- 1Department of Biological Sciences, Institute of Environment, Florida International University, Miami, FL, United States
- 2Department of Biology, Montclair State University, Montclair, NJ, United States
- 3Van Hall Larenstein University of Applied Sciences, Leeuwarden, Netherlands
- 4Marine Animal Ecology Group, Wageningen University and Research, Wageningen, Netherlands
- 5School of Forest, Fisheries, and Geomatics Sciences, Institute of Food and Agricultural Sciences, University of Florida, Apollo Beach, FL, United States
- 6Florida Fish and Wildlife Conservation Commission, Fish and Wildlife Research Institute, Marathon, FL, United States
- 7Elizabeth Moore International Center for Coral Reef Research & Restoration, Mote Marine Laboratory, Summerland Key, FL, United States
- 8Institute for Socio-Ecological Research, Lajas, Puerto Rico
That coral reefs are in decline worldwide, particularly in the Caribbean, will come as no surprise. This decades-long decline has reached a potential tipping point as the weight of the effects of climate change have come decidedly to bear on the planet’s most diverse marine ecosystem. Whether coral reefs can persist without restorative intervention is debatable, which has prompted a surge in coral reef restoration projects focusing primarily on the cultivation and transplantation of coral fragments onto degraded reefs. But that widespread approach does little to address the underlying causes of coral loss, one of which is the proliferation of macroalgae that are deleterious to corals. An emerging solution to this problem is the enhancement of herbivory on coral reefs through improved management of herbivores, artificial enhancement of herbivore settlement, or their mariculture and subsequent stocking. This review explores the nuances of the biology of well-studied Caribbean coral reef herbivores (fishes, sea urchins, and crabs) as it relates to their mariculture and investigates the promise of herbivore stocking onto coral reefs as a restoration strategy. Fish, urchin, and crab herbivores differ appreciably in life histories, which confers advantages and disadvantages with respect to their mariculture and effectiveness as grazers. Mariculture of herbivorous marine fish for reef restoration is essentially non-existent so the reestablishment of grazing fish abundance on coral reefs focuses primarily on their protection through fishery regulations, but only at a few locations in the Caribbean. Mariculture of herbivorous urchins and crabs for restoration purposes is in its infancy, but promising especially for crabs whose larval rearing is less difficult. Perhaps the biggest challenge for the mariculture of either taxon is “scaling-up” from research settings to large-scale mariculture needed for stocking. Numerous studies extol the benefits of functional redundancy and complementarity for coral reef ecosystem stability, but whether this principal applies to the restoration of grazing function is untested. We identify gaps in our knowledge of best practices for the restoration of grazing function on coral reefs and conclude with some practical guidance on the establishment of targets for macroalgal reduction, along with strategic advice on grazer stocking in a given reef habitat.
Caribbean reefs and herbivory
For 200 million years, scleractinian corals have created and maintained coral reefs - the largest, most complex biogenic structures on Earth that support >25% of the current global marine biodiversity (Kiessling, 2009; Kiessling et al., 2010). This diversity, in turn, contributes to a range of critical ecosystem services to humans including food provisioning, coastal defense, tourism, and recreational activities (Moberg and Folke, 1999). However, coral reefs have slid into a precipitous trajectory of decline during the current Anthropocene epoch (Done, 1992; Jackson, 1997). Worldwide, corals suffer from bleaching events caused by the increasing temperature of the ocean and compromised rates of calcification due to ocean acidification, both a consequence of climate change (Hughes et al., 2018; Godefroid et al., 2022). The synergistic effects of a rapidly changing climate along with local stressors such as hurricanes, eutrophication, overfishing, disease, and other disturbances have triggered the widespread loss of corals and a decline in the biological and architectural complexity of these ecosystems (Gardner et al., 2005; Alvarez-Filip et al., 2009; Harborne et al., 2017). The problems are so severe that the viability of coral reef ecosystems is now in question (Alvarez-Filip et al., 2015; Agudo-Adriani et al., 2019; McKay et al., 2022; Hoegh-Guldberg et al., 2023).
Coral reef degradation often manifests in a dramatic phase shift in benthic taxonomic dominance from corals to fleshy macroalgae (Done, 1992; Mumby, 2006a). This change, at least on Caribbean reefs, appears to represent a shift to an alternate stable state (Mumby and Steneck, 2008; Mumby et al., 2013). Macroalgae are natural components of coral reef ecosystems that serve beneficial ecological functions (Ortega et al., 2019; Fulton et al., 2020), but their overgrowth of reefs is indicative of degradation (Morand and Briand, 1996). Competition with macroalgae has a range of negative consequences for coral demographics, among them: reduced fecundity, inhibition of recruitment, suppression of growth, and reduced survival, all with deleterious cascading effects on ecosystem health (Rasher et al., 2011; Rasher and Hay, 2014; van Woesik et al., 2018; Monteil et al., 2020; Smith et al., 2022). The negative effects of macroalgae on corals result from direct interactions (e.g., shading, space pre-emption) as well as indirect mechanisms such as allelopathy (Birrell et al., 2008). Furthermore, once a reef becomes macroalgal dominated, a range of feedback mechanisms make it difficult for a reef to recover (Mumby and Steneck, 2008). For example, loss of three-dimensional structure limits grazing fish abundances, and fishes may avoid reef areas with high macroalgal density because of predation risks (Hoey and Bellwood, 2011; Dell et al., 2016; Davis, 2018). Thus on macroalgal-covered reefs, the sustainability of corals and their resilience to disturbance are compromised, particularly in the Caribbean (Mumby et al., 2007a; Roff and Mumby, 2012).
The importance of herbivory as a critical driver of macroalgal assemblages and, in turn, of coral assemblages was perhaps first recognized in the early 1960s (Randall, 1961). By the 1970s, sea urchins and several groups of reef fishes (e.g., parrotfishes, Scaridae; surgeonfishes, Acanthuridae) were identified as the major controllers of coral reef macroalgae (Figure 1; Sammarco et al., 1974; Ogden, 1976; Ogden and Lobel, 1978). Research in the 1980s explored coral-algae-herbivore relationships, demonstrating the positive indirect effects of herbivory on corals (Sammarco, 1980; Hay, 1981; Steneck and Watling, 1982; Steneck, 1983), exemplified by the sudden Caribbean-wide die-off of a prominent urchin grazer (Diadema antillarum) in 1983–84 that demonstrated the functional importance of herbivores on coral reefs (Lessios, 2016). The mass mortality of D. antillarum sparked an explosion of research on reef herbivory as evident in the threefold increase in publications following that event (Lessios, 2005). Still, there was scientific debate regarding the primacy of herbivory versus eutrophy in driving macroalgae abundance on coral reefs (Hughes, 1994; Lapointe, 1997; Hughes et al., 1999; Lapointe, 1999). Moreover, the importance of herbivory for the resilience of Pacific coral reefs is less clear than in the Caribbean (Roff and Mumby, 2012).
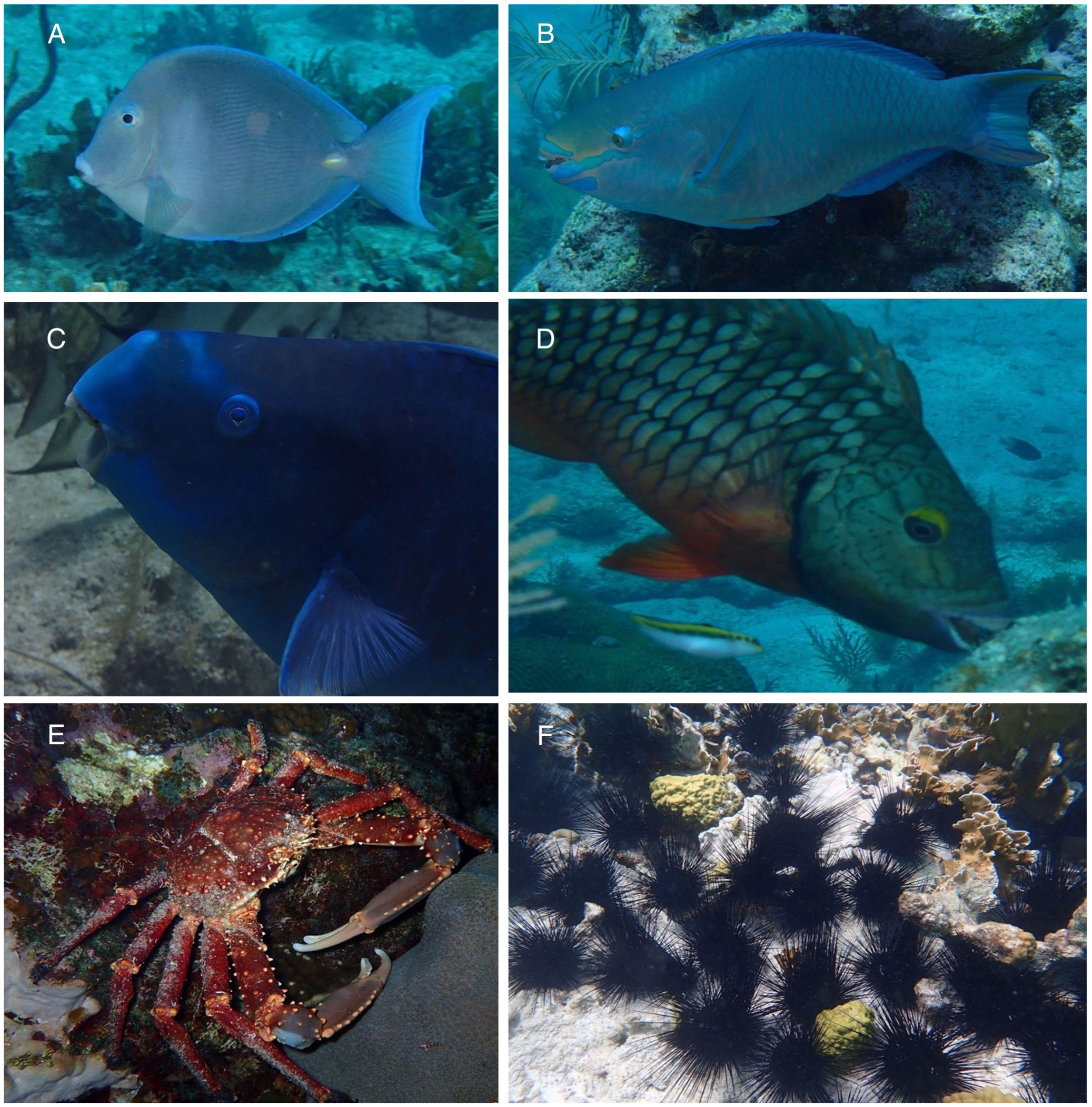
Figure 1 Photos of common Caribbean reef herbivores. (A) Blue Tang, Acanthurus coeruleus; Acanthuridae (photo credit: Alain Duran). (B) Queen Parrotfish, Scarus vetula; Scaridae (photo credit: Alain Duran). (C) Blue Parrotfish, Scarus coeruleus; Scaridae (photo credit: Alain Duran). (D) Stoplight Parrotfish (initial phase), Sparisoma viride; Scaridae (photo credit: Alain Duran). (E) Caribbean King Crab, Maguimithrax spinosissimus; Mithracidae (photo credit: AJ Spadaro). (F) Long-spined Sea Urchin, Diadema antillarum; Diadematidae (photo credit: Alwin Hylkema).
Although the importance of grazing as a key driver of reef resilience seems unequivocal (Mumby et al., 2007a), abundant herbivores may not guarantee high coral cover or low macroalgal cover. For example, in the absence of urchins even relatively unexploited parrotfish populations do not provide sufficient grazing pressure if nutrient levels are elevated or hurricane disturbances are frequent (Mumby et al., 2006a). Similarly, when coral cover becomes very low, even unexploited parrotfish populations may not provide sufficient grazing pressure to shift reefs toward coral-dominated states without additional grazing and improved coral recruitment (Mumby et al., 2007a). Finally, although models of Caribbean resilience are relatively well parameterized and tested for mid-depth forereefs, they may not necessarily apply to other habitats, such as shallow lagoonal patch reefs or sub-tropical reefs.
Responding to the growing threats to coral reefs, coral restoration programs have sprung up around the world with the intention of rebuilding the spawning stocks of reef-building corals by out-planting colonies grown in in situ nurseries or land-based “ex situ” facilities (Boström-Einarsson et al., 2020). The strategy of transplanting new corals onto degraded reefs has met with varying degrees of success (Boström-Einarsson et al., 2020), especially where macroalgae are abundant and retard the reestablishment of both transplanted and naturally recruiting corals.
In short, absent the proper benthic habitat conditions, the transplantation of corals to ‘jump-start’ reef recovery is doomed to failure (Arnold and Steneck, 2011). In response to this challenge, restoration science turned to two complementary objectives to increase reef herbivory: (1) regulate and better manage the fishing of piscine herbivores, and (2) restock invertebrate herbivores where their abundance has diminished. The latter alternative naturally centered on the re-establishment of D. antillarum, whose density in most locales never recovered to pre die-off levels (Lessios, 2016). In recent years, the potential importance of herbivory by a suite of other reef invertebrates has emerged, including urchins other than D. antillarum and a guild of herbivorous crabs in the Mithracidae family (Coen, 1988a, 1988b; Stachowicz and Hay, 1999a; Butler and Mojica, 2012; Francis et al., 2019; Spadaro and Butler, 2021; Zeinert et al., 2021). These findings have naturally led to consideration of invertebrate herbivores as agents of reef recovery through stock enhancement.
In contrast, the restocking of herbivorous fishes on reefs has few champions. Fish stock enhancement relying on aquaculture of young fishes, largely funded by recreational fishing fees, has a long history in freshwater, estuarine, and coastal marine systems (Lorenzen et al., 2010). But fishing of herbivorous reef fishes is generally not a recreational fishing activity. Instead, herbivorous fishes are landed by subsistence fishers, mostly in developing countries, or constitute bycatch in commercial trap fisheries (Shantz et al., 2020). So, the recreational fishery stock enhancement model is not easily transferred to coral reef systems.
The conditions required for the mariculture of reef fishes from eggs to the stocking of juveniles or adults are also considerably more complex and expensive than for most freshwater or coastal fishes that are stocked for recreational angling. This disparity due primarily to the longer pelagic larval duration and the open sea environment required for the successful larval development of most reef fishes. As an alternative, a few studies have investigated the potential use of postlarval fishes captured from the nearshore plankton as a means of assisting the restoration of fish assemblages, though not herbivorous fishes in particular (Heenan et al., 2009; Abelson et al., 2016; Cortés-Useche et al., 2021). This approach is based on the fact that fishes experience high mortality during the settlement and postsettlement phases of their life cycle, so their capture and ex situ rearing to a safer stocking size avoids that demographic bottleneck and the difficult task of rearing them from captive broodstocks. This methodology is still in its infancy but may become more feasible with advances in mariculture.
Instead, replenishing herbivorous reef fishes has focused on reducing their mortality from fishing through traditional fishery regulations or by establishing marine protected areas (Mumby et al., 2006a; O’Farrell et al., 2015), A controversial suggestion for solving the Caribbean’s problem with macroalgal overgrowth is the intentional introduction of Indo-Pacific herbivores (e.g., Pacific rabbitfish) into the Caribbean (Bellwood and Goatley, 2017), but support for this approach is lacking because the introduction of non-indigenous species often poses significant ecological harm to marine ecosystems (Benkwitt, 2015; Alidoost Salimi et al., 2021).
Given our current state of knowledge, along with a glimmer of hope that political interest in the problem and adequate funding exist to advance this area of research, we offer a review and comparison of the biological and logistical advantages and disadvantages of the restoration of herbivores on coral reefs. We focus on the western Atlantic because many reefs in this region are targeted for restoration, much of the work on reef invertebrate aquaculture has occurred here, and the important role of herbivores on Caribbean coral reef ecosystems has been more clearly defined.
Coral reef herbivores: fishes, urchins, and … crabs?
Fishes
The pathogen-driven reduction in populations of D. antillarum on Caribbean reefs in the 1980s fundamentally changed herbivory patterns in the region (Jackson, 2001; Lessios, 2016). Herbivorous fishes that previously competed with D. antillarum (Carpenter, 1990) became key grazers of macroalgae (Hay and Taylor, 1985; Robertson, 1991). Parrotfishes (Scaridae; Figure 1), in particular, were identified as playing a primary role in regulating the abundance of macroalgae and turf algae and limiting the development of juvenile macroalgae into larger forms (Frias-Torres and van de Geer, 2015; Adam et al., 2018). However, parrotfishes are also bioeroders of coral reefs. Foraging by species such as Scarus guacamaia and S. coelestinus leave only superficial scars on the substrate and so are described as ‘scrapers’ (sensu Bellwood and Choat, 1990). But ‘excavator’ species (sensu Bellwood and Choat, 1990), such as Sparisoma viride, are important bioeroders because their mode of feeding removes portions of the underlying carbonate reef structure (Clements et al., 2016; Ruttenberg et al., 2019). Recent data from the Pacific suggests that at least some parrotfishes may be microphages that consume reef-bound microorganisms (Clements et al., 2016), but this work has not been replicated in the Caribbean. Despite nuances in the feeding preferences and patterns among species of parrotfish, combined they serve a powerful functional role in removing macroalgae from reefs.
Surgeonfishes (Acanthuridae; Figure 1) are another key family of grazing fishes, although they are much less well-studied than parrotfishes. Surgeonfishes mostly target turf algal assemblages (Duran et al., 2016), although the proportion of energy they derive from turfs, cyanobacteria, and other algae intermixed within the turf algae is unclear. A few species of damselfishes (family Pomacentridae) are also considered herbivorous (e.g., Stegastes planifrons), but their presence tends to increase rather than decrease benthic algae communities because they are “gardener species” that create and vigorously maintain small (~0.1 m2) macroalgal patches on reefs (Sammarco and Carleton, 1982; Klumpp et al., 1987; Santodomingo et al., 2002). Because of their unique role in modifying macroalgal assemblages rather than removing them, we do not consider herbivory by damselfishes further in this review.
The degree of functional redundancy among herbivore fishes is far from clear, but the trophic differentiation, even among congeneric parrotfishes, suggests the importance of a diverse assemblage of species on healthy reefs (Bonaldo et al., 2014; Adam et al., 2015). For example, when both parrotfishes and surgeonfishes are present, benthic algal assemblages are mostly turf algae rather than macroalgae-dominated, which translates into associated benefits to corals (Burkepile and Hay, 2010). Even when targeting different food sources, herbivorous fishes often feed in separate reef areas. For example, some parrotfishes spend most of their time feeding in reef habitats that comprise <20% of the total reef area (Streit et al., 2018). The loss of large-bodied parrotfishes also appears particularly detrimental to the control of macroalgal cover (Steneck et al., 2014; Shantz et al., 2020; but see Kuempel and Altieri, 2017 for an example of the importance of small-bodied herbivores on lagoonal reefs).
One study of purported Caribbean herbivorous fishes suggests that some do not consume macroalgae but instead target epibionts on macroalgae (Dell et al., 2020). That study revealed that only three fishes (surgeonfish, Acanthurus coeruleus; red-band parrotfish, Sparisoma aurofrenatum; chubs, Kyphosus spp.) dominated consumption of experimental outplants of two common species of macroalgae (Dell et al., 2020). Moreover, one of those fishes (chubs) is not generally considered an herbivore. That finding echoes results from an experiment conducted a decade earlier on Australian coral reefs in which the primary consumption of macroalgae was by a “sleeping functional group” (Bellwood et al., 2006). Using caging experiments, the authors observed that the rapid reversal from a degraded, macroalgal-dominated reef to a coral- and epilithic algal-dominated state was the result of herbivory by a single batfish species (Platax pinnatus), previously considered an invertebrate feeder (Bellwood et al., 2006). Recently, the triggerfish Melichthys niger was reported as a consumer of macroalgae in the western Atlantic (Tebbett et al., 2020). More work is needed on the foraging of herbivores, but the multi-faceted dynamics of grazing by reef fishes argues that the restoration of fish herbivory must include a multitude of taxa.
Urchins
Five sea urchin genera (Diadema, Echinometra, Tripneustes, Eucidaris and Lytechinus) are commonly found on Caribbean coral reefs, and they all consume macroalgae (Figure 1). Given its historical high densities (Lessios, 1984) and generalist macroalgal-based diet (Solandt and Campbell, 2001; Herrera-Lopez et al., 2004), D. antillarum is considered one of the most important herbivorous sea urchins in the Caribbean. When D. antillarum is present in sufficient densities, algal cover declines and coral recruitment improves (Edmunds and Carpenter, 2001; Carpenter and Edmunds, 2006; Myhre and Acevedo-Gutiérrez, 2007; Idjadi et al., 2010). After the 1983–84 collapse of D. antillarum populations throughout the Caribbean, macroalgae increased (Lessios, 2016). Subsequently, in the few places (e.g., Jamaica, Curacao) where urchins recovered macroalgal cover decreased with positive effects on coral communities (Edmunds and Carpenter, 2001; Debrot and Nagelkerken, 2006). In the decades after the die-off, D. antillarum recovery was very slow on many reefs and densities were estimated at 12% of pre die-off densities 2015 (Lessios, 2016). In 2022, a new mass mortality occurred in 25 territories across the Caribbean, resulting in up to 99% mortality (Hylkema et al., 2023). Although the causative agent of the 2022 D. antillarum die-off was identified as a scuticociliate (Hewson et al., 2023), it is not known if this micro-organim is invasive or has always been present. Similarly, little is known about the environmental conditions that resulted in the outbreak. As a result of this recent die-off, D. antillarum densities have diminished in some areas of the Eastern Caribbean and Greater Antilles but the condition has not been observed in large parts of the Western Caribbean (Hylkema et al., 2023).
At least one species of Echinometra (E. viridis) is also found in large numbers on coral reefs (up to 35 ind./m2; Shulman, 2020) and has the capacity to consume substantial amounts of macroalgae (Sangil and Guzman, 2016). However, the foraging range of E. viridis is small relative to other grazers (Table 1), rendering high densities necessary to provide significant macroalgae removal. Paired with the observation that high E. viridis densities can also negatively impact reefs through bioerosion (Brown-Saracino et al., 2007), questions about the trade-off between herbivory and bioerosion must be addressed before it is a viable candidate for reef restoration efforts. Tripneustes ventricosus, another urchin, also occurs on Caribbean reefs where they forage nocturnally on macroalgae at rates exceeding 0.19 g/hr, but their low abundance translates into minimal overall rates of macroalgal removal (Francis et al., 2019). These studies point to urchins as a significant component of the grazer guild on coral reefs and a potential target for inclusion in coral reef restoration. The diets of D. antillarum, E. lucunter, and E. viridis do not overlap appreciably, suggesting niche partitioning of food resources (Rodríguez-Barreras et al., 2016), perhaps driven by interspecific aggression (Shulman, 1990). This partitioning underscores the need for a better understanding of competitive interactions among echinoids so as to develop a co-restocking strategy.
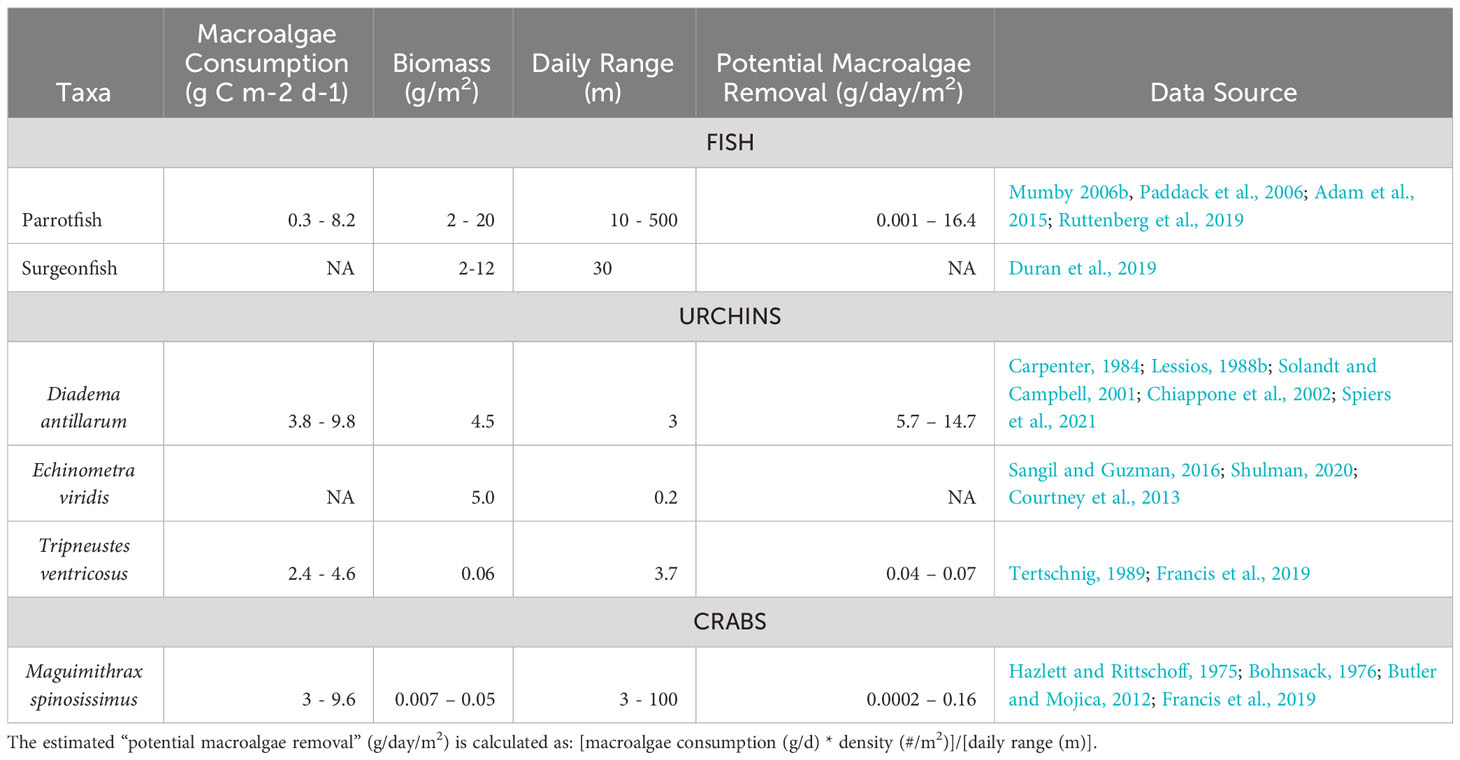
Table 1 A comparison of the estimated mean daily biomass of macroalgae removed per day per m2 for various Caribbean reef herbivores based on measures of their mean daily consumption of macroalgae, density, and foraging range.
Crabs
Most crabs are not herbivorous and the few that are also consume animal tissue, but herbivorous crabs have emerged as another character in the cast of coral reef grazers. In the Caribbean and western Atlantic, spider crabs (Mithracidae) comprise a diverse guild of macroalgae grazers (Figure 1). Coen (1988a, 1988b) was perhaps the first to recognize the importance of Mithracid crab grazing for coral communities. Working in Belize, he showed that grazing by Mithraculus spp. reduced the cover of algae epibionts in Porites divaricata thickets from 75% to 10% and suggested that this grazing may have pronounced positive effects on corals. A decade later, studies on Mithraculus sculptus revealed a facultative mutualism between the grazing crabs and their coralline algal hosts (Stachowicz and Hay, 1996). Stachowicz and Hay (1999a) later reported a similar mutualistic relationship between Mithraculus forceps and a coral host (Oculina arbuscula). The crab attained shelter among the branches of the coral and grew faster in association with natural as compared to artificial corals, presumably by feeding on the lipid-rich coral mucus. The coral in turn benefitted from reduced fouling by algae.
The previously mentioned Caribbean Mithracid crabs are diminutive, but among them exists a “giant” with profound grazing effects and mariculture potentia. The Channel Clinging Crab or Caribbean King Crab (Maguimithrax spinosissimus; formerly Damithrax, formerly Mithrax; Windsor and Felder, 2014; Klompmaker et al., 2015) is the largest crab in the Caribbean and western Atlantic (Figure 1). Large males can weigh more than 3 kg with carapace widths (CW) >190 mm, although adults more typically range in size from 50 to >100 mm CW (Rathbun, 1925; Creswell et al., 1989; Iglehart et al., 1989; Baeza et al., 2012). Maguimithrax spinosissimus is widespread throughout the Caribbean Sea, Gulf of Mexico, and tropical and sub-tropical western Atlantic (Rathbun, 1925; Williams, 1984), where they are found in crevice-rich habitats, including coral reefs at depths ranging from <1 m to >200 m. Although edible, there are only a few scattered, mostly artisanal, fisheries for M. spinosissimus (Rubino and Stoffle, 1989, 1990; Guzman and Tewfik, 2004), as they are difficult to exploit commercially due to their local scarcity, daytime occupancy deep within rocky crevices, and herbivorous diet which makes them difficult to target with traps.
Maguimithrax spinosissimus graze on a wide variety of macroalgae and algal turfs, including chemically and physically defended macroalgae (e.g., Amphiroa, Halimeda, Dictyota) that most urchin and fish grazers avoid (Adey, 1987; Butler and Mojica, 2012; Spadaro, 2019, but see Adam et al., 2018; Duran et al., 2019). The crabs also opportunistically consume benthic fauna (Wilber and Wilber, 1989; Creswell, 2011), which improves their growth as compared to a diet composed entirely of algae (Wilber and Wilber, 1989, 1991). Their grazing rate exceeds that of urchins and they consume more algae per gram of body mass than nearly all Caribbean parrotfishes (Butler and Mojica, 2012). Although M. spinosissimus occurs naturally on coral reefs, their overall grazing effects are diminished by their low density: 0.005 to 0.01 crabs/m2 (Butler and Mojica, 2012; Francis et al., 2019). However, Francis et al. (2019) estimated that macroalgal consumption achieved by doubling M. spinosissimus density on coral reefs in The Bahamas could alone exceed macroalgae production. In two separate experiments in the Florida Keys (Florida, USA), Spadaro and Butler (2021) reported that stocking ~1 crab/m2 onto coral patch reefs overgrown by macroalgae (primarily Halimeda and Dictyota) reduced macroalgal cover by 50 to 85%. Furthermore, the crabs maintained a low cover of macroalgae for the entirety of both year-long studies (Spadaro and Butler, 2021). This resulted in a significant increase in the density of juvenile corals and the richness and abundance of reef-associated fishes (Spadaro, 2019; Spadaro and Butler, 2021), demonstrating the potential use of this crab for coral reef restoration.
Restoration of herbivory
Effective approaches to restoring grazing function on coral reefs depend on herbivore identity. The culture and stocking of herbivorous fishes is not a widely advocated solution to restoring grazing on coral reefs (see Rinkevich, 2014; Abelson et al., 2016; Obolski et al., 2016). Restoration of herbivorous reef fish through proper fishery management and enforcement is generally considered a superior approach and has seen some success with respect to parrotfish recovery in places such as Belize and Bermuda (Cox et al., 2013; O’Farrell et al., 2015). Furthermore, in the absence of restocking programs, fish grazers will likely benefit from establishing no-take marine reserves, a widely used spatial management tool (Mumby et al., 2006b). In contrast, mariculture-based solutions show some promise for the recovery of urchins and crabs (Spadaro, 2019; Pilnick et al., 2021; Spadaro and Butler, 2021; Wijers et al., 2023). In this section, the various approaches to recover and sustain herbivores are reviewed.
Herbivorous fishes: management rather than mariculture
The establishment of marine reserves has repeatedly resulted in increases in herbivorous fish, particularly parrotfishes, that can both aid natural reef recovery (Mumby et al., 2007a) and restoration by reducing coral-algal competition and biofouling of structures used for coral attachment (reviewed by Seraphim et al., 2020). For example, meta-analyses demonstrate the benefits of spatial fishing bans to multiple groups of fishes, including herbivores, with a more significant effect where fish are most heavily exploited (Micheli et al., 2004). Similarly, a global analysis of the effect of protection on piscine browsers, scraper/excavators, and grazers demonstrated rapid recovery of their functional roles on reefs (MacNeil et al., 2015). The impact on parrotfishes and their functional role on reefs protected from fishing is clear from a case study in the large, long-established Exuma Cays and Land and Sea Park (ECLSP) in The Bahamas. Despite an increase in predatory groupers, an absence of traps inside the ECLSP led to a significant rise in parrotfish biomass and grazing and a reduction in macroalgal cover (Mumby et al., 2006b). This reduction in macroalgae in turn resulted in increased coral recruitment (Mumby et al., 2007b) and, ultimately, an increase in coral cover (Mumby and Harborne, 2010). By maintaining populations of large-bodied groupers, marine reserves may also benefit populations of herbivorous damselfishes that are susceptible to predation by smaller-bodied groupers (Mumby et al., 2012). However, recent studies in the Pacific suggest that increases in the abundance of grazers may be sensitive to food availability along with a reduction in fishing pressure, meaning that the impacts of marine reserves may be challenging to predict on reefs after disturbance events (Russ et al., 2015; Graham et al., 2020; Fidler et al., 2021).
Although marine reserves simplify the management of reef fisheries, the challenges of their establishment mean that tools such as herbivore-specific fishing bans should also be considered (Rogers et al., 2015), especially with parrotfish fisheries increasing in the Caribbean (Callwood, 2021). For example, in 2009 Belize established a national ban on catching herbivorous fish and, despite some parrotfishes still being found in markets, the ban appears to have reduced harvest (Cox et al., 2013). Parrotfishes have limited susceptibility to hook-and-line fishing and are readily identified by spear fishers so bans on traps, in particular, significantly increase parrotfish populations (O’Farrell et al., 2015). Despite the benefits of management practices for adult fish grazers, concurrent increases in meso-predators may disrupt the expected stock-recruitment relationship (O’Farrell et al., 2015). Moreover, trap bans may not be effective on shallow, highly productive patch reef habitats where other fishing methods predominate (McClanahan and Muthiga, 2020). Implementing changes in fishing methods can be challenging (Agar et al., 2019), but bans on the fishing of herbivores, perhaps in combination with other management tools, could significantly increase the resilience of Caribbean reefs and aid their recovery (Steneck et al., 2019; Mumby et al., 2021b).
In addition to reducing fish abundance and their functional role, over-exploitation of herbivores can have devastating effects on fish reproductive output that persist over generations even among highly fecund species (Sadovy, 2001). For gonochoric, protogynous species where terminal males mate with several females, a reduction in size as a result of overfishing can be particularly detrimental to population sustainability. Hawkins and Roberts (2003) reported order of magnitude declines in terminal male size and even the total absence of Sparisoma viride and Scarus vetula with increasing fishing across several Caribbean islands. Surgeonfishes might be more resilient to fishing than parrotfishes, given their rapid growth, early maturity, and short life spans (Robertson et al., 2005). Typically, herbivorous fish communities respond quickly to effective fishing regulation (e.g., no-take areas). Their size and number can double in less than five years (Hawkins and Roberts, 2003; O’Farrell et al., 2015) under proper management, which can also reestablish natural sex ratios (O’Farrell et al., 2016). Fish grazing rates are strongly dependent on fish size, therefore maintaining or restoring large fishes to populations can have important consequences on rates of macroalgae removal.
Surgeonfishes and parrotfishes reproduce and recruit year-round and most species display peaks of reproductive activity during the warmest months (Munro et al., 1973), likely related to higher food availability (Clifton, 1995). The pelagic period of the larvae is estimated around 45–70 days for surgeonfishes and 60 days for parrotfishes with some variation depending upon factors such as current speed (Rocha et al., 2002; Valles, 2017). Currently, we are unaware of any attempt to rear surgeonfish or parrotfish for restoration purposes. Anecdotal reports describe aquarist culture of convict surgeonfish (Acanthurus triostegus) from wild-spawned eggs, and wild postlarvae capture, culture, and release may hold promise for some reef species (Richardson et al., 2023). There is still much to be done in this field, but the best option appears to be protecting existing stocks from fishing.
Urchins and crabs: mariculture to the rescue?
Recent progress in the mariculture and stocking of urchins and crabs provides promise for the restoration of herbivory on coral reefs in the Caribbean. Table 2 summarizes some biological characteristics most pertinent to the mariculture and stocking success of Caribbean herbivorous fishes, urchins, and crabs. The urchin D. antillarum has been experimentally spawned from broodstock and reared through settlement in captivity (Pilnick et al., 2021; Wijers et al., 2023). The Caribbean King crab (M. spinosissimus) has all of the biological hallmarks for mariculture: the species possesses a short, non-feeding larval period of <1 wk, exhibits rapid growth on a mostly herbivorous diet, and has high post-stocking survival when transplanted at sizes >30 mm CW (Spadaro and Butler, 2021). Researchers have successfully reared M. spinosissimus in captivity as a potential seafood product, and new mariculture projects in Florida, Belize, and Mexico are exploring the efficacy of rearing crabs in captivity for release onto coral reefs to manage macroalgal overgrowth. This section reviews the current progress in the mariculture and stocking of herbivorous urchins and crabs on coral reefs.
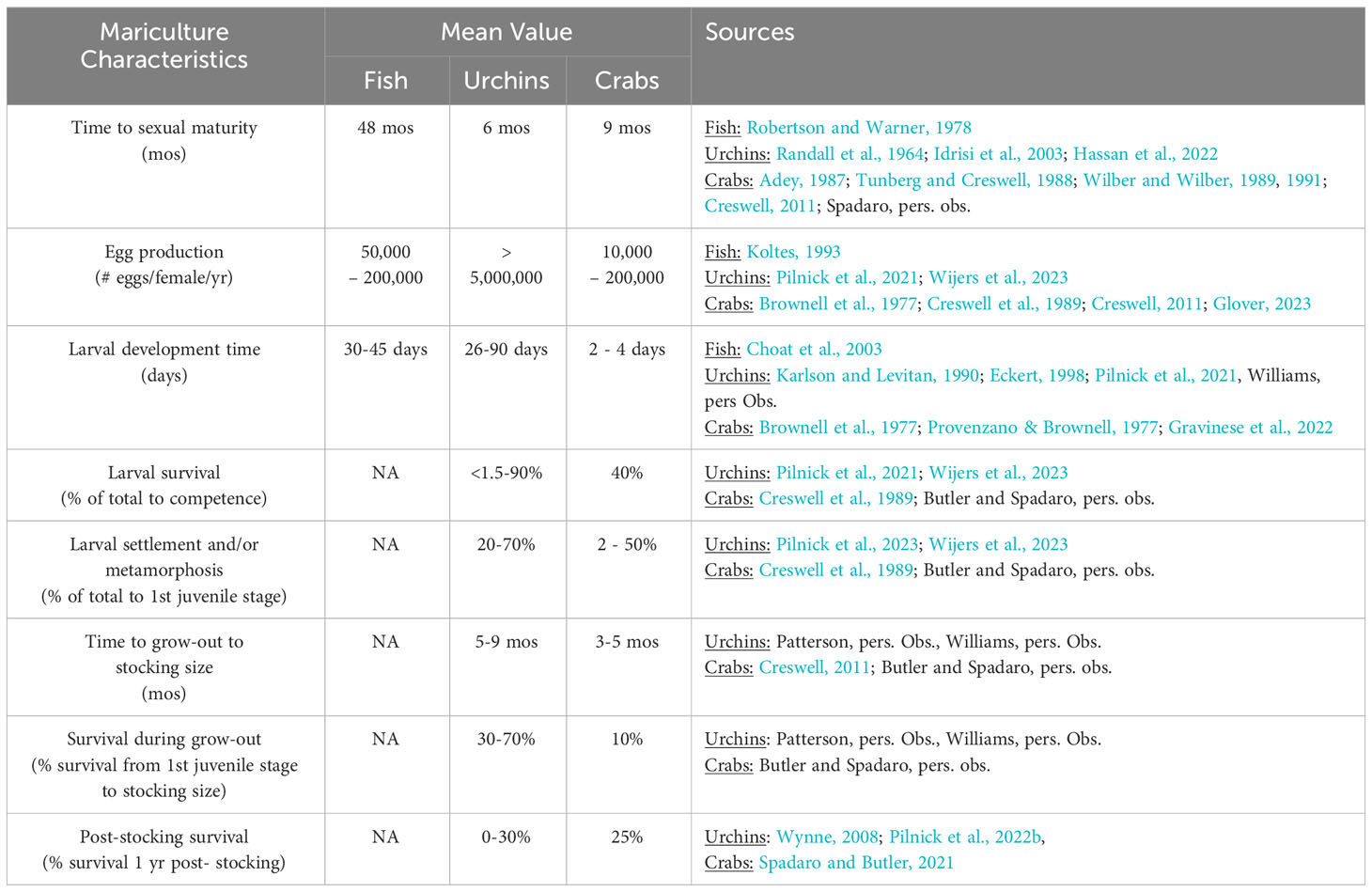
Table 2 A summary of biological characteristics pertinent to mariculture and stocking success of Caribbean herbivorous fishes, urchins, and crabs.
A. Spawning stocks
Urchins
Adult D. antillarum can be maintained in recirculating aquaculture systems (Pilnick et al., 2021; Wijers et al., 2023) and in tropical coastal areas with adequate water quality. Culturists have also had success holding and rearing D. antillarum juveniles and adults in systems receiving natural seawater (Sharp et al., 2018; Williams, 2022). For ease of transport and genetic considerations, acquiring animals from local source populations is preferable. However, a Florida Keys-level population study by Chandler et al. (2017) found a homogenous D. antillarum genetic structure across the sampled area (Dry Tortugas to Key Biscayne) and good genetic representation in two locally sourced broodstock populations of mixed ancestry. Paired with a lengthy planktonic larval duration (35–90 days; Levitan, 1991; Eckert, 1998), these results suggest that broodstock does not need to originate from the specific reefs that are the restocking target.
Crabs
Most restoration-focused crab mariculture operations rely on the collection of wild ovigerous females rather than both male and female broodstock. However, M. spinosissimus will mate and extrude viable clutches of eggs in captivity with appropriate environmental conditions and ample space and food. Spawning stocks are typically collected from reef or backreef habitats by hand and at night. It is possible to collect some gravid females as bycatch in trap fisheries (e.g., Brownell et al., 1977), but catch rates are typically low. For example, Munro (1983) reported landings of ~27 crabs per 1,000 trap nights off Pedro Bank, Jamaica. Husbandry requirements for broodstock are minimal: the crabs require good water quality, appropriate and ample diet, and a low male:female broodstock ratio. Attempts at culturing the species have been carried out either in situ in cages (Adey, 1987) or in laboratory seawater systems (Brownell et al., 1977; Provenzano and Brownell, 1977; Tunberg and Creswell, 1991; Wilber and Wilber, 1991).
Given the short planktonic larval duration of M. spinosissimus (~5 d), larval dispersal is presumably limited suggesting that there should be high levels of genetic differentiation among subpopulations in the Caribbean. Simulation modeling, along with an analysis of molecular variance and pairwise FST values of crabs collected in Costa Rica, Mexico, and the Florida Keys (USA), indeed suggest low, multi-generational or stepping-stone connectivity among natural populations of M. spinosissimus (Baeza et al., 2019). With this in mind, it is prudent that broodstock be sourced from populations near the intended release site to avoid genomic mixing of potential subpopulations while still retaining the genomic heterogeneity of wild stocks so as not to limit adaptive potential in response to changing environmental conditions (Texeira and Huber, 2021).
B. Spawning, fertilization, and larval production
Urchins
Echinoids have a complex life history involving the external fertilization of planktonic eggs, development of planktotrophic (feeding) larvae, metamorphosis, settlement, and recruitment of larvae to a benthic habitat followed by maturation of recruits into adults (Smith, 1997). D. antillarum reaches sexual maturity at 25–30 mm test diameter (Levitan, 1991). The species spawns throughout the year in much of the Caribbean (Lessios, 1981; Steiner and Williams, 2006; Williams et al., 2009). Settlement studies around Puerto Rico and St. Eustatius revealed peak settlement from May through September (Williams et al., 2009; Hylkema et al., 2022a), indicating that spawning occurs mostly in spring. In the Florida Keys, peak spawning occurs a few months earlier in November (Bauer, 1976).
Spawning in D. antillarum can be induced with heat shock (Leber et al., 2009; Pilnick et al., 2021), which is less invasive as compared to the often-used method of injecting a KCl solution into the coelomic cavity (Rahman et al., 2012). Spawning of T. ventricosus can be induced by gently rocking or shaking the individual while out of the water (Williams, pers. observ.). If maintained in a stable environment with continuous access to food, urchins can spawn monthly year-round in the laboratory (Pilnick et al., 2021; Wijers et al., 2023).
The mean egg diameter of D. antillarum is 72.7 ± 2.4 μm, which is slightly larger than T. ventricosus at 61 ± 2 μm (Guete-Salazar et al., 2021). In a hatchery setting, fecundity is high (i.e., several million eggs regularly spawned per female) and fertilization rates are generally >90% with no evidence of polyspermy (see Pilnick et al., 2021). Embryo development proceeds rapidly within a fertilization membrane that disappears in the blastula stage, followed by the prism and early pluteus stages within two days at 27°C (Eckert, 1998). The larvae of D. antillarum grow into an echinopluteus (first described by Mortensen, 1921; Figure 2), which is unique to urchins in the Diadematidae family and is a morphology that presents challenges for larviculture.
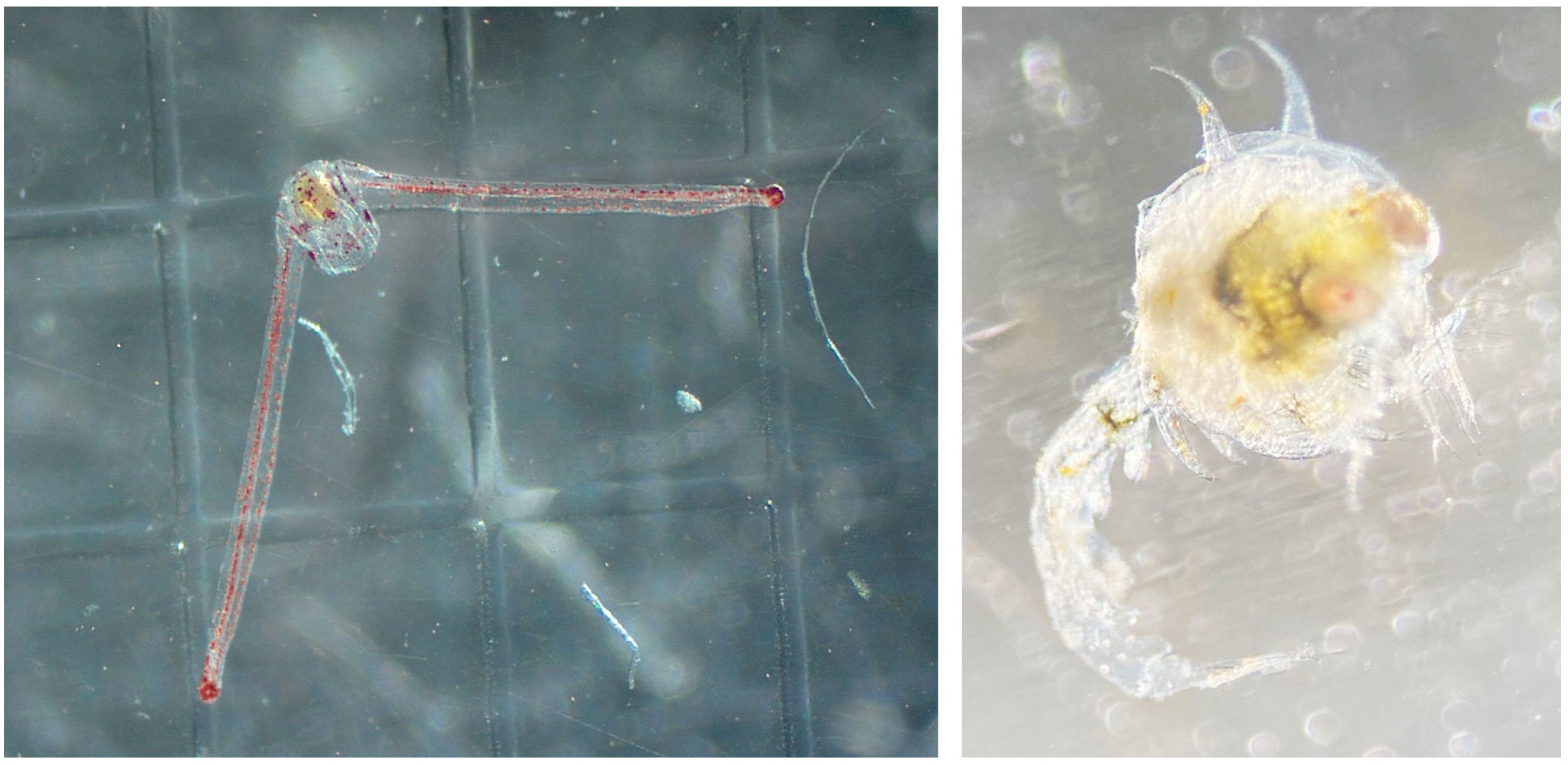
Figure 2 (Left) Photo of a D. antillarum echinopluteus larva reared in the laboratory (photo credit: A. Hylkema). (Right) Photo of a M. spinosissimus megalopa larvae reared in the laboratory (photo credit: L. Cifers).
Larval functional performance depends on larval size and shape, which influence susceptibility to predation (Eckert, 1998), swimming speeds (Emlet, 1983), metabolic requirements (McEdward, 1984), and clearance capacity (Hart and Strathmann, 1995). The pluteus arm span of D. antillarum is larger than most tropical echinoid larvae and can grow to over 4.5 mm long for a total arm span of over 9 mm (Eckert, 1998). Eckert (1998) stated that with this large arm span, D. antillarum larvae may not be as vulnerable to predators as other echinoid larvae. Also, larvae with larger arm lengths may maximize the capability of locomotion and feeding (Hart and Strathmann, 1994). However, in the culture environment these long arms are fragile and subject to physical damage, which may lead to energetic expenditure for repair and/or opportunistic infection.
Eckert (1998) was the first to successfully culture D. antillarum from eggs to a few juveniles. More small-scale cultures followed (Idrisi et al., 2003; Nedimyer and Moe, 2006; Leber et al., 2009; Pilnick et al., 2021), but large-scale culture has not yet been achieved. Larval D. antillarum are extremely sensitive to many environmental parameters, bacterial infection, and are described by Bielmyer et al. (2005) as among the marine organisms most sensitive to dissolved metal toxicity. The larvae of D. antillarum need constant, gentle water movement during culture because they are negatively buoyant (Leber et al., 2009; Pilnick et al., 2021; Wijers et al., 2023). The microalgae Rhodomonas (either R. lens or R. salina) seems essential for rearing D. antillarum at a larval density between 0.1 and 2 larvae/mL (Eckert, 1998; Nedimyer and Moe, 2006; Pilnick et al., 2022a; Wijers et al., 2023). Improving survival of urchins during their sensitive planktonic larval and early benthic juvenile stages will be necessary for large-scale production of juvenile D. antillarum for restoration.
Crabs
There are no published data on mating dynamics or fertilization success in M. spinosissimus, but crabs in nearshore habitats of the Florida Keys attain sexual maturity at ~45 mm CW in males (Baeza et al., 2012) and 50–70 mm CW in females (Baeza et al., 2012; Glover, 2022). They are reproductively active year-round in the Caribbean (Munro, 1974; Craig et al., 1989; Iglehart et al., 1989), with females producing successive clutches from a single mating approximately every three to six weeks (Adey, 1987; Creswell, 2011). Clutches are incubated for 2–3 weeks before hatching, and fecundity estimates vary widely from tens of thousands (Brownell et al., 1977; Creswell et al., 1989) to ~100,000 eggs/clutch (Creswell, 2011; Glover, 2022). Egg mortality in females held under laboratory conditions varies widely (0%–100%) but is unrelated to female body size (Baeza et al., 2015).
Provenzano and Brownell (1977) were the first to describe the early life history and culture of M. spinosissimus and to successfully rear larvae; in their case within static containers with daily water changes of natural seawater. A new description of the larval development was recently published by Turini et al. (2021). After hatching, larvae spend a short time in the plankton with two swimming zoeal stages lasting 8–24 hours each, followed by a single benthic post-larval megalopa stage lasting 3–4 days (Brownell et al., 1977; Provenzano and Brownell, 1977; Tunberg and Creswell, 1988; Gravinese et al., 2022) (Figure 2). Both zoeal larval stages and the post-larval megalopa stage are lecithotrophic (non-feeding) (Creswell, 2011). As with many taxa, the larvae are sensitive to water quality and require well-oxygenated seawater for successful development. However, Gravinese et al. (2022) report that larval and juvenile M. spinosissimus are tolerant to seawater conditions associated with climate change predictions.
C. Grow-out
Urchins
D. antillarum requires ~1 year to grow from settler to reproductive adult. However, growth rates depend on the space available for the urchin to grow and the amount and type of feed provided. Feed used for grow-out includes macroalgae such as Ulva sp. and Dictyota sp., but also dried nori or pellets marketed for herbivorous fish (Hassan et al., 2022). Idrisi et al. (2003) found an average growth rate of 3 mm/mo for juveniles (Figure 3) reared in a flow-through seawater system and fed solely with the macroalgae Gracilaria ferox. Under ideal circumstances, higher growth rates can be expected, as Randall et al. (1964) reported growth rates of up to 6.7 mm/mo for caged juveniles in field experiments. In a laboratory recirculating seawater system, Hassan et al. (2022) measured average increases in test diameter of up to 8.3 mm/mo over six weeks for hatchery-propagated juvenile urchins at an average starting test diameter of 5.5 mm. By both test diameter and live weight metrics, Hassan et al. (2022) observed improved growth in animals fed dried nori rather than an equivalent weight of a commercial herbivore pellet.
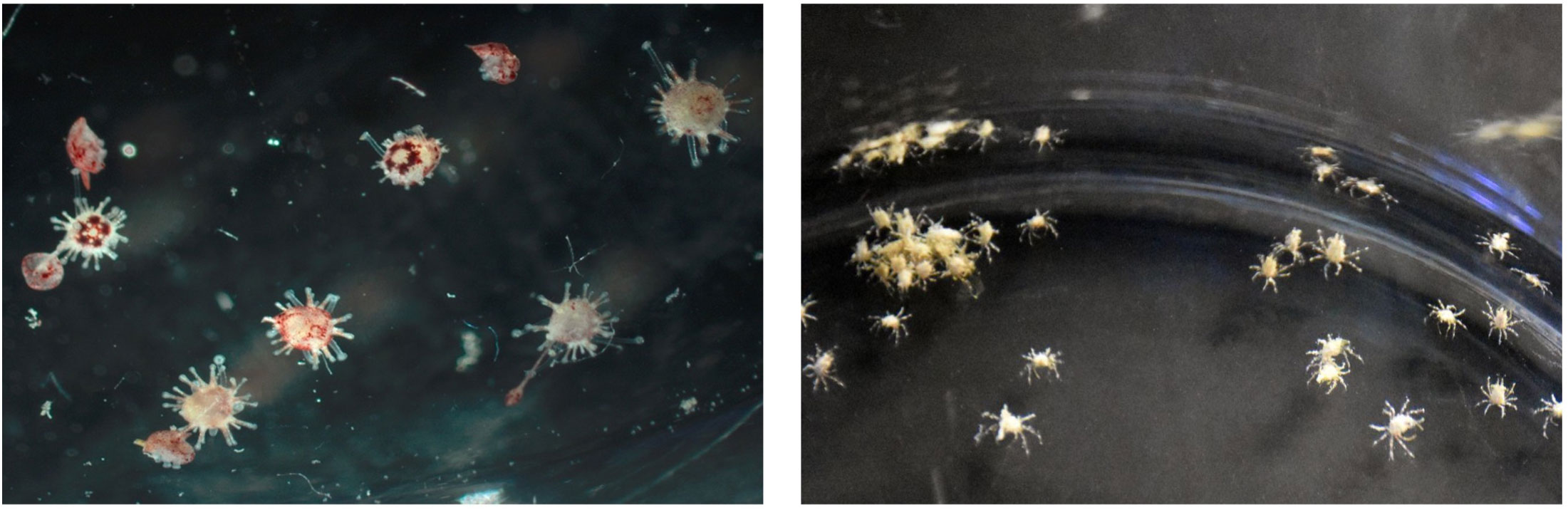
Figure 3 (Left) Photo of recently settled D. antillarum juveniles hatched in the laboratory (photo credit: T. Wijers). (Right) Photo of recently settled M. spinosissimus hatched in the laboratory (photo credit: AJ Spadaro).
Crabs
Early juveniles of M. spinosissimus feed primarily on filamentous turf algae and benthic diatoms (Brownell et al., 1977; Tunberg and Creswell, 1988; Lellis, 1992) (Figure 3). Wilber and Wilber (1989) reported that juvenile growth and survival both increased significantly with the addition of animal protein to the diet. The crabs have been successfully reared in situ within mesh cages fouled by algal turfs and moored in backreef habitats (Adey, 1987), as well as under controlled laboratory settings (Tunberg and Creswell, 1988, 1991; Gravinese et al., 2022). Tunberg and Creswell (1988) noted that, when provided with good water exchange, early benthic juvenile crabs could be maintained at a high stocking density (>22,500 ind./m2), whereas Wilber and Wilber (1989, 1991) found that survival and growth were both directly correlated with holding space and inversely correlated with crab density. In the laboratory, Provenzano and Brownell (1977) reared M. spinosissimus to a CW of 20 mm in 175 days after hatch, whereas Creswell (2011) reared them to ~30 mm CW in about 180 days. Based on juvenile growth trajectories, Adey (1987) estimated that crabs could be cultured to marketable adult size (~140 mm CW) in just over 400 days.
Adult M. spinosissimus consume algae and animal protein (Winfree and Weinstein, 1989). Recent physiological evidence suggests that the gut chemistry of M. spinosissimus changes through ontogeny, with protein increasing in importance in the diet of adult crabs (Chávez-Rodríguez et al., 2020). Nevertheless, Mithracid crabs are hearty consumers of macroalgae. In laboratory feeding trials conducted by Coen (1988a), two crabs related to M. spinosissimus (Mithraculus sculptus and M. coryphe) ate corticated (e.g., Laurencia, Acanthophora) and leathery (e.g., Padina, Lobophora ruffled form) macroalgae, except where algal secondary metabolites reduced consumption (e.g., Lobophora decumbent form, Stypopodium). Crab feeding preferences were negatively correlated with measures of algal quality, perhaps reflecting their aversion to high caloric but chemically defended macroalgae. Later studies with M. spinosissimus (Butler and Mojica, 2012; Spadaro, 2019) show that, unlike its congeners, the species consumed a wide range of macroalgae, including chemically defended algae (e.g., Dictyota sp; Laurencia sp.) as well as physically defended macroalgae (e.g., Halimeda spp.) but diet preferences were weak, suggesting that the species is a generalist grazer.
D. Post-release mortality, growth, and emigration
Urchins
Out-planting smaller (juvenile) D. antillarum is expected to be more cost effective than out-planting adults, but with the possible trade-off of higher post-stocking mortality. It is therefore important to determine the best size for restocking to optimize cost and retention. The size of restocked D. antillarum may affect retention, as size determines the use of shelters and avoidance of predation. Restocking experiments with D. antillarum have been conducted throughout the Caribbean and the Florida Keys (Table 3). Studies have differed greatly in methodology, duration, reef type and size, and D. antillarum size and source and so cannot be easily compared; yet some general conclusions are possible.
Overall, predation (7 out of 10 studies) and emigration (6 out of 10 studies) were the main explanations for low retention of restocked urchins. High predation pressure and low shelter availability appear to be the two main factors determining predation rates (The Nature Conservancy, 2004; Chiappone et al., 2006; Nedimyer and Moe, 2006; Burdick, 2008; Wynne, 2008; de Breuyn et al., 2023), whereas emigration seems most dependent on the size of the restocked D. antillarum and shelter availability (Maciá et al., 2007; Wynne, 2008; Williams, 2018; Pilnick et al., 2022b). Retention of stocked D. antillarum on reefs varies greatly among studies, from 0% (The Nature Conservancy, 2004; Burdick, 2008; Dame, 2008) to as much as 20%–75% after one year (Chiappone et al., 2006; Nedimyer and Moe, 2006; Nedimyer and Moe, 2006; Maciá et al., 2007; Wynne, 2008; Delgado and Sharp, 2021; Pilnick et al., 2022b). Most restocking studies were performed on relatively shallow reefs (<10 m), the two restocking studies that were performed on deeper reefs had low retention that was attributed to high predation pressure (Burdick, 2008; de Breuyn et al., 2023). In the Florida Keys, Delgado and Sharp (2021) found that adult D. antillarum (60 mm test diameter) had significantly higher survival when tethered with artificial crevice structures on a severely degraded reef previously devoid of appropriately scaled natural shelter. Any effort to stock D. antillarum on reefs must consider habitat quality, which is location-specific and can be susceptible to natural disturbances (Kobelt et al., 2019),and must either target sites with appropriate shelter for the urchins or artificially enhance shelter prior to stocking to reduce post-stocking predation.
Juvenile D. antillarum are preyed upon by many fishes, including: queen triggerfish (Balistes vetula), Spanish hogfish (Bodianus rufus), pudding wife (Halichoeres radiatus), black margate (Anisotremus surinamensis), Spanish grunt (Haemulon macrostomum) and jolthead porgy (Calamus bajonado) (Randall et al., 1964). Spiny lobsters (Panulirus argus, Panulirus guttatus) also consume D. antillarum of many sizes (Butler and Kintzing, 2016), and chemical odors from P. guttatus elicited shelter seeking behavior and reduced foraging in D. antillarum in the laboratory (Kintzing and Butler, 2014). The batwing coral crab (Carpilius corallinus) and helmet shells (Cassis spp.) also prey on D. antillarum (Randall et al., 1964; Sharp and Reckenbeil, 2022). The presence of damselfish that tend algae gardens, especially the three-spot damselfish, also reduces retention of D. antillarum (Williams, 2022). Damselfish do not prey on D. antillarum, but they induce the urchins to flee by pecking at their spines (Williams, 2022).
Stocking of herbivores is only useful if their density is high enough to reduce macroalgae cover and, in the case of D. antillarum, optimal restocking density depends on the rugosity of the reef, which affects urchin movement and predation (Olmeda-Saldaña et al., 2021; Pilnick et al., 2022b). Burdick (2008) suggests avoiding small patch reefs (<8 m2) when restocking urchins because larger reefs improve retention and reduce emigration by providing more shelters. Two studies, one conducted on large patch reefs (44 to 96 m2) and another on contiguous reefs, arrived at similar estimates of D. antillarum retention (23%–30%) after 267–365 days (Wynne, 2008; Pilnick et al., 2022b). Olmeda-Saldaña et al. (2021) showed that for more rugose reefs, the optimum density for stocking D. antillarum is about 2–5 urchin/m2. Pilnick et al. (2022b) noted a significant decline in macroalgae cover (~27%) on reefs stocked with D. antillarum when urchin densities were above 0.15 urchin/m2 but observed no effect on macroalgae at urchin densities of 0.04 urchin/m2.
Crabs
Stachowicz and Hay (1999b) postulated that high site fidelity and predation risk are associated with a broad generalist diet and compensatory feeding in marine crabs. Still, only three studies have been published on the movement of M. spinosissimus in the wild. Using external tags, Hazlett and Rittschoff (1975) followed the daily movement of a wild population of crabs living along the sides of a man-made canal in the Florida Keys for four months. In that essentially linear expanse of continuous rocky crevice habitat, adult M. spinosissimus generally moved <10 m/d with the majority remaining within just a few meters of their daytime crevice den. Male crabs moved further from their site of capture (58 m) than females (32 m) and, when at higher density, crabs moved further. Bohnsack (1976) also conducted a mark-recapture study with externally tagged crabs in two rocky canals in the Florida Keys. During the 12-month study, Bohnsack (1976) recovered over 50% of the tagged crabs within the 100 m long survey areas and noted that crabs often used the same daytime den repeatedly over time. Spadaro and Butler (2021) found that tagged crabs stocked on coral patch reefs (~3 to 5 m diameter) that were isolated by expanses (~10 m) of sand and open hardbottom typically remained on the patch reefs where they were released, with 40% remaining after 6 months and 25% after a year. More detailed studies on the factors that influence crab movement and home range in more contiguous and architecturally complex coral reef habitats are needed to develop appropriate, perhaps context-dependent stocking densities for reef restoration.
Tethering of various sizes of crabs (10–90 mm CW) on coral reefs and other habitats (e.g., saltwater quarries, coastal hardbottom) indicates that crab mortality is highest among small crabs on coral reefs (Spadaro and Butler, 2021; Glover, 2023). Male and female crabs appear to suffer similar rates of mortality, but mortality declines precipitously once crabs reach ~30 mm CW, representing a potential target size at which crabs can be stocked in the field with substantially lower risk of predation. Analysis of the stomach contents of the reef-dwelling spotted spiny lobster (Panulirus guttatus) confirms that a large portion of their diet is small juvenile crabs (Butler and Kintzing, 2016). Spadaro and Butler (2021) reported a strong effect of M. spinosissimus on macroalgae cover (50%–85% removal) and coral reef community composition with a stocking density of 1 crab/m2, but optimal restocking densities for M. spinosissimus have yet to be determined. Similarly, optimal restocking sex ratios are unknown. Large male M. spinosissimus appear to be territorial and defend a harem of females against other large males (Bohnsack, 1976). This territoriality will likely present a challenge to restocking efforts aimed at maintaining crab density through time. Table 3 summarizes data on the attempts to release and monitor the persistence of urchins and crabs on coral reefs.
E. Captivity-related genomic or behavioral selection?
Urchins
Animals cultured and raised in captivity often exhibit traits that are suboptimal for survival in the wild (Näslund, 2021). Wild D. antillarum flee from predator chemical cues (e.g., Kintzing and Butler, 2014) and largely remain in shelter during the daytime, a behavior typical of individuals under predation pressure, whereas hatchery-produced urchins may not. Sharp et al. (2018) suggested that hatchery-produced D. antillarum possess innate predator recognition and that shelter-seeking behavior could be increased by laboratory-based habituation. Indeed, refinements in grow-out conditions of newly-settled, cultured D. antillarum (e.g., natural light cycles, access to appropriately scaled shelters, and reduced handling; Sharp et al., 2018; Pilnick et al., 2021) have resulted in individuals that exhibit diurnal shelter-seeking behavior similar to that of wild individuals (Hassan et al., 2022; Sharp et al., 2023).
In Florida, resource managers require the development of a veterinarian health certification protocol for any captive reared animals to be released in the wild. Accordingly, a framework has been adopted for the culture and release of D. antillarum in Florida based upon the development of diagnostic methods for the comprehensive health assessment for the species (Francis-Floyd, 2020) and a description of its captivity-related genomics (Chandler et al., 2017).
Crabs
There is no published information on captivity-related effects on M. spinosissimus genomics or behavior. This presents a challenge to restocking efforts and is a substantial knowledge gap, but researchers and resource managers are currently developing a health certification protocol for M. spinosissimus.
F. Reproductive success of stocked individuals and potential for self-recruitment?
Urchins
Following the Caribbean-wide disease-induced mass mortality of D. antillarum in the 1980’s, recovery has been restricted in part by low fertilization success at low population density (Lessios, 2005; Chiappone et al., 2013; Feehan et al., 2016), so stocking may be necessary to reestablish viable spawning populations. Diadema antillarum exhibits lunar periodicity in spawning (Bauer, 1976; but see Levitan, 1988) and aggregation during spawning is posited as an adaptation to increase fertilization success (Randall et al., 1964; Bauer, 1976; Younglao, 1987). An advection-diffusion and fertilization-kinetics model was used to estimate D. antillarum fertilization success as a function of various site-specific biophysical factors (Feehan et al., 2016). The model indicated that fertilization success varies indirectly with current velocity and directly with urchin density, urchin aggregation size, and the linear extent of the population in the direction of mean flow (Feehan et al., 2016). This information provides a reference point for assessing how stocking density, the subsequent survival and behavior of stocked individuals, and local physical characteristics may affect reproductive output and the potential for post-stocking self-recruitment. An understanding of the broadscale source-sink population dynamics of D. antillarum across the Caribbean, which is currently lacking, also will be important for understanding future population growth.
Natural recruitment of D. antillarum at stocked sites may act to supplement stocked populations if sites contain appropriate settlement habitat. Given the relatively long planktonic larval duration of D. antillarum, larval dispersal could in theory occur at scales of up to 1000s of km (Feehan et al., 2019). However, biophysical dispersal models for many taxa demonstrate the strong effects of regional oceanography and larval behavior on dispersal that often increase retention (Cowen et al., 2006; Williams et al., 2009; Butler et al., 2011; Feehan et al., 2019). Moreover, the settlement of larval D. antillarum around the Caribbean and in specific regions is highly variable in space and time (Hernández et al., 2006; Miller et al., 2009; Williams et al., 2010; Hylkema et al., 2022a).
The relationship between larval supply and recruitment can be decoupled by larval and post-settlement mortality. For example, on reefs in the Florida Keys, larval settlement on artificial substrate did not translate into measurable recruitment to the benthic population, likely due to a lack of appropriate natural settlement substrate or habitat structure to protect from predators (Feehan et al., 2019). The spine canopy of adult D. antillarum can provide a local refuge to juveniles from predators (Miller et al., 2007). Hence, adult urchin restocking could enhance the post-settlement survival of natural recruits and is an area for future research.
Crabs
The extraordinarily brief planktonic larval duration of M. spinosissimus portends a high probability of limited dispersal and self-recruitment, which is supported by population genetics information and connectivity modeling (Baeza et al., 2019). Therefore, it stands to reason that increasing breeding stocks by an order of magnitude or greater through stocking could increase local larval supply. As an example of local recruitment, populations of M. spinosissimus have been discovered in land-locked saltwater quarries in the Florida Keys that are physically isolated from the sea. Those crab populations have reproductive patterns and fecundity identical to those in wild habitats and, therefore, are almost certainly self-recruiting (Glover, 2023). Furthermore, unpublished data indicate that crab populations in quarries are genetically distinct from nearby wild populations and are consistent with a “founder effect” genetic structure (Whitaker, 2023). Despite the potential for self-recruitment, wherever M. spinosissimus occurs naturally their density is low suggesting either limited larval supply or high larval and post-settlement mortality (Butler and Kintzing, 2016), so studies that disentangle those explanations will shed light on the possibility of enhancing local recruitment through stocking.
G. Enhancement of natural recruitment
Urchins
Many species of sea urchins respond to bare substrate or those with new biofilms as cues for settlement (Bak, 1985; Pearce and Scheibling, 1991; Rahim et al., 2004; Williams et al., 2011), but the spatiotemporal patterns of settlement by D. antillarum vary appreciably among locations (Bak, 1985; Miller et al., 2009; Vermeij et al., 2010; Williams et al., 2010, 2011; Feehan et al., 2019; Maldonado-Sánchez et al., 2019; Hylkema et al., 2022a). Recent laboratory experiments by Pilnick et al. (2023) and Wijers et al. (2024) revealed a lack of settlement by competent D. antillarum larvae exposed to a sterile seawater control, but settlement rates increased to ~50% in response to two types of calcareous macroalgae (Pilnick et al., 2023; Wijers et al., 2024) and to natural biofilms (Wijers et al., 2024). If settlement hotspots are identified it is possible to collect high numbers of D. antillarum settlers during certain months of the year (Williams et al., 2010, 2011; Hylkema et al., 2022a; Klokman and Hylkema, 2024). Settlement collectors are preferably deployed in mid-water (Williams et al., 2010; Hylkema et al., 2022a) at a depth of around 9 m (Williams et al., 2011). They have been constructed of many materials (e.g., plastic doormats; bio balls; rope; artificial turf) but plastic doormats and bio balls appear best (Williams et al., 2010; Hylkema et al., 2022a). The collection of D. antillarum postlarvae appears to be a viable option for naturally enhancing populations given the difficulties in rearing D. antillarum larvae in the laboratory. For example, over 6500 D. antillarum juveniles collected from the field have been successfully reared in the laboratory and restocked to multiple reefs in Puerto Rico (Williams, 2022).
Hylkema et al. (2022b) introduced a new approach of D. antillarum restoration, termed “Assisted Natural Recovery” (ANR) in which settlers are not collected, but their recruitment facilitated on the reef. Strings of plastic bio balls, which are normally used in aquaculture filters but also work well as D. antillarum settlement collectors (Hylkema et al., 2022a), were attached to the reef at the onset of the settlement season to provide additional settlement substrate and shelter availability. Without further modifications and without the need to raise urchins ex situ, reefs with bio ball streamers had significantly more D. antillarum recruits than control reefs without the urchin larval collectors after six months, showing that a lack of settlement substrate is one of the factors constraining natural recovery. However, in that same study, subsequent recruit retention was relatively low, which was attributed to high predation pressure (Hylkema et al., 2023).
Crabs
There have been no assessments of post-stocking reproductive success for M. spinosissimus nor mention of in situ larval collection of M. spinosissimus in the literature. Although culture of wild larvae collected in the field is theoretically feasible, the cost (labor and time) involved in obtaining and separating M. spinosissimus larvae from the plankton would likely outweigh the potential benefits. Natural settlement and recruitment of early benthic phase M. spinosissimus has not been reported, but their density is likely limited due to high larval and post-settlement mortality (Butler and Kintzing, 2016; Spadaro and Butler, 2021; Glover, 2022).
Similarly, there have been no published studies on settlement patterns or habitat preference for the various life stages of the species. Identifying the cues and conditions that limit or promote settlement and recruitment of M. spinosissimus are of obvious importance. Such studies may also offer insight into physical or chemical interventions that can be made to restoration sites to improve the likelihood of crab settlement and recruitment, thus reducing the potential need for repeated stocking events.
Cost of herbivore restoration?
Estimating the cost of large-scale restoration efforts is imprecise and changes with economic conditions (e.g., labor, utilities, and materials costs). In the case of coral reef restoration, costs are largely unknown because few if any large-scale programs have been completed and, therefore, actual expenses incurred are undetermined (but see Bayraktarov et al., 2016 for estimated costs of various forms of marine coastal restoration). Perhaps the most thorough estimation of the cost of large-scale coral reef restoration was crafted by NOAA for the “Mission: Iconic Reefs” restoration project in the Florida Keys (NOAA, 2021). For each of the seven reef areas to be restored, estimates were made of the daily effort and cost required for site preparation, coral additions, grazer additions, monitoring, maintenance, and adaptive management. This process arrived at an overall cost of approximately $97M US spread over a 10-year timeframe to restore about 27 hectares of coral reef habitat. Stocking >400,000 grazers (D. antillarum and M. spinosissimus) on those 27 hectares is expected to cost approximately $14M US or an estimated $35 per grazer (compared to $259 per restored coral). Grazer additions account for only about 7% of the estimated total cost of restoration and represent only about 10% of the estimated cost of reintroducing corals (~$61M US). How accurate such a priori estimates may be is unknown and most of the funding for the project is yet to be acquired.
Unintended or non-beneficial effects of increasing grazers on coral reefs
One of the aims of coral out-planting is to ‘kick start’ natural coral reproduction and larval settlement. A potential concern of increasing herbivore populations during the early stages of restoration efforts is that they may negatively affect other ecological processes on coral reefs. For example, parrotfishes eat juvenile corals, either deliberately or while grazing for other food sources, so increasing their abundance may increase the mortality of small corals. Still, the evidence suggests that the positive effect of parrotfishes in reducing macroalgal cover outweighs any negative impacts on coral demographics (Mumby, 2009). Herbivores may also increase the mortality of outplanted corals either directly via predation or through increases in algal gardens by territorial damselfishes (Schopmeyer and Lirman, 2015; Koval et al., 2020).
When urchins form unnaturally large populations or dense aggregations in the absence of predators (e.g., triggerfishes, spiny lobsters), they can increase the mortality rates of coral recruits, damage established coral colonies (Bak and van Eys, 1975; Sammarco, 1980), and increase reef bioerosion (Ogden, 1977; Bak, 1994; Brown-Saracino et al., 2007). However, we anticipate that most restoration efforts will be paired with some level of protection of fishes and, thus, unnaturally large populations of Caribbean urchins are unlikely because of the presence and conservation of their predators. Indeed, the effectiveness of Caribbean fish predators has been invoked as one reason why urchin populations have not recovered naturally (Lessios, 1988a; Harborne et al., 2009).
There are no known negative effects of increasing the density of Mithracid crabs on coral reefs. Indeed, some Mithracid crabs dwell symbiotically with corals and improve coral growth and health by removing detritus and algae from their coral hosts (Coen, 1988a; Stachowicz and Hay, 1996, 1999a). The positive indirect effects of crab consumption of macroalgae on coral growth and recruitment are also well documented (Coen, 1988a; Spadaro and Butler, 2021). But crabs also consume small, soft-bodied benthic fauna opportunistically (Wilber and Wilber, 1989; Creswell and Tunberg, 2000; Chávez-Rodríguez et al., 2020). As described earlier, juvenile Mithracid crabs are prominent in the guts of one species of reef-dependent spiny lobster (P. guttatus; Butler and Kintzing, 2016) and the discarded remnants of the exoskeletons of larger crabs are frequent evidence of octopus and triggerfish predation (Spadaro, 2019). However, the implications of these predatory interactions on coral reef food webs are unknown.
The question of how restocking herbivores will affect the wider food webs at restored sites is difficult to predict. Reef food webs are complex and typically contain a large number of weak trophic links (Bascompte et al., 2005). If the aim is the restoration of grazers to approximate ‘natural’ levels of macroalgae abundance, any negative effects on reef trophodynamics are likely to be modest or nil. Still, the long-term implications of restoring grazers on reefs represents a challenging knowledge gap. Looking ahead to large-scale coral reef restoration efforts whose plans include the simultaneous stocking of corals and different grazer species (e.g., “Mission: Iconic Reefs” program in Florida Keys) though desirable from a restoration perspective, will not alone permit assessment of the unintended effects of grazer reintroductions on coral reef ecosystems. Ferraro et al. (2023) argue that natural resource management, including restoration, be conducted in an experimental format so as to simultaneously fill-in important knowledge gaps. We believe this is sound advice as it pertains to the restoration of coral reef herbivores. For example, the “Mission: Iconic Reefs” restoration program mentioned above would benefit from a design that includes further in-situ and modeling research on trophic networks under different restocking scenarios.
Ramifications of climate change for the restoration of herbivores
Higher levels of CO2 expected under near-future climate change is likely to increase the growth of macroalgae, further exacerbating the present overgrowth of macroalgae on coral reefs (Fong and Paul, 2011). But the effects of climate change on grazers is unclear. Bodmer et al. (2017) caution that predator avoidance behavior by D. antillarum may be compromised under near-future ocean warming scenarios due to reduced function in the light-detecting melanophores of urchins at elevated temperatures, leading to less spine movement in response to predator shadows. In the closely related sea urchin D. africanum, reduced pH resulted in lower fertilization and development rates, which were partially ameliorated by higher temperatures (García et al., 2018). Similarly, reduced pH resulted in a lower skeleton density, weaker spines, and increased predation on stocked D. africanum (Rodríguez et al., 2017). For other sea urchins, ocean acidification reduces fertilization success (Havenhand et al., 2008) and has negative effects on larval development (Sheppard Brennand et al., 2010), settlement, and post-settlement survival. Wittmann and Pörtner (2013) reviewed all existing studies on the subject and concluded that most sea urchin species were negatively affected by moderate climate change scenarios although much will be dependent on the speed and extent of global warming and ocean acidification.
In general, fish size – especially for tropical fishes – is predicted to decline in response to the higher temperatures and lower oxygen concentrations expected with climate change (Cheung et al., 2013), and there is empirical evidence in support of this. Comparisons of reef fish assemblages in the Persion Gulf and Gulf of Oman between sites that differ in the effects of climate change suggest that overall fish abundance, richness, biomass, and size are likely to decrease when subject to climate change (Feary et al., 2010). However, there is evidence that the abundance of small herbivorous fish in particular may increase on reefs subject to climate change due to the proliferation of macroalgae (Feary et al., 2010; Thibaut et al., 2012; Graham et al., 2020).
Diseases in decapod crustaceans are generally expected to rise in response to elevated temperatures, acidification, and salinity change which are all host stressors associated with climate change (Shields, 2019). However, no diseases have been described in M. spinosissimus, which also appears relatively robust to climate change. Laboratory experiments exposing larval and juvenile M. spinosissimus to the elevated temperatures and lower pH revealed that the crabs are tolerant to changes associated with climate change (Gravinese et al., 2022). The tolerance of M. spinosissimus to such climate stressors suggests that it could be particularly beneficial to restoration efforts aimed at making coral reefs more resilient to increasingly warm and acidic seas.
Guiding principles for herbivore restoration
Coral reef restoration has largely focused on out-planting a few species of framework-building corals with the aim of reestablishing sufficient coral populations to rebuild reef architecture and reestablish lost biodiversity and ecological function. The goal is lofty considering the ecological headwinds posed by climate change, disease, and habitat degradation fueled by eutrophication, siltation, and the proliferation of macroalgae. The latter problem is the one most tractable at local scales given emerging capacities for rearing and stocking native grazers, the promise of which is reviewed here.
As one might expect, Caribbean fish, urchin, and crab herbivores differ appreciably in life histories, which confer advantages and disadvantages in the ease of their mariculture (Table 2) and their suitability for specific restoration scenarios. As noted by others (Burkepile and Hay, 2009; Mumby, 2021a), studies of herbivory on coral reefs have repeatedly taught us that reliance on just one or a few species creates ecosystem instability. Even taxa with seemingly similar ecological functions are rarely identical (e.g., diet preferences, reproductive periodicity, resistance to disease, mobility) and their respective populations fluctuate. This variability implies that a restoration approach that embraces functional redundancy and complementarity in the management and restoration of grazing function, coupled with the enhancement of coral abundance, diversity, and recruitment is likely to be the most effective.
Macroalgal coverage targets
Using grazers as part of a reef restoration plan begs the question of what is the target in terms of a reduction in macroalgae on reefs? Restoration targets for macroalgae abundance could be based on historical data for a region assuming those data exist and there is a clear indication of how far back to reset the ecological clock. However, a historical approach to setting such a baseline ignores the myriad of environmental changes that have occurred since then that influence the coral-algae relationship. Therefore, addressing the dilemma of “how much grazing is enough” in the modern context requires consideration of two additional questions:
(1) At what level of abundance does macroalgae negatively affect corals?
(2) Which and how many herbivores are needed to keep reefs below this threshold?
With respect to question one, data (Butler; unpublished data) from 29 coral reefs surveyed (by divers using 0.25m2 photo-quadrats) throughout the Florida Keys in 2021 suggest a negative relationship between total macroalgae abundance and coral abundance (both expressed as % cover), but the correspondence breaks down when macroalgae cover falls below about 25% cover at which point coral cover varies widely (Figure 4A). This suggests that below this threshold or “tipping point”, coral community dynamics may be driven by other factors unrelated to macroalgae abundance. If so, this represents a logical target for the reduction of macroalgae that can be accomplished through the stocking of grazers. Yet, such relationships – if they exist – are likely to be idiosyncratic to ecoregions because of the importance of local driving factors. This appears to be the case in the Florida Keys. A separate data set (Butler; unpublished data) focusing on a similar number of survey sites conducted within a smaller ecoregion (Lower Florida Keys) in 2021 still implies that there is a tipping point where macroalgae cover hampers coral cover. But at this smaller scale the tipping point is reached at a higher level of macroalgal cover of approximately 55% (Figure 4B).
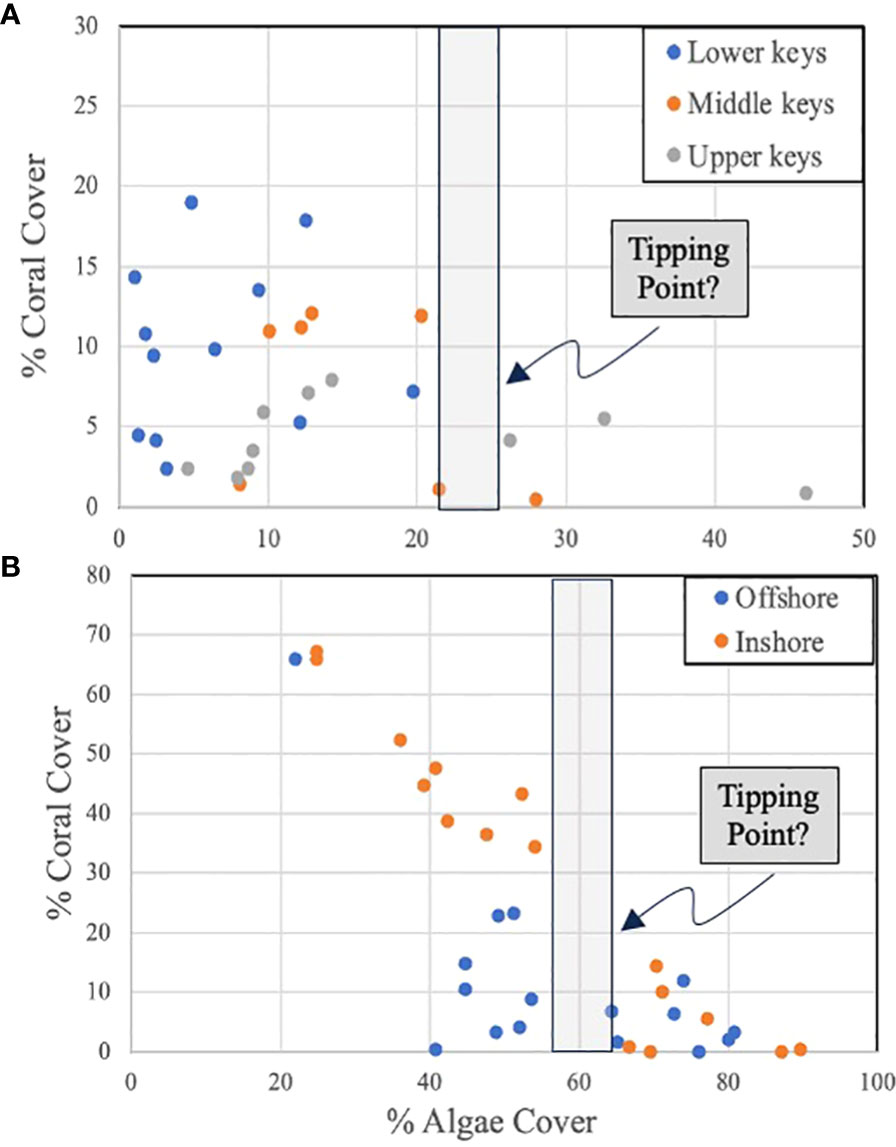
Figure 4 (A) Plot of percent coral cover versus percent macroalgae cover on 28 coral reef sites surveyed in 2021 throughout the lower, middle, and upper Florida Keys, Florida (USA). (B) Plot of percent coral cover versus percent macroalgae cover on 32 coral reef sites within the lower Florida Keys, Florida (USA) surveyed in 2021. The green bar in both graphs represents an inflection in the data that may represent a “tipping point” in the relationship. Graphics in Figure 4 are adapted with permission from the Integration and Application Network, University of Maryland Center for Environmental Science (ian.umces.edu/imagelibrary/).
As the above example demonstrates, despite the known negative effects of macroalgae on corals the relationship between their respective abundances on a particular reef is far from precise (hence the variance in Figure 4) and fraught with complexity. All macroalgae are not the same in terms of their effects on corals, coral species or individuals differ in their susceptibility to the insults posed by macroalgae, and casting this question so simply ignores other important considerations such as coral larval supply, the effects of climate change, coral diseases, and so on.
More sophisticated approaches to predicting how much grazing is ‘enough’ to ensure a reef’s resilience have been proposed based on estimates of coral cover and grazing intensity (Mumby et al., 2007a) and such calculations have been made for mid-depth forereefs using fish grazers (Mumby et al., 2006a; Mumby, 2006b; Mumby et al., 2007a). For example, there are algorithms for calculating the grazing intensity of parrotfishes based on species identity, life phase, and size (e.g., Mumby, 2006b; Ruttenberg et al., 2019) that when combined with estimates of density, allow for the quantification of grazing intensity, which is a key input into models of reef resilience (Mumby et al., 2007a).
With proper parameterization, such models could be extended to other piscine herbivores (e.g., surgeonfishes; Duran et al., 2019) as well as invertebrate grazers (i.e., urchins, crabs). Important herbivore inputs to such models include size- or phase-specific grazing rates, algal preference, and foraging areas such as those summarized in Table 1. But to extend the generality of this approach, those models must also be modified to account for physical aspects of reefs (e.g., depth, wave exposure, water quality) that modify herbivory and algal growth rates (Mumby et al., 2006a; Mumby and Hastings, 2008; Graham et al., 2015). Synergistic (e.g., grazing complementarity), inhibitory (e.g., inter-specific competition), and non-consumptive interactions (e.g., behavioral modification of grazing) are also possible with multi-species stocking – even likely – which mandates careful empirical studies and model accommodation of these unknown consequences of multi-species stocking of reef grazers. Again, sufficient data at an appropriate local scale may not be available to make such an approach practical at the multitude of localities around the Caribbean where reef restoration is desired. Is a more basic “blueprint” possible that can help guide restoration practitioners in situations where quantitative data and historical records are lacking?
A grazer restoration decision framework
Developing a decision framework for restoration practitioners to estimate which and how many grazers need to be stocked to make their targeted reefs more resilient represents an important advance for increasing the effectiveness and cost-efficiency of reef restoration work.
In Figure 5, we present a decision tree that can help guide the implementation of a grazer stocking plan based on the general attributes of the reef system in question. This framework lacks recommendations for the stocking density of grazers, which may be idiosyncratic to local conditions. For example, more isolated reefs surrounded by open sand bottom But that issue can be addressed based on consideration of the details we provide in terms of rates of herbivory and foraging areas of fish, urchins, and crabs contained in this review.
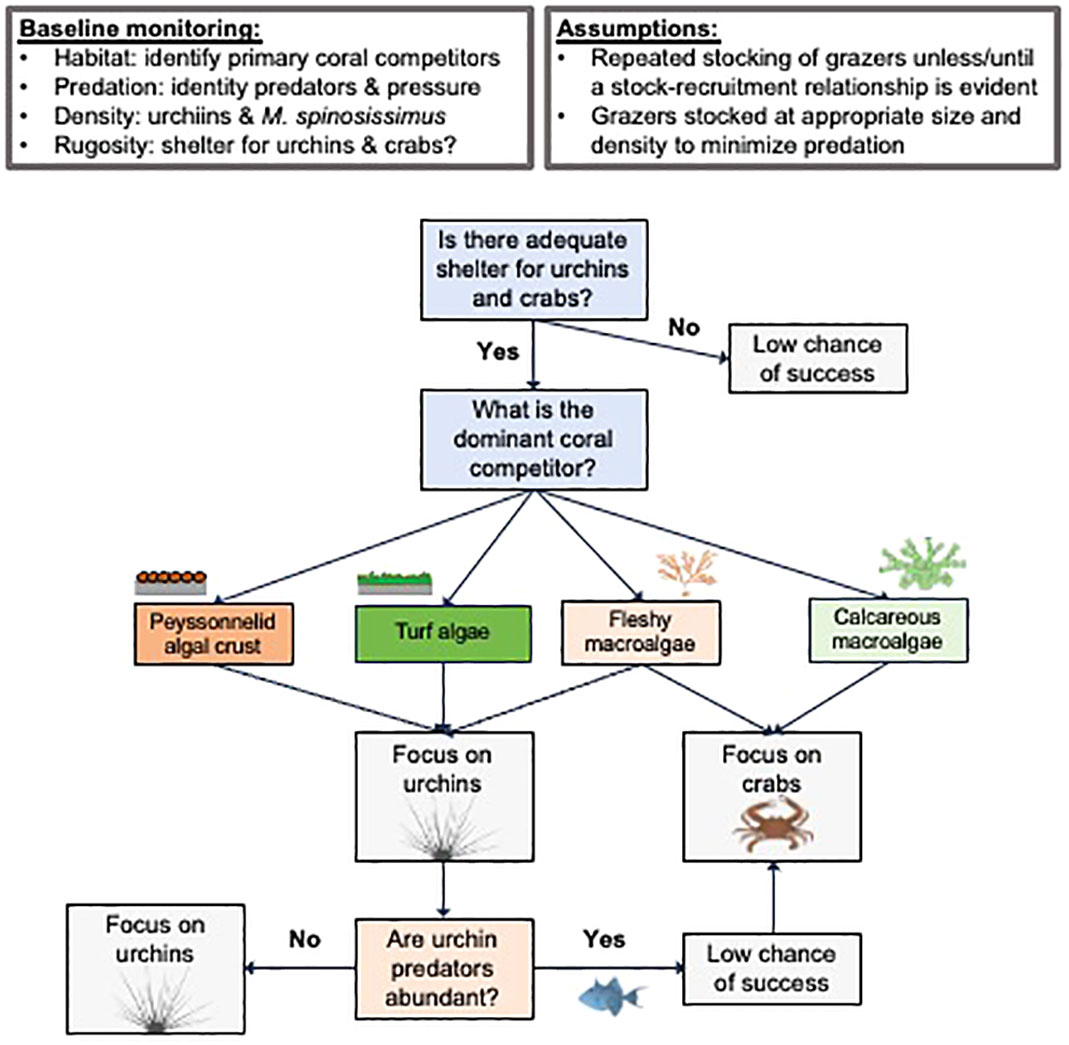
Figure 5 Decision tree specifying which reef herbivore type is recommended for a given coral reef restoration effort depending upon shelter availability, type of macroalgae overgrowth, and predation pressure.
Restoration of grazer assemblages is expected to yield the most durable outcome for the management of macroalgae on Caribbean coral reefs. Hence, we recommend restoration of multiple invertebrate grazers for which mariculture is presently considered feasible: the Long-Spined Sea Urchin D. antillarum and the Caribbean King Crab M. spinosissimus. Yet, there will be circumstances in which stocking success is unlikely for one or both species and efforts should be focused on other locations or the one species deemed most likely to succeed.
Prior to initiation of such a program, we recommend that baseline monitoring of the proposed sites be conducted to identify characteristics of the target reefs that are key to the successful stocking of grazers. We also assume that stocking of herbivores will be an on-going task, at least until (or if) local increases in grazer recruitment and survival are demonstrated by a stock-recruitment relationship. It is also important that grazers be stocked at sizes large enough to minimize post-release predation, which may vary with local predator assemblages. Other relevant factors to consider when reaching a stocking decision include: the availability of shelters for urchins and crabs, the dominant coral competitor present, and the types of predators that are locally abundant. The availability of shelters of the right dimension for use by stocked grazers is crucial to reduce predation that can decimate stocked invertebrates. The type of nuisance macroalgae that is locally abundant is another important factor to consider because their palatability to urchins and crabs differs, as will the desired grazing effect of each herbivore.
Conclusions
Whether coral reefs as humans have appreciated, studied, and exploited them for tens of thousands of years will survive the Anthropocene in their current or diminished state is a matter of conjecture (Norström et al., 2016; Bellwood et al., 2019). Reefs in the Caribbean are particularly in peril. Restoration of corals lost on reefs has been successful in some cases and a miserable failure in others, owing largely to the background conditions into which corals are out-planted. Along with climate change and disease, the over-abundance of macroalgae is a common culprit in the degradation of coral reefs and a persistent roadblock to the return of corals to their former prominence. The large-scale mariculture and stocking of native herbivores onto reefs to counterbalance the proliferation of macroalgae will require a herculean effort. But doing so is feasible and early studies indicate that it can be an effective means of restoring coral reefs to some semblance of their previous state. There are gaps, identified here, in our knowledge of some of the best practices needed for successful coral reef habitat restoration by stocking grazers. However, the biggest challenge is the funding necessary to scale-up the mariculture and stocking of grazers from its current research development stage to the level necessary for meaningful coral reef restoration.
We conclude by acknowledging that any conservation measure must be considered within the context of climate change, and the restoration of herbivores is no different. It is clear that unless significant steps are taken to reduce the use of fossil fuels, coral reefs are one of the most threatened ecosystems on the planet and no amount of restoration will maintain coral dominance without constant outplanting (Bellwood et al., 2019). While global solutions remain challenging it is clear that local actions, such as restocking or managing herbivores, can at least ‘buy time’ for reefs and make them more resilient to temperature induced mortality events (Kennedy et al., 2013). Over the short term, this makes research into the relative impacts of increased temperatures on macroalgal growth rates versus the physiology and energetic demands, and hence grazing rates, of all herbivores all the more critical. But we must recognize that the reduction or removal of major stressors to coral reefs underpins the long-term future of reefs and must allocate conservation resources accordingly.
Author contributions
MB: Writing – original draft, Supervision, Investigation, Funding acquisition, Conceptualization. AD: Writing – review & editing, Investigation. CF: Writing – review & editing, Investigation. ARH: Writing – review & editing, Investigation. AH: Writing – review & editing, Investigation. JP: Writing – review & editing, Investigation. WS: Writing – review & editing, Investigation. AS: Writing – review & editing, Investigation. TW: Writing – review & editing, Investigation. SW: Writing – review & editing, Investigation.
Funding
The author(s) declare that no financial support was received for the research, authorship, and/or publication of this article.
Acknowledgments
This paper stems from discussions and contributions from participants at the “Reef Grazer Workshop” held in Miami, Florida (USA) in April 2022 and sponsored by the Walter and Rosalie Goldberg Endowment for Tropical Ecology at Florida International University. The authors are grateful for the insights offered by all of the participants at the workshop. This is contribution #1682 from the Institute of Environment at Florida International University.
Conflict of interest
The authors declare that the research was conducted in the absence of any commercial or financial relationships that could be construed as a potential conflict of interest.
Publisher’s note
All claims expressed in this article are solely those of the authors and do not necessarily represent those of their affiliated organizations, or those of the publisher, the editors and the reviewers. Any product that may be evaluated in this article, or claim that may be made by its manufacturer, is not guaranteed or endorsed by the publisher.
References
Abelson A., Obolski U., Regoniel P., Hadany L. (2016). Restocking herbivorous fish populations as a social-ecological restoration tool in coral reefs. Front. Mar. Sci. 3. doi: 10.3389/fmars.2016.00138
Adam T. C., Duran A., Fuchs C., Roycroft M., Rojas. M., Ruttenberg B. I., et al. (2018). Comparative analysis of diet, foraging behavior and bite mechanisms reveals high functional diversity among Caribbean parrotfishes. Mar. Ecol. Prog. Ser. 597, 207–220. doi: 10.3354/meps12600
Adam T. C., Kelley M., Ruttenberg B. I., Burkepile D. E. (2015). Resource partitioning along multiple niche axes drives functional diversity in parrotfishes on Caribbean coral reefs. Oecologia 179, 1173–1185. doi: 10.1007/s00442-015-3406-3
Adey W. H. (1987). Food production in low nutrient seas: Bringing tropical ocean deserts to life. Aquaculture 37, 340–348. doi: 10.2307/1310690
Agar J. J., Fleming C. S., Tonioli F. (2019). The net buyback and ban in St. Croix, U.S. Virgin Islands. Ocean Coast. Manage. 167, 262–270. doi: 10.1016/j.ocecoaman.2018.10.019
Agudo-Adriani E. A., Cappelletto J., Cavada-Blanco F., Cróquer A. (2019). Structural complexity and benthic cover explain reef-scale variability of fish assemblages in los roques national park, Venezuela. Front. Mar. Sci. 6. doi: 10.3389/fmars.2019.00690
Alidoost Salimi P., Creed J. C., Esch M. M., Fenner D., Jaafar Z., Levesque J. C., et al. (2021). A review of the diversity and impact of invasive non-native species in tropical marine ecosystems. Mar. Biodiversity Records 14, 1–19. doi: 10.1186/s41200-021-00206-8
Alvarez-Filip L., Dulvy N. K., Gill J. A., Côté I. M., Watkinson A. R. (2009). Flattening of Caribbean coral reefs: region-wide declines in architectural complexity. Proc. R. Soc. B-Biological Sci. 276, 3019–3025. doi: 10.1098/rspb.2009.0339
Alvarez-Filip L., Paddack M. J., Collen B. B., Robertson D. R., I.M. Côté I. M. (2015). Simplification of caribbean reef-fish assemblages over decades of coral reef degradation. PloS One 10, e0126004. doi: 10.1371/journal.pone.0126004
Arnold S. N., Steneck R. S. (2011). Settling into an increasingly hostile world: the rapidly closing ‘‘Recruitment window’’ for corals. PloS One 6, e28681. doi: 10.1371/journal.pone.0028681
Baeza J. A., Anderson J. R., Spadaro A. J., Behringer D. C. (2012). Sexual dimorphism, allometry, and size at first maturity of the Caribbean King Crab, Mithrax spinosissimus, in the Florida Keys. J. Shellfish Res. 31, 909–916. doi: 10.2983/035.031.0401
Baeza J. A., Holstein D., Umaña-Castro R., Mejía-Ortíz L. M. (2019). Population genetics and biophysical modeling inform metapopulation connectivity of the Caribbean king crab Maguimithrax spinosissimus. Mar. Ecol. Prog. Ser. 610, 83–97. doi: 10.3354/meps12842
Baeza J. A., Simpson L., Ambrosio L. J., Gureon R. (2015). Reproductive investment in a phyletic giant, the Caribbean King Crab Damithrax spinosissimus: exploring egg production costs in large brooding marine invertebrates. J. Shellfish Res. 34, 1049–1056. doi: 10.2983/035.034.0331
Bak R. P. M. (1985). “Recruitment patterns and mass mortalities in the sea urchin Diadema antillarum,” in Proceedings 5th International Coral Reef Congress (Moorea, French Polynesia: Antenne Museum -- EPHE), Vol. 5. 267–272.
Bak R. P. M. (1994). Sea urchin bioerosion on coral reefs: place in the carbonate budget and relevant variables. Coral reefs 13, 99–103. doi: 10.1007/BF00300768
Bak R. P., van Eys G. (1975). Predation of the sea urchin Diadema antillarum Philippi on living coral. Oecologia. 20, 111–115. doi: 10.1007/BF00369023
Bascompte J., Melián C. J., Sala E. (2005). “Interaction strength combinations and the overfishing of a marine food web,” in Proceedings of the National Academy of Sciences of the United States of America (Washington, DC: Kenneth R. Fulton (PNAS)), Vol. 102. 5443–5447.
Bauer J. C. (1976). Growth, aggregation, and maturation in the echinoid, Diadema antillarum. Bull. Mar. Sci. 26, 273–277.
Bayraktarov E., Saunders M. I., Abdullah S., Mills M., Beher J., Possingham H. P., et al. (2016). The cost and feasibility of marine coastal restoration. Ecol. Appl. 26, 1055–1074. doi: 10.1890/15-1077
Bellwood D. R., Choat J. H. (1990). A functional analysis of grazing in parrotfishes (family Scaridae): the ecological implications. Environ. Biol. Fishes 28, 189–214. doi: 10.1007/BF00751035
Bellwood D. R., Goatley C. H. R. (2017). Can biological invasions save Caribbean coral reefs? Curr. Biol. 27, R1–R18. doi: 10.1016/j.cub.2016.11.018
Bellwood D. R., Hughes T. P., Hoey A. S. (2006). Sleeping functional group drives coral-reef recovery. Curr. Biol. 16, 2434–2439. doi: 10.1016/j.cub.2006.10.030
Bellwood D. R., Pratchett M. S., Morrison T. H., Gurney G. G., Hughes T. P., Álvarez-Romero J. G., et al. (2019). Coral reef conservation in the Anthropocene: Confronting spatial mismatches and prioritizing functions. Biol. Conserv. 236, 604–615. doi: 10.1016/j.biocon.2019.05.056
Benkwitt C. E. (2015). Non-linear effects of invasive lionfish density on native coral-reef fish communities. Biol. Invasions 17, 1383–1395. doi: 10.1007/s10530-014-0801-3
Bielmyer G. K., Brix K. V., Capo T. R., Grosell M. (2005). The effects of metals on embryo-larval and adult life stages of the sea urchin, Diadema antillarum. Aquat. Toxicol. 74, 254–263. doi: 10.1016/j.aquatox.2005.05.016
Birrell C. L., McCook L. J., Willis B. L., Diaz-Pulido G. A. (2008). Effects of benthic algae on the replenishment of corals and the implications for the resilience of coral reefs. Oceanography Mar. Biology: Annu. Rev. 46, 25–63. doi: 10.1201/9781420065756-4
Bodmer M. D. V., Wheeler P. M., Hendrix A. M., Cesarano D. N., East A. S., Exton D. A. (2017). Interacting effects of temperature, habitat and phenotype on predator avoidance behaviour in Diadema antillarum: implications for restorative conservation. Mar. Ecol. Prog. Ser. 566, 105–115. doi: 10.3354/meps12034
Bohnsack J. A. (1976). The spider crab, Mithrax spinosissimus: an investigation including commercial aspects. Florida Scientist 30, 254–258.
Bonaldo R. M., Hoey A. S., Bellwood D. R. (2014). The ecosystem roles of parrotfishes on tropical reefs. Oceanogr. Mar. Biol. Annu. Rev. 52, 81–132. doi: 10.1201/b17143
Boström-Einarsson L., Babcock R. C., Bayraktarov E., Ceccarelli D., Cook N., Ferse S. C., et al. (2020). Coral restoration–A systematic review of current methods, successes, failures and future directions. PloS One 15, e0226631. doi: 10.1371/journal.pone.0226631
Brownell W. N., Provenzano A. J., Martinez M. (1977). “Culture of the West Indian spider crab (Mithrax spinosissimus) at Los Roques, Venezuela,” in Proceedings of the Annual Meeting World Mariculture Society (Los Roques, Venezuela: World Aquaculture Society), Vol. 8. 157–168. doi: 10.1111/j.1749-7345.1977.tb00114.x
Brown-Saracino J., Peckol P., Allen Curran H., Robbart M. L. (2007). Spatial variation in sea urchins, fish predators, and bioerosion rates on coral reefs of Belize. Coral Reefs 26, 71–78. doi: 10.1007/s00338-006-0159-9
Burdick D. R. (2008). “The effectiveness of macroalgal reduction and Diadema antillarum addition in limiting macroalgal growth and facilitating coral recovery,” in Proceedings of the 11th International Coral Reef Symposium (Davie, Florida: Nova Southeastern University National Coral Reef Institute).
Burkepile D. E., Hay M. E. (2009). Nutrient versus herbivore control of macroalgal community development and coral growth on a Caribbean reef. Mar. Ecol. Prog. Ser. 389, 71–84. doi: 10.3354/meps08142
Burkepile D. E., Hay M. E. (2010). Impact of herbivore identity on algal succession and coral growth on a Caribbean reef. PloS One 5 (1), e8963. doi: 10.1371/journal.pone.0008963
Butler M. J. IV, Kintzing M. D. (2016). An exception to the rule: top-down control of a coral reef macroinvertebrate community by a tropical spiny lobster. Bull. Mar. Sci. 92, 137–152. doi: 10.5343/bms.2015.1045
Butler M. J. IV, Mojica A. M. (2012). Effect of the herbivorous channel clinging crab on patch reef algal communities in Florida. Mar. Biol. 159, 2697–2706. doi: 10.1007/s00227-012-2027-1
Butler M. J. IV, Paris C. B., Goldstein J. S., Matsuda H., Cowen R. K. (2011). Behavior constrains the dispersal of long-lived spiny lobster larvae. Mar. Ecol. Prog. Ser. 422, 223–237. doi: 10.3354/meps08878
Callwood K. A. (2021). Examining the development of a parrotfish fishery in The Bahamas: Social considerations & management implications. Global Ecol. Conserv. 28, e01677. doi: 10.1016/j.gecco.2021.e01677
Carpenter R. C. (1984). Predator and population density control of homing behavior in the Caribbean echinoid Diadema antillarum. Mar. Biol. 82, 101–108. doi: 10.1007/BF00392768
Carpenter R. C. (1990). Mass mortality of Diadema antillarum: II. Effects on population densities and grazing intensity of parrotfishes and surgeonfishes. Mar. Biol. 104, 79–86. doi: 10.1007/BF01313160
Carpenter R. C., Edmunds P. J. (2006). Local and regional scale recovery of Diadema promotes recruitment of scleractinian corals. Ecol. Lett. 9, 271–280. doi: 10.1111/j.1461-0248.2005.00866.x
Chandler L. M., Walters L. J., Sharp W. C., Hoffman E. A. (2017). Genetic structure of natural and broodstock populations of the long-spined sea urchin, Diadema antillarum, throughout the Florida Keys. Bull. Mar. Sci. 93, 881–889. doi: 10.5343/bms.216.1101
Chávez-Rodríguez L., Rodríguez-Viera L., Montero-Alejo V., Perdomo-Morales R., Mancera J. M., Perera E. (2020). A very active α-amylase and an inhibitor-based control of proteinases are key features of digestive biochemistry of the omnivorous Caribbean king crab Maguimithrax spinosissimus. J. Evolutionary Biochem. Physiol. 56, 550–564. doi: 10.1134/S0022093020060083
Cheung W., Sarmiento J., Dunne J., Frölicher T. L., Lam V. W. Y., Palomares M. L. D., et al. (2013). Shrinking of fishes exacerbates impacts of global ocean changes on marine ecosystems. Nat. Clim Change 3, 254–258. doi: 10.1038/nclimate1691
Chiappone M., Rutten L. M., Miller S. L., Swanson D. W. (2013). Recent trends, (1999–2011) in population density and size of the echinoid Diadema antillarum in the Florida Keys. Florida Scientist 67, 23–35.
Chiappone M., Swanson D. W., Miller S. L. (2002). Density, spatial distribution and size structure of sea urchins in Florida Keys coral reef and hard-bottom habitats. Mar. Ecol. Prog. Ser. 235, 117–126. doi: 10.3354/meps235117
Chiappone M., Swanson D. I., Miller S. L. (2006) One-year Response of Florida Keys Patch Reef Communities to Translocation of Long-spined Sea Urchins (Diadema antillarum). Available online at: https://api.semanticscholar.org/CorpusID:85722543.
Choat J., Ackerman J. L., Juan P. (2003). An age-based demographic analysis of the Caribbean stoplight parrotfish Sparisoma viride. Mar. Ecol. Prog. Ser. 246, 265–277. doi: 10.3354/meps246265
Clements K. D., German D. P., Piche J., Tribollet A., Choat J. H. (2016). Integrating ecological roles and trophic diversification on coral reefs: multiple lines of evidence identify parrotfishes as microphages. Biol. J. Linn. Soc. 120, 729–751. doi: 10.1111/bij.12914
Clifton K. E. (1995). Asynchronous food availability of neigboring Caribbean coral reefs determines seasonal patterns of growth and reproduction for the herbivorous parrotfish Scarus iserti. Mar. Ecol. Prog. Ser. 116, 39–46. doi: 10.3354/meps116039
Coen L. D. (1988a). Herbivory by Caribbean majid crabs: feeding ecology and plant susceptibility. J. Exp. Mar. Biol. Ecol. 122, 257–276. doi: 10.1016/0022-0981(88)90127-X
Coen L. D. (1988b). Herbivory by crabs and the control of algal epibionts on Caribbean host corals. Oecologia 75, 198–203. doi: 10.1007/BF00378597
Cortés-Useche C., Reyes-Gamboa W., Cabrera-Pérez J. L., Calle-Triviño J., Cerón-Flores A., Raigoza-Figueras R., et al. (2021). Capture, culture and release of postlarvae fishes: proof-of-concept as a tool approach to support reef management. Front. Mar. Sci. 8. doi: 10.3389/fmars.2021.718526
Courtney T., Westfield I., Ries J. B. (2013). CO2-induced ocean acidification impairs calcification in the tropical urchin echinometra viridis. J. Exp. Mar. Biol. Ecol. 440, 169–175. doi: 10.1016/j.jembe.2012.11.013
Cowen R. K., Paris C. B., Srinivasan A. (2006). Scaling of connectivity in marine populations. Science 311, 522–527. doi: 10.1126/science.1122039
Cox C. E., Jones C. D., Wares J. P., Castillo K. D., McField M. D., Bruno J. F. (2013). Genetic testing reveals some mislabeling but general compliance with a ban on herbivorous fish harvesting in Belize. Conserv. Lett. 6, 132–140. doi: 10.1111/j.1755-263X.2012.00286.x
Craig M. P., Porter K. L., Ruark R. V., Iglehart J. M. (1989). “Reproductive biology, fecundity and embryonic development of Mithrax spinosissimus,” in The Biology, Ecology, and Mariculture of Mithrax spinosissimus utilizing cultured algal turfs (The Mariculture Institute, Los Angeles, CA).
Creswell R. L. (2011). “The cultivation of marine invertebrates indigenous to the Wider Caribbean Region: established culture techniques and research needs for crustaceans,” in A regional shellfish hatchery for the Wider Caribbean: Assessing its feasibility and sustainability, vol. 19 . Eds. Lovatelli A., Sarkis S. (FAO Regional Technical Workshop, Kingston, Jamaica), 105–117.
Creswell R. L., Tunberg B. (2000). “Crab culture: West Indian red spider crab,” in Encyclopedia of Aquaculture. Ed. Stickney R. R. (New York, New York: John Wiley & Sons), 180–184.
Creswell R., Tunberg B. G., Winfree R. A. (1989). “Mariculture of the Caribbean king crab, Mithrax spinosissimus (Lamarck), in the Caribbean region: Progress and constraints,” in Proceedings of the 39th Gulf and Caribbean Fisheries Institute. (Fort Pierce, Florida: Gulf and Caribbean Fisheries Institute), 469–476.
Dame E. A. (2008). Assessing the effect of artificial habitat structure on translocation of the long-spined sea urchin, Diadema antillarum, in Curaçao (Netherlands Antilles). Bull. Mar. Sci. 82, 247–254.
Davis S. (2018). Associational refuge facilitates phase shifts to macroalgae in a coral reef ecosystem. Ecosphere 9, e02272. doi: 10.1002/ecs2.2272
de Breuyn M., van der Last A. J., Klokman O. J., Hylkema A. (2023). Diurnal predators of restocked lab-reared and wild Diadema antillarum near artificial reefs in Saba. PeerJ 11, e16189. doi: 10.7717/peerj.16189
Debrot A. O., Nagelkerken I. (2006). Recovery of the long spined sea urchin Diadema antillarum in Curacao (Netherlans Antilles) linked to Lagonal and wave shelter in shallow rocky habitats. Bull. Mar. Sci. 79, 415–424.
Delgado G. A., Sharp W. C. (2021). Does artificial shelter have a place in Diadema antillarum restoration in the Florida Keys? Tests of habitat manipulation and sheltering behavior. Global Ecol. Conserv. 26, e01502. doi: 10.1016/j.gecco.2021.e01502
Dell C. L. A., Longo G. O., Burkepile D. E., Manfrino C. (2020). Few herbivore species consume dominant macroalgae on a caribbean coral reef. Front. Mar. Sci. 7. doi: 10.3389/fmars.2020.00676
Dell C. L. A., Longo G. O., Hay M. E. (2016). Positive feedbacks enhance macroalgal resilience on degraded coral reefs. PloS One 11, e0155049. doi: 10.1371/journal.pone.0155049
Done T. J. (1992). Phase-shifts in coral reef communities and their ecological significance. Hydrobiologia 247, 121–132. doi: 10.1007/BF00008211
Duran A., Adam T. C., Palma L., Moreno S., Collado-Vides L., Burkepile D. E. (2019). Feeding behavior in Caribbean surgeonfishes varies across fish size, algal abundance, and habitat characteristics. Mar. Ecol. 40, e12561. doi: 10.1111/maec.12561
Duran A., Collado-Vides L., Burkepile D. E. (2016). Seasonal regulation of herbivory and nutrient effects on macroalgal recruitment and succession in a Florida coral reef. PeerJ. 4, e2643. doi: 10.7717/peerj2643
Eckert G. L. (1998). Larval development, growth and morphology of the sea urchin Diadema antillarum. Bull. Mar. Sci. 63, 443–451.
Edmunds P. J., Carpenter R. C. (2001). Recovery of Diadema antillarum reduces macroalgal cover and increases abundance of juvenile corals on a Caribbean reef. PNAS 98, 5067–5071. doi: 10.1073/pnas.071524598
Emlet R. B. (1983). Locomotion, drag, and the rigid skeleton of larval echinoderms. Biol. Bull. 164, 433–445. doi: 10.2307/1541253
Feary D. A., Burt J. A., Bauman A. G., Usseglio P., Sale P. F., Cavalcante G. H. (2010). Fish communities on the world’s warmest reefs: what can they tell us about the effects of climate change in the future? Fish Biol. 77, 1931–1947. doi: 10.1111/j.1095-8649.2010.02777.x
Feehan C. J., Brown M. S., Sharp W. C., Lauzon-Guay J.-S., Adams D. K. (2016). Fertilization limitation of Diadema antillarum on coral reefs in the Florida Keys. Ecology 97, 1897–1904. doi: 10.1002/ecy.1461
Feehan C. J., Sharp W. C., Miles T. N., Brown M. S., Adams D. K. (2019). Larval influx of Diadema antillarum to the Florida Keys linked to passage of a Tortugas Eddy. Coral Reefs 38, 387–393. doi: 10.1007/s00338-019-01786-9
Ferraro P. J., Cherry T. L., Shogren J. F., Vossler C. A., Cason T. N., Flint H. B., et al. (2023). Create a culture of experiments in environmental programs. Science 381, 735–737. doi: 10.1126/science.adf7774
Fidler R. Y., Andradi-Brown D. A., Awaludinnoer D., Purwanto P., Hidayat N. I., Ahmadia G. N., et al. (2021). The importance of biophysical context in understanding marine protected area outcomes for coral reef fish populations. Coral Reefs 40, 791–805. doi: 10.1007/s00338-021-02085-y
Fong P., Paul V. J. (2011). “Coral Reef Algae,” in Coral Reefs: An Ecosystem in Transition. Eds. Dubinsky Z., Stambler N. (Springer, Dordrecht). doi: 10.1007/978-94-007-0114-4_17
Francis F. T., Filbee-Dexter K., Yan H. F., Cote I. M. (2019). Invertebrate herbivores: Overlooked allies in the recovery of degraded coral reefs? Global Ecol. Conserv. 17, e00593 . doi: 10.1016/j.gecco.2019.e00593
Francis-Floyd R. (2020). Diagnostic methods for the comprehensive health assessment of the long-spined Sea urchin, Diadema antillarum: VM244/VM244, 05/2020. Vol. 2020 (Gainesville, Florida: EDIS).
Frias-Torres S., van de Geer C. (2015). Testing animal-assisted cleaning prior to transplantation in coral reef restoration. PeerJ. 3, e1287. doi: 10.7717/peerj.1287
Fulton C. J., Berkstrom C., Wilson S. K., Abesamis R. A., Bradley M., Kerlund C., et al. (2020). Macroalgal meadow habitats support fish and fisheries in diverse tropical seascapes. Fish Fisheries 21, 700–717. doi: 10.1111/faf.12455
García E., Hernández J. C., Clemente S. (2018). Robustness of larval development of intertidal sea urchin species to simulated ocean warming and acidification. Mar. Environ. Res. 139, 35–45. doi: 10.1016/j.marenvres.2018.04.011
Gardner T. A., Cote I. M., Gill J. A., Grant A., Watkinson A. R. (2005). Hurricanes and Caribbean coral reefs: Impact, recovery patterns, and the role in long-term decline. Ecology 86, 174–184. doi: 10.1890/04-0141
Glover S. (2022). An evaluation of the potential use of saltwater quarries for the semi-wild mariculture of Caribbean King Crab (Maguimithrax spinosissimus). Old Dominion University, Norfolk, Virginia, USA, 59.
Glover S. (2023). “Evaluating the potential use of saltwater quarries in the florida keys for the semi-wild mariculture of Caribbean King Crab (Maguimithrax spinosissimus)” M.S. Thesis. Norfolk, Virginia USA: Old Dominion University, 75pp.
Godefroid M., Dupont S., Metian M., Hédouin L. (2022). Two decades of seawater acidification experiments on tropical scleractinian corals: Overview, meta-analysis and perspectives. Mar. pollut. Bull. 178, 113552. doi: 10.1016/j.marpolbul.2022.113552
Graham N. A. J., Jennings S., MacNeil M. A., Mouillot D., Wilson S. K. (2015). Predicting climate-driven regime shifts versus rebound potential in coral reefs. Nature 518, 94–97. doi: 10.1038/nature14140
Graham N. A. J., Robinson J. P. W., Smith S. E., Govinden R., Gendron G., Wilson S. K. (2020). Changing role of coral reef marine reserves in a warming climate. Nat. Commun. 11, 2000. doi: 10.1038/s41467-020-15863-z
Gravinese P. M., Perry S. A., Spadaro A. J., Boyd A. E., Enochs I. C. (2022). Caribbean king crab larvae and juveniles show tolerance to ocean acidification and ocean warming. Mar. Biol. 169, 65. doi: 10.1007/s00227-022-04053-8
Guete-Salazar C., Barros J., Velasco L. A. (2021). Spawning, larval culture, settlement and juvenile production of the west Indian Sea egg, Tripneustes ventricosus (Lamarck 1816), under hatchery conditions. Aquaculture 544, 737059. doi: 10.1016/j.aquaculture.2021.737059
Guzman H. M., Tewfik A. (2004). Population Characteristics and Co-occurrence of three exploited decapods (Panulirus argus, P. guttatus, Mithrax spinosissimus) in Bocas del Toro, Panama. J. Shellfish Res. 23 (2), 575–580.
Harborne A. R., Renaud P. G., Tyler E. H. M., Mumby P. J. (2009). Reduced density of the herbivorous urchin Diadema antillarum inside a Caribbean marine reserve linked to increased predation pressure by fishes. Coral Reefs 28, 783–791. doi: 10.1007/s00338-009-0516-6
Harborne A. R., Rogers A., Bozec Y.-M., Mumby P. J. (2017). Multiple stressors and the functioning of coral reefs. Annu. Rev. Mar. Sci. 9, 445–468. doi: 10.1146/annurev-marine-010816-060551
Hart M. W., Strathmann R. R. (1994). Functional consequences of phenotypic plasticity in echinoid larvae. Biol. Bull. 186, 291–299. doi: 10.2307/1542275
Hart M. W., Strathmann R. R. (1995). “Mechanisms and rates of suspension feeding,” in Ecology of marine invertebrate larvae (Chicago, Illinois: CRC Press), 193–221.
Hassan M. M., Pilnick A. R., Petrosino A. M., Harpring J., Schwab C. J., O’Neil K. L., et al. (2022). Growth and foraging behavior of hatchery propagated long-spined sea urchins, Diadema antillarum: Implications for aquaculture and restocking. Aquaculture Rep. 26, 101298. doi: 10.1016/j.aqrep.2022.101298
Havenhand J. N., Buttler F. R., Thorndyke M. C., Williamson J. E. (2008). Near-future levels of ocean acidification reduce fertilization success in a sea urchin. Curr. Biol. 18, R651–R652. doi: 10.1016/j.cub.2008.06.015
Hawkins J. P., Roberts C. M. (2003). Effects of fishing on sex-changing Caribbean parrotfishes. Biol. Conserv. 115, 213–226. doi: 10.1016/S0006-3207(03)00119-8
Hay M. E. (1981). Spatial patterns of grazing intensity on a Caribbean barrier reef: herbivory and algal distribution. Aquat. Bot. 11, 97–109. doi: 10.1016/0304-3770(81)90051-6
Hay M. E., Taylor P. R. (1985). Competition between fishes and urchins on Caribbean reefs. Oecologia 65, 591–598. doi: 10.1007/BF00379678
Hazlett B., Rittschoff D. (1975). Daily movements and home range in Mithrax spinosissimus (Majidae, Decapoda). Mar. Behav. Physiol. 3, 101–118. doi: 10.1080/10236247509378500
Heenan A., Simpson S. D., Meekan M. G., Healy S. D., Braithwaite V. A. (2009). Restoring depleted coral-reef fish populations through recruitment enhancement: a proof of concept. Fish Biol. 75, 1857–1867. doi: 10.1111/j.1095-8649.2009.02401.x
Hernández J. C., Brito A., Cubero E., García N., Girard D., González-Lorenzo G., et al. (2006). Temporal patterns of larval settlement of Diadema antillarum (Echinodermata: Echinoidea) in the Canary Islands using an experimental larval collector. Bull. Mar. Sci. 78, 271–279.
Herrera-Lopez G., Cruz-Reyes A., Hernandez J., Gonzalez-Lorenzo J. G., Garcia-Hernandez N. (2004). Alimentación y diversidad algal en la dieta del erizo Vol. 2 (La Laguna: Academia Canaria de Ciencias), 129–141.
Hewson I., Ritchie I. T., Evans J. S., Altera A., Behringer D., Bowman E., et al. (2023). A scuticociliate causes mass mortality of diadema antillarum in the caribbean sea. Sci. Adv. 9 (16). doi: 10.1126/sciadv.adg3200
Hoegh-Guldberg O., Skirving W., Dove S. G., Spady B. L., Norrie A., Geiger E. F., et al. (2023). Coral reefs in peril in a record-breaking year: Climate change and its impacts on coral reefs have reached unchartered territory. Science 382, 1238–1240. doi: 10.1126/science.adk4532
Hoey A. S., Bellwood D. R. (2011). Suppression of herbivory by macroalgal density: a critical feedback on coral reefs? Ecol. Lett. 14, 267–273. doi: 10.1111/j.1461-0248.2010.01581.x
Hughes T. P. (1994). Catastrophes, phase shits, and large-scale degradation of a Caribbean coral reef. Science 265, 1547–1551. doi: 10.1126/science.265.5178.1547
Hughes T. P., Anderson K. D., Connolly S. R., Heron S. F., Kerry J. T., Lough J. M., et al. (2018). Spatial and temporal patterns of mass bleaching of coral in the Anthropocene. Science 359, 80–83. doi: 10.1126/science.aan8048
Hughes T. P., Szmant A. M., Steneck R., Carpenter R., Miller S. (1999). Algal blooms on coral reefs: what are the causes? Limnology Oceanography 44, 1583–1586. doi: 10.4319/lo.1999.44.6.1583
Hylkema A., Debrot A. O., Pistor M., Postma E., Williams S. M., Kitson-Walters K. (2022a). High peak settlement of Diadema antillarum on different artificial collectors in the Eastern Caribbean. J. Exp. Mar. Biol. Ecol. 549, 151–693. doi: 10.1016/j.jembe.2022.151693
Hylkema A., Debrot A. O., Van De Pas E. E., Osinga R., Murk A. J. (2022b). Assisted natural recovery: a novel approach to enhance Diadema antillarum recruitment. Front. Mar. Sci. 9, 1160. doi: 10.3389/fmars.2022.929355
Hylkema A., Kitson-Walters K., Kramer P. R., Patterson J., Roth L., Sevier M. L., et al. (2023). The 2022 Diadema antillarum die-off event: Comparisons with the 1983-1984 mass mortality. Front. Mar. Sci. 9, 2654. doi: 10.3389/fmars.2022.1067449
Idjadi J. A., Haring R. N., Precht W. F. (2010). Recovery of the sea urchin Diadema antillarum promotes scleractinian coral growth and survivorship on shallow Jamaican reefs. Mar. Ecol. Prog. Ser. 403, 91–100. doi: 10.3354/meps08463
Idrisi N., Capo T. R., Serafy J. E. (2003). Postmetamorphic growth and metabolism of long-spined black sea urchin (Diadema antillarum) reared in the laboratory. Mar. Freshw. Behav. Physiol. 36, 87–95. doi: 10.1080/1023624031000140003
Iglehart J. M., Ruark R. V., Koltes K. H. (1989). Population density and structure of Mithrax spinosissimus. The Biology, Ecology, and Mariculture of Mithrax spinosissimus utilizing cultured algal turfs (Los Angeles, CA: The Mariculture Institute).
Jackson J. B. C. (2001). What was natural in the coastal oceans? PNAS 98, 5411–5418. doi: 10.1073/pnas.091092898
Karlson R. H., Levitan D. R. (1990). Recruitment-limitation in open populations of Diadema antillarum: an evaluation. Oecologia 82, 40–44. doi: 10.1007/BF00318531
Kennedy E. V., Perry C. T., Halloran P. R., Iglesias-Prieto R., Schönberg C. H. L., Wisshak M., et al. (2013). Avoiding coral reef functional collapse requires local and global action. Curr. Biol. 23, 912–918. doi: 10.1016/j.cub.2013.04.020
Kiessling W. (2009). Geologic and biologic controls on the evolution of reefs. Annu. Rev. Evol. Systematics 40, 173–192. doi: 10.1146/annurev.ecolsys.110308.120251
Kiessling W., Simpson C., Foote M. (2010). Reefs as cradles of evolution and sources of biodiversity in the Phanerozoic. Science. 327, 196–198. doi: 10.1126/science.1182241
Kintzing M. D., Butler M. J. IV (2014). Effects of predation upon the long-spined sea urchin Diadema antillarum by the spotted spiny lobster Panulirus guttatus. Mar. Ecol. Prog. Ser. 495, 185–191. doi: 10.3354/meps10568
Klokman O. J., Hylkema A. (2024). “Accepted pending minor revisisons,” in Spatial distribution of settlement of Diadema antillarum around Saba(Dutch Caribbean).
Klompmaker A. A., Portell R. W., Klier A. T., Prueter V., Tucker A. L. (2015). Spider crabs of the Western Atlantic with special reference to fossil and some modern Mithracidae. PeerJ 3, e1301. doi: 10.7717/peerj.1301
Klumpp D. W., McKinnon D., Daniel P. (1987). Damselfish territories: zones of high productivity on coral reefs. Mar. Ecol. Prog. Ser. 40, 41–51. doi: 10.3354/meps040041
Kobelt J. N., Sharp W. C., Miles T. N., Feehan C. J. (2019). Localized impacts of Hurricane Irma on Diadema antillarum and coral reef community structure. Estuaries Coasts 43, 1133–1143. doi: 10.1007/s12237-019-00665-4
Koltes K. (1993). Aspects of the Reproductive Biology and Social Structure of the Stoplight Parrotfish Sparisoma viride, at Grand Turk, Turks and Caicos Islands, B.W.I. Bull. Mar. Sci. 52, 792–805.
Koval G., Rivas N., D’Alessandro M., Hesley D., Santos R., Lirman D. (2020). Fish predation hinders the success of coral restoration efforts using fragmented massive corals. PeerJ 8, e9978. doi: 10.7717/peerj.9978
Kuempel C. D., Altieri A. H. (2017). The emergent role of small-bodied herbivores in pre-empting phase shifts on degraded coral reefs. Nat. Sci. Rep. 7, 39670. doi: 10.1038/srep39670
Lapointe B. E. (1997). Nutrient threshold for bottom-ip control of macroalgal blooms on coral reefs in Jamaica and southeast Florida. Limnology Oceanography 42, 1119–1131. doi: 10.4319/lo.1997.42.5_part_2.1119
Lapointe B. E. (1999). Simultaneous top-down and bottom-up forces control macroalgal blooms on coral reefs (reply to the comment by Hughes et al.). Limnology Oceanography 44, 1586–1592. doi: 10.4319/lo.1999.44.6.1586
Leber K., Lorenzen K., Main K., Moe M., Vaughan D., Capo T., et al. (2009). Developing Restoration Methods to Aid in Recovery of a Key Herbivore, Diademia antillarum, on Florida Coral Reefs. (Summerland Key, Florida: Mote Marine Laboratory Technical Report No. 1347).
Lellis W. A. (1992). A standard reference diet for crustacean nutrition research VI. Response of postlarval stages of the Caribbean king crab Mithrax spinosissimus and the spiny lobster Panulirus argus. J. World Aquaculture Soc. 23, 1–7. doi: 10.1111/j.1749-7345.1992.tb00744.x
Lessios H. A. (1981). Reproductive periodicity of the echinoids Diadema and Echinometra on the two coasts of Panama. J. Exp. Mar. Biol. Ecol. 50, 47–61. doi: 10.1016/0022-0981(81)90062-9
Lessios H. A. (1984). Possible prezygotic reproductive isolation in sea urchins separated by the Isthmus of Panama. Evolution 38, 1144–1148. doi: 10.2307/2408446
Lessios H. A. (1988a). Mass mortality of Diadema antillarum in the Caribbean: What have we learned? Annu. Rev. Ecol. Systematics 19, 371–393. doi: 10.1146/annurev.es.19.110188.002103
Lessios H. A. (1988b). Population dynamics of Diadema antillarum (Echinodermata: Echinoidea) following mass mortality in Panamá. Mar. Biol. 99, 515–526. doi: 10.1007/BF00392559
Lessios H. A. (2005). Diadema antillarum populations in Panama twenty years following mass mortality. Coral Reefs 24, 125–127. doi: 10.1007/s00338-004-0443-5
Lessios H. A. (2016). The great Diadema antillarum die-off: 30 years later. Annu. Rev. Mar. Sci. 8, 267–283. doi: 10.1146/annurev-marine-122414-033857
Levitan D. R. (1991). Influence of body size and population density on fertilization success and reproductive output in a free-spawning invertebrate. Biol. Bull. 181, 261–268. doi: 10.2307/1542097
Levitan D. R. (1988). Density-dependent size regulation and negative growth in the sea urchin Diadema antillarum philippi. Oecologia 76, 627–629. doi: 10.1007/BF00397880
Lorenzen K., Leber K. M., Blankenship H. L. (2010). Responsible approach to marine stock enhancement: an update. Rev. Fisheries Sci. 18, 189–210. doi: 10.1080/10641262.2010.491564
Maciá S., Robinson M. P., Nalevanko A. (2007). Experimental dispersal of recovering Diadema antillarum increases grazing intensity and reduces macroalgal abundance on a coral reef. Mar. Ecol. Prog. Ser. 348, 173–182. doi: 10.3354/meps06962
MacNeil M. A., Graham N. A. J., Cinner J. E., Wilson S. K., Williams I. D., Maina J., et al. (2015). Recovery potential of the world’s coral reef fishes. Nature 520, 341–344. doi: 10.1038/nature14358
Maldonado-Sánchez J., Mariño-Tapia I., Teresa Herrera-Dorantes M., Ardisson P. L. (2019). Hydrodynamic conditions that favor the settlement of Diadema antillarum to a western Caribbean coral reef. Bull. Mar. Sci. 95, 251–264. doi: 10.5343/bms.2018.0001
McClanahan T. R., Muthiga N. A. (2020). Change in fish and benthic communities in Belizean patch reefs in and outside of a marine reserve, across a parrotfish capture ban. Mar. Ecol. Prog. Ser. 645, 25–40. doi: 10.3354/meps13357
McEdward L. R. (1984). Morphometric and metabolic analysis of the growth and form of an echinopluteus. J. Exp. Mar. Biol. Ecol. 82, 259–287. doi: 10.1016/0022-0981(84)90109-6
McKay D. I. A., Staal A., Abrams J. F., Winkelmann R., Sakschewski B., Loriani S., et al. (2022). Exceeding 1.5°C global warming could trigger multiple climate tipping points. Science 377, 1171–1182. doi: 10.1126/science.abn7950
Micheli F., Halpern B. S., Botsford L. W., Warner R. R. (2004). Trajectories and correlates of community change in no-take marine reserves. Ecol. Appl. 14, 1709–1723. doi: 10.1890/03-5260
Miller M. W., Kramer K. L., Williams S. M., Johnston L., Szmant A. M. (2009). Assessment of current rates of Diadema antillarum larval settlement. Coral Reefs 28, 511–515. doi: 10.1007/s00338-008-0458-4
Miller R. J., Adams A. J., Ebersole J. P., Ruiz E. (2007). Evidence for positive density-dependent effects in recovering diadema antillarum populations. J. Exp. Mar. Biol. Ecol. 349, 215–222. doi: 10.1016/j.jembe.2007.05.014
Moberg F., Folke C. (1999). Ecological goods and services of coral reef ecosystems. Ecol. Economics 29, 215–233. doi: 10.1016/S0921-8009(99)00009-9
Monteil Y., Teo A., Fong J., Bauman A. G., Todd P. A. (2020). Effects of macroalgae on coral fecundity in a degraded coral reef system. Mar. Pollut. Bull. 151, 110890. doi: 10.1016/j.marpolbul.2020.110890
Morand P., Briand X. (1996). Excessive growth of macroalgae: a symptom of environmental disturbance. Botanica Marina 39, 491–516. doi: 10.1515/botm.1996.39.1-6.491
Mortensen T. (1921). Studies of the development and larval forms of echinoderms (Copenhagen, Denmark: GEC Gad).
Mumby P. J. (2006a). Phase shifts and the stability of macroalgal communities on Caribbean coral reefs. Coral Reefs 28, 761–773. doi: 10.1007/s00338-009-0506-8
Mumby P. J. (2006b). The impact of exploiting grazers (Scaridae) on the dynamics of Caribbean coral reefs. Ecol. Appl. 16, 747–769. doi: 10.1890/1051-0761(2006)016[0747:tioegs]2.0.co;2
Mumby P. J. (2009). Herbivory versus corallivory: are parrotfish good or bad for Caribbean coral reefs? Coral Reefs 28, 683–690. doi: 10.1007/s00338-009-0501-0
Mumby P. J. (2021a). Ecology: returning caribbean coral reefs to their former glory. Curr. Biol. 31, R186–R214. doi: 10.1016/j.cub.2020.11.068
Mumby P., Dahlgren C., Harborne A., Kappel C., Micheli F., Brumbaugh D., et al. (2006b). Fishing, trophic cascades, and the process of grazing on coral reefs. Science 311, 98–101. doi: 10.1126/science.1121129
Mumby P. J., Harborne A. R. (2010). Marine reserves enhance the recovery of corals on Caribbean reefs. PloS One 5, e8657. doi: 10.1371/journal.pone.0008657
Mumby P. J., Harborne A. R., Williams J., Kappel C. V., Brumbaugh D. R., Micheli F., et al. (2007b). “Trophic cascade facilitates coral recruitment in a marine reserve,” in Proceedings of the National Academy of Sciences of the United States of America. (Washington, DC: Kenneth R. Fulton (PNAS), Vol. 104. 8362–8367.
Mumby P. J., Hastings A. (2008). The impact of ecosystem connectivity on coral reef resilience. J. Appl. Ecol. 45, 854–862. doi: 10.1111/j.1365-2664.2008.01459.x
Mumby P. J., Hastings A., Edwards H. J. (2007a). Thresholds and the resilience of Caribbean coral reefs. Nature 450, 98–101. doi: 10.1038/nature06252
Mumby P. J., Hedley J. D., Zychaluk K., Harborne A. R., Blackwell P. G. (2006a). Revisiting the catastrophic die-off of the urchin Diadema antillarum on Caribbean coral reefs: fresh insights on resilience from a simulation model. Ecol. Model. 196, 131–148. doi: 10.1016/j.ecolmodel.2005.11.035
Mumby P. J., Steneck R. S. (2008). Coral reef management and conservation in light of the rapidly evolving ecological paradigms. TREE 23, 555–563. doi: 10.1016/j.tree.2008.06.011
Mumby P. J., Steneck R. S., Edwards A. J., Ferrari R., Coleman R., Harborne A. R., et al. (2012). Fishing down a Caribbean food web relaxes trophic cascades. Mar. Ecol. Prog. Ser. 445, 13–24. doi: 10.3354/meps09450
Mumby P. J., Steneck R. S., Hastings A. (2013). Evidence for and against the existence of alternate attractors on coral reefs. Oikos 122, 481–491. doi: 10.1111/j.1600-0706.2012.00262.x
Mumby P. J., Steneck R. S., Roff G., Paul V. J. (2021b). Marine reserves, fisheries ban, and 20 years of positive change in a coral reef ecosystem. Conserv. Biol. 35, 1473–1483. doi: 10.1111/cobi.13738
Munro J. L. (1974). The biology, ecology, exploitation and management of Caribbean reef fishes. Part V. The biology, ecology and bionomics of Caribbean reef fishes: Crustaceans (spiny lobster and crabs). Res. Rept Zool Dept Univ West Indies 3, 39–48.
Munro J. L. (1983). “Caribbean coral reef fishery resources,” in Ed. Munro J. L.(Manila, Philippines: International Center for Living Aquatic Resources Management), 218–222.
Munro J. L., Gaut V. C., Thompson R., Reeson P. H. (1973). The spawning seasons of the Caribbean reef fishes. J. Fisheries Biol. 5, 69–84. doi: 10.1111/j.1095-8649.1973.tb04431.x
Myhre S., Acevedo-Gutiérrez A. (2007). Recovery of sea urchin Diadema antillarum populations is correlated to increased coral and reduced macroalgal cover. Mar. Ecol. Prog. Ser. 329, 205–210. doi: 10.3354/meps329205
Näslund J. (2021). Reared to become wild-like: addressing behavioral and cognitive deficits in cultured aquatic animals destined for stocking into natural environments—a critical review. Bull. Mar. Sci. 97, 489–538. doi: 10.5343/bms.2020.0039
Nedimyer K., Moe M. A. (2006). Techniques Development for the Reestablishment of the Long-spined Sea Urchin, Diadema antillarum, on Two Small Patch Reefs in the upper Florida Keys. (Marathon, Florida: Florida Keys National Marine Sanctuary), 285–318.
NOAA. (2021). Restoring Seven Iconic Reefs: A Mission to Recover the Coral Reefs of the Florida Keys. Available online at: https://media.fisheries.noaa.gov/dammigration/restoringseveniconicreefsamissiontorecoverthecoralreefsoftheFloridaKeys.pdf.
Norström A. V., Nyström M., Jouffray J. B., Folke C., Graham N. A., Moberg F., et al. (2016). Guiding coral reef futures in the Anthropocene. Front. Ecol. Environ. 14, 490–498. doi: 10.1002/fee.1427
Obolski U., Hadany L., Abelson A. (2016). Potential contribution of fish restocking to the recovery of deteriorated coral reefs: an alternative restoration method? Peer J. 4, e1732. doi: 10.7717/peerj.1732
O’Farrell S., Harborne A. R., Bozec Y.-M., Luckhurst B. E., Mumby P. J. (2015). Protection of functionally important parrotfishes increases their biomass but fails to deliver enhanced recruitment. Mar. Ecol. Prog. Ser. 522, 245–254. doi: 10.3354/meps11134
O’Farrell S., Luckhurst B. E., Box S. J., Mumby P. J. (2016). Parrotfish sex ratios recover rapidly in Bermuda following a fishing ban. Coral Reefs 35, 421–425. doi: 10.1007/s00338-015-1389-5
Ogden J. C. (1976). Some aspects of herbivore-plant relationships on Caribbean reefs. Aquat. Bot. 2, 103–116. doi: 10.1016/0304-3770(76)90013-9
Ogden J. C. (1977). Carbonate-sediment production by parrot fish and sea urchins on Caribbean reefs. Stud. Geology 4, 281–288. Available at: https://www.researchgate.net/publication/284099575_Carbonate-sediment_production_by_parrot_fish_and_sea_urchins_on_Caribbean_reefs.
Ogden J. C., Lobel P. S. (1978). The role of herbivorous fishes and sea urchins in coral reef communities. Environ. Biol. Fishes 3, 49–63. doi: 10.1007/BF00006308
Olmeda-Saldaña M., Williams S. M., Weil E., Cruz-Motta J. J. (2021). Experimental evaluation of Diadema antillarum herbivory effects on benthic community assemblages. J. Exp. Mar. Biol. Ecol. 541, 151566. doi: 10.1016/j.jembe.2021.151566
Ortega A., Geraldi N. R., Alam I., Kamau A. A., Acinas S. G., Logares R., et al. (2019). Important contribution of macroalgae to oceanic carbon sequestration. Nat. Geosci. 12, 748–754. doi: 10.1038/s41561-019-0421-8
Paddack M. J., Cowen R. K., Sponaugle S. (2006). Grazing pressure of herbivorous coral reef fishes on low coral-cover reefs. Coral Reefs 25, 461–472. doi: 10.1007/s00338-006-0112-y
Pearce C. M., Scheibling R. E. (1991). Effect of macroalgae, microbial films, and conspecifics on the induction of metamorphosis of the green sea urchin Strongylocentrotus droebachiensis (Müller). J. Exp. Mar. Biol. Ecol. 147, 147–162. doi: 10.1016/0022-0981(91)90179-Z
Pilnick A. R., Henry J. A., Hesley D., Akins J. L., Patterson J. T., Lirman D. (2022b). Long−term retention and density−dependent herbivory from Diadema antillarum following translocation onto a reef restoration site. Coral Reefs 42, 629–634. doi: 10.1007/s00338-023-02369-5
Pilnick A. R., O’Neil K., DiMaggio M., Patterson J. T. (2022a). Development of larviculture protocols for the long-spined sea urchin (Diadema antillarum) and enhanced performance with diets containing the cryptophyte Rhodomonas lens. Aquaculture Int. 30, 3017–3034. doi: 10.1007/s10499-022-00945-0
Pilnick A. R., O’Neil K. L., Moe M., Patterson J. T. (2021). A novel system for intensive Diadema antillarum propagation as a step towards population enhancement. Sci. Rep. 11, 1–13. doi: 10.1038/s41598-021-90564-1
Pilnick A. R., Petrosino A., Hassan M. M., Patterson J. T. (2023). Cue selection and ontogeny reveal larval settlement dynamics of the long-spined sea urchin Diadema antillarum, a keystone coral reef herbivore. Mar. Biol. 170, 139. doi: 10.1007/s00227-023-04290-5
Provenzano A. J., Brownell W. N. (1977). “Larval and early post-larval stages of the West Indian spider crab, Mithrax spinosissimus (Lamarck) (Decapoda: Majidae),” in Proceedings Biological Society Washington (Lawrence, Kansas: Allen Press Inc.), Vol. 90. 735–752.
Rahim S. A. K. A., Li J. Y., Kitamura H. (2004). Larval metamorphosis of the sea urchins, Pseudocentrotus depressus and Anthocidaris crassispina in response to microbial films. Mar. Biol. 144, 71–78. doi: 10.1007/s00227-003-1171-z
Rahman M. A., Yusoff F. M., Arshad A., Shamsudin M. N., Amin S. M. N. (2012). Embryonic, larval, and early juvenile development of the tropical sea urchin, Salmacis sphaeroides (Echinodermata: Echinoidea). Sci. World J. 2012, 938482. doi: 10.1100/2012/938482
Randall J. E. (1961). Overgrazing of algae by herbivorous marine fishes. Ecology 42, 812. doi: 10.2307/1933510
Randall J. E., Schroeder R. E., Starck W. A. (1964). Notes on the biology of the echinoid Diadema antillarum. Caribbean J. Sci. 4, 421–433. Available at: https://www.researchgate.net/publication/246980787_Notes_on_the_biology_of_the_echinoid_Diadema_antillarum.
Rasher D. B., Hay M. E. (2014). Competition induces allelopathy but suppresses growth and anti-herbivore defence in a chemically rich seaweed. Proc. R. Soc. B: Biol. Sci. 281, 20132615. doi: 10.1098/rspb.2013.2615
Rasher D. B., Stout E. P., Engel S., Kubanek J., Hay M. E. (2011). Macroalgal terpenes function as allelopathic agents against reef corals. Proc. Natl. Acad. Sci. 108, 17726–17731. doi: 10.1073/pnas.1108628108
Rathbun M. J. (1925). The spider crabs of America. United States Natl. Museum Bull. 129, 1–599. doi: 10.5479/si.03629236.129.i
Richardson L. E., Lenfant P., Clarke L., Fontcuberta A., Gudefin A., Lecaillon G., et al. (2023). Examining the potential of wild post-larvae capture, culture, and release (PCCR) for fisheries enhancement. Front. Mar. Sci. 9, 1058497. doi: 10.3389/fmars.2022.1058497
Rinkevich B. (2014). Rebuilding coral reefs: does active reef restoration lead to sustainable reefs? Curr. Opin. Environ. Sustainability 7, 28–36. doi: 10.1016/j.cosust.2013.11.018
Robertson D. R. (1991). Increases in surgeonfish populations after mass mortality of the sea urchin Diadema antillarum in Panama indicate food limitation. Mar. Biol. 111, 437–444. doi: 10.1007/BF01319416
Robertson D. R., Choat J. H., Posada J. M., Pitt J., Ackerman J. L. (2005). Ocean surgeonfish Acanthurus bahianus. II Fishing effects on longevity, size and abundance? Mar. Ecol. Prog. Ser. 295, 25–256. doi: 10.3354/meps295245
Robertson D. R., Warner R. R. (1978). Sexual patterns in the labroid fishes of the western caribbean, II: the parrotfishes. Smithsonian Contribution to Zoology No. 255, 1–26. doi: 10.5479/si.00810282.255
Rocha L. A., Bass A. L., Robertson D. R., Bowen B. W. (2002). Adult habitat preferences, larval dispersal, and the comparative phylogeography of three Atlantic surgeonfishes (Teleostei: Acanthuridae). Mol. Ecol. 11, 243–252. doi: 10.1046/j.0962-1083.2001.01431.x
Rodríguez A., Hernández J. C., Brito A., Clemente S. (2017). Effects of ocean acidification on juveniles sea urchins: predator-prey interactions. J. Exp. Mar. Biol. Ecol. 493, 31–40. doi: 10.1016/j.jembe.2017.04.005
Rodríguez-Barreras R., Cuevas E., Cabanillas-Terán N., Branoff B. (2016). Understanding trophic relationships among Caribbean sea urchins. Rev. Biología Trop. 64, 837–848. doi: 10.15517/rbt.v64i2.19366
Roff G., Mumby P. J. (2012). Global disparity in the resilience of coral reefs Trends in Ecology and Evolution. Trends Ecol. Evol. 27, 404–413. doi: 10.1016/j.tree.2012.04.007
Rogers A., Harborne A. R., Brown C. J., Bozec Y. M., Castro C., Chollett I., et al. (2015). Anticipative management for coral reef ecosystem services in the 21st century. Global Change Biol. 21, 504–514. doi: 10.1111/gcb.12725
Rubino M. C., Stoffle R. W. (1989). “Caribbean Mithrax crab mariculture and traditional seafood distribution,” in Proceedings of the Gulf and Caribbean Fisheries Institute. (Fort Pierce, Florida: Gulf and Caribbean Fisheries Institute), Vol. 39. 134–145.
Rubino M. C., Stoffle R. W. (1990). Who will control the blue revolution? Economic and social feasibility of Caribbean crab mariculture. Hum. Organ. 49, 386–394. doi: 10.17730/humo.49.4.r1201168042n1t68
Russ G. R., Questel S. L. A., Rizzari J. R., Alcala A. C. (2015). The parrotfish-coral relationship: refuting the ubiquity of a prevailing paradigm. Mar. Biol. 162, 2029–2045. doi: 10.1007/s00227-015-2728-3
Ruttenberg B. I., Adam T. C., Duran A., Burkepile D. E. (2019). Diversity of coral reef herbivores drives variation in ecological processes over multiple spatial scales. Ecol. Appl. 29, e01893. doi: 10.1002/eap.1893
Sadovy Y. (2001). The thread of fishing to highly fecund fishes. J. Fisheries Biol. 59, 90–108. doi: 10.1111/j.1095-8649.2001.tb01381.x
Sammarco P. W. (1980). Diadema and its relationship to coral spat mortality: grazing, competition, and biological disturbance. J. Exp. Mar. Biol. Ecol. 45, 245–272. doi: 10.1016/0022-0981(80)90061-1
Sammarco P. W., Carleton J. H. (1982). “Damselfish territoriality and coral community structure: reduced grazing, coral recruitment, and effects on coral spat,” in Proceedings of the fourth international coral reef symposium, May 1981 Manila, vol. 2 . Eds. Gomez E., Birkeland D., Buddemeier C. E., Johannes R. E., Marsh J. A. Jr., Tsuda R. T. (Marine Sciences Center, University of the Philippines, Diliman, Quezon City), 525–535.
Sammarco P. W., Levinton J. S., Ogden J. C. (1974). Grazing and control of coral reef community structure by Diadema antillarum Philippi (Echinodermata: Echinoidea): a preliminary study. J. Mar. Res. 32, 47–53. Available at: https://www.researchgate.net/publication/284054060_Grazing_and_control_of_coral_reef_community_structure_by_Diadema_antillarum_Philippi_Echinodermata_Echinoidea_a_preliminary_study.
Sangil C., Guzman H. M. (2016). Assessing the herbivore role of the sea-urchin Echinometra viridis: Keys to determine the structure of communities in disturbed coral reefs. Mar. Environ. Res. 120, 202–213. doi: 10.1016/j.marenvres.2016.08.008
Santodomingo N., Rodriguez-Ramirez A., Garzon-Ferreira J. (2002). Territorios del pez Stegastes planifrons en formaciones coralinas del parquet nacional natural Tayrona, Caribe Colombiano: un panorama general. Boletín Investigaciones Marinas y Costeras-INVEMAR 31, 65–84. Available at: http://hdl.handle.net/1834/3125.
Schopmeyer S. A., Lirman D. (2015). Occupation dynamics and impacts of damselfish territoriality on recovering populations of the threatened staghorn coral, Acropora cervicornis. PloS One 10, e0141302. doi: 10.1371/journal.pone.0141302
Seraphim M. J., Sloman K. A., Alexander M. E., Janetski N., Jompa J., Ambo-Rappe R., et al. (2020). Interactions between coral restoration and fish assemblages: implications for reef management. J. Fish Biol. 97, 633–655. doi: 10.1111/jfb.14440
Shantz A. A., Ladd M. C., Burkepile D. E. (2020). Overfishing and the ecological impacts of extirpating large parrotfish from Caribbean coral reefs. Ecol. Monogr. 90, e01403. doi: 10.1002/ecm.1403
Sharp W. C., Delgado G. A., Hart J. E., Hunt J. H. (2018). Comparing the behavior and morphology of wild-collected and hatchery-propagated long-spined urchins (Diadema antillarum): implications for coral reef ecosystem restoration. Bull. Mar. Sci. 94, 103–122. doi: 10.5343/bms.2017.1068
Sharp W. C., Delgado G. A., Pilnick A., Patterson J. (2023). Diurnal sheltering behavior of hatchery-propagated long-spined urchins (Diadema antillarum): A reexamination following refinements to the husbandry process. Bull. Mar. Sci. 99, 97–108. doi: 10.5343/bms.2022.0054
Sharp W. C., Reckenbeil B. A. (2022). Predation on a newly-stocked long-spined sea urchin (Diadema antillarum) by the batwing coral crab (Carpilius corallinus) on a coral reef restoration site. Bull. Mar. Sci. 98, 99–100. doi: 10.5343/bms.2021.0032
Sheppard Brennand H., Soars N., Dworjanyn S. A., Davis A. R., Byrne M. (2010). Impact of ocean warming and ocean acidification on larval development and calcification in the sea urchin Tripneustes gratilla. PloS One 5, e11372. doi: 10.1371/journal.pone.0011372
Shields J. D. (2019). Climate change enhances disease processes in crustaceans: case studies in lobsters, crabs, and shrimps. J. Crustacean Biol. 39, 673–683. doi: 10.1093/jcbiol/ruz072
Shulman M. J. (1990). Aggression among sea urchins on Caribbean coral reefs. J. Exp. Mar. Biol. Ecol. 140, 197–207. doi: 10.1016/0022-0981(90)90127-X
Shulman M. J. (2020). Echinometra sea urchins on Caribbean coral reefs: Diel and lunar cycles of movement and feeding, densities, morphology. J. Exp. Mar. Biol. Ecol. 530-531, 151430. doi: 10.16/j.jembe.2020.151430
Smith A. B. (1997). Echinoderm larvae and phylogeny. Annu. Rev. Ecol. systematics 28, 219–241. doi: 10.1146/annurev.ecolsys.28.1.219
Smith H. A., Brown D. A., Arjunwadkar C. V., Fulton S. E., Whitman T., Hermanto B., et al. (2022). Removal of macroalgae from degraded reefs enhances coral recruitment. Restor. Ecol. 30, e13624. doi: 10.1111/rec.13624
Solandt J. L., Campbell A. C. (2001). Macroalgal feeding characteristics of the sea urchin Diadema antillarum Philippi at discovery bay, Jamaica. Caribbean J. Sci. 37, 227–238. Available at: https://www.researchgate.net/publication/266479307_Macroalgal_feeding_characteristics_of_the_sea_urchin_Diadema_antillarum_Philippi_at_Discovery_Bay_Jamaica.
Spadaro A. J. (2019). Cryptic herbivorous invertebrates restructure the composition of degraded coral reef communities in the Florida Keys, Florida, USA (Norfolk, Virginia: Old Dominion University), 190.
Spadaro A. J., Butler M. J. (2021). Herbivorous crabs reverse the seaweed dilemma on coral reefs. Curr. Biol. 31, 853–859. doi: 10.1016/j.cub.2020.10.097
Spiers L. J., Harrison S. J., Deutsch N. G., Paul V. J. (2021). The role of algal chemical defenses in the feeding preferences of the long-spined sea urchin Diadema antillarum. Aquat. Ecol. 55, 941–953. doi: 10.1007/s10452-021-09873-2
Stachowicz J. J., Hay M. E. (1996). Facultative mutualism between an herbivorous crab and a coralline alga: advantages of eating noxious seaweeds. Oecologia 105, 377–387. doi: 10.1007/BF00328741
Stachowicz J. J., Hay M. E. (1999a). Mutualism and coral persistence: the role of herbivore resistance to algal chemical defense. Ecology 80, 2085–2101. doi: 10.1890/0012-9658(1999)080[2085:MACPTR]2.0.CO;2
Stachowicz J. J., Hay M. E. (1999b). Reduced mobility is associated with compensatory feeding and increased diet breath of marine crabs. Mar. Ecol. Prog. Ser. 188, 169–178. doi: 10.3354/meps188169
Steiner S. C. C., Williams S. M. (2006). The density and size distribution of Diadema antillarum in Dominica (Lesser Antilles): 2001–2004. Mar. Biol. 149, 1071–1078. doi: 10.1007/s00227-006-0279-3
Steneck R. S. (1983). “Quantifying herbivory on coral reefs: just scratching the surface and still biting off more than we can chew,” in The ecology of deep and shallow coral reefs, vol. 1 . Ed. Reaka L. (National Oceanic and Atmospheric Administration, Rockville, Maryland, USA), 103–111.
Steneck R. S., Arnold S. N., Boenish R., de León R., Mumby P. J., Rashers D. B., et al. (2019). Managing recovery resilience in coral reefs against climate-induced bleaching and hurricanes: A 15 year case study from Bonaire, Dutch Caribbean. Front. Mar. Sci. 6, 265. doi: 10.3389/fmars.2019.00265
Steneck R. S., Arnold S. N., Mumby P. J. (2014). Experiment mimics fishing on parrotfish: insights on coral reef recovery and alternative attractors. Mar. Ecol. Prog. Ser. 506, 115–127. doi: 10.3354/meps10764
Steneck R. S., Watling L. (1982). Feeding capabilities and limitation of herbivoues molluscs: a functional group approach. Mar. Biol. 68, 299–319. doi: 10.1007/BF00409596
Streit R. P., Cumming G. S., Bellwood D. R. (2018). Patchy delivery of functions undermines functional redundancy in a high diversity system. Funct. Ecology. 33, 1144–1155. doi: 10.1111/1365-2435.13322
Tebbett S. B., Hoey A. S., Depczynski M., Wismer S., Bellwood D. R. (2020). Macroalgae removal on coral reefs: realised ecosystem functions transcend biogeographic locations. Coral Reefs 39, 203–214. doi: 10.1007/s00338-019-01874-w
Tertschnig W. P. (1989). Diel activity patterns and foraging dynamics of the sea urchin Tripneustes ventricosus in a tropical seagrass community and a reef environment (Virgin Islands). Mar. Ecol. 10, 3–21. doi: 10.1111/j.1439-0485.1989.tb00063.x
Texeira J. C., Huber C. D. (2021). The inflated significance of neutral genetic diversity in conservation genetics. Proc. Natl. Acad. Sci. U.S.A. 118, e2015096118. doi: 10.1073/pnas.2015096118
Thibaut L. M., Connolly S. R., Sweatman H. P. A. (2012). Diversity and stability of herbivorous fishes on coral reefs. Ecology 93, 891–901. doi: 10.1890/11-1753.1
Tunberg B. G., Creswell R. L. (1988). Early growth and mortality of the Caribbean king crab Mithrax spinosissimus reared in the laboratory. Mar. Biol. 98, 337–343. doi: 10.1007/BF00391109
Tunberg B. G., Creswell R. L. (1991). Development, growth, and survival in the juvenile Caribbean king crab Mithrax spinosissimus (Lamarck) reared in the laboratory. J. Crustacean Biol. 11, 138–149. doi: 10.2307/1548551
Turini T., Colavite J., Bolaños J. A., Hernández J. E., Baeza J. A., Santana W. (2021). Larval development of the Caribbean king crab Maguimithrax spinosissimus (Lamarck 1818), the largest brachyuran in the western Atlantic (Crustacea: Decapoda: Majoidea). J. Mar. Biol. Assoc. United Kingdom 101, 577–589. doi: 10.1017/S0025315421000515
Valles H. (2017). Parrotfish recruitment revisited: a key role for sea surface currents. Environ. Biol. Fishes 100, 1649–1657. doi: 10.1007/s10641-017-0673-3
van Woesik R., Ripple K., Miller S. L. (2018). Macroalgae reduces survival of nursery-reared Acropora corals in the Florida reef tract. Restor. Ecol. 26, 563–569. doi: 10.1111/rec.12590
Vermeij M. J. A., Debrot A. O., van der Hal N., Bakker J., Bak R. P. M. (2010). Increased recruitment rates indicate recovering populations of the sea urchin Diadema antillarum on Curaçao. Bull. Mar. Sci. 86, 719–725. Available at: https://landbouwwagennld-library-ingentaconnect-com.ezproxy.library.wur.nl/content/umrsmas/bullmar/2010/00000086/00000003/art00011#.
Whitaker N. (2023). Genetic diversity and connectivity of the Caribbean king crab (Maguimithrax spinosissimus) in the Florida Keys (Miami, FL: Florida International University).
Wijers T., Hylkema A., Pilnick A. R., Murk A. J., Patterson J. T. (2023). Novel shaker bottle cultivation method for the long spined sea urchin (Diadema antillarum; Philippi 1845) results in high larval survival and settlement rates. Aquaculture 562, 738855. doi: 10.1016/j.aquaculture.2022.738855
Wijers T., van Herpen B., Mattijssen D., Murk A. J., Patterson J. T., Hylkema A. (2024). Implications of changing Caribbean coral reefs on Diadema antillarum larvae settlement. Mar. Biol. 171, 48. doi: 10.1007/s00227-023-04368-0
Wilber D. H., Wilber T. P. (1989). The effects of holding space and diet on the growth of the West Indian spider crab Mithrax spinosissimus (Lamarck). J. Exp. Mar. Biol. Ecol. 131, 215–222. doi: 10.1016/0022-0981(89)90113-5
Wilber D. H., Wilber T. P. (1991). Environmental influences on the growth and survival of West Indian spider crabs Mithrax spinosissimus (Lamarck) in culture. J. Exp. Mar. Biol. Ecol. 146, 27–38. doi: 10.1016/0022-0981(91)90253-S
Williams A. B. (1984). Shrimps, lobsters, and crabs of the Atlantic coast of the eastern United States, Maine to Florida. (Washington, DC: Smithsonian Institute Press), 550.
Williams S. M. (2018). The control of algal abundance on coral reefs through the reintroduction of Diadema antillarum. Final Report (San Juan, Puerto Rico: Department of Natural and Environmental Resource of Puerto Rico).
Williams S. (2022). The reduction of harmful algae on Caribbean coral reefs through the reintroduction of a keystone herbivore, the long-spined sea urchin Diadema antillarum. Restor. Ecol. 30, e13475. doi: 10.1111/rec.13475
Williams S. M., García-Sais J. R., Capella J. (2009). Temporal variation of early larval stages of the long-spined sea urchin Diadema antillarum in La Parguera, Puerto Rico. Caribbean J. Sci. 45, 110–117. doi: 10.18475/cjos.v45i1.a14
Williams S. M., García-Sais J. R., Yoshioka P. M. (2011). Spatial variation of Diadema antillarum settlement in La Parguera, Puerto Rico. Bull. Mar. Sci. 87, 531–540. doi: 10.5343/bms.2010.1041
Williams S. M., Yoshioka P. M., García Sais J. R. (2010). Recruitment pattern of Diadema antillarum in La Parguera, Puerto Rico. Coral Reefs 29, 809–812. doi: 10.1007/s00338-010-0633-2
Windsor A. M., Felder D. L. (2014). Molecular phylogenetics and taxonomic reanalysis of the family Mithracidae MacLeay (Decapoda: Brachyura: Majoidea). Invertebrate Systematics 28, 145–173. doi: 10.1071/IS13011
Winfree R. A., Weinstein S. (1989). Food habits of the Caribbean King Crab Mithrax spinosissimus (Lamarck). Proc. Gulf Caribbean Fisheries Institute 39, 459–464. Available at: http://hdl.handle.net/1834/28.
Wittmann A. C., Pörtner H. O. (2013). Sensitivities of extant animal taxa to ocean acidification. Nat. Climate Change 3, 995–1001. doi: 10.1038/nclimate1982
Wynne S. (2008). Diadema antillarum Transloction Study in Anguilla, British West Indies Department of Fisheries and Marine Resources (The Valley, Anguilla: Department of Fisheries and Marine Resources of the Government of Anguilla).
Younglao D. (1987). Spawning, aggregation and recruitment in the black sea urchin Diadema antillarum. [dissertation/master's thesis]. [Montreal, (Canada)]: McGill University.
Keywords: coral reef, grazer, herbivore, restoration, Caribbean
Citation: Butler MJ IV, Duran A, Feehan CJ, Harborne AR, Hykema A, Patterson JT, Sharp WC, Spadaro AJ, Wijers T and Williams SM (2024) Restoration of herbivory on Caribbean coral reefs: are fishes, urchins, or crabs the solution? Front. Mar. Sci. 11:1329028. doi: 10.3389/fmars.2024.1329028
Received: 27 October 2023; Accepted: 22 February 2024;
Published: 18 March 2024.
Edited by:
Jesús Ernesto Arias González, Center for Research and Advanced Studies, National Polytechnic Institute of Mexico (CINVESTAV), MexicoReviewed by:
Miriam Reverter, University of Plymouth, United KingdomSalvador Zarco-Perello, University of New Hampshire, United States
Copyright © 2024 Butler, Duran, Feehan, Harborne, Hykema, Patterson, Sharp, Spadaro, Wijers and Williams. This is an open-access article distributed under the terms of the Creative Commons Attribution License (CC BY). The use, distribution or reproduction in other forums is permitted, provided the original author(s) and the copyright owner(s) are credited and that the original publication in this journal is cited, in accordance with accepted academic practice. No use, distribution or reproduction is permitted which does not comply with these terms.
*Correspondence: Mark J. Butler IV, bWJ1dGxlcmlAZml1LmVkdQ==