- 1Center for Research on Offshore Wind and the Environment, Biodiversity Research Institute, Portland, ME, United States
- 2British Trust for Ornithology, The Nunnery, Thetford, United Kingdom
- 3Northern Rocky Mountain Science Center, US Geological Survey, Bozeman, MT, United States
- 4Center for Avian Population Studies, Cornell Lab of Ornithology, Ithaca, NY, United States
- 5Actions, New York, NY, United States
- 6National Renewable Energy Laboratory, National Wind Technology Center, Golden, CO, United States
- 7U.S. Fish and Wildlife Service, Division of Migratory Birds, Charlestown, RI, United States
- 8New Jersey Audubon, Center for Research and Education, Cape May Court House, NJ, United States
- 9Department of Ecoscience, Aarhus University, Rønde, Denmark
- 10Stantec Consulting Services Inc., Topsham, ME, United States
- 11Department of Environmental Research, New York State Energy Research and Development Authority, Albany, NY, United States
- 12Center for Waterbird Studies, Biodiversity Research Institute, Portland, ME, United States
Offshore wind energy development (OWED), while a key strategy for reducing carbon emissions, has potential negative effects to wildlife that should be examined to inform decision making and adaptive management as the industry expands. We present a conceptual framework to guide the long-term study of potential effects to birds and bats from OWED. This framework includes a focus on exposure and vulnerability as key determinants of risk. For birds and bats that are exposed to OWED, there are three main effects of interest that may impact survival and productivity: 1) collision mortality, 2) behavioral responses, including avoidance, displacement, and attraction, and 3) habitat-mediated effects to prey populations. If these OWED effects cause changes in survival and/or breeding success (e.g., fitness), they have the potential for population-level consequences, including changes in population size and structure. Understanding the influence of ecological drivers on exposure and effect parameters can help to disentangle the potential impacts of OWED from other stressors. We use this theoretical framework to summarize existing relevant knowledge and identify current priority research questions (n=22) for the eastern United States, where large-scale development of OWED is primarily in the planning and early construction phase. We also identify recommendations for study design and further prioritization of research topics.
1 Introduction
Reliance on renewable energy, including offshore wind energy, is an essential strategy to reduce the rate of climate change (IPCC, 2011) and achieve net positive global impacts to wildlife and their ecosystems (Jedele et al., 2023). However, offshore wind energy development (OWED) also has the potential to negatively affect wildlife that use marine habitats, including marine airspace (Largey et al., 2021). In the offshore environment, many marine birds have the potential to interact with OWED during all parts of their life cycle. Terrestrial species, including songbirds, shorebirds, and bats, may interact with offshore wind infrastructure during migration (Loring et al., 2020a; Lagerveld et al., 2021; Brust and Hüppop, 2022). To develop offshore wind energy at the necessary scale in an environmentally responsible way, further research is needed to help decision makers understand the industry’s effects on birds and bats, identify the consequences of those effects, and inform adaptive management and mitigation for these taxa, where needed.
The rapidity and scale of planned development of the offshore wind industry in the United States, which is mirrored in many other places around the globe, indicates the importance of establishing a framework to set focal environmental priorities and direct support towards a cohesive and effective research approach. In the United States, the Bureau of Ocean Energy Management (BOEM) has leased over 2.2 million acres of the Atlantic Outer Continental Shelf (OCS) for OWED to date, with further leases along the Pacific coast and in the Gulf of Mexico. These leases are all located in federal waters greater than 5.5 km from shore. The Biden-Harris administration has set targets of 30 gigawatts (GW) of traditional offshore wind energy by 2030 and 15 GW of floating offshore wind by 2035, enough to power up to 15 million homes (Daly and McDermott, 2022). As of 2024, several commercial-scale offshore wind projects are in construction along the Atlantic coast of the United States, with at least 20 more in the planning stages.
The U.S. regulatory environment directs attention towards threatened and endangered species of birds and bats and is evolving with the offshore wind industry to further protect other migratory species. Under the National Environmental Policy Act of 1969 (NEPA; 42 U.S.C. §§4321-4370h), BOEM and other relevant federal regulatory agencies are required to conduct environmental impact assessments of construction and operations plans for proposed offshore developments. The U.S. Fish and Wildlife Service (FWS) oversees legal protections of bird and bat species under the Endangered Species Act of 1973 (ESA; 16 U.S.C. §§1531-1544). On the Atlantic coast of the United States, these protected species include roseate tern (Sterna dougallii), piping plover (Charadrius melodus), red knot (Calidris canutus rufa), northern long-eared bat (Myotis septentrionalis) and Indiana bat (Myotis sodalis). Anticipated take of endangered species requires an incidental take statement prepared in consultation with the FWS, and any requirements to minimize impacts are incorporated into OWED permits. Most other bird species in the United States are protected under the Migratory Bird Treaty Act (MBTA; 16 U.S.C. §§703–712). In summary, effects on birds and bats must be considered under the ESA and MBTA as part of the NEPA process. However, limited information is currently available to inform these assessments.
Given these regulatory requirements and the nascent state of offshore wind energy development in the eastern United States, stakeholders have identified the need for guidance to inform research efforts to better understand the potential influence of OWED on birds and bats. Recent research frameworks have identified priorities for the study of other wildlife taxa in relation to OWED (e.g., Kraus et al., 2019; Williams et al., 2023). This framework draws from these efforts, and the general exposure-conditions and stimuli-vulnerability framework outlined here is broadly applicable to both aquatic and above-water taxa. However, while some hypothesized changes to bird/bat behaviors, distributions, and prey populations in response to OWED are also applicable to other taxa (Kraus et al., 2019; Williams et al., 2023) the potential stimuli, or hazards, posed to birds and bats by OWED vary substantially from those posed to aquatic taxa. Primary risks to marine mammals, for example, are thought to relate to underwater sound during the pre-construction and construction phases of development, as well as vessel activity (and associated collision risk) associated with all phases of development (Bailey et al., 2014). Nevertheless, frameworks such as the Population Consequences of Disturbance (PCoD; Pirotta et al., 2018) and related models, which were developed for marine mammals, have partially informed the framework presented in this paper. Further work is needed to develop so-called consequence models for other taxa such as fishes (Williams et al., 2023).
By identifying a conceptual framework for effect pathways that in turn informs key questions and potential study methods, we aim to improve understanding of the effects of OWED construction and operations on birds and bats. This includes consideration of potential short-term, long-term, and population-level effects for all species of birds and bats that use the offshore environment and, therefore, have the potential to interact with OWED in U.S. Atlantic federal waters. The taxonomic focus includes marine birds that breed, migrate, or overwinter in the U.S. Atlantic (focusing on federal waters of the Mid-Atlantic Bight from Massachusetts to North Carolina), as well as terrestrial species of both birds and bats that pass through this area during spring or fall migration. Although OWED pre-construction or construction activities may affect bird and bat species, the primary focus of this framework is on the decades-long post-construction period of offshore wind energy development, with the intent of generating research that may inform the siting and adaptive management of future offshore wind projects. Although this particular effort builds on the regulatory process emerging in the U.S. Atlantic, the offshore wind industry is rapidly expanding in other regions of the globe where OWED stakeholders and regulators will encounter similar challenges. Thus, the goal of this framework is to inform future research efforts and funding needs in the U.S. Atlantic and elsewhere facing similar proposed developments.
This broad framework draws from existing frameworks for other wildlife taxa, where applicable (e.g., Kraus et al., 2019; Williams et al., 2023), and identifies key questions and study goals to inform the development of future detailed study plans for OWED, both in the U.S. Atlantic and globally. The end goal of the research framework is to understand cumulative and population-level effects to birds and bats, thereby informing our understanding of what mitigation measures may be needed to allow OWED to create net positive global impacts for bird and bat populations and ecosystems.
2 Methods
In early 2020, the New York State Environmental Technical Working Group (E-TWG) convened a facilitated stakeholder workshop that included scientists and representatives of environmental non-governmental organizations, regulators, and offshore wind developers. Based in part on similar previous efforts for marine mammals and sea turtles in southern New England (Kraus et al., 2019), this workshop focused on: 1) reviewing the existing knowledge on the influence of OWED on birds and bats (drawn primarily from studies of the European offshore wind industry, as well as other industries where appropriate), 2) generating priority questions and hypotheses for the U.S. Atlantic (focused on the Mid-Atlantic Bight from Massachusetts to North Carolina), and 3) identifying potential study methods. The scientific research framework (hereafter ‘framework’) presented here builds on outputs from this workshop (NYSERDA New York State Energy Research and Development Authority, 2020) as well as efforts by related workgroups to prioritize research topics specific to understanding the cumulative effects of OWED on birds (Cook et al., 2021) and bats (Hein et al., 2021), with additional input from a small group of subject matter experts with taxonomic, methodological, and statistical expertise. For purposes of discussion, “offshore” areas are beyond 5.5 km from shore (e.g., waters in which offshore wind leasing is typically controlled by the federal government), while “nearshore” or “coastal” areas are within 5.5 km of shore (and are under state jurisdiction). While the focus of workshop discussions was the offshore context, discussions also included consideration of primarily coastal species and locations, including potential wildlife interactions with aspects of offshore wind facility infrastructure located in state waters, such as transmission cables.
Research priorities were identified via an iterative discussion of research needs and data gaps during both breakout groups and full group discussions, beginning with the larger workshop group. Following these initial discussions, workshop participants were divided into groups structured first around study methods, then by different taxa and types of impacts (e.g., avian displacement, avian collisions, avian attraction, avian habitat effects, and effects to bats), to begin refining the list of research questions. This list was refined further by the smaller expert group by (1) combining similar questions (i.e. questions posed about different specific taxa that were focused on the same underlying exposure or effect), (2) considering the availability of existing data to fill gaps, and (3) identifying where existing evidence suggests that specific questions might be lower priority than other topics on the list, due to expected low incidence of effects, low levels of impacts, or other factors. These judgements relied in large part on the existing scientific literature for offshore wind energy, as well as studies of related anthropogenic activities, such as offshore oil and gas exploration and terrestrial wind energy development.
The bird and bat workgroups from the 2020 State of the Science Workshop on Wildlife and Offshore Wind Energy (Cook et al., 2021; Hein et al., 2021), which overlapped substantially in membership with that of the 2020 stakeholder workshop, met virtually several times in 2020–2021 to prioritize research topics specific to understanding the cumulative effects of OWED for birds and bats. These discussions were also influential in the refinement of this research framework and research questions.
3 Framework for understanding offshore wind energy effects
Potential impacts to birds and bats from OWED are determined by a combination of exposure, hazard, and vulnerability (Crichton, 1999; Goodale and Milman, 2014). In this risk framework, exposure describes how the spatiotemporal patterns of space use by animals overlap with that of wind energy development (Figure 1). This may include overlap in the distribution, abundance, and/or flight altitudes of a species with OWED rotor-swept zones. Animals that are exposed to OWED may be affected by specific conditions and stimuli in the wind facility and its surroundings (Southall et al., 2021; conditions and stimuli are also commonly called “hazards” or “stressors”). These are attributes of the OWED such as the physical presence of structures, associated lighting and vessel traffic, and changes in habitat, resources, or foraging conditions of benthic and pelagic ecosystems. These conditions and stimuli may have either positive or negative effects on animals, and may change over the construction and operational period of an offshore wind farm. The third aspect determining OWED effects is the vulnerability of exposed animals to specific stimuli. Vulnerability encompasses 1) individual sensitivity to effects, which is driven by factors such as behavior (e.g., flight height, time budgets), morphology (size, maneuverability), response to disturbance, and habitat flexibility, among other factors, and 2) population sensitivity, defined by the population characteristics, such as conservation status and demographic parameters, that determine how OWED effects on individual animals may translate into population-level impacts (Figure 1). The vulnerability of animals to OWED stimuli is partially dictated by site-level characteristics that may influence responses (Fox and Petersen, 2019).
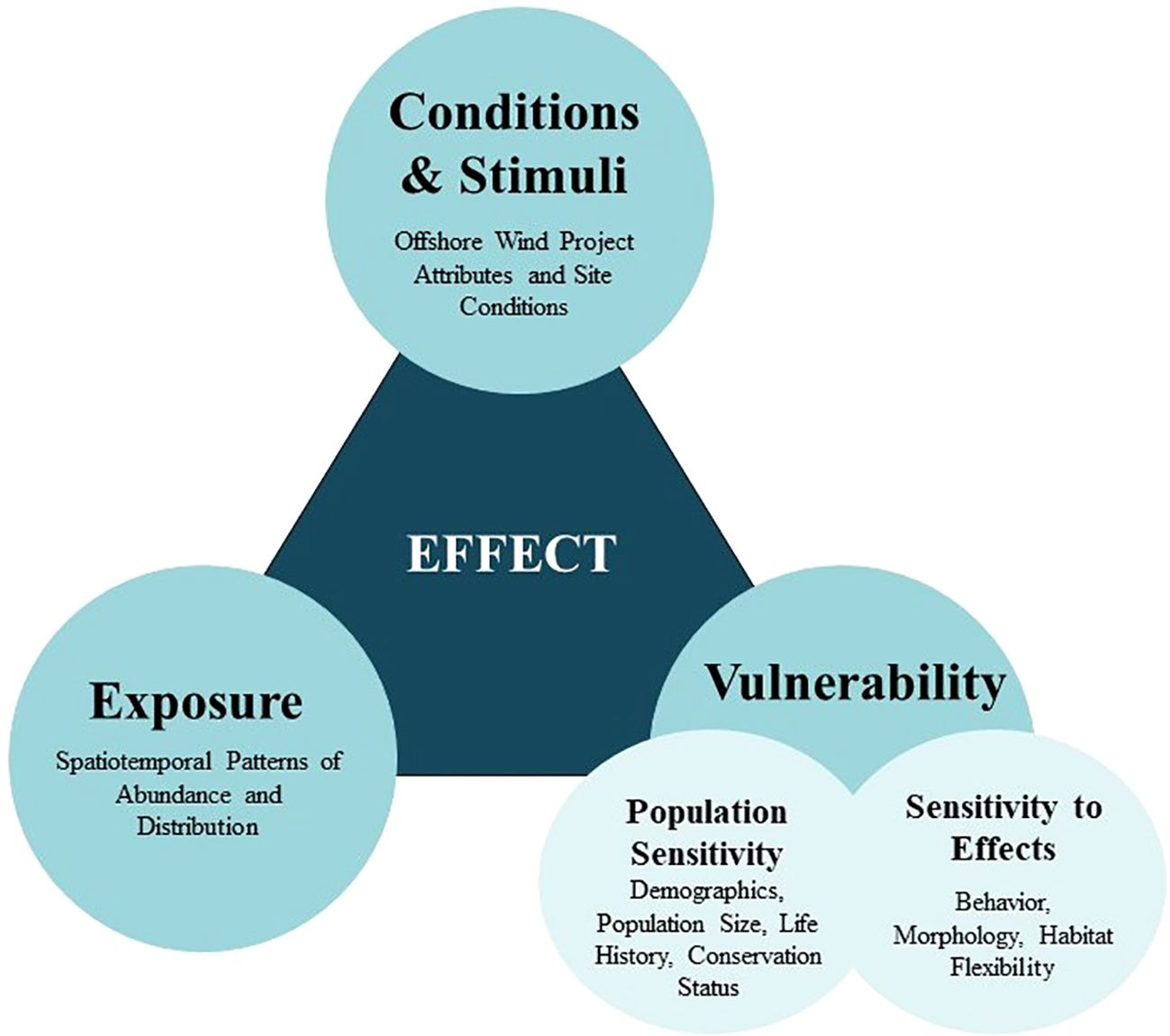
Figure 1 Main factors that determine the impacts to birds and bats from offshore wind energy development. Impacts are determined based on a combination of exposure, conditions and stimuli, and vulnerability (modified from Crichton, 1999; Goodale and Milman, 2014), where exposure primarily relates to spatiotemporal patterns of species distributions and abundance, conditions and stimuli are the attributes of offshore wind farms that drive wildlife responses, and vulnerability is dictated by the sensitivity of exposed animals to these stimuli, which is determined by a combination of individual and population factors.
For birds and bats, there are three main effects of interest from OWED: (1) collision mortality, (2) behavioral responses, and (3) habitat-mediated effects (Figure 2). For collision mortality, vulnerability is partially driven by avoidance behaviors (i.e. any action taken by an individual when in proximity to an operational wind farm to prevent collision). Avoidance may occur at multiple spatial scales, including macro-avoidance, whereby individuals avoid the entire wind farm; meso-avoidance, where individuals maneuver within a wind farm to avoid turbine vicinities; and micro-avoidance, where individuals make last-minute maneuvers (<10 m distant) to avoid the turbine blades (Cook et al., 2018). Avoidance, as well as displacement and attraction, is also a potential behavioral effect of OWED. Displacement is a subset of avoidance behaviors that occurs when individuals adjust their habitat use (e.g., foraging, roosting, or breeding habitat) due to a new anthropogenic feature or disturbance, causing effective habitat loss. In contrast, some bird and bat species may be attracted to wind farms due to increased roosting or foraging opportunities on and around foundations of structures, or due to lighting on structures (Croll et al., 2022; Guest et al., 2022). Attraction may be beneficial, or it may be maladaptive such that it increases collision risk. Habitat-mediated effects relate to changes in habitat and prey resources through the introduction of new hard substrates (i.e. reef effects; Methratta and Dardick, 2019), modification of human pressures (i.e. changes in fishing activities; Hammar et al., 2016), disturbance and change to substrates from the installation of turbines and cables, and changes in oceanographic dynamics (Gill et al., 2020; van Berkel et al., 2020). Behavioral effects are direct responses to OWED structures or activities; habitat-mediated effects may also indirectly lead to behavioral responses, for example as seabirds respond to changes in the distributions of their prey.
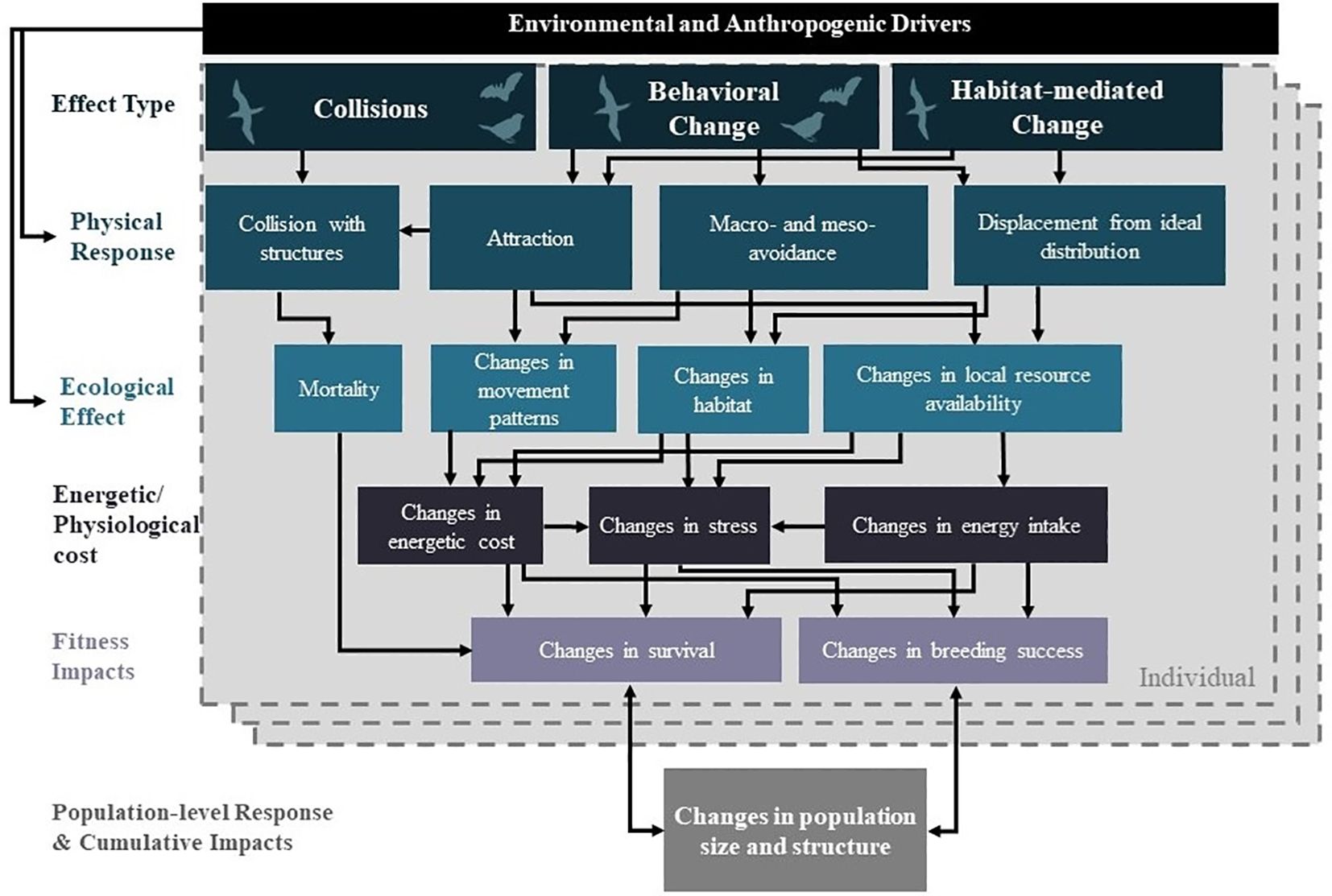
Figure 2 Conceptual framework for understanding potential effects of offshore wind energy development on birds and bats (modified from (Fox et al., 2006). Symbols in the “Effect Type” section of the diagram indicate applicability to marine birds, diurnal and nocturnal avian migrants, and bats. Effects include collisions, behavioral change, and habitat-mediated change, each of which may cause one or more physical responses by birds or bats that in turn result in ecological, energetic/physiological, and fitness effects on individuals. These individual-level effects can then be scaled up to understand the potential for population-level or cumulative response. Ecological drivers (e.g., environmental variability, resource distributions) and anthropogenic drivers (e.g., OWED turbine size and spacing) can influence and introduce variability into physical response, as well as effects.
Each of the three effects (collision, behavioral, habitat-mediated) and the physical responses by animals can lead, individually or collectively, to a range of ecological effects, energetic and physiological costs, and fitness consequences (Figure 2). While collisions can lead to direct mortality of individuals, most other effects are indirect, with potential energetic costs or changes in prey availability or foraging success that may have downstream impacts on fitness. By understanding energetic and physiological costs and fitness effects to individuals and groups, we can begin to understand potential population-level consequences, including changes in population size and structure. There are also environmental drivers, including oceanography, meteorology, and climatology, as well as baseline distributions of prey and other resources, which can influence aspects of this framework in diverse and complex ways. Understanding the influence of these drivers can help to disentangle potential impacts of OWED from other stressors, such as the effects of climate change. While not explicitly included in this framework, interactive changes among individuals or populations, such as changes in competition, may also be important considerations to fully understand how OWED might affect populations and ecosystems.
The following summary of existing knowledge and key research questions is structured to follow the two-part conceptual effects framework outlined above, focusing on the areas with the greatest and/or most urgent gaps in knowledge (see Methods). We begin with the importance of understanding exposure, since animals that are not exposed logically cannot be affected by OWED conditions and stimuli. The three main types of effects (collisions, behavioral change, and habitat change) are then discussed with regard to existing knowledge about vulnerability of taxa to these effects. And finally, we examine how the scope and degree of effects, coupled with population sensitivity, determines population-level response and cumulative effects.
4 Exposure
4.1 Current knowledge
4.1.1 Marine birds
Marine birds use the offshore environment of the eastern United States to forage, rest, and travel. The spatial scale of this habitat use varies substantially by species, life history stage, and other factors. Marine birds that breed in the region include terns, gulls, and cormorants (Brinker et al., 2007; Nisbet et al., 2013); however, migrant and wintering marine birds are more numerous than breeders in U.S. Atlantic waters (Nisbet et al., 2013), and thus may be more exposed to OWED in many locations. Many species that breed in the Arctic and sub-Arctic use the coastal and offshore environments of the eastern United States as wintering grounds, including auks, sea ducks, gannets, storm-petrels, and loons (Winship et al., 2018). In addition, some species, such as great shearwaters (Ardenna gravis), sooty shearwaters (A. grisea), and Wilson’s storm-petrels (Oceanites oceanicus), breed in the Southern Hemisphere and frequent the region during their non-breeding season in the austral winter/boreal summer (Nisbet et al., 2013; Powers et al., 2020).
Some of the best available information about the at-sea distribution of marine birds in the U.S. Atlantic comes from visual sightings and digital (photography/video) surveys conducted aboard boats and aircraft (Winship et al., 2018). Recent Marine-life Data and Analysis Team (MDAT) spatial modeling efforts used these data to estimate seasonal patterns of relative densities from 49 marine bird species across the Atlantic OCS (Winship et al., 2023). Other baseline studies have examined marine bird distribution and abundance in various subregions offshore of the eastern United States (Williams et al., 2015; Veit et al., 2016; Palka et al., 2017; Normandeau Associates and APEM, 2019; Robinson Willmott et al., 2021), and recent tracking efforts also contribute to our understanding of habitat use and movement patterns throughout the Atlantic OCS (Williams et al., 2015; Loring et al., 2018, 2019; Loring et al., 2020a; Loring et al., 2020b; Stenhouse et al., 2020).
Oceanographic processes regulate prey availability and predictability, which in turn drive patterns of habitat use for marine predators (Scales et al., 2014). Prey populations are often difficult to measure directly, so marine bird distribution patterns are more often studied in relation to environmental proxies (e.g., sea surface temperature, chlorophyll a; Belkin, 2021). Water depth and distance to shore are important explanatory and predictive variables for many species in the region (Winiarski et al., 2014; Williams et al., 2015; Goyert et al., 2016; Winship et al., 2018). Dynamic variables, like chlorophyll a concentrations, also influence patterns for some species, such as red-throated loons (Gavia stellata), common loons (G. immer), northern gannets (Morus bassanus), and long-tailed ducks (Clangula hyemalis). While existing survey and tracking data are informative of species distributions, abundance, and movements, substantial gaps remain in these datasets. Moreover, we often lack the spatial and temporal resolution to determine environmental drivers of distributions and movements, and little is known about how these spatiotemporal patterns are changing over time, particularly in relation to climate change.
4.1.2 Diurnal and nocturnal avian migrants
In addition to marine birds, other diurnal and nocturnal avian migrants use the airspace above the marine environment during migration, including terrestrial songbirds and near-passerines, shorebirds, raptors, and wading birds. Radar can be used to detect such movements (Hüppop et al., 2019). For example, Dokter et al. (2018) estimated migration traffic over the water in the western Atlantic Ocean at 219 ± 63 million birds during autumn. BirdCast (birdcast.info), a project employing methods to extract bird migration signals from weather surveillance radar to observe, characterize, and predict bird migration intensity aloft (Dokter et al., 2018; Van Doren and Horton, 2018; Lin et al., 2019), offers nearshore rather than offshore spatial coverage but can provide information on potential coastal departure and arrival points for migrants, broad-scale information on fluctuations in migration activity, and flight heights in relation to weather conditions. However, such data tend to be reliable at larger spatial scales than that of individual wind farms, and thus may not be ideal for assessing exposure on an individual OWED project basis.
Some land birds appear to be obligate offshore migrants (DeLuca et al., 2019), while others use offshore habitats more facultatively (Shamoun-Baranes and van Gasteren, 2011). Much of our understanding of the offshore occurrence of individual species is derived anecdotally from sightings from boats and offshore platforms, though the advent of smaller tracking technologies has provided new opportunities to improve our understanding of offshore movements. For example, recent geolocator tracking studies show that semipalmated sandpipers (Calidris pusilla), blackpoll warblers (Setophaga striata), bobolinks (Dolichonyx oryzivorus), and Connecticut warblers (Oporornis agilis) depart the U.S. Atlantic coast and engage in multi-day nonstop flights over the ocean during fall migration (Deluca et al., 2015; Brown et al., 2017; McKinnon et al., 2017; Perlut, 2018). Tracking studies of piping plovers and red knots (Loring et al., 2020a), and whimbrel (Numenius phaeopus; (Watts et al., 2022), as well as peregrine falcons (Falco peregrinus) and merlins (F. columbarius; DeSorbo et al., 2019), have also revealed migratory use of the Atlantic OCS. Additional data comes from primarily terrestrial sources, such as eBird, which relies on community science observations (Sullivan et al., 2009).
Given the diversity of this species assemblage, a variety of factors drive the migratory patterns and offshore occurrence of diurnal and nocturnal avian migrants. Flights over water, though not fully characterized, occur with uneven spatial and temporal distributions (Farnsworth et al., 2016). Season, location, migration strategy, local weather, and regional climate indices influence the timing of migration, among other factors (Van Buskirk et al., 2009; Deppe et al., 2015; La Sorte et al., 2015; Horton et al., 2016; Van Doren and Horton, 2018; Brust et al., 2019; Loring et al., 2020a; Cohen et al., 2021). Offshore migratory activity tends to occur episodically in relation to conditions conducive to offshore movements and is especially prevalent in fall, when many bird species move from breeding grounds in Greenland, Canada, and the northern United States, to wintering grounds in the southern United States, Caribbean, Central America, and South America. Given limitations in tracking technology for small-bodied species, we often lack the spatial resolution and sample sizes needed to understand spatiotemporal patterns of fine-scale offshore movements on a species level, hindering our understanding of population-level exposure.
4.1.3 Bats
In addition to birds, nine species of insectivorous bats occur in the U.S. mid-Atlantic region. Migratory tree-roosting bats, including the eastern red bat (Lasiurus borealis), hoary bat (L. cinereus), and silver-haired bat (Lasionycteris noctivagans), tend to be solitary and can migrate long distances. Cave-roosting species migrate short distances between summer roosts and winter hibernacula. These species include the northern long-eared bat, Indiana bat, little brown bat (M. lucifugus), eastern small-footed bat (M. leibii), big brown bat (Eptesicus fuscus), and tri-colored bat (Perimyotis subflavus).
Much of what we know of potential bat exposure to wind energy impacts comes from the terrestrial wind industry. Substantial numbers of bats collide with terrestrial turbines, with nearly 80% of reported fatalities belonging to the migratory tree-roosting species (Arnett and Baerwald, 2013; Allison and Butryn, 2020). This disparity may be related to how different species respond to, or interact with, wind turbines. Several attraction hypotheses have been suggested, mostly related to bats perceiving wind turbines as a resource for roosting, foraging, or mating (Guest et al., 2022). Bat activity and mortality at terrestrial wind farms tend to be highest during fall migration under low wind speed conditions (Rydell et al., 2010; Arnett et al., 2013). Similar temporal patterns in activity have been observed along the Atlantic coast (Stantec, 2016) and at offshore sites in Europe (Lagerveld et al., 2021).
Eastern red bats represent a majority of coastal and offshore bat detections in the northwest Atlantic (Hatch et al., 2013), and bats have been detected in all offshore areas that have been surveyed in the Gulf of Maine and mid-Atlantic United States (Peterson et al., 2014; Solick and Newman, 2021). However, the overall activity levels of bats offshore are lower relative to terrestrial settings. Moreover, it is unclear whether bats will interact with offshore wind turbines in the same manner as with terrestrial wind turbines. Therefore, the level of exposure and potential collision risk of bats from OWED remains uncertain (Hein et al., 2021).
4.2 Research gaps
Several research questions were identified as priorities to address current gaps in knowledge about the potential exposure of marine birds, avian migrants, and bats, to OWED in the mid-Atlantic OCS (Table 1). Several of these questions focus specifically on marine birds, and one on bats; the first question is relevant to all three groups. All four questions require data to be collected at regional scales to inform our understanding of distribution and abundance patterns and inform estimates of exposure (including changes in exposure over time and three-dimensional space).
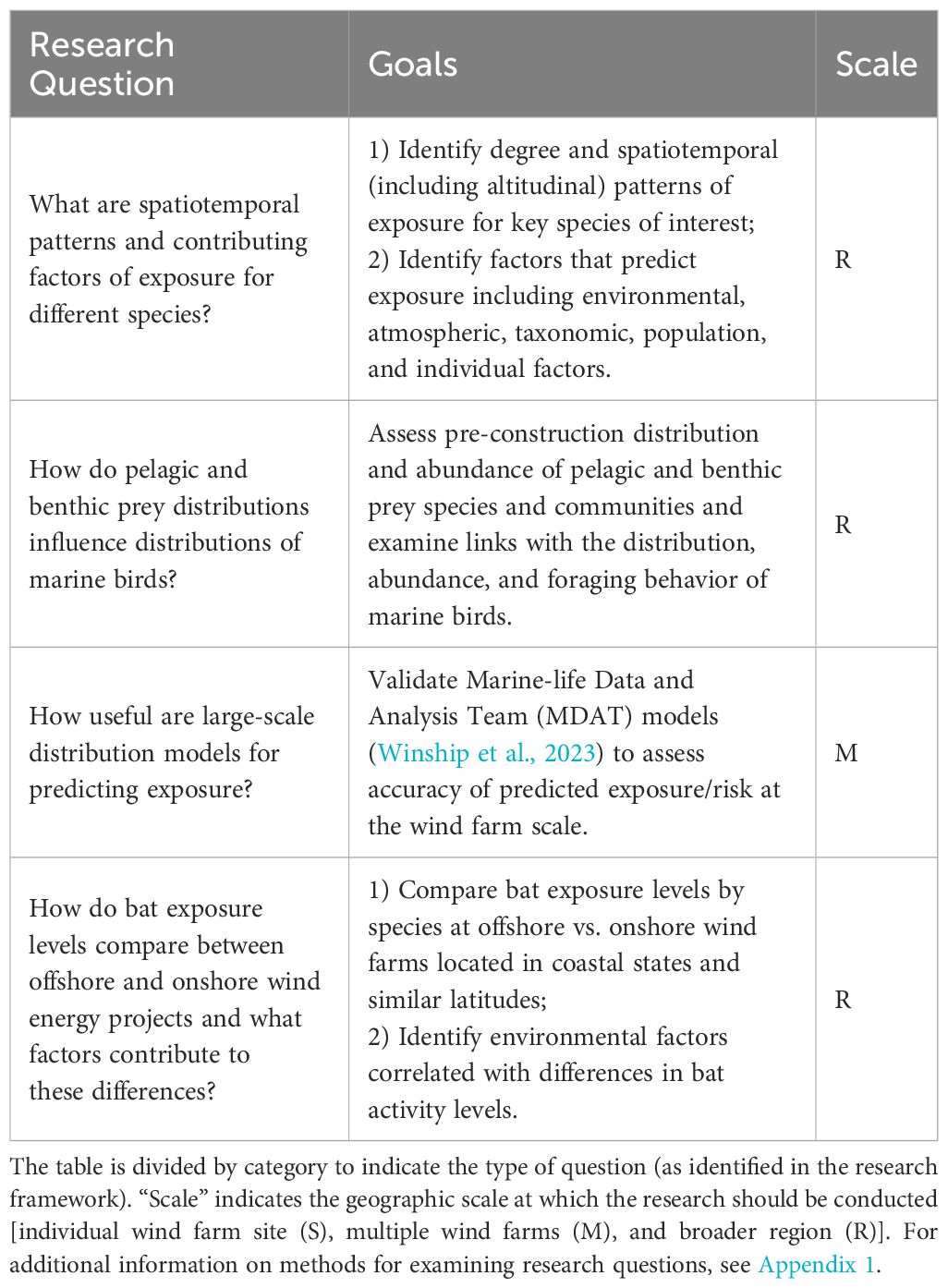
Table 1 Research questions/goals to improve understanding of potential exposure of birds and bats to offshore wind energy development in the U.S. Atlantic.
5 Collision-related effects from offshore wind development
5.1 Current knowledge
Collisions of birds and bats with wind turbine structures are high-profile effects due to the potential to cause direct mortality and the frequency of collisions reported at some terrestrial wind farms. It is thought that collisions offshore are less common than at wind farms in terrestrial systems (Cook et al., 2018; Skov et al., 2018), and there are few records to date of confirmed collisions with offshore turbines (Pettersson, 2005; Desholm, 2006; Newton and Little, 2009; Skov et al., 2018; Tjørnløv et al., 2023). However, collisions are also much more difficult to detect offshore, as it requires technology that can measure levels of avoidance, detect collisions, identify species, and monitor a large enough area of turbines over time to obtain adequate sample sizes to inform an understanding of effects. While birds are also known to collide with a range of other anthropogenic structures, including marine oil and gas platforms (Ellis et al., 2013), these data are of little utility for predicting risk from OWED, given the limited nature of these data (Ellis et al., 2013) and the substantial differences in the collision-related stressors posed by offshore wind energy vs. offshore oil and gas extraction.
Information on collisions and avoidance at offshore wind turbines comes primarily from several recent large-scale studies in Europe (Skov et al., 2018; Tjørnløv et al., 2023), which used different combinations of methods, including observers with range finders, radar systems, and visual/thermal detection tracking systems. Skov et al. (2018) detected 6 collisions in 20 months of monitoring an offshore turbine, and concluded that marine birds, including northern gannets, black-legged kittiwakes, and large gulls, exhibited high levels of macro-, meso- and micro-avoidance. More recently, Tjørnløv et al. (2023) detected no collisions in 14 months of diurnal monitoring at an offshore wind farm in Scottish waters, despite tracking >3,000 marine birds (including gannets, gulls, procellarids, skuas, and others) through the wind farm via a combined marine radar and visual camera system.
There are a variety of other collision detection technologies in development that detect collision events and classify the animals involved to some level of taxonomic specificity (Dirksen, 2017; Courbis et al. 2024). Several such technologies have been deployed on turbines at offshore wind farms around the world (Carbon Trust, 2022), though third-party validation of system capabilities remains rare (Courbis et al. 2024).
Given the lack of validated technologies to directly measure collisions until recently, and given the high cost of such studies, collision risk models (CRMs) have been developed. Most CRMs use a variation on the Band model (Band, 2012), which was first developed for use onshore and has since been expanded for offshore use (Masden and Cook, 2016), and focuses primarily on marine birds. CRMs allow for a standardized and transparent estimation of collision risk by incorporating information about the bird species of interest and wind farm specifications. The primary model output is an estimate of annual numbers of collisions by species for an offshore wind farm.
There is substantial uncertainty around the accuracy of the structure of CRMs for describing the factors influencing collision rates, as well as uncertainty around individual values of CRM parameters. There has been very limited empirical validation of CRMs, and terrestrial studies of raptors in Spain found low correlation between actual collisions and predicted collision risk from CRMs (De Lucas et al., 2008). The models are particularly sensitive to avoidance rates; avoidance can occur at multiple spatial and temporal scales and is difficult to measure quantitatively, though advances in technology, such as GPS and the above-mentioned combined radar/camera systems, are providing new insights (Johnston et al., 2022; Tjørnløv et al., 2023). Recognizing the potential variation in many CRM input parameters, there have been efforts to develop stochastic models to better reflect uncertainty and incorporate spatiotemporal variation (McGregor et al., 2018). However, there are factors, such as time of day and weather conditions, that still are not incorporated into CRMs despite their capacity to influence behavior.
5.2 Research gaps
Five collision-related questions were identified to improve our understanding of the relationship between exposure and vulnerability, determine the influence of environmental conditions on collision risk, parameterize collision risk models, and assess the accuracy of collision risk models (Table 2). Studies could be designed to help answer these questions at individual wind farms, but multiple studies from different locations will be needed to fully address these questions.
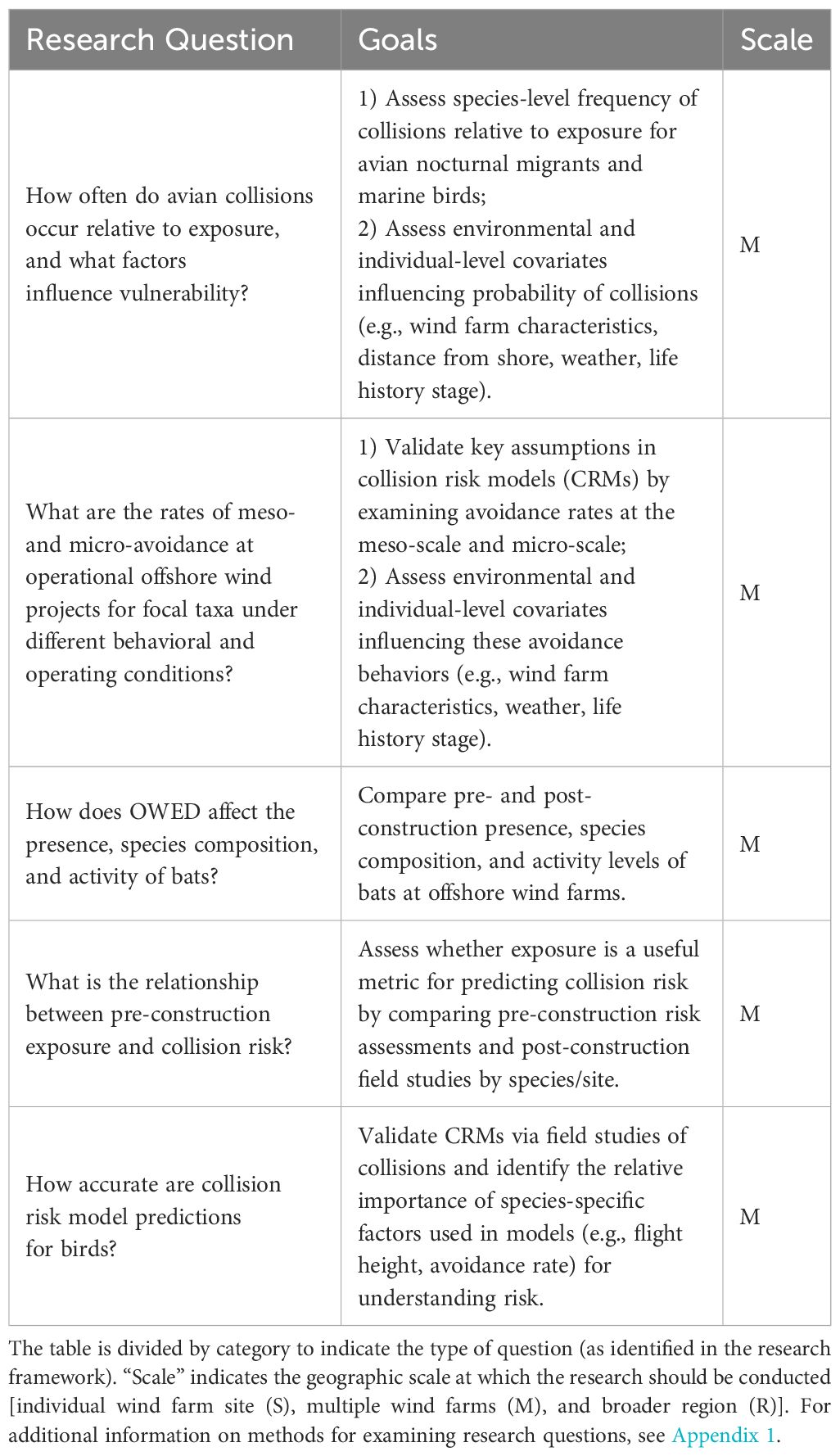
Table 2 Research questions/goals to improve understanding of collisions of birds and bats with offshore wind energy development in the U.S. Atlantic.
6 Behavioral effects from offshore wind development
6.1 Current knowledge
In addition to mortality, offshore wind construction and operational activities may directly affect bird and bat behavior in a variety of ways. (Indirect effects on behavior are discussed in Section 7, below). Not all effects are necessarily negative for all species (Dierschke et al., 2016), but behavioral changes may lead to modifications in energy expenditures and/or energy intake, as well as physiological changes, such as stress responses, that in turn are hypothesized to have the potential for population-level consequences in some species (e.g., Dierschke et al., 2017).
6.1.1 Displacement
Displacement has been widely studied at European offshore wind projects, though the scale, degree, and duration of response can vary greatly (Dierschke et al., 2016). Red-throated loons have consistently exhibited displacement up to 15 km from offshore wind farms in Germany (Dorsch et al., 2019; Mendel et al., 2019; Heinänen et al., 2020) and Denmark (Petersen et al., 2006, 2014). Other marine bird species displaced from offshore wind farms include common scoters (Melanitta nigra), long-tailed ducks, northern fulmars (Fulmaris glacialis), northern gannets, common murres (Uria aalge), and razorbills (Alca torda; (Petersen et al., 2006; Leopold et al., 2011; Petersen et al., 2014; Welcker and Nehls, 2016). There are many factors that may be influencing spatiotemporal patterns of displacement, including the presence and spacing of structures, increases in boat traffic, and changes in habitat characteristics. Little is known about habituation over time; a degree of habituation has been observed in some sea duck species after 2–5 years in Denmark, thought to be related to food resource availability, but no habituation has been observed in other species (Drewitt and Langston, 2006; Petersen et al., 2006; Leonhard et al., 2013).
6.1.2 Avoidance
Macro-scale avoidance behavior may lead to changes in movement patterns (e.g., flying around rather than through a wind farm). Wind farms may act as barriers for migratory or feeding movements (e.g., the movements of central place foragers). There have been studies demonstrating macro-scale avoidance in pink-footed geese (Anser brachyrhychus; (Plonczkier and Simms, 2012), common eiders (Somateria mollissima; (Masden et al., 2009), northern gannets, common murres and razorbills (Vanermen et al., 2015), and scoters (Dierschke and Garthe, 2006), among other taxa. These changes to movement patterns can increase energy expenditures; efforts to model this additional cost suggest that the energetic effects to long-distance migrants, such as common eiders, are generally minor, though the highest energetic costs likely occur in species with high wing-loading, such as cormorants and auks, and those conducting frequent foraging flights, such as breeding terns (Masden et al., 2010b).
6.1.3 Attraction
Some marine bird species, such as gulls and cormorants, may increase their use of operational OWED areas to use turbine structures as perching sites (Leopold et al., 2011; Welcker and Nehls, 2016). In Europe, bats have also been found to roost on offshore wind turbines (Ahlén et al., 2009; Lagerveld et al., 2014). Lighted offshore oil and gas platforms are known to attract nocturnally migrating birds, which can increase energy expenditures and potentially lead to starvation or collision-related mortality (Hope Jones, 1980; Russell, 2005; Hüppop et al., 2006). While lighting regimes on operational turbines in the United States are designed to minimize attraction risk for birds and other animals (BOEM, 2021), flood lighting during construction (especially lighting at night during migration seasons) has been identified as a concern (Bird and Bat Specialist Committee, 2020).
Species that may be particularly vulnerable to lighting-related effects include nocturnally active seabirds (Montevecchi, 2006) and nocturnal avian migrants (Hüppop et al., 2006; Van Doren et al., 2017). Other factors, including weather conditions, cloud cover, and moon phase (Montevecchi, 2006; Kerlinger et al., 2010; Ronconi et al., 2015), as well as characteristics of the light (e.g., color, intensity, duration; Evans, 2007; Poot et al., 2008; Gehring et al., 2009; Cook et al., 2011), influence vulnerability to lighting-related effects.
6.2 Research gaps
Seven key research questions were identified around the topic of behavioral change, with a primary focus on developing an improved understanding of displacement and its fitness consequences in marine birds (Table 3). Two attraction-related questions were also identified, including one for bats and one in relation to maladaptive attraction of nocturnally active birds to artificial lighting. Quantifying the fitness consequences of avoidance and attraction to OWED will inform mitigation measures that may be needed to achieve net positive global impacts to wildlife and ecosystems.
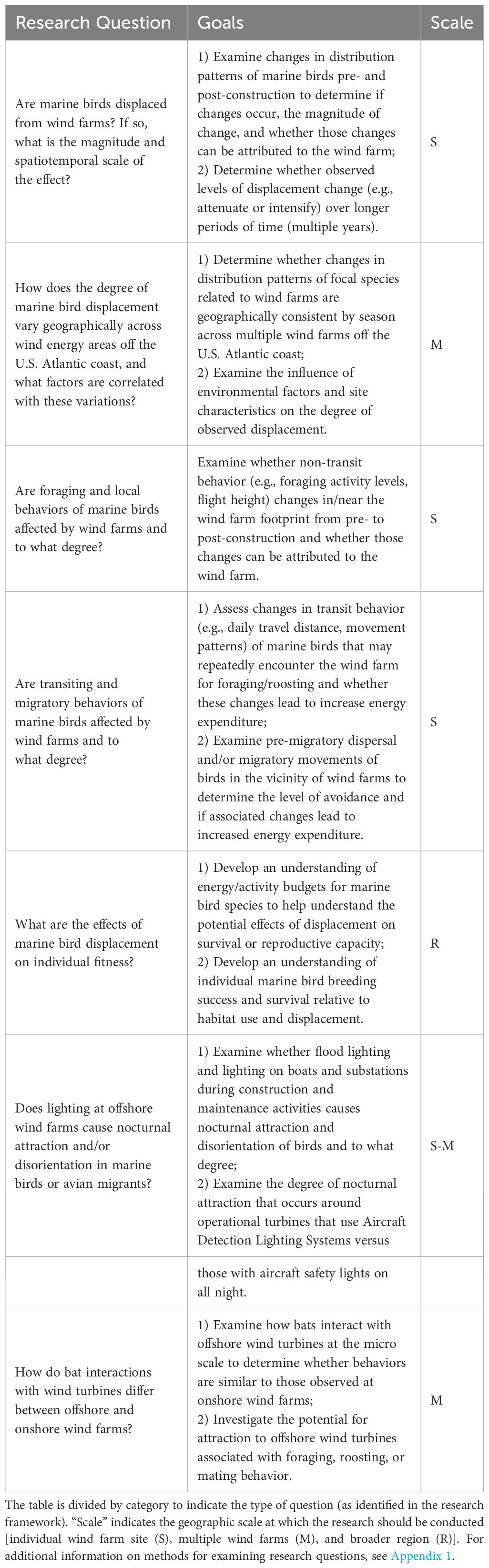
Table 3 Research questions/goals to improve understanding of behavioral changes (e.g., displacement, attraction, avoidance) of birds and bats in relation to offshore wind energy development in the U.S. Atlantic.
7 Habitat-mediated effects from offshore wind development
7.1 Current knowledge
Reef effects from offshore wind farms have been found to increase localized primary productivity, as well as the local density, biodiversity, and biomass of benthic organisms and fishes (Methratta and Dardick, 2019; Slavik et al., 2019; Dannheim et al., 2020; Zupan et al., 2023). The reduction of trawling activity in a wind farm footprint has also been found to result in changes in the macrobenthic community (Coates et al., 2016). While offshore wind energy areas change the structure of local food webs and the function of benthic ecosystems (Zupan et al., 2023), and increase food provisions for at least some species (Mavraki et al., 2020), it remains unclear the degree to which these changes may result in an increase in biomass of upper-trophic-level species versus concentrating existing biomass from the surrounding area (Wilhelmsson and Malm, 2008; Inger et al., 2009). There is some evidence that offshore oil and gas structures support more fish production than surrounding hard habitats, but this has not yet been demonstrated for OWED structures (Gill et al., 2020). New studies in Europe are examining the links between changes in lower trophic levels and marine bird distributions1. Regardless of whether OWED facilities support increased biomass of predators such as seabirds or merely aggregate these animals, attraction to new foraging and perching opportunities at OWED facilities may indirectly lead to changes in fitness via such mechanisms as increased energy intake (due to increased prey availability), decreased energy expenditure (due to perching opportunities in new locations), or increased mortality (if attracted animals use altitudes in the rotor-swept zone of turbines as well as near the water’s surface).
Offshore wind development is also predicted to alter the local physical environment through changes in stratification (Floeter et al., 2017; Schultze et al., 2020), suspended sediment (Vanhellemont and Ruddick, 2014), and wind- and ocean-wake effects (Djath et al., 2018; Platis et al., 2018; Schultze et al., 2020), which can influence lower-trophic-level species (e.g., benthos, fishes) and in turn mediate changes in the resource availability and distribution of marine birds. The ecological implications of oceanographic change around offshore wind structures are not well explored (van Berkel et al., 2020; Carpenter et al., 2021). However, wake dynamics around tidal energy turbines have led to localized and persistent foraging opportunities for terns, suggesting that localized changes in oceanographic conditions may play a role in resource availability (Lieber et al., 2021).
7.2 Research gaps
Three research questions were identified in this topic area, focusing on the effects of benthic and oceanographic change on marine birds (Table 4). These changes may be short-term (e.g., in relation to disturbance from cable-laying or other construction activities) but are generally thought to be much longer-term in their potential effects, especially given that successional changes to benthic communities appear to continue at least 11 years following commencement of OWED operations (Zupan et al., 2023). The likely changes in foraging habitat in relation to OWED (e.g., via artificial reef effects and wake effects) may attract marine birds as well as potentially changing the carrying capacity of the ecosystem, both of which have implications for understanding the net effects of OWED on wildlife.
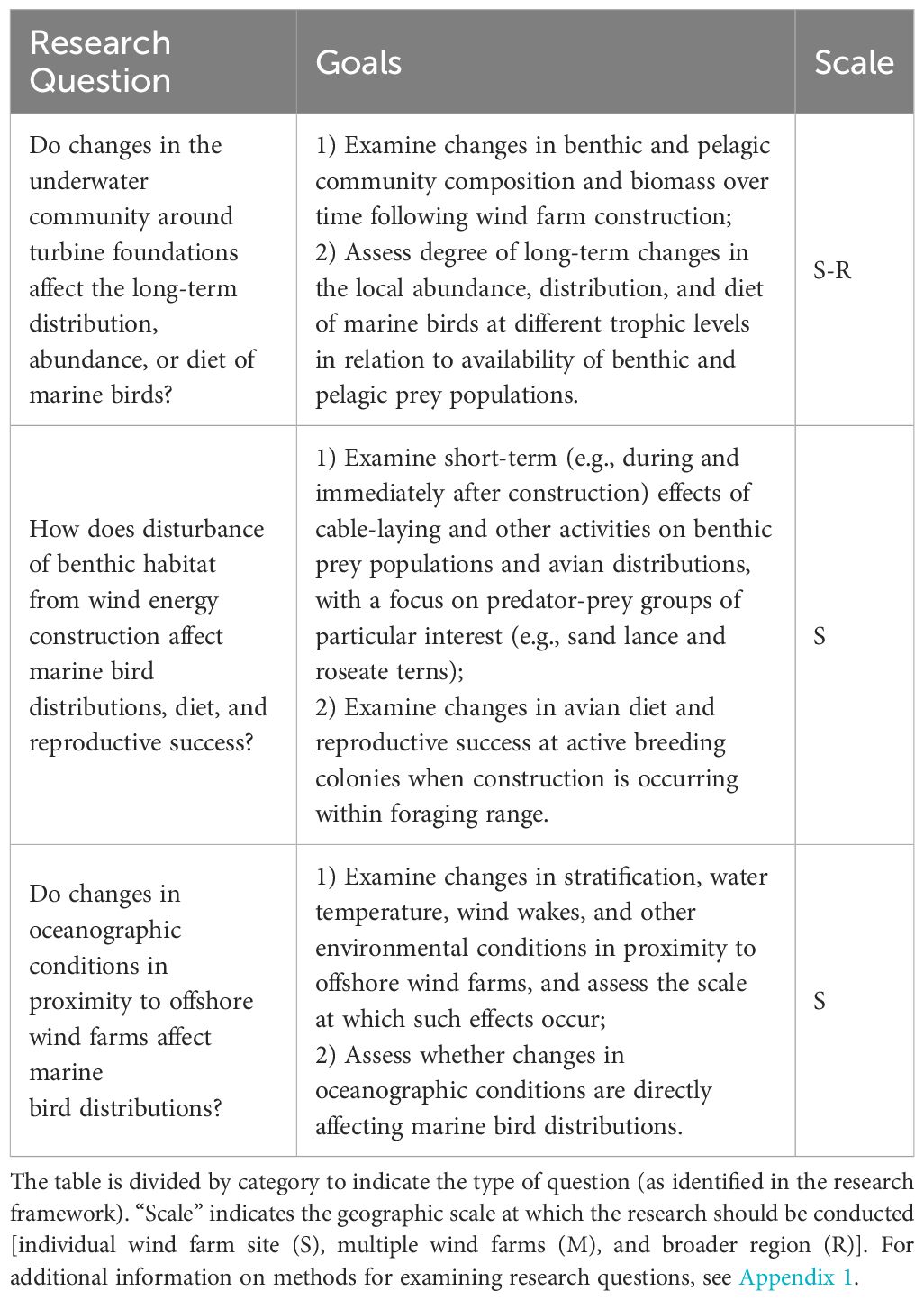
Table 4 Research questions/goals to improve understanding of habitat-mediated effects to birds and bats from offshore wind energy development in the U.S. Atlantic.
8 Population-level responses and cumulative effects from offshore wind development
8.1 Current knowledge
OWED effects on birds and bats that cause changes in survival and/or breeding success may result in population-level consequences. Collisions are direct mortality events, and so can directly influence population size; this is particularly true for species with small populations, where losses of even relatively few individuals can have significant consequences for the resilience of the population. Changes in habitat and behavior may affect fitness less directly (i.e. by affecting reproductive success, body condition/stress, carry-over effects between seasons and life history stages) leading to changes in population size and structure that are difficult to quantify. Life history strategy affects the population sensitivity, and thus the vulnerability, of species to different types of fitness effects. For example, species with high adult survival and low reproductive rates may be particularly vulnerable to collision mortality that directly affects adult survival rates (Allison et al., 2019), whereas species reliant on high reproductive outputs may be more vulnerable to effects on reproductive success.
8.1.1 Marine birds
Our current understanding of population-level and demographic consequences from OWED is largely based on population models, with a focus on marine birds. Estimates for population-level collision impacts have been modeled in Europe (Brabant et al., 2015), though understanding the consequences of such mortality levels can be difficult, as identification of acceptable thresholds for population decline will depend on species-specific population dynamics (Furness et al., 2013). Tools such as SeabORD (Searle et al., 2018) estimate population-level consequences of displacement by predicting time and energy budgets of marine birds, which are then translated into projections of adult annual survival and productivity. Population Viability Assessments (PVAs) have also been used as a tool to better understand potential population-level impacts of displacement (Cook and Robinson, 2017). There are ongoing efforts to examine the energetic consequences of displacement (e.g., Duckworth et al., 2020), which will help to validate these models.
8.1.2 Diurnal and nocturnal avian migrants
Some efforts have examined the potential for cumulative collision impacts to avian migrants across offshore wind farms in the North Sea. For example, Brabant et al. (2015) used visual survey and radar data to estimate parameters for a CRM for thrushes (Turdus spp.), using a mix of taxon-specific parameter estimates and general values for parameters, such as micro-avoidance, where no thrush-specific data were available. They extrapolated wind-farm-specific collision risk estimates from this CRM to a buildout scenario of 10,000 turbines in the North Sea and estimated >5,000 collisions of thrushes could occur during a single night of heavy migration. Similarly, in Belgium, it was estimated that 761 songbird collisions would potentially occur during 14 hours of heavy migration during a single fall season across all wind farms in the country (Degraer et al., 2021). Such estimates rely on the accuracy of CRMs, which have not been validated for any songbird species in the offshore environment. The effects of such levels of mortality on songbird population dynamics have not been calculated; however, similar assessments for terrestrial wind energy in the United States have concluded that collision mortality with wind turbines is generally not a population-level concern for passerines (Erickson et al., 2014). Mortality for some raptor species, given their higher adult survival rates, has been a more substantial management concern for terrestrial wind energy in the United States (Allison et al., 2019) and globally (Watson et al., 2018).
8.1.3 Bats
There have been efforts to investigate the degree to which fatalities from terrestrial wind farms could impact population viability for particular species, such as the hoary bat (Frick et al., 2017; Friedenberg and Frick, 2021) as well as examinations of regional-scale population dynamics (Rodhouse et al., 2019). Assessing the cumulative impacts to bats from OWED will first require an improved understanding of exposure and effects in this taxon in the offshore environment.
8.1.4 Effects of multiple stressors
If U.S. OWED goals are achieved, bird and bat populations will be cumulatively exposed to thousands of turbines (Goodale and Milman, 2016). Thus, understanding cumulative impacts is ecologically important and required in U.S. environmental impact assessments [40 C.F.R. §1508.25]. There have been some efforts to develop cumulative impact frameworks (Masden et al., 2010a; Goodale and Milman, 2016) as well as efforts to understand cumulative impacts for particular species (Goodale and Milman, 2020) and types of effects (Busch et al., 2013), and to develop research priorities to improve our understanding of cumulative impacts (Cook et al., 2021; Hein et al., 2021). Efforts to model cumulative impacts across species of birds and bats in the southern North Sea suggests that gull species and northern gannets may be most vulnerable to cumulative effects and potential population declines with full build-out of offshore wind farms in that region (Leopold et al., 2015; Potiek et al., 2022). However, limitations exist in these efforts due to a lack of information on exposure and population size for many species, as well as uncertainty around number, sizing, and spacing of turbines for future projects. Moreover, there is currently a poor understanding of how the impacts of OWED may interact with those of other stressors (e.g., commercial fishing, climate change).
8.2 Research gaps
Several questions were identified to help better understand potential population-level responses and cumulative effects to birds and bats from OWED (Table 5). Gaining an improved understanding of these cumulative effects is the primary goal of the research framework presented in this paper, but is challenging to achieve. It requires demonstration of individual effects, an understanding of the variation in those effects across space and time, and knowledge of how different types of effects may interact. In addition, those individual effects must then be translated into impacts to individual fitness and, by extension, to populations, given population size, structure, and other demographic parameters. As a result, we identify relatively few questions in this category as immediate priorities, recognizing that answers to basic questions on exposure and effects (Table 1) are in many cases necessary to adequately assess population-level cumulative effects. However, this does not imply that understanding population-level effects and cumulative impacts is less urgent or important, but rather that this research gap culminates from fundamental gaps in basic research needs.
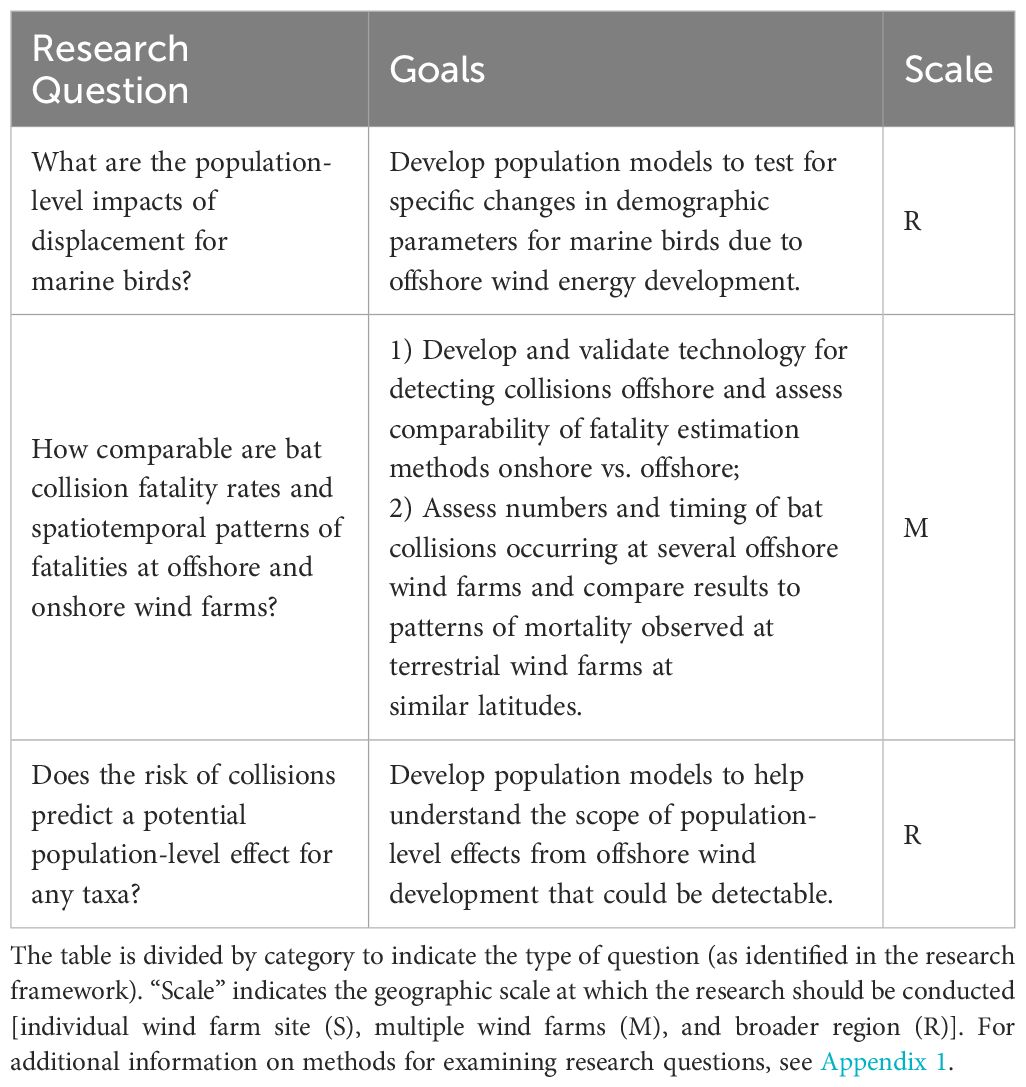
Table 5 Research questions/goals to improve understanding of potential population-level responses and cumulative effects to birds and bats from offshore wind energy development in the U.S. Atlantic.
9 Recommendations and conclusions
9.1 Suggestions for further prioritization of research
Based on the above framework and existing knowledge, we have identified 22 key research gaps, to be addressed to understand potential effects of OWED on birds and bats in the U.S. Atlantic (Tables 1–5). Different management decisions require different priorities, but in general (and in no particular order), further prioritization and the development of research studies on these topics should consider the degree to which studies:
Are feasible and practicable. Study designs should be based on available methods and the scope of the question. Consideration should focus on whether the available methods and study designs will provide an effective test of the hypothesis and answer the question with reasonable confidence.
Inform adaptive monitoring and management. Results should help to inform decisions for existing or future projects via permitting decisions, operational implications, mitigation actions, incorporating new technologies into monitoring programs, or other means.
Address urgent information needs. Research that will be most likely to effectively improve our understanding, address permitting risk, and inform decision making should be prioritized.
Focus on stressors with the greatest likely effect. This includes stressors with the greatest likelihood of causing a population-level decline, which may be species- or community-specific.
Leverage existing resources. There is value in promoting regional coordination and maximizing logistical and cost efficiencies.
Balance needs at different spatial and temporal scales, including a focus on studies to better understand cumulative effects. There is a trade-off between research with a clear nexus for short-term action (e.g., permitting, mitigation) and broad-scale, population-wide strategic studies. The latter approach is particularly important in the context of other stressors, including climate change, and in examining cumulative impacts. Both types of research are important to ensure the best long-term outcomes for wildlife and the offshore wind industry. Where possible, utilizing consistent methods for data collection, storage, and metadata among short-term research efforts can increase utility of these data to inform broad-scale assessments.
Focus on priority species. Some questions are species-specific, including those driven by the ESA regulatory process; others are more generally focused on understanding the types and degree of effects expected for various taxa. Thus, priority species can be defined in several ways:
o Species of conservation concern. Species listed under the U.S. ESA and under endangered species acts within various U.S. states are clear regulatory priorities. Species with other designations could also be prioritized, including FWS Birds of Conservation Concern and species listed in State Wildlife Action Plans as Species of Greatest Conservation Need. As it may be difficult to obtain sufficient sample sizes of endangered or declining species for robust analysis, the use of surrogate species may be considered, but should only be used with caution (Caro et al., 2005; Murphy et al., 2011) and after careful consideration of ecological niche, morphology, and behavior, as well as the type and scale of the question.
o Species most likely to be affected, as determined by local conditions and existing knowledge from other regions or industries. Red-throated loons, gannets, terns, auks, and migratory tree-roosting bats are examples of species and taxa likely to be affected based on current knowledge.
o Species for which we have substantial existing knowledge. Effective study design, particularly to understand population-level impacts, may be more achievable for well-studied, data-rich populations. This may include common species, like some gulls, and species for which we have a high level of baseline information on population dynamics and demography, such as species nesting at regularly monitored colonies (e.g., northern gannets) or highly managed colonies (e.g., some tern populations).
o Species for which little is known about potential impacts. By focusing on data-poor species, such as migrant songbirds, shorebirds, and bats, we could expand our understanding of the taxa affected by OWED.
o Species that play a key role in the structure and function of the food web or ecosystem. It may be beneficial to focus on “foundation species” that play key roles given that effects to these species may have cascading or ecosystem-wide effects (Lamy et al., 2020; Ansley et al., 2023).
Research questions (Table 1) are grouped by category of response. Potential effects are contingent on exposure; thus, we begin with questions relating to exposure, and in cases where exposure occurs, the subsequent key questions are relevant to understand potential effects and impacts relating to collision, behavioral change, habitat change, and population-level consequences. Geographic scale is categorized as (1) individual wind farm (including cable route as well as the wind farm footprint), (2) multiple wind farms, or (3) regional scale (including locations that may not be directly associated with any offshore wind development). A more detailed description of the goals of each research question, as well as potential methods for addressing these questions, are included in Appendix 1.
9.2 General study design recommendations
Carefully designed studies can deal with the highly variable marine environment and help ensure sufficient power to differentiate the effects of OWED from other sources of variation. While this can be both challenging and costly, investments in such research early in the buildout of the offshore wind industry have great potential to inform future decision making and minimize or mitigate offshore wind energy’s effects to wildlife and their habitats. Regardless of focus, it would be beneficial for research studies relating to the effects of OWED on birds and bats to:
Identify an appropriate scale of study. Testable hypotheses require identification of an appropriate geographic and temporal scale. Rather than thinking at scales relevant to bird and bat populations, the U.S. regulatory framework is focused at the offshore wind project level. However, understanding effects and scaling those effects to potential impacts requires research at broader scales and coordination across projects. Some research questions can be answered at the spatial scale of an individual offshore wind project, while others may only be addressed through data aggregation across multiple projects and over time, or by conducting regional-scale studies that include data collection both within and outside of project areas.
Identify appropriate sample size. Sample size and statistical power are key considerations when designing studies. The sample size necessary to have an appropriate level of inference will inform the level of funding and data collection effort required to make meaningful conclusions. Power analysis should be used to inform the choice of sample size, incorporating existing data wherever possible. Insomuch as existing data allow, the choice of effect size value (e.g., for power analysis) should take into consideration sources of variability, including taxonomy, seasonal and annual fluctuations, and spatial variability.
Identify informative parameters to reduce uncertainty. Population-level impacts may manifest through changes to productivity, survival, or other demographic parameters before there are detectable changes in population abundance. Thus, we should think critically about the factors driving change, and identify informative population or ecosystem parameters to be measured. Indirect effects, such as those related to habitat or prey populations, may be more difficult to quantify than direct effects, and will require particularly careful consideration of appropriate approaches to detect change, determine causes, and understand the potential for population-level consequences. In some cases, we lack the necessary information to develop sound hypotheses about these connections. We must work to fill those gaps in our basic understanding such that we can better examine these indirect effects.
Design studies to differentiate effects from disparate sources. Attributing effects to offshore wind development or other stressors can be challenging, particularly given high levels of natural variability in the marine ecosystem, ongoing changes in the system related to climate change, highly migratory species that cross multiple jurisdictions, effects that may vary across the annual cycle, and the potential for carry-over effects between life history periods. Baseline population and ecosystem monitoring, including pre-construction data collection at project sites at appropriate temporal and geographic scales of coverage for comparison to the post-construction period, is necessary to understand pre-existing environmental and population fluctuations prior to development and be able to identify changes that are due to offshore wind energy activities (see Maclean et al., 2013; Mendel et al., 2019). Before-After Gradient (BAG) designs and other distance-based sampling designs are more appropriate than Before-After Control-Impact (BACI) designs for many types of studies examining OWED-related changes to communities or ecosystems (Methratta, 2021).
Integrate study methods into OWED operations to improve inference. In some cases, particularly in relation to directly measuring collisions in the offshore environment, we lack the technologies needed to answer some research questions (due to a combination of technological limitations and cost). We must continue to fund and prioritize the development and validation of these technologies to help validate CRMs and improve our ability to address research questions. Integration of different technologies and study methods is important to take advantage of each approach’s strengths and minimize their limitations. Safe, effective integration of wildlife monitoring technologies into OWED infrastructure is essential to deploy these technologies on the scale that will be needed to answer key questions.
Standardize data collection and management. As increasing resources are channeled into environmental monitoring, well-considered and coordinated data collection is becoming increasingly important (NYSERDA 2021). The collection, reporting, and management of data in a consistent manner is essential to collate data across projects to assess the consistency of effects and address regional-scale research questions and large-scale needs, including understanding cumulative impacts. Several recent guidelines have been developed for standardized data collection in relation to OWED (e.g., Loring et al., 2022).
Prioritize data sharing and transparency. It is beneficial for both raw data and derived data products to be made publicly available as soon as feasible following quality assurance and quality control. This includes effort data and other metadata to maximize the data’s utility in informing future analyses, including regional-scale assessments and meta-analyses (NYSERDA 2021).
10 Conclusions
A stakeholder workshop and expert workgroup process was implemented to develop this research framework. Despite several challenges (e.g., the COVID-19 pandemic), this process was effective in identifying research needs and developing a broader framework for considering OWED impacts to bird and bat taxa. The success of this effort was due to the involvement of scientists and resource managers with wide-ranging expertise, including methodological, statistical, OWED-specific, and taxonomic specialization, as well as financial support for workshop facilitation and for the development of this product by the authors. Expert knowledge from European collaborators, who brought a wealth of knowledge from previous OWED-focused studies in the North Sea and Baltic Sea, was also essential to ensure the primarily U.S.-based group was kept informed of existing data and research with relevance to the emerging U.S. context.
This framework aims to identify key research questions to understand exposure, collision-related effects, behavioral effects, and resource-mediated effects of OWED on birds and bats in the U.S. Atlantic. Many data gaps exist despite knowledge gained from the European offshore wind industry, other industries, and initial studies in the U.S. OWED context. We lack understanding of how responses and effects may differ in the U.S. context as compared to the North Sea and Baltic Sea, where much of the world’s OWED research has occurred to date. There are substantial differences between these regions in relation to wind farm characteristics, substrate types, oceanographic conditions, community composition, life history stages at which birds are most commonly interacting with offshore structures, and ecosystem function, among other factors. For example, much of the European research on birds/bats and OWED to date has focused on breeding seabirds, while in many parts of the world (including the U.S. Atlantic), nonbreeding and migrating taxa may be much more prevalent and exposed to development activities. Thus, we must take care to design and implement new research to improve our collective understanding of the effects of offshore wind development on birds and bats, both in the eastern United States as well as other regions around the globe where the OWED industry is growing.
Advanced planning, coordination, and careful study design will help ensure that data collection can meaningfully answer questions and feed into a broader understanding of cumulative anthropogenic impacts to birds and bats from a range of sources. We can then use this knowledge to inform decision-making for current and future offshore wind energy projects, including siting projects to avoid impacts to sensitive species, more accurately address risks posed by proposed projects, and determine the need for mitigation efforts to more effectively conserve imperiled species. Quantification of OWED’s effects to birds and bats, as well as potential fitness consequences, will be essential to inform mitigation measures that may be needed to achieve net positive global impacts of OWED on wildlife and ecosystems.
Collaboration will be key to maximize resources and collect data to answer long-term and regional-scale questions. This framework can act as a starting point for regional efforts to develop detailed plans and implement research related to impacts from offshore wind development on birds and bats in the U.S. Atlantic and elsewhere.
Author contributions
KW: Conceptualization, Formal analysis, Investigation, Methodology, Project administration, Validation, Writing – original draft, Writing – review & editing. JG: Conceptualization, Formal analysis, Investigation, Methodology, Writing – original draft, Writing – review & editing. AC: Investigation, Writing – review & editing. RH: Investigation, Writing – review & editing. AF: Investigation, Methodology, Writing – review & editing. HG: Investigation, Writing – review & editing. CH: Investigation, Writing – review & editing. PL: Investigation, Writing – review & editing. DM: Investigation, Writing – review & editing. IP: Investigation, Writing – review & editing. TP: Investigation, Writing – review & editing. KP: Conceptualization, Funding acquisition, Investigation, Project administration, Writing – review & editing. IS: Investigation, Writing – review & editing.
Funding
The author(s) declare financial support was received for the research, authorship, and/or publication of this article. This study was funded by the New York State Energy Research and Development Authority (NYSERDA) under Agreements #118972 and #179237. This work was authored in part by the National Renewable Energy Laboratory, operated by Alliance for Sustainable Energy, LLC, for the U.S. Department of Energy (DOE) under Contract No. DE-AC36-08GO28308, with funding provided by U.S. Department of Energy Office of Energy Efficiency and Renewable Energy Wind Energy Technologies Office. This work was also authored in part by the Center for Avian Population Studies, Cornell Lab of Ornithology, with support from the Leon Levy Foundation, Lyda Hill Philanthropies, and the National Science Foundation (Award #1927743).
Acknowledgments
The authors would like to thank participants in a 2020 workshop in Manhattan, including A. Webb, A. Winship, B. Woeck, C. Mostello, C. Bowes, C. Guy, C. Cooper-Mullin, C. Folsom O’Keefe, C. Smalling, D. Allen, D. Bigger, D. Phillips, E. Craig, E. Argos, G. George, G. Harker-Klimeš, J. Stucker, J. Liner, J. Walsh, J. Brown-Saracino, J. Wilmott, L. Welch, L. Brzuzy, M. Hall, M. Lin, M. Robertson, M. Whitby, N. Lewandowski, S. Johnston, S. Huber, S. Papa, S. von Oettingen, T. Allison, and T. White; full names and affiliations may be found in Appendix B of the workshop report at www.nyetwg.com/bird-bat-research-framework. We would also like to thank the members of the 2020 State of the Science Workshop on Wildlife and Offshore Wind bird and bat workgroups (listed in Appendix A of their respective workgroup reports, available at www.nyetwg.com/2020-workgroups), P. Field (Consensus Building Institute), E. Adams, A. Gilbert, W.M. Goodale (Biodiversity Research Institute), T.J. Zenzal (U.S. Geological Survey), and several reviewers for feedback on partial or complete earlier drafts of this paper, and B. Kinlan for introducing K.A.W. to the concept of the risk triangle.
Conflict of interest
Author TP was employed by Stantec Consulting Services Inc.
The remaining authors declare that the research was conducted in the absence of any commercial or financial relationships that could be construed as a potential conflict of interest.
Publisher’s note
All claims expressed in this article are solely those of the authors and do not necessarily represent those of their affiliated organizations, or those of the publisher, the editors and the reviewers. Any product that may be evaluated in this article, or claim that may be made by its manufacturer, is not guaranteed or endorsed by the publisher.
Author disclaimer
Any opinions expressed in this report are those of the co-authors and do not necessarily reflect those of NYSERDA or the State of New York. Likewise, the views expressed in the article do not necessarily represent the views of the DOE, USFWS, or the U.S. Government. The U.S. Government retains and the publisher, by accepting the article for publication, acknowledges that the U.S. Government retains a nonexclusive, paid-up, irrevocable, worldwide license to publish or reproduce the published form of this work, or allow others to do so, for U.S. Government purposes. Any use of trade, firm, or product names is for descriptive purposes only and does not imply endorsement by the U.S. Government.
Supplementary material
The Supplementary Material for this article can be found online at: https://www.frontiersin.org/articles/10.3389/fmars.2024.1274052/full#supplementary-material
SUPPLEMENTARY APPENDIX A | Methodological information and recommendations for collecting data to answer research questions.
Footnotes
- ^ PREDICT Project. https://eri.ac.uk/predict/
References
Ahlén I., Baagøe H. J., Bach L. (2009). Behavior of Scandinavian bats during migration and foraging at sea. J. Mammal 90, 1318–1323. doi: 10.1644/09-MAMM-S-223R.1
Allison T. D., Butryn R. (2020). 2nd edition: Summary of Bat Fatality Monitoring Data Contained in AWWIC (Washington, DC: American Wind Wildlife Institute Technical Report), 27 pp.
Allison T. D., Diffendorfer J. E., Baerwald E. F., Beston J. A., Drake D., Hale A. M., et al. (2019). Impacts to wildlife of wind energy siting and operation in the United States. Issues In Ecol. 21, 23.
Ansley R. J., Rivera-Monroy V. H., Griffis-Kyle K., Hoagland B., Emert A., Fagin T., et al. (2023). Assessing impacts of climate change on selected foundation species and ecosystem services in the South-Central USA. Ecosphere 14, e4412. doi: 10.1002/ecs2.4412
Arnett E. B., Baerwald E. F. (2013). “Impacts of Wind Energy Development on Bats: Implications for Conservation,” in Bat Evolution, Ecology, and Conservation. Eds. Adams R. A., Pedersen S. C. (Springer Science+Business Media, New York, NY), 435–456. doi: 10.1007/978-1-4614-7397-8
Arnett E. B., Johnson G. D., Erickson W. P., Hein C. D. (2013). A Synthesis of Operational Mitigation Studies to Reduce Bat Fatalities at Wind Energy Facilities in North America (Austin, TX: Bat Conservation International). doi: 10.1016/j.jallcom.2014.09.009
Bailey H., Brookes K. L., Thompson P. M. (2014). Assessing environmental impacts of offshore wind farms: lessons learned and recommendations for the future. Aquat. Biosyst. 10, 8. doi: 10.1186/2046-9063-10-8
Band W. (2012). Using a collision risk model to assess bird collision risk for offshore windfarms. SOSS-02. Report to The Crown Estate Commission (London U.K: Strategic Ornithological Support Services (SOSS)), 62 pp.
Belkin I. M. (2021). Review remote sensing of ocean fronts in marine ecology and fisheries. Remote Sens. (Basel) 13, 1–22. doi: 10.3390/rs13050883
Bird and Bat Specialist Committee (2020). Summary of Discussions from the Bird and Bat Specialist Committee of the Environmental Technical Working Group (E-TWG) (Albany, New York: Environmental Technical Working Group).
BOEM (2021). Guidelines for Lighting and Marking of Structures Supporting Renewable Energy Development (Sterling, VA: Department of the Interior, Bureau of Ocean Energy Management).
Brabant R., Vanermen N., Stienen E. W. M., Degraer S. (2015). Towards a cumulative collision risk assessment of local and migrating birds in North Sea offshore wind farms. Hydrobiologia 756, 63–74. doi: 10.1007/s10750-015-2224-2
Brinker D. F., McCann J. M., Williams B., Watts B. D. (2007). Colonial-nesting seabirds in the Chesapeake Bay region: Where have we been and where are we going? Waterbirds 30, 93–104. doi: 10.1675/1524-4695(2007)030
Brown S., Gratto-Trevor C., Porter R., Weiser E. L., Mizrahi D., Bentzen R., et al. (2017). Migratory connectivity of Semipalmated Sandpipers and implications for conservation. Condor 119, 207–224. doi: 10.1650/CONDOR-16-55.1
Brust V., Hüppop O. (2022). Underestimated scale of songbird offshore migration across the south-eastern North Sea during autumn. J. Ornithol. 163, 51–60. doi: 10.1007/s10336-021-01934-5
Brust V., Michalik B., Hüppop O. (2019). To cross or not to cross - Thrushes at the German North Sea coast adapt flight and routing to wind conditions in autumn. Mov Ecol. 7, 1–10. doi: 10.1186/s40462-019-0173-5
Busch M., Kannen A., Garthe S., Jessopp M. (2013). Consequences of a cumulative perspective on marine environmental impacts: Offshore wind farming and seabirds at North Sea scale in context of the EU Marine Strategy Framework Directive. Ocean Coast. Manag. 71, 213–224. doi: 10.1016/j.ocecoaman.2012.10.016
Carbon Trust (2022). Offshore Renewables Joint Industry Programme (ORJIP) for Offshore Wind: Review of seabird monitoring technologies for offshore wind farms (London, United Kingdom: Carbon Trust).
Caro T., Eadie J., Sih A. (2005). Use of substitute species in conservation biology. Conserv. Biol. 19, 1821–1826. doi: 10.1111/j.1523-1739.2005.00251.x
Carpenter J. R., Williams K. A., Jenkins E. (2021). Environmental Stratification Workgroup Report for the State of the Science Workshop on Wildlife and Offshore Wind Energy 2020: Cumulative Impacts (Albany, NY: Report to the New York State Energy Research and Development Authority (NYSERDA). 14 pp.
Coates D. A., Kapasakali D. A., Vincx M., Vanaverbeke J. (2016). Short-term effects of fishery exclusion in offshore wind farms on macrofaunal communities in the Belgian part of the North Sea. Fish Res. 179, 131–138. doi: 10.1016/j.fishres.2016.02.019
Cohen E. B., Horton K. G., Marra P. P., Clipp H. L., Farnsworth A., Smolinsky J. A., et al. (2021). A place to land: spatiotemporal drivers of stopover habitat use by migrating birds. Ecol. Lett. 24 (1), 38–49. doi: 10.1111/ele.13618
Cook A. S. C. P., Humphreys E. M., Bennet F., Masden E. A., Burton N. H. K. (2018). Quantifying avian avoidance of offshore wind turbines: Current evidence and key knowledge gaps. Mar. Environ. Res. 140, 278–288. doi: 10.1016/j.marenvres.2018.06.017
Cook A. S. C. P., Robinson R. A. (2017). Towards a framework for quantifying the population-level consequences of anthropogenic pressures on the environment: The case of seabirds and windfarms. J. Environ. Manage 190, 113–121. doi: 10.1016/j.jenvman.2016.12.025
Cook A. S. C. P., Ross-Smith V. H., Roos S., Burton N. H. K., Beale N., Coleman C., et al. (2011). Identifying a range of options to prevent or reduce avian collision with offshore wind farms using a UK-based case study. Research Report No. 580 (Thetford, UK: British Trust for Ornithology: Environmental Technical Working Group), 197 pp.
Courbis S., Williams K., Stepanuk J., Etter H., McManus M., Campoblanco F., et al. (2024). Assessment of Technology Gaps for Statistically Robust Data and Integration of Monitoring of Birds and Marine Mammals into Equipment and Operations of Offshore Windfarms. Report 418160-42106. Final Report prepared by Worley, Inc. and Biodiversity Research Institute for the National Offshore Wind Research and Development Consortium. 44 pp. Available at: https://nationaloffshorewind.org/projects/technologydevelopment-priorities-for-scientifically-robust-and-operationally-compatible-wildlife-monitoring-and-adaptive-management/.
Cook A., Williams K., Jenkins E., Gulka J., Liner J. (2021). Bird Workgroup Report for the State of the Science Workshop on Wildlife and Offshore Wind Energy 2020: Cumulative Impacts (Albany, NY). 37 pp.
Crichton D. (1999). “The risk triangle,” in Natural Disaster Management. Ed. Ingleton J. (Tudor Rose Holdings Ltd, London, UK).
Croll D. A., Ellis A. A., Adams J., Cook A. S. C. P., Garthe S., Goodale M. W., et al. (2022). Framework for assessing and mitigating the impacts of offshore wind energy development on marine birds. Biol. Conserv. 276, 109795. doi: 10.1016/j.biocon.2022.109795
Daly M., McDermott J. (2022). Biden plans floating platforms to expand offshore wind power (Washington, DC: Associated Press).
Dannheim J., Bergstrom L., Birchenough S. N. R., Brzana R., Boon A. R., Coolen J. W. P., et al. (2020). Benthic effects of offshore renewables: identification of knowledge gaps and urgently needed research. ICES J. Mar. Sci. 77, 1092–1108. doi: 10.1093/icesjms/fsz018
Degraer S., Brabant R., Rumes B., Vigin L. (2021). Environmental impacts of offshore wind farms in the Belgian part of the North Sea. Attraction, avoidance and habitat use at various spatial scales (Brussels, Belgium: Royal Belgian Institute of Natural Sciences, OD Natural Environment, Marine Ecology and Management), 104 pp.
DeLuca W. V., Woodworth B. K., Mackenzie S. A., Newman A. E. M., Cooke H. A., Phillips L. M., et al. (2019). A boreal songbird’s 20,000 km migration across North America and the Atlantic Ocean. Ecology 100, 1–4. doi: 10.1002/ecy.2651
Deluca W. V., Woodworth B. K., Rimmer C. C., Marra P. P., Taylor P. D., Mcfarland K. P., et al. (2015). Transoceanic migration by a 12g songbird. Biol. Lett. 11, 1–4. doi: 10.1098/rsbl.2014.1045
De Lucas M., Janss G. F. E., Whitfield D. P., Ferrer M. (2008). Collision fatality of raptors in wind farms does not depend on raptor abundance. J. Appl. Ecol. 45, 1695–1703. doi: 10.1111/j.1365-2664.2008.01549.x
Deppe J. L., Ward M. P., Bolus R. T., Diehl R. H., Celis-Murillo A., Zenzal T. J., et al. (2015). Fat, weather, and date affect migratory songbirds’ departure decisions, routes, and time it takes to cross the Gulf of Mexico. Proc. Natl. Acad. Sci. U.S.A. 112, E6331–E6338. doi: 10.1073/pnas.1503381112
Desholm M. (2006). Wind farm related mortality – a remote sensing study and model analysis. [PhD dissertation] Copenhagen, Denmark: University of Copenhagen.
DeSorbo C. R., Persico C. P., Gilpatrick L., Gilbert A., Dalton A., Burton M. (2019). Studying migrant raptors using the Atlantic Flyway: Block Island Raptor Research Station, Block Island, RI: 2018 season. BRI Report # 2019-10 submitted to The Nature Conservancy, Block Island, Rhode Island, and The Bailey Wildlife Foundation, Cambridge, Massachusetts (Portland, Maine: Biodiversity Research Institute). 33 pp plus appendices.
Dierschke V., Furness R. W., Garthe S. (2016). Seabirds and offshore wind farms in European waters: Avoidance and attraction. Biol. Conserv. 202, 59–68. doi: 10.1016/j.biocon.2016.08.016
Dierschke V., Garthe S. (2006). “Literature review of offshore wind farms with regards to seabirds,” in Ecological Research on Offshore Wind Famrs: International Exchange of Experiences. Eds. Zucco C., Wende W., Merck T., Kochling I., Koppel J. (Bonn, Germany: Bundesamt für Naturschutz).
Dierschke R. W., Gray C. E., Petersen I. K., Schmutz J., Zydelis R., Daunt V. F., et al. (2017). Possible Behavioural, Energetic and Demographic Effects of Displacement of Red-throated Divers. JNCC Report No. 605 (Peterborough: JNCC).
Dirksen S. (2017). Review of methods and techniques for field validation of collision rates and avoidance amongst birds and bats at offshore wind turbines (Report by Sjoerd Dirksen Ecology to Rijkswaterstaat WVL). 47 pp.
Djath B., Schulz-Stellenfleth J., Cañadillas B. (2018). Impact of atmospheric stability on X-band and C-band synthetic aperture radar imagery of offshore windpark wakes. J. Renewable Sustain. Energy 10, 043301. doi: 10.1063/1.5020437
Dokter A. M., Farnsworth A., Fink D., Ruiz-Gutierrez V., Hochachka W. M., La Sorte F. A., et al. (2018). Seasonal abundance and survival of North America’s migratory avifauna determined by weather radar. Nat. Ecol. Evol. 2, 1603–1609. doi: 10.1038/s41559-018-0666-4
Dorsch M., Burger C., Heinanen S., Kleinschmidt B., Morkunas J., Nehls G., et al. (2019). DIVER: German tracking study of seabirds in areas of planned Offshore Wind Farms at the example of divers. FKZ 0325747A/B (Husum, Germany: Report to the Federal Ministry of Economics and Energy), 282 pp.
Drewitt A. L., Langston R. H. W. (2006). Assessing the impacts of wind farms on birds. Ibis 148, 29–42. doi: 10.1111/j.1474-919X.2006.00516.x
Duckworth J., Green J., Daunt F., Johnson L., Lehikoinen P., Okill D., et al. (2020). Red-throated diver energetics project: Preliminary results from 2018/2019 (Peterborough, UK: JNCC Report No. 638). 31 pp.
Ellis J. I., Wilhelm S. I., Hedd A., Fraser G. S., Robertson G. J., Rail J.-F., et al. (2013). Mortality of migratory birds from marine commercial fisheries and offshore oil and gas production in Canada. Avian Conserv. Ecol. 8, 4. doi: 10.5751/ACE-00589-080204
Erickson W. P., Wolfe M. M., Bay K. J., Johnson D. H., Gehring J. L. (2014). A comprehensive analysis of small-passerine fatalities from collision with turbines at wind energy facilities. PloS One 9, e107491. doi: 10.1371/journal.pone.0107491
Evans R. (2007). Response of night-migrating songbirds in cloud to colored and flashing light. North Am. Birds 60, 476–488.
Farnsworth A., Van Doren B. M., Hochachka W. M., Sheldon D., Winner K. (2016). A characterization of autumn nocturnal migration detected by weather surveillance radars in the northeastern USA. Ecol. Appl. 26, 752–770. doi: 10.1890/15-0023
Floeter J., van Beusekom J. E. E., Auch D., Callies U., Carpenter J. J. J., Dudeck T., et al. (2017). Pelagic effects of offshore wind farm foundations in the stratified North Sea. Prog. Oceanogr. 156, 154–173. doi: 10.1016/j.pocean.2017.07.003
Fox A. D., Desholm M., Kahlert J., Christensen T. K., Petersen I. K. (2006). Information needs to support environmental impact assessment of the effects of European marine offshore wind farms on birds. Ibis 148, 129–144. doi: 10.1111/j.1474-919X.2006.00510.x
Fox A. D., Petersen I. K. (2019). Offshore wind farms and their effects on birds. Dansk Orn. Foren. Tidsskr. 113, 86–101.
Frick W. F., Baerwald E. F., Pollock J. F., Barclay R. M. R., Szymanski J. A., Weller T. J., et al. (2017). Fatalities at wind turbines may threaten population viability of a migratory bat. Biol. Conserv. 209, 172–177. doi: 10.1016/j.biocon.2017.02.023
Friedenberg N. A., Frick W. F. (2021). Assessing fatality minimization for hoary bats amid continued wind energy development. Biol. Conserv. 262, 109309. doi: 10.1016/j.biocon.2021.109309
Furness R. W., Wade H. M., Masden E. A. (2013). Assessing vulnerability of marine bird populations to offshore wind farms. J. Environ. Manage 119, 56–66. doi: 10.1016/j.jenvman.2013.01.025
Gehring J., Kerlinger P., Manville A. M. (2009). Communication towers, lights, and birds: successful methods of reducing the frequency of avian collisions. Ecol. Appl. 19, 505–514. doi: 10.1890/07-1708.1
Gill A. B., Degraer S., Lipsky A., Mavraki N., Methratta E., Brabant R. (2020). Special issue on understanding the effects of offshore wind energy development on fisheries setting the context for offshore wind development effects on fish and fisheries. Oceanography 33, 118–127. doi: 10.5670/oceanog
Goodale M. W., Milman A. (2014). Cumulative adverse effects of offshore wind energy development on wildlife. J. Environ. Plann. Manage. 59 (1), 1–21. doi: 10.1080/09640568.2014.973483
Goodale M. W., Milman A. (2016). Cumulative adverse effects of offshore wind energy development on wildlife. J. Environ. Plann. Manage. 59, 1–21. doi: 10.1080/09640568.2014.973483
Goodale M. W., Milman A. (2020). Assessing cumulative exposure of northern gannets to offshore wind farms. Wildl. Soc. Bull. 44, 252–259. doi: 10.1002/wsb.1087
Goyert H. F., Gardner B., Sollmann R., Veit R. R., Gilbert A. T., Connelly E. E., et al. (2016). Predicting the offshore distribution and abundance of marine birds with a hierarchical community distance sampling model. Ecol. Appl. 26, 1797–1815. doi: 10.1890/15-1955.1
Guest E. E., Stamps B. F., Durish N. D., Hale A. M., Hein C. D., Morton B. P., et al. (2022). An updated review of hypotheses regarding bat attraction to wind turbines. Animals 12, 343. doi: 10.3390/ani12030343
Hammar L., Perry D., Gullström M. (2016). Offshore wind power for marine conservation. Open J. Mar. Sci. 06, 66–78. doi: 10.4236/ojms.2016.61007
Hatch S. K., Connelly E. E., Divoll T. J., Stenhouse I. J., Williams K. A. (2013). Offshore observations of eastern red bats (Lasiurus borealis) in the Mid-Atlantic United States using multiple survey methods. PloS One 8, e83803. doi: 10.1371/journal.pone.0083803
Hein C., Williams K. A., Jenkins E. (2021). Bats Workgroup Report for the State of the Science Workshop on Wildlife and Offshore Wind Energy 2020: Cumulative Impacts (Albany, NY: Report to the New York Energy Research and Development Authority (NYSERDA). 21 pp.
Heinänen S., Žydelis R., Kleinschmidt B., Dorsch M., Burger C., Morkūnas J., et al. (2020). Satellite telemetry and digital aerial surveys show strong displacement of red-throated divers (Gavia stellata) from offshore wind farms. Mar. Environ. Res. 160, 104989. doi: 10.1016/j.marenvres.2020.104989
Horton K. G., Van Doren B. M., Stepanian P. M., Farnsworth A., Kelly J. F. (2016). Where in the air? Aerial habitat use of nocturnally migrating birds. Biol. Lett. 12, 4–8. doi: 10.1098/rsbl.2016.0591
Hüppop O., Ciach M., Diehl R., Reynolds D. R., Stepanian P. M., Menz M. H. M. (2019). Perspectives and challenges for the use of radar in biological conservation. Ecography 42, 912–930. doi: 10.1111/ecog.04063
Hüppop O., Dierschke J., Exo K.-M., Fredrich E., Hill R. (2006). Bird migration studies and potential collision risk with offshore wind turbines. Ibis 148, 90–109. doi: 10.1111/j.1474-919X.2006.00536.x
Inger R., Attrill M. J., Bearhop S., Broderick A. C., James Grecian W., Hodgson D. J., et al. (2009). Marine renewable energy: Potential benefits to biodiversity? An urgent call for research. J. Appl. Ecol. 46, 1145–1153. doi: 10.1111/j.1365-2664.2009.01697.x
Intergovernmental Panel on Climate Change (2011). IPCC Special Report on Renewable Energy Sources and Climate Change Mitigation (New York, NY: Cambridge University Press).
Jedele T., Migliaccio E., Wyman J. (2023). Can offshore wind development have a net positive impact on biodiversity? Regulatory and scientific perspectives and considerations. (Bristol, Rhode Island: The Marine Affairs Institute at Roger Williams University School of Law and The Nature Conservancy), 41 pp.
Johnston D. T., Thaxter C. B., Boersch-Supan P. H., Humphreys E. M., Bouten W., Clewley G. D., et al. (2022). Investigating avoidance and attraction responses in lesser black-backed gulls Larus fuscus to offshore wind farms. Mar. Ecol. Prog. Ser. 686, 187–200. doi: 10.3354/meps13964
Kerlinger P., Gehring J. L., Erickson W. P., Curry R., Jain A., Guarnaccia J. (2010). Night migrant fatalities and obstruction lighting at wind turbines in North America. Wilson J. Ornithol. 122, 744–754. doi: 10.1676/06-075.1
Kraus S. D., Kenney R. D., Thomas L. (2019). A Framework for Studying the Effects of Offshore Wind Development on Marine Mammals and Turtles (Boston, MA: Massachusetts Clean Energy Center and the Bureau of Ocean Energy Management).
Lagerveld S., Poerink B. J., Geelhoed S. C. V. (2021). Offshore occurrence of a migratory bat, pipistrellus nathusii, depends on seasonality and weather conditions. Animals 11, 1–14. doi: 10.3390/ani11123442
Lagerveld S., Poerink B. J., Haselager R., Verdaat H. (2014). Bats in Dutch offshore wind farms in autumn 2012. Lutra 57, 61–69.
Lamy T., Koenigs C., Holbrook S. J., Miller R. J., Stier A. C., Reed D. C. (2020). Foundation species promote community stability by increasing diversity in a giant kelp forest. Ecology 101, e02987. doi: 10.1002/ecy.2987
Largey N., Cook A. S. C. P., Thaxter C. B., McCluskie A., Stokke Bå.G., Wilson B., et al. (2021). Methods to quantify avian airspace use in relation to wind energy development. Ibis 163, 747–764. doi: 10.1111/ibi.12913
La Sorte F. A., Hochachka W. M., Farnsworth A., Sheldon D., Fink D., Geevarghese J., et al. (2015). Migration timing and its determinants for nocturnal migratory birds during autumn migration. J. Anim. Ecol. 84, 1202–1212. doi: 10.1111/1365-2656.12376
Leonhard S. B., Pedersen J., Gron P. N., Skov H., Jansen J., Topping C., et al. (2013). “Wind farms affect common scoter and red-throated diver behaviour,” in Danish Offshore Wind: Key Environmental Issues - A Follow-up (The Environment Group: The Danish Energy Agency (The Danish Nature Agency, DONG Energy and Vattenfall), 70–93.
Leopold M. F., Boonman M., Collier M. P., Davaasuren N., Fijn R. C., Gyimesi A., et al. (2015). A first approach to deal with cumulative effects on birds and bats of offshore wind farms and other human activities in the Southern North Sea. IMARES Report C166/14 (IJmuiden, Netherlands: IMARES Wageningen UR for Rijkswaterstaat), 188 pp.
Leopold M. F., Dijkman E. M., Teal L., OWEZ-Team (2011). Local Birds in and around the Offshore Wind Farm Egmond aan Zee (OWEZ) (IJmuiden, Netherlands: Report by the Institute for Marine Resources & Ecosystem Studies to NoordzeeWind), 176 pp.
Lieber L., Langrock R., Nimmo-Smith W. A. M. (2021). A bird’s-eye view on turbulence: seabird foraging associations with evolving surface flow features. Proc. R. Soc. B: Biol. Sci. 288, 20210592. doi: 10.1098/rspb.2021.0592
Lin T.-Y., Winner K., Bernstein G., Mittal A., Dokter A. M., Horton K. G., et al. (2019). MistNet: Measuring historical bird migration in the US using archived weather radar data and convolutional neural networks. Methods Ecol. Evolution2 10, 1908–1922. doi: 10.1111/2041-210X.13280
Loring P. H., Carlson E., Gobeille D., Deluca R., Makenzie S., Berrigan L., et al. (2022). Monitoring Framework for Automated Radio Telemetry at Offshore Wind Projects in the U.S (Albany, New York: Atlantic).
Loring P., Lenske A., McLaren J., Aikens M., Anderson A., Aubrey Y., et al. (2020b). Tracking Movements of Migratory Shorebirds in the US Atlantic Outer Continental Shelf Region. OCS Study BOEM 2021-008 (Sterling, VA: Department of the Interior, Bureau of Ocean Energy Management). 104 pp.
Loring P. H., Mclaren J. D., Goyert H. F., Paton P. W. C. (2020a). Supportive wind conditions influence offshore movements of Atlantic Coast Piping Plovers during fall migration. Condor 122, 1–16. doi: 10.1093/condor/duaa028
Loring P. H., McLaren J. D., Smith P. A., Niles L. J., Koch S. L., Goyert H. F., et al. (2018). Tracking Movements of Threatened Migratory rufa Red Knots in U.S. Atlantic Outer Continental Shelf Waters. OCS Study BOEM 2018-046 (Sterling (VA: US Department of the Interior, Bureau of Ocean Energy Management). 145 pp.
Loring P., Paton P., McLaren J., Bai H., Janaswamy R., Goyert H., et al. (2019). Tracking Offshore Occurrence of Common Terns, Endangered Roseate Terns, and Threatened Piping Plovers with VHF Arrays. OCS Study (Sterling, Virginia: US Department of the Interior, Bureau of Ocean Energy Management).
Maclean I., Rehfisch M. M., Skov H., Thaxter C. B. (2013). Evaluating the statistical power of detecting changes in the abundance of seabirds at sea. Ibis 155, 113–126. doi: 10.1111/j.1474-919X.2012.01272.x
Masden E. A., Cook A. S. C. P. (2016). Avian collision risk models for wind energy impact assessments. Environ. Impact Assess. Rev. 56, 43–49. doi: 10.1016/j.eiar.2015.09.001
Masden E. A., Fox A. D., Furness R. W., Bullman R., Haydon D. T. (2010a). Cumulative impact assessments and bird/wind farm interactions: Developing a conceptual framework. Environ. Impact Assess. Rev. 30, 1–7. doi: 10.1016/j.eiar.2009.05.002
Masden E. A., Haydon D. T., Fox A. D., Furness R. W. (2010b). Barriers to movement: Modelling energetic costs of avoiding marine wind farms amongst breeding seabirds. Mar. pollut. Bull. 60, 1085–1091. doi: 10.1016/j.marpolbul.2010.01.016
Masden E. A., Haydon D. T., Fox A. D., Furness R. W., Bullman R., Desholm M. (2009). Barriers to movement: impacts of wind farms on migrating birds. ICES J. Mar. Sci. 66, 746–753. doi: 10.1093/icesjms/fsp031
Mavraki N., Degraer S., Moens T., Vanaverbeke J. (2020). Functional differences in trophic structure of offshore wind farm communities: A stable isotope study. Mar. Environ. Res. 157, 104868. doi: 10.1016/j.marenvres.2019.104868
McGregor R. M., King S., Donovan C. R., Caneco B., Webb A. (2018). A stochastic collision risk model for seabirds in flight. HC0010-400-001., Report by HiDef and BioConsult SH to Marine Scotland. (HiDef Aerial Surveying Limited and BioConsult SH), 59 pp.
McKinnon E. A., Artuso C., Love O. P. (2017). The mystery of the missing warbler. Ecology 98, 1970–1972. doi: 10.1002/ecy.1844
Mendel B., Schwemmer P., Peschko V., Müller S., Schwemmer H., Mercker M., et al. (2019). Operational offshore wind farms and associated ship traffic cause profound changes in distribution patterns of Loons (Gavia spp.). J. Environ. Manage 231, 429–438. doi: 10.1016/j.jenvman.2018.10.053
Methratta E. T. (2021). Distance-based sampling methods for assessing the ecological effects of offshore wind farms: Synthesis and application to fisheries resource studies. Front. Mar. Sci. 8, 1062. doi: 10.3389/fmars.2021.674594
Methratta E. T., Dardick W. R. (2019). Meta-analysis of finfish abundance at offshore wind farms. Rev. Fish. Sci. Aquacult. 27, 242–260. doi: 10.1080/23308249.2019.1584601
Montevecchi W. A. (2006). “Influences of artificial light on marine birds,” in Ecological Consequences of Artificial Night Lighting. Eds. Rich C., Longcore T. (Island Press, Washington, D.C), 94–113. doi: 10.1111/bph.13539
Murphy D. D., Weiland P. S., Cummins K. W. (2011). A critical assessment of the use of surrogate species in conservation planning in the Sacramento-San Joaquin Delta, California (U.S.A.). Conserv. Biol. 25, 873–878. doi: 10.1111/j.1523-1739.2011.01711.x
Newton I., Little B. (2009). Assessment of wind-farm and other bird casualties from carcasses found on a Northumbrian beach over an 11-year period. Bird Study 56, 158–167. doi: 10.1080/00063650902787767
Nisbet I. C., Veit R. R., Auer S. A., White T. P. (2013). Marine birds of the eastern United States and the Bay of Fundy: Distribution, numbers, trends, threats, and management. Ed. Buckley P. A. (Cambridge, MA: Nuttall Ornithological Club).
Normandeau Associates, APEM (2019). Digital aerial baseline survey of marine wildlife in support of offshore wind energy. Data for the New York State Energy Research and Development Authority. Report by Normandeau Associates and APEM Ltd to the New York State Research and Development Authority, Albany, New York.
NYSERDA New York State Energy Research and Development Authority (2020). Stakeholder workshop: Scientific research framework to understand the effects of offshore wind energy development on birds and bats in the eastern United States, Building Energy Exchange, March 4-6, 2020. NYSERDA Report Number 20-26. Eds. Gulka J., Williams K. (Portland ME: Biodiversity Research Institute).
NYSERDA New York State Energy Research and Development Authority (2021). Wildlife Data Standardization and Sharing: Environmental Data Transparency for New York State Offshore Wind Energy. NYSERDA Report 21-11. Eds. Jenkins E., Williams K. (Portland ME: Biodiversity Research Institute). 41 pp.
Palka D. L., Chavez-Rosales S., Josephson E., Cholewiak D., Haas H. L., Garrison L., et al. (2017). Atlantic Marine Assessment Program for Protected Species: 2010-2014. OCS Study BOEM 2017-071 (Washington DC: U.S. Department of the Interior, Bureau of Ocean Energy Management). 211 pp.
Perlut N. G. (2018). Prevalent transoceanic fall migration by a 30-gram songbird, the Bobolink. Auk 135, 992–997. doi: 10.1642/AUK-18-56.1
Petersen I. K., Christensen T. K., Kahlert J., Desholm M., Fox A. D. (2006). Final results of bird studies at the offshore wind farms at Nysted and Horns Rev, Denmark. Report by The National Environmental Research Institute to DONG energy and Vattenfall A/S. (Aarhus, Denmark: National Environmental Research Institute), 161 pp.
Petersen I. K., Nielsen R. D., Mackenzie M. L. (2014). Post-construction evaluation of bird abundances and distributions in the Horns Rev 2 offshore wind farm area 2011 and 2012. Report by Aarhus University Danish Centre for Environment and Energy to DONG Energy. (Aarhus, Denmark: Aarhus University Danish Centre for Environment and Energy), 51 pp.
Peterson T. S., Pelletier S. K., Boyden S. A., Watrous K. S. (2014). Offshore acoustic monitoring of bats in the Gulf of Maine. Northeast Nat. (Steuben) (Aarhus, Denmark: Aarhus University Danish Centre for Environment and Energy). 21, 154–163. doi: 10.1656/045.021.0107
Pettersson J. (2005). The impact of offshore wind farms on bird life in Southern Kalmar Sound Sweden final report based on studies 1999-2003 (Lund, Sweden: Report by the Department of Animal Ecology, Lund University, Sweden for the Swedish Energy Agency), 124 pp.
Pirotta E., Booth C. G., Costa D. P., Fleishman E., Kraus S. D., Lusseau D., et al. (2018). Understanding the population consequences of disturbance. Ecol. Evol. 8, 9934–9946. doi: 10.1002/ece3.4458
Platis A., Siedersleben S., Bange J., Lampert A., Bärfuss D., Hankers R., et al. (2018). First in situ evidence of wakes in the far field behind offshore wind farms. Sci. Rep. 8, 2163. doi: 10.1038/s41598-018-20389-y
Plonczkier P., Simms I. C. (2012). Radar monitoring of migrating pink-footed geese: behavioural responses to offshore wind farm development. J. Appl. Ecol. 49, 1187–1194. doi: 10.1111/j.1365-2664.2012.02181.x
Poot H., Ens B. J., de Vries H., Donners M. A. H., Wernand M. R., Marquenie J. M. (2008). Green light for nocturnally migrating birds. Ecol. Soc. 13, 47. doi: 10.5751/ES-02720-130247
Potiek A., Leemans J. J., Middelveld R. P., Gyimesi A. (2022). Cumulative impact assessment of collisions with existing and planned offshore wind turbines in the southern North Sea: Analysis of additional mortality using collision rate modelling and impact assessment based on population modelling for the KEC 4.0. Report nr 21-205 (Culemborg: Bureau Waardenburg).
Powers K. D., Wiley D. N., Robuck A. R., Olson Z. H., Welch L. J., Thompson M. A., et al. (2020). Spatiotemporal characterization of non-breeding Great Shearwaters Ardenna gravis within their wintering range. Mar. Ornithol. 48, 215–229.
Robinson Willmott J., Clerc J., Vukovich M., Pembroke A. (2021). Digital aerial baseline survey of marine wildlife in support of offshore wind energy. Overview and Summary. Report by Normandeau Associates and APEM Ltd to the New York State Research and Development Authority, Albany, New York. Contract no. 95764, 60 pp.
Rodhouse T. J., Rodriguez R. M., Banner K. M., Ormsbee P. C., Barnett J., Irvine K. M. (2019). Evidence of region-wide bat population decline from long-term monitoring and Bayesian occupancy models with empirically informed priors. Ecol. Evol. 9, 11078–11088. doi: 10.1002/ece3.5612
Ronconi R. A., Allard K. A., Taylor P. D. (2015). Bird interactions with offshore oil and gas platforms: Review of impacts and monitoring techniques. J. Environ. Manage 147, 34–45. doi: 10.1016/j.jenvman.2014.07.031
Russell R. W. (2005). Interactions between migrating birds and offshore oil and gas platforms in the northern Gulf of Mexico: Final report. OCS Study MMS 2005-009 (New Orleans, LA: U.S. Deptartment of the Interior, Minerals Management Service). 348 pp.
Rydell J., Bach L., Dubourg-Savage M.-J., Green M., Rodrigues L., Hedenström A. (2010). Bat mortality at wind turbines in northwestern Europe. Acta Chiropt 12, 261–274. doi: 10.3161/150811010X537846
Scales K. L., Miller P. I., Embling C. B., Ingram S. N., Pirotta E., Votier S. C. (2014). Mesoscale fronts as foraging habitats: Composite front mapping reveals oceanographic drivers of habitat use for a pelagic seabird. J. R Soc. Interface 11, 20140679. doi: 10.1098/rsif.2014.0679
Schultze L. K., Merckelbach L. M., Horstmann J., Raasch S., Carpenter J. R. (2020). Increased mixing and turbulence in the wake of offshore wind farm foundations. J. Geophys. Res. Oceans 125, e2019JC015858. doi: 10.1029/2019JC015858
Searle K. R., Mobbs D. C., Butler A., Furness R. W., Trinder M. N., Daunt F. (2018). Finding out the fate of displaced birds. Scottish Mar. Freshw. Sci. 9, 1–161. doi: 10.7489/12118-1
Shamoun-Baranes J., van Gasteren H. (2011). Atmospheric conditions facilitate mass migration events across the North Sea. Anim. Behav. 81, 691–704. doi: 10.1016/j.anbehav.2011.01.003
Skov H., Heinanen S., Norman T., Ward R. M., Mendez-Roldan S., Ellis I. (2018). ORJIP Bird Collision and Avoidance Study. Final Report - April 2018. Report by NIRAS and DHI to The Cabon Trust (U.K). 247 pp.
Slavik K., Lemmen C., Zhang W., Kerimoglu O., Klingbeil K., Wirtz K. W. (2019). The large scale impact of offshore wind farm structures on pelagic primary productivity in the southern North Sea. Hydrobiologia 845, 35–53. doi: 10.1007/s10750-018-3653-5
Solick D. I., Newman C. M. (2021). Oceanic records of North American bats and implications for offshore wind energy development in the United States. Ecol. Evol. 11, 14433–14447. doi: 10.1002/ece3.8175
Southall B., Morse L., Williams K. A., Jenkins E. (2021). Marine Mammals Workgroup Report for the State of the Science Workshop on Wildlife and Offshore Wind Energy 2020: Cumulative Impacts (Albany, NY: Report to the New York State Energy Research and Development Authority (NYSERDA). 50 pp.
Stantec (2016). Long-term bat monitoring on islands, offshore structures, and coastal sites in the Gulf of Maine, mid-Atlantic, and Great Lakes - Final Report. Report by Stantec COnsulting Services Inc. to U.S. Department of Energy, Topsham, Maine, 171 pp.
Stenhouse I. J., Berlin A. M., Gilbert A. T., Goodale M. W., Gray C. E., Montevecchi W. A., et al. (2020). Assessing the exposure of three diving bird species to offshore wind areas on the U.S. Atlantic Outer Continental Shelf using satellite telemetry. Divers. Distrib 26 (12), 1703–1714. doi: 10.1111/ddi.13168
Sullivan B. L., Wood C. L., Iliff M. J., Bonney R. E., Fink D., Kelling S. (2009). eBird: A citizen-based bird observation network in the biological sciences. Biol. Conserv. 142, 2282–2292. doi: 10.1016/j.biocon.2009.05.006
Tjørnløv R. S., Skov H., Armitage M., Barker M., Jørgensen J. B., Mortensen L. O., et al. (2023). Resolving Key Uncertainties of Seabird Flight and Avoidance Behaviours at Offshore Wind Farms: Final report for the study period 2020-2021. Report by DHI A/S to Vattenfall, Horsholm, Denmark. Project No. 11820296.
van Berkel J., Burchard H., Christensen A., Mortensen L. O., Petersen O. S., Thomsen F. (2020). The effects of offshore wind farms on hydrodynamics and implications for fishes. Oceanography 33, 108–117. doi: 10.5670/oceanog
Van Buskirk J., Mulvihill R. S., Leberman R. C. (2009). Variable shifts in spring and autumn migration phenology in North American songbirds associated with climate change. Glob Chang. Biol. 15, 760–771. doi: 10.1111/j.1365-2486.2008.01751.x
Van Doren B. M., Horton K. G. (2018). A continental system for forecasting bird migration. Science (1979) 361, 1115–1118. doi: 10.1126/science.aat7526
Van Doren B. M., Horton K. G., Dokter A. M., Klinck H., Elbin S. B., Farnsworth A. (2017). High-intensity urban light installation dramatically alters nocturnal bird migration. Proc. Natl. Acad. Sci. U.S.A. 114, 11175–11180. doi: 10.1073/pnas.1708574114
Vanermen N., Onkelinx T., Verschelde P., Courtens W., Van de Walle M., Verstraete H., et al. (2015). Assessing seabird displacement at offshore wind farms: power ranges of a monitoring and data handling protocol. Hydrobiologia 756, 155–167. doi: 10.1007/s10750-014-2156-2
Vanhellemont Q., Ruddick K. (2014). Turbid wakes associated with offshore wind turbines observed with Landsat 8. Remote Sens. Environ. 145, 105–115. doi: 10.1016/j.rse.2014.01.009
Veit R. R., White T. P., Perkins S. A., Curley S. (2016). Abundance and distribution of seabirds off Southeastern Massachusetta 2011-2015 Final Report. OCS Study BOEM 2016-067. (Sterling VA: U.S. Department of the Interior, Bureau of Ocean Energy Management). 82 pp.
Watson R. T., Kolar P. S., Ferrer M., Nygård T., Johnston N., Hunt W. G., et al. (2018). Raptor interactions with wind energy: case studies from around the world. J. Raptor Res. 52, 1–18. doi: 10.3356/JRR-16-100.1
Watts B. D., Hines C., Duval L., Wilke A. L. (2022). Exposure of Whimbrels to offshore wind leases during departure from and arrival to a major mid-Atlantic staging site. Avian Conserv. Ecol. 17, 31. doi: 10.5751/ACE-02312-170231
Welcker J., Nehls G. (2016). Displacement of seabirds by an offshore wind farm in the North Sea. Mar. Ecol. Prog. Ser. 554, 173–182. doi: 10.3354/meps11812
Wilhelmsson D., Malm T. (2008). Fouling assemblages on offshore wind power plants and adjacent substrata. Estuar. Coast. Shelf Sci. 79, 459–466. doi: 10.1016/j.ecss.2008.04.020
Williams K., Connelly E., Johnson S., Stenhouse I. (2015). Wildlife Densities and Habitat Use Across Temporal and Spatial Scales on the Mid-Atlantic Outer Continental Shelf: Final Report to the Department of Energy EERE Wind & Water Power Technologies Office (Portland, Maine: Biodiversity Research Institute). 715, Award Number: DE-EE0005362. Report BRI 2015-11, Biodiv.
Williams K. A., Popper A. N., Hice-Dunton L., Higgs D. M., Jenkins E., Krebs J. M., et al. (2023). “Sound-related effects of offshore wind energy on fishes and aquatic invertebrates: research recommendations,” in The Effects of Noise on Aquatic Life: Principles and Considerations. Eds. Popper, An. N., Sisneros J., Hawkins A. D., Thomsen F. (Springer Cham). doi: 10.1007/978-3-031-10417-6
Winiarski K. J., Miller D. L., Paton P. W. C., McWilliams S. R. (2014). A spatial conservation prioritization approach for protecting marine birds given proposed offshore wind energy development. Biol. Conserv. 169, 79–88. doi: 10.1016/j.biocon.2013.11.004
Winship A., Kinlan B., White T., Leirness J., Christensen J. (2018). Modeling at-sea density of marine birds to support Atlantic marine renewable energy planning. OCS Study BOEM 2018-010. (Sterling, VA: U.S. Department of the Interior, Bureau of Ocean Energy Management). 67 pp.
Winship A. J., Leirness J. B., Coyne M., Howell J., Saba V. S., Christensen J., et al (2023). Modeling the distributions of marine birds at sea to inform planning of energy development on the US Atlantic Outer Continental Shelf. OCS Study BOEM 2023-060 (Sterling, VA: U.S. Department of the Interior, Bureau of Ocean Energy Management), 413 p.
Keywords: bird, bat, offshore wind energy, framework, research priorities, collision, displacement
Citation: Williams KA, Gulka J, Cook ASCP, Diehl RH, Farnsworth A, Goyert H, Hein C, Loring P, Mizrahi D, Petersen IK, Peterson T, Press KM and Stenhouse IJ (2024) A framework for studying the effects of offshore wind energy development on birds and bats in the Eastern United States. Front. Mar. Sci. 11:1274052. doi: 10.3389/fmars.2024.1274052
Received: 07 August 2023; Accepted: 19 April 2024;
Published: 03 May 2024.
Edited by:
Emeline Pettex, La Rochelle University – Cohabys, FranceReviewed by:
David Ainley, H.T. Harvey & Associates, United StatesSarah Klain, Utah State University, United States
Copyright © 2024 Williams, Gulka, Cook, Diehl, Farnsworth, Goyert, Hein, Loring, Mizrahi, Petersen, Peterson, Press and Stenhouse. This is an open-access article distributed under the terms of the Creative Commons Attribution License (CC BY). The use, distribution or reproduction in other forums is permitted, provided the original author(s) and the copyright owner(s) are credited and that the original publication in this journal is cited, in accordance with accepted academic practice. No use, distribution or reproduction is permitted which does not comply with these terms.
*Correspondence: Kathryn A. Williams, a2F0ZS53aWxsaWFtc0Bicml3aWxkbGlmZS5vcmc=
†Present address: Aonghais S. C. P. Cook, The Biodiversity Consultancy, 3E King’s Parade, Cambridge, United Kingdom
‡These authors share first authorship