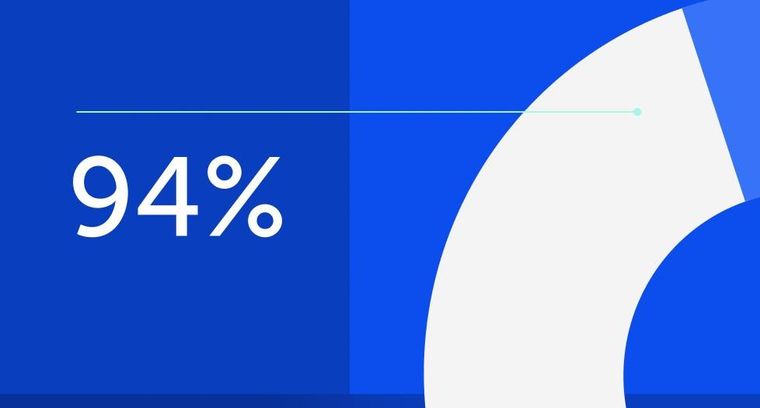
94% of researchers rate our articles as excellent or good
Learn more about the work of our research integrity team to safeguard the quality of each article we publish.
Find out more
ORIGINAL RESEARCH article
Front. Mar. Sci., 15 February 2024
Sec. Aquatic Physiology
Volume 11 - 2024 | https://doi.org/10.3389/fmars.2024.1334937
Introduction: The transplantation of seagrass fragments with shoots and rhizomes is the most common method for the ecological restoration of damaged seagrass meadows.
Methods: The aim of this study was to explore the effects of exogenous addition (10 mg per month for 3 months) of three commonly used plant growth regulators (PGRs), including indoleacetic acid (IAA), gibberellin (GA3), and paclobutrazol (PP333) on the growth and physiology of transplanted Thalassia hemprichii fragments (TTFs), with and without the rhizome apex (RA), using a simulation test. [Results] IAA and GA3 elevated the photosynthetic capacity and growth rate of TTF leaves but did not significantly alter leaf length and width. In contrast, PP333 reduced the leaf photosynthetic capacity and growth rate, while greatly increasing the leaf width and root viability. Additionally, PP333 treatment led to an increase in antioxidant enzyme activities (peroxidase [POD], superoxide dismutase [SOD], and catalase [CAT]), as well as malondialdehyde (MDA) and total phenol contents in TTFs, indicating some stress effects on the seagrass. Furthermore, IAA and GA3 decreased soluble sugar and protein contents and increased starch content in TTF tissues, whereas PP333 treatment elevated both nonstructural carbohydrate and soluble protein contents. The presence of RA positively affected the growth and physiology of T. hemprichii compared with TTFs without RA. There was a significant interaction between the PGRs and RA on leaf growth rate, chlorophyll fluorescence variables, and photosynthetic pigment content of the TTFs (p < 0.05).
Discussion: This study provides guidelines for the application of PGRs during the transplantation of T. hemprichii fragments for the restoration of seagrass meadows.
Seagrasses are the only angiosperms that exclusively inhabit seawater (Waycott et al., 2009; Reynolds et al., 2016). These underwater ecosystems often form extensive seagrass meadows. Seagrass meadows have important ecological functions, as they oxygenate the water column (Hoang et al., 2021), regulate nutrients (Jacob et al., 2013), stabilize sediments (Giulia et al., 2021), provide nursery grounds for important recreational fisheries (Jones et al., 2018), and serve as important food sources and habitats for dugongs and sea turtles (Samantha et al., 2017). Unfortunately, seagrass meadows face serious threats due to global climate change and human activities, leading to accelerated degradation processes (Holon et al., 2018; James et al., 2023). Research has shown that seagrass meadow areas have been declining at a rate of approximately 7% per year, and up to 35% of seagrass meadows have been under severe stress since 1990 (Duarte et al., 2013). Similar to most seagrass meadows worldwide, the area of seagrass meadows around Hainan Island in China is shrinking due to a variety of factors (environmental, biological, and climatic) (Glenn et al., 2016).
Efforts to restore and rehabilitate seagrass meadows have been ongoing since the mid-20th century (Maite et al., 2010; Yue et al., 2019). Three types of methods are commonly used to restore the damaged seagrass meadows: i) habitat improvement (Fonseca et al., 2000; van Katwijk et al., 2009); 2) asexual reproduction, including transplantation of seagrass patches or rhizomes (Horn et al., 2009; Li et al., 2010); 3) sexual reproduction, including seed planting, seedling transplantation, etc. (Jarvis and Moore, 2010; Tanner and Parham, 2010). However, due to complex factors, successful precedents in this field have been relatively limited (Statton et al., 2012). Currently, the main method for ecologically restoring seagrass meadows involves transplanting seagrass fragments, as this method minimizes damage to the source seagrass meadows (Park et al., 2021). However, due to the inherent genetic characteristics of seagrasses (Pazzaglia et al., 2021), as well as the inadequate adaptation of the transplanted seagrass fragments to the hydrodynamic, temperature, salinity, nutrient levels, etc. of the new environment, a slower growth rate, mortality or substantial losses often occur, leading to the failure of seagrass meadow restoration programs (Moreno et al., 2001). To optimize the techniques and methods for transplanting seagrass fragments, some researchers have treated seagrasses with plant growth regulators (PGRs) to promote the growth of shoots and roots, enhancing the survival rate of transplanted seagrass fragments and the overall success of seagrass meadow restoration (Balestri and Bertini, 2003; Giulia et al., 2021).
PGRs are synthesized based on an understanding of the structure and mechanisms of natural plant hormones, exhibiting physiological and biological effects similar to those of plant hormones (Guzmán et al., 2021). PGRs can be used not only in terrestrial plants, but also in some marine plants, such as mangroves, seagrasses, and seaweeds, to promote growth and development (Balestri and Bertini, 2003; Hong et al., 2018; Ali et al., 2020). For example, research has shown that treating Posidonia oceanica seedlings with α-naphthalene acetic acid and indole-3-butyric acid accelerated root formation and leaf growth (Balestri and Bertini, 2003). Both cytokinins and gibberellin (GA3) can induce leaf and rhizome growth in Cymodocea nodosa explants and can be used either alone or in combination (Balestri and Lardicci, 2006; García-Jiménez et al., 2006; Maite et al., 2010).
Thalassia hemprichii is one of the dominant species of seagrass meadows in the Western Pacific-Indian Ocean marine region (Waycott et al., 2009). They typically occupy the coastal area from intertidal to partially subtidal zones (Saewong et al., 2022), forming either a single population or a mixed seagrass community with other seagrasses (e.g., Enhalus acoroides, Cymodocea rotundata) (Rocío et al., 2018; Liu et al., 2022). Although some studies have investigated the effects of PGRs on seagrasses, limited information exists regarding the application of PGRs during the transplantation of the tropical seagrass T. hemprichii (Balestri and Lardicci, 2006; Yu et al., 2023).
Indoleacetic acid (IAA) is a plant growth promoter that is widely used in agriculture and forestry because it effectively promotes the elongation and division of plant cells (Glasby et al., 2015; Zhang et al., 2016). GA3, another commonly used plant growth regulator, regulates leaf extension, root development, the shedding of plant organs (Muniandi et al., 2018; Shan et al., 2021). In contrast, paclobutrazol (PP333) is a plant growth retardant that inhibits the elongation of plant internodes and promotes short and strong plant forms (Liu et al., 2018; Zhang et al., 2023). In this study, our primary objective was to explore how these three PGRs (IAA, GA3, and PP333) affect the growth, morphology, and physiology of transplanted T. hemprichii fragments (TTFs).
Furthermore, it has been shown that the rhizome apex (RA) serves as the meristematic zone of seagrass roots, containing numerous meristematic cells that effectively promote the growth and elongation of seagrass roots and rhizomes. These cells also play a pivotal role in regulating the growth and development of the entire seagrass plant by secreting various endogenous plant hormones (Narayan et al., 2005; Zhukovskaya et al., 2020). Therefore, the secondary objective of this study was to investigate potential differences in the growth and physiology of TTFs with and without RA in the context of PGR application.
T. hemprichii and sediment samples were collected during low tide in March 2022 from the seagrass distribution area in Xincun Bay, Hainan Province (18°24’26”N, 109°59’05”E). Subsequently, the collected T. hemprichii samples were transported to the laboratory, where dead and senescent leaves and epiphytes were carefully removed. T. hemprichii plants were then dissected using a precision knife to obtain standardized TTFs with RA (RA) or without RA (NRA) (Figure 1). All TTFs were inserted into sediments, which were laid flat in an incubator, with seawater covering the seagrass leaves. These incubators, housing the TTFs, were placed in a greenhouse made of glass for 2 weeks of pre-cultivation for acclimation, after which the main experiment commenced. During pre-cultivation, natural light was applied, and water curtains and atomization devices were used to control the temperature inside the greenhouse to not exceed 42 °C. The salinity of the seawater (drawn from the sea near the seagrass source) was controlled within the range of 32–34 ‰. To maintain water circulation, a moderate wave pump was used, and the seawater was replaced every 3 days.
Figure 1 The standardized T. hemprichii fragment for transplantation with (A) or without (B) rhizome apex. Each standardized fragment includes a completed T. hemprichii shoot and a segment of rhizome approximately 10 cm.
Three different PGRs were used in this study: IAA, GA3, and PP333 (all purchased from Shanghai McLean Biochemical Science and Technology Co., Ltd.). A control group (CK) was established without the application of any PGR. The TTFs were divided into two categories: NRA and RA. Therefore, eight treatment groups were created for this study. Each group was subjected to three replicate experiments.
Ten pre-cultured TTFs (NRA or RA) were transplanted into a polyethylene incubator (37 cm × 25 cm × 22 cm) with a flat (5 cm thick) layer of sediment. Seawater was added until all seagrass leaves were submerged (15 cm deep). To allow the PGR to be better absorbed by the seagrass root system and ensure its long-term efficacy, an ethanol solution (2 mL) containing 10 mg of PGR was diluted with 50 mL of seawater. The entire mixture was slowly injected into the sediment at multiple points using a syringe. The CK was injected with an equal volume of seawater.
After PGR application, all incubators were placed in the greenhouse for incubation under the same conditions as the pre-cultivation. The incubation process lasted for 90 days. During the process, a Temperature-light Data Logger (HOBO-UA-64, USA) was used to monitor and record the temperature and photon flux density inside the greenhouse, with a frequency of every 60 minutes. The results are shown in Supplementary Figure S1. Additional doses of 10 mg of PGRs were injected into the sediments on days 31 and 61 after the start of incubation.
During the final 10 days of incubation, five T. hemprichii plants were randomly selected from each treatment group to determine their growth rate using the pinhole method (Zieman, 1974). All other morphological and physiological indices of T. hemprichii were measured at the end of the incubation period.
To determine leaf area, five mature T. hemprichii leaves were randomly selected from each treatment group and the length and width of the leaves were measured. Leaf area was calculated as leaf length × leaf width. Three seagrass plants were randomly selected, and their root viability was determined according to a previously described method (Kulikova et al., 2011).
Chlorophyll fluorescence was measured in vivo using a Diving-PAM-II (Heinz Walz, Effeltrich, Germany) (Lu et al., 2020). Rapid light curves (RLC) of relative electron transport rate (rETR) through photosystem II (PSII) versus irradiance were obtained by subjecting leaves to light intensities ranging from 0–1200 μmol photons m-2 s-1 for 10 s per step. The rETR was calculated from the RLC data using the formula rETR = ΔF/Fm′ × PAR × 0.5 × 0.84. The maximum rETR (rETRmax) and photosynthetic light-harvesting efficiency (α, the initial slope of the RLC) were obtained by fitting the RLC to the equation of Eilers and Peeters (1988) using the least squares method.
Pulse-amplitude-modulated fluorescence measurements were employed to accurately assess the photosynthetic properties of plants and reflect the photosynthetic responses of seagrass to experimental treatments. Before measurement, the plants were dark-adapted by shading for 30 min, and three leaves were randomly selected. Under a photosynthetically active radiation of 131 μmol photons m-2 s-1 (635 nm), the minimum fluorescence (Fo) and maximum fluorescence (Fm) of seagrass leaves were measured. Additional parameters such as the maximum photochemical efficiency (Fv/Fm), photochemical burst coefficient (qP), non-photochemical burst coefficient (NPQ), and actual photochemical quantum yield (ΦPSII) of PSII were calculated using equations provided by Jiang et al. (2010).
The chlorophyll (chlorophyll a, Chla; chlorophyll b, Chlb) and carotenoid contents in the leaves of T. hemprichii were determined using the acetone extraction method (Jung et al., 2016), and the total photosynthetic pigment content was calculated as the sum of the above three components. Peroxidase (POD), superoxide dismutase (SOD), and catalase (CAT) activities, as well as malondialdehyde (MDA) content of T. hemprichii tissues (leaves and roots) were determined using the corresponding kits (Nanjing Jiancheng Institute of Bioengineering). Non-structural carbohydrates, including soluble sugars and starch, were quantified using the anthrone and colorimetric methods described by Jiang et al. (2012). Soluble protein content was determined using the Coomassie Brilliant Blue method (Wu and Shen, 2021). Total phenol content was determined using the forintol colorimetric method (Tosun et al., 2009).
Data were analyzed using SPSS software (version 26.0; IBM Corp., Armonk, NY, USA), with LSD (least significant difference) post hoc tests for pairwise comparisons. All measurements were performed on randomly selected samples, and the data are presented as the mean ± standard deviation (SD) of at least three replicates. One-way analysis of variance (ANOVA) and multiple comparisons were used to analyze different PGRs with the same TTF morphology (RA or NRA). Independent-sample t-tests were used to analyze different TTFs (RA and NRA) within the same PGR group. Furthermore, interaction analyses between different factors were performed using two-way or three-way ANOVA. Graphs were created using GraphPad Prism software. Statistical significance was set at p < 0.05.
As shown in Figure 2A, three months after transplantation, the leaf growth rates of TTFs under different treatments ranged from 0.33 ± 0.12 to 1.48 ± 0.12 mm d-1. The addition of IAA and GA3 significantly enhanced the leaf growth rate of TTFs, regardless of the presence or absence of RA (p < 0.05). In contrast, PP333 significantly reduced the seagrass growth rate (p < 0.05). The TTFs with RA that were treated with PP333 had a significantly higher growth rate than those without RA (p < 0.01), whereas the other treatments (CK, IAA, and GA3) showed no significant difference in growth rate between the two TTF types (p > 0.05).
Figure 2 Effects of PGRs on leaf growth rate (A), leaf length (B), leaf width (C), and leaf area (D) of transplanted T. hemprichii fragments. NRA, transplanted T. hemprichii fragments without rhizome apex; RA, transplanted T. hemprichii fragments with rhizome apex; CK, control; IAA, indoleacetic acid; GA3, gibberellin; PP333, paclobutrazol. Different lowercase letters indicate significant differences (p < 0.05) in transplanted T. hemprichii fragments without rhizome apex applied with different PGRs treatments; different uppercase letters indicate significant differences (p < 0.05) in transplanted T. hemprichii fragments with rhizome apex applied with different PGRs treatments. * and ** indicate significant (p < 0.05) or highly significant (p < 0.01) differences between treatments of transplanted T. hemprichii fragments with and without rhizome apexes, respectively, when the same PGRs were applied.
For TTFs without RA, the PP333 treatment resulted in significantly shorter leaf lengths compared to the other treatments (p < 0.05; Figure 2B), whereas for TTFs with RA, the PP333 treatment had significantly (p < 0.05) shorter leaf length than the GA3 treatment. Compared with the CK, the GA3 treatment significantly decreased the leaf width of TTFs without RA (p < 0.05; Figure 2C), whereas the PP333 treatment significantly increased leaf width (p < 0.05). For TTFs with RA, PP333 treatment resulted in significantly greater leaf width compared to all other treatments (p < 0.05). When comparing the TFFs with and without RA, leaf length was significantly enhanced by PP333 (p < 0.05) and leaf width was significantly enhanced by GA3 (p < 0.01).
For TTFs without RA, the leaf area was significantly greater in the IAA treatment than in the GA3 treatment (p < 0.05); however, there were no significant differences among the other treatments (p > 0.05, Figure 2D).
Two-way ANOVA (Supplementary Table S1) showed that PGRs significantly affected the leaf growth rate, length, and width of TTFs (p < 0.01), but there was no significant effect on leaf area (p > 0.05). The presence or absence of RA significantly affected the leaf growth rate and leaf area (p < 0.01) of TTFs, as well as leaf width (p < 0.05); however, it had no significant effect on leaf length (p > 0.05). Furthermore, PGR and RA had significant (p < 0.01) interactions with leaf growth rate, but not with the other variables (p > 0.05).
As shown in Figure 3, for TTFs without RA, the GA3 treatment significantly reduced root vitality compared to the other treatments (p < 0.05), whereas the PP333 treatment led to significantly higher root vitality (p < 0.05). In contrast, for TTFs with RA, the IAA and PP333 treatments significantly increased root viability compared to the CK and GA3 treatments (p < 0.05). The presence of RA significantly enhanced root vitality of the TTFs in the CK, IAA, and GA3 groups (p < 0.05). Moreover, the most significant enhancement was observed in the TTFs treated with PP333 (p < 0.01). Both PGR and RA significantly affected the root vitality of the TTFs (p < 0.01), but there was no significant association between them (Supplementary Table S1).
Figure 3 Effect of PGRs on root vitality of transplanted T. hemprichii fragments. NRA, transplanted T. hemprichii fragments without rhizome apex; RA, transplanted T. hemprichii fragments with rhizome apex; CK, control; IAA, indoleacetic acid; GA3, gibberellin; PP333, paclobutrazol. Different lowercase letters indicate significant differences (p < 0.05) in transplanted T. hemprichii fragments without rhizome apex applied with different PGRs treatments; different uppercase letters indicate significant differences (p < 0.05) in transplanted T. hemprichii fragments with rhizome apex applied with different PGRs treatments. * and ** indicate significant (p < 0.05) or highly significant (p < 0.01) differences between treatments of transplanted T. hemprichii fragments with and without rhizome apexes, respectively, when the same PGRs were applied.
As shown in Figure 4, the parameters of RLC, α, and rETRmax, were increased in the IAA group compared to the CK group, but significantly decreased in the PP333 group (p < 0.05). Moreover, α and rETRmax were significantly higher for TTFs with RA than for TTFs without RA when PP333 was applied (p < 0.05 or p < 0.01). The type of PGR had a significant effect on α and rETRmax (p < 0.01), and RA had a significant effect on rETRmax (p < 0.01). However, there was no significant interaction between the PGR and RA (Supplementary Table S2).
Figure 4 Effect of PGRs on the rapid light curve (RLC) fitting parameters α (initial slope of the RLC, (A) and rETRmax (maximum rETR, rETR is the relative electron transport rate through PSII, (B) in leaves of transplanted T. hemprichii fragments. NRA, transplanted T. hemprichii fragments without rhizome apex; RA, transplanted T. hemprichii fragments with rhizome apex; CK, control; IAA, indoleacetic acid; GA3, gibberellin; PP333, paclobutrazol. Different lowercase letters indicate significant differences (p < 0.05) in transplanted T. hemprichii fragments without rhizome apex applied with different PGRs treatments; different uppercase letters indicate significant differences (p < 0.05) in transplanted T. hemprichii fragments with rhizome apex applied with different PGRs treatments. * and ** indicate significant (p < 0.05) or highly significant (p < 0.01) differences between treatments of transplanted T. hemprichii fragments with and without rhizome apexes, respectively, when the same PGRs were applied.
The chlorophyll fluorescence parameters measured in the leaves of the TTFs after dark adaptation are shown in Table 1. The results showed that IAA decreased Fo and NPQ and increased Fv/Fm, qP, and ΦPSII of the TTF leaves compared to CK. Conversely, PP333 increased Fo and NPQ and decreased Fm, Fv/Fm, qP, and ΦPSII. GA3 had minimal effect on the dark-responsive chlorophyll fluorescence parameters, except for qP and NPQ. The results of the two-way ANOVA (Supplementary Table S2) showed that the PGR type had a significant effect on all dark-responsive chlorophyll fluorescence parameters (p < 0.01), while RA had a significant effect on all parameters except Fv/Fm (p < 0.01). Both PGR and RA had significant interaction effects on Fo, qP, and NPQ (p < 0.05 or p < 0.01).
Table 1 Effect of PGRs on chlorophyll fluorescence parameters of leaves of transplanted T. hemprichii fragments.
As shown in Figure 5, the IAA treatment enhanced Chla, carotenoid, and total photosynthetic pigment contents of the leaves of the TTFs. However, PP333 treatment reduced Chla and total photosynthetic pigment content in the TTF leaves. GA3 had no significant effect on the leaves (p > 0.05). The presence of RA significantly enhanced Chla, Chlb, and total photosynthetic pigment content in the GA3 and PP333 treatments. The results of the two-way ANOVA (Supplementary Table S2) revealed that PGR type had a significant effect on the photosynthetic pigment content of the TTFs leaves (p < 0.05 or p < 0.01). The presence of RA had a significant effect on Chla, Chlb, and total photosynthetic pigment content (p < 0.05 or p < 0.01). Furthermore, there was a significant interaction between the two factors on Chla and total photosynthetic pigment content (p < 0.05).
Figure 5 Effects of PGRs on chlorophyll a (A), chlorophyll b (B), carotenoid (C), and total photosynthetic pigment (D) contents of leaves of transplanted T. hemprichii fragments. NRA, transplanted T. hemprichii fragments without rhizome apex; RA, transplanted T. hemprichii fragments with rhizome apex; CK, control; IAA, indoleacetic acid; GA3, gibberellin; PP333, paclobutrazol. Different lowercase letters indicate significant differences (p < 0.05) in transplanted T. hemprichii fragments without rhizome apex applied with different PGRs treatments; different uppercase letters indicate significant differences (p < 0.05) in transplanted T. hemprichii fragments with rhizome apex applied with different PGRs treatments. * and ** indicate significant (p < 0.05) or highly significant (p < 0.01) differences between treatments of transplanted T. hemprichii fragments with and without rhizome apexes, respectively, when the same PGRs were applied.
As shown in Figure 6, IAA and GA3 had minimal effect on POD and SOD activities of TTF tissues (leaves and roots) but reduced their CAT activities to varying degrees compared to CK. PP333 significantly elevated the activity of all three enzymes (p < 0.05). The presence of RA elevated all three enzyme activities in the roots of TTFs, but had no significant effect on the antioxidant enzyme activities in the leaves. PGR application elevated MDA content in the leaves of TTFs, especially in TTFs with RA. The root MDA content was significantly elevated by PP333 treatment TTFs with RA (p < 0.05).
Figure 6 Effect of PGRs on leaf and root peroxidase [POD (A)], superoxide dismutase [SOD (B)], catalase [CAT (C)] activities and malondialdehyde [MDA (D)] content of transplanted T. hemprichii fragments. NRA, transplanted T. hemprichii fragments without rhizome apex; RA, transplanted T. hemprichii fragments with rhizome apex; CK, control; IAA, indoleacetic acid; GA3, gibberellin; PP333, paclobutrazol. Different lowercase letters indicate significant differences (p < 0.05) in transplanted T. hemprichii fragments without rhizome apex applied with different PGRs treatments; different uppercase letters indicate significant differences (p < 0.05) in transplanted T. hemprichii fragments with rhizome apex applied with different PGRs treatments. * and ** indicate significant (p < 0.05) or highly significant (p < 0.01) differences between treatments of transplanted T. hemprichii fragments with and without rhizome apexes, respectively, when the same PGRs were applied.
The results of the three-way ANOVA of the effects of the TTF part, PGR type, and RA on POD, SOD, and CAT activities and MDA content are presented in Supplementary Table S3. The results showed that TTF part, PGR type, and RA had significant effects on the activities of all three antioxidant enzymes and MDA content (p < 0.01). There was no significant interaction between the three treatments on POD activity (p > 0.05), a weak interaction on MDA content, and a strong interaction on SOD and CAT activities.
The contents of soluble sugars, starches, soluble proteins, and total phenols in TTF tissues under different PGR treatments are shown in Figure 7. These metabolite contents varied with PGR type, TTF part, and RA presence. The soluble sugar content was much higher in the roots of TTFs than in the leaves (Figure 7A). The application of IAA and GA3 reduced the soluble sugar content to varying degrees in both the leaves and roots. In contrast, the soluble sugar content of PP333-treated TTFs was elevated, particularly in the TTFs without RA. Across all treatments, the starch content was slightly higher in the roots than in the leaves (Figure 7B). PGR treatment resulted in elevated starch content in seagrass tissues. The presence of RA had no significant effect on the soluble sugar and starch content of the TTFs (p > 0.05). Similar to soluble sugars, soluble protein content in TTF tissues was decreased by IAA and GA3 treatments and increased by PP333 treatment (Figure 7C). The presence of RA drastically elevated soluble protein content in T. hemprichii tissues, especially in roots. As shown in Figure 7D, the total phenol content in the roots of the TTFs was much lower than that in the leaves. The total phenol content in the tissues of TTFs with RA was lower than that in the TTFs without RA. PP333 application elevated total phenol content in the tissues.
Figure 7 Effects of PGRs on the content of soluble sugars (A), starch (B), soluble proteins (C), and total phenols (D) in the leaves and roots of transplanted T. hemprichii fragments. NRA, transplanted T. hemprichii fragments without rhizome apex; RA, transplanted T. hemprichii fragments with rhizome apex; CK, control; IAA, indoleacetic acid; GA3, gibberellin; PP333, paclobutrazol. Different lowercase letters indicate significant differences (p < 0.05) in transplanted T. hemprichii fragments without rhizome apex applied with different PGRs treatments; different uppercase letters indicate significant differences (p < 0.05) in transplanted T. hemprichii fragments with rhizome apex applied with different PGRs treatments. * and ** indicate significant (p < 0.05) or highly significant (p < 0.01) differences between treatments of transplanted T. hemprichii fragments with and without rhizome apexes, respectively, when the same PGRs were applied.
Three-way ANOVA (Supplementary Table S4) showed that TTF part and PGR type had significant effects on the contents of the four metabolites in the TTFs (p < 0.01). Moreover, the presence of RA had a significant effect on soluble protein and total phenol content (p < 0.01). There was no significant interaction effect between the TTF part, PGR type, and RA presence on soluble sugar content (p > 0.05). However, TTF part and PGR type had a significant interaction effect on starch content (p < 0.05), while TTF part and RA had a significant interaction effect on soluble protein and total phenol content.
At the end of the experiment, the leaf growth rate of TTFs ranged from 0.33 ± 0.12 to 1.48 ± 0.12 mm d-1 (Figure 2A). Compared to the CK, the IAA treatment increased the growth rate of seagrass leaves by 31.3% (NRA) and 45.0% (RA). GA3 treatment increased the growth rate of seagrass leaves by 41.1% (NRA) and 32.1% (RA), indicating that both treatments were effective in promoting the growth rate of seagrass leaves. This is consistent with the results of previous studies (Balestri and Lardicci, 2006; García-Jiménez et al., 2006). However, while IAA and GA3 accelerated leaf growth, they did not effectively promote an increase in leaf length (Figure 2B). This indicated that their application accelerated leaf turnover (0.0103 ± 0.0011 mm d-1 for CK; 0.0131 ± 0.0015 and 0.0128 ± 0.0015 mm d-1 for IAA and GA3 treatments, respectively). We also found that for the TTFs without RA, leaf width was significantly greater in the IAA treatment group than in the GA3 treatment group (p < 0.05; Figure 2C). This resulted in a larger leaf area in the IAA-treated seagrass than in the GA3-treated seagrass (Figure 2D). This suggests that IAA and GA3 have different mechanisms of action in regulating the growth of seagrass leaves. IAA promotes cell division and differentiation (Glendon et al., 2009; Yu et al., 2017), while GA3 promotes cell elongation (Kou et al., 2021).
Conversely, PP333, a plant growth retardant, functions by reducing the level of endogenous hormones in plants (Ren et al., 2003; Zhang et al., 2023). This inhibits cell division and elongation within plant meristematic tissues, effectively moderating the overall growth rate of the plant. As a result, it leads to reduced nutrient uptake and contributes to the dwarfing of the plant. In this study, PP333 treatment not only reduced the growth rate of the leaves of the TTFs by 66.7% (NRA) and 33.7% (RA) compared to CK (Figure 2A), but also reduced their leaf length (Figure 2B) and leaf turnover (0.0059 ± 0.0020 mm d-1). Moreover, leaf width in the PP333 treatment group was significantly greater than that of the other treatments (p < 0.05; Figure 2C). Studies have shown that PP333 application reduces plant leaf area (Misra et al., 2005; Kumar and Sharma, 2016; Liu et al., 2018). However, the results of the present study showed that there was no significant difference in the leaf area of TTFs in the PP333 treatment group compared to the other treatments (p > 0.05; Figure 2D).
Correlation analysis of leaf growth rate and leaf length and width (Figure 8A), as well as leaf length and leaf width (Figure 8B) of TTFs for all treatments revealed interesting relationships. There was a significant positive correlation between leaf length and growth rate (p < 0.01), while leaf width was significantly negatively correlated with leaf length (p < 0.05). Additionally, there was a significant negative correlation between leaf width and growth rate (p < 0.05). This suggests that regardless of PGR application or type, accelerated leaf growth rate led to thinner and longer leaves, and vice versa.
Figure 8 Correlation of leaf growth rate with leaf length and width (A) and correlation between leaf length and leaf width (B) in transplanted T. hemprichii fragments. *, correlation is significant at the 0.05 level (2-tailed); **, correlation is significant at the 0.01 level (2-tailed).
The rate of photosynthesis and the efficiency of light energy utilization play a critical role in plant growth (Semesi et al., 2009; Liao et al., 2022). Currently, research on both terrestrial (Hussain et al., 2020) and aquatic plants (Balestri and Bertini, 2003; Balestri and Lardicci, 2006) supports the conclusion that IAA and GA3 application improves plant photomorphogenesis and promotes efficient photosynthesis by leaves. We found that the application of IAA and GA3 enhanced rETRmax, Fm, Fv/Fm, qP, ΦPSII, and photosynthetic pigment content in the leaves of TTFs, and reduced their Fo and NPQ (Figures 4 and 5; Table 1). Overall, IAA was more effective than GA3 in enhancing photosynthesis in T. hemprichii. Furthermore, many studies have found that the PP333 application enhances the chlorophyll content and photosynthetic efficiency of plants (Cheng et al., 2002; Zhang et al., 2023). However, we found that several important chlorophyll fluorescence parameters (rETRmax, Fv/Fm, and ΦPSII) and leaf photosynthetic pigment content were lower in the PP333 treatment than in other treatments (Figures 4 and 5; Table 1). This discrepancy may be attributed to distinct responses of different plants to PP333 (Liu et al., 2018). Additionally, inappropriate dosage or application of PP333 in the present study may have contributed to its ineffectiveness.
Antioxidant enzymes play a crucial role in protecting plants from environmental stress by scavenging reactive oxygen species (ROS) (Halder et al., 2018). Previous studies have shown that the application of PGRs significantly increases the antioxidant enzyme activities in plant tissues and enhances the scavenging of ROS (Wang et al., 2016; Jahan et al., 2019; Rajput et al., 2021). However, we observed no significant elevation in the activities of POD, SOD, and CAT in the IAA and GA3 treatments compared to CK (Figure 6). This indicates that the application of these two PGRs did not cause any significant stress effects on the TTFs and that T. hemprichii was in a good growth condition. However, the antioxidant enzyme activities and MDA (a product of lipid peroxidation of plant cell membranes) content were higher in the tissues (leaves and roots) of the PP333-treated TTFs compared to the other treatments (Figure 6). This indicates that PP333 potentially induced stress in TTFs, leading to lipid peroxidation and damage to the membrane system (Cristina et al., 2016; Mahfuza et al., 2017). This may be attributed to the improper use and dosage of PP333 used in this study. Nevertheless, the antioxidant enzyme activities and MDA content of PP333-treated TTFs were not significantly increased, indicating that the stress and damage were not severe.
Furthermore, the content of non-structural carbohydrates (soluble sugars and starch) in the TTF tissues was influenced by PGR treatments (Figures 7A, B). IAA and GA3 treatments decreased the soluble sugar content and increased starch content in leaves and roots. This may be attributed to the ability of these two PGRs to regulate carbohydrate allocation between soluble sugars and starch (Ren et al., 2022). It has been shown that the application of IAA and GA3 can enhance the metabolism and output rate of soluble sugars in plant tissues by inducing the expression of sugar metabolism-related enzyme genes. This promotes leaf growth, starch accumulation, and provides the necessary carbon source for overall plant growth and development (Jiang et al., 2012; Zhang et al., 2021). Additionally, these PGRs reduce the inhibitory effect of excessive sugar content on photosynthesis as demonstrated in vivo (Rafael et al., 2017; Ren et al., 2022). Conversely, both soluble sugar and starch contents in the tissues of PP333-treated TTFs exhibited an increasing trend compared to CK. This increase may be attributed to the slow growth and metabolism of T. hemprichii in the PP333 treatment group, leading to the accumulation of photosynthetic products within the plant. Similar to the soluble sugars, the soluble proteins in the leaves and roots of the TTFs also decreased in the IAA and GA3 treatments compared with CK, but slightly increased in the PP333 treatment (Figure 7C). This indicates consistency in the carbon and nitrogen metabolism of T. hemprichii.
Phenolic compounds are important secondary metabolites in plants (Trevathan-Tackett et al., 2015; Martina et al., 2023). Elevated levels phenolic compounds often indicate that plants are experiencing some degree of stress effects (Stamp, 2003; Fan et al., 2020). Consistent with the antioxidant enzyme activities and MDA performance, the total phenolic content of PP333-treated TTFs was elevated compared to that of the other treatments (Figure 7D), further proving that the treated T. hemprichii experienced some degree of stress.
Studies have shown that the application of PGRs such as IAA, GA3, and PP333 promotes root development in plants (Yoon et al., 2021). For example, the application of PGRs to plant cuttings during cutting promotes the production of adventitious roots (Balestri and Lardicci, 2006; de Andrade et al., 2023). However, we did not observe similar adventitious root production near the cuttings of severed T. hemprichii rhizomes, regardless of whether PGR application. Moreover, we did not observe significant elongation of seagrass rhizomes. This may be attributed to the fact that the root development of T. hemprichii is insensitive to PGRs, to the difficulty in forming adventitious roots, and the inherently slower growth rate of T. hemprichii rhizomes (Abadie et al., 2019). However, the application of IAA and PP333 enhanced the root vitality of TTFs to some extent (Figure 3).
The results of this study indicated that the presence of RA had a significant effect on leaf growth rate, leaf area, root vitality, most of the chlorophyll fluorescence variables, and chlorophyll content of TTFs (p < 0.01; Supplementary Tables S1 and S2). Additionally, the presence of RA had a significant effect on antioxidant enzyme activities and MDA content of T. hemprichii tissues (leaves and roots) (p < 0.01; Supplementary Table S3), as well as soluble proteins and total phenol content (Supplementary Table S4). The RA is the most active site for seagrass root growth (de Battisti et al., 2021), where endogenous hormones such as growth hormones (e.g., IAA and GA3) can be synthesized and transported through polar transport to the phloem distribution area of the plant to promote nutrient propagation and expand ecological niche space (Ewan et al., 2020). Therefore, TTFs with RA benefitted from the presence of endogenous hormones synthesized by RA and the absorption of exogenous PGRs through roots and leaves, resulting in a more significant effect on growth and physiological enhancement. Conversely, TTFs without RA had weakened abilities to produce endogenous hormones and relied solely on exogenous PGRs for growth regulation; thus, their effects were more limited. Owing to differences in the mechanisms of action of different types of endogenous hormones and exogenous PGRs on seagrass growth and physiology, there were significant interaction effect of PGRs and RA on leaf growth rate (Supplementary Table S1), chlorophyll fluorescence variables, and photosynthetic pigment content (Supplementary Table S2) in TTFs (p < 0.05 or p < 0.01).
The effects of exogenous PGRs on plant growth and development are complex, and their regulatory effects can vary significantly depending on factors such as application concentrations and methods for different seagrass species. Therefore, the findings of this study cannot blindly be extrapolated to seagrass species beyond T. hemprichii. Furthermore, we cannot assume that the application of PGRs will yield favorable results in all scenarios, such as ecological restoration in intertidal seagrass meadows. Additionally, it is well known that the effects of PGRs highly dose-dependent (Ren et al., 2003; Balestri and Lardicci, 2006). For example, IAA typically functions as a plant growth promoter at low concentrations but can inhibit growth at high concentrations (Zhang et al., 2016). Notably, this study represents a preliminary exploration of the effects of three PGRs under specific conditions. A uniform dose (10 mg per incubator per month) was used, and the optimal dosage of the PGRs was not investigated. Besides that, it is necessary to apply RT-PCR or metabolomics to explore the molecular mechanisms underlying the physiological effects of PGRs on seagrasses. Therefore, rigorous long-term field trials and molecular mechanism research are necessary to elucidate the effectiveness of these PGRs in seagrass meadow ecological restoration practices.
We draw several noteworthy conclusions from this study. The growth and physiological responses of TTFs exhibited varying degrees of improvement following a single application of the three PGRs. These improvements were generally consistent with the functional expectations of the PGRs. This provides us with confidence to continue the application of PGRs in seagrass meadow restoration in the future. Moreover, the application of PGRs had contrasting effects on the leaf length and width of TTFs. GA3 reduced the leaf width of T. hemprichii, resulting in an elongated and streamlined leaf morphology, while PP333 reduced the leaf length and enhanced the root vitality. This reduces the probability of TTFs being washed away by currents or waves during high sea state conditions and enhances their resilience to scouring. Finally, the presence of RA had a positive effect on the growth and physiological conditions of TTFs, underscoring the importance of safeguarding this region when restoring damaged seagrass meadows through rhizome fragment transplantation.
The original contributions presented in the study are included in the article/Supplementary Material. Further inquiries can be directed to the corresponding authors.
ZL: Writing – original draft, Writing – review & editing. YS: Writing – original draft, Writing – review & editing. MZ: Investigation, Writing – review & editing. ZS: Data curation, Formal analysis, Writing – review & editing. HL: Data curation, Writing – review & editing. JC: Investigation, Writing – review & editing. QH: Formal analysis, Writing – review & editing.
The author(s) declare financial support was received for the research, authorship, and/or publication of this article. This work was sponsored by the Key Research and Development Project of Hainan Province (ZDYF2023SHFZ100) and the Major Science and Technology Program Project of the Yazhou Bay Innovation Research Institute of Hainan Tropical Ocean University (2022CXYZD002).
We thank Drs. Zhen Ni and Mingzhong Liu for their comments and suggestions, which have greatly improved our manuscript.
The authors declare that the research was conducted in the absence of any commercial or financial relationships that could be construed as a potential conflict of interest.
All claims expressed in this article are solely those of the authors and do not necessarily represent those of their affiliated organizations, or those of the publisher, the editors and the reviewers. Any product that may be evaluated in this article, or claim that may be made by its manufacturer, is not guaranteed or endorsed by the publisher.
The Supplementary Material for this article can be found online at: https://www.frontiersin.org/articles/10.3389/fmars.2024.1334937/full#supplementary-material
Abadie A., Richir J., Lejeune P., Leduc M., Gobert S. (2019). Structural changes of seagrass seascapes driven by natural and anthropogenic factors: A multidisciplinary approach. Front. Ecol. Evol. 7. doi: 10.3389/fevo.2019.00190
Ali M. K. M., Critchley A. T., Hurtado A. Q. (2020). Micropropagation and sea-based nursery growth of selected commercial Kappaphycus species in Penang, Malaysia. J. Appl. Phycol. 32, 1301–1309. doi: 10.1007/s10811-019-02003-4
Balestri E., Bertini S. (2003). Growth and development of Posidonia oceanica seedlings treated with plant growth regulators: possible implications for meadow restoration. Aquat. Bot. 76, 291–297. doi: 10.1016/S0304-3770(03)00074-3
Balestri E., Lardicci C. (2006). Stimulation of root formation in Posidonia oceanica cuttings by application of auxins (NAA and IBA). Mar. Biol. 149, 393–400. doi: 10.1007/s00227-005-0193-0
Cheng S. P., Ren F., Grosse W., Wu Z. B. (2002). Effects of cadmium on chlorophyll content, photochemical efficiency, and photosynthetic intensity of Canna indica Linn. Int. J. Phytoremediat. 4, 239–246. doi: 10.1080/15226510208500085
Cristina O. V., Stefan B., Ángel B. S., Flor M., Luis E. H. (2016). In vivo ROS and redox potential fluorescent detection in plants: Present approaches and future perspectives. Methods 109, 92–104. doi: 10.1016/j.ymeth.2016.07.009
de Andrade F. H. A., Ferreira A. M. O., Azevedo L. M., Meline D. O. S., Gladyston R. C., Resende M. L. V., et al. (2023). IBA and melatonin increase trigonelline and caffeine during the induction and initiation of adventitious roots in Coffea arabica L. cuttings. Sci. Rep. 13, 15151. doi: 10.1038/s41598-023-41288-x
de Battisti D., Balestri E., Pardi G., Menicagli V., Lardicci C. (2021). Substrate type influences the structure of epiphyte communities and the growth of Posidonia oceanica seedlings. Front. Plant Sci. 12. doi: 10.3389/fpls.2021.660658
Duarte C. M., Kennedy H., Marba N., Hendriks I. (2013). Assessing the capacity of seagrass meadows for carbon burial: Current limitations and future strategies. Ocean Coast. Manage. 83, 32–38. doi: 10.1016/j.ocecoaman.2011.09.001
Eilers P., Peeters J. (1988). A model for the relationship between light intensity and the rate of photosynthesis in phytoplankton. Ecol. Model. 42, 199–215. doi: 10.1016/0304-3800(88)90057-9
Ewan T., Grégoire T. G., Cindy C. C., Pierre F. (2020). Valuation of coastal ecosystem services in the Large Marine Ecosystems of Africa. Environ. Dev. 36, 100584. doi: 10.1016/j.envdev.2020.100584
Fan X. D., Cao X., Zhou H. R., Hao L. H., Dong W., He C. L., et al. (2020). Carbon dioxide fertilization effect on plant growth under soil water stress associates with changes in stomatal traits, leaf photosynthesis, and foliar nitrogen of bell pepper (Capsicum annuum L.). Environ. Exper. Bot. 179, 104203. doi: 10.1016/j.envexpbot.2020.104203
Fonseca M. S., Julius B. E., Kenworthy W. J. (2000). Integrating biology and economics in seagrass restoration: How much is enough and why? Ecol. Eng. 15, 227–237. doi: 10.1016/S0925-8574(00)00078-1
García-Jiménez P., Navarro E., Santana C., Luque A., Robaina R. R. (2006). Anatomical and nutritional requirements for induction and sustained growth in vitro of Cymodocea nodosa (Ucria) Ascherson. Aquat. Bot. 84, 79–84. doi: 10.1016/j.aquabot.2005.07.006
Giulia F., Tim M. G., Alistair G. B., Callaghan C. T., Graham P. H., Madelaine L., et al. (2021). Naturally-detached fragments of the endangered seagrass Posidonia australis collected by citizen scientists can be used to successfully restore fragmented meadows. Biol. Conserv. 262, 109308. doi: 10.1016/j.biocon.2021.109308
Glasby T. M., Taylor S. L., Housefield G. P. (2015). Factors influencing the growth of seagrass seedlings: A case study of Posidonia australis. Aquat. Bot. 120, 251–259. doi: 10.1016/j.aquabot.2014.09.003
Glendon D. A., Ondřej N., Aleš P., Jakub R., Miroslav S., John E. E., et al. (2009). Hormonal and cell division analyses in Watsonia lepida seedlings. J. Plant Physiol. 166, 1497–1507. doi: 10.1016/j.jplph.2009.03.009
Glenn A. H., Kenneth L. H., Adriana V., Euan S. H., Gary A. K., Paul S. L., et al. (2016). Accelerating tropicalization and the transformation of temperate seagrass meadows. Bio. Sci. 66, 938–948. doi: 10.1093/biosci/biw111
Guzmán Y., Pugliese B., González C. V., Travaglia C., Bottini R., Berli F. (2021). Spray with plant growth regulators at full bloom may improve quality for storage of ‘Superior Seedless’ table grapes by modifying the vascular system of the bunch. Postharvest Biol. Tec. 176, 111522. doi: 10.1016/j.postharvbio.2021.111522
Halder T., Upadhyaya G., Basak C., Das A., Chakraborty C., Ray S. (2018). Dehydrins impart protection against oxidative stress in transgenic tobacco plants. Front. Plant Sci. 9. doi: 10.3389/fpls.2018.00136
Hoang C. T., Bui T. P., Duong V. H. (2021). Species biodiversity and distribution of seagrass beds in several coastal areas of central Vietnam. Reg. Stud. Mar. Sci. 41, 101531. doi: 10.1016/j.rsma.2020.101531
Holon F., Marre G., Parravicini V., Mouquet N., Bockel T., Descamp P., et al. (2018). A predictive model based on multiple coastal anthropogenic pressures explains the degradation status of a marine ecosystem: Implications for management and conservation. Biol. Conserv. 222, 125–135. doi: 10.1016/j.biocon.2018.04.006
Hong L., Su W., Zhang Y., Ye C., Shen Y. J., Li Q. S. (2018). Transcriptome profiling during mangrove viviparity in response to abscisic acid. Sci. Rep. 8, 770. doi: 10.1038/s41598-018-19236-x
Horn L. E., Paling E. I., Keulen M. V. (2009). Photosynthetic recovery of transplanted Posidonia sinuosa, Western Australia. Aquat. Bot. 90, 149–156. doi: 10.1016/j.aquabot.2008.08.002
Hussain K., Anwer S., Nawaz K., Malik M. F., Zainab N., Nazeer A., et al. (2020). Effect of foliar applications of IAA and GA3 on growth, yield and quality of pea (Pisum sativum L.). Pak. J. Bot. 52, 447–460. doi: 10.30848/PJB2020-2(32
Jacob E. A., Lauren A. Y., Craig A. L. (2013). Consumers regulate nutrient limitation regimes and primary production in seagrass ecosystems. Ecology 94, 521–529. doi: 10.1890/12-1122.1
Jahan M. S., Wang Y., Shu S., Zhong M., Chen Z., Wu J. Q., et al. (2019). Exogenous salicylic acid increases the heat tolerance in Tomato (Solanum lycopersicum L.) by enhancing photosynthesis efficiency and improving antioxidant defense system through scavenging of reactive oxygen species. Sci. Hortic. 247, 421–429. doi: 10.1016/j.scienta.2018.12.047
James R. K., Keyzer L. M., van de Velde S. J., Herman P. M. J., van Katwijk M. M., Bouma T. J. (2023). Climate change mitigation by coral reefs and seagrass beds at risk: How global change compromises coastal ecosystem services. Sci. Total Environ. 857, 159576. doi: 10.1016/j.scitotenv.2022.159576
Jarvis J. C., Moore K. A. (2010). The role of seedlings and seed bank viability in the recovery of Chesapeake Bay, USA, Zostera marina populations following a large-scale decline. Hydrobiologia 649, 55–68. doi: 10.1007/s10750-010-0258-z
Jiang Z. J., Huang X. P., Zhang J. P. (2010). Effects of CO2 enrichment on photosynthesis, growth, and biochemical composition of seagrass Thalassia hemprichii (Ehrenb.) Aschers. J. Integr. Plant Biol. 52, 904–913. doi: 10.1111/j.1744-7909.2010.00991.x
Jiang Z. J., Huang X. P., Zhang J. P. (2012). Effect of environmental stress on non-structural carbohydrates reserves and transfer in seagrasses. Acta Ecol. Sin. 32, 6242–6250. doi: 10.5846/stxb201108311275
Jones B. L., Cullen-Unsworth L., Robert C. H., Richard K. F. (2018). Complex yet fauna-deficient seagrass ecosystems at risk in southern Myanmar. Bot. Mar. 61, 193–203. doi: 10.1515/bot-2017-0082
Jung D. H., Kim D., Yoon H. I. (2016). Modeling the canopy photosynthetic rate of romaine lettuce (Lactuca sativa L.) grown in a plant factory at varying CO2 concentrations and growth stages. Hortic. Environ. Biotechnol. 57, 487–492. doi: 10.1007/s13580-016-0103-z
Kou E., Huang X., Zhu Y., Su W., Liu H. C., Sun G. W., et al. (2021). Crosstalk between auxin and gibberellin during stalk elongation in flowering Chinese cabbage. Sci. Rep. 11, 3976. doi: 10.1038/s41598-021-83519-z
Kulikova A. L., Kuznetsova N. A., Kholodova V. P. (2011). Effect of copper excess in environment on soybean root viability and morphology. Russ. J. Plant Physiol. 58, 836–843. doi: 10.1134/S102144371105013X
Kumar A., Sharma N. (2016). Effect of growth retardants on growth, flowering and physiological characteristics of olive cultivar Leccino under rain-fed conditions of Himachal Pradesh, India. Indian J. Agric. Res. 50, 487–490. doi: 10.18805/ijare.v0iOF.10778
Li W. T., Kim J. H., Park J. I., Lee K. S. (2010). Assessing establishment success of Zostera marina transplants through measurements of shoot morphology and growth. Estuar. Coast. Shelf S. 88, 377–384. doi: 10.1016/j.ecss.2010.04.017
Liao Z. Q., Zeng H. L., Fan J. L., Lai Z. L., Zhang C., Zhang F. C., et al. (2022). Effects of plant density, nitrogen rate and supplemental irrigation on photosynthesis, root growth, seed yield and water-nitrogen use efficiency of soybean under ridge-furrow plastic mulching. Agric. Water Manage. 268, 107688. doi: 10.1016/j.agwat.2022.107688
Liu P. J., Chang H. F., Mayfield A. B. (2022). Assessing the effects of ocean warming and acidification on the seagrass Thalassia hemprichii. J. Mar. Sci. Eng. 10, 714–723. doi: 10.3390/jmse10060714
Liu L., Zheng S. L., Chen F. B., Li J. H., Liao M., Lin L. J., et al. (2018). Effects of paclobutrazol (PP333) on lead and zinc accumulations in Pseudostellaria maximowicziana. Chem. Ecol. 34, 412–421. doi: 10.1080/02757540.2018.1437149
Lu J., Yin Z., Lu T., Yang X., Wang F., Qi M., et al. (2020). Cyclic electron flow modulate the linear electron flow and reactive oxygen species in tomato leaves under high temperature. Plant Sci. 292, 110387. doi: 10.1016/j.plantsci.2019.110387
Mahfuza P., Takashi A., Rashid M. H. (2017). Hydrogen sulfide induced growth, photosynthesis and biochemical responses in three submerged macrophytes. Flora 230, 1–11. doi: 10.1016/j.flora.2017.03.005
Maite Z. E., Nieves G. H., Pilar G. J., Robaina R. R. (2010). Restoration of Cymodocea nodosa seagrass meadows through seed propagation: germination in vitro, seedling culture and field transplants. Bot. Mar. 53, 173–181. doi: 10.1515/bot.2010.019
Martina K., Anna J., Marketa D., Monika Z., Pavel C., Olga K., et al. (2023). Role of secondary metabolites in distressed microalgae. Environ. Res. 224, 115392. doi: 10.1016/j.envres.2023.115392
Misra A., Srivastava N. K., Kumar R., Khan A. (2005). Effect of palcobutrazol (PP333) on flower quality and quantity of Rosa damascene. Commun. Soil Sci. Plant Anal. 36, 477–486. doi: 10.1081/CSS-200043219
Moreno D., Aguilera P. A., Castro H. (2001). Assessment of the conservation status of seagrass (Posidonia oceanica) meadows: implications for monitoring strategy and the decision-making process. Biol. Conserv. 102, 325–332. doi: 10.1016/S0006-3207(01)00080-5
Muniandi S. K. M., Hossain M. A., Abdullah M. P., Shukor N. A. A. (2018). Gibberellic acid (GA3) affects growth and development of some selected kenaf (Hibiscus cannabinus L.) cultivars. Ind. Crop Prod. 118, 180–187. doi: 10.1016/j.indcrop.2018.03.036
Narayan M. S., Thimmaraju R., Bhagyalakshmi N. (2005). Interplay of growth regulators during solid-state and liquid-state batch cultivation of anthocyanin producing cell line of Daucus carota. Process Biochem. 40, 351–358. doi: 10.1016/j.procbio.2004.01.009
Park H. J., Park T. H., Kang H. Y., Lee K., Kim Y. K., Kang C. K. (2021). Assessment of restoration success in a transplanted seagrass bed based on isotopic niche metrics. Ecol. Eng. 166, 106239. doi: 10.1016/j.ecoleng.2021.106239
Pazzaglia J., Nguyen H. M., Santillán-Sarmiento A., Ruocco M., Procaccini G. (2021). The genetic component of seagrass restoration: What we know and the way forwards. Water 13, 829. doi: 10.3390/w13060829
Rafael V. R., Eduardo C. M., José R. M. F., Ana K. M. L., Márcio O. M., Joaquim A. G. S., et al. (2017). Increased sink strength offsets the inhibitory effect of sucrose on sugarcane photosynthesis. J. Plant Physiol. 208, 61–69. doi: 10.1016/j.jplph.2016.11.005
Rajput V. D., Singh R. K., Verma K. K., Sharma L., Quiroz-Figueroa F. R., Meena M., et al. (2021). Recent developments in enzymatic antioxidant defence mechanism in plants with special reference to abiotic stress. Biology 10, 267. doi: 10.3390/BIOLOGY10040267
Ren X. Q., Liang H. W., Chen B. Q., Ji M. Y. (2003). Dwarfing effects of plant growth regulators on narcissi. J. Forestry Res. 14, 339–341. doi: 10.1007/BF02857865
Ren R. H., Wan Z. W., Chen H. W., Zhang Z. W. (2022). The effect of inter-varietal variation in sugar hydrolysis and transport on sugar content and photosynthesis in Vitis vinifera L. leaves. Plant Physiol. Bioch. 189, 1–13. doi: 10.1016/j.plaphy.2022.07.031
Reynolds L. K., Waycott M., McGlathery K. J., Orth R. J. (2016). Ecosystem services returned through seagrass restoration. Restor. Ecol. 24, 583–588. doi: 10.1111/rec.12360
Rocío J. R., Fernando G. B., Luis G. E., Juan J. V. (2018). Food choice effects on herbivory: Intra-specific seagrass palatability and inter-specific macrophyte palatability in seagrass communities. Estuar. Coast. Shelf Sci. 204, 31–39. doi: 10.1016/j.ecss.2018.02.016
Saewong C., Soonthornkalump S., Buapet P. (2022). Combined effects of high irradiance and temperature on the photosynthetic and antioxidant responses of Thalassia hemprichii and Halophila ovalis. Bot. Mar. 65, 325–335. doi: 10.1515/bot-2022-0014
Samantha J. T., Jessie C. J., Paul H. Y., Alana G., Bradley C. C., Robert G. C. (2017). Long distance biotic dispersal of tropical seagrass seeds by marine mega-herbivores. Sci. Rep-UK. 7, 4458. doi: 10.1038/s41598-017-04421-1
Semesi I. S., Beer S., Bjoerk M. (2009). Seagrass photosynthesis controls rates of calcification and photosynthesis of calcareous macroalgae in a tropical seagrass meadow. Mar. Ecol. Prog. Ser. 382, 41–47. doi: 10.3354/meps07973
Shan F., Zhang R., Zhang J., Wang C., Lyu X., Xin T., et al. (2021). Study on the regulatory effects of GA3 on soybean internode elongation. Plants 10, 1737. doi: 10.3390/plants10081737
Stamp N. (2003). Out of the quagmire of plant defense hypotheses. Q. Rev. Biol. 78, 23–55. doi: 10.1111/j.1365-2028.2007.00919.x
Statton J., Dixon K. W., Hovey R. K., Kendrick G. A. (2012). A comparative assessment of approaches and outcomes for seagrass revegetation in Shark Bay and Florida Bay. Mar. Freshw. Res. 63, 984–993. doi: 10.1071/mf12032
Tanner C. E., Parham T. (2010). Growing Zostera marina (eelgrass) from seeds in land-based culture systems for use in restoration projects. Restor. Ecol. 18, 527–537. doi: 10.1111/j.1526-100X.2010.00693.x
Tosun M., Ercisli S., Sengul M., Ozer H., Polat T., Ozturk E. (2009). Antioxidant properties and total phenolic content of eight Salvia species from Turkey. Biol. Res. 42, 175–181. doi: 10.1016/j.aquabot.2014.12.005
Trevathan-Tackett S. M., Lane A. L., Bishop N. (2015). Metabolites derived from the tropical seagrass Thalassia testudinum are bioactive against pathogenic. Labyrinthula sp[J]. Aquat Bot, 122(1), 1–8.
van Katwijk M. M., Bos A. R., de Jonge V. N., Hanssen L. S. A. M., Hermus D. C. R., de Jong D. J. (2009). Guidelines for seagrass restoration: importance of habitat selection and donor population, spreading of risks, and ecosystem engineering effects. Mar. pollut. Bull. 58, 179–188. doi: 10.1016/j.marpolbul.2008.09.028
Wang W., Xia M. X., Chen J., Yuan R., Deng F. N., Shen F. F. (2016). Gene expression characteristics and regulation mechanisms of superoxide dismutase and its physiological roles in plants under stress. Biochem. 81, 465–480. doi: 10.1134/S0006297916050047
Waycott M., Duarte C. M., Tim J. B. C., Orth R. J., Dennison. W. C., Olyarnik S., et al. (2009). Accelerating loss of seagrasses across the globe threatens coastal ecosystems. Proc. Natl. Acad. Sci. U. S. A. 106, 12377–12381. doi: 10.1073/pnas.0905620106
Wu Y., Shen Y. (2021). Dormancy in Tilia miqueliana is attributable to permeability barriers and mechanical constraints in the endosperm and seed coat. Braz. J. Bot. 44, 725–740. doi: 10.1007/s40415-021-00749-1
Yoon A., Oh H. E., Kim S. Y., Park Y. (2021). Plant growth regulators and rooting substrates affect growth and development of Salix koriyanagi cuttings. Rhizosphere 20, 100437. doi: 10.1016/j.rhisph.2021.100437
Yu X., Li Y., Cui Y., Liu R., Li Y., Chen Q., et al. (2017). An indoleacetic acid-producing Ochrobactrum sp. MGJ11 counteracts cadmium effect on soybean by promoting plant growth. J. Appl. Microbiol. 122, 987–996. doi: 10.1111/jam.13379
Yu B., Yang Q. W., Zhang Y. H., Li H. C., Zhang P. D. (2023). Effects of gibberellin and weak acid on seed gemination and physiological characteristics of the eelgrass. Zostera marina. Prog. Fish. Sci. 4, 26–34. doi: 10.19663/j.issn2095-9869.20230331002
Yue S., Zhang Y., Zhou Y., Xu S. C., Xu S., Zhang X. M., et al. (2019). Optimal long-term seed storage conditions for the endangered seagrass Zostera japonica: implications for habitat conservation and restoration. Plant Methods 15, 158. doi: 10.1186/s13007-019-0541-6
Zhang H., Fan J. J., Peng Q., Song W., Zhou W. H., Xia C. L., et al. (2023). Effects of chlormequat chloride and paclobutrazol on the growth and chlorophyll fluorescence kinetics of Daphne genkwa. Russ. J. Plant Physiol. 70, 159. doi: 10.1134/S1021443723900016
Zhang P. D., Wu X. X., Xu Q., Fang C. (2016). Studies on promotion of exogenous auxin to Zostera marina growth. Period. Ocean Univ. China 11, 99–107. doi: 10.16441/j.cnki.hdxb.20160269
Zhang Y. X., Yuan Y. C., Liu Z. J., Zhang T., Li F., Liu C. Y., et al. (2021). GA3 is superior to GA4 in promoting bud endodormancy release in tree peony (Paeonia suffruticosa) and their potential working mechanism. BMC. Plant Biol. 21, 323. doi: 10.1186/s12870-021-03106-2
Zhukovskaya N. V., Bystrova E. I., Lunkova N. F. (2020). Root growth at the cellular level in plants of different species: comparative analysis. Russ. J. Plant Physiol. 67, 618–625. doi: 10.1134/S1021443720040214
Keywords: plant growth regulator, Thalassia hemprichii, transplantation, indoleacetic acid, gibberellin, paclobutrazol, rhizome apex
Citation: Li Z, Shi Y, Zhao M, Shi Z, Luo H, Cai J and Han Q (2024) Plant growth regulators improve the growth and physiology of transplanted Thalassia Hemprichii fragments. Front. Mar. Sci. 11:1334937. doi: 10.3389/fmars.2024.1334937
Received: 08 November 2023; Accepted: 30 January 2024;
Published: 15 February 2024.
Edited by:
Vikash Kumar, Central Inland Fisheries Research Institute (ICAR), IndiaReviewed by:
Miriam Ruocco, Anton Dohrn Zoological Station Naples, ItalyCopyright © 2024 Li, Shi, Zhao, Shi, Luo, Cai and Han. This is an open-access article distributed under the terms of the Creative Commons Attribution License (CC BY). The use, distribution or reproduction in other forums is permitted, provided the original author(s) and the copyright owner(s) are credited and that the original publication in this journal is cited, in accordance with accepted academic practice. No use, distribution or reproduction is permitted which does not comply with these terms.
*Correspondence: Yunfeng Shi, c2hpeXVuZmVuZzgxODlAc2luYS5jb20=; Muqiu Zhao, emhhb211cWl1QHNpbmEuY29t
Disclaimer: All claims expressed in this article are solely those of the authors and do not necessarily represent those of their affiliated organizations, or those of the publisher, the editors and the reviewers. Any product that may be evaluated in this article or claim that may be made by its manufacturer is not guaranteed or endorsed by the publisher.
Research integrity at Frontiers
Learn more about the work of our research integrity team to safeguard the quality of each article we publish.