- 1Ocean Biogeosciences, National Oceanography Centre, Southampton, United Kingdom
- 2Ocean and Earth Science, University of Southampton, Southampton, United Kingdom
- 3The Lyell Centre, Heriot-Watt University, Edinburgh, United Kingdom
- 4Norwegian Research Centre, Bergen, Norway
- 5Turneffe Atoll Sustainability Association, Belize City, Belize
- 6UK Centre for Ecology and Hydrology, Bangor, United Kingdom
- 7Emirates Nature - World Wide Fund for Nature, Abu Dhabi, United Arab Emirates
- 8Faculty of Science and Technology, University of Belize, Belmopan, Belize
- 9Coastal Zone Management Authority and Institute, Belize City, Belize
Introduction: Seagrass sediments are important ‘blue carbon’ reservoirs which store climatically significant quantities of organic carbon (Corg) at the global scale. Seagrass meadows that overly these sediments also provide a range of critical ecosystem services including shoreline stabilization, storm surge protection, and fisheries nursery grounds. However, the controls over accumulation and the sources of organic C to these sediments beds are highly variable and poorly understood with the relative importance of hydrodynamic setting, species composition and canopy density being unclear.
Methods: Here we address these questions using the first observation-based estimates of Corg stocks and provenance on Turneffe Atoll, Belize, made via remotely-sensed habitat extent, local Corg data and isotopic data. Sedimentary Corg was highest in sediments underlying the most sheltered meadows and decreased with increasing exposure to wind and wave energy with the seagrass meadows in the central lagoon containing an extensive deposit of mangrove derived organic carbon, stabilized and protected by the overlying seagrass meadow.
Results: The influence of species composition appeared weak with the ubiquitous species T. testudinum occurring across a wide range of hydrodynamic regimes ranging from the most sheltered to the most energetic and being associated with a wide range of sedimentary organic C concentrations. Importantly from the perspective of remote sensing, org C concentrations were unrelated to canopy density. We hypothesize that this decoupling of organic C concentration from seagrass canopy cover reflects a much longer timescale for carbon storage in the sediments than the lifespan of the seagrass plants themselves and/or a substantial non seagrass derived organic C burden in seagrass sediments. Overall, we conservatively estimate that the top 30cm of sediments underlying the seagrass meadows overlying carbonate sediments on the atoll exterior store 0.58 x 106 Mg Corg, most of which is seagrass-derived, whilst the sediments underlying the meadows within the central lagoon store an additional 1.28 x 106 Mg Corg. When the maximum possible extent of seagrass is considered, this estimate increases to 3.54 x 106 Mg Corg. Substantial Corg stocks extending >1m depth were observed across all sites, and so these inventories are considered conservative.
Discussion: A preliminary ‘cost of loss’ for sedimentary Corg in the top 30 cm of Turneffe Atoll’s seagrass meadows, based on a carbon trading value of €60 tCO2 (eq), is estimated at €42 million for the outer atoll, increasing to €136 million when the mangrove-derived sediments of the central atoll are considered and €260 million when turbid areas are assumed to contain seagrass.
1 Introduction
Since the industrial revolution, fossil fuel combustion and land use change have resulted in substantial emissions of carbon dioxide (CO2) to the atmosphere. Between 1850 and 2020, anthropogenic CO2 emissions totaled 2420 ± 240 Gt, equivalent to the amount of carbon stored in terrestrial ecosystems (2500 Gt; IPCC, 2023). Around 50% of this emitted CO2 remains in the atmosphere today, where it has been linked to increased radiative forcing, rapid climate change, an increase in global average temperatures, and a suite of associated ecological, social, and economic consequences (e.g. Huckelba and Van Lange, 2020 #15). In response, efforts to quantify and enhance natural C sequestration have increased, particularly at local scales where management and auditing can be straightforward, and where C sequestration objectives do not compete with critical land uses including agriculture and urban settlement (Freedman et al., 2009).
As pressure for space intensifies on land, interest in the carbon storage potential of marine environments has intensified (e.g. Nellemann and Corcoran, 2009; Mcleod et al., 2011; Macreadie et al., 2017; Lovelock and Duarte, 2019). A particular focus is on vegetated coastal ‘blue carbon’ ecosystems, which include mangrove forests, saltmarshes, and seagrass meadows where marine angiosperms can fix and store carbon more efficiently than many of their terrestrial counterpart ecosystems (Mcleod et al., 2011). These ecosystems also provide multiple ecosystem services, including storm surge protection, mitigation of sea level rise, fisheries nursery grounds, water clarity, and habitats for numerous endangered species (de los Santos et al., 2020), yet are among the most threatened ecosystems on the planet with around 50% of their historic extent having already been lost (Duarte et al., 2013).
Seagrass meadows are capable of sequestering carbon much faster per unit area than rainforests on mineral soil, and account for 10 - 18% of oceanic carbon burial, storing between 27 and 55 Tg organic carbon (Corg) yr-1 despite covering just 0.1% of the seafloor (Duarte et al., 2005; Mcleod et al., 2011; Fourqurean et al., 2012). Multiple factors affect Corg burial rates in seagrass ecosystems, including canopy cover, proximity to additional carbon sources such as forests and mangrove swamps, and hydrodynamic conditions (Mazarrasa et al., 2018). Generalized estimates suggest that around half of the organic material stored within seagrass meadows comes from the seagrass itself, whilst the rest comes from the surrounding environment, for example from adjacent mangrove forests (Kennedy et al., 2010). Different sources of organic material typically exhibit contrasting elemental stoichiometry that can reflect varying biogeochemical reactivities (e.g. varying organic C:N). Thus, proximity to alternative carbon sources can be an important determiner of sedimentary Corg storage and burial efficiency. Particle capture from overlying waters has been shown to be positively correlated with seagrass canopy density, and is also influenced by local hydrodynamic regimes whereby seagrass in lower energy environments tends to be more efficient at carbon sequestration and storage (Hendriks et al., 2008).
The importance of accurately quantifying and understanding the Corg stored within seagrass meadows, and within blue carbon habitats more widely, has come to the fore in recent years due to increasing interest in its exploitation via carbon finance (e.g. Friess et al., 2022) and payment for ecosystem services (PES) initiatives (e.g. Locatelli et al., 2014), and in its inclusion within Nationally Determined Contributions (NDCs; Dencer-Brown et al., 2022). Belize is one of the country’s leading the way towards integration of seagrass blue carbon into NDCs, evidenced by their COP26 pledge to develop and implementation a national seagrass policy which includes enhanced protection measures. To the best of our knowledge, however, no published data exists for carbon storage within the nation’s extensive seagrass meadows (229,146 ha; Grimm et al., 2023).
An estimated 16% of Belize’s seagrass exists within the shallow waters around the Turneffe Atoll Marine Reserve (Fedler, 2018), a marine protected area located approximately 32 km offshore of Belize. In this paper we combine recent, high resolution estimates of local seagrass extent (Carpenter et al., 2022) with site-specific Corg data to estimate seagrass Corg storage within the reserve. We use these data to explore how sedimentary Corg storage is influenced by (1) site energetics; (2) seagrass canopy cover; (3) Corg source and (4) the species composition of the seagrass assemblage.
2 Materials and methods
2.1 Site description
Turneffe Atoll is a 16 x 32 km reef structure that has developed on a north-south structural high, approximately 32 km off-shore of Belize, Central America (Figure 1). The Atoll resides atop a fault escarpment, which runs parallel to the coast on a complex underwater platform constructed of Pleistocene limestone, and formed as coral reef growth kept pace with gradual subsidence of the fault system and local sea level rise (McCloskey and Liu, 2013). The visible atoll mainly comprises mangrove cays, interspersed with extensive seagrass meadows. These meadows are dominated by turtle- (Thalassia testudinum), manatee- (Syringodium filiforme), and shoal- grass (Halodule wrightii). The close proximity of mangroves to seagrass meadows and the sheltered nature of the central lagoon means that the provenance of the organic matter across the system is likely to be highly variable, leading to the choice of geochemical and isotope methods detailed later. The atoll is a designated marine reserve and an ecological hotspot, with established breeding populations of endangered species including crocodile, manatee, and sea turtle. The cays are largely uninhabited, except for a few boutique dive resorts and a research station.
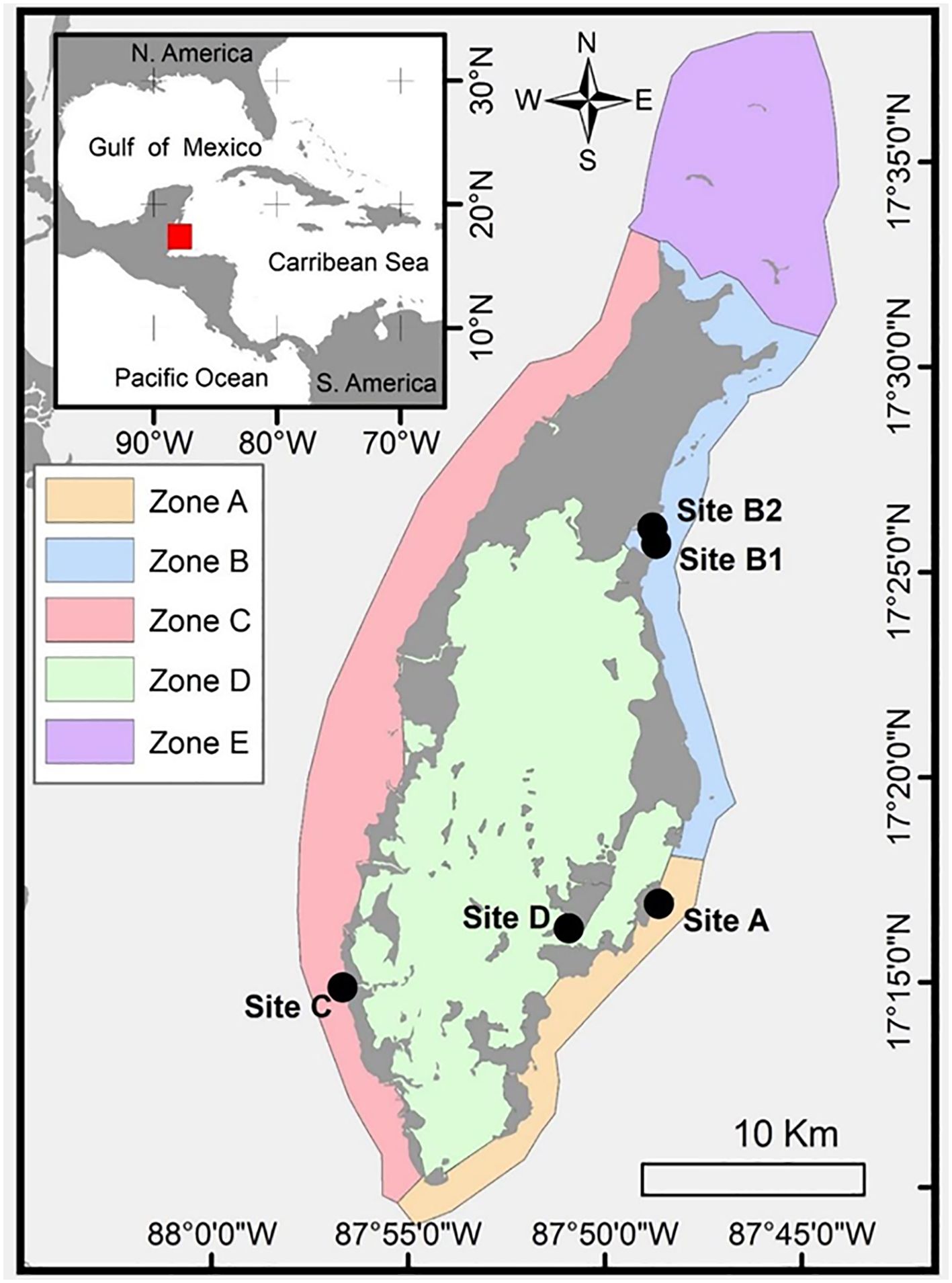
Figure 1. Map of Turneffe Atoll showing the location of the study area (inset) and the location of Zones A – E and Sites A – D). Grey regions denote the atoll land mass, which is almost entirely comprised of mangrove cayes.The location of Belize in the wider region is shown by the red square in the inset box.
2.2 Site selection
In January 2019, we undertook a week-long field study of seagrass meadows on Turneffe Atoll. In consultation with local ecosystem managers, we split the atoll into five zones according to aspect, capturing differences in exposure to the prevailing hydro-energetic regime (Figure 1).
Zones A and B are located on the seaward side of the atoll, which is regularly exposed to strong prevailing winds, wave action, and storm events. The dominant current flow comes from the south and moves anti-clockwise around the Atoll, meaning that Zone A is typically subject to greater hydrodynamic energy than Zone B. Zone C is located on the more sheltered landward side of the atoll where wind speeds and wave action tend to be lower and storm events less impactful. Zone D includes the Atolls central lagoon, which is sheltered on all fronts by mangrove forest and fringe reefs. Zone E lies to the north of the Atoll in open water, where relatively sparse seagrass is interspersed with extensive patch reefs of coral. It experiences minimal sheltering and is thought to be the most energetic region of the atoll.
We used local knowledge obtained from local fishermen, divers, ecosystem managers, and academics with knowledge of the area to select a sampling site within each Zone which was considered representative of typical hydrodynamic and sedimentary conditions. A second site was added within Zone B in order to check the potential importance of environmental vs. energetic setting, specifically proximity to mangrove forest.
- Site A was located in a mixed-species meadow containing both T. testudinum and S. filiforme in a sandy embayment off Calabash Caye.
- Sites B1 and B2 were located within a T. testudinum meadow in Northern Bogue Inlet, which connects the northern area of the Central Lagoon to the eastern (seaward) margin of the atoll. These sites are< 200 m apart, but Site B1 bordered a sand cay and Site B2 bordered a mangrove cay.
- Site C was in a H. wrightii meadow located next to another inlet known as Jones’ Hole, which connects the Central Lagoon to the western (leeward) margin of the atoll. This site is relatively sheltered, and prevailing currents supply a steady input of material from the lagoon.
- Site D was in a T. testudinum meadow located in a protected, relatively sheltered embayment within the Central Lagoon, to the south of Shag Cay.
- Site A was also used as representative of Zone E, which we were unable to sample due to inclement weather.
2.3 Sample collection
Sampling took place between 22nd and 27th January 2019. At each site, we placed nine 50 cm x 50 cm quadrats at random within a 50 x 50 m survey zone, except at Site C where we only placed four quadrats prior to our survey being halted for health and safety reasons (the arrival of an American crocodile in the adjacent mangrove forest), and where n = 4. For each quadrat, we recorded water depth, seagrass species, and a visual estimate of seagrass canopy cover (%). Underwater photographs were taken of the quadrat from above, and used to refine and confirm our canopy cover estimates, with the final value being the mean of seven independent estimates made by researchers with field experience. Such methods (e.g. https://naturalhistory.si.edu/sites/default/files/media/file/seagrass-monitoring-activity.pdf) are commonly used in similar field surveys.
To quantify sedimentary organic carbon content and its origin, we took a short sediment core from a randomized location within each quadrat by inserting an open-barrel PVC pipe (40 cm length, 6 cm diameter) to a depth of ~ 30 cm. A rubber stopper was inserted into the top of the pipe to provide suction as the cores were withdrawn, keeping the sediment in place and maintaining structural integrity. A second stopper was used to seal the pipe at the bottom for transport to shore, where the cores were extruded using a home-made extrusion tool which comprised a rubber stopper attached to a metal pole which was inserted into the top of the core and used to manually push the core out of the bottom end, onto a PVC sheet.2 cm thick sections were cut from the top (0-2 cm), middle (~14-16 cm), and bottom (~26-28 cm) of the cores using a sharp, clean blade. Living plant material (above and below ground biomass) was manually removed before these sections were bagged and frozen. The effects of species composition on these pools was unfortunately beyond the scope of this study, although clearly important, and will form the basis of a subsequent publication. Compaction during sampling was determined by measuring core depth, length of core within the core tube, and length of the extruded core, and was minimal (< 5%).
To obtain insight into longer-term Corg storage and historic site conditions, we also collected ‘long’ cores from locations within each site: two from Sites A, B1 and C and three from Sites B2 and D. The locations from which these longer cores were collected was determined upon visual inspection of the shorter cores collected at that site, with positions selected to be as representative as possible of typical site conditions. A larger open-barrel PVC pipe (1.5 m length, 25 cm diameter) which had been sharpened at the bottom end was manually inserted as far into the sediment as possible, and rubber drain stoppers were inserted at the top to provide suction during removal. On shore, the sediments were extruded using a larger version of the home-made extrusion device described above. For these larger cores, the extruder was pushed into the core tube using a mallet, and the extruded core was caught in a halved core tube. These larger cores were split horizontally, photographed, and a detailed core log was taken. The core log included a photo of the core and a written description of the sediment type, any visible features, and depths at which these features occurred. We then cut 2 cm thick sediment sections every 10 cm along the core length, again using a clean, sharp blade. Again, living plant material was removed before bagging and freezing the samples. Compaction during coring was more substantial in these longer cores (mean = 18%, range = -8% to 39%), and was corrected for using standard methods (Howard et al., 2014).
One additional long core was collected from each site for archiving and analysis of grain size, sediment characterization, and dry bulk density (DBD). At Site D we encountered two contrasting sedimentary environments (silty clay vs. organic rich), and so we collected archive cores representative of both.
2.4 Measurement and analysis of Corg and δ13Corg
Each bagged sample was homogenized and an approximately 10 g sub-sample oven dried at 60°C to a constant weight (within 5%; 24 hours for Sites A – C; 48 hrs for Site D). Carbon content and stable isotope ratios were determined using a Flash 2000 Elemental Analyzer (EA) coupled to a Delta V Advantage Isotope ratio mass spectrometer (IRMS) attached to a ConFlo IV Continuous Flow interface (Both Thermo Fisher Scientific). After first measuring total carbon (Ctot), the inorganic carbon (Cinorg) fraction was removed from a duplicate sub-sample using 3 M hydrochloric acid. The remaining organic carbon (Corg) and its isotopic composition (δ13Corg) were then analyzed, and Cinorg calculated by subtracting Corg from Ctot. The isotopic ratio of Cinorg was then calculated from the contents and isotopic ratio of the two carbon fractions δ13Corg data are reported in the conventional delta notation relative to a Vienna Pee Dee Belemnite (V-PDB) standard. Analytical precision based on standard reproducibility for Corg and Ctot was 0.34 wt. %, and for δ13Corg it was ±0.12 ‰. The final tally of cores analyzed for Corg and δ13Corg was 11 from Site A, 10 from Site B1, 12 from Site B2, 6 from Site C, and 12 from Site D.
Seagrass and mangrove Corg contributions were estimated using a two-endmember stable isotope model (Equations 1, 2). As no local seagrass and mangrove data were available, we used literature values of -12.8 ‰ for seagrass Corg (Lin et al., 1991) and -29.5 ‰ for mangrove Corg (Ray et al., 2018) as model endmembers.
2.5 Dry bulk density, grain size, and sediment characterization
Previous work has demonstrated that the highly porous nature of carbonate biogenic sediments typically results in DBDs which are consistent both between and within cores (Miyajima et al., 2015). DBD was therefore measured for three sub-samples taken within the top 30 cm of each archive core at 10 cm intervals. Samples were collected with 50 mL cut-off plastic syringe, weighed before and after collection of the sediment. The volume of the sample in the syringe was recorded, and the syringe was placed in an oven at 60°C for 24 hours. After a further 24 hrs in the oven, the syringes were reweighed once more to check their contents were completely dry (taken as a difference of less than 5% mass between 24 and 48 hrs). Any not completely dry were returned to the oven and weighed every 24 hrs until dry. DBD was calculated from the dry sample weight divided by the volume of the sub-sample. The mean within-core standard deviation (SD) was 15%. We also checked the method uncertainty by measuring DBD at each depth in triplicate for one site (B2) and found a SD of 6%.
Analyses of grain size distribution was undertaken on each archive core at 1 cm depth intervals using a Malvern Mastesizer 2000 laser microgranulometer. In addition, grain composition was manually described for each archive core using a binocular microscope. To ensure archive cores were representative, further grain size and composition analysis was undertaken at 10 cm intervals on a selection field-cut core samples, with no notable difference observed. As such, only archive core data are presented for these parameters.
2.6 Carbon stock calculations
Seagrass extent (ha) and canopy cover (%) across Turneffe Atoll and within each of the energetic zones mapped in Figure 1 were estimated using drone and satellite mapping according to the methods and results set out in Price et al. (2022) and Carpenter et al. (2022), respectively.
The Intergovernmental Panel on Climate Change have set out a tiered approach for blue carbon quantification at national scale (IPCC, 2014) which can also be applied at regional and site level, allowing local ecosystem managers to identify key carbon storage regions and conservation priorities outside of national-scale initiatives. Tier 1 estimates are the most basic, obtained by applying habitat-specific global averages for carbon storage to the extent of a given habitat within national borders. These estimates carry a high level of uncertainty but can be a useful starting point. Tier 2 estimates reduce uncertainty by using country-specific carbon storage data. Tier 3 estimates require monitoring at sufficient temporal resolution and at a diversity of locations within a country, but can be prohibitively costly, particularly in parts of the world where science funding and/or capacity are limited. The seagrass sedimentary Corg stock of Turneffe Atoll was calculated at atoll scale using globally averaged (Tier 1) and locally derived (Tier 2) Corg storage values.
2.6.1 Tier 1 estimate
We multiplied total seagrass extent by the global mean (108 Mg Corg ha-1) and maximum (829 Mg Corg ha-1) Corg storage values provided by the Blue Carbon Initiative (Howard et al., 2014). These values are for the top 1 m of sediment. We also scaled the resultant Corg stock to the top 30 cm of sediment by assuming a constant relationship with depth. This was done to standardize Tier 1 and Tier 2 estimates, with 30 cm chosen based on the depth at which most field data had been obtained.
2.6.2 Tier 2 estimate
We averaged Corg (% wt) and DBD across the top 30 cm of each core and multiplied the results to calculate a value for Corg (g cm-3). These values were scaled to the top 30 cm of sediment (g cm-2) and converted to Mg Corg ha-1, which is the standard unit typically used in blue carbon assessments. Mean Corg (in Mg Corg ha-1) at each site was multiplied by the associated zone’s individual seagrass extent to give an estimate of Corg storage within that zone. As no Corg data were available within Zone E, we used data from the zone with the closest energetics (Zone A). We then summed the estimates for each zone (A – E) to give an estimate for atoll-wide Corg storage.
2.7 Statistical method
All data investigation, plotting, and statistical analysis was conducted in R (v. 4.2.0; R Core Team, 2020). Statistical significance was investigated using Analysis of Variance (ANOVA) and Tukey HSD tests, using an α = 0.05.
3 Results
3.1 Seagrass extent and canopy cover
Total seagrass extent on Turneffe Atoll was estimated as 26,700 ha, split into zones as per Table 1 and visualized in Figure 2.
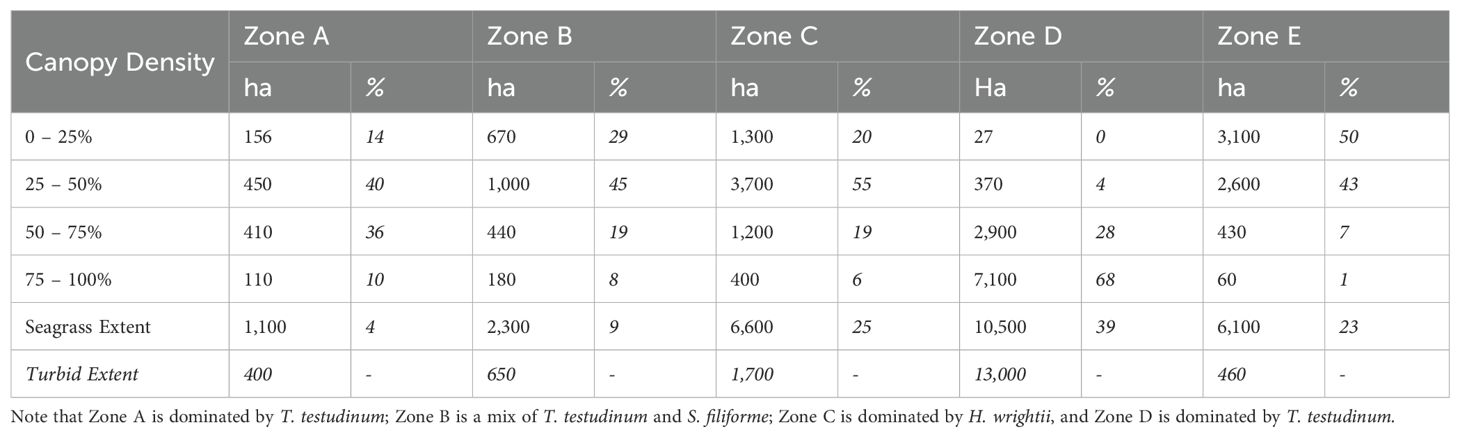
Table 1. Mapped seagrass extent within each zone, split according to modeled seagrass canopy cover (2 significant figures).
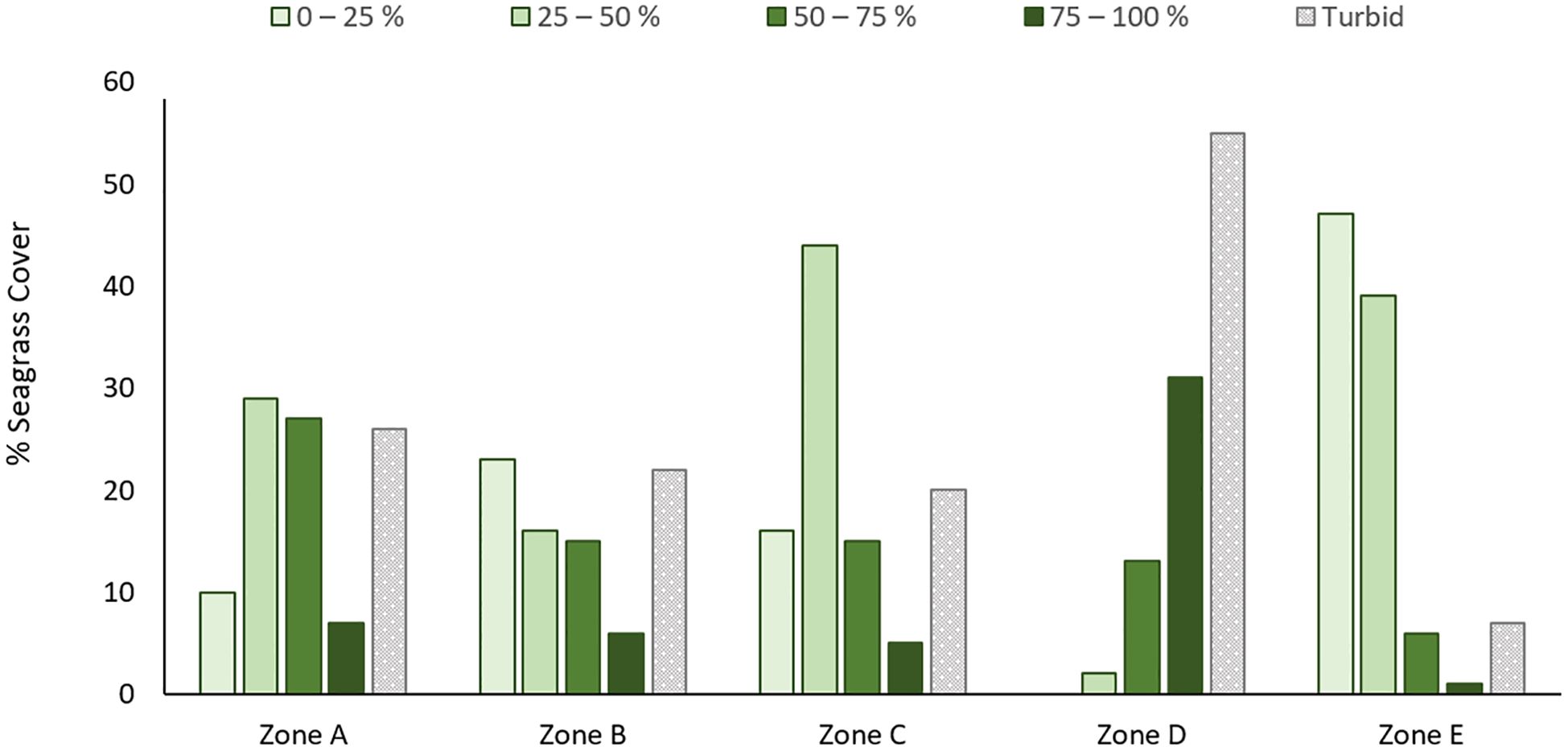
Figure 2. Bar chart showing the distribution of % seagrass cover within Zones A – E. Turbid regions (where seagrass cover could not be adequately assessed via drone) are shown as dotted grey bars. These areas are assumed to be high density seagrass, based on manual ground truthing visits.
Most seagrass was found within the central lagoon (Zone D; 39%), where 96% seagrass was found to be high (> 50%) density, with 68% found in the highest (> 75%) density band. At the other extreme, 93% of seagrass found in the northern atoll (Zone E; 23%) grew at low (< 50%) densities, split fairly evenly between the lowest two density bands (0 – 25% = 50%; 25 – 50% = 43%). Most seagrass in the near-shore (Zone C; 25%) was found in the mid-range (25 – 50% = 55%) and its shoulder bands (0 – 25% = 20%; 50 – 75% = 19%), with only 6% in the highest density band. This pattern was echoed on the seaward side of the atoll within Zones A (4%) and B (9%).
Over half of the Central Lagoon’s seagrass cover was partially-obscured by high turbidity (55%), making it difficult to determine % canopy cover remotely. We investigated these high turbidity regions by boat and found that the turbid zones contained high density seagrass cover. Based on these field observations, we estimate that the true extent of Zone D seagrass is closer to 23,500 ha, 86% of which is growing at the highest density (75 – 100%). We did not have time to investigate the turbid regions within other zones, and so cannot infer seagrass presence or density for them. However, our extent estimates for Zones A, B, C, and E could be underestimated by up to 22, 27, 20, and 7% if all turbid cells contained seagrass. If all turbid areas contained seagrass, the actual habitat extent would be 42,500 ha.
3.2 Sediment characterization
Sites on the atoll exterior (Sites A – C) were dominated by calcareous bioclastic material. Where visible macerals of mangrove peat were observed (i.e., darker, finer deposits, partly with mangrove roots still visible, Figure 2 lower panel), these represented ~ 5% of recovered material at Sites A and C, and< 2% of recovered material at Sites B1 and B2. In contrast, sediments in the Central Lagoon (Side D) contained a majority (< 60%) of materials which were mangrove derived. We encountered a secondary sedimentary environment within Site D dominated by calcareous materials, with mangrove material representing< 20%. This was only observed in one core next to a small creek, suggesting localized deposition. Typical examples of cores obtained from the outer and inner atoll respectively are shown in Figure 3.
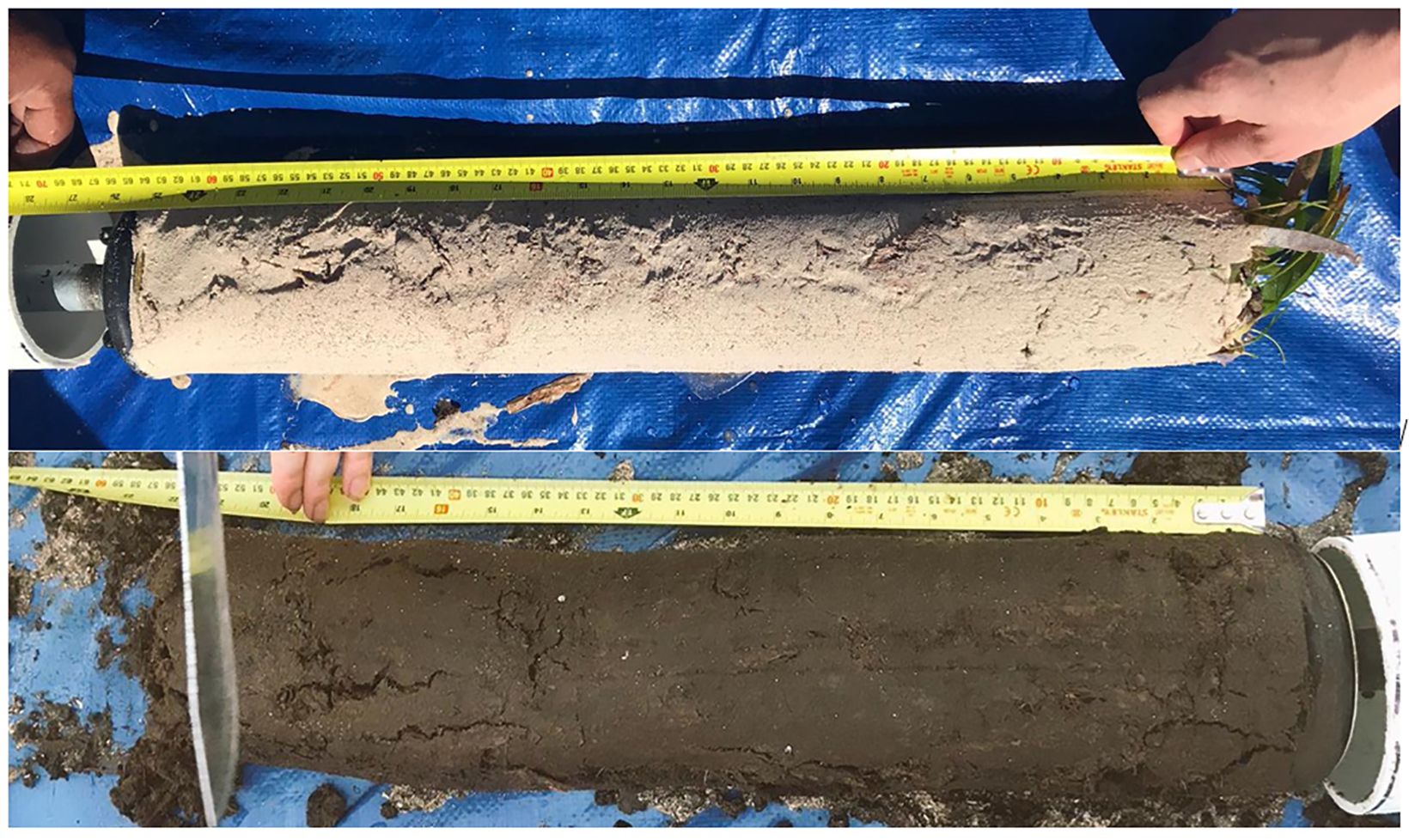
Figure 3. Typical cores from the atoll exterior (in this case Site A, upper panel) and interior (Site D, lower panel).
Sediments at Site A were dominated by coarse sands, with an average grain size of > 430 µm and no grains< 80 µm. Sediments at B1 and B2 were generally less coarse than those found at Site A, with average grain sizes 280 µm and 320 µm, respectively, and bimodal distributions suggesting rapid deposition followed by more careful sorting. At Site C, sediments were finer still with an average grain size of 155 µm. At Site D, where mangrove peat dominated, any calcareous sands observed were composed of crushed bioclasts, with an average grain size of 270 µm (Figure 4). These data support our initial classification, based on location and current direction, that Site A is the most energetic site, followed by Sites B1 and B2 (with B2 experiencing more rapid deposition than B1, owing to its slightly more sheltered location), Site C and then Site D.
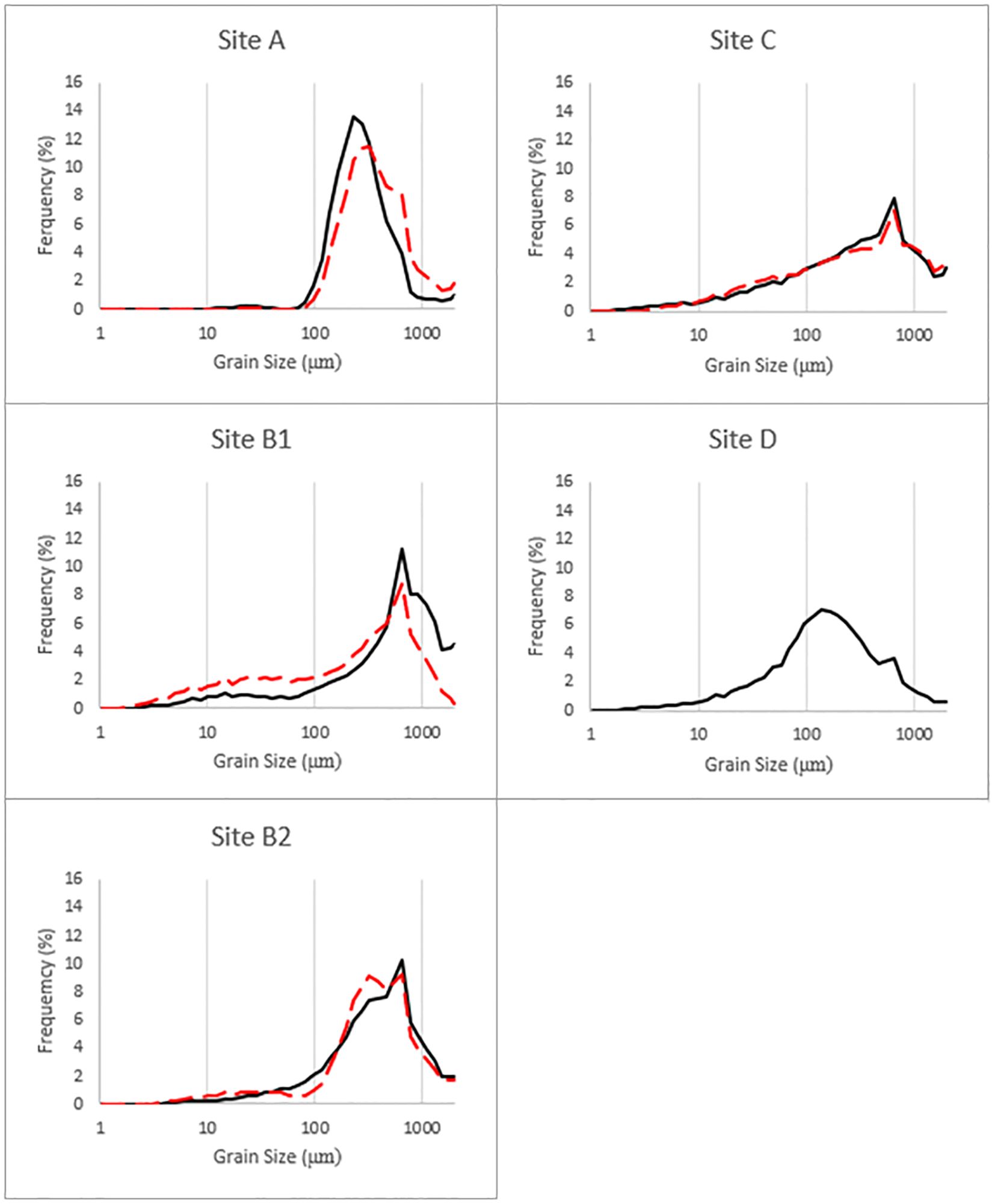
Figure 4. Grain size distribution sediments at Sites A–D. Data presented have been averaged across the top 30 cm of each core. Duplicate cores were measured at each site (shown as solid vs. dashed lines), excepting Site D where only one core was measured.
3.3 Dry bulk density, Corg and δ13Corg
On the outer atoll (Sites A – C), Corg content ranged from 0.04% to 4.72%, DBD ranged from 7.78 mg cm-3 to 42.38 mg cm-3, and δ13Corg ranged from -28.54 ‰ to -9.26 ‰. Within the Central Lagoon (Site D), Corg values ranged from 3.7% to 37.85%, DBD ranged from 12.01 mg cm-3 to 225 mg cm-3, and δ13Corg values ranged from -27.4 ‰ to -15.20 ‰. Corg stored in the top 30 cm ranged from 0.35 g cm-2 to 59.96 g cm-2 on the outer atoll, and from 16.5 g cm-2 to 2570g cm-2 within the Central Lagoon (Figure 5A).
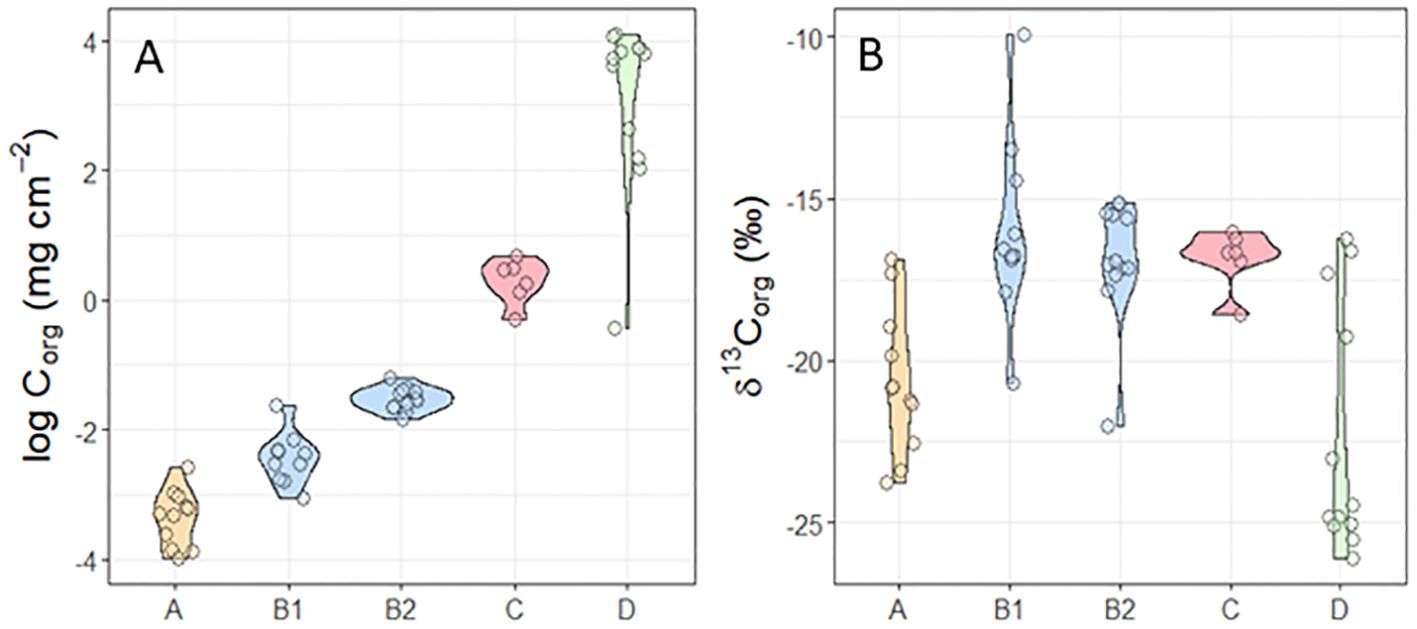
Figure 5. Violin plots of (A) Corg concentrations in the top 30cm (mg cm-2) and (B) mean δ13Corg signatures (‰) at Sites A–D. Corg is displayed as a natural log to show vastly different values on one plot.
According to our two-endmember Corg source model, the mean contribution of seagrass-derived material to the sedimentary Corg stock was 49% Site A, 80% at Site B1, 72% at Site B2, 72% at Site C, and 39% at Site D (Figure 5B).
3.4 Corg storage: physical setting, canopy cover and species composition
Figure 6 shows that there is no relationship between above ground canopy cover obtained from field observations and below ground Corg, whether as content (% wt), concentration (mg cm-3), or stock (mg cm-2) at site or atoll scale. Individual sites had rather similar organic C concentrations regardless of percentage cover with variability in physical setting appearing to drive most of the variation in organic C concentration - Figure 6 shows clearly that the high energy site a has the lowest variation organic C concentrations and the sheltered site d has the highest. The influence of species composition appeared weak with the ubiquitous species T. Testudinum being present across the atoll across the whole range of hydrodynamic conditions and organic C concentrations, leading to a very wide range of organic C concentrations associated with this keystone species (57 +/- 58.83 Mg Org C Ha-1).
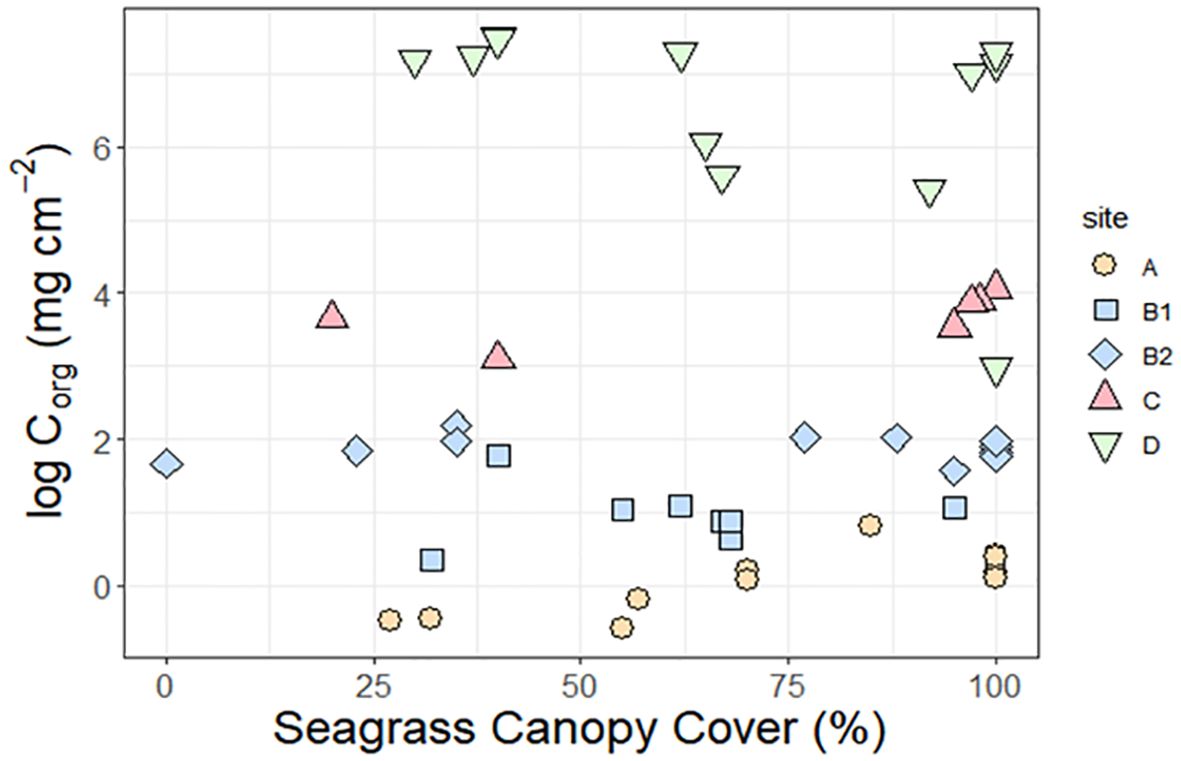
Figure 6. Corg concentrations in the top 30 cm of Turneffe atoll sediments as a function of percent seagrass canopy cover, with data split according to site. Corg is presented as a natural log in order to show all sites side by side. Each data point represents a separate sample.
3.5 Corg stock assessment
Applying the recommended Tier 1 average value of 108 Mg ha-1 to our derived seagrass extent produced an estimated seagrass Corg stock of 0.86 x 106 Mg Corg in the top 30 cm of the seagrass sediments (Table 2), which would be equivalent to 3.17 Mt CO2 (eq) if released. Applying the minimum (10 Mg ha-1) and maximum (829 Mg ha-1) values for Tier 1 assessment produced a range of between 0.08 x 106 and 5.10 x 106 Mg Corg, with an associated potential CO2 release of between 0.29 and 24.3 Mt CO2 (eq). Tier 2 assessment using site specific values produced an estimated stock of 1.86 x 106 Mg Corg, equivalent to 6.82 Mt CO2 (eq), which is more than double that obtained using the global average value, but towards the bottom of the full range.
Most of this estimate was associated with Zone D (69%) where most Corg appeared to be mangrove rather than seagrass derived (Figure 5B), and so we repeated the assessment for the outer atoll only. This produced a much smaller estimated stock of 0.58 x 106 Mg or 2.14 Mt CO2 (eq). Repeating the Tier 1 assessment for the same area produced an estimate of 0.52 x 106 Mg (range = 0.05 x 106 – 4.03 x 106 Mg) with a GHG potential of 1.93 Mt CO2 (eq) (range = 0.18 – 14.79 CO2 (eq)). Thus, when focusing on seagrass-dominated sediments, our Tier 2 estimate is broadly similar to the Tier 1 estimate achieved using the global average value.
4 Discussion
4.1 Seagrass Corg on Turneffe Atoll
To the best of our knowledge, this is the first study to quantify Corg storage within Belize’s seagrass meadows using local data, and therefore represents an important step forward for both national and global blue carbon datasets.
4.2 Comparison with previous estimate
Fedler (2018) previously reached a Tier 1 estimate of 5.52 x 106 Mg Corg, using a literature derived Corg value of 150.9 Mg ha-1. This was for the top 1m of sediment, however, which we can scale to our working depth of 30 cm to get a comparable estimate of 1.67 x 106 Mg Corg (relative to our Tier 2 estimate of 1.86 x 106 Mg Corg). Fedler’s estimate was obtained using a greater habitat extent (36,643 ha vs. 26,700 ha), presumably because they included areas we identified as highly turbid. Thus, it seems highly likely that areas of turbidity in our habitat mapping did indeed overly seagrass beds. This would render ours an underestimate and suggest that Turneffe Atoll contains more Corg within its seagrass sediments than previously thought.
To investigate the effect of habitat extent, we repeated our assessment under the assumption that all turbid areas contain seagrass (as was true in Zone D). The results of this re-assessment are shown in Table 3.
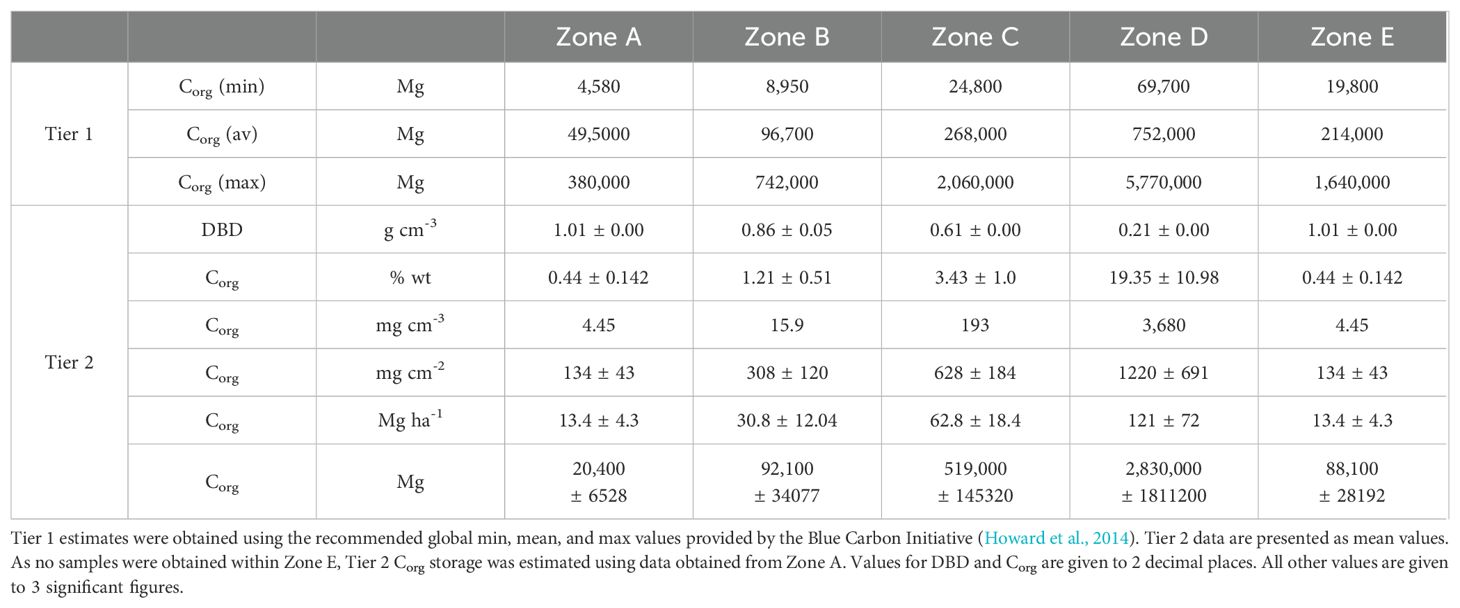
Table 3. Estimated Corg stored within the top 30 cm of sediment within each zone, in a scenario where all turbid areas contain seagrass.
This gives Tier 1 estimates ranging from 0.13 x 106 Mg Corg to 10.59 x 106 Mg Corg and a Tier 2 value of 3.54 x 106 Mg Corg. This represents our maximum estimate for Corg within Turneffe Atoll’s seagrass meadows and is more than double Fedler’s literature based 1.67 x 106 Mg Corg.
We can also compare the data found here to other systems (Table 4). The results suggest that the storage values given here are broadly comparable to those found elsewhere in tropical environments at a similar latitude. Clearly physical setting is important, as shown here, and it is likely that some of this variability reflects the wide range of hydrodynamic conditions sampled.
4.3 Validity of the zoning approach
Seagrass meadows are highly heterogenous environments, and Corg storage is controlled by a number of environmental conditions including canopy cover, proximity to additional carbon sources such as forests and mangrove swamps, and hydrodynamic conditions (Mazarrasa et al., 2018). The net effect of which can be difficult to predict. Turneffe Atoll exists in a data poor region, meaning that our sampling scheme was largely built around local knowledge. This approach suggested that site energetics were the overwhelming environmental factor at play. The data presented in this paper (seagrass density and distribution, sediment characteristics, Corg storage, and δ13Corg) collectively suggest that each site represents a different sedimentary environment, and that those environments reflect the foundational local knowledge and initial zone assignment used to guide their selection. Thus, while more spatial resolution would undoubtedly be desirable, we believe our zoning approach to be a reasonable first step towards a comprehensive Tier 2 assessment of Turneffe Atoll’s seagrass Corg carbon stores.
4.4 The exterior Atoll vs. the Central Lagoon
Sedimentary Corg storage on Turneffe Atoll is dominated by Zone D, however this was due to the accumulation of an extremely organic rich layer of mangrove peat rather which we do not associate with seagrass presence per se. Indeed, we observed a clear stratigraphy within the ‘large’ cores taken at Site D which suggests these sediments are related to the presence of mangroves, which have since been removed, rather than accrued by seagrass particle capture. Thus, while the inner lagoon encapsulated within Zone D undoubtedly represents a substantial carbon store, its existence cannot be attributed directly to the presence of overlying seagrass meadows and its inclusion in any valuation of seagrass blue carbon would likely be inappropriate. A follow-up study to investigate the sedimentary environment within Zone D (unpublished) confirmed that this peat-rich sedimentary environment is wide-spread within the Central Lagoon. This lends confidence to our relatively large estimate of Corg storage within this zone.
4.5 Isotope mixing model
Our two-endmember mixing model could be improved by obtaining endmember values from local vegetation rather than from the literature. Nonetheless, whilst the absolute values for mangrove and seagrass Corg contributions are not absolute, the substantial differences predicted by the model can be considered to reflect reality. Field observations and laboratory sediment type classification lends confidence to this. Converting isotopically derived % contributions to Corg quantities provides would provide additional insight into e.g. the amount of mangrove-derived Corg being captured within seagrass sediments.
Sites B1 and B2 were selected to investigate how proximity to mangrove influenced Corg storage in seagrass sediments, being located in similar energetic settings but next to a sandy beach and a mangrove forest, respectively. These had statistically indistinguishable proportions of mangrove-derived material (Figure 5B) and mean Corg content (Figure 5A) implying the this difference in setting was rather unimportant.
Despite Site A being a highly exposed, seagrass dominated site with minimal carbon storage, the site appears to be strongly influenced by mangrove-derived carbon (Figure 5B). An alternative interpretation is that there is an additional carbon source not accounted for in our model which has a similarly low 13C value to mangrove carbon, for instance a strong terrestrial signal. Our model could therefore be improved by including other Corg sources, such as algal and epiphytic production.
4.6 Relationship between Corg and canopy cover
We did not find any relationship between above ground canopy density and below ground Corg storage, in disagreement with the documented relationship between canopy cover and particle capture (Mazarrasa et al., 2018). At Zone D, it is likely that the extremely high carbon content of the relic peat on top of which seagrass is growing would overwhelm any contemporary relationship between canopy cover and carbon storage, but we would expect to see any relationship between these two parameters in Zone A – C if such a relationship exists on the atoll. It is possible that the highly exposed nature of the atoll means that even the lowest energetic regime studied was subject to sufficiently high energy as to overwhelm the expected relationship. It is also possible that this relationship is unreliable (Ricart et al., 2020). This demonstrates the need for more data collection across a range of diverse environments before assigning universal relationships to these complex, dynamic environments.
4.7 Valuing Turneffe Atoll’s seagrass Corg
A preliminary ‘cost of loss’ for sedimentary Corg in the top 30 cm of Turneffe Atoll’s seagrass meadows, based on an approximate carbon trading value of €60 tCO2 (eq), is estimated at €42 million for the outer atoll. This valuation increases to €136 million when the mangrove-derived sediments of the central atoll are considered, and €260 million when turbid areas are assumed to contain seagrass.
As noted above, we observed a high degree of turbidity at Site D which was mirrored across much of the associated Zone D (Table 1). This may indicate erosion of these mangrove-derived sediments and their associated Corg, the cost of the which could already be substantial. At the same time, any recapture of this sediment by seagrass beds within Zone D or, indeed, on the outer Atoll, may be important for limiting the overall rate of carbon loss. Future work is ongoing to investigate the causes and magnitude of this apparent loss of mangrove-derived carbon.
It is important to note that this approximate valuation does not consider restoration value, the efficacy of which has recently been called into question (Williamson and Gattuso, 2022). Instead, we specifically focus on the value of the existing seagrass carbon store. Previous work has demonstrated that loss of existing seagrass meadows leads to erosion of the underlying sediment carbon stock, producing a net source of CO2 to the atmosphere (e.g. Greiner et al., 2013), thus reinforcing the merit of conservation efforts where seagrass meadows are known to overlie substantial Corg stores. The value expressed in this study therefore represents the cost of seagrass degradation and subsequent sediment disturbance, rather than any additive benefit. This value also assumes that the entire top 30 cm of carbon is vulnerable to loss, and does not take into account differences in lability. There have been in the past significant hurricane events in Belize and the vulnerability of seagrass carbon stocks to these are unknown but potentially significant. One consequence of such a loss could be the exposure and erosion of the carbonate coral reef underlying the organic deposits. The probability of this occurring is hard to judge but it is clearly a hypothesis worthy of further study.
4.8 Limitations
As with all such studies, the dynamic and heterogeneous nature of seagrass habitats means that more sites will always be desirable. In this study, we took a pragmatic approach to maximize the usefulness of the available data but accept that sampling across a wider range of sites would help to build confidence in our zone assignments and refine our overall Corg storage estimate.
Sampling in this study focused on relatively shallow waters which could be easily accessed. Studies have shown that seagrass Corg capture can vary with water depth, but the direction of change depends upon many factors and so is difficult to predict (Mazarrasa et al., 2018). For example, seagrass in deeper waters can be sheltered from surface storms (increasing storage) but receive less light (decreasing production and hence storage). Future work would benefit from including deeper water sampling to assess this variability across the atoll.
We were unable to obtain suitable blank samples as part of this study (i.e., sediment cores from an area without seagrass influence). This was because seagrass coverage on Turneffe Atoll is extensive, and we could not find a suitable, accessible location in which to core. However, the fact that our Corg values are physically and environmentally sensible (i.e., Corg stocks increase as site energetics decrease) suggest that our overall findings have not been substantially altered by this.
This study does not account for C stored in above and below ground seagrass biomass, which can be significant. We did not measure biomass Corg, but can estimate it here using data presented in Fourqurean et al. (2012) for the Tropical Western Atlantic. That study presents 44 data points from the region, the mean of which is 0.85 Mg ha-1. Scaling this estimate to the habitat extent used in our study (including turbid regions) adds approximately 39 x 103 Mg C to our estimate of seagrass blue carbon, compared to estimates in the x106 for sedimentary Corg. This suggests that seagrass biomass stores are minimal, relative to our estimate of sedimentary Corg storage, but future work could aim to aim to confirm this.
We could not sample Zone E due to inclement weather. As a result, our estimate of Corg storage in this zone is speculative. The sparser seagrass coverage observed in Zone E coupled with local knowledge of smaller seagrass species and more open, higher energy waters is likely to result in limited Corg accrual. This will, in turn, limit Corg storage. Corg stocks in Zone E may be better approximated using a value closer to the Tier 1 minimum value, producing a stock estimate of 19,800 Mg Corg as opposed to our Tier 2 estimate of 88,100 Mg Corg. Further sampling is required to verify this, but we note that this difference equates to just 2% of our Tier 2 estimate for Turneffe Atoll as a whole (Zones A – D) and< 10% of our estimate for the outer atoll (Zones A – C). Thus, whilst uncertainty around Corg storage within Zone E is significant, refinement of our estimate with field data is unlikely to substantially alter our findings.
Irrespective of a possible over-estimate Corg storage within Zone E, our assessment of seagrass blue carbon on is likely to be an underestimate as it only accounts for the top 30 cm of seagrass sediment, whereas we know that these sediments can extend several meters underground. Whilst we do not know how deep this may be on Turneffe, we do know that it extends at least as deep as our ‘long’ cores (i.e., > 1 m). Future studies should aim to better constrain Corg storage at depths > 30 cm, and to determine the total depth of seagrass sediments on Turneffe Atoll. Finally, the impact of black refractory carbon in the areas is unknown and could be quantified further.
5 Conclusions
In this study we present data from five seagrass meadows on Turneffe Atoll, Belize, and test several hypotheses regarding the drivers of seagrass Corg storage. As expected, a δ13Corg mixing model confirmed that proximity to mangroves increased the proportion of mangrove carbon within seagrass sediments, and sedimentary Corg storage was found to increase as site energetics decreased. However, in disagreement with the prevailing literature, we found no relationship between seagrass canopy cover and sedimentary Corg storage.
We estimated Corg storage within the top 30 cm of seagrass sediments at atoll scale, and conservatively estimate that the seagrass meadows of Turneffe Atoll store 0.58 x 106 Mg or 2.14 Mt CO2 (eq) on the atoll exterior, with an additional 1.28 x 106 Mg Corg or 4.68 Mt CO2 (eq), stored within the Central Lagoon, most of which is likely not seagrass derived. This translates to a tentative present-day ‘cost for loss’ value of around €42 million for the predominantly seagrass-derived sediments of the outer atoll, with an additional €94 million stored within the central lagoon, most of which is mangrove-derived. We also provide a maximum estimate of 3.54 x 106 Mg Corg for the entire atoll, where (a) the Central Lagoon is included; and (b) all turbid areas are assumed to contain seagrass. This translates to an approximate valuation of €260 million.
The data contained in this paper represent an important step forward for blue carbon research in Belize, and will augment and strengthen the global dataset, having been collected in a region which is relatively data poor, and where carbon sequestration and storage may operate at different rates to the regions which dominate the global data set (i.e., Australia and North America).
Data availability statement
The data presented in the study are deposited in the British Oceanographic Data Repository (BODC) repository, accession number 10.5285/2357af23-c6be-a412-e063-7086abc04245.
Author contributions
SF: Conceptualization, Data curation, Formal analysis, Investigation, Methodology, Visualization, Writing – original draft, Writing – review & editing. RS: Conceptualization, Investigation, Methodology, Supervision, Writing – original draft, Writing – review & editing. VA: Methodology, Resources, Writing – review & editing. CB: Investigation, Methodology, Writing – review & editing. HB: Investigation, Writing – review & editing. SC: Data curation, Formal analysis, Investigation, Methodology, Writing – review & editing. AC: Writing – review & editing. EC: Investigation, Writing – review & editing. CDE: Supervision, Writing – review & editing. JH: Methodology, Writing – review & editing. AL: Investigation, Methodology, Writing – review & editing. DM: Supervision, Writing – review & editing. KP: Data curation, Formal analysis, Writing – review & editing. DP: Formal analysis, Investigation, Methodology, Visualization, Writing – review & editing. FR: Methodology, Writing – review & editing. AY: Conceptualization, Funding acquisition, Resources, Writing – review & editing. CE: Conceptualization, Data curation, Funding acquisition, Project administration, Resources, Writing – review & editing.
Funding
The author(s) declare financial support was received for the research, authorship, and/or publication of this article. This work was supported by the UK government through the Commonwealth Marine Economies Programme, which aims to enable safe and sustainable marine economies across the Commonwealth Small Island Developing States. SF received additional support from the National Environment Research Council (NERC) funded SPITFIRE Doctoral Training Programme (grant number NE/L002531/1). CDE was supported by the Natural Environmental Research Council (NERC) Independent Research Fellowship NE/M018806/1.
Acknowledgments
The authors wish to thank colleagues at the Turneffe Atoll Sustainability Association (TASA), Belize’s Coastal Zone Management Institute (CZMAI), and the University of Belize (UB) for access to laboratory facilities and logistical support during fieldwork. Deserving of special mention are Ellis Requena, Jayron Young, and Miss Estelle.
Conflict of interest
The authors declare that the research was conducted in the absence of any commercial or financial relationships that could be construed as a potential conflict of interest.
Publisher’s note
All claims expressed in this article are solely those of the authors and do not necessarily represent those of their affiliated organizations, or those of the publisher, the editors and the reviewers. Any product that may be evaluated in this article, or claim that may be made by its manufacturer, is not guaranteed or endorsed by the publisher.
References
Carpenter S., Byfield V., Felgate S. L., Price D. M., Andrade V., Cobb E., et al. (2022). Using unoccupied aerial vehicles (UAVs) to map seagrass cover from Sentinel-2 imagery. Remote Sens. 14, 477. doi: 10.3390/rs14030477
de los Santos C. B., Scott A., Arias-Ortiz A., Jones B., Kennedy H., Mazarrasa I., et al. (2020). “Seagrass ecosystem services: Assessment and scale of benefits,” in Out of the Blue: The Value of Seagrasses to the Environment and to People (Nairobi: UNEP), 19–21.
Dencer-Brown A. M., Shilland R., Friess D., Herr D., Benson L., Berry N. J., et al. (2022). Integrating blue: How do we make nationally determined contributions work for both blue carbon and local coastal communities? Ambio 51, 1978–1993. doi: 10.1007/s13280-022-01723-1
Duarte C. M., Losada I. J., Hendriks I. E., Mazarrasa I., Marbà N. (2013). The role of coastal plant communities for climate change mitigation and adaptation. Nat. Climate Change 3, 961–968. doi: 10.1038/nclimate1970
Duarte C. M., Middelburg J. J., Caraco N. (2005). Major role of marine vegetation on the oceanic carbon cycle. Biogeosciences 2, 1–8. doi: 10.5194/bg-2-1-2005
Fedler T. (2018). The Value of Turneffe Atoll Blue Carbon. (Florida: Human Dimensions Consulting, Gainesville).
Fourqurean J. W., Duarte C. M., Kennedy H., Marbà N., Holmer M., Mateo M. A., et al. (2012). Seagrass ecosystems as a globally significant carbon stock. Nat. Geosci. 5, 505–509. doi: 10.1038/ngeo1477
Freedman B., Stinson G., Lacoul P. (2009). Carbon credits and the conservation of natural areas. Environ. Rev. 17, 1–19. doi: 10.1139/a08-007
Friess D. A., Howard J., Huxham M., Macreadie P. I., Ross F. (2022). Capitalizing on the global financial interest in blue carbon. PloS Climate 1, e0000061. doi: 10.1371/journal.pclm.0000061
Greiner J. T., McGlathery K. J., Gunnell J., McKee B. A. (2013). Seagrass restoration enhances “blue carbon” sequestration in coastal waters. PloS One 8, e72469. doi: 10.1371/journal.pone.0072469
Grimm K. E., Archibald J. L., Bonilla-Anariba S. E., Bood N., Canty S. W. (2023). Framework for fostering just and equitable seagrass policy, management, and social-ecological outcomes: Lessons learned from Belizean marine resource managers. Mar. Policy 152, 105606. doi: 10.1016/j.marpol.2023.105606
Hendriks I. E., Sintes T., Bouma T. J., Duarte C. M. (2008). Experimental assessment and modeling evaluation of the effects of the seagrass Posidonia oceanica on flow and particle trapping. Mar. Ecol. Prog. Ser. 356, 163–173. doi: 10.3354/meps07316
Howard J., Hoyt S., Isensee K., Telszewski M., Pidgeon E. (2014). Coastal blue carbon: methods for assessing carbon stocks and emissions factors in mangroves, tidal salt marshes, and seagrasses. (Arlington, Virginia, USA: Conservation International, Intergovernmental Oceanographic Commission of UNESCO, International Union for Conservation of Nature).
Huckelba A. L., Van Lange P. A. (2020). The silent killer: Consequences of climate change and how to survive past the year 2050. Sustainability 12, 3757. doi: 10.3390/su12093757
IPCC (2014). 2013 supplement to the 2006 IPCC guidelines for national greenhouse gas inventories: Wetlands.
IPCC (2023). Climate Change 2023: Synthesis Report, Summary for Policymakers. Contribution of Working Groups I, II and III to the Sixth Assessment Report of the Intergovernmental Panel on Climate Change.
Kennedy H., Beggins J., Duarte C. M., Fourqurean J. W., Holmer M., Marbà N., et al. (2010). Seagrass sediments as a global carbon sink: Isotopic constraints. Global biogeochemical cycles 24 (4). doi: 10.1029/2010gb003848
Lin G., Banks T., O’Reilly L.d. S.L. (1991). Variation in δ13C values for the seagrass Thalassia testudinum and its relations to mangrove carbon. Aquatic Botany 40 (4), 333–341.
Locatelli T., Binet T., Kairo J. G., King L., Madden S., Patenaude G., et al. (2014). Turning the tide: how blue carbon and payments for ecosystem services (PES) might help save mangrove forests. Ambio 43, 981–995. doi: 10.1007/s13280-014-0530-y
Lovelock C. E., Duarte C. M. (2019). Dimensions of blue carbon and emerging perspectives. Biol. Lett. 15, 20180781. doi: 10.1098/rsbl.2018.0781
Macreadie P. I., Nielsen D. A., Kelleway J. J., Atwood T. B., Seymour J. R., Petrou K., et al. (2017). Can we manage coastal ecosystems to sequester more blue carbon? Front. Ecol. Environ. 15, 206–213. doi: 10.1002/fee.1484
Macreadie P. I., Wartman M., Roe P., Hodge J. M., Helber S. B., Waryszak P., et al. (2024). Seagrasses produce most of the soil blue carbon in three Maldivian islands. Front. Mar. Sci. 11. doi: 10.3389/fmars.2024.1359779
Mazarrasa I., Samper-Villarreal J., Serrano O., Lavery P. S., Lovelock C. E., Marbà N., et al. (2018). Habitat characteristics provide insights of carbon storage in seagrass meadows. Mar. pollut. Bull. 134, 106–117. doi: 10.1016/j.marpolbul.2018.01.059
McCloskey T. A., Liu K.-b. (2013). Sedimentary history of mangrove cays in Turneffe Islands, Belize: evidence for sudden environmental reversals. J. Coast. Res. 29, 971–983. doi: 10.2112/jcoastres-d-12-00156.1
Mcleod E., Chmura G. L., Bouillon S., Salm R., Björk M., Duarte C. M., et al. (2011). A blueprint for blue carbon: toward an improved understanding of the role of vegetated coastal habitats in sequestering CO2. Front. Ecol. Environ. 9, 552–560. doi: 10.1890/110004
Miyajima T., Hori M., Hamaguchi M., Shimabukuro H., Adachi H., Yamano H., et al. (2015). Geographic variability in organic carbon stock and accumulation rate in sediments of East and Southeast Asian seagrass meadows. Global biogeochemical cycles 29, 397–415. doi: 10.1002/2014gb004979
Nellemann C., Corcoran E. (2009). Blue carbon: the role of healthy oceans in binding carbon: a rapid response assessment (UNEP/Earthprint).
Price D. M., Felgate S. L., Huvenne V. A., Strong J., Carpenter S., Barry C., et al. (2022). Quantifying the intra-habitat variation of seagrass beds with unoccupied aerial vehicles (UAVs). Remote Sens. 14, 480. doi: 10.3390/rs14030480
Ray R., Michaud E., Aller R., Vantrepotte V., Gleixner G., Walcker R., et al. (2018). The sources and distribution of carbon (DOC, POC, DIC) in a mangrove dominated estuary (French Guiana, South America). Biogeochemistry 138, 297–321. doi: 10.1007/s10533-018-0447-9
R Core Team (2020). R: A language and environment for statistical computing In (Version 4.2.0) (R Foundation for Statistical Computing).
Ricart A. M., York P. H., Bryant C. V., Rasheed M. A., Ierodiaconou D., Macreadie P. I. (2020). High variability of Blue Carbon storage in seagrass meadows at the estuary scale. Scientific reports, 10.
Rowlands G. P., Antat S., Baez S. K., Cupidon A., Faure A., Harlay J., et al. (2024). The Seychelles Seagrass Mapping and Carbon Assessment. A report to be submitted to the Government of Seychelles, Ministry of Agriculture. Climate Change Environ. (MACCE)., p1–60.
Serrano O., Gómez-López D. I., Sánchez-Valencia L., Acosta-Chaparro A., Navas-Camacho R., González-Corredor J., et al. (2021). Seagrass blue carbon stocks and sequestration rates in the Colombian Caribbean. Sci. Rep. 11, 11067. doi: 10.1038/s41598-021-90544-5
Stankovic M., Ambo-Rappe R., Carly F., Dangan-Galon F., Fortes M. D., Shawkat Hossain M., et al. (2021). Quantification of blue carbon in seagrass ecosystems of Southeast Asia and their potential for climate change mitigation. Sci. Total Environ. 783, 146858. doi: 10.1016/j.scitotenv.2021.146858
Keywords: blue carbon, seagrass, organic carbon, sediment carbon, stable isotopes, mangroves, atoll, Belize
Citation: Felgate SL, Sanders R, Andrade V, Barry CDG, Brittain H, Carpenter S, Carrias A, Cobb E, Evans CD, Hunt J, Lichtschlag A, Mayor DJ, Peel K, Price DM, Radford F, Young A and Evans C (2024) Quantifying sedimentary ’blue carbon’ in relation to canopy cover in the seagrass meadows of Turneffe Atoll, Belize. Front. Mar. Sci. 11:1371162. doi: 10.3389/fmars.2024.1371162
Received: 15 January 2024; Accepted: 11 September 2024;
Published: 15 November 2024.
Edited by:
Liyang Yang, Fuzhou University, ChinaReviewed by:
Amrit Mishra, James Cook University, AustraliaAntoine De Ramon N’Yeurt, University of the South Pacific, Fiji
Copyright © 2024 Felgate, Sanders, Andrade, Barry, Brittain, Carpenter, Carrias, Cobb, Evans, Hunt, Lichtschlag, Mayor, Peel, Price, Radford, Young and Evans. This is an open-access article distributed under the terms of the Creative Commons Attribution License (CC BY). The use, distribution or reproduction in other forums is permitted, provided the original author(s) and the copyright owner(s) are credited and that the original publication in this journal is cited, in accordance with accepted academic practice. No use, distribution or reproduction is permitted which does not comply with these terms.
*Correspondence: Stacey L. Felgate, cy5mZWxnYXRlQGh3LmFjLnVr
†Present addresses: Daniel J. Mayor, Biosciences, University of Exeter, Exeter, United KingdomDavid M. Price, Departamento de Geociencias Marinas, Instituto de Ciencias del Mar, Consejo Superior de Investigaciones Científicas (CSIC), Barcelona, Spain