- 1Department of Marine Ecology, Faculty of Oceanography and Geography, University of Gdańsk, Gdynia, Poland
- 2Department of Chemistry, Life Sciences and Environmental Sustainability, University of Parma, Parma, Italy
- 3Department of Integrative Marine Ecology, Stazione Zoologica Anton Dohrn - National Institute of Marine Biology, Ecology and Biotechnology, Genoa Marine Center, Genoa, Italy
Estuaries can remove and/or retain land-derived nitrogen (N) and act as filters buffering N loads to the open sea. The N coastal filter can be seasonally variable depending on water temperature and transported loads, two factors acting in synergy and strongly influenced by climate change. The capacity of sediments to mitigate riverine N loads was investigated at four sites in the Vistula River plume area (Gulf of Gdańsk, Southern Baltic Sea). Samplings were carried out in two contrasting seasons: spring and summer, characterized by different water temperatures and nitrate (NO3-) levels. Inorganic N fluxes, and rates of denitrification and dissimilatory nitrate reduction to ammonium (DNRA) were measured in intact sediment cores by means of dark incubations and 15N-nitrate concentration-series experiments. Sampling sites were selected along a gradient of depth (5 to 24 m), that was also a gradient of sediment organic matter content. In both seasons, denitrification rates increased along with depth and from spring (6.5 ± 7.0 µmol m-2 h-1) to summer (20.4 ± 15.4 µmol m-2 h-1), despite lower NO3- concentrations in summer. In spring, at higher NO3- loading, denitrification was likely limited by low water temperature, and elevated sediment oxygen penetration. Coupled denitrification-nitrification prevailed over denitrification of water column NO3- across all sites and seasons, contributing to over 80% of the total denitrification. Notably, no anammox was detected at the sampling sites. DNRA exhibited low to undetectable rates in spring, especially at the shallowest sites. However, during summer, N recycling via DNRA increased and ranged from 0.7 to 14.9 µmol m-2 h-1. The denitrification efficiency (DE), calculated as the ratio between molecular nitrogen (N2) flux and dissolved inorganic N effluxes from sediments, ranged from 0 to 37% in spring, whereas in summer DE did not exceed 16%. Despite the dominance of denitrification over DNRA, the analyzed sediments acted as weak N buffers under in situ dark conditions. However, concentration-series experiments suggested high potential denitrification capacity, exceeding 400 µmol m-2 h-1, in response to short-term, large riverine inputs of NO3-.
1 Introduction
Coastal zones and estuaries receive and process large nutrient loads of terrestrial origin, buffering their impact on marine ecosystems through multiple biogeochemical processes (Asmala et al., 2017). The so-called coastal filters permanently remove or temporarily retain nutrients and organic matter via abiotic and biotic processes such as sedimentation, precipitation, burial, primary producers’ uptake and microbial transformations (Bouwman et al., 2013; Carstensen et al., 2020). The effectiveness of the coastal filter depends upon factors like the timing of nutrient and organic matter transport, water residence time, temperature and dissolved oxygen (O2), and the diversity and richness of primary producers and benthic organisms; most of these factors are, in turn, affected by climate change (Rabalais et al., 2009; Lunau et al., 2013; Magri et al., 2022).
Nitrogen (N) is an important nutrient in water bodies controlling primary and secondary production and is considered a limiting element in marine ecosystems (Howarth, 1988). However, anthropogenic activities (e.g., the intensive use of fertilizers in modern agriculture), together with industrial and wastewater treatment plant inputs, have increased N loads delivered to the coastal zones by rivers, leading to widespread eutrophication phenomena (Lunau et al., 2013; Paerl et al., 2014; Asmala et al., 2017). Nitrogen can be removed permanently from the coastal ecosystems through the production of molecular nitrogen (N2) via two processes: denitrification, which is the reduction of nitrate (NO3-) or nitrite (NO2-) to N2, and the anaerobic ammonium (NH4+) oxidation (anammox) (Canfield et al., 2005). Alternatively, the processes that increase retention and recycling of N within coastal ecosystems include the uptake into biomass, ammonification, nitrification and dissimilatory nitrate reduction to ammonium (DNRA) (Canfield et al., 2005). In the coastal zones the regulation of both denitrification and DNRA generally depends upon temperature, the availability of NO3-, organic carbon input, and the availability of reductants (Burgin and Hamilton, 2007; Kessler, 2018). Benthic macrofauna has also a demonstrated important role in the biogeochemical functioning of sediments, particularly in regulating the N cycle (Kristensen et al., 2012). Macrofauna affects benthic N biogeochemical processes directly through its physiology (e.g., via respiration, feeding or excretion) (Berezina et al., 2019; Zilius et al., 2022). Additionally, benthic animals can influence sediment through their activity (movement, burrowing or ventilation), stimulating microbial and meiofauna communities and N transformations (Gilbertson et al., 2012; Bonaglia et al., 2014a; Moraes et al., 2018). Laboratory experiments have demonstrated that burrowing macrofauna stimulates permanent N loss via denitrification, either by increasing sediment nitrification or by directly enhancing the availability of NO3- in sub-surface sediments via burrow ventilation and irrigation (Samuiloviene et al., 2019; Zilius et al., 2022). Comparatively, a few studies have analyzed the effects of macrofauna on nitrate ammonification (Bonaglia et al., 2013).
Processes leading to N removal and recycling co-occur and can be measured simultaneously, for example by means of intact cores incubation, in order to contrast the relative importance of the two pathways in benthic N cycling. Aggregated indices, such as the denitrification efficiency, allow the calculation of the fraction of ammonified organic N that is permanently lost via denitrification (Eyre and Ferguson, 2009; Bartoli et al., 2012). The ratio between N permanent loss and N recycling can vary spatially and temporally, depending on the timing of loads and the transported chemical forms of N, as well as factors like temperature, organic sedimentary loads, the presence of bioturbating fauna and climate change-related issues. Ongoing climate change increases the frequency of flash floods and prolonged drought periods (Paerl et al., 2014; Magri et al., 2022). Short-term, intense precipitation events increase the transfer velocity and the amount of N loads generated in heavily fertilized agricultural basins or bypassing wastewater treatment plants that reach the coastal zones, mainly in the form of NO3- (Rabalais et al., 2009; Baron et al., 2013). Different authors indeed report positive correlations between river discharge and nitrate concentrations (Johannsen et al., 2008; Oeurng et al., 2010; Pastuszak et al., 2012; Vybernaite-Lubiene et al., 2018). During flash floods, large inputs of N-rich freshwater and short residence time in the coastal zone may result in limited processing of N loads by primary producers and communities of denitrifying bacteria, leading to direct export to the open sea (Nixon et al., 1996; Magri et al., 2020). Moreover, frequent and prolonged periods of water scarcity are expected to increase water residence time in the coastal zones, resulting in water stratification, deoxygenation and an increase in salinity and sulphate (Rabalais et al., 2009). Under these circumstances sulphate reduction becomes a dominant microbial respiration pathway in anoxic sediments and the produced sulphides may accumulate in pore water depressing processes as nitrification and denitrification and favoring processes like nitrate ammonification (Murphy et al., 2020). Hydrological extremes are also expected to impact benthic macrofauna diversity and abundance; the onset of anoxia due to prolonged drought and water stratification determines the loss of benthic macrofauna and associated biogeochemical ecosystem processes and services (Rabalais et al., 2009). Ultimately, climate-related drought conditions, stratification and deoxygenation are expected to favor internal N recycling, mainly in the form of NH4+ (Magri et al., 2020; Murphy et al., 2020). Decreased N removal and increased recycling lead to a higher bioavailability of reactive N in the coastal systems (Giblin et al., 2013), which can stimulate the growth of primary producers resulting in positive feedback to eutrophication (McGlathery et al., 2007; Rabalais et al., 2009).
While the estuarine and coastal N cycle has been extensively studied, only a few investigations have explored the interactive impacts of eutrophication and climate change on the N estuarine or coastal filter, particularly on processes involved in N removal or recycling. The aim of this work was to investigate the capacity of coastal sediments to recycle or permanently remove N in a eutrophic area of the Gulf of Gdańsk (Southern Baltic Sea, Poland) influenced by the plume of the Vistula River. Experiments were carried out in 2022 during two contrasting seasons: spring that is generally characterized by high precipitation, riverine discharge and N loads, and summer that is characterized by drought, low riverine discharge and N loads. In this study, net inorganic N fluxes and in situ denitrification and DNRA rates were measured in intact sediment cores. Additionally, rates of denitrification and DNRA were measured by adding increasing concentrations of NO3- to the water phase of intact cores. This approach mimics riverine flash floods and hydrologic extremes in two seasons, leading to large NO3- delivery to the coastal zone. The concentration-series experiments enabled the identification of unknown threshold concentrations that saturate processes. The range of NO3- concentrations tested included values far beyond those typically recorded during high discharge events. This allowed to explore the potential denitrification capacity of reactive sediments affected by river plumes.
2 Materials and methods
2.1 Study area and samples collection
Samplings were carried out at four sites located in the coastal area of the Gulf of Gdańsk (southern Baltic Sea) influenced by the Vistula River plume (Figure 1). The Vistula is one of the largest rivers flowing into the Baltic Sea, contributing to its high nutrient load and trophic status. Over a multi-annual perspective, the average discharge of the Vistula is 33.7 km3 per year, with higher outflow in the semester November-April as compared to the May-October period (Bajkiewicz-Grabowska et al., 2019).
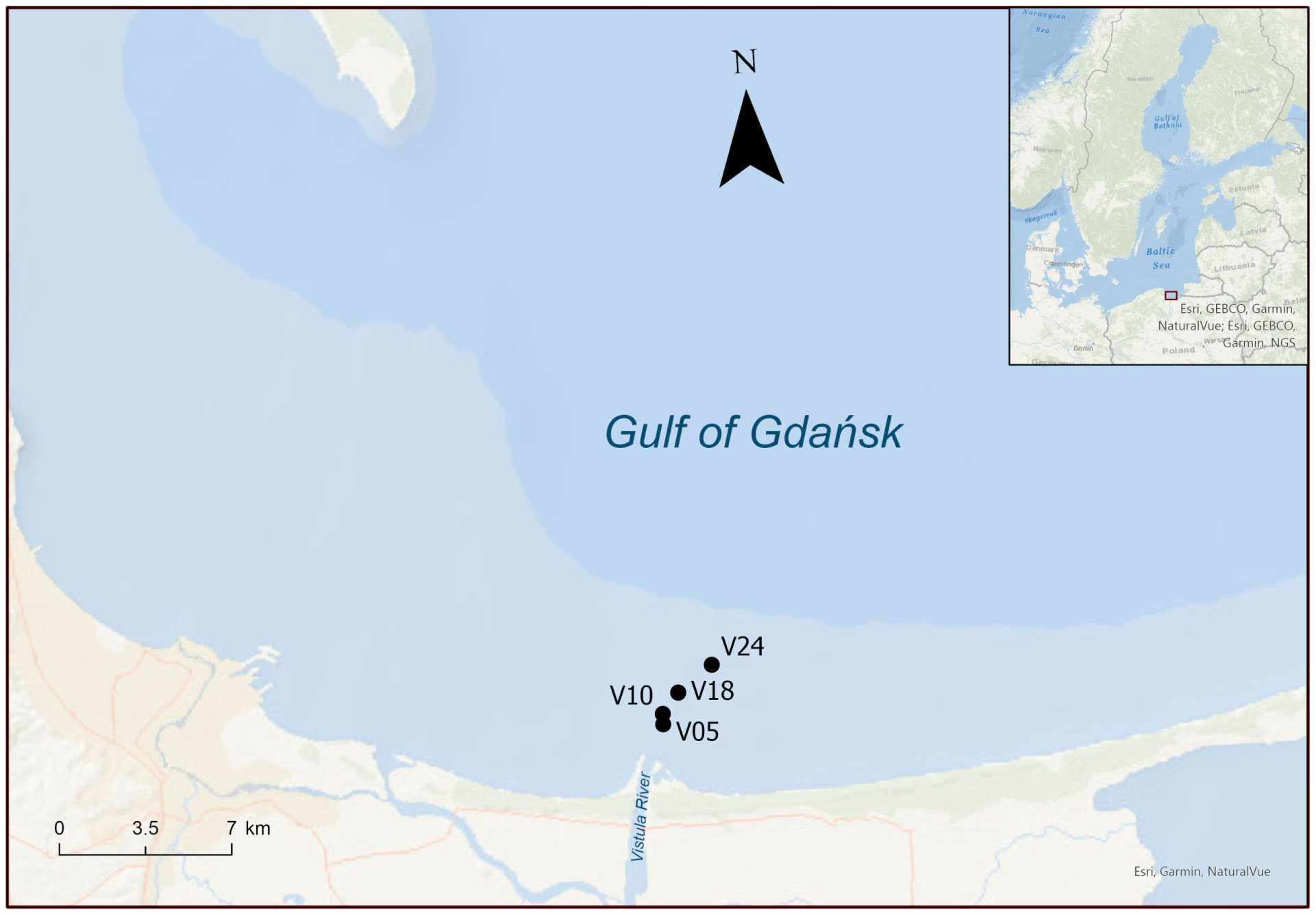
Figure 1 Map of the sites sampled in spring and summer 2022. Numbers in the sites name indicate the depth of the water column.
The Gulf of Gdańsk is a coastal system strongly affected by a variable and wind-driven plume from the Vistula River. In the gulf, parameters such as salinity, N compounds and chlorophyll a concentrations change with both distance from the river mouth and depth (Kruk-Dowgiałlo and Szaniawska, 2008; Włodarska-Kowalczuk et al., 2016). Indeed, Bartl et al. (2018) found nitrite and nitrate concentrations significantly higher in the Vistula River plume compared to the coastal surface water, with values in the river plume up to 200 µM recorded in spring. Nitrate concentrations in the Vistula River (<5 to >300 µM) show positive correlation with water discharge and undergo large seasonal variations, with nearly undetectable values during summer and winter peaks, as reported by Pastuszak et al. (2012) and references therein, and for other large rivers in the same area (Vybernaite-Lubiene et al., 2018). In the Vistula basin, a seasonal thermocline is present at a depth of about 30-40 m and a halocline is observed at a depth of about 60-80 m. Bottom sediments in the Gulf of Gdańsk display a patchy distribution, with various sands or mixed muddy-sand sediments in shallow waters and muds in the central part of the gulf (Uścinowicz, 1997).
Sediment sampling sites were aligned in front of the Vistula River mouth. Sites are named according to the depth at which sediments were collected: 5, 10, 18 and 24 m. Two cruises were carried out in 2022 onboard r/v Oceanograf (research vessel owned by the University of Gdańsk). The first cruise took place in March, while the second occurred in July. In spring, the historical average discharge of the Vistula River (~1,500 m3 s-1) nearly doubled compared to the summer average (~800 m3 s-1) (Pastuszak et al., 2018). Prior to coring, a CTD (Sea-Bird 25plus), equipped with a circular rosette of Niskin bottles (12 × 10 L), was deployed to determine water column characteristics at the time of sampling, such as dissolved O2 saturation, temperature and salinity, and to collect bottom water. Sediment samples were collected by box-corer (20 x 30 cm). Intact sediment cores were obtained by hand, subsampling from the box-corer via transparent Plexiglass liners (i.d. 4.6 cm, height 20 cm, n=22 per site). The sediment inside the liners was levelled to achieve a sediment and water column height of 7 and 8 cm, respectively. A Teflon-coated magnetic bar of 3 cm was suspended in each core approximated 5 cm above the sediment-water interface. The sediment cores were then transferred to a temperature-controlled room and submerged uncapped in tanks filled with unfiltered in situ water. Each tank was equipped with a cooling system to maintain a stable in situ water temperature (4 and 18°C in spring and summer, respectively) and with aquarium pumps and air stones to maintain O2 concentration at 100% saturation, mirroring in situ bottom water conditions. Cores were preincubated overnight in the dark to allow for re-equilibration. During the whole preincubation period the magnetic bars, driven by an external motor at 40 rpm, gently stirred the bottom water to ensure water exchange between the tank and the core, preventing water stratification and sediment resuspension (Magri et al., 2020).
2.2 Measurements of benthic fluxes at the sediment-water interface
The morning after sampling, the sediment cores underwent two dark sequential incubations. The duration of the incubations varied in the two seasons depending on the bottom water temperature to keep O2 concentration within 20-30% of initial values and started when gas-tight lids were positioned on the top of the cores. Dissolved O2 concentration was measured in each core with a microelectrode (OX-100, Unisense A/S, DK) at the beginning and at the end of the incubations. Water samples were collected in quadruplicates from each tank at the beginning of the incubation and from the water phase of each core at the end of the incubation. In both cases, an aliquot of water was filtered (Whatman GF/F glass fiber filters) and transferred to scintillation vials to analyze dissolved inorganic N compounds via standard spectrophotometric techniques. Ammonium concentration was determined using salicylate and hypochlorite in the presence of sodium nitroprussiate (Bower and Holm-Hansen, 1980). Nitrate concentration was determined after reduction to NO2− in the presence of cadmium, and NO2− was determined using sulphanilamide and N-(1-naphthyl)ethylenediamine with a continuous flow analyzer (San++, Skalar, sensitivity 0.3 μM) at the Marine Research Institute (Klaipeda University, Lithuania) (Golterman et al., 1978; APHA, 1992). Hereafter, the sum of NO3- and NO2- will be expressed as NOx-. Oxygen and nutrient fluxes at the sediment-water interface were calculated according to the equation below:
Where Fx is the flux of the chemical species x expressed in µmol m-2 h-1, Ci and Cf (µM) are concentration values of the chemical species x at the beginning and at the end of incubation, respectively, V is the water column volume (L), A (m2) is the sediment surface, and t (h) is the incubation time.
From measured O2 fluxes a theoretical ammonification rate (TA), which is a proxy of the total amount of NH4+ produced within surface sediments, was calculated. TA was obtained by the conversion of dark O2 fluxes into organic carbon oxidation rate using site-specific respiratory quotients (RQ) calculated from surface sediment C/N molar ratio, as detailed in Jørgensen et al. (2022). Calculated RQ values ranged from a minimum of 0.75 to a maximum of 1.06. The obtained carbon oxidation rates were then divided by the sediment C/N ratio to calculate TA.
2.3 Determination of denitrification and DNRA rates
After net flux measurements, the water in the tanks was renewed and the cores were left open and submerged for a few hours in in situ and well-mixed water. Thereafter, the cores were incubated in the darkness to quantify denitrification and DNRA rates with the revised isotope pairing technique (r-IPT, Risgaard-Petersen et al., 2003). To this purpose, the water in the tanks was lowered just below the top of the cores and 15NO3- from a 20 mM 15NO3- stock solution (Na15NO3, ≥ 98 atom % 15N Sigma Aldrich) was added to the water phase of each core. For each site in the Gulf of Gdańsk, a time-series experiment and a concentration-series experiment were carried out. For the time-series, a set of six cores was added with labelled NO3- to have a fixed, final 15NO3- concentration of 100 µM; incubation times varied from 1 to 24 hours in spring and from 1 to 9 hours in summer. For the concentration-series, a set of ten cores was added with increasing amounts of 15NO3- to the water phase of each core to reach a final concentration ranging from 10 to 2,000 µM. We acknowledge that a concentration of 2,000 µM largely exceeds, by a factor of 7, average annual NO3- concentrations reported for the closing section of the Vistula River (Pastuszak et al., 2012). However, such NO3- concentration was intentionally tested in order to saturate the NO3- reduction capacity of the different sites and to identify potential denitrification thresholds. The incubation time of this second set of cores was nearly 24 hours in spring and between 3 and 9 hours in summer. A water sample was collected from each core before and after the 15NO3- addition to determine the 15N-enrichment of the NO3- pools. Thereafter, cores were capped with rubber lids and incubated as described for nutrient flux measurements in order to keep O2 concentration within 20-30% of the initial value. At the end of the time- and concentration-series incubations, just after O2 measurements, the whole sediment column in each core was gently mixed with the water column and homogenized to a slurry. An aliquot of the slurry was transferred to 12-mL exetainers, allowing abundant overflow, and fixed with 200 µL of 7 M ZnCl2 solution to inhibit further microbial activity. The abundance of 29N2 and 30N2 was determined via a membrane inlet mass spectrometer (MIMS, Bay instrument, MD, USA). Total denitrification rates (denitrification of 14NO3- and 15NO3-) were calculated from the production of 29N2 and 30N2, assuming a binomial distribution of 28N2, 29N2, and 30N2. The denitrification of NO3- diffusing from the water column (Dw) and the coupled nitrification-denitrification (Dn) were calculated as described by Nielsen (1992). A second aliquot of the slurry (30 mL) was sampled to determine DNRA rates from the production of 15NH4+. The sample was transferred to 50-mL falcon tubes and treated with 2 g of KCl. Tubes were shaken, centrifuged (2,000 rpm for 5 minutes) and the supernatant was filtered into 20-mL scintillation vials. These samples were purged with air for 10 minutes, to eliminate 29N2 and 30N2 pools produced during the incubations and transferred to 12-mL exetainers added with 200 µL of alkaline hypobromite solution to oxidize NH4+ to N2 (Warembourg, 1993). The abundance of 29N2 and 30N2 was determined via MIMS. Total DNRA rates were calculated assuming that DNRA occurs in the same sediment horizon as denitrification. Total DNRA rates were divided into DNRA of water column NO3- (DNRAw) and DNRA of NO3- produced by nitrification (DNRAn) as reported in Risgaard-Petersen and Rysgaard (1995). All calculations are detailed in Magri et al. (2020).
From the results of two sequential dark incubations, denitrification efficiency (DE) was calculated according to Eyre and Ferguson (2009) as:
where Dtot is total denitrification (Dw+Dn) and DIN represents the sum of dissolved inorganic N effluxes (NH4++NO2-+NO3-) directed from the sediment to the water column.
The response of total denitrification to NO3- addition was fitted to a Michaelis-Menten type function:
where Vmax is the maximum denitrification rate (µmol m-2 h-1), x is the concentration of NO3- expressed in µM, and Km is the concentration of NO3- at which denitrification rate is half maximal (Vmax/2) expressed in µM. Values of Vmax and Km were estimated through the nonlinear least-squares regression using R (R Core Team, 2022).
2.4 Macrofauna analysis
At the end of the incubations, sediments from each core were sieved (1 mm mesh size) to retrieve, identify and weigh macrofauna. Until the analysis, the fauna was preserved in 70% ethanol. Organisms were sorted and identified to the species level with the exception of Oligochaeta, Marenzelleria spp. polychaetes and Chironomidae insect larvae, then taxa were counted to determine their abundance, and after drying for 48 h at 65°C weighed to determine their biomass (shell-free dry mass) per square meter. For statistical purposes, to analyze the potential role of macrofauna bioturbation as a co-regulator of the benthic N cycle, the retrieved organisms were divided into three groups. Groups identified the macrofauna burial depth and burial type in shallow burrowers, deep burrowers (burrow/tube dwellers) and free-living deep burrowers (Thoms et al., 2018; Miernik et al., 2023).
2.5 Sediment characterization
Another set of intact sediment cores (i.d. 4.6 cm, length 20 cm, n=6 per site) was collected at each site and season for the physical properties characterization. Cores were extruded and sliced into five layers: 0-1, 1-2, 2-3, 3-5 and 5-10 cm. Slices were homogenized, and subsamples of 5 mL were collected using cut-off syringes to measure physical properties. Bulk density was determined as the ratio between wet weight and volume (5 mL) of sediment. Sediment porosity was determined from the loss of wet weight after 48 h at 70°C. Organic matter content (OM) was measured as a percentage of weight loss on ignition (450°C, 6 h) from dried, powdered sediment. Later, sediments were analyzed for carbon (C) and N content and their isotopic composition in the upper 0-1 cm sediment layer with a mass spectrometer (Thermo Scientific Delta V) coupled with an element analyzer (FlashEA 1112, Thermo Electron Corporation) at the Center for Physical Sciences and Technology (Lithuania). Before measurements sediment samples were ground and acidified with 1 N HCl in order to remove carbonates.
2.6 Statistical analysis
A two-way ANOVA was used to test the effects of the factors: season (spring/summer) and site (including their interactive effects) on sediment features. Data normality was checked with the Shapiro-Wilk test and homogeneity of variance was checked using Levene’s median test; if needed data were transformed to meet normality. For significant factors, a pairwise multiple comparison was carried out with the post hoc Tukey HSD test.
As oxygen and nutrient fluxes, and denitrification and DNRA rates were not normally distributed, two-way PERMANOVA was applied to test the significance of the factors season, site, and of their interactive effects. For significant factors, a pairwise test was performed (Anderson et al., 2008).
Distance-based linear models (DistLM) were used to examine simultaneously the effect of environmental and biological variables on measured fluxes and process rates (Anderson et al., 2008). This model can provide a better understanding of the factors affecting ecosystem functioning and processes (Gammal et al., 2017; Kauppi et al., 2017). Firstly, the relationships between variables were examined and highly (Pearson’s r ≥ 0.80) co-correlated environmental or biological variables were excluded from the analysis. Temperature and macrofauna biomass were log(x+1) transformed. The following environmental variables and biological traits were selected to examine their influence on fluxes and rates: OM, C/N, δ13C, temperature, and biomass of shallow burrowers, biomass of deep tube dwellers and biomass of free-living deep burrowers.
Multivariate oxygen and nutrient fluxes, and denitrification and DNRA rates were analyzed together at all sites and in the two seasons. The flux and rate data were normalized to ensure the equal importance of all data. Resemblance matrices were based on the Euclidean distances. The stepwise selection and the AICc stopping criterion were used in the DistLM to determine the relative importance of predictors. The marginal test indicates the proportion of the variation explained by predictors when fitted individually, while the results from the sequential test indicate the proportion added by the predictor to the cumulative total proportion explained.
Statistical analyses were performed with R and PRIMER 7 + PERMANOVA (PRIMER-E Ltd) and graphs were made with ggplot2 package (Wickham, 2016; Xu et al., 2021) in R. For all tests significance was set at p< 0.05.
3 Results
3.1 Environmental and biological characteristics of the benthic system
Bottom water temperatures varied between 4.4 and 4.8°C in spring and from 17.6 to 18.5°C in summer. Salinity fell within the range of 7.0 and 7.6 and it was comparable between the two seasons. Salinity was lowest at site V05, closest to the river mouth, and increased towards site V24. In both sampling periods bottom water was oxygen saturated. Nitrate concentrations were higher in spring than in summer at the four sites, with values averaging 10±2 µM in spring and 2±1 µM in summer (Table 1).
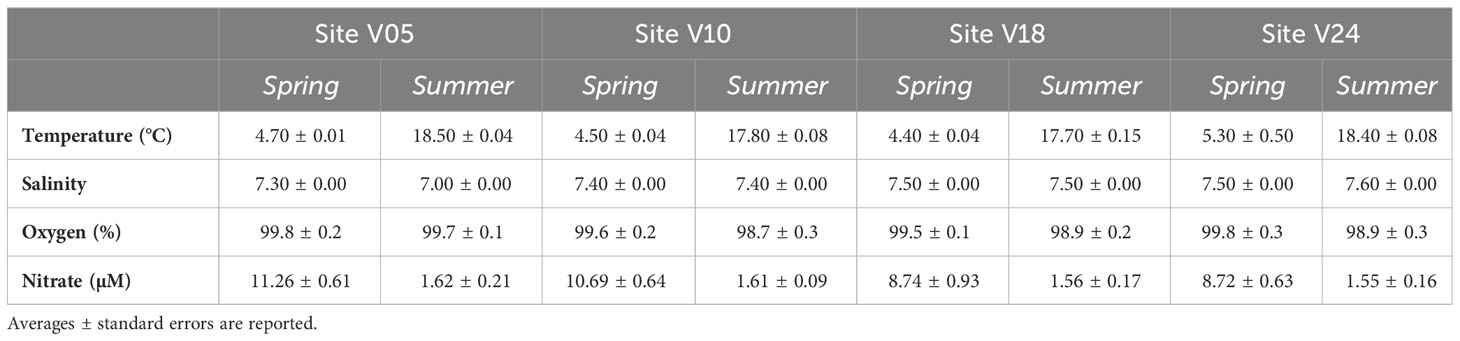
Table 1 Characteristics of the bottom water at the four sites (V05, V10, V18, V24) in the two seasons (Spring and Summer).
Sediments significantly differed among sites and between seasons for all the physical properties, except density, which remained constant across sites (Table 2). The interaction of sites and seasons did not affect the C/N ratio, leading to the selection of the additive model. As the depth increased, the sedimentary organic matter content also increased by a factor of 10. The shallowest site (V05) exhibited the lowest organic matter content. The sediment C/N ratio was higher in spring than in summer at all sites, while an opposite trend was found for δ13C and δ15N. Site V10 in summer displayed higher porosity, water content, OM percentage, and C/N ratio, suggesting that this sampling area was influenced by a terrestrial input from the Vistula River, as evidenced by the C and N isotopic composition in sediments (-29.31 ± 0.09‰ δ13C and 7.69 ± 0.06‰ δ15N).
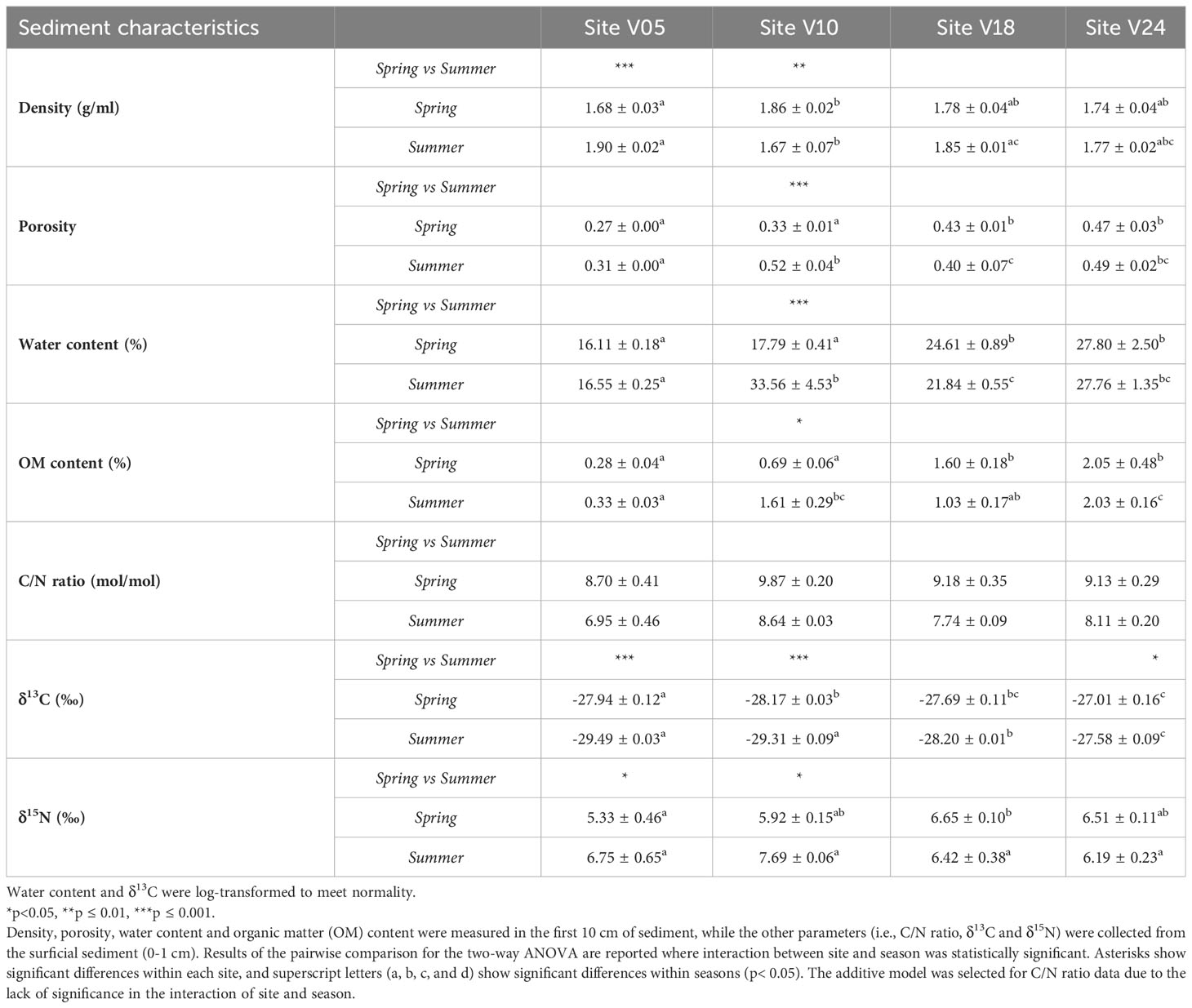
Table 2 Averages ± standard errors of the sediment characteristics at the four sites (V05, V10, V18, V24) and for the two seasons (Spring and Summer).
Macrofauna at all sampling sites and in both seasons was represented by a total of 11 taxa (Supplementary Table S1). In spring, no macrofauna was recorded at the two shallowest sites, and 5 and 7 taxa were present at sites V18 and V24, respectively. In summer, macrozoobenthos was found at all sites with variable species richness, from 2 to 7 taxa. Benthos biomass at the sites also differed between seasons: in summer it was higher and ranged from 0.04 gDW m-2 (site V05) to 47.27 gDW m-2 (site V18) (Figure 2, Supplementary Table S1). Macrofauna had higher biomass at the deepest sites (V18 and V24). At all sites, the biomass of fauna consisted of shallow burrowing organisms and deep burrowers, mostly polychaetes, living in tubes or dwellers. At the deepest sites, there were also free-living bivalves burrowing deep into the sediment as Mya arenaria and Macoma baltica, constituting more than 50% of the benthic biomass (Figure 2).
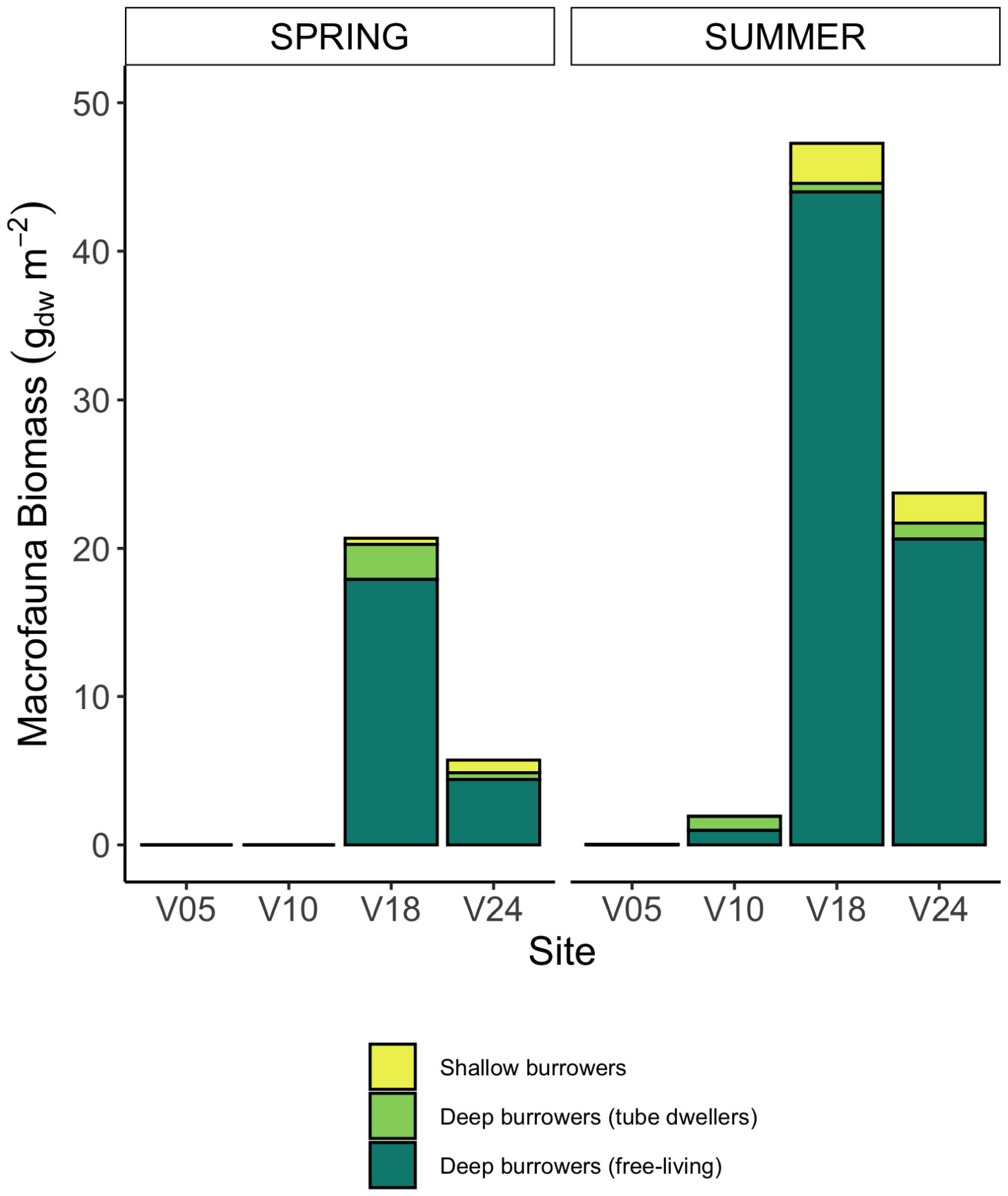
Figure 2 Average benthic fauna biomass retrieved at the four sites in the two seasons (gDW m-2) and grouped in three functional groups: shallow bioturbating organisms, deep burrowing taxa living in fixed tubes or dwellers, and deep burrowing taxa free-living.
3.2 Net O2 and nutrient fluxes at the sediment-water interface
Total benthic O2 uptake was significantly higher in summer compared to spring and differed among sites, but there was an interaction between the two factors (Figure 3A). In spring, O2 fluxes ranged from -96.2 ± 8.3 to -446.8 ± 78.4 µmol m-2 h-1 (average ± standard error), while in summer, they ranged from -540.4 ± 26.8 to -3,345.0 ± 221.7 µmol m-2 h-1. The lowest O2 consumption rates were measured at site V05 in both seasons. In summer, sediments at site V10 displayed a peak in O2 fluxes that was almost two-fold higher than at sites V18 and V24, although the macrofauna biomass was much lower (Supplementary Table S1).
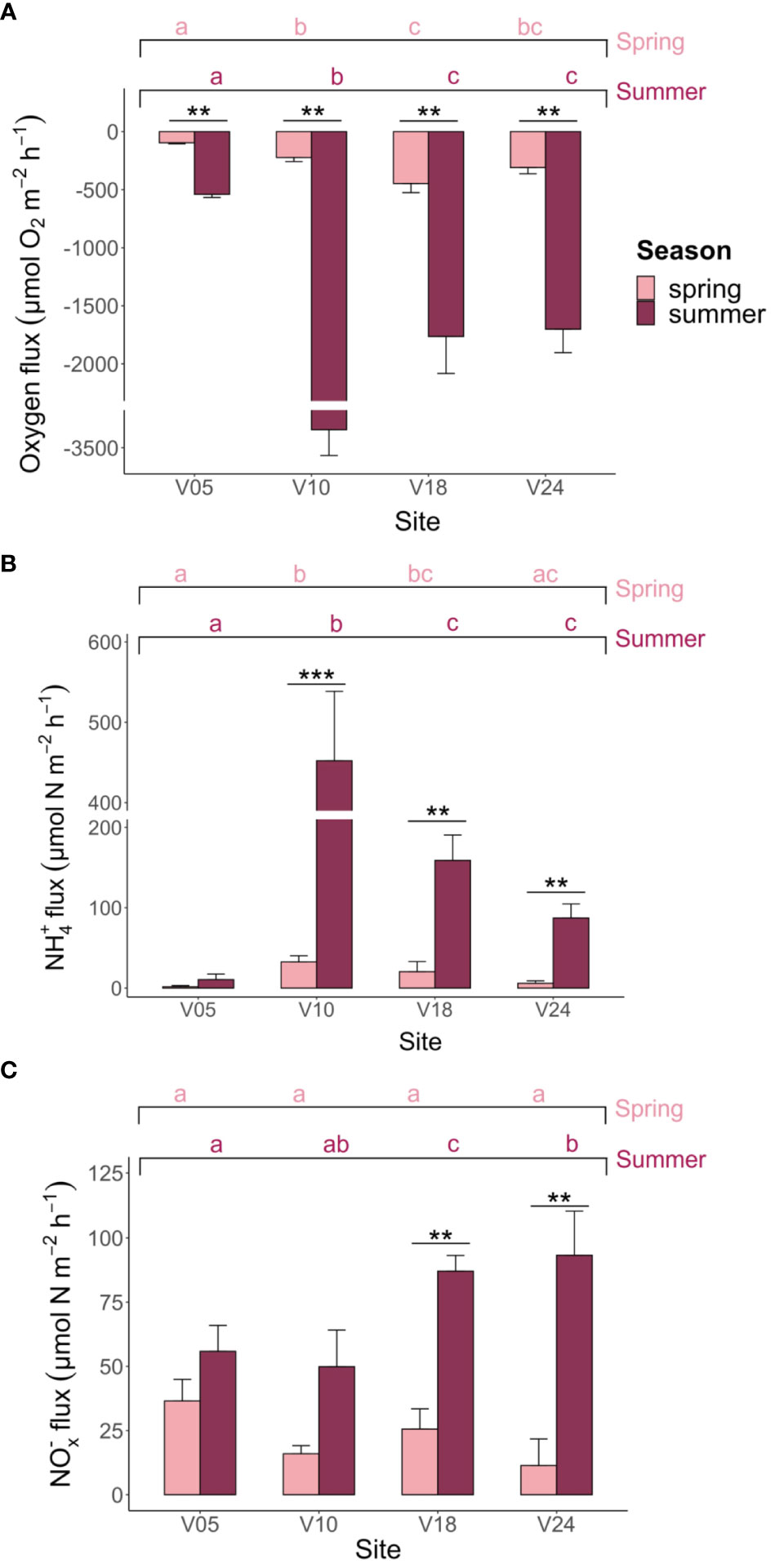
Figure 3 Net (A) O2, (B) NH4+ and (C) NOx- (NO3-+NO2-) fluxes measured at the four sites in spring and summer. Averages (n=6) ± standard errors are reported. The graphs present the outcomes of the pairwise comparison for the two-way PERMANOVA, focusing on the interaction between season and site where interaction effect was statistically significant (p< 0.05). Asterisks denote significant differences within each site, highlighting variations attributed to season. Meanwhile, letters denote significant differences within seasons, emphasizing site-related variations.
Dark ammonium fluxes were always positive, suggesting that variable fractions of ammonified N were not retained within sediments or completely oxidized to NOx-. Ammonium fluxes were significantly higher in summer compared to spring and differed among sites as shown by the two-way PERMANOVA (Figure 3B). In spring, fluxes ranged from 1.7 ± 1.4 to 32.5 ± 7.7 µmol m-2 h-1, while in summer, they ranged from 10.6 ± 6.9 to 452.2 ± 86.3 µmol m-2 h-1. Sediments at sites V05 and V10 displayed the lowest and the largest NH4+ fluxes in both seasons, respectively.
Theoretical ammonification rates were generally higher than measured NH4+ fluxes. Since C/N ratios were rather similar across different sites, TA displayed a pattern that overlapped with that of O2 fluxes. TA varied between 10 ± 1 and 48 ± 10 µmol m-2 h-1 in spring and between 58 ± 7 and 357 ± 25 µmol m-2 h-1 in summer.
Fluxes of NOx- were consistently directed to the water column, indicating an excess of nitrification over nitrate reduction. In spring, they ranged from 11.4 ± 10.3 to 36.5 ± 8.4 µmol m-2 h-1, while in summer, they ranged from 49.9 ± 14.3 to 93.3 ± 17.2 µmol m-2 h-1 (Figure 3C). Although NOx- fluxes differed between the two seasons, they did not show significant differences among sites (Figure 3C). Notably, NOx- fluxes were mostly relevant in the N inorganic exchanges at sites V05 and V24 in both seasons, while NH4+ fluxes dominated at site V10 in both seasons. Site V18 shifted from NOx- to NH4+ fluxes dominance from spring to summer.
3.3 Rates of denitrification and DNRA
The time-series incubations revealed linearity in N2 production with time at both seasons, with the exception of sites V05 and V10 in spring when denitrification rates were below the detection limits of the method employed (< 0.5 µmol m-2 h-1). Where the production of labelled N2 was measurable, it was possible to calculate the denitrification of 15NO3- (D15) and of 14NO3- (D14). The latter, which is the genuine 28N2 production, was always independent from the concentration of 15NO3- added to the water phase, indicating that N2 was mostly produced by denitrification. The concentration-series experiments suggested that anammox was not present at any site and in any season, and that the IPT assumptions and calculations could have been applied (Nielsen, 1992; Robertson et al., 2019). Total denitrification rates (Dtot = Dw + Dn) were higher in summer compared to spring at all sites and increased from the shallowest to the deepest site. Both Dw and Dn differed among sites and between seasons (Figures 4A, B). Total denitrification rates ranged from< 0.5 to 10.5 ± 5.2 µmol m-2 h-1 in spring and from 2.3 ± 0.2 to 35.1 ± 4.4 µmol m-2 h-1 in summer, at sites V05 and V24, respectively. At all sites where denitrification was measurable and in both seasons, Dn was the dominant nitrate reduction pathway, accounting for more than 80% of the total denitrification.
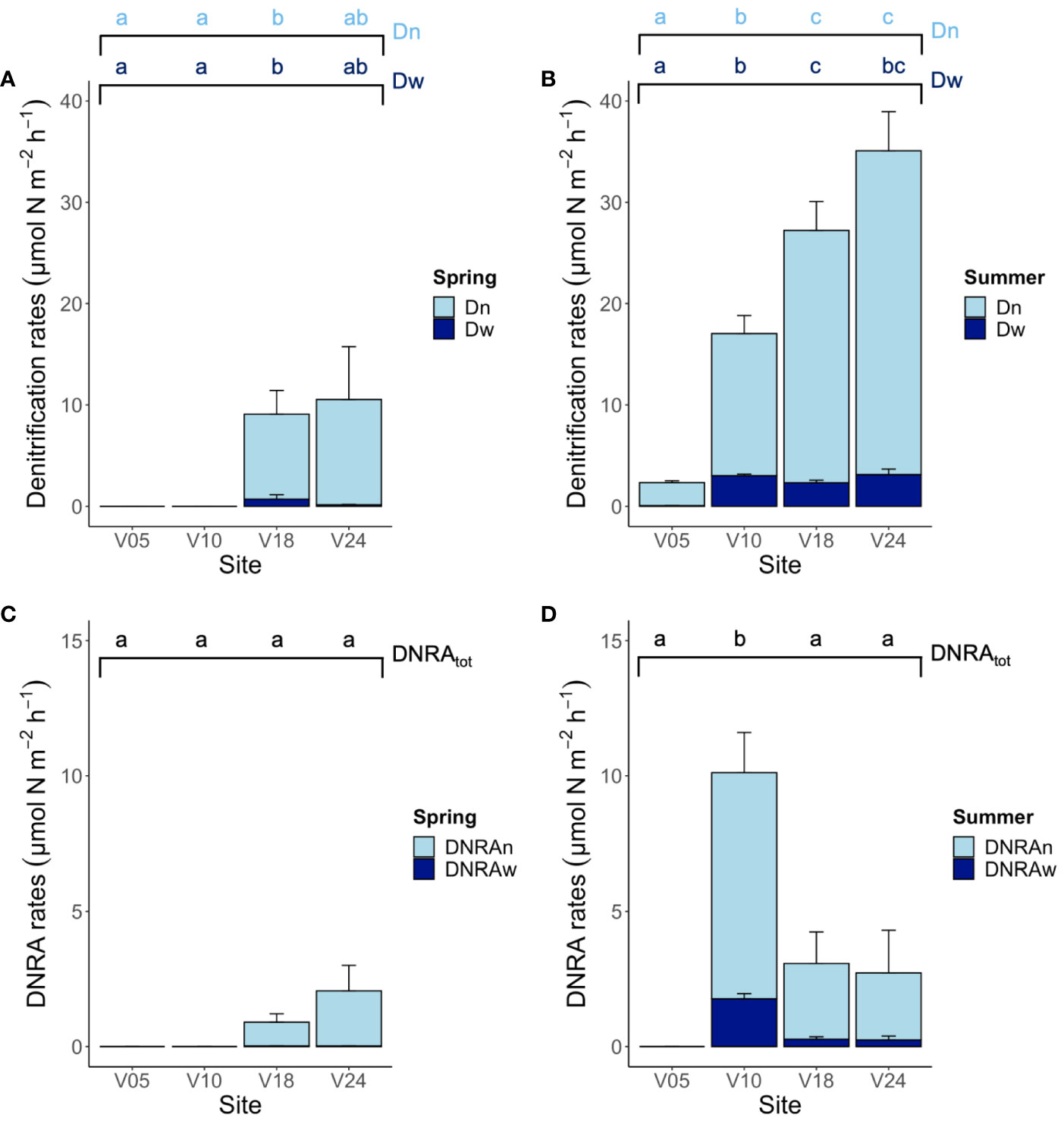
Figure 4 The staked bar chart in the upper panel depicts the rates of denitrification of nitrate produced via nitrification (Dn) and of nitrate diffusing into the sediment from the water column (Dw) measured at the four sites in (A) spring and (B) summer. The staked bar chart in the below panel depicts total DNRA rates divided into the DNRA coupled to nitrification (DNRAn) and DNRA of water column nitrate (DNRAw) measured at the four sites in (C) spring and (D) summer. Averages (n=6) ± standard errors are reported. Note the different scales on y-axis in the two panels. The graph presents the results of the pairwise comparison for the two-way PERMANOVA, investigating the interaction between season and site. Letters indicate statistically significant differences among sites within season (p< 0.05).
DNRA rates differed significantly among the four sites and tended to be higher in summer compared to spring (Figures 4C, D). However, the only significant seasonal difference was found at site V10 that also exhibited the highest rate of nitrate ammonification (10.1 ± 1.6 µmol m-2 h-1, corresponding to 37% of the total dissimilative NO3- reduction). At the other sites, in both seasons, the contribution of DNRA to microbial nitrate reduction was low to negligible and never above 20%. As for denitrification, the production of NOx- via nitrification in the sediment was always the main nitrate source to DNRA.
Concentration-series experiments revealed that the addition of large amounts of NO3- to the water column produced contrasting effects at the four investigated sites (Figure 5). In spring, at the shallowest V05 site the addition of NO3- produced negligible stimulation of Dw as compared to in situ rates. In summer, rates increased significantly to a plateau of ~56 µmol m-2 h-1, but the process was half saturated (Km) at NO3- concentration of 350 µM. At site V10, NO3- addition produced a limited effect on Dw in spring, but a much larger response of the microbial community occurred in summer when the saturation of denitrification was reached at 475 µmol m-2 h-1 and the Km was found at a NO3- concentration of 306 µM. At the deepest sites, the addition of NO3- resulted in significant stimulation of Dw both in spring and summer, with the highest rates measured in the warm period, up to 400 µmol m-2 h-1. Comparing all the sites, site V18 displayed the highest Vmax and Km values, estimated in 688 µmol m-2 h-1 and 859 µM, respectively.
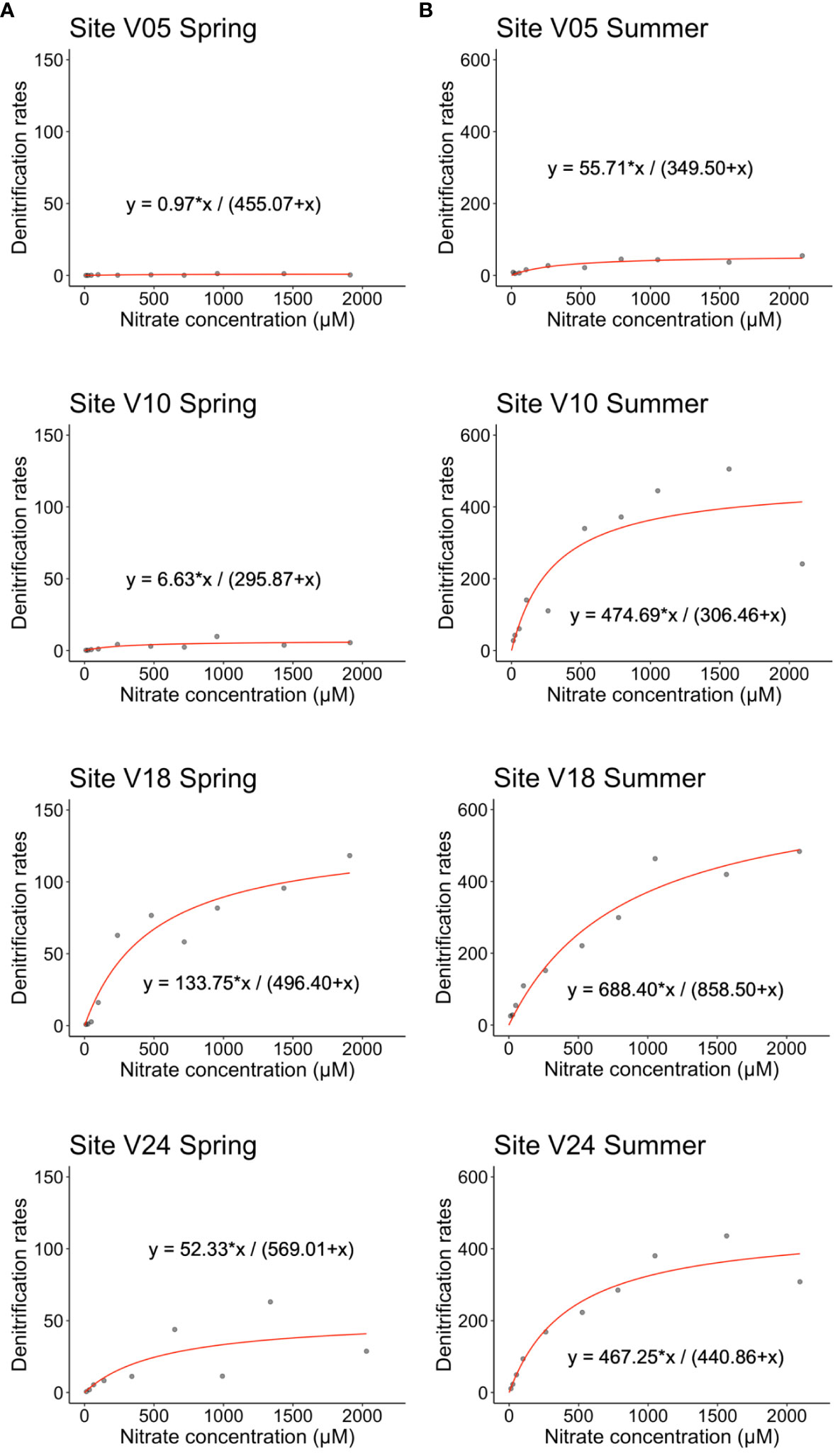
Figure 5 Hourly rates of denitrification (µmol m-2 h-1) measured at the four sampling sites in (A) spring and (B) summer as a function of NO3− concentration (µM). The equation of the Michaelis-Menten model is reported for each site and season. Note different scales on the y-axis for the two seasons.
In situ rates of denitrification and DNRA were combined with net NH4+ and NOx- fluxes and with TA rates to reconstruct seasonal benthic N cycling at the four sites (Supplementary Figure S1). The reconstructions reveal slight variations in benthic N cycling among seasons and sites, with ammonification increasing from spring to summer at all sites, mirroring the patterns observed for oxygen, and denitrification efficiency increasing along depth. The schematics illustrate that, at all sites and in both seasons, a major fraction of the ammonified organic nitrogen (ranging from 53 to 100%) is recycled to the water column as NH4+ or NOx-. Nitrification plays a key role in converting a major fraction of the NH4+ produced within sediments, but the coupling of nitrification with denitrification or DNRA remains low, as depicted in 7 out of 8 schematics in Supplementary Figure S1. Here, only 0 to 31% of the NO3- produced within sediments is reduced to N2 or NH4+. In the case of V24 during spring, this percentage increases to 52%. As a net result, benthic N regeneration exceeds rates of permanent N loss, and denitrification efficiency consistently remains below 40%. The regulation of the benthic N cycle at the four sites in the two seasons is discussed in paragraph 4.2.
3.4 Effect of environmental and biological variables on solute fluxes and dissimilative N reduction
Environmental and biological variables were both drivers of solute fluxes and nitrate reduction rates in spring, whereas in summer biological variables played a minor role (Table 3). In spring, three predictors (δ13C, the biomass of shallow and deep burrowers) explained 53% of the measured fluxes and N processes, whereas in summer environmental variables alone explained 69% of the total variation (Table 3). In the marginal tests, all factors were significant and explain ≥10% of the variation in both studied seasons, with spring C/N sediment ratio and water temperature as only exceptions.
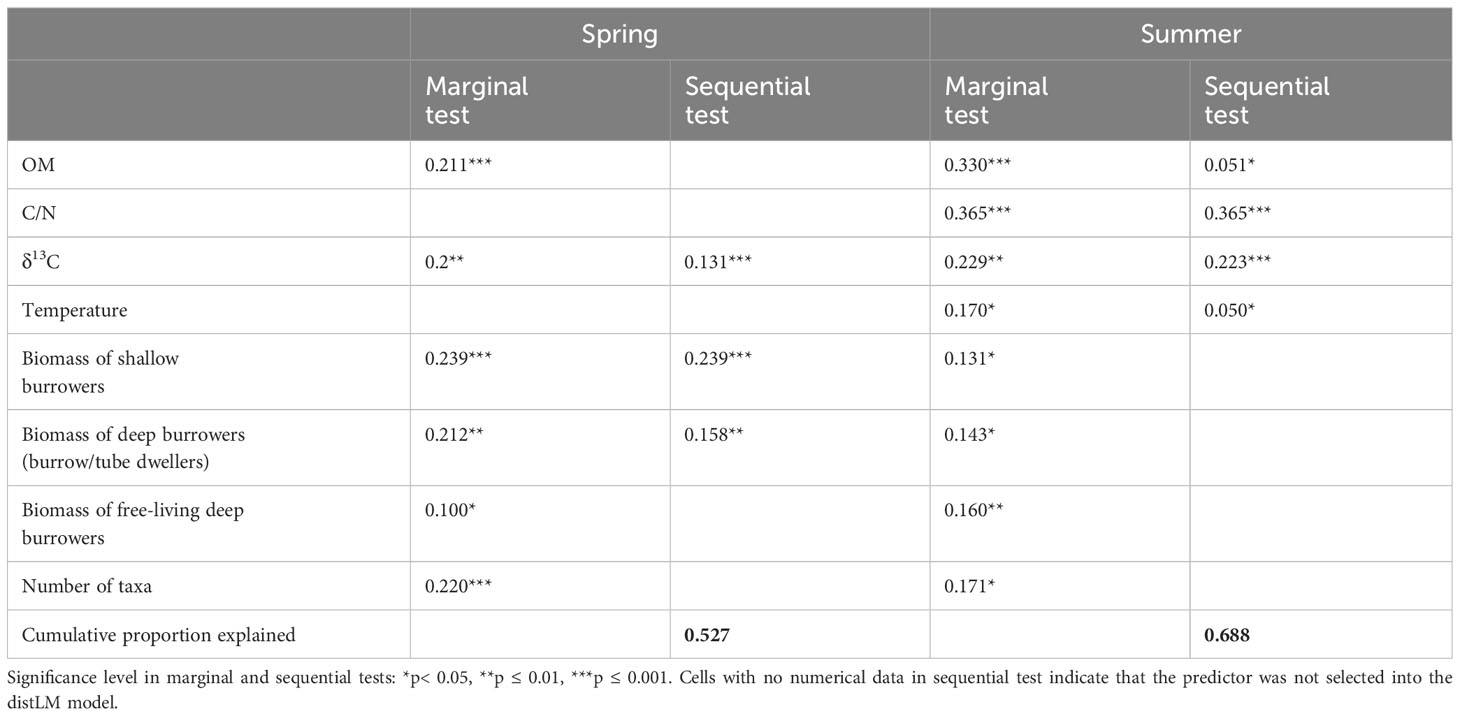
Table 3 DistLM results between environmental and biological predictors and the combined oxygen and nutrient fluxes and processes rates as a measure of ecosystem functioning for the study sites in spring and summer.
4 Discussion
4.1 Effects of river plumes on sediment properties and instability, and benthic communities
Sediments at the study area were mainly characterized by fine-grained sand with organic matter content increasing from the shallower to the deeper sites (Thoms et al., 2018; Miernik et al., 2023). Indeed, sedimentary material discharged by the Vistula River is initially deposited in the shallower area and is subsequently remobilized and transported to deeper areas (Damrat et al., 2013). The rates of O2 consumption in sediments showed strong seasonality, with a significant increase recorded at all sites from the spring to the summer (Figure 3A). Indeed, respiration rates varied by factors of nearly 4 (V18) to >10 (V10), coinciding with warmer bottom water temperatures (by nearly 12°C) and an increase in macrofauna biomass and its contribution to aerobic respiration. In summer, the sediment at site V10 was characterized by the highest O2 consumption and OM content, which significantly differed from the same site sampled in spring. This suggests a strong seasonality in sediment transport or locally different sedimentation rates in the Vistula River plume area (Uścinowicz, 1997). Aerobic respiration rates reported in the present study are in the same order of magnitude as those from analogous studies carried out at similar temperatures in the southern Baltic Sea (Thoms et al., 2018; Janas et al., 2019; Kendzierska et al., 2020; Silberberger et al., 2022).
In both seasons and at all sites, the δ13C signature of sedimentary organic carbon suggested the dominance of terrestrial inputs and very weak trend of δ13C along depth gradient, slightly increasing at higher depths only in summer (Table 2). Taken together, these results allow to deduce that sediments from the analyzed sites are unstable during high discharge and high-energy periods, such as spring, especially at shallow depths. This instability can limit the development of macrofauna communities. Indeed, the shallowest site displayed low diversity and biomass of macrofauna and low aerobic respiration rates. This instability decreases during summer when lower discharge, more stable sediment, and higher temperature favor the development of macrofauna communities. Results also suggest a minor effect of seasonality on the origin of the organic matter, which is predominantly terrestrial, regardless of river discharge and water temperatures. The slight increase in δ13C along depth recorded during summer is likely due to a small contribution of marine phytoplankton growth and sedimentation to the organic pool, coinciding with lower discharge and warmer water temperatures (Thoms et al., 2018; Szczepanek et al., 2022). Additionally, the values of δ15N were rather conservative among sites and seasons (Table 2).
The results from this study align with findings reported for other coastal areas affected by freshwater plumes that are demonstrated to stimulate the activity of meiofauna, macrofauna and microbial communities (Manini et al., 2004; Hermand et al., 2008; Carstensen et al., 2020). Such stimulation is expected due to the fertilization exerted by plumes in the pelagic compartment, enhancing the activity of primary producers and, consequently, the input of labile organic matter to sediments (Cloern et al., 2014). However, it can also occur through the direct supply to the sediment of organic matter and inorganic nutrients from terrestrial sources (Voss et al., 2021). As our data suggest, the area influenced by river plumes is highly dynamic due to variable river discharge, resulting in different extensions of the pelagic volumes and benthic surfaces affected by plume fertilization. Previous studies have demonstrated that the benthic compartment displays very rapid responses to variable nutrient inputs, either in terms of sedimentary features (e.g., organic matter content and macromolecular composition), distribution and composition of meiofaunal and macrofaunal assemblages, bacterial biomass, and associated enzymatic activities (Danovaro et al., 2000; Forrest et al., 2007; Nasi et al., 2020; Voss et al., 2021). Manini et al. (2004) conducted microcosm experiments exposing sediments to inorganic N-rich plume water, revealing that bacterial abundance in the sediment doubled, and enzymatic activities increased by over 50% within 12-24 h.
4.2 Seasonality of nitrate reduction rates in sediments within the Vistula River plume area
Denitrification rates in coastal systems exhibit significant spatial and seasonal variability influenced by various factors. These include the fluctuating availability of NO3- in the water column, which tends to be higher during colder periods, variations in macrofauna diversity, abundance, and biomass, diverse organic matter inputs from the water column, and varying water temperatures (Bonaglia et al., 2014b; Bartoli et al., 2021; Zilius et al., 2022). Results from this study suggest that the rise in water temperature played a significant role in the elevated denitrification rates observed during the summer in the Vistula plume area (Figure 4). This occurred even in the presence of lower NO3- availability in the water column. The warmer temperatures of summer resulted in detectable denitrification rates at shallower sites, where they were previously undetectable in spring, and exhibited rates three times larger at the deeper sites. A positive correlation between denitrification rates and temperature was also found in other estuaries in the Baltic Sea (Bonaglia et al., 2014b; Hellemann et al., 2017; Bartl et al., 2019). Indeed, elevated water temperatures stimulate aerobic microbial respiration, leading to a decrease in O2 penetration depth within sediments and shortening the pathlength required for NO3- to reach the anaerobic horizon where denitrification takes place (Seitzinger, 1988; Piña-Ochoa and Alvarez-Cobelas, 2006; Deutsch et al., 2010). Increased respiration rates further contribute to higher ammonification rates in sediments, leading to increased NH4+ availability for the community of nitrifiers. The seasonal oxygen fluxes reported in this study displayed a large summer increase, exceeding spring values by a factor of 10. This significant increase undoubtedly led to diminished O2 penetration depth in sediments, particularly pronounced at site V10, and enhanced production of NH4+ and NO3-.
In the sediments of the Vistula plume area, elevated temperatures led to increased N loss via denitrification, primarily driven by a stimulation of nitrification and coupled nitrification-denitrification (Dn). This is supported by the NOx- fluxes measured at the 4 sites, which showed an increase from spring to summer and consistently flowed toward the water column, indicating the dominance of nitrification over nitrate-consuming processes. In comparison, the contribution of Dw to total denitrification was relatively minor at the study site due to relatively low NO3- concentrations in bottom water, never exceeding 10 µM. In other coastal areas, the large dilution of riverine nitrate inputs results in limited rates of Dw and the dominance of Dn (Hietanen and Kuparinen, 2008; Zilius et al., 2022). Dn has a complex regulation that depends on the availability of O2 and NH4+ in the pore water, which impacts nitrification rates and the proximity of the denitrification zone. Moreover, it is influenced by the quantity and quality of organic matter in sediments, which in turn affects heterotrophic aerobic and anaerobic microbial activity, including ammonification rates (Seitzinger, 1994; Bartoli et al., 2021). Additionally, macrofaunal activity may stimulate both Dw and Dn, by transporting water column NO3- or favoring its production and consumption in subsurface sediments, where thin aerobic burrow walls are in close proximity to anaerobic sediment layers (Pelegri and Blackburn, 1994; Bartoli et al., 2000; Nizzoli et al., 2007; Moraes et al., 2018; Zilius et al., 2022). Polychaetes such as Marenzelleria spp. and Hediste diversicolor can dig up to several centimeters into the sediment in the Gulf of Gdańsk (Miernik et al., 2023). They impact the processes not only by influencing sediment structure and pore water chemistry, but also by affecting the microbial diversity, and also as holobionts (Dale et al., 2019; Zilius et al., 2022). In spring, deep burrowers likely stimulated Dw rates by increasing fluxes of water column NO3- into the sediments via bioirrigation (Pelegri and Blackburn, 1994; Bartoli et al., 2000; Nizzoli et al., 2007; Moraes et al., 2018; Zilius et al., 2022). Results of the DistLM model support such interpretation, as both environmental and biological factors significantly explain the seasonal and site-specific variability of fluxes and N-related processes.
During summer, the coastal zones are generally characterized by low NO3- concentrations in the water column, and the main source of NO3- within sediments is nitrification of NH4+ produced by oxidation of organic matter (Seitzinger, 1988; Bartl et al., 2019). Based on calculations of theoretical ammonification rates, we reconstructed N cycling at the four sites in spring and summer (Supplementary Figure S1). From this reconstruction of the benthic N cycle, we infer that at all sites from 50 to 70% of NH4+ produced by ammonification within sediments was nitrified, with the exception of site V10 in summer, where only 20% was nitrified. The partial oxidation of the ammonified N is supported by net NH4+ effluxes measured at all sites in both seasons. In turn, only a fraction of the produced NO3- was denitrified, suggesting only partially coupled ammonification, nitrification, and denitrification. Indeed, part of the produced NOx- was released to the water column, resulting in positive NO3- fluxes measured at all sites, in particular during summer. Some DIN recycling was also evidenced in spring but to a minor extent. In both seasons, denitrification efficiency was low, with dominance of DIN regeneration to the water column against net N loss to the atmosphere. In spring, denitrification at the shallowest sites V05 and V10 was not measurable and denitrification efficiency was 0, suggesting 100% recycling of the oxidized organic N, released as NH4+ and NO3- to the water column. The deepest sites V18 and V24 instead were characterized by higher denitrification rates and lower N recycling, resulting in DE of 17 and 38%, respectively. However, the recycling of inorganic forms was dominant over N removal. In summer, bottom water was always saturated with O2 and the high availability of NH4+ produced by the mineralization of organic matter enhanced the process of nitrification (Canfield et al., 2005). However, NH4+ effluxes were measured at all sites, with values up to 452.2 ± 86.3 µmol m-2 h-1 at site V10 (Figure 3B). This efflux was not sustained by macrofauna excretion or bioturbation, as in summer the biomass of invertebrates at this site was lower than at V18 and V24 (Figure 2). We can assume that factors other than those we measured are responsible for this high flux, such as the macromolecular quality of organic matter (Pusceddu et al., 2009). Overall, site V05 displayed the lowest denitrification rates in both seasons, likely due to the combination of the highest O2 penetration depth in sediments (up to 10.3 mm measured in spring, data not shown) and lowest OM content, whereas V24 showed the highest denitrification rates and the thinnest oxidized layer (2.3 mm) with the highest OM content. In both seasons, coupled denitrification-nitrification was the main process of NO3- reduction, and likely driven by environmental features such as O2 availability, NH4+ concentration in pore water, and quality and quantity of organic matter (Bartoli et al., 2021). Dn rates could also be driven by the presence of shallow burrowing (i.e., Peringia ulvae) and deep free-living macrofauna (M. baltica and M. arenaria), affecting microorganisms and increasing the oxic-anoxic microniches within sediment layers (Gilbertson et al., 2012; Moraes et al., 2018; Camillini et al., 2019). Total denitrification rates found in this study are similar to those found by Bartl et al. (2019) in the same estuary (3-15 µmol m-2 h-1), with the dominance of Dn over Dw. Furthermore, rates reported in this study are comparable to those found at similar depths by Deutsch et al. (2010) in the open Baltic Proper (from 0.5 to 28.7 µmol m-2 h-1). Comparatively analyzing the microbial processes that reduce NO3-, DNRA was less relevant than denitrification at the study sites, with the highest contribution at site V10 in summer (37% of the total measured NO3- reduction, Figure 4D). DNRA rates reported in this study (range < 0.5 to 10.1 µmol m-2 h-1) are comparable to those measured in other sediments from the Baltic Sea (Bonaglia et al., 2014b; Hellemann et al., 2020; Zilius et al., 2022). Sediments collected from the four sites in the two seasons always appeared rather oxidized, with light brown to grey color in the upper 10 cm, and never smelled sulphides. This, together with the net production of nitrate both in spring and summer, suggests oxidized conditions in the upper sediment layers and no accumulation of anaerobic metabolism end-products. In turn, low organic C content and absence of free sulphides in pore water can explain the low rates of DNRA measured in these sediments (Burgin and Hamilton, 2007; Kessler, 2018). However, despite the dominance of denitrification over DNRA, the analyzed sediments recycled a large amount of DIN and acted as weak N buffers.
Denitrification and DNRA measured in the Vistula plume area displayed low rates compared to inner lagoon areas, either due to high dilution of NO3- loads, resulting in low water column concentrations, low organic matter content in sediments and much larger availability of other electron acceptors like O2 (Dong et al., 2000; Bartoli et al., 2012, 2021; Magri et al., 2022). In both sampling periods, dissolved O2 in bottom water was close to theoretical saturation, with concentrations averaging 400 and 280 µM in spring and summer, respectively. Nitrate concentrations in bottom water averaged 10 and 2 µM, resulting in O2 to NO3- ratios of 40 and 140 in spring and summer, respectively. At the four investigated sites, the ratios between O2 respiration and denitrification were generally lower in summer than in spring, contrary to O2 and NO3- concentration ratios. This is likely due to different diffusion pathlengths to reach the anaerobic zone and much higher nitrification rates in sediments during summer. The lowest O2 respiration to denitrification ratio was nearly 50, calculated in the summer for the deepest site where denitrification peaked. Such a ratio suggests that even in the highly reactive sediment area affected by the Vistula River plume, under a low salinity regime, denitrification as a respiratory process is responsible for a minor fraction of organic carbon oxidation. This result aligns with other studies in the coastal zone of the Baltic Sea, where the ratios between O2 consumption and N2 production are in the range of 30-60 (Bonaglia et al., 2014b; Zilius et al., 2022).
4.3 The denitrification potential and saturation of Vistula plume sediments under varying NO3- availability
The addition of labelled nitrate to final concentrations largely exceeding those measured in situ produced contrasting results at the four sites (Figure 5). At the shallowest sites V05, denitrification rates of labelled NO3- were from negligible to low in both seasons, likely due to the limited availability of organic matter and large O2 penetration within sediments. At site V10, the response of the community of denitrifiers to NO3- addition varied significantly between seasons. Possible explanations for such different seasonal responses include water temperature, organic matter content and quality, and O2 penetration in surface sediments. At sites V18 and V24, the denitrification potential was higher in both seasons, especially in summer, and the process was stimulated by NO3- addition. While in situ rates are limited by low water column nitrate concentrations, at deeper sites the organic matter pool and the presence of burrowing macrofauna provide the potential for higher N removal rates.
Taken together, these results suggest that hydrological extremes leading to larger NO3- inputs in colder months would result in a limited increase in NO3- removal via denitrification, whereas larger inputs during summer would produce much larger N removal. They also suggest that any increase in NO3- production in sediments would stimulate summer denitrification at all sites and in 3 out of 4 sites during spring, as sediments have a higher, partially expressed, denitrification potential (Figure 5). We remark that these experiments were carried out by adding NO3- to final concentrations that largely exceed any realistic in situ increase in NO3- even under extreme conditions. The reasons for the concentration-series experiments are to address the response of the community of denitrifiers, its limiting factors, and its NO3- reduction potential and saturation. In the Gulf of Gdańsk, the plume from the Vistula River can display much higher NO3- concentrations during winter and spring (up to 200 µM of NOx-) compared to those measured in the present study but much lower than those simulated (Bartl et al., 2018). However, monitoring agencies and the international literature report NO3- concentrations in the Vistula River seasonally varying between <50 and >300 µM, with peaks up to 1,000 µM (Dojlido, 1997). Concentrations >1,000 µM are also reported for other European rivers (Dong et al., 2000) and are of the same order of magnitude as the highest concentrations tested in this work.
Rates of DNRA along variable NO3- availability in the water column were also measured but are not reported, as they consistently remained much lower to negligible when compared to denitrification rates and did not display any significant trend. This result is supported by the hypothesis by Tiedje (1988), who suggested the inhibition of DNRA in presence of high NO3- concentration. The only exception is represented by site V10 in summer that displayed potential DNRA rates up to 200 µmol m-2 h-1, likely due to higher rate of O2 consumption and C content in the sediments of this site.
5 Conclusions
Results from this study confirm that sediments influenced by river plumes are unstable and dynamic systems. The sedimentary environment of 4 sites within the Vistula River plume area underwent pronounced seasonal changes in their metabolism and N cycling, reflecting variations in water temperatures, sedimentary variables and macrofauna biomass. During spring, generally characterized by high discharge and low temperatures, nitrate concentrations were 5-fold higher than in summer when discharge halved, and water temperatures increased. Low temperatures limited microbial activity, including O2 and NO3- respiration. Simultaneously, high discharge affected sediment features and limited the development of macrofauna community, in particular in shallower areas closer to river inputs. During summer, O2 and NO3- respirations increased significantly at all sites, despite lower O2 and NO3- concentrations in bottom water. Lower discharge likely favored the development of more structured and abundant macrofauna communities at all sites. Warmer temperatures and higher metabolic rates resulted in large rates of ammonification, partially coupled with nitrification. The latter was also partly coupled with denitrification, and the net result of such uncoupling was the simultaneous regeneration of both NH4+ and NO3- to the water column, decreasing the efficiency of denitrification. Climate change, among other effects, can lead to a significant increase in water temperature and the results of the present study suggest a stimulation of both N removal and N recycling under higher temperature regimes, with the latter process showing a comparatively greater level of stimulation than the former. Climate change can also produce sudden increase in NO3- inputs to the coastal zone, and results from the present study suggest an increase in denitrification rates under increasing NO3- levels. Indeed, denitrification potentials are saturated at NO3- concentrations that largely exceed in situ nitrate levels. Results also suggest that, in the Vistula plume area, sedimentary DNRA is a minor NO3- reduction pathway compared to denitrification. We deduce that the response of sediments in terms of denitrification rates to sudden NO3- inputs and efficiency varies seasonally and between shallower and deeper sites. More experimental studies are needed, together with biogeochemical models capable of upscaling these results. This investigation, despite being limited to two seasons and to four sites within a single plume area, suggests higher N recycling under higher temperature regimes and lower N removal under cold, high discharge periods.
Data availability statement
The datasets generated for this study can be found in the RepOD (https://repod.icm.edu.pl) at the following doi: https://doi.org/10.18150/VWEYM9.
Ethics statement
The manuscript presents research on animals that do not require ethical approval for their study.
Author contributions
SB: Conceptualization, Data curation, Formal analysis, Funding acquisition, Investigation, Project administration, Writing – original draft, Writing – review & editing. MB: Conceptualization, Supervision, Writing – original draft, Writing – review & editing. MM: Investigation, Writing – review & editing, Writing – original draft. RB: Investigation, Writing – review & editing. HK: Resources, Writing – review & editing. KS-O: Investigation, Writing – review & editing. UJ: Formal analysis, Funding acquisition, Writing – review & editing, Project administration.
Funding
The author(s) declare that financial support was received for the research, authorship, and/or publication of this article. The research leading to these results has received funding from the Norwegian Financial Mechanism 2014-2021 under Project No 2020/37/K/NZ8/02782.
Acknowledgments
The authors thank the captains and the crew of R/V Oceanograf for their friendly hospitality and professional support during the cruises. Thanks to Zuzanna Czenczek, Natalia Anna Gintowt and Agnieszka Zimoch for their dedicated support in the field and in the lab. The authors would also like to thank two reviewers for their comments and suggestions.
Conflict of interest
The authors declare that the research was conducted in the absence of any commercial or financial relationships that could be construed as a potential conflict of interest.
Publisher’s note
All claims expressed in this article are solely those of the authors and do not necessarily represent those of their affiliated organizations, or those of the publisher, the editors and the reviewers. Any product that may be evaluated in this article, or claim that may be made by its manufacturer, is not guaranteed or endorsed by the publisher.
Supplementary material
The Supplementary Material for this article can be found online at: https://www.frontiersin.org/articles/10.3389/fmars.2024.1333707/full#supplementary-material
Supplementary Figure 1 | Graphical representation of benthic N cycle in (A) spring and (B) summer at the four sampling sites. Solute fluxes and rates are derived from direct measurements and calculations, and have been rounded to the unit. Net O2 fluxes were converted into theoretical rates of organic N ammonification (TA). TA was obtained by the conversion of dark O2 fluxes into organic carbon oxidation rates using site-specific respiratory quotients calculated from surface sediment C/N molar ratio, as detailed in Jørgensen et al. (2022). The obtained carbon oxidation rates were then divided by the sediment C/N ratio to calculate TA. Nitrification rates were estimated from the sum of NOx- effluxes, Dn and DNRAn. Mean rates (averages ± standard errors) are expressed in μmol m-2 h-1. Denitrification efficiency was calculated as the ratio between dinitrogen (N2) flux and the sum of N2 and DIN effluxes. See materials and methods section for more details.
Supplementary Table 1 | Macrofauna retrieved from the intact cores collected at each site in the two seasons expressed in gDW m-2. Averages ± standard errors are reported.
References
Anderson M. J., Gorley R. N., Clarke K. R. (2008). PERMANOVA+ for PRIMER: guide to software and statistical methods (Plymouth, UK: PRIMER-E Ltd.).
APHA (American Public Health Association) (1992). Standard methods for the examination of water and wastewaters. 18th ed (Washington DC: American Public Health Association, 1015 Eighteenth Street NW).
Asmala E., Carstensen J., Conley D. J., Slomp C. P., Stadmark J., Voss M. (2017). Efficiency of the coastal filter: Nitrogen and phosphorus removal in the Baltic Sea. Limnol. Oceanogr. 62, S222–S238. doi: 10.1002/lno.10644
Bajkiewicz-Grabowska E., Zalewski M., Kobusińska M. E., Niemirycz E. (2019). Sezonowa struktura odpływu rzeki Wisły do Morza Bałtyckiego. Technol. Wody. 6, 8–15.
Baron J. S., Hall E. K., Nolan B. T., Finlay J. C., Bernhardt E. S., Harrison J. A., et al. (2013). The interactive effects of excess reactive nitrogen and climate change on aquatic ecosystems and water resources of the United States. Biogeochemistry 114, 71–92. doi: 10.1007/s10533-012-9788-y
Bartl I., Hellemann D., Rabouille C., Schulz K., Tallberg P., Hietanen S., et al. (2019). Particulate organic matter controls benthic microbial N retention and N removal in contrasting estuaries of the Baltic Sea. Biogeosciences 16, 3543–3564. doi: 10.5194/bg-16-3543-2019
Bartl I., Liskow I., Schulz K., Umlauf L., Voss M. (2018). River plume and bottom boundary layer–Hotspots for nitrification in a coastal bay? Estuar. Coast. Shelf. Sci. 208, 70–82. doi: 10.1016/j.ecss.2018.04.023
Bartoli M., Castaldelli G., Nizzoli D., Viaroli P. (2012). Benthic primary production and bacterial denitrification in a Mediterranean eutrophic coastal lagoon. J. Exp. Mar. Biol. Ecol. 438, 41–51. doi: 10.1016/j.jembe.2012.09.011
Bartoli M., Nizzoli D., Welsh D. T., Viaroli P. (2000). Short-term influence of recolonisation by the polychaete worm Nereis succinea on oxygen and nitrogen fluxes and denitrification: a microcosm simulation. Hydrobiologia 431, 165–174. doi: 10.1023/A:1004088112342
Bartoli M., Nizzoli D., Zilius M., Bresciani M., Pusceddu A., Bianchelli S., et al. (2021). Denitrification, nitrogen uptake, and organic matter quality undergo different seasonality in sandy and muddy sediments of a turbid estuary. Front. Microbiol. 11. doi: 10.3389/fmicb.2020.612700
Berezina N. A., Maximov A. A., Vladimirova O. M. (2019). Influence of benthic invertebrates on phosphorus flux at the sediment-water interface in the easternmost Baltic Sea. Mar. Ecol. Prog. Ser. 608, 33–43. doi: 10.3354/meps12824
Bonaglia S., Bartoli M., Gunnarsson J. S., Rahm L., Raymond C., Svensson O., et al. (2013). Effect of reoxygenation and Marenzelleria spp. bioturbation on Baltic Sea sediment metabolism. Mar. Ecol. Prog. Ser. 482, 43–55. doi: 10.3354/meps10232
Bonaglia S., Deutsch B., Bartoli M., Marchant H. K., Brüchert V. (2014b). Seasonal oxygen, nitrogen and phosphorus benthic cycling along an impacted Baltic Sea estuary: regulation and spatial patterns. Biogeochemistry 119, 139–160. doi: 10.1007/s10533-014-9953-6
Bonaglia S., Nascimento F. A., Bartoli M., Klawonn I., Brüchert V. (2014a). Meiofauna increases bacterial denitrification in marine sediments. Nat. Commun. 5, 5133. doi: 10.1038/ncomms6133
Bouwman A., Bierkens M., Griffioen J., Hefting M., Middelburg J., Middelkoop H., et al. (2013). Nutrient dynamics, transfer and retention along the aquatic continuum from land to ocean: Towards integration of ecological and biogeochemical models. Biogeosciences 10, 1–22. doi: 10.5194/bg-10-1-2013
Bower C. E., Holm-Hansen T. (1980). A salicylate-hypochlorite method for determining ammonia in seawater. Can. J. Fish. Aquat. Sci. 37, 794–798. doi: 10.1139/f80-106
Burgin A. J., Hamilton S. K. (2007). Have we overemphasized the role of denitrification in aquatic ecosystems? A review of nitrate removal pathways. Front. Ecol. Environ. 5, 89–96. doi: 10.1890/1540-9295(2007)5[89:HWOTRO]2.0.CO;2
Camillini N., Larsen M., Glud R. N. (2019). Behavioural patterns of the soft-shell clam Mya arenaria: implications for benthic oxygen and nitrogen dynamics. Mar. Ecol. Prog. Ser. 622, 103–119. doi: 10.3354/meps13004
Canfield D. E., Kristensen E., Thamdrup B. (2005). “The nitrogen cycle,” in Advances in Marine Biology, vol. 48. (Cambridge: Academic Press), 205–267.
Carstensen J., Conley D. J., Almroth-Rosell E., Asmala E., Bonsdorff E., Fleming-Lehtinen V., et al. (2020). Factors regulating the coastal nutrient filter in the Baltic Sea. Ambio 49, 1194–1210. doi: 10.1007/s13280-019-01282-y
Cloern J. E., Foster S. Q., Kleckner A. E. (2014). Phytoplankton primary production in the world’s estuarine-coastal ecosystems. Biogeosciences 11, 2477–2501. doi: 10.5194/bg-11-2477-2014
Dale H., Taylor J. D., Solan M., Lam P., Cunliffe M. (2019). Polychaete mucopolysaccharide alters sediment microbial diversity and stimulates ammonia-oxidising functional groups. FEMS Microbiol. Ecol. 95, fiy234. doi: 10.1093/femsec/fiy234
Damrat M., Zaborska A., Zajaczkowski M. (2013). Sedimentation from suspension and sediment accumulation rate in the River Vistula prodelta, Gulf of Gdańsk (Baltic Sea). Oceanologia 55, 937–950. doi: 10.5697/oc.55-4.937
Danovaro R., Gambi C., Manini E., Fabiano M. (2000). Meiofauna response to a dynamic river plume front. Mar. Biol. 137, 359–370. doi: 10.1007/s002270000353
Deutsch B., Forster S., Wilhelm M., Dippner J., Voss M. (2010). Denitrification in sediments as a major nitrogen sink in the Baltic Sea: An extrapolation using sediment characteristics. Biogeosciences 7, 3259. doi: 10.5194/bg-7-3259-2010
Dojlido J. (1997). Water quality in the Vistula basin. Int. River Water Qual. Best G., Bogacka T., Niemirycz E. (E & FN Spon, London), 21–31.
Dong L. F., Thornton D. C. O., Nedwell D. B., Underwood G. J. C. (2000). Denitrification in sediments of the River Colne estuary. England. Mar. Ecol. Prog. Ser. 203, 109–122. doi: 10.3354/meps203109
Eyre B. D., Ferguson A. J. (2009). “Denitrification efficiency for defining critical loads of carbon in shallow coastal ecosystems,” in Eutrophication in Coastal Ecosystems. Developments in Hydrobiology (Dordrecht: Springer) 207. doi: 10.1007/978-90-481-3385-7_12
Forrest B. M., Gillespie P. A., Cornelisen C. D., Rogers K. M. (2007). Multiple indicators reveal river plume influence on sediments and benthos in a New Zealand coastal embayment. N. Z. J. Mar. Freshw. Res. 41, 13–24. doi: 10.1080/00288330709509892
Gammal J., Norkko J., Pilditch C. A., Norkko A. (2017). Coastal hypoxia and the importance of benthic macrofauna communities for ecosystem functioning. Estuaries. Coast. 40, 457–468. doi: 10.1007/s12237-016-0152-7
Giblin A. E., Tobias C. R., Song B., Weston N., Banta G. T., Rivera-Monroy V. H. (2013). The importance of dissimilatory nitrate reduction to ammonium (DNRA) in the nitrogen cycle of coastal ecosystems. Oceanography 26, 124–131. doi: 10.5670/oceanog.2013.54
Gilbertson W. W., Solan M., Prosser J. I. (2012). Differential effects of microorganism–invertebrate interactions on benthic nitrogen cycling. FEMS Microbiol. Ecol. 82, 11–22. doi: 10.1111/j.1574-6941.2012.01400.x
Golterman H. L., Clymo L. S., Ohnstand M. A. M. (1978). Methods for physical and chemical analysis of freshwaters (Oxford). In Handbook, Blackwell Science, 2nd ed. (Oxford, UK) 8.
Hellemann D., Tallberg P., Aalto S. L., Bartoli M., Hietanen S. (2020). Seasonal cycle of benthic denitrification and DNRA in the aphotic coastal zone, northern Baltic Sea. Mar. Ecol. Prog. Ser. 637, 15–28. doi: 10.3354/meps13259
Hellemann D., Tallberg P., Bartl I., Voss M., Hietanen S. (2017). Denitrification in an oligotrophic estuary: a delayed sink for riverine nitrate. Mar. Ecol. Prog. Ser. 583, 63–80. doi: 10.3354/meps12359
Hermand R., Salen-Picard C., Alliot E., Degiovanni C. (2008). Macrofaunal density, biomass and composition of estuarine sediments and their relationship to the river plume of the Rhone River (NW Mediterranean). Estuar. Coast. Shelf. Sci. 79, 367–376. doi: 10.1016/j.ecss.2008.04.010
Hietanen S., Kuparinen J. (2008). Seasonal and short-term variation in denitrification and anammox at a coastal station on the Gulf of Finland, Baltic Sea. Hydrobiologia 596, 67–77. doi: 10.1007/s10750-007-9058-5
Howarth R. W. (1988). Nutrient limitation of net primary production in marine ecosystems. Annu. Rev. Ecol. Evol. Syst. 19, 89–110. doi: 10.1146/annurev.es.19.110188.000513
Janas U., Burska D., Kendzierska H., Pryputniewicz-Flis D., Łukawska-Matuszewska K. (2019). Importance of benthic macrofauna and coastal biotopes for ecosystem functioning – oxygen and nutrient fluxes in the coastal zone. Estuar. Coast. Shelf. Sci. 225, 106238. doi: 10.1016/j.ecss.2019.05.020
Johannsen A., Dähnke K., Emeis K. (2008). Isotopic composition of nitrate in five German rivers discharging into the North Sea. Org. Geochem. 39, 1678–1689. doi: 10.1016/j.orggeochem.2008.03.004
Jørgensen B. B., Wenzhöfer F., Egger M., Glud R. N. (2022). Sediment oxygen consumption: Role in the global marine carbon cycle. Earth-Sci. Rev. 228, 103987. doi: 10.1016/j.earscirev.2022.103987
Kauppi L., Norkko J., Ikonen J., Norkko A. (2017). Seasonal variability in ecosystem functions: quantifying the contribution of invasive species to nutrient cycling in coastal ecosystems. Mar. Ecol. Prog. Ser. 572, 193–207. doi: 10.3354/meps12171
Kendzierska H., Łukawska-Matuszewska K., Burska D., Janas U. (2020). Benthic fluxes of oxygen and nutrients under the influence of macrobenthic fauna on the periphery of the intermittently hypoxic zone in the Baltic Sea. J. Exp. Mar. Biol. Ecol. 530-531, 151439. doi: 10.1016/j.jembe.2020.151439
Kessler A. J. (2018). Biogeochemical controls on the relative importance of denitrification and dissimilatory nitrate reduction to ammonium in estuaries. Global Biogeochem. Cycles. 1, 1045–1057. doi: 10.1029/2018GB005908
Kristensen E., Penha-Lopes G., Delefosse M., Valdemarsen T., Quintana C. O., Banta G. T. (2012). What is bioturbation? The need for a precise definition for fauna in aquatic sciences. Mar. Ecol. Prog. Ser. 446, 285–302. doi: 10.3354/meps09506
Kruk-Dowgiałlo L., Szaniawska A. (2008). “Gulf of Gdańsk and Puck Bay,” in Ecology of baltic coastal waters (Springer Berlin Heidelberg., Berlin, Heidelberg), 139–165.
Lunau M., Voss M., Erickson M., Dziallas C., Casciotti K., Ducklow H. (2013). Excess nitrate loads to coastal waters reduces nitrate removal efficiency: mechanism and implications for coastal eutrophication. Environ. Microbiol. 15, 1492–1504. doi: 10.1111/j.1462-2920.2012.02773.x
Magri M., Benelli S., Bonaglia S., Zilius M., Castaldelli G., Bartoli M. (2020). The effects of hydrological extremes on denitrification, dissimilatory nitrate reduction to ammonium (DNRA) and mineralization in a coastal lagoon. Sci. Total. Environ. 740, 140169. doi: 10.1016/j.scitotenv.2020.140169
Magri M., Benelli S., Castaldelli G., Bartoli M. (2022). The seasonal response of in situ denitrification and DNRA rates to increasing nitrate availability. Estuar. Coast. Shelf. Sci. 271, 107856. doi: 10.1016/j.ecss.2022.107856
Manini E., Luna G. M., Danovaro R. (2004). Benthic bacterial response to variable estuarine water inputs. FEMS Microbiol. Ecol. 50, 185–194. doi: 10.1016/j.femsec.2004.06.011
McGlathery K. J., Sundbäck K., Anderson I. C. (2007). Eutrophication in shallow coastal bays and lagoons: the role of plants in the coastal filter. Mar. Ecol. Prog. Ser. 348, 1–18. doi: 10.3354/meps07132
Miernik N. A., Janas U., Kendzierska H. (2023). Role of macrofaunal communities in the vistula river plume, the Baltic sea—Bioturbation and bioirrigation potential. Biology 12, 147. doi: 10.3390/biology12020147
Moraes P. C., Zilius M., Benelli S., Bartoli M. (2018). Nitrification and denitrification in estuarine sediments with tube-dwelling benthic animals. Hydrobiologia 819, 217–230. doi: 10.1007/s10750-018-3639-3
Murphy A. E., Bulseco A. N., Ackerman R., Vineis J. H., Bowen J. L. (2020). Sulphide addition favours respiratory ammonification (DNRA) over complete denitrification and alters the active microbial community in salt marsh sediments. Environ. Microbiol. 22, 2124–2139. doi: 10.1111/1462-2920.14969
Nasi F., Auriemma R., Relitti F., Bazzaro M., Cassin D., Cibic T. (2020). Structural and functional response of coastal macrofaunal community to terrigenous input from the Po River (northern Adriatic Sea). Estuar. Coast. Shelf. Sci. 235, 106548. doi: 10.1016/j.ecss.2019.106548
Nielsen L. P. (1992). Denitrification in sediment determined from nitrogen isotope pairing. FEMS Microbiol. Lett. 86, 357–362. doi: 10.3354/meps137181
Nixon S. W., Ammerman J. W., Atkinson L. P., Berounsky V. M., Billen G., Boicourt W. C., et al. (1996). The fate of nitrogen and phosphorus at the land-sea margin of the North Atlantic Ocean. Biogeochemistry 35, 141–180. doi: 10.1007/BF02179826
Nizzoli D., Bartoli M., Cooper M., Welsh D. T., Underwood G. J., Viaroli P. (2007). Implications for oxygen, nutrient fluxes and denitrification rates during the early stage of sediment colonisation by the polychaete Nereis spp. in four estuaries. Estuar. Coast. Shelf. Sci. 75, 125–134. doi: 10.1016/j.ecss.2007.03.035
Oeurng C., Sauvage S., Sánchez-Pérez J. M. (2010). Temporal variability of nitrate transport through hydrological response during flood events within a large agricultural catchment in south-west France. Sci. Total. Environ. 409, 140–149. doi: 10.1016/j.scitotenv.2010.09.006
Paerl H. W., Hall N. S., Peierls B. L., Rossignol K. L. (2014). Evolving paradigms and challenges in estuarine and coastal eutrophication dynamics in a culturally and climatically stressed world. Estuaries. Coast. 37, 243–258. doi: 10.1007/s12237-014-9773-x
Pastuszak M., Bryhn A. C., Håkanson L., Stålnacke P., Zalewski M., Wodzinowski T. (2018). Reduction of nutrient emission from Polish territory into the Baltic Sea, (1988–2014) confronted with real environmental needs and international requirements. Oceanol. Hydrobiol. Stud. 47, 140–166. doi: 10.1515/ohs-2018-0015
Pastuszak M., Stalnacke P., Pawlikowski K., Witek Z. (2012). Response of Polish rivers (Vistula, Oder) to reduced pressure from point sources and agriculture during the transition period, (1988–2008). J. Mar. Syst. 94, 157–173. doi: 10.1016/j.jmarsys.2011.11.017
Pelegri S. P., Blackburn T. H. (1994). Bioturbation effects of the amphipod Corophium volutator on microbial nitrogen transformations in marine sediments. Mar. Biol. 121, 253–258. doi: 10.1007/BF00346733
Piña-Ochoa E., Alvarez-Cobelas M. (2006). Denitrification in aquatic environments: a cross-system analysis. Biogeochemistry 81, 111–130. doi: 10.1007/s10533-006-9033-7
Pusceddu A., Dell’Anno A., Fabiano M., Danovaro R. (2009). Quantity and bioavailability of sediment organic matter as signatures of benthic trophic status. Mar. Ecol. Prog. Ser. 375, 41–52. doi: 10.3354/meps07735
Rabalais N. N., Turner R. E., Díaz R. J., Justić D. (2009). Global change and eutrophication of coastal waters. ICES. J. Mar. Sci. 66, 1528–1537. doi: 10.1093/icesjms/fsp047
R Core Team (2022). R: A language and environment for statistical computing (Vienna, Austria: R Foundation for Statistical Computing).
Risgaard-Petersen N., Nielsen L. P., Rysgaard S., Dalsgaard T., Meyer R. L. (2003). Application of the isotope pairing technique in sediments where anammox and denitrification coexist. Limnol. Oceanogr-Meth. 1, 63–73. doi: 10.4319/lom.2003.1.63
Risgaard-Petersen N., Rysgaard S. (1995). “Nitrate reduction in sediments and waterlogged soil measured by 15N techniques,” in Methods in applied soild microbiology and biochemistry. Eds. Alef K., Nannipieri P. (Cambridge: Academic Press), 287–295.
Robertson E. K., Bartoli M., Brüchert V., Dalsgaard T., Hall P. O. J., Hellemann D., et al. (2019). Application of the isotope pairing technique in sediments: use, challenges, and new directions. Limnol. Oceanogr. 17, 112–136. doi: 10.1002/lom3.10303
Samuiloviene A., Bartoli M., Bonaglia S., Cardini U., Vybernaite-Lubiene I., Marzocchi U., et al. (2019). The effect of chironomid larvae on nitrogen cycling and microbial communities in soft sediments. Water 11, 1931. doi: 10.3390/w11091931
Seitzinger S. P. (1988). Denitrification in freshwater and coastal marine ecosystems: ecological and geochemical significance. Limnol. Oceanogr. 33, 702–724. doi: 10.4319/lo.1988.33.4part2.0702
Seitzinger S. P. (1994). Linkages between organic matter mineralization and denitrification in eight riparian wetlands. Biogeochemistry 25, 19–39. doi: 10.1007/BF00000510
Silberberger M. J., Koziorowska-Makuch K., Borawska Z., Szczepanek M., Kędra M. (2022). Disentangling the drivers of benthic oxygen and dissolved carbon fluxes in the coastal zone of the southern Baltic Sea. Estuaries. Coast. 45, 2450–2471. doi: 10.1007/s12237-022-01074-w
Szczepanek M., Silberberger M. J., Koziorowska-Makuch K., Kędra M. (2022). Utilization of riverine organic matter by macrobenthic communities in a temperate prodelta. Front. Mar. Sci. 9. doi: 10.3389/fmars.2022.974539
Thoms F., Burmeister C., Dippner J. W., Gogina M., Janas U., Kendzierska H., et al. (2018). Impact of macrofaunal communities on the coastal filter function in the Bay of Gdansk, Baltic Sea. Front. Mar. Sci. 5. doi: 10.3389/fmars.2018.00201
Tiedje J. M. (1988). “Ecology of denitrification and dissimilatory nitrate reduction to ammonium,” in Environmental microbiology of anaerobes. Ed. Zehnder A. J. B. (New York: John Wiley & Sons, N.Y.), 179–244.
Voss M., Asmala E., Bartl I., Carstensen J., Conley D. J., Dippner J. W., et al. (2021). Origin and fate of dissolved organic matter in four shallow Baltic Sea estuaries. Biogeochemistry 154, 385–403. doi: 10.1007/s10533-020-00703-5
Vybernaite-Lubiene I., Zilius M., Saltyte-Vaisiauske L., Bartoli M. (2018). Recent trends, (2012–2016) of N, Si, and P export from the Nemunas River Watershed: Loads, unbalanced stoichiometry, and threats for downstream aquatic ecosystems. Water 10, 1178. doi: 10.3390/w10091178
Warembourg F. R. (1993). Nitrogen fixation in soil and plant systems, Nitrogen Isotope Techniques (Cambridge: Academis Press). doi: 10.1016/b978-0-08-092407-6.50010
Włodarska-Kowalczuk M., Mazurkiewicz M., Jankowska E., Kotwicki L., Damrat M., Zajączkowski M. (2016). Effects of fluvial discharges on meiobenthic and macrobenthic variability in the Vistula River prodelta (Baltic Sea). J. Mar. Syst. 157, 135–146. doi: 10.1016/j.jmarsys.2015.12.009
Xu S., Chen M., Feng T., Zhan L., Zhou L., Yu G. (2021). Use ggbreak to effectively utilize plotting space to deal with large datasets and outliers. Front. Genet. 12. doi: 10.3389/fgene.2021.774846
Keywords: estuary, Baltic Sea, buffer capacity, denitrification, DNRA
Citation: Benelli S, Bartoli M, Magri M, Brzana R, Kendzierska H, Styrcz-Olesiak K and Janas U (2024) Spatial and seasonal pattern of microbial nitrate reduction in coastal sediments in the Vistula River plume area, Gulf of Gdańsk. Front. Mar. Sci. 11:1333707. doi: 10.3389/fmars.2024.1333707
Received: 05 November 2023; Accepted: 19 February 2024;
Published: 05 March 2024.
Edited by:
Jun Sun, China University of Geosciences, ChinaCopyright © 2024 Benelli, Bartoli, Magri, Brzana, Kendzierska, Styrcz-Olesiak and Janas. This is an open-access article distributed under the terms of the Creative Commons Attribution License (CC BY). The use, distribution or reproduction in other forums is permitted, provided the original author(s) and the copyright owner(s) are credited and that the original publication in this journal is cited, in accordance with accepted academic practice. No use, distribution or reproduction is permitted which does not comply with these terms.
*Correspondence: Sara Benelli, c2FyYS5iZW5lbGxpQHVnLmVkdS5wbA==