- Red Sea Research Center (RSRC), King Abdullah University of Science and Technology (KAUST), Thuwal, Saudi Arabia
Despite being exposed to extreme water temperatures and solar irradiances, Red Sea corals are relatively resistant to bleaching. While their thermal tolerance is well described, little is known about their resistance to ultraviolet-B radiation (UVB). Here, we performed a short-term (2 days) UVB-removal incubation with Stylophora pistillata, and in situ measurements with Pocillopora verrucosa complemented by a long-term (46 days) transplantation and UVB-removal experiment. Using a suite of physiological parameters (effective quantum yield (Fv’/Fm’), oxidative stress (lipid peroxidation, LPO), and primary production), we assessed the impacts of UVB on the physiology and acclimation capacity of Red Sea corals. Shielding S. pistillata from UVB did not change the gross primary production or Fv’/Fm’, and respiration and LPO in the host remained unaffected. In situ, P. verrucosa exhibited less varying and significantly higher Fv’/Fm’ in 8 m depth (0.61 ± 0.04) than in 4 m (0.52 ± 0.06), 2 m (0.51 ± 0.09), and 0.5 m (0.50 ± 0.11), where water temperatures ranged from 30.5–33.4, 30.6–34.0, 30.8–34.5, 30.6–37.3°C and daily UVB exposures averaged 0.9, 2.9, 11.8 and 21.4 kJ m-2, respectively. Fv’/Fm’ correlated the strongest with UVB (-0.57), followed by PAR (-0.54) and temperature (-0.40), suggesting that UVB is a key determinant of photosynthetic efficiency. Fv’/Fm’ of upward transplanted specimens (T 1m) was initially decreased but gradually increased and reached the same values as shallow corals (1 m) after 44 days. UVB removal significantly increased the Fv’/Fm’ of transplanted corals in the first 20 days. Oxidative stress was initially highest in T 1m samples under full sunlight but equalized with 1 m specimens by day 46, whereas oxidative stress was significantly reduced by day 4 in T 1m corals sheltered from UVB. Overall, UVB-removal generally had little impact on the physiology of shallow-water S. pistillata and P. verrucosa but considerably accelerated the acclimation of upward transplanted corals. Our study highlights that UVB is a crucial stressor governing the photoacclimation capacity of these Red Sea coral species.
1 Introduction
To preserve biodiversity and for coral reef management to be successful, it is crucial to understand ecosystem functioning in general, and the effects of environmental conditions on the physiology and health status of the coral holobiont (Hughes et al., 2017a).
Ecophysiology-focused reef studies have traditionally investigated the biological responses of corals to individual environmental stresses, such as temperature- (Yakovleva et al., 2009; Oliver and Palumbi, 2011; Sawall et al., 2015) and salinity-extremes (Gardner et al., 2016; Gegner et al., 2019), ocean acidification (Hoegh-Guldberg et al., 2007; Grinblat et al., 2018), and deoxygenation (Altieri et al., 2017; Johnson et al., 2018; Hughes et al., 2020). However, in recent years, an increasing number of studies have pointed out that a broader understanding of coral functioning can only be gained by including the complex interactions between various ecosystem stressors (Dunne, 2010; Ban et al., 2014; Cote et al., 2016; Ellis et al., 2019; Jin et al., 2019). In the context of coral reef science, obtaining a better understanding of the interplay between different stresses could aid to disentangle the mechanisms responsible for the phenomenon of coral bleaching, i.e., the loss of photosymbionts and photosynthetic pigments, which presently threatens coral reefs worldwide (Hoegh-Guldberg, 1999; Hughes et al., 2003; Hoegh-Guldberg and Bruno, 2010).
Several studies have concluded that the onset of coral bleaching is predominantly attributed to prolonged or closely recurring periods of extreme water temperatures (Fitt et al., 2001; Krueger et al., 2015; Hughes et al., 2017b). Yet, some reports have suggested that the concurrence of thermal stress and extreme irradiance can further deteriorate the corals’ health or intensify the thermally induced bleaching response (Lesser et al., 1990; Anthony et al., 2007; Brown and Dunne, 2008; Nesa et al., 2012; Courtial et al., 2017). Detrimental impacts of light on scleractinian corals, however, have mainly been studied for solar radiation as a whole (Brown et al., 1994; Lesser and Farrell, 2004; Anthony and Kerswell, 2007; Richier et al., 2008; Bellworthy and Fine, 2017) or the photosynthetically active part of the solar spectrum (i.e., PAR, 400–700 nm) (Fitt et al., 2000; Warner et al., 2002; Ulstrup et al., 2008), although some studies have acknowledged the importance of spectral composition (Mass et al., 2010), particularly ultraviolet radiation (UVR, 280–400 nm).
UVR may affect a broad array of functional levels in marine organisms such as corals, ranging from molecular to behavioral responses (Banaszak and Lesser, 2009), such as disrupted reproduction and larvae recruitment (Kuffner, 2001; Gleason et al., 2006; Torres et al., 2008; Zhou et al., 2016), reduced growth rates (Torres-Perez and Armstrong, 2012) and symbiont densities (Zhou et al., 2017), increased accumulation of mycosporine-like amino acids (MAAs) (Shick et al., 2003; Shick et al., 2005), impaired photosynthetic efficiency (Kinzie, 1993; Ferrier-Pages et al., 2007), damage to the genetic material (Anderson et al., 2001; Baruch et al., 2005; Torregiani and Lesser, 2007), while, the responses of coral holobionts to UV remain understudied and consequently not well understood (van de Water et al., 2018).
A further effect of UVR exposure is an increased production of reactive oxygen species (ROS) (Lesser, 1996). ROS, which include superoxide anion (O2–), hydroxyl radicals (•OH), and hydrogen peroxide (H2O2), are produced by organisms in low to moderate concentrations due to normal cellular metabolism and physiological cell processes (Birben et al., 2012). Under environmental stress, however, ROS production is enhanced, leading to adverse modifications to the corals’ cell components, such as lipids, proteins, and DNA (Downs et al., 2002). Reported impacts include elevated lipid peroxidation levels, increased antioxidant enzyme activity, and the breakdown of the corals’ photo-symbiosis (Levy et al., 2006; Csaszar et al., 2009; Linan-Cabello et al., 2010). To protect against ROS-induced damage, organisms can produce enzymatic ROS scavengers (e.g. superoxide dismutase, catalase) or non-enzymatic antioxidants (e.g. vitamins, β-carotene) (Birben et al., 2012).
The severity of UV impacts depends on the intensity and duration of solar UV exposure. Daily UV exposure ranges greatly between seasons at sea level due to the variation in the intensity of incoming solar radiation and the number of daylight hours. For example, daily UVB exposure in the central Red Sea was reported to range from 21.6 kJ m-2 d-1 in December to 48.3 kJ m-2 d-1 in June (Overmans and Agusti, 2020). In the water column, daily UV exposure is also governed by the depth at which an organism resides and the bio-optical properties of the water column. Due to the proximity of coral reefs to the equator and the high transparency of oligotrophic tropical waters (e.g. Red Sea min. Kd(305nm): ~0.16 m–1) (Overmans and Agusti, 2019), corals in shallow parts of tropical reefs are typically exposed to extreme UV irradiances of > 30 kJ UVB m-2 d-1 in 1 m depth (Zepp et al., 2008; Downs et al., 2013; Overmans and Agusti, 2020).
The Red Sea is known to receive intense solar UV radiation (~ 50 kJ m-2 d-1) (McKenzie et al., 2007; Smyth, 2011), while the UVB attenuation coefficient is exceptionally low (min. Kd(305nm): 0.168 m–1) due to negligible UVB absorption by chromophoric dissolved organic matter (min. aCDOM(305 nm): 0.128 m–1) and minimal chlorophyll-a concentrations (0.07 mg m–3), characteristic of ultraoligotrophic waters (Stambler, 2005; Overmans and Agusti, 2019; Overmans and Agusti, 2020).
Most of the existing studies that aimed to elucidate the ecological effects of UVB have examined the stress responses of corals in the laboratory using artificially supplied PAR and UVB without providing UVA (315–400 nm), despite the latter being known to have beneficial effects (Häder et al., 2015). The few in situ studies mostly tested stress responses of corals following upward transplantations without any additional manipulation of the light conditions, which makes it challenging to disentangle the impacts of increased UVB from those of increased PAR and UVA. Upward transplanted corals exhibited reduced fecundity, symbiosis breakdown, or died within 20 days of transplantation (Vareschi and Fricke, 1986; Richier et al., 2008; Torres et al., 2008; Cohen et al., 2013).
Due to the intense levels of incident UV and the high transparency of reef waters, we hypothesize that UV, and especially the highly energetic wavelengths of the UVB spectrum (280–315 nm), can induce severe stress on Red Sea corals and select for resistant organisms, as previously suggested (Agusti et al., 2015). We further hypothesize that these corals have developed acclimation responses to natural solar UVB.
We tested this hypothesis by determining the acclimation potential to natural solar UVB radiation and measuring physiological stress on the host and symbiont metabolism (oxidative stress, photochemical efficiency, and net primary production) of the two Pocilloporidae coral species, Pocillopora verrucosa and Stylophora pistillata, which are common in the Red Sea. We did so as part of a short-term (2 days) incubation experiment with S. pistillata, as well as in situ measurements with P. verrucosa. Subsequently, we performed a long-term (46 days) transplant experiment to test the acclimation capacity of P. verrucosa to increased UVB irradiances following transplantation from subsurface (5 m) to surface (1 m) waters.
2 Materials and methods
2.1 Stylophora pistillata incubation experiment (short-term)
Immediate effects of a short-term reduction or removal of solar UVB on the coral S. pistillata were investigated by performing a 2-day UVB-removal experiment onboard R/V Thuwal as part of the Threats oceanographic cruise (Overmans and Agusti, 2019). On 5th October 2016, S. pistillata specimens were sampled at an offshore reef in the far north of the Red Sea (27.686162° N, 35.231405° E) close to Almuwaylih (Figure 1). A total of 40 broken off coral fragments (~10–15 cm length) of >15 recently storm-damaged shallow water colonies were collected from the reef flat zone (~ 1.5 m depth) in the early morning and immediately transported back to the vessel in shaded 10 L containers filled with seawater. The specimens were transferred into an open-top incubator (LxWxH: 150x80x50 cm) supplied with flow-through seawater to maintain in situ water temperature (29.0–30.8°C; see experimental setup Supplementary Figure 1). Incident PAR and UVB irradiances inside the incubator were monitored during the incubations with a PMA2100 data-logging radiometer (Solar Light, USA) fitted with a PAR (400–700 nm, model PMA2132-WP) and a UVB sensor (model PMA2106-WP). The coral fragments remained under those conditions for 20 h to allow some recovery time from sampling-induced stress.
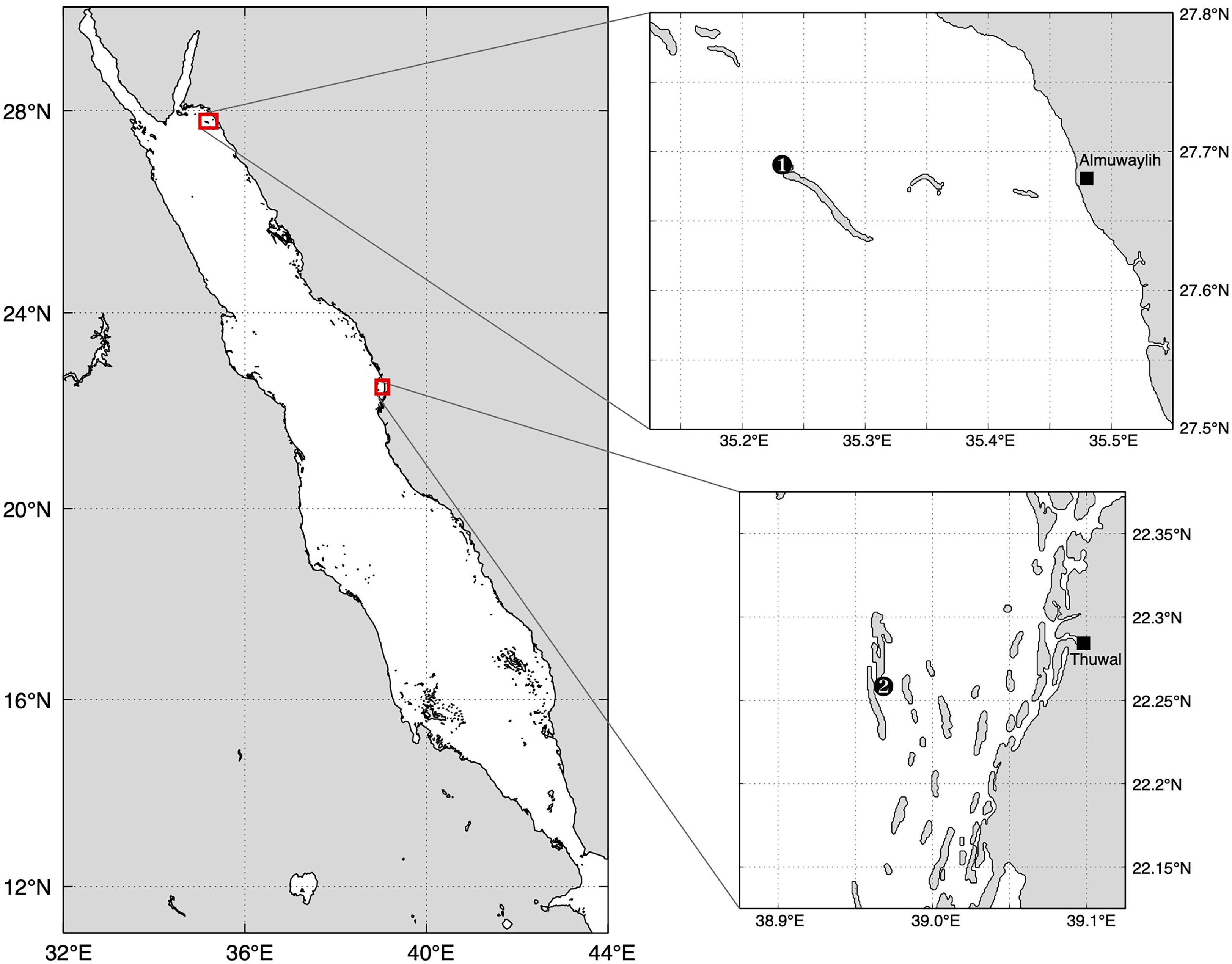
Figure 1 Red Sea map indicating the study locations of the Stylophora pistillata experiment (1) in the northern Red Sea, and study site of the Pocillopora verrucosa experiment in the central Red Sea (2).
The following morning, 12 fragments were transferred into 12 individual 2-L quartz glass Erlenmeyer flasks (UVB transmission: 88%, one fragment per flask) (Supplementary Figure 1) containing filtered (0.2 µm) seawater to prevent interference of microorganisms with metabolism measurements. The flasks were sealed and kept in the incubator at in situ temperatures. The samples were subdivided into three treatment groups (n=4 per treatment): PAR+UVA+full UVB, PAR+UVA+medium UVB, and PAR+UVA only, termed high UVB (H-UVB), medium UVB (M-UVB), and UVB removal (0-UVB), respectively. The three treatments were achieved by covering the flasks with different optical plastic screens. The M-UVB and 0-UVB treatment flasks were wrapped in foils that transmitted 54% (PE foil) and < 1% (Mylar foil) of the total UVB irradiance, respectively. The H-UVB flasks were covered in a coarse shade cloth (75% transmission) to create similar PAR conditions to the other two treatments (see Table 1; Supplementary Figure 2).
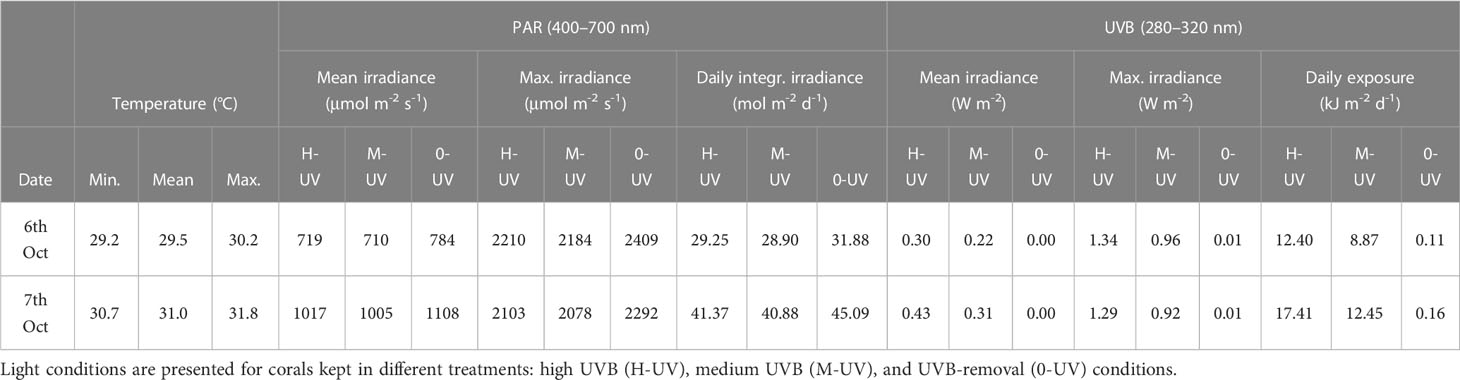
Table 1 Water temperature, and PAR and UVB conditions during the Stylophora pistillata UV-experiment, conducted in October 2016 in the northern Red Sea as part of the THREATS cruise.
The oxygen evolution inside the flasks was monitored for 35 h (see section 2.4). Afterwards, the samples were shock-frozen in liquid nitrogen and stored at -80°C until analysis of lipid peroxidation (MDA) and total protein content (see 2.6).
The remaining coral fragments (n=24) were kept in an open-top incubator with sea water flow-through for 31 h (9 am–4 pm) at the three light treatments mentioned above (n=8 per treatment) by installing the optical screens directly above the fragments. The effective quantum yield (Fv’/Fm’) of fragments was determined at the onset of the experiment, and after 3 h, 7 h, and 31 h (see section 2.5).
2.2 Pocillopora verrucosa in situ measurements and UVB dosimeter calibration
The photosynthetic efficiency of Red Sea Pocillopora verrucosa in different depths (0.5, 2, 4, 8 m) during summer (22nd July and 09th August 2018) was investigated by determining their Fv’/Fm’ ratios using a Diving PAM fluorometer (Supplementary Figure 1, see section 2.5). P. verrucosa colonies were located on the inshore side of Al Fahal reef (22.254232 N, 38.960779 E) in the central Red Sea, ~10 km offshore from Thuwal, Saudi Arabia (Figure 1).
In each of the four sampling depths, one waterproofed and pre-calibrated UVB dosimeter (Scienterra, Oamaru, New Zealand) and one HOBO Pendant® data logger (Onset Computer Corporation, USA) were installed to record UVB (280–315 nm) irradiances, and water temperature (accuracy at 0–50°C: ± 0.53°C) and lux, respectively, in 10-minute intervals between 5th July and 12th August 2018. Lux values were converted to PAR using a previously described conversion factor (1 μmol quanta m−2 s−1 = 52.0 lux) (Tilstra et al., 2018). The loggers were visited every 2-3 days to remove biofouling or to be replaced.
The UVB dosimeters were calibrated against spectrally-integrated, unweighted UVB (280–315 nm) irradiance data measured with a stationary UVB radiometer (model SUV-B, Kipp & Zonen, Netherlands). For the calibration, the dosimeters and radiometer recorded data in 60-second intervals from dawn until mid-day at the KAUST CMOR pier (22.304639°N, 39.102167°E) on a cloudless day (10th June 2018). Each dosimeter data set was plotted against the radiometer data, and a second-order polynomial curve in the form y = A*x2 + B*x + C was fitted, which provided a high coefficient of determination (R2 > 0.99). The coefficients A, B, and C were subsequently used to convert raw dosimeter data to calibrated UVB values (in W m-2).
In situ temperature data were used to identify periods when the coral bleaching thermal threshold of the Jeddah region (i.e., 32.1°C) was exceeded (Osman et al., 2018).
2.3 Pocillopora verrucosa transplant experiment (long-term)
To determine UVB exposure effects on the coral Pocillopora verrucosa, a long-term (46 days) in situ transplantation experiment was performed. Specimens were either kept at natural UVB irradiances, under UVB removal conditions, or exposed to elevated UVB irradiances (fragments transplanted from 5 m to 1 m depth). The study site was the inshore side of Al Fahal reef (22.254232 N, 38.960779 E), located in the central Red Sea ~10 km offshore from Thuwal, Saudi Arabia (Figure 1). On 28th April 2019, four P. verrucosa colonies were collected from the reef flat (0.5–1 m depth), and a further four colonies were sampled in ~ 4.5–5 m on the inshore side of the reef. Each colony was fragmented into 24 similarly sized (~ 10 cm long) nubbins (n= 96 fragments per sampling depth). Fragments from the same depth were randomized (to minimize colony effects) and attached to ~2 m long cords (8 cords, 12 fragments per cord) using small cable ties. Subsequently, the coral samples were returned to their original sampling depth for a fragmentation recovery period of 4 days.
On 2nd May 2019, the coral nubbins were recovered and equally distributed between two experimental treatments, for which two stainless steel frames (height: 1 m, base area: 1.58 m2, top area: 5.62 m2) were installed in the lagoon zone of the reef in 2 ± 0.1 m depth (tide-dependent) (see experimental setup in Supplementary Figures 1, 3). One frame, used for the full-sunlight (FS) treatment, was covered with a wire mesh fence on all sides to prevent predators from entering. The second fence, used for the UVB-removal treatment (-UVB), was secured with fencing at the sides, however, the top was covered with Mylar foil (UVB transmission: < 1%) (Supplementary Figure 2).
In each frame, 48 fragments from each of the two sampling depths (1 m and 5 m) were kept immediately below the top of the frames (1.0 ± 0.1 m depth), thereby generating four experimental groups: corals collected from 1 m depth under full sunlight (1 m FS) or UVB-removal conditions (1 m -UVB), and corals from 5 m depth transplanted to 1 m depth kept in full sunlight (T 1m FS) or with UVB removed (T 1m -UVB). As such, samples that were collected in 1 m were either exposed to the same PAR and UVB conditions or the same PAR but without UVB while corals originating from 5 m depth were exposed to either increased PAR and UVB levels or elevated PAR but without UVB (see 3.2.1 for details).
In each sampling depth (1 m and 5 m), one HOBO Pendant® data logger (Onset Computer Corporation, USA) was installed to record water temperature and PAR conditions in 10-minute intervals. A waterproofed and pre-calibrated UVB dosimeter (Scienterra, Oamaru, New Zealand) was deployed to record UVB (280–315 nm) irradiances every 10 minutes. The frames were visited every 2-3 days to remove biofouling and replace the loggers.
During the experimental phase (2nd May–16th June), the effective quantum yield (Fv’/Fm’) of the fragments was measured at the onset of the experiment and after 4, 7, 11, 14, 17, 20, and 24 days (see 2.5.). Additional Fv’/Fm’ measurements were recorded for the FS fragments after 46 days.
At the start of the experiment and after 4, 11, and 20 days, 12 coral fragments per treatment (1m FS, 1m -UVB, T 1m FS, T 1m -UVB) were collected, plus an additional 12 fragments from the two FS groups after 46 days. The samples were shock-frozen in liquid nitrogen and stored at -80°C until further analysis of lipid peroxidation and total protein content (see 2.6.).
2.4 Primary production measurements
As part of the UVB-removal experiment with Stylophora pistillata, the oxygen evolution inside the sample flasks was assessed using a non-intrusive fluorescence-based method. A fiber optic Witrox Oxygen meter with oxygen mini-sensors (optodes) (Loligo Systems, Tjele, Denmark) fixed to the flasks were used to measure oxygen concentrations. In the mornings, a two-point calibration of the oxygen meter was performed according to the manufacturer’s instructions. Oxygen concentrations (in µmol O2 l-1) inside each flask were measured on 6th October at 10 am and at 3 pm, 5 pm, and 6 pm. After the 6 pm measurement, the water inside the flasks was replaced with freshly filtered seawater to remove metabolic waste products. Further oxygen measurements were performed at 8 pm and on the following day at 6 am, 10.30 am, 3.30 pm, 6 pm, and 9.30 pm. Oxygen data were recorded, and salinity- and temperature-corrected (based on the SeaBird CTD measurements of the cruise stations; see Lopez-Sandoval et al. (2021)) using the software WitroxView (v.2.0.1). The changes in oxygen concentration (in µmol O2 l-1 h-1) during the light (10 am–6 pm) and dark periods (8 pm–6 am on day 1; 6 pm–9.9.30 pm on day 2) were used to calculate gross primary production (GPP), respiration (R), and net primary production (NPP). The change in oxygen concentration during the day informed about the NPP rate, while the rate of oxygen decrease during the night represents the respiration rate. GPP was calculated as GPP = NPP + R. Each of the three parameters was normalized by the coral fragments’ surface area and reported as µmol O2 cm-2 h-1. To quantify the surface area, three photos per fragment were taken from different angles, the main dimensions were measured with the image processing software ImageJ2 (v. 2.0.0) and the total surface area (in cm–2) was calculated using the surface area formula of a cylinder (A = 2πr(r + h)).
2.5 Diving PAM measurements
UVB-induced damage to the photosynthetic apparatus of the corals’ symbionts was evaluated by measuring the variable chlorophyll fluorescence of photosystem II (PSII) with a Diving Pulse Amplitude Modulation (Diving PAM) fluorometer (Heinz Walz GmbH, Germany). The effective quantum yield of coral samples (Fv’/Fm’) was determined as the ratio of variable fluorescence (Fv’) to maximal fluorescence (Fm’) under actinic light (Genty et al., 1989; Baker, 2008). For the Stylophora pistillata experiment, fluorescence measurements (MI: 9–11, SI: 8, Sat width: 0.8 s, Gain 2–4, Damp: 2) were performed on eight fragments (n=8) per light treatment at the abovementioned sampling points. For the in situ measurements on Pocillopora verrucosa, ten fluorescence measurements (n = 10) per depth were recorded on each sampling day. During the long-term experiment with P. verrucosa, fluorescence measurements of ten fragments per light treatment were recorded per day. All measurements were recorded between 08:15 am and 10:45 am.
2.6 Lipid peroxidation (MDA), in vivo absorbance & total protein
Samples were processed according to standard protocols, with slight modifications (Krueger et al., 2015) (see Supplementary Figure 4). Each coral fragment was airbrushed and the tissue collected in 5 mL of ice-cold Phosphate buffer (50mM). The resulting tissue slurry was blended on ice for 30 s (3 x 10 s) and centrifuged at 3000 g and 4°C for 15 min to separate host tissue from symbiont cells. The resulting supernatant, containing enriched host cells, was analyzed for its malondialdehyde (MDA) content and absorbance in the UV and PAR spectra.
MDA was determined colorimetrically with an assay kit (Sigma-Aldrich, cat. # MAK085). Briefly, the samples were centrifuged at 13,000 g for 10 min to remove insoluble material. 200 µL of the supernatant from each homogenized sample was placed into a microcentrifuge tube, and 600 µL of TBA (thiobarbituric acid) solution was added to each standard or sample vial to form the MDA-TBA adduct. The mixtures were incubated (95°C for 60 min) and cooled to room temperature on ice for 10 min before the absorbances of samples at 532 nm (A532) were measured with a microplate reader (SpectraMax® Paradigm®, Molecular Devices, USA). Absorbances were converted to MDA concentrations using a standard curve.
To compare the relative contribution of MAAs to UVB absorption, the absorbance spectra of coral tissues were measured (Rossbach et al., 2020). Briefly, 2 mL of the supernatant containing coral tissue were added to 2 mL 100% HPLC grade methanol, kept in the dark at 4°C for 24 h and subsequently centrifuged at 3000 g and 16°C for 5 min. The samples were transferred into a 1-mL quartz cuvette and their absorbance between 280–750 nm was assessed against a methanol blank, using a UV/Vis/NIR Spectrophotometer (Lambda 1050, PerkinElmer, USA), and normalized by subtracting the absorbance at 750 nm, where absorbance was minimal.
Lipid peroxidation and absorbance were normalized by the total protein content. Protein contents were determined using a Micro-BCA™ Protein Assay Kit (Thermo Fisher, cat. # 23235). MDA concentrations were expressed as nmol MDA mg-1 protein, while absorbances were presented as absorption coefficients (m-1 mg-1 protein).
2.7 Statistical analysis
To test for normal distribution of the data and equal variances, Shapiro–Wilk tests and Levene’s tests were performed, respectively. For the S. pistillata experiment, one-way ANOVAs followed by Tukey’s post hoc tests were performed to identify if mean gross primary production (GPP), respiration (R), net primary production (NPP), quantum yields (Fv’/Fm’), MDA concentrations and absorption coefficients at 330 nm varied significantly (p <0.05) between radiation treatments. For the P. verrucosa in situ measurements, correlation analyses were performed using the Fv’/Fm’ values of P. verrucosa colonies and the environmental parameters water temperature, PAR, and UVB measured in 0.5–8.0 m at Al Fahal reef between 15th July–09th August 2018. For temperature, PAR, and UVB, average values of the 10 min before each Fv’/Fm’ measurement were used. For the P. verrucosa transplantation experiment, Student’s t-tests were performed to identify whether mean temperatures in 1 m and 5 m were significantly different and test whether Fv’/Fm’ and MDA concentrations differed significantly between the P. verrucosa fragments from the two sampling depths and radiation treatments. To establish at what rate (in % per day) MDA concentrations and Fv’/Fm’ of the P. verrucosa corals from the three manipulative treatments (1 m -UVB, T 1m FS, T 1m -UVB) changed compared to the 1 m FS samples, a linear regression analysis was performed, whereby the slope of the regression line informed about the change in Fv’/Fm’ and MDA concentration day–1. All data were analyzed and visualized using either JMP Pro 15.1.0 (SAS Institute Inc., Cary, North Carolina, USA) or GraphPad Prism 8.4.2 (GraphPad Software Inc., CA, USA).
3 Results
3.1 Stylophora pistillata incubation experiment (short-term)
3.1.1 Light conditions and primary production
Across the three treatments, mean PAR irradiances varied from 710–784 µmol m-2 s-1 on 6th Oct and from 1005–1108 on 7th Oct, reaching maximum midday PAR values of 2210–2409 µmol m-2 s-1 (6th Oct) and 2078–2292 µmol m-2 s-1 (7th Oct) (Table 1; Supplementary Figure 5). UVB irradiances reached maxima of 1.34 W m-2 and 1.29 W m-2 in the full sunlight treatment on the first and second day of the experiment, while we measured UVB irradiances of up to 0.96 and 0.92 W m-2 in the M-UVB treatment during the two days. UVB irradiances in the UVB-removal treatment were negligible (0.01 W m-2).
The pattern and absolute change in oxygen concentration showed no significant differences between the three radiation treatments (Supplementary Figure 6). On the first day, gross primary production (GPP) was on average lowest in the H-UVB treatment and highest in the M-UVB treatment, with GPP rates (mean ± SD) of 0.377 ± 0.021 µmol O2 cm-2 h-1 (H-UVB), 0.406 ± 0.091 µmol O2 cm-2 h-1 (0-UVB), and 0.455 ± 0.094 µmol O2 cm-2 h-1 (M-UVB) (Figure 2). The respiration (R) rates did not differ significantly between the three treatments (H-UVB: -0.186 ± 0.014 µmol O2 cm-2 h-1, M-UVB: -0.194 ± 0.041 µmol O2 cm-2 h-1, 0-UVB: -0.190 ± 0.035 µmol O2 cm-2 h-1). Mean NPP rates were very similar between the H-UVB (0.190 ± 0.026 µmol O2 cm-2 h-1) and 0-UVB (0.216 ± 0.066 µmol O2 cm-2 h-1) treatments and highest in the M-UVB treatment samples (0.261 ± 0.066 µmol O2 cm-2 h-1), although neither treatment exhibited a significantly different NPP. On the second day, the average GPP was higher in all three treatments, and more similar between groups (H-UVB: 0.535 ± 0.090 µmol O2 cm-2 h-1, M-UVB: 0.531 ± 0.027 µmol O2 cm-2 h-1, 0-UVB: 0.511 ± 0.132 µmol O2 cm-2 h-1) compared to the previous day. The same pattern could be observed for the respiration rates, with mean rates varying from -0.256 ± 0.038 µmol O2 cm-2 h-1 (M-UVB) to -0.275 ± 0.072 µmol O2 cm-2 h-1 (0-UVB), and a higher variability in respiration of H-UVB fragments (-0.272 ± 0.079 µmol O2 cm-2 h-1) compared to day 1 (Figure 2). The mean NPP rates were highly similar between the corals of all three light treatments (H-UVB: 0.263 ± 0.024 µmol O2 cm-2 h-1, M-UVB: 0.276 ± 0.064 µmol O2 cm-2 h-1, 0-UVB: 0.236 ± 0.091 µmol O2 cm-2 h-1).
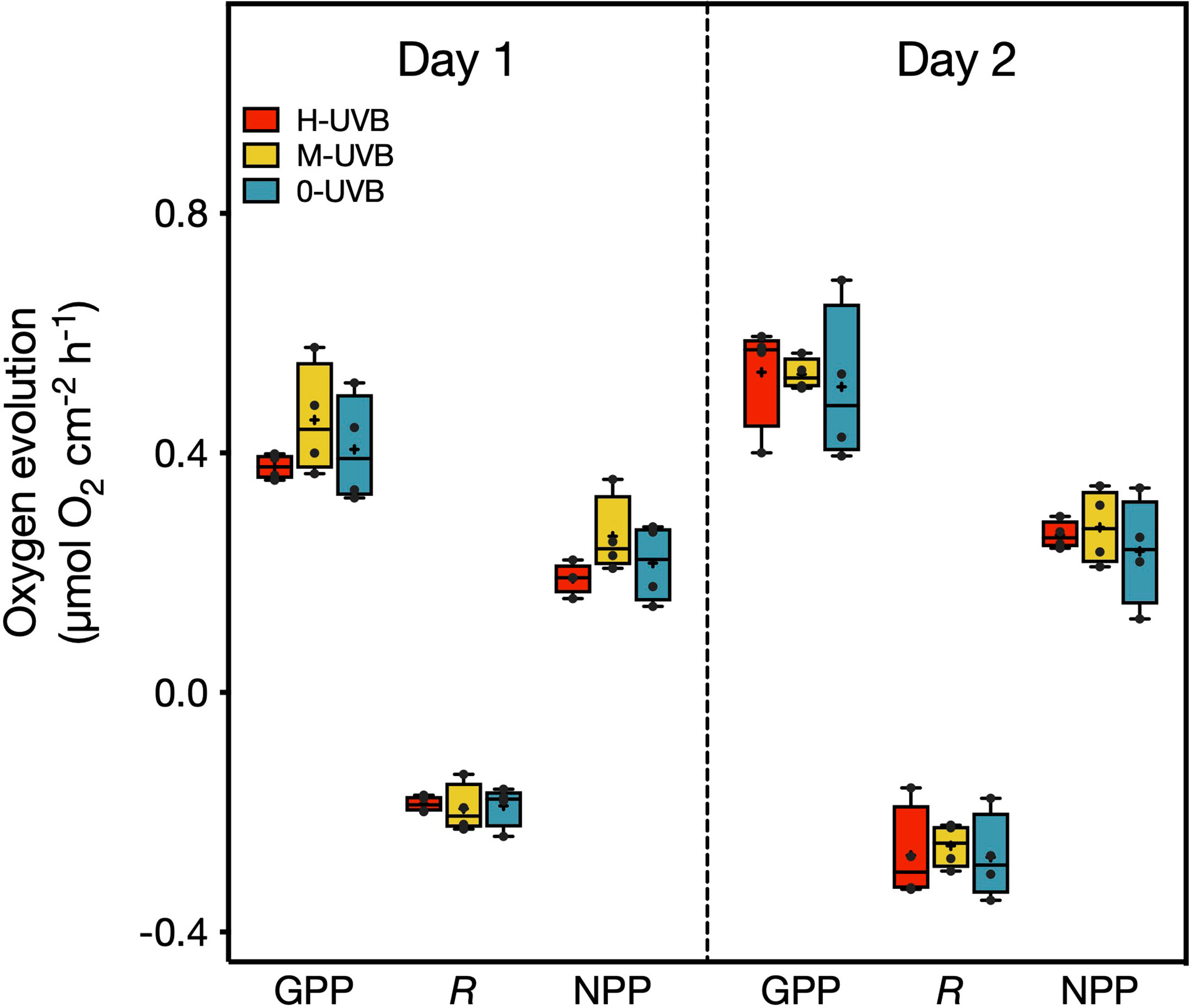
Figure 2 Oxygen evolution/consumption (µmol O2 cm-2 h-1) measured during the incubations as part of the Stylophora pistillata experiment on 6th (Day 1) and 7th October 2016 (Day 2). Displayed are the gross primary production (GPP), respiration (R), and net primary production (NPP). Samples were incubated in either full UVB (red), medium UVB (yellow), or under UVB-removal (blue) conditions. Horizontal lines inside the boxplots indicate median values, and crosses the means. Black dots represent individual data points. One-way ANOVAs and subsequent Tukey’s post hoc tests identified no significant differences (p < 0.05) between treatments for any of the three parameters on either day.
3.1.2 Effective quantum yield (Fv’/Fm’)
Effective quantum yields (Fv’/Fm’) of Stylophora pistillata ranged broadly from 0.11–0.51 (Figure 3). The Diving PAM measurements revealed that on day 1, the coral fragments from the two treatments where UVB was either reduced or removed, showed on average the lowest Fv’/Fm’ values at midday (M-UVB: 0.22 ± 0.10 (mean ± SD), 0-UVB: 0.20 ± 0.09), while the fragments of the full sunlight, i.e., H-UVB, group had the highest Fv’/Fm’ at that time (0.27 ± 0.09) (Figure 3). Towards the afternoon, Fv’/Fm’ of the M-UVB fragments increased on average by 0.04 (mean: 0.25 ± 0.06), whereas in the 0-UVB corals, the mean Fv’/Fm’ increased by 0.11 (mean: 0.31 ± 0.12) compared to 12 pm.
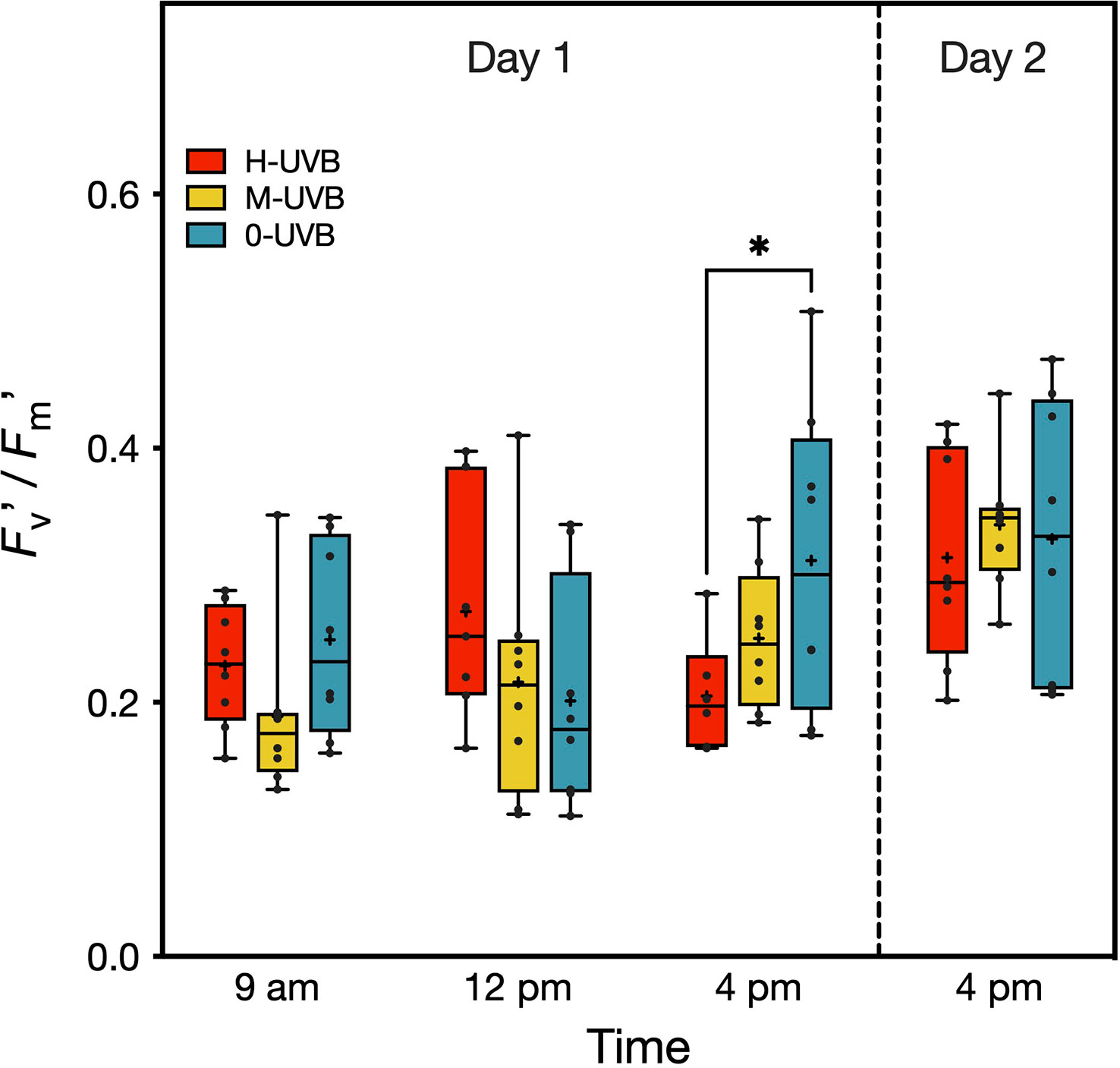
Figure 3 Effective quantum yield (Fv’/Fm’) of Stylophora pistillata fragments, measured during the experiment on 6th (Day 1) and 7th October 2016 (Day 2). Coral fragments were incubated in either full UVB (red), medium UVB (yellow), or UVB removal (blue) conditions. Boxplots indicate 95% CIs. The horizontal lines inside the boxplots represent medians, while crosses indicate means (n=8). Tukey’s pairwise comparison results are shown above boxplots (* indicates p < 0.05).
On both days, the highest recorded Fv’/Fm’ at 4 pm were from fragments of the 0-UVB treatment (day 1: 0.51, day 2: 0.47), while the Fv’/Fm’ values of the H-UVB corals did not exceed 0.33 and 0.42 on days 1 and 2, respectively. On the other hand, Fv’/Fm’ ratios were the most similar between groups at the last measurement on day 2, with mean Fv’/Fm’ of 0.33 ± 0.11, 0.34 ± 0.05, and 0.31 ± 0.08 for the 0-UVB, M-UVB, and H-UVB treatment, respectively.
3.1.3 Lipid peroxidation (MDA)
MDA concentrations in the Stylophora pistillata coral tissues varied from 199.2 to 241.9 nmol mg-1 protein, depending on the radiation treatment (Figure 4). The highest recorded concentration was found in a fragment from the H-UVB treatment, whereas a sample from the 0-UVB treatment exhibited the lowest degree of lipid peroxidation. Mean MDA concentrations gradually decreased from samples of the full sunlight treatment to those that were not exposed to UVB, with mean ± SD values of 229.4 ± 12.9, 220.5 ± 10.1, and 213.0 ± 14.9 nmol mg-1 protein for the H-UVB, M-UVB, and 0-UVB treatment, respectively.
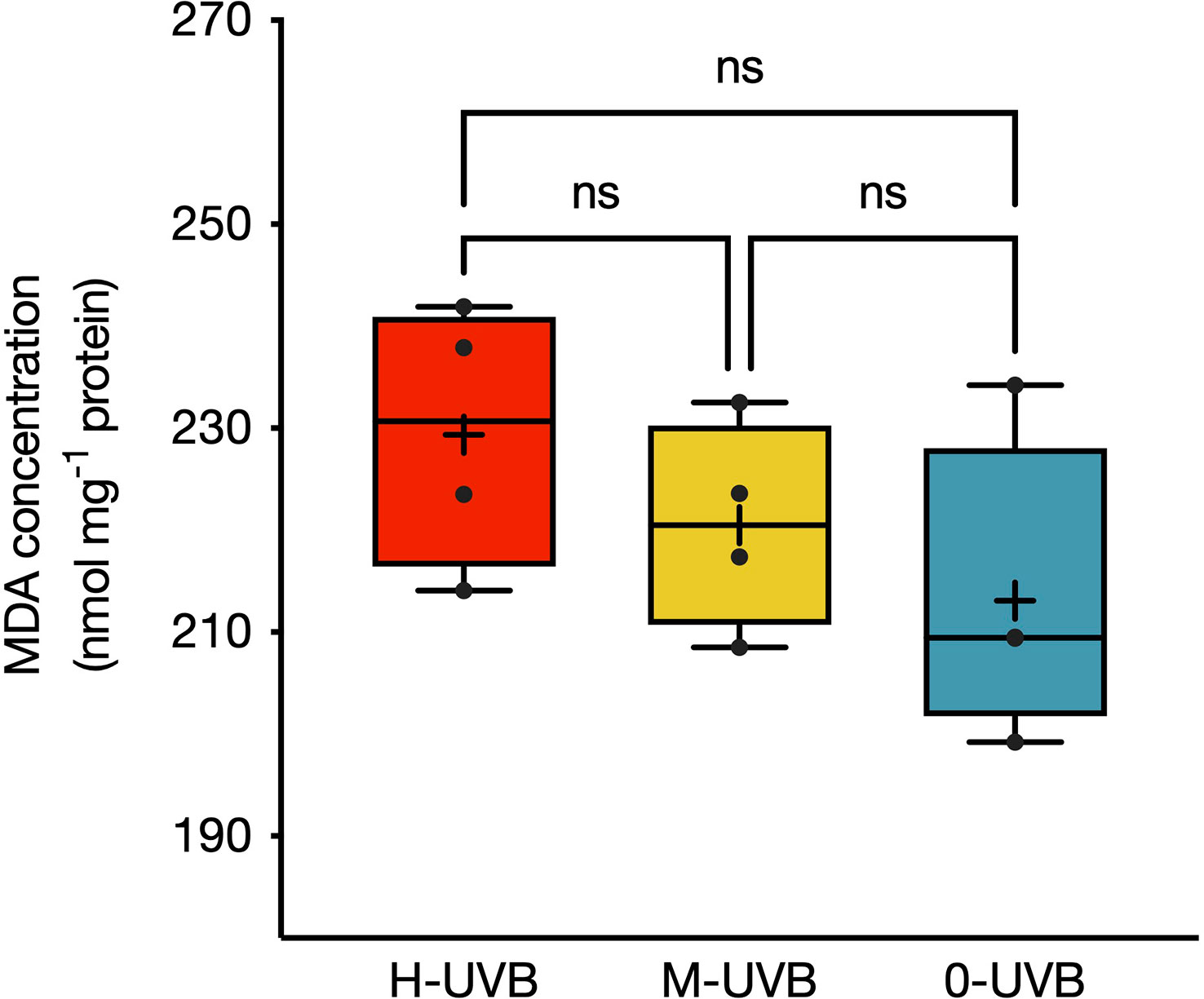
Figure 4 MDA concentrations (in nmol mg-1 protein) in Stylophora pistillata fragments at the end of the experiment performed on 6–7th October 2016. Coral fragments were incubated in either full UVB (red), medium UVB (yellow), or UVB removal (blue) conditions. Boxplots indicate 95% CIs. Horizontal lines inside the boxplots represent medians, while crosses indicate means (n=4). Tukey’s pairwise comparison results are indicated above boxplots (ns = non-significant).
3.2 Pocillopora verrucosa in situ measurements
3.2.1 Temperature and solar radiation
Between 5th July and 12th August 2018, the temperature at Al Fahal reef varied considerably between depths, and daily fluctuations were most pronounced in the shallows. Specifically, water temperatures ranged from 30.6–37.3°C (0.5 m), 30.8–34.5°C (2 m), 30.6–34.0°C (4 m), and 30.5–33.4°C (8 m) (Supplementary Figure 7A; Table 2), which indicates that minimum temperatures were almost identical between the four depths, whereas maximum temperatures varied greatly. Overall, we determined that daily average temperatures in 0.5, 2, 4, and 8 m depth were 33.1 ± 1.2°C, 32.6 ± 0.7°C, 32.4 ± 0.6°C, and 32.3 ± 0.6°C, respectively. We did not detect any apparent signs of coral bleaching during our measurements, even though the bleaching threshold temperature of 32.1°C was exceeded during the daytime for the entire duration of the measurement period (5th July–12th August) in 0.5 and 2 m depth. In 4 m and 8 m depth, daytime temperatures exceeded the threshold after the 7th and 14th July, respectively. In 0.5 m, temperatures generally fell below the threshold temperature during the night, except between 31st July and 3rd August. In 4 m depth, the longest time that temperatures consistently remained above the critical threshold was from 31st July until 2nd August and from 4th–6th August. In contrast, the bleaching threshold temperature was exceeded in 2 m and 8 m depth for a prolonged period, i.e., from 30th July and 3rd August, respectively, until 15th August.
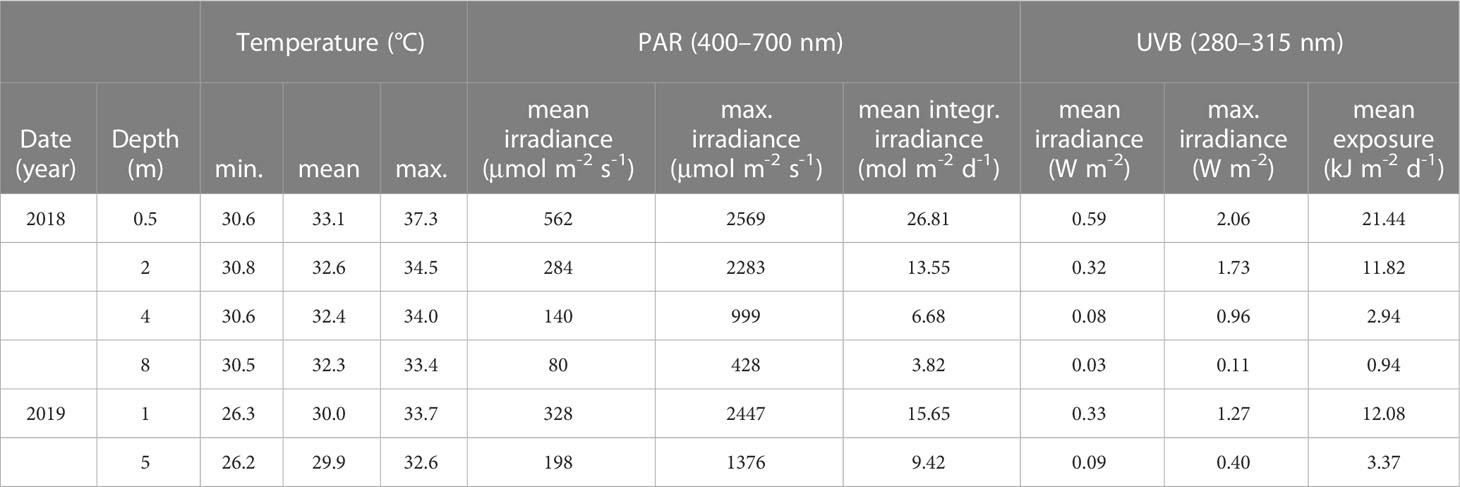
Table 2 Water temperature and solar radiation (PAR and UVB) in different depths at Al Fahal reef in the central Red Sea between 5th July–12th August 2018, and during the Pocillopora verrucosa transplant experiment from 28th April–16th June 2019.
PAR irradiances reached midday maxima of 2569, 2283, 999, and 428 µmol m-2 s-1 in 0.5, 2, 4, and 8 m depth, respectively, while the average integrated PAR irradiances received in those depths per day were 26.81, 13.55, 6.68, and 3.82 mol m-2 d-1 (Table 2; Supplementary Figure 7B).
The maximum recorded UVB (280–315 nm) irradiances at noon were 2.06, 1.73, 0.96, and 0.11 W m-2 in 0.5, 2, 4, and 8 m, respectively (Table 2; Supplementary Figure 7C). The average daily UVB exposure measured in 2, 4, and 8 m was 11.82, 2.94, and 0.94 kJ m-2, approximately 55, 14, and 4% of the daily UVB exposure received in 0.5 m depth (21.44 kJ m-2) (Table 2).
3.2.2 Effective quantum yield (Fv’/Fm’) in situ
The range of effective quantum yields (Fv’/Fm’) was greatest in the shallows (0.5 m) and less variable towards the deepest sampling depth (8 m), with Fv’/Fm’ ranging from 0.30–0.68, 0.31–0.66, 0.38–0.63, and 0.49–0.67 in colonies from 0.5, 2, 4, and 8 m depth, respectively (Figure 5). Overall, Fv’/Fm’ varied significantly between the P. verrucosa coral fragments from the four sampling depths (F(3, 312) = 29.55, p < 0.0001), with the mean Fv’/Fm’ of the corals at 8 m depth (Mean ± SD= 0.61 ± 0.04) being significantly higher than the means of the corals in shallower depths, including those at 4 m (0.52 ± 0.06, p< 0.0001), 2 m (0.51 ± 0.09, p< 0.0001), and 0.5 m (0.50 ± 0.11, p< 0.0001).
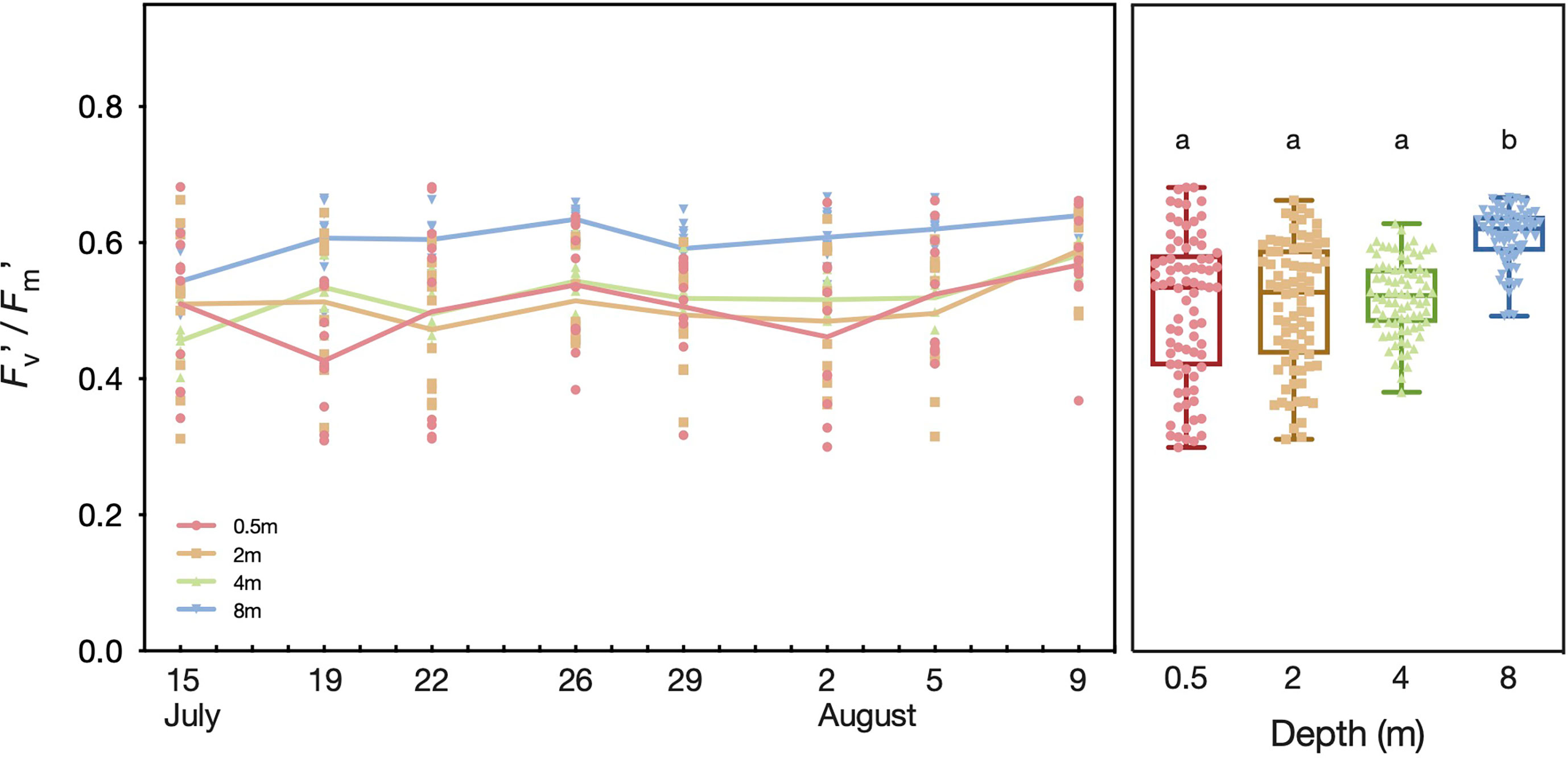
Figure 5 Effective quantum yield (Fv’/Fm’) of Pocillopora verrucosa colonies in different depths at Al Fahal reef in the central Red Sea between 15th July–09th August 2018 on individual days (left panel) and combined for all days (right panel). The colored lines connect mean Fv’/Fm’ values. Letters above the boxplots show the results of the Tukey’s post hoc test, whereby plots that do not share the same letter had significantly different means at a level of p < 0.05.
We found a negative correlation between water temperature and Fv’/Fm’ (r = -0.40), although the lowest and highest Fv’/Fm’ were both recorded at intermediate temperatures (Figure 6). For PAR, we found an even stronger correlation with Fv’/Fm’ (r = -0.54), and the highest Fv’/Fm’ were recorded when PAR was only ~100 µmol m-2 s-1, while the lowest Fv’/Fm’ was determined when PAR was ~ 800 µmol m-2 s-1. However, the strongest correlation was found between UVB and Fv’/Fm’ (r = -0.57). It was evident that the maximum Fv’/Fm’ ratios were all measured in the near absence of UVB while the minimum ratio was recorded when UVB was 0.52 W m-2.
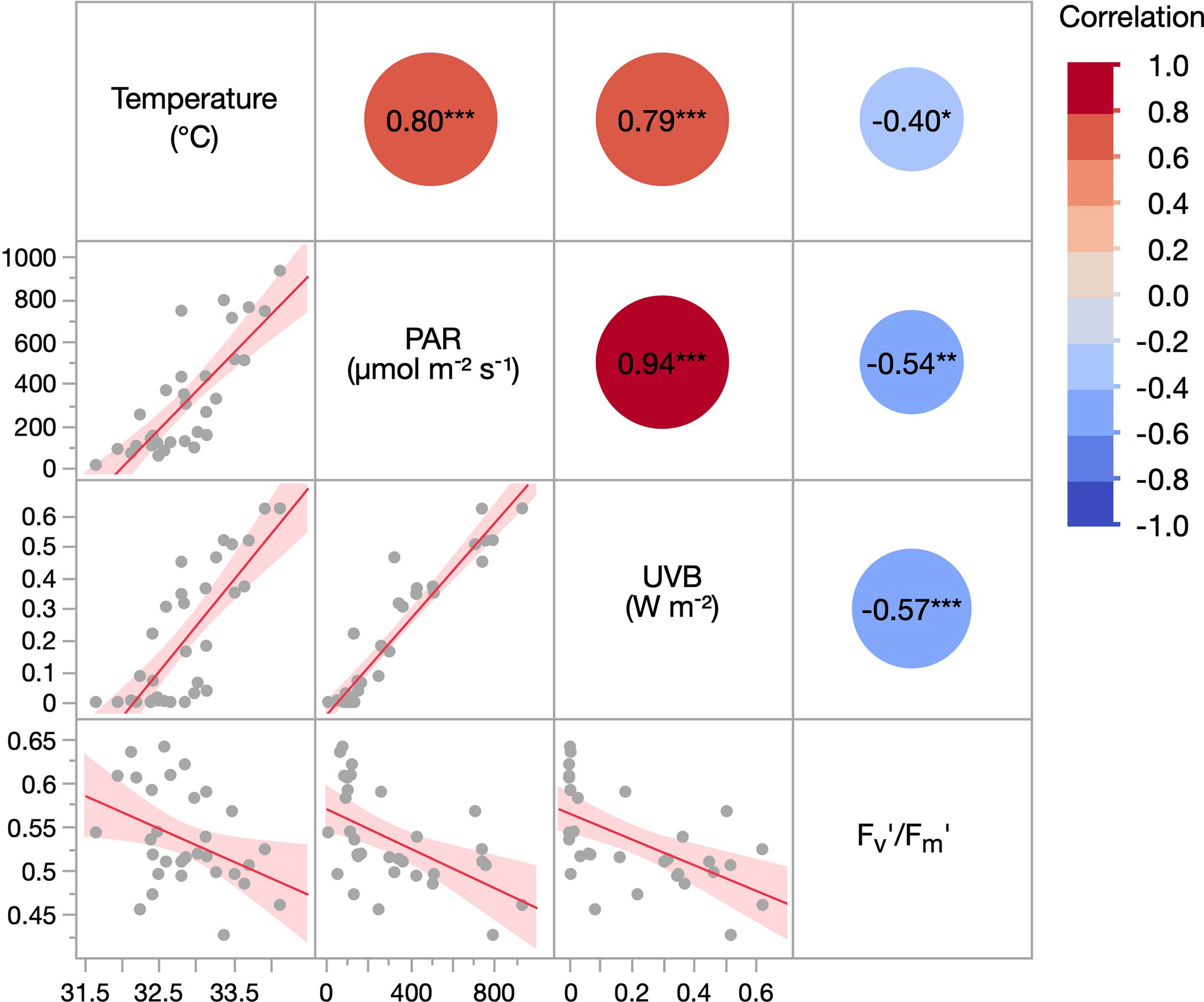
Figure 6 Correlation matrix of the environmental parameters water temperature, PAR, and UVB, as well as the effective quantum yield (Fv’/Fm’) of Pocillopora verrucosa colonies. Measurements were performed in 0.5–8.0m at Al Fahal reef in the central Red Sea between 15th July–09th August 2018. Temperature, PAR, and UVB values are averages of the 10 minutes before each Fv’/Fm’ measurement. The red line and shaded area represent the correlation line and 95% CIs, respectively. Values in the circles indicate the Pearson correlation coefficient (r). Asterisks show p < 0.05 (*), p < 0.01 (**) and p < 0.001 (***).
3.3 Pocillopora verrucosa transplant experiment (long-term)
3.3.1 Temperature and solar radiation
During the experimental period, water temperatures gradually increased from 02nd May until 3rd June, after which temperatures started to decrease again (Supplementary Figure 8A). Overall, mean water temperatures were significantly higher in 1 m (30.0 ± 1.6°C) than in 5 m depth (29.8 ± 1.6°C) (t(27401)= -8.62, p < 0.0001) (Table 2). Corals that were upward transplanted experienced an average temperature increase of 0.1°C. However, the maximum temperature was 1.1°C higher in 1 m (33.7°C) than in 5 m depth (32.6°C). In both depths, the critical bleaching threshold temperature of 32.1°C was only exceeded during the daytime. Specifically, this threshold temperature was surpassed between 26th May and 8th June (in 1 m depth) and from 29th May–3rd June and 5th–8th June (in 5 m).
Between 28th April and 16th June, PAR irradiance reached midday maxima of 2447 µmol m-2 s-1 in 1 m, and 1376 µmol m-2 s-1 in 5 m (Table 2; Supplementary Figure 8B), while the integrated PAR irradiance received per day was on average 66% higher in 1 m (15.65 mol m-2 d-1) than in 5 m (9.42 mol m-2 d-1). At midday, the maximum recorded UVB (280–315 nm) irradiances were 1.27 and 0.40 W m-2 in 1 and 5 m, respectively (Table 2; Supplementary Figure 8C). Daily UVB exposure was over 3.5-fold higher in the shallows (12.08 kJ m-2) than in 5 m depth (3.37 kJ m-2).
3.3.2 Effective quantum yield (Fv’/Fm’)
Overall, the effective quantum yields (Fv’/Fm’) of the 1 m P. verrucosa coral fragments had a significantly higher mean Fv’/Fm’ ratio (0.62 ± 0.03) than the 5 m fragments (0.59 ± 0.03) (t(283)= -7.52, p < 0.0001), and varied significantly between the four different treatment groups (F(3, 280) = 29.64, p < 0.0001) (Figure 7A). Fv’/Fm’ of the T 1m FS corals (Mean ± SD, 0.58 ± 0.04) was significantly lower compared to any other treatment group, including the T 1m -UVB fragments (0.61 ± 0.03) (p < 0.0001). Yet, the discrepancy in Fv’/Fm’ between those two groups increased over time. On day 4 of the experiment, there was a marginal (< 2%) difference between the mean Fv’/Fm’ of the 1 m FS (0.61 ± 0.02) and 1 m -UVB corals (0.60 ± 0.03). On day 14 there was a sharp decrease in Fv’/Fm’ across all treatments. After 20 days, the Fv’/Fm’ of the 1 m -UVB corals (0.65 ± 0.01) was significantly higher than that of the 1 m FS specimens (0.63 ± 0.02) (p = 0.0115).
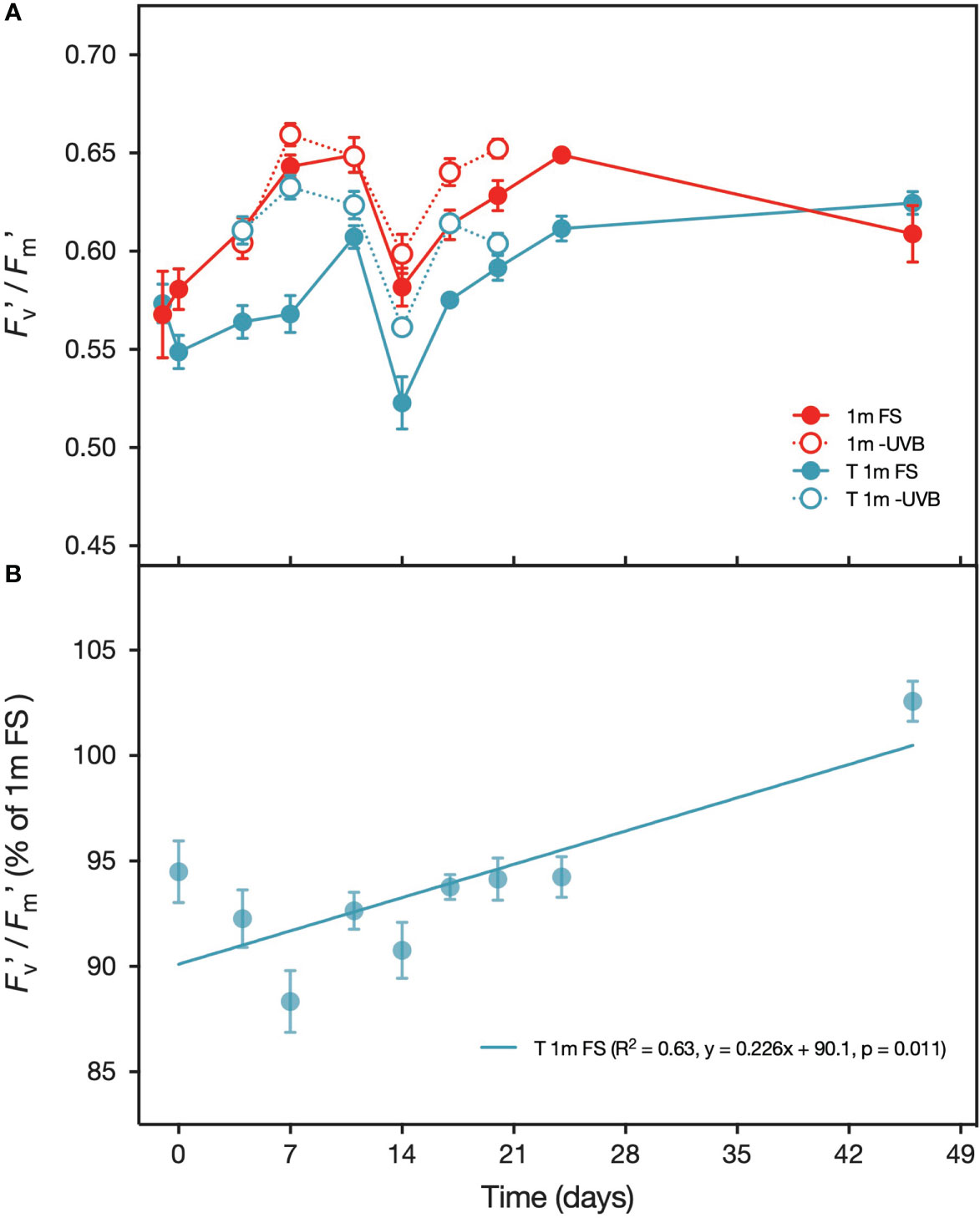
Figure 7 (A) Effective quantum yield (Fv’/Fm’) of Pocillopora verrucosa fragments from 1 m (red) and T 1m (transplanted from 5 m) (blue) exposed to either full sunlight (filled circles) or with UVB-removed (open circles) during the transplantation experiment performed between 2nd May – 16th June 2019. Also displayed is the Fv’/Fm’ of colonies in situ on the day before the experiment (T = -1 days). (B) Effective quantum yield (Fv’/Fm’) of T 1 m (transplanted from 5 m) Pocillopora verrucosa specimens under FS, displayed as percentage of the Fv’/Fm’ of the FS corals kept at their original sampling depth (1 m). Values are means (n=10) ± SEM.
Furthermore, the time required by the T 1m corals to reach the same Fv’/Fm’ as the 1 m corals was shortened under UVB-removal conditions. The mean Fv’/Fm’ of the 1 m FS and T 1 m FS corals was significantly different at each sampling point during the first 24 days. At the onset of the experiment, Fv’/Fm’ of the corals from the T 1 m FS treatment was approximately 9.9% lower than of the 1 m FS fragments, but this difference decreased by 0.226% per day (y = 0.226x + 90.1, p = 0.011) (Figure 7B), which means the two treatments should have the same Fv’/Fm’ after ~44 days. Indeed, after 46 days the mean Fv’/Fm’ of the 1 m FS group (0.61 ± 0.05) was very similar to that of the T 1 m FS group (0.62 ± 0.02) (t(17)= 0.97, p > 0.05). In comparison, UVB removal significantly increased Fv’/Fm’ in the T 1 m corals so that by day 4, there was no difference between the T 1 m -UVB corals and those from the 1 m FS treatment.
3.3.3 Lipid peroxidation (MDA)
The malondialdehyde (MDA) concentration inside the coral tissue ranged from 37.1–140.0 nmol mg-1 protein (Figure 8) and varied significantly between the four treatment groups (F(3, 116) = 26.29, p < 0.0001). Overall, T 1m FS corals had significantly higher MDA concentrations (86.7 ± 23.4 nmol mg-1 protein (mean ± SD) than 1m FS corals (55.5 ± 23.5 nmol mg-1 protein) (p< 0.0001), although this was not the case on day 46. However, when upward transplanted corals were kept under UVB removal conditions, mean MDA concentrations were significantly reduced (T 1m -UVB: 48.0 ± 6.6 nmol mg-1 protein) (p< 0.0001), and the concentrations were similar to those determined in the 1m FS samples.
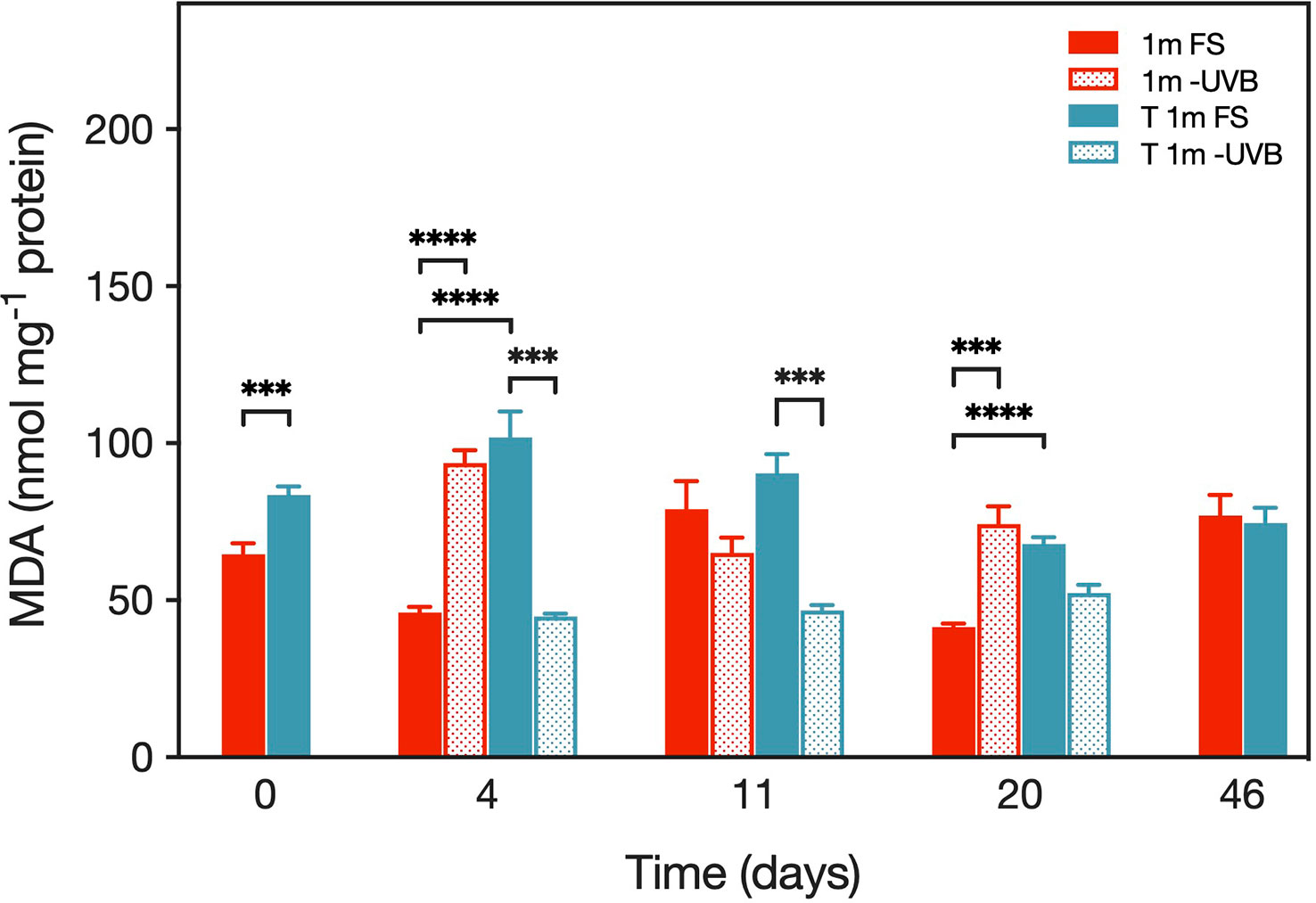
Figure 8 MDA concentrations (in nmol mg-1 protein) in transplanted Pocillopora verrucosa fragments during the experiment in May/June 2019. Values are means (n=10) ± SEM. Asterisks indicate cases where the Tukey’s post hoc test identified significantly different means between treatments at levels p < 0.001 (***) and p < 0.0001 (****).
The difference in mean MDA concentrations between the two upward transplanted coral treatments decreased during the experiment, i.e., on day 4, MDA levels in the T 1m -UVB fragments (44.8 ± 26.2 nmol mg-1 protein) were significantly lower than in the T 1m FS corals (101.9 ± 26.2 nmol mg-1 protein) (p< 0.0001) (Figure 8). The concentrations were no longer significantly different between the T 1m -UVB (52.3 ± 26.2 nmol mg-1 protein) and the T 1m -FS corals (67.9 ± 6.9 nmol mg-1 protein) on day 20 (p > 0.05). Also, on day 46, mean MDA concentrations did no longer differ between the shallow and the transplanted corals (t(18)= -0.30, p > 0.05).
3.3.4 In vivo UV absorption
All absorbance spectra of the coral tissue samples showed the highest absorbance in the wavelength range 320–340 nm (Supplementary Figure 9). However, while the absorbance determined for S. pistillata had a distinct peak at 330 nm which decreases from 330 nm to 290 nm, the absorbance by P. verrucosa was relatively constant in the range 330–290 nm. The absorption coefficient at 330 nm i.e., a(330), was approximately 2–3 orders higher in S. pistillata than in P. verrucosa (Figure 9). The ANOVA found no difference in mean absorption at 330 nm between S. pistillata from the three radiation treatments. P. verrucosa 1m FS fragments initially had a significantly higher mean a(330) (33.2 ± 5.0 m-1 mg-1 protein) than corals that were upward transplanted from 5 m depth (17.9 ± 2.3 m-1 mg-1 protein) (t(8)= -2.79, p < 0.05). The opposite pattern could be observed on day 46, with corals from 1 m exhibiting a significantly lower a(330) (9.5 ± 0.6 m-1 mg-1 protein) than 5 m corals (14.8 ± 2.0 m-1 mg-1 protein) (t(8)= 2.60, p < 0.05).
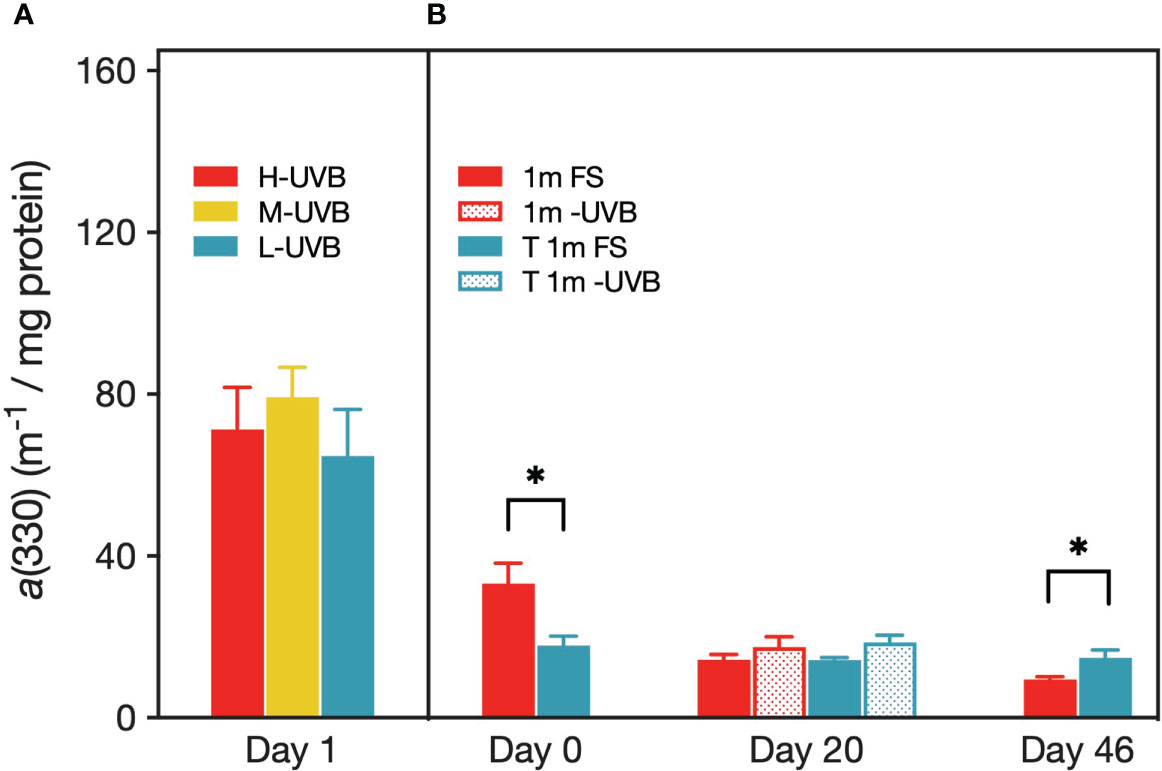
Figure 9 Absorption coefficient at 330 nm (m-1 per mg protein) of coral host tissue from Stylophora pistillata (A) and Pocillopora verrucosa (B). Values are means (n=5) ± SEM. Asterisks indicate cases where the Tukey’s post hoc test identified significantly different means between treatments at a level of p < 0.05.
4 Discussion
We observed that shallow specimens of the Red Sea coral Pocillopora verrucosa showed fast responses in their physiology while the LPO in Stylophora pistillata changed under UVB reduction, suggesting both corals were well acclimated to the high UVB in situ. However, in situ data from Pocillopora verrucosa indicated that deeper (8 m) specimens showed significantly higher and more constant effective quantum yields (Fv’/Fm’) values than specimens at shallow depths, and the strong correlation between Fv’/Fm’ and UVB irradiances suggests a considerable decrease of UVB stress with depth. We identified photo-physiological changes and the requirement of long-term acclimation responses of Pocillopora verrucosa specimens after their transplantation to shallower depths, suggesting that these corals optimize their photon capture and photochemical processes in response to new solar radiation conditions.
During the short-term UVB removal experiment with S. pistillata fragments, the reduction or removal of UVB did not result in an immediate, significant increase in effective quantum yields (Fv’/Fm’) of the algal symbionts nor enhance gross primary production (GPP) of the holobiont. Instead, both photosynthetic parameters remained equal between the three treatments during the experiment. In contrast, distinct signs of coral symbiont photoinhibition were reported from other manipulative experiments, following either a transplantation of the corals to shallower waters (Masuda et al., 1993; Shick et al., 1995; Lesser, 2000; Tamir et al., 2020) or the exposure of corals specimens to elevated levels of (solar) radiation (Gorbunov et al., 2001; Jones and Hoegh-Guldberg, 2001; Yakovleva and Hidaka, 2004; Brown and Dunne, 2008; Bellworthy and Fine, 2017) (Table 3). For example, S. pistillata fragments exposed to UV radiation had a significantly lower Fv/Fm than specimens exposed to PAR-only after 5 h of incubation irrespective of the incubation temperature; and Fv/Fm in the UV treatment was still significantly reduced after three days (Ferrier-Pages et al., 2007). Even after 17 days of incubation, Fv/Fm had not recovered to its initial value. However, it is noteworthy that the UV treatment in the before-mentioned experiment consisted of exposure to total UV, i.e., UVA and UVB, while the control samples were only exposed to PAR. In contrast, we either reduced or removed UVB radiation in the present study, whereas UVA was always present.
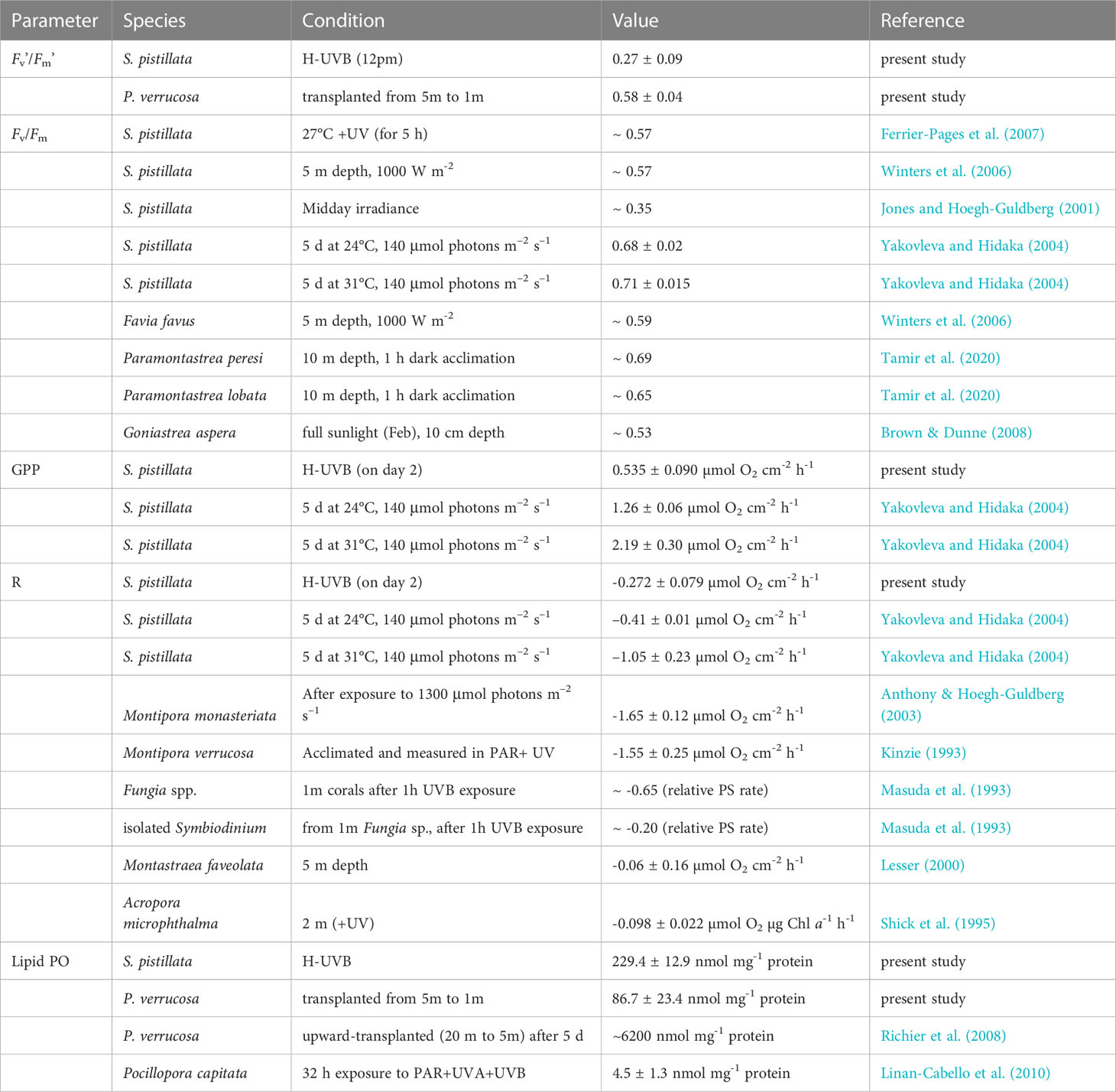
Table 3 Physiological parameters of Stylophora pistillata and Pocillopora verrucosa measured in the present study in comparison to previously reported values from the same species as well as other coral species.
Therefore, our result that neither GPP nor effective quantum yields were affected upon UVB reduction/removal is perhaps due to the presence of UVA in all treatments. Whereas UVA can have positive effects when received in moderate doses, extreme UVA exposure can have detrimental effects (Häder et al., 2015). For example, UVA exposure had a major impact on the quantum yields of Montipora verrucosa but the addition of UVB did not result in a further reduction of the quantum yield, highlighting the strong impact of UVA on the photophysiology (Torregiani and Lesser, 2007).
Regarding the corals’ respiration, we found that reduced UVB exposure during the day, or the complete absence thereof, did not result in a significant change in the respiration rates of S. pistillata. This result was surprising since previous studies found that corals exposed to bright sunlight would have higher respiration rates than corals exposed to low light or only PAR because of the increased metabolic costs entailed in maintaining the array of UV-protection and repair mechanisms (Anthony and Hoegh-Guldberg, 2003). However, we found no difference in respiration between coral from those two treatments, which is in line with reports on Montipora verrucosa where dark respiration of the corals was not affected by the light conditions (PAR or PAR+UV) immediately before the dark period (Kinzie, 1993). Similarly, respiration rates of intact corals collected at 1 m and 30 m depth did not change after exposure to UVB radiation (Masuda et al., 1993). In contrast, the same authors established that the respiration rates of isolated zooxanthellae were significantly reduced by UVB, indicating that zooxanthellae are protected against UVB damage when living in symbiosis. In contrast, UVB irradiation likely damages the mitochondria of zooxanthellae in isolation (Masuda et al., 1993).
We established that the concentrations of malondialdehyde (MDA), i.e., a product of lipid peroxidation and thus a proxy for oxidative stress, varied only marginally in S. pistillata between the radiation treatments. While we found that mean MDA concentrations gradually increased with increasing UVB exposure (0-UVB: 213.0, M-UVB 220.5, H-UVB: 229.4 nmol mg-1 protein), the concentrations were not significantly different, suggesting that UVB exposure had only minor effects on the coral host.
In comparison, lipid peroxidation in P. verrucosa colonies from the western Indian Ocean (11°30 S’, 47°20 E’) was unchanged after 5 days of enhanced or reduced irradiance levels, following an upward- (from 20 m to 5 m depth) or downward transplantation (5 m to 20 m), respectively (Richier et al., 2008). However, Richier et al. (2008) reported ~ 15 to 35-fold higher mean MDA concentrations than the concentrations measured in the present study, suggesting a higher resistance of P. verrucosa from the Red Sea. MDA concentrations of Pocillopora capitata specimens exposed to PAR and those exposed to PAR+UVB+UVA (predominantly UVB, λmax: 310 nm) for 32 h were not significantly different (Linan-Cabello et al., 2010). However, when the data from all sampling points were pooled, UV-treated corals had significantly higher levels of lipid peroxidative damage (4.5 ± 1.3 nmol mg-1 protein) than PAR-treated specimens (3.4 ± 0.8 nmol mg-1 protein), which in our study was not evident for S. pistillata, but significant differences were observed for transplanted P. verrucosa after four days.
It is well known that corals possess specific physiological characteristics that allow them to cope with variations in solar radiation, one of these being the expression of green fluorescent proteins (GFPs) (Richier et al., 2008). The accumulation of GFPs has been described as a photoprotective mechanism in shallow-water corals that dissipates highly energetic wavelengths, thereby providing photoprotection to both the coral host and its symbiotic algae (Salih et al., 2000; Scucchia et al., 2020). Additionally, corals can synthesize another group of UV-absorbing compounds, known as mycosporine-like amino acids (MAAs) (Shick et al., 1995; Dunlap and Shick, 1998). Shallow-water colonies of the coral S. pistillata were found to contain unusually large amounts of MAAs (Shick, 2004; Ferrier-Pages et al., 2007), which is in line with the high UV absorption we found and the high UV resistance observed during our short-term experiment with this coral species.
It has previously been reported that S. pistillata is exceptionally thermotolerant (Bellworthy and Fine, 2017; Krueger et al., 2017), which could explain the relatively minor physiological changes in both the host and the endosymbionts upon UVB reduction or removal. We did not identify the symbionts associated with S. pistillata or P. verrucosa as part of our study. However, it is widely reported that shallow-water specimens of both species in the Red Sea are predominantly associated with Symbiodinium sp. clade A (Arrigoni et al., 2016; Ziegler et al., 2017; Bednarz et al., 2018), which is known to be thermotolerant (Sawall et al., 2014). The coral specimens were sampled from a shallow depth (~ 1 m) characterized by high solar irradiances, suggesting that the specimens were exceptionally well adapted or acclimated to extreme UV irradiances. Indeed, we identified a distinct peak in absorption by the coral at 320–340 nm, so it may be that the existing UV-protection strategies of shallow-water S. pistillata are so well developed that even under ambient UVB, the symbionts can photosynthesize at the highest possible rate. Consequently, reducing or removing UVB exposure could not further enhance their photosynthetic capacity.
The in situ measurements with Pocillopora verrucosa identified that the Fv’/Fm’ of colonies between 0.5 and 4 m depths were very similar. In contrast, colonies at 8 m depth had a significantly higher Fv’/Fm’ (Figure 5). This result might be due to the reduced PAR or UVA, the near-absence of UVB in 8 m depths, or due to the lower and more stable temperatures in deeper depths (Supplementary Figure 7). The strong correlation between these three environmental parameters in natural waters (see Figure 6) presents a challenge when trying to disentangle the effects of individual stressors. However, the correlation analysis strongly suggests that UVB (r = -0.57) is a crucial determinant for the photosynthetic efficiency of coral symbionts in situ; and possibly more so than PAR (r = -0.54) or water temperature (r = -0.40), which is in line with the finding that high solar irradiances reduce Fv/Fm in corals at least as much as high temperatures (Winters et al., 2006).
We did not observe any bleaching or mortality in any of the depths during our experiment. The critical bleaching threshold temperature of 32.1°C was frequently exceeded in the extreme shallows during the daytime. However, rapid cooling in the evening meant that temperatures quickly dropped again below the threshold, alleviating the corals from thermal stress, which may explain their high bleaching resistance (Safaie et al., 2018). Additionally, our findings confirm the observation that shallow-water corals on the wave-sheltered side of central Red Sea reefs experience extreme diurnal temperature variations in summer (> 5°C) (Davis et al., 2011), which may further contribute to their high bleaching resistance (Sammarco et al., 2006; Oliver and Palumbi, 2011).
Our transplantation experiment with P. verrucosa found that low-light adapted corals, i.e., those from deeper depths, have a lower initial tolerance to high irradiance conditions, particularly UVB. Specifically, we found that P. verrucosa fragments transplanted from 5 m to 1 m and kept under full sunlight conditions initially had significantly lower Fv’/Fm’ than their shallow-water conspecifics adapted to high irradiance. Besides experiencing higher PAR (Δ mean: + 131 μmol m-2 s-1, Δ max: +1071 μmol m-2 s-1) and UVB (Δ mean: + 0.24 W m-2, Δ max: +0.87 W m-2) irradiances, the upward transplanted corals were also exposed to increased temperatures (Table 2). The latter is important since several studies have described that the photosynthetic efficiency of corals can be severely reduced under elevated temperatures (Lesser, 1996; Bellworthy and Fine, 2017; Voolstra et al., 2020). However, the temperature increase due to the upward transplantation was relatively minor in the present study (Δ mean: + 0.1°C, Δ max: +1.1°C), so that the temperature increase did probably not contribute significantly to the observed inhibition of the photosynthetic efficiency. This hypothesis is further supported by the fact that a few days after the upward transplantation, there was no significant difference between the Fv’/Fm’ of corals from 1 m and 5 m when UVB was removed, indicating that UVB rather than temperature or PAR is the key determinant. Four days after their transplantation, the removal of UVB in corals originating from 5 m depth also led to a significantly higher Fv’/Fm’ compared to their full sunlight conspecifics, which lasted until day 20. In fact, for the entire experiment duration, the Fv’/Fm’ of the UVB-removal specimens originating from 5 m was the same as that of the 1 m samples, which highlights the importance of UVB as photoinhibitor. This finding is in line with the report that Montipora verrucosa corals acclimated to PAR + UV displayed higher photosynthesis under full sunlight conditions than corals acclimated to PAR-only (Kinzie, 1993). However, no difference in the photosynthetic parameters was found when photosynthesis was measured in the light regime to which the corals had been acclimated, which agrees with our Fv’/Fm’ results of corals in situ, from extreme shallows down to at least 4 m depth (see Figure 5).
The discrepancy in Fv’/Fm’ between full sunlight samples from 1 m and those upward transplanted from 5 m gradually decreased throughout the experiment, and both groups performed equally well by the end of the experiment. Although differences in Fv’/Fm’ values were small, this equalization suggests a slow acclimation potential of Pocillopora verrucosa from deeper waters to changes in solar UVB conditions. In contrast, the fast adaptation of transplanted P. verrucosa fragments when UVB was removed, highlights that elevated UVB was a primary factor limiting the acclimation response, as opposed to other changes such as increased temperature or PAR. This hypothesis is further supported by our findings that Fv’/Fm’ exhibited the strongest correlation (r = -0.57) with the factor UVB, and that the highest Fv’/Fm’ were measured when UVB irradiances were negligible (Figure 6).
The upward transplantation of 5 m corals to shallower waters may have initially caused an enhanced accumulation of intracellular oxygen that could have led to 1) damage to photosystem II and ultimately photoinhibition in the first few days because of the higher PAR and UVB photon flux in shallower waters, and 2) to the production of reactive oxygen species (ROS), which can damage chloroplasts (Finelli et al., 2006; Mass et al., 2010) and result in programmed cell-death and degradation (LeTissier and Brown, 1996; Franklin et al., 2004). ROS can also impact cellular adhesive proteins (Davies, 1987) leading to the expulsion of endosymbionts (Dunn et al., 2002). Therefore, the production of ROS initiates negative feedback by reducing the concentration of symbiont cells, thereby lowering the evolution rate of oxygen molecules that could become toxic. We, therefore, expect that the exposure of the 5 m corals to elevated UVB invoked by the transplantation triggered the abovementioned ROS-induced photoacclimation, which supports the conclusion that UVB radiation acts as a photoacclimatory enhancer (Cohen et al., 2013).
The presence of MAAs is particularly prominent in shallow-water specimens (Banaszak et al., 1998; Shick and Dunlap, 2002), which agrees with our finding that P. verrucosa from 1m exhibited a higher absorption at 330 nm than corals from 5 m. Torres et al. (2007) reported a 40-fold increase in total MAA concentration in transplanted Acropora cervicornis corals (from 20 m to 1 m depth) after one month of enhanced UV exposure and an 88-fold increase in MAAs after a further three months. However, in the present study, in vivo absorption at 330 nm in upward transplanted corals did not change over 46 days. Therefore, the drastic improvement in the photosynthetic efficiency of transplanted corals in full sunlight after ~5 weeks was likely not linked to a change in MAAs concentrations. In the T 1 m FS corals, the acclimation of the symbionts’ photosynthetic apparatus and the equilibration of MDA levels in the coral host occurred within a similar time frame of 1–1.5 months. While several studies have reported that high temperatures can cause an increased occurrence of lipid peroxidation (LPO) in corals (Flores-Ramirez and Linan-Cabello, 2007; Yakovleva et al., 2009), in our upward transplanted corals, the average temperature increase was only marginal (mean ΔT = + 0.1°C) compared to temperature increments of ≥2°C in previous reports, which suggest that other factors such as elevated solar irradiances likely caused the increased peroxidation of host lipids.
When we compared LPO between our experiments, we found that mean MDA concentrations in the full sunlight treated S. pistillata were approximately four-fold higher than in P. verrucosa despite the near-identical UVB conditions. This finding may suggest that 1) S. pistillata suffered more damage to lipids due to the stress of transferring the corals and keeping them in shallow incubators, or 2) that P. verrucosa possesses exceptionally well-developed, species-specific UVB coping-mechanisms. Also, the sensitivity of corals to UVB was found to be strongly dependent on the corals’ prior history of UV exposure (Siebeck, 1981; Torregiani and Lesser, 2007). For example, when Plerogyra sinuosa specimens were upward transplanted from 25 m (where UVB was almost non-detectable) to 5 m depth, the mortality rate of UV-unshielded samples was 90% after one month (Vareschi and Fricke, 1986). In comparison, we did not detect any mortality nor signs of coral bleaching during our transplantation experiment, which is likely linked to the fact that corals in 5 m depth are already exposed to relatively high levels of UV and therefore possess a reasonably well baseline protection against UVB.
Additionally, it has been suggested that the ability to contend with substantial light variations is strongly species-specific (Nakamura and Yamasaki, 2008). For example, the discrepancy in mortality rates between the study of Vareschi and Fricke (1986) and ours might stem from the morphological differences between the two coral species. Plerogyra sinuosa has large vesicle-like polyps that often protrude from the skeleton structure, whereas P. verrucosa consists of small polyps that are mostly retracted into the corallites (Veron, 2000). This difference is of relevance since coral skeletons can 1) absorb UV radiation and dissipate excess energy as yellow fluorescence, resulting in less DNA damage in the coral tissue, and 2) reflect PAR wavelengths to the overlying tissue, which benefits the photosynthesis of the endosymbionts and might be another factor contributing to the reasonably quick acclimation of P. verrucosa to enhanced UVB without any mortality incidences (Reef et al., 2009).
A recent study found that maximum incident UVB irradiances in the central Red Sea can be observed earlier in the year (May/June) than peak water temperatures (July/August) (Overmans and Agusti, 2020). However, it remains to be seen how S. pistillata, P. verrucosa, and other shallow-water Red Sea corals will fare in the near future since both temperature (Chaidez et al., 2017; Osman et al., 2018) and UVB (Watanabe et al., 2011; Meul et al., 2016; Bais et al., 2019) are predicted to increase over the next few decades, which will likely result in longer periods when both environmental parameters are above their critical threshold for corals (Overmans and Agusti, 2020). This prognosis is of particular relevance for specimens in shallow waters, where UVB exposure was already extreme (> 20 kJ m-2) and water temperatures surpassed the bleaching threshold temperature during the daytime for > 5 weeks. We could observe that shallow-water P. verrucosa showed a stronger inhibition of Fv’/Fm’, indicative of higher stress, possibly due to the combination of both environmental stressors. Similarly, the high UVB exposure in 1 m reduced the acclimation response to changing environmental conditions, suggesting that the acclimation response could be further decelerated in future climates under enhanced temperatures and UVB. Protecting S. pistillata from UVB did not change the gross primary production or Fv’/Fm’, and respiration and LPO in the host remained unaffected, highlighting that S. pistillata possesses well-developed UVB coping mechanisms and hints that this species might have a high capacity to tolerate the predicted elevated UVB.
Previous studies have identified that elevated temperature and extreme ultraviolet can act synergistically, thereby leading to impacts of multiplicative magnitude (Ban et al., 2014; Jin et al., 2019). For instance, elevated temperature and UVB irradiances were found to act synergistically on the symbiont density (Drohan et al., 2005) and the survival and photosynthetic efficiency (Ferrier-Pages et al., 2007; Zeevi Ben-Yosef and Benayahu, 2008) of tropical reef cnidarians, which bears the question if Red Sea scleractinian corals will be able to acclimate to the increased temperatures and UVB exposure in future environmental conditions.
5 Conclusion
In summary, our study demonstrated that, overall, Red Sea corals showed a large acclimation capacity and resistance to UVB radiation, although we could observe species-specific tolerance capacities. Shielding shallow-water specimens from UVB did neither change their respiration nor enhance their photosynthetic efficiency, suggesting that shallow-water specimens can maximize photon capture and their photosynthetic activity, even when UVB irradiances are high. P. verrucosa in deeper waters (8 m) exhibited less varying and higher Fv’/Fm’ than those in 4 m depth and above, where water temperatures, PAR and UVB were considerably higher. Upward transplanted corals exhibited slow acclimation under full sunlight and required 2-4 weeks to acclimate while under UVB removal conditions the acclimation took ≤ 4 d, which highlights that UVB, besides temperature, is indeed a crucial stressor governing the physiology of P. verrucosa. Overall, however, P. verrucosa from 5 m were well protected against elevated UVB and temperature since neither bleaching nor mortality was detected after transplantation.
We conclude that elevated UVB exposure may serve as a trigger for corals to enhance their photoacclimation when exposed to increased solar irradiances. Furthermore, it would be interesting to test if a reduction or removal of UVB can help alleviate thermally induced stress in corals and ultimately lead to a higher resistance against coral bleaching or an accelerated recovery thereof.
Data availability statement
The original contributions presented in the study are included in the article/Supplementary Material. Further inquiries can be directed to the corresponding author.
Author contributions
SA and SO conceptualized the study. SO collected the data. SO and SA analyzed the data. SO generated tables and figures. SO wrote the manuscript with a substantial contribution from SA. Both authors contributed to the article and approved the final version.
Funding
The research reported in this publication was supported by baseline funding from KAUST to SA under award number BAS/1/1072-01-01.
Acknowledgments
We would like to express special thanks to Daffne Celeste López-Sandoval, Susann Rossbach, Katherine Rowe, Francisco Luis Aparicio Bernat, Amr Gusti, and Ricardo Alves, the team of the Coastal and Marine Resources Core Lab (CMR) at KAUST, and the captain and crew of R/V Thuwal for their expert support and assistance.
Conflict of interest
The authors declare that the research was conducted in the absence of any commercial or financial relationships that could be construed as a potential conflict of interest.
Publisher’s note
All claims expressed in this article are solely those of the authors and do not necessarily represent those of their affiliated organizations, or those of the publisher, the editors and the reviewers. Any product that may be evaluated in this article, or claim that may be made by its manufacturer, is not guaranteed or endorsed by the publisher.
Supplementary material
The Supplementary Material for this article can be found online at: https://www.frontiersin.org/articles/10.3389/fmars.2023.847559/full#supplementary-material
References
Agusti S., Llabres M., Carreja B., Fernandez M., Duarte C. M. (2015). Contrasting sensitivity of marine biota to UV-b radiation between southern and northern hemispheres. Estuaries Coasts 38 (4), 1126–1133. doi: 10.1007/s12237-014-9790-9
Altieri A. H., Harrison S. B., Seemann J., Collin R., Diaz R. J., Knowlton N. (2017). Tropical dead zones and mass mortalities on coral reefs. Proc. Natl. Acad. Sci. U.S.A. 114 (14), 3660–3665. doi: 10.1073/pnas.1621517114
Anderson S., Zepp R., Machula J., Santavy D., Hansen L., Mueller E. (2001). Indicators of UV exposure in corals and their relevance to global climate change and coral bleaching. Hum. Ecol. Risk Assess. 7 (5), 1271–1282. doi: 10.1080/20018091094998
Anthony K. R. N., Connolly S. R., Hoegh-Guldberg O. (2007). Bleaching, energetics, and coral mortality risk: Effects of temperature, light, and sediment regime. Limnology Oceanography 52 (2), 716–726. doi: 10.4319/lo.2007.52.2.0716
Anthony K. R. N., Hoegh-Guldberg O. (2003). Variation in coral photosynthesis, respiration and growth characteristics in contrasting light microhabitats: an analogue to plants in forest gaps and understoreys? Funct. Ecol. 17 (2), 246–259. doi: 10.1046/j.1365-2435.2003.00731.x
Anthony K. R. N., Kerswell A. P. (2007). Coral mortality following extreme low tides and high solar radiation. Mar. Biol. 151 (5), 1623–1631. doi: 10.1007/s00227-006-0573-0
Arrigoni R., Benzoni F., Terraneo T. I., Caragnano A., Berumen M. L. (2016). Recent origin and semi-permeable species boundaries in the scleractinian coral genus stylophora from the red Sea. Sci. Rep. 6, 34612. doi: 10.1038/srep34612
Bais A. F., Bernhard G., McKenzie R. L., Aucamp P. J., Young P. J., Ilyas M., et al. (2019). Ozone-climate interactions and effects on solar ultraviolet radiation. Photochem. Photobiol. Sci. 18 (3), 602–640. doi: 10.1039/c8pp90059k
Baker N. R. (2008). Chlorophyll fluorescence: a probe of photosynthesis in vivo. Annu. Rev. Plant Biol. 59, 89–113. doi: 10.1146/annurev.arplant.59.032607.092759
Ban S. S., Graham N. A., Connolly S. R. (2014). Evidence for multiple stressor interactions and effects on coral reefs. Glob. Chang. Biol. 20 (3), 681–697. doi: 10.1111/gcb.12453
Banaszak A. T., Lesser M. P. (2009). Effects of solar ultraviolet radiation on coral reef organisms. Photochem. Photobiol. Sci. 8 (9), 1276–1294. doi: 10.1039/b902763g
Banaszak A. T., Lesser M. P., Kuffner I. B., Ondrusek M. (1998). Relationship between ultraviolet (UV) radiation and mycosporine-like amino acids (MAAS) in marine organisms. Bull. Mar. Sci. 63 (3), 617–628.
Baruch R., Avishai N., Rabinowitz C. (2005). UV Incites diverse levels of DNA breaks in different cellular compartments of a branching coral species. J. Exp. Biol. 208 (Pt 5), 843–848. doi: 10.1242/jeb.01496
Bednarz V. N., Naumann M. S., Cardini U., van Hoytema N., Rix L., Al-Rshaidat M. M. D., et al. (2018). Contrasting seasonal responses in dinitrogen fixation between shallow and deep-water colonies of the model coral stylophora pistillata in the northern red Sea. PloS One 13 (6), e0199022. doi: 10.1371/journal.pone.0199022
Bellworthy J., Fine M. (2017). Beyond peak summer temperatures, branching corals in the gulf of aqaba are resilient to thermal stress but sensitive to high light. Coral Reefs 36 (4), 1071–1082. doi: 10.1007/s00338-017-1598-1
Birben E., Sahiner U. M., Sackesen C., Erzurum S., Kalayci O. (2012). Oxidative stress and antioxidant defense. World Allergy Organ J. 5 (1), 9–19. doi: 10.1097/WOX.0b013e3182439613
Brown B. E., Dunne R. P. (2008). Solar radiation modulates bleaching and damage protection in a shallow water coral. Mar. Ecol. Prog. Ser. 362, 99–107. doi: 10.3354/meps07439
Brown B. E., Dunne R. P., Scoffin T. P., Le Tissier M. D. A. (1994). Solar damage in intertidal corals. Mar. Ecol. Prog. Ser. 105 (3), 219–230. doi: 10.3354/meps105219
Chaidez V., Dreano D., Agusti S., Duarte C. M., Hoteit I. (2017). Decadal trends in red Sea maximum surface temperature. Sci. Rep. 7 (1), 8144. doi: 10.1038/s41598-017-08146-z
Cohen I., Dishon G., Iluz D., Dubinsky Z. (2013). UV-B as a photoacclimatory enhancer of the hermatypic coral stylophora pistillata. Open J. Mar. Sci. 3 (02), 15. doi: 10.4236/ojms.2013.32A003
Cote I. M., Darling E. S., Brown C. J. (2016). Interactions among ecosystem stressors and their importance in conservation. Proc. Biol. Sci. 283 (1824), 20152592. doi: 10.1098/rspb.2015.2592
Courtial L., Roberty S., Shick J. M., Houlbreque F., Ferrier-Pages C. (2017). Interactive effects of ultraviolet radiation and thermal stress on two reef-building corals. Limnology Oceanography 62 (3), 1000–1013. doi: 10.1002/lno.10481
Csaszar N. B. M., Seneca F. O., van Oppen M. J. H. (2009). Variation in antioxidant gene expression in the scleractinian coral Acropora millepora under laboratory thermal stress. Mar. Ecol. Prog. Ser. 392, 93–102. doi: 10.3354/meps08194
Davies K. J. (1987). Protein damage and degradation by oxygen radicals. i. general aspects. J. Biol. Chem. 262 (20), 9895–9901. doi: 10.1016/S0021-9258(18)48018-0
Davis K. A., Lentz S. J., Pineda J., Farrar J. T., Starczak V. R., Churchill J. H. (2011). Observations of the thermal environment on red Sea platform reefs: A heat budget analysis. Coral Reefs 30 (1), 25–36. doi: 10.1007/s00338-011-0740-8
Downs C. A., Fauth J. E., Halas J. C., Dustan P., Bemiss J., Woodley C. M. (2002). Oxidative stress and seasonal coral bleaching. Free Radic. Biol. Med. 33 (4), 533–543. doi: 10.1016/s0891-5849(02)00907-3
Downs N. J., Schouten P. W., Parisi A. V. (2013). Seasonal variations in the subsurface ultraviolet-b on an inshore pacific coral reef ecosystem. Photochem. Photobiol. 89 (5), 1234–1243. doi: 10.1111/php.12101
Drohan A. F., Thoney D. A., Baker A. C. (2005). Synergistic effect of high temperature and ultraviolet-b radiation on the gorgonian eunicea tourneforti (Octocorallia : Alcyonacea : Plexauridae). Bull. Mar. Sci. 77 (2), 257–266.
Dunlap W. C., Shick J. M. (1998). Ultraviolet radiation-absorbing mycosporine-like amino acids in coral reef organisms: A biochemical and environmental perspective. J. Phycology 34 (3), 418–430. doi: 10.1046/j.1529-8817.1998.340418.x
Dunn S. R., Bythell J. C., Le Tissier M. D. A., Burnett W. J., Thomason J. C. (2002). Programmed cell death and cell necrosis activity during hyperthermic stress-induced bleaching of the symbiotic sea anemone aiptasia sp. J. Exp. Mar. Biol. Ecol. 272 (1), 29–53. doi: 10.1016/S0022-0981(02)00036-9
Dunne R. P. (2010). Synergy or antagonism-interactions between stressors on coral reefs. Coral Reefs 29 (1), 145–152. doi: 10.1007/s00338-009-0569-6
Ellis J. I., Jamil T., Anlauf H., Coker D. J., Curdia J., Hewitt J., et al. (2019). Multiple stressor effects on coral reef ecosystems. Glob. Chang. Biol. 25 (12), 4131–4146. doi: 10.1111/gcb.14819
Ferrier-Pages C., Richard C., Forcioli D., Allemand D., Pichon M., Shick J. M. (2007). Effects of temperature and UV radiation increases on the photosynthetic efficiency in four scleractinian coral species. Biol. Bull. 213 (1), 76–87. doi: 10.2307/25066620
Finelli C. M., Helmuth B. S. T., Pentcheff N. D., Wethey D. S. (2006). Water flow influences oxygen transport and photosynthetic efficiency in corals. Coral Reefs 25 (1), 47–57. doi: 10.1007/s00338-005-0055-8
Fitt W. K., Brown B. E., Warner M. E., Dunne R. P. (2001). Coral bleaching: interpretation of thermal tolerance limits and thermal thresholds in tropical corals. Coral Reefs 20 (1), 51–65. doi: 10.1007/s003380100146
Fitt W. K., McFarland F. K., Warner M. E., Chilcoat G. C. (2000). Seasonal patterns of tissue biomass and densities of symbiotic dinoflagellates in reef corals and relation to coral bleaching. Limnology Oceanography 45 (3), 677–685. doi: 10.4319/lo.2000.45.3.0677
Flores-Ramirez L. A., Linan-Cabello M. A. (2007). Relationships among thermal stress, bleaching and oxidative damage in the hermatypic coral, pocillopora capitata. Comp. Biochem. Physiol. C. Toxicol. Pharmacol. 146 (1-2), 194–202. doi: 10.1016/j.cbpc.2006.09.008
Franklin D. J., Hoegh-Guldberg P., Jones R. J., Berges J. A. (2004). Cell death and degeneration in the symbiotic dinoflagellates of the coral stylophora pistillata during bleaching. Mar. Ecol. Prog. Ser. 272, 117–130. doi: 10.3354/meps272117
Gardner S. G., Nielsen D. A., Laczka O., Shimmon R., Beltran V. H., Ralph P. J., et al. (2016). Dimethylsulfoniopropionate, superoxide dismutase and glutathione as stress response indicators in three corals under short-term hyposalinity stress. Proc. Biol. Sci. 283 (1824), 20152418. doi: 10.1098/rspb.2015.2418
Gegner H. M., Radecker N., Ochsenkuhn M., Barreto M. M., Ziegler M., Reichert J., et al. (2019). High levels of floridoside at high salinity link osmoadaptation with bleaching susceptibility in the cnidarian-algal endosymbiosis. Biol. Open 8 (12), bio045591. doi: 10.1242/bio.045591
Genty B., Briantais J. M., Baker N. R. (1989). The relationship between the quantum yield of photosynthetic electron-transport and quenching of chlorophyll fluorescence. Biochim. Et Biophys. Acta 990 (1), 87–92. doi: 10.1016/S0304-4165(89)80016-9
Gleason D. F., Edmunds P. J., Gates R. D. (2006). Ultraviolet radiation effects on the behavior and recruitment of larvae from the reef coral porites astreoides. Mar. Biol. 148 (3), 503–512. doi: 10.1007/s00227-005-0098-y
Gorbunov M. Y., Kolber Z. S., Lesser M. P., Falkowski P. G. (2001). Photosynthesis and photoprotection in symbiotic corals. Limnology Oceanography 46 (1), 75–85. doi: 10.4319/lo.2001.46.1.0075
Grinblat M., Fine M., Tikochinski Y., Loya Y. (2018). Stylophora pistillata in the red Sea demonstrate higher GFP fluorescence under ocean acidification conditions. Coral Reefs 37 (1), 309–320. doi: 10.1007/s00338-018-1659-0
Häder D. P., Williamson C. E., Wangberg S. A., Rautio M., Rose K. C., Gao K., et al. (2015). Effects of UV radiation on aquatic ecosystems and interactions with other environmental factors. Photochem. Photobiol. Sci. 14 (1), 108–126. doi: 10.1039/c4pp90035a
Hoegh-Guldberg O. (1999). Climate change, coral bleaching and the future of the world's coral reefs. Mar. Freshw. Res. 50 (8), 839–866. doi: 10.1071/Mf99078
Hoegh-Guldberg O., Bruno J. F. (2010). The impact of climate change on the world's marine ecosystems. Science 328 (5985), 1523–1528. doi: 10.1126/science.1189930
Hoegh-Guldberg O., Mumby P. J., Hooten A. J., Steneck R. S., Greenfield P., Gomez E., et al. (2007). Coral reefs under rapid climate change and ocean acidification. Science 318 (5857), 1737–1742. doi: 10.1126/science.1152509
Hughes D. J., Alderdice R., Cooney C., Kuhl M., Pernice M., Voolstra C. R., et al. (2020). Coral reef survival under accelerating ocean deoxygenation. Nat. Climate Change 10 (4), 296–307. doi: 10.1038/s41558-020-0737-9
Hughes T. P., Baird A. H., Bellwood D. R., Card M., Connolly S. R., Folke C., et al. (2003). Climate change, human impacts, and the resilience of coral reefs. Science 301 (5635), 929–933. doi: 10.1126/science.1085046
Hughes T. P., Barnes M. L., Bellwood D. R., Cinner J. E., Cumming G. S., Jackson J. B. C., et al. (2017a). Coral reefs in the anthropocene. Nature 546 (7656), 82–90. doi: 10.1038/nature22901
Hughes T. P., Kerry J. T., Alvarez-Noriega M., Alvarez-Romero J. G., Anderson K. D., Baird A. H., et al. (2017b). Global warming and recurrent mass bleaching of corals. Nature 543 (7645), 373–377. doi: 10.1038/nature21707
Jin P., Overmans S., Duarte C. M., Agustí S. (2019). Increasing temperature within thermal limits compensates negative ultraviolet-b radiation effects in terrestrial and aquatic organisms. Global Ecol. Biogeography 28 (11), 1695–1711. doi: 10.1111/geb.12973
Johnson M. D., Rodriguez L. M., Altieri A. H. (2018). Shallow-water hypoxia and mass mortality on a Caribbean coral reef. Bull. Mar. Sci. 94 (1), 143–144. doi: 10.5343/bms.2017.1163
Jones R. J., Hoegh-Guldberg O. (2001). Diurnal changes in the photochemical efficiency of the symbiotic dinoflagellates (Dinophyceae) of corals: Photoprotection, photoinactivation and the relationship to coral bleaching. Plant Cell Environ. 24 (1), 89–99. doi: 10.1046/j.1365-3040.2001.00648.x
Kinzie R. A. (1993). Effects of ambient levels of solar ultraviolet-radiation on zooxanthellae and photosynthesis of the reef coral montipora-verrucosa. Mar. Biol. 116 (2), 319–327. doi: 10.1007/Bf00350022
Krueger T., Hawkins T. D., Becker S., Pontasch S., Dove S., Hoegh-Guldberg O., et al. (2015). Differential coral bleaching-contrasting the activity and response of enzymatic antioxidants in symbiotic partners under thermal stress. Comp. Biochem. Physiol. A Mol. Integr. Physiol. 190, 15–25. doi: 10.1016/j.cbpa.2015.08.012
Krueger T., Horwitz N., Bodin J., Giovani M. E., Escrig S., Meibom A., et al. (2017). Common reef-building coral in the northern red Sea resistant to elevated temperature and acidification. R Soc. Open Sci. 4 (5), 170038. doi: 10.1098/rsos.170038
Kuffner I. B. (2001). Effects of ultraviolet (UV) radiation on larval settlement of the reef coral pocillopora damicornis. Mar. Ecol. Prog. Ser. 217, 251–261. doi: 10.3354/meps217251
Lesser M. P. (1996). Elevated temperatures and ultraviolet radiation cause oxidative stress and inhibit photosynthesis in symbiotic dinoflagellates. Limnology Oceanography 41 (2), 271–283. doi: 10.4319/lo.1996.41.2.0271
Lesser M. P. (2000). Depth-dependent photoacclimatization to solar ultraviolet radiation in the Caribbean coral montastraea faveolata. Mar. Ecol. Prog. Ser. 192, 137–151. doi: 10.3354/meps192137
Lesser M. P., Farrell J. H. (2004). Exposure to solar radiation increases damage to both host tissues and algal symbionts of corals during thermal stress. Coral Reefs 23 (3), 367–377. doi: 10.1007/s00338-004-0392-z
Lesser M. P., Stochaj W. R., Tapley D. W., Shick J. M. (1990). Bleaching in coral reef anthozoans: effects of irradiance, ultraviolet radiation, and temperature on the activities of protective enzymes against active oxygen. Coral Reefs 8 (4), 225–232. doi: 10.1007/bf00265015
LeTissier M. D. A., Brown B. E. (1996). Dynamics of solar bleaching in the intertidal reef coral goniastrea aspera at ko phuket, Thailand. Mar. Ecol. Prog. Ser. 136 (1-3), 235–244. doi: 10.3354/meps136235
Levy O., Achituv Y., Yacobi Y. Z., Stambler N., Dubinsky Z. (2006). The impact of spectral composition and light periodicity on the activity of two antioxidant enzymes (SOD and CAT) in the coral favia favus. J. Exp. Mar. Biol. Ecol. 328 (1), 35–46. doi: 10.1016/j.jembe.2005.06.018
Linan-Cabello M. A., Flores-Ramirez L. A., Cobo-Diaz J. F., Zenteno-Savin T., Olguin-Monroy N. O., Olivos-Ortiz A., et al. (2010). Response to short term ultraviolet stress in the reef-building coral pocillopora capitata (Anthozoa: Scleractinia). Rev. Biol. Trop. 58 (1), 103–118. doi: 10.15517/rbt.v58i1.5197
Lopez-Sandoval D. C., Duarte C. M., Agusti S. (2021). Nutrient and temperature constraints on primary production and net phytoplankton growth in a tropical ecosystem. Limnology Oceanography 66 (7), 2923–2935. doi: 10.1002/lno.11849
Mass T., Kline D. I., Roopin M., Veal C. J., Cohen S., Iluz D., et al. (2010). The spectral quality of light is a key driver of photosynthesis and photoadaptation in stylophora pistillata colonies from different depths in the red Sea. J. Exp. Biol. 213 (Pt 23), 4084–4091. doi: 10.1242/jeb.039891
Masuda K., Goto M., Maruyama T., Miyachi S. (1993). Adaptation of solitary corals and their zooxanthellae to low-light and UV-radiation. Mar. Biol. 117 (4), 685–691. doi: 10.1007/Bf00349781
McKenzie R. L., Aucamp P. J., Bais A. F., Bjorn L. O., Ilyas M. (2007). Changes in biologically-active ultraviolet radiation reaching the earth's surface. Photochem. Photobiol. Sci. 6 (3), 218–231. doi: 10.1039/b700017k
Meul S., Dameris M., Langematz U., Abalichin J., Kerschbaumer A., Kubin A., et al. (2016). Impact of rising greenhouse gas concentrations on future tropical ozone and UV exposure. Geophysical Res. Lett. 43 (6), 2919–2927. doi: 10.1002/2016gl067997
Nakamura T., Yamasaki H. (2008). Flicker light effects on photosynthesis of symbiotic algae in the reef-building coral acropora digitifera (Cnidaria : Anthozoa : Scleractinia). Pacific Sci. 62 (3), 341–350. doi: 10.2984/1534-6188(2008)62[341:Fleopo]2.0.Co;2
Nesa B., Baird A. H., Harii S., Yakovleva I., Hidaka M. (2012). Algal symbionts increase DNA damage in coral planulae exposed to sunlight. Zoological Stud. 51 (1), 12–17.
Oliver T. A., Palumbi S. R. (2011). Do fluctuating temperature environments elevate coral thermal tolerance? Coral Reefs 30 (2), 429–440. doi: 10.1007/s00338-011-0721-y
Osman E. O., Smith D. J., Ziegler M., Kurten B., Conrad C., El-Haddad K. M., et al. (2018). Thermal refugia against coral bleaching throughout the northern red Sea. Glob Chang Biol. 24 (2), e474–e484. doi: 10.1111/gcb.13895
Overmans S., Agusti S. (2019). Latitudinal gradient of UV attenuation along the highly transparent red Sea basin. Photochem. Photobiol. 95 (5), 1267–1279. doi: 10.1111/php.13112
Overmans S., Agusti S. (2020). Unraveling the seasonality of UV exposure in reef waters of a rapidly warming (Sub-)tropical Sea. Front. Mar. Sci. 7 (111). doi: 10.3389/fmars.2020.00111
Reef R., Kaniewska P., Hoegh-Guldberg O. (2009). Coral skeletons defend against ultraviolet radiation. PloS One 4 (11), e7995. doi: 10.1371/journal.pone.0007995
Richier S., Cottalorda J. M., Guillaume M. M. M., Fernandez C., Allemand D., Furla P. (2008). Depth-dependant response to light of the reef building coral, pocillopora verrucosa: Implication of oxidative stress. J. Exp. Mar. Biol. Ecol. 357 (1), 48–56. doi: 10.1016/j.jembe.2007.12.026
Rossbach S., Overmans S., Kaidarova A., Kosel J., Agusti S., Duarte C. M. (2020). Giant clams in shallow reefs: UV-resistance mechanisms of tridacninae in the red Sea. Coral Reefs 39 (5), 1345–1360. doi: 10.1007/s00338-020-01968-w
Safaie A., Silbiger N. J., McClanahan T. R., Pawlak G., Barshis D. J., Hench J. L., et al. (2018). High frequency temperature variability reduces the risk of coral bleaching. Nat. Commun. 9 (1), 1671. doi: 10.1038/s41467-018-04074-2
Salih A., Larkum A., Cox G., Kuhl M., Hoegh-Guldberg O. (2000). Fluorescent pigments in corals are photoprotective. Nature 408 (6814), 850–853. doi: 10.1038/35048564
Sammarco P. W., Winter A., Stewart J. C. (2006). Coefficient of variation of sea surface temperature (SST) as an indicator of coral bleaching. Mar. Biol. 149 (6), 1337–1344. doi: 10.1007/s00227-006-0318-0
Sawall Y., Al-Sofyani A., Banguera-Hinestroza E., Voolstra C. R. (2014). Spatio-temporal analyses of symbiodinium physiology of the coral pocillopora verrucosa along Large-scale nutrient and temperature gradients in the red Sea. PloS One 9 (8), e103179. doi: 10.1371/journal.pone.0103179
Sawall Y., Al-Sofyani A., Hohn S., Banguera-Hinestroza E., Voolstra C. R., Wahl M. (2015). Extensive phenotypic plasticity of a red Sea coral over a strong latitudinal temperature gradient suggests limited acclimatization potential to warming. Sci. Rep. 5, 8940. doi: 10.1038/srep08940
Scucchia F., Nativ H., Neder M., Goodbody-Gringley G., Mass T. (2020). Physiological characteristics of stylophora pistillata larvae across a depth gradient. Front. Mar. Sci. 7. doi: 10.3389/fmars.2020.00013
Shick J. M. (2004). The continuity and intensity of ultraviolet irradiation affect the kinetics of biosynthesis, accumulation, and conversion of mycosporine-like amino acids (MAAS) in the coral stylophora pistillata. Limnology Oceanography 49 (2), 442–458. doi: 10.4319/lo.2004.49.2.0442
Shick J. M., Dunlap W. C. (2002). Mycosporine-like amino acids and related gadusols: biosynthesis, acumulation, and UV-protective functions in aquatic organisms. Annu. Rev. Physiol. 64, 223–262. doi: 10.1146/annurev.physiol.64.081501.155802
Shick J. M., Ferrier-Pages C., Allemand D. (2003). UV-Induced biosynthesis of algal mycosporine-like amino acids (MAAs) and their conversion to secondary MAAs in the zooxanthellate coral, stylophora pistillata. Integr. Comp. Biol. 43 (6), 819–819.
Shick J. M., Ferrier-Pages C., Grover R., Allemand D. (2005). Effects of starvation, ammonium concentration, and photosynthesis on the UV-dependent accumulation of mycosporine-like amino acids (MAAs) in the coral stylophora pistillata. Mar. Ecol. Prog. Ser. 295, 135–156. doi: 10.3354/meps295135
Shick J. M., Lesser M. P., Dunlap W. C., Stochaj W. R., Chalker B. E., Won J. W. (1995). Depth-dependent responses to solar ultraviolet-radiation and oxidative stress in the zooxanthellate coral acropora-microphthalma. Mar. Biol. 122 (1), 41–51. doi: 10.1007/Bf00349276
Siebeck O. (1981). Photoreactivation and depth-dependent UV-tolerance in reef coral in the great barrier-reef Australia. Naturwissenschaften 68 (8), 426–428. doi: 10.1007/Bf01079713
Smyth T. J. (2011). Penetration of UV irradiance into the global ocean. J. Geophysical Research-Oceans 116(C11). doi: 10.1029/2011jc007183
Stambler N. (2005). Bio-optical properties of the northern red Sea and the gulf of eilat (Aqaba) during winter 1999. J. Sea Res. 54 (3), 186–203. doi: 10.1016/j.seares.2005.04.006
Tamir R., Ben-Zvi O., Eyal G., Kramer N., Loya Y. (2020). Reciprocal-transplantation between shallow and mesophotic stony corals. Mar. Environ. Res. 161, 105035. doi: 10.1016/j.marenvres.2020.105035
Tilstra A., van Hoytema N., Cardini U., Bednarz V. N., Rix L., Naumann M. S., et al. (2018). Effects of water column mixing and stratification on planktonic primary production and dinitrogen fixation on a northern red Sea coral reef. Front. Microbiol. 9 2351. doi: 10.3389/fmicb.2018.02351
Torregiani J. H., Lesser M. P. (2007). The effects of short-term exposures to ultraviolet radiation in the Hawaiian coral montipora verrucosa. J. Exp. Mar. Biol. Ecol. 340 (2), 194–203. doi: 10.1016/j.jembe.2006.09.004
Torres J. L., Armstrong R. A., Corredor J. E., Gilbes F. (2007). Physiological responses of acropora cervicornis to increased solar irradiance. Photochem. Photobiol. 83 (4), 839–850. doi: 10.1562/2006-09-01-RA-1025
Torres J. L., Armstrong R. A., Weil E. (2008). Enhanced ultraviolet radiation can terminate sexual reproduction in the broadcasting coral species acropora cervicornis lamarck. J. Exp. Mar. Biol. Ecol. 358 (1), 39–45. doi: 10.1016/j.jembe.2008.01.022
Torres-Perez J. L., Armstrong R. A. (2012). Effects of UV radiation on the growth, photosynthetic and photoprotective components, and reproduction of the Caribbean shallow-water coral porites furcata. Coral Reefs 31 (4), 1077–1091. doi: 10.1007/s00338-012-0927-7
Ulstrup K. E., Hill R., van Oppen M. J. H., Larkum A. W. D., Ralph P. J. (2008). Seasonal variation in the photo-physiology of homogeneous and heterogeneous symbiodinium consortia in two scleractinian corals. Mar. Ecol. Prog. Ser. 361, 139–150. doi: 10.3354/meps07360
van de Water J. A., Courtial L., Houlbreque F., Jacquet S., Ferrier-Pagès C. (2018). Ultra-violet radiation has a limited impact on seasonal differences in the acropora muricata holobiont. Front. Mar. Sci. 5, 275. doi: 10.3389/fmars.2018.00275
Vareschi E., Fricke H. (1986). Light responses of a scleractinian coral (Plerogyra-sinuosa). Mar. Biol. 90 (3), 395–402. doi: 10.1007/Bf00428563
Veron J. E. (2000). Corals of the world (Townsville, Australia: Australian Institute of Marine Science), 1–3.
Voolstra C. R., Buitrago-Lopez C., Perna G., Cardenas A., Hume B. C. C., Radecker N., et al. (2020). Standardized short-term acute heat stress assays resolve historical differences in coral thermotolerance across microhabitat reef sites. Glob Chang Biol. 26 (8), 4328–4343. doi: 10.1111/gcb.15148
Warner M. E., Chilcoat G. C., McFarland F. K., Fitt W. K. (2002). Seasonal fluctuations in the photosynthetic capacity of photosystem II in symbiotic dinoflagellates in the Caribbean reef-building coral montastraea. Mar. Biol. 141 (1), 31–38. doi: 10.1007/s00227-002-0807-8
Watanabe S., Sudo K., Nagashima T., Takemura T., Kawase H., Nozawa T. (2011). Future projections of surface UV-b in a changing climate. J. Geophysical Research-Atmospheres 116(D16). doi: 10.1029/2011jd015749
Winters G., Loya Y., Beer S. (2006). In situ measured seasonal variations in f-v/F-m of two common red Sea corals. Coral Reefs 25 (4), 593–598. doi: 10.1007/s00338-006-0144-3
Yakovleva I. M., Baird A. H., Yamamoto H. H., Bhagooli R., Nonaka M., Hidaka M. (2009). Algal symbionts increase oxidative damage and death in coral larvae at high temperatures. Mar. Ecol. Prog. Ser. 378, 105–112. doi: 10.3354/meps07857
Yakovleva I., Hidaka M. (2004). Different effects of high temperature acclimation on bleaching-susceptible and tolerant corals. Symbiosis 37 (1-3), 87–105.
Zeevi Ben-Yosef D., Benayahu Y. (2008). Synergistic effects of UVR and temperature on the survival of azooxanthellate and zooxanthellate early developmental stages of soft corals. Bull. Mar. Sci. 83 (2), 401–414.
Zepp R. G., Shank G. C., Stabenau E., Patterson K. W., Cyterski M., Fisher W., et al. (2008). Spatial and temporal variability of solar ultraviolet exposure of coral assemblages in the Florida keys: Importance of colored dissolved organic matter. Limnology Oceanography 53 (5), 1909–1922. doi: 10.4319/lo.2008.53.5.1909
Zhou J., Fan T. Y., Beardall J., Gao K. (2016). Incident ultraviolet irradiances influence physiology, development and settlement of larva in the coral pocillopora damicornis. Photochem. Photobiol. 92 (2), 293–300. doi: 10.1111/php.12567
Zhou J., Huang H., Beardall J., Gao K. (2017). Effect of UV radiation on the expulsion of symbiodinium from the coral pocillopora damicornis. J. Photochem. Photobiol. B 166, 12–17. doi: 10.1016/j.jphotobiol.2016.11.003
Keywords: Red Sea, UVB radiation, Pocilloporidae corals, Pocillopora, Stylophora, primary production (PP), maximum photochemical efficiency (Fv’/Fm’), lipid peroxidation (LPO)
Citation: Overmans S and Agustí S (2023) Differential susceptibility of Red Sea Pocilloporidae corals to UVB highlights photoacclimation potential. Front. Mar. Sci. 10:847559. doi: 10.3389/fmars.2023.847559
Received: 02 January 2022; Accepted: 27 February 2023;
Published: 13 March 2023.
Edited by:
Noga Stambler, Bar-Ilan University, IsraelReviewed by:
Guilhem Banc-Prandi, EPFL, SwitzerlandShinya Shikina, National Taiwan Ocean University, Taiwan
Copyright © 2023 Overmans and Agustí. This is an open-access article distributed under the terms of the Creative Commons Attribution License (CC BY). The use, distribution or reproduction in other forums is permitted, provided the original author(s) and the copyright owner(s) are credited and that the original publication in this journal is cited, in accordance with accepted academic practice. No use, distribution or reproduction is permitted which does not comply with these terms.
*Correspondence: Sebastian Overmans, c2ViYXN0aWFuLm92ZXJtYW5zQGthdXN0LmVkdS5zYQ==