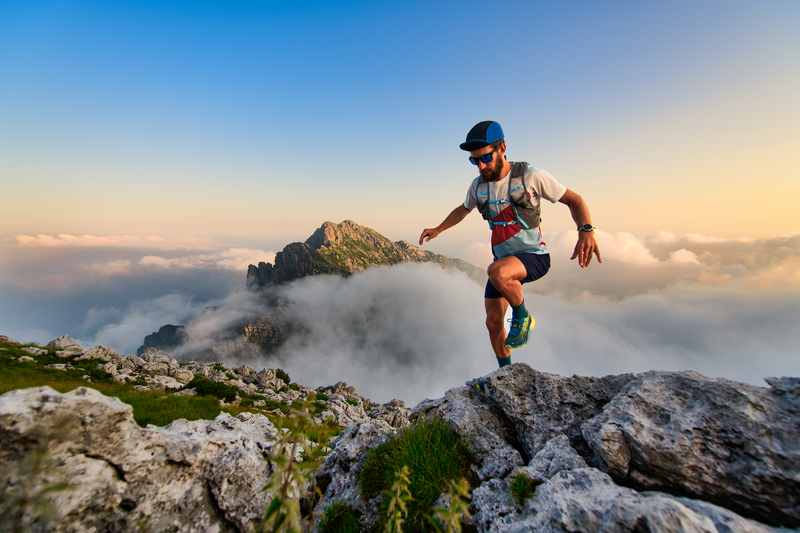
95% of researchers rate our articles as excellent or good
Learn more about the work of our research integrity team to safeguard the quality of each article we publish.
Find out more
METHODS article
Front. Mar. Sci. , 27 March 2024
Sec. Marine Biogeochemistry
Volume 11 - 2024 | https://doi.org/10.3389/fmars.2024.1356801
Compounds containing one carbon atom or no carbon-carbon bond (C1 compounds), such as trimethylamine and methanol, are important climate relevant gases in the atmosphere and play key roles in global warming. The ocean is a significant source or sink of such compounds, while the concentrations of trimethylamine and methanol in seawater remain largely unconstrained due to the analytical challenges involved. Therefore, it is necessary to establish a continuous, rapid and sensitive method for the determination of these compounds with high polarity, volatility or solubility at low seawater concentrations. Here we developed a purge and trap system, coupled to a gas chromatography equipped with dual nitrogen phosphorus detector (NPD) and flame ionization detector (FID) for the simultaneous online analysis of trimethylamine and methanol at nanomolar range using a small sample volume (~ 10 mL). The dual detection of trimethylamine and methanol with NPD or FID was achieved by installing a capillary flow splitter between the capillary column and detectors. After modification and optimization of the setup and conditions, excellent linearity (R2 > 0.99) and repeatability (< 6%) were obtained for both compounds; the detection limits for trimethylamine and methanol were 0.3 nM and 17.6 nM, respectively. Using this method, water samples collected from coastal and open ocean were analyzed; trimethylamine and methanol concentrations ranged from 0.6 to 18.8 nM and 26.0 to 256.2 nM, respectively. Collectively, this method allowed for online, rapid, sensitive and simultaneous quantification of trace trimethylamine and methanol concentrations with low-cost instrumentation and small sample volume, which makes it promising for further application in volatile compounds analysis in marine environments.
One carbon (C1) compounds, defined as molecules with no carbon-carbon bond, such as methane, methanol, methyl sulfides and methyl amines, are widespread on earth and play key roles in atmospheric chemistry and global warming (Yang et al., 2013; Masson-Delmotte et al., 2018; Sun et al., 2019; Mao et al., 2022; Hopkins et al., 2023; Bange et al., 2024). While methane and dimethyl sulfide have been intensively studied due to their impact on climate change (Charlson et al., 1987; Dean et al., 2018; Zhuang et al., 2018a; Forster et al., 2021; Mao et al., 2021), little is known about the biogeochemical cycling of other C1 compounds, such as trimethylamine and methanol (Van Neste et al., 1987; King, 1988; Singh et al., 2001; Zhuang et al., 2019; Fitzsimons et al., 2023). Gaseous amines in the atmosphere, including trimethylamine, can enhance particle nucleation as well as growth and affect secondary organic aerosol formation (Yao et al., 2018). Methanol is one of the most abundant oxygenated volatile organic compounds that can contribute to the formation of atmospheric ozone (Heikes et al., 2002).
Seawater represents an important sink and source of C1 compounds with respect to the atmosphere. Trimethylamine can be released by phytoplankton from the degradation of quaternary amines (e.g., glycine betaine) and derived from the excretion or decay of marine animals and microorganisms (Carpenter et al., 2012; Mausz and Chen, 2019). Containing both carbon and nitrogen, trimethylamine can be used as carbon, nitrogen and energy sources for marine microbes (Taubert et al., 2017; Zhuang et al., 2018b). Similar to trimethylamine, methanol is ubiquitous in marine systems and largely produced by phytoplankton (Yang et al., 2013; Mincer and Aicher, 2016). Methanol is predominately used as a microbial energy source for CO2 production (Zhou et al., 2023), and was also observed as an important carbon source channeled into biomass in highly productive areas (Dixon et al., 2013b; Zhuang et al., 2018b). Therefore, the metabolism of trimethylamine and methanol constitute an important part of the marine carbon and nitrogen cycles.
Despite the significance of trimethylamine and methanol in the ocean, large uncertainty remains for global sea-air exchange fluxes due to limited measurements of their concentrations. A few studies reported that trimethylamine and methanol concentrations were within the nanomolar range in seawater (Beale et al., 2011, 2013; Cree et al., 2018; Wu et al., 2020). Determination of trimethylamine and methanol at such low concentrations is challenging and an efficient enrichment for preconcentration is usually required before chromatographic (GC) or mass spectrometric (MS) analysis. The basic nature and high polarity lead to the strong adsorption of trimethylamine to the surface of glassware and instruments, while the high solubility of methanol makes it difficult to be extracted from seawater. Several approaches have been developed to determine the low concentrations of trimethylamine or methanol in seawater, for example, solid-phase microextraction with gas chromatography method and dopant-assisted atmospheric pressure photoionization time-of-flight mass spectrometry method for trimethylamine and inlet-proton transfer reaction/mass spectrometer method for methanol (Beale et al., 2011; Cree et al., 2018; Wu et al., 2020). However, the time-consuming pretreatment with manipulative steps and requirement of a large sample volume, or the employment of high-cost instruments, has limited the routine application of these methods in marine research. Furthermore, the purge and trap (P&T) pretreatment with gas chromatography has been widely used for the trace analysis of marine volatile compounds (Zhang et al., 2004, Zhang et al., 2015; Yang et al., 2010, Yang et al., 2011; Zhong et al., 2012) due to its versatility with easy operation, high extraction efficiency, and the requirement of low-cost instrumentation. Recently, we have successfully developed P&T-GC-MS or P&T-GC methods to analyze concentrations of trimethylamine or methanol in sedimentary pore waters with 5 – 6 mL samples (Zhuang et al., 2014, 2017).
In this study, we sought to establish a P&T-GC method for the simultaneous determination of seawater trimethylamine and methanol. To achieve this, the two compounds were separated by a single column but detected using dual nitrogen-phosphorus detector (NPD) and flame-ionization detector (FID). We further optimized the P&T conditions (e.g., purge flow rate, purge temperature and trap temperature) to improve the sensitivity of the method. After optimization, we assessed the performance and applied this method to determine natural seawater samples collected from the coastal waters of Jiaozhou Bay and the open ocean of the western Pacific.
Trimethylamine hydrochloride (98%) and methanol (analytical reagent) were purchased from Merck (KGaA, Germany) and Sinopharm Chemical Reagent, Ltd (Shanghai, China), respectively. The primary stocks of trimethylamine (1.2 mM) and methanol (12.3 mM) were prepared separately in double-distilled water and both stock solutions were stored < 1 week in a 50 mL serum vial without headspace at 4°C in the dark. Standard solutions for calibration (trimethylamine: 0.5 – 7.2 nM; methanol: 24.0 – 360.0 nM) were made by mixing and diluting the trimethylamine and methanol primary stocks to desired concentrations. Ammonium chloride (> 99.5%) and sodium hydroxide (> 96%) were purchased from Sinopharm Chemical Reagent Co., Ltd (Shanghai, China).
A modified P&T system (Acrichi PTC, Beijing Juxin Zhuifeng Technology, Ltd, China) was used for the preconcentration of trimethylamine and methanol before GC analysis. The P&T system includes a purge unit to extract the volatiles from the seawater, a dehydration unit to remove the moisture, and a trap unit to concentrate the volatile gases (Figure 1). Briefly, 10 mL seawater was introduced into a 40 mL glass vial and capped with a silicone/PTFE septum. After injecting 400 μL of 5 M NaOH and 200 μL of 20 mM NH4Cl through the septum, the 40 mL glass vial, used as the bubbling chamber, was placed on a heating bracket at 85°C. The addition of NaOH helps to release trimethylamine from the non-volatile hydrochloride form at seawater pH ~ 8.2 and NH4Cl would reduce the adsorption of trimethylamine on the surface of labware and column (Dalene et al., 1981). A sparging needle pierced through the septum, and then the sample was purged with nitrogen gas. The evolved gas passed through an inert hollow condenser pipe electronically set at −5°C to condense the water, and then entered a cryogenic trap packed with Tenax TA, which was electronically controlled at −25°C. After 10 min of purging and trapping, the cold trap was heated to 220°C for 2 min and the vaporized analytes were channeled to the GC with high-purity nitrogen. After the introduction of gas into GC, the P&T system was purged with high-purity nitrogen (99.999%) for 3 min to eliminate moisture and the cryogenic trap was heated to 300°C to avoid carryover between samples. All tubes, components, and connectors of the P&T system were deactivated and maintained at 180°C to minimize adsorption.
Figure 1 P&T-GC-NPD&FID system for trimethylamine and methanol analysis in seawater. 1: Nitrogen 2: Toggle valve 3: Gas controller 4: Pressure gauge 5: Purge syringe 6: Bubbling chamber 7: Heater block 8: Dehydration unit 9: Cold trap 10: Six port valve 11: Injection port 12: Capillary flow splitter 13: FID 14: NPD.
The quantification of trimethylamine and methanol was performed using an Agilent 8890 GC equipped with NPD and FID. This is achieved through a splitter based on the capillary flow technology of Agilent, which was installed between the capillary column and detectors and connected the NPD and FID in parallel (Figure 1). Volatile compounds were separated with a HP-PLOT Q column (30 m × 0.32 mm, Agilent Technologies Inc., USA). The optimized GC conditions included: split ratio, 0.5:1; injection temperature, 70°C; oven temperature started at 40°C for 2 min, ramped to 240°C at 35°C min–1 and held at 240°C for 3 min. Carrier gas flow rates were optimized to increase the analytes response (5.5 mL min–1, see section 3.1). The NPD temperature was set at 320°C and the flow rates of hydrogen and air were 10 mL min–1 and 60 mL min–1. The temperature of the FID was 300°C, with a hydrogen and air flow of 30 mL min–1 and 400 mL min–1, respectively. Parameters for the P&T system were detailed in Supplementary Table S1.
To test for potential contamination from the laboratory, a procedural blank of non-spiked water was measured using the same procedure as the standards. While trimethylamine was not detected in non-spiked double-distilled water, there was a consistent background presence of methanol in the water. Additional tests confirmed that the P&T system did not introduce trimethylamine and methanol blank by purging an empty vial without adding water. Therefore, the calibration for methanol was conducted by subtracting the blank from the water. To prevent methanol contamination, the septa used to seal the bubbling chamber were baked at 75°C for 72 h. Furthermore, all measurements were conducted in a laboratory free of trimethylamine and methanol, as confirmed by exposing blank water in the lab for two weeks (Zhuang et al., 2014).
Surface seawaters were collected from shallow waters of Jiaozhou Bay and open ocean of the western Pacific (Supplementary Figure S1) during May 2022 and August 2022, respectively. Seawater samples from Jiaozhou Bay and the western Pacific Ocean were collected using a 5 L plexiglass water sampler and using 12 L Niskin bottles deployed on a Seabird 911 conductivity-temperature-depth rosette system, respectively. After collection, seawater was immediately transferred into 60 mL serum vials and sealed with thick butyl rubber stoppers to minimize gas loss and air contamination. All samples were stored at −80°C immediately after sampling (< 10 min) on board to prevent biological loss and potential degradation before analysis (Zhuang et al., 2018b), as methylamines can be consumed quickly without preservation (Cree et al., 2018). Samples were not filtered before analysis as the filtering process could lead to the release of organic volatiles or introduce contaminations (Beale et al., 2011). Therefore, our measurements represented the total concentrations of TMA and methanol in the environments, including dissolved and particulate fractions. However, it should be noted that separating dissolved and particulate phases of TMA through filtration is necessary for quantifying the fluxes of TMA to the atmosphere, which is an important measurement to assess the residence time in seawater once produced. All glass bottles for sampling were soaked in hydrochloric acid (3% v/v) for > 24 h and rinsed with double-distilled water three times before use.
One-way analysis of variance (One-way ANOVA) was performed on Origin 2023 (OriginLab Corporation, Northampton, MA, USA) and the significance of differences during optimization was tested with a significance level of 0.05. Sampling sites and the distribution of trimethylamine and methanol were mapped using Ocean Data View 5.3.0 (Schlitzer, Reiner, Ocean Data View, odv.awi.de, 2022).
Given the different responses of volatile compounds to specific detectors, trimethylamine and methanol were analyzed with the NPD and FID, respectively. As such, a splitter based on capillary flow technology was installed between the capillary column and detectors to split carrier gas from the same column to the NPD and FID. Accordingly, continuous online quantification of trimethylamine and methanol could be achieved with single injection after P&T preconcentration using a small sample volume (~ 10 mL). This not only significantly reduced running time but was particularly important for volume-limited samples (e.g., pore water and microlayer samples).
The split of carrier gas into two streams would result in an over 50% reduction in the response of trimethylamine and methanol. The decrease could be attributed to the disparity in textures between the capillary and inertia tee-junction. This can be improved by increasing the carrier gas flow, but high flow would also lead to the elution of the column’s stationary phase. Therefore, optimal flow rates were examined between 3.0 to 6.0 mL min–1, and a final flow of 5.5 mL min–1 was used to yield a satisfactory response without compromising the column performance. After optimization, trimethylamine and methanol were separated with good peak shapes and detected simultaneously with NPD and FID (Figure 2).
Figure 2 Chromatogram of trimethylamine (A, 12.0 nM) and methanol (B, 1.2 μM) in double-distilled water measured with P&T-GC-NPD&FID.
To preconcentrate the target compounds more efficiently, a series of P&T conditions were optimized. We assessed the effect of purge parameters, such as purge flow, purge time and purge temperature on the extraction efficiency (Zhuang et al., 2014, 2017).
Purge time and flow reflect the total gas volume used for degassing, which help the volatiles escape to the headspace. For methanol, the responses generally increased with purge flow from 50 to 90 mL min–1 (Figure 3A). Similarly, higher flow (> 70 mL min–1) also resulted in elevated response for trimethylamine (Figure 3A). Considering that higher flow would cause the loss of adsorbent in the trap and large purging gas volume would elute the analyte compounds from the adsorbents, we selected a flow rate of 90 mL min–1 for 10 min.
Figure 3 The effects of (A) purge flow, (B) purge temperature, (C) trap temperature, (D) NaOH addition and (E) NH4Cl addition on the response of trimethylamine and methanol measured with P&T-GC-NPD&FID. In each panel, 100% relative intensity corresponds to the highest signal observed. Error bars represent the standard deviation of triplicate measurements, and ultimate conditions are denoted with * in each panel.
Temperature is also important for extraction and both trimethylamine and methanol signals increased significantly from ambient to 85°C (Figure 3B). Heating at high temperature introduced more moisture to the cold absorbent trap. To avoid clogging, a hollow condenser pipe at −5°C was used for dehydration before trapping, which removed moisture from the gas stream without significant loss of methanol. For the temperature of the adsorbent trap, while methanol was not affected, the recovery of trimethylamine decreased at −15°C compared to other lower temperature (Figure 3C). Based on these facts, our samples were heated at 85°C during purging, dried at −5°C, and finally trapped with Tenax TA at −25°C.
We tested the effect of NaOH and NH4Cl on the recovery of trimethylamine and methanol. As expected, the signal of trimethylamine without NaOH was only 8.1% of those purged with 400 µL 5 M NaOH (Figure 3D). This is because trimethylamine, with a pKb value of 4.19, exists mostly in non-volatile hydrochloride form under neutral or seawater condition (pH ~ 8.2). The addition of NH4Cl significantly improves the response of trimethylamine, since NH3 reduces the adsorption of trimethylamine by competing for sites on the surface of the P&T system (Figure 3E). Moreover, methanol response was also improved with the addition of NaOH, which could be attributed to the “salting-out effect” (Peng and Wan, 1998). Ultimately, 200 µL 20 mM NH4Cl and 400 µL 5 M NaOH were added to the sample to release trimethylamine and reduce adsorption.
Under the optimum conditions, we assessed the performance of the method. A six-point calibration curve was conducted for trimethylamine (0.5 – 7.2 nM) and methanol (24.0 – 360.0 nM), and excellent linearity was observed with R2 > 0.99 (Figure 4). The repeatability, calculated from the relative standard deviation (RSD) of repeated measurements of the standard solution (n = 7) was better than 6%, suggesting the good stability of the method (Table 1). Good reproducibility was also observed for the seawater sample (RSD < 8%), which was measured repeatedly seven times.
Figure 4 Six-point calibration curves for trimethylamine (0.5 – 7.2 nM) and methanol (24.0 – 360.0 nM). Error bars represent the standard deviation of triplicate measurements.
Table 1 Linearity, repeatability, and detection limits for trimethylamine and methanol analysis by P&T-GC-NPD&FID.
The method detection limit (MDL) was calculated according to Equation 1.
where δ is the standard deviation of replicate measurements (n = 7) of standards spiked with low concentrations of analytes according to EPA. Using this method, the calculated MDL for trimethylamine and methanol was 0.3 nM and 17.6 nM, respectively (Table 1). Compared to previous methods, this is a significant improvement on the detection of trimethylamine and methanol with a 10 mL sample volume. Taking the preconcentration and GC running time into account, the total process time for each sample is 20 min (Table 2). The short processing time, the high sensitivity of detection, the relatively low requirement of sample volume, and the capability to analyze two C1 compounds simultaneously, make our method a suitable assay for rapid and sensitive analysis of trimethylamine and methanol in marine environments.
Table 2 Comparison of different methods used for the analysis of trimethylamine and methanol in marine system.
The application of the developed method for trimethylamine and methanol analysis was evaluated using seawater samples retrieved from Jiaozhou Bay and the western Pacific Ocean. Both trimethylamine and methanol were detected in the seawater at all sampling sites (Supplementary Table S2). In Jiaozhou Bay, the concentrations of trimethylamine varied from 1.3 to 18.8 nM (5.2 ± 4.4 nM). Methanol concentrations ranged from 26.0 to 256.2 nM, with an average of 93.2 ± 62.1 nM. These values were comparable to the measured concentrations in coastal waters (Table 3). The highest concentrations of trimethylamine and methanol were observed at site D4 of the east bay mouth, which was close to Qingdao Cruise Port (Figure 5). The elevated concentration at this site suggested that anthropogenic activity might influence marine productivity and also the production of trimethylamine and methanol. In the Pacific Ocean, the concentrations of trimethylamine were much lower, ranging from 0.6 to 2.3 nM (1.1 ± 0.7 nM). In seawater, trimethylamine is mainly derived from the degradation of its precursors (trimethylamine N-oxide, glycine betaine, carnitine) that serve as osmolytes in marine organisms (Jameson et al., 2016). Given the dominance of biological source for trimethylamine, it is not surprising that the abundance of trimethylamine was higher in coastal water than in the open ocean due to the high primary production.
Figure 5 Distributions of trimethylamine (A, C; nM) and methanol (B, D; nM) in the surface seawater of Jiaozhou Bay and the western Pacific Ocean.
By comparison, methanol concentrations in oceanic waters (122.8 ± 48.0 nM) were comparable to those measured in Jiaozhou Bay and were also generally within the wide range reported in the Atlantic, and Pacific oceans (Table 3). In contrast to trimethylamine, the sources of methanol can be more complex, such as phytoplankton production, photochemical decomposition of organic matter, and atmosphere deposition (Schink and Zeikus, 1980; Yang et al., 2013; Dixon et al., 2013a; Mincer and Aicher, 2016). In the Atlantic Ocean, methanol is mostly derived from biological production, while photoproduction seemed to be negligible for gross in situ production (Dixon et al., 2013a). Although production rates can be higher in coastal waters, rapid consumption of methanol in near-shore waters would maintain methanol concentration at a low level. In the Northwest Pacific Ocean, atmospheric deposition is also a source of methanol and it accounted for 22.7% of microbial methanol consumption in the mixed layer (Zhou et al., 2023). Collectively, the in situ concentrations of trimethylamine and methanol reflected the relative importance of production and consumption processes involved in their cycling in marine environments.
Investigating the concentrations and distributions of trimethylamine and methanol is a prerequisite to understand their biogeochemical cycling in the ocean. In this study, we established a rapid and sensitive GC approach for online and simultaneous analysis of trimethylamine and methanol in seawater. After preconcentration with a P&T setup, trimethylamine and methanol were analyzed with GC equipped with dual NPD and FID. We optimized GC and P&T conditions and obtained good performance of linearity, repeatability and detection limit. The application of this approach was validated using the seawater samples collected from Jiaozhou Bay and the western Pacific Ocean, and trimethylamine and methanol were detected at all sites with spatial variability. Overall, this method is simple, relatively fast with high sensitivity and small sample volume for simultaneous analysis of trimethylamine and methanol using a GC. Furthermore, this method could be extended for the analysis of other methylamines or alcohols with optimizations, not only in the seawater, but possibly in air samples, which would be helpful for future investigation of C1 or other volatile organics in marine environments.
The original contributions presented in the study are included in the article/Supplementary Material. Further inquiries can be directed to the corresponding author.
FJ: Data curation, Formal analysis, Investigation, Methodology, Software, Validation, Visualization, Writing – original draft, Writing – review & editing. ZZ: Data curation, Formal analysis, Investigation, Methodology, Software, Validation, Visualization, Writing – original draft, Writing – review & editing. J-YW: Supervision, Visualization, Writing – original draft, Writing – review & editing. W-JG: Methodology, Software, Writing – review & editing. L-GH: Resources, Writing – review & editing. X-BL: Writing – review & editing. G-CZ: Conceptualization, Funding acquisition, Methodology, Project administration, Resources, Software, Supervision, Validation, Writing – original draft, Writing – review & editing.
The author(s) declare financial support was received for the research, authorship, and/or publication of this article. This work was supported by the National Key Research and Development Program of China (Grant 2022YFE0136300), the National Natural Science Foundation of China (Grant 42076031), the Taishan Scholars Program of Shandong Province (Grant tsqn201909057).
We thank Agilent for providing technical support during the method development. We acknowledge the captain, crew, and scientists of the R/V “Dongfanghong 3” for their kind assistance and cooperation during sampling. We sincerely thank the editor and reviewers for their constructive suggestions for improving the overall quality of our manuscript.
Author L-GH was employed by the company Beijing Juxin Zhuifeng Technology, Ltd.
The remaining authors declare that the research was conducted in the absence of any commercial or financial relationships that could be construed as a potential conflict of interest.
All claims expressed in this article are solely those of the authors and do not necessarily represent those of their affiliated organizations, or those of the publisher, the editors and the reviewers. Any product that may be evaluated in this article, or claim that may be made by its manufacturer, is not guaranteed or endorsed by the publisher.
The Supplementary Material for this article can be found online at: https://www.frontiersin.org/articles/10.3389/fmars.2024.1356801/full#supplementary-material
Abdul-Rashid M. K., Riley J. P., Fitzsimons M. F., Wolff G. A. (1991). Determination of volatile amines in sediment and water samples. Anal. Chim. Acta 252, 223–226. doi: 10.1016/0003-2670(91)87219-W
Bange H. W., Mongwe P., Shutler J. D., Arévalo-Martínez D. L., Bianchi D., Lauvset S. K., et al. (2024). Advances in understanding of air–sea exchange and cycling of greenhouse gases in the upper ocean. Elem Sci. Anthd 12, 44. doi: 10.1525/elementa.2023.00044
Beale R., Dixon J. L., Arnold S. R., Liss P. S., Nightingale P. D. (2013). Methanol, acetaldehyde, and acetone in the surface waters of the Atlantic Ocean. J. Geophys. Res. Oceans 118, 5412–5425. doi: 10.1002/jgrc.20322
Beale R., Dixon J. L., Smyth T. J., Nightingale P. D. (2015). Annual study of oxygenated volatile organic compounds in UK shelf waters. Mar. Chem. 171, 96–106. doi: 10.1016/j.marchem.2015.02.013
Beale R., Liss P. S., Dixon J. L., Nightingale P. D. (2011). Quantification of oxygenated volatile organic compounds in seawater by membrane inlet-proton transfer reaction/mass spectrometry. Anal. Chim. Acta 706, 128–134. doi: 10.1016/j.aca.2011.08.023
Carpenter L. J., Archer S. D., Beale R. (2012). Ocean-atmosphere trace gas exchange. Chem. Soc Rev. 41, 6473–6506. doi: 10.1039/c2cs35121h
Charlson R. J., Lovelock J. E., Andreae M. O., Warren S. G. (1987). Oceanic phytoplankton, atmospheric sulphur, cloud albedo and climate. Nature 326, 655–661. doi: 10.1038/326655a0
Cree C. H. L., Airs R., Archer S. D., Fitzsimons M. F. (2018). Measurement of methylamines in seawater using solid phase microextraction and gas chromatography. Limnol. Oceanogr. Methods 16, 411–420. doi: 10.1002/lom3.10255
Dalene M., Mathiasson L., Jönsson J.Å. (1981). Trace analysis of free amines by gas-liquid chromatography. J. Chromatogr. A 207, 37–46. doi: 10.1016/S0021-9673(00)82690-4
Dean J. F., Middelburg J. J., Röckmann T., Aerts R., Blauw L. G., Egger M., et al. (2018). Methane feedbacks to the global climate system in a warmer world. Rev. Geophys. 56, 207–250. doi: 10.1002/2017RG000559
Dixon J. L., Beale R., Nightingale P. D. (2013a). Production of methanol, acetaldehyde, and acetone in the Atlantic Ocean. Geophys. Res. Lett. 40, 4700–4705. doi: 10.1002/grl.50922
Dixon J. L., Sargeant S., Nightingale P. D., Colin Murrell J. (2013b). Gradients in microbial methanol uptake: productive coastal upwelling waters to oligotrophic gyres in the Atlantic Ocean. ISME J. 7, 568–580. doi: 10.1038/ismej.2012.130
Fitzsimons M. F., Tilley M., Cree C. H. L. (2023). The determination of volatile amines in aquatic marine systems: A review. Anal. Chim. Acta 1241, 340707. doi: 10.1016/j.aca.2022.340707
Forster P., Storelvmo T., Armour K., Collins W., Dufresne J.-L., Frame D., et al. (2021). “The earth’s energy budget, climate feedbacks, and climate sensitivity,” in Climate change 2021: the physical science basis. Contribution of working group I to the sixth assessment report of the intergovernmental panel on climate change (Cambridge University Press, Cambridge, United Kingdom and New York, NY, USA), 923–1054. doi: 10.1017/9781009157896.009
Gibb S. W., Hatton A. D. (2004). The occurrence and distribution of trimethylamine-n-oxide in antarctic coastal waters. Mar. Chem. 91, 65–75. doi: 10.1016/j.marchem.2004.04.005
Gibb S. W., Mantoura R. F. C., Liss P. S. (1995). Analysis of ammonia and methylamines in natural waters by flow injection gas diffusion coupled to ion chromatography. Analytica Chimica Acta 316, 291–304. doi: 10.1016/0003-2670(95)00372-7
Gibb S. W., Mantoura R. F. C., Liss P. S., Barlow R. G. (1999). Distributions and biogeochemistries of methylamines and ammonium in the arabian sea. Deep-Sea Res. II: Top. Stud. Oceanogr. 46, 593–615. doi: 10.1016/S0967-0645(98)00119-2
Gibb S. W., Wood J. W., Fauzi R., Mantoura C. (1995). Automation of flow injection gas diffusion-ion chromatography for the nanomolar determination of methylamines and ammonia in seawater and atmospheric samples. J. Automatic Chem. 17, 205–212. doi: 10.1155/S1463924695000320
Heikes B. G., Chang W., Pilson M. E. Q., Swift E., Singh H. B., Guenther A., et al. (2002). Atmospheric methanol budget and ocean implication. Glob. Biogeochem. Cycles. 16, 80-1-80–13. doi: 10.1029/2002GB001895
Hopkins F. E., Archer S. D., Bell T. G., Suntharalingam P., Todd J. D. (2023). The biogeochemistry of marine dimethylsulfide. Nat. Rev. Earth Environ. 4, 361–376. doi: 10.1038/s43017-023-00428-7
Jameson E., Doxey A. C., Airs R., Purdy K. J., Murrell J. C., Chen Y. (2016). Metagenomic data-mining reveals contrasting microbial populations responsible for trimethylamine formation in human gut and marine ecosystems. Microb. Genomics 2, e000080. doi: 10.1099/mgen.0.000080
Kameyama S., Tanimoto H., Inomata S., Tsunogai U., Ooki A., Takeda S., et al. (2010). High-resolution measurement of multiple volatile organic compounds dissolved in seawater using equilibrator inlet–proton transfer reaction-mass spectrometry (EI–PTR-MS). Mar. Chem. 122, 59–73. doi: 10.1016/j.marchem.2010.08.003
King G. M. (1988). Methanogenesis from methylated amines in a hypersaline algal mat. Appl. Environ. Microbiol. 54, 130–136. doi: 10.1128/aem.54.1.130-136.1988
Mao S.-H., Zhang H.-H., Zhuang G.-C., Li X.-J., Liu Q., Zhou Z., et al. (2022). Aerobic oxidation of methane significantly reduces global diffusive methane emissions from shallow marine waters. Nat. Commun. 137309. doi: 10.1038/s41467-022-35082-y
Mao S.-H., Zhuang G.-C., Liu X.-W., Jin N., Zhang H.-H., Montgomery A., et al. (2021). Seasonality of emethylated sulfur compounds cycling in north China mar-ginal seas. Mar. pollut. Bull. 170, 112635. doi: 10.1016/j.marpolbul.2021.112635
Masson-Delmotte V., Zhai P., Pörtner H.-O., Roberts D., Skea J., Shukla P. R., et al. (2018). “An IPCC Special Report on the impacts of global warming of 1.5°C above pre-industrial levels and related global greenhouse gas emission pathways, in the context of strengthening the global response to the threat of climate change, sustainable development, and efforts to eradicate poverty. Summary for Policymakers,” in Global warming of 1.5°C (Cambridge University Press, Cambridge, UK and New York, NY, USA), 3–24. doi: 10.1017/9781009157940.001
Mausz M. A., Chen Y. (2019). Microbiology and ecology of methylated amine metabolism in marine ecosystems. Curr. Issues Mol. Biol. 33, 133–148. doi: 10.21775/cimb.033.133
Mincer T. J., Aicher A. C. (2016). Methanol production by a broad phylogenetic array of marine phytoplankton. PloS One 11, e0150820. doi: 10.1371/journal.pone.0150820
Peng J., Wan A. (1998). Effect of ionic strength on Henry’s constants of volatile organic compound. Chemosphere 36, 2731–2740. doi: 10.1016/S0045-6535(97)10232-6
Schink B., Zeikus J. G. (1980). Microbial methanol formation: a major end product of pectin metabolism. Curr. Microbiol. 4, 387–389. doi: 10.1007/BF02605383
Singh H., Chen Y., Staudt A., Jacob D., Blake D., Heikes B., et al. (2001). Evidence from the Pacific troposphere for large global sources of oxygenated organic compounds. Nature 410, 1078–1081. doi: 10.1038/35074067
Sun J., Mausz M. A., Chen Y., Giovannoni S. J. (2019). Microbial trimethylamine metabolism in marine environments. Environ. Microbiol. 21, 513–520. doi: 10.1111/1462-2920.14461
Taubert M., Grob C., Howat A. M., Burns O. J., Pratscher J., Jehmlich N., et al. (2017). Methylamine as a nitrogen source for microorganisms from a coastal marine environment. Environ. Microbiol. 19, 2246–2257. doi: 10.1111/1462-2920.13709
Van Neste A., Duce R. A., Lee C. (1987). Methylamines in the marine atmosphere. Geophys. Res. Lett. 14, 711–714. doi: 10.1029/GL014i007p00711
Williams J., Holzinger R., Gros V., Xu X., Atlas E., Wallace D. W. R. (2004). Measurements of organic species in air and seawater from the tropical atlantic. Geophys. Res. Lett. 31. doi: 10.1029/2004GL020012
Wohl C., Brown I., Kitidis V., Jones A. E., Sturges W. T., Nightingale P. D., et al. (2020). Underway seawater and atmospheric measurements of volatile organic compounds in the southern ocean. Biogeosciences 17, 2593–2619. doi: 10.5194/bg-17-2593-2020
Wu C., Wen Y., Hua L., Jiang J., Xie Y., Cao Y., et al. (2020). Rapid and highly sensitive measurement of trimethylamine in seawater using dynamic purge-release and dopant-assisted atmospheric pressure photoionization mass spectrometry. Anal. Chim. Acta 1137, 56–63. doi: 10.1016/j.aca.2020.08.060
Yang G.-P., Lu X.-L., Song G.-S., Wang X.-M. (2010). Purge-and-trap gas chromatography method for analysis of methyl chloride and methyl bromide in seawater. Chin. J. Anal. Chem. 38, 719–722. doi: 10.1016/S1872-2040(09)60046-3
Yang G.-P., Zhang H.-H., Zhou L.-M., Yang J. (2011). Temporal and spatial variations of dimethylsulfide (DMS) and dimethylsulfoniopropionate (DMSP) in the East China Sea and the Yellow Sea. Cont. Shelf Res. 31, 1325–1335. doi: 10.1016/j.csr.2011.05.001
Yang M., Nightingale P. D., Beale R., Liss P. S., Blomquist B., Fairall C. (2013). Atmospheric deposition of methanol over the Atlantic Ocean. Proc. Natl. Acad. Sci. 110, 20034–20039. doi: 10.1073/pnas.1317840110
Yang M., Beale R., Liss P., Johnson M., Blomquist B., Nightingale P. (2014). Air–sea fluxes of oxygenated volatile organic compounds across the atlantic ocean. Atmos. Chem. Phys. 14, 7499–7517. doi: 10.5194/acp-14-7499-2014
Yang X.-H., Lee C., Scranton M. I. (1993). Determination of nanomolar concentrations of individual dissolved low molecular weight amines and organic acids in seawater. Anal. Chem. 65, 572–576. doi: 10.1021/ac00053a014
Yang X.-H., Scranton M., Lee C. (1994). Seasonal variations in concentration and microbial uptake of methylamines in estuarine waters. Mar. Ecol. Prog. Ser. 108, 303–312. doi: 10.3354/meps108303
Yao L., Garmash O., Bianchi F., Zheng J., Yan C., Kontkanen J., et al. (2018). Atmospheric new particle formation from sulfuric acid and amines in a Chinese megacity. Science 361, 278–281. doi: 10.1126/science.aao4839
Zhang H.-H., Li J.-L., Yang G.-P., Song Y.-C., Jin N. (2015). Purge-trap gas chromatography and mass spectrometric method for analysis of isoprene in natural waters. Chin. J. Anal. Chem. 43, 333–337. doi: 10.1016/S1872-2040(15)60812-X
Zhang G. L., Zhang J., Kang Y. B., Liu S. M. (2004). Distributions and fluxes of methane in the East China Sea and the Yellow Sea in spring. J. Geophys. Res. Oceans 109, C07011. doi: 10.1029/2004JC002268
Zhong Z., Li G., Luo Z., Zhu B. (2012). Multi-channel purge and trap system coupled with ion chromatography for the determination of alkylamines in cosmetics. Anal. Chim. Acta 715, 49–56. doi: 10.1016/j.aca.2011.12.017
Zhou Z., Zhuang G.-C., Mao S.-H., Liu J., Li X.-J., Liu Q., et al. (2023). Methanol concentrations and biological methanol consumption in the Northwest Pacific Ocean. Geophys. Res. Lett. 50, e2022GL101605. doi: 10.1029/2022GL101605
Zhuang G.-C., Heuer V. B., Lazar C. S., Goldhammer T., Wendt J., Samarkin V. A., et al. (2018a). Relative importance of methylotrophic methanogenesis in sediments of the Western Mediterranean Sea. Geochim. Cosmochim. Acta 224, 171–186. doi: 10.1016/j.gca.2017.12.024
Zhuang G.-C., Lin Y.-S., Bowles M. W., Heuer V. B., Lever M. A., Elvert M., et al. (2017). Distribution and isotopic composition of trimethylamine, dimethylsulfide and dimethylsulfoniopropionate in marine sediments. Mar. Chem. 196, 35–46. doi: 10.1016/j.marchem.2017.07.007
Zhuang G.-C., Lin Y.-S., Elvert M., Heuer V. B., Hinrichs K.-U. (2014). Gas chromatographic analysis of methanol and ethanol in marine sediment pore waters: validation and implementation of three pretreatment techniques. Mar. Chem. 160, 82–90. doi: 10.1016/j.marchem.2014.01.011
Zhuang G.-C., Montgomery A., Joye S. B. (2019). Heterotrophic metabolism of C1 and C2 low molecular weight compounds in northern Gulf of Mexico sediments: Controlling factors and implications for organic carbon degradation. Geochim. Cosmochim. Acta 247, 234–260. doi: 10.1016/j.gca.2018.10.019
Keywords: trimethylamine, methanol, purge and trap, gas chromatography, dual detectors
Citation: Jiang F, Zhou Z, Wang J-Y, Guan W-J, Han L-G, Lin X-B and Zhuang G-C (2024) Simultaneous determination of seawater trimethylamine and methanol by purge and trap gas chromatography using dual nitrogen-phosphorus detector and flame-ionization detector. Front. Mar. Sci. 11:1356801. doi: 10.3389/fmars.2024.1356801
Received: 16 December 2023; Accepted: 12 March 2024;
Published: 27 March 2024.
Edited by:
Zhaohui Zhang, Zhejiang University, ChinaReviewed by:
Mark Francis Fitzsimons, University of Plymouth, United KingdomCopyright © 2024 Jiang, Zhou, Wang, Guan, Han, Lin and Zhuang. This is an open-access article distributed under the terms of the Creative Commons Attribution License (CC BY). The use, distribution or reproduction in other forums is permitted, provided the original author(s) and the copyright owner(s) are credited and that the original publication in this journal is cited, in accordance with accepted academic practice. No use, distribution or reproduction is permitted which does not comply with these terms.
*Correspondence: Guang-Chao Zhuang, emdjQG91Yy5lZHUuY24=
†These authors have contributed equally to this work and share first authorship
Disclaimer: All claims expressed in this article are solely those of the authors and do not necessarily represent those of their affiliated organizations, or those of the publisher, the editors and the reviewers. Any product that may be evaluated in this article or claim that may be made by its manufacturer is not guaranteed or endorsed by the publisher.
Research integrity at Frontiers
Learn more about the work of our research integrity team to safeguard the quality of each article we publish.