- 1Marine Biology Research Group, Ghent University, Gent, Belgium
- 2Institute of Natural Sciences, Operational Directorate Natural Sciences, Marine Ecology and Management, Brussels, Belgium
- 3Department of Estuarine and Delta Systems, Royal Netherlands Institute for Sea Research (NIOZ), Yerseke, Netherlands
- 4Instituto Antártico Argentino, Buenos Aires, Argentina
- 5Centro Austral de Investigaciones Científicas, Ushuaia, Tierra del Fuego, Argentina
- 6Universidad Nacional de Tierra del Fuego, Ushuaia, Tierra del Fuego, Argentina
Most coastal glaciers on the West Antarctic Peninsula are in retreat. Glacial ice scouring and lithogenic particle runoff increase turbidity and shape soft sediment benthic communities. This, in turn, has the potential to induce a shift in these systems from an autotrophic to a heterotrophic state. In this study, we investigated the influence of glacial runoff on carbon flows in the soft-sediment food web of Potter Cove, a well-studied shallow fjord located in the northern region of the West Antarctic Peninsula. We constructed linear inverse food web models using a dataset that includes benthic carbon stocks as well as carbon production and respiration rates. The dataset offers detailed spatial information across three locations and seasonal variations spanning three seasons, reflecting different degrees of disturbance from glacial melt runoff. In these highly resolved food web models, we quantified the carbon flows from various resource compartments (phytoplankton detritus, macroalgae, microphytobenthos, sediment detritus) to consumers (ranging from prokaryotes to various functional groups in meio- and macrofauna). Locations and seasons characterized by high glacial melt runoff exhibited distinct patterns of carbon flow compared to those with low glacial melt runoff. This difference was primarily driven by a less pronounced benthic primary production pathway, an impaired microbial loop and a lower secondary production of the dominant bivalve Aequiyoldia eightsii and other infauna in the location close to the glacier. In contrast, the bivalve Laternula elliptica and meiofauna had the highest secondary production close to the glacier, where they are exposed to high glacial melt runoff. This study shows how the effects of glacial melt propagate from lower to higher trophic levels, thereby affecting the transfer of energy in the ecosystem.
1 Introduction
The West Antarctic Peninsula (WAP) is experiencing rapid and significant warming since the second half of the 20th century (Turner et al., 2019). In addition to a shortening of the sea ice season by about 100 days (Stammerjohn et al., 2012; Ducklow et al., 2013), 87% of coastal glaciers are in retreat (Rückamp et al., 2011; Cook et al., 2016). The effects of glacial retreat are most pronounced in coastal ecosystems of the WAP (Henley et al., 2019).
When tide-water glaciers retreat on land, coastal and sub-glacial runoff of lithogenic particles increase water column turbidity (Monien et al., 2017) and reduce photosynthetic active radiation levels, hampering primary production of phytoplankton (Kim et al., 2018), macroalgae (Deregibus et al., 2016) and benthic diatoms (Campana et al., 2018; Hoffmann et al., 2019). Benthic communities further have to cope with increased ice scour frequency (Smale et al., 2008), which may lead to benthos mortality (Gutt, 2001; Smale et al., 2007; Barnes and Souster, 2011), stress for filter feeders due to sediment resuspension (Torre et al., 2012), and consequently, a restructuring of benthic assemblages in terms of community composition (Pasotti et al., 2015a; Sahade et al., 2015) and trophic interactions (Pasotti et al., 2015b; Alurralde et al., 2020). This results in a shift in benthic community functioning from being net autotrophic to net heterotrophic (Braeckman et al., 2021).
The expansion of macroalgal biomass on the newly exposed hard substrates resulting from retreating glaciers (Quartino et al., 2013; Amsler et al., 2023; Deregibus et al., 2023) leads to a shift in the dominance of primary producer communities and adds substantial amounts of macroalgal detritus to the benthic compartment (Braeckman et al., 2019; Quartino et al., 2020). A recent study demonstrated the strong microbial degradation of this macroalgal detritus in shallow Antarctic sediments (Aromokeye et al., 2021). Alterations in the carbon flow through the food web can therefore be expected as a result of the increased macroalgal biomass in the benthic system.
Qualitative networks of food webs in polar marine ecosystems are increasingly being used to infer information on food web structure and stability (Gillies et al., 2012; De Santana et al., 2013; Kortsch et al., 2015; Pasotti et al., 2015b; Marina et al., 2018; Michel et al., 2019; Rodriguez et al., 2022). However, these networks primarily remain descriptive in nature, with limited attention given to quantifying the impact of climate-induced changes to the cryosphere on the food web (Gillies et al., 2012; Pasotti et al., 2015b; Michel et al., 2019; Alurralde et al., 2020). Yet, fluctuations in composition and magnitude of the organic carbon input from primary producers and alterations in the composition and stocks of secondary (and higher order) consumers have been shown to change food web structure and stability (Forest et al., 2011; Sailley et al., 2013). For instance, a regional warming-induced stratification of the water column lead to a shift from diatom to cryptophyte production (Mendes et al., 2018), potentially explaining the observed shifts in the krill:salp ratio on the mid-WAP, with negative feedbacks on higher trophic levels (Moline et al., 2004).
The quantification of trophic interactions in a food web is needed to evaluate the magnitude of the energy flows between food web compartments, but these studies are often limited by a lack of data. For example, the first attempt to quantify energy flows within an Antarctic benthic food web associated with macroalgal beds was presented in a preliminary trophic model, but lacked incorporation of an important resource (benthic primary production by microphytobenthos) and focused on austral summer only (Ortiz et al., 2016). Linear inverse modelling (van Oevelen et al., 2010) is a technique that addresses this lack of data challenge resolving the carbon (C) flows in a food web using various sources of quantitative data (e.g. biomass, respiration rates, physiological rates) and a topological flow network (Vézina and Platt, 1988). Network indices calculated based on the quantified food web allow to capture aspects of food web functioning, such as total system throughput (sum of all C flows in the food web) and Finn’s cycling index (proportion of recycled C in the system, reflecting efficiency of C usage) (Kones et al., 2009). The technique has been successfully applied to investigate the impact of stressors on food webs, e.g. the regional warming-induced shift from multivorous to microbial pelagic food webs with impact on Adélie penguin populations on the WAP (Sailley et al., 2013). Additionally, its application in investigating the effect of sediment disturbance on deep-sea benthos (De Jonge et al., 2020) showed a reduced C cycling and cycling efficiency in the benthic food web in response to sediment disturbance and resuspension by deepsea trawling. This reduction was related to a lower microbial loop strength (both lower prokaryotic biomass and C production). Similarly, the ice scouring in the vicinity of glacier fronts in shallow Antarctic fjords can therefore be expected to influence C cycling and cycling efficiency in the sediment.
Here, we investigate the influence of glacial melt on carbon flows in the benthic food web of Potter Cove, a well-studied fjord in the North-WAP. The qualitative structure of the Potter Cove food web has been extensively described in earlier works: An analysis of binary predator-prey relationships in this shallow fjord, including hard substrate fauna and flora, pelagic food sources and fish, characterized the food web as relatively low in complexity (i.e. low linkage density, low connectance) (Marina et al., 2018), comparable to other polar food webs (De Santana et al., 2013). The food web has further been evaluated as relatively stable, with a high degree of omnivory (Rodriguez et al., 2022), and showing no clear risk of collapse upon simulated species loss (Cordone et al., 2020, 2018). Moreover, a qualitative study on the effect of glacial melt disturbance and availability of new substrate after glacial retreat on benthic trophic interactions in Potter Cove, described the benthic food web transfer at the oldest ice-free site Creek as more compact (in terms of isotopic niches) and more efficient in terms of biomass transfer. In contrast, the food web was wider (i.e. broader diet) at the new ice-free site close to the glacier Isla D (Pasotti et al., 2015b).
In this study, we use a dataset on benthic carbon (C) stocks, C production and respiration rates with detailed spatial and seasonal resolution (3 locations x 3 seasons) reflecting different degrees of glacial melt (Braeckman et al., 2021) to construct nine food web models. The hypothesized changes in the benthic food web characteristics with respect to glacial melt disturbance are:
(1) Reduced benthic primary production pathway, as reflected by lower C flows mediated by microphytobenthos,
(2) Altered contribution of the microbial loop, as reflected in changes in C flows within the microbial loop in high glacial melt runoff zones/times,
(3) Reduced secondary productivity across different trophic groups,
(4) Reduced C cycling and recycling efficiency, reflected by a reduced total system throughput (T..) and Finn’s cycling index (FCI), respectively, and
(5) Increased C burial owing to higher sediment deposition.
2 Methods
2.1 Study site
We constructed nine benthic food webs for Potter Cove, a ~3 km long and 1.2 km wide, shallow, fjord-like bay situated in the south-west of King George Island/Isla 25 de Mayo, an island located at the tip of the Antarctic Peninsula (Figure 1). The Cove receives freshwater from the Fourcade Glacier (Rückamp et al., 2011) and from seasonal meltwater discharge as a consequence of permafrost and snow melt. Sediment accumulation from turbid meltwater plumes discharging in Potter Cove (Schloss et al., 2012) has almost tripled since the 1940s (< 0.15 g cm−2 yr−1 to 0.15–0.45 g cm−2 yr−1) (Monien et al., 2011), during the Fourcade Glacier transition from a tidewater to a land-terminated glacier (Monien et al., 2017). A dominant clock-wise circulation, with an average current speed of 0.03 m s-1 (Lim, 2014), transports suspended matter out of the Cove. In the shallowest areas of Potter Cove, water column turbidity is further sustained through wind- or wave-induced sediment resuspension (Kim et al., 2018). The three locations included in the present study (6–9 m water depth, Figure 1; Supplementary Table 1) are located in the inner part of the Cove, mainly characterized by the presence of a soft sediment bottom (Wölfl et al., 2014; Pasotti et al., 2015a).
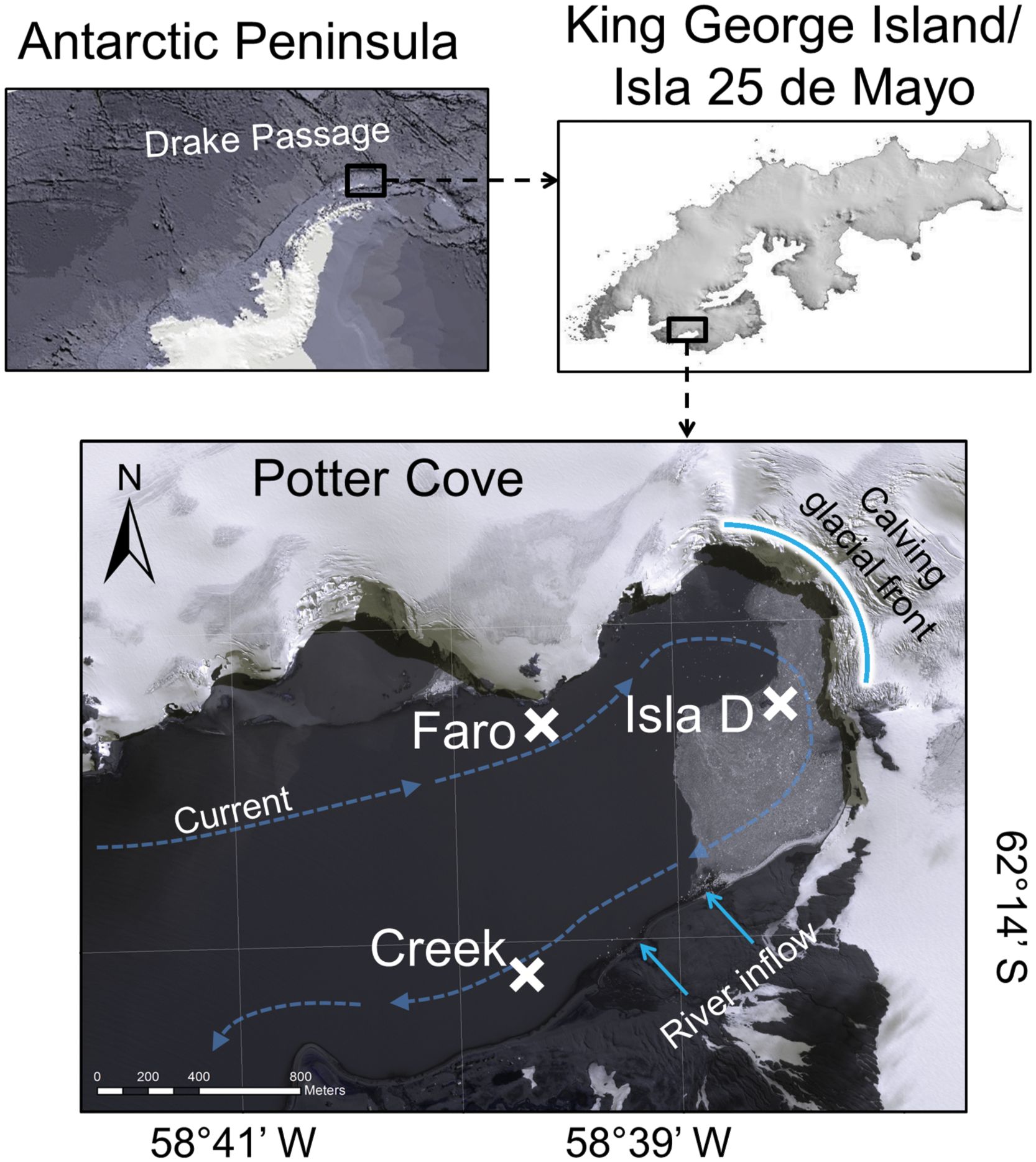
Figure 1 Study site. Potter Cove study locations Faro, Creek, and Isla D (positions are marked with a cross). The curved, bright blue line marks the front of the Fourcade Glacier. The bright blue arrows indicate river runoffs from the creeks, supplied mainly by melting glacier, permafrost and snow. The dashed blue arrows indicate the direction of the main current in Potter Cove. Satellite picture from Potter Cove taken on 3 November 2013 from Digital Globe (Digital Globe, 2014).
The locations (Faro, Isla D and Creek) are all glacial ice-free (Rückamp et al., 2011), but are regularly covered by sea ice during winter (Schloss et al., 2012). All investigated sites can be classified as meltwater fjord habitats (Jerosch et al., 2018) but owing to their location and the current system in Potter Cove, they are permanently and naturally exposed to contrasting intensities of disturbance, a consequence of the turbidity and the sediment accumulation regime (Pasotti et al., 2015a; Deregibus et al., 2016). The amount of suspended particulate matter in the water column is highest at Isla D and Creek and lowest at Faro (Monien et al., 2017). As a result, sediment accumulation is highest at Isla D, intermediate at Creek and lowest at Faro (Pasotti et al., 2015a; Seefeldt et al., 2017).
Samples were collected at the Argentinian Carlini scientific research station during the austral summer and spring of 2015 and in the spring of 2016. These seasons are characterized by strongly different glacial melt conditions. Glacial melt is typically highest during the summer (Falk et al., 2018). However, following a warm and rainy winter in 2016, glacial melt was exceptionally high in the following spring, approximately 4.5 times higher than the maxima recorded from 2010 to 2015. This resulted in a Suspended Particulate Matter (SPM) peak in the water column during the spring of 2016 that was more than twice as large as the peak observed in the spring 2015 (characterized by a cold winter) (Braeckman et al., 2021) ( Supplementary Table 2).
Data from each location – season combination (9 combinations in total) was used to construct 9 food web models. Data was retrieved from the literature as location-specific as possible. Through long-standing European-Argentinean scientific collaborations, Potter Cove has one of the most comprehensive datasets on complementary aspects of the marine ecosystem along the WAP. These include carbon stocks in different benthic compartments, meio- and macrofauna community composition, sedimentary chl a and total organic carbon content (Figure 2; Supplementary Table 3), sediment respiration and microphytobenthos production (Table 1), which in this study were considered for the three study sites in three mentioned seasons, representing both spatial and temporal variability in glacial melt runoff (Braeckman et al., 2021). Data on nematode trophic community structure from the three study sites are available for summer 2011 (Pasotti et al., 2015a), while 13C data from consumers and food sources from several locations in Potter Cove for summer 2012 (Pasotti et al., 2015b) and summer 2015 (Braeckman et al., 2019). Maximum organic carbon deposition rates were estimated from deposition rates based on sediment trap data between 2009 and 2012 (Monien et al., 2017), and the particulate organic carbon in the water column, for several periods (Schloss et al., 1999, 2012). The resulting average organic carbon deposition estimates were: 89 (Faro), 395 (Isla D) and 318 (Creek) mmol C m-2 d-1. This complementary dataset makes the site highly suitable for quantitative estimates of carbon flows in the benthic food web through linear inverse models.
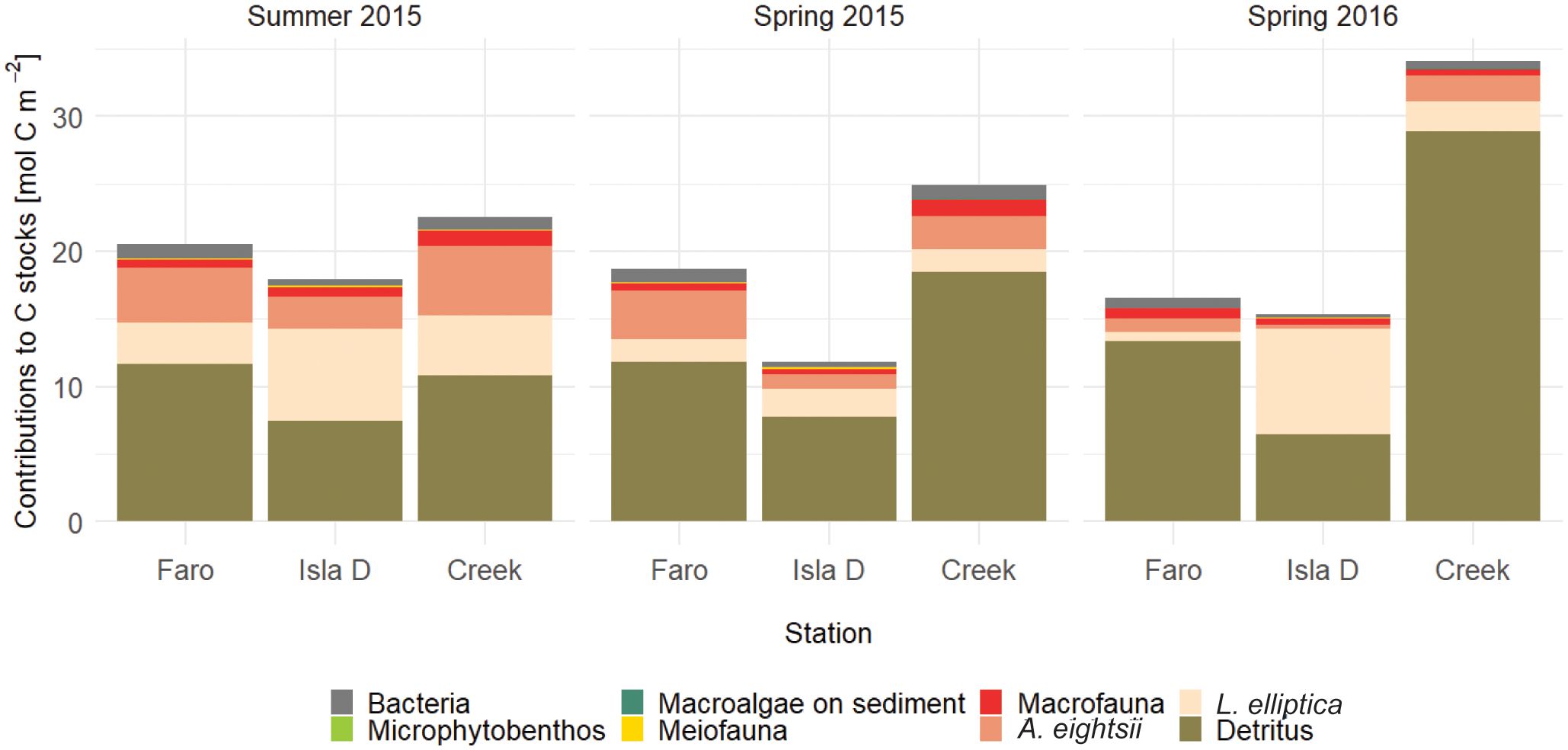
Figure 2 Overview of average measured carbon stocks at each study site and season. Largest carbon stocks are detritus pools in the sediment and stocks of the large bivalve species Laternula elliptica and Aequiyoldia eightsii.
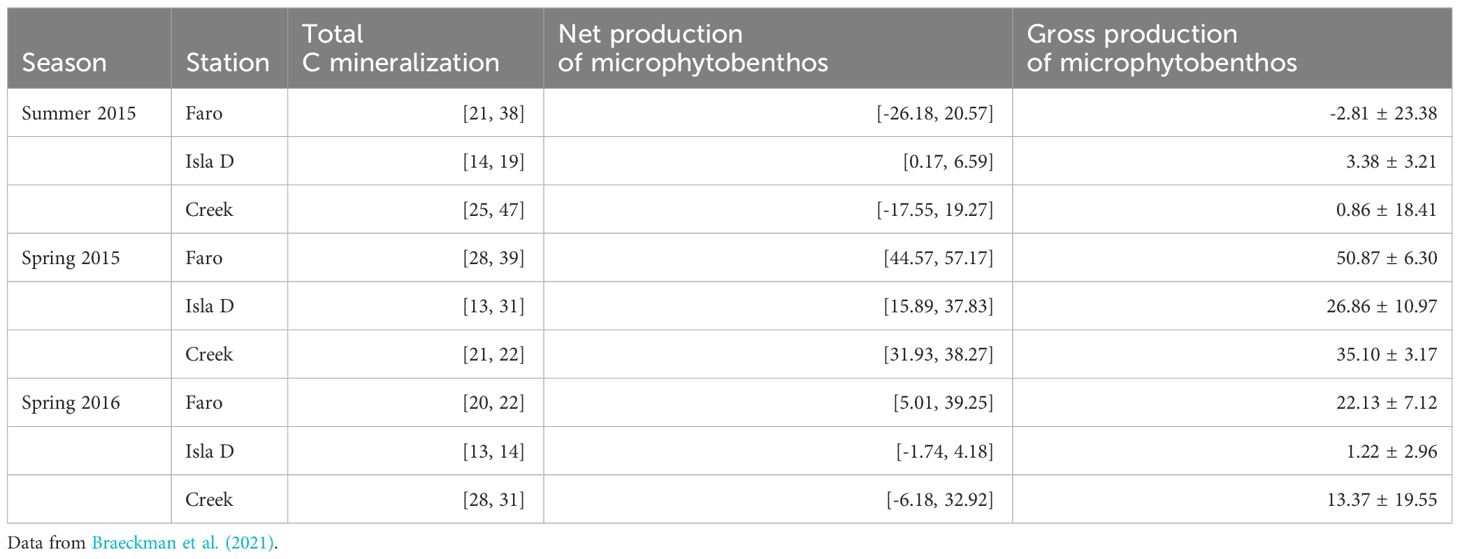
Table 1 Data on carbon fluxes (mmol C m-2 d-1) that were fed into the model as inequalities [minimum, maximum].
2.2 Food web structure
Organic carbon input into Potter Cove arises from various sources in both the water column and the sediment. In the water column, labile detritus (lDet_w), semi-labile detritus (sDet_w) and macroalgal fragments (Malg_wc) originating from the macroalgae beds on the rocky shore (Malg_hs) are deposited onto the seafloor as detritus (Malg_ps). The sediment non-faunal food-web compartments include prokaryotes (BAC), microphytobenthos (MPB), macroalgal fragments (Malg_ps), labile detritus (lDet), semi-labile detritus (sDet) and refractory detritus (rDet) sensu (Van Oevelen et al., 2011).
Faunal compartments in the food web were defined using size-classes (Meiofauna, represented by numerically dominant Nematodes NEM and other Meiofauna deposit feeders MEIODF; and macrofauna MAC) and feeding types (Nematodes: selective feeders NEMSF, non-selective feeders NEMNF, predator-omnivores NEMPO; Macrofauna: surface deposit feeders MACSDF, deposit feeders MACDF, suspension feeders MACSF and predator-scavengers MACPS). The burrowing bivalve Aequiyoldia eightsii (YOL) is a deposit-feeder and Laternula elliptica (LAT) a suspension-feeder. These bivalve species were considered separately due to their dominance in terms of biomass.
Nematode classification in feeding types was based on mouth morphology as described by Wieser (1953). However, epistrate feeders and selective deposit-feeders were pooled into one group, NEMSF. ‘Other Meiofauna Deposit feeders’ included small polychaetes, Cumacea and harpacticoid Copepoda. Macrofauna taxa included Amphipoda, Bivalvia, Cumacea, Gastropoda, Isopoda, Nemertea, Oligochaeta, Ostracoda, Pennatulacea, Polychaeta, Priapulida and Tanaidacea. The classification into feeding types was based on reported feeding ecologies in peer-reviewed literature (Supplementary Table 4). The macrobenthic deposit-feeders MACDF included the polychaete families Cirratulidae, Maldanidae, Opheliidae, Orbiniidae and Travisiidae, the oligochaetes and the bivalve family Nuculidae. The surface deposit feeders MACSDF included the polychaete families Terebellidae, Ampharetidae, Gastropods, Cumacea, Tanaidacea and the bivalve genus Mysella. Macrobenthic suspension feeders MACSF consisted of Ostracoda, Pennatulacea and the bivalve family Thyasiridae. Macrobenthic predators and scavengers MACPS were composed of the polychaete families Nephtydae, Polynoidae, the amphipod families Phoxocephalidae, Eusiridae, Oedicerotidae, Dexaminidae, Stenothoidae and Lysianassidae, the isopod families Munnidae, Serolidae, Priapulidae and Nemertea (Braeckman et al., 2021).
Carbon outflows from the food web are respiration to dissolved inorganic carbon (DIC), burial of rDet and export of macrobenthos (e.g. consumption by fish) and macroalgal fragments in the water column (physical drift out of Potter Cove). To keep the model in steady-state condition, an additional ‘C outflow’ had to be added, in which C accumulated in microphytobenthos biomass at sites or during seasons of high benthic primary production. This accumulation term also has a biological meaning: it indicates that more microphytobenthos biomass is produced than is disappearing through grazing or decay for a certain unit of time. In this case, a temporal ‘food bank’ for grazers is created. However, in the food web model description, herbivores only feed on the microphytobenthos stock, not on the accumulating microphytobenthos biomass term, since it is exactly this excess biomass that remains after herbivory.
2.3 Food web links
Carbon transfer links in the food web were implemented as exemplified in Figure 3. Within the food web, all sedimentary detritus pools are considered to be hydrolyzed, therefore contributing to the dissolved organic carbon pool in the sediment (DOC_s). They are further grazed upon by meiofauna (MeioDF, NemSF, NemDF and NemPS) and MacSDF, MacDF, MacSF, MacPS, Yol and Lat. DOC_s is also released by macroalgal fragments in the sediment or exuded by microphytobenthos and taken up by prokaryotes and NemNF. Predatory feeding links are primarily defined based on size class; prokaryotes are consumed by the meiofauna and non-suspension-feeding macrofaunal compartments. Microphytobenthos is grazed upon by meiofauna, non-suspension-feeding macrofauna, A. eightsii and L. elliptica. Macroalgal fragments in the sediment are consumed by prokaryotes, meiofauna and all non-suspension-feeding macrofauna, including A. eightsii and L. elliptica. Deposit-feeding meiofauna (incl. nematodes) are consumed by predatory nematodes, all meiofauna groups are consumed by non-suspension-feeding macrofaunal compartments including A. eightsii and L. elliptica. Macrofaunal compartments MacSDF, MacDF and MacSF are preyed upon by predatory macrofauna MacPS. Part of the sources ingested by the faunal compartments is not assimilated and expelled as feces. The non-assimilated labile (e.g. labile detritus, prokaryotic, macroalgal and faunal compartments) and semi-labile (semi-labile detritus) carbon enter the semi-labile and refractory detritus, respectively. Respiration by faunal compartments is defined as the sum of maintenance respiration (biomass-specific respiration) and growth respiration (overhead on new biomass production). Prokaryotic mortality is defined as a flux to DOC_s; microphytobenthos and faunal mortality is defined as a flux to labile detritus in the sediment.
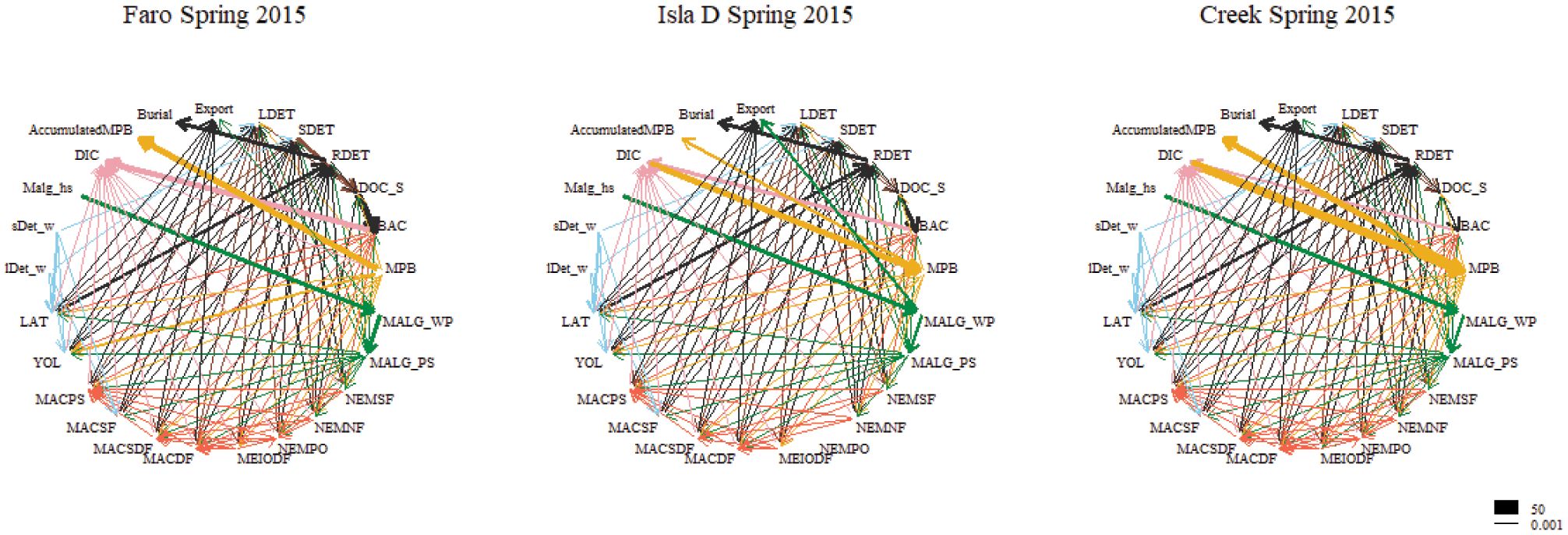
Figure 3 Food web models for Spring 2015 at the three study locations. Food web models for all seasons and full compartment names can be viewed in Supplementary Figure 1. Carbon flows in mmol C m-2 d-1.
2.4 Linear inverse food web models
The structure of the nine benthic food webs in Potter Cove was described by means of a linear inverse model (LIM). This modelling technology is explained extensively in Soetaert and van Oevelen (2009) and van Oevelen et al. (2010). In short, this model is expressed as a set of linear equality equations and a set of inequality equations. The equality equations contain the mass balance of each compartment of the food web and the flow rate data:
Where vector x contains the unknown fluxes, vectors B and H contain empirical equality and inequality data respectively, and the coefficients in matrices A and G specify the combination of unknown fluxes that should meet the requirements defined in vectors B and H.
The inequality equations are used to constrain the model output by placing upper and lower boundaries on single flows or combinations of flows. The empirical data on total respiration and microphytobenthos Gross Primary Production (GPP) were used in the constraint equation, while the stable isotope mass balances (see van Oevelen et al., 2006) were implemented in the equality equation. Furthermore, biologically realistic boundaries were placed on unmeasured fluxes (implemented as inequality equations) using literature data (Table 2).
The nine food webs contained each a total of 139 unknown C flows, 10 equations (isotope equations, see further), 18 components (mass balances) and 159 inequalities (constraints) and the implicit assumption that each flow should be ≥ 0. The number of unknown C flows outnumbers the number of data equations (10). Therefore, the model solution is underdetermined, as there exists an infinitely large set of solutions that fit the matrix equations. A likelihood approach to solving the model was taken (Van den Meersche et al., 2009; van Oevelen et al., 2010). Instead of selecting a single solution from the infinite set of solutions, the approach here uses a sampling method to retrieve the distribution of flow values in the solution set. A “best” flow value and its associated uncertainty can be inferred from all sampled food web solutions (van Oevelen et al., 2010). A total of 1000 different model solutions was sampled from the infinite possible solutions. Each of these solutions is consistent with the equality and inequality equations of the matrix, and used to calculate the mean and standard deviation of each flow. The variance on the standard deviation of 1000 iterations of a flow solution was < 10% of the variance on the standard deviation of 50 randomly sampled solutions of a flow. This means that the 1000 solutions ensure sufficient convergence of the mean and standard deviation estimate for each carbon flow (van Oevelen et al., 2011).
2.5 Data availability
2.5.1 Carbon stocks of food web compartments
Data on carbon stocks was available for all compartments (Braeckman et al., 2021) (Figure 2), except for DOC in the sediment and macroalgal fragments in the water column. Sediment was sampled with replicated 3.6 cm diameter cores by scientific divers (detritus, prokaryotes, microphytobenthos, meiobenthos) of which the top 5 cm were vertically split in sections of 1 cm and further analyzed. Labile detritus was defined as all carbon associated with chlorophyll a in the top 1 cm. Chlorophyll a concentrations were converted to carbon units by assuming a carbon to chlorophyll a ratio of 55 (van Oevelen et al., 2012, 2006). Semi-labile detritus was defined as the fraction of total organic carbon in the top 4 cm minus that of the bottom cm (4-5 cm). Refractory detritus was defined as the total OC stock in the lowest layer of the top 5 cm of the sediment (4-5 cm). Prokaryotic and microphytobenthos carbon stocks were inferred from cell counts and cell volumes (Braeckman et al., 2021). The biomass of macroalgal fragments was estimated from images within frames of 45x45 cm taken by scientific divers. Biomass in the sediment was determined based on percent cover as analyzed with ImageJ. A surface-algal-dry weight conversion factor of 0.6 g dry weight dm-2 was used for Saccharina latissima (Broch and Slagstad, 2012). For Desmarestia anceps and Palmaria decipiens an organic carbon fraction of 25% of the dry weight was considered (Peters et al., 2005; Braeckman et al., 2019). The species composition of the macroalgal fragments in the sediments was more diverse, and the tissue not always a flat surface. Therefore, the macroalgal fragment biomass estimates are considered conservative. These images also served to estimate the biomass of L. elliptica through siphon counts and conversion from siphon width to ash-free dry weight (Hoffmann et al., 2018). The biomass of meiofauna in the top 5 cm and other macrofauna including A. eightsii was taken from Braeckman et al. (2021). Carbon biomass for each of the compartments used in the models is displayed in Figure 2 and Supplementary Table 3.
2.5.2 Inequality constraints
Total oxygen exchange was measured in transparent and dark in situ benthic chambers over the course of 22-24h (Braeckman et al., 2021). Total respiration was derived from the total oxygen uptake (TOU) rates measured in in situ dark benthic chambers, while GPP by microphytobenthos was estimated from the sum of Net Community Metabolism (NCM), measured in transparent benthic chambers, and Respiration (R) (Table 2). Total respiration includes that of bacteria, microphytobenthos, meiofauna and macrofauna, including respiration of A. eightsii and L. elliptica. The minimum and maximum respiration rates of the benthic primary producers were set at 5 and 30% of GPP, respectively (Vézina and Platt, 1988). An additional number of general inequality constraints was taken from the literature to constrain degradation rates of the labile, semi-labile and refractory detritus pools (Supplementary Table 5), prokaryote growth efficiency, assimilation efficiency of all faunal compartments, net growth efficiency of all faunal compartments, production and mortality rates of all faunal compartments (Table 2, Supplementary Table 6). Since measurements of assimilation and growth efficiencies of Antarctic benthos are very rare, we relied on a literature review of temperate benthos (van Oevelen et al., 2006) as basis for these constraints. Biomass-specific maintenance respiration of meiofaunal and macrofaunal compartments was defined as 0.01 d-1 at 20°C, while for the large bivalves A. eightsii and L. elliptica, 0.001 d-1 at 20°C was assumed (see references in van Oevelen et al., 2006). These respiration rates were corrected to respiration estimates at the in situ temperature, using a temperature-correction factor (Tlim) for each season, assuming a Q10 temperature coefficient of 2 (Supplementary Table 7).
2.6 Implementation of natural abundance isotope and isotope tracer data
Assimilation rates of macroalgal C by prokaryotes, meiofauna and macrofauna including A. eightsii and L. elliptica (Table 3) were assessed in a laboratory setting by conducting in situ pulse-chase experiments with 13C-labelled Desmarestia anceps and Palmaria decipiens (Braeckman et al., 2019). These phytodetritus C incorporation rates (minimum and maximum rates) were integrated in the linear inverse model to further constrain C flows (van Oevelen et al., 2012, 2006).
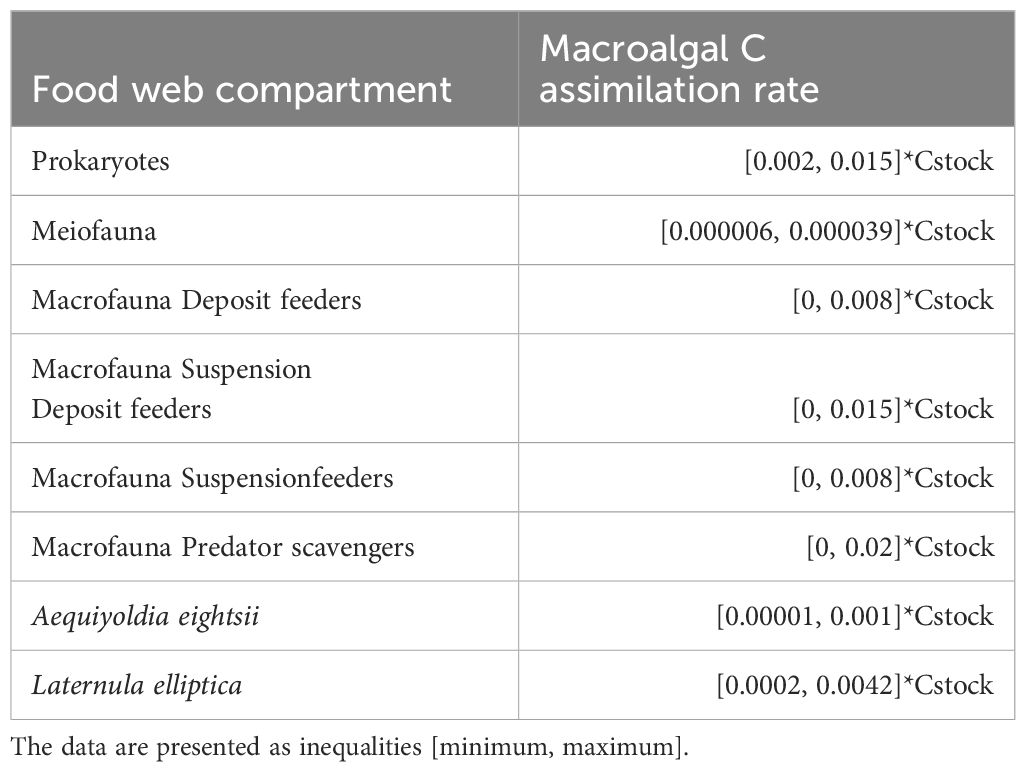
Table 3 Macroalgal C assimilation rates I (mmol C m-2 d-1) in prokaryotes, meio- and macrofauna based on pulse-chase experiments by Braeckman et al. (2019).
Natural isotope abundance data (δ13C) were available for all primary food sources and the faunal compartments in Potter Cove from summer 2012 and 2015 (Pasotti et al., 2015b; Braeckman et al., 2019) (Supplementary Table 8). Hence, a seasonal distinction could not be imposed by implementing the natural 13C abundance signature data in the LIM. Also, the spatial resolution at the scale of the three investigated sites was not available for the primary food sources, nor for every faunal compartment. However, for those taxa that were sampled at the three study sites, no spatial difference in δ13C was found. The natural abundance δ13C data were implemented in the LIM as equality equations in the form of mixing models (10 equations, 1 equation for each consumer compartment of meiofauna and macrofauna) (van Oevelen et al., 2006).
2.7 Model calibration
The accuracy of the model estimates is inherently dependent on both the quality of the input data and model assumptions. For this reason, we focused on soft-substrate communities, constraining the models with as much site - and season-specific biological and biogeochemical data as possible, using site-specific experimental pulse-chase and natural abundance stable isotope data and literature sources to constrain unmeasured C flows. An advantage of the linear inverse modelling technique is that the data are checked for internal consistency, meaning that if the data (i.e. carbon stocks and turnover rates) are not compatible, the model cannot be solved.
To make the models solvable, the ranges of some physiological constraints found in literature (Table 2) had to be slightly adjusted. This was the case for the minimum growth rate of macrofauna and the minimum ingestion rate and net growth efficiency of L. elliptica, while the upper boundary on the mortality rates of L. elliptica and A. eightsii had to be increased. While the adjustments for macrofauna in general were limited (growth rate of macrofauna was lowered with 3% compared to literature values), the ranges of the physiological rates of the large bivalves had to be expanded more substantially. In this sense, the minimum ingestion rate had to be lowered to 42% of what was reported as minimum for the sister species L. marilina (Supplementary Table 6), and maximum mortality rates had to be increased by 20% for L. elliptica and multiplied by 20 for A. eightsii (Supplementary Table 6). This indicates that the carbon input estimated by the model was not sufficient to sustain bivalve populations that are fast growing and long-lived. An explanation for this incompatibility can be that the physiological rates found in literature originate from experimental studies (van Oevelen et al., 2006) and that a temperature correction was not sufficient to reduce the metabolic rate. The lower mortality rates could originate from populations that are or were less affected by glacial melt disturbance at the time of study (Peck and Bullough, 1993; Urban and Mercuri, 1998). The feeding preference of macrofauna predators and scavengers was originally 0.75 (van Oevelen et al., 2011), meaning that these organisms were feeding for 75% selectively on other organisms or carrion. However, this value had to be lowered to 0.3, indicating a higher degree of omnivory, which is consistent with a recent food web topology study demonstrating the importance of omnivory in Potter Cove (Rodriguez et al., 2022). In all, adjusting these physiological constraints from literature to values that make the model solvable, informs us on more realistic site-specific values for the populations in our study area. It also informs us about the strong influence of the high biomass of the L. elliptica and A. eightsii populations on the carbon fluxes in the benthic food web of Potter Cove. The values of the constraints on the physiology of these two bivalve species had to be adjusted to decrease the carbon cycling in these species. As literature sources reported higher carbon cycling rates, our use of lower rate estimates means that the contribution of these two large bivalve species to carbon cycling in Potter Cove might be underestimated.
Finally, the fractionation factor of 13C applied in the isotope mixing models integrated in the LIMs, had to be increased from 1 to 2 for the grazing of A. eightsii on microphytobenthos (incl. benthic diatoms) and to 3 for macrofauna suspension feeding on water column detritus and macroalgal fragments. The reason for this might be that natural abundance stable isotope data were obtained in summer (Pasotti et al., 2015b), whereas the LIMs were also constructed for spring seasons. In spring, high growth rates of diatoms lead to reduced isotope fractionation with respect to the inorganic carbon source, but subsequent lower growth rates increase the isotope fractionation again (Laws et al., 1995). These fluctuations might have contributed to the mismatch in 13C signature between food source and consumer, hence the higher fractionation factor.
Phytoplankton production was not included in the models, as no data were available for the three different seasons in 2015-2016. Since phytoplankton biomass and production can vary an order of magnitude interseasonally – and interannually (Latorre et al., 2023; Antoni et al. in review), we did not include phytoplankton data from other years. Nevertheless, glacier melting limits phytoplankton as well (Schloss et al., 2002), but benthic primary production is considered more important for benthic organisms than phytoplankton production (Ahn et al., 1993).
Foraminifera were not included, because no biomass data for this compartment were available. These organisms graze on diatoms, bacteria and consume DOC and their grazing rates in Antarctic sediment can attain the same order of magnitude as those of metazoans (Rivkin and DeLaca, 1990).
No epifauna was included, although ascidians, limpets and sponges clearly play an important role in Potter Cove (Sahade et al., 2015; Lagger et al., 2018). Nevertheless, these organisms were not observed in our image analysis of ~150 pictures of Potter Cove sediments, hence their absence from the models.
The predation flow from infauna to consumers was not considered in the present food web models. Species preying on the sediment infauna would include Nototheniid fish and starfish (Moreno and Osorio, 1977; Casaux, 1998; Dunton, 2001; Moreira et al., 2014). However, there are no standardized density and/or biomass data for Nototheniid fish, which are usually sampled with trammel nets (Moreira et al., 2014). The carbon flow from infaunal taxa to their predators was therefore included in the ‘Export’ term. This term also includes physical export of C biomass away from the study site.
2.8 Network indices
Network indices “Total system throughput” (T..), i.e., the sum of all C flows in the food web and “Finns’ Cycling Index” (FCI), i.e. the proportion of the total system of cycled throughflow, were calculated with the R package NetIndices v.1.4.4 (Kones et al., 2009). for each of the 1000 model solutions and summarized as mean ± sd.
2.9 Intermodel comparison of results
To test the five hypotheses, the variables resulting from the nine models were compared by calculating the fraction of which the randomized set of 1000 results of one model is larger than that of another model. For example, when this fraction is 0.90, this implies that 90% of the values of model 1 are larger than the ones of model 2 (and consequently, 10% of the values are lower). We define differences of 90% and 10% as significant difference and 95% and 5% as highly significant difference.
3 Results
3.1 Carbon stocks
Carbon stocks in the soft-substrate benthic ecosystem of Potter Cove were dominated by detritus (the sum of the labile, semi-labile and refractory compartments made up 42 – 85% of the total carbon stocks), followed by the large bivalves L. elliptica (4 – 51%) and A. eightsii (2 – 20%) (Figure 2; Supplementary Table 3). Bacterial organic carbon and that of other macrofauna constituted both 1 – 5% of the total organic carbon stock. Meiofauna (0.1 – 1%), microphytobenthos and macroalgal fragments on the sediment (all 0 – 0.1%) were the smallest components of the organic carbon stock. Total carbon stocks were smallest at the glacier station (Isla D) and largest at the downstream station (Creek). Close to the glacier, the organic detritus, A. eightsii and other macrofauna stocks were smaller, while the meiofauna and L. elliptica stock was larger.
3.2 Carbon flows
The model-derived carbon flows in the food webs of each of the study sites and seasons are exemplified in Figure 3 and fully depicted in Supplementary Figure 1. The largest carbon flows were related to burial of refractory detritus in the sediment, microphytobenthos production and accumulation of microphytobenthic organisms, the carbon flow of labile detritus in the water column to L. elliptica and A. eightsii and the export of macroalgae particles in the water column to the pool of macroalgae fragments in the sediment (Figure 3). The maximum flow magnitude was primary production by microphytobenthos in Faro in Spring 2015 (50 mmol m-2 d-1).
3.2.1 Carbon input
The total input of carbon, that is the sum of the release of macroalgae from hard substrates as fragments into the water column, the deposition of labile and semi-labile detritus from the water column onto the sediment, suspension feeding and gross microphytobenthos production) was significantly lower at Isla D in Summer 2015 than in the other two stations (Figure 4A; Supplementary Table 9). Total carbon input at Faro and Isla D was significantly higher in Spring 2015 (57 – 84 mmol m-2 d-1) compared to Summer 2015 and Spring 2016 (30 – 61 mmol m-2 d-1). At Creek, total carbon input was also significantly higher in Spring 2015 compared to Spring 2016 (Figure 4A). The main contributor to these differences was the higher gross microphytobenthos production found in Spring 2015 (41 – 59% of the total carbon input) than in Summer 2015 and Spring 2016 (14 – 35%). This represented the highest number of ‘significantly different’ results in model intercomparison encountered (Supplementary Table 9). Macroalgae input into the system contributed 19 - 40% to the total input, suspension feeding accounted for 14 to 49%, and water column detritus for 4 – 14%.
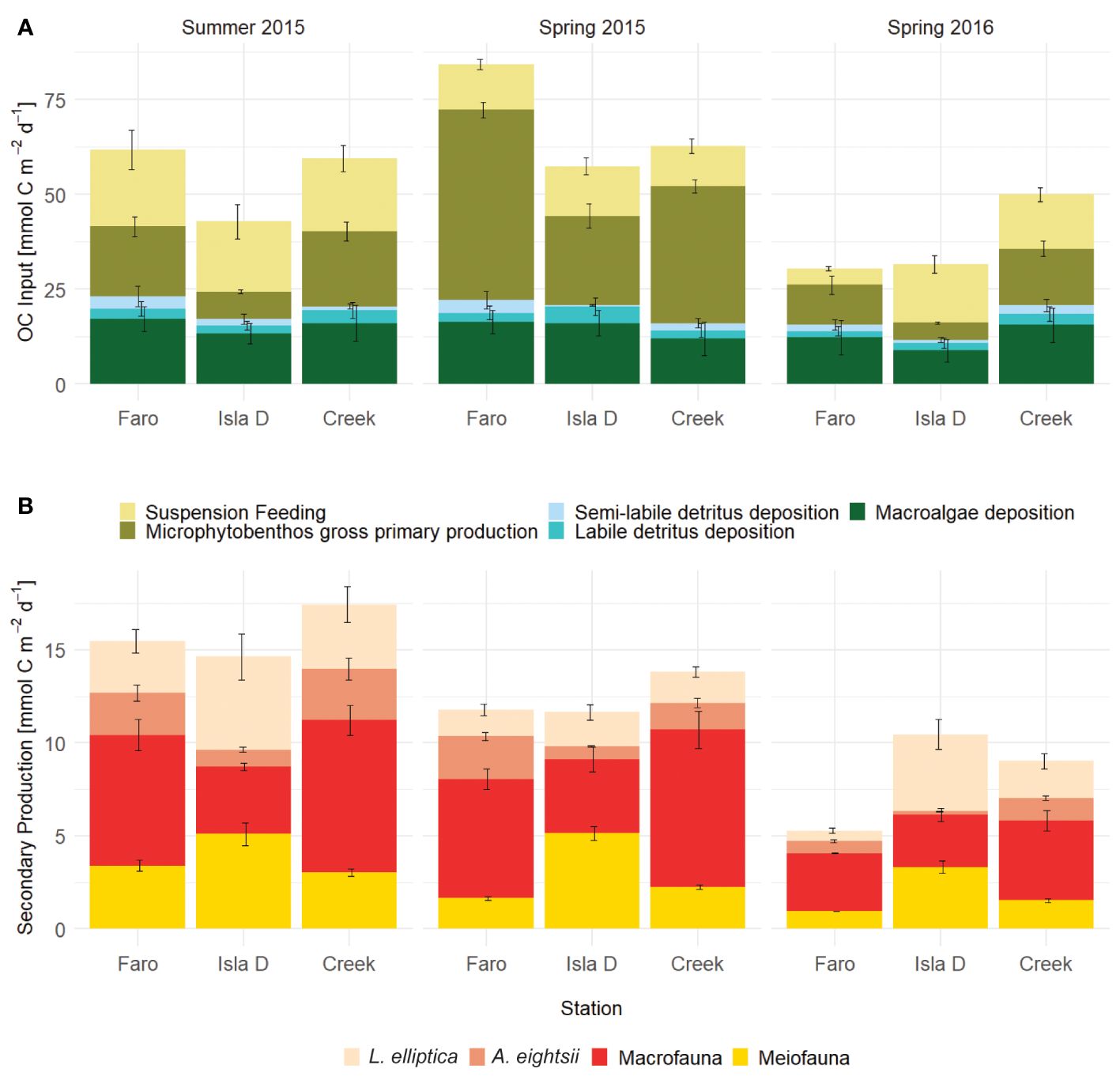
Figure 4 (A) Contributions to carbon input in Potter Cove (Macroalgae from hard substrates to water column, deposition of labile and semi-labile detritus in the water column on the sediment, suspension feeding and gross microphytobenthos production). (B) Contributions to secondary production (meiofauna, large burrowing bivalves Laternula elliptica, Aequiyoldia eightsii and other macrofauna). Means and standard deviations of 1000 simulations.
3.2.2 Carbon outflow
Model carbon outflow consists of respiration represented as flows to DIC, accumulation in microphytobenthos biomass, export (physical export out of Potter Cove and predation by higher trophic levels such as Nototheniid fish) and burial (Table 4). Respiration was the largest outflow (34 – 69% of total C outflow), and was significantly lower at Isla D in Summer 2015 and Spring 2016 (14 and 19 mmol C m-2 d-1 respectively) than in other seasons and sites (21 – 40 mmol C m-2 d-1) (Table 4; Supplementary Table 9). Bacteria were the largest contributors to total respiration (34 – 63% of total respiration), followed by L. elliptica (6 – 28%) and macrobenthic predator-scavengers (6 – 17%) (Supplementary Table 10).
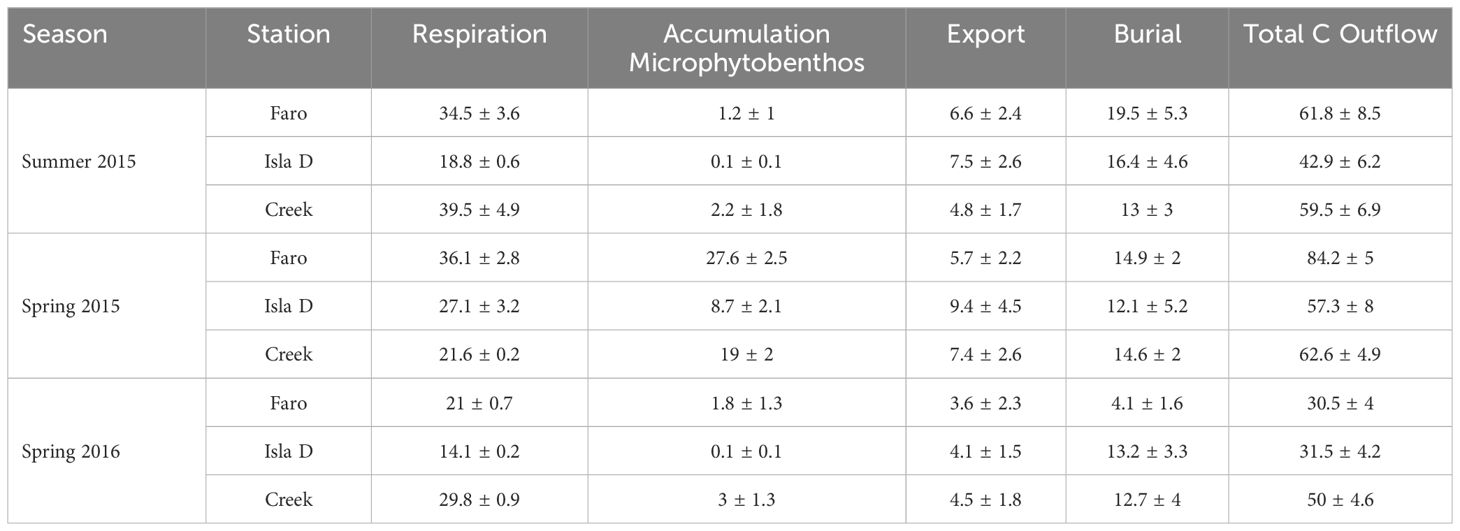
Table 4 Outflows in the Potter Cove benthic food web, average ± sd of 1000 solutions (mmol C m-2 d-1).
Burial (i.e. the carbon flow from refractory detritus to long-term burial in the sediment) was the second largest C outflow (13 – 42% of C outflow), with rates ranging between 4 and 19.5 mmol C m-2 d-1 (Table 4).
Export (7 – 17% of C outflows) was composed of export of macrofaunal biomass (24 – 84% of C outflow) and macroalgal fragments in the water column (15 – 75%) (Supplementary Table 11). The contribution of export to C outflows was highest at Isla D (13 – 17% of C outflows). Model intercomparisons did not indicate a consistent influence of glacial melt on C burial nor export (Supplementary Table 9).
Accumulation of carbon in the microphytobenthos pool was small at all stations in Summer 2015 and Spring 2016 (0.2 – 6% of C outflow), but was significantly higher in Spring 2015, ranging from 15% at Isla D to 33% of the carbon outflow at Faro (Table 4; Supplementary Table 9).
3.2.3 Carbon ingestion
Deposit feeding on sediment detritus (23 – 34% of the carbon that was ingested) and suspension feeding (22 – 43%, mainly by L. elliptica) were the dominant feeding modes (Table 5). Grazing on microphytobenthos was important in Faro and Creek (22 – 30% of carbon ingestion pathways) and significantly lower at Isla D (Supplementary Table 9). There were no other major differences in carbon ingestion pathways with regard to glacial melt impact, but carbon ingestion in Spring 2016 was consistently lower than in the other seasons (Table 5). Predation (4 – 8%) and grazing on macroalgal fragments in the sediment (2 – 5%) and on bacteria (4 – 9%) were the smallest carbon ingestion flows.
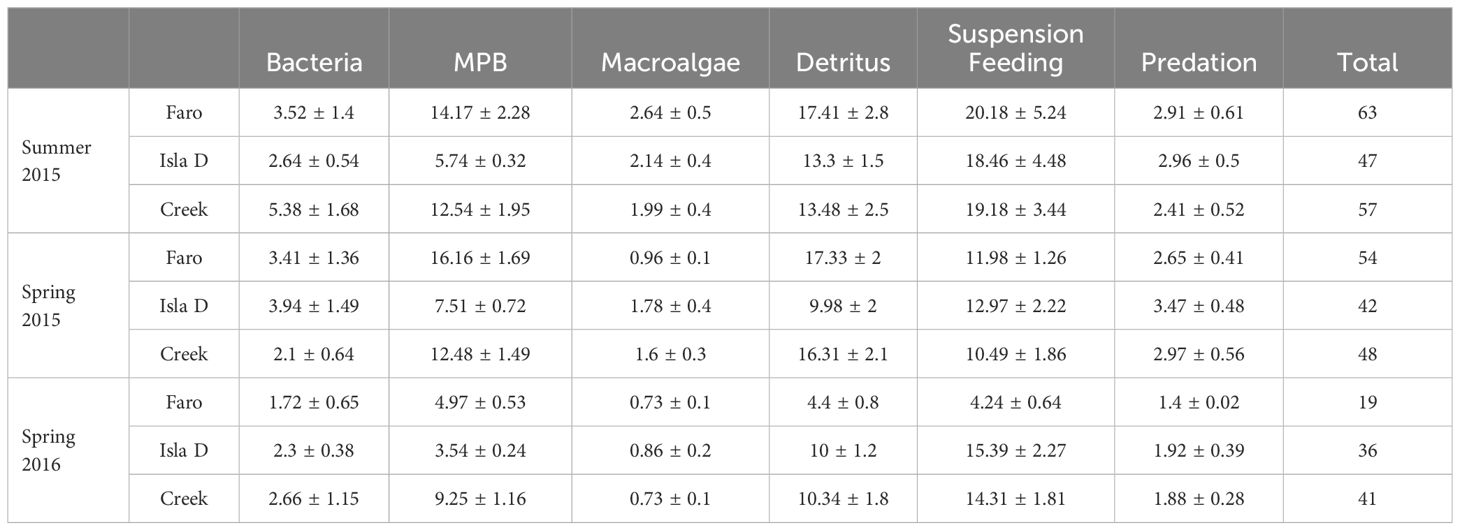
Table 5 Ingestion modes in the Potter Cove benthic food web, average ± sd of 1000 solutions, in mmol C m-2 d-1.
3.2.4 Secondary production
Total secondary production did not show any influence of glacial melt disturbance (Figure 4B). A significantly reduced secondary production close to the glacier was mainly observed for the burrowing bivalve A. eightsii and other macrofauna (Figure 4B; Supplementary Table 9). In contrast, the large burrowing bivalve species, L. elliptica, showed a significantly higher secondary production close to the glacier (Isla D) than at Faro in summer 2015 or Faro and Creek in Spring 2015.
Meiofauna secondary production was highest close to the glacier compared to the stations further away from the glacier in all three seasons (Figure 4B; Supplementary Table 9). In spring 2016, a season with strong glacial melt runoff, total secondary production was even higher close to the glacier (Isla D) compared to the site upstream of the glacier (Faro), governed by the significantly higher secondary production of L. elliptica and meiofauna (Supplementary Table 9).
3.2.5 Network indices
The average total system throughput (summed carbon flows, in mmol C m-2 d-1) in Summer 2015 was 32 and 36% lower at the site closest to the glacier (Isla D) than at Creek and Faro, respectively. In Spring 2015, total system throughput was highest at Faro, but similar in the two other locations, while in Spring 2016, Creek was characterized by a significantly higher total system throughput than the other two stations (Figure 5; Supplementary Table 9).
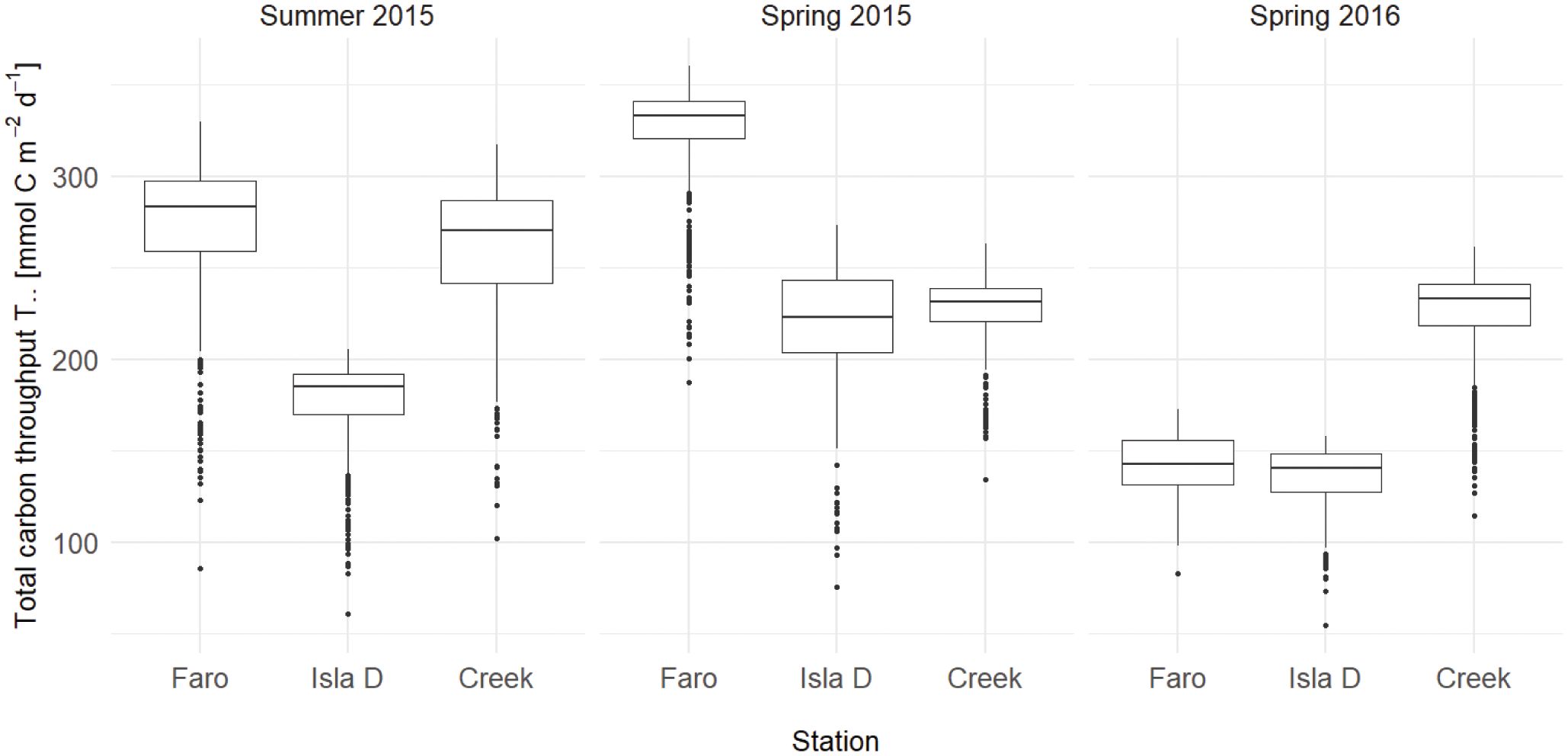
Figure 5 Network index total carbon throughput (T..) in the Potter Cove benthic food webs. Boxplot of 1,000 simulations.
The cycling index FCI was low at the three sites. About 5 to 8% of the carbon was recycled in the benthic system.
3.3 Specific carbon pathways
3.3.1 Herbivorous pathways
The benthic primary production pathway consists of net primary production by microphytobenthos, accumulation of carbon in microphytobenthos biomass, DOC production and respiration by microphytobenthos and grazing on microphytobenthos. This pathway was of significantly lower magnitude closest to the glacier (Isla D) and in the seasons experiencing strongest glacial runoff (Summer 2015 and Spring 2016) (Figure 6A; Supplementary Table 9).
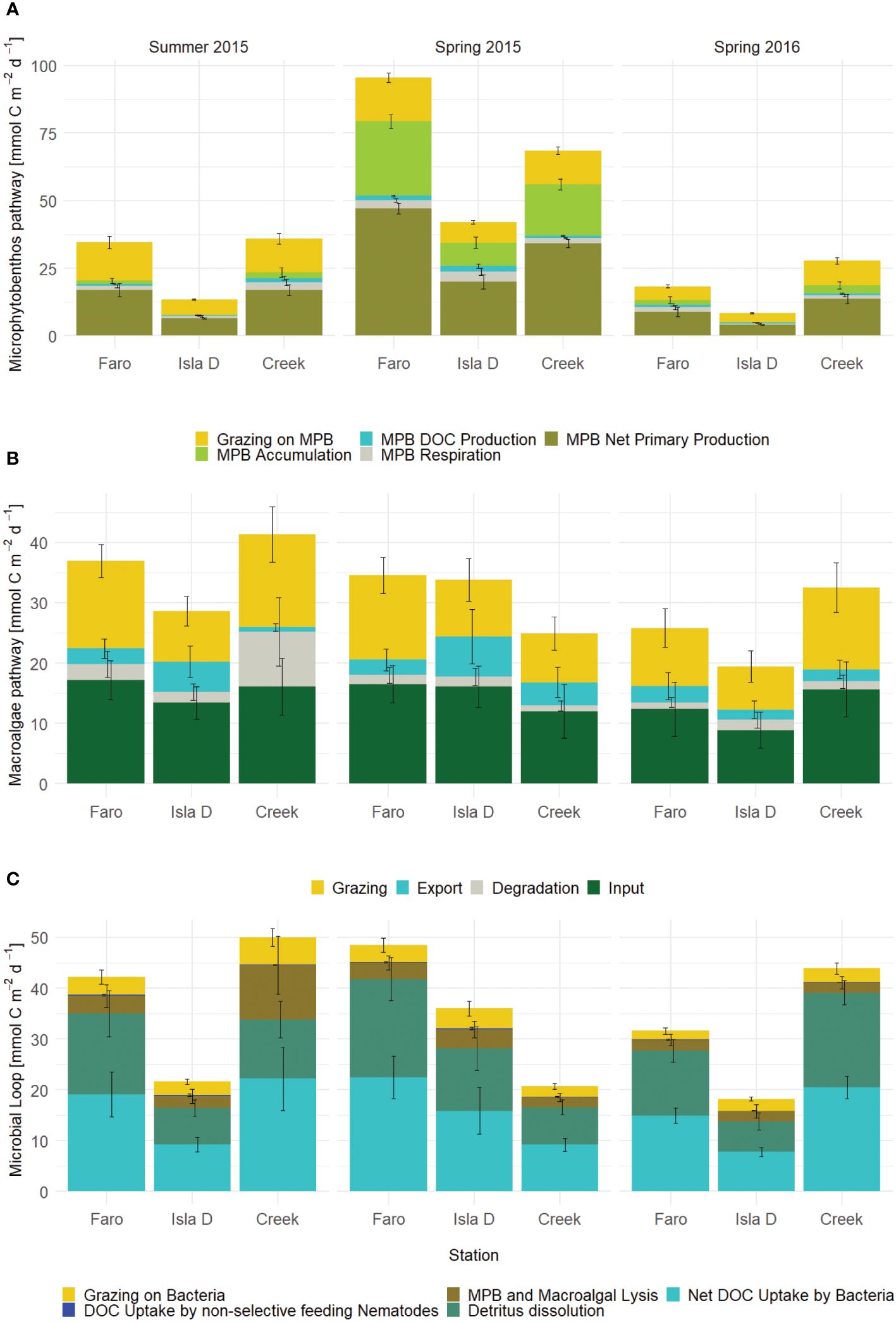
Figure 6 Contributions to three pathways at the three locations and three seasons with different glacial melt impact: (A) Microphytobenthos (MPB) pathway, (B) Macroalgae pathway and (C) Microbial loop. Means and standard deviations of 1000 simulations.
The macroalgae pathway consists of the input of organic matter from hard substrates into the water column through fragmentation, export of these water column fragments out of Potter Cove, grazing by bacteria, meiofauna and macrofauna, including large bivalves in the sediment, and degradation as dissolution of macroalgal material to DOC in the sediment. In general, grazing was the dominant fate of the macroalgal fragments (Figure 6B). The fraction of macroalgae that was exported ranged between 4 and 37% of the macroalgae carbon outflow. The macroalgae pathway and its individual flows were not impacted by glacial runoff (no significant differences shown in Supplementary Table 9).
3.3.2 Microbial loop
The microbial loop was significantly reduced close to the glacier during seasons with high glacial disturbance (Figure 6C; Supplementary Table 9). However, due to a higher detritus dissolution and high net DOC uptake by bacteria close to the glacier (Isla D), this was not the case in Spring 2015.
4 Discussion
Here, we present the first quantitative study on carbon flows in an Antarctic benthic food web attempting to quantify the effect of glacial melt disturbance on the benthic food web. Our comprehensive dataset on soft-substrate benthos and carbon turnover rates (Braeckman et al., 2021, 2019; Pasotti et al., 2015b) has been instrumental in this endeavor, complemented by insights from numerous site-specific literature sources. The presented benthic food web models also allow us to estimate C flows that were not directly measured, such as the carbon flow of macroalgae detritus to benthos and the export of macroalgae fragments in the water column, including the associated uncertainty. This adds considerable detail to our understanding of the fate of detached macroalgae in Potter Cove, for which a rough budget has been recently presented (Quartino et al., 2020).
4.1 Glacial melt impact on the benthic primary production pathway (H1)
We hypothesized a reduced benthic primary production pathway under glacial melt disturbance (H1). The benthic primary production pathway is indeed less intense in the site closest to the glacier (Isla D) and in the seasons experiencing the strongest glacial runoff (Summer 2015 and Spring 2016) (Figure 6B). In addition to the low light levels in the water column close to the glacier, the high sedimentation rate close to the glacier buries the benthic diatoms. Although these organisms have the capacity to migrate to the surface, the repeated disturbance affects their energy allocation, hence lowering their production potential (Hoffmann et al., 2019). In addition, the scouring of ice growlers dropping down from the glacier frequently resuspends the benthic diatoms into the overlying water, excluding them from the contribution to the microphytobenthos C stock and benthic primary production. Although in spring 2015, the microphytobenthos pathway was more intense in all three study sites in Potter Cove, the food web at the site closest to the glacier (Isla D) still had the least intense microphytobenthos pathway. This means that the frequent disturbance of the sediments close to the glacier poses a continuous limit on the primary production by the microphytobenthos community, both through a reduction in light availability and the resuspension of benthic diatoms. The resuspended benthic diatoms would still be available to filter-feeding macrofauna extending siphons in the water column, such as L. elliptica (Ahn et al., 1993).
In contrast, when glacial runoff was reduced in Spring 2015, a clear build-up of microphytobenthos biomass was observed as increased values of the accumulation term. This would indicate that when glacial disturbance is low, microphytobenthos biomass remains longer available to grazers in the area. Strong evidence for the existence of such ‘food banks’ that persist during winter months has been shown for Antarctic shelf sediments (Smith et al., 2012).
4.2 Glacial melt impact on the microbial loop (H2)
The microbial loop was hypothesized to change under glacial melt impact (H2). The direction of change was not specified in the hypothesis, since the response of the microbial community could be net positive or negative. A negative impact of sediment disturbance and resuspension on the benthic microbial loop have been observed in a deep-sea trawling experiment, probably because of slow regrowth of microbial biomass (De Jonge et al., 2020). Similarly, lower bacterial abundance but increased C turnover rates were observed in microcosm experiments simulating resuspension events (Pusceddu et al., 2005). In Potter Cove sediments, we observed that the microbial loop was indeed less intense close to the glacier during high runoff seasons (Figure 6C). However, during the low runoff season (Spring 2015), the microbial loop close to the glacier was nearly as strong as in the sediments underlying clear waters further from the glacier (Faro). Most likely, this reflects to a large extent the intense recycling of new biomass produced by benthic diatoms and/or the DOC they exudate, the higher the detritus dissolution and net DOC uptake by Bacteria. Also, degradation of macroalgal detritus might contribute to the microbial loop strength. A strong positive influence of macroalgal detritus on the microbial loop has recently been demonstrated in Potter Cove (Aromokeye et al., 2021).
4.3 Glacial melt impact on secondary production (H3)
Hypothesis 3, i.e. a reduced secondary production of different trophic groups, was corroborated, in particular for the burrowing bivalve A. eightsii and other macrofauna, but not for total secondary production. A reduced secondary production of A. eightsii is in agreement with smaller size and age of A. eightsii populations that are regularly disturbed by ice scouring (Peck and Bullough, 1993). Lower food availability close to the glacier (i.e. lower concentration of benthic diatoms or detritus more diluted in high sediment deposits) is a potential explanation for reduced fitness in Nuculanid bivalves (Bascur et al., 2020), to which A. eightsii belongs. A lower fitness might result in slower growth under high glacial runoff.
The opposite is true for the secondary production of L. elliptica and meiofauna, who seem to thrive close to the glacier. The secondary production of this bivalve close to the glacier (Isla D) was often higher compared to the sites less impacted by glacial runoff (Faro, Creek). Notably, the L. elliptica population near the glacier was dominated by small individuals (Braeckman et al., 2021). Smaller individuals feature a faster (re)burying activity and recovery from injury than larger specimens, which renders them better adapted to frequent scouring disturbance (Philipp et al., 2011). L. elliptica was considered to graze primarily on phytoplankton and resuspended benthic diatoms (Ahn, 1997; Mercuri et al., 1998). Under increased glacial melt scenarios, high turbidity and/or frequent sediment resuspension may limit the supply of unicellular microalgae (pelagic/benthic), whereas macroalgae detritus input to the benthos is expected to increase. The diet plots (Supplementary Figure 2) indicate that, although L. elliptica can feed on macroalgal detritus in the sediment (Braeckman et al., 2019), its main diet consists of detritus from pelagic origin. While populations close to the glacier have adapted to frequent ice scouring, L. elliptica might become food-limited if it does not diversify its diet. In upcoming realistic ocean acidification scenarios, L. elliptica has been shown difficulties in calcification and whole-organism functioning that are likely energetically difficult to maintain in the long term (Cummings et al., 2011). Potential changes in food availability may compromise energy supply for L. elliptica, which might even deteriorate its calcification rates, impacting the sturdiness of the shell and hence their natural protection against physical damage from ice scouring.
4.4 Glacial melt impact on carbon flows (H4)
We expected a reduced total system throughput and cycling index under high glacier impact (H4). The total system throughput was indeed 32 - 36% lower at the site closest to the glacier (Isla D) than at the other two sites in Summer 2015, but not during the other two seasons. This reflects a stronger benthic primary production and microbial loop pathway in sediments close to the glacier during weak glacial runoff (Spring 2015) or the subsequent year (Spring 2016) in which the benthic community could still benefit from the previous productive year (cf. food bank built up during Spring 2015).
Finn’s cycling index FCI was, however, consistently low at the three sites and seasons. For comparison, in Fildes Bay, a neighboring bay on King George Island, an FCI of 1.09% was found (Ortiz et al., 2016). In the shallow northern Bering Sea, FCI values ranging from 0.48 to 0.60% have been reported (Lovvorn et al., 2015). A low FCI indicates that the primary produced material has a short residence time in Potter Cove sediments. Combined with a high system throughput, this indicates that Potter Cove relies on newly produced carbon to sustain a high level of throughflow (Fath et al., 2019). As long as sufficient fresh organic carbon is produced by phytoplankton, microphytobenthos and macroalgae, this carbon input condition should be met on the long-term.
4.5 Glacial melt impact on carbon burial (H5)
The estimated carbon burial, representing the flow from refractory detritus to a buried carbon pool that is not further degraded, did not follow any patterns related to increased glacially-derived sediment deposition. This might at first sight seem remarkable, since increased sediment accumulation rates (SAR) have been observed in a gradient toward the Fourcade Glacier (Monien et al., 2017). However, we did not impose constraints on burial rates of organic carbon to the model due to the absence of site-specific data for the three stations on SAR and refractory organic carbon (organic carbon content in deep sediments). Spatial resolution in organic carbon burial estimates with respect to the glacier was for this reason not observed. Nevertheless, the range of our carbon burial rates specific for Potter Cove (4 - 20 mmol C m-2 d-1) can be compared to earlier estimates for Potter Cove and other Antarctic fjord environments. In Potter Cove, surface sediments show an estimate of 0.13% TOC (Braeckman et al., 2021), and an average SAR of 0.47 g cm-2 y-1 in sediments at a distance to the glacier comparable to our Faro site up to 1.8 g cm-2 y-1, as estimated from sediment traps in the melt water stream outflow (Monien et al., 2017). These values suggest an organic carbon burial rate of 1.4 – 5.3 mmol m-2 d-1. In inner Andvord Bay, further south on the WAP, an estimated burial rate of 3.9 mmol TOC m-2 d-1 has been reported (Eidam et al., 2019), while in South Georgia, organic carbon burial rates range between 1.0 – 2.8 mmol TOC m-2 d-1 (Berg et al., 2021). Our estimates for Potter Cove are thus on the high side. However, burial acts on longer time scales than the two years targeted with the LIM. Hence, while the order of magnitude is potentially correct, the temporal and spatial dynamics are probably less well resolved.
4.6 Carbon ingestion flows
Suspension feeding, predominantly by L. elliptica, and deposit feeding on detritus were the main pathways of the organic carbon flow into soft-substrate benthos. This is not surprising, since most of the infaunal species are deposit or suspension feeders (Supplementary Table 4). The contribution of predation was low, but this is an underestimation, since predation by fish (e.g. Nothoteniids) and mobile epibenthos has not been quantified, although it has been considered in the term ‘Export’.
The substantial biomass of macroalgae has been suggested to contribute significantly to the feeding of benthic communities in Potter Cove (Quartino and de Zaixso, 2008; Seefeldt et al., 2017; Braeckman et al., 2019; Alurralde et al., 2020) and other shallow coastal systems on the WAP (Corbisier et al., 2004; Ortiz et al., 2016). Macroalgae are expanding on the new hard substrate areas that are becoming available due to glacial retreat (Quartino et al., 2013; Amsler et al., 2023; Deregibus et al., 2023). Our quantitative benthic food web models suggest that, although the deposition of macroalgal detritus on the sediments can contribute up to 37% of the total carbon input into the system, grazing on macroalgal fragments by infaunal benthos is a pathway that currently contributes< 5% to the diet of benthic meio- and macrofauna communities of soft substrates (Table 5). Grazing on microphytobenthos, which constituted 10 - 30% of carbon ingestion flows, is more important, at least in these soft sediment communities where benthic diatoms are abundant. In the hard-substrate food web, macroalgae are clearly a much more important food source for mobile amphipods and Nototheniid fish (Amsler et al., 2012; Aumack et al., 2017; Cordone et al., 2020). With increasing glacial melt disturbance, there could be a shift in the balance of food sources for infauna towards grazing on macroalgal detritus. This is because macroalgae usually grow under better light conditions on the hard substrate than microphytobenthos on the sediment and are likely better adapted to increased turbidity resulting from glacial disturbance in terms of production (Deregibus et al., 2016; Hoffmann et al., 2019). However, not all macroalgae species are palatable to benthic species (Amsler et al., 2012), hence a shift in the composition of the primary producer community will likely affect secondary production of consumers and energy transfer to higher trophic levels.
4.7 Outlook to energy transfer in shallow WAP food webs
Potter Cove is often considered as an example of the future of shallow ecosystems further south on the WAP. The Fourcade Glacier once covered a substantial part of Potter Cove until the 1950s, but has been retreating since then at a rate of 40 m y-1 (Rückamp et al., 2011) until its front position on land in 2016 (Latorre et al., 2023). With ongoing glacier retreat along the WAP, disturbance by ice growlers is expected to increase (Deregibus et al., 2017), and the discharge of sediment-loaded meltwater streams will continue to increase turbidity of the shallow and mixed waters, disturbing both pelagic and benthic unicellular primary producers (Braeckman et al., 2021). Macroalgae seem to profit from the expansion of new hard substrate with glacial retreat (Quartino et al., 2013; Amsler et al., 2023; Deregibus et al., 2023). As evidenced in several aspects of our food web analysis, increased glacial runoff leads to a less intense benthic primary production pathway, a weaker microbial loop, and reduced secondary production of infaunal species that are not adapted to continual sediment deposition (e.g. filter feeders, but even large deposit-feeding bivalves such as A. eightsii) (Figure 7). The lower secondary production of these infaunal species may limit the transfer of energy to higher trophic levels, such as benthic fish (e.g (Moreno and Osorio, 1977; Casaux, 1998; Moreira et al., 2014). and starfish (Dunton, 2001) that feed on these species.
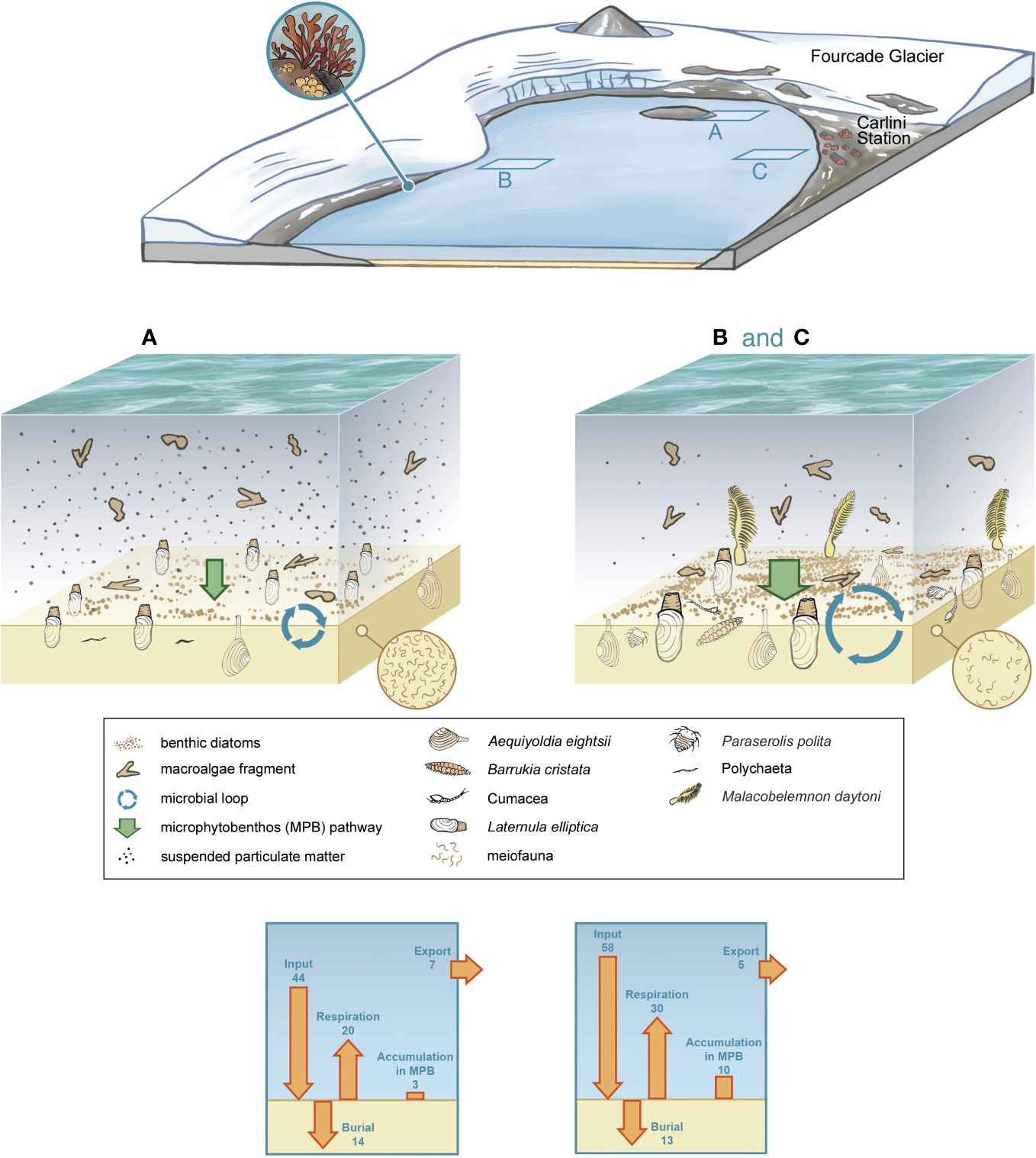
Figure 7 Summary of alterations to carbon flows in the benthic food web as a result of glacial melt disturbance. Location A: Isla D; Location B and C: Faro and Creek, respectively. Carbon flows in mmol C m-2 d-1.
4.8 Conclusion
The linear inverse food web models applied in this study were instrumental in the quantification of carbon transfer among the benthic food web compartments, providing the first estimates on carbon flows that had so far only been speculated about (e.g. export and deposition of macroalgal detritus to Potter Cove seabed). We show that high glacial melt runoff can reduce the carbon throughput (i.e., the sum of all carbon flows in the food web), mainly through a more limited benthic primary production pathway, an impaired microbial loop, and lower secondary production of the bivalve Aequiyoldia eightsii and other infauna. In contrast, the bivalve Laternula elliptica and meiofauna seemed to thrive in the sediments close to the glacier, as evidenced by their highest secondary production at this location. This study shows how glacial melt effects have the potential to propagate from the lower to the higher trophic levels and, as such, affect the transfer of energy in the ecosystem.
Data availability statement
The original contributions presented in the study are included in the article/Supplementary Material. Further inquiries can be directed to the corresponding author.
Ethics statement
The manuscript presents research on animals that do not require ethical approval for their study.
Author contributions
UB: Conceptualization, Data curation, Formal analysis, Funding acquisition, Investigation, Methodology, Project administration, Resources, Validation, Visualization, Writing – original draft, Writing – review & editing. KS: Conceptualization, Methodology, Software, Supervision, Writing – original draft, Writing – review & editing. FP: Investigation, Writing – original draft, Writing – review & editing. MQ: Investigation, Writing – original draft, Writing – review & editing. AV: Resources, Writing – original draft, Writing – review & editing. LS: Supervision, Writing – original draft, Writing – review & editing. IS: Supervision, Writing – original draft, Writing – review & editing. DV: Conceptualization, Methodology, Software, Supervision, Writing – original draft, Writing – review & editing.
Funding
The author(s) declare financial support was received for the research, authorship, and/or publication of this article. The first author was a senior postdoctoral research fellow at Research Foundation-Flanders (FWO Belgium) during the model elaboration phase (grant no. 1201720N). This manuscript contributes to the Belgian Science Policyfunded TANGO project (Estimating Tipping points in habitability of ANtarctic benthic ecosystems under GlObal future climate change scenarios, No B2/212/P1/TANGO) and the CoastCarb project, funded by the European Union’s Horizon 2020 research and innovation programme under the Marie Skłodowska-Curie grant agreement No 87269. This study contributes to the BELSPO FED-tWIN METRIC project: Marine EcosysTem Responses In a multiple pressures Context.
Acknowledgments
The authors wish to honor the memory of Prof. Dr. Doris Abele through this work. Doris Abele was instrumental in fostering the Argentinean-German scientific partnership since 1995. She facilitated fieldwork in Potter Cove under challenging conditions and inspired numerous productive collaborations, leaving a lasting legacy as a brilliant scientist. The schematic summary in is the work of Dr. Hendrik Gheerardyn (www.hendrikgheerardyn.com).
Conflict of interest
The authors declare that the research was conducted in the absence of any commercial or financial relationships that could be construed as a potential conflict of interest.
Publisher’s note
All claims expressed in this article are solely those of the authors and do not necessarily represent those of their affiliated organizations, or those of the publisher, the editors and the reviewers. Any product that may be evaluated in this article, or claim that may be made by its manufacturer, is not guaranteed or endorsed by the publisher.
Supplementary material
The Supplementary Material for this article can be found online at: https://www.frontiersin.org/articles/10.3389/fmars.2024.1359597/full#supplementary-material
References
Ahn I.-Y. (1997). “Feeding ecology of the Antarctic lamellibranch bivalve Laternula elliptica (Laternulidae) in Marian Cove and vicinity, King George Island during one austral summer,” in Antarctic Communities: Species, Structure and Survival (Cambridge University Press, London), 142–151.
Ahn I.-Y., Kang J.-S., Kang S.-H. (1993). Primary food sources for shallow-water benthic fauna in Marian Cove, King George Island during an austral summer. J Exp Mar Biol Ecol. 171 (1), 75–90. doi: 10.1016/0022-0981(93)90141-A
Alurralde G., Fuentes V. L., De Troch M., Tatián M. (2020). Suspension feeders as natural sentinels of the spatial variability in food sources in an Antarctic fjord: A stable isotope approach. Ecol. Indic. 115, 106378. doi: 10.1016/j.ecolind.2020.106378
Amsler C. D., Amsler M. O., Klein A. G., Galloway A. W. E., Iken K., McClintock J. B., et al. (2023). Strong correlations of sea ice cover with macroalgal cover along the Antarctic Peninsula: Ramifications for present and future benthic communities. Elem. Sci. Anthr. 11, 20. doi: 10.1525/elementa.2023.00020
Amsler C. D., Mcclintock J. B., Baker B. J. (2012). Palatability of living and dead detached Antarctic macroalgae to consumers. Antarct. Sci. 24, 589. doi: 10.1017/S0954102012000624
Aromokeye D. A., Willis-Poratti G., Wunder L. C., Yin X., Wendt J., Richter-Heitmann T., et al. (2021). Macroalgae degradation promotes microbial iron reduction via electron shuttling in coastal Antarctic sediments. Environ. Int. 156, 106602. doi: 10.1016/j.envint.2021.106602
Aumack C. F., Lowe A. T., Amsler C. D., Amsler M. O., McClintock J. B., Baker B. J. (2017). Gut content, fatty acid, and stable isotope analyses reveal dietary sources of macroalgal-associated amphipods along the western Antarctic Peninsula. Polar Biol. 40, 1371–1384. doi: 10.1007/s00300-016-2061-4
Barnes D. K., Souster T. (2011). Reduced survival of Antarctic benthos linked to climate-induced iceberg scouring. Nat. Clim. Change 1, 365. doi: 10.1038/nclimate1232
Bascur M., Muñoz-Ramírez C., Román-González A., Sheen K., Barnes D. K. A., Sands C. J., et al. (2020). The influence of glacial melt and retreat on the nutritional condition of the bivalve Nuculana inaequisculpta (Protobranchia: Nuculanidae) in the West Antarctic Peninsula. PloS One 15, e0233513. doi: 10.1371/journal.pone.0233513
Berg S., Jivcov S., Kusch S., Kuhn G., White D., Bohrmann G., et al. (2021). Increased petrogenic and biospheric organic carbon burial in sub-Antarctic fjord sediments in response to recent glacier retreat. Limnol. Oceanogr. 66, 4347–4362. doi: 10.1002/lno.11965
Braeckman U., Pasotti F., Hoffmann R., Vázquez S., Wulff A., Schloss I. R., et al. (2021). Glacial melt disturbance shifts community metabolism of an Antarctic seafloor ecosystem from net autotrophy to heterotrophy. Commun. Biol. 4, 1–11. doi: 10.1038/s42003-021-01673-6
Braeckman U., Pasotti F., Vázquez S., Zacher K., Hoffmann R., Elvert M., et al. (2019). Degradation of macroalgal detritus in shallow coastal Antarctic sediments. Limnol. Oceanogr. 64, 1423–1441. doi: 10.1002/lno.11125
Broch O. J., Slagstad D. (2012). Modelling seasonal growth and composition of the kelp Saccharina latissima. J. Appl. Phycol. 24, 759–776. doi: 10.1007/s10811-011-9695-y
Campana G. L., Zacher K., Deregibus D., Momo F. R., Wiencke C., Quartino M. L. (2018). Succession of Antarctic benthic algae (Potter Cove, South Shetland Islands): structural patterns and glacial impact over a four-year period. Polar Biol. 41, 377–396. doi: 10.1007/s00300-017-2197-x
Casaux R. (1998). The contrasting diet of Harpagifer antarcticus (Notothenioidei, Harpagiferidae) at two localities of the South Shetland Islands, Antarctica. Polar Biol. 19, 283–285. doi: 10.1007/s003000050246
Cook A. J., Holland P. R., Meredith M. P., Murray T., Luckman A., Vaughan D. G. (2016). Ocean forcing of glacier retreat in the western Antarctic Peninsula. Science 353, 283–286. doi: 10.1126/science.aae0017
Corbisier T. N., Petti M. A. V., Skowronski R. S. P., Brito T. A. S. (2004). Trophic relationships in the nearshore zone of Martel Inlet (King George Island, Antarctica): δ13C stable-isotope analysis. Polar Biol. 27, 75–82. doi: 10.1007/s00300-003-0567-z
Cordone G., Marina T. I., Salinas V., Doyle S. R., Saravia L. A., Momo F. R. (2018). Effects of macroalgae loss in an Antarctic marine food web: applying extinction thresholds to food web studies. PeerJ 6, e5531. doi: 10.7717/peerj.5531
Cordone G., Salinas V., Marina T. I., Doyle S. R., Pasotti F., Saravia L. A., et al. (2020). Green vs brown food web: Effects of habitat type on multidimensional stability proxies for a highly-resolved Antarctic food web. Food Webs 25, e00166. doi: 10.1016/j.fooweb.2020.e00166
Cummings V., Hewitt J., Van Rooyen A., Currie K., Beard S., Thrush S., et al. (2011). Ocean Acidification at High Latitudes: Potential Effects on Functioning of the Antarctic Bivalve Laternula elliptica. PloS One 6, e16069. doi: 10.1371/journal.pone.0016069
De Jonge D. S., Stratmann T., Lins L., Vanreusel A., Purser A., Marcon Y., et al. (2020). Abyssal food-web model indicates faunal carbon flow recovery and impaired microbial loop 26 years after a sediment disturbance experiment. Prog. Oceanogr. 189, 102446. doi: 10.1016/j.pocean.2020.102446
Deregibus D., Quartino M. L., Campana G. L., Momo F. R., Wiencke C., Zacher K. (2016). Photosynthetic light requirements and vertical distribution of macroalgae in newly ice-free areas in Potter Cove, South Shetland Islands, Antarctica. Polar Biol. 39, 153–166. doi: 10.1007/s00300-015-1679-y
Deregibus D., Quartino M. L., Zacher K., Campana G. L., Barnes D. K. (2017). Understanding the link between sea ice, ice scour and Antarctic benthic biodiversity–the need for cross-station and international collaboration. Polar Rec. 53, 143–152. doi: 10.1017/S0032247416000875
Deregibus D., Campana G. L., Neder C., Barnes D. K. A., Zacher K., Piscicelli J. M., et al. (2023). Potential macroalgal expansion and blue carbon gains with northern Antarctic Peninsula glacial retreat. Mar. Environ. Res. 189, 106056. doi: 10.1016/j.marenvres.2023.106056
De Santana C. N., Rozenfeld A. F., Marquet P. A., Duarte C. M. (2013). Topological properties of polar food webs. Mar. Ecol. Prog. Ser. 474, 15–26. doi: 10.3354/meps10073
Digital Globe (2014) WorldView-2 scene 103001001F612100, Image Courtesy of / Copyright © DigitalGlobe - Longmont, Colorado. All rights reserved, Catalog ID: 103001001F612100. Sensor:WV02, Band Info: Pan_MS1_MS2, Resolution 0.5∗0.5m (Accessed November 3, 2013).
Ducklow H. W., Fraser W. R., Meredith M. P., Stammerjohn S. E., Doney S. C., Martinson D. G., et al. (2013). West Antarctic Peninsula: an ice-dependent coastal marine ecosystem in transition. Oceanography 26, 190–203. doi: 10.5670/oceanog
Dunton K. H. (2001). δ15N and δ13C measurements of Antarctic Peninsula fauna: trophic relationships and assimilation of benthic seaweeds. Am. Zool. 41, 99–112. doi: 10.1093/icb/41.1.99
Eidam E. F., Nittrouer C. A., Lundesgaard Ø., Homolka K. K., Smith C. R. (2019). Variability of sediment accumulation rates in an Antarctic Fjord. Geophys. Res. Lett. 46, 13271–13280. doi: 10.1029/2019GL084499
Falk U., López D. A., Silva-Busso A. (2018). Multi-year analysis of distributed glacier mass balance modelling and equilibrium line altitude on King George Island, Antarctic Peninsula. Cryosphere 12, 1211–1232. doi: 10.5194/tc-12-1211-2018
Fath B. D., Asmus H., Asmus R., Baird D., Borrett S. R., de Jonge V. N., et al. (2019). Ecological network analysis metrics: The need for an entire ecosystem approach in management and policy. Ocean Coast. Manage. 174, 1–14. doi: 10.1016/j.ocecoaman.2019.03.007
Forest A., Tremblay J.-É., Gratton Y., Martin J., Gagnon J., Darnis G., et al. (2011). Biogenic carbon flows through the planktonic food web of the Amundsen Gulf (Arctic Ocean): A synthesis of field measurements and inverse modeling analyses. Prog. Oceanogr. 91, 410–436. doi: 10.1016/j.pocean.2011.05.002
Gillies C. L., Stark J. S., Johnstone G. J., Smith S. D. A. (2012). Carbon flow and trophic structure of an Antarctic coastal benthic community as determined by δ13C and δ15N. Estuar. Coast. Shelf Sci. 97, 44–57. doi: 10.1016/j.ecss.2011.11.003
Gutt J. (2001). On the direct impact of ice on marine benthic communities, a review. Polar Biol. 24, 553–564. doi: 10.1007/s003000100262
Henley S. F., Schofield O. M., Hendry K. R., Schloss I. R., Steinberg D. K., Moffat C., et al. (2019). Variability and change in the west Antarctic Peninsula marine system: Research priorities and opportunities. Prog. Oceanogr. 173, 208–237. doi: 10.1016/j.pocean.2019.03.003
Hoffmann R., Al-Handal A. Y., Wulff A., Deregibus D., Quartino M. L., Wenzhöfer F., et al. (2019). Implications of glacial melt-related processes on the potential primary production of a microphytobenthic community in Potter Cove (Antarctica). Front. Mar. Sci. 6, 655. doi: 10.3389/fmars.2019.00655
Hoffmann R., Pasotti F., Vázquez S., Lefaible N., Torstensson A., MacCormack W., et al. (2018). Spatial variability of biogeochemistry in shallow coastal benthic communities of Potter Cove (Antarctica) and the impact of a melting glacier. PloS One 13, e0207917. doi: 10.1371/journal.pone.0207917
Jerosch K., Pehlke H., Monien P., Scharf F., Weber L., Kuhn G., et al. (2018). Benthic meltwater fjord habitats formed by rapid glacier recession on King George Island, Antarctica. Phil Trans. R Soc. A 376, 20170178. doi: 10.1098/rsta.2017.0178
Kim H., Ducklow H. W., Abele D., Barlett E. M. R., Buma A. G., Meredith M. P., et al. (2018). Inter-decadal variability of phytoplankton biomass along the coastal West Antarctic Peninsula. Phil Trans. R Soc. A 376, 20170174. doi: 10.1098/rsta.2017.0174
Kones J. K., Soetaert K., van Oevelen D., Owino J. O. (2009). Are network indices robust indicators of food web functioning? A Monte Carlo approach. Ecol. Model. 220, 370–382. doi: 10.1016/j.ecolmodel.2008.10.012
Kortsch S., Primicerio R., Fossheim M., Dolgov A. V., Aschan M. (2015). Climate change alters the structure of arctic marine food webs due to poleward shifts of boreal generalists. Proc. R. Soc B Biol. Sci. 282, 20151546. doi: 10.1098/rspb.2015.1546
Lagger C., Nime M., Torre L., Servetto N., Tatián M., Sahade R. (2018). Climate change, glacier retreat and a new ice-free island offer new insights on Antarctic benthic responses. Ecography 41, 579–591. doi: 10.1111/ecog.03018
Latorre M. P., Iachetti C. M., Schloss I. R., Antoni J., Malits A., de la Rosa F., et al. (2023). Summer heatwaves affect coastal Antarctic plankton metabolism and community structure. J. Exp. Mar. Biol. Ecol. 567, 151926. doi: 10.1016/j.jembe.2023.151926
Laws E. A., Popp B. N., Bidigare R. R., Kennicutt M. C., Macko S. A. (1995). Dependence of phytoplankton carbon isotopic composition on growth rate and [CO2) aq: Theoretical considerations and experimental results. Geochim. Cosmochim. Acta 59, 1131–1138. doi: 10.1016/0016-7037(95)00030-4
Lim C. (2014). Modelling waves and currents in Potter Cove,King George Island, Antarctica, PhD Thesis. Ed. von Ossietzky C. (Oldenburg: Universität Oldenburg).
Lovvorn J. R., Jacob U., North C. A., Kolts J. M., Grebmeier J. M., Cooper L. W., et al. (2015). Modeling spatial patterns of limits to production of deposit-feeders and ectothermic predators in the northern Bering Sea. Estuar. Coast. Shelf Sci. 154, 19–29. doi: 10.1016/j.ecss.2014.12.020
Marina T. I., Salinas V., Cordone G., Campana G., Moreira E., Deregibus D., et al. (2018). The food web of Potter Cove (Antarctica): complexity, structure and function. Estuar. Coast. Shelf Sci. 200, 141–151. doi: 10.1016/j.ecss.2017.10.015
Mendes C. R. B., Tavano V. M., Dotto T. S., Kerr R., de Souza M. S., Garcia C. A. E., et al. (2018). New insights on the dominance of cryptophytes in Antarctic coastal waters: A case study in Gerlache Strait. Deep Sea Res. Part II Top. Stud. Oceanogr. 149, 161–170. doi: 10.1016/j.dsr2.2017.02.010
Mercuri G., Iken K., Ledesma B., Dubois R. F. (1998). On the distribution patterns and density of the Antarctic infaunal bivalve Laternula elliptica in Potter Cove, King George Island., in: The Potter Cove Coastal Ecosystem, Antarctica. Berichte Polarforschung 299, 137–143.
Michel L. N., Danis B., Dubois P., Eleaume M., Fournier J., Gallut C., et al. (2019). Increased sea ice cover alters food web structure in East Antarctica. Sci. Rep. 9, 8062. doi: 10.1038/s41598-019-44605-5
Moline M. A., Claustre H., Frazer T. K., Schofield O., Vernet M. (2004). Alteration of the food web along the Antarctic Peninsula in response to a regional warming trend. Glob. Change Biol. 10, 1973–1980. doi: 10.1111/j.1365-2486.2004.00825.x
Monien D., Monien P., Brünjes R., Widmer T., Kappenberg A., Busso A. A. S., et al. (2017). Meltwater as a source of potentially bioavailable iron to Antarctica waters. Antarct. Sci. 29, 277–291. doi: 10.1017/S095410201600064X
Monien P., Schnetger B., Brumsack H.-J., Hass H. C., Kuhn G. (2011). A geochemical record of late Holocene palaeoenvironmental changes at King George Island (maritime Antarctica). Antarct. Sci. 23, 255–267. doi: 10.1017/S095410201100006X
Moreira E., Juáres M., Barrera-Oro E. (2014). Dietary overlap among early juvenile stages in an Antarctic notothenioid fish assemblage at Potter Cove, South Shetland Islands. Polar Biol. 37, 1507–1515. doi: 10.1007/s00300-014-1545-3
Moreno C. A., Osorio H. H. (1977). Bathymetric food habit changes in the antarctic fish, Notothenia gibberifrons Lönnberg. (Pisces: Nototheniidae). Hydrobiol. 55, 139–144. doi: 10.1007/BF00021055
Ortiz M., Berrios F., González J., Rodríguez-Zaragoza F., Gómez I. (2016). Macroscopic network properties and short-term dynamic simulations in coastal ecological systems at Fildes Bay (King George Island, Antarctica). Ecol. Complex. 28, 145–157. doi: 10.1016/j.ecocom.2016.06.003
Pasotti F., Manini E., Giovannelli D., Wölfl A.-C., Monien D., Verleyen E., et al. (2015a). Antarctic shallow water benthos in an area of recent rapid glacier retreat. Mar. Ecol. 36, 716–733. doi: 10.1111/maec.12179
Pasotti F., Saravia L. A., De Troch M., Tarantelli M. S., Sahade R., Vanreusel A. (2015b). Benthic trophic interactions in an Antarctic shallow water ecosystem affected by recent glacier retreat. PloS One 10, e0141742. doi: 10.1371/journal.pone.0141742
Peck L. S., Bullough L. W. (1993). Growth and population structure in the infaunal bivalve Yoldia eightsi in relation to iceberg activity at Signy Island, Antarctica. Mar. Biol. 117, 235–241. doi: 10.1007/BF00345668
Peters K. J., Amsler C. D., Amsler M. O., McClintock J. B., Dunbar R. B., Baker B. J. (2005). A comparative analysis of the nutritional and elemental composition of macroalgae from the western Antarctic Peninsula. Phycologia 44, 453–463. doi: 10.2216/0031-8884(2005)44[453:ACAOTN]2.0.CO;2
Philipp E. E., Husmann G., Abele D. (2011). The impact of sediment deposition and iceberg scour on the Antarctic soft shell clam Laternula elliptica at King George Island, Antarctica. Antarct. Sci. 23, 127–138. doi: 10.1017/S0954102010000970
Pusceddu A., Fiordelmondo C., Danovaro R. (2005). Sediment resuspension effects on the benthic microbial loop in experimental microcosms. Microb. Ecol. 50, 602–613. doi: 10.1007/s00248-005-5051-6
Quartino M. L., Deregibus D., Campana G. L., Latorre G. E. J., Momo F. R. (2013). Evidence of macroalgal colonization on newly ice-Free areas following glacial retreat in potter cove (South Shetland Islands), Antarctica. PloS One 8, e58223. doi: 10.1371/journal.pone.0058223
Quartino M. L., de Zaixso A. B. (2008). Summer macroalgal biomass in Potter Cove, South Shetland Islands, Antarctica: its production and flux to the ecosystem. Polar Biol. 31, 281–294. doi: 10.1007/s00300-007-0356-1
Quartino M. L., Saravia L. A., Campana G. L., Deregibus D., Matula C. V., Boraso A. L., et al. (2020). Production and biomass of seaweeds in newly ice-free areas: implications for coastal processes in a changing Antarctic environment. Antarct. Seaweeds Divers. Adapt. Ecosyst. Serv., 155–171.
Rivkin R. B., DeLaca T. E. (1990). Trophic dynamics in antarctic benthic communities. I. In situ ingestion of microalgae by Foraminifera and metazoan meiofauna. Mar. Ecol. Prog. Ser. 64, 129–136. doi: 10.3354/meps064129
Rodriguez I. D., Marina T. I., Schloss I. R., Saravia L. A. (2022). Marine food webs are more complex but less stable in sub-Antarctic (Beagle Channel, Argentina) than in Antarctic (Potter Cove, Antarctic Peninsula) regions. Mar. Environ. Res. 174, 105561. doi: 10.1016/j.marenvres.2022.105561
Rückamp M., Braun M., Suckro S., Blindow N. (2011). Observed glacial changes on the King George Island ice cap, Antarctica, in the last decade. Glob. Planet. Change 79, 99–109. doi: 10.1016/j.gloplacha.2011.06.009
Sahade R., Lagger C., Torre L., Momo F., Monien P., Schloss I., et al. (2015). Climate change and glacier retreat drive shifts in an Antarctic benthic ecosystem. Sci. Adv. 1, e1500050. doi: 10.1126/sciadv.1500050
Sailley S. F., Ducklow H. W., Moeller H. V., Fraser W. R., Schofield O. M., Steinberg D. K., et al. (2013). Carbon fluxes and pelagic ecosystem dynamics near two western Antarctic Peninsula Adélie penguin colonies: an inverse model approach. Mar. Ecol. Prog. Ser. 492, 253–272. doi: 10.3354/meps10534
Schloss I. R., Abele D., Moreau S., Demers S., Bers A. V., González O., et al. (2012). Response of phytoplankton dynamics to 19-year, (1991–2009) climate trends in Potter Cove (Antarctica). J. Mar. Syst. 92, 53–66. doi: 10.1016/j.jmarsys.2011.10.006
Schloss I., Ferreyra G., Mercuri G., Kowalke J. (1999). Potential food availability for benthic filter feeders in an Antarctic coastal shallow environment: a sediment trap study. Magellan-Antarct. Ecosytems Drifted Apart Sci. Mar. 63, 99–111.
Schloss I. R., Ferreyra G. A., Ruiz-Pino D. (2002). Phytoplankton biomass in Antarctic shelf zones: a conceptual model based on Potter Cove, King George Island. J. Mar. Syst. 36, 129–143. doi: 10.1016/S0924-7963(02)00183-5
Seefeldt M. A., Campana G. L., Deregibus D., Quartino M. L., Abele D., Tollrian R., et al. (2017). Different feeding strategies in Antarctic scavenging amphipods and their implications for colonisation success in times of retreating glaciers. Front. Zool. 14, 59. doi: 10.1186/s12983-017-0248-3
Smale D. A., Barnes D. K. A., Fraser K. P. P. (2007). The influence of ice scour on benthic communities at three contrasting sites at Adelaide Island, Antarctica. Austral Ecol. 32, 878–888. doi: 10.1111/j.1442-9993.2007.01776.x
Smale D. A., Brown K. M., Barnes D. K., Fraser K. P., Clarke A. (2008). Ice scour disturbance in Antarctic waters. Science 321, 371–371. doi: 10.1126/science.1158647
Smith C. R., DeMaster D. J., Thomas C., Sr\vsen P., Grange L., Evrard V., et al. (2012). Pelagic-benthic coupling, food banks, and climate change on the West Antarctic Peninsula Shelf. Oceanography 25, 188–201. doi: 10.5670/oceanog
Soetaert K., van Oevelen D. (2009). Modeling food web interactions in benthic deep-sea ecosystems: A practical guide. Oceanography 22, 128–143. doi: 10.5670/oceanog
Stammerjohn S., Massom R., Rind D., Martinson D. (2012). Regions of rapid sea ice change: An inter-hemispheric seasonal comparison. Geophys. Res. Lett. 39. doi: 10.1029/2012GL050874
Torre L., Servetto N., Eöry M. L., Momo F., Tatián M., Abele D., et al. (2012). Respiratory responses of three Antarctic ascidians and a sea pen to increased sediment concentrations. Polar Biol. 35, 1743–1748. doi: 10.1007/s00300-012-1208-1
Turner J., Marschall G., Clem K., Colwell S., Phillips T., Lu H. (2019). Antarctic temperature variability and change from station data - Turner - - - Wiley Online Library. Int. J. Climatol. 40 (6), 2986–3007. doi: 10.1002/joc.6378
Urban H.-J., Mercuri G. (1998). Population dynamics of the bivalve Laternula elliptica from Potter cove, King George Island, South Shetland islands. Antarct. Sci. 10, 153–160. doi: 10.1017/S0954102098000200
Van den Meersche K., Van Rijswijk P., Soetaert K., Middelburg J. J. (2009). Autochthonous and allochthonous contributions to mesozooplankton diet in a tidal river and estuary: Integrating carbon isotope and fatty acid constraints. Limnol. Oceanogr. 54 (1), 62–74. doi: 10.4319/lo.2009.54.1.0062
van Oevelen D., Bergmann M., Soetaert K., Bauerfeind E., Hasemann C., Klages M., et al. (2011). Carbon flows in the benthic food web at the deep-sea observatory HAUSGARTEN (Fram Strait). Deep Sea Res. Part Oceanogr. Res. Pap. 58, 1069–1083. doi: 10.1016/j.dsr.2011.08.002
Van Oevelen D., Soetaert K., Garcia R., De Stigter H. C., Cunha M. R., Pusceddu A., et al. (2011). Canyon conditions impact carbon flows in food webs of three sections of the Nazaré canyon. Deep Sea Res. Part II Top. Stud. Oceanogr. 58, 2461–2476. doi: 10.1016/j.dsr2.2011.04.009
van Oevelen D., Soetaert K., Heip C. (2012). Carbon flows in the benthic food web of the Porcupine Abyssal Plain: The (un) importance of labile detritus in supporting microbial and faunal carbon demands. Limnol. Ocean. 57, 645–664. doi: 10.4319/lo.2012.57.2.0645
van Oevelen D., Soetaert K., Middelburg J. J., Herman P. M. J., Moodley L., Hamels I., et al. (2006). Carbon flows through a benthic food web: Integrating biomass, isotope and tracer data. J. Mar. Res. 64, 453–482. doi: 10.1357/002224006778189581
van Oevelen D., Van den Meersche K., Meysman F. J. R., Soetaert K., Middelburg J. J., Vézina A. F. (2010). Quantifying food web flows using linear inverse models. Ecosystems 13, 32–45. doi: 10.1007/s10021-009-9297-6
Vézina A. F., Platt T. (1988). Food web dynamics in the ocean. 1. Best-estimates of flow networks using inverse methods. Mar. Ecol. Prog. Ser. 42, 269–287. doi: 10.3354/meps042269
Wieser W. (1953). Die Beziehung zwischen Mundh\öhlengestalt, Ern\ährungsweise und Vorkommen bei freilebenden marinen Nematoden. Eine \ökologisch-morphologische Studie. Arktik Zool. 4, 439–483.
Wölfl A.-C., Lim C. H., Hass H. C., Lindhorst S., Tosonotto G., Lettmann K. A., et al. (2014). Distribution and characteristics of marine habitats in a subpolar bay based on hydroacoustics and bed shear stress estimates—Potter Cove, King George Island, Antarctica. Geo Mar. Lett. 34, 435–446. doi: 10.1007/s00367-014-0375-1
Keywords: Antarctica coastal ecology, glacial melt runoff, linear inverse model, carbon flow analysis, food web alteration
Citation: Braeckman U, Soetaert K, Pasotti F, Quartino ML, Vanreusel A, Saravia LA, Schloss IR and van Oevelen D (2024) Glacial melt impacts carbon flows in an Antarctic benthic food web. Front. Mar. Sci. 11:1359597. doi: 10.3389/fmars.2024.1359597
Received: 21 December 2023; Accepted: 07 March 2024;
Published: 27 March 2024.
Edited by:
Donata Melaku Canu, National Institute of Oceanography and Applied Geophysics, ItalyReviewed by:
Natalia Venturini, Universidad de la República, UruguayIrene Martins, University of Porto, Portugal
Copyright © 2024 Braeckman, Soetaert, Pasotti, Quartino, Vanreusel, Saravia, Schloss and van Oevelen. This is an open-access article distributed under the terms of the Creative Commons Attribution License (CC BY). The use, distribution or reproduction in other forums is permitted, provided the original author(s) and the copyright owner(s) are credited and that the original publication in this journal is cited, in accordance with accepted academic practice. No use, distribution or reproduction is permitted which does not comply with these terms.
*Correspondence: Ulrike Braeckman, dWxyaWtlLmJyYWVja21hbkB1Z2VudC5iZQ==