- 1Ecosystems Team, British Antarctic Survey, Cambridge, United Kingdom
- 2Plymouth Marine Laboratory, Prospect Place, The Hoe, Plymouth, United Kingdom
- 3Association of Responsible Krill Harvesting Companies, Toronto, ON, Canada
- 4Forest Research, Northern Research Station, Roslin, United Kingdom
- 5Southern Ocean Persistent Organic Pollutants Program, Centre for Planetary Health and Food Security, Griffith University, Nathan, QLD, Australia
- 6College of Earth, Ocean, and Atmospheric Sciences, Oregon State University, Corvallis, OR, United States
- 7Australian Antarctic Division, Kingston, TAS, Australia
- 8Virginia Institute of Marine Science, William & Mary, Williamsburg, VA, United States
- 9Polar Biological Oceanography Division, Alfred Wegener Institute Helmholtz Centre for Polar and Marine Research, Bremerhaven, Germany
- 10National Institute of Water and Atmospheric Research, Auckland, New Zealand
- 11Antarctic Ecosystem Research Division, Southwest Fisheries Science Centre, National Marine Fisheries Service, National Oceanic and Atmospheric Administration, La Jolla, CA, United States
- 12Institute of Marine Science, University of California Santa Cruz, Santa Cruz, CA, United States
- 13Centre for Ecology and Conservation, University of Exeter, Penryn, United Kingdom
- 14Mediterranean Institute for Advanced Studies, Esporles, Spain
- 15Plankton Department, Institute of Marine Research, Bergen, Norway
- 16Departamento Científico, Instituto Antártico Chileno, Punta Arenas, Chile
- 17Division of Ocean Sciences, Korea Polar Research Institute, Incheon, Republic of Korea
- 18Institute for Chemistry and Biology of the Marine Environment, Carl von Ossietzky University of Oldenburg, Oldenburg, Germany
- 19Helmholtz Institute for Functional Marine Biodiversity (HIFMB), University of Oldenburg, Oldenburg, Germany
- 20Department of Earth, Ocean & Atmospheric Sciences (EOAS), Institute for the Oceans and Fisheries and the Institute for the Oceans and Fisheries, University of British Columbia, Vancouver, BC, Canada
- 21Marine Biological Association, The Laboratory, Plymouth, United Kingdom
- 22Institute of Marine and Limnological Sciences, Austral University of Chile, Valdivia, Chile
- 23Department of Oceanography and Coastal Sciences Louisiana State University, 93 South Quad Dr. Room 1239 Energy, Coast & Environment Building, Baton Rouge, LA, United States
- 24Ross Analytics, Hobart, TAS, Australia
- 25Dirección Nacional del Antártico, Instituto Antártico Argentino, San Martín, Buenos Aires, Argentina
- 26University of Plymouth, School of Geography, Earth and Environmental Sciences, Plymouth, United Kingdom
- 27Environmental Studies Program, University of Colorado Boulder, Boulder, CO, United States
- 28National Institute of Polar Research, Tokyo, Japan
- 29Institute for Marine and Antarctic Studies, University of Tasmania, Hobart, TAS, Australia
- 30University of Coimbra, Marine and Environmental Sciences Centre/ARNET - Aquatic Research Network, Department of Life Sciences, Coimbra, Portugal
- 31Key Laboratory of Marine Ecology and Environmental Sciences, Institute of Oceanology, Chinese Academy of Sciences, Qingdao, China
Understanding and managing the response of marine ecosystems to human pressures including climate change requires reliable large-scale and multi-decadal information on the state of key populations. These populations include the pelagic animals that support ecosystem services including carbon export and fisheries. The use of research vessels to collect information using scientific nets and acoustics is being replaced with technologies such as autonomous moorings, gliders, and meta-genetics. Paradoxically, these newer methods sample pelagic populations at ever-smaller spatial scales, and ecological change might go undetected in the time needed to build up large-scale, long time series. These global-scale issues are epitomised by Antarctic krill (Euphausia superba), which is concentrated in rapidly warming areas, exports substantial quantities of carbon and supports an expanding fishery, but opinion is divided on how resilient their stocks are to climatic change. Based on a workshop of 137 krill experts we identify the challenges of observing climate change impacts with shifting sampling methods and suggest three tractable solutions. These are to: improve overlap and calibration of new with traditional methods; improve communication to harmonise, link and scale up the capacity of new but localised sampling programs; and expand opportunities from other research platforms and data sources, including the fishing industry. Contrasting evidence for both change and stability in krill stocks illustrates how the risks of false negative and false positive diagnoses of change are related to the temporal and spatial scale of sampling. Given the uncertainty about how krill are responding to rapid warming we recommend a shift towards a fishery management approach that prioritises monitoring of stock status and can adapt to variability and change.
1 Introduction
Pelagic animals are key components of marine ecosystems. In this paper we use the term “pelagic animals” to refer to species from zooplankton to small pelagic fish that tend to form dense aggregations and are mainly sampled in the upper few hundred metres of the water column. These animals support biodiversity at higher trophic levels, carbon export and important commercial fisheries, functions that are changing in response to a variety of pressures such as climate change (Siegel and Watkins, 2016; Cavan et al., 2019; Meyer et al., 2020). Understanding of such changes is based on large-scale spatial and temporal observations (Benway et al., 2019) but the approaches, scales and technology used to sample pelagic animals are also changing. It has long been possible to acquire high volumes of data including in situ images, multi-frequency acoustics and molecular samples, but only recently have we gained the computer processing power and machine learning capabilities to effectively process the terabytes of data involved. Concurrently it has become increasingly difficult to maintain large-scale and long-term sampling programs using traditional nets and bottles, with humans identifying the individual species including their ontogenetic stages (Benway et al., 2019). A key issue is that research vessels have significant financial and environmental costs (Kintisch, 2013) and lower cost, lower carbon platforms are preferred. Here we use the specific case of Antarctic krill (Euphausia superba, hereafter “krill”), which shows signs of long-term change (Atkinson et al., 2022) and is the target of the largest fishery in the Southern Ocean (SO) (Meyer et al., 2020), to assess how evolving sampling technology maps onto the information requirements for understanding and protecting a globally important pelagic species.
Krill has an enormous biomass, perhaps 400 Mt (Siegel and Watkins, 2016), and plays a central role in pelagic foodwebs (Figure 1), as a key prey species for a diverse range of predators including many SO endemics (McCormack et al., 2020; Cavanagh et al., 2021b), and as a major grazer (Yang et al., 2022). The huge faecal production (tens of Mt C yr-1) resulting from this grazing contributes to carbon export to deep water and therefore long-term removal from the atmosphere (Cavan et al., 2019). Krill are also important in the regeneration and translocation of limiting nutrients (Schmidt et al., 2016). Krill fishery catch has tripled since 2000 and now accounts for c. 97% of the SO catch of all species, although it represents an apparently small (<1%) fraction of krill biomass. The fishery is concentrated in the Southwest Atlantic sector of the SO which is also a major krill population centre and the area where most krill sampling has occurred but has experienced rapid warming and sea ice loss (Meredith et al., 2019) (Figure 2).
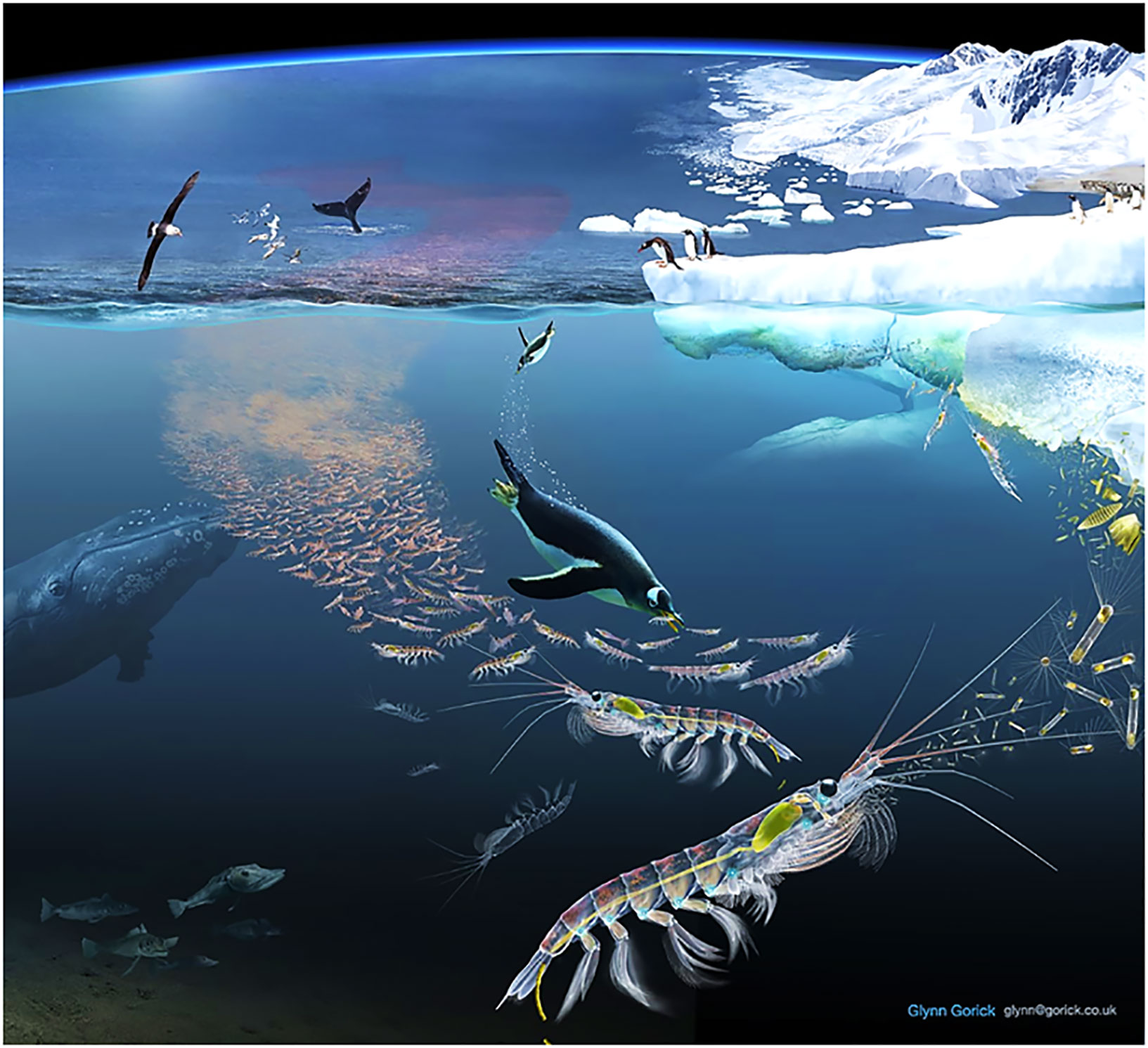
Figure 1 Traditional view of the krill-based food web. This schematic highlights an anthropocentric emphasis on organisms that are beautiful (e.g. diatoms, albatrosses), large (e.g. whales), charismatic (e.g. penguins) or land breeding and slightly easier to sample (e.g. seals). By contrast the ectothermic predators, illustrated bottom right by icefish species, are perhaps the major krill consumers in this food web, but the relative contributions of the various groups (icefish, Antarctic cod, myctophids, and squid) is poorly known. Sampling to detect changes within this krill-based food web is therefore challenged by the fact that we have an insufficient overview of the web itself. Previous over-exploitation of a small subset of major krill predators (fur seals, then whales then fish) has added to the effects of climate in perturbing this food web. While it is clear that some of the links in the food web are changing over time, the reasons are not yet always clear.
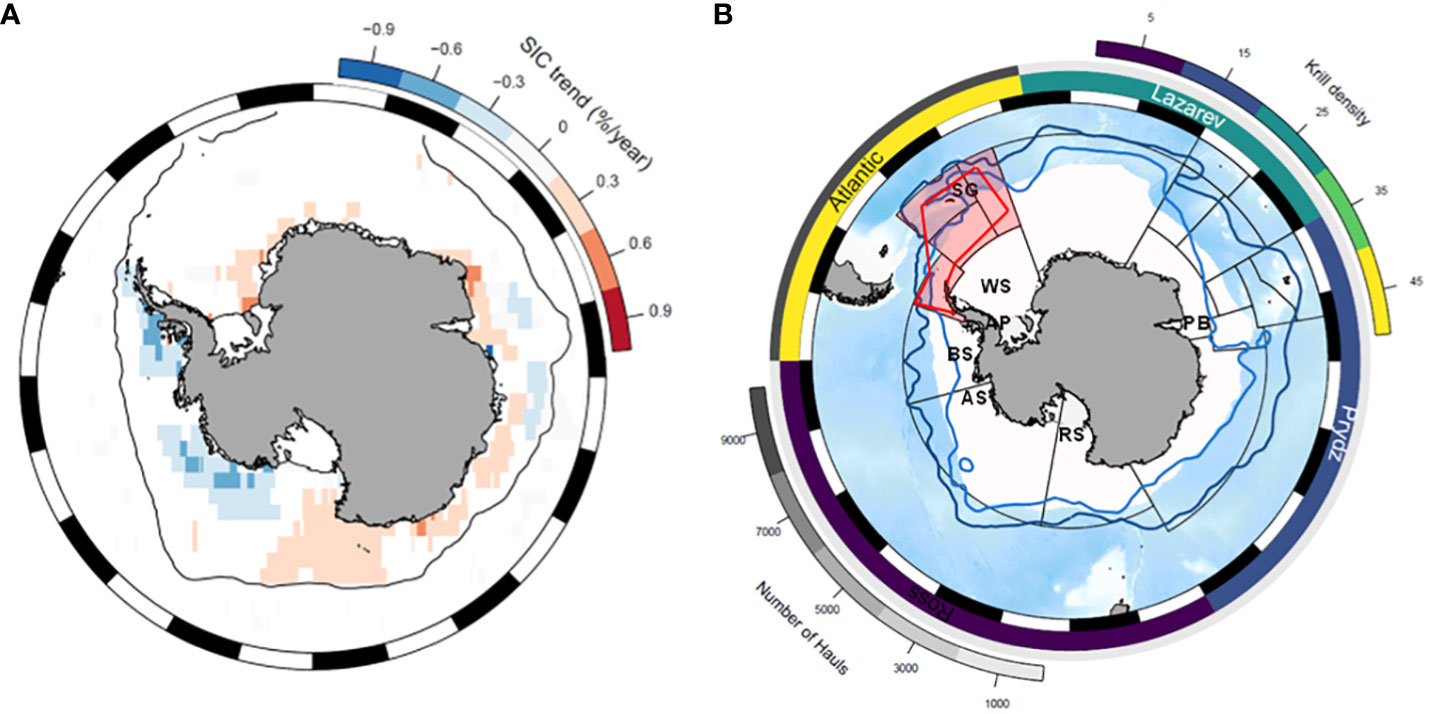
Figure 2 (A) Significant (p<0.05) interannual trends in sea-ice concentration (1978-11-01 to 2021-12-01). Calculated from monthly averages of daily gridded sea-ice concentration from NSIDC (https://nsidc.org/data/G02202/versions/3), and binned to a 1x1 degree grid. Trends were calculated for each grid cell by fitting monthly SIC using a GAMM with two terms: (1) a periodic spline in day of the year to capture the seasonal trend; and (2) a fixed linear term for the index in the time series to capture the multi-year trend; and also including an AR1 correlation coefficient for year. If the linear interannual trend was significant, then the slope of the trend (in %/year) is shown in the colour ramp for that grid cell. This figure was produced in the R Statistical Computing Environment (RCoreTeam, 2021) using the SOmap package (Maschette et al., 2019) and the NSIDC sea ice files were accessed using the raadtools package (Sumner, 2018). (B) Long-term sampling effort relative to krill numerical density observed in four sectors of the Southern Ocean. The grey scale (outermost) annulus shows the number of net hauls recorded in the KRILLBASE dataset, and the coloured annulus shows the average krill numerical density (number per m2) indicated by these net hauls. Grey lines are CCAMLR management units. The four subareas in the Southwest Atlantic sector are shaded pink and the approximate outer boundaries of the large scale-acoustic survey conducted in 2000 are shown in red. The dark blue and light blue lines represent the Polar Front and the southern Antarctic Circumpolar Front. SG: South Georgia; WS: Weddell Sea; AP: Antarctic Peninsula; BS: Bellingshausen Sea; AS: Amundsen Sea; RS: Ross Sea; PB: Prydz Bay.
While the ecological and economic importance of krill ensure that it is a focus of SO research and monitoring (Siegel and Watkins, 2016), understanding of krill populations, including how their biomass changes between years, remains highly uncertain. Catch limits for the fishery are based on historic catches rather than any estimate of population status (Siegel and Watkins, 2016; Meyer et al., 2020). Meanwhile there is a debate over whether long-term population change has occurred in the Southwest Atlantic (Cox et al., 2018; Atkinson et al., 2019; Cox et al., 2019; Hill et al., 2019; Candy, 2021). Given the central role that krill plays in the SO pelagic ecosystem and the services it provides (Cavanagh et al., 2021a), understanding krill population dynamics is critical to gauge the ecosystem response to climate change. It is also a major information requirement for improved fishery management (Nicol and Foster, 2016).
The Scientific Committee on Antarctic Research Krill Action Group (SKAG), an international community of Antarctic krill scientists, which has now become an Expert Group (SKEG), held an online workshop in April 2021 to evaluate existing and emerging sampling technologies against the information requirements for understanding population change and improving fishery management. The workshop was attended by 137 krill researchers from 19 countries (Atkinson et al., 2021) and its outcomes and recommendations are reported here. The general nexus of SO ecosystems, krill and climate change impacts and projections has been well covered by a suite of reviews over the last decade (Flores et al., 2012; Constable et al., 2014; Siegert et al., 2019; Rogers et al., 2020; Johnston et al., 2022; Kawaguchi et al., 2024), and we do not repeat these here.
We provide context for our overview of sampling methods through a brief recap on the krill fishery and climate change (section 2) and a survey of workshop participant views on priority issues for understanding krill populations (section 3). We then describe the strengths and limitations of each sampling method (sections 4-6). Those methods that have been used to sample krill have been applied over different timescales, in different locations, and often for different purposes. This precludes detailed analysis of their relative performance. However, we were able to compare the methods in two ways. First, we asked workshop participants to score the suitability of each method for addressing key current problems in the understanding of krill populations (section 7). Second, we assessed the characteristics of methods used to infer stability or change in the Southwest Atlantic krill stock to identify the relationship between the conclusion reached and the sampling approach used (section 8). We conclude with a series of tractable recommendations for the SO research community and its funders (section 9).
2 Krill fishing and environmental change
Antarctic krill catches have varied over the past 50 years, peaking at 528,000 t in 1982 before declining to 66,000 t in 1993. Catches have steadily increased over the past decade to reach a three-decade peak of 450,000t in 2020 (the catch was 416,000t in 2022). In the 1980s fishing occurred in the Atlantic, Pacific and Indian Ocean sectors but since the 1990s it has occurred almost exclusively in the Southwest Atlantic sector. The fishery is managed by the Commission for the Conservation of Antarctic Marine Living Resources (CCAMLR) which is also responsible for managing the impact of the fishery on the wider state of the ecosystem (Constable, 2001). To date, this responsibility has largely been focused on the protection of land-based krill predators, especially penguins and fur seals and has resulted in catch limits much lower than those in other fisheries for high-biomass species (Hill et al., 2020). Nonetheless catches in the Southwest Atlantic have never reached the effective catch limit set in 1991, indicating limited demand.
Management of the krill fishery in the Southwest Atlantic sector (Figure 2) includes three incremental levels of precaution. Only one of these, the nominal catch limit (5.6 million t yr-1), is based on sampling of the krill stock, specifically during a single survey in 2000 (Hewitt et al., 2004). The nominal catch limit divides long-term krill production at the large scale (c. 3.5 million km2) between the fishery, predators and recruitment to the krill stock, but a lower effective catch limit (620,000 t yr-1) applies until a system for managing the risks associated with spatially-concentrated fishing has been agreed. There are also lower catch limits (<279,000 t yr-1) for four subareas (c. 0.5 to 1 million km2) within the sector, intended as an interim measure to manage these risks (Hill et al., 2016). This measure expired in 2021 but CCAMLR has maintained it on a temporary basis while working on a more robust alternative (SC-CCAMLR, 2023).
CCAMLR recognises that progress is “urgently needed” as current catch limits “are not related to the status of the stock” and it identifies “feedback management” as a mechanism to “improve future management of krill, and the spatial allocation of krill catches” (CCAMLR, 2021). Thus, the intention within CCAMLR is to develop a management approach based on regularly updated information on the status of the krill stock (SC-CCAMLR, 2019).
Changes in the krill fishery have occurred against a backdrop of rapid environmental change within the Southwest Atlantic sector. This has had a notable effect on fishing patterns, with catches at the Antarctic Peninsula becoming increasingly concentrated in the austral winter and to the south of the South Shetland Islands following declines in sea ice extent and duration. Various potential environmental influences on krill populations and distribution have been reported (Meredith et al., 2019; Henley et al., 2020; Morley et al., 2020), including a strong link between the Southern Annular Mode (SAM), the leading mode of Southern Hemisphere atmospheric circulation variability, and krill recruitment (Atkinson et al., 2019). Critically krill is a cold-water species which is sustained by localised high primary production and which has a lifecycle that includes significant associations with sea ice, and apparent dependence on a limited number of spawning sites (Siegel and Watkins, 2016; Meyer et al., 2020; Atkinson et al., 2022). Prognostic studies generally suggest that krill populations and habitat extent are likely to be negatively impacted by future climate change. A series of studies suggest that there have already been climate-related changes to the distribution and, possibly, abundance of the krill population in the Southwest Atlantic. More detail, including on the drivers of change, is available in recent reviews (Meredith et al., 2019; Morley et al., 2020; Cavanagh et al., 2021b; Johnston et al., 2022).
3 Prioritisation of key topics and actions for change
The 137 participants in the 2021 SKAG workshop were mainly active krill researchers, including about one-third early career scientists, with some stakeholders from the krill fishery, management and NGOs (Atkinson et al., 2021). This engagement, enabled by the novel wholly-online format, provided a good sample size to assess expert opinion on scientifically-based management of the krill fishery in a changing climate. We asked participants via online multiple-choice polling, repeated at the start and end of the workshop, to identify priorities to improve krill fishery management (Figure 3). Only 3% of respondents supported “no real need for change” with the majority indicating an appetite for change in how the krill fishery is managed.
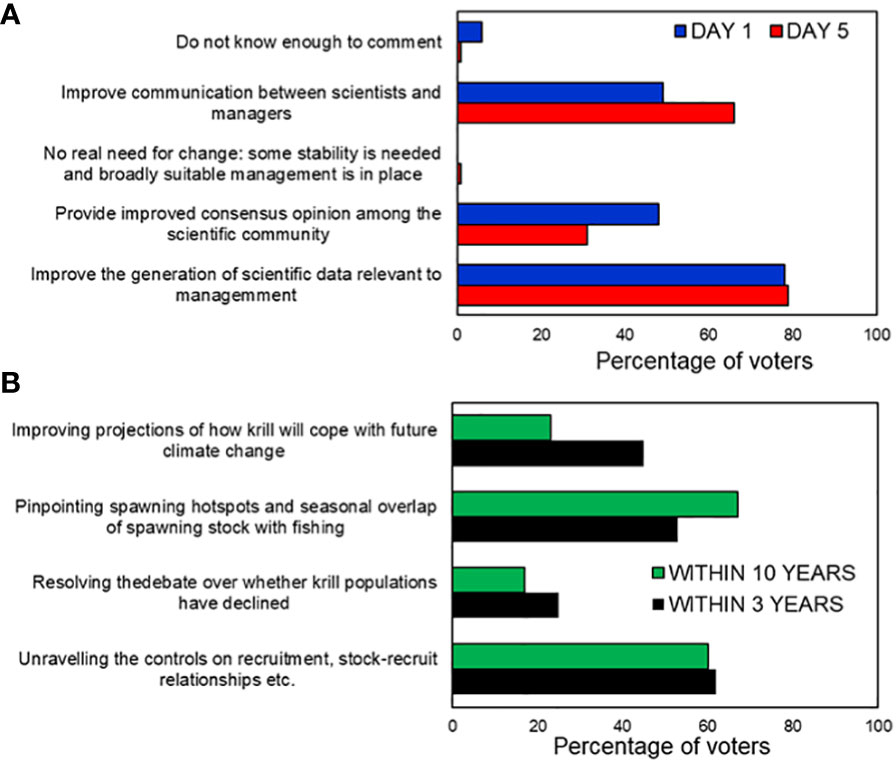
Figure 3 Results of online polling among approximately 100 workshop participants with mainly scientific backgrounds. Respondents were asked to vote for up to two responses and the results are expressed as percentages of voters who chose each option, rather than percentage of votes. (A) Polling at the start and end of the workshop in response to the question “What do you think are the most attainable ways to improve management of the krill fishery over the next five years?”. (B) Previous work (text box 2 in Meyer et al., (2020)) has identified four main scientific priorities for helping towards improved management of the krill fishery. The question posed was “which of these are most important to prioritise over a timescale of 3 years and over the next decade?”.
In both polls, around 80% of replies supported improved generation of scientific data relevant to management, and on the final day, 66% also supported improved communication between scientists and managers. Support for “improved consensus among the scientific community” fell from 50% to 31%, after the meeting. This followed discussion in which the opinions of some appeared to shift from seeing uncertainty over past krill stock declines as an impediment to management to seeing it as an indicator of the need for precaution.
Previous work identified four major research foci to help krill fishery management (Meyer et al., 2020) (Improving projections of how krill will cope with climate change; Pinpointing spawning hotspots and seasonal overlap of spawning stock and fishing; Resolving the debate over whether krill populations have declined; and Unravelling the controls on recruitment). We asked participants to identify which of these to prioritise over timescales of 3 and 10 years (Figure 3). Participants strongly support prioritisation of two of the foci (pinpointing spawning hotspots and unravelling the controls on recruitment) on both timescales. Pinpointing spawning was particularly well supported (67%) over the shorter timescale as it represents a potential quick win, whereby spatial protection could be targeted at a vulnerable life-history stage. Understanding recruitment processes was likewise identified as a priority in a workshop a decade ago (Flores et al., 2012).
These research priorities require direct collection of krill from the sea as this is the only approach currently available for determining their life stage. Sampling of krill with nets has greatly declined in recent years. Acoustic surveys have also been impacted and there is an active shift to newer approaches which will take years to develop time series. These alternative methods present additional challenges such as the small spatial scales covered by individual moorings and gliders, or the limited size range sampled by commercial fishing nets. The workshop addressed this issue by evaluating the strengths and weaknesses of traditional and emerging methods (Sections 4 to 6) – and mapping them onto the priority issues (Section 7). The diversity of krill sampling methods is illustrated schematically in Figure 4 and described below.
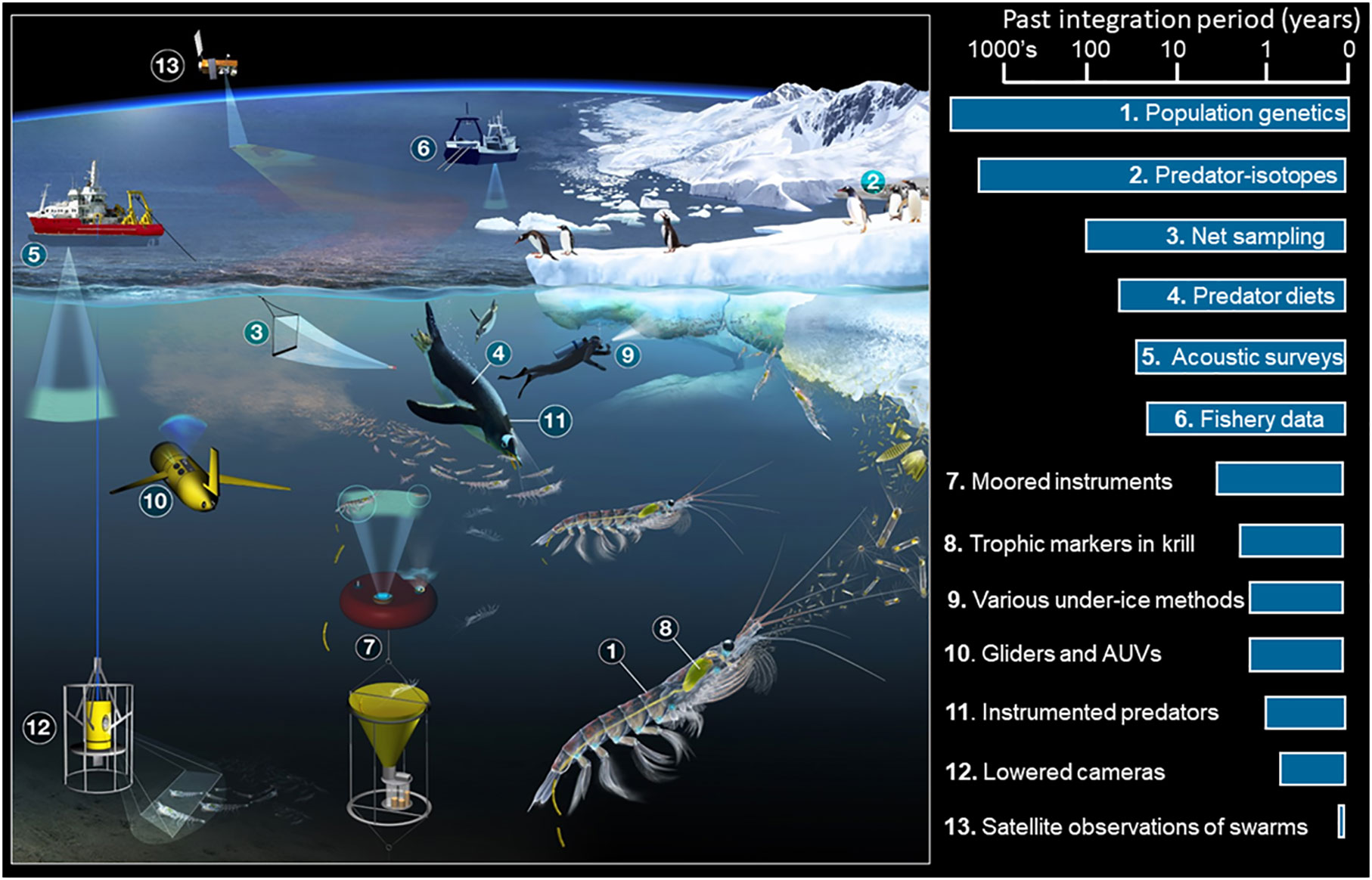
Figure 4 Traditional, emerging and future approaches to detect change in the krill-based food web. Illustrated are single-image depictions of groups of approaches; for example, the diver used to depict under-ice observations (No. 9) represents a suite of methods including diver-observation and sampling, a suite of net types and pumps, ROVs and AUVs. Moored instrumentation can also sample under ice and likewise includes various approaches including ADCPs and sediment traps. The fishery includes data from observers and from fishery statistics as well as newly developing opportunities (for example to sample krill in similar ways to research vessels). Imaging systems are also typically deployed from ships and other surface platforms, and can also be mounted on AUVs. For these reasons the right-hand scale can show only an approximate timescale over which these approaches can integrate. Importantly, the newer technology and approaches may be better at providing more direct, high-resolution observation, from ice crevices to abyssal seabeds, but so far the time scales are too short to yield evidence of multi-decadal change. Conversely, longer time series have greater statistical power but can face issues of interpretation of the trends observed.
4 Insights on change over multiple decades
4.1 Molecular approaches
Molecular genetics is an emerging approach for investigating ecosystems, which is compatible with a wide range of sampling methods. For example, krill DNA can be obtained from scientific or commercial nets, predator diets, archive specimens or the environment. One application relevant to krill population dynamics has been to determine whether there are distinct breeding stocks. While some studies have suggested weak stock structure (Zane et al., 1998; Batta-Lona et al., 2011), there is no conclusive evidence of genetically separate groups at any scale from swarm to circumpolar (Deagle et al., 2015; Jarman and Deagle, 2016; Dong et al., 2019). By contrast, genetic analyses of the bacteria associated with krill exoskeletons have shown geographically distinct assemblages (Clarke et al., 2019). This indicates separation of host populations over a timescale of months and suggests that the method might be useful for investigating separation over longer timescales. DNA sequence data has also been used to infer past population sizes. This suggests that the population generally increased over the last 400,000 years but levelled off or declined in the most recent 20,000 years, although uncertainty is high (Goodall-Copestake et al., 2010; Dong et al., 2019). All of these analyses have been hindered by krill’s unusually large and repetitive genome (Jeffery, 2012; Deagle et al., 2015). RNA sequencing of krill has also been applied to investigate responses to the environment, with early results showing effects of CO2 levels, light, and food availability (Martins et al., 2015; Hunt et al., 2017; Sales et al., 2017).
Improvements in DNA sequencing technology, decreasing cost and increasingly robust bioinformatic tools should give higher resolution to address questions of population size and structure, including identifying any subtler structure only detectable in genomic regions under selection. Use of environmental DNA to measure the presence or abundance of krill in an area through DNA in water samples is potentially a high temporal and spatial resolution technique for monitoring krill populations, and problems of quantification (Yamahara et al., 2019) may be reduced by developing appropriate standards. Likewise, monitoring of krill transcriptional activity (RNA), feeding, and/or symbionts may develop into a useful rapid indicator of stresses on krill populations, able to detect perturbations before effects are measured at the level of stock sizes.
4.2 Millennial, centennial, and decadal time paleo reconstructions
Paleoenvironmental proxies are commonly used to reconstruct krill-based food web dynamics prior to the era of direct krill sampling. The microscopic, geochemical, isotopic, and molecular analyses of ice cores, marine and limnological sediments, ornithogenic soils, and historic museum collections provide insights on SO climate and food-web dynamics over millennial, centennial, and decadal timescales. Collectively, these provide proxy records of past climate [e.g. isotopic and chemical analysis of ice cores (Mulvaney et al., 2012)], sea ice conditions [e.g. marine diatom sedimentary records (Taylor and Sjunneskog, 2002)], oceanic productivity [e.g. biogenic silica sedimentary records (Johnson et al., 2021)], sea-ice algal productivity [e.g. highly branched isoprenoid sedimentary records (Vorrath et al., 2020)] shifts in krill predator populations [e.g. bio-element, faecal sterol, and radiocarbon analyses of ornithogenic soils (Emslie et al., 2014)], and changes in krill predator diets over time [e.g. bulk tissue and compound-specific stable isotope analyses (McMahon et al., 2019; Kalvakaalva et al., 2020)].
While these paleoenvironmental proxies provide insights into how krill-based food webs have changed, no single proxy directly measures krill abundance over time. They are innately patchy in time and space and their use requires an understanding of the relationships between climate, productivity, krill abundance, and predator population and foraging dynamics. For example, multiple paleoenvironmental proxies in the Ross Sea were used to show that changes in atmospheric circulation and oceanographic conditions between ∼1600 and ∼1850 AD increased primary productivity, krill and silverfish (Pleuragramma antarctica) abundance, providing greater open-water access for krill predators relative to today (Yang et al., 2018). Compound-specific stable isotope analyses provided support for the hypothesis that historic anthropogenic exploitation and recent climate change reduced the availability of krill to their predators in the Antarctic Peninsula over the past century (Johnson et al., 2021).
The development and validation of zooplankton and possibly krill-specific paleoenvironmental biomarkers in sedimentary and ornithogenic archives represent an important future research need (Yang et al., 2021a). This could allow future studies to better relate variation in krill abundance over time with past climate, productivity, and krill-predator dynamics to understand longer-term changes in krill-based food webs.
4.3 Time series from net surveys
Net sampling is the longest-running sampling method for krill, with the earliest available data coming from the Discovery Investigations in 1926. These data provide our foundational understanding of krill (Fraser, 1936; Marr, 1962; Mackintosh, 1972, 1973). Net surveys are unique in their ability to provide quantitative data on different krill life stages, length-frequency distributions, and physical samples for laboratory-based research[e.g (Conroy et al., 2020; Steinke et al., 2021)]. However, net sampling comes with disadvantages such as mesh selectivity (Siegel, 1986), net avoidance (Wiebe et al., 2004), escapement, damage and integration (Watkins, 2000), and net feeding (Hirota, 1984). Nearly a century of circumpolar net-catch data are available in the standardised, composite KRILLBASE database (Atkinson et al., 2017), a historical reference to which new data can be added. These data have been used for a variety of purposes, for example to estimate krill biomass and annual production (Atkinson et al., 2009) and to identify habitat partitioning between life stages (Perry et al., 2019).
Analyses of net time series reveal spatial differences in multidecadal krill abundance trends. This topic has raised some controversy and section 8 enlarges upon the issue of detecting time trends in krill populations from a series of sampling methods including nets. Importantly, scientific netting remains the most viable method to capture and quantify the pelagic early larval stages of krill (eggs to furcilia) which indicate spawning, nursery and recruitment areas (Perry et al., 2019; Rombolá et al., 2021; Atkinson et al., 2022). However, the recent decline in net sampling surveys, in part due to competing demands for ship time, is expected to continue and presents a major challenge for detecting long-term change in krill populations.
4.4 Scientific ship-based acoustics
Acoustic instruments, deployed on research or fishing vessels, have been the main tools used by CCAMLR to assess krill density and distribution since they were first used to survey krill biomass in the 1980s (Siegel and Watkins, 2016). Large scale (i.e. ≥0.5 million km2), often multi-vessel, surveys require significant ship time and as a result happen infrequently (Krafft et al., 2021). Smaller-scale, single-vessel surveys (103 - 105 km2) have provided annual assessments of krill biomass in three areas in the SW Atlantic sector. These are the US-AMLR time series, north of the tip of the Antarctic Peninsula [1996 – 2012 (summer), 2014-2016 (winter), with subsequent surveys conducted by a Chinese fishing vessel (2013-present)] (Reiss et al., 2008, 2017) (WG-ASAM, 2019) the Norwegian South Orkney time series (2011 – present) of krill biomass in a krill fishing hotspot (Krafft et al., 2018), and the Western Core Box area north of South Georgia has been sampled by the British Antarctic Survey annually since summer 1997 (Fielding et al., 2014). Each survey provides a snapshot of biomass, but a time series built on such snapshots might be sensitive to phenology, amongst other factors. These time series tend to show high inter-annual variability in krill biomass and no evidence for an overall trend (see section 8).
Annual acoustic surveys provide key datasets contributing to the understanding of inter-annual variability in krill populations. Additional data such as net samples and predator observations are collected at the same time. Historically these surveys have been undertaken by research vessels but research fleet sizes are declining (Kintisch, 2013). Subsequent sections describe parallel acoustics from commercial fishing vessels, autonomous vehicles and gliders, and the approaches needed to ensure that estimates from different platforms are comparable, and trends can be detected.
4.5 Data from the krill fishery
The krill fishery has reported catches and operational details for nearly five decades. Haul-level data from the fishery can be used as proxies for describing krill horizontal and vertical distribution, and catch per unit of effort (CPUE), calculated at the haul-level and averaged per year over appropriate spatial units provides an indirect indicator of krill stock state (Ribic et al., 2008; Santa Cruz et al., 2022). The capacity to process catches sets an upper limit on CPUEs beyond which they are insensitive to increases in abundance. Conversely, fished species can maintain high densities within aggregations, maintaining high CPUEs even as overall abundance falls. CPUE can also be affected by shifts in location. These issues are compounded by the fact that the krill fishery is experiencing radical shifts in technology and strategy, and becoming increasingly concentrated in areas of reliable catches (Santa Cruz et al., 2018; Krüger, 2019). Nonetheless, there is some correspondence between spatially and temporally averaged CPUE time series and acoustic series for similar locations (Ribic et al., 2008). Decreases in spatially averaged CPUE were observed as the fishery expanded southwards in the Antarctic Peninsula region in the last two decades and fishing operations also shifted temporally to begin later in the summer-autumn season (Santa Cruz et al., 2018; Krüger, 2019; Santa Cruz et al., 2022). These changes also resulted in increased fishing depth (Krüger, 2019). Disentangling the various influences on CPUE data will require comparison with independent estimates of biomass, which could be based on acoustic transects provided by fishing vessels following scientific protocols.
The presence of scientific observers on krill vessels has increased from low levels in the 1990s to the current 100% coverage and this provides a valuable source of information on biological characteristics, especially krill size. Nonetheless, these data must be interpreted cautiously since sampling locations, swarm sizes, depths and biological characteristics such as body condition and feeding status are selected to suit fishery objectives, and commercial nets are too coarse to quantitatively sample smaller (<30mm) individuals.
4.6 Monitoring diet and foraging behaviour of krill predators
Long-term studies on central-place krill predators, including penguins and seals, provide the majority of data to CCAMLR’s ecosystem monitoring programme which monitors the life history parameters of these foragers to help detect changes in the abundance of harvested species, especially krill, and determine whether such changes are driven by fishing (Agnew, 1997).
The availability of krill to these predators is a function of krill biomass and its accessibility. Unravelling predator responses to changes in krill availability therefore requires consideration of factors beyond biomass estimates from traditional net or acoustic surveys. For example, accessibility of krill can vary due to diel vertical migration, tides and near-shore currents, prey depletion, annual on-shelf migrations, and episodic krill recruitment events (Wilson et al., 1993; Ribic et al., 2008; Ballance et al., 2009; Lowther et al., 2018; Nardelli et al., 2021). It is increasingly recognized that availability is a multivariate phenomenon and that understanding predator responses to changes in prey availability benefits from integrated sampling approaches (Boyd et al., 2017; Waggitt et al., 2018).
Despite these caveats, studies monitoring predator diets and foraging effort reveal predator sensitivity to changes in krill availability. For example, diet samples from krill predators reveal regional-scale coherence in krill sizes with foraging trip durations that correlate negatively with mean krill sizes (Hinke et al., 2007). Furthermore, years with longer foraging trips exhibit lower offspring production and growth (US AMLR, unpublished data). At Bird Island, South Georgia, where krill abundance is more dependent on advection/migration (Murphy et al., 2004), periodic variability in its availability, in turn correlated with variation in the physical environment, correlates with subsequent fluctuation in vital rates of predators, particularly those that are more dependent on krill (Murphy et al., 2007; Forcada et al., 2008). Together, the weight of evidence helps to link changes in size structure of the krill population with predator foraging behaviour and vital rates and reaffirms the utility of predator monitoring for understanding changes in krill populations.
5 Emerging and novel approaches to observe and monitor krill
5.1 Moored instrument arrays
Mooring arrays offer the potential to deliver temporally extensive (sub-daily and monthly) data on krill and, with concurrent oceanographic data, can help us understand intra- and inter-annual variations in their density, behaviour and population dynamics (Brierley, 2006). For example, moored echosounders and Acoustic Doppler Current Profilers at South Georgia have revealed a seasonal cycle in krill density, with peaks in summer and troughs in winter, which may be associated with seasonality in temperature, swarming behaviour and/or depth distribution (Saunders, 2007; Saunders et al., 2007). However, methodological challenges with this approach include ground-truthing and robust species identification, which may be improved using new multi-frequency echosounders. Innovative methods using moored sediment traps that collect krill moults can also provide alternative approaches to measure krill density, population structure, recruitment and carbon flux (Manno et al., 2020). Elsewhere, acoustic moorings in the Amundsen Sea coastal polynya have revealed seasonal and climate-related trends in zooplankton vertical migration behaviour (La et al., 2015, 2019).
In addition to identifying the scatterers, a major challenge with moorings for monitoring krill is the requirement to set their small-scale, point observations into a broader spatial context. To address this, integration of mooring-based observations with larger scale observations will be required.
5.2 Gliders and autonomous underwater vehicles
Remotely piloted and self-propelled Autonomous Underwater Vehicles (AUVs) are able to sample marine ecosystems autonomously for long periods of time, even in inaccessible, inhospitable and challenging regions (Testor et al., 2019). The current summary focuses on the buoyancy-driven glider (Webb et al., 2001; Schofield et al., 2007), while the later “under ice” section considers Remote Operating Vehicles (ROVs) dedicated to observing the ice-water interface.
Gliders can sample across a wide range of scales, from micro to macro, in both space and time, but there are limitations to where they can operate, especially in near-shore environments. Multiple gliders can simultaneously sample multiple locations and scales. Gliders outfitted with echosounders can measure krill distribution and biomass in a comparable manner to ship-based acoustic surveys (Reiss et al., 2021) while additional sensors for parameters including temperature, salinity and fluorescence (Testor et al., 2019) help to resolve relationships between krill distribution and the physical environment at scales not achievable from ship-based surveys (Nardelli et al., 2021). These scales range from sub-meso (10s of kilometres and days to weeks) (Cimino et al., 2016; Hann, 2021; Nardelli et al., 2021), to macro (100s of kilometres and months to years) (Reiss et al., 2021) (Guihen et al., 2014). Glider-borne acoustic surveys of Antarctic krill have illuminated predator-prey interactions (Cimino et al., 2016), examined krill diel vertical migration patterns (Nardelli et al., 2021), and assessed krill-salp distribution overlap (Hann, 2021). Acoustic data obtained from glider-borne echosounders are often less noisy than data collected from vessels (Hann, 2021) and, because they traverse the water column, clear acoustic signals can be detected at great depths, which are limited to lower frequency echosounders in ship-based surveys.
Target validation and length frequency determination from net tows pose a challenge for gliders. However, new techniques are being developed to overcome this, including the use of imaging (Ohman et al., 2019). Acquiring and maintaining a glider fleet is costly and logistically challenging and might limit the scales of the questions that can be addressed by any single research group. However, by pooling resources to develop programs that coordinate AUV fleets, a research community can begin to conduct truly transformative science (Testor et al., 2019).
5.3 Instrumented air-breathing predators
Instrumented predators can provide valuable insight into the distribution, availability, and density of krill. Predators such as penguins and seals consume large amounts of krill, concentrating their foraging efforts in the areas and depths where krill occur in high densities. Recent developments in biologging (the study of marine life via animal-borne instruments) have allowed us to monitor the movements, diving behaviour, feeding rates, and prey field of these predators at multiple spatial (<1 – 100s km) and temporal (minutes to decades) scales (Hindell et al., 2020).
Recent successful uses of biologgers include a study of crabeater seals, a highly-specialized krill predator, showing how their foraging effort related to sea ice, water temperature, and bathymetry across the western Antarctic Peninsula (WAP) (Hückstädt et al., 2020). Forward projection of seal habitat use indicated that krill distribution will expand offshore and towards southern sectors of the WAP in response to future environmental conditions. A study on Adelie penguins in East Antarctica found that their feeding rates while diving for krill (a putative indicator of krill density) were higher in a year of less extensive fast-ice than in extensive fast-ice years (Watanabe et al., 2020).
These studies suggest that changes in krill distribution, density and availability, and their links to environmental fluctuations, can be inferred from instrumented predators, although this approach is biased towards areas where these predators aggregate (e.g., breeding colonies). While long time series of predator behaviour data from biologgers are not yet available, simultaneous monitoring of feeding behaviour and in-situ environmental characteristics will expand our ability to understand fine-scale links between the environment, krill, and their predators (Keates et al., 2020; Kokubun et al., 2021).
5.4 Developments in under ice krill sampling
Sea ice is an important habitat for early life stages of krill, and its study provides insight into their life cycle, recruitment and population dynamics. A variety of methods have been used to sample at different spatial and temporal scales.
SCUBA divers (O’Brien, 1987; Meyer et al., 2009, 2017) and ROVs help us understand how krill use sea ice on a scale of meters (Marschall, 1988; Nordhausen, 1994). Divers can also provide data on stage structure and condition, and are unique in their ability to collect pristine live krill (Meyer et al., 2009). Specialized nets for sampling under ice, such as the Surface and Under-ice Trawl (SUIT), can survey across the water/ice interface on the kilometre scale while providing samples for assessing stage and condition (van Franeker et al., 2009). Traditional oblique Rectangular Midwater Trawl (RMT) and vertical nets (Bongo) deployed from a ship in ice provide similar information but vertically integrate the water column (Nordhausen, 1994; Schaafsma et al., 2016; Reiss et al., 2020). Acoustic methods can integrate biomass over many km2 but are limited in their ability to assess the water/ice interface (Brierley et al., 2002; Reiss et al., 2020). In a recent study synthesizing different methods, abundance estimates were 2-3 orders of magnitude higher in the same location using acoustic and diver methods than SUIT and vertical net methods and one-order of magnitude higher than RMT or ROV methods (Meyer et al., 2017). The methods used reflect the research questions and available time. While nets can be deployed relatively quickly from research vessels, divers and ROVs require great logistical efforts, for instance over weeks from winter ice camps on stable ice floes (Meiners et al., 2017; Meyer et al., 2017).
5.5 Direct visual observations of krill
Direct observations of krill behaviour in the water column, particularly through the use of divers and various camera systems, have provided much information about how individual krill behave (Hamner and Hamner, 2000; Nicol and Brierley, 2010; Atkinson et al., 2012; Tarling and Fielding, 2016). While krill behaviour within surface swarms was described from the Discovery Investigations (Marr, 1962), divers provided some of the first observations of krill behaviours in the water column and continue to be valuable under sea ice (Kils, 1981; Hamner et al., 1983; O’Brien, 1987; Stretch et al., 1988; Meyer et al., 2017). Acoustic systems are ideal for observing collective krill behaviour over larger spatial scales (De Robertis et al., 2003; Klevjer and Kaartvedt, 2003; 2006; 2011; Lawson et al., 2008; Klevjer and Kaartvedt, 2011), but cannot currently resolve the finer-scale behaviours of individual krill (Kils, 1981; Kawaguchi et al., 2011). Camera systems, deployed either on static rigs, ROVs, AUVs, ships, or even krill predators (Kawaguchi et al., 1986; Jaffe et al., 1998; Kokubun et al., 2013; Letessier et al., 2013; Watanabe and Takahashi, 2013; Kubilius et al., 2015; Schmid et al., 2016; Handley et al., 2018; Kane et al., 2018) provide these finer-scale observations, and can be adapted to determine the abundance of zooplankton and possibly other pelagic organisms (Jaffe et al., 1998; Letessier et al., 2013; Kubilius et al., 2015; Schmid et al., 2016; Kane et al., 2018, 2021). They can be used at deeper depths than scuba divers or acoustics can operate (Kawaguchi et al., 1986; Gutt and Siegel, 1994; Letessier et al., 2013; Kubilius et al., 2015; Kane et al., 2018; Zabroda et al., 2020; Kane et al., 2021), but importantly, can also be used to ground-truth acoustics data.
Direct observations of individual krill from divers and camera systems, as well as indirect observations from acoustics, have enabled us to document krill anti-predatory behaviours (O’Brien, 1987), seasonal changes in movement (Kane et al., 2018, 2021), interactions between individual krill (Hamner and Hamner, 2000; Kawaguchi et al., 2011), and with their environment. Camera observations of their behaviour at the seabed have brought about a paradigm shift in our understanding of krill-seabed interactions, showing that krill regularly interact with the seabed, in contrast to the earlier assumption that such interactions were rare (Gutt and Siegel, 1994; Clarke and Tyler, 2008; Kawaguchi et al., 2011; Schmidt et al., 2011; Zabroda et al., 2020; Kane et al., 2021).
Despite these advantages, direct, in situ observations of individual krill remain scarce, which hampers our ability to generalise their individual behaviours, including swimming, feeding, and mating. Nevertheless, improvements in image capture and processing technologies will enable us to better observe krill interactions with each other and their environment at small scales. These observations will allow laboratory results to be placed into better context, and help to elucidate the relationship between individuals and population dynamics.
5.6 Remote detection of krill swarms
The biomass and distribution of near-surface swarms of krill are poorly quantified by conventional sampling, and remote detection by satellites or aerial vehicles offers one potential solution. Historical records indicate that large surface swarms can change the water colour, so ocean colour satellites, which measure the surface ocean synoptically, should theoretically allow measurement of near-surface krill swarms at high spatial and temporal resolution. Such data have already been used to detect aggregations of the pelagic copepod Calanus finmarchicus and global patterns of diurnal vertical migration (Basedow et al., 2019; Behrenfeld et al., 2019). A pilot study (Belcher et al., 2021) has shown that water containing krill indeed has a different reflectance spectrum, thus providing proof of concept for remote detection of surface swarms.
To develop earth observation methods for krill swarms, more ground-truthing is needed. This requires the use of unmanned aerial vehicles mounted with spectroradiometers and/or cameras, in concert with surface net tows from a ship under cloud-free conditions. Cloud cover presents a challenge for ocean colour satellite measurements, thus the use of LIDAR from autonomous aerial vehicles must also be considered. Nevertheless, remote detection has the potential to provide unparalleled resolution of near-surface krill swarm distribution, which could facilitate a step-change in our ability to map and monitor this species over large areas of the SO.
6 Detecting change in the krill-based food web
The methods described in previous sections provide indices of numerical or biomass density or availability to predators over various time and space scales. This section describes other complementary indices of change in the krill-based food web, namely in recruitment, population structure and krill trophic position.
6.1 Changes in krill recruitment and population structure
Indices of krill recruitment, the replenishment of the adult population by approximately one-year old animals, are generally based on length-frequency distributions from multi-decadal series of length measurements based on net tows (Atkinson et al., 2019) and predator diet data (Saba et al., 2014). Such data are mainly available for the Atlantic sector, with some coverage in other areas such as the Indian sector (Nicol et al., 2000; Kawaguchi et al., 2010). Recruitment indices provide critical information on krill population dynamics, including on possible causes of large interannual variations in stock size. Nonetheless, there are potential biases in such data due to potential size-selectivity of sampling and uneven distribution of recruits (Ducklow et al., 2007).
Analyses of trends in recruitment are sensitive to scale and location. At sector-wide scales, strong declines in recruit density, related to summer, autumn and winter SAM anomalies emerge (Atkinson et al., 2019), but as the spatial scales of study decline, recruitment time series become increasingly fragmented (Ducklow et al., 2007; Veytia et al., 2021), and conclusions are often dependent on the region and time-period of study. For example, in the early 21st century, relationships between localised recruitment indices and sea ice extent or duration (Quetin and Ross, 2001; Wiedenmann et al., 2009), supported findings of southward shifts in recruitment with declining sea ice (Ross et al., 2014). However more recent observations suggest that the processes underpinning recruitment are regionally variable (Jia et al., 2016; Meyer et al., 2017; Walsh et al., 2020), or changing to become less reliant on sea ice habitats (Walsh et al., 2020).
Understanding of krill recruitment requires progress in data analysis, including integrated modelling (Kinzey et al., 2018) to handle the statistical challenges of comparing proportional and absolute recruitment data. It also requires an improved sampling strategy to coordinate across science programmes and target the spatiotemporal scales at which krill recruitment plays out. This implies a role for modelling to optimize sampling design (Peel et al., 2013). Because measuring recruitment requires direct sampling and identification of small (~20 mm) krill, which are the same size as the adults of other euphausiid species, there is a continuing need for traditional net sampling and identification skills until reliable alternative methods are available.
6.2 Tracing krill diet and resource allocation
Analyses of krill diet and body condition provide insights into the processes affecting population change. A suite of methods have shown that krill exploit a broad range of food items from phytoplankton blooms and sea ice algae, micro- and mesozooplankton, to seabed detritus (Hopkins et al., 1993a, Hopkins et al., 1993b; Martin et al., 2006; Passmore et al., 2006; Schmidt et al., 2011; Cleary et al., 2018; Schmidt et al., 2018; Pauli et al., 2021). Krill also accumulate substantial lipid reserves for overwintering (Hagen et al., 2001), but importantly for understanding recruitment, overwintering strategies vary between sectors and latitudes (Schmidt et al., 2014). At an interannual scale, differences in sea ice dynamics and phytoplankton abundance are reflected in krill diet, body conditions and fecundity (Quetin and Ross, 2001; Jia et al., 2016; Ericson et al., 2018; Bernard et al., 2019; Walsh et al., 2020). However, we are not aware of any long time series of krill diet/body condition that accompanies those of recruitment or density.
As krill sampling methods evolve in future, two types of approach may prove promising. First, creating biomarker time series on frozen or formalin-preserved krill to understand temporal-spatial changes in the krill food web. Studies elsewhere have shown that biomarkers such as bulk stable isotopes (δ15N, δ13C), amino acid δ15N, highly branched isoprenoids and even DNA of preserved tissue can provide robust data that reflect changes in trophic level or diet in relation to climatic events (Rau et al., 2003). As scientific sampling with nets diminishes, the krill fishery can provide a valuable source of material; for example, frozen winter-caught specimens have been used for lipid content and various diet and condition indices (Schmidt et al., 2014). Secondly, a wider perspective on how krill-based food webs are faring under climate change can also be obtained by sampling other plankton alongside krill to understand changing body composition of “winners” and “losers” under varying climatic conditions.
7 Mismatch between key research areas for krill and emerging sampling methods
Thirty-three of the workshop participants, spanning discipline areas, career stage and experience, contributed to Table 1 which maps methods of sampling and analysing krill onto key areas in current research. Table 1 identifies a mismatch between priority issues identified for improving management (e.g. larger scale and longer term population trends, sampling of larval or newly-recruited krill) and the emerging methods that in many cases are partially replacing traditional ship-based scientific surveys. Paradoxically, the new methods allow us to sample krill on ever smaller scales of space and time, while the pressing issues of climate change and habitat occupancy concern much larger scales. All of the new methods received lower support than ship-based methods for their ability to assess long-term population trends, and only gliders/AUVs received a similar level of support to ship-based methods for their ability to assess large-scale distribution.
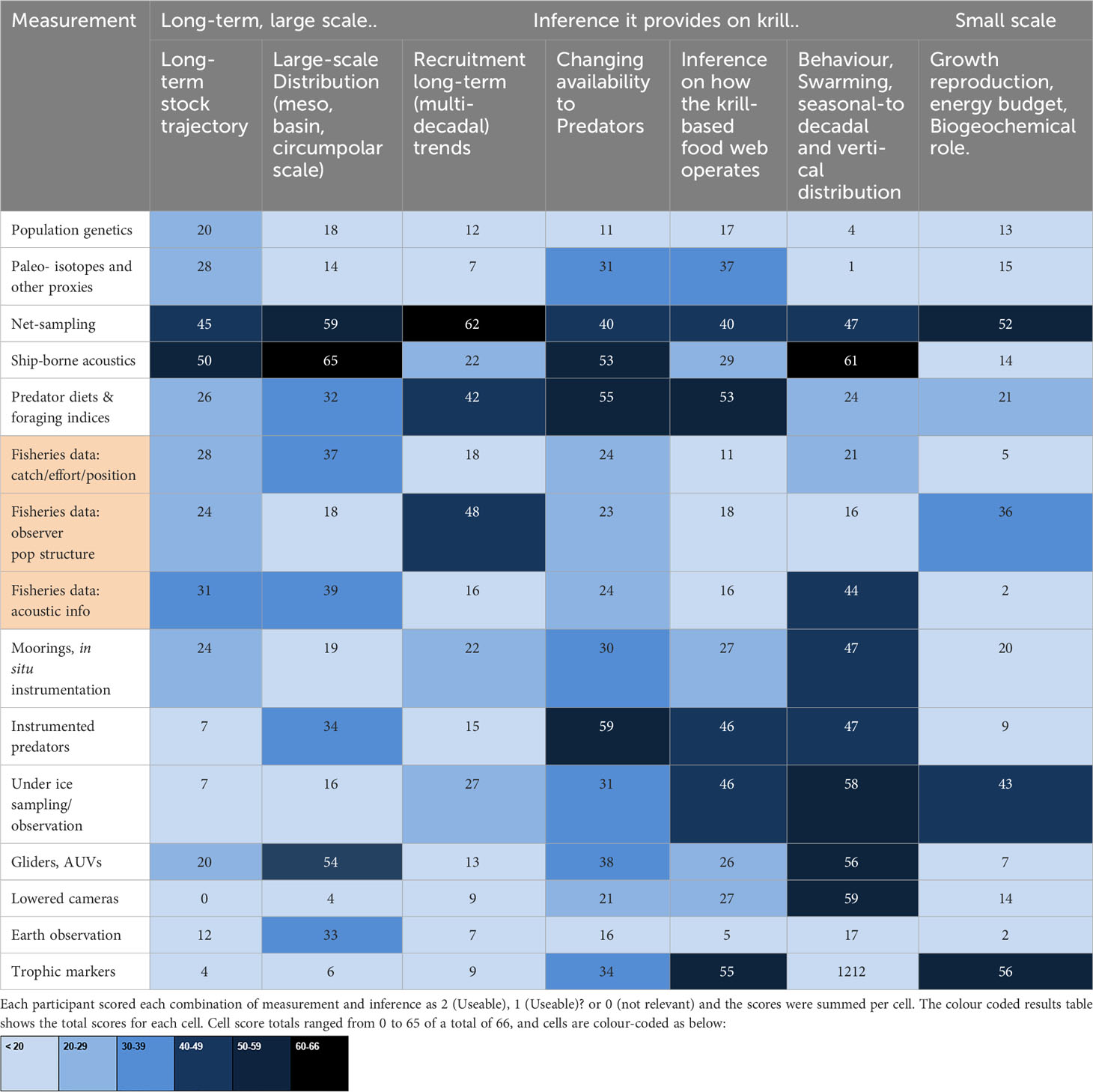
Table 1 Mapping established and developing krill sampling methods onto some of the key current research areas.
8 The influence of sampling approach on perceptions of change
A debate about whether krill have declined in the last 50 years within the SW Atlantic sector (Cox et al., 2018; Atkinson et al., 2019; Cox et al., 2019; Hill et al., 2019; Candy, 2021) provides an opportunity to examine the influence of sampling scale and location and time series length on perceptions of change in a pelagic population. Resolving this debate has previously been identified as a key step towards better understanding of future change in krill populations (Meyer et al., 2020). This controversy results, in part, from a lack of large-scale monitoring during the 1980s, the main period of the reported decline. Just one large-scale survey (0.5 million km2) was conducted during this period (Siegel and Watkins, 2016) and two further surveys (2 million km2) were conducted, in 2000 and 2019 (Krafft et al., 2021). This lack of large-scale monitoring has led to attempts to fill the information gaps, including the CCAMLR Ecosystem Monitoring Programme (Agnew, 1997) and the compilation of KRILLBASE data (Atkinson et al., 2017).
We compiled and compared analyses reporting changes or stability in the krill population over multiple years (Figure 5 and Supplementary Table 1). This shows that many of the apparently conflicting interpretations can be explained by sampling differences. Suggestions of a decline are based on data series that have one or more of the following features: a) net or predator-based data; b) a start date before the late 1980s; c) more than 20 years of observations; d) spatial coverage that includes the northern (warmer) part of the range of krill, particularly north of 60°S. Conversely, most studies which report an absence of directional trends are based on combinations of a) acoustic or fisheries methods; b) data series starting in the 1990s or later; c) fewer than 20 years of data; d) sampling only south of 60°S.
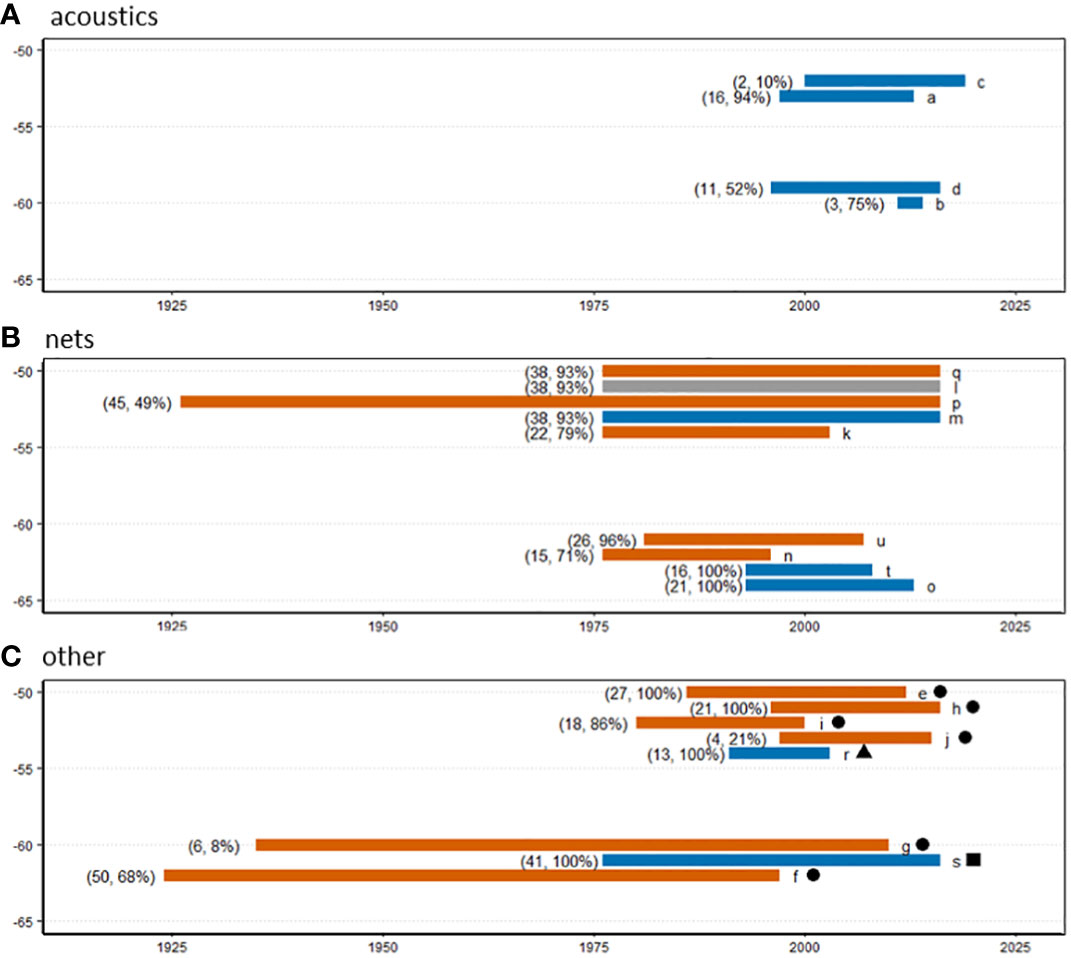
Figure 5 Summary of studies reporting on krill trends within the SW Atlantic sector, showing the time span, northern latitude (vertical axis: 5° bins) and findings of the study (orange = a negative trend, blue = no trend, grey = ambiguous). Panels group studies by data source: (A)=acoustics, (B)=nets, (C)=other methods indicated by the symbol: solid circle = availability to predators, solid triangle = fishery CPUE, and solid square = integrated assessment of multiple data sources. Additional values in parentheses are the number of years of data and the percentage of years sampled in the time span of the study. The study reference (Supplementary Table 1) is indicated by a letter on the right of each bar. In our synthesis of evidence, we have included only studies which report previously unreported data or present analyses that provide direct information about changes in indices of krill population size (including population density, biomass and recruitment strength). We have not included reviews, opinions expressed in papers without supporting data and papers only reporting trends in indirect indicators such as predator population parameters.
Beyond these factors, repeated analyses of the net-based data in KRILLBASE have either supported (Atkinson et al., 2004, 2019; 2022; Candy, 2021; Yang et al., 2021b) or not supported (Cox et al., 2018; Candy, 2021) a decline in the late twentieth century. This partly reflects differences in the selection of data for inclusion in the analysis, the covariates included, and the statistical method used. A recent analysis (Candy, 2021) presents a series of models in which the significance of a decline reported north of 60°S9 varies from highly significant (P<0.001) to non-significant (P>0.05) depending on the formulation of the model. Likewise, the statistical significance of the decline depends on whether larval or post-larval data are used and whether they are aggregated by year or multi-year period, and presented as mean densities or swarm frequency (Atkinson et al., 2022). The finding that declining mean densities of krill were reflected in declining frequencies of high (swarm) densities (Atkinson et al., 2022) requires further study, because it supports earlier suggestions (Brierley and Cox, 2015) that changes in density of aggregating species like krill are associated with changes in swarm frequency rather than swarm size.
Importantly, none of the various sampling methods provides a direct estimate of the biomass or abundance of the whole population. Predator and fishery-based indices are likely to be highly non-linear indicators, as explained in section 4. Acoustic and net surveys often miss krill dispersed in mid-water layers and at the seabed (Kane et al., 2021). Nor is there a simple relationship between numerical density (from nets) and biomass density (from acoustics). Net sampling suggests that a dramatic decline in small (~20 mm) krill over 20 years resulted in a 40% increase in mean mass of individuals, due to a significant increase in mean krill length (Atkinson et al., 2019). Thus, declines in abundance could occur with little or no parallel change in biomass. In addition, acoustic methods [typically using transducers with frequencies in the range 38-120 kHz (Krafft et al., 2021)] and net sampling have different selectivities for krill in the affected size range. Each of these factors might contribute to discrepancies between krill trends observed with acoustics and nets (Figure 5).
When trends have been reported they explain only a fraction of the observed variance (e.g. 8% (Atkinson et al., 2019)). Great inter-annual variability in recruitment translates into significant, possibly cyclic, interannual fluctuations in post-larval density. Periods of rapid change punctuated by periods of relative stability are also possible. This high variation makes secular trends difficult to evaluate statistically without long data series. The spatial scale of sampling is also important because changes in krill distribution, from inshore-offshore migration (Cleary et al., 2018) to longer-term range shifts (Atkinson et al., 2022) could mask or exaggerate changes in population size.
While we did not seek consensus on the interpretation of these data, the prevailing view of workshop participants was that uncertainty over krill trends strengthens the case for management based on repeated sampling of the krill stock. Importantly, short data series tend to have a higher risk of type II error (falsely assuming that lack of statistical support for a trend means stability). Sampling at scales much smaller than the population carries risks of both type I (falsely identifying a trend, or falsely assuming that a trend applies to the whole population) and type II error. Conversely the debate over krill trends indicates a concern that analyses of large-scale krill population trends carry a high risk of type I error. These risks are important and need to be communicated to fisheries managers. An abrupt shift to new methods could mean starting afresh with very short data series and exacerbating the type II risk.
9 Future outlook and recommendations
Workshop participants supported change in how the krill fishery is managed, with the majority identifying improved communication and the provision of data as tractable steps towards more scientifically-based management. A key priority is improving understanding of the combined impacts of climate change and fishing on krill. Other priorities, including better understanding of recruitment, spawning areas and time trends were articulated a decade ago (Flores et al., 2012) but remain largely unresolved. Consensus on time trends seems unlikely but better quantification and explanation of uncertainty in reports of both time trends and stability is required. The scale of this uncertainty is a strong argument for management that is able to adapt to change and a key requirement for such management is regularly updated information on the state of the krill stock.
There are clear benefits in new sampling methods, which can be applied to krill and other pelagic animals. These methods diversify the types of data obtained, require fewer personnel, and often have lower costs and carbon footprints than research vessel surveys. However, newer sampling methods generally focus on finer scales of space and time while tracking change, for management or ecosystem monitoring, requires larger-scale and longer-term data. Many methods could potentially be scaled up to provide such coverage, for example by developing coordinated fleets of gliders. There is a clear need to plan the transition to new methods to minimise the risk of a loss of coverage. A key risk is a lack of comparability between old and new data to the extent that it becomes impossible to disentangle ecological change from methodological change.
Coupled to these issues is the changing skill set among the scientific community. Only 65% of established researchers had handled live krill. The percentage was lower (58%) amongst early career researchers and the ability to identify pre-adult life stages was lower still (13%). Multiple complementary approaches are useful for studying a behaviourally flexible species and it is important to retain fundamental field biology skills even as science becomes more reliant on new technology. We have shown here that diversification of approaches has the added benefit of providing complementary (and sometimes alternative) insights into long-term change in the krill-based food web.
To meet these challenges, Box 1 provides a series of key solutions arising from our workshop. Continued ship-based monitoring, which includes net-sampling of live animals, is necessary to minimize the risks associated with a transition to new methods, improve understanding of demographics and physiology, and ensure that researchers are able to learn and practice handling and identification skills. This requires ship-time and experimental facilities, the cost of which has led to some key monitoring programmes (e.g. the summer AMLR surveys) being discontinued. Several acoustics-based monitoring programmes using moorings and AUVs have started in the last decade, but these use very different techniques to ship-based surveys, so lengthy intercalibration periods are necessary to allow continuity and comparability between old and new methods.
Box 1.
Potential solutions to mismatches between the information requirements for managing the krill fishery and the capabilities of new sampling methods
Improve overlap and intercalibration of new and existing methods: Despite challenges of expense and availability of research vessels, it is imperative that traditional capture of krill with nets is maintained, alongside scientific acoustic survey techniques. With acoustic krill monitoring, for example from gliders and moorings, we need to verify acoustic target identification and provide intercalibration time series. Given the importance of understanding recruitment and spawning hotspots it is vital to sample with sufficiently fine mesh nets to capture larval and juvenile krill, and that experience is retained in the community to handle live krill and to identify these stages.
Harmonise, network and upscale the capacity of national sampling programmes: Despite challenges of administrative load, national preferences and history of sampling approaches, we urgently need to scale up localised data collection efforts (for example glider tracks or survey grids, fixed moorings) to the ocean basin scales occupied by krill stocks. Multiple programmes are much easier to compare if sampling methods are similar, and looking forward, key targets for harmonising to provide better comparability of study are: mesh size and type of nets; acoustic frequencies; sampling depths of nets and moorings. Some of these best practice procedures are already in place for zooplankton (Harris et al., 2000) or exist within the Ocean Best Practices System (OBPS); these can be adapted for krill by using existing networks such as ICED or SKEG. Likewise, efforts to combine and network data (for example CCAMLR observer programme, and BIOMASS, COPEPOD and KRILLBASE databases) should be supported and funded, with open provision and FAIR (https://www.go-fair.org/fair-principles/) access to data being the default.
Make greater use of the fishing industry, as a provider of data and as a research platform: Fishing vessels are not optimised for science, have competing commercial interests and use nets too coarse for catching juvenile krill. Their great advantages, however, are in the fact that they are a cheaper method of collecting large amounts of krill year-round, year after year and the data provided by the fishery have a wide variety of uses. Currently, through the Science-Industry Forum (SIF), there are also opportunities to use fishing vessels in a more scientific capacity, for example to repeat scientific acoustic survey transects year after year (Krafft et al., 2021) and to collect krill with finer mesh nets alongside environmental data.
Cross-cutting recommendations to achieve these solutions:
Coordination: Multiple institutes and nations have Antarctic interest, typically with each country working in “their” sector. Given the circumpolar and multinational issues around krill all three of our proposed solutions require multinational organisations, including SKEG, to take the lead in coordinating research to advertise ship opportunities, promote inter-lab visits, training, harmonisation of efforts and networking of data coverage.
Calibration: many of the debates about krill highlighted in this synthesis arise from use of different methods and approaches and for all three of our proposed solutions it is imperative that the multiple methods are used alongside each other for substantial periods of time, or at the very least that objective approaches are used to compare and to intercalibrate them to better understand their strengths and weaknesses.
Training: with a shift in approaches to sample krill we need to retain traditional skills, for example in identification and ability to handle live krill for experiments. Likewise, skills in handling the large volumes of new data may need to embrace rapidly developing fields: for example, machine learning. For all these skills the training of early career researchers is paramount.
Alignment of funding: the funding routes for national research programmes around krill are diverse, varied and often short-term. Achievement of the research to enable CCAMLR to manage the fishery safely requires a much more joined-up and longer-term approach.
An ongoing issue, exacerbated by the smaller scales of newer methods, is that fieldwork focusses on localised areas, while the vast majority of the pelagic habitat is poorly sampled. To help rectify this, workshop participants strongly supported developing strategies to network individual observations and allow for easier and effective international collaboration and sharing of resources. A newly created Science-Industry Forum (SIF) aims to coordinate the use of fishing vessels as science platforms, improving access to an underused resource. Nonetheless the capabilities of fishing vessels are currently limited by the available gear and facilities and the operational priorities of the industry. Consequently, there remains a need for the science community to better coordinate its own resources. An internationally coordinated approach to addressing the major gaps in our knowledge on key pelagic species could include developing standardised methods approved through the Global Ocean Observing System (GOOS) Ocean Best Practices System (OBPS) and specialised training of ECRs. Antarctic krill research is generally funded by national governments, so there is also a need for higher-level intergovernmental agreements that support collaborative research and sharing of polar facilities. Similar agreements will be needed for other pelagic species whose distributions typically extend across political borders and into areas beyond national jurisdiction.
Author contributions
SLH: Conceptualization, Formal analysis, Funding acquisition, Methodology, Project administration, Visualization, Writing – original draft, Writing – review and editing. AA: Conceptualization, Formal analysis, Funding acquisition, Methodology, Project administration, Visualization, Writing – original draft, Writing – review and editing. JA: Writing – original draft, Writing – review and editing. AB: Writing – original draft, Writing – review and editing. SB: Writing – review and editing. KB: Writing – original draft, Writing – review and editing. AC: Writing – original draft, Writing – review and editing. JC: Writing – original draft, Writing – review and editing. RD: Writing – original draft, Writing – review and editing. SF: Writing – original draft, Writing – review and editing. HF: Writing – review and editing. JF: Writing – original draft, Writing – review and editing. SH: Writing – review and editing. JH: Writing – original draft, Writing – review and editing. LH: Writing – original draft, Writing – review and editing. NJ: Writing – review and editing. MK: Writing – original draft, Writing – review and editing. SK: Writing – original draft, Writing – review and editing. BK: Writing – original draft, Writing – review and editing. LK: Writing – original draft, Writing – review and editing. HL: Writing – original draft, Writing – review and editing. CL: Visualization, Writing – original draft. BM: Writing – original draft, Writing – review and editing. EM: Writing – original draft, Writing – review and editing. EP: Writing – review and editing. FP: Writing – original draft, Writing – review and editing. AP: Writing – original draft, Writing – review and editing. MP: Writing – original draft, Writing – review and editing. KR: Writing – original draft, Writing – review and editing. CR: Writing – original draft, Writing – review and editing. ER: Writing – review and editing. RS: Writing – original draft, Writing – review and editing. KS: Writing – original draft, Writing – review and editing. ZS: Writing – review and editing. AT: Writing – original draft, Writing – review and editing. GT: Writing – original draft, Writing – review and editing. PT: Writing – review and editing. DV: Visualization, Writing – original draft, Writing – review and editing. GW: Writing – original draft, Writing – review and editing. JX: Writing – review and editing. GY: Writing – original draft, Writing – review and editing.
Funding
The author(s) declare financial support was received for the research, authorship, and/or publication of this article. The workshop on which this MS is based, and the joint lead authors (SLH and AA) were supported by WWF UK. SLH, JF, SF, NJ, EM, GT, AB, and PT were supported by the Natural Environment Research Council (NERC) British Antarctic Survey (BAS) ALI-Science Southern Ocean Ecosystems project. KB was supported by the US National Science Foundation Office for Polar Programs, grants 1753101 and 2038145. AC is supported by the European Union’s Horizon 2020 research and innovation programme under the Marie Sklodowska-Curie grant agreement No 887760 (ParaKrill). JC was supported by the U.S. National Science Foundation Office of Polar Programs, award 2026045. HF was supported by the Helmholtz Research Program Changing Earth – Sustaining our Future, Research Field Earth & Environment, Topic 6, subtopic 6.1. LH was supported by the US National Science Foundation Office for Polar Programs, grants 2042032 and 2054963. SK was supported by the Australian Antarctic Program Project #4636. BK was supported by the IMR project KRILL (no. 14246). LK was supported by Areas Marinas Protegidas program of the Instituto Ant´rtico Chileno (AMP 24 03 052) and by Instituto Milenio BASE (ICN_2021_002). HL was supported by a Korea Polar Research Institute grant (PE22110) and the Ministry of Oceans and Fisheries (KIMST 20220547). BM was supported by the Helmholtz Research Program Changing Earth – Sustaining our Future, Research Field Earth & Environment, Topic 6, subtopic 6.1. EP was supported by Natural Sciences and Engineering Research Council (NSERC) Discovery Grant (RGPIN-2014-05107). AP was supported by ANID-FONDECYT 1210988. MP was supported by the US National Science Foundation Office for Polar Programs, award # 1443585. KS was funded through NERC Grant NE/S002502/1 (MOSAiC-SYM-PEL). AT was supported by Japan Society for the Promotion of Science Kakenhi grant 22K18432. DV was the recipient of a Tasmania Graduate Research Scholarship provided by the University of Tasmania. JX was supported by national funds through Fundação para a Ciência e Tecnologia (FCT) under the project LA/P/0069/2020 granted to the Associate Laboratory ARNET and by the MARE grant MARE UIDB/04292/2020.
Acknowledgments
The authors thank Glynn Gorick for his patience during the provision of the infographics.
Conflict of interest
The authors declare that the research was conducted in the absence of any commercial or financial relationships that could be construed as a potential conflict of interest.
Publisher’s note
All claims expressed in this article are solely those of the authors and do not necessarily represent those of their affiliated organizations, or those of the publisher, the editors and the reviewers. Any product that may be evaluated in this article, or claim that may be made by its manufacturer, is not guaranteed or endorsed by the publisher.
Supplementary material
The Supplementary Material for this article can be found online at: https://www.frontiersin.org/articles/10.3389/fmars.2024.1307402/full#supplementary-material
References
Agnew D. J. (1997). Review - the CCAMLR ecosystem monitoring programme. Antarctic Sci. 9, 235–242. doi: 10.1017/S095410209700031X
Atkinson A., Hill S. L., Pakhomov E. A., Siegel V., Anadon R., Chiba S., et al. (2017). KRILLBASE: a circumpolar database of Antarctic krill and salp numerical densities 1926–2016. Earth System Sci. Data 9, 193–210. doi: 10.5194/essd-9-193-2017
Atkinson A., Hill S. L., Pakhomov E. A., Siegel V., Reiss C. S., Loeb V. J., et al. (2019). Krill (Euphausia superba) distribution contracts southward during rapid regional warming. Nat. Clim. Change 9, 142. doi: 10.1038/s41558-018-0370-z
Atkinson A., Hill S. L., Reiss C. S., Pakhomov E. A., Beaugrand G., Tarling G. A., et al. (2022). Stepping stones towards Antarctica: Switch to southern spawning grounds explains an abrupt range shift in krill. Glob. Change Biol. 28, 1359–1375. doi: 10.1111/gcb.16009
Atkinson A., Meyer B., Kawaguchi S., Hill S., Arata J. A., Driscoll R., et al. (2021). Evaluating change within the krill-based food web and developing solutions for the future sampling of krill: Report of the online SCAR Krill Action Group (SKAG) workshop, 26-30 April 2021 (SCAR Krill Action Group (SKAG) workshop). doi: 10.5281/zenodo.4776335
Atkinson A., Nicol S., Kawaguchi S., Pakhomov E., Quetin L., Ross R., et al. (2012). Fitting Euphausia superba into Southern Ocean food-web models: a review of data sources and their limitations. CCAMLR Sci. 19, 219–245.
Atkinson A., Siegel V., Pakhomov E. A., Jessopp M. J., Loeb V. (2009). A re-appraisal of the total biomass and annual production of Antarctic krill. Deep Sea Res. I 56, 727–740. doi: 10.1016/j.dsr.2008.12.007
Atkinson A., Siegel V., Pakhomov E. A., Rothery P. (2004). Long-term decline in krill stock and increase in salps within the Southern Ocean. Nature 432, 100–103. doi: 10.1038/nature02996
Ballance L. T., Ainley D. G., Ballard G., Barton K. (2009). An energetic correlate between colony size and foraging effort in seabirds, an example of the Adélie penguin Pygoscelis adeliae. J. Avian Biol. 40, 279–288. doi: 10.1111/j.1600-048X.2008.04538.x
Basedow S. L., McKee D., Lefering I., Gislason A., Daase M., Trudnowska E., et al. (2019). Remote sensing of zooplankton swarms. Sci. Rep. 9, 686. doi: 10.1038/s41598-018-37129-x
Batta-Lona P. G., Bucklin A., Wiebe P. H., Patarnello T., Copley N. J. (2011). Population genetic variation of the Southern Ocean krill, Euphausia superba, in the Western Antarctic Peninsula region based on mitochondrial single nucleotide polymorphisms (SNPs). Deep Sea Res. Part II 58, 1652–1661. doi: 10.1016/j.dsr2.2010.11.017
Behrenfeld M. J., Gaube P., Della Penna A., O’malley R. T., Burt W. J., Hu Y., et al. (2019). Global satellite-observed daily vertical migrations of ocean animals. Nature 576, 257–261. doi: 10.1038/s41586-019-1796-9
Belcher A., Fielding S., Gray A., Biermann L., Stowasser G., Fretwell P., et al. (2021). Experimental determination of reflectance spectra of Antarctic krill (Euphausia superba) in the Scotia Sea. Antarctic Sci. 33, 402–414. doi: 10.1017/S0954102021000262
Benway H. M., Lorenzoni L., White A. E., Fiedler B., Levine N. M., Nicholson D. P., et al. (2019). Ocean time series observations of changing marine ecosystems: an era of integration, synthesis, and societal applications. Front. Mar. Sci. 6. doi: 10.3389/fmars.2019.00393
Bernard K. S., Gunther L. A., Mahaffey S. H., Qualls K. M., Sugla M., Saenz B. T., et al. (2019). The contribution of ice algae to the winter energy budget of juvenile Antarctic krill in years with contrasting sea ice conditions. ICES J. Mar. Sci. 76, 206–216. doi: 10.1093/icesjms/fsy145
Boyd C., Grünbaum D., Hunt G. L. Jr., Punt A. E., Weimerskirch H., Bertrand S. (2017). Effects of variation in the abundance and distribution of prey on the foraging success of central place foragers. J. Appl. Ecol. 54, 1362–1372. doi: 10.1111/1365-2664.12832
Brierley A. S. (2006). Use of moored acoustic instruments to measure short-term variability in abundance of Antarctic krill. Limnol. Oceanogr. Methods 4, 18–29. doi: 10.4319/lom.2006.4.18
Brierley A. S., Cox M. J. (2015). Fewer but not smaller schools in declining fish and krill populations. Curr. Biol. 25, 75–79. doi: 10.1016/j.cub.2014.10.0622015
Brierley A. S., Fernandes P. G., Brandon M. A., Armstrong F., Millard N. W., McPhail S. D., et al. (2002). Antarctic krill under sea ice: elevated abundance in a narrow band just south of ice edge. Science 295, 1890–1892. doi: 10.1126/science.1068574
Candy S. G. (2021). Long-term trend in mean density of Antarctic krill (Euphausia superba) uncertain. Annu. Res. Rev. Biol. 36, 27–43. doi: 10.9734/arrb/2021/v36i1230460
Cavan E., Belcher A., Atkinson A., Hill S., Kawaguchi S., McCormack S., et al. (2019). The importance of Antarctic krill in biogeochemical cycles. Nat. Commun. 10, 1–13. doi: 10.1038/s41467-019-12668-7
Cavanagh R. D., Melbourne-Thomas J., Grant S. M., Barnes D. K. A., Hughes K. A., Halfter S., et al. (2021a). Future risk for southern ocean ecosystem services under climate change. Front. Mar. Sci. 7. doi: 10.3389/fmars.2020.615214
Cavanagh R. D., Trathan P. N., Hill S. L., Melbourne-Thomas J., Meredith M. P., Hollyman P., et al. (2021b). Utilising IPCC assessments to support the ecosystem approach to fisheries management within a warming Southern Ocean. Mar. Policy 131, 104589. doi: 10.1016/j.marpol.2021.104589
Cimino M. A., Moline M. A., Fraser W. R., Patterson-Fraser D. L., Oliver M. J. (2016). Climate-driven sympatry may not lead to foraging competition between congeneric top-predators. Sci. Rep. 6, 18820. doi: 10.1038/srep18820
Clarke L. J., Suter L., King R., Bissett A., Deagle B. E. (2019). Antarctic krill are reservoirs for distinct southern ocean microbial communities. Front. Microbiol. 9. doi: 10.3389/fmicb.2018.03226
Clarke A., Tyler P. A. (2008). Adult Antarctic krill feeding at abyssal depths. Curr. Biol. 18, 282–285. doi: 10.1016/j.cub.2008.01.059
Cleary A. C., Durbin E. G., Casas M. C. (2018). Feeding by Antarctic krill Euphausia superba in the West Antarctic Peninsula: differences between fjords and open waters. Mar. Ecol. Progr. Ser. 595, 39–54. doi: 10.3354/meps12568
Conroy J. A., Reiss C. S., Gleiber M. R., Steinberg D. K. (2020). Linking Antarctic krill larval supply and recruitment along the Antarctic Peninsula. Integr. Comp. Biol. 60, 1386–1400. doi: 10.1093/icb/icaa111
Constable A. (2001). The ecosystem approach to managing fisheries: Achieving conservation objectives for predators of fished species. CCAMLR Sci. 8, 37–64.
Constable A. J., Melbourne-Thomas J., Corney S. P., Arrigo K., Barbraud C., Barnes D., et al. (2014). Climate Change and Southern Ocean ecosystems I: How changes in physical habitats directly affect marine biota. Glob. Change Biol. 20, 3004–3025. doi: 10.1111/gcb.12623
Cox M. J., Candy S., de la Mare W. K., Nicol S., Kawaguchi S., Gales N. (2018). No evidence for a decline in the density of Antarctic krill Euphausia superba Dana 1850, in the Southwest Atlantic sector between 1976 and 2016. J. Crustacean Biol. 38, 656–661. doi: 10.1093/jcbiol/ruy072
Cox M. J., Candy S., de la Mare W. K., Nicol S., Kawaguchi S., Gales N. (2019). Clarifying trends in the density of Antarctic krill Euphausia superba Dana 1850 in the South Atlantic. A response to Hill et al. J. Crustacean Biol. 39, 323–327. doi: 10.1093/jcbiol/ruz010
Deagle B. E., Faux C., Kawaguchi S., Meyer B., Jarman S. N. (2015). Antarctic krill population genomics: apparent panmixia, but genome complexity and large population size muddy the water. Mol. Ecol. 24, 4943–4959. doi: 10.1111/mec.13370
De Robertis A., Schell C., Jaffe J. S. (2003). Acoustic observations of the swimming behavior of the euphausiid Euphausia pacifica Hansen. ICES J. Mar. Sci. 60, 885–898. doi: 10.1016/S1054-3139(03)00070-5
Dong Y., Qi G., Feng C., Wang W., Zhang F., Zhao M., et al. (2019). Genetic diversity and structure of Euphausia superba in the South Shetland Islands using the mitochondrial ND6 gene. Crustaceana 92, 1295–1309. doi: 10.1163/15685403-00003950
Ducklow H. W., Baker K., Martinson D. G., Quetin L. B., Ross R. M., Smith R. C., et al. (2007). Marine pelagic ecosystems: the West Antarctic Peninsula. Philos. T. R. Soc Lon. B 362, 67–94. doi: 10.1098/rstb.2006.1955
Emslie S. D., Polito M., Brasso R., Patterson W., Sun L. (2014). Ornithogenic soils and the paleoecology of pygoscelid penguins in Antarctica. Quat. Int. 352, 4–15. doi: 10.1016/j.quaint.2014.07.031
Ericson J. A., Hellessey N., Nichols P. D., Kawaguchi S., Nicol S., Hoem N., et al. (2018). Seasonal and interannual variations in the fatty acid composition of adult Euphausia superba Dana 1850 (Euphausiacea) samples derived from the Scotia Sea krill fishery. J. Crustacean Biol. 38, 662–672. doi: 10.1093/jcbiol/ruy032
Fielding S., Watkins J. L., Trathan P. N., Enderlein P., Waluda C. M., Stowasser G., et al. (2014). Interannual variability in Antarctic krill (Euphausia superba) density at South Georgia, Southern Ocean: 1997–2013. ICES J. Mar. Sci. 71, 2578–2588. doi: 10.1093/icesjms/fsu104
Flores H., Atkinson A., Kawaguchi S., Krafft B. A., Milinevsky G., Nicol S., et al. (2012). Impact of climate change on Antarctic krill. Mar. Ecol. Progr. Ser. 458, 1–19. doi: 10.3354/meps09831
Forcada J., Trathan P. N., Murphy E. J. (2008). Life history buffering in Antarctic mammals and birds against changing patterns of climate and environmental variation. Global Change Biol. 14, 2473–2488. doi: 10.1111/j.1365-2486.2008.01678.x
Fraser F. C. (1936). On the development and distribution of the young stages of krill (Euphausia superba). Disc. Rep. 14, 1–192. doi: 10.5962/bhl.part.3886
Goodall-Copestake W., Perez-Espona S., Clark M., Murphy E., Seear P., Tarling G. (2010). Swarms of diversity at the gene cox1 in Antarctic krill. Heredity 104, 513–518. doi: 10.1038/hdy.2009.188
Guihen D., Fielding S., Murphy E. J., Heywood K. J., Griffiths G. (2014). An assessment of the use of ocean gliders to undertake acoustic measurements of zooplankton: the distribution and density of Antarctic krill (Euphausia superba) in the Weddell Sea. Limnol. Oceanogr.: Meth. 12, 373–389. doi: 10.4319/lom.2014.12.373
Gutt J., Siegel V. (1994). ). Benthopelagic aggregations of krill (Euphausia superba) on the deeper shelf of the Weddell Sea (Antarctic). Deep Sea Res. Part I 41, 169–178. doi: 10.1016/0967-0637(94)90031-0
Hagen W., Kattner G., Terbrüggen A., Van Vleet E. (2001). Lipid metabolism of the Antarctic krill Euphausia superba and its ecological implications. Mar. Biol. 139, 95–104. doi: 10.1007/s002270000527
Hamner W. M., Hamner P. P. (2000). Behavior of Antarctic krill (Euphausia superba): schooling, foraging, and antipredatory behavior. Can. J. Fish. Aquat. Sci. 57, 192–202. doi: 10.1139/f00-195
Hamner W. M., Hamner P. P., Strand S. W., Gilmer R. W. (1983). Behavior of Antarctic krill, Euphausia superba: chemoreception, feeding, schooling, and molting. Science 220, 433–435. doi: 10.1126/science.220.4595.433
Handley J. M., Thiebault A., Stanworth A., Schutt D., Pistorius P. (2018). Behaviourally mediated predation avoidance in penguin prey: in situ evidence from animal-borne camera loggers. R. Soc Open Sci. 5, 171449. doi: 10.1098/rsos.171449
Hann A. (2021). Southern ocean science and society: case studies at the nexus of research, education, and policy (Oregon State University, USA: Master of Science thesis).
Harris R., Wiebe P., Lenz J., Skjoldal H.-R., Huntley M. (2000). ICES zooplankton methodology manual. (London: Elsevier Academic Press).
Henley S. F., Cavan E. L., Fawcett S. E., Kerr R., Monteiro T., Sherrell R. M., et al. (2020). Changing biogeochemistry of the Southern Ocean and its ecosystem implications. Front. Mar. Sci. 7. doi: 10.3389/fmars.2020.00581
Hewitt R. P., Watkins J., Naganobu M., Sushin V., Brierley A. S., Demer D., et al. (2004). Biomass of Antarctic krill in the Scotia Sea in January/February 2000 and its use in revising an estimate of precautionary yield. Deep Sea Res. II 51, 1215–1236. doi: 10.1016/S0967-0645(04)00076-1
Hill S., Atkinson A., Darby C., Fielding S., Krafft B. A., Godo O., et al. (2016). Is current management of the Antarctic krill fishery in the Atlantic sector of the Southern Ocean precautionary? CCAMLR Sci. 23, 31–51.
Hill S. L., Atkinson A., Pakhomov E. A., Siegel V. (2019). Evidence for a decline in the population density of Antarctic krill Euphausia superba still stands. A comment on Cox et al. J. Crustacean Biol. 39, 316–322. doi: 10.1093/jcbiol/ruz004
Hill S. L., Hinke J., Bertrand S., Fritz L., Furness R. W., Ianelli J. N., et al. (2020). Reference points for predators will progress ecosystem-based management of fisheries. Fish Fisheries 21, 368–378. doi: 10.1111/faf.12434
Hindell M. A., Reisinger R. R., Yan Ropert-Coudert Y., Hückstädt L. A., Trathan P. N., Bornemann H., et al. (2020). Tracking of marine predators to protect Southern Ocean ecosystems. Nature 580, 87–92. doi: 10.1038/s41586-020-2126-y
Hinke J. T., Salwicka K., Trivelpiece S. G., Watters G. M., Trivelpiece W. Z. (2007). Divergent responses of Pygoscelis penguins reveal a common environmental driver. Oecologia 153, 845. doi: 10.1007/s00442-007-0781-4
Hirota Y. (1984). Feeding of euphausiids in the plankton net after capture. Bull. Plankton Soc Japan 31, 53–60.
Hopkins T. L., Ainley D. G., Torres J. J., Lancraft T. M. (1993a). Trophic structure in open waters of the marginal ice zone in the Scotia-Weddell confluence region during spring, (1983). Polar Biol. 13, 389–397. doi: 10.1007/BF01681980
Hopkins T. L., Lancraft T. M., Torres J. J., Donnelly J. (1993b). Community structure and trophic ecology of zooplankton in the Scotia Sea marginal ice zone in winter, (1988). Deep Sea Res. Part I 40, 81–105. doi: 10.1016/0967-0637(93)90054-7
Hückstädt L. A., Piñones A., Palacios D. M., McDonald B. I., Dinniman M. S., Hofmann E. E., et al. (2020). Projected shifts in the foraging habitat of crabeater seals along the Antarctic Peninsula. Nat. Clim. Change 10, 472–477. doi: 10.1038/s41558-020-0745-9
Hunt B. J., Özkaya Ö., Davies N. J., Gaten E., Seear P., Kyriacou C. P., et al. (2017). The Euphausia superba transcriptome database, Superba SE: An online, open resource for researchers. Ecol. Evol. 7, 6060–6077. doi: 10.1002/ece3.3168
Jaffe J., Ohman M., De Robertis A. (1998). OASIS in the sea: measurement of the acoustic reflectivity of zooplankton with concurrent optical imaging. Deep Sea Res. II 45, 1239–1253. doi: 10.1016/S0967-0645(98)00030-7
Jarman S. N., Deagle B. E. (2016). “Genetics of antarctic krill,” in Biology and Ecology of Antarctic Krill. (Switzerland: Springer), 247–277.
Jeffery N. W. (2012). The first genome size estimates for six species of krill (Malacostraca, Euphausiidae): large genomes at the north and south poles. Polar Biol. 35, 959–962. doi: 10.1007/s00300-011-1137-4
Jia Z., Swadling K. M., Meiners K. M., Kawaguchi S., Virtue P. (2016). The zooplankton food web under East Antarctic pack ice–A stable isotope study. Deep Sea Res. II 131, 189–202. doi: 10.1016/j.dsr2.2015.10.010
Johnson K. M., McKay R. M., Etourneau J., Jiménez-Espejo F. J., Albot A., Riesselman C. R., et al. (2021). Sensitivity of Holocene East Antarctic productivity to subdecadal variability set by sea ice. Nat. Geosci. 14, 762–768. doi: 10.1038/s41561-021-00816-y
Johnston N. M., Murphy E. J., Atkinson A., Constable A. J., Cotté C., Cox M., et al. (2022). Status, change, and futures of zooplankton in the southern ocean. Front. Ecol. Evol. 9. doi: 10.3389/fevo.2021.624692
Kalvakaalva R., Clucas G., Herman R. W., Polito M. J. (2020). Late Holocene variation in the Hard prey remains and stable isotope values of penguin and seal tissues from the Danger Islands, Antarctica. Polar Biol. 43, 1571–1582. doi: 10.1007/s00300-020-02728-w
Kane M. K., Atkinson A., Menden-Deuer S. (2021). Lowered cameras reveal hidden behaviors of Antarctic krill. Curr. Biol. 31, R237–R238. doi: 10.1016/j.cub.2021.01.091
Kane M. K., Yopak R., Roman C., Menden-Deuer S. (2018). Krill motion in the Southern Ocean: quantifying in situ krill movement behaviors and distributions during the late austral autumn and spring. Limnol. Oceanogr. 63, 2839–2857. doi: 10.1002/lno.11024
Kawaguchi S., Atkinson A., Bahlburg D., Bernard K. S., Cavan E. L., Cox M. J., et al. (2024). Climate change impacts on Antarctic krill behaviour and population dynamics. Nat. Rev. Earth Environ. 5, 43–58. doi: 10.1038/s43017-023-00504-y
Kawaguchi S., Kilpatrick R., Roberts L., King R. A., Nicol S. (2011). Ocean-bottom krill sex. J. Plankton Res. 33, 1134–1138. doi: 10.1093/plankt/fbr006
Kawaguchi K., Matsuda O., Ishikawa S., Naito Y. (1986). A light trap to collect krill and other micronektonic and planktonic animals under the Antarctic coastal fast ice. Polar Biol. 6, 37–42. doi: 10.1007/BF00446238
Kawaguchi S., Nicol S., Virtue P., Davenport S. R., Casper R., Swadling K. M., et al. (2010). Krill demography and large-scale distribution in the Western Indian Ocean sector of the Southern Ocean (CCAMLR Division 58.4. 2) in Austral summer of 2006. Deep Sea Res. II 57, 934–947. doi: 10.1016/j.dsr2.2008.06.014
Keates T. R., Kudela R. M., Holser R. R., Hückstädt L. A., Simmons S. E., Costa D. P. (2020). Chlorophyll fluorescence as measured in situ by animal-borne instruments in the northeastern Pacific Ocean. J. Mar. Syst. 203, 103265. doi: 10.1016/j.jmarsys.2019.103265
Kils U. (1981). Swimming behaviour, swimming performance and energy balance of Antarctic krill Euphausia superba. Biomass Sci. Ser. 3, 1–121.
Kintisch E. (2013). The new generation of sea scientist. Science 339, 1141–1141. doi: 10.1126/science.339.6124.1141
Kinzey D., Watters G. M., Reiss C. S. (2018). Parameter estimation using randomized phases in an integrated assessment model for Antarctic krill. PloS One 13, e0202545. doi: 10.1371/journal.pone.0202545
Klevjer T. A., Kaartvedt S. (2003). Split-beam target tracking can be used to study the swimming behaviour of deep-living plankton in situ. Aquat. Living Resour. 16, 293–298. doi: 10.1016/S0990-7440(03)00013-5
Klevjer T. A., Kaartvedt S. (2006). In situ target strength and behaviour of northern krill (Meganyctiphanes norvegica). ICES J. Mar. Sci. 63, 1726–1735. doi: 10.1016/j.icesjms.2006.06.013
Klevjer T. A., Kaartvedt S. (2011). Krill (Meganyctiphanes norvegica) swim faster at night. Limnol. Oceanogr. 56, 765–774. doi: 10.4319/lo.2011.56.3.0765
Kokubun N., Kim J.-H., Takahashi A. (2013). Proximity of krill and salps in an Antarctic coastal ecosystem: evidence from penguin-mounted cameras. Polar Biol. 36, 1857–1864. doi: 10.1007/s00300-013-1400-y
Kokubun N., Tanabe Y., Hirano D., Mensah V., Tamura T., Aoki S., et al. (2021). Shoreward intrusion of oceanic surface waters alters physical and biological ocean structures on the Antarctic continental shelf during winter: Observations from instrumented seals. Limnol. Oceanogr. 66, 3740–3753. doi: 10.1002/lno.11914
Krafft B. A., Krag L. A., Knutsen T., Skaret G., Jensen K. H. M., Krakstad J. O., et al. (2018). Summer distribution and demography of Antarctic krill Euphausia superba Dana 1850 (Euphausiacea) at the South Orkney Islands 2011–2015. J. Crustacean Biol. 38, 682–688. doi: 10.1093/jcbiol/ruy061
Krafft B. A., Macaulay G. J., Skaret G., Knutsen T., Bergstad O. A., Lowther A., et al. (2021). Standing stock of Antarctic krill (Euphausia superba Dana 1850)(Euphausiacea) in the Southwest Atlantic sector of the Southern Ocean 2018–19. J. Crustacean Biol. 41, ruab046. doi: 10.1093/jcbiol/ruab071
Krüger L. (2019). Spatio-temporal trends of the Krill fisheries in the Western Antarctic Peninsula and Southern Scotia Arc. Fish. Manage. Ecol. 26, 327–333. doi: 10.1111/fme.12363
Kubilius R., Ona E., Calise L. (2015). Measuring in situ krill tilt orientation by stereo photogrammetry: examples for Euphausia superba and Meganyctiphanes norvegica. ICES J. Mar. Sci. 72, 2494–2505. doi: 10.1093/icesjms/fsv077
La H., Ha H. K., Kang C. Y., Wåhlin A., Shin H. C. (2015). Acoustic backscatter observations with implications for seasonal and vertical migrations of zooplankton and nekton in the Amundsen shelf (Antarctica). Estuar. Coast. Shelf Sci. 152, 124–133. doi: 10.1016/j.ecss.2014.11.020
La H. S., Park K., Wåhlin A., Arrigo K. R., Kim D. S., Yang E. J., et al. (2019). Zooplankton and micronekton respond to climate fluctuations in the Amundsen Sea polynya, Antarctica. Sci. Rep. 9, 1–7. doi: 10.1038/s41598-019-46423-1
Lawson G. L., Wiebe P. H., Ashjian C. J., Stanton T. K. (2008). Euphausiid distribution along the Western Antarctic Peninsula—Part B: distribution of euphausiid aggregations and biomass, and associations with environmental features. Deep Sea Res. II 55, 432–454. doi: 10.1016/j.dsr2.2007.11.014
Letessier T. B., Kawaguchi S., King R., Meeuwig J. J., Harcourt R., Cox M. J. (2013). A robust and economical underwater stereo video system to observe Antarctic krill (Euphausia superba). Open J. Mar. Sci. 3, 148–153. doi: 10.4236/ojms.2013.33016
Lowther A. D., Trathan P., Tarroux A., Lydersen C., Kovacs K. M. (2018). The relationship between coastal weather and foraging behaviour of chinstrap penguins, Pygoscelis Antarctica. ICES J. Mar. Sci. 75, 1940–1948. doi: 10.1093/icesjms/fsy061
Mackintosh N. A. (1972). Life cycle of Antarctic krill in relation to ice and water conditions. Discovery Rep. 36, 1–94.
Mackintosh N. A. (1973). Distribution of post-larval krill in the Antarctic. Discovery Rep. 36, 95–156.
Manno C., Fielding S., Stowasser G., Murphy E., Thorpe S., Tarling G. (2020). Continuous moulting by Antarctic krill drives major pulses of carbon export in the north Scotia Sea, Southern Ocean. Nat. Commun. 11, 1–8. doi: 10.1038/s41467-020-19956-7
Marr J. W. S. (1962). The natural history and geography of the Antarctic krill (Euphausia superba Dana). Discovery Rep. 32, 33–464.
Marschall H.-P. (1988). The overwintering strategy of Antarctic krill under the pack-ice of the Weddell Sea. Polar Biol. 9, 129–135. doi: 10.1007/BF00442041
Martin D. L., Ross R. M., Quetin L. B., Murray A. E. (2006). Molecular approach (PCR-DGGE) to diet analysis in young Antarctic krill Euphausia superba. Mar. Ecol. Progr. Ser. 319, 155–165. doi: 10.3354/meps319155
Martins M. J. F., Lago-Leston A., Anjos A., Duarte C. M., Agusti S., Serrão E. A., et al. (2015). A transcriptome resource for Antarctic krill (Euphausia superba Dana) exposed to short-term stress. Mar. Genomics 23, 45–47. doi: 10.1016/j.margen.2015.04.008
Maschette D., Sumner M., Raymond B. (2019). SOmap: Southern Ocean maps. Available online at: https://github.com/AustralianAntarcticDivision/SOmapVersion.
McCormack S. A., Melbourne-Thomas J., Trebilco R., Blanchard J. L., Constable A. (2020). Alternative energy pathways in Southern Ocean food webs: Insights from a balanced model of Prydz Bay, Antarctica. Deep Sea Res. Part II 174, 104613. doi: 10.1016/j.dsr2.2019.07.001
McMahon K. W., Michelson C. I., Hart T., McCarthy M. D., Patterson W. P., Polito M. J. (2019). Divergent trophic responses of sympatric penguin species to historic anthropogenic exploitation and recent climate change. Proc. Natl. Acad. Sci. U.S.A. 116, 25721–25727. doi: 10.1073/pnas.1913093116
Meiners K., Arndt S., Bestley S., Krumpen T., Ricker R., Milnes M., et al. (2017). Antarctic pack ice algal distribution: Floe-scale spatial variability and predictability from physical parameters. Geophys. Res. Lett. 44, 7382–7390. doi: 10.1002/2017GL074346
Meredith M., Sommerkorn M., Cassota S., Derksen C., Ekaykin A., Hollowed A., et al. (2019). “Polar regions,” in IPCC special report on the ocean and cryosphere in a changing climate. Eds. Pörtner D.C.R.H.-O., Masson-Delmotte V., Zhai P., Tignor M., Poloczanska E., Mintenbeck K., Alegría A., Nicolai M., Okem A., Petzold J., Rama B., Weyer. N. M. (Cambridge University Press, Cambridge, UK and New York, NY, USA), 203–320.
Meyer B., Atkinson A., Bernard K. S., Brierley A. S., Driscoll R., Hill S. L., et al. (2020). Successful ecosystem-based management of Antarctic krill should address uncertainties in krill recruitment, behaviour and ecological adaptation. Commun. Earth Environ. 1, 1–12. doi: 10.1038/s43247-020-00026-1
Meyer B., Freier U., Grimm V., Groeneveld J., Hunt B. P., Kerwath S., et al. (2017). The winter pack-ice zone provides a sheltered but food-poor habitat for larval Antarctic krill. Nat. Ecol. Evol. 1, 1853–1861. doi: 10.1038/s41559-017-0368-3
Meyer B., Fuentes V., Guerra C., Schmidt K., Atkinson A., Spahic S., et al. (2009). Physiology, growth, and development of larval krill Euphausia superba in autumn and winter in the Lazarev Sea, Antarctica. Limnology Oceanography 54, 1595–1614. doi: 10.4319/lo.2009.54.5.1595
Morley S. A., Abele D., Barnes D. K., Cárdenas C. A., Cotté C., Gutt J., et al. (2020). Global drivers on Southern Ocean ecosystems: changing physical environments and anthropogenic pressures in an earth system. Front. Mar. Sci. 7. doi: 10.3389/fmars.2020.547188
Mulvaney R., Abram N. J., Hindmarsh R. C., Arrowsmith C., Fleet L., Triest J., et al. (2012). Recent Antarctic Peninsula warming relative to Holocene climate and ice-shelf history. Nature 489, 141–144. doi: 10.1038/nature11391
Murphy E. J., Trathan P. N., Watkins J. L., Reid K., Meredith M. P., Forcada J., et al. (2007). Climatically driven fluctuations in Southern Ocean ecosystems. P. R. Soc B 274, 3057–3067. doi: 10.1098/rspb.2007.1180
Murphy E., Watkins J., Meredith M., Ward P., Trathan P., Thorpe S. (2004). Southern Antarctic Circumpolar Current Front to the northeast of South Georgia: horizontal advection of krill and its role in the ecosystem. J. Geophys. Res. 109. doi: 10.1029/2002JC001522
Nardelli S. C., Cimino M. A., Conroy J. A., Fraser W. R., Steinberg D. K., Schofield O. (2021). Krill availability in adjacent Adélie and gentoo penguin foraging regions near Palmer Station, Antarctica. Limnol. Oceanogr. 66, 2234–2250. doi: 10.1002/lno.11750
Nicol S., Brierley A. S. (2010). Through a glass less darkly—new approaches for studying the distribution, abundance and biology of euphausiids. Deep Sea Res. Part II 57, 496–507. doi: 10.1016/j.dsr2.2009.10.002
Nicol S., Foster J. (2016). “The fishery for Antarctic krill: Its current status and management regime,” in Biology and Ecology of Antarctic Krill. Ed. Siegel. V. (Cham. Switzerland: Springer), 387–421.
Nicol S., Kitchener J., King R., Hosie G., William K. (2000). Population structure and condition of Antarctic krill (Euphausia superba) off East Antarctica (80–150 E) during the Austral summer of 1995/1996. Deep Sea Res. II 47, 2489–2517. doi: 10.1016/S0967-0645(00)00033-3
Nordhausen W. (1994). Winter abundance and distribution of Euphausia superba, E. crystallorophias, and Thysanoessa macrura in Gerlache Strait and Crystal Sound, Antarctica. Mar. Ecol. Progr. Ser. 109, 131–131. doi: 10.3354/meps109131
O’Brien D. (1987). Description of escape responses of krill (Crustacea: Euphausiacea), with particular reference to swarming behavior and the size and proximity of the predator. J. Crustacean Biol. 7, 449–457. doi: 10.2307/1548294
Ohman M. D., Davis R. E., Sherman J. T., Grindley K. R., Whitmore B. M., Nickels C. F., et al. (2019). Zooglider: an autonomous vehicle for optical and acoustic sensing of zooplankton. Limnol. Oceanogr.- Meth. 17, 69–86. doi: 10.1002/lom3.10301
Passmore A., Jarman S., Swadling K., Kawaguchi S., McMinn A., Nicol S. (2006). DNA as a dietary biomarker in Antarctic krill, Euphausia superba. Mar. Biotechnol. 8, 686–696. doi: 10.1007/s10126-005-6088-8
Pauli N.-C., Metfies K., Pakhomov E. A., Neuhaus S., Graeve M., Wenta P., et al. (2021). Selective feeding in Southern Ocean key grazers—diet composition of krill and salps. Commun. Biol. 4, 1–12. doi: 10.1038/s42003-021-02581-5
Peel D., Bravington M., Kelly N., Wood S. N., Knuckey I. (2013). A model-based approach to designing a fishery-independent survey. J. Agric. Biol. Environ. Stat. 18, 1–21. doi: 10.1007/s13253-012-0114-x
Perry F. A., Atkinson A., Sailley S. F., Tarling G. A., Hill S. L., Lucas C. H., et al. (2019). Habitat partitioning in Antarctic krill: Spawning hotspots and nursery areas. PloS One 14, e0219325. doi: 10.1371/journal.pone.0219325
Quetin L. B., Ross R. M. (2001). Environmental variability and its impact on the reproductive cycle of Antarctic krill. Am. Zool. 41, 74–89. doi: 10.1093/icb/41.1.74
Rau G. H., Ohman M. D., Pierrot-Bults A. (2003). Linking nitrogen dynamics to climate variability off central California: a 51 year record based on 15N/14N in CalCOFI zooplankton. Deep Sea Res. Part II 50, 2431–2447. doi: 10.1016/S0967-0645(03)00128-0
RCoreTeam. (2021). R: a language and environment for statistical computing. (R Foundation for Statistical Computing, Viena R Core Team). Available at: https://www.R-project.org/.
Reiss C. S., Cossio A. M., Loeb V., Demer D. A. (2008). Variations in the biomass of Antarctic krill (Euphausia superba) around the South Shetland Islands 1996–2006. ICES J. Mar. Sci. 65, 497–508. doi: 10.1093/icesjms/fsn033
Reiss C. S., Cossio A., Santora J. A., Dietrich K. S., Murray A., Mitchell B. G., et al. (2017). Overwinter habitat selection by Antarctic krill under varying sea-ice conditions: implications for top predators and fishery management. Mar. Ecol. Prog. Ser. 568, 1–16. doi: 10.3354/meps12099
Reiss C. S., Cossio A. M., Walsh J., Cutter G. R., Watters G. M. (2021). Glider-Based estimates of meso-zooplankton biomass density: a fisheries case study on antarctic krill (Euphausia superba) around the northern antarctic peninsula. Front. Mar. Sci. 8. doi: 10.3389/fmars.2021.604043
Reiss C. S., Hinke J. T., Watters G. M. (2020). Demographic and maturity patterns of Antarctic krill (Euphausia superba) in an overwintering hotspot. Polar Biol. 43, 1233–1245. doi: 10.1007/s00300-020-02704-4
Ribic C. A., Chapman E., Fraser W. R., Lawson G. L., Wiebe P. H. (2008). Top predators in relation to bathymetry, ice and krill during austral winter in Marguerite Bay, Antarctica. Deep Sea Res. Part II 55, 485–499. doi: 10.1016/j.dsr2.2007.11.006
Rogers A., Frinault B., Barnes D., Bindoff N., Downie R., Ducklow H., et al. (2020). Antarctic futures: an assessment of climate-driven changes in ecosystem structure, function, and service provisioning in the Southern Ocean. Annu. Rev. Mar. Sci. 12, 87–120. doi: 10.1146/annurev-marine-010419-011028
Rombolá E., Franzosi C., Tosonotto G., Vivequin S., Alder V., Marschoff E. (2021). Density and distribution of euphausiid larvae in the Scotia Sea in the 2011 summer. Polar Biol. 44, 783–794. doi: 10.1007/s00300-021-02836-1
Ross R. M., Quetin L. B., Newberger T., Shaw C. T., Jones J. L., Oakes S. A., et al. (2014). Trends, cycles, interannual variability for three pelagic species west of the Antarctic Peninsula 1993-2008. Mar. Ecol. Progr. Ser. 515, 11–32. doi: 10.3354/meps10965
Saba G. K., Fraser W. R., Saba V. S., Iannuzzi R. A., Coleman K. E., Doney S. C., et al. (2014). Winter and spring controls on the summer food web of the coastal West Antarctic Peninsula. Nat. Commun. 5, 4318. doi: 10.1038/ncomms5318
Sales G., Deagle B. E., Calura E., Martini P., Biscontin A., De Pittà C., et al. (2017). KrillDB: A de novo transcriptome database for the Antarctic krill (Euphausia superba). PloS One 12, e0171908. doi: 10.1371/journal.pone.0171908
Santa Cruz F., Ernst B., Arata J. A., Parada C. (2018). Spatial and temporal dynamics of the Antarctic krill fishery in fishing hotspots in the Bransfield Strait and South Shetland Islands. Fish. Res. 208, 157–166. doi: 10.1016/j.fishres.2018.07.020
Santa Cruz F., Krüger L., Cárdenas C. A. (2022). Spatial and temporal catch concentrations for Antarctic krill: Implications for fishing performance and precautionary management in the Southern Ocean. Ocean Coast. Manage. 223, 106146. doi: 10.1016/j.ocecoaman.2022.106146
Saunders R. A. (2007). Ecological investigations of euphausiids at high latitudes (PhD, University of St. Andrews, St. Andrews, Scotland).
Saunders R., Brierley A., Watkins J. L., Reid K., Murphy E. J., Enderlein P., et al. (2007). Intra-annual variability in the density of Antarctic krill (Euphausia superba) at South Georgia 2002–2005: within-year variation provides a new framework for interpreting previous A’nnual’estimates of krill density. CCAMLR Sci. 14, 27–41.
SC-CCAMLR (2019). CCAMLR report of the thirty-eight meeting of the scientific committee, of the commission for the conservation of antarctic marine living resources, CCAMLR (Hobart, Australia).
SC-CCAMLR (2023). CCAMLR report of the fortieth meeting of the scientific committee, of the commission for the conservation of antarctic marine living resources, CCAMLR (Hobart, Australia).
Schaafsma F., David C., Pakhomov E., Hunt B. P., Lange B., Flores H., et al. (2016). Size and stage composition of age class 0 Antarctic krill (Euphausia superba) in the ice–water interface layer during winter/early spring. Polar Biol. 39, 1515–1526. doi: 10.1007/s00300-015-1877-7
Schmid M. S., Aubry C., Grigor J., Fortier L. (2016). The LOKI underwater imaging system and an automatic identification model for the detection of zooplankton taxa in the Arctic Ocean. Methods Oceanogr. 15, 129–160. doi: 10.1016/j.mio.2016.03.003
Schmidt K., Atkinson A., Pond D. W., Ireland L. C. (2014). Feeding and overwintering of Antarctic krill across its major habitats: The role of sea ice cover, water depth, and phytoplankton abundance. Limnol. Oceanogr. 59, 17–36. doi: 10.4319/lo.2014.59.1.0017
Schmidt K., Atkinson A., Steigenberger S., Fielding S., Lindsay M. C., Pond D. W., et al. (2011). Seabed foraging by Antarctic krill: Implications for stock assessment, bentho-pelagic coupling, and the vertical transfer of iron. Limnol. Oceanogr. 56, 1411–1428. doi: 10.4319/lo.2011.56.4.1411
Schmidt K., Brown T. A., Belt S. T., Ireland L. C., Taylor K. W., Thorpe S. E., et al. (2018). Do pelagic grazers benefit from sea ice? Insights from the Antarctic sea ice proxy IPSO 25. Biogeosci. 15, 1987–2006. doi: 10.5194/bg-15-1987-2018
Schmidt K., Schlosser C., Atkinson A., Fielding S., Venables H. J., Waluda C. M., et al. (2016). Zooplankton gut passage mobilizes lithogenic iron for ocean productivity. Curr. Biol. 26, 2667–2673. doi: 10.1016/j.cub.2016.07.058
Schofield O., Kohut J., Aragon D., Creed L., Graver J., Haldeman C., et al. (2007). Slocum gliders: Robust and ready. J. Field Robot. 24, 473–485. doi: 10.1002/rob.20200
Siegel V. (1986). Untersuchungen zur Biologie des antarktischen Krill, Euphausia superba, im Bereich der Bransfield Strasse und angrenzender Gebiete (Braunschweig: Institut für Seefischerei der Bundesforschungsanstalt für Fischerei).
Siegel V., Watkins J. L. (2016). “Distribution, biomass and demography of Antarctic krill, Euphausia superba,” in The Biology and Ecology of Antarctic Krill, Euphausia superba. Ed. Siegel. V. (Switzerland: Springer), 21–100.
Siegert M., Atkinson A., Banwell A., Brandon M., Convey P., Davies B., et al. (2019). The Antarctic Peninsula under a 1.5 C global warming scenario. Front. Environ. Sci. 7. doi: 10.3389/fenvs.2019.00102
Steinke K., Bernard K., Ross R., Quetin L. (2021). Environmental drivers of the physiological condition of mature female Antarctic krill during the spawning season: implications for krill recruitment. Mar. Ecol. Prog. Ser. 669, 65–82. doi: 10.3354/meps13720
Stretch J. J., Hamner P. P., Hamner W. M., Michel W. C., Cook J., Sullivan C. W. (1988). Foraging behavior of Antarctic krill Euphausia superba on sea ice microalgae. Mar. Ecol. Prog. Ser. 44, 131–139. doi: 10.3354/meps044131
Sumner M. D. (2018). Raadtools: Tools for synoptic environmental spatial data. Available online at: https://github.com/AustralianAntarcticDivision/raadtools.
Tarling G. A., Fielding S. (2016). “Swarming and behaviour in Antarctic krill,” in Biology and Ecology of Antarctic Krill. Ed. Siegel. V. (Switzerland: Springer), 279–319.
Taylor F., Sjunneskog C. (2002). Postglacial marine diatom record of the palmer deep, antarctic peninsula (odp leg 178, site 1098) 2. Diatom assemblages. Paleoceanogr. 17. doi: 10.1029/2000PA000564
Testor P., de Young B., Rudnick D., Glenn S., Hayes D., Lee C., et al. (2019). OceanGliders: a component of the integrated GOOS. Frontiers in Marine Science. Front. Mar. Sci. 6. doi: 10.3389/fmars.2019.00422
van Franeker J. A., Flores H., Van Dorssen M. (2009). “The surface and under ice trawl (SUIT),” in Frozen desert alive - the role of sea ice for pelagic macrofauna and its predators. Ed. Flores. H. (PhD thesis. University of Groningen, Groningen, Netherlands), 181–188.
Veytia D., Bestley S., Kawaguchi S., Meiners K. M., Murphy E. J., Fraser A. D., et al. (2021). Overwinter sea-ice characteristics important for Antarctic krill recruitment in the southwest Atlantic. Ecol. Indic. 129, 107934. doi: 10.1016/j.ecolind.2021.107934
Vorrath M.-E., Müller J., Rebolledo L., Cárdenas P., Shi X., Esper O., et al. (2020). Sea ice dynamics in the Bransfield Strait, Antarctic Peninsula, during the past 240 years: a multi-proxy intercomparison study. Clim. Past 16, 2459–2483. doi: 10.5194/cp-16-2459-2020
Waggitt J. J., Cazenave P. W., Howarth L. M., Evans P. G., van der Kooij J., Hiddink J. G. (2018). Combined measurements of prey availability explain habitat selection in foraging seabirds. Biol. Lett. 14, 20180348. doi: 10.1098/rsbl.2018.0348
Walsh J., Reiss C. S., Watters G. M. (2020). Flexibility in Antarctic krill Euphausia superba decouples diet and recruitment from overwinter sea-ice conditions in the northern Antarctic Peninsula. Mar. Ecol. Prog. Ser. 642, 1–19. doi: 10.3354/meps13325
Watanabe Y. Y., Ito K., Kokubun N., Takahashi A. (2020). Foraging behavior links sea ice to breeding success in Antarctic penguins. Sci. Adv. 6, eaba4828. doi: 10.1126/sciadv.aba4828
Watanabe Y. Y., Takahashi A. (2013). Linking animal-borne video to accelerometers reveals prey capture variability. Proc. Nat. Acad. Sci. U.S.A. 110, 2199–2204. doi: 10.1073/pnas.1216244110
Watkins J. (2000). “Aggregation and vertical migration,” in Krill: biology, ecology and fisheries. Ed. Everson. I. (Wiley-Blackwell, United Kingdom), 80–102.
Webb D. C., Simonetti P. J., Jones C. P. (2001). SLOCUM: An underwater glider propelled by environmental energy. IEEE J. Ocean. Eng. 26, 447–452. doi: 10.1109/48.972077
WG-ASAM (2019). Report of the meeting of the subgroup on acoustic survey and analysis methods (Hobart, Australia: CCAMLR).
Wiebe P. H., Ashjian C. J., Gallager S. M., Davis C. S., Lawson G. L., Copley N. J. (2004). Using a high-powered strobe light to increase the catch of Antarctic krill. Mar. Biol. 144, 493–502. doi: 10.1007/s00227-003-1228-z
Wiedenmann J., Cresswell K. A., Mangel M. (2009). Connecting recruitment of Antarctic krill and sea ice. Limnol. Oceanogr. 54, 799–811. doi: 10.4319/lo.2009.54.3.0799
Wilson R. P., Puetz K., Bost C. A., Culik B. M., Bannasch R., Reins T., et al. (1993). Diel dive depth in penguins in relation to diel vertical migration of prey: whose dinner by candlelight? Mar. Ecol. Prog. Ser. 94, 101–104. doi: 10.3354/meps094101
Yamahara K. M., Preston C. M., Birch J., Walz K., Marin R. III, Jensen S., et al. (2019). In situ autonomous acquisition and preservation of marine environmental DNA using an autonomous underwater vehicle. Front. Mar. Sci. 6. doi: 10.3389/fmars.2019.00373
Yang G., Atkinson A., Hill S. L., Guglielmo L., Granata A., Li C. (2021b). Changing circumpolar distributions and isoscapes of Antarctic krill: Indo-Pacific habitat refuges counter long-term degradation of the Atlantic sector. Limnol. Oceanogr. 66, 272–287. doi: 10.1002/lno.11603
Yang G., Atkinson A., Pakhomov E. A., Hill S. L., Racault M.-F. (2022). Massive circumpolar biomass of Southern Ocean zooplankton: Implications for food web structure, carbon export, and marine spatial planning. Limnol. Oceanogr. 67, 2516–2530. doi: 10.1002/lno.12219
Yang D., Haisheng Z., Han Z., Han X., Zang Y., Chen W., et al. (2021a). Biomarker records of D5-6 columns in the eastern Antarctic Peninsula waters: responses of planktonic communities and bio-pump structures to sea ice global warming in the past centenary. Adv. Polar Sci. 32, 28–41. doi: 10.13679/j.advps.2020.0025
Yang L., Sun L., Emslie S. D., Xie Z., Huang T., Gao Y., et al. (2018). Oceanographic mechanisms and penguin population increases during the Little Ice Age in the southern Ross Sea, Antarctica. Earth Planet. Sci. Lett. 481, 136–142. doi: 10.1016/j.epsl.2017.10.027
Zabroda P., Pshenichnov L., Marichev D. (2020). Benthic wildlife underwater video recording during longline survey in Weddell Sea. Ukrainian Antarctic J. 2), 75–83. doi: 10.33275/1727-7485.2.2020.655
Keywords: ecosystem monitoring, population change, Antarctic kill, fishery management, new technologies
Citation: Hill SL, Atkinson A, Arata JA, Belcher A, Nash SB, Bernard KS, Cleary A, Conroy JA, Driscoll R, Fielding S, Flores H, Forcada J, Halfter S, Hinke JT, Hückstädt L, Johnston NM, Kane M, Kawaguchi S, Krafft BA, Krüger L, La HS, Liszka CM, Meyer B, Murphy EJ, Pakhomov EA, Perry F, Piñones A, Polito MJ, Reid K, Reiss C, Rombola E, Saunders RA, Schmidt K, Sylvester ZT, Takahashi A, Tarling GA, Trathan PN, Veytia D, Watters GM, Xavier JC and Yang G (2024) Observing change in pelagic animals as sampling methods shift: the case of Antarctic krill. Front. Mar. Sci. 11:1307402. doi: 10.3389/fmars.2024.1307402
Received: 04 October 2023; Accepted: 19 February 2024;
Published: 08 March 2024.
Edited by:
Stelios Katsanevakis, University of the Aegean, GreeceReviewed by:
Tosca Ballerini, Independent researcherChristian Briseño-Avena, University of North Carolina Wilmington, United States
Copyright © 2024 Hill, Atkinson, Arata, Belcher, Nash, Bernard, Cleary, Conroy, Driscoll, Fielding, Flores, Forcada, Halfter, Hinke, Hückstädt, Johnston, Kane, Kawaguchi, Krafft, Krüger, La, Liszka, Meyer, Murphy, Pakhomov, Perry, Piñones, Polito, Reid, Reiss, Rombola, Saunders, Schmidt, Sylvester, Takahashi, Tarling, Trathan, Veytia, Watters, Xavier and Yang. This is an open-access article distributed under the terms of the Creative Commons Attribution License (CC BY). The use, distribution or reproduction in other forums is permitted, provided the original author(s) and the copyright owner(s) are credited and that the original publication in this journal is cited, in accordance with accepted academic practice. No use, distribution or reproduction is permitted which does not comply with these terms.
*Correspondence: Simeon L. Hill, c2loQGJhcy5hYy51aw==
†Present addresses: John A. Conroy, Ocean Sciences Department, University of California, Santa Cruz, Santa Cruz, CA, USA
Frances Perry, Department of Biosciences, University of Exeter, Exeter, UK
Keith Reid, Institute for Marine and Antarctic Studies, University of Tasmania, Hobart, TAS, Australia
‡These authors have contributed equally to this work