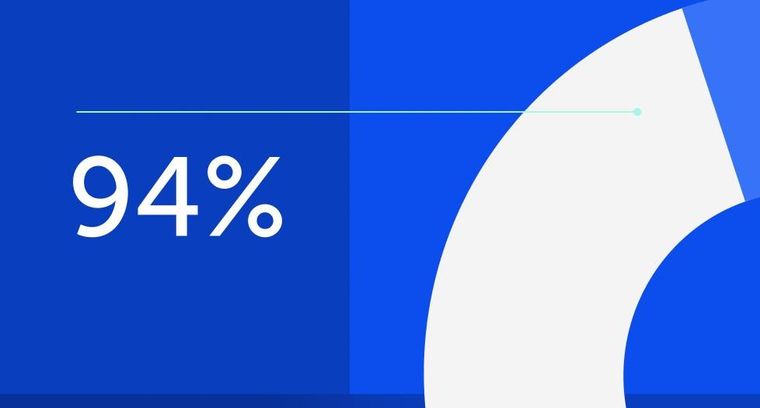
94% of researchers rate our articles as excellent or good
Learn more about the work of our research integrity team to safeguard the quality of each article we publish.
Find out more
ORIGINAL RESEARCH article
Front. Mar. Sci., 26 January 2024
Sec. Marine Fisheries, Aquaculture and Living Resources
Volume 10 - 2023 | https://doi.org/10.3389/fmars.2023.1305192
This article is part of the Research TopicFunctional Feed Additives: Current TrendsView all 8 articles
Our study investigated the effects of dietary arginine supplementation on the growth performance, antioxidant capacity, intestinal digestive enzyme activity, muscle transcriptome, and gut health of Chinese perch (Siniperca chuatsi). Four isonitrogenous and isolipidic diets (50% crude protein, 11% crude lipid) with graded levels (2.61%, 2.99%, 3.37%, and 3.82%) of arginine were formulated and processed. A total of 360 Chinese perch (approximately 45–46 g) were randomly divided into four groups with triplicates of 30 fish per cage in each group. Our results revealed that although there was no significant improvement in the growth performance of Chinese perch, their antioxidant capacity and nutritional metabolism were significantly improved. Compared to the control group (without arginine supplementation, 2.61% group), feeding graded arginine levels could significantly (P < 0.05) decrease serum glucose and malondialdehyde (MDA) contents, as well as increase total superoxide dismutase (TSOD) activity. Fish fed a 3.37% arginine diet had the highest TSOD activity in the serum and liver. The intestinal morphological structure was clearer and more uniform in the 2.99% and 3.37% arginine groups. Fish fed with 3.82% arginine had significantly decreased (P < 0.05) muscle valine, glutamate, tyrosine, and total non-essential amino acid contents, with decreased (P < 0.05) intestinal amylase activity. Transcriptome analysis showed that graded arginine levels significantly (P < 0.05) influenced muscle gene expression involved in the Kyoto Encyclopedia of Genes and Genomes (KEGG) pathways of energy metabolism (amino acid, glucose, fatty acid), signaling pathways (mTOR, PI3K-Akt, MAPK, foxO, and insulin pathway), etc. The microbial community structure did not change significantly. Mycoplasma showed the highest abundance in the control group, and Cetobacterium had the highest abundance in the dietary arginine supplementation groups. Network analysis showed that the network complexity of the control group was higher than those of arginine groups. Our finding would help advance the field of arginine nutrition and guide the development of future fish feeds. Based on antioxidant and intestinal health indicators, the optimal dietary arginine requirement for Chinese perch was 2.99%–3.37% of the dry diet (6.08%–6.79% of dietary protein).
Arginine, an amino acid primarily used in protein biosynthesis, is an indispensable amino acid for fish because of their limited ability to endogenously biosynthesize arginine (Andersen et al., 2016). The ability of arginine to impact nutrient metabolism, influence insulin release, modulate immune and antioxidant response, and improve disease resistance in fish has been well demonstrated (Wang Q. et al., 2021). Arginine could stimulate the secretion of insulin-like growth factor 1 (IGF-1) and activate the TOR signaling pathway to promote protein synthesis for the fish body (Levine et al., 2006; Wei et al., 2021). Studies have shown that 3.61% dietary arginine would significantly improve the weight gain and specific growth rate of rice-field eel (Monopterus albus) (Huo et al., 2023). Furthermore, 2.52% dietary arginine would significantly increase the gene expression of Nrf2 in largemouth bass (Micropterus salmoides) (Yu et al., 2023). Like other animals, lack of arginine may interfere with the fish body normal function of riboflavin, which is not conducive to the synthesis of heme and niacin, as well as decrease resistance against pathogen challenges (Huo et al., 2023). At present, the requirements for dietary arginine have been reported for several fish species, including largemouth bass (Yu et al., 2023), hybrid snakehead (Channa maculata ♀ × Channa argus) (Li et al., 2022), spotted seabass (Lateolabrax maculatus) (Cheng et al., 2021), tiger puffer (Takifugu rubripes) (Wei et al., 2021), and rainbow trout (Oncorhynchus mykiss) (Wang et al., 2022). Arginine function may vary with dose, fish species, fish size, fish health status, and environmental conditions (Azeredo et al., 2015; Hoseini et al., 2020). Therefore, the optimum arginine supplementation level and its physiological function in formulated diets should be carefully evaluated for specific fish species, especially carnivorous fish.
Chinese perch (Siniperca chuatsi) is a temperate perch from the order Perciformes and the genus Siniperca. As a highly valued carnivorous fish, China’s S. chuatsi aquaculture output increased from 281,500 tons in 2012 to 374,000 tons in 2021 (CFSY (China Fishery Statistical Yearbook), 2021), with an annual output value of more than 20 billion yuan (Peng et al., 2022). This shows the increasing importance of Chinese perch in China’s aquaculture. In recent years, the requirements and roles of several dietary functional nutrients for Chinese perch were investigated, including exogenous probiotics (Feng et al., 2021; Zhang et al., 2022a), host probiotics (Ji et al., 2023), bile acids (Zhang et al., 2022b), pyridoxine (Xie et al., 2023), zinc (Peng et al., 2023), and feed attractants (Peng et al., 2022). However, the influence of arginine on the physiological function in Chinese perch had not been explored hitherto. To close this gap, this study evaluated the influence of dietary arginine on Chinese perch based on growth performance, antioxidant capacity, intestinal digestive enzyme activity, and gut health. In addition, transcriptome analysis was conducted to examine the influence of arginine on muscle function in Chinese perch. This study would contribute to an in-depth understanding of arginine’s physiological function in Chinese perch.
The experiment was conducted in pond cages with dimensions of 1.0 m × 1.0 m × 2 m with a net cover. At the beginning of the experiment, the acclimated Chinese perch were starved for 24 h, and the healthy-looking ones with an initial weight of 45–46 g were distributed into 12 cages at 30 fish per cage. The stocked cages were then randomly assigned to the test diets in triplicate. Four isonitrogenous and isolipidic diets (50% crude protein, 11% crude lipid) were formulated with incremental levels of arginine (L-arginine hydrochloride, purity ≥99%, purchased from Hebei Huayang Group Co., Ltd.). The control group was fed the basal diet without arginine supplementation, and the other three treatment groups were supplemented with 0.5%, 1%, and 1.5% arginine, respectively. The analyzed arginine supplementation levels in the diets were 2.61% (the control group), 2.99%, 3.37%, and 3.82%, respectively. The formulation and proximate composition of the experimental diets are shown in Table 1, and the amino acid composition is shown in Table 2.
The feeding period lasted for 56 days, with the fish fed to apparent satiation twice a day (6:00 and 18:00). Water quality was tested regularly: the temperature ranged 26–32°C, ammonia nitrogen was less than 0.5 mg/L, and the pH ranged 7.5–8.5. The water was continuously aerated to keep dissolved oxygen at 7.0–9.3 mg/L.
After the feeding period, the fish were starved for 24 h and then anesthetized with MS222 for weighing and counting. A total of 12 fish were randomly taken from each tank to measure the individual body length and weight. Blood was collected from the tail vein, and the fish were dissected for liver, intestine, and muscle samples. Intestines from six fish were put in sterilized EP tubes and stored at −80°C for analysis of enzyme activities. Intestines from three fish were placed in a cryopreservation tube filled with Bonn reagent in advance and stored in a 4°C refrigerator for intestinal histology observation. Muscle samples were obtained for amino acid analysis. The remaining intestine, liver, and muscle samples were stored at −80°C for further analysis. The percentage weight gain (WG), specific growth rate (SGR), feed conversion ratio (FCR), feed intake (FI), survival rate (SR), condition factor (CF), hepatosomatic index (HSI), and viscerosomatic index (VSI) were determined as follows:
WG (%) = 100 × (final weight − initial weight)/initial weight
SGR (%/d) = 100 × (Ln final weight − Ln initial weight)/test days
FCR = dry feed consumed/(final weight − initial weight)
FI (g/100 g/days) = dry feed consumed/[(final fish weight + initial fish weight)/2]/feeding days
SR (%) = final fish number/initial fish number × 100
CF (g/cm3) = 100 × weight/body length3
HSI = 100 × liver weight/body weight
VSI = 100 × visceral weight/weight.
The nutrition compositions of the experimental diets were determined according to AOAC (Association of Official Analytical Chemists) (1995). The muscle and experimental diet amino acid compositions were determined using the high-performance liquid chromatography (HPLC) method described by a previous study (Wang et al., 2015). Samples were digested with 6 M HCl for 22 h, and precolumn derivatization was performed using HPLC (Agilent 1090 system, Palo Alto, CA). Serum glucose was determined with an automatic biochemistry analyzer (Hitachi 7600-110 Ltd., Japan). Total superoxide dismutase (TSOD) and malondialdehyde (MDA) in serum, liver, and intestines, as well as digestive enzyme activities (trypsin, lipase, and amylase), were measured using diagnostic reagent kits (Nanjing Jiancheng Bioengineering Institute, Jiangsu, China) according to the manufacturer’s instructions.
After removing the midgut sample from Bouin’s solution, it was subjected to ethanol gradient dehydration, xylene transparency, and paraffin embedding, and the sample tissue was sliced using a slicing machine to a thickness of 5 μm. Hematoxylin–eosin (HE) staining was used to prepare intestinal tissue sections. The slices were observed under a light microscope, using an imaging microscope or the CaseViewer 2.2 software to capture maps of different typical lesions.
Total RNA was extracted using TRIzol® reagents (Invitrogen, Waltham, MA, USA), and genomic DNA was removed using DNase I (Takara). RNA quality and quantity were determined using a 2100 Bioanalyzer (Agilent) and an ND 2000 (NanoDrop Technologies), respectively. Subsequently, messenger RNA (mRNA) was isolated and fragmented for complementary DNA (cDNA) synthesis. A sequencing library was constructed and sequenced by Web Shenzhen Huada Gene Technology Co., Ltd with the Illumina HiSeq PE 2X150bp read length.
After trimming the raw paired-end reads, the cleaned data were de novo assembled using Trinity before proteins were identified for annotation of functions. The fragments per kilobase of exon per million mapped reads (FPKM) method was used to calculate the expression value of each transcript, and the RSEM software package was used for quantification of isoform abundances and genes. The threshold for gene expression significance was set at P < 0.05 and log2(fold change).
PrimeScript RT Reagent Kit (Takara, Japan) was used to reverse-transcribe 1 μg RNA into cDNA following the manufacturer’s instruction, and eight related genes were selected for quantitative PCR (qPCR) validation. The qPCR was performed with a Bio-Rad CFX-96 real-time PCR system, using a total volume of 20 μL containing SYBR Green Real-time PCR Master Mix (10 μL), primers (0.2 μL, 10 μm), diluted cDNA template (1 μL), and ultrapure water (8.6 μL). The PCR program involved 95°C for 30 s, 40 cycles of 95°C for 5 s, and 60°C for 30 s. The comparative Ct method was used to calculate the relative expression levels of target genes with β-actin as the reference gene. All reactions were performed in triplicate, and the results are presented as mean ± SD.
PowerSoil DNA Kit (Mo Bio Laboratories, Carlsbad, CA) was used to extract DNA from the fish gut before using a NanoDrop photometer (Thermo Fisher Scientific, USA) for measuring the DNA quality and concentration. The V4 region was targeted with primers 515F and 806R and sequenced with an Illumina MiSeq (Magigene, China). After the raw data were cleaned with Fastp (version 0.14.1), the clean reads were clustered as amplicon sequence variants (ASVs) using Divisive Amplicon Denoising Algorithm 2 (DADA2), and ASVs fewer than 2 were filtered (Hu et al., 2021).
IBM® SPSS (version 26.0, Chicago, IL, USA) was used for statistical analysis. All the quantitative data are shown as mean ± standard deviation (SD) (n = 3). The data were tested for normality and homoscedasticity before one-way ANOVA was used to determine statistical significance (P < 0.05) using Tukey’s multiple range test, and a Kruskal–Wallis test was performed in the absence of normality. A co-occurrence network was constructed to determine potential microbial interaction using the sparse correlations for compositional (SparCC) method (Feng et al., 2019). The nodes indicated each ASV, and the edge indicated the correlations. The topological indexes for the constructed co-occurrence network, including the average degree (avgK), average path distance (GD), and geodesic efficiency (E), were calculated by the R package “ggClusterNet”.
Table 3 shows the effect of dietary arginine levels on the growth performance of S. chuatsi. Although the FBW, WG, SGR, HSI, VSI, and CF increased initially and then decreased with increasing arginine supplementation levels, no significant difference was observed (P > 0.05). There was no significant variation in FCR, FI, and SR among all groups (P > 0.05); the highest SR (96.67%) occurred with 3.37% dietary arginine levels.
Table 3 Effect of dietary arginine levels on the growth performance of Chinese perch (Siniperca chuatsi).
Dietary arginine levels had no significant effect on intestinal trypsin and lipase activities (P > 0.05) (Table 4). With dietary arginine levels increased, the activity of intestinal amylase shows a decreasing trend; fish fed a diet with 3.82% arginine had the lowest amylase activity, which was significantly lower than that of the control group (P < 0.05).
Table 4 Effect of dietary arginine levels on digestive enzyme activities of Chinese perch (Siniperca chuatsi).
Table 5 shows the effect of dietary arginine levels on antioxidant enzyme capacity and serum glucose content in S. chuatsi. Dietary arginine levels did significantly influence TSOD activity in the serum, liver, and intestines of S. chuatsi (P < 0.05); fish fed a 3.37% arginine diet had the highest TSOD activity in the serum and liver, which was significantly higher than those of the control group and 3.82% arginine group (P < 0.05). The MDA content was also significantly affected by the dietary arginine levels; fish fed a 3.37% arginine diet had the lowest liver MDA content (P < 0.05).
Table 5 Effect of dietary arginine levels on antioxidant enzyme activities and serum glucose of Chinese perch (Siniperca chuatsi).
Dietary arginine levels did significantly influence the serum glucose content; the serum glucose content decreased significantly with the increase of dietary arginine levels, and that of the control group was significantly higher than those of the other groups (P < 0.05).
As shown in Figure 1, there were no significant pathological changes in the structure of the midgut tissue of each group, with clear mucosal folds, mucosal layer, submucosal layer, muscular layer, and serosal layer. Compared with the control group, the density of intestinal villi increased, and the morphological structure was clearer and more uniform in the 2.99% and 3.37% arginine groups.
Figure 1 Intestinal morphology of Chinese perch fed diets containing different levels of arginine for 56 days: (A) 2.61%; (B) 2.99%; (C) 3.37%; (D) 3.82%. SL, serosal layer; MS, muscular layer; ML/V, mucosal layer/villi; MF, mucosal folds (HE staining; original magnification ×40).
Table 6 shows the effect of dietary arginine levels on amino acid contents in the muscle of Chinese perch. The muscle arginine content was significantly increased by dietary arginine supplementation (P < 0.05). Fish fed a diet with a 3.82% arginine concentration had significantly lower muscle valine, glutamate, tyrosine, and total non-essential amino acid contents than the control group (P < 0.05).
Table 6 Effect of dietary arginine levels on muscle amino acid contents of Chinese perch (Siniperca chuatsi).
The data have been uploaded to NCBI (https://www.ncbi.nlm.nih.gov) (No. RJNA1029502). As shown in Table 7, four cDNA libraries were constructed. In this experiment, approximately 92.08%–92.86% of the reads were mapped onto the reference genome. The percent of sequenced bases with Q phred > 30 was higher than 90.63%, and that with Q phred > 20 was higher than 96.05%. These showed that good-quality sequence data were obtained for the bioinformatics analysis.
As shown in Table 8, compared with the control group, 140 genes were upregulated and 148 genes were downregulated in the 2.99% arginine group. A total of 135 genes were upregulated and 114 genes were downregulated in the 3.37% arginine group. A total of 421 genes were upregulated and 440 genes were downregulated in the 3.82% arginine group.
Figure 2 shows that biological processes, including metabolic processes, growth, localization, locomotion, multicellular organismal processes, immune system processes, and response to stimulus, were significantly influenced by arginine supplementation (P < 0.05). Cellular components, including cellular anatomical entities and protein-containing complexes, were significantly influenced by dietary arginine supplementation (P < 0.05). Molecular functions, including ATP-dependent activity, binding, catalytic activity, cytoskeletal motor activity, molecular carrier activity, molecular transducer activity, structural molecular activity, and transporter activity, were significantly influenced by dietary arginine supplementation (P < 0.05).
As shown in Supplementary Material 1, compared with the control group, differentially expressed genes were clustered (P < 0.05) in the Kyoto Encyclopedia of Genes and Genomes (KEGG) pathways of glycine, serine, and threonine metabolism; one carbon pool by folate; biosynthesis of unsaturated fatty acids; biosynthesis of nucleotide sugars; cell adhesion molecules; renin–angiotensin system; circadian rhythm; and hippo signaling pathway in the 2.99% arginine group. Feeding fish with 3.37% arginine significantly (P < 0.05) affected genes involved in hematopoietic cell lineage, the PI3K-Akt signaling pathway, extracellular matrix (ECM)–receptor interaction, regulation of the actin cytoskeleton and MAPK signaling pathway, etc. Feeding fish with 3.82% arginine significantly (P < 0.05) affected genes involved in the PI3K-Akt signaling pathway, the MAPK signaling pathway, tight junctions, the Rap1 signaling pathway, focal adhesion, the Ras signaling pathway, regulation of the actin cytoskeleton, cell adhesion molecules, leukocyte transendothelial migration, etc.
Five related differentially expressed genes, namely, MCOLN3B, EME2, PTER, OTX5, and OSMA6l, were selected for validation using quantitative real-time PCR (qRT-PCR). The fold changes obtained from qRT-PCR were compared to those obtained from the differential gene expression (DGE) analysis. The results showed a correlation between the trends of qPCR and RNA sequencing (RNA-Seq) data, indicating the accuracy of the deep-sequencing data (Figure 3). The primers for the different genes are shown in Table 9.
High-throughput sequencing was further used to reveal the changes in gut bacteria (Figure 3). The data have been uploaded to NCBI (https://www.ncbi.nlm.nih.gov) (No. RJNA1029319). Results of principal coordinates analysis (PCoA) indicated that arginine supplementation would not significantly disturb the gut bacterial community (P > 0.05) (Figure 4A). As there was no significant difference between the bacterial communities among fish fed different arginine levels, the samples were divided into two groups, namely, the control group and arginine group (Figure 4B). Mycoplasma and Cetobacterium were the dominant genera in both the control and arginine groups. Mycoplasma had higher abundance in the control group, approximately 1.4 times higher than that in the arginine group, while Cetobacterium was more abundant in the arginine group, 5.4 times higher than in the control group. Furthermore, the results of STAMP analysis showed that 11 genera, including Cetobacterium and Mycoplasma, were significantly influenced by arginine supplementation (Figure 4C).
Figure 4 Succession of bacteria communities. (A) PCoA. (B) Relative abundance of the top 10 genera. (C) STAMP analysis.
Network analysis (Figure 5) showed that the network of the control group had 52 nodes and 166 edges, while the arginine group had only 14 nodes and 18 edges. This correlated with the higher avg k in the control group. The control group had higher negative correlations (41.6%) than the arginine group (0%), and the arginine group had a higher E value and a lower GD value than the control group.
To further explore the benefits of dietary arginine to important aquaculture species, this study observed that supplementing arginine in diets of S. chuatsi from 2.61% to 3.82% did not have any significant impact on growth performance, indicating that 2.61% dietary arginine satisfied the basic growth requirements of S. chuatsi. Studies on largemouth bass (Yu et al., 2023) and hybrid snakehead (Li et al., 2022) have shown that their growth performance decreases with further increased dietary arginine levels, possibly due to the antagonistic effects between arginine and lysine. In the present study, the HSI, VSI, and CF were not significantly different between the different dietary groups, like rice-field eel (Huo et al., 2023) and golden pompano (Trachinotus ovatus) (Lin et al., 2015). The dietary arginine requirement varies with different fish species, nutrition levels, weights, and breeding densities. The requirement of most fish species was previously reported to range from 1.20% to 1.50% (dry matter) except in Pacific salmon (2.04%, dry matter) (Ball et al., 2007). Further studies later revealed a much higher arginine requirement of over 2.0% in other fish species, especially carnivores (Hoseini et al., 2020; Wang Q. et al., 2021), such as largemouth bass (2.40%–2.63%, dry matter) (Yu et al., 2023), rice-field eel (3.16%–3.63%, dry matter) (Huo et al., 2023), hybrid snakehead (2.95%, dry matter) (Li et al., 2022), and spotted seabass (2.53%, dry matter) (Cheng et al., 2021).
Digestive enzyme activity is an important indicator of how diets affect intestinal health (Roxas, 2008). Although dietary arginine supplementation did not influence the intestinal trypsin and lipase activities, interestingly, amylase activity in S. chuatsi significantly decreased when the dietary arginine supplementation level reached 3.82%. This provides a new perspective on the interaction of l-arginine with digestive enzymes in fish species. Similarly, L-arginine was shown to inhibit the activity of α-amylase by decreasing β-sheets and increasing random coils (Ye et al., 2022).
Lack of balance between reactive oxygen species (ROS) and the antioxidant defense system in animals can lead to oxidative stress (Hoseinifar et al., 2020). To reduce oxidative stress, superoxide dismutase reacts against ROS and catalyze the reaction converting O2− into O2, and H2O2 is further converted into water with CAT or GSH-Px (Zengin, 2021). An increased MDA level is an indication of lipid peroxidation from oxidative stress (Garcia et al., 2020). Our study showed that S. chuatsi fed a 3.37% arginine diet had a significantly increased serum TSOD activity and a decreased MDA content, which indicates that arginine could improve the antioxidant capacity in S. chuatsi. Similar results were reported in juvenile blunt snout bream (1.62% and 1.96% arginine levels) (Liang et al., 2018a) and juvenile Chinese mitten crab (2.7%–3.7% arginine level) (Qi et al., 2019). Arginine has been shown to increase antioxidant capacity by stimulating GSH synthesis and modulating the Nrf2-Keap1 pathway (Liang et al., 2018b).
As a precursor for nitric oxide (NO) synthesis, arginine is very essential in regulating energy metabolism. It also plays a role in several physiological processes like degradation of amino acids and fatty acid and glucose syntheses (Tan et al., 2011; Wang Q. et al., 2021). This study showed that the muscle arginine content of S. chuatsi increased with graded dietary arginine levels. The accuracy of the transcriptome data was confirmed by verification of the expression profiles of selected genes [differentially expressed genes (DEGs)] using qRT-PCR. The clustering of muscle DEGs in arginine biosynthesis and the mTOR signaling pathway supported this result. The mTOR signaling pathway controls many metabolic processes and is regulated by amino acid signals, especially arginine (Gai et al., 2016). Muscle DEGs clustered in the mTOR signaling pathway were observed in fish fed diets with arginine inclusion. Muscle valine, glutamate, tyrosine, and total non-essential amino acid contents were significantly decreased in fish fed 3.82% dietary arginine in this study. Although muscle DEGs clustered in biosynthesis of amino acids were observed with dietary arginine supplementation, the influence of arginine on the metabolism of other amino acids remains unknown. In addition, KEGG pathway analysis showed a dose-dependent arginine function. Different arginine supplementation levels influenced different genes and their functions in S. chuatsi. The PI3K-Akt signaling pathway, MAPK signaling pathway, FoxO signaling pathway, and insulin pathway were clustered under arginine supplementation. Insulin is the core substance of glucose metabolism, and it can strictly control the utilization of glucose. Currently, there are at least two known insulin signal transduction pathways: the PI3K-Akt signaling pathway and MAPK signaling pathway (Guan et al., 2021). PI3K and Akt are two major nodes downstream of insulin receptor substrate 1 (IRS-1), which could regulate the proliferation and differentiation of skeletal muscle myoblasts and modulate the absorption of sugar and amino acids in tissues (Taniguchi et al., 2006). Arginine methylation of FOXO transcription factors inhibits their phosphorylation by Akt (Yamagata et al., 2008). MAPK signaling could regulate insulin sensitivity to control glucose metabolism (Zhang et al., 2011). In parallel with that, the serum glucose content decreased with arginine supplementation in S. chuatsi. Studies have reported that a 2.31% dietary arginine level resulted in the upregulation of the relative gene expression of IRS-1, PI3K, and Akt in the insulin signaling pathway (improving glycolysis), while a 2.70% dietary arginine level led to inhibition of these genes (high plasma glucose) in juvenile blunt snout bream Megalobrama amblycephala (Liang et al., 2017). Our result showed that an arginine supplementation level of approximately 2.99%–3.82% could lead to serum glucose concentration, which was closely associated with muscle glucose metabolism in S. chuatsi. Lipid metabolism-associated pathways were also influenced by the arginine supplementation level in our study. Similarly, dietary L-arginine supplementation (1%–2%) in tilapia reduced high fat diet-induced fat deposition and fatty acid composition in the liver by regulating the expression of genes for lipid metabolism (Li et al., 2020).
Gut microbes play an essential role in regulating immune response, nutritional status, and metabolic homeostasis (Lai et al., 2020). According to the results from this study, arginine supplementation modulated the bacterial community of Chinese perch. Mycoplasma was the predominant genus in the gut microbes of Chinese perch from the control group, while Cetobacterium dominated in the dietary arginine supplementation groups probably due to the ability of arginine to regulate glucose homeostasis in fish (Wang A. et al., 2021). The low carbohydrate utilization ability of carnivorous fish can lead to postprandial hyperglycemia in high-carbohydrate diets (Hemre et al., 2002). The enhancement of Cetobacterium by arginine can be explored to ameliorate the deleterious effects of high carbohydrate in diets. The enrichment of Cetobacterium could also be observed with additional probiotic treatment (Wang et al., 2020). Cetobacterium is an anaerobic indigenous bacterium present in the gut of most freshwater fish (Tang et al., 2022), and it also acts a sensor of health. The abundance of Cetobacterium would sharply decrease in infected Aeromonas veronii (Liu et al., 2020). Meanwhile, Ofek et al. (2022) also confirmed that the abundance of Cetobacterium was lower in infected tilapia than in healthy tilapia. The enhancement of Cetobacterium by arginine can be explored to ameliorate the deleterious effects of high carbohydrate in diets. Results of network analysis highlighted that adding arginine had a marked impact on the interaction between bacteria taxa, rather than relative abundance. Adding arginine reduced the network complexity by more than 73.1%, which implies microbial interaction. However, it promoted the ratio of positive correlations and led to 100.0% in its network. These results indicated that adding arginine could eliminate microbial competition and Cetobacterium has the potential to produce secondary metabolites to strengthen microbial cooperation due to the weaker carbohydrate metabolism in the gut microbes of carnivorous fish. Cetobacterium might act as the key species in maintaining fish health and providing protection against some pathogenic bacteria. Cetobacterium is a unique species capable of synthesizing vitamin B12 (Qi et al., 2023), and over 80% of microbes require vitamin B12 for their growth (Zhao et al., 2023). Overall, the increase of Cetobacterium might strengthen interactions among gut microbes and improve host resistance to pathogen infections. These results imply that arginine supplementation can proliferate Cetobacterium to improve microbial cooperation and eliminate competition, thus improving the growth of Chinese perch.
Our results revealed that although growth performance was not significantly influenced by dietary arginine supplementation, antioxidant capacity, intestinal digestion, and nutritional metabolism were significantly impacted in Chinese perch. Further transcriptome analysis result showed that graded arginine levels significantly influenced muscle gene expression involved in KEGG pathways of energy metabolism (amino acid, glucose, fatty acid), signaling pathways (mTOR, PI3K-Akt signaling pathway, MAPK signaling pathway, FoxO signaling pathway, and insulin pathway), etc. Microbial analysis showed that adding arginine reduced the network complexity of potential interactions between bacterial taxa and eliminated microbial competition. Based on antioxidant and intestinal health indicators, the optimal dietary arginine requirement for Chinese perch was 2.99%–3.37% of the dry diet (6.08%–6.79% of dietary protein).
The original contributions presented in the study are included in the article / supplementary material. Further inquiries can be directed to the corresponding. The RNA sequencing data has been uploaded to NCBI NO. PRJNA1029502 (https://www.ncbi.nlm.nih.gov/). The high-throughput sequencing data has been uploaded to NCBINO. PRJNA1029319 (https://www.ncbi.nlm.nih.gov/).
The animal study was approved by The Animal Care and Use Committee of Jiangxi Fisheries Research Institute. The study was conducted in accordance with the local legislation and institutional requirements.
LD: Data curation, Investigation, Writing – original draft. JC: Data curation, Investigation, Methodology, Writing – original draft. FH: Formal analysis, Investigation, Writing – original draft. QC: Project administration, Supervision, Writing – review & editing. YL: Supervision, Writing – review & editing. WC: Funding acquisition, Writing – review & editing.
The author(s) declare financial support was received for the research, authorship, and/or publication of this article. This research was supported by the Key Research and Development Program of Jiangxi (20232BBF60011), China Agriculture Research System (CARS-46), the earmarked fund for Jiangxi Agriculture Research System (JXARS-03), Jiangxi Province Joint Breeding Research Project (2023yyzygg-02), Fujian Key Laboratory of Special Aquatic Formula Feed (Fujian Tianma Science and Technology Group Co., Ltd.) (TMKJZ2001), and High Level and Skilled Leading Talent Special Support Project of Jiangxi.
Authors JC and QC were employed by the company Fujian Tianma Science and Technology Group Co., Ltd.
The remaining authors declare that the research was conducted in the absence of any commercial or financial relationships that could be construed as a potential conflict of interest.
All claims expressed in this article are solely those of the authors and do not necessarily represent those of their affiliated organizations, or those of the publisher, the editors and the reviewers. Any product that may be evaluated in this article, or claim that may be made by its manufacturer, is not guaranteed or endorsed by the publisher.
The Supplementary Material for this article can be found online at: https://www.frontiersin.org/articles/10.3389/fmars.2023.1305192/full#supplementary-material
Andersen S. M., Waagbo R., Espe M. (2016). Functional amino acids in fish nutrition, health and welfare. Front. Biosci. (Elite Ed) 8 (1), 143–169. doi: 10.2741/757
AOAC (Association of Official Analytical Chemists) (1995). Official Methods of Analysis. 17th ed (Arlington, VA, USA: Association of Official Analytical Chemists).
Azeredo R., Perez-Sanchez J., Sitja-Bobadilla A., Fouz B., Tort L., Aragao C., et al. (2015). European sea bass (Dicentrarchus labrax) immune status and disease resistance are impaired by arginine dietary supplementation. PloS One 10 (10), e0139967. doi: 10.1371/journal.pone.0139967
Ball R. O., Urschel K. L., Pencharz P. B. (2007). Nutritional consequences of interspecies differences in arginine and lysine metabolism. J. Nutr. 137 (6 Suppl 2), 1626S–1641S. doi: 10.1093/jn/137.6.1626S
Cheng Y., Li X., Wang L., Lu K., Song K., Ai Q., et al. (2021). Effects of dietary arginine levels on growth, immune function of physical barriers and serum parameters of spotted seabass (Lateolabrax maculatus) reared at different water temperatures. Aquaculture 541, 736812. doi: 10.1016/j.aquaculture.2021.736812
Feng K., Zhang Y., He Z., Ning D., Deng Y. (2019). Interdomain ecological networks between plants and microbes. Mol. Ecol. Resour. 19 (6), 1565–1577. doi: 10.1111/1755-0998.13081
Feng H., Zhang Y., Liang X. F., He S., Li L. (2021). Dietary supplementation of exogenous probiotics reduces excessive liver lipid deposition in Chinese perch (Siniperca chuatsi). Aquaculture Res. 52 (11), 5430–5440. doi: 10.1111/are.15413
Gai Z., Wang Q., Yang C., Wang L., Deng W., Wu G. (2016). Structural mechanism for the arginine sensing and regulation of CASTOR1 in the mTOR C1 signaling pathway. Cell Discovery 2, 16051. doi: 10.1038/celldisc.2016.51
Garcia D., Lima D., da Silva D. G. H., de Almeida E. A. (2020). Decreased malondialdehyde levels in fish (Astyanax altiparanae) exposed to diesel: Evidence of metabolism by aldehyde dehydrogenase in the liver and excretion in water. Ecotoxicol Environ. Saf. 190, 110107. doi: 10.1016/j.ecoenv.2019.110107
Guan D. Y., Sun H. W., Wang J. T., Wang Z., Li Y., Han H. J., et al. (2021). Rosiglitazone promotes glucose metabolism of GIFT tilapia based on the PI3K/Akt signaling pathway. Physiol. Rep. 9 (5), e14765. doi: 10.14814/phy2.14765
Hemre G. I., Mommsen T. P., Krogdahl Å. (2002). Carbohydrates in fish nutrition: effects on growth, glucose metabolism and hepatic enzymes. Aquaculture Nutr. 8 (3), 175–194. doi: 10.1046/j.1365-2095.2002.00200.x
Hoseini S. M., Ahmad Khan M., Yousefi M., Costas B. (2020). Roles of arginine in fish nutrition and health: insights for future researches. Rev. Aquaculture 12 (4), 2091–2108. doi: 10.1111/raq.12424
Hoseinifar S. H., Yousefi S., Van Doan H., Ashouri G., Gioacchini G., Maradonna F., et al. (2020). Oxidative stress and antioxidant defense in fish: the implications of probiotic, prebiotic, and synbiotics. Rev. Fisheries Sci. Aquaculture 29 (2), 198–217. doi: 10.1080/23308249.2020.1795616
Hu J., Zhao Y., Yao X., Wang J., Zheng P., Xi C., et al. (2021). Dominance of comammox Nitrospira in soil nitrification. Sci. Total Environ. 780, 146558. doi: 10.1016/j.scitotenv.2021.146558
Huo H., Peng M., Yu M., Zhang Y., Xiong L., Zhou Q. (2023). Effects of dietary arginine level on growth performance and immune response in monopterus albus. Aquaculture Res. 2023, 8371801. doi: 10.1155/2023/8371801
Ji Z., Zhu C., Zhu X., Ban S., Yu L., Tian J., et al. (2023). Dietary host-associated Bacillus subtilis supplementation improves intestinal microbiota, health and disease resistance in Chinese perch (Siniperca chuatsi). Anim. Nutr. 13, 197–205. doi: 10.1016/j.aninu.2023.01.001
Lai K. P., Lin X., Tam N., Ho J. C. H., Wong M. K. S., Gu J., et al. (2020). Osmotic stress induces gut microbiota community shift in fish. Environ. Microbiol. 22 (9), 3784–3802. doi: 10.1111/1462-2920.15150
Levine A. J., Feng Z., Mak T. W., You H. (2006). Coordination and communication between the p53 and IGF-1–AKT–TOR signal transduction pathways. Genes Dev. 20 (3), 267–275. doi: 10.1101/gad.1363206
Li P., Hou D., Zhao H., Peng K., Chen B., Guo H., et al. (2022). Effects of dietary arginine levels on intestinal morphology, digestive enzyme activity, antioxidant capacity and intestinal flora of hybrid snakehead (Channa maculata♀× Channa argus♂). Aquaculture Rep. 25, 101244. doi: 10.1016/j.aqrep.2022.101244
Li S., Zhang Y., Liu N., Chen J., Guo L., Dai Z., et al. (2020). Dietary L-arginine supplementation reduces lipid accretion by regulating fatty acid metabolism in Nile tilapia (Oreochromis niloticus). J. Anim. Sci. Biotechnol. 11, 82. doi: 10.1186/s40104-020-00486-7
Liang H., Habte-Tsion H. M., Ge X., Ren M., Xie J., Miao L. (2017). Dietary arginine affects the insulin signaling pathway, glucose metabolism and lipogenesis in juvenile blunt snout bream Megalobrama amblycephala. Sci. Rep. 7 (1), 7864. doi: 10.1038/s41598-017-06104-3
Liang H., Ji K., Ge X., Ren M., Liu B., Xi B., et al. (2018a). Effects of dietary arginine on antioxidant status and immunity involved in AMPK-NO signaling pathway in juvenile blunt snout bream. Fish Shellfish Immunol. 78, 69–78. doi: 10.1016/j.fsi.2018.04.028
Liang M., Wang Z., Li H., Cai L., Pan J., He H., et al. (2018b). l-Arginine induces antioxidant response to prevent oxidative stress via stimulation of glutathione synthesis and activation of Nrf2 pathway. Food Chem. Toxicol. 115, 315–328. doi: 10.1016/j.fct.2018.03.029
Lin H., Tan X., Zhou C., Niu J., Xia D., Huang Z., et al. (2015). Effect of dietary arginine levels on the growth performance, feed utilization, non-specific immune response and disease resistance of juvenile golden pompano Trachinotus ovatus. Aquaculture 437, 382–389. doi: 10.1016/j.aquaculture.2014.12.025
Liu Z., Li A., Wang Y., Iqbal M., Zheng A., Zhao M., et al. (2020). Comparative analysis of microbial community structure between healthy and Aeromonas veronii-infected Yangtze finless porpoise. Microbial Cell factories 19 (1), 1–12. doi: 10.1186/s12934-020-01383-4
Ofek T., Lalzar M., Izhaki I., Halpern M. (2022). Intestine and spleen microbiota composition in healthy and diseased tilapia. Anim. Microbiome 4 (1), 50. doi: 10.1186/s42523-022-00201-z
Peng D., Peng B., Li J., Zhang Y., Luo H., Xiao Q., et al. (2022). Effects of three feed attractants on the growth, biochemical indicators, lipid metabolism and appetite of Chinese perch (Siniperca chuatsi). Aquaculture Rep. 23, 101075. doi: 10.1016/j.aqrep.2022.101075
Peng D., Yang L., Liang X. F., Chai F. (2023). Dietary zinc levels affect growth, appetite, and lipid metabolism of Chinese perch (Siniperca chuatsi). Fish Physiol. Biochem. 49, 1017–1030. doi: 10.1007/s10695-023-01238-w
Qi C., Wang X., Han F., Jia Y., Lin Z., Wang C., et al. (2019). Arginine supplementation improves growth, antioxidant capacity, immunity and disease resistance of juvenile Chinese mitten crab, Eriocheir sinensis. Fish Shellfish Immunol. 93, 463–473. doi: 10.1016/j.fsi.2019.07.082
Qi X., Zhang Y., Zhang Y., Luo F., Song K., Wang G., et al. (2023). Vitamin B12 produced by Cetobacterium somerae improves host resistance against pathogen infection through strengthening the interactions within gut microbiota. Microbiome 11 (1), 135. doi: 10.1186/s40168-023-01574-2
Roxas M. (2008). The role of enzyme supplementation in digestive disorders. Altern. Med. Rev. 13 (4), 307–314.
Tan B., Yin Y., Liu Z., Tang W., Xu H., Kong X., et al. (2011). Dietary L-arginine supplementation differentially regulates expression of lipid-metabolic genes in porcine adipose tissue and skeletal muscle. J. Nutr. Biochem. 22 (5), 441–445. doi: 10.1016/j.jnutbio.2010.03.012
Tang X., Ma S., Sun L., Li Y., Yang Q., Yu X., et al. (2022). Isolation, identification, and positive effects of potential probiotics on Carassius auratus. Aquaculture 548, 737668. doi: 10.1016/j.aquaculture.2021.737668
Taniguchi C. M., Emanuelli B., Kahn C. R. (2006). Critical nodes in signalling pathways: insights into insulin action. Nat. Rev. Mol. Cell Biol. 7 (2), 85–96. doi: 10.1038/nrm1837
Wang B., Liu Y., Feng L., Jiang W. D., Kuang S. Y., Jiang J., et al. (2015). Effects of dietary arginine supplementation on growth performance, flesh quality, muscle antioxidant capacity and antioxidant-related signalling molecule expression in young grass carp (Ctenopharyngodon idella). Food Chem. 167, 91–99. doi: 10.1016/j.foodchem.2014.06.091
Wang Y., Wang C. A., Liu S., Zhang S., Lu S., Zhang Y. (2022). Effects of dietary arginine on growth performance, digestion, absorption ability, antioxidant capability, gene expression of intestinal protein synthesis, and inflammation-related genes of triploid juvenile Oncorhynchus mykiss fed a low-fishmeal diet. Aquaculture Nutr. 2022, 1–14. doi: 10.1155/2022/3793727
Wang Q., Xu Z., Ai Q. (2021). Arginine metabolism and its functions in growth, nutrient utilization, and immunonutrition of fish. Anim. Nutr. 7 (3), 716–727. doi: 10.1016/j.aninu.2021.03.006
Wang M., Yi M., Lu M., Gao F., Liu Z., Huang Q., et al. (2020). Effects of probiotics Bacillus cereus NY5 and Alcaligenes faecalis Y311 used as water additives on the microbiota and immune enzyme activities in three mucosal tissues in Nile tilapia Oreochromis niloticus reared in outdoor tanks. Aquaculture Rep. 17, 100309. doi: 10.1016/j.aqrep.2020.100309
Wang A., Zhang Z., Ding Q., Yang Y., Bindelle J., Ran C., et al. (2021). Intestinal Cetobacterium and acetate modify glucose homeostasis via parasympathetic activation in zebrafish. Gut Microbes 13 (1), 1–15. doi: 10.1080/19490976.2021.1900996
Wei Y., Zhang Q., Jia L., Xu H., Liang M. (2021). Effects of dietary arginine levels on growth, intestinal peptide and amino acid transporters, and gene expressions of the TOR signaling pathway in tiger puffer, Takifugu rubripes. Aquaculture 532, 736086. doi: 10.1016/j.aquaculture.2020.736086
Xie R. P., Liang X. F., Peng D., Zhang Q. W., Wu D. L., Zeng M. (2023). Dietary supplementation of pyridoxine can enhance the growth performance and improve the protein, lipid utilization efficiency of mandarin fish (Siniperca chuatsi). Fish Physiol. Biochem. 49, 1063–1078. doi: 10.1007/s10695-023-01223-3
Yamagata K., Daitoku H., Takahashi Y., Namiki K., Hisatake K., Kako K., et al. (2008). Arginine methylation of FOXO transcription factors inhibits their phosphorylation by Akt. Mol. Cell 32 (2), 221–231. doi: 10.1016/j.molcel.2008.09.013
Ye Y. T., Zhang H., Deng J. L., Li M. Z., Chen Z. X. (2022). l-Arginine inhibits the activity of alpha-amylase: Rapid kinetics, interaction and functional implications. Food Chem. 380, 131836. doi: 10.1016/j.foodchem.2021.131836
Yu Y., Huang D., Zhang L., Chen X., Wang Y., Liang H. (2023). Dietary arginine levels affect growth performance, intestinal antioxidant capacity and immune responses in largemouth bass (Micropterus salmoides). Aquaculture Rep. 32, 101703. doi: 10.1016/j.aqrep.2023.101703
Zengin H. (2021). The effects of feeding and starvation on antioxidant defence, fatty acid composition and lipid peroxidation in reared Oncorhynchus mykiss fry. Sci. Rep. 11 (1), 16716. doi: 10.1038/s41598-021-96204-y
Zhang W., Thompson B. J., Hietakangas V., Cohen S. M. (2011). MAPK/ERK signaling regulates insulin sensitivity to control glucose metabolism in Drosophila. PloS Genet. 7 (12), e1002429. doi: 10.1371/journal.pgen.1002429
Zhang Y., Liang X. F., He S., Feng H., Li L. (2022a). Dietary supplementation of exogenous probiotics affects growth performance and gut health by regulating gut microbiota in Chinese Perch (Siniperca chuatsi). Aquaculture 547, 737405. doi: 10.1016/j.aquaculture.2021.737405
Zhang Y., Feng H., Liang X. F., He S., Lan J., Li L. (2022b). Dietary bile acids reduce liver lipid deposition via activating farnesoid X receptor, and improve gut health by regulating gut microbiota in Chinese perch (Siniperca chuatsi). Fish Shellfish Immunol. 121, 265–275. doi: 10.1016/j.fsi.2022.01.010
Keywords: arginine, antioxidant capacity, intestinal digestion, muscle transcriptome, intestinal microbiota, Siniperca chuatsi
Citation: Ding L, Chen J, He F, Chen Q, Li Y and Chen W (2024) Effects of dietary arginine supplementation on growth performance, antioxidant capacity, intestinal digestive enzyme activity, muscle transcriptome, and gut health of Siniperca chuatsi. Front. Mar. Sci. 10:1305192. doi: 10.3389/fmars.2023.1305192
Received: 01 October 2023; Accepted: 22 December 2023;
Published: 26 January 2024.
Edited by:
Amina Zuberi, Quaid-i-Azam University, PakistanCopyright © 2024 Ding, Chen, He, Chen, Li and Chen. This is an open-access article distributed under the terms of the Creative Commons Attribution License (CC BY). The use, distribution or reproduction in other forums is permitted, provided the original author(s) and the copyright owner(s) are credited and that the original publication in this journal is cited, in accordance with accepted academic practice. No use, distribution or reproduction is permitted which does not comply with these terms.
*Correspondence: Yiyi Li, bGl5aXlpMjFAbWFpbHMudWNhcy5hYy5jbg==; Wenjing Chen, d2pjaGVuODdAMTI2LmNvbQ==
†These authors have contributed equally to this work
Disclaimer: All claims expressed in this article are solely those of the authors and do not necessarily represent those of their affiliated organizations, or those of the publisher, the editors and the reviewers. Any product that may be evaluated in this article or claim that may be made by its manufacturer is not guaranteed or endorsed by the publisher.
Research integrity at Frontiers
Learn more about the work of our research integrity team to safeguard the quality of each article we publish.