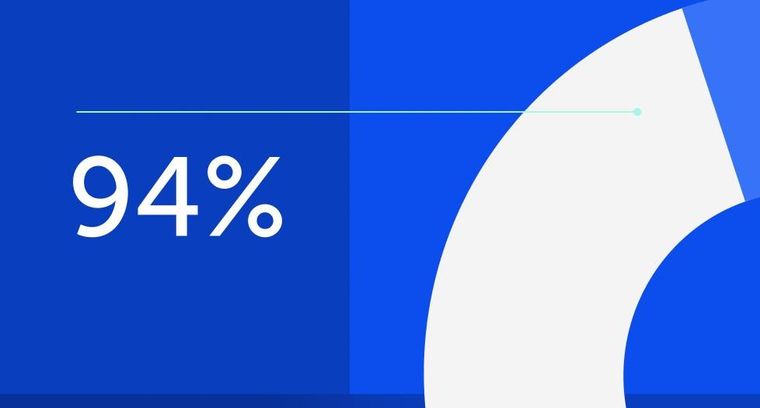
94% of researchers rate our articles as excellent or good
Learn more about the work of our research integrity team to safeguard the quality of each article we publish.
Find out more
ORIGINAL RESEARCH article
Front. Mar. Sci., 21 March 2024
Sec. Marine Biology
Volume 11 - 2024 | https://doi.org/10.3389/fmars.2024.1336024
This article is part of the Research TopicCurrent and Future Threats to Marine Zooplankton in Changing Polar Oceans and Their Potential for Adaption and CopingView all 5 articles
Introduction: Hyperiid amphipods of the genus Themisto are a key polar zooplankton group in terms of biomass and play an important role as prey for higher trophic levels. They are prone to undergo changes in abundance and distribution in the course of the ongoing environmental changes. In the Southern Ocean, Themisto gaudichaudii is predicted to expand its distribution poleward. In the Arctic, the boreal-Atlantic T. abyssorum increases in abundance, resulting in an increased competition with a genuine polar congener. It is not known, however, whether T. gaudichaudii and T. abyssorum have the potential to efficiently adapt to changing water temperatures at their current distribution range or whether they will be shifting their ranges poleward.
Methods: We exposed the two Themisto species from different geographic populations to temperature-change experiments, a cold treatment and a heat-shock treatment. After that, we carried out transcriptome sequencing to compare gene expression patterns in the different treatments and species.
Results: We show that under similar heat conditions, T. gaudichaudii differentially expressed more genes (26-fold change) than T. abyssorum. Furthermore, we observed qualitative differences between genetic clusters in T. gaudichaudii.
Discussion: The differences observed between genetic clusters in T. gaudichaudii suggest that evolutionary divergence can be linked to changes in the regulatory pathways involved in temperature stress. These could influence the capacity of each genetic cluster to cope differently with temperature changes. In contrast to its congeneric species, T. abyssorum showed a pronounced adaptive flexibility to thermal stress; it appears to have the ability to continue its poleward expansion but may also cope with increasing temperatures in its current environments. Our findings contribute to understand the response of two range-shifting Themisto species to thermal stress in view of the environmental gradients they encounter throughout their current or future distribution ranges.
Climate change, proceeding at an unprecedented pace, is currently redistributing life on Earth (Pecl et al., 2017). Its impacts are strongest in polar regions and both the Arctic and Southern Oceans are experiencing rapid environmental changes. In the Arctic Ocean, warming of the atmosphere and upper ocean layer has occurred at nearly four times the global average (Rantanen et al., 2022) resulting in a significant decline in sea-ice extent and thickness (Krumpen et al., 2016; Belter et al., 2021) with a projection of nearly ice-free summers by the middle of the century (Overland and Wang, 2013; Notz and Stroeve, 2018). Over the last two decades, the inflow of warmer Atlantic waters into the high-Arctic has increased, with the Arctic transitioning into a state resembling more closely that of Atlantic waters. This phenomenon is referred to as the “Atlantification” of the Arctic Ocean (Polyakov et al., 2017; Ingvaldsen et al., 2021). In the Southern Ocean, the SW Atlantic sector in particular has been drastically affected over the last 30 years (Meredith et al., 2019) and the most obvious changes include increasing ocean temperatures, poleward shifts of hydrographic fronts, and changes in sea-ice distribution and extent (Meredith and King, 2005; Böning et al., 2008; Hobbs et al., 2016).
In the course of these changes, range shifts of a wide spectrum of marine taxa are ongoing and predicted. Boreal or cold-temperate species are forced to respond to warming waters with a general shift to cooler waters (e.g., Parmesan, 2006; Beaugrand, 2009; Descamps et al., 2017; Hop et al., 2021), whereas polar and ice-dependent species face increasing competition by invaders of Atlantic origin with higher ecological fitness at increasing temperatures (Renaud et al., 2011; Kaiser et al., 2022). These polar species are thus not only facing population decline and reduction in distribution range because of changing abiotic conditions (temperature increase, disappearance of sea ice) but also due to increased competition with species invading from lower latitudes.
In the Arctic, the inflow of warmer Atlantic waters, via the West Spitsbergen Current, carries along increasing amounts of boreal species into the Arctic (Wassmann et al., 2015; Andrews et al., 2019). As a consequence, zooplankton communities in the Arctic have undergone structural and functional changes, due to sub-Arctic and boreal-Atlantic species expanding, including phytoplankton (Neukermans et al., 2018), copepods (Weydmann et al., 2014), amphipods (Schröter et al., 2019), euphausiids (Buchholz et al., 2009) and fishes (Fossheim et al., 2015), as well as poleward contractions of genuine polar species (Basedow et al., 2018).
In the Southern Ocean, Antarctic krill Euphausia superba declined over the last decades, concomitant with a poleward range expansion of salps, increasing in biomass in waters near the Antarctic Peninsula (Atkinson et al., 2004, 2019). Such shifts in both polar oceans will result in system-wide reorganizations of regional food webs with novel interactions between incoming and resident species, affecting upper trophic levels, but also energy flow and biogeochemical processes (Grebmeier, 2012; Kortsch et al., 2015). Hence, understanding the range-shifting dynamics of native and non-indigenous species is crucial for predicting changes in communities and ecosystem functioning (Pecl et al., 2017).
Hyperiid amphipods of the genus Themisto (Guérin, 1825) play important roles in high-latitude pelagic ecosystems, where they represent a major trophic link between zooplankton secondary production and higher trophic levels such as squid, fish, seabirds and marine mammals (reviewed in Havermans et al., 2019). Their key role as prey in high-latitude pelagic communities has been described to be similar to that of krill or copepods (Dalpadado, 2002; Padovani et al., 2012). In the Arctic Ocean, two sympatric species occur: Themisto abyssorum (Boeck, 1871), considered a sub-Arctic, boreal-Atlantic species, and Themisto libellula (Mandt, 1822), a genuine polar cold-water species. T. abyssorum can be found throughout the Arctic Ocean, albeit linked to the presence of warmer Atlantic water masses, hence it is likely to benefit from the ongoing “Atlantification” of the Arctic. It has been suggested that although Arctic species are physiologically more tolerant to ocean warming than previously suggested, they might be outcompeted by North Atlantic species such as T. abyssorum above a certain temperature threshold in areas of strong distribution overlap (Kaiser et al., 2022). A third species, Themisto compressa (Goes, 1865), recently shifted in from the North Atlantic, and reproducing populations were reported in the Atlantic gateway to the Arctic Ocean, i.e. Fram Strait, albeit in lower abundances (Kraft et al., 2013). In the Southern Ocean, a single widespread species, Themisto gaudichaudii (Guérin, 1825), occurs from sub-tropical to polar latitudes, with 17.5°C being the upper limit of temperature tolerance (Auel and Ekau, 2009). Despite their dominance, these hyperiid species are too often overlooked in molecular and ecological studies to predict future changes in the polar ecosystems (Johnston et al., 2022). This leaves many questions unaddressed about their population dynamics and connectivity, their trophic role (Havermans et al., 2019), their capacity to undergo range shifts and the impact these may have on polar ecosystems.
T. gaudichaudii and T. abyssorum have their distribution centers or hydrographic affinities associated with sub-Antarctic and sub-Arctic latitudes, respectively (Havermans et al., 2019). For both species, poleward distributional shifts have been anticipated or observed, together with an increase in abundance (Mackey et al., 2012; CAFF, 2017). A better understanding of the genetic and physiological factors predisposing these species to undergo poleward range expansions or regional changes in population size in response to climate change is urgently needed. Previous studies on the genetic diversity, investigated with the DNA barcoding fragment of the mitochondrial cytochrome c oxidase I (COI) gene, indicated a very high haplotypic diversity in T. abyssorum, which is not geographically structured (Murray et al., 2023), as well as in T. gaudichaudii, for which three genetic clusters could be identified (Havermans, 2015). The mitochondrial genetic diversity within T. gaudichaudii is not explained by its morphological diversity, nor by its geographic distribution (Havermans, 2015). However, it is known that geographic variation in the thermal environment impacts a broad range of biochemical and physiological processes (Schoville et al., 2012). RNA sequencing, or whole-transcriptome sequencing, can reveal differences at the gene expression levels of different genes in response to external stimuli and stressors, allowing to detect whether populations are locally adapted across an environmental gradient (Guzman and Conaco, 2016).
This study is the first to compare the thermal response of two range-expanding marine sister species at the transcriptome level, across an environmental gradient both in the Arctic and the Southern Ocean. Our major goal is to identify gene expression patterns and physiological/metabolic pathways that may predispose these species to poleward range shifts into colder waters, and reveal geographic and genotypic divergence in these patterns. In this study, we performed a transcriptome-wide analysis with RNA sequencing of T. abyssorum and T. gaudichaudii under heat and cold treatment, to investigate how further predicted ocean warming and range shifts into colder waters may influence these two Themisto species.
Both species cover a wide range of temperature gradients across their areas of distribution, which extend into (sub-)polar waters. T. gaudichaudii’s distribution is centered in ice-free subpolar waters, but currently expanding into subzero polar waters near the Antarctic Peninsula, whereas T. abyssorum has colonized the entire Arctic Ocean. Despite the latter species being associated with Atlantic water masses in the area, it is likely to generally experience strong temperature gradients, as Arctic subzero surface waters overlay deeper, warmer Atlantic water masses (ranging from 5-9°C; Kaiser et al., 2022). Since T. abyssorum populations are locally exposed to a steeper gradient of temperatures, our hypothesis is that individuals of this species will experience a weaker molecular response to thermal stress than T. gaudichaudii individuals.
Individuals of Themisto abyssorum were collected during the R/V Polarstern expedition PS100 in Fram Strait in 2016; T. gaudichaudii amphipods were obtained during the R/V Polarstern expedition PS103 to the Southern Ocean in 2016 (Havermans et al., 2017). Details on the different sampling stations are given in Table 1 and sampling localities are indicated in Figure 1. Macrozooplankton samples, including Themisto spp., were collected from a depth range from max. 300 m to the surface using oblique hauls with towed bongo nets (mesh size 500 µm, max. towing speed 1.5 kn) equipped with a non-filtering cod-end. Zooplankton individuals were sorted alive immediately after the catch in a temperature-controlled laboratory container, set at the same (in situ) temperature as the seawater, in which the amphipods were sampled (T0) (see Table 1 for the respective in situ temperatures). Adult specimens of Themisto spp. of similar size were gently removed with a large spoon containing the individual and a quantity of surrounding water, to avoid physical damage and minimize the stress imposed on the organisms.
Table 1 Locations and dates (month and year) where Themisto amphipods were sampled and subjected to thermal stress experiments (in blue: cold treatment; in red: heat-shock).
Figure 1 Sampling areas of the Themisto amphipods analyzed in this study, with details on the stations and the in-situ water temperatures of the sampling localities; (A) T. abyssorum in the Arctic, (B) T. gaudichaudii in the Southern Ocean.
Individuals sampled from populations inhabiting warmer than average waters within both species’ distributions (north of the Antarctic Polar Front for T. gaudichaudii, at 5°C, and in the eastern Greenland Sea for T. abyssorum, at 8°C) were exposed to a cold treatment. We carried out this cold treatment to test the impact on the thermal response in the context of a poleward range expansion of these populations. In the cold treatment of T. gaudichaudii, the temperature was decreased from 5°C to 1.5°C, and for T. abyssorum, the cold treatment implied a change from 8°C to 5°C. Individuals sampled from populations living more poleward and in colder than average waters (0°C) were exposed to a heat treatment, which implied a temperature increase of 10°C for T. abyssorum and 11°C for T. gaudichaudii (see Table 1 for details). Individuals of T. gaudichaudii for the heat treatment were sampled south of the Antarctic Polar Front and T. abyssorum was sampled on the East Greenland shelf.
Specimens were distributed over several aquaria containing ca. 300 ml seawater. Three aquaria (controls) were maintained under in situ temperature and contained five animals each, while five aquaria were used for the treatment settings, also containing five animals each. The required temperature was reached by keeping/transferring the aquaria in three different temperature-controlled laboratory containers on board R/V Polarstern: 1) set at the in situ temperature (T0) of the sampling localities (see Table 1 for their respective temperatures), 2) at a temperature increased to 10-11°C, and 3) at a temperature decreased by 3-3.5°C compared to the in situ temperature. The experienced temperatures are within their temperature tolerance limits and correspond to locally experienced temperature fluctuations in their environment (T. abyssorum) or to gradients known within their distribution centers (T. gaudichaudii). During the experiments, no mortality was observed and animals remained active. After 24 h of acclimation at in situ temperature, the animals serving as control (T0) were removed from the aquaria, preserved in RNAlater, and immediately frozen at -80°C. The other animals were either transferred to 1) another laboratory container set at a lower temperature (cold treatment) or 2) put into an aquarium with warmer seawater (approx. 10°C higher than in situ). Small fluctuations of water temperature in the different aquaria ( ± 0.5°C) may have occurred in the temperature-controlled laboratory containers. After being exposed for 32 h to the cold or heat treatment, these sets of animals were preserved in RNAlater and frozen at -80°C.
Since three genetically highly divergent, but morphologically indistinguishable lineages have been detected previously within T. gaudichaudii (Havermans, 2015), we dissected and “barcoded” the individuals before RNA extraction. For all individuals used for the control and temperature treatments, genomic DNA was isolated from 2-4 of their pleopods, using the Qiagen DNeasy Blood & Tissue Kit (Qiagen), following the manufacturer’s instructions. We used 20 µl of proteinase K for the digestion of the tissues, and 100 µl of AE buffer for the final elution. The DNA content of the different samples was measured with a Nanodrop ND-1000 (Thermo Fisher Scientific) and diluted to approximately 50 ng/µl of DNA for performing the Polymerase Chain Reaction (PCR). PCR amplification of a 658 bp fragment of the mitochondrial cytochrome oxidase subunit 1 (COI) gene was carried out using the LCO1490 and HCO2198 primers (Folmer et al., 1994). PCR reactions consisted of 0.02 U/µl 5 PRIME HotMaster Taq polymerase (QuantaBio), 0.2 mM dNTPs, 0.5 µM of forward and reverse primers, 1x PCR buffer, and 1 µl (about 30 ng) of template DNA filled with molecular grade water to 25 µl. PCR conditions were: initial denaturation at 94°C for 2 min, followed by 36 cycles of 94°C for 20 s, annealing at 42°C for 20 s, extension at 65°C for 1 min, and a final extension at 65°C for 15 min. The quality and length of the PCR products were assessed with electrophoresis (110 V, 45 min) on a GelRed-stained 2%-agarose gel in a TRIS-Borat-EDTA buffer and visualized with the Biovision camera system and the software Vision Capt v.15.08 (PeqLab, Erlangen, Germany). Both forward and reverse strands were sequenced by Eurofins Genomics (Germany) with an ABI 3730XL sequencer (Applied Biosystems, Life Technologies GmbH, Darmstadt, Germany). Chromatograms of the COI sequences were manually checked for ambiguous base calls and stop codons in the amino acid translations, using the software CodonCode Aligner 8.0.2 (CodonCode Corporation). Primers were removed, and all sequences were trimmed to a fragment of 658 bp. A consensus of all forward and reverse amplicons were created and sequences were aligned with the ClustalW algorithm (Thompson et al., 1994) in CodonCode Aligner. The assignment of each sequence to one of the three clusters was done based on the Neighbor-Joining tree (Saitou and Nei, 1987) generated with the Mega X software (Kumar et al., 2018). Parameters used were the Kimura 2-parameter (K2P) method with pairwise deletion, and 2000 bootstrap replicates (Felsenstein, 1985). We used the existing COI sequence dataset of Havermans (2015) to assign the sequences to one of the three clusters so far detected in T. gaudichaudii.
Samples of amphipods stored in RNAlater and frozen at -80°C were used for isolating RNA; the entire specimen was used for the extraction (no mass was determined). Cell lysis was performed in 0.6 ml lysis buffer RLT (Qiagen) with beta-mercaptoethanol using the Precellys CKMix (KTO3961-1-009.2) with 16 s shaking at 6500 rpm. Subsequently, samples were centrifuged at 16,000 g for 10 min and then transferred into a clean 2 ml Eppendorf tube. Total RNA was isolated using RNeasy Mini Kit spin columns (Qiagen) following the manufacturer’s instructions. DNase digestion was carried out twice (Qiagen). RNA quantity was analyzed using a NanoDrop spectrometer (PeqLab) and RNA quality was determined by microfluidic electrophoresis in a Bioanalyzer (Agilent Technologies, USA) and by a Nanodrop ND-1000 (Thermo Fisher Scientific) spectrophotometer.
A total of 83 libraries (44 of T. gaudichaudii and 39 of T. abyssorum) were created and utilized for the transcriptome assembly of both species. The libraries were subsequently used for differential gene expression analyses, which were based on the comparison of individuals within experimental groups to characterize the thermal response of both species.
The construction of the cDNA libraries was done following the TrueSeq Stranded mRNA sample prep LS protocol (Illumina) starting from 1 μg of total RNA. The Poly-A-containing mRNA molecules were purified with poly-T oligo-attached magnetic beads. Subsequently, the mRNA was fragmented using divalent cations under elevated temperatures. The short RNA fragments were utilized as templates for first-strand cDNA with reverse transcriptase and random primers. This was followed by second-strand cDNA synthesis employing DNA Polymerase I, RNase H, and dUTP instead of dTTP to achieve strand specificity and to remove the RNA template. Following adapter ligation, products were enriched by PCR and purified to create the cDNA library. All RNA libraries were checked in a Labchip GX Touch (PerkinElmer, USA). Magnetic beads were utilized in all the samples to remove the remaining primer content. The final cDNA concentration was determined in a Labchip GX Touch (PerkinElmer, USA). Libraries were denatured with 5 ml 0.2N NaOH for 5 min at RT and diluted in HT-1 buffer. The samples were sequenced using the NextSeq High Output Kit v2 (150 cycles) on an Illumina NextSeq 500 sequencer.
Trimmomatic software in paired-end mode version 0.32 (Bolger et al., 2014) was used for quality-filtering of the raw RNA reads. The quality-filtering parameters included a sliding window size of 5 bases, with an average quality of 20 and a minimum length of 36 bases. The quality of the filtered datasets was determined with the fastQC software (version 0.11.7, http://www.bioinformatics.babraham.ac.uk/projects/fastqc/). After the filtering process, clean reads were used to perform the de novo assembly of both species with Trinity genome-independent transcriptome assembler version 2.8.5 (Grabherr et al., 2011) with a minimum contig length of 200 bases.
Differential expression analysis was carried out by comparing the differentially down- or up-regulated genes according to the thermal treatments (Table 2). Differential expression analysis involved the alignment of the reads of each sample separately against the de novo reference transcriptome using Bowtie2 version 2.3.4.1 (Langmead et al., 2009). For T. gaudichaudii, samples with different genotypes were pooled for the gene expression analysis, but were analyzed separately in a second step. Differential expression of genes was assessed using a test based on the negative binomial distribution as integrated with the Bioconductor R package DESeq2 (Love et al., 2014), with a standard level of p ≤ 0.001. Trinity package version 2.8.5 was used to execute the tools. Finally, functional annotation of the differentially expressed genes was performed using the Trinotate functional annotation suite version 3.1.1 (Grabherr et al., 2011) including a homology search against the UniProt Swiss-Prot database.
Table 2 Number of Themisto individuals in the thermal treatments used for the gene expression analysis.
The Illumina sequencing of Themisto abyssorum resulted in 392.2 million sequences (average of sequences per library: 10.05 million), of which, after quality filtering, 391.8 million sequences were used for the de novo transcriptome assembly of T. abyssorum. For T. gaudichaudii, Illumina sequencing resulted in 449.4 million sequences (average of sequences per library: 10.21 million), of which, after filtering, we used 449.0 million sequences for the de novo transcriptome assembly of T. gaudichaudii (Figure 2). For T. abyssorum, a total of 107,369 genes (217,988 transcripts) were assembled with an average length of 896 bp; for T. gaudichaudii, 122,686 genes (275,566 transcripts) were assembled with an average length of 785 bp (Table 3).
Figure 2 Flow chart of the analysis of Themisto abyssorum (left) and T. gaudichaudii (right) samples. 1. Raw reads from all the libraries. 2. The adapter sequences and other artifacts were trimmed and only those with high quality were assembled. 3. The assembled data was used for two different gene expression analyses in each of the species, one with the cold-treated samples and the other with the heat-treated samples. 4. Those genes differentially expressed were annotated in step 5.
Table 3 Basic statistics of the de novo assembled transcriptomes of Themisto abyssorum and T. gaudichaudii.
For T. gaudichaudii we used the species’ reference transcriptome generated in this study (from 44 libraries) for the differential expression analysis. The samples of the cold treatment (7 controls against 19 experimental) as well as the samples of the heat treatment were compared (6 controls against 12 experimental). The pairwise comparison between the samples of T. gaudichaudii under heat treatment (11°C) and their control (0°C) resulted in 2880 differentially expressed genes (DEG) in contrast to 37 DEGs between samples under cold treatment (1.5°C) and their control (5°C) (Figure 2). Annotation of the DEGs was performed against the databases Swiss-Prot-Uniprot (bastx), Swiss-Prot-Uniprot (bastp), TrEMBL-Uniprot (bastx) and TrEMBL-Uniprot (bastp). For cold-treated individuals of T. gaudichaudii, 8.1% from the initial 37 DGEs were successfully annotated, while 10.6% of the 2,880 DEGs were annotated in the heat treatment, resulting in 305 DEGs successfully annotated genes (Figure 2).
In T. gaudichaudii, the number of DEGs in response to heat exposure (Figure 3B) was significantly higher than their response to cold temperatures (Figure 3A). In both cold- and heat-treatments, the difference between controls and treated samples was very clear: In the cold-treated samples, some of the successfully annotated DEGs correspond to cytochrome c oxidase (up-regulation >3fold change) and cuticle protein, the latter one was up-regulated (>4fold change) in comparison with the control. In the heat-treated samples the highly up-regulated and successfully annotated DEGs were ATP synthetase (>21fold change), glutamine synthetase (>9fold change), cytochrome c oxidase (>6fold change), and cuticle proteins (>5fold change). Heat shock protein 60A in T. gaudichaudii was down regulated (>2.5fold change) and also mitochondrial heat shock proteins (>3fold change) in response to heat. For a complete list of the annotated DEGs of T. gaudichaudii under heat treatments we refer to the complementary data (Table 4 Supplementary Information).
Figure 3 Heatmap with the differentially expressed genes (A) under cold-treatment in Themisto gaudichaudii and (B) under heat treatment in T. gaudichaudii. Control conditions in red and experimental conditions in blue. A color code indicates up-regulated and down-regulated expression levels (top left).
According to the barcoding analysis of T. gaudichaudii, this study included individuals belonging to two of the three existing genetic clusters (cluster 2 & 3). Differential expression of 28 genes was found between these two clusters, of which only four DEGs were successfully annotated. Three of the four annotated genes corresponded to cytochrome c oxidase, and were up-regulated in cluster 3 in comparison with cluster 2. The fourth gene corresponded to ATP synthase subunit a (Figure 4).
Figure 4 Heatmap with the differentially expressed genes under heat treatment in Themisto gaudichaudii. Color code indicates the two genetic clusters based on mtDNA.
The differential expression analysis of cold-treated individuals involved 20 libraries of T. abyssorum (10 controls against 10 cold-treated) using as a reference the newly generated transcriptome of T. abyssorum created in this study (from 39 libraries). Differential expression analysis also involved 19 libraries of the heat treatment (9 controls against 10 heat treatments), again using as a reference the newly generated transcriptome of T. abyssorum. In both cases, pairwise differential expression analysis was performed between control and treatment. The pairwise comparison between the individuals of the cold treatment resulted in 151 DEGs, while the heat treatment of T. abyssorum resulted in 110 DEGs (Figure 2). The annotation of DEGs in the cold-treatment of T. abyssorum resulted in 12 annotated genes, 7.9% of the initial 151 genes that were differentially expressed. For the heat treatment, 10 out of 110 DEGs were annotated.
The gene expression heatmap in Figure 5A shows DEGs of T. abyssorum in response to low- temperature exposure (5°C) compared to their control (8°C), and in Figure 5B it displays the DEGs in response to heat exposure (10°C) compared to control exposure (0°C). In the heatmap with responses from cold-treated individuals, no clear patterns appeared in the DEGs between control and treatment, since several DEGs were up-regulated only in some samples exposed to cold temperatures, but not in all of the samples. Between those individuals with a clear response to cold-temperature exposure, we found the expression of genes involved in RNA processing, inflammatory response, and apoptotic processes. Contrary to the cold treatment, in the heat treatment heatmap of T. abyssorum the observed DEGs between control and treatment showed a clear pattern, with a group of 35 highly differentially expressed genes among the heat-exposed individuals (Figure 5B). Not all of those DEGs were successfully annotated, however, among those genes highly up-regulated in T. abyssorum we found the cuticle proteins that were upregulated in the cold-treated samples (>11fold change) and in the heat-treated samples (>6fold change) in comparison with their respective controls. No significant up- or down-regulations were detected for any of the heat-shock proteins for any of the treatments in T. abyssorum.
Figure 5 Heatmap with the differentially expressed genes (A) under cold-treatment in Themisto abyssorum and (B) under heat treatment in T. abyssorum. Control conditions in red and experimental conditions in blue. A color code indicates up-regulated and down-regulated expression levels (top left).
This study investigated the whole-transcriptomic response of the Southern Ocean amphipod Themisto gaudichaudii and the Arctic Themisto abyssorum in the context of global warming and associated poleward range shifts. We applied a cold and heat treatment on adult individuals from populations inhabiting subpolar water masses in both hemispheres, to investigate how continued ocean warming as predicted may shape their future distribution. Differential expression analysis detected a total of 3178 gene transcripts that exhibited a significant change in expression across all treatments (p ≤ 0.001). This response was variable between species and treatments, however, in T. gaudichaudii under temperature increase, this response was remarkably high.
Although T. abyssorum and T. gaudichaudii showed molecular responses to thermal stress, the highest number of differentially regulated genes was observed between the control and the individuals in the heat treatments in T. gaudichaudii. This response greatly exceeded its response to cold temperature and also the responses of T. abyssorum in both scenarios. Hence, our hypothesis was confirmed.
Taking into consideration the level of substantial changes in gene expression triggered by the high-temperature exposure in T. gaudichaudii, we assume that this species exhibits a stronger reaction, which can be interpreted as a stress reaction to thermal changes. This could be explained by the fact that T. gaudichaudii has not yet colonized high-Antarctic water masses, contrary to T. abyssorum, which is widely established throughout the Arctic region (Mumm et al., 1998). During the heat shock stress, (11°C) T. gaudichaudii was far below its upper thermal limit (17.5°C) and it corresponds to temperature gradients experienced throughout its distribution center. The response of T. gaudichaudii to thermal stress resulted in the down-regulation of 53% of its DEGs. These findings are consistent with transcriptome-wide studies of other crustaceans e.g., the crayfish Cherax quadricarinatus (Wu et al., 2019) and Procambarus clarkii (Luo L. et al., 2021) and the crab Charybdis japonica (Lou F. et al., 2022). These suggests that under stressful conditions down-regulation is a conserved phenomenon, which may be an adaptive mechanism reflecting the redirection of resources towards the maintenance and repair of cellular machinery. The distribution of gene ontology terms of the DEGs (Figure 6), particularly in the “cellular component” and “molecular function” categories, supports that this is the case for T. gaudichaudii under heat treatment as well. The results presented here indicate that in T. gaudichaudii the heat treatment induced far more metabolic responses than the cold treatment. Since heat stress can be lethal in a short time, an immediate physiological response to higher temperatures may be critical to survival. Therefore, exposure to temperatures at the upper extreme of the thermal niche is generally more stressful than exposure to low temperatures (Bennett and Lenski, 1997). However, it is important to mention that in this study the temperature increase was much more extreme and abrupt (10°C and 11°C higher than the in situ temperature) compared to the cold treatment (3.5°C lower than the in situ temperature), which may explain the stronger response to heat treatment. Furthermore, predicted increases in seawater temperature are far lower than those applied in our temperature treatments.
Figure 6 Distribution of gene ontology (GO) terms of DEGs in heat treatment in Themisto gaudichaudii. The most represented GO terms were divided into three main categories: biological processes (green), cellular components (blue) and molecular functions (orange).
From our analyses, cuticle proteins represented an important element in the temperature response of both species. These proteins were highly up-regulated during the heat treatment in both species and also during the cold treatment in T. gaudichaudii. Hence, we suggest that the expression of genes involved in cuticle formation plays an important role in the thermal response of both species. Up-regulation of cuticle proteins after thermal stress has also been demonstrated in populations of the intertidal copepod Tigriopus californicus, but their specific role during acute thermal stress is not yet understood (Schoville et al., 2012). Moreover, cuticle genes are up-regulated in many invertebrate species during diapause (MacRae, 2010), presumably to reduce cuticle permeability, prevent water loss and promote survival (Baker and Russell, 2009). It is plausible that the regulation of cuticle genes in the two Themisto species may control the cuticle permeability of individuals under thermal stress.
Furthermore, cytochrome c oxidase regulation was greatly affected in T. gaudichaudii by temperature changes, as shown by the pronounced up-regulation in cold- and heat-treated specimens in comparison with their controls. Hence, respiratory electron transport in mitochondria may play a key role in thermal stress in T. gaudichaudii. This hypothesis is supported by the differential expression found in cytochrome c oxidase between the two clusters of T. gaudichaudii. Furthermore, due the high number of annotated genes, it is possible to visualize a scatterplot of genes involved in biological processes, cellular components and molecular functions in heat-treated T. gaudichaudii. It showed that down-regulated genes are primarily involved in cellular iron ion homeostasis, iron ion transport and ferric iron binding, whereas up-regulated genes are involved in hydrogen ion transmembrane transport (Figure 7). This suggests a thermal response in the osmoregulation mechanism of the individuals. The extracellular ion concentrations of the amphipods Eulimnogammarus cyaneus and Gammarus lacustris were positively correlated with temperature (Jakob et al., 2016).
Figure 7 Scatterplot of genes involved in biological process, cellular component and molecular function in T. gaudichaudii individuals under heat treatment. Circle size is proportional to the frequency of the GO term, whereas color indicates the log10 p-value for the enrichment, and the two ends of the colors are red and blue, representing lower and higher p-values, respectively.
The response of T. gaudichaudii to the low-temperature treatment was weaker in comparison to that of the high-temperature treatment. In the amphipods Eulimnogammarus verrucosus and Eulimnogammarus cyaneus, a small response to low temperature has been attributed to post-transcriptional and translational processes, including protein modification and protein stability/turnover (Lipaeva et al., 2021). This could also be the case for T. gaudichaudii, however, due to the low number of annotated genes from the cold treatment, it is difficult to confirm.
The distribution of T. gaudichaudii in the southern hemisphere ranges from sub-tropical latitudes such as the Benguela upwelling system (Auel and Ekau, 2009) via areas of high biomass on the Patagonian shelf (Padovani et al., 2012) and sub-Antarctic regions such as South Georgia to waters around the Antarctic Peninsula (Havermans et al., 2019). Similarly, T. abyssorum occurs over a wide latitudinal and environmental range from the Atlantic Ocean to the central Arctic Ocean, where it is associated with warmer Atlantic water masses. It is, however, unclear whether these widespread Themisto populations are connected by gene flow or whether heat tolerances are maintained throughout the species’ range and its diverse gene pool.
Following the strong response to the heat treatment, we analyzed the differential gene expression in the different clusters of T. gaudichaudii. According to the genotyping carried out in this study, the individuals used in the treatments belong to two of the three genetic clusters (clusters 2 and 3) that occur in T. gaudichaudii (Havermans, 2015). The heatmap with DEGs between these two clusters showed a distinct thermal stress response. Although only four of the 28 differentially regulated genes could be annotated (three corresponding to cytochrome c oxidase and one to ATP synthase subunit a), these qualitative differences between genetic clusters in T. gaudichaudii suggest an evolutionary divergence in the regulatory pathways involved in temperature stress. The three clusters of T. gaudichaudii previously documented have been found in sympatry throughout a wide range of the species’ distribution (Havermans, 2015). However, individuals occurring near the Antarctic Peninsula, the region where T. gaudichaudii is expanding its range, appeared to belong exclusively to cluster 1 (Havermans, 2015). Since the clusters deviate in thermal response, this may indicate a particular predisposition of one lineage only to undergo a range expansion. However, since we did not test any individuals belonging to that particular lineage, this statement must be taken with caution.
Contrary to T. gaudichaudii, the gene expression in T. abyssorum showed only a moderate response to thermal stress. The species also exhibited a higher response in the heat treatment in comparison with that of the cold treatment. However, the differential gene expression of T. abyssorum individuals under cold treatment was not homogeneous, 60% of the analyzed individuals showed almost no response, while the other 40% showed moderate responses. This might be related to the high haplotype diversity of this species (Murray et al., 2023). However, in the present study, the genetic diversity of T. abyssorum was not analyzed and further studies combining genomic and transcriptomic patterns are needed to understand the heterogeneous response of T. abyssorum observed under the cold treatment.
Surprisingly and contrary to T. gaudichaudii, cytochrome c oxidase regulation was not affected in heat- nor cold-treated T. abyssorum. Another contrasting difference was the absence of regulation of heat-shock proteins in T. abyssorum in either of the treatments. A lack of an HSP70 heat shock response has also been observed in several Antarctic marine species, such as the sea star Odontaster validus and the gammarid Paraceradocus gibber (Clark et al., 2008, 2017), and in the Arctic crab Hyas araneus (Harms et al., 2014). It has been proposed that the initial down-regulation of certain heat-shock genes is due to the initial shock response followed by a return to equilibrium as the animals acclimated (Clark et al., 2008). As both Themisto species were exposed for 32 h to the heat treatment, it is not possible to determine, if the heat shock protein genes were up-regulated in the initial phase of the thermal shock and then returned to equilibrium, as the animals acclimated to the new temperature. It is, however, very unlikely that the animals acclimated so quickly to such a high increase in temperature. Another explanation may be that T. abyssorum may rely on a constitutive rather than an inducible heat-shock response. Several species that inhabit cold environments have been found to accumulate the gene product at a constant rate that is independent from an environmental trigger, presumably to be able to mitigate damage associated with protein folding at low temperatures (Buckley et al., 2004; Kaiser et al., 2022).
The most likely explanation for the lack of heat shock protein regulation in T. abyssorum is the possibility that the temperature increase in this study did not reach the upper limit of the thermal window of T. abyssorum. T. abyssorum inhabits dynamic oceanographic environments, carrying out diel vertical migrations with temperature gradients of ca. ten degrees Celsius, between Arctic, surface waters at subzero temperatures and deeper, Atlantic waters. The lack of regulation in catalase-related genes supports this explanation. In other amphipod species, such as Eulimnogammarus verrucosus, a threefold increase in heat shock protein levels (HSP70) was detected together with a doubling of catalase activity at the upper limit of the thermal window, indicating that physiological and biochemical transition phases go hand in hand (Axenov-Gribanov et al., 2016). There are other indications that the T. abyssorum specimens were not reaching the upper limit of their thermal window during this experiment: this species only showed a small increase in respiration rates, when exposed to a temperature increase from 0 to 10°C, suggesting a lower metabolic activity in comparison to co-occurring Arctic species such as Themisto libellula (Kaiser et al., 2022). The observed moderate response to thermal stress in T. abyssorum and the missing differential expression of key protein genes indicates that for T. abyssorum an increase of 10°C is not sufficient to trigger an important stress response.
It is important to emphasize that of the 3,178 DEGs, only 330 were annotated, and most of them could be assigned to the heat treatment in T. gaudichaudii. Hence, additional studies are needed to elucidate the functions of the unannotated genes, and future improved annotations will provide more insight into the functions associated with this large set of as yet unannotated genes.
Although both Themisto species were exposed to similar temperature increases of 10 to 11°C, their response was very different. For T. gaudichaudii, 2,880 genes were differentially expressed, in contrast to only 110 genes for T. abyssorum. More than half of these 2,880 DEGs in T. gaudichaudii were down-regulated, which can be explained by a redirection of resources to maintenance and repair instead of activating certain metabolic processes.
One of the reasons for the difference in thermal response between these two species may be that T. gaudichaudii is characterized by more locally adapted lineages, some of them more cold-adapted than others along its latitudinal gradient. In contrast, T. abyssorum likely experiencing a higher gene flow (Murray et al., 2023) may be adapted to more pronounced temperature fluctuations across its geographic and vertical range (Havermans et al., 2019). The long-term ability of a population to respond genetically and evolve adaptive responses to thermally stressful environments may be crucial to its persistence, which can be advantageous particularly during a period of warming climates (Bennett and Lenski, 1997). Our results indicate that T. abyssorum exhibits a pronounced adaptive flexibility under thermal stress; it appears to have the ability to continue its poleward expansion, but may also cope with increasing temperatures in its current environments. While T. gaudichaudii’s response under thermal stress suggests that this species is more likely to move poleward into warming Antarctic regions than to remain in its core distribution area (with higher temperatures). Further studies integrating gene expression with physiological responses and functional traits are necessary to complement these data and elucidate, if and how these species can adapt efficiently and cope with warming polar oceans. In addition, future studies will need to expand this autecological approach focusing on temperature adaptation and integrate food availability and interspecific competition in order to gain a deeper understanding of future distribution of species and composition of faunal communities.
The datasets presented in this study can be found in online repositories. The names of the repository/repositories and accession number(s) can be found below: https://www.ncbi.nlm.nih.gov/, PRJNA949550, https://www.ncbi.nlm.nih.gov/, PRJNA952103.
The requirement of ethical approval was waived by Sampling permits: the Government of Greenland with a non-exclusive license n° G22-049 for the utilization of Greenland resources, with prior informed consent under the Biological Diversity Convention and Nagoya Protocol. A permit for sampling in the Southern Ocean south of 60°S under the Antarctic Treaty was obtained from the German Environmental Agency (UBA, 2016) with the number II 2.8-94003-3/382. Animals are very abundant invertebrate species, not threatened, not regulated, so no other permits were needed for the experiments. The studies were conducted in accordance with the local legislation and institutional requirements.
DM-A: Conceptualization, Investigation, Methodology, Writing – original draft, Writing – review & editing, Data curation, Formal Analysis. CHe: Conceptualization, Methodology, Writing – review & editing, Resources. LH: Data curation, Formal Analysis, Methodology, Writing – review & editing. HA: Conceptualization, Investigation, Methodology, Resources, Writing – review & editing. WH: Conceptualization, Project administration, Resources, Writing – review & editing. CHa: Conceptualization, Funding acquisition, Investigation, Methodology, Project administration, Resources, Supervision, Writing – original draft, Writing – review & editing.
The author(s) declare financial support was received for the research, authorship, and/or publication of this article. This study was funded by the Deutsche Forschungsgemeinschaft (DFG, German Research Foundation) with the projects HA 7627/1-1 and HA 7627/1-2, granted to CHa within the Priority Program 1158 on Antarctic Research with Comparable Investigations in Arctic Sea Ice Areas. DM-A is currently funded by the European Union’s Horizon 2020 research and innovation program under the Marie Sklodowska Curie grant agreement (101023801) at the French National Centre for Scientific Research (CNRS). CHa is currently supported by the Helmholtz Young Investigator Group “ARJEL - Arctic Jellies” (project number VH-NG-1400) at the Alfred Wegener Institute Helmholtz Centre for Polar and Marine Research.
We greatly acknowledge the skillful support of the captain, crews, chief scientists, and scientific teams on PS100 and PS1303. This work is a result of the Polarstern Grant-Nos: AWI_PS100_07 (project “AMICA”, PI Holger Auel) and AWI_PS103_03 (project “InterPelagic”, PI CHa). Special thanks to Andrea Eschbach for her technical assistance in the laboratory.
The authors declare that the research was conducted in the absence of any commercial or financial relationships that could be construed as a potential conflict of interest.
All claims expressed in this article are solely those of the authors and do not necessarily represent those of their affiliated organizations, or those of the publisher, the editors and the reviewers. Any product that may be evaluated in this article, or claim that may be made by its manufacturer, is not guaranteed or endorsed by the publisher.
The Supplementary Material for this article can be found online at: https://www.frontiersin.org/articles/10.3389/fmars.2024.1336024/full#supplementary-material
Andrews A. J., Christiansen J. S., Bhat S., Lynghammar A., Westgaard J. I., Pampoulie C., et al. (2019). Boreal marine fauna from the Barents Sea disperse to Arctic Northeast Greenland. Sci. Rep. 9, 5799. doi: 10.1038/s41598-019-42097-x
Atkinson A., Hill S. L., Pakhomov E. A., Siegel V., Reiss C. S., Loeb V. J., et al. (2019). Krill (Euphausia superba) distribution contracts southward during rapid regional warming. Nat. Climate Change 9, 142–147. doi: 10.1038/s41558-018-0370-z
Atkinson A., Siegel V., Pakhomov E., Rothery P. (2004). Long-term decline in krill stock and increase in salps within the Southern Ocean. Nature 432, 100–103. doi: 10.1038/nature02996
Auel H., Ekau W. (2009). Distribution and respiration of the high-latitude pelagic amphipod Themisto gaudichaudi in the Benguela Current in relation to upwelling intensity. Prog. Oceanography 83, 237–241. doi: 10.1016/j.pocean.2009.07.040
Axenov-Gribanov D., Bedulina D., Shatilina Z., Jakob L., Vereshchagina K., Lubyaga Y., et al. (2016). Thermal preference ranges correlate with stable signals of universal stress markers in Lake Baikal endemic and holarctic amphipods. PloS One 11, e0164226. doi: 10.1371/journal.pone.0164226
Baker D. A., Russell S. (2009). Gene expression during Drosophila melanogaster egg development before and after reproductive diapause. BMC Genomics 10, 242. doi: 10.1186/1471-2164-10-242
Basedow S. L., Sundfjord A., von Appen W.-J., Halvorsen E., Kwasniewski S., Reigstad M. (2018). Seasonal variation in transport of zooplankton into the Arctic Basin through the Atlantic Gateway, Fram Strait. Front. Mar. Sci. 5. doi: 10.3389/fmars.2018.00194
Beaugrand G. (2009). Decadal changes in climate and ecosystems in the North Atlantic Ocean and adjacent seas. Deep-Sea Res. Part II: Topical Stud. Oceanography 56, 656–673. doi: 10.1016/j.dsr2.2008.12.022
Belter H. J., Krumpen T., von Albedyll L., Alekseeva T. A., Birnbaum G., Frolov S. V., et al. (2021). Interannual variability in Transpolar Drift summer sea ice thickness and potential impact of Atlantification. Cryosphere 15, 2575–2591. doi: 10.5194/tc-15-2575-2021
Bennett A. F., Lenski R. E. (1997). “Phenotypic and evolutionary adaptation of a model bacterial system to stressful thermal environments,” in Environmental Stress, Adaptation and Evolution, vol. 83 . Eds. Bijlsma R., Loeschcke V. (Birkhäuser, Basel). doi: 10.1007/978-3-0348-8882-0_8
Boeck A. (1871). Crustacea amphipoda borealia et arctica. Forhandlinger i Videnskabs-Selskabet i Christiania. 1870, 83–280.
Bolger A. M., Lohse M., Usadel B. (2014). Trimmomatic: a flexible trimmer for Illumina sequence data. Bioinformatics 30, 2114–2120. doi: 10.1093/bioinformatics/btu170
Böning C. W., Dispert A., Visbeck M., Rintoul S. R., Schwarzkopf F. U. (2008). The response of the Antarctic Circumpolar Current to recent climate change. Nat. Geosci. 1, 864–869. doi: 10.1038/ngeo362
Buchholz F., Buchholz C., Weslawski J. M. (2009). Ten years after: krill as indicator of changes in the macro-zooplankton communities of two Arctic fjords. Polar Biol. 33, 101–113. doi: 10.1007/s00300-009-0688-0
Buckley B. A., Place S. P., Hofmann G. E. (2004). Regulation of heat shock genes in isolated hepatocytes from an Antarctic fish, Trematomus bernacchii. J. Exp. Biol. 207, 3649–3656. doi: 10.1242/jeb.01219
CAFF (2017). State of the Arctic Marine Biodiversity Report (Akureyri, Iceland: Conservation of Arctic Flora and Fauna International Secretariat).
Clark M. S., Fraser K. P. P., Peck L. S. (2008). Lack of an HSP70 heat shock response in two Antarctic marine invertebrates. Polar Biol. 31, 1059–1065. doi: 10.1007/s00300-008-0447-7
Clark M. S., Sommer U., Sihra J. K., Thorne M. A., Morley S. A., King M., et al. (2017). Biodiversity in marine invertebrate responses to acute warming revealed by a comparative multi-omics approach. Global Change Biol. 23, 318–330. doi: 10.1111/gcb.13357
Dalpadado P. (2002). Inter-specific variations in distribution, abundance and possible life-cycle patterns of Themisto spp. (Amphipoda) in the Barents Sea. Polar Biol. 25, 656–666. doi: 10.1007/s00300-002-0390-y
Descamps S., Aars J., Fuglei E., Kovacs K. M., Lydersen C., Pavlova O., et al. (2017). Climate change impacts on wildlife in a high Arctic archipelago - Svalbard, Norway. Glob Chang Biol. 23, 490–502. doi: 10.1111/gcb.13381
Felsenstein J. (1985). Confidence limits on phylogenies: an approach using the bootstrap. Evolution 39, 783–791. doi: 10.2307/240867
Folmer, Black M., Hoeh W., Lutz R., Vrijenhoek R. (1994). DNA primers for amplification of mitochondrial cytochrome c oxidase subunit I from diverse metazoan invertebrates. Mol. Mar. Biol. Biotechnol. 3, 294–299.
Fossheim M., Primicerio R., Johannesen E., Ingvaldsen R. B., Aschan M. M., Dolgov A. V. (2015). Recent warming leads to a rapid borealization of fish communities in the Arctic. Nat. Climate Change 5, 673–677. doi: 10.1038/nclimate2647
Goes A. T. (1865). Crustacea amphipoda maris spetsbergiam alluentis cum speciebus aliis arcticis enumerat. Öfversigt af Kongelige Vetenskaps-Akademiens Förhandlingar 8, 517–536.
Grabherr M. G., Haas B. J., Yassour M., Levin J. Z., Thompson D. A., Amit I., et al. (2011). Full-length transcriptome assembly from RNA-Seq data without a reference genome. Nat. Biotechnol. 29, 644–652. doi: 10.1038/nbt.1883
Grebmeier J. M. (2012). Shifting patterns of life in the pacific arctic and sub-arctic seas. Annu. Rev. Mar. Sci. 4, 63–78. doi: 10.1146/annurev-marine-120710-100926
Guérin F. E. (1825). “Encyclopédie méthodique histoire naturelle,” in Entomologie, ou histoire naturelle des crustacés, des arachnides et des insectes par m. latreille, vol. 10. (Paris: Tome).
Guzman C., Conaco C. (2016). Gene expression dynamics accompanying the sponge thermal stress response. PloS One 11, e0165368. doi: 10.1371/journal.pone.0165368
Harms L., Frickenhaus S., Schiffer M., Mark F. C., Storch D., Held C., et al. (2014). Gene expression profiling in gills of the great spider crab Hyas araneus in response to ocean acidification and warming. BMC Genomics 15, 1–7. doi: 10.1186/1471-2164-15-789
Havermans C. (2015). “Report COMNAP fellowship 2013/2014,” in The impact of environmental changes on a key component of pelagic food webs in the Southern Ocean: the amphipod Themisto gaudichaudii (Crustacea: Hyperiidea).
Havermans C., Auel H., Hagen W., Held C., Ensor N. S., Tarling G. A. (2019). Predatory zooplankton on the move: Themisto amphipods in high-latitude marine pelagic food webs. Adv. Mar. Biol. 82, 51–92. doi: 10.1016/bs.amb.2019.02.002
Havermans C., Schobinger S., Shroter F. (2017). Interactions between key players of the Southern Ocean zooplankton: amphipods, copepods, krill and salps. In Boebel, O. (Ed.). The Expedition PS103 of the Research Vessel POLARSTERN to the Weddell Sea in 2016/2017. Rep. Polar Mar. Res. 710, 1–160. doi: 10.2312/BzPM_0710_2017
Hobbs W. R., Massom R., Stammerjohn S., Reid P., Williams G., Meier W. (2016). A review of recent changes in Southern Ocean sea ice, their drivers and forcings. Global Planetary Change 143, 228–250. doi: 10.1016/j.gloplacha.2016.06.008
Hop H., Vihtakari M., Bluhm B. A., Daase M., Gradinger R., Melnikov I. A. (2021). Ice-associated amphipods in a pan-arctic scenario of declining sea ice. Front. Mar. Sci. 8. doi: 10.3389/fmars.2021.743152
Ingvaldsen R. B., Assmann K. M., Primicerio R., Fossheim M., Polyakov I. V., Dolgov A. V. (2021). Physical manifestations and ecological implications of Arctic Atlantification. Nat. Rev. Earth Environ. 2, 874–889. doi: 10.1038/s43017-021-00228-x
Jakob L., Axenov-Gribanov D. V., Gurkov A. N., Ginzburg M., Bedulina D. S., Timofeyev M. A., et al. (2016). Lake Baikal amphipods under climate change: thermal constraints and ecological consequences. Ecosphere 7, e01308. doi: 10.1002/ecs2.1308
Johnston E. L., Clark G. F., Bruno J. F. (2022). The speeding up of marine ecosystems. Climate Change Ecol. 3, 100055. doi: 10.1016/j.ecochg.2022.100055
Kaiser P., Hagen W., Bode-Dalby M., Auel H. (2022). Tolerant but facing increased competition: Arctic zooplankton versus Atlantic invaders in a warming ocean. Front. Mar. Sci. 9. doi: 10.3389/fmars.2022.908638
Kortsch S., Primicerio R., Fossheim M., Dolgov A. V., Aschan M. (2015). Climate change alters the structure of arctic marine food webs due to poleward shifts of boreal generalists. Proc. R. Soc. B Biol. Sci. 282, 20151546-20151546. doi: 10.1098/rspb.2015.1546
Kraft A., Nöthig E. M., Bauerfeind E., Wildish D. J., Pohle G. W., Bathmann U. V., et al. (2013). First evidence of reproductive success in a southern invader indicates possible community shifts among Arctic zooplankton. Mar. Ecol. Prog. Ser. 493, 291–296. doi: 10.3354/meps10507
Krumpen T., Gerdes R., Haas C., Hendricks S., Herber A., Selyuzhenok V., et al. (2016). Recent summer sea ice thickness surveys in Fram Strait and associated ice volume fluxes. Cryosphere 10, 523–534. doi: 10.5194/tc-10-523-2016
Kumar S., Stecher G., Li M., Knyaz C., Tamura K. (2018). MEGA X: molecular evolutionary genetics analysis across computing platforms. Mol. Biol. Evol. 35, 1547–1549. doi: 10.1093/molbev/msy096
Langmead B., Trapnell C., Pop M., Salzberg S. L. (2009). Ultrafast and memory-efficient alignment of short DNA sequences to the human genome. Genome Biol. 10, R25. doi: 10.1186/gb-2009-10-3-r25
Lipaeva P., Vereshchagina K., Drozdova P., Jakob L., Kondrateva E., Lucassen M., et al. (2021). Different ways to play it cool: Transcriptomic analysis sheds light on different activity patterns of three amphipod species under long-term cold exposure. Mol. Ecol. 30, 5735–5751. doi: 10.1111/mec.16164
Lou F., Wang Y., Han Z., Shui B. (2022). Comparative transcriptome reveals the molecular regulation mechanism of Charybdis japonica to high- and low-temperature stresses. Front. Mar. Sci. 9. doi: 10.3389/fmars.2022.849485
Love M. I., Huber W., Anders S. (2014). Moderated estimation of fold change and dispersion for RNA-seq data with DESeq2. Genome Biol. 15, 550. doi: 10.1186/s13059-014-0550-8
Luo L., Huang J.-H., Liu D.-L., Jiang S.-G., Zhou F.-L., Jiang S., et al. (2021). Transcriptome reveals the important role of metabolic imbalances, immune disorders and apoptosis in the treatment of Procambarus clarkii at super high temperature. Comp. Biochem. Physiol. Part D: Genomics Proteomics 37, 100781. doi: 10.1016/j.cbd.2020.100781
Mackey A. P., Atkinson A., Hill S. L., Ward P., Cunningham N. J., Johnston N. M., et al. (2012). Antarctic macrozooplankton of the southwest Atlantic sector and Bellingshausen Sea: Baseline historical distributions (Discovery Investigations 1928–1935) related to temperature and food, with projections for subsequent ocean warming. Deep-Sea Res. Part II: Topical Stud. Oceanography 59-60, 130–146. doi: 10.1016/j.dsr2.2011.08.011
MacRae T. H. (2010). Gene expression, metabolic regulation and stress tolerance during diapause. Cell. Mol. Life Sci. 67, 2405–2424. doi: 10.1007/s00018-010-0311-0
Mandt M. W. (1822). “Observationes in historiam naturalem et anatomiam comparatam in itinere groenlandico factae,” in Dissertatio inauguralis quam consensu et auctoritate gratiosi micorum ordinis in universitate literaria berolinensi ut summi in medicina et chirurgia honores rite, 40.
Meredith M. P., King J. C. (2005). Rapid climate change in the ocean west of the Antarctic Peninsula during the second half of the 20th century. Geophysical Res. Lett. 32. doi: 10.1029/2005gl024042
Meredith M., Sommerkorn M., Cassotta S., Derksen C., Ekaykin A., Hollowed A., et al. (2019). “Polar Regions,” in IPCC Special Report on the Ocean and Cryosphere in a Changing Climate. Eds Poürtner H.-O., Roberts D. C., Masson-Delmotte V., Zhai P., Tignor M., Poloczanska E., Mintenbeck K., Alegriía A., Nicolai M., Okem A., Petzold J., Rama B., Weyer N. M.. IPCC. pp. 1–173.
Mumm N., Auel H., Hanssen H., Hagen W., Richter C., Hirche H.-J. (1998). Breaking the ice: large-scale distribution of mesozooplankton after a decade of Arctic and transpolar cruises. Polar Biol. 20, 189–197. doi: 10.1007/s003000050295
Murray A., Praebel K., Desiderato A., Auel H., Havermans C. (2023). Phylogeography and molecular diversity of two highly abundant Themisto amphipod species in a rapidly changing Arctic Ocean. Ecol. Evol. 13, e10359. doi: 10.1002/ece3.10359
Neukermans G., Oziel L., Babin M. (2018). Increased intrusion of warming Atlantic water leads to rapid expansion of temperate phytoplankton in the Arctic. Global Change Biol. 24, 2545–2553. doi: 10.1111/gcb.14075
Notz D., Stroeve J. (2018). The trajectory towards a seasonally ice-free Arctic Ocean. Curr. Climate Change Rep. 4, 407–416. doi: 10.1007/s40641-018-0113-2
Overland J. E., Wang M. (2013). When will the summer Arctic be nearly sea ice free? Geophysical Res. Lett. 40, 2097–2101. doi: 10.1002/grl.50316
Padovani L. N., Viñas M. D., Sánchez F., Mianzan H. (2012). Amphipod-supported food web: Themisto gaudichaudii, a key food resource for fishes in the southern Patagonian Shelf. J. Sea Res. 67, 85–90. doi: 10.1016/j.seares.2011.10.007
Parmesan C. (2006). Ecological and evolutionary responses to recent climate change. Annual Review of Ecology. Evolution Systematics 37, 637–669. doi: 10.1146/annurev.ecolsys.37.091305.110100
Pecl G. T., Araujo M. B., Bell J. D., Blanchard J., Bonebrake T. C., Chen I. C., et al. (2017). Biodiversity redistribution under climate change: Impacts on ecosystems and human well-being. Science 355, eaai9214. doi: 10.1126/science.aai9214
Polyakov I. V., Pnyushkov A. V., Alkire M. B., Ashik I. M., Baumann T. M., Carmack E. C., et al. (2017). Greater role for Atlantic inflows on sea-ice loss in the Eurasian Basin of the Arctic Ocean. Science 356, 285–291. doi: 10.1126/science.aai8204
Rantanen M., Karpechko A. Y., Lipponen A., Nordling K., Hyvärinen O., Ruosteenoja K., et al. (2022). The Arctic has warmed nearly four times faster than the globe since 1979. Commun. Earth Environ. 3, 168. doi: 10.1038/s43247-022-00498-3
Renaud P. E., Berge J., Varpe Ø., Lønne O. J., Nahrgang J., Ottesen C., et al. (2011). Is the poleward expansion by Atlantic cod and haddock threatening native polar cod, Boreogadus saida? Polar Biol. 35, 401–412. doi: 10.1007/s00300-011-1085-z
Saitou N., Nei M. (1987). The neighbor-joining method: A new method for reconstructing phylogenetic trees. Mol. Biol. Evol. 4, 406–425. doi: 10.1093/oxfordjournals.molbev.a040454
Schoville S. D., Barreto F. S., Moy G. W., Wolff A., Burton R. S. (2012). Investigating the molecular basis of local adaptation to thermal stress: population differences in gene expression across the transcriptome of the copepod Tigriopus californicus. BMC Evolutionary Biol. 12, 170. doi: 10.1186/1471-2148-12-170
Schröter F., Havermans C., Kraft A., Knüppel N., Beszczynska-Möller A., Bauerfeind E., et al. (2019). Pelagic amphipods in the eastern Fram Strait with continuing presence of Themisto compressa based on sediment trap time series. Front. Mar. Sci. 6. doi: 10.3389/fmars.2019.00311
Thompson J. D., Higgins D. G., Gibson T. J. (1994). CLUSTAL W: improving the sensitivity of progressive multiple sequence alignment through sequence weighting, position-specific gap penalties and weight matrix choice. Nucleic Acids Res. 22, 4673–4680. doi: 10.1093/nar/22.22.4673
Wassmann P., Kosobokova K. N., Slagstad D., Drinkwater K. F., Hopcroft R. R., Moore S. E., et al. (2015). The contiguous domains of Arctic Ocean advection: Trails of life and death. Prog. Oceanography 139, 42–65. doi: 10.1016/j.pocean.2015.06.011
Weydmann A., Carstensen J., Goszczko I., Dmoch K., Olszewska A., Kwasniewski S. (2014). Shift towards the dominance of boreal species in the Arctic: inter-annual and spatial zooplankton variability in the West Spitsbergen Current. Mar. Ecol. Prog. Ser. 501, 41–52. doi: 10.3354/meps10694
Keywords: Themisto gaudichaudii, Themisto abyssorum, range shifts, Atlantification, Arctic, Antarctic
Citation: Martinez-Alarcón D, Held C, Harms L, Auel H, Hagen W and Havermans C (2024) Race to the poles: the thermal response of the transcriptome of two range-expanding pelagic amphipod species. Front. Mar. Sci. 11:1336024. doi: 10.3389/fmars.2024.1336024
Received: 10 November 2023; Accepted: 05 March 2024;
Published: 21 March 2024.
Edited by:
Doreen Kohlbach, UiT The Arctic University of Norway, NorwayReviewed by:
Ajit Kumar Mohanty, Indira Gandhi Centre for Atomic Research (IGCAR), IndiaCopyright © 2024 Martinez-Alarcón, Held, Harms, Auel, Hagen and Havermans. This is an open-access article distributed under the terms of the Creative Commons Attribution License (CC BY). The use, distribution or reproduction in other forums is permitted, provided the original author(s) and the copyright owner(s) are credited and that the original publication in this journal is cited, in accordance with accepted academic practice. No use, distribution or reproduction is permitted which does not comply with these terms.
*Correspondence: Charlotte Havermans, Y2hhcmxvdHRlLmhhdmVybWFuc0Bhd2kuZGU=
Disclaimer: All claims expressed in this article are solely those of the authors and do not necessarily represent those of their affiliated organizations, or those of the publisher, the editors and the reviewers. Any product that may be evaluated in this article or claim that may be made by its manufacturer is not guaranteed or endorsed by the publisher.
Research integrity at Frontiers
Learn more about the work of our research integrity team to safeguard the quality of each article we publish.