- 1School of Earth Sciences, University of Western Australia, Perth, WA, Australia
- 2Oceans Institute, University of Western Australia, Perth, WA, Australia
- 3School of Molecular and Life Sciences, Curtin University, Perth, WA, Australia
- 4Mina and Everard Goodman Faculty of Life Sciences, Bar- Ilan University, Ramat Gan, Israel
- 5School of Biological Sciences, University of Western Australia, Perth, WA, Australia
Predicted future increases in both local and global stressors are expected to lead to elevated turbidity levels and an expansion of the geographical range of turbid coral reefs. Corals typically respond to elevated turbidity by increasing their rates of heterotrophy as means of compensating for low energy levels from reduced light and photosynthesis. We analysed Acropora tenuis energy acquisition along a natural turbidity gradient over two time points in Exmouth Gulf, Western Australia, using in-situ environmental data with coral physiology attributes and stable isotopes to assess trophic strategy. Our hypothesis was that as turbidity levels increased, so too would heterotrophy rates. Both δ13C and δ15N values decreased from the clear-water to the turbid sites, which along with Bayesian analysis revealed that all A. tenuis communities along the turbidity gradient are on a mixotrophic-heterotrophic feeding strategy scale. We propose that the low δ15N levels at the most turbid site may result from a combination of Acropora physiological limitations (e.g., reduced feeding capacity) and highly variable turbidity levels. In contrast, the higher δ15N at the clear-water site likely results from increased nutrient availability from additional sources such as upwelling. Our findings suggest that increased heterotrophy by coral hosts in turbid coral reef areas is not a universal pattern. Importantly, the loss of carbon in the turbid sites is not supplemented by nitrogen intake, which might suggest that Exmouth Gulfs Acropora communities are more vulnerable to future climate stressors and bleaching.
1 Introduction
Turbid coral reefs are light-limited coral habitats characterized by elevated turbidity, high sedimentation, and variable nutrient concentrations (Larcombe and Woolfe, 1999; Zweifler et al., 2021). It is predicted that future increases in local and global stressors will elevate turbidity levels and expand the geographic range of turbid coral reefs (Oppenheimer et al., 2019; Cartwright et al., 2021). To date, turbid reefs have been under-studied due to the logistical difficulties associated with working in low visibility conditions both directly (in-situ) and indirectly using remote sensing technologies (Morgan et al., 2017), resulting in less data and collective knowledge on turbid reef systems (Zweifler et al., 2021). In addition, in turbid coral reefs, increased light attenuation with depth typically confine them to shallow inshore water settings (<10 m depth) (Morgan et al., 2016; Luo et al., 2022) where higher and more variable sea surface temperatures (SST’s) are likely to elevate the frequency and severity of coral bleaching (Brown et al., 2019). Yet, over the last decade, field observations suggested that turbid coral reefs are potentially more resilient to bleaching than clear-water reefs (Morgan et al., 2017; Browne et al., 2019; Teixeira et al., 2019; Sully & van Woesik, 2020).
Reef-building corals obtain nutrition autotrophically through their symbiotic algae, Symbiodiniaceae, which utilize sunlight and carbon dioxide to produce organic carbon and oxygen (Muscatine and Porter, 1977; Page et al., 2019). Additionally, corals can acquire nutrition heterotrophically through particle capture (Houlbrèque and Ferrier-Pagès, 2009). It is has been observed that coral in turbid reefs exhibit high rate of mixotrophy and/or heterotrophy as a physiological adaptation (Fox et al., 2018; Travaglione et al., 2023). These feeding strategies can compensate for energy deficits caused by the reduced light and photosynthesis (Anthony et al., 2009; Houlbrèque and Ferrier-Pagès, 2009; Wooldridge, 2014). In turbid reefs, the combination of increased heterotrophic feeding (Anthony and Fabricius, 2000; Anthony et al., 2007; Piniak and Storlazzi, 2008) and reduced UV stress from accelerated light attenuation (Luo et al., 2022) may enhance coral survival during heat stress events which often lead to coral bleaching (Grottoli et al., 2006; Browne, 2012; Hughes and Grottoli, 2013). Coral bleaching is initiated by the synergistic effect of high SST with high UV irradiance, leading to the photoinhibition of the symbiotic algae (Iglesias-Prieto et al., 1992; Warner et al., 1999).
Many studies have shown that shifts from autotrophy to heterotrophy can occur within species across a wide range of water depths (Palardy et al., 2005), turbidity (Anthony and Fabricius, 2000), or availability of nutrition resources (Fox et al., 2019). However, not all corals have the same ability to switch between autotrophy and heterotrophy (Houlbrèque and Ferrier-Pagès, 2009). Corals (e.g., Platygyra, Turbinaria) that are able to effectively switch to heterotrophy may have larger polyps to capture larger particulates in the water column, have lower surface area to volume ratios and/or are able to produce mucus which traps particulates as they settle on the coral (Porter, 1976; Conti-Jerpe et al., 2020). Although a review of heterotrophy in corals by Houlbrèque & Ferrier-Pagès (2009) concluded that feeding rates (and success) were a result of increased feeding effort rather than polyp size or morphology. Regardless, corals that are less effective at trapping particulates may acclimate to low light levels through other mechanisms such as increased symbiont densities and/or chlorophyll concentrations (Titlyanov & Titlyanova, 2002; Cooper et al., 2008). This enables the coral to increase its light capturing efficiencies and therefore maintain photosynthesis. These types of acclimatory responses have been observed in corals on turbid reefs such as Singapore (Browne et al., 2015) and nearshore Great Barrier reef (Rocker et al., 2017).
The effect of turbidity on coral physiology and their potential adaptation mechanisms has been investigated under both natural (Ferrier-Pagès et al., 2011; Browne et al., 2015) and experimental (Bessell-browne, 2017; Luter et al., 2021) conditions, mostly through coral physiology measurements such as photosynthesis, respiration, growth and feeding rates (Jones et al., 2020; Roitman et al., 2020; Tisthammer et al., 2020; Bollati et al., 2021). These studies provide estimations of energy sources under varying turbidity regimes. For example, Anthony (2000), showed under experimental conditions using sediment labelled with 14C that the rates of sediment ingestion are a linear function of sediment load, with an assimilation efficiency of 50-80% in the coral Acropora millepora. However, the feeding strategies of the coral host and its symbiont algae (i.e., autotrophy, mixotrophy or heterotrophy) may vary in accordance with local environmental conditions, including nutrient concentrations, light availability, and temperature (Baumann et al., 2014; Krueger et al., 2018), as well as nutritional conditions such as the isotopic values of the dissolved inorganic carbon and nitrogen (Hoegh-Guldberg et al., 2004; Nahon et al., 2013; Seemann et al., 2013; Price et al., 2021). Hence, assessing feeding strategies under experimental conditions may not represent the absolute incorporation of the various nutritional sources by the coral holobiont. This is also the case when relying solely on direct measurements of photosynthesis and feeding rates. However, by employing the assessment of stable carbon (δ13C) and nitrogen (δ15N) isotopes natural abundance in both the coral host and symbiont algae, using Bayesian ellipses metrics (Jackson et al., 2011), one can effectively identify the coral trophic strategy and isotopic niche (Ferrier-Pagès et al., 2011; Fox et al., 2018; Radice et al., 2019a; Conti-Jerpe et al., 2020). Despite the potential benefits of Bayesian approach in stable isotopes analysis, they have not been widely used for this purpose in coral research (Price et al., 2021).
The north-west coast of Western Australia (WA) provides a key location to explore the differences in coral energy acquisition between clear and turbid water reefs. This region is home to both Ningaloo Marine Park (NMP), a clear water nearshore coral reef situated on the western flank of Cape Range, and Exmouth Gulf, which hosts turbid water inshore reefs. Importantly, the area’s remoteness limits local anthropogenic impacts, resulting in a naturally occurring east – west turbidity gradient along the same latitude (Dee et al., 2020; Cartwright et al., 2021). The NMP also experiences less frequent and more localised coral bleaching events compared to the Great Barrier Reef (Great Barrier Reef Marine Park Authority, 2019), with the last major heat-wave event recorded in 2011-2013 (Gilmour et al., 2019). This event caused several coral reefs to bleach along the WA coast, including Bundegi reef situated on the border between Ningaloo and Exmouth Gulf (Depczynski et al., 2013; Gilmour et al., 2019). Unfortunately, no data was found in the literature on coral bleaching within the eastern to southern turbid reefs of Exmouth Gulf.
In this study we chose to work on the coral Acropora tenuis, from four sites along a natural turbidity gradient in Exmouth Gulf, WA and across two time points. Acropora is an important reef building coral genus characterized by high growth rate, complex structure that serves as habitat for various organisms, and widespread distribution across diverse habitats, reefs and regions (Lewis, 1984; Wallace and Willis, 1994). Acropora tenuis is common on both clear-water reefs where corals are exposed to comparatively high light levels, as well as on inshore turbid reefs where colonies are typically exposed to high levels of suspended particulate matter and nutrients (Anthony & Connolly, 2004; Anthony, 2006), suggesting high acclimation potential to variable environmental conditions (Conlan et al., 2017; Rocker et al., 2017; Strahl et al., 2019, 2019). To better quantify sources of energy and strategies for resource acquisition under different turbidity regimes, we combined (1) in-situ environmental data (e.g., turbidity, temperature) with (2) physiology attributes (e.g., chlorophyll a and symbiont density) and (3) stable isotope data for trophic strategy assessments (heterotrophy, mixotrophy or autotrophy). By combining analysis of coral physiological and trophic strategies, we provide a detailed evaluation of the coral holobiont energy sourcing, thereby increasing our broader understanding of physiological trade-offs in a naturally turbid versus clear-water setting (Mydlarz et al., 2010; Rocker et al., 2017). This knowledge is fundamental to improving our understanding of coral health and resilience status, which heavily influences the vulnerability of Acropora corals to extreme environmental changes. In addition, understanding of the variability in heterotrophic capacity relative to environmental conditions is important when determining which coral communities are more likely to survive future climate change events. Therefore, this information should be incorporated in coral reef management and conservation efforts.
2 Materials and methods
2.1 Regional geography
Exmouth Gulf is a shallow (mean depth 11.9 m) and large (∼3000 km2 area), subtropical inverse estuarine embayment influenced by a strong tidal cycle (Figure 1) (Twiggs and Collins, 2010). Throughout the Gulf, coral reefs are predominantly found as small shallow fringing reefs that typically surround small reef islands (Bonesso et al., 2022). Turbidity conditions are caused by: (1) runoff due to high rainfall from the rivers (e.g. Ashburton River) flowing north to the Gulf that deliver a pulse of sediments and nutrients into the nearshore environment (Cartwright et al., 2021), (2) bottom sediment resuspension and transport by wind waves and strong tidal currents (Dee et al., 2020), (3) cyclones (90 km-h), which occur on average five times per year (Australian Bureau of Meteorology, 2022) delivering an influx of red siliciclastic sediment from the hinterland, and (4) commercial prawn trawling that causes a sediment disturbance (Lough, 1998; Orpin et al., 1999). The fringing Ningaloo Reef is a UNESCO World Heritage Site that extends for 300 km along the western side of Cape Range national park (Collins et al., 2003). The Ningaloo reef flat is separated from shore by a wide, ~2 to 4 m deep lagoon where surface waves drive a cross-reef flow with water driven into the lagoon and returning to the ocean through reef channels all year round (Wyatt et al., 2010). During summer it is dominated by the Ningaloo Current along with wind-driven equatorial surface current on the continental shelf that drives upwelling on the shelf slope (Hanson et al., 2005). Upwelling of deep water generates fluxes of inorganic nutrients that increase the particulate resources across the reef and are important source for the coral primary productivity and growth (Stuhldreier et al., 2015; Radice et al., 2019b). In contrast, Exmouth Gulf, located on the Pilbara continental shelf is highly dominated by the Leeuwin Current, an oligotrophic eastern boundary current that flows strongest during autumn–winter and La Nina years (Feng et al., 2003). By generating large-scale downwelling, it transports tropical water poleward and carries warm, low salinity, low-nutrient water to the Exmouth Gulf, thus, limiting productivity and species distribution (Smith et al., 1991; Hanson et al., 2005; Twiggs & Collins, 2010).
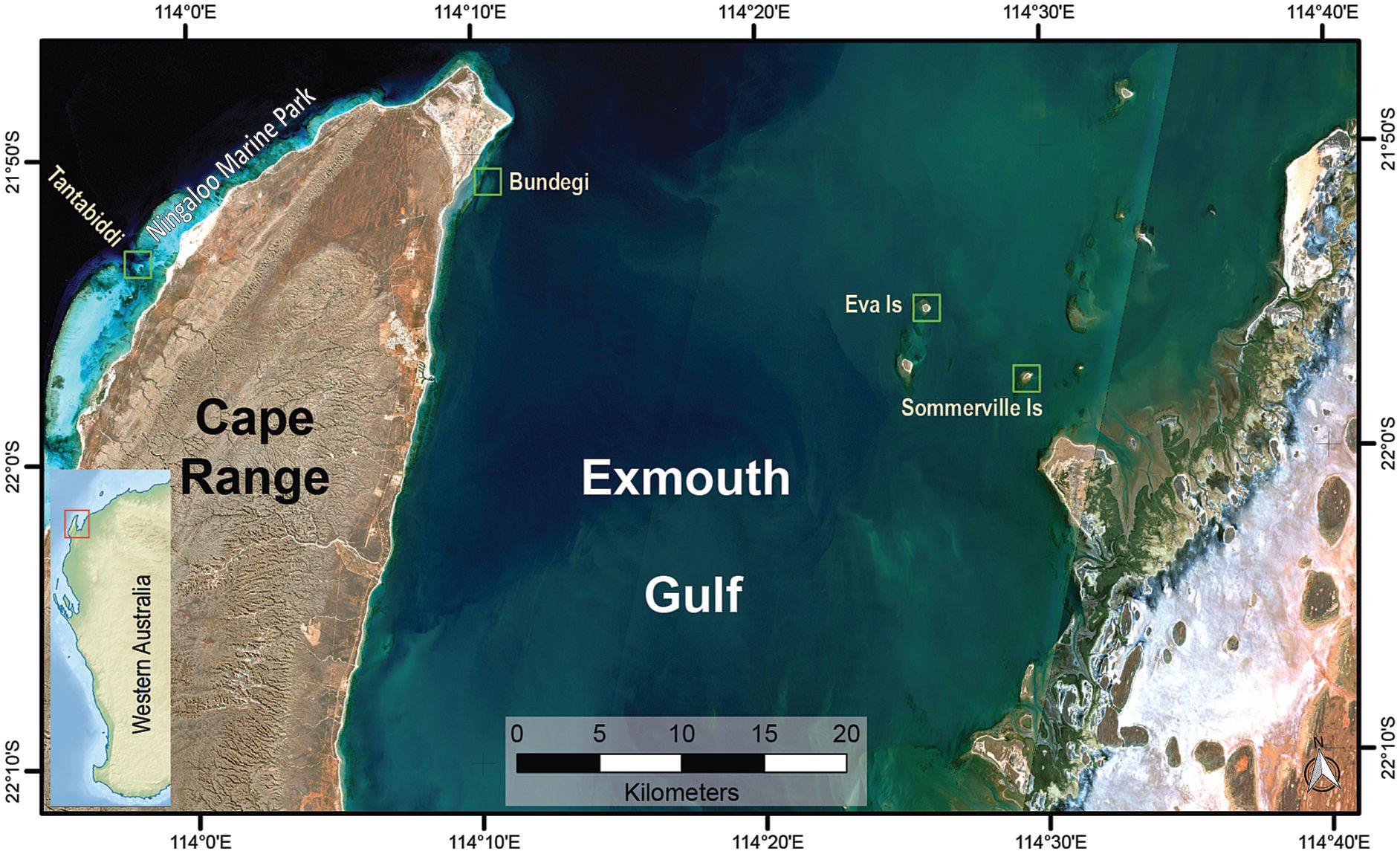
Figure 1 Map of the study sites at Exmouth Gulf and the Ningaloo Marine Park (green rectangles). Exmouth Gulf location on Western Australian coast (red rectangle in the small map, left hand side).
2.2 Study sites
This study took place at four shallow (2-4 m) sites across a turbidity gradient from Exmouth Gulf to the adjacent Ningaloo Marine Park (NMP) (Figure 1). Somerville (21°57’27.9”S 114°29’35.3”E) and Eva (21°55’02.1”S 114°25’56.9”E) Islands, located on the eastern side of the Gulf, have similar fringing reef morphology and are naturally fluctuating turbid reefs that experience episodic (daily to monthly) severe turbidity (>150 NTU or approximately >50 mg L−1) events interspersed by periods of low turbidity (<15 NTU or approximately <5 mg L−1) or clear water (<2 NTU or approximately <0.5 mg L-1) (Dee et al., 2020; Zweifler et al., 2021). Bundegi Reef (21°50’07.7”S 114°10’40.8”E), located at the north-western tip of the Gulf, is at the boundary of the NMP, and is a low turbidity patchy fringing reef (Speed et al., 2013; Doropoulos et al., 2022). In contrast, Tantabiddi (21°52’50.8”S 113°58’52.3”E) is part of the fringing reef of the NMP on the western side of Cape Range characterised by clear waters (Collins et al., 2003).
2.3 Environmental conditions
Turbidity and temperature data were logged in-situ at each site using data loggers (NTU-LPT, In-Situ Marine Optics, Bibra Lake, WA) that recorded the average of ten measurements every thirty minutes. Loggers were deployed horizontally at 30 cm above the seabed on a metal frame from May 2020 to October 2021. Turbidity, measured in nephelometric turbidity units (NTU), is the incident light scattered at right angles from suspended particles in the water column. Logger optics were cleaned every hour to avoid organic growth using an integrated wiper. Batteries were recharged, and data downloaded every 3-4 months. Raw data underwent a cleaning process to remove anomalously high values that exceeded the trend observed at a single time point prior to calculating the daily (24hr) average and plotting (Figure 2). We also obtained one day composite data of diffuse attenuation (Kd490; MODIS) used as a proxy for turbidity, and multi-scale ultra-high resolution (MUR) of sea surface temperatures (SST). These remote data sources provided an alternative when the in-situ data loggers failed (June-October 2021), allowing us to verify spatial differences in turbidity and temperature (Australian Bureau of Meteorology, 2022b).
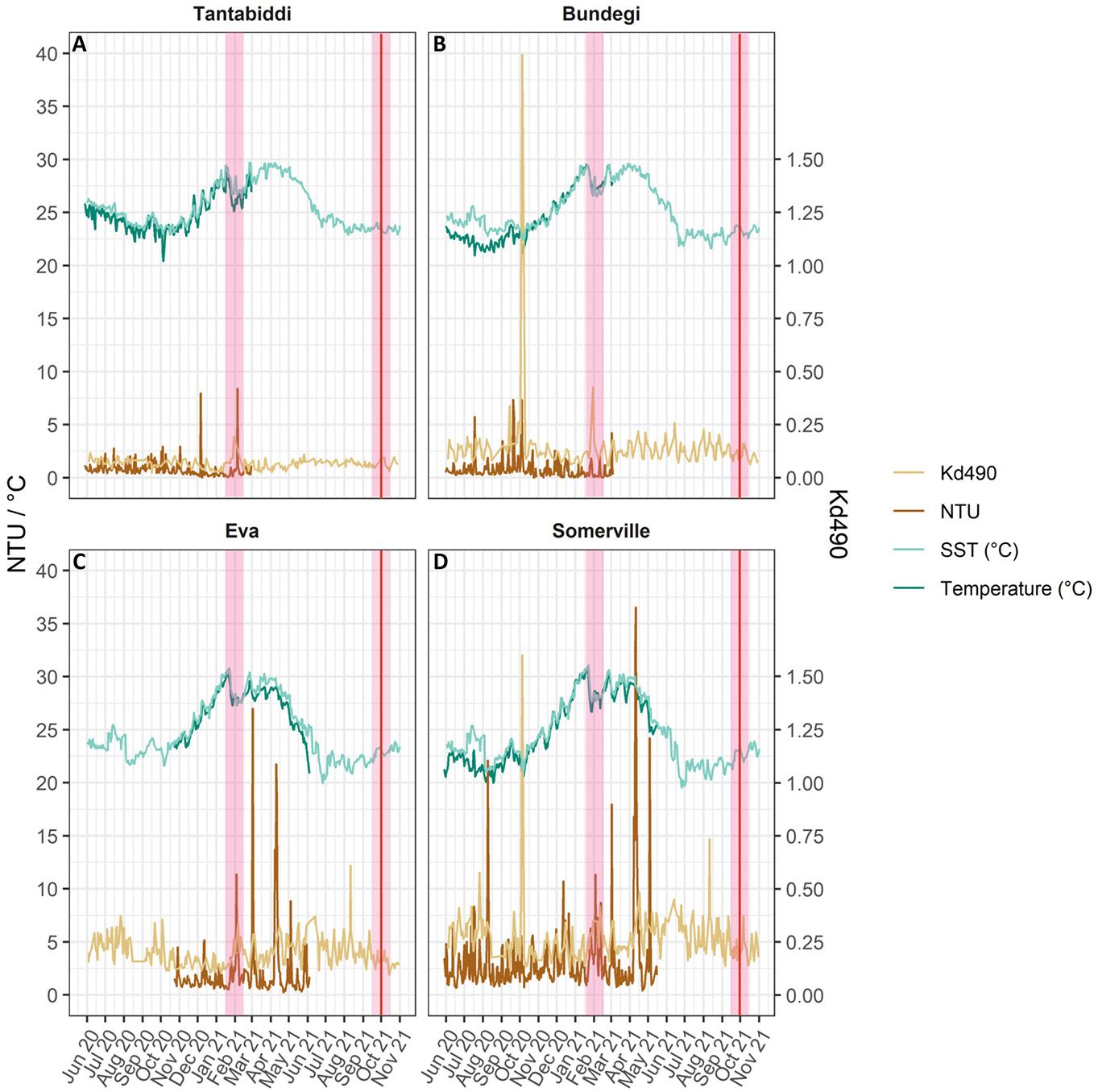
Figure 2 Left Y axis: Daily mean of NTU (brown), in-situ temperature °C (NTU data loggers; dark green) and SST °C (MUR; light green). Right Y axis: Daily Kd490 (Aqua MODIS; light brown). (A) Tantabiddi, (B) Bundegi, (C) Eva and (D) Somerville. Pink vertical lines highlight coral sampling times, red line indicate water sampling time.
2.4 Sample collection
Coral samples were collected to measure physiological attributes (chlorophyll a and symbiont density) and for stable isotope analysis. Between six to eight Acropora tenuis colonies (> 20 cm in diameter) within a ~15 m radius were tagged at each site. Each coral colony was sampled (3-5 fragments of ~5cm long) in February 2021 (end of summer) and October 2021 (end of winter) by SCUBA. Samples were placed in tagged bags with seawater and transferred to shore, where each individual sample was wrapped with aluminum foil before being snap frozen in liquid nitrogen (Grottoli et al., 2021). Samples were transferred in a dry shipper to a -80 freezer in the Indian Ocean Marine Research Centre (IOMRC) at the University of Western Australia until further analysis.
In October 2021, water samples were collected for the analysis of zooplankton (>167 µm) and phytoplankton (>67 µm) stable isotopes to identify potential carbon and nitrogen sources. A single sample, of approximately 1000 L of seawater from each site, was acquired using a bilge pump and preserved at -20°C prior to filtration. In the laboratory, duplicates from the defrosted samples were filtered using a pre-combusted (550°C for 4hr) 0.7 micron 47 mm glass fiber filters. Filters were than dried in an oven at 60°C for 48 hr and stored in a Ziplock bag with desiccant dehumidifier beads until further analysis. Due to technical issues no water samples were taken in February 2021.
2.5 Coral physiology
Two coral fragments from each tagged colony were processed to assess coral symbiont density and chlorophyll a (Chl a) concentration. Coral tissue was removed from the frozen coral fragments using an airbrush and filtered (0.22 uM) seawater (FSW) producing 15 ml tissue slurry. Skeletons were dried and kept for surface area measurement using the wax dipping technique (Stimson and Kinzie, 1991). Coral tissue slurry was homogenized for 2 minutes using an electrical tissue homogenizer (TissueRuptor, QIAGEN) and centrifuged at 3,000 g for 5 minutes to separate host and symbiont fractions. Additional centrifugation at 3,000 g for 5 minutes and washing with FSW was performed to isolate the symbiont for cell count and photosynthetic pigment extraction for Chlorophyll a concentration. Symbiont cells were counted in replicates of six using a Neubauer haemocytometer under a microscope and normalized to the coral surface area (cell cm-2). Pigments were extracted for 24 h in 90% acetone at 4°C in the dark and Chl a concentration was measured at 630, 664, and 750 nm with a spectrophotometer (UV/VIS SP8001, Metertech Inc, Taiwan). Chl a concentration was determined using the equations offered by (Jeffrey and Humphrey, 1975) and normalized to surface area (µg cm-2).
2.6 Stable isotopes
Stable isotope (δ15N and δ13C) analysis was conducted separately for coral host tissue and symbiont in duplicates from five of the eight tagged colonies at each site. Samples were prepared for analysis following Rosenberg et al. (2022). Coral tissue was removed, and the homogenate was centrifuged to separate the algae from the host tissue at 3,000 g for 5 minutes at 4°C. Supernatant containing host tissue was transferred to a 50 ml falcon tube. The remaining pellet containing the algae was re-suspended in 1 ml of FSW and transferred to a 1.5 ml Eppendorf tube. To eliminate all other remaining cells, symbiont samples were centrifuged at 4,000 g for 5 minutes and host tissue at 13,500 g for 10 minutes, both at 4°C. The remaining supernatant was discarded, and the pellet was re-suspended in 1 ml of deionized water (DIW). 0.1 ml of 1N HCL was added to all samples (host and symbiont), which were then left with cap off for 10 minutes at room temperature. Symbiont and host samples were then centrifuged at 4,000 and 13,500 g, respectively, for 5 minutes at 4°C. Supernatant was discarded and pellets were re-suspended in 1 ml DIW. This process was repeated two more times before a final centrifugation (5 min at 4°C) at 5,000 g for symbiont samples and 15,000 g for host samples. Once the supernatant was discarded, samples were dried in a freeze-dryer for 48 hours.
Signatures of δ15N and δ13C for all samples (symbiont, host and water) were analysed using a continuous flow system consisting of a Delta V Plus mass spectrometer connected with a Thermo Flush 1112 via Conflo IV (Thermo-Finnigan, Germany) at the West Australian Biogeochemistry Centre (WABC), School of Biological Sciences, University of Western Australia. All δ13C and δ15N values are given in per mil [‰, Vienne Pee Dee Beleminte] and [‰, Air], respectively (Skrzypek, 2013). Multi-points normalization was used on the raw isotopic data and compared to the international reference scale (Skrzypek, 2013). Normalization was done based on international standards provided by the International Atomic Energy Agency (IAEA) (δ13C - NBS22, USGS24, IAEA600, USGS40; and δ15N - N1, N2, USGS40, USGS32) and laboratory standards. The external error of analysis (1σsd) was δ13C = 0.10 ‰, δ15N = 0.10 ‰, and C/N=0.1.
2.7 Statistical analysis
All statistical analysis and plotting of the data were conducted in R software version 4.1.1 (R Core Team, 2021). Means were produced by first averaging the two duplicates of each colony and then calculating colony mean per site. Data were tested for normality using the Shapiro–Wilk test with QQ-plots and homogeneity of variance with Levene’s test. When the statistical test assumptions were not met, a Log10 transformation was performed on symbiont density and Square root transformation on Chl a data. The environmental data (i.e., NTU, temperature, SST, Kd490) monthly mean, site and site by time interaction were analysed using Two-way ANOVA with a Tukey’s post-hoc test to identify differences among the sites and between sampling times at each site. A Pearson’s correlation (Wei et al., 2021) test was also used to detect if there were similar trends between the in-situ (NTU and temperature) and the remotely sensed (Kd490 and SST) environmental data. A linear mixed effects model was used with the package nlme (Pinheiro et al., 2022) to assess potential differences in coral physiology parameters (symbiont density, Chl a) and coral host and symbiont algae stable isotopes (δ13C, δ15N) between sites, time and the site by time interaction, with individual coral colonies treated as a random effect. Where appropriate, a pairwise Tukey’s post-hoc comparisons of marginal estimated means was performed using the package emmeans (Lenth et al., 2022). We recognize the limitations associated with temporal pseudoreplication in this analysis, as colony replicates between sampling times lack statistical independence. The average of the duplicated water samples from each site was used to assess the potential contribution by phytoplankton and zooplankton to the coral holobiont diet at each site. Typically, these sources have lower δ13C values compared to Symbiodiniaceae by at least 4-6 ‰ (Alamaru et al., 2009). Consequently, increased heterotrophic nutrition may result in reduction in coral host δ13C (Fox et al., 2018). Therefore, the relative difference between host and symbiont (Δ13C = δ13Chost-symbiont) can be used to provide an assessment of the relative effects of the photosynthetic fractionation and incorporation of heterotrophic carbon. Further, it can also indicate if there are deviations from a fully autotrophic diet and track gradients in resource availability (Fox et al., 2018). Differences between host and symbiont δ15N values (Δ15N = δ15Nhost-symbiont) were calculated to evaluate the autotrophy to heterotrophy spectrum, where higher Δ15N values indicate increased heterotrophy (Ferrier-Pagès et al., 2011; Conti-Jerpe et al., 2020; Price et al., 2021). Further, the stable isotopic signature shift between each coral host and its associated symbiont algae along with the phytoplankton and zooplankton isotope values per site were used to determine sources of energy (autotrophic, heterotrophic, mixed).
To explore differences in isotopic niches, for each site and time, host and symbiont fractions were fitted with 40% and 95% variation ellipses to their δ13C and δ15N values and plotted on an isotope biplot. The standard ellipse area (‰2) of their distribution was also estimated correcting for sample size (SEAC, a proxy for isotopic niche) using the Stable Isotope Bayesian Ellipses in R (SIBER) (Jackson et al., 2011; Jackson & Parnell, 2021). A method that enables direct comparison of isotopic niches across communities. Applying both ellipse sizes (0.4 and 0.95) provide a means to determine whether the coral holobiont is autotrophic (with the 40% ellipse), or heterotrophic (with the 95% ellipse). To determine whether the relative placements of the host and symbiont niche differed, the Euclidean distance between the centroids (means) of the two was calculated with p.interval = 0.95. A residual permutation procedure and Hotelling T2 test were also used to evaluate significance, with p < 0.05 indicating that niches occupy significantly different isotopic space, an indication for heterotrophic feeding strategy (Turner et al., 2010). The ellipse overlap (p.interval = 0.95) of coral host and symbiont cut-off was eventually used to determine trophic strategy where ≥70% overlap indicates autotrophy, ≤10% indicates heterotrophy and overlap between >10% and < 70% indicates mixotrophy (Conti-Jerpe et al., 2020).
3 Results
3.1 Environmental monitoring
NTU data show a distinct turbidity gradient across the four study sites (ANOVA, F=49, p<0.001) from the most turbid site, Somerville, with monthly (May 2020-May 2021) levels of 3.2 ± 3 NTU (mean ± sd), to the clearest site, Tantabiddi, with 0.8 ± 0.6 NTU (monthly, May 2020-March 2021, mean ± sd) at the western side of Cape Range (Figure 2; Supplementary Table S1, Figure S1). Fluctuating high turbidity events at Somerville and Eva occurred all year round with a daily average max of 36.5 NTU at Somerville (11/04/2021) and 27 NTU at Eva (02/03/2021). In contrast, turbidity at Tantabiddi (monthly, May 2020-March 2021) and Bundegi (monthly, May 2020-March 2021) is less variable, typically ranging from 0 to 2.5 NTU with occasional peaks of 4 to 8 NTU (Figure 2). Although Turbidity (NTU) and Kd490 satellite derived data were not significantly correlated (Pearson’s correlation, r <0.5, p>0.05; Supplementary Figure S2A, Table S2), Kd490 was still used separately as an estimate of in-water turbidity conditions during the three months (June – November 2021) when the loggers failed to record data. Temporal differences in NTU mean values were significant at all sites (ANOVA, F=10.15, p<0.0001), while Kd490 mean values presented significant interaction between site and time (ANOVA, F=2.24, p<0.0001). Temperature recorded by our in-situ data loggers was strongly positively correlated with remote sensed SST (Pearson’s correlation, r>0.85, p<0.0001; Supplementary Table S2, Figure S2B) and both parameters produced significant differences at each site between times (ANOVA, p<0.0001; Supplementary Table S1). The highest SST monthly mean ± sd was recorded in Somerville (29.3 ± 1.3 °C) during January 2021 with a max of 30.5 °C while Tantabiddi recorded 27.4 ± 1.1°C with a max of 29 °C (Figure 2). The lowest temperatures recorded were also at Somerville (20.9 ± 0.7 °C) during August 2021 while Tantabiddi monthly mean ± sd at that time was 23.3 ± 0.6 °C. Somerville SST (°C) varied significantly (ANOVA, p<0.0001) by 12 °C between the warmest (31 °C, January 2021) and the coldest (19.5 °C, June 2021) months, compared to Tantabiddi where temperature range was 7°C degrees between 29.6 °C (February 2021) to 22.8 °C (August 2021) (ANOVA, T=397, P<0.0001).
3.2 Coral physiology
Across all sites and sampling times, mean symbiont density was 46 ± 22.8 (105 cell cm-2) with the highest mean found at Tantabiddi (53.7 ± 22, 105 cell cm-2; Figure 3A; Supplementary Table S3). Symbiont densities were greater at Tantabiddi and lower at Eva in both times, however, the only significant difference observed was between Tantabiddi and Bundegi and between Tantabiddi and Eva(Tukey’s HSD, p=0.006 and p=0.003, respectively; Supplementary Table S4, S5) in October 2021. No significant temporal differences in symbiont density within a site were observed, despite an increase at Tantabiddi, from 52.1 ± 19 in February 2021 to 79.8 ± 22.5 (105 cell cm-2) in October 2021.
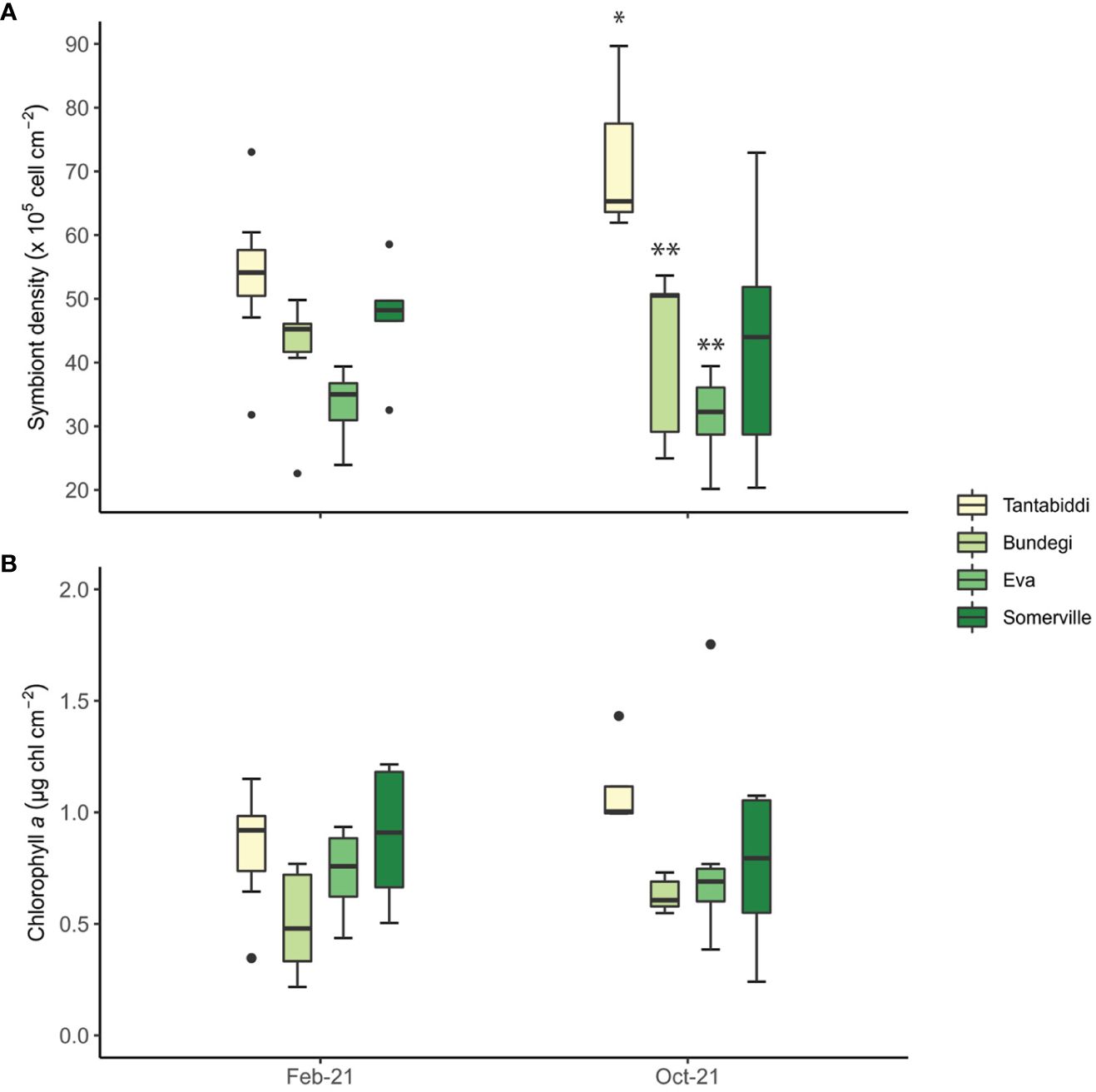
Figure 3 (A) Symbiont density (105 cell cm-2) and (B) chlorophyll a (µg Chl cm-2) collected at each site during two sampling times. Whiskers represent minimum and maximum values, of the lower and upper quartile, respectively; dots represent outliers. n=6-8 colonies, two duplicate samples from each colony were analysed (Supplementary Table S3). Asterisks (*) indicate statistical differences between the sites.
Mean Chl a values in the Acropora tenuis colonies across all sites and sampling times were 0.7 ± 0.3 (µg chl cm-2; Figure 3B; Supplementary Table S3). Chl a content was significantly different between sampling times with lower values in February 2021 (ANOVA, p<0.05; Supplementary Table S4). Highest Chl a values were observed at Tantabiddi 0.77 ± 0.35 (µg chl cm-2) and lowest values were recorded at Bundegi 0.58 ± 0.21 (µg chl cm-2). Still, we found no significant differences in Chl a values between sites.
3.3 δ13C and δ15N variability along the turbidity gradient
δ13C signatures differed significantly between the host and its symbiont only at Somerville in February 2021 (Tukey’s HSD, p=0.003; Supplementary Table S7, S8) with lower values in the host (-15.78 ± 1.24) compared to the symbiont (-14.94 ± 1.71). In contrast, δ15N values were significantly higher in the host compared to the its symbiont across all sites in both sampling times (Tukey’s HSD, P ≤ 0.0001; Supplementary Table S7, S8).
Between the sites, coral host tissue δ13C signatures (Figure 4A; Supplementary Table S6) show a significant trend from higher values at the clear water sites, Tantabiddi and Bundegi, (Tukey’s HSD, P ≤ 0.0001) compared to lower values at the turbid Eva and Somerville sites. Coral host tissue δ13C signatures were also significantly lower in October 2021 across all sites (Tukey’s HSD, p ≤ 0.001; Supplementary Table S10, S11). Similarly, symbiont δ13C signatures at the clear-water sites were significantly higher than at the turbid water sites (Tukey’s HSD, p<0.0001). However, a significant difference between sampling times (Tukey’s HSD, p=0.005; Supplementary Table S10, S11) was only present at Somerville with lower symbiont δ13C signature in October 2021 (-17.69 ± 1.22) compared to February 2021 (-14.94 ± 1.71). As with δ13C values, coral host tissue δ15N levels show a significant decreasing trend from the clear-water Tantabiddi and Bundegi to the turbid Eva and Somerville (Tukey’s HSD, p ≤ 0.0001) across the two sampling times. However, unlike δ13C values, coral host δ15N did not vary significantly between sampling times at each site. These spatial and temporal patterns were also observed in the symbiont δ15N values (Figure 4B; Supplementary Table S10, S11).
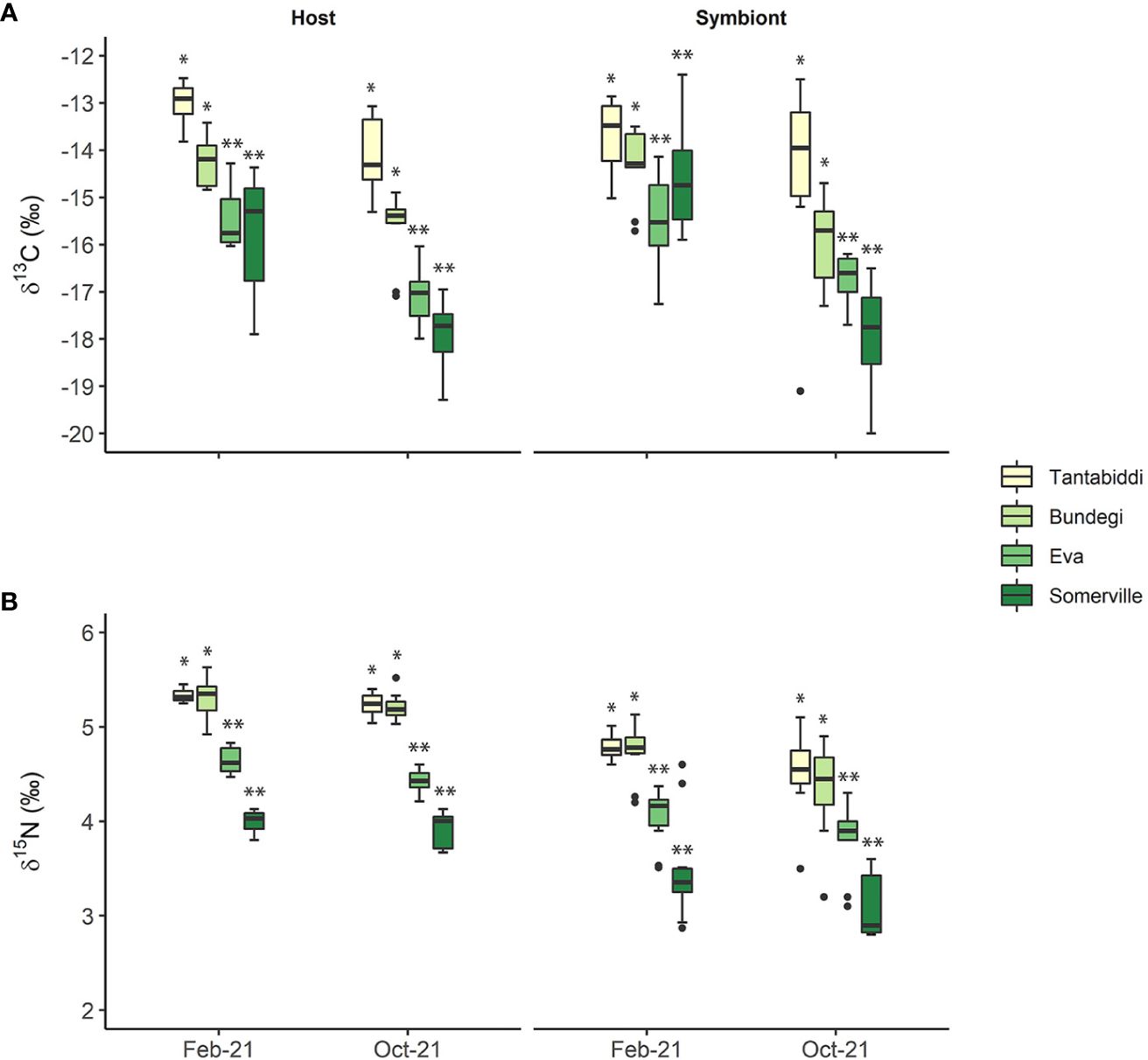
Figure 4 (A) δ13C and (B) δ15N signature values of the coral host tissue and the symbiont algae in the four study sites over two sampling times. Whiskers represent minimum and maximum values, of the lower and upper quartile, respectively; dots represent outliers. n=5 colonies, two duplicate samples from each colony were analysed. Asterisks (*) indicate statistical differences between the sites.
Interestingly, spatial differences in zooplankton and phytoplankton δ13C and δ15N values from the sites water samples, did not always reflect the spatial differences in the coral host and symbiont δ13C and δ15N values (Supplementary Figure S2, Table S9). δ13C in the zooplankton and phytoplankton were higher at the clear-water sites where δ13C was also higher in the coral tissue and symbiont. However, zooplankton and phytoplankton δ15N levels were typically higher at the turbid water sites whose coral and symbiont had relatively lower δ15N than at the clearwater sites.
3.4 Δ13C(= δ13Chost-symbiont) and Δ15N (= δ15Nhost-symbiont) along the turbidity gradient
Δ13C values were low ranging between -0.96 ± 2 (mean ± sd) at Eva and 0.09 ± 1.64 (mean ± sd) at Tantabiddi, both in October 2021 (Figure 5A). Despite these observations, there were no significant differences in Δ13C over space and time. Nevertheless, Somerville is found with the greatest range of Δ13C from -0.84 ± 0.87 in February 2021 to -0.21 ± 0.56 in October 2021. Similarly, Δ15N values did not differ significantly between sites and sampling times, although Δ15N values at Somerville were consistently higher at 0.70 ± 0.18 and 0.81 ± 0.22 in February 2021 and October 2021, respectively (Figure 5B). Lowest Δ15N values were recorded in February 2021 at Bundegi (0.43 ± 0.19) and Eva (0.45 ± 0.14). Across all sites there was a non-significant increase in Δ15N levels from February 2021 to October 2021 (Supplementary Table S12).
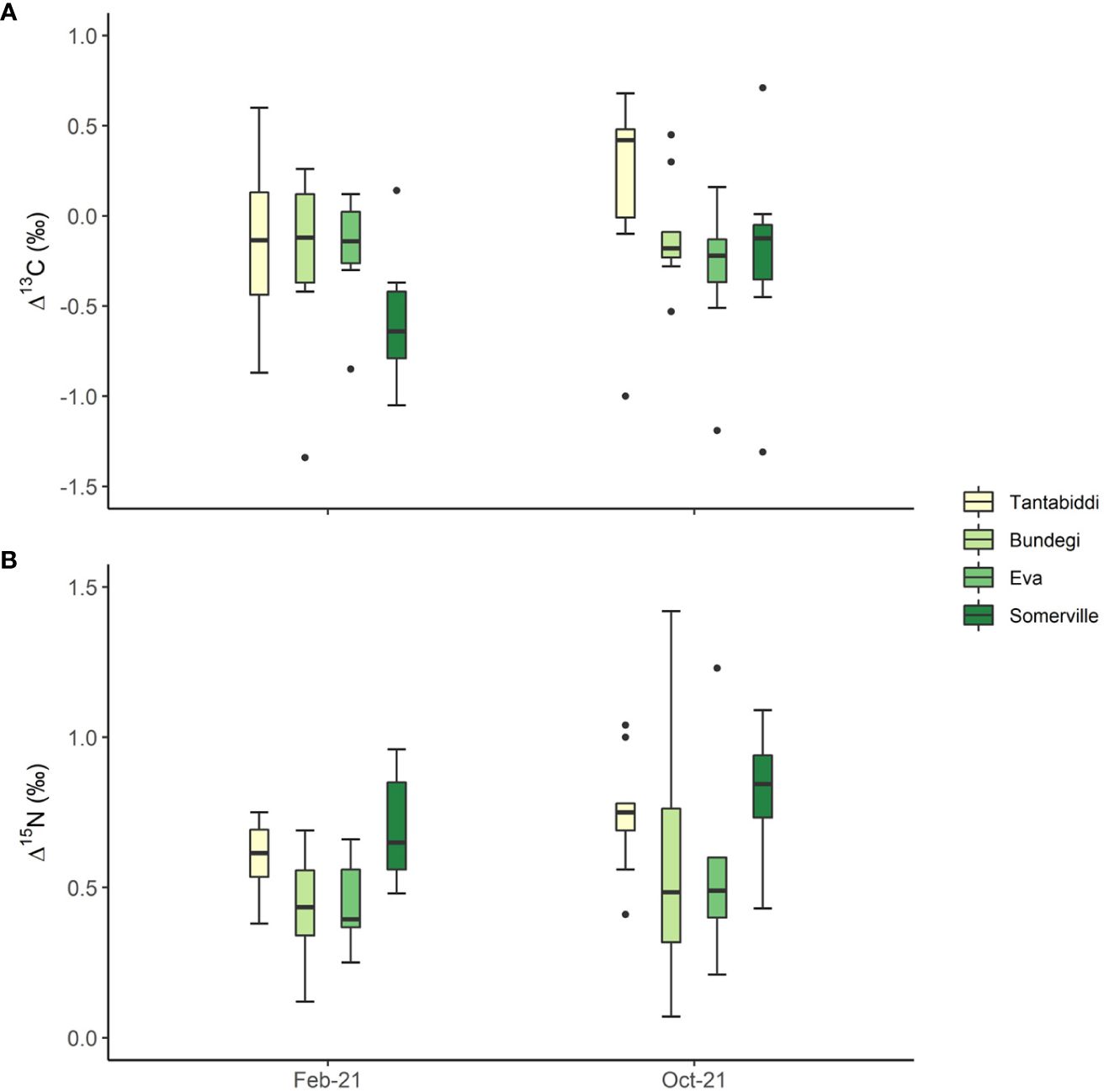
Figure 5 (A) Δ13C ( δ13Chost-symbiont) and (B) Δ15N (δ15Nhost-symbiont) in the four study sites from two sampling times. Whiskers represent minimum and maximum values, of the lower and upper quartile, respectively; dots represent outliers. n=5 colonies, two duplicate samples from each colony were analysed.
3.5 SIBER analysis
Plotting both the 40% (p.interval) and 95% (p.interval) SEAC ellipses for the coral host and symbionts revealed 0% overlap at the 40% (p.interval) SEAC across all sites and sampling times (Figure 6). A broader investigation of the 95% (p.interval) SEAC overlap found the Acropora tenuis coral community at Tantabiddi with 0% overlap between coral host and symbiont in February 2021 (summer) and 11% in October 2021 (winter) (Figures 6A, B; Supplementary Table S13, S14). In contrast, Bundegi corals displayed the greatest level of overlap (39% and 52%, in February 2021 and October 2021, respectively; Figures 6C, D). Similarly, at Eva, overlap between host and symbiont was 22% and 19% in February 2021 and October 2021, respectively (Figure 6E, F). At Somerville, the A.tenuis coral community displayed 23% overlap in February 2021 and 0% overlap in October 2021 (Figures 6G, H; Supplementary Table S13, S14).
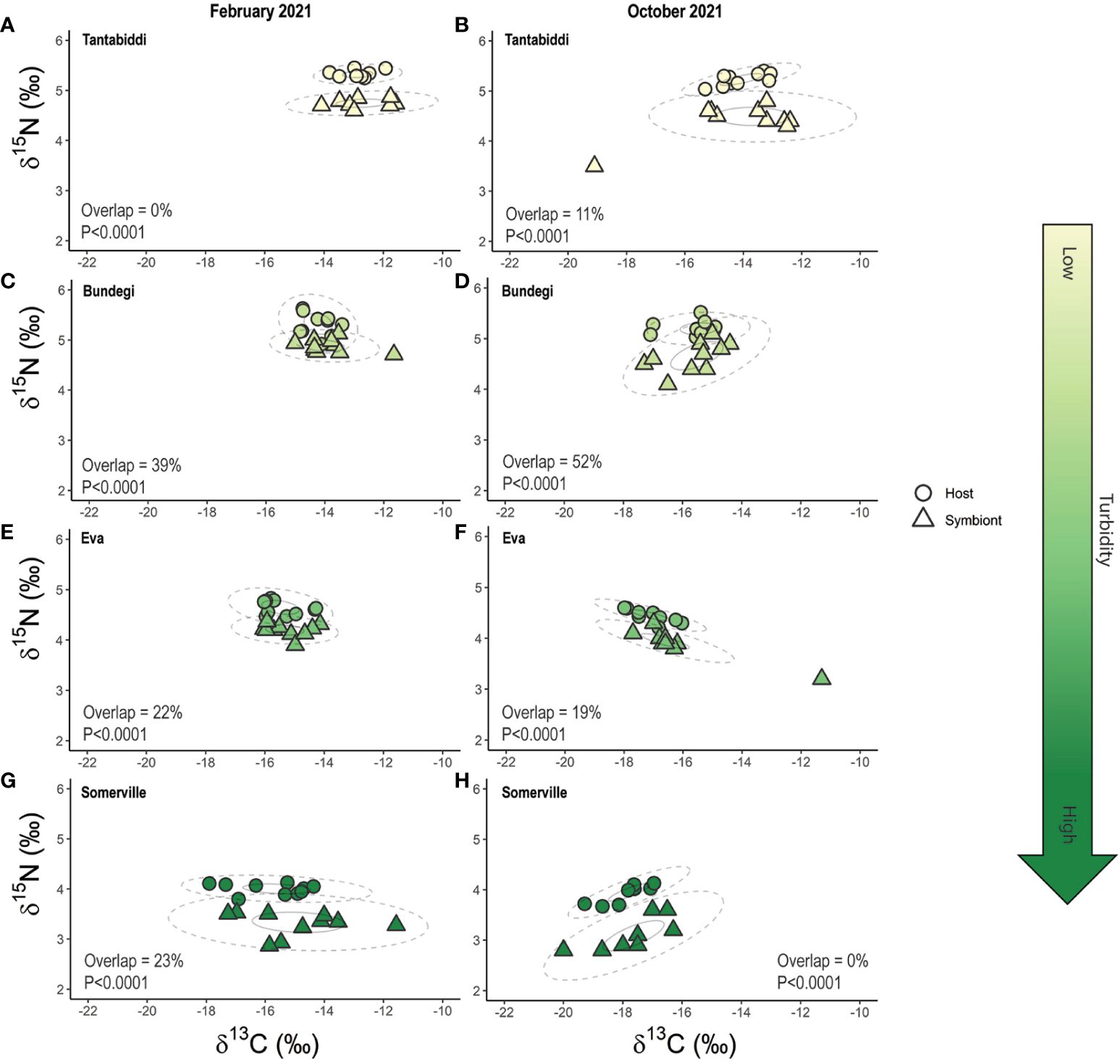
Figure 6 Isotope biplot of paired coral host and its algal symbiont δ13C and δ15N stable isotope measured in February 2021 (left column) and October 2021 (right column) at Tantabiddi (A,B), Bundegi (C, D), Eva (E, F) and Somerville (G, H). Dotted (p.interval = 0.95) and solid (p.interval = 0.4) lines represent Standard Ellipse Areas corrected for sample size (SEAC), analysed using SIBER r package. Overlap = 0.95 p.interval ellipse overlap between host and symbiont. p<0.05 is an indication of heterotrophic feeding strategy. n=5 colonies, two duplicate samples from each colony were analysed.
4 Discussion
The main aim of this study was to identify Acropora tenuis physiological attributes and energy acquisition strategies in response to varying turbidity levels at four sites and two sampling times (February and October). We expected to find increased heterotrophy rates to compensate for lower levels of light with increasing turbidity levels. In addition, coral physiology parameters were expected to vary significantly among sites, with higher amounts of Symbiodiniaceae and Chl a at the turbid sites, while the clear-water site were expected to have increased autotrophic feeding. The results of this study contradict our hypothesis and instead suggest that the physiological behavior of Acropora tenuis does not directly correlate to turbidity but may be influenced by a wider range of environmental conditions that are unique to each site.
4.1 Coral physiology
Symbiont densities and Chl a showed no significant trend across the turbidity gradient. However, we did see a decline in these parameters from the low turbidity site Tantabiddi to the mid-turbid site Eva before they increased at the most turbid site Somerville. Typically, symbiont density and Chl a concentrations increase with increasing turbidity levels, most likely to compensate for decreasing light levels. For example, in the Burdekin region in the GBR, Acropora tenuis sampled from a turbid nearshore reef site was found to have a symbiont density of ~15 (105 cell cm-2), which was three times higher compared to the most offshore remote reef site (Rocker et al., 2017). Similarly, Strahl et al. (2019) found that total chlorophyll of Acropora tenuis (7 µg chl cm-2) was also higher at turbid sites with levels decreasing as water quality increased. Jacquemont et al. (2022) reported values of ~12 Chl a (µg chl cm-2) and symbiont density of ~ 30 (105 cell cm-2) in Acropora tenuis from the Bouraké in New-Caladonia, a reef site noted for its marginal environmental conditions, including high turbidity (Maggioni et al., 2021). The atypical trend observed in this study could be due to additional environmental drivers (e.g., nutrients) influencing symbiont densities (and Chl a). For example, in a study of A.tenuis health with water quality on the GBR, dissolved organic carbon (DOC) was also positively correlated with symbiont densities (Rocker et al., 2017). Overall, symbiont densities were well within optimum ranges (10 to 30 x 105 cells per cm2) suggested by Woolridge (2020) under non-ideal environmental conditions. However, Chl a levels were comparatively low (0.5 to 1 µg chl cm-2), indicating low Chl a concentration per symbiont. These trends partially explain the pattern observed in δ13C values (explained below).
4.2 Coral trophic strategy
The coral energy acquisition strategy was assessed by analysing the stable isotope values of the coral host and its symbiont algae, and the overlap between their fractions (SIBER). Here we found that the variations in turbidity levels among sites had a stronger impact on trophic strategy than temporal differences within a site. As expected, δ13C values, a product of the photosynthesis process (Anthony and Fabricius, 2000), significantly decreased with increasing turbidity (Anthony and Fabricius, 2000). A similar trend in δ13C values with turbidity was also observed by Nahon et al. (2013) who found coral host tissue in A. hyacinthus from Moorea Island, French Polynesia, to be the most δ13C depleted at the site with the highest levels of suspended particulate matter. Yet, δ13C values in two other Acropora species sampled in their study (A. cytherea and A. pulchra) were similar across sites and seasons, regardless of differences in environmental conditions including suspended particulate matter. In contrast, Porites spp. (P. rus, P. verrucose, P. cactus) δ13C values were found to vary with environmental change demonstrating large plasticity in their physiology. This suggests that there is a variation in physiological adaptation within and between coral genera, and that some species, including A. tenuis are more adaptable to varying turbidity and light levels. Counterintuitively, δ15N values were also found to be highest at the clear-water site and lowest at the most turbid site. Ingestion of organic matter may represent an important source of nitrogen for corals living in shallow inshore waters (Houlbrèque and Ferrier-Pagès, 2009). As heterotrophic contribution rises, the δ15N values of corals are expected to align with those of the nitrogen contributors and potentially reach levels of 2.5-3.5 ‰ higher than their food source when engaged in fully heterotrophic feeding (Muscatine and Kaplan, 1994; Reynaud et al., 2002). Thus, typically, δ15N values will increase with increasing turbidity as the trophic strategy shifts from autotrophy to heterotrophy (Anthony and Fabricius, 2000; Nahon et al., 2013).
The outcomes of the SIBER analysis and the difference between coral host and symbiont, identified the A. tenuis communities in this study to be mixotrophic to heterotrophic communities. We observed that the most significant factor effecting the trophic niche was the consistently higher δ15N values in the host compared to the symbiont across all sites and in both sampling times. This highlights the crucial role of nitrogen in the coral symbiosis (Conti-Jerpe et al., 2020). Similar δ15N values of the coral host and its symbiont imply a shared nitrogen source that potentially involves nitrogen recycling within the holobiont (Tanaka et al., 2006; Gustafsson et al., 2013). This underscores the limiting role of nitrogen on reefs (Muscatine and Porter, 1977) and illustrates how corals have evolved symbiosis as an adaptive response to oligotrophic conditions (Conti-Jerpe et al., 2020). Generally, autotrophic coral will have higher δ15N levels and lower δ13C in the host tissue compared to their symbiont algae as a result of isotopic fractionation and mutual exchanges of carbon and nitrogen between the coral host and its symbiont algae (Muscatine et al., 1989; Muscatine & Kaplan, 1994; Swart et al., 2005a). When heterotrophy is the more dominant feeding strategy used by the coral, the difference in δ13C values between the coral hosts and their symbiont algae will be greater and the host signatures will more closely resemble the δ13C values of their heterotrophic feeding sources (i.e., zooplankton δ13C <-16‰) (Muscatine et al., 1989). We see these associations in A. tenuis at the more turbid sites, Somerville and Eva, where coral host δ13C average is -16.7 ± 0.8, and phytoplankton and zooplankton δ13C signature was -17. However, this was less evident at Tantabiddi, were δ13C signature of phyto/zooplankton was -7 whereas in the coral host tissue it was -13.4 ± 0.6. The reduced association between host signatures and feeding sources is likely due to other important factors such as the isotopic values of the dissolved inorganic carbon and nitrogen sources (Heikoop et al., 2000; Risk et al., 2009), nutrient concentrations (Hoegh-Guldberg et al., 2004; Fox et al., 2019), respiration rates (Swart et al., 2005b) and light availability (Grottoli and Wellington, 1999), all of which influence δ13C and δ15N values of Scleractinia corals. In summary, despite the dominance of a heterotrophic energy acquisition strategy across all sites, the lack of an increase with rising turbidity could be explained by one or a combination of the following:
1) Acropora physiology
The ability to capture particulate organic matter, along with feeding rate and strategy vary among species potentially in relation to their polyp size and surface area (Palardy et al., 2005), as well feeding rates (Houlbrèque and Ferrier-Pagès, 2009). The Acroporidea family when compared to other families such as Montiporidae or Pocilloporidae, likely has a limited heterotrophic capacity due to small polyps (<2 mm) and short tentacles, which limits their ability to feed on larger organic matter (Palardy et al., 2008; Conti-Jerpe et al., 2020; Sangmanee et al., 2020). In contrast, those species with larger polyps (e.g., Galaxea sp., Lobophyllia sp., Euphyllia sp.) are found to be more efficient at heterotrophic feeding (Anthony and Fabricius, 2000). Consequently, the lower than expected coral host δ15N values at the more turbid sites may not be due to the lack of nitrogen sources available in the water column, but rather from the reduced ability of A. tenuis to capture the particles. Further, turbidity levels at Somerville were highly variable characterised by extreme high turbidity events of 36 NTU followed by very low turbidity levels of ~1 NTU the following day. These highly variable conditions for a coral species that are less tolerant to sediments, may further stress these corals resulting in reduced nitrogen intake via heterotrophy. Alternatively, it could be that the periodic extreme turbidity events in Somerville do not reach the required threshold to increase heterotrophic feeding in A.tenuis. This suggests that sustained high levels of turbidity pressure may be necessary for a shift in its feeding strategy.
2) Variable sediment loads and composition
Both Somerville and Eva experience elevated turbidity and resuspension events all year round with a weak pattern of higher values during winter and lower values during summer (Cartwright et al., 2021). This sediment is mostly composed of quartz and calcite sand that are recycled and resuspended locally due to strong wind driven waves and tidal regime in the area (Massel et al., 1997; Brunskill et al., 2001). Sediment loads at certain times of the year may be exceeding heterotrophic thresholds of A. tenuis. In Somerville, host-symbiont SEAC overlap indicated transition from mixotrophy (23% overlap) in February2021, the wet season (rainfall = 2 mm, <11.3 NTU, <0.41 Kd490), to heterotrophy (0% overlap) in October 2021, the dry season (rainfall = 0 mm, <0.35 Kd490). These data suggest that during the wet season when more sediments are delivered to coastal waters, the sediment stress threshold for A.tenuis has been exceeded resulting in a decline in heterotrophy. The observed δ15N enrichment of coral host and symbiont in Tantabiddi suggests that turbidity is not the only driver effecting trophic levels but also supports the notion that the isotopic composition of nitrogen sources differs between sites (Heikoop et al., 2000; Baker et al., 2011).
3) Nutrient sources
Heterotrophy by corals can be enhanced by the increase of available particulate nutrient sources in turbid environments to counteract the reduction in autotrophy by the photosymbionts and allow the corals to maintain a positive energy budget (Anthony, 2000). Here, Somerville corals were found to acquire most of their energy from heterotrophy as indicated by the range in Δ13C (-0.84 ± 0.87 in February 2021 and -0.21 ± 0.56 in October 2021), as well as the highest Δ15N in both sampling times. Still, the fact that corals were the most δ15N enriched at Tantabiddi compared to corals from Somerville, Δ15N variability among sites was not significant, and Δ13C at Somerville remained small, suggests no additional increase in heterotrophic feeding strategy at the turbid sites (Einbinder et al., 2009; Nahon et al., 2013). Importantly, Tantabiddi experiences the Ningaloo current which leads to upwelling during the summer months. Deep-water upwelling is essential in generating fluxes of inorganic nutrients, contributing to increased reef-wide primary productivity and the availability of particulate resources (Leichter and Genovese, 2006; Stuhldreier et al., 2015; Radice et al., 2019b). Consequently, the variability in nutrient and particulate concentrations can impact the trophic strategies adopted by symbiotic corals (Porter, 1976). This may explain increasing nitrogen levels and nutrient influx to the NMP waters and might be a contributing factor to why the A.tenius community at the clear water site is the most heterotrophic, with SEAC 0% overlap between coral host and symbiont in February 2021 (summer) and with 11% in October 2021 (winter). In contrast, Somerville and Eva are found in a more coastal – protected location, with little supply of freshwater from land or terrestrial runoff, and thus nutrient supply may be very low and limited (Brunskill et al., 2001).
5 Conclusion
Although limited in distribution, corals from naturally extreme environments are invaluable to understanding the mechanisms that support higher tolerance to future climate change. The spatial variability in turbidity of the shallow inshore waters of Exmouth Gulf provided the opportunity to assess how an important coral species can adapt to variable light and sediment conditions. We assumed that as turbidity increased, corals would increase their heterotrophic feeding rates to compensate for the reduction in carbon from photosynthesis. However, our results suggest that increased heterotrophy by coral hosts in turbid coral reef areas is not a universal pattern. Stable isotopic compositions of several coral species show variability through space and time, suggesting that adjustments in the heterotrophic pathway is a species-specific phenomenon.
A.tenuis was able to acquire additional energy through non-photosynthetic means and, as such, was classed as a mixotroph. However, highly variable and elevated sediment loads at the most turbid site negatively influenced A. tenuis ability to ingest sources of nitrogen by decreasing rates of heterotrophy during the most turbid months. Moreover, it seems that the loss of carbon due to low light at the turbid sites is not accompanied by nitrogen intake, which might suggest lower coral growth and reproduction as well as increased vulnerability to climate stressors. The main finding of this study is that A. tenuis feeding pattern varied mostly spatially rather than between the two sampling times, being more heterotrophic (than mixotrophic) at times of lower turbidity on sites within the Exmouth Gulf, and at the Ningaloo site during times of likely higher nutrient supply. This highlights the fact that Exmouth Gulf is a dynamic environment with variable environmental conditions and nutrient supply, which in combination has led to an opportunistic A. tenuis coral community that frequently changes its feeding strategy in response to varying turbidity and light levels. Yet, it is crucial to acknowledge that this conclusion may be influenced by varying environmental conditions at different study sites.
Despite the limitations posed by small sample sizes, the incorporation of both carbon and nitrogen isotope values in SIBER facilitated the exploration of trophic dynamics for both nutrients within the coral holobiont. To enhance our understanding of trophic strategies of corals in turbid environments, future research should consider a larger sample size and a broader range of taxa. The diverse findings reported in other studies from various regions, highlight the constraints associated with transferring results between different systems (Travaglione et al., 2023). Therefore, conservation and management plans should aim to include site-specific data on coral energy acquisition mechanisms along with long term environmental monitoring and examination of the different energy investment mechanisms of the coral to provide improved predictions of coral reef health under varying climate change scenarios.
Data availability statement
The original contributions presented in the study are included in the article/Supplementary Material. Further inquiries can be directed to the corresponding author.
Ethics statement
Ethical approval was not required for the study involving animals in accordance with the local legislation and institutional requirements because all necessary permits were obtained to conduct the research described in this manuscript. Coral sampling in Exmouth Gulf was conducted under permit 3619 from the Department of primary industries and regional development, Australia. Sampling at the Ningaloo Marine Park was conducted under permit FO25000277 from the Department of Biodiversity, Conservation and Attractions.
Author contributions
AZ: Conceptualization, Data curation, Formal analysis, Funding acquisition, Investigation, Methodology, Project administration, Resources, Software, Supervision, Validation, Visualization, Writing – original draft, Writing – review & editing. NB: Conceptualization, Project administration, Supervision, Validation, Writing – original draft, Writing – review & editing. OL: Conceptualization, Writing – review & editing. RH: Methodology, Writing – review & editing. MO: Conceptualization, Funding acquisition, Investigation, Methodology, Visualization, Writing – review & editing.
Funding
The author(s) declare that financial support was received for the research, authorship, and/or publication of this article. This work was funded by the Holsworth Wildlife Research Endowment, the Ecological Society of Australia (PG51006700, AZ) and by the Robson and Robertson award granted to AZ through the Jock Clough Marine Foundation, the Oceans Institute, University of Western Australia. Additional support was provided by NB, DECRA Fellowship DE180100391 from Curtin University, Perth.
Acknowledgments
We would like to thank the Minderoo-Exmouth Research Laboratory (MERL) operated by the Minderoo Foundation and its staff for their in-kind support of using their facility. A special appreciation to Shannon Dee for her significant part in conducting field work. We also thank Natalie Travaglione, Jurgen Valckenaere, Kesia Savill, Jack Henharen, Brooke Pyke, Michael Cuttler and Dafne Sahin for assisting with field work. To Greg Skrzypek from WABC, School of Plant Biology, the University of Western Australia, for his help in analysing and interpreting the data. We would like to extend our thanks to Yaeli Rosenberg from Bar-Ilan University, Israel and to Celeste Wale and Mary Lee from CELLCentral, School of Human Sciences, the University of Western Australia, for their technical support and advice.
Conflict of interest
The authors declare that the research was conducted in the absence of any commercial or financial relationships that could be construed as a potential conflict of interest.
Publisher’s note
All claims expressed in this article are solely those of the authors and do not necessarily represent those of their affiliated organizations, or those of the publisher, the editors and the reviewers. Any product that may be evaluated in this article, or claim that may be made by its manufacturer, is not guaranteed or endorsed by the publisher.
Supplementary material
The Supplementary Material for this article can be found online at: https://www.frontiersin.org/articles/10.3389/fmars.2024.1288296/full#supplementary-material
References
Alamaru A., Loya Y., Brokovich E., Yam R., Shemesh A. (2009). Carbon and nitrogen utilization in two species of Red Sea corals along a depth gradient: Insights from stable isotope analysis of total organic material and lipids. Geochimica Cosmochimica Acta 73, 5333–5342. doi: 10.1016/j.gca.2009.06.018
Anthony K. R. N. (2000). Enhanced particle-feeding capacity of corals on turbid reefs (Great Barrier Reef, Australia). Coral Reefs 19, 59–67. doi: 10.1007/s003380050227
Anthony K. R. N. (2006). Enhanced energy status of corals on coastal, high-turbidity reefs. Mar. Ecol. Prog. Ser. 319, 111–116. doi: 10.3354/meps319111
Anthony K. R. N., Connolly S. R. (2004). Environmental limits to growth: Physiological niche boundaries of corals along turbidity-light gradients. Oecologia 141, 373–384. doi: 10.1007/s00442-004-1647-7
Anthony K. R. N., Connolly S. R., Hoegh-Guldberg O. (2007). Bleaching, energetics, and coral mortality risk: Effects of temperature, light, and sediment regime. Limnology Oceanography 52, 716–726. doi: 10.4319/lo.2007.52.2.0716
Anthony K. R. N., Fabricius K. E. (2000). Shifting roles of heterotrophy and autotrophy in coral energetics under varying turbidity. J. Exp. Mar. Biol. Ecol. 252, 221–253. doi: 10.1016/S0022-0981(00)00237-9
Anthony K. R. N., Hoogenboom M. O., Maynard J. A., Grottoli A. G., Middlebrook R. (2009). Energetics approach to predicting mortality risk from environmental stress: a case study of coral bleaching. Funct. Ecol. 23, 539–550. doi: 10.1111/j.1365-2435.2008.01531.x
Australian Bureau of Meteorology (2022) Australian tropical cyclone season outlook. Available online at: http://www.bom.gov.au/climate/cyclones/Australia/ (Accessed 1 July 2022).
Baker D. M., Kim K., Andras J. P., Sparks J. P. (2011). Light-mediated 15N fractionation in Caribbean gorgonian octocorals: implications for pollution monitoring. Coral Reefs 30, 709–717. doi: 10.1007/s00338-011-0759-x
Baumann J., Grottoli A. G., Hughes A. D., Matsui Y. (2014). Photoautotrophic and heterotrophic carbon in bleached and non-bleached coral lipid acquisition and storage. J. Exp. Mar. Biol. Ecol. 461, 469–478. doi: 10.1016/j.jembe.2014.09.017
Bessell-browne P. (2017). Lethal and sub-lethal impacts of dredge related stressors on corals. Centre Microscopy Characterisation Anal. Doctor, 181.
Bollati E., Rosenberg Y., Simon‐Blecher N., Tamir R., Levy O., Huang D., et al. (2021). Untangling the molecular basis of coral response to sedimentation. Mol. Ecol. 31, 1–18. doi: 10.1111/mec.16263
Bonesso J. L., Browne N. K., Murley M., Dee S., Cuttler M. V. W.W., Paumard V., et al. (2022). Reef to island sediment connections within an inshore turbid reef island system of the eastern Indian Ocean. Sedimentary Geology 436, 106177. doi: 10.1016/j.sedgeo.2022.106177
Brown B. E., Dunne R. P., Somerfield P. J., Edwards A. J., Simons W. J. F., Phongsuwan N., et al. (2019). Long-term impacts of rising sea temperature and sea level on shallow water coral communities over a ~40 year period. Sci. Rep. 9, 1–12. doi: 10.1038/s41598-019-45188-x
Browne N. K. (2012). Spatial and temporal variations in coral growth on an inshore turbid reef subjected to multiple disturbances. Mar. Environ. Res. 77, 71–83. doi: 10.1016/j.marenvres.2012.02.005
Browne N., Braoun C., McIlwain J., Nagarajan R., Zinke J. (2019). Borneo coral reefs subject to high sediment loads show evidence of resilience to various environmental stressors. PeerJ 7, e7382. doi: 10.7717/peerj.7382
Browne N. K., Tay J. K. L., Low J., Larson O., Todd P. A. (2015). Fluctuations in coral health of four common inshore reef corals in response to seasonal and anthropogenic changes in water quality. Mar. Environ. Res. 105, 39–52. doi: 10.1016/j.marenvres.2015.02.002
Brunskill G. J., Orpin A. R., Zagorskis I., Woolfe K. J., Ellison J. (2001). Geochemistry and particle size of surface sediments of Exmouth Gulf, northwest shelf, Australia. Continental Shelf Res. 21, 157–201. doi: 10.1016/S0278-4343(00)00076-5
Cartwright P. J., Fearns P. R. C. S., Branson P., Cutler M. V. W., O’Leary M., Browne N. K., et al. (2021). Identifying metocean drivers of turbidity using 18 years of MODIS satellite data: implications for marine ecosystems under climate change. Remote Sens. 13, 3616. doi: 10.3390/rs13183616
Collins L. B., Zhu Z. R., Wyrwoll K. H., Eisenhauer A. (2003). Late Quaternary structure and development of the northern Ningaloo Reef, Australia. Sedimentary Geology 159:81–94. doi: 10.1016/S0037-0738(03)00096-4
Conlan J. A., Humphrey C. A., Severati A., Francis D. S. (2017). Influence of different feeding regimes on the survival, growth, and biochemical composition of Acropora coral recruits. PloS One 12, e0188568. doi: 10.1371/journal.pone.0188568
Conlan J. A., Humphrey C. A., Severati A., Parrish C. C., Francis D. S. (2019). Elucidating an optimal diet for captive Acropora corals. Aquaculture 513, 734420. doi: 10.1016/j.aquaculture.2019.734420
Conti-Jerpe I. E., Thompson P. D., Wong C. W. M., Oliveira N. L., Duprey N. N., Moynihan M. A., et al. (2020). Trophic strategy and bleaching resistance in reef-building corals. Sci. Adv. 6, 2–4. doi: 10.1126/sciadv.aaz5443
Cooper T. F., Ridd P. V., Ulstrup K. E., Humphrey C., Slivkoff M., Fabricius K. E. (2008). Temporal dynamics in coral bioindicators for water quality on coastal coral reefs of the Great Barrier Reef. Mar. Freshw. Res. 59, 703–716. doi: 10.1071/MF08016
Dee S., Cuttler M., O’Leary M., Hacker J., Browne N. (2020). The complexity of calculating an accurate carbonate budget. Coral Reefs 39, 1525–1534. doi: 10.1007/s00338-020-01982-y
Depczynski M., Gilmour J. P., Ridgway T., Barnes H., Heyward A. J., Holmes T. H., et al. (2013). Bleaching, coral mortality and subsequent survivorship on a West Australian fringing reef. Coral Reefs 32, 233–238. doi: 10.1007/s00338-012-0974-0
Doropoulos C., Gómez‐Lemos L. A., Salee K., McLaughlin M. J., Tebben J., van Koningsveld M., et al. (2022). Limitations to coral recovery along an environmental stress gradient. Ecol. Appl. 32, 1–16. doi: 10.1002/eap.2558
Einbinder S., Mass T., Brokovich E., Dubinsky Z., Erez J., Tchernov D. (2009). Changes in morphology and diet of the coral Stylophora pistillata along a depth gradient. Mar. Ecol. Prog. Ser. 381, 167–174. doi: 10.3354/meps07908
Feng M., Meyers G., Pearce A., Wijffels S. (2003). Annual and interannual variations of the Leeuwin Current at 32°S. J. Geophysical Res. C: Oceans 108, 19–11. doi: 10.1029/2002JC001763
Ferrier-Pagès C., Peirano A., Abbate M., Cocito S., Negri A., Rottier C., et al. (2011). Summer autotrophy and winter heterotrophy in the temperate symbiotic coral Cladocora caespitosa. Limnology Oceanography 56, 1429–1438. doi: 10.4319/lo.2011.56.4.1429
Fox M. D., Williams G. J., Johnson M. D., Radice V. Z., Zgliczynski B. J., Kelly E. L. A., et al. (2018). Gradients in primary production predict trophic strategies of mixotrophic corals across spatial scales. Curr. Biol. 28, 3355–3363.e4. doi: 10.1016/j.cub.2018.08.057
Fox M. D., Smith E. A. E., Smith J. E., Newsome S. D. (2019). Trophic plasticity in a common reef-building coral: Insights from δ13C analysis of essential amino acids. Funct. Ecol. 33, 2203–2214. doi: 10.1111/1365-2435.13441
Gilmour J. P., Cook K. L., Ryan N. M., Puotinen M. L., Green R. H., Shedrawi G., et al. (2019). The state of Western Australia’s coral reefs. Coral Reefs 38, 651–667. doi: 10.1007/s00338-019-01795-8
Great Barrier Reef Marine Park Authority 2019 (2019). Great Barrier Reef Outlook Report (GBRMPA, Townsville: GBRMPA, Townsville).
Grottoli A. G., Toonen R. J., van Woesik R., Vega Thurber R., Warner M. E., McLachlan R. H., et al. (2021). Increasing comparability among coral bleaching experiments. Ecol. Appl. 31, 1–17. doi: 10.1002/eap.2262
Grottoli A. G., Rodrigues L. J., Palardy J. E. (2006). Heterotrophic plasticity and resilience in bleached corals. Nature 440, 1186–1189. doi: 10.1038/nature04565
Grottoli A. G., Wellington G. M. (1999). Effect of light and zooplankton on skeletal δ 13 C values in the eastern Pacific corals Pavona clavus and Pavona gigantea. Coral Reefs 18, 29–41. doi: 10.1007/s003380050150
Gustafsson M. S. M., Baird M. E., Ralph P. J. (2013). The interchangeability of autotrophic and heterotrophic nitrogen sources in Scleractinian coral symbiotic relationships: A numerical study. Ecol. Model. 250, 183–194. doi: 10.1016/j.ecolmodel.2012.11.003
Hanson C. E., Pattiaratchi C. B., Waite A. M. (2005). Sporadic upwelling on a downwelling coast: Phytoplankton responses to spatially variable nutrient dynamics off the Gascoyne region of Western Australia. Continental Shelf Res. 25, 1561–1582. doi: 10.1016/j.csr.2005.04.003
Heikoop J. M., Dunn J. J., Risk M. J., Tomascik T., Schwarcz H. P., Sandeman I. M., et al. (2000). δ15 N and δ13 C of coral tissue show significant inter-reef variation. Coral Reefs 19, 189–193. doi: 10.1007/s003380000092
Hoegh-Guldberg O., Muscatine L., Goiran C., Siggaard D., Marion G. (2004). Nutrient-induced perturbations to δ13C and δ15N in symbiotic dinoflagellates and their coral hosts. Mar. Ecol. Prog. Ser. 280, 105–114. doi: 10.3354/meps280105
Houlbrèque F., Ferrier-Pagès C. (2009). Heterotrophy in tropical scleractinian corals. Biol. Rev. 84, 1–17. doi: 10.1111/j.1469-185X.2008.00058.x
Hughes A. D., Grottoli A. G. (2013). Heterotrophic compensation: A possible mechanism for resilience of coral reefs to global warming or a sign of prolonged stress? PloS One 8, e81172. doi: 10.1371/journal.pone.0081172
Iglesias-Prieto R., Matta J. L., Robins W. A., Trench R. K. (1992). Photosynthetic response to elevated temperature in the symbiotic dinoflagellate Symbiodinium microadriaticum in culture. Proc. Natl. Acad. Sci. 89, 10302–10305. doi: 10.1073/pnas.89.21.10302
Jackson A. L., Inger R., Parnell A. C., Bearhop S. (2011). Comparing isotopic niche widths among and within communities: SIBER - Stable Isotope Bayesian Ellipses in R. J. Anim. Ecol. 80, 595–602. doi: 10.1111/jane.2011.80.issue-3
Jackson A., Parnell A. (2021) SIBER: Stable Isotope Bayesian Ellipses in R. Available online at: https://cran.r-project.org/web/packages/SIBER/ (Accessed 15 March 2022).
Jacquemont J., Houlbrèque F., Tanvet C., Rodolfo-Metalpa R. (2022). Long-term exposure to an extreme environment induces species-specific responses in corals’ photosynthesis and respiration rates. Mar. Biol. 169, 1–15. doi: 10.1007/s00227-022-04063-6
Jeffrey S. W., Humphrey G. F. (1975). New spectrophotometric equations for determining chlorophylls a, b, c1 and c2 in higher plants, algae and natural phytoplankton. Biochemie und Physiologie der Pflanzen 167, 191–194. doi: 10.1016/S0015-3796(17)30778-3
Jones R., Giofre N., Luter H. M., Neoh T. L., Fisher R., Duckworth A. (2020). Responses of corals to chronic turbidity. Sci. Rep. 10, 1–13. doi: 10.1038/s41598-020-61712-w
Krueger T., Bodin J., Horwitz N., Loussert-Fonta C., Sakr A., Escrig S., et al. (2018). Temperature and feeding induce tissue level changes in autotrophic and heterotrophic nutrient allocation in the coral symbiosis – A NanoSIMS study. Sci. Rep. 8, 1–15. doi: 10.1038/s41598-018-31094-1
Larcombe P., Woolfe K. J. (1999). Increased sediment supply to the Great Barrier Reef will not increase sediment accumulation at most coral reefs. Coral Reefs 18, 163–169. doi: 10.1007/s003380050174
Leichter J. J., Genovese S. J. (2006). Intermittent upwelling and subsidized growth of the scleractinian coral Madracis mirabilis on the deep fore-reef slope of Discovery Bay, Jamaica. Mar. Ecol. Prog. Ser. 316, 95–103. doi: 10.3354/meps316095
Lenth R. V., Buerkner P., Herve M., Love J., Fernando Riebl M., Hannes Singmann H. (2022) emmeans: Estimated Marginal Means, aka Least-Squares Means. Available online at: https://cran.r-project.org/web/packages/emmeans/index.html (Accessed 15 March 2022).
Lewis J. B. (1984). Coral reefs the acropora inheritance: A reinterpretation of the development of fringing reefs in Barbados, west indies. Coral Reefs 3, 117–122. doi: 10.1007/BF00301955
Lough J. M. (1998). Coastal climate of northwest Australia and comparisons with the Great Barrier Reef: 1960 to 1992. Coral Reefs 17, 351–367. doi: 10.1007/s003380050139
Luo Y., Huang L., Lei X., Yu X., Liu C., Jiang L., et al. (2022). Light availability regulated by particulate organic matter affects coral assemblages on a turbid fringing reef. Mar. Environ. Res. 177, 105613. doi: 10.1016/j.marenvres.2022.105613
Luter H. M., Pineda M.-C., Ricardo G., Francis D. S., Fisher R., Jones R. (2021). Assessing the risk of light reduction from natural sediment resuspension events and dredging activities in an inshore turbid reef environment. Mar. pollut. Bull. 170, 112536. doi: 10.1016/j.marpolbul.2021.112536
Maggioni F., Pujo-Pay M., Aucan J., Cerrano C., Calcinai B., Payri C., et al. (2021). The Bouraké semi-enclosed lagoon (New Caledonia) – a natural laboratory to study the lifelong adaptation of a coral reef ecosystem to extreme environmental conditions. Biogeosciences 18, 5117–5140. doi: 10.5194/bg-18-5117-2021
Massel R., Mason L., Bode L. ,. S. B. (1997). “Water circulation and waves in Exmouth Gulf,” in Proceedings of the Australian Physical Oceanography Conference, Sydney, Australia. 48.
Morgan K. M., Perry C. T., Johnson J. A., Smithers S. G. (2017). Nearshore turbid-zone corals exhibit high bleaching tolerance on the Great Barrier Reef following the 2016 ocean warming event. Front. Mar. Sci. 4. doi: 10.3389/fmars.2017.00224
Morgan K. M., Perry C. T., Smithers S. G., Johnson J. A., Gulliver P. (2016). Transitions in coral reef accretion rates linked to intrinsic ecological shifts on turbid-zone nearshore reefs. Geology 44, 995–998. doi: 10.1130/G38610.1
Muscatine L., Kaplan I. R. (1994). Resource Partitioning by Reef Corals as Determined from Stable Isotope Composition II. ~l5N of Zooxanthellae and Animal Tissue versus Depth l. PACIFIC Sci. 48, 9. doi: 10.1007/BF00391957
Muscatine L., Porter J. W. (1977). Reef corals: mutualistic symbioses adapted to nutrient-poor environments. BioScience 27, 454–460. doi: 10.2307/1297526
Muscatine L., Porter J. W., Kaplan I. R. (1989). Resource partitioning by reef corals as determined from stable isotope composition - I. δ13C of zooxanthellae and animal tissue vs depth. Mar. Biol. 100, 185–193. doi: 10.1007/BF00391957
Mydlarz L. D., McGinty E. S., Harvell C. D. (2010). What are the physiological and immunological responses of coral to climate warming and disease? J. Exp. Biol. 213, 934–945. doi: 10.1242/jeb.037580
Nahon S., Richoux N. B., Kolasinski J., Desmalades M., Pages C. F., Lecellier G., et al. (2013). Spatial and temporal variations in stable carbon (δ13C) and nitrogen (δ15N) isotopic composition of symbiotic scleractinian corals. PloS One 8, 81247. doi: 10.1371/journal.pone.0081247
Oppenheimer M., Glavovic B. C., Hinkel J., Wal R., van de Magnan A. K., Abd-Elgawad A., et al. (2019). “Sea Level Rise and Implications for Low Lying Islands, Coasts and Communities,” in IPCC Special Report on the Ocean and Cryosphere in a Changing Climate. Cambridge, UK and New York, NY, USA: Cambridge University Press.
Orpin A. R., Haig D. W., Woolfe K. J. (1999). Sedimentary and foraminiferal facies in Exmouth Gulf, in arid tropical northwestern Australia. Aust. J. Earth Sci. 46, 607–621. doi: 10.1046/j.1440-0952.1999.00728.x
Page C. E., Leggat W., Heron S. F., Choukroun S. M., Lloyd J., Ainsworth T. D. (2019). Seeking resistance in coral reef ecosystems: the interplay of biophysical factors and bleaching resistance under a changing climate: the interplay of a reef’s biophysical factors can mitigate the coral bleaching response. BioEssays 41, 1–12. doi: 10.1002/bies.201800226
Palardy J. E., Grottoli A. G., Matthews K. A. (2005). Effects of upwelling, depth, morphology and polyp size on feeding in three species of Panamanian corals. Mar. Ecol. Prog. Ser. 300, 79–89. doi: 10.3354/meps300079
Palardy J. E., Rodrigues L. J., Grottoli A. G. (2008). The importance of zooplankton to the daily metabolic carbon requirements of healthy and bleached corals at two depths. J. Exp. Mar. Biol. Ecol. 367, 180–188. doi: 10.1016/j.jembe.2008.09.015
Pinheiro J., et al. (2022) nlme: Linear and Nonlinear Mixed Effects Models. Available online at: https://cran.r-project.org/web/packages/nlme/index.html (Accessed 15 March 2022).
Piniak G. A., Storlazzi C. D. (2008). Diurnal variability in turbidity and coral fluorescence on a fringing reef flat: Southern Molokai, Hawaii. Estuarine Coast. Shelf Sci. 77, 56–64. doi: 10.1016/j.ecss.2007.08.023
Porter J. W. (1976). Autotrophy , Heterotrophy , and Resource Partitioning in Caribbean Reef-Building Corals Vol. 110. Ed. James W. (The University of Chicago Press for The American Society of Naturalists), 731–742. Available at: https://www.jstor.org/stable/2460081.
Price J. T., McLachlan R. H., Jury C. P., Toonen R. J., Grottoli A. G. (2021). Isotopic approaches to estimating the contribution of heterotrophic sources to Hawaiian corals. Limnology Oceanography 66, 2393–2407. doi: 10.1002/lno.11760
Radice V. Z., Brett M. T., Fry B., Fox M. D., Hoegh-Guldberg O., Dove S. G. (2019a). Evaluating coral trophic strategies using fatty acid composition and indices. PloS One 14, e0222327. doi: 10.1371/journal.pone.0222327
Radice V. Z., Hoegh-Guldberg O., Fry B., Fox M. D., Dove S. G. (2019b). Upwelling as the major source of nitrogen for shallow and deep reef-building corals across an oceanic atoll system. Funct. Ecol. 33, 1120–1134. doi: 10.1111/1365-2435.13314
Reynaud S., Ferrier-Pagès C., Sambrotto R., Juillet-Leclerc A., Jaubert J., Gattuso J.-P. (2002). Effect of feeding on the carbon and oxygen isotopic composition in the tissues and skeleton of the zooxanthellate coral Stylophora pistillata. Mar. Ecol. Prog. Ser. 238, 81–89. doi: 10.3354/meps238081
Risk M. J., Lapointe B. E., Sherwood O. A., Bedford B. J. (2009). The use of δ15N in assessing sewage stress on coral reefs. Mar. pollut. Bull. 58, 793–802. doi: 10.1016/j.marpolbul.2009.02.008
Rocker M. M., Francis D. S., Fabricius K. E., Willis B. L., Bay L. K. (2017). Variation in the health and biochemical condition of the coral Acropora tenuis along two water quality gradients on the Great Barrier Reef, Australia. Mar. pollut. Bull. 119, 106–119. doi: 10.1016/j.marpolbul.2017.03.066
Roitman S., López-Londoño T., Joseph Pollock F., Ritchie K. B., Galindo-Martínez C. T., Gómez-Campo K., et al. (2020). Surviving marginalized reefs: assessing the implications of the microbiome on coral physiology and survivorship. Coral Reefs 39, 795–807. doi: 10.1007/s00338-020-01951-5
Rosenberg Y., Simon‐Blecher N., Lalzar M., Yam R., Shemesh A., Alon S., et al. (2022). Urbanization comprehensively impairs biological rhythms in coral holobionts. Global Change Biol 00:1–16. doi: 10.1111/gcb.16144
Sangmanee K., Casareto B. E., Nguyen T. D., Sangsawang L., Toyoda K., Suzuki T., et al. (2020). Influence of thermal stress and bleaching on heterotrophic feeding of two scleractinian corals on pico-nanoplankton. Mar. pollut. Bull. 158, 111405. doi: 10.1016/j.marpolbul.2020.111405
Seemann J., Berry K. L., Carballo-Bolaños R., Struck U., Leinfelder R. R. (2013). The use of 13C and 15N isotope labeling techniques to assess heterotrophy of corals. J. Exp. Mar. Biol. Ecol. 442, 88–95. doi: 10.1016/j.jembe.2013.01.004
Skrzypek G. (2013). Normalization procedures and reference material selection in stable HCNOS isotope analyses: an overview. Analytical Bioanalytical Chem. 2012 405:9 405, 2815–2823. doi: 10.1007/s00216-012-6517-2
Smith R. L., Huyer A., Godfrey J. S., Church J. A. (1991). The leeuwin current off western Australia 1986-1987. Phys. Oceaonagraphy 21, 323–345. doi: 10.1175/1520-0485(1991)021<0323:TLCOWA>2.0.CO;2
Speed C. W., Babcock R. C., Bancroft K. P., Beckley L. E., Bellchambers L. M., Depczynski M., et al. (2013). Dynamic stability of coral reefs on the west Australian coast. PloS One 8, e69863. doi: 10.1371/journal.pone.0069863
Stimson J., Kinzie R. A. (1991). The temporal pattern and rate of release of zooxanthellae from the reef coral Pocillopora damicornis (Linnaeus) under nitrogen-enrichment and control conditions. J. Exp. Mar. Biol. Ecol. 153, 63–74. doi: 10.1016/S0022-0981(05)80006-1
Strahl J., Rocker M. M., Fabricius K. E. (2019). Contrasting responses of the coral Acropora tenuis to moderate and strong light limitation in coastal waters. Mar. Environ. Res. 147, 80–89. doi: 10.1016/j.marenvres.2019.04.003
Stuhldreier I., Sánchez-Noguera C., Roth F., Cortés J., Rixen T., Wild C. (2015). Upwelling increases net primary production of corals and reef-wide gross primary production along the pacific coast of Costa Rica. Front. Mar. Sci. 2. doi: 10.3389/fmars.2015.00113
Sully S., van Woesik R. (2020). Turbid reefs moderate coral bleaching under climate-related temperature stress. Global Change Biol. 26, 1367–1373. doi: 10.1111/gcb.14948
Swart P. K., Saied A., Lamb K. (2005a). Temporal and spatial variation in the δ15N and δ13C of coral tissue and zooxanthellae in Montastraea faveolata collected from the Florida reef tract. Limnology Oceanography 50, 1049–1058. doi: 10.4319/lo.2005.50.4.1049
Swart P. K., Szmant A., Porter J. W., Dodge R. E., Tougas J. I., Southam J. R. (2005b). The isotopic composition of respired carbon dioxide in scleractinian corals: Implications for cycling of organic carbon in corals. Geochimica Cosmochimica Acta 69, 1495–1509. doi: 10.1016/j.gca.2004.09.004
Tanaka Y., Miyajima T., Koike I., Hayashibara T., Ogawa H. (2006). Translocation and conservation of organic nitrogen within the coral-zooxanthella symbiotic system of Acropora pulchra, as demonstrated by dual isotope-labeling techniques. J. Exp. Mar. Biol. Ecol. 336, 110–119. doi: 10.1016/j.jembe.2006.04.011
Teixeira C. D., Leitão R. L. L., Ribeiro F., Moraes F. C., Neves L. M., Bastos A. C., et al. (2019). Sustained mass coral bleaching, (2016–2017) in Brazilian turbid-zone reefs: taxonomic, cross-shelf and habitat-related trends. Coral Reefs 38, 801–813. doi: 10.1007/s00338-019-01789-6
Tisthammer K. H., Timmins-Schiffman E., Seneca F. O., Nunn B. L., Richmond R. H. (2020). Surviving in high stress environments: Physiological and molecular responses of lobe coral indicate nearshore adaptations to anthropogenic stressors. bioRxiv, 786673. doi: 10.1101/786673v3
Titlyanov E. A., Titlyanova T. V. (2002). Reef-building corals—Symbiotic autotrophic organisms: 1. General structure, feeding pattern, and light-dependent distribution in the shelf. Russian J. Mar. Biol. 28, S1–S15. doi: 10.1023/A:1021836204655
Travaglione N., Evans R., Moustaka M., Cuttler M., Thomson D. P., Tweedley J., et al. (2023). Scleractinian corals rely on heterotrophy in highly turbid environments. Coral Reefs 42:997–1010. doi: 10.1007/s00338-023-02407-2
Turner T. F., Collyer M. L., Krabbenhoft T. J. (2010). A general hypothesis-testing framework for stable isotope ratios in ecological studies. Ecology 91, 2227–2233. doi: 10.1890/09-1454.1
Twiggs E. J., Collins L. B. (2010). Development and demise of a fringing coral reef during Holocene environmental change, eastern Ningaloo Reef, Western Australia. Mar. Geology 275, 20–36. doi: 10.1016/j.margeo.2010.04.004
Wallace C. C., Willis B. L. (1994). Systematics of the coral genus acropora: implications of new biological findings for species concepts. Annu. Rev. Ecol. Systematics 25, 237–262. doi: 10.1146/annurev.es.25.110194.001321
Warner M. E., Fitt W. K., Schmidt G. W. (1999). Damage to photosystem II in symbiotic dinoflagellates: A determinant of coral bleaching. Proc. Natl. Acad. Sci. 96, 8007–8012. doi: 10.1073/pnas.96.14.8007
Wei T., et al. (2021) CRAN - Package corrplot. Available online at: https://cran.r-project.org/web/packages/corrplot/index.html (Accessed 2 June 2022).
Wooldridge S. A. (2014). Formalising a mechanistic linkage between heterotrophic feeding and thermal bleaching resistance. Coral Reefs 33, 1131–1136. doi: 10.1007/s00338-014-1193-7
Wooldridge S. A. (2020). Excess seawater nutrients, enlarged algal symbiont densities and bleaching sensitive reef locations: 1. Identifying thresholds of concern for the Great Barrier Reef, Australia. Marine Pollution Bulletin 152. doi: 10.1016/j.marpolbul.2016.04.054
Wyatt A. S. J., Lowe R. J., Humphries S., Waite A. M. (2010). Particulate nutrient fluxes over a fringing coral reef: relevant scales of phytoplankton production and mechanisms of supply. Mar. Ecol. Prog. Ser. 405, 113–130. doi: 10.3354/meps08508
Keywords: turbidity, coral reef, physiology, stable isotopes, feeding strategy
Citation: Zweifler A, Browne NK, Levy O, Hovey R and O’Leary M (2024) Acropora tenuis energy acquisition along a natural turbidity gradient. Front. Mar. Sci. 11:1288296. doi: 10.3389/fmars.2024.1288296
Received: 04 September 2023; Accepted: 24 April 2024;
Published: 14 May 2024.
Edited by:
Charles Alan Jacoby, University of South Florida St. Petersburg, United StatesReviewed by:
Ajit Kumar Mohanty, Indira Gandhi Centre for Atomic Research (IGCAR), IndiaTali Mass, University of Haifa, Israel
Copyright © 2024 Zweifler, Browne, Levy, Hovey and O’Leary. This is an open-access article distributed under the terms of the Creative Commons Attribution License (CC BY). The use, distribution or reproduction in other forums is permitted, provided the original author(s) and the copyright owner(s) are credited and that the original publication in this journal is cited, in accordance with accepted academic practice. No use, distribution or reproduction is permitted which does not comply with these terms.
*Correspondence: Adi Zweifler, YWRpLnp2aWZsZXJAcmVzZWFyY2gudXdhLmVkdS5hdQ==