- 1Department of Ecology, Evolution & Behavior, The Alexander Silberman Institute of Life Sciences, The Hebrew University of Jerusalem, Jerusalem, Israel
- 2The Interuniversity Institute of Marine Sciences in Eilat, Eilat, Israel
Consumption of pelagic zooplankton plays a vital role in the functioning of benthic communities such as coral reefs and kelp forests. Many fish that consume zooplankton in those habitats are site attached, foraging for drifting prey while maintaining a fixed position close to a shelter such as a branching coral or a perforated rock. Therefore, the flow, in which their planktonic prey drifts, is expected to affect their foraging movements. However, most attributes of those movements are poorly understood- a gap that our study seeks to fulfil. Our experiments were carried out in a laboratory flume with 4 common coral-reef site-attached species. Their movements were recorded in 3D, using two orthogonal video cameras. Different fishes exhibited similar trends despite noticeable differences in their body size, their morphology, the type of shelters they use, and the typical size of the groups in which they reside. In all species, the strike distance decreased with increasing flow speed. Similarly, the distance between the fish and prey at the moment of strike initiation (“Reactive Distance”) decreased with increasing flow speed, as well as the angle between that “Reactive Distance” and flow direction. Surprisingly, striking speeds (relative to Earth) remained nearly unchanged under different flows speeds. However, faster strikes occurred when oriented at wider angles relative to the flow. Taken together, the fish appear to determine the speed and angle of their strikes based on a cognitive ability to assess the prey’s drifting speed and path in order to reach on time the intercepting point. A rough estimate of the time it takes the fish to decide on the strike’s orientation and speed, would suggest a few hundred of milliseconds. Using published data on the fishes’ feeding rates, we found that the fish significantly differed in their feeding efficiencies, defined as the percent of prey they captured from those passing through their actual foraging space. That difference may explain inter-specific differences in the habitats the fish use and their group size.
Introduction
Coral-reef fishes live in rich communities within complex environmental conditions in which their abundance and distribution is thought to be tightly related to their feeding ecology (Bellwood et al., 2017). Many planktivorous fish in that habitat are site-attached (Kiflawi and Genin, 1997), capturing drifting zooplankton while maintaining quasi-stationary positions next to a shelter. The shelters can be coral heads, large rocks or other complex substrates. In some places, those fish are extremely abundant, titled by Hamner et al. (1988) “a wall of mouths”. The concentration of pelagic zooplankton strongly declines in the waters down-current of that “wall”. A substantial import of allochthonous nutrients via such predation was proposed as an explanation of the “Paradox of the Reef” (Erez, 1990; Genin et al., 2009; Wyatt et al., 2010; Wyatt et al., 2013; Morais and Bellwood, 2019), referring to the unknown source(s) of nutrients needed to support the exceptionally high productivity of the reef. Coral reefs are typically surrounded by oligotrophic waters with low abundance of zooplankton. The constraint in zooplankton availability requires planktivorous fish to capture individual prey (Confer and Blades, 1975; Werner, 1977; Vinyard, 1980; Kiflawi and Genin, 1997), rather than filter-feed (Nonacs et al., 1994). Prey capture in site-attached fishes consists of three steps: (i) a quasi-stationary wait-and-search interval, where the fish faces the on-coming current, waiting for a drifting prey to reach a sufficiently close range to be visually detected (ii) a strike, characterized by rapid swimming toward the prey, and (iii) capturing the prey using ram-jaw suction (Coughlin and Strickler, 1990; Wainwright et al., 2007; Jacobs and Holzman, 2018; Olsson et al., 2020).
A visual detection of zooplanktonic prey and the use of suction to ingest it, is shared by numerous adult fish and larvae (Turingan et al., 2005; China and Holzman, 2014; Holzman et al., 2015). Unlike adult fish, small larvae frequently miss prey. A low success rate is mostly due to the larvae’s minute size relative to their prey, their limited swimming ability, and lack of experience (Krebs and Turingan, 2003; Turingan et al., 2005; China et al., 2017). The effects of the above factors are greatly reduced in the larger, faster, and experienced adults (Coughlin and Strickler, 1990; Olsson et al., 2020). In their work with coral-reef fishes in a flume, Genin et al. (submitted1) found 100% success rate in fish striking a non-evasive prey (Artemia nauplii). The rates of feeding, however, also depends on external factors such as the concentration of the prey, current speed, and light (Kiflawi and Genin, 1997; Manatunge and Asaeda, 1998; Clarke et al., 2005; Rickel and Genin, 2005; Clarke et al., 2009; Palstra et al., 2015; Khrizman et al., 2018; Ishikawa et al., 2022; Genin et al., submitted).
The mechanisms through which site-attached zooplanktivorous fish strike their prey in the flow are not fully understood and warrant further investigations. For example, Kiflawi and Genin (1997) characterized the effects of flow and prey density on zooplankton capture, but their study used only 2 individuals from one species and a single individual from another. McFarland and Levin (2002) studied the effect of flow on the fish’s striking strategies, but focused on juveniles, not adults. Studies by Holzman and colleagues (Holzman et al., 2012; Jacobs and Holzman, 2018; Olsson et al., 2020) focused only on the final step in the fish’s strikes, the ram-jaw suction without addressing the other components of the strikes. Clarke et al. (2009) and Finelli et al. (2009) studied 2 species of gobies that differ from the common site-attached fishes since their strikes are intermittent, having to emerge from shelter in order to strike a passing-by prey. Therefore, the gobies’ feeding rate is much slower than that of common site-attached fishes (1-3 min-1 vs. 0.5-2 s-1, respectively; Kiflawi and Genin, 1997; Clarke et al., 2009; Genin et al., submitted).
Current speed appears to be a key factor in determining the foraging behavior and feeding rates in site-attached fish (Kiflawi and Genin, 1997; O’Brien et al., 2001; Clarke et al., 2009; Khrizman et al., 2018; Ishikawa et al., 2022). As those fish typically have a fusiform or compressed-fusiform shape (Fulton, 2007; Cano-Barbacil et al., 2020; Siqueira et al., 2020), they lack the ability to swim sideward, as puffers and boxfish do (Gordon et al., 1996). To reduce the area projected perpendicular to the flow, thereby reducing pressure drag (Khrizman et al., 2018), the fish narrow down the angle between the side of their body and the direction of the current (Kiflawi and Genin, 1997). Narrower angles under stronger flows may explain the surprising absence of increased feeding rates under higher flow speed, expected to occur under higher prey flux (Kiflawi and Genin, 1997; Videler and Wardle, 1991; Genin et al., submitted). Alternatively, McFarland and Levin (2002) showed that flow speed can change the fish’s striking strategy, in which under weak flows (10–14 cm/s) the fish swim directly toward the prey but under stronger currents they tend to fall back with the flows and capture the prey during their drift.
Large variations in body forms and fin functions occur in site-attached fishes. Such variations are expected to affect the fish’s striking motions and their ability to capture fast-drifting prey (Hobson and Chess, 1978; Webb, 1984; Clarke et al., 2005). The ratio of body length to height (fineness ratio) is considered to be a key factor determining the fish ability to maneuver and overcome drag forces (Webb and Weihs, 1983; Webb, 1984). However, later studies (Walker and Westneat, 2002; Blake, 2004; Fulton et al., 2005; Lauder et al., 2012; Fulton et al., 2013; Heatwole and Fulton, 2013; Walker et al., 2013; Schakmann and Korsmeyer, 2023) revised that view, emphasizing, in addition, the role of fin shape and motion in determining maneuvrability. For example, paired motions of pelvic and pectoral fins are commonly used to perform the maneuvers required to capture a drifting prey (Fulton et al., 2013; Engel et al., 2021).
The objectives of this study are: (1) to quantitatively characterize the key attributes of striking movements in 4 species of site-attached coral-reef fishes, and (2) to test the alleged relationships between feeding rates and flow-driven changes in the fish’s body orientation. Our study also allows an approximation of the fish’s cognitive ability in determining its striking attributes.
Methods
Study site
Our field work was carried out in the coral reef in front of the Interuniversity Institute for Marine Sciences (IUI), Gulf of Eilat/Aqaba, Red Sea. A detailed description of the reef and its environment is found in Yahel et al. (1998); Biton and Gildor (2011), and Shaked and Genin (2022), and references therein. Briefly, this fringing reef is located on a steep slope. A diverse guild of stony corals, consisting of branching and massive corals cover 20-50% of the rocky substrate (Fishelson, 1971; Benayahu and Loya, 1977; Shaked and Genin, 2022). The currents are weak, with a mean speed of 10 cm/s and the waves are small most of the time (Genin et al., 1994; Reidenbach et al., 2006). The Gulf of Eilat/Aqaba is oligotrophic with clear waters; visibility typically extends tens of meters. The fish community in the reef includes >260 species, including piscivores, zooplanktivores, and herbivores (Brokovich, 2001). Within this highly diverse community, zooplanktivorous fishes comprise >40% of the total (Shaked and Genin, 2022).
Fish
Four species of common site-attached, zooplanktivorous fish were studied: the damselfishes Neopomacentrus miryae (hereafter Nm; mean ± SD fineness ratio of 2.54 ± 0.143; N = 5), Chromis viridis (Cv; 2.32 ± 0.11; N = 8), and Dascyllus marginatus (Dm; 1.49 ± 0.07; N = 6), and the serranid Pseudanthias squamipinnis (Ps; 2.68 ± 0.072; N = 5). Those species share a similar lifestyle, including the formation of social groups and a long-term fidelity to their shelters (Allen and Randall, 1980; Smith and Heemstra, 1986; Myers, 1989; Lieske and Myers, 1994; Baensch and Riehl, 1997). A detailed description of three species (Nm, Dm, Ps) is given in Genin et al. (submitted). Dm and Cv are usually associated with corals of the genus Acropora. Typical group size in Cv is substantially larger than in Dm (tens vs several, respectively; Supplementary Table 1). The four species are diurnal, using vision to detect and capture meso-zooplankton. Their diet is diverse, reflecting the wide taxonomic composition of the planktonic community in the surrounding waters (Smith and Heemstra, 1986; Karpestam et al., 2007; Genin et al., submitted). In captivity, those fish readily feed on live substitute prey, such as nauplii of Artemia salina (Genin et al., submitted).
Adult fish were used in all our experiments. Females were used in Ps, while the gender of Cv, Dm and Nm in our experiment remained indeterminate due an undifferentiated morphology of the two sexes.
The fish were collected in the IUI reef at 6-15 m depth. Cv and Dm were collected with small hand nets at their home corals after partially anaesthetizing the fish with clove oil. Ps and Nm were collected using a gill net, into which the fish were carefully scared by divers. The collected fish were rapidly (<15 min) transported to an acclimation tank (50-60 L in volume) in the laboratory. This acclimation period lasted 1-3 weeks. A fish was considered acclimated once it started to readily feed upon prey that was added to the tank by a person standing nearby. Acclimated fish were transferred to the flume a day or more prior to the beginning of trials. During the entire acclimation periods the fish were fed ad libitum with nauplii of brine shrimps. A light/darkness cycle identical to the natural cycle was maintained in the tank and flume.
All fish were returned to the reef, preferably to the exact sites where they had been collected. A total of ≥5 individuals were used per species. The methods used to collect and handle the fish were done under a permit from Israel Nature & Parks Authority and fully complied with the ethical rules of animal treatment at the Hebrew University of Jerusalem.
Prey
Live, 1-day old nauplii of brine shrimps (Artemia salina) were used as prey. The nauplii were similar in size (length mean ± SD of 0.60 ± 0.07 mm, n = 50) and transparency to the natural prey the fishes feed on in the reef. Unlike most zooplankters, Artemia nauplii are poor swimmers and lack any form of escape behavior (Trager et al., 1994). However, those differences can be considered advantageous for a comparative study seeking to experimentally isolate the effects of prey density and flow from other factors.
Flume
Our experiments were carried out in the recirculating flume (Supplementary Figure 2) that was used by Kiflawi and Genin (1997) and Genin et al. (submitted). The flume contained 330 L of water. The transparent work section was 200x30x30 cm in size. Fish movement in the flume was restricted to a 50 cm long experimental arena in the downstream part of the work section by coarse plastic-coated grid. A shelter (branching coral skeleton or a small pipe) was placed near the flume’s down-current end. Sea water in the tank was replenished between trials. The replenishing water was pumped from the nearby reef at 30 m depth. Natural zooplankton was removed from the pumped water using a 65 µm plankton net. The flume was thoroughly cleaned, removing fouling organisms and dirt, at least 3 times a week. Water temperature was maintained at 24° ± 1° C. A single Metal Halide and 4 fluorescent lamps were used to illuminate the work section with intensity of 2700 Lx, similar to that prevailing during mid-day at the fish’s natural habitat.
The flow in the flume was generated using a controlled propeller. Effective flow straighteners provided nearly uniform flow profiles across the flume. The uniformity of the flow was verified using 3D tracking of suspended particles (see below) and a 1 MHz acoustic velocity meter (ADV, Nortek, Norway). An examination of the boundary layer made during the construction of the flume by Kiflawi and Genin (1997), revealed a ~1 cm-thick boundary layer over the walls and bottom. The fish rarely foraged within that layer. Similarly, the fish always foraged up-current of their shelter, away from the zone where it affected the water motion. The flow speeds used in our experiments were 5, 10, 15, and 20 cm/s, covering the typical range of currents at the local coral reef.
Two high-resolution, orthogonally-oriented video cameras (UI-3070CP-C-HQ Rev.2, IDS, Obersulm, Germany) were used to record fish strikes in 3D. A down-looking camera with a 16 mm lens (IDS-8M118-C1620) was positioned 50 cm above the flume and a side-looking camera with 25 mm lens (IDS-8M118-C2520) was positioned 75 cm from the flume’s wall oriented directly to the center of the water column. The cameras recorded frames at a rate of 100 fps, synchronized to within one frame ( ± 3ms). Calibration and quality control of the 3D data were performed with the EasyWand Camera Calibration tool (Theriault et al., 2014), using 1840 frames of a calibration stick that was manually moved across the camera field of view. Calibration records were processed using MATLAB DLTdv8a digitizing tool, indicating a spatial precision of ±2 mm.
Work protocol
A single fish was used in each trial. The fish was not fed during the 12 hrs preceding the trial. A day of trials always started by cleaning the flume water and closing the valve to the freshly-pumped seawater. Suspended particles that sometimes remained in the flume were filtered out using a 20 µm plankton net. The net, having a frame that tightly fit inside the flume’s cross section, was inserted inside the flume and the flow speed was raised to 25 cm/s for a few minutes, assuring several filtering cycles of the water. Approximately 10 min before the start, a few (5-8) nauplii were added to the flume, verifying that the fish readily started to capture the prey. Then, 10-12 nauplii were gradually ejected to the flume using a syringe positioned above the propeller, while keeping the fish in its shelter. Thereby, the prey was nearly homogeneously distributed along the flume. The low density of the prey and its homogenous distribution assured that the strikes would be well-separated, so that each strike would start with the fish waiting for a prey to drift into its detection space. Video recording started as soon as the operator left and entered a control room, hidden from the flume with a dark, separation curtain. The recording session lasted a few minutes, after which recording was stopped and another cycle of prey ejection started. At least 30 strikes were recorded for each flow speed for each individual. Each work day consisted of several trials using different, haphazardly-selected flow speeds. Once the trials covering all flow speeds with one fish were completed, it was replaced by another fish (after thoroughly cleaning the flume). The total number of individuals per species were 5 for Ps and Nm, and 6 for Cv and Dm. The corresponding total numbers of recorded strikes for each species were 434, 538, 369, and 496, respectively.
“Strike distance” (Supplementary Figure 3) was measured based on the clearly-observed points of the strike initiation (the steady, direct, and rapid swim toward the prey) and ram-jaw capture. “Strike angle” (β in Supplementary Figure 3) was defined as the angle between the direction of the flow and the strike path. “Reactive Distance” was defined as the distance between the fish and the prey at the instance the strike was initiated. It was computed considering the duration of the strike and a back-calculation of the location at which the passively drifting prey was found when the strike was initiated (i.e., up-current of the capture point). “Detection Angle” (α in Supplementary Figure 3) was the angle between the flow direction and the imaginary line extended between the fish snout and the distal point of the Reactive Distance. For each Detection Angle we also calculated its horizontal component (αH), referring to a 2D distance on the horizontal plane perpendicular to the flow direction. In order to test the effect of flow speed on Reactive Distance independently of possible effects of Detection Angle, we limited the Detection Angles we used to ≤45°. Based on purely geometric considerations, under equal flow speeds and striking distance, the reactive distance under 45° is 14% shorter than under 0°.
In order to assess the relationships between Detection Angles and the strike attributes, values of αH were sorted into 3 categories: “narrow” (αH<10), “mid” (10≤αH<20), and “wide” (αH≥20). Both strike and Detection Angles are presented below using absolute values, disregarding their left or right direction with respect to the flow.
Statistical analysis
The effect of flow speed and species on the strike’s distance, speed, duration, and angle, and the effect of flow on Reactive Distance and Detection Angle were tested using Repeated Measures ANOVA. The measured parameter was the dependent variable (measured repeatedly for the same individual under all flow speeds) and the species was used for testing the Between Subject Effect. Sphericity and Homogeneity of Variance were verified prior to testing. Tukey post hoc assessed the differences between pairs of species in cases where the aforementioned ANOVA indicated significant species effects. To test the Interaction among factors, we used Compare Mean Effects with Bonferroni for confident interval adjustment. Values of the aforementioned strike parameters that were measured through replicated trials (N=28 ± 15) were averaged, yielding a single mean value for each individual for each flow speed, which was then used as the input variable for the Repeated Measures ANOVA.
Due to non-homogeneous variances, the effects of the three αH categories (wide, mid, and narrow) on strike attributes were tested using Bootstrap with 10,000 samplings and Biased corrected acceleration, with flow speed and species as fixed Factor.
Repeated Measures ANOVA and post hoc tests were performed using R Studio (packages “agricolae”,”emmeans”). Bootstrap tests were performed using SPSS (v. 28).
Results
Flow and strike attributes
Mean 3D strike distances ranged ~1.7 to 5.9 cm, depending on species and flow-speed. The horizontal (perpendicular to the flow) and vertical components ranged ~0.9-4.1 and ~1-2.4 cm, respectively (Figure 1). In all species, the total strike distance and the horizontal and vertical components decreased with increasing flow speeds (Repeated Measures ANOVA, total distance- F(2)=43 P<0.0001; horizontal component- F(2)=23.7 P<0.0001; vertical component- F(2)=30.6 P<0.0001). The extent of that decrease in the total and horizontal components was steeper in Cv and Ps than in the other two species when the flow intensified from 10 to 15 cm/s (Figures 1A, B). The decrease in all distance components when the flow changed from 15 to 20 cm/s was relatively small and similar in all species (Figure 1). The former inter-species difference was reflected in the significant interaction between species and flow (total distance-F(6)=2.7 P<0.03; horizontal component-F(6)=2.7 P<0.03), although the effect of species alone was insignificant. On the other hand, a significant effect of species was found for the strikes’ vertical component (F(3)=3.8 P<0.03), with a post hoc test indicating that Nm (higher vertical component) and Dm (lower vertical component) significantly differed one from another (Tukey, P<0.03).
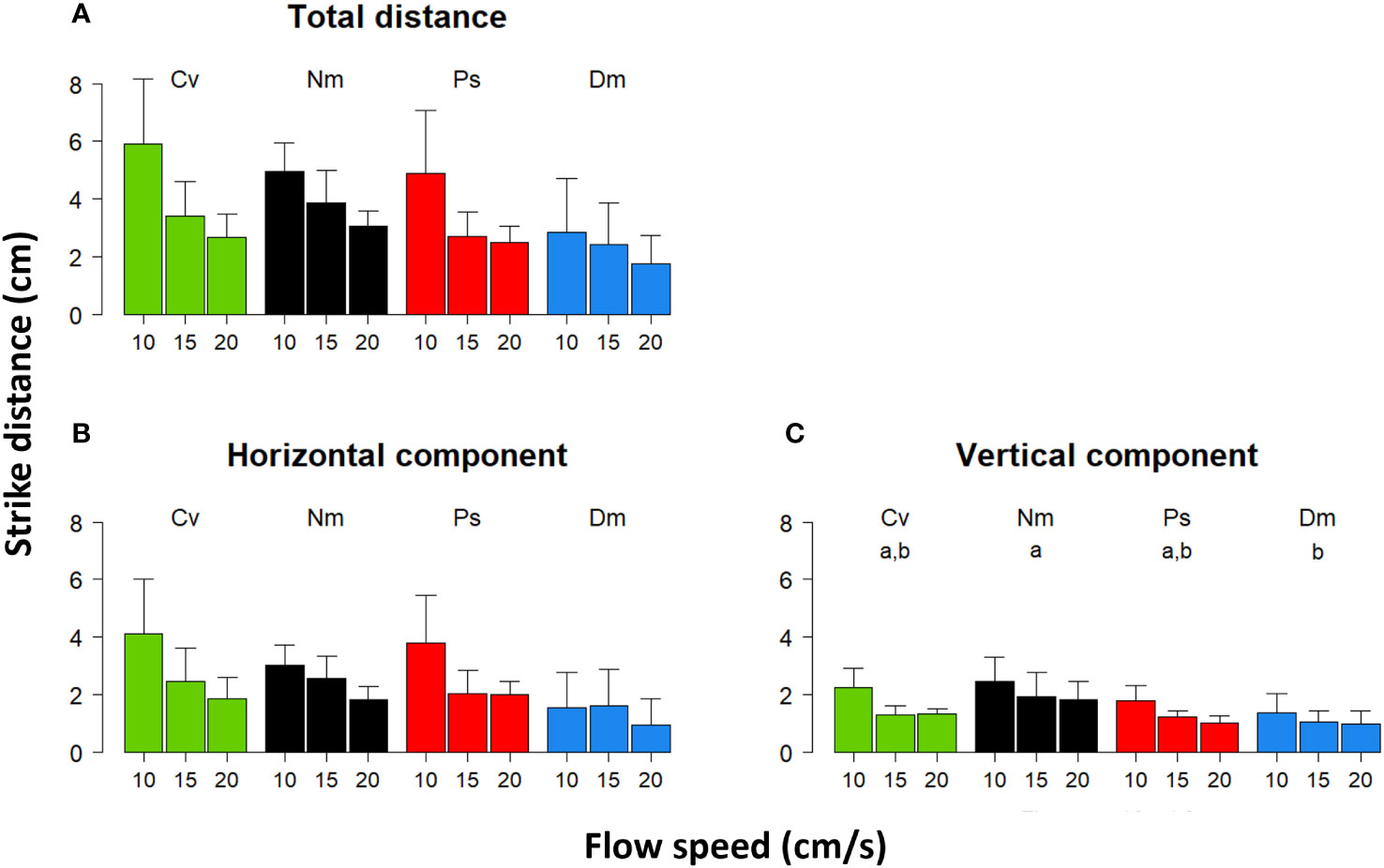
Figure 1 Strike distance vs. flow speed. Mean (± SD) of the (A) - total strike distance in 3D, (B) - the horizontal component of the strike, and (C) - the vertical component of the strike under flow speeds of 10, 15, and 20 cm/s. Species are color coded (Cv-green, Nm-black, Ps-red, Dm-blue) and their acronyms indicated above the bars. Small letters above the bars in (C) - indicate the results of post hoc testing of inter-specific differences, so that pair of species that significantly differed (P<0.05) are indicated using different letters.
The shorter strike distances under stronger flow corresponded to a significant decrease in the strike duration (Figure 2, Repeated Measures ANOVA, F(2)=163 P<0.0001). Also here, the effect of species was significant (F(3)=9 P<0.0008) with a post hoc test indicating that the strike duration in Cv was significantly shorter than in all other species (Tukey, P<0.03). The interaction between species and flow was significant (F(6)=5 P<0. 0008), reflecting a weaker flow effect on Cv, compared with all other species.
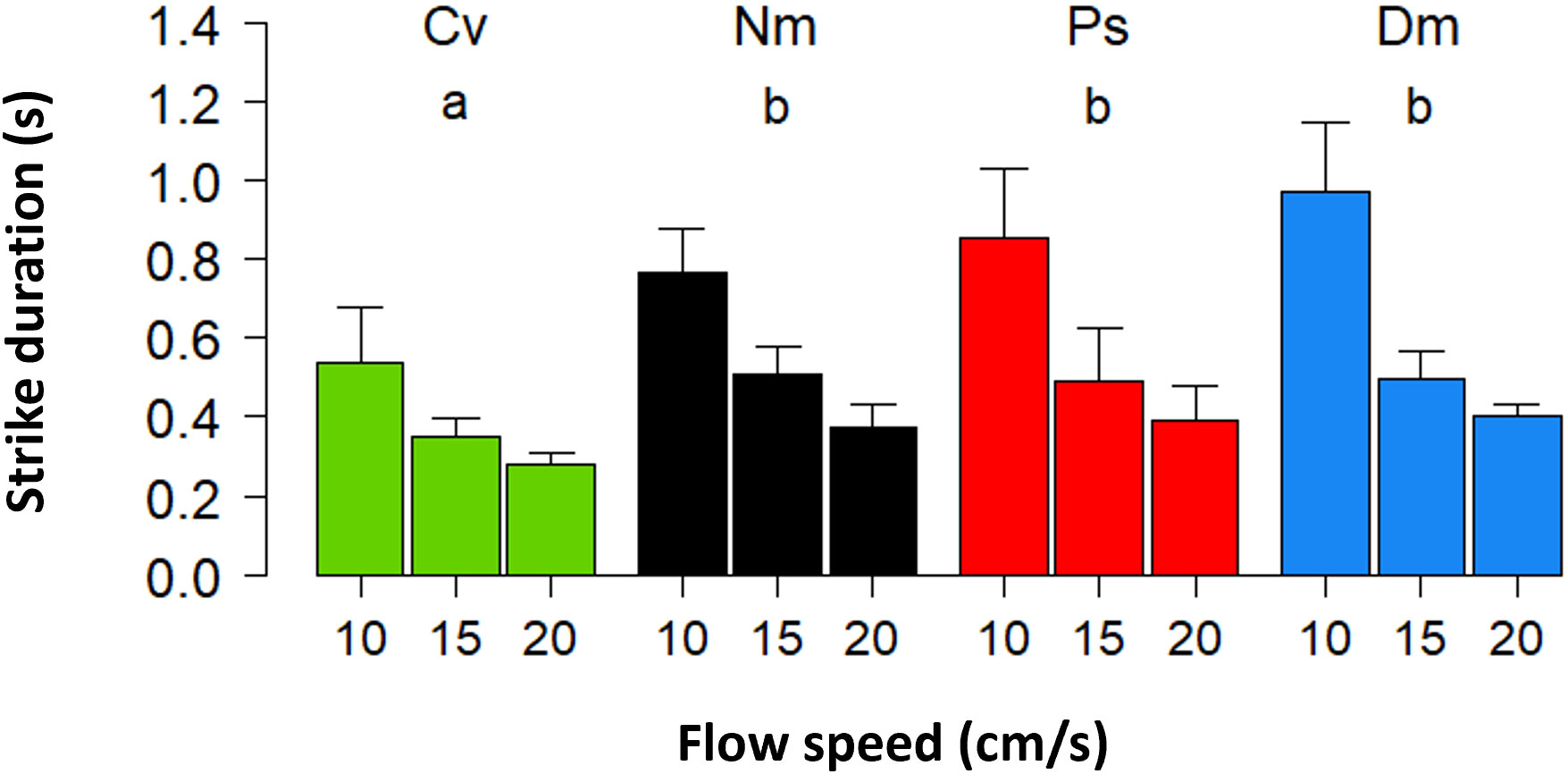
Figure 2 Strike duration vs. flow speed. Mean (± SD) duration of strikes. Different species and indications of statistics are coded as in Figure 1.
The effects of flow on the strike’s distance and duration were balanced so that no significant effect on strike speed (relative to Earth) was found (Figure 3A, Repeated Measures ANOVA, F(2)=0.9 P=0.42). Conversely, the strike speed relative to the water became significantly faster with increasing flow speed (Figure 3B, Repeated Measures ANOVA, F(2)=877.4 P<0.0001). Different species significantly differed in their striking speeds relative to both Earth and water (F(3)=10.3 P<0.0004; F(3)=10.7 P<0.0003, respectively), with a post hoc test indicating that strikes by Cv were significantly faster relative to the Earth (P<0.02) and water (P<0.003) than those of Ps and Dm. The strikes of Dm were significantly slower relative to Earth than those of Nm (P<0.04). A significant interaction between flow speed and species was found relative to both Earth and water (F(6)=3.5 P<0.01; F(6)=5.5 P<0.0004, respectively).
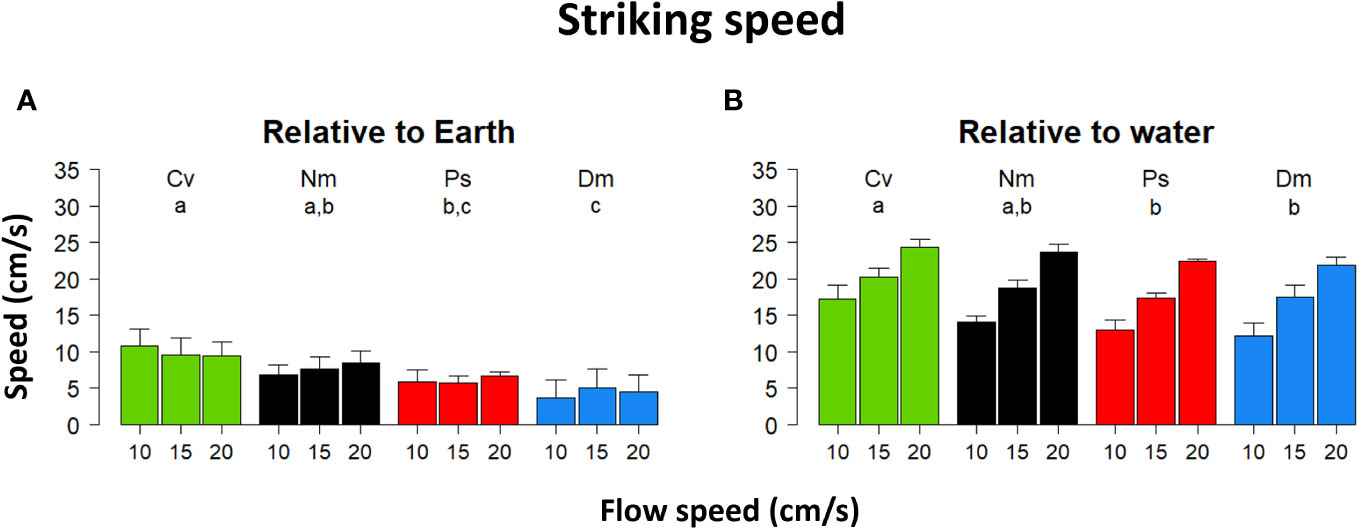
Figure 3 Striking speed vs. flow speed. Mean (± SD) striking speed relative to the (A) – Earth, (B) - the flowing water. Species codes and statistics are as in Figure 1.
Strike angles in 3D slightly but significantly widened under faster flows (Figure 4B, Repeated Measures ANOVA, F(2)=7 P<0.003). Despite this widening, the horizontal angles were not significantly affected by flow speed (Figure 4C, Repeated Measures ANOVA, F(2)=2.9 P=0.07), indicating that the significant effect on the total (3D) angels was mostly due to the corresponding changes of the vertical angles (Figure 4D, Repeated Measures ANOVA, F(2)=12 P<0.0001). Inter-specific differences of the strike and horizontal angles (Figures 4B, C; F(3)=4.4 P<0.02; F(3)=18 P<0.0001, respectively) showed that both the total and horizontal angles were widest in Ps.
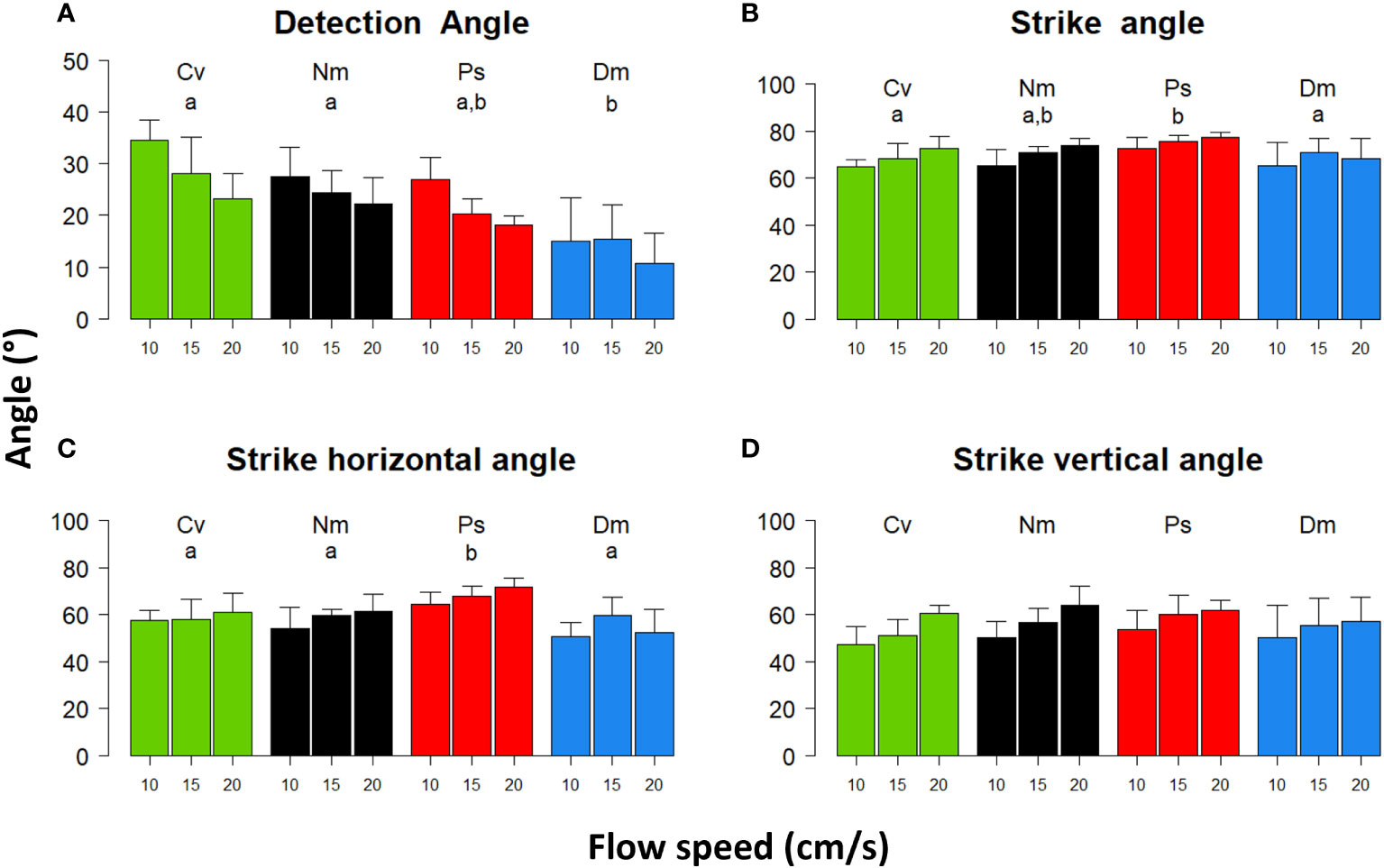
Figure 4 Detection and striking angles vs. flow speed. Shown are mean (± SD) angles with respect to the flow direction of (A) - Reactive Distance vector, (B) - strike angle in 3D, (C) - the strike angle along the horizontal plane perpendicular to the flow direction, and (D) - the strike angle along the vertical plane perpendicular to the flow direction. Species and statistics are coded as in Figure 1.
Flow and reactive distance
In all species the Reactive Distance, that is, the distance to the prey at the moment the strike started, significantly decreased with increasing flow speed (Figure 5, Repeated Measures ANOVA, F(2)=28.6 P<0.001) with non-significant interaction of species and flow. A post hoc test indicated that the decrease in Reactive Distance was significant only when the flow intensified from 10 cm/s to 15 (or 20) cm/s (Bonferroni, P<0.001), with a non-significant difference between 15 and 20 cm/s.
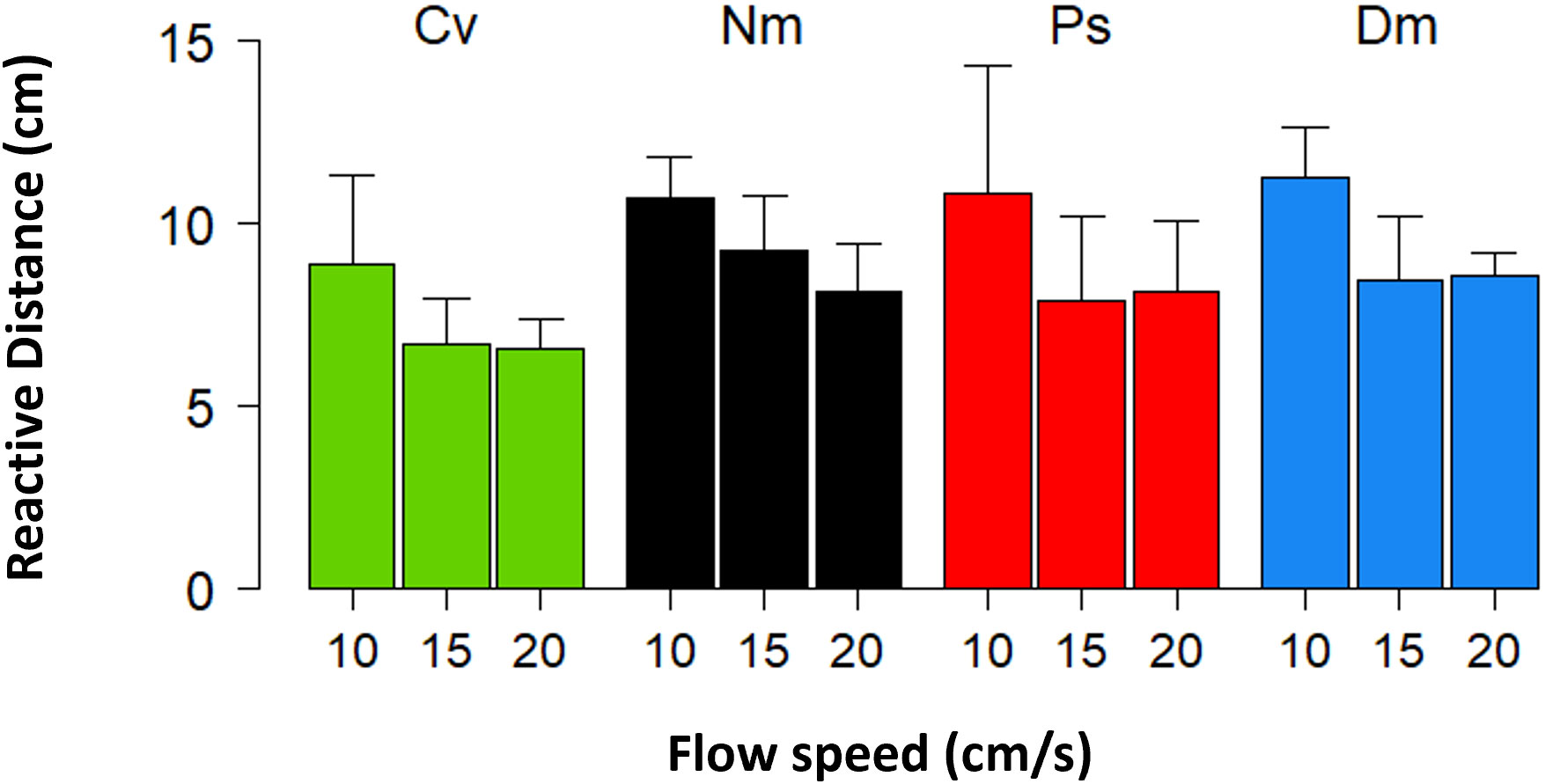
Figure 5 Reactive Distance vs. flow speed. Mean (± SD) Reactive Distances for cases in which the prey drifted almost directly (≤45°) toward the fish. Species are coded as in Figure 1.
Under stronger flows, Detection Angles significantly narrowed down (Figure 4A, Repeated Measures ANOVA, F(2)=28 P<0.001) with a significant effect of species (F(3)=9.7, P<0.001). A post hoc test indicated that the Detection Angles of Dm were significantly narrower (P<0.001) than those of Cv and Nm.
Body orientation and strike attribute
Most strike attributes appeared to depend on the horizontal Detection Angle (αH). In all species, the frequency distribution of αH was strongly skewed to the left (Figure 6), reflecting a preference to strike prey that was drifting nearly head-on toward the fish. Using the categorial sorting of αH to narrow (N), mid (M), and wide (W) angles, we found that in all species and under all levels of flow speeds, the total strike distance significantly increased with the widening αH (Figure 7; bootstrap, flow: F(2)=88.9, P<0.001 angle: F(2)=231.2, P<0.001 species: F(3)= 16.1, P<0.001). The strikes were approximately twice as long under a wide αH than under narrow αH (Figure 7). Despite a general decrease of strike duration with increasing flow speed (Figure 2), the within-speed effect of αH on strike duration was significant (bootstrap, flow: F(2)=332.3, P<0.001 angle: F(2)=8.5, P<0.001 species: F(3)=56.3, P<0.001). Though, no consistent trend was apparent. That is, under some changes in flow speed the strike duration increased in others it decreased (Figure 8). Nevertheless, in all species, the striking speed relative to both Earth and water significantly increased with widening of αH (Figures 9, 10, bootstrap, flow: F(2)=49.2, P<0.001 angle: F(2)=513.5, P<0.001 species: F(3)=119.3, P<0.001; flow: F(2)=2335, P<0.0001 angle: F(2)=140.3, P<0.001 species: F(3)=113.9, P<0.001, respectively). Noteworthy is the finding that despite the significant effects of αH on strike distance, duration, and speed, its effect on Reactive Distance was insignificant (Supplementary Figure 5, bootstrap, flow: F(2)=34.8, P<0.001 angle: F(2)=0.6, P=0.5 species: F(3)=26.2, P<0.001).
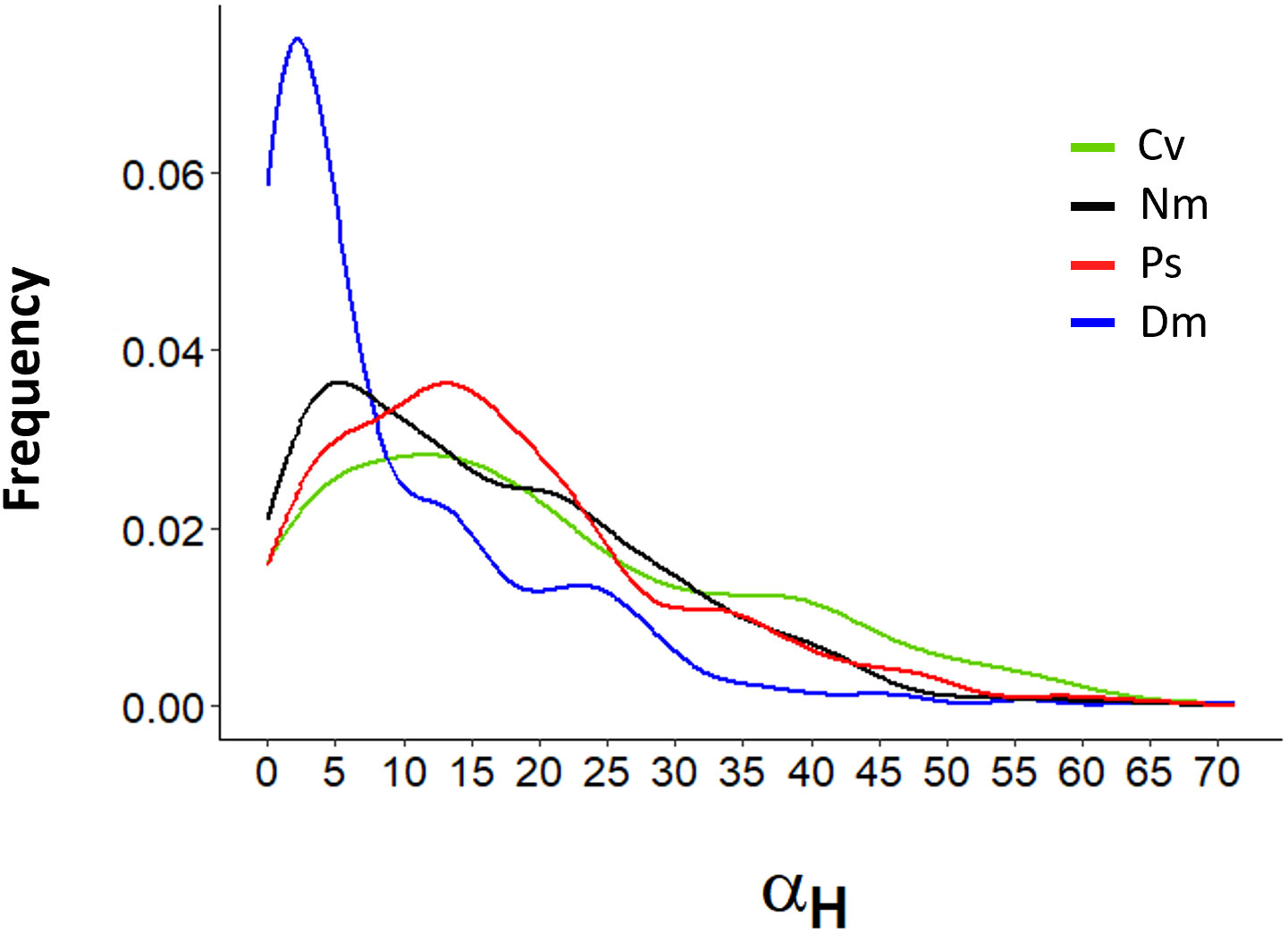
Figure 6 Frequency distribution of αH. Detection Angles (αH) were measured on the horizontal plane perpendicular to the flow direction. Lines are plotted based on measurements made every 1 cm/s increment. Species are color coded as in Figure 1.
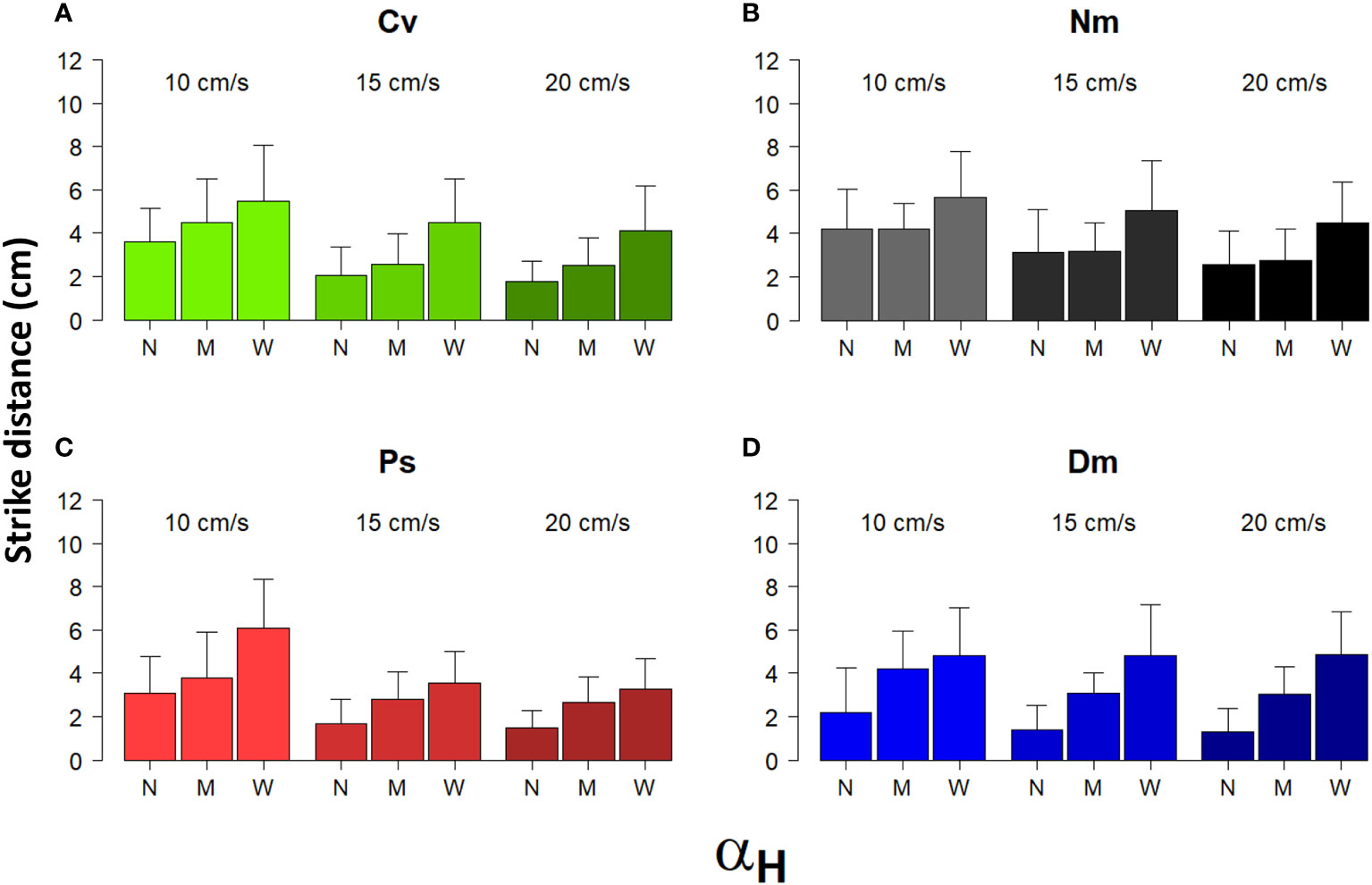
Figure 7 Strike distance under different categories of αH and different flow speed. Shown are the mean (± SD) distances in 3D of strikes performed under different flow speeds (between bar triplicates) in the three different categories of αH: N-narrow, M-mid, and W-wide (within bar triplicates) for the 4 different species (A)-Cv, (B)-Nm, (C)-Ps, and (D)-Dm (color coded as in Figure 1).
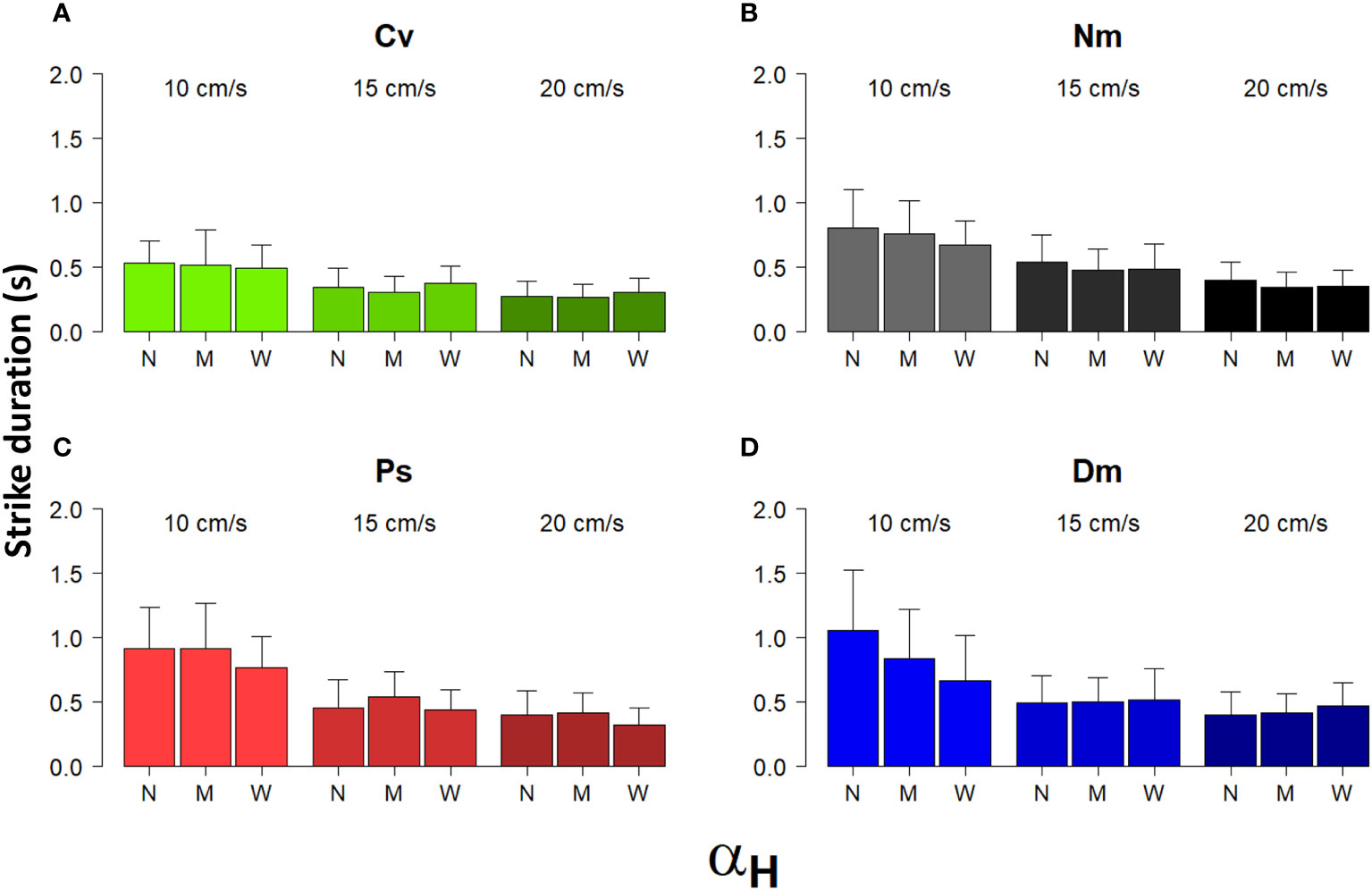
Figure 8 Strike duration vs. αH and flow speed. Shown are the means (± SD) of the duration of strikes performed under different flow speeds (between bar triplicates) in the three different categories of αH: N-narrow, M-mid, and W-wide (within bar triplicates) for the 4 different species (A)-Cv, (B)-Nm, (C)-Ps, and (D)-Dm (color coded as in Figure 1).
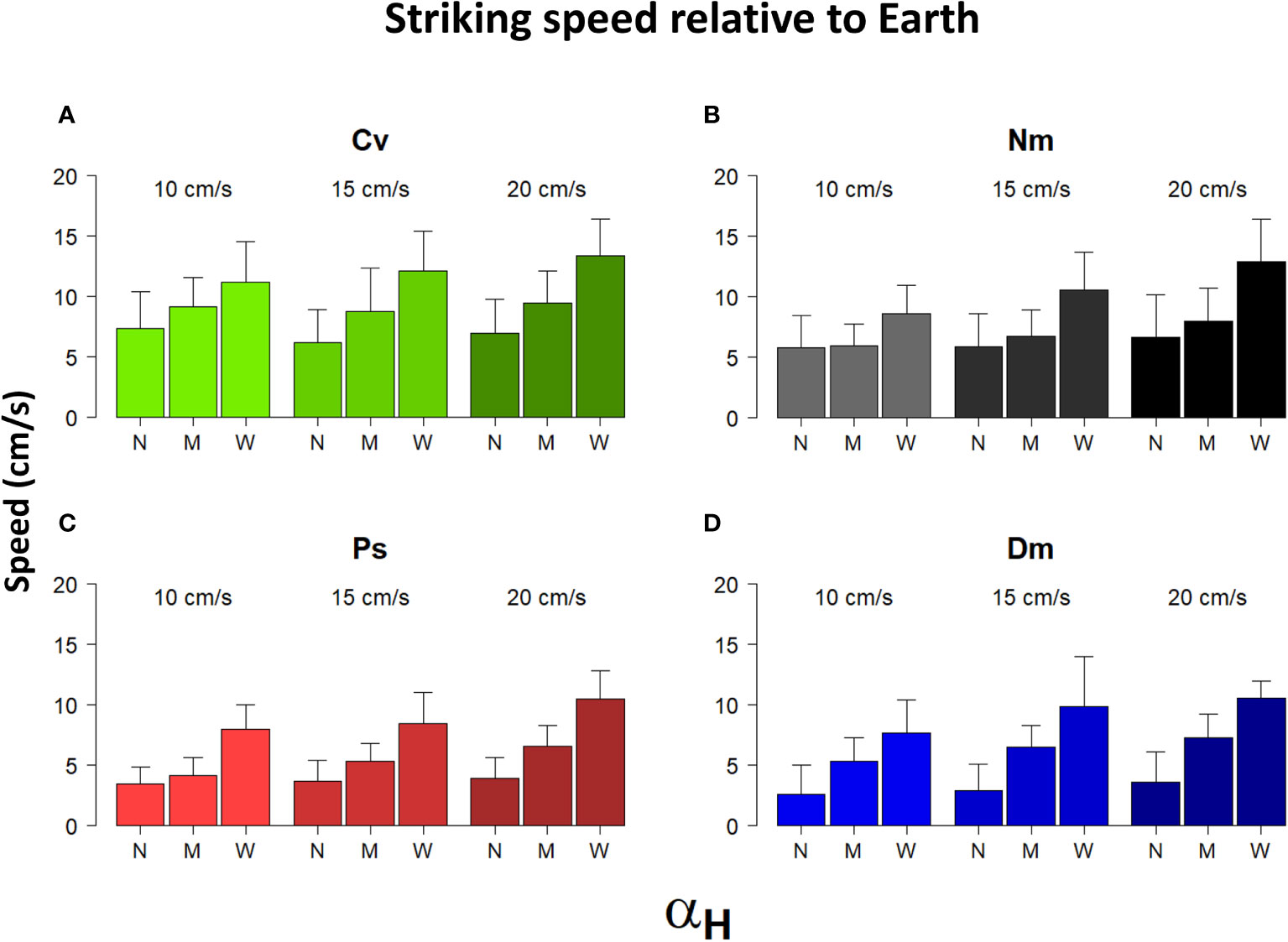
Figure 9 Striking speed relative to Earth vs. αH and flow speed. Shown are the means (± SD) of the speed of strikes in Earth coordinates performed under different flow speeds (between bar triplicates), in the three different categories of αH: N-narrow, M-mid, and W-wide (within bar triplicates) for the 4 different species (A)-Cv, (B)-Nm, (C)-Ps, and (D)-Dm (color coded as in Figure 1).
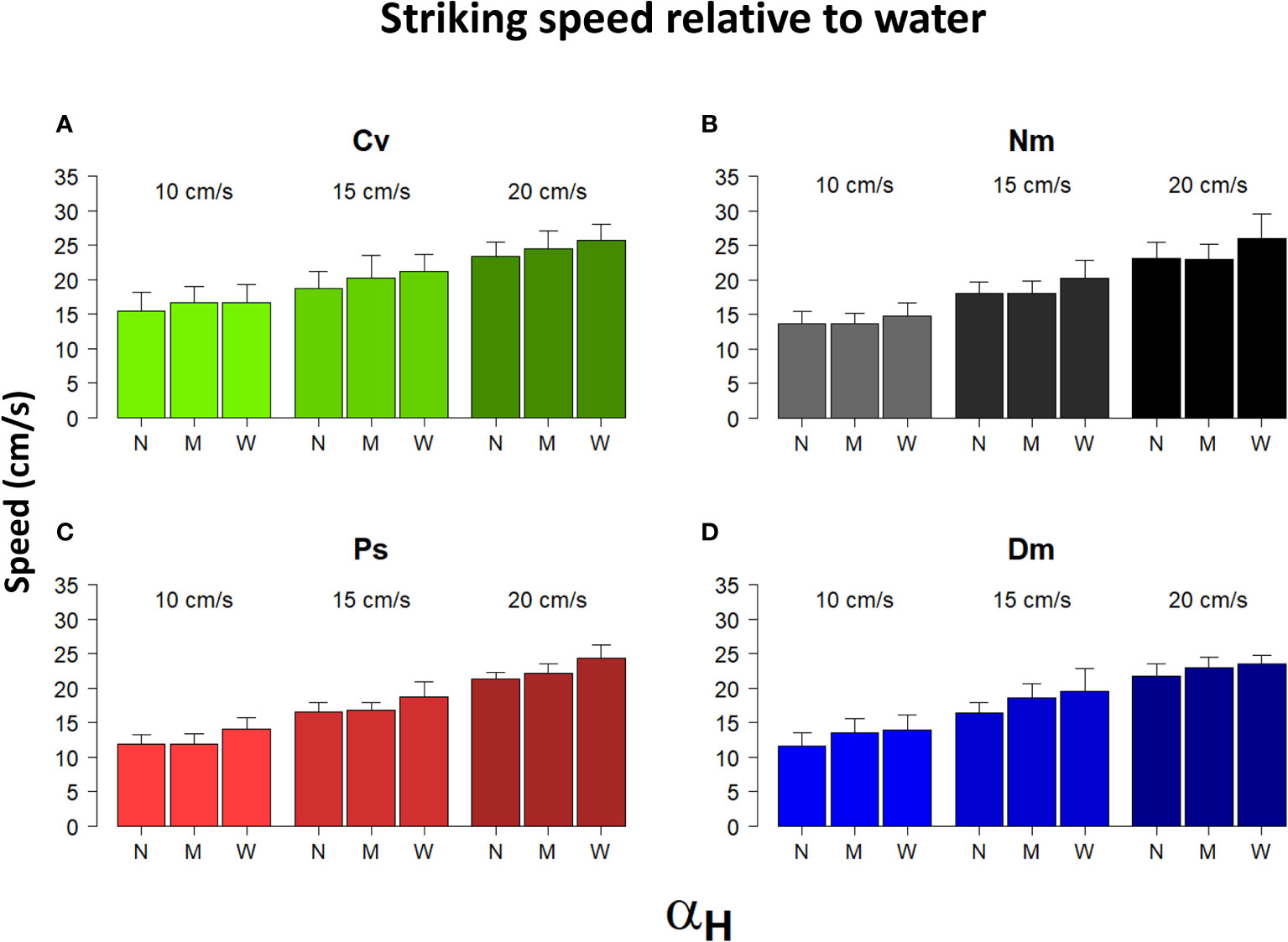
Figure 10 Striking speed relative to water vs. αH and flow speed. Shown are the means (± SD) of the speed of strikes relative to water performed under different flow speeds (between bar triplicates) in the three different categories of αH: N-narrow, M-mid, and W-wide (within bar triplicates) for the 4 different species (A)-Cv, (B)-Nm, (C)-Ps, and (D)-Dm (color coded as in Figure 1).
Discussion
To forage for drifting zooplankters while keeping a position near a fixed shelter, site-attached fish steadily swim against the current. Our observations show that their mean striking speed relative to the water was always positive and faster than the flow speed (Figure 3B), indicating that in order to capture prey, the fish mostly swam forward into the flow. (Note that rare occurrences of down-stream strikes were omitted from our database.) Moreover, with increasing flow speed, the fish striking speed relative to the water became significantly faster (Figure 3B), but not relative to Earth, where it remained nearly unchanged (Figure 3A). Similar findings, namely, an absence of flow effect on striking speed relative to Earth, was reported by Piccolo et al. (2008) for freshwater (stream) fish. However, our results do not agree with their conclusion that in order to strike, the fish use their maximum sustainable speed. Had they done so, the striking speed should have been slower under conditions of stronger head currents, as seen, for example, in competitive bike-riding, where maximum sustainable paddling force is typically used (Atkinson et al., 2003). Instead, the fish modulated their striking speed based on the ambient flow, on the prey’s distance, and on the angle at which the prey was located at the moment of strike initiation (Figures 7, 9, 10). We suggest that the striking speed in the fish we studied is determined by, and based on cognitive decisions (see below).
The studied species are fusiform, morphologically adapted to swim forward, rather than sideway as non-fusiform boxfishes and pufferfishes do (Videler and Wardle, 1991; Wardle et al., 1995; Gordon et al., 1996; Gordon et al., 2000; Siqueira et al., 2020). To lower the pressure-drag that pushes the fish down-current (Khrizman et al., 2018), the fish gradually narrow down their angle to the flow under stronger currents (Schakmann et al., 2020; Schakmann and Korsmeyer, 2023; Figure 7 in Genin et al., submitted). In doing so, the fish reduce the chance of being swept down-current and the ensuing risk of being captured by predators. The orientation to the flow also reduces the energetic cost of maintaining a fixed position near a shelter. Unexpectedly, the narrowing-down of the body orientation while foraging, as observed by Genin et al. (submitted), was observed by us for Detection Angles (Figures 4A, 6; Supplementary Figure 6), not strike angles (Figure 4B). Based on geometry alone, at a given Detection Angle, under stronger flows, the prey would reach closer to the position of strike initiation. Therefore, under stronger flows, the strike’s total, vertical, and horizontal angles were expected to become wider (Supplementary Figure 3). The fact that the horizontal strike angles remain unchanged (Figure 4C) is likely an outcome of the fish’s preference to strike prey that have lower Detection Angles as the flow becomes stronger. Noteworthy is the observation that the strike’s vertical angles do follow the expectation that follows the above geometric considerations, becoming larger with increasing flow speed (Figure 4D). This dichotomy between the horizontal and vertical movements may indicate a cognitive ability by the fish to decide which prey to strike and which to give up, based on the prey’s location and drifting speed.
Among the four species we studied, the morphology of Dm was the least “hydrodynamic”, having the smallest fineness ratio and a non-forked caudal fin (see Method Fish and Supplementary Figure 1). Accordingly, Dm exhibited slower striking speeds relative to Earth, and its Detection Angles were narrower than those of Nm and Cv (Figures 3A, 4A, 6). Furthermore, its striking speed relative to water was slower than that of Cv (Figure 4B). The non-forked caudal fin of Dm, compared with a forked fin in the other species, may limit the swimming speed and require higher energetic cost (Sambilay, 1990; Lauder et al., 2012; Xin and Wu, 2013). Likewise, Cv, the fish with the largest fineness ratio, exhibited faster striking speeds than those of Ps and Dm (relative to both Earth and water; Figure 3, and Method Fish).
Noteworthy is the inter-specific difference in the vertical component of the strikes, which was higher in Nm than in Dm (Figure 1C). Higher vertical vectors, hence larger space in which prey may be captured, could explain the higher feeding rates of Nm (Genin et al., submitted). Moreover, Genin et al. (submitted) hypothesized that despite the occurrence of higher prey fluxes under faster flow (with unchanged prey concentrations), the corresponding feeding rates did not increase because of the gradual narrowing down of the fish’s body orientation with respect to the flow direction. That is, as the flow becomes stronger, the “true” prey flux (hereafter “Actual Flux”) was not the one passing through a fixed area (e.g., the flume’s cross section), but through a gradually shrinking area defined by the narrowing down angle of detection (Figures 11B–D; Table 1). To explain the unchanged feeding rates when the flow becomes stronger, we defined an “Effective Efficiency”- the percent of prey captured off forming the Actual Flux. If the narrowing down of the foraging angle explains the absence of higher feeding rates under stronger flows, we expect the Effective Efficiency to remain constant. In order to test this hypothesis, we used the feeding rates measured by Genin et al. (submitted) for Ps, Dm, and Nm, who measured predation rates in the same flume and under the same experimental setting. Our findings (Figure 11A; Table 1) refute that hypothesis for Nm and Ps, for which the Effective Efficiency decreased or increased, respectively. Dm was the only species in which the Effective Efficiency remained nearly constant in the range of 9-15 cm/s, though it decreased at 21 cm/s.
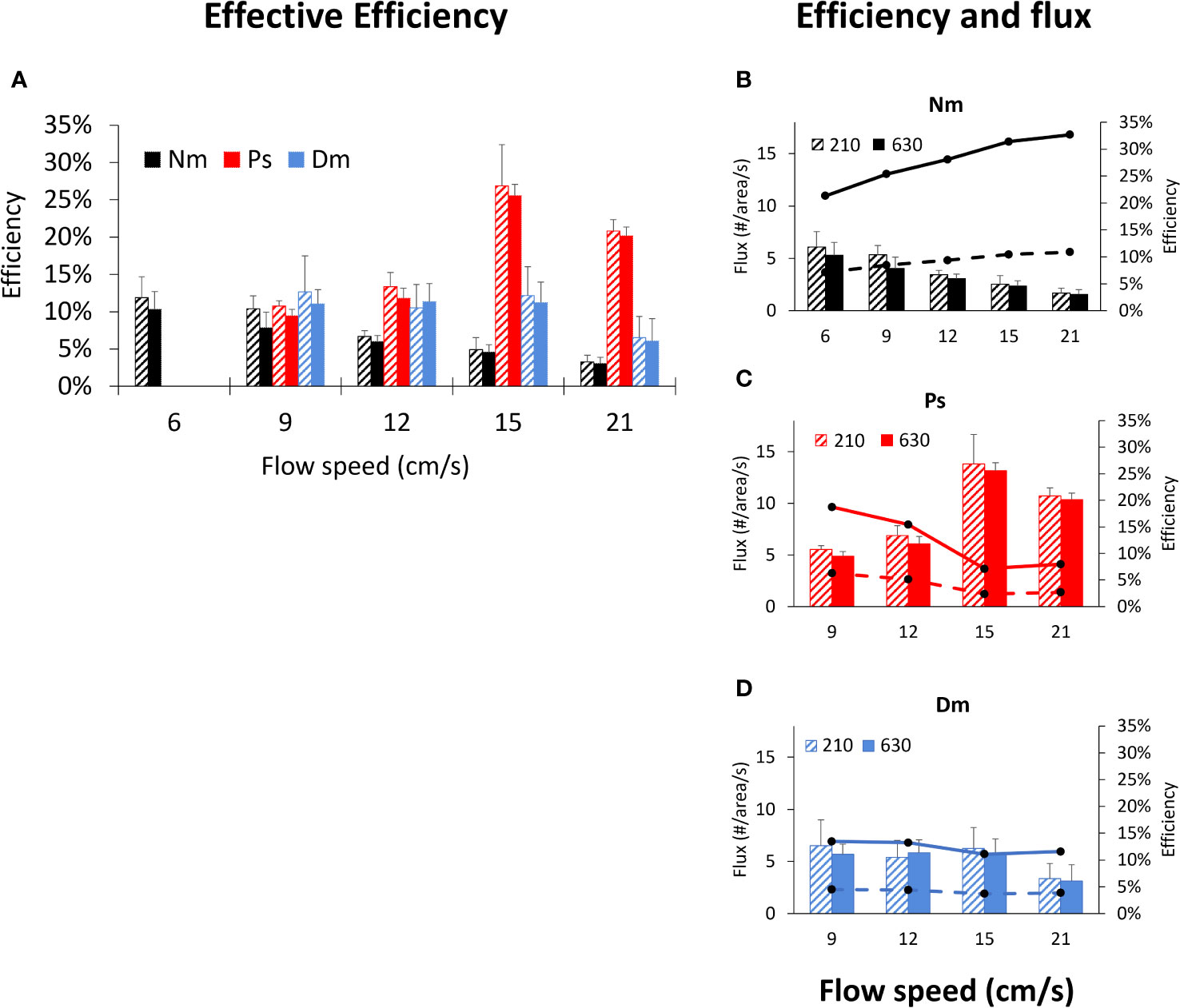
Figure 11 Effective Efficiencies vs. flow speed. (A) - Mean (± SD) Effective Efficiency under different flow speeds for 3 different species (color coded as in Figure 1) under conditions of prey densities of 210 prey m-3 (hatched bars) and 630 prey m-3 (full bars). (B–D): as in (A) with added lines indicating actual prey fluxes under prey densities of 210 prey m-3 (dashed lines) and 630 prey m-3 (full lines). Calculations are based on feeding rates reported by Genin et al. (submitted; see text). The foraging area used to calculate “Effective Efficiencies” and “Actual Fluxes” are taken from our measurements. The distal area of the feeding space, through which potentially-captured prey pass, was calculated as an ellipse in which the longer and shorter axes were twice the maximum horizontal and maximum vertical components of the Reactive Distance, respectively. Since those dimensions were calculated for Dm and Ps at 10, 15, and 20 cm/s (and 5 cm/s for Nm), and since feeding rates were measured by Genin et al. (submitted) at 3, 6, 9, 12, 15, and 21 cm/s, the dimensions measured in this study were linearly interpolated or extrapolated to the flow speeds for which feeding rates are available (Figure 2 in Genin et al., submitted): 9, 12, 15, and 21 cm/s (and 6 cm/s for Nm). The corresponding statistics are reported in Table 1, showing that the effect of flow speed, but not of prey density, was significant and the different species significantly differed one from another.
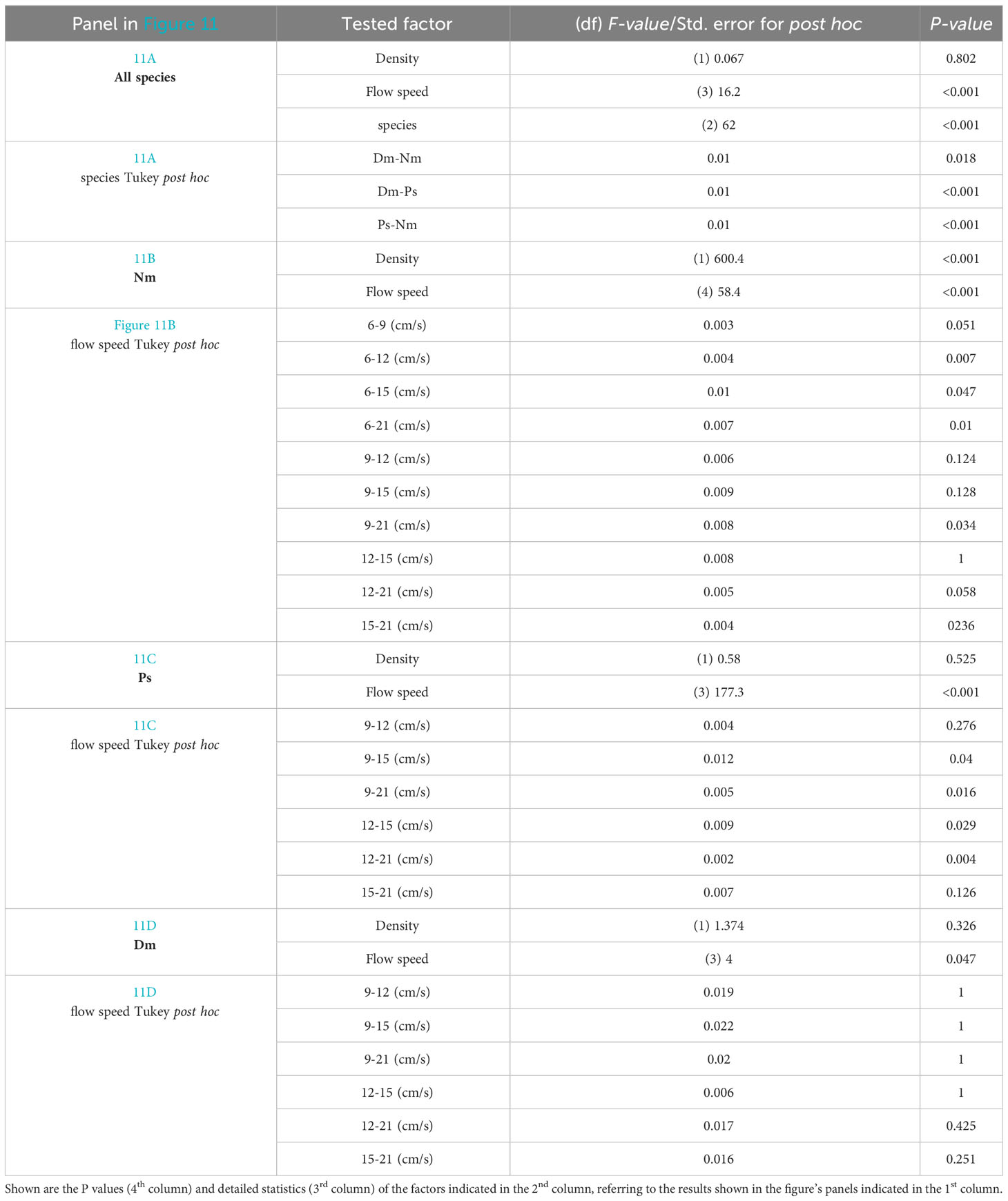
Table 1 Statistics of the trends presented in Figure 11.
Noteworthy is the finding that in Ps, the Effective Efficiency increased despite a decrease in Actual Flux (Figure 11C), indicating that in the narrower foraging space Ps was able to catch relatively more prey. In other words, this species is well-adapted to living at sites exposed to strong currents. Conversely, in Nm, a decreasing Effective Efficiency under stronger flow (Figure 11B), indicated a poor functional response to intensifying flows. Indeed, our extensive survey of exposed coral reefs along the Gulf of Aqaba (unpublished) agree well with those inter-specific differences: Ps, the species that appears well-adapted to strong flows, is extremely abundant at sites exposed to strong currents (up to ~100 cm/s, mean of 40 cm/s; Genin et al., 1994), whereas Nm is totally absent. Both species are common at bays and sheltered sites in the Gulf, where the currents are much weaker (mean of 10 cm/s; Genin and Paldor, 1998).
Among the four species we studied, Dm and Cv use branching corals as shelters, whereas Ps and Nm favor large protruding knolls and complex rocks. Therefore, Dm and Cv usually forage at lower heights above the bottom than the latter ones. However, despite the use of similar shelters by Cv and Dm, the former is found in groups that are ~4 times more crowded than Dm (Supplementary Table 1; Brokovich, 2008). This difference agrees with the occurrence of significantly shorter strike durations, faster striking speeds (relative to Earth and water), and wider Detection Angle in Cv, allowing Cv to maintain higher feeding rates and efficiencies. Indeed, observations reported in Figure 2 of Kiflawi and Genin (1997) for a single Cv and two Dm suggest higher feeding rates in the former species.
Note that the average Effective Efficiencies in the fish we studied ranged 3 to 27%, considerably lower than 100% (Figure 11). Namely, much of the prey passing through the fish’s foraging space is not captured. Therefore, the wider Detection Angles and faster striking speed in Cv may allow this species to maintain relatively higher feeding rates despite crowding. Unfortunately, feeding rates by Cv have not yet been quantified.
Under unchanged strike durations, geometric calculations show that for wider Detection Angles, longer strike distances are expected (Supplementary Figure 4). Indeed, in all species, strike distances under wide Detection Angles were approximately twice as long compared with narrow angles (Figure 7). Moreover, significantly higher swimming speeds were exhibited for wider Detection Angles in all species (Figures 9, 10). While the fish have no control on the angle at which the prey approaches them, they are free to decide which angle to use for the strike, and, in turn, choose the swimming speed needed to reach the intercept point on time. The decision on the combination of angle and speed, where the speed depends on the angle, is chosen out of a wide repertoire of possible values (as indicated by high SD in Figures 3, 4B). Hence, the occurrence of those decisions likely indicates a cognitive ability in those fish.
The broad definition of cognition refers to the way animals acquire information through their senses, process that information, and decide to act on it (Shettleworth, 2001; Bshary and Triki, 2022). Spatial cognition refers to the ability to make such decisions based on environmental information (Poucet, 1993). For example, spatial cognition was demonstrated in goldfish (Carassius auratus), referring to their ability to obtain food in a four-arm maze (Rodriguez et al., 1994) and effective navigation in complex settings (Givon et al., 2022). The suggested occurrence of cognitive behavior in the fish we studied refers to the individual ability to visually sense the location of its drifting prey, to process its movement velocities, and, based on that information, to decide which speed and direction to use in its strikes. Under a null hypothesis where the fish have no cognitive behavior, the fish would always strike the approaching prey at the same speed. For example, a use of the maximum speed should increase the chance of reaching the prey before a neighboring fish does. However, Figure 3B shows a dependency of the strike speed on the ambient current: under stronger currents faster strikes are made. Similarly, under the null hypothesis, one would not expect the striking speed to become faster when the angle to the prey is wider (Figure 9). These observations indicate the occurrence of cognitive decisions among foraging zooplanktivorous fish.
A cognitive ability of zooplanktivorous fish was also shown by McFarland and Levin (2002), demonstrating that the three species of site-attached fishes they studied were able to change their striking strategy based on flow speed. While under weak flows, those fish instantaneously struck the prey, when the flow speed exceeded a threshold of 10-14 cm/s, the fish deferred their strikes until the drifting prey got closer to them. An indication that a similar threshold occurred in 3 of the 4 species we studied (Ps, Dm, and Cv) is shown in Figure 5. A relatively long Reactive Distance was found in all species under a weak flow (10 cm/s), becoming shorter and statistically unchanged when the flow speed exceeded that level.
How long does it take the fish to initiate a strike? That decision must be made during the time elapsed between the moment of prey detection to the time the strike is initiated. Alas, we have no information on the moment of detection. Here we suggest an indirect way to determine the upper limit of the decision time (hereafter: Information Processing Time, or IPT). We assume that a strike is initiated immediately after IPT without delay (for other considerations such as energetic costs). During IPT, the planktonic prey continues to drift toward the fish, thereby gradually closing the gap to the fish. Due to that drift, a shortening of Reactive Distance occurs. For example, let’s consider a flow speed of 10 cm/s, oriented directly onto the fish, a detection distance of 8 cm, and an IPT of 100 msec. Simple calculations indicate that by the end of IPT, the prey had advanced 1 cm, and is found 7 cm up-current of the fish when the strike starts. Note that this is a maximum bound of the predator-prey gap because delays in initiating the strike beyond IPT should further shorten that gap. Indeed, Figure 5 shows a significant decrease in Reactive Distance with increasing flow speed. For unknown reasons, for all species, the decrease of Reactive Distance was greater for the change from 10 to 15 cm/s than from 15 to 20 cm/s. Perhaps the change to flows stronger than 10 cm/s require longer IPT that is associated with a shift to a different mode of strikes (McFarland and Levin, 2002). Our calculations of the upper bound of IPT, referring to the greatest decline of Reactive Distance between 10 and 15 cm/s (Figure 5), indicate 429 msec. This value is of the same order of magnitude as that reported for cognitive-based decisions in birds (Pomeroy and Heppner, 1977) and mammals (Proctor and Brosnan, 2013). Interestingly, also in humans, that decision time is approximately 400 ms (Thorpe et al., 1996). While zooplanktivorous, site-attached fishes provide an appealing way to calculate IPT, studies are still needed in order to further develop this idea.
Why does it take longer for the fish to initiate a strike when the flow speed is faster (Figure 5)? We propose that a likely explanation is the well-known association between flow speed and turbulence, especially over the rough topography of coral reefs (Lowe et al., 2008; Asher and Shavit, 2019). A stronger turbulence means that the zooplankton’s drifting path is more erratic, making it more difficult for the fish to predict the precise location of the intercept point. By deferring the strike initiation until the prey is closer, the fish can improve its ability to correctly predict the intercept point.
Several caveats should be considered. First, the type of prey we used was Artemia nauplii. Unlike copepods, the dominant taxon in the fish’s natural diet (Noda et al., 1992), those nauplii are poor swimmers and lack an escape response (Trager et al., 1994). However, poor swimming and the absence of escape response are unlikely to have a considerable effect of the strike parameters that we measured. Secondly, in nature, the fish we studied live in social groups, whereas in the flume we always used single fish. Our attempts to concurrently use more than one fish in the flume failed due to extreme aggressive interactions among the fish. A third caveat is that our experiments were carried out in a laboratory flume (Supplementary Figure 2), where conditions are different from nature and the fish movements are constrained by the walls. Hence, our results should be evaluated in a comparative sense, comparing one species or one flow speed to another. Extra caution should be applied if an extrapolation of our results natural conditions is sought.
Overall, our flume study presents for the first time some of the key attributes of prey strikes by site-attached coral-reef fishes. In planning their strikes, including the initiation, orientation, and speed of striking, the fish appear to use their cognitive ability to perceive the location and drifting speed of their prey. Decisions are made within a few hundred of milliseconds. Inter-specific differences of strike attributes may explain the corresponding differences in their feeding rates and their preferred habitats, as well as inter-specific differences in group sizes in two of the studied species.
Data availability statement
The raw data supporting the conclusions of this article will be made available by the authors, without undue reservation.
Ethics statement
The animal study was approved by The Hebrew University of Jerusalem, Ethics Committee for Maintenance and Experimentation on Laboratory Animals, Permit # NS-24540-08. The study was conducted in accordance with the local legislation and institutional requirements.
Author contributions
HE: Conceptualization, Data curation, Formal Analysis, Investigation, Methodology, Project administration, Software, Visualization, Writing – original draft, Writing – review & editing. AG: Conceptualization, Data curation, Formal Analysis, Investigation, Methodology, Project administration, Software, Visualization, Writing – original draft, Writing – review & editing.
Funding
The author(s) declare financial support was received for the research, authorship, and/or publication of this article. This study was funded by Israel Science Foundation (ISF) grant #1422-19 to AG.
Acknowledgments
We thank Moti Ohevia for his ingenious assistance with the flume and video cameras and Daniela Genin for thoroughly editing our manuscript. Nachumi Sela professionally assisted our diving operations, both above- and under-water. He, Michal Sela, and Aharon Adam kindly and effectively helped with the collection of fish in the reef. We are indebted to Irena Kolesnikov for her help with diverse undertakings at the lab. We thank the IUI staff for our use of the institutional facilities.
Conflict of interest
The authors declare that the research was conducted in the absence of any commercial or financial relationships that could be construed as a potential conflict of interest.
Publisher’s note
All claims expressed in this article are solely those of the authors and do not necessarily represent those of their affiliated organizations, or those of the publisher, the editors and the reviewers. Any product that may be evaluated in this article, or claim that may be made by its manufacturer, is not guaranteed or endorsed by the publisher.
Supplementary material
The Supplementary Material for this article can be found online at: https://www.frontiersin.org/articles/10.3389/fmars.2023.1327581/full#supplementary-material
Footnotes
- ^ Genin, A., Rickel, S., Zarubin, M., and Kiflawi, M. (submitted)Effects of flow speed and prey density on the rate and efficiency of prey capture in 4 species of zooplanktivorous coral-reef fishes. Submitt. to Front. Mar. Sci.
References
Allen G. R., Randall J. E. (1980). A review of the damselfishes (Teleostei: Pomacentridae) of the red sea. Isr. J. Zool 29, 1–98. doi: 10.1080/00212210.1980.10688486
Asher S., Shavit U. (2019). The effect of water depth and internal geometry on the turbulent flow inside a coral reef. J. Geophys. Res. Ocean. 124, 3508–3522. doi: 10.1029/2018JC014331
Atkinson G., Davison R., Jeukendrup A., Passfield L. (2003). Science and cycling: Current knowledge and future directions for research. J. Sports Sci. 21, 767–787. doi: 10.1080/0264041031000102097
Bellwood D. R., Goatley C. H. R., Bellwood O. (2017). The evolution of fishes and corals on reefs: Form, function and interdependence. Biol. Rev. 92, 878–901. doi: 10.1111/brv.12259
Benayahu Y., Loya Y. (1977). Space partitioning by stony corals soft corals and benthic algae on the coral reefs of the northern Gulf of Eilat (Red Sea). Helgoländer Wissenschaftliche Meeresuntersuchungen 30, 362–382. doi: 10.1007/BF02207848
Biton E., Gildor H. (2011). The general circulation of the Gulf of Aqaba (Gulf of Eilat) revisited: The interplay between the exchange flow through the Straits of Tiran and surface fluxes. J. Geophys. Res. Ocean. 116. doi: 10.1029/2010JC006860
Blake R. W. (2004). Fish functional design and swimming performance. J. Fish Biol. 65, 1193–1222. doi: 10.1111/j.0022-1112.2004.00568.x
Brokovich E. (2001). The community structure and biodiversity of reef fishes at the northern Gulf of Aqaba (Red Sea) with relation to their habitat. Fac. Life Sci. Tel Aviv Univ. Isr. 116.
Brokovich E. (2008). Coral reef fish assemblages in the upper twilight zone (< 65 m). Aqaba-Eilat, improbable Gulf Environ (Jerusalem: Biodivers. Preserv. Magnes Press), 255–266.
Bshary R., Triki Z. (2022). Fish ecology and cognition: insights from studies on wild and wild-caught teleost fishes. Curr. Opin. Behav. Sci. 46. doi: 10.1016/j.cobeha.2022.101174
Cano-Barbacil C., Radinger J., Argudo M., Rubio-Gracia F., Vila-Gispert A., García-Berthou E. (2020). Key factors explaining critical swimming speed in freshwater fish: a review and statistical analysis for Iberian species. Sci. Rep. 10, 1–12. doi: 10.1038/s41598-020-75974-x
China V., Holzman R. (2014). Hydrodynamic starvation in first-feeding larval fishes. Proc. Natl. Acad. Sci. U. S. A. 111, 8083–8088. doi: 10.1073/pnas.1323205111
China V., Levy L., Liberzon A., Elmaliach T., Holzman R. (2017). Hydrodynamic regime determines the feeding success of larval fish through the modulation of strike kinematics. Proc. R. Soc B Biol. Sci. 284. doi: 10.1098/rspb.2017.0235
Clarke R. D., Buskey E. J., Marsden K. C. (2005). Effects of water motion and prey behavior on zooplankton capture by two coral reef fishes. Mar. Biol 146, 1145–1155. doi: 10.1007/s00227-004-1528-y
Clarke R. D., Finelli C. M., Buskey E. J. (2009). Water flow controls distribution and feeding behavior of two co-occurring coral reef fishes: II. Laboratory experiments. Coral Reefs 28, 475–488. doi: 10.1007/s00338-009-0479-7
Confer J. L., Blades P. I. (1975). Omnivorous zooplankton and planktivorous fish. Limnol. Oceanogr 20 (4), 571–579. doi: 10.4319/lo.1975.20.4.0571
Coughlin D. J., Strickler J. R. (1990). Zooplankton capture by a coral reef fish: an adaptive response to evasive prey. Environ. Biol. Fishes 29, 35–42. doi: 10.1007/BF00000566
Engel A., Reuben Y., Kolesnikov I., Churilov D., Nathan R., Genin A. (2021). In situ three-dimensional video tracking of tagged individuals within site-attached social groups of coral-reef fish. Limnol. Oceanogr. Methods 19, 579–588. doi: 10.1002/lom3.10444
Erez J. (1990). On the importance of food sources in coral-reef ecosystems. Ecosyst. World 25, 411–418.
Finelli C. M., Clarke R. D., Robinson H. E., Buskey E. J. (2009). Water flow controls distribution and feeding behavior of two co-occurring coral reef fishes: I. Field measurements. Coral Reefs 48, 461–473. doi: 10.1007/s00338-009-0481-0
Fishelson L. (1971). Ecology and distribution of the benthic fauna in the shallow waters of the Red Sea. Mar. Biol. 10, 113–133.
Fulton C. J. (2007). Swimming speed performance in coral reef fishes: Field validations reveal distinct functional groups. Coral Reefs 26, 217–228. doi: 10.1007/s00338-007-0195-0
Fulton C. J., Bellwood D. R., Wainwright P. C. (2005). Wave energy and swimming performance shape coral reef fish assemblages. Proc. R. Soc B Biol. Sci. 272, 827–832. doi: 10.1098/rspb.2004.3029
Fulton C. J., Johansen J. L., Steffensen J. F. (2013). Energetic extremes in aquatic locomotion by coral reef fishes. PloS One 8. doi: 10.1371/journal.pone.0054033
Genin A., Karp L., Miroz A. (1994). Effects of flow on competitive superiority in scleractinian corals. Limnol. Oceanogr. 39, 913–924. doi: 10.4319/lo.1994.39.4.0913
Genin A., Monismith S. G., Reidenbach M. A., Yahel G., Koseff J. R. (2009). Intense benthic grazing of phytoplankton in a coral reef. Limnol. Oceanogr. 54, 938–951. doi: 10.4319/lo.2009.54.3.0938
Genin A., Paldor N. (1998). Changes in the circulation and current spectrum near the tip of the narrow, seasonally mixed Gulf of Elat. Isr. J. Earth Sci. 47, 87–92.
Givon S., Samina M., Ben-Shahar O., Segev R. (2022). From fish out of water to new insights on navigation mechanisms in animals. Behav. Brain Res. 419, 113711. doi: 10.1016/j.bbr.2021.113711
Gordon M. S., Hove J. R., Webb P. W., Weihs D. (2000). Boxfishes as unusually well-controlled autonomous underwater vehicles. Physiol. Biochem. Zool. 73, 663–671. doi: 10.1086/318098
Gordon M. S., Plaut I., Kim D. (1996). How puffers (Teleostei: Tetraodontidae) swim. J. Fish Biol. 49, 319–328. doi: 10.1111/J.1095-8649.1996.TB00026.X
Hamner W. M., Jones M. S., Carleton J. H., Hauri I. R., Williams D. M. (1988). Zooplankton, planktivorous fish, and water currents on a windward reef face: Great Barrier Reef, Australia. Bull. Mar. Sci. 42, 459–479.
Heatwole S. J., Fulton C. J. (2013). Behavioural flexibility in reef fishes responding to a rapidly changing wave environment. Mar. Biol. 160, 677–689. doi: 10.1007/s00227-012-2123-2
Hobson E., Chess J. (1978). Trophic relationships among fishes and plankton in the lagoon at Enewetak Atoll, Marshall Islands. Fish Bull. 76, 133–153.
Holzman R., China V., Yaniv S., Zilka M. (2015). “Hydrodynamic constraints of suction feeding in low reynolds numbers, and the critical period of larval fishes,” in Integrative and Comparative Biology (Oxford University Press), 48–61. doi: 10.1093/icb/icv030
Holzman R., Collar D. C., Mehta R. S., Wainwright P. C. (2012). An integrative modeling approach to elucidate suction-feeding performance. J. Exp. Biol. 215, 1–13. doi: 10.1242/jeb.057851
Ishikawa K., Wu H., Mitarai S., Genin A. (2022). Effects of prey density and flow speed on plankton feeding by garden eels: A flume study. J. Exp. Biol. 225, 1–10. doi: 10.1242/jeb.243655
Jacobs C. N., Holzman R. (2018). Conserved spatio-temporal patterns of suction-feeding flows across aquatic vertebrates: A comparative flow visualization study. J. Exp. Biol 221 (7). doi: 10.1242/jeb.174912
Karpestam B., Gustafsson J., Shashar N., Katzir G., Kröger R. H. H. (2007). Multifocal lenses in coral reef fishes. J. Exp. Biol. 210, 2923–2931. doi: 10.1242/jeb.002956
Khrizman A., Ribak G., Churilov D., Kolesnikov I., Genin A. (2018). Life in the flow: Unique adaptations for feeding on drifting zooplankton in garden eels. J. Exp. Biol 221 (16). doi: 10.1242/jeb.179523
Kiflawi M., Genin A. (1997). Prey flux manipulation and the feeding rates of reef-dwelling planktivorous fish. Ecology 78, 1062–1077. doi: 10.1890/0012-9658(1997)078[1062:PFMATF]2.0.CO;2
Krebs J. M., Turingan R. G. (2003). Intraspecific variation in gape-prey size relationships and feeding 561 success during early ontogeny in red drum, Sciaenops ocellatus.
Lauder G. V., Flammang B., Alben S. (2012). Passive robotic models of propulsion by the bodies and caudal fins of fish. Integr. Comp. Biol. 52, 576–587. doi: 10.1093/icb/ics096
Lieske E., Myers R. F. (1994). Coral reef fishes: caribbean, indian ocean and pacific ocean including 565 the red sea.
Lowe R. J., Shavit U., Falter J. L., Koseff J. R., Monismith S. G. (2008). Modeling flow in coral communities with and without waves: A synthesis of porous media and canopy flow approaches. Limnol. Oceanogr. 53, 2668–2680. doi: 10.4319/lo.2008.53.6.2668
Manatunge J., Asaeda T. (1998). Optimal foraging as the criteria of prey selection by two centrarchid fishes. Hydrobiologia 391, 223–240.
McFarland W., Levin S. (2002). Modelling the effects of current on prey acquisition in planktivorous fishes. Mar. Freshw. Behav. Physiol. 35, 69–85. doi: 10.1080/10236240290025626
Morais R. A., Bellwood D. R. (2019). Pelagic subsidies underpin fish productivity on a degraded coral reef. Curr. Biol. 29, 1521–1527. doi: 10.1016/j.cub.2019.03.044
Myers R. (1989) Micronesian Reef Fishes: A Practical Guide to the Identification of the Coral Reef Fishes of the Tropical Central and Western Pacific. Available at: https://api.semanticscholar.org/CorpusID:140187433.
Noda M., Kawabata K., Gushima K., Kakuda S. (1992). Importance of zooplankton patches in foraging ecology of the planktivorous reef fish Chromis chrysurus (Pomacentridae) at Kuchinoerabu Island, Japan. Mar. Ecol. Prog. Ser. 87, 251–263. doi: 10.3354/meps087251
Nonacs P., Smith P. E., Bouskila A., Luttbeg B. (1994). Modeling the behavior of the northern anchovy, Engraulis mordax, as a schooling predator exploiting patchy prey. Deep. Res. Part II 41, 147–169. doi: 10.1016/0967-0645(94)90065-5
O’Brien W. J., Barfield M., Sigler K. (2001). The functional response of drift-feeding Arctic grayling: the effects of prey density, water velocity, and location efficiency. Can. J. Fish. Aquat. Sci. 58, 1957–1963. doi: 10.1139/cjfas-58-10-1957
Olsson K. H., Martin C. H., Holzman R. (2020). Erratum: Hydrodynamic simulations of the performance landscape for suctioneeding fishes reveal multiple peaks for different prey types (Integrative and Comparative Biology DOI: 10.1093/icb/icaa021). Integr. Comp. Biol 60 (5), 1251–1267. doi: 10.1093/icb/icaa122
Palstra A. P., Mes D., Kusters K., Roques J. A. C., Flik G., Kloet K., et al. (2015). Forced sustained swimming exercise at optimal speed enhances growth of juvenile yellowtail kingfish (Seriola lalandi). Front. Physiol. 6. doi: 10.3389/fphys.2014.00506
Piccolo J. J., Hughes N. F., Bryant M. D. (2008). Water velocity influences prey detection and capture by drift-feeding juvenile coho salmon (Oncorhynchus kisutch) and steelhead (Oncorhynchus mykiss irideus). Can. J. Fish. Aquat. Sci. 65, 266–275. doi: 10.1139/F07-172
Pomeroy H., Heppner F. (1977). Laboratory determination of startle reaction time of the starling (Sturnus vulgaris). Anim. Behav. 25, 720–725. doi: 10.1016/0003-3472(77)90121-X
Poucet B. (1993). Spatial cognitive maps in animals: new hypotheses on their structure and neural mechanisms. Psychol. Rev. 100, 163–182. doi: 10.1037/0033-295x.100.2.163
Proctor D., Brosnan S. F. (2013). Visual Processing Speed in Capuchin Monkeys (Cebus apella) and Rhesus Macaques (Macaca mulatta). Int. J. Comp. Psychol. 26, 166–175. doi: 10.46867/ijcp.2013.26.02.01
Reidenbach M. A., Monismith S. G., Koseff J. R., Yahel G., Genin A. (2006). Boundary layer turbulence and flow structure over a fringing coral reef. Limnol. Oceanogr. 51, 1956–1968. doi: 10.4319/lo.2006.51.5.1956
Rickel S., Genin A. (2005). Twilight transitions in coral reef fish: The input of light-induced changes in foraging behaviour. Anim. Behav. 70, 133–144. doi: 10.1016/J.ANBEHAV.2004.10.014
Rodriguez F., Duran E., Vargas J. P., Torres B., Salas C. (1994). Performance of goldfish trained in allocentric and egocentric maze procedures suggests the presence of a cognitive mapping system in fishes. Anim. Learn. Behav. 22, 409–420. doi: 10.3758/BF03209160
Sambilay J. V. (1990). Interrelationships between swimming speed, caudal fin aspect ratio and body length of fishes. Fishbyte 8, 16–20. Available at: https://hdl.handle.net/20.500.12348/3181.
Schakmann M., Korsmeyer K. E. (2023). Fish swimming mode and body morphology affect the energetics of swimming in a wave-surge water flow. J. Exp. Biol. 226. doi: 10.1242/jeb.244739
Schakmann M., Steffensen J. F., Bushnell P. G., Korsmeyer K. E. (2020). Swimming in unsteady water flows: Is turning in a changing flow an energetically expensive endeavor for fish? J. Exp. Biol. 223. doi: 10.1242/jeb.212795
Shaked Y., Genin A. (2022). The Israel National Monitoring Program in the Northern Gulf of Aqaba – scientific report. Isr. Minist. Environ. Prot. Available at: https://iui-eilat.huji.ac.il/uploaded/NMP/reports/NMP%20Report%202022.pdf.
Shettleworth S. J. (2001). Animal cognition and animal behaviour. Anim. Behav. 61, 277–286. doi: 10.1006/anbe.2000.1606
Siqueira A. C., Morais R. A., Bellwood D. R., Cowman P. F. (2020). Trophic innovations fuel reef fish diversification. Nat. Commun. 11, 1–11. doi: 10.1038/s41467-020-16498-w
Theriault D. H., Fuller N. W., Jackson B. E., Bluhm E., Evangelista D., Wu Z., et al. (2014). A protocol and calibration method for accurate multi-camera field videography. J. Exp. Biol. 217, 1843–1848. doi: 10.1242/jeb.100529
Thorpe S., Fize D., Mralot C. (1996). Speed of processing in the human visual system. Nature 381, 520–522. doi: 10.1038/381520a0
Trager G., Achituv Y., Genin A. (1994). Effects of prey escape ability, flow speed, and predator feeding mode on zooplankton capture by barnacles. Mar. Biol. 120, 251–259. doi: 10.1007/BF00349685
Turingan R. G., Beck J. L., Krebs J. M., Licamele J. D. (2005). “Development of feeding mechanics in marine fish larvae and the swimming behavior of zooplankton prey: implications for rearing marine fishes,” in Copepods in Aquaculture (Copepods in Aquaculture, Hoboken, New Jersey: Blackwell Publishing Professional), 119–132. doi: 10.1002/9780470277522.ch10
Videler J. J., Wardle C. S. (1991). Fish swimming stride by stride: speed limits and endurance. Rev. Fish Biol. Fish. 1, 23–40. doi: 10.1007/BF00042660
Vinyard G. L. (1980). Differential Prey Vulnerability and Predator Selectivity: Effects of Evasive Prey on Bluegill ( Lepomis macrochirus ) and Pumpkinseed ( L . gibhosus ) Predation. Can. J. Fish. Aquat. Sci 37 (12), 2294–2299. doi: 10.1139/f80-276
Wainwright P., Carroll A. M., Collar D. C., Day S. W., Higham T. E., Holzman R. A. (2007). Suction feeding mechanics, performance, and diversity in fishes. Integr. Comp. Biol 47 (1), 96–106. doi: 10.1093/icb/icm032
Walker J. A., Alfaro M. E., Noble M. M., Fulton C. J. (2013). Body fineness ratio as a predictor of maximum prolonged-swimming speed in coral reef fishes. PloS One 8, 1–13. doi: 10.1371/journal.pone.0075422
Walker J. A., Westneat M. W. (2002). Performance limits of labriform propulsion and correlates with fin shape and motion. J. Exp. Biol. 205, 177–187. doi: 10.1242/jeb.205.2.177
Wardle C. S., Videler J. J., Altringham J. D. (1995). Tuning in to fish swimming waves: body form, swimming mode and muscle function. J. Exp. Biol. 198, 1629–1636. doi: 10.1242/jeb.198.8.1629
Webb P. W. (1984). Form and function in fish swimming. Sci. Am. 251 (1), 72–83. doi: 10.1038/scientificamerican0784-72
Webb P., Weihs D. (1983) Fish biomechanics. Available at: https://cir.nii.ac.jp/crid/1130000797018149888. (Accessed October 22, 2023).
Werner E. E. (1977). Species packing and niche complementarity in three sunfishes. Am. Nat. 111, 553–578. doi: 10.1086/283184
Wyatt A. S. J., Lowe R. J., Humphries S., Waite A. M. (2010). Particulate nutrient fluxes over a fringing coral reef: Relevant scales of phytoplankton Production and mechanisms of supply. Mar. Ecol. Prog. Ser 405, 113–130. doi: 10.3354/meps08508
Wyatt A. S. J., Lowe R. J., Humphries S., Waite A. M. (2013). Particulate nutrient fluxes over a fringing coral reef: Source-sink dynamics inferred from carbon to nitrogen ratios and stable isotopes. Limnol. Oceanogr. 58, 409–427. doi: 10.4319/lo.2013.58.1.0409
Xin Z. Q., Wu C. J. (2013). Shape optimization of the caudal fin of the three-dimensional self-propelled swimming fish. Sci. China Physics Mech. Astron. 56, 328–339. doi: 10.1007/s11433-013-4994-8
Keywords: coral-reef, planktivores, foraging, movement, Red Sea
Citation: Ella H and Genin A (2024) Capture of zooplankton by site-attached fish: striking dynamics under different flow speeds and prey paths. Front. Mar. Sci. 10:1327581. doi: 10.3389/fmars.2023.1327581
Received: 25 October 2023; Accepted: 11 December 2023;
Published: 04 January 2024.
Edited by:
Stuart Humphries, University of Lincoln, United KingdomReviewed by:
Paul Carl Sikkel, University of Miami, United StatesRoy Harpaz, Harvard University, United States
Copyright © 2024 Ella and Genin. This is an open-access article distributed under the terms of the Creative Commons Attribution License (CC BY). The use, distribution or reproduction in other forums is permitted, provided the original author(s) and the copyright owner(s) are credited and that the original publication in this journal is cited, in accordance with accepted academic practice. No use, distribution or reproduction is permitted which does not comply with these terms.
*Correspondence: Hadar Ella, Hadar.ella@mail.huji.ac.il