- 1Conservation Ecology Group, Groningen Institute for Evolutionary Life Sciences, University of Groningen, Groningen, Netherlands
- 2Department of Coastal Systems, Royal Netherlands Institute of Sea Research (NIOZ), Den Hoorn, Netherlands
- 3BESE, Waardenburg Ecology, Culemborg, Netherlands
Extensive subtidal eelgrass (Zostera marina) meadows (~150 km2) once grew in the Dutch Wadden Sea, supporting diverse species communities, but disappeared in the 1930s and have been absent ever since. Identifying the most critical bottlenecks for eelgrass survival is a crucial first step for reintroduction through active restoration measures. Seagrasses are ecosystem engineers, inducing self-facilitating feedbacks that ameliorate stressful conditions. Consequently, once seagrass, including its self-facilitating feedbacks, is lost, reintroduction can be challenging. Therefore, we aimed to test whether 1) sediment stabilization and 2) hydrodynamic stress relief would facilitate eelgrass survival in a field experiment replicated at two sites in the Dutch Wadden Sea. We induced feedbacks using biodegradable root-mimicking structures (BESE-elements) and sandbag barriers. Root mimics had a significant positive effect, increasing the chances of short-term survival by +67% compared to controls. Contrary to our expectations, barriers decreased short-term survival probabilities by -26%, likely due to hydrodynamic turbulence created by the barrier edges, leading to high erosion rates (-14 cm). Site selection proved crucial as short-term survival was entirely negated on one of the two study sites after five weeks due to high floating and epiphytic macroalgae loads. No long-term survival occurred, as plants died at the other site two weeks later. Overall, we found that sediment stabilization by root-mimicking structures was promising, whereas manipulating hydrodynamic forces using sandbag barriers had adverse effects. A mechanistic understanding of transplant failures is required before attempting large-scale restoration. Our study indicates that for seagrass restoration in the Wadden Sea, one should carefully consider 1) the reintroduction of positive feedbacks through restoration tools, 2) donor population choice and transplantation timing, and 3) site selection based on local biotic and abiotic conditions. Optimizing these restoration facets might lower additive stress to a degree that allows long-term survival.
1 Introduction
Seagrasses are rooted marine flowering plants that provide many important ecosystem services (Nordlund et al., 2016 and sources within). They improve coastal protection by reducing hydrodynamic forcing (Ondiviela et al., 2014) and improve water quality by acting as a filter (de los Santos et al., 2020; Prystay et al., 2023). Many economically relevant species like cod and herring (Havinga, 1954; Schaper, 1962) rely on complex seagrass beds as habitat (Cullen-Unsworth et al., 2014), nurseries (Boström et al., 2014; Unsworth et al., 2019), food source, and foraging grounds (Martin et al., 2010), underlining the ecological value of seagrass beds as biodiverse and productive ecosystems (Hemminga and Duarte, 2000; Duffy, 2006). Despite their socio-economic importance, seagrass systems are under pressure and severely threatened by anthropogenic stressors like coastal development and ecological degradation (Duffy, 2006; Orth et al., 2006). While in some bioregions, these negative trends have recently stabilized or even reversed (de los Santos et al., 2019), the global losses of 29% since initial seagrass recordings in 1879 (Waycott et al., 2009) still outweigh gains (Dunic et al., 2021).
Seagrasses are autogenic ecosystem engineers (Jones et al., 1994), which ameliorate stressful conditions with their physical structures (Luhar et al., 2017). Their aboveground structures attenuate waves (Fonseca and Cahalan, 1992; Christianen et al., 2013) and slow down current flow (Fonseca et al., 1982; van Keulen and Borowitzka, 2002; Lacy and Wyllie-Echeverria, 2011), ameliorating hydrodynamic stress and preventing plants from becoming dislodged or broken. Additionally, suspended organic matter, nutrients (McGlathery et al., 2007), and fine sediment particles (Gacia et al., 2003) are trapped, leading to improved water clarity (van der Heide et al., 2011). Their belowground structures increase sediment stability and thereby decrease erosion (Gacia and Duarte, 2001; Gacia et al., 2003; Marin-Diaz et al., 2020), further improving water clarity (Maxwell et al., 2017). These are examples of so-called positive or self-facilitating feedback loops where seagrass induces better growing conditions for itself through scale- and density-dependent feedbacks. These scale and density dependences result from emergent traits, which are not expressed by individual plants or small clones but only emerge when expressed on a population level while organized as a group or large clone (Smaldino, 2014). However, once a meadow is lost, reintroduction can be challenging, as rather than on a patch or meadow scale, the full force of the stressor acts upon individual transplants or seedlings, which do not have the density and numbers to produce self-facilitating feedbacks. Therefore, firstly, the consideration of scale is crucial when designing the setup of a seagrass restoration attempt (van Katwijk et al., 2016; Gräfnings et al., 2023b). Large-scale restoration gains cumulative value. However, when ecosystem responses to treatments are unpredictable, treatments should be tested on a smaller scale. This ensures a knowledge-based approach and limits the risk of over-extending valuable resources like finances, effort, time (Gann et al., 2019), and donor plant material. Secondly, the application of restoration tools that bridge establishment thresholds by mimicking emergent traits (Temmink et al., 2020; Fivash et al., 2021) should be considered.
In the 1930s, an estimated area of 65 to 150 km2 of subtidal eelgrass (Zostera marina) beds (van Goor, 1919) were permanently lost in the Dutch Wadden Sea (Oudemans et al., 1870; den Hartog and Polderman, 1975) due to the outbreak of the wasting disease (Rasmussen, 1977) and the coinciding construction of the enclosure dam (“Afsluitdijk”) (Giesen et al., 1990a). The former inland sea (Zuiderzee) was now closed off from the Wadden Sea, and the construction works of the 32 km long dam greatly affected hydrological processes and sediment dynamics. The tidal range in the Wadden Sea increased up to 50 cm (de Jonge and de Jong, 1992), leading to 1.2 to 2.9 times higher water transport rates (Thijsse, 1972). An increase in current velocities has led to erosion and, at least, to a temporal increase in water turbidity (de Jonge et al., 1996), negatively affecting underwater light conditions for seagrasses. It has been estimated that today, due to the absence of the seagrass and its self-facilitating mechanisms in some of the former seagrass habitats in the Dutch Wadden Sea, water turbidity has increased by six-fold (van den Hoek et al., 1979; Giesen et al., 1990a; van der Heide et al., 2007, 2006; van Katwijk et al., 2009). Since its disappearance in the 1930s, subtidal eelgrass has not managed to recover, and the substantial distance (>400 km) to the closest natural subtidal population makes natural recovery by seed or vegetative fragment dispersion highly unlikely. In addition, once the self-facilitating feedback loops induced by seagrass presence have been disrupted, natural recolonization may be impossible (Suding et al., 2004; Suykerbuyk et al., 2012). Substantial intervention can be required in degraded systems that lack natural recovery potential (Gann et al., 2019). Here, the active restoration measure of species reintroduction seems necessary to restore the subtidal eelgrass population in the Dutch Wadden Sea.
Self-facilitating feedbacks can be artificially induced by applying restoration tools (Maxwell et al., 2017). For instance, underwater barriers can reduce wave force (van Zuidam et al., 2022), and thereby facilitate seagrass restoration. Barriers can reduce orbital velocities that act on the seagrass shoot, and thus, more waves of the same magnitude are required to dislodge a single-shoot transplant (Kamperdicks et al., pers. comm.1). Other feedback-inducing measures are increasing sediment stability through buried root mimics (Temmink et al., 2020; Fivash et al., 2021), plant anchorage (Moksnes et al., 2016; Cronau et al., 2022; Lange et al., 2022), or artificial seagrass deployment (Campbell and Paling, 2003; Carus et al., 2022, 2021; T. Fauvel, pers. comm., Jan. 31, 2024). In this study, we investigated if the reintroduction of self-facilitating feedbacks can aid the restoration of subtidal eelgrass in a dynamic coastal ecosystem that has been absent for almost 100 years. Specifically, we tested the effects of 1) increased sediment stability by applying root-mimicking mats with buried three-dimensional biopolymer structures and 2) decreased hydrodynamic forcing through the deployment of barriers made of sandbags on the survival of seagrass transplants.
2 Materials and methods
2.1 Study site
The Wadden Sea is the world’s most extensive intertidal flat system and stretches along the coasts of the Netherlands, Germany, and Denmark (Figures 1A, B). This shallow coastal sea has been awarded UNESCO World Heritage status, reflecting its high ecological and economic importance. We performed the experiment on two separate study sites in the Western Dutch Wadden Sea: Zachte Bed Oost (53°6’25.664’’N, 5°9’11.538’’E) and Vlakte van Kerken (53°6’21.168’’N, 4°55’11.982’’E) (Figure 1C), with annual mean salinities of 26.4 PSU (± 3.9 SD), and 28.6 PSU (± 2.5 SD), respectively (van Weerdenburg and Vroom, 2021). According to the procedure for reintroduction measures defined by the Standards of the Society for Ecological Restoration, suggesting reintroducing an organism in its original range from which it has disappeared (Gann et al., 2019), we chose sites located in or close to former eelgrass beds. Zachte Bed Oost is located in an area that had been populated by subtidal eelgrass before it vanished in the 1930s, and Vlakte van Kerken is located close to a former eelgrass bed (Figure 1C, green polygons). Both locations fall under the Natura 2000 protection, as the entire Dutch Wadden Sea has this protection status. In addition, Zachte Bed Oost is within an area closed off to mussel seed fishery since 2015. Vlakte van Kerke is closed off for hand-cockle fishery and in close proximity (~200 m) to an area closed off to shrimp and mussel seed fishery, which are the most prominent bottom-disturbing fisheries in the Dutch Wadden Sea. Considering legal restrictions, like marine protection status, improves efficient habitat selection (Pogoda et al., 2020). Furthermore, the sites’ shallow bathymetries make it physically impossible for mussel seed- and shrimp fishers to fish on both sites. Potential study sites were pre-selected based on suitable bathymetry, sediment grain size, and position within areas partially closed to fisheries. In the scope of a system analysis combined with field measurements in 2020 and 2021, we searched for locally low to moderate flow velocities, relatively high underwater light availability (measured as photosynthetically active radiation (PAR)), and minor wave height. Based on their promising sets of abiotic conditions, we chose Zachte Bed Oost and Vlakte van Kerken as our two study sites. Their shallow bathymetric positions (-135 cm and -130 cm below mean water level (MWL), respectively) ensure that seagrass remains permanently submerged and lays well within the depth range of former seagrass beds of the 1930s, which were growing at depths between -50 cm and -230 cm below MWL (van der Heide et al., 2007). A shallow water column during low tide might enable more light to reach the plants, leading to less light limitation than in deeper areas. Light limitation is currently one of the most pressing bottlenecks for subtidal eelgrass restoration in the Dutch Wadden Sea (van der Heide et al., 2007, 2006). For light-saturated growth, subtidal eelgrass requires 7 mol photons m-2 day-1 (Thom et al., 2008). In June 2021, we measured a mean photosynthetic photon flux density (PPFD) of 7.0 mol photons m-2 d-1 at Zachte Bed Oost and 9.0 mol photons m-2 d-1 at Vlakte van Kerken. These values are at or above this saturation limit, indicating that the sites might meet the light requirements.
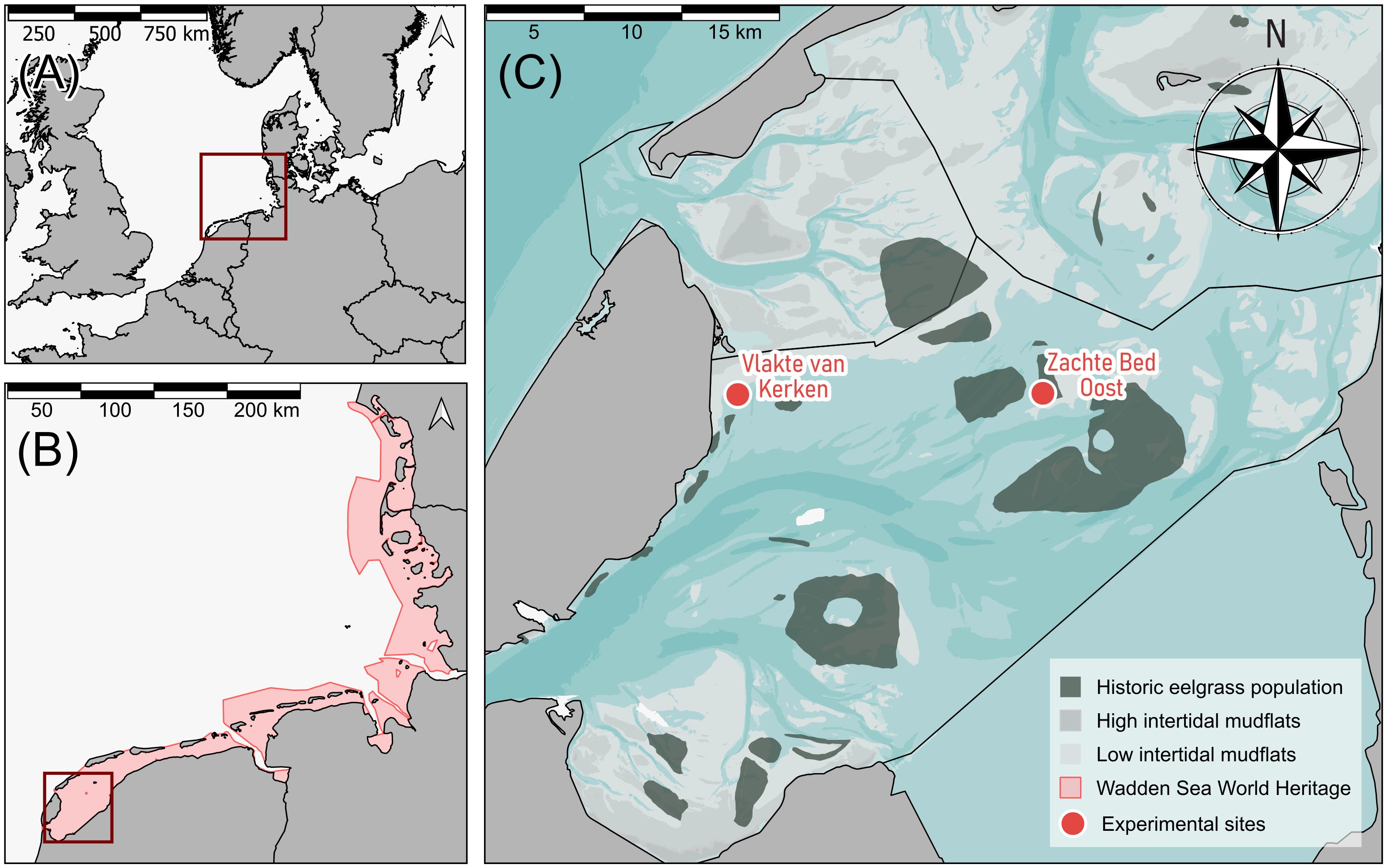
Figure 1 Maps of the study area. (A) Map depicting northwest Europe with the Wadden Sea highlighted in dark red. (B) The Dutch, German, and Danish coastline with the trilateral Wadden Sea UNESCO World Heritage is highlighted in red with the study area, i.e., the western Dutch Wadden Sea, depicted in a dark red box. (C) Zoomed-in area of the study site in the western Dutch Wadden Sea, with dark green areas indicating the location and extent of historic eelgrass beds in 1925 (van der Heide et al., 2007), and red dots indicating our study sites Vlakte van Kerken and Zachte Bed Oost. The underlying blue layer (de Kruif, 2001) depicts the bathymetry, with darker blue shades indicating deeper parts of the seafloor and brighter areas depicting more shallow areas. Dark gray areas with black borders represent land, and brighter gray shades intertidal mudflats. Black lines depict the tidal basins.
2.2 Study species and plant processing
Eelgrass grows in two morphologically distinct varieties: flexible intertidal and robust subtidal (van Katwijk and van Tussenbroek, 2023 and sources within). In 2022, 650 hectares of intertidal eelgrass grew in the Dutch Wadden Sea due to a long-term research project through seed-based restoration (Gräfnings et al., 2023b). While remarkable restoration success has been accomplished for the intertidal variety, the subtidal variety, which grows under permanently submerged conditions, is still absent. For the subtidal eelgrass variety, one unsuccessful restoration attempt was carried out in 1950 (Korringa, unpublished data). Since then, this study has been the first to investigate restoration possibilities further within the scope of a field experiment. Due to COVID-19 border restrictions, we could not access donor plants from the nearest preferred donor site in Limfjord, Denmark. Instead, the collection of approximately 900 seagrass shoots took place in June 2021 in the bay of Eckernförde (54°27’49.9’’N, 9°56’42.0’’E), Germany, at 2 m depth (~14 PSU). Multiple shoot counts were performed by placing a 0.5 × 0.5 m metal frame on randomly selected patches within the meadow, resulting in a conservative estimation of 235 shoots m-2. Based on recent satellite images, the donor meadow covered approximately 12,000 m2. Hence, our harvest impact was estimated to have affected less than 0.05% of the meadow. To minimize the impact on the donor meadow, apical shoots, including rhizomes and roots, were carefully collected by hand at the edge of multiple patches. To fulfill permit requirements to limit the risk of unwanted transport of seagrass-associated species, sediment was removed entirely from the belowground structures, followed by a thorough rinse of aboveground plant structures, removing all visibly attached species. This step was a requirement of the Directorate General for Public Works and Water Management (Rijkswaterstaat) (registration nr. RWSZ2021–00011036). During transportation, seagrasses were kept moist and cool. Overnight storage of the plants took place in aerated artificial seawater with higher salinity (Tropic Marine Classic, 20 PSU) to aid the acclimatization of the plants from the Baltic Sea to the higher salinity levels of the Wadden Sea. We constructed transplant units (TPUs) out of three seagrass shoots with 10 cm long rhizome fragments (mean wet weight = 15.2 gr, SE = 5.6), which we anchored with a 20-gram metal nail with thin metal wire (0.5 mm), according to the method established in Denmark by Lange et al. (2022). Transplant units were constructed one day after harvest and kept in shaded Wadden Sea surface water for 1.5 days until planted.
2.3 Experimental setup
We performed the experiment at two sites: Vlakte van Kerken and Zachte Bed Oost (Figure 1C). We subjected the seagrass transplants to two treatments: altered hydrodynamic forcing (barrier vs. control) and altered sediment stability (BESE vs. control stability). The treatments were applied in a full-factorial design, resulting in four treatments replicated 12 times at each study site (n = 12).
For the first treatment, to manipulate hydrodynamic forcing, we constructed two barriers per site with jute bags filled with ~33 kg sand. We used mortar sand (overall grain size ≤ 3mm), which has sharp edges and a more complex structure, increasing the stability of the bags. The bags were piled up (L:H:W: ~7:0.5:0.6 m) in a slightly bent curve with an arc length of 7.2 m at a radius of 7.0 m and 59°central angle (Figure 2A). The direction of the barriers was perpendicular to the predominant directions of current flow and waves to shelter the seagrasses from the incoming tidal current. Predominant wind direction data from the Royal Netherlands Meteorological Institute (KNMI) was used as a proxy for predominant wave direction. We measured the predominant heading of the current flow with tilt current meters (Lowell Instruments LLC, East Falmouth, United States) three months before the experiment. Per site, the two barriers and bare controls were arranged alternating in a line next to each other (Figure 2C). We stacked three layers of 1 × 10 m coconut fiber mesh (Ø 10 mm; mesh size = 2 cm) under each of the four barriers and secured them with four L-shaped rebars (l = 50 cm; Ø 10 mm). One meter behind each barrier or bare control, we semi-randomly arranged twelve plots in two rows containing equal numbers of plants treated with increased or control sediment stability (Figure 3).
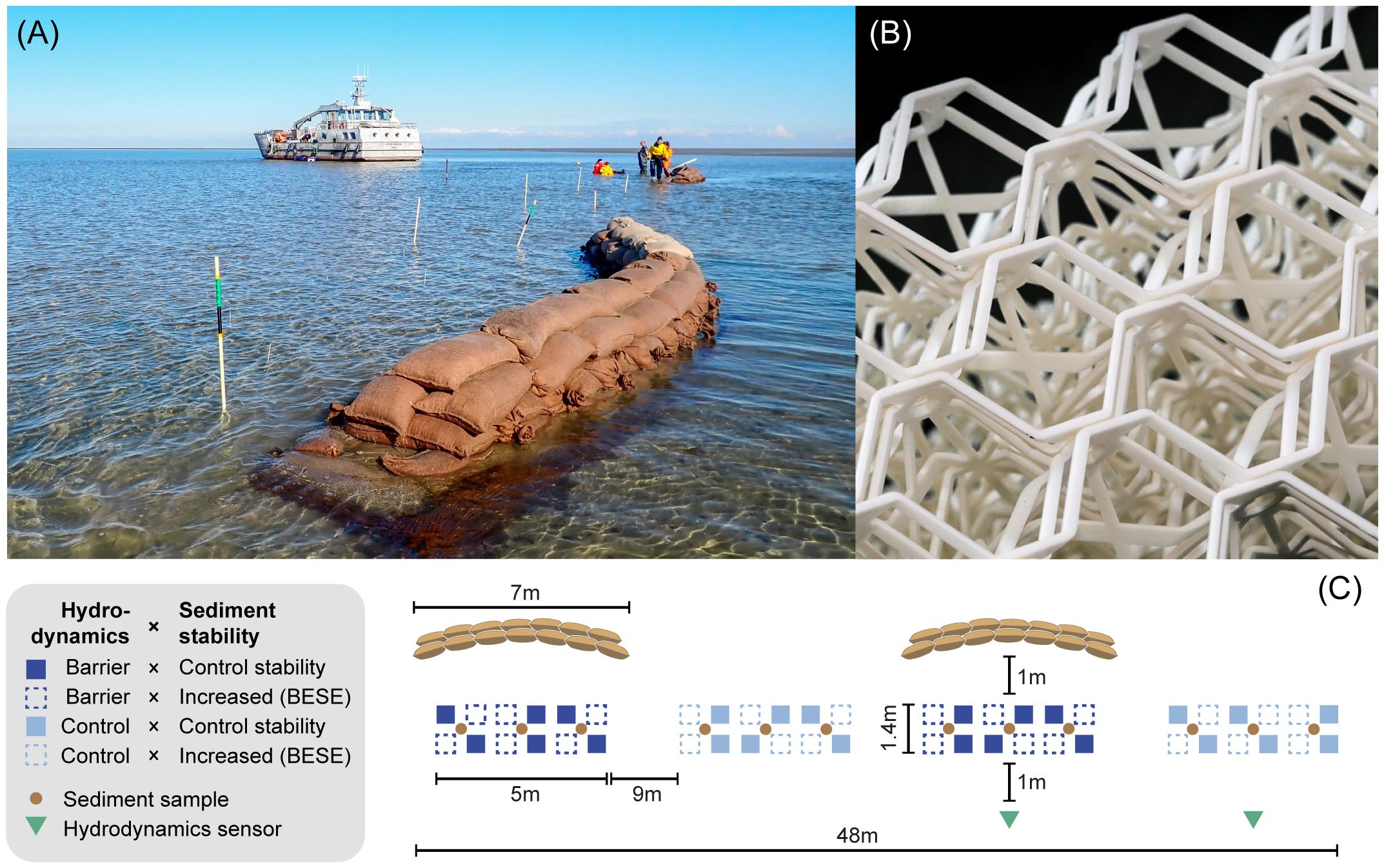
Figure 2 (A) Experimental setup of two 7-m long barriers consisting of sandbags piled on an anti-erosion coco fiber mat. PVC tubes indicate areas in which seagrass transplants were planted. (B) Biodegradable root mimics (BESE) were buried to increase the sediment stability. (C) Schematic illustration of the setup. All squares indicate seagrass plots with three transplant units. Textured plots were treated with sediment-stabilizing root mimics (BESE), while filled squares were kept at control sediment stability. Dark blue squares (textured and filled) indicate the hydrodynamic barrier treatment, and light blue squares (textured and filled) indicate the hydrodynamic control treatment. Green triangles indicate field loggers for wave data and current flow velocity, and brown circles indicate where sediment samples were taken. Representation not to scale.
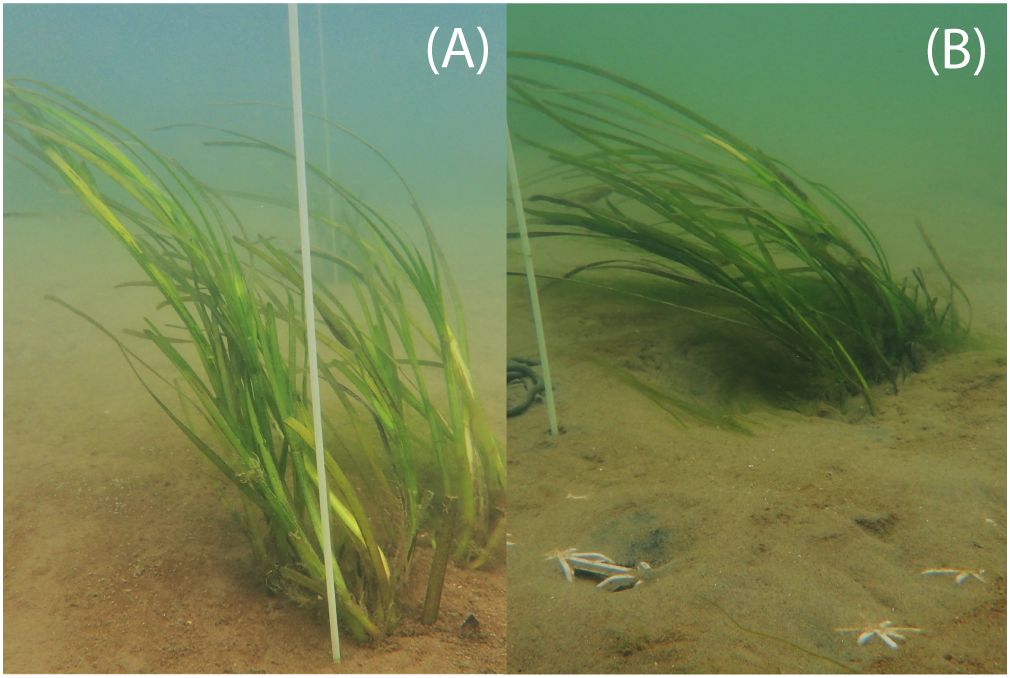
Figure 3 Seagrass transplant units planted (A) without and (B) with buried sediment-stabilizing BESE-elements. The white glass-fiber sticks were deployed only during monitoring and removed afterward.
For the second treatment, to increase sediment stability, we buried three-dimensional artificial structures, mimicking seagrass root mats, completely sub-surface. The structures consisted of Biodegradable Elements for Starting Ecosystems (BESE-elements, http://www.bese-products.com/, Figure 2B) (BESE BV, Culemborg, Netherlands), composed of a carbon neutral biodegradable polymer made from potato-waste Solanyl C1104M (Rodenburg Biopolymers, Oosterhout, the Netherlands) (Temmink et al., 2020; Marin-Diaz et al., 2021). We stacked two layers of BESE (45 cm × 45 cm), resulting in a 4 cm high 3-D honeycomb-shaped matrix with a 12 cm diameter circular planting hole cut in the center. Each structure was secured with three L-shaped rebars (l = 50 cm; Ø 10 mm). The control sediment stability plots (45 cm × 45 cm) consisted of bare sediment. The experiment was set up (i.e., barrier construction and sediment-stabilizing structures burial) one month before planting to allow sediment to settle around the restoration tools. To start the experiment, we manually planted three seagrass transplant units just below the sediment surface in the center of each plot within a diameter of 12 cm. For this, we lifted the sediment with an elongated trowel and gently pushed the units into the gap at an angle. The experiment was terminated thirteen weeks after planting.
2.4 Monitoring: biotic conditions
We monitored the seagrass transplants while snorkeling during daylight low tides, which occurred approximately monthly. At Zachte Bed Oost, we monitored 1, 5, 8, and 13 weeks after planting. Similar monitoring timing occurred at Vlakte van Kerken, except that the 8-week sample was impossible due to high turbidity that impaired underwater visibility. Plants did not grow sufficiently to determine growth parameters like expansion or elongation rate, so we assessed whether the transplant units still contained alive shoots. We checked whether the three transplant units held live shoots at each plot. We recorded the death of a transplant unit when all leaves of the same transplant unit were absent or completely discolored or when the entire transplant unit was missing. To monitor transplant dislodgement, we recorded the presence or absence of transplant anchors (i.e., nails) in week five at both locations. We took pictures of all plants during monitoring events. Additionally, we visually estimated the epiphyte cover when visibility and duration of the low tide during monitoring allowed it. We estimated the percentage of epiphyte cover of the seagrass leaves twice at Zachte Bed Oost (weeks five and eight) and once at Vlakte van Kerken (week five). The experiment was terminated at the last monitoring event, after thirteen weeks, when no surviving shoots were present anymore.
2.5 Monitoring: abiotic conditions
We monitored the abiotic conditions of current flow velocity and heading direction with tilt current meters (Lowell Instruments LLC, East Falmouth, United States). The loggers recorded current data at a sampling rate of 8 Hz and bursting duration of 10 seconds per ten minutes. To prevent the heading and current velocity measurements from being expressed relative to the magnetic north, we corrected for the magnetic declination, which is the angle between the magnetic north and true north. We determined the declination of 1.48° east with the “National Centers for Environmental Information (NOAA) Declination Calculator” (https://www.ngdc.noaa.gov/geomag/calculators/magcalc.shtml), according to the experimental site’s locations in the western Wadden Sea. The current reduction occurred during incoming tides through the barriers’ position regarding the seagrass plots. Therefore, we categorized the records’ heading degrees into incoming (Vlakte van Kerken: ~20°; Zachte Bed Oost: ~40°) and outgoing tides (Vlakte van Kerken: ~180°; Zachte Bed Oost: ~230°), for the velocity reduction calculations. For both sites, data of barriers and controls was aligned based on time stamps and expressed as percentual current velocity reduction or current attenuation. The maximum wave height was measured with wave gauge sensors (Ocean Sensor Systems Inc., Coral Springs, United States) at a sampling rate of 10 Hz and bursting length of 7 minutes per 15 minutes. We derived the minimum and maximum pressure values per measurement moment. From that, we calculated the maximum wave height per burst. Light intensity was monitored as photosynthetically active radiation (PAR) throughout the experiment, approximately 50 m next to the experimental setup with Odyssey Integrating PAR Sensors (Dataflow Systems PTY Limited, Christchurch, New Zealand). Loggers were cleaned off barnacle and algae biofouling during monitoring events, and only PAR data collected within three weeks after cleaning events were used in analyses to ensure the reliability of the data, as the PAR sensors were especially susceptible to biofouling. In addition, eight weeks after setting up the barriers, hence four weeks after planting, sediment surface samples were taken by extracting the top 5 cm layer with a 50 ml syringe (∅ 3 cm). We took three samples between seagrass plots behind each barrier and control, totaling 24 samples per site (Figure 2C, brown circles). Samples were analyzed for median sediment grain size and silt percentage (% with sediment grain size < 63 μm). Freeze-dried sediment samples were analyzed by the Royal Netherlands Institute for Sea Research (NIOZ, Texel) with a particle size analyzer (Beckman Coulter LS 13 320, Aqueous Liquid Module) using Polarization Intensity Differential Scattering (PIDS) technology. We took drone pictures of the experimental setup eight weeks after planting. Bathymetric information of the exact transplanting position and large-scale changes in bathymetry within the experimental setup were collected using the Trimble® R8 GNSS System (Global Navigation Satellite System) rtk-dGPS with a vertical resolution of 15 mm, one week and four weeks after planting by either measuring at the plots directly or in an approximately 1.5 × 1.5 m resolution around the setup. Due to the unexpected nature of the large bathymetry change next to the barriers, we did not take repeated bathymetry measurements in the scour holes. Bathymetry differences over time are, therefore, likely an underestimation.
2.6 Statistical analyses
Statistical analyses were performed with R version 4.3.2 (R Core Team 2014). Throughout this study, we refer to the standard error with SE. We predicted the odds of shoot survival with a Generalized Linear Mixed-Effect Model (GLME) using the lme4 package (Bates et al., 2015). We converted the survival data into a binomial distribution. We considered a plot as having survived (1) if at least one of the three seagrass transplant units still had at least one alive shoot. On the other hand, we noted death (0) if none of the three seagrass transplants contained living shoots. We set hydrodynamic treatment, sediment stability treatment, experimental site, time (in days after transplantation), and interaction between time after transplantation and study site as fixed effects. Transplant unit ID and plot ID were applied as nested random effects. Model estimates on a logit scale were back-transformed by exponentiation to generate survival odds. We computed 95% Confidence Intervals (CIs) and calculated p-values using a Wald z-distribution approximation. For the abiotics, we fitted linear mixed-effect (LME) models with the nlme package (Pinheiro et al., 2023) to predict the effect of the hydrodynamic barriers on current flow and wave height, with the hydrodynamic treatment as a fixed effect and time as a random effect. Models were run separately for both locations. Additionally, we fitted a linear model with the median grain size as the dependent variable and hydrodynamic treatment and experimental site as independent variables. The difference in silt content between the study sites was analyzed non-parametrically for barrier and control treatments using a Kruskal-Wallis test, as assumptions for normality were not met. We validated model assumptions by plotting residuals versus fitted values to verify homogeneity and assessing Q–Q plots of the residuals to check for normality and residuals versus each explanatory variable to check for independence. Additionally, the Shapiro-Wilks test (α >.05) was used to test for normality of variance, and Levene’s test (α >.05) was used for testing homogeneity of variance. Changes in bathymetry depending on the presence of the barriers were tested with a Welch two-sample t-test due to unequal variances of the groups.
3 Results
3.1 Transplant survival
We investigated if the reintroduction of self-facilitating feedbacks can facilitate the restoration of subtidal eelgrass in a dynamic ecosystem. For this, we tested the effects of increased sediment stability and the manipulation of hydrodynamic forcing on seagrass survival. The reported survival probabilities are not absolute but relative survival chances. They are expressed in relation to the survival probabilities of the other level of the same treatment. In line with our hypothesis, we found that short-term survival chances of eelgrass transplants treated with sediment stabilizing root mimics were +67% higher than the bare controls (GLME, beta = -1.11, 95% CI [-1.96, -0.26], p <.01) (Supplementary Table 1). The mean survival of the four different treatment combinations was similar at the same monitoring event, with mean survival peaking at 15%. At Zachte Bed Oost, treatment effects were visible after five weeks, with increased sediment stability resulting in increased survival. We found a highly significant interaction effect between transplantation time and study site, as shoots survived longer at Zachte Bed Oost (beta = 0.18, 95% CI [0.11, 0.25], p <.001) (Figure 4). Interestingly, the overall seagrass survival at Zachte Bed Oost was still higher after eight weeks than after five weeks at Vlakte van Kerken, so seagrass transplants survived for a more extended period at Zachte Bed Oost. After five weeks, we discovered that the survival rate of plants treated with the barriers at Vlakte van Kerken was merely 8% of the survival rate observed at Zachte Bed Oost. This suggests a stark difference in survival rates between the two locations, with Vlakte van Kerken showing lower plant survival relative to Zachte Bed Oost. Contrasting to our expectations, barriers decreased transplant survival probabilities by -74% (beta = -1.33, 95% CI [-2.29, -0.36], p <.01).
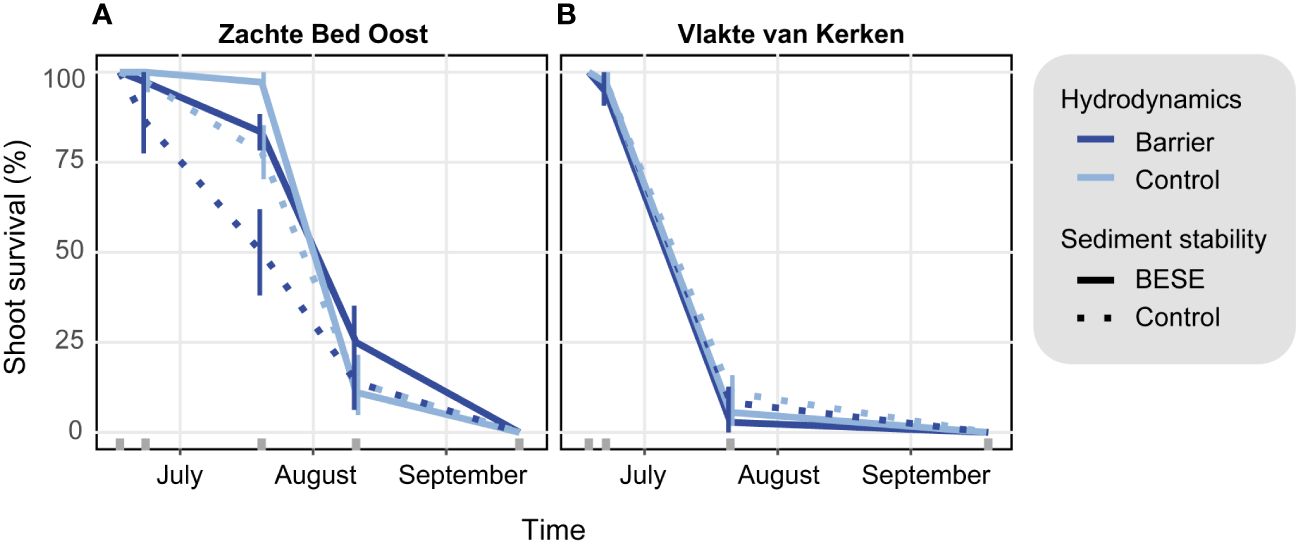
Figure 4 Relationship between experimental treatments and survival of subtidal eelgrass transplants throughout time for plants at (A) Zachte Bed Oost and (B) Vlakte van Kerken. Color indicates the hydrodynamic treatment, with red lines representing the hydrodynamic barrier treatment, while blue lines represent the hydrodynamic control treatment. Line type indicates the sediment stability treatment: Solid lines represent transplant survival of transplants treated with control sediment stability, and dotted lines increased sediment stability by adding BESE. Error bars represent standard errors, and gray ticks above the x-axis indicate monitoring events.
All seagrass shoots died in the course of three months after transplantation, so there was no long-term survival of the transplant units. We noticed that many shoots and leaves broke off at a straight edge, approximately 3 cm close to the ground, where leaves split from the leaf sheath (Supplementary Figures 1A, B). The remnants were often overgrown with epiphytes (see Supplementary Figure 1A for a representative photo) and/or discolored entirely. We found that the proportion of mortality caused by dislodgement was relatively low in most treatment groups (Supplementary Figure 2). At Vlakte van Kerken, the median percentage of mortality caused by dislodgement was 16.7%, while at Zachte Bed Oost, it was 4.2%. Interestingly, at Zachte Bed Oost, we recorded both the highest and lowest proportion of mortality due to uprooting. The BESE treatment without a barrier resulted in 0% mortality due to dislodgement, while the barrier treatment without BESE resulted in more than 30%.
3.2 Abiotic conditions
The abiotic conditions at both sites did not differ substantially. We found a tidal range of ~1.9 m at Zachte Bed Oost and ~1.7 m at Vlakte van Kerken. The median sediment grain sizes at the two study sites were 176 μm (SE = 2.4) and 174 μm (SE = 2.4), respectively. From June to August 2021, the average current flow velocities without barriers (16.15 cm s-1, SE = 0.09) were significantly higher at Zachte Bed Oost compared to the average at Vlakte van Kerken (14.72 cm s-1, SE = 0.09) (beta = 0.76, SE = 0.17, t(18426) = 4.51, p <.001). We recorded maximum current flow velocities of 42.63 cm s-1 and 42.21 cm s-1 and mean wave heights of 11.26 cm (SE = 0.11) and 8.55 cm (SE = 0.08) (Supplementary Table 2) at Zachte Bed Oost (Figure 5A) and Vlakte van Kerken (Figure 5B), respectively.
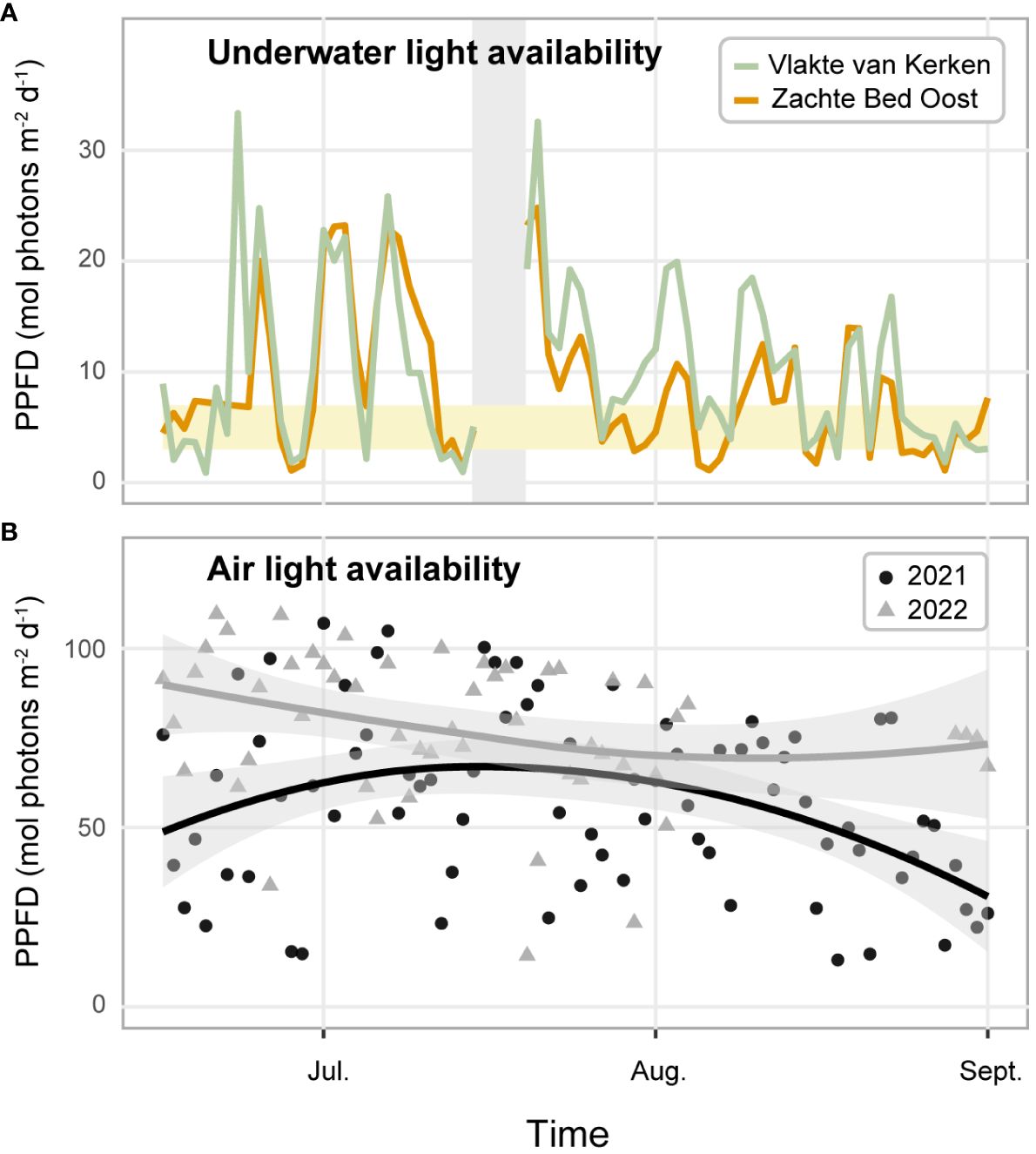
Figure 5 Maximum wave height (cm) throughout time at (A) Zachte Bed Oost and (B) Vlakte van Kerken. The dark blue graphs represent the maximum wave height measured behind a hydrodynamic barrier, while the light blue graphs depict measurements at the hydrodynamic controls.
We measured the light irradiance on land and underwater as Photosynthetic Photon Flux Density (PPFD), the sum of all recorded photosynthetically active radiation on a day. The land light irradiance when we conducted the experiment was relatively low, as the 2021 mean (55.32 mol photons m-2 day-1; SE = 2.75) was 28% lower than the 2022 mean (76.95 mol photons m-2 day-1; SE = 2.96) (Figure 6B). Our underwater measurements (Figure 6A) revealed that the monthly mean PPFD in June, July, and August 2021 at Zachte Bed Oost (7.0; 12.3; 5.9 mol photons m-2 d-1, respectively) and Vlakte van Kerken (9.0; 12.4; 9.2 mol photons m-2 d-1, respectively) laid well above the threshold for long-term survival of established meadows of 3 mol photons m-2 d-1. 75% of the recordings at Zachte Bed Oost and 86% of the records at Vlakte van Kerken exceeded the long-term survival threshold. Furthermore, measurements drew close to or exceeded the light requirement for light-saturated growth of 7 mol photons m-2 d-1 (Thom et al., 2008), as 48% and 58% exceeded the threshold for light-saturated growth at Zachte Bed Oost and Vlakte van Kerken, respectively (Figure 6A). It is important to address the difference between the measured and the actual light availability, as light-competing species (epiphytes and macroalgae) likely reduced the light availability (Sand-Jensen, 1977; Hauxwell et al., 2003).
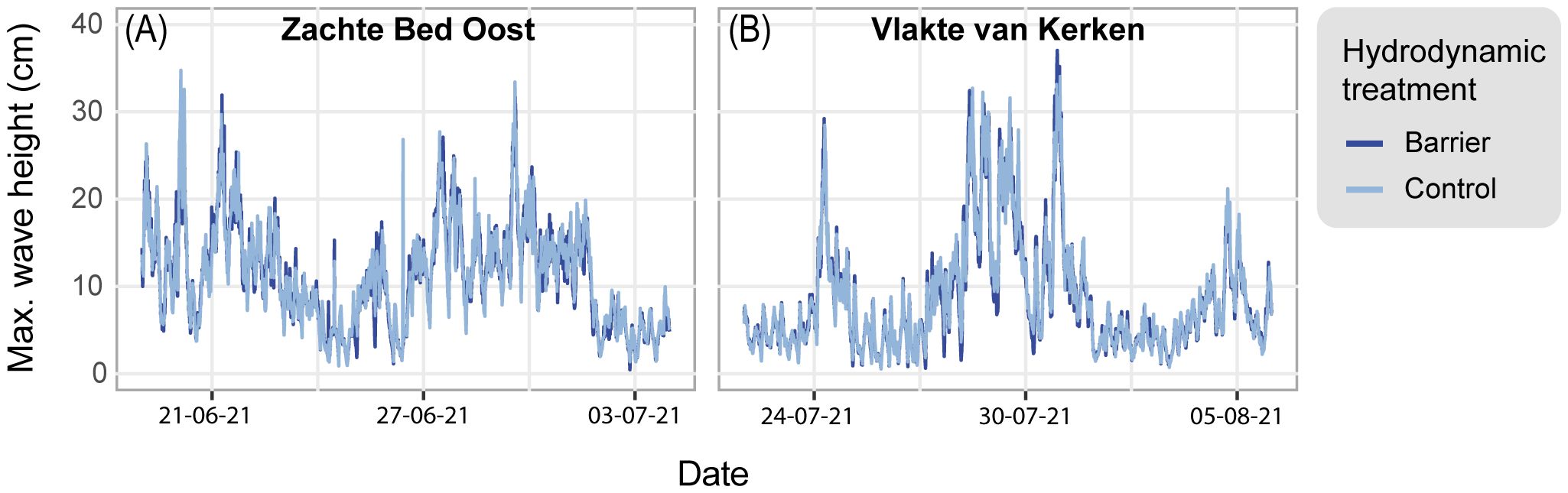
Figure 6 (A) Underwater light availability was measured as Photosynthetic Photon Flux Density (PPFD) (mol photons m-2 d-1) from June to August 2021 with measurements at Zachte Bed Oost (green line) and Vlakte van Kerken (orange line). The lower border of the bright orange ribbon represents the eelgrass’ light requirement for long-term survival (3 mol photons m-2 d-1), and the upper border the light requirement for light-saturated growth (7 mol photons m-2 d-1) (Thom et al., 2008). The light gray ribbon indicates where data has been filtered out due to biofouling. (B) Land light availability measured as Photosynthetic Photon Flux Density (PPFD) (mol photons m-2 d-1) from June to August 2021 (black triangles) and 2022 (gray triangles) on the Wadden Sea island Texel. The black and gray lines represent locally estimated scatterplot smoothing regressions with gray ribbons indicating 95% confidence intervals.
3.3 Biotic conditions
At Vlakte van Kerken, very high loads of macroalgae, predominantly sea lettuce (Ulva lactuca), grew and floated throughout the water column. Sea lettuce attached in very high quantities to the barriers, sensor poles (Supplementary Figure 3), and base of the seagrass transplant units. The high weight of the floating macroalgae suffocated the seagrass. At Zachte Bed Oost, only a small amount of floating macroalgae was present. In our drone picture (Figure 7), the quantity of macroalgae can be seen. It did not seem to affect the seagrass negatively.
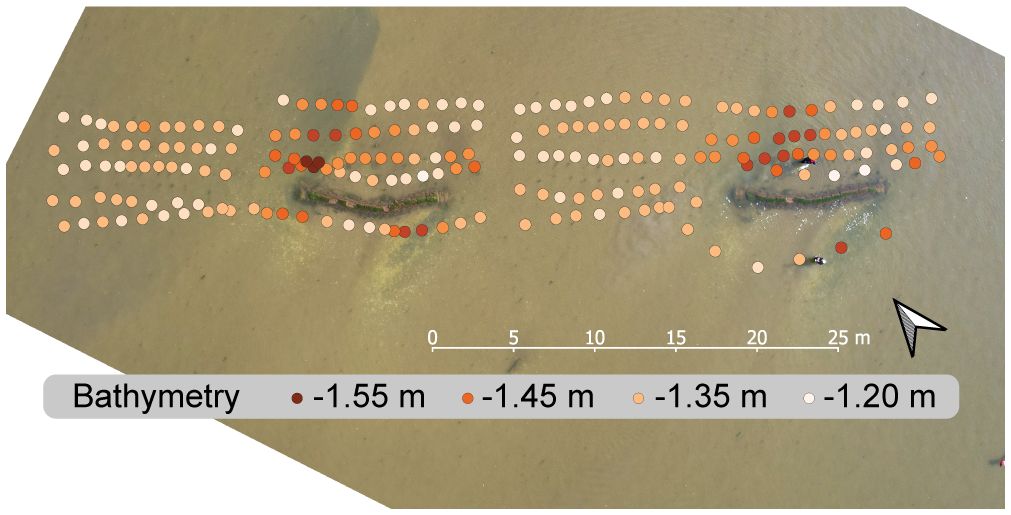
Figure 7 Drone picture of the experimental setup at Zachte Bed Oost, eight weeks after constructing the barriers with an overlying spatial layer depicting dGPS measurements of the bathymetry around the setup, with bathymetry shown in meters to MWL.
We quantified the epiphyte cover on seagrasses once at Vlakte van Kerken after five weeks and twice at Zachte Bed Oost after five and eight weeks. We found that after five weeks, at Vlakte van Kerken, the seagrass epiphyte cover was significantly higher than at Zachte Bed Oost (F1,1 = 25.96, p <.01). Here, only approximately half of the epiphyte cover was detected. Comparing the cover at Zachte Bed Oost at 5 and 8 weeks reveals no statistically detectable difference between these monitoring events (F1,1 = 2.78, p = .17) (Figure 8). Epiphyte volume (Supplementary Figure 1A) and weight added considerable drag to the plants leading to breakage of the leaves.
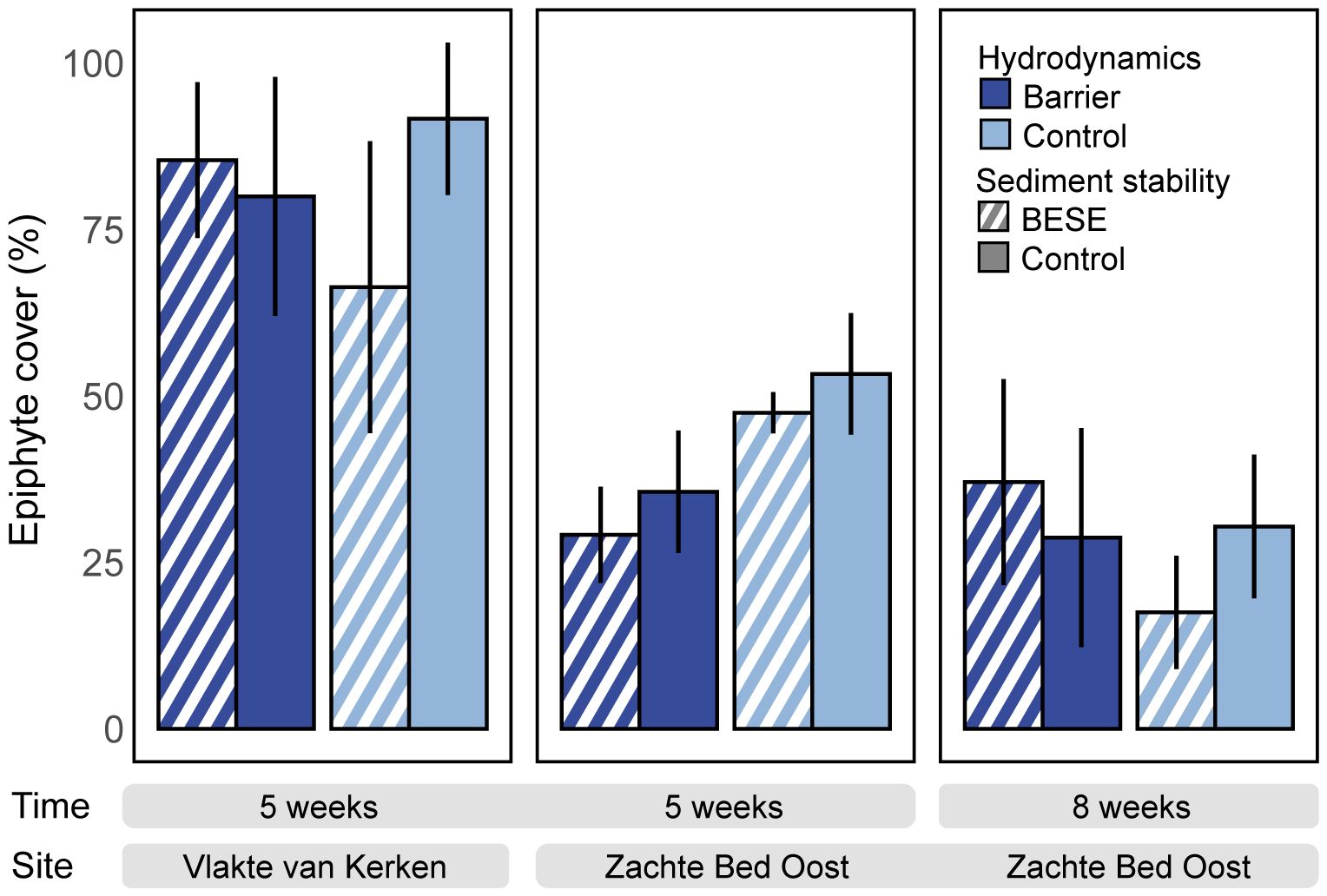
Figure 8 Percentage of the epiphyte cover on seagrass transplants. Dark blue bars represent the hydrodynamic barrier treatment, and bright blue is the hydrodynamic control treatment. Pattern fill (striped) indicates increased sediment stability (BESE), and no pattern fill (plain) is the control sediment stability treatment. Error bars represent standard errors.
3.4 Effect of the barriers
3.4.1 Effect of the barriers on hydrodynamics
The barriers significantly decreased the current flow velocities of the incoming tides, with mean reductions of -1.5% at Zachte Bed Oost and -1.3% at Vlakte van Kerken. Even though they were statistically significant, these reductions were probably not high enough to be ecologically relevant (beta = -6.91, SE = 0.17, t(18426) = -41.07, p <.001). The maximum decreases at Zachte Bed Oost and Vlakte van Kerken were -9.2% and -8.9%, respectively. The percentage of current flow reduction was correlated to the current flow velocity, with higher velocities being more reduced (Supplementary Figure 4).
At Zachte Bed Oost, the maximum wave heights were 24% higher (mean = 11.26 cm, SE = 0.11) than at Vlakte van Kerken (mean = 8.55 cm, SE = 0.08). At both sites, the barriers did not significantly decrease wave height (linear model, p = .15, Supplementary Tables 3, 4). This could also be seen in the almost identical intercept and slope of the fitted linear model in Supplementary Figure 5, which shows the difference in wave height with and without barrier throughout time. These results likely underestimate the barriers’ effect, as we only monitored the hydrodynamic conditions 3.4 m behind the barrier.
3.4.2 Effect of the barriers on sediment dynamics
We found a pronounced edge effect with dGPS bathymetry measurements eight weeks after planting; bathymetry was up to 14 cm deeper next to the barrier than surrounding sediment and controls in the experimental setup. This spatial bathymetry difference (Figure 7) likely resulted from erosion near the barriers’ edges. It seems likely that turbulence created by the sandbag edges, where current velocities were increased, had led to high erosion rates while sedimentation occurred behind the barriers. Repeated dGPS measurements from June and July at Zachte Bed Oost compared changes in bathymetry behind barriers (mean = 4.34 cm, SE = 0.94) to controls (mean = 0.98 cm, SE = 0.38). Unfortunately, we did not take repeated measurements at the edges of the barriers, where most erosion had occurred. We found that the change in bathymetry behind barriers was significantly higher than behind controls. For this, we used a Welch unequal variances t-test (t(22.40) = 3.315, p = .003). Furthermore, we noticed that the data variation was higher behind barriers, as bathymetry changes occurred here in a range of 17 cm. In comparison, the range behind controls was only 5.6 cm (Supplementary Figure 6).
There was no significant difference in the median sediment grain size at the two study sites (linear model, F1,21 = 0.02, p = .89) (Vlakte van Kerken: median = 172.84 μm, SE = 4.46; Zachte Bed Oost: median = 173.18 μm, SE = 7.52). At both sites, the median sediment grain size did not differ between hydrodynamic treatments (F1,21 = 3.23, p = .09). At Vlakte van Kerken, the barriers did not have a significant effect on the silt content (barriers: mean = 5.93%, SE = 1.70; controls: mean = 5.35%, SE = 0.62) (Chi2 = 0.23, df = 1, p = .63). Contrastingly, at Zachte Bed Oost, sediment behind barriers had a +44% higher silt content (mean = 9.71%, SE = 5.02) than at the controls (mean = 5.21%, SE = 2.09) (Chi2 = 5.03, df = 1, p = .02).
4 Discussion
For the first time since 1950 (Korringa, unpublished data), subtidal eelgrass (Zostera marina) was planted in the Dutch Wadden Sea, where this perennial variety has been absent for almost a century. We tested the potential of using restoration tools that mimic self-facilitating feedbacks to bridge settlement thresholds. In our study, we show that short-term survival (5 weeks) can be increased (+67%) by generating self-facilitating feedback through the application of belowground root mimics (BESE-elements). The hydrodynamic barriers decreased transplant survival (-74%). This decline was likely the result of enhanced sediment erosion created by turbulence. Vegetation survival up to a point where plants are established and can induce their own self-facilitating feedbacks is required (Campbell and Paling, 2003; Carus et al., 2022), and reintroducing the feedback of hydrodynamic stress reduction should therefore be further explored. Unfortunately, all transplants died within three months after transplanting. We recommend continuing to explore restoration possibilities through field studies. In disturbance-driven systems like the Wadden Sea, a combination of several possible bottlenecks, including yearly varying light availability, orbital velocities, and suboptimal sediment stability, makes shifting between stable states challenging (Balke et al., 2014).
4.1 Sediment stability increases short-term survival
When growing in low densities, sparse seagrass rhizome networks may not be able to stabilize the sediment (Suykerbuyk et al., 2016). When reintroducing this self-facilitating feedback with transplants in a bare system, very high quantities would be required to initiate the sediment-stabilizing feedback. Artificially inducing the same feedback can minimize the need for donor material (Temmink et al., 2020). Thus, the root-mimicking structures that we used for the sediment treatment were designed to induce this stabilizing feedback. Seagrass restoration can be aided by overcoming the settlement threshold of low sediment stability with BESE structures (Gagnon et al., 2021). Studies in Sweden and the US found increased restoration success for subtidal eelgrass transplants by applying the buried root mimics (Temmink et al., 2020; van der Heide et al., 2021). However, the restoration success can be conditional (van der Heide et al., 2021), as too little hydrodynamics rendered the BESE unnecessary, while too strong hydrodynamics overpowered the effect of the root mimics. Apparently, the hydrodynamic conditions in the Wadden Sea, where our study was carried out, lay within the windows where BESE is useful. It also means that if our barriers had worked in the anticipated way, the relative effect of the root mimics would likely have been smaller, and these measures have the potential to interact. Here, we found that BESE increased short-term survival probabilities, meaning that seagrass restoration success in the Dutch Wadden Sea may benefit from artificially induced sediment-stabilizing feedbacks.
4.2 Barriers do not relieve hydrodynamical stress
Contrary to our expectations, the 7-m hydrodynamic sandbag barriers did not have the intended effect of creating a calmer growth environment for seagrass transplants. Furthermore, many BESE-elements were washed clear, reversing their effect. The barriers were ineffective in reducing maximum wave height and reduced current velocities by less than 10%. Here, one should keep in mind that these values might be underestimated, as we only measured 3.4 m behind the barriers. Regardless, the barriers did not have their desired effect on seagrass survival, and their ameliorating effect was seemingly too low for ecological relevance. In a mussel reef restoration experiment in the Dutch Oosterschelde estuary, breakwaters did not increase the mussel coverage, probably because the barriers were too shallow or narrow to sufficiently reduce hydrodynamic stress (Schotanus et al., 2020). In our study, an increased barrier height might have asserted ecologically relevant hydrodynamic stress amelioration. Because the barriers deflected water, scour holes formed along the edges, likely due to locally increased current flow and resulting sediment transport. The pronounced edge effect would probably be relatively smaller if barriers were longer. Furthermore, an alternative barrier design might result in a lower edge effect, as, for instance, a semi-permeable barrier would not deflect as high volumes of water.
Installing functional underwater barriers is challenging in practice because of the high hydrodynamic forces they are supposed to ameliorate. Even though several attempts in close-by systems were made to establish structures targeted specifically at seagrass restoration, only a few results have been published. In the Danish estuary Vejle Fjord, barrier boulder reefs were installed to facilitate seagrass restoration within the ongoing “Sund Vejle Fjord” project. Similar to our observations, the barriers considerably affected sediment dynamics after installation. The effects on eelgrass restoration attempts cannot be quantified before a new steady state of the sediment dynamics is established (T. Banke, pers. comm., Feb. 7, 2024). In the Dutch Lake Grevelingen (R. Cronau, pers. comm., Feb. 9, 2024), the Dutch intertidal Wadden Sea (L. Govers, pers. comm., Jan. 24, 2024), and the German Baltic Sea (L. Kamperdicks & M. Paul, pers. comm., Feb. 1, 2024), underwater barriers were dislodged due to the hydrodynamic forcing. In the Dutch Lake Markermeer, a series of breakwaters were successfully used to relieve hydrodynamical stress and stimulate the growth of submerged macrophytes. However, this restoration effect occurred ten years after the installation, attributed to underwater light limitations (van Zuidam et al., 2022). These examples highlight the challenges and the necessity of applying restoration tools to reduce hydrodynamic stress below its limiting threshold.
The current velocity maxima for established eelgrass meadows range for instance between 0.5 – 1.8 m s-1 (Koch, 2001 and sources within) and 1.2 – 1.5 m s-1 (Fonseca et al., 1983). We conducted field measurements in the summer of 2022 and found mean current velocities of 0.27 m s-1 at Zachte Bed Oost and 0.26 m s-1 at Vlakte van Kerken, values well below the stated threshold. However, dislodgement of unanchored transplants may start at lower hydrodynamic threshold values of 0.16 – 0.23 m s-1 (Carus et al., 2022), as transplants may be more vulnerable to dislodgement and the adverse effects of high current speeds than established meadows. This was why our experimental design included, firstly, shoot anchorage and, secondly, the barrier treatment to mimic the hydrodynamic forcing reducing feedback of an established meadow. Even though the monitoring values during our experimental phase were lower than in 2022, the hydrodynamic stress, a combination of waves and currents, still seemed too high.
4.3 Biotics limit site suitability
Conducting transplantation efforts across different sites leads to spreading the risk, which is important in dynamic coastal systems like the Wadden Sea (van Katwijk et al., 2009). Our study further evidences this, as we found significant differences in survival between the two study sites. We showed that the survival probabilities when planted at Vlakte van Kerken were 77% of the survival probabilities at Zachte Bed Oost. Depending on the treatment, seagrass at Vlakte van Kerken declined from 100% to 3 – 11% in the course of only five weeks. The second site, Zachte Bed Oost, showed higher and longer short-term survival, but survival percentages dropped to similar lows three weeks later. While the abiotic conditions of both sites seem quite similar (Supplementary Table 2), the biotic conditions deviated strongly.
The presence of antagonistic species like fast-growing pleustophytic macroalgae can compromise the success of transplantation activities (Sfriso et al., 2023). Vlakte van Kerken functions as a basin where high macroalgae loads accumulate. The border to the next tidal basin is 2 km north of the site (Figure 1C), and 1.4 km to the west lies the barrier island Texel. Combined with the incoming tides from the south from the tidal basin “Marsdiep”, this leads to macroalgae accumulation. The algae biomass (predominantly Ulva lactuca) attached to the barriers and transplants (Supplementary Figure 3) seemed to have suffocated the seagrass. This observation aligns with previous studies in the Wadden Sea (Bos et al., 2004, 2005; van Katwijk et al., 2009). Other adverse effects of floating algae are competition for light (Hauxwell et al., 2001, 2003) and unfavorable biogeochemical conditions like lowered redox conditions and potentially toxic concentrations of ammonium (NH4+) (Hauxwell et al., 2001).
For our site selection, a system analysis was performed based on abiotic factors like bathymetry, sediment grain size, position within areas partially closed to fisheries, flow velocity, underwater light availability, and wave height. To effectively choose an optimal site, it is essential to consider not only abiotic factors but also to incorporate biotic data into the decision-making process. Next to floating and epiphytic macroalgae, examples of species that could affect seagrass habitat suitability in the Wadden Sea are bioturbators like blow lugworms (Arenicola marina) (Philippart, 1994), grazers like mud snails (Peringia ulvae) (Gräfnings et al., 2023a), and herbivores of seedlings like the European green crab (Carcinus maenas) (Infantes et al., 2016). Taking the occurrence and the species’ antagonistic or mutualistic effect into account could further improve effective site selection.
4.4 Understanding the lack of long-term survival
Even though we found treatment effects on short-term survival, no long-term survival occurred. When seagrasses are exposed to single or multiple stressors, the plants’ carbon budget undergoes alterations: the carbon acquisition can be lowered, and the reserves can be drained. Energy can be allocated to defense or repair processes instead of growth, reducing growth and increasing mortality (Moreno-Marín et al., 2018). Abiotic differences between donor and transplantation sites led to sudden changes in water composition (salinity mean and fluctuation) and light availability. The roots of the single shoots were physically disconnected from the surrounding nutrient-providing sediment. Using rhizome fragments, shoots were disconnected from their extensive rhizome network and access to energy through carbon storage. Furthermore, as the transplantation occurred relatively late in the growing season (i.e., mid-June), the timing probably also adversely affected the survival. Lastly, light was limited due to fairly low irradiance during the experimental period, with epiphytic growth on the seagrass leaves further limiting light availability. Especially at Vlakte van Kerken, the high floating and epiphytic macroalgae loads overshadowed the treatment effects of the barriers and root mimics. We hypothesize that the high exposure to cumulative stress caused seagrass die-off in the scope of this experiment.
4.4.1 Salinity
Restoring eelgrass is challenging in practice (van Katwijk et al., 2016). Donor seagrass plants should be recruited from populations in comparable environments (e.g., van Katwijk et al., 2009, 1998). Therefore, transplants are often harvested from meadows near the transplantation site (Moksnes et al., 2016). Alternatively, populations with resilient features can be used (McDonald et al., 2020). All subtidal eelgrass populations in the entire trilateral Wadden Sea are extinct. Therefore, the population from the Limfjord, a Danish sound with an inlet from the North Sea and located more than 600 km from the western Dutch Wadden Sea, is spatially the closest indirectly connected donor population. Here (22 PSU), the water is of a more comparable salinity to the Dutch Wadden Sea (28 PSU). However, COVID-19 border restrictions hindered access to the preferred Danish meadows. Using seagrass from the Baltic Sea (14 PSU) instead may have posed a limitation, as the salinity differed substantially, with the Dutch water being twice as saline. While it is well known from literature that Z. marina can tolerate an extensive range of salinities (e.g., Nejrup and Pedersen, 2008; Boström et al., 2014; Spalding et al., 2014), the sudden relocation from a brackish environment into a saline environment has most likely posed additional stress to the plants. If using plants from similar donor plants is impossible, an added phase in which plants can gradually acclimatize to a large salinity difference might increase the chances of long-term survival.
4.4.2 Transplantation technique
The anchored single-shoot method has succeeded in other systems, for instance, Horsens Fjord in Denmark (Lange et al., 2022) and Lake Grevelingen in the Netherlands (Cronau et al., 2022). In the former, donor and transplantation sites were nearby, which indicates environmental similarity. In the latter, the transplantation site seems less dynamic as the marine lake is wave-dominated, unlike the Wadden Sea, which is wave- and tide-dominated. We used metal nails as anchors to overcome the loss of the anchorage function of an intact root mat. Unanchored single-shoot dislodgement starts at current flow velocities of 16 cm s-1 (Carus et al., 2022). Average current flow velocities in our system were slightly below or at that threshold. Still, maximum velocities exceeded this threshold by more than three-fold (Supplementary Table 2). Anchorage, which is another form of reintroduced positive feedback, therefore seems necessary.
Five weeks after planting, we still found high numbers of transplant anchors where no surviving plants were detected (Supplementary Figure 2). We ascribed the reason for mortality in plots with no anchors to dislodgement. At Vlakte van Kerken, the mortality due to dislodgement (median = 16.7%) was almost 4-fold higher than at Zachte Bed Oost (median = 4.2%). This was probably the result of the high loads of floating macroalgae present at Vlakte van Kerken, as the floating biomass attached to the transplants added drag. At both sites, mortality due to dislodgement was lower than 25%, except for the bare sediment group at Zachte Bed Oost planted behind a barrier, where more than 30% of the mortality was ascribed to uprooting. Here, the high levels of erosion probably washed clear the transplant units. Simultaneously, high levels of sedimentation might have buried other transplants, including their anchors. Overall, most transplant anchors stayed put. This indicates that the anchoring process worked well despite the high levels of hydrodynamic forcing in the system.
Handling single shoots comes with certain challenges. Plants are disconnected from their belowground nutrient supply, and single rhizome fragments might be more vulnerable to physical damage than a network. Furthermore, seagrasses with large amounts of stored carbohydrates in their rhizomes can optimize their carbon balance by moving these energy resources to stressed shoots (Alcoverro et al., 1999). Therefore, the anchored single-shoot method may be unsuitable for a system as dynamic as the Wadden Sea. As multiple simultaneous stressors act upon vulnerable single shoots, an alternative transplantation technique might prolong survival in future restoration activities. Sediment-intact techniques like sods, cores, or plugs leave the root and rhizome system relatively unimpaired (Phillips, 1990; Fonseca et al., 1998), meaning that the positive feedback of anchorage is still maintained. Furthermore, transplanting with the plant’s intact rhizosphere might lead to more root biomass in the first two weeks (Wang et al., 2021), which is a critical time for the plant to establish.
4.4.3 Timing of the transplantation
The timing of transplantation plays a crucial role in seagrass restoration success and should be carefully considered when planning restoration activities (van Katwijk et al., 2009). Temperate seagrasses generally have a biomass peak during the growing season and a substantial decrease in biomass in winter (Duarte, 1989). Carbohydrates are accumulated and stored during periods with a positive carbon balance, which occurs typically in summer and autumn (Zimmerman and Alberte, 1996; Olivé et al., 2007; Vichkovitten et al., 2007). In the winter, less light availability leads to lower photosynthesis rates and, thus, to a reduced carbon gain. When the carbon demand through respiration and growth becomes negative (Alcoverro et al., 2001), seagrasses depend on the reserves in their rhizomes (Zimmerman et al., 1995; Alcoverro et al., 1999; Vichkovitten et al., 2007). According to these seasonal changes in energy reserves, we tried to harvest and transplant at the beginning of the growing season (i.e., late spring). Transplanting in this period might benefit the carbon balance, as the respiratory demand decreases at lower temperatures (Lee et al., 2007), and high growth rates might help the seagrass to recover faster from the transplantation (Govers et al., 2015). However, our efforts were postponed to June because we were restricted due to COVID-19 regulations and strict border restrictions. This late transplantation might have resulted in dependency on carbon reserves and might have contributed to the die-off once these reserves were depleted (Olesen and Sand-Jensen, 1993; Ralph et al., 2007; Silva et al., 2013). Plants might not have been able to gain a positive carbon balance due to the low light availability that was further decreased by epiphytes. It is difficult to unravel if the lack of long-term survival is due to fundamentally unsuitable conditions or if the conditions were temporarily unsuitable due to temporal variation in conditions.
4.4.4 Light limitations through irradiance and epiphytes
Water turbidity and resulting low light availability pose an establishment and survival limitation for eelgrass in general and smaller rhizome fragments in particular (Carr et al., 2010). Seagrasses have to maintain their belowground biomass, which embodies a large portion of non-photosynthetic tissue. This leads to a relatively high minimum light requirement (Kenworthy and Fonseca, 1996). Tidal and wind-induced currents often have a large impact on the water turbidity of shallow coastal environments and estuaries through the suspension of particles, together with phytoplankton in the water column (Postma, 1961; Colijn, 1982; Giesen et al., 1990a; Kemp et al., 2005; van der Heide et al., 2007). van der Heide et al. (2007) found that in the Wadden Sea, phytoplankton has a neglectable effect on turbidity, leaving suspended sediment as the main factor. Turbidity through sediment dynamics is mainly caused by sediment resuspension on the mudflats and sediment transport between the mudflats and channels (e.g., Postma, 1961; Janssen-Stelder, 2000; Christiansen et al., 2006). Seagrass effectively filters suspended sediment particles from the water column (Gacia et al., 2003). Therefore, a restored seagrass meadow in the Wadden Sea might improve water clarity to such an extent that it allows for sufficient light penetration, enabling a positive carbon balance. Seagrasses can improve the underwater light regime by trapping sediment particles (e.g., Barcelona et al., 2023) and filtering nutrients (e.g., Prystay et al., 2023).
Still, limitations in periods with low irradiance pose a severe threat, especially when combined with additional stressors. When we carried out the experiment, the light regime in 2021 (Figure 6B, in black) was relatively low. We measured -22% less photosynthetic photon flux density compared to the same period from mid-June to September 2022 (Figure 6B, in black). In the 1930s, the subtidal eelgrass die-off occurred in the Dutch Wadden Sea due to coinciding events. Dull and very dull growing seasons were recorded in 1931 and 1932. These reduced light conditions likely affected the vitality of eelgrass stands (Giesen et al., 1990b). Subsequently, in 1932, the turbidity increased due to the construction of the enclosure dam (Giesen et al., 1990b). This series of low light events coincided with the epidemic wasting disease (Den Hartog, 1987; Giesen et al., 1990a; Giesen, 1990c). Therefore, in the past, insufficient light during critical periods impaired seagrass survival.
Thresholds for the long-term survival of established eelgrass meadows range from 3 (Thom et al., 2008) to 3.7 mol photons m-2 day-1 (Léger-Daigle et al., 2022), and for light-saturated growth, 7 mol photons m-2 day-1 are required (Thom et al., 2008). It should be noted that the actual values for the used donor plants might deviate, as eelgrass can photo-acclimatize to local light regimes. The site-specific temperature influences the light demand, as increased water temperatures promote higher respiration relative to photosynthesis and decreased photosynthetic efficiency (Marsh et al., 1986; Dennison, 1987). Over 75% of the recorded days at both sites exceeded the 3 mol photons m-2 day-1 threshold. While these values suggest that light availability was high enough, it should be noted that the light requirements for adult transplants with short rhizome segments are higher compared to established meadows, as they cannot translocate energy reserves or carbohydrates to support stressed shoots (Harrison, 1978; Ralph et al., 2007). Furthermore, 58% of the light recordings reached or exceeded the light-saturated 7 mol photons m-2 day-1 mark at Vlakte van Kerken. At the same time, only less than half of the recordings (48%) at Zachte Bed Oost reached this mark. Transplantation likely imposes high stress levels on plants and might increase their light requirements (Moreno-Marín et al., 2018). The fact that the seagrass shoots were not established yet and could not fall back on energy reserves in extensive rhizome networks (Alcoverro et al., 1999) might have made them susceptible to low light conditions.
Another critical factor affecting light availability is the competition for light. Epiphytes can reduce photosynthetic rates by blocking carbon uptake and light intensity (Sand-Jensen, 1977). Therefore, the considerably high epiphytic growth on the seagrass leaves, especially at Vlakte van Kerken, likely reduced the light availability. Another known adverse effect of epiphytes on seagrasses is impeding radial oxygen loss into the sediment (Brodersen et al., 2015). We found almost double the epiphyte coverage on seagrass leaves at Vlakte van Kerken (+193%) compared to the coverage on the seagrass leaves at Zachte Bed Oost during the monitoring event after five weeks. High epiphyte loads at Vlakte van Kerken could indicate possibly stress-induced nutrient leakage of the plants, as nitrogen, carbon (McRoy and Goering, 1974), and phosphorous (Penhale and Thayer, 1980) can leak from seagrass leaves, where epiphytes take them up. Seagrass leaves with high loads of epiphyte cover were reported to become more brittle and break off (Heijs, 1985; Borowitzka and Lethbridge, 1989). The increased volume and weight exerted a considerable drag on the plants, seemingly contributing to the breakage of leaves (Supplementary Figure 1A). The cover at Zachte Bed Oost decreased within three weeks. However, this was likely related to the substantial decrease in seagrass biomass and the resulting decrease in growth medium for epiphytes during that period (Figure 8).
4.5 Lessons learned and implications for practice
Our study is an important first step towards restoring the once vast (~150 km2) subtidal eelgrass meadows in the Dutch Wadden Sea. This study identified several current bottlenecks and insights for knowledge-based decision-making for future restoration activities. We show that artificially reintroducing positive feedback, i.e., sediment stabilization, positively affected short-term survival and suggest incorporating measures that induce this feedback in future restoration activities. Even though the second treatment was unsuccessful in inducing the positive feedback of creating shelter from hydrodynamic forces, we remain convinced that reducing hydrodynamic forces could be an important measure to bridge establishment thresholds for seagrass (Temmink et al., 2020). The installation of inter- and subtidal barriers is challenging due to the high hydrodynamic forces they are designed to ameliorate (R. Cronau, pers. comm., Feb. 9, 2024; L. Govers, pers. comm., Jan. 24, 2024; L. Kamperdicks & M. Paul, pers. comm., Feb.1, 2024) and altered sediment dynamics (T. Banke, pers. comm., Feb. 7, 2024). The barriers need sufficient height and length to ameliorate the hydrodynamic forces to an ecologically relevant degree (Schotanus et al., 2020). A positive effect can be overshadowed by other bottlenecks like low light availability (van Zuidam et al., 2022) or the presence of antagonistic species (Sand-Jensen, 1977; Hauxwell et al., 2001). It should be further investigated if barriers with an adjusted design (e.g., altered height, length, permeability) can reduce hydrodynamic forcing to an ecologically relevant degree. Another aspect that requires further research, as the sudden translocation to a system with twice the salinity levels (14 vs. 28 PSU) likely posed additional stress, is whether donor plants can tolerate the Wadden Sea salinity fluctuations and if an acclimatization phase with gradual salinity increases promotes field survival.
We recommend lowering high cumulative stress levels by carefully considering the following aspects when planning future restoration activities. Firstly, an eelgrass population from a donor meadow with more similar abiotic Wadden Sea conditions should be used (van Katwijk et al., 2009). Secondly, the annual variations of carbon reserves (Vichkovitten et al., 2007) should be considered when planning transplantation activities. Lastly, we found that the site selection played a major role in the survival of seagrass transplants, as macroalgae (Supplementary Figure 3) and epiphytes (Figure 8; Supplementary Figure 1A) competed for light (Sand-Jensen, 1977; Hauxwell et al., 2001). They added drag and physical stress to the plants on one of our experimental sites (Vlakte van Kerken). This added drag resulted in higher dislodgement of the transplant units. To effectively choose an optimal site, it is essential to consider a habitat suitability map based on human impact and abiotic factors (Pogoda et al., 2023, 2020) and incorporate biotic data into the decision-making process.
To conclude, for seagrass restoration in the Wadden Sea, one should carefully consider 1) the reintroduction of positive feedbacks through restoration tools, 2) lowering high cumulative stress levels regarding donor population choice and timing, and 3) site selection, taking both local biotic (e.g., floating macroalgae) and abiotic conditions (e.g., light conditions) into account. Optimizing these restoration facets might lower the additive stress that eelgrass transplants face to the degree that allows long-term survival in the Dutch Wadden Sea.
Data availability statement
Original datasets are available in a publicly accessible repository: The original contributions presented in the study are publicly available. This data can be found here: https://doi.org/10.34894/XIHQEA.
Author contributions
KR, LG, TH, SH, and OF contributed to the conception and design of the study. KR, LG, SH, TH, OF, and KM conducted the fieldwork. KR performed the statistical analysis and wrote the first draft of the manuscript. All authors contributed to the article and approved the submitted version.
Funding
The author(s) declare financial support was received for the research, authorship, and/or publication of this article. The study was part of project “Waddentools: habitatheterogeniteit” (also known as “Waddenmozaïek”), registered under reference number WF2018–187059, and funded by the Waddenfonds, the Directorate General for Public Works and Water Management (Rijkswaterstaat) and the provinces of Noord-Holland, Fryslân and Groningen.
Acknowledgments
The authors thank Wim Jan Boon, Jouke van der Meulen, Willem-Anton Schagen, and Jouke Visser, skippers of the vessels Stern, Ambulant, Texel 96, and Harlingen 2, respectively, for logistic facilitation and support in the field. Marta Ferraro and Evaline van Weerlee are acknowledged for their help analyzing the sediment samples. We are grateful to Maite Vogel, Max Gräfnings, and all volunteers for assistance in the field and lab and to Hannah Charan-Dixon for constructive criticism of the manuscript. Lastly, we thank all three reviewers for their helpful comments and valuable suggestions that improved this manuscript.
Conflict of interest
Authors KD and WL were employed by the company Waardenburg Ecology (BESE).
The remaining authors declare that the research was conducted in the absence of any commercial or financial relationships that could be construed as a potential conflict of interest.
Publisher’s note
All claims expressed in this article are solely those of the authors and do not necessarily represent those of their affiliated organizations, or those of the publisher, the editors and the reviewers. Any product that may be evaluated in this article, or claim that may be made by its manufacturer, is not guaranteed or endorsed by the publisher.
Supplementary material
The Supplementary Material for this article can be found online at: https://www.frontiersin.org/articles/10.3389/fmars.2024.1253067/full#supplementary-material
Footnotes
- ^ Kamperdicks, L., Lattuada, M., O’ Corcora, T., Schlurmann, T., and Paul, M. (n.d). Resilience Against Waves: Enhancing Seagrass Restoration Success through Facilitators, Rooting Periods, and Plant Traits.
References
Alcoverro T., Manzanera M., Romero J. (2001). Annual metabolic carbon balance of the seagrass Posidonia oceanica: The importance of carbohydrate reserves. Mar. Ecol. Prog. Ser. 211, 105–116. doi: 10.3354/meps211105
Alcoverro T., Zimmerman R. C., Kohrs D. G., Alberte R. S. (1999). Resource allocation and sucrose mobilization in light-limited eelgrass Zostera marina. Mar. Ecol. Prog. Ser. 187, 121–131. doi: 10.3354/meps187121
Balke T., Herman P. M. J., Bouma T. J. (2014). Critical transitions in disturbance-driven ecosystems: Identifying windows of opportunity for recovery. J. Ecol. 102, 700–708. doi: 10.1111/1365-2745.12241
Barcelona A., Colomer J., Serra T. (2023). Spatial sedimentation and plant captured sediment within seagrass patches. Mar. Environ. Res. 188, 105997. doi: 10.1016/j.marenvres.2023.105997
Bates D., Mächler M., Bolker B., Walker S. (2015). Fitting linear mixed-effects models using lme4. J. Stat. Softw 67, 1–48. doi: 10.18637/jss.v067.i01
Borowitzka M., Lethbridge R. (1989). “Seagrass Epiphytes,” in Biology of Seagrasses: A Treatise on the Biology of Seagrasses with Special Reference to the Australian Region. Eds. Larkum A., McComb A., Shepherd S. (Elsevier, Amsterdam), 458–499.
Bos A., Dankers N., Groeneweg A., Hermus D., Jager Z., de Jong D., et al. (2005). “Eelgrass (Zostera marina L.) in the western Wadden Sea: monitoring, habitat suitability model, transplantations and communication,” in Proceedings ‘Dunes and Estuaries 2005’ – International Conference on Nature Restoration Practices in European Coastal Habitats. Eds. Herrier J.-L., Mees J., Salman A. S., van Nieuwenhuyse H., Dobbelaere I. (VLIZ Special Publication, Koksijde, Belgium), 95–109.
Bos A., Hermus D., Vugteveen P., van Katwijk M. (2004). Herintroductie van Zostera marina in de westelijke Waddenzee, (2002–2006). Resultatenrapportage 2004 (Nijmegen, Netherlands: University of Nijmegen, Department of environmental science).
Boström C., Baden S., Bockelmann A. C., Dromph K., Fredriksen S., Gustafsson C., et al. (2014). Distribution, structure and function of Nordic eelgrass (Zostera marina) ecosystems: Implications for coastal management and conservation. Aquat Conserv. 24, 410–434. doi: 10.1002/aqc.2424
Brodersen K. E., Lichtenberg M., Paz L. C., Kühl M. (2015). Epiphyte-cover on seagrass (Zostera marina L.) leaves impedes plant performance and radial O2 loss from the below-ground tissue. Front. Mar. Sci. 2. doi: 10.3389/fmars.2015.00058
Campbell M. L., Paling E. I. (2003). Evaluating vegetative transplant success in Posidonia australis: A field trial with habitat enhancement. Mar. Pollut. Bull. 46, 828–834. doi: 10.1016/S0025-326X(03)00093-6
Carr J., D’Odorico P., McGlathery K., Wiberg P. (2010). Stability and bistability of seagrass ecosystems in shallow coastal lagoons: Role of feedbacks with sediment resuspension and light attenuation. J. Geophys Res. Biogeosci 115, 1-14. doi: 10.1029/2009JG001103
Carus J., Arndt C., Bouma T. J., Schröder B., Paul M. (2022). Effect of artificial seagrass on hydrodynamic thresholds for the early establishment of Zostera marina. J. Ecohydraulics 7, 17–27. doi: 10.1080/24705357.2020.1858197
Carus J., Arndt C., Schröder B., Thom M., Villanueva R., Paul M. (2021). Using artificial seagrass for promoting positive feedback mechanisms in seagrass restoration. Front. Mar. Sci. 8. doi: 10.3389/fmars.2021.546661
Christianen M. J. A., van Belzen J., Herman P. M. J., van Katwijk M. M., Lamers L. P. M., van Leent P. J. M., et al. (2013). Low-canopy seagrass beds still provide important coastal protection services. PloS One 8, 1-8. doi: 10.1371/journal.pone.0062413
Christiansen C., Vølund G., Lund-Hansen L. C., Bartholdy J. (2006). Wind influence on tidal flat sediment dynamics: Field investigations in the Ho Bugt, Danish Wadden Sea. Mar. Geol 235, 75–86. doi: 10.1016/j.margeo.2006.10.006
Colijn F. (1982). Light absorption in the waters of the Ems-Dollard estuary and its consequences for the growth of phytoplankton and microphytobenthos. Netherlands J. Sea Res. 15, 196–216. doi: 10.1016/0077-7579(82)90004-7
Cronau R. J. T., de Fouw J., van Katwijk M. M., Bouma T. J., Heusinkveld J. H. T., Hoeijmakers D., et al. (2022). Seed- versus transplant-based eelgrass (Zostera marina L.) restoration success in a temperate marine lake. Restor. Ecol 31, 1-10. doi: 10.1111/rec.13786
Cullen-Unsworth L. C., Nordlund L. M., Paddock J., Baker S., McKenzie L. J., Unsworth R. K. F. (2014). Seagrass meadows globally as a coupled social-ecological system: implications for human wellbeing. Mar. Pollut. Bull. 83, 387–397. doi: 10.1016/j.marpolbul.2013.06.001
de Jonge V. N., de Jong D. J. (1992). Role of tide, light and fisheries in the decline of Zostera marina L. in the Dutch Wadden Sea. Biol. Conserv. 65, 188. doi: 10.1016/0006–3207(93)90478-J
de Jonge V. N., de Jong D. J., van den Bergs J. (1996). Reintroduction of eelgrass (Zostera marina) in the Dutch Wadden Sea; review of research and suggestions for management measures. J. Coast. Conserv. 2, 149–158. doi: 10.1007/BF02905200
de Kruif A. C. (2001). Bodemdieptegegevens van het Nederlandse kustsysteem, beschikbare digitale data en een overzicht van aanvullende analoge data (Netherlands: Ministerie van Verkeer en Waterstaat, Rijkswaterstaat, Rijksinstituut voor Kust en Zee (RWS, RIKZ)).
de los Santos C. B., Krause-Jensen D., Alcoverro T., Marbà N., Duarte C. M., van Katwijk M. M., et al. (2019). Recent trend reversal for declining European seagrass meadows. Nat. Commun 10, 1-8. doi: 10.1038/s41467-019-11340-4
de los Santos C. B., Olivé I., Moreira M., Silva A., Freitas C., Araújo Luna R., et al. (2020). Seagrass meadows improve inflowing water quality in aquaculture ponds. Aquaculture 528, 1-9. doi: 10.1016/j.aquaculture.2020.735502
Den Hartog C. (1987). “Wasting disease” and other dynamic phenomena in Zostera beds. Aquat Bot. 27, 3–14. doi: 10.1016/0304-3770(87)90082-9
den Hartog C., Polderman P. J. G. (1975). Changes in the seagrass populations of the Dutch Waddenzee. Aquat Bot. 1, 141–147. doi: 10.1016/0304-3770(75)90019-4
Dennison W. C. (1987). Effects of light on seagrass photosynthesis, growth and depth distribution. Aquat Bot. 27, 15–26. doi: 10.1016/0304-3770(87)90083-0
Duarte C. (1989). Temporal biomass variability and production/biomass relationships of seagrass communities. Mar. Ecol. Prog. Ser. 51, 269–276. doi: 10.3354/meps051269
Duffy J. (2006). Biodiversity and the functioning of seagrass ecosystems. Mar. Ecol. Prog. Ser. 311, 233–250. doi: 10.3354/meps311233
Dunic J. C., Brown C. J., Connolly R. M., Turschwell M. P., Côté I. M. (2021). Long-term declines and recovery of meadow area across the world’s seagrass bioregions. Glob Chang Biol. 27, 4096–4109. doi: 10.1111/gcb.15684
Fivash G. S., Temmink R. J. M., D’Angelo M., van Dalen J., Lengkeek W., Didderen K., et al. (2021). Restoration of biogeomorphic systems by creating windows of opportunity to support natural establishment processes. Ecol. Appl. 31, 1-16. doi: 10.1002/eap.2333
Fonseca M. S., Cahalan J. A. (1992). A preliminary evaluation of wave attenuation by four species of seagrass. Estuar. Coast. Shelf Sci. 35, 565–576. doi: 10.1016/S0272-7714(05)80039-3
Fonseca M. S., Fisher J. S., Zieman J. C., Thayerd G. W. (1982). Influence of the Seagrass, Zostera marina L., on current flow. Estuar. Coast. Shelf Sci. 15, 351–364. doi: 10.1016/0272-7714(82)90046-4
Fonseca M. S., Kenworthy W. J., Thayer G. W. (1998). Guidelines for the conservation and restoration of seagrasses in the United States and adjacent waters. NOAA Coast. Ocean Program 12, 113-115.
Fonseca M. S., Zieman J. C., Thayer G. W., Fisher J. S. (1983). The role of current velocity in structuring eelgrass (Zostera marina L.) meadows. Estuar. Coast. Shelf Sci. 17, 367–380. doi: 10.1016/0272-7714(83)90123-3
Gacia E., Duarte C. M. (2001). Sediment retention by a mediterranean posidonia oceanica meadow: the balance between deposition and resuspension. Estuar. Coast. Shelf Sci. 52, 505–514. doi: 10.1006/ecss.2000.0753
Gacia E., Duarte C. M., Marbà N., Terrados J., Kennedy H., Fortes M. D., et al. (2003). Sediment deposition and production in SE-Asia seagrass meadows. Estuar. Coast. Shelf Sci. 56, 909–919. doi: 10.1016/S0272-7714(02)00286-X
Gagnon K., Christie H., Didderen K., Fagerli C. W., Govers L. L., Gräfnings M. L. E., et al. (2021). Incorporating facilitative interactions into small-scale eelgrass restoration—challenges and opportunities. Restor. Ecol. 29, 1-11. doi: 10.1111/rec.13398
Gann G. D., McDonald T., Walder B., Aronson J., Nelson C. R., Jonson J., et al. (2019). International principles and standards for the practice of ecological restoration. Second edition. Restor. Ecol. 27, S1–S46. doi: 10.1111/rec.13035
Giesen W. B. (1990c). Wasting disease and present eelgrass condition (Nijmegen: University of Nijmegen, Laboratory of aquatic ecology).
Giesen W. B., van Katwijk M. M., den Hartog C. (1990a). Temperature, salinity, insolation and wasting disease of eelgrass (Zostera marina L.) in the Dutch Wadden Sea in the 1930’s. Netherlands J. Sea Res. 25, 395–404. doi: 10.1016/0077-7579(90)90047-K
Giesen W. B., van Katwijk M. M., den Hartog C. (1990b). Eelgrass condition and turbidity in the Dutch Wadden Sea. Aquat Bot. 37, 71–85. doi: 10.1016/0304-3770(90)90065-S
Govers L. L., Suykerbuyk W., Hoppenreijs J. H. T., Giesen K., Bouma T. J., Van Katwijk M. M. (2015). Rhizome starch as indicator for temperate seagrass winter survival. Ecol. Indic 49, 53–60. doi: 10.1016/j.ecolind.2014.10.002
Gräfnings M. L. E., Govers L. L., Heusinkveld J. H. T., Silliman B. R., Smeele Q., Valdez S. R., et al. (2023a). Macrozoobenthos as an indicator of habitat suitability for intertidal seagrass. Ecol. Indic 147, 1-9. doi: 10.1016/j.ecolind.2023.109948
Gräfnings M. L. E., Heusinkveld J. H. T., Hijner N., Hoeijmakers D. J. J., Smeele Q., Zwarts M., et al. (2023b). Spatial design improves efficiency and scalability of seed-based seagrass restoration. J. Appl. Ecol 00, 1–11. doi: 10.1111/1365–2664.14405
Harrison P. G. (1978). Patterns of uptake and translocation of 14C by Zostera americana. Aquat Bot. 5, 93–97. doi: 10.1016/0304-3770(78)90050-5
Hauxwell J., Cebrián J., Furlong C., Valiela I. (2001). Macroalgal canopies contribute to eelgrass (Zostera marina) decline in temperate estuarine ecosystems. Ecology 82, 1007–1022. doi: 10.1890/0012-9658(2001)082[1007:MCCTEZ]2.0.CO;2
Hauxwell J., Cebrián J., Valiela I. (2003). Eelgrass Zostera marina loss in temperate estuaries: Relationship to land-derived nitrogen loads and effect of light limitation imposed by algae. Mar. Ecol. Prog. Ser. 247, 59–73. doi: 10.3354/meps247059
Havinga B. (1954). Veranderingen in de flora en fauna van de Zuiderzee (thans Ijsselmeer) na de afsluiting in 1932. Ed. Beaufort L. (Vissen. Den Helder:Nederlandse Dierkundige Vereniging), 253–267.
Heijs F. M. L. (1985). The seasonal distribution and community structure of the epiphytic algae on Thalassia hemprichii (Ehrenb.) Aschers. from Papua New Guinea. Aquat Bot. 21, 295–324. doi: 10.1016/0304–3770(85)90074–9
Hemminga M. A., Duarte C. M. (2000). Seagrass Ecology (The Pitt Building, Trumpington Street, Cambridge, United Kingdom: Cambridge University Press). doi: 10.1017/CBO9780511525551
Infantes E., Crouzy C., Moksnes P. O. (2016). Seed predation by the shore crab carcinus maenas: A positive feedback preventing eelgrass recovery? PloS One (Sweden: Swedish Agency for Marine and Water Management) 11, 1-19. doi: 10.1371/journal.pone.0168128
Janssen-Stelder B. (2000). The effect of different hydrodynamic conditions on the morphodynamics of a tidal mudflat in the Dutch Wadden Sea. Cont Shelf Res. 20, 1461–1478. doi: 10.1016/S0278-4343(00)00032-7
Jones C. G., Lawton J. H., Shachak M. (1994). Organisms as ecosystem engineers. Oikos 69, 373. doi: 10.2307/3545850
Kemp W., Boynton W., Adolf J., Boesch D., Boicourt W., Brush G., et al. (2005). Eutrophication of Chesapeake Bay: historical trends and ecological interactions. Mar. Ecol. Prog. Ser. 303, 1–29. doi: 10.3354/meps303001
Kenworthy W. J., Fonseca M. S. (1996). Light Requirements of Seagrasses Halodule wrightii and Syringodium filiforme Derived from the Relationship between Diffuse Light Attenuation and Maximum Depth Distribution. Estuaries 19, 740. doi: 10.2307/1352533
Koch E. W. (2001). Beyond light: physical, geological, and geochemical parameters as possible submersed aquatic vegetation habitat requirements. Estuaries 24, 1. doi: 10.2307/1352808
Lacy J. R., Wyllie-Echeverria S. (2011). The influence of current speed and vegetation density on flow structure in two macrotidal eelgrass canopies. Limnology Oceanography: Fluids Environments 1, 38–55. doi: 10.1215/21573698-1152489
Lange T., Oncken N. S., Svane N., Steinfurth R. C., Kristensen E., Flindt M. R. (2022). Large-scale eelgrass transplantation: a measure for carbon and nutrient sequestration in estuaries. Mar. Ecol. Prog. Ser. 685, 97–109. doi: 10.3354/meps13975
Lee K. S., Park S. R., Kim Y. K. (2007). Effects of irradiance, temperature, and nutrients on growth dynamics of seagrasses: A review. J. Exp. Mar. Biol. Ecol 350, 144–175. doi: 10.1016/j.jembe.2007.06.016
Léger-Daigle R., Noisette F., Bélanger S., Cusson M., Nozais C. (2022). Photoacclimation and light thresholds for cold temperate seagrasses. Front. Plant Sci. 13. doi: 10.3389/fpls.2022.805065
Luhar M., Infantes E., Nepf H. (2017). Seagrass blade motion under waves and its impact on wave decay. J. Geophys Res. Oceans 122, 3736–3752. doi: 10.1002/2017JC012731
Marin-Diaz B., Bouma T. J., Infantes E. (2020). Role of eelgrass on bed-load transport and sediment resuspension under oscillatory flow. Limnol Oceanogr 65, 426–436. doi: 10.1002/lno.11312
Marin-Diaz B., Fivash G. S., Nauta J., Temmink R. J. M., Hijner N., Reijers V. C., et al. (2021). On the use of large-scale biodegradable artificial reefs for intertidal foreshore stabilization. Ecol. Eng. 170, 1-9. doi: 10.1016/j.ecoleng.2021.106354
Marsh J. A., Dennison W. C., Alberte R. S. (1986). Effects of temperature on photosynthesis and respiration in eelgrass (Zostera marina L.). Mar. Biol. Ecol 101, 257-267. doi: 10.1016/0022-0981(86)90267-4
Martin P., Sébastien D., Gilles T., Isabelle A., de Montaudouin X., Emery É, et al. (2010). Long-term evolution, (1988–2008) of Zostera spp. meadows in Arcachon Bay (Bay of Biscay). Estuar. Coast. Shelf Sci. 87, 357–366. doi: 10.1016/j.ecss.2010.01.016
Maxwell P. S., Eklöf J. S., van Katwijk M. M., O’Brien K. R., de la Torre-Castro M., Boström C., et al. (2017). The fundamental role of ecological feedback mechanisms for the adaptive management of seagrass ecosystems – a review. Biol. Rev. 92, 1521–1538. doi: 10.1111/brv.12294
McDonald A. M., Christiaen B., Major K. M., Cebrian J. (2020). The influence of seagrass donor source on small-scale transplant resilience. Aquat Conserv. 30, 730–742. doi: 10.1002/aqc.3283
McGlathery K. J., Sundbäck K., Anderson I. C. (2007). Eutrophication in shallow coastal bays and lagoons: The role of plants in the coastal filter. Mar. Ecol. Prog. Ser 348, 1–18. doi: 10.3354/meps07132
McRoy P. C., Goering J. J. (1974). Nutrient transfer between the seagrass Zostera marina and its epiphytes. Nature 248, 173–174. doi: 10.1038/248173a0
Moksnes P., Gipperth L., Eriander L., Laas K., Cole S., Infantes E. (2016). Handbook for restoration of eelgrass in Sweden - National guideline. (Sweden: Swedish Agency for Marine and Water Management).
Moreno-Marín F., Brun F. G., Pedersen M. F. (2018). Additive response to multiple environmental stressors in the seagrass Zostera marina L. Limnol Oceanogr 63, 1528–1544. doi: 10.1002/lno.10789
Nejrup L. B., Pedersen M. F. (2008). Effects of salinity and water temperature on the ecological performance of Zostera marina. Aquat Bot. 88, 239–246. doi: 10.1016/j.aquabot.2007.10.006
Nordlund L. M., Koch E. W., Barbier E. B., Creed J. C. (2016). Seagrass ecosystem services and their variability across genera and geographical regions. PloS One 11, 1-23. doi: 10.1371/journal.pone.0163091
Olesen B., Sand-Jensen K. (1993). Seasonal acclimatization of eelgrass Zostera marina growth to light. Mar. Ecol. Prog. Ser. 94, 91–99. doi: 10.3354/meps094091
Olivé I., Brun F. G., Vergara J. J., Pérez-Lloréns J. L. (2007). Effects of light and biomass partitioning on growth, photosynthesis and carbohydrate content of the seagrass Zostera noltii Hornem. J. Exp. Mar. Biol. Ecol. 345, 90–100. doi: 10.1016/j.jembe.2007.02.008
Ondiviela B., Losada I. J., Lara J. L., Maza M., Galván C., Bouma T. J., et al. (2014). The role of seagrasses in coastal protection in a changing climate. Coast. Eng. 87, 158–168. doi: 10.1016/j.coastaleng.2013.11.005
Orth R. J., Carruthers T. J. B., Dennison W. C., Duarte C. M., Fourqurean J. W., Heck K. L., et al. (2006). A global crisis for seagrass ecosystems. Bioscience 56, 987–996. doi: 10.1641/0006-3568(2006)56[987:AGCFSE]2.0.CO;2
Oudemans C. A. J. A., Conrad J. F. W., Maats P., Bouricius L. J. (1870). "Verslag der Staatscommissie inzake de wiermaayerij," in Verslag aan den Koning over de Openbare Werken in het Jaar 1869. Algemeene Landsdrukkerij, ’s-Gravenhage. pp. 199-231.
Penhale P. A., Thayer G. W. (1980). Uptake and transfer of carbon and phosphorus by eelgrass (Zostera marina L.) and its epiphytes. Mar. Biol. Ecol. 42, 113–123. doi: 10.1016/0022-0981(80)90170-7
Philippart C. J. M. (1994). Interactions between Arenicola marina and Zostera noltii on a tidal flat in the Wadden Sea. Mar. Ecol. Prog. Ser. 111, 251–257. doi: 10.3354/meps111251
Phillips R. C. (1990). “Transplant methods,” in Seagrass Research Methods. Eds. Phillips R. C., McRoy C. P. (United Nations Educational, Scientific and Cultural Organization, Paris), 51–54.
Pinheiro J., Bates D., DebRoy S., Sarkar D., Heisterkamp S., Van Willigen B., et al. (2023). Fit and compare Gaussian linear and nonlinear mixed-effects models.
Pogoda B., Hausen T., Rothe M., Bakker F., Hauser S., Colsoul B., et al. (2023). Come, tell me how you live: Habitat suitability analysis for Ostrea edulis restoration. Aquat Conserv. 33, 678–695. doi: 10.1002/aqc.3928
Pogoda B., Merk V., Colsoul B., Hausen T., Peter C., Pesch R., et al. (2020). Site selection for biogenic reef restoration in offshore environments: The Natura 2000 area Borkum Reef Ground as a case study for native oyster restoration. Aquat Conserv. 30, 2163–2179. doi: 10.1002/aqc.3405
Postma H. (1961). Transport and accumulation of suspended matter in the Dutch Wadden Sea. Netherlands J. Sea Res. 1, 148–190. doi: 10.1016/0077-7579(61)90004-7
Prystay T. S., Sipler R. E., Foroutani M. B., Le Bris A. (2023). The role of boreal seagrass meadows in the coastal filter. J. Geophys Res. Biogeosci 128, 1-16. doi: 10.1029/2023JG007537
Ralph P. J., Durako M. J., Enríquez S., Collier C. J., Doblin M. A. (2007). Impact of light limitation on seagrasses. J. Exp. Mar. Biol. Ecol. 350, 176–193. doi: 10.1016/j.jembe.2007.06.017
Rasmussen E. (1977). “The wasting disease of eelgrass (Zostera marina) and its effects on environmental factors and fauna,” in Seagrass Ecosystems: A Scientific Perspective. Eds. McRoy C., Helfferich C. (Marcel Dekker, Inc., New York), 1–51.
Sand-Jensen K. (1977). Effect of epiphytes on eelgrass photosynthesis. Aquat Bot. 3, 55–63. doi: 10.1016/0304-3770(77)90004-3
Schaper A. (1962). De Ijsselmeervisserij (Kemink en Zoon NV, Utrecht, the Netherlands: University of Amsterdam).
Schotanus J., Capelle J. J., Paree E., Fivash G. S., van de Koppel J., Bouma T. J. (2020). Restoring mussel beds in highly dynamic environments by lowering environmental stressors. Restor. Ecol. 28, 1124–1134. doi: 10.1111/rec.13168
Sfriso A. A., Sciuto K., Mistri M., Munari C., Juhmani A.-S., Buosi A., et al. (2023). Where, when, how and what seagrass to transplant for long lasting results in transitional water systems: the cases of Cymodocea nodosa, Zostera marina, Zostera noltei and Ruppia cirrhosa. Front. Mar. Sci 10. doi: 10.3389/fmars.2023.1299428
Silva J., Barrote I., Costa M. M., Albano S., Santos R. (2013). Physiological responses of Zostera marina and Cymodocea nodosa to light-limitation stress. PloS One 8, 1-9. doi: 10.1371/journal.pone.0081058
Smaldino P. E. (2014). The cultural evolution of emergent group-level traits. Behav. Brain Sci. 37, 243–254. doi: 10.1017/S0140525X13001544
Spalding M., Taylor M., Ravilious C., Short F., Green E. (2014). The distribution and status of seagrasses, in: World Atlas of Seagrasses (California, USA: University of California Press), 5–26.
Suding K. N., Gross K. L., Houseman G. R. (2004). Alternative states and positive feedbacks in restoration ecology. Trends Ecol. Evol. 19, 46-53. doi: 10.1016/j.tree.2003.10.005
Suykerbuyk W., Bouma T. J., van der Heide T., Faust C., Govers L. L., Giesen W. B. J. T., et al. (2012). Suppressing antagonistic bioengineering feedbacks doubles restoration success. Ecol. Appl. 22, 1224–1231. doi: 10.1890/11–1625.1
Suykerbuyk W., Govers L. L., Bouma T. J., Giesen W. B. J. T., de Jong D. J., van de Voort R., et al. (2016). Unpredictability in seagrass restoration: analysing the role of positive feedback and environmental stress on Zostera noltii transplants. J. Appl. Ecol. 53, 774–784. doi: 10.1111/1365-2664.12614
Temmink R. J. M., Christianen M. J. A., Fivash G. S., Angelini C., Boström C., Didderen K., et al. (2020). Mimicry of emergent traits amplifies coastal restoration success. Nat. Commun. 11, 1-9. doi: 10.1038/s41467-020-17438-4
Thijsse J. (1972). Een halve eeuw Zuiderzeewerken 1920 -1970, Hydraulic Engineering Reports (the Netherlands: Groningen).
Thom R. M., Southard S. L., Borde A. B., Stoltz P. (2008). Light requirements for growth and survival of eelgrass (Zostera marina L.) in pacific northwest (USA) estuaries. Estuaries Coasts 31, 969–980. doi: 10.1007/s12237-008-9082-3
Unsworth R. K. F., Nordlund L. M., Cullen-Unsworth L. C. (2019). Seagrass meadows support global fisheries production. Conserv. Lett. 12, 1-8. doi: 10.1111/conl.12566
van den Hoek C., Admiraal W., Colijn F., de Jonge V. (1979). “The role of algae and seagrasses in the ecosystem of the Wadden Sea, a review,” in Flora and Vegetation of the Wadden Sea. Ed. Wolff W. (Wadden Sea Working Group, Leiden), 9–118.
van der Heide T., Temmink R. J. M., Fivash G. S., Bouma T. J., Boström C., Didderen K., et al. (2021). Coastal restoration success via emergent trait-mimicry is context dependent. Biol. Conserv. 264, 1-9. doi: 10.1016/j.biocon.2021.109373
van der Heide T., van Katwijk M. M., Geerling G. W. (2006). Een verkenning van de groeimogelijkheden van ondergedoken Groot zeegras (Zostera marina) in de Nederlandse Waddenzee (Netherlands: Ministerie van Verkeer en Waterstaat, Rijkswaterstaat, Rijksinstituut voor Kust en Zee (RWS, RIKZ)).
van der Heide T., van Nes E. H., Geerling G. W., Smolders A. J. P., Bouma T. J., Van Katwijk M. M. (2007). Positive feedbacks in seagrass ecosystems: Implications for success in conservation and restoration. Ecosystems 10, 1311–1322. doi: 10.1007/s10021-007-9099-7
van der Heide T., van Nes E. H., van Katwijk M. M., Olff H., Smolders A. J. P. (2011). Positive feedbacks in seagrass ecosystems - Evidence from large-scale empirical data. PloS One 6, 1-7. doi: 10.1371/journal.pone.0016504
van Goor A. (1919). Het zeegras (Zostera marina L.) en zijn beteekenis voor het leven der visschen. Rapp Verh RijksinstVisscherij I. (Ijmuiden, Natherlands: Rijksinstituut voor Visscherijonderzoek), 415–498.
van Katwijk M. M., Bos A. R., de Jonge V. N., Hanssen L. S. A. M., Hermus D. C. R., de Jong D. J. (2009). Guidelines for seagrass restoration: Importance of habitat selection and donor population, spreading of risks, and ecosystem engineering effects. Mar. Pollut. Bull. 58, 179–188. doi: 10.1016/j.marpolbul.2008.09.028
van Katwijk M. M., Schmitz G. H., Hanssen L. S., den Hartog C. (1998). Suitability of Zostera marina populations for transplantation to the Wadden Sea as determined by a mesocosm shading experiment. Aquat. Bot 60, 283–305. doi: 10.1016/S0304-3770(98)00053-9
van Katwijk M. M., Thorhaug A., Marbà N., Orth R. J., Duarte C. M., Kendrick G. A., et al. (2016). Global analysis of seagrass restoration: The importance of large-scale planting. J. Appl. Ecol. 53, 567–578. doi: 10.1111/1365-2664.12562
van Katwijk M. M., van Tussenbroek B. I. (2023). Facultative annual life cycles in seagrasses. Plants 12, 1-15. doi: 10.3390/plants12102002
van Keulen M., Borowitzka M. A. (2002). Comparison of water velocity profiles through dissimilar seagrasses measured with a simple and inexpensive current meter. Bull. Mar. Sci. 71, 1257–1267.
van Weerdenburg R., Vroom J. (2021). Modelparameters Ecotopenkaart Waddenzee. 11206799–003-ZKS-0003 (Delft: Deltares).
van Zuidam B. G., Bakker E. S., van Geest G. J., Peeters E. T. H. M. (2022). Submerged vegetation colonizes behind artificial wave shelter after a 10-year time-lag and persists under high grazing pressure by waterbirds. Aquat Bot. 181, 1-10. doi: 10.1016/j.aquabot.2022.103541
Vichkovitten T., Holmer M., Frederiksen M. S. (2007). Spatial and temporal changes in non-structural carbohydrate reserves in eelgrass (Zostera marina L.) in Danish coastal waters. Botanica Marina 50, 75–87. doi: 10.1515/BOT.2007.009
Wang L., English M. K., Tomas F., Mueller R. S. (2021). Recovery and Community Succession of the Zostera marina Rhizobiome after Transplantation. Aquatic Toxicology 240, 1-12. doi: 10.1128/AEM
Waycott M., Duarte C. M., Carruthers T. J. B., Orth R. J., Dennison W. C., Olyarnik S., et al. (2009). Accelerating loss of seagrasses across the globe threatens coastal ecosystems. PNAS 106, 12377–12381. doi: 10.1073/pnas.0905620106
Zimmerman R. C., Alberte R. S. (1996). Effect of light/dark transition on carbon translocation in eelgrass Zostera marina seedlings. Mar. Ecol. Prog. Ser. 136, 305–309. doi: 10.3354/meps136305
Keywords: Zostera marina, seagrass restoration, positive feedbacks, Wadden Sea, subtidal eelgrass, self-facilitation, BESE-elements, hydrodynamic barrier
Citation: Rehlmeyer K, Franken O, van der Heide T, Holthuijsen SJ, Meijer KJ, Olff H, Lengkeek W, Didderen K and Govers LL (2024) Reintroduction of self-facilitating feedbacks could advance subtidal eelgrass (Zostera marina) restoration in the Dutch Wadden Sea. Front. Mar. Sci. 11:1253067. doi: 10.3389/fmars.2024.1253067
Received: 04 July 2023; Accepted: 10 May 2024;
Published: 28 May 2024.
Edited by:
Bernadette Pogoda, Alfred Wegener Institute Helmholtz Centre for Polar and Marine Research (AWI), GermanyReviewed by:
Kasper Elgetti Brodersen, University of Copenhagen, DenmarkJennifer Li Ruesink, University of Washington, United States
Copyright © 2024 Rehlmeyer, Franken, van der Heide, Holthuijsen, Meijer, Olff, Lengkeek, Didderen and Govers. This is an open-access article distributed under the terms of the Creative Commons Attribution License (CC BY). The use, distribution or reproduction in other forums is permitted, provided the original author(s) and the copyright owner(s) are credited and that the original publication in this journal is cited, in accordance with accepted academic practice. No use, distribution or reproduction is permitted which does not comply with these terms.
*Correspondence: Katrin Rehlmeyer, ay5yZWhsbWV5ZXJAcnVnLm5s
†Present address: Sander J. Holthuijsen, Rijkswaterstaat, Ministry of Infrastructure and Water Management, The Hague, Netherlands