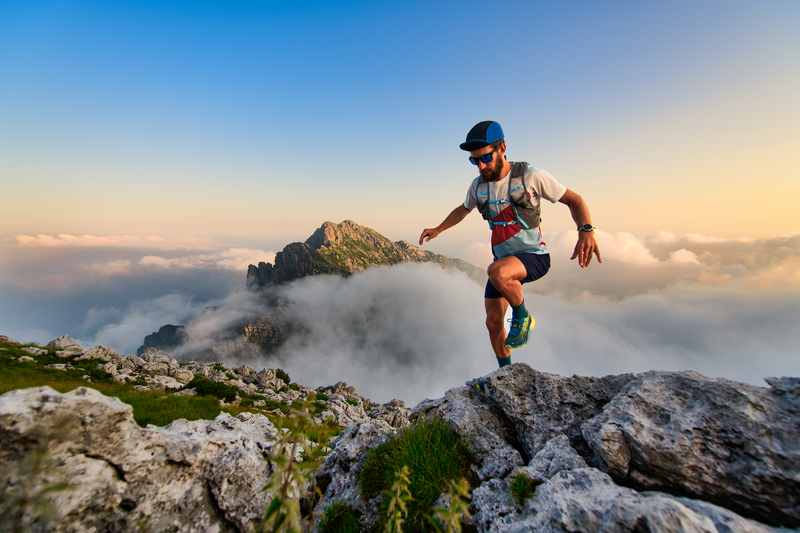
95% of researchers rate our articles as excellent or good
Learn more about the work of our research integrity team to safeguard the quality of each article we publish.
Find out more
ORIGINAL RESEARCH article
Front. Mar. Sci. , 23 January 2024
Sec. Coastal Ocean Processes
Volume 11 - 2024 | https://doi.org/10.3389/fmars.2024.1346275
This article is part of the Research Topic Impact of Ocean Forcing on the Coastal Hydrology, Environment and Freshwater Resources View all 12 articles
Management aquifer recharge (MAR) technology is widely applied to solve seawater intrusion caused by groundwater overexploitation in coastal areas. However, MAR creates an important pathway for microplastics (particle size< 5 mm) to enter groundwater. To explore the clogging potential of microplastics in aquifer media, a series of laboratory-scale column experiments were conducted in this study. The hydraulic conductivity of porous media and deposition amount of microplastics were investigated under different experimental conditions. In our study, most of the microplastics were intercepted in the sand column’s surface layer. The difference of particle size in porous media greatly influence the clogging development. The hydraulic conductivity of the aquifer media decreased as the microplastic particle size decreased. When the particle size of microplastic was larger than 300 mm, most of the microplastics deposits on the surface of the porous media, forming a “microplastic accumulation layer”. Microplastics are affected by particle size, flow shear stress and preferential flow during migration. The migration ability of microplastics increased significantly with the increase of hydraulic head difference and decreased with the increase of sand column depth. The bacteria microorganisms are projected to be a new biological control strategy in conjunction with MAR. The study of clogging risk of microplastics particles in porous media during artificial recharge provides novel and unique insights for the management and control of microplastic pollution in groundwater systems.
Groundwater plays vital role in drinking, agricultural, domestic, and industrial uses worldwide (Aeschbach-Hertig and Gleeson, 2012; Gorelick and Zheng, 2015). Groundwater overexploitation cannot be neglected worldwide (Fang et al., 2022a); which leads to a series of environmental problems, such as seawater intrusion, soil salinization, ground subsidence, etc (Fang et al., 2022b). MAR technology can effectively control seawater intrusion by replenishing groundwater, and form an underground freshwater curtain in the coastal region to prevent further seawater intrusion. In addition, groundwater pollution is one of the environmental problems that cannot be neglected (Re, 2019). Major sources of groundwater pollution include agricultural runoff (Abdalla and Khalil, 2018), reuse of wastewater (Luo et al., 2014), biosolids (Gago et al., 2016), and industrial activities (Xu et al., 2021). Besides, groundwater can be contaminated by a wide variety of emerging pollutants related to anthropogenic activities (Lapworth et al., 2012; Hoellein et al., 2021; Bayabil et al., 2022; Khan, 2022) such as per- and poly-fluoroalkyl substances (PFASs) (Szabo et al., 2018; Hepburn et al., 2019) and pharmaceuticals (Sui et al., 2015). This prevents the beneficial use of groundwater resources and requires costly and technically challenging remediation (Smith et al., 2014).
Plastic products are widely used in human life and industry production due to their low cost, high ductility and stable properties (Paul-Pont et al., 2018; Murawski et al., 2022). Plastics can be classified into five types according to diameter: macroplastics (>25 mm), mesoplastics (1–25 mm), large microplastics (1–5 mm), small microplastics (1–1000 μm), and nanoplastics(<1 μm) (Eriksen et al., 2014; Dris et al., 2015; Alimi et al., 2018; Hanvey, 2023). Microplastics refer to fragments of plastic particles with size smaller than 5 mm (Chae et al., 2023; Leusch et al., 2023). As an emerging pollutant, microplastics have attracted great attention worldwide (Alimi et al., 2018) due to the large amount and wide distribution. From 1950 to 2017, global production of plastics increased from 1.5 million tons to 320 million tons and is expected to double within the next 20 years (Alimi et al., 2018; Wanner, 2021). However, case of the low recovery rate of plastics and difficult to decompose, it leads to serious environmental pollution. Microplastics are abundant in freshwater, marine and terrestrial environments, from beaches to the deepest part of the Mariana Trench (Cincinelli et al., 2021) and from cities to remote areas (Zhang et al., 2016), even in locations far from point sources such as Mount Everest (Napper et al., 2021). It is estimated that 71% of plastics produced globally were discarded and ended up in the environment (Geyer et al., 2017; Hohn et al., 2020). About 275 million metric tons of plastic trash from 192 coastal regions/nations entered the sea, totaling 4.8–12.7 million metric tons (Pirsaheb et al., 2020). In 2017-2018, 3.5 million tons of plastic were produced in Australia, with only 11% (38.5 thousand tons) of the plastics being recycled (Samandra, 2022). In recent years, the presence of microplastics in groundwater has been verified (Alnahdi et al., 2023). The maximum concentration of microplastics was 15.2 particles/L detected in karst aquifers in Illinois, USA (Panno et al., 2019). Microplastics (concentration of 0-7 microplastics/m3) with a particle size of 50-150 μm were detected in groundwater in northern Germany (Mintenig et al., 2019). The average amount of microplastics detected across all sites in groundwater bores from Australia was 38 ± 8 microplastics/L (Samandra, 2022). Panno et al. (2019) proposed that microplastics are transported to karst groundwater through two potential ways: (1) Fractures and crevices in underlying aquifers in karst systems. (2) Groundwater contamination by septic systems and sinkholes in rural areas, leaky municipal sewage lines, and surface runoff. This confirms that hydrogeologic connections from the surface to the underlying aquifers provide a potential pathway for microplastics from the ground surface to groundwater.
In recent years, managed aquifer recharge (MAR) technology has been used in a large number of engineering applications to combat various environmental problems caused by groundwater overexploitation, such as seawater intrusion, which provides a potential pathway for microplastics to enter the groundwater environment (Wang H. et al., 2023; Wang et al., 2020). Some researchers have proposed that more studies are needed to investigate the presence of microplastics (MPs) in soils and groundwater. Microplastics possess stable carbon-hydrogen bonds. The chemical stability of microplastics and its resistance to degradation (Krueger et al., 2015; Geyer et al., 2017) make it face long degradation duration in natural environment. Long degradation duration and relatively large specific surface area of microplastics (Cole et al., 2011) allow it to easily adsorb some toxic substances, such as heavy metals (Ashton et al., 2010), endocrine disrupting chemicals (Ng and Obbard, 2006)and persistent organic pollutants (POPs) (Andrady, 2011; Guo et al., 2012). Some of the chemicals that microplastics contains are also threats to surrounding environment, such as plasticizers (Koelmans et al., 2019), bisphenol A (BPA), nonylphenol (NP), etc. Due to the small size, microplastics can be ingested by plants and animals; In fact, microplastic fragments have been commonly found in the intestines of fish (Tanaka and Takada, 2016), the digestive tracts of shellfish (Van Cauwenberghe and Janssen, 2014; Rochman et al., 2015), the feces of birds (Provencher et al., 2018), marine top predators such as whales (Nelms et al., 2018), and soil organisms, such as earthworms. Microplastics with small particle sizes are then likely to accumulate in organisms, causing physical damage such as ruptures in the gut and digestive tract (Yang X et al., 2022), abrasions and clogging (Guo et al., 2020). The ineligible harm of microplastic pollution to environment and organisms have attracted great attention.
Existing studies on microplastic contamination in the subsurface environment mainly focus on summarizing its sources and distribution, separation and detection methods, and ecotoxicity. Some researchers have paid attention to the impact of microplastics on saturation zone. For example, it has been reported that the penetration rate of microplastics in saturated sand increased as the particle size of polystyrene (PS) microplastics decreased from 2.0 mm to 0.8 mm (Dong, 2018). This indicates that the physicochemical properties of microplastics influence their ability of transport in porous media. The physical properties of porous media, such as particle size and non-homogeneity, also play an important role in the transport behavior of microplastics. Macroscopic inhomogeneity can cause preferential flow, enabling microplastics to be transported to deeper media. (Majdalani et al., 2008). Microscopic inhomogeneities, such as media surface roughness, can control the transport behavior of particles by affecting the forces between the media surface and particles (Li et al., 2019). The pore water flow rate in porous media is also an important factor affecting the deposition and release of microplastics on the surface of aqueous media (Tong and Johnson, 2006). Research on the transport behavior of microplastics in porous media has increased significantly; however, the migration of microplastic particles in porous media mainly focuses on small particle sizes (Yan et al., 2020).There are still many physical and chemical factors that have not been taken into account, such as the particle size of porous media, the types of microplastic particles, as well as hydraulic condition, which require further research. There are still research gaps in microplastics migration and impact of microplastics on groundwater that need to be explored deeply. During artificial recharge process which was conducted to solve the problem of seawater intrusion, the transport behavior of microplastics from subsurface water to groundwater influenced by these factors lack of investigation.
In this study, the clogging risk of microplastics in aqueous media was investigated by a laboratory experiment, which simulate the artificial recharge process. The effects of different influencing factors, such as particle size (aqueous medium and microplastics) and hydrodynamic conditions, on the clogging of microplastics was explored in porous media during artificial recharge. The temporal and spatial variation of hydraulic conductivity influenced by particle size (aqueous media and microplastics) were investigated. The potential clogging risk of microplastics was evaluated. The preventive and controlled measures of microplastic clogging were proposed to guide MAR engineering applications.
The experimental device consisted of a column, water pump, waterhead constant device, water tank (including stirring device), and piezometer plate. The column was made of plexiglass with a height of 15 cm and an inner diameter of 4 cm. An overflow port was set 2 cm from the top of the column. Piezometer ports were placed at intervals of 3 cm along the column, numbered Y1, Y2, Y3, Y4, Y5 from top to bottom on the right side. Five sampling holes were set on the left side of the column at intervals of 3 cm and numbered A, B, C, D, E from top to bottom. The schematic diagram of the experimental device is presented in Figure 1.
Standard quartz sand (loss on ignition ≤ 0.40%, SiO2 > 96%, Xiamen ISO Standard sand Co., Ltd, China) was used as porous media. The porous media was rinsed several times and soaked in 0.25 mol/L HCl for 12 h to remove metal impurities. The sand was rinsed with water to neutral pH and dried at 105 °C. After that, the sand was cauterized in a muffle furnace at 550 °C for 2 h to remove organic matter and sterilized by ultraviolet light before use. The simulated recharge water was prepared with PS microsphere (100 mg/L). PS microspheres was washed and dried at 25 °C before staining treatment with 1 μg/ml Nile Red-methanol solution.
The amount of microplastic deposition on the aqueous media surface of the entire sand column was calculated using mass method. Sterilized sand samples were packed into the Plexiglas column according to the wet method with equal volume weight (1.63 g/cm3). Sterile water was slowly passed from the bottom of the sand column to saturate the sand column for 24 h to remove any remaining bubbles. Recharge water containing microplastics was introduced to the sand column from the top of the sand column. The values of the pressure plate and the flow rate at the outlet were recorded over time.
Column experiments were designed to simulate the process of artificial recharge in laboratory under conditions of continuous water supply. The influential factors, such as particle size of the aqueous media (AM) and microplastics (MP), and the hydrodynamic conditions (HC), were taken into consideration to investigate the clogging risk of microplastic particles. To minimize the possibility of accidental errors, our experiment was conducted in triplicate. The main groups and parameter designs were listed in Table 1.
Hydraulic conductivity K was calculated according to Darcy’s law, shown as Equation 1.
Q is the flow rate at the outlet (m3/d); “Δx” is the distance between any two pressure measuring tubes (m); “Δh” is the head difference between the two pressure measuring tubes (m); “d” is the inner diameter of the sand column (m).
In order to reflect the variation of the hydraulic conductivity of the sand column more intuitively, the relative hydraulic conductivity K’ is introduced in this study (Equation 2).
Where K0 is initial hydraulic conductivity of the sand layer (m/d); Kt is hydraulic conductivity of any sand layer at any moment (m/d). In this research, K’ between 0-0.30 is considered as “significant clogging”, K’ between 0.30-0.80 is defined as “moderate clogging”, and K’ between 0.80-1.00 is regarded as “mild clogging”.
The amount of microplastic deposition on the aqueous media of the entire sand column was calculated using mass method. At the end of the experiment, the sand column was carefully placed horizontally and the wire mesh was removed. The sand sample was collected and dried until constant weight. The mass was recorded as m0 (g).
The saturated NaCl solution was added to the sand samples and treated with an ultrasonic machine for 2 min. The sample was stirred with magnetic stirrer for 5 min. Then the microplastics was filtered repeatedly until the aqueous medium is clean. The constant weight of the sand sample is recorded as md (g). The amount of deposition SS (g) is obtained using Equation 3.
Microplastics with diameter of 100 μm, 300 μm, and 500 μm were visualized by a fluorescence microscope (Panalytical Axios FAST, Freebo International Co., Ltd, Netherlands), as shown in Figure 2. Fluorescent particles in all fields of view are captured and photographed by a camera inside the fluorescence microscope. The size of the particles detected by the fluorescence microscope is calculated based on the square root of the particle area. The area was analyzed and measured using ImageJ software.
Figure 3 describes the change in overall relative hydraulic conductivity (overall K’) of entire sand column and the change in relative hydraulic conductivity (K’) of each seepage layer over time during recharge (AB: 0-3 cm, BC: 3-6 cm, CD: 6-9 cm, DE: 9-12 cm). The overall K’ in different experimental groups has different changing trends over time. Within the first 25 h, the overall K’ of the sand column in AM groups changed similarly, all dropping to about 0.90. However, as the recharge continued, the differences of overall K’ gradually became apparent. The overall K’ values of sand column in AM-C and AM-M both decreased to around 0.60 at 40 h, which was more significant than that in AM-F (dropped to 0.90). After 66 h, the overall K’ values in AM-C dropped to 0.36, which decreased by 63.8%, similar to that in AM-F which decreased by 64.0% with a value of 0.36. The overall K’ values in AM-M decreased most significantly to 0.18, with a rate decreasing by 81.0%.
Figure 3 Spatial and temporal variation of hydraulic conductivity. (A) Overall hydraulic conductivity variation in experimental groups with different grain size (B) hydraulic conductivity variation of each seepage layer in coarse sand (AM-C) (C) hydraulic conductivity variation of each seepage layer of medium sand (AM-M) (D) hydraulic conductivity variation of each seepage layer of fine sand (AM-F).
Figure 4 shows the relationship between the deposition amount of microplastics in AM groups and the overall K’ after 66 h of recharge. It can be seen that the overall K’ of the sand column in AM-M is lowest among AM groups, and the deposition amount is up to 6.00 g. The deposition amount in AM-F is 4.69 g, which is similar to that in AM-C group with a value of 4.67 g. The deposition amount of microplastics is closely related to the hydraulic conductivity of the sand column. Figures 3B-D shows the change of K’ in each seepage layer for different particle size media in AM groups. The relative hydraulic conductivity of surface layers (AB layer) continues to decrease during the whole recharge process. This indicates that microplastics have a certain degree of deposition in the surface layer of the aqueous media, which caused the occurrence of clogging. However, the degree of surface clogging among these groups was obvious different. In this study, the clogging occurring in the surface layers (AB:0-3 cm) of the sand column was defined as the surface clogging, and the clogging occurred in other layers of the sand column (BC, CD, DE layers) was regarded as the internal clogging. This is consistent with others’ studies. Huang (2021) observed that suspended particles mostly settle in the surface layer of the medium, causing more serious surface clogging than deep clogging in sand columns. Moreover, due to the different relative diameter ratios (dp/Dp) between suspended particles and porous media, the clogging degree of porous media in different layers and has difference in the same layer among three experimental groups. The relative diameter ratio of aqueous medium was larger in AM-C than that in the other two groups. When recharge process begins, microplastic particles migrate downward with water flow through larger pores in the medium, and a small part stays in the BC layer causing clogging. However, most of the microplastic particles migrate deeper into sand columns until DE layer and then migrate out of the sand columns. Among the AM groups, the pore throat diameter in AM-F group was the smallest, and the clogging of surface layer (AB layer) developed the fastest. The overall K’ values of AM-F dropped to 0.88 within 10 h. However, there is a delayed drop trend of K’ after 11 h and a continuous drop after 40 h due to accumulation of microplastics deposition in sand columns. Due to severe surface clogging in AM-F, most microplastic particles are trapped by AB layer and BC layer. The change of K’ in deep layers (CD layer and DE layer) was not obvious in AM-F. The K’ of each layer in AM-M exhibits a downward trend with varying degrees. It indicates that microplastic particles are intercepted and deposited in each seepage section of AM-M, which causes cake-filtration clogging on the surface layer and internal clogging inside sand columns. As a result, the most severe overall clogging development and the largest overall K’ decrease of porous media occurred in AM-M.
Figure 4 Relationship between the deposition amount of microplastics in different media grain size group and the overall relative hydraulic conductivity K’ after end of recharge.
Based on the results above, when recharge water contained with 100 μm microplastics particles (100 mg/L), medium size has greater impact on the microplastic transport and clogging behavior in porous media. The hydraulic conductivity in different experimental groups (AM-C, AM-M and AM-F) all showed a downward trend. However, the effect of different particle size of sand media on microplastic migration was obviously different. When the pore size of the coarse sand medium is larger, it is easier for microplastics to migrate through pore channels. However, the effect of particle size on microplastic migration was obviously different. The pore size of the coarse sand medium is larger, which is easier for microplastics to migrate through pore channels. Thus, the clogging development in AM-C is slower than the other two groups. The pore size of sand media in AM-F is smaller than that in the other two groups, forming a “cake-like” clogging on the surface of the medium. Under the combined action of gravity and hydrodynamics, the pressure on the particles continues to increase. When the pressure exceeds the limit that maintains the original stable equilibrium, the surface layer is compacted and microplastic particles are forced to migrate to deeper pore channels, resulting in periodic exacerbations of clogging. The overall clogging of the sand column also develops slowly in fine sand group. While the pore size of sand media in AM-M is moderate, part of the microplastics accumulated in the surface layer of the sand column to form surface clogging. Part of the microplastics penetrate into the deep sand column through medium pores and form internal clogging. Due to the development of internal-surface double clogging, clogging in AM-M is most severe.
To explore the influence of microplastic particle size on the hydraulic conductivity, the overall K’ of the sand column and K’ of different layers (AB: 0-3 cm, BC: 3-6 cm, CD: 6-9 cm, DE: 9-12 cm) with time are shown in Figure 5. According to Figure 5A, it was observed that particle size of microplastic has significant effect on the clogging of porous media. At the end of recharge, K’ of the aqueous media is 0.18 and the final hydraulic conductivity decreases by 81.9% in MP-100. However, the overall K’ fluctuates around 1.00 after 66 h of recharge in MP-300 and MP-500. It can be concluded that, microplastics with a particle size of 100 μm cause significant clogging, while microplastics with particle sizes of 300 μm and 500 μm hardly cause clogging. One possible explanation is that particles with smaller sizes have greater migration ability than the bigger ones. The migration distance of microplastics increases as the particle size decreases. When the particle size of microplastics increases to a certain extent, it is difficult for microplastics to penetrate into the porous medium. Thus, large amount of microplastics accumulates on the surface of the aqueous medium to form a “microplastic accumulation medium layer”.
Figure 5 Spatial and temporal variation of hydraulic conductivity. (A) Overall hydraulic conductivity variation in experimental groups with different microplastics size (B) hydraulic conductivity variation of each seepage layer of 100 μm microplastics (MP-100) (C) hydraulic conductivity variation of each seepage layer of 300 μm microplastics (MP-300) (D) hydraulic conductivity variation of each seepage layer of 500 μm microplastics (MP-500).
Figures 5B-D show that the surface layer (AB layer) is clogged when microplastic particle size is 100 μm. At the same time, microplastic particles migrate downward and are intercepted in BC and CD layers to form internal clogging. Therefore, there is a certain decrease in hydraulic conductivity of BC and CD layers. And K’ of AB layer has a slight decrease under the 300 μm microplastic recharge, and the changes in K’ of other seepage sections are not obvious over time. When recharging with 500 μm microplastics particle, hydraulic conductivity of porous media remains basically unchanged. The particle sizes of microplastics in our experimental groups are much larger compared to the size of porous media, which makes it difficult for microplastics to migrate into porous media and can only deposit on the surface layer of experimental sand column. After the end of recharge, the amount of microplastic particles deposited in the quartz sand column both reached about 7.5 g in MP-300 and MP-500. The deposition amount of microplastic particles in MP-100 was 5.8 g, which was less than that in MP-300 and MP-500. The quartz sand media acts as a filter, allowing water to pass through while larger microplastic particles trapped. This process contributes to the formation of the accumulation layer. Due to the restriction of particle size, a “microplastic accumulation layer” was formed on the surface layer of porous media. A new type of plastic material in the environment-plastic-rock complexes was formed when plastic debris irreversibly sorbs onto the quartz-dominated after historical flooding events (Wang L. et al., 2023). Due to the limitation of experimental conditions, this plastic−rock complex was not observed in this study, but the effect of microplastic particles on particles in aqueous media can also be observed.
In Figure 6, although there is a large amount of 300 μm and 500 μm microplastic deposition, hydraulic conductivity does not decrease correspondingly. This also indicates that there are large amounts of microplastics deposition in MP-300 and MP-500. However, almost all of the microplastics deposit on surface layer of porous media to form “microplastic accumulation medium layer” without migrating into sand column through pore channels. Therefore, although there is a large amount deposition of microplastics in MP-300 and MP-500 groups, there are no decrease occurred in hydraulic conductivity, which is a very interesting phenomenon. In further studies, the complex interactions of physical adhesion, chemical binding and microbial colonization can be investigated during the development of “microplastic accumulation layer”.
Figure 6 Relationship between the deposition amount of microplastics in different microplastics size group and the overall relative hydraulic conductivity K’ after end of recharge.
Under different hydraulic heads (ΔH=5 cm, 10 cm, 15 cm), effects of microplastics on the hydraulic conductivity were studied. The overall K’ of the sand column and K’ of different seepage layers (AB: 0-3 cm, BC: 3-6cm, CD: 6-9 cm, DE: 9-12 cm) were measured over time, as shown in Figure 7. It is shows that the overall K’ of the sand column decreased over time under three different hydraulic conditions. At the end of recharge, the overall K’ decreased most rapidly and significantly under HC-5, dropping to 18.0% of its initial value. The K’ decreased by 22.0% to 0.78 under HC-10 and only decreased by 10.0% to 0.91 under HC-15, respectively. The effect of microplastic particles on the overall K’ of sand column decreases with the increase of hydraulic heads. This trend is associated with the increased water flow velocity resulting from the increased hydraulic heads. Figures 7B-D show that the hydraulic conductivity of the surface layer (AB layer) decreased most significantly under all hydraulic conditions with the greatest decrease observed under HC-5. The K’ in the surface layer (AB layer) of the sand column decreases to about 0.50 in group HC-10 and group HC-15. Microplastic particles have a substantial impact on the permeability of the surface layer of the aqueous media under the condition with different hydraulic heads. With higher hydraulic heads, microplastics do not cause clogging in the surface layer but instead migrate deeper into the sand column. The hydraulic conductivity of BC and CD layer all decreased to around 0.80 at the end of the experiment under HC-5. Under the condition of HC-10, K’ decreased to around 0.90 in both in BC and CD layer. This indicates that microplastic particles exhibit higher deposition in BC and DE layer in the HC-5 group. This indicates that the K’ of BC, CD, and DE layer under different hydraulic conditions has different change patterns, with a general trend of decreasing change as hydraulic head increases. This is closely related to the migration of microplastics in each layer of the sand column under different hydrodynamic conditions.
Figure 7 Spatial and temporal variation of hydraulic conductivity. (A) Overall hydraulic conductivity variation in experimental groups with different hydrodynamic conditions (B) hydraulic conductivity variation of each seepage layer of DH= 5cm (HC-5) (V) (C) hydraulic conductivity variation of each seepage layer of DH=10cm (HC-10) (D) hydraulic conductivity variation of each seepage layer of DH=15cm (HC-15).
Combined with deposition amount shown in Figure 8, it was seen that microplastic deposition in the sand column was about four to five times higher under HC-5 than that under HC-10 or HC-15. Under lower hydraulic head conditions, the water flow velocity is relatively slow, resulting in a higher possibility of microplastic particles being trapped and deposited in the aqueous media. Under conditions with higher hydraulic heads, microplastics deposited less in the sand column because they had a greater migration ability due to higher flow rates. Besides that, during recharge process, microplastic particles did not have enough time to deposit in pore throats due to water shear forces and were forced to migrate downward until they exited the sand column. This effect increases with increasing hydraulic head difference and decreases with increasing sand column depth.
Figure 8 Relationship between the deposition amount of microplastics in different hydraulic head group and the overall relative hydraulic conductivity K’ after end of recharge.
Particle migration is influenced by many factors, such as particle properties, medium properties, hydraulic conditions, physicochemical property of water. Figure 9 shows the main mechanisms of microplastic particles settling along the water flow in our study. Microplastics have the characteristics of large specific surface area, long persistence and difficult degradation, which is significant different from traditional physical particles in water. (Wu P. et al., 2019; Maguire and Gardner, 2023; Yang H. et al., 2022). As typical organic synthetic polymer, microplastics have high hydrophobicity (Li et al., 2020). The properties of microplastics directly affect its deposition, migration and transformation of degradation (He et al., 2022). Due to the high hydrophobicity between microplastic particles, the double electric layer of microplastic particles is compressed and thinned under certain conditions, and the surface charge is shielded (Wang et al., 2022). As the electrostatic repulsion between particles decreases, it is easier for microplastic particles to undergo the coagulation (Wu J. et al., 2019). The coagulated microplastic agglomerates have larger particle size than the single one, which is not conducive to their transport in medium pores and increases the possibility of microplastic particle clogging (Jiang et al., 2022). The larger the medium particle size, the more conducive it is for microplastic particle to move out. Porous media with smaller particle sizes have smaller pore throats and permeability (He et al., 2023). This is well proved by the difference in hydraulic conductivity of aquifer medium among AM-F, AM-M and AM-C groups in this study. The interception of particles by the aqueous media plays a vital role during recharge process. Therefore, the migration and deposition processes of microplastics in porous media are a result of the interaction of media particle size, pore structure, and microplastic particle properties (Li and Prigiobbe, 2018; Li et al., 2023).
In our study, when the diameter of microplastic particles is larger than the pore diameter of the sand medium, the microplastic particles will collide with the pore wall and be filtered out. It caused a large amount of microplastic particles to deposit on the surface of porous medium and formed a “cake-like” clogging phenomenon and “microplastic accumulation layer”. Besides the interception effect, gravity deposition and bridging are also important effect influence the microplastic mitigation and deposition (Bydalek et al., 2023). Microplastic particles bridged with each other and accumulate in pores influenced by gravity and water flow. In addition, the flow rate of pore water in porous media is also an important factor affecting the deposition and release of microplastics on media surfaces. With increasing water flow, microplastics are subjected to stronger shear stress during migration process. This leads to shorter deposition time of microplastics and makes it more difficult to deposit on media surface (Li et al., 2023). In natural environment, differences in the particle size of the media particles, the influence of animals and plants, and other factors create preferential channels through which water can pass through at a rapid rate (Bianchi et al., 2011).
Under preferential flow, water pass through the column with higher flow rate and may greatly influence the migration behavior of microplastics (Li and Prigiobbe, 2018). Thus, microplastics mainly migrate through preferential channels instead of being deposited throughout the porous medium uniformly. This preferential effect usually becomes more significant with increasing water flow. In our study, the particles in the aqueous medium mainly filter and deposit microplastic particles. However, in MP group, the large microplastic particles deposit on surface layer of porous media to form “microplastic accumulation medium layer” without migrating into sand column through pore channel; Under conditions with higher hydraulic heads, microplastic particles did not have enough time to deposit in pore throats due to shear forces and were forced to migrate downward until they exited the sand column. The deposition of microplastics in porous media is a complex process that takes into account multiple factors, such as microplastic particle characteristics, media characteristics, and hydrodynamic conditions (He et al., 2020). An intensive analysis of these mechanisms was beneficial for more accurately assess and the effective management of microplastics and provide a scientific basis for the development of more effective prevention and control strategies (Jiang et al., 2022).
In an effort to mitigate the clogging risk of microplastics in the management of artificial aquifers, a range of preventative and remedial approaches have been implemented in engineering practice. Although recharge water can be pretreated via sedimentation, coagulation, filtration, advanced oxidation, and disinfection prior to artificial recharge (Jeong et al., 2018), which lessens the clogging potential. These traditional pretreatment methods may be not applied well to microplastics. Similarly, rehabilitation methods include backwashing, surging, jetting, under-reaming, acidification, and biocide, which help restore the artificial recharge system may not function well in recharge system contained microplastics (Jeong et al., 2018). Due to the high chemical inertness and durability of microplastics, they are resistant to traditional biodegradation or chemical treatment, resulting in longer persistence and greater accumulation effects when clogging occurs with microplastics (Guo and Wang, 2019). Under certain hydrodynamic conditions, microplastic particles exhibit higher adsorption ability, lower density and stronger compressive than other particles, which facilitate clogging caused by microplastics to form larger aggregates, hinder their discharge from the aquifer, and alter the pore structure and permeability of the medium (Lim et al., 2022). Traditional treatments of media clogging have limited effectiveness in addressing clogging caused by microplastics. Clogging by microplastics not only compromises the technical feasibility and economic feasibility of MAR to solve seawater intrusion, but also poses potential risks to the environment and human health (Peng et al., 2017). For instance, microplastics may carry toxic or harmful substances or pathogens into aquatic environment, causing physiological or behavioral interference or damage to organisms (Pereao et al., 2020; Yang X. et al., 2022). These risks may be amplified over time. It has demonstrated that microplastics have transported into the aqueous media layer, forming a “plastid-rock complex” by interaction of physical adhesion, chemical binding and microbial colonization (Wang L et al., 2023). In this study, it is observed that the large plastic particles form a “microplastic accumulation layer” and affect the permeability of the aquifer medium.
Therefore, in-depth research and discussion on the clogging behavior of microplastics are needed during managed aquifer recharge (MAR). The generation and discharge of microplastics should be controlled from the source water. The monitoring and analysis of various types and sources of microplastics should be strengthened. The main sources and pathways should be identified and the effective emission reduction measures and policies should be formulated. This requires using more strict quality standards and more advanced treatment technologies. For example, certain microorganisms are verified to be able to degrade microplastic. Over 90 microorganisms, including bacteria and fungi, have been known to degrade petroleum-based plastics (Jumaah et al., 2017) mostly in vitro condition. Galleria melonella have been found capable of hydrolyzing polyethylene (PE) (Yang et al., 2014) such as Bacillus spp. (Sudhakar et al., 2008; Abrusci et al., 2013), Rhodococcus spp. (Bonhomme et al., 2003; Gilan et al., 2004; Fontanella et al., 2010), and Pseudomonas spp. (Rajandas et al., 2012). These microorganisms are expected to be combined with artificial aquifer management in the future to solve the problem of microplastic clogging as a new biological control method. At the same time, future research can focus on the migration behavior and clogging treatment of microplastics by disclosing the mechanism and estimating their influence and risk on MAR.
This study focuses on the transport behavior of microplastic particles and simulates the clogging of microplastics during artificial recharge by sand column experiments in laboratory. Most of the microplastics were intercepted in the sand column’s surface layer. Internal clogging was more prevalent in the coarse sand group, while surface clogging was more prevalent in the fine sand group. Both types of clogging occurred in the medium sand. The hydraulic conductivity of the aqueous medium remains almost unaltered with a “microplastic accumulation medium layer” developed when the microplastic particle size are larger than 300 mm. The increase in water head difference increased the inflow velocity, which decreased the deposition period of microplastics on the medium’s surface and hence enhanced their ability to migrate. This effect increases as the water head difference grows and reduces as the sand column depth increases. In MAR projects, to alleviate the problem of microplastic clogging in the future, bacteria microorganisms with the ability to degrade microplastics have broad application prospect in conjunction with artificial aquifer management. Further systematic and in-depth research is required to elucidate the extent and impact of different factors.
The raw data supporting the conclusions of this article will be made available by the authors, without undue reservation.
HW: Conceptualization, Funding acquisition, Investigation, Writing – original draft. Writing – review & editing. JZ: Data curation, Validation, Writing – original draft. YC: Supervision, Writing – review & editing. YX: Validation, Writing – review & editing. PJ: Investigation, Methodology, Writing – review & editing. HL: Methodology, Writing – review & editing.
The author(s) declare financial support was received for the research, authorship, and/or publication of this article. This study was supported by the National Natural Science Foundation of China (42207064), Guangxi Science and Technology Planning Project (Grant No. GuiKe-AD 21220079), and the National Natural Science Foundation of Guangxi Province, China (Grant No. 2022GXNSFBA035577).
The authors declare that the research was conducted in the absence of any commercial or financial relationships that could be construed as a potential conflict of interest.
All claims expressed in this article are solely those of the authors and do not necessarily represent those of their affiliated organizations, or those of the publisher, the editors and the reviewers. Any product that may be evaluated in this article, or claim that may be made by its manufacturer, is not guaranteed or endorsed by the publisher.
Abdalla F., Khalil R. (2018). Potential effects of groundwater and surface water contamination in an urban area, Qus City, Upper Egypt. J. Afr. Earth Sci. 141, 164–178. doi: 10.1016/j.jafrearsci.2018.02.016
Abrusci C., Pablos J. L., Marín I., Espí E., Corrales T., Catalina F. (2013). Comparative effect of metal stearates as pro-oxidant additives on bacterial biodegradation of thermal- and photo-degraded low density polyethylene mulching films. Int. Biodeterior. Biodegrad. Biodeterior. 83, 25–32. doi: 10.1016/j.ibiod.2013.04.002
Aeschbach-Hertig W., Gleeson T. (2012). Regional strategies for the accelerating global problem of groundwater depletion. Nat. Geosci. 5, 853–861. doi: 10.1038/ngeo1617
Alimi O. S., Budarz J. F., Hernandez L. M., Tufenkji N. (2018). Microplastics and nanoplastics in aquatic environments: aggregation, deposition, and enhanced contaminant transport. Environ. Sci. Technol. 52, 1704–1724. doi: 10.1021/acs.est.7b05559
Alnahdi K. A., Alali L. W., Suwaidan M. K., Akhtar M. K. (2023). Engineering a microbiosphere to clean up the ocean – inspiration from the plastisphere. Front. Mar. Sci. 10. doi: 10.3389/fmars.2023.1017378
Andrady A. L. (2011). Microplastics in the marine environment. Mar. pollut. Bull. 62, 1596–1605. doi: 10.1016/j.marpolbul.2011.05.030
Ashton K., Holmes L., Turner A. (2010). Association of metals with plastic production pellets in the marine environment. Mar. pollut. Bull. 60, 2050–2055. doi: 10.1016/j.marpolbul.2010.07.014
Bayabil H. K., Teshome F. T., Li Y. C. (2022). Emerging contaminants in soil and water. Front. Environ. Sci. 10. doi: 10.3389/fenvs.2022.873499
Bianchi M., Zheng C., Wilson C., Tick G. R., Liu G., Gorelick S. M. (2011). Spatial connectivity in a highly heterogeneous aquifer: From cores to preferential flow paths. Water Resour. Res. 47, W05524. doi: 10.1029/2009WR008966
Bonhomme S., Cuer A., Delort A. M., Lemaire J., Sancelme M., Scott G. (2003). Environmental biodegradation of polyethylene. Polymer Degrad. Stabil. 81, 441–452. doi: 10.1016/S0141-3910(03)00129-0
Bydalek F., Ifayemi D., Reynolds L., Barden R., Kasprzyk-Hordern B., Wenk J. (2023). Microplastic dynamics in a free water surface constructed wetland. Sci. Total Environ. 858, 160113. doi: 10.1016/j.scitotenv.2022.160113
Chae B., Oh S., Lee D. G. (2023). Is 5 mm still a good upper size boundary for microplastics in aquatic environments? Perspectives on size distribution and toxicological effects. Mar. pollut. Bull. 196, 115591. doi: 10.1016/j.marpolbul.2023.115591
Cincinelli A., Scopetani C., Chelazzi D., Martellini T., Pogojeva M., Slobodnik J. (2021). Microplastics in the black sea sediments. Sci. Total Environ. 760, 143898. doi: 10.1016/j.scitotenv.2020.143898
Cole M., Lindeque P., Halsband C., Galloway T. S. (2011). Microplastics as contaminants in the marine environment: A review. Mar. Mar. pollut. Bull. 62, 2588–2597. doi: 10.1016/j.marpolbul.2011.09.025
Dong Z. (2018). Size-dependent transport and retention of micron-sized plastic spheres in natural sand saturated with seawater. Water Res. 143, 518–526. doi: 10.1016/j.watres.2018.07.007
Dris R., Imhof H., Sanchez W., Gasperi J., Galgani F., Tassin B., et al. (2015). Beyond the ocean: contamination of freshwater ecosystems with (micro-)plastic particles. Environ. Chem. 12, 539. doi: 10.1071/EN14172
Eriksen M., Lebreton L. C. M., Carson H. S., Thiel M., Moore C. J., Borerro J. C., et al. (2014). Plastic Pollution in the World’s Oceans: More than 5 Trillion Plastic Pieces Weighing over 250,000 Tons Afloat at Sea. PloS One 9, e111913. doi: 10.1371/journal.pone.0111913
Fang Y., Zheng T., Guo B., Zhan H., Wang H., Zheng X., et al. (2022a). Transformation in the stability of tide-induced upper saline plume driven by transient external forcing. Water Resour. Res. 58, e2021WR031331. doi: 10.1029/2021WR031331
Fang Y., Zheng T., Wang H., Zheng X., Walther M. (2022b). Influence of dynamically stable-unstable flow on seawater intrusion and submarine groundwater discharge over tidal and seasonal cycles. J. Geophys. Res.: Oceans 127, e2021JC018209. doi: 10.1029/2021JC018209
Fontanella S., Bonhomme S., Koutny M., Husarova L., Brusson J.-M., Courdavault J.-P., et al. (2010). Comparison of the biodegradability of various polyethylene films containing pro-oxidant additives. Polymer Degrad. Stabil. 95, 1011–1021. doi: 10.1016/j.polymdegradstab.2010.03.009
Gago J., Galgani F., Maes T., Thompson R. C. (2016). Microplastics in seawater: recommendations from the marine strategy framework directive implementation process. Front. Mar. Sci. 3. doi: 10.3389/fmars.2016.00219
Geyer R., Jambeck J. R., Law K. L. (2017). Production, use, and fate of all plastics ever made. Sci. Adv. 3, e1700782. doi: 10.1126/sciadv.1700782
Gilan I., Hadar Y., Sivan A. (2004). Colonization, biofilm formation and biodegradation of polyethylene by a strain of Rhodococcus ruber. Appl. Microbiol. Biotechnol. 65, 97–104. doi: 10.1007/s00253-004-1584-8
Gorelick S. M., Zheng C. (2015). Global change and the groundwater management challenge. Water Resour. Res. 51, 3031–3051. doi: 10.1002/2014WR016825
Guo J.-J., Huang X.-P., Xiang L., Wang Y.-Z., Li Y.-W., Li H., et al. (2020). Source, migration and toxicology of microplastics in soil. Environ. Int. 137, 105263. doi: 10.1016/j.envint.2019.105263
Guo X., Wang J. (2019). The chemical behaviors of microplastics in marine environment: A review. Mar. pollut. Bull. 142, 1–14. doi: 10.1016/j.marpolbul.2019.03.019
Guo X., Wang X., Zhou X., Kong X., Tao S., Xing B. (2012). Sorption of four hydrophobic organic compounds by three chemically distinct polymers: role of chemical and physical composition. Environ. Sci. Technol. 46, 7252–7259. doi: 10.1021/es301386z
Hanvey I. (2023). Glass children: the lived experiences of siblings with a disability or chronic illness. Pediatr. Pulmonol. 58, S11–S12. doi: 10.1002/casp.2602
He B., Wijesiri B., Ayoko G. A., Egodawatta P., Rintoul L., Goonetilleke A. (2020). Influential factors on microplastics occurrence in river sediments. Science of The Total Environment 738, 139901. doi: 10.1016/j.scitotenv.2020.139901
He S., Jia M., Xiang Y., Song B., Xiong W., Cao J., et al. (2022). Biofilm on microplastics in aqueous environment: Physicochemical properties and environmental implications. J. Hazard. Mater. 424, 127286. doi: 10.1016/j.jhazmat.2021.127286
He H., Wu T., Chen Y.-F., Yang Z. (2023). A pore-scale investigation of microplastics migration and deposition during unsaturated flow in porous media. Sci. Total Environ. 858, 159934. doi: 10.1016/j.scitotenv.2022.159934
Hepburn E., Madden C., Szabo D., Coggan T. L., Clarke B., Currell M. (2019). Contamination of groundwater with per- and polyfluoroalkyl substances (PFAS) from legacy landfills in an urban re-development precinct. Environ. pollut. 248, 101–113. doi: 10.1016/j.envpol.2019.02.018
Hoellein T., Rovegno C., Uhrin A. V., Johnson E., Herring C. (2021). Microplastics in invasive freshwater mussels (Dreissena sp.): spatiotemporal variation and occurrence with chemical contaminants. Front. Mar. Sci. 8. doi: 10.3389/fmars.2021.690401
Hohn S., Acevedo-Trejos E., Abrams J. F., de Moura J. F., Spranz R., Merico A. (2020). The long-term legacy of plastic mass production. Sci. Total Environ. 746, 141115. doi: 10.1016/j.scitotenv.2020.141115
Huang J. (2021). Microplastic pollution in soils and groundwater: Characteristics, analytical methods and impacts. Chem. Eng. J. 425, 131870. doi: 10.1016/j.cej.2021.131870
Jeong H. Y., Jun S.-C., Cheon J.-Y., Park M. (2018). A review on clogging mechanisms and managements in aquifer storage and recovery (ASR) applications. Geosci. J. 22, 667–679. doi: 10.1007/s12303-017-0073-x
Jiang Y., Zhou S., Fei J., Qin Z., Yin X., Sun H., et al. (2022). Transport of different microplastics in porous media: Effect of the adhesion of surfactants on microplastics. Water Res. 215, 118262. doi: 10.1016/j.watres.2022.118262
Jumaah O. S. (2017). Screening of Plastic Degrading Bacteria from Dumped Soil Area. IOSR JESTFT 11, 93–98. doi: 10.9790/2402-1105029398
Khan S. (2022). Emerging contaminants of high concern for the environment: Current trends and future research. Environ. Res. 207, 112609. doi: 10.1016/j.envres.2021.112609
Koelmans A. A., Mohamed Nor N. H., Hermsen E., Kooi M., Mintenig S. M., De France J. (2019). Microplastics in freshwaters and drinking water: Critical review and assessment of data quality. Water Res. 155, 410–422. doi: 10.1016/j.watres.2019.02.054
Krueger M. C., Harms H., Schlosser D. (2015). Prospects for microbiological solutions to environmental pollution with plastics. Appl. Microbiol. Biotechnol. 99, 8857–8874. doi: 10.1007/s00253-015-6879-4
Lapworth D. J., Baran N., Stuart M. E., Ward R. S. (2012). Emerging organic contaminants in groundwater: A review of sources, fate and occurrence. Environ. pollut. 163, 287–303. doi: 10.1016/j.envpol.2011.12.034
Leusch F., Lu H.-C., Perera K., Neale P. A., Ziajahromi S. (2023). Analysis of the literature shows a remarkably consistent relationship between size and abundance of microplastics across different environmental matrices. Environ. pollut. 319, 120984. doi: 10.1016/j.envpol.2022.120984
Li M., He L., Hsieh L., Rong H., Tong M. (2023). Transport of plastic particles in natural porous media under freeze–thaw treatment: Effects of porous media property. J. Hazard. Mater. 442, 130084. doi: 10.1016/j.jhazmat.2022.130084
Li Q., Prigiobbe V. (2018). Numerical simulations of the migration of fine particles through porous media. Transp. Porous Med. 122, 745–759. doi: 10.1007/s11242-018-1024-3
Li J., Song Y., Cai Y. (2020). Focus topics on microplastics in soil: Analytical methods, occurrence, transport, and ecological risks. Environ. pollut. 257, 113570. doi: 10.1016/j.envpol.2019.113570
Li X., Xu H., Gao B., Sun Y., Shi X., Wu J. (2019). Transport of a PAH-degrading bacterium in saturated limestone media under various physicochemical conditions: Common and unexpected retention and remobilization behaviors. J. Hazard. Mater. 380, 120858. doi: 10.1016/j.jhazmat.2019.120858
Lim C., Kim N., Lee J., Yoon Y. (2022). Potential of adsorption of diverse environmental contaminants onto microplastics. Water 14, 4086. doi: 10.3390/w14244086
Luo Y., Guo W., Ngo H. H., Nghiem L. D., Hai F. I., Zhang J., et al. (2014). A review on the occurrence of micropollutants in the aquatic environment and their fate and removal during wastewater treatment. Sci. Total Environ., 473–474, 619–641. doi: 10.1016/j.scitotenv.2013.12.065
Maguire L. W., Gardner C. M. (2023). Fate and transport of biological microcontaminants bound to microplastics in the soil environment. Sci. Total Environ. 892, 164439. doi: 10.1016/j.scitotenv.2023.164439
Majdalani S., Michel E., Di-Pietro L., Angulo-Jaramillo R. (2008). Effects of wetting and drying cycles on in situ soil particle mobilization. Eur. J. Soil Sci. 59, 147–155. doi: 10.1111/j.1365-2389.2007.00964.x
Mintenig S. M., Löder M. G. J., Primpke S., Gerdts G. (2019). Low numbers of microplastics detected in drinking water from ground water sources. Sci. Total Environ. 648, 631–635. doi: 10.1016/j.scitotenv.2018.08.178
Murawski J., She J., Frishfelds V. (2022). Modeling drift and fate of microplastics in the Baltic Sea. Front. Mar. Sci. 9. doi: 10.3389/fmars.2022.886295
Napper I. E., Baroth A., Barrett A. C., Bhola S., Chowdhury G. W., Davies B. F. R., et al. (2021). The abundance and characteristics of microplastics in surface water in the transboundary Ganges River. Environ. pollut. 274, 116348. doi: 10.1016/j.envpol.2020.116348
Nelms S. E., Galloway T. S., Godley B. J., Jarvis D. S., Lindeque P. K. (2018). Investigating microplastic trophic transfer in marine top predators. Environ. pollut. 238, 999–1007. doi: 10.1016/j.envpol.2018.02.016
Ng K. L., Obbard J. P. (2006). Prevalence of microplastics in Singapore’s coastal marine environment. Mar. pollut. Bull. 52, 761–767. doi: 10.1016/j.marpolbul.2005.11.017
Panno S. V., Kelly W. R., Scott J., Zheng W., McNeish R. E., Holm N., et al. (2019). Microplastic contamination in karst groundwater systems. Groundwater 57, 189–196. doi: 10.1111/gwat.12862
Paul-Pont I., Tallec K., Gonzalez-Fernandez C., Lambert C., Vincent D., Mazurais D., et al. (2018). Constraints and priorities for conducting experimental exposures of marine organisms to microplastics. Front. Mar. Sci. 5. doi: 10.3389/fmars.2018.00252
Peng J., Wang J., Cai L. (2017). Current understanding of microplastics in the environment: Occurrence, fate, risks, and what we should do. Integrated Environ. Assess. Manage. 13, 476–482. doi: 10.1002/ieam.1912
Pereao O., Opeolu B., Fatoki O. (2020). Microplastics in aquatic environment: characterization, ecotoxicological effect, implications for ecosystems and developments in South Africa. Environ. Sci. pollut. Res. 27, 22271–22291. doi: 10.1007/s11356-020-08688-2
Pirsaheb M., Hossini H., Makhdoumi P. (2020). Review of microplastic occurrence and toxicological effects in marine environment: Experimental evidence of inflammation. Process Saf. Environ. Prot. 142, 1–14. doi: 10.1016/j.psep.2020.05.050
Provencher J. F., Vermaire J. C., Avery-Gomm S., Braune B. M., Mallory M. L. (2018). Garbage in guano? Microplastic debris found in faecal precursors of seabirds known to ingest plastics. Sci. Total Environ. 644, 1477–1484. doi: 10.1016/j.scitotenv.2018.07.101
Rajandas H., Parimannan S., Sathasivam K., Ravichandran M., Yin L. S. (2012). A novel FTIR-ATR spectroscopy based technique for the estimation of low-density polyethylene biodegradation. Polymer Testing 31, 1094–1099. doi: 10.1016/j.polymertesting.2012.07.015
Re V. (2019). Shedding light on the invisible: addressing the potential for groundwater contamination by plastic microfibers. Hydrogeol. J. 27, 2719–2727. doi: 10.1007/s10040-019-01998-x
Rochman C. M., Tahir A., Williams S. L., Baxa D. V., Lam R., Miller J. T., et al. (2015). Anthropogenic debris in seafood: Plastic debris and fibers from textiles in fish and bivalves sold for human consumption. Sci. Rep. 5, 14340. doi: 10.1038/srep14340
Samandra S. (2022). Microplastic contamination of an unconfined groundwater aquifer in Victoria, Australia. Sci. Total Environ. 802, 149727. doi: 10.1016/j.scitotenv.2021.149727
Smith B., Siegel D., Neslund C., Carter C. (2014). Organic contaminants in portland cements used in monitoring well construction. Groundwater Monit. Remediation 34, 102–111. doi: 10.1111/gwmr.12082
Sudhakar M., Doble M., Murthy P. S., Venkatesan R. (2008). Marine microbe-mediated biodegradation of low- and high-density polyethylenes. Int. Biodeterior. Biodegrad. 61, 203–213. doi: 10.1016/j.ibiod.2007.07.011
Sui Q., Wang D., Zhao W., Huang J., Yu G., Cao X., et al. (2015). Pharmaceuticals and consumer products in four wastewater treatment plants in urban and suburb areas of Shanghai. Environ. Sci. pollut. Res. 22, 6086–6094. doi: 10.1007/s11356-014-3793-8
Szabo D., Coggan T. L., Robson T. C., Currell M., Clarke B. O. (2018). Investigating recycled water use as a diffuse source of per- and polyfluoroalkyl substances (PFASs) to groundwater in Melbourne, Australia. Sci. Total Environ. 644, 1409–1417. doi: 10.1016/j.scitotenv.2018.07.048
Tanaka K., Takada H. (2016). Microplastic fragments and microbeads in digestive tracts of planktivorous fish from urban coastal waters. Sci. Rep. 6, 34351. doi: 10.1038/srep34351
Tong M., Johnson W. P. (2006). Excess colloid retention in porous media as a function of colloid size, fluid velocity, and grain angularity. Environ. Sci. Technol. 40, 7725–7731. doi: 10.1021/es061201r
Van Cauwenberghe L., Janssen C. R. (2014). Microplastics in bivalves cultured for human consumption. Environ. pollut. 193, 65–70. doi: 10.1016/j.envpol.2014.06.010
Wang L., Bank M. S., Rinklebe J., Hou D. (2023). Plastic–rock complexes as hotspots for microplastic generation. Environ. Sci. Technol. 57, 7009–7017. doi: 10.1021/acs.est.3c00662
Wang H., Xin J., Zheng X., Fang Y., Zhao M., Zheng T. (2023). Effect of biofilms on the clogging mechanisms of suspended particles in porous media during artificial recharge. J. Hydrol. 619, 129342. doi: 10.1016/j.jhydrol.2023.129342
Wang H., Xin J., Zheng X., Li M., Fang Y., Zheng T. (2020). Clogging evolution in porous media under the coexistence of suspended particles and bacteria: Insights into the mechanisms and implications for groundwater recharge. J. Hydrol. 582, 124554. doi: 10.1016/j.jhydrol.2020.124554
Wang Y., Xu L., Chen H., Zhang M. (2022). Retention and transport behavior of microplastic particles in water-saturated porous media. Sci. Total Environ. 808, 152154. doi: 10.1016/j.scitotenv.2021.152154
Wanner P. (2021). Plastic in agricultural soils - A global risk for groundwater systems and drinking water supplies? - A review. Chemosphere 264, 128453. doi: 10.1016/j.chemosphere.2020.128453
Wu P., Huang J., Zheng Y., Yang Y., Zhang Y., He F., et al. (2019). Environmental occurrences, fate, and impacts of microplastics. Ecotoxicol. Environ. Saf. 184, 109612. doi: 10.1016/j.ecoenv.2019.109612
Wu J., Jiang R., Lin W., Ouyang G. (2019). Effect of salinity and humic acid on the aggregation and toxicity of polystyrene nanoplastics with different functional groups and charges. Environ. pollut. 245, 836–843. doi: 10.1016/j.envpol.2018.11.055
Xu Y., Gong H., Chen B., Zhang Q., Li Z. (2021). Long-term and seasonal variation in groundwater storage in the North China Plain based on GRACE. Int. J. Appl. Earth Observ. Geoinform. 104, 102560. doi: 10.1016/j.jag.2021.102560
Yan X., Yang X., Tang Z., Fu J., Chen F., Zhao Y., et al. (2020). Downward transport of naturally-aged light microplastics in natural loamy sand and the implication to the dissemination of antibiotic resistance genes. Environ. pollut. 262, 114270. doi: 10.1016/j.envpol.2020.114270
Yang X., Man Y. B., Wong M. H., Owen R. B., Chow K. L. (2022). Environmental health impacts of microplastics exposure on structural organization levels in the human body. Sci. Total Environ. 825, 154025. doi: 10.1016/j.scitotenv.2022.154025
Yang H., Yan Y., Yu Y., He Y., Fu B., Wang J. (2022). Distribution, sources, migration, influence and analytical methods of microplastics in soil ecosystems. Ecotox. Ecotoxicol. Environ. Saf. 243, 114009. doi: 10.1016/j.ecoenv.2022.114009
Yang J., Yang Y., Wu W.-M., Zhao J., Jiang L. (2014). Evidence of polyethylene biodegradation by bacterial strains from the guts of plastic-eating waxworms. Environ. Sci. Technol. 48, 13776–13784. doi: 10.1021/es504038a
Keywords: microplastics, groundwater artificial recharge, hydraulic conductivity, clogging risk, porous media
Citation: Wang H, Zhang J, Chen Y, Xia Y, Jian P and Liang H (2024) Clogging risk of microplastics particles in porous media during artificial recharge: a laboratory experiment. Front. Mar. Sci. 11:1346275. doi: 10.3389/fmars.2024.1346275
Received: 29 November 2023; Accepted: 03 January 2024;
Published: 23 January 2024.
Edited by:
Chengji Shen, Hohai University, ChinaReviewed by:
Lu Xia, Shandong University of Science and Technology, ChinaCopyright © 2024 Wang, Zhang, Chen, Xia, Jian and Liang. This is an open-access article distributed under the terms of the Creative Commons Attribution License (CC BY). The use, distribution or reproduction in other forums is permitted, provided the original author(s) and the copyright owner(s) are credited and that the original publication in this journal is cited, in accordance with accepted academic practice. No use, distribution or reproduction is permitted which does not comply with these terms.
*Correspondence: Yudao Chen, Y3lkMDA1NkB2aXAuc2luYS5jb20=; Yuan Xia, eGlheXVhbkBnbHV0LmVkdS5jbg==
Disclaimer: All claims expressed in this article are solely those of the authors and do not necessarily represent those of their affiliated organizations, or those of the publisher, the editors and the reviewers. Any product that may be evaluated in this article or claim that may be made by its manufacturer is not guaranteed or endorsed by the publisher.
Research integrity at Frontiers
Learn more about the work of our research integrity team to safeguard the quality of each article we publish.