- 1Pacific Biological Station, Fisheries and Oceans Canada, Nanaimo, BC, Canada
- 2Centre for Shellfish Research, Vancouver Island University, Nanaimo, BC, Canada
In recent years, Pacific oyster growers in British Columbia (BC), Canada have experienced devastating losses due to summer mortality syndrome. While anecdotal evidence suggests that intertidally-grown oysters may fare better during mass mortality events than deep-water counterparts, there remains a lack of research examining how different culture conditions may influence severity. To address this, we compared growth, condition, histopathology, reproductive status, and survival between intertidally- and deep-water-cultured oysters over 2 years at three oyster farms in Baynes Sound (BC). A reciprocal transplant was carried out after 1 year to test the use of the intertidal as a mechanism for promotion of physiological resilience prior to deep-water deployment. Field trial results showed significantly higher final survival in oysters transferred from the intertidal to deep water (83.5%) compared to those maintained in deep water (63.6%), but only at one farm, likely as a consequence of varying physical and/or biological characteristics associated with particular farm locations. Histopathology showed little role of disease with regards to varying survival among treatments, though higher occurrence of Viral Gametocytic Hypertrophy was observed in Year 1 oysters under deep-water (62.2%) versus intertidal (37.8%) conditions. Additionally, after 2 years, there was no significant difference in oyster size nor condition index between oysters transplanted from the intertidal to deep water and those solely cultured in deep water. A laboratory-challenge experiment determined significantly different survival curves of Year 1 intertidally- and deep-water-cultured oysters under immersion/emersion and warming conditions, with final survival of 88% and 64%, respectively, under conditions of high temperature (25°C) and immersion. Likewise, Year 2 (i.e. post-transfer) intertidally- and deep-water-cultured oysters showed significantly different survival curves under laboratory-based Vibrio challenge conditions (16°C) with final survival of 63% and 34%, respectively. Results suggest that partial culture in the intertidal at some farms may be an effective method for conferring resilience to summer mortality in Pacific oysters.
1 Introduction
Pacific oyster (Crassostrea gigas) growers across the globe continue to contend with mass mortality events during summer months. In recent years, this widespread and recurrent phenomenon has caused increasing annual losses (20–100%) at farms spanning North America, Europe, Asia, and Australia (Malham et al., 2009; Cotter et al., 2010; Dégremont et al., 2010; Green et al., 2019; King et al., 2019; Lafont et al., 2019; Ashton et al., 2020; Yang et al., 2021). The shellfish aquaculture industry is a large global employer and provides an increasingly important dietary protein source for human populations (FAO, 2022). Likewise, the industry contributes greatly to international markets, global exports of bivalve mollusks being worth approximately USD 4.3 billion in 2020 (FAO, 2022). Accordingly, there is a critical need for the development of practical mitigation methods to reduce summer mortality in order to support regional economies and safeguard global food security.
Summer mortality of Pacific oysters is associated with a complex set of interrelated and interactive physical and biological factors. While events are primarily driven by high seawater temperatures (i.e. >19−20°C) (Green et al., 2019; Petton et al., 2021), there is a range of additional key contributory factors including reproductive status, genetics, growth and condition, and viral/bacterial pathogenic infection (Li et al., 2009; Barbosa Solomieu et al., 2015; Petton et al., 2021; Cowan et al., 2023). Other environmental factors, including low salinity events (Brown and Hartwick, 1988) and harmful algal blooms (Cassis et al., 2011), have also been associated with increased levels of mortality during the summer.
The Pacific oyster has broad environmental tolerance limits and consequently is successfully cultured under a wide range of growing conditions. Culture environments extend from the intertidal zone, where diurnal tidal emersion events generate large and regular environmental fluctuations, to deep-water (i.e. suspended culture) conditions where animals experience constant immersion and relatively stable growing conditions. The distinctive exposures associated with intertidal and deep-water culture environments drive contrasting magnitudes and frequencies of related stress responses with direct repercussions for growth/condition, reproduction, and survival (Cotter et al., 2010; Pernet et al., 2012, 2014; Petton et al., 2021; Cowan et al., 2023).
Species inhabiting the intertidal zone contend with daily fluctuations in physical (e.g. temperature, dissolved oxygen, pH) and biological (e.g. feed availability, predators) parameters. This is particularly relevant for oysters and clams, which, as non-motile thermo-conformers, cannot directly avoid such conditions. To combat this, intertidal bivalves have evolved a number of adaptative mechanisms that allow them to tolerate diurnal and seasonal variations in physical conditions (e.g. wide thermal fluctuations) and to survive short-term stressor events (e.g. hypoxia/anoxia, osmotic stress, heat stress) (Clark et al., 2018; Masanja et al., 2023). These include a well-developed antioxidant defense system, the ability to enter a metabolically-depressed state (including shifting to anaerobic glycolysis metabolism), mitochondrial adjustments, episodic shell gaping to access atmospheric oxygen, protective burrowing behaviors, and changes in shell morphology (Somero, 2002; Abele et al., 2009; Tagliarolo et al., 2012; Li et al., 2013; Zhang et al., 2016; Meng et al., 2018; Lafont et al., 2020; Amorim et al., 2021). Additionally, gene expression profiles of individuals from intertidal populations tend to be markedly different in comparison to those from subtidal ones, the former’s characterized by an upregulation in genes involved in respiration, antioxidant production, protein degradation, DNA repair, and cytoskeleton pathways, reflecting the stressor conditions associated with the intertidal environment (Li et al., 2018; Clark et al., 2019). While animals in the intertidal face regular stress events (e.g. high atmospheric temperature, increased oxidative stress) (Pörtner, 2012; Zhang et al., 2016), deep-water counterparts largely experience less challenging physical conditions whilst also benefiting from increased availability of feed and reduced predation pressure.
Gene-environment interactions represent a major shaping force of the intertidal zone and lead to considerable intra-specific variation in the evolved responses of marine invertebrates across the intertidal environmental gradient. The interplay between genetics and the surrounding environment dictates phenotypic plasticity, which serves as an important mechanism by which intertidal species may respond to changing environmental conditions (Li et al., 2018; Clark et al., 2019). Indeed, phenotypic plasticity has been observed in an extensive range of bivalve traits including metabolism and shell structure (Tagliarolo et al., 2012; Clark et al., 2018), gene expression (Hamdoun et al., 2003; Clark et al., 2018), energy allocation (Ernande et al., 2004), and feeding behavior (Bayne, 2004), with responses encompassing whole-organism to tissue-, cellular-, and molecular-level systems (Somero, 2002). A number of recent investigations have also demonstrated that phenotypic plasticity may be adaptively-favored by intertidal organisms (Clark et al., 2018; Li et al., 2018; Wang et al., 2021, 2023).
Anecdotal evidence from oyster growers (British Columbia Shellfish Growers Association, pers. comm.) and previous research (Peeler et al., 2012; Pernet et al., 2019) suggest that intertidally-grown oysters may fare more favorably during mass mortality events compared to counterparts in deep-water culture conditions. The higher mortality rates observed at deep-water sites compared to intertidal sites may be due to several factors. Firstly, as previously discussed, deep-water cultured oysters lack the exposure to short-term stress events regularly experienced by animals in the intertidal, which could serve as important physiological triggers for the development of resilience to more extreme stressor events (Wang et al., 2012; Zhang et al., 2016; Meng et al., 2018). Secondly, shore-based culture may promote an oyster microbiome that is better suited to contend with various pathogens (e.g. Vibrio) (King et al., 2019; Offret et al., 2020). As well, the reduced feeding duration of intertidal animals may result in lowered reproductive condition and reduced spawning, thus decreasing the stress associated with the latter (Cotter et al., 2010; Huvet et al., 2010; Cowan et al., 2023). In contrast, deep-water cultured animals may have to contend with an extended presence of seasonally-unfavorable seawater conditions that may be the result of environmental variations (e.g. high seasonal water temperatures, harmful algal blooms) (Evans et al., 2019) or due to high-density culture within oyster trays/stacks (e.g. reduced flow rates, low dissolved oxygen concentration, high dissolved waste concentration) (Campbell and Hall, 2019). Additionally, intertidal and deep-water culture conditions may vary in terms of altered host–pathogen interactions as intertidal host species spend less time underwater so benefit from reduced exposure to seawater pathogens (Pernet et al., 2012, 2014). The proliferation of pathogens in host oysters is also likely to vary according to site-driven differences in metabolism and growth (Pernet et al., 2014).
There remains a lack of research examining how the varying physical conditions and oyster physiologies associated with deep-water and intertidal culture sites may promote or reduce the severity of summer mortality events. To address this, the present study compared growth, condition, histopathology, reproductive status, and survival between intertidally- and deep-water-cultured oysters over one full growth cycle (i.e. 2 years, initial deployment to harvest). Additionally, we tested the use of the intertidal zone as a mechanism for promotion of physiological resilience prior to deep-water deployment via a reciprocal transplant between intertidal and deep-water sites after the first year. We also examined site-based responses to specific summer mortality stressors via controlled temperature and pathogen challenge experiments within a laboratory setting. Results will contribute to the collective understanding of drivers of Pacific oyster summer mortality and advance the development of practical mitigation strategies in order to promote the sustainability of this important global food resource.
2 Methodology
2.1 Field experiment
In March 2021, juvenile (~8 months old, mean shell height (± SD): 26.22 ± 3.30 mm, N = 30) Pacific oysters were placed in “surface” float bags (black Vexar® 9-mm mesh bags, L x W x H = ~100 x 50 x 15 cm). Approximately 500 individuals were placed in every bag (N = 180), representing commercial stocking densities, prior to deployment at an intertidal site and a deep-water site at each of three commercial farms: Deep Bay (DB), Denman Island (DN), and Fanny Bay (FB), located within Baynes Sound, British Columbia (BC), Canada (Figure 1). Baynes Sound represents the most intensely farmed shellfish area in BC (BCMSRM, 2002) and farms across the region have experienced considerable summer mortality events in recent years (Cowan, 2020; Cowan et al., 2023). Stocking of float bags with oysters and deployment of bags were carried out by an industry partner (Taylor Shellfish Canada) according to industry protocols. Two lines of 30 float bags (~15 cm between bags) were positioned near a main oyster growing area within each farm with one line positioned in a subtidal area and the other established in the intertidal zone. In the subtidal sites (hereafter referred to as deep-water sites), float bags remained floating in surface seawater (~50 cm depth) for the duration of the field trial, thus providing continuous immersion, irrespective of tidal phase. At the intertidal sites, float bags were positioned along the 6-foot tidal mark as per industry protocol, thus providing regular (i.e. diurnal) periods of emersion/immersion according to tidal phases. The distance between intertidal and deep-water sites was <300 m at all three farms. In August 2021, the density in all bags was reduced by 50% to approximately 250 animals per bag to ensure optimal growing densities, as per industry protocol. Bags were then left over the autumn and winter months (i.e. October through April) with periodic visits made to check the integrity of the float bags and the general condition of animals.
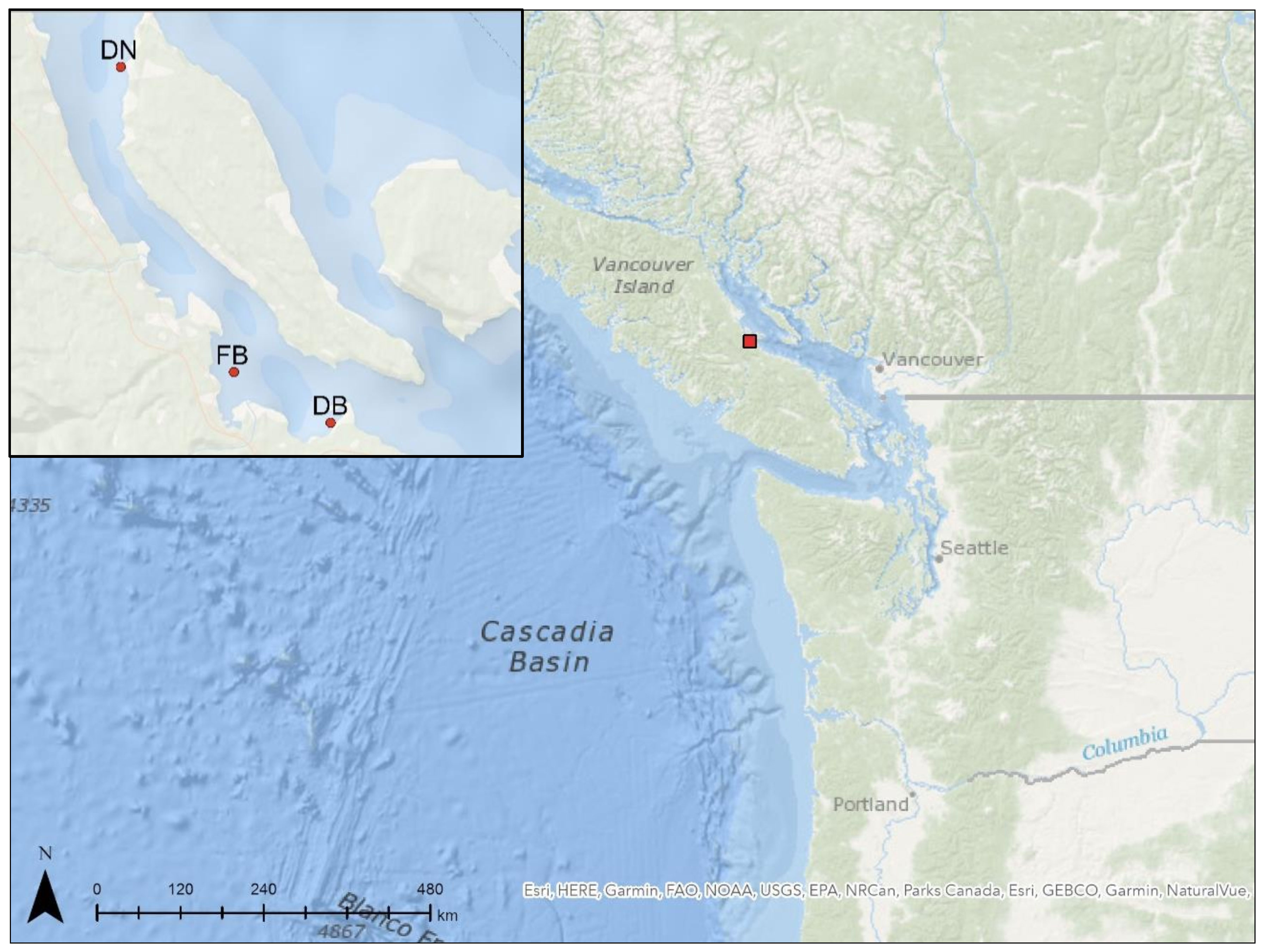
Figure 1 Maps of study location. Main map shows location of Baynes Sound (red square) in British Columbia, Canada. Inset map shows location of the three farms (red dots) where the experiment took place: Deep Bay (DB), Denman Island (DN), and Fanny Bay (FB). At each farm, oysters were deployed at an intertidal site and a deep-water suspended site in close proximity to one another. Maps produced using Canada Marine Planning Atlas interactive mapping tool and contain information licensed under the Open Government Licence - Canada.
In May 2022, a reciprocal transplant of 15 bags from the deep-water site and 15 bags from the intertidal site was carried out at each farm. Alternating bags from each line were transferred and re-attached to the corresponding deep-water/intertidal line. This resulted in four experimental treatment groups: (1) animals solely cultured in deep-water (Deep), (2) animals initially cultured in deep water and then transplanted to the intertidal (Deep→Intertidal), (3) animals solely cultured in the intertidal (Intertidal), and (4) animals initially cultured in the intertidal and then transplanted to deep water (Intertidal→Deep). In early June 2022, all bag densities were reduced by 50% to approximately 100 animals per bag to ensure optimal growing densities, as per industry protocol.
2.2 Survival assessment
Survival assessments were carried out across all three farms on a bi-weekly (i.e. every 2 weeks) basis from May 2021 to September 2021 (Year 1: T01−T08). From April 2022 to September 2022 (Year 2: T09−T16), bi-weekly assessment was limited to two farms (DB, DN) due to winter damage occurring at the FB farm. In Year 1, survival was assessed in six bags per line (n = 6, N = 12 counts per farm) at each time point as the percent of the 500 (or 250, after thinning) original animals that were alive, as the shells of dead oysters were easily broken down/lost between sampling events due to the small size of animals. Counts were conducted according to a blocked design along the line (i.e. one bag counted per block of five bags). In Year 2, survival percent was assessed via live and dead counts on a sub-set of five bags from each treatment group (n = 5, N = 20 counts per farm). As in Year 1, counts were conducted according to a blocked design along the line (i.e. one bag of each treatment counted per block of six bags). Dead or moribund (determined as those displaying continuous gape) animals and any empty shells were recorded as “dead”. Following counts, all live and dead animals and empty shells were replaced in the bags to mimic normal farm growing conditions.
2.3 Sampling
During survival assessment, animals were sampled for analyses of condition index, growth, reproductive status, and histopathology. In Year 1, a single animal was haphazardly sampled from every bag (N = 30) and a randomly chosen sub-set was then used for condition index (18) and histology (12). Due to sampling constraints in Year 2, the routine was reduced to haphazardly sampling five animals from bags that were used for survival assessment (N = 5). A randomly chosen sub-set was then used for condition index (3) and histopathological analyses (2). Sampled animals were placed into labelled bags and stored in cool insulated boxes for transport. Histology sections (one per sampled animal) were taken and fixed in Davidson’s solution within 24 hours of collection (see details below). Animals to be used for measurement of condition index were frozen and stored at -80°C until subsequent processing.
2.4 Seawater monitoring
Sea surface temperatures (SST) at intertidal and deep-water sites, and air temperature during tidal emersion at intertidal sites, were tracked over the two summer periods (June−August) of the project. HOBO pendant loggers (ONSET, Bourne, MA, USA) were added to bags situated within each site (N = 3 per line) at each farm, with readings taken at 30-minute intervals. SST and salinity were also monitored via a ProQuatro hand-held multiparameter probe (YSI Incorporated, Yellow Springs, OH, USA) during sampling events at deep-water sites only. Triplicate readings were taken along each line of float bags (at end-points and mid-point) within the top 30 cm of surface waters. In addition, in Year 2, dissolved oxygen was monitored between May and August at DB and DN deep-water sites. U26-001 dissolved oxygen loggers (ONSET, Bourne, MA, USA) were positioned at two points across each line with readings taken at 15-minute intervals.
2.5 Reproductive status and histopathology
Tissue cross sections were processed using routine histological techniques (Marty et al., 2006). In brief, 5-μm-thick tissue sections were cut from paraffin-embedded samples, stained with Harris’s modified hematoxylin and eosin (H&E), and then examined under light microscopy for assessment of reproductive stage (i.e. gonad maturity) and histopathology. Assessment was restricted to summer months (June − August) in both years of the project. Reproductive stage was determined following a qualitative classification (six stages: 0 to 5; Supplementary Table 1), based on Mann (1979), Normand et al. (2008), and Steele and Mulcahy (1999). Sex ratio was determined as the ratio of females to males (F:M) excluding hermaphrodites and undifferentiated oysters. Histopathology included screening for Diffuse Haemocyte Infiltration (DHI), Focalized Haemocyte Infiltration (FHI), Rickettsia-like inclusion (RLP-like), Metaplasia of Digestive Gland Tubules (MDGT), and Viral Gametocytic Hypertrophy (VGH).
2.6 Condition index and growth
Condition index (CI) was determined according to Rainer and Mann (1992): CI = (P1 × 100)/P2, where P1 equals the dry weight (g) of soft tissues and P2 equals the dry weight (g) of the shell. Shell length, width, and height were taken with Vernier digital calipers to 0.01 mm. Wet and dry weights (DW) of tissues and shells were measured to 0.001 g (Pioneer PX, Ohaus, Parsippany, NJ, USA). DWs were taken after a 7-day drying period at 60°C, ensuring drying to constant weight. Mean growth values were determined as final shell height and tissue DW at the end of each growing season (T08, T15). At deployment, mean (± SD) CI, shell height, and tissue DW were 9.1 ± 2.1, 26.22 ± 3.30 mm, and 0.09 ± 0.03 g, respectively (N = 30).
2.7 Laboratory-challenge experiments
Challenge experiments were carried out to compare the survival of deep-water- and intertidally-cultured oysters under separate and/or coinciding high-temperature and Vibrio aestuarianus stress conditions. Juvenile oysters for the experiment were taken from treatment groups at the DB farm and were collected when bag densities were reduced in Year 1 (using excess individuals) and at the end of Year 2. Animals were then used in laboratory-challenge experiments carried out at Vancouver Island University’s Centre for Shellfish Research (CSR).
2.7.1 Heatwave challenge (year 1)
In Year 1, following 8 months of culture under deep-water or intertidal conditions at DB, approximately 300 animals from each of the two treatment groups (Deep, Intertidal) were collected for use in a laboratory-challenge experiment comparing survival under high-temperature stress conditions. Ten haphazardly-selected animals from each treatment group were placed in each of fifteen 20-L glass tanks with 5 L of 80 µM-filtered seawater and held at 15, 20, or 25°C (n = 5 tanks per treatment level, N = 10 tanks per temperature level) via temperature-controlled rooms. Temperatures were chosen to reflect average summer temperatures (15−16°C, Cowan et al., 2023) and natural warming events (~25°C, present study) for the region, and the approximate temperature associated with the onset of summer mortality (19−20°C, Go et al., 2017). Salinity was approximately 30 ppt and a constant air supply was provided to each tank via an air stone. Oysters were acclimated to temperatures over a 24-hour period. No feed was administered over the experiment in order to prevent altered algal concentrations across temperature treatments and to avoid issues with bacterial growth. An additional emersion challenge (i.e. dry conditions) at the same temperatures was carried out in parallel (n = 5 per treatment, 10 animals per replicate) along bench tops in the temperature-controlled rooms. Animals were left under constant emersion conditions with no recovery period (i.e. immersion in seawater) over the entire exposure period. The authors emphasize that the emersion challenge was not carried out to simulate natural conditions but strictly posed as a fitness test for intertidally- versus deep-water-cultured oysters. Mortality in all treatments was assessed on a daily basis over a 10-day exposure period. Moribund animals and/or those displaying a lack of response to stimulus (i.e. continuous gape) were reported as “dead” and removed from the tanks/bench tops.
2.7.2 Heatwave and Vibrio challenge (year 2)
In Year 2, 5 months after the reciprocal transfer, approximately 250 animals from each of the deep-water treatment groups (Deep, Intertidal→Deep) at the DB farm were collected for use in a second laboratory-challenge experiment comparing survival rates under separate and/or coinciding high-temperature and V. aestuarianus stress conditions. The specific farm and treatment groups were selected for the challenge due to pre-observed differences in survival during the field trial, and as part of a continued investigation of whether time in the intertidal zone confers increased resilience to summer mortality stressors post-transfer to deep-water conditions, respectively. Vibrio aestuarianus was used for the pathogen challenge as has been previously isolated from moribund oysters displaying signs of summer mortality syndrome (Labreuche et al., 2006; Cowan et al., 2023). Prior to the challenge, all animals were notched on the distal portion of the shell (adjacent to the adductor muscle) for later inoculation. Following, 11 haphazardly-selected animals from each treatment group were placed in each of twenty 20-L glass tanks (N = 40) with 5 L of 80 µM-filtered seawater (i.e. static) and held overnight at 16°C. Salinity was approximately 30 ppt and a constant air supply was provided to each tank via an air stone. The following day, a 2x2 fully factorial design (high/low temperature x Vibrio/no Vibrio) was applied with animals from both treatment groups (Deep, Intertidal→Deep) (n = 5 tanks per treatment level, N = 20 tanks per temperature level). All Vibrio treatment oysters were inoculated with 50 µL of V. aestuarianus solution via injection into the adductor muscle as per Mackenzie et al. (2022). Vibrio aestuarianus was grown overnight with constant agitation at 21°C in tryptic soy broth containing 2% NaCl (TSB + 2% NaCl) with inocula taken from frozen glycerol stocks and tested for purity prior to use. Following, cells were washed and re-suspended to OD600 = 1.9 with concentration based on pilot studies carried out using subsets of individuals from each treatment group. The relationship between OD and colony-forming units (CFUs) was determined by serial dilution plating (OD600 = 2.50 per 50-µL dose; CFUs = 5.09 x 108 ml-1). All pathogen control (i.e. no exposure to Vibrio) animals were injected with 50 µL of autoclaved seawater and placed in separate tanks from Vibrio treatment animals. Seawater temperature was then maintained at 16°C (temperature control) or raised to 24°C. Temperature conditions were maintained via temperature-controlled rooms. As in Year 1, no feed was administered in order to prevent altered algal concentrations across temperature treatments and to avoid issues with bacterial growth. Mortality was assessed on a daily basis over a 2-week exposure period according to the same criteria as previously described. After 8 days, the high temperature (24°C) challenge was ended due to ≥50% mortality across all treatment groups. The control temperature (16°C) challenge continued for an additional week until ≥50% mortality was observed in the Vibrio treatment groups.
2.8 Data analyses
Three-way ANOVAs were applied to examine the effect of culture site/treatment, farm, timepoint, and all interactions on survival, condition index, and oyster size (shell height and tissue DW). Percent survival data were logit-transformed prior to ANOVA. Where significant differences were detected, Tukey’s HSD method for multiple comparisons was applied. Additionally, one-way and two-way ANOVAs with post-hoc testing were carried out to compare treatment groups at a particular farm across time or at specific timepoints across farms. All data were tested to ensure assumptions of normality (Kolmogorov-Smirnov, p < 0.05) and homogeneity of variances (Levene’s Test, p < 0.05) were met prior to analyses. All ANOVAs were carried out in RStudio (v4.2.2; R Core Team, 2022). Means ± SDs are presented.
Kaplan-Meier survival curves of treatment groups under laboratory-based heatwave and/or Vibrio challenges were generated in RStudio and compared via log-rank testing. Cox proportionate hazards analyses were carried out to investigate associations between survival time and predictor variables.
3 Results
3.1 Seawater monitoring
In April to August of Year 1 (2021), SST ranged between 7.5 and 30.8°C (DB), 7.6 and 26.8°C (DN), and 6.0 and 28.1°C (FB) at deep-water sites, and between 8.9 and 37.5°C (DB), 6.6 and 37.7°C (DN), and 6.4 and 44.8°C (FB) at intertidal sites (Figure 2). Mean salinities over sampling events for DB, DN, and FB over the same period ranged from 24.5 to 28.1 ppt, 24.5 to 27.7 ppt, and 23.8 to 25.2 ppt, respectively. Mean monthly SSTs, salinities, and DO levels measured during sampling events are provided in Supplementary Table 2. Of note, an acute warming event took place in late June 2021 lasting for approximately 1 week, during which average SSTs were 22.0 ± 2.4°C (DB), 21.5 ± 2.0°C (DN), and 21.2 ± 2.6°C (FB) at deep-water sites, and 23.3 ± 4.3°C (DB), 22.0 ± 4.1°C (DN), and 22.5 ± 4.6°C (FB) at intertidal sites (Figure 2). Mean SSTs at DB and DN from September 2021 through April 2022 were 8.2 ± 3.6°C and 8.4 ± 3.2°C, respectively.
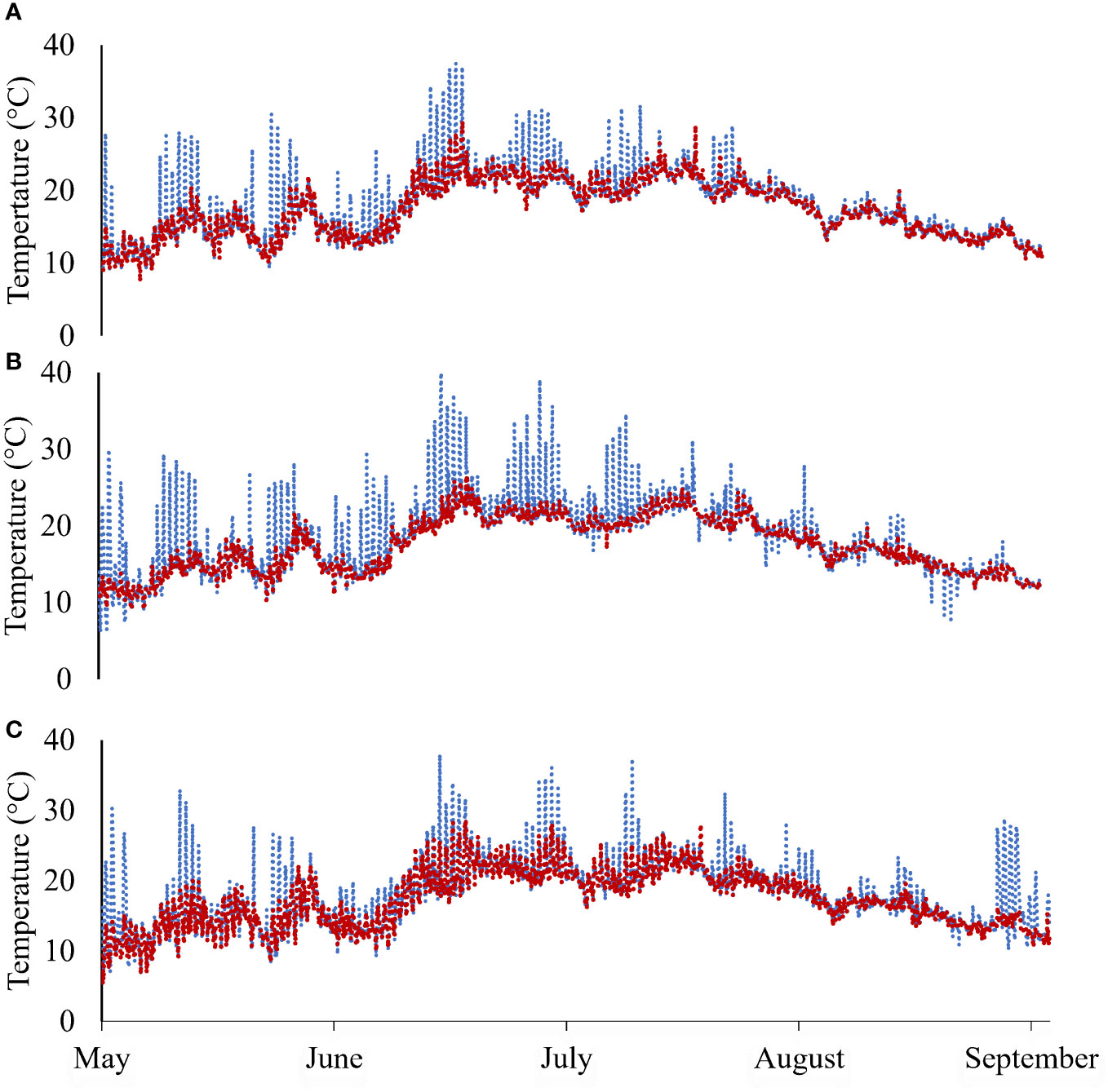
Figure 2 Continuous temperature data for deep-water sites (red-dashed line) and intertidal sites (blue-dashed line) at (A) Deep Bay, (B) Denman Island, and (C) Fanny Bay farms in Year 1 (May−September 2021).
In May to August of Year 2 (2022), SST ranged between 6.3 and 25.0°C (DB) and 7.9 and 27.5°C (DN) at deep-water sites, and between 9.2 and 35.0°C (DB) and 9.6 and 34.3°C (DN) at intertidal sites. Mean salinities at DB and DN over the same period were 24.4 ± 1.6 ppt and 23.9 ± 1.5 ppt, respectively. Of note, an acute warming event took place in late July 2022 lasting for approximately 1 week, during which average SSTs were 21.9 ± 1.1°C (DB) and 22.2 ± 1.2°C (DN) at deep-water sites, and 22.8 ± 2.5°C (DB) and 22.9 ± 2.6°C (DN) at intertidal sites. Maximum SSTs during this event were 25.0°C (DB) and 25.7°C (DN) at deep-water sites, and 32.9°C (DB) and 34.3°C (DN) at intertidal sites. In May to August of Year 2, DO levels at DB and DN showed a gradual decline over time with increasing daily temperature, and a clear increase in DO fluctuations (between hypoxia and normoxia) was observed following the warming event at the end of July 2021, with daily DO ranging from approximately 2 to 15 mg L-1 (Figure 3).
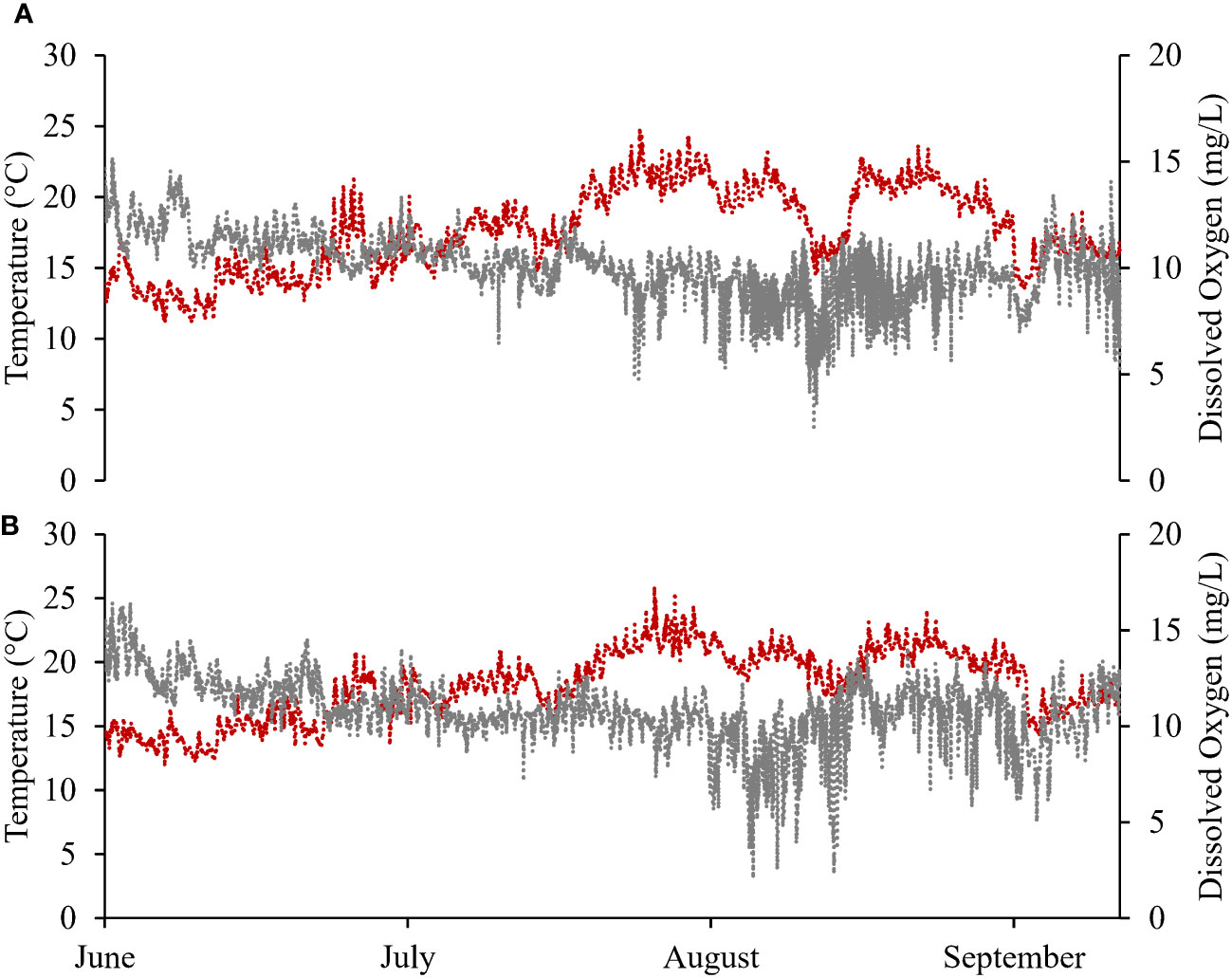
Figure 3 Continuous temperature (red-dashed line) and dissolved oxygen (grey-dashed line) data for deep-water sites at (A) Deep Bay and (B) Denman Island farms in Year 2 (June−September 2022).
3.2 Survival
In Year 1, mean percent survivals in August (T07) for Deep and Intertidal treatment groups at DB, DN, and FB were 86.4 ± 6.1%, 91.7 ± 2.8%, and 96.6 ± 5.0% (Deep), and 89.5 ± 14.1%, 84.4 ± 9.8%, and 96.1 ± 4.3% (Intertidal), respectively (Figure 4). Collectively across all farms and sites, a 3-way ANOVA detected a significant difference in percent survival among sampling times (F(6,245) = 10.14, p = 0.003), but no significant effect of farm nor site, or any interaction between/among factors. For the time factor, only one pairwise comparison was significant, early May (T01) having significantly higher percent survival than early August (T07).
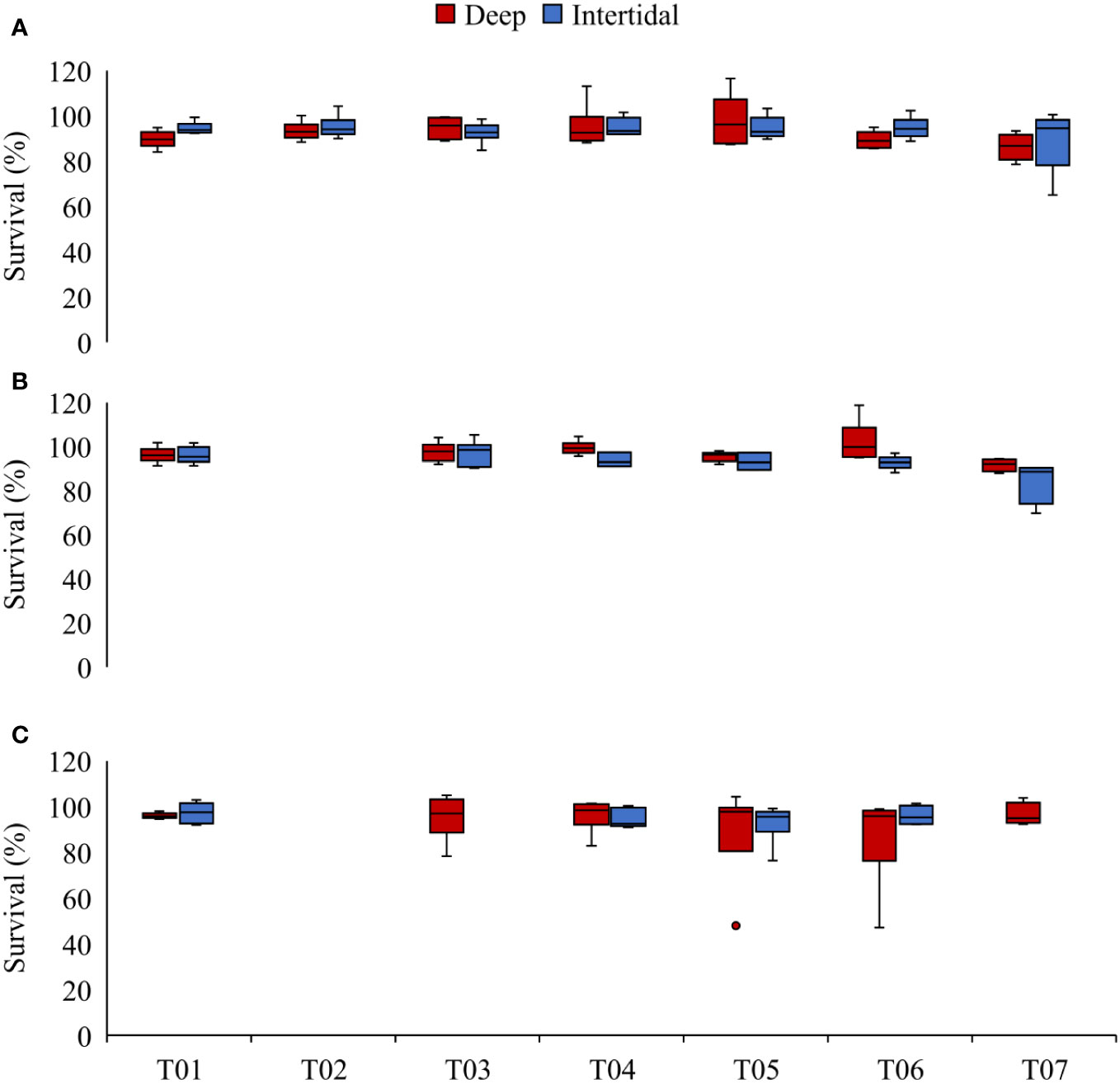
Figure 4 Box plots of survival (%) in Deep and Intertidal (blue) sites at (A) Deep Bay, (B) Denman Island, and (C) Fanny Bay farms in Year 1 (T01−T07). T01 = early May, T02 = late May, T03 = early June, T04 = late June, T05 = early July, T06 = late July, and T07 = early August. Due to inclement sea conditions, sampling could not take place at T02 at Denman Island (Deep and Intertidal sites) and Fanny Bay (Deep and Intertidal sites) nor at T07 at Fanny Bay (Intertidal site). T08 is not included as bag splitting (number of oysters per bag reduced from 500 to 250) occurred at this timepoint.
In Year 2, mean percent survivals in September in the four treatments at DB and DN farms were: 63.6 ± 10.2% and 70.6 ± 10.1% (Deep), 68.9 ± 14.4% and 65.4 ± 11.6% (Deep→Intertidal), 86.6 ± 10.7% and 61.2 ± 15.7% (Intertidal), and 83.5 ± 11.0% and 70.6 ± 13.1% (Intertidal→Deep), respectively (Figure 5). A three-way ANOVA showed a significant effect of time (F(7,273) = 8.51, p < 0.001) and treatment (F(3,276) = 13.15, p < 0.001), but no significant effect of farm (F(1,278) = 1.67, p = 0.197). There were, however, significant interactions between time and farm (F(7,217) = 3.76, p < 0.001) and treatment and farm (F(3,217) = 22.39, p < 0.001). A one-way ANOVA examining final percent survival at DB showed a significant treatment effect (F(3,16) = 11.56, p < 0.001). Post-hoc testing revealed the following: (Intertidal = Intertidal→Deep) > (Deep→Intertidal = Deep), demonstrating that oysters spending their first year in the intertidal zone had significantly increased percent survival in comparison to those spending Year 1 in deep water, regardless of Year 2 culture conditions. In contrast, a one-way ANOVA examining final percent survival at DN showed no significant treatment effect.
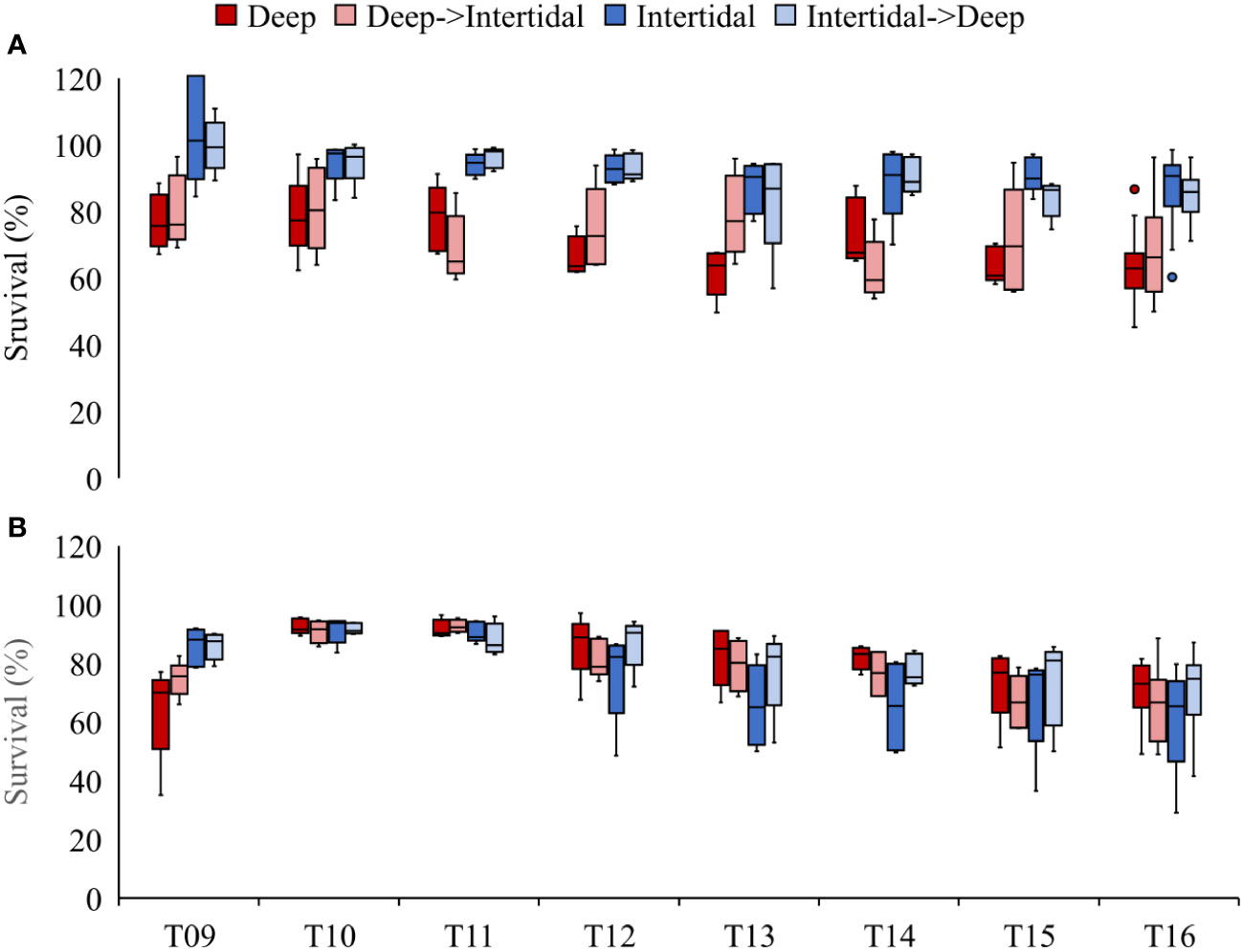
Figure 5 Box plots of survival (%) in Deep (dark red), Deep→Intertidal (light red), Intertidal (dark blue), and Intertidal→Deep (light blue) treatments at (A) Deep Bay and (B) Denman Island farms in Year 2 (T09−T16). T09 = late May, T10 = late June, T11 = early July, T12 = late July, T13 = early August, T14 = late August, T15 = mid-September, and T16 = late September. Bag splitting (number of oysters per bag reduced from 250 to 100) occurred between T09 and T10.
3.3 Reproductive status
In Year 1, the highest proportion of females for a given timepoint, irrespective of farm/site, occurred in early August (T07) (83.3%, F:M = 6.1). When farms and sites were considered separately, the highest proportion of females occurring in Deep and Intertidal groups within each farm also occurred at T07. At DB, a higher proportion of females was reported in the Deep group (91.6%; F:M = 11.0) compared to the Intertidal group (83.3%, F:M = 10.0) at T07. In contrast, at DN and FB farms, higher proportions of females were reported in Intertidal groups (DN: 100%, F:M = n/a; FB: 83.5%, F:M = 5) than in Deep groups (DN: 75%, F:M = 3; FB: 66.7%, F:M = 2.7) at T07. Across all farms and sites, the majority of males and females were classed as Stage 3 maturity (Mature/Ripe) from mid-June through August.
In Year 2, the highest proportion of females for a given timepoint, irrespective of farm/treatment, occurred in late August (T14) (90.3%, F:M = 9.3). When farms and treatments were considered separately, the highest proportion of females occurred in late July (T12) at both DB and DN, but under varying treatments. At DB, the highest proportion of females occurred in late July at the Intertidal site (100%, F:M = n/a), followed by Deep→Intertidal (90.0%, F:M = 9.0), Intertidal→Deep (88.9%, F:M = 8.0), and Deep (70.0%, F:M = 7.0). By late August (T14), this pattern had generally reversed with the Deep treatment having the highest proportion of females (80.0%, F:M = 4.0). The majority of DB males and females, irrespective of treatment group, were classed at Stage 3 (Mature/Ripe) or Stage 4 (Partially Spawned) from late June (T10) through to late July (T12), thereafter largely shifting to Stage 4 through to late August.
At DN, the highest proportion of females in Year 2 also occurred in late July (T12) in the following treatment order: Deep→Intertidal (90%, F:M = 9), Intertidal→Deep (66.7%, F:M = 2), Intertidal (66.7%, F:M = 2), and Deep (62.5%, F:M = 1.7). Of note, there was also an elevated preponderance of females in the Deep treatment in late June (T10) (80%), which dropped to 62.4% by late July (T12) before steadily increasing again. The majority of males and females in the Deep and Intertidal→Deep treatments were classed at Stage 3−4 in late June (T10), shifting to mostly Stage 4 by early July (T11) and throughout August. The DN Intertidal and Deep→Intertidal treatments were largely classed at Stage 4 in late June (T10), shifting to Stages 4−5 by early July (T11), suggesting an earlier/faster maturation status at the intertidal site in comparison to deep water. By late July (T12), the DN intertidal treatment groups were generally classified as Stage 3 or 4, shifting to Stage 4 with some Stage 5 by late August (T14), suggesting that the DN intertidal treatment groups may have had multiple spawning events.
3.4 Histopathology
In general, there were minimal histopathological conditions observed in Year 1 samples. However, of note, VGH was observed in 37/330 (11.2%) of screened animals with more cases observed in oysters from deep-water (23/37, 62.2%) than intertidal sites (14/37, 37.8%). The highest proportion of infected individuals for a given timepoint, irrespective of farm or site, occurred in mid-August (T08) (9/37, 24.3%). Comparison of infection incidence across farms, irrespective of sites and timepoints, showed slightly higher incidence at FB (13/37, 35.1%) and DN (13/37, 35.1%) than at DB (11/37, 29.7%). In contrast, in Year 2, VGH was observed in only 13/358 (2.3%) of screened animals with slightly higher proportion of infected individuals in Deep (4/13, 30.8%) and Deep→Intertidal treatments (4/13, 30.8%) compared to Intertidal (3/13, 23.1%) and Intertidal→Deep (2/13, 15.4%) treatments. The highest proportion of infected individuals for a given timepoint, irrespective of farm or treatment, occurred in early August (T13) (6/13, 46.2%). The majority of infected animals were observed at the DB farm (7/13, 53.8%). A number of other histopathological conditions were reported in Year 2 samples including localized FHI and DHI (in various tissues), MDGT, and ciliate infection, but generally conditions were reported as minor or light infections with no associated pathogens. It should be noted that due to poor fixation, the histopathology of a large number of samples from late July (T12) and early August (T13) of Year 2 (2022) sampling periods could not be properly assessed.
3.5 Condition index and growth
3.5.1 Condition index
In Year 1, mean CIs at DB, DN, and FB were 11.9 ± 3.9, 12.8 ± 4.4, and 9.6 ± 2.0, respectively (Figure 6). Maximum CI in Deep and Intertidal sites at each farm occurred at T03 and T03 (DB), T03 and T04 (DN), and T08 and T06 (FB), respectively. Mean CIs at the end of Year 1 in Deep and Intertidal sites at each farm were 11.1 ± 3.1 and 10.9 ± 2.7 (DB), 10.7 ± 2.2 and 9.3 ± 2.1 (DN), and 10.3 ± 1.6 and 9.0 ± 1.8 (FB), respectively.
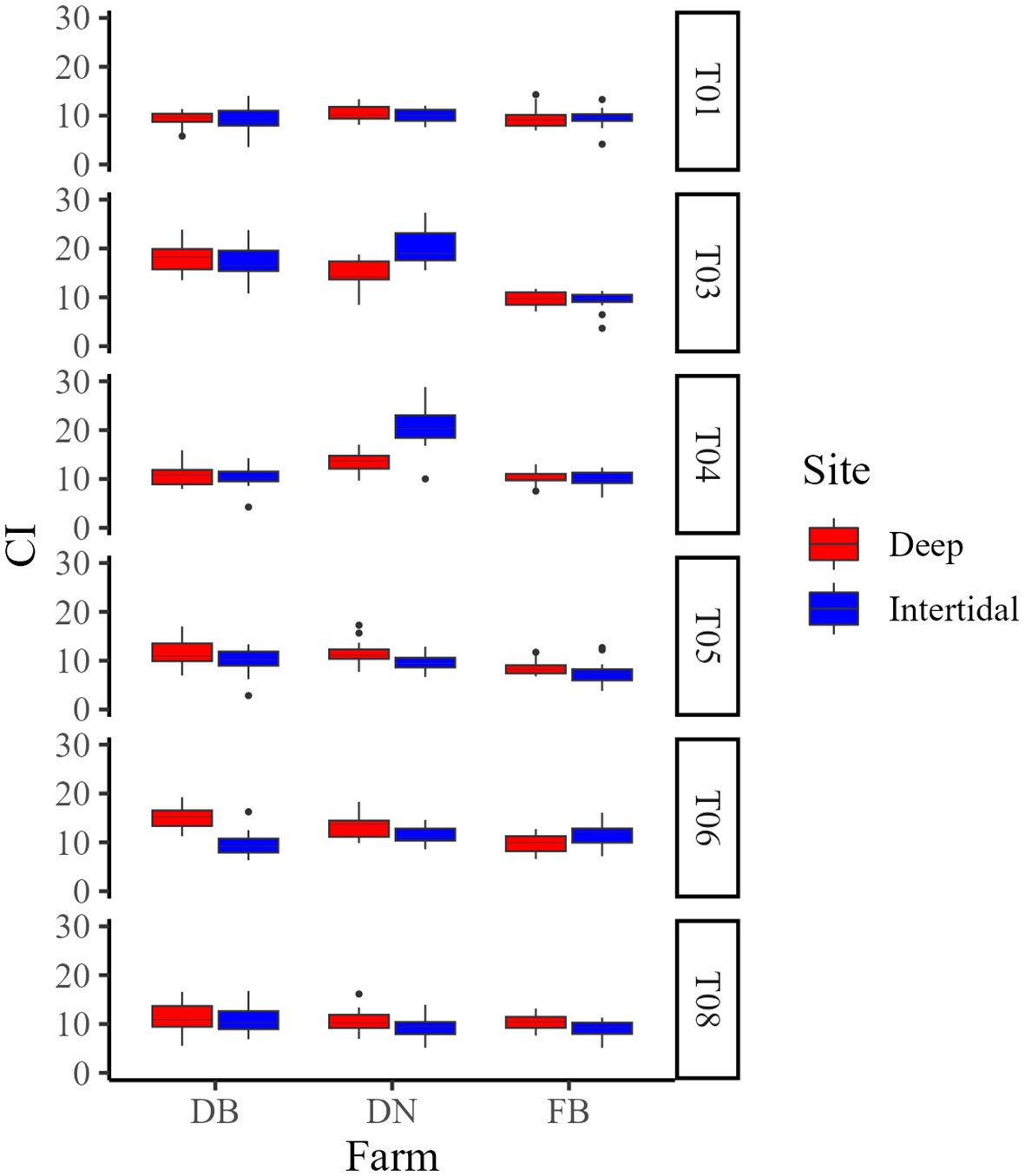
Figure 6 Box plots of condition index (CI) at Deep (red) and Intertidal (blue) sites at Deep Bay (DB), Denman Island (DN), and Fanny Bay (FB) farms in Year 1 (T01−T08). T01 = early May, T03 = early June, T04 = late June, T05 = early July, T06 = late July, and T08 = late August. T02 and T07 are not shown as no samples were collected due to logistical constraints.
In Year 2, mean CIs at DB and DN were 9.7 ± 2.1 and 12.3 ± 3.0, respectively (Figure 7). Maximum CI in Deep, Deep→Intertidal, Intertidal, and Intertidal→Deep treatment groups at each farm occurred at: T09 and T14, T14, T13, and T15 (DB), and T10, T09, T09, and T10 (DN), respectively. Mean CIs at the end of the field trial in Deep, Deep→Intertidal, Intertidal, and Intertidal→Deep treatment groups at each farm were 11.1 ± 1.2, 7.3 ± 1.9, 7.4 ± 3.0, and 10.9 ± 3.1 (DB), and 11.5 ± 2.3, 9.1 ± 3.2, 10.4 ± 2.3, and 12.1 ± 2.3 (DN), respectively.
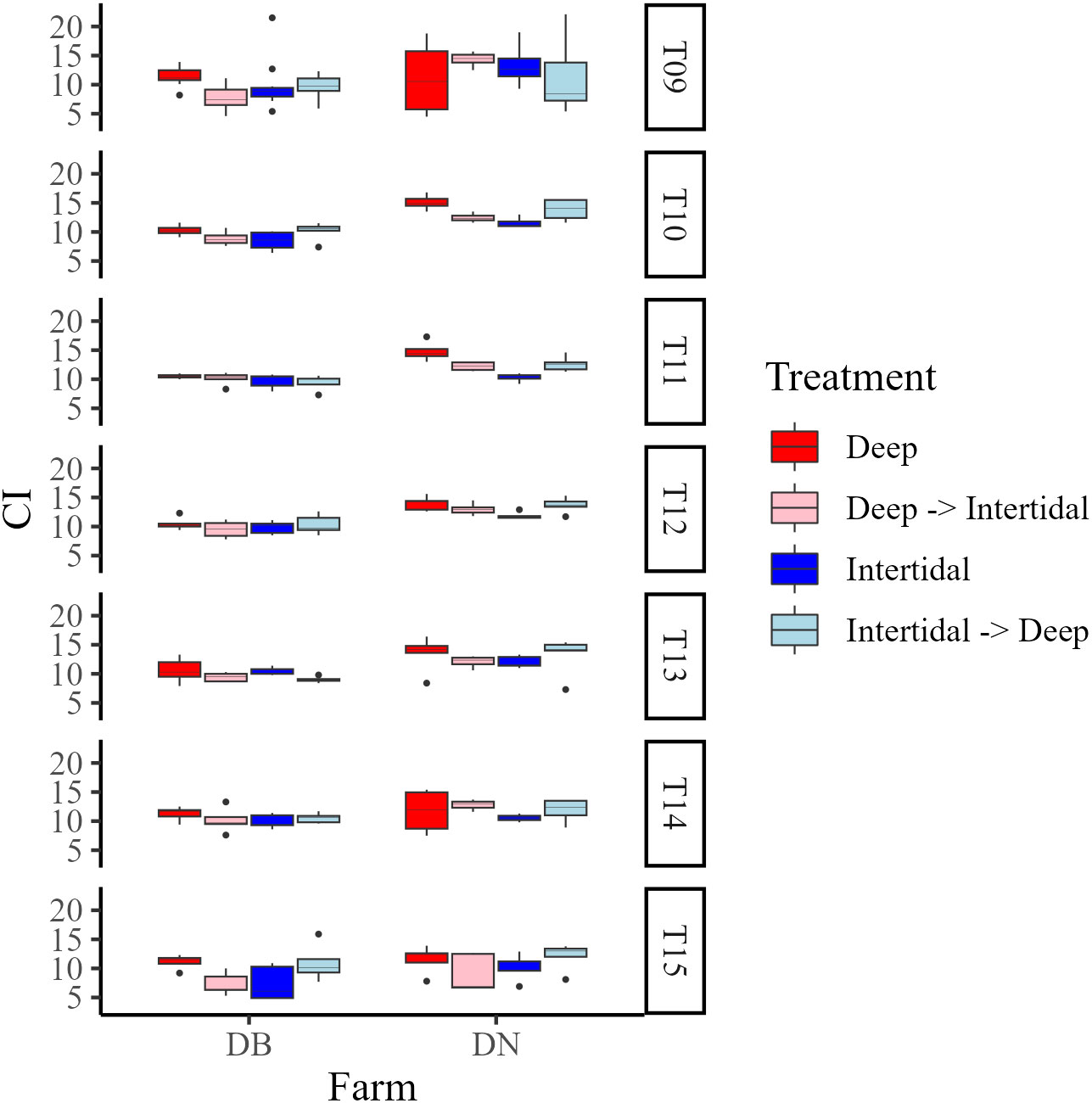
Figure 7 Box plots of condition index (CI) at Deep (dark red), Deep→Intertidal (light red), Intertidal (dark blue), and Intertidal→Deep (light blue) treatments at Deep Bay (DB) and Denman Island (DN) farms in Year 2 (T09−T15). T09 = late May, T10 = late June, T11 = early July, T12 = late July, T13 = early August, T14 = late August, and T15 = mid-September.
Two-way ANOVAs looking at the effect of site/treatment and farm on CI at the end of each growing season [i.e. late August 2020 (T08), mid-September 2021 (T15)] showed an influence of site (F(1,102) = 4.69, p < 0.05) and farm (F(2,102) = 3.39, p < 0.05) on CI at the end of Year 1 (T08), and an effect of treatment only (F(3,32) = 4.56, p < 0.01) on CI in Year 2 (T15), with no significant interactions between factors at either timepoint. One-way ANOVAs of CI between sites/treatments within each farm at the end of each growing season showed an effect of site (F(1,34) = 5.18, p < 0.05) at the end of Year 1 (T08) at FB only, with Deep oysters having a significantly greater CI than Intertidal oysters, and a significant effect of treatment (F(3,16) = 3.77, p < 0.05) on CI at the end of Year 2 (T15) at DB only. However, post-hoc testing detected no significant differences in CI between any treatment groups at the end of Year 2 at DB.
3.5.2 Growth
In Year 1, mean shell heights by late August (T08) at Deep and Intertidal sites at each farm were 78.10 ± 7.19 mm and 74.35 ± 4.73 mm (DB), 73.69 ± 4.78 mm and 64.68 ± 4.95 mm (DN), and 70.06 ± 6.32 mm and 72.07 ± 7.43 mm (FB), respectively (Figure 8A). Mean tissue DWs for the same timepoint (T08) at Deep and Intertidal sites at each farm were 1.64 ± 0.58 g and 1.55 ± 0.54 g (DB), 1.36 ± 0.45 g and 1.07 ± 0.29 g (DN), and 1.47 ± 0.41 g and 1.49 ± 0.39 g (FB), respectively (Figure 8B).
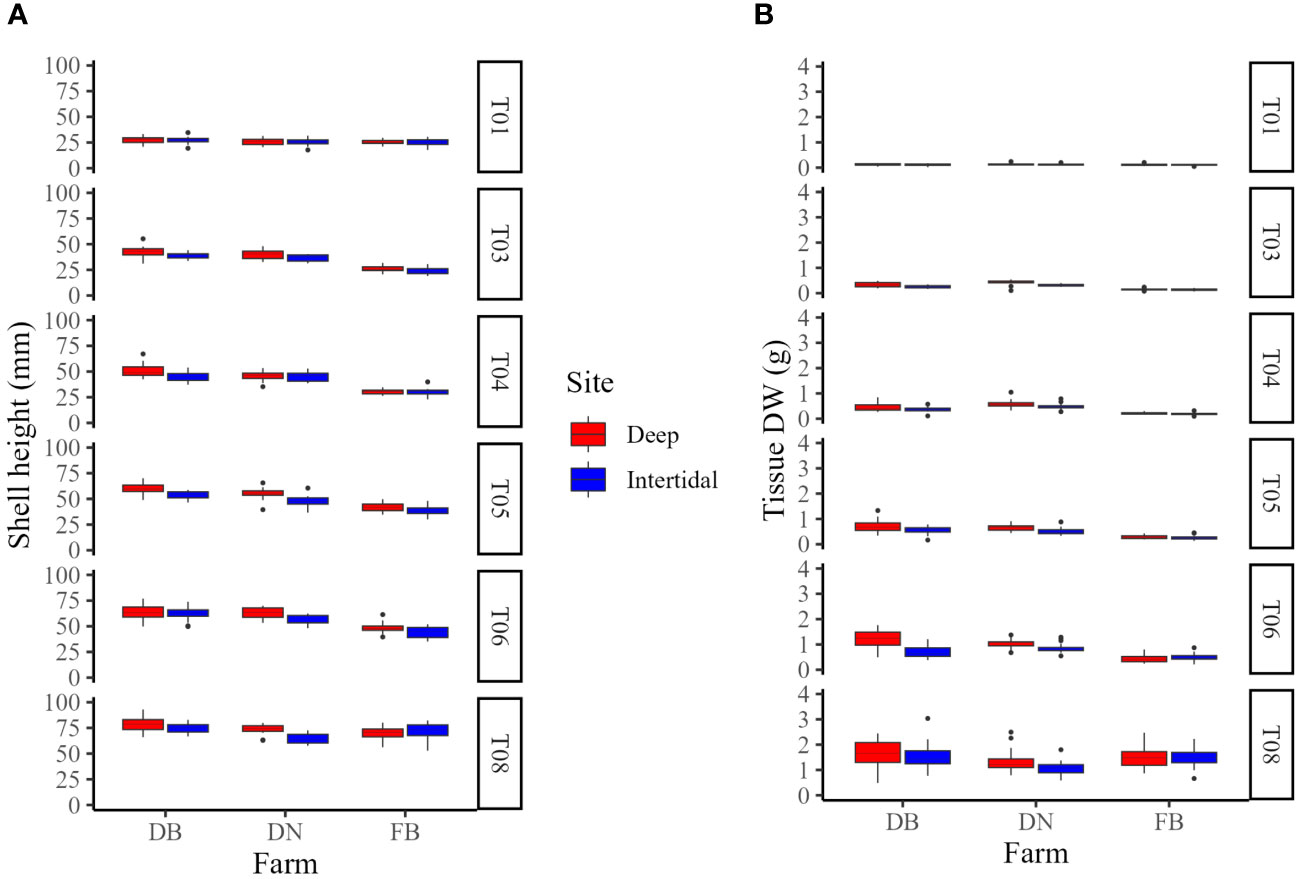
Figure 8 Box plots of (A) shell height and (B) tissue dry weight (DW) at Deep (red) and Intertidal (blue) sites at Deep Bay (DB), Denman Island (DN), and Fanny Bay (FB) farms in Year 1 (T01−T08). T01 = early May, T03 = early June, T04 = late June, T05 = early July, T06 = late July, and T08 = late August. T02 and T07 are not shown as no samples were collected due to logistical constraints.
In Year 2, mean shell heights at the end of the field trial (T15) in Deep, Deep→Intertidal, Intertidal, and Intertidal→Deep treatment groups at each farm were 90.34 ± 6.88 mm, 94.66 ± 5.76 mm, 86.68 ± 3.03 mm, and 94.60 ± 9.28 mm (DB), and 95.00 ± 8.73 mm, 93.38 ± 2.43 mm, 93.02 ± 5.67 mm, and 87.20 ± 4.77 mm (DN), respectively (Figure 9A). Mean tissue DWs for the same timepoint (T15) in Deep, Deep→Intertidal, Intertidal, and Intertidal→Deep treatment groups at each farm were 4.52 ± 1.07 g, 3.37 ± 1.45 g, 2.43 ± 1.01 g, and 4.75 ± 1.46 g (DB), and 6.18 ± 2.28 g, 5.06 ± 1.88 g, 5.44 ± 1.74 g, and 6.16 ± 1.73 g (DN), respectively (Figure 9B).
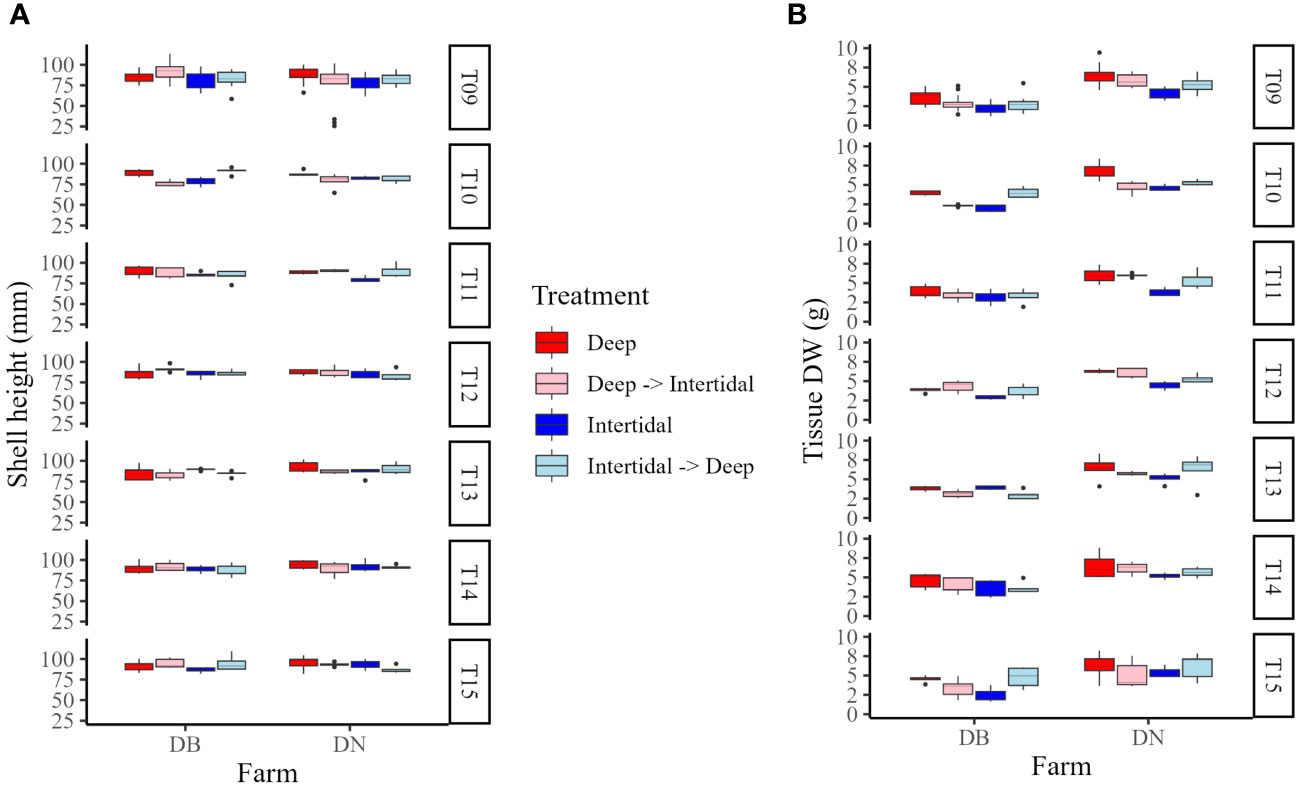
Figure 9 Box plots of (A) shell height and (B) tissue dry weight (DW) in Deep (dark red), Deep→Intertidal (light red), Intertidal (dark blue), and Intertidal→Deep (light blue) treatment groups at DB and DN farms in Year 2 (T09−T15). T09 = late May, T10 = late June, T11 = early July, T12 = late July, T13 = early August, T14 = late August, and, T15 = mid-September.
Two-way ANOVAs looking at the effect of site/treatment and farm on shell height at the end of each growing season [i.e. late August 2020 (T08), mid-September 2021 (T15)] showed a significant influence of site (F(1,102) = 9.60, p < 0.01) and farm (F(2,102) = 13.24, p < 0.001), and an interaction effect (F(2,204) = 7.59, p < 0.001) at the end of Year 1 (T08), but no significant effect of treatment or farm, nor interaction, at the end of Year 2 (T15). One-way ANOVAs of shell height between sites within each farm at the end of each growing season showed a significant effect of site (F(1,34) = 30.9, p < 0.001) at the end of Year 1 (T08) at DN only, with Deep oysters having a significantly greater shell height than Intertidal oysters, but no significant effect of treatment at the end of Year 2 (T15) at either farm.
Two-way ANOVAs looking at the effect of site/treatment and farm on tissue DW at the end of each growing season [i.e. late August 2020 (T08), mid-September 2021 (T15)] showed a significant influence of farm (F(2,102) = 6.72, p < 0.01) at the end of Year 1 (T08), and significant effects of treatment (F(3,32) = 3.63, p < 0.05) and farm (F(1,32) = 22.69, p < 0.001) at the end of Year 2 (T15), with no significant interactions between factors at either timepoint. One-way ANOVAs of tissue DW between sites/treatments within each farm at the end of each growing season showed a significant effect of site (F(1,34) = 5.42, p < 0.05) at the end of Year 1 (T08) at DN only, with Deep oysters having significantly greater tissue DW than the Intertidal oysters, and a significant effect of treatment (F(3,16) = 5.57, p < 0.01) at the end of Year 2 (T15) at DB only, with Deep and Deep→Intertidal oysters having a significantly greater tissue DW than Intertidal oysters. Of note, there were no significant differences in the tissue DW of Intertidal→Deep and Deep treatments at either farm at the end of the field trial (T15).
3.6 Laboratory-challenge experiments
3.6.1 Heatwave challenge (year 1)
Under an immersion challenge at 15°C, final percent survivals were 100% and 98% in oysters from Intertidal and Deep sites, respectively, while under emersion at 15°C, final percent survivals were only 30% and 10%, respectively (Figure 10A). Under emersion conditions, log-rank testing showed a significant difference (χ2 = 8.4, df = 1, p = 0.004) in survival curves of oysters from Intertidal and Deep sites, and hazards analyses determined a significantly decreased hazard (HR = 0.53, 95% CI = 0.34−0.83, p = 0.006) in oysters from the Intertidal site in comparison to those from the Deep site (Figure 10A).
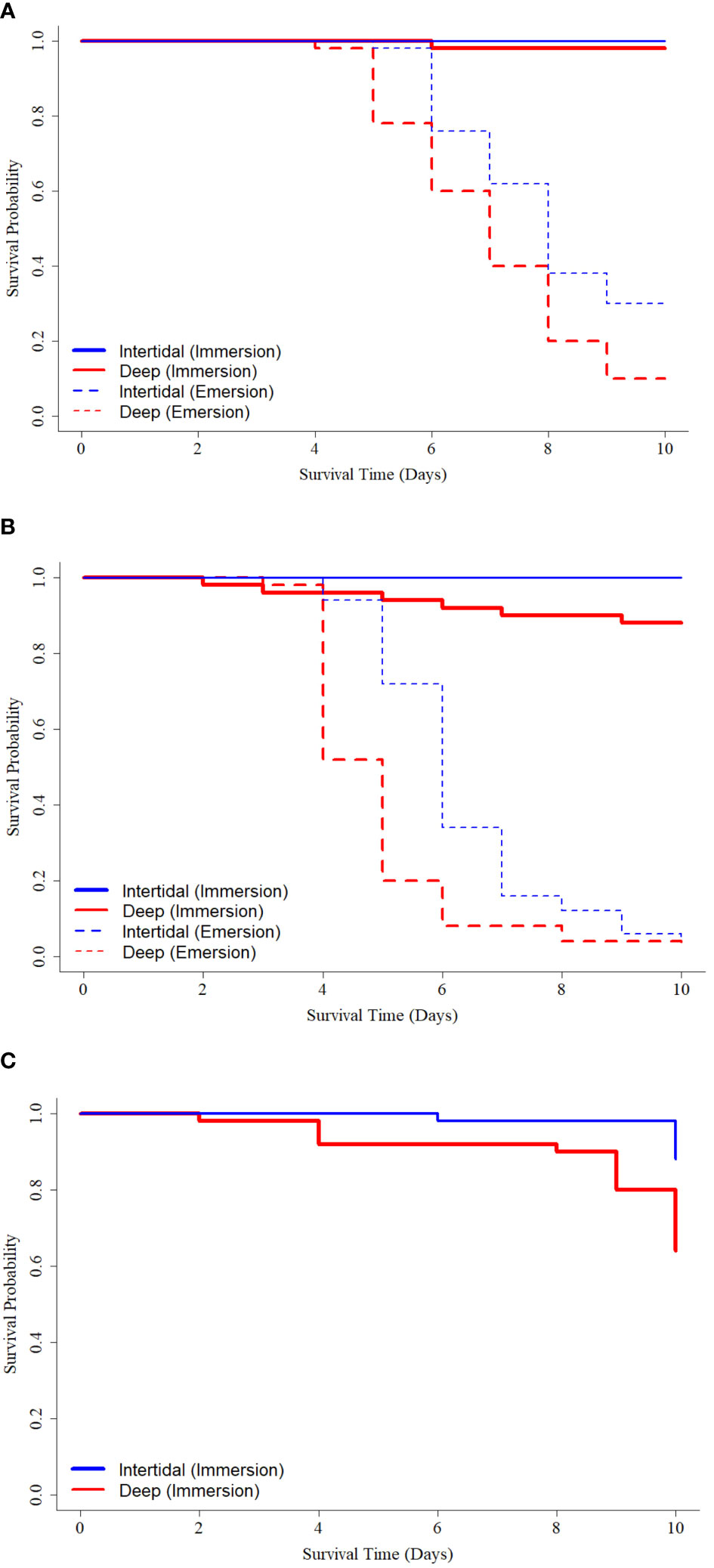
Figure 10 Kaplan-Meier survival curves of oysters from Deep (red lines) and Intertidal (blue lines) sites under immersion (solid lines) or emersion (dashed lines) exposures at (A) control (15°C) or (B, C) simulated heatwave (20 and 25°C, respectively) conditions.
Under an immersion challenge at 20°C, final percent survivals were 100% and 88% in oysters from Intertidal and Deep sites, respectively (Figure 10B). Log-rank testing showed a significant difference (χ2 = 6.3, df=1, p = 0.010) in survival curves. Hazards analyses could not be carried out for this comparison due to total survival of oysters in the Intertidal treatment group. Under an emersion challenge at 20°C, final percent survivals were 4% and 2% in oysters from the Intertidal and Deep sites, respectively. Log-rank testing of oysters emersed at 20°C showed a significant difference (χ2 = 19.2, df = 1, p < 0.001) in survival curves of oysters from Deep and Intertidal sites, and hazards analyses determined a significantly decreased hazard (HR = 0.43, 95% CI = 0.28−0.64, p < 0.001) in oysters from the Intertidal site in comparison to those from the Deep site (Figure 10B).
Under an immersion challenge at 25°C, final percent survivals were 88% and 64% in oysters from Intertidal and Deep sites, respectively (Figure 10C). Log-rank testing showed a significant difference (χ2 = 8.5, df=1, p = 0.004) in survival curves of oysters from Deep and Intertidal sites, and hazards analyses determined a significantly decreased hazard (HR = 0.28, 95% CI = 0.11–0.70, p = 0.007) in oysters from the Intertidal site in comparison to those from the Deep site (Figure 10C). Under an emersion challenge at 25°C, the majority of animals from both sites spawned within 48 hours so the challenge was ended.
Under all temperature and immersion/emersion conditions, Deep oysters experienced earlier and greater mortality than their Intertidal counterparts. This was most obvious at 20 and 25°C under emersion challenge, where Deep oysters experienced some morality at 2 days (at both 20 and 25°C) compared with Intertidal counterparts that showed zero mortality (at 20°C) or delayed mortality until 6 days (at 25°C).
3.6.2 Heatwave and Vibrio challenge (year 2)
Under 16°C and control (no Vibrio) conditions, final percent survivals were 100% for oysters from both Intertidal→Deep and Deep treatments (Figure 11A). Under 16°C and a Vibrio challenge, final percent survivals were 63% and 34% for oysters from Intertidal→Deep and Deep treatments, respectively. Log-rank testing showed a significant difference (χ2 = 4.4, df = 1, p = 0.040) in survival curves and hazards analyses determined a significantly decreased hazard (HR = 0.49, 95% CI = 0.25−0.97, p = 0.041) for oysters from the Intertidal→Deep treatment in reference to those from the Deep treatment (Figure 11A).
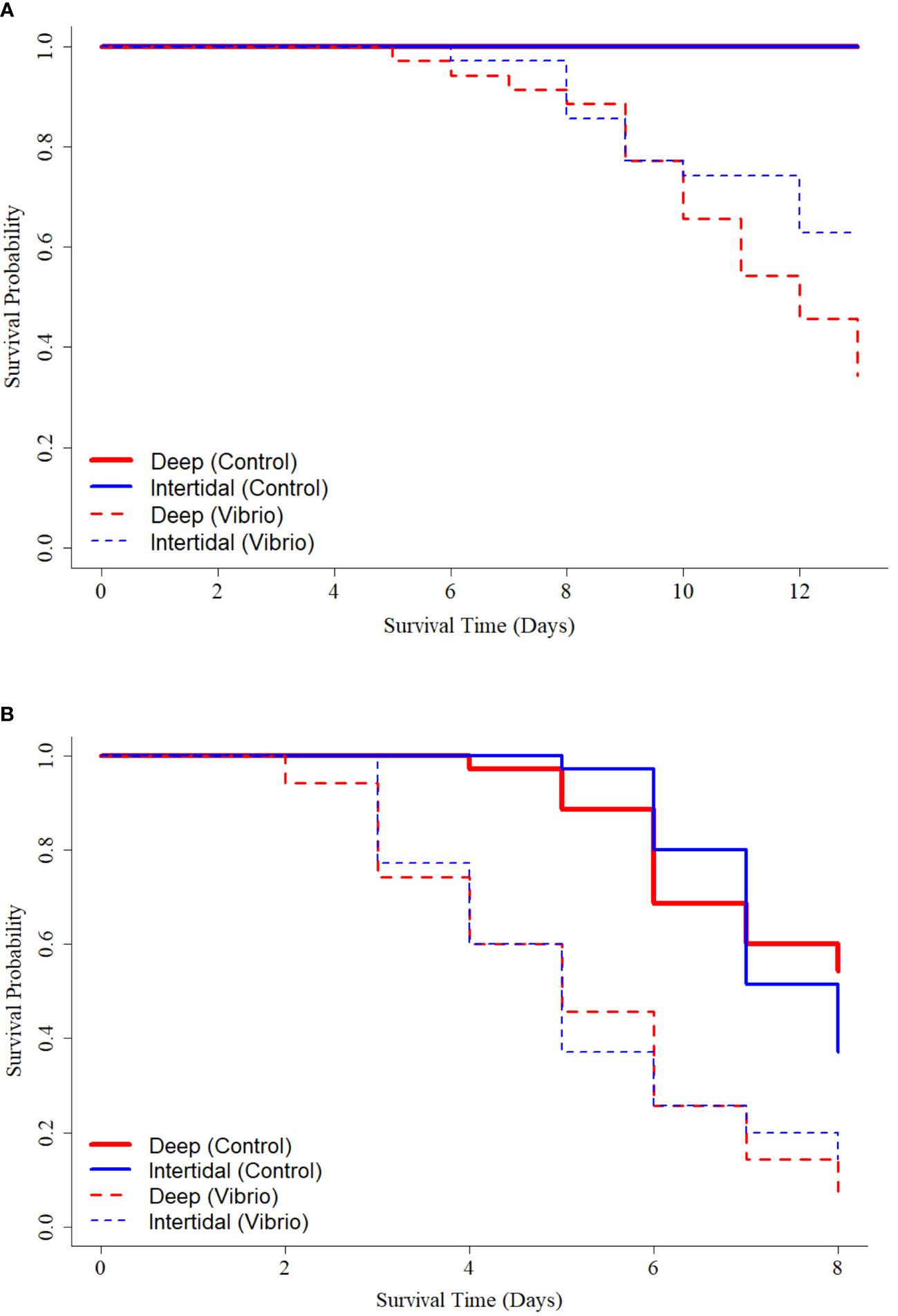
Figure 11 Kaplan-Meier survival curves for oysters from Intertidal→Deep (blue lines) and Deep (red lines) treatments under Vibrio (dashed lines) and control (solid lines) exposures at (A) control (16°C) or (B) simulated heatwave (24°C) conditions.
Under 24°C and control (no Vibrio) conditions, final percent survivals were 54% and 37% for oysters from Deep and Intertidal→Deep treatments, respectively (Figure 11B). However, log-rank testing showed no significant difference (χ2 = 0.8, df = 1, p = 0.400) in survival curves and hazards analyses showed an insignificant decrease in hazard (HR = 1.40, 95% CI = 0.71−2.60, p = 0.348) for oysters from the Intertidal→Deep treatment in reference to those from the Deep treatment (Figure 11B).
Under 24°C and Vibrio challenge, final percent survivals were 14% and 6% for oysters from Intertidal→Deep and Deep treatments, respectively (Figure 11B). Log-rank testing showed no significant difference (χ2 = 0.2, df = 1, p = 0.600) in survival curves and hazards analyses showed an insignificant decrease in hazard (HR = 0.88, 95% CI = 0.54−1.50, p = 0.626) for oysters from the Intertidal→Deep treatment in reference to those from the Deep treatment (Figure 11B).
As observed in the Year 1 challenge experiment, Deep treatment oysters experienced earlier mortality than their Intertidal→Deep treatment counterparts. Under Vibrio conditions at both 16 and 24°C, and under 24°C (no Vibrio) conditions, Deep treatment oysters experienced mortality 1 day earlier than Intertidal→Deep treatment oysters.
4 Discussion
Results suggest that partial or full culture of oysters in the intertidal zone over a 2-year growth period does confer some resilience to summer mortality stressor conditions at some locations and should be considered as one tool under a collective approach for mitigation of Pacific oyster summer mortality. However, given that oyster summer mortality is a multi-faceted phenomenon (Dégremont et al., 2010; Barbosa Solomieu et al., 2015; Cowan et al., 2023), it is acknowledged that the specific physical and biological factors associated with any given farm will play contributory roles. Here we discuss how the intertidal zone may improve oyster resilience to summer mortality stressors and consider how farm-specific factors in the present study may have shaped responses.
We propose that the high level of phenotypic plasticity associated with intertidal oysters confers to them an increased level of resilience to summer mortality in comparison to deep-water counterparts. In the present study, laboratory-challenge experiments demonstrated increased survival time and reduced mortality in intertidally-cultured oysters (Year 1: Intertidal, Year 2: Intertidal→Deep) as compared to deep-water-cultured oysters, which was paralleled under field settings at one farm site (DB). Corporeau et al. (2022) also reported a substantial (>1 week) delay in mortality in intertidal Pacific oysters compared with subtidal counterparts during a summer mortality event. Likewise, intertidal Pacific oysters have shown improved survival over subtidal equivalents during emersion stress (Meng et al., 2018).
Pre-exposure of oysters to stressor conditions may lead to improved resilience that is also maintained after introduction to a less dynamic/stressful environment. In the present study, the benefits conferred by time in the intertidal appeared to be maintained several months after transplantation to the subtidal environment, as evidenced by the increased survival of oysters from Intertidal and Intertidal→Deep treatments in the field (at the DB site only) and of those from the Intertidal→Deep treatment during the laboratory temperature/Vibrio challenge. We suggest that the persistence of phenotypic plasticity associated with intertidal conditions may account for improved survival after transplant. The extension of mechanisms of resilience from dynamic to more stable environments may be facilitated by epigenetic processes such as DNA acetylation and methylation, histone modifications, and non-coding RNAs (Hofmann, 2017; Li et al., 2018), which collectively allow phenotypes that confer resilience in one environment to be transferred to novel settings and/or later life stages (Jablonka and Lamb, 2002). Indeed, a number of prior studies have demonstrated that intertidal populations of bivalve species (including Pacific oysters) show increased genome methylation and acetylation compared to subtidal counterparts during exposure to novel conditions (Clark et al., 2018; Li et al., 2018). Wang et al. (2021) also reported a greater proportion of differentially-expressed genes and significantly greater methylation variation in intertidal Pacific oysters in comparison to subtidal equivalents under a high temperature challenge. Similarly, continued upregulation of genes involved in antioxidation processes was observed in intertidal populations of Antarctic limpets (Nacella concinna) after transplant to the subtidal zone (Clark et al., 2018).
Adult bivalves may also maintain some degree of resilience to environmental stressors when there is a history of parental or early-life-stage stress exposure (Reid et al., 2019; Dang et al., 2023). For example, F2 generation intertidal Pacific oysters were shown to maintain differentially-methylated genes associated with the thermal responses of the F0 generation (Wang et al., 2023). Similarly, adult Hong Kong oysters (C. hongkongensis) exposed to ocean acidification as larvae demonstrated a beneficial carry-over effect associated with the methylation of metabolic and endocytic genes under novel stressor conditions (Dang et al., 2023). In the same way, epigenetic mechanisms could account for the maintained resilience of intertidally-cultured oysters after transplant to the subtidal environment, as observed in the present study.
Other environmentally-driven physiological differences between intertidal and subtidal groups could also account for differences in survival. For example, intertidally-cultured animals have been observed to develop thicker shells and larger adductor muscles than their subtidal counterparts (Lewis and Seed, 1969; Hickman and Illlingworth, 1980; Tagliarolo et al., 2012), which may support improved resilience to temperature and pathogen stressors. Likewise, the intertidal environment may modify host-pathogen interactions via promotion of microbiomes, metabolism, inflammation, and innate immunity that collectively grant improved resistance to pathogen infections (King et al., 2019; Offret et al., 2020; Corporeau et al., 2022). Intertidal oysters may also fare better under stressor conditions due to an improved ability to enact larger energy metabolism depressions and earlier anaerobic glycolysis responses (Meng et al., 2018).
In the present study, while time in the intertidal was associated with improved survival (at the DB site) and largely equivalent condition across treatment groups, intertidal treatment groups did show decreases in growth metrics. Specifically, in Year 1, intertidal oysters were significantly smaller in shell height and tissue DW than deep-water cultured individuals within the same farm at a number of timepoints. The observed reductions in growth were likely a result of reduced feeding opportunities in the intertidal zone, but, importantly, could support higher survival during summer months as a result of associated delays in reproductive development and/or reduced spawning (Cotter et al., 2010; Huvet et al., 2010; Cowan et al., 2023). Moreover, it has been suggested that individuals demonstrating plasticity in order to tolerate a range of environmental conditions at the expense of normal physiological function and energetics, may show a rebound in growth and survival when under more optimal conditions (Barbosa et al., 2022). This could be the case in the present study, as growth discrepancies were no longer apparent by the end of Year 2 (i.e. at harvest), shell height, tissue DW, and CI being generally comparable across Deep and Intertidal→Deep treatments.
Site-related differences may have also had a significant effect on the subsequent performance of oysters in terms of growth and maturity, and could account for the differing patterns of survival observed among farm sites in the present study. Differing levels of nutrients and phytoplankton across the study’s spatial extent, for example, could result in varying growth rates, reproductive development, and overall condition (Malham et al., 2009; Cotter et al., 2010), all of which represent key factors that shape susceptibility to summer mortality. While we did not observe obvious differences in physical parameters (temperature, salinity, and dissolved oxygen) among farm sites, variation in patterns of reproductive development, CI, and growth across the farms/sites does suggest temporal and spatial variations in levels of feed and nutrient availability.
Results of histopathological screening in the present study suggested a minimal role of disease in driving observed differences in survival between treatments. However, of note, there was a greater incidence of VGH observed in deep-water oysters compared to intertidal counterparts in Year 1, suggesting that oysters under suspended culture conditions have increased exposure risk and/or susceptibility to VGH infection. It is also of interest that increased VGH incidence was detected in August of both years, suggesting a link with increased temperatures, as has been previously observed (Choi et al., 2004). Historically, VGH infections have occurred at low frequency and intensity and lack correlation with increased mortality rates, cumulatively suggesting that the VGH virus poses little overall threat to oysters. However, in recent years, a number of oyster-producing regions across the globe have reported an increasing prevalence of the virus, including higher infection rates within populations and a wider distribution range (Garcia et al., 2006). Results here could also suggest that VGH poses an increased threat under future climate change conditions, but further investigation is warranted.
We propose the use of intertidal culture in Year 1 as part of a practical mitigative approach in order to combat summer mortality in Pacific oysters and improve resilience to climate change stressors. Partial culture in the intertidal conferred improved resilience to summer mortality stressor conditions both in the field (at one farm) and under laboratory conditions, with no obvious detriment to harvest size or condition. These findings may inform industry practice under the context of a changing climate, though additional examination is needed. Future work should include re-testing the intertidal culture approach across an increased number of farms, incorporating other mitigative approaches (e.g. selective breeding programs), examining multiple grow-out gear types, and conducting comprehensive physical and biological monitoring at farm sites.
Summer mortality events and climate change are long-term and accelerating challenges that pose serious threats to the shellfish aquaculture sector. An improved understanding of impacts and a sustained focus on developing practical methods that promote resilience in oysters are critical for safe-guarding this important global resource.
Data availability statement
The raw data supporting the conclusions of this article will be made available by the authors, without undue reservation.
Ethics statement
The animal study was approved by the Pacific Region Animal Care Committee of Fisheries and Oceans Canada. The study was conducted in accordance with the local legislation and institutional requirements.
Author contributions
CM: Conceptualization, Formal analysis, Funding acquisition, Investigation, Methodology, Project administration, Supervision, Visualization, Writing – original draft. MR: Formal analysis, Investigation, Project administration, Supervision, Visualization, Writing – original draft. SL: Investigation, Project administration, Writing – review & editing. CW: Investigation, Writing – review & editing. TG: Investigation, Project administration, Resources, Supervision, Writing – review & editing. EK: Investigation, Writing – review & editing. EM: Investigation, Writing – review & editing. SG: Investigation, Writing – review & editing. AL: Project administration, Resources, Supervision, Writing – review & editing. CP: Conceptualization, Funding acquisition, Methodology, Project administration, Resources, Supervision, Writing – review & editing.
Funding
The author(s) declare financial support was received for the research, authorship, and/or publication of this article. Funding for this work was provided by Fisheries and Oceans Canada’s Aquaculture Collaborative Research and Development Program (ACRDP 21-P-02) and Taylor Shellfish Canada ULC.
Acknowledgments
The authors gratefully acknowledge the contributions of Alex Munro (Taylor Shellfish Canada ULC) and the staff, students, and volunteers of the Deep Bay Marine Field Station (Vancouver Island University).
Conflict of interest
The authors declare that the research was conducted in the absence of any commercial or financial relationships that could be construed as a potential conflict of interest.
This project was carried out under Fisheries and Oceans Canada’s Aquaculture Collaborative Research and Development Program with Taylor Shellfish Canada ULC as the industry partner. Taylor Shellfish Canada ULC provided project funds and in-kind contributions in support of the project. Two of the three field sites included in the study (DN, FB) are commercial farms of Taylor Shellfish Canada ULC.
Publisher’s note
All claims expressed in this article are solely those of the authors and do not necessarily represent those of their affiliated organizations, or those of the publisher, the editors and the reviewers. Any product that may be evaluated in this article, or claim that may be made by its manufacturer, is not guaranteed or endorsed by the publisher.
Supplementary material
The Supplementary Material for this article can be found online at: https://www.frontiersin.org/articles/10.3389/fmars.2024.1345493/full#supplementary-material
References
Abele D., Brey T., Philipp E. (2009). Bivalve models of aging and the determination of molluscan lifespans. Exp. Gerontol. 44, 307. doi: 10.1016/j.exger.2009.02.012
Amorim K., Piontkivska H., Zettler M. L., Sokolov E., Hinzke T., Nair A. M., et al. (2021). Transcriptional response of key metabolic and stress response genes of a nuculanid bivalve, Lembulus bicuspidatus from an oxygen minimum zone exposed to hypoxia-reoxygenation. Comp. Biochem. Physiol. Part B: Biochem. Mol. Biol. 256, 110617. doi: 10.1016/j.cbpb.2021.110617
Ashton E. C., Guist S., Roberts D., Sigwart J. D. (2020). Effects of environmental factors and husbandry practices on summer mortality events in the cultivated Pacific oyster Crassostrea gigas in the North of Ireland. J. Shellfish Res. 39, 13–20. doi: 10.2983/035.039.0102
Barbosa M., Schwaner C., Pales Espinosa E., Allam B. (2022). A transcriptomic analysis of phenotypic plasticity in Crassostrea virginica larvae under experimental acidification. Genes 13, 1529. doi: 10.3390/genes13091529
Barbosa Solomieu V., Renault T., Travers M. A. (2015). Mass mortality in bivalves and the intricate case of the Pacific oyster, Crassostrea gigas. J. Invertebrate Pathol. 131, 2–10. doi: 10.1016/j.jip.2015.07.011
Bayne B. L. (2004). Phenotypic flexibility and physiological tradeoffs in the feeding and growth of marine bivalve molluscs. Integr. Comp. Biol. 44, 425–432. doi: 10.1093/icb/44.6.425
BCMSRM (2002). The Baynes Sound coastal plan for shellfish aquaculture (Victoria, BC, Canada: British Columbia Ministry of Sustainable Resource Management, Coast & Marine Planning Branch).
Brown J. R., Hartwick E. B. (1988). Influences of temperature, salinity and available food upon suspended culture of the Pacific oyster, Crassostrea gigas. I. Absolute and allometric growth. Aquaculture 70, 231–251. doi: 10.1016/0044-8486(88)90099-3
Campbell M. D., Hall S. G. (2019). Hydrodynamic effects on oyster aquaculture systems: a review. Rev. Aquac. 11, 896–906. doi: 10.1111/raq.12271
Cassis D., Pearce C. M., Maldonado M. T. (2011). Effects of the environment and culture depth on growth and mortality in juvenile Pacific oysters in the Strait of Georgia, British Columbia. Aquac. Environ. Interact. 1, 259–274. doi: 10.3354/aei00025
Choi D. L., Lee N. S., Choi H. J., Park M. A., McGladdery S. E., Mi S. P. (2004). Viral gametocytic hypertrophy caused by a papova-like virus infection in the Pacific oyster Crassostrea gigas in Korea. Dis. Aquat. Organ. 59, 205–209. doi: 10.3354/dao059205
Clark M. S., Suckling C. C., Cavallo A., Mackenzie C. L., Thorne M. A. S., Davies A. J., et al. (2019). Molecular mechanisms underpinning transgenerational plasticity in the green sea urchin Psammechinus miliaris. Sci. Rep. 9 (1), 952. doi: 10.1038/s41598-018-37255-6
Clark M. S., Thorne M. A. S., King M., Hipperson H., Hoffman J. I., Peck L. S. (2018). Life in the intertidal: cellular responses, methylation and epigenetics. Funct. Ecol. 32, 1982–1994. doi: 10.1111/1365-2435.13077/SUPPINFO
Corporeau C., Petton S., Vilaça R., Delisle L., Quéré C., Le Roy V., et al. (2022). Harsh intertidal environment enhances metabolism and immunity in oyster (Crassostrea gigas) spat. Mar. Environ. Res. 180, 105709. doi: 10.1016/j.marenvres.2022.105709
Cotter E., Malham S. K., O’Keeffe S., Lynch S. A., Latchford J. W., King J. W., et al. (2010). Summer mortality of the Pacific oyster, Crassostrea gigas, in the Irish Sea: the influence of growth, biochemistry and gametogenesis. Aquaculture 303, 8–21. doi: 10.1016/j.aquaculture.2010.02.030
Cowan M. (2020). Exploring the mechanisms of Pacific oyster summer mortality in Baynes Sound aquaculture. MSc Thesis. Victoria, Canada: University of Victoria.
Cowan M. W., Pearce C. M., Finston T., Meyer G. R., Marshall R., Evans W., et al. (2023). Role of the Vibrio community, reproductive effort, and environmental parameters in intertidal Pacific oyster summer mortality in British Columbia, Canada. Aquaculture 565, 739094. doi: 10.1016/j.aquaculture.2022.739094
Dang X., Lim Y. K., Li Y., Roberts S. B., Li L., Thiyagarajan V. (2023). Epigenetic-associated phenotypic plasticity of the ocean acidification-acclimated edible oyster in the mariculture environment. Mol. Ecol. 32, 412–427. doi: 10.1111/mec.16751
Dégremont L., Bédier E., Boudry P. (2010). Summer mortality of hatchery-produced Pacific oyster spat (Crassostrea gigas). II. Response to selection for survival and its influence on growth and yield. Aquaculture 299, 21–29. doi: 10.1016/j.aquaculture.2009.11.017
Ernande B., Boudry P., Clobert J., Haure J. (2004). Plasticity in resource allocation based life history traits in the Pacific oyster, Crassostrea gigas. I. Spatial variation in food abundance. J. Evol. Biol. 17, 342–356. doi: 10.1046/j.1420-9101.2003.00674.x
Evans O., Kan J. Z. F., Pathirana E., Whittington R. J., Dhand N., Hick P. (2019). Effect of emersion on the mortality of Pacific oysters (Crassostrea gigas) infected with Ostreid herpesvirus-1 (OsHV-1). Aquaculture 505, 157–166. doi: 10.1016/j.aquaculture.2019.02.041
FAO (2022). The State of World Fisheries and Aquaculture 2022. Towards Blue Transformation (Rome: FAO). doi: 10.4060/cc0463en
Garcia C., Robert M., Arzul I., Chollet B., Joly J. P., Miossec L., et al. (2006). Viral gametocytic hypertrophy of Crassostrea gigas in France: from occasional records to disease emergence? Dis. Aquat. Organ. 70, 193–199. doi: 10.3354/dao070193
Go J., Deutscher A. T., Spiers Z. B., Dahle K., Kirkland P. D., Jenkins C. (2017). Mass mortalities of unknown aetiology in Pacific oysters Crassostrea gigas in Port Stephens, New South Wales, Australia. Dis. Aquat. Org. 125 (3), 227–242. doi: 10.3354/dao03146
Green T. J., Siboni N., King W. L., Labbate M., Seymour J. R., Raftos D. (2019). Simulated marine heat wave alters abundance and structure of Vibrio populations associated with the Pacific oyster resulting in a mass mortality event. Microb. Ecol. 77, 736–747. doi: 10.1007/s00248-018-1242-9
Hamdoun A. M., Cheney D. P., Cherr G. N. (2003). Phenotypic plasticity of HSP70 and HSP70 gene expression in the Pacific oyster (Crassostrea gigas): implications for thermal limits and induction of thermal tolerance. Biol. Bull. 205, 160–169. doi: 10.2307/1543236
Hickman R. W., Illlingworth J. (1980). Condition cycle of the green-lipped mussel Perna canaliculus in New Zealand. Mar. Biol. 60, 27–38. doi: 10.1007/BF00395603
Hofmann G. E. (2017). Ecological epigenetics in marine metazoans. Front. Mar. Sci. 4. doi: 10.3389/fmars.2017.00004
Huvet A., Normand J., Fleury E., Quillien V., Fabioux C., Boudry P. (2010). Reproductive effort of Pacific oysters: a trait associated with susceptibility to summer mortality. Aquaculture 304, 95–99. doi: 10.1016/j.aquaculture.2010.03.022
Jablonka E., Lamb M. J. (2002). The changing concept of epigenetics. Ann. N.Y. Acad. Sci. 981, 82–96. doi: 10.1111/j.1749-6632.2002.tb04913.x
King W. L., Jenkins C., Go J., Siboni N., Seymour J. R., Labbate M. (2019). Characterisation of the Pacific oyster microbiome during a summer mortality event. Microb. Ecol. 77, 502–512. doi: 10.1007/s00248-018-1226-9
Labreuche Y., Lambert C., Soudant P., Boulo V., Huvet A., Nicolas J. L. (2006). Cellular and molecular hemocyte responses of the Pacific oyster, Crassostrea gigas, following bacterial infection with Vibrio aestuarianus strain 01/32. Microbes Infect. 8, 2715–2724. doi: 10.1016/j.micinf.2006.07.020
Lafont M., Goncalves P., Guo X., Montagnani C., Raftos D., Green T. (2019). Transgenerational plasticity and antiviral immunity in the Pacific oyster (Crassostrea gigas) against Ostreid herpesvirus 1 (OsHV-1). Dev. Comp. Immunol. 91, 17–25. doi: 10.1016/j.dci.2018.09.022
Lafont M., Vergnes A., Vidal-Dupiol J., De Lorgeril J., Gueguen Y., Haffner P., et al. (2020). A sustained immune response supports long-term antiviral immune priming in the Pacific oyster Crassostrea gigas. mBio 11 (2), 10–1128. doi: 10.1128/mBio.02777-19
Lewis J., Seed R. (1969). Morphological variations in Mytilus from south-west England in relation to the occurrence of M. galloprovincialis Lamarck. Cahiers Biologie Mar. 10, 231–253.
Li L., Li A., Song K., Meng J., Guo X., Li S., et al. (2018). Divergence and plasticity shape adaptive potential of the Pacific oyster. Nat. Ecol. Evol. 2, 1751–1760. doi: 10.1038/s41559-018-0668-2
Li Y., Qin J. G., Li X., Benkendorff K. (2009). Monthly variation of condition index, energy reserves and antibacterial activity in Pacific oysters, Crassostrea gigas, in Stansbury (South Australia). Aquaculture 286, 64–71. doi: 10.1016/j.aquaculture.2008.09.004
Li Q., Zhao X., Kong L., Yu H. (2013). Transcriptomic response to stress in marine bivalves. Invertebrate Survival J. 10, 84–93.
Mackenzie C. L., Pearce C. M., Leduc S., Roth D., Kellogg C. T. E., Clemente-Carvalho R. B. G., et al. (2022). Impacts of seawater pH buffering on the larval microbiome and carry-over effects on later-life disease susceptibility in Pacific oysters. Appl. Environ. Microbiol. 88 (22), e01654-22. doi: 10.1128/aem.01654-22
Malham S. K., Cotter E., O’Keeffe S., Lynch S., Culloty S. C., King J. W., et al. (2009). Summer mortality of the Pacific oyster, Crassostrea gigas, in the Irish Sea: the influence of temperature and nutrients on health and survival. Aquaculture 287, 128–138. doi: 10.1016/j.aquaculture.2008.10.006
Mann R. (1979). Some biochemical and physiological aspects of growth and gametogenesis in Crassostrea gigas and Ostrea edulis grown at sustained elevated temperatures. J. Mar. Biol. Assoc. United Kingdom 59, 95–110. doi: 10.1017/S0025315400046208
Marty G. D., Bower S. M., Clarke K. R., Meyer G., Lowe G., Osborn A. L., et al. (2006). Histopathology and a real-time PCR assay for detection of Bonamia ostreae in Ostrea edulis cultured in western Canada. Aquaculture 261, 33–42. doi: 10.1016/j.aquaculture.2006.07.024
Masanja F., Yang K., Xu Y., He G., Liu X., Xu X., et al. (2023). Impacts of marine heat extremes on bivalves. Front. Mar. Sci. 10, 1159261. doi: 10.3389/fmars.2023.1159261
Meng J., Wang T., Li L., Zhang G. (2018). Inducible variation in anaerobic energy metabolism reflects hypoxia tolerance across the intertidal and subtidal distribution of the Pacific oyster (Crassostrea gigas). Mar. Environ. Res. 138, 135–143. doi: 10.1016/j.marenvres.2018.04.002
Normand J., Le Pennec M., Boudry P. (2008). Comparative histological study of gametogenesis in diploid and triploid Pacific oysters (Crassostrea gigas) reared in an estuarine farming site in France during the 2003 heatwave. Aquaculture 282, 124–129. doi: 10.1016/j.aquaculture.2008.06.026
Offret C., Paulino S., Gauthier O., Chateau K., Bidault A., Corporeau C., et al. (2020). The marine intertidal zone shapes oyster and clam digestive bacterial microbiota. FEMS Microbiol. Ecol. 96, 78. doi: 10.1093/femsec/fiaa078
Peeler E. J., Reese R. A., Cheslett D. L., Geoghegan F., Power A., Thrush M. A. (2012). Investigation of mortality in Pacific oysters associated with Ostreid herpesvirus-1 μVar in the Republic of Ireland in 2009. Prev. Vet. Med. 105, 136–143. doi: 10.1016/j.prevetmed.2012.02.001
Pernet F., Barret J., Le Gall P., Corporeau C., Dégremont L., Lagarde F., et al. (2012). Mass mortalities of Pacific oysters Crassostrea gigas reflect infectious diseases and vary with farming practices in the Mediterranean Thau lagoon, France. Aquac. Environ. Interact. 2, 215–237. doi: 10.3354/aei00041
Pernet F., Gachelin S., Stanisiere J.-Y., Petton B., Fleury E., Mazurié J. (2019). Farmer monitoring reveals the effect of tidal height on mortality risk of oysters during a herpesvirus outbreak. ICES J. Mar. Sci. 76, 1816–1824. doi: 10.1093/icesjms/fsz074
Pernet F., Lagarde F., Le Gall P., D’Orbcastel E. R. (2014). Associations between farming practices and disease mortality of Pacific oyster Crassostrea gigas in a Mediterranean lagoon. Aquac. Environ. Interact. 5, 99–106. doi: 10.3354/aei00096
Petton B., Destoumieux-Garzón D., Pernet F., Toulza E., de Lorgeril J., Degremont L., et al. (2021). The Pacific oyster mortality syndrome, a polymicrobial and multifactorial disease: state of knowledge and future directions. Front. Immunol. 12, 630343. doi: 10.3389/fimmu.2021.630343
Pörtner H. O. (2012). Integrating climate-related stressor effects on marine organisms: unifying principles linking molecule to ecosystem-level changes. Mar. Ecol. Prog. Ser. 470, 273–290. doi: 10.3354/meps10123
Rainer J. S., Mann R. (1992). A comparison of methods for calculating condition index in Eastern oysters, Crassostrea virginica (Gmelin 1791). J. Shellfish Res. 11, 55–58.
R Core Team (2022). R: A language and environment for statistical computing (Vienna, Austria: R Foundation for Statistical Computing).
Reid G. K., Gurney-Smith H. J., Flaherty M., Garber A. F., Forster I., Brewer-Dalton K., et al. (2019). Climate change and aquaculture: considering adaptation potential. Aquac. Environ. Interact. 11, 603–624. doi: 10.3354/aei00333
Somero G. N. (2002). Thermal physiology and vertical zonation of intertidal animals: optima, limits, and costs of living. Integr. Comp. Biol. 42, 780–789. doi: 10.1093/icb/42.4.780
Steele S., Mulcahy M. F. (1999). Gametogenesis of the oyster Crassostrea gigas in southern Ireland. J. Mar. Biol. Assoc. United Kingdom 79, 673–686. doi: 10.1017/S0025315498000836
Tagliarolo M., Clavier J., Chauvaud L., Koken M., Grall J. (2012). Metabolism in blue mussel: intertidal and subtidal beds compared. Aquat. Biol. 17, 167–180. doi: 10.3354/ab00464
Wang X., Cong R., Li A., Wang W., Zhang G., Li L. (2023). Transgenerational effects of intertidal environment on physiological phenotypes and DNA methylation in Pacific oysters. Sci. Total Environ. 871, 162112. doi: 10.1016/j.scitotenv.2023.162112
Wang X., Li A., Wang W., Que H., Zhang G., Li L. (2021). DNA methylation mediates differentiation in thermal responses of Pacific oyster (Crassostrea gigas) derived from different tidal levels. Heredity 126, 10–22. doi: 10.1038/s41437-020-0351-7
Wang J., Zhang G., Fang X., Guo X., Li L., Luo R., et al. (2012). The oyster genome reveals stress adaptation and complexity of shell formation. Nature 490, 49–54. doi: 10.1038/nature11413
Yang B., Zhai S., Li X., Tian J., Li Q., Shan H., et al. (2021). Identification of Vibrio alginolyticus as a causative pathogen associated with mass summer mortality of the Pacific oyster (Crassostrea gigas) in China. Aquaculture 535, 736363. doi: 10.1016/j.aquaculture.2021.736363
Keywords: food security, heatwaves, mitigation, Pacific oyster (Crassostrea gigas), shellfish aquaculture, summer mortality syndrome
Citation: Mackenzie CL, Raap MR, Leduc S, Walker CYV, Green TJ, Kim E, Montgomery EM, Gray SLM, Long A and Pearce CM (2024) Development of a nature-based solution for mitigation of Pacific oyster summer mortality: use of the intertidal zone to improve resilience to environmental stressors. Front. Mar. Sci. 11:1345493. doi: 10.3389/fmars.2024.1345493
Received: 27 November 2023; Accepted: 19 February 2024;
Published: 12 March 2024.
Edited by:
Tangtian He, Hong Kong Polytechnic University, Hong Kong SAR, ChinaReviewed by:
Liqiang Zhao, Guangdong Ocean University, ChinaZhe Zheng, Guangdong Ocean University, China
Copyright © 2024 Mackenzie, Raap, Leduc, Walker, Green, Kim, Montgomery, Gray, Long and Pearce. This is an open-access article distributed under the terms of the Creative Commons Attribution License (CC BY). The use, distribution or reproduction in other forums is permitted, provided the original author(s) and the copyright owner(s) are credited and that the original publication in this journal is cited, in accordance with accepted academic practice. No use, distribution or reproduction is permitted which does not comply with these terms.
*Correspondence: Clara L. Mackenzie, Q2xhcmEuTWFja2VuemllQGRmby1tcG8uZ2MuY2E=