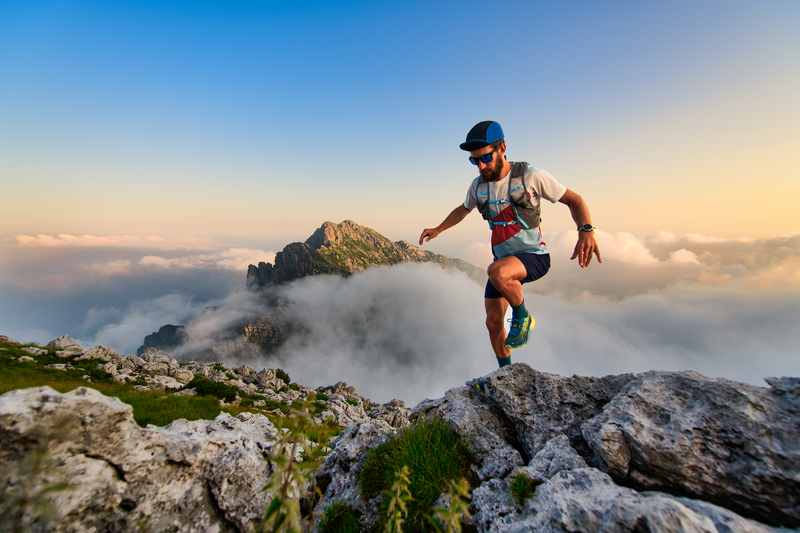
94% of researchers rate our articles as excellent or good
Learn more about the work of our research integrity team to safeguard the quality of each article we publish.
Find out more
ORIGINAL RESEARCH article
Front. Mar. Sci. , 14 June 2024
Sec. Marine Ecosystem Ecology
Volume 11 - 2024 | https://doi.org/10.3389/fmars.2024.1337080
This article is part of the Research Topic Antarctic Krill and Interactions in the East Antarctic Ecosystem View all 16 articles
Antarctic krill (Euphausia superba) aggregations are important in the Southern Ocean ecosystem; however, the amount of energy that krill swarms contain is unknown. We assessed the population structure of E. superba swarms in East Antarctica for two different sectors corresponding to the study areas of the ENRICH (2019) and TEMPO (2021) voyages. We also estimated swarm energy content based on calorific values for whole animals and key tissues (gonads, digestive gland, and muscle). A relationship between the energy content of muscle and gonads in gravid and adult females was detected: The higher the energy content in muscle tissue, the lower the energy content in the gonad tissue. This relationship was consistent for different female maturity stages, suggesting a trade-off in energy allocation between somatic growth and reproduction. Females will redirect energy to gonad development at the expense of somatic growth. Total swarm energy was calculated using the volumetric density of each swarm estimated from an acoustic survey. Four swarm types (A-D) were determined based on the body length and maturity stage of the individuals. The highest energy contents were found in Type B swarms, composed predominantly of adult males and females, and Type D swarms, comprising mostly gravid females. Trends in swarm demographic composition and energy content were consistent between surveys. We recommend swarm energy be incorporated into ecosystem models to represent energy transfer to top predators.
Antarctic krill (Euphausia superba, hereafter “krill”) is a vital component of the Southern Ocean ecosystem, having both ecological and commercial importance. Krill plays a central role in carbon export (Cavan et al., 2019) and is a key species in the food web south of the Polar Front in the East Antarctic ecosystem, providing a link between primary production and higher trophic levels. Gaining knowledge about the energy availability and flow from mid-trophic to top trophic levels is critical for ecosystem modelling and management. Krill has a high lipid content (up to 40% of dry mass; Atkinson et al., 2002), and it has been determined that, on average, an adult individual has an energy content between 15.24 and 22.6 KJ g dry mass-1 during the summer season (Schaafsma et al., 2018). Furthermore, krill energy content varies with size, sex, and maturity stage (Färber-Lorda et al., 2009; Färber-Lorda and Ceccaldi, 2020).
Krill form large aggregations commonly called swarms, which is a key behavioral and ecological trait. Swarming behavior has been previously attributed to environmental cues such as temperature, oxygen saturation, chlorophyll a concentration and/or water column dynamics (Ritz, 2011; Leonori et al., 2017). The formation of swarms has further been linked to predator avoidance, reproduction, and improved fitness by increasing swimming speeds and saving energy for other physiological activities (Hamner and Hamner, 2000; Brierley and Cox, 2010). Physical characteristics of swarms have been at the center of previous classification schemes (e.g. Tarling et al., 2009; Bestley et al., 2018), and studies have primarily focused on attributes such as swarm shape (Cox et al., 2009), packing density, position in the water column and size (Kohut et al., 2019; Miller et al., 2019). However, other characteristics can be useful. For example, the sex ratio or stage composition of swarms can vary from 100% adults to 50:50 adults:juveniles, or an even distribution of juveniles, gravid females, adult males, and sub-adults (Watkins et al., 1986, 1990, 1992). It has been theorized that the physical characteristics of swarms might vary in accordance with the biological characteristics of the krill within a swarm (Amakasu et al., 2011); e.g., swarm shape and packing density might differ for different maturity stages (Tarling et al., 2009). Furthermore, as energy content varies between different developmental stages, it is reasonable to hypothesize that different swarms will have different energy content, not only based on size but also their demographic composition.
The relationships of krill with its predators have been studied from multiple angles, including predator avoidance in space and time (Alonzo and Mangel, 2001; Alonzo et al., 2003), predator energy intake (Friedlaender et al., 2019), and physical characteristics of swarms being targeted (Tarling et al., 2009). Despite extensive research on krill biology and ecology, some aspects with respect to energy availability have been overlooked. Although it is known that krill are a key species in the Southern Ocean food web (Alurralde et al., 2019), the amount of energy that krill swarms contribute to this complex system is unknown. Important questions around this topic are how much energy krill has to offer predators, and how swarm composition affects energy content? That is, what are the specific characteristics of krill swarms that most influence their energy content? Firstly, this study describes the population structure of krill swarms and calculates their energy content. Secondly, it utilizes swarm demographic composition to distinguish between swarm types to ultimately examine which swarm type offers the most energy to predators. As energy content varies between developmental stages, and swarms of equal demographic composition are yet to be found, here it is hypothesized that different swarms have different energy content not only based on swarm size but also their demographic composition.
This study used krill acoustic surveys and biological samples collected during two voyages to the east Antarctic. The Euphausiids and Nutrient Recycling in Cetacean Hotspots (ENRICH) research voyage was carried out between 19 January and 6 March, 2019, with the goal of studying the availability of Antarctic krill to large predators and their role in biogeochemical recycling in the Southern Ocean. The Trends in Euphausiids of Mawson, Predators, and Oceanography (TEMPO) research voyage occurred between 29 January and 24 March, 2021 (Figure 1). The area of operation spanned from 64°S to 63°S and 138°E to 155°E for ENRICH and from 62°S to 68°S and 55°E to 80°E for TEMPO. To detect krill swarms, active acoustic data were collected using a calibrated downward-facing split-beam echosounder (Simrad EK60) operating at 38 and 120 kHz. In this paper we use the term ‘swarm’ as a broad reference to each patch detected in the acoustic survey and the aggregations sampled during trawls.
Figure 1 Map of study areas (A) ENRICH and (B) TEMPO. X and y axes on panels (A, B) correspond to degrees of longitude and latitude, respectively. Points represent trawl sampling stations. There was a combination of targeted (T) and routine (R) trawls during both voyages; target trawls represent responsive fishing when krill-like echoes were observed through echosounders, +and routine trawls are set locations.
Biological samples were collected from a total of 41 trawls during ENRICH and 59 during TEMPO along the pre-determined survey transects (for both voyages). Krill samples were collected using a Rectangular Midwater Trawl (RMT) towed for 7–15 min, with RMT 1 + 8 nets (8 m2: mesh size 4.5 mm; 1 m2; mesh size 300 μm) (Everson and Bone, 1986). Once on board, each individual krill was sexed and staged (classified as adult female (not gravid), adult male, sub-adult female, sub-adult male, spent female, gravid female, or juvenile). Finally, body morphometric characteristics (total length (mm), carapace length (mm), wet mass (g) and length of the digestive gland (mm)) were measured (Makarov and Denys, 1984). All specimens were stored in individual vials, snap-frozen in liquid nitrogen, and then stored at -86 °C for later use.
Krill were chosen from six randomly selected trawls (stations) to perform the energetic measurements. Thirty individuals were randomly chosen from each trawl, representing the population of the sampled swarm. First, all individuals were sexed, and the maturity stage was identified using a Makarov key (Makarov and Denys, 1984). The wet mass was recorded with an analytical balance (GR-200, 0.01 mg precision), and morphological measurements were made using a slide caliper (0.01 mm precision) following the descriptions for standard length 1 in Morris et al. (1988). The samples were defrosted at room temperature for 60 to 80 minutes. Using a dissecting microscope (Leica M12), each individual was then dissected to obtain samples of the following tissues: gonad, digestive gland, muscle, and remaining tissue (further detail in Annex 1). Lastly, the tissues were freeze-dried at -50°C for 48 hours.
Bomb calorimetry was used to determine the calories (kilojoules per gram) in each tissue sample. A semi-micro bomb calorimeter (Parr 6725) equipped with a semi-micro-oxygen combustion vessel (Parr 1109A) and a thermometer (Parr 6722) was used for the energy measurements. Prior to each measurement the bomb was calibrated using calorimeter standard benzoic acid pellets (Parr 3418). Dried samples were pooled according to their trawl, sex, stage, and tissue. Then, micro pellets were made using a pellet press (Parr 2811). The pellet size was chosen based on the total dry mass of the samples. 25 mg of dry sample was used for each digestive gland and gonad pellet, 50 mg for the muscle samples and 100 mg for the remaining tissue.
Adult krill are known for their high calorific content (Hagen et al., 2001). However, when dissected, the capacity of some tissues to produce heat decreases causing incomplete sample combustion and leading to invalid results. To overcome this, benzoic acid spikes were used to ensure that complete combustion was achieved for each sample. To ensure the correct weight of spike was used, samples of different sizes were combusted with varying weights of spike, and then a linear regression was fitted to the data (Annex 4). No relationship was found between spike weight and sample heat of combustion, therefore, based on this information, a benzoic acid standard for calorimetry powder (Parr 3402) was used to supplement the spiked pellets, bringing the ‘total sample’ mass (krill tissue plus spike) to 250 mg. The estimates of gross heat or calorific value (Hc;KJ g-1) were made using the following:
where W is the energy equivalent of the calorimeter in use, T is the observed temperature rise, e1 is the heat produced by burning the nitrogen portion of the air trapped in the bomb to form nitric acid, e2 is the heat produced by the combustion of the fuse wire, e3 corresponds to the fuse wire correction, m corresponds to the mass of the sample, Hcs is the heat of combustion of the spike and Ms the mass of the spike.
Equation 1 is based on the substitution procedure, which compares the heat obtained from the sample with the heat obtained by the combustion of a known material. The energy released by the sample during combustion is absorbed within the calorimeter and the resulting temperature change within the medium is recorded. The heat of combustion of the sample is then calculated by multiplying the temperature rise in the calorimeter by the known heat equivalent of the benzoic acid standards. The formula makes any correction needed based on sample weight, accounting for fuse wire and spike combustion heat using the length of the remaining fuse wire after combustion and the weight of the spike used (further details in Parr 6725 semi-micro calorimeter instruction manual).
For both surveys, hierarchical clustering was used to group stations with similar biometric and demographic characteristics based on krill total body length and development stage. Total body length, sex and maturity stage were used to determine the extent of similarity between the different stations. The cluster analysis was performed following the methods of Kawaguchi et al. (2010), using Ward distance and posterior multidimensional scaling (MDS) and Sammon mapping to project each station within the correspondent clusters using R and RStudio (RStudio, 2022; R version 4.2.2; packages: Wickham, 2023; MASS Venables and Ripley, 2002).
The mean energy measured in key tissues (gonad, digestive gland, and muscle) was used to calculate the energy content for each individual, grouped by stage, station, and cluster. One-way ANOVA and Tukey HSD post hoc tests were performed to evaluate the differences in energy content in tissues and total gross heat between the different stages. Linear regression models were used to assess the relationships between muscle and gonad energy content and total body length for the female stages. The mean energy of each cluster was calculated using the proportional mean energy of each stage, with their proportional standard errors, in units of kilojoules per gram of wet mass (KJ g-1 wet mass). This method takes into consideration the percentage of each stage within a swarm. Wet mass energy estimations were calculated based on the linear regression equation (y = a + bx) between whole dry mass and whole wet mass measurements.
To identify the geometrical attributes of swarms/trawls in accordance with their corresponding cluster, polygons were selected by grouping the acoustic line transects into lengths with similar demographics based on the hierarchical clustering results. This was achieved by constructing polygons encompassing areas that enclosed trawls with similar krill demographics. More than one polygon could exist for each cluster. The geometrical attributes of the polygons were described using QGIS (version 3.32.3 Lima), and acoustic transects were then matched to the polygon geometry to classify the acoustic data into clusters. Swarm detection and density estimations within the acoustic survey transects were undertaken as per Cox et al. (2011). In short, aggregations were classified as krill or non-krill using the SHAPES algorithm (Watkins and Brierley, 2002), following the mean volume backscatter strength (ΔSv) technique with threshold value ΔSv=120–38 kHz (Reiss et al., 2008), based on the length frequency collected from the net samples. Once an aggregation was determined to be a swarm, the ΔSv = 120 kHz data were used to calculate the wet mass of krill per m3 in each swarm (ρv) following Equation 2 (Demer and Conti, 2005).
where Sv is the mean volume backscatter in units of dB re 1 m-1, and TS is the krill target strength in units of dB re 1 kg.
Swarm volumetric density (ρv) (g m-3) was estimated following Cox et al. (2022), ρv was then used to calculate the biomass as grams of wet mass for each swarm, estimating volume by assuming that a swarm was detected by the echosounder along its longest length and that swarm shape was cylindrical. Biomass data were interpolated with the proportional mean energy data previously estimated for each swarm type. Swarms within the geometrical area of the polygon were used to determine the total proportional energy of each swarm in megajoules per swarm (MJ swarm-1).
Cluster analysis for ENRICH grouped the data into four clusters based on the developmental stage and body length of the individuals sampled in each trawl (Annex 2). All clusters contained the seven maturity stages examined in this study (sub-adult female and male, adult females and males, juveniles, spent females and gravid females), although in different proportions and body length distributions (Figure 2; Annex 3). Cluster 1 was composed predominately of sub-adult males (59%), with an average size of 44.8 ± 5.0 mm (average for sub-adult females and males). Cluster 2 was primarily composed of early life stages, sub-adult females (26%) and adult females (25%) and comprised a wide range of krill lengths as they were composed of all life stages from small juveniles to large gravid females. Cluster 3 was dominated by spent females (62%), and cluster 4 by 60% gravid females. As each cluster was composed of all stages, albeit in different proportions, body length ranged from small juveniles to large adults and gravid females. Cluster 4 was represented by larger individuals, with a mean body length (± standard deviation) of 48.6 ± 4.4 mm (range: 23.8 to 59.7 mm), while cluster 3 had a mean body length of 47.6 ± 4.3 mm (range: from 35.3 to 56.8 mm). Smaller sized krill were found in clusters 1 and 2, with averages of 43.1 ± 3.9 mm (range: 32.2 to 54.1 mm) and 40.9 ± 5.0 mm (range: 19.5 to 55.8 mm), respectively.
Figure 2 Krill stage composition of each cluster for ENRICH (top panel) and TEMPO (bottom panel). Each bar represents one cluster and the sections within a bar represent the percentage of each stage within a given cluster.
Similar results were found for the TEMPO dataset (Annex 2) but body length distribution for TEMPO swarms was overall smaller than observed for the ENRICH dataset, particularly the gravid females (46.1 ± 3.5 mm and 49.5 ± 3.9 mm, respectively). A different average swarm composition was found in cluster 3 for TEMPO. Juveniles with a mean size of 32.9 ± 5.4 mm and sub-adult stages (36.7 ± 3.7 mm females, 39.1 ± 4.8 mm males) dominated the swarm composition. This cluster did not fall into the categories (clusters) described for the ENRICH survey.
All stages had significantly different individual energy content (Figure 3E) (one-way ANOVA; p = 0.0021). Gravid females had a higher mean energy content than other stages, with a mean value of 3.8 KJ ind dry mass-1. Adult males (3.2 KJ ind dry mass-1) had a statistically significant lower energy content than adult females (3.4 KJ ind dry mass-1) (Tukey’s post hoc test, p = 0.0241). Juveniles had the lowest mean energy content (2.4 KJ ind dry mass-1) among all stages. Finally, spent females had the lowest energy content among all female stages (3.2 KJ ind dry mass-1). We standardized the energy content and expressed the results in mean kilojoules per gram of dry mass and found that adult females (21.19 KJ g dry mass-1) had a higher energy content than other stages, followed by juveniles (21.15 KJ g dry mass-1), adult males (21.14 KJ g dry mass-1), spent females (20.37 KJ g dry mass-1) and gravid females (20.36 KJ g dry mass-1) (Figure 3F; Annex 6). The mean energy content for sub-adult females and males are 21.01 and 21.29 KJ g dry mass-1, respectively.
Figure 3 Mean energy content of key tissues in each developmental stage of krill for the ENRICH survey: (A) muscle, (B) digestive gland, (C) gonad, (D) remaining tissue, and (E) mean total gross heat by individual and by (F) gram of dried mass for the five stages. p and F values correspond to one-way ANOVA results, except for panel C where the values correspond to post hoc Tukey tests comparing the female stages only. Boxes represent the interquartile range, whiskers represent minimum and maximum values, horizontal line corresponds to the median, black dots correspond to outliers and blue dots represent the mean value. All units are in kilojoules per mass of dry weight, except for panel E, which is energy per individual. .
Energy allocation varied between the tissues of all stages (see details in Figures 3A–D and values in Annex 6 and 7). Gravid females partitioned more energy into the gonads, while adult females contained proportionally more energy in their digestive glands. In contrast to adult females, gravid females had higher mean energy in their gonads than their muscles when body mass was taken into consideration. For gravid females, the gonad represented 6.5% of the total body energy and the muscle 23%, while for adult females 4.5% corresponded to gonad energy and 29% to muscle. Only 0.3% of the energy in spent females was from the gonad, while muscle accounted for 29% and 8.5% was allocated to the digestive gland. The linear regression model showed a direct relationship between the muscle and the gonad energy content (linear regression p = 0.004, Annex 5): the higher the energy content of the gonad, the lower the energy content of the muscle. This trend was also observed in the dry mass of these tissues.
Clusters 1 and 2 had the highest mean energy, 5.41 ± 0.1 and 5.44 ± 0.1 KJ g wet mass-1, respectively. Clusters 3 and 4 had similar mean energy contents but were lower than the other clusters: 5.32 ± 0.1 and 5.25 ± 0.1 KJ g wet mass-1, respectively. A one-way ANOVA was performed on the swarm energy content data, showing significant differences between all cluster mean energy contents (p = 0.0321), validating the idea that different stage compositions of swarms translate to different energy contents of the swarms.
To enhance clarity and streamline the discussion of our findings, from this section we transition from referring to clusters numbered 1 through 4 to identifying them as swarm types A, B, C, and D, respectively. This renaming is intended to make our findings easier to understand and convey because of the unique makeup of swarms, which is defined by the length and maturity stages of the individuals within each cluster.
1,675 and 4,219 krill swarms fell within the polygons selected using the krill acoustic survey area of ENRICH and TEMPO, respectively. Table 1 summarizes the number of swarms detected for each type, as well as the volume and energy of each swarm type. Type D swarms had both higher mean energy and higher overall total energy (sum of total energy of all swarm) of all four swarm types. Further, there was no correlation (Pearson, corr = -0.003, p=0.8122) between swarm biomass and swarm total energy. Swarm energy content varied in a geographical setting according to the volumetric density of the aggregations. Swarms with high energy content (MJ) were identified closer to the continental shelf (Figure 4).
Table 1 Summary of mean swarm dimensions (height1 and length2), volumetric density (ρv) g wet mass/m33, mean swarm energy4, and sum of total energy among swarms of the same type 5.
Figure 4 Maps of volumetric density (ρv(gm-3)) (top) and proportional total swarm energy (MJ per swarm) (bottom) for ENRICH (left) and TEMPO (right). Colors represent different clusters. Swarms located near the shelf break contained greater energy content; these swarms correspond to those of type D (C4).
Krill swarms were grouped into four categories based on the size of the individuals and the proportion of the different stages within swarms. Higher energy contents (average mean energy per swarm type) were found in small swarms of types D and B, which were composed primarily of gravid females and equal proportions of all stages, respectively. This evidence shows that energy content in krill swarms is driven by the stage composition and not by the physical size of the swarm.
This study is the first to report on energy allocation for both sexes and all main maturity stages. The assessment of the energy allocation showed that the female stages, both gravid and immature adults, have the highest energy content among all the measured life stages. The energy contents measured in the present study were similar to those in previous studies for female and male individuals (Ichii et al., 2007; Ruck et al., 2014; Schaafsma et al., 2018). However, energy content varied significantly between life stages. This has been suggested before by Schaafsma et al. (2018) and Clarke (1984), due to variations in the biochemical compositions of krill at different stages. By comparing results from this study with previous reports of energy content in different krill stages, we infer that the krill energy content might be related to geographical distribution and time of year. For example, Ichii et al. (2007) reported an energy value of 20.08 KJ g-1 and 17.41 KJ g-1 for gravid females and immature adult females in the vicinity of Elephant Island, while Schaafsma et al. (2018) reported 22.27 KJ g-1 for immature adult females in the Lazarev Sea. The present study estimated that immature adult females had a mean energy value of 21.08 KJ g-1 dry mass and gravid females 20.36 KJ g-1 dry mass during the summer season in east Antarctica. Krill populations undergo seasonal ontogenetic migration, therefore seasonal distribution of maturity composition and individual? size vary considerably, which has implications for the dynamics of energy content of krill populations. In addition to this, we believe this study was the first to measure krill energy content in all sex and maturity stages of krill for one geographical area in the same time span, giving us an understanding of the variability between stages.
Trade-offs are part of the evolutionary history of all animals (Garland, 2014). Growth patterns, reproductive investments, and longevity are all qualities that may be shaped by trade-offs (Ou et al., 2020). However, as far as we are aware, reproductive trade-offs have only been reported twice before for krill: Male krill reduce lipid storage to use all incoming energy in reproduction, thereby increasing their mortality rate (Virtue et al., 1996), while Steinke et al. (2022) found through controlled laboratory experiments that the physiological condition of juvenile female krill declined in response to high metabolic requirements. The energetic cost of reproduction is high for both females and males (Ross and Quetin, 2000), and this cost in krill has been attributed primarily to the generation of lipid-rich oocytes (Quentin et al., 1994). As a result, lipid levels and composition vary significantly according to sex and maturation stage. Female reproductive krill can have up to twice the amount of lipid as males (Clarke, 1984) and female krill lose 34% of their body mass after laying eggs (Nicol et al., 1995). It has been estimated that phytoplankton concentration in the Southern Ocean is not high enough to support the energetic demand of reproductive female krill (Nicol et al., 1995). We observed that spent females allocated the highest energy content to their digestive gland coupled with low energy content in muscle tissues. This could be interpreted as females trying to increase their lipid reserves at the expense of muscle tissue post-spawning and in preparation to produce more eggs. Similar to our findings, Steinke et al. (2022) found that juveniles allocate their energy towards reproductive development in winter when their lipid levels and reproductively important fatty acids supersede what is necessary for survival. Sexual development comes at a cost, as more mature juveniles were found to be in worse physiological condition. The trade-off between reproductive development and condition in juvenile krill is significant, as early maturation may better prepare krill for the spawning season but may also leave them more susceptible to suboptimal conditions in the environment. Juvenile krill has the capacity to utilize stored lipids throughout the autumn and winter, which can be used to initiate early reproductive development (Steinke et al., 2022). To confirm our trade-off hypothesis and to further understand the energy requirements of krill, the energy allocation of female krill at different times of the reproductive cycle needs to be evaluated.
Previous research has suggested that krill sometimes form swarms of individuals of the same maturity stage and that this composition changes depending on the season (Watkins, 1986; Watkins et al., 1992; Atkinson et al., 2006). However, our results differ as the swarms evaluated in this study were composed of all maturity stages. This may help increase fitness, whereby larger individuals can maintain faster swimming speeds (Ritz, 2011). It could be speculated that smaller individuals would also benefit by aggregating with larger krill due to the possibility that if the swarm moves faster, juveniles within a swarm composed of adults could also increase their swimming speed. This study proposes a classification of krill swarms based on their biological makeup. Larger gravid females dominated swarms of type D, and most swarms detected corresponded to this type. This is congruent with the time of the survey being peak breeding season. However, individuals of all stages were identified in all krill swarms. Tarling et al. (2009) previously classified krill swarms into two categories based on their density and horizontal length: type I swarms are those with densities below 10 ind m-3 and 50 m in length, and type II swarms are those above type I limits. If this classification system is applied to the swarms observed in the present study, type B and D swarms fall into Tarling et al.’s (2009) larger swarms (type II) and swarms A and C into type I. Based on our results and the previous swarm classification, larger swarms, defined as swarms with the longest height and length, correspond to swarms with high gravid female composition.
High energy swarms were found on the shelf break in this study. As there are no previous studies that estimate swarm or krill population energy content, we can put our results into context with previous estimations of krill density. High density values were found near the shelf break in both Kawaguchi et al. (2010) and the present study. On the other hand, much lower density values were found offshore, consistent with results for the Lazarev Sea reported by Siegel et al. (2016). The shelf break is of great importance to krill and its predators. For example, Adélie penguins forage at the shelf break during the breeding season (Alonzo et al., 2003), while dense aggregations of whales are found within the vicinities of the shelf break (Friedlaender et al., 2006), and some fish species also use the area all year round as a foraging ground (Hoddell et al., 2000). The shelf break is a region of high phytoplankton biomass, as upwelling in this region increases primary production (Wright and van den Enden, 2000), consequently boosting krill abundance (Atkinson et al., 2008) and leading to high swarm densities and high swarm energy content.
There are two important points in this study that should be carefully considered when interpreting the results. Firstly, while a swarm-based approach is suitable when managing krill fisheries, the way that biomass measurements are currently made could be biased as they assume that the swarm shape is a cylinder when obtaining volumetric density estimates. In the same way, our study also assumed swarm shapes to be cylindrical when calculating the volumetric density. Future research should focus on finding a way to estimate swarm volumetric density more accurately, perhaps by reconstructing the swarm shape detected with acoustic methods using multiple geometrical shapes.
Secondly, the swarm energy results from this study (even the individual energy contents) are only valid for the summer season in the east Antarctic. Both season and location should be considered as krill varies over temporal and spatial scales. Physical processes in the Southern Ocean also vary over time and space, although this study only focuses on one habitat of krill. Krill also resides under sea ice and may have different energy contents compared to krill from open waters. Moreover, different species of krill predators will be present in sea ice areas and their energetic needs could be different to those predators living and feeding in open waters. Understanding the transfer of energy from krill swarms to marine predators will require this information to be incorporated into ecosystem models.
This was the first study of its kind to describe the population structure of krill swarms coupled with energy. Swarms with high energy contents were identified, aiding in our understanding of the biological characteristics of krill swarms. This paper examines an important factor that should be considered when assessing Antarctic krill swarms: the maturity-stage composition of the swarms. Moreover, this paper reveals a reproductive trade-off in E. superba, suggesting that female krill invest most of their energy into gonadal development at the expense of muscle tissue. This paper is the first to quantify the energy content of krill individuals at different sex and maturity stages for the same region in the same time span. Measuring energy density using bomb calorimetry and proximate composition is a time-consuming process. Increased information on the relationships between energy density and other, easier to measure parameters could help in reducing the effort when estimating krill swarm energy density. If a sustained relationship between the physical, demographic, and energetic characteristics of krill swarms can be identified, then the makeup and energy content of the swarms could be estimated directly for swarms found with acoustic surveys without needing substantial laboratory work.
Krill raw energy measurements are available in an open-source data set: https://data.aad.gov.au/metadata/SCAR_Diet_Energetics.
The manuscript presents research on animals that do not require ethical approval for their study.
CCM: Conceptualization, Data curation, Formal analysis, Investigation, Methodology, Visualization, Writing – original draft, Writing – review & editing. SK: Supervision, Writing – review & editing. MJC: Formal analysis, Methodology, Supervision, Writing – review & editing. JM: Data curation, Writing – review & editing. VR: Data curation, Writing – review & editing. KMS: Conceptualization, Funding acquisition, Investigation, Supervision, Writing – review & editing.
The author(s) declare financial support was received for the research, authorship, and/or publication of this article. This project was funded by the Institute for Marine and Antarctic Studies at the University of Tasmania in collaboration with the Australian Antarctic Division. This project was supported and funded by Australian Antarctic Science Project 4512 and 4636 and received grant funding from the Australian Government as part of the Antarctic Science Collaboration Initiative program. This project also received financial support from Pew Charitable Trust and Antarctic Science Foundation. We acknowledge the use of the CSIRO Marine National Facility (https://ror.org/01mae9353) and grant of sea time on RV Investigator in undertaking this research.
This work could not have been accomplished without the support of the crew and scientists on the TEMPO and ENRICH voyages.
The authors declare that the research was conducted in the absence of any commercial or financial relationships that could be construed as a potential conflict of interest.
All claims expressed in this article are solely those of the authors and do not necessarily represent those of their affiliated organizations, or those of the publisher, the editors and the reviewers. Any product that may be evaluated in this article, or claim that may be made by its manufacturer, is not guaranteed or endorsed by the publisher.
The Supplementary Material for this article can be found online at: https://www.frontiersin.org/articles/10.3389/fmars.2024.1337080/full#supplementary-material
Alonzo S. H., Mangel M. (2001). Survival strategies and growth of krill: avoiding predators in space and time. Mar. Ecol. Prog. Ser. 209, 203–217. doi: 10.3354/meps209203
Alonzo S. H., Switzer P. V., Mangel M. (2003). Ecological games in space and time: The distribution and aundance of Antarctic krill and penguins. Ecology 84, 1598–1607. doi: 10.1890/0012-9658(2003)084[1598:EGISAT]2.0.CO;2
Alurralde G., Fuentes V. L., Maggioni T., Movilla J., Olariaga A., Orejas C., et al. (2019). Role of suspension feeders in antarctic pelagic-benthic coupling: Trophic ecology and potential carbon sinks under climate change. Mar. Environ. Res. 152, 104790. doi: 10.1016/j.marenvres.2019.104790
Amakasu K., Ono A., Hirano D., Moteki M., Ishimaru T. (2011). Distribution and density of Antarctic krill (Euphausia superba) and ice krill (E. crystallorophias) off Adélie Land in austral summer 2008 estimated by acoustical methods. Polar Sci. 5, 187–194. doi: 10.1016/j.polar.2011.04.002
Atkinson A., Meyer B., Stuϋbing D., Hagen W., Schmidt K., Bathmann U. V. (2002). Feeding and energy budgets of Antarctic krill Euphausia superba at the onset of winter—II. Juveniles and adults. Limnol. Oceanogr. 47, 953–966. doi: 10.4319/lo.2002.47.4.0953
Atkinson A., Shreeve R. S., Hirst A. G., Rothery P., Tarling G. A., Pond D. W., et al. (2006). Natural growth rates in Antarctic krill (Euphausia superba): II. Predictive models based on food, temperature, body length, sex, and maturity stage. Limnol. Oceanogr. 51, 973–987. doi: 10.4319/lo.2006.51.2.0973
Atkinson A., Siegel V., Pakhomov E. A., Rothery P., Loeb V., Ross R. M., et al. (2008). Oceanic circumpolar habitats of Antarctic krill. Mar. Ecol. Prog. Ser. 362, 1–23. doi: 10.3354/meps07498
Bestley S., Raymond B., Gales N. J., Harcourt R. G., Hindell M. A., Jonsen I. D., et al. (2018). Predicting krill swarm characteristics important for marine predators foraging off East Antarctica. Ecography 41, 996–1012. doi: 10.1111/ecog.03080
Brierley A. S., Cox M. J. (2010). Shapes of krill swarms and fish schools emerge as aggregation members avoid predators and access oxygen. Curr. Biol. 20, 1758–1762. doi: 10.1016/j.cub.2010.08.041
Cavan E. L., Belcher A., Atkinson A., Hill S. L., Kawaguchi S., McCormack S., et al. (2019). The importance of Antarctic krill in biogeochemical cycles. Nat. Commun. 10, 4742. doi: 10.1038/s41467-019-12668-7
Clarke A. (1984). Lipid content and composition of Antarctic Krill, Euphausia Superba Dana. J. Crustacean Biol. 4, 285–294. doi: 10.1163/1937240X84X00660
Cox M., Demer D., Warren J., Cutter G., Brierley A. (2009). Multibeam echosounder observations reveal interactions between Antarctic krill and air-breathing predators. Mar. EcologyProgress Ser. 378, 199–209. doi: 10.3354/meps07795
Cox M. J., Macaulay G., Brasier M. J., Burns A., Johnson O. J., King R., et al. (2022). Two scales of distribution and biomass of Antarctic krill (Euphausia superba) in the eastern sector of the CCAMLR Division 58.4.2 (55°E to 80°E). PloS One 17, e0271078. doi: 10.1371/journal.pone.0271078
Cox M. J., Watkins J. L., Reid K., Brierley A. S. (2011). Spatial and temporal variability in the structure of aggregations of Antarctic krill (Euphausia superba) around South Georgia 1997–1999. Ices J. Mar. Sci. 68, 489–498. doi: 10.1093/icesjms/fsq202
Demer D. A., Conti S. G. (2005). New target-strength model indicates more krill in the Southern Ocean. Ices J. Mar. Sci. 62, 25–32. doi: 10.1016/j.icesjms.2004.07.027
Everson I., Bone D. G. (1986). Effectiveness of the RMT8 system for sampling krill (Euphausia superba) swarms. Polar Biol. 6, 83–90. doi: 10.1007/BF00258257
Färber-Lorda J., Ceccaldi H. J. (2020). Relationship of morphometrics, total carotenoids, and total lipids with activity and sexual and spatial features in Euphausia superba. Sci. Rep. 10, 13177. doi: 10.1038/s41598–020-69780–8
Färber-Lorda J., Gaudy R., Mayzaud P. (2009). Elemental composition, biochemical composition and caloric value of Antarctic krill: Implications in energetics and carbon balances. J. Mar. Syst. 78, 518–524. doi: 10.1016/j.jmarsys.2008.12.021
Friedlaender A. S., Bowers M. T., Cade D., Hazen E. L., Stimpert A. K., Allen A. N., et al. (2019). The advantages of diving deep: Fin whales quadruple their energy intake when targeting deep krill patches. Funct. Ecol. 34, 497–506. doi: 10.1111/1365-2435.13471
Friedlaender A. S., Halpin P., Qian S., Lawson G. L., Wiebe P. H., Thiele D., et al. (2006). Whale distribution in relation to prey abundance and oceanographic processes in shelf waters of Western Antarctic Peninsula. Mar. Ecol. Prog. Ser. 317, 297–310. doi: 10.3354/meps317297
Hagen W., Kattner G., Terbrüggen A., Van Vleet E. S. (2001). Lipid metabolism of the Antarctic krill Euphausia superba and its ecological implications. Mar. Biol. 139, 95–104. doi: 10.1007/s002270000527
Hamner W. M., Hamner P. P. (2000). Behavior of Antarctic krill (Euphausia superba): schooling, foraging, and antipredatory behavior. Can. J. Fisheries Aquat. Sci. 57, 192–202. doi: 10.1139/f00-195
Hoddell R. J., Crossley A. C., Williams R., Hosie G. W. (2000). The distribution of Antarctic pelagic fish and larvae (CCAMLR division 58.4.1). Deep Sea Res. Part II: Topical Stud. Oceanogr. 47, 2519–2541. doi: 10.1016/S0967–0645(00)00034–5
Ichii T., Bengston J., Boveng P., Takao Y., Jansen J., Hiruki-Raring L., et al. (2007). Provisioning strategies of Antarctic fur seals and chinstrap penguins produce different responses to distribution of common prey and habitat. Mar. Ecology-Progress Ser. 344, 277–297. doi: 10.3354/meps06873
Kawaguchi S., Nicol S., Virtue P., Davenport S. R., Casper R., Swadling K. M., et al. (2010). Krill demography and large-scale distribution in the Western Indian Ocean sector of the Southern Ocean (CCAMLR Division 58.4.2) in Austral summer of 2006. Deep Sea Res. Part II: Topical Stud. Oceanogr. 57, 934–947. doi: 10.1016/j.dsr2.2008.06.014
Kohut J., Statscewich H., Oliver M., Fredj E., Klinck J., Dinniman M., et al. (2019). Project SWARM: The application of an integrated polar ocean observing system to map the physical mechanisms driving food web focusing in an Antarctic biological hotspot. OCEANS 2019 MTS/IEEE SEATTLE, 1–7. doi: 10.23919/OCEANS40490.2019
Leonori I., De Felice A., Canduci G., Costantini I., Biagiotti I., Giuliani G., et al. (2017). Krill distribution in relation to environmental parameters in mesoscale structures in the Ross Sea. J. Mar. Syst. 166, 159–171. doi: 10.1016/j.jmarsys.2016.11.003
Makarov R., Denys C. J. (1984). “Stages of sexual maturity of Euphausia superba Dana,” in BIOMASS Handbook, vol. 11. (BIOMASS Handbook, Oban, United Kingdom), 1–11.
Miller E., Potts J., Cox M., Miller B., Calderan S., Leaper R., et al. (2019). The characteristics of krill swarms in relation to aggregating Antarctic blue whales. Sci. Rep. 9 (1), 16487. doi: 10.1038/s41598–019-52792–4
Morris D., Watkins J. L., Ricketts C., Buchholz F., Priddle J. (1988). An assessment of the merits of length and weight measurements of Antarctic krill Euphausia superba. Br. Antarctic Survey Bull. 79), 27–50.
Nicol S., de la Mare W. K., Stolp M. (1995). The energetic cost of egg production in Antarctic krill (Euphausia superba Dana). Antarctic Sci. 7, 25–30. doi: 10.1017/S0954102095000058
Ou Q., Vannier J., Yang X., Chen A., Mai H., Shu D., et al. (2020). Evolutionary trade-off in reproduction of Cambrian arthropods. Sci. Adv. 6, eaaz3376. doi: 10.1126/sciadv.aaz3376
Quentin L. B., Ross R. M., Clarke A. (1994). Krill energetics: seasonal and environmental aspects of the physiology of Euphausia superba. South. Ocean Ecol. Biomass perspective, 165–184.
Reiss C. S., Cossio A. M., Loeb V., Demer D. A. (2008). Variations in the biomass of Antarctic krill (Euphausia superba) around the South Shetland Islands 1996–2006. Ices J. Mar. Sci. 65, 497–508. doi: 10.1093/icesjms/fsn033
Ritz D. (2011). Is social aggregation in aquatic crustaceans a strategy to conserve energy? Can. J. Fisheries Aquat. Sci. 57, 59–67. doi: 10.1139/f00-170
Ross R. M., Quetin L. B. (2000). “Reproduction in Euphausiacea,” in Krill. Ed. Everson I. (Blackwell Science Ltd, Oxford, UK), 150–181. doi: 10.1002/9780470999493.ch6
Ruck K. E., Steinberg D. K., Canuel E. A. (2014). Regional differences in quality of krill and fish as prey along the Western Antarctic Peninsula. Mar. Ecol. Prog. Ser. 509, 39–55. doi: 10.3354/meps10868
Schaafsma F. L., Cherel Y., Flores H., van Franeker J. A., Lea M.-A., Raymond B., et al. (2018). Review: the energetic value of zooplankton and nekton species of the Southern Ocean. Mar. Biol. 165, 129. doi: 10.1007/s00227-018-3386-z
Siegel V. (2016). Biology and ecology of Antarctic krill (Cham, Switzerland: Springer International Publishing AG). doi: 10.1007/978-3-319-29279-3
Steinke K. B., Bernard K. S., Fontana J. M., Copeman L. A., Garcia L. M. (2022). The energetic cost of early reproductive development in juvenile Antarctic krill at the Western Antarctic Peninsula. Front. Mar. Sci. 9. doi: 10.3389/fmars.2022.1009385
Tarling G. A., Klevjer T., Fielding S., Watkins J., Atkinson A., Murphy E., et al. (2009). Variability and predictability of Antarctic krill swarm structure. Deep Sea Res. Part I: Oceanographic Res. Papers 56, 1994–2012. doi: 10.1016/j.dsr.2009.07.004
Venables W. N., Ripley B. D. (2002). Modern applied statistics with S. 4th ed. (New York: Springer), ISBN: ISBN 0–387-95457–0.
Virtue P., Nichols P. D., Nicol S., Hosie G. (1996). Reproductive trade-off in male Antarctic krill, Euphausia superba. Mar. Biol. 126, 521–527. doi: 10.1007/BF00354634
Watkins J. L. (1986). Variations in the size of Antarctic krill, Euphausia superba Dana, in small swarms. Mar. Ecol. Prog. Ser. 31, 67–73. doi: 10.3354/meps031067
Watkins J. L., Brierley A. S. (2002). Verification of the acoustic techniques used to identify Antarctic krill. ICES J. Mar. Sci. 59, 1326–1336. doi: 10.1006/jmsc.2002.1309
Watkins J. L., Buccholz F., Priddle J., Morris D. J., Ricketts C. (1992). Variation in reproductive status of Antarctic krill swarms; evidence for a size-related sorting mechanism? Mar. Ecol. Prog. Ser. 82, 163–174. doi: 10.3354/meps082163
Watkins J. L., Morris D. J., Ricketts C., Murray A. W. A. (1990). Sampling biological characteristics of krill: Effect of heterogeneous nature of swarms. Mar. Biol. 107, 409–415. doi: 10.1007/BF01313422
Watkins J. L., Morris D. J., Ricketts C., Priddle J. (1986). Differences between swarms of Antarctic krill and some implications for sampling krill populations. Mar. Biol. 93, 137–146. doi: 10.1007/BF00428662
Wickham H., François R., Henry L., Müller K. (2023). dplyr: A Grammar of Data Manipulation_ (R package version 1.0.9). Available at: https://CRAN.R-project.org/package=dplyr.
Wright S. W., van den Enden R. L. (2000). Phytoplankton community structure and stocks in the East Antarctic marginal ice zone (BROKE survey, January–March 1996) determined by CHEMTAX analysis of HPLC pigment signatures. Deep Sea Research Part II: Topical Studies in Oceanography 2000. 47 (12), 2363–2400. doi: 10.1016/S0967-0645(00)00029-1
Keywords: bomb calorimetry, energy content, swarm, summer, partitioning
Citation: Cataldo-Mendez C, Kawaguchi S, Cox MJ, Melvin J, Rae V and Swadling KM (2024) The energy content and demographic composition of Antarctic krill (Euphausia superba) swarms in East Antarctica. Front. Mar. Sci. 11:1337080. doi: 10.3389/fmars.2024.1337080
Received: 12 November 2023; Accepted: 30 May 2024;
Published: 14 June 2024.
Edited by:
Ibon Galparsoro, Technological Center Expert in Marine and Food Innovation (AZTI), SpainReviewed by:
Geraint A. Tarling, British Antarctic Survey (BAS), United KingdomCopyright © 2024 Cataldo-Mendez, Kawaguchi, Cox, Melvin, Rae and Swadling. This is an open-access article distributed under the terms of the Creative Commons Attribution License (CC BY). The use, distribution or reproduction in other forums is permitted, provided the original author(s) and the copyright owner(s) are credited and that the original publication in this journal is cited, in accordance with accepted academic practice. No use, distribution or reproduction is permitted which does not comply with these terms.
*Correspondence: Camila Cataldo-Mendez, Y2FtaWxhLmNhdGFsZG9tZW5kZXpAdXRhcy5lZHUuYXU=
Disclaimer: All claims expressed in this article are solely those of the authors and do not necessarily represent those of their affiliated organizations, or those of the publisher, the editors and the reviewers. Any product that may be evaluated in this article or claim that may be made by its manufacturer is not guaranteed or endorsed by the publisher.
Research integrity at Frontiers
Learn more about the work of our research integrity team to safeguard the quality of each article we publish.