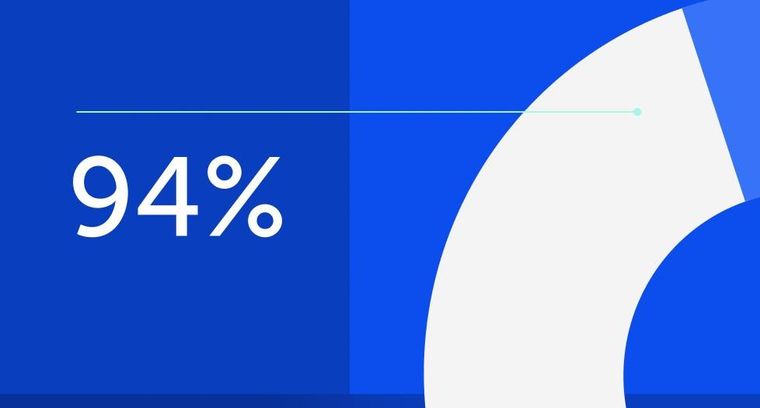
94% of researchers rate our articles as excellent or good
Learn more about the work of our research integrity team to safeguard the quality of each article we publish.
Find out more
ORIGINAL RESEARCH article
Front. Mar. Sci., 08 August 2024
Sec. Coral Reef Research
Volume 11 - 2024 | https://doi.org/10.3389/fmars.2024.1361137
In the Caribbean, reef-building scleractinian corals have declined precipitously and octocorals have emerged as one of their main successors. The success of octocorals and the formation of octocoral forests has been attributed to their continuing recruitment to reef habitats, as well as tolerance to pollution, reduced direct competition with scleractinians, and resistance and resilience to climatic events. Benthic grazers on coral reefs can facilitate the growth and recruitment of corals by reducing the abundance of competitive algal turfs and macroalgae. However, grazing can also hinder corals through sublethal damage to coral tissue and predation of recruits. We assessed the effects of grazing by fishes and the sea urchin Diadema antillarum as well as predation by mesofauna on octocoral recruitment through a series of manipulative in situ and ex situ experiments. Exposure to fish and urchin grazing significantly reduced the post-settlement survival of octocoral recruits, while turf-associated mesofauna did not significantly affect recruitment. We also found a positive relationship between octocoral recruitment and the abundance of turf algae, which may reflect the deleterious effect of grazing on both turf algae and octocoral recruits. These data suggest that grazers and predators mediate the bottleneck characteristic of recruitment, primarily through their effect on post-settlement survival. Thus, the declines in the abundance of grazing fishes and urchins throughout the Caribbean may have contributed to the increase in Caribbean octocoral abundance, concurrent with the loss of scleractinians.
Many taxa, including plants, algae, and sessile invertebrates, exhibit complex life histories characterized by dispersive propagules and sessile adults. Propagules must locate and select a permanent location where all subsequent stages can grow and survive. Settlement location and the subsequent post-settlement mortality can have major implications on population dynamics and ecosystem function (James et al., 2011; Chou et al., 2018). For many species, mortality of recruits (i.e., the newly established individuals) is high, creating a bottleneck in their demographics, and even when recruit survival is not the key factor determining species abundance (e.g., Zuidema and Franco, 2001), the magnitude of the mortality can exert potent selective pressures. Identifying and quantifying the factors that drive early selection and subsequent community structure is critical to understanding the dynamics of contemporary populations and how those populations will respond to anthropogenic environmental changes.
Understanding the dynamics of early life stages is particularly crucial in ecosystems such as Caribbean coral reefs, where reef-building scleractinian corals have declined precipitously (Gardner et al., 2003; Bellwood et al., 2004; Hughes et al., 2018) and octocorals have emerged as one of their major successors (Ruzicka et al., 2013; Lenz et al., 2015; Sánchez et al., 2019). Octocoral forests (sensu Rossi et al., 2017) provide some of the same functions as scleractinian reefs, such as creating refuges for grazing fishes and improving local coral recruitment (e.g., Privitera-Johnson et al., 2015; Tsounis et al., 2020). Unlike scleractinians (Gardner et al., 2003), octocorals are resilient (Lasker et al., 2020) and sometimes resistant (Yoshioka and Yoshioka, 1987) to climatic events like hurricanes. Additionally, octocoral success has been attributed to higher fecundity, greater juvenile survivorship and recruitment, tolerance to pollution, resilience to bleaching events, and reduced direct competition with scleractinians (Lenz et al., 2015; Tsounis and Edmunds, 2017; Lasker et al., 2020; Martínez-Quintana and Lasker, 2021; Coffroth et al., 2023; Rey-Villiers et al., 2023).
Scleractinian corals are particularly sensitive to the post-settlement survival bottleneck. Although recruits can settle in high densities, they frequently do not develop into adult colonies (Chong-Seng et al., 2014; Edmunds, 2021). Macroalgae are often highlighted as a threat to juvenile recruitment of both scleractinians and octocorals (Kuffner et al., 2006; Wells et al., 2021), and the decline of scleractinians in the Caribbean has been associated with the increase in macroalgae abundance (Hughes, 1994). Proposed mechanisms for this negative interaction include allelopathic competition (Kuffner et al., 2006), algae serving as reservoirs for virulent disease (Nugues et al., 2004), microscale alterations of conditions in the benthic boundary layer (Carpenter and Williams, 1993), and physical interactions between polyps and algae (River and Edmunds, 2001). Wells et al. (2021) suggested that turf algae may also negatively impact octocoral recruits by providing habitat to corallivorous mesofauna.
While octocorals and scleractinians are both negatively affected by algal competition (Lasker and Kim, 1996; Lirman, 2001; Kuffner et al., 2006; Hughes et al., 2007; Wells et al., 2021), their response to grazing is more complex. Grazers frequently facilitate scleractinian growth, fecundity, and recruitment by removing algae (Lirman, 2001; Nugues and Bak, 2006; Stockton and Edmunds, 2021). However, urchin grazing can reduce recruitment and growth by direct consumption of coral tissue (Bak and van Eys, 1975; Davies et al., 2013). Some particularly palatable coral species are excluded from reef habitats by parrotfish through direct grazing (Littler et al., 1989). Fish can reduce competition between recruits and macroalgae by directly consuming algae, but this can result in incidental recruit predation. Doropoulos et al. (2016) found that scleractinian corals settled within crevices on exposed surfaces where fish removed algal competitors but could not access the recruits.
The impact of grazers on octocorals is less studied, but the available observations suggest grazing negatively affects octocoral recruitment. For example, octocoral recruitment increased two orders of magnitude in Puerto Rican reefs in 1984, concurrent with a mass mortality of the grazing sea urchin Diadema antillarum (Yoshioka and Yoshioka, 1989). Yoshioka and Yoshioka (1989) postulated that the loss of D. antillarum led to a consolidation of loose sediments by algae, leading to less frequent burial of octocoral recruits. Urchin grazing also affected octocoral recruit distribution in the temperate Gulf of Maine; recruits were more often found among adult conspecifics where they were consumed far less frequently by urchins than those that settled outside adult aggregations (Sebens, 1983b). In several octocoral recruitment studies, exposure to fish grazing led to reduced octocoral recruitment (Lasker et al., 1998; Evans et al., 2013; Wells et al., 2021), primarily through their grazing activities, although predation was never directly observed.
This study investigates the factors influencing recruitment of octocorals, specifically focusing on predation by mesofauna, grazing fishes, and sea urchins. To achieve this, we used field experiments to quantify fish and urchin impacts on newly-settled octocorals by excluding large herbivorous fish with cages and caging D. antillarum with octocoral polyps. Additionally, we quantified the effects of mesofauna on recruitment in a laboratory experiment by naturally and experimentally manipulating the abundance of mesofauna. We hypothesized that grazers and mesofauna would negatively affect octocoral recruitment through incidental or intentional predation of primary polyps.
The effects of grazing and predation on octocoral recruitment were assessed through a series of sequential experiments using unglazed terracotta settlement tiles (Supplementary Figure 1 and Supplementary Figure 2). Settlement tiles (15 × 15 × 1 cm, n = 40) were initially deployed and conditioned on an octocoral-dominated reef in Grootpan Bay, St. John, U.S. Virgin Islands (18.309° N, 64.719° W) on April 12, 2021. The tile undersides had 22 3-mm deep vertical grooves providing refugia for algae and invertebrate recruits. Tiles were attached to the reef with 10-cm long stainless-steel rods with the grooved side down, positioned 2-5 cm above the substratum.
To investigate the impact of excluding fish predators on octocoral recruitment, we caged tiles on the reef and allowed octocorals to settle. At the start of the experiment, the tiles had been conditioned for two months. Any octocorals that settled during the conditioning period (12 individuals) were removed at that time. The experiment coincided with summer spawning of plexaurid octocorals (Kahng et al., 2011), and was designed to generate seasoned tiles for the subsequent invertebrate removal experiment. At the start of the experiment, 30 tiles were caged in polyvinyl chloride-coated steel mesh cages (1.3 × 1.3 cm square mesh, 25 × 25 × 15 cm) to exclude large invertebrates and fish from feeding on algae and invertebrates. Ten tiles were left uncaged to allow grazing. After seven weeks, we counted octocoral recruits that had settled on each tile and mapped their positions, so that we could identify individuals throughout subsequent experiments.
At completion of the initial caging experiment, the tiles were transferred to sea tables with flowing sea water at the Virgin Islands Environmental Research Station to test the effect of invertebrate predation on octocoral recruitment. The tiles were removed from the reef six days after the July full moon so that larvae collected from the field would be competent to settle during the experiment. We applied one of three treatments to the previously caged tiles: (1) left in a sea table with ten non-terminal phase bluehead wrasse Thalassoma bifasciatum (8-11 cm standard length, herein referred to as “fish removal” tiles) for three days, which removed most of the motile invertebrates; (2) soaked in 7.5% magnesium chloride for 30 minutes, shaken briefly to dislodge motile invertebrates, and then large solitary invertebrates were removed by hand (herein referred to as “manual removal” tiles); or (3) left alone (herein referred to as “caged” tiles). The ten tiles left outside cages in the field were not manipulated and are referred to as “open” tiles. The amphinomid worm Hermodice carunculata, when present, was removed from all treatments as it is a voracious predator of coral recruits (Wolf and Nugues, 2013) and would likely hide the effect of other invertebrate predators. Invertebrates removed from three of the manual removal tiles were preserved in 70% ethanol and their identity and abundances quantified. Invertebrates from one tile of each treatment were identified and counted to determine the effectiveness of each invertebrate removal treatment by soaking them in magnesium chloride for 30 minutes, shaking them, and then manually picking any remaining invertebrates off the tile. These method-testing tiles were not used in the settlement experiment, leaving 36 tiles (9 per treatment). The undersides of the tiles, where octocorals primarily settle (Martínez-Quintana and Lasker, 2021; Wells et al., 2021), were photographed to categorize benthic cover after treatments were applied. Photographs of the submerged tiles were taken with an Olympus Tough TG-6 (12 megapixels) held 15 cm from the tile. Turf height was measured in five locations on the underside of the tiles with a Vernier caliper.
After three days in the laboratory, tiles were placed in 14 L clear plastic containers, one tile per container, refuge side down, and elevated by a ring of mesh as in Wells et al. (2021). Water circulation in each container was provided by bubbling air from a glass Pasteur pipette attached to an air pump. Water movement was slow, but circulation was observed throughout the container. Ambient sunlight was provided from large south facing windows and the temperature of the room was maintained at 28°C. Every day, 25% of the water in each container was changed.
Octocoral planulae were collected from the water column within five meters of the surface at Grootpan Bay on August 1, 2021 (nine days after the full moon). Fifty-three competent octocoral planulae were added to each container (n = 36, nine containers per treatment). Planulae settled over the following ten days, after which all polyps on the tiles were mapped again, providing an assessment of settlement in the different treatments. Polyps present before the experiment began were identifiable by their previously mapped position and size relative to newly settled polyps. As size has may offer greater resistance to predation by mesofauna, individuals that settled during the initial caging experiment were excluded from analyses.
To assess the effects of urchin and fish grazing on octocoral survival and recruitment in the field, we redeployed the tiles onto steel rods in Grootpan Bay, eleven days after we introduced planulae to the tiles in the laboratory experiment. Of the 27 tiles that were caged in the initial caging experiment, the three tiles with the fewest octocoral settlers were dropped and the remaining 24 were placed back into cages to exclude fish. The 9 non-caged treatment tiles were redeployed uncaged. One small (1.5-2.5 cm test diameter) long-spined sea urchin Diadema antillarum was added to half of the cages to test the effect of urchin grazing on field settlement and survival. Survival of the recruits settled in the lab as well as settlement and survival of exogenous octocorals were tracked over 18 days, with observations on days 0, 3, 6, 12, and 18. Octocorals are difficult to identify before the axial skeleton develops, which precluded species or genus level identification of the polyps in this experiment. Photographs of the undersides of the tiles were taken on days 0, 6, 12, and 18 to compare settlement and survival with benthic cover.
Benthic cover on tiles throughout the laboratory and field experiments was quantified from photographs using CoralNet, a cloud-based website and platform for semi-automatic analysis of benthic images (Beijbom et al., 2015). The EfficientNet feature extractor was used as it was trained on more data and is generally 2-3% more accurate on average than the previous VGG16 feature extractor (Chen et al., 2021). Initially, 25 randomly distributed points were overlaid on each image and benthic cover was manually annotated. These data (4250 annotations across 170 images) were used to train an automatic annotator using the computer vision algorithm. The same images were then overlaid with an 18 × 18 grid of points (324 points per image). These points were automatically annotated by the vision algorithm when the algorithm was ≥84% confident in its identification (95% accurate). A 5% reduction in annotation accuracy has marginal impacts on cover estimates (Beijbom et al., 2015). Points in which the algorithm was <84% confident were manually annotated. Benthic cover was characterized using the following categories: bare space, colonial and solitary ascidians, bryozoans, crustose coralline algae, non-coralline encrusting algae, upright algae, sponges, turf algae, coral polyps, mollusk eggs, calcareous worm tubes, and proteinaceous worm tubes. These broad categories were chosen to characterize general shifts in community composition on the tiles (e.g., increasing turf cover) without the destructive sampling that would be required to identify taxa at higher resolution.
The effects of initial fish exclusion via caging, invertebrate removal, and sea urchin inclusion/fish exclusion on octocoral recruitment were tested with generalized linear models (GLM) with treatment as a fixed factor using Poisson error distributions in R version 4.2.1 (R Core Team, 2022). Equidispersion was checked, and when models were not equidispersed, a negative binomial error distribution was used (R package MASS 7.3-57, Venables and Ripley, 2002). 95% bias-corrected and accelerated bootstrap confidence intervals were calculated with 100,000 replicates (R package bcaboot 0.2-3, Efron and Narasimhan, 2021).
Benthic cover in each experiment was visualized with non-metric multidimensional scaling (nMDS) using a Bray-Curtis dissimilarity index with 9999 random starts to find a stable solution with two dimensions (R package vegan 2.6-2, Oksanen et al., 2022). When the final solution had a stress over 0.18, three dimensions were used. The effect of individual benthic cover types on recruitment was assessed for cover types with more than 1% of the total cover using smoothing functions in generalized additive models (GAMs, R package mgcv 1.8-40, Wood, 2021). To determine the effect of turf height on octocoral recruitment in the invertebrate removal experiment, an additional GAM was run with turf height as a smoothing function and recruitment as the response variable. In the urchin inclusion and fish exclusion experiment, the effect of exposure to predation by fish and urchins on recruitment was analyzed using a GAM with sampling day as a smoothing function and treatment as a fixed factor. Variance components were estimated by restricted maximum likelihood with penalized cubic regression splines for smoothing, three knots for basis construction, and a negative binomial error distribution. Basis dimension choices were assessed for oversmoothing and where basis functions were too small, we added more knots, up to ten. Additionally, we tested for concurvity in smooths.
Time-dependent Cox proportional hazard (CPH) models were used to determine the effect of exposure to urchins, fish, and benthic cover on survival of octocoral polyps (R package survival 3.3-1, Therneau, 2022). The assumption that the relative effect of the hazard is proportional was tested prior to the analysis. When this assumption failed, piece-wise exponential additive mixed models (PAMM) were used (R package pammtools 0.5.8, Bender et al., 2018). PAMMs allow for time-varying covariates such as benthic cover and allow for the calculation of hazard ratios without the need for proportional hazards (Bender et al., 2018). Hazard ratios were calculated to compare treatments and levels of cover types with a significant effect on survival. Hazard ratios, in this case, depict the probability of mortality between time-steps for each treatment relative to the probability of mortality between time-steps for either a reference category for discrete variables or a reference value for continuous variables. For these models, we used either “caged” tiles as the reference category or median cover for benthic cover analyses. Hazard ratios significantly greater than 1.0 indicate that level is more hazardous than the reference, while those below 1.0 indicate it is less hazardous.
In the initial caging experiment, 74 octocoral polyps recruited across the 40 tiles in 47 days. Recruitment was patchy; many tiles had no recruits (15 of 30 caged tiles and 6 of 10 open tiles). One caged tile had an exceptionally high recruitment of 24 polyps, but with no a priori reason to remove it, it was included in the analysis. Recruitment was significantly higher on tiles within cages compared to uncaged tiles (Figure 1, GLM, df = 38, deviance = 5.37, p = 0.02). Mean recruitment on caged tiles was 2.1 polyps × tile-1 (95% CI [1.2 – 5.1]) whereas open cages had a mean recruitment of 1.0 polyps × tile-1 (95% CI [0.2 – 2.0]). nMDS characterizations of composition on the undersides of the tiles greatly overlapped among treatments (Supplementary Figure 3). Octocoral recruitment was positively associated with increased cover of turf algae (Figure 2A, GAM, edf = 0.95, χ2 = 3.81, p = 0.03) and negatively associated with increased cover of colonial ascidians (Figure 2B, GAM, edf = 1.08, χ2 = 6.05, p < 0.01).
Figure 1 Effect of tile treatment on the number of recruited octocorals. Error bars are bootstrapped 95% confidence intervals of the means.
Figure 2 Effect of (A) turf algae and (B) colonial ascidian cover on octocoral recruitment on the underside of settlement tiles in the initial caging experiment. Bold lines are fitted predictions from a generalized additive model with 95% confidence intervals. Predictor variables are shown by rug plots on each abscissa.
In the invertebrate removal experiment 606 of 1908 octocoral planulae (32%) settled and metamorphosed across the 36 tiles. Octocoral recruitment did not vary significantly between removal treatments (Figure 3, GLM, deviance = 0.63, F3,32 = 0.21, p = 0.89). Mean recruitment across all treatments was 5.8 polyps × tile-1 (95% CI [4.9 – 6.7]).
Figure 3 Effect of invertebrate removal treatment on the number of recruited octocorals in the laboratory experiment. Error bars are bootstrapped 95% confidence intervals of the means.
The manual removal treatment was effective at removing annelid worms, arthropods, mollusks, and nematodes (Supplementary Table 1). At the end of the experiment, invertebrate abundance was lower across all treatments, except for copepods, cephalocaridians, and nematodes in the fish removal treatment; nematodes in the manual removal treatment; and isopods in the reef removal treatment (Supplementary Table 2). Bare space on the tiles was more abundant at the end of the experiment although tiles that were originally caged in the initial caging experiment maintained higher levels of turf throughout the laboratory experiment (Supplementary Figure 4). Octocoral recruitment in the lab experiment did not significantly respond to any benthic cover category (GAM, χ2 ≤ 1.38, p ≥ 0.12) nor turf height (GAM, edf = 2.13, χ2 = 5.29, p = 0.12).
The 33 tiles that were outplanted to the reef following the laboratory settlement contained a total of 587 polyps, and 312 (53%) of those polyps survived to day 18. The urchin inclusion and non-caged treatments were significantly more hazardous to octocoral polyps than the caged treatment (CPH, z ≥ 2.09, p ≤ 0.03) with a 35% higher chance of dying in the next time step when exposed to an urchin and a 67% higher chance of dying in the next time step when exposed to fish (Figure 4A). Increased cover of coralline algae, calcified worm tubes, and turf algae were associated with reduced survival of octocoral polyps (PAMM, z ≥ 2.09, p ≤ 0.04). For every 1% increase in coralline algae, calcified worm tubes, and turf algae cover, there was a respective 4.0%, 2.5%, and 1.7% increase in the likelihood of dying in the next time step.
Figure 4 Effect of (A) experimental treatment, (B) coralline algae cover, (C) calcified worm tube cover, and (D) turf cover on hazard ratio for polyps on tiles redeployed to the field. The hazard ratio is the probability of mortality in the next time step relative to the probability of mortality of the reference value (the red point). Reference values for benthic cover types is the median cover. Error bars and shaded areas are 95% confidence intervals of the hazard ratio.
Over the course of the 18-day urchin inclusion and fish exclusion experiment, 191 additional polyps recruited to the tiles. While these polyps were unidentifiable by visual characteristics, it was clear that several species of octocorals were settling on the tiles based on initial size of the polyps and color. Octocoral recruitment did not have a significant response to the urchin inclusion and fish exclusion treatments (Figure 5A, GLM, deviance = 0.70, F2,30 = 0.35, p = 0.71) but was related to sample day, with more recruitment at the beginning and end of the experiment than in the middle (Figure 5B, GAM, edf = 1.94, χ2 = 70.6, p < 0.01). High settlement occurred during periods when many planulae were observed in the water column. nMDS characterizations of composition on the undersides of the tile greatly overlapped among treatments and did not change greatly over time (Supplementary Figure 5). Octocoral recruitment on the undersides of the tiles was negatively associated with increased levels of coralline algae (Figure 5C, GAM, edf = 1.11, χ2 = 4.61, p = 0.02).
Figure 5 Effect of (A) experimental treatment, (B) sampling day, and (C) coralline algae cover on exogenous octocoral settlement on tiles redeployed to the field. (A) Error bars are bootstrapped 95% confidence intervals of the means and data points are jitter along both axes for visibility. (B) The bold line is the fitted prediction from a generalized additive model with shaded 95% confidence intervals. (C) Predictor variables are shown by a rug plot on the abscissa.
Grazers on coral reefs play a crucial role in shaping benthic community dynamics by either facilitating settlement and the subsequent survival of settlers through the removal of competitors, such as turf algae, or by reducing survival through direct consumption of coral tissue. Our experiments reflect both effects. Exposure to fish and urchin grazing significantly reduced survival of octocoral recruits (Figures 1, 4A), highlighting the negative impact of these grazers on octocoral recruits. In contrast, we did not observe differences in recruitment across treatments in the lab experiment indicating that mesofauna did not significantly affect recruitment (Figure 3), underscoring the dominant influence of larger grazers and predators. We also found significant relationships between octocoral recruitment and benthic cover in our field experiments (Figures 2, 4, 5), but these correlations likely reflect exposure to grazing instead of facilitatory and inhibitory interactions. The interplay between grazer abundance and recruitment dynamics underscores the importance of grazers in mediating the mortality bottleneck characteristic of early octocoral life stages. Consequently, the observed declines in grazing fishes and urchins throughout the Caribbean may be a key factor contributing to the recent increases in octocoral populations, particularly in the context of declining scleractinian corals.
Exposure to D. antillarum urchin grazing led to decreased survival (Figure 4A), indicating that D. antillarum can have both a direct negative effect (this study) and an indirect negative effect, as previously shown by Yoshioka and Yoshioka (1989) by preventing algal growth that binds otherwise mobile sediments. Within our urchin inclusion cages, sections of the tiles were denuded of live cover, except for coralline crusts and calcium carbonate worm tubes. Octocoral recruits within those areas were all removed. While it is unknown whether removal of newly-settled octocorals was via direct consumption or incidental dislodgement as urchins grazed on the benthic community, the effect is the same. These findings likely reflect natural uncaged interactions between newly-settled octocorals and urchins, as the grazing behavior we observed was typical of D. antillarum (i.e., areas denuded of live cover) and the urchin density in our experiments was comparable to densities documented prior to the epidemic (Lessios, 2016).
Urchins negatively affected recruit survival but did not affect octocoral settlement (Figure 5A). Settlement on tiles caged with urchins was similar to that on tiles in cages without urchins or tiles outside of cages. Settlement in the field was only related to the sampling day (Figure 5B). We observed elevated settlement approximately ten days after the full moon, which is approximately five days after predicted spawning for a number of octocoral species (Kahng et al., 2011). This timing matches the findings of Tonra et al. (2021) and Lasker and Kim (1996), where Caribbean plexaurid octocorals took five days for median competency. Plexaurids were the most abundant family of octocorals that spawned during the time of this study (Kahng et al., 2011).
Similar experiments examining the interactions between urchins and scleractinian corals have found the relationship between the two taxa to be facilitatory in some circumstances and detrimental in others. Generally, urchin grazing improves recruitment of scleractinians by removing macroalgae and exposing crustose coralline algae (Edmunds and Carpenter, 2001). However, urchins at high densities can reduce survival of particularly palatable scleractinian species on surfaces without grazing refugia (Sammarco, 1980; Davies et al., 2013). In a series of manipulative experiments, Sammarco (1980) found that coral survival was highest at intermediate levels of D. antillarum density, and that at a density of 16 adult urchins × m-2 scleractinian recruitment was reduced by an order of magnitude compared to replicates without D. antillarum. In our experiments, a single small D. antillarum (≤ 2.5 cm test diameter) was present in each replicate (density = 16 urchins × m-2) with 3 mm deep crevices as potential refugia. This density of urchins was too high or the refugia were insufficient to protect octocorals from grazing pressure. Urchin grazing capabilities should also scale with urchin size. Indeed, small urchins (1 cm Mespilia globulus), in an urchin-coral co-culturing experiment, improved growth and survival of scleractinians by reducing algal competition, even at high urchin densities (75 urchins × m-2, Craggs et al., 2019). Additionally, grazing rates can vary among species of urchin. The Caribbean reef urchin Echinometra viridis also grazes on reefs, but unlike D. antillarum, they do not exert control over local algal cover. In fact, E. viridis abundance was positively correlated with algal abundance in Belize (McClanahan, 1999), rather than inversely correlated as has been observed with D. antillarum (Hughes, 1994).
Prior to the catastrophic die-off of D. antillarum in 1983-1984, octocoral recruitment was likely reduced both directly and indirectly by urchins. After the die-off, this pressure was relieved, leading to the impressive recruitment pulse observed by Yoshioka and Yoshioka (1989). In the decades since the first die-off, D. antillarum recovery has been modest (reviewed in Lessios, 2016) with some small-scale restoration efforts finding success (e.g., Maciá et al., 2007). However, the effects of D. antillarum on modern reefs are a fraction of their effects 40 y ago and another epidemic is currently reducing D. antillarum abundance across the Caribbean once again (Hylkema et al., 2023). Unlike the first epidemic, the decline of the relatively low-density populations on contemporary reefs is unlikely to improve octocoral recruitment. In fact, the effect of losing the few remaining D. antillarum may be detrimental to octocoral recruitment through increased competition with algae as was seen in the first epidemic with scleractinian corals (e.g., Sammarco, 1980), a topic for future research.
Similar to the effect of urchins, exposure to fish decreased survival, but not settlement, of octocorals (Figures 4A, 5). While predation was never directly observed, fourspot butterfly fish (Chaetodon capistratus) were frequently seen inspecting the open tiles, presumably looking for cnidarian polyps and annelids, their preferred foods (Liedke et al., 2018). In addition, parrotfish (scarids) and tangs (acanthurids) were observed grazing on the tiles and grazing scars from fish were abundant, especially on the upper surfaces, similar to observations made by Wells et al. (2021). In a similar fish exclusion study, octocoral recruit survival was significantly improved within cages where fish predation was reduced (Evans et al., 2013). In the same study, scleractinian recruit survival and fish exposure were not related. Scleractinian recruits seem to be avoided by grazing fishes (Birkeland, 1977), although grazing fishes with relatively large gapes and less discriminating grazing behavior can remove scleractinian recruits (Christiansen et al., 2009). In some locations, reduced scleractinian recruitment has been associated with elevated grazing pressure (Penin et al., 2010). Scleractinian recruits are partially protected from predation by their calcium carbonate skeleton, which octocorals lack. Octocorals employ secondary metabolites and calcium carbonate sclerites as an antipredator deterrent (Sammarco and Coll, 1992; Van Alstyne et al., 1992). Chemical defenses can lead both specialist and naïve predators to reject larvae (Harvell et al., 1996), but if a grazer or predator inadvertently consumed an octocoral recruit but rejected it as unpalatable, the result is still death for the recruit.
Octocoral recruitment was not reduced by mesofauna in the laboratory experiment (Figure 3). Wells et al. (2021) hypothesized that the reduced survival in turf algae could have been caused by elevated levels of mesofauna within the turf. Our results demonstrate that mesofauna did not affect survival, at least when measured over a 10-d experiment. While mesofauna did not reduce recruitment, there are several invertebrates that actively consume octocorals such as the amphinomid worm H. carunculata and ovulid gastropods in the genus Cyphoma (Lasker and Coffroth, 1988; Vreeland and Lasker, 1989). Both of these taxa were excluded in the laboratory experiments. Caged treatments in the field did not exclude H. carunculata. Larger Cyphoma spp. should have been excluded by the cages, and we never observed small Cyphoma on any of the tiles. The effects of predation on recruits may even be greater than measured in our experiments if predation by these two groups is considered. Furthermore, fishes feed on both of these octocoral predators and decreased fish abundance has been shown to increase the abundance of Cyphoma gibbosum (Burkepile and Hay, 2007; Ladd and Shantz, 2016).
In the initial caging experiment, tiles with more turf algae on their undersides had higher octocoral recruitment (Figure 2A). However, it is important to note that turf algae are a multispecies community and different species within this group could differentially influence octocoral recruitment. Coral planulae can use mechano- and chemoreception to identify suitable areas for settlement (Müller and Leitz, 2002) suggesting that the specific species composition of turf algae may play a role in this process. Conversely, octocoral recruitment was reduced on tiles with more colonial ascidians (Figure 2B). Neither cover category dominated the undersides. Both were less than 9% of the total benthic cover and there was sufficient empty tile space for octocoral settlement (11%). In addition, there was an average of 35% crustose coralline algae cover, a preferred settlement location for some octocorals (Lasker and Kim, 1996). One hypothesis for this relationship is that these two benthic groups, turf algae and colonial ascidians, were indicator taxa for how grazed the underside of a tile was with more grazed tiles having less turf and more colonial ascidians. Interestingly, Wells et al. (2021) found that more octocorals recruited and then survived longer on tiles with less turf algae, opposite of this study. Perhaps, at low levels of turf cover, the competition between octocoral recruits and turf algae is less detrimental to octocoral recruitment than the presence of potential grazers and so both taxa increase in abundance together controlled by a similar mechanism. We observed several instances of sponges and colonial ascidians overgrowing octocoral recruits, but generally these interactions were limited to a few recruits that settled directly next to these taxa on a few tiles.
Unexpectedly, in the urchin inclusion and fish exclusion experiment, when moved back to the reef, tiles with more crustose coralline algae had less settlement (Figure 5C) and tiles with more coralline algae were more hazardous to recruits (Figure 4B). The abundant coralline algae, may be a product of heavier grazing pressure, similar to turf algae and colonial ascidians in the initial caging experiment. The negative relationship between polyp survival and calcified worm tubes (Figure 4C) suggests this is the most likely explanation as there are no studies that indicate calcified worm tubes negatively affect coral recruits. In fact, some cnidarians can be induced to settle when presented with calcified worm tubes (Chia and Spaulding, 1972). Calcified worm tubes were present in similar abundances on all tiles but were only visible for cover analysis from photographs if they were not covered by other benthic cover groups, such as turf algae. Additionally, the negative relationship between recruitment and coralline algal cover is in contrast to many studies indicating the importance of coralline algae on settlement of octocorals (e.g., Sebens, 1983a; Lasker and Kim, 1996). However, not all corals are induced to settle by coralline algae and not all coralline algae induce settlement of corals (Morse et al., 1988; Heyward and Negri, 1999). Additionally, coralline algae can actively deter settlement and defend themselves from being fouled by shedding epithelial cells, overgrowing organisms that grow on them, and exuding allelopathic chemicals (Sebens, 1986; Keats et al., 1997; Jorissen et al., 2020). Thus, the inverse relationship between successful recruitment and coralline algal cover could also represent an interaction between the two taxa. Opposite to the initial caging experiment, but similar to findings of Wells et al. (2021), turf algae reduced survival in the urchin inclusion and fish exclusion experiment (Figure 4D). This disparity may be due to the difference in ranges of turf cover in the two experiments. In the initial caging experiment, turf algae were always less than 21% cover whereas in the urchin inclusion and fish exclusion experiment the turf cover ranged from 0 to 59%. At higher levels of turf cover, competition with turf algae may become more detrimental, reducing survival.
Our experiments indicate that post-settlement survival of Caribbean octocorals is mediated by urchin and fish grazing, and not by mesofaunal invertebrates. Our experiments did not include the effects of specialist predators like ovulid snails and amphinomid worms and the role of grazing and predation may be greater still if those taxa are considered. Our observations suggest that the rise in octocoral abundance in the Caribbean is partly due to the decline of herbivorous fishes, through overfishing, and urchins, which have been affected by a pair of epidemics, and is not solely linked to their resilience to climate change and bleaching (e.g., Coffroth et al., 2023).
Regardless of whether a recruit is a colonial invertebrate or a seedling, their survival is controlled by a complex interplay of direct and indirect effects. Our examination of grazing and predation on octocoral recruits illustrates how different classes of predators can have varied and often nonlinear effects on survival. In the case of Caribbean octocorals, natural events such as the D. antillarum epidemic and anthropogenic changes in the abundance of fishes have undoubtedly changed recruit success. These findings underscore the importance of considering multiple ecological factors when predicting future changes in increasingly stressed ecosystems. As global ocean conditions continue to change, understanding these dynamics will be crucial for predicting and managing the resilience and composition of coral reef communities.
The raw data supporting the conclusions of this article will be made available by the authors, without undue reservation. Data will also be available on Biological and Chemical Oceanography Data Management Office (https://www.bco-dmo.org/).
The animal study was approved by Institutional Animal Care and Use Committee, University at Buffalo. The study was conducted in accordance with the local legislation and institutional requirements.
CW: Conceptualization, Data curation, Formal analysis, Investigation, Methodology, Project administration, Validation, Visualization, Writing – original draft, Writing – review & editing. JB: Conceptualization, Investigation, Writing – original draft, Writing – review & editing. KT: Conceptualization, Investigation, Writing – original draft, Writing – review & editing. EA: Conceptualization, Investigation, Writing – original draft, Writing – review & editing. HL: Conceptualization, Investigation, Methodology, Writing – original draft, Writing – review & editing.
The author(s) declare financial support was received for the research, authorship, and/or publication of this article. This research was funded by the National Science Foundation (OCE 17-56381).
This work was completed under a permit from the Virgin Islands National Park (VIIS-2021-SCI-0008). We thank the Virgin Islands Environmental Research Station and the University of the Virgin Islands for laboratory space and P.J. Edmunds for reading and commenting on the manuscript. This manuscript has previously appeared online as a preprint (Wells et al., 2022).
The authors declare that the research was conducted in the absence of any commercial or financial relationships that could be construed as a potential conflict of interest.
All claims expressed in this article are solely those of the authors and do not necessarily represent those of their affiliated organizations, or those of the publisher, the editors and the reviewers. Any product that may be evaluated in this article, or claim that may be made by its manufacturer, is not guaranteed or endorsed by the publisher.
The Supplementary Material for this article can be found online at: https://www.frontiersin.org/articles/10.3389/fmars.2024.1361137/full#supplementary-material
Bak R. P. M., Van Eys G. (1975). Predation of the sea urchin Diadema antillarum Philippi on living coral. Oecologia 20, 111–115. doi: 10.1007/BF00369023
Beijbom O., Edmunds P. J., Roelfsema C., Smith J., Kline D. I., Neal B. P., et al. (2015). Towards automated annotation of benthic survey images: Variability of human experts and operational modes of automation. PloS One 10, e0130312. doi: 10.1371/journal.pone.0130312
Bellwood D. R., Hughes T. P., Folke C., Nyström M. (2004). Confronting the coral reef crisis. Nature 429, 827–833. doi: 10.1038/nature02691
Bender A., Groll A., Scheipl F. (2018). A generalized additive model approach to time-to-event analysis. Stat. Model. 18, 299–321. doi: 10.1177/1471082X17748083
Birkeland C. (1977). “The importance of rate of biomass accumulation in early successional stages of benthic communities to the survival of coral recruits,” in Proceedings of the third international symposium on coral reefs. ed. Taylor D. L.. (Miami, FL, USA: School of Marine and Atmospheric Sciences, University of Miami). 15–21.
Burkepile D. E., Hay M. E. (2007). Predator release of the gastropod Cyphoma gibbosum increases predation on gorgonian corals. Oecologia 154, 167–173. doi: 10.1007/s00442-007-0801-4
Carpenter R. C., Williams S. L. (1993). Effects of algal turf canopy height and microscale substratum topography on profiles of flow speed in a coral forereef environment. Limnol. Oceanogr. 38, 687–694. doi: 10.4319/lo.1993.38.3.0687
Chen Q., Beijbom O., Chan S., Bouwmeester J., Kriegman D. (2021). “A new deep learning engine for CoralNet,” in Proceedings of the IEEE/CVF International Conference on Computer Vision (ICCV) Workshops. (Montreal, BC, Canada: Institute of Electrical and Electronics Engineers). doi: 10.1109/ICCVW54120.2021.00412
Chia F.-S., Spaulding J. G. (1972). Development and juvenile growth of the sea anemone, Tealia crassicornis. Biol. Bull. 142, 206–218. doi: 10.2307/1540225
Chong-Seng K. M., Graham N. A. J., Pratchett M. S. (2014). Bottlenecks to coral recovery in the Seychelles. Coral Reefs 33, 449–461. doi: 10.1007/s00338-014-1137-2
Chou C. B., Hedin L. O., Pacala S. W. (2018). Functional groups, species and light interact with nutrient limitation during tropical rainforest sapling bottleneck. J. Ecol. 106, 157–167. doi: 10.1111/1365-2745.12823
Christiansen N. A., Ward S., Harii S., Tibbetts I. R. (2009). Grazing by a small fish affects the early stages of a post-settlement stony coral. Coral Reefs 28, 47–51. doi: 10.1007/s00338-008-0429-9
Coffroth M. A., Buccella L. A., Eaton K. M., Lasker H. R., Gooding A. T., Franklin H. (2023). What makes a winner? Symbiont and host dynamics determine Caribbean octocoral resilience to bleaching. Sci. Adv. 9, eadj6788. doi: 10.1126/sciadv.adj6788
Craggs J., Guest J., Bulling M., Sweet M. (2019). Ex situ co culturing of the sea urchin, Mespilia globulus and the coral Acropora millepora enhances early post-settlement survivorship. Sci. Rep. 9, 12984. doi: 10.1038/s41598-019-49447-9
Davies S. W., Matz M. V., Vize P. D. (2013). Ecological complexity of coral recruitment processes: Effects of invertebrate herbivores on coral recruitment and growth depends upon substratum properties and coral species. PloS One 8, e72830–e72830. doi: 10.1371/journal.pone.0072830
Doropoulos C., Roff G., Bozec Y.-M., Zupan M., Werminghausen J., Mumby P. J. (2016). Characterizing the ecological trade-offs throughout the early ontogeny of coral recruitment. Ecol. Monogr. 86, 20–44. doi: 10.1890/15-0668.1
Edmunds P. J. (2021). Recruitment hotspots and bottlenecks mediate the distribution of corals on a Caribbean reef. Biol. Lett. 17, 20210149. doi: 10.1098/rsbl.2021.0149
Edmunds P. J., Carpenter R. C. (2001). Recovery of Diadema antillarum reduces macroalgal cover and increases abundance of juvenile corals on a Caribbean reef. Proc. Natl. Acad. Sci. 98, 5067–5071. doi: 10.1073/pnas.071524598
Efron B., Narasimhan B. (2021). bcaboot: Bias corrected bootstrap confidence intervals (R package version 0.2-3 ed).
Evans M. J., Coffroth M. A., Lasker H. R. (2013). Effects of predator exclusion on recruit survivorship in an octocoral (Briareum asbestinum) and a scleractinian coral (Porites astreoides). Coral Reefs 32, 597–601. doi: 10.1007/s00338-012-1001-1
Gardner T. A., Côté I. M., Gill J. A., Grant A., Watkinson A. R. (2003). Long-term region-wide declines in Caribbean corals. Science 301, 958–960. doi: 10.1126/science.1086050
Harvell C. D., West J. M., Griggs C. (1996). Chemical defense of embryos and larvae of a West Indian gorgonian coral, Briareum asbestinum. Invertebrate Reprod. Dev. 30, 239–247. doi: 10.1080/07924259.1996.9672550
Heyward A. J., Negri A. P. (1999). Natural inducers for coral larval metamorphosis. Coral Reefs 18, 273–279. doi: 10.1007/s003380050193
Hughes T. P. (1994). Catastrophes, phase shifts, and large-scale degradation of A caribbean coral reef. Science 265, 1547–1551. doi: 10.1126/science.265.5178.1547
Hughes T. P., Anderson K. D., Connolly S. R., Heron S. F., Kerry J. T., Lough J. M., et al. (2018). Spatial and temporal patterns of mass bleaching of corals in the Anthropocene. Science 359, 80–83. doi: 10.1126/science.aan8048
Hughes T. P., Rodrigues M. J., Bellwood D. R., Ceccarelli D., Hoegh-Guldberg O., Mccook L., et al. (2007). Phase shifts, herbivory, and the resilience of coral reefs to climate change. Curr. Biol. 17, 360–365. doi: 10.1016/j.cub.2006.12.049
Hylkema A., Kitson-Walters K., Kramer P. R., Patterson J. T., Roth L., Sevier M. L. B., et al. (2023). The 2022 Diadema antillarum die-off event: Comparisons with the 1983-1984 mass mortality. Front. Mar. Sci. 9, 1067449. doi: 10.3389/fmars.2022.1067449
James J. J., Svejcar T. J., Rinella M. J. (2011). Demographic processes limiting seedling recruitment in arid grassland restoration. J. Appl. Ecol. 48, 961–969. doi: 10.1111/jpe.2011.48.issue-4
Jorissen H., Baumgartner C., Steneck R. S., Nugues M. M. (2020). Contrasting effects of crustose coralline algae from exposed and subcryptic habitats on coral recruits. Coral Reefs 39, 1767–1778. doi: 10.1007/s00338-020-02002-9
Kahng S. E., Benayahu Y., Lasker H. R. (2011). Sexual reproduction in octocorals. Mar. Ecol. Prog. Ser. 443, 265–283. doi: 10.3354/meps09414
Keats D. W., Knight M. A., Pueschel C. M. (1997). Antifouling effects of epithallial shedding in three crustose coralline algae (Rhodophyta, Corallinales) on a coral reef. J. Exp. Mar. Biol. Ecol. 213, 281–293. doi: 10.1016/S0022-0981(96)02771-2
Kuffner I. B., Walters L. J., Becerro M. A., Paul V. J., Ritson-Williams R., Beach K. S. (2006). Inhibition of coral recruitment by macroalgae and cyanobacteria. Mar. Ecol. Prog. Ser. 323, 107–117. doi: 10.3354/meps323107
Ladd M. C., Shantz A. A. (2016). Novel enemies – previously unknown predators of the bearded fireworm. Front. Ecol. Environ. 14, 342–343. doi: 10.1002/fee.1305
Lasker H. R., Coffroth M. A. (1988). Temporal and spatial variability among grazers: Variability in the distribution of the gastropod Cyphoma gibbosum on octocorals. Mar. Ecol. Prog. Ser. 43, 285–295. doi: 10.3354/meps043285
Lasker H. R., Kim K. (1996). Larval development and settlement behavior of the gorgonian coral Plexaura kuna (Lasker, Kim and Coffroth). J. Exp. Mar. Biol. Ecol. 207, 161–175. doi: 10.1016/S0022-0981(96)02625-1
Lasker H. R., Kim K., Coffroth M. A. (1998). Production, settlement, and survival of plexaurid gorgonian recruits. Mar. Ecol. Prog. Ser. 162, 111–123. doi: 10.3354/meps162111
Lasker H. R., Martínez-Quintana Á., Bramanti L., Edmunds P. J. (2020). Resilience of octocoral forests to catastrophic storms. Sci. Rep. 10, 4286. doi: 10.1038/s41598-020-61238-1
Lenz E. A., Bramanti L., Lasker H. R., Edmunds P. J. (2015). Long-term variation of octocoral populations in St. John, US Virgin Islands. Coral Reefs 34, 1099–1109. doi: 10.1007/s00338-015-1315-x
Lessios H. A. (2016). The great Diadema antillarum die-off: 30 years later. Annu. Rev. Mar. Sci. 8, 267–283. doi: 10.1146/annurev-marine-122414-033857
Liedke A. M. R., Bonaldo R. M., Segal B., Ferreira C. E. L., Nunes L. T., Burigo A. P., et al. (2018). Resource partitioning by two syntopic sister species of butterflyfish (Chaetodontidae). J. Mar. Biol. Assoc. United Kingdom 98, 1767–1773. doi: 10.1017/S0025315417001321
Lirman D. (2001). Competition between macroalgae and corals: effects of herbivore exclusion and increased algal biomass on coral survivorship and growth. Coral Reefs 19, 392–399. doi: 10.1007/s003380000125
Littler M. M., Taylor P. R., Littler D. S. (1989). Complex interactions in the control of coral zonation on a Caribbean reef flat. Oecologia 80, 331–340. doi: 10.1007/BF00379034
Maciá S., Robinson M. P., Nalevanko A. (2007). Experimental dispersal of recovering Diadema antillarum increases grazing intensity and reduces macroalgal abundance on a coral reef. Mar. Ecol. Prog. Ser. 348, 173–182. doi: 10.3354/meps06962
Martínez-Quintana Á., Lasker H. R. (2021). Early life-history dynamics of Caribbean octocorals: The critical role of larval supply and partial mortality. Front. Mar. Sci. 8, 705563. doi: 10.3389/fmars.2021.705563
Mcclanahan T. R. (1999). Predation and the control of the sea urchin Echinometra viridis and fleshy algae in the patch reefs of Glovers Reef, Belize. Ecosystems 2, 511–523. doi: 10.1007/s100219900099
Morse D. E., Hooker N., Morse A. N. C., Jensen R. A. (1988). Control of larval metamorphosis and recruitment in sympatric agariciid corals. J. Exp. Mar. Biol. Ecol. 116, 193–217. doi: 10.1016/0022-0981(88)90027-5
Müller W. A., Leitz T. (2002). Metamorphosis in the cnidaria. Can. J. Zool. 80, 1755–1771. doi: 10.1139/z02-130
Nugues M. M., Bak R. P. M. (2006). Differential competitive abilities between Caribbean coral species and a brown alga: A year of experiments and a long-term perspective. Mar. Ecol. Prog. Ser. 315, 75–86. doi: 10.3354/meps315075
Nugues M. M., Smith G. W., Van Hooidonk R. J., Seabra M. I., Bak R. P. M. (2004). Algal contact as a trigger for coral disease. Ecol. Lett. 7, 919–923. doi: 10.1111/j.1461-0248.2004.00651.x
Oksanen J., Simpson G. L., Blanchet F. G., Kindt R., Legendre P., Minchin P. R., et al. (2022). vegan: Community ecology package (R package version 2.6-2 ed).
Penin L., Michonneau F., Baird A. H., Connolly S. R., Pratchett M. S., Kayal M., et al. (2010). Early post-settlement mortality and the structure of coral assemblages. Mar. Ecol. Prog. Ser. 408, 55–64. doi: 10.3354/meps08554
Privitera-Johnson K., Lenz E. A., Edmunds P. J. (2015). Density-associated recruitment in octocoral communities in St. John, US Virgin Islands. J. Exp. Mar. Biol. Ecol. 473, 103–109. doi: 10.1016/j.jembe.2015.08.006
R Core Team (2022). “R: A language and environment for statistical computing,” in R FOUNDATION FOR STATISTICAL COMPUTING (R Foundation for Statistical Computing, Vienna, AT).
Rey-Villiers N., Sánchez A., González-Díaz P., Álvarez-Filip L. (2023). Morphometric responses of two zooxanthellate octocorals along a water quality gradient in the Cuban northwestern coast. PloS One 18, e0290293. doi: 10.1371/journal.pone.0290293
River G. F., Edmunds P. J. (2001). Mechanisms of interaction between macroalgae and scleractinians on a coral reef in Jamaica. J. Exp. Mar. Biol. Ecol. 261, 159–172. doi: 10.1016/S0022-0981(01)00266-0
Rossi S., Bramanti L., Gori A., Orejas C. (2017). “Animal forests of the world: An overview,” in Marine Animal Forests: The Ecology of Benthic Biodiversity Hotspots. Eds. Rossi S., Bramanti L., Gori A., Orejas C. (Springer International Publishing, Cham).
Ruzicka R. R., Colella M. A., Porter J. W., Morrison J. M., Kidney J. A., Brinkhuis V. I. P., et al. (2013). Temporal changes in benthic assemblages on Florida Keys reefs 11 years after the 1997/1998 El Niño. Mar. Ecol. Prog. Ser. 489, 125–141. doi: 10.3354/meps10427
Sammarco P. W. (1980). Diadema and its relationship to coral spat mortality: Grazing, competition, and biological disturbance. J. Exp. Mar. Biol. Ecol. 45, 245–272. doi: 10.1016/0022-0981(80)90061-1
Sammarco P. W., Coll J. C. (1992). Chemical adaptations in the Octocorallia: Evolutionary considerations. Mar. Ecol. Prog. Ser. 88, 93–104. doi: 10.3354/meps088093
Sánchez J. A., Gómez-Corrales M., Gutierrez-Cala L., Vergara D. C., Roa P., González-Zapata F. L., et al. (2019). Steady decline of corals and other benthic organisms in the SeaFlower Biosphere Reserve (Southwestern Caribbean). Front. Mar. Sci. 6, 73. doi: 10.3389/fmars.2019.00073
Sebens K. P. (1983a). The larval and juvenile ecology of the temperate octocoral Alcyonium siderium Verrill. I. Substratum selection by benthic larvae. J. Exp. Mar. Biol. Ecol. 71, 73–89. doi: 10.1016/0022-0981(83)90105-3
Sebens K. P. (1983b). The larval and juvenile ecology of the temperate octocoral Alcyonium siderium Verrill. II. Fecundity, survival, and juvenile growth. J. Exp. Mar. Biol. Ecol. 72, 263–285. doi: 10.1016/0022-0981(83)90111-9
Sebens K. P. (1986). Spatial relationships among encrusting marine organisms in the New England subtidal zone. Ecol. Monogr. 56, 73–96. doi: 10.2307/2937271
Stockton L., Edmunds P. J. (2021). Spatially aggressive peyssonnelid algal crusts (PAC) constrain coral recruitment to Diadema grazing halos on a shallow Caribbean reef. J. Exp. Mar. Biol. Ecol. 541, 151569. doi: 10.1016/j.jembe.2021.151569
Tonra K. J., Wells C. D., Lasker H. R. (2021). Spawning, embryogenesis, settlement, and post-settlement development of the gorgonian Plexaura homomalla. Invertebrate Biol. 140, e12319. doi: 10.1111/ivb.12319
Tsounis G., Edmunds P. J. (2017). Three decades of coral reef community dynamics in St. John, USVI: A contrast of scleractinians and octocorals. Ecosphere 8, e01646. doi: 10.1002/ecs2.1646
Tsounis G., Steele M. A., Edmunds P. J. (2020). Elevated feeding rates of fishes within octocoral canopies on Caribbean reefs. Coral Reefs 39, 1299–1311. doi: 10.1007/s00338-020-01963-1
Van Alstyne K. L., Wylie C. R., Paul V. J., Meyer K. (1992). Antipredator defenses in tropical Pacific soft corals (Coelenterata: Alcyonacea). I. Sclerites as defenses against generalist carnivorous fishes. Biol. Bull. 182, 231–240. doi: 10.2307/1542116
Venables W. N., Ripley B. D. (2002). Modern applied statistics with S (New York, NY, USA: Springer).
Vreeland H. V., Lasker H. R. (1989). Selective feeding of the polychaete Hermodice carunculata Pallas on Caribbean gorgonians. J. Exp. Mar. Biol. Ecol. 129, 265–277. doi: 10.1016/0022-0981(89)90108-1
Wells C. D., Benz J., Tonra K. J., Anderson E. R., Lasker H. R. (2022). Grazers and predators mediate the post-settlement bottleneck in Caribbean octocoral forests. bioRxiv. doi: 10.1101/2022.08.09.503401
Wells C. D., Martínez-Quintana Á., Tonra K. J., Lasker H. R. (2021). Algal turf negatively affects recruitment of a Caribbean octocoral. Coral Reefs 40, 1045–1053. doi: 10.1007/s00338-021-02103-z
Wolf A. T., Nugues M. M. (2013). Predation on coral settlers by the corallivorous fireworm Hermodice carunculata. Coral Reefs 32, 227–231. doi: 10.1007/s00338-012-0969-x
Wood S. N. (2021). Mixed GAM computation vehicle with automatic smoothness estimation (R package version 1.8-38 ed).
Yoshioka P. M., Yoshioka B. B. (1987). Variable effects of Hurricane David on the shallow water gorgonians of Puerto Rico. Bull. Mar. Sci. 40, 132–144.
Yoshioka P. M., Yoshioka B. B. (1989). Effects of wave energy, topographic relief and sediment transport on the distribution of shallow-water gorgonians of Puerto Rico. Coral Reefs 8, 145–152. doi: 10.1007/BF00338270
Keywords: gorgonian, recruitment dynamics, coral settlement, early-life history, U.S. Virgin Islands, planulae, fish-coral interactions
Citation: Wells CD, Benz J, Tonra KJ, Anderson ER and Lasker HR (2024) Grazers and predators mediate the post-settlement bottleneck in Caribbean octocoral forests. Front. Mar. Sci. 11:1361137. doi: 10.3389/fmars.2024.1361137
Received: 25 December 2023; Accepted: 08 July 2024;
Published: 08 August 2024.
Edited by:
Aldo Cróquer, The Nature Conservancy, Dominican RepublicReviewed by:
Danielle M. DeLeo, Florida International University, United StatesCopyright © 2024 Wells, Benz, Tonra, Anderson and Lasker. This is an open-access article distributed under the terms of the Creative Commons Attribution License (CC BY). The use, distribution or reproduction in other forums is permitted, provided the original author(s) and the copyright owner(s) are credited and that the original publication in this journal is cited, in accordance with accepted academic practice. No use, distribution or reproduction is permitted which does not comply with these terms.
*Correspondence: Christopher D. Wells, Y2hyaXN0b3BoZXIud2VsbHMuMjNAZ21haWwuY29t
Disclaimer: All claims expressed in this article are solely those of the authors and do not necessarily represent those of their affiliated organizations, or those of the publisher, the editors and the reviewers. Any product that may be evaluated in this article or claim that may be made by its manufacturer is not guaranteed or endorsed by the publisher.
Research integrity at Frontiers
Learn more about the work of our research integrity team to safeguard the quality of each article we publish.