- 1Marine Biodiversity and Environmental Assessment Research Center (BioEnv), Research Institute for Global Change (RIGC), Japan Agency for Marine-Earth Science and Technology (JAMSTEC), Yokosuka, Japan
- 2Institute for Extra-cutting-edge Science and Technology Avant-garde Research (X-star), Japan Agency for Marine-Earth Science and Technology (JAMSTEC), Yokosuka, Japan
Polychlorinated biphenyls (PCBs) persistently pollute marine ecosystems even though their production has been discontinued decades ago. Deep-sea organisms have been shown to accumulate PCBs and other persistent organic compounds; however, the extent of contamination and its effects remain poorly understood. Here, we measured PCB concentrations in separated organs of non-feeding bivalve clams belonging to Phreagena, a taxon representative of deep-sea chemosynthetic ecosystems. The highest concentrations of PCBs were detected in the ovaries. We also examined surface sediments of the clam habitat and observed concentrations comparable to those in the sediments of the seas around Japan, including shallow waters. The results showed that PCBs from the environment penetrate Phreagena clams through the body surface and accumulate in the female reproductive system. This raises concerns about a potentially severe direct impact on the survival of vulnerable and highly endemic deep-sea chemosynthetic bivalves.
1 Introduction
Marine ecosystems face constant exposure to environmental pollution, emphasizing the need for effective conservation measures. Persistent organic pollutants (POPs) are resistant to environmental decomposition, accumulate in living organisms, and exhibit high biological toxicity and potential for environmental contamination over long distances (Ashraf, 2017). For decades, polychlorinated biphenyls (PCBs) have attracted considerable attention because of their ubiquity and toxicity to biota and humans (Ohkouchi et al., 2016). These compounds originate from biphenyl chlorination, encompassing a theoretical range of 10–209 homologs contingent on the number and configuration of chlorine atoms (Yuan et al., 2015). In the late 1920s, PCBs were initially manufactured in various countries for use as insulating oils in electrical transformers and capacitors and as additives in paints and plastics owing to their considerable electrical insulation, chemical resistance, and non-flammability properties. However, in the majority of developed nations, including Japan, their production and utilization were banned in the 1970s, following the recognition of their environmental persistence and toxicity (Erickson and Kaley, 2011). Global initiatives for stricter regulations were initiated amidst reports of PCB contamination spreading to untouched regions, such as in the Arctic Circle. The Stockholm Convention on POPs, adopted in 2001, mandates the cessation of PCB use in any equipment by 2025 and enforces stringent management practices until 2028. Furthermore, polybrominated diphenyl ethers (PBDEs) are important environmental contaminants, which are brominated aromatic hydrocarbons with structural variations similar to PCBs and are frequently used as flame retardants in plastics (Talsness, 2008). Under the Stockholm Convention, the production, use, and import of these substances have been prohibited. Despite international endeavors, however, these substances persistently contaminate diverse biota worldwide (Domingo and Bocio, 2007; Lee and Kim, 2015).
PCBs, like most other POPs, are hydrophobic and lipophilic, and thus readily adsorb onto organic particles, such as algae, suspended sediment, and micro- or nano-plastics present in aquatic environments (Ghosh et al., 2003; Yamashita et al., 2019). They can enter the body of animals primarily via ingestion through the digestive tract and also through absorption across the body surfaces (e.g., gills and epidermis). They subsequently accumulate in adipose tissue and lipid-rich organs, such as the liver, and also accumulate throughout the food chain (Matthews and Dedrick, 1984). Exposed organisms may experience a spectrum of toxic effects of PCBs, including hepatomegaly, skin toxicity, immunosuppressive effects, reproductive and developmental toxicity, carcinogenesis, other genotoxic responses, modulation of diverse endocrine-derived pathways, neurotoxicity, and acute lethality (Safe, 1994). Among various aquatic organisms, filter-feeding bivalves are considered reliable indicators of environmental pollution because they accumulate various POPs from surrounding waters (Chiesa et al., 2018), and the uptake of PCBs by filter-feeding bivalves such as mussels and oysters has been well documented (Chu et al., 2003).
POPs are vertically transported into the deep sea via a variety of biogeochemical processes (Takahashi et al., 2014). Understanding the behavior and effects of POPs on living organisms helps determine the extent of anthropogenic impacts on deep-sea ecosystems. Organisms with a prolonged lifespan, slow growth, and slow maturation are particularly vulnerable to environmental disturbances (Nakajima et al., 2019), and some species may face extinction because they would require long periods of time to recover from such disturbances (Llodra and Billett, 2006). In recent years, reports of POPs contaminating deep-sea organisms other than fish have been presented (Ikuta et al., 2021). Chemosynthetic ecosystems in deep-sea hydrothermal and seep sites are often inhabited by non-feeding animals that rely on symbiotic bacteria colonizing their bodies for nutrition. Bivalves belonging to the genus Phreagena are deep-sea endobenthic clams, representative of non-feeding chemosynthetic symbionts, harbor obligate symbiotic bacteria in their gill cells which they inherit through maternal transmission (Ikuta et al., 2016) (Figure 1A). The degree of accumulation of organic contaminants in aquatic organisms depends on the biotic or abiotic rate processes involved in the uptake, distribution, and elimination of contaminants (Bjork, 1995). In aquatic environments, PCBs are either slightly dissolved in water or adsorbed onto organic particles. In some filter-feeding bivalves, the dietary uptake of contaminants associated with these particles has been suggested to be the predominant route of contamination (Bjork, 1995). However, in non-feeding chemosynthetic bivalves, gill filtration serves purely respiratory purposes, and contamination from the gastrointestinal tract does not occur. A recent report regarding PCB contamination in Phreagena clams raised concerns about potential effects on highly endemic and vulnerable deep-sea organisms, even if they are non-feeding (Ikuta et al., 2021). However, contamination pathways and the biological and physiological effects on chemosynthetic bivalves remain unclear.
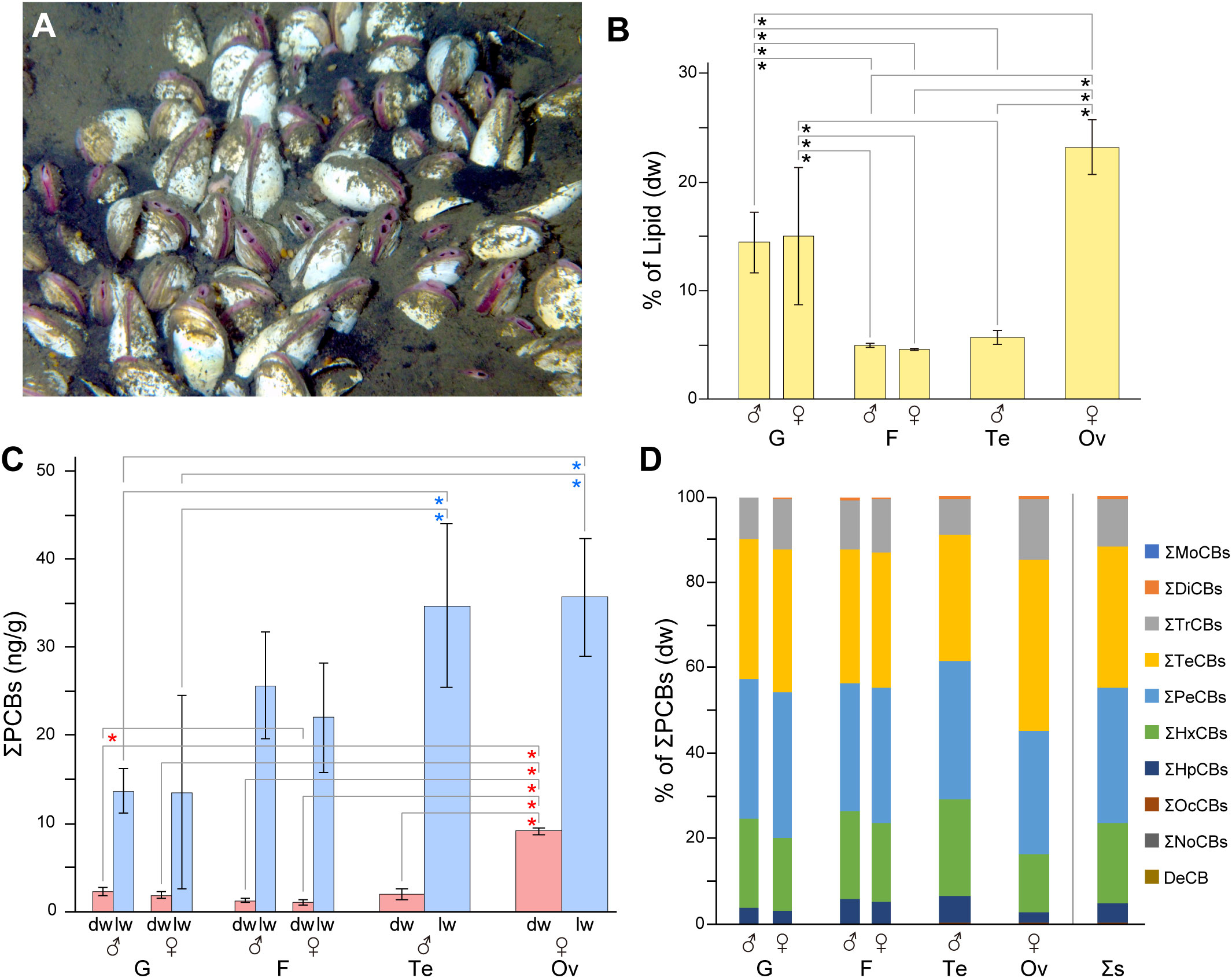
Figure 1 Polychlorinated biphenyl (PCB) concentrations in the organs of clams. (A) Phreagena clams burrowing into sediments in the Sagami Bay habitat. (B) Lipid content per dry weight of each organ. Asterisks indicate statistically significant differences (P< 0.05). Error bars indicate the standard deviation (SD; n = 3). G, gill; F, foot; Te, testis; Ov, ovary. (C) Mean total PCB concentration (ng/g) per organ from three individuals. Red and blue asterisks indicate statistically significant differences (P< 0.05) in values per dry weight (dw) and per lipid weight (lw). Error bars indicate the standard deviation (SD; n = 3). (D) Mean congener composition of PCBs in each organ. The concentrations are based on the dw. Σs, total samples.
Assessments of PCB contamination pathways and their effects on deep-sea organisms such as Phreagena clams are challenging because of the difficulty in maintaining these organisms in a laboratory; however, they can be inferred by assessing the contamination levels of the organism’s habitats and distribution within their bodies. In this study, we separately measured the PCB concentrations in the gills, feet, and gonads of male and female Phreagena clams collected off Hatsushima Island in Sagami Bay, where PCB contamination was reported previously (Ikuta et al., 2021). Sediments from clam colonies were collected, and the concentrations of PCBs and PBDEs were also analyzed. Because PBDEs were not detected in the most recently collected clams in our previous study (Ikuta et al., 2021), the habitat sediment was examined for the presence of PBDEs in the current study. Additionally, sediments were collected from a location at least 10 m away from the colony and sediments from the Myojin Knoll, which is located 300 miles from the populated area where PCB contamination of the resident Bathymodiolus mussels had previously been reported (Ikuta et al., 2021). Based on the results, we explored the potential effects and pathways of PCB contamination in chemosynthetic bivalves.
2 Methods
2.1 Sample collection
P. okutanii were collected from a hydrocarbon seep site off Hatsushima Island in Sagami Bay, Japan, at a depth of 858 m (Dive KM-ROV#112) during the KM20-09 cruise (November 14–22, 2020) using the R/V Kaimei-operated remotely operated vehicle (ROV) KM-ROV (Japan Agency of Marine-Earth Science and Technology; JAMSTEC). The clams were immediately dissected onboard using dry heat-sterilized stainless-steel trays. The shells were carefully removed using a sterile stainless-steel scalpel, to excise the gills, feet, gonads, and mantle (Supplementary Figure 1). The samples were placed in sterile aluminum containers and stored at −80°C until analysis. Three male and three female clams were prepared for analysis. Sex determination was performed according to a previously described gonad morphological method (Igawa-Ueda et al., 2021). All individuals were confirmed to be fully sexually mature, with shell lengths greater than 100 mm (Table 1). Species identification was performed using a previously established multiplex PCR method (Watanabe et al., 2013) using template DNA extracted from the mantles with a DNeasy Blood and Tissue Kit (Qiagen, Hilden, Germany). Using a push corer, surface sediment samples were collected at the margins of the clam colony off Hatsushima Island and at a minimal distance of 10 m from the colony. Sediment samples from within the colony could not be collected with push corers because of the abundant shells; therefore, sediment samples were collected from the margins (described as sediments from the colony). The samples were collected at depths of 1,190 and 1,183 m (Dive 6K#1602) during the YK21-18C cruise (September 28–October 16, 2021) using the R/V Yokosuka-operated (JAMSTEC) human-occupied vehicle Shinkai 6500, at 859 and 853 m depth (Dive KM-ROV#159) during the KM21-08 cruise (October 22–24, 2021), and at 847 and 856 m (Dive KM-ROV#188) during the KM22-15 cruise (December 3–20, 2022) using the R/V Kaimei-operated KM-ROV. Additionally, surface sediment samples were collected using a push corer at Myojin Knoll at a depth of 1,352 m (Dive KM-ROV#196 and #197) during the KM22-15 cruise. The outer part of the sediment cores that had been in contact with the polycarbonate tube during sampling was scrapped off using a sanitized spatula. Sediment samples at a depth of 0–7 cm were placed in sterile glass jars, sealed with combusted aluminum foil, and stored at −30°C until analysis.
2.2 Chemical analysis
Chemical analyses were conducted in the laboratory of IDEA Consultants (Tokyo, Japan). The analytical method followed previously described procedures (Ikuta et al., 2021) and the manual published by the United States Environmental Protection Agency (United States Environmental Protection Agency (USEPA) 2003; United States Environmental Protection Agency (USEPA) 2010). For the clam samples, following the addition of an internal standard solution TPCB-LCS-A500 (Wellington Laboratories Japan, Tokyo, Japan) to the homogenized samples (approximately 2 g wet weight), an acetone/hexane (1:2) mixture was added, and two 2-min stirred extractions were conducted using a homogenizer. The centrifuged extract was treated with sulfuric acid and purified using multilayer silica gel-column chromatography following acetonitrile/hexane partitioning. After purification, the sample volume was standardized to 20 µL, and syringe spikes (Wellington Laboratories Japan) were added. For the sediment samples, dried and homogenized samples (approximately 15 g wet weight) were subjected to sonication following the addition of an internal spike (Wellington Laboratories Japan) and acetone. Subsequently, the samples were suction-filtered, and residues were extracted using toluene in a Soxhlet apparatus for 18 h while concentrating the flow-through. Following treatment with sulfuric acid, both fractions were purified using multilayer silica gel-column chromatography. The resulting extracts were subsequently separated for PCB and PBDE analysis. For PCB analysis, following acetonitrile/hexane partitioning, the sample volume was standardized to 20 µL, and syringe spikes (Wellington Laboratories Japan) were added. For PBDE analysis, the sample was further purified using activated carbon-dispersed silica gel-column chromatography. The sample volume was standardized to 20 µL, followed by the addition of syringe spikes (Wellington Laboratories Japan). All instruments used in the analysis were cleaned with tap water and detergent, and subsequently with hexane and acetone to remove the chemicals.
The samples were analyzed for PCBs and PBDEs concentrations through gas chromatography–mass spectrometry using an Agilent 6890 GC (Agilent Technologies, Santa Clara, CA, USA) equipped with an AutoSpec-Ultima mass selective detector (Waters Corporation, Milford, MA, USA). Calibration of the instruments was performed at the time of each measurement. For PCBs, 10 homologs (mono–deca- CBs) and 14 coplanar PCBs were assessed. For the PBDEs, seven homologs (tetra–deca- BDEs) and six congeners listed in Annex A of the Stockholm Convention were assessed. One procedural blank was run for every 10 samples, with no effect on sample concentrations. The method detection limit (MDL) for each congener or homolog concentration was calculated using the following equation:
where t(n−1, 0.05) is Student’s t value at an α level of 0.05, with n−1 degrees of freedom; s is the standard deviation of the blank measurements in seven replicates. The total lipid content of the samples was determined using a single-step extraction technique based on a conventional chloroform-methanol extraction method (Daugherty and Lento, 1983). The results were expressed as either ng/g lipid weight (lw) or ng/g dry weight (dw), calculated based on the weight of water loss from the freeze-dried samples. Statistical comparisons of PCB and lipid quantities were performed using the Tukey–Kramer test (P< 0.05) with Excel 2011 (Microsoft, Redmond, WA, USA) and the add-on software Statcel4 (OMS publishers, Tokyo, Japan). In order to examine differences in the PCB components among the sediments and bivalves, hierarchical cluster analysis using the group average method was performed on the PCB abundance data using Bray-Curtis similarity (Bray and Curtis, 1957). A similarity profile test was used as a prerequisite for defining the PCB composition zones. Ordinations in reduced spaces were subsequently generated by non-metric multidimensional scaling. All multivariate analyses were performed using PRIMER, version 7 (Primer-e, Plymouth, UK) software.
The total organic carbon (TOC) content of the sediment was analyzed using an elemental analyzer (Micro Corder JM10, J-Science Lab, Kyoto, Japan). Each crushed sediment sample (approximately 1 g) was dried at 100–105°C, treated with 1N HCl to remove carbonates, re-dried, and analyzed. The TOC content/g dw was calculated by adjusting the initial dry sample through weight loss after HCl treatment based on the carbon content obtained in the analysis. The biota-sediment accumulation factor (BSAF) was calculated using the following equation (Ankley et al., 1992):
where Co is the mean PCB concentration (dw) in Phreagena clams collected in 2019, as described previously (Ikuta et al., 2021); Fl is the mean lipid concentration (fraction) in the clams (dw), and Cs is the mean PCB concentration in the sediment (dw) analyzed in this study.
3 Results
The gills, feet, and gonads (testes and ovaries) of male and female Phreagena clams (three each) were excised and individually analyzed for PCB content. The ovaries exhibited the highest lipid content per dry sample among all organs, averaging 23%, followed by the gills (males, 14%; females, 15%), testes (5.7%), and feet (males, 5.0%; females, 4.6%) (Figure 1B, Table 1). PCBs were detected in all samples, with ovaries exhibiting the highest total PCB (ΣPCB) content among all organs, i.e., 8.8–9.6 ng/g dw (mean = 9.1 ng/g) and 30–43 ng/g lw (mean = 36 ng/g). The gills, feet, and testes exhibited significantly lower PCB content per dw compared to the ovaries, at a range of 1.5–2.8 ng/g dw (mean = 2.3 ng/g; for males, 1.9 ng/g for females), 0.79–1.5 ng/g dw (mean = 1.2 ng/g for males, 1.0 ng/g for females), and 1.6–2.7 ng/g dw (mean = 2.0 ng/g), respectively. In contrast, the gills exhibited significantly lower PCB content per lw than the ovaries, with a range of 5.3–26 ng/g lw (mean = 1.4 ng/g for both males and females) (Figure 1C, Table 1). Based on the PCB homolog percentages in the ΣPCBs of each organ, tetra- (mean = 33%, dw), penta- (mean = 32%, dw), and hexa-CBs (mean = 19%, dw) dominated the biological samples, followed by tri- (mean = 11%, dw) and hepta-CBs (mean = 5%, dw) (Figure 1D). The amounts of other homologs were notably small, with no detection of mono-, nona-, and deca-CBs. Additionally, the percentages of the 14 coplanar PCBs in the ΣPCBs ranged from 8.1%–14% (dw) for all organ samples (Supplementary Table 1).
PCBs were detected in all sediment samples, with total concentrations ranging from 0.74 to 13 ng/g dw (Table 2). No statistically significant differences in the ΣPCBs were detected among samples collected from the clam colony in Sagami Bay, those collected at a distance from the colony, and those from the Myojin Knoll (Table 2). The percentages of the 14 coplanar PCBs in the ΣPCBs ranged from 6.4% to 12% (dw) for all sediment samples (Supplementary Table 2).
PCB homolog percentage comparisons were analyzed between sediment and biological samples using previous study data from intact Phreagena clam bodies (Ikuta et al., 2021). The results demonstrated that habitat sediments were dominated by tetra- (mean = 26%, dw), penta- (mean = 21%, dw), and tri-CBs (mean = 20%, dw), whereas the clams were dominated by tetra- (mean = 35%, dw), penta- (mean = 31%, dw), and hexa-CBs (mean = 16%, dw), indicating a trend towards higher-chlorinated PCB homologs in the clams. This trend was slightly stronger in the mussel Bathymodiolus japonicus, living sympatrically with the clams, and the PCB compositions were dominated by penta- (mean = 31%, dw), hexa- (mean = 29%, dw), and tetra-CBs (mean = 24%, dw) (Figure 2A) (Ikuta et al., 2021). The PCB compositions in the sediments of the clam colony, those in sediment from outside the colony, and those within the intact bodies of clams and mussels were separated by 85% similarity, with no statistically significant difference (Figure 2B). Additionally, a comparison of PCB compositions was conducted between the Myojin Knoll sediments and intact bodies of another mussel (B. septemdierum) from a previous study (Ikuta et al., 2021). The sediments were dominated by tetra- (mean = 39%, dw), tri- (mean = 34%, dw), and penta-CBs (mean = 10%, dw), and the mussels were dominated by penta- (mean = 32%, dw), hexa- (mean = 32%, dw), and tetra-CBs (mean = 21%, dw), indicating a tendency towards higher-chlorinated PCB homologs in the mussels. The two were separated by 80% similarity; however, no statistically significant differences were observed (Supplementary Figure 2). The BSAF value of P. okutanii for the total ΣPCBs was 0.66 (Supplementary Table 3).
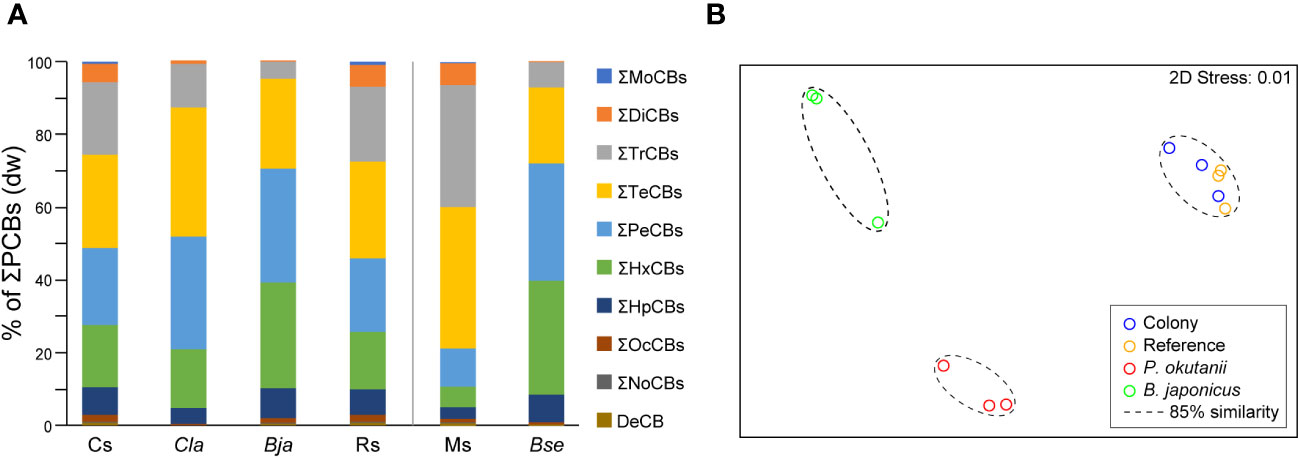
Figure 2 PCB composition comparison in bivalves and habitat sediments. Intact body concentrations of bivalves (collected in 2019) are based on data obtained from a previous study (Ikuta et al., 2021). (A) Mean congener composition of PCBs in bivalves and habitat sediments. The concentrations are based on the dry weight (dw). Cs, sediments from the clam colony; Cla, intact bodies of Phreagena clams; Bja, intact bodies of Bathymodiolus japonicus; Rs, sediments at a distance from the colony; Ms, sediments from the Myojin Knoll; and Bse, intact bodies of Bathymodiolus septemdierum. (B) Multidimensional scaling (MDS) plot demonstrating both bivalve colony and reference sediments in Sagami Bay placed in distinct clusters (85% similarity) from the two bivalve species.
We also analyzed the PBDE content in the sediment samples. PBDEs were detected in every sample, and the total concentrations were between 0.24–13 ng/g dw (Table 2). No statistically significant differences in the ΣPBDEs were detected among samples collected from the clam colony in Sagami Bay, those collected at a distance from the colony, and the Myojin Knoll samples; however, the Myojin Knoll samples exhibited the three lowest values (Table 2). Based on the PBDE homolog percentages in the ΣPBDEs, de-BDE accounted for the majority of PBDE content (mean = 83%, dw), followed by nona- (mean = 12%, dw) and octa-BDEs (mean = 2.5%, dw, Supplementary Figure 3).
4 Discussion
In this study, we determined the PCB concentrations in the gills, feet, and gonads of the deep-sea chemosynthetic clam P. okutanii. A positive correlation was identified between the lipid content and PCB concentrations across all biological samples, and the ovaries exhibited the highest PCB concentrations and elevated lipid content. The buoyancy of P. okutanii eggs and numerous lipid droplets in the cytoplasm of the vegetal hemisphere (Ikuta et al., 2016) indicate that these droplets may be responsible for the accumulation of PCBs in eggs. Filter-feeding bivalves such as mussels and oysters absorb PCBs with their diet, and biomagnification of PCBs through trophic transfer has also been reported. PCBs affect various biological processes, including immune, endocrine, and reproductive functions (Ngoubeyou et al., 2022), across various organisms. Moreover, experimental studies have assessed their accumulation and effects on bivalves. For example, in Crassostrea virginica oyster, PCBs mixed with algae in the diet accumulated predominantly in the gonads, thus affecting reproductive functions (Chu et al., 2003). Similar experiments have demonstrated that PCBs accumulate in oysters and are transferred to eggs (Chu et al., 2000). In the hard clam Mercenaria, exposure to Aroclor 1254, a prevalent commercial PCB mixture primarily composed of penta- (71.44%) and hexa-CBs (21.97%), during development resulted in concentration-dependent larval morphological abnormalities (Ryan et al., 2001). Freshwater clam, Corbicula fluminea exposed to water containing Aroclor 1260, a commercial PCB mixture primarily composed of penta- (43.35%) and hexa-CBs (38.54%), exhibit pronounced gonadal atrophy, effacement of gonad architecture, and inflammation and necrosis in the digestive gland and foot processes. This confirms the considerable threat of PCB exposure to bivalve reproduction (Lehmann et al., 2007). Our previous study demonstrated that non-feeding deep-sea chemosynthetic bivalves were contaminated with PCBs (Ikuta et al., 2021). PCB contamination levels in the intact Phreagena clam bodies collected off Hatsushima Island in Sagami Bay were considerably lower compared to the significantly higher PCB concentrations detected in shallow-water mussels collected near the densely populated regions of Japan; however, this was comparable to the levels detected in shallow-water bivalves across various international sites (Ikuta et al., 2021). Additionally, this was comparable to or slightly lower than those of other deep-sea organisms at lower trophic levels collected from Sagami Bay, including pelagic fishes, crustaceans, and zooplankton (Toyoshima et al., 2009; Ikuta et al., 2021). In the present study, the PCB concentrations detected in the ovaries of Phreagena clams were considerably lower than those in the ovaries of oysters in experiments using administration of PCB-contaminated algae (3,490 ng/g, dw), as described above (Chu et al., 2003). The level of contamination that may cause harmful effects on Phreagena clams remains unclear. Examining the maturity status and PCB concentrations of clams at different growth stages will provide insight into the effects of PCBs on clam maturity. However, PCB contamination in Phreagena clams at this site has been present for over 30 years (Ikuta et al., 2021), which complicates the determination of any histological and functional abnormalities compared to uncontaminated samples. The current study revealed significantly higher levels of PCB contamination in the ovaries, indicating a potential effect on reproductive functions. Among the coplanar PCBs examined in this study, PCB118, which had the highest content in both biological and sediment samples, is known to cause reproductive abnormalities in male and female mammals (Kuriyama and Chahoud, 2004; Ge et al., 2022), although its effects on bivalves are unknown. Thus, our results raise concerns regarding a severe direct effect on the survival of this vulnerable and highly endemic species.
Our chemical analyses showed PCBs in all sediment samples (mean = 3.9 ng/g dw). This value aligns with PCB concentrations in surface sediments collected from various bays around Japan, including shallow waters (Shimizu et al., 2008) and the deep sea (Ohkouchi et al., 2016). Comparisons of each homolog composition, including intact body data from previous studies of Phreagena clams and Bathymodiolus mussels (Ikuta et al., 2021), demonstrated a distinct clustering of sediment data from the bivalve data. The bivalve bodies contained PCBs with higher-chlorinated compounds than those in the sediments, similar to those observed in shallow-water clams (Choi et al., 2010). In general, less-chlorinated PCBs are metabolized more readily than highly chlorinated congeners, which exhibit a lower tendency for in vivo metabolism and a higher tendency to remain in the animal body (Agency for Toxic Substances and Disease Registry (ATSDR) 2014). Therefore, PCBs that accumulate in organisms exhibit a higher-chlorinated composition than those in the environment. However, how extensively persistent PCBs are metabolically degraded in bivalves at low deep-sea temperatures of approximately 4°C remains to be investigated. As an alternative explanation, the examined bivalves may take up highly chlorinated PCBs. Our recent findings indicate that both deep-sea bivalves absorb plastic particles through their body surface into their gill cells by phagocytosis (Ikuta et al., 2022). Highly chlorinated and hydrophobic PCBs are more readily adsorbed onto organic particles, such as microplastics, in the environment (Colabuono et al., 2010). In line with our results, if these particles are taken up from the body surface, they may contribute to the accumulation of highly chlorinated PCBs in the bivalve bodies. The current study demonstrated that the BSAF value of P. okutanii for PCBs, which indicates the biological potential for PCB accumulation from the environment, was comparable to that of some shallow-water bivalves (Tracey and Hansen, 1996). Therefore, the continuous accumulation of microplastics and other organic particles containing PCBs and other toxic substances on the deep-sea floor can markedly affect these deep-sea clams. Substantial amounts of microplastic have already been detected in the same clam colony site, suggesting that microplastics can be carriers of POPs in this habitat, although other organic particles are also responsible for this phenomenon (Tsuchiya et al., 2023). In a previous study, no PBDEs were detected in the bodies of clams collected in 2019 (Ikuta et al., 2021); however, in the present study, PBDEs were detected in sediments from clam habitats at levels comparable to those of PCBs. The interpretation of these results is difficult because the BSAF of PBDEs in shallow-water mussels was reportedly higher than that of PCBs, indicating higher bioaccumulation of PBDEs in mussels than of PCBs (Debruyn et al., 2009). Examining PBDE concentrations in other chemosynthetic organisms, such as tubeworms living sympatrically with the clams, may provide further insight.
In conclusion, our results demonstrate that non-feeding Phreagena clams, a representative taxon in the deep-sea chemosynthetic ecosystem, take up environmental PCBs through their body surface and accumulate them predominantly in their ovaries. These results indicate a potential direct effect on their reproductive function. Recent findings have indicated a reduction in PCB contamination within Phreagena clams in recent years in Sagami Bay; however, the current study identified PCBs in the habitat sediments. Contaminated marine sediments act as a secondary source of contaminants for the benthic communities they inhabit and other organisms through food web bioaccumulation. Additionally, the increasing presence of plastics and other organic particles with adsorbed contaminants is anticipated to persistently increase and accumulate in the deep sea (Wayman and Niemann, 2021). It is necessary to take approaches such as examining PCBs associated with particles at the habitat site and PCBs dissolved in seawater to determine whether these PCB-adsorbed organic particles are the primary source of contamination. In the future, the results of the present study can serve as a foundation for monitoring the status of marine pollution in vulnerable deep-sea ecosystems.
Data availability statement
The original contributions presented in the study are included in the article/Supplementary Material. Further inquiries can be directed to the corresponding author.
Ethics statement
The manuscript presents research on animals that do not require ethical approval for their study.
Author contributions
TI: Formal analysis, Investigation, Methodology, Visualization, Writing – original draft. HN: Funding acquisition, Resources, Writing – review & editing. RN: Formal analysis, Writing – review & editing.
Funding
The author(s) declare financial support was received for the research, authorship, and/or publication of this article. This work was partly supported by the New Energy and Industrial Technology Development Organization (NEDO) under Project JPNP18016.
Acknowledgments
We are grateful to Dr. Tomo Kitahashi (JAMSTEC), the captains and crew of the R/V Kaimei and R/V Yokosuka, and the operation teams of ROV KM-ROV and HOV Shinkai 6500, for their assistance with sampling. We also thank Editage [http://www.editage.com] for editing and reviewing the language used in this manuscript.
Conflict of interest
The authors declare that the research was conducted in the absence of any commercial or financial relationships that could be construed as a potential conflict of interest.
Publisher’s note
All claims expressed in this article are solely those of the authors and do not necessarily represent those of their affiliated organizations, or those of the publisher, the editors and the reviewers. Any product that may be evaluated in this article, or claim that may be made by its manufacturer, is not guaranteed or endorsed by the publisher.
Supplementary material
The Supplementary Material for this article can be found online at: https://www.frontiersin.org/articles/10.3389/fmars.2024.1358774/full#supplementary-material
References
Agency for Toxic Substances and Disease Registry (ATSDR). (2014). Case studies in environmental medicine polychlorinated biphenyls (PCBs) toxicity. Washington, DC: U.S. Department of Health and Human Services.
Ankley G. T., Cook P. M., Carlson A. R., Call D. J., Swenson J. A., Corcoran H. F., et al. (1992). Bioaccumulation of PCBs from sediments by oligochaetes and fishes: Comparison of laboratory and field studies. Can. J. Fish. Aquat. Sci. 49, 2080–2085. doi: 10.1139/f92-231
Ashraf M. A. (2017). Persistent organic pollutants (POPs): a global issue, a global challenge. Environ. Sci. pollut. Res. 24, 4223–4227. doi: 10.1007/s11356-015-5225-9
Bjork M. (1995). Bioavailability and uptake of hydrophobic organic contaminants in bivalve filter-feeders. Ann. Zool. Fenn. 32, 237–245.
Bray J. R., Curtis J. T. (1957). An ordination of the upland forest communities of Southern Wisconsin. Ecol. Monogr. 27, 325–349. doi: 10.2307/1942268
Chiesa L. M., Nobile M., Malandra R., Pessina D., Panseri S., Labella G. F., et al. (2018). Food safety traits of mussels and clams: distribution of PCBs, PBDEs, OCPs, PAHs and PFASs in sample from different areas using HRMS-Orbitrap (R) and modified QuEChERS extraction followed by GC-MS/MS. Food Addit. Contam. Part A Chem. Anal. Control Expo. Risk Assess. 35, 959–971. doi: 10.1080/19440049.2018.1434900
Choi J.-Y., Yang D.-B., Hong G.-H. (2010). Bioaccumulation of polychlorinated biphenyls (PCBs) and organochlorine pesticides in manila clams (Ruditapes philippinarum) collected from the mid-western coast of Korea. Ocean Polar Res. 32, 237–245. doi: 10.4217/opr.2010.32.3.237
Chu F. L., Soudant P., Cruz-Rodriguez L. A., Hale R. C. (2000). PCB uptake and accumulation by oysters (Crassostrea virginica) exposed via a contaminated algal diet. Mar. Environ. Res. 50, 217–221. doi: 10.1016/s0141-1136(00)00070-2
Chu F.-L. E., Soudant P., Hale R. C. (2003). Relationship between PCB accumulation and reproductive output in conditioned oysters Crassostrea virginica fed a contaminated algal diet. Aquat. Toxicol. 65, 293–307. doi: 10.1016/s0166-445x(03)00152-8
Colabuono F. I., Taniguchi S., Montone R. C. (2010). Polychlorinated biphenyls and organochlorine pesticides in plastics ingested by seabirds. Mar. pollut. Bull. 60, 630–634. doi: 10.1016/j.marpolbul.2010.01.018
Daugherty C. E., Lento H. G. (1983). Chloroform-methanol extraction method for determination of fat in foods: collaborative study. J. Assoc. Off. Anal. Chem. 66, 927–932. doi: 10.1093/jaoac/66.4.927
Debruyn A. M., Meloche L. M., Lowe C. J. (2009). Patterns of bioaccumulation of polybrominated diphenyl ether and polychlorinated biphenyl congeners in marine mussels. Environ. Sci. Technol. 43, 3700–3704. doi: 10.1021/es900472k
Domingo J. L., Bocio A. (2007). Levels of PCDD/PCDFs and PCBs in edible marine species and human intake: a literature review. Environ. Int. 33, 397–405. doi: 10.1016/j.envint.2006.12.004
Erickson M. D., Kaley R. G. (2011). Applications of polychlorinated biphenyls. Environ. Sci. pollut. Res. 18, 135–151. doi: 10.1007/s11356-010-0392-1
Ge C., Geng T., Cheng L., Zhang Y. (2022). Gestational exposure to PCB-118 impairs placental angiogenesis and fetal growth. Environ. Sci. pollut. Res. 29, 49126–49135. doi: 10.1007/s11356-022-19240-9
Ghosh U., Zimmerman J. R., Luthy R. G. (2003). PCB and PAH speciation among particle types in contaminated harbor sediments and effects on PAH bioavailability. Environ. Sci. Technol. 37, 2209–2217. doi: 10.1021/es020833k
Igawa-Ueda K., Ikuta T., Tame A., Yamaguchi K., Shigenobu S., Hongo Y., et al. (2021). Symbiont transmission onto the cell surface of early oocytes in the deep-sea clam Phreagena okutanii. Zoolog. Sci. 38, 140–147. doi: 10.2108/zs200129
Ikuta T., Igawa K., Tame A., Kuroiwa T., Kuroiwa H., Aoki Y., et al. (2016). Surfing the vegetal pole in a small population: extracellular vertical transmission of an 'intracellular' deep-sea clam symbiont. R. Soc Open Sci. 3, 160130. doi: 10.1098/Rsos.160130
Ikuta T., Nakajima R., Tsuchiya M., Chiba S., Fujikura K. (2021). Interdecadal distribution of persistent organic pollutants in deep-sea chemosynthetic bivalves. Front. Mar. Sci. 8. doi: 10.3389/fmars.2021.751848
Ikuta T., Tame A., Takahashi T., Nomaki H., Nakajima R. (2022). Microplastic particles are phagocytosed in gill cells of deep-sea and coastal mussels. Front. Mar. Sci. 9. doi: 10.3389/fmars.2022.1034950
Kuriyama S. N., Chahoud I. (2004). In utero exposure to low-dose 2,3',4,4',5-pentachlorobiphenyl (PCB 118) impairs male fertility and alters neurobehavior in rat offspring. Toxicology 202, 185–197. doi: 10.1016/j.tox.2004.05.006
Lee H. J., Kim G. B. (2015). An overview of polybrominated diphenyl ethers (PBDEs) in the marine environment. Ocean Sci. J. 50, 119–142. doi: 10.1007/s12601-015-0010-8
Lehmann D. W., Levine J. F., McHugh Law J. (2007). Polychlorinated biphenyl exposure causes gonadal atrophy and oxidative stress in Corbicula fluminea clams. Toxicol. Pathol. 35, 356–365. doi: 10.1080/01926230701230288
Llodra E. R., Billett D. S. M. (2006). “Deep-sea ecosystems: pristine biodiversity reservoir and technological challenges,” in The Exploration of Marine Biodiversity: Scientific and Technological Challenges. Ed. Duarte C. M. (Bilbao, Spain: Fundacion BBVA), 63–92.
Matthews H. B., Dedrick R. L. (1984). Pharmacokinetics of PCBs. Annu. Rev. Pharmacol. Toxicol. 24, 85–103. doi: 10.1146/annurev.pa.24.040184.000505
Nakajima R., Chen C., Iwase R., Yamamoto H., Fujikura K. (2019). Clams after storms: the impact of multiple disturbances on seep vesicomyid clams revealed by long-term monitoring. Mar. Biol. 166, 35. doi: 10.1007/s00227-019-3484-6
Ngoubeyou P. S. K., Wolkersdorfer C., Ndibewu P. P., Augustyn W. (2022). Toxicity of polychlorinated biphenyls in aquatic environments - A review. Aquat. Toxicol. 251, 106284. doi: 10.1016/j.aquatox.2022.106284
Ohkouchi N., Shibata H., Chikaraishi Y., Nomaki H., Ogawa N. O., Nagata T., et al. (2016). A monitoring result of polychlorinated biphenyls (PCBs) in deep-sea organisms and sediments off Tohoku during 2012–2014: temporal variation and the relationship with the trophic position. J. Oceanogr. 72, 629–639. doi: 10.1007/s10872-016-0359-z
Ryan R. L., Lachmayr K. L., Jay J. A., Ford T. E. (2001). Developmental effects of PCBs on the hard clam (Mercenaria mercenaria). J. Environ. Sci. Health A 36, 1571–1578. doi: 10.1081/Ese-100106242
Safe S. H. (1994). Polychlorinated-biphenyls (PCBs): environmental-impact, biochemical and toxic responses, and implications for risk assessment. Crit. Rev. Toxicol. 24, 87–149. doi: 10.3109/10408449409049308
Shimizu J., Nobuchi K., Miura Y., Tomohisa T. (2008). Temporal trends of the concentrations of pollutants in the surface sediments from the major bays of Japan. Rep. Hydrographic Oceanographic Res. 44, 57–66.
Takahashi S., Karri R., Tanabe S. (2014). “Contamination by persistent organic pollutants and related compounds in deep-sea ecosystems along frontal zones around Japan,” in Chemical Oceanography of Frontal Zones. Ed. Belkin L. M. (Berlin: Springer-Verlag), 1–36.
Talsness C. E. (2008). Overview of toxicological aspects of polybrominated diphenyl ethers: a flame-retardant additive in several consumer products. Environ. Res. 108, 158–167. doi: 10.1016/j.envres.2008.08.008
Toyoshima S., Isobe T., Ramu K., Miyasaka H., Omori K., Takahashi S., et al. (2009). “Organochlorines and brominated flame retardants in deep-sea ecosystem of Sagami Bay,” in Interdisciplinary Studies on Environmental Chemistry – Environmental Research in Asia. Eds. Oshihoi T., Isobe T., Subramanian A., Suzuki S., Tanabe S. (Tokyo: Terra Pub), 83–90.
Tracey G. A., Hansen D. J. (1996). Use of biota-sediment accumulation factors to assess similarity of nonionic organic chemical exposure to benthically coupled organisms of differing trophic mode. Arch. Environ. Contam. Toxicol. 30, 467–475. doi: 10.1007/BF00213397
Tsuchiya M., Kitahashi T., Nakajima R., Oguri K., Kawamura K., Nakamura A., et al. (2023). Distribution of microplastics in bathyal- to hadal-depth sediments and transport process along the deep-sea canyon and the Kuroshio Extension in the Northwest Pacific. Mar. pollut. Bull. 199, 115466. doi: 10.1016/j.marpolbul.2023.115466
United States Environmental Protection Agency (USEPA). (2003). Method 1668, revision a chlorinated biphenyl congeners in water, soil, sediment, biosolids, and tissue by HRGC/HRMS. Washington, DC: United States Environmental Protection Agency (USEPA).
United States Environmental Protection Agency (USEPA). (2010). Method 1614A, brominated diphenyl ethers in water soil, sediment and tissue by HRGC/HRMS. Washington, DC: United States Environmental Protection Agency (USEPA).
Watanabe H., Seo E., Takahashi Y., Yoshida T., Kojima S., Fujikura K., et al. (2013). Spatial distribution of sister species of vesicomyid bivalves Calyptogena okutanii and Calyptogena soyoae along an environmental gradient in chemosynthetic biological communities in Japan. J. Oceanogr. 69, 129–134. doi: 10.1007/S10872-012-0155-3
Wayman C., Niemann H. (2021). The fate of plastic in the ocean environment - a minireview. Environ. Sci. Process. Impacts 23, 198–212. doi: 10.1039/d0em00446d
Yamashita R., Tanaka K., Yeo B. G., Takada H., van Franeker J. A., Dalton M., et al. (2019). “Hazardous chemicals in plastics in marine environments: International Pellet Watch,” in Handbook of Environmental Chemistry (Berlin Heidelberg: Springer Verlag), 163–183.
Yuan X. T., Yang X. L., Na G. S., Zhang A. G., Mao Y. Z., Liu G. Z., et al. (2015). Polychlorinated biphenyls and organochlorine pesticides in surface sediments from the sand flats of Shuangtaizi Estuary, China: levels, distribution, and possible sources. Environ. Sci. pollut. Res. 22, 14337–14348. doi: 10.1007/s11356-015-4688-z
Keywords: persistent organic pollutant, polychlorinated biphenyl, bioaccumulation, deep-sea, chemosynthesis, Phreagena
Citation: Ikuta T, Nomaki H and Nakajima R (2024) Accumulation of polychlorinated biphenyls in the ovaries of deep-sea chemosynthetic clam Phreagena okutanii. Front. Mar. Sci. 11:1358774. doi: 10.3389/fmars.2024.1358774
Received: 20 December 2023; Accepted: 24 January 2024;
Published: 09 February 2024.
Edited by:
Matteo Oliva, Centro Interuniversitario di Biologia Marina ed Ecologia Applicata “G. Bacci” di Livorno (CIBM), ItalyReviewed by:
Jingqian Xie, Shanghai Ocean University, ChinaChunming Dong, Ministry of Natural Resources, China
Copyright © 2024 Ikuta, Nomaki and Nakajima. This is an open-access article distributed under the terms of the Creative Commons Attribution License (CC BY). The use, distribution or reproduction in other forums is permitted, provided the original author(s) and the copyright owner(s) are credited and that the original publication in this journal is cited, in accordance with accepted academic practice. No use, distribution or reproduction is permitted which does not comply with these terms.
*Correspondence: Tetsuro Ikuta, dGVpa3V0YUBqYW1zdGVjLmdvLmpw