- 1Geographic Information Systems (GIS) and Spatial Ecology Laboratory, Halmos College of Arts and Sciences, Nova Southeastern University, Dania Beach, FL, United States
- 2Department of Marine and Environmental Sciences, Nova Southeastern University, Dania Beach, FL, United States
The presence and abundance of reef-building corals are crucial to the long-term existence of Caribbean coral reef ecosystems, providing both direct and indirect, local and global, ecological, economic, and social benefits. In 2014, stony coral tissue loss disease (SCTLD) was first identified in southeast Florida and remains endemic to the region, while continuing to spread throughout the Caribbean. Effective in situ intervention treatments using antibiotic paste can halt lesion progression on Montastraea cavernosa up to 90% of the time. This study investigated intervention activities over a three-year period to identify efficiencies in disease response. Since May 2019, 1,037 corals, >85% of which were M. cavernosa, were treated during disease intervention dives in southeast Florida. Treated coral density, the number of treated corals per meter along a dive track, was significantly higher in the first year compared to subsequent years and displayed annual peaks in late summer each year. Season significantly influenced treatment density, leading to higher values in the wet season across all years, 2019 to 2022. Areas of highest treatment density were identified between Haulover Inlet and Government Cut near Miami and Hillsboro Inlet in northern Broward County. Areas with the highest treatment density were only identified in the first year, suggesting that broadscale interventions may have decreased disease prevalence in subsequent years. Results indicate that in endemic areas with sporadic and dynamic disease prevalence, intervention efforts should be weighted proportionally across space and time to maximize intervention efficiency. This study provides optimistic results for the potential of interventions reducing disease prevalence and supports that disease interventions are an effective coral restoration tool that can decrease the increasing burden on post hoc coral restoration.
Introduction
Stony coral tissue loss disease (SCTLD) is one of the most destructive Caribbean coral diseases. Its persistence, virulence, mortality rate, and previously documented high disease prevalence (61%) compared to other white tissue loss diseases (<5%) (Precht et al., 2016; Aeby et al., 2019) have decimated coral populations in southeast Florida (SEFL), leaving some species with >98% tissue loss (Hayes et al., 2022). SCTLD was first reported in September 2014 near Miami, Florida in the Kristin Jacobs Coral Reef Ecosystem Conservation Area (Coral ECA) although the exact location and date of its origins are questionable as multiple diseased Dendrongyra cylindrus colonies displaying similar symptoms were documented in February 2014 further north (Jones et al., 2021). The disease onset coincided with and may have been exacerbated by a massive sedimentation event caused by the 2013-2015 dredging in the Port of Miami (Barnes et al., 2015; Cunning et al., 2019) and the 2014-2016 summer thermal stress-driven coral bleaching events (Manzello, 2015; Precht et al., 2016; Aeby et al., 2019; Jones et al., 2020).
As of November 2023, the causative agent of SCTLD remains unknown. Data support that the disease is associated with dysbiosis of the zooxanthellae living within the coral tissue, followed by a secondary bacterial infection directly affecting the coral tissue itself (Landsberg et al., 2020; Work et al., 2021), while other studies suggest it to be predominantly bacterial or influenced mainly by bacterial interactions (Iwanowicz et al., 2020; Rosales et al., 2023). SCTLD differs from other white plagues and syndromes in that it often displays lytic necrosis of the coral tissue along the disease margin (Aeby et al., 2021; Cróquer et al., 2021). Diseased colonies are often found in clusters among the reef in SEFL, suggesting a contagious mode of transmission (Muller et al., 2020). Dobbelaere et al. (2020) found that disease spread matched that of a neutrally buoyant particle, suggesting that it is water-borne (Aeby et al., 2019) and currents likely play an important role in its spread. SCTLD has been documented to infect over 24 of the estimated 45 species of stony corals found within Florida’s Coral Reef (FCR), making it wide-ranging and highly destructive to the majority of reef-building species (Walton et al., 2018; Aeby et al., 2021; Florida Coral Disease Response Research & Epidemiology Team, 2018).
Because of SCTLD’s continued persistence, high prevalence among reefs, wide range of susceptible species, and high mortality rate of infected individuals (Aeby et al., 2019; Estrada-Saldívar et al., 2020; Spadafore et al., 2021; Alvarez-Filip et al., 2022), efforts were undertaken to develop disease interventions to stop the spread of SCTLD. However, stony coral disease identification and intervention methods have been historically limited and effective methods and materials were and remain underdeveloped. Many previous attempts were limited to ex situ scenarios where colonies were treated in aquaria. For example, Sweet et al. (2014) found that ampicillin and paromomycin, two common antibiotics used to treat gram-positive bacterial infections, completely arrested white band disease in Acropora, a genus of branching corals, while two other antibiotics had little to no effect on these disease lesions. Although effective, ex situ methods are limited in scale because colonies must be removed, transported, treated for a period of time and then replanted on the reef.
Previous in situ treatments tested on various common coral diseases have included shading, aspiration, trenching of coral tissue, removal of microbial material and more, with success varying by method and disease. In corals presenting with yellow band disease, a trench cut into the coral tissue and skeleton had high short-term success of ~70% but long-term success of only ~10% (Randall et al., 2018). Black band disease in the Looe Key National Marine Sanctuary in the late 1980s, was successfully treated by in situ removal of the microbial mat followed by the application of clay to seal the lesion edge, halting the spread of the lesion (Hudson, 2000; Teplitski and Ritchie, 2009). Although effective, coral colonies were reinfected ~30% of the time (Hudson, 2000). This method was highly time-consuming and exhibited the need for a wide-reaching and efficient intervention method that decreased the probability of disease re-emerging along the reef (Hudson, 2000). Phage therapy has been tested on corals with white plague in the Red Sea, and was successful when used in the early stages of infection (Efrony et al., 2009). In 2015, topical application of a mixture of marine epoxy and chlorine powder were used to successfully halt black band disease in Hawaii (Aeby et al., 2015). This method did not require extensive equipment, time, or strict permitting and was relatively inexpensive, leading to its adoption for the first broadscale treatments of SCTLD. The first SCTLD treatments with epoxy and chlorine powder were monitored extensively to understand their efficacy and showed moderate success on Orbicella spp. (77%) but not for Montastraea cavernosa (<40%) (Walker et al., 2021c).
Concomitantly, to increase the applicability and viability of in situ disease treatments, a team of scientists formed Ocean Alchemists LLC and developed CoralCure™ Base2b, a silicone-based paste, to be used in conjunction with powdered antibiotics, as these had shown success in ex situ treatments of corals presenting with SCTLD lesions. The paste allowed for proper lesion adhesion and drug release over three days. Amoxicillin, an antibiotic effective against gram-positive bacteria, was selected for these treatments based on its accessibility, low cost, and frequent applicability in medicine which showed promise for treating SCTLD, as the causative agent remained unknown. The CoralCure™ treatment consisted of amoxicillin and base in a 1:8 ratio (Neely, 2018). Once permits were approved for emergency treatment usage, disease intervention teams started treating lesions across FCR in 2018 (Neely et al., 2020; Neely et al., 2021; Shilling et al., 2021; Walker et al., 2021b; Walker et al., 2022a). Walker et al. (2021b) found that CoralCure™ treatment paste, at the disease lesion, combined with a ‘disease break’ filled with CoralCure™, cut about 5 cm from the disease lesion and 1cm deep on the live tissue side, was the most effective in situ intervention method halting disease lesions on Montastraea cavernosa at a rate of 91.2% within the first year of treatment in SEFL. Additionally, CoralCure™ treatments showed long term success in which 95% of treated corals across 5 species, Orbicella faveolata, M. cavernosa, Colpophyllia natans, Psuedodiploria strigosa, and Diploria labyrinthiformis, showed no signs of disease after 2 years in the Florida Keys (Neely et al., 2021). This highly effective treatment method diminished the need to return to monitor, therefore increasing the efficiency of broad scale interventions while reducing disease loads on the reef.
The effectiveness of broad scale disease interventions relies not only on treatment efficacy, but also on the ability to find and treat as many diseased individuals as possible throughout the seascape, requiring extensive planning and field work. High-density coral reefs naïve to a new disease may have many diseased corals in close proximity, making these treatment dives highly efficient (high density of corals requiring treatment per search area). However, once disease moves through the highly susceptible species and individuals in an area, it becomes spatially and temporally sporadic, making disease intervention efforts less efficient and more costly. For example, between January 2019 and August 2022, 3,765 corals were treated in the Florida Keys National Marine Sanctuary (FKNMS) and 1,037 corals were treated in the Coral ECA. Divers in the FKNMS typically treated 25-50 corals per dive, about one coral every 13.5 minutes, whereas Coral ECA divers treated 2-5 corals per dive, one coral every 37.2 minutes on dives where at least one coral was treated (Neely, unpublished data). This disparity in treated corals between regions might be attributed to variation in the SCTLD highly susceptible coral densities between regions, with higher disease-susceptible coral density in FKNMS (Toth et al., 2023). The Coral ECA reefs have historically had lower species diversity, coral cover, and reduced coral populations than the FKNMS (Veron, 1995) and the endemicity of SCTLD has further reduced coral density and reduced live tissue cover by 59% in the Coral ECA (Walton et al., 2018; Hayes et al., 2022). Thus, disease interventions in the Coral ECA require covering longer distances or larger areas to locate diseased colonies than in the FKNMS.
Although significantly less expensive than the restoration required to replace corals lost to disease, regional scale in situ disease interventions are daunting and expensive, especially since current interventions do not provide an apparent long-term benefit to getting reinfected. Coral diseases have previously been associated with thermal stress (Bruno et al., 2007; Howells et al., 2020; Williams et al., 2011) and terrestrial runoff (Bruno et al., 2003; Haapkylä et al., 2011), thus disease intervention efficiencies in endemic locations might be enhanced by targeting certain times of the year or areas that receive more terrestrial influences. SCTLD has been reported to be affected by thermal stress, with increasing prevalence during warmer, wetter periods (Walker et al., 2022b), but also complete quiescence during bleaching (Meiling et al., 2020). Therefore, we analyzed differences in efficiency of broadscale disease interventions within the Coral ECA over a 3-year period to maximize future coral treatments, providing a roadmap for ongoing and future disease responses in endemic zones to evaluate and improve their efficiency and effectiveness at halting disease, saving live coral tissue, and reducing the need for post hoc restoration.
Materials and methods
Broad-scale disease interventions reported herein occurred between May 2019 and April 2022 in the southern portion of the Coral ECA bounded by Hillsboro Inlet to the North and Cape Florida Channel to the South on FCR (Figure 1). Disease interventions have continued through the publication of this study but are not included because they did not encompass a full year at the start of the analysis. With limited resources at hand, the focus of the disease response was to treat as many diseased individuals as possible to reduce disease loads, stop lesion progression, and preserve coral tissue coverage. Treatment dive sites were initially chosen using data from the Southeast Florida reef-wide post-hurricane Irma coral disease surveys that collected the number of coral species, density, and percent cover at 62 sites in 2017 (Walker, 2018). Benthic habitat maps and bathymetry data (Walker et al., 2008; Walker, 2012) were used in ArcGIS to identify high topographic relief areas indicative of reef habitat to fill spatial gaps. The mapping data ensured that divers spent time surveying reef habitats rather than seagrass, sand, or rubble areas. Additionally, the SCTLD presence data during the 2020 NOAA Coral Reef Conservation Program (CRCP) National Coral Reef Monitoring Program (CRCP project #743) benthic and fish surveys were used to identify habitats where SCTLD was more likely to be present: the shallow habitats in the Broward-Miami ecoregion (Walker et al., 2021a).
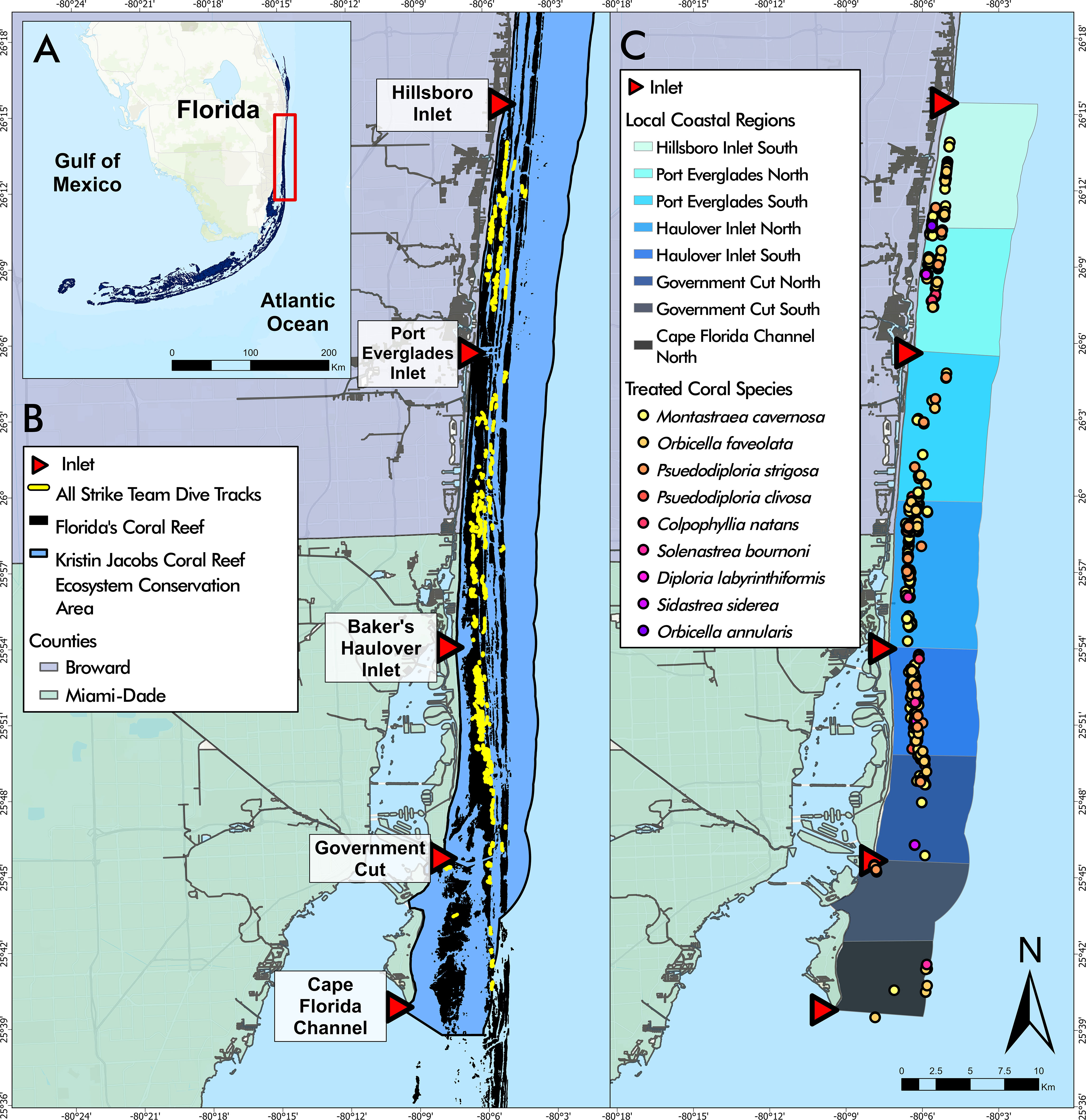
Figure 1 (A) Florida’s Coral Reef in southeast Florida, the study area outlined in red. (B) Disease intervention dive tracks from May 2019 to April 2022 overlaying Florida’s Coral Reef within the Kristin Jacobs Coral Reef Ecosystem Conservation Area. (C) Disease intervention treated corals by species from May 2019 to April 2022 overlaying Local Coastal Regions (LCRs). Coral species listed in order of most frequently treated to least frequently treated.
Dives occurred on reef habitats of Broward and Miami-Dade counties in depths ranging from 3 to 15 m. During each dive, scuba divers spread out near the bottom to within visible range of each other, typically covering a breadth of approximately 15 meters, heading with the current while following the reef topography. If divers swam upon a non-hardbottom area or an area without stony corals for more than 5 minutes, they would end their dive and move to a new location, to increase the likelihood of finding corals with lesions, heretofore referred to as ‘diseased coral’. Once a diseased coral was found, divers visually assessed the lesion to ensure it was consistent with SCTLD. Disease lesions were considered to be SCTLD if lesions had distinct tissue loss, clear margins of newly exposed bare skeleton, and/or appeared to be focal or multi-focal (Florida Coral Disease Response Research & Epidemiology Team, 2018). If so, divers proceeded to tag, identify, assess, measure, and photograph each coral before treatment. Treatment consisted of applying CoralCure™ with amoxicillin (1:8 weight ratio) to the disease lesion, sometimes combined with a disease break, a 1 cm trench cut with a submersible angle grinder about 5 cm inward from the lesion to isolate visually diseased areas from the remaining live tissue. The most time-consuming part of each coral treatment is the tagging, assessment and documentation and therefore the size of the colony, length of treatment paste applied, and number of lesions were not factored into effort. Colonies were not revisited to assess treatment success, however if a previously treated colony was encountered with disease, it was retreated and documented.
A floating DGPS WAAS unit time synced to a dive computer was used to attain each treated coral’s location and the entire spatial and temporal dive extent. The diver recorded the time at the start and end of the dive and at each treated coral. GPS point and line data collected from each dive were obtained using DNR Garmin (Version 6.1.0.6, Minnesota Department of Natural Resources, 2011). Both line and point data were input to ArcGIS Pro (Version 3.1.2, Esri Inc, 2023). GPS points associated with the time recorded at each treated coral were extracted to a separate database. The line data were trimmed to ensure they represented the underwater survey time. These data were used to calculate the total number of treated colonies divided by the linear distance covered. This metric was termed treatment density and used as a measure of efficiency.
Coastal spatial variation within the study area was analyzed using a layer depicting Local Coastal Regions (LCRs). Each LCR was bounded by the middle of each inlet mouth and the midpoint between two consecutive inlets (Figure 1). The LCRs were named according to the inlet and relative location (“Port Everglades North” to designate the area between Port Everglades and the midpoint of Port Everglades and Hillsboro Inlet).
Data analysis was conducted in ArcGIS Pro (Version 3.1.2, Esri Inc., 2023) and R (Version 4.1.0, R Core Team, 2021). A spatial autocorrelation test (Global Moran’s I) showed that treated corals (Moran’s Index = 1.00157, z = 59.43677, p = 0.00) and dive track locations (Moran’s Index = 0.997269, z = 38.743816, p = 0.00) were spatially autocorrelated, but treatment density was not (Moran’s Index = 0.021719, z = 1.940706, p = 0.052294). Thus, a kernel density estimation was performed to visually represent treatment density spatial patterns using the geometric center of the dive tracks. Statistical spatial analyses were limited to the LCR analyses conducted in R (Version 4.1.0, R Core Team, 2021), where none of the LCR treatment density values were normally distributed except the Cape Florida Channel North region. A negative binomial GLM was used to compare treatment density between LCRs to one another.
Dive tracks and treated coral data were categorized by each project year beginning in May and continuing through the following April. The project years were as follows: A: May 1st 2019- April 30th 2020, B: May 1st 2020 - April 20th 2021, C: May 1st 2021 - April 30th 2022. Seasons were categorized as wet from May 1st to October 30th and dry from November 1st to April 30th based on historic climate data (Davis and Ogden, 1994). The dry season is associated with less rainfall and cooler water and air temperatures, and the wet season is associated with more rainfall and warmer water and air temperatures. Shapiro-Wilkes test and distribution plots of each variable determined that none of the factors were normal. Therefore, negative binomial GLMs were used for analyses of treatment density, by seasons and project years, independently. Additional negative binomial GLMs were conducted to inspect relationships between project year and LCR, season and project year, as well as season and LCR. All negative binomial GLMs were conducted using coral treatments and a logarithmic offset term of dive distance to represent the values of treatment density. For all negative binomial GLMs, where applicable, pairwise comparisons were completed using the “emmeans” package (Lenth et al., 2022), the “pairs” and “contrast” functions, and the “pairwise” method (Spadafore et al., 2021) to determine the significance between levels of factors.
Results
Disease intervention teams conducted 333 dives (Table 1) across 120 days throughout the Coral ECA from May 2019 to April 2022 covering roughly 250 km of reef (Figure 1B). On average, each field day consisted of three dives covering an average linear distance of 722.89 m on each dive. During the project period, 1,037 corals were treated, however only 941 of these corals were included in analysis due to missing location information. The average number of corals treated per dive was 2.81 corals. Corals treated during the study period comprised nine species including 888 (86%) Montastraea cavernosa, 76 (7%) Orbicella faveolata, 27 (3%) Pseudodiploria strigosa, 19 (2%) Pseudodiploria clivosa, 15 (1.5%) Colpophyllia natans, 5 (<1%) Solenastrea bournoni, 4 (<1%) Diploria labryinthiformis, 2 (<1%) Siderastrea siderea, and 1 (<1%) Orbicella annularis indicating that highly and intermediately susceptible species were found with active lesions throughout the study period (Figure 1C).
Since disease intervention activities were not randomized or normalized by region or time of year and were not standardized by time, length, or survey area, the treated corals were standardized to the dive length (treated corals per m) to get an estimate of effort per dive. A polynomial regression of treated corals and dive distance determined that coral treatments were a significant, but minor function of dive distance (p = 5.59 x 10-5, Adjusted R2 = 0.05758), suggesting that dives covering more distance were not guaranteed to have more diseased corals and other factors were mostly responsible for the variation of the number of corals treated on any given dive.
The number of dives and treated corals varied by project year (Table 1). Year A (May 2019 – April 2020) had the fewest number of dives (99) but most coral treatments (402). Similar numbers of coral treatments (262 and 277, respectively) were conducted in Year B (May 2020 – April 2021) and Year C (May 2021 – April 2022), but 32 more dives were conducted in Year C. A negative binomial GLM showed that project year was a significant predictor of treatment density per dive (LR χ2 = 21.863, df = 2, p = 1.789 x 10-5, respectively). Treatment density was significantly higher in Project Year A than in Project Years B and C (p = 0.0102 and p < 0.0001, respectively; Figure 2). Additionally, a negative binomial GLM showed that dive distance was not significantly different by year (LR χ2 = 4.5937, df = 2, p = 0.1006).
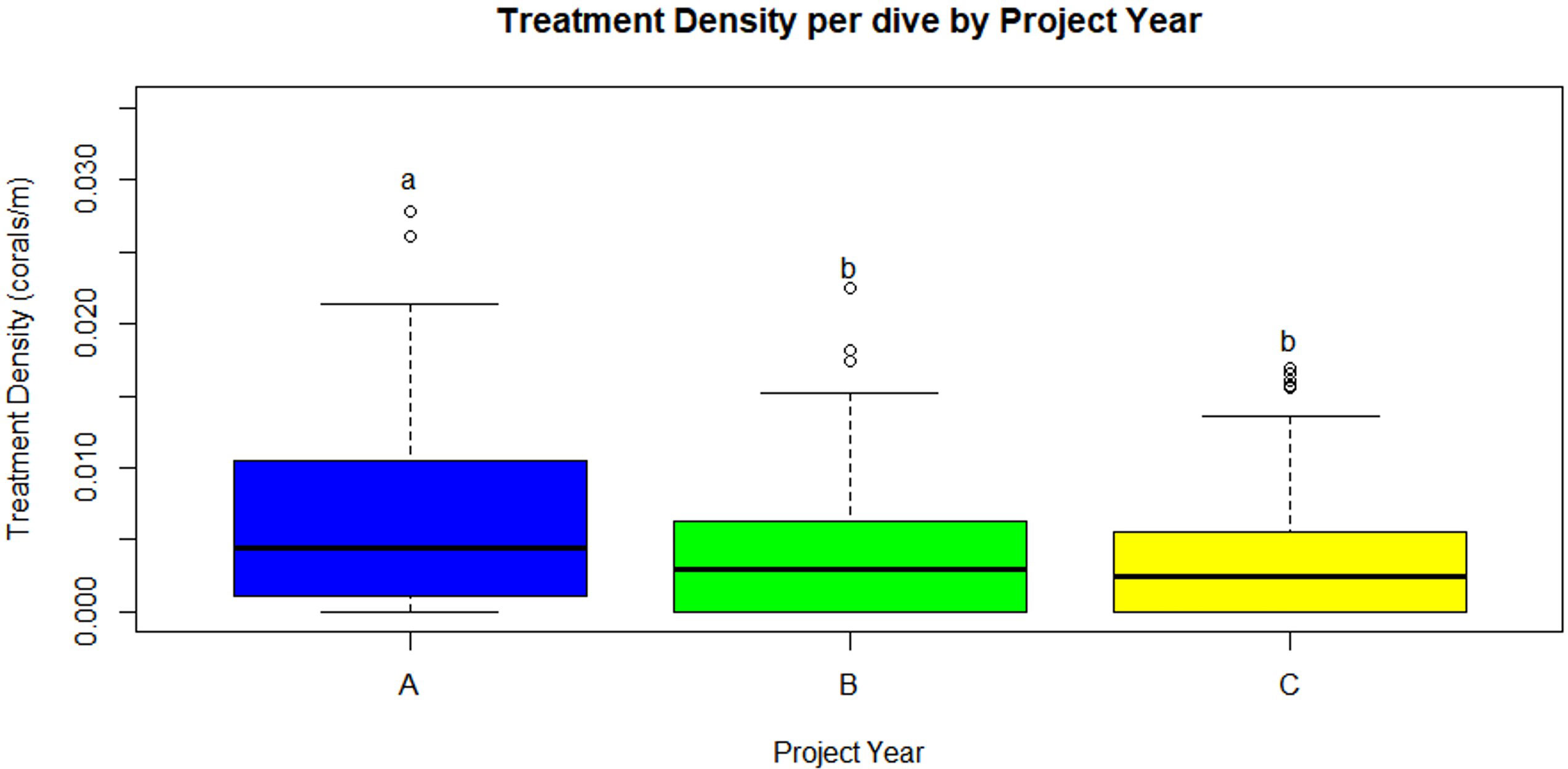
Figure 2 Treatment density per dive (treated corals/dive distance (m), median) was significantly higher in Year A than Years B and C (p=0.0102 and p<0.0001, respectively). Statistical significance is represented by letters. (Project Year classification: A- May 2019 through April 2020, B- May 2020 through April 2021, C- May 2021 through April 2022).
Total number of dives and treated corals varied by LCR (Table 2). The number of dives was lowest in Cape Florida Channel North (8) and highest in Haulover South (107). The number of treated corals was highest in Haulover South (333), Haulover North (290), and Hillsboro South (136). Treatment density per dive was not significantly correlated to latitude (z = -0.18352, p = 0.8544), however, a negative binomial GLM showed that LCRs were a significant predictor of treatment density (LR χ2 = 47.249, df = 7, p = 4.992 x 10-8, Figure 3A). The Haulover North region was a significant predictor of the model (z = 2.169, p = 0.0301). The regions with significant positive effects were Haulover North, Haulover South, and Hillsboro South, predicting higher treatment densities than other LCRs (p < 0.0001, p = 0.0001, p = 0.0031, respectively). The region with significant negative effects was Government Cut North, predicting lower treatment densities than other LCRs (p = 0.0131). The kernel density estimation for treatment density per dive exhibited a large area of high kernel density in Haulover South and medium values in Hillsboro South (Figure 4).
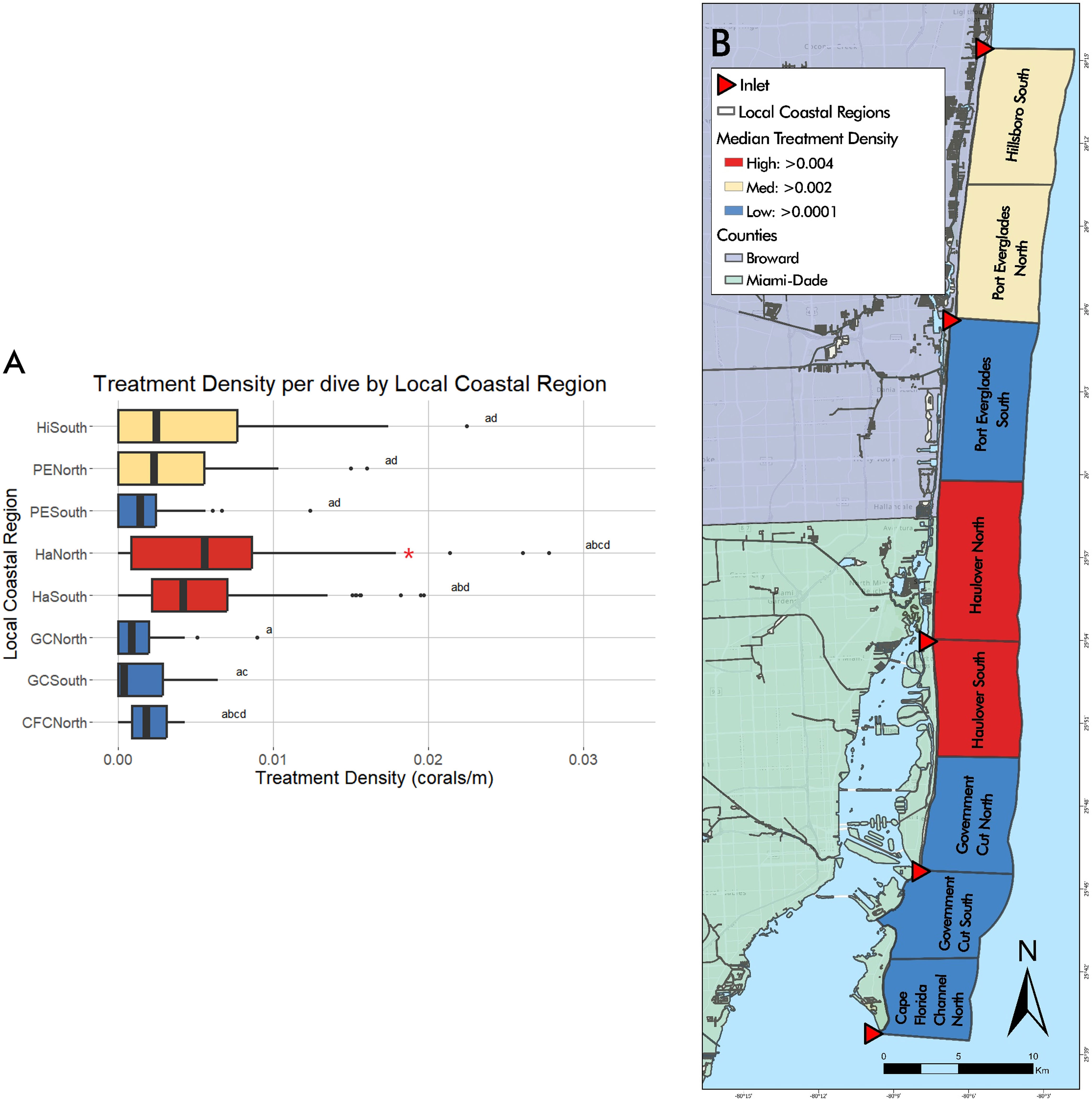
Figure 3 (A) Graphical representation of treatment density per dive by LCRs from May 2019 to April 2022. The highest median treatment density was found in Haulover North and South regions, followed by Hillsboro South and Port Everglades North. Red asterisks represent significant predictors of treatment density. Statistical significance is represented by letters. (B) Visual representation of median treatment density per dive by LCRs from May 2019 to April 2022. Median treatment density per dive by Local Coastal Region shown through gradient, high values shown in red, moderate in yellow, and low values in blue.
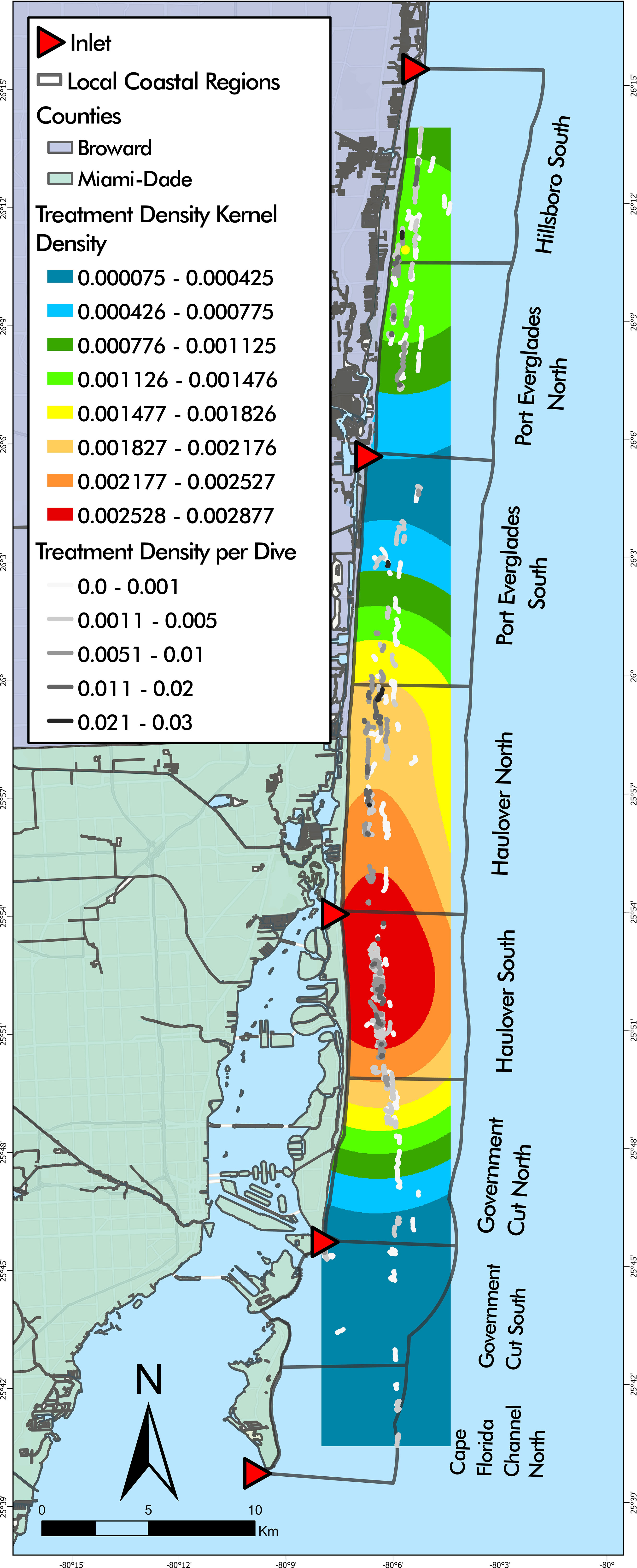
Figure 4 Disease intervention dive tracks overlaying treatment density kernel density estimation. The highest kernel density of treatment densities was found largely in Haulover South with a small portion in Haulover North.
LCR and project year were also identified as significant predictors of treatment density per dive (LR χ2 = 54.266, df = 7, p = 2.083 x 10-9 and LR χ2 = 22.422, df = 2, p = 1.352 x 10-5, respectively) (Figure 5). Haulover South was the only region with significant positive effects across all three years, predicting higher treatment densities than other LCRs (p < 0.0001, p = 0.001, p = 0.0162). No regions had significant negative effects across all three years. Haulover North, Hillsboro South, and Port Everglades North had significant positive effects in Year A, predicting higher treatment densities than other LCRs (p < 0.0001, p < 0.0001, and p = 0.0225, respectively). No regions had significant negative effects in Year A. Haulover North had significant positive effects in Year B (p = 0.0162). Government Cut North and Hillsboro South had significant negative effects in Year B, predicting lower treatment densities than all other LCRs (p = 0.0012 and p = 0.0162, respectively). Government Cut North had significant negative effects in Year C, predicting lower treatment densities than other LCRs (p = 0.0001). Haulover South was the only region with significant positive effects in Year C (p = 0.0162).
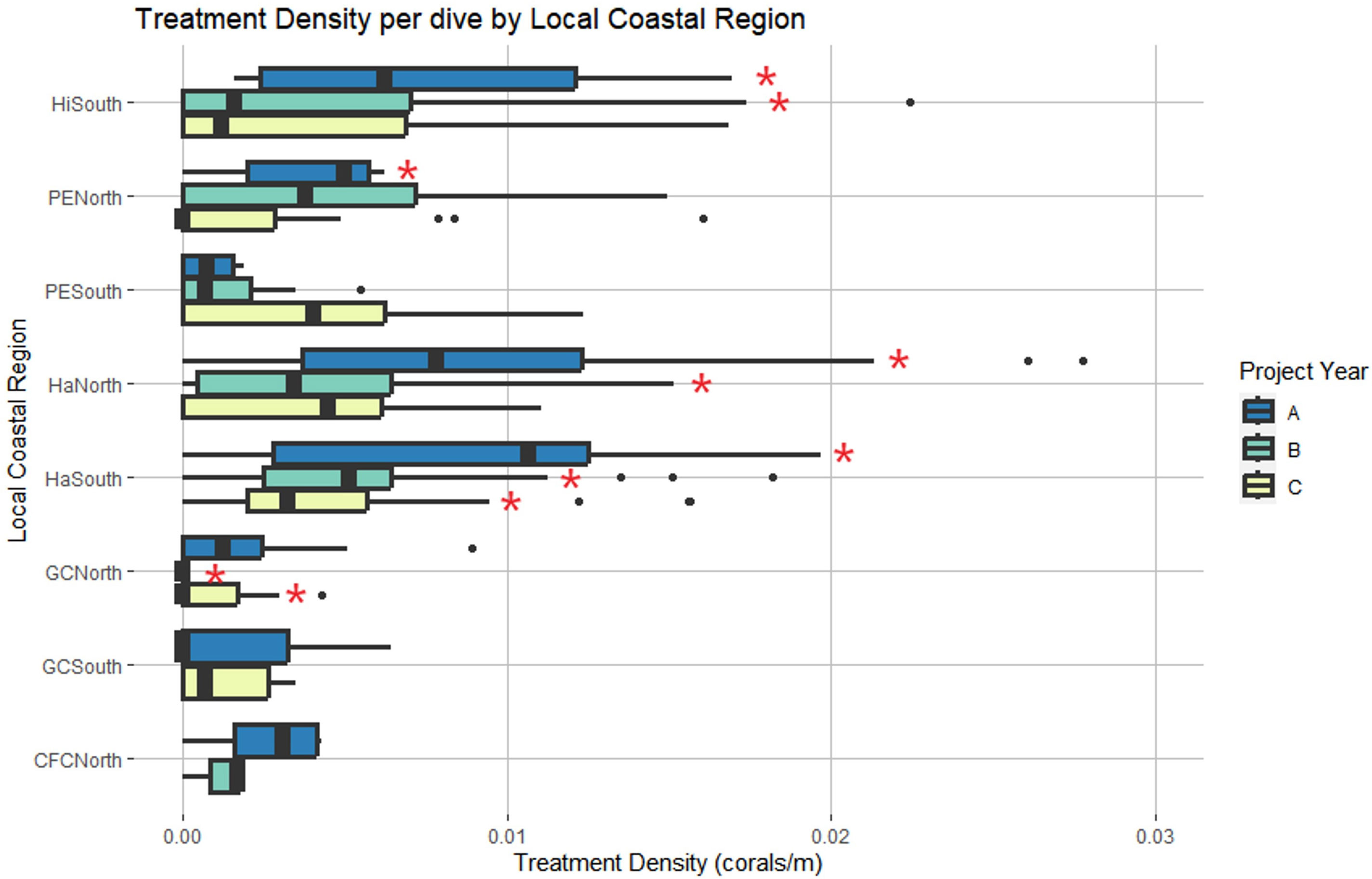
Figure 5 Local Coastal Region and project year were identified as significant predictors of treatment density per dive. Red asterisks represent region and year combinations that had significant effects on the model. Haulover South was the only region with significant effects across all three years. (Project Year classification: A- May 2019 through April 2020, B- May 2020 through April 2021, C- May 2021 through April 2022).
The kernel density estimation by project year showed that Year A had the highest clustering of high treatment density dives in Haulover North and Port Everglades South (Figure 6A). Year B was relatively consistent throughout the study area (Figure 6B) and did not indicate areas with higher or lower treatment density while Year C showed higher treatment density in Haulover South and Port Everglades South (Figure 6C). Interestingly, many Year B dives in Haulover North and South and Year C dives in Hillsboro South yielded low treatment densities, unlike Year A.
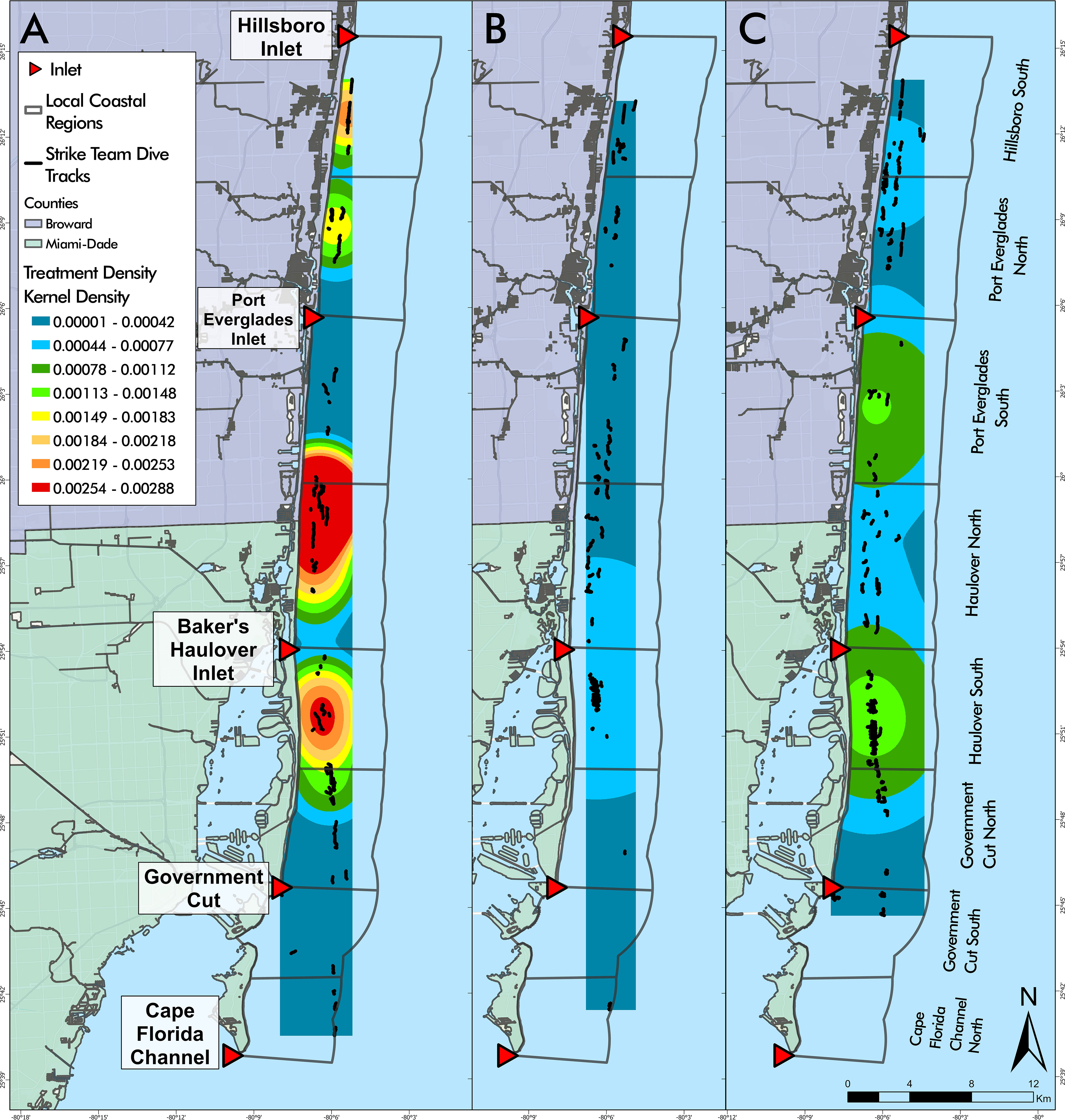
Figure 6 Disease intervention dive tracks by year overlaying kernel density estimations of treatment density per dive for each project year (A–C). Year (A) was the only year with high kernel density areas identified (red) (A). There was little variability of kernel density in Years (B, C). (Project Year classification: A- May 2019 through April 2020, B- May 2020 through April 2021, C- May 2021 through April 2022).
Seasonal dives and coral treatments varied over the project duration. The wet season had 2.5 times the number of treated corals (673) than the dry season (268) despite only having 1.6 times more dives (206 versus 127) (Table 3). Treatment density per dive was significantly higher in the wet season than in the dry season (p = 0.0001) and season and project year were significant predictors of treatment density each year (χ2 = 19.534, df =1, p = 9.885 x 10-6 and χ2 = 21.984, df = 2, p =1.684 x 10-5, respectively) (Figure 7). Treatment density in the wet season of Year A was significantly higher than that of the wet season of Year C (p < 0.001), however, treatment density of the wet season of Year B was not significantly different from the treatment density of Year A or Year C (p = 0.4218 and p = 0.0867, respectively). An F-test confirmed that season explained the variance in treatment density and had the same relative effect each year of the project in which wet season treatment densities were significantly higher than those in the dry season each year (F = 0.61069, p = 0.002786).
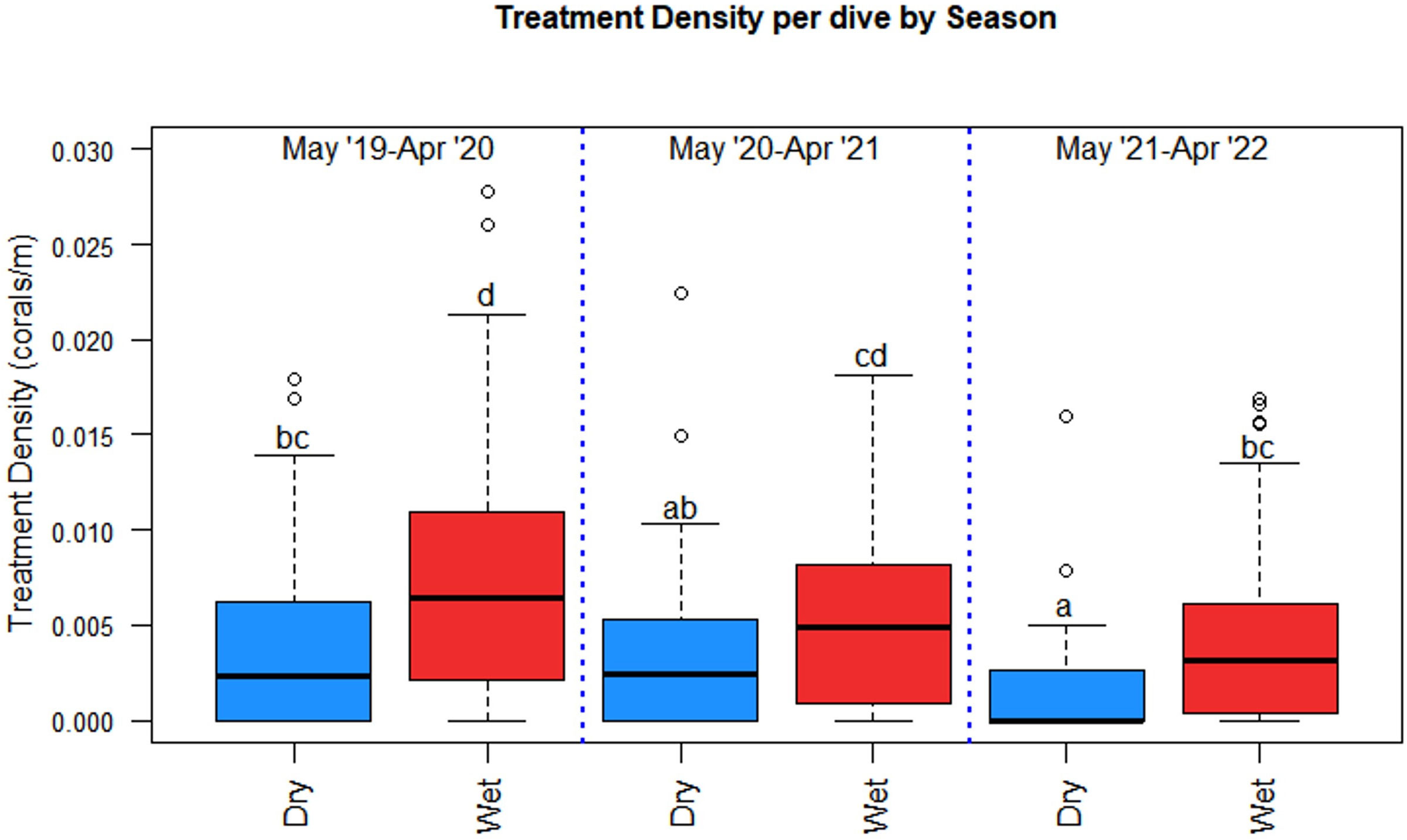
Figure 7 Season and project year were significant predictors of treatment density per dive where season had the same effect each project year. The wet season had significantly higher treatment densities than the dry season each year. Statistical significance is represented by letters.
A negative binomial GLM identified LCR and season as significant predictors of treatment density per dive (LR χ2 = 44.279, df = 7, p = 1.887 cx 10-7 and LR χ2 = 11.271, df = 1, p = 7.872 x 10-4, respectively) (Figure 8). Haulover North was the only region with significant positive effects across both wet and dry seasons, predicting higher treatment densities than other LCRs, no matter the season (p = 0.0187, p < 0.0001, respectively). No regions had significant negative effects across both seasons. Hillsboro South had significant but differing effects across wet and dry seasons despite having similar median treatment density values in both seasons (Figure 9). The region had significant negative effects in the wet season (p < 0.0001) and significant positive effects in the dry season (p = 0.0421), the opposite of the general seasonal trend seen within the data. Haulover South had significant positive effects in the wet season, predicting higher treatment densities than other LCRs (p < 0.0001). Port Everglades North had significant negative effects in the wet season (p = 0.0421). Government Cut North, Government Cut South, and Port Everglades South had significant negative effects in the dry season, predicting lower treatment densities than other LCRs (p = 0.0020, p = 0.0421, and p = 0.0187). The kernel density estimation of treatment density per dive by season showed distinct differences in each season (Figure 9). The highest values identified in the wet season occurred in the middle of Haulover South with a relatively high area identified on the border of Haulover North and Port Everglades South. The highest values identified in the dry season occurred at the border of Port Everglades north and Hillsboro South, an area where low values were seen in the wet season. However, all kernel density estimation values of treatment density in the dry season (November to April) were lower than all values estimated in the wet season (May to October).
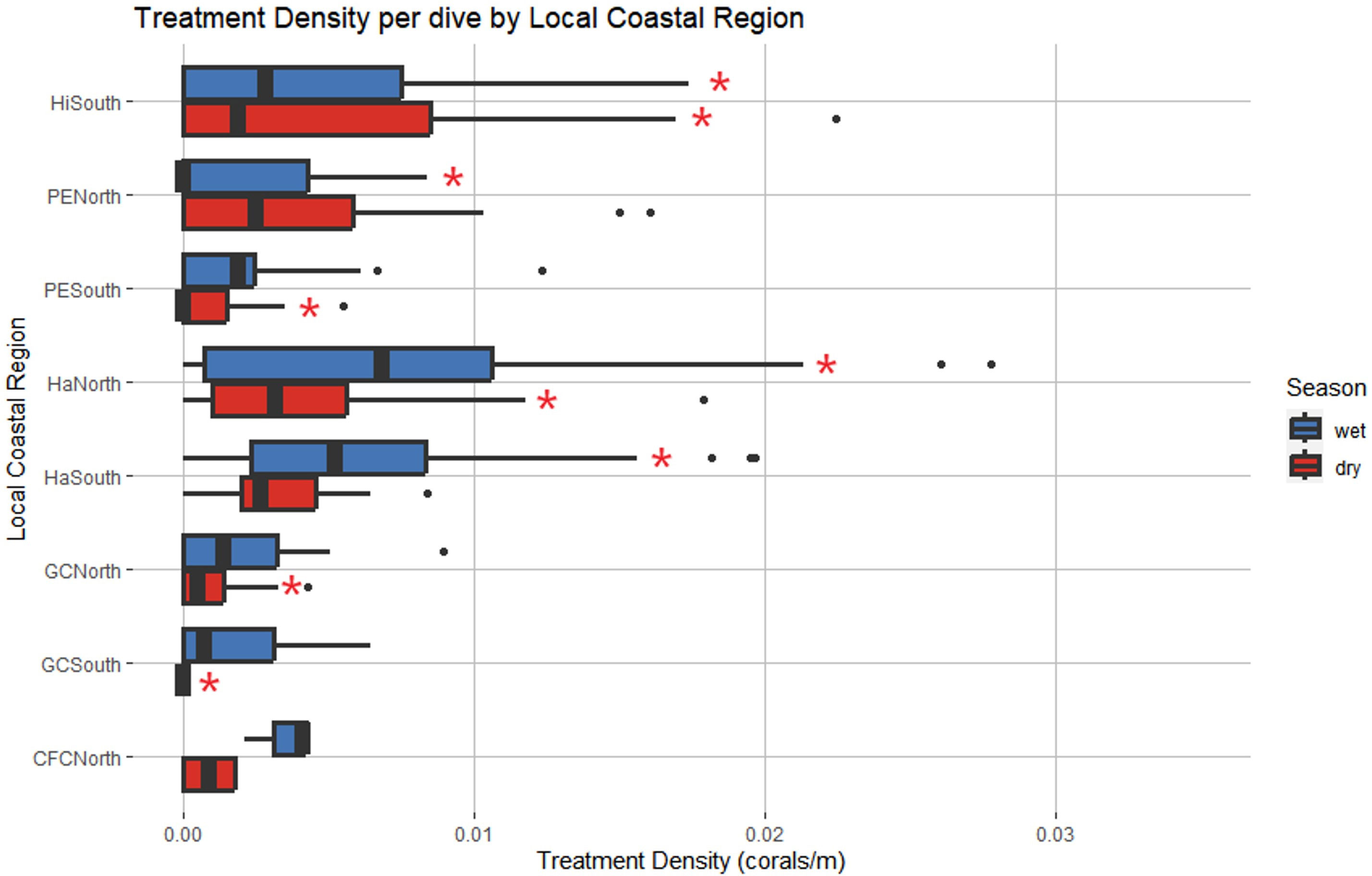
Figure 8 Local Coastal Region and season were identified as significant predictors of treatment density per dive. Red asterisks represent levels that had significant effects on the model. Haulover North was the only region with significant positive effects across both seasons. Hillsboro South had significant negative effects in the wet season and significant positive effects in the dry season, an opposing pattern to that identified in Figure 7. (Wet Season: May to October, Dry Season: November to April).
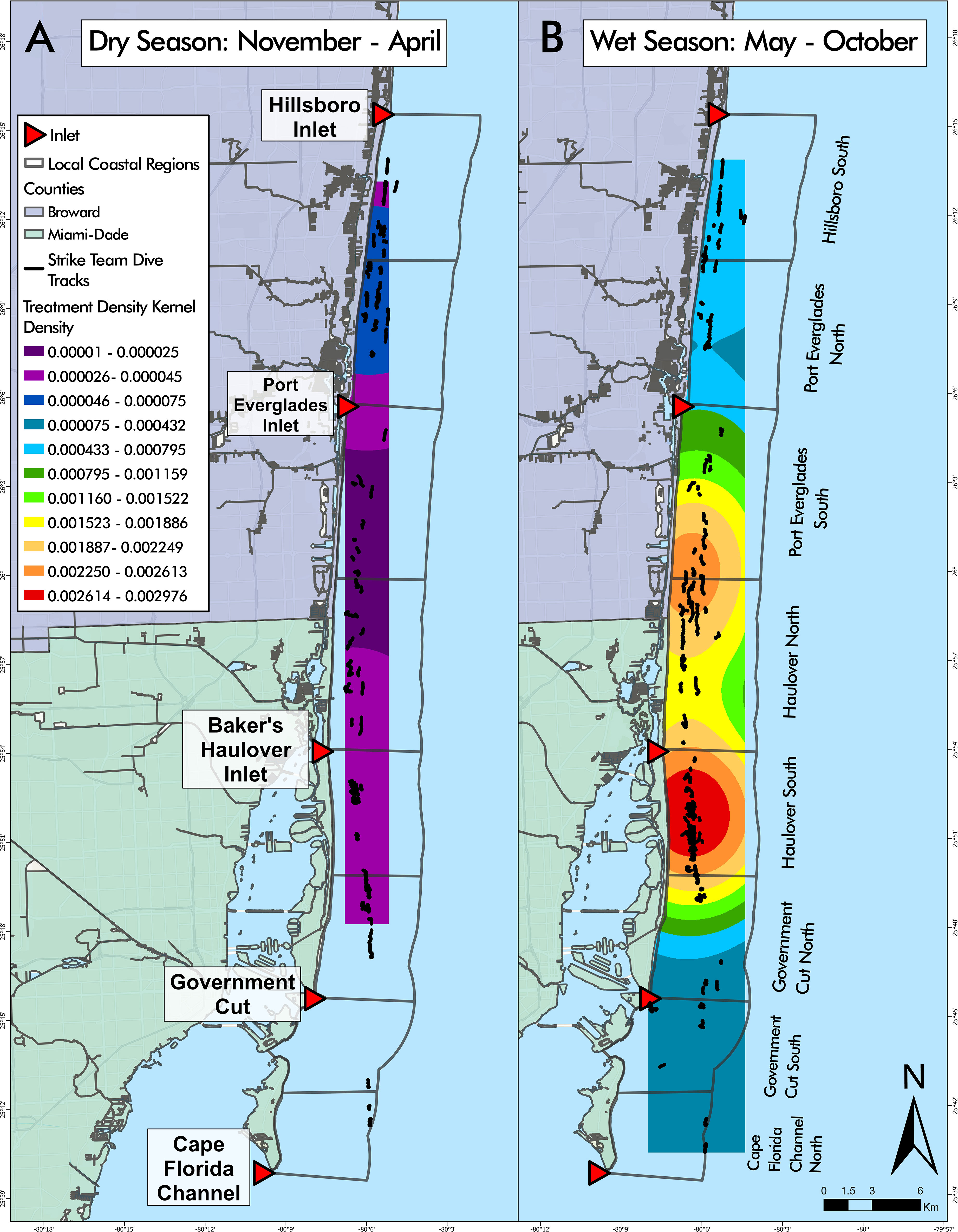
Figure 9 Disease intervention dive tracks overlaying seasonal kernel density estimations of treatment density per dive. Kernel density estimation values during the dry season, from November to April (A), were lower than all values estimated in the wet season, from May to October (B). Kernel density estimations showed that Hillsboro South and Port Everglades North were relatively consistent throughout seasons, with the highest values in the dry season and lowest values in the wet season. Conclusions regarding kernel density cannot be made for Cape Florida Channel North or Government Cut South due to the limited number of dives conducted during the dry season.
Discussion
Caribbean coral reef ecosystems are experiencing increased stressors and threats, endangering their long-term existence and their ability to provide numerous relied upon benefits (Lirman et al., 2018; Moberg and Folke 1999). SCTLD, potentially the most damaging threat to reefs to date, is spreading throughout many Caribbean and Mesoamerican locales threatening the future of Caribbean coral reef existence and functionality (Kramer et al., 2019; Lee Hing et al., 2022; Walker et al., 2021c). The number of treated corals in this study illustrates that SCTLD continued to infect reef building corals in endemic regions eight years after initial onset. The information provided herein can aid in planning disease intervention response in other locales.
Finding efficiencies in disease interventions helps optimize disease responses faced with limited available resources. This study provides guidance to increase SCTLD intervention efficiencies, especially once it becomes endemic. Disease interventions using CoralCure™, are highly effective at stopping lesions (Neely et al., 2021; Walker et al., 2021b), particularly when using a disease break, and should continue as long as SCTLD persists to save stony coral genetic diversity and decrease reliance on post hoc restoration (e.g. microfragging and outplanting). Saving existing corals maintains many ecological functions that may take many years to recover from post hoc restoration, if ever. This study supports that highly effective interventions weighted proportionally across space and time can optimize broadscale intervention efficiency. However, because spatiotemporal patterns of disease are often dynamic, periodic analyses may be needed to inform future efficiencies.
Although our study lacked comparable untreated control sites to specifically test the observed pattern, the spatiotemporal patterns of treatment densities herein are likely indicative of SCTLD spatial and temporal patterns. Treatment density was the number of treated corals standardized by the linear distance of a dive. The number of treated corals was not simply a function of longer dives because dive length only explained 5.8% of the variance in treatment density. Therefore, it is likely that higher treatment density was generally indicative of higher disease prevalence. Treated corals (Table 1) and treatment densities decreased throughout this study (Figure 6). Year A had the highest number of treated corals with fewer dives than Years B and C while more dives were needed in Year C to match the number of treatments in Year B and was not attributed to a decrease in dive distance. These variations perhaps indicate a decrease in disease prevalence, increase in disease-driven mortality, or both. Although the specific fate of each coral was not evaluated in this effort, regional coral cover, density, and live tissue area remained stable throughout the study period (Hayes et al., 2022; Viehman et al., 2023), indicating that disease-driven mortality was not a major factor. Generally, coral disease prevalence is strongly influenced by exposure, virulence, susceptibility, and colony health (Moriarty et al., 2020; Vega Thurber et al., 2020; Sweeny and Albery, 2022). As long as the source and etiology of SCTLD remains unknown, we can only speculate about disease exposures. However, disease interventions stop disease lesions (Neely et al., 2020; Neely et al., 2021; Walker et al., 2021b) and have the potential to decrease local pathogen load and reduce transmission to nearby individuals, as shown in other marine diseases (Atad et al., 2012; Groner et al., 2016). Mean treatment density decreased significantly after the initial year of intervention activity, from 0.0063 treated corals/m to 0.0038 treated corals/m meaning increased area was required to treat the same number of corals. Returning to previous reef areas of higher treatment density did not yield the same treatment density, suggesting that disease prevalence did not stay consistently high over time. These results align with Forrester et al. (2022) who found reduced SCTLD prevalence, lesion severity, and mortality at treatment sites in the British Virgin Islands. Although only few individuals at each treatment site received treatment, such sites exhibited community-wide benefits such as decreased disease prevalence over time and an increase in cover of some highly susceptible species. Due to the lack of control sites within our study, we cannot explicitly conclude sites with treated corals exhibited similar patterns to Forrester et al. (2022), but the decrease in disease prevalence throughout the study suggests this as a plausible explanation.
Abundance and virulence of coral diseases are known to fluctuate through space and time with varying environmental factors (Vega Thurber et al., 2014), and disease prevalence can be exacerbated by increased sea surface temperatures and weekly sea surface temperature anomalies (Burke et al., 2023). SEFL has two distinct seasons which coincide with temperature, wherein the wet season is the warmest and the dry season the coolest. A number of studies show temporal fluctuations of SCTLD in the Coral ECA with the highest metrics during the warm, wet season (Walker et al., 2021c; Walker et al., 2022b). Mean temperature, mean rainfall and flow out of the inlets over various temporal windows explained 63.1% of the variation in the number of SCTLD treatments and 66.2% of the total number of corals with lesions on the large Orbicella faveolata population. Treatment densities exhibited distinct seasonal and spatial patterns throughout each year and were significantly higher in the hotter wet season every year (Figure 7). These results indicate that a larger proportion of disease intervention effort should be prioritized during the hotter wet season in the Coral ECA. However, a lower proportion of disease intervention activity still must be conducted in the dry season since disease remains year-round and corals can die in a few weeks (Aeby et al., 2019; Neely et al., 2021; Walker et al., 2021b).
Treatment densities were spatially variable along the coast, however the lack of correlation with latitude suggests that disease differences were likely driven by other factors (Figure 4). Our results indicate that disease interventions should be spatially allocated proportionally to focus more effort in regions of previously higher efficiency, while still maintaining lower effort (perhaps less frequent) across all areas. Haulover North, Haulover South, and Hillsboro South had the highest treatment densities, which may mean that disease prevalence was higher in these areas (Figure 3). Most of the coral reefs in the Coral ECA occur within three miles of the coast (Walker, 2012), making them highly influenced by coastal activities such as beach restoration (Jordan et al., 2010), dredging and shipping (Walker, 2012), runoff from land, and discharge from inlets and outflow pipes (Wear and Thurber, 2015). Anthropogenically induced water quality decline has been linked to increased coral disease prevalence elsewhere (Bruno et al., 2003) making it an important consideration for SCTLD. Staley et al. (2017) found that in southern Coral ECA sites, near Haulover Inlet and Government Cut, reef water and coral mucus prokaryotic communities were more influenced by outfall source communities than more northern sites, near Port Everglades and Hillsboro Inlet, which were more influenced by ocean water source communities. These source communities likely influence the coral microbiome, potentially shifting community structure and impacting the coral’s resistance to pathogens (Pollock et al., 2019). Interestingly, the regions with highest treatment densities mirror the spatial epidemiological probability models of the spread of SCTLD which found peaks near Haulover Inlet and Hillsboro Inlet in 2016 (Muller et al., 2020). Although the relationship between nutrients and SCTLD bears more research focus, other countries experiencing SCTLD should consider the presence and location of inlets, outfalls, and water sources, and their impacts, in disease monitoring and response.
Seasonal increases of rainfall and runoff may exacerbate water quality decline. The general inland water flow in 2019 was southwestward throughout Broward and northern Miami-Dade Counties (Abtew et al., 2019). However, near the midpoint of Miami-Dade County, the water flow turns eastward, aligning latitudinally with Haulover Inlet (Abtew et al., 2019). This increased water flow and subsequent runoff intensified in the wet season may help to explain the heightened number of diseased corals and treatment density in the Haulover regions because nutrient loading/enrichment has the potential to lower the resistance of corals to disease pathogens (Bruno et al., 2003; Vega Thurber et al., 2014). Statistical models of water quality data at reef sites between 2018 and 2021 found that inlet flow, rainfall, and wind predictors explained 79% of the model variation of orthophosphates and 55% of nitrates (Walker et al., 2022b). Since rain and inlet flow also correlate with increased SCTLD (Walker et al., 2022b), it is possible that SCTLD is exacerbated seasonally by excessive nutrients during increased rainfall and inlet flow rates.
Higher coral disease prevalence can be associated with higher host density (Bruno et al., 2007; Walton et al., 2018; Hayes et al., 2022), but this can depend on site diversity (Muller et al., 2020; Costa et al., 2021), exposure (Studivan et al., 2022), stress (Precht et al., 2016), and species susceptibility (Precht et al., 2016; Florida Coral Disease Response Research & Epidemiology Team, 2018). The scope of our efforts was too large to account for varying coral density between dives because counting all corals along a 724 m x 15 m search area was not feasible. However, coral density of the nearshore habitats within the study area is fairly homogenous on a regional scale (Burman et al., 2012; Klug, 2015). Given the haphazard nature of the dives, the amount of reef habitat covered per dive, and replication between years and regions, differences in coral densities between dives probably did not have a significant effect on our results.
Identifying and periodically revisiting high coral density areas can increase intervention efficiency because it allows more colonies to be assessed over a dive. Interventions at high-density locations may have an added benefit of increasing the likelihood of successful natural reproduction by attempting to save corals already located nearby one another. This is especially important at restoration sites where corals are planted at higher densities, such as spawning hubs (Gilliam et al., 2021). Spawning hubs are areas where corals of targeted restoration species are brought in close spatial proximity to increase local densities with the hopes of increasing fertilization success and natural reef recovery (Gilliam et al., 2021). Costa et al. (2021) found an increase in SCTLD disease prevalence with increased species diversity in epidemic (2-6 months) and endemic (>9 months) zones, however coral cover declines were less apparent because of the rarity of highly susceptible species. Since disease prevalence can be related to host density and diversity, disease monitoring and interventions at restoration sites are necessary to attempt to reduce local disease loads and save the live tissue of costly, valuable relocated corals.
Continued research is necessary to fully identify the disease-causing agent and specific stressors leading to the development of SCTLD lesions to advance treatment methods specific to stony coral tissue loss disease. However, disease intervention has been shown to be an effective tool in helping to conserve live coral tissue and thus decreasing the burden and cost of post hoc reef restoration. By increasing disease intervention activity efficiency, resources can be used to broaden the scope of disease responses.
Data availability statement
The original contributions presented in the study are included in the article/Supplementary material, further inquiries can be directed to the corresponding author.
Ethics statement
The manuscript presents research on animals that do not require ethical approval for their study.
Author contributions
KT: Formal Analysis, Investigation, Methodology, Validation, Visualization, Writing – original draft, Writing – review & editing. SB: Data curation, Methodology, Project administration, Writing – review & editing. HN: Methodology, Project administration, Writing – review & editing. KN: Writing – review & editing. BW: Conceptualization, Funding acquisition, Methodology, Resources, Supervision, Writing – review & editing.
Funding
The author(s) declare financial support was received for the research, authorship, and/or publication of this article. Funding for disease intervention activities was provided by the Florida Department of Environmental Protection (FDEP) Award No. B96800. The funders had no role in data collection and analysis, decision to publish, or preparation of this manuscript.
Acknowledgments
We would like to thank the Florida Department of Environmental Protection for funding the disease intervention activities conducted under Award No. B96800. We also thank members of the Department of Regulatory and Economic Resources, Environmental Resources Management Restoration & Enhancement Section for conducting extensive disease intervention dives and treatments throughout the project. Without this support, our local reefs would have suffered even further damage from stony coral tissue loss disease. We also thank members of the GIS and Spatial Ecology Lab at Nova Southeastern University who have helped to collect and manage the study data.
Conflict of interest
The authors declare that the research was conducted in the absence of any commercial or financial relationships that could be construed as a potential conflict of interest.
Publisher’s note
All claims expressed in this article are solely those of the authors and do not necessarily represent those of their affiliated organizations, or those of the publisher, the editors and the reviewers. Any product that may be evaluated in this article, or claim that may be made by its manufacturer, is not guaranteed or endorsed by the publisher.
Supplementary material
The Supplementary Material for this article can be found online at: https://www.frontiersin.org/articles/10.3389/fmars.2023.1302697/full#supplementary-material
References
Abtew W., Qiu C., Ciuca V. (2019). “Chapter 2: South Florida Hydrology and Water Management,” in 2019 South Florida Environmental Report- Volume I (West Palm Beach, Florida: South Florida Water Management District).
Aeby G., Ushijima B., Bartels E., Walter C., Kuehl J., Jones S., et al. (2021). Changing stony coral tissue loss disease dynamics through time in montastraea cavernosa. Front. Mar. Sci. 8 (September). doi: 10.3389/fmars.2021.699075
Aeby G. S., Ushijima B., Campbell J. E., Jones S., Williams G. J., Meyer J. L., et al. (2019). Pathogenesis of a tissue loss disease affecting multiple species of corals along the Florida reef tract. Front. Mar. Sci. 6 (November). doi: 10.3389/fmars.2019.00678
Aeby G. S., Work T. M., Runyon C. M., Shore-Maggio A., Ushijima B., Videau P., et al. (2015). First record of black band disease in the hawaiian archipelago: response, outbreak status, virulence, and a method of treatment. PloS One 10 (3), e0120853. doi: 10.1371/journal.pone.0120853
Alvarez-Filip L., González-Barrios F.J., Pérez-Cervantes E., Molina-Hernández A., Estrada-Saldívar N. (2022). Stony coral tissue loss disease decimated Caribbean coral populations and reshaped reef functionality. Commun. Biol. 5 (1), 440. doi: 10.1038/s42003-022-03398-6
Atad I., Zvuloni A., Loya Y., Rosenberg E. (2012). Phage therapy of the white plague-like disease of Favia favus in the Red Sea. Coral Reefs 31, 665–670. doi: 10.1007/s00338-012-0900-5
Barnes B. B., Hu C., Kovach C., Silverstein R. N. (2015). Sediment plumes induced by the port of miami dredging: analysis and interpretation using landsat and MODIS data. Remote Sens. Environ. 170 (December), 328–339. doi: 10.1016/j.rse.2015.09.023
Bruno J. F., Petes L. E., Harvell C.D., Hettinger A. (2003). Nutrient enrichment can increase the severity of coral diseases: effect of nutrients on coral disease severity. Ecol. Lett. 6 (12), 1056–1061. doi: 10.1046/j.1461-0248.2003.00544.x
Bruno J. F., Selig E. R., Casey K. S., Page C. A., Willis B. L., Harvell C.D., et al. (2007). Thermal stress and coral cover as drivers of coral disease outbreaks. PloS Biol. 5 (6), e124. doi: 10.1371/journal.pbio.0050124
Burke S., Pottier P., Lagisz M., Macartney E. L., Ainsworth T., Drobniak S. M., et al. (2023). The impact of rising temperatures on the prevalence of coral diseases and its predictability: A global meta-analysis. Ecol. Lett. 26, 1466–1481. doi: 10.1111/ele.1426
Burman S., Aronson R., Woesik R. v. (2012). Biotic homogenization of coral assemblages along the Florida reef tract. Mar. Ecol. Prog. Ser. 467, 89–96. doi: 10.3354/meps09950
Costa S. V., Hibberts S. J., Olive D. A., Budd K. A., Long A. E., Meiling S. S., et al. (2021). Diversity and disease: the effects of coral diversity on prevalence and impacts of stony coral tissue loss disease in saint thomas, U.S. Virgin islands. Front. Mar. Sci. 8. doi: 10.3389/fmars.2021.682688
Cróquer A., Weil E., Rogers C. S. (2021). Similarities and differences between two deadly caribbean coral diseases: white plague and stony coral tissue loss disease. Front. Mar. Sci. 8 (1331). doi: 10.3389/fmars.2021.709544
Cunning R., Silverstein R. N., Barnes B. B., Baker A. C. (2019). Extensive coral mortality and critical habitat loss following dredging and their association with remotely-sensed sediment plumes. Mar. pollut. Bull. 145 (August), 185–199. doi: 10.1016/j.marpolbul.2019.05.027
Davis S., Ogden J. C. (1994). Everglades: The Ecosystem and Its Restoration. 1st ed (Delray Beach, Florida: CRC Press). doi: 10.1201/9781466571754
Dobbelaere T., Muller E. M., Gramer L. J., Holstein D. M., Hanert E. (2020). Coupled epidemio-hydrodynamic modeling to understand the spread of a deadly coral disease in Florida. Front. Mar. Sci. 7 (December). doi: 10.3389/fmars.2020.591881
Efrony R., Atad I., Rosenberg E. (2009). Phage Therapy of Coral White Plague Disease: Properties of Phage BA3. Curr. Microbiol. 58 (2), 139–145. doi: 10.1007/s00284-008-9290-x
Esri Inc (2023). ArcGIS Pro (Version 3.1.2) (Esri Inc). Available at: https://www.esri.com/en-us/arcgis/products/arcgis-pro/overview.
Estrada-Saldívar N., Molina-Hernández A., Pérez-Cervantes E., Medellín-Maldonado F., González-Barrios F.J., Alvarez-Filip L. (2020). Reef-scale impacts of the stony coral tissue loss disease outbreak. Coral Reefs 39, 861–866. doi: 10.1007/s00338-020-01949-z
Florida Coral Disease Response Research & Epidemiology Team (2018) NOAA Stony coral tissue loss disease case definition. Available at: https://floridadep.gov/sites/default/files/Copy%20of%20StonyCoralTissueLossDisease_CaseDefinition%20final%2010022018.pdf.
Forrester G. E., Arton L., Horton A., Nickles K., Forrester L. M. (2022). Antibiotic treatment ameliorates the impact of stony coral tissue loss disease (SCTLD) on coral communities. Front. Mar. Sci. 9. doi: 10.3389/fmars.2022.859740
Gilliam D. S., Figueiredo J., VanWynen C. (2021). Stony Coral Spawning Hubs in the Southeast Florida Coral Reef Ecosystem Conservation Area: Phase 1 Final Report (Miami Beach, FL: Florida DEP), 29.
Groner M. L., Maynard J., Breyta R., Carnegie R. B., Dobson A., Friedman C. S., et al. (2016). Managing marine disease emergencies in an era of rapid change. Philos. Trans. R. Soc. B: Biol. Sci. 371 (1689). doi: 10.1098/rstb.2015.0364
Haapkylä J., Unsworth R. K. F., Flavell Mike, Bourne D. G., Schaffelke B., Bette L W. (2011). Seasonal rainfall and runoff promote coral disease on an inshore reef. PloS One 6 (2), e16893. doi: 10.1371/journal.pone.0016893
Hayes N. K., Walton C. J., Gilliam D. S. (2022). Tissue loss disease outbreak significantly alters the southeast Florida stony coral assemblage. Front. Mar. Sci. 9 (August). doi: 10.3389/fmars.2022.975894
Lee Hing C., Guifarro Z., Dueñas D., Ochoa G., Nunez A., Forman K., et al. (2022). Management Responses in Belize and Honduras, as Stony Coral Tissue Loss Disease Expands Its Prevalence in the Mesoamerican Reef. Front. Mar. Sci. 9, 883062. doi: 10.3389/fmars.2022.883062
Howells E., Vaughan G., Work T., Burt J., Abrego D. (2020). Annual outbreaks of coral disease coincide with extreme seasonal warming. Coral Reefs 39. doi: 10.1007/s00338-020-01946-2
Hudson J. H. (2000). First aid for massive corals infected with black band disease, Phormidium corallyticum: an underwater aspirator and post-treatment sealant to curtail reinfection. . Diving science AAUS 20th symposium proceedings;, 10–11.
Iwanowicz D. D., Schill W. B., Woodley C. M., Bruckner A., Neely K., Briggs K. M. (2020). Exploring the stony coral tissue loss disease bacterial pathobiome. bioRxiv 2020.05.27.120469. doi: 10.1101/2020.05.27.120469
Jones N. P., Figueiredo J., Gilliam D. S. (2020). Thermal stress-related spatiotemporal variations in high-latitude coral reef benthic communities. Coral Reefs 39 (6), 1661–1673. doi: 10.1007/S00338-020-01994-8
Jones N. P., Kabay L., Lunz K. S., Gilliam D. S. (2021). Temperature stress and disease drives the extirpation of the threatened pillar coral, Dendrogyra cylindrus, in southeast Florida. Sci. Rep. 11 (1), 14113. doi: 10.1038/s41598-021-93111-0
Jordan L., Banks K. W., Fisher L. E., Walker B. K., Gilliam D. S. (2010). Elevated sedimentation on coral reefs adjacent to a beach nourishment project. Mar. pollut. Bull. 60 (2), 261–271. doi: 10.1016/j.marpolbul.2009.08.032
Klug K. (2015). Cross-Shelf and Latitudinal Benthic Community Investigation in the Nearshore Habitats of the Northern Florida Reef Tract (Dania Beach, Florida: Nova Southeastern University. NSUWorks). Available at: http://nsuworks.nova.edu/occ_stuetd/386.
Kramer P. R., Roth L., Lang J. (2019) Map of Stony Coral Tissue Loss Disease Outbreak in the Caribbean. Available at: https://www.agrra.org/coral-disease-outbreak/ (Accessed 14, 2022).
Landsberg J. H., Kiryu Y., Peters E. C., Wilson P. W., Perry N., Waters Y., et al. (2020). Stony coral tissue loss disease in florida is associated with disruption of host–zooxanthellae physiology. Front. Mar. Sci. 7 (December). doi: 10.3389/fmars.2020.576013
Lenth R. V., Buerkner P., Herve M., Love J., Miguez F., Riebl H., et al. (2022). Package ‘emmeans’: Estimated marginal means, aka least-squares means. Version 1 (7), 5. https://cran.r-project.org/web/packages/emmeans/emmeans.pdf.
Lirman D., Ault J. S., Fourqurean J. W., Lorenz J. J. (2018). The Coastal Marine Ecosystem of South Florida, United States. World Seas: Environ. Eval. Volume I: Europe Americas West Afr., 427–444. doi: 10.1016/B978-0-12-805068-2.00021-8
Manzello D. P. (2015). Rapid recent warming of coral reefs in the florida keys. Sci. Rep. 5 (1), 16762. doi: 10.1038/srep16762
Meiling S., Muller E. M., Smith T. B., Brandt M. E. (2020). 3D photogrammetry reveals dynamics of stony coral tissue loss disease (SCTLD) lesion progression across a thermal stress event. Front. Mar. Sci. 7. doi: 10.3389/fmars.2020.597643
Minnesota Department of Natural Resources (2011). DNRGPS. Version 6.1.0.6. ABOUT — DNRGPS 6.0 documentation (state.mn.us).
Moberg F., Folke C. (1999). Ecological Goods and Services of Coral Reef Ecosystems. Ecol. Economics 29 (2), 215–233. doi: 10.1016/S0921-8009(99)00009-9
Moriarty T., Leggat W., Huggett M. J., Ainsworth T. D. (2020). Coral disease causes, consequences, and risk within coral restoration. Trends Microbiol. 28 (10), 793–807. doi: 10.1016/j.tim.2020.06.002
Muller E. M., Sartor C., Alcaraz N. I., Woesik R. v. (2020). Spatial epidemiology of the stony-coral-tissue-loss disease in florida. Front. Mar. Sci. 7 (March). doi: 10.3389/fmars.2020.00163
Neely K. L., Macaulay K. A., Hower E. K., Dobler M. A. (2020). Effectiveness of topical antibiotics in treating corals affected by stony coral tissue loss disease. PeerJ 6). doi: 10.7717/peerj.9289
Neely K. L., Shea C. P., Macaulay K. A., Hower E. K., Dobler M. A. (2021). Short- and long-term effectiveness of coral disease treatments. Front. Mar. Sci. 8 (August). doi: 10.3389/fmars.2021.675349
Pollock F.J., Lamb J. B., de Water J. A.J.M., Smith H. A., Schaffelke B., Willis B. L., et al. (2019). Reduced diversity and stability of coral-associated bacterial communities and suppressed immune function precedes disease onset in corals. R. Soc Open Sci. 6, 190355. doi: 10.1098/rsos.190355
Precht W. F., Gintert B. E., Robbart M. L., Fura R., Robert Van W. (2016). Unprecedented disease-related coral mortality in Southeastern Florida. Sci. Rep. 6, 1–11. doi: 10.1038/srep31374
Randall C. J., Whitcher E. M., Code T., Pollock C., Lundgren I., Hillis-Starr Z., et al. (2018). Testing Methods to Mitigate Caribbean Yellow-Band Disease on Orbicella Faveolata. PeerJ 6, e4800. doi: 10.7717/peerj.4800
R Core Team (2021). Version 4.1.0. R: “A language and environment for statistical computing (Vienna, Austria: R Foundation for Statistical Computing). Available at: https://www.R-project.org/.
Rosales S. M., Huebner L. K., Evans J. S., Apprill A., Baker C. C., et al. (2023). A meta-analysis of the stony coral tissue loss disease microbiome finds key bacteria in unaffected and lesion tissue in diseased colonies. ISME Commun 3, 19. doi: 10.1038/s43705-023-00220-0
Shilling E. N., Combs I. R., Voss J. D. (2021). Assessing the effectiveness of two intervention methods for stony coral tissue loss disease on montastraea cavernosa. Sci. Rep. 11 (1). doi: 10.1038/s41598-021-86926-4
Spadafore R., Fura R., Precht W. F., Vollmer S. V. (2021). Multi-variate analyses of coral mortality from the 2014–2015 stony coral tissue loss disease outbreak off Miami-Dade County, Florida. Front. Mar. Sci. 8 (September). doi: 10.3389/fmars.2021.723998
Staley C., Kaiser T., Gidley M. L., Enochs I. C., Jones P. R., Goodwin K. D., et al. (2017). Differential impacts of land-based sources of pollution on the microbiota of Southeast Florida coral reefs. Appl. Environ. Microbiol. 83, e03378–e03316. doi: 10.1128/AEM.03378-16
Studivan M. S., Rossin A. M., Rubin E., Soderberg N., Holstein D. M., Enochs I. C. (2022). Reef sediments can act as a stony coral tissue loss disease vector. Front. Mar. Sci. 8 (January). doi: 10.3389/fmars.2021.815698
Sweeny A. R., Albery G. F. (2022). Exposure and susceptibility: The Twin Pillars of infection. Funct. Ecol. 36, 1713–1726. doi: 10.1111/1365-2435.14065
Sweet M. J., Croquer A., Bythell J. C. (2014). Experimental antibiotic treatment identifies potential pathogens of white band disease in the endangered caribbean coral acropora cervicornis. Proc. R. Soc. B: Biol. Sci. 281 (1788). doi: 10.1098/rspb.2014.0094
Teplitski M., Ritchie K. (2009). How Feasible Is the Biological Control of Coral Diseases? Trends Ecol. Evol. 24 (7), 378–385. doi: 10.1016/j.tree.2009.02.008
Toth L. T., Courtney T. A., Colella M. A., Ruzicka R. R. (2023). Stony coral tissue loss disease accelerated shifts in coral composition and declines in reef accretion potential in the Florida Keys. Front. Mar. Sci. 10. doi: 10.3389/fmars.2023.1276400
Vega Thurber R. L., Burkepile D. E., Fuchs C., Shantz A. A., McMinds R., Zaneveld J. R. (2014). Chronic nutrient enrichment increases prevalence and severity of coral disease and bleaching. Glob Change Biol. 20, 544–554. doi: 10.1111/gcb.12450
Vega Thurber R., Mydlarz L. D., Brandt M., Harvell D., Weil E., Raymundo L., et al. (2020). Deciphering coral disease dynamics: integrating host, microbiome, and the changing environment. Front. Ecol. Evol. 8. doi: 10.3389/fevo.2020.575927
Veron J. E. N. (1995). Coral in space and time: The biogeography and evolution of the Scleractinia (Ithaca, London: NSW University Press).
Viehman T. S, Grove L. J.W., Blondeau J., Cain E., Edwards K. F., Groves S. H., et al. (2023). National coral reef monitoring program, biological monitoring summary – Florida : 2022. NOAA Technical Memorandum NOS CRCP, 48. doi: 10.25923/e6xg-1989
Walker B. K. (2012). Spatial analyses of benthic habitats to define coral reef ecosystem regions and potential biogeographic boundaries along a latitudinal gradient. PloS One 7 (1), e30466. doi: 10.1371/journal.pone.0030466
Walker B. (2018). Southeast Florida Reef-Wide Post-Irma Coral Disease Surveys (Miami, FL: Florida DEP), 1–37.
Walker B., Noren H., Sharkey R., Buckley S. (2022a). 2021-2022 SE FL ECA Reef-Building-Coral Disease Intervention and Preparation for Restoration Progress Report (Miami, FL.: Florida DEP). 33p.
Walker B. K., Riegl B., Dodge R. E. (2008). Mapping coral reef habitats in southeast Florida using a combined technique approach. J. Coast. Res. (West Palm Beach, Florida) 24 (5), 1138–1150. http://www.allenpress.com/pdf/coas_24_313_1138_1150.pdf.
Walker B. K., Turner N. R., Brunelle A., Noren H., Buckley S. (2021a). 2020-2021 Coral ECA Reef-Building-Coral Disease Intervention and Preparation for Restoration Final Report (Miami, FL.: Florida DEP). 22p.
Walker B. K., Turner N. R., Noren H. K. G., Buckley S. F., Pitts K. A. (2021b). Optimizing stony coral tissue loss disease (SCTLD) intervention treatments on montastraea cavernosa in an endemic zone. Front. Mar. Sci. 8. doi: 10.3389/fmars.2021.666224
Walker B. K., Williams G. J., Aeby G. S., Maynard J. A., Whitall D. (2022b). Environmental and human drivers of stony coral tissue loss disease (SCTLD) incidence within the Southeast Florida Coral Reef Ecosystem Conservation Area, 2021-22. Final Report (Miami, FL.: Florida DEP). 36 p.
Walker B. K., Williams G. J., Maynard J. A. (2021c). 2020-2021 Coral ECA Reef-Building Coral Disease Intervention, Statistical Modelling, and Preparation for Restoration Task 6 Final Report: Environmental drivers of stony coral tissue loss disease (Miami, FL.: Florida DEP). 122p.
Walton C. J., Hayes N. K., Gilliam D. S. (2018). Impacts of a regional, multi-year, multi-species coral disease outbreak in Southeast Florida. Front. Mar. Sci. 5 (SEP). doi: 10.3389/fmars.2018.00323
Wear S. L., Thurber R. V. (2015). Sewage pollution: mitigation is key for coral reef stewardship. Ann. New York Acad. Sci. 1355 (1), 15–30. doi: 10.1111/nyas.12785
Williams G., Knapp I., Work T., Conklin E. J. (2011). Outbreak of Acropora white syndrome following a mild bleaching event at Palmyra Atoll, Northern Line Islands, Central Pacific. Coral Reefs. 30, 621–621. doi: 10.1007/s00338-011-0762-2
Keywords: coral tissue loss disease, SCTLD, disease response, Montastraea cavernosa, Orbicella faveolata, amoxicillin, lesion treatment
Citation: Toth KA, Buckley SF, Noren H, Neely KL and Walker BK (2024) Broadscale coral disease interventions elicit efficiencies in endemic disease response. Front. Mar. Sci. 10:1302697. doi: 10.3389/fmars.2023.1302697
Received: 26 September 2023; Accepted: 26 December 2023;
Published: 16 January 2024.
Edited by:
Lorenzo Alvarez-Filip, National Autonomous University of Mexico, MexicoReviewed by:
Victor Rodriguez-Ruano, Florida Institute of Technology, United StatesSonora Meiling, University of the Virgin Islands, US Virgin Islands
Copyright © 2024 Toth, Buckley, Noren, Neely and Walker. This is an open-access article distributed under the terms of the Creative Commons Attribution License (CC BY). The use, distribution or reproduction in other forums is permitted, provided the original author(s) and the copyright owner(s) are credited and that the original publication in this journal is cited, in accordance with accepted academic practice. No use, distribution or reproduction is permitted which does not comply with these terms.
*Correspondence: Kathryn A. Toth, a2FydG90aDk4QGdtYWlsLmNvbQ==