- 1Department of Life and Environmental Sciences, Università Politecnica delle Marche, Ancona, Italy
- 2National Biodiversity Future Center (NBFC), Palermo, Italy
- 3Enalia Physis Environmental Research Centre, Nicosia, Cyprus
- 4Ghent University, Ghent, Belgium
- 5Energy, Environmental and Water Research Center, The Cyprus Institute, Nicosia, Cyprus
Chemical pollution in marine ecosystems is a factor of stress interacting in multiple and complex ways with other major causes of deterioration, such as warming seas due to climate change. Here we surveyed epibenthic communities from a shipwreck in the Levantine Basin for temporal and spatial changes in the community in relation to chronic oil pollution, comparing results collected from an area of the wreck characterized by chronic oil leakage with another area not affected by oil. Polycyclic aromatic hydrocarbons (PAHs) bioaccumulation analyses were integrated with characterization of the efficiency of xenobiotics biotransformation processes and antioxidant network of the scleractinian coral Madracis pharensis, chosen as bioindicator species. Results highlighted the two areas hosting different epibenthic communities over a period of 11 years. Significant changes in the percentage cover of M. pharensis could be the result of recent mass mortality associated to Marine Heat Waves. Biological investigation conducted in M. pharensis tissues revealed an increased content of PAHs in specimens collected from the oil-impacted area, coupled with an increased capability of oxyradicals scavenging capacity and a lower functionality of phase II biotransformation mechanisms associated to glutathione S-transferase. Overall, the results suggest that M. pharensis has the capability to develop cellular and physiological adaptations to chemical-mediated stress, with yet unknown possible energy trade-offs to sustain stress response.
Introduction
Epibenthic communities from shipwrecks are studied intensively due to the characteristics that make the wreck an artificial reef. Knowledge about the ecological processes operating on these assemblages (e.g., recruitment, competition, predation) assist in the elaboration of management options for the restoration of degraded habitats, population dynamics of invasive species, to concentrate human activities in specific areas, and more often than not, for the enhancement or creation of habitat (Brockinton et al., 2022; Hughes et al., 2023). Behind the apparent benefits of shipwrecks to the general biodiversity of an area, that is, by providing free substrate for epibenthic species that are fast and successful colonizers (Hoeksema et al., 2023) and refuge for mobile species increasing thus the biodiversity (Monchanin et al., 2021), wrecks might also contribute to local pollution by leaking chemicals (e.g., van der Schyff et al., 2020). Such is the case with Zenobia shipwreck in Cyprus, where rich epibenthic communities of corals and sponges live closely related to chronic leakage of oil, noticeable in various sections of the wreck’s structure (Jimenez et al., 2017a).
In general, scleractinian corals are good indicators of chemical pollution (Guzmán and Jiménez, 1992), and corals from shipwrecks have been reported to show higher concentrations of pollutants, e.g. heavy metals, compared to corals from other substrates sampled in areas away from the wrecks (van der Schyff et al., 2020). Coral species’ capability to accumulate PAHs from surrounding environments with diverse degree of contamination has been extensively reported, and studies on PAHs effects in Scleractinia under laboratory conditions highlighted a plethora of alterations ranging from the lower levels of biological organization, i.e. changes in gene expression, genotoxicity, to the higher ones, i.e. physiological and morphological alterations, mortality (Menezes et al., 2023 and references therein; Turner and Renegar, 2017 and references therein). On the other hand, studies on the sub-lethal alterations at cellular level, mechanisms of stress-response and adaptations in coral species under real environmental scenarios of chronic oil pollution are yet globally underrepresented (Turner and Renegar, 2017).
Once within organisms, chemical pollutants may exert disturbance at various levels of biological organization, and pathways of chemicals toxicity strictly depend on their typology, which determines initiating events at cellular level (Allen et al., 2016). It is now well recognized that PAHs toxicity arise from the metabolic processes aimed to breakdown these chemicals and allow for their elimination. Indeed, PAHs, as polychlorobiphenyls (PCBs) and dioxins, bind the cytosolic aryl hydrocarbon receptor (AhR), and form a ligand-receptor complex that once transported to the nucleus binds to xenobiotic metabolism specific regions of the DNA, known as dioxin responsive element (DRE), through the interaction with ARNT, AhR nuclear translocator (Regoli and Giuliani, 2014; Wang et al., 2020). This molecular initiating event activates the transcription of several genes involved in the biotransformation and metabolism of xenobiotics, as CYP1 and CYP1B. Such biotransformation reactions of xenobiotics are aimed to decrease their lipophilicity, but these may not be sufficient and organic xenobiotics metabolism may proceed toward phase II conjugation reactions, catalysed by glutathione S-transferase, UDP- glucuronosyl transferases (UGTs) and sulfotransferases (SULTs) which use endogenous cofactors as reduced glutathione (GSH), glucuronic acid and sulfate, respectively, and are as well modulated through the Ah-gene battery.
PAHs toxicity partly derives from their bioactivation through these reactions, which can produce metabolites that show higher toxicity compared to parent compounds and damage cellular macromolecules, e.g. forming DNA adducts (as 7,8-dihydrodiol-9,10-oxide, the DNA-adduct forming metabolite of benzo(a)pyrene responsible for its carcinogenicity, Regoli and Giuliani, 2014). At the same time, PAHs metabolism is also recognized as one of the main processes causing the release of reactive oxygen species (ROS), consequential to electron transport uncoupling occurring during the slow oxidation of substrates and to “redox cycling” of metabolites (Schlezinger et al., 2006; Shakunthala, 2010; Regoli and Giuliani, 2014).
Cells are able to neutralize the negative effects of oxyradicals through a complex network of low molecular weight scavengers and enzymes, collectively recognized as antioxidant system, universally developed to counteract the naturally occurring production of ROS from several cellular pathways of aerobic metabolism, as mitochondrial electron transport chain and active phagocytosis (Benedetti et al., 2015, 2022; Lushchak, 2011; Regoli and Giuliani, 2014). Reduced glutathione, ascorbic and uric acid scavenge ROS by directly acting as reducing agents on oxyradicals, but are also fundamental co-factors for the functioning of other antioxidants, enzymes that catalyze highly specific reactions, as superoxide dismutase (SOD), catalase (CAT), glutathione reductase (GR), glutathione peroxidases (GPx) and glutathione S-transferases (GST), the main involved (Lushchak, 2011). In this respect, the efficiency of antioxidant defenses is especially fundamental in symbiotic organisms, as sclearactinians and sponges, which show physiological adaptations to counteract the toxicity of photosynthetically produced oxygen, which can easily undergo univalent reduction to form O2-, H2O2 and HO• (Regoli et al., 2004a; Regoli et al., 2004b; Lesser, 2006).
Due to the sensitivity of the antioxidant defenses, their functionality has been extensively studied in marine species under chemical exposure and more in general abiotic stress, especially when aiming to understand mechanisms behind the onset of oxidative stress and cellular damages. Activities of SOD, CAT, GR, GST, GPx, as well as cellular homeostasis of glutathione have been demonstrated to be either increased or decreased by exogenous cellular stressors as chemical pollutants, namely trace metals, PAHs, dioxins, microplastics and active pharmaceutical ingredients (Lushchak, 2011; Benedetti et al., 2022). On the other hand, specific methodologies have been developed to quantify and characterize the overall capability and functionality of cellular antioxidant network, as the total oxyradical scavenging capacity assay (TOSCA, Regoli and Winston, 1998; Gorbi and Regoli, 2003). This methodology, compared to the evaluation of single antioxidant defenses, provide a more comprehensive picture of cellular redox status, and therefore health. Such approach has been successfully applied in several field and laboratory investigations, to characterize marine species antioxidant defenses, their capability to neutralize oxyradicals and prevent oxidative damage when subjected to exogenous stressors as chemical pollutants, algal toxins, thermal stress and hypercapnia (Regoli et al., 2004a; Regoli et al., 2004b; Gorbi et al., 2012; Gorbi et al. 2014; Nardi et al., 2017; Nardi et al., 2018; Iannello et al., 2021).
In this study we aimed to characterize the composition of epibenthic communities, as well as PAHs bioaccumulation, antioxidant network and xenobiotics biotransformation efficiency of a selected scleractinian coral species, Madracis pharensis, at two sites of the Zenobia wreck, one under the influence of oil leakages and one considered free from this environmental stressor. Obtained results were intended to further elucidate aspects of Scelractinians’ biology and their mechanisms of tolerance and responsiveness to chemical stress, as well as and deepen our understanding on the capability of coral species to adapt to chronic subtle pollution.
Materials and methods
Study site
The large ferry MS Zenobia (10,000ton, 172m length) loaded with vehicles, lorries and cargo capsized in 1980 in Larnaka Bay, Cyprus (34° 55.128’N, 33° 39.354’E; Figure 1A). Since then, Zenobia has been resting horizontally on its portside (Figure 1B) in about 43m depth. The sea bottom of Larnaka Bay is characterized by scattered, small-sized rocky outcrops and extensive sandy and muddy areas. Given the large dimension of Zenobia, the low deterioration of its hull, complexity and heterogeneity of the metal structure, it is not surprising to find a rich biodiversity associated with the shipwreck. For example, the high abundance and diversity of fish and colorful epibenthic communities are well known among the international diving community that ranks Zenobia among the most popular recreational dives sites of the world (Scuba, 2022). A more detailed description of the shipwreck and the biotic and environmental factors that operate in the Larnaka Bay area can be found in Jimenez et al. (2017a). For this study, two contrasting environments of the wreck were selected: the Car Deck and the Smokestacker areas, both at 25m depth (Figure 1B). In the Car Deck area, constant leakage of oil from the wreck accumulates in “ponds” between spaces on the bulwark of the starboard side, which corresponds to the “upper” shaded surface. Organisms in this area live in close contact with those stagnant “oil ponds”, corals in particular (Figure 1C). On the contrary, the epibenthic communities in the Smokestacker area are not affected by oil leakages (Figure 1D). In both sites, epibenthic biodiversity was assessed, and an in-depth focus on pollutants bioaccumulation and cellular alterations was conducted on the scleractinian M. pharensis, as described below.
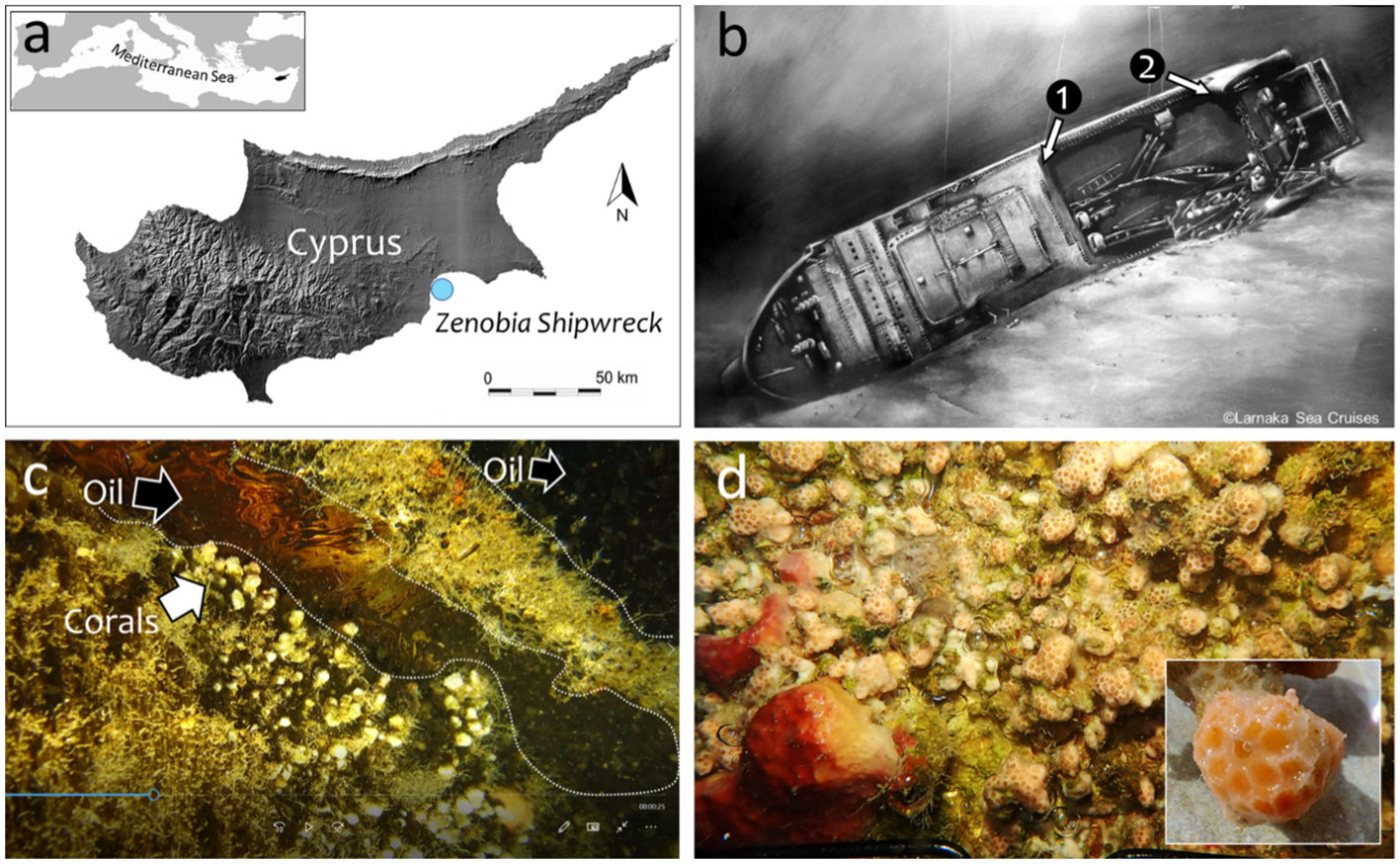
Figure 1 Location of the Zenobia shipwreck in Cyprus (A). Sampling stations along the starboard side of the shipwreck (25-27m depth): 1=inside the ”Car Deck” (oil); 2=below the “Smokestack” (no oil) (B). Madracis pharensis coral colonies from station 1 in contact with oil “ponds”; snapshot from a video (C). Community of M. pharensis and sponges from station 2; insert=detail of a coral sample (D).
Epibenthic biodiversity assessment
The diverse epibenthic communities of Zenobia thrive at the shaded undersides of the wreck’s external structures and inside the hull, where the high heterogeneity of surfaces, relatively open areas (e.g., cabins, corridors), and multitude crannies offer shelter from direct exposure to the sunlight. The benthic cover (>25% on average) is mainly composed by sponges, corals, bryozoans, and other organisms (Jimenez et al., 2017a).
To assess the status of the epibenthic communities, the percentage of benthic cover was measured from photo-quadrats following the protocol of Jimenez et al. (2017a). Succinctly, 15 photos from each area were taken consecutively, avoiding overlap, with a frame (13cm x 19cm) at a fixed distance from the digital camera (Olympus Tough equipped with strobes). Analysis of the photos was made using Coral Point Count program (Kohler and Gill, 2006) with a systematic lay-out of 225 points. The pre-existing code files were modified with the following seven categories: live coral, dead coral, macro algae, calcareous algae, porifera, other (e.g., serpulid polychaetes, bivalves, bryozoans, cyanobacteria), and substrate (not occupied by organisms). The selection of this categories was based on other similar studies in Cyprus (Jimenez et al., 2017a, Jimenez et al., 2017b; Jimenez et al., 2022). The percentage of cover from multiple image frames was grouped together for single site evaluation and to allow comparisons between the two sites (see below).
Madracis pharensis sampling and tissue collection
Samples of the scleractinian coral M. pharensis (Heller 1868) were collected at the Car Deck and Smokestacker sites for the analysis of PAHs bioaccumulation, total oxyradical scavenging capacity assay and biotransformation processes. This species is a facultative zooxanthellate coral that is abundant in Cyprus and can be found up to a depth of 100m. In general, colonies of M. pharensis can exhibit encrusting or knobby morphologies. This species was chosen due to its abundance on the shipwreck and simplicity of collection. Four small knobby-shaped colonies were collected from each site by gently removing the coral from the substrate with a thin metal chisel and small hammer and transported to the support vessel in seawater-filled plastic containers. Onboard, the seawater was decanted from the containers and the coral samples were placed in dry ice and transported to the laboratory, where they were stored into a -80° C freezer.
PAHs bioaccumulation, detoxification mechanisms and total oxyradical scavenging capacity in Madracis pharensis
For the analysis of polycyclic aromatic hydrocarbons (PAHs), about 3 g (wet weight) of coral samples (soft tissue and skeleton) were extracted in 5 mL 0.5 M potassium hydroxide in methanol using a microwave system at 55°C for 20 min (800 Watt) (Mars6, CEM Corporation, Matthews NC). After centrifugation at 3,000 × g for 10 min, the supernatants (methanolic solution) were concentrated using a rotational vacuum concentrator (RVC 2-18 Cdplus, CHRIST) and purified with solid-phase extraction (Octadecyl C18, 500 mg × 6 mL, Merck). A final volume of 1 mL was recovered with pure, analytical HPLC gradient grade acetonitrile, and HPLC analyses were carried out in a water and acetonitrile gradient by fluorimetric and diode array detection. The PAHs were identified according to the retention times of an appropriate pure standards solution (EPA 610 Polynuclear Aromatic Hydrocarbons Mix), and classified as low molecular weight (LMW: naphthalene, acenaphthylene, 1-methyl naphthalene, 2-methyl naphthalene, acenaphthene, fluorene, phenanthrene, anthracene) or high molecular weight (HMW: fluoranthene, pyrene, benzo(a)anthracene, chrysene, 7,12-dimethyl benzo(a)anthracene, benzo(b)fluoranthene, benzo(k)fluoranthene, benzo(a)pyrene, dibenzo(a,h)anthracene, benzo(g,h,i)perylene, indeno(1,2,3,c,d)pyrene). Quality assurance and quality control were done by processing blank and reference samples (freeze-dried mussel tissues SRM 2974a, NIST), and concentrations obtained for the SRM were always within the 95% confidence interval of certified value. The water content in tissues was determined in all the samples and concentrations of PAHs expressed as ng/g dw (Iannello et al., 2021).
Coral samples for biological analyses were gently washed with PBS buffer, ground powdered in liquid nitrogen and then homogenized with a homogenization ratio 1:5 (w:v) in a 100 mM K-phosphate buffer pH 7.5, with 2.5% NaCl, 0.1 mM phenyl-methyl sulphonyl fluoride (PMSF), 0.008 trypsin inhibitor unit (TIU) mL−1 aprotinin, 1 ng mL−1 leupeptin, 0.5 ng mL−1 pepstatin, and 0.1 mg mL−1 bacitracin. Sample homogenates were centrifuged at 12,000 x g for 70 min at 4° C, and the zooxanthellae-free supernatant collected, divided into aliquots and stored at −80° C until analysis (Yakovleva et al., 2004; Tang et al., 2018).
Protein content in each sample was measured through a modification of the Lowry’s method (Lowry et al., 1951), and used to normalize the results of biological investigations described after. Briefly, samples aliquots were diluted 100 times, and 500 µL of these were incubated in tubes with 2.5 mL of reaction medium (0.01% CuSO4, 0.02% Na3C6H5O7, 2% Na2CO3, and 0.4% NaOH in water). After 25 minutes, 250 µL of a solution made of Folin-Ciocalteau:water (1:1) was added to each sample, and these were left in incubation for 50 minutes. Protein standards prepared with albumin from bovine serum (BSA) at different concentrations (0, 20, 50, 100, 200, 400 µg/mL) were treated with the same procedure. At the end of the second incubation, the absorbance of standards and samples was acquired with triplicate readings at the spectrophotometer (ƛ=750 nm). The absorbance values of standards were plotted for creating the standard curve used to fit the absorbance of samples and calculate the proteins’ concentration of each sample.
Glutathione S-transferases activity was spectrophotometrically determined at 340 nm using 1-chloro-2,4-dinitrobenzene as substrate (CDNB). The assay consists in measuring the increment of the product of conjugation of 1.5 mM CDNB with 1 mM GSH in 100 mM K-phosphate buffer pH 6.5, GS-DNB. Enzymatic activity is expressed as µmol GS-DNB/min/mg protein, with ε = 9.6 mM−1 cm−1 (Habig and Jakoby, 1981).
The overall capability of cellular antioxidants to neutralize peroxyl (ROO•) and hydroxyl (HO•) radicals was measured through the total oxyradical scavenging capacity (TOSC) assay, by evaluating the inhibition of 0.2 mM α-keto-γ-methiolbutyric acid (KMBA) oxidation to ethylene gas (Regoli and Winston, 1998). Thermal homolysis in 100 mM phosphate buffer pH 7.4 of 20 mM 2-2-azo-bis-(2-methylpropionamide)-dihydrochloride (ABAP), and Fenton reaction of 1.8 μM iron-3.6 μM EDTA plus 180 μM ascorbate were used to generate peroxyl (ROO•) and hydroxyl (HO•) radicals, respectively. For each sample, ethylene formation was evaluated with GC-method at 10 min intervals in sample and blank reaction and used to calculate the total oxyradical scavenging capacity (TOSC) value with the equation: TOSC = 100−(∫SA/∫BA × 100), where ∫SA and ∫BA are the integral areas calculated from the ethylene kinetic curve measured during the reaction, for sample and blank reactions, respectively. The obtained specific TOSC value was normalized on the relative protein concentration measured with the Lowry method, and results expressed as U TOSC/µg protein.
Statistical analyses
The 2022 epibenthic community composition from Car Deck and Smokestacker stations was statistically analyzed after the inclusion of data from a previous survey conducted in 2011 (Jimenez et al., 2017a). The analysis tested for any temporal (2011 vs. 2022) or spatial (between stations) similarities between the two communities. To visualize the dissimilarity in the community compositions of the two stations, metaMDS and ordination plots, with Bray Curtis dissimilarity measure, were plotted in R (Version Ri386 3.4.4) and statistically compared with ANOSIMs (Analysis Of SIMlarities; permutations = 9999, distance = Bray) and SIMPER analysis (Similarity Percentage; permutation = 999). Furthermore, for the metaMDS, an envfit analysis, which indicates which species may be driving the distribution patterns observed, was also run. Since corals were the main focus, further analysis of the data was concentrated on this category (e.g., live and dead coral percentages), and more specifically with the species M. pharensis, which was also selected for the investigation of cellular effects. Linearized models (LMs; Supplementary Table S1) were run in R to investigate the changes in (i) the percentage of coral species’ cover, and (ii) percentage of mortality, across the two stations and the two time periods. Significance was determined using the p-values (significance level= 0.05) from the ANOVA and t-tests. Linearized models were applied to investigate differences among corals sampled at the two stations in terms of PAHs bioaccumulation, detoxification mechanisms and total oxyradical scavenging capacity.
Results
Epibenthic biodiversity assessment
The community composition between the two stations (Car Deck and Smokestaker; Supplementary Figure S1) was different in 2011 (ANOSIM; R2 = 0.44, p < 0.001) and in 2022 (ANOSIM; R2 = 0.15, p < 0.001). The dissimilarity in the two stations in 2011 was due to the organism groups ‘Other organisms’ (p = 0.02), ‘Substrate’ (p = 0.001), and ‘Calcareous organisms’ (p = 0.001), while the dissimilarity in the 2022 data where due to the organism groups ‘Substrate’ (p = 0.001) and ‘Porifera’ (p = 0.03) (SIMPER; Supplementary Materials, Supplementary Table S5).
Similarly, the composition was statistically different when pooling stations (Figure 2; ANOSIM, R2 = 0.15, p < 0.001) and years (Figure 2; ANOSIM, R2 = 0.33, p < 0.001) into a single analysis. The NMDS (dark grey clusters), shows that the community composition between two stations, ‘Car Deck’ and ‘Smokestacker’ are not completely dissimilar, but have differences. The organism groups responsible for the dissimilarity between the two stations were ‘Substrate’ (p = 0.001) and ‘Porifera’ (p = 0.041) (SIMPER; Supplementary Materials, Supplementary Table S5). As with the stations, year in the NMDS (light grey clusters) illustrates that there are differences in the community composition across the year 2011 and 2022. For year the dissimilarities were due to a bigger range of groups including ‘Corals’ (p = 0.001), ‘Substrate’ (p = 0.001), ‘Other organisms’ (p = 0.001), ‘Calcareous algae’ (p = 0.02), and ‘Algae’ (p = 0.004) (SIMPER; Supplementary Materials, Supplementary Table S5). Moreover, the envfit (Supplementary Materials, Supplementary Table S6), which tells us which species may be driving the distribution patterns observed in the NMDS, imply that all organism groups are responsible (Corals, Porifera, Other organism, and substrate have a p = 0.001; Algae p = 0.006, and Calcareous algae p = 0.004).
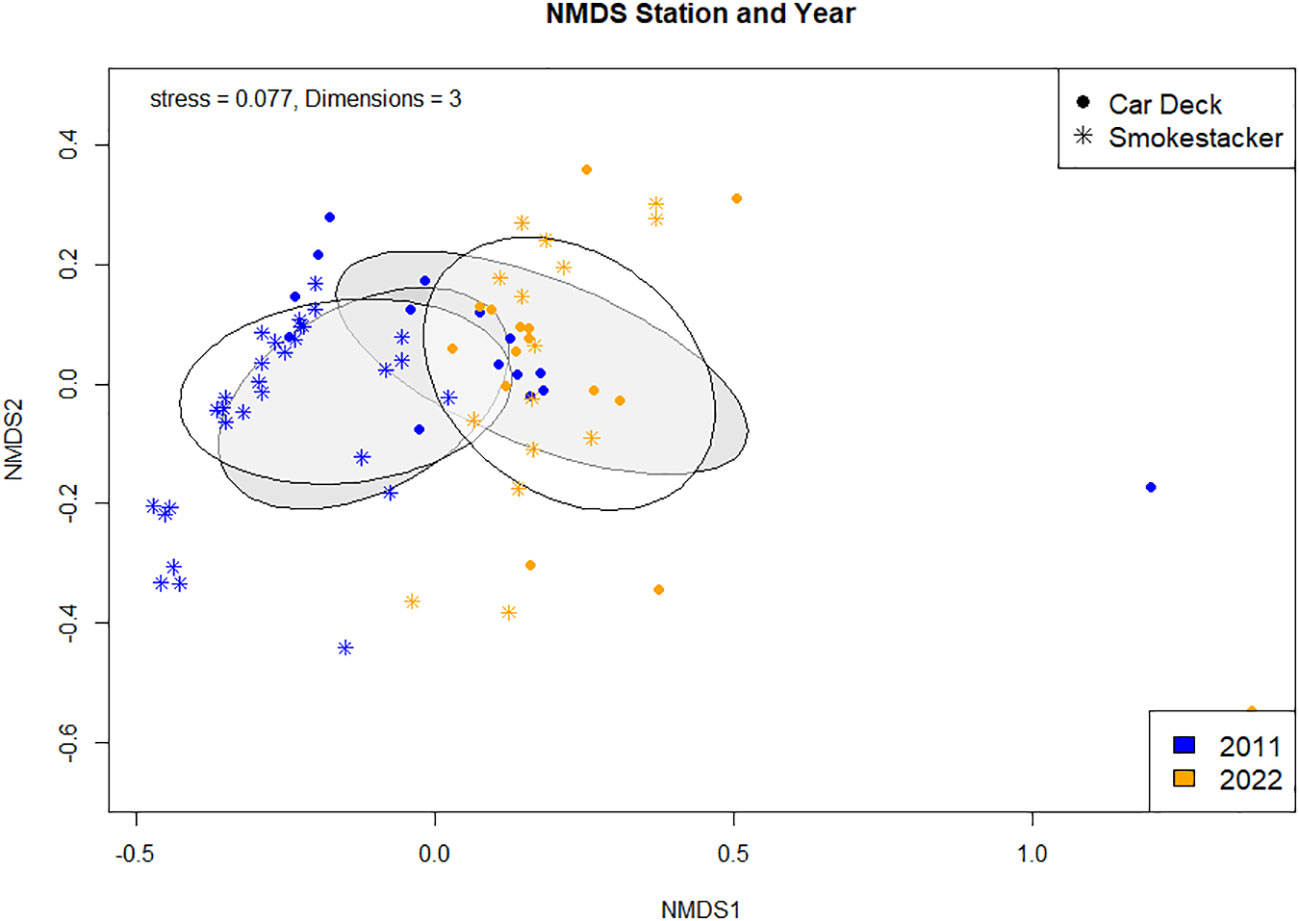
Figure 2 metaMDS ordination with Bray Curtis Dissimilarity distance calculation comparing community composition between stations and years.
The percentage cover of three coral species (Caryophyllia inornata, Phyllangia mouchezii and Polycyathus muellerae) found in the two stations did not show significant changes across years and locations, with the exception of M. pharensis (Figure 3; Supplementary Table S1). This species experienced an increment in the percentage of cover from the year 2011 to 2022, range 9.6% to 12.3% and 24.4% to 26.7%, respectively (T-test, t=2.298, p=0.022; Figure 3A, Supplementary Table S2), but the increase was proportional across the two stations (Figure 3B, Supplementary Table S2). Overall, the percentage of cover of dead and living corals (all species polled, Supplementary Table S3) was similar in both stations (range 4.4% to 7.1% and 10.1% and 30.4%, respectively) but there were more living corals in the 2022 survey compared to 2011 (Figure 4; T-test, t=2.041, p=0.04; Supplementary Table S4).
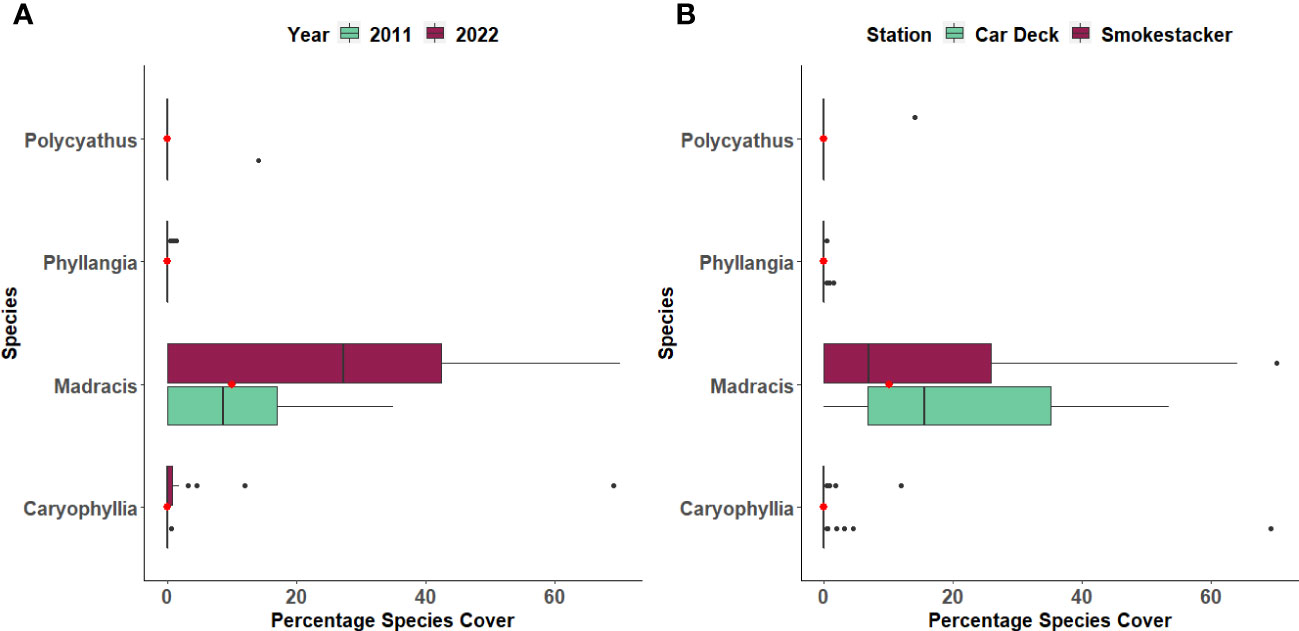
Figure 3 Percentage cover of four coral species across (A) Years and (B) Stations. The boxplots indicate the median (horizontal line), interquartile range (boxes), minimum/maximum values (whiskers), outliers (black points), as well as the combined median of both groups (red point).
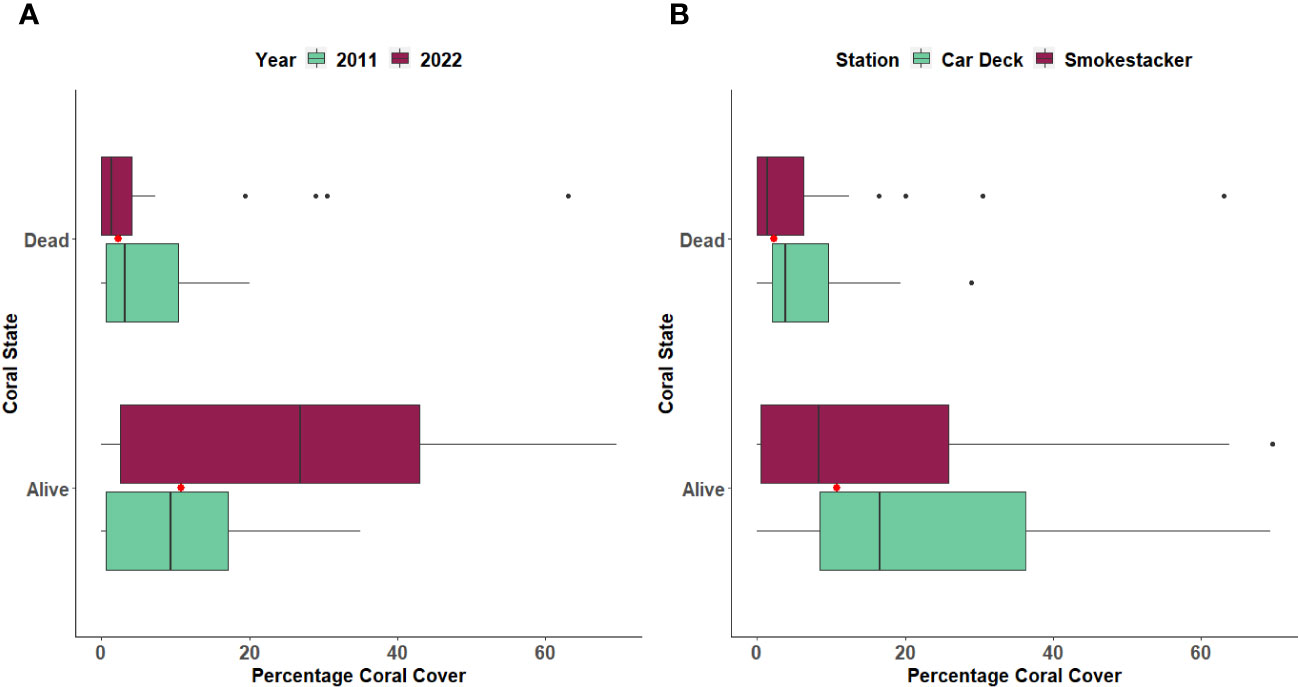
Figure 4 Percentage of cover of Dead and Living corals between (A) Years and (B) Stations. The boxplots indicate the median (horizontal line), interquartile range (boxes), minimum/maximum values (whiskers), outliers (black points), as well as the combined median of both groups (red point).
PAHs bioaccumulation in Madracis pharensis
Analyses of PAHs in corals samples confirmed the exposure of M. pharensis to PAHs, with significantly higher levels of total PAHs (Figure 5A) bioaccumulated in organisms collected at the Car Deck site (273.2 ± 166.9 ng/g d.w.) compared to those collected at Smokestacker site (34.9 ± 21.8 ng/g d.w.). Overall, both low molecular and high molecular weight PAHs significantly contributed to the observed differences (Figures 5B, C): low molecular PAHs were 33.6 ± 22.2 and 70.9 ± 2.19 ng/g d.w. at Smokestacker and Car Deck sites, respectively, while high molecular PAHs were 1.32 ± 0.45 and 202.3 ± 169.1 ng/g d.w. at Smokestacker and Car Deck sites, respectively. On the other hand, high molecular weight PAHs accounted for roughly 75% of the total PAHs in organisms sampled at Car Deck site, while these represented almost only 10% of total PAHs in tissues of organisms sampled at Smokestacker site.
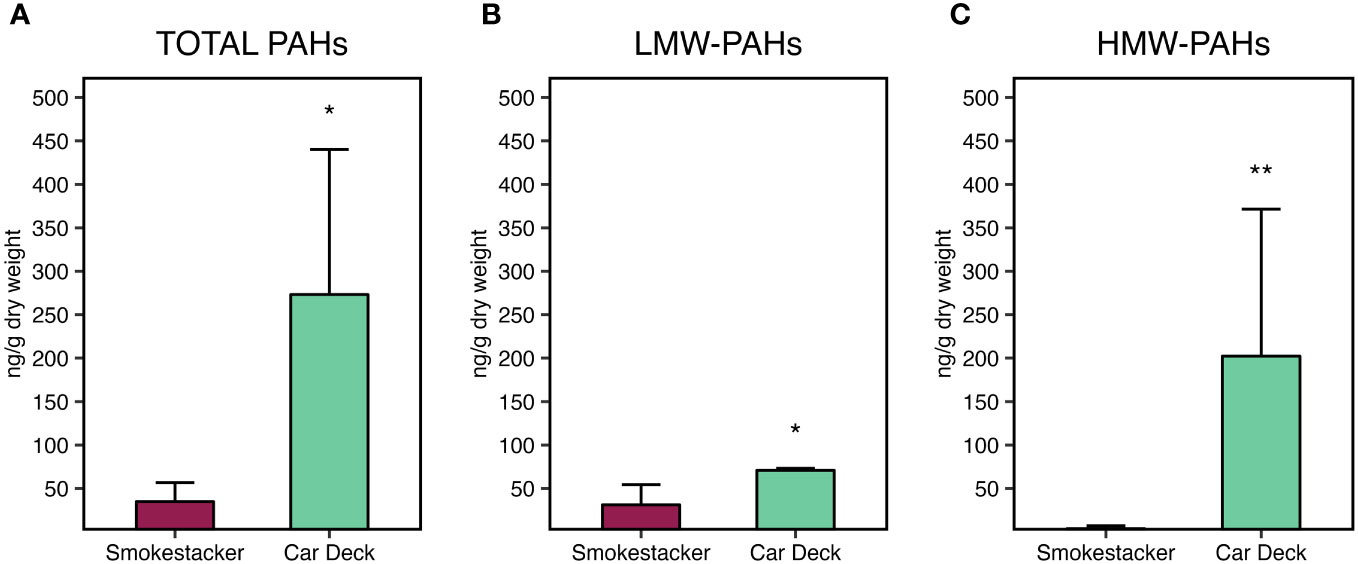
Figure 5 Bioaccumulation of (A) total PAHs, (B) low molecular weight PAHs, and (C) high molecular weight PAHs, in M. pharensis samples collected from Smokestacker and Car Deck sites, respectively. Results are given as mean ± standard deviation (n=4), asterisks denote significant differences between the two sites (*: p < 0.05; **: p < 0.01; ***: p < 0.001).
Among the contributors to these differences, 1-methylnaphthylene was the main low molecular weight PAH congener presenting different levels in organisms sampled in the two sites, while pyrene, chrysene, 7,12-dimethylbenz(a)anthracene, benzo(b)fluoranthene and benzo(k)fluoranthene were the main high molecular weight PAH congeners supporting the differences in PAHs bioaccumulation in M. pharensis sampled the two sites (Supplementary Materials, Supplementary Table S7).
Detoxification mechanisms and total oxyradical scavenging capacity in Madracis pharensis
A statistically significant decrease of glutathione S-transferases activity (Figure 6A) was measured in tissues of M. pharensis organisms sampled at the Car Deck site (8.25 ± 0.57 nmol/min/mg protein) compared to those collected at Smokestacker site (13.21 ± 2.27 nmol/min/mg protein).
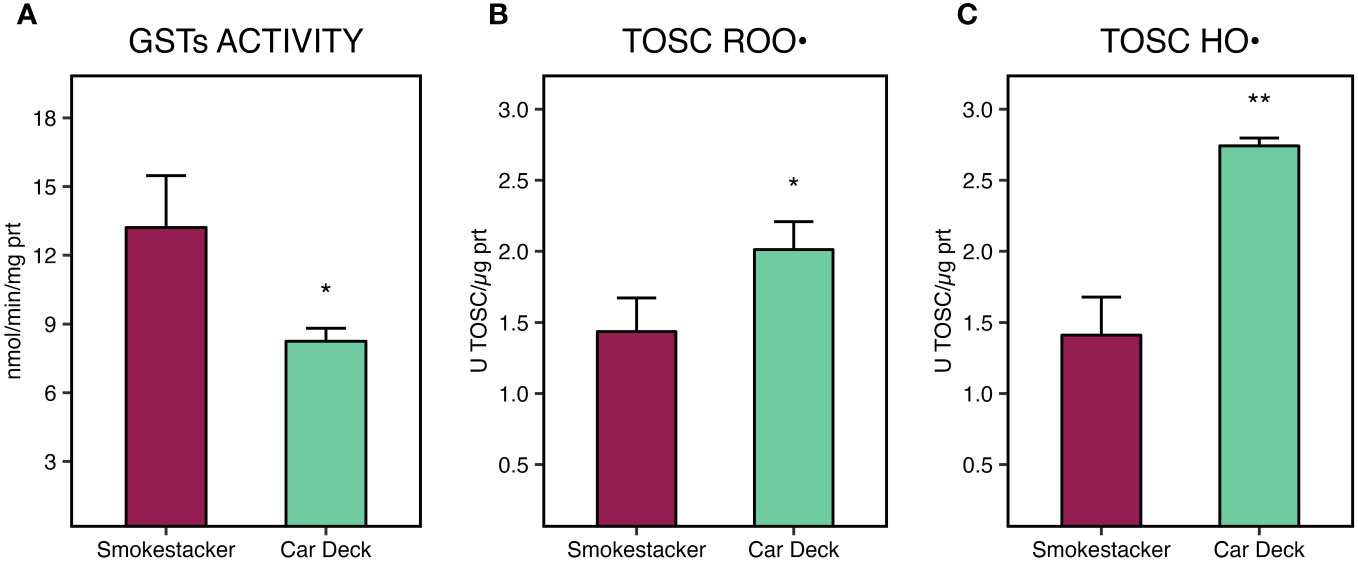
Figure 6 Glutathione S-transferases (GSTs) activity (A), total oxyradical scavenging capacity (TOSC) towards peroxyl (B) and hydroxyl radicals (C), in M. pharensis samples collected from Smokestacker and Car Deck sites, respectively. Results are given as mean ± standard deviation (n=4), asterisks denote significant differences between the two sites (*: p < 0.05; **: p < 0.01; ***: p < 0.001).
On the other hand, significant differences of total oxyradical scavenging capacity towards both peroxyl (TOSC ROO•) and hydroxyl radicals (TOSC HO•) were measured in M. pharensis, with lower values in organisms collected from Car Deck site compared to specimens collected from Smokestacker site (Figures 6B, C). Values were 1.44 ± 0.24 and 2.01 ± 0.20 U TOSC ROO•/µg protein for M. pharensis sampled at Smokestacker and Car Deck sites, respectively, while 1.41 ± 0.27 and 2.74 ± 0.06 U TOSC HO•/µg protein for M. pharensis sampled at Smokestacker and Car Deck sites, respectively.
Discussions
The communities’ composition of the studied areas of the Zenobia shipwreck were distinct between sites and changed over time, from the first survey in 2011 to the recent study in 2022. These findings are not unexpected given the particularities of each area and the major ecological changes that have occurred in Cyprus during those 11 years: population collapse of key species, such as habitat forming corals, bryozoans, seagrasses, and echinoid grazers (Garrabou et al., 2022; see below). Considering the spatial component, the communities of the Car Deck are under limiting conditions in contrast to the Smokestacker. Hydrodynamism, availability of light, substrate and structural features (i.e., heterogeneity) are among the main abiotic factors affecting epibenthic communities and ecological processes on shipwrecks (González-Duarte et al., 2018; Hill et al., 2021; Achilleos et al., 2023; Jimenez et al., 2023). To the best of our knowledge, there is only one source of information regarding oceanographic conditions at the shipwreck (i.e., Jimenez et al., 2017a). However, we can complement that limited amount of data with unpublished observations stemming from our regular (seasonal) visits to the shipwreck since 2010. In the Car Deck area, the penetration of light and the water circulation decrease just a few meters from the gateway. The sector where the sampling was made can be considered a semi-dark zone of confined hydrological conditions. The restricted water circulation allows the accumulation of positively buoyant debris from the wreck itself (e.g., plastic containers from the cargo, shreds of carpeting material), including the oil leaking from the piping system. These almost still-conditions also affect the arrival of nutrients, exchange of gases, dispersion of larvae, etc. that are highly dependent on water circulation. The epibenthic communities at the Smokestacker are under radically different conditions. Even though the sampling site is under the large structure, it is a well-lit environment of high hydrodynamism (days with very strong currents are common, making diving there difficult). In consequence, the differences in composition between the communities from both sampling locations are product of the species’ adaptation to site-related characteristics.
Regarding the ecological changes experienced in Cyprus during the last decade, five mass mortalities of epibenthic organisms occurred around the island since the first survey in 2011 of the Zenobia wreck (Jiménez et al., 2016; Garrabou et al., 2022, Jimenez unpublished data). These mortality events are strongly related to the increase in frequency and intensity of Marine Heat Waves (MHWs) affecting Cyprus and the Mediterranean in general (Garrabou et al., 2022; Stipcich et al., 2023). But it is during the period 2018-2021 that the Zenobia wreck showed prolonged (ca. 18 months) and wide-spread (almost in all sections of the wreck, from 18m to 44m depth) mortalities during the MHWs (Jimenez unpublished data). Massive sponges were among the most affected epibenthic species; independently from the location, entire necrotic colonies (from 2-3cm to 80cm in diameter) decayed for weeks until only spongin fibers remained. Other sponges in various stages of necrosis fell on the sandy bottom from the vertical walls or “ceiling” surface of the starboard side of the wreck’s structure. The net result of the disappearance of massive sponges was the increase in available substrate (open space) for colonization, expansion of “creeping” species that first grow expanding branches along the surface, such as Madracis, and certainly the reduction of competition for resources in the epibenthic communities. Competition for space between corals and sponges is a classic example of a biological factor affecting species’ assemblages in natural and artificial substrates, in the Mediterranean and elsewhere (Chadwick and Morrow, 2011; Kayal and Adjeroud, 2022). Several life-traits allow sponges to thrive and outcompete other species in limiting environments such as those found in shipwrecks; for example, sciaphilic species and physical and chemical defenses against other benthic organisms (Wulff, 2006, González-Murcia et al., 2023). We believe that less competition with sponges is probably the main reason behind the increase in Madracis’ percentage of cover at both sampling sites in the 2022 survey. During the 2010-2012 MHWs, this coral species was observed to be less affected than others, such as Cladocora caespitosa, P. mouchezii, and P. muellerae (Jiménez et al., 2016), also in the 2022 survey, “creeping branches” of Madracis were observed extending in an almost rivulet-like fashion. It is also possible that the disappearance of massive sponges facilitates the visual counting in the photo quadrats; but we support the competition-release explanation in view of the low mortality experienced by Madracis.
Despite M. pharensis coverage increased in both investigated stations from 2011 to 2022, corals living closely to “oil ponds” at Car Deck site showed to be threatened by chemical stress, causing the onset of cellular stress responses. Indeed, despite the origin and chemical composition of these “oil ponds” has not been addressed in this study, the investigation revealed a consistent accumulation of PAHs in organisms’ sampled at Car Deck site, with levels significantly higher than the ones sampled at Smokestacker, namely high molecular weight PAHs (HMW-PAHs). We hypothesize that HMW-PAHs accumulation may be the result of the exposure to petroleum fuels and lubricants, composed by noticeable amounts of such congeners, which have low biodegradation rates, high lipophilicity, and long persistence (Head et al., 2006; Beolchini et al., 2010; Goto et al., 2021). This has also been suggested in a mesocosm study of Yamada et al., 2003, in which a simulated dispersion of the water-available fraction of heavy residual oil highlighted HMW PAHs to persist longer than low molecular weight compounds. PAHs levels comparable to those measured in this study have been observed in tissues of corals sampled from reefs of South China Sea (Han et al., 2020), and in massive corals of the Sanya coral reef regions (Xiang et al., 2018), both being coastal areas impacted by anthropogenic activities.
Noticeably, HMW-PAHs such as chrysene, 7,12-dimethylbenz(a)anthracene, benzo(b)fluoranthene and benzo(k)fluoranthene, found to be present at high concentrations in organisms from Car Deck site, have been addressed as potentially carcinogenic for vertebrates (Baird et al., 2005; Honda and Suzuki, 2020). Indeed, biotransformation and detoxification processes of PAHs generate reactive metabolites which can bind DNA, triggering the formation of adducts and the onset of genotoxic effects (Benedetti et al., 2015). Benzo(b)fluoranthene and benzo(k)fluoranthene are also listed in the priority group of substances to be monitored in EU water bodies with the Water Framework Directive 2000/60/EC (European Commission, 2000).
While organic xenobiotics metabolism is well characterized in vertebrates, and known to be inducible through AhR by different typologies of planar organic chemicals as PAHs, PCB, and dioxins (Regoli and Giuliani, 2014; Benedetti et al., 2015), the specificity of AhR binding has been proposed to be restricted to chordates (Reitzel et al., 2014) and other xenobiotic biotranformation and detoxification pathways can be induced in marine invertebrates, as carboxylesterases and glutathione S-transferases (Regoli and Giuliani, 2014; Solé et al., 2021). Interestingly, in this study GSTs activity was significantly lower in corals sampled at Car Deck site compared to those sampled at Smokestack, and thus negatively correlated with PAHs in corals tissue; this result is of particular interest when compared to other findings on corals under organic xenobiotic stress: indeed, early induction of GSTs activity has been described in the coral Montastraea faveolata under acute short-term exposure to 0.1 ppm benzo[a]pyrene for 72h (Ramos and García, 2007), and similarly GST-pi gene induction was observed in Pocillopora damicornis after 24h under different concentrations of water available fraction of oil (Rougée et al., 2006). To date, a negative effect on GSTs activity was described in the reef-building coral Orbicella flaveolata affected by the Carribean yellow band disease under anthracene exposure (Montilla et al., 2016), and in the scleractinian P. damicornis exposed to polystyrene microplastics (Tang et al., 2018). The observed inverse relationship between GSTs activity and PAHs in corals tissues allows to hypothesize different possible mechanisms causing the lower activity of this detoxification process. The activity of GST might be directly disrupted by the PAHs accumulated in the cellular milieu, as previously observed in the mussels Dreissena polymorpha and Mytilus galloprovincialis exposed to PAHs- and PCB-contaminated sediments and benzo(a)pyrene, respectively (Akcha et al., 2000; Osman et al., 2007); on the other hand, a lower activity of the enzyme could be the consequence of a decreased availability of its main co-factor, reduced glutathione (GSH), either consumed by other enzymes of the antioxidants’ network (e.g. glutathione peroxidases, glyoxalase) or directly involved in oxyradicals scavenging, and not promptly replenished (Karami et al., 2011; Regoli and Giuliani, 2014; Benedetti et al., 2015; Vašková et al., 2023). Nonetheless, the accumulation of other chemicals, not assessed in this study, might have contributed to the GST activity decrease, as previously described for graphene oxide, flame retardants, insecticides, and pesticides (Özaslan et al., 2018; Josende et al., 2020; Bellas et al., 2022).
During xenobiotic metabolism, certain chemicals and their metabolites may undergo redox cycling (Cohen and D'Arcy Doherty, 1987; Regoli and Giuliani, 2014; Benedetti et al., 2015) resulting in the production of reactive oxygen species (ROS) and in redox homeostasis unbalance (Regoli and Giuliani, 2014; Benedetti et al., 2015). This oxidative pathway of chemicals toxicity often leads to the activation of the antioxidant system through the up-regulation of genes controlled by the antioxidant responsive element (ARE), coding for enzymes as catalase, superoxide dismutase, glutathione peroxidases, glutathione S-transferases, among others (Regoli and Giuliani, 2014).
In this study, we characterized for the first time the efficiency of the antioxidant system of M. pharensis through the evaluation of the total oxyradical scavenging capacity towards peroxyl and hydroxyl radicals: our results suggest that M. pharensis possess an efficient cellular antioxidant defense network, as shown to other marine symbiotic species (Dykens and Shick, 1982; Dykens et al., 1992; Regoli et al., 2004b; Liñán-Cabello et al., 2010; Liñán-Cabello et al., 2011). Indeed, symbiotic species have been indicated to possess a relevant antioxidant machinery to balance the significant pro-oxidant challenge generated by the photosintetically-mediated cellular hyperoxic conditions (Lesser, 2006). Specimens of M. pharensis sampled at the “Smokestack” site, not impacted by pollution, showed TOSC levels consistent with those measured in other symbiotic cnidarian species, such as the sea anemone Bunodosoma cavernatum and the sponges Petrosia ficiformis and Haliclona dancoi, when in concomitant with increased temperature, symbiont density and photosynthetically-mediated boost of pro-oxidative pressure (Regoli and Winston, 1998; Regoli et al., 2004a, b). Moreover, M. pharensis presented, in average, 2 to 5 times higher TOSC levels that other marine species, as the bivalves M. galloprovincialis, Flexopecten glaber, Ruditapes philippinarum, polychaetes Platynereis dumerilii, Polyophthalmus pictus, Syllis prolifera (Nardi et al., 2017; Iannello et al., 2021, 2018; Ricevuto et al., 2015).
Regarding organisms sampled at the Car Deck site, these exhibited an increased oxyradicals scavenging capacity towards ROO• and HO•, allowing to suggest an increased ROS production at cellular level mediated by the high levels of bioaccumulated PAHs, which results in a sustained activation of the response toward oxidative stress. A similar response has been previously described in the cnidarian Nematostella vectensis following short-term exposure to crude oil (Tarrant et al., 2014), in the scleractinians P. damicornis following an acute laboratory exposure to fuel oil (Rougée et al., 2006) and in Porites lobata sampled 3 months after an oil spill in the Federated States of Micronesia (Downs et al., 2006); nonetheless, a generalized increase of the total antioxidant capacity was observed in the coral Mussismilia harttii and in the hydrocoral Millepora alcicornis sampled in Abrolhos Reef Banks (Brazil) 2 and 4 months after the rupture of a mine dam (Dal Pizzol et al., 2022). Noteworthy, Rougée et al. (2006) and Downs et al. (2006) described a significant increase of GST-pi protein content in corals subjected to chemical pollution. As previously discussed, in this study GST enzymatic activity was significantly reduced in corals with higher PAHs content, which reinforces the discussed hypothesis that the lower enzymatic activity may result from chemicals-mediated effects on the functionality of this detoxification mechanism under chronic pollution conditions rather than from a lower expression at transcriptional level.
These overall findings suggest that despite our study revealed a sustained presence and growth of M. pharensis in both investigated sites, those living in close contact with oil ponds may be subjected to increased environmental stress, with possible implications on long-term species distribution at local scale. Indeed, sustaining the antioxidant defenses network under chronic stress conditions is an energetic cost adaptation, that could lead to energy trades-off, reducing the amount of energy available to be allocated to physiological processes other than stress-response and thus leading to suboptimal growth and reduced reproductive rates (Sokolova, 2013; Lachs et al., 2023). These aspects, along to a more in-depth characterization of Madracis pharensis stress response dynamics at transcriptional, physiological, and ecological levels, deserve further studies to fully elucidate the mechanisms underlying the capability of this species to survive under a chronic chemical stress.
Data availability statement
The raw data supporting the conclusions of this article will be made available by the authors, without undue reservation.
Author contributions
AN: Conceptualization, Data curation, Formal analysis, Writing – original draft, Writing – review & editing. VR: Data curation, Investigation, Writing – review & editing. MP: Visualization, Writing – original draft, Writing – review & editing, Formal analysis. MDC: Investigation, Writing – original draft, Data curation. HV: Formal analysis, Investigation, Writing – original draft. FR: Writing – review & editing, Supervision. SG: Conceptualization, Writing – original draft, Writing – review & editing, Project administration. CJ: Conceptualization, Funding acquisition, Investigation, Writing – original draft, Writing – review & editing, Project administration.
Funding
The author(s) declare that no financial support was received for the research, authorship, and/or publication of this article.
Acknowledgments
Author would like to thank Marios Papageorgiou (ENALIA) for his advice during the study but also for his administrative and logistical support, Constantina Stylianou (ENALIA) and Valentina Fossati (ENALIA) for assisting with administrative and analytical tools, respectively. The International Master of Science in Marine Biological Resources provided support to HV.
Conflict of interest
The authors declare that the research was conducted in the absence of any commercial or financial relationships that could be construed as a potential conflict of interest.
Publisher’s note
All claims expressed in this article are solely those of the authors and do not necessarily represent those of their affiliated organizations, or those of the publisher, the editors and the reviewers. Any product that may be evaluated in this article, or claim that may be made by its manufacturer, is not guaranteed or endorsed by the publisher.
Supplementary material
The Supplementary Material for this article can be found online at: https://www.frontiersin.org/articles/10.3389/fmars.2024.1330894/full#supplementary-material
References
Achilleos K., Jimenez C., Petrou A. (2023). “Wreck site formation process: the use of bryozoans,” in The Kyrenia Ship Final Excavation Report, Volume I: History of the Excavation, Amphoras, Ceramics, Coins and Evidence for Dating. Eds. Womer Katzev S., Wylde Swiny H. (Oxford: Oxbow Books), 166–171.
Akcha F., Izuel C., Venier P., Budzinski H., Burgeot T., Narbonne J.-F. (2000). Enzymatic biomarker measurement and study of DNA adduct formation in benzo[a]pyrene-contaminated mussels, Mytilus galloprovincialis. Aquat. Toxicol. 49, 269–287. doi: 10.1016/S0166-445X(99)00082-X
Allen T. E. H., Goodman J. M., Gutsell S., Russell P. J. (2016). A history of the molecular initiating event. Chem. Res. Toxicol. 29, 2060–2070. doi: 10.1021/acs.chemrestox.6b00341
Baird W. M., Hooven L. A., Mahadevan B. (2005). Carcinogenic polycyclic aromatic hydrocarbon-DNA adducts and mechanism of action. Environ. Mol. Mutagenesis 45, 106–114. doi: 10.1002/em.20095
Bellas J., Rial D., Valdés J., Vidal-Liñán L., Bertucci J. I., Muniategui S., et al. (2022). Linking biochemical and individual-level effects of chlorpyrifos, triphenyl phosphate, and bisphenol A on sea urchin (Paracentrotus lividus) larvae. Environ. Sci. pollut. Res. 29, 46174–46187. doi: 10.1007/s11356-022-19099-w
Benedetti M., Giuliani M. E., Mezzelani M., Nardi A., Pittura L., Gorbi S., et al. (2022). Emerging environmental stressors and oxidative pathways in marine organisms: Current knowledge on regulation mechanisms and functional effects. Biocell 46, 37–49. doi: 10.32604/biocell.2022.017507
Benedetti M., Giuliani M. E., Regoli F. (2015). Oxidative metabolism of chemical pollutants in marine organisms: Molecular and biochemical biomarkers in environmental toxicology. Ann. New York Acad. Sci. 1340, 8–19. doi: 10.1111/nyas.12698
Beolchini F., Rocchetti L., Regoli F., Dell'Anno A. (2010). Bioremediation of marine sediments contaminated by hydrocarbons: Experimental analysis and kinetic modeling. J. Hazard. Maters 182, 403–407. doi: 10.1016/j.jhazmat.2010.06.047
Brockinton E. E., Peterson M. R., Wang H.-H., Grant W. E. (2022). Importance of anthropogenic determinants of tubastraea coccinea invasion in the northern gulf of Mexico. Water 14, 1365. doi: 10.3390/w14091365
Chadwick N. E., Morrow K. M. (2011). “Competition Among Sessile Organisms on Coral Reefs,” in Coral Reefs: An Ecosystem in Transition. Eds. Dubinsky Z., Stambler N. (Springer, Dordrecht). doi: 10.1007/978-94-007-0114-4_20
Cohen G. M., D'Arcy Doherty M. (1987). Free radical mediated cell toxicity by redox cycling chemicals. Br. J. Cancer 55, 46–52.
Dal Pizzol J. L., Marques J. A., da Silva Fonseca J., Costa P. G., Bianchini A. (2022). Metal accumulation induces oxidative stress and alters carbonic anhydrase activity in corals and symbionts from the largest reef complex in the South Atlantic ocean. Chemosphere 290, 133216. doi: 10.1016/j.chemosphere.2021.133216
Dykens J. A., Shick J. M. (1982). Oxygen production by endosymbiotic algae controls superoxide dismutase activity in their animal host. Nature 297, 579–580. doi: 10.1038/297579a0
Dykens J. A., Shick J. M., Benoit C., Buettner G. R., Winston G. W. (1992). Oxygen radical production in the sea anemone anthopleura elegantissima and its endosymbiotic algae. J. Exp. Biol. 168, 219–241. doi: 10.1242/jeb.168.1.219
Downs C. A., Richmond R. H., Mendiola W. J., Rougée L., Ostrander G. K. (2006). Cellular physiological effects of the MV Kyowa Violet fuel-oil spill on the hard coral, Porites lobata. Environ. Toxicol. Chem. 25 (12), 3171–3180. doi: 10.1897/05-509R1.1
European Commission (2000). Directive 2000/60/EC of the European Parliament and of the Council of 23 October 2000 establishing a framework for Community action in the field of water policy. Offic. J. Eur. Commun. L 327, 1.
Garrabou J., Gómez-Gras D., Medrano A., Cerrano C., Ponti M., Schlegel R., et al. (2022). Marine heatwaves drive recurrent mass mortalities in the Mediterranean Sea. Global Change Biol. 28 (19), 5708–5725. doi: 10.1111/gcb.16301
González-Duarte M. M., Fernández-Montblanc T., Bethencourt M., Izquierdo A. (2018). Effects of substrata and environmental conditions on ecological succession on historic shipwrecks. Estuarine Coast. Shelf Sci. 200, 301–310. doi: 10.1016/j.ecss.2017.11.014
González-Murcia S., Ekins M., Bridge T. C. L., Battershill C. N., Jones G. P. (2023). Substratum selection in coral reef sponges and their interactions with other benthic organisms. Coral Reefs 42, 427–442. doi: 10.1007/s00338-023-02350-2
Gorbi S., Bocchetti R., Binelli A., Bacchiocchi S., Orletti R., Nanetti L., et al. (2012). Biological effects of palytoxin-like compounds from Ostreopsis cf. ovata: A multibiomarkers approach with mussels Mytilus galloprovincialis. Chemosphere 89, 623–632. doi: 10.1016/j.chemosphere.2012.05.064
Gorbi S., Giuliani M. E., Pittura L., d'Errico G., Terlizzi A., Felline S., et al. (2014). Could molecular effects of Caulerpa racemosa metabolites modulate the impact on fish populations of Diplodus sargus? Mar. Environ. Res. 96, 2–11. doi: 10.1016/j.marenvres.2014.01.010
Gorbi S., Regoli F. (2003). Total oxyradical scavenging capacity as an index of susceptibility to oxidative stress in marine organisms. Comments Toxicol. 9, 303–322. doi: 10.1080/08865140390450395
Goto Y., Nakamuta K., Nakata H. (2021). Parent and alkylated PAHs profiles in 11 petroleum fuels and lubricants: Application for oil spill accidents in the environment. Ecotoxicol. Environ. Saf. 224, 112644. doi: 10.1016/j.ecoenv.2021.112644
Guzmán H. M., Jiménez C. E. (1992). Contamination of coral reefs by heavy metals along the Caribbean coast of Central America (Costa Rica and Panama). Mar. pollut. Bull. 24, 554–561. doi: 10.1016/0025-326X(92)90708-E
Habig W. H., Jakoby W. B. (1981). Assays for differentiation of glutathione-S-transferase. Methods Enzymol. 77, 398–405. doi: 10.1016/s0076-6879(81)77053-8
Han M., Zhang R., Yu K., Li A., Wang Y., Huang X. (2020). Polycyclic aromatic hydrocarbons (PAHs) in corals of the South China Sea: Occurrence, distribution, bioaccumulation, and considerable role of coral mucus. J. Hazard. Maters 384, 121299. doi: 10.1016/j.jhazmat.2019.121299
Head I. M., Jones D. M., Röling W. F. (2006). Marine microorganisms make a meal of oil. Nat. Rev. Microbiol. 4, 173–182. doi: 10.1038/nrmicro1348
Hill C. E. L., Lymperaki M. M., Hoeksema B. W. (2021). A centuries-old manmade reef in the Caribbean does not substitute natural reefs in terms of species assemblages and interspecific competition. Mar. Pollut. Bull. 169, 112576. doi: 10.1016/j.marpolbul.2021.112576
Hoeksema B. W., zu Schlochtern M. P. M., Samimi-Namin K., McFadden C. S. (2023). In the aftermath of Hurricane Irma: Colonization of a 4-year-old shipwreck by native and non-native corals, including a new cryptogenic species for the Caribbean. Mar. pollut. Bull. 188, 114649. doi: 10.1016/j.marpolbul.2023.114649
Honda M., Suzuki N. (2020). Toxicities of polycyclic aromatic hydrocarbons for aquatic animals. Int. J. Environ. Res. Public Health 17, 1363. doi: 10.3390/ijerph17041363
Hughes T. P., Baird A. H., Morrison T. H., Torda G. (2023). Principles for coral reef restoration in the anthropocene. One Earth 6, 656–665. doi: 10.1016/j.oneear.2023.04.008
Iannello M., Mezzelani M., Dalla Rovere G., Smits M., Patarnello T., Ciofi C., et al. (2021). Long-lasting effects of chronic exposure to chemical pollution on the hologenome of the Manila clam. Evolution. Appl. 14, 2864–2880. doi: 10.1111/eva.13319
Jimenez C., Achilleos K., Petrou A., Hadjioannou L. (2023). “Tales from taphonomic amphoras: marine biofouling as interpretive ecological tool on wreck-site formation,” in The Kyrenia Ship Final Excavation Report, Volume I: History of the Excavation, Amphoras, Ceramics, Coins and Evidence for Dating. Eds. Womer Katzev S., Wylde Swiny H. (Oxford: Oxbow Books), 156–165.
Jimenez C., Andreou V., Evriviadou M., Munkes B., Hadjioannou L., Petrou A., et al. (2017a). Epibenthic communities associated with unintentional artificial reefs (modern shipwrecks) under contrasting regimes of nutrients in the Levantine Sea (Cyprus and Lebanon). PloS One 12, e0182486. doi: 10.1371/journal.pone.0182486
Jimenez C., Hadjioannou L., Petrou A., Andreou V., Georgiou A. (2017b). Fouling communities of two accidental artificial reefs (Modern shipwrecks) in Cyprus (Levantine sea). Water 9, 1–11. doi: 10.3390/w9010011
Jiménez C., Hadjioanou L., Petrou A., Nikolaides A., Evriviadou M., Lange M. A. (2016). Mortality of the scleractinian coral Cladocora caespitosa during a warming event in the Levantine Sea (Cyprus). Region. Environ. Change 16, 1963. doi: 10.1007/s10113-014-0729-2
Jimenez C., Petrou A., Papatheodoulou M., Resaikos V. (2022). “No one is safe: destruction of coralligenous concretions and other benthic sciaphilic communities in the MPA Cape Greco (Cyprus),” in 4th Mediterranean Symposium on the conservation of Coralligenous & other Calcareous Bio-Concretions, Genoa, Italy, 20-21 September 2022. 74–79.
Josende M. E., Nunes S. M., de Oliveira Lobato R., González-Durruthy M., Kist L. W., Bogo M. R., et al. (2020). Graphene oxide and GST-omega enzyme: An interaction that affects arsenic metabolism in the shrimp Litopenaeus vannamei. Sci. Total Environ. 716, 136893. doi: 10.1016/j.scitotenv.2020.136893
Karami A., Christianus A., Ishak Z., Syed M. A., Courtenay S. C. (2011). The effects of intramuscular and intraperitoneal injections of benzo[a]pyrene on selected biomarkers in Clarias gariepinus. Ecotoxicol. Environ. Saf. 74, 1558–1566. doi: 10.1016/j.ecoenv.2011.05.012
Kayal M., Adjeroud M. (2022). The war of corals: patterns, drivers and implications of changing coral competitive performances across reef environments. R. Soc. Open Sci. 9, 220003. doi: 10.1098/rsos.220003
Kohler K. E., Gill S. M. (2006). Coral Point Count with Excel extensions (CPCe): A Visual Basic program for the determination of coral and substrate coverage using random point count methodology. Comput. Geosci. 32, 1259–1269. doi: 10.1016/j.cageo.2005.11.009
Lachs L., Humanes A., Pygas D. R., Bythell J. C., Mumby P. J., Ferrari R., et al. (2023). No apparent trade-offs associated with heat tolerance in a reef-building coral. Commun. Biol. 6 (1), art. no. 400. doi: 10.1038/s42003-023-04758-6
Lesser M. P. (2006). Oxidative stress in marine environments: Biochemistry and physiological ecology. Annu. Rev. Physiol. 68, 253–278. doi: 10.1146/annurev.physiol.68.040104.110001
Liñán-Cabello M. A., Flores-Ramírez L. A., Zenteno-Savin T., Olguín-Monroy N. O., Sosa-Avalos R., Patiño-Barragan M., et al. (2010). Seasonal changes of antioxidant and oxidative parameters in the coral Pocillopora capitata on the Pacific coast of Mexico. Mar. Ecol. 31, 407–417. doi: 10.1111/j.1439-0485.2009.00349.x
Liñán-Cabello M. A., Lesser M. P., Flores-Ramírez L. A., Zenteno-Savín T., Reyes-Bonilla H. (2011). Oxidative stress in coral-photobiont communities. Oxid. Stress Aquat. Ecosyst. 127–137. doi: 10.1002/9781444345988.ch9
Lowry O. H., Rosebrough N. J., Farr A. L., Randall R. J. (1951). Protein measurement with the Folin phenol reagent. J. Biol. Chem. 193, 265–275. doi: 10.1016/S0021-9258(19)52451-6
Lushchak V. I. (2011). Environmentally induced oxidative stress in aquatic animals. Aquat. Toxicol. 101, 13–30. doi: 10.1016/j.aquatox.2010.10.006
Menezes N., Cruz I., da Rocha G. O., de Andrade J. B., Leão Z. M. A. N. (2023). Polycyclic aromatic hydrocarbons in coral reefs with a focus on Scleractinian corals: A systematic overview. Sci. Total Environ. 877, 162868. doi: 10.1016/j.scitotenv.2023.162868
Monchanin C., Mehrotra R., Haskin E., Scott C. M., Plaza P. U., Allchurch A., et al. (2021). Contrasting coral community structures between natural and artificial substrates at Koh Tao, Gulf of Thailand. Mar. Environ. Res. 172, 105505. doi: 10.1016/j.marenvres.2021.105505
Montilla L. M., Ramos R., García E., Cróquer A. (2016). Caribbean yellow band disease compromises the activity of catalase and glutathione S-transferase in the reef-building coral Orbicella faveolata exposed to anthracene. Dis. Aquat. Organ. 119, 153–161. doi: 10.3354/dao02980
Nardi A., Benedetti M., Fattorini D., Regoli F. (2018). Oxidative and interactive challenge of cadmium and ocean acidification on the smooth scallop Flexopecten glaber. Aquat. Toxicol. 196, 53–60. doi: 10.1016/j.aquatox.2018.01.008
Nardi A., Mincarelli L. F., Benedetti M., Fattorini D., d'Errico G., Regoli F. (2017). Indirect effects of climate changes on cadmium bioavailability and biological effects in the Mediterranean mussel Mytilus galloprovincialis. Chemosphere 169, 493–502. doi: 10.1016/j.chemosphere.2016.11.093
Osman A. M., Van Den Heuvel H., Van Noort P. C. M. (2007). Differential responses of biomarkers in tissues of a freshwater mussel, Dreissena polymorpha, to the exposure of sediment extracts with different levels of contamination. J. Appl. Toxicol. 27, 51–59. doi: 10.1002/jat.1183
Özaslan M. S., Demir Y., Aksoy M., Küfrevioğlu Ö.I., Beydemir Ş. (2018). Inhibition effects of pesticides on glutathione-S-transferase enzyme activity of Van Lake fish liver. J. Biochem. Mol. Toxicol. 32, e22196. doi: 10.1002/jbt.22196
Ramos R., García E. (2007). Induction of mixed-function oxygenase system and antioxidant enzymes in the coral Montastraea faveolata on acute exposure to benzo(a)pyrene. Comp. Biochem. Physiol. - C Toxicol. Pharmacol. 144, 348–355. doi: 10.1016/j.cbpc.2006.11.006
Regoli F., Cerrano C., Chierici E., Chiantore M. C., Bavestrello G. (2004a). Seasonal variability of prooxidant pressure and antioxidant adaptation to symbiosis in the Mediterranean demosponge Petrosia ficiformis. Mar. Ecol. Prog. Ser. 275, 129–137. doi: 10.3354/meps275129
Regoli F., Giuliani M. E. (2014). Oxidative pathways of chemical toxicity and oxidative stress biomarkers in marine organisms. Mar. Environ. Res. 93, 106–117. doi: 10.1016/j.marenvres.2013.07.006
Regoli F., Nigro M., Chierici E., Cerrano C., Schiapparelli S., Totti C., et al. (2004b). Variations of antioxidant efficiency and presence of endosymbiotic diatoms in the Antarctic porifera Haliclona dancoi. Mar. Environ. Res. 58, 637–640. doi: 10.1016/j.marenvres.2004.03.055
Regoli F., Winston G. W. (1998). Applications of a new method for measuring the total oxyradical scavenging capacity in marine invertebrates. Mar. Environ. Res. 46, 439–442. doi: 10.1016/S0141-1136(97)00119-0
Reitzel A. M., Passamaneck Y. J., Karchner S. I., Franks D. G., Martindale M. Q., Tarrant A. M., et al. (2014). Aryl hydrocarbon receptor (AHR) in the cnidarian Nematostella vectensis: Comparative expression, protein interactions, and ligand binding. Dev. Genes Evol. 224, 13–24. doi: 10.1007/s00427-013-0458-4
Ricevuto E., Benedetti M., Regoli F., Spicer J. I., Gambi M. C. (2015). Antioxidant capacity of polychaetes occurring at a natural CO2 vent system: Results of an in situ reciprocal transplant experiment. Mar. Environ. Res. 112, 44–51. doi: 10.1016/j.marenvres.2015.09.005
Rougée L., Downs C. A., Richmond R. H., Ostrander G. K. (2006). Alteration of normal cellular profiles in the scleractinian coral (Pocillopora damicornis) following laboratory exposure to fuel oil. Environ. Toxicol. Chem. 25, 3181–3187. doi: 10.1897/05-510R2.1
Schlezinger J. J., Struntz W. D. J., Goldstone J. V., Stegeman J. J. (2006). Uncoupling of cytochrome P450 1A and stimulation of reactive oxygen species production by co-planar polychlorinated biphenyl congeners. Aquat. Toxicol. 77, 422–432. doi: 10.1016/j.aquatox.2006.01.012
Scuba (2022) The Best Shipwreck Dive Sites in the World. Available online at: https://www.scuba.com/blog/best-shipwreck-dive-sites/ (Accessed 20 September, 2023).
Shakunthala N. (2010). New cytochrome P450 mechanisms: Implications for understanding molecular basis for drug toxicity at the level of the cytochrome. Expert Opin. Drug Metab. Toxicol. 6, 1–15. doi: 10.1517/17425250903329095
Solé M., Freitas R., Rivera-Ingraham G. (2021). The use of an in vitro approach to assess marine invertebrate carboxylesterase responses to chemicals of environmental concern. Environ. Toxicol. Pharmacol. 82, 103561. doi: 10.1016/j.etap.2020.103561
Stipcich P., Beca-Carretero P., Álvarez-Salgado X. A., Apostolaki E. T., Chartosia N., Efthymiadis P. T., et al. (2023). Effects of high temperature and marine heat waves on seagrasses: Is warming affecting the nutritional value of Posidonia oceanica? Mar. Environ. Res. 184, 105854. doi: 10.1016/j.marenvres.2022.105854
Sokolova I. M. (2013). Energy-limited tolerance to stress as a conceptual framework to integrate the effects of multiple stressors. Integr. Comp. Biol. 53 (4), 597–608. doi: 10.1093/icb/ict028
Tang J., Ni X., Zhou Z., Wang L., Lin S. (2018). Acute microplastic exposure raises stress response and suppresses detoxification and immune capacities in the scleractinian coral Pocillopora damicornis. Environ. Pollut. 243, 66–74. doi: 10.1016/j.envpol.2018.08.045
Tarrant A. M., Reitzel A. M., Kwok C. K., Jenny M. J. (2014). Activation of the cnidarian oxidative stress response by ultraviolet radiation, polycyclic aromatic hydrocarbons and crude oil. J. Exp. Biol. 217, 1444–1453. doi: 10.1242/jeb.093690
Turner N. R., Renegar D. A. (2017). Petroleum hydrocarbon toxicity to corals: A review. Mar. pollut. Bull. 119, 1–16. doi: 10.1016/j.marpolbul.2017.04.050
van der Schyff V., du Preez M., Blom K., Kylin H., Choong N. S., Yive K., et al. (2020). Impacts of a shallow shipwreck on a coral reef: a case study from st. Brandon’s Atoll, Mauritius, Indian Ocean. Mar. Environ. Res. 156, 104916. doi: 10.1016/j.marenvres.2020.104916
Vašková J., Kočan L., Vaško L., Perjési P. (2023). Glutathione-related enzymes and proteins: A review. Molecules 28, 1447. doi: 10.3390/molecules28031447
Wang H., Pan L., Zhang X., Ji R., Si L., Cao Y. (2020). The molecular mechanism of AhR-ARNT-XREs signaling pathway in the detoxification response induced by polycyclic aromatic hydrocarbons (PAHs) in clam Ruditapes philippinarum. Environ. Res. 183, 109165. doi: 10.1016/j.envres.2020.109165
Wulff J. L. (2006). Ecological interactions of marine sponges. Can. J. Zool. 84, 146–166. doi: 10.1139/z06-019
Xiang N., Jiang C., Yang T., Li P., Wang H., Xie Y., et al. (2018). Occurrence and distribution of Polycyclic aromatic hydrocarbons (PAHs) in seawater, sediments and corals from Hainan Island, China. Ecotoxicol. Environ. Saf. 152, 8–15. doi: 10.1016/j.ecoenv.2018.01.006
Yakovleva I., Bhagooli R., Takemura A., Hidaka M. (2004). Differential susceptibility to oxidative stress of two scleractinian corals: Antioxidant functioning of mycosporine-glycine. Comp. Biochem. Physiol. - B Biochem. Mol. Biol. 139, 721–730. doi: 10.1016/j.cbpc.2004.08.016
Yamada M., Takada H., Toyoda K., Yoshida A., Shibata A., Nomura H., et al. (2003). Study on the fate of petroleum-derived polycyclic aromatic hydrocarbons (PAHs) and the effect of chemical dispersant using an enclosed ecosystem, mesocosm. Mar. Pollut. Bull. 47, 105–113. doi: 10.1016/S0025-326X(03)00102-4
Keywords: pollution, PAHs, epibenthic biodiversity, detoxification, oxidative stress, Levantine Sea
Citation: Nardi A, Resaikos V, Papatheodoulou M, Di Carlo M, Vedhanarayanan H, Regoli F, Gorbi S and Jimenez C (2024) Cellular adaptations of the scleractinian coral Madracis pharensis to chronic oil pollution in a Mediterranean shipwreck. Front. Mar. Sci. 11:1330894. doi: 10.3389/fmars.2024.1330894
Received: 31 October 2023; Accepted: 25 January 2024;
Published: 07 March 2024.
Edited by:
Ranjeet Bhagooli, University of Mauritius, MauritiusReviewed by:
Adán Guillermo Jordán-Garza, Universidad Veracruzana, MexicoInês Martins, University of the Azores, Portugal
Copyright © 2024 Nardi, Resaikos, Papatheodoulou, Di Carlo, Vedhanarayanan, Regoli, Gorbi and Jimenez. This is an open-access article distributed under the terms of the Creative Commons Attribution License (CC BY). The use, distribution or reproduction in other forums is permitted, provided the original author(s) and the copyright owner(s) are credited and that the original publication in this journal is cited, in accordance with accepted academic practice. No use, distribution or reproduction is permitted which does not comply with these terms.
*Correspondence: Stefania Gorbi, cy5nb3JiaUB1bml2cG0uaXQ=; Carlos Jimenez, Yy5qaW1lbmV6QGVuYWxpYXBoeXNpcy5vcmcuY3k=