- 1MARUM - Center for Marine Environmental Sciences, University of Bremen, Bremen, Germany
- 2Department of Geosciences, University of Bremen, Bremen, Germany
- 3Constructor University, Department of Physics and Earth Sciences, Bremen, Germany
- 4Department of Earth and Environmental Sciences, University of Minnesota, Minneapolis, MN, United States
The increasing threat of anthropogenic environment and climate change amplifies the urgency to investigate the effect of these changes on marine ecosystems. We provide information about the export flux of organic-walled dinoflagellate cysts between 2003 and 2020 in the upwelling ecosystem off Cape Blanc (Mauritania), one of the world’s most productive regions. We compared the cyst export flux with variability in environmental parameters, such as wind speed, wind direction, dust emission, sea surface temperature (SST), SST difference between trap location and open ocean (SSTa), and chlorophyll-a concentration. This information is valuable to determine the ecological signal of dinoflagellate cysts that could be applied in recent and paleo records. The total export production of dinoflagellate cysts fluctuated between 0 - 1.18 x 105 cysts m-2 d-1 for the heterotrophs and 0 - 1.06 x 104 cysts m-2 d-1 for the photo-/mixotrophs. The export productions of both groups were in line with changes in upwelling intensity, which in most years, intensified in spring - summer. Dinoflagellate cyst association was dominated by heterotrophic taxa that formed an average of 94% of the association throughout the sediment trap record. A strong interannual variation in the cyst export fluxes, as well as the association composition was observed in the record. We identified five groups that showed comparable variability in export production with changes in environmental conditions: (1) maximal upwelling; Echinidinium delicatum/granulatum, E. transparantum/zonneveldiae, Echinidinium spp., Trinovantedinium spp., and Protoperidinium latidorsale, (2) combined maximal upwelling and dust input; Archaeperidinium spp., P. americanum, P. stellatum, and P. subinerme, (3) upwelling relaxation; Gymnodinium spp. and L. polyedra, (4) warm surface waters; Bitectatodinium spongium and Protoceratium reticulatum, (5) species with no specific relationship to the studied environmental variables; Brigantedinium spp., E. aculeatum, Impagidinium aculeatum, P. conicum, P. monospinum, Pentapharsodinium dalei, and Spiniferites spp. The sediment trap record documented a gradual shift in the cyst taxa association that co-occurred with the gradual increase of Saharan dust input to the region, notably after 2008. The cyst association contained five photo-/mixotrophic taxa that were formed by potentially toxic dinoflagellates. The latter could cause threats to the socio-economy of coastal communities.
1 Introduction
Along with diatoms and coccolithophorids, dinoflagellates are among the major eukaryotic primary producers in marine environments (e.g., Dale and Dale, 1992; Rochon et al., 1999). Dinoflagellates include species with various feeding strategies: some are photosynthetic, heterotrophic, and many are mixotrophic (e.g., Schnepf and Elbrächter, 1992; Taylor et al., 2008; Jeong et al., 2010). The composition of the dinoflagellate community is strongly influenced by the upper water column environmental and oceanographic conditions (Rochon et al., 1999; Taylor et al., 2008; Gómez, 2012). Within the scope of the concern about anthropogenic impact on climate and marine ecosystems, changes in the dinoflagellate community can be used as a key indicator to study the impact of these changes on marine ecosystems (e.g., Kremp et al., 2016; Brosnahan et al., 2020).
Around 15% of the extant dinoflagellate species are known to produce fossilisable cysts that have a species-specific morphology (e.g., Dale, 1976; Head, 1996; Dale et al., 2002; Bravo and Figueroa, 2014; Luo et al., 2019). After production in the upper water column, cysts sink to the ocean floor, where they can form a seed bank (e.g., Head, 1996; Matsuoka and Head, 2013; Bravo and Figueroa, 2014). Cysts of some species can remain viable in the sediment for up to a century (Lundholm et al., 2011; Ellegaard and Ribeiro, 2018; Delebecq et al., 2020). Dinoflagellate cysts in sedimentary archives are widely used for environmental, oceanographic, and climatic reconstruction and provide insight into past dinoflagellate bloom dynamics (e.g., Matsuoka and Head, 2013; Zonneveld et al., 2018; Sala-Pérez et al., 2020). To establish an adequate reconstruction of past environmental conditions, detailed information about cyst-forming dinoflagellates ecology is essential. Several dinoflagellate species can produce biotoxins or can form Harmful Algal Blooms (HAB), which can be a significant threat to marine ecosystems and human health (e.g., Balech, 1985; Amorim et al., 2001; Figueroa et al., 2018; Anderson et al., 2021). To understand the bloom dynamics of dinoflagellate species, including potentially toxic ones, information about the relationship between upper water column conditions and long-term variability in cyst export production of these species is required.
The number of studies on the ecology of dinoflagellate cysts has increased notably over the last decades (e.g., de Vernal et al., 2020; García-Moreiras et al., 2021; Likumahua et al., 2021; Rodríguez–Villegas et al., 2022; Zonneveld et al., 2022b; Obrezkova et al., 2023). However, only limited information is available about the seasonal, intra-, and interannual variability in dinoflagellate cyst production and their species composition. A method to investigate these aspects is to study sediment trap records (e.g., Dale and Dale, 1992; Harland and Pudsey, 1999; Zonneveld and Brummer, 2000; Fujii and Matsuoka, 2006; Pitcher and Joyce, 2009; Pospelova et al., 2010; Price and Pospelova, 2011; Bringué et al., 2013; Pospelova et al., 2018; Romero et al., 2020). Previous sediment trap studies showed highly variable cyst production and taxa composition over time. Studying a long time series is essential to obtain a better insight into the interannual variability. Until now, decade-long studies are limited, with only one record from the Cariaco Basin covering 12.5 years (Bringué et al., 2019). Here, we enhance the information about the ecology and long-term variability of dinoflagellate cysts by providing a record of cyst export production in the Cape Blanc upwelling region over 18 years.
Cape Blanc in the Canary-Current upwelling system is one of the world’s most noteworthy marine ecosystems, forming part of the Eastern Boundary Upwelling Ecosystems (EBUEs). Although covering a small portion of the Earth’s ocean surface (ca. 10%), EBEUs are marine diversity hotspots and contribute significantly to marine primary production (e.g., Pauly and Christensen, 1995; Arístegui et al., 2009). In the study area, primary production is enhanced by permanent upwelling and import of trace elements by Saharan dust (e.g., Mittelstaedt, 1983; van Camp et al., 1991; Hagen, 2001). Upwelling on the Atlantic Coast of Mauritania is supported by the coastal wind system, favourable shelf morphology, and surface currents that can transport nutrient-rich water farther offshore (e.g., Mittelstaedt, 1983; Hagen, 2001). Although upwelling occurs all year long, its strongest intensity is observed during boreal winter (winter and spring seasons) (e.g., Lathuilière et al., 2008; Cropper et al., 2014). A similar trend is shown by the dust emission from the Sahara into the Cape Blanc oceanic area by the winds at low altitudes (0 - 3km) (Stuut et al., 2005; Adams et al., 2012; Skonieczny et al., 2013). The strength of upwelling and dust emission in winter and spring is influenced by the Inter Tropical Convergence Zone (ITCZ), which migrates southward to the equator realm during boreal winter and northward during boreal summer (Mittelstaedt, 1991; Ben-Ami et al., 2009; Adams et al., 2012; Yu et al., 2019).
Here, we present records of the export flux of organic-walled dinoflagellate cysts in the Cape Blanc upwelling area collected by a sediment trap covering the time span from 2003 until 2020 with temporal resolution between 3.5 and 22 days. The trap was moored close to the position of active upwelling cells at a location where upwelling filaments frequently pass the upper waters. We expanded the five years (2003-2008) dataset of Romero et al. (2020) with data from the following 13 years (Table 1). To obtain insight into abiotic factors that influence the export production of the photo-/mixotrophic and heterotrophic cyst species we compared dinoflagellate cyst fluxes and the association composition with wind speed, wind direction, atmospheric dust concentration, sea surface temperature (SST), SST difference between trap location and open ocean (SSTa), and sea surface chlorophyll-a concentration (Chl-a) at or in the vicinity of the trap location.
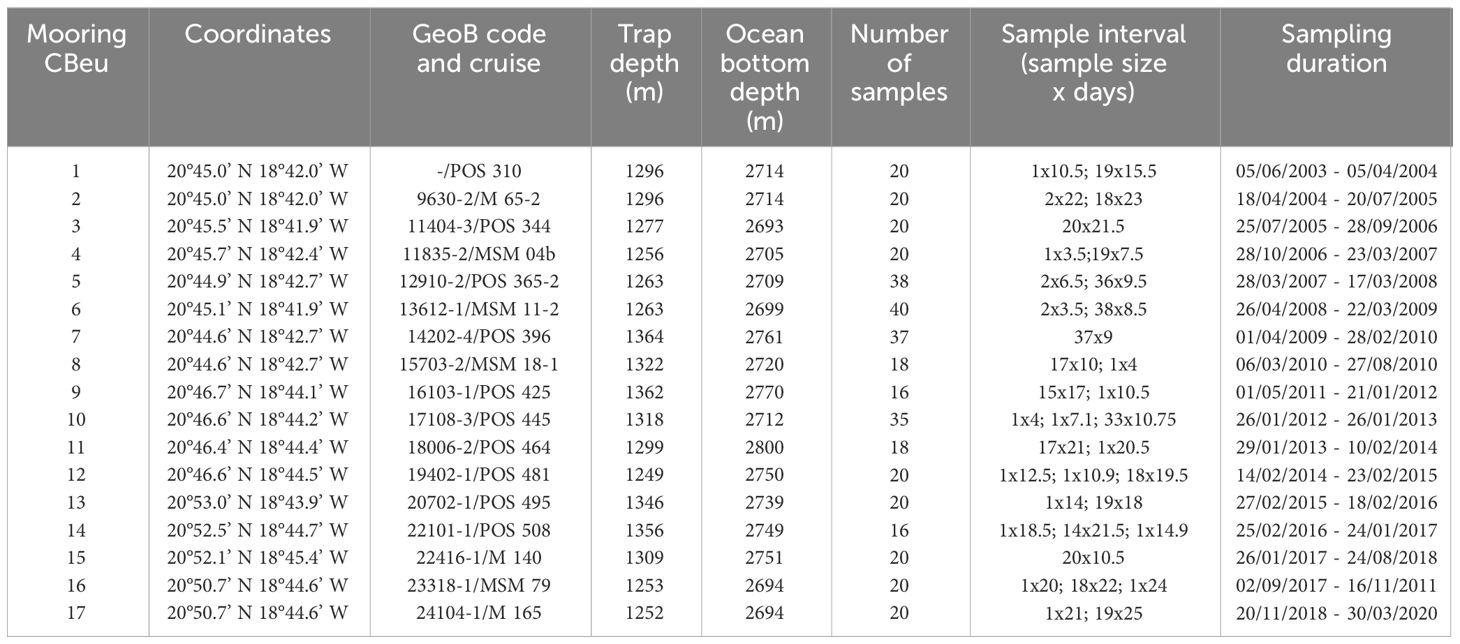
Table 1 Detailed information of CBeu sediment trap deployments and recoveries during multiple research expeditions off Cape Blanc (Mauritania, NW Africa).
2 Materials and methods
2.1 Oceanography of the study area
The current research was carried out in the Canary Current Eastern Boundary Upwelling Ecosystem (CC-EBUEs) located at the eastern border of the North Atlantic Subtropical Gyre (e.g., Arístegui et al., 2009; Cropper et al., 2014). The region is characterised by permanent upwelling driven by the equatorward wind trade system and frequent input of Saharan dust. Both upwelling and dust fertilize the upper ocean with the input of nutrients and trace elements, resulting in the CC-EBUEs being one of the most productive oceanic regions in the world (Mittelstaedt, 1983; van Camp et al., 1991; Hagen, 2001; Lathuilière et al., 2008). The surface currents in this area consist of the Canary Current (CC) and Mauritanian Current (MC) (Figure 1). The CC flows southwards along the northern coast of NW Africa, where it detaches from the shelf between 7° - 20° N, supplying cool coastal upwelled water to the North Equator Current (NEC) (Mittelstaedt, 1991; Alves et al., 2002). The MC gradually flows northward parallel to the Mauritanian coast towards 20° N, transporting warm waters of southern origin northwards (Mittelstaedt, 1983). MC intensifies in summer when it is pushed to the shelf by the North Equatorial Counter Current but weakens and is gradually replaced by the CC in late autumn due to increasing trade wind intensity south of 20° N (Zenk et al., 1991). The movement of both surface currents creates a substantial horizontal shear in the surface layer, forming a convergence zone called the Cape Verde Frontal Zone (CVFZ) (Zenk et al., 1991).
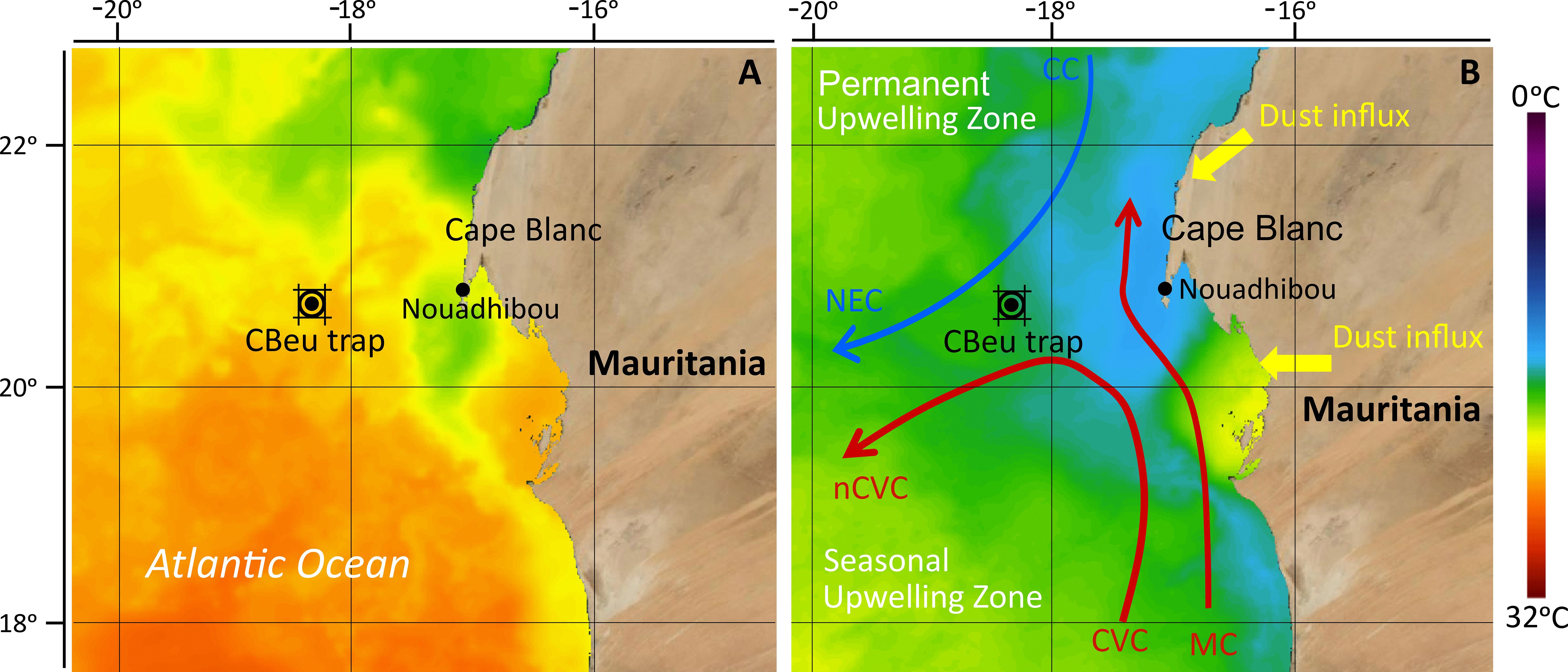
Figure 1 The location of the CBeu sediment trap (double black circle) and the surface currents in the studied area adapted after Mittelstaedt (1983, 1991) and Zenk et al. (1991). Blue arrow: relatively cold waters of the Canary Current (CC) and North Equatorial Current (NEC). Red arrows: warm waters of the Mauritanian Current (MC), Cape Verde Current (CVC), and north Cape Verde Current (nCVC). Satellite image depicted from NASA “State Of The Ocean (SOTO)” showing sea surface temperature during: (A) low upwelling intensity in autumn 2020 and; (B) high upwelling intensity in spring 2021.
Although coastal upwelling off Cape Blanc is a permanent feature, its maximal southward extension and intensity vary depending on the strength and direction of the surface winds (Lathuilière et al., 2008; Cropper et al., 2014). Maximal upwelling intensity is mainly observed in winter and spring, whereas lower intensity is often observed in summer and autumn (Fischer et al., 2016). The annual trend of the surface wind trade system variation is orchestrated by the seasonal migration of the Inter-Tropical Convergence Zone (ITCZ) (Mittelstaedt, 1991).
Upwelled water in the region is sourced from subsurface waters, either from the southward-flowing North Atlantic Central Water (NACW) or the northward-flowing South Atlantic Central Water (SACW) (Mittelstaedt, 1991; Meunier et al., 2012; Olivar et al., 2016). NACW originates from the North Atlantic carrying warmer, more saline, and nutrient-poorer water compared to SACW (Sarmiento et al., 2004). SACW originates from the Southern Ocean, moving north (Sarmiento et al., 2004). Upwelled waters north of Cape Blanc mainly consist of NACW, south of Cape Blanc they are mainly formed by SACW. Off Cape Blanc, NACW forms the major source of upwelling waters at times of maximal upwelling intensity, whereas larger amounts of SACW form the upwelled waters at times of low upwelling intensity (Sarmiento et al., 2004).
Dust emission also plays an important role in the vast growth of plankton in the region. Sahara, as the world’s largest contributor of aeolian mineral dust, influences the studied area by providing essential elements, such as iron and phosphorus (Kolber et al., 1994; Jickells et al., 2005; Huneeus et al., 2011). The maximum dust emission in this research area occurs in winter, with dust transported by surface winds at altitudes between 0 - 3 km (Stuut et al., 2005; Adams et al., 2012; Skonieczny et al., 2013; Fomba et al., 2014; Fischer et al., 2016). In summer, dust emissions mainly occur at more distal locations (e.g. the Caribbean) due to Saharan Air Layer (SAL), transporting dust at higher altitudes (5 - 7 km) (Prospero, 1990; Stuut et al., 2005; Adams et al., 2012; Prospero et al., 2014). The occurrence and intensity of dust storms in the Sahara are strongly influenced by the ITCZ, which controls the aridity of the continent and the direction and strength of the wind as an aerosol-carrier to the ocean (Ben-Ami et al., 2009; Adams et al., 2012; Yu et al., 2019).
2.2 Mooring site and sample treatment
Dinoflagellate cysts were collected between June 2003 and March 2020 at the Cape Blanc eutrophic mooring (CBeu) station (Figure 1). The trap was located between 20°44.6’ - 20°53.0’ N and 18°41.9’ - 18°45.4’ W at water depths between 1249 - 1364 m (Table 1). Samples were collected by a classical cone-shaped trap with a surface opening area of 0.5 m2 (Kiel SMT 230/234, Kremling 1998). Particle collection time varied between 3.5 and 22 days. The sampling cups contained mercury chloride (HgCl2) to prevent biochemical degradation and alteration of the captured organic material. More details on the sample processing were given in Mollenhauer et al. (2015); Romero and Fischer (2017), and Fischer et al. (2019). A total of 369 samples were collected and used throughout the 18 years of study.
Upon recovery, the materials were evenly divided into 1/125 fractions for various purposes using a Mc Lane splitter (Romero and Fischer, 2017; Fischer et al., 2019; Romero et al., 2020). Initially, the larger nektonic plankton, such as the copepods, were manually removed. Samples were filtered with a sieve of 1 mm pore size to isolate the target protists from coarser particles. The samples were transferred into sampling bottles and stored in the dark at the MARUM core repository for further treatment. They were kept at 4°C to prevent degradation of the organic content.
2.3 Dinoflagellate cysts extraction and taxonomic identification
For every sample, a 1/125 fraction of the material was sieved with distilled water over a 100 μm and a 20 μm high-precision metal sieve (Storck-Veco) to remove the remaining poisonous HgCl2. The washed samples were ultrasonically treated and sieved successively over a high-precision sieve with a pore size 20 μm. The remaining material was transferred to Eppendorf tubes, diluted to 1 mL, and homogenized. A known volume of the well-mixed sample was embedded in glycerin gelatin that was placed on a microscope slide, covered with a cover slip, and isolated from the air with wax (Zonneveld et al., 2010, 2022b). Organic dinoflagellate cysts were studied by light microscopy (Zeiss Axiovert, 400x magnification). The dinoflagellate export flux (cyst m-2 day-1) was calculated by dividing the counted specimens with the initial split fraction (1/125), the volume of the counted sample, the trap collection area, and the sampling collecting duration. Taxonomic identification of dinoflagellate cysts and the motile affinity were based on Zonneveld and Pospelova (2015); Mertens et al. (2020), and van Nieuwenhove et al. (2020). Cysts identified at species level with well-established cyst-theca relationships were referred to by their theca names following the statement of Elbrächter et al. (2023). For the motile affinity of cysts produced by toxin-producing dinoflagellates, see Wall and Dale (1968), Sarjeant (1970), Anderson et al. (1988), Dodge (1989), Head (1996), and Ellegaard and Moestrup (1999).
2.4 Environmental parameters
Dinoflagellate cyst fluxes and associations composition were compared to wind speed, wind direction, atmospheric dust concentration, sea surface temperature (SST), SST difference between trap location and open ocean (SSTa), and sea surface chlorophyll-a concentration (Chl-a). Wind speed (m s-1), wind direction, and occurrences of dust input were derived from Nouadhibou Airport (20°56’ N and 17°2’ W) meteorological report, the land-based location near the sediment trap (Figure 1). The decoded synoptic values were calculated to determine vector values of wind strength relative to the measured wind direction according to the equations below (Grange, 2014):
calculated vector wind from north
calculated vector wind from east
calculated resultant vector average of the wind direction
where is wind speed (m s-1) and is wind direction (°). Atmospheric dust concentration was defined by the minimal distance of horizontal visibility. Enhanced atmospheric dust concentration was detected when the visibility distance values were below 60 km.
SST and Chlorophyll-a data were obtained from ERDDAP daily optimum interpolation (OI), AVHRR dataset (Dataset ID ncdcOisst21Agg_Lon PM 180), the database of the National Oceanic and Atmospheric Administration (NOAA) for a 4 km grid at the trap location (20° 22.5 ’N and 18° 22.5 ’W). Sea surface temperature anomaly (SSTa) represents the difference between the daily values of SST at the trap location and 200 km offshore at the same latitude located outside the influence of upwelling and offshore drifting upwelling filaments (Cropper et al., 2014). More negative SSTa values represent the presence of colder waters at the trap site, which in the region is caused by the presence of upwelled water, as such SSTa represents the upwelling intensity. The daily values of all environmental parameters were calculated as lag and average of 10 days for the region’s estimated sinking duration of the dinoflagellate cyst in the water column (Fischer and Karakaş, 2009; Iversen and Ploug, 2013).
2.5 Statistical analyses
Dinoflagellate cysts and environmental parameter data were analysed by multivariate analyses that can independently explain the relationship between multiple variables, using the software package Canoco-5 (Šmilauer and Lepš, 2014). For the analyses, species occurring only sporadically in low numbers in the dataset were excluded from this analysis (Tables 2, 3). Multivariate analyses were performed on the relative abundances by the excluding samples that contained less than 100 specimens. Detrended Correspondence Analysis (DCA) was carried out to analyse the length of the gradient to verify the species response curves in the dataset (ter Braak and Prentice, 1988; ter Braak and Šmilauer, 2002). Additionally, a Canonical Correspondence Analysis (CCA) was used to determine the relationship between the cyst distribution patterns and the above-described environmental parameters.
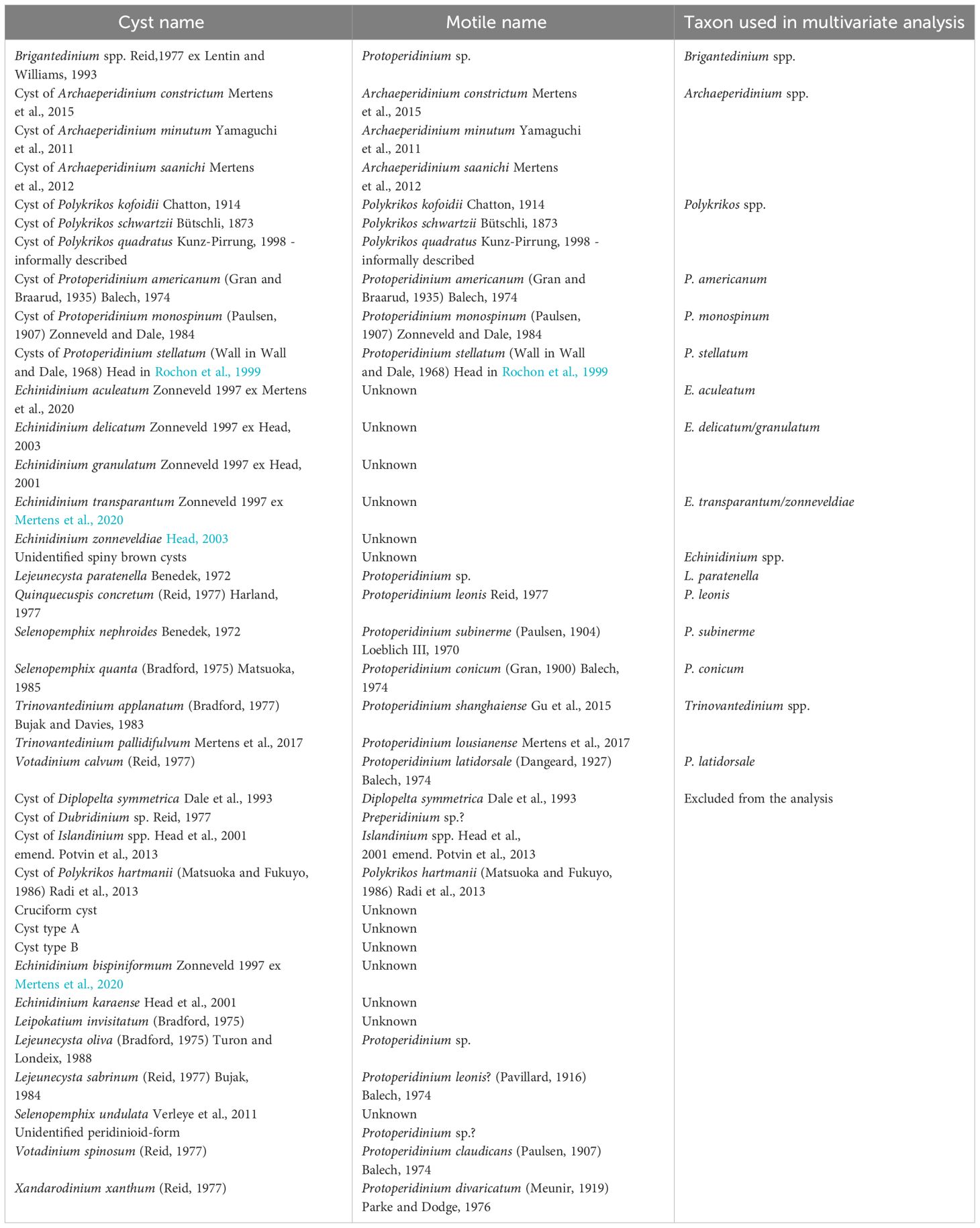
Table 2 The list of identified heterotrophic dinoflagellate cyst (paleontological) taxa in the studied area corresponds to their respective motile cell (biological) names and grouping of cysts used in statistical analysis.
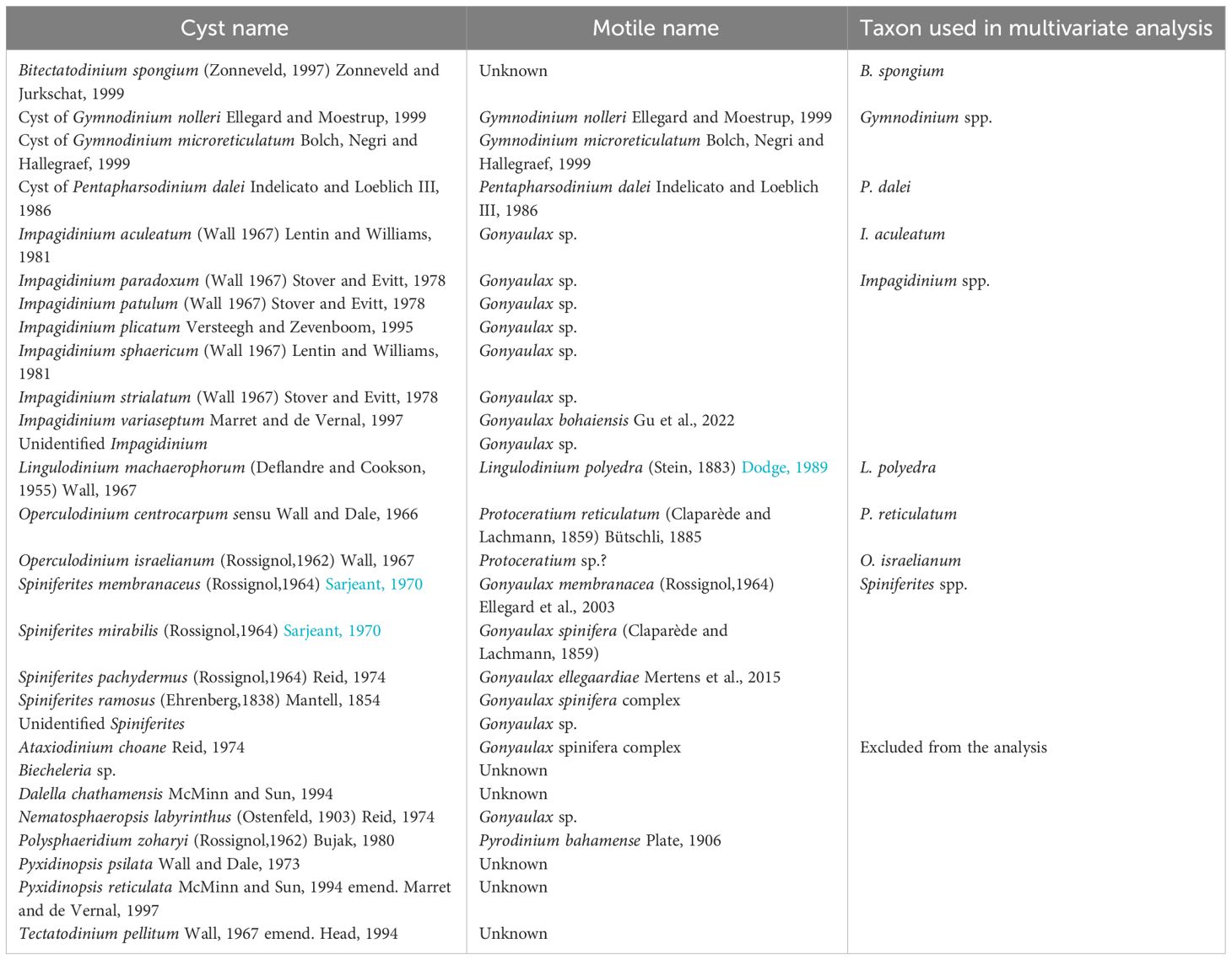
Table 3 The list of identified photo-/mixotrophic dinoflagellate cysts (paleontological) taxa in the studied area corresponds to their respective motile cell (biological) names and grouping of cysts used in statistical analysis.
3 Results
3.1 Dinoflagellate cysts flux
Throughout 18 years, the cyst export flux of heterotrophic dinoflagellates largely exceeded the photo-/mixotrophic dinoflagellates. On average, heterotrophic taxa formed ca. 94% of the total cyst record. The export flux of heterotrophic dinoflagellate cysts showed large interannual fluctuations with minimal fluxes occurring in spring - summer 2018 when no export flux was observed and maximal flux in winter 2011/2012 (1.18 x 105 cysts m-2 d-1) (Figure 2B). A malfunctioning of the sediment trap led to these minimal fluxes, but the samples could still be recovered from the cups. Over the years, the flux showed a trend from relatively low values between 2003 and 2005 to higher values between 2006 – 2013 and declined again after 2014. Exceptions were noted in 2009 and 2010, characterised by lower export fluxes than in the previous and later years. Throughout the years, the flux showed a more or less regular seasonal pattern, with maximal flux occurring in spring at times of maximal wind speed (Figure 2A). The duration of the high flux period varied; sometimes, it started in late winter or extended to summer. In other years, it was restricted to spring. Few exceptions occurred in 2005 and 2018, where the maximal fluxes occurred in autumn.
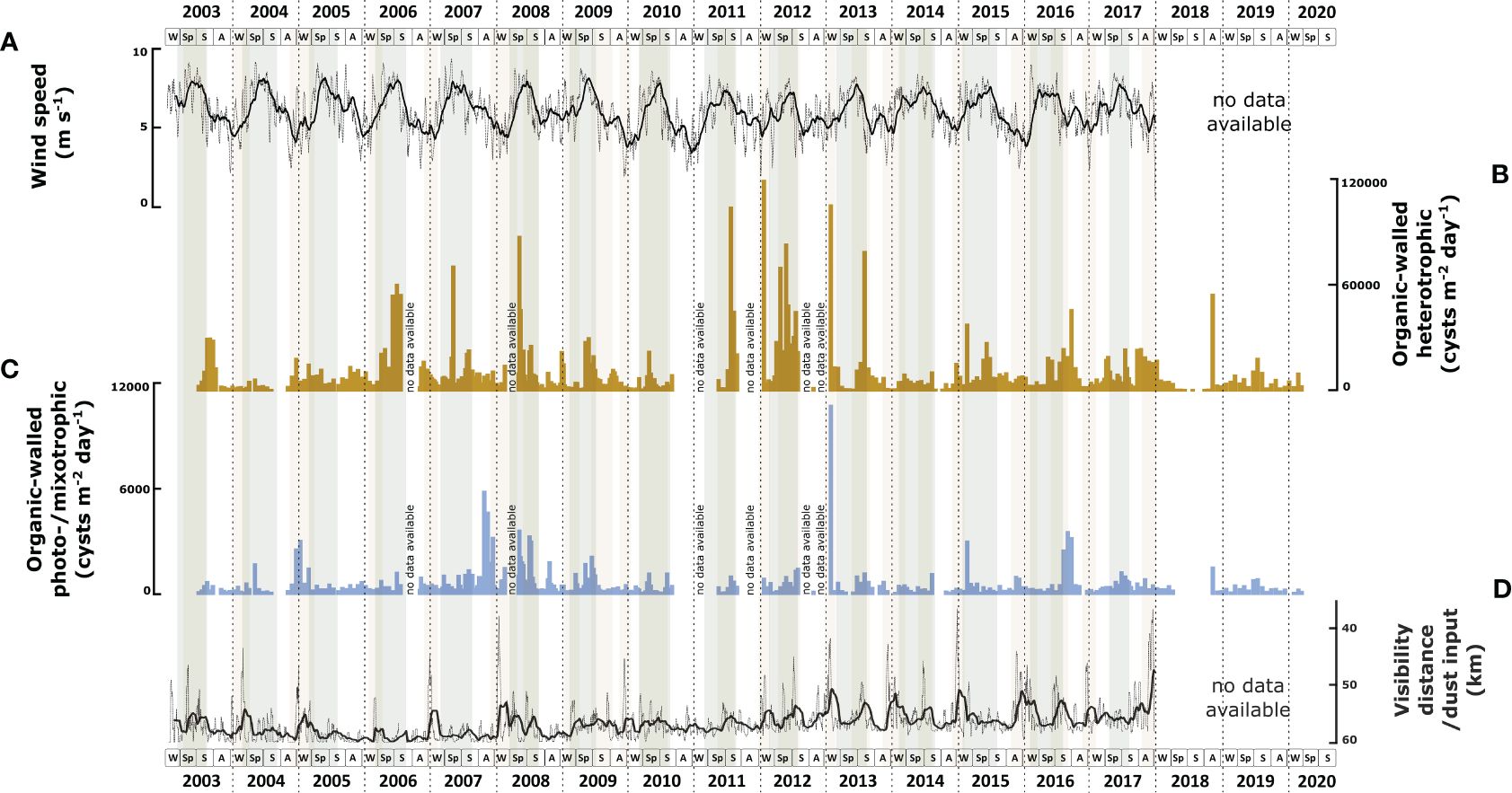
Figure 2 Correlation of environmental parameters with the total export fluxes of organic-walled dinoflagellate cysts based on 18 years CBeu sediment trap study: (A) daily wind speed in 10-running points average (the background grey line connects daily data points and the thicker black line represents the 10-point mean); (B) heterotrophic dinoflagellate cysts (cysts m-2 d-1, dark yellow bars); (C) photo-/mixotrophic dinoflagellate cysts (cysts m-2 d-1, blue bars). (D) daily visibility distance indicating dust input in 10-running points average (the background grey line connects the daily data points and the thicker black line represents the 10-point mean). The boxes in the lower panels refer to seasons (W, winter; Sp, spring; S, summer; A, autumn). Grey shades indicate maximal upwelling intensity, brown shades indicate maximum dust input, and the horizontal dashed lines indicate calendar year separation.
The export flux of cysts produced by photo-/mixotrophic dinoflagellates also showed strong interannual variability varying from no observed export flux in spring - summer 2018 to a maximal flux of 1.06 x 104 cysts m-2 d-1 in winter 2012/2013 (Figure 2C). The flux was relatively low in 2003 and increased steadily until 2008. From 2008 on, the photo-/mixotrophic cyst export flux decreased except for 2013, 2015, and 2016, when high export fluxes occurred. In contrast to the heterotrophic taxa, the cyst fluxes of photo-/mixotrophic dinoflagellates did not show a clear seasonal pattern. In several years, such as in 2008, 2009, 2016, and 2017, the highest fluxes occurred in spring - summer with intervals of intensified wind speed. In 2004 and 2007, the highest export fluxes occurred during weakened wind speed. In 2013 and 2015, the maximal export flux of cysts of photo/mixotrophic species occurred in winter during maximal Saharan dust input into the North Atlantic (Figure 2D).
3.2 Association of dinoflagellates cysts
In the analysed samples, 67 cyst taxa were identified, of which 39 belonged to heterotrophic species (Figure 3A–I). The most dominant taxon was Brigantedinium spp., accounting for ca. 60% of the cyst association (Figure 3A). This genus was present in the majority of samples throughout the time series, even when cyst recovery was extremely low. The annual and seasonal export flux of Brigantedinium spp. followed the trend of the total cyst export flux. Species of Echinidinium were the second most abundant, forming an average of 15% of the association (Figures 3G, H). Of this genus, Echinidinium transparantum/zonneveldiae was the most abundant species (5.9%), followed by E. aculeatum (4.3%), and E. delicatum/granulatum (4.3%). The relative abundance of Echinidinium species increased at times of increased total cyst export flux that usually occurred in spring. However, the Echinidinium association composition varied between the years (Figure 4A). E. aculeatum dominated in spring-summer 2009 and 2016, E. delicatum/granulatum was abundant in spring 2011 and 2019, and spring-summer 2012 and 2017. E. transparantum/zonneveldiae dominated in spring-summer 2010 and from 2013 to 2015.
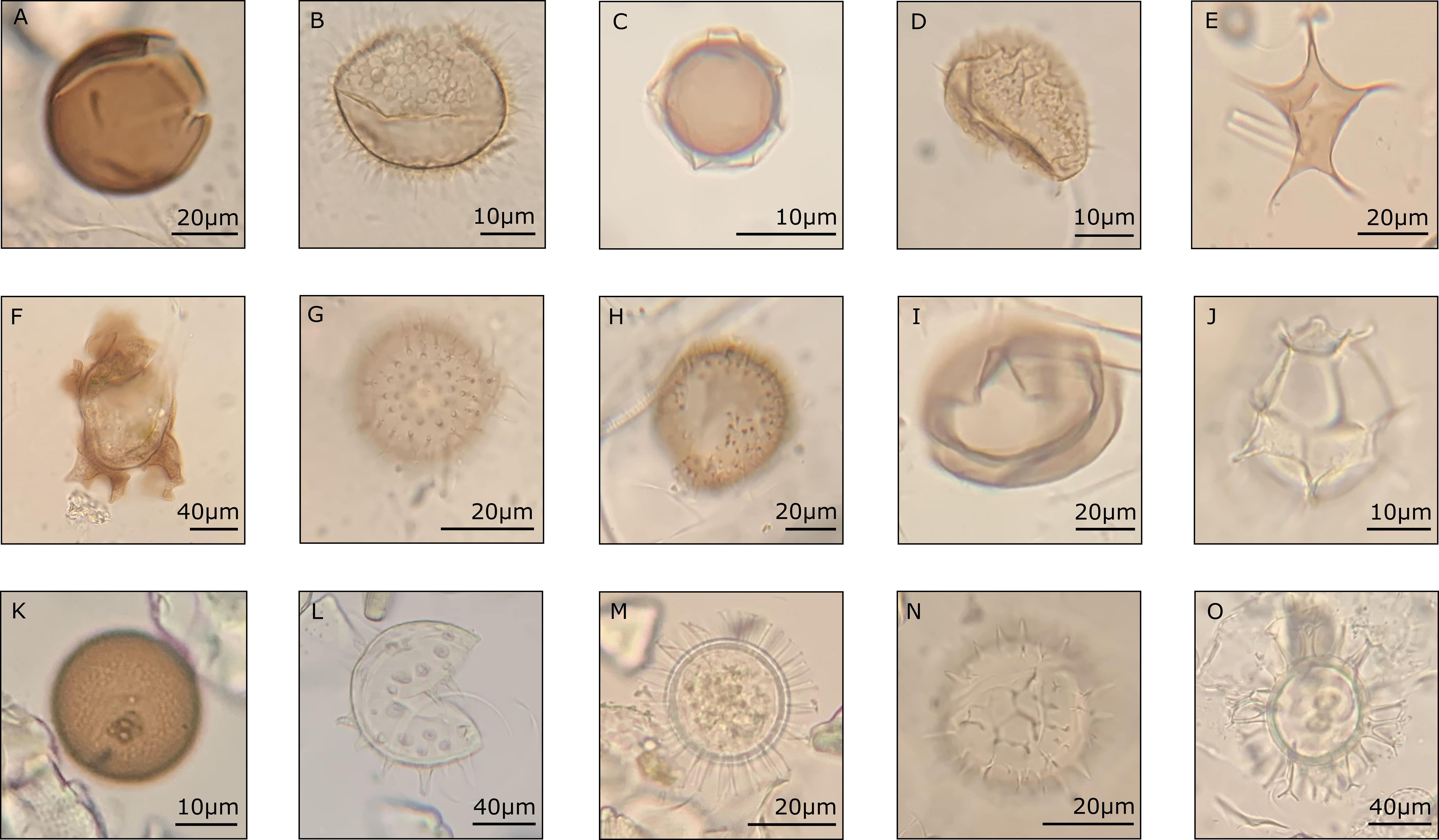
Figure 3 Some of the identified dinoflagellate cysts taxa from the CBeu trap. Heterotrophic taxa: (A) Brigantedinium spp., (B) Archaeperidinium constrictum (uploaded to modern dinocyst key by Zonneveld and Pospelova (2015) online catalogue), (C) Protoperidinium americanum, (D) P. monospinium, (E) P. stellatum, (F) Polykrikos quadratus, (G) Echinidinium granulatum, (H) E. zonneveldiae, and (I) P. subinerme. Photo-/mixotrophic taxa: (J) Impagidinium aculeatum, (K) Gymnodinium spp., (L) Lingulodinium polyedra, (M) Protoceratium reticulatum, (N) Pyrodinium bahamense, and (O) Spiniferites mirabilis.
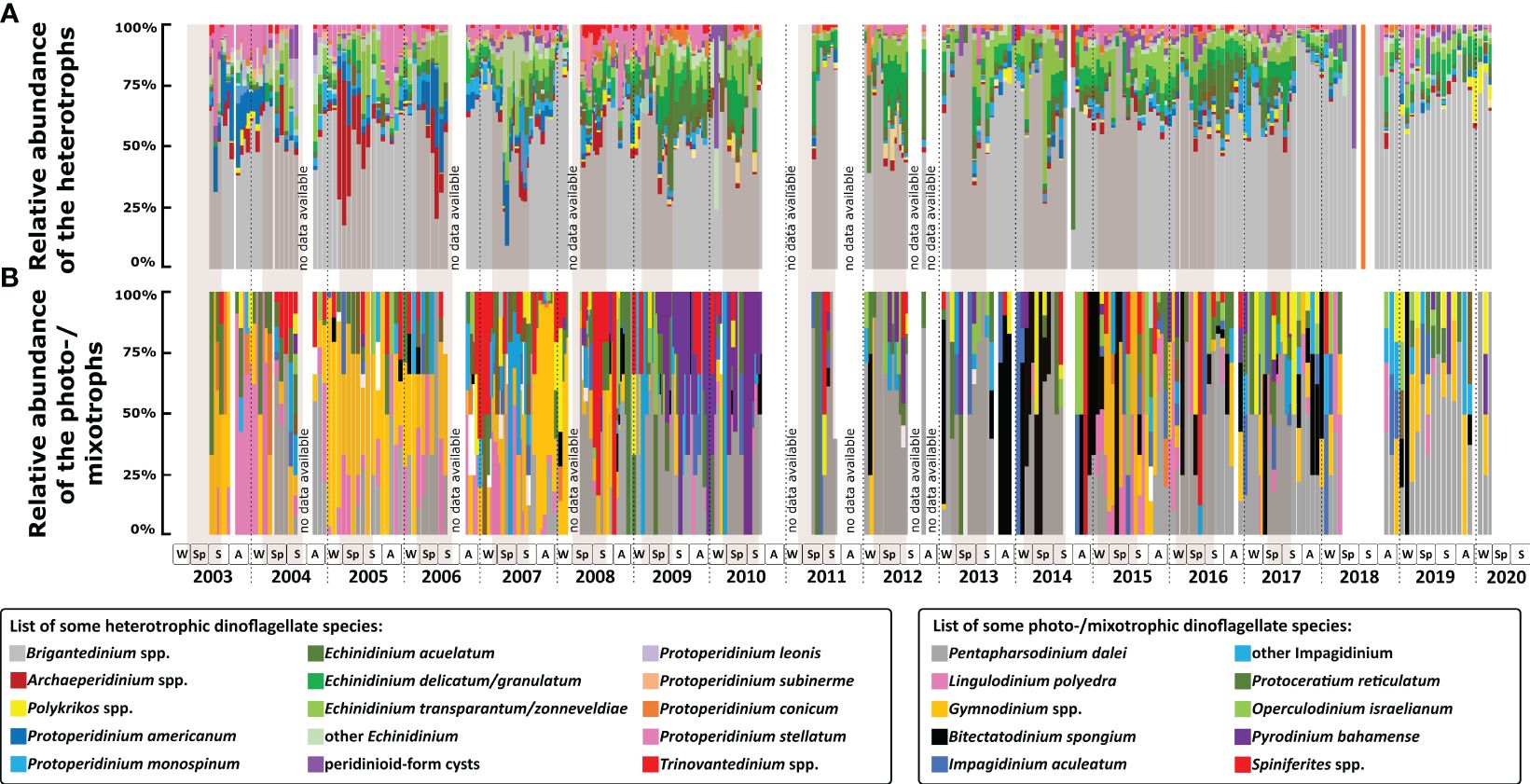
Figure 4 Relative abundance of dinoflagellate cyst species: (A) heterotrophic dinoflagellate taxa; (B) photo-/mixotrophic dinoflagellate taxa. Two boxes below the graphs show the name of important species found in Cape Blanc, one colour indicates one taxon. The gradation of colours indicates several species in the same genus/group. The squares in the lower panels refer to seasons (W, winter; Sp, spring; S, summer; A, autumn). Brown shades indicate maximal upwelling intensity and the vertical dashed lines indicate calendar year separation.
Other cyst taxa observed in most samples were Protoperidinium stellatum (2.7%), P. americanum (2.2%), Archaeperidinium spp. (2.2%), and P. monospinum (2.2%) (Figures 3B–E). The maximal relative abundance of these species varied strongly over the years (Figure 4A). P. stellatum had the highest relative abundance in summer 2003, winter 2003/2004, autumn 2005, and spring to autumn 2008. Archaeperidinium spp. peaked once in spring-summer 2006, while P. monospinum peaked in summer 2007. P. americanum had the highest relative abundance in autumn 2003, winter 2003/2004, and spring 2006. The irregular abundance of these species documented a strong inter-annual variability of the cyst association in the studied area.
Cysts of photo-/mixotrophic dinoflagellates formed only about 6.8% on average of the total association (Figures 3J–O). Of this group, Pentapharsodinium dalei showed the highest relative abundance (2.1%), followed by Gymnodinium spp. (1.3%). Prior to 2008, Gymnodinium spp. was the association most abundant photo/mixotrophic taxon. From then on, P. dalei showed an increased relative abundance and sustained its abundance until the end of the time series in 2020. P. dalei were observed almost every year with maximal relative abundances in spring - summer. Relative abundance of Gymnodinium spp. peaked in 2003 and from 2005 to 2007, mainly in autumn – winter association. Other photo-/mixotrophic cyst species that were commonly observed were Lingulodinium polyedra (Lingulodinium machaerophorum) (0.8%), Protoceratium reticulatum (Operculodinium centrocarpum) (0.5%), species of Spiniferites (0.3%), Bitectatodinium spongium (0.3%), and Impagidinium aculeatum (0.2%). L. polyedra was observed in higher relative abundances in autumn - winter 2003. B. spongium had high relative abundances from autumn 2013 until spring 2014, winter 2015, and autumn 2017. High relative abundances of Spiniferites species were observed in spring 2004, winter 2006/2007, spring 2008, and autumn - winter 2009. P. reticulatum, I. aculeatum, and the other photo-/mixotrophic dinoflagellate taxa were sporadically present throughout the 18 years record and formed a minor part of the total association (Figure 4B).
3.3 Multivariate analyses
The DCA ordination revealed a gradient length of 2.2, indicating a unimodal structure of the dataset. Therefore, CCA was the preferred method to study the relationship between cyst taxa and studied environmental parameters. The CCA analysis indicated that the wind system was the dominant factors influencing cyst export production. Wind speed explained 23.5% of the total variance, followed by Chl-a (22.8%), wind direction (18.8%), dust input (15.6%), and SSTa (9.2%). The least prominent parameter (SST) corresponded to 8.4% of the variance (Table 4). The CCA analysis identified five cyst groups based on similar taxa responses to the analysed environmental parameters (Figure 5).

Table 4 Result values of detrended correspondence analysis (DCA) and canonical correspondence analysis (CCA) executed with the software package Canoco 5 (ter Braak and Šmilauer, 2012; Šmilauer and Lepš, 2014).
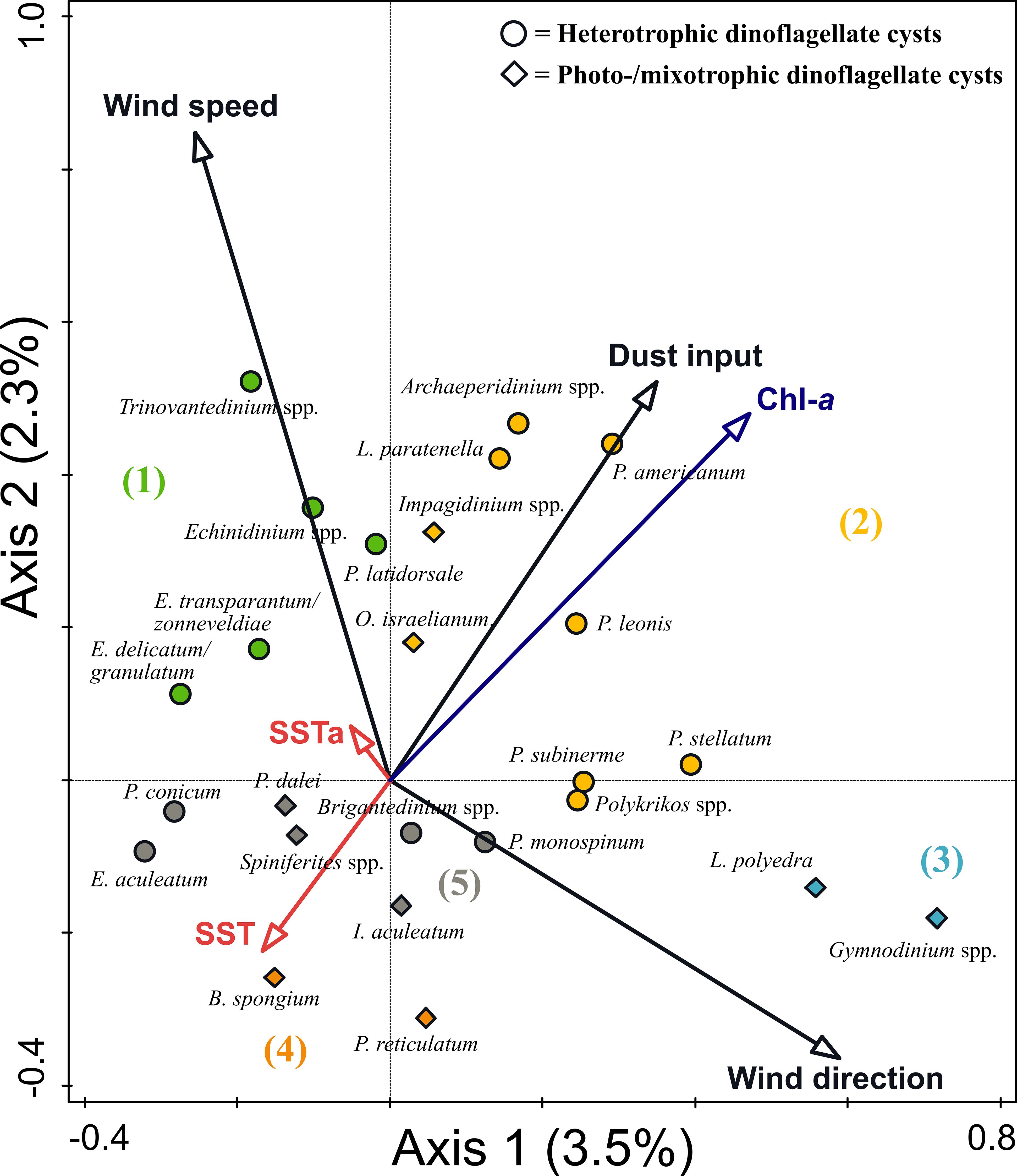
Figure 5 Result of Canonical Correspondence Analysis (CCA) of dinoflagellate cyst species at the CBeu trap from June 2003 and March 2020. Ordination assigned five species groups: (1) upwelling indicators, (2) upwelling and dust indicators, (3) upwelling relaxation indicators, (4) eutrophic and warm water indicators, and (5) species with no specific indication.
Group 1: Echinidinium spp., Echinidinium delicatum/granulatum, Echinidinium transparantum/zonneveldiae, Trinovantedinium spp., and Protoperidinium latidorsale (Votadinium calvum). These taxa were ordinated on the positive side of the wind speed and SSTa and the negative side of the wind direction (northeastern origin).
Group 2: Archaeperidinium spp., Lejeunecysta paratenella, Polykrikos spp., Protoperidinium americanum, Protoperidinium leonis (Quinquecuspis concretum), Protoperidinium subinerme (Selenopemphix nephroides), and Protoperidinium stellatum as well as the two photo-/mixotrophic taxa; Impagidinium spp. and Operculodinium israelianum. These taxa were ordinated on the positive side of the dust input and Chl-a concentration, the intermediate value of wind direction, and the negative side of the SST.
Group 3: Gymnodinium spp. and Lingulodinium polyedra were ordinated on the most positive side of the wind direction and negative side of the wind speed and SSTa.
Group 4: Bitectatodinium spongium and Protoceratium reticulatum. Taxa of this group were ordinated on high SST values, intermediate wind direction values, and negative side of Chl-a and dust input.
Group 5: Brigantedinium spp., Echinidinium aculeatum, Protoperidinium monospinum, and Protoperidinium conicum (Selenopemphix quanta), as well as three photo-/mixotrophic taxa (Pentapharsodinium dalei, Impagidinium aculeatum, and Spiniferites spp.). These taxa were ordinated on intermediate values of all studied environmental parameters.
4 Discussion
4.1 Physical condition of the upper water column
In the studied area, coastal upwelling was driven by surface winds from the north and northeast with intensities that were strongly related to the wind speed (Cropper et al., 2014; Fischer et al., 2016; Romero et al., 2020) (Figures 6C, D). Coastal upwelling transported colder, nutrient-rich intermediate water to the upper water column and successively carried them offshore toward the sediment trap area via large filaments (Fischer et al., 2009). When these filaments crossed the trap mooring site, local surface water temperatures decreased compared to the surrounding offshore waters, causing enhanced sea surface temperature anomaly (SSTa) (Figures 6A, B). In contrast, at times of a weakened upwelling intensity (upwelling relaxation), surface waters at the trap were unaffected by upwelling filaments, resulting in the SST of the trap location being similar to that of more offshore waters (low SSTa). We observed enhanced crossing of upwelling filaments at the trap sites, mainly in spring until early summer. The upwelling relaxation at the location mainly occurred in late summer and autumn. Besides upwelling fertilization of the upper water, additional micronutrients were carried into this region by aerosol dust from the Sahara (Jickells et al., 2005; Skonieczny et al., 2013; Fischer et al., 2016). Throughout the record, we observed enhanced dust input mainly in winter to early spring in this studied area, coinciding with the surface winds from the northeast. From 2008, the maximum dust emission increased in winter and summer (Figure 6H).
4.2 Dinoflagellates cyst export fluxes
The strong dominance of heterotrophic taxa in the organic-walled dinoflagellate cyst export flux was also reported from sediment trap observations in other upwelling ecosystems such as Somali Basin (Northwest Arabian Sea), Benguela (Southwest Africa), NE Pacific, and Cariaco Basin (Caribbean Sea) (Zonneveld and Brummer, 2000; Pitcher and Joyce, 2009; Pospelova et al., 2010; Bringué et al., 2013, 2018 and 2019). In all of these regions, upwelled nutrient-rich waters stimulated phytoplankton production in surface waters, which in turn formed the food source of heterotrophic dinoflagellates (Jacobson and Anderson, 1986; Jeong, 1999; Pitcher et al., 2010; Anderson et al., 2018). We also observed that the export fluxes of dinoflagellate cysts in our studied area were in the same range as those in the other upwelling ecosystems, for instance, the Arabian Sea (Zonneveld and Brummer, 2000), off Southern California (Bringué et al., 2013) and the Caribbean Sea (Bringué et al., 2018 and 2019). An exception was formed by the Benguela upwelling region, where the cyst fluxes were assumed to be a factor of 100 higher (Pitcher and Joyce, 2009). The latter might be a calculation error in the study of Pitcher and Joyce as compared to all other studies from the upwelling area; not only the maximal flux but also the variation in fluxes between upwelling and upwelling relaxation phases was similar to our study even though the temporal occurrence and duration of active or strong upwelling varied across different upwelling regions.
To compare the dinoflagellate cyst data with environmental parameters in the upper water column, we have considered a time lag between cyst production in the upper water column and the time of recovery in the sediment trap. Around the mooring site, the sinking velocities of particles in the water column have been estimated between 75 - 150 m day-1 (Fischer and Karakaş, 2009; Iversen and Ploug, 2013). Iversen and Ploug (2013) showed that sinking velocities of diatoms increased linearly to the size of aggregated diatoms, resulting in a mean sinking velocity of about 150 m day-1. Dinoflagellate cysts and diatoms sank down to the ocean floor as part of aggregates or concentrated in faecal pellets. In the upwelling area off East Africa in the Arabian Sea, cyst associations changed simultaneously in sediment trap material collected at 1030 and 3045 m depth within 14 days collection intervals, leading to a minimal sinking velocity of 140 m day-1 (Zonneveld and Brummer, 2000). Higher sinking velocities of up to 274 m day-1 were estimated in Cape Blanc by comparing the cyst associations in sediment trap samples collected at 730 and 3557 m depths at a location more offshore to the present trap site (Zonneveld et al., 2010). Based on the results of 140 m day-1 and 274 m day-1 sinking velocities and deployment depths between 1249 – 1364 m, a time lag of 4.6 to ten days was estimated. When a maximal time lag of ten days was applied, the cyst export flux was enhanced when upwelling filaments crossed the trap location, and enhanced dust input was observed in the trap samples, increasing the upper water nutrient concentrations (Figure 6E). Furthermore, the highest export flux of dinoflagellate cysts in the winter of 2012 coincided with a long period of strong upwelling in the region during the years 2011 and 2012 and enhanced lithogenic influx in the trap samples related to increased Saharan dust emissions recorded in 2012 and 2013 (Fischer et al., 2019). This finding agreed with earlier observations of increased cyst production during high nutrient concentrations in upper waters supporting the assumption that of cyst sinking rates are the same order of magnitude as those of diatoms (e.g., Zonneveld et al., 2022a).
Lateral transport of particulate organic matter in the water column can occur in the region in nepheloid layers of subsurface and intermediate water depths as well as just above the sea floor (e.g., Karakaş et al., 2006, 2009; Zonneveld et al., 2018). Lateral transport of particles can alter the rate and composition of the export flux collected by the sediment traps (Inthorn et al., 2006; Asper and Smith, 2019; Romero et al., 2021; Romero and Ramondenc, 2022). Dinoflagellate cysts laterally transported in intermediate and bottom water nepheloid layers extended up to 130 km off the Cape Blanc shelf break (Zonneveld et al., 2018). As such, part of the observed cysts collected in the traps did not originate from the upper waters at the trap site. However, recent investigations showed that the resuspension of shelf material and the position of nepheloid layers in the region were not permanent (Zonneveld et al., 2022a; 2022b). Clear nepheloid layers were observed in the water column in November 2015 and 2018 and were almost absent in the summer of 2020 and November 2021 (see cruise reports of Zonneveld et al., 2016, 2019a, 2020, 2022c). Romero and Ramondenc (2022) investigated the diatom export flux in the same sediment trap. They documented that throughout the time series, benthic diatoms from the shelf sediments formed a considerable part of the association. However, microscopic observations of upper water plankton samples collected in November 2018 revealed that many benthic diatoms could have colonized larger pelagic diatoms (Zonneveld and Versteegh, pers. obs). Therefore, it was unclear if the observed benthic diatoms collected in the sediment trap originated from the shelf or local sources. Unfortunately, we have no method to determine the ratio between the autochthonous and allochthonous association recovered in our trap samples. Nevertheless, we observed a strong correlation of cyst fluxes and association composition variabilities with changing environmental conditions in the upper waters at the trap site with a time lag of 10 days. Therefore, we assumed that most of the recovered dinoflagellate cysts in the trap represented cysts originating from the upper water column in the vicinity of the trap site.
4.3 Dinoflagellate cyst groups according to environmental parameters
Based on the CCA analyses mentioned in the result and the visual observation of the absolute abundances of the main contributor species, five groups of dinoflagellate cysts that showed similar relationships to the studied environmental parameters could be distinguished:
4.3.1 Species group 1 (maximal upwelling)
CCA group 1 consisted of heterotrophic taxa that were ordinated on the positive side of the wind speed and SSTa. In the region, strong northwestern winds triggered maximal upwelling intensity with the filaments reaching far into the open ocean. These filaments were characterised by relatively stratified surface waters with high nutrient availability. This condition correlated with the highest relative and absolute abundances of Echinidinium delicatum/granulatum, Echinidinium spp., Echinidinium transparantum/zonneveldiae, Trinovantedinium spp., and Protoperidinium latidorsale (Figures 6F, 7).
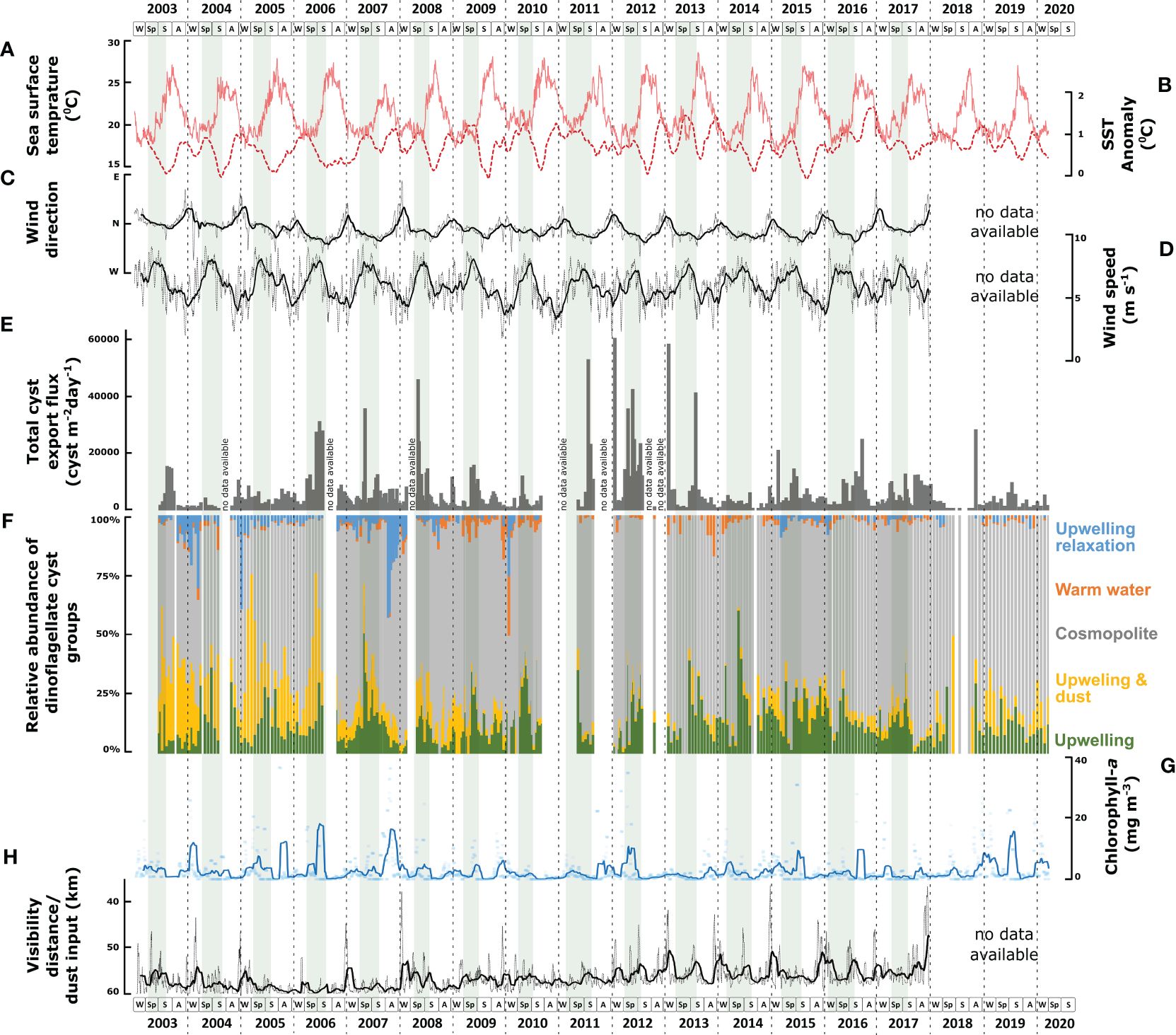
Figure 6 Comparison between several environmental parameters and the concentration as well as the relative contributions of six dinoflagellate cyst species groups. (A) sea surface temperature (SST); (B) sea surface temperature anomaly (SSTa); (C) wind direction; (D) wind speed; (E) total export fluxes (cysts m-2 d-1) of dinoflagellate cysts; (F) relative contribution (%) of each dinoflagellate species group according to CCA analysis; (G) concentration of Chlorophyll-a; (H) visibility distance indicating dust input. Grey shades in the background indicate maximal upwelling intensity.
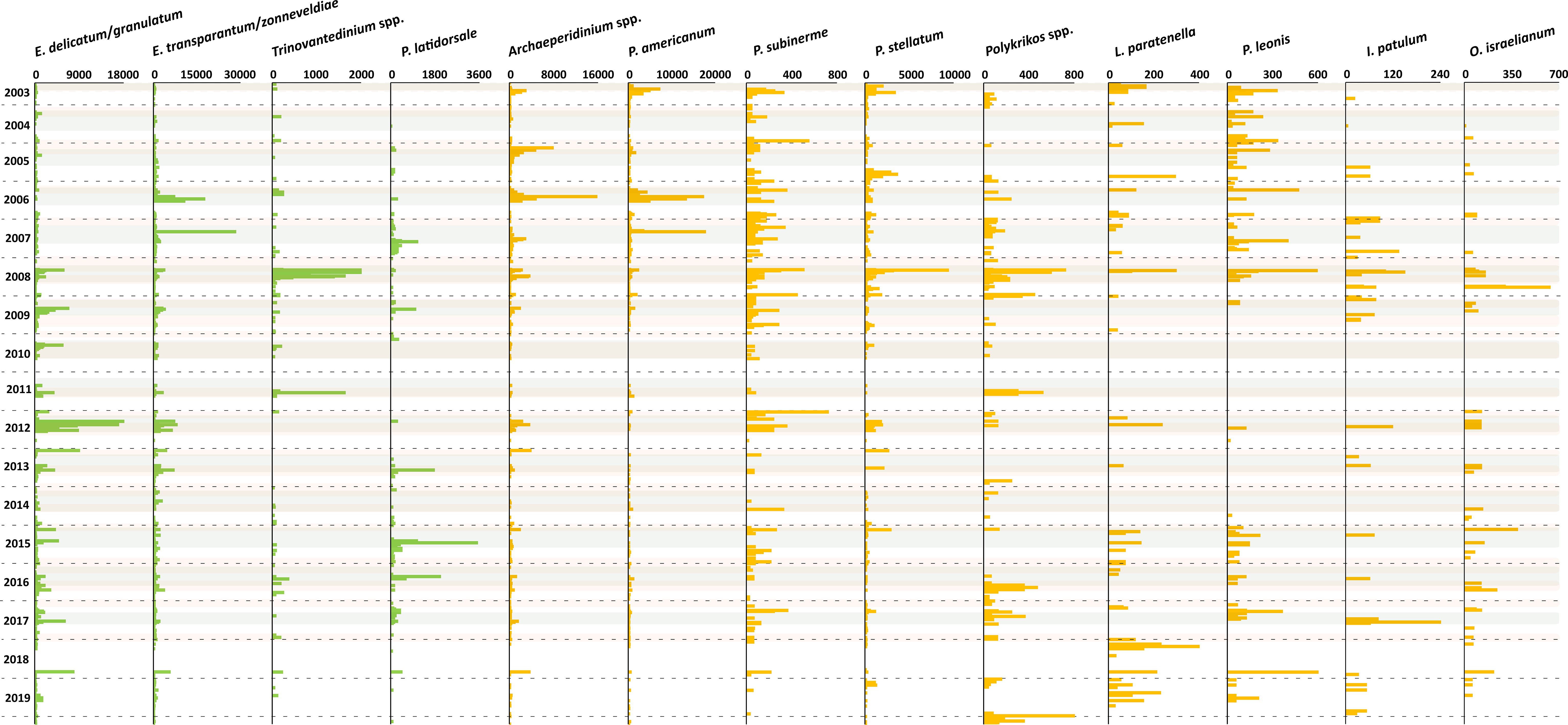
Figure 7 Flux rate of dinoflagellate cyst taxa (cysts m-2 d-1). Fluxes in green bars are species group 1 and fluxes in yellow bars are species group 2. Grey shades indicate maximal upwelling intensity, brown shades indicate maximum dust input, and the horizontal dashed lines indicate calendar year separation.
This result was also observed in drifting trap studies in our study area, which revealed that a higher export flux of Echinidinium species occurred in and at the rim of active upwelling cells (Zonneveld et al., 2022b). Furthermore, sediment trap and surface sediment studies reported high concentrations of E. delicatum/granulatum and E. transparantum/zonneveldiae at times of enhanced active upwelling at a more offshore position compared to our trap site (Zonneveld et al., 2010) and in other areas with a similar system such as the Arabian Sea, the Benguela upwelling area, along the North American Pacific coast, along the southwest Mexican coast, the Cariaco Basin, the Portugal Bay, and off the west coast of Iberian Peninsula (Zonneveld and Brummer, 2000; Radi and de Vernal, 2004; Pospelova et al., 2008; Ribeiro and Amorim, 2008; Bouimetarhan et al., 2009; Limoges et al., 2010; Zonneveld et al., 2010; Bringué et al., 2019; García-Moreiras et al., 2021). E. zonneveldiae was described by Head (2003) and successively documented in the upwelling regions of the Santa Barbara Basin, Cariaco Basin, and off Cape Blanc. Bringué et al. (2019) reported that E. granulatum was more abundant at the weaker stage of upwelling. However, this species was recorded during maximal upwelling in the other regions. Seasonal production of Echinidinium was also observed in areas with stratified upper water columns where the high nutrient concentrations were provided by river discharge or transported coastal upwelling waters (Pospelova et al., 2010; Price and Pospelova, 2011). The ambient water conditions of these regions were often characterised by some variability in the water column stratification. A strong stratification appeared when fresh (river) waters covered the salty marine waters while turbulence occurred at the freshwater - saltwater interface.
Trinovantedinium spp. was observed only sporadically in our record, and the connection of high concentration of this genus with maximal upwelling was recorded for a few years only. In other upwelling regions, this genus was often grouped with other peridinioid cysts because of its low export flux or its sporadic occurrence showed no clear temporal pattern (Zonneveld and Brummer, 2000; Ribeiro and Amorim, 2008). The same holds for P. latidorsale. Nevertheless, in the Santa Barbara Basin sediment traps, an enhanced flux of P. latidorsale showed a positive correlation to the active upwelling phase (Bringué et al., 2013). In the Northwest Arabian Sea, Saanich Inlet, and Cariaco Basin, the cyst export production of this species was not strictly linked to the presence of an active upwelling phase but could be linked to the availability of its food source (Zonneveld and Brummer, 2000; Price and Pospelova, 2011; Bringué et al., 2019). Therefore, we assume these species were related to upwelled waters in the research area.
4.3.2 Species group 2 (maximal upwelling and dust input)
CCA group 2 consisted of heterotrophic and photo-/mixotrophic taxa that were ordinated on the positive side of dust input, Chl-a concentration, and intermediate wind speed values. Throughout the years, the relative abundance and export fluxes of group 2 increased when dust input and upwelling intensified (Figures 6F, 7). Dust contains several trace elements, and although the exact ways in which dust input fertilizes the ocean are not well understood, high dust input can enhance phytoplankton production (Erickson et al., 2003; Stuut et al., 2005; Duarte et al., 2006; Skonieczny et al., 2013; Fischer et al., 2016). This latter was supported by our observation of a close relationship between Chl-a concentration and enhanced dust input in CCA analyses (Figure 5). Group 2 contained the heterotrophic taxa; Archaeperidinium spp., Lejeunecysta paratenella, Polykrikos spp., Protoperidinium americanum, Protoperidinium stellatum, and Protoperidinium subinerme, and the photo-/mixotrophic species; Impagidinium spp. and Operculodinium israelianum (Figure 7).
The seasonal production of Archaeperidinium species has not been well investigated except in Price and Pospelova (2011), where A. saanichi is listed as Cyst type L. This condition could be caused by taxonomic difficulties and often low concentrations of this genus in sediment trap samples from other regions. In this study, the cyst export production of this genus, notably A. saanichi and A. constrictum had relatively high export production until 2008, after which it declined. A similar trend was observed in P. americanum. This species seasonal export flux was more pronounced in the first half of the trap time series and declined after 2007. A strong positive correlation between the production of P. americanum and seasonal upwelling was observed in the NW Arabian Sea (Zonneveld and Brummer, 2000). However, a relation to enhanced dust input was not reported before the present study.
The overall trend of these species export flux seemed contradictory to the enhanced dust input. The long-term trend of dust input showed a gradual increase rather than a decrease. A possible explanation for this is that the dust composition changed over the years, which could influence the composition of the phytoplankton community (Friese et al., 2017; Lu et al., 2017; Yang et al., 2019). Shifts in prey availability might lead to the declining production of these cyst taxa. Unfortunately, no record was available to show if the dust composition in this location has changed over the trap time series. Future assessments of the dust composition from these sediment trap samples are required to clarify this hypothesis.
A positive relationship between the export fluxes of P. stellatum and P. subinerme (Figure 3I) with the presence of upwelling has also been observed in sediment trap samples from the Northwest Arabian Sea and Cariaco Basin (Zonneveld and Brummer, 2000; Bringué et al., 2019). However, the influence of dust input on the respective cyst fluxes had not yet been recorded. This latter holds as well for the other species within this group. It might be caused by taxonomic problems (some species generally being grouped) and the lack of studies investigating the role of terrestrial mineral input on the production of cysts. Therefore, this study proposes implementing terrestrial minerals as potential steering factors for cyst production in future studies.
4.3.3 Species group 3 (upwelling relaxation)
CCA group 3 was formed by the photo-/mixotrophic taxa Lingulodinium polyedra and Gymnodinium spp. (Figures 6F, 8). These taxa were ordinated on the positive side of the wind direction and the negative side of the wind speed and SSTa (Figure 5). This represents northwestern winds, which led to weaker upwelling in the region, mainly in late summer - autumn. The overall export flux of both species was higher from 2003 to early 2009, strongly fell in the following years, and mildly recovered in 2015.
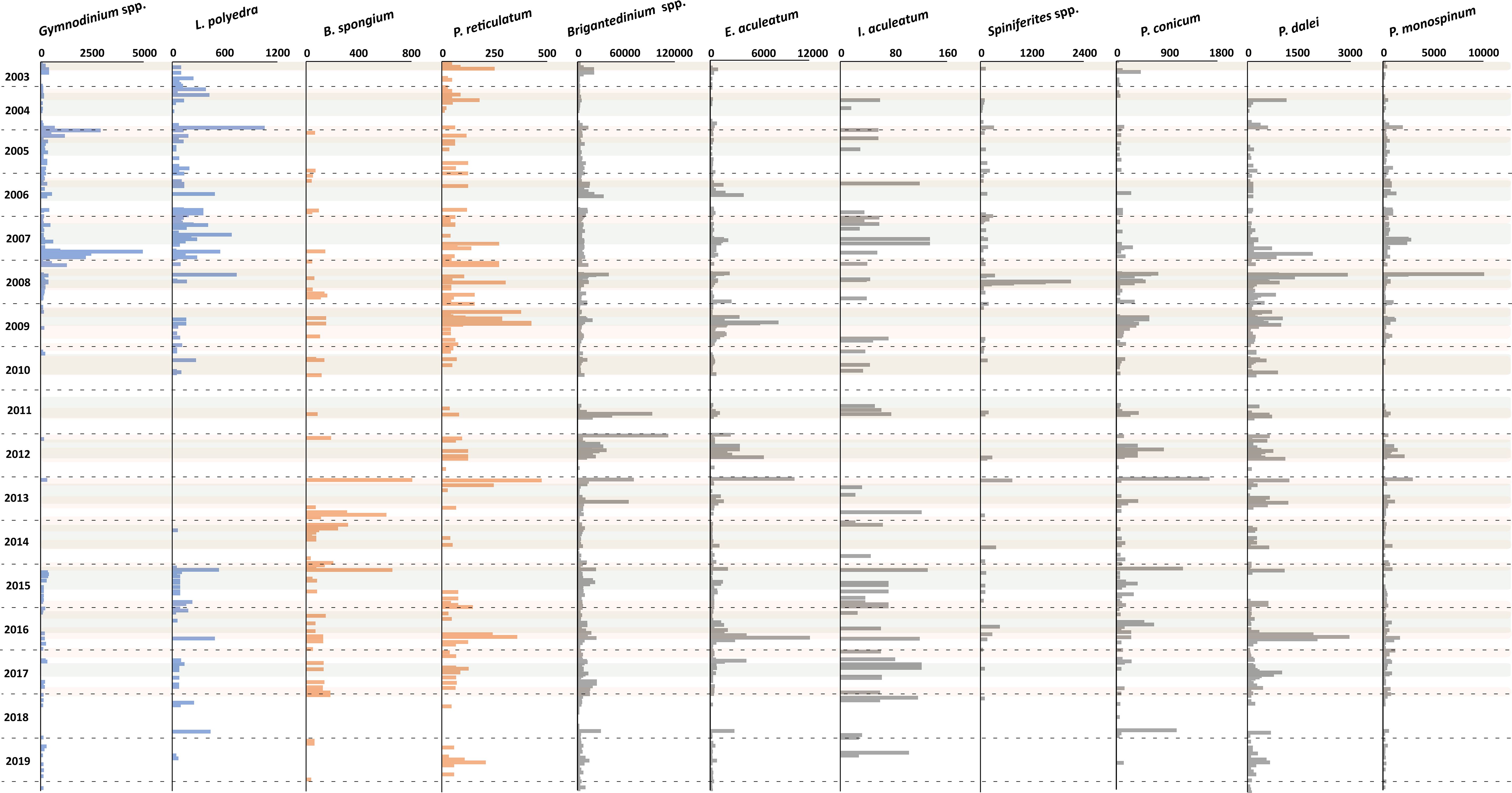
Figure 8 Flux rate of dinoflagellate cyst species (cysts m-2 d-1). Blue bars represent species of group 3, orange bars represent species group 4, and grey bars represent species group 5. Grey shades indicate maximal upwelling intensity, brown shades indicate maximum dust input, and the horizontal dashed lines indicate calendar year separation.
The increased cyst flux of L. polyedra and Gymnodinium spp. during less intense upwelling, in line with previous studies in the region and other upwelling areas. Sediment trap studies in the Santa Barbara and Cariaco Basins showed higher export production of L. polyedra during phases of upwelling relaxation (Bringué et al., 2013, 2019). A drifting trap survey in an upwelling cell off Cape Blanc demonstrated that L. polyedra was formed during the transition from active upwelling towards more stratified conditions in offshore drifting filaments (Zonneveld et al., 2020). Sediment surface studies in Northeast Brazil, Northeast Pacific, West Africa, Black Sea corridor and the West Coast of Iberian Peninsula documented that cyst production of this species increased under warm, stratified, and nutrient-rich waters (e.g., Vink et al., 2000; Pospelova et al., 2008; Radi and de Vernal, 2008; Bouimetarhan et al., 2009; Leroy et al., 2013; Mudie et al., 2017; García-Moreiras et al., 2021, 2023). In our record, the export flux of L. polyedra was not restricted to upwelling relaxation phases. We observed enhanced export flux of L. polyedra during maximal upwelling in a few years. At times of maximal upwelling of this area, the upper water conditions, such as the rate of stratification, can change fast in small spatial and temporal scales. Studies on the life cycle of L. polyedra have shown that this species can produce both asexual and sexual cysts (Figueroa and Bravo, 2005). Although the possible role of sexual and asexual cysts has not been established, asexual cysts have often been linked to the ability of the species to adapt quickly to fast-changing environments (Figueroa and Bravo, 2005). In river plume areas and fjord systems that are characterised by a strong variability in upper water stratification, high concentrations of L. polyedra were observed (e.g., Lewis, 1988; Blanco, 1995; Smayda and Trainer, 2010; Zonneveld et al., 2013; García-Moreiras et al., 2023). Therefore, we assume that in our research area, L. polyedra benefited from the changing environmental conditions in upper water at transition phases from active upwelling to more stratified conditions.
Unfortunately, it was not possible to differentiate unequivocally between cysts of G. catenatum, G. microreticulatum, and G.nolleri as the size of all brown microreticulate cysts observed in the Cape Blanc region fell in the overlapping size range of the three species. Furthermore, it was generally difficult to identify the exact number of cingular vesicles in the observed specimens. Consequently, we had to group these species as Gymnodinium spp. The export fluxes of Gymnodinium spp. were higher during the weaker upwelling phases in most of the years but comparable to L. polyedra, the enhanced cyst export flux of Gymnodinium spp. was not completely restricted to phases of upwelling weakening. Nevertheless, our observations are largely in line with surface sediments studies conducted in NW Iberian Peninsula, where G. catenatum had a positive relationship with more stratified upper water conditions (Bravo et al., 2010; Pitcher et al., 2010; Ribeiro et al., 2012; García-Moreiras et al., 2021). In the Cariaco Basin sediment trap study, the concentration of G. nolleri cysts increased during “secondary upwelling” marked by the weakening of upwelling winds (Bringué et al., 2018). A 7-day in-situ observation of cyst production in an active upwelling cell offshore Cape Blanc also documented that L. polyedra and Gymnodinium spp. were generally produced when the water column became more stratified due to upwelled waters forming an offshore drifting upwelling filament (Zonneveld et al., 2022b). Therefore, we suggest that the species of group 3 can be used as indicators for the upwelling relaxation in our studied area.
4.3.4 Species group 4 (warm surface waters)
CCA group 4 was formed by species that ordinated on high values of the SST, intermediate values of the wind direction, and negative values of the dust input and Chl-a concentration. Despite the insignificant impact of the SST (p-value<0.05) in this system (Table 5), the relative abundance of this group showed a higher percentage at times of warmer SST that was usually observed during upwelling relaxation (Figure 6F). The main contributors of this group are Protoperidinium reticulatum and Bitectatodinium spongium (Figure 8).
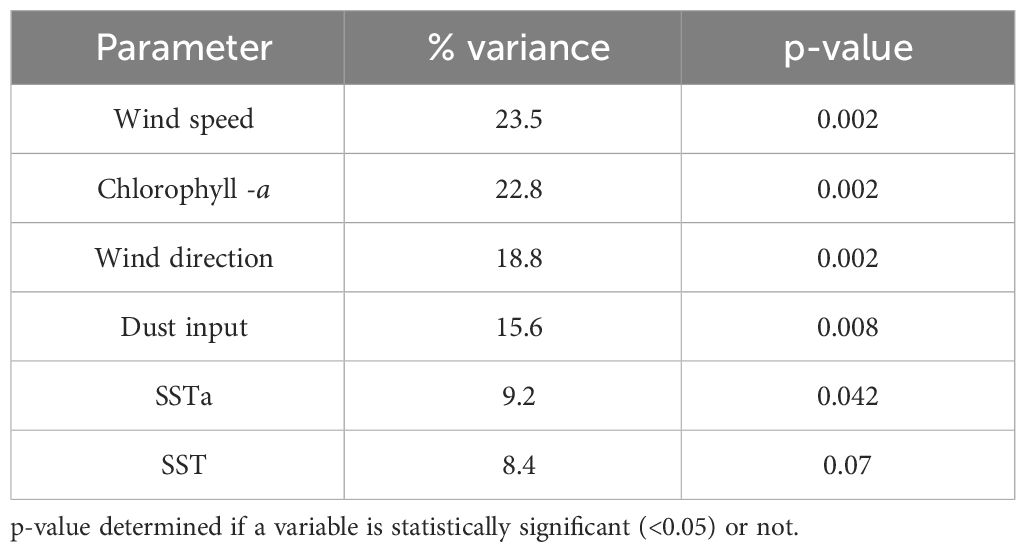
Table 5 Result of canonical correspondence analysis (CCA) measuring contribution values of environmental parameters in the studied area.
Although P. reticulatum was observed in the majority of our samples, its occurrences still showed a positive relationship with warm surface waters at times of weaker upwelling. This result suggested that this species could tolerate variable environmental conditions, which was corroborated by the global geographic distribution of P. reticulatum in surface sediment samples, showing that it has a cosmopolitan distribution (e.g., Zonneveld et al., 2013; Marret et al., 2020). The sediment trap study from the Cariaco Basin, P. reticulatum showed higher export flux during upwelling relaxation when surface waters were more stratified (Bringué et al., 2018 and 2019). Furthermore, in the Northeast Pacific and Southwest/West African surface sediments, high relative abundances of these cysts were observed in the more offshore regions of the coastal upwelling areas (Radi and de Vernal, 2004; Holzwarth et al., 2007; Pospelova et al., 2008; Bouimetarhan et al., 2009).
In contrast, B. spongium is distributed strictly in tropical and sub-tropical regions (e.g., Zonneveld et al., 2013; Marret et al., 2020). In the Cariaco Basin, the highest production of B. spongium was observed during active upwelling (Bringué et al., 2018; 2019). However, in other regions, the production of B. spongium was not restricted to the presence of upwelling. Although in the Northwest Arabian Sea, the highest export flux was observed during the southwest monsoon at times of active upwelling, cysts of this species were observed as well in samples collected during the northeast monsoon when warm stratified upper waters were present (Zonneveld and Brummer, 2000). The highest absolute and relative abundances of B. spongium was found in the Indus Fan (Northwest Arabian Sea), a region without upwelling but with warm and nutrient-rich surface waters (Zonneveld and Jurkschat, 1999). Therefore, we interpret that warm eutrophic surface waters is required for the occurrence of B. spongium rather than the mixing of upper waters.
4.3.5 Species group 5 (no relationship with local environmental conditions)
CCA group 5 consisted of heterotrophic and photo-/mixotrophic taxa that were ordinated on the intermediate values of all studied parameters. The main contributors in this group were Brigantedinium spp., Echinidinium aculeatum, Pentapharsodinium dalei, Protoperidinium conicum, and Protoperidinium monospinum (Figure 8). Additionally, this group includes two taxa that occurred sporadically in the record: Impagidinium aculeatum and Spiniferites spp.
Brigantedinium spp. dominated the dinoflagellate cyst association in most samples. Although its concentration followed the upwelling intensity, its relative abundance remained high during upwelling relaxation. The accumulation rate of Brigantedinium spp. was related to enhanced upwelling in many regions such as the Arabian Sea, Benguela upwelling, Canary Current Upwelling, West Coast of Iberian Peninsula, and Northeast Pacific (e.g., Zonneveld and Brummer, 2000; Radi and de Vernal, 2004; Sprangers et al., 2004; Holzwarth et al., 2007; Pospelova et al., 2008; Bouimetarhan et al., 2009; Limoges et al., 2010; García-Moreiras et al., 2021). Sediment trap studies in Omura Bay (Japan), Saanich Inlet (Canada), and the Mauritanian upwelling region (NW Africa) documented a positive correlation between export production of Brigantedinium spp. with several primary producers such as diatoms, coccolithophores, and photo-/mixotrophic dinoflagellates (Fujii and Matsuoka, 2006; Pospelova et al., 2010; Zonneveld et al., 2010; Price and Pospelova, 2011; Bringue et al., 2013). These results indicated that cyst export flux of Brigantedinium spp. is strongly related to the abundance of their prey.
Although the relative abundance of E. aculeatum did not show a positive relationship with specific environmental parameters, the highest annual export fluxes of E. aculeatum usually occurred at times of maximal upwelling and sometimes during high dust input and when upwelling weakened (indicated by higher SST). The export flux of E. aculeatum was not always higher during enhanced upwelling or dust input. However, our data still suggested that enhanced availability of nutrients in the upper water might increase its cyst export production. In surface sediments from the Benguela upwelling area and off Southwest Mexico, a higher abundance of E. aculeatum was observed in regions characterised by coastal upwelling (Zonneveld et al., 2001; Holzwarth et al., 2007; Limoges et al., 2010). In the sediment traps of the Santa Barbara and Cariaco Basins, E. aculeatum showed increased export production during the later stages of active upwelling until upwelling relaxation set in (Bringué et al., 2013, 2018, 2019). The combination of this information and our results indicated that export production of E. aculeatum might be positively influenced by the availability of nutrients in the upper water column that was not always triggered by upwelling, as observed in Saanich Inlet (Price and Pospelova, 2011).
A similar pattern was observed for P. dalei, the most dominant photo-/mixotrophic species in our dataset. However, Li et al. (2020) reported another species (Pentapharsodinium imarense) with similar morphology features. It is possible that P. imarense was identified as P. dalei since they are better distinguished through molecular identification. Although no significant annual trend was observed in the export flux of P. dalei, its high cyst flux was observed at maximal upwelling intensity and enhanced dust input, notably in winter. In Northeast Pacific, Portugal Bay, and Red Sea surface sediments, high concentrations of cysts of P. dalei were observed in regions characterised by colder upper waters (Radi and de Vernal, 2004; Pospelova et al., 2008; Ribeiro and Amorim, 2008; Elshanawany and Zonneveld, 2016). Also, sediment trap observation in the Cariaco Basin documented increased P. dalei export flux during low SST and active upwelling (Bringué et al., 2018; 2019). However, it was argued that lower temperatures rather than upwelling enhanced export fluxes of P. dalei in this region. In our record, the occurrence of P. dalei could not be linked to low temperatures but rather to nutrient availability.
Even though the overall export flux of I. aculeatum was low, it occurred in the samples throughout the trap time series. I. aculeatum was generally observed in high relative abundances in surface sediment samples of oligotrophic regions and was often interpreted as a typical oligotrophic species (Vink et al., 2000; Sprangers et al., 2004; Pospelova et al., 2008; Radi and de Vernal, 2008; Bouimetarhan et al., 2009; Elshanawany et al., 2010; Zumaque et al., 2017). However, cyst degradation studies showed that this species is more resistant to aerobic degradation than many other dinoflagellate cysts (Zonneveld et al., 2019b). This species high relative abundance in oligotrophic areas was generally the result of the high bottom water oxygen concentrations that prevail in oligotrophic regions, leading to the post-depositional degradation of the other cyst species (e.g., Zonneveld et al., 2019b). Upwelling is a permanent feature and dust reaches this region regularly, leading to the upper water column trophic states never becoming oligotrophic. Thus, our results suggested that I. aculeatum could tolerate limited nutrient availability but was not restricted to it.
Only little information has been obtained on the relation between the export production of P. monospinum and environmental conditions. It could be that this species was often combined with the other spiny peridinioid cysts due to its rarity and low concentration in other locations. Sediment trap studies in the distal extension of the upwelling filaments of Cape Blanc showed that cyst production of P. monospinum increased when filaments reached the trap location (Susek et al., 2005; Zonneveld et al., 2010). However, at our trap location, the export production of this species could not be linked to any variation in environmental conditions. This was also the case for P. conicum, with a positive relationship between increasing upwelling and increasing export flux of P. conicum only reported in the Cariaco Basin (Bringué et al., 2019). In Omura Bay (Japan), Lisbon Bay (Portugal), and the central Strait of Georgia (Canada), the production of P. conicum was high throughout the year with no clear seasonal trend (Susek et al., 2005; Fujii and Matsuoka, 2006; Ribeiro and Amorim, 2008; Pospelova et al., 2010; Price and Pospelova, 2011). Therefore, our result implies that strong upwelling was not the only driving factor influencing the cyst production of P. conicum.
4.4 Long-term variations in the cyst association
The result of the CBeu sediment trap study in the Mauritanian upwelling system provided a long and almost continuous record of the seasonal, annual, and multi-annual variability in the dinoflagellate cyst association. As expected, the upwelling intensity was triggered by the strength of upwelling winds that control the annual seasonality of dinoflagellate cyst export flux. However, we observed that each upwelling episode showed differences in the dominance and composition of the species (Figure 4A). Since the beginning of the record, no species, except for Brigantedinium spp., could sustain its dominance during maximal upwelling throughout all years. Sporadic occurrences and irregular increases of some species added to the complexity of dinoflagellate response to episodes of more intense upwelling in the studied area. For instance, the short-term occurrence of Protoperidinium lousianense (Trinovantedinium pallidifulvum) in 2008 and increased export fluxes of many Spiniferites species in 2008 and B. spongium in 2014/2015, demonstrated this heterogeneity (Figure 8). The Chl-a concentration was the only parameter that showed strong inter-annual variations similar to the dinoflagellate cyst association composition and export flux. These findings suggested that biological factors such as intra- and inter-species interactions were responsible for the changes in this ecosystem, coherent with changes found in other ecosystems (Daly and Smith, 1993; Naujokaitis-Lewis and Fortin, 2016; Rollwagen-Bollens and Bollens, 2020). Inter-species and group competition, predation, and variation of prey might have determined the dominance and composition of dinoflagellate cysts taxa in every upwelling episode (Daly and Smith, 1993; Rollwagen-Bollens and Bollens, 2020). This outcome agreed with observations from in-situ pump and drifting trap surveys in the region. Here, it was observed that the export flux of cysts in individual active upwelling cells contained different unique species associations (Zonneveld et al., 2022a, 2022b).
The 18-year sediment trap record also documented a significant change in the composition of dinoflagellate cyst association. A turnover was marked by the shift in dominance from Archaeperidinium and Protoperidinium in favour of Echinidinium species in the maximum upwelling phase of 2007. The dominance of Echinidinium species became more pronounced after 2009 (Figure 4A). Around the same time, a shift in dominance of the photo-/mixotrophic association was observed from Gymnodinium spp. to Pentapharsodinium dalei (Figure 4B). These changes in the association coincided with the enhancement of the frequency of dust input and a shift in its seasonality recorded since 2008. The dust transported offshore in the Cape Blanc area has different sources in different seasons (Friese et al., 2017). In the summer of 2013 - 2015, dust contained ferroglaucophane and zeolite were transported from Mauritania, Mali, and Libya. In the winter, the dust contained fluellite, indicating that it had been transported from Western Sahara. Furthermore, the aerosol dust carried not only micronutrients but also toxic metals such as Cadmium (Cd), Copper (Cu), and Zinc (Zn). A high concentration of those heavy metals in seawater could threaten phytoplankton growth and alter their community; for instance, cyanobacteria (Synechococcus), haptophytes (Emiliania huxleyi), chrysophytes (Hymenomonus corterae) and dinoflagellates (Alexandrium sp. Gonyaulax sp. and Protocentrum sp.) (Lu et al., 2017; Yang et al., 2019; Zhou et al., 2021). Cyst production of L. polyedra was more resistant to metal contamination, but there was a threshold that negatively influenced the cyst production of this species (Lu et al., 2017). A shift in diatoms association was detected from the same sediment trap in 2006 but was not linked to dust input or upwelling changes (Romero and Ramondenc, 2022). The shifts in phytoplankton would eventually influence the zooplankton, in this case, heterotrophic dinoflagellates. However, no report has specified the prey selections of Archaeperidinium and Protoperidinium species identified in this study, and the motile affinities of Echinidinium species are still unknown (see Table 2). There is also limited information about the potential influence of dust input and its composition on the dinoflagellate cyst community and production. However, our results suggested that the mineral composition of aerosol dust might be a factor influencing the cyst export production of several dinoflagellate taxa in this study. Consequently, more research is needed to study the effect of terrestrial mineral particle input on the production of dinoflagellate cysts. This new information is valuable to interpret changes in the dinoflagellate cyst association throughout time, such as in sediment cores, since the source or changes of nutrient composition have not often been addressed as driving factors.
4.5 Cysts of potentially toxic dinoflagellates
Several marine cyst-forming dinoflagellates can produce toxins, with their blooms sometimes accompanied by discoloration of the upper water column (e.g., Amorim et al., 2001; Quijano-Scheggia et al., 2012; Liu et al., 2020; Terenko and Krakhmalnyi, 2021). Blooms of these species can cause severe health problems and/or can have a large economic impact (e.g. Holmes and Teo, 2002; Starr et al., 2017; Pitcher and Louw, 2021). As their cysts can form a seed bank in sediments, the upper water population can be revived, it is important to obtain insight into their cyst export production dynamics (Anderson et al., 2021). We recovered cysts of five potentially toxic dinoflagellates (Figures 3L–O), they were cysts of Gymnodinium spp., Lingulodinium polyedra, Protoceratium reticulatum, Pyrodinium bahamense, and members of the Gonyaulax spinifera complex.
G. spinifera, L. polyedra, and P. reticulatum produce yessotoxins, whereas species of Gymnodinium (notably G. catenatum) and P. bahamense secrete saxitoxins (Costa et al., 2015; Liu et al., 2017; Morquecho, 2019). These toxins are the primary cause of high mortality in marine organisms and can cause human health problems as well, such as Diarrhetic Shellfish Poisoning (DSP) and Paralytic Shellfish Poisoning (PSP) (Holmes and Teo, 2002; Starr et al., 2017). So far, only minimal information was available about the impact of these species off Cape Blanc (e.g., Hernández et al., 1998; Reyero et al., 1999). In our study, these species either occurred only in a few years or were present in a certain time interval. For instance, Gymnodinium spp. and L. polyedra occurred in the association before 2009 and after 2015, while P. bahamense was notably present between 2009 and 2012. However, north of this region, on the Atlantic coast in the region north off Cape Yubi, frequent recordings of red tides and high toxin concentrations in mussels and oysters were documented, as well as high concentrations of these cysts in surface sediments (Taleb et al., 2003; Holzwarth et al., 2010; Pitcher and Fraga, 2015). Even further north in the upwelling region off the Iberian Peninsula, occurrences of L. polyedra were typically observed in sediments of transitional environments between coastal upwelling water and warmer offshore waters whereas cysts of G. catenatum were typically observed below the mid-shelf upwelling fronts (García-Moreiras et al., 2021).
Compared to our study region, the upwelling in these northern regions was not permanent but intensified in summer/fall, and waters were more stratified in boreal winter (Arístegui et al., 2009; Cropper et al., 2014). Our study showed a correlation with earlier observations; these potentially toxic species thrive best at times of upwelling relaxation, where the water column becomes more stratified but still shows high nutrient availability (Smayda, 2002; Smayda and Trainer, 2010). Due to the non-permanent character of the upwelling episodes in the regions north of Cape Blanc, it was likely that these conditions occurred more frequently, which might explain the higher abundance of these species in these areas. In the sediment trap record, Gymnodinium spp. and L. polyedra were among the taxa that went down in production after the dust input enhancement in 2008 (Figure 8). The cause might be the composition and intensity of dust emitted in the upper water column, as explained in the previous section. The methodology applied in this study could not detect a significant change in the upwelling wind speed or direction. therefore, no conclusion could be made from this parameter. However, this study still provided information about the ecology of these harmful species and the potential driving factor of their production dynamics. Hopefully, it will enrich our knowledge about future toxic blooms in the Cape Blanc area and nearby fishing grounds off Mauritania or another location with a similar environmental setting.
5 Conclusions
The dinoflagellate cyst export flux recovered by a sediment trap off Cape Blanc, Mauritania between, 2003 and 2020 was dominated by heterotrophic species. Throughout this period the upper water environment was influenced by permanent upwelling, the intensity in which was controlled by the speed of the coastal wind originating from the north and northeast. Stronger upwelling occurred in most years in spring – summer, resulting in large offshore drifting filaments transporting cool and nutrient-rich across the location of our sediment trap. By estimating a phase lag of maximal ten days, the annual high export production of dinoflagellate cysts correlated to the maximum upwelling phase even though the intensities varied over 18 years. Enhanced export fluxes of dinoflagellate cysts were also observed when the Saharan dust input to the Atlantic Ocean intensified in the winter prior to 2008 as well as in both winter and summer after 2008. Indication of laterally transported dinoflagellate cysts could not be confirmed in the trap samples. However, the variations of dinoflagellate cyst export fluxes indicated a positive relationship with the environmental parameters.
Results of Canonical Correspondence Analysis (CCA) demonstrated the strong impact of wind systems on the dinoflagellate cysts export production. On this basis, taxa with similar export flux patterns were grouped. These groups consisted of taxa that had their maximal export flux during (1) maximal upwelling intensity: Echinidinium spp., E. delicatum/granulatum, E. transparantum/zonneveldiae, Trinovantedinium spp., and Protoperidinium latidorsale; (2) maximal upwelling and times of increased dust input: Archaeperidinium spp., P. americanum, P. stellatum, P. subinerme, Impagidinium spp., and Operculodinium israelianum; (3) upwelling relaxation: Gymnodinium spp. and Lingulodinium polyedra; (4) warm surface waters: Bitectatodinium spongium and Protoceratium reticulatum; (6) dinoflagellate cysts with no specific relation to all studied parameters: Brigantedinium spp., E. aculeatum, I. aculeatum, Pentapharsodinium dalei, P. conicum, P. monospinium, and Spiniferites spp.
Although Brigantedinium spp. dominated the association throughout the entire sediment trap time series, the composition and total flux of every species varied strongly between episodes of enhanced upwelling. We suggest that biological factors such as intra- and inter-species interactions might have caused this strong variation in the cyst export flux. A long-term variation occurred in 2007 and became more pronounced in 2009. It was indicated by a domination shift from Archaeperidinium and Protoperidinium, to Echinidinium species in the heterotrophic species association as well as a change in domination from Gymnodinium spp. to P. dalei in the photo-/mixotrophic species association. These changes coincided with the increase of dust input and the shift of dust seasonality since 2008, suggesting that different dust compositions due to changes in dust sources might have influenced the cyst export flux off Cape Blanc.
We observed cysts of five potentially toxic dinoflagellate species in our trap material: Gymnodinium spp., L. polyedra, Protoceratium reticulatum, Pyrodinium bahamense, and members of the Gonyaulax spinifera complex. Although the concentration of these cysts was low and their occurrence was infrequent, their presence in our sediment trap suggests a potential risk for future toxic blooms in the Cape Blanc area and nearby fishing grounds off Mauritania.
Data availability statement
Supplementary data of this study is available in PANGAEA repository data, access to the dataset: doi.pangaea.de/10.1594/PANGAEA.963113.
Author contributions
SR: Formal analysis, Investigation, Methodology, Visualization, Writing – original draft. GV: Formal analysis, Investigation, Methodology, Writing – review & editing. VP: Data curation, Formal analysis, Methodology, Visualization, Writing – review & editing. KZ: Conceptualization, Data curation, Formal analysis, Funding acquisition, Investigation, Methodology, Project administration, Resources, Supervision, Validation, Visualization, Writing – review & editing.
Funding
The author(s) declare financial support was received for the research, authorship, and/or publication of this article. This comprehensive research is supported by the funding from German Research Foundation (DFG) through MARUM Excellence Cluster “The Ocean in the Earth System”.
Acknowledgments
The authors thank the captains and crew members of RV Poseidon, RV METEOR, and RV Maria S. Merian, the MARUM sediment trap team for deploying and recovering the sediment trap, and all institutions and individuals who have participated and contributed throughout this research. The authors are also thankful of the support from German, Moroccan, and Mauritanian authorities. The work was supported by the Hanse-Wissenschaftskolleg (HWK) senior research fellowship in marine and climate research to Dr. Pospelova during her 2016 sabbatical at the Institute for Advanced Study (Germany) with Prof. Zonneveld group at the University of Bremen.
Conflict of interest
The authors declare that the research was conducted in the absence of any commercial or financial relationships that could be construed as a potential conflict of interest.
Publisher’s note
All claims expressed in this article are solely those of the authors and do not necessarily represent those of their affiliated organizations, or those of the publisher, the editors and the reviewers. Any product that may be evaluated in this article, or claim that may be made by its manufacturer, is not guaranteed or endorsed by the publisher.
References
Adams A. M., Prospero J. M., Zhang C. (2012). CALIPSO-Derived three-dimensional structure of aerosol over the Atlantic Basin and adjacent continents. J. Clim. 25, 6862–6879. doi: 10.1175/JCLI-D-11-00672.1
Alves M., Gaillard F., Sparrow M., Knoll M., Giraud S. (2002). Circulation patterns and transport of the Azores Front-Current system. Deep Sea Res. Part 2 Top. Stud. Oceanogr. 49, 3983–4002. doi: 10.1016/S0967-0645(02)00138-8
Amorim A., Palma A. S., Sampayo M. A., Moita M. T. (2001). On a Lingulodinium polyedrum bloom in Setúbal bay, Portugal. Harmful Algal Blooms 2000. 133–136.
Anderson D. M., Fachon E., Pickart R. S., Lin P., Fischer A. D., Richlen M. L., et al. (2021). Evidence for massive and recurrent toxic blooms of Alexandrium catenella in the Alaskan Arctic. Proc. Natl. Acad. Sci. 118, e2107387118. doi: 10.1073/pnas.2107387118
Anderson D. M., Jacobson D. M., Bravo I., Wrenn J. H. (1988). The unique, microreticulate cyst of the naked dinoflagellate Gymnodinium catenatum. J. Phycol. 24, 255–262. doi: 10.1111/j.1529-8817.1988.tb00085.x
Anderson E. E., Wilson C., Knap A. H., Villareal T. A. (2018). Summer diatom blooms in the eastern North Pacific gyre investigated with a long-endurance autonomous surface vehicle. PeerJ 6, e5387. doi: 10.7717/peerj.5387
Arístegui J., Barton E. D., Álvarez-Salgado X. A., Santos A. M. P., Figueiras F. G., Kifani S., et al. (2009). Sub-regional ecosystem variability in the Canary Current upwelling. Prog. Oceanogr. 83, 33–48. doi: 10.1016/j.pocean.2009.07.031
Asper V. L., Smith W. O. (2019). Variations in the abundance and distribution of aggregates in the Ross Sea, Antarctica. Elementa (Wash. DC) 7, 23. doi: 10.1525/elementa.355
Balech E. (1985). The genus Alexandrium or Gonyaulax of the tamarensis group. Toxic Dinoflagellates, 33–38.
Ben-Ami Y., Koren I., Altaratz O. (2009). Patterns of North African dust transport over the Atlantic: winter vs. summer, based on CALIPSO first year data. Atmos. Chem. Phys. 9, 7867–7875. doi: 10.5194/acp-9-7867-2009
Blanco J. (1995). The distribution of dinoflagellate cysts along the Galician (NW Spain) coast. J. Plankton Res. 17, 283–302. doi: 10.1093/plankt/17.2.283
Bouimetarhan I., Marret F., Dupont L., Zonneveld K. A. F. (2009). Dinoflagellate cyst distribution in marine surface sediments off West Africa (17–6 N) in relation to sea-surface conditions, freshwater input and seasonal coastal upwelling. Mar. Micropaleontology 71, 113–130. doi: 10.1016/j.marmicro.2009.02.001
Bravo I., Figueroa R. (2014). Towards an ecological understanding of dinoflagellate cyst functions. Microorganisms 2, 11–32. doi: 10.3390/microorganisms2010011
Bravo I., Fraga S., Isabel Figueroa R., Pazos Y., Massanet A., Ramilo I. (2010). Bloom dynamics and life cycle strategies of two toxic dinoflagellates in a coastal upwelling system (NW Iberian Peninsula). Deep Sea Res. Part 2 Top. Stud. Oceanogr. 57, 222–234. doi: 10.1016/j.dsr2.2009.09.004
Bringué M., Pospelova V., Pak D. (2013). Seasonal production of organic-walled dinoflagellate cysts in an upwelling system: A sediment trap study from the Santa Barbara Basin, California. Mar. Micropaleontol. 100, 34–51. doi: 10.1016/j.marmicro.2013.03.007
Bringué M., Pospelova V., Tappa E. J., Thunell R. C. (2019). Dinoflagellate cyst production in the Cariaco Basin: A 12.5 year-long sediment trap study. Prog. Oceanogr. 171, 175–211. doi: 10.1016/j.pocean.2018.12.007
Bringué M., Thunell R. C., Pospelova V., Pinckney J. L., Romero O. E., Tappa E. J. (2018). Physico-chemical and biological factors influencing dinoflagellate cyst production in the Cariaco Basin. Biogeosciences 15, 2325–2348. doi: 10.5194/bg-15-2325-2018
Brosnahan M. L., Fischer A. D., Lopez C. B., Moore S. K., Anderson D. M. (2020). Cyst-forming dinoflagellates in a warming climate. Harmful Algae 91, 101728. doi: 10.1016/j.hal.2019.101728
Costa P. R., Robertson A., Quilliam M. A. (2015). Toxin profile of Gymnodinium catenatum (Dinophyceae) from the Portuguese coast, as determined by liquid chromatography tandem mass spectrometry. Mar. Drugs 13, 2046–2062. doi: 10.3390/md13042046
Cropper T. E., Hanna E., Bigg G. R. (2014). Spatial and temporal seasonal trends in coastal upwelling off Northwest Africa 1981–2012. Deep Sea Res. Part I 86, 94–111. doi: 10.1016/j.dsr.2014.01.007
Dale B. (1976). Cyst formation, sedimentation, and preservation: Factors affecting dinoflagellate assemblages in recent sediments from Trondheimsfjord, Norway. Rev. Palaeobot. Palynol. 22, 39–60. doi: 10.1016/0034-6667(76)90010-5
Dale B., Dale A. L. (1992). “Dinoflagellate contributions to the open ocean sediment flux,” in Dinoflagellate contributions to the deep sea. Ed. Honjo S. (Massachusetts, United States: Woods Hole Oceanographic Institution Woods Hole), 1–73.
Dale B., Dale A. L., Jansen J. H. F. (2002). Dinoflagellate cysts as environmental indicators in surface sediments from the Congo deep-sea fan and adjacent regions. Palaeogeogr. Palaeoclimatol. Palaeoecol. 185, 309–338. doi: 10.1016/S0031-0182(02)00380-2
Daly K. L., Smith W. O. (1993). Physical-biological interactions influencing marine plankton production. Annu. Rev. Ecol. Syst. 24, 555–585. doi: 10.1146/annurev.es.24.110193.003011
Delebecq G., Schmidt S., Ehrhold A., Latimier M., Siano R. (2020). Revival of ancient marine dinoflagellates using molecular biostimulation. J. Phycol. 56, 1077–1089. doi: 10.1111/jpy.13010
de Vernal A., Radi T., Zaragosi S., Van Nieuwenhove N., Rochon A., Allan E., et al. (2020). Distribution of common modern dinoflagellate cyst taxa in surface sediments of the Northern Hemisphere in relation to environmental parameters: The new n= 1968 database. Mar. Micropaleontol. 159, 101796. doi: 10.1016/j.marmicro.2019.101796
Dodge J. D. (1989). Some Revisions of the Family Gonyaulacaceae (Dinophyceae) Based on a Scanning Electron Microscope Study. Botanica Marina. 32, 275–298. doi: 10.1515/botm.1989.32.4.275
Duarte C. M., Dachs J., Llabrés M., Alonso-Laita P., Gasol J. M., Tovar-Sánchez A., et al. (2006). Aerosol inputs enhance new production in the subtropical northeast Atlantic. J. Geophys. Res. 111. doi: 10.1029/2005JG000140
Elbrächter M., Gottschling M., Hoppenrath M., Keupp H., Kusber W.-H., Streng M., et al. (2023). Proposals to eliminate contradiction between Articles 11.7 and 11.8 and to equate non-fossil with fossil names of dinophytes for purposes of priority. Taxon 72, 258–260. doi: 10.1002/tax.12947
Ellegaard M., Moestrup Ø. (1999). Fine structure of the flagellar apparatus and morphological details of Gymnodinium nolleri sp. nov. (Dinophyceae), an unarmored dinoflagellate producing a microreticulate cyst. Phycologia 38, 289–300. doi: 10.2216/i0031-8884-38-4-289.1
Ellegaard M., Ribeiro S. (2018). The long-term persistence of phytoplankton resting stages in aquatic “seed banks”. Biol. Rev. Camb. Philos. Soc 93, 166–183. doi: 10.1111/brv.12338
Elshanawany R., Zonneveld K. A. F. (2016). Dinoflagellate cyst distribution in the oligotrophic environments of the Gulf of Aqaba and northern Red Sea. Mar. Micropaleontol. 124, 29–44. doi: 10.1016/j.marmicro.2016.01.003
Elshanawany R., Zonneveld K., Ibrahim M. I., Kholeif S. E. A. (2010). Distribution patterns of recent organic-walled dinoflagellate cysts in relation to environmental parameters in the Mediterranean Sea. Palynology 34, 233–260. doi: 10.1080/01916121003711665
Erickson D. J. III, Hernandez J. L., Ginoux P., Gregg W. W., McClain C., Christian J. (2003). Atmospheric iron delivery and surface ocean biological activity in the Southern Ocean and Patagonian region. Geophys. Res. Lett. 30. doi: 10.1029/2003GL017241
Figueroa R. I., Bravo I. (2005). Sexual reproduction and two different encystment strategies of Lingulodinium polyedrum (Dinophyceae) in culture1. J. Phycol. 41, 370–379. doi: 10.1111/j.1529-8817.2005.04150.x
Figueroa R. I., Estrada M., Garcés E. (2018). Life histories of microalgal species causing harmful blooms: Haploids, diploids and the relevance of benthic stages. Harmful Algae 73, 44–57. doi: 10.1016/j.hal.2018.01.006
Fischer G., Karakaş G. (2009). Sinking rates and ballast composition of particles in the Atlantic Ocean: Implications for the organic carbon fluxes to the deep ocean. Biogeosciences 6, 85–102. doi: 10.5194/bg-6-85-2009
Fischer G., Reuter C., Karakas G., Nowald N., Wefer G. (2009). Offshore advection of particles within the Cape Blanc filament, Mauritania: Results from observational and modelling studies. Prog. Oceanogr. 83, 322–330. doi: 10.1016/j.pocean.2009.07.023
Fischer G., Romero O., Merkel U., Donner B., Iversen M., Nowald N., et al. (2016). Deep ocean mass fluxes in the coastal upwelling off Mauritania from 1988 to 2012: Variability on seasonal to decadal timescales. Biogeosciences 13, 3071–3090. doi: 10.5194/bg-13-3071-2016
Fischer G., Romero O., Toby E., Iversen M., Donner B., Mollenhauer G., et al. (2019). Changes in the dust-influenced biological carbon pump in the Canary Current system: Implications from a coastal and an offshore sediment trap record off Cape Blanc, Mauritania. Global Biogeochem. Cycles 33, 1100–1128. doi: 10.1029/2019GB006194
Fomba K. W., Müller K., van Pinxteren D., Poulain L., van Pinxteren M., Herrmann H. (2014). Long-term chemical characterization of tropical and marine aerosols at the Cape Verde Atmospheric Observatory (CVAO) from 2007 to 2011. Atmospheric Climate Phys. 14, 8883–8904. doi: 10.5194/acp-14-8883-2014
Friese C. A., van Hateren J. A., Vogt C., Fischer G., Stuut J.-B. W. (2017). Seasonal provenance changes in present-day Saharan dust collected in and off Mauritania. Atmos. Chem. Phys. 17, 10163–10193. doi: 10.5194/acp-17-10163-2017
Fujii R., Matsuoka K. (2006). Seasonal change of dinoflagellates cyst flux collected in a sediment trap in Omura Bay, West Japan. J. Plankton Res. 28, 131–147. doi: 10.1093/plankt/fbi106
García-Moreiras I., Oliveira A., Santos A. I., Oliveira P. B., Amorim A. (2021). Environmental factors affecting spatial dinoflagellate cyst distribution in surface sediments Off Aveiro-Figueira da Foz (Atlantic Iberian Margin). Front. Mar. Sci. 8. doi: 10.3389/fmars.2021.699483
García-Moreiras I., Vila Costas S., García-Gil S., Muñoz Sobrino C. (2023). Organic-walled dinoflagellate cyst assemblages in surface sediments of the Ría de Vigo (Atlantic margin of NW Iberia) in relation to environmental gradients. Mar. Micropaleontol. 180, 102217. doi: 10.1016/j.marmicro.2023.102217
Gómez F. (2012). A quantitative review of the lifestyle, habitat and trophic diversity of dinoflagellates (Dinoflagellata, Alveolata). System. Biodivers. 10, 267–275. doi: 10.1080/14772000.2012.721021
Grange S. K. (2014). Technical report: Averaging wind speeds and directions. (Auckland, New Zealand: University of Auckland), 12. doi: 10.13140/RG.2.1.3349.2006
Hagen E. (2001). Northwest African upwelling scenario. Oceanol. Acta 24, 113–128. doi: 10.1016/S0399-1784(00)01110-5
Harland R., Pudsey C. J. (1999). Dinoflagellate cysts from sediment traps deployed in the Bellingshausen, Weddell and Scotia seas, Antarctica. Mar. Micropaleontol. 37, 77–99. doi: 10.1016/S0377-8398(99)00016-X
Head M. J. (1996). “Modern dinoflagellate cysts and their biological affinities,” in Palynology: principles and applications. Eds. Jansonius J., McGregor D. C. (Texas, United States: American Association of Stratigraphic Palynologists), 1197–1248.
Head M. J. (2003). Echinidinium zonneveldiae sp. nov., a dinoflagellate cyst from the Late Pleistocene of the Baltic Sea, northern Europe. J. Micropalaeontology 21, 169–173. doi: 10.1144/jm.21.2.169
Hernández M., Robinson I., Aguilar A., González L. M., López-Jurado L. F., Reyero M. I., et al. (1998). Did algal toxins cause monk seal mortality? Nature 393, 28–29. doi: 10.1038/29906
Holmes M. J., Teo S. L. M. (2002). Toxic marine dinoflagellates in Singapore waters that cause seafood poisonings. Clin. Exp. Pharmacol. Physiol. 29, 829–836. doi: 10.1046/j.1440-1681.2002.03724.x
Holzwarth U., Esper O., Zonneveld K. (2007). Distribution of organic-walled dinoflagellate cysts in shelf surface sediments of the Benguela upwelling system in relationship to environmental conditions. Mar. Micropaleontol. 64, 91–119. doi: 10.1016/j.marmicro.2007.04.001
Holzwarth U., Meggers H., Esper O., Kuhlmann H., Freudenthal T., Hensen C., et al. (2010). NW African climate variations during the last 47,000 years: evidence from organic-walled dinoflagellate cysts. Palaeogeogr. Palaeoclimatol. Palaeoecol. 291, 443–455. doi: 10.1016/j.palaeo.2010.03.013
Huneeus N., Schulz M., Balkanski Y., Griesfeller J., Prospero J., Kinne S., et al. (2011). Global dust model intercomparison in AeroCom phase I. Atmos. Chem. Phys. 11, 7781–7816. doi: 10.5194/acp-11-7781-2011
Inthorn M., Mohrholz V., Zabel M. (2006). Nepheloid layer distribution in the Benguela upwelling area offshore Namibia. Deep Sea Res. Part I 53, 1423–1438. doi: 10.1016/j.dsr.2006.06.004
Iversen M. H., Ploug H. (2013). Temperature effects on carbon-specific respiration rate and sinking velocity of diatom aggregates – potential implications for deep ocean export processes. Biogeosciences 10, 4073–4085. doi: 10.5194/bg-10-4073-2013
Jacobson D. M., Anderson D. M. (1986). Thecate heterotrophic dinoflagellates: Feeding behavior and mechanisms. J. Phycol. 22, 249–258. doi: 10.1111/j.1529-8817.1986.tb00021.x
Jeong H. J. (1999). The ecological roles of heterotrophic dinoflagellates in marine planktonic community. J. Eukaryot. Microbiol. 46, 390–396. doi: 10.1111/j.1550-7408.1999.tb04618.x
Jeong H. J., Yoo Y. D., Kim J. S., Seong K. A., Kang N. S., Kim T. H. (2010). Growth, feeding and ecological roles of the mixotrophic and heterotrophic dinoflagellates in marine planktonic food webs. Ocean Sci. J. 45, 65–91. doi: 10.1007/s12601-010-0007-2
Jickells T. D., An Z. S., Andersen K. K., Baker A. R., Bergametti G., Brooks N., et al. (2005). Global iron connections between desert dust, ocean biogeochemistry, and climate. Science 308, 67–71. doi: 10.1126/science.1105959
Karakaş G., Nowald N., Blaas M., Marchesiello P., Frickenhaus S., Schlitzer R. (2006). High-resolution modeling of sediment erosion and particle transport across the northwest African shelf. J. Geophys. Res. 111. doi: 10.1029/2005jc003296
Karakaş G., Nowald N., Schäfer-Neth C., Iversen M., Barkmann W., Fischer G., et al. (2009). Impact of particle aggregation on vertical fluxes of organic matter. Prog. Oceanogr. 83, 331–341. doi: 10.1016/j.pocean.2009.07.047
Kolber Z. S., Barber R. T., Coale K. H., Fitzwateri S. E., Greene R. M., Johnson K. S., et al. (1994). Iron limitation of phytoplankton photosynthesis in the equatorial Pacific Ocean. Nature 371, 145–149. doi: 10.1038/371145a0
Kremp A., Oja J., LeTortorec A. H., Hakanen P., Tahvanainen P., Tuimala J., et al. (2016). Diverse seed banks favour adaptation of microalgal populations to future climate conditions. Environ. Microbiol. 18, 679–691. doi: 10.1111/1462-2920.13070
Lathuilière C., Echevin V., Lévy M. (2008). Seasonal and intraseasonal surface chlorophyll-a variability along the northwest African coast. J. Geophys. Res. 113. doi: 10.1029/2007jc004433
Leroy S. A. G., Lahijani H. A. K., Reyss J.-L., Chalié F., Haghani S., Shah-Hosseini M., et al. (2013). A two-step expansion of the dinocyst Lingulodinium machaerophorum in the Caspian Sea: the role of changing environment. Quat. Sci. Rev. 77, 31–45. doi: 10.1016/j.quascirev.2013.06.026
Lewis J. (1988). Cysts and Sediments: Gonyaulax machaerophorum (Lingulodinium machaerophorum) in Loch Creran. J. Mar. Biol. Assoc. U. K. 68, 701–714. doi: 10.1017/S0025315400028812
Li Z., Mertens K. N., Gottschling M., Gu H., Söhner S., Price A. M., et al. (2020). Taxonomy and molecular phylogenetics of Ensiculiferaceae, fam. nov. (Peridiniales, Dinophyceae), with consideration of their life-history. Protist 171, 125759. doi: 10.1016/j.protis.2020.125759
Likumahua S., Sangiorgi F., de Boer M. K., Tatipatta W. M., Pelasula D. D., Polnaya D., et al. (2021). Dinoflagellate cyst distribution in surface sediments of Ambon Bay (eastern Indonesia): Environmental conditions and harmful blooms. Mar. pollut. Bull. 166, 112269. doi: 10.1016/j.marpolbul.2021.112269
Limoges A., Kielt J.-F., Radi T., Ruíz-Fernandez A. C., de Vernal A. (2010). Dinoflagellate cyst distribution in surface sediments along the south-western Mexican coast (14.76° N to 24.75°N). Mar. Micropaleontol. 76, 104–123. doi: 10.1016/j.marmicro.2010.06.003
Liu M., Gu H., Krock B., Luo Z., Zhang Y. (2020). Toxic dinoflagellate blooms of Gymnodinium catenatum and their cysts in Taiwan Strait and their relationship to global populations. Harmful Algae 97, 101868. doi: 10.1016/j.hal.2020.101868
Liu L., Wei N., Gou Y., Li D., Liang Y., Xu D., et al. (2017). Seasonal variability of Protoceratium reticulatum and yessotoxins in Japanese scallop Patinopecten yessoensis in northern Yellow Sea of China. Toxicon 139, 31–40. doi: 10.1016/j.toxicon.2017.09.015
Lu X., Wang Z., Guo X., Gu Y., Liang W., Liu L. (2017). Impacts of metal contamination and eutrophication on dinoflagellate cyst assemblages along the Guangdong coast of southern China. Mar. pollut. Bull. 120, 239–249. doi: 10.1016/j.marpolbul.2017.05.032
Lundholm N., Ribeiro S., Andersen T. J., Koch T., Godhe A., Ekelund F., et al. (2011). Buried alive – germination of up to a century-old marine protist resting stages. Phycologia 50, 629–640. doi: 10.2216/11-16.1
Luo Z., Mertens K. N., Nézan E., Gu L., Pospelova V., Thoha H., et al. (2019). Morphology, ultrastructure and molecular phylogeny of cyst-producing Caladoa arcachonensis gen. et sp. nov. (Peridiniales, Dinophyceae) from France and Indonesia. Eur. J. Phycol. 54, 235–248. doi: 10.1080/09670262.2018.1558287
Marret F., Bradley L., de Vernal A., Hardy W., Kim S.-Y., Mudie P., et al. (2020). From bi-polar to regional distribution of modern dinoflagellate cysts, an overview of their biogeography. Mar. Micropaleontol. 159, 101753. doi: 10.1016/j.marmicro.2019.101753
Matsuoka K., Head M. J. (2013). “Clarifying cyst–motile stage relationships in dinoflagellates,” in Biological and geological perspectives of dinoflagellates. Eds. Lewis J. M., Marret F., Bradley L. (The Micropalaeontological Society, Special Publications. Geological Society, London), 325–350.
Mertens K. N., Gu H., Gurdebeke P. R., Takano Y., Clarke D., Aydin H., et al. (2020). A review of rare, poorly known, and morphologically problematic extant marine organic-walled dinoflagellate cyst taxa of the orders Gymnodiniales and Peridiniales from the Northern Hemisphere. Mar. Micropaleontol. 159, 101773. doi: 10.1016/j.marmicro.2019.101773
Meunier T., Barton E. D., Barreiro B., Torres R. (2012). Upwelling filaments off Cap Blanc: Interaction of the NW African upwelling current and the Cape Verde frontal zone eddy field? J. Geophys. Res. C: Oceans 117. doi: 10.1029/2012JC007905
Mittelstaedt E. (1983). The upwelling area off Northwest Africa—A description of phenomena related to coastal upwelling. Prog. Oceanogr. 12, 307–331. doi: 10.1016/0079-6611(83)90012-5
Mittelstaedt E. (1991). The ocean boundary along the northwest African coast: Circulation and oceanographic properties at the sea surface. Prog. Oceanogr. 26, 307–355. doi: 10.1016/0079-6611(91)90011-A
Mollenhauer G., Basse A., Kim J.-H., Damste J. S. S., Fischer G. (2015). A four-year record of UK′-37-and TEX86-derived sea surface temperature estimates from sinking particles in the filamentous upwelling region off Cape Blanc, Mauritania. Deep Sea Res. Part I 97, 67–79. doi: 10.1016/j.dsr.2014.11.015
Morquecho L. (2019). Pyrodinium bahamense one the most significant harmful dinoflagellate in Mexico. Front. Mar. Sci. 6. doi: 10.3389/fmars.2019.00001
Mudie P. J., Marret F., Mertens K. N., Shumilovskikh L., Leroy S. A. G. (2017). Atlas of modern dinoflagellate cyst distributions in the Black Sea Corridor: from Aegean to Aral Seas, including Marmara, Black, Azov and Caspian Seas. Mar. Micropaleontol. 134, 1–152. doi: 10.1016/j.marmicro.2017.05.004
Naujokaitis-Lewis I., Fortin M.-J. (2016). Spatio-temporal variation of biotic factors underpins contemporary range dynamics of congeners. Glob. Change Biol. 22, 1201–1213. doi: 10.1111/gcb.13145
Obrezkova M. S., Pospelova V., Kolesnik A. N. (2023). Diatom and dinoflagellate cyst distribution in surface sediments of the Chukchi Sea in relation to the upper water masses. Mar. Micropaleontol. 178, 102184. doi: 10.1016/j.marmicro.2022.102184
Olivar M. P., Sabatés A., Pastor M. V., Pelegrí J. L. (2016). Water masses and mesoscale control on latitudinal and cross-shelf variations in larval fish assemblages off NW Africa. Deep Sea Res. Part I 117, 120–137. doi: 10.1016/j.dsr.2016.10.003
Pauly D., Christensen V. (1995). Primary production required to sustain global fisheries. Nature 374, 255–257. doi: 10.1038/374255a0
Pitcher G. C., Figueiras F. G., Hickey B. M., Moita M. T. (2010). The physical oceanography of upwelling systems and the development of harmful algal blooms. Prog. Oceanogr. 85, 5–32. doi: 10.1016/j.pocean.2010.02.002
Pitcher G. C., Fraga S. (2015) Harmful algal bloom events in the Canary Current large marine ecosystem. Available online at: https://aquadocs.org/handle/1834/9187.
Pitcher G. C., Joyce L. B. (2009). Dinoflagellate cyst production on the southern Namaqua shelf of the Benguela upwelling system. J. Plankton Res. 31, 865–875. doi: 10.1093/plankt/fbp040
Pitcher G. C., Louw D. C. (2021). Harmful algal blooms of the Benguela eastern boundary upwelling system. Harmful Algae 102, 101898. doi: 10.1016/j.hal.2020.101898
Pospelova V., de Vernal A., Pedersen T. F. (2008). Distribution of dinoflagellate cysts in surface sediments from the northeastern Pacific Ocean (43-25 N) in relation to sea-surface temperature, salinity, productivity and coastal upwelling. Mar. Micropaleontol. 68, 21–48. doi: 10.1016/j.marmicro.2008.01.008
Pospelova V., Esenkulova S., Johannessen S. C., O’Brien M. C., Macdonald R. W. (2010). Organic-walled dinoflagellate cyst production, composition and flux from 1996 to 1998 in the central Strait of Georgia (BC, Canada): A sediment trap study. Mar. Micropaleontol. 75, 17–37. doi: 10.1016/j.marmicro.2010.02.003
Pospelova V., Zonneveld K. A. F., Heikkilä M., Bringué M., Price A. M., Esenkulova S., et al. (2018). Seasonal, annual, and inter-annual Spiniferites cyst production: a review of sediment trap studies. Palynology 42, 162–181. doi: 10.1080/01916122.2018.1465738
Price A. M., Pospelova V. (2011). High-resolution sediment trap study of organic-walled dinoflagellate cyst production and biogenic silica flux in Saanich Inlet (BC, Canada). Mar. Micropaleontol. 80, 18–43. doi: 10.1016/j.marmicro.2011.03.003
Prospero J. M. (1990). “Mineral-aerosol transport to the north atlantic and north pacific: the impact of african and asian sources,” in The long-range atmospheric transport of natural and contaminant substances. Eds. Knap A. H., Kaiser M.-S., Kaiser M.-S. (Springer Netherlands, Dordrecht), 59–86. doi: 10.1007/978-94-009-0503-0_4
Prospero J. M., Collard F.-X., Molinié J., Jeannot A. (2014). Characterizing the annual cycle of African dust transport to the Caribbean Basin and South America and its impact on the environment and air quality. Global Biogeochem. Cycles 28, 757–773. doi: 10.1002/2013GB004802
Quijano-Scheggia S., Olivos-Ortiz A., Bustillos-Guzmán J. J., Garcés E., Gaviño-Rodríguez J. H., Galicia-Pérez M. A., et al. (2012). Bloom of gymnodinium catenatum in bahía santiago and bahía manzanillo, colima, Mexico. Rev. Biol. Trop. 60, 173–186. doi: 10.15517/rbt.v60i1.2750
Radi T., de Vernal A. (2004). Dinocyst distribution in surface sediments from the northeastern Pacific margin (40–60°N) in relation to hydrographic conditions, productivity and upwelling. Rev. Palaeobot. Palynol. 128, 169–193. doi: 10.1016/S0034-6667(03)00118-0
Radi T., de Vernal A. (2008). Dinocysts as proxy of primary productivity in mid–high latitudes of the Northern Hemisphere. Mar. Micropaleontol. 68, 84–114. doi: 10.1016/j.marmicro.2008.01.012
Reyero M., Cacho E., Martínez A., Vázquez J., Marina A., Fraga S., et al. (1999). Evidence of saxitoxin derivatives as causative agents in the 1997 mass mortality of monk seals in the Cape Blanc Peninsula. Nat. Toxins 7, 311–315. doi: 10.1002/1522-7189(199911/12)7:6<311::aid-nt75>3.0.co;2-i
Ribeiro S., Amorim A. (2008). Environmental drivers of temporal succession in recent dinoflagellate cyst assemblages from a coastal site in the North-East Atlantic (Lisbon Bay, Portugal). Mar. Micropaleontol. 68, 156–178. doi: 10.1016/j.marmicro.2008.01.013
Ribeiro S., Amorim A., Andersen T. J., Abrantes F., Ellegaard M. (2012). Reconstructing the history of an invasion: the toxic phytoplankton species Gymnodinium catenatum in the Northeast Atlantic. Biol. Invasions 14, 969–985. doi: 10.1007/s10530-011-0132-6
Rochon A., Vernal A., Turon J.-L., Matthießen J., Head M. J. (1999). Distribution of recent dinoflagellate cysts in surface sediments from the North Atlantic Ocean and adjacent seas in relation to sea-surface parameters. Am. Assoc. Stratigraphic Palynologists Contribution Ser. 35, 1–146.
Rodríguez–Villegas C., Díaz P. A., Salgado P., Tomasetti S. J., Díaz M., Marín S. L., et al. (2022). The role of physico-chemical interactions in the seasonality of toxic dinoflagellate cyst assemblages: The case of the NW Patagonian fjords system. Environ. pollut. 311, 119901. doi: 10.1016/j.envpol.2022.119901
Rollwagen-Bollens G., Bollens S. (2020). Biotic vs. abiotic forcing on plankton assemblages varies with season and size class in a large temperate estuary. J. Plankton Res. 42, 221–237. doi: 10.1093/plankt/fbaa010
Romero O. E., Baumann K.-H., Zonneveld K. A. F., Donner B., Hefter J., Hamady B., et al. (2020). Flux variability of phyto- and zooplankton communities in the Mauritanian coastal upwelling between 2003 and 2008. Biogeosciences 17, 187–214. doi: 10.5194/bg-17-187-2020
Romero O. E., Fischer G. (2017). Shift in the species composition of the diatom community in the eutrophic Mauritanian coastal upwelling: Results from a multi-year sediment trap experiment, (2003–2010). Prog. Oceanogr. 159, 31–44. doi: 10.1016/j.pocean.2017.09.010
Romero O. E., Ramondenc S. (2022). A 17-year time-series of diatom populations ‘flux and composition in the Mauritanian coastal upwelling. Front. Mar. Sci. 9. doi: 10.3389/fmars.2022.1006345
Romero O. E., Ramondenc S., Fischer G. (2021). A 2-decade, (1988–2009) record of diatom fluxes in the Mauritanian coastal upwelling: Impact of low-frequency forcing and a two-step shift in the species composition. Biogeosciences 18, 1873–1891. doi: 10.5194/bg-18-1873-2021
Sala-Pérez M., Lattuada M., Flecker R., Anesio A., Leroy S. A. G. (2020). Dinoflagellate cyst assemblages as indicators of environmental conditions and shipping activities in coastal areas of the Black and Caspian Seas. Reg. Stud. Mar. Sci. 39, 101472. doi: 10.1016/j.rsma.2020.101472
Sarjeant W. A. S. (1970). The Genus Spiniferites Mantell, 1850 (Dinophyceae). Grana 10, 74–78. doi: 10.1080/00173137009429857
Sarmiento J. L., Gruber N., Brzezinski M. A., Dunne J. P. (2004). High-latitude controls of thermocline nutrients and low latitude biological productivity. Nature 427, 56–60. doi: 10.1038/nature02127
Schnepf E., Elbrächter M. (1992). Nutritional strategies in dinoflagellates: A review with emphasis on cell biological aspects. Eur. J. Protistol. 28, 3–24. doi: 10.1016/S0932-4739(11)80315-9
Skonieczny C., Bory A., Bout-Roumazeilles V., Abouchami W., Galer S. J. G., Crosta X., et al. (2013). A three-year time series of mineral dust deposits on the West African margin: Sedimentological and geochemical signatures and implications for interpretation of marine paleo-dust records. Earth Planetary Sci. Lett. 364, 145–156. doi: 10.1016/j.epsl.2012.12.039
Smayda T. J. (2002). Adaptive ecology, growth strategies and the global bloom expansion of dinoflagellates. J. Oceanogr. 58, 281–294. doi: 10.1023/A:1015861725470
Smayda T. J., Trainer V. L. (2010). Dinoflagellate blooms in upwelling systems: Seeding, variability, and contrasts with diatom bloom behaviour. Prog. Oceanogr. 85, 92–107. doi: 10.1016/j.pocean.2010.02.006
Šmilauer P., Lepš J. (2014). Multivariate Analysis of Ecological Data using CANOCO 5 (Cambridge, United Kingdom: Cambridge University Press). Available at: https://play.google.com/store/books/details?id=3hkmAwAAQBAJ. doi: 10.1017/CBO9781139627061
Sprangers M., Dammers N., Brinkhuis H., van Weering T. C. E., Lotter A. F. (2004). Modern organic-walled dinoflagellate cyst distribution offshore NW Iberia; tracing the upwelling system. Rev. Palaeobot. Palynol. 128, 97–106. doi: 10.1016/S0034-6667(03)00114-3
Starr M., Lair S., Michaud S., Scarratt M., Quilliam M., Lefaivre D., et al. (2017). Multispecies mass mortality of marine fauna linked to a toxic dinoflagellate bloom. PloS One 12, e0176299. doi: 10.1371/journal.pone.0176299
Stuut J. B., Zabel M., Ratmeyer V., Helmke P., Schefuß E., Lavik G., et al. (2005). Provenance of present-day eolian dust collected off NW Africa. J. Geophysical Res. 110. doi: 10.1029/2004JD005161
Susek E., Zonneveld K. A. F., Fischer G., Versteegh G. J. M., Willems H. (2005). Organic-walled dinoflagellate cyst production in relation to upwelling intensity and lithogenic influx in the Cape Blanc region (off north-west Africa). Phycological Res. 53, 97–112. doi: 10.1111/j.1440-1835.2005.tb00362.x
Taleb H., Vale P., Blaghen M. (2003). Spatial and temporal evolution of PSP toxins along the Atlantic shore of Morocco. Toxicon 41, 199–205. doi: 10.1016/S0041-0101(02)00277-5
Taylor F. J. R., Hoppenrath M., Saldarriaga J. F. (2008). Dinoflagellate diversity and distribution. Biodivers. Conserv. 17, 407–418. doi: 10.1007/s10531-007-9258-3andcasa_token=AKcfDUykj90AAAAA:2UXWn00fflL95fEwUUT5Qo6WbnFHBn8AYQ2Q8oKvDfGa0XCaIJi0yJ6GdCjZ2qzoa2MsbZgRRD5d5xlWEg
ter Braak C. J. F., Prentice I. C. (1988). “A theory of gradient analysis,” in Advances in ecological research. Eds. Begon M., Fitter A. H., Ford E. D., Macfadyen A. (Massachusetts, United States: Academic Press), 271–317. doi: 10.1016/S0065-2504(08)60183-X
ter Braak C. J. F., Šmilauer P. (2002). CANOCO reference manual and canoDraw for windows user’s guide: software for canonical community ordination (version 4.5) (Itacha, NY, USA: Microcomputer Power).
Terenko G., Krakhmalnyi A. (2021). Red tide of Lingulodinium polyedrum (Dinophyceae) in Odesa Bay (Black Sea). Turkish J. Fisheries Aquat. Sci. 22. doi: 10.4194/1303-2712
van Camp L., Nykjaer L., Mittelstaedt E., Schlittenhardt P. (1991). Upwelling and boundary circulation off Northwest Africa as depicted by infrared and visible satellite observations. Prog. Oceanogr. 26, 357–402. doi: 10.1016/0079-6611(91)90012-B
van Nieuwenhove N., Head M. J., Limoges A., Pospelova V., Mertens K. N., Matthiessen J., et al. (2020). An overview and brief description of common marine organic-walled dinoflagellate cyst taxa occurring in surface sediments of the Northern Hemisphere. Mar. Micropaleontol. 159, 101814. doi: 10.1016/j.marmicro.2019.101814
Vink A., Zonneveld K. A. F., Willems H. (2000). Organic-walled dinoflagellate cysts in western equatorial Atlantic surface sediments: distributions and their relation to environment. Rev. Palaeobot. Palynol. 112, 247–286. doi: 10.1016/S0034-6667(00)00046-4
Wall D., Dale B. (1968). Modern dinoflagellate cysts and evolution of the Peridiniales. Micropaleontology 14, 265–304. doi: 10.2307/1484690
Yang T., Chen Y., Zhou S., Li H. (2019). Impacts of aerosol copper on marine phytoplankton: a review. Atmosphere 10, 414. doi: 10.3390/atmos10070414
Yu H., Tan Q., Chin M., Remer L. A., Kahn R. A., Bian H., et al. (2019). Estimates of African dust deposition along the trans-Atlantic transit using the decade-long record of aerosol measurements from CALIOP, MODIS, MISR, and IASI. J. Geophys. Res. 124, 7975–7996. doi: 10.1029/2019JD030574
Zenk W., Klein B., Schroder M. (1991). Cape verde frontal zone. Deep Sea Res. A 38, S505–S530. doi: 10.1016/S0198-0149(12)80022-7
Zhou W., Li Q. P., Wu Z. (2021). Coastal phytoplankton responses to atmospheric deposition during summer. Limnol. Oceanogr. 66, 1298–1315. doi: 10.1002/lno.11683
Zonneveld K. A. F., Albert M., Boom L., Donner B., Ebersbach F., Friese C., et al. (2016). Aerobic degradation of particulate organic matter and benthic microbial turnover rates reflecting ocean redox conditions off NW Africa (ADOMIS) Vol. 48 (Maria S. Merian MSM), 65.
Zonneveld K. A. F., Baumann K.-H., Boersen B., Bösche J., Decker C., de J.-D., et al. (2019a). A deglacial record of carbon release from thawing permafrost of the European tundra (EUROTHAW) - Marine Carbon Production, Export, relocation and degradation under varying ocean redox conditions off NW Africa (MACPEI) (Maria S. Merian MSM79), 64.
Zonneveld K. A. F., Brummer G. A. (2000). (Palaeo-)ecological significance, transport and preservation of organic-walled dinoflagellate cysts in the Somali Basin, NW Arabian Sea. Deep Sea Res. Part 2 Top. Stud. Oceanogr. 47, 2229–2256. doi: 10.1016/S0967-0645(00)00023-0
Zonneveld K. A. F., Coulibaly O., Flintrop C., Grotheer H., Klann M., Knoke M., et al. (2020). Marine Particles off NW Africa, from source to sink (Cruise report R.V. Meteor M165), 49.
Zonneveld K. A. F., Ebersbach F., Maeke M., Versteegh G. J. M. (2018). Transport of organic-walled dinoflagellate cysts in nepheloid layers off Cape Blanc (N-W Africa). Deep Sea Res. Part I 139, 55–67. doi: 10.1016/j.dsr.2018.06.003
Zonneveld K. A. F., Gray D. D., Kuhn G., Versteegh G. J. M. (2019b). Postdepositional aerobic and anaerobic particulate organic matter degradation succession reflected by dinoflagellate cysts: The Madeira Abyssal Plain revisited. Mar. Geol. 408, 87–109. doi: 10.1016/j.margeo.2018.11.010
Zonneveld K. A. F., Grotheer H., Versteegh G. J. M. (2022a). Dinoflagellate cysts production, excystment and transport in the upwelling off Cape Blanc (NW Africa). Front. Mar. Sci. 9. doi: 10.3389/fmars.2022.915755.10.1016/s0079-6611(00)00047-1
Zonneveld K. A. F., Jurkschat T. (1999). Bitectatodinium spongium (Zonneveld 1997) Zonneveld et Jurkschat, comb. nov. from modern sediments and sediment trap samples of the Arabian Sea (northwestern Indian Ocean): taxonomy and ecological affinity. Rev. Palaeobot. Palynol. 106, 153–169. doi: 10.1016/S0034-6667(99)00007-X
Zonneveld K. A. F., Marret F., Versteegh G. J. M., Bogus K., Bonnet S., Bouimetarhan I., et al. (2013). Atlas of modern dinoflagellate cyst distribution based on 2405 data points. Rev. Palaeobot. Palynol. 191, 1–197. doi: 10.1016/j.revpalbo.2012.08.003
Zonneveld K. A. F., Meilland J., Donner B., Versteegh G. J. M. (2022b). Export flux succession of dinoflagellate cysts and planktonic foraminifera in an active upwelling cell off Cape Blanc (NW Africa). Eur. J. Phycol. 57, 29–47. doi: 10.1080/09670262.2021.1885066
Zonneveld K. A. F., Mundanatt A. A., Bolte A., Eldering D., de Visser J.-D., Gottwald J., et al. (2022c). Sinking Particles, their production, transfer and preservation Vol. 104 (Cruise Report R.V. Maria S. Merian MSM), 51.
Zonneveld K. A. F., Pospelova V. (2015). A determination key for modern dinoflagellate cysts. Palynology 39, 387–409. doi: 10.1080/01916122.2014.990115
Zonneveld K. A. F., Susek E., Fischer G. (2010). Seasonal variability of the organic-walled dinoflagellate cyst production in the coastal upwelling region off Cape Blanc (Mauritania): A five-year survey1. J. Phycol. 46, 202–215. doi: 10.1111/(ISSN)1529-8817
Zonneveld K. A.F., Hoek R. P., Brinkhuis H., Willems H. (2001). Geographical distributions of organic-walled dinoflagellate cysts in surficial sediments of the Benguela upwelling region and their relationship to upper ocean conditions. Progress in Oceanography 48, 25–72. doi: 10.1016/s0079-6611(00)00047-1
Keywords: dinoflagellate cysts, ecology, coastal upwelling, Saharan dust, interannual variability, ecosystem change
Citation: Roza SEV, Versteegh GJM, Pospelova V and Zonneveld KAF (2024) Environmental control of interannual and seasonal variability in dinoflagellate cyst export flux over 18 years in the Cape Blanc upwelling region (Mauritania). Front. Mar. Sci. 11:1284425. doi: 10.3389/fmars.2024.1284425
Received: 28 August 2023; Accepted: 22 February 2024;
Published: 15 April 2024.
Edited by:
Maria Luisa Machain-Castillo, National Autonomous University of Mexico, MexicoReviewed by:
Iria García-Moreiras, University of Vigo, SpainMaija Heikkilä, University of Helsinki, Finland
Copyright © 2024 Roza, Versteegh, Pospelova and Zonneveld. This is an open-access article distributed under the terms of the Creative Commons Attribution License (CC BY). The use, distribution or reproduction in other forums is permitted, provided the original author(s) and the copyright owner(s) are credited and that the original publication in this journal is cited, in accordance with accepted academic practice. No use, distribution or reproduction is permitted which does not comply with these terms.
*Correspondence: Surya Eldo V. Roza, ZXJvemFAbWFydW0uZGU=