- 1University of South Bohemia in Ceske Budejovice, Faculty of Fisheries and Protection of Waters, South Bohemian Research Center of Aquaculture and Biodiversity of Hydrocenoses, Vodňany, Czechia
- 2University of South Bohemia in Ceske Budejovice, Faculty of Science, Ceske, Budejovice, Czechia
- 3Lallemand SAS, 19 rue des Briquetiers, Blagnac, France
- 4Departamento de Biología Animal, Edafología y Geología, Universidad de La Laguna, La Laguna, Spain
Lactic acid bacteria (LAB) probiotics were evaluated for their impact on the microbiota and development of pikeperch (Sander lucioperca) larvae during their initial feeding stage (first 21 days). Pikeperch larvae were exposed to LAB probiotics in two ways: (1) via the live-feed only (Treatment 1, live-feed) or (2) via the live-feed and the larval culture water (Treatment 2, probiotic) in comparison to a control group without LAB supplementation. Total length (TL), myomere height (MH), and survival rate were significantly increased in the probiotic compared the Control group. The administration of probiotics significantly positively influenced the microbiome’s diversity. Specifically, the relative abundance of Cytophagales decreased and that of several other taxa increased in both probiotic treatments although differences between treatments became statistically insignificant by day 21. Furthermore, the different treatments had distinct and significant impacts on digestive enzyme development such as protease and lipase, with the most prominent differences occurring at seven days post-hatching (dph). Taken together, these results indicate that the use of LAB in both the live-feed and rearing water has a positive impact on the larvae microbiota and digestive enzyme development in turn positively impacting their development and viability under intensive rearing conditions.
1 Introduction
Pikeperch (Sander lucioperca) is a sought-after freshwater and brackish water fish belonging to the Percidae family. It greatly appeals to recreational fishermen and the gastronomic industry (Kestemont et al., 2015). Due to its high demand and high value, pikeperch is currently a focal species in the European Union’s efforts to diversify inland brackish aquaculture. However, the development of larval rearing in recirculating aquaculture systems (RAS) faces several challenges including low-stress resistance, nutritional deficiencies, and cannibalism, which collectively result in low survival rates at the larval stage (Steenfeldt et al., 2015; Policar et al., 2019).
To ensure adequate nutrition of the larvae, live feeds such as rotifers and artemia are essential (Imentai et al., 2019a, b; Yanes-Roca et al., 2018, 2020a, 2020b). Nevertheless, using live feeds carries the risk of introducing pathogenic bacteria into the closed system (Lubzens et al., 1989). Probiotics are live microbial supplements that positively influence the host animal by promoting a healthy balance of intestinal microbes (Gatesoupe, 1999; Balcázar et al., 2006). Exposing fish larvae to probiotics has been demonstrated to enhance their overall health status and support their resilience against specific pathogens and diseases (Avella et al., 2010; Vanbelle et al., 1990). This effect is primarily attributed to the reliance of gastrointestinal microbiota on external environmental conditions (Carnevali et al., 2017). Probiotics also compete with harmful pathogens for nutrients and attachment sites, thereby bolstering the immune system (Hai, 2015).
The defense mechanisms crucial for the underdeveloped immune system of fish larvae, as noted by Hai (2015), highlight the importance of supplementing live feeds with probiotic bacteria. This supplementation provides advantages such as enhanced nutritional content, improved growth performance, and increased survival for larval fish in Recirculating Aquaculture Systems (RAS), as suggested by studies by Borges et al. (2021) and Nayak (2010).
Most probiotic microorganisms belong to the category of lactic acid bacteria (LAB) which are characterized as gram-positive, generally non-motile, non-sporulating bacteria known for their production of lactic acid as a major product of fermentative metabolism (Klein et al., 1998; Ljung and Wadström Torkel, 2018). Among these, Pediococcus acidilactici CNCM I-4622 (MA 18/5M) is one of the most documented LAB strains in aquaculture, with positive findings in several finfish larvae in particular (Gatesoupe, 2002; Zacarias-Soto et al., 2011; Lamari et al., 2013; Ringø et al., 2018).
Recent studies in pikeperch have provided evidence of the beneficial effects of incorporating LAB probiotics into their diet (Ljubobratovic et al., 2017, 2019; Yanes-Roca et al., 2020b), but such studies lack a closer look into the effects over the digestive enzymes and the microbiome.
The aim of the study was to assess the impact of P. acidilactici CNCM I-4622 (MA 18/5M) on the time-development of the intestinal microbiome and of key digestive enzyme activities in relation to the morphological development and larvae-rearing performance of pikeperch during the initial 21 days post-hatching (dph).
2 Materials and methods
2.1 Experimental fish and system
The experiment took place at the University of South Bohemia’s Facility of Fisheries and Protection of Waters in the Czech Republic (USB, FFPW), where from fertilized eggs were also sourced as follows. Locally managed broodstock were hormonally induced to spawn using Human Chorionic Gonadotropin (hCG; Chorulon, Intervet International B.V., Ljubljana, Slovenia) as previously documented (Křištan et al., 2013; Blecha et al., 2015, 2016) and broodstock allowed to naturally nest spawn as outlined by Malinovskyi et al (Malinovskyi et al., 2018, 2019). After laying the naturally fertilized eggs, the broodstock was removed, and the eggs were left in the spawning tank for incubation at a consistent water temperature of 16 ± 0.5°C for 8 days until hatching.
The larval rearing system consisted of an indoor RAS equipped with mechanical and biological filtration, UV-disinfection, aeration, photo- and thermo-control and consisting of 12 rectangular tanks (3 liter/tank) maintained under a constant 12:12 h light: dark photoperiod. During the trial, water quality parameters were maintained within a suitable range for the species and averaged: salinity (3.0 ± 0.5 ppt), dissolved oxygen (8.0 ± 1 mg/L), temperature (17.1 ± 0.2°C); ammonia (0.20 ± 0.05 mg/L), nitrite (0.02 ± 0.01 mg/L) and nitrate (0.10 ± 0.03 mg/L).
2.2 Experimental design and rearing protocol
At 3 dph and prior to the start of exogenous feeding, newly hatched larvae were transferred from the incubation tank and randomly stocked into the larval rearing system (12 tanks; density of 100/L) when the trial started.
The trial tested 3 experimental groups in quadruplicate as follows: A Control group without probiotic administration (Control) and two probiotic groups managed identically but with either 1) Probiotic administered via the live feed only (treatment 1; live feed) or 2) Probiotic administration via the live feed and by direct application into the larvae rearing water (Treatment 2, probiotic). The test probiotic was P. acidilactici CNCM I-4622 supplied in the commercial lyophilized form (Bactocell Aqua 100; 100 × 109; Lallemand, Blagnac, France) applied over the trial’s duration at a daily dose of 1 g/m3 (1 × 105 CFU/mL) in the live feed culture tank as well as in the larval rearing water. To do so and 12 hours prior to the first feeding of the day, the required quantity of the probiotic was diluted directly into the harvested live feed, as well as into 1 L of larval rearing water prior to spreading the mixture at the tank surface.
The live-feed feeding schedule (Table 1) followed a previously established protocol (Yanes-Roca et al., 2020b). In brief, larvae were fed 3 times a day (0800, 1130, and 1530) with enriched saltwater rotifers (Brachionus plicatilis) using Nannochloropsis occulata (1mL/L enrichment; Nanno 3600, Reed Mariculture, Campbell, USA) from 4 dph until 15 dph. Artemia feeding commenced at 12 dph using unenriched Artemia until the end of the trial at 21 dph. Live feed input was adjusted based on residual counts performed before each meal to achieve the target live prey densities (Table 1). The flow rates within each rearing tank were set at 100 mL/min at the start of the trial and progressively increased to 250 mL/min. (Table 1). To maximize larval feeding efficiency, the water flow was temporarily halted for 2 hours at each meal.
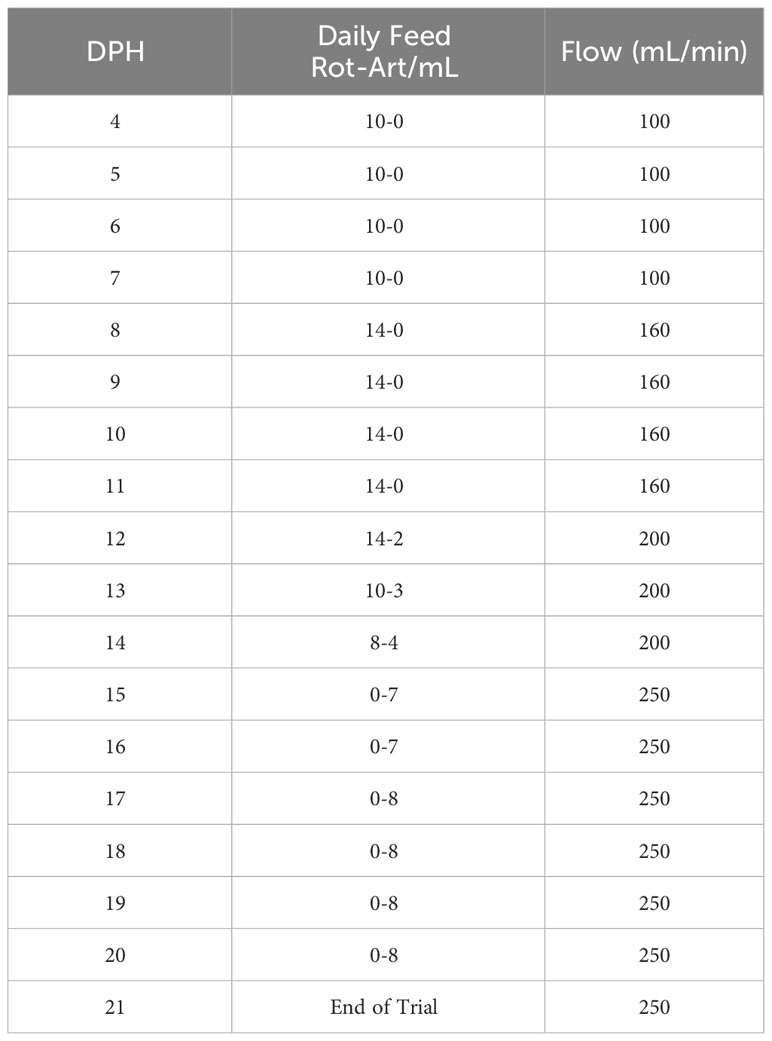
Table 1 Experiment husbandry schedule. Amount of daily feed offered and recirculation flow changes with time are shown.
The trial was terminated at 21 dph before initiating the transition to inert feed and to the emergence of cannibalistic behaviors.
2.3 Larval development and survival
At 3 (trial start), 12 and 16 dph, 10 larvae/tank/time point were randomly collected using a 300 microns net. Total length (TL), myomere height (MH), and eye diameter (ED) were measured, and air bladder inflation was assessed following a standardized methodology (Yanes-Roca et al., 2018) using a microscope equipped with a digital camera and an imaging software (Olympus cellSens, v 1.3). At day 21 dph, 25 larvae/tank were collected for morphometric analysis as done at prior time-points, and survival rates per tank were evaluated by volumetric counting of larvae.
2.4 Microbiome analysis
At 4, 7, 14, and 21 dph, a minimum of 5 larvae/tank/time-point were collected along with triplicates of water samples (500 mL) from the larval culture tanks. Larvae were pooled per tank within a dry-aseptic vial and immediately snap-frozen using liquid nitrogen. Pellets resulting from the immediate centrifugation of water samples were processed likewise. Altogether, 80 samples were then stored at -80°C prior to DNA extraction. Using Micro (Qiagen, Hilden, Germany), DNA templates were extracted from 24 water samples, 55 individual larvae and a single gut dissected from a 21-day old individual used as an internal control with the potential to distinguish between fish gut and surface microbiome (all sample metadata available in Supplementary Table 1).
2.4.1 Amplicon library preparation and sequencing
The amplification of the 16S rRNA gene was carried out according to Earth Microbiome Project standards (EMP, http://earthmicrobiome.org/protocols-and-standards/16s/) as described previously (Brown et al., 2020). Four negative and four positive controls were amplified along with the samples. The negative controls included one blank extraction control and three blank PCR controls. The positive controls, employed to assess amplification bias and the detection limit, consisted of commercially supplied gDNA templates, ATCC® MSA-1000™ and ATCC® MSA-1001™ (ATCC, Virginia, USA), both comprising the same 10 bacterial species with different distribution. The purified and equimolarly pooled amplicons were sequenced on Illumina MiSeq using v2 chemistry with 2 x 250 paired ends.
2.4.2 Analysis of the amplicon data
Initially, amplicons were quality-checked using FastQC (Andrews, 2010). Downstream processing, i.e. demultiplexing, merging, trimming, quality filtering, and OTU (operational taxonomic units) clustering, was performed by implementing corresponding scripts from USEARCH v9.2.64 (Edgar, 2013). OTU taxonomy was generated using BLASTn searches of representative sequences against the SILVA_139_SSUREF_Nr99_tax database (Quast et al., 2013). On average, we have retrieved 7816 ± 4956 reads per a sample, 18682 ± 4783 reads per a positive control, and 1080 ± 1187 reads per a negative control. The OTU profiles of the negative controls, containing from 0 to 2346 reads, were inspected for potential contaminants. Altogether, 5 OTUs were filtered out from the OTU table. Four were found consistently across 3 negative controls (Sphingomonas, Staphylococcus, Acidovorax, and Cutibacterium), and two (Acetobacter and Streptococcus) exceeded the abundance of 90 reads in any of the negative controls (Supplementary Table 1).
2.5 Digestive enzyme activities
Samples of pikeperch larvae at four-time points (hundred larvae at 4 dph (25 per tank), twenty 7 dph-larvae (5 per tank), twenty 14 dph-larvae (5 per tank), and four 21 dph-larvae (1 per tank)) were pooled and homogenized in triplicates in 10 volumes (v/w) of ice-cold Milli-Q water using an Ultra-Turrax T8 (IKA©-Werke, Germany). Homogenates were centrifuged at 3,300 x g for 3 min at 4°C, and the supernatant was collected and kept at -80°C until the analysis of pancreatic (α-amylase, bile salt-activated lipase, total alkaline proteases) and gastric (pepsin) enzyme activities (Solovyev and Gisbert, 2016). Whole body samples were used for 4, 7, and 14 dph larvae, while heads and tails were removed for 21 dph larvae.
Alkaline proteases activity was spectrophotometrically assayed at 366 nm using azocasein as substrate, according to García-Carreño and Haard (1993). One unit of activity was defined as 1 μmol of azo dye released per min per mL.
Alpha-amylase (E.C. 3.2.1.1) was analyzed by incubation with 0.3% soluble starch, and the increase in absorbance of the supernatant was determined at 580 nm (Métais and Bieth, 1968). Alpha-amylase activity corresponded to the mg of starch hydrolyzed at 37°C per 30 min and per mL.
Bile salt-activated lipase (BAL, E.C. 3.1.1) activity was measured by incubating the extracts with p-nitrophenyl myristate and reading the absorbance of the supernatant at 405 nm (Iijima et al., 1998). BAL activity was defined as the μmol of myristate hydrolyzed per min per mL.
Lastly, pepsin (E.C. 3.4.23.1) was quantified by incubation with a 2% hemoglobin solution. The absorbance was measured at 280 nm (Worthington Biochemical Corporation, 1972). One unit of activity corresponded to the μmol of tyrosine released per min per mL.
Soluble protein of extracts was analyzed using bovine serum albumin as standard (Bradford, 1976). Absorbance was read in a spectrophotometer (Beckman Coulter DU800, Fullerton, CA), and specific activity is expressed as mU or U mg protein-1.
2.6 Statistical analysis
Differences between the body morphometry were evaluated with linear mixed models (LMM, package lme4, version 1.1-7); (Bates et al., 2015) testing the effect of the probiotic treatment on fish TL, MH, and ED (response variables). The tank was included as a random effect. Prior to LMM, the different response variables were transformed with the Box-Cox transformation, which gives the best power estimate for each variable (package car, version 2.1.2; Fox and Weisberg, 2011). After that, multiple pairwise comparisons between treatments were obtained using Tukey’s all-pair comparisons, applying the Bonferroni correction to adjust the p-values (package multcomp, version 1.3-3; Fox and Weisberg, 2011).
For digestive enzyme activity data, the one-sample Shapiro-Wilk test and the Levene tests were performed prior to data analysis to verify the data normality and homogeneity of variance, respectively. The one-way ANOVA followed by a Tukey HSD multiple comparison test was used to determine statistical differences between treatments for each digestive enzyme and larval age and between ages for each digestive enzyme and treatment. If normal distribution or homoscedasticity were not achieved, data were transformed using logarithm or arcsine square root. When transformations did not succeed, the Welch test was performed, followed by the T3 Dunnet for no homoscedastic data. In contrast, the Kruskall-Wallis non-parametric test was applied in the case of no normal distribution, followed by pair-wise Mann-Whitney test comparisons with Bonferroni correction.
Survival rate was compared between treatments using a generalized linear mixed model (GLMM) with survival (i.e., the proportion of alive fish at 21 dph as a response variable) fitted with a binomial error structure and with enrichment as a fixed effect and the tank as a random effect. After GLMM, pairwise comparisons were obtained with Tukey’s all-pair comparison test. A Bonferroni correction was applied to adjust the p-values of multiple comparisons (Hothorn et al., 2008).
Microbiome analyses, data visualization, and statistical tests were performed in the R environment using MicroEco package and its dependencies (https://github.com/Russel88/MicEco/tree/v0.9.15). The decontaminated OTU table was initially cleaned from archaeal, mitochondrial, chloroplast, and unclassified taxa and rarefied. Differences between microbiome diversity measures were statistically evaluated for the control group across the 4 analyzed time points using the Kruskal-Wallis Rank Sum Test. The alpha diversity indices were calculated and the pair differences between different treatments were statistically evaluated using Dunn’s Kruskal-Wallis Multiple Comparisons.
3 Results
3.1 Larval growth
At the start of the trial, there was no difference in pikeperch larval TL (5.25 ± 0.5 mm) between groups. At 12 dph and at 21 dph (end of trial), TL was significantly greater in the probiotic compared to the live-feed and Control groups (Figure 1A; LMM, p-value <0.05), although no significant differences were detected at 16 dph. In contrast, MH from the Probiotic group was significantly higher at 16 dph compared to the live feed group. Figure 1B; LMM p-value <0.001). There was no treatment effect on ED (LMM, p-value >0.05, data not shown).
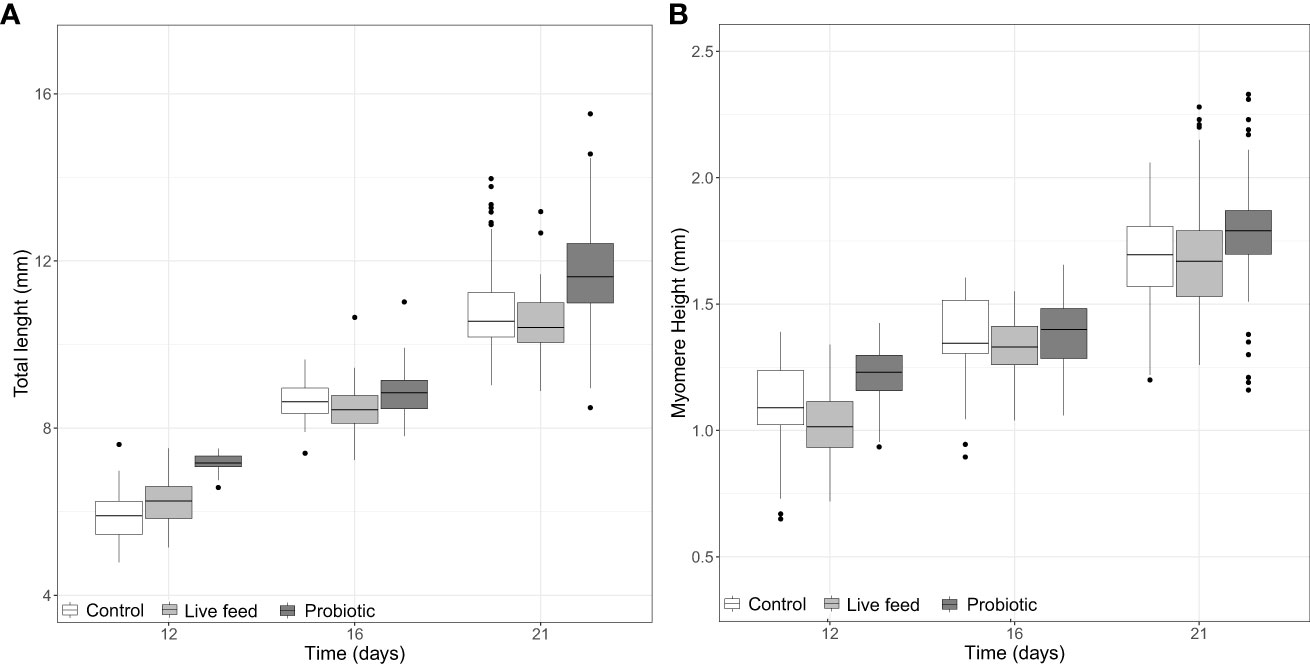
Figure 1 Boxplots of pikeperch larval (A) total length and (B) myomere height per group at days 12 (n = 40), 16 (n = 40), and 21 dph (n = 100). Whiskers indicate the maximum and minimum values excluding out layers (black dots), the line in the middle of the box is the median value, and the upper and lower quartiles are the ends of the box. Statistically significant differences between treatments are marked with an asterisk.
3.2 Survival
At the end of the trial (21 dph), survival was significantly improved in the probiotic compared to the control and live-feed groups (Figure 2; GLMM and pairwise comparisons; p < 0.001). Survival of the probiotic group was 1.7 times higher than that of the control group and 1.5 times higher than that of the live-feed group, with no significant differences between live-feed and control groups.
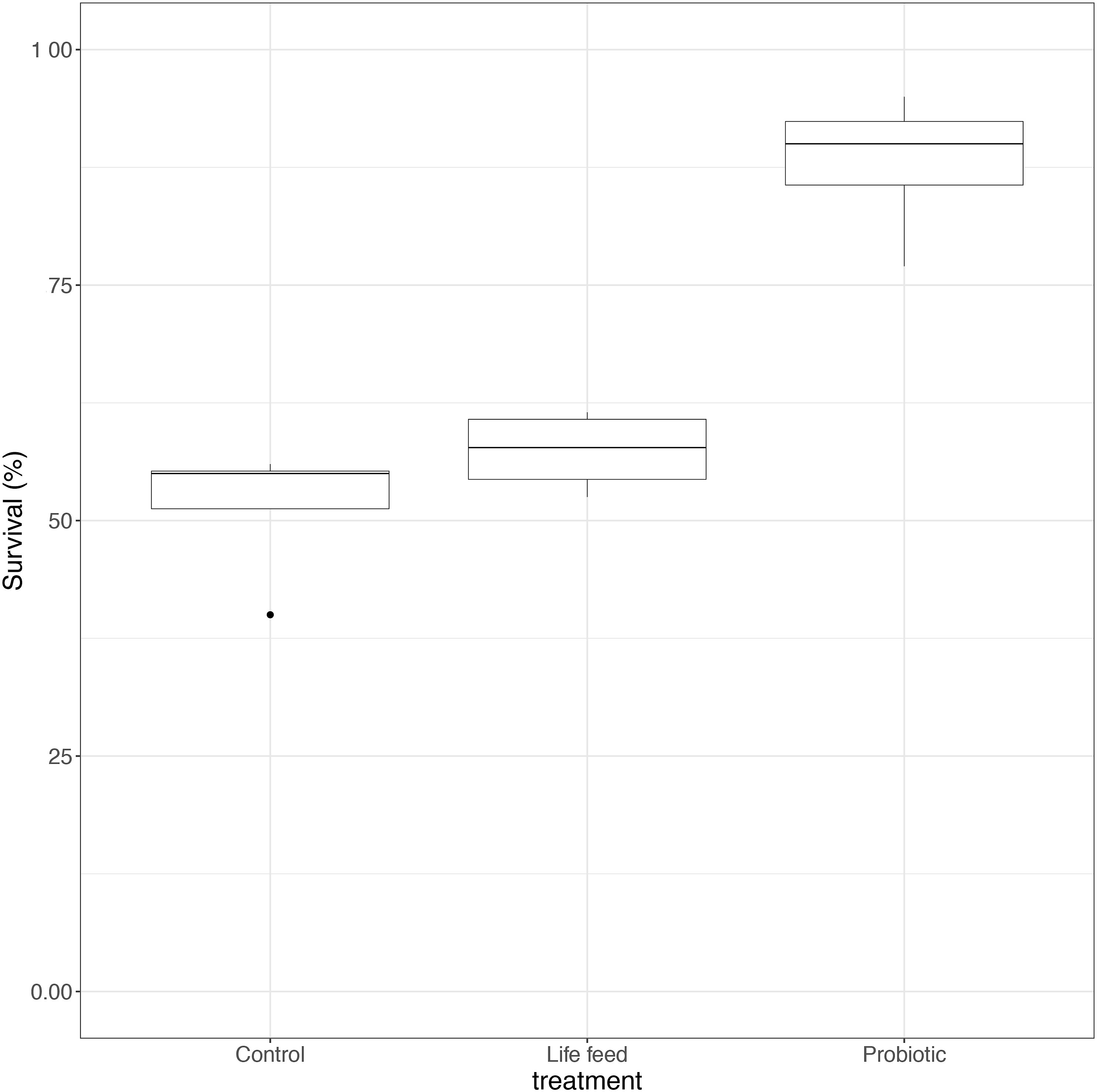
Figure 2 Box plot of survival rate per experimental group at the end of the trial (21 dph; n = 4). Whiskers indicate the maximum and minimum values excluding out layers (black dots), the line in the middle of the box is the median value, and the upper and lower quartiles are the ends of the box. Statistically significant differences between treatments are marked with an asterisk.
3.3 Microbiome
The microbiome of pikeperch larvae across experimental groups and sampling points was clearly dominated by a Cytophagales OTU, which was detected in low relative abundance (0.1%) in a single water sample (Figure 3A). Besides the order Cytophagales, the larvae-specific microbiome included families such as Rhizobiaceae, Parachlamydiaceae, and Peptostreptococcaceae (Figure 3A).
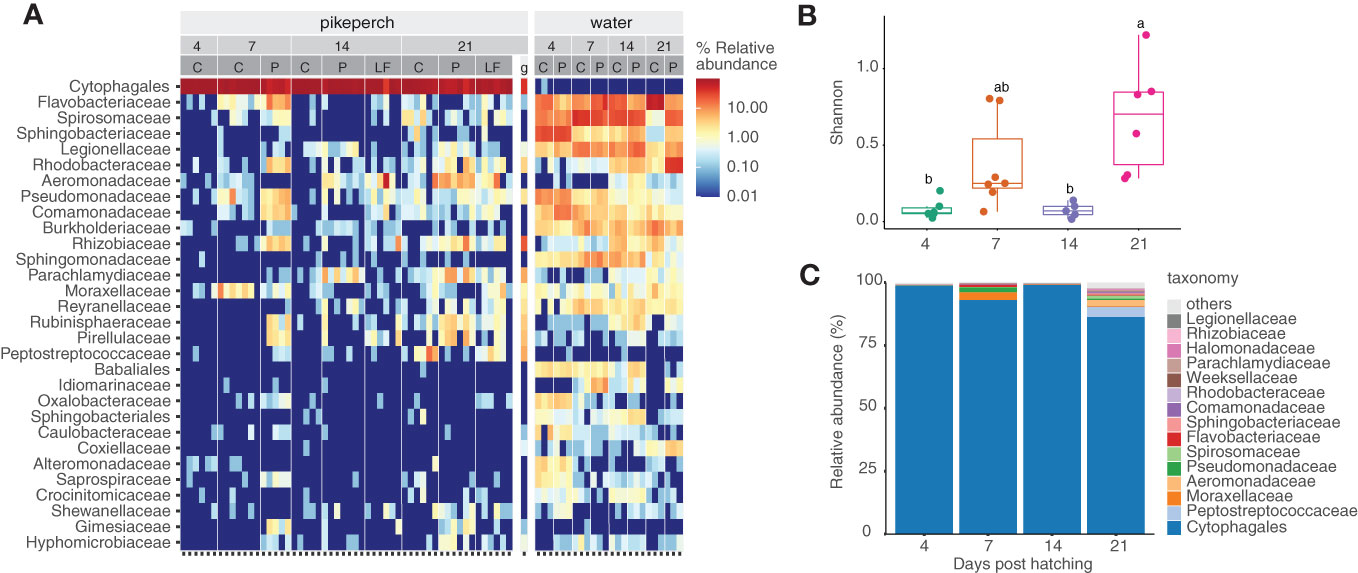
Figure 3 Water and Pikeperch larvae microbiome abundance per experimental groups; each column stands for an individual; numbers stand for time points, experimental groups (C, Control; LF, live-feed; P, probiotic) per time point (4, 7, 14 and 21 dph) (A). Development of the gut microbiome of the control group in time: alpha diversity measure, letters stand for anova results (B). Microbiome content averaged per a time point using 15 most abundant bacterial families from the control group (C).
3.3.1 Microbiome dynamics in early pikeperch ontogeny
Microbiome development of the control group displayed a pronounced diversity dynamic across time-points among which the microbiome alpha diversity significantly varied (Kruskal-Wallis Rank Sum Test, p< 0.0021, Figure 3B; Supplementary Table 2). The control group sampled at 4 dph showed a very low mean microbiome diversity (Richness: 9.50 ± 5.24, Shannon index: 0.10 ± 0.09; Supplementary Table 2), possibly reflecting the limited feeding ability of the early larvae and thus the minimal influx of environmental bacteria. Following a live-feed introduction at 4 dph, the microbiome diversity in the control group at 7 dph rapidly increased (Richness: 58.40 ± 14.76, Shannon index: 1.73 ± 0.61; Supplementary Table 3). This was followed by a diversity decrease at 14 dph (Richness: 9.00 ± 3.70, Shannon index: 0.09 ± 0.04; Supplementary Table 3). At the last sampled time point (21 dph), the microbiome diversity reached higher values again (Richness: 31.83 ± 9.15; Shannon index: 0.70 ± 0.37; Supplementary Table 3).
3.3.2 Effects of administrated probiotics on the microbiome of pikeperch larvae
Probiotic administration significantly affected microbiome diversity. Early larvae (7 dph) from the probiotic group had significantly higher microbiome diversity than the control group (Kruskal-Wallis Rank Sum Test, p< 0.012, Figure 4A; Supplementary Table 3). At 14 dph, significant increases in microbiome Shannon diversity in both probiotic-administered groups compared to the Control were observed (Dunn’s Kruskal-Wallis Multiple Comparisons, p<0.03, Figure 4A; Supplementary Data 2). In particular, the relative abundance of Cytophagales decreased in both probiotic treatments at the benefit of several other minor taxa which increased in proportion, e.g. Aeromonadaeae, Parachlamydiaceae, Legionellaceae, and Pirellulaceae (Figure 4C). There was no more statistical treatment effect on microbiome diversity at 21 dph (Figures 4A, B; Supplementary Data 2), which was marked, in particular, by an increased diversity in the Control group compared to the prior time point. However, at that time-point, the microbiome diversity was visible higher in the probiotic compared to the live-feed and Control group, and this was again associated with a decreased prevalence of Cytophagales. Finally, there was no effect of probiotic administration on the microbiome diversity of the larval-rearing water.
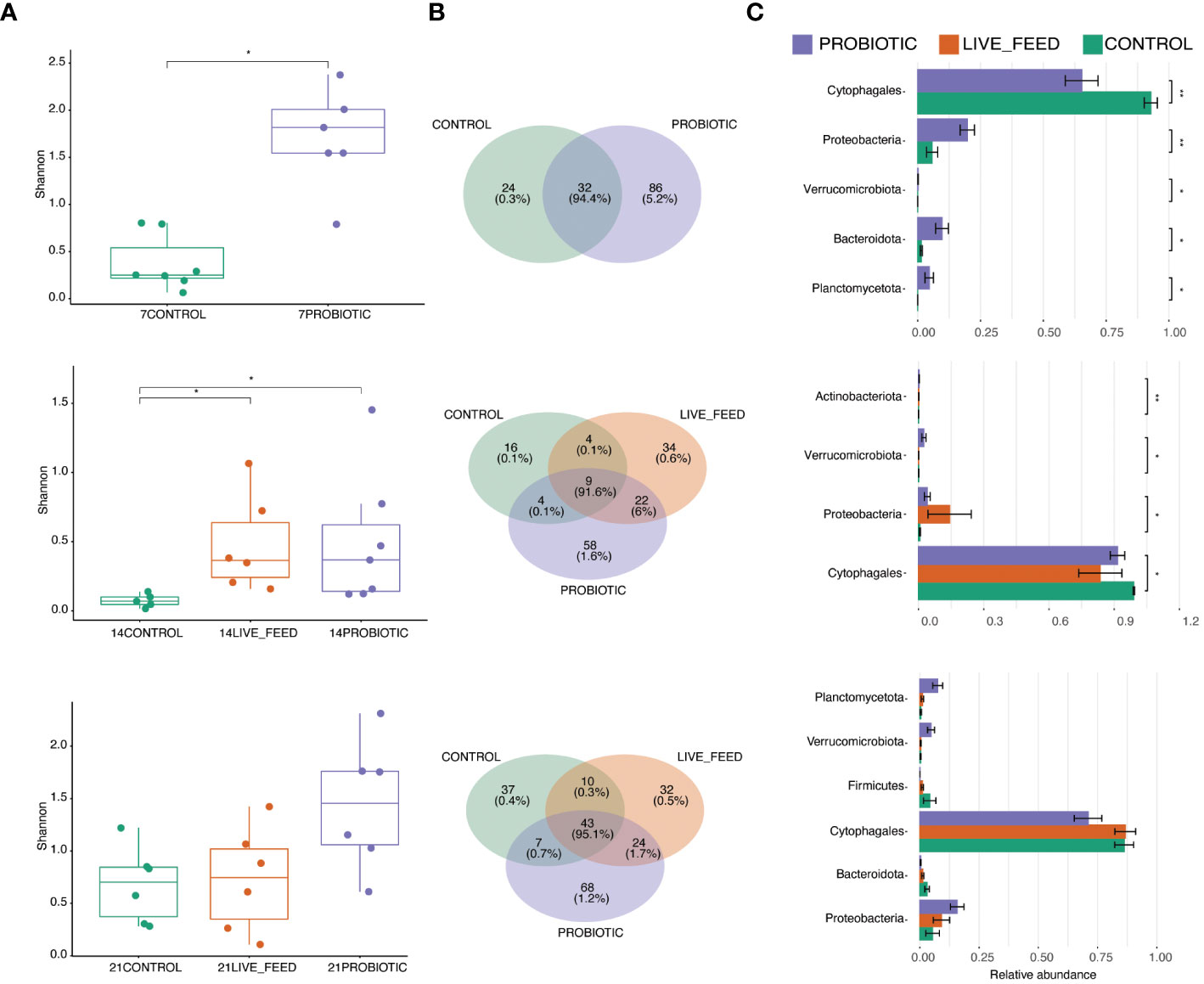
Figure 4 Microbiome characteristics among different experimental groups within three time points (7, 14 and 21 dph). Differences in alpha diversity measures between the experimental groups evaluated using Dunn’s Kruskal-Wallis Multiple Comparisons (A). Venn diagrams showing the numbers of shared and unique OTUs for the experimental groups; the percentage stands for number of reads (B). Differential analysis on the taxonomic abundance of shared taxa; the taxa are ordered according to qvalue from low to high (C). Asterisks stand for significant differences between pairs. Non-significant values are not depicted.
3.3.3 Administration of probiotics in the water
When looking at the water samples analysis results, it can be observed the Flavobacteriaceae, Chitinophagaceae, Saprospiraceae, and Spirosomaceae families were more prevalent in the probiotic group compared to the control. Simultaneously, a higher abundance of Rhodobacteraceae and Sphingobacteriaceae in the rearing water of the probiotic compared to the control group at the 21-day mark was observed (Figure 3C).
3.4 Enzymes activity
Alkaline proteases specific activity increased in both experimental treatments compared to the control at 7 dph (Table 2). Thus, probiotic larvae showed the highest activity at 7 dph, followed by live feed and control fish (9.27 ± 1.81, 6.23 ± 0.66, and 2.13 ± 0.83 mU/mg protein, respectively; p<0.05). However, these differences were compensated with age, where live feed treatment reported the lowest activity, although these differences were not significant at 21 dph. Moreover, lipase activity was higher in probiotic-larvae with respect to control fish at 21 dph (10.71 ± 2.84 vs. 5.39 ± 1.89 mU/mg protein; p<0.05) whereas live feed-larvae resulted in intermediate values (6.22 ± 1.75 mU/mg protein). Pepsin activity seems to increase with age in both experimental treatments. By contrast, α-amylase activity was not affected by experimental treatments despite a tendency to be higher in probiotic larvae throughout the whole experimental period. The activities of the alkaline proteases, α-amylase and lipase increased with age in the control larvae but not in the experimental fish, with a peak of activity registered for alkaline proteases and lipase at 14 dph and at 21 dph for α-amylase (Table 2).

Table 2 Specific activity (U/mg protein for α-amylase and mU/mg protein for alkaline proteases, bile salt-activated lipase and pepsin) of digestive enzymes of 4, 7, 14 and 21 dph pikeperch larvae under the different experimental conditions (Control, probiotic, live feed).
4 Discussion
The study aimed at characterizing the development of the intestinal microbiome and digestive enzyme activities in first-feeding pikeperch larvae in relation to development and survival; and its potential modulation by probiotic intervention. The study documented the predominance of phylum Cytophagales prior to and over the first-feeding phase of pikeperch larvae and the progressive, albeit fluctuating, increase in the diversity and richness of the larvae microbiome. Interestingly, at this early life stage, the larvae microbiome was apparently not associated with the water microbiota makeup. Still, its diversity is positively modulated by the administration of the probiotic P. acidilactici CNCM I-4622 (MA 18/5M). Probiotic administration was also associated with enhanced activity of some digestive enzymes, larval growth, and survival. Taken together, the study contributes to knowledge on pikeperch larvae microbiome and highlight the potential contribution of P. acidilactici CNCM I-4622 (MA 18/5M) administration on the maturation of the gut microbiome and digestive capacity and, as a result, on the growth and robustness of first-feeding pikeperch larvae.
The factors responsible for microbiome differences, whether biotic (such as nutrition or immunity) or abiotic, are of key importance. Like in many fish species, pikeperch larvae exhibit substantial microbiota diversity among individuals (Ljubobratovic et al., 2017; Dulski et al., 2018).
4.1 Growth and survival
There was a positive effect on growth of pikeperch larvae with the application of P. acidilactici CNCM I-4622 (MA 18/5M) during the first 21 days post-hatching, especially between the treatments that were exposed to probiotics versus the control treatment; such positive results were also observed in other species such as Nile tilapia (Oreochromis niloticus) and swordtail (Xiphophorus Heller; Lara-Flores et al., 2003; Dharmaraj and Dhevendaran, 2010) and may be attributed to enhanced intestinal maturation and digestive capacities upon probiotic supplementation.
Simultaneously, the use of this commercial probiotic on the live feed cultures as well as in the larval rearing water (probiotic treatment) significantly increased larval survival. Such increases in survival rate upon probiotics administration in the water have been previously described in the shrimp Penaeus vannamei (Zhou et al., 2009). In prior studies, probiotics applied in the live feed instead of in water were more effective for improving growth and survival in Sparus aurata and Dicentrarchus labrax larvae (Carnevali et al., 2004, 2006; Suzer et al., 2008). This study did not compare probiotic administration strategies but documented the superior benefit of applying the probiotic in the live-feed and directly in the larval-rearing water. Probiotic administration in the larval rearing water could have increased its uptake hence direct benefit to the larvae. Still, it may also have positively modulated the rearing water quality inherent to the multifaceted mode of action and benefits of probiotics. Although some studies have documented an effect of LABs supplementation on water biochemistry (Eissa et al., 2022), no statistical differences in ammonia, nitrate, or nitrite levels were observed between the treatments in this study.
Such direct effects of probiotics on larval growth and survival are directly connected to the gastrointestinal colonization by the probiotic, as highlighted in studies by Balcázar et al. (2006) and Fuller (1989), and specifically documented for P. acidilactici CNCM I-4622 (MA 18/5M) (Merrifield et al., 2009; Fergusson et al., 2010; Harper et al., 2011).
4.2 Effect on microbiome
The utilization of probiotics in the present study has proven effective in controlling bacterial levels and enhancing microbiome diversity in larvae, in accordance with prior studies (Hines et al., 2021, Hines et al., 2022; Walburn et al., 2019; Rasmussen et al., 2022). In RAS, the proliferation of undesirable bacteria is a common issue, and various studies have emphasized the critical importance of managing bacterial populations in live feed to mitigate adverse effects (Ringø, 1999; Verschuere et al., 2000; Villamil et al., 2003; Rurangwa and Verdegem, 2015; Bentzon-Tilia et al., 2016). Such effects were observed during the trial when looking at the effect on the abundance of Cytophagales, which was significantly lower than the control, confirming the effect on bacterial population control. The limited larvae microbiome diversity (Shannon index) at 4 dph compared to the water microbiome is possibly reflecting the limited influx of the bacteria from the environment at an early larval ontogeny stage, in which they rely on their oil droplet and lack a functional digestive tract.
Regarding gut-specific microbiomes such as Rhizobacteriaceae, Parachlamydiaceae, and Peptostreptococacea, the effect on microbiome abundance after days 4 dph was significantly higher from both treatments when compared to the control, matching results from several studies such as in Asian seabass (Lates calcarifer) and roach (Rutilus rutilus caspicus) (Tarkhani et al., 2020; Ghanei-Motlagh et al., 2021).
The omnipresence across the developmental time points and treatments of OTU1 suggests the taxon possibly represents a functionally important member of the pikeperch gut microbiome. We found its taxonomical assignment (Candidatus Campbel lbacteria (Patescibacteria) based on SILVA) misleading since the blastn hits against the Patescibacteria group (taxid:1783273) retrieved nucleotide identity lower than 85%. Additional blastn searches against nt database pointed out a high similarity (97.49%-99.69%) of this predominant taxon to the order Cytophagales (Bacteroidetes/Chlorobi group). The internal controls, i.e., water samples and a dissected gut from 21-day old individual (see Materials and methods), further allowed for the identification of gut and environment-specific bacteria.
When examining the water samples collected over the course of 21 days, notable differences in the abundance of certain families become evident. Specifically, Flavobacteriaceae, Chitinophagaceae, Saprospiraceae, and Spirosomaceae were more prevalent in the probiotic group compared to the control. These species are well-known for their ability to degrade polymeric organic substances (Leahy and Colwell, 1990; Raj and Maloy, 1990). Additionally, there was a higher abundance of Sphingobacteriaceae at the 21-day mark in the probiotic group. This particular species is recognized for its fermentative capabilities concerning carbohydrates and its possession of Menaquinone, also known as vitamin K2. Vitamin K plays essential roles in blood coagulation and bone mineralization in fish; its deficiency can lead to severe consequences, including increased mortality, anemia, prolonged blood clotting times, and histopathological changes in the liver and gills (Yabuuchi et al., 1983; Krossøy et al., 2011). While no specific analysis on Vitamin K was conducted, the variance in the abundance of this family among the treatments could potentially serve as a critical factor affecting the survival outcomes. Further research to test this hypothesis is required. Another noteworthy observation is the higher abundance of Rhodobacteraceae in the rearing water of the probiotic compared to the control group at the 21-day mark. This family’s capacity for demineralizing nitrogen in the form of ammonium (Lidbury et al., 2015), and its potential role in N2O reduction (Choi et al., 2021) may have influenced the results. Rhodobacteraceae is recognized as a dominant family in bioreactor environments (Chen et al., 2019), and may play a significant role in nitrogen conversion within RAS (Attramadal et al., 2014). Conducting more comprehensive investigations into the influence of Rhodobacteraceae will provide valuable insights into whether their contributions significantly impact overall larval fitness.
The probiotic treatment exhibited slightly lower ammonia (NH3 = 0.18 ± 0.03 mg/L) and nitrate (NO3 = 0.08 ± 0.04 mg/L) levels than the live feed and control treatments. These distinctions may be attributed to the fact that larvae in the probiotic treatment were exposed to the probiotic through both the live feed and the culture water, potentially resulting in superior water conditions compared to the live feed treatment, where the water wasn’t exposed to P. acidilactici MA 18/5M.
Water addition of LAB is indeed more clearly associated with modulation of the microbial quality of the water via the direct suppression of potential pathogens in rearing water, the microbiological maturation of water, and displacement of opportunistic bacteria (Verschuere et al., 2000; Al-Dohail et al., 2009).
In general, the larval microbiome exhibited significant differences that most likely directly enhanced digestive enzyme activity, aligning with findings reported in seabass and Nile tilapia studies (Lara-Flores et al., 2003; Tovar-Ramırez et al., 2004). This effect can be observed in the trial results when examining the development of digestive enzymes over the course of 21 days.
4.3 Effect on digestive enzymes
It was previously documented that probiotics produce and stimulate specific and total activities of digestive enzymes (Sáenz de Rodrigáñez, 2009). As a consequence, an enhancement in larval digestive processes, growth, and survival should be expected by the use of these microorganisms in larval rearing protocols. In our present experiment, alkaline proteases activity was improved in pikeperch larvae during the first larval development stages (7dph) by adding probiotics to the feeding protocol in both probiotic and live feed treatments compared to the control. This improvement might be attributed to larval gut-colonization by the probiotic enhancing digestibility as previously reported by Suzer et al. (2008) in Sparus aurata. With age, the reported differences in activity were compensated with the normal increase in tissue proteins in rapid-growing larval tissues (Hamza et al., 2016; Pérez et al., 2020).
The marked difference in protease activity observed at 7 dph species in the probiotic group coincided with a higher prevalence of Pseudomonas and Flavobacterium, both of which are capable of producing proteases (Solanki et al., 2021). This finding corresponds with earlier research conducted in various species, including grey mullet (Hamid et al., 1979), salmon (Hoshino et al., 1997), arabesque greenling (Morita et al., 1998), and roach (Skrodenyte-Arbaciauskiene, 2007). Furthermore, Pseudomonas species have been identified as producers of lipase in arctic char and European seabass larvae by Ringø et al. (1995) and Gatesoupe et al. (1997), which is consistent with the results observed here at 7 dph. Finally, De Schryver et al. (2010) have documented an increase in pepsin activity in the European seabass in relation to alterations in microbiome abundance, providing a potential correlation with the findings on day 7 post-hatching. Another interesting finding was the significantly higher abundance of Idiomarinaceae at 21 dph in the larval microbiota of the probiotic compared to the control group. Idiomarinaceae, a gram-negative bacteria, is known for distinctive traits such as its primary reliance on amino acid catabolism for carbon and energy rather than sugar fermentation (Hou et al., 2014). This family of bacteria is also recognized for its production of diverse enzymes, including lipase (Li et al., 2014) again suggesting a link between microbiome modulation and digestive enzyme capacity upon administration of the test probiotic.
5 Conclusions
Our results indicate a positive effect of Pediococcus acidilactici CNCM I-4622 (MA 18/5M) application in live-feed and water. Pikeperch larval microbiota, increased in microbiome abundance as well as in digestive enzyme activities at earlier stages. Furthermore, larval growth and survival was improved. However, elucidating the interactions among the aforementioned factors will be crucial for advancing our knowledge and achieving greater progress. Novel insights into pikeperch larvae’s intestinal microbiota and host-microbiota relationships could lead to the development of effective microbiota-based methods to enhance their health, performance, and resilience. Furthermore, future research in metabolic analyses of IGF receptors and binding proteins similar to those in pikeperch larvae is recommended.
Data availability statement
The original contributions presented in the study are publicly available. This data can be found here: NCBI BioProject, PRJNA1074290.
Ethics statement
The animal study was approved by EU-harmonized Animal Welfare Act of the Czech Republic. RTD capacity permits issued to No. 58672/2020-MZE-18134 and No. 33446/2020-MZE-18134. The study was conducted in accordance with the local legislation and institutional requirements.
Author contributions
CY-R: Conceptualization, Formal analysis, Funding acquisition, Investigation, Methodology, Writing – original draft, Writing – review & editing. EN: Data curation, Methodology, Software, Writing – review & editing. EL: Resources, Writing – review & editing. LV: Data curation, Formal analysis, Methodology, Writing – original draft. AG: Formal analysis, Methodology, Writing – original draft. JP: Writing – review & editing. TPe: Data curation, Writing – original draft. TPo: Funding acquisition, Resources, Supervision, Writing – review & editing.
Funding
The author(s) declare financial support was received for the research, authorship, and/or publication of this article. Ministry of Agriculture of the Czech Republic, project NAZV QK 23020002: Support all the work done at the main experimental facility and the personnel costs: Caja site supported A. Galindo labor costs. Ministerio de Ciencia, Innovación y Universidades and Catalina Ruiz Programme funded by Consejería de Economía, Conocimiento y Empleo and FSE. finance the laboratory cost from the enzyme analysis. Lallemand: supplied the Lactic Acid bacteria.
Conflict of interest
Author EL was employed by company Lallemand SAS.
The remaining authors declare that the research was conducted in the absence of any commercial or financial relationships that could be construed as a potential conflict of interest.
Publisher’s note
All claims expressed in this article are solely those of the authors and do not necessarily represent those of their affiliated organizations, or those of the publisher, the editors and the reviewers. Any product that may be evaluated in this article, or claim that may be made by its manufacturer, is not guaranteed or endorsed by the publisher.
Supplementary material
The Supplementary Material for this article can be found online at: https://www.frontiersin.org/articles/10.3389/fmars.2024.1363522/full#supplementary-material
Abbreviations
dph, Days post hatch; RAS, Recirculation aquaculture systems; TL, Total length; BW, Body weight; MH, Myomere height; ED, Eye diameter; FFPW, Faculty of Fisheries and Protection of Waters; USB, University of South Bohemia; LMM, Linear mixed model; GLMM, Generalized linear mixed models; LAB, Lactic acid bacteria.
References
Al-Dohail M. A., Hashim R., Aliyu-Paiko M. (2009). Effects of the probiotic, Lactobacillus acidophilus, on the growth performance, haematology parameters and immunoglobulin concentration in African Catfish (Clarias gariepinus, Burchell 1822) fingerling. Aquaculture Res. 40 (14), 1642–1652.
Andrews S. (2010). FastQC: A Quality Control Tool for High Throughput Sequence Data. Available online at: http://www.bioinformatics.babraham.ac.uk/projects/fastqc/.
Attramadal K. J., Truong T. M. H., Bakke I., Skjermo J., Olsen Y., Vadstein O. (2014). RAS and microbial maturation as tools for K-selection of microbial communities improve survival in cod larvae. Aquaculture 432, 483–490. doi: 10.1016/j.aquaculture.2014.05.052
Avella M. A., Gioacchini G., Decamp O., Makridis P., Bracciatelli C., Carnevali O. (2010). Application of multi-species of Bacillus in sea bream larviculture. Aquaculture 305, 12–19. doi: 10.1016/j.aquaculture.2010.03.029
Balcázar J. L., de Blas I., Ruiz-Zarzuela I., Cunningham D., Vendrell D., Múzquiz J. L. (2006). The role of probiotics in aquaculture. Vet. Microbiol. 114, 173–186. doi: 10.1016/j.vetmic.2006.01.009
Bates D., Mächler M., Bolker B., Walker S. (2015). Fitting linear mixed-effects models using lme4. J. Stat. Softw. 67 (1), 1–14. doi: 10.18637/jss.v067.i01.
Bentzon-Tilia M., Sonnenschein E. C., Gram L. (2016). Monitoring and managing microbes in aquaculture - Towards a sustainable industry. Microbial. Biotechnol. 9, 576–584. doi: 10.1111/1751-7915.12392
Blecha M., Kristan J., Samarin A. M., Rodina M., Policar T. (2015). Quality and quantity of pikeperch (Sander lucioperca) spermatozoa after varying cold water treatments. J. Appl. Ichthyol. 31, 75–78. doi: 10.1111/jai.12853
Blecha M., Samarin A. M., Křišťan J., Policar T. (2016). Benefits of hormone treatment of both sexes in semi-artificial reproduction of pikeperch (Sander lucioperca L.). Czech J. Anim. Sci. 61, 2016–2203. doi: 10.17221/60/2015-CJAS
Borges A. K. M., Oliveira T. P. R., Rosa I. L., Braga-Pereira F., Ramos H. A. C., Rocha L. A., et al. (2021). Caught in the (inter) net: online trade of ornamental fish in Brazil. Biol. Conserv. 263, 109344.
Bradford M. (1976). A rapid and sensitive method for the quantitation of microgram quantities of protein utilizing the principle of protein-dye binding. Anal. Biochem. 72, 248–254. doi: 10.1016/0003-2697(76)90527-3
Carnevali O., Claudia M., Sulpizio Z. R., Rollo A., Nardi M., Orpianesi C., et al. (2004). Administration of probiotic strain to improve sea bream wellness during development. Aquacult. Int. 12, 377–386. doi: 10.1023/B:AQUI.0000042141.85977.bb
Carnevali O., Maradonna F., Gioacchini G. (2017). Integrated control of fish metabolism, wellbeing and reproduction: The role of probiotics. Aquaculture 472, 144–155. doi: 10.1016/j.aquaculture.2016.03.037
Carnevali O., Vivo L., Sulpizio R., Gioacchini G., Olivotto I., Silvi S., et al. (2006). Growth improvement by probiotic in European sea bass juveniles (Dicentrarchus labrax, L.), with particular attention to IGF-1, myostatin and cortisol gene expression. Aquaculture 258, 430–438. doi: 10.1016/j.aquaculture.2006.04.025
Chen Z., Chang Z., Zhang L., Jiang Y., Ge H., Song X., et al. (2019). Effects of water recirculation rate on the microbial community and water quality in relation to the growth and survival of white shrimp (Litopenaeus vannamei). BMC Microbiol. 19, 1–15. doi: 10.1186/s12866-019-1564-x
Choi M. J., Oh Y. D., Kim Y. R., Lim H. K., Kim J. M. (2021). Intestinal microbial diversity is higher in Pacific abalone (Haliotis discus hannai) with slower growth rates. Aquaculture 537, 736500.
De Schryver P., Sinha A. K., Kunwar P. S., Baruah K., Verstraete W., Boon N. (2010). Poly-beta-hydroxybutyrate (PHB) increases growth performance and intestinal bacterial rangeweighted richness in juvenile European sea bass (Dicentrarchus labrax). Appl. Microbiol. Biotechnol. 86, 1535–1541. doi: 10.1007/s00253-009-2414-9
Dharmaraj S., Dhevendaran K. (2010). Evaluation of Streptomyces as a probiotic feed for the growth of ornamental fish Xiphophorus helleri. Food Technol. Biotechnol. 48, 497–504.
Dulski T., Zakęś Z., Ciesielski S. (2018). Characterization of the gut microbiota in early life stages of pikeperch (Sander lucioperca). J. Fish Biol. 92, 94–104. doi: 10.1111/jfb.13496
Edgar R. C. (2013). UPARSE: highly accurate OTU sequences from microbial amplicon reads. Nat. Methods 10, 996–998. doi: 10.1038/nmeth.2604
Eissa E. H., Ahmed N. H., El-Badawi A. A., Munir M. B., Abd Al-Kareem O. M., Eissa M. E. H., et al. (2022). Assessing the influence of the inclusion of Bacillus subtilis AQUA-GROW® as feed additive on the growth performance, feed utilization, immunological responses and body composition of the Pacific white shrimp, Litopenaeus vannamei. Aquaculture Res. 53 (18), 6606–6615. doi: 10.1111/are.16129
Ferguson R. M., Merrifield D. L., Harper G. M., Rawling M. D., Mustafa S., Picchietti S., et al. (2010). The effect of Pediococcus acidilactici on the gut microbiota and immune status of on-growing red tilapia (Oreochromis niloticus). J. Appl. Microbiol. 109 (3), 851–862.
Fox J., Weisberg S. (2011). An R Companion to Applied Regression, Second (Thousand Oaks, CA, USA: Sage).
Fuller R. (1989). Probiotics in man and animals. J. Appl. Bacteriol. 66, 365–378. doi: 10.1111/j.1365-2672.1989.tb05105.x
García-Carreño F. L., Haard N. F. (1993). Characterization of proteinase classes in langostilla (Pleuroncodes planipes) and crayfish (Pacifastacus astacus) extracts. J. Food Biochem. 17, 97–113. doi: 10.1111/j.1745-4514.1993.tb00864.x
Gatesoupe F. J. (1999). The use of probiotics in aquaculture. Aquaculture 180, 147–165. doi: 10.1016/S0044-8486(99)00187-8
Gatesoupe F. J. (2002). Probiotic and formaldehyde treatments of Artemia nauplii as food for larval pollack, Pollachius pollachius. Aquaculture 212, 347–360. doi: 10.1016/S0044-8486(02)00138-2
Gatesoupe F. J., Zambonino Infante J.-L., Chu C., Quazuguel P. (1997). Early weaning of sea bass larvae, Dicentrarchus labrax: the effect of microbiota, with particular attention to iron supply and exoenzymes. Aquaculture 158, 117–127. doi: 10.1016/S0044-8486(97)00179-8
Ghanei-Motlagh R., Mohammadian T., Gharibi D., Khosravi M., Mahmoudi E., Zarea M., et al. (2021). Quorum quenching probiotics modulated digestive enzymes activity, growth performance, gut microflora, haemato-biochemical parameters and resistance against Vibrio harveyi in Asian seabass (Lates calcarifer). Aquaculture 531, 735–874. doi: 10.1016/j.aquaculture.2020.735874
Hai N. V. (2015). The use of probiotics in aquaculture. J. Appl. Microbiol. 119, 917–935. doi: 10.1111/jam.12886
Hamid A., Sakata T., Kakimoto D. (1979). Microflora in the alimentary tract of grey mullet. IV. Estimation of enzyme activities of the intestinal bacteria. Bull. Japan. Soc. Sci. Fish. 45, 99–106. doi: 10.2331/suisan.45.99
Hamza A., Fdhila K., Zouiten D., Masmoudi A. S. (2016). Virgibacillus proomii and Bacillus mojavensis as probiotics in sea bass (Dicentrarchus labrax) larvae: Effects on growth performance and digestive enzyme activities. Fish Physiol. Biochem. 42, 495–507. doi: 10.1007/s10695-015-0154-6
Harper G. M., Monfort M., Saoud I. P., Emery M., Mustafa S., Rawling M., et al. (2011). An ex vivo approach to studying the interactions of probiotic Pediococcus acidilactici and Vibrio (Listonella) anguillarum in the anterior intestine of rainbow trout Oncorhynchus mykiss. J. Aquaculture Res. Dev. 2 (Special issue).
Hines I. S., Ferguson C. S., Bushman T. J., Gatlin D. M., Jensen R. V., Smith S. A. (2021). Impact of a yeast-based dietary supplement on the intestinal microbiome of rainbow trout, Oncorhynchus mykiss. Aquacult. Res. 52, 1594–1604. doi: 10.1111/are.15011
Hines I. S., Santiago-Morales K. D., Ferguson C. S., Clarington J., Thompson M., Rauschenbach M., et al. (2022). Steelhead trout (Oncorhynchus mykiss) fed probiotic during the earliest developmental stages have enhanced growth rates and intestinal microbiome bacterial diversity. Front. Mar. Sci. 9, 1021647.
Hoshino T., Ishizaki K., Sakamoto T., Kumeta H., Yumoto I., Matsuyama H., et al. (1997). Isolation of a Pseudomonas species from fish intestine that produces a protease active at low temperature. Lett. Appl. Microbiol. 25, 70–72. doi: 10.1046/j.1472-765X.1997.00183.x
Hothorn T., Bretz F., Westfall P. (2008). Simultaneous inference in general parametric models. Biometric. J. 50, 346–363. doi: 10.1002/bimj.200810425
Hou H., Ding J., Zhang G., Chen L. (2014). Bacterial flora in turbot Scophthalmus maximus cultured in deepwell seawater of Liaodong Peninsula. J. Aquat. Food product Technol. 23 (6), 530–541.
Iijima N., Tanaka S., Ota Y. (1998). Purification and characterization of bile-salt activated lipase from the hepatopancreas of red sea bream, Pagrus major. Fish Physiol. Biochem. 18, 59–69. doi: 10.1023/A:1007725513389
Imentai A., Yanes-Roca C., Malinovskyi O., Policar T. (2019a). Effect of Brachionus plicatilis density on pikeperch (Sander lucioperca L.) larva performance at first feeding. J. Appl. Ichthyol. 35, 1292–1294. doi: 10.1111/jai.13963
Imentai A., Yanes-Roca C., Steinbach C., Policar T. (2019b). Optimized application of rotifers Brachionus plicatilis for rearing pikeperch Sander lucioperca L. larvae. Aquacult. Int. 27, 1137–1149. doi: 10.1007/s10499-019-00420-3
Kestemont P., Dabrowski K., Summerfelt R. C. (2015). Biology and Culture of Percid Fishes: Principles and Practices (Dordrecht, The Netherlands: Springer). doi: 10.1007/978-94-017-7227-3
Klein G., Pack A., Bonaparte C., Reuter G. (1998). Taxonomy and physiology of probiotic lactic acid bacteria. Int. J. Food Microbiol. 41, 103–125. doi: 10.1016/S0168-1605(98)00049-X
Křištan J., Alavi J., Stejskal S. M. H., Policar T. (2013). Hormonal induction of ovulation in pikeperch (Sander lucioperca L.) using human chorionic gonadotropin (hCG) and mammalian GnRH analogue. Aquacult. Int. 21, 811–818. doi: 10.1007/s10499-012-9572-y
Krossøy C., Waagbø R., Ørnsrud R. (2011). Vitamin K in fish nutrition. Aquacult. Nutr. 17, 585–594. doi: 10.1111/anu.2011.17.issue-6
Lamari F., Castex M., Larcher T., Ledevin M., Mazurais D., Bakhrouf A., et al. (2013). Comparison of the effects of the dietary addition of two lactic acid bacteria on the development and conformation of sea bass larvae, Dicentrarchus labrax, and the influence on associated microbiota. Aquaculture 376, 137–145. doi: 10.1016/j.aquaculture.2012.11.016
Lara-Flores M., Olvera-Novoa M. A., Guzmán-Méndez B. E., López-Madrid W. (2003). Use of the bacteria Streptococcus faecium and Lactobacillus acidophilus, and the yeast Saccharomyces cerevisiae as growth promoters in Nile tilapia (Oreochromis niloticus). Aquaculture 216, 193–201. doi: 10.1016/S0044-8486(02)00277-6
Leahy J. G., Colwell R. R. (1990). Microbial degradation of hydrocarbons in the environment. Microbiol. Rev. 54, 305–315. doi: 10.1128/mr.54.3.305-315.1990
Li J., Ni J., Li J., Wang C., Li X., Wu S., et al. (2014). Comparative study on gastrointestinal microbiota of eight fish species with different feeding habits. J. Appl. Microbiol. 117 (6), 1750–1760.
Lidbury I., Murrell J. C., Chen Y. (2015). Trimethylamine and trimethylamine N-oxide are supplementary energy sources for a marine heterotrophic bacterium: implications for marine carbon and nitrogen cycling. ISME J. 9, 760–769. doi: 10.1038/ismej.2014.149
Ljubobratovic U., Kosanovic D., Demény F. Z., Krajcsovics A., Vukotic G., Stanisavljevic N., et al. (2017). Supplementation of lactobacilli improves growth, regulates microbiota composition, and suppresses skeletal anomalies in juvenile pike-perch (Sander lucioperca) reared in recirculating aquaculture system (RAS): A pilot study. Res. Vet. Sci. 115, 451–462. doi: 10.1016/j.rvsc.2017.07.018
Ljubobratovic U., Kosanovic D., Vukotic G., Molnar Z., Stanisavljevic N., Ristovic T., et al. (2019). The effect of live and inert feed treatment with lactobacilli on weaning success in intensively reared pike-perch larvae. Aquaculture 516, 734608. doi: 10.1371/journal.pone.0219558
Lubzens E., Tandler A., Minkoff G. (1989). Rotifers as food in aquaculture. Hydrobiologia 186, 387–400. doi: 10.1007/BF00048937
Malinovskyi O., Kolářová J., Blecha M., Stará A., Velíšek J., Křišťan J., et al. (2019). 'Behavior and physiological status of pond-cultured pikeperch (Sander lucioperca) broodstock affected by sexual interactions throughout semi-artificial reproduction. Aquacult. Int. 27, 1093–1107. doi: 10.1007/s10499-019-00401-6
Malinovskyi O., Veselý L., Blecha M., Křišťan J., Policar T. (2018). The substrate selection and spawning behaviour of pikeperch (Sander lucioperca L.) broodstock under pond conditions. Aquacult. Res. 49, 3541–3547. doi: 10.1111/are.2018.49.issue-11
Merrifield D. L., Dimitroglou A., Bradley G., Baker R. T. M., Davies S. J. (2009). Soybean meal alters autochthonous microbial populations, microvilli morphology and compromises intestinal enterocyte integrity of rainbow trout, Oncorhynchus mykiss (Walbaum). J. Fish Dis. 32 (9), 755–766.
Métais P., Bieth J. (1968). Détermination de l’amylase par une microtechnique. Annales Biol. Clinique 26, 133–142.
Morita Y., Hasan Q., Sakaguchi T., Murakami Y., Yokoyama K., Tamiya E. (1998). Properties of a cold-active protease from psychrotrophic Flavobacterium balustinum P104. Appl. Microbiol. Biotechnol. 50, 669–675. doi: 10.1007/s002530051349
Nayak S. K. (2010). Role of gastrointestinal microbiota in fish. Aquaculture Res. 41 (11), 1553–1573.
Pérez J. A., Papadakis I., Papandroulakis N., Cruces L., Cotou E., Gisbert E., et al. (2020). The ontogeny of greater amberjack digestive and antioxidant defense systems under different rearing conditions: A histological and enzymatic approach. Aquacult. Nutr. 26, 1908–1925. doi: 10.1111/anu.13128
Policar T., Schaefer F. J., Panana E., Meyer S., Teerlinck S., Toner D., et al. (2019). Recent progress in European percid fish culture production technology—Tackling bottlenecks. Aquacult. Int. 27, 1151–1174. doi: 10.1007/s10499-019-00433-y
Quast C., Pruesse E., Yilmaz P., Gerken J., Schweer T., Yarza P., et al. (2013). The SILVA ribosomal RNA gene database project: improved data processing and web-based tools. Nucleic Acids Res. 41, D590–D596. doi: 10.1093/nar/gks1219
Raj H. D., Maloy S. R. (1990). Family Spirosomaceae: gram-negative ring-forming aerobic bacteria. Crit. Rev. Microbiol. 17, 329–364. doi: 10.3109/10408419009114761
Rasmussen J. A., Villumsen K. R., Ernst M., Hansen M., Forberg T., Gopalakrishnan S. (2022). A multi-omics approach unravels metagenomic and metabolic alterations of a probiotic and synbiotic additive in rainbow trout (Oncorhynchus mykiss). Microbiome 10, 21. doi: 10.1186/s40168-021-01221-8
Ringø E. (1999). Intestinal microflora of fish larvae and fry. Aquacult. Res. 30, 73. doi: 10.1046/j.1365-2109.1999.00302.x
Ringø E., Hoseinifar S. H., Ghosh K., van Doan H., Beck B. R., Song S. K. (2018). Lactic acid bacteria in finfish—An update. Front. Microbiol. 9, 1818.
Ringø E., Strøm E., Tabachek J. A. (1995). Intestinal microflora of salmonids: a review. Aquacult. Res. 26, 773–789. doi: 10.1111/j.1365-2109.1995.tb00870.x
Rurangwa E., Verdegem M. C. (2015). Microorganisms in recirculating aquaculture systems and their management. Rev. Aquacult. 7, 117–130. doi: 10.1111/raq.12057
Sáenz de Rodrigáñez M. A. (2009). Effect of dietary administration of probiotics on growth and intestine functionality of juvenile Senegalese sole (Solea Senegalensis, Kaup 1858). Aquacult. Nutr. 15, 177–185. doi: 10.1111/j.1365-2095.2008.00581.x
Skrodenyte-Arbaciauskiene V. (2007). Enzymatic activity of intestinal bacteria in roach (Rutilus rutilus L.). Fish. Sci. 73, 964–966. doi: 10.1111/j.1444-2906.2007.01421.x
Solanki P., Putatunda C., Kumar A., Bhatia R., Walia A. (2021). Microbial proteases: ubiquitous enzymes with innumerable uses. 3 Biotech. 11, 428. doi: 10.1007/s13205-021-02928-z
Solovyev M., Gisbert E. (2016). Influence of time, storage temperature and freeze/thaw cycles on the activity of digestive enzymes from gilthead sea bream (Sparus aurata). Fish Physiol. Biochem. 42, 1383–1394. doi: 10.1007/s10695-016-0226-2
Steenfeldt S., Fontaine P., Overton J. L., Policar T., Toner D., Falahatkar B., et al. (2015). “Current status of Eurasian percid fishes aquaculture,” in Biology and Culture of Percid Fishes. (London: Springer), 817–841.
Suzer C., Çoban D., Kamaci H. O., Saka Ş., Firat K., Otgucuoğlu Ö., et al. (2008). Lactobacillus spp. bacteria as probiotics in gilthead sea bream (Sparus aurata, L.) larvae: effects on growth performance and digestive enzyme activities. Aquaculture 280, 140–145. doi: 10.1016/j.aquaculture.2008.04.020
Tarkhani R., Imani A., Hoseinifar S. H., Ashayerizadeh O., Sarvi Moghanlou K., Manaffar R., et al. (2020). Comparative study of host-associated and commercial probiotic effects on serum and mucosal immune parameters, intestinal microbiota, digestive enzymes activity and growth performance of roach (Rutilus rutilus caspicus) fingerlings. Fish Shell. Immunol. 98, 661–669. doi: 10.1016/j.fsi.2019.10.063
Tovar-Ramırez D., Zambonino I. J., Cahu C., Gatesoupe F. J., Vazquez-Ju´rez R. (2004). Influence of dietary live ´ yeast on European sea bass (Dicentrarchus labrax) larval development. Aquaculture 234, 415–427. doi: 10.1016/j.aquaculture.2004.01.028
Vanbelle M., Teller E., Focant M. (1990). Probiotics in animal nutrition: A review. Arch. für Tierernährung 40, 543–567. doi: 10.1080/17450399009428406
Verschuere L., Rombaut G., Sorgeloos P., Verstraete W. (2000). Probiotic bacteria as biological control agents in aquaculture. Microbiol. Rev. 64, 655–671. doi: 10.1128/MMBR.64.4.655-671.2000
Villamil L., Figueras A., Planas M., Novoa B. (2003). Control of Vibrio alginolyticus in Artemia culture by treatment with bacterial probiotics. Aquaculture 219, 43–56. doi: 10.1016/S0044-8486(02)00515-X
Walburn W. J., Wemheuer B., Thomas T., Copeland E., O’Connor W., Booth M., et al. (2019). Diet and diet-associated bacteria shape early microbiome development in yellowtail kingfish (Seriola lalandi). Microbial. Biotechnol. 12, 275–288. doi: 10.1111/1751-7915.13323
Yabuuchi E., Kaneko T., Yano I., Moss C. W., Miyoshi N. (1983). Sphingobacterium gen. nov., Sphingobacterium spiritivorum comb. nov., Sphingobacterium multivorum comb. nov., Sphingobacterium mizutae sp. nov., and Flavobacterium indologenes sp. nov.: glucose-nonfermenting Gram-negative rods in CDC groups IIk-2 and IIb. Int. J. System. Bacteriol. 33, 580–598. doi: 10.1099/00207713-33-3-580
Yanes-Roca C., Leclercq E., Vesely L., Malinovskyi O., Policar T. (2020a). Use of lactic acid bacteria during pikeperch (Sander lucioperca) larval rearing. Microorganisms 8 (2).
Yanes-Roca C., Mráz J., Born-Torrijos A., Holzer A. S., Imentai A., Policar T. (2018). Introduction of rotifers (Brachionus plicatilis) during pikeperch first feeding. Aquaculture 497, 260–268.
Yanes-Roca C., Mráz J., Vesely L., Malinovskyi O., Holzer A. S., Policar T. (2020b). Live feed enrichment alternatives for Pike-Perch first feeding. Animals 10 (3), 401.
Zacarias-Soto M., Lazo J. P., Viana M. T. (2011). Effect of three probiotics administered through live feed on digestive enzyme activity in California halibut, paralichthys californicus, larvae. J. World Aquacult. Soc. 42, 321–331. doi: 10.1111/j.1749-7345.2011.00470.x
Keywords: probiotics, live feed, first feeding, Sander lucioperca larvae, lactic acid bacteria
Citation: Yanes-Roca C, Novakova E, Leclercq E, Vesely L, Galindo A, Pérez JA, Penka T and Policar T (2024) Pikeperch (Sander lucioperca) larval rearing optimization: utilization of lactic acid bacteria for improving microbiome diversity and digestive enzyme activity. Front. Mar. Sci. 11:1363522. doi: 10.3389/fmars.2024.1363522
Received: 30 December 2023; Accepted: 05 March 2024;
Published: 27 March 2024.
Edited by:
Khor Waiho, University of Malaysia Terengganu, MalaysiaReviewed by:
Jingjing Tian, Chinese Academy of Fishery Sciences, ChinaLaura Ballesteros Redondo, University of Rostock, Germany
Copyright © 2024 Yanes-Roca, Novakova, Leclercq, Vesely, Galindo, Pérez, Penka and Policar. This is an open-access article distributed under the terms of the Creative Commons Attribution License (CC BY). The use, distribution or reproduction in other forums is permitted, provided the original author(s) and the copyright owner(s) are credited and that the original publication in this journal is cited, in accordance with accepted academic practice. No use, distribution or reproduction is permitted which does not comply with these terms.
*Correspondence: Carlos Yanes-Roca, Y3lhbmVzcm9jYUBqY3UuY3o=