- 1Scripps Institution of Oceanography, University of California, San Diego, La Jolla, CA, United States
- 2Paua Marine Research Group, San Diego, CA, United States
- 3The Nature Conservancy, Los Angeles, CA, United States
- 4Department of Biology, San Diego State University, San Diego, CA, United States
- 5California Department of Fish and Wildlife, Santa Rosa, CA, United States
- 6The Bay Foundation, Los Angeles, CA, United States
The high ecological and economic value of seagrass has been long recognized, with these foundational habitats providing myriad ecosystem services. Yet through cumulative anthropogenic impacts, seagrasses are exhibiting extensive declines globally. A litany of studies and active restoration trials have demonstrated practical methodologies to restore seagrass habitats and effectively return critical habitat functions to degraded coastal zone systems worldwide. Seagrass loss along the U.S. West Coast has precipitated decades of seagrass protection, conservation, and restoration efforts. Yet, mitigation transplanting efforts have prioritized Zostera marina (narrow-leaved eelgrass) in shallow, protected environments, while a dearth of information is available on species inhabiting offshore islands and exposed mainland coasts. In this study, we conducted a novel transplant of Zostera pacifica, a wide-leaved species found in depths of 7 – 20 m along the offshore islands and mainland coast of California. Transplants were conducted at three geographically distinct sites in Santa Monica Bay, coupled with continuous monitoring of biophysical parameters providing insight into physical drivers at transplant and donor sites. Utilizing in situ data, and environmental thresholds adapted from the literature for Z. marina, we performed exposure analyses to evaluate factors influencing Z. pacifica transplant performance. Exceedances of threshold values for environmental parameters, specifically, wave exposure and near-bed flow speeds (Hrms > 0.59 m and Urms > 0.1 m s-1), photosynthetically active radiation (< 3 and > 5 mol m-2 day-1) and dissolved oxygen (< 3 mg O2 L-1) exposure impacted transplant survivorship. These results suggest Z. pacifica persist in biophysically dynamic conditions and are sensitive to exceedances of thresholds, underlining the importance of pre-transplant site-selection processes to this species. These data represent the first holistic study of Z. pacifica transplanting on an exposed mainland coast, which provides a view into the baseline environmental envelopes within existing Z. pacifica habitat, and further, may serve as a model for investigating scalable open coast seagrass restoration for temperate regions.
1 Introduction
Seagrasses are a suite of submerged aquatic angiosperms found globally and constitute critical foundational species in near-shore, shallow water marine systems (Duarte, 2002; Short et al., 2007; Unsworth and Cullen-Unsworth, 2014). Seagrasses provide myriad ecosystem services, supporting diverse fish and invertebrate species (Hoffman, 1986; Irlandi et al., 1999; Allen et al., 2002; Pondella et al., 2003; Tanner et al., 2019) and buffering against coastal erosion (Hansen and Reidenbach, 2012; Duarte et al., 2013). Seagrasses act as ‘blue carbon’ systems, capable of mitigating local impacts of ocean acidification (Kapsenberg and Hofmann, 2016; Ricart et al., 2021), and contributing to biological carbon fixation (Fourqurean et al., 2012; Duarte and Krause-Jensen, 2017; Ward et al., 2021). Regardless of the ecological function, value, and importance of seagrasses (Barbier et al., 2011), these habitats are experiencing stark rates of decline at ~ 30% loss globally (Orth et al., 2006; Waycott et al., 2009; Krause-Jensen et al., 2020). The shallow coastal zones colonized by seagrasses are often jointly exposed to multiple local stressors (e.g., sedimentation, eutrophication, habitat fragmentation) (Obaza et al., 2015; Adams et al., 2016; Livernois et al., 2017) compounded by cumulative global pressures (e.g., elevated sea surface temperatures, sea level rise) (Turschwell et al., 2021; Unsworth et al., 2022). The vast benefits of seagrass ecosystems, coupled with ongoing threats to habitat, have precipitated diverse lines of inquiry into drivers of ecosystem structure, function, and the capacity for habitat restoration (Short and Wyllie-Echeverria, 1996; McKenzie et al., 2020).
Seagrass spatial coverage and habitat function are controlled by complex abiotic and biotic environmental factors (Munsch et al., 2023). Biological top-down controls can positively impact seagrass health (Hughes et al., 2013; Foster et al., 2021), or as is the case with herbivory, cause direct consumptive loss to the seagrass bed (Altstatt, 2003; Heck and Valentine, 2006; Jiménez-Ramos et al., 2021; Valentine and Heck, 2021). High ocean temperatures can change photosynthetic efficiencies, deteriorate disease resistance, alter community structure, and reduce growth and survival of seagrasses growing near species-specific thermal tolerances (Johnson et al., 2003; York et al., 2013; Moore et al., 2014; Jakobsson-Thor et al., 2020; Strydom et al., 2020). Likewise, dissolved oxygen is a vital factor influencing habitat function (Parnell et al., 2020; Tassone et al., 2022), with hypoxia and anoxia precipitating reduced growth and survival rates in seagrasses (Holmer and Bondgaard, 2001; Greve et al., 2003; Pulido and Borum, 2010). The two most consequential drivers influencing seagrass distribution at local scales are wave action and light attenuation (Zimmerman et al., 1997; Short and Coles, 2001; Duarte, 2002). Physical damage via wave exposure can break shoots and uproot plants, often setting the shallow limit of seagrass beds (Fonseca and Bell, 1998; Kopp, 1999; Koch, 2001; Short et al., 2002). Hydrodynamics can disrupt critical seagrass ecological processes, impacting nutrient uptake (Cornelisen and Thomas, 2004; El-Hacen et al., 2019; Dahl et al., 2020) and altering ‘blue carbon’ capabilities through POC and DOC resuspension (Egea et al., 2023). Light regimes influence the photosynthetic capabilities of seagrass, and often constrain the depth limit of successful colonization (Ostenfeld, 1905; Phillips, 1964; Backman and Barilotti, 1976; Dennison and Alberte, 1982; Duarte, 1991; Nielsen et al., 2002; Adams et al., 2016; Beca-Carretero et al., 2021; Krumhansl et al., 2021; Jiménez-Ramos et al., 2023). Stochastic environmental events such as storms alter seagrass survival and can precipitate cascading declines in regional and local seagrass coverage (Rasmussen, 1977; Pollard and Greenway, 2013). The elucidation of species-specific biophysical limitations remains a critical component needed to enact appropriate and effective conservation measures and restorative actions (Aoki et al., 2020).
Along the Pacific Coast of North America, Zostera marina (L.) (narrow-leaved eelgrass) is the largest distributed seagrass species in the region inhabiting coastal estuaries and bays (Short et al., 2007; Blok et al., 2018). A second principal eelgrass species, the larger congener Zostera pacifica (wide-leaved eelgrass) (Watson, 1890), is present in just the Southern California Bight (SCB), inhabiting coastal shelf habitats and offshore islands in water depths of 7 – 20 m (Cottam and Munro, 1954; State Water Resources Control Board (SWRCB), 1979; Engle and Miller, 2005; Coyer et al., 2008; Olsen et al., 2014; Obaza et al., 2022). Seagrass habitats along the West Coast of the USA, especially in California, are subject to significant anthropogenic impacts that have precipitated substantial losses of Zostera spp. (National Oceanic and Atmospheric Administration, 2014; Kelly et al., 2019), in some cases as severe as 95% loss in a 10-year period (O’Leary et al., 2021). To combat these losses, regulatory agencies have enacted ‘no net loss’ guidelines which necessitate compensatory mitigation when coastal development degrades Zostera spp. habitat (Bernstein et al., 2011). As such, restoration efforts to alleviate the loss of Zostera spp. habitat have been on-going for nearly 50 years (Phillips, 1964), and in the SCB, for more than 30 years (Ward and Beheshti, 2023). These compensatory mitigations and active restoration projects have remained relatively small and have utilized a multitude of transplant techniques, including single shoot, bundle shoot, transplant frames (TERFS), among others, and each project resulted in varying degrees of project success (Williams, 2001; Altstatt, 2003; Ward and Beheshti, 2023).
Restoration of estuarine and protected seagrass systems have garnered recognizable successes in restoring seagrass extent and habitat function (Orth et al., 2020; Beheshti et al., 2022; Gräfnings et al., 2023). Yet, global attempts at exposed open coast seagrass restoration are limited, with successful projects confined primarily to Western Australia (Paling et al., 2001; Paling et al., 2003; Paling et al., 2007; Tan et al., 2020), though additional examples of successful projects exists in Portugal and Tanzania (Paulo et al., 2019; Wegoro et al., 2022). Open coast seagrass habitats, especially on the West Coast of the U.S., are plagued by inconsistent, outdated, or entirely absent data; congruent with the challenges highlighted by global assessments seeking to gauge seagrass coverage more accurately (McKenzie et al., 2020). To date, nearly all seagrass restoration projects in the SCB have focused on Z. marina following impacts from coastal development projects occurring in depths from 1 to ~6 m in protected bays, lagoons, and estuaries (Altstatt, 2003; and e.g., Hoffman, 1986; Pondella et al., 2006; Merkel & Associates, Inc, 2010; Obaza et al., 2015; MBC Applied Environmental, 2016), a disparity evidenced in a meta-analysis that identified 43 out of 44 transplant projects occurring in the SCB from 1989 to 2020 focused on Z. marina in protected embayments and estuaries (Ward and Beheshti, 2023).
Santa Monica Bay (SMB) constitutes the expanse from the rocky headland of Point Dume to the Palos Verdes Peninsula and includes much of the Los Angeles watershed (Figure 1). SMB comprises a vast diversity of intertidal and subtidal habitats that are critically important for the ecological, economic, and cultural wellbeing of the region, earning the designation as one of the USA EPA’s 28 National Estuary Programs (NEP). Historic accounts show that along with vast sandy habitats as well as rocky reefs and kelp forests, Zostera spp. were prevalent within the SMB NEP boundaries (Egstrom, 1974). Urbanization (i.e., coastal development, eutrophication, and pollution) associated with the growth of Los Angeles contributed to widespread degradation of the local marine environment (North, 1963; State Water Resources Control Board (SWRCB), 1979; Wilson and North, 1983; Williams et al., 2021). Contemporary assessments, though scattered and incomplete, of Zostera spp. in SMB reveal patchy and ephemeral beds predominately along the north-western shores near Malibu (Egstrom, 1974; Bernstein et al., 2011), in addition to robust and stable beds on offshore islands (Engle and Miller, 2005). This paucity of information specifically regarding Z. pacifica habitat hinders conservation efforts (but see Engle and Miller, 2005; Coyer et al., 2008; Altstatt et al., 2014; Olsen et al., 2014; Obaza et al., 2022).
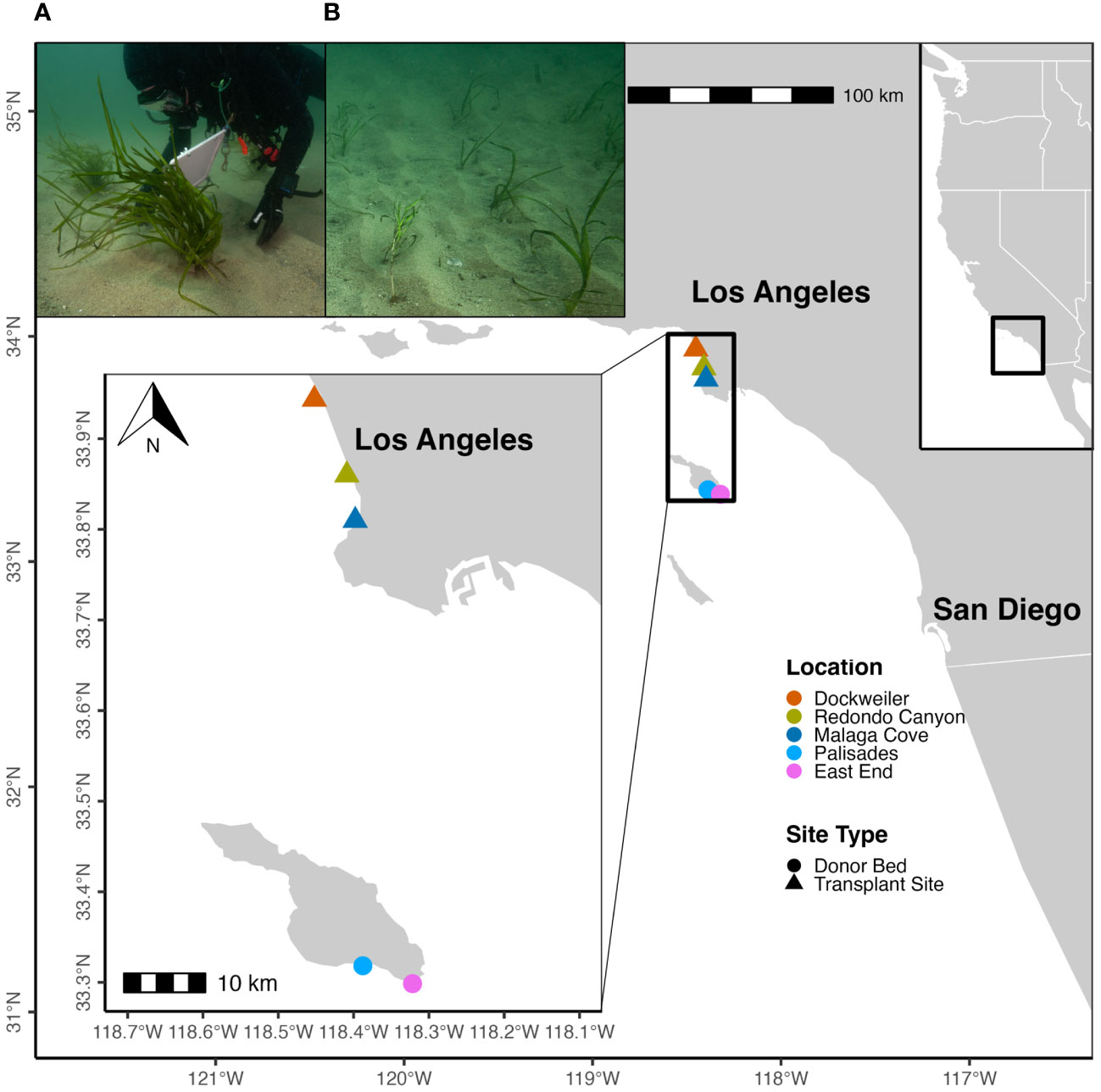
Figure 1 Locations of Zostera pacifica (wide-leaved eelgrass) transplant sites and donor bed sites within the Southern California Bight. Sites are indicated by color and site types are designated by shape. Photos from transplant sites of the (A) bundle shoot transplant method and (B) single shoot transplant method are displayed.
The primary goals of this study were to apply prior successful Zostera spp. transplant techniques to a novel environment (i.e., exposed mainland coast) to advance methods for Z. pacifica restoration, and subsequently, assess the efficacy and scalability of open coast restoration in the SCB. In particular, the purpose of our study was to (1) track the temporal survivorship of transplanted Z. pacifica across three geographically distinct transplant sites within SMB, (2) quantify a suite of environmental parameters likely to be key to open coast seagrass survival, and (3) elucidate the conditions within extant open coast Z. pacifica beds. To evaluate causative factors impacting transplant performance, continuous in situ measurements of biophysical oceanographic metrics (temperature, wave characterizations, photosynthetically active radiation, and dissolved oxygen) were recorded at discrete transplant and extant donor sites from 2021 through 2023. These data can provide scientists, resource managers, and restoration practitioners a lucid view into the realized environmental niche of Z. pacifica and can substantially improve our ability to protect and restore this regionally significant foundational coastal habitat. And further, upscaling Zostera spp. restoration throughout an expanded scope of available habitat (i.e., open coast), allows for a greater range of improved habitat function. This study advances our foundational understanding of temperate open coast seagrass habitats by disentangling the biophysical oceanographic dynamics and species-specific requirements conducive to Z. pacifica proliferation, further aiding to inform effective restoration.
2 Materials and methods
2.1 Study sites
The Southern California Bight (SCB) spans ~1200 km of coastline from Ensenada, Mexico to Point Conception, California and consists of a multitude of subtidal habitats, including rocky reef, kelp forest, and seagrass habitats (Williams et al., 2022). This project was focused along the mainland coast of Santa Monica Bay (SMB) and offshore Catalina Island (Figure 1). Three transplant sites were within coastal SMB, while the two donor bed sites (two largest extant Z. pacifica beds in the region) were located ~ 40km away on the south-eastern side of Catalina Island. Each site was ~0.5km offshore of the local coastline (mainland or Catalina). All transplant sites were established at 10 – 12 m depth, typical of natal Z. pacifica beds within the SCB (Bernstein et al., 2011; Obaza et al., 2022). Z. pacifica transplanting at the three mainland sites occurred in July 2021, during the primary growing season (March through October) established by the 2014 National Oceanic and Atmospheric Administration California Eelgrass Mitigation Policy (CEMP). Donor material was collected at Catalina and transplanting occurred at Redondo Canyon on 20 July 2021, at Malaga Cove on 22 July 2021, and at Dockweiler on 27 July 2021. Dockweiler, Redondo Canyon, and Malaga Cove received 520, 486, and 526 shoots, respectively. A total of 1532 shoots were collected and transplanted to the three sites, constituting the largest Z. pacifica transplant reported to date.
2.2 Donor material collection
The donor shoots were collected from two robust extant beds on the windward side of Catalina Island, Palisades and East End, with areas and densities of 27.74 hectares and 112 shoots m-2, and 10.48 hectares and 114 shoots m-2, respectively (Obaza et al., 2022). The donor bed sites ranged from 11 – 22 m depth. While numerous extant Z. pacifica beds exist closer to the mainland within SMB, their ephemeral nature and small patchy distribution excluded them as adequate donor beds for transplant experiments. We instead selected sites at Catalina Island characterized by extensive and healthy Z. pacifica populations with decadal stability (Engle and Miller, 2005).
Material was collected from donor beds, for both the single shoot and bundle shoot transplant methodologies, in a systematic fashion, utilizing a thinning approach to avoid the creation of noticeable (> 0.5 m-2) bare patches, and minimizing fragmentation. For the single shoot method, divers selected individual shoots and gently maneuvered the shoot and rhizome out of the sediment such that at least three internodal segments (~100 mm) of rhizome were attached to the apical shoot (Altstatt, 2003). Rhizomes with a single shoot were selectively used for this method, but per Paling et al. (2007) sediment was not actively removed from the rhizomes. Material for the bundle shoot method was collected similarly, although rhizomes with several shoots attached were also included along with a small amount of sediment. Divers selected shoots from the shallow edge, middle, and deep edge of the site to minimize fragmentation impact on the donor bed, while likely increasing clonal genetic diversity following Olsen et al. (2014). To complete the bundle approach (Zhou et al., 2014), multiple shoots were connected with biodegradable twine on the research vessel during the transit to the transplant sites. Donor material was temporarily stored in large coolers with flow-through seawater and kept shaded for up to four hours between collecting and transplanting.
2.3 Transplant
Collecting donor material and transplanting for single shoot and bundle shoot methodology occurred on the same day for each transplant site to minimize exposure stress. At each transplant location, SCUBA divers established a 30 m line at 10 to 12 m depth with individual experimental plots dispersed on either side. Each transplant site consisted of seven experimental plots, four bundle shoot plots (each 12 m-2) and three single shoot plots (each 9 m-2), ensuring inter-site replication along a single depth strata. Material was planted in a grid pattern within each plot and meter sticks were used to maintain transplant spacing. For the bundle shoot method, between 10 – 13 bundles (consisting of 80 – 110 total shoots) were planted at 1 m intervals within the four replicate plots at each site (see Figure 1A). For each plot, divers excavated a small hole in the sediment, and the entire rhizome mass of the bundle was placed in the excavated hole along with a wooden tongue depressor, which was secured to the rhizome mass and positioned parallel to the substrate to act as an anchor. Single shoot planting was conducted using methods adapted from Altstatt (2003). Between 40 – 46 shoots were planted at 0.5 m intervals within the three replicate single shoot plots at each site (see Figure 1B). Divers used a trowel to excavate sediment and gently maneuvered a single rhizome into the hole, placing a small gardening stake over the rhizome to act as an anchor. Sediment was pushed back over the rhizome so that it was completely buried, leaving the shoot naturally situated above the sediment.
2.4 Biological monitoring
All biological monitoring was conducted via SCUBA. Fish and eelgrass structural surveys were conducted at donor beds prior to (June 2021), and after (August 2021, July 2022, March 2023), collection of transplant material. Fish surveys were conducted at each site, where divers swam < 1 m above the substrate and identified the species, counted the abundance, and determined the size of each fish within a 1 m high x 2 m wide survey window along the timed roving diver fish surveys (per Pondella et al., 2006; Looby and Ginsburg, 2021; Obaza et al., 2022). Collection of morphometrics (length, width, and density) of Z. pacifica in donor beds followed methods in McCune et al. (2020) and Obaza et al. (2022).
Divers performed pre-transplant site surveys at each transplant location, and subsequently conducted monitoring to assess the biological characteristics of the transplants at intervals of 1-day, 1-week, 2-weeks, 1-month and quarterly thereafter through June 2023. Survivorship was defined as the number of remaining planting units (i.e., single shoots or bundle shoots) within plots (Wegoro et al., 2022). Inter-plot survivorship was assessed by enumerating single shoots and bundle shoots in each experimental plot at each transplant site during each timepoint (Altstatt et al., 2014). Divers temporarily placed flagging stakes next to each shoot to aid in accurate counts and to increase efficacy during monitoring events.
2.5 Environmental monitoring
The establishment of habitat and species-specific threshold values is a critical tool in ecosystem management regimes, as exceedances of threshold values can catalyze abrupt responses, often leading to habitat decline or loss (Uhrin and Turner, 2018). Threshold values of biophysical parameters impacting Z. marina have been expounded in numerous studies across its geographical distribution, both ex situ and in situ (Short et al., 2002; Lee et al., 2007; Moore and Jarvis, 2008; Thom et al., 2008). No critical threshold tolerances have been investigated for Z. pacifica; thus, biophysical thresholds established in this study have been adapted either from data collected near Z. pacifica habitat or from applicable Z. marina literature.
We measured temperature, light, dissolved oxygen, and wave exposure at each site, as these biophysical parameters can result in adverse impact to seagrass health, function, and survival (Fonseca and Bell, 1998; Holmer and Bondgaard, 2001; Johnson et al., 2003; Duarte et al., 2007). Temperature was measured from July 2021 – June 2023. Wave dynamics were measured across four deployments October 2020, July – August 2021, September – December 2021, and February – June 2022. Light and dissolved oxygen sensors were added from May 2022 – June 2023. The number of days with acceptable data in the time series varied among the sites and environmental metrics (Table 1).
2.5.1 Temperature
Following known adverse effects of elevated temperature on seagrass survival (Hammer et al., 2018), this study adapted a temperature threshold based on data collected near a Z. pacifica bed on Santa Cruz Island, California (Kapsenberg and Hofmann, 2016) and studies presented in a review by Lee et al. (2007). The upper temperature induced growth inhibition threshold for this study was set at 20°C.
In situ bottom temperature was measured with thermistor instruments deployed continuously throughout the project. HOBO Temperature/Light Data Logger (Onset UA-002-64 loggers) set to 10-minute sampling interval were deployed at < 1 m above the substrate in the transplant sites from July 2021 – July 2022. HOBO loggers were phased out of the project and from May 2022 – June 2023, transplant and donor beds had bottom mounted (< 1 m above substrate) continuous temperature data recorded by miniPAR data loggers (Precision Measurement Engineering) set to 10-minute sample interval from May – October 2022 and 5-minute sample interval from November 2022 – June 2023).
2.5.2 Wave exposure
Wave action can cause dislodgment and physical damage to seagrass (Koch, 2001). Open Wave Height Loggers (OWHLs) are an inexpensive open-source design pressure transducer and digital recorder capable of continuous, multi-month deployment, with data quality comparable to commercially available products (Lyman et al., 2020). OWHLs are a cost-effective approach for site selection and optimization projects necessitating multi-site wave observations. OWHLs (4 Hz continuously recording) were deployed < 1 m from the substrate in each donor bed and transplant site across multiple seasons. An initial deployment in October 2020 (n = 19 days) was conducted at the Palisades donor site to characterize wave conditions at a donor bed. The second deployment occurred July – August 2021 (n = 44 days) at the three sites in SMB, providing data on inter-transplant site differences prior to and during the initial transplant period. Based on the findings from this deployment, notably that Dockweiler experienced significantly higher magnitude and longer sustained exposure to wave conditions than the other two transplant sites (see results below), the Dockweiler transplant site was excluded from subsequent OWHL deployments, Instead opting to reallocate the four OWHL sensors to concomitantly monitor both donor bed sites (Palisades and East End) and transplant sites (Redondo Canyon and Malaga Cove). The third (n = 95 days) and fourth (n= 108 days) OWHL deployments occurred from September – December 2021 and February – June 2022, respectively, in order to characterize wave conditions throughout the transplant and post-transplant time period to understand the role wave exposure might play in survivorship and persistence.
Wave spectra and derived quantities including root-mean-square wave heights, peak period, and wave orbital velocity at the bottom (seabed), were calculated from the OWHL pressure time series in MATLAB (The MathWorks Inc, 2022). First, to correct for attenuation of the pressure signal with depth, a pressure response factor (e.g., Dean and Dalrymple, 1991) was applied to the discrete Fourier transform calculated for each 30-min interval. From the corrected sea surface time series, the power spectral density (hereafter ‘the wave spectrum’), was then calculated every 30 minutes. The root-mean-square wave height (Hrms) is representative of the energy of the sea state (swell and wind waves) and is calculated as the square root of eight multiplied by the variance of the surface elevation. Note that in some studies wave energy is reported as a significant wave height, Hs, which is proportional to Hrms so that . The peak period (Tp) is the inverse of the frequency containing the highest wave energy found from the wave spectrum (e.g., Holthuijsen, 2007). Particle trajectories under a wave follow an orbital motion (Dean and Dalrymple, 1991), where the horizontal velocity Urms was calculated following Svendsen (2005) and Monismith et al. (2013).
Short et al. (2002) established a threshold value for wave exposure limiting Z. marina transplant beds as the mean of the conditions at the donor bed(s) plus two standard deviations. In our study, data from each donor bed site across all deployments was aggregated to calculate mean + 2SD, resulting in a threshold Hrms value of 0.59 m and a Urms value of 0.1 m s-1.
2.5.3 Photosynthetically active radiation
Light availability is critical to seagrass growth and survival (Duarte, 1991). Photosynthetically active radiation (PAR) was measured continuously in situ as photosynthetic photon flux density (PPFD) using optical time-series instruments equipped with cleaning wipers deployed from May 2022 – June 2023. The fixed vertical sensor arrays were deployed < 1 m from the substrate. The miniPAR light sensor (Precision Measurement Engineering) sampled at 10-minute intervals from May – October 2022 and 5-minute intervals from November 2022 – June 2023, is equipped with a LI-192 Underwater Quantum Sensor (manufactured by LI-COR), which can detect a wavelength range of 400 – 700 nm. To reduce the noise created by surface light flicker, the value measured by the miniPAR sensor is an average of 30 readings taken over a 5 second period. The anti-fouling cleaning wiper was set to wipe the sensor at 3-hour intervals throughout the deployments. The PPFD data (µmol m-2 s-1) were integrated over the course of each sampling day, creating daily integrated PAR (mol quantum m-2 day-1) following Dunic and Côté (2023).
Thom et al. (2008) conducted ex situ and in situ research on light requirements for Z. marina in the Pacific Northwest region of the U.S. and found 3 mol m-2 day-1 to be growth and survival limiting, while 5 mol m-2 day-1 was found to induce strong growth rates with long-term survival. This study has adapted these values as light thresholds.
2.5.4 Dissolved oxygen
Accelerated seagrass mortality and reduced growth rates are common impacts associated with low dissolved oxygen (DO) conditions (Holmer and Bondgaard, 2001). DO was measured in situ using a submersible miniDOT instrument (Precision Measurement Engineering) sampling at 10-minute intervals from May – October 2022 and 1-minute sample interval from November 2022 – June 2023. The miniDOT contains an optode that detects oxygen concentrations in the water column across a sensing foil membrane and records values in mg O2 L-1. Temperature is measured concurrently with DO values. A 33.50 ppt salinity correction factor, representative of the long-term average for the region (Carter et al., 2022), was applied to the raw data following the manufacturer’s recommendation. Further, miniDOT instruments record a ‘Q value’ corresponding to the quality of the individual measurement, where a value > 0.7 is indicative of proper sensor operation. A copper plate and copper mesh package were utilized as anti-fouling devices.
Moore and Jarvis (2008) identified water column DO values of < 3 mg O2 L-1 to induce tissue degradation in Z. marina, thus, we set a threshold of 3 mg O2 L-1.
2.6 Statistical analysis
Differences in the composition of fish assemblages were compared by creating a Bray-Curtis dissimilarity matrix from average relative species-specific encounter rates across individual surveys completed at transplant and donor beds from 2021 – 2023. All species with < 2% mean encounter rates across all surveys were removed. The dissimilarity matrix was used to create non-metric multi-dimensional scaling plots to visualize changes in fish assemblages across transplant and donor beds. Assemblage differences were tested using permutation multivariate analysis of variance (PERMANOVA) (McCune and Grace, 2002). Differences in group dispersion were tested using the ‘betadisper’ function in the ‘vegan’ package and were not significant; therefore, no additional transformations on these data were conducted. To determine species-specific differences in fish assemblage, a similarity percentages (SIMPER) procedure was performed with treatment as a factor. All multivariate statistical approaches were conducted using the ‘vegan’ package in R (Oksanen et al., 2019).
Environmental data were analyzed and graphed in R (RCore Team, 2021, v. 4.1.2). Data corresponding to sensor malfunction and extreme biofouling were removed during quality control and were excluded from analysis (following Ricart et al., 2021). Standardization of the timeseries date range was applied to environmental data for statistical analysis and was based on the latest data start date and the earliest data end date across all sites for each biophysical parameter. Exposure analysis was conducted utilizing the ‘heatwaveR’ package in R (Schlegel and Smit, 2018). Exposure calculations were performed based on daily exceedance (or negative exceedance, i.e., below) of established parameter thresholds. Empirical cumulative distribution function (eCDF) plots were employed to differentiate patterns of the environmental metrics among sites (Beheshti et al., 2022). Environmental data lacked variance homogeneity and were therefore analyzed using a non-parametric Kruskal-Wallis test and Dunn’s post hoc test with a Holm-Bonferroni correction to critical p-value due to application of multiple tests of significance among sites (Pulido and Borum, 2010; Campbell et al., 2018). A p-value < 0.05 represents a significant difference detected between sites, indicating a rejection of the ‘similarity of sites’ null hypothesis.
3 Results
3.1 Transplant survivorship
Transplant sites experienced minor loss immediately post-transplanting, followed by a slight expansion in total shoots observed at two of the three sites around 20 days post-transplant, until complete mortality was observed across all sites after day 315 (Figure 2). Survivorship varied across sites, with poor survivorship at Dockweiler (DW), better survivorship at Redondo Canyon (RC), and longest survivorship at Malaga Cove (MC). At DW and RC, 1.9 and 2.1% of material was lost between transplanting and the 24-hour survey respectively, while MC did not lose any transplanted material until day 15. Highlighting site-specific divergence, survivorship at DW was only 35.8% by day 37, while RC and MC experienced 85.4% and 80.4% survivorship respectively. By day 90, DW, RC, and MC had 23.3%, 67.9%, and 68.1% survivorship, but by day 197 complete mortality occurred at DW and RC, and survivorship at MC had declined to 30%.
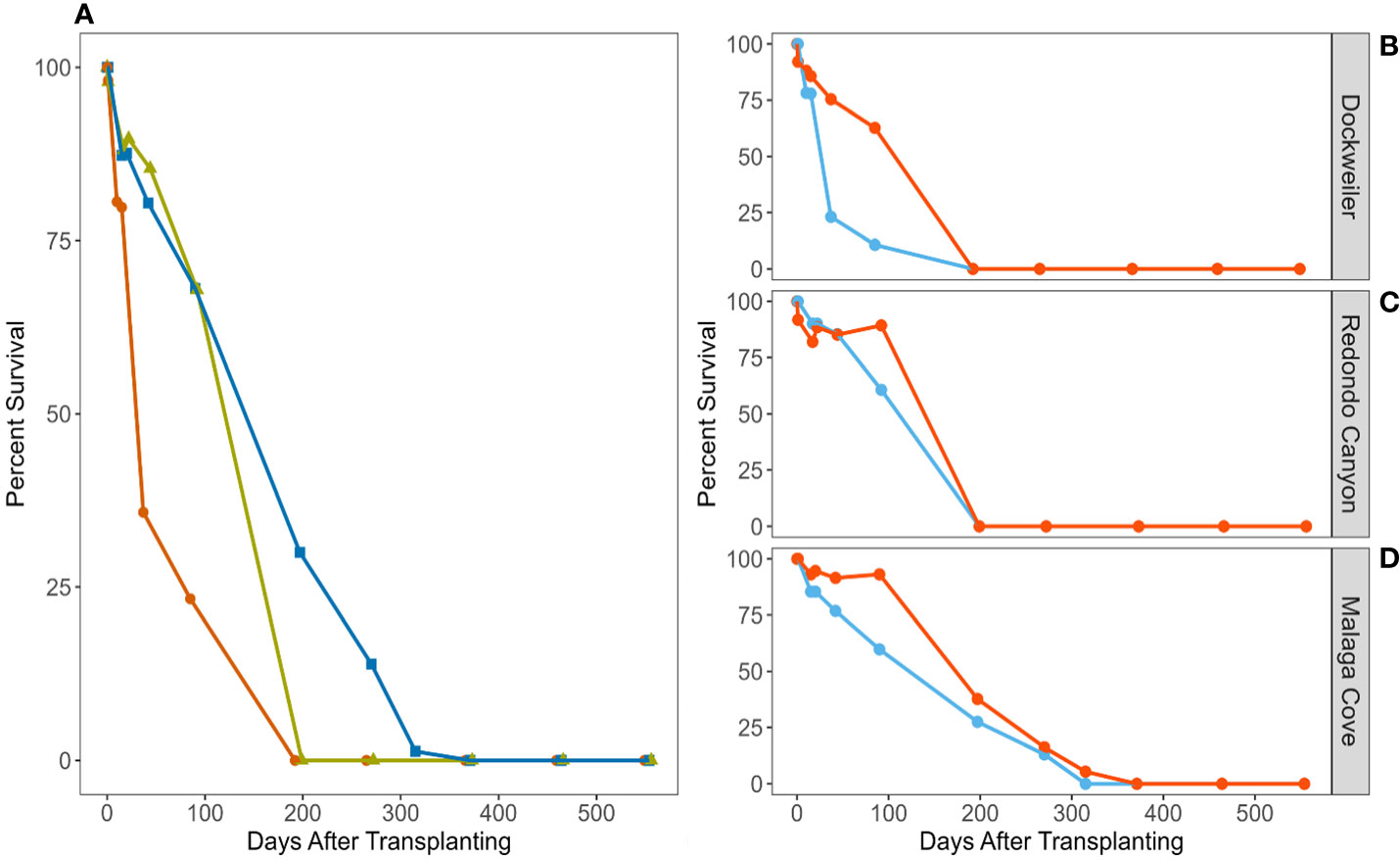
Figure 2 Zostera pacifica (wide-leaved eelgrass) transplant survivorship from July 2021 – June 2023, expressed in days after transplanting. Percent survivorship by site (A), color and shape designate site: orange circles (Dockweiler), gold triangles (Redondo Canyon), blue squares (Malaga Cove). Percent survivorship by transplanting method per site (B-D), color designates transplant method: blue (bundle shoot) and orange (single shoot).
Further survival disparity was pronounced among transplant methods, with the single shoot method outperforming bundle shoot method across all sites (Figure 2). Methodological survival differences were most prominent at DW, whereby day 37 bundle shoot survivorship was reduced to 23.1%, while single shoot survivorship was substantially higher at 75.4%. Differences in survival among transplant methods were also evident at other sites, whereby day 90 RC single shoot survivorship was 89.3% while bundle shoot survivorship was 60.7%; and at MC single shoot survivorship was 93.1% while bundle shoot survivorship was 59.8%. At MC (the best performing site) 5.4% of single shoots remained on site until after day 315, while bundle shoots suffered prior complete mortality.
3.2 Fish surveys
A total of 3,692 fishes from 26 species were recorded over 139 transects across all five sites. The donor bed sites had greater species richness than transplant sites throughout the study period and greater species encounter rates for all fishes as well (Figure 3). No fish encounters were observed during pre-transplant site selection surveys, and there were no significant increases in encounter rate before and after transplant activities at transplant sites. No meaningful relationships of species richness or encounter rate were detected between the three transplant sites. PERMANOVA results indicated that the composition of fish assemblages observed at donor beds and transplant sites were significantly different from one another (F1,24 =10.14, p = 0.001), with no overlap in the NMDS plot (Figure 3). According to results from the SIMPER analysis, differences were driven by greater relative abundance of sanddab (Citharichthys stigmaeus) and California halibut (Paralichthys californicus) at transplant sites and greater relative abundance of kelp bass (Paralabrax clathratus), rock wrasse (Halichoeres semicinctus), señorita (Oxyjulis californica), and salema (Xenistius californiensis) at donor bed sites.
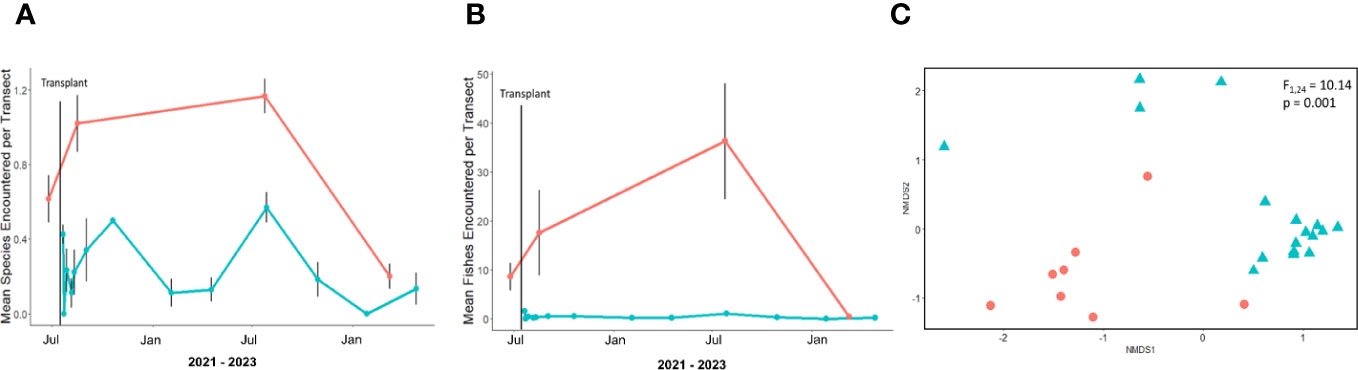
Figure 3 Blue color represents transplant sites and red color represents donor bed sites. (A) Species richness at transplant and donor bed sites. Points are averages of all transects at each survey and error bars are standard error. (B) Encounter rates for all fishes at transplant and donor bed sites. Points are averages of all transects at each survey and error bars are standard error. (C) NMDS plot of fish assemblages in transplant and donor bed sites. Each point represents the mean relative encounter rates across all transects during a single survey event at a transplant or donor bed site. Surveys took place from 2021 to 2023. The points remain separate despite passage of time, suggesting relative fish encounters remained different across transplant and donor bed sites. Stress was 0.033. Permutation multivariate analysis of variance (PERMANOVA) results reported on plot.
3.3 Temperature exposure
Temperature exposure above the 20°C threshold was not a crucial indicator of transplant performance as values fell well within, and even below, the exposure conditions at the donor bed sites (Figure 4, Tables 1, 2). Transplant site high temperature exposure percentages were DW (2.06%), RC (2.34%), and MC (3.30%) and were less exposed than the donor bed sites Palisades (PAL) (11.54%) and East End (EE) (17.07%). The longest continual exposure period above the threshold for the sites were 10, 11, 11, 11, and 26 days for DW, RC, MC, PAL, and EE respectively. The highest temperature recorded at the transplant sites was 22.39°C and 23.09°C at the donor bed sites. From August 2021 – January 2023 for transplant sites (n = 514 days), DW was significantly different from MC (p < 0.05) and MC was significantly different from RC (p < 0.05), though DW was not significantly different from RC (p = 0.76). For continuity of date range across all transplant and donor sites, from May 2022 – January 2023 (n = 221 days) significant differences were detected between comparisons of transplant sites and donor bed sites (p < 0.05), though temperatures at the two donor bed sites were not significantly different from one another (p = 0.28).
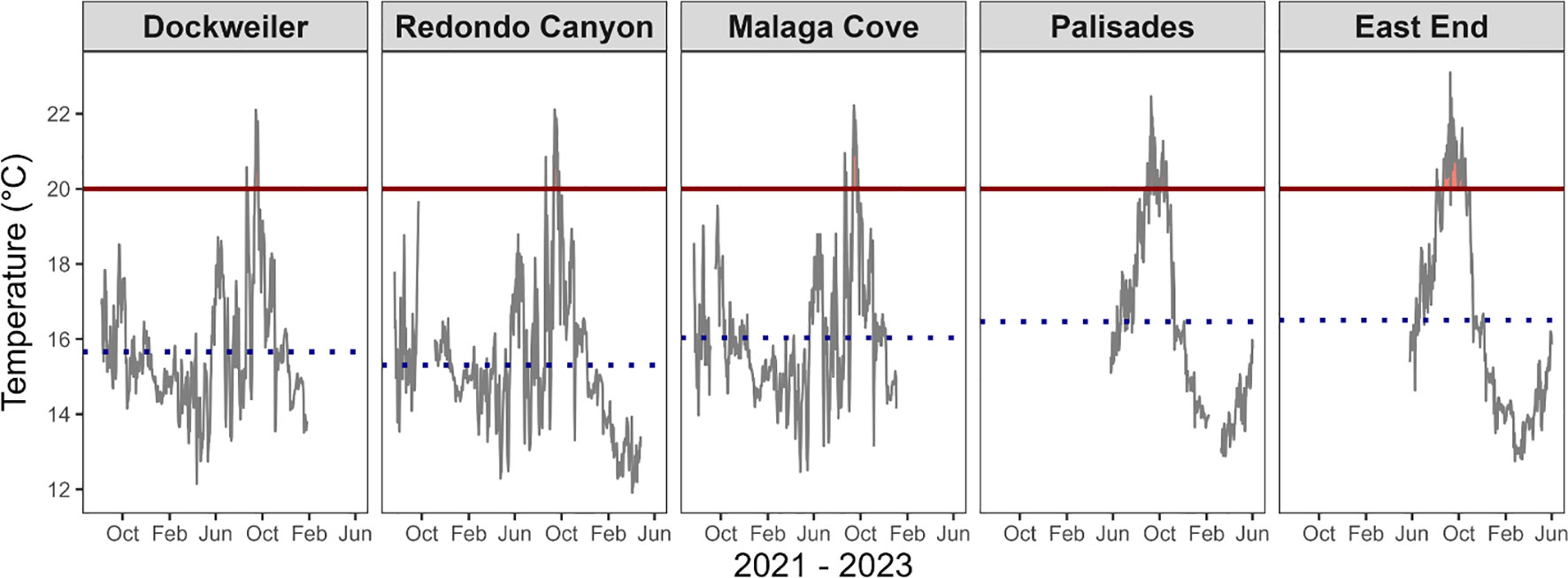
Figure 4 Temperature exposure timeseries across all transplant and donor sites from July 2021 – June 2023. Solid black line represents mean daily temperature timeseries, while dotted blue line represents site average across the span of the timeseries. Red horizontal line represents temperature threshold set at 20 °C. Gaps in data correspond to sensor malfunctions. Temperature data was unavailable at donor beds until May 2022. Dockweiler (DW), Redondo Canyon (RC), and Malaga Cove (MC) are transplant sites. Palisades (PAL) and East End (EE) are donor beds. Site average ± SD in °C: DW (15.66 ± 1.58), RC (15.30 ± 1.82), MC (16.03 ± 1.69), PAL (16.46 ± 2.41), and EE (16.50 ± 2.64). See Table 2 for percent time each site was above the threshold.
3.4 Wave exposure
Exposure exceeding the wave thresholds was common from July – August 2021 (Figure 5, Tables 1, 2). Transplant site exposure percentages above the Hrms threshold were DW (6.82%), RC (0%), and MC (2.27%), while exposure percentages above the Urms threshold were DW (86.36%), RC (13.64%), and MC (36.36%). The longest continual exposure period above the Urms threshold for the sites were 28, 3, and 8 days for DW, RC and MC respectively. Over the July – August 2021 deployment (n = 44 days), DW exhibited significantly different Hrms values from RC and from MC (p < 0.05), while RC and MC were not significantly different (p = 0.22). The Urms data were significantly different from one another for all sites (p < 0.05).
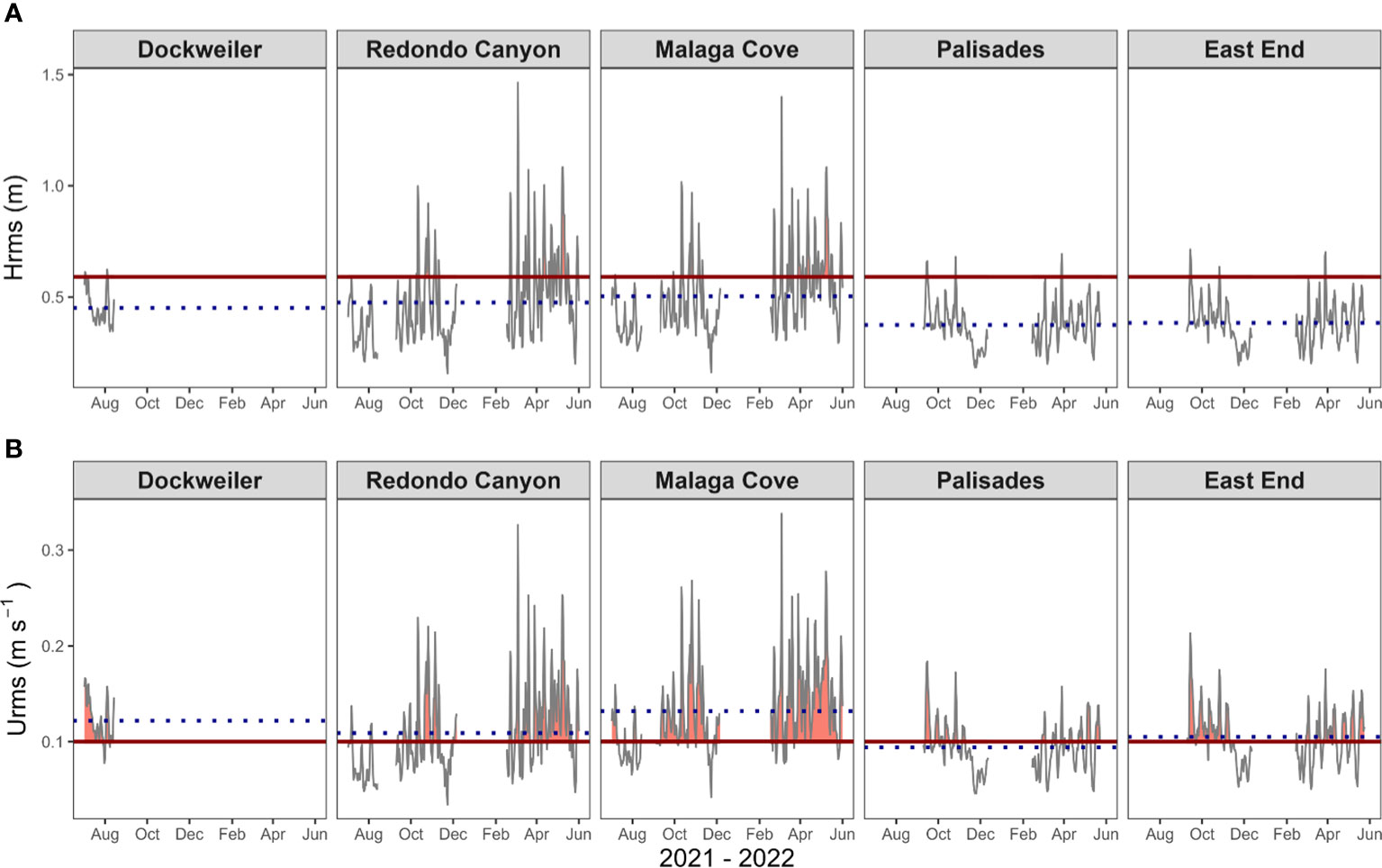
Figure 5 Wave characterization, with Hrms (A) and Urms (B), exposure timeseries at the three transplant sites: Dockweiler (DW), Redondo Canyon (RC), and Malaga Cove (MC) and two donor beds Palisades (PAL) and East End (EE). Deployments spanned from July – August 2021 (n = 44 days), September – December 2021 and February – June 2022 (n = 195 days). Note, July – August 2021 sensors were only deployed at transplant sites, and Dockweiler was excluded from the subsequent two deployments while donor beds were added. Solid black line represents daily mean wave data timeseries, while dotted blue line represents site average across the span of the timeseries. Red horizontal line represents Hrms threshold of 0.59 m, and Urms threshold of 0.1 m s-1. Hrms site average ± SD in m: DW (0.45 ± 0.08), RC (0.48 ± 0.20), MC (0.50 ± 0.18), PAL (0.37 ± 0.09), and EE (0.38 ± 0.10). Urms site average ± SD in m s-1: DW (0.12 ± 0.02), RC (0.11 ± 0.05), MC (0.13 ± 0.05), PAL (0.09 ± 0.03), and EE (0.11 ± 0.03). See Table 2 for percent time each site was above threshold values.
Based on early results, DW was excluded from the subsequent two deployments, opting rather to monitor better performing transplant sites (RC and MC) and donor bed sites (PAL and EE) concurrently. RC, MC, PAL, and EE had continuous in situ wave characterizations from September – December 2021 and February – June 2022. These two deployments were aggregated for analysis (n = 185 days). Again, it is evident that transplant sites were exposed to higher degrees of Hrms and Urms values and for longer durations than donor bed sites (Figure 5, Tables 1, 2). Site exposure percentages above the Hrms threshold were RC (28.50%), MC (31.09%), PAL (2.56%), and EE (2.56%), while exposure percentages above the Urms threshold were RC (58.55%), MC (83.42%), PAL (34.36%), and EE (55.90%). The highest daily mean Hrms and Urms values recorded at the transplant sites were 1.46 m and 0.34 m s-1, while the values at the donor beds were 0.71 m and 0.21 m s-1. Hrms values at the transplant sites MC and RC were significantly different (p = 0.05), but donor bed sites PAL and EE were not significantly different from one another (p = 0.44). All Hrms comparisons between transplant sites and donor bed sites were significantly different (p < 0.05). The Urms data shows that RC and EE were not significantly different (p = 0.09), though all other site comparisons were significantly different from one another (p < 0.05).
3.5 Photosynthetically active radiation exposure
PAR exposure below the 3 mol m-2 day-1 growth limited, non-survival threshold, as well as exposure (or lack thereof) above the upper survival-supportive threshold of 5 mol m-2 day-1 was prevalent during the project period (Figure 6, Tables 1, 2). Exposure below the growth limited, non-survival threshold is especially prevalent in the late fall and winter months. Transplant site exposure percentages below 3 mol m-2 day-1 were DW (96.13%), RC (91.85%), and MC (87.44%) and exhibited greater exposure times than the donor bed sites PAL (62.72%) and EE (47.43%). The longest continual exposure period below the non-survival supportive threshold for the sites were 141, 203, 100, 56, and 75 days for DW, RC, MC, PAL, and EE respectively. Donor bed sites PAL (21.01%) and EE (31.44%) experienced sustained exposure in exceedance of the 5 mol m-2 day-1, while MC only had 0.93% and both DW and RC had zero exposure above the survival-supportive threshold. The two donor bed sites each spent considerable time from spring through fall (June 2022 – October 2023) above the survival-supportive threshold (12-day continual exposure at PAL and 29-day continual exposure at EE) and both sites rarely dip below the growth limited, non-survival threshold. The highest daily integrated PAR value recorded at the transplant sites was 6.10 mol m-2 day-1 while the highest value at the donor beds was 11.56 mol m-2 day-1. Over the timeseries from July 2022 – January 2023 (n = 159 days), DW was significantly different from all other transplant and donor bed sites (p < 0.05), while RC and MC were not significantly different from each other (p = 0.88) but were significantly different from the donor bed sites (p < 0.05). Donor bed sites were not significantly different from each other (p = 0.18).
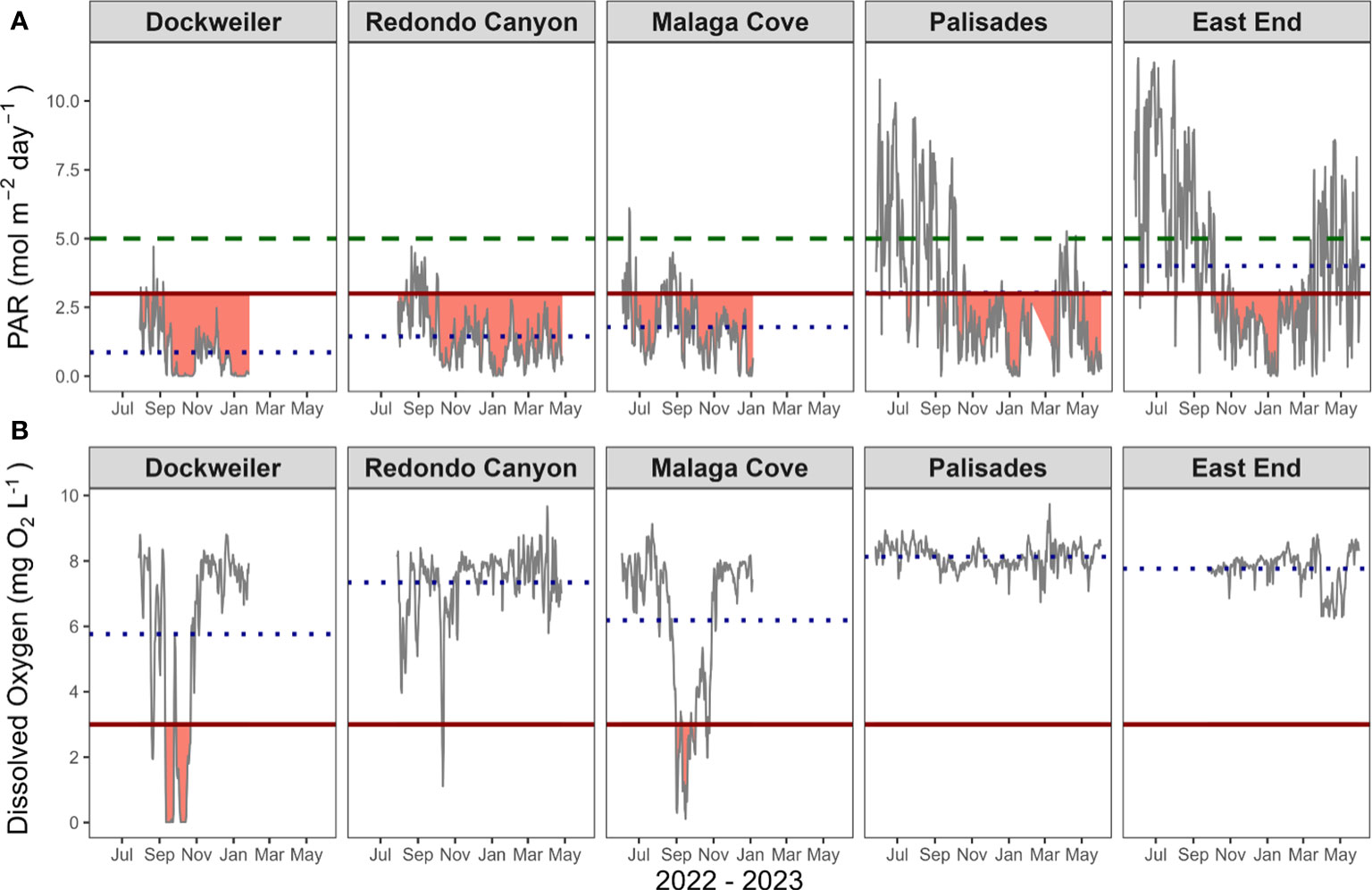
Figure 6 Photosynthetically active radiation (PAR) (A) and dissolved oxygen (DO) (B) exposure timeseries across all transplant and donor sites from May 2022 – June 2023. Solid black line represents integrated daily PAR and mean daily DO timeseries. Dotted blue line represents site average. Red horizontal line represents thresholds. For PAR: non-survival threshold of 3 mol m-2 day-1; green dashed line survival-supportive threshold of 5 mol m-2 day-1. For DO: tissue degradation threshold of 3 mg O2 L-1. Gaps in data correspond to sensor malfunctions. Dockweiler, Redondo Canyon, and Malaga Cove are transplant sites. Palisades and East End are donor beds. Site average ± SD for PAR in mol m-2 day-1: DW (0.87 ± 0.92), RC (1.44 ± 1.00), MC (1.78 ± 1.09), PAL (3.02 ± 2.51), and EE (4.01 ± 2.88). Site average ± SD for DO in mg O2 L-1: DW (5.77 ± 2.95), RC (7.34 ± 1.04), MC (6.19 ± 2.30), PAL (8.13 ± 0.37), and EE (7.76 ± 0.55). See Table 2 for percent time each site was exposed for each threshold.
3.6 Dissolved oxygen exposure
DO exposure below the 3 mg O2 L-1 tissue degradation threshold occurred (Figure 6, Tables 1, 2). Transplant site exposure percentages below 3 mg O2 L-1 were DW (23.20%), RC (1.11%), and MC (15.35%) and exhibited consequential exposure times compared to the donor bed sites PAL (0%) and EE (0%). The longest continual exposure period below the tissue degradation threshold for the sites were 24, 3, and 14 days for DW, RC and MC respectively, while PAL and EE experienced zero exposure periods. The lowest daily mean DO value recorded at the transplant sites was 0.01 mg O2 L-1 while the lowest value at the donor beds was 6.24 mg O2 L-1. A DO timeseries from September 2022 – January 2023 (n = 102 days), indicated transplant sites were not significantly different from one another (DW – RC p = 0.48; DW – MC p = 0.33; RC – MC p = 0.11). Likewise, the oxygen exposure at donor beds were not significantly different (p = 0.05). All comparisons between transplant sites and donor bed sites were significantly different (p < 0.05).
4 Discussion
4.1 Evaluation of transplant success
This study conducted the first ever mainland Zostera pacifica transplant, with methods and results that can allow for further technique development of Z. pacifica restoration in the Southern California Bight (SCB). From the in situ biophysical monitoring, this study has developed clear linkages pertaining to Z. pacifica environmental constrains, enabling advancements in site selection procedures that may serve as a model for open coast seagrass restoration. At all three sites the majority of transplants initially established, and at two sites, experienced evidence of growth and expansion. However, beyond 315 days there was complete mortality at the coastal transplant sites, most likely due to predominantly unfavorable environmental conditions associated with high wave exposure, low light, and periods of hypoxia. Thus, in the most conspicuous definition of success, or even that from the NOAA California Eelgrass Mitigation Policy (CEMP) (2014) criteria requiring 85% areal coverage two years post transplanting, the transplants were not successful. Transplanting and restoring seagrass beds is notably difficult, and quantification of seagrass transplant performance is far more nuanced and complex than simply considering total survival as the sole metric for success. Contextualizing, in a meta-analysis of 82 seagrass restoration projects on the West Coast of the U.S., 32 – 60% of projects were unsuccessful (Ward and Beheshti, 2023), yet ‘unsuccessful’ projects certainly can still contribute to advancing methods in restoration science (Zhang et al., 2021). As such, other metrics beyond survivorship (i.e., ecosystem services) should also be considered in the evaluation of transplant success (Ward and Beheshti, 2023).
The assessment of ecosystem services (fish community dynamics, carbon stocks, turbidity, etc.) in restored sites compared to donor beds, can serve as valuable performance metrics and motivations for larger scale restoration projects (Orth et al., 2020; Lange et al., 2022). Zostera spp. (eelgrass) beds have high fish biomass and diversity compared to unvegetated sediments (Duffy, 2006), and fish biomass can be a useful comparative metric between transplant and donor beds (Beheshti et al., 2022). Utilizing fish surveys adapted from Obaza et al. (2022), dissimilarity in fish communities is evident between transplant sites and donor bed sites (Figure 3). The small amount of low-density eelgrass followed by bare soft-bottom in the transplant sites as compared with extensive Z. pacifica donor beds on Catalina Island is likely the driving factor in fish assemblage differences (Pihl et al., 2006). Older aged, structured seagrass habitat offers more robust ecosystem function (i.e., fish diversity) compared to younger aged seagrass beds (i.e., transplant sites) (McGlathery et al., 2012; Orth et al., 2020; Hardison et al., 2023). The species more commonly encountered at transplant sites were flatfish, as would be expected on soft-bottom habitats in the region (Craig et al., 2004; O’Leary et al., 2021), while those more regularly recorded at donor bed sites are usual inhabitants of open coast Z. pacifica (Obaza et al., 2022). Fish surveys associated with this project quantitatively support the conclusion that the eelgrass transplants did not create meaningful structure for nearshore fishes, nor restore that ecosystem function, during the study period.
The only other known Z. pacifica transplant occurred in 2002 at a sheltered cove off Anacapa Island where 450 shoots were transplanted to an area of 300 m-2 and resulted in a successful and stable Z. pacifica population on the island (Altstatt et al., 2014). Over 95% mortality was recorded on day 155 in that study, suggesting early mortality may be the norm, even in successful efforts. Altstatt et al. (2014) provides context to our study’s ~30% survivorship on day 197 at Malaga Cove (Figure 2) and illustrates the benefit of this effort – affirming the present study represents an advancement on prior restoration efforts for Z. pacifica. Studies of open coast seagrass restoration conducted elsewhere globally report similar findings to those in this study, as open coast environments are inherently dynamic, leading to higher degrees of initial transplant mortality (Paulo et al., 2019; Wegoro et al., 2022).
This project highlights insight into the efficacy of exposed mainland coast transplanting methodology, with initial survival differences of the single shoot method over the bundle shoot method (Figure 2). In the exposed mainland coast environment, transplants can be subject to periods of intense wave action and high near bottom velocity (Figure 5; van Katwijk et al., 2009), where the smaller profile single shoots may induce less drag and thus, experience reduced impact compared to bundle shoots (Orth et al., 1999). These insights may contribute to future transplanting efforts, as the bundle shoot method requires a greater degree of harvest investment compared to the single shoot method. Focusing transplanting efforts on the single shoot method allows for a redistribution of the harvest material, spreading the transplanting risk across a greater area and number of ‘planting units’ (Orth et al., 2006; van Katwijk et al., 2009). The results of this study, in concert with findings from Altstatt et al. (2014), reaffirm that methodological approach is critical (Fonseca et al., 1998), as the single shoot method is linked to higher survivorship along exposed coastlines in the SCB. However, during the harvesting and transplanting process, the donor material experiences stress (Lange et al., 2022), catalyzing initial mortality or decreased function. Therefore, future studies on the open coast should aim to gauge the effects of transplanting stress and methodology on survival by also transplanting back into donor beds. These efforts may further disentangle the efficacy of transplant methodologies in the absence of environmental conditions that vary from those experienced at the natal donor beds.
4.2 Causative factors of transplant mortality
Stochastic events are prevalent in seagrass beds and can result in adverse transplant performance (Lange et al., 2022). Unpredictable deleterious singular events may have influenced transplant performance, as numerous acute events occurred across transplant sites. The Dockweiler transplant site is located ~2km from the largest wastewater treatment facility in Los Angeles, and from July 2021 – May 2022, 60 effluent limit violations occurred because of an unprecedented > 12-million-gallon raw sewage discharge from a pipe 1.6 km offshore, resulting in numerous algal blooms (LA Water Board, 2023). A large body of literature speaks to the negative impacts of eutrophication on seagrasses (Burkholder et al., 2007; Hughes et al., 2013). Further, during a post-transplanting survey at Redondo Canyon, located at the head of an active submarine canyon, divers observed macroalgae detritus engulfing transplant units. Macroalgae inundation is known to decrease health and survival of Zostera spp. by altering light regimes and decreasing photosynthetic capabilities (Huntington and Boyer, 2008), a factor that likely contributed to declines in transplanted Z. pacifica survivorship. The pairing of high wave characterizations (Hrms and Urms values) and macroalgae inundation at the Redondo Canyon site may have contributed to physical damage – a concept further supported by diver observations of dislodged transplanting units. Hydrodynamical forces and nutrient regimes can negatively impact the biomechanical properties (i.e., shoot strength, elasticity) of seagrasses (Patterson et al., 2001; La Nafie et al., 2013; Risandi et al., 2023), and it is likely that the hydrodynamic conditions and acute eutrophication events impacted the transplant sites, though the quantification of biomechanical properties is outside the scope of this study.
The biophysical characterizations reveal a more compelling and consistent case for deleterious site conditions, as a mosaic of unsuitable environmental conditions occurred at transplant sites. And while biologic controls are undoubtably important to seagrass systems, regionally along the West Coast of the USA, physical drivers are principally responsible for eelgrass transplant success or decline (Ward and Beheshti, 2023). Yet surprisingly, many Zostera spp. restoration projects that offer suspected reasons for transplant success or failure lack quantitative in situ environmental data, instead relying on observational data to infer physical drivers (Ward and Beheshti, 2023). In this study, generally, PAR, DO, and temperature were lower, and wave dynamics (Hrms and Urms) higher in transplanted sites compared to donor bed sites (Figures 4–6). Indeed, empirical cumulative distribution function (eCDF) curves of transplant sites compared to donor beds depict a pronounced decoupling between transplant and donor bed sites and show only partial overlap, confirming that conditions at transplanting locations were inapt for Z. pacifica long-term survival (Figure 7; e.g., Beheshti et al., 2022). The transplant study approach herein showcases an avenue to discretely monitor in situ data and quantify known physical controls on seagrass habitat function.
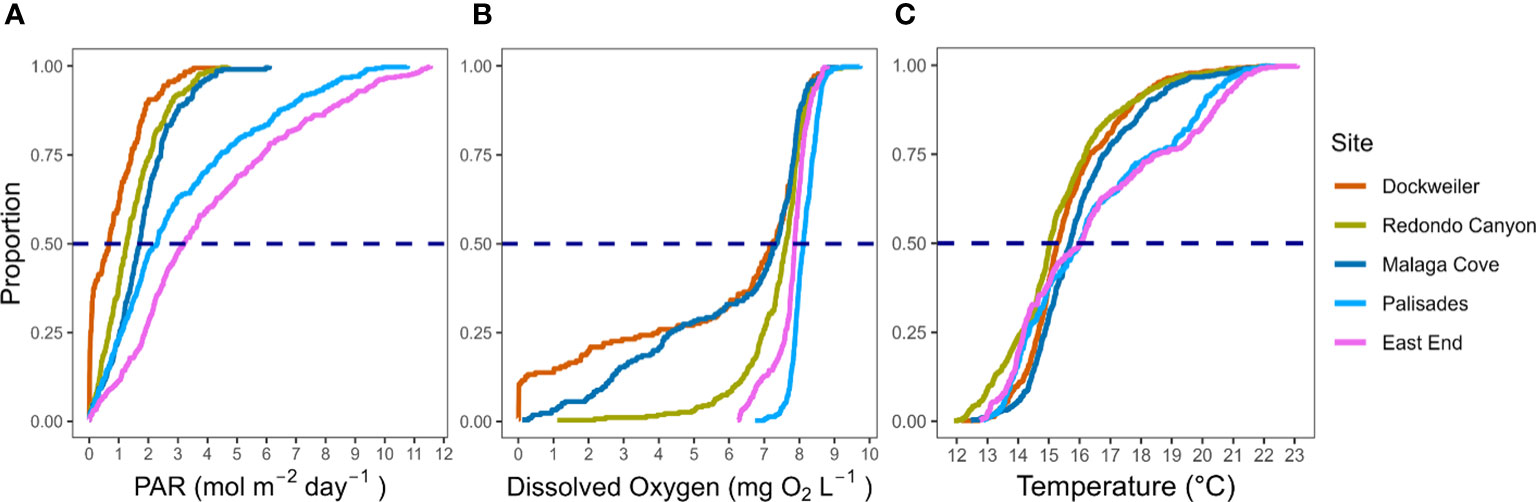
Figure 7 Empirical cumulative distribution function (eCDF) curves for (A) photosynthetically active radiation (mol m-2 day-1), (B) dissolved oxygen (mg O2 L-1), and (C) temperature (°C) at transplant and donor sites. Plotted PAR values are daily integrated data, while DO and temperature values are mean daily data. The intersection between eCDF and the horizontal blue dashed line represents median (50%) value at each site. Dockweiler, Redondo Canyon, and Malaga Cove are transplant sites. Palisades and East End are donor beds.
Minimum colonization depth is often restrained by wave orbital velocity and large wave exposure directly correlates with transplant mortality (Bos and van Katwijk, 2007; de Boer, 2007). Wave characterizations were of paramount importance for transplanting on the exposed mainland coast of the SCB. Inter-site comparisons amongst solely transplant sites from initial nascent stages of the transplant revealed disparities between sites, namely at Dockweiler where exceedances of threshold values corresponded with large drops in survivorship (Figure 5). Coupling survivorship and wave characterizations allow for insights to be gleaned, as periods of similar Hrms and Urms values at transplant and donor bed sites reveal stabilization in survivorship (September – October 2021), while deviations above threshold values (November 2021 – June 2022) correspond with transplant mortality (Figures 2, 5). The paucity of wave characterization for exposed mainland coast seagrass beds makes quantitative comparisons challenging. Hansen and Reidenbach (2012) investigated significant wave heights in extant Z. marina beds, finding maximum values around 0.18 m – far smaller wave heights than those values reported from extant beds in this study. Further, the wave characterizations of our study are conceptually supported by Hansen and Reidenbach (2013), who investigated near bottom wave velocity inside and outside Z. marina beds in lower Chesapeake Bay, finding flows to be significantly higher in the unvegetated site (0.09 m s-1) than within the seagrass site (0.026 m s-1). Additional studies have found, while uncommon in extant beds, maximum velocities of 0.5 m s-1 were incurred for brief periods by Z. marina (Fonseca et al., 1983; Koch, 2001). Though perhaps the most supportive of our results comes from a study conducted on survival of two western Mediterranean seagrasses at water depths of 12 and 18 m, concluding that near-bottom velocities tolerated were between 0.18 – 0.39 m s-1 (Infantes et al., 2011). These depths and velocities more aptly compare to those experienced in an exposed mainland coastal shelf environment occupied by Z. pacifica, evidencing that this species inhabits areas with sustained exposure above values typically tolerated by Z. marina, and other seagrasses, in protected environments.
Light availability constrains seagrass growth at the deep edge of the bed, but sufficient quantities are required to maintain health throughout the entirety of the local distribution (Drew, 1979; Dennison, 1987; Duarte et al., 2007). Based on the adapted non-survival light threshold from Thom et al. (2008), transplant sites did not receive sufficient light to satisfy growth requirements. The values (< 3 mol m-2 day-1) experienced at the transplant sites, and the ensuing mortality, are supported by results from a field study that found drastic mortality in Z. marina transplants exposed to PAR below 3 mol m-2 day-1 (Moore et al., 1997), as well as by a meta-analysis reporting similar deleterious impacts of values below the established PAR threshold (Dunic and Côté, 2023). Our study also shows that the donor bed sites average below those thresholds from November 2022 – April 2023 and did not induce mortality. During light limiting conditions, Zostera spp. can facilitate survival through the metabolization of carbohydrates (sugar and starch content) stored in the rhizomes and leaves (Zimmerman et al., 1995; Burke et al., 1996; Ralph et al., 2007; Vichkovitten et al., 2007; Bertelli and Unsworth, 2018). Research from Baja, Mexico supports this notion that carbohydrate reserves allow Zostera spp. to survive in short-term adverse light conditions but noted that mortality occurred when carbohydrate reserves decreased ~85% following three weeks of nearly zero PAR values (Cabello-Pasini et al., 2002). It follows then that lower light limits are not binary, rather eelgrass light requirements are nuanced, requiring sufficient light during growing seasons to build carbohydrate reserves needed to draw down during light limiting conditions encountered when irradiance levels are naturally lower (i.e., winter months) (Govers et al., 2015; Wong et al., 2020; Wong et al., 2021). The donor bed sites in our study did not experience near zero PAR conditions for an extended duration and did receive sufficient growing season light levels, and thus, Z. pacifica at these sites were likely able to draw on ample reserves to survive during brief light limiting conditions. Further, while acclimatization of transplanted Z. marina is documented (Krumhansl et al., 2021), the ability to build carbohydrate reserves is diminished under 3 mol m-2 day-1 light regimes (Eriander, 2017). The transplant sites in our study may have adapted to a new light regime initially, but when PAR values were reduced to ~ 0 mol m-2 day-1 for extended periods of time, an insufficient carbohydrate storage likely prevented survival in these adverse light conditions.
Low DO values impact Z. marina growth and can accelerate mortality (Holmer and Bondgaard, 2001). Hypoxic values (< 2 mg O2 L-1) and values nearing anoxia (< 0.01 mg O2 L-1) are confirmed in other systems containing submerged aquatic vegetation (Tassone et al., 2022). DO values in donor bed sites did not drop below 6.24 mg O2 L-1, but transplant sites, primarily Dockweiler and Malaga Cove, underwent multiple periods of extended hypoxia, and at Dockweiler, even values approaching anoxia (Figure 6). Low dissolved oxygen concentrations are reported as contributors of Zostera decline in other systems in the USA, such as the lower Chesapeake Bay (Moore and Jarvis, 2008), an Oregon estuary (Magel et al., 2023), and two separate California estuaries (Walter et al., 2018; Beheshti et al., 2022). A buildup in concentration of sulfides in the rhizome can occur during periods of hypoxia (Goodman et al., 1995), and may have significantly impacted transplant performance, though a quantification of sediment composition in our study is absent. The rapid impact of low DO can manifest in 12 hours and increases with duration, with absolute mortality occurring with anoxic exposure for 36 hours (Pulido and Borum, 2010; Raun and Borum, 2013). Continuous hypoxic conditions for 24 days at Dockweiler and 14 days at Malaga Cove is a salient contributor to transplant failure.
Heat stress induced mortality, decreased photosynthetic efficiency, or reduced growth rate, are common occurrences amongst Zostera spp. (Moore et al., 2014; Hammer et al., 2018; Serrano et al., 2021). Our study shows that temperature stress at the transplant sites was not a key variable influencing transplant site performance, as extant Z. pacifica donor beds experienced higher overall temperatures and for longer periods of time. The limited temperature threshold exposure in our study is supported by data taken in the proximity of a Z. pacifica bed off Santa Cruz Island, California (Kapsenberg and Hofmann, 2016), highlighting that extant open coast Zostera spp. proliferate within the range of temperatures reported in our study. Climate change models predict warming ocean temperatures will impact seagrasses (Carr et al., 2012), though the extant Z. pacifica beds in our study do not appear to be growing near their physiological temperature tolerances and may be less exposed to climate change related temperature stress compared to other temperate foundational species (i.e., Macrocystis pyrifera, see Cavanaugh et al., 2019 and Leichter et al., 2023). Yet, Zostera spp. are susceptible to both pulse extreme heat events and episodic heat exposure which can alter local resilience to additional stressors (DuBois et al., 2022), therefore it follows that the increasing magnitude, frequency, and duration of episodic events (i.e., ENSO) may coincide with baseline climatic warming to induce deleterious impacts on seagrasses in the SCB (Johnson et al., 2003; Echavarria-Heras et al., 2006).
An amalgamation of synergistic stressors, whether short-term stochastic or chronic, ultimately resulted in conditions unfavorable for Z. pacifica proliferation at transplant sites. While the survivorship of this project initially performed better than the Anacapa Island transplant conducted by Altstatt et al. (2014), fundamentally, the hydrodynamic data in our study from November 2021 – June 2022, coupled with water quality data from Spring 2022 – Spring 2023 portray an unambiguous unsuitability for long-term Z. pacifica survival at these sites. In opposition, the Anacapa transplant site characteristics proved hospitable for Z. pacifica expansion following a reduction in grazers responsible for the initial mortality (Altstatt et al., 2014). The present study represents the first quantification of physical parameters within Z. pacifica beds on the open coast, distinguishing from the less turbulent conditions of shallow, protected, estuarine Z. marina habitat.
5 Conclusion
These data mark a preludial effort to establish in situ tolerances and responses for open coast temperate seagrasses along California, and reaffirms the importance of site selection, noting that for hydrodynamically exposed regions, it may be the cynosure factor for transplant consideration, and necessitate discrete pre-transplant environmental sampling efforts. Though the transplant sites in this study did not exhibit sustained survival, as much of the nearshore coastal shelf environment within the SCB consists of the requisite substrate necessitated for Z. pacifica, the establishment of a transplanting regime could be of regional significance given the ecological and ‘blue carbon’ benefits of seagrass restoration (Postlethwaite et al., 2018; Lange et al., 2022; Shayka et al., 2023).
Considering the results of this study, merged with a current scarcity of environmental time-series data from Z. pacifica beds, we strongly encourage further research, both ex situ and in situ, on Z. pacifica. Building upon the physical and biological monitoring conducted in this study can spur the expansion of monitoring efforts to other Z. pacifica beds in the region, beds that persist on the mainland (as opposed to offshore islands such as the donor beds in this study), which may illuminate ranges of biophysical metrics that more closely reflect transplant site conditions. To facilitate greater efficacy of open coast restoration, a balance must be struck between conservative resource management policies and allocating adequate donor material to overcome critical thresholds of population density needed to induce positive feedback loops and reduce transplant risk on the open coast (Adams et al., 2016; Suykerbuyk et al., 2016; van Katwijk et al., 2016; Paulo et al., 2019; Orth et al., 2020; Valdez et al., 2020). Investigating the use of artificial seagrass structures to baffle strong hydrodynamic conditions (Carus et al., 2022) may reveal applied benefits for transplanting on the open coast. Burgeoning research indicates utilizing seeds may be a preferred restoration approach (Orth et al., 2020; Gräfnings et al., 2023), and has garnered success in high-energy sites (Unsworth et al., 2019), making it a technique worth investigating along the open coast of California.
Ultimately, the further elucidation of the realized niche Z. pacifica inhabits will provide essential information for more targeted open coast site selection and scalable restoration in the SCB. Species-specific research may disentangle long-held assumptions based on shallow protected Z. marina habitat, and provide clarity to seagrass resiliency in the region, particularly relevant in the face of climate change and episodic climactic events (i.e., ENSO) which have pervasive impacts on the Pacific Coast of North America (Tegner and Dayton, 1987; Chavez et al., 2002; Fumo et al., 2020). Teasing apart fish community dynamics, species-specific ecosystem services, distributional patterns, and threshold tolerances are fundamental to a holistic comprehension for academics, resource managers, and restoration practitioners alike.
Data availability statement
The raw data supporting the conclusions of this article will be made available by the authors, without undue reservation.
Author contributions
RS: Conceptualization, Data curation, Formal analysis, Funding acquisition, Investigation, Methodology, Supervision, Visualization, Writing – original draft, Validation, Writing – review & editing, Software. AO: Conceptualization, Data curation, Formal analysis, Investigation, Methodology, Visualization, Writing – review & editing, Resources, Supervision, Validation. BG: Conceptualization, Data curation, Investigation, Methodology, Writing – review & editing. ML: Data curation, Formal analysis, Visualization, Writing – review & editing, Software, Validation. LM: Formal analysis, Investigation, Methodology, Resources, Software, Supervision, Writing – review & editing, Validation. KE: Conceptualization, Formal analysis, Investigation, Methodology, Resources, Software, Supervision, Writing – review & editing, Data curation, Validation. OC: Conceptualization, Data curation, Investigation, Methodology, Writing – review & editing, Resources, Validation. TF: Conceptualization, Funding acquisition, Investigation, Methodology, Project administration, Writing – review & editing, Resources. JL: Conceptualization, Formal analysis, Funding acquisition, Investigation, Methodology, Resources, Supervision, Validation, Writing – review & editing.
Funding
The author(s) declare financial support was received for the research, authorship, and/or publication of this article. Funding and resources for this study provided to TF by the California Coastal Conservancy (grant no. LALSP SCC 18-077) and Restore America’s Estuaries Coastal Watershed Program (grant no. NEPCWG-20-SMBF). Additional support was provided to RS through The Mia Tegner fellowship at Scripps Institution of Oceanography.
Acknowledgments
The authors thank Heather Burdick, Amanda Bird, and Dr. David Ginsburg for assisting with field work and for their continued support of this research. Authors extend their gratitude to Mike Anghera and Dr. David Witting for truly supporting active scientific diving programs. The authors value the partnership with Vantuna Research Group and appreciate their assistance in mapping open coast seagrass beds. Emily Nixon provided critical input on early manuscript drafts.
Conflict of interest
The authors declare that the research was conducted in the absence of any commercial or financial relationships that could be construed as a potential conflict of interest.
The author(s) declared that they were an editorial board member of Frontiers, at the time of submission. This had no impact on the peer review process and the final decision.
Publisher’s note
All claims expressed in this article are solely those of the authors and do not necessarily represent those of their affiliated organizations, or those of the publisher, the editors and the reviewers. Any product that may be evaluated in this article, or claim that may be made by its manufacturer, is not guaranteed or endorsed by the publisher.
References
Adams M. P., Hovey R. K., Hipsey M. R., Bruce L. C., Ghisalberti M., Lowe R. J., et al. (2016). Feedback between sediment and light for seagrass: Where is it important? Limnol. Oceanogr. 61 (6), pp.1937–1955. doi: 10.1002/lno.10319
Allen L. G., Findlay A. M., Phalen C. M. (2002). Structure and standing stock of the fish assemblages of San Diego Bay, California from 1994 to 1999. Bulletin-Southern. California. Acad. Sci. 101 (2), 49–85.
Altstatt J. (2003). “Restoration of a historic eelgrass (Zostera marina) bed at Frenchy’s Cove, Anacapa Island,” in Proceedings of the Sixth California Islands Symposium (Arcata: Institute for Wildlife Studies), 397–404.
Altstatt J., Ambrose R., Carroll J., Coyer J., Wible J., Engle J. (2014). Eelgrass meadows return to Frenchy’s Cove, Anacapa Island: recovery ten years after successful transplantation. Monogr. Western. North Am. Nat. 7 (1), 500–517. doi: 10.3398/042.007.0139
Aoki L. R., McGlathery K. J., Wiberg P. L., Al-Haj A. (2020). Depth affects seagrass restoration success and resilience to marine heat wave disturbance. Estuaries. Coasts. 43, 316–328. doi: 10.1007/s12237-019-00685-0
Backman T. W., Barilotti D. C. (1976). Irradiance reduction: effects on standing crops of the eelgrass Zostera marina in a coastal lagoon. Mar. Biol. 34, 33–40. doi: 10.1007/BF00390785
Barbier E. B., Hacker S. D., Kennedy C., Koch E. W., Stier A. C., Silliman B. R. (2011). The value of estuarine and coastal ecosystem services. Ecol. Monogr. 81 (2), 169–193. doi: 10.1890/10-1510.1
Beca-Carretero P., Azcárate-García T., Julia-Miralles M., Stanschewski C. S., Guihéneuf F., Stengel D. B. (2021). Seasonal acclimation modulates the impacts of simulated warming and light reduction on temperate seagrass productivity and biochemical composition. Front. Mar. Sci. 8, 731152. doi: 10.3389/fmars.2021.731152
Beheshti K. M., Williams S. L., Boyer K. E., Endris C., Clemons A., Grimes T., et al. (2022). Rapid enhancement of multiple ecosystem services following the restoration of a coastal foundation species. Ecol. Appl. 32 (1), p.e02466. doi: 10.1002/eap.2466
Bernstein B., Merkel K., Chesney B., Sutula M. (2011). Recommendations for a southern California regional eelgrass monitoring program. South. California. Coast. Water Res. Project. 632, 45. Southern California Coastal Water Research Project, Costa Mesa, CA, USA.
Bertelli C. M., Unsworth R. K. (2018). Light stress responses by the eelgrass, Zostera marina (L). Front. Environ. Sci. 6, 39. doi: 10.3389/fenvs.2018.00039
Blok S. E., Olesen B., Krause-Jensen D. (2018). Life history events of eelgrass Zostera marina L. populations across gradients of latitude and temperature. Mar. Ecol. Prog. Ser. 590, 79–93. doi: 10.3354/meps12479
Bos A. R., van Katwijk M. M. (2007). Planting density, hydrodynamic exposure and mussel beds affect survival of transplanted intertidal eelgrass. Mar. Ecol. Prog. Ser. 336, 121–129. doi: 10.3354/meps336121
Burke M. K., Dennison W. C., Moore K. A. (1996). Non-structural carbohydrate reserves of eelgrass Zostera marina. Mar. Ecol. Prog. Ser. 137, 195–201. doi: 10.3354/meps137195
Burkholder J. M., Tomasko D. A., Touchette B. W. (2007). Seagrasses and eutrophication. J. Exp. Mar. Biol. Ecol. 350 (1-2), 46–72. doi: 10.1016/j.jembe.2007.06.024
Cabello-Pasini A., Lara-Turrent C., Zimmerman R. C. (2002). Effect of storms on photosynthesis, carbohydrate content and survival of eelgrass populations from a coastal lagoon and the adjacent open ocean. Aquat. Bot. 74 (2), 149–164. doi: 10.1016/S0304-3770(02)00076-1
Campbell M. L., Heppenstall L. D., Hendry R., Martin R., Sørensen S., Rubenstein A. N., et al. (2018). Niche partitioning of intertidal seagrasses: evidence of the influence of substrate temperature. New Phytol. 217 (4), 1449–1462. doi: 10.1111/nph.14944
Carr J. A., D’Odorico P., McGlathery K. J., Wiberg P. L. (2012). Stability and resilience of seagrass meadows to seasonal and interannual dynamics and environmental stress. J. Geophys. Res.: Biogeosci. 117 (G1). doi: 10.1029/2011JG001744
Carter M. L., Flick R. E., Terrill E., Beckhaus E. C., Martin K., Fey C. L., et al. (2022). “Shore Stations Program, La Jolla - Scripps Pier (La Jolla Archive 2022-10-24),” in Shore Stations Program Data Archive: Current and Historical Coastal Ocean Temperature and Salinity Measurements from California Stations (California, USA: UC San Diego Library Digital Collections). doi: 10.6075/J06T0K0M
Carus J., Arndt C., Bouma T. J., Schröder B., Paul M. (2022). Effect of artificial seagrass on hydrodynamic thresholds for the early establishment of Zostera marina. J. Ecohydraulics. 7 (1), 17–27. doi: 10.1080/24705357.2020.1858197
Cavanaugh K. C., Reed D. C., Bell T. W., Castorani M. C., Beas-Luna R. (2019). Spatial variability in the resistance and resilience of giant kelp in southern and Baja California to a multiyear heatwave. Front. Mar. Sci. 6, 413. doi: 10.3389/fmars.2019.00413
Chavez F. P., Pennington J. T., Castro C. G., Ryan J. P., Michisaki R. P., Schlining B., et al. (2002). Biological and chemical consequences of the 1997–1998 El Niño in central California waters. Prog. Oceanogr. 54 (1-4), pp.205–pp.232. doi: 10.1016/S0079-6611(02)00050-2
Cornelisen C. D., Thomas F. I. (2004). Ammonium and nitrate uptake by leaves of the seagrass Thalassia testudinum: impact of hydrodynamic regime and epiphyte cover on uptake rates. J. Mar. Syst. 49 (1-4), 177–194. doi: 10.1016/j.jmarsys.2003.05.008
Cottam C., Munro D. A. (1954). Eelgrass status and environmental relations. J. Wildlife. Manage. 18 (4), 449–460. doi: 10.2307/3797080
Coyer J. A., Miller K. A., Engle J. M., Veldsink J., Cabello-Pasini A., Stam W. T., et al. (2008). Eelgrass meadows in the California Channel Islands and adjacent coast reveal a mosaic of two species, evidence for introgression and variable clonality. Ann. Bot. 101 (1), 73–87. doi: 10.1093/aob/mcm288
Craig M. T., Fodrie F. J., Hastings P. A. (2004). The nearshore fish assemblage of the Scripps coastal reserve, San Diego, California. Coast. Manage. 32 (4), 341–351. doi: 10.1080/08920750490487188
Dahl M., Asplund M. E., Björk M., Deyanova D., Infantes E., Isaeus M., et al. (2020). The influence of hydrodynamic exposure on carbon storage and nutrient retention in eelgrass (Zostera marina L.) meadows on the Swedish Skagerrak coast. Sci. Rep. 10 (1), 13666. doi: 10.1038/s41598-020-70403-5
Dean R. G., Dalrymple R. A. (1991). Water Wave Mechanics for Engineers and Scientists Vol. 2 (Singapore: Advanced Series on Ocean Engineering). doi: 10.1142/1232
de Boer W. F. (2007). Seagrass–sediment interactions, positive feedbacks and critical thresholds for occurrence: a review. Hydrobiologia 591, 5–24. doi: 10.1007/s10750-007-0780-9
Dennison W. C. (1987). Effects of light on seagrass photosynthesis, growth and depth distribution. Aquat. Bot. 27 (1), 15–26. doi: 10.1016/0304-3770(87)90083-0
Dennison W. C., Alberte R. S. (1982). Photosynthetic responses of Zostera marina L.(eelgrass) to in situ manipulations of light intensity. Oecologia 55, 137–144. doi: 10.1007/BF00384478
Drew E. A. (1979). Physiological aspects of primary production in seagrasses. Aquat. Bot. 7, 139–150. doi: 10.1016/0304-3770(79)90018-4
Duarte C. M. (1991). Seagrass depth limits. Aquat. Bot. 40 (4), 363–377. doi: 10.1016/0304-3770(91)90081-F
Duarte C. M. (2002). The future of seagrass meadows. Environ. Conserv. 29 (2), 192–206. doi: 10.1017/S0376892902000127
Duarte C. M., Krause-Jensen D. (2017). Export from seagrass meadows contributes to marine carbon sequestration. Front. Mar. Sci. 4, 13. doi: 10.3389/fmars.2017.00013
Duarte C. M., Losada I. J., Hendriks I. E., Mazarrasa I., Marbà N. (2013). The role of coastal plant communities for climate change mitigation and adaptation. Nat. Climate Change 3 (11), 961–968. doi: 10.1038/nclimate1970
Duarte C. M., Marbà N., Krause-Jensen D., Sánchez-Camacho M. (2007). Testing the predictive power of seagrass depth limit models. Estuaries. Coasts. 30, 652–656. doi: 10.1007/BF02841962
DuBois K., Pollard K. N., Kauffman B. J., Williams S. L., Stachowicz J. J. (2022). Local adaptation in a marine foundation species: Implications for resilience to future global change. Global Change Biol. 28 (8), 2596–2610. doi: 10.1111/gcb.16080
Duffy J. E. (2006). Biodiversity and the functioning of seagrass ecosystems. Mar. Ecol. Prog. Ser. 311, 233–250. doi: 10.3354/meps311233
Dunic J. C., Côté I. M. (2023). Management thresholds shift under the influence of multiple stressors: Eelgrass meadows as a case study. Conserv. Lett. 16 (2), e12938. doi: 10.1111/conl.12938
Echavarria-Heras H. A., Solana-Arellano E., Franco-Vizcaíno E. (2006). The role of increased sea surface temperature on eelgrass leaf dynamics: onset of El Niño as a proxy for global climate change in San Quintín Bay, Baja California. Bull. South. California. Acad. Sci. 105 (3), 113–127. doi: 10.3160/0038-3872(2006)105[113:TROISS]2.0.CO;2
Egea L. G., Infantes E., Jiménez-Ramos R. (2023). Loss of POC and DOC on seagrass sediments by hydrodynamics. Sci. Total. Environ. 901, 165976. doi: 10.1016/j.scitotenv.2023.165976
Egstrom G. H. (1974). The Los Angeles County Underwater Resource Inventory, Oct. 1972-Sept. 1974 (California, USA: Underwater Laboratory, Dept. of Kinesiology, University of California). Available at: https://search-library.ucsd.edu/permalink/01UCS_SDI/1vtf07t/cdi_proquest_miscellaneous_17950184 (Accessed: 5 September 2023).
El-Hacen E. H. M., Bouma T. J., Govers L. L., Piersma T., Han O. (2019). Seagrass sensitivity to collapse along a hydrodynamic gradient: Evidence from a pristine subtropical intertidal ecosystem. Ecosystems 22, 1007–1023. doi: 10.1007/s10021-018-0319-0
Engle J. M., Miller K. A. (2005). “Distribution and morphology of eelgrass (Zostera marina L.) at the California Channel Islands,” in Proceedings of the sixth California Islands symposium. Eds. Garcelon D. K., Schwemm C. A., (California, USA: Ventura) 405–414.
Eriander L. (2017). Light requirements for successful restoration of eelgrass (Zostera marina L.) in a high latitude environment–acclimatization, growth and carbohydrate storage. J. Exp. Mar. Biol. Ecol. 496, 37–48. doi: 10.1016/j.jembe.2017.07.010
Fonseca M. S., Bell S. S. (1998). Influence of physical setting on seagrass landscapes near Beaufort, North Carolina, USA. Mar. Ecol. Prog. Ser. 171, 109–121. doi: 10.3354/meps171109
Fonseca M. S., Kenworthy W.J., Thayer G. W. (1998). Guidelines for the Conservation and Restoration of Seagrasses in the United States and Adjacent Waters. NOAA, Coastal Ocean Program, Decision Analysis Series No. 12 (Silver Spring, MD: U.S. Department of Commerce, NOAA, Coastal Ocean Office), 222pp. Available at: https://repository.library.noaa.gov/view/noaa/1672.
Fonseca M. S., Zieman J. C., Thayer G. W., Fisher J. S. (1983). The role of current velocity in structuring eelgrass (Zostera marina L.) meadows. Estuarine. Coast. Shelf. Sci. 17 (4), 367–380. doi: 10.1016/0272-7714(83)90123-3
Foster E., Watson J., Lemay M. A., Tinker M. T., Estes J. A., Piercey R., et al. (2021). Physical disturbance by recovering sea otter populations increases eelgrass genetic diversity. Science 374 (6565), 333–336. doi: 10.1126/science.abf2343
Fourqurean J. W., Duarte C. M., Kennedy H., Marbà N., Holmer M., Mateo M. A., et al. (2012). Seagrass ecosystems as a globally significant carbon stock. Nat. Geosci. 5 (7), 505–509. doi: 10.1038/ngeo1477
Fumo J. T., Carter M. L., Flick R. E., Rasmussen L. L., Rudnick D. L., Iacobellis S. F. (2020). Contextualizing marine heatwaves in the Southern California bight under anthropogenic climate change. J. Geophys. Res.: Oceans. 125 (5), e2019JC015674. doi: 10.1029/2019JC015674
Goodman J. L., Moore K. A., Dennison W. C. (1995). Photosynthetic responses of eelgrass (Zostera marina L.) to light and sediment sulfide in a shallow barrier island lagoon. Aquat. Bot. 50 (1), 37–47. doi: 10.1016/0304-3770(94)00444-Q
Govers L. L., Suykerbuyk W., Hoppenreijs J. H., Giesen K., Bouma T. J., van Katwijk M. M. (2015). Rhizome starch as indicator for temperate seagrass winter survival. Ecol. Indic. 49, 53–60. doi: 10.1016/j.ecolind.2014.10.002
Gräfnings M. L., Heusinkveld J. H., Hijner N., Hoeijmakers D. J., Smeele Q., Zwarts M., et al. (2023). Spatial design improves efficiency and scalability of seed-based seagrass restoration. J. Appl. Ecol. 60 (6), 967–977. doi: 10.1111/1365-2664.14405
Greve T. M., Borum J., Pedersen O. (2003). Meristematic oxygen variability in eelgrass (Zostera marina). Limnol. Oceanogr. 48 (1), 210–216. doi: 10.4319/lo.2003.48.1.0210
Hammer K. J., Borum J., Hasler-Sheetal H., Shields E. C., Sand-Jensen K., Moore K. A. (2018). High temperatures cause reduced growth, plant death and metabolic changes in eelgrass Zostera marina. Mar. Ecol. Prog. Ser. 604, 121–132. doi: 10.3354/meps12740
Hansen J. C., Reidenbach M. A. (2012). Wave and tidally driven flows in eelgrass beds and their effect on sediment suspension. Mar. Ecol. Prog. Ser. 448, 271–287. doi: 10.3354/meps09225
Hansen J. C., Reidenbach M. A. (2013). Seasonal growth and senescence of a Zostera marina seagrass meadow alters wave-dominated flow and sediment suspension within a coastal bay. Estuaries. Coasts. 36, 1099–1114. doi: 10.1007/s12237-013-9620-5
Hardison S. B., McGlathery K. J., Castorani M. C. (2023). Effects of seagrass restoration on coastal fish abundance and diversity. Conserv. Biol. 37 (6), e14147. doi: 10.1111/cobi.14147
Heck K. L. Jr., Valentine J. F. (2006). Plant–herbivore interactions in seagrass meadows. J. Exp. Mar. Biol. Ecol. 330 (1), 420–436. doi: 10.1016/j.jembe.2005.12.044
Hoffman R. S. (1986). Fishery utilization of eelgrass (Zostera marina) beds and non-vegetated shallow water areas in San Diego Bay (California, USA: Southwest Region, NOAA, National Marine Fisheries Service).
Holmer M., Bondgaard E. J. (2001). Photosynthetic and growth response of eelgrass to low oxygen and high sulfide concentrations during hypoxic events. Aquat. Bot. 70 (1), 29–38. doi: 10.1016/S0304-3770(00)00142-X
Holthuijsen L. G. (2007). Waves in Oceanic and Coastal Waters (Cambridge University Press), 387 pp. doi: 10.1017/CBO9780511618536
Hughes B. B., Eby R., Van Dyke E., Tinker M. T., Marks C. I., Johnson K. S., et al. (2013). Recovery of a top predator mediates negative eutrophic effects on seagrass. Proc. Natl. Acad. Sci. 110 (38), 15313–15318. doi: 10.1073/pnas.1302805110
Huntington B. E., Boyer K. E. (2008). Effects of red macroalgal (Gracilariopsis sp.) abundance on eelgrass Zostera marina in Tomales Bay, California, USA. Mar. Ecol. Prog. Ser. 367, 133–142. doi: 10.3354/meps07506
Infantes E., Orfila A., Bouma T. J., Simarro G., Terrados J. (2011). Posidonia oceanica and Cymodocea nodosa seedling tolerance to wave exposure. Limnol. Oceanogr. 56 (6), 2223–2232. doi: 10.4319/lo.2011.56.6.2223
Irlandi E. A., Orlando B. A., Ambrose W. G. Jr. (1999). Influence of seagrass habitat patch size on growth and survival of juvenile bay scallops, Argopecten irradians concentricus (Say). J. Exp. Mar. Biol. Ecol. 235 (1), 21–43. doi: 10.1016/S0022-0981(98)00185-3
Jakobsson-Thor S., Brakel J., Toth G. B., Pavia H. (2020). Complex interactions of temperature, light and tissue damage on seagrass wasting disease in Zostera marina. Front. Mar. Sci. 7, 575183. doi: 10.3389/fmars.2020.575183
Jiménez-Ramos R., Brun F. G., Pérez-Lloréns J. L., Vergara J. J., Delgado-Cabezas F., Sena-Soria N., et al. (2023). Resistance and recovery of benthic marine macrophyte communities to light reduction: Insights from carbon metabolism and dissolved organic carbon (DOC) fluxes, and implications for resilience. Mar. pollut. Bull. 188, 114630. doi: 10.1016/j.marpolbul.2023.114630
Jiménez-Ramos R., Egea L. G., Vergara J. J., Brun F. G. (2021). Factors modulating herbivory patterns in Cymodocea nodosa meadows. Limnol. Oceanogr. 66 (6), 2218–2233. doi: 10.1002/lno.11749
Johnson M. R., Williams S. L., Lieberman C. H., Solbak A. (2003). Changes in the abundance of the seagrasses Zostera marina L.(eelgrass) and Ruppia maritima L.(widgeongrass) in San Diego, California, following and El Niño Event. Estuaries 26, 106–115. doi: 10.1007/BF02691698
Kapsenberg L., Hofmann G. E. (2016). Ocean pH time-series and drivers of variability along the northern Channel Islands, California, USA. Limnol. Oceanogr. 61 (3), 953–968. doi: 10.1002/lno.10264
Kelly J. J., Orr D., Takekawa J. Y. (2019). Quantification of damage to eelgrass (Zostera marina) beds and evidence-based management strategies for boats anchoring in San Francisco Bay. Environ. Manage. 64 (1), 20–26. doi: 10.1007/s00267-019-01169-4
Koch E. W. (2001). Beyond light: physical, geological, and geochemical parameters as possible submersed aquatic vegetation habitat requirements. Estuaries 24, 1–17. doi: 10.2307/1352808
Kopp B. S. (1999) Effects of nitrate fertilization and shading on physiological and biomechanical properties of eelgrass (Zostera marina L.) (Order No. 9945210). Available at: https://www.proquest.com/dissertations-theses/effects-nitrate-fertilization-shading-on/docview/304522955/se-2.
Krause-Jensen D., Archambault P., Assis J., Bartsch I., Bischof K., Filbee-Dexter K., et al. (2020). Imprint of climate change on pan-Arctic marine vegetation. Front. Mar. Sci. 7, p.617324. doi: 10.3389/fmars.2020.617324
Krumhansl K. A., Dowd M., Wong M. C. (2021). Multiple metrics of temperature, light, and water motion drive gradients in eelgrass productivity and resilience. Front. Mar. Sci. 8, 597707. doi: 10.3389/fmars.2021.597707
La Nafie Y. A., de los Santos C. B., Brun F. G., Mashoreng S., van Katwijk M. M., Bouma T. J. (2013). Biomechanical response of two fast-growing tropical seagrass species subjected to in situ shading and sediment fertilization. J. Exp. Mar. Biol. Ecol. 446, 186–193. doi: 10.1016/j.jembe.2013.05.020
Lange T., Oncken N. S., Svane N., Steinfurth R. C., Kristensen E., Flindt M. R. (2022). Large-scale eelgrass transplantation: a measure for carbon and nutrient sequestration in estuaries. Mar. Ecol. Prog. Ser. 685, 97–109. doi: 10.3354/meps13975
LA Water Board (2023) Administrative Civil Liability Complaint NO. R4-2023-0007 (Los Angeles Region: California Regional Water Quality Control Board). Available at: https://www.waterboards.ca.gov/losangeles/water_issues/programs/enforcement/acl_docs/2023/ACLC_No_R4-2023-0007_Hyperion_ADAchecked.pdf (Accessed 5 September 2023).
Lee K. S., Park S. R., Kim Y. K. (2007). Effects of irradiance, temperature, and nutrients on growth dynamics of seagrasses: a review. J. Exp. Mar. Biol. Ecol. 350 (1-2), 144–175. doi: 10.1016/j.jembe.2007.06.016
Leichter J. J., Ladah L. B., Parnell P., Stokes M. D., Costa M. T., Fumo J., et al. (2023). Persistence of southern California Giant Kelp beds and alongshore variation in nutrient exposure driven by seasonal upwelling and internal waves. Front. Mar. Sci. 10, 339. doi: 10.3389/fmars.2023.1007789
Livernois M. C., Grabowski J. H., Poray A. K., Gouhier T. C., Hughes A. R., O’Brien K. F., et al. (2017). Effects of habitat fragmentation on Zostera marina seed distribution. Aquat. Bot. 142, 1–9. doi: 10.1016/j.aquabot.2017.05.006
Looby A., Ginsburg D. W. (2021). Nearshore species biodiversity of a marine protected area off Santa Catalina Island, California. Western. North Am. Nat. 81 (1), 113–130. doi: 10.3398/064.081.0110
Lyman T. P., Elsmore K., Gaylord B., Byrnes J. E., Miller L. P. (2020). Open Wave Height Logger: An open source pressure sensor data logger for wave measurement. Limnol. Oceanogr.: Methods 18 (7), 335–345. doi: 10.1002/lom3.10370
Magel C. L., Hacker S. D., Chan F., Helms A. R. (2023). Eelgrass and macroalgae loss in an oregon estuary: consequences for ocean acidification and hypoxia. Ocean-land-atmosphere. Res. 2, 0023. doi: 10.34133/olar.0023
MBC Applied Environmental (2016). Orange County Parks Newport Bay Eelgrass Mitigation Project (California, USA: Prepared for Orange County Parks). Available at: https://www.ecoatlas.org/upfiles/5020/60%20Month%20Eelgrass%20Survey.pdf (Accessed: 5 September 2023).
McCune K., Gillett D. J., Stein E. D. (2020). Methods and guidance on assessing the ecological functioning of submerged aquatic vegetation in Southern California estuaries and embayments. Technical Report No. 1136 (Costa Mesa, CA: Southern California Coastal Water Research Project).
McCune B., Grace J. B. (2002). Analysis of ecological communities. MjM Software Design: Glenden Beach, Oregon, USA. pp. 304.
McGlathery K. J., Reynolds L. K., Cole L. W., Orth R. J., Marion S. R., Schwarzschild A. (2012). Recovery trajectories during state change from bare sediment to eelgrass dominance. Mar. Ecol. Prog. Ser. 448, 209–221. doi: 10.3354/meps09574
McKenzie L. J., Nordlund L. M., Jones B. L., Cullen-Unsworth L. C., Roelfsema C., Unsworth R. K. (2020). The global distribution of seagrass meadows. Environ. Res. Lett. 15 (7), 074041. doi: 10.1088/1748-9326/ab7d06
Merkel & Associates, Inc (2010). 84 Month Post Transplant Survey at the Eelgrass Mitigation Site in Support of the Pier 300 Expansion Project at the Port of Los Angeles, California. California, USA. Available at: https://www.ecoatlas.org/upfiles/4493/84%20Month%20Eelgrass%20Survey.pdf (Accessed: 5 September 2023).
Monismith S. G., Herdman L. M., Ahmerkamp S., Hench J. L. (2013). Wave transformation and wave-driven flow across a steep coral reef. J. Phys. Oceanogr. 43 (7), 1356–1379. doi: 10.1175/JPO-D-12-0164.1
Moore K. A., Jarvis J. C. (2008). Environmental factors affecting recent summertime eelgrass diebacks in the lower Chesapeake Bay: implications for long-term persistence. J. Coast. Res. 10055), 135–147. doi: 10.2112/SI55-014
Moore K. A., Shields E. C., Parrish D. B. (2014). Impacts of varying estuarine temperature and light conditions on Zostera marina (Eelgrass) and its interactions with Ruppia maritima (Widgeongrass). Estuaries. Coasts. 37, 20–30. doi: 10.1007/s12237-013-9667-3
Moore K. A., Wetzel R. L., Orth R. J. (1997). Seasonal pulses of turbidity and their relations to eelgrass (Zostera marina L.) survival in an estuary. J. Exp. Mar. Biol. Ecol. 215 (1), 115–134. doi: 10.1016/S0022-0981(96)02774-8
Munsch S. H., Beaty F. L., Beheshti K. M., Chesney W. B., Endris C. A., Gerwing T. G., et al. (2023). Northeast Pacific eelgrass dynamics: interannual expansion distances and meadow area variation over time. Mar. Ecol. Prog. Ser. 705, 61–75. doi: 10.3354/meps14248
National Oceanic and Atmospheric Administration (2014). California eelgrass mitigation policy and implementing guidelines (Santa Rosa, CA: NOAA Fisheries). Available at: https://media.fisheries.noaa.gov/dam-migration/cemp_oct_2014_final.pdf.
Nielsen S. L., Sand-Jensen K., Borum J., Geertz-Hansen O. (2002). Depth colonization of eelgrass (Zostera marina) and macroalgae as determined by water transparency in Danish coastal waters. Estuaries 25, 1025–1032. doi: 10.1007/BF02691349
North W. J. (1963). Ecology of the rocky nearshore environment in southern California and possible influences of discharged wastes. Air. Water pollut. 7, 721–736.
Obaza A. K., Bird A., Sanders R., Ware R., Ginsburg D. W. (2022). Variable fish habitat function in two open-coast eelgrass species. Mar. Ecol. Prog. Ser. 696, 15–27. doi: 10.3354/meps14135
Obaza A., Hoffman R., Clausing R. (2015). Long-term stability of eelgrass fish assemblages in two highly developed coastal estuaries. Fisheries. Manage. Ecol. 22 (3), 224–238. doi: 10.1111/fme.12119
Oksanen J., Blanchet F. G., Friendly M., Kindt R., Legendre P., McGlinn D., et al. (2019). Package ‘vegan’. Community ecology package, R package version 2.5-6. https://cran.r-project.org/web/packages/vegan/index.html.
O’Leary J. K., Goodman M. C., Walter R. K., Willits K., Pondella D. J., Stephens J. (2021). Effects of estuary-wide seagrass loss on fish populations. Estuaries. Coasts. 44, 2250–2264. doi: 10.1007/s12237-021-00917-2
Olsen J. L., Coyer J. A., Chesney B. (2014). Numerous mitigation transplants of the eelgrass Zostera marina in southern California shuffle genetic diversity and may promote hybridization with Zostera pacifica. Biol. Conserv. 176, 133–143. doi: 10.1016/j.biocon.2014.05.001
Orth R. J., Carruthers T. J., Dennison W. C., Duarte C. M., Fourqurean J. W., Heck K. L., et al. (2006). A global crisis for seagrass ecosystems. Bioscience 56 (12), pp.987–pp.996. doi: 10.1641/0006-3568(2006)56[987:AGCFSE]2.0.CO;2
Orth R. J., Harwell M. C., Fishman J. R. (1999). A rapid and simple method for transplanting eelgrass using single, unanchored shoots. Aquat. Bot. 64 (1), 77–85. doi: 10.1016/S0304-3770(99)00007-8
Orth R. J., Lefcheck J. S., McGlathery K. S., Aoki L., Luckenbach M. W., Moore K. A., et al. (2020). Restoration of seagrass habitat leads to rapid recovery of coastal ecosystem services. Sci. Adv. 6 (41), p.eabc6434. doi: 10.1126/sciadv.abc6434
Ostenfeld C. H. (1905). Preliminary remarks on the distribution and biology of the Zostera of the Danish seas. Bot. Tidsskr. 27, 123–125.
Paling E. I., Van Keulen M., Tunbridge D. J. (2007). Seagrass transplanting in Cockburn Sound, Western Australia: a comparison of manual transplantation methodology using Posidonia sinuosa Cambridge et Kuo. Restor. Ecol. 15 (2), 240–249. doi: 10.1111/j.1526-100X.2007.00207.x
Paling E. I., Van Keulen M., Wheeler K. D., Phillips J., Dyhrberg R. (2003). Influence of spacing on mechanically transplanted seagrass survival in a high wave energy regime. Restor. Ecol. 11 (1), 56–61. doi: 10.1046/j.1526-100X.2003.00072.x
Paling E. I., Van Keulen M., Wheeler K. D., Phillips J., Dyhrberg R., Lord D. A. (2001). Improving mechanical seagrass transplantation. Ecol. Eng. 18 (1), 107–113. doi: 10.1016/S0925-8574(01)00065-9
Parnell P. E., Levin L. A., Navarro M. O. (2020). Gauging oxygen risk and tolerance for the megafauna of the Southern California shelf based on in situ observation, species mobility, and seascape. ICES. J. Mar. Sci. 77 (5), 1941–1952. doi: 10.1093/icesjms/fsaa088
Patterson M. R., Harwell M. C., Orth L. M., Orth R. J. (2001). Biomechanical properties of the reproductive shoots of eelgrass. Aquat. Bot. 69 (1), 27–40. doi: 10.1016/S0304-3770(00)00133-9
Paulo D., Cunha A. H., Boavida J., Serrão E. A., Gonçalves E. J., Fonseca M. (2019). Open coast seagrass restoration. Can we do it? Large scale seagrass transplants. Front. Mar. Sci. 6, 52. doi: 10.3389/fmars.2019.00052
Phillips R. C. (1964). Comprehensive bibliography of Zostera marina (No. 79) (District of Columbia, USA: US Fish and Wildlife Service).
Pihl L., Baden S., Kautsky N., Rönnbäck P., Söderqvist T., Troell M., et al. (2006). Shift in fish assemblage structure due to loss of seagrass Zostera marina habitats in Sweden. Estuarine. Coast. Shelf. Sci. 67 (1-2), 123–132. doi: 10.1016/j.ecss.2005.10.016
Pollard P. C., Greenway M. (2013). Seagrasses in tropical Australia, productive and abundant for decades decimated overnight. J. Biosci. 38, 157–166. doi: 10.1007/s12038-013-9299-6
Pondella D. J., Allen L. G., Craig M. T., Gintert B. (2006). Evaluation of eelgrass mitigation and fishery enhancement structures in San Diego Bay, California. Bull. Mar. Sci. 78 (1), 115–131.
Pondella D. J., Craig M. T., Franck J. P. (2003). The phylogeny of Paralabrax (Perciformes: Serranidae) and allied taxa inferred from partial 16S and 12S mitochondrial ribosomal DNA sequences. Mol. Phylogenet. Evol. 29 (1), 176–184. doi: 10.1016/S1055-7903(03)00078-2
Postlethwaite V. R., McGowan A. E., Kohfeld K. E., Robinson C. L., Pellatt M. G. (2018). Low blue carbon storage in eelgrass (Zostera marina) meadows on the Pacific Coast of Canada. PloS One 13 (6), e0198348. doi: 10.1371/journal.pone.0198348
Pulido C., Borum J. (2010). Eelgrass (Zostera marina) tolerance to anoxia. J. Exp. Mar. Biol. Ecol. 385 (1-2), 8–13. doi: 10.1016/j.jembe.2010.01.014
Ralph P. J., Durako M. J., Enriquez S., Collier C. J., Doblin M. A. (2007). Impact of light limitation on seagrasses. J. Exp. Mar. Biol. Ecol. 350 (1-2), 176–193. doi: 10.1016/j.jembe.2007.06.017
Rasmussen E. (1977). “The wasting disease of eelgrass (Zostera marina) and its effects on environmental factors and fauna,” in Seagrass Ecosystems: A Scientific Perspective, eds McRoy C. P., Helfferich C. (New York: Marcel Dekker), 1–52.
Raun A. L., Borum J. (2013). Combined impact of water column oxygen and temperature on internal oxygen status and growth of Zostera marina seedlings and adult shoots. J. Exp. Mar. Biol. Ecol. 441, 16–22. doi: 10.1016/j.jembe.2013.01.014
RCore Team (2021). R: A language and environment for statistical computing (Vienna, Austria: R Foundation for Statistical Computing). Available at: https://www.R-project.org/.
Ricart A. M., Ward M., Hill T. M., Sanford E., Kroeker K. J., Takeshita Y., et al. (2021). Coast-wide evidence of low pH amelioration by seagrass ecosystems. Global Change Biol. 27 (11), 2580–2591. doi: 10.1111/gcb.15594
Risandi J., Rifai H., Lukman K. M., Sondak C. F., Hernawan U. E., Quevedo J. M. D., et al. (2023). Hydrodynamics across seagrass meadows and its impacts on Indonesian coastal ecosystems: A review. Front. Earth Sci. 11, 1034827. doi: 10.3389/feart.2023.1034827
Schlegel R. W., Smit A. J. (2018). heatwaveR: A central algorithm for the detection of heatwaves and cold-spells. J. Open Source Software. 3 (27), 821. doi: 10.21105/joss.00821
Serrano O., Arias-Ortiz A., Duarte C. M., Kendrick G. A., Lavery P. S. (2021). “Impact of marine heatwaves on seagrass ecosystems,” in Ecosystem Collapse and Climate Change (Cham: Springer International Publishing), 345–364.
Shayka B. F., Hesselbarth M. H., Schill S. R., Currie W. S., Allgeier J. E. (2023). The natural capital of seagrass beds in the Caribbean: evaluating their ecosystem services and blue carbon trade potential. Biol. Lett. 19 (6), 20230075. doi: 10.1098/rsbl.2023.0075
Short F., Carruthers T., Dennison W., Waycott M. (2007). Global seagrass distribution and diversity: a bioregional model. J. Exp. Mar. Biol. Ecol. 350 (1-2), 3–20. doi: 10.1016/j.jembe.2007.06.012
Short F. T., Coles R. G. (Eds.) (2001). Global seagrass research methods (London: Elsevier). doi: 10.1016/B978-0-444-50891-1.X5000-2
Short F. T., Davis R. C., Kopp B. S., Short C. A., Burdick D. M. (2002). Site-selection model for optimal transplantation of eelgrass Zostera marina in the northeastern US. Mar. Ecol. Prog. Ser. 227, 253–267. doi: 10.3354/meps227253
Short F. T., Wyllie-Echeverria S. (1996). Natural and human-induced disturbance of seagrasses. Environ. Conserv. 23 (1), 17–27. doi: 10.1017/S0376892900038212
State Water Resources Control Board (SWRCB) (1979) California Marine Waters Areas of Special Biological Significance: Mugu Lagoon to Latigo Point Reconnaissance Survey Report. Available at: https://www.waterboards.ca.gov/publications_forms/publications/general/docs/asbs_mugu_a.pdf.
Strydom S., Murray K., Wilson S., Huntley B., Rule M., Heithaus M., et al. (2020). Too hot to handle: Unprecedented seagrass death driven by marine heatwave in a World Heritage Area. Global Change Biol. 26 (6), 3525–3538. doi: 10.1111/gcb.15065
Suykerbuyk W., Govers L. L., Bouma T. J., Giesen W. B., de Jong D. J., van de Voort R., et al. (2016). Unpredictability in seagrass restoration: analyzing the role of positive feedback and environmental stress on Zostera noltii transplants. J. Appl. Ecol. 53 (3), 774–784. doi: 10.1111/1365-2664.12614
Svendsen I. A. (2005). Introduction to nearshore hydrodynamics. World Sci., 722 pp. doi: 10.1142/5740
Tan Y. M., Dalby O., Kendrick G. A., Statton J., Sinclair E. A., Fraser M. W., et al. (2020). Seagrass restoration is possible: insights and lessons from Australia and New Zealand. Front. Mar. Sci. 7, 617. doi: 10.3389/fmars.2020.00617
Tanner R. L., Obaza A. K., Ginsburg D. W. (2019). Secondary Production of Kelp Bass Paralabrax clathratus in Relation to Coastal Eelgrass Zostera marina Habitat in a Southern California Marine Protected Area. Bull. South. California. Acad. Sci. 118 (3), 158–172. doi: 10.3160/0038-3872-118.3.158
Tassone S. J., Besterman A. F., Buelo C. D., Walter J. A., Pace M. L. (2022). Co-occurrence of aquatic heatwaves with atmospheric heatwaves, low dissolved oxygen, and low PH events in estuarine ecosystems. Estuaries. Coasts. 45 (3), 707–720. doi: 10.1007/s12237-021-01009-x
Tegner M. J., Dayton P. K. (1987). El Niño effects on southern California kelp forest communities. Adv. Ecol. Res. 17, 243–279. doi: 10.1016/S0065-2504(08)60247-0
The MathWorks Inc (2022). MATLAB version: 9.13.0 (R2022b) (Natick, Massachusetts: The MathWorks Inc). Available at: https://www.mathworks.com.
Thom R. M., Southard S. L., Borde A. B., Stoltz P. (2008). Light requirements for growth and survival of eelgrass (Zostera marina L.) in Pacific Northwest (USA) estuaries. Estuaries. Coasts. 31, 969–980. doi: 10.1007/s12237-008-9082-3
Turschwell M. P., Connolly R. M., Dunic J. C., Sievers M., Buelow C. A., Pearson R. M., et al. (2021). Anthropogenic pressures and life history predict trajectories of seagrass meadow extent at a global scale. Proc. Natl. Acad. Sci. 118 (45), e2110802118. doi: 10.1073/pnas.2110802118
Uhrin A. V., Turner M. G. (2018). Physical drivers of seagrass spatial configuration: the role of thresholds. Landscape Ecol. 33, 2253–2272. doi: 10.1007/s10980-018-0739-4
Unsworth R. K., Bertelli C. M., Cullen-Unsworth L. C., Esteban N., Jones B. L., Lilley R., et al. (2019). Sowing the seeds of seagrass recovery using hessian bags. Front. Ecol. Evol. 311. doi: 10.3389/fevo.2019.00311
Unsworth R. F. K., Cullen-Unsworth L. C. (2014). Biodiversity, ecosystem services, and the conservation of seagrass meadows. Coast. Conserv. 19, 95. doi: 10.1017/CBO9781139137089.005
Unsworth R. K., Cullen-Unsworth L. C., Jones B. L., Lilley R. J. (2022). The planetary role of seagrass conservation. Science 377 (6606), 609–613. doi: 10.1126/science.abq6923
Valdez S. R., Zhang Y. S., van der Heide T., Vanderklift M. A., Tarquinio F., Orth R. J., et al. (2020). Positive ecological interactions and the success of seagrass restoration. Front. Mar. Sci. 7, 91. doi: 10.3389/fmars.2020.00091
Valentine J. F., Heck K. L. Jr. (2021). Herbivory in seagrass meadows: an evolving paradigm. Estuaries. Coasts. 44 (2), 491–505. doi: 10.1007/s12237-020-00849-3
van Katwijk M. M., Bos A. R., De Jonge V. N., Hanssen L. S. A. M., Hermus D. C. R., De Jong D. J. (2009). Guidelines for seagrass restoration: importance of habitat selection and donor population, spreading of risks, and ecosystem engineering effects. Mar. pollut. Bull. 58 (2), 179–188. doi: 10.1016/j.marpolbul.2008.09.028
van Katwijk M. M., Thorhaug A., Marbà N., Orth R. J., Duarte C. M., Kendrick G. A., et al. (2016). Global analysis of seagrass restoration: the importance of large-scale planting. J. Appl. Ecol. 53 (2), 567–578. doi: 10.1111/1365-2664.12562
Vichkovitten T., Holmer M., Frederiksen M. (2007). Spatial and temporal changes in non-structural carbohydrate reserves in eelgrass (Zostera marina L.) in Danish coastal waters. Botanica Marina. 50 (2), 75–87. doi: 10.1515/BOT.2007.009
Walter R. K., Rainville E. J., O’Leary J. K. (2018). Hydrodynamics in a shallow seasonally low-inflow estuary following eelgrass collapse. Estuarine. Coast. Shelf. Sci. 213, 160–175. doi: 10.1016/j.ecss.2018.08.026
Ward M., Beheshti K. (2023). Lessons learned from over thirty years of eelgrass restoration on the US West Coast. Ecosphere 14 (8), e4642. doi: 10.1002/ecs2.4642
Ward M. A., Hill T. M., Souza C., Filipczyk T., Ricart A. M., Merolla S., et al. (2021). Blue carbon stocks and exchanges along the California coast. Biogeosciences 18 (16), 4717–4732. doi: 10.5194/bg-18-4717-2021
Watson S. (1890). Contributions to american botany. Proc. Am. Acad. Arts. Sci. 26, 124–163. doi: 10.2307/20013481
Waycott M., Duarte C. M., Carruthers T. J., Orth R. J., Dennison W. C., Olyarnik S., et al. (2009). Accelerating loss of seagrasses across the globe threatens coastal ecosystems. Proc. Natl. Acad. Sci. 106 (30), 12377–12381. doi: 10.1073/pnas.0905620106
Wegoro J., Pamba S., George R., Shaghude Y., Hollander J., Lugendo B. (2022). Seagrass restoration in a high-energy environment in the Western Indian Ocean. Estuarine. Coast. Shelf. Sci. 278, 108119. doi: 10.1016/j.ecss.2022.108119
Williams S. L. (2001). Reduced genetic diversity in eelgrass transplantations affects both population growth and individual fitness. Ecol. Appl. 11 (5), 1472–1488. doi: 10.1890/1051-0761(2001)011[1472:RGDIET]2.0.CO;2
Williams J. P., Claisse J. T., Pondella D. J. II, Williams C. M., Robart M. J., Scholz Z., et al. (2021). Sea urchin mass mortality rapidly restores kelp forest communities. Mar. Ecol. Prog. Ser. 664, 117–131. doi: 10.3354/meps13680
Williams C. M., Eagleton J. L., Pondella D. J., Claisse J. T. (2022). Habitat type and environmental conditions influence the age and growth of a temperate marine damselfish. Front. Mar. Sci. 9, 988158. doi: 10.3389/fmars.2022.988158
Wilson K. C., North W. J. (1983). A review of kelp bed management in southern California. J. World Maricult. Soc. 14 (1-4), 345–359. doi: 10.1111/j.1749-7345.1983.tb00089.x
Wong M. C., Griffiths G., Vercaemer B. (2020). Seasonal response and recovery of eelgrass (Zostera marina) to short-term reductions in light availability. Estuaries. Coasts. 43, 120–134. doi: 10.1007/s12237-019-00664-5
Wong M. C., Vercaemer B. M., Griffiths G. (2021). Response and recovery of eelgrass (Zostera marina) to chronic and episodic light disturbance. Estuaries. Coasts. 44, 312–324. doi: 10.1007/s12237-020-00803-3
York P. H., Gruber R. K., Hill R., Ralph P. J., Booth D. J., Macreadie P. I. (2013). Physiological and morphological responses of the temperate seagrass Zostera muelleri to multiple stressors: investigating the interactive effects of light and temperature. PloS One 8 (10), e76377. doi: 10.1371/journal.pone.0076377
Zhang Y. S., Gittman R. K., Donaher S. E., Trackenberg S. N., van der Heide T., Silliman B. R. (2021). Inclusion of intra-and interspecific facilitation expands the theoretical framework for seagrass restoration. Front. Mar. Sci. 8, 645673. doi: 10.3389/fmars.2021.645673
Zhou Y., Liu P., Liu B., Liu X., Zhang X., Wang F., et al. (2014). Restoring eelgrass (Zostera marina L.) habitats using a simple and effective transplanting technique. PloS One 9 (4), e92982. doi: 10.1371/journal.pone.0092982
Zimmerman R. C., Kohrs D. G., Steller D. L., Alberte R. S. (1997). Impacts of CO2 enrichment on productivity and light requirements of eelgrass. Plant Physiol. 115 (2), 599–607. doi: 10.1104/pp.115.2.599
Keywords: eelgrass, transplant, Zostera pacifica, PAR, DO, wave, thresholds
Citation: Sanders RD, Obaza AK, Grime BC, Lindhart M, Miller LP, Elsmore KE, Carmack OC, Ford TK and Leichter JJ (2024) Wave, light, and dissolved oxygen exposures drive novel coastal eelgrass (Zostera pacifica) transplant performance. Front. Mar. Sci. 11:1355449. doi: 10.3389/fmars.2024.1355449
Received: 13 December 2023; Accepted: 25 January 2024;
Published: 09 February 2024.
Edited by:
Richard K. F. Unsworth, Swansea University, United KingdomReviewed by:
W. Judson Kenworthy, Independent Researcher, Beaufort, SC, United StatesLuis G. Egea, University of Cádiz, Spain
Copyright © 2024 Sanders, Obaza, Grime, Lindhart, Miller, Elsmore, Carmack, Ford and Leichter. This is an open-access article distributed under the terms of the Creative Commons Attribution License (CC BY). The use, distribution or reproduction in other forums is permitted, provided the original author(s) and the copyright owner(s) are credited and that the original publication in this journal is cited, in accordance with accepted academic practice. No use, distribution or reproduction is permitted which does not comply with these terms.
*Correspondence: Rilee D. Sanders, cmRzYW5kZXJzQHVjc2QuZWR1