- 1School of Fishery, Zhejiang Ocean University, Zhoushan, China
- 2Zhejiang Marine Fisheries Research Institute, Key Lab of Mariculture and Enhancement of Zhejiang Province, Zhoushan, China
This study investigated the effects of levofloxacin (LEV) and norfloxacin (NOR) on Skeletonema costatum, focusing on cell growth, chlorophyll a (Chla) content, maximal quantum yield of PSII (Fv/Fm), protein content, enzyme activities of superoxide dismutase (SOD), glutathione reductase (GR), and glutathione peroxidase (GSH-PX), and the membrane lipid peroxidation product malondialdehyde (MDA) content were conducted to analyze the responses of S. costatum under LEV and NOR exposure. Cell growth, Chla content, Fv/Fm, protein content, enzyme activities, and MDA content were assessed to elucidate physiological changes. Both LEV and NOR inhibited S. costatum growth, except for 10 mg/L NOR, which promoted growth. Algal cells exhibited higher sensitivity to LEV, with 96h-IC50 values of 14.770 mg/L for LEV and 44.250 mg/L for NOR. Low NOR concentration (10 mg/L) increased Chla content, while high antibiotic concentrations (>20 mg/L for LEV, >100 mg/L for NOR) decreased Chla content and Fv/Fm, indicating an impact on photosynthesis. Elevated LEV and NOR levels reduced protein and MDA content but increased GR, SOD, and GSH activities, indicating induced oxidative stress. The study provides a comprehensive analysis of LEV and NOR effects on marine microalgae growth and underlying physiological mechanisms, shedding light on potential ecological risks posed by antibiotics in marine ecosystems.
1 Introduction
Antibiotics are extensively employed globally in sectors like animal husbandry, aquaculture, and human healthcare (Zhang et al., 2023). In natural water bodies, the transformation of antibiotics mainly occurs through hydrolysis, photolysis, absorption, adsorption, and biodegradation (Martinez, 2009; Zhang et al., 2020a; Leung et al., 2012). Organisms are unable to fully absorb antibiotics and approximately 90% of antibiotics consumed are excreted by humans and livestock in their original or metabolized form, ultimately entering the environment directly or after wastewater treatment (Huang et al., 2020). Existing research has confirmed that Antibiotics present in the environment may pose a risk to the health of both humans and animal, as well as ecosystem integrity through the food chain and food web (Huang et al., 2014). Data on antibiotics reported from 2009 to 2022 indicate that in China, antibiotic concentrations have been detected at the micrograms per liter (μg/L) level in two-thirds of marine areas, with overall concentrations ranging from 0.04 to 6,800 ng/L (Gao et al., 2023). Quinolone antibiotics have been detected in the seas of Bohai, East China, and South China. Ofloxacin (OFX) and norfloxacin (NOR) are commonly found antibiotics in the Bohai Sea, with concentrations varying between 3 and 5,100 ng/L, 3and 6,800 ng/L, respectively (Zou et al., 2011). Additionally, in 2017, the highest concentration of NOR, reaching a maximum of 1,990 ng/L, was detected in water samples collected along China’s approximately 18,000 km coastline (Lu et al., 2018).
Levofloxacin (LEV) is the levorotatory enantiomer of OFX and is used more widely due to its stronger antibacterial properties compared with OFX. Research on LEV is primarily concentrated within the medical realm (Liou et al., 2016; White et al., 2019), with relatively little research on its effects on microalgae (Pan et al., 2009; Wan et al., 2014). Most research on NOR has focused on clarifying its short-term toxicity to large Daphnia, freshwater fish, and freshwater algae (Gonzalez-Pleiter et al., 2013; Liang et al., 2015; Pan et al., 2017; Xiong et al., 2017a; Eluk et al., 2021), with relatively less research on the impacts of NOR on marine microalgae (Aderemi et al., 2018; Dow et al., 2020).
Marine microalgae serve as primary producers within marine aquatic ecosystems, exerting a profound influence on the system’s structure and function. The diverse array of microalgae, coupled with their significant primary productivity, profoundly influences the dynamics of the entire aquatic ecosystem (Nie et al., 2013; Wan et al., 2015). Although antibiotics in the marine environment are typically found at low concentrations, they can still affect certain highly sensitive aquatic organisms. As antibiotics accumulate, they may impact the intricate community dynamics within marine ecosystems. Studies have shown that antibiotics affect the photosynthetic capability, cellular reproduction, and algae growth through impeding chloroplast formation, protein synthesis, and damaging to chlorophyll. As a result, the levels of Chla and maximal quantum yield of PSII (Fv/Fm) in algal cells are diminished to varying extents (Pinckney et al., 2013; Liu et al., 2018; Mao et al., 2021). Low concentrations of antibiotics may stimulate the expression of genes related to DNA replication, exhibiting a promotive effect (Jiang et al., 2021). This response may differ based on the type of antibiotics or the species of microalgae (Chen and Guo, 2012; Zhang et al., 2021b). The growth and photosynthesis of algal cells are impacted by antibiotics, leading to the production of reactive oxygen species (ROS), which in turn inflict damage upon the cells. During this process, malondialdehyde (MDA) is produced, and its production directly reflects the cell’s oxidative stress level (Koussevitzky et al., 2007). To mitigate the harmful effects caused by ROS, algal cells activate antioxidant defense systems, comprising superoxide dismutase (SOD), glutathione reductase (GR) and glutathione peroxidase (GSH-PX) in the antioxidant enzyme system, as well as MDA in the non-enzyme system, to jointly eliminate and regulate the overall ROS level (Teisseire and Guy, 2000; Sun et al., 2017; Liu et al., 2021). Once ROS is overproduced, it induces oxidative damage, resulting in protein degradation, DNA damage, and lipid peroxidation, ultimately leading to algal cell death (Sies, 1997; Wang et al., 2012; Tang et al., 2016).
S. costatum, a planktonic diatom that is widely distributed in coastal waters around the world, often representing a dominant species in coastal areas (Ma et al., 2023). The current study employed two quinolone antibiotics, LEV and NOR, as target pollutants to investigate the impact of varying concentrations of these antibiotics on S. costatum. The biological mechanism of their effect on S. costatum was clarified through analyzing the growth, photosystem activity and enzyme activity within algal cells. This study provides a reference for evaluating the potential ecological hazard of antibiotics in marine ecosystems.
2 Materials and methods
2.1 Materials and culture condition
Natural seawater was collected from Changzhi Island in Zhoushan, China (E122°11′03.36″, N29°57′27.13″). After being filtered using a nitrocellulose membrane (0.45-µm pore size), and undergoing sterilization via high-pressure steam (120°C, 20 min), the seawater had a salinity of 27 ± 0.5 and a pH value of 8.10 ± 0.01. S. costatum was acquired from Shanghai Guangyu Biotechnological Co., Ltd. (Shanghai, China) and inoculated into a 2,000 mL Erlenmeyer flask filled with 1,000 mL f/2 (+Si) culture medium. The culture was performed at a light intensity of 4,000 lx, following a 12-hour light-on, 12-hour light-off photoperiod, and a constant temperature of 23 ± 0.1°C. The flask underwent regular shaking three times daily.
LEV and NOR were acquired from Shanghai Aladdin Biochemical Technology Co., Ltd. (Shanghai, China). Weighed 0.5g each of LEV and NOR and placed them in separate 50 mL sterilized beakers. Slowly added 0.1mol/L HCl until LEV and NOR were completely dissolved. The consumed amounts of 0.1% HCl were 8 mL and 15 mL, respectively. Transferred the completely dissolved LEV and NOR to 500 mL volumetric flasks and make up to the volume with sterilized seawater. This resulted in a mother liquor concentration of 1g/L. sterilized using the Biosharp vacuum filtration system (Biosharp Life Sciences, Hefei, Anhui, China), and then stored in a 4°C refrigerator. Before utilizing the mother liquor in the experiment, adjusted the pH using 0.1mol/L NaOH.
2.2 Experimental methods
2.2.1 Growth experiment
Cultured microalgae in the logarithmic growth phase growing in sterilized seawater containing f/2 medium and antibiotics were added in certain proportions into 2,000 mL Erlenmeyer flasks to reach a culture volume totaling 1,200 mL. The concentrations of LEV were 0 (blank control), 5, 10, 20, 40, and 60 mg/L. Concentrations of LEV exceeding 20 mg/L were classified as the high-concentration group. The concentrations of NOR were 0 (blank control), 10, 50, 100, 200, 400 mg/L. Concentrations of NOR over 100 mg/L were considered the high-concentration group. Each concentration was tested three times in replicates. The initial algae density after inoculation was 5×105 cells·mL−1. The cultured microalgae were sampled 4 h after inoculation on the first day. After that, the microalgae were sampled regularly every 24 h for 10 days. The algal cells were enumerated utilizing a plankton counting chamber (Zhang et al., 2021b), following the inhibition rate (IR, %) and relative growth rate were calculated. The formula used to calculate the IR (%) is as follows (Equation 1) :
where N0 and Nt represent the density of microalgae at the beginning of the experiment and at day t of the experiment, respectively.
The equation used to determine the relative growth rate (μ) is as follows (Equation 2) (Zhang et al., 2021b):
where μ denotes the relative growth rate, h−1; Xn represents the cell density at time tn; and X(n−1) is the cell density at time t(n−1) (Zhang et al., 2021b).
2.2.2 Measurement of Fv/Fm and Chla
For each sampling, 20 mL of algae suspension was collected from all treatment groups. Following a 15 min adaptation period, the Fv/Fm were measured utilizing a hand-held chlorophyll fluorometer, AquaPen-C AP-C 100 (Photon Systems Instruments, The Czech Republic). The algae suspension was filtered with a 0.45 μm nitrocellulose membrane. Subsequently, the filtrate was transferred into a centrifuge tube, followed by the addition of 10 mL of 90% acetone. After incubation at 4°C for 24 hours, the centrifuge tube underwent centrifugation at 3,000 rpm for 10 minutes. Subsequently, the absorbance of the supernatant was assessed at wavelengths of 750, 664, 647, and 630 nm, with 90% acetone serving as the control solution. The Chla content was quantified utilizing the formula (Equation 3) improved by Jeffrey and Humphrey in 1975:
where Chla represents the Chla concentration (μg/L); v signifies the volume of extraction (ml); V represents the actual amount of the seawater sample (L); L represents the cuvette path length in centimeters (cm); and E750, E664, E647, and E630 correspond to the absorbance values at the wavelengths of 750, 664, 647, and 630 nm, respectively.
2.2.3 Measurement of enzyme activity
From each treatment, 50 mL suspension of algae was collected and subsequently centrifuged at 3,000 rpm for 10 minutes. The supernatant was removed, and the precipitated cells were subsequently collected. The protein content, SOD activity, MDA content, GSH-PX activity, and GR activity were quantified by the corresponding reagent kits (A045-2-2 for protein content, A001-1-2 for SOD, A003-1-3 for MDA, A005-1-2 for GSH-PX, and A062-1-1 for GR) purchased from Nanjing Jiancheng Bioengineering Research Institute (Nanjing, China).
2.3 Data processing
The experimental data were organized and plotted utilizing Origin 9.2. One-way analysis of variance (ANOVA) was conducted using SPSS 26.0.0, with statistical significance defined at p< 0.05. Additionally, GraphPad Prism 9.5.0 software was employed to determine the 96-h half-maximal inhibitory concentrations (96h-IC50).
3 Results and analysis
3.1 Effect of antibiotics on microalgae growth
As the treatment duration and antibiotic concentration escalated, the growth of algal cells was increasingly suppressed, showing a clear dose-dependent relationship (Figure 1). In the presence of LEV, the relative growth rate of S. costatum exhibited a significant decrease compared to the control group (p< 0.05), with a gradual decline observed as the antibiotic concentration increased. During the experimental period, the groups with concentrations including 5, 10, and 20 mg/L initially exhibited higher growth rates before gradually decreasing. In all treatment groups, the relative growth rates became negative after 168 h (Table 1). LEV in all treatment groups exhibited inhibitory effects at 4 h into the experiment. The 40 and 60 mg/L groups both showed inhibition rates exceeding 25%, and the 60 mg/L group displayed significant inhibition (p< 0.05) with an inhibition rate of 50.1%. At 72 h, the 5 mg/L group stimulated algal cell growth, whereas the groups treated containing 10 and 20 mg/L exhibited inhibition rates of less than 50%. At 96 h, the 5 mg/L group once again exhibited inhibitory effects, with an inhibition rate of 39.1%, and the 10 mg/L group had an inhibition rate of 39.2%. The high-concentration groups showed significant inhibition (p< 0.05), with inhibition rates of 50.1%, 89.7%, and 93.4% for treatments with 20, 40, and 60 mg/L LEV, respectively. At 144 h, the 60 mg/L group had an inhibition rate of 96.6%, and thereafter, the inhibition rate remained relatively stable. After 192 h, the inhibition rates in all treatment groups were greater than 50% (Figure 1).
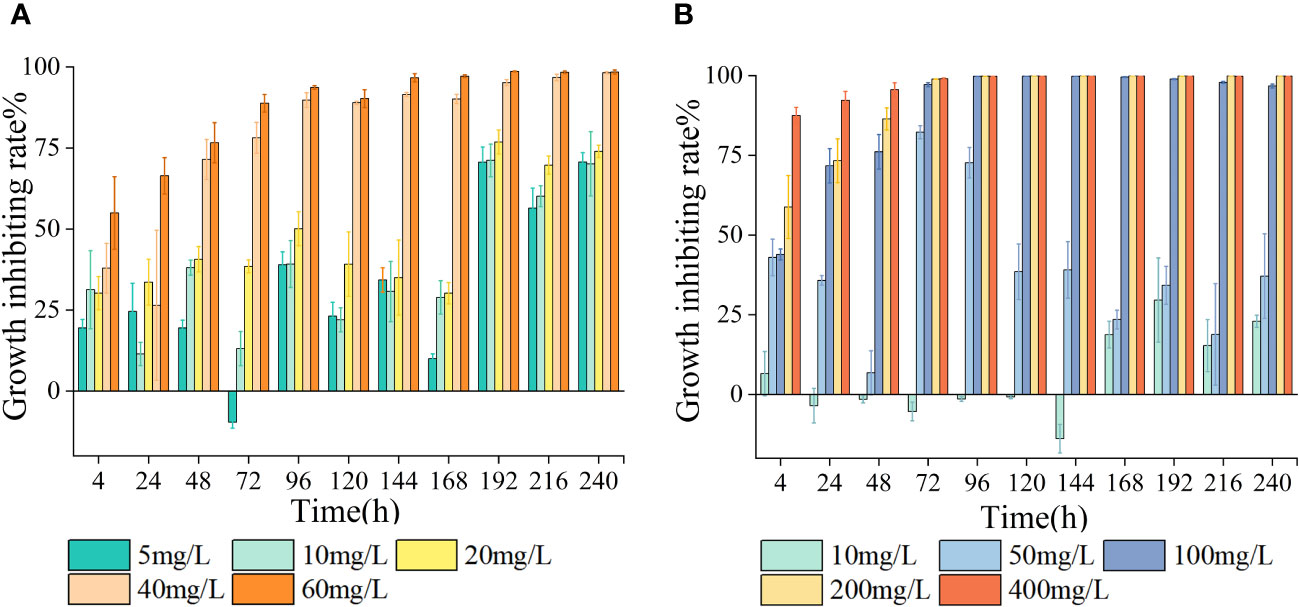
Figure 1 Effects of LEV and NORon chlorophyll a(Chla) content (A, C) and maximal quantum yield of PS II (Fv/Fm) (B, D) of Skeletonema costatum.
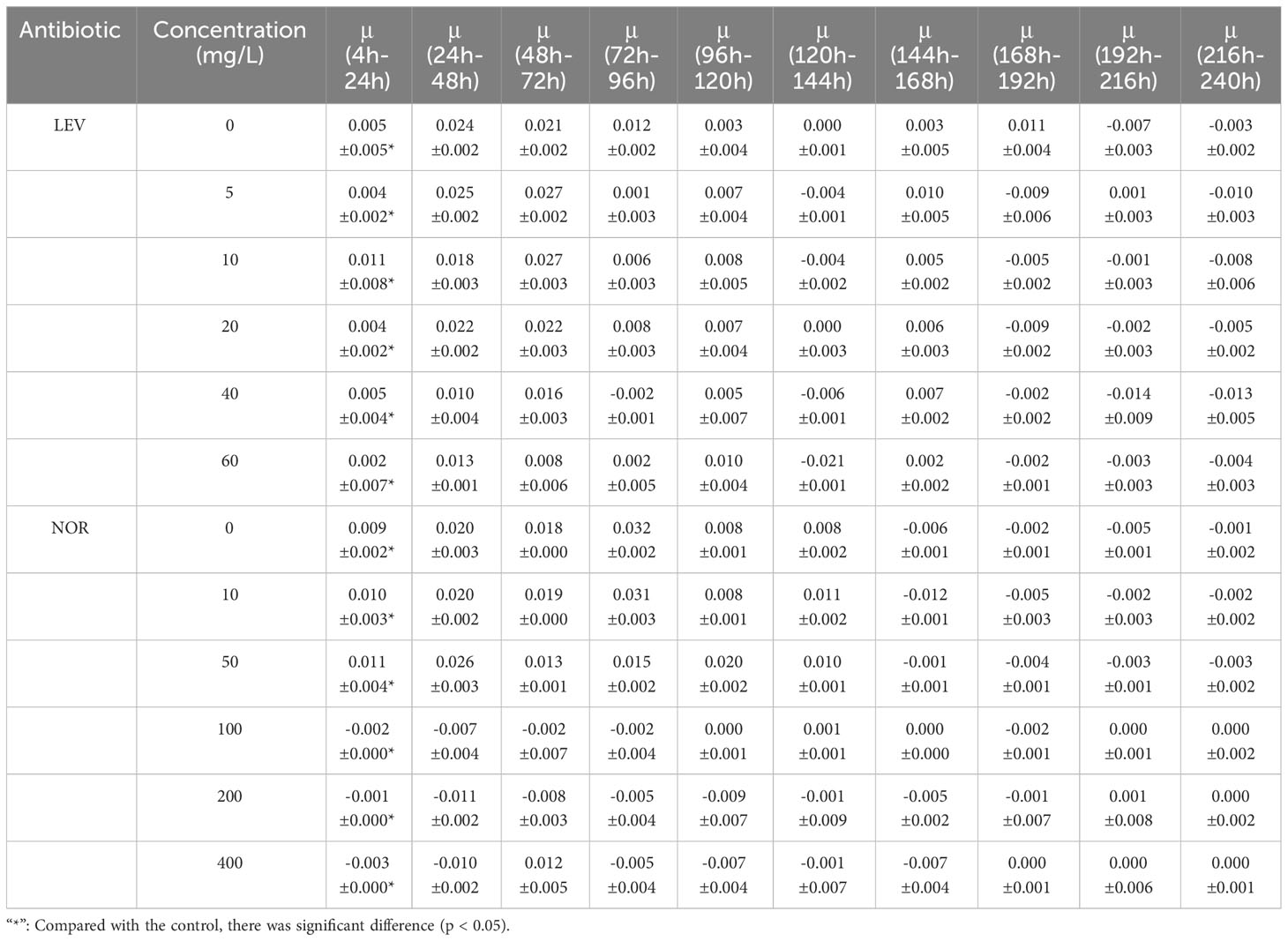
Table 1 The relative growth rate of Skeletonema costatum under the influence of different concentrations of antibiotics(μ) (h-1).
Under the influence of NOR, the relative growth rate of S. costatum exhibited significant differences compared to the control group (p< 0.05). In the low-treatment groups, growth resumed after 24 h. However, as the treatment duration increased, the relative growth rates gradually decreased. In groups with treatments exceeding 100 mg/L, the relative growth rates were either zero or negative. After 144 h, all treatment groups exhibited negative relative growth rates (Table 1). At 24 h, the 10 mg/L concentration promoted the growth of algal cells, the 20 mg/L group had an inhibition rate of less than 50%, and the other three treatment groups all exhibited significant inhibition (p< 0.05). At 96 h, the group treated with 5 mg/L exhibited a negative growth rate, and the other four treatment groups all exhibited significant inhibition, with the 50 mg/L group having an inhibition rate of 73%. The inhibition rates gradually decreased thereafter. The high-concentration groups had inhibition rates of 99.8%, 99.9%, and 99.8% for 100, 200, and 400 mg/L NOR, respectively, with inhibition rates maintaining stability thereafter. At 168 h, the 10 mg/L group showed an increase in the inhibition rate, reaching 23.5% (Figure 2).
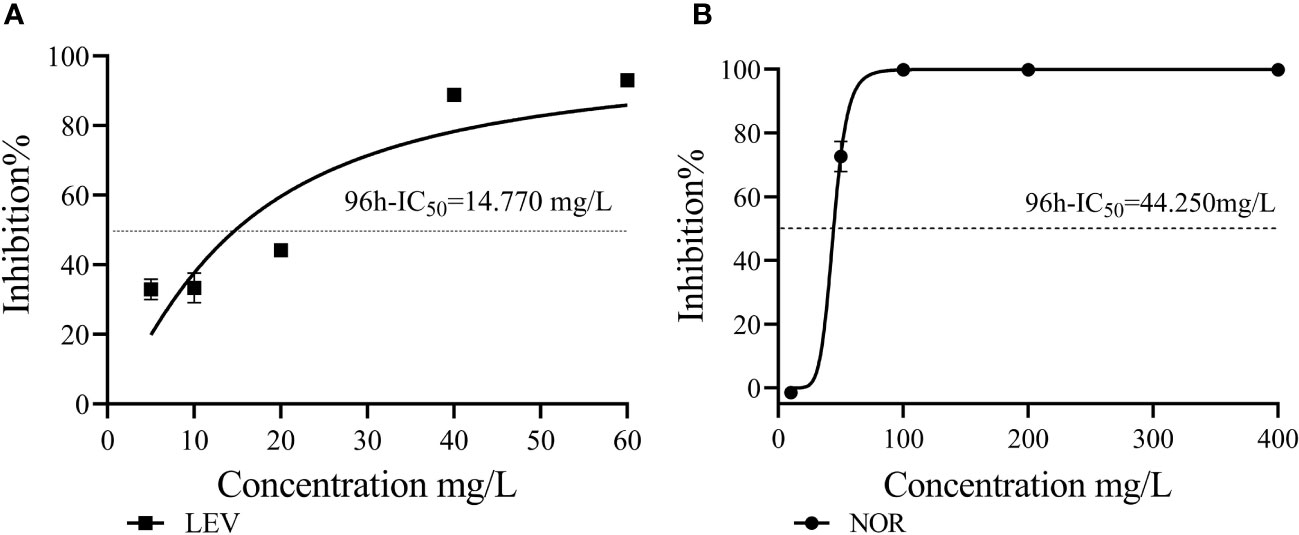
Figure 2 The 96h-IC50 values of LEV (A) and NOR (B) were compared with that of Skeletonema costatum.
LEV exerted stronger inhibitory effects on S. costatum compared with NOR. When comparing the growth inhibition rates of the two antibiotics at the same concentration (10 mg/L) under different culture durations, LEV consistently showed higher inhibition rates than NOR. Based on the dose–response curves obtained through nonlinear fitting for algal cell inhibition rates, the half-maximal inhibitory concentration (IC50) was calculated. The 96h-IC50 for LEV was 14.770 mg/L, while the 96h-IC50 for NOR was 44.250 mg/L. Therefore, the order of inhibitory effects of these two antibiotics on S. costatum was LEV > NOR.
3.2 Effect of antibiotics on the Chla and Fv/Fm
As the concentration of LEV increased, the Chla content gradually decreased compared to the control group (Figure 3). At 24 h, the Chla contents in all treatment groups were lower compared to the control group, with the 40 mg/L and 60 mg/L groups showing significantly decreased Chla levels (p< 0.05). After 48 h, the 5 mg/L group showed slightly higher Chla content compared to the control group, while the groups treated with 10, 20, 40, and 60 mg/L all contained significantly lower Chla contents (p< 0.05). After 96 h, both the groups treated with 5 mg/L and 10 mg/L showed an upward trend in Chla content, albeit remaining significantly lower than the control group (p< 0.05). For Fv/Fm, all treatment groups initially showed an increase followed by a decreasing trend, resulting in lower Fv/Fm values compared to the control group. After 24 h, the 20, 40, and 60 mg/L groups all showed significantly lower Fv/Fm values compared to the control group (p< 0.05). After 96 h, all groups, except for the 5 mg/L group, exhibited significantly lower Fv/Fm values compared to the control group (p< 0.05). At 240 h, The Fv/Fm value for the group exposed to 40 mg/L decreased to 0.003 ± 0.006, and that of the 60 mg/Ltreatment dropped to zero. The trend observed in Fv/Fm values after 24 h was consistent with that of Chla content.
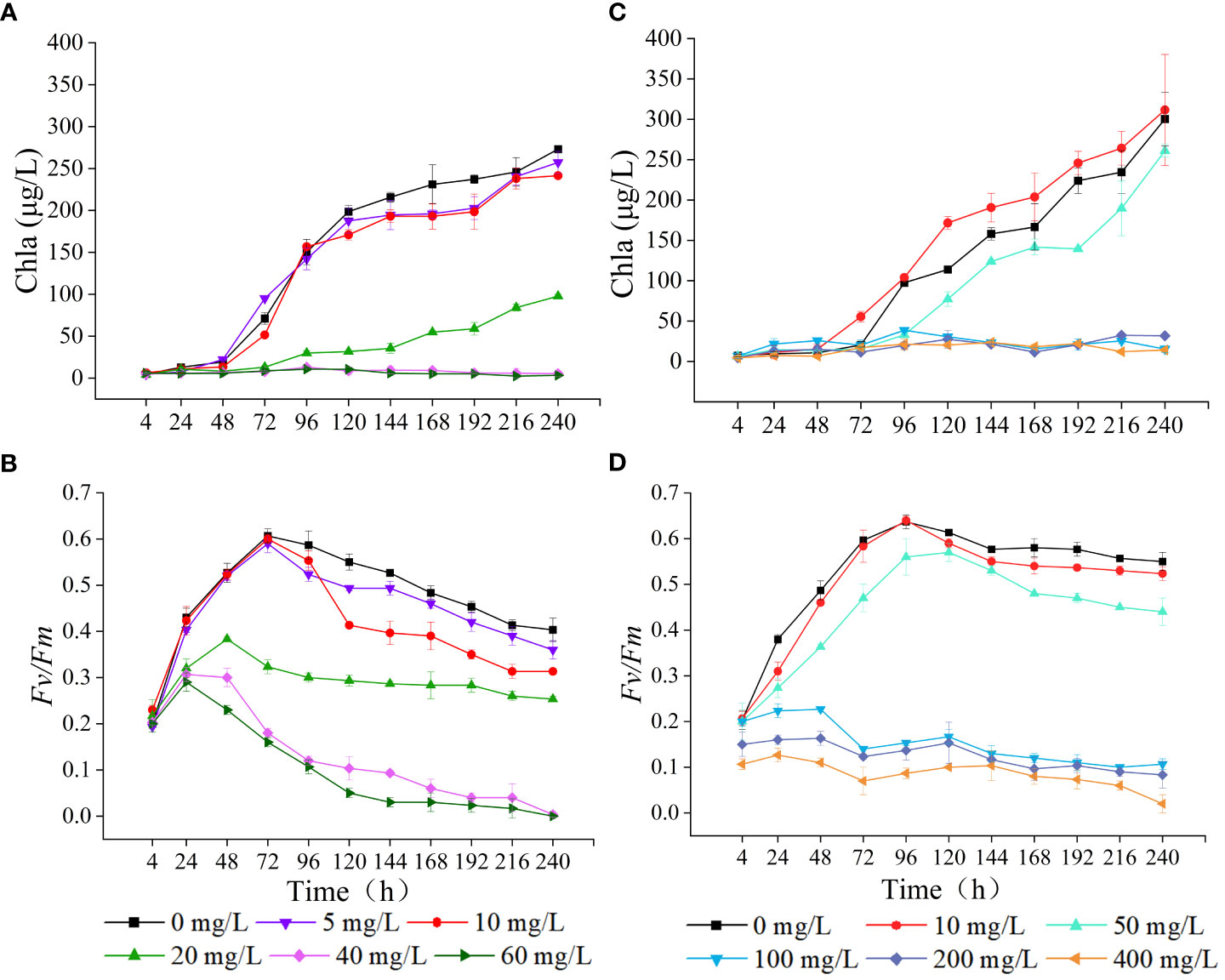
Figure 3 Effects of LEV and NOR on chlorophyll a(Chla) content (A, C) and maximal quantum yield of PSII (Fv/Fm) (B, D) of Skeletonema costatum.
Under the influence of NOR, the Chla content exhibited a trend of enhancement in low concentrations and decrease in high concentrations (Figure 3). At 24 h, NOR concentrations of 10, 50, 100, and 200 mg/L all had higher Chla content than the control group. After 24 h, Chla gradually increased in the low-concentration group, and at 72 h, the group exposed to 10 mg/L exhibited a markedly elevated Chla content compared to the control group (p< 0.05). However, at 96 h, the group treated with 50 mg/L had notably lower Chla content than the untreated group (p< 0.05). After 72 h, the Chla content in the groups treated with 100, 200, and 400 mg/L gradually decreased. By 96 h, the Chla content in each group was notably lower compared to the control group (p< 0.05). At 4 h, At 4 hours, the Fv/Fm values of the groups treated with 200 mg/L and 400 mg/L were notably lower compared to the control group (p< 0.05). After 24 h, the Fv/Fm in all treatment groups gradually increased. The groups exposed to 10 mg/L and 50 mg/L exhibited an initial increase followed by a decreasing trend, with the 10 mg/L group showing relatively small differences compared to the control. The high concentrations all showed significantly lower Chla content compared to the control (p< 0.05). After 48 h, the Fv/Fm initially declined in the groups with NOR concentrations above 100 mg/L, and then gradually increased, with the final Fv/Fm values being lower than 0.100. At 240 h, the Fv/Fm value decreased to 0.083 ± 0.029 in the 200 mg/L group, dropped to 0 in the 400 mg/L group, and remained at 0.020 ± 0.020 in the control group.
3.3 Effects of antibiotics on protein content and enzyme activity
In the group exposed to high concentrations of LEV, the protein concentration of algal cells, MDA content, and activities of SOD, GSH-PX, GR were significantly altered compared to the control group. In both the low concentrations and the control group, only the MDA content exhibited a significant difference (Figure 4). Compared to the control, the protein concentration and MDA content in the low concentrations showed a gradual increase, surpassing those of the control group. The MDA levels in the low-concentration group showed a significant increase compared to those in the control group at 96 h (p< 0.05) and gradually decreased after reaching the highest value at 120 h. In comparison to the control group, the high-concentration groups exhibited notably lower levels of protein concentration and MDA content at 96 hours and 4 hours, respectively (p< 0.05). Compared to the control, the levels of SOD, GR, GSH-PX in all treatment groups initially exhibited an increase followed by a subsequent decrease. As the treatment duration increased, in the low-concentrations the SOD, GR, and GSH-PX activities gradually became notably lower compared to high-concentrations. At 48 h, the 10 and 20 mg/L groups had significantly higher SOD activity compared to the control (p< 0.05). In both low-concentration group and the 20 mg/L group, SOD activity peaked at 72 hours before gradually declining. After 120 h, the SOD activity in both the control group and the low-concentration group significantly decreased compared to the high concentrations, reaching significance at 144 h (p< 0.05). At 96 h, the low concentrations exhibited significantly higher GR activity compared to the control group (p< 0.05). Similarly, at 120 hours, the high concentrations demonstrated significantly elevated GR activity relative to the control (p< 0.05). At 4 h, all treatments exhibited lower GSH-PX compared to the control. However, after 24 h, the GSH-PX activity in all treatments gradually increased, and in the low concentrations it reached the highest value at 72 h. After 96 h, the GSH-PX activity gradually decreased but remained higher compared to the control. In the high concentrations, the activity of GSH-PX peaked at 96 hours, surpassing significantly that of the control group beyond this time point (p< 0.05).
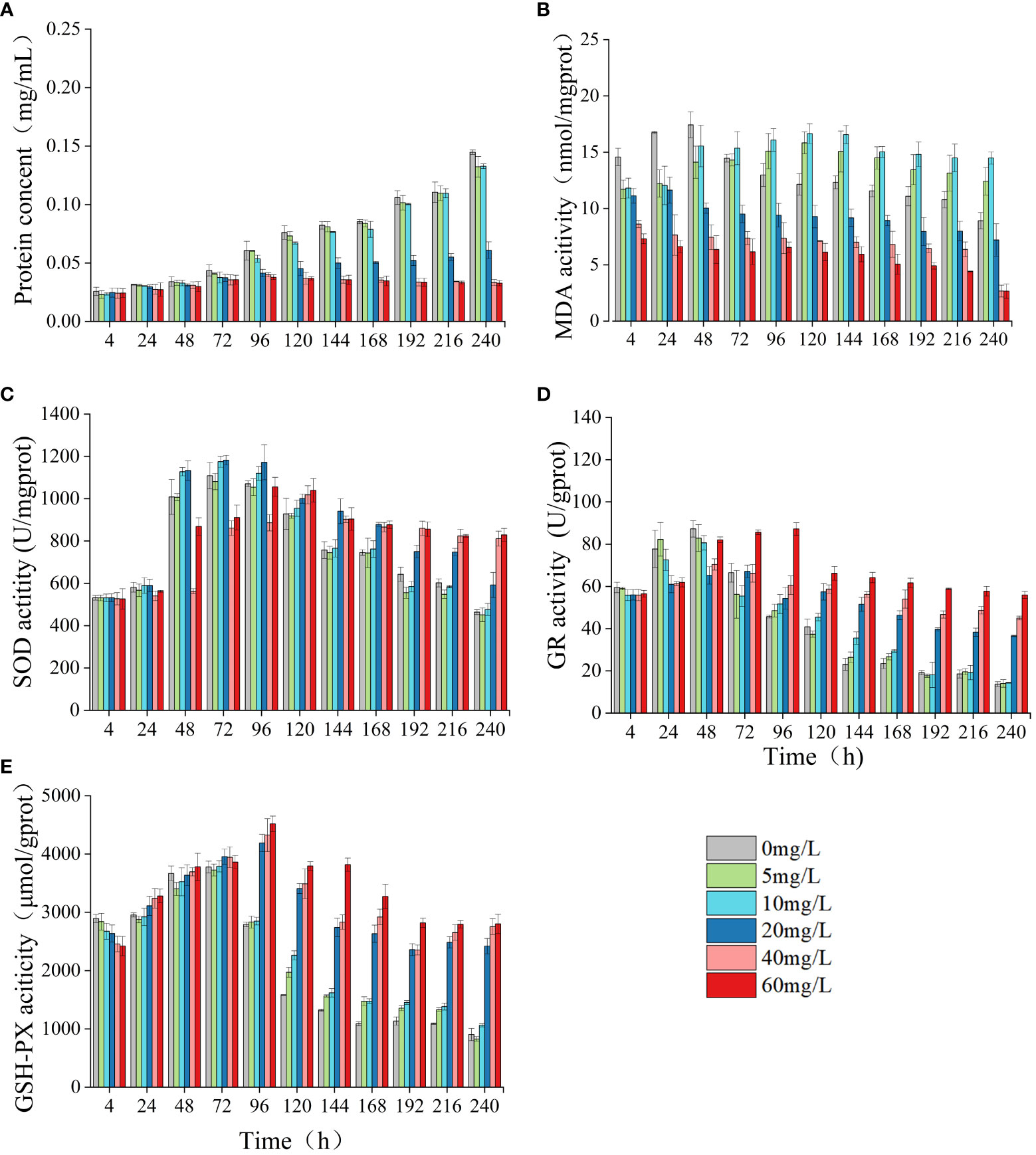
Figure 4 Effect of LEV on protein concent and antioxidant system of Skeletonema costatum. Protien concent (A), MDA (B), SOD (C), GR (D) and GSH-PX (E).
Compared to the control group, NOR had a significant impact on the algal cell protein concentration, MDA content, and the activity levels of SOD, GSH-PX, GR (Figure 5). In the low concentrations, there was a gradual increase in protein concentration, which became significantly higher than that of the control group by 168 h (p< 0.05). In the high-concentration group, the protein concentration exhibited a significant increase compared to the control group at 24 h (p< 0.05), but notably decreased relative to the control group after 96 h (p< 0.05). At 240 h, the high-concentration group exhibited a protein concentration merely one-third of that observed in the control group. The MDA content in the low concentrations exhibited an initial increase followed by a subsequent decrease. By 168 h, the MDA content in the 50 mg/L group was notably higher compared to the control (p< 0.05). In the high concentrations, the MDA content was notably lower compared to the control group at 4 h (p< 0.05) and decreased overall. The activities of SOD, GR, and GSH-PX all initially increased and then decreased. At 4 h, the SOD activities in the 50 mg/L group and the high concentrations were significantly elevated compared to those in the control group (p< 0.05). The GR and GSH-PX activities of the low-concentration group were notably elevated compared to those in the control group at 48 h and 72 h, respectively (p< 0.05). In the high-concentration group, the GR and GSH-PX levels were elevated compared to those in the control at 120 h and 96 h, respectively (p< 0.05).
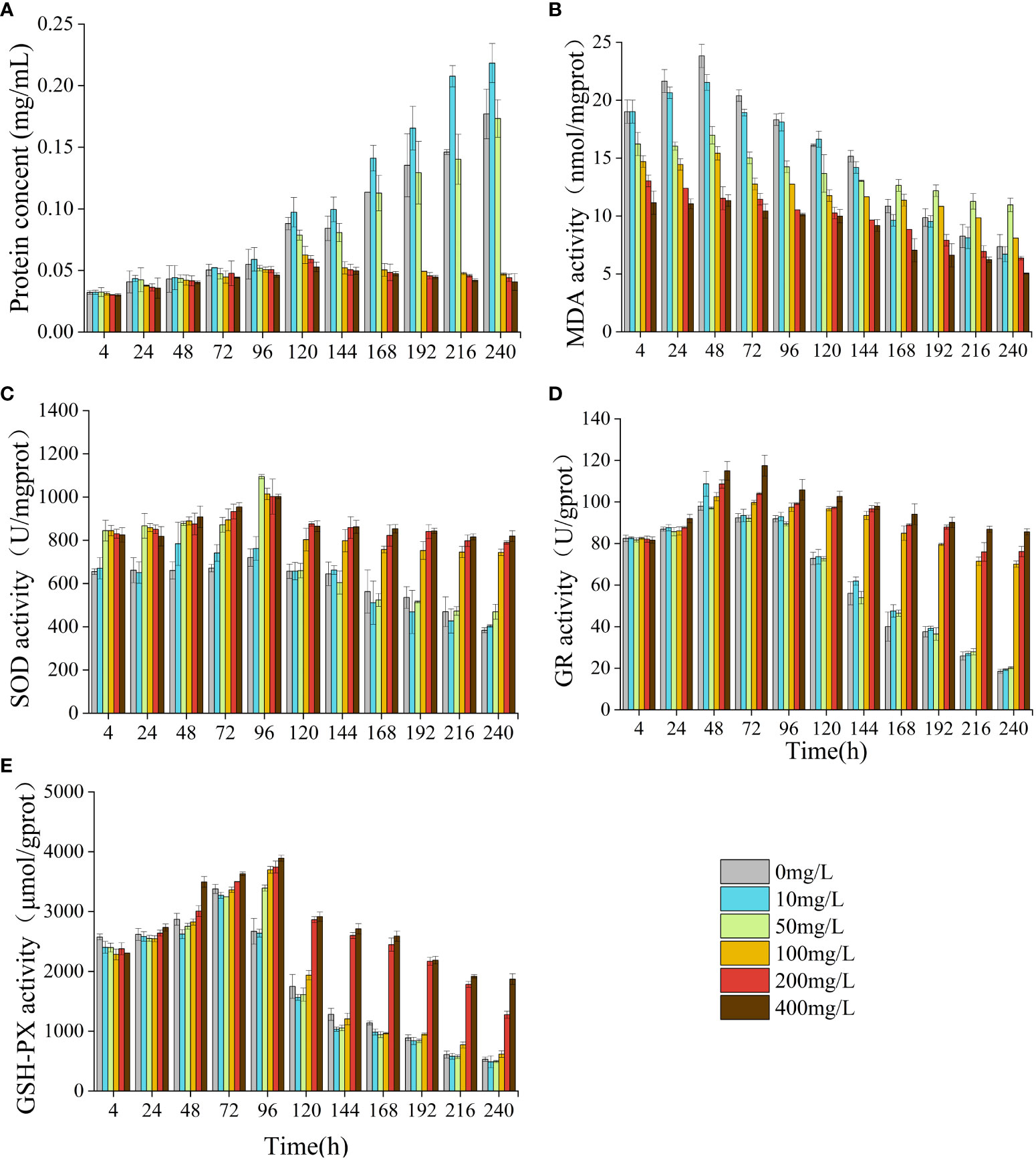
Figure 5 Effect of NOR on protein concent and antioxidant system of Skeletonema costatum. Protien concent (A), MDA (B), SOD (C), GR (D) and GSH-PX (E).
4 Discussion
4.1 Influence of LEV and NOR on the S. costatum growth
Cell density and mean specific growth rate are commonly utilized metrics for quantifying the growth of microalgae (Qian et al., 2018). the calculated inhibition rate can indicate the degree to which microalgae are inhibited under the influence of pollutants (Kurade et al., 2016). In the current study, NOR had dual impacts on S. costatum. The 10 mg/L NOR group showed a growth-promoting effect, while the other treatment groups exhibited varying degrees of inhibition. A previous study showed 1 mg/L of enrofloxacin (ENR) facilitated the growth of Ourococcus multisporus,as well as Micractinium resseri, but ENR concentrations in excess of 20 mg/L led to different levels of inhibition on O. multisporus and M. resseri (Xiong et al., 2017a). For moxifloxacin (MOX) and gatifloxaci (GAT), after being used in culture for 48 h, MOX concentrations greater than 5 mg/L significantly inhibited Chlamydomonas reinhardtii, Moreover, the inhibitory effects of both MOX and gatifloxacin (GAT) increased with prolonged culture duration (Wan et al., 2022). The presence of antibiotics in marine environments can have a dual impact on microalgae. On one hand, antibiotics may stimulate the growth of microalgae, thereby increasing primary productivity levels. However, on the other hand, they may also contribute to outbreaks of red tide. This phenomenon highlights the complex relationship between antibiotics and marine ecosystems, as they can both enhance and disrupt the delicate balance of primary productivity in the marine.
Through nonlinear fitting, it was determined that the 96h-IC50 values for LEV and NOR on algal cells were 14.770 mg/L and 44.250 mg/L, respectively. This finding suggested algal cells were more sensitive to LEV than to NOR. Therefore, algae have varying sensitivities to different antibiotics. Previous studies have shown that Pseudokirchneriella subcapitata and Anabaena subcapitata are more sensitive to LEV than NOR, with A. subcapitata being more sensitive than P. subcapitata. The median effective concentration (EC50) values for LEV and NOR in A. subcapitata were 4.8 mg/L and 5.6 mg/L, respectively (Gonzalez-Pleite et al., 2013). Other quinolone antibiotics have varying half-maximal inhibitory concentrations on microalgae. For example, for Selenastrum capricornutum, ciprofloxacin (CIP) reached EC50 at 11.3 mg/L (Magdaleno et al., 2015), for Chlorella vulgaris,ENR reached EC50 at EC50 at 124.5 mg/L (Wang et al., 2019a), and the IC50 values were found to be 5.18 mg/L for Raphidocelis subcapitata and 10.6 mg/L for Ankistrodesmus fusiformis (Carusso et al., 2018).
4.2 Impact on the Chla and Fv/Fm
Chlorophyll is essential for capturing light, converting it into energy, and facilitating energy transfer within plants. The suppression of algal growth is frequently associated with the adverse effects of toxic substances on chlorophyll synthesis (Koussevitzky et al., 2007; Nie et al., 2008). Previous research have indicated that low-concentration of antibiotics can stimulate an increase in Chla, whereas high-concentration of antibiotics can inhibit Chla and reduce the Fv/Fm (Chen et al., 2020a; Li et al., 2023). Additionally, it has been reported that antibiotics caused the elevation of photosynthetic pigments in algal cells. For example, 60 mg/L and 100 mg/L of CIP resulted in a 38% and 19% elevation, respectively, in chlorophyll content in Chlamydomonas cells (Xiong et al., 2017b). It was also found that quinolone antibiotics inhibited the photosynthesis of diatoms (Dow et al., 2020). In this study, the two antibiotics in the groups exposed to low concentrations caused the gradual increase of the Chla content of microalgae, with the Chla content of the 10 mg/L NOR group consistently surpassing that of the control group after 48 h, while at high concentrations, both antibiotics significantly inhibited the Chla content. Low doses of antibiotics increased the Chla levels possibly because the antibiotics triggered the protective mechanism of algal cells to remove ROS accumulated in chloroplasts and alter some proteins in chloroplasts to delay and reduce the damage to photosynthetic organs (Shinkai et al., 2002; Wan et al., 2014). When antibiotic concentrations exceed the self-regulation range of algal cells, their inhibition effect is strengthened, leading to structural damage in the algal cells. As a result, photosynthesis is negatively affected. Excessive ROS in algal cell membrane lipids disrupt the structure of the microalgal chloroplasts and prevent the localization of chlorophyll, ultimately resulting in the degradation of free chlorophyll in the chloroplasts, thus inhibiting algal growth and reducing the chlorophyll content (Liu et al., 2011; Wang et al., 2019b).
Maximal quantum yield of PSII (Fv/Fm) is a critical indicator of plant photosynthetic efficiency, particularly under stressful conditions (Krause, 1988). Processes such as the absorption of light, transmission of energy, and initiation of primary photochemical reactions during photosynthesis in microalgae are closely related to changes in the photosynthetic pigments. Therefore, the composition and content of photosynthetic pigments directly determine the efficiency of light energy capture. Under the influence of two low-concentration antibiotics in this study, the Fv/Fm of microalgae exhibited an initial increase followed by a subsequent decrease, whereas the high-concentration group demonstrated markedly lower Fv/Fm values compared to the control group at the 4 h. This result indicated that with an increase in the duration of treatment and antibiotic concentration, the damage to the algal cell photosynthetic system worsened, making normal photosynthesis impossible. Therefore, under the influence of antibiotics, the Fv/Fm values of algal cells vary due to factors such as the treatment duration, antibiotic concentration, and microalgae species (Chen et al., 2020b; Jia et al., 2023; Li et al., 2023).
4.3 Effects on the protein content and enzyme activity
The protein content in algal cells increases in response to external stress to maintain normal cellular growth and metabolic activities (Hu et al., 2019). MDA serves as the ultimate outcome of peroxidation reactions. Its concentration can reflect the extent of microalgae cell membrane damage caused by antibiotics at different concentrations (Ke et al., 2010). In addition, antibiotic-induced oxidative stress in microalgae activates various antioxidant defense systems, such as the SOD and GR of the enzymatic system and the GSH-PX of the non-enzymatic system, all of which can counteract and regulate excessive ROS production (Jubany-Marí et al., 2010; Nie et al., 2013; Guo et al., 2020). Under the influence of low concentrations of LEV and NOR, the protein content of algae cells was significantly elevated compared to the control group. However, exposure to high concentrations resulted in a notable decrease in protein content relative to the control group. This indicated that low concentrations of LEV and NOR stimulated S. costatum to increase protein production. The elevation in protein content could stem from ae physiological response induced by external stress. This stimulation prompted the metabolic pathways within microalgae cells, thereby protecting important cellular components and biological membranes (Guzmán-Murillo et al., 2007; Hu et al., 2019). In the current study, as the concentration of both antibiotics increased, there was a significant decrease in protein content observed in the high-concentration group. This phenomenon can be attributed to oxidative damage inflicted upon microalgal cells, which triggers the excessive production of reactive oxygen species (ROS) and subsequently leads to protein oxidation (Xiong et al., 2021). The trend of MDA content mirrored that that of protein content, exhibiting a notable rise in MDA levels in the low-concentration LEV group and a gradual increase in the 50 mg/L NOR group compared to the control group, indicating that both antibiotics promoted membrane peroxidation. In contrast, the MDA levels within the high concentrations consistently decreased, suggesting that irreversible damage had been caused to the algal cells. Antibiotics induce oxidative damage to algal cells as the malondialdehyde (MDA) generated by free radicals can cross-link with proteins, phospholipids, and nucleic acids, thereby disrupting DNA synthesis (Zhang et al., 2020b; Hena et al., 2021). Under the respective influence of the t antibiotics, the changes in SOD, GR, and GSH-PX activities in algal cells all demonstrated a pattern of initially increasing followed by decreasing. In the high-concentration group, although there was a decreasing trend with increasing treatment duration, the SOD, GR, and GSH-PX activities remained higher compared to both the control and the low-concentration group. This indicated that as the concentration of antibiotics rise, the activities of SOD and GR were not sufficient to clear the accumulated excess ROS in the algae, and GSH-PX was unable to neutralize the large amount of H2O2 produced under stress, inducing an increase in antioxidant activity (Das and Roychoudhury, 2014). The high-concentration group exhibited a higher level of oxidative stress than the low-concentration group. Therefore, in combination with the results regarding the protein and antioxidant systems, both LEV and NOR affected the enzyme activity of S. costatum, caused oxidative stress to its cells, and activated its defense system to reduce oxidative damage. Continuous stress disrupted the algal defense system, consequently damaging both the structure and function of algal cells, thereby inhibiting algal growth. This result was consistent with the behavior of algal cells under the influence of other antibiotics and pollutants. For instance, the enzyme activities of SOD, GR, and GSH-PX exhibited significant elevation in Chlorella sp., Microcystis flosaquae, C. reinhardtii, Scenedesmus obliquus, and Merismopedia subcapitata under the influence of CIP, sulfamethoxazole (SMZ), erythromycin (ERY), polystyrene microplastics, aluminum, and chlorpyrifos (Nie et al., 2013; Wan et al., 2015; Chen et al., 2016; Ameri et al., 2020; Chen et al., 2020b). In addition, under the influence of ENR or benzo[a]pyrene, the MDA content decreased in O. multisporus and Isochrysis zhanjiangensis (Shen et al., 2016; Xiong et al., 2017a).
5 Conclusion
In this investigation, we explored the impacts of two quinolone antibiotics, LEV and NOR, on S. costatum. Through an analysis of their effects on microalgal cell growth, Chla contents, Fv/Fm, protein content, antioxidant enzyme systems (SOD, GR, and GSH-PX), and non-enzymatic system (MDA), it was found that both LEV and NOR inhibited the growth of microalgae, with the cells being more sensitive to LEV. These antibiotics might affect photosynthesis through influencing the algal photosynthetic system. High doses of LEV and NOR induced significant oxidative stress in microalgae cells. This study provides a comprehensive analysis of the impacts of LEV and NOR on marine microalgae growth and elucidates the underlying physiological mechanisms. These findings significantly contribute to the assessment of ecological risks associated with antibiotics in marine environments, furnishing a theoretical foundation for the development of environmental monitoring and management strategies aimed at controlling antibiotic pollution.
Data availability statement
The original contributions presented in the study are included in the article/supplementary material, further inquiries can be directed to the corresponding author/s.
Author contributions
YL: Data curation, Software, Writing – original draft. TL: Funding acquisition, Resources, Supervision, Writing – review & editing. YZ: Data curation, Methodology, Project administration, Writing – review & editing.
Funding
The author(s) declare financial support was received for the research, authorship, and/or publication of this article. This work was supported by the Zhejiang Provincial Natural Science Foundation of China for Distinguished Young Scientists under grant No. LR21C190001.
Conflict of interest
The authors declare that the research was conducted in the absence of any commercial or financial relationships that could be construed as a potential conflict of interest.
Publisher’s note
All claims expressed in this article are solely those of the authors and do not necessarily represent those of their affiliated organizations, or those of the publisher, the editors and the reviewers. Any product that may be evaluated in this article, or claim that may be made by its manufacturer, is not guaranteed or endorsed by the publisher.
References
Aderemi A. O., Novais S. C., Lemos M. F. L., Alves L. M., Hunter C., Pahl O. (2018). Oxidative stress responses and cellular energy allocation changes in microalgae following exposure to widely used human antibiotics. Aquat. Toxicol. 203, 130–139. doi: 10.1016/j.aquatox.2018.08.008
Ameri M., Baron-Sola A., Khavari-Nejad R. A., Soltani N., Najafi F., Bagheri A., et al. (2020). Aluminium triggers oxidative stress and antioxidant response in the microalgae Scenedesmus sp. J. Plant Physiol. 246-247, 153114. doi: 10.1016/j.jplph.2020.153114
Carusso S., Juarez A. B., Moretton J., Magdaleno A. (2018). Effects of three veterinary antibiotics and their binary mixtures on two green alga species. Chemosphere 194, 821–827. doi: 10.1016/j.chemosphere.2017.12.047
Chen J. Q., Guo R. X. (2012). Access the toxic effect of the antibiotic cefradine and its UV light degradation products on two freshwater algae. J. Hazard. Mater. 209-210, 520–523. doi: 10.1016/j.jhazmat.2012.01.041
Chen S., Chen M., Wang Z., Qiu W., Wang J., Shen Y., et al. (2016). Toxicological effects of chlorpyrifos on growth, enzyme activity and chlorophyll a synthesis of freshwater microalgae. Environ. Toxicol. Pharmacol. 45, 179–186. doi: 10.1016/j.etap.2016.05.032
Chen S., Zhang W., Li J., Yuan M., Zhang J., Xu F., et al. (2020a). Ecotoxicological effects of sulfonamides and fluoroquinolones and their removal by a green alga (Chlorella vulgaris) and a cyanobacterium (Chrysosporum ovalisporum). Environ. Pollut. 263, 114554. doi: 10.1016/j.envpol.2020.114554
Chen S., Wang L., Feng W., Yuan M., Li J., Xu H., et al. (2020b). Sulfonamides-induced oxidative stress in freshwater microalga Chlorella vulgaris: Evaluation of growth, photosynthesis, antioxidants, ultrastructure, and nucleic acids. Sci. Rep. 10, 8243. doi: 10.1038/s41598-020-65219-2
Das K., Roychoudhury A. (2014). Reactive oxygen species (ROS) and response of antioxidants as ROS-scavengers during environmental stress in plants. Front. Environ. Sci. 2. doi: 10.3389/fenvs.2014.00053
Dow L., Stock F., Peltekis A., Szamosvari D., Prothiwa M., Lapointe A., et al. (2020). The multifaceted inhibitory effects of an alkylquinolone on the diatom Phaeodactylum tricornutum. Chembiochem 21, 1206–1216. doi: 10.1002/cbic.201900612
Eluk D., Nagel O., Gagneten A., Reno U., Althaus R. (2021). Toxicity of fluoroquinolones on the cladoceran Daphnia magna. Water Environ. Res. 93, 2914–2930. doi: 10.1002/wer.1631
Gao H., Li B., Yao Z. (2023). Advances in research on the presence and environmental behavior of antibiotics in the marine environment. Eviron. Chem. 42, 779–791. doi: 10.7524/j.issn.0254-6108.2022103107
Gonzalez-Pleiter M., Gonzalo S., Rodea-Palomares I., Leganes F., Rosal R., Boltes K., et al. (2013). Toxicity of five antibiotics and their mixtures towards photosynthetic aquatic organisms: implications for environmental risk assessment. Water Res. 47, 2050–2064. doi: 10.1016/j.watres.2013.01.020
Guo J., Peng J., Lei Y., Kanerva M., Li Q., Song J., et al. (2020). Comparison of oxidative stress induced by clarithromycin in two freshwater microalgae Raphidocelis subcapitata and Chlorella vulgaris. Aquat. Toxicol. 219, 105376. doi: 10.1016/j.aquatox.2019.105376
Guzmán-Murillo M. A., López-Bolaños C. C., Ledesma-Verdejo T., Roldan-Libenson G., Cadena-Roa M. A., Ascencio F. (2007). Effects of fertilizer-based culture media on the production of exocellular polysaccharides and cellular superoxide dismutase by Phaeodactylum tricornutum (Bohlin). J. Appl. Phycol. 19, 33–41. doi: 10.1007/s10811-006-9108-9
Hena S., Gutierrez L., Croué J. P. (2021). Removal of pharmaceutical and personal care products (PPCPs) from wastewater using microalgae: A review. J. Hazard. Mater. 403, 124041. doi: 10.1016/j.jhazmat.2020.124041
Hu H., Zhou Q., Li X., Lou W., Du C., Teng Q., et al. (2019). Phytoremediation of anaerobically digested swine wastewater contaminated by oxytetracycline via Lemna aequinoctialis: Nutrient removal, growth characteristics and degradation pathways. Bioresour. Technol. 291, 121853. doi: 10.1016/j.biortech.2019.121853
Huang F., An Z., Moran M. J., Liu F. (2020). Recognition of typical antibiotic residues in environmental media related to groundwater in China, (2009-2019). J. Hazard. Mater. 399, 122813. doi: 10.1016/j.jhazmat.2020.122813
Huang D. J., Hou J. H., Kuo T. F., Lai H. T. (2014). Toxicity of the veterinary sulfonamide antibiotic sulfamonomethoxine to five aquatic organisms. Environ. Toxicol. Pharmacol. 38, 874–880. doi: 10.1016/j.etap.2014.09.006
Jeffrey S. W., Humphrey G. F. (1975). New spectrophotometric equations for determining chlorophylls a, b, c1and c2 in higher plants, Algae and Natural Phytoplankton. Biochemie und Physiologie der Pflanzen 167, 191–194. doi: 10.1016/S0015-3796(17)30778-3
Jia Y., Lu J., Wang M., Qin W., Chen B., Xu H., et al. (2023). Algicidal bacteria in phycosphere regulate free-living Symbiodinium fate via triggering oxidative stress and photosynthetic system damage. Ecotoxicol. Environ. Saf. 263, 115369. doi: 10.1016/j.ecoenv.2023.115369
Jiang Y., Liu Y., Zhang J. (2021). Mechanisms for the stimulatory effects of a five-component mixture of antibiotics in Microcystis aeruginosa at transcriptomic and proteomic levels. J. Hazard. Mater. 406, 124722. doi: 10.1016/j.jhazmat.2020.124722
Jubany-Marí T., Munné-Bosch S., Alegre L. (2010). Redox regulation of water stress responses in field-grown plants. Role of hydrogen peroxide and ascorbate. Plant Physiol. Biochem. 48, 351–358. doi: 10.1016/j.plaphy.2010.01.021
Ke L., Luo L., Wang P., Luan T., Tam N. F. Y. (2010). Effects of metals on biosorption and biodegradation of mixed polycyclic aromatic hydrocarbons by a freshwater green alga Selenastrum capricornutum. Bioresour. Technol. 101, 6950–6961. doi: 10.1016/j.biortech.2010.04.011
Koussevitzky S., Stanne T. M., Peto C. A., Giap T., Sjgren L. L. E., Zhao Y., et al. (2007). An Arabidopsis thaliana virescent mutant reveals a role for ClpR1 in plastid development. Plant Mol. Biol. 63, 85–96. doi: 10.1007/s11103-006-9074-2
Krause G. H. (1988). Photoinhibition of photosynthesis. An evaluation of damaging and protective mechanisms. Physiol. Plant 74, 566–574. doi: 10.1111/j.1399-3054.1988.tb02020.x
Kurade M. B., Kim J. R., Govindwar S. P., Jeon B.-H. (2016). Insights into microalgae mediated biodegradation of diazinon by Chlorella vulgaris: Microalgal tolerance to xenobiotic pollutants and metabolism. Algal Res. 20, 126–134. doi: 10.1016/j.algal.2016.10.003
Leung H. W., Minh T. B., Murphy M. B., Lam J. C. W., So M. K., Martin M., et al. (2012). Distribution, fate and risk assessment of antibiotics in sewage treatment plants in Hong Kong, South China. Environ. Int. 42, 1–9. doi: 10.1016/j.envint.2011.03.004
Li Z., Gao X., Bao J., Li S., Wang X., Li Z., et al. (2023). Evaluation of growth and antioxidant responses of freshwater microalgae Chlorella sorokiniana and Scenedesmus dimorphus under exposure of moxifloxacin. Sci. Total Environ. 858, 159788. doi: 10.1016/j.scitotenv.2022.159788
Liang X., Wang L., Ou R., Nie X., Yang Y. F., Wang F., et al. (2015). Effects of norfloxacin on hepatic genes expression of P450 isoforms (CYP1A and CYP3A), GST and P-glycoprotein (Pgp) in Swordtail fish (Xiphophorus Helleri). Ecotoxicology 24, 1566–1573. doi: 10.1007/s10646-015-1457-1
Liou J. M., Bair M. J., Chen C. C., Lee Y. C., Chen M. J., Chen C. C., et al. (2016). Levofloxacin Sequential Therapy vs Levofloxacin Triple Therapy in the Second-Line Treatment of Helicobacter pylori: A Randomized Trial. Am. J. Gastroenterol. 111, 381–387. doi: 10.1038/ajg.2015.439
Liu Y., Gu Y., Lou Y., Wang G. (2021). Response mechanisms of domoic acid in Pseudo-nitzschia multiseries under copper stress. Environ. pollut. 272, 115578. doi: 10.1016/j.envpol.2020.115578
Liu B., Liu W., Nie X., Guan C., Yang Y., Wang Z., et al. (2011). Growth response and toxic effects of three antibiotics on Selenastrum capricornutum evaluated by photosynthetic rate and chlorophyll biosynthesis. J. Environ. Sci. (China). 23, 1558–1563. doi: 10.1016/S1001-0742(10)60608-0
Liu L., Wu W., Zhang J., Lv P., Xu L., Yan Y. (2018). Progress of research on the toxicology of antibiotic pollution in aquatic organisms. Acta Ecol. Sin. 38, 36–41. doi: 10.1016/j.chnaes.2018.01.006
Lu J., Wu J., Zhang C., Zhang Y., Lin Y., Luo Y. (2018). Occurrence, distribution, and ecological-health risks of selected antibiotics in coastal waters along the coastline of China. Sci. Total Environ. 644, 1469–1476. doi: 10.1016/j.scitotenv.2018.07.096
Ma Q., Chen L., Zhang L. (2023). Effects of phosphate on the toxicity and bioaccumulation of arsenate in marine diatom Skeletonema costatum. Sci. Total Environ. 857, 159566. doi: 10.1016/j.scitotenv.2022.159566
Magdaleno A., Saenz M. E., Juarez A. B., Moretton J. (2015). Effects of six antibiotics and their binary mixtures on growth of Pseudokirchneriella subcapitata. Ecotoxicol. Environ. Saf. 113, 72–78. doi: 10.1016/j.ecoenv.2014.11.021
Mao Y., Yu Y., Ma Z., Li H., Yu W., Cao L., et al. (2021). Azithromycin induces dual effects on microalgae: Roles of photosynthetic damage and oxidative stress. Ecotoxicol. Environ. Saf. 222, 112496. doi: 10.1016/j.ecoenv.2021.112496
Martinez J. L. (2009). Environmental pollution by antibiotics and by antibiotic resistance determinants. Environ. pollut. 157, 2893–2902. doi: 10.1016/j.envpol.2009.05.051
Nie X. P., Liu B. Y., Yu H. J., Liu W. Q., Yang Y. F. (2013). Toxic effects of erythromycin, ciprofloxacin and sulfamethoxazole exposure to the antioxidant system in Pseudokirchneriella subcapitata. Environ. pollut. 172, 23–32. doi: 10.1016/j.envpol.2012.08.013
Nie X., Wang X., Chen J., Zitko V., An T. (2008). Response of the freshwater alga Chlorella vulgaris to trichloroisocyanuric acid and ciprofloxacin. Environ. Toxicol. Chem. 27, 168–173. doi: 10.1897/07-028.1
Pan Y., Liu C., Li F., Zhou C., Yan S., Dong J., et al. (2017). Norfloxacin disrupts Daphnia magna induced colony formation in Scenedesmus quadricauda and facilitates grazing. Ecol. Eng. 102, 255–261. doi: 10.1016/j.ecoleng.2017.02.037
Pan X., Zhang D., Chen X., Mu G., Li L., Bao A. (2009). Effects of levofloxacin hydrochloride on photosystem II activity and heterogeneity of Synechocystis sp. Chemosphere 77, 413–418. doi: 10.1016/j.chemosphere.2009.06.051
Pinckney J. L., Hagenbuch I. M., Long R. A., Lovell C. R. (2013). Sublethal effects of the antibiotic tylosin on estuarine benthic microalgal communities. Mar. pollut. Bull. 68, 8–12. doi: 10.1016/j.marpolbul.2013.01.006
Qian L., Qi S., Cao F., Zhang J., Zhao F., Li C., et al. (2018). Toxic effects of boscalid on the growth, photosynthesis, antioxidant system and metabolism of Chlorella vulgaris. Environ. Pollut. 242, 171–181. doi: 10.1016/j.envpol.2018.06.055
Shen C., Miao J., Li Y., Pan L. (2016). Effect of benzo[a]pyrene on detoxification and the activity of antioxidant enzymes of marine microalgae. J. Ocean Univ. China. 15, 303–310. doi: 10.1007/s11802-016-2771-9
Shinkai K., Mohrs M., Locksley R. M. (2002). Helper T cells regulate type-2 innate immunity in vivo. Nature 420, 825–829. doi: 10.1038/nature01202
Sies H. (1997). Oxidative stress: oxidants and antioxidants. Exp. Physiol. 82, 291–295. doi: 10.1113/expphysiol.1997.sp004024
Sun M., Lin H., Guo W., Zhao F., Li J. (2017). Bioaccumulation and biodegradation of sulfamethazine in Chlorella pyrenoidosa. J. Ocean Univ. China. 16, 1167–1174. doi: 10.1007/s11802-017-3367-8
Tang S., Yin H., Chen S., Peng H., Chang J., Liu Z., et al. (2016). Aerobic degradation of BDE-209 by Enterococcus casseliflavus: Isolation, identification and cell changes during degradation process. J. Hazard. Mater. 308, 335–342. doi: 10.1016/j.jhazmat.2016.01.062
Teisseire H., Guy V. (2000). Copper-induced changes in antioxidant enzymes activities in fronds of duckweed (Lemna minor). Plant Sci. 153, 65–72. doi: 10.1016/S0168-9452(99)00257-5
Wan J., Guo P., Peng X., Wen K. (2015). Effect of erythromycin exposure on the growth, antioxidant system and photosynthesis of Microcystis flos-aquae. J. Hazard. Mater. 283, 778–786. doi: 10.1016/j.jhazmat.2014.10.026
Wan J., Guo P., Zhang S. (2014). Response of the cyanobacterium Microcystis flos-aquae to levofloxacin. Environ. Sci. pollut. Res. Int. 21, 3858–3865. doi: 10.1007/s11356-013-2340-3
Wan L., Wu Y., Zhang Y., Zhang W. (2022). Toxicity, biodegradation of moxifloxacin and gatifloxacin on Chlamydomonas reinhardtii and their metabolic fate. Ecotoxicol. Environ. Saf. 240, 113711. doi: 10.1016/j.ecoenv.2022.113711
Wang C., Dong D., Zhang L., Song Z., Hua X., Guo Z. (2019b). Response of freshwater biofilms to antibiotic florfenicol and ofloxacin stress: role of extracellular polymeric substances. Int. J. Environ. Res. 16, 715. doi: 10.3390/ijerph16050715
Wang Z. H., Nie X. P., Yue W. J., Li X. (2012). Physiological responses of three marine microalgae exposed to cypermethrin. Environ. Toxicol. 27, 563–572. doi: 10.1002/tox.20678
Wang G., Zhang Q., Li J., Chen X., Lang Q., Kuang S. (2019a). Combined effects of erythromycin and enrofloxacin on antioxidant enzymes and photosynthesis-related gene transcription in Chlorella vulgaris. Aquat. Toxicol. 212, 138–145. doi: 10.1016/j.aquatox.2019.05.004
White M., Lenzi K., Dutcher L. S., Saw S., Morgan S. C., Binkley S., et al. (2019). 124. Impact of levofloxacin MIC on outcomes with levofloxacin step-down therapy in enterobacteriaceae bloodstream infections. Open Forum Infect. Dis. 6, S10. doi: 10.1093/ofid/ofz360.199
Xiong Q., Hu L. X., Liu Y. S., Zhao J. L., He L. Y., Ying G. G. (2021). Microalgae-based technology for antibiotics removal: From mechanisms to application of innovational hybrid systems. Environ. Int. 155, 106594. doi: 10.1016/j.envint.2021.106594
Xiong J. Q., Kurade M. B., Jeon B. H. (2017a). Ecotoxicological effects of enrofloxacin and its removal by monoculture of microalgal species and their consortium. Environ. pollut. 226, 486–493. doi: 10.1016/j.envpol.2017.04.044
Xiong J. Q., Kurade M. B., Kim J. R., Roh H. S., Jeon B. H. (2017b). Ciprofloxacin toxicity and its co-metabolic removal by a freshwater microalga Chlamydomonas mexicana. J. Hazard. Mater. 323, 212–219. doi: 10.1016/j.jhazmat.2016.04.073
Zhang Y., He D., Chang F., Dang C., Fu J. (2021a). Combined effects of sulfamethoxazole and erythromycin on a freshwater microalga, Raphidocelis subcapitata: toxicity and oxidative stress. Antibiotics (Basel). 10, 576. doi: 10.3390/antibiotics10050576
Zhang Y., Li M., Chang F., Yi M., Ge H., Fu J., et al. (2023). The distinct resistance mechanisms of cyanobacteria and green algae to sulfamethoxazole and its implications for environmental risk assessment. Sci. Total Environ. 854, 158723. doi: 10.1016/j.scitotenv.2022.158723
Zhang L., Shen L., Qin S., Cui J., Liu Y. (2020a). Quinolones antibiotics in the Baiyangdian Lake, China: Occurrence, distribution, predicted no-effect concentrations (PNECs) and ecological risks by three methods. Environ. pollut. 256, 113458. doi: 10.1016/j.envpol.2019.113458
Zhang Y., Xu N., Li Y. (2021b). Effect of the extracts of Sargassum fusiforme on red tide microalgae in East China sea. Front. Mar. Sci. 2021. doi: 10.3389/fmars.2021.628095
Zhang Y., Zhang X., Guo R., Zhang Q., Cao X., Suranjana M., et al. (2020b). Effects of florfenicol on growth, photosynthesis and antioxidant system of the non-target organism Isochrysis galbana. Comp. Biochem. Physiol. C Toxicol. Pharmacol. 233, 108764. doi: 10.1016/j.cbpc.2020.108764
Keywords: levofloxacin, norfloxacin, Skeletonema costatum, photosynthesis, antioxidant system
Citation: Lin Y, Li T and Zhang Y (2024) Effects of two typical quinolone antibiotics in the marine environment on Skeletonema costatum. Front. Mar. Sci. 11:1335582. doi: 10.3389/fmars.2024.1335582
Received: 09 November 2023; Accepted: 28 February 2024;
Published: 18 March 2024.
Edited by:
Kun Chen, Jiangsu University, ChinaReviewed by:
Yao Zheng, Chinese Academy of Fishery Sciences (CAFS), ChinaFutian Li, Jiangsu Ocean University, China
Copyright © 2024 Lin, Li and Zhang. This is an open-access article distributed under the terms of the Creative Commons Attribution License (CC BY). The use, distribution or reproduction in other forums is permitted, provided the original author(s) and the copyright owner(s) are credited and that the original publication in this journal is cited, in accordance with accepted academic practice. No use, distribution or reproduction is permitted which does not comply with these terms.
*Correspondence: Yurong Zhang, eXVyb25nemhhbmcyMDA4QDE2My5jb20=