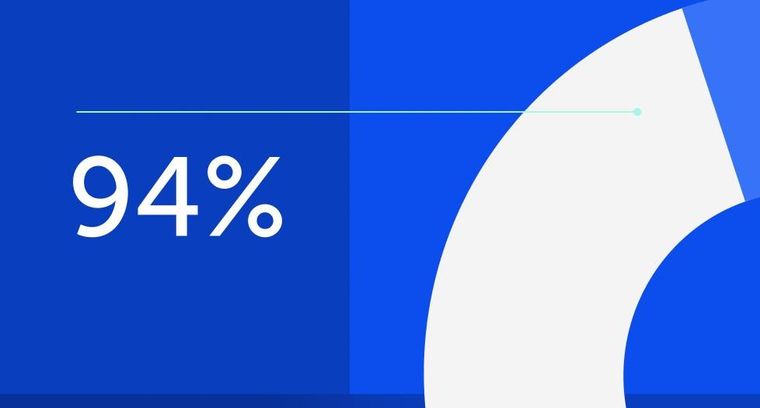
94% of researchers rate our articles as excellent or good
Learn more about the work of our research integrity team to safeguard the quality of each article we publish.
Find out more
ORIGINAL RESEARCH article
Front. Mar. Sci., 24 October 2023
Sec. Marine Megafauna
Volume 10 - 2023 | https://doi.org/10.3389/fmars.2023.689953
Northern elephant seals engage in large-scale foraging migrations traveling up to 15,000 Km over 8 months in the northeast Pacific. While traditionally considered solitary migrants, we demonstrate here that female seals migrate in a surprisingly coherent manner, for individual northern elephant seals traveling in over such a large region of the ocean. Animal movement remained coherent, in terms of the direction of individual swimming relative to group movement, throughout much of their migrations. Movement coherence remained well above the value expected if the movement was independent until the migrating seals were further than 1,000 Km from the colony, beyond which movement coherence declined. Migrating seals presented regional aggregations consisting of female seals traveling within the center of the aggregation, closely following the main migration pathway, with individuals isolated on the extremes of the aggregation. These formations were preserved in the out-and-return migration trips. Animals at the edges of the group show an absence of correlation in their movement with the rest of animals. The observed movements exhibited a lag in the group movement patterns that was greater for female animals > 1,000 Km apart. A model that reproduced movement based on the average individual movement properties failed to reproduce the observed movement patterns. In turn, when a parameter was introduced that reflected group behavior, the resulting modelled movement conformed to the observed patterns, thereby demonstrating the presence of coherent, or synchronized, movement. Whereas the duration of female migration is ultimately constrained by reproductive biology, the coherent movement may involve both endogenous and exogenous cues determining the timing of the initiation of return across 25 million Km2 in the northeast Pacific.
Satellite tracking studies have revealed that large marine animals often have home ranges spanning thousands of kilometers (Block et al., 2011; Hussey et al., 2015; Sequeira et al., 2018). Yet, they often maintain a strong fidelity to key components of their habitat, including reproductive sites, particularly for animals that reproduce on land, such as a high number of pinniped species, to which they return at specific times of the year. This requires accurate time keeping and navigation skills using a range of clues, such as Earth magnetic fields (Lohmann et al., 2004; Lohmann, 2010; Cresci et al., 2017; Naisbett-Jones et al., 2017), to migrate along persistent oceanic corridors or highways (Rose, 1993; Shaffer et al., 2006; Horton et al., 2017; Iwasa-Arai et al., 2017). Migration highways are more often observed in strongly social animals, such as marine mammals, in contrast to solitary animals, such as turtles and sharks (Queiroz et al., 2016), (Broderick et al., 2007).
However, despite the increase in the number of animals tagged in tracking studies over time (Block et al., 2011; Hussey et al., 2015; Sequeira et al., 2018), most analyses to-date focus on detailed analyses of individual animals, with collective movement behaviors of animals remaining poorly understood. Recent studies, involving southern (Mirounga leonina, Rodriguez et al., 2017) and northern elephant seals (Mirounga angustirostris, Duarte et al., 2018; Beltran et al., 2022), examined their collective movement patterns to define the partitioning of the space during their feeding migration, and providing evidence, based on a sonification of movement, of group behavior that requires the perception of space and time (Beltran et al., 2022).
Northern elephant seals conduct two migrations per year, modulated by their requirement to return to land twice each year, to molt and to breed (Stewart and DeLong, 1995). The short post-breeding feeding migration in early spring precedes molting and mating. Following mating female seals take off on the long, 8-month foraging trip to acquire enough energy to complete reproduction and give birth upon their arrival back to the colony (Robinson et al., 2012; Beltran et al., 2022). Both migrations are characterized by segregation by sex during both migrations (Stewart and DeLong, 1995), with male elephant seals performing generally shorter migrations and more variable in duration (Stewart and DeLong, 1995). Female northern elephant seals dive continually to depths up to 500 m during both migrations and travel linear distances of about 20,000 Km during their 250 to 300 days at sea, the longest annual migrations yet recorded for individual mammals (Stewart and DeLong, 1995).
A sonification of the migration of female northern elephant seals, suggested they migrate in a coherent manner (Duarte et al., 2018). Specifically, female animals depart the colony in waves, exhibiting fluctuations in the dispersion of the wave followed by the coalescence of the wave prior to initiating return (Duarte et al., 2018). These observations suggested that both the initiation of the migration trip and the return are coherent (Duarte et al., 2018). This is a remarkable possibility, as these animals engage in migrations where individual animals may be separated over thousands of kilometers from one another (Le Boeuf et al., 2000; Robinson et al., 2012. Duarte et al., 2018).
Here we further explore the patterns of coherent foraging migration of female northern elephant seals across the northeast Pacific, where they cover an area exceeding 25 million Km2. We use “coherent” to reflect the group movement features detected following Duarte et al. (2018), and following the acception of the adjective in the Merryan-Webster Dictionary of “having the quality of holding together” (www.merriam-webster.com). We examine the coherence of these movements. We do so on the basis of a 10-years data set involving 321 tagged female northern elephant seals, encompassing more than one million positions.
The female northern elephant seal population tagged here showed a consistent migration pathway during their long migration, which has been shown to correspond to the Earth’s magnetic field (Horton et al., 2017), but with higher variability in pathways during their shorter annual trip (Figure 1). Indeed, the individuals display coherence in their movements spread across vast distances. The distance between all pairs and nearest-neighbor pairs of tagged individuals at the time the furthest distance from the colony for the group (median and maximum = 1,430 km and 4,124 km for all pairs, median and maximum = 580 km and 2,526 km for nearest-neighbor pairs, respectively), was orders of magnitude above the distances for olfactory or visual detection and likely beyond the range of acoustic exchange. Yet, the animal movement remains coherent, in terms of the direction of individual swimming relative to group movement, throughout much of their migrations (Figure 1). Movement coherence remained well above the value expected if the movement was independent until the migrating seals were further than 1,000 Km from the colony, beyond which movement coherence declined (Figure 1; Supplementary Figures 1 and 2).
Figure 1 Analyses of movement of female seals. (A) Heatmap of all short trip movement of 10 years. The tracks with different color denote the center of mass of short trip of 10 years. (B). Heatmap of all long trip movement of 10 years. The tracks with different color denote the center of mass of long trip of 10 years. Embedded figure shows the distance from the origin (colonies) to the center of mass with respect to time. The inserts show the mean ± SD group spread and coherence of movement by week (i.e., an average year) and the relationship between the mean ± SD coherence, averaged across log10 distance classes, and the distance from the center of mass of the group to the colony during the migration.
Further evidence of coherent movement is provided by comparing a naive model, where individual female seals follow trajectories sampled randomly from their observed individual direction and speed distributions, with the observed pattern. The naïve model adequately represents the heat map of seal distribution during the short trip, but is unable to reproduce the further penetration in the central Pacific that characterizes the long trip (Figures 2A, C). We then introduced a simple rule reflecting coherent movement. This was done by allowing the simulated animals to swim with the distribution of speed sampled from that observed for the aggregated seals but with a direction sampled randomly from those relative to the center of mass observed at each time interval (6 h). This additional, simple rule resulted in the distribution of seals conforming closely to the observed one (Figures 2A, B) for the long trip, but was tighter than observed in the case of the short trip (Figures 2A, B). The simulated pattern of coherence in swimming with distance from the colony also matched closely the observed pattern, while the null-hypothesis, naive simulation showed low coherence (< 0.4, Supplementary Figure 3). Hence, the patterns observed cannot be reproduced in the absence of coherent swimming, which requires, as a parsimonious set of rules, coherence of the track followed, the maximum group spread, and the timing of return. The track followed has been recently shown to correspond to Earth magnetic clues (Horton et al., 2017), whereas group spread and the timing of return are unlikely to be guided by spatial futures and requires either endogenous or exogenous temporal clues that drive coherence in the return among animals. What clues female elephant seals use to determine the timing of their return, months prior to giving birth at the return from the long migration, is unknown, as the animals are scattered across different time zones and latitudes, thereby resulting in differential sunrise and sunset times.
Figure 2 Compared simulation results. Position heat-maps summarizing the distribution of observed (A) and simulated trajectories for the female northern elephant seals long (left panels) and short (right panels) migration, including simulations where the female seals move around the observed center of mass for each trip (B), which suffices to reproduce the observed, coordinated trajectories; and (C) simulations where the animals move with speed and direction sampled from the observed individual distributions, and therefore lack any coordination.
Detailed analyses of group movement for each of the trips (Supplementary Video S1 representing year 2012, Supplementary Videos S2-S10 for the complete set) revealed that the relative position of the animals was highly conserved throughout the migrations (Figure 3), despite animals being separated by hundreds of kilometers from one another. There appeared to be coherent aggregations of female seals that exhibited a higher level of coherence (Figure 3; Supplementary Video S1 representing year 2012, Supplementary Videos S2-S10 for the complete set). These regional aggregations exhibited internal structure that is groups of female seals traveling within the center of the aggregation, closely following the main migration pathway. In turn, there were individuals in the early aggregations that flanked those in the center with individuals isolated on the extremes of the aggregation (Figure 3 showing year 2012, Supplementary Figure 4 for the complete set). These patterns were preserved in the way out and return trips (Figure 3). Animals at the edges of the group show an absence of correlation in their movement with the rest of animals (Figure 3).
Figure 3 Correlation and ranking. Hierarchical clustering correlation matrix (A) and female seals’ ranking (B) of year 2012 (numbers represent the id of individual tagged female northern elephant seals engaged in the same group migration) showing coordinated movement and rank position by animal subgroups. (A) shows the correlation coefficients of the movements of female seals in year 2012 (darker color represents stronger correlation), and the resulting hierarchical clustering of the female seals into groups. There are generally four groups of female seals, Group 1 including seals with id’s 238, 237 and 239; Group 2 including seals with id’s 246 and 250; Group 3 including seals with id’s 240 and 241; and Group 4 including seals with id’s 242, 247, 244, 245 and 249. (B) shows the ranking of female seals migrating in year 2012, according to their proximity to the center of mass of the group, during the migratory trip. Dark blue indicates seals close to the center, while light yellow indicates organisms distant from the center. Seals belonging to Group 4 (cluster in (A)) traveled all close to the center, while the two seals with strongly correlated movement in Group 2 have very similar ranking patterns (close to the center during going outbound trip and far away from center during return trip). Seals in Group 1 and Group 3 also converse similar ranking during the migration. Individuals 243 and 248 are not correlated to any seal (A) and remain consistently distant from the center of mass (B).
Inspection of group movement (Supplementary Video S1 representing year 2012, Supplementary Videos S2–S10 for the complete set) also showed that the initiation of return is relatively simultaneous across female seals and occurs over a relatively narrow time span, with a median lag time among nearest neighbors of 1 day (4 days for the entire group) (Figure 4). If the animals were swimming following independent external or internal clues, we would expect the relative differences in initiation of migration to be conserved along the trip, so the individuals departing last would also be arriving last back to the colony. However, analyses of lag times showed that any history of departure sequence was lost once the animals reached the furthest point in the migration (r = 0.0154, P > 0.05, Figure 4), so that differences in the arrival time were independent of the sequence of trip initiation (r = 0.2059, P > 0.05, Figure 4). Examination of return dates (Supplementary Table 1), for both migrations, revealed that female northern elephant seals initiate their return at days, with median across the 10 years of data, March 28 (SD 5) and September 7 (SD 10) for the short and long migration, respectively, which correspond closely to the equinox dates (March 20/21 and September 22/23, respectively). The equinoxes, the two days in the year when day and night have similar lengths, can be indeed an appropriate clue as these dates are independent of position on the planet.
Figure 4 Time lags analysis. Cumulative distribution of time-lags of all paired female seals across all years (blue line), and relative to their nearest neighbor (orange line) at 2 days prior to their return point. The inset shows the distribution of time lags (days), as error-bar plots showing the mean (center line), and the standard error, with the distance between each possible pair of female seals from initiating the return to arrival. The text in the inset shows the correlation coefficient between four time-lags across all years, showing that arrival times are independent of departure times for individual female seals.
The distribution of lag times with distance between pairs of female seals, showed these distributions remain relatively uniform until the animals are > 2,000 Km away from each other, when median lag times in initiating the return trip increase from two weeks to about three weeks, with the longest lag times, of > 10 weeks, for animals > 3,700 Km away.
The analysis presented here provides compelling evidence for coherent movement of female northern elephant seals migrating across the North Pacific, as hypothesized from the features of sonification of the movement of these animals (Duarte et al., 2018). Our results show that female northern elephant seals show a coherent pattern of migration for the long migration while they swim more independently for the short migration. This analysis is focused on female elephant seals, as data available for male elephant seals is too limited to support a comparable analysis.
A parsimonious representation of the group movement of female northern elephant seals requires, therefore, three parameters, one being the overall migration pathway, as depicted by the center of mass, the second being the distribution of individual swimming speed and angles relative to that pathway, and the last one a signal, endogenous, exogenous or both, indicating the initiation of return. The existence of a migration pathway for female California northern elephant seals has been demonstrated recently to correspond to Earth magnetic fields (Horton et al., 2017) and their behavior has been interpreted to involve the capacity for the perception of space and time (Beltran et al., 2022). Selection could operate for the seals to use a robust and reliable environmental cue or a suite of cues, and such as the Earth magnetic field and the equinox, which is independent of the animal’s position along the Pacific Ocean. These cues would enable the perception of space and time (Beltran et al., 2022), respectively, supporting the navigational skills of female northern elephant seals. Our analysis suggests that a possible exogeneous cue animals may use is the spring and fall equinox as a signal to initiate the return for both migrations. Astronomic navigation is widespread in animals (Mouritsen, 2018) and is, therefore, possible. Endogenous, factors, such as hormonal changes, and vocalization, which is both endogenous (emissary) and exogenous (receptor), may also be involved in supporting coherent migrations, but there is currently no evidence supporting either possibility. Memory, an endogenous archive of exogenous cues, may play a role, but this cannot explain that naïve female seals follow the same pattern. Synchronized movement has been reported for other marine species. For instance, Olive Ridley sea turtles synchronize the movement of reproductive females to nesting beaches, known as “arribadas” (Mishra et al., 2021). For whales, Chambault et al. (2021) reported that 70% of the migrant sperm whales studied, which were mostly mature females, showed a surprisingly synchronized departure from Mauritius in mid-December. Long-finned pilot whales were also shown to show synchronized migration as a common behavior reflected in maintaining close proximity and the capacity for rapid coordinated response of individuals, with the multiple functions of showing affiliation and reacting to disturbance (Senigaglia et al., 2012). However, the possible benefits of coherent movement over such large scales, across such different habitats is unclear for northern elephant seals. Specifically, it is not evident what relevant information could be stored or transmitted about foraging conditions across such great distances, especially when individual foraging behavior is associated with mesoscale features on the scale of kilometers, not thousands of kilometers (Bost et al., 2009; Robinson et al., 2012; Abrahms et al., 2018).
The existence of a few animals, located in the periphery of the group showing a loose correlation with the group movement, exploring locations far away from the main axis of migration, is characteristic of group foraging, as a small percent of organisms showing an independent, maverick behavior has been found to be essential to find new resources for social animals, best studied in ants (e.g. (Traniello, 1989)), exploiting resources containing some degree of stochastic distribution in space (Bell, 1990). Indeed, this strategy is also used to best explore the search space to parametrize algorithms (Dorigo et al., 1996) and develop optimization algorithms (He et al., 2009), and represents the essence of swarm algorithms for artificial intelligence applications (Bansal and Pal, 2019).
The pattern of increasing lag-time in initiating the return with distance from the group center cannot be readily explained by the existence of endogenous cues or astronomical cues, such as equinoxes. A hypothetical driver of coherent movement consistent with increasing lag in return dates with increasing distance from the group center would be the use of vocal clues to trigger this event by the animal(s) located near the group center of mass. Whereas all animals may be able to independently determine the equinox, this would result in lag times between pairs of animals that would be independent of the distance between them. However, we found that lag times in initiating the return increased at distances > 1,000 km, and were particularly long for animals separated across distances > 2,000 Km. It is well documented that large cetaceans are capable of using low frequency sounds to communicate across vast reaches of the ocean using low frequency sounds (Payne and McVay, 1971). The communication range between Northern Elephant Seals depends on the frequency at which they communicate and the properties of the sea water. Studies of Northern Elephant Seals show the emission of sounds at a frequency in the range 150 to 300 Hz (Southall et al., 2003; Casey et al., 2018) and a cochlear sensitivity compatible with this range (Kastak and Schusterman, 1999). Sound absorption in the Pacific Ocean at 100 Hz is of the order of 10-3 dB km-1 in the Pacific Ocean (Ainslie and McColm, 1998), resulting in a characteristic length for the communication range, obtained as the inverse of the sound absorption, of the order of about one thousand kilometers. This is consistent with the finding of increased lag times for return times for animals separated by distance > 1,000 Km. Northern elephant seals have significant vocalization and auditory skills, which allow animals to detect acoustic signals at relatively low signal-to-noise ratios underwater (Kastak and Schusterman, 1998; Southall et al., 2000) and recognize individual voices (Casey et al., 2015). A hypothetical vocal cue for the initiation of the return is, therefore, consistent with the capacities of the animals, sound propagation in the Pacific Ocean, and the observed increasing lag-time in initiating the return with distances > 1,000 Km between pairs of animals. However, early studies of acoustic behavior of the elephant seals did not detect seal vocalizations during migratory swimming (Fletcher et al., 1996) and additional recordings collected over the entire migration similarly did not record any elephant initiating vocalizations (Costa unpublished data). Thus, the possibility of vocal cues is rejected since vocal communication during migrations has not been documented for northern elephant seals. As an alternative, soundscape cues not emitted by the animals may be present in the region that could be used to guide coherent movement and result in the lagged responses with distance.
However, no single cue or mechanism will enable highly migratory animals to navigate with pinpoint accuracy over thousands of kilometers, which requires multiscale and multisensory cue integration in the brain (Mouritsen, 2018). Long-distance migration involves three phases: (1) a long-distance phase; (2) a narrowing-in or homing phase; and (3) a pinpointing-the-goal phase (Mouritsen, 2018). Framing northern female elephant seal migration in this context suggests that naïve migrants inherit skills enabling the long-distance phase, which requires sophisticated magnetic receptors (Mouritsen, 2018). Since all tagged elephant seals found their way to the colony, they must have acquired the capacity to enable phase (3), which may involve, for instance, olfactory cues (Mouritsen, 2018). However, phase (2) will be required to initiate the return, which is learned from experience (Mouritsen, 2018), suggesting animals at greater distances from the center of gravity of the group may either be naïve animals or be less skilled at phase (3). It is possible that the regional clusters of group behavior detected when analyzing movement patterns reflect regional variation in oceanographic features such as frontal zones, eddies and filaments where prey availability is greatest (Bost et al., 2009; Abrahms et al., 2018), that affect the coherence of movement patterns with increasing distance from the center of gravity of the group.
This highly coherent migration pattern is likely to be associated with the timing of the return of females to the breeding colony. The breeding system of elephant seals is highly polygamous (Leboeuf, 1972; Reiter et al., 1981) with one male breeding with many females. This system has a high environmental potential for polygyny (EPP) (Emlen and Oring, 1977), facilitated by females aggregating into a harem defended by a male (Bartholomew, 1970). The high EPP is made possible by the very tight timing of females coming ashore synchronizing their estrous (Bartholomew, 1970). Greater variation in the timing of arrival would lead to a decrease in polygyny (Twiss et al., 2007). Such a highly polygynous breeding system selects for females to arrive so they get the best males for their offspring and to reduce the marginal male effect. Older more experienced females tend to arrive first, so they can pup in the middle of the harem, ensuring that they are in the best possible location to have a pup. They rely on the best male to reduce the level of disturbance (Reiter et al., 1981), which involves risks of the pup being to be separated from the mother, reducing milk transfer efficiency and, in some cases, resulting in pup death (Morejohn and Briggs, 1973). Coherent movement patterns observed during the long migration likely synchronize female return to maximize competition to insure they get the best male in the colony (Bartholomew, 1970; Reiter et al., 1981). The lesser coherence observed during the shorter post-breeding trip, follows this scenario as the females are returning to shore to molt and, as breeding is not taking place, there is no competition on the colony and, thus, little or no advantage in synchronizing the timing of arrival and departure.
The swimming speed of elephant seals may be controlled intrinsically, by hydrodynamic and energy balances of the animals, embedded in their biology and form (Hassrick et al., 2007). However, this would not preclude the group from spreading beyond the observed range, as is the case for male seeds. Indeed, our results show that female group spread is, counterintuitively, more tightly controlled in the long than in the short migration, as independent movement patterns would lead to the expectation of looser group spread, resulting from diffusive spread operating over longer time scales, in the long, compared to the short migration. Most importantly, our analysis shows that (a) female seals keep their relative position in the group throughout their 8 months migration, including a clear subgroup structure in their travel position within the group, and (b) the distribution of lag times in initiating the return among animals is distance dependent. The animals must be using the same cues, endogenous or exogenous, to maintain the coherence in their migration.
Northern elephant seals have been long believed to engage in solitary migrations, which apply for male seals. However, females present remarkable skills at large-scale and long-distance coherent foraging, including route fidelity, the maintenance of regional aggregations maintaining tighter regional synchronization, and the use of cues that synchronize their return to the colony. The migratory voyages are driven by foraging needs (Adachi et al., 2017), so the coherent movement demonstrated here is expected to provide advantages in term of foraging outcomes. In contrast, the return trip is driven by reproductive needs and, thus, could be expected to display a different behavior. However, we found outbound and inbound trips to follow similar paths and display similar group organization and coherence, thereby suggesting that the drivers, and possible benefits, of coherent movement do not differ between the outbound and inbound migration.
The skills of female northern elephant seals at synchronizing their return to the breeding colony is particularly remarkable as these animals were at the brink of extinction, with a minimum population size of< 20 individuals by the end of the 19th century, until restrictions on hunting allowed the populations to surge to its current estimate of about 200,000 individuals (Hoelzel et al., 1993). The expected genetic bottlenecks do not seem to have impaired their remarkable skills at group migrations (Hoelzel et al., 1993). The finding of coherent movements across the North Pacific by female northern elephant seals opens a new path to interrogate data on the movement of other animals migrating alone in the ocean for large-scale foraging behavior.
The animals studied were adult female northern elephant seals (Mirounga angustirostris) instrumented at two breeding colonies: Año Nuevo state reserve, California, USA (37° 5′ N, 122° 16′ W; n = 277) and Islas San Benito, Mexico (28° 18′ N, 115° 22′ W; n = 20). The animals were immobilized following standard techniques (Robinson et al., 2012) and equipped with a 0.5W ARGOS satellite transmitter (Wildlife Computers, Belleview, WA, USA: SPOT4, SPOT5, MK10-AF; or Sea Mammal Research Unit, St. Andrews, Scotland: SRDL-CTD) using a ∼45 s repetition rate, a time-depth recorder (Wildlife Computers MK9, MK10) sampling at least once every 8 s, and a VHF transmitter attached to assist in locating the animals on the beach upon their return (MM170B and MM230B, ATS, Isanti, MN, USA) (Duarte et al., 2018). At each tagging period, healthy adult female seals were randomly selected among the animals fitted with flipper tags, which provided information on the age and prior history of the animals. Most seals (78%) were of known age and ranged from 4 to 17 years old. Many of the seals (38%) were instrumented for more than one trip to sea. All analyses and visualizations were corrected to ensure equal representation from each seal when appropriate: mixed models were run with individual as a random effect and kernel densities were down-weighted for repeat deployments. In the 2010 post-breeding season, we intentionally biased animal selection toward an even mix of seals that used coastal and oceanic habitats, based on tracking data from previous deployments, as part of a concurrent study.The tagging of the animals took place from 2004 to 2010 and included both annual foraging migrations. The animals were chemically immobilized to attach and recover the sensors. We equipped each seal with a 0.5W ARGOS satellite transmitter (Wildlife Computers, Belleview, WA, USA: SPOT4, SPOT5, MK10-AF; or Sea Mammal Research Unit, St. Andrews, Scotland: SRDL-CTD) using a ∼45 s repetition rate, a time-depth recorder (Wildlife Computers MK9, MK10; or Lotek, St. John’s, NL, Canada: 2310) sampling at least once every 8 s, and a VHF transmitter (MM170B and MM230B, ATS, Isanti, MN, USA). Further details on the animals tagged, tagging procedures and data processing are provided in (Le Bouef et al., 2000; Robinson et al., 2012).
The dataset includes 297 tagged female elephant seals. Each record of the movement contains four parameters, which are animal ID, latitude and longitude of the position, and timestamp. We interpolated, using a linear interpolation, the records when needed to fill missing data points. We further smoothed the movement trajectory, calculating the average position (latitude, longitude) along a six-hour moving time window.
Detailed Methods
We calculated the coherence of animals traveling in a group, indicating the consistency of the direction of the movement of all animals in a time interval defined as:
Where Ct denotes the coherence for time interval t, nt denotes the number of animals in time interval t, and θt refers to the angle between the direction of movement and the line of latitude.
The movement dynamics of the animals was reflected by the temporal evolution of the distance between the origin (colonies) and the center of mass of the group during the migration, which is calculated as the average position of all animals at a given time. The latitude and longitude of center of mass μ of a group for time interval t was calculated as:
Where CM_Latt and CM_Lont are latitude and longitude of center of mass for time interval t, nt denotes the number of animals in time intervalt, Lati and Loniare latitude and longitude of animal i in time interval t.
Next, we can calculate the distance between origin and a center of mass on the Earth surface. We compared the trajectory of the center of mass of the long and short migration trips and checked if the center of mass showed a similar trajectory during 10 years in both migrations.
Group spread, which denotes the area covered by the group of animals at any time point during movement, can be calculated as the geodesic variance of the (Lont, Latt) positions of animals in a group. We define group spread as:
Where ||.||geo refers to the calculation of the geodesic distance between two points on the surface of earth, and μt denotes the center of mass at time interval t. pt(t) denotes the (Lont(t), Latt(t)) position of animal i in this group in the time interval t.
The role of an individual in a group may be expressed by their relative position relative to the center of mass. The rank can be simply defined as:
Where dt denotes the distance between animal i and the center of mass for this group. We used linear correlation coefficients between the longitude position vectors among pairs of animals traveling as a group in each long migration trip and used hierarchical clustering (Johnson, 1967) to cluster the correlation between each pair of animals and computed correlation time-lags to explore the timeline of coordination in movement, with a particular attention to time lags in the time to initiate the return to the colony.
We designed a simulation model that generates, as a null hypothesis, movements of each animal according to their individual statistics, without any consideration of group movement. Also, we designed a simulation model that generates movements of each animal according to their group’s statistics which denotes the distributions of speed, and select angles according to the variance of the center of mass of movement, so the group would move along with the center of mass calculated from original data. The difference is consistency between the two models and the observed data provide a test of the contribution of group coordination to observed movement patterns.
From the observed data, we first obtain two distributions for each animal: one is about the speed, the other one is about the variance of the travelling angle. Next, we calculated the trip length distribution for all animals. For simulating the random movement of each animal, we sample values for speed and angle from their corresponding distribution, where the value appeared with high probability will be sampled with high probability and vice versa. The outgoing stage and return stage are simulated separately.
The procedure of simulation is as follows:
1. All animals start their trip from the true initial locations (obtained from the real data).
2. For each animal, sample an outgoing (return) period length from outgoing (return) stage length distribution.
3. Starting from time t=1, for each animal, initialize the speed/angle variable by sampling from the animal’s outgoing (return) speed/angle distribution.
4. Continue for each animal at t = t + 1;
5. Sample a speed value from its corresponding outgoing (return) speed distribution with probability P<0.3; otherwise, take the speed value at t and continuously use it for t + 1.
6. Sample an angle value from its corresponding outgoing (return) angle distribution with probability P<0.3; otherwise, take the angle value at t and continuously use it for t + 1.
Until the number of samples equals to the sampled outgoing (return) period length.
By observing the tracks of the center of mass, we find that a long trip has three stages: outgoing, middle, and return stage. Therefore, we sample speed values from three different speed distributions for long trips at different stages, while we consider only two stages (outgoing and return stage) for short trips. The length of different stages is sampled from a distribution obtained from all animals. Since the simulation is based on the known center of mass, the angle will be determined by driving the group of animals to move along with the observed center of mass.
The procedure of simulation is as follows:
1. All animals start their trip from the true initial locations (obtained from the real data).
2. For each animal, sample an outgoing (middle, or return) period length from outgoing (middle, or return) stage length distribution (three stages in the long trip and two stages in the short trip).
3. Starting from time t = 1, for each animal, initialize the speed variable by sampling from the animal’s outgoing (middle, or return) speed distribution. The initial angle of the center of mass is denoted as A.
4. Continue for each animal at t = t + 1;
5. Sample a speed value from the animal’s outgoing (middle, or return) speed distribution with probability P< 0.3; otherwise, take the speed value at t and continuously use it for t + 1.
6. For an angle value,
7. during the outgoing stage, for the first 50 time intervals, sample an angle uniformly from [A - 30, A + 30]; during the rest part of the outgoing stage, sample an angle uniformly from [A - 90, A + 90].
8. during the middle stage, sample an angle uniformly from [0, 360] with probability P< 0.5, otherwise take the angle value at time t and use it for t + 1.
9. during the return stage, we assume that either the group or some animals in this group know the end location. Denoting the angle to the end point as B, we sample an angle from [B - 30, B + 30].
Until the number of samples equals to the sampled outgoing (middle, or return) period length.
Publicly available datasets were analyzed in this study. The data that support the findings of this study are available from the Earthdata program of NASA (https://cmr.earthdata.nasa.gov/search/concepts/C1214591971-SCIOPS).
The animal study was approved by University of California at Santa Cruz Institutional Animal Care and Use Committee and was authorized under National Marine Fisheries Service permits: #786-1463 and #87-143. The study was conducted in accordance with the local legislation and institutional requirements.
CD, XZ, VE, and DC conceived the research. DC, PR, and SK generated the data. SP and XZ analyzed the data. SP, XZ, CD, and VE wrote the manuscript. All authors contributed to the article and approved the submitted version.
This research was conducted as part of the Tagging of Pacific Predators (TOPP) program and was supported in part by the National Ocean Partnership Program (N00014–02-1–1012); the Office of Naval Research (N00014–00-1-0880, N00014–03-1–0651, N00014–08-1–1195), the E&P Sound and Marine Life Joint Industry Project of the International Association of Oil and Gas Producers (JIP2207–23); the University of California Natural Reserve System the Moore, Packard, and Sloan Foundations; and the Sooy Graduate Fellowship and King Abdullah University of Science and Technology through the base-line funding to CD and XZ. VE acknowledges IKERBASQUE and María de Maeztu Excellence Unit 2023-2027 Ref. CEX2021-001201-M, funded by MCIN/AEI /10.13039/501100011033.
The authors declare that the research was conducted in the absence of any commercial or financial relationships that could be construed as a potential conflict of interest.
All claims expressed in this article are solely those of the authors and do not necessarily represent those of their affiliated organizations, or those of the publisher, the editors and the reviewers. Any product that may be evaluated in this article, or claim that may be made by its manufacturer, is not guaranteed or endorsed by the publisher.
The Supplementary Material for this article can be found online at: https://www.frontiersin.org/articles/10.3389/fmars.2023.689953/full#supplementary-material
Abrahms B., Scales K. L., Hazen E. L., Bograd S. J., Schick R. S., Robinson P. W., et al. (2018). Mesoscale activity facilitates energy gain in a top predator. Proc. R. Soc. B-Biological Sci. 285, 1885. doi: 10.1098/rspb.2018.1101
Adachi T., Costa D. P., Robinson P. W., Peterson S. H., Yamamichi M., Naito Y., et al. (2017). Searching for prey in a three-dimensional environment: hierarchical movements enhance foraging success in northern elephant seals. Funct. Ecol. 31, 361–369. doi: 10.1111/1365-2435.12686
Ainslie M. A., McColm J. G. (1998). A simplified formula for viscous and chemical absorption in sea water. J. Acoustical Soc. America 103, 1671–1672. doi: 10.1121/1.421258
Bansal J. C., Pal N. R. (2019). “Swarm and Evolutionary Computation,” in Evolutionary and Swarm Intelligence Algorithms. Eds. Bansal J. C., Singh P. K., Pal N. R. (Cham: Springer International Publishing), 1–9. doi: 10.1007/978-3-319-91341-4_1
Bartholomew G. A. (1970). A model for the evolution of pinniped polygyny. Evolution 24, 546–559. doi: 10.2307/2406835
Beltran R. S., Yuen A. L., Condit R., Robinson P. W., Czapanskiy M. F., Crocker D. E., et al. (2022). Elephant seals time their long-distance migrations using a map sense. Curr. Biol. 32, R156–R157. doi: 10.1016/j.cub.2022.01.031
Block B. A., Jonsen I. D., Jorgensen S. J., Winship A. J., Shaffer S. A., Bograd S. J., et al. (2011). Tracking apex marine predator movements in a dynamic ocean. Nature 475, 86–90. doi: 10.1038/nature10082
Bost C. A., Cotte C., Bailleul F., Cherel Y., Charrassin J. B., Guinet C., et al. (2009). The importance of oceanographic fronts to marine birds and mammals of the southern oceans. J. Mar. Syst. 78, 363–376. doi: 10.1016/j.jmarsys.2008.11.022
Broderick A. C., Coyne M. S., Fuller W. J., Glen F., Godley B. J. (2007). Fidelity and over-wintering of sea turtles. Proc. Biol. Sci. 274, 1533–1538. doi: 10.1098/rspb.2007.0211
Casey C., Charrier I., Mathevon N., Reichmuth C. (2015). Rival assessment among northern elephant seals: evidence of associative learning during male-male contests. R Soc. Open Sci. 2, 150228. doi: 10.1098/rsos.150228
Casey C., Reichmuth C., Costa D. P., Le Boeuf B. (2018). The rise and fall of dialects in northern elephant seals. Proc. R. Soc. B-Biological Sci. 285, 20182176. doi: 10.1098/rspb.2018.2176
Chambault P., Fossette S., Heide-Jørgensen M. P., Jouannet D., MiM.chel Vély. (2021). Predicting seasonal movements and distribution of the sperm whale using machine learning algorithms. Ecol. Evol. 11, 1432–1445. doi: 10.1002/ece3.7154
Cresci A., Paris C. B., Durif C. M. F., Shema S., Bjelland R. M., Skiftesvik A. B., et al. (2017). Glass eels (Anguilla Anguilla) have a magnetic compass linked to the tidal cycle. Sci. Adv. 3, e1602007. doi: 10.1126/sciadv.1602007
Dorigo M., Maniezzo V., Colorni A. (1996). Ant system: optimization by a colony of cooperating agents. IEEE Trans. Syst. Man Cybern B Cybern 26, 29–41. doi: 10.1109/3477.484436
Duarte C. M., Riker P., Srinivasan M., Robinson P. W., Gallo-Reynoso J. P., Costa D. P. (2018). Sonification of animal tracks as an alternative representation of multi-dimensional data: A northern elephant seal example. Front. Mar. Sci. 5. doi: 10.3389/fmars.2018.00128
Emlen S. T., Oring L. W. (1977). Ecology, sexual selection, and the evolution of mating systems. Science 197, 215–223. doi: 10.1163/156853972X00167
Fletcher S., Le Boeuf B. J., Costa D. P., Tyack P. L., Blackwell S. B. (1996). Onboard acoustic recording from diving northern elephant seals. J. Acoust Soc. Am. 100, 2531–2539. doi: 10.21236/ada327199
Hassrick J. L., Crocker D. E., Zeno R. L., Blackwell S. B., Costa D. P., Le Boeuf B. J. (2007). Swimming speed and foraging strategies of northern elephant seals. Deep-Sea Res. Part Ii-Topical Stud. Oceanography 54, 369–383. doi: 10.1016/j.dsr2.2006.12.001
He S., Wu Q. H., Saunders J. R. (2009). Group search optimizer: an optimization algorithm inspired by animal searching behavior. IEEE Trans. Evolutionary Comput. 13, 973–990. doi: 10.1109/tevc.2009.2011992
Hoelzel A. R., Halley J., Obrien S. J., Campagna C., Arnbom T., Leboeuf B., et al. (1993). Elephant seal genetic-variation and the use of simulation-models to investigate historical population bottlenecks. J. Heredity 84, 443–449. doi: 10.1093/oxfordjournals.jhered.a111370
Horton T. W., Hauser N., Zerbini A. N., Francis M. P., Domeier M. L., Andriolo A., et al. (2017). Route fidelity during marine megafauna migration. Front. Mar. Sci. 4. doi: 10.3389/fmars.2017.00422
Hussey N. E., Kessel S. T., Aarestrup K., Cooke S. J., Cowley P. D., Fisk A. T., et al. (2015). Ecology. Aquat. Anim. telemetry: A panoramic window into underwater world. Sci. 348, 1255642. doi: 10.1126/science.1255642
Iwasa-Arai T., Siciliano S., Serejo C. S., Rodriguez-Rey G. T. (2017). Life history told by a whale-louse: a possible interaction of a southern right whale Eubalaena australis calf with humpback whales Megaptera novaeangliae. Helgoland Mar. Res. 71. doi: 10.3389/fmars.2017.00422
Johnson S. C. (1967). Hierarchical clustering schemes. Psychometrika 32 (3), 241–254. doi: 10.1007/BF02289588
Kastak D., Schusterman R. J. (1998). Low-frequency amphibious hearing in pinnipeds: methods, measurements, noise, and ecology. J. Acoust Soc. Am. 103, 2216–2228. doi: 10.1121/1.421367
Kastak D., Schusterman R. J. (1999). In-air and underwater hearing sensitivity of a northern elephant seal (Mirounga angustirostris). Can. J. Zoology-Revue Can. Zoologie 77, 1751–1758. doi: 10.1139/z99-151
Leboeuf B. J. (1972). Sexual behavior in the Northern Elephant seal Mirounga angustirostris. Behaviour 41, 1–26. doi: 10.1163/156853972X00167
Le Boeuf B. J., Crocker D. E., Costa D. P., Blackwell S. B., Webb P. M., Houser D. S. (2000). Foraging ecology of northern elephant seals. Ecol. Monogr. 70, 353–382. doi: 10.2307/2657207
Lohmann K. J. (2010). Q&A animal behaviour magnetic-field perception. Nature 464, 1140–1142. doi: 10.1038/4641140a
Lohmann K. J., Lohmann C. M. F., Ehrhart L. M., Bagley D. A., Swing T. (2004). Animal behaviour - Geomagnetic map used in sea-turtle navigation. Nature 428, 909–910. doi: 10.1038/428909a
Mishra M., Acharyya T., Kar D., Debanath M., Santos C. A. G., da Silva R. M., et al. (2021). Geo-ecological cues for mass nesting synchronization of Olive Ridley turtles along Rushikulya estuary in Odisha, east coast of India. Mar. pollut. Bull. 172, 112881. doi: 10.1016/j.marpolbul.2021.112881
Morejohn G. V., Briggs K. T. (1973). Post-mortem studies of northern elephant seal pups. J. Zool. Lond. 171, 67–77. doi: 10.1111/j.1469-7998.1973.tb07516.x
Mouritsen H. (2018). Long-distance navigation and magnetoreception in migratory animals. Nature 558, 50–59. doi: 10.1038/s41586-018-0176-1
Naisbett-Jones L. C., Putman N. F., Stephenson J. F., Ladak S., Young K. A. (2017). A magnetic map leads juvenile european eels to the gulf stream. Curr. Biol. 27, 1236–1240. doi: 10.1126/sciadv.1602007
Payne R. S., McVay S. (1971). Songs of humpback whales. Science 173, 585–597. doi: 10.1126/science.173.3997.585
Queiroz N., Humphries N. E., Mucientes G., Hammerschlag N., Lima F. P., Scales K. L., et al. (2016). Ocean-wide tracking of pelagic sharks reveals extent of overlap with longline fishing hotspots. Proc. Natl. Acad. Sci. U.S.A. 113, 1582–1587. doi: 10.1073/pnas.1510090113
Reiter J., Panken K. J., Leboeuf B. J. (1981). Female competition and reproductive success in northern elephant seals. Anim. Behav. 29, 670–687. doi: 10.1016/S0003-3472(81)80002-4
Robinson P. W., Costa D. P., Crocker D. E., Gallo-Reynoso J. P., Champagne C. D., Fowler M. A., et al. (2012). Foraging behavior and success of a mesopelagic predator in the northeast Pacific Ocean: insights from a data-rich species, the northern elephant seal. PloS One 7, e36728. doi: 10.1098/rspb.2018.1101
Rodriguez J. P., Fernandez-Gracia J., Thums M., Hindell M. A., Sequeira A. M., Meekan M. G., et al. (2017). Big data analyses reveal patterns and drivers of the movements of southern elephant seals. Sci. Rep. 7, 112. doi: 10.1038/s41598-017-00165-0
Rose G. A. (1993). Cod spawning on a migration highway in the north-west atlantic. Nature 366. doi: 10.3389/fmars.2017.00422
Senigaglia V., de Stephanis R., Verborgh P., Lusseau D. (2012). The role of synchronized swimming as affiliative and anti-predatory behavior in long-finned pilot whales. Behav. processes 91, 8–14. doi: 10.1016/j.beproc.2012.04.011
Sequeira A. M. M., Rodriguez J. P., Eguiluz V. M., Harcourt R., Hindell M., Sims D. W., et al. (2018). Convergence of marine megafauna movement patterns in coastal and open oceans. Proc. Natl. Acad. Sci. U.S.A. 115, 3072–3077. doi: 10.1073/pnas.1716137115
Shaffer S. A., Tremblay Y., Weimerskirch H., Scott D., Thompson D. R., Sagar P. M., et al. (2006). Migratory shearwaters integrate oceanic resources across the Pacific Ocean in an endless summer. Proc. Natl. Acad. Sci. U.S.A. 103, 12799–12802. doi: 10.1073/pnas.0603715103
Southall B. L., Schusterman R. J., Kastak D. (2000). Masking in three pinnipeds: underwater, low-frequency critical ratios. J. Acoust Soc. Am. 108, 1322–1326. doi: 10.1121/1.1288409
Southall B. L., Schusterman R. J., Kastak D. (2003). Auditory masking in three pinnipeds: Aerial critical ratios and direct critical bandwidth measurements. J. Acoustical Soc. America 114, 1660–1666. doi: 10.1121/1.1587733
Stewart B. S., DeLong R. L. (1995). Double migrations of the northern elephant seal, Mirounga angustirostris. J. mammalogy 76, 196–205. doi: 10.2307/1382328
Traniello J. F. A. (1989). Foraging strategies of ants. Annu. Rev. Entomology 34, 191–210. doi: 10.1146/annurev.ento.34.1.191
Keywords: northern elephant seal, foraging migration, northeast Pacific, coherent movement, acoustics, solar equinox
Citation: Pei S, Zhang X, Eguíluz VM, Kienle SS, Robinson PW, Costa DP and Duarte CM (2023) Coherent movement patterns of female northern elephant seals across the NE Pacific Ocean. Front. Mar. Sci. 10:689953. doi: 10.3389/fmars.2023.689953
Received: 01 April 2021; Accepted: 03 October 2023;
Published: 24 October 2023.
Edited by:
Nuno Queiroz, Centro de Investigacao em Biodiversidade e Recursos Geneticos (CIBIO-InBIO), PortugalReviewed by:
Fernando Elorriaga-Verplancken, Centro Interdisciplinario de Ciencias Marinas (IPN), MexicoCopyright © 2023 Pei, Zhang, Eguíluz, Kienle, Robinson, Costa and Duarte. This is an open-access article distributed under the terms of the Creative Commons Attribution License (CC BY). The use, distribution or reproduction in other forums is permitted, provided the original author(s) and the copyright owner(s) are credited and that the original publication in this journal is cited, in accordance with accepted academic practice. No use, distribution or reproduction is permitted which does not comply with these terms.
*Correspondence: Carlos M. Duarte, Y2FybG9zLmR1YXJ0ZUBrYXVzdC5lZHUuc2E=
†These authors have contributed equally to this work
Disclaimer: All claims expressed in this article are solely those of the authors and do not necessarily represent those of their affiliated organizations, or those of the publisher, the editors and the reviewers. Any product that may be evaluated in this article or claim that may be made by its manufacturer is not guaranteed or endorsed by the publisher.
Research integrity at Frontiers
Learn more about the work of our research integrity team to safeguard the quality of each article we publish.