) and food waste from Chinese restaurants (Li et al., 2023) were also proved to be excellent feedstock alternatives for DHA production of thraustochytrids. Future endeavors utilizing simple structural, widely sourced one-carbon (methanol, formic acid) and two-carbon (acetic acid) substrates for thraustochytrid fermentation may yield even more exciting outcomes.
In addition, the downstream process of thraustochytrids based biorefinery including lipid extraction and the refining procedure had been developed increasingly. Several extraction methods of lipids containing high levels of DHA were established including enzymatic ethanolysis (He et al., 2019), high shear-assisted solvent extraction (Kwak et al., 2019), supercritical CO2 extraction (de Melo et al., 2020) and ultrasound-assisted extraction (Li et al., 2021). Remarkably, plenty of recent researches demonstrated that feeding supplementation of biomass or extracts of thraustochytrids was beneficial for aquatic animals such as Nile tilapia (dos Santos et al., 2019), golden pompano (Xie et al., 2019), Pacific white shrimp (Xu et al., 2022) and Penaeus monodon larvae (Visudtiphole et al., 2021), as well as Japanese quail (Gladkowski et al., 2014), layer hen (Moran et al., 2020) and steers (Carvalho et al., 2018) in improving their growth performance, survival, immunity, anti-inflammatory effect, and regulating the blood cells and gut microbiota (Souza et al., 2020). Presumably, thraustochytrids will become an important source of DHA in animal originated foods in our daily diet, and thus improve human health (Figure 1).
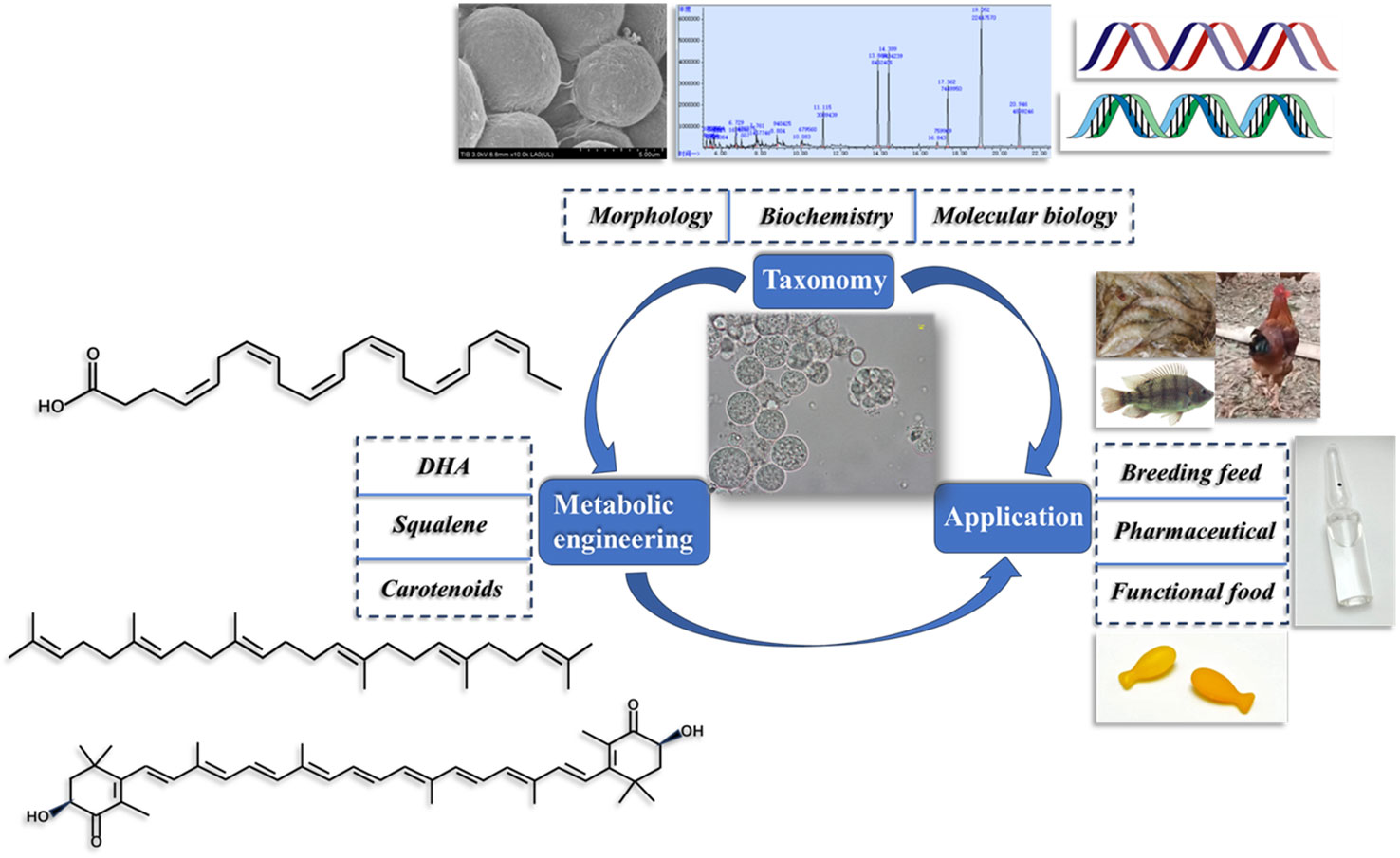
Figure 1 Efforts surrounding thraustochytrid that need more attention include taxonomy, metabolic engineering and application research.
2 Taxonomy challenges of thraustochytrids: advancements and issues in classification methods
Considering the great contribution of thraustochytrids to marine ecosystem and humans, it is necessary to figure out the taxonomy characters of Thraustochytriaceae sp. in order to take better advantage of this kind of protists. In the past decades, increasing novel thraustochytrid strains were progressively obtained along with the development of isolation and culture techniques for marine microorganism. However, due to the morphological characters of thraustochytrids vary with their habitats, the traditional classification system based on morphological data are facing many challenges. Besides, the molecular biological method which was widely applied in microorganism identification, also led to confused taxonomy of thraustochytrids because of these disordered reference sequences in database. Moreover, the biochemical markers such as fatty acid profiles had been used as classification features of thraustochytrids. In the following paragraphs, we summarized the development and current issues in the morphological, biochemical and molecular phylogeny methods for thraustochytrid classification (Table 1). Additionally, we discussed polyphasic taxonomy in order to establish an accurate and scientific taxonomic method.
2.1 Morphological features: challenges and evolution in thraustochytrid identification and classification
At present, the characteristics of life cycle, morphology, ultrastructure, biochemical markers and phylogenetic analysis were typical characteristics for the taxonomy of thraustochytrids. Except for the phylogeny, all the other features are traditional taxonomic features and usually fail to correspond to the phylogenetic relationship of thraustochytrids (Marchan et al., 2017; Dellero et al., 2018a). The life cycle and morphological development of thraustochytrids are complex. And several attempts have been made to redefine the taxonomic status and phylogenetic relationship of them (Yokoyama and Honda, 2007; Yokoyama et al., 2007; Doi and Honda, 2017; Dellero et al., 2018b). The taxonomic features at the genus and species level were usually dependent on the specific morphological features such as zoospore production (Doi and Honda, 2017), and biochemical characteristics such as the production of specific fatty acids and pigment compounds (Honda et al., 1998). However, differences existing in the life cycle and metabolic profiling with the variation of culture conditions deepened the burden on homogenizing and systematically defining taxa only using phenotypic traits (Raghukumar, 1988). And this thus led to an excessive criteria for identifying and describing specific taxa of thraustochytrids (Raghukumar, 1988). When Aurantiochytrium limacinum was grown in artificial medium, the cell body accumulating large amounts of triglycerides at the end of the growth stage produced no zoospores (Dellero et al., 2018a). While large amounts of zoospores produced in oligotrophic medium such as artificial seawater (Dellero et al., 2018a). Besides, the zoospores could swim for at least six days in the absence of organic carbon consuming intracellular triglycerides for energy (Dellero et al., 2018a). While the addition of glucose rapidly triggered the maturation of the zoospores (Dellero et al., 2018a). Therefore, it is necessary to specify the medium for life cycle observation in the identification of thraustochytrid strains (Raghukumar, 1988).
Presently, the most controversial taxonomic species of thraustochytrids exist in the genus Thraustochytrium, a typical genus of the family Thraustochytriaceae. Species Thraustochytrium. sp. are characterized by the formation of zoospores, including the cell wall shape of sporangium and the presence or absence of proliferates. However, several taxonomic problems remain in this genus, including the overlap of polyphyletic and interspecific taxonomic features (Gao et al., 2013; Doi and Honda, 2017). For example, the first and second species of this genus, T. proliferum and T. globosum, were described by observing the morphological characteristics of natural specimens without culture (Doi and Honda, 2017). The cellular morphology of T. globosum was first observed on the surface of green algae and was clearly distinguished from the typical species T. proliferum by morphological characteristics, namely the proliferates, zoospores and ectoplasms features (Kobayashi and Ookubo, 1953). However, this strain has never been registered in cultured specimens previously, so the taxonomic characteristics cannot be recognized under artificial conditions (Sparrow, 1936). It was believed that the genus Thraustochytrium was composed of heterogeneous species (Sparrow, 1969). Later, Doi and Honda (2017) successfully isolated a thraustochytrid strain NBRC 112723, which had typical classification characteristics of T. globosum in natural condition (Doi and Honda, 2017). But under culture condition it showed similar taxonomic features with other thraustochytrid species (Doi and Honda, 2017). Finally, combined with its molecular phylogeny and the taxonomic criteria of typical species T. proliferum, the genus Thraustochytrium was rearranged for T. globosum. Thus, a new genus Monorhizochytrium was established to clearly distinguish T. globosum (Doi and Honda, 2017).
2.2 Biochemical indicators: incorporating biochemical characteristics for enhanced identification
Biochemical characteristics such as lipids and carotenoids, were proposed to be included into the taxonomy of thraustochytrids (Huang et al., 2003; Yokoyama and Honda, 2007; Yokoyama et al., 2007). In the early stage, thraustochytrids newly isolated from coastal areas of Japan and Fiji had been divided into five main groups (DHA/DPAω6、DHA/DPAω6/EPA, DHA/EPA, DHA/DPAω6/EPA/ARA, DHA/DPAω6/EPA/ARA/DTA) based on PUFA profiles, which were allocated into a single cluster based on the 18S rRNA gene (Huang et al., 2003). However, this method failed to determine the PUFA profiles of a specific genus, and they were used as biochemical markers for thraustochytrids instead (Huang et al., 2003). Later, two secondary biochemical markers, i.e. the PUFA and carotenoid profiles were combined with the 18S rRNA marker, and were successfully used for the identification of new thraustochytrid genera (Yokoyama and Honda, 2007; Yokoyama et al., 2007). This provided a more robust phylogenetic mechanism for a single phylogenetic branch of Thraustochytriaceae sp. with the exception of genus Thraustochytrium. The genus Thraustochytrium might contain subgenera. Because it did not form a single phylogenetic group based on 18S rRNA and did not exhibit a common PUFA or carotenoid spectrum between species (Huang et al., 2003). Therefore, it is expected that new genera will be proposed in the future.
2.3 Molecular phylogeny: advancements and challenges in thraustochytrid identification
Recently, morphological taxonomic features of thraustochytrids have been largely replaced by molecular phylogenetic analysis (Pan et al., 2017). However, the names of many taxa have not been effectively reported because no designated type is specified in the molecule database. Besides, multiple sequences for a single strain will inevitably affect species identification and may lead to an overestimation of genetic diversity. At present, molecular classification based on the 18S rRNA gene has been widely used in different biological systems. Recent studies had shown that no genetic polymorphism existed in the mitochondrial cytochrome C oxidase subunit I (COI) sequence of A. sp., which was conducive to phylogenetic analysis (Nishitani and Yoshida, 2018). Besides, primers designed for this gene can successfully amplify the COI sequences of 16 thraustochytrid strains belonging to five genera, i.e., Thraustochytrium, Aurantiochytrium, Schizochytrium, Botrychytrium and Parietichytrium, with clear single strips (Nishitani and Yoshida, 2018). Moreover, this mitochondrial gene was successfully sequenced without gene cloning, indicating that the mitochondrial region can be used for the identification and genetic diversity analysis of the related genera (Nishitani and Yoshida, 2018). This great facilitated the development of molecular phylogeny of thraustochytrids with multigene locus. Thus, it was beneficial for the clear clarification of natural evolutionary relationship between thraustochytrid species of different genera, enabling their accurate identification.
2.4 Polyphasic taxonomy: integrating phenotype and molecular traits for genus reclassification
The taxonomic characteristics of Labyrinthulomycetes at the genera level are mainly based on the cell morphology at different growth stages. And the influence of culture conditions on morphological characteristics enhances the instability of morphological classification. However, based on 18S rRNA and protein-coding sequence data, species in family Thraustochytriaceae tend to form polyphyletic groups (Wang et al., 2019). The phylogenetic system constructed using 18S rRNA gene sequences showed that the genera Schizochytrium and Ulkenia were divided into three and four branches, respectively. Combined with the distribution characteristics of cell morphology, PUFAs and carotenoids, they were individually reclassified into two new genera and three new genera respectively (Yokoyama and Honda, 2007; Yokoyama et al., 2007). In the constructed molecular phylogenetic system, the genus Thraustochytrium is divided into at least seven different branches (Yokoyama and Honda, 2007; Yokoyama et al., 2007). However, this genus has not been reclassified so far (Marchan et al., 2017). In addition, the molecular phylogenetic relationship between two newly isolated thraustochytrid strains were similar, but there were differences in growth cycle, morphology and biochemical characteristics (Dellero et al., 2018a). And they were finally divided into two different genera, i.e., Aurantiochytrium (CCAP 4062/1) and Hondaea gen. nov (CCAP 4062/3) (Dellero et al., 2018a). Therefore, phenotypic or molecular traits solely have obvious deficiencies in the classification of T. sp. And the polyphase taxonomic method combining phenotypic and molecular traits has great feasibility for the reclassification of this genus.
2.5 Parasitic thraustochytrids in Labyrinthulomycetes taxonomy: pathogenicity and host interactions
Thraustochytrids coupled with labyrinthulids had been preliminarily reported to be associated with pathological condition in the Lesser Octopus Eledone cirrhosa, with symptoms of progressive ulceration of the skin and followed by oedema of body tissues and death (Polglase, 1980). In addition, Quahog Parasite Unknown (QPX), a protistan, had caused high mortalities of wild hard clams Mercenaria mercenaria in various regions of Canada and America (Susan et al., 2002). And they were identified to be a member of phylum Labyrinthulomycota based on the morphological and ultramicrostructure features as well as 18S rRNA gene sequences (Maas et al., 1999; Ragan et al., 2000). However, all the QPX protists isolated from infected hard clams from Duxbury, Massachusetts, were proved to be the same species employing phylogenetic analysis (Stokes et al., 2002). Furthermore, possibility existed that the molecular genetic and physiological variation exists among QPX isolates responsible for outbreaks of QPX disease in different areas (Qian et al., 2007). And the variation could most probably be found at the site that directly involved in and potently under selective pressure for the virulence (Qian et al., 2007). Moreover, thraustochytrid had been isolated from the surface mucus of the hermatypic coral Fungia granulosa, which established a symbiotic relationship with corals through secreting metabolites such as fatty acids and degradable enzymes (Harel et al., 2008). Future research on the isolation, physiological and biochemical characteristics of parasitic thraustochytrid strains will undoubtedly enrich our understanding of these Labyrinthulomycetes protists.
3 Composition analysis of thraustochytrids: unveiling bioactive components and ecological significance
3.1 Biotechnological exploitation of thraustochytrids: advancements in metabolic product research and enzymatic potential
Marine microorganisms, which have long thrived in extreme environments of low temperature and high pressure, continually adjust their metabolic activities to adapt to external conditions. Marine microorganisms represent a treasure trove for the discovery of natural bioactive metabolic products. And researchers have obtained many potential medicinal active substances from these organisms. Thraustochytrids are most notably recognized for their rich content of natural bioactive components such as PUFAs, squalene and carotenoids, demonstrating immense application potential in industries such as food, pharmaceuticals, feed and cosmetics. Additionally, the presence of substantial amounts of long-chain saturated fatty acids and monounsaturated fatty acids in thraustochytrids makes them a promising candidate for biodiesel production. Furthermore, the lipid and terpenoid components in thraustochytrids can be simultaneously extracted through fractionation, enhancing the efficiency of biomass resource utilization. Research on the metabolic products of thraustochytrids is focused on increasing the yield of these high-value products through biotechnological methods. It also aims at identifying substrates that are efficiently utilized by thraustochytrids, which are widely available and cost-effective, in order to enhance their commercial application potential by reducing production costs. Additionally, employing Raman spectroscopy to characterize the PUFAs and carotenoids in thraustochytrids offers the ability to monitor the composition within individual cells and variations among cells (Sasaki et al., 2023). This method, with its short analysis time and non-destructive nature, holds great potential for application in the production of thraustochytrid metabolic products and molecular domestication breeding (Sasaki et al., 2023).
Due to the high yield and wide application, the intracellular fatty acids and terpenoids in thraustochytrids currently constitute a research hotspot in the biotechnology field. However, the extracellular polysaccharides and enzymes of thraustochytrids, detected in lower quantities, have attracted the attention of only a few researchers. Given their natural habitat and heterotrophic lifestyle, thraustochytrids are capable of degrading extracellular macromolecules like proteins and polysaccharides, thus possessing a variety of extracellular enzyme synthesis and secretion capabilities. The potential and activity of thraustochytrid extracellular enzymes might be underestimated, and related research lacks in-depth study. Limited studies indicate that nitrogen nutrient limitation can induce protease activity in S. aggregatum ATCC 28209, suggesting that nutrient limitation strategies could be an effective means to stimulate high-level synthesis of extracellular enzymes (Man et al., 2022).
3.2 Discovery and potential of low-abundance compounds in thraustochytrids: advances in marine biopharmaceuticals
In recent years, components in thraustochytrids present in extremely low quantities have garnered increasing attention from scientists. A new acidic morpholine derivative containing glyceride was isolated and identified from A. sp., accounting for about 0.1~0.4% of the freeze-dried cells (Kaya et al., 2021). Its polar part is 2-hydroxy-3-oxomorpholino propionic acid, which may play a significant role in the lifecycle of thraustochytrids and other organisms (Kaya et al., 2021). Morpholine, a nitrogen and oxygen-containing six-membered heterocyclic compound, is extremely rare in biological compositions (Kaya et al., 2021). Besides, it is an important raw material in many industrial and organic syntheses, often used in the field of pharmaceutical chemistry (Kaya et al., 2021). From 2 kilograms of biomass of A. mangrovei BT3, 20 milligrams of (24R)-4α-methyl-5α-stigmasta-7,22-dien-3β-ol (AME1) with 94% purity was obtained with a lipid-lowering effect (Hoang et al., 2022). Besides, a new sterol and 16 known compounds were isolated and identified from the methanol extract of T. pachydermum TSL10 (Thuy et al., 2023). Among them, sterols 1-6 showed significant antibacterial activity against Gram-positive bacteria (Enterococcus faecium TCC29212, Staphylococcus aureus ATCC25923 and Bacillus cereus ATCC14579) and yeast (Candida albicans ATCC10231), serving as potential antibacterial drugs against Gram-positive bacteria (Thuy et al., 2023). With the saturation of researches on medicinal plant resources, it is promising to explore natural active pharmaceuticals from marine protists like thraustochytrids which has truly begun. However, the extremely low yield of active products is one of the significant challenges especially for some secondary metabolites, with products at microgram or milligram levels. Microbial fermentation is one of the key methods for rapidly obtaining related resources. But there are various limitations in the actual fermentation process. Despite this, microbial fermentation has become one of the important solutions for sourcing marine pharmaceutical development.
3.3 Ecological insights: unraveling the compositional complexity of thraustochytrids
Additionally, analyzing the compositional components of thraustochytrids can provide new perspectives on revealing their ecological roles. Laboratory-cultured marine thraustochytrids including A. sp., Botryochytrium radiatum and S. sp., can produce methyl halides that catalyzing the depletion of stratospheric ozone, potentially serving as sources of methyl halides in marine environments (Sato et al., 2018). Additionally, analyses of the C, N, H and S elemental composition and cell mass quantification of seven thraustochytrid strains isolated from different marine habitats revealed that the proportions of C and H elements were significantly higher in the stationary phase than in the exponential phase (Sen et al., 2021). While the proportions of N and S were the opposite, implying the role of thraustochytrids in the cycling of these elements (Sen et al., 2021). Furthermore, this study also discovered that the carbon density of thraustochytrids cells has been underestimated, suggesting that thraustochytrids contribute more significantly to the oceanic carbon pool than previously thought, surpassing bacteria in this regard (Sen et al., 2021). Consequently, future analyses of the chemical composition of thraustochytrids will provide more insights into their ecological functions and potential biotechnological applications.
4 Metabolic engineering strategies in thraustochytrids: boosting production of lipids, squalene and carotenoids
4.1 Optimizing fatty acid synthesis in thraustochytrids with molecular engineering strategies
Thraustochytrids, which are significant marine oleaginous microorganisms, could accumulate saturated, monounsaturated and PUFAs, constituting over 50% of their dry cell weight. The saturated fatty acids primarily include palmitic acid (C16:0), myristic acid (C14:0) and stearic acid (C18:0). While the monounsaturated ones mainly consist of C16:1 and C18:1. Both saturated and monounsaturated fatty acids are crucial for the synthesis of biodiesel. Polyunsaturated fatty acids, composed of ω-3 fatty acids such as DHA, EPA and arachidonic acid, hold significant economic value and are a key focus in the biotechnological research of thraustochytrids.
Early researchers improved intracellular lipid production by optimizing the fermentation conditions for thraustochytrids. They found that stress conditions such as low temperature (Ma et al., 2017), low or high dissolved oxygen (Sun et al., 2016; Heggeset et al., 2019) and nitrogen starvation (Chen et al., 2022) could stimulate the accumulation of intracellular lipids. Laboratory domestication and phased cultivation strategies (Wang et al., 2021a; Chen et al., 2022) have been developed. Additionally, the inclusion of lipase inhibitors (Orlistat) (Ma et al., 2021), antioxidants (Zhang et al., 2020) and plant hormones (Yu et al., 2016) in the culture medium can influence PUFAs metabolism and promote lipid accumulation. While fermentation optimization could increase the lipid yields, the targeted modification and alteration of key genes in the fatty acid synthesis pathway represent a rational design approach in molecular breeding with tremendous potential for application (Table 2A). For example, overexpressing glucose-6-phosphate dehydrogenase (Cui et al., 2016) and disrupting the 2,4-dienoyl-CoA reductase (Liang et al., 2022) had both been shown to enhance the accumulation of PUFAs in thraustochytrids. By knocking out a copy of the fatty acid synthase gene to deactivate the competitive pathway of DHA biosynthesis, and overexpressing acetyl-CoA carboxylase and diacylglycerol acyltransferase to enhance substrate supply and triglyceride synthesis, mutant strains of thraustochytrids have been developed (Wang et al., 2021b). The DHA purity in the intracellular lipids of these mutants can account for 61% of the total fatty acids, offering a significant approach for the production of high-purity DHA in thraustochytrids (Wang et al., 2021b). Many scholars have also experimented with non-rational design through mutagenesis and selection, obtaining mutant strains with excellent traits and significant application potential (Qi et al., 2017; Liu et al., 2020b; Nazir et al., 2022). Furthermore, researchers are continually seeking new effective molecular targets to provide novel insights into the rational design of thraustochytrid strains and the construction of thraustochytrid cell factories (Lan et al., 2021; Chi et al., 2022).
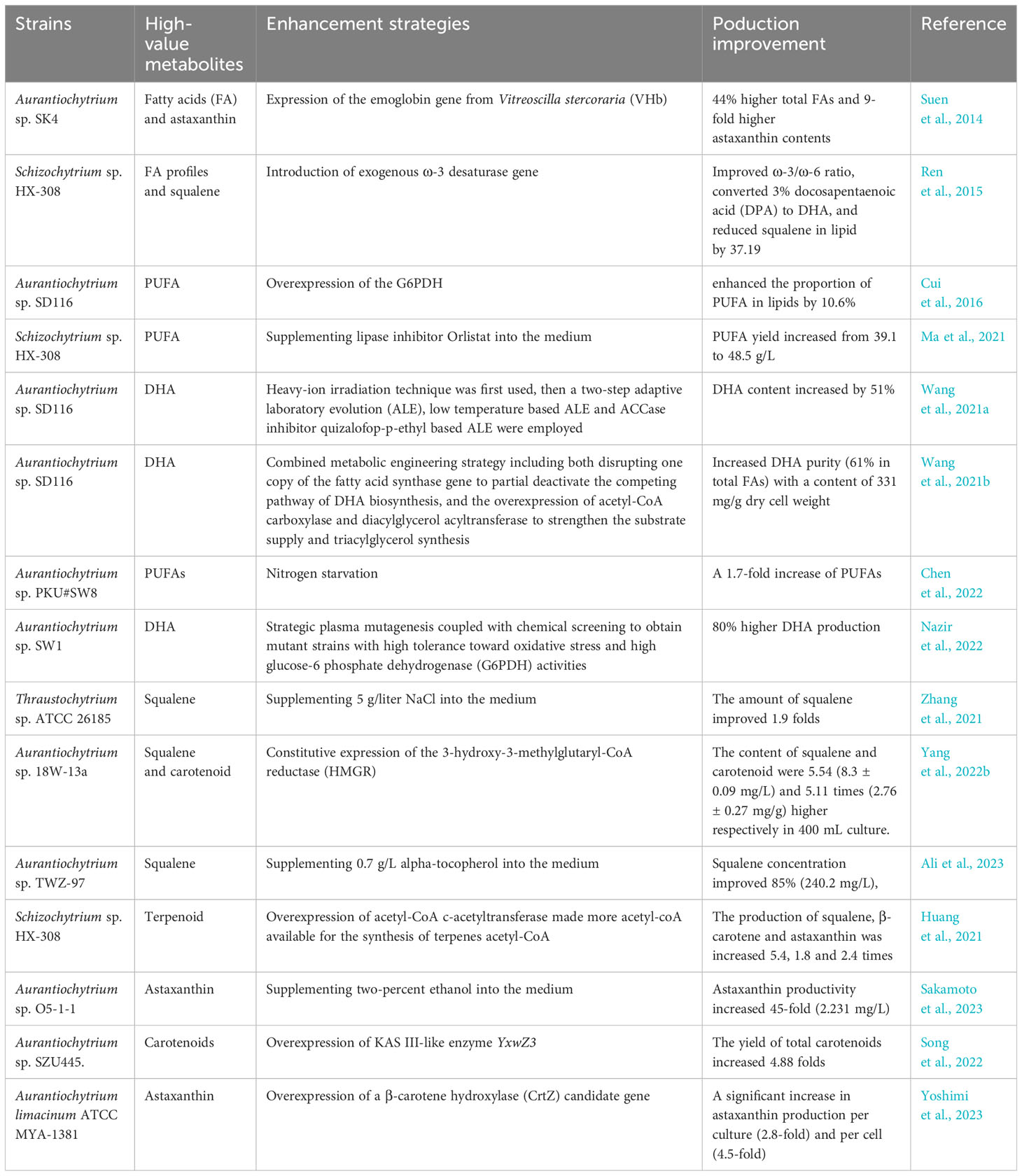
Table 2 Studies on significantly enhancing the production of high-value metabolites in thraustochytrids through fermentation optimization and genetic modification.
4.2 Strategies for enhancing squalene production in thraustochytrids through metabolic engineering
Squalene is a class of terpenoid hydrocarbons, an important precursor in cholesterol biosynthesis, present in many microorganisms, higher plants and animals. In thraustochytrids, the accumulation of squalene can account for about 20% of the cell dry weight (Kaya et al., 2011; Patel et al., 2022). The biosynthetic pathways of squalene in organisms include the mevalonate (MVA) and methyl-Derythritol 4-phosphate (MEP) pathways. In thraustochytrids, the MVA pathway is predominant and is characterized by low flux. The peak accumulation of squalene usually occurs on the second to third day of cultivation, when most strains are in the logarithmic growth phase. Further extending the cultivation period drastically reduces the squalene yield, to the point where it may not be detectable. Additionally, thraustochytrid strains in squalene synthesis research are mainly focused on the Aurantiochytrium and Schizochytrium genera. The intracellular accumulation of squalene can be improved by optimizing fermentation culture parameters or using metabolic engineering strategies (Table 2B). The bioactive substance α-tocopherol significantly increases the squalene production in A. urantiochyum sp. TWZ-97 by reducing the levels of reactive oxygen species and upregulating key genes in central carbon metabolism pathways (Ali et al., 2023). Compared to sodium-free media, the addition of sodium chloride to the culture medium of T. sp. ATCC 26185 doubled the concentration of squalene to 123.6 mg/L (Zhang et al., 2021). This is due to sodium induction shifting energy production from carbohydrate to lipid oxidation and increasing ATP generation. Thereby enhancing squalene synthesis by overcoming thermodynamic constraints in low flux pathways through increasing ATP consumption (Zhang et al., 2021). Moreover, the expression and overexpression of type-2 Acyl-CoA:diacylglycerol acyltransferases (DGAT2) genes, T66ASATb in A. sp. T66 and its homologs AlASATb in A. limacinum SR21, could improve squalene accumulation by up to 88% in A. sp. SR21 (Rau et al., 2022). Additionally, the expression of 3-hydroxy-3-methylglutaryl-coenzyme A reductase 29 (HMGR) is the bottleneck of squalene synthesis, and its constitutive expression could improve squalene production in A. sp. 18W-13a (Yang et al., 2022b). Furthermore, the discovery of new molecular targets and the development of efficient gene editing tools will accelerate the construction of thraustochytrid squalene cell factories (Huang et al., 2021).
4.3 Enhancing carotenoids production in thraustochytrids with genetic manipulation strategies
Carotenoids are valuable tetraterpene pigments, and could be synthesized by various organisms such as microorganisms, plants and animals. The majority of organisms capable of synthesizing carotenoids, such as cyanobacteria and microalgae, are phototrophs. However, the non-phototrophic protists like the labyrinthulids can acquire the ability to synthesize carotenoids through horizontal gene transfer from Actinobacteria, Proteobacteria and archaea (Rius et al., 2023). Thraustochytrids can accumulate high levels of astaxanthin and are characterized by short growth cycles and simple astaxanthin extraction processes, making them a promising source of this compound (Table 2C). The synthesis pathway of astaxanthin in thraustochytrids is not fully understood. But overexpression of the β-carotene hydroxylase gene (crtZ) in A. limacinum significantly increases astaxanthin content per culture volume (ng/mL) and per cell (ng/mL * OD) by 2.8 and 4.5 times, respectively (Yoshimi et al., 2023). Furthermore, extended light exposure increases astaxanthin yield in wild-type strains and decreases β-carotene content, indicating that light duration is a critical factor influencing astaxanthin synthesis (Yoshimi et al., 2023). Under light conditions, A. limacinum reduces ATP consumption to decrease the reactive oxygen species (ROS) occurrence in mitochondria while accumulating astaxanthin to prevent ROS damage (Kubo et al., 2022). The overexpression of the 3-ketoacyl-ACP synthase (KAS) III-like gene YxwZ3 increases carotenoids production in A. sp. SZU445, including β-carotene (37.96 μg/g), phytoene, β-cryptoxanthin, antheraxanthin, α-cryptoxanthin and echinenone (Song et al., 2022). This results in the reduced accumulation of fatty acids by increasing the consumption of malonyl-CoA through the MVA pathway of carotenoid synthesis (Song et al., 2022). The geranylgeranyl pyrophosphate synthase encoding gene (AlGGPPS) and isopentenyl pyrophosphate isomerase encoding gene (AlIDI) from A. limacinum MYA-1381 were integrated into the de novo carotene biosynthetic pathway in Escherichia coli, which increased the carotenoid by 2.99-fold compared with the initial strain (Shi et al., 2023). This indicated the participation of the two novel genes functioned coordinately in the carotene biosynthesis and provided novel functional elements for carotenoid engineering improvements (Shi et al., 2023). These studies provide vital references for biosynthesis research of carotenoids and other metabolic products in different thraustochytrid species.
4.4 Challenges in the development of genetic tools for thraustochytrids
Compared to model microorganisms such as Escherichia coli and Saccharomyces cerevisiae, non-model microorganisms like thraustochytrids often exhibit more complex phenotypic characteristics. This offers significant potential advantages for future scale production of biofuels and chemicals (Riley and Guss, 2021). Developing precise genetic editing tools for these non-model microorganisms is crucial. As it allows for rational optimization of their metabolic pathways, and thereby enhances the efficiency of target product synthesis (Riley and Guss, 2021). Metabolic engineering techniques enable microorganisms to meet the titer, rate and yield requirements for industrial applications and to synthesize a diverse array of metabolic products (Riley and Guss, 2021). However, the research and application of non-model microorganisms are limited by the lack of efficient genetic manipulation tools, hindering their widespread use and development of high-performance strains (Riley and Guss, 2021). Research has been conducted on genetic tools for marine protists across different taxonomic groups, yet no universally applicable solutions have been found (Faktorová et al., 2020). The diversity in genomic sequence features as well as differences in cell structure and morphology lead to significant variations in the strategies for exogenous DNA transformation and selection of DNA elements among different microorganisms. Consequently, the genetic manipulation schemes developed for thraustochytrids have largely been restricted to one or a few strains (Rau and Ertesvåg, 2021). However, advances in sequencing technology and cost reductions, along with the increase of omics analysis data and DNA synthesis technology, have made it more convenient to obtain genomic sequence information and identify gene editing targets. This facilitates the development of genetic tools and the optimization of metabolic engineering strategies (Rau and Ertesvåg, 2021). Furthermore, it is essential to establish an accurate species classification system based on the physiological, biochemical and molecular biological characteristics of thraustochytrids. This will provide a more practical reference for developing genetic tools for new strains and related species within the same genus or species.
5 Potential applications in nutritional supplementation and pharmaceutical industry
For the abundant amounts of high-value-added metabolites, especially unsaturated fatty acids (DHA, EPA), squalene and astaxanthin, thraustochytrids had been proposed to be applied in various fields, such as Feed, pharmacological and cosmetics industries. Most of the applicational researches for thraustochytrids were focused on the animal feeds of aquatic animals, poultry and livestock with improved growth and physiological indicators, as well as the higher DHA contents in their bodies or eggs (Yamasaki et al., 2007; Carvalho et al., 2018; dos Santos et al., 2019; Keegan et al., 2019). Besides, some natural products such as terpenoids in thraustochytrids could exert antioxidant effect, thus substitute for the antioxidants and pigments chemicals in cosmetics. In addition, thraustochytrids had exhibited anti-inflammatory and anti-cancer activities (Shakeri et al., 2017; Takahashi et al., 2019), and were proposed to be used to develop oral vaccines for their beneficial characters (Dahmen et al., 2023). The active lipids and defatted by-products of thraustochytrids with important nutritional value, have great potential in the development of functional food (Paulo et al., 2020; Reboleira et al., 2021).
5.1 Thraustochytrid supplementation strategies in enhancing feed nutrition
5.1.1 Enhancing fish nutrition with thraustochytrid-based feeds
Ω-3 long chain polyunsaturated fatty acids (LC-PUFAs) especially DHA and EPA, were proposed to exert vital effects in not only regulating normal physiological activities but the resistance to inflammation related diseases, which were thus suggested to be supplemented into human daily diets (Liu et al., 2014a). As is known, deep-sea fish accumulating large amounts of LC-PUFAs by preying on primary marine microalgae and microorganisms with PUFAs metabolic pathways, was the traditional sources. In the last decades, thraustochytrids have been considered as potential alternatives of DHA to traditional fish oils for several beneficial characters in growth and metabolism. On the other hand, marine animals were supposed to utilize and store LC-PUFAs in their bodies through diet supplementation and simultaneously exhibited excellent growth and physiological indicators. Besides, the feasibility of substitution of thraustochytrid oils for fish oils in full or partially has been demonstrated in several different marine animals.
In the aquatic feed industry, significant efforts had been made on exploring sustainable and environmental alternatives for fishmeal and fish oils (Torrecillas et al., 2017). Terrestrial meals and vegetable oils with high protein and lipid contents were excluded for undesired and adverse effects, for example, absorption suppression for amino acids, vitamins and minerals, as well as the deficiency in LC-PUFAs (Krogdahl et al., 2010; Tocher, 2015). Meanwhile, focus was paid on marine microalgae and microorganism, which was considered as desirable baits with certain physiological active substances. Among them, marine heterotrophic thraustochytrids attracted the most attention for absolute advantages e.g. fast growth, high DHA content and wide-sourced substrates.
Generally, dietary supplementation of thraustochytrids belonging mainly to two genera, namely Aurantiochytrium and Schizochytrium, could improve growth performance and PUFAs content of fishes. To investigate the feasibility of complete replacement of fish oil with plant oil mixtures (canola oil and palm oil) and A. sp. in juvenile yellowtail Seriola quinqueradiata, A. sp. supplementation at 0% (AM0), 1% (AM1), 2% (AM2), 3% (AM3) and 4% (AM4) into the mixtures of canola oil and palm oil was studied (Fukada et al., 2019). Results showed that the AM2 group exhibited significant higher final body weight than the AM0 group, thus indicated the beneficial effect of A. sp. on yellowtail aquaculture (Fukada et al., 2019). In addition, Nile tilapia juveniles fed A. sp. meal had significantly greater weight gains when compared to fish fed cod liver oil (CLO) diet, so as the DHA, EPA and LC-PUFAs contents (Nobrega et al., 2019). Similar results were obtained as for shrimp. A. limacinum BCC52274 (AL) enriched by instar-II Artemia benefited the growth performance of post-larval (PL) shrimp with an increasing trend of PL biomass gain as percent of the enriched meals increased (Visudtiphole et al., 2018).
Generally, thraustochytrid-based feed has been shown to improve growth, metabolism, digestion, immunity and reproductive indices in farmed fish species such as Nile tilapia (Batista et al., 2021; Nobrega et al., 2022; Luc et al., 2023), European sea bass (Soudant et al., 2022), Micropterus salmoides (Habte-Tsion et al., 2020), Salmo salar (Wei et al., 2021), Cyprinus carpio (Eljasik et al., 2020), humpback grouper (Cromileptes altivelis) (Sun et al., 2019) and Oncorhynchus mykiss (Sevgili et al., 2019). And it is applicable in the nutrition of larval feeds such as rotifers and Artemia nauplii and in DHA enrichment of fish flesh (Yamasaki et al., 2007). Recent transcriptomic approaches have explored the positive impacts of thraustochytrids supplementation on growth, metabolism, immunity and gonadal development in zebrafish, a model organism and provide a new molecular perspective for the application of thraustochytrids feed (Yang et al., 2022a; Huang et al., 2023). Furthermore, compared to other PUFAs sources, such as marine microalgae and oilseed crops, thraustochytrids, with their heterotrophic nature, rapid growth and high-density fermentation capabilities, offer unparalleled advantages. The addition forms of thraustochytrids in feed primarily include lipid extracts and freeze-dried biomass. Lipid extracts in feed are probably more readily absorbed and utilized by animals. While due to the thick cell walls of the organisms, the freeze-dried biomass is not easily digested in the animal gut. Therefore, broken-cell freeze-dried biomass appears more advantageous for simplifying feed preparation processes and improving food utilization rates. In summary, to further explore the potential of thraustochytrids as a sustainable feed resource, future research could focus on the effects of feed supplementation at different aquaculture stages, application in various aquaculture systems, long-term and short-term impacts on farmed animals, as well as its effects on aquaculture water environments. Furthermore, expanding the application of thraustochytrids feed to a broader range of aquatic animals could significantly contribute to the development of the aquaculture industry.
5.1.2 The role of thraustochytrid supplements in growth, health and environmental sustainability in shrimp aquaculture
Long-chain PUFAs play a crucial role in the growth, development and health maintenance of shrimps, which have limited capacity to synthesize these essential fatty acids and rely on dietary intake from feed. Firstly, the incorporation of thraustochytrid biomass has been found to promote the healthy growth of shrimps and PUFAs accumulation in their tissues. Utilizing A. mangrovei fiku008 as a juvenile shrimp feed supplement has been shown to enhance growth rate, survival rate and tissue PUFA content in Penaeus semisulcatus (Yilmaz et al., 2023). Additionally, direct supplementation of A. limacinum BCC52274 in feed significantly improved growth rate and survival of Litopenaeus vannamei juveniles and enhancing their lipid profile meanwhile (Visudtiphole et al., 2021). Furthermore, replacing fish oil entirely with S. sp. in shrimp feed did not adversely affect growth performance, survival rate and muscle fatty acid composition (Allen et al., 2019). Secondly, supplementation of thraustochytrid biomass can enhance the antioxidant capacity of shrimps. Low-fishmeal diets supplemented with S. limacinum significantly improved the antioxidant capacity of Litopenaeus vannamei, altering its transcriptomic expression patterns, particularly genes related to antioxidant and immune functions, thus enhancing the health status of the shrimp (Xu et al., 2022). Moreover, the biofloc technology is an emerging and environmentally friendly aquaculture method. A biofloc system integrated S. sp. with Lactobacillus plantarum maintained suitable water quality conditions, promoted shrimp growth and reduced concentrations of common pathogens (e.g., Vibrio spp.) (Pacheco-Vega et al., 2018). It is thus demonstrated to have obvious competitive advantage in maintaining water quality and promoting shrimp growth (Pacheco-Vega et al., 2018). Future developments in encapsulation technologies for thraustochytrids, such as microencapsulated bacterial feed (Willer et al., 2020), and the development of thraustochytrid-based probiotic consortia, are significant for developing sustainability aquaculture feeds.
5.1.3 Enhancing nutritional value of poultry products through thraustochytrid supplementation
Chicken meat and eggs, being among the most consumed protein sources in human diets, can benefit the public health improvement from enhanced nutritional value, particularly in increasing ω-3 fatty acid content. Compared to traditional ω-3 sources such as salmon oil (SO) and flaxseed oil (FO), the supplementation of Schizochytrium powder (SP) in chicken feed could significantly increasing DHA content in thigh meat (Jeon et al., 2022). While SO and FO treatments showed notable increases in EPA and linolenic acid (ALA), respectively (Jeon et al., 2022). Furthermore, the tissue DHA concentrations increased by adding A. limacinum throughout the whole life and fattening periods of broiler chickens (Keegan et al., 2019). Though DHA enrichment levels supplemented in two different period were similar, the fat deposition was unrelated to increased ω-3 PUFA levels, likely influenced by other nutritional and genetic factors (Keegan et al., 2019). Adding A. limacinum as ω-3 fatty acid sources significantly altered certain skeletal characteristics of ISA Brown and Shaver white breeders and progeny, influencing the bone mineralization process in chicken embryos, with varying impacts on the skeletal health of pullet of the two breeds (Kakhki et al., 2020). Therefore, the enrichment of PUFAs in chicken eggs and tissues is related to factors such as the DHA source and dose, feeding duration and chicken breed.
Except for DHA, thraustochytrids also contain antioxidants such as carotenoids and squalene, stabilizing DHA in its non-oxidized state. Supplementation with DHA-rich thraustochytrids significantly increased the DHA content and other bioactive components, such as carotenoids and tocopherols in broilers meat, effectively improving the nutritional value of the meat (Kalogeropoulos et al., 2010). Adding 1.0% A. sp. to layer chicken feed significantly increased DHA content in egg yolks within approximately 30 days, reduced the ω-6/ω-3 ratio and improved the anti-atherogenic and anti-thrombotic index of egg yolks (Liu et al., 2020a). Additionally, carotenoid supplementation from thraustochytrids enhanced egg yolk color, helped to slow down lipid oxidation and increased the lipid oxidation stability of DHA-rich egg yolks (Liu et al., 2020b). Future studies are anticipated to further investigate the impact of long-term storage and refrigeration conditions on DHA retention in egg yolks. Additionally, It is expected to explore the mechanisms of absorption and conversion of DHA and carotenoids in egg yolks, which are expected to enhance the nutritional value of eggs.
5.1.4 Omega-3 levels in livestock and insect feeds with thraustochytrid supplements
Meat from the beef, lamb and pork are also staple delicacies in the public daily diet. Although the addition of ω-3 fatty acids to the feed of these large livestock can increase the ω-3 fatty acid content in the meat, the impact on growth, meat color and flavor cannot be overlooked. The supplementation of S. limacinum in cattle feed increased the ω-3 fatty acid content in beef, but also increased the degree of meat oxidation, adversely affecting color stability and flavor (Phelps et al., 2016). Adding S. sp. to steer feed improved the ω-3 fatty acid content in beef and enhanced insulin sensitivity, but had no significant effect on the growth and carcass characteristics (Carvalho et al., 2018). Feeding pigs with DHA-rich Schizochytrium dry biomass improved the growth efficiency, lowered blood triglyceride levels and promoted the expression of fat oxidation genes (Jon Meadus et al., 2011). The longissimus dorsi muscle of pigs, a prime part of pork, showed increased DHA content with the increasing addition of A. limacinum in the diet (Moran et al., 2017). And the maximum values were 2.7 and 4 folds higher than the control in pig longissimus dorsi and back fat, respectively, with a greater increase in sows compared to boars (Moran et al., 2017). When fed with S. sp., the lambs showed slower growth rates but did not affect carcass weight and GR fat content (Burnett et al., 2016). Overall, research on thraustochytrid supplementation in feed for large livestock is relatively limited. This may be due to the larger size of livestock, requiring a greater amount of thraustochytrids biomass, minor positive effects on growth and DHA enrichment and economic costs, have not shown a clear advantage. Additionally, the black soldier fly, Hermetia illucens (HI), due to its high reproduction rate and nutritional value, is a valuable alternative ingredient for feed and food production. A substrate containing 10% biomass of S. sp. for insect rearing increased the ω-3 PUFAs content of HI pre-pupae, while higher proportions of S. sp. did not provide additional nutritional value (Truzzi et al., 2020). Therefore, future research could focus more on feed addition of thraustochytrid biomass or lipid extracts for insect, to develop novel functional feed and food nutrition components derived from insects.
5.2 Harnessing thraustochytrids for nutraceutical and functional food advancements
Existing research has demonstrated that thraustochytrid PUFAs or intracellular lipid extracts possess anti-inflammatory (Takahashi et al., 2019), antioxidant (Hien et al., 2022; Kalidasan et al., 2022; Kaliyamoorthy et al., 2023), neuroprotective (Hien et al., 2022) and anticancer properties (Shakeri et al., 2017; Kalidasan et al., 2022), highlighting their significant medicinal value (Table 3). Dietary supplementation with DHA has been proven to effectively elevate plasma total DHA levels (reaching 65 μM) (Mayer et al., 2003). Additionally, terpenoids like squalene and carotenoids also exhibit potent antioxidant, anticancer and anti-inflammatory activities. However, when co-produced with PUFAs in thraustochytrids, they are often emphasized as high-value by-products to reduce production costs. Among all thraustochytrid species, Schizochytrium was the only genus approved for commercial production of DHA-rich oils primarily for food and nutraceutical industries with completed feed safety evaluations for animals during 2001-2003 (Hammond et al., 2001a, b, c; Abril et al., 2003). It’s worth noting that currently only DHA-enriched oils extracted from S. sp. are permitted for use as nutritional additives, but not whole cells. Moreover, oils with high DHA content produced from genetically modified thraustochytrids are not accepted by the food and pharmaceutical industries in many countries (Wang et al., 2021a). Upon the European Commission’s request, the European Food Safety Authority (EFSA) Panel on Nutrition, Novel Foods and Food Allergens (NDA) evaluated the safety of S. sp. oil, specifically strains WZU477, FCC-3204, TKD-1 and CABIO-A-2, all belonging to the species S. limacinum, as novel foods under Regulation (EU) 2015/2283 (Turck et al., 2020; Turck et al., 2021a, b; Turck et al., 2023a, b). These oils, intended for use in infant and follow-on formulae with DHA at a level of 20-50 mg/100 kcal based on Regulation (EU) 2016/127 guidelines (Turck et al., 2020; Turck et al., 2021a; Turck et al., 2023a, b). And strain FCC-3204 was added for food supplements at up to 1 g DHA/day for adults (excluding pregnant and lactating women) (Turck et al., 2021b). These additions were deemed safe despite the absence of strain-specific toxicological studies. This conclusion was drawn from existing toxicological data on Schizochytrium-derived oils, their Qualified Presumption of Safety (QPS) status, free from marine biotoxins and viable cells production process. However, the oil from strain S. sp. ATCC-20889 was not confirmed safe due to insufficient species-level characterization and a potential unknown toxin risk (Turck et al., 2022). It highlighted the EFSA’s cautious approach to ensuring food safety and the necessity for detailed data on specific strains.
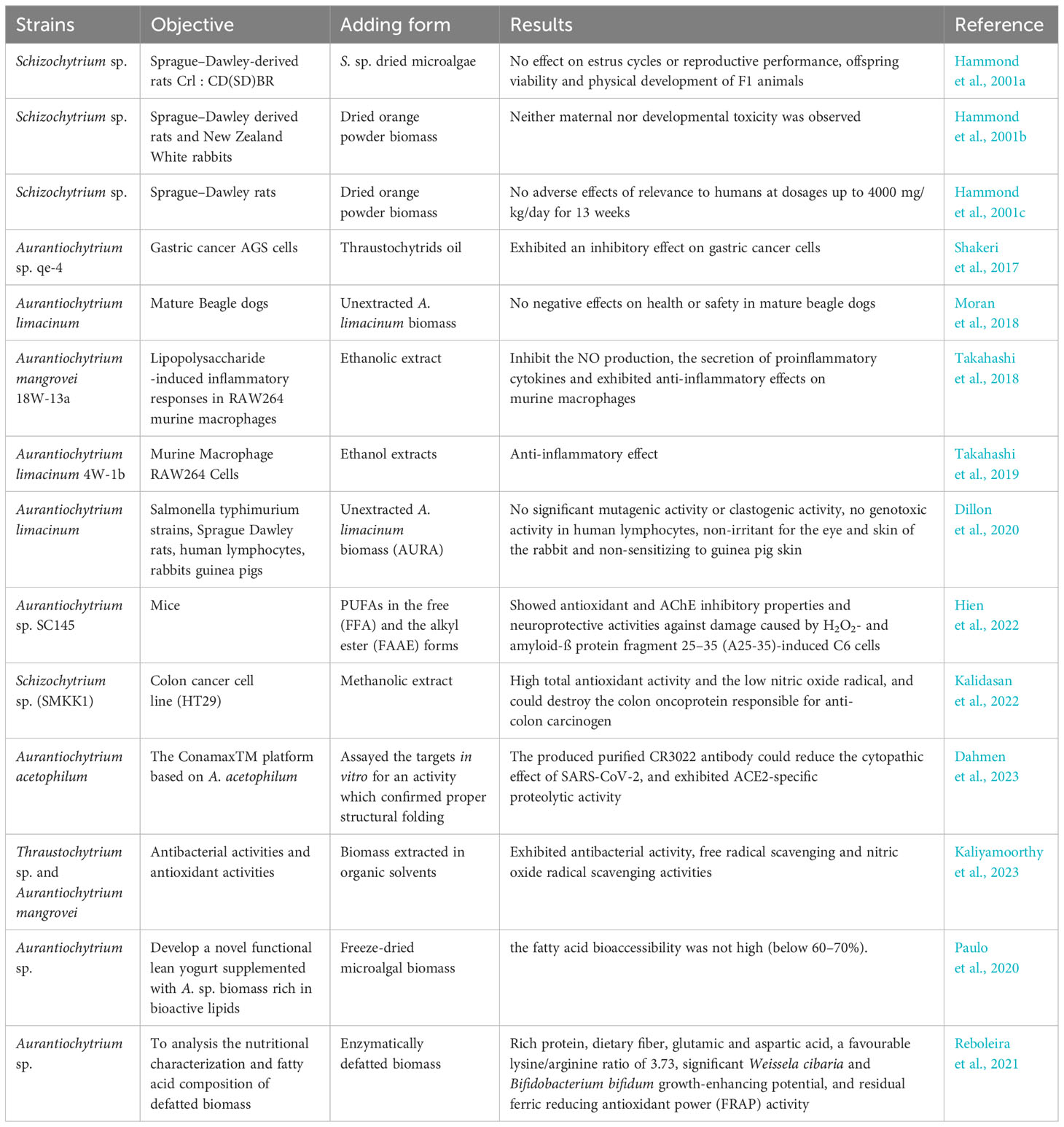
Table 3 Summaries of studies on the pharmacological activity and functional food application of thraustochytrids.
Furthermore, the genus Aurantiochytrium, closely related evolutionarily to Schizochytrium, exhibits rapid growth, high DHA content and physiological activities such as anti-inflammation, antioxidant and neuroprotection, becoming a significant target in pharmacological research. However, studies on the dietary safety and toxicology of Aurantiochytrium for animals are currently scarce. Limited research indicates that unextracted Aurantiochytrium biomass, when added to dog food at recommended levels (5 times) and fed for 15 days, showed no adverse effects on the health and safety of adult Beagles (Moran et al., 2018). Studies evaluating the toxicological potential of unextracted A. limacinum biomass as a food additive observed no significant mutagenic activity, genotoxic activity, induction of genotoxicity in human lymphocytes and death or acute toxicity (Dillon et al., 2020). Additionally, scientists have already initiated attempts to produce anti-SARS-CoV-2 antibodies related to interventions for Coronavirus disease 2019 (COVID-19) using A. acetophilum as a host (Dahmen et al., 2023). Furthermore, the development of novel functional foods enriched with DHA, particularly a yogurt enriched with A. sp. biomass (Paulo et al., 2020), appears to underscore the advantages of thraustochytrid-sourced DHA. Unlike DHA derived from fish oil, which has a pronounced fishy taste, DHA from thraustochytrids is more palatable. Moreover, the presence of potential functional nutritional components in the defatted biomass waste of thraustochytrids, such as abundant protein, dietary fiber, glutamic and aspartic acid, makes the biorefinery process of utilizing these defatted by-products for the development of food supplements more feasible (Reboleira et al., 2021). Overall, future research in the pharmacological study of the genus Aurantiochytrium, as well as the development of functional foods, is poised to further enhance the role of thraustochytrids in biotechnological development and functional food and pharmaceutical manufacturing.
6 Conclusions and prospects
As a marine heterotrophic protist, thraustochytrid contribute significantly to marine ecosystems and human welfare. Understanding their taxonomic characteristics is crucial for better utilization of these protists. However, current research on thraustochytrid taxonomy necessitates a more in-depth and systematic approach, incorporating physiological, biochemical and molecular biology methods, particularly genomics, to establish an accurate and scientific polyphasic taxonomy system. The diversity of metabolic products in thraustochytrids renders them a biotechnological resource with enormous potential. Future use of rapid detection technologies, including Raman spectroscopy, as well as natural product isolation and identification methods, will enrich our understanding of high-value metabolites and low-concentration components in thraustochytrids. Additionally, fermentation optimization and molecular genetic modifications can significantly enhance the accumulation of key bioactive substances including PUFAs, squalene and carotenoids. These will promote the construction of thraustochytrid cell factory.
Currently, studies on the metabolic engineering and application of thraustochytrids primarily utilizes strains from the genera Schizochytrium and Aurantiochytrium. However, thraustochytrid belonging to other genera and their close relatives Labyrinthula sp., could accumulate significant amounts of DHA as well. Future studies with different strains might yield better feed utilization results. Moreover, existing studies on thraustochytrids supplementation to enhance the PUFAs content of meat or egg yolks have overlooked the utilization and transformation of other active components (squalene, carotenoids, proteins and substances in low quantities) in them.
In summary, future challenges and opportunities lie in unlocking the full potential of thraustochytrids through advanced research tools and exploitation of species resources. Innovations in metabolic engineering, cultivation techniques and biorefinery approaches will be critical to increase the efficiency and sustainability of thraustochytrid-based production systems. Furthermore, expanding the understanding of thraustochytrid taxonomy and genetics can facilitate the discovery and optimization of strains with superior production capacities. Besides, regulatory approval and market acceptance will hinge on demonstrating the safety, efficacy and economic viability of thraustochytrid-derived products. As we delve deeper into the capabilities of these protists, we stand on the brink of a new era in biotechnology. Then, thraustochytrids could play a pivotal role in meeting global demands for sustainable and health-enhancing commodities.
Author contributions
QW: Conceptualization, Formal Analysis, Funding acquisition, Project administration, Supervision, Writing – original draft, Writing – review & editing. YZ: Investigation, Writing – original draft, Writing – review & editing. RH: Investigation, Writing – original draft. YXZ: Investigation, Writing – original draft.
Funding
The author(s) declare financial support was received for the research, authorship, and/or publication of this article. This research was funded by the National Natural Science Foundation of China (No. 32100003) and Natural Science Foundation of Hebei Province of China (No. D2019204215).
Conflict of interest
The authors declare that the research was conducted in the absence of any commercial or financial relationships that could be construed as a potential conflict of interest.
Publisher’s note
All claims expressed in this article are solely those of the authors and do not necessarily represent those of their affiliated organizations, or those of the publisher, the editors and the reviewers. Any product that may be evaluated in this article, or claim that may be made by its manufacturer, is not guaranteed or endorsed by the publisher.
References
Abril R., Garrett J., Zeller S. G., Sander W. J., Mast R. W. (2003). Safety assessment of DHA-rich microalgae from Schizochytrium sp. Part V: target animal safety/toxicity study in growing swine. Regul. Toxicol. Pharmacol. 37, 73–82. doi: 10.1016/S0273-2300(02)00030-2
Ali M. K., Liu X., Li J., Zhu X., Sen B., Wang G. (2023). Alpha-tocopherol significantly improved squalene production Yield of Aurantiochytrium sp. TWZ-97 through lowering ROS levels and up-regulating key genes of central carbon metabolism pathways. Antioxidants 12, 1034. doi: 10.3390/antiox12051034
Allen K. M., Habte-Tsion H. M., Thompson K. R., Filer K., Tidwell J. H., Kumar V. (2019). Freshwater microalgae (Schizochytrium sp.) as a substitute to fish oil for shrimp feed. Sci. Rep. 9, 6178. doi: 10.1038/s41598-019-41020-8
Batista R. O., Nobrega R. O., Schleder D. D., Pettigrew J. E., Fracalossi D. M. (2021). Aurantiochytrium sp. meal Improved body fatty acid profile and morphophysiology in Nile Tilapia reared at Low Temperature. Fishes 6, 45. doi: 10.3390/fishes6040045
Bongiorni L., Jain R., Raghukumar S., Aggarwal R. K. (2005). Thraustochytrium gaertnerium sp. nov.: a new thraustochytrid stramenopilan protist from mangroves of Goa, India. Protist 156, 303–315. doi: 10.1016/j.protis.2005.05.001
Burnett V. F., Jacobs J. L., Norng S., Ponnampalam E. N. (2016). Feed intake, liveweight gain and carcass traits of lambs offered pelleted annual pasture hay supplemented with flaxseed (Linum usitatissimum) flakes or algae (Schizochytrium sp.). Anim. Prod. Sci. 57, 877. doi: 10.1071/AN15230
Carvalho J. R. R., Brennan K. M., Ladeira M. M., Schoonmaker J. P. (2018). Performance, insulin sensitivity, carcass characteristics, and fatty acid profile of beef from steers fed microalgae. J. Anim. Sci. 96, 3433–3445. doi: 10.1093/jas/sky210
Chen X., He Y., Liu L., Zhu X., Sen B., Wang G. (2022). Nitrogen starvation enhances the production of saturated and unsaturated fatty acids in Aurantiochytrium sp. PKU#SW8 by regulating key biosynthetic genes. Mar. Drugs 20, 621. doi: 10.3390/md20100621
Chi G., Cao X., Li Q., Yao C., Lu F., Liu Y., et al. (2022). Computationally guided enzymatic studies on Schizochytrium-sourced malonyl-CoA: ACP Transacylase. J. Agric. Food Chem. 70, 13922–13934. doi: 10.1021/acs.jafc.2c05447
Chi Z., Pyle D., Wen Z., Frear C., Chen S. (2007). A laboratory study of producing docosahexaenoic acid from biodiesel-waste glycerol by microalgal fermentation. Process Biochem. 42, 1537–1545. doi: 10.1016/j.procbio.2007.08.008
Cui G., Ma Z., Liu Y. J., Feng Y., Sun Z., Cheng Y., et al. (2016). Overexpression of glucose-6-phosphate dehydrogenase enhanced the polyunsaturated fatty acid composition of Aurantiochytrium sp. SD116. Algal Res. 19, 138–145. doi: 10.1016/j.algal.2016.08.005
Dahmen J., Vermeulen A., Payne S., Lippmeier C. (2023). Thraustochytrid hosts for expression of proteins relevant to SARS-CoV-2 intervention. PloS One 18, e0283592. doi: 10.1371/journal.pone.0283592
Damare V. S., D’Costa P. M., Shivaramu M. S., Borges V., Fernandes M., Fernandes C., et al. (2020). Preliminary study on the response of marine fungoid protists, the thraustochytrids, to lipid extracts of diatoms. Aquat. Ecol. 54, 355–367. doi: 10.1007/s10452-020-09747-z
Damare V. S., Raghukumar S. (2012). Marine aggregates and transparent exopolymeric particles (TEPs) as substrates for the stramenopilan fungi, the thraustochytrids: roller table experimental approach. Kavaka 40, 22–31.
de la Broise D., Ventura M., Chauchat L., Guerreiro M., Michez T., Vinet T., et al. (2022). Scale-up to pilot of a non-axenic culture of thraustochytrids using digestate from methanization as nitrogen source. Mar. Drugs 20, 499. doi: 10.3390/md20080499
Dellero Y., Cagnac O., Rose S., Seddiki K., Cussac M., Morabito C., et al. (2018a). Proposal of a new thraustochytrid genus Hondaea gen. nov. and comparison of its lipid dynamics with the closely related pseudo-cryptic genus Aurantiochytrium. Algal Res. 35, 125–141. doi: 10.1016/j.algal.2018.08.018
Dellero Y., Rose S., Metton C., Morabito C., Lupette J., Jouhet J., et al. (2018b). Ecophysiology and lipid dynamics of a eukaryotic mangrove decomposer. Environ. Microbiol. 20, 3057–3068. doi: 10.1111/1462-2920.14346
de Melo M. M. R., Sapatinha M., Pinheiro J., Lemos M. F. L., Bandarra N. M., Batista I., et al. (2020). Supercritical CO2 extraction of Aurantiochytrium sp. biomass for the enhanced recovery of omega-3 fatty acids and phenolic compounds. J. CO2 Util. 38, 24–31. doi: 10.1016/j.jcou.2020.01.014
Dillon G. P., Keegan J. D., Moran C. A. (2020). Toxicological evaluation of an unextracted Aurantiochytrium limacinum biomass, a novel docosahexaenoic acid rich feed ingredient. Food Chem. Toxicol. 141, 111397. doi: 10.1016/j.fct.2020.111397
Doi K., Honda D. (2017). Proposal of Monorhizochytrium globosumgen. nov., comb. nov. (Stramenopiles, Labyrinthulomycetes) for former Thraustochytrium globosumbased on morphological features and phylogenetic relationships. Psychol. Res. 65, 188–201. doi: 10.1111/pre.12175
dos Santos S. K. A., Schorer M., Moura G., d. S., Lanna E. A. T., Pedreira M. M. (2019). Evaluation of growth and fatty acid profile of Nile tilapia (Oreochromis niloticus) fed with Schizochytrium sp. Aquac. Res. 50, 1068–1074. doi: 10.1111/are.2019.50.issue-4
Eljasik P., Panicz R., Sobczak M., Sadowski J., Barbosa V., Marques A., et al. (2020). Plasma biochemistry, gene expression and liver histomorphology in common carp (Cyprinus carpio) fed with different dietary fat sources. Food Chem. Toxicol. 140, 111300. doi: 10.1016/j.fct.2020.111300
Faktorová D., Nisbet R. E. R., Fernández Robledo J. A., Casacuberta E., Sudek L., Allen A. E., et al. (2020). Genetic tool development in marine protists: emerging model organisms for experimental cell biology. Nat. Methods 17, 481–494. doi: 10.1038/s41592-020-0796-x
Fukada H., Kitagima R., Shinagawa J., Morino H., Masumoto T. (2019). Effects of complete replacement of fish oil with plant oil mixtures and algal meal on growth performance and fatty acid composition in juvenile yellowtail Seriola quinqueradiata. Fish. Sci. 86, 107–118. doi: 10.1007/s12562-019-01361-9
Gao M., Song X., Feng Y. (2013). Isolation and characterization of Aurantiochytrium species: high docosahexaenoic acid (DHA) production by the newly isolated microalga, Aurantiochytrium sp. SD116. J. Oleo. Sci. 62, 143–151. doi: 10.5650/jos.62.143
Gladkowski W., Kiełbowicz G., Chojnacka A., Bobak Ł., Spychaj R., Dobrzański Z., et al. (2014). The effect of feed supplementation with dietary sources ofn-3 polyunsaturated fatty acids, flaxseed and algae Schizochytrium sp., on their incorporation into lipid fractions of Japanese quail eggs. Int. J. Food Sci. Technol. 49, 1876–1885. doi: 10.3390/molecules22101771
Graham A., Naye A., Akinbobye O., Sepper O., Karim A., Tulp O., et al. (2021). Effects of DHA and EPA on cardiovascular indices. FASEB J. 35. doi: 10.1096/fasebj.2021.35.S1.01815
Guesnet P., Alessandri J. M. (2011). Docosahexaenoic acid (DHA) and the developing central nervous system (CNS) - Implications for dietary recommendations. Biochimie 93, 7–12. doi: 10.1016/j.biochi.2010.05.005
Habte-Tsion H. M., Kolimadu G. D., Rossi W. Jr., Filer K., Kumar V. (2020). Effects of Schizochytrium and micro-minerals on immune, antioxidant, inflammatory and lipid-metabolism status of Micropterus salmoides fed high- and low-fishmeal diets. Sci. Rep. 10, 7457. doi: 10.1038/s41598-020-64286-9
Hammond B. G., Mayhew D. A., Holson J. F., Nemec M. D., Mast R. W., Sander W. J. (2001a). Safety assessment of DHA-rich microalgae from Schizochytrium sp. Regul. Toxicol. Pharmacol 33, 205–217. doi: 10.1006/rtph.2001.1459
Hammond B. G., Mayhew D. A., Naylor M. W., Ruecker F. A., Mast R. W., Sander W. J. (2001b). Safety assessment of DHA-rich microalgae from Schizochytrium sp. Regul. Toxicol. Pharmacol. 33, 192–204. doi: 10.1006/rtph.2001.1458
Hammond B. G., Mayhew D. A., Robinson K., Mast R. W., Sander W. J. (2001c). Safety assessment of DHA-rich microalgae from Schizochytrium sp. Regul. Toxicol. Pharmacol. 33, 356–362. doi: 10.1006/rtph.2001.1477
Harel M., Ben-Dov E., Rasoulouniriana D., Siboni N., Kramarsky-Winter E., Loya Y., et al. (2008). A new Thraustochytrid, strain Fng1, isolated from the surface mucus of the hermatypic coral Fungia granulosa. FEMS Microbiol. Ecol. 64, 378–387. doi: 10.1111/fem.2008.64.issue-3
He Y., Wang X., Zhang Y., Guo Z., Jiang Y., Chen F. (2019). Enzymatic ethanolysis subjected to Schizochytrium biomass: Sequential processing for DHA enrichment and biodiesel production. Energy. Convers. Manage. 184, 159–171. doi: 10.1016/j.enconman.2019.01.051
Heggeset T. M. B., Ertesvag H., Liu B., Ellingsen T. E., Vadstein O., Aasen I. M. (2019). Lipid and DHA-production in Aurantiochytrium sp. - Responses to nitrogen starvation and oxygen limitation revealed by analyses of production kinetics and global transcriptomes. Sci. Rep. 9, 19470. doi: 10.1038/s41598-019-55902-4
Hien H. T. M., Thom L. T., Ha N. C., Tam L. T., Thu N. T. H., Nguyen T. V., et al. (2022). Characterization and optimization of culture conditions for Aurantiochytrium sp. SC145 isolated from Sand Cay (Son Ca) island, Vietnam, and antioxidative and neuroprotective activities of its polyunsaturated fatty acid mixture. Mar. Drugs 20, 780. doi: 10.1007/s10529-017-2345-y
Hoang T. M. H., Luu T. T., Ngo T. H. T., Nguyen T. M. H., Tran H. G., Nguyen T. T. O., et al. (2022). Hypolipidaemic effects of (24R)-4α-methyl-5α-stigmasta-7,22-dien-3β-ol derived from aurantiochytrium mangrovei BT3 in the HEPG2 cell line. Appl. Biochem. Microbiol. 58, 286–293. doi: 10.1134/S0003683822030048
Honda D., Yokochi T., Nakahara T., Erata M., Higashihara T. (1998). Schizochytrium limacinum sp. nov., a new thraustochytrid from a mangrove area in the west Pacific Ocean. Mycol. Res. 102, 439–448. doi: 10.1017/S0953756297005170
Honda D., Yokochi T., Nakahara T., Raghukumar S., Nakagiri A., Schaumann K., et al. (1999). Molecular phylogeny of labyrinthulids and thraustochytrids based on the sequencing of 18S ribosomal RNA gene. J. Eukanot. Mirrobiol. 46, 637–647. doi: 10.1111/j.1550-7408.1999.tb05141.x
Huang J., Aki T., Yokochi T., Nakahara T., Honda D., Kawamoto S., et al. (2003). Grouping newly isolated docosahexaenoic acid-producing thraustochytrids based on their polyunsaturated fatty acid profiles and comparative analysis of 18S rRNA genes. Mar. Biotechnol. (NY). 5, 450–457. doi: 10.1007/s10126-002-0110-1
Huang P.-W., Xu Y.-S., Sun X.-M., Shi T.-Q., Gu Y., Ye C., et al. (2021). Development of an efficient gene editing tool in schizochytrium sp. and improving its lipid and terpenoid biosynthesis. Front. Nutr. 8. doi: 10.3389/fnut.2021.795651
Huang Y., Yang H., Li Y., Guo Y., Li G., Chen H. (2023). Comparative transcriptome analysis reveals the effect of Aurantiochytrium sp. on gonadal development in zebrafish. Animals 13, 2482. doi: 10.3390/ani13152482
Jeon J. J., Kim H. J., Kang H. K., Kim C. H., Kim H. S., Hong E. C., et al. (2022). Effects of dietary thraustochytrid Schizochytrium sp. and other omega-3 sources on growth performance, carcass characteristics, and meat quality of broilers. Animals 12, 1166. doi: 10.3390/ani12091166
Jon Meadus W., Duff P., Rolland D., Lynn Aalhus J., Uttaro B., Russell Dugan M. E. (2011). Feeding docosahexaenoic acid to pigs reduces blood triglycerides and induces gene expression for fat oxidation. Can. J. Anim. Sci. 91, 601–612. doi: 10.4141/cjas2011-055
Kakhki R. A. M., Price K. R., Moats J., Bedecarrats G., Karrow N. A., Kiarie E. G. (2020). Impact of feeding microalgae (Aurantiochytrium limacinum) and co-extruded mixture of full-fat flaxseed as sources of n-3 fatty acids to ISA brown and Shaver white breeders and progeny on pullet skeletal attributes at hatch through to 18 weeks of age. Poult. Sci. 99, 2087–2099. doi: 10.1016/j.psj.2019.12.016
Kalidasan K., Dufossé L., Manivel G., Senthilraja P., Kathiresan K. (2022). Antioxidant and anti-Colorectal cancer properties in methanolic extract of mangrove-derived Schizochytrium sp. J. Mar. Sci. Eng. 10, 431. doi: 10.3390/jmse10030431
Kaliyamoorthy K., Chavanich S., Kandasamy K., Ponnuvel M., Kamlangdee N., Taoka Y., et al. (2023). PUFA and carotenoid producing thraustochytrids and their anti-microbial and antioxidant activities. Front. Mar. Sci. 10. doi: 10.3389/fmars.2023.1126452
Kalogeropoulos N., Chiou A., Gavala E., Christea M., Andrikopoulos N. K. (2010). Nutritional evaluation and bioactive microconstituents (carotenoids, tocopherols, sterols and squalene) of raw and roasted chicken fed on DHA-rich microalgae. Food Res. Int. 43, 2006–2013. doi: 10.1016/j.foodres.2010.05.018
Kaya K., Nakazawa A. A., Matsuura H., Honda D., Inouye I., Watanabe M. M. (2011). Thraustochytrid Aurantiochytrium sp. 18W-13a accummulates high amounts of squalene. Biosci. Biotechnol. Biochem. 75, 2246–2248. doi: 10.1271/bbb.110430
Kaya K., Shiraishi F., Iida T., Yamada M., Sano T. (2021). An acidic morpholine derivative containing glyceride from thraustochytrid, Aurantiochytrium. Sci. Rep. 11. doi: 10.1038/s41598-021-85636-1
Keegan J. D., Fusconi G., Morlacchini M., Moran C. A. (2019). Whole-life or fattening period only broiler feeding strategies achieve similar levels of omega-3 fatty acid enrichment using the DHA-rich protist, Aurantiochytrium limacinum. Animals 9, 327. doi: 10.3390/ani9060327
Kobayashi Y., Ookubo M. (1953). Studies on the marine phycomycetes. Bull. Natl. Sci. Mus. Tokyo. 33, 53–65.
Krogdahl A., Penn M., Thorsen J., Refstie S., Bakke A. M. (2010). Important antinutrients in plant feedstuffs for aquaculture: an update on recent findings regarding responses in salmonids. Aquac. Res. 41, 333–344. doi: 10.1111/j.1365-2109.2009.02426.x
Kubo Y., Morimoto D., Shiroi M., Yoshimi T., Ohara K., Higashine T., et al. (2022). Transcriptional responses of Aurantiochytrium limacinum under light conditions. J. Appl. Microbiol. 132, 4330–4337. doi: 10.1111/jam.15527
Kwak M., Roh S., Yang A., Lee H., Chang Y. K. (2019). High shear-assisted solvent extraction of lipid from wet biomass of Aurantiochytrium sp. KRS101. Sep. Purif. Technol. 227, 115666. doi: 10.1016/j.seppur.2019.06.004
Laddha H., Pawar P. R., Prakash G. (2021). Bioconversion of waste acid oil to docosahexaenoic acid by integration of “ex novo’’ and “de novo’’ fermentation in Aurantiochytrium limacinum. Bioresour. Technol. 332, 125062. doi: 10.1016/j.biortech.2021.125062
Lan C., Wang S., Zhang H., Wang Z., Wan W., Liu H., et al. (2021). Cocktail biosynthesis of triacylglycerol by rational modulation of diacylglycerol acyltransferases in industrial oleaginous Aurantiochytrium. Biotechnol. Biofuels. 14. doi: 10.1186/s13068-021-02096-5
Lee G.-I., Shin W.-S., MoonGeun Jung S., Kim W., Lee C., Kwon J.-H. (2020). Effects of soybean curd wastewater on growth and DHA production in Aurantiochytrium sp. Lwt 134, 110245. doi: 10.1016/j.lwt.2020.110245
Lee Chang K. J., Dumsday G., Nichols P. D., Dunstan G. A., Blackburn S. I., Koutoulis A. (2013). High cell density cultivation of a novel Aurantiochytrium sp. strain TC 20 in a fed-batch system using glycerol to produce feedstock for biodiesel and omega-3 oils. Appl. Microbiol. Biotechnol. 97, 6907–6918. doi: 10.1007/s00253-013-4965-z
Li W., Bian Y., Chai Y., Ding H., Sheng S., Wu F., et al. (2021). Ultrasound-assisted extraction ameliorates the physicochemical properties of defatted mulberry seed protein to promote lipid production in Schizochytrium sp. SR21. Biomass. Convers. Bior. 11, 489–502. doi: 10.1007/s13399-020-00626-z
Li X., Yu X., Liu Q., Zhang Y., Wang Q. (2023). Lipid production of Schizochytrium sp. HBW10 isolated from coastal waters of Northern China cultivated in food waste hydrolysate. Microorganisms 11, 2714. doi: 10.3390/microorganisms11112714
Liang S., Yang X., Zhu X., Ibrar M., Liu L., Li S., et al. (2022). Metabolic engineering to improve docosahexaenoic acid production in marine protist Aurantiochytrium sp. by disrupting 2,4-Dienoyl-CoA reductase. Front. Mar. Sci. 9. doi: 10.3389/fmars.2022.939716
Liu Y., Chen L. Y., Sokolowska M., Eberlein M., Alsaaty S., Martinez-Anton A., et al. (2014a). The fish oil ingredient, docosahexaenoic acid, activates cytosolic phospholipase A(2) via GPR120 receptor to produce prostaglandin E(2) and plays an anti-inflammatory role in macrophages. Immunology 143, 81–95. doi: 10.1111/imm.12296
Liu L., Hu Z., Li S., Yang H., Li S., Lv C., et al. (2020b). Comparative transcriptomic analysis uncovers genes responsible for the DHA enhancement in the mutant Aurantiochytrium sp. Microorganisms 8. doi: 10.3390/microorganisms8040529
Liu Y., Singh P., Liang Y., Li J., Xie N., Song Z., et al. (2017). Abundance and molecular diversity of thraustochytrids in coastal waters of southern China. FEMS Microbiol. Ecol. 93, fix070. doi: 10.1093/femsec/fix070
Liu Y., Singh P., Sun Y., Luan S., Wang G. (2014b). Culturable diversity and biochemical features of thraustochytrids from coastal waters of Southern China. Appl. Microbiol. Biotechnol. 98, 3241–3255. doi: 10.1007/s00253-013-5391-y
Liu B., Zhou Q., Zhu J., Lin G., Yu D., Ao T. (2020a). Time course of nutritional and functional property changes in egg yolk from laying hens fed docosahexaenoic acid-rich microalgae. Poult. Sci. 99, 4616–4625. doi: 10.1016/j.psj.2020.06.007
Luc Q. C., Ncho C. M., Dhahbi S., Olowe O. S. (2023). Mitigation of cold stress in Nile tilapia (Oreochromis niloticus) through dietary lipids supplementation: a preliminary network meta-analysis. Fish Physiol. Biochem 50, 209–223. doi: 10.1007/s10695-023-01217-1
Ma Z., Tian M., Tan Y., Cui G., Feng Y., Cui Q., et al. (2017). Response mechanism of the docosahexaenoic acid producer Aurantiochytrium under cold stress. Algal Res. 25, 191–199. doi: 10.1016/j.algal.2017.05.021
Ma W., Wang Y.-Z., Nong F.-T., Du F., Xu Y.-S., Huang P.-W., et al. (2021). An emerging simple and effective approach to increase the productivity of thraustochytrids microbial lipids by regulating glycolysis process and triacylglycerols’ decomposition. Biotechnol. Biofuels. 14. doi: 10.1186/s13068-021-02097-4
Maas P. A. Y., Kleinschuster S. J., Dykstra M. J., Smolowitz R., Parent J. (1999). Molecular characterization of QPX (Quahog Parasite Unknown), a pathogen of Mercenaria mercenaria. J. Shellf. Res. 18, 561–567.
Man C. H., Shimura Y., Suzuki I. (2022). Identification of extracellular proteases induced by nitrogen-limited conditions thraustochytrids Schizochytrium aggregatum ATCC 28209. Mar. Biotechnol. (NY). 24, 243–254. doi: 10.1007/s10126-022-10103-5
Marchan L. F., Lee Chang K. J., Nichols P. D., Mitchell W. J., Polglase J. L., Gutierrez T. (2018). Taxonomy, ecology and biotechnological applications of thraustochytrids: A review. Biotechnol. Adv. 36, 26–46. doi: 10.1016/j.biotechadv.2017.09.003
Marchan L. F., Lee Chang K. J., Nichols P. D., Polglase J. L., Mitchell W. J., Gutierrez T. (2017). Screening of new British thraustochytrids isolates for docosahexaenoic acid (DHA) production. J. Appl. Phycol. 29, 2831–2843. doi: 10.1007/s10811-017-1149-8
Mayer K., Gokorsch S., Fegbeutel C., Hattar K., Rosseau S., Walmrath D., et al. (2003). Parenteral nutrition with fish oil modulates cytokine response in patients with sepsis. Am. J. Respir. Crit. Care Med. 167, 1321–1328. doi: 10.1164/rccm.200207-674OC
Moran C. A., Fusconi G., Morlacchini M., Jacques K. A. (2017). Docosahexaenoic acid content in longissimus dorsi and backfat tissues of grow-finish pigs fed diets containing 0, 0.25 or 0.5% heterotrophically grown algae: Study 2. J. Anim. Sci. 95, 58–59. doi: 10.2527/asasmw.2017.124
Moran C. A., Morlacchini M., Keegan J. D., Rutz F., Fusconi G. (2020). Docosahexaenoic acid enrichment of layer hen tissues and eggs through dietary supplementation with heterotrophically grown Aurantiochytrium limacinum. J. Appl. Poult. Res. 29, 152–161. doi: 10.1016/j.japr.2019.10.002
Moran C., Reinemeyer C., Keegan J., Groenewegen P., Jacques K. (2018). A crossover target animal safety study of unextracted Aurantiochytrium microalgal biomass (AURA) as a dietary source of docosahexaenoic acid in mature dogs. J. Anim. Sci. 96, 156. doi: 10.1093/jas/sky404.341
Nazir Y., Phabakaran P., Halim H., Mohamed H., Naz T., Abdul Hamid A., et al. (2022). Strategic development of Aurantiochytrium sp. mutants with superior oxidative stress tolerance and glucose-6-phosphate dehydrogenase activity for enhanced DHA production through plasma mutagenesis coupled with chemical screening. Front. Nutr. 9. doi: 10.3389/fnut.2022.876649
Nishitani G., Yoshida M. (2018). A new primer set to amplify the mitochondrial cytochrome C oxidase subunit I (COI) gene in the DHA-rich microalgae, the genus Aurantiochytrium. Microbes Environ. 33, 227–229. doi: 10.1264/jsme2.ME17145
Nobrega R. O., Batista R. O., Corrêa C. F., Mattioni B., Filer K., Pettigrew J. E., et al. (2019). Dietary supplementation of Aurantiochytrium sp. meal, a docosahexaenoic-acid source, promotes growth of Nile tilapia at a suboptimal low temperature. Aquaculture 507, 500–509. doi: 10.1016/j.aquaculture.2019.04.030
Nobrega R. O., Dafre A. L., Corrêa C. F., Mattioni B., Batista R. O., Pettigrew J. E., et al. (2022). Oxidative damage in Nile tilapia, Oreochromis niloticus, is mainly induced by water temperature variation rather than Aurantiochytrium sp. meal dietary supplementation. Fish. Physiol. Biochem. 48, 85–99. doi: 10.1007/s10695-021-01025-5
Pacheco-Vega J. M., Cadena-Roa M. A., Leyva-Flores J. A., Zavala-Leal O. I., Pérez-Bravo E., Ruiz-Velazco J. M. J. (2018). Effect of isolated bacteria and microalgae on the biofloc characteristics in the Pacific white shrimp culture. Aquac. Rep. 11, 24–30. doi: 10.1016/j.aqrep.2018.05.003
Pan J., Del Campo J., Keeling P. J. (2017). Reference tree and environmental sequence diversity of labyrinthulomycetes. J. Eukaryot Microbiol. 64, 88–96. doi: 10.1111/jeu.12342
Patel A., Bettiga M., Rova U., Christakopoulos P., Matsakas L. (2022). Microbial genetic engineering approach to replace shark livering for squalene. Trends Biotechnol. 40, 1261–1273. doi: 10.1016/j.tibtech.2022.03.008
Patel A., Rova U., Christakopoulos P., Matsakas L. (2020). Assessment of fatty acids profile and omega-3 polyunsaturated fatty acid production by the oleaginous marine thraustochytrid Aurantiochytrium sp. T66 cultivated on volatile fatty acids. Biomolecules 10, 694. doi: 10.3390/biom10050694
Paulo M. C., Marques J., Cardoso C., Coutinho J., Gomes R., Gomes-Bispo A., et al. (2020). The development of a novel functional food: bioactive lipids in yogurts enriched with Aurantiochytrium sp. biomass. Food Funct. 11, 9721–9728. doi: 10.1039/D0FO01884H
Phelps K. J., Drouillard J. S., O’Quinn T. G., Burnett D. D., Blackmon T. L., Axman J. E., et al. (2016). Feeding microalgae meal (All-G Rich (TM); Schizochytrium limacinum CCAP 4087/2) to beef heifers. I: Effects on longissimus lumborum steak color and palatibility. J. Anim. Sci. 94, 4016–4029. doi: 10.2527/jas.2016-0487
Polglase J. L. (1980). A preliminary report on the thraustochytrid(s) and labyrinthulid(s) associated with a pathological condition in the lesser octopus eledone cirrllOsa. Botanica Marina XXlIl, 699–706. doi: 10.1515/botm-1980-1106
Qi F., Zhang M., Chen Y., Jiang X., Lin J., Cao X., et al. (2017). A lignocellulosic hydrolysate-tolerant Aurantiochytrium sp. mutant strain for docosahexaenoic acid production. Bioresour. Technol. 227, 221–226. doi: 10.1016/j.biortech.2016.12.011
Qian H., Liu Q., Allam B., Collier J. L. (2007). Molecular genetic variation within and among isolates of QPX (Thraustochytridae), a parasite of the hard clam Mercenaria mercenaria. Dis. Aquat. Organ. 77, 159–168. doi: 10.3354/dao01848
Ragan M. A., MacCallum G. S., Murphy C. A., Cannone J. J., Gutell R. R., McGladdery S. E. (2000). Protistan parasite QPX of hard-shell clam Mercenaria mercenaria is a member of Labyrinthulomycota. Dis. Aquat Org. 42, 185–190. doi: 10.3354/dao042185
Raghukumar S. (1988). Schizochytrium mangrovei sp. nov., a thraustochytrid from mangroves in India. Trans. Br. Mycol. Soc 90, 627–631. doi: 10.1016/S0007-1536(88)80068-8
Rau E. M., Bartosova Z., Kristiansen K. A., Aasen I. M., Bruheim P., Ertesvåg H. (2022). Overexpression of two new acyl-coA : diacylglycerol acyltransferase 2-like acyl-coA : sterol acyltransferases enhanced squalene accumulation in aurantiochytrium limacinum. Front. Microbiol. 13. doi: 10.3389/fmicb.2022.822254
Rau E. M., Ertesvåg H. (2021). Method development progress in genetic engineering of thraustochytrids. Mar. Drugs 19, 515. doi: 10.3390/md19090515
Reboleira J., Félix R., Félix C., de Melo M. M. R., Silva C. M., Saraiva J. A., et al. (2021). Evaluating the potential of the defatted by-product of Aurantiochytrium sp. industrial cultivation as a functional food. Foods 10, 3058. doi: 10.3390/foods10123058
Ren L., Zhuang X., Chen S., Ji X., Huang H. (2015). Introduction of omega-3 desaturase obviously changed the fatty acid profile and sterol content of Schizochytrium sp. J. Agric. Food Chem. 63, 9770–9776. doi: 10.1021/acs.jafc.5b04238
Riley L. A., Guss A. M. (2021). Approaches to genetic tool development for rapid domestication of non−model microorganisms. Biotechnol. Biofuels. 14, 30. doi: 10.1186/s13068-020-01872-z
Rius M., Rest J. S., Filloramo G. V., Novák Vanclová A. M. G., Archibald J. M., Collier J. L., et al. (2023). Horizontal gene transfer and fusion spread carotenogenesis among diverse heterotrophic protists. Genome Biol. Evol. 15. doi: 10.1093/gbe/evad029
Russo G. L., Langellotti A. L., Verardo V., Martín-García B., Di Pierro P., Sorrentino A., et al. (2021). Formulation of new media from dairy and brewery wastes for a sustainable production of DHA-rich oil by Aurantiochytrium mangrovei. Mar. Drugs 20, 39. doi: 10.3390/md20010039
Ryu B. G., Kim K., Kim J., Han J. I., Yang J. W. (2013). Use of organic waste from the brewery industry for high-density cultivation of the docosahexaenoic acid-rich microalga, Aurantiochytrium sp. KRS101. Bioresour. Technol. 129, 351–359. doi: 10.1016/j.biortech.2012.11.049
Sakamoto T., Ikeda Y., Masuda N., Sakuradani E. (2023). Ethanol enhances astaxanthin production by Aurantiochytrium sp. O5-1-1. J. Oleo Sci. 72, 441–446. doi: 10.5650/jos.ess22308
Sasaki R., Toda S., Sakamoto T., Sakuradani E., Shigeto S. (2023). Simultaneous imaging and characterization of polyunsaturated fatty acids, carotenoids, and microcrystalline guanine in single Aurantiochytrium limacinum cells with linear and nonlinear Raman microspectroscopy. J. Phys. Chem. B. 127, 2708–2718. doi: 10.1021/acs.jpcb.3c00302
Sato N., Hamamoto K., Kurihara M., Abe M., Hashimoto S. (2018). Methyl halide production by cultures of marine thraustochytrids, Aurantiochytrium sp., Botryochytrium radiatum, and Schizochytrium sp. Mar. Chem. 208, 95–102. doi: 10.1016/j.marchem.2018.11.009
Sen B., Li J., Lu L., Bai M., He Y., Wang G. (2021). Elemental composition and cell mass quantification of cultured thraustochytrids unveil their large contribution to marine carbon pool. Mar. Drugs 19, 493. doi: 10.3390/md19090493
Sevgili H., Sezen S., Yılayaz A., Aktaş Özgür, Pak F., Aasen I. M., et al. (2019). Apparent nutrient and fatty acid digestibilities of microbial raw materials for rainbow trout (Oncorhynchus mykiss) with comparison to conventional ingredients. Algal Res. 42, 101592. doi: 10.1016/j.algal.2019.101592
Shakeri S., Amoozyan N., Fekrat F., Maleki M. (2017). Antigastric cancer bioactive Aurantiochytrium oil rich in docosahexaenoic acid: From media optimization to cancer cells cytotoxicity assessment. J. Food Sci. 82, 2706–2718. doi: 10.1111/1750-3841.13925
Shi S., Chang Y., Yu J., Chen H., Wang Q., Bi Y. (2023). Identification and functional analysis of two novel genes—geranylgeranyl pyrophosphate synthase gene (AlGGPPS) and isopentenyl pyrophosphate isomerase gene (AlIDI)—from Aurantiochytrium limacinum significantly enhance de novo β-carotene biosynthesis in Escherichia coli. Mar. Drugs 21, 249. doi: 10.3390/md21040249
Song X., Ma Z., Tan Y., Zhang H., Cui Q. (2017). Wastewater recycling technology for fermentation in polyunsaturated fatty acid production. Bioresour. Technol. 235, 79–86. doi: 10.1016/j.biortech.2017.03.034
Song Y., Zhu X., Wang B., Ibrar M., Hu Z., Li S., et al. (2022). Overexpression of the KAS III-like gene YxwZ3 increases carotenoids production in Aurantiochytrium sp. SZU445. Ind. Crops Prod. 187, 115435. doi: 10.1016/j.indcrop.2022.115435
Soudant P., Ventura M., Chauchat L., Guerreiro M., Mathieu-Resuge M., Le Grand F., et al. (2022). Evaluation of Aurantiochytrium mangrovei biomass grown on digestate as a sustainable feed ingredient of Sea Bass, Dicentrarchus labrax, Juveniles and Larvae. Sustainability 14, 14573. doi: 10.3390/su142114573
Souza F. P., Lima E. C. S., Urrea-Rojas A. M., Suphoronski S. A., Facimoto C. T., Bezerra Junior J. D. S., et al. (2020). Effects of dietary supplementation with a microalga (Schizochytrium sp.) on the hemato-immunological, and intestinal histological parameters and gut microbiota of Nile tilapia in net cages. PloS One 15, e0226977. doi: 10.1371/journal.pone.0226977
Sparrow F. K. (1936). Biological observations on the marine fungi of Woods Hole waters. Biol. Bull. 70, 236–263. doi: 10.2307/1537470
Sparrow F. K. (1969). Zoosporic marine fungi from the Pacific Northwest (U.S.A.). Arch. Mikrobiol. 66, 129–146. doi: 10.1007/BF00410220
Stokes N. A., Ragone Calvo L. M., Reece K. S., Burreson E. M. (2002). Molecular diagnostics, field validation, and phylogenetic analysis of Quahog Parasite Unknown (QPX), a pathogen of the hard clam Mercenaria mercenaria. Dis. Aquat. Organ. 52, 233–247. doi: 10.3354/dao052233
Suen Y. L., Tang H., Huang J., Chen F. (2014). Enhanced production of fatty acids and astaxanthin in Aurantiochytrium sp. by the expression of Vitreoscilla hemoglobin. J. Agric. Food Chem. 62, 12392–12398. doi: 10.1021/jf5048578
Sun X. M., Ren L. J., Ji X. J., Chen S. L., Guo D. S., Huang H. (2016). Adaptive evolution of Schizochytrium sp. by continuous high oxygen stimulations to enhance docosahexaenoic acid synthesis. Bioresour. Technol. 211, 374–381. doi: 10.1016/j.biortech.2016.03.093
Sun Y., Xiang Y., He M., Zhang X., Wang S., Guo W., et al. (2019). Evaluation of Lactococcus lactis HNL12 combined with Schizochytrium limacinum algal meal in diets for humpback grouper (Cromileptes altivelis). Fish Shellf. Immunol. 94, 880–888. doi: 10.1016/j.fsi.2019.09.059
Susan E. F., John N. K., Robert D. B., George M. (2002). Aquaculture-associated factors in QPX disease of hard clams: density and seed source. Aquaculture 208, 23–38. doi: 10.1016/S0044-8486(01)00795-5
Takahashi S., Sakamaki M., Ferdousi F., Yoshida M., Demura M., Watanabe M. M., et al. (2018). Ethanol extract of Aurantiochytrium mangrovei 18W-13a strain possesses anti-inflammatory effects on murine macrophage RAW264 cells. Front. Physiol. 9. doi: 10.3389/fphys.2018.01205
Takahashi S., Yoshida M., Watanabe M. M., Isoda H. (2019). Anti-inflammatory effects of Aurantiochytrium limacinum 4W-1b ethanol extract on murine macrophage RAW264 cells. Biomed. Res. Int. 2019, 3104057. doi: 10.1155/2019/3104057
Thuy N. T. T., Minh Hien H. T., Ha N. C., Thom L. T., Hong D. D., Thinh N. V., et al. (2023). Chemical constituents from the marine microalgae Thraustochytrium pachydermum. Nat. Prod. Commun. 18, 1934578X2311571. doi: 10.1177/1934578X231157145
Tocher D. R. (2015). Omega-3 long-chain polyunsaturated fatty acids and aquaculture in perspective. Aquaculture. 449, 94–907. doi: 10.1016/J.AQUACULTURE.2015.01.010
Torrecillas S., Mompel D., Caballero M. J., Montero D., Merrifield D., Rodiles A., et al. (2017). Effect of fishmeal and fish oil replacement by vegetable meals and oils on gut health of European sea bass (Dicentrarchus labrax). Aquaculture 468, 386–398. doi: 10.1016/j.aquaculture.2016.11.005
Truzzi C., Giorgini E., Annibaldi A., Antonucci M., Illuminati S., Scarponi G., et al. (2020). Fatty acids profile of black soldier fly (Hermetia illucens): Influence of feeding substrate based on coffee-waste silverskin enriched with microalgae. Anim. Feed Sci. Technol. 259, 114309. doi: 10.1016/j.anifeedsci.2019.114309
Turck D., Bohn T., Castenmiller J., De Henauw S., Hirsch-Ernst K. I., Maciuk A., et al. (2022). Safety of oil from Schizochytrium sp. (strain ATCC 20889) for use in infant and follow-on formula as a novel food pursuant to Regulation (EU). EFSA J. 20, 7083–7097. doi: 10.2903/j.efsa.2022.7083
Turck D., Bohn T., Castenmiller J., De Henauw S., Hirsch-Ernst K. I., Maciuk A., et al. (2023a). Safety of oil from Schizochytrium limacinum (strain TKD-1) for use in infant and follow-on formula as a novel food pursuant to Regulation (EU) 2015/2283. EFSA J. 21, e8414. doi: 10.2903/j.efsa.2023.8414
Turck D., Bohn T., Castenmiller J., De Henauw S., Hirsch-Ernst K. I., Maciuk A., et al. (2023b). Safety of oil from Schizochytrium sp. (strain CABIO-A-2) for use in infant and follow-on formula as a novel food pursuant to Regulation (EU) 2015/2283. EFSA J. 21, e8415. doi: 10.2903/j.efsa.2023.8415
Turck D., Castenmiller J., De Henauw S., Hirsch-Ernst K. I., Kearney J., Maciuk A., et al. (2020). Safety of Schizochytrium sp. oil as a novel food pursuant to Regulation (EU) 2015/2283(a). EFSA J. 18, 6242. doi: 10.2903/j.efsa.2020.6242
Turck D., Castenmiller J., De Henauw S., Hirsch-Ernst K. I., Kearney J., Maciuk A., et al. (2021a). Safety of oil from Schizochytrium limacinum (strain FCC-3204) for use in infant and follow-on formula as a novel food pursuant to Regulation (EU) 2015/2283. EFSA J. 19, 6344. doi: 10.2903/j.efsa.2021.6344
Turck D., Castenmiller J., De Henauw S., Hirsch-Ernst K. I., Kearney J., Maciuk A., et al. (2021b). Safety of oil from Schizochytrium limacinum (strain FCC-3204) for use in food supplements as a novel food pursuant to Regulation (EU) 2015/2283. EFSA J. 19, 6345. doi: 10.2903/j.efsa.2021.6345
Ueda M., Nomura Y., Doi K., Nakajima M., Honda D. (2015). Seasonal dynamics of culturable thraustochytrids (Labyrinthulomycetes, Stramenopiles) in estuarine and coastal waters. Aquat. Microb. Ecol. 74, 187–204. doi: 10.3354/ame01736
Villarroel Hipp M. P., Silva Rodriguez D. (2018). Bioremediation of piggery slaughterhouse wastewater using the marine protist, Thraustochytrium kinney VAL-B1. J Adv. Res. 12, 21–26. doi: 10.1016/j.jare.2018.01.010
Visudtiphole V., Khudet J., Chaitongsakul P., Plaisen S., Siriwattano J., Laiphrom S., et al. (2021). Growth and lipidomic analyses of Penaeus monodon larvae supplemented with Aurantiochytrium limacinum BCC52274. Front. Mar. Sci. 8. doi: 10.3389/fmars.2021.771929
Visudtiphole V., Phromson M., Tala S., Bunphimpapha P., Raweeratanapong T., Sittikankaew K., et al. (2018). Aurantiochytrium limacinum BCC52274 improves growth, hypo-salinity tolerance and swimming strength of Penaeus vannamei post larvae. Aquaculture 495, 849–857. doi: 10.1016/j.aquaculture.2018.06.066
Wang S., Wan W., Wang Z., Zhang H., Liu H., Arunakumara K. K. I. U., et al. (2021a). A two-stage Adaptive laboratory evolution strategy to enhance docosahexaenoic acid synthesis in oleaginous thraustochytrid. Front. Nutr. 8. doi: 10.3389/fnut.2021.795491
Wang Z., Wang S., Feng Y., Wan W., Zhang H., Bai X., et al. (2021b). Obtaining high-purity docosahexaenoic acid oil in thraustochytrid Aurantiochytrium through a combined metabolic engineering strategy. J. Agric. Food Chem. 69, 10215–10222. doi: 10.1021/acs.jafc.1c03781
Wang S. K., Wang X., Tian Y. T., Cui Y. H. (2020). Nutrient recovery from tofu whey wastewater for the economical production of docosahexaenoic acid by Schizochytrium sp. S31. Sci. Total Environ. 710, 136448. doi: 10.1016/j.scitotenv.2019.136448
Wang Q., Ye H., Xie Y., He Y., Sen B., Wang G. (2019). Culturable diversity and lipid production profile of Labyrinthulomycete protists isolated from coastal mangrove habitats of China. Mar. Drugs 17, 268. doi: 10.3390/md17050268
Watanabe K., Nishijima M., Mayuzumi S., Aki T. (2022). Utilization of sugarcane bagasse as a substrate for lipid production by Aurantiochytrium sp. J. Oleo Sci. 71, 1493–1500. doi: 10.5650/jos.ess22206
Wei M., Parrish C. C., Guerra N. I., Armenta R. E., Colombo S. M. (2021). Extracted microbial oil from a novel Schizochytrium sp. (T18) as a sustainable high DHA source for Atlantic salmon feed: Impacts on growth and tissue lipids. Aquaculture 534, 736249. doi: 10.1016/j.aquaculture.2020.736249
Willer D. F., Furse S., Aldridge D. C. (2020). Microencapsulated algal feeds as a sustainable replacement diet for broodstock in commercial bivalve aquaculture. Sci. Rep. 10, 12577. doi: 10.1038/s41598-020-69645-0
Xie N., Bai M., Liu L., Li J., He Y., Collier J. L., et al. (2022). Patchy blooms and multifarious ecotypes of Labyrinthulomycetes protists and their implication in vertical carbon export in the pelagic Eastern Indian Ocean. Microbiol. Spectr. 10. doi: 10.1128/spectrum.00144-22
Xie J., Fang J., Liao S., Guo T., Yin P., Liu Y., et al. (2019). Study on Schizochytrium sp. improving the growth performance and non-specific immunity of golden pompano (Trachinotus ovatus) while not affecting the antioxidant capacity. Fish Shellf. Immun. 95, 617–623. doi: 10.1016/j.fsi.2019.10.028
Xu J., Yao X., Lin Y., Chi S., Zhang S., Cao J., et al. (2022). Schizochytrium limacinum altered antioxidant capacity and transcriptome profiles in Pacific white shrimp fed a low-fishmeal diet. Aquac. Rep. 27, 101399. doi: 10.1016/j.aqrep.2022.101399
Yamasaki T., Aki T., Mori Y., Yamamoto T., Shinozaki M., Kawamoto S., et al. (2007). Nutritional enrichment of larval fish feed with thraustochytrid producing polyunsaturated fatty acids and xanthophylls. J. Biosci. Bioeng. 104, 200–206. doi: 10.1263/jbb.104.200
Yang H., Huang Y., Li Z., Guo Y., Li S., Huang H., et al. (2022a). Effects of dietary supplementation with Aurantiochytrium sp. on zebrafish growth as determined by transcriptomics. Animals 12, 2794. doi: 10.3390/ani12202794
Yang T. J., Juntila D. J., Fujihara N., Inada T., Yoneda K., Suzuki I. (2022b). Enhancement of squalene production by constitutive expression of the 3-hydroxy3-methylglutaryl-CoA reductase in Aurantiochytrium sp. 18W-13a. Mar. Biotechnol. (NY). 24, 733–743. doi: 10.1007/s10126-022-10139-7
Yilmaz H. A., Kumlu M., Evliyaoglu E., Praiboon J., Duman M. B., Ak Cimen B., et al. (2023). Effects of the alga Aurantiochytrium mangrovei FIKU008-enriched Artemia on early stages of the Green Tiger Shrimp, Penaeus semisulcatus. Turk. J. Fish. Aquat. Sci. 23. doi: 10.4194/1303-2712
Yokoyama R., Honda D. (2007). Taxonomic rearrangement of the genus Schizochytrium sensu lato based on morphology, chemotaxonomic characteristics, and 18S rRNA gene phylogeny (Thraustochytriaceae, Labyrinthulomycetes): emendation for Schizochytrium and erection of Aurantiochytrium and Oblongichytrium gen. nov. Mycoscience 48, 199–211. doi: 10.1007/S10267-006-0362-0
Yokoyama R., Salleh B., Honda D. (2007). Taxonomic rearrangement of the genus Ulkenia sensu lato based on morphology, chemotaxonomical characteristics, and 18S rRNA gene phylogeny (Thraustochytriaceae, Labyrinthulomycetes): emendation for Ulkenia and erection of Botryochytrium, Parietichytrium, and Sicyoidochytrium gen. nov. Mycoscience 48, 329–341. doi: 10.1007/S10267-007-0377-1
Yoshimi T., Hashimoto S., Kubo Y., Takeuchi M., Morimoto D., Nakagawa S., et al. (2023). Improvement of astaxanthin production in Aurantiochytrium limacinum by overexpression of the beta-carotene hydroxylase gene. Appl. Biochem. Biotechnol. 195, 1255–1267. doi: 10.1007/s12010-022-04172-4
Yu X. J., Sun J., Zheng J. Y., Sun Y. Q., Wang Z. (2016). Metabolomics analysis reveals 6-benzylaminopurine as a stimulator for improving lipid and DHA accumulation of Aurantiochytrium sp. J. Chem. Technol. Biotechnol. 91, 1199–1207. doi: 10.1002/jctb.4869
Yu X. J., Wang Z. P., Liang M. J., Wang Z., Liu X. Y., Hu L., et al. (2020). One-step utilization of inulin for docosahexaenoic acid (DHA) production by recombinant Aurantiochytrium sp. carrying Kluyveromyces marxianus inulinase. Bioprocess Biosyst. Eng. 43, 1801–1811. doi: 10.1007/s00449-020-02371-z
Zhang S., He Y., Sen B., Wang G. (2020). Reactive oxygen species and their applications toward enhanced lipid accumulation in oleaginous microorganisms. Bioresour. Technol. 307, 123234. doi: 10.1016/j.biortech.2020.123234
Keywords: thraustochytrid, protist, taxonomy, ω-3 polyunsaturated fatty acid, squalene, pharmacological activity, functional food
Citation: Wang Q, Zhang Y, Hui R and Zhu Y (2024) Marine thraustochytrid: exploration from taxonomic challenges to biotechnological applications. Front. Mar. Sci. 11:1371713. doi: 10.3389/fmars.2024.1371713
Received: 17 January 2024; Accepted: 12 March 2024;
Published: 22 March 2024.
Edited by:
Chiara Lauritano, Anton Dohrn Zoological Station Naples, ItalyReviewed by:
Xia Wan, Chinese Academy of Agricultural Sciences, ChinaJianhua Fan, East China University of Science and Technology, China
Copyright © 2024 Wang, Zhang, Hui and Zhu. This is an open-access article distributed under the terms of the Creative Commons Attribution License (CC BY). The use, distribution or reproduction in other forums is permitted, provided the original author(s) and the copyright owner(s) are credited and that the original publication in this journal is cited, in accordance with accepted academic practice. No use, distribution or reproduction is permitted which does not comply with these terms.
*Correspondence: Qiuzhen Wang, cXF6ejE5OTBAMTYzLmNvbQ==