- 1Yantai Graduate School, Harbin Engineering University, Yantai, China
- 2College of Information Science and Engineering, Hunan City University, Yiyang, China
The development of solar energy is one of the most effective means to deal with the environmental and energy crisis. The floating photovoltaic (PV) system is an attractive type because of its multiple advantages and has been well developed based on fresh water areas on land. This paper focuses on the expansion of this sector towards the ocean, offshore floating PV plants, which is the new growth point with huge potential for the future PV sector. For this new field, the technology readiness level is really low and research to understand the interaction between offshore floating PV plants and marine environment are proceeding. In this paper, we aim to discuss the technological feasibility of offshore floating PV plants as well as analyze potential impacts on the marine environment during the life cycle of PV from manufacturing until disposal.
1 Introduction
In response to the increasingly serious energy and environmental crisis, the development and utilization of clean and renewable energy has always been a hot spot in the world (Kabir et al., 2018; Massa et al., 2021). Among all kinds of new energy, photovoltaic (PV) solar energy is regarded as one of the most promising and fastest-growing renewable (see Figure 1). And, as an important component of PV solar energy, the floating PV systems are growing at an accelerated pace (see Figure 1) (Spencer et al., 2019; Wang and Lund, 2022; Zhang et al., 2023). In terms of environmental impact, the life cycle of PV systems (from the manufacturing stage to installation and operation, decommission and disposal or recycling of solar PV equipment) was equivalent to 4.5% of that of the current coal-based electrical power system (Xie et al., 2018). A well-ordered Life-Cycle Assessment Method (LAC), which has been accomplished by maintaining the ISO (International Organization for Standards) standards14040:2006 and 14044:2006 (Mahmud et al., 2018). It has been used by following the four basic steps (goal and scope definition, life-cycle inventory, Life-cycle environmental-impact evaluation as well as impact outcome interpretation) to assess the environmental impacts of solar technologies like solar PV and solar-thermal systems and compare their effects on the environment using sixteen impact indicators.
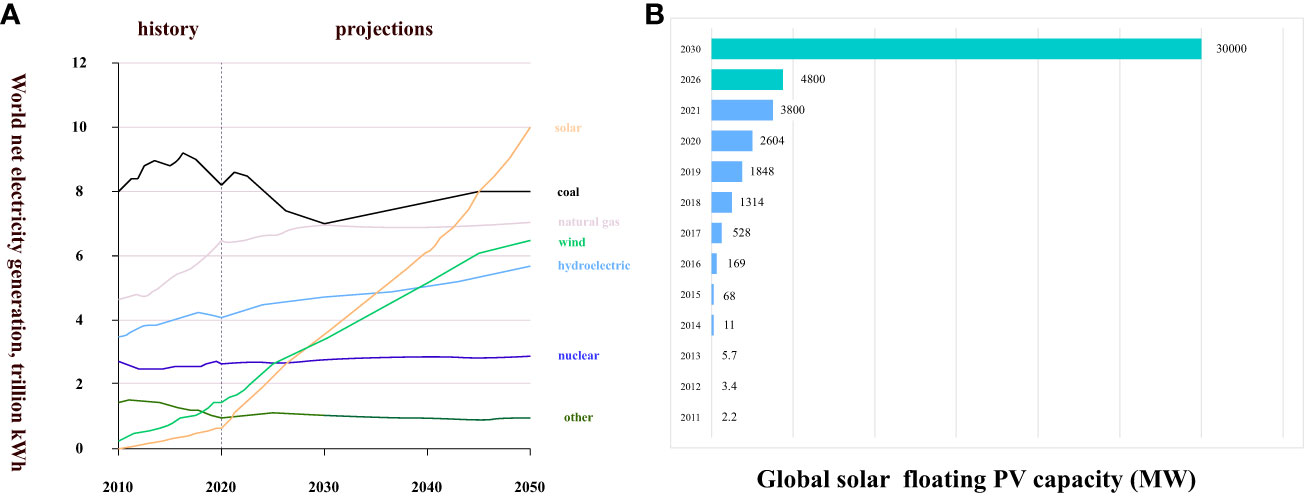
Figure 1 (A) World net electricity generation (trillion kWh) (Zhang et al., 2023); (B) Growth trend of cumulative global installed capacity (MW) of floating PV during 2011-2030. (Silalahi and Blakers, 2023).
PV systems are mainly classified as ground-mounted, roof, and floating ones. Due to the low power density of sunlight, PV system requires much space, which has significantly limited the onshore PV expansion (Trapani and Redón Santafé, 2014; Vervloesem et al., 2022). Considering a PV panel efficiency of 15%, setting up a 1 MWp power station needs 10,000 m2 of land area (Ghosh, 2023). Since 71% of the Earth’s surface area is occupied by the ocean, this has been provided to be an ideal location for renewable energy power plants such as offshore wind and photovoltaic farms (Fan et al., 2022). There is an increasing interest from industries to expand PV to oceans, where the PV systems have endless space, less dust, more light and lower temperature (Tina et al., 2018; Oliveira-Pinto and Stokkermans, 2020; Pouran et al., 2022; Wang and Lund, 2022). A detailed comparative analysis of various marine renewable energies (wave, tide, current, thermal energy conversion, salinity gradient, wind and solar) from several aspects of the theoretical and technical energetic potential, levelised cost of energy, total installed capacity worldwide and power density has been summarized in Table 1. According to the above table, for the moment, offshore wind power is considered to be the most mature marine renewable energy, which possesses the lowest levelised cost of energy (115 euro/MWh for 2018) and the largest total installed capacity worldwide (58 GW for 2021). For floating PV plants, it is not difficult to see that freshwater PV plant with levelised cost of energy of 25-51euro/MWh is much more mature than offshore one (354 euro/MWh for 2020). At the same time, the unparalleled theoretical energetic potential (220,000-1,000,000 TWh/yr) and the predictable and rapidly declining levelised cost of energy (354 euro/MWh for 2020 and 40 euro/MWh for 2050) highlight the huge development space of offshore PV power generation systems. The offshore PV modules possess higher efficiencies (an increase of 5 to 15%) due to the cooling effect of the water and higher wind speed (Trapani and Millar, 2014; Claus and López, 2022; Pouran et al., 2022; Jathar et al., 2023). Although, for the moment, the harsh marine environment still is an obstacle to the development of offshore PV plants in terms of technology and cost, the offshore PV plants always are attractive and there may be no choice but to consider to reduce carbon emissions and maintain energy security for small island nations (Malta and Singapore, etc.) or nations with comparatively large coastal areas (e.g. Netherlands) (Golroodbari et al., 2023). Some offshore PV plants are summarized in Table 2.
In general, offshore PV systems can be roughly divided into two categories: fixed pile-based PV systems and floating PV systems (Wang and Lund, 2022). The fixed pile-based PV systems are stationary PV systems in offshore or tidal areas, where the water depth is less than 5 meters. This kind of system is characterized by relatively lower technical difficulty, and higher safety, but also a higher initial investment. This financial benefit of such a bottom-fixed solution significantly decreases with increasing water depth due to the largely increased piling cost. The floating ones refer to the use of floating materials and anchoring systems to make photovoltaic modules, inverters and other power generation equipment floating in the ocean for power generation (Rosa-Clot et al., 2010). It is believed that offshore floating PV has a wider range of applications, but the current technology maturity is relatively low from materials to structural design to operations and maintenance to cope with the marine environment, which is much harsher than the freshwater environment on land (Claus and López, 2022; Wang and Lund, 2022). Relatively mature design and build programs have been established for freshwater-based floating PV systems due to their early development. The recommended practice of freshwater-based floating PV systems will be useful to refer to, but offshore PV plants have much higher technical requirements and we cannot copy these practices or guidelines of freshwater-based PV systems. To promote the commercialization of the sector and protect the marine ecology environment to the greatest extent, it is necessary to discuss the technical development of offshore floating PV plants.
This paper aims to analyze potential impacts on the marine environment during the life cycle of PV from manufacturing until disposal, as well as discussing the corresponding technical solution to eliminate or attenuate the aforementioned impacts. In addition, we try to highlight existing production and design technologies and investigate the possible solutions in terms of the commercialization of offshore floating PV plants. The paper is organized, in order of the life cycle of offshore floating PV plants as follows. The manufacture and design of the offshore floating PV plants from overall structural to individual components are summarized in Section 2. The assemblage and installation of the PV plants are presented in Section 3. Degradation, and Operation and maintenance systems are detailed in Section 4. Section 5 presents the decommissioning and disposal of the PV equipment.
2 Manufacture and design
Although suffering from harsh environments such as saltwater corrosion, great wind and wave, UV degradation and bio-fouling, the designed service life of offshore floating PV plants is not less than twenty-five years (Sahu and Sudhakar, 2019). Therefore, reasonable structural design and material selection are particularly important (see Figure 2). Due to the immaturity of marine PV plants, there is no specific standard for this sector (Wu et al., 2019). As the freshwater floating PV plant started earlier than offshore one and is much more mature, a comparative study of the two types of floating PV plants will play a positive role in the development of offshore photovoltaics. Table 3 illustrated the comparative analysis of the freshwater floating PV plants and the offshore ones. In terms of resistance to environmental loads and corrosion, construction and maintenance, the technical requirements and cost investment of offshore PV systems are much higher than those of freshwater PV.
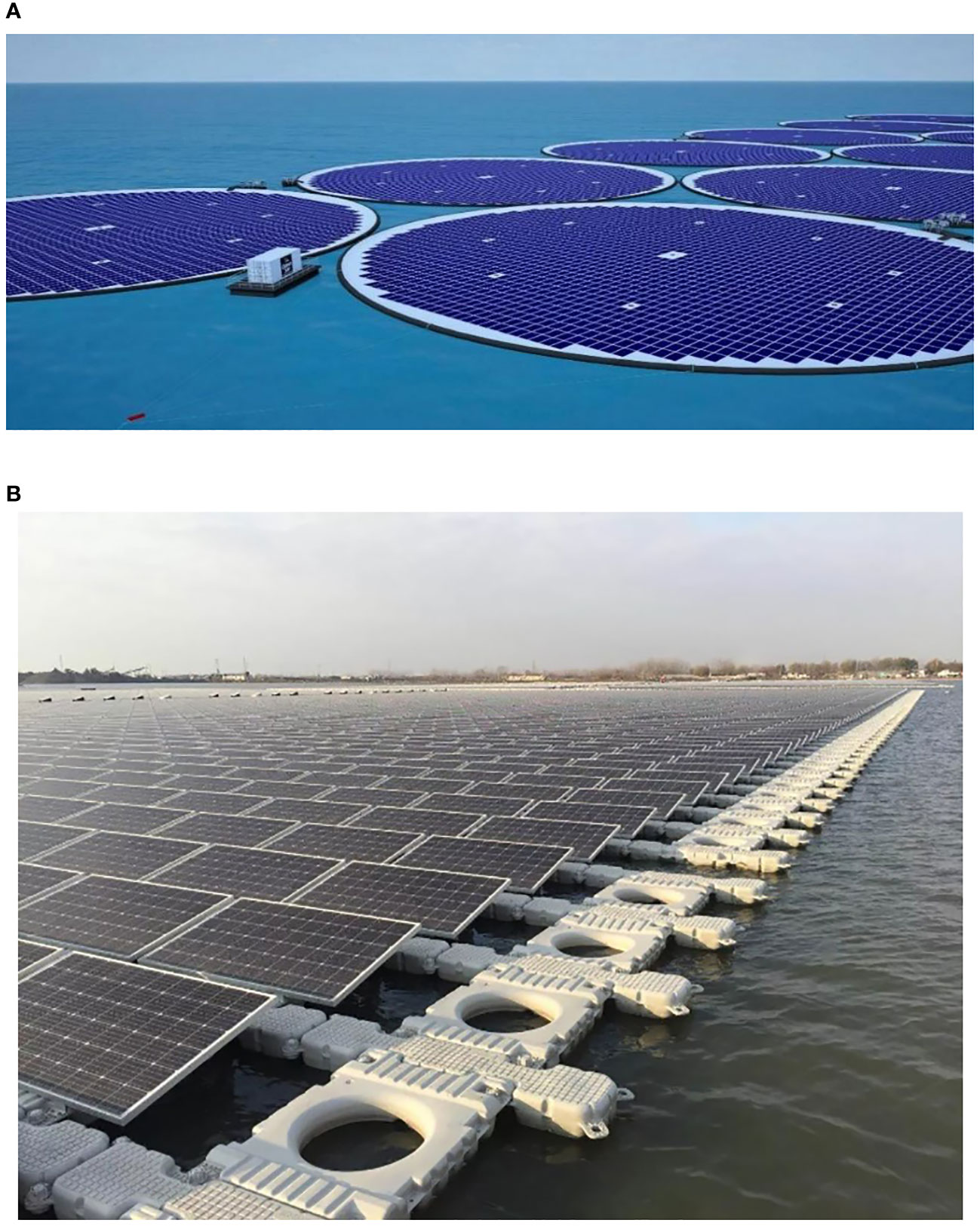
Figure 2 Flexible design of offshore floating PV: (A) Modified solar modules mounted on a flexible floating membrane (Ocean Sun, 2022); (B) Rigid offshore PV modules with hinged connectors.
In 2021, DNV provided the recommended practices for freshwater floating solar design (DNV, 2021). It is certain that the recommended practice will be useful to refer to, but offshore PV plants have much higher technical requirements. For example, the sea-based floating PV systems have to bear a higher overall load (2135 kN) than the lake-based one (1245 kN) possesses a three times bigger capacity (Ikhennicheu et al., 2021; Kumar et al., 2021). In addition, it is claimed that the energy loss to moving modules due to waves can range from 3% for medium wave intensity up to 9% for extreme wave intensity (Golroodbari et al., 2023). Since many design aspects are common, drawing on the standards of mature sectors such as offshore oil and gas and even the ones of the relatively advanced offshore wind power sector become an effective compromise (DNV, 2021). For example, the Eurocodes (Eurocode 0 to 4 for concrete, steel or composite steel and concrete structures) specify how structural design of infrastructures should be conducted within the European Union (Claus and López, 2022). It is noteworthy that offshore floating PV plants possess less weight per wet surface and more deck area than other offshore energy plants. Thus, when drawing on the standards of other mature sectors, the destructive power of wave load including resonance and fatigue damage needs to be fully considered (Ranjbaran et al., 2019).
The floating PV plant normally consists of the PV module, the float and the anchoring and mooring systems (see Figure 3). When exposed to harsh marine environments, the key criteria for evaluating the PV plants from overall structures to individual components are the cost, the robustness and the environment impact.
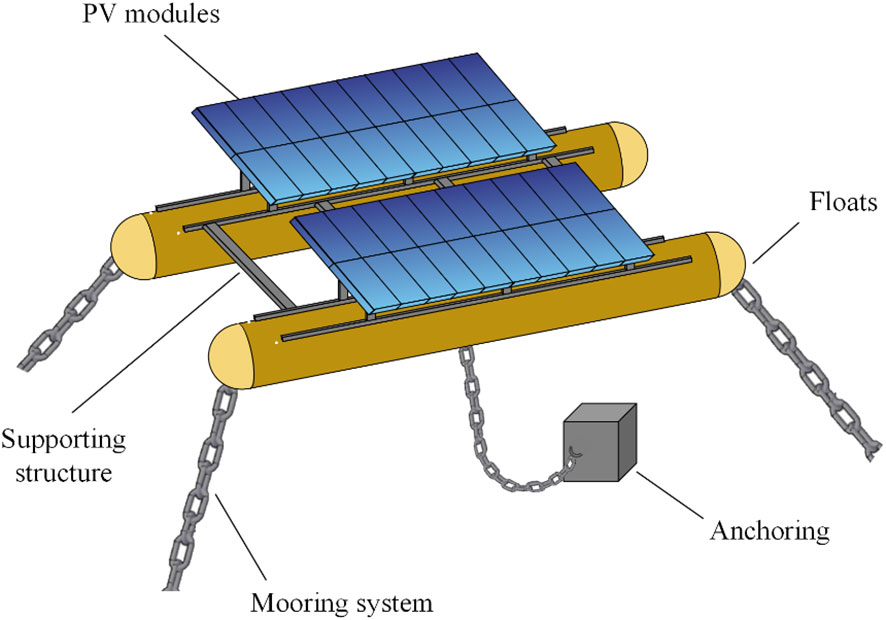
Figure 3 Components of a generic offshore floating PV system (Claus and López, 2022).
2.1 PV module
From the perspective of photovoltaic technology, it is roughly divided into five types: crystalline silicon (Mono-Si, Multi-Si, etc.), thin-film [amorphous-Si (a-Si), CdTe (cadmium telluride), etc.], hybrid PV [Heterojunction Intrinsic Thin-Layer (HIT), Perovskite], dye-sensitized and organic PV (single- layered, multi-layered) (Kumar and Kumar, 2017) (see Figure 4). Currently, large-scale floating PV installations have mainly employed crystalline silicon wafer-based modules (Claus and López, 2022). Finding the most suitable PV technology for offshore PV plants is difficult and complex. This is due to the following reasons: there is limited comparative data reported in the literature on the performance of different PV technologies on the water surface; data collection of the performance of PV technologies on the seawater surface is short-term (less than 2 years) and lacks the support of long-term data; the performance of different PV technologies fluctuates greatly depending on the local climate of the area (temperature, humidity, light, wind speed, etc.) in which they are installed. IEC 61724 provides some metrics to analyze the performance of PV systems or technologies under local climatic conditions (IEC, 2021). From the performance point of view, preliminary comparisons between different technologies have been implemented (Table 4). The authors reported the better performance of HIT and CdTe technologies as compared to multi-Si. Additionally, the degradation rate of these PV technologies is also found better than the multi-Si technology on the water surface (Kumar and Kumar, 2019; Kumar et al., 2020). The performance of c-Si-based technologies such as HIT and multi-Si modules is found to be lower on the water surface than the corresponding PV technologies on the ground surface. However, a thin-film technology such as CdTe has higher performance on the water surface than the corresponding CdTe technology on the ground surface (Kumar and Kumar, 2019).
From the greenhouse gas (GHG) emissions point of view, among different PV systems, GHG emission factors from thin-film technology (7.4-35 gCO2 eq./kWh) are sensibly lower-carbon than those of silicon-based PV (23-83 gCO2 eq./kWh) (Zhang et al., 2023) (Table 4). From the toxic metal content point of view, the content of Cd in CdTe PV cell is significantly higher than that in crystalline silicon (c-Si), amorphous silicon (a-Si), and copper-indium-gallium-selenide (CIGS) PV cells, while lead (Pb) content in c-Si is relatively high (Table 4). The highly toxic elements, such as Pb and Cd, are carcinogens and hazardous at even low doses. If not properly deposed and recycled, toxic metals utilized in the PV cell materials such as Pb and Cd could be released. As reported, from the performance point of view alone, thin-film technology carries less environmental life cycle impact than the first generation, including multi-Si and mono-Si technology, does. (Kumar et al., 2021) In order to eliminate the impacts of toxic metals in PV modules on the marine environment: 1. Developing packaging technology and protective coatings to ensure that no leaching and leakage of heavy metal ions occurs during operation; 2. New generation PV technologies such as dye-sensitized solar panels, perovskite and organic solar panels should be developed (Nain and Kumar, 2020). Bella et al. (Bella et al., 2016) reported an effective polymeric dye-sensitized solar cells based floating PV system, which can be made transparent and low-density, leading to good bio-friendliness, flexibility and floating capacity.
As a popular and fast-growing PV technology, bifacial technology is a non-negligible candidate for developing offshore floating PV plants. As the name suggests, bifacial modules can concurrently absorb light from both front and back sides, which endows this technology with significant benefits, compared to mono-facial ones, such as higher power generation efficiency, smaller area consumption for same watt-peak installation (Ziar et al., 2020; Kumar et al., 2021). Compared with monofacial modules, it has been proved that the energy gain of bifacial modules was increased by 13.5% with a smoother daily power curve (Gibbons, 2016; Ziar et al., 2020). Therefore, in terms of energy yield potential, the application of this technology will contribute to the further development of offshore floating PV plants. However, to combine them effectively, for the moment, there are some potential issues that need to be considered (Table 5). Mounting PV modules at steep angles will be conducive for the back sides of modules to effectively capture sunlight. Given harsh marine environments that possess much larger environmental loads than those of freshwater bodies and there is no shelter from wind, the tilt angle of PV modules as well as the height of modules from the water have to be significantly compromised to ensure safety. Additionally, the power generation of the PV module backside depends on the intensity of reflected light from the water surface, which varies with wind speed, wave motion and solar altitude angle. Albedo refers to the fraction of solar energy reflected from various surfaces in forms of direct and diffuse radiation (Rosa-Clot and Tina, 2018). Based on some studies, the albedo of the water surface (5-8%) is lower than that of the ground surface (13-15%) (Liu et al., 2018; Ziar et al., 2020). This indicates that reflectors are necessary for offshore floating PV plants to effectively combine bifacial technology. The cooling effect of water is one benefit of floating PV to lead to an average of 5% increase in power generation efficiency. To take advantage of this, the module should be mounted at low angles and near the water surface, which will be detrimental to the absorption of light on the backside of the PV panel.
While the above issues need to be fully explored, it doesn’t negate the fact that the offshore floating bifacial PV technology is a viable option in the future. Although the data of the long-term field performance of this technology is lacking, scientists have made some meaningful explorations. Ziar et al. (Ziar et al., 2020) simulated the various orientation and tilt angles for the bifacial PV module and the optimum orientation and tilt angle have opted for the installation with or without reflectors on the water bodies. In the case of using reflector, to maximize the total radiation, the reflector should be installed under the bifacial PV module at right distance but not too close. Taking into account the most important geometrical parameters of the floating bifacial PV module such as tilt angle, pitch, height from the water and the ratio of pitch to module length, Tina et al. (Tina et al., 2021) proposed the optimum design configuration. According to the study, the increasing of tilt angle, height from the water in a certain limit will be beneficial to increase the efficiency of energy generation. Hasan et al. (Hasan and Dincer, 2020) have simulated the floating bifacial PV systems and the results indicated that PV modules aligned in north/south directions could produce a maximum of 55% growth in exposure to irradiance in comparison with conventional modules and the growth of the ones aligned in east/west directions was estimated to be 33%.
Manufacturing of photovoltaic modules for marine environments has to take into account the higher environmental loads and effects of salt deposition and its corrosive nature. The component and panel evaluations have to be performed in real and simulated floating conditions, which includes component salt spray, panel vibration, corrosion, oxidation, immersion and UV exposure tests. The modules for ocean installation are needed to undergo a Salt Mist Corrosion test according to the IEC 61,701 standards for special certification (Sahu et al., 2016). Gretkowska et al. (Gretkowska, 2018), recommended that PV modules employed in floating projects must be protected from direct contact with saline water, and the selection of adequate PV technology is critical as Copper Indium Gallium Selenide (CIGS) PV technology showed the most impact from the salt deposition. In another effort, Setiawan et al. (Setiawan et al., 2019), explored the behavior of PV panels under the off-shore floating conditions by splashing seawater on the modules. The accumulation of salt particles after three days of treatment could result in a drop of 1.3778 W and 0.948 in power and efficiency respectively.
Minimizing seawater adhesion by increasing the hydrophobicity and optimizing the geometrical shape of the PV panel surface deserves further research. Given that nearly any metal will corrode over time and therefore alternatives to standard aluminium frames and mounts with polymer-based ones are desired. Ethylene-vinyl acetate is a polymer that can isolate PV cells from the flow of electrons, structural strength and defend the external face by substantially avoiding water accumulation at its joining parts (Kim et al., 2021). Mechanical properties can be enhanced by increasing panel stiffness or by mounting strings and cells on the neutral axis. The crack formation can be partially mitigated using encapsulants with lower elasticity and rectangular or half-cut cells (Claus and López, 2022).
2.2 Floating systems
Floating systems provide foundation support or platform for all working components of PV plants as well as human accessibility. Currently, available structure for floating PV systems includes structure 1, 2 and 3 (Figure 5). For structure 1, the floating systems include high-density polyethylene (HDPE) or fiber-reinforced plastic (FRP) based floating pipes consists of aluminium and steel rafts. For structure 2, the floating and rafts are made with HDPE. Structure 3 contains a main float supporting for PV and a second float for maintenance. The floating pontoon can connect together to form a large single-unit structure to hold a PV module (Kim, 2017). In response to long-term exposure to harsh marine environments, the selection of appropriate materials thus becomes crucial. Nowadays, the most common material used in floating PV plants is HDPE, which is characterized as corrosion-resistant, UV-resistant, maintenance free and has high tensile strength (Sahu and Sudhakar, 2019; Kumar et al., 2021). In addition, there are other materials available, such as medium- density polyethylene (MDPE), fiber-reinforced plastic (FRP), and ferro-cement (Sahu et al., 2016; Gorjian et al., 2021). The floats, in case of sinking, will have a lasting damaging effect on the marine environment. So, it can be filled with polystyrene foam, which decreases the risk of sinking even if damaged (Kumar et al., 2021). From an environmental point of view, there is a potential risk of slowly releasing organic hydrocarbon pollutants. Another risk to consider is the generation of micro-nano plastic particles, which may cause unpredictable ecological risks (Andrady, 2011; Claus and López, 2022). They can be accumulated in aquatic organisms to bring various physical injuries, and may eventually reverberate humans through the food chain (Kumar et al., 2021). To date, as offshore floating PV plant is in its infancy, there has been no systematic assessment of the environmental impact of the above materials. To protect the marine ecological environment, developing new environmentally friendly floating and coating materials is necessary.
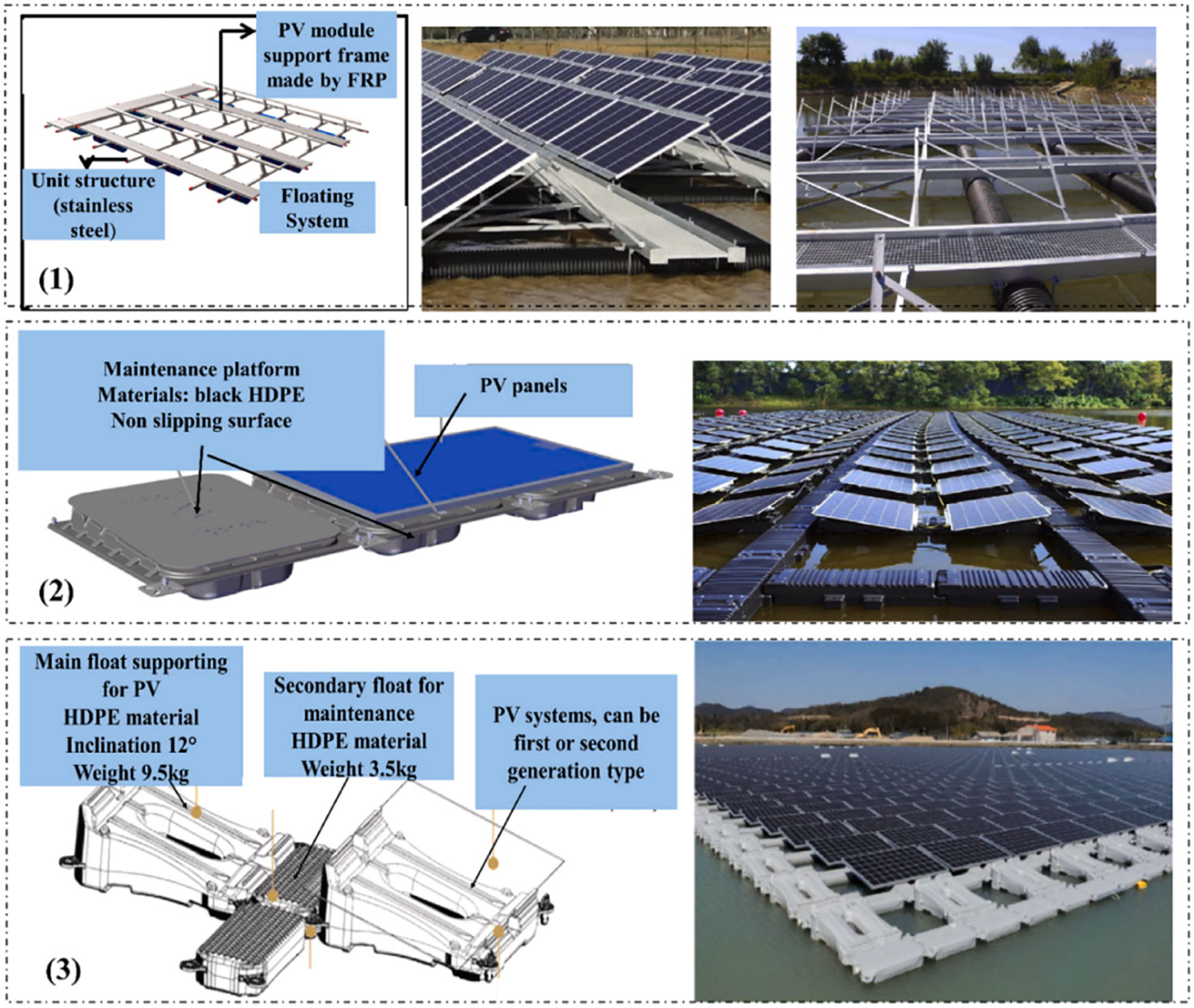
Figure 5 Commercially available three different types of structures employed for floating PV application (Kim, 2017; Ghosh, 2023).
Compared to freshwater bodies, there are much larger environmental loads in harsh marine environments, such as wind loads, wave loads, current loads, etc. As the most common threat to the offshore environment, wind loads have evaluation systems based on wind tunnel testing, digital simulation as well as the method proposed by DNVGL-RP-C205 (DNV, 2021), etc. In addition, the wind loads on floating PV structures were also analytically estimated (Kim et al., 2017; Ikhennicheu et al., 2021) and numerically analyzed (Choi et al., 2021). Thus, offshore floating PV plants can be reasonably designed to minimize the wind loads, especially the shape of the floating body and the incidence angle of the wind. It is not difficult to imagine that the smaller the tilt of the PV panels, the smaller the wind loads. However, a balance needs to be found for optimizing illumination and minimizing wind loads (Gorjian et al., 2021). By the way, in terms of current loads, it also can be estimated by the method proposed by DNVGL-RP-C205 (DNV, 2021).
To cope with the wave loads, flexible structures including PV modules and floats are applicable for large-area offshore floating PV arrays. (Trapani and Millar, 2014; Cazzaniga et al., 2018) The wave loads can be greatly dissolved by moving with the wave rather than directly withstanding its force. Logically, the above flexible structure design also reduces the load on the mooring systems. The common flexible types currently include thin-film flexible modules, crystalline modules backed with flexible foam as well as rigid modules with hinged connectors (see Figure 2). The characteristics of the above three flexible structures are summarized in Table 6. Thin-film flexible modules are designed to float on water bodies with the aid of air pockets and eliminate the pontoon structure, which leads to a water contact-induced cooling and cleaning nature. The self-cooling is beneficial for power generation efficiency (increasing to between 5 and 15%). However, this flexible structure is unable to tilt modules to optimally determine the inclination of PV panels and the alignment of the modules is always changing, which leads to reduced energy absorption (Trapani and Millar, 2014; Kougias et al., 2016). From an economic perspective, the thin-film floating plants benefit from less material usage, lighter structural weight, lower mooring load and fewer components (Trapani and Millar, 2015). In the case of crystalline modules backed with flexible foam, designed by Ocean Sun, the main components include buoyancy rings, membranes, and PV modules. The operating temperature of the module is reduced due to the cool water below the buoyancy rings, leading to higher yields than air-cooled systems. The obvious advantage of this type is that connectors are almost avoided, which are usually the most vulnerable parts. As a result, the stability of the entire structure will be significantly improved. In addition, the customizability of flexible membranes is also an advantage to adapt to different environments and construction needs. Linking rigid modules with hinged connectors to form flexible large arrays is another flexible design of floating PV plants. As the most critical component and the long-standing challenge of this structure, the connectors need to be rationally designed (e.g., rigid, semi-rigid, and flexible) based on multi-analysis such as coupling analysis to meet project requirements and optimize production costs (Jiang et al., 2021).
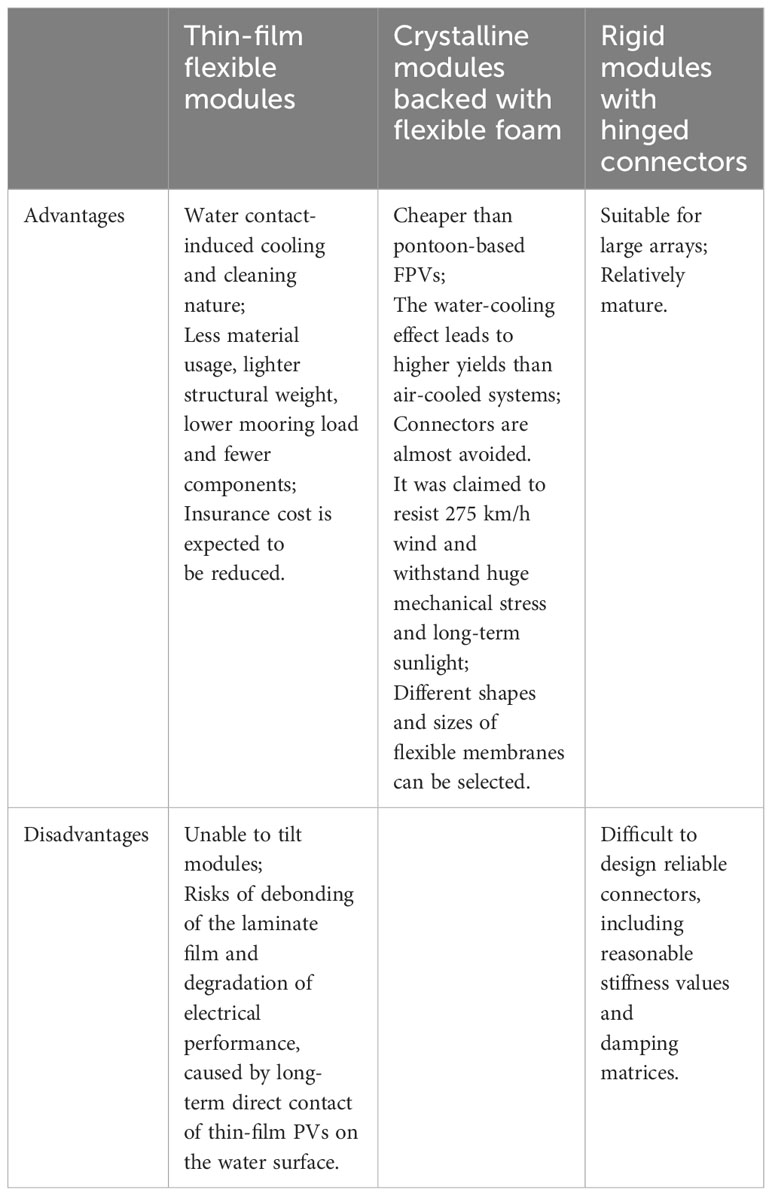
Table 6 Comparative analysis of the three common flexible types of offshore floating PV plants (Shi et al., 2023).
To cope with wind and wave loads, another intuitive design is submerged rigid PV plants, which avoid the direct impact of wind and waves by sinking to a certain depth below the water surface (see Figure 6). Although the concept was proposed by Stachiw decades ago, it has not achieved large applications of PV plants due to some issues. In order to ensure sufficient light intensity, the depth of the PV dive should generally not exceed 0.5 meters and this will significantly limit the avoidance effect of wave loads (Shi et al., 2023). It is true that the operating temperature of the device is reduced due to cool seawater, the prolonged immersion will place high technical demands on the stability of the equipment to deal with the corrosion of seawater and the adhesion and corrosion of organisms and thus increase costs. In any case, the positive aspects of this concept cannot be denied, especially its emergency sheltering potential against extreme weather, if controlled dives can be achieved.
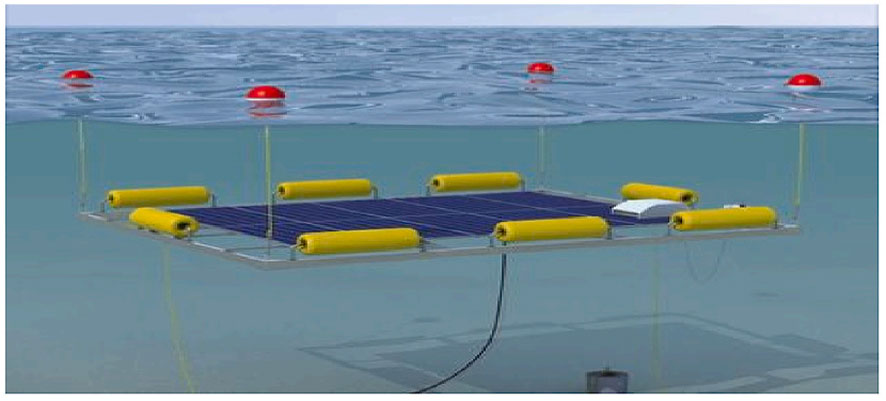
Figure 6 Submerged rigid PV plants (Cazzaniga et al., 2018).
2.3 Mooring and anchor
As the cornerstone of the entire offshore floating PV plant, the stability of the mooring system must be ensured. In 2021, DNV has recommended practice for designing floating PV mooring systems in freshwater (DNV, 2021). This could be a good technical reference, but significantly larger environmental loads on the marine environment needed to be fully taken into account. Ikhennicheu et al. (Ikhennicheu et al., 2021) found that the wave load can contribute significantly to the total load (~ 50%) on floating PV plants in offshore locations, based on a quasi-static analytical method. This means that the stability of mooring systems under wave action is very important.
As mooring lines, the marine ones are usually made of steel chains or wire ropes to resist harsher environments (Sahu et al., 2016). The use of steel chains or wire ropes is mainly due to its safety and certainty (DNV, 2021). As the most commonly used material in engineering construction, chain and wire ropes can be tested by various standards or methods such as Staad and ProStructures software, to ensure their sufficient bearing capacity. However, in order to commercialize this sector, the research and development of new alternative materials is also very necessary. This is because of the corrosion problem of the steel itself in the ocean and the cost. Given larger water surface variations due to tides and storm surges and the need to avoid slack to produce sudden jerks for the mooring points, the elastic mooring systems based on rubber with good ductility have been proposed, such as superflex rope (Huang et al., 2018). As an important complement to traditional mooring systems, the elastic mooring system still does not solve the cost problem. In addition, its safety and certainty to resist harsh environments of marine lack long-term data support. A comparative analysis of the above two systems is shown in Table 7. Zeng et al. (Zeng et al., 2023) reported a novel adaptive barrier-mooring system for coastal floating PV plants, consists of perimeter pontoons, barriers, clump weights, mooring lines and anchors (Figure 7). It is cheaper in materials and maintenance as well as more wave-stable (improved by 40% under wave action), compared to mooring systems using elastic cables. In addition, the new mooring system enables the floating solar farm to adapt up to 36% of water depth without introducing slack in the mooring cables.
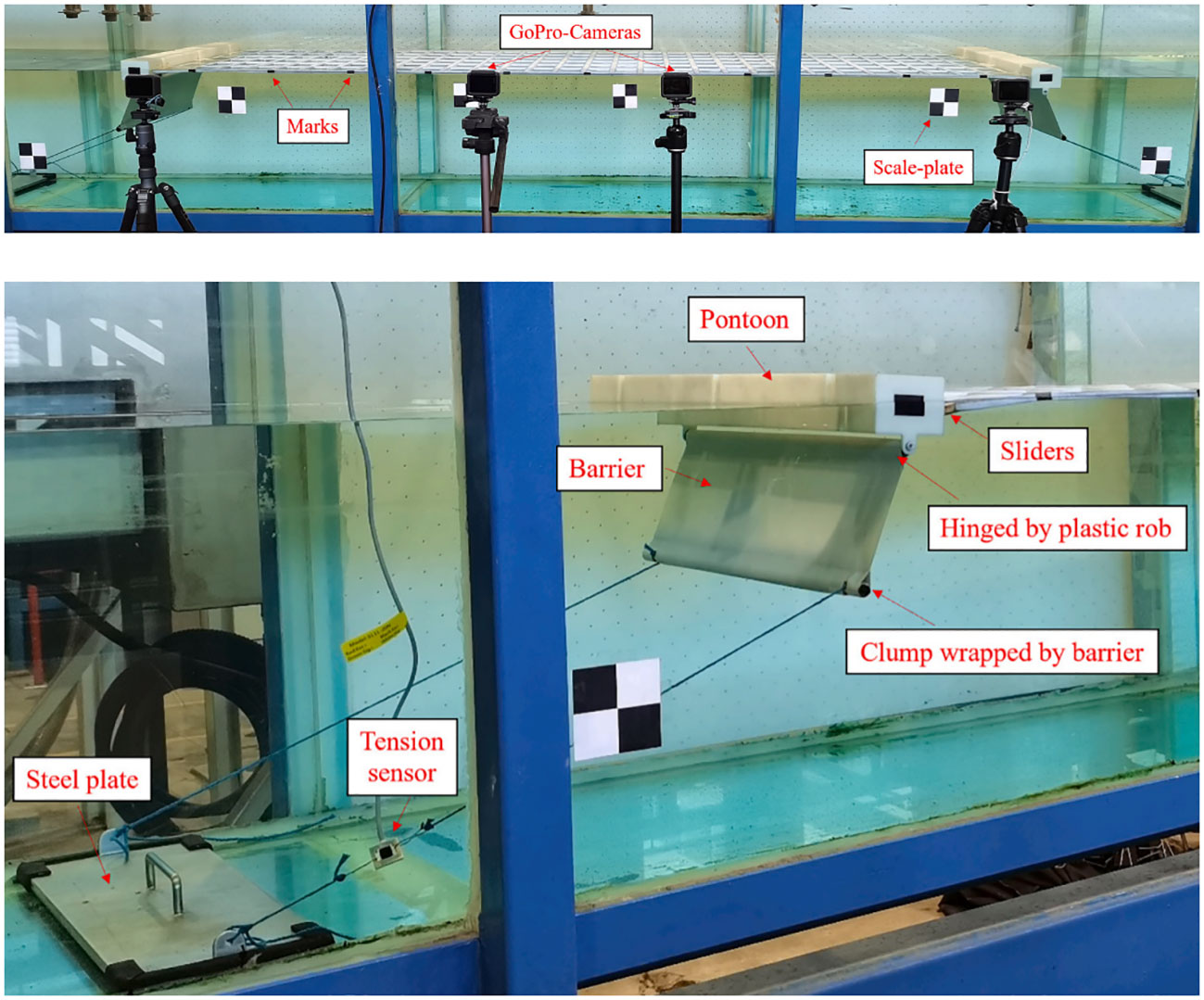
Figure 7 The novel adaptive barrier-mooring system in the experiments (Zeng et al., 2023).
From the perspective of structural design, four anchoring modes, catenary, compliant, taut and rigid can be referred to (Hicks and Culley, 2005) (see Figure 8). Since the catenary mooring systems only use their self-weight to provide a spring rate to the float, whether it can withstand the ocean winds and currents is worrying. Compliant moorings are catenary moorings that use floats and weights to adjust the layout of the mooring lines. They can increase the weight of the mooring line and reduce the mooring radius by connecting the buoy (submerged or surface) and sinker. In the case of the taut ones, a more significant restoring force can be provided, but the water level variation due to tides can be problematic unless elastic mooring lines are adopted. The installation and maintenance of taut moorings are complex for deep water compared with excessively heavy catenary. The rigid systems seem guaranteed but are only economically reasonable for shallow water, as anchorage structures attached to the seabed are essential (Claus and López, 2022).
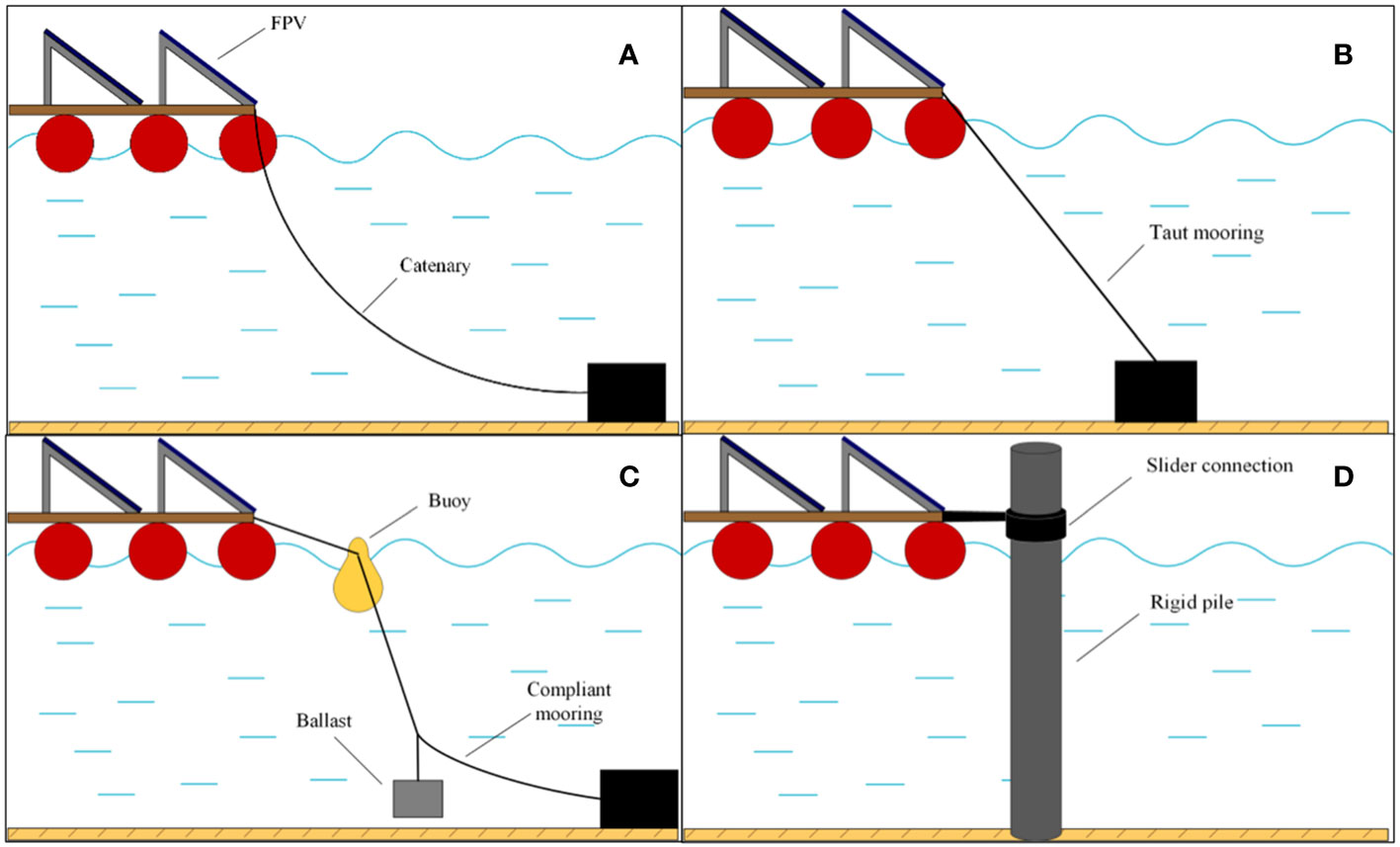
Figure 8 Examples of mooring layouts for floating PV systems (A) catenary, (B) taut mooring, (C) compliant mooring, and (D) rigid mooring (Claus and López, 2022).
Normally, to well control the floating platform, especially for the one with lightweight, a relatively rigid mooring system is preferable. However, too rigid mooring system will impact the flexible structure of floating platforms to resist environment loads such as wave loads. The balance between the above aspects is the focus of the structural design of the mooring system.
Until now, anchoring systems designed for the floating marine PV industry segment remain a challenge. The general consensus is to draw on the traditional marine structures designs and from other marine renewable energy (MRE) technologies (Xu et al., 2019). According to traditional marine anchoring systems, dead weights, drag anchors, embedded anchors or suction foundations are all taken into account for the offshore floating PV plants (see Figure 9). Although the dead weight anchor is easy to set up, regardless of the seabed structure, and will not cause any penetrating damage to the seabed, it is too inefficient. The drag anchor is preferable for the soft seabed and the embedded depth depends on loads. For the hard seabed, the plate anchor is suitable.
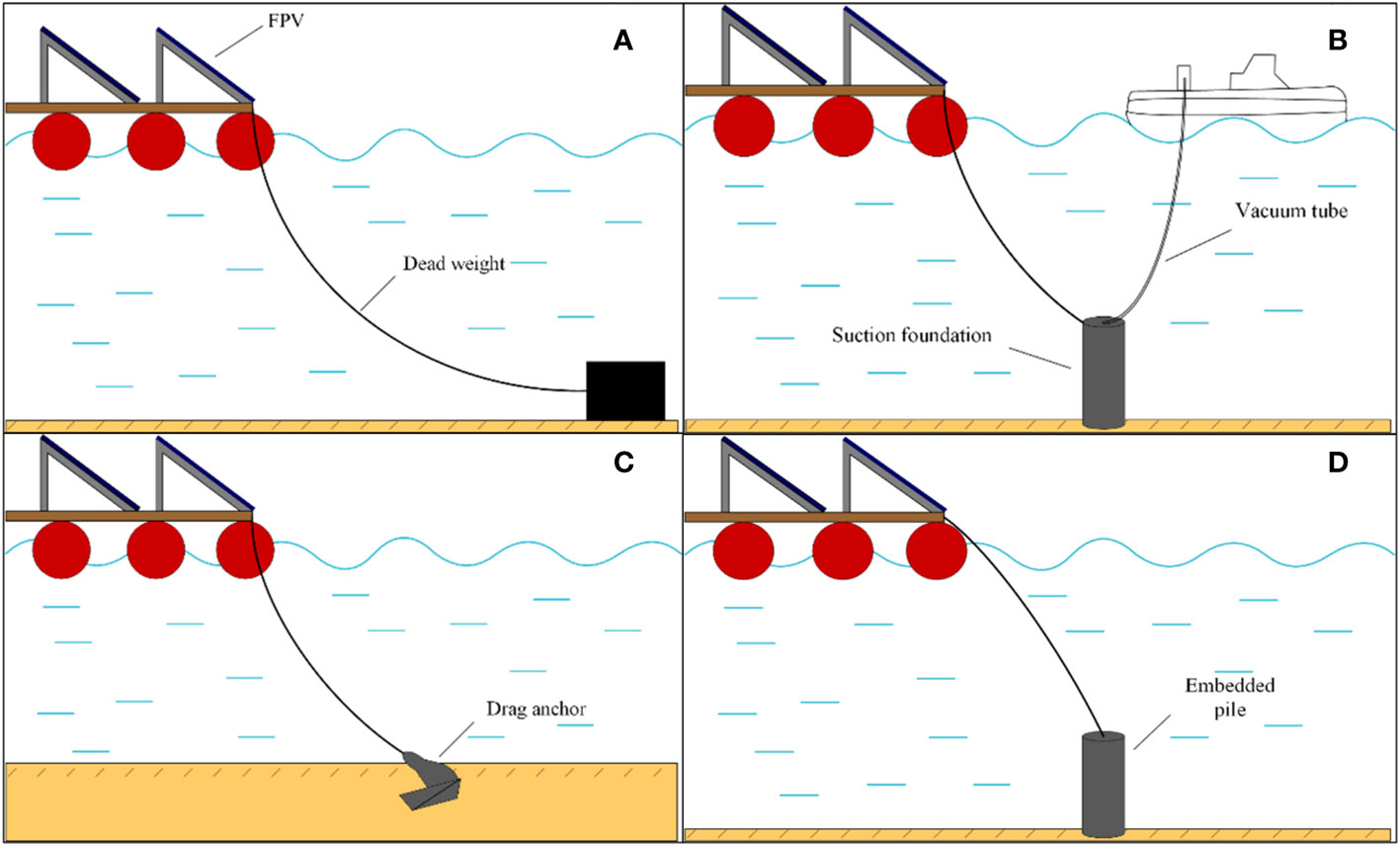
Figure 9 Examples of anchoring systems for the marine environment layouts for floating PV systems (A) dead weight, (B) suction foundation, (C) drag anchor, (D) embedded anchor (Claus and López, 2022).
3 Assemblage and installation
Due to the harsh and challenging character of the open sea environment such as great wind and wave, weak capacity of lifting, towing, maneuvering and positioning of heavy structures, the assembly and installation of floating PV plants is expensive and complicated (Oliveira-Pinto and Stokkermans, 2020). Until now, there is no commercial technology available for this sector, let alone standards that can be directly consulted to use the best-fit equipment and operation process. The trade-off, but most effective, approach is to learn from several mature sectors such as offshore oil & gas, and offshore wind. Benefiting from these industrialized solutions that have already been established, the marine floating PV sector will be able to find safe and low-cost construction solutions more efficiently. For example, (DNV, 2021) the offshore standards for planning and execution of marine operations, mainly developed for offshore oil & gas industries.
From the perspective of marine ecological and hydrological environmental protection, referring to the on-land PV plants (Tawalbeh et al., 2021), we put forward some suggestions.
1. During the construction period, the concomitant domestic sewage, mechanical equipment flushing wastewater and oily sewage should be well collected and then treated onshore. Considering the predictable impacts of the loud noise generated by large machinery on sensitive marine life, it is strongly recommended to avoid the migratory and breeding period of birds, fish and the like.
2. The large-scale layout of floating PV plants will significantly prevent sunlight from hitting the sea surface. This will seriously or even fatally affect a variety of organisms, including seagrasses and coral reefs. In terms of the layout density of the solar panel array, it should strike a balance between making full use of ocean space and allowing more sunlight to shine into the sea. The impeded photosynthesis may further affect the content of dissolved oxygen. Although given the marine environment, this possibility is slim. Therefore, when selecting a site for construction, these vulnerable areas should be avoided as much as possible. Baradei et al. (Baradei and Sadeq, 2020) have analyzed the impact of PV systems on the water quality of the 50 km long channel of a canal in Egypt. The dissolved oxygen concentration and algae concentration can be significantly effected, especially in the case of high coverage of photovoltaic panels (Figure 10).
3. The possible scour between mooring cables and the seabed, caused by tidal or great wind and waves, may seriously impact the seagrass beds (Hooper et al., 2021). This issue should be taken into account at the initial design stage of mooring systems. The number of anchor points can be minimized by optimizing the overall design of offshore floating PV plants. For example, DNV Kema developed the hexagonal-shaped floating PV plants, inspired by a spider web structure, to minimize the number of anchor points. However, the hydrodynamic performance of DNV’s FPV concept should be fully studied in detail before any potential application.
4. Given the experience of offshore wind construction, the possible risk of species invasion should be fully considered for the construction of offshore floating PV plants (Adams et al., 2014; Loxton et al., 2017). In order to minimize ecological damage, the transportation of floating PV infrastructure should avoid being dragged directly from the harbor to the deployment site, since ports are often highly contaminated with non-native species.
5. For the marine ecological environment, the existence of offshore floating PV plants is not all negative. By design, its role as an artificial reef can be intentionally expanded and enhanced. The establishment of a new ecosystem will compensate for the original affected ecological environment. For this, we can take inspiration from other more mature marine renewable energy such as the offshore wind sector, for which the nature-inclusive design is a must in some countries of Europe. As a mounted platform and accompanied by an operation and maintenance system, the offshore floating PV plants play the role of marine ecological environment monitoring.
6. Another potential solution to deal with high construction costs and possible ecological environmental impacts is the co-location of offshore floating PV with other marine renewable energy (MRE) plants (Pérez-Collazo et al., 2015; Loxton et al., 2017), especially offshore wind plants, which is considered to be the most mature marine renewable energy and natural complementary with offshore floating PV from summertime to wintertime (Oliveira-Pinto and Stokkermans, 2020; Silalahi and Blakers, 2023; see Figure 11). By sharing resources such as logistics, power grid infrastructure, etc., the construction and operation costs of offshore floating PV will be greatly reduced (Pérez-Collazo et al., 2015).
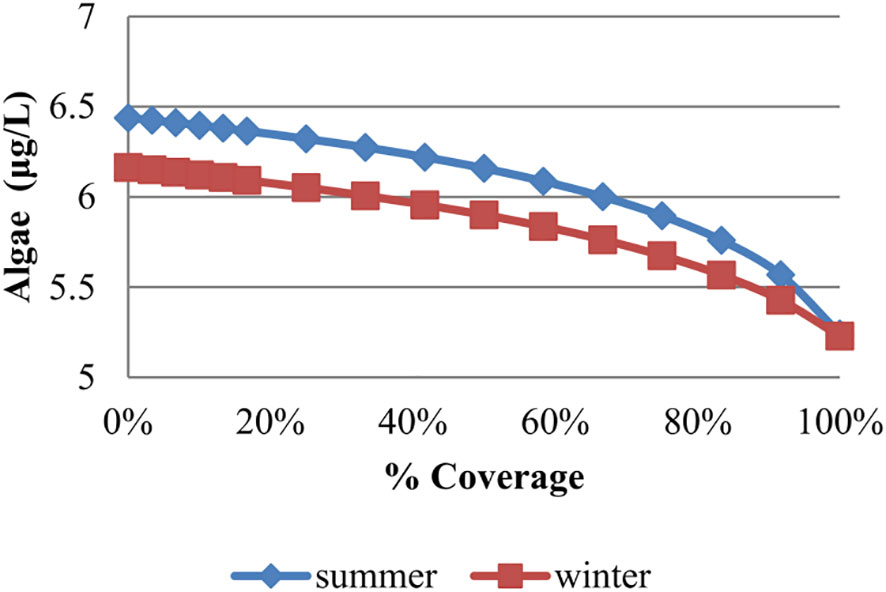
Figure 10 Curve of algae concentration as a function of coverage of PV panels (Baradei and Sadeq, 2020).
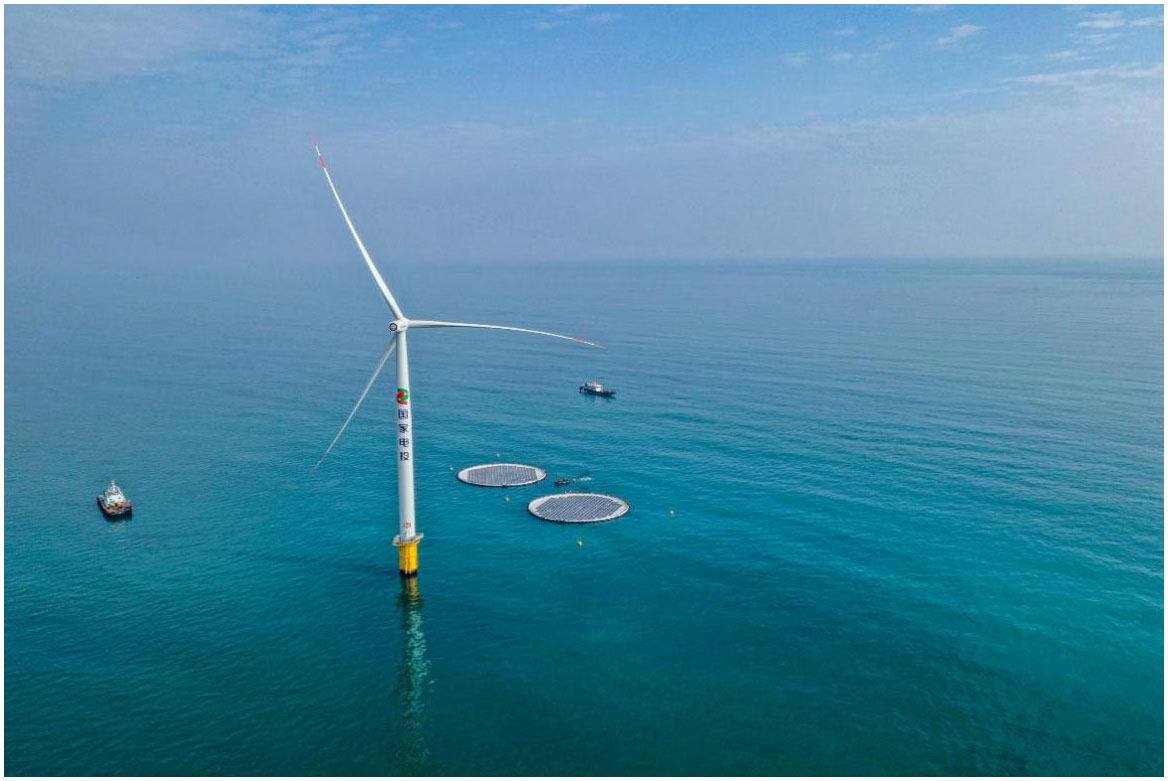
Figure 11 Shan Dong Peninsula deep-sea “wind +solar” project (Ocean Sun, 2022).
Normally, there are two ways to achieve the hybrid: space sharing and platform sharing. space sharing means the rational use of space resources to improve the power generation per unit of marine area (Golroodbari et al., 2021). The integration of offshore floating PV plants and offshore wind plants is ideal (Silalahi and Blakers, 2023). The natural complementarity of them from summertime to wintertime is conducive to the relative stability of the current output. In addition, the presence of wind turbines will provide a certain degree of protection for offshore floating PV plants by reducing the wind speed. Platform sharing indicates the integration of different MRE types into a platform. This deep integration approach will make full use of the respective points and maximize cost reduction. But at present, this technology is not mature and lacks engineering experience.
4 Degradation, and operation and maintenance
When the marine floating PV plants are built and put into use, it will be inevitable to face various types of degradation driven by harsh environments, including discoloration, corrosion, breakages and micro-cracks, soiling loss, hot spots, salt deposition as well as delamination and bubbles (Sahu et al., 2016; Mustafa et al., 2020). It will not only negatively impact the performance of PV panels but also cause the occurrence of short circuits, fires, etc. (Jathar et al., 2023). Among them, the soling loss is one of the most common types of degradation to weaken the performance of PV panels, caused by dust accumulation, soil cementation, dirt, bird droppings, soiling and other particles. In the marine environment, the only source of dust is from air and thus it seems that the dust effect is smaller than that of onshore PV panels. However, the highly humid environment promotes soil cementation and dust accumulation on the module surface. Salt mist can cause corrosion of the PV frames and metal wire boxes and may accelerate potential-induced degradation (Shi et al., 2023). By attenuating the absorption of light, the salt deposition on PV panels will further degrade the power generation performance of PV modules (Figure 12). The effects of salt accumulation on PV panels have been investigated by simulating floating PV modules working in a marine environment for 30 days. The power performance shrunk by 14-28% and 13-25% respectively for simulation procedures of immersion and dripping (Gorjian et al., 2021). Suzuki et al. (Suzuki et al., 2015) reported that the combined exposure of salt spray followed by high voltage can easily lead to the degradation of generated power of PV modules, possibly due to sodium ions penetrating the encapsulation of the modules. The corrosion caused by salt spray also has a negative impact on the silicone adhesives that seal the edges of the modules (Zaharia et al., 2017). In addition, because floating offshore PV plants can be regarded as artificial reefs, bird activities are very frequent. Thus, bird dropping becomes a serious issue that not only shades the PV panel but also leads to hotspots (Wang et al., 2022). Although using bird deterrents such as sound systems can be effective in reducing the impact of bird droppings, this is not feasible in terms of minimizing environmental impact of the offshore PV systems. Biofouling is also a very common and tricky issue during the operation of marine and freshwater floating PV plants. It will roughen the surface of the structure, obstruct the dissipation of heat from cables and other components, enhance corrosion mooring lines as well as weaken light transmittance (Nall et al., 2017). Hooper et al. (Hooper et al., 2021) reported that fouling could reduce the fatigue life of wave energy mooring lines by 20%. Therefore, the development of new materials or processes that are resistant to biofouling and do not cause negative effects on the environment will be a necessary requirement.
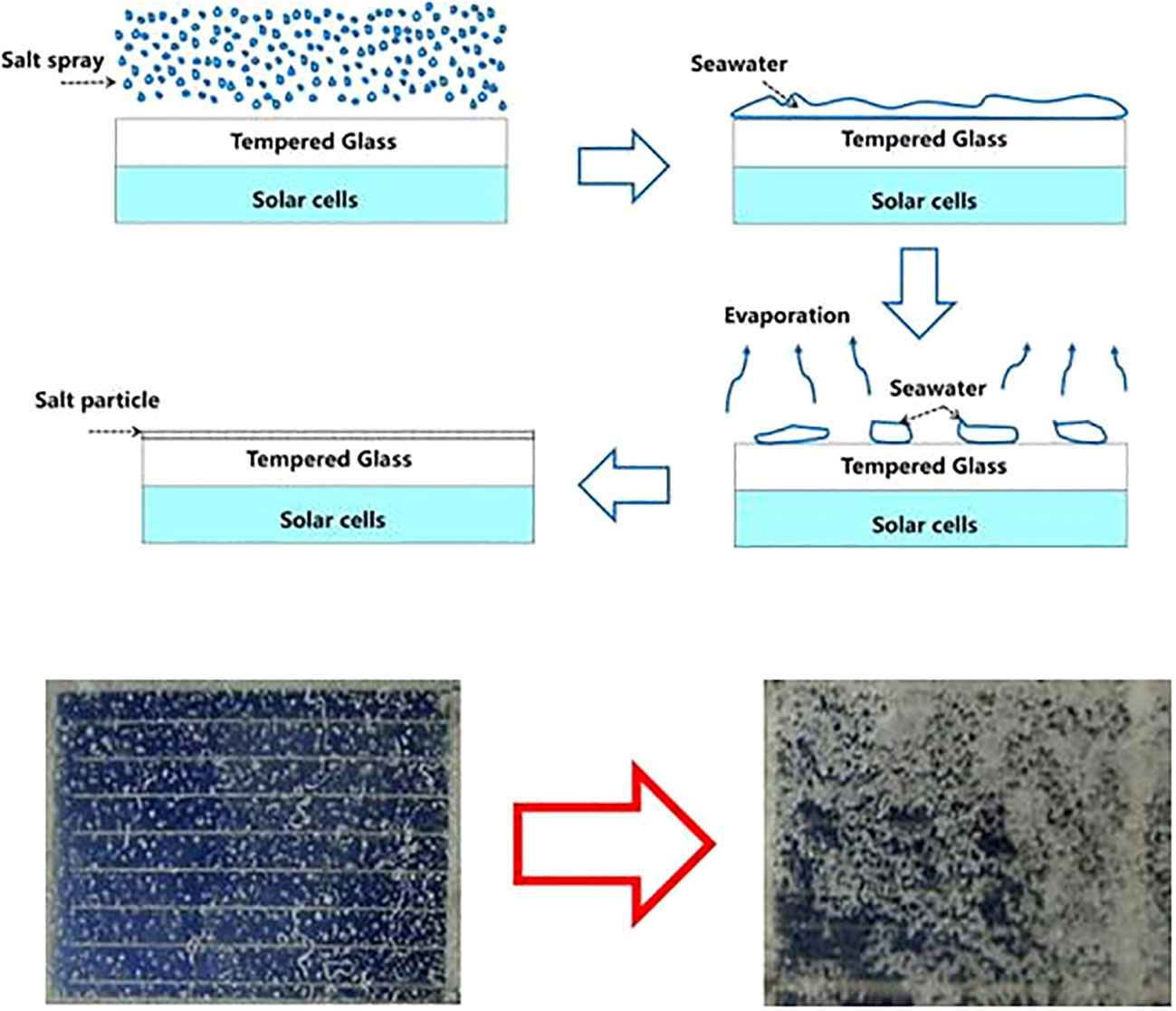
Figure 12 The process salt deposition on PV panels (Zhang and Yuan, 2021).
Effective and efficient methods to determine the degradation of PV systems are essential and the accurate and early detection of degradation will be useful for proper planning, estimation and life cycle analysis. Under normal circumstances, commercialized PV systems shall have a service period of not less than 20 years, the degradation rates of the solar panels must be well-defined and be below 0.8% per year (Kim et al., 2021). As reported by the National Renewable Energy Laboratory, the degradation modes in modules for the last 10 years were hot spots (33%) followed by ribbon discoloration (20%), glass breakage (12%), encapsulant discoloration (10%), cell breakage (9%), and potential-induced degradation (8%) (Jordan et al., 2017; Kim et al., 2021). For the moment, a long-term reliability assessment for floating PV systems has yet to be established. For offshore floating PV systems, the higher environmental loads, higher humidity and UV radiation as well as salt deposition will significantly influence the degradation process. Sun et al. (Sun et al., 2018) monitored the module current and voltage and used the data to calculate the degradation of PV modules using MPPT. Lyu et al. (Lyu et al., 2019), used a fluorescence imaging technique to accurately determine the degradation rate of PV systems. The accelerated aging test is one of the major analyses that the predictive model is based on (Jordan and Kurtz, 2011). Kim et al. (Kim et al., 2021) discussed different types of degradation, accelerated-stress tests, levels, and prioritization to expand the life expectancy of the PV module by means of IEC 61215 (The standard qualification test programs for terrestrial photovoltaic modules). Mannino et al. (Mannino et al., 2023), reported the preliminary study of evaluating the influence of marine environmental variables on the degradation trend of photovoltaic modules. Onshore and offshore environmental variables are used as input for the degradation forecast model and subjected to numerical simulations to obtain quantitative results. Two methods for PV degradation forecast Kaaya and Sumbramaniyan have been used.
All in all, an efficient and environmentally friendly Operation and maintenance (O&M) system is essential for this sector. O&M system is also very important to maintain the marine environment, because it will curb the occurrence of short circuits, fires, sinking and other accidents that can cause serious environmental impacts.
4.1 Commercial PV O&M systems
Since the 21st century, international PV O&M systems including intelligent monitoring, unmanned aerial vehicle (UAV) inspection, self-cleaning and machine learning have made great progress, and there are now various types of O&M products suitable for different platform applications. In terms of intelligent monitoring systems, Germany Solar-Log and SMA have been in the global market dominance, followed by Austria Fronius, Israel SolarEdge and Japan OMRON and other companies. These products are approaching maturity after years of development and practice, and can help photovoltaic power generation system operators to achieve real-time monitoring and data analysis (Kalay et al., 2022). In terms of UAV inspection technology, the AirRobot in Germany, Solmetric in the United States, DJ-innovation in China as well as Heliolytics in Canada are world-renowned (Bhatt et al., 2018). In terms of self-cleaning technology, the market sales of SunBrush Mobil in Germany, Ecoppia in Israel, Heliotex in the United States, DENKA and YAMABIKO in Japan are very large (Syafiq et al., 2018). In terms of machine learning technology, the products of Verdigris, Terabase Energy, Aurora Solar, SunPower, TÜV Rheinland, Fraunhofer ISE and other companies occupy the main market (Wang et al., 2017). In general, the PV O&M systems of the United States, Japan and some European countries have been quite mature, occupying the leading position and countries such as Canada and Israel have their own expertise in some fields. These countries have continuously developed and applied advanced technologies such as infrared, laser, ultrasonic, reverse thermal inertia, image recognition and artificial intelligence to PV O&M systems, which has greatly improved the power generation efficiency and reliability of photovoltaic systems and promoted the continuous development of the photovoltaic industry (Osmani et al., 2020).
4.2 Offshore floating PV O&M systems
Due to the constraints of the marine environment, the maintenance of offshore floating PV plants will become difficult in timely cleaning by manpower, troubleshooting of faults, time-consuming and labor-intensive, especially for the ones far from the coast. The development of O&M systems aiming at offshore floating PV plants should be intelligent, unmanned and remote-controlled.
Using UAVs to regularly patrol photovoltaic arrays can reduce labor costs and improve detection efficiency. At the same time, the UAV can also collect high-resolution image and video data, analyze the condition of photovoltaic panels, accurately identify and locate component defects, and give accurate maintenance recommendations (Alsafasfeh et al., 2018). Limited by the endurance of UAVs, generally one to four hours, monitoring is incoherent and cumbersome, especially for power plants far from the coast (Ahmed et al., 2020). It will be attractive to develop wireless charging technology suitable for offshore PV plants to achieve in-situ charging of UAVs (see Figure 13).
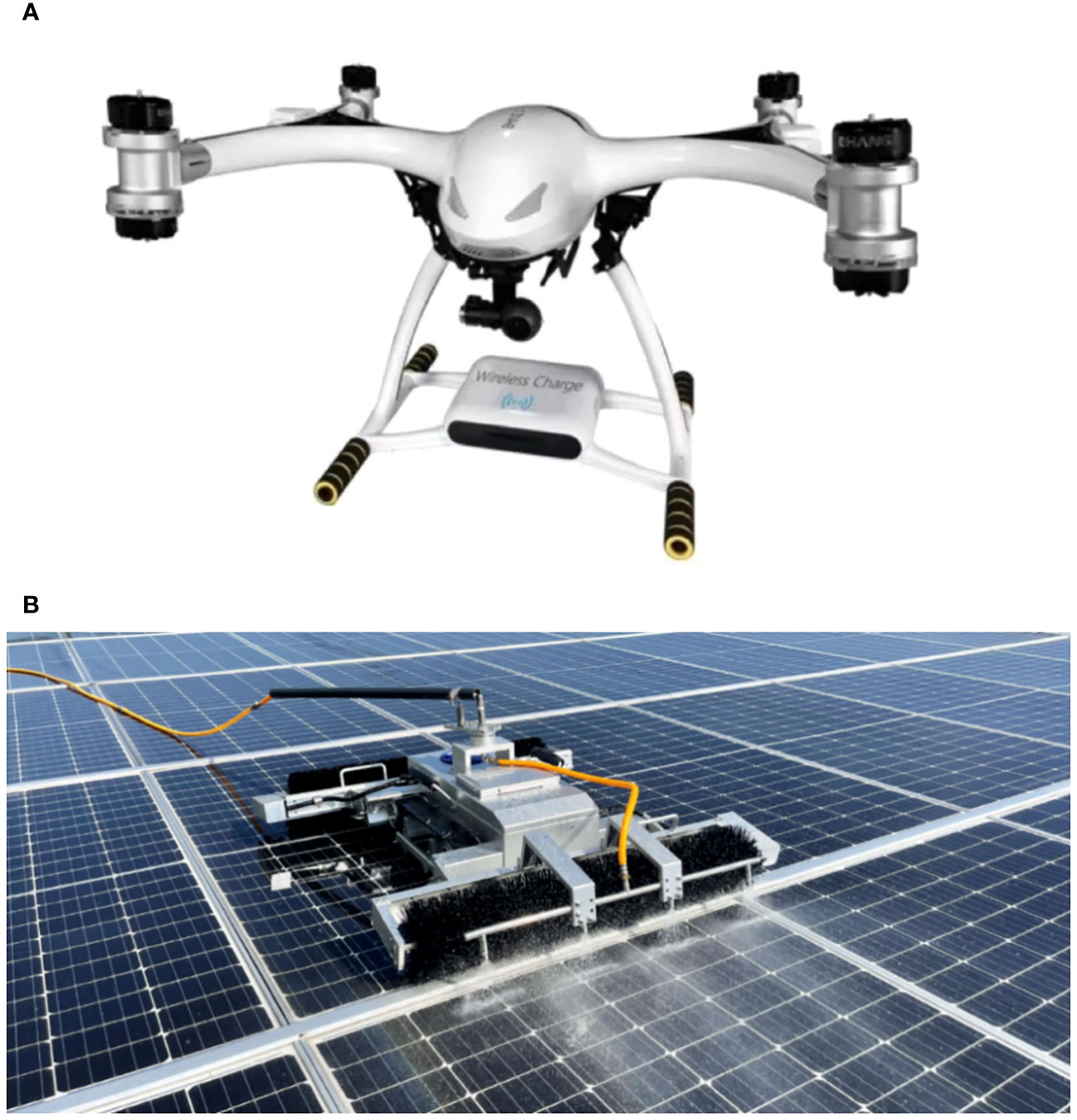
Figure 13 (A) Wireless charging technology of UAVs (Chu Shan, 2022); (B) Robot cleaning system (Kwunphi, 2022).
This method of indirectly circumventing the range limitation of UAV not only greatly improves the efficiency and automation, but also reduces O&M costs. The development of efficient self-cleaning technology is essential to deal with the aforementioned issues such as dust, bird droppings, etc. (Syafiq et al., 2018) Cooperating with UAV inspection to realize on-demand cleaning of the self-cleaning system and optimizing the design of water flow controls, rotating brushes, drives, etc., to improve clear efficiency will be the direction (see Figure 13). From the perspective of marine ecological environmental protection, the use of any environmentally unfriendly products should be avoided in the cleaning process (Rosa-Clot, 2020).
Through the analysis and processing of the operation data, machine learning algorithms can mine potential fault characteristics and trends, improve the accuracy of prediction, and provide support for subsequent operation and maintenance. Some U.S. companies integrate technologies such as machine learning, the Internet of Things, and cloud computing to enable real-time data collection and analysis to optimize performance and long-term reliability (Benkercha and Moulahoum, 2018).
As emerging technologies including cloud computing, big data, the internet of things and mobile internet continue to evolve, the predictable development trend of marine PV plants O&M platform is mainly as follows: 1. Using cloud computing technology to realize a centralized platform integrating analysis, supervision and production under multi-level control mode. 2. Using big data technology to create a big data-driven O&M model and enter the era of intelligent O&M.
5 Decommission and disposal
Abandoning retired offshore PV directly into the ocean will cause marine ecological disasters. With the rapid expansion of the photovoltaic industry, the recycling of retired PV modules is a very critical step in line with an attitude of ecological responsibility (Aman et al., 2015). Efficient recycling technology will significantly reduce costs and minimize environmental impact. As predicted by the International Renewable Energy Agency (IRENA) and the International Energy Agency (IEA), there would be 60-78 million tons of retired PV modules by 2050. These retired PV modules would have contained highly toxic heavy metals such as cadmium (Cd), tellurium (Te), selenium (Se), lead (Pd) and gallium (Ga), etc., because they are widely utilized during PV cells’ manufacturing process. Other essential raw materials such as plastics, polymers, etc. will release toxic hydrocarbons and produce micro-nano plastic particles. Since 2012, the EU has established the requirements for recycling waste PV panels, based on the initial Waste Electrical and Electronic Equipment (WEEE) regulation. Since then, many countries in the world have carried out technological research in this field and some solutions have been commercialized. As reported, 90% of the materials can be fully recycled. However, most of these methods are quite complex, not only high energy consumption, but also accompanied by the use of a large number of chemical reagents (Tao and Yu, 2015). Until now, recycling seems to be challenging and the development of new technologies is imminent. We recommend that the government develop policies and evaluation systems to ensure that the future PV industry is greener. Encouraging companies to try more green production and recycling methods through government subsidies.
6 Conclusion
The offshore floating PV plants will become a new growth point with huge potential for the future PV sector due to their higher power generation efficiency, much broader layout space, and non-occupation of precious land resources. However, for this sector towards the ocean with harsh environment, the technology readiness level is really low and commercial application is currently in the exploration stage. This paper discusses the technological feasibility of commercialization from the perspective of a life cycle of offshore floating PV plants, emphasizing the protection of the marine ecological environment. The main conclusions are as follows.
I. The path to commercialization in any industry is based on the upgrading of technology and cost control. Although offshore PV systems are believed to be one of the most promising types, the enormous environmental loads imposed by the harsh marine environment is a huge challenge. For now, efforts are mainly focused on achieving the stability and safety of offshore floating PV plants. 1. By learning from these industrialized solutions that have already been established offshore oil & gas, offshore wind, the marine floating PV sector will be able to find safe and low-cost construction solutions more efficiently. 2. In the selection of materials and the overall structural design, the huge marine environmental loads should be fully considered. Flexible structures are applicable for large-area offshore floating PV arrays to weaken the wave loads. The wind loads can be evaluated based on wind tunnel testing, digital simulation, and can be minimized by reasonably designed especially the shape of the floating body and the incidence angle of the wind. 3. The structural design of the mooring system takes into account the actual environmental loads and the seabed structure. Conventional stainless-steel chains and wires are used as much as possible to ensure safety to ensure that the mooring system has sufficient bearing capacity for the entire power plant. 4.The intelligent O&M systems based on cloud computing, big data, internet of things and mobile internet, will be a strong guarantee for the safe operation of the offshore floating PV plants. 5. By sharing resources such as logistics, power grid infrastructure with offshore oil & gas, offshore wind, the construction and operation costs of offshore floating PV will be greatly reduced.
II. The impact on the marine environment of the entire life cycle of offshore floating PV from manufacture to installation to operation and finally to retirement is currently unclear. There is an extreme lack of relevant long-term data to convincingly reveal the extent and law of impact on the ocean from ecology to hydrology and to geology. In this paper, we refer to the existing marine engineering construction standards, photovoltaic components production and manufacturing processes and some new environmental protection concepts to summarize and propose some solutions to minimize the potential impact on the marine environment. They are detailed as follows. 1.The construction of PV plants in the marine environment needs to strictly follow the corresponding technical standards, and the associated oil pollution, noise, invasive species and seabed damage should be strictly controlled. 2. In order to cope with the possible heavy metal pollution to the marine environment, micro-nano plastic pollution, etc., a new generation of heavy metal-free photovoltaic cells such as organic photovoltaics are being developed, new environmentally friendly floating materials, anti-corrosion coatings are also being developed, and the environment-friendly production process is also being further upgraded. In addition, efficient recycling technology will significantly minimize environmental impact. However, most existing recycling methods are inefficient, complex, and accompanied by secondary pollution, and the development of new technologies is imminent. 3.The co-location and nature-inclusive concepts are valuable for minimizing environmental impact. By grafting onto existing infrastructure such as wind power and offshore oil and gas, new construction can be avoided as much as possible. To the main body of the power plant, the design of auxiliary for biological reproduction is added, so as to compensate for the affected ecological environment.
Author contributions
SW: Writing – original draft, Writing – review & editing, Conceptualization, Investigation, Data curation, Resources. NJ: Writing – original draft, Writing – review & editing. SZ: Methodology, Writing – original draft, Writing – review & editing, Formal Analysis, Visualization. PPZ: Writing – original draft. PZ: Writing – review & editing. YL: Writing – original draft. YW: Supervision, Writing – original draft, Writing – review & editing, Validation.
Funding
The author(s) declare financial support was received for the research, authorship, and/or publication of this article. This work was supported by National Key R&D Program of China No.2022YFB4200704.
Conflict of interest
The authors declare that the research was conducted in the absence of any commercial or financial relationships that could be construed as a potential conflict of interest.
Publisher’s note
All claims expressed in this article are solely those of the authors and do not necessarily represent those of their affiliated organizations, or those of the publisher, the editors and the reviewers. Any product that may be evaluated in this article, or claim that may be made by its manufacturer, is not guaranteed or endorsed by the publisher.
References
Adams T. P., Miller R. G., Aleynik D., Burrows M. T., Frederiksen M. (2014). Offshore marine renewable energy devices as stepping stones across biogeographical boundaries. J. Appl. Ecol. 51 (2), 330–338. doi: 10.1111/1365-2664.12207
Ahmed S., Chowdhury M.Z., Jang Y.M. (2020). Energy-efficient UAV-to-user scheduling to maximize throughput in wireless networks. IEEE Access 8, 21215–21225. doi: 10.1109/ACCESS.2020.2969357
Alsafasfeh M., Abdel-Qader I., Bazuin B., Alsafasfeh Q., Su W.C. (2018). Unsupervised fault detection and analysis for large photovoltaic systems using drones and machine vision. Energies 11 (9), 2252. doi: 10.3390/en11092252
Aman M. M., Solangi K. H., Hossain M. S., Badarudin A., Jasmon G. B., Mokhlis H., et al. (2015). A review of Safety, Health and Environmental (SHE) issues of solar energy system. Renewable Sustain. Energy Rev. 41, 1190–1204. doi: 10.1016/j.rser.2014.08.086
Andrady A. L. (2011). Microplastics in the marine environment. Mar. pollut. Bull. 62 (8), 1596–1605. doi: 10.1016/j.marpolbul.2011.05.030
Arias F. J., Heras S. D. L. (2020). Ocean salt basins energy harvesting. Appl. Ocean Res. 98, 102074. doi: 10.1016/j.apor.2020.102074
Baradei S. E., Sadeq M. A. (2020). Effect of solar canals on evaporation, water quality, and power production: an optimization study. Water 12 (8), 2103. doi: 10.3390/w12082103
Bella F., Lamberti A., Bianco S., Tresso E., Gerbaldi C., Pirri C. F., et al. (2016). Floating, flexible polymeric dye-sensitized solar-cell architecture: the way of near-future photovoltaics. Advanced Materials Technol. 1 (2), 1600002. doi: 10.1002/admt.201600002
Benkercha R., Moulahoum S. (2018). Fault detection and diagnosis based on C4.5 decision tree algorithm for grid connected PV system. Solar Energy 173, 610–634. doi: 10.1016/j.solener.2018.07.089
Bhatt K., Pourmand A., Sikka N. (2018). Targeted applications of unmanned aerial vehicles (Drones) in telemedicine. Telemed J. E Health 24 (11), 833–838. doi: 10.1089/tmj.2017.0289
Cazzaniga R., Cicu M., Rosa-Clot M., Rosa-Clot P., Tina G. M., Ventura C., et al. (2018). Floating photovoltaic plants: Performance analysis and design solutions. Renewable Sustain. Energy Rev. 81, 1730–1741. doi: 10.1016/j.rser.2017.05.269
CHN ENERGY (2022). Available at: https://www.chnenergy.com.cn/.
Choi S. M., Lee G. R., Park C. D., Cho S. H., Lim B. J. (2021). Wind load on the solar panel array of a floating photovoltaic system under extreme hurricane conditions. Sustain. Energy Technol. Assessments 48, 101616. doi: 10.1016/j.seta.2021.101616
Chu Shan (2022). Available at: http://www.chushantech.com/default.aspx.
CIMC RAFFLES (2023). Available at: http://www.cimc-raffles.com/.
Claus R., López M. (2022). Key issues in the design of floating photovoltaic structures for the marine environment. Renewable Sustain. Energy Rev. 164, 112502. doi: 10.1016/j.rser.2022.112502
DNV GL (2021). Available at: https://www.dnv.com/.
Du T., Jing Z., Wu L., Wang H., Chen Z., Ma X., et al. (2022). Growth of ocean thermal energy conversion resources under greenhouse warming regulated by oceanic eddies. Nat. Commun. 13 (1), 7249. doi: 10.1038/s41467-022-34835-z
Emmott C. J. M., Moia D., Sandwell P., Ekins-Daukes N., Hösel M., Lukoschek L., et al. (2016). In-situ, long-term operational stability of organic photovoltaics for off-grid applications in Africa. Solar Energy Materials Solar Cells 149, 284–293. doi: 10.1016/j.solmat.2016.01.036
Fan Q. X., Wang X., Yuan J., Liu X., Hu H., Lin P. (2022). A review of the development of key technologies for offshore wind power in China. J. Mar. Sci. Eng. 10 (7), 929. doi: 10.3390/jmse10070929
Ghosh A. (2023). A comprehensive review of water-based PV: Flotavoltaics, under water, offshore & canal top. Ocean Eng. 281, 115044. doi: 10.1016/j.oceaneng.2023.115044
Gibbons J. (2016). Kernels, in a nutshell. J. Logical Algebraic Methods Programming 85 (5), 921–930. doi: 10.1016/j.jlamp.2015.10.006
Global info research (2023). Available at: https://www.globalinforesearch.com/.
Golroodbari S. Z. M., Vaartjes D. F., Meit J. B. L., van Hoeken A. P., Eberveld M., Jonker H., et al. (2021). Pooling the cable: A techno-economic feasibility study of integrating offshore floating photovoltaic solar technology within an offshore wind park. Solar Energy 219, 65–74. doi: 10.1016/j.solener.2020.12.062
Golroodbari S. Z., Ayyad A. W. A., van Sark W. (2023). Offshore floating photovoltaics system assessment in worldwide perspective. Prog. Photovoltaics: Res. Appl. 31 (11), 1061–1077. doi: 10.1002/pip.3723
Gorjian S., Sharon H., Ebadi H., Kant K., Scavo F. B., Tina G. M., et al. (2021). Recent technical advancements, economics and environmental impacts of floating photovoltaic solar energy conversion systems. J. Cleaner Production 278, 124285. doi: 10.1016/j.jclepro.2020.124285
Gretkowska L. (2018). A study on the performance of solar photovoltaic modules exposed to salt water. (Master's thesis, University of Malta). Available at: https://www.um.edu.mt/library/oar/handle/123456789/37502
Hasan A., Dincer I. (2020). A new performance assessment methodology of bifacial photovoltaic solar panels for offshore applications. Energy Conversion Manage. 220, 112972. doi: 10.1016/j.enconman.2020.112972
Heliofloat (2016). Available at: https://www.heliofloat.com/index.php?id=17.
Hicks B. J., Culley S. J. (2005). An integrated modelling environment for the computer-based embodiment of engineering systems with standard components. Proc. Institution Mechanical Engineers Part B: J. Eng. Manufacture 218 (2), 159–168. doi: 10.1243/095440504322886488
Hooper T., Armstrong A., Vlaswinkel B. (2021). Environmental impacts and benefits of marine floating solar. Solar Energy 219, 11–14. doi: 10.1016/j.solener.2020.10.010
Huang S., Sheng S. W., You Y., Gerthoffert A., Wang W. S., Wang Z. P., et al. (2018). Numerical study of a novel flex mooring system of the floating wave energy converter in ultra-shallow water and experimental validation. Ocean Eng. 151, 342–354. doi: 10.1016/j.oceaneng.2018.01.017
IEC (2021). Available at: https://www.iec.ch/homepage.
Ikhennicheu M., Danglade B., Pascal R., Arramounet V., Trébaol Q., Gorintin F., et al. (2021). Analytical method for loads determination on floating solar farms in three typical environments. Solar Energy 219, 34–41. doi: 10.1016/j.solener.2020.11.078
Jathar L. D., Ganesan S., Awasarmol U., Nikam K., Shahapurkar K., Soudagar M. E. M., et al. (2023). Comprehensive review of environmental factors influencing the performance of photovoltaic panels: Concern over emissions at various phases throughout the lifecycle. Environ. pollut. 326, 121474. doi: 10.1016/j.envpol.2023.121474
Jiang D., Tan K. H., Wang C. M., Dai J. (2021). Research and development in connector systems for Very Large Floating Structures. Ocean Eng. 232, 109150. doi: 10.1016/j.oceaneng.2021.109150
Jordan D. C., Silverman T. J., Wohlgemuth J. H., Kurtz S. R., VanSant K. T. (2017). Photovoltaic failure and degradation modes. Prog. Photovoltaics: Res. Appl. 25 (4), 318–326. doi: 10.1002/pip.2866
Jordan D. C., Kurtz S. R. (2011). Photovoltaic degradation rates—an analytical review. Prog. Photovoltaics: Res. Appl. 21 (1), 12–29. doi: 10.1002/pip.1182
Kabir E., Kumar P., Kumar S., Adelodun A. A., Kim K. (2018). Solar energy: Potential and future prospects. Renewable Sustain. Energy Rev. 82, 894–900. doi: 10.1016/j.rser.2017.09.094
Kalay M. Ş., Kılıç B., Sağlam Ş. (2022). Systematic review of the data acquisition and monitoring systems of photovoltaic panels and arrays. Solar Energy 244, 47–64. doi: 10.1016/j.solener.2022.08.029
KHNP (2021). Available at: https://home.kepco.co.kr/kepco/EN/G/htmlView/ENGCHP00202.do?menuCd=EN07040202.
Kim D. K. (2017). Nanomedicine for inner ear diseases: A review of recent in vivo studies. BioMed. Res. Int. 2017, 3098230. doi: 10.1155/2017/3098230
Kim S.-H., Yoon S.-J., Choi W. (2017). Design and construction of 1 MW class floating PV generation structural system using FRP members. Energies 10 (8), 1142. doi: 10.3390/en10081142
Kim J., Rabelo M., Padi S. P., Yousuf H., Cho E.-C., Yi J. (2021). A review of the degradation of photovoltaic modules for life expectancy. Energies 14 (14), 4278. doi: 10.3390/en14144278
Kini G. P., Jeon S.J., Moon D.K. (2021). Latest progress on photoabsorbent materials for multifunctional semitransparent organic solar cells. Advanced Funct. Materials 31 (15), 2007931. doi: 10.1002/adfm.202007931
Kougias I., Szabó S., Monforti-Ferrario F., Huld T., Bódis K. (2016). A methodology for optimization of the complementarity between small-hydropower plants and solar PV systems. Renewable Energy 87, 1023–1030. doi: 10.1016/j.renene.2015.09.073
Kumar M., Kumar A., Gupta R. (2020). Comparative degradation analysis of different photovoltaic technologies on experimentally simulated water bodies and estimation of evaporation loss reduction. Prog. Photovoltaics: Res. Appl. 29 (3), 357–378. doi: 10.1002/pip.3370
Kumar M., Mohammed N. H., Gupta R. (2021). Challenges and opportunities towards the development of floating photovoltaic systems. Solar Energy Materials Solar Cells 233, 111408. doi: 10.1016/j.solmat.2021.111408
Kumar M., Kumar A. (2017). Performance assessment and degradation analysis of solar photovoltaic technologies: A review. Renewable Sustain. Energy Rev. 78, 554–587. doi: 10.1016/j.rser.2017.04.083
Kumar M., Kumar A. (2019). Experimental characterization of the performance of different photovoltaic technologies on water bodies. Prog. Photovoltaics: Res. Appl. 28 (1), 25–48. doi: 10.1002/pip.3204
Kwunphi (2022). Available at: https://www.kwunphi.com/.
Limmanee A., Udomdachanut N., Songtrai S., Kaewniyompanit S., Sato Y., Nakaishi M., et al. (2016). Field performance and degradation rates of different types of photovoltaic modules: A case study in Thailand. Renewable Energy 89, 12–17. doi: 10.1016/j.renene.2015.11.088
Liu H. H., Krishna V., Lun L. J., Reindl T., Zhao L., et al. (2018). Field experience and performance analysis of floating PV technologies in the tropics. Prog. Photovoltaics: Res. Appl. 26 (12), 957–967. doi: 10.1002/pip.3039
Lizin S., VanPassel S., DeSchepper E., Maes W., Lutsenm L., Manca J., et al. (2013). Life cycle analyses of organic photovoltaics: a review. Energy & Environmental Science 6 (11), 3136.
Loxton J., Macleod A. K., Nall C. R., McCollin T., Machado I., Simas T., et al. (2017). Setting an agenda for biofouling research for the marine renewable energy industry. Int. J. Mar. Energy 19, 292–303. doi: 10.1016/j.ijome.2017.08.006
Lyu Y., Fairbrother A., Kim J. H., Gong M. Y., Sung L.-P., Watson S. S., et al. (2019). Fluorescence imaging analysis of depth-dependent degradation in photovoltaic laminates: insights to the failure. Prog. Photovoltaics: Res. Appl. 28 (2), 122–134. doi: 10.1002/pip.3212
Ma C., Liu Z. (2022). Water-surface photovoltaics: Performance, utilization, and interactions with water eco-environment. Renewable Sustain. Energy Rev. 167, 112823. doi: 10.1016/j.rser.2022.112823
Mahmud M., Huda N., Farjana S., Lang C. (2018). Environmental impacts of solar-photovoltaic and solar-thermal systems with life-cycle assessment. Energies 11 (9), 2346. doi: 10.3390/en11092346
Mannino G., Tina G. M., Cacciato M., Merlo L., Cucuzza A. V., Bizzarri F., et al. (2023). Photovoltaic module degradation forecast models for onshore and offshore floating systems. Energies 16 (5), 2117. doi: 10.3390/en16052117
Massa G., Pappalardo D., Acunzo R., Oliviero M. (2021). “An overview on artificial intelligence techniques for advanced operation and maintenance of photoVoltaic power plants,” in 2021 5th International Conference on Electrical, Electronics, Communication, Computer Technologies and Optimization Techniques (ICEECCOT), Mysuru, India. 734–740. doi: 10.1109/ICEECCOT52851.2021.9707940
Moss Maritime (2022). Available at: https://www.mossww.com/clean-energy-solutions/.
Mühlberger M. M., Kopp S., Deyett A. A., Pribyl M., Haslinger M. J., Siegel A. M., et al. (2023). Nanoimprinted hierarchical micro-/nanostructured substrates for the growth of cardiomyocyte fibers. Nanomanufacturing 3 (4), 416–433. doi: 10.3390/nanomanufacturing3040026
Mustafa R. J., Gomaa M. R., Al-Dhaifallah M., Rezk H. (2020). Environmental impacts on the performance of solar photovoltaic systems. Sustainability 12 (2), 608. doi: 10.3390/su12020608
Nain P., Kumar A. (2020). Ecological and human health risk assessment of metals leached from end-of-life solar photovoltaics. Environ. pollut. 267, 115393. doi: 10.1016/j.envpol.2020.115393
Nall C. R., Schlappy M. L., Guerin A. J. (2017). Characterisation of the biofouling community on a floating wave energy device. Biofouling 33 (5), 379–396. doi: 10.1080/08927014.2017.1317755
Oceans of Energy (2022). Available at: https://oceansofenergy.blue/.
Ocean Sun (2022). Available at: https://oceansun.no/.
Oliveira-Pinto S., Stokkermans J. (2020). Marine floating solar plants: an overview of potential, challenges and feasibility. Proc. Institution Civil Engineers - Maritime Eng. 173 (4), 120–135. doi: 10.1680/jmaen.2020.10
Osmani K., Haddad A., Lemenand T., Castanier B., Ramadan M. (2020). A review on maintenance strategies for PV systems. Sci. Total Environ. 746, 141753. doi: 10.1016/j.scitotenv.2020.141753
Pérez-Collazo C., Greaves D., Iglesias G. (2015). A review of combined wave and offshore wind energy. Renewable Sustain. Energy Rev. 42, 141–153. doi: 10.1016/j.rser.2014.09.032
Pouran H. M., Padilha C. L. M., Nogueira T., Alves C. B. D., Sheng Y., et al. (2022). Environmental and technical impacts of floating photovoltaic plants as an emerging clean energy technology. iScience 25 (11), 105253. doi: 10.1016/j.isci.2022.105253
PV-magazine (2022). Available at: https://www.pv-magazine.com/.
Ranjbaran P., Yousefi H., Gharehpetian G. B., Astaraei F. R. (2019). A review on floating photovoltaic (FPV) power generation units. Renewable Sustain. Energy Rev. 110, 332–347. doi: 10.1016/j.rser.2019.05.015
Rawat R., Kaushik S. C., Sastry O. S., Singh Y. K., Bora B., et al. (2016). Energetic and exergetic performance analysis of CdS/CdTe based photovoltaic technology in real operating conditions of composite climate. Energy Conversion Manage. 110, 42–50. doi: 10.1016/j.enconman.2015.11.069
Rosa-Clot P. (2020). FPV and environmental compatibility. Floating PV Plants, 101–118. doi: 10.1016/B978-0-12-817061-8.00009-9
Rosa-Clot M., Rosa-Clot P., Tina G. M., Scandura P. F. (2010). Submerged photovoltaic solar panel: SP2. Renewable Energy 35 (8), 1862–1865. doi: 10.1016/j.renene.2009.10.023
Rosa-Clot M., Tina G. M. (2018). The Floating PV Plant. 89–136. doi: 10.1016/B978-0-12-812149-8.00005-3
Rosa-Clot M., Tina G. M. (2020). “Levelized cost of energy (LCOE) analysis,” in Floating PV Plants, 119–127. doi: 10.1016/B978-0-12-817061-8.00010-5
Sahu A., Yadav N., Sudhakar K. (2016). Floating photovoltaic power plant: A review. Renewable Sustain. Energy Rev. 66, 815–824. doi: 10.1016/j.rser.2016.08.051
Sahu A. K., Sudhakar K. (2019). Effect of UV exposure on bimodal HDPE floats for floating solar application. J. Materials Res. Technol. 8 (1), 147–156. doi: 10.1016/j.jmrt.2017.10.002
Samsó R., Crespin J., García-Olivares A., Solé J. (2023). Examining the potential of marine renewable energy: A net energy perspective. Sustainability 15 (10), 8050. doi: 10.3390/su15108050
Setiawan F., Dewi T., Yusi S. (2019). Sea Salt Deposition Effect on Output and Efficiency Losses of the Photovoltaic System; a case study in Palembang, Indonesia. J. Physics: Conf. Ser. 1167, 012028. doi: 10.1088/1742-6596/1167/1/012028
Shi W., Yan C. J., Ren Z. R., Yuan Z. M., Liu Y. Y., Zheng S. M., et al. (2023). Review on the development of marine floating photovoltaic systems. Ocean Eng. 286, 115560. doi: 10.1016/j.oceaneng.2023.115560
Silalahi D. F., Blakers A. (2023). Global atlas of marine floating solar PV potential. Solar 3 (3), 416–433. doi: 10.3390/solar3030023
SolarDuck (2022). Available at: https://solarduck.tech/.
Spencer R. S., Macknick J., Aznar A., Warren A., Reese M. O. (2019). Floating photovoltaic systems: assessing the technical potential of photovoltaic systems on man-made water bodies in the continental United States. Environ. Sci. Technol. 53 (3), 1680–1689. doi: 10.1021/acs.est.8b04735
Sun X. S., Chavali R. V. K., Alam M. A. (2018). Real-time monitoring and diagnosis of photovoltaic system degradation only using maximum power point—the Suns-Vmp method. Prog. Photovoltaics: Res. Appl. 27 (1), 55–66. doi: 10.1002/pip.3043
Sunseap (2022). Available at: https://www.sunseap.com/sg/.
Suzuki S., Nishiyama N., Yoshino S., Ujiro T., Watanabe S., Doi T., et al. (2015). Acceleration of potential-induced degradation by salt-mist preconditioning in crystalline silicon photovoltaic modules. Japanese J. Appl. Phys. 54 (8S1), 08kg08. doi: 10.7567/JJAP.54.08KG08
Swimsol (2014). Available at: https://swimsol.com/.
Syafiq A., Pandey A. K., Adzman N. N., Rahim N. A. (2018). Advances in approaches and methods for self-cleaning of solar photovoltaic panels. Solar Energy 162, 597–619. doi: 10.1016/j.solener.2017.12.023
Tao J., Yu S. (2015). Review on feasible recycling pathways and technologies of solar photovoltaic modules. Solar Energy Materials Solar Cells 141, 108–124. doi: 10.1016/j.solmat.2015.05.005
Taveira-Pinto F., Rosa-Santos P., Fazeres-Ferradosa T. (2020). Marine renewable energy. Renewable Energy 150, 1160–1164. doi: 10.1016/j.renene.2019.10.014
Tawalbeh M., Al-Othman A., Kafiah F., Abdelsalam E., Almomani F., Alkasrawi M., et al. (2021). Environmental impacts of solar photovoltaic systems: A critical review of recent progress and future outlook. Sci. Total Environ. 759, 143528. doi: 10.1016/j.scitotenv.2020.143528
Tina G., Cazzaniga R., Rosa-Clot M., Rosa-Clot P. (2018). Geographic and technical floating photovoltaic potential. Thermal Sci. 22 (Suppl. 3), 831–841. doi: 10.2298/TSCI170929017T
Tina G. M., Bontempo S. F., Merlo L., Bizzarri F. (2021). Comparative analysis of monofacial and bifacial photovoltaic modules for floating power plants. Appl. Energy 281, 116084. doi: 10.1016/j.apenergy.2020.116084
Tractebel (2023). Available at: https://tractebel-engie.com/.
Trapani K., Millar D. L. (2013). Proposing offshore photovoltaic (PV) technology to the energy mix of the Maltese islands. Energy Conversion Manage. 67, 18–26. doi: 10.1016/j.enconman.2012.10.022
Trapani K., Millar D. L. (2014). The thin film flexible floating PV (T3F-PV) array: The concept and development of the prototype. Renewable Energy 71, 43–50. doi: 10.1016/j.renene.2014.05.007
Trapani K., Millar D. L. (2015). Floating photovoltaic arrays to power the mining industry: A case study for the McFaulds lake (Ring of Fire). Environ. Prog. Sustain. Energy 35 (3), 898–905. doi: 10.1002/ep.12275
Trapani K., Redón Santafé M. (2014). A review of floating photovoltaic installations: 2007–2013. Prog. Photovoltaics: Res. Appl. 23 (4), 524–532. doi: 10.1002/pip.2466
Vervloesem J., Marcheggiani E., Choudhury M. A. M., Muys B. (2022). Effects of photovoltaic solar farms on microclimate and vegetation diversity. Sustainability 14 (12), 7493. doi: 10.3390/su14127493
Wang F., Zhen Z., Wang B., Mi Z. Q. (2017). Comparative study on KNN and SVM based weather classification models for day ahead short term solar PV power forecasting. Appl. Sci. 8 (1), 28. doi: 10.3390/app8010028
Wang D. L., Li C., Li M.S., Zhang T. Y., Zhu R. (2022). Thermal spot detection of photovoltaic modules based on deep convolutional neural network. J. Solar Energy 43 (01), 412–417. doi: 10.19912/j.0254-0096.tynxb.2020-0953
Wang J., Lund P. D. (2022). Review of recent offshore photovoltaics development. Energies 15 (20), 7462. doi: 10.3390/en15207462
Weiss C. V. C., Guanche R., Ondiviela B., Castellanos O. F., Juanes J., et al. (2018). Marine renewable energy potential: A global perspective for offshore wind and wave exploitation. Energy Conversion Manage. 177, 43–54. doi: 10.1016/j.enconman.2018.09.059
Wen Y., Lin P. (2022). Exploitation potential of tidal current energy in Southern China seas. Energy Conversion Manage. 267, 115901. doi: 10.1016/j.enconman.2022.115901
Wu Y., Li W. Y., Song Z. X., Lin X. S. (2019). Risk assessment on offshore photovoltaic power generation projects in China based on a fuzzy analysis framework. J. Cleaner Production 215, 46–62. doi: 10.1016/j.jclepro.2019.01.024
Xie M., Ruan J. L., Bai W. N., Qiao Q., Bai L., Zhang J., et al. (2018). Pollutant payback time and environmental impact of Chinese multi-crystalline photovoltaic production based on life cycle assessment. J. Cleaner Production 184, 648–659. doi: 10.1016/j.jclepro.2018.02.290
Xu S., Wang S., Guedes S. C. (2019). Review of mooring design for floating wave energy converters. Renewable Sustain. Energy Rev. 111, 595–621. doi: 10.1016/j.rser.2019.05.027
Yang S. B., Fan L. L., Duan S. H., Zheng C. W., Li X. F., Li H. Y., et al. (2019). Long-term assessment of wave energy in the China Sea using 30-year hindcast data. Energy Explor. Exploitation 38 (1), 37–56. doi: 10.1177/0144598719878777
Zaharia S. M., Pop M. A., Chicos L. A., Lancea C., Semenescu A., Florea B., et al. (2017). An investigation on the reliability and degradation of polycrystalline silicon solar cells under accelerated corrosion test. Materiale Plastice 54 (3), 466–472. doi: 10.37358/MP.17.3.4872
Zeng F. X., Bi C., Sree D., Huang G. X., Zhang N.C., Law A. W. K., et al. (2023). An adaptive barrier-mooring system for coastal floating solar farms. Appl. Energy 348, 121618. doi: 10.1016/j.apenergy.2023.121618
Zhang H., Yu Z., Zhu C., Yang R., Yan B., Jiang G., et al. (2023). Green or not? Environmental challenges from photovoltaic technology. Environ. pollut. 320, 121066. doi: 10.1016/j.envpol.2023.121066
Zhang Y., Yuan C. Q. (2021). Effects of marine environment on electrical output characteristics of PV module. Renewable Sustain. Energy 13 (5), 053701. doi: 10.1063/5.0060201
Keywords: floating photovoltaic, offshore, marine environment protection, technological feasibility, life cycle of photovoltaic, potential impacts
Citation: Wu S, Jiang N, Zhang S, Zhang P, Zhao P, Liu Y and Wang Y (2024) Discussion on the development of offshore floating photovoltaic plants, emphasizing marine environmental protection. Front. Mar. Sci. 11:1336783. doi: 10.3389/fmars.2024.1336783
Received: 11 November 2023; Accepted: 29 January 2024;
Published: 05 March 2024.
Edited by:
Mario López Gallego, University of Oviedo, SpainReviewed by:
Nitin Agarwala, National Maritime Foundation, IndiaGiuseppe Marco Tina, University of Catania, Italy
Tiago Ferradosa, University of Porto, Portugal
Copyright © 2024 Wu, Jiang, Zhang, Zhang, Zhao, Liu and Wang. This is an open-access article distributed under the terms of the Creative Commons Attribution License (CC BY). The use, distribution or reproduction in other forums is permitted, provided the original author(s) and the copyright owner(s) are credited and that the original publication in this journal is cited, in accordance with accepted academic practice. No use, distribution or reproduction is permitted which does not comply with these terms.
*Correspondence: Yanhui Wang, d2FuZ3loMTk4N0BocmJldS5lZHUuY24=