- 1Guangdong Ocean University, Southern Marine Science and Engineering Guangdong Laboratory (Zhuhai), Zhanjiang, China
- 2Guangdong Province Engineering Laboratory for Marine Biological Products, Guangdong Provincial Engineering Technology Research Center of Seafood, College of Food Science and Technology, Guangdong Ocean University, Zhanjiang, China
- 3College of Coastal Agriculture Sciences, Guangdong Ocean University, Zhanjiang, Guangdong, China
The genus Asparagopsis has garnered escalating attention in the spheres of marine biology and biotechnology due to its diverse chemical composition and promising biological capabilities. This all-encompassing review is dedicated to conducting an exhaustive inquiry into the chemical identification and biological importance of Asparagopsis species. By meticulously dissecting the array of chemical compounds found in genus Asparagopsis, encompassing polysaccharides, lipids, proteins, sterols, and bromoform. We unveil their potential utility in realms such as biomedicine, biotechnology, and the conservation of the environment. Furthermore, we delve into the bioactive attributes inherent in these compounds, encompassing effects such as antioxidative, antimicrobial, and anti-inflammatory properties, as well as their conceivable role in cancer treatments. Furthermore, this review underscores the environmental pertinence of genus Asparagopsis, particularly its capacity to mitigate climate change through the generation of compounds that alleviate greenhouse gas effects. Additionally, we delve into the economic facets of this genus, spanning from its integration into food additives to its contributions in cosmetics and sustainable agriculture. This comprehensive review furnishes a multi-faceted comprehension of Asparagopsis, illuminating its chemical diversity and biological significance, thereby paving the way for further explorations into its potential contributions across a spectrum of sectors.
1 Introduction
The marine environment holds a priceless collection of diverse and plentiful components, with marine algae playing a particularly vital role in this ecological treasure trove. The world’s oceans harbor an astonishing diversity of life forms, and marine algae, commonly known as seaweeds, stand out as a notable and renewable resource (Wei et al., 2013; Wells et al., 2017). In light of the persistent challenges presented by issues like overfishing, deforestation, and the depletion of terrestrial resources, the sustainable management and utilization of marine resources, including marine algae, become of utmost importance (Duarte et al., 2022). Marine algae, distinguished organisms in their own merit, serve as essential elements within marine ecosystems, offering a broad range of ecological and economic benefits (Hossain et al., 2021). Within the wider classification of marine algae, the genus Asparagopsis is situated within the family Bonnemaisoniaceae (Neethu et al., 2017). These algae are categorized within the Rhodophyta division, which also houses various other widely recognized species of red algae (Nahor et al., 2022).
The significance of the genus Asparagopsis derives from its intricate chemical composition and the potential utility of its constituents (Ponte et al., 2022). Asparagopsis species have been documented to produce an extensive array of bioactive compounds, polysaccharides, lipids, proteins, sterols, and bromoform (Félix et al., 2021). These compounds manifest a diverse spectrum of biological functions, encompassing antimicrobial, antioxidant, prebiotic, anti-inflammatory, and antitumor properties, among others (Silva et al., 2021; Zanolla et al., 2022). The diverse biological attributes displayed by genus Asparagopsis compounds make them notably intriguing, with applications spanning a broad spectrum, including cosmetics, pharmaceuticals, functional foods, agriculture, aquaculture, and the promotion of environmental sustainability (Hafting et al., 2015; Peñalver et al., 2020; Glasson et al., 2022). Activity and application of Asparagopsis as shown in Figure 1. The ingredients and chemical composition of genus Asparagopsis has been summarized in Table 1.
In the contemporary age, the exploration of natural compounds derived from marine algae has gained paramount importance owing to the progressively recognized untapped potential they encompass across a spectrum of sectors. Using the genus Asparagopsis as an illustrative instance, marine algae, known for their extensive diversity and distinctive biochemical composition, offer a myriad of bioactive molecules with significant potential to tackle modern challenges. Amid the world’s grappling with mounting concerns like chronic ailments, antibiotic resistance, environmental degradation, and the imperative for sustainable resources, marine algae stand out as a valuable repository of innovative solutions (Fernando et al., 2016; Carreira-Casais et al., 2021; Yao et al., 2022). In recent times, the role of an unhealthy diet in contributing substantially to a wide range of afflictions, spanning both physical and metabolic disorders, has come into prominence. Contemporary dietary trends characterized by excessive consumption of processed edibles, sugary drinks, saturated fats, and refined carbohydrates have led to a disconcerting surge in obesity, cardiovascular illnesses, type 2 diabetes, and various chronic afflictions (DeFronzo et al., 2015; Furman et al., 2019). Such eating habits frequently lack essential nutrients and dietary fiber, undermining overall well-being and diminishing the immune system’s robustness (Pahwa and Sharan, 2022; Qiu et al., 2022). In this context, the importance of incorporating marine seaweed into our diets has captured attention as a potential remedy. These nutrient-abundant marine plants not only have low caloric content but are also enriched with essential nutrients and dietary fiber (Lopez-Santamarina et al., 2020). By harnessing the potential of natural compounds sourced from marine algae, researchers and industries are not solely opening doors to pioneering breakthroughs but also pushing the frontiers of science, sustainability, and human well-being.
This investigation’s primary aim is to conduct a thorough investigation into the chemical identification and characterization of the genus Asparagopsis, with a primary objective of consolidating the latest progress in this sphere of research. By amalgamating the existing corpus of literature, this review seeks to construct a comprehensive overview of the wide array of chemical compounds inherent in Asparagopsis species. Through a rigorous exploration of the bioactive qualities inherent within these compounds, our goal is to elucidate their potential applicability across various domains, including functional foods, pharmaceuticals, and biotechnology. This, in turn, stands poised to provide invaluable insights that can guide forthcoming research and development endeavors. Furthermore, the purview of this review extends to embrace the environmental consequences of genus Asparagopsis, particularly its role in mitigating the repercussions of climate change. By grappling with both challenges and opportunities interwoven with the chemical identification of genus Asparagopsis and its multifaceted applications, this review aspires to render substantial contributions toward an enriched understanding of marine biodiversity and the advancement of sustainable resource utilization.
2 Historical background and taxonomy of genus Asparagopsis
The Asparagopsis species generally include Asparagopsis armata, Asparagopsis taxiformis, and Asparagopsis svedelii. Within the realm of biological taxonomy, they are classified as members of the Plantae kingdom, belonging to the Phylum Rhodophyta, Order Bonnemaisoniales, and Family Bonnemaisoniaceae (Bonin and Hawkes, 1987), an inclusive domain encompassing a wide variety of multicellular photosynthetic organisms (Zanolla et al., 2022). These specific species find their place within the phylum Rhodophyta, colloquially known as red algae due to their distinct pigmentation (Zanolla et al., 2014). The categorization of A. armata, A. taxiformis, and A. svedelii within the broader lineage of red algae emphasizes their shared evolutionary history with other members of this group. It also highlights their unique characteristics and adaptations that distinguish them from other red algae species. Comprehending their taxonomic stance equips researchers and scientists with valuable insights into their morphological, physiological, and genetic characteristics, consequently enriching our broader understanding of their ecological functions, adaptive traits, and prospective applications (Andreakis et al., 2004; Clark et al., 2018).
A. armata, commonly recognized as “Harpoon Weed”, exhibits a distribution predominantly centered around the Mediterranean region and western Pacific Ocean, with its principal habitats situated along the coastlines of Australia, New Zealand, and adjacent islands (Pacios et al., 2011). This distribution profile underscores its proclivity for temperate, warm-temperate and subtropical waters (Chualáin et al., 2004). Flourishing within these specific ecological contexts, A. armata establishes itself within rocky substrates, thriving proficiently in both subtidal and intertidal zones (Kraan and Barrington, 2005). In contrast, A. taxiformis, colloquially referred to as “Limu kohu” displays a distribution inherently confined to the Southern Hemisphere, manifesting notably in regions such as Australia, New Zealand, and South Africa (Dijoux et al., 2014; Clark et al., 2018). This distributional tendency underscores its inclination toward temperate and subtropical waters specifically within these designated regions. Within its natural habitat, A. taxiformis predominantly occupies shallow rocky environments, predominately inhabiting both intertidal and subtidal zones. A. svedelii represents the original description of a unique specimen discovered at a depth of 55 meters in the Galapagos Islands by W.R. Taylor in 1934 (Zanolla et al., 2022).
The realm of cultivating A. armata and A. taxiformis has captured considerable attention, as sustained endeavors are being channeled toward the exploration of methodologies that can facilitate the growth of these algae within controlled environments. The central objective revolves around establishing a reliable and sustainable broodstock of biomass, one that can cater to a multitude of applications. Delving into cultivation studies entails an in-depth exploration of the most optimal growth circumstances, encapsulating variables such as temperature, light intensity, nutrient availability, and water quality (Zhu et al., 2021). Diligent efforts by researchers are dedicated to unraveling the complexities surrounding the life cycle, growth rates, and reproductive behaviors of these species, all aimed at crafting effective cultivation strategies. This endeavor encompasses a comprehensive strategy, encompassing both laboratory-based experimentation and field trials, with the intent of replicating the natural habitat conditions that these algae thrive in.
3 Lipids
Lipids represent a heterogeneous group of biomolecules characterized by their hydrophobic nature (low solubility in water) and high solubility in organic solvents. This category encompasses a diverse range of compounds, including fats, oils, sterols, and phospholipids. Within biological systems, lipids play indispensable roles, serving essential functions in energy storage, cell membrane integrity, cellular signaling, and acting as precursors for various bioactive molecules (Hamsanathan and Gurkar, 2022). Genus Asparagopsis shows great promise as a rich source of lipids, offering numerous applications in industries such as food, pharmaceuticals, and cosmetics (Félix et al., 2021).
Fatty acids are fundamental components of lipids and they are consisting of hydrocarbon chains with a carboxylic acid group at one end. They can be classified into two main categories: saturated fatty acids, which lack double bonds in their hydrocarbon chain, and unsaturated fatty acids, which contain one or more double bonds. The total fatty acids content in A. armata was approximately 60.23 mg/g dry weight, comprising saturated fatty acids (SFAs) at 27.10 mg/g dry weight, monounsaturated fatty acids (MUFAs) at 18.90 mg/g dry weight, polyunsaturated fatty acids (PUFAs) at 2.21 mg/g dry weight, and highly unsaturated fatty acids (HUFAs) at 12.03 mg/g dry weight (Rocha et al., 2021).
Genus Asparagopsis is distinguished by its notable fatty acids, especially ω-3 fatty acids, such as eicosapentaenoic acid and docosahexaenoic acid (Rocha et al., 2021). Marine algae offer a sustainable and eco-friendly alternative to conventional marine sources like fish oil, meeting the increasing demand for ω-3 supplements and functional foods. These essential fatty acids are renowned for their cardiovascular benefits, cognitive support, and anti-inflammatory properties, making them vital for human health (Lordan et al., 2017). Furthermore, ω-3 fatty acids derived from genus Asparagopsis show significant promise in the aquaculture industry. These fatty acids can be added to the diets of fish and bivalve mollusks, enhancing the nutritional profile of farmed fish and increasing their ω-3 content (Morais et al., 2020). This sustainable approach ensures that farmed fish provide a rich source of essential fatty acids without depleting wild fish populations, thus promoting a more environmentally responsible approach to aquaculture (Adarme-Vega et al., 2014).
Asparagopsis species display significant concentrations of unsaturated fatty acids, with ω-3 fatty acids being particularly prominent. This observation is further corroborated by their low ω-6/ω-3 ratios, which are approximately 0.12 (Ragonese et al., 2014; Rocha et al., 2021). While ω-6 fatty acids, including linoleic acid and arachidonic acid, play pivotal roles in immune response and cell signaling, maintaining a balanced intake of these essential fatty acids is crucial for optimal health. To support proper neuronal development and prevent chronic disorders, it is recommended to aim for a target ratio of around 4:1 or lower between ω-6 and ω-3 (Jauregibeitia et al., 2020). This ratio significantly impacts body fat metabolism, influencing processes like adipogenesis, lipid homeostasis, adipose tissue browning, and systemic inflammation (Patel et al., 2022). An increased ω-6 to ω-3 fatty acids ratio has been linked to obesity, attributed to elevated phospholipids in red blood cell membranes (Jauregibeitia et al., 2020). Such changes in lipid composition within cell membranes can disrupt cell signaling, alter membrane fluidity, and potentially affect the function and circulation of red blood cells. In the typical Western diet, these recommended ratios are often exceeded, with estimates showing ratios as high as 20:1, largely due to the prevalence of ω-6-rich oils, processed foods, and meat (Patel et al., 2022). To achieve a more balanced ratio, individuals can focus on incorporating sources of ω-3 fatty acids into their diet, such as fatty fish (salmon, mackerel, sardines), flaxseeds, chia seeds, walnuts, and marine algae. By promoting a healthier ω-6 to ω-3 ratio, individuals may enhance their overall health and reduce the risk of chronic diseases associated with imbalanced essential fatty acid intake (Artemis and James, 2016).
4 Bromoform and greenhouse gas mitigation
Bromoform, chemically known as CHBr3, falls under the category of halogenated hydrocarbons and functions as a volatile organic compound that naturally emerges as a secondary metabolite in certain algae species, notably red seaweed (Magnusson et al., 2020). Its structural formula is shown in Figure 2. The infrequent occurrence of bromoform in these specific red seaweed species has fascinated scientists and aroused their interest in this intriguing natural occurrence. Accurate quantification of bromoform is essential to assess its concentration in genus. Researchers. evaluated the potential applications of Asparagopsis extracts and successfully quantified bromoform using GC-MS (Romanazzi et al., 2021). The bromoform content was found to be 2.04 mg/g in A. taxiformis and 1.39 mg/g in A. armata (Romanazzi et al., 2021). In A. taxiformis, bromoform, a brominated halomethane, dominated with the highest content at approximately 1723 μg/g dry weight, followed by dibromochloromethane, which exhibited the second-highest content at around 15.8 μg/g dry weight (Machado et al., 2016b).
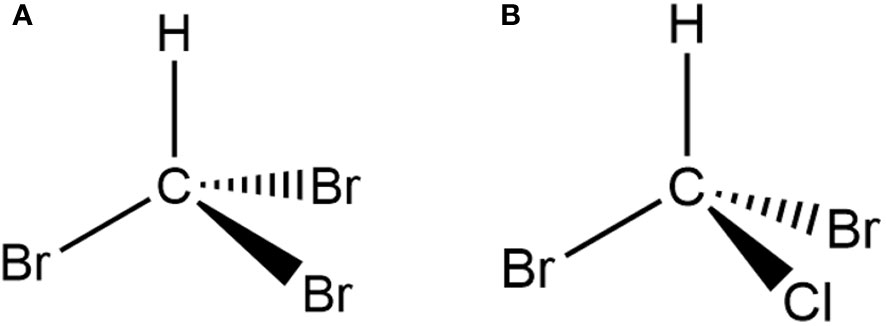
Figure 2 Chemical structures of (A) bromoform and (B) dibromochloromethane identified in A. taxiformis.
Bromoform derived from genus Asparagopsis has generated significant interest in environmental conservation endeavors due to its potential as a natural remedy for mitigating greenhouse gas emissions (Muizelaar et al., 2021). Recent research has highlighted that when introduced in small quantities to livestock feed, genus Asparagopsis can efficiently decrease methane production in ruminant animals, including cattle (Roque et al., 2021). Consistent findings demonstrate that the inclusion of Asparagopsis in a 2% organic matter supplement with bromoform considerably curbs in vitro methane production. Notably, these additions have no adverse effects on substrate degradability and do not influence the production of total volatile fatty acids (Machado et al., 2016b). In a similar vein, Machado et al. discovered that a 5 μmol/L concentration of bromoform led to over 99% reduction in methane production compared to a basal substrate-only control (Machado et al., 2018). Their investigation underscored the in vitro antimethanogenic properties of bromoform, effectively inhibiting the growth of Methanobacteriales, Methanomassiliicoccales, and Methanomicrobiales (Machado et al., 2018), the archaea responsible for microbial methane production (Choi et al., 2021). Given that methane is a potent greenhouse gas, the application of genus Asparagopsis bromoform as a supplement offers an innovative strategy for combatting climate change.
5 Sterols
Sterols are fundamental constituents of cell membranes, playing a significant role in enhancing their stability and flexibility. They are vital in controlling membrane permeability and upholding the structural integrity of cells (Dufourc, 2008). As a group of lipids, sterols perform indispensable roles in diverse biological processes across all living organisms, spanning from bacteria to humans (Wang et al., 2021). The importance of sterols, both in structure and function, is apparent across various life domains. They share a common molecular structure characterized by a sterane backbone and a hydrocarbon side chain (Summons et al., 2022). The total sterol content in A. armata ranged from 0.15 to 0.32 mg/g dry weight (Pellegrini, 1969), with the associated sterols listed in Table 2. This content exhibits similarity with other red seaweeds, like Gracilaria gracilis (approximately 0.45 mg/g dry weight) (Francavilla et al., 2013) and Palmaria sp. (varying between 0.187 and 0.337 mg/g dry weight) (Sánchez-Machado et al., 2004). Notably, Asparagopsis species have been identified as prolific producers of a wide range of sterols (Alves et al., 2020), each exhibiting a distinctive chemical structure and potential biological activities. These include desmosterol (A), cholesterol (B), stigmasterol (C), 25-hydroxy-24-methylcholesterol (D), avenasterol (E), 22-dehydrocholesterol (F), 25-hydroxycholesterol (G), and cholestanol (H) (Pinto et al., 2022; Ponte et al., 2022), whose structural formulas are depicted in Figure 3.
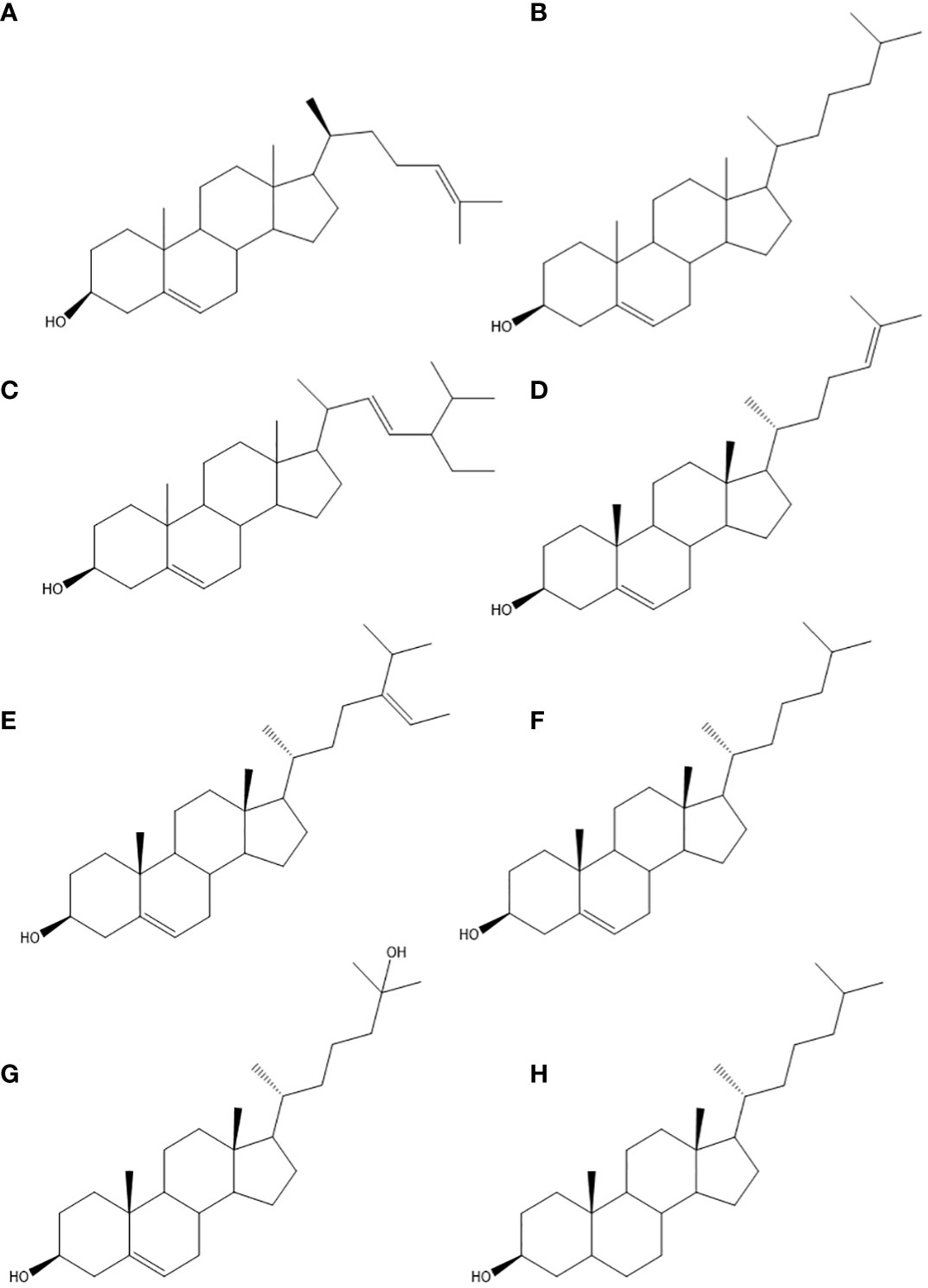
Figure 3 Structural formula for sterols. Desmosterol (A), cholesterol (B), stigmasterol (C), 25-hydroxy-24-methylcholesterol (D), avenasterol (E), 22-dehydrocholesterol (F), 25-hydroxy cholesterol (G), and cholestanol (H).
Sterols derived from red algae offer a promising combination of natural origin and potential health-supporting properties, rendering them subjects of keen scientific interest and exploration. Numerous studies have underscored their anti-inflammatory, antioxidant, and anticancer activities (Hannan et al., 2020; Sohn et al., 2021). Of these sterols, desmosterol emerges as a prominent component in A. armata (Lopes et al., 2011). It plays a critical role in regulating lipid metabolism and maintaining cell membrane integrity, serving as a crucial intermediate in cholesterol biosynthesis (Spann et al., 2012). Acting as a substrate for cholesterol biosynthetic enzymes, desmosterol facilitates the production of cholesterol, vital for the synthesis of steroid hormones, bile acids, and vitamin D (Zhang et al., 2021). Furthermore, its potential involvement in brain health and neuroprotection has been investigated. Desmosterol exhibits a high concentration in brain tissues, particularly in regions associated with memory and cognition (Cenik et al., 2022). In contrast, fucosterol showcases potent antioxidant characteristics, acting as an effective scavenger of free radicals and reducing oxidative stress, which is linked to various diseases (Abdul et al., 2016). By neutralizing harmful reactive oxygen species, fucosterol safeguards cells and tissues from oxidative damage, contributing to overall health and well-being. Moreover, fucosterol displays anti-inflammatory effects by inhibiting the production of pro-inflammatory molecules like cytokines and chemokines, effectively mitigating inflammation (Meinita et al., 2021). Another noteworthy sterol identified in A. taxiformis is stigmasterol (El-Baroty et al., 2007). Stigmasterol present potential anticancer properties and have demonstrated its ability to impede the growth of cancer cells, trigger apoptosis (programmed cell death), and hinder angiogenesis (formation of new blood vessels) (Bakrim et al., 2022).
Asparagopsis sterols exhibit considerable potential for diverse therapeutic applications in medicine, cosmeceuticals, and nutraceuticals. Their bioactive attributes, encompassing antioxidant, anti-inflammatory, and anticancer properties, position them as promising contenders for innovative pharmaceutical interventions. In the medical realm, genus Asparagopsis sterols hold great promise in addressing chronic inflammatory conditions like rheumatoid arthritis and inflammatory bowel disease (Rocha et al., 2022). Their anti-inflammatory effects aid in symptom management and hinder disease progression. Within the cosmeceutical industry, genus Asparagopsis sterols present exciting prospects. Their antioxidant prowess effectively safeguards the skin from oxidative damage caused by environmental factors, contributing to anti-aging effects. By countering the impact of free radicals, genus Asparagopsis sterols may reduce wrinkles and enhance overall skin health (Ferreira et al., 2021). Moreover, their potential anti-inflammatory effects make them beneficial in managing various skin conditions, including dermatitis and acne. This renders genus Asparagopsis sterols attractive for developing skincare products aimed at anti-aging, moisturization, and skin health enhancement. In the realm of nutraceuticals, genus Asparagopsis sterols have gained recognition for their cholesterol-lowering capabilities (Máximo et al., 2018). The phytosterols in genus Asparagopsis can reduce the absorption of low-density lipoprotein cholesterol, making them valuable functional ingredients in cholesterol-lowering food products. Incorporating genus Asparagopsis sterols into the diet may lead to improved cardiovascular health and a reduced risk of heart disease (Cho et al., 2022). Furthermore, methane, a potent greenhouse gas released during the digestive processes of ruminant animals like cattle, contributes to climate change (Króliczewska et al., 2023). Research has shown that incorporating genus Asparagopsis seaweeds into the diets of these animals can significantly diminish their methane emissions. In a study conducted by Machadoet al., utilizing a semi-continuous in vitro rumen system, the inclusion of A. taxiformis at a 5% organic matter inclusion rate resulted in a remarkable 95% reduction in methane production from enteric fermentation in dairy cattle (Machado et al., 2016a). Importantly, this reduction occurred without any apparent negative impacts on volatile fatty acid production. The study also observed a substantial decrease in the relative abundance of methanogens (Roque et al., 2019a). Similarly, the inclusion of 1% A. armata in cow diets led to a reduction of up to 67% in methane production. While there was a decrease in milk protein percentage, no significant differences were found in other milk composition characteristics (Stefenoni et al., 2021). A study by Roque et al., 2019b reported that an inclusion rate of 0.5% did not affect milk composition, except for a decrease in lactose concentration (Roque et al., 2019b). These findings hold great promise for mitigating the environmental consequences of livestock farming and emphasize the potential of genus Asparagopsis protein in fostering a more sustainable and environmentally friendly food system. these sterols hold potential as functional ingredients in other nutraceutical formulations, such as dietary supplements, promoting overall health and well-being.
6 Protein
The protein obtained from the Asparagopsis genus has recently attracted considerable attention in the health and nutrition sector due to its potential as an innovative plant-based protein source. The Asparagopsis genus stands out for its exceptional protein content, approximately 17.55 dw/100 g, surpassing that of other species such as Chondrus crispus, Galaxaura rugosa, Grateloupia lanceola, and Nemalion elminthoides, which range from 2.80-9.41 dw/100 g. This notable protein content of the genus Asparagopsis has the potential to contribute approximately 31.34% of the recommended daily intake. Moreover, the protein derived from the genus Asparagopsis exhibits a wide range of advantageous properties, including anti-inflammatory, antioxidant, anti-tumor, anti-aging, and protective activities (Ismail et al., 2020; El-Beltagi et al., 2022). These properties position it as a promising candidate for the prevention and treatment of various conditions, such as neurodegenerative diseases, cancers, gastric ulcers, DNA replication, response to stimuli, molecular transport, and the facilitation of biochemical reactions (Catanesi et al., 2021).
In contrast, genus Asparagopsis protein provides a diverse range of essential amino acids, which are fundamental constituents of proteins and play vital roles in the body. Essential amino acids are of great importance because they cannot be synthesized by the body and must be acquired through dietary sources (Church et al., 2020). The composition and nutritional quality of proteins in food have direct implications for processes such as digestion, absorption, and the potential health effects associated with proteins and amino acids. According to the Food and Agriculture Organization (FAO), protein quality is determined by the amino acid composition and bioavailability, which is closely linked to the digestibility of the proteins consumed (Lee et al., 2016). Significantly, genus Asparagopsis protein contains all the essential amino acids necessary for optimal human health. These essential amino acids, such as histidine, isoleucine, leucine, lysine, methionine, phenylalanine, threonine, tryptophan, and valine, play distinct roles in the body (83, 84). A. armata and A. taxiformis are particularly rich in valine (0.95 g/100 g), leucine (0.91 g/100 g), threonine (0.76 g/100 g), and lysine (0.46 g/100 g) (Torres et al., 2021; De Bhowmick and Hayes, 2022). Notably, many plant-based protein sources lack lysine (Leinonen et al., 2019). Valine, leucine, and threonine are vital for muscle metabolism, tissue repair, nitrogen balance maintenance, and immune function (Wu, 2009; Wu, 2010), as well as energy production and blood sugar level regulation (Fan et al., 2022). The presence of other essential amino acids supports these processes, facilitating post-exercise recovery and muscle health (Kamei et al., 2020). Hence, genus Asparagopsis protein holds significant potential for the development of functional pharmaceuticals, nutraceuticals, and cosmeceuticals.
The macroalgae protein nowadays can be effectively utilized in the creation of protein bars, energy balls, and other snack items. Its high protein content and neutral taste allow it to harmonize with other ingredients, providing a nutritional punch to these portable and convenient treats (Geada et al., 2021). When it comes to cooked dishes, the versatility of macroalgae protein becomes evident. Its ability to retain moisture and provide a pleasing texture makes it a suitable replacement for traditional animal-based proteins in a variety of recipes (Thornton et al., 2023). It can be used as an ingredient in vegetarian burgers, meatballs, and patties, offering a plant-based alternative that is both tasty and protein-rich (Tso et al., 2021). Additionally, it can be incorporated into stir-fries, curries, and pasta dishes, adding a nutritional boost without overpowering the flavors of the dish (Kadam and Prabhasankar, 2010). Marine macroalgae protein can also be employed in sweet creations. It can be added to smoothie bowls, yogurt parfaits, and baked goods to increase their protein content (O'Sullivan et al., 2016). By incorporating macroalgae protein into desserts, individuals can enjoy guilt-free treats that not only satisfy their sweet tooth but also provide valuable nutrition.
7 Polysaccharides
Polysaccharides are complex carbohydrates consisting of repeated units of monosaccharides linked together by glycosidic bonds. Figure 4 shows the structural formula of polysaccharides. They are widely abundant in diverse natural sources, including plants, algae, fungi, bacteria, and animals (Yang et al., 2022; Wang and Cheong, 2023; Wu et al., 2023). The chemical composition of polysaccharides obtained from marine algae differs significantly from those found in land-based plants, particularly in terms of their sulfate content. The polysaccharides belonging to the genus Asparagopsis are typically sulfated galactans. These polysaccharides are formed through the repetitive alternation of α(1,3)- and β(1,4)-linked galactopyranoses (Haslin et al., 2000). They demonstrate variation in the degree and pattern of substitution by sulfate, methoxyl, pyruvate groups, and sugar side chains, as well as in the proportion of 1,4-linked 3,6-anhydro-α-galactose. The extraction yield of polysaccharides from A. armata was determined to be 20% (Haslin et al., 2000), whereas that of A. taxiformis was found to be 0.18% (Arunkumar et al., 2021). The extraction yield of A. armata polysaccharide was significantly higher when compared to other Rhodophyta species, such as Pyropia haitanensis (4.10%) (Xu et al., 2019) and Gelidium amansii (7.80%) (Xu et al., 2018), but lower than that of Gracilariopsis lemaneiformis (34.84%) (Zhang et al., 2020) and Gracilaria cornea (21.4%) (Melo et al., 2002).
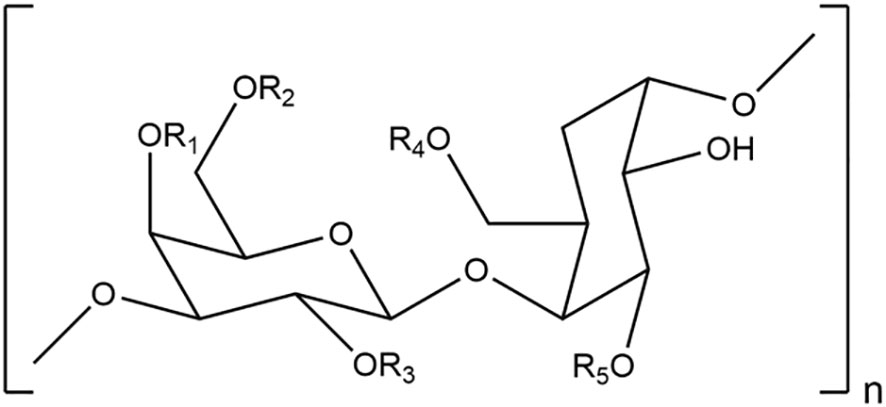
Figure 4 Representative structural formula of sulfated galactan derived from the genus Asparagopsis. R1-4: SO3- or H; R5: H.
The level of sulphation and uronic acids content were about 10%, and 15.9%, respectively, which determined by colorimetric method (Haslin et al., 2000). Unlike other marine algae polysaccharides isolated from seaweeds such as Fucus vesiculosus and Ascophyllum nodosum had an high content of sulfate with low content of uronic acids (Fletcher et al., 2017), while polysaccharides derived from genus Asparagopsis had an important amount of uronic acids, which could be considered as an indicator reflecting its potent biological activities. The average molecular weight of A. armata was estimated using a size exclusion chromatography and showed that approximately 355.01 kDa (Feki et al., 2023).
The significance of polysaccharides lies in their ability to combat oxidative stress, which arises from an imbalance between the production of reactive oxygen species and the body’s defense mechanisms against them. Research has shown that polysaccharides derived from A. armata and A. taxiformis possess antioxidant properties. These polysaccharides demonstrate the capacity to scavenge hydroxyl, 1,1’-diphenyl-2-picrylhydrazyl, and 2,2’-azino-bis(3-ethylbenzothiazoline-6-sulfonic acid) radicals, as well as chelate metal ions and enhance the body’s natural antioxidant systems (Arunkumar et al., 2021; Feki et al., 2023). Consequently, polysaccharides play a crucial role in shielding cells from oxidative damage. This cellular protection is pivotal in preventing lipid peroxidation, DNA damage, and protein oxidation. Additionally, the benefits of antioxidants extend to the prevention of chronic ailments, including cardiovascular disorders, neurodegenerative conditions, and cancer (Willcox et al., 2004).
Polysaccharides play a vital role in maintaining the delicate equilibrium of blood coagulation by exerting anticoagulant activity. Excessive formation of blood clots can give rise to thrombosis, which carries substantial health hazards, including deep vein thrombosis, pulmonary embolism, and stroke (Chapin and Hajjar, 2015). Polysaccharides with anticoagulant properties contribute to the prevention of thrombosis by impeding clot formation. The polysaccharides extracted from A. armata and A. taxiformis exhibit potential in averting abnormal blood clotting and the associated complications (Adarshan et al., 2024). They achieve this preventive effect by extending the clotting times of activated partial thromboplastin time, prothrombin time, and thrombin time. The elongated clotting times indicate that these polysaccharides disrupt specific clotting factors or inhibit the activity of thrombin (Manilal et al., 2012; Feki et al., 2023). By diminishing the risk of anomalous blood clotting, the polysaccharides not only foster cardiovascular well-being but also reduce the likelihood of heart attacks and other cardiovascular incidents (Zaporozhets and Besednova, 2016).
In addition to the existing body of research on the antioxidant and anticoagulant properties of polysaccharide from genus Asparagopsis, future advancements in this field offer considerable potential, prompting the need to explore further research areas. One crucial aspect that merits deeper investigation is the expansion of in vivo studies. While in vitro examinations provide valuable insights, in vivo investigations are indispensable for fully comprehending the physiological and pharmacological effects of polysaccharides (Veeraperumal et al., 2020). These studies would yield valuable information regarding crucial factors such as absorption, metabolism, bioavailability, and potential adverse reactions. By conducting in vivo studies, researchers can amass comprehensive data on the therapeutic effectiveness and long-term consequences of polysaccharides in relevant disease models or clinical contexts. Moreover, there is a burgeoning interest in exploring the prebiotic activity of marine algal polysaccharides and their impact on gastrointestinal health (Cheong et al., 2022). Prebiotics have the unique ability to selectively stimulate the growth and activity of beneficial gut microorganisms, resulting in enhanced composition and functionality of the gut microbiota (Lopez-Santamarina et al., 2020; Xie and Cheong, 2022). Marine algal polysaccharides exhibit promise as prebiotic agents (Zheng et al., 2020), nourishing the proliferation of advantageous gut bacteria and exerting positive influences on gut health (Yu et al., 2023). Therefore, future research should prioritize the investigation of polysaccharides’ prebiotic properties, encompassing studies on fermentation patterns, metabolite production, and modulation of the gut microbiota.
8 Future trends and conclusion
The inherent potency of bioactive compounds found within the genus Asparagopsis resonates across an extensive range of domains, spanning from biotechnology to the realm of environmental science. The revelations stemming from research within this genus bear the potential to initiate transformative impacts in a multitude of sectors. Within the biotechnology domain, the bioactive compounds derived from the genus Asparagopsis unfurl a panorama of potentialities. These compounds, endowed with an array of antioxidant, antimicrobial, and anticancer attributes, harbor the capability to inaugurate a fresh epoch of pioneering pharmaceuticals and cosmeceuticals. Consequently, this has the potential to reshape the landscape of medical treatments and skincare regimens, engendering innovative advancements. Many studies provide empirical evidence supporting the biological activities of genus Asparagopsis compounds, enhancing the credibility of the findings. However, limitations exist, such as the lack of standardized methods across studies, hindering comparability of results. Additionally, mechanistic insights into the biological activities of genus Asparagopsis compounds remain limited, and gaps persist in our understanding of its ecological roles and long-term effects. Future research should focus on standardizing methods, elucidating molecular mechanisms, investigating ecological interactions, and assessing long-term effects to address these limitations and advance our understanding of genus Asparagopsis.
Moreover, the competency of genus Asparagopsis compounds in attenuating greenhouse gas emissions underscores their momentous relevance in addressing the predicament of climate change. The specific compounds’ adeptness in intervening within methane production among ruminant animals carries far-reaching implications for mitigating methane emissions linked to livestock. These applications serve as a testament to the capacity of Asparagopsis ‘ chemical diversity to transcend disciplinary confines, bestowing fields with resolutions that seamlessly align with the imperatives of our contemporary world. The sustained pursuit of delving deeper into its chemical composition and biological attributes holds the potential to yield groundbreaking revelations that could extend their consequences extensively.
In conclusion, this review presents a thorough examination of the diverse chemical composition and potential applications inherent in this remarkable genus. As the awareness of the genus Asparagopsis ‘ environmental and biological significance continues to grow, this review holds a significant place as an indispensable point of reference for researchers and industries endeavoring to unlock the full potential of this marine macroalgae. As advancements unfold in this field, the ongoing investigation within this domain holds the promise of enhancing our understanding of marine biodiversity and the prudent utilization of sustainable resources.
Author contributions
KC: Writing – original draft, Writing – review & editing, Conceptualization, Investigation. GL: Data curation, Methodology, Writing – review & editing. MW: Investigation, Visualization, Writing – review & editing. YY: Conceptualization, Supervision, Writing – review & editing. SZ: Conceptualization, Funding acquisition, Supervision, Writing – review & editing.
Funding
The author(s) declare that financial support was received for the research, authorship, and/or publication of this article. This study was supported in part by the Southern Marine Science and Engineering Guangdong Laboratory (Zhuhai) (SML2023SP237), Key-Area Research and Development Program of Guangdong Province (2020B1111030004), The Innovative Team Program of High Education of Guangdong Province (2021KCXTD021), and The Program for Scientific Research Start-up Funds of Guangdong Ocean University (060302042302).
Conflict of interest
The authors declare that the research was conducted in the absence of any commercial or financial relationships that could be construed as a potential conflict of interest.
Publisher’s note
All claims expressed in this article are solely those of the authors and do not necessarily represent those of their affiliated organizations, or those of the publisher, the editors and the reviewers. Any product that may be evaluated in this article, or claim that may be made by its manufacturer, is not guaranteed or endorsed by the publisher.
References
Abdul Q. A., Choi R. J., Jung H. A., Choi J. S. (2016). Health benefit of fucosterol from marine algae: a review. J. Sci. Food Agric. 96, 1856–1866. doi: 10.1002/jsfa.7489
Adarme-Vega T. C., Thomas-Hall S. R., Schenk P. M. (2014). Towards sustainable sources for omega-3 fatty acids production. Curr. Opin. Biotechnol. 26, 14–18. doi: 10.1016/j.copbio.2013.08.003
Adarshan S., Sree V. S., Muthuramalingam P., Nambiar K. S., Sevanan M., Satish L., et al. (2024). Understanding macroalgae: A comprehensive exploration of nutraceutical, pharmaceutical, and omics dimensions. Plants 13, 113. doi: 10.3390/plants13010113
Alves E., Dias M., Lopes D., Almeida A., Domingues M., Rey F. (2020). Antimicrobial lipids from plants and marine organisms: An overview of the current state-of-the-art and future prospects. Antibiotics 9, 441. doi: 10.3390/antibiotics9080441
Andreakis N., Procaccini G., Kooistra W. H. C. F. (2004). Asparagopsis taxiformis and Asparagopsis armata (Bonnemaisoniales, Rhodophyta): genetic and morphological identification of Mediterranean populations. Eur. J. Phycol. 39, 273–283. doi: 10.1080/0967026042000236436
Artemis P. S., James J. D. (2016). The importance of a balanced ω-6 to ω-3 ratio in the prevention and management of obesity. Open Heart 3, e000385. doi: 10.1136/openhrt-2015-000385
Arunkumar K., Raja R., Kumar V. B. S., Joseph A., Shilpa T., Carvalho I. S. (2021). Antioxidant and cytotoxic activities of sulfated polysaccharides from five different edible seaweeds. J. Food Measurement Characterization 15, 567–576. doi: 10.1007/s11694-020-00661-4
Bakrim S., Benkhaira N., Bourais I., Benali T., Lee L.-H., El Omari N., et al. (2022). Health benefits and pharmacological properties of stigmasterol. Antioxidants 11, 1912. doi: 10.3390/antiox11101912
Bonin D. R., Hawkes M. W. (1987). Systematics and life histories of New Zealand Bonnemaisoniaceae (Bonnemaisoniales, Rhodophyta): I. The genus Asparagopsis. N. Z. J. Bot. 25, 577–590. doi: 10.1080/0028825X.1987.10410088
Carreira-Casais A., Otero P., Garcia-Perez P., Garcia-Oliveira P., Pereira A. G., Carpena M., et al. (2021). Benefits and drawbacks of ultrasound-assisted extraction for the recovery of bioactive compounds from marine algae. Int. J. Env. Res. Public Health 18, 9153. doi: 10.3390/ijerph18179153
Catanesi M., Caioni G., Castelli V., Benedetti E., d’Angelo M., Cimini A. (2021). Benefits under the sea: The role of marine compounds in neurodegenerative disorders. Mar. Drugs 19, 24. doi: 10.3390/md19010024
Cenik B., Palka J. M., Thompson B. M., McDonald J. G., Tamminga C. A., Cenik C., et al. (2022). Desmosterol and 7-dehydrocholesterol concentrations in post mortem brains of depressed people: The role of trazodone. Trans. Psychiatry 12, 139. doi: 10.1038/s41398-022-01903-3
Chapin J. C., Hajjar K. A. (2015). Fibrinolysis and the control of blood coagulation. Blood Rev. 29, 17–24. doi: 10.1016/j.blre.2014.09.003
Cheong K.-L., Yu B., Chen J., Zhong S. (2022). A comprehensive review of the cardioprotective effect of marine algae polysaccharide on the gut microbiota. Foods 11, 3550. doi: 10.3390/foods11223550
Cho C.-H., Lu Y.-A., Kim M.-Y., Jeon Y.-J., Lee S.-H. (2022). Therapeutic potential of seaweed-derived bioactive compounds for cardiovascular disease treatment. Appl. Sci. 12, 1025. doi: 10.3390/app12031025
Choi Y., Lee S. J., Kim H. S., Eom J. S., Jo S. U., Guan L. L., et al. (2021). Effects of seaweed extracts on in vitro rumen fermentation characteristics, methane production, and microbial abundance. Sci. Rep. 11, 24092. doi: 10.1038/s41598-021-03356-y
Chualáin F. N., Maggs C. A., Saunders G. W., Guiry M. D. (2004). The invasive genus Asparagopsis (Bonnemaisoniaceae, Rhodophyta): Molecular systematics, morphology, and ecophysiology of Falkenbergia isolates. J. Phycol. 40, 1112–1126. doi: 10.1111/j.1529-8817.2004.03135.x
Church D. D., Hirsch K. R., Park S., Kim I.-Y., Gwin J. A., Pasiakos S. M., et al. (2020). Essential amino acids and protein synthesis: Insights into maximizing the muscle and whole-body response to feeding. Nutrients 12, 3717. doi: 10.3390/nu12123717
Clark B. R., Mizobe M., Kaluhiwa J. L. M., Leong J.-A., Borris R. P. (2018). Chemical and genetic differences between Hawaiian lineages of the alga Asparagopsis taxiformis. J. Appl. Phycol. 30, 2549–2559. doi: 10.1007/s10811-018-1474-6
De Bhowmick G., Hayes M. (2022). In vitro protein digestibility of selected seaweeds. Foods 11, 289. doi: 10.3390/foods11030289
DeFronzo R. A., Ferrannini E., Groop L., Henry R. R., Herman W. H., Holst J. J., et al. (2015). Type 2 diabetes mellitus. Nat. Rev. Dis. Primers 1, 15019. doi: 10.1038/nrdp.2015.19
Dijoux L., Viard F., Payri C. (2014). The more we search, the more we find: discovery of a new lineage and a new species complex in the genus asparagopsis. PloS One 9, e103826. doi: 10.1371/journal.pone.0103826
Duarte C. M., Bruhn A., Krause-Jensen D. (2022). A seaweed aquaculture imperative to meet global sustainability targets. Nat. Sustainability 5, 185–193. doi: 10.1038/s41893-021-00773-9
Dufourc E. J. (2008). Sterols and membrane dynamics. J. Chem. Biol. 1, 63–77. doi: 10.1007/s12154-008-0010-6
El-Baroty G., Moussa M., Shallan M., Ali M., Sabh A., Shalaby E. (2007). Contribution to the aroma, biological activities, minerals, protein, pigments and lipid contents of the red alga: Asparagopsis taxiformis (Delile) Trevisan. J. Appl. Sci. Res. 3, 1825–1834.
El-Beltagi H. S., Mohamed A. A., Mohamed H. I., Ramadan K. M. A., Barqawi A. A., Mansour A. T. (2022). Phytochemical and potential properties of seaweeds and their recent applications: A review. Mar. Drugs 20, 342. doi: 10.3390/md20060342
Fan J., Yuan Z., Burley S. K., Libutti S. K., Zheng X. F. S. (2022). Amino acids control blood glucose levels through mTOR signaling. Eur. J. Cell Biol. 101, 151240. doi: 10.1016/j.ejcb.2022.151240
Feki A., Cherif B., Sellem I., Naifar M., Ben Amar I., Ben Azaza Y., et al. (2023). Biomedical applications of polysaccharide derived from tetrasporophyte tufts of Asparagopsis armata (Falkenbergia rufolanosa): Focus on antioxidant, anti-inflammatory, anti-coagulant and hepato-protective activities. Algal Res. 69, 102958. doi: 10.1016/j.algal.2022.102958
Félix R., Dias P., Félix C., Cerqueira T., Andrade P. B., Valentão P., et al. (2021). The biotechnological potential of Asparagopsis armata: What is known of its chemical composition, bioactivities and current market? Algal Res. 60, 102534. doi: 10.1016/j.algal.2021.102534
Fernando I. P. S., Nah J.-W., Jeon Y.-J. (2016). Potential anti-inflammatory natural products from marine algae. Environ. Toxicol. Pharmacol. 48, 22–30. doi: 10.1016/j.etap.2016.09.023
Ferreira M. S., Resende D. I. S. P., Lobo J. M. S., Sousa E., Almeida I. F. (2021). Marine ingredients for sensitive skin: Market overview. Mar. Drugs 19, 464. doi: 10.3390/md19080464
Fletcher H. R., Biller P., Ross A. B., Adams J. M. M. (2017). The seasonal variation of fucoidan within three species of brown macroalgae. Algal Res. 22, 79–86. doi: 10.1016/j.algal.2016.10.015
Francavilla M., Franchi M., Monteleone M., Caroppo C. (2013). The red seaweed Gracilaria gracilis as a multi products source. Mar. Drugs 11, 3754–3776. doi: 10.3390/md11103754
Furman D., Campisi J., Verdin E., Carrera-Bastos P., Targ S., Franceschi C., et al. (2019). Chronic inflammation in the etiology of disease across the life span. Nat. Med. 25, 1822–1832. doi: 10.1038/s41591-019-0675-0
Geada P., Moreira C., Silva M., Nunes R., Madureira L., Rocha C. M. R., et al. (2021). Algal proteins: Production strategies and nutritional and functional properties. Bioresour. Technol. 332, 125125. doi: 10.1016/j.biortech.2021.125125
Glasson C. R. K., Kinley R. D., de Nys R., King N., Adams S. L., Packer M. A., et al. (2022). Benefits and risks of including the bromoform containing seaweed Asparagopsis in feed for the reduction of methane production from ruminants. Algal Res. 64, 102673. doi: 10.1016/j.algal.2022.102673
Hafting J. T., Craigie J. S., Stengel D. B., Loureiro R. R., Buschmann A. H., Yarish C., et al. (2015). Prospects and challenges for industrial production of seaweed bioactives. J. Phycol. 51, 821–837. doi: 10.1111/jpy.12326
Hamsanathan S., Gurkar A. U. (2022). Lipids as regulators of cellular senescence. Front. Physiol. 13. doi: 10.3389/fphys.2022.796850
Hannan M. A., Sohag A. A. M., Dash R., Haque M. N., Mohibbullah M., Oktaviani D. F., et al. (2020). Phytosterols of marine algae: Insights into the potential health benefits and molecular pharmacology. Phytomedicine 69, 153201. doi: 10.1016/j.phymed.2020.153201
Haslin C., Lahaye M., Pellegrini M. (2000). Chemical composition and structure of sulphated water-soluble cell-wall polysaccharides from the gametic, carposporic and tetrasporic stages of Asparagopsis armata Harvey (Rhodophyta, Bonnemaisoniaceae). Bot. Mar. 43, 475–482. doi: 10.1515/BOT.2000.048
Hossain M. S., Sharifuzzaman S. M., Nobi M. N., Chowdhury M. S. N., Sarker S., Alamgir M., et al. (2021). Seaweeds farming for sustainable development goals and blue economy in Bangladesh. Mar. Policy 128, 104469. doi: 10.1016/j.marpol.2021.104469
Ismail M. M., Alotaibi B. S., EL-Sheekh M. M. (2020). Therapeutic uses of red macroalgae. Molecules 25, 4411. doi: 10.3390/molecules25194411
Jauregibeitia I., Portune K., Rica I., Tueros I., Velasco O., Grau G., et al. (2020). Fatty acid profile of mature red blood cell membranes and dietary intake as a new approach to characterize children with overweight and obesity. Nutrients 12, 3446. doi: 10.3390/nu12113446
Kadam S. U., Prabhasankar P. (2010). Marine foods as functional ingredients in bakery and pasta products. Food Res. Int. 43, 1975–1980. doi: 10.1016/j.foodres.2010.06.007
Kamei Y., Hatazawa Y., Uchitomi R., Yoshimura R., Miura S. (2020). Regulation of skeletal muscle function by amino acids. Nutrients 12, 261. doi: 10.3390/nu12010261
Kraan S., Barrington K. A. (2005). Commercial farming of Asparagopsis armata (Bonnemaisoniceae, Rhodophyta) in Ireland, maintenance of an introduced species? J. Appl. Phycol. 17, 103–110. doi: 10.1007/s10811-005-2799-5
Króliczewska B., Pecka-Kiełb E., Bujok J. (2023). Strategies used to reduce methane emissions from ruminants: Controversies and issues. Agriculture 13, 602. doi: 10.3390/agriculture13030602
Lee W. T. K., Weisell R., Albert J., Tomé D., Kurpad A. V., Uauy R. (2016). Research approaches and methods for evaluating the protein quality of human foods proposed by an FAO expert working group in 2014. J. Nutr. 146, 929–932. doi: 10.3945/jn.115.222109
Leinonen I., Iannetta P. P. M., Rees R. M., Russell W., Watson C., Barnes A. P. (2019). Lysine supply is a critical factor in achieving sustainable global protein economy. Front. Sustain. Food Syst. 3. doi: 10.3389/fsufs.2019.00027
Lopes G., Sousa C., Bernardo J., Andrade P. B., Valentão P., Ferreres F., et al. (2011). Sterol profiles in 18 macroalgae of the Portuguese coast. J. Phycol. 47, 1210–1218. doi: 10.1111/j.1529-8817.2011.01028.x
Lopez-Santamarina A., Miranda J. M., Mondragon A., Lamas A., Cardelle-Cobas A., Franco C. M., et al. (2020). Potential use of marine seaweeds as prebiotics: A review. Molecules 25, 1004. doi: 10.3390/molecules25041004
Lordan R., Tsoupras A., Zabetakis I. (2017). Phospholipids of animal and marine origin: Structure, function, and anti-inflammatory properties. Molecules 22, 1964. doi: 10.3390/molecules22111964
Machado L., Magnusson M., Paul N. A., Kinley R., de Nys R., Tomkins N. (2016a). Dose-response effects of Asparagopsis taxiformis and Oedogonium sp. on in vitro fermentation and methane production. J. Appl. Phycol. 28, 1443–1452. doi: 10.1007/s10811-015-0639-9
Machado L., Magnusson M., Paul N. A., Kinley R., de Nys R., Tomkins N. (2016b). Identification of bioactives from the red seaweed Asparagopsis taxiformis that promote antimethanogenic activity in vitro. J. Appl. Phycol. 28, 3117–3126. doi: 10.1007/s10811-016-0830-7
Machado L., Tomkins N., Magnusson M., Midgley D. J., de Nys R., Rosewarne C. P. (2018). In vitro response of rumen microbiota to the antimethanogenic red macroalga Asparagopsis taxiformis. Microb. Ecol. 75, 811–818. doi: 10.1007/s00248-017-1086-8
Magnusson M., Vucko M. J., Neoh T. L., de Nys R. (2020). Using oil immersion to deliver a naturally-derived, stable bromoform product from the red seaweed Asparagopsis taxiformis. Algal Res. 51, 102065. doi: 10.1016/j.algal.2020.102065
Manilal A., Sujith S., Selvin J., Panikkar M. V. N., George S. (2012). Anticoagulant potential of polysaccharide isolated from Indian red alga, Asparagopsis taxiformis (Delile) Trevisan. Thalassas 28, 9–15.
Máximo P., Ferreira L. M., Branco P., Lima P., Lourenço A. (2018). Secondary metabolites and biological activity of invasive macroalgae of southern Europe. Mar. Drugs 16, 265. doi: 10.3390/md16080265
Meinita M. D. N., Harwanto D., Tirtawijaya G., Negara B. F. S. P., Sohn J.-H., Kim J.-S., et al. (2021). Fucosterol of marine macroalgae: Bioactivity, safety and toxicity on organism. Mar. Drugs 19, 545. doi: 10.3390/md19100545
Melo M. R. S., Feitosa J. P. A., Freitas A. L. P., de Paula R. C. M. (2002). Isolation and characterization of soluble sulfated polysaccharide from the red seaweed Gracilaria cornea. Carbohydr. Polym. 49, 491–498. doi: 10.1016/S0144-8617(02)00006-1
Morais T., Inácio A., Coutinho T., Ministro M., Cotas J., Pereira L., et al. (2020). Seaweed potential in the animal feed: A review. J. Mar. Sci. Eng. 8, 559. doi: 10.3390/jmse8080559
Muizelaar W., Groot M., van Duinkerken G., Peters R., Dijkstra J. (2021). Safety and transfer study: Transfer of bromoform present in Asparagopsis taxiformis to milk and urine of lactating dairy cows. Foods 10, 584. doi: 10.3390/foods10030584
Nahor O., Luzzatto-Knaan T., Israel Á. (2022). A new genetic lineage of Asparagopsis taxiformis (Rhodophyta) in the Mediterranean Sea: As the DNA barcoding indicates a recent Lessepsian introduction. Front. Mar. Sci. 9. doi: 10.3389/fmars.2022.873817
Neethu P. V., Suthindhiran K., Jayasri M. A. (2017). Antioxidant and antiproliferative activity of asparagopsis taxiformis. Pharmacognosy Res. 9, 238–246. doi: 10.4103/pr.pr_128_16
O'Sullivan A. M., O'Grady M. N., O'Callaghan Y. C., Smyth T. J., O'Brien N. M., Kerry J. P. (2016). Seaweed extracts as potential functional ingredients in yogurt. Innov. Food Sci. Emerg. Technol. 37, 293–299. doi: 10.1016/j.ifset.2016.07.031
Pacios I., Guerra-García J. M., Baeza-Rojano E., Cabezas M. P. (2011). The non-native seaweed Asparagopsis armata supports a diverse crustacean assemblage. Mar. Environ. Res. 71, 275–282. doi: 10.1016/j.marenvres.2011.02.002
Pahwa H., Sharan K. (2022). Food and nutrition as modifiers of the immune system: A mechanistic overview. Trends Food Sci. Technol. 123, 393–403. doi: 10.1016/j.tifs.2022.03.017
Patel A., Desai S. S., Mane V. K., Enman J., Rova U., Christakopoulos P., et al. (2022). Futuristic food fortification with a balanced ratio of dietary ω-3/ω-6 omega fatty acids for the prevention of lifestyle diseases. Trends Food Sci. Technol. 120, 140–153. doi: 10.1016/j.tifs.2022.01.006
Pellegrini M. (1969). Contribution à l’étude chimique des Algues Méditerranéennes. Bot. Mar. 12, 179–184. doi: 10.1515/botm.1969.12.1-4.179
Peñalver R., Lorenzo J. M., Ros G., Amarowicz R., Pateiro M., Nieto G. (2020). Seaweeds as a functional ingredient for a healthy diet. Mar. Drugs 18, 301. doi: 10.3390/md18060301
Pinto D. C. G. A., Lesenfants M. L., Rosa G. P., Barreto M. C., Silva A. M. S., Seca A. M. L. (2022). GC- and UHPLC-MS profiles as a tool to valorize the red alga Asparagopsis armata. Appl. Sci. 12, 892. doi: 10.3390/app12020892
Ponte J. M. S., Seca A. M. L., Barreto M. C. (2022). Asparagopsis genus: What we really know about its biological activities and chemical composition. Molecules 27, 1787. doi: 10.3390/molecules27061787
Qiu S.-M., Aweya J. J., Liu X., Liu Y., Tang S., Zhang W., et al. (2022). Bioactive polysaccharides from red seaweed as potent food supplements: a systematic review of their extraction, purification, and biological activities. Carbohydr. Polym. 275, 118696. doi: 10.1016/j.carbpol.2021.118696
Ragonese C., Tedone L., Beccaria M., Torre G., Cichello F., Cacciola F., et al. (2014). Characterisation of lipid fraction of marine macroalgae by means of chromatography techniques coupled to mass spectrometry. Food Chem. 145, 932–940. doi: 10.1016/j.foodchem.2013.08.130
Regal A. L., Alves V., Gomes R., Matos J., Bandarra N. M., Afonso C., et al. (2020). Drying process, storage conditions, and time alter the biochemical composition and bioactivity of the anti-greenhouse seaweed Asparagopsis taxiformis. Eur. Food Res. Technol. 246, 781–793. doi: 10.1007/s00217-020-03445-8
Rocha C. P., Pacheco D., Cotas J., Marques J. C., Pereira L., Gonçalves A. M. M. (2021). Seaweeds as valuable sources of essential fatty acids for human nutrition. Int. J. Env. Res. Public Health 18, 4968. doi: 10.3390/ijerph18094968
Rocha D. H. A., Pinto D. C. G. A., Silva A. M. S. (2022). Macroalgae specialized metabolites: Evidence for their anti-inflammatory health benefits. Mar. Drugs 20, 789. doi: 10.3390/md20120789
Romanazzi D., Sanchez-Garcia C., Svenson J., Mata L., Pes K., Hayman C. M., et al. (2021). Rapid analytical method for the quantification of bromoform in the red seaweeds Asparagopsis armata and Asparagopsis taxiformis using gas chromatography–mass spectrometry. ACS Agric. Sci. Technol. 1, 436–442. doi: 10.1021/acsagscitech.1c00161
Roque B. M., Brooke C. G., Ladau J., Polley T., Marsh L. J., Najafi N., et al. (2019a). Effect of the macroalgae Asparagopsis taxiformis on methane production and rumen microbiome assemblage. Anim. Microbiome 1, 3. doi: 10.1186/s42523-019-0004-4
Roque B. M., Salwen J. K., Kinley R., Kebreab E. (2019b). Inclusion of Asparagopsis armata in lactating dairy cows’ diet reduces enteric methane emission by over 50 percent. J. Cleaner Production 234, 132–138. doi: 10.1016/j.jclepro.2019.06.193
Roque B. M., Venegas M., Kinley R. D., de Nys R., Duarte T. L., Yang X., et al. (2021). Red seaweed (Asparagopsis taxiformis) supplementation reduces enteric methane by over 80 percent in beef steers. PloS One 16, e0247820. doi: 10.1371/journal.pone.0247820
Sánchez-Machado D. I., López-Hernández J., Paseiro-Losada P., López-Cervantes J. (2004). An HPLC method for the quantification of sterols in edible seaweeds. Biomed. Chromatogr. 18, 183–190. doi: 10.1002/bmc.316
Silva C. O., Simões T., Félix R., Soares A. M. V. M., Barata C., Novais S. C., et al. (2021). Asparagopsis armata exudate cocktail: the quest for the mechanisms of toxic action of an invasive seaweed on marine invertebrates. Biology 10, 223. doi: 10.3390/biology10030223
Sohn S.-I., Rathinapriya P., Balaji S., Jaya Balan D., Swetha T. K., Durgadevi R., et al. (2021). Phytosterols in seaweeds: An overview on biosynthesis to biomedical applications. Int. J. Mol. Sci. 22, 12691. doi: 10.3390/ijms222312691
Spann N. J., Garmire L. X., McDonald J. G., Myers D. S., Milne S. B., Shibata N., et al. (2012). Regulated accumulation of desmosterol integrates macrophage lipid metabolism and inflammatory responses. Cell 151, 138–152. doi: 10.1016/j.cell.2012.06.054
Stefenoni H. A., Räisänen S. E., Cueva S. F., Wasson D. E., Lage C. F. A., Melgar A., et al. (2021). Effects of the macroalga Asparagopsis taxiformis and oregano leaves on methane emission, rumen fermentation, and lactational performance of dairy cows. J. Dairy Sci. 104, 4157–4173. doi: 10.3168/jds.2020-19686
Summons R. E., Welander P. V., Gold D. A. (2022). Lipid biomarkers: molecular tools for illuminating the history of microbial life. Nat. Rev. Microbiol. 20, 174–185. doi: 10.1038/s41579-021-00636-2
Thornton P., Gurney-Smith H., Wollenberg E. (2023). Alternative sources of protein for food and feed. Curr. Opin. Environ. Sustainability 62, 101277. doi: 10.1016/j.cosust.2023.101277
Torres R., Mata L., Santos R., Alexandre A. (2021). Nitrogen uptake kinetics of an enteric methane inhibitor, the red seaweed Asparagopsis armata. J. Appl. Phycol. 33, 4001–4009. doi: 10.1007/s10811-021-02604-y
Tso R., Lim A. J., Forde C. G. (2021). A critical appraisal of the evidence supporting consumer motivations for alternative proteins. Foods 10, 24. doi: 10.3390/foods10010024
Veeraperumal S., Qiu H.-M., Zeng S.-S., Yao W.-Z., Wang B.-P., Liu Y., et al. (2020). Polysaccharides from Gracilaria lemaneiformis promote the HaCaT keratinocytes wound healing by polarised and directional cell migration. Carbohydr. Polym. 241, 116310. doi: 10.1016/j.carbpol.2020.116310
Wang M., Cheong K.-L. (2023). Preparation, structural characterisation, and bioactivities of fructans: A review. Molecules 28, 1613. doi: 10.3390/molecules28041613
Wang W., Liu X., Govers F. (2021). The mysterious route of sterols in oomycetes. PloS Path. 17, e1009591. doi: 10.1371/journal.ppat.1009591
Wei N., Quarterman J., Jin Y.-S. (2013). Marine macroalgae: an untapped resource for producing fuels and chemicals. Trends Biotechnol. 31, 70–77. doi: 10.1016/j.tibtech.2012.10.009
Wells M. L., Potin P., Craigie J. S., Raven J. A., Merchant S. S., Helliwell K. E., et al. (2017). Algae as nutritional and functional food sources: revisiting our understanding. J. Appl. Phycol. 29, 949–982. doi: 10.1007/s10811-016-0974-5
Willcox J. K., Ash S. L., Catignani G. L. (2004). Antioxidants and prevention of chronic disease. Crit. Rev. Food Sci. Nutr. 44, 275–295. doi: 10.1080/10408690490468489
Wu G. (2009). Amino acids: metabolism, functions, and nutrition. Amino Acids 37, 1–17. doi: 10.1007/s00726-009-0269-0
Wu G. (2010). Functional amino acids in growth, reproduction, and health. Adv. Nutr. 1, 31–37. doi: 10.3945/an.110.1008
Wu D.-T., Wang J., Li J., Hu J.-L., Yan H., Zhao J., et al. (2023). Physicochemical properties and biological functions of soluble dietary fibers isolated from common and Tartary buckwheat sprouts. LWT 183, 114944. doi: 10.1016/j.lwt.2023.114944
Xie X.-T., Cheong K.-L. (2022). Recent advances in marine algae oligosaccharides: structure, analysis, and potential prebiotic activities. Crit. Rev. Food Sci. Nutr. 62, 7703–7717. doi: 10.1080/10408398.2021.1916736
Xu S.-Y., Aweya J. J., Li N., Deng R.-Y., Chen W.-Y., Tang J., et al. (2019). Microbial catabolism of Porphyra haitanensis polysaccharides by human gut microbiota. Food Chem. 289, 177–186. doi: 10.1016/j.foodchem.2019.03.050
Xu S.-Y., Kan J., Hu Z., Liu Y., Du H., Pang G.-C., et al. (2018). Quantification of neoagaro-oligosaccharide production through enzymatic hydrolysis and its anti-oxidant activities. Molecules 23, 1354. doi: 10.3390/molecules23061354
Yang W., Zhao P., Li X., Guo L., Gao W. (2022). The potential roles of natural plant polysaccharides in inflammatory bowel disease: A review. Carbohydr. Polym. 277, 118821. doi: 10.1016/j.carbpol.2021.118821
Yao W., Qiu H.-M., Cheong K.-L., Zhong S. (2022). Advances in anti-cancer effects and underlying mechanisms of marine algae polysaccharides. Int. J. Biol. Macromol. 221, 472–485. doi: 10.1016/j.ijbiomac.2022.09.055
Yu B., Wang M., Teng B., Veeraperumal S., Cheung P. C.-K., Zhong S., et al. (2023). Partially acid-hydrolyzed porphyran improved dextran sulfate sodium-induced acute colitis by modulation of gut microbiota and enhancing the mucosal barrier. J. Agric. Food. Chem. 71, 7299–7311. doi: 10.1021/acs.jafc.2c08564
Zanolla M., Carmona R., de la Rosa J., Salvador N., Sherwood A. R., Andreakis N., et al. (2014). Morphological differentiation of cryptic lineages within the invasive genus Asparagopsis (Bonnemaisoniales, Rhodophyta). Phycologia 53, 233–242. doi: 10.2216/13-247.1
Zanolla M., Carmona R., Mata L., de la Rosa J., Sherwood A., Barranco C. N., et al. (2022). Concise review of the genus Asparagopsis Montagn. J. Appl. Phycol. 34, 1–17. doi: 10.1007/s10811-021-02665-z
Zaporozhets T., Besednova N. (2016). Prospects for the therapeutic application of sulfated polysaccharides of brown algae in diseases of the cardiovascular system: review. Pharm. Biol. 54, 3126–3135. doi: 10.1080/13880209.2016.1185444
Zhang X., Aweya J. J., Huang Z.-X., Kang Z.-Y., Bai Z.-H., Li K.-H., et al. (2020). In vitro fermentation of Gracilaria lemaneiformis sulfated polysaccharides and its agaro-oligosaccharides by human fecal inocula and its impact on microbiota. Carbohydr. Polym. 234, 115894. doi: 10.1016/j.carbpol.2020.115894
Zhang X., McDonald J. G., Aryal B., Canfrán-Duque A., Goldberg E. L., Araldi E., et al. (2021). Desmosterol suppresses macrophage inflammasome activation and protects against vascular inflammation and atherosclerosis. Proc. Natl. Acad. Sci. 118, e2107682118. doi: 10.1073/pnas.2107682118
Zheng L.-X., Chen X.-Q., Cheong K.-L. (2020). Current trends in marine algae polysaccharides: The digestive tract, microbial catabolism, and prebiotic potential. Int. J. Biol. Macromol. 151, 344–354. doi: 10.1016/j.ijbiomac.2020.02.168
Keywords: genus Asparagopsis, chemical composition, sterols, bromoform, polysaccharides
Citation: Cheong K-L, Li G, Wang M, Yang Y and Zhong S (2024) Unraveling the chemical identification and biological potential of the genus Asparagopsis: a comprehensive review. Front. Mar. Sci. 11:1360425. doi: 10.3389/fmars.2024.1360425
Received: 23 December 2023; Accepted: 26 February 2024;
Published: 15 March 2024.
Edited by:
Puja Kumari, Scottish Association For Marine Science, United KingdomReviewed by:
Leonel Pereira, University of Coimbra, PortugalAlok Arun, Inter American University of Puerto Rico, United States
Kusum Khatri, Ben-Gurion University of the Negev, Israel
Copyright © 2024 Cheong, Li, Wang, Yang and Zhong. This is an open-access article distributed under the terms of the Creative Commons Attribution License (CC BY). The use, distribution or reproduction in other forums is permitted, provided the original author(s) and the copyright owner(s) are credited and that the original publication in this journal is cited, in accordance with accepted academic practice. No use, distribution or reproduction is permitted which does not comply with these terms.
*Correspondence: Saiyi Zhong, emhvbmdzeUBnZG91LmVkdS5jbg==; Yufeng Yang, dHl5ZkBqbnUuZWR1LmNu