- 1Climate Change Cluster, University of Technology Sydney, Ultimo, NSW, Australia
- 2KAUST Reefscape Restoration Initiative (KRRI) and Red Sea Research Centre (RSRC), King Abdullah University of Science and Technology, Thuwal, Saudi Arabia
- 3Mars Sustainable Solutions, Cairns, QLD, Australia
- 4GBR Biology, Cairns, QLD, Australia
Coral carbonate production is fundamental to reef accretion and, consequently, the preservation of essential reef ecosystem services, such as wave attenuation and sustained reef biodiversity. However, the unprecedented loss of coral reefs from anthropogenic impacts has put these valuable ecosystem services at risk. To counteract this loss, active rehabilitation of degraded reef sites has accelerated globally. A variety of restoration practices exist, tailored to local site needs and reef types. For sites where there is a significant unconsolidated substrate, Mars Assisted Reef Restoration System (MARRS, or “Reef Stars”) has been utilised to contribute toward rubble stabilisation and reef accretion. However, the effect of the Reef Stars on the local carbonate budgets and structural complexity has not been assessed. For that purpose, we assess coral cover and reef complexity through a census-based approach to identify the contribution of carbonate producers and eroders alongside studying coral skeletal properties to estimate current carbonate budgets on a rehabilitated site compared to natural unrehabilitated reef and rubble patches on the mid-Great Barrier Reef. Our research identified positive ecological processes and ecological functions such as increased carbonate budget, coral cover and structural complexity at the restored site compared to the non-intervened reef and rubble patches. In general, no impacts on skeletal rigour relative to this active reef restoration were found for two key coral species and the Acropora rubble for most of the skeletal traits. However, Pocillopora damicornis hardness seemed to decrease on the restored site compared to the other sites, demonstrating different performances of coral species during restoration activities that should be considered to maximise return-on-effort of restoration activities. Overall, our data demonstrate that consideration of carbonate budgets is important for measuring success of coral restoration initiatives and that coral restoration can be a relevant tool to recover lost local carbonate budgets.
Introduction
Declines in live coral cover since the 1970s have dramatically slowed reef accretion on a global scale. Mean rates of contemporary reef carbonate production for the Caribbean are below mean historical and geological levels (Gardner et al., 2003; Alvarez-Filip et al., 2009; Perry et al., 2012). Similarly, Indo-Pacific reefs have been driven into net negative accretion states and reef structural collapse following coral mortality (Bak, 1990; Eakin, 1996), including recent bleaching-driven coral die-offs (Perry and Morgan, 2017). On the Great Barrier Reef (GBR), coral cover has been declining since the mid-1980s (Sweatman et al., 2011; De’Ath et al., 2012) from the combined effects of both local (e.g., coastal development; Halpern et al., 2008) and global (e.g., climate change; Hughes et al., 2018) stressors. Recent recurrent episodes of mass bleaching (i.e., four major events between 2016 and 2022), corresponding with extreme thermal anomalies in 2016, 2017, 2020, and notably the first documented mass bleaching event under La Niña cool conditions in 2022, have driven coral cover to all-time lows (e.g., Hughes et al., 2017; Hughes et al., 2021). Despite catastrophic coral loss, only a few studies have investigated changes to reef carbonate budgets (i.e., balance between rates of carbonate production and erosion) for the GBR, often focussing on a particular organism, process, or environmental condition, such as the calcareous green alga Halimeda (Rees et al., 2007; Castro-Sanguino et al., 2020), bioerosion activity (Tribollet and Golubic, 2005; Aline, 2008), highly turbid reefs (Browne et al., 2013), and island geomorphological habitats (Brown et al., 2021). So far, few studies have focussed on carbonate budgets in the Southern and Middle GBR regions; therefore, knowledge of the entire GBR is wholly lacking.
Hard (scleractinian) corals are the main contributors to the structure and function of coral reef ecosystems, providing (1) reef accretion (Perry et al., 2013), (2) habitat structure (Graham et al., 2006; Alvarez-Filip et al., 2009), and (3) biogeochemical cycling (Goreau et al., 1979; Wild et al., 2004; Cole et al., 2008). Fundamental to the provision of these features is carbonate production. Scleractinian corals undergo skeletogenesis (Al-Horani, 2015) to rapidly precipitate calcium carbonate (CaCO3) (reviewed in McCulloch et al., 2017) that — on a community level — results in the reef framework. CaCO3 production is continuously exposed to both biological (associated with fish and invertebrate grazing and endolithic borers) and physical (e.g., storm disturbance) agents of reef bioerosion (Perry and Hepburn, 2008) that ultimately comprise the net three-dimensional structure and function of the reef (Leggat et al., 2019). Healthy-functioning reef systems in effect self-repair, balancing growth (carbonate production) with erosion processes (UNEP-WCMC, 2006); consequently, stressors that tip the balance in carbonate production versus loss will inevitably undermine the many ecosystem services that reef structure provides (Pratchett et al., 2021), both directly as wave attenuation and shoreline protection for coastal communities (Yates et al., 2017; Storlazzi et al., 2018) and indirectly via reef habitat structure (Perry et al., 2013; Perry and Morgan, 2017) as fisheries productivity (Bell et al., 2013; Rogers et al., 2014; Rogers et al., 2018) and biodiversity (Wilson et al., 2006; Stuart-Smith et al., 2018).
To counteract rapidly declining coral cover, global management approaches are expanding beyond traditional protection (and stress mitigation) practices to include proactive interventions (e.g., Shaver et al., 2022; Suggett and van Oppen, 2022) aimed at recovering reef ecosystem services (Hein et al., 2021). Measurements of restoration success have conventionally been evaluated via two coarse metrics of coral growth and survivorship (Hein et al., 2017; Boström-Einarsson et al., 2020; Nuñez Lendo et al., 2023), even though restoration goals are often centred on recovering broader ecosystem service value attributes (Hein et al., 2021; Shaver et al., 2022). As such, how restoration activities may impact healthy reef functioning — in terms of long-term consequences for different ecosystem service attributes — remains hard to ascertain (Hughes et al., 2023). Maintaining desirable features such as high coral cover, structural complexity, and fish biomass are common conservation objectives. Even so, the processes underpinning these features (including calcium carbonate dynamics) are poorly defined and understood (Brandl et al., 2019). For example, trade-offs in resource acquisition and partitioning that potentially regulate growth versus survival may yield very different outcomes towards factors governing reef accretion and structural integrity (Nuñez Lendo et al., 2023). For reef restoration programs focused on regaining reef structure and function, resolving goal-relevant metrics, such as carbonate production and coral skeletal traits, will therefore be critical to actually evaluate success.
Reef restoration methods span multiple disciplines ranging from coral biology and ecology to ecological modelling and geoengineering (Suggett and van Oppen, 2022). Where reef substrates have become unconsolidated — through blast fishing or framework collapse following mass bleaching or cyclone disturbance (Harmelin-Vivien, 1994; Leggat et al., 2019) — restoration often requires substrate enhancement to initiate regrowth (Ceccarelli et al., 2020). For example, practices that use mesh or netting over the rubble to boost natural binding and cementation processes (Raymundo et al., 2007), or rock piles placed on rubble fields to stabilise loose reef substrate (Fox et al., 2019). Such activities can significantly boost coral cover compared to neighbouring non-stabilised reefs (Fox et al., 2019). One example of substrate stabilisation that has recently gained popularity is the so-called MARRS (Mars Assisted Reef Restoration System, or “Reef Stars”), which are hexagonal-shaped structures made of reinforcing steel rods that each enclose an area of 0.337 m2, and are interconnected with cable ties in a web to cover large areas (Figure S1; Williams et al., 2019). Reef Stars have been successfully used in Indonesia following physical damage caused by blast fishing and are in one of the world’s largest restored reef efforts to date (Williams et al., 2019; Lamont et al., 2022). This successful example of a restored degraded area initially extended to 7,000 km2 of stabilised coral rubble by using around 198,000 coral fragments on 11,000 Reef Stars (Williams et al., 2019).
Reef Stars were first installed on the GBR as part of a broader restoration plan for Moore Reef (central GBR) to stabilise an accumulated coral rubble field produced from the 2011 Cyclone Yasi. A set of 87 Reef Stars (named also “Block A2” to differentiate from other installations) holding corals of opportunity was installed in October 2020. By March 2023, a total of 439 Reef Stars have been completed, with 6,471 coral fragments of opportunity. Whilst ecological recovery from this and other Reef Stars installations is already evident (Figure 1) — and is the focus of other ongoing research — the impact towards changes in reef accretion and structural rigour (i.e., coral density, porosity, and hardness) remains unknown. As such, we examined the Moore Reef installation as a novel means to identify how this reef stabilisation approach impacts the rate of reef-structure gain (or loss) compared to neighbouring non-restored reef controls via the balance of constructional (i.e., calcification) and de-constructional (i.e., erosion) processes (Stearn et al., 1977; Scoffin et al., 1980; Hubbard et al., 1990; Perry et al., 2012) and therefore resolve carbonate budgets (Lange et al., 2020). We specifically document spatial variability in carbonate budgets using a census-based approach (see a schematic view in Figure 2; Perry et al., 2012) in which rates of carbonate production and erosion were estimated for three reef sites (i.e., at a 16 month restored reef site using the Reef Stars; a nearby representative natural reef; and a degraded area represented by coral rubble). We further studied the skeletal properties — bulk volume, biomineral density, bulk density, pore volume, apparent (internal) porosity, and hardness— of the dominant calcifying biota (i.e., coral), focussing on the main coral species in Moore Reef (Acropora intermedia and Pocillopora damicornis) alongside branching Acropora rubble (which was dominantly present in the study area). For this approach, we identified how reef stabilisation can enhance accretion by 6-29% compared to unrestored sites, and we discuss how this approach potentially provides critical means for practitioners to evaluate wider ecosystem service values attained through restoration.
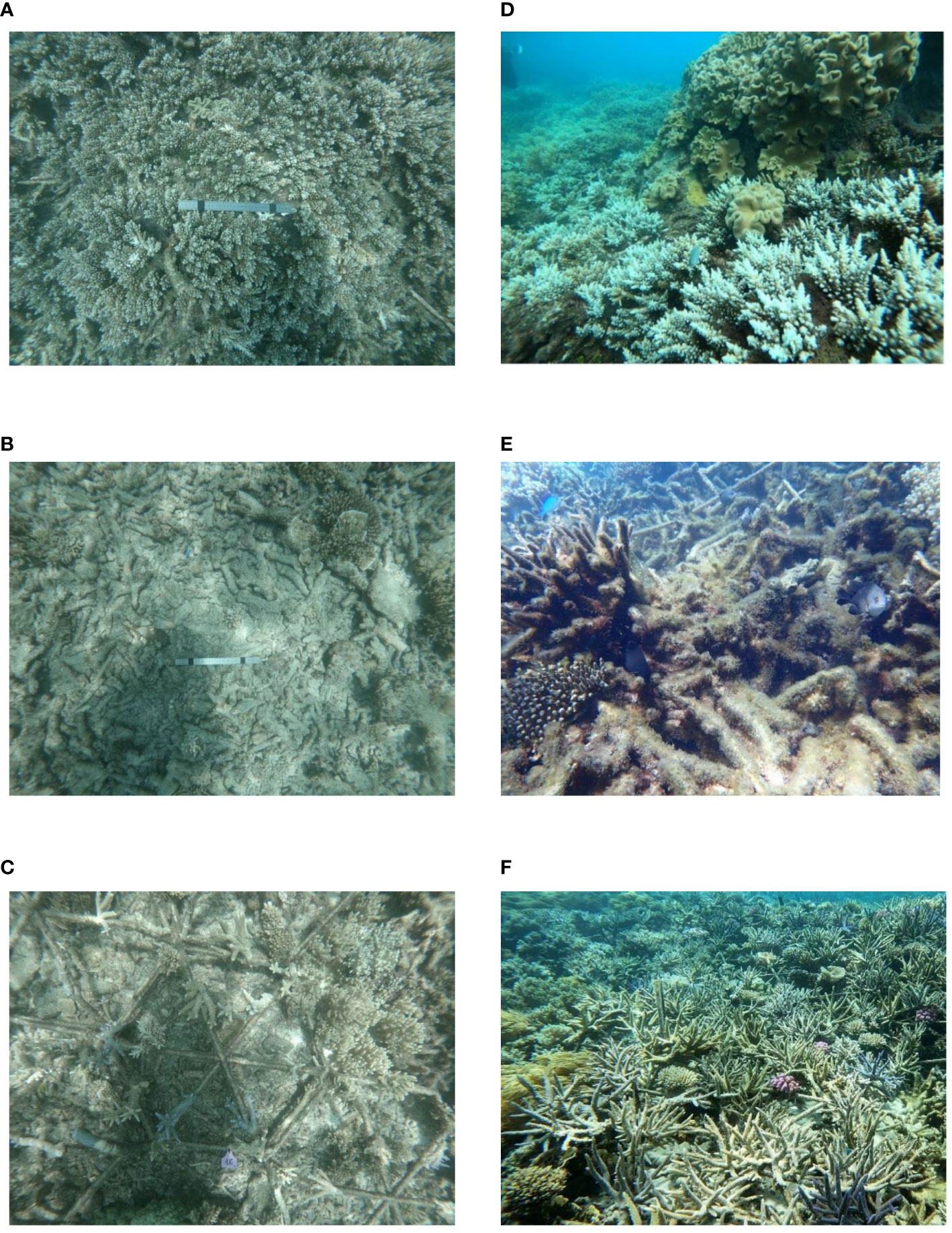
Figure 1 Moore Reef study sites. In February 2021, (A) Natural healthy-looking representative neighbouring reef with high coral cover (positive control, ‘PC’) (B) View of a 11-year-old rubble field, created by Cyclone Yasi without rehabilitation (negative control, ‘NC’) (C) Stabilisation reef plot, showing the Reef Stars structures holding corals of opportunity (4 months post-installation). Approximately 11 months later (December 2021), same reef sites ‘PC’, ‘NC’, and ‘E’ (D–F). Note the high coral cover observed on the Reef Stars in the intervened rubble site ‘E’. Credits: Nuñez Lendo, C.I., and Mars Sustainable Solutions.
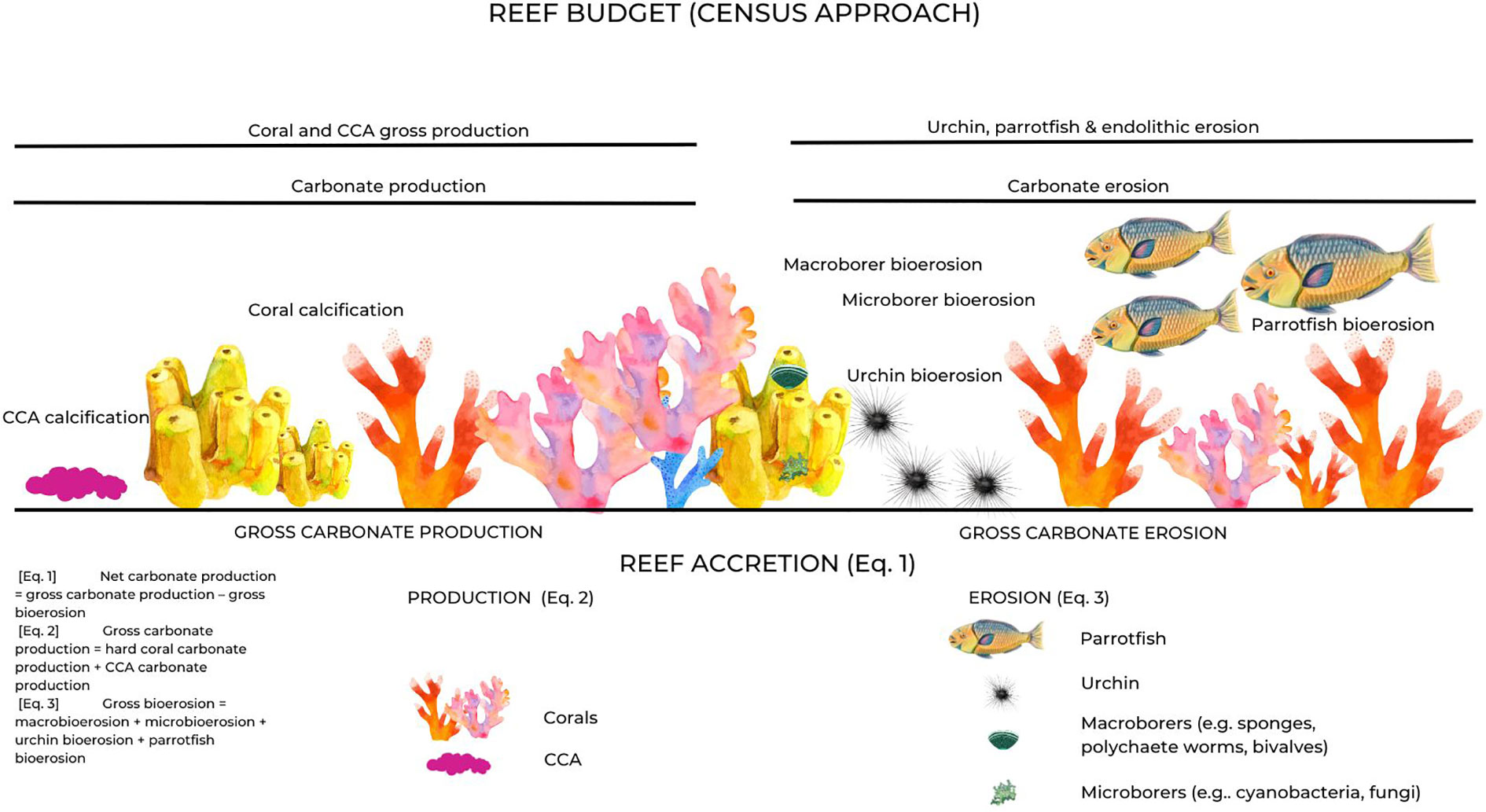
Figure 2 Schematic view of the methodology used to estimate coral reef carbonate budgets on the three studied Moore Reef sites. Our study was based on the Reef Budget methodology from Perry et al. (2012). Positive and negative contributions to reef accretion (production and erosion, respectively) are visualised. Specifically, net carbonate production [Equation 1], gross carbonate production [Equation 2], and gross bioerosion [Equation 3], and the main contributors to production and erosion processes. Credits: Nuñez Lendo, C.I.
Materials and methods
Study location
MARRS Reef Stars were installed at Moore Reef (3-5 m depth), a mid-shelf patch reef located 50 km east of Cairns, in the central Great Barrier Reef (GBR), Australia (146° 14’ 40.9’’E, 16°51’ 59.0’’S). Our study location (~2,000 m2) is an area divided into three sites (30 x 20 m), where each site is marked with star pickets at the corners corresponding to (i) a stabilised substrate section using the Reef Stars (Experimental, “E”), and two nearby sites of (ii) a rubble patch where no Reef Stars were deployed (negative control, “NC”), and (iii) a non-intervened healthy-looking representative neighbouring reef area with high coral cover and diversity (positive control, “PC”) (Figure S2). Herein we refer to Reef Stars, rubble, and reef site, as their abbreviations, E, NC and PC. Two months post-deployment hard coral cover on all sites was below 50% (25.8, 32.3 and 39.3% for the NC, PC, and E sites) (AM, FN, and EEF, pers. comm.).
On October 22, 2020, Mars Sustainable Solutions and partners deployed a set of 87 Reef Stars (the so-called Block A2 with ~29.32 m2) with attached coral fragments (i.e., corals of opportunity, mainly Acropora and Pocillopora spp.). Whilst additional Reef Stars installations have been conducted, COVID-19 restrictions meant that our study site could only be first accessed in February 2021 (six months post-installation), and during this time, we took initial imagery data for coral growth. We revisited the study site after a 12-month period (February 2022) to examine the Reef Stars’ effects on reef accretion (including hard coral cover, rugosity, carbonate budgets, skeletal properties of key coral taxa, and growth).
Temperature HOBO™ loggers (1-h logging interval) were deployed in February 2021 to track key environmental variance over the 12 months of growth but unfortunately failed after 8 months. Therefore, additional environmental data for the entire 12-month study period were extracted from GIOVANNI online system for satellite-derived data maintained by NASA (https://giovanni.gsfc.nasa.gov/giovanni/). Sea surface temperature (SST) was obtained using monthly area-averaging bounded to 146° 14’ 40.9’’E, 16°51’ 59.0’’S (Moore Reef) between the beginning of the monitoring (February 2021) and the end of the experiment (February 2022). SST data from HOBO™ loggers for the reef and MARRS/rubble sites was therefore overlayed onto that derived from Giovanni SST data for Moore Reef (Figure S3).
Experimental design
In February 2022, a commonly applied census-based approach was undertaken to characterise benthos and determine the carbonate budget for the E, NC, and PC sites (i.e., restored, negative, and positive control sites; Perry et al., 2012). The Reef Budget methodology is detailed in the section below. In addition, coral fragments (< 5 cm; two coral species, n = 5 each) were collected from the inner side (avoiding cutting eroded fragments from the outer edge) of randomly selected colonies using steel pliers and stored in individual Ziploc bags. Once on board the research vessel, the collected fragments were firstly immersed in freshwater followed by sodium hypochlorite to remove coral tissue for subsequent analysis of skeletal properties of bulk volume, biomineral density, bulk density, pore volume, apparent (internal) porosity, and hardness (Fantazzini et al., 2015; Madin et al., 2016; Leggat et al., 2019). Data on Acropora spp. coral growth was obtained by underwater photographs (Siebeck et al., 2006; Lirman et al., 2014) in February 2021 and 2022 (1-year growth period). The various methods for these attributes are detailed in the following sections.
Coral reef carbonate budgets and benthic characterisation
We developed carbonate budgets using an adapted version of the Reef Budget protocol (Perry et al., 2012), a census-based approach that quantifies cover/abundance of carbonate- (CaCO3) producing (corals and crustose coralline algae (CCA)) and bioeroding taxa (urchins, parrotfish and micro- and macro-endolithic taxa), and combines these data with already published measures of species/genera CaCO3 production and erosion rates to calculate net carbonate budget.
We estimated both gross carbonate production and bioerosion rates, and the resultant net carbonate production (G = kg CaCO3 m−2 yr−1) on each site (E, NC, and PC) in February 2022 using 3 x 10 m transects, each separated by 5-10 m, with which to collect all relevant data (except parrotfish data). Along each transect, benthic classes (hard/soft corals, CCA, rubble, other calcareous encrusters, and eroders) were determined following the Reef Budget taxa-specific codes (Appendix A, Reef Budget, Perry et al., 2012). Categories were photographed with an Olympus Stylus TOUGH TG-4 digital camera, and codes were noted on an underwater notebook. Within each meter of the 10 m transect, the distance (cm) covered by each benthic component immediately beneath the transect was measured using a short (~1 m) flexible tape. Sea urchin bioerosion was determined from the abundance and size of bioeroding urchins of the family Diadematidae (Diadema spp., and Echinothrix spp.) and the genera Echinometra, Echinostrephus, and Eucidaris, which was tallied 2 m either side of each 10 m transect (and hence a 40 m2 belt) (Bak, 1990; Bak, 1994). To complement bioerosion rates, we also collected data on fish abundance at each survey site by deploying additional belt transects (30 x 5 m, n = 3 separated by 5-10 m at each site) (Reef Budget, Perry et al., 2012). Initial and developmental phases of parrotfish species were tallied across nine size classes as assigned based on fish fork length: 8–10 for the juvenile phase, 11– 20, 21–30, 31–40 for the initial phase, and 11–20, 21–30, 31–40, 41–50, and 51–60 for the terminal phase. At site E only, due to the reduced size being 30 m x 20 m, the fish transect was placed in an S-shaped to ensure the surveys were only conducted on that habitat.
Net (total) carbonate production (i.e., the carbonate budget) was estimated as the balance between gross carbonate production and gross bioerosion (see equations).
Within this methodology, carbonate production is driven by hard corals and CCA, whereas macro- and microbioerosion are related to the substrate (dead reef substrate, sponge and CCA cover for macrobioerosion, or rock, sand and seagrass cover for microbioerosion) available for internal bioeroders (e.g., reef-dwelling organisms such as worms, bivalves and sponges) (Tribollet et al., 2002; Rice et al., 2020). External bioeroders, such as parrotfish and urchins, are also major contributors to the bioerosion of the reefs and, together with macro- and microbioerosion, constitute the gross bioerosion.
In February 2022, benthic characterisation was also carried as part of the Reef Budget protocol (Perry et al., 2012) for each site, and this included metrics such as the benthic cover of major functional categories (hard and soft coral, CCA, sediment producers, macro- and turf algae, rubble, rock, limestone pavement, sand, and others, alongside estimating the rugosity (R; Equation 4) which is an index of surface roughness that is a common measure for quantifying landscape structural complexity (i.e., topographic heterogeneity) (McCormick, 1994).
Coral survival and growth
Since most of the corals seeded on the Reef Stars in October 2020 belong to the genus Acropora (> 95%; compared to < 5% for Pocillopora spp.; Mars Sustainable Solutions pers. comm.), coral survival and growth was followed on the same Acropora fragments (n = 15) for 12 months. Coral fragments were photographed in situ using a GoPro Hero 8 digital camera together with a scale reference by SCUBA in February 2021 (T0) and 2022 (T12) on site E. Survival rate as the proportion of all initial coral individuals remaining over time (%) and growth rates for each coral were determined as the change in size (areal and linear extension) over this 12-month period (ΔG; cm2 y-1 and cm y-1) and averaged.
Coral sampling and skeletal properties
Two key coral taxa (Acropora intermedia and Pocillopora damicornis) at Moore Reef (and used to originally seed the Reef Stars) were targeted to study their skeletal properties. Whilst P. damicornis was present at all sites, A. intermedia was not present in the rubble patch (NC) where this site was predominantly covered by dead branching Acropora spp. or rubble. Therefore, we collected Acropora “rubble” instead (its homolog but in a non-living state). Fragments (< 5 cm in length) of A. intermedia, P. damicornis and Acropora rubble were thus collected (n = 5 each) as follows: A. intermedia at the E and PC sites, P. damicornis at the E, PC, NC sites, and Acropora rubble only from the rubble patch (NC site). See Table S1 for details of the coral collection).
Hardness testing was conducted using a Shore D Hardness Tester (TE-271) calibrated using reference material in accordance with the manufacturer’s recommendation (Vander Voort, 1999) as per Leggat et al. (2019) on a coral fragment (< 2 cm) which was previously cleaned and dried. Hardness was consistently determined on the base of the branch for all fragments, with 10 measurements performed for each. In addition, the buoyant method applied for corals (adapted from Jokiel et al., 1978; Bucher et al., 1998), combined with the Archimedes principles, were used to test bulk volume, biomineral density, bulk density, pore volume, and apparent (internal) porosity on a second coral sample (< 2 cm) (Fantazzini et al., 2015). Briefly, the fragment was previously weighed to obtain the dry weight (DW; g) before being inserted in a glass vessel in a 50°C water bath for two h under vacuum. The glass vessel was filled with Mili-Q to obtain the saturated weight (SW; g) of the coral, followed by the measurement of the buoyant weight (BW; g). Skeletal properties were extracted using the previous measurements and the density of water (ρH2O) at 20°C (0.9982 g cm-3) as per the following equations:
Statistical analysis
Data analyses were performed using Rstudio (version 1.4.1717) and GraphPad Prism (version 9.1.2). Assumptions of normality were assessed visually via QQ plots and a Shapiro-Wilk’s test, and equal variances were assessed using Brown-Forsythe test (Shapiro and Wilk, 1965). Significance was set at p < 0.05 (for all tests), and the mean ± the standard error of the mean (SEM) was reported unless expressly noted. A series of analysis of variance (ANOVA) (or its non-parametric homolog, Kruskal-Wallis tests) with post hoc tests (Tukey or Dunn) were undertaken to compare each coral class (A. intermedia, P. damicornis and Acropora rubble) across sites (E, NC, and PC) for the skeletal traits, as well as comparing wild A. intermedia and P. damicornis fragments (from the PC site — the nearby healthy-looking-reef —) to observe potential natural interspecific differences.
Results
Benthic cover and rugosity
Three well-differentiated sites were studied on Moore Reef, (i) a stabilised substrate area using the MARRS Reef Stars (Experimental, “E”), and two nearby sites of (ii) a coral rubble substrate where no Reef Stars were installed (negative control, “NC”), and (iii) a non-intervened healthy-looking representative neighbouring reef zone with high coral cover and diversity (positive control, “PC”). All sites contained variations in substrate type including rubble cover, live and dead coral cover, primary or major secondary carbonate producers (i.e., corals, and CCA), and coral morphology. In February 2022, the benthic cover of major functional categories (e.g., hard and soft coral, crustose coralline algae (CCA), turf, rubble, limestone pavement; Figure S4) was estimated (Reef Budget, Perry et al., 2012). Relatively high hard and soft coral cover (47.5 ± 3.8 and 22.5 ± 4.0%, n = 3 each) alongside turf algae mats (25.1 ± 7.2%, n = 3) were observed in the non-intervened reef site (PC). The non-restored impacted site (NC) was characterised by an open framework of rubble (84.7 ± 2.5%, n = 3) and low hard coral cover (14.9 ± 2.4%, n = 3). In contrast to both control sites, the MARRS restored site (E) had a high hard coral cover of 98.0 ± 2.0% (n = 3), where the initial rubble patch (NC) became negligible (2.0 ± 2.0%, n = 3) after 16 months of Reef Stars installation (Figure 3A). High coral cover present in the site E consisted mainly of Acropora spp. (95.5 ± 3.9%, n = 3) (in descending order, branching (47.7 ± 23.9%, n = 3), corymbose/digitate (37.8 ± 30.5%, n = 3), tabular (5.1 ± 3.2%, n = 3), and hispidiose/arborescent (4.9 ± 3.2%, n = 3) growth forms). Coral diversity was also dominated by Acropora spp. on the site PC (64.7 ± 11.9%, n = 3), where 37.7 ± 0.8% (n = 3) were corymbose/digitate, followed by 25.5 ± 12.8% (n = 3) hispidiose/arborescent, tabular (1.2 ± 1.2%, n = 3) and branching (0.3 ± 0.3%, n = 3) morphologies. The low hard coral cover present at the site NC consisted of 11.8 ± 1.9% Acropora spp., where 6.7 ± 0.4, 4.5 ± 2.3, and 0.6 ± 0.6 (n = 3 each) corresponded to hispidiose/arborescent, corymbose/digitate, and branching morphologies, respectively.
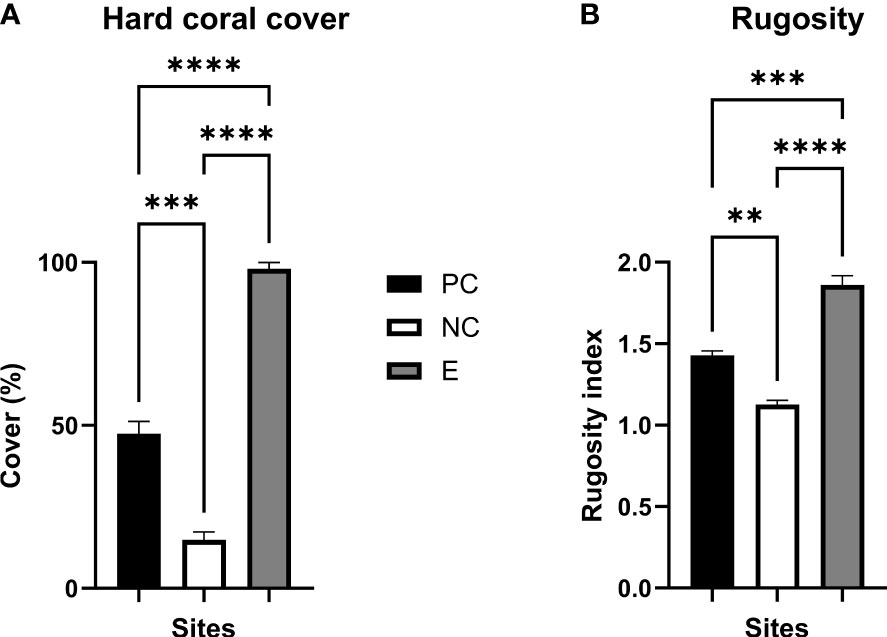
Figure 3 Average hard coral cover and rugosity. (A) Hard coral cover (%) and (B) rugosity index were estimated following the Reef Budget methodology (Perry et al., 2012) at the three studied sites, (i) Reef Stars (Experimental, ‘E’), (ii) a rubble patch (negative control, ‘NC’), and (iii) a non-intervened healthy-looking representative neighbouring reef zone (positive control, ‘PC’) in February 2022 (16 months post-deployment of the Reef Stars). Means (n = 3 per reef site) (± SEM; standard error of the mean) were compared by analysis of variance (ANOVA) with post hoc Tukey tests (see main text) where **, ***, and **** indicate p ≤ 0.01, 0.001, and 0.0001, respectively.
Rugosity (dimensionless) was higher at the Reef Stars restored site (E) compared to the two control sites (PC and NC). In increasing order, the rugosity index was as follows: 1.13 ± 0.03, 1.43 ± 0.03, and 1.86 ± 0.06 for sites NC, PC, and E, respectively (Figure 3B; one-way ANOVA, F(2,6) = 0.5, p < 0.0001; Tukey’s test PC–NC, PC–E, and NC–E, p = 0.0039, 0.0006, and < 0.0001, respectively).
Survivorship and growth
Acropora spp. survivorship after 12 months was 100% ± 0.0 at the Reef Stars (E; n = 15) alongside a moderate (close to 50%) relative areal growth rate (ΔGA; % growth in cm2 y-1) over 12 months (43.9 ± 19.3) (Figure S5A). This outcome was also observed in the relative increase in area covered by coral tissue (%), i.e., final areal size/initial starting size x 100 (143.9 ± 19.3). However, the observed relative linear growth rate (ΔGL; % growth in cm y-1) appeared to be < 50% (27.5 ± 11.0) for this 12-month period. Of the 15 fragments examined, the highest areal growth rates were consistently observed for fragment #9 (from 27.6 to 78.6 cm2 in the same period; see example in Figures S5B, C).
Net carbonate production
Calculating net (total) carbonate production (i.e., the carbonate budget, G; kg CaCO3 m−2 yr−1, Figure 4A) using the Reef Budget census-based approach in February 2022, was estimated as the balance between gross carbonate production and gross bioerosion. Net carbonate production rates were significantly higher on reef site E compared to NC and PC sites. The unstabilised rubble patch (NC) was in a net negative carbonate budget state (-3.7 ± 1.8), and hence carbonate production processes for this area were highly susceptible to bioerosion processes. The same site, but that underwent a restoration intervention using the Reef Stars (E), exhibited much higher net carbonate production (25.3 ± 1.0), a rate that was also higher than the nearby natural coral reef (PC), which had a net carbonate budget of 4.3 ± 3.4.
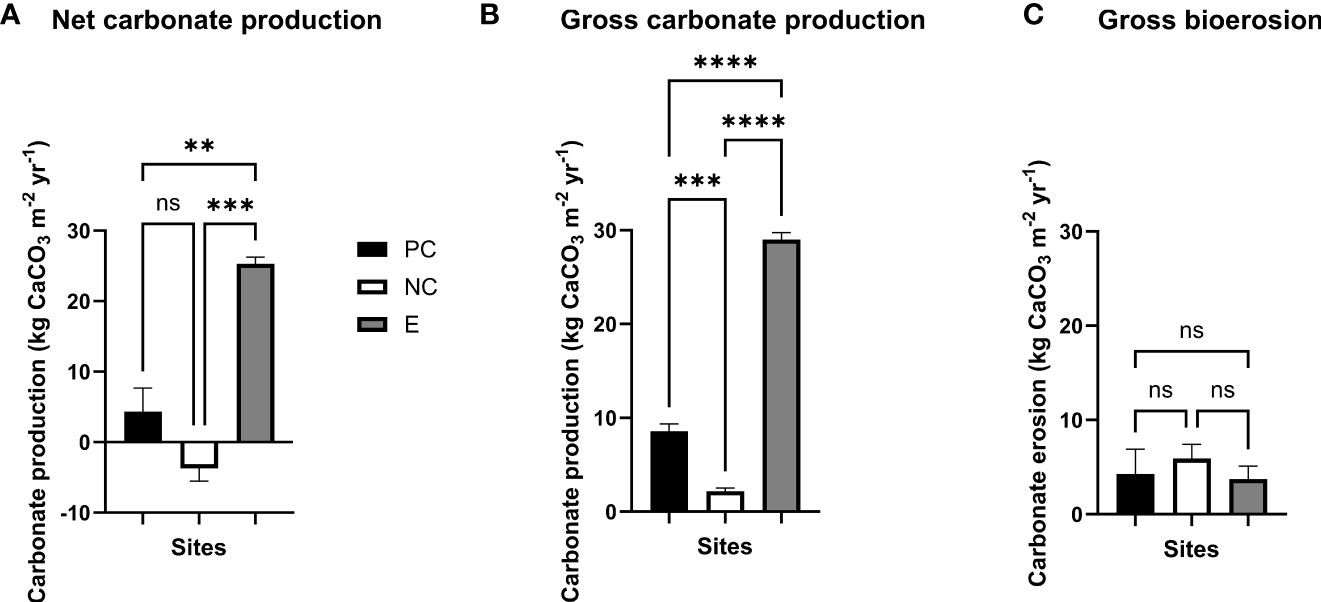
Figure 4 Average (A) net and (B) carbonate production, and (C) gross bioerosion expressed in kg CaCO3 m−2 yr−1 (following the Reef Budget methodology, Perry et al., 2012) across Moore Reef studied sites, (i) Reef Stars (Experimental site, ‘E’), (ii) the negative control ‘NC’ constituted of unconsolidated coral rubble substrate, and (iii) the positive control ‘PC’ represented by a natural healthy-looking neighbouring reef area, in February 2022 (16 months post-installation of the Reef Stars). Means (n = 3 per site) were compared by analysis of variance (ANOVA) with post hoc Tukey tests (see main text) where ns indicates no statistical significance, and **, ***, and **** indicate p ≤ 0.01, 0.001, and 0.0001, respectively.
Gross carbonate production
Higher gross carbonate production (G; kg CaCO3 m−2 yr−1) was evident for the site E (29.0 ± 0.7), followed by the PC (8.6 ± 0.8) and NC (2.2 ± 0.3) sites. Carbonate production of the Reef Stars restored site (E) was 3.4 and 13.2 times higher than PC and NC sites, respectively, after 16 months post-deployment. This contrast was revealed as significant differences in gross carbonate production among sites (one-way ANOVA, F(2,6) = 488.4, p < 0.0001; Tukey’s test PC–NC, PC–E, and NC–E, p = 0.0009, < 0.0001, and < 0.0001, respectively, Figure 4B). Thus, even in the absence of bio-erosion, carbonate production remained much lower for the two control sites than compared to the Reef Star site, presumably reflecting the different extent of coral cover/diversity amongst the sites.
Gross bioerosion
Bioerosion processes encompassed internal (micro- and macro-bioerosion) and external bioeroders (urchin and parrotfish bioerosion) (G; kg CaCO3 m−2 yr−1). In general, micro- and macro-borer erosion when combined was similar for all sites at ~0.5 (Figure S6A) (one-way ANOVA, F(2,6) = 1.1, p = 0.4046). However, when considered individually, significant differences were evident for both erosion categories between sites (macrobioerosion, one-way ANOVA, F(2,6) = 46.1, p = 0.0002; Tukey’s test PC–NC, PC–E, and NC–E, p = 0.0028, 0.0227, and 0.0002, respectively, and microbioerosion, one-way ANOVA, F(2,6) = 88.0, p < 0.0001; Tukey’s test PC–NC, PC–E, and NC–E, p = 0.0039, 0.0006, and < 0.0001, respectively; Figures S6B, C). The Reef Star site (E) showed the highest microbioeroding rates (0.4877 ± 0.0149), followed by PC and NC sites (0.3744 ± 0.0071 and 0.2952 ± 0.0068, respectively). In contrast, higher macrobioerosion rates were found at the rubble site NC (0.2001 ± 0.0086), followed by negligible levels on sites PC and E (0.0833 ± 0.0217 and 0.0082 ± 0.0081, respectively).
Urchins were not observed across sites during the daytime surveys (0.0 individual/m2), and hence did not contribute to our gross bioerosion rates. The contribution of parrotfish to bioerosion was similar across sites and estimated, in ascending order, at 3.2 ± 1.3, 3.8 ± 2.6, and 5.4 ± 1.5 for the sites E, PC, and NC, respectively (one-way ANOVA, F(2,6) = 0.3, p = 0.7202; Figure S6D). Lastly, an overall gross bioerosion rate (the sum of parrotfish, urchin, macro- and microbioerosion rates, G; kg CaCO3 m−2 yr−) was calculated to be 3.7 ± 1.4, 4.3 ± 2.6, and 5.9 ± 1.5 for E, PC, and NC, respectively. Despite the slightly higher gross bioerosion rates at NC, no significant differences were found among sites (one-way ANOVA, F(2,6) = 0.3, p = 0.7223; Figure 4C).
Skeletal properties
Skeletal traits (bulk volume, bulk density, biomineral density, pore volume, apparent (internal) and porosity) for both A. intermedia (living and non-living forms) and P. damicornis were generally consistent across the three experimental sites (Figures 5A–E). The exception was hardness (HD) (one-way ANOVA, F(5,24) = 14.8, p < 0.0001). Fragments of P. damicornis had the lowest HD at the E site, where they were on the Reef Stars (36.3 ± 1.1) compared to those inhabiting the reef at the PC and NC sites (50.3 ± 1.4 and 44.1 ± 0.8; Tukey’s test PC–NC, PC–E, and NC–E, p = 0.0297, < 0.0001, and 0.0041, respectively; Figure 5F). Furthermore, the dead Acropora spp. rubble showed the lowest hardness (35.9 ± 2.4) compared to the alive A. intermedia fragments on the PC and E sites (42.0 ± 1.4 and 42.3 ± 0.7; Tukey’s test PC–NC and NC–E, p = 0.0342, and 0.0223, respectively). Differences in pore volume (cm3) and apparent (internal) porosity (%) among wild A. intermedia and P. damicornis from the natural-healthy looking reef (PC) were also observed (0.8 ± 0.1 vs 0.4 ± 0.0, and 80.2 ± 7.3 vs 31.9 ± 1.8, for A. intermedia vs P. damicornis, respectively; Tukey’s test, p = 0.0016 and 0.0099) alongside differences in HD (42.0 ± 1.4 vs 50.3 ± 1.4, respectively; Tukey’s test, p = 0.0021). Thus, overall, the Reef Stars did not appear to have an impact on coral skeletal properties examined, except for P. damicornis where HD was lowered.
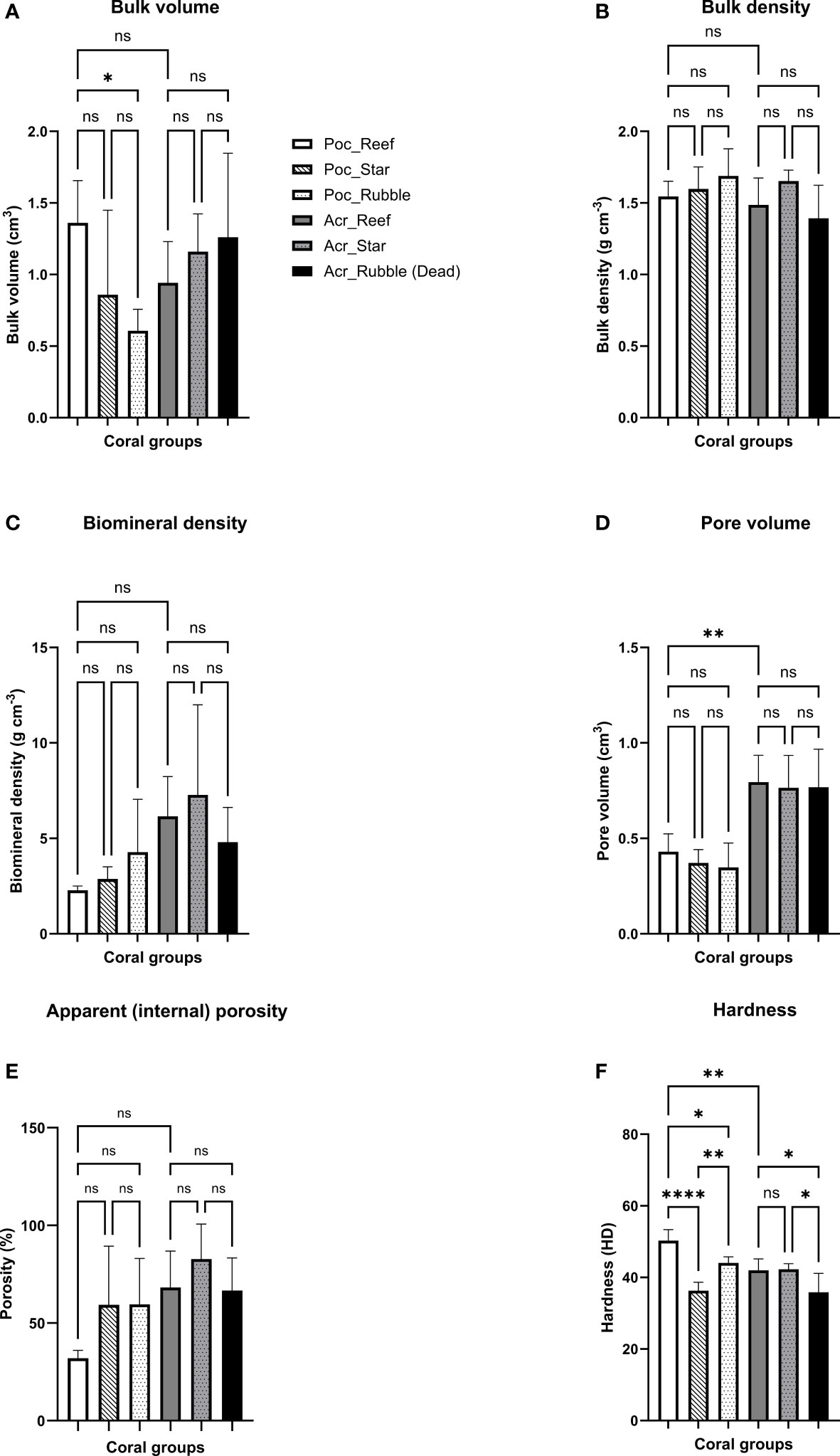
Figure 5 Mean (± SEM) skeletal (A) bulk volume (cm3), (B) bulk density (g cm-3), (C) biomineral density (g cm-3), (D) pore volume (cm3), (E) apparent (internal) porosity (%), and (F) hardness (HD) of three dominant coral classes (Acropora intermedia, Acropora rubble, and Pocillopora damicornis) across sites: E, NC, and PC). All data are fragments (n = 5 for each group). Means (n = 3 per reef site) were compared by one-way analysis of variance (ANOVA) with post hoc Tukey tests (see main text) where ns indicates no statistical significance, and *, **, and **** indicate p ≤ 0.05, p ≤ 0.01, and p ≤ 0.0001.
Discussion
Reef stabilisation approaches are becoming increasingly popular as means to aid reef recovery where reef structure has become unconsolidated (e.g., Ceccarelli et al., 2020); however, how these approaches potentially enhance carbonate budgets and the means for reefs to enhance accretion rates remains untested. Our results using Reef Stars provide new evidence that such practices can increase carbonate budgets, from our example of a degraded mid-shelf patch reef on the central Great Barrier Reef (GBR), in turn, highlighting the importance of carbonate budgets as a metric to evaluate coral restoration success. In doing so, we also present new estimates for carbonate budgets for representative Australian reef environments.
Carbonate budgets (G = kg CaCO3 m−2 yr−1) we observed for the representative “healthy” control reef site (PC; 4.3 ± 3.4) were similar to values reported across the Indian Ocean (3.7; Perry et al., 2018) but surprisingly lower than turbid reefs on the central GBR (6.9–12.3 for Middle Reef and Paluma Shoals, respectively, Browne et al., 2013) and higher than for Western Australia (2.5 for Ningaloo Reef; Perry et al., 2018). All of these Australian reefs (including our studied reef) are characterised by similar gross carbonate production and hard coral cover but different coral community structure at the time of the study (Browne et al., 2013; Perry et al., 2018). However, gross bioerosion rates (mainly driven by parrotfish eroding activity), were doubled in Moore Reef compared to the two turbid reef zones (4.3 vs 1.51-1.62), which seems to explain the lower carbonate budget observed in our studied reef site. Whilst values for the carbonate production in Ningaloo Reef are considered amongst the highest contemporary budgets for the Indian Ocean (alongside those from Mozambique, Perry et al., 2018), they are lower than our study sites (and accompanied by ~3 times lower bioerosion rates). However, this lower bioerosion for Ningaloo may reflect that the sites examined were deeper (8 m) compared to ours (3-5 m) and the associated coral community composition and associated fauna.
At the restored site (E), the highly concentrated (e.g., 15 coral fragments per Reef Star, and so ~750 corals in 600 m2) deployment of typically fast-growing (e.g., branching Acropora spp.) corals resulted in a very high net carbonate budget (25.3 ± 1.0), and ~6 times higher than the carbonate budget of our representative “healthy” control site (PC; 4.3 ± 3.4). By including the NC rubble site within our experimental design, we demonstrated that without intervention, the rubble site — which is still evident post Cyclone Yasi 2011 — is in a state of net erosion (a net negative carbonate budget; -3.7 ± 1.8). Furthermore, rugosity, which is an important metric to describe reef habitat complexity (i.e., the structural changes that reefs undergo from coral renewal and mortality and balance between rates of carbonate bioerosion and production; Alvarez-Filip et al., 2009), was half that of the restored site (E). While our study only assesses the first 16 months of deployment, our data demonstrate that restoration structures, such as the MARRS Reef Stars, can positively enhance not only carbonate budgets and structural complexity (and the services they support, such as wave energy dissipation and habitat provision; Alvarez-Filip et al., 2011; Ferrario et al., 2014) on local reef sites but boost coral reef recovery that is often very low on rubble areas (Johns et al., 2018; Viehman et al., 2018; Fox et al., 2019; Kenyon et al., 2020). In the case of the NC site in our study, 11 years after Cyclone Yasi hit Moore Reef, the recovery trajectory of this unconsolidated reef patch (as seen by the number of small colonies and recruits on the rubble, and derived low carbonate production, 2.2 ± 0.3) cannot cope with the gross bioerosion processes (e.g., parrotfish erosion activity is intensified on the remaining/new small colonies; Huertas et al., 2021) or natural mortality of the corals when rubble moves (Kenyon et al., 2022). This situation highlights that the balance in carbonate production versus loss will inevitably undermine the ecosystem services the reef structure provides (Pratchett et al., 2021) on Moore Reef unless a restoration intervention such as MARRS Reef Stars is taken place. To note that our study focused on a degraded reef originated by a cyclone, but there are more risks to coral calcification, and thus reef accretion, in the Anthropocene, including ocean acidification (Hoegh-Guldberg et al., 2017) and increased potential substrate loss post coral bleaching (Leggat et al., 2019). Thus, efforts to restore reefs and sustain CaCO3 production are important to be considered in restoration programs (Hughes et al., 2023).
An important consideration for restoration activities is the selection of coral species. As observed for natural reef recovery (Lange and Perry, 2019), the community composition used in restoration will clearly influence the carbonate budget, rugosity and, ultimately, ecosystem service provision. Currently, the restored Moore Reef site (E) has disproportionate fast-growing Acropora species that provide a high carbonate production and rugosity, but the lack of other coral growth forms could potentially come with other functional costs (e.g., Madin et al., 2023). For example, acroporids have been observed to be generally less heat-tolerant than both massive and encrusting growth forms (e.g., Porites spp., the non-scleractinian Heliopora spp., Leptastrea spp.) during past bleaching events (Marshall and Baird, 2000; Loya et al., 2001; McClanahan et al., 2002; Pratchett et al., 2013; Carroll et al., 2017). However, restoring with slower growing (and architecturally less complex) taxa is likely less beneficial for sites in need of fast recovery in terms of carbonate budgets (e.g., Tortolero-Langarica et al., 2019) and sustaining biodiversity (Lirman et al., 2010; Xin et al., 2016; Nithyanandan et al., 2018; Tiddy et al., 2021), but also where Acropora spp. were preferentially lost during recent bleaching of the GBR (Hoogenboom et al., 2017). Including heat-resistant variants of coral species may still come at a cost to growth (Cornwell et al., 2021) within the restoration strategy but could potentially confer reef resilience and maintain reef framework while allowing the more susceptible coral species to recover as well as still providing (modified but not lost) services such as coastal protection and fish productivity (e.g., Tortolero-Langarica et al., 2019). Moreover, rugosity as a proxy for reef habitat complexity can increase fish diversity (Santoso et al., 2022) but not necessarily cryptofauna. Several studies have highlighted enhanced invertebrate density and diversity in coral rubble compared to sites with high live coral cover (Enochs et al., 2011; Nelson et al., 2016; Fraser et al., 2021; Stella et al., 2022; Wolfe et al., 2023). Therefore, restorative interventions through structures that support ecological communities (e.g., Reef Stars) should match the niche of target species, and this also includes the micro-habitat for reef-associated organisms (besides macro-habitat for coral species), thus, promoting habitat heterogeneity to enhance reef biodiversity and ecological functions (Bishop et al., 2022).
Another factor that may ultimately drive the enhanced carbonate budgets observed for the Reef Stars (E) is presumably the size of material used for restoration. When a sexually mature coral colony is fragmented below a certain size, resources are directed towards regrowth instead of reproduction (Lirman, 2000; Zakai et al., 2000). As such, the size and life stage (age) of corals used for restoration will inevitably impact the carbonate budgets retrieved as freshly fragmented coral show higher growth/regeneration (i.e., following trauma, corals undergo rapid tissue and skeletal repair; Loya, 1976; Bak, 1983; Chadwick and Loya, 1990; Meesters et al., 1994) than well-established adult coral colonies, which above a size threshold, shift resources from growth to sexual reproduction (Babcock, 1991; Soong, 1993; Kojis and Quinn, 2001). Larger coral size classes will further exercise more inter- and intraspecific competition for resources (e.g., space, food, light), thus limiting potential coral growth and survival (Box and Mumby, 2007; Evensen et al., 2015).
Most structural property traits examined in our study were not compromised within the experimental restored setting (E) for either A. intermedia or P. damicornis, yet a reduction in hardness for P. damicornis was detected when they were on the Reef Stars (E). Whilst the cause of the reduced hardness cannot be ascertained from our current data set, it potentially indicates a trade-off in resource acquisition and partitioning towards mineral deposition from an altered resource landscape on the Reef Stars (E). For example, calcium carbonate dissolution (not measured with the used Reef Budget methodology) reduces coral skeletal hardness and density after a marine heatwave event (Leggat et al., 2019) and varies according to reef environmental conditions (inshore vs offshore reefs, Aline, 2008). Coral restoration efforts in the Caribbean have also shown reduced coral skeletal density (while calcification rate was conserved and growth was enhanced) attributed to the grow-out method used (thus nursery environment) (Kuffner et al., 2017). Taken together, this evidence would suggest that species-specific trade-offs in physiological properties require measurements of success to include structural properties to robustly resolve whether ecosystem service value (e.g., reef accretionary potential) will be retained. Importantly, hardness was lower in Acropora spp. rubble compared to its living form (A. intermedia). New coral rubble (dead coral) is known to be very breakable (Davies and Hutchings, 1983; Scoffin, 1992; Greenstein and Pandolfi, 2003), and hardness increases when crustose coralline algae (CCA) colonises the surface of the dead coral fragment. As such, 11 years post-cyclone, CCA colonisation on the coral rubble may still be in progress, again highlighting the importance of restoration interventions on reef sites with poor recovery trajectory.
Macroerosion was expected to be low in the restored site (E) because of the lack of substrate susceptible to being eroded compared to the rubble patch (NC). Our study showed that macrobioerosion was lower at the restored site (E; contrary to microbioerosion, which was higher) (0.0082 ± 0.0081 and 0.4877 ± 0.0149, respectively), but combined rates and parrotfish erosion rates (and hence gross bioerosion rates) were similar across reef sites suggesting that bioeroders did not drive differences amongst net carbonate budgets we observed. However, our results should be taken with caution as eroding activity has been observed to be intensified on the scarce new small coral colonies due to a lack of larger coral size classes (Huertas et al., 2021). Despite parrotfish bioerosion being similar across sites, the unique coral composition of each reef site is intimately related to unique fish assemblages (Chong-Seng et al., 2012), which will have different effects on the reef community. Also note that the lack of urchins during our daytime census might not fully resolve erosion rates since urchins are more active at night (Young and Bellwood, 2011).
The census-based approach of the Reef Budget methodology (i.e., calculated from biota cover/abundance and taxa- or species-specific rates of growth/erosion) employed in our study is a frequent and globally used method to determine coral reefs carbonate budgets, and hence a useful means to inter-compare observations. Yet the methodology has assumptions that are acknowledged (e.g., potential error from using abundance snapshots, unconsidered lithification or dissolution processes, and limited spatial scale and depth; Lange et al., 2020). In the case of census-based net carbonate budgets (i.e., gross carbonate production – gross bioerosion) and chemistry-based net calcifications (i.e., balance between calcification and calcium carbonate dissolution), they quantify different processes at different spatial and temporal scales. Several studies have highlighted the significance of calcium carbonate dissolution within the reef framework and sediments in driving shifts in reef communities (Tribble et al., 1990; Andersson et al., 2009; Cyronak et al., 2013). Hence, coupling biogeochemical monitoring of coral reef state variables such as analysing the unique stoichiometry of elements (the so-called “elementome” Peñuelas et al., 2019; Grima et al., 2022) or through chemistry-based net calcification (Broecker and Takahashi, 1966; Smith and Key, 1975; Chisholm and Gattuso, 1991) is highly recommended to improve census based assumptions (Courtney et al., 2022).
Despite not having a tailor-made Reef Budget methodology for the GBR, the approach can (i) determine the relative contribution of different carbonate producers/eroders, (ii) allow spatial comparisons of different reef environments, and (iii) support other indices of reef functionality (e.g., rugosity) as has been successfully demonstrated at sites around the world (Perry et al., 2018; Courtney et al., 2022), and here for the first time in a restoration setting on the GBR. As such, within the context of reef restoration, and despite the need to tailor the methodology, there is clearly a benefit in expanding “best practice” to optimise return-on-effort by integrating this method into restoration guides so practitioners can consider carbonate assessments from the onset of their restorative interventions.
In 2019, the United Nations Environment Assembly adopted Resolution 4/13 on sustainable coral reefs management, requesting the United Nations Environment Programme (UNEP) and the International Coral Reef Initiative (ICRI) to better define best practices for coral restoration for the maintenance of ecosystem goods and services. However, reporting of success has primarily focused on a few metrics (e.g., coral growth and survival — see Suggett et al., 2019) rather than metrics related to ecosystem function and health (Hein et al., 2017; Boström-Einarsson et al., 2020; Nuñez Lendo et al., 2023), making it difficult to assess the success of restorative interventions in maintaining or restoring desirable ecosystem service value. Therefore, understanding the carbonate budget of both natural and restored reefs will become increasingly important as they continue to experience environmental stress (e.g., tropical cyclones/hurricanes and changes in sea-surface temperature; Glynn, 1984; Done, 1992) in order to evaluate their capacity to provide essential ecosystem goods and services (e.g., coastal protection and fish nursery areas).
In conclusion, our finding highlights the relevance of using more goal-oriented metrics (e.g., carbonate budgets, rugosity, and skeletal properties) to evaluate restoration success depending on the desired returned ecosystem service (e.g., coastal protection and habitat provision). In doing this, we can assess if a restoration intervention is maintaining or restoring reef functioning and associated ecosystem services, which is the ultimate goal of restoration, and optimised return-on-effort.
Data availability statement
The datasets generated and analysed during the current study are available from the corresponding author upon request.
Author contributions
CINL: Conceptualization, Data curation, Formal analysis, Investigation, Methodology, Project administration, Validation, Visualization, Writing – original draft, Writing – review & editing, Funding acquisition, Resources. DJS: Conceptualization, Writing – review & editing, Supervision, Funding acquisition, Resources. CB: Methodology, Writing – review & editing. AM: Funding acquisition, Writing – review & editing. FN: Funding acquisition, Writing – review & editing. EEF: Resources, Writing – review & editing. DS: Funding acquisition, Writing – review & editing. EFC: Conceptualization, Supervision, Writing – review & editing, Funding acquisition, Resources.
Funding
The author(s) declare financial support was received for the research, authorship, and/or publication of this article. This research was supported by an International Research Scholarship (IRS) and UTS President’s Scholarship (UTSP) to CINL. The contribution of EFC was supported by an Australian Research Council Discovery Early Career Research Award (DE190100142). Access to sites and coral material, as well as contributions of DJS, EFC, CB, AM, FN, DS and EEF to writing and manuscript preparation, was facilitated through funding to the Coral Nurture Program from the Australian & Queensland Governments “Solving the bottleneck of reef rehabilitation through boosting coral abundance: Miniaturising and mechanising coral out-planting” in 2020; and the partnership between the Australian Government’s Reef Trust and the Great Barrier Reef Foundation in 2021; also, the many in-kind contributions from Reef Magic and GBR Biology crew who have tirelessly supported the work at Moore Reef since 2020. The Moore Reef MARSS installation and all coral material were collected under permits G20/42902.1 (2020) and G21/45224.1 (2021), respectively, from the Great Barrier Reef Marine Park Authority.
Acknowledgments
We wish to thank the past, present, and emerging Gadigal of the Eora Nation, Yidinji, Gunggandji, Yirrganydji, and Kuku Yalanji Traditional Owners of the of the land and sea country where our research took place. Also, to the partners Mars Sustainable Solutions, James Cook University, Yirrganydji Land and Sea Country Rangers, Biopixel, and Citizens of the GBR, who participated alongside the main project managers Reef Magic and GBR Biology to install the initial set of Reef Stars on Moore Reef. A special thanks to the dive masters Penny McCracken (faculty technical staff) who supervised my diving work and safety, Daniela Berenice Martı́nez Leon from Wavelength Reef Cruises who accompanied me during the February 2021 fieldwork; and representing Reef Magic and GBR Biology, Tessa Concannon and Justin Bovery-Spencer who ensured my diving safety during February 2022; and Caitlin Younis who helped me to contextualise my results with Moore Reef trajectory and characteristics.
Conflict of interest
The authors declare that the research was conducted in the absence of any commercial or financial relationships that could be construed as a potential conflict of interest.
The author(s) declared that they were an editorial board member of Frontiers, at the time of submission. This had no impact on the peer review process and the final decision.
Publisher’s note
All claims expressed in this article are solely those of the authors and do not necessarily represent those of their affiliated organizations, or those of the publisher, the editors and the reviewers. Any product that may be evaluated in this article, or claim that may be made by its manufacturer, is not guaranteed or endorsed by the publisher.
Supplementary material
The Supplementary Material for this article can be found online at: https://www.frontiersin.org/articles/10.3389/fmars.2023.1298411/full#supplementary-material
References
Al-Horani F. A. (2015). “Physiology of skeletogenesis in scleractinian coral,” in Diseases of Coral. Eds. Woodley C. M., Downs C. A., Bruckner A. W., J. W. P., S. B. G. 192–205. doi: 10.1002/9781118828502.ch12
Aline T. (2008). Dissolution of dead corals by euendolithic microorganisms across the northern Great Barrier Reef (Australia). Microb. Ecol. 55, 569–580. doi: 10.1007/s00248-007-9302-6
Alvarez-Filip L., Dulvy N. K., Gill J. A., Côté I. M., Watkinson A. R. (2009). Flattening of Caribbean coral reefs: region-wide declines in architectural complexity. Proc. R. Soc. B Biol. Sci. 276, 3019–3025. doi: 10.1098/RSPB.2009.0339
Alvarez-Filip L., Gill J. A., Dulvy N. K. (2011). Complex reef architecture supports more small-bodied fishes and longer food chains on Caribbean reefs. Ecosphere 2 (10), 1–17. doi: 10.1890/ES11-00185.1
Andersson A. J., Kuffner I. B., MacKenzie F. T., Jokiel P. L., Rodgers K. S., Tan A. (2009). Net Loss of CaCO3 from a subtropical calcifying community due to seawater acidification: Mesocosm-scale experimental evidence. Biogeosciences 6, 1811–1823. doi: 10.5194/bg-6-1811-2009
Babcock R. C. (1991). Comparative demography of three species of scleractinian corals using age- and size-dependent classifications. Ecol. Monogr. 61, 225–244. doi: 10.2307/2937107
Bak R. (1990). Patterns of echinoid bioerosion in two Pacific coral reef lagoons. Mar. Ecol. Prog. Ser. 66, 272. doi: 10.3354/meps066267
Bak R. P. M. (1983). Neoplasia, regeneration and growth in the reef-building coral Acropora palmata. Mar. Biol. 77, 221–227. doi: 10.1007/BF00395810
Bak R. P. M. (1994). Sea urchin bioerosion on coral reefs: place in the carbonate budget and relevant variables. Coral Reefs 13, 99–103. doi: 10.1007/BF00300768
Bell G., Gehrke H., Hoegh-Guldberg, Johnson J., Borgne L., Lehodey L., et al. (2013). “Vulnerability of fisheries and aquaculture to climate change in Pacific Island countries and territories,” in Priority Adaptations to Climate Change for Pacific Fisheries and Aquaculture: Reducing Risks an Capitalizing on Opportunities. Eds. Johnson J., Bell J., D. Y. C. (Rome: FAO), 25–100.
Bishop M. J., Vozzo M. L., Mayer-Pinto M., Dafforn K. A. (2022). Complexity–biodiversity relationships on marine urban structures: reintroducing habitat heterogeneity through eco-engineering. Philos. Trans. R. Soc. 377, 20210393. doi: 10.1098/RSTB.2021.0393
Boström-Einarsson L., Babcock R. C., Bayraktarov E., Ceccarelli D., Cook N., Ferse S. C. A., et al. (2020). Coral restoration – A systematic review of current methods, successes, failures and future directions. PloS One 15, e0226631. doi: 10.1371/journal.pone.0226631
Box S. J., Mumby P. J. (2007). Effect of macroalgal competition on growth and survival of juenile Caribbean corals. Mar. Ecol. Prog. Ser. 342, 139–149. doi: 10.3354/meps342139
Brandl S. J., Rasher D. B., Côté I. M., Casey J. M., Darling E. S., Lefcheck J. S., et al. (2019). Coral reef ecosystem functioning: eight core processes and the role of biodiversity. Front. Ecol. Environ. 17, 445–454. doi: 10.1002/fee.2088
Broecker W. S., Takahashi T. (1966). Calcium carbonate precipitation on the Bahama Banks. J. Geophys. Res. 71, 1575–1602. doi: 10.1029/jz071i006p01575
Brown K. T., Bender-Champ D., Achlatis M., van der Zande R. M., Kubicek A., Martin S. B., et al. (2021). Habitat-specific biogenic production and erosion influences net framework and sediment coral reef carbonate budgets. Limnol. Oceanogr. 66, 349–365. doi: 10.1002/lno.11609
Browne N. K., Smithers S. G., Perry C. T. (2013). Carbonate and terrigenous sediment budgets for two inshore turbid reefs on the central Great Barrier Reef. Mar. Geol. 346, 101–123. doi: 10.1016/j.margeo.2013.08.011
Bucher D. J., Harriott V. J., Roberts L. G. (1998). Skeletal micro-density, porosity and bulk density of acroporid corals. J. Exp. Mar. Bio. Ecol. 228, 117–136. doi: 10.1016/S0022-0981(98)00020-3
Carroll A. G., Harrison P. L., Adjeroud M. (2017). Susceptibility of coral assemblages to successive bleaching events at Moorea, French Polynesia. Mar. Freshw. Res. 68, 760–771. doi: 10.1071/MF15134
Castro-Sanguino C., Bozec Y. M., Mumby P. J. (2020). Dynamics of carbonate sediment production by Halimeda: implications for reef carbonate budgets. Mar. Ecol. Prog. Ser. 639, 91–106. doi: 10.3354/MEPS13265
Ceccarelli D. M., McLeod I. M., Boström-Einarsson L., Bryan S. E., Chartrand K. M., Emslie M. J., et al. (2020). Substrate stabilisation and small structures in coral restoration: State of knowledge, and considerations for management and implementation. PloS One 15, e0240846. doi: 10.1371/journal.pone.0240846
Chadwick N. E., Loya Y. (1990). Regeneration after experimental breakage in the solitary reef coral Fungia granulosa Klunzinger 1879. J. Exp. Mar. Bio. Ecol. 142, 221–234. doi: 10.1016/0022-0981(90)90093-R
Chisholm J. R. M., Gattuso J.-P. (1991). Validation of the alkalinity anomaly technique for investigating calcification of photosynthesis in coral reef communities. Limnol. Oceanogr. 36, 1232–1239. doi: 10.4319/lo.1991.36.6.1232
Chong-Seng K. M., Mannering T. D., Pratchett M. S., Bellwood D. R., Graham N. A. J. (2012). The influence of coral reef benthic condition on associated fish assemblages. PloS One 7, e42167. doi: 10.1371/journal.pone.0042167
Cole A. J., Pratchett M. S., Jones G. P. (2008). Diversity and functional importance of coral-feeding fishes on tropical coral reefs. Fish Fish 9, 286–307. doi: 10.1111/j.1467-2979.2008.00290.x
Cornwell B., Armstrong K., Walker N., Lippert M., Nestor V., Golbuu Y., et al. (2021). Widespread variation in heat tolerance and symbiont load are associated with growth tradeoffs in the coral Acropora hyacinthus in Palau. Elife 10, e64790. doi: 10.7554/ELIFE.64790
Courtney T. A., Barkley H. C., Chan S., Couch C. S., Kindinger T. L., Oliver T. A., et al. (2022). Rapid assessments of Pacific Ocean net coral reef carbonate budgets and net calcification following the 2014-2017 global coral bleaching event. Limnol. Ocean. 67, 1687–1700. doi: 10.1002/lno.12159
Cyronak T., Santos I. R., Eyre B. D. (2013). Permeable coral reef sediment dissolution driven by elevated pCO 2 and pore water advection. Geophys. Res. Lett. 40, 4876–4881. doi: 10.1002/grl.50948
Davies P. J., Hutchings P. A. (1983). Initial colonization, erosion and accretion of coral substrate. Coral Reefs 2, 27–35. doi: 10.1007/bf00304729
De’Ath G., Fabricius K. E., Sweatman H., Puotinen M. (2012). The 27-year decline of coral cover on the Great Barrier Reef and its causes. Proc. Natl. Acad. Sci. U.S.A. 109, 17995–17999. doi: 10.1073/pnas.1208909109
Done T. J. (1992). Effects of tropical cyclone waves on ecological and geomorphological structures on the Great Barrier Reef. Cont. Shelf Res. 12, 859–872. doi: 10.1016/0278-4343(92)90048-O
Eakin C. M. (1996). Where have all the carbonates gone? A model comparison of calcium carbonate budgets before and after the 1982-1983 El Niño at Uva Island in the eastern Pacific. Coral Reefs 15, 109–119. doi: 10.1007/BF01771900
Enochs I. C., Toth L. T., Brandtneris V. W., Afflerbach J. C., Manzello D. P. (2011). Environmental determinants of motile cryptofauna on an eastern Pacific coral reef. Mar. Ecol. Prog. Ser. 438, 105–118. doi: 10.3354/meps09259
Evensen N. R., Edmunds P. J., Sakai K. (2015). Effects of pCO2 on spatial competition between the corals Montipora aequituberculata and Porites lutea. Mar. Ecol. Prog. Ser. 541, 123–134. doi: 10.3354/meps11512
Fantazzini P., Mengoli S., Pasquini L., Bortolotti V., Brizi L., Mariani M., et al. (2015). Gains and losses of coral skeletal porosity changes with ocean acidification acclimation. Nat. Commun. 6, 7785. doi: 10.1038/ncomms8785
Ferrario F., Beck M. W., Storlazzi C. D., Micheli F., Shepard C. C., Airoldi L. (2014). The effectiveness of coral reefs for coastal hazard risk reduction and adaptation. Nat. Commun. 5, 3794. doi: 10.1038/ncomms4794
Fox H. E., Harris J. L., Darling E. S., Ahmadia G. N., Estradivari, Razak T. B. (2019). Rebuilding coral reefs: success (and failure) 16 years after low-cost, low-tech restoration. Restor. Ecol. 27, 862–869. doi: 10.1111/rec.12935
Fraser K. M., Stuart-Smith R. D., Ling S. D., Edgar G. J. (2021). High biomass and productivity of epifaunal invertebrates living amongst dead coral. Mar. Biol. 168, 102. doi: 10.1007/s00227-021-03911-1
Gardner T. A., Côté I. M., Gill J. A., Grant A., Watkinson A. R. (2003). Long-term region-wide declines in caribbean corals. Science 301, 958–960. doi: 10.1126/science.1086050
Glynn P. W. (1984). Widespread coral mortality and the 1982–83 el niño warming event. Environ. Conserv. 11, 133–146. doi: 10.1017/S0376892900013825
Goreau T. F., Goreau N. I., Goreau T. J. (1979). Corals and coral reefs. Sci. Am. 241, 124–137. doi: 10.1038/scientificamerican0879-124
Graham N. A. J. J., Wilson S. K., Jennings S., Polunin N. V. C. C., Bijoux J. P., Robinson J. (2006). Dynamic fragility of oceanic coral reef ecosystems. Proc. Natl. Acad. Sci. U.S.A. 103, 8425–8429. doi: 10.1073/pnas.0600693103
Greenstein B. J., Pandolfi J. M. (2003). Taphonomic alteration of reef corals: Effects of reef environment and coral growth form II: The Florida Keys. Palaios 18, 495–509. doi: 10.1669/0883-1351(2003)018<0495:TAORCE>2.0.CO;2
Grima A. J., Clases, David, Gonzalez De Vega R., Nitschke M. R., Goyen S., et al. (2022). Species-specific elementomes for scleractinian coral hosts and their associated Symbiodiniaceae. Coral Reefs 1, 1–16. doi: 10.1007/S00338-022-02259-2
Halpern B. S., Walbridge S., Selkoe K. A., Kappel C. V., Micheli F., D’Agrosa C., et al. (2008). A global map of human impact on marine ecosystems. Science 319, 948–952. doi: 10.1126/science.1149345
Harmelin-Vivien M. (1994). The effects of storms and cyclones on coral reefs: A review on JSTOR. J. Coast. Res. 12, 211–231.
Hein M., Vardi T., Shaver E. C., Pioch S., Boström-Einarsson L., Ahmed M., et al. (2021). Perspectives on the use of coral reef restoration as a strategy to support and improve reef ecosystem services. Front. Mar. Sci. 0. doi: 10.3389/FMARS.2021.618303
Hein M., Willis B. L., Beeden R., Birtles A. (2017). The need for broader ecological and socioeconomic tools to evaluate the effectiveness of coral restoration programs. Restor. Ecol. 25, 873–883. doi: 10.1111/rec.12580
Hoegh-Guldberg O., Poloczanska E. S., Skirving W., Dove S. (2017). Coral reef ecosystems under climate change and ocean acidification. Front. Mar. Sci. 4. doi: 10.3389/fmars.2017.00158
Hoogenboom M. O., Frank G. E., Chase T. J., Jurriaans S., Álvarez-Noriega M., Peterson K., et al. (2017). Environmental drivers of variation in bleaching severity of Acropora species during an extreme thermal anomaly. Front. Mar. Sci. 4. doi: 10.3389/FMARS.2017.00376/BIBTEX
Hubbard D., Miller A., Scaturo D. (1990). Production and cycling of calcium carbonate in a shelf-edge reef system (St. Croix, US Virgin Islands); applications to the nature of reef systems in the fossil record. J. Sediment. Res. 60, 335–360. doi: 10.1306/212F9197-2B24-11D7-8648000102C1865D
Huertas V., Morais R. A., Bonaldo R. M., Bellwood D. R. (2021). Parrotfish corallivory on stress-tolerant corals in the Anthropocene. PloS One 16, e0250725. doi: 10.1371/JOURNAL.PONE.0250725
Hughes T. P., Baird A. H., Morrison T. H., Torda G. (2023). Principles for coral reef restoration in the anthropocene. One Earth 6, 656–665. doi: 10.1016/j.oneear.2023.04.008
Hughes T. P., Kerry J. T., Álvarez-Noriega M., Álvarez-Romero J. G., Anderson K. D., Baird A. H., et al. (2017). Global warming and recurrent mass bleaching of corals. Nature 543, 373–377. doi: 10.1038/nature21707
Hughes T. P., Kerry J. T., Baird A. H., Connolly S. R., Dietzel A., Eakin C. M., et al. (2018). Global warming transforms coral reef assemblages. Nature 556, 492–496. doi: 10.1038/s41586-018-0041-2
Hughes T. P., Kerry J. T., Connolly S. R., Álvarez-Romero J. G., Eakin C. M., Heron S. F., et al. (2021). Emergent properties in the responses of tropical corals to recurrent climate extremes. Curr. Biol. 31, 5393–5399. doi: 10.1016/j.cub.2021.10.046
Johns K. A., Emslie, Michael J., Hoey, Andrew S., Osborne, et al. (2018). Macroalgal feedbacks and substrate properties maintain a coral reef regime shift. Ecosphere 9, e02349. doi: 10.1002/ECS2.2349
Jokiel P. L., Maragos J., Franzisket L. (1978). “Coral growth: buoyant weight technique," in Coral Reefs: Research Methods. Eds. Stoddart D. R., Johannes R. E. (UNESCO), 529–541.
Kenyon T. M., Doropoulos C., Dove S., Webb G. E., Newman S. P., Sim C. W. H., et al. (2020). The effects of rubble mobilisation on coral fragment survival, partial mortality and growth. J. Exp. Mar. Bio. Ecol. 533, 151467. doi: 10.1016/j.jembe.2020.151467
Kenyon T. M., Doropoulos C., Wolfe K., Webb G. E., Dove S., Harris D., et al. (2022). Coral rubble dynamics in the Anthropocene and implications for reef recovery. Limnol. Oceanogr. 9999, 1–38. doi: 10.1002/LNO.12254
Kojis B. L., Quinn N. J. (2001). The importance of regional differences in hard coral recruitment rates for determining the need for coral restoration. Bull. Mar. Sci. 69, 967–974.
Kuffner I. B., Bartels E., Stathakopoulos A., Enochs I. C., Kolodziej G., Toth L. T., et al. (2017). Plasticity in skeletal characteristics of nursery-raised staghorn coral, Acropora cervicornis. Coral Reefs 36, 679–684. doi: 10.1007/s00338-017-1560-2
Lamont T. A. C., Williams B., Chapuis L., Prasetya M. E., Seraphim M. J., Harding H. R., et al. (2022). The sound of recovery: Coral reef restoration success is detectable in the soundscape. J. Appl. Ecol. 59, 742–756. doi: 10.1111/1365-2664.14089
Lange I. D., Perry C. T. (2019). Bleaching impacts on carbonate production in the Chagos Archipelago: influence of functional coral groups on carbonate budget trajectories. Coral Reefs 36, 679–684. doi: 10.1007/s00338-019-01784-x
Lange I. D., Perry C. T., Alvarez-Filip L. (2020). Carbonate budgets as indicators of functional reef “health”: A critical review of data underpinning census-based methods and current knowledge gaps. Ecol. Indic. 110, 105857. doi: 10.1016/j.ecolind.2019.105857
Leggat W. P., Camp E. F., Suggett D. J., Heron S. F., Fordyce A. J., Gardner S., et al. (2019). Rapid coral decay is associated with marine heatwave mortality events on reefs. Curr. Biol. 29, 2723–2730. doi: 10.1016/j.cub.2019.06.077
Lirman D. (2000). Fragmentation in the branching coral Acropora palmata (Lamarck): Growth, survivorship, and reproduction of colonies and fragments. J. Exp. Mar. Bio. Ecol. 251, 41–57. doi: 10.1016/S0022-0981(00)00205-7
Lirman D., Schopmeyer S., Galvan V., Drury C., Baker A. C., Baums I. B. (2014). Growth dynamics of the threatened caribbean staghorn coral acropora cervicornis: Influence of host genotype, symbiont identity, colony size, and environmental setting. PloS One 9, e107253. doi: 10.1371/journal.pone.0107253
Lirman D., Thyberg T., Herlan J., Hill C., Young-Lahiff C., Schopmeyer S., et al. (2010). Propagation of the threatened staghorn coral Acropora cervicornis: Methods to minimize the impacts of fragment collection and maximize production. Coral Reefs 29, 729–735. doi: 10.1007/s00338-010-0621-6
Loya Y. (1976). Skeletal regeneration in a Red Sea scleractinian coral population. Nature 261, 490–491. doi: 10.1038/261490a0
Loya Y., Sakai K., Yamazato K., Nakano Y., Sambali H., van Woesik R. (2001). Coral bleaching: the winners and the losers. Ecol. Lett. 4, 122–131. doi: 10.1046/j.1461-0248.2001.00203.x
Madin J. S., Hoogenboom M. O., Connolly S. R., Darling E. S., Falster D. S., Huang D., et al. (2016). A trait-based approach to advance coral reef science. Trends Ecol. Evol. 31, 419–428. doi: 10.1016/j.tree.2016.02.012
Madin J. S., McWilliam M., Quigley K., Bay L. K., Bellwood D., Doropoulos C., et al. (2023). Selecting coral species for reef restoration. J. Appl. Ecol. 60, 1537–1544. doi: 10.1111/1365-2664.14447
Marshall P. A., Baird A. H. (2000). Bleaching of corals on the Great Barrier Reef: Differential susceptibilities among taxa. Coral Reefs 19, 155–163. doi: 10.1007/s003380000086
McClanahan T., Maina J., Pet-Soede L. (2002). “Effects of the 1998 coral morality event on Kenyan coral reefs and fisheries. Ambio 31, 543–550. doi: 10.1579/0044-7447-31.7.543
McCormick M. I. (1994). Comparison of field methods for measuring surface tomography and their associations with a tropical reef fish assemblage. Mar. Ecol. Prog. Ser. 112, 87–96. doi: 10.3354/meps112087
McCulloch M. T., D’Olivo J. P., Falter J., Holcomb M., Trotter J. A. (2017). Coral calcification in a changing World and the interactive dynamics of pH and DIC upregulation. Nat. Commun. 8, 15686. doi: 10.1038/ncomms15686
Meesters E. H., Noordeloos M., Bak R. P. M. (1994). Damage and regeneration: Links to growth in the reef-building coral Montastrea annularis. Mar. Ecol. Prog. Ser. 112, 119–128. doi: 10.3354/meps112119
Nelson H. R., Kuempel C. D., Altieri A. H. (2016). The resilience of reef invertebrate biodiversity to coral mortality. Ecosphere 7, e01399. doi: 10.1002/ecs2.1399
Nithyanandan M., Le Vay L., Raja D. K., Kesavan R., Pereira D. (2018). Coral nursery and transplantation of the staghorn coral, Acropora downingi in Sabah Al-Ahmad Sea City, Kuwait, Arabian Gulf. Cogent Environ. Sci. 4, 1480334. doi: 10.1080/23311843.2018.1480334
Nuñez Lendo C. I., Camp E. F., Edmondson J., Hughes D. H., Kuzhiumparambil U., Clases D., et al. (2023). Multiple trait approach to inform ecosystem service value of corals propagated for restoration on the Great Barrier Reef. Res. Sq. doi: 0.21203/rs.3.rs-2030847/v1
Peñuelas J., Fernández-Martínez M., Ciais P., Jou D., Piao S., Obersteiner M., et al. (2019). The bioelements, the elementome, and the biogeochemical niche. Ecology 100, e02652. doi: 10.1002/ecy.2652
Perry C. T., Alvarez-Filip L., Graham N. A. J., Mumby P. J., Wilson S. K., Kench P. S., et al. (2018). Loss of coral reef growth capacity to track future increases in sea level. Nature 558, 396–400. doi: 10.1038/s41586-018-0194-z
Perry C. T., Edinger E. N., Kench P. S., Murphy G. N., Smithers S. G., Steneck R. S., et al. (2012). Estimating rates of biologically driven coral reef framework production and erosion: A new census-based carbonate budget methodology and applications to the reefs of Bonaire. Coral Reefs 31, 853–868. doi: 10.1007/s00338-012-0901-4
Perry C. T., Hepburn L. J. (2008). Syn-depositional alteration of coral reef framework through bioerosion, encrustation and cementation: Taphonomic signatures of reef accretion and reef depositional events. Earth-Science Rev. 86, 106–144. doi: 10.1016/j.earscirev.2007.08.006
Perry C. T., Morgan K. M. (2017). Bleaching drives collapse in reef carbonate budgets and reef growth potential on southern Maldives reefs. Sci. Rep. 7, 40581. doi: 10.1038/srep40581
Perry C. T., Murphy G. N., Kench P. S., Smithers S. G., Edinger E. N., Steneck R. S., et al. (2013). Caribbean-wide decline in carbonate production threatens coral reef growth. Nat. Commun. 4, 1402. doi: 10.1038/ncomms2409
Pratchett M. S., Heron S. F., Scott F., Mellin C., Cumming G. S. (2021). “Recurrent mass-bleaching and the potential for ecosystem collapse on Australia’s great barrier reef,” in Ecosystem Collapse and Climate Change. Ecological Studies, Vol 241. Eds. Canadell J. G., Jackson R. B. (Cham: Springer), 265–289. doi: 10.1007/978-3-030-71330-0_10
Pratchett M. S., McCowan D., Maynard J. A., Heron S. F. (2013). Changes in bleaching susceptibility among corals subject to ocean warming and recurrent bleaching in moorea, French Polynesia. PloS One 8, e70443. doi: 10.1371/JOURNAL.PONE.0070443
Raymundo L. J., Maypa A. P., Gomez E. D., Cadiz P. (2007). Can dynamite-blasted reefs recover? A novel, low-tech approach to stimulating natural recovery in fish and coral populations. Mar. Pollut. Bull. 54, 1009–1019. doi: 10.1016/j.marpolbul.2007.02.006
Rees S. A., Opdyke B. N., Wilson P. A., Henstock T. J. (2007). Significance of Halimeda bioherms to the global carbonate budget based on a geological sediment budget for the Northern Great Barrier Reef, Australia. Coral Reefs 26, 177–188. doi: 10.1007/s00338-006-0166-x
Rice M. M., Maher R. L., Correa A. M. S., Moeller H. V., Lemoine N. P., Shantz A. A., et al. (2020). Macroborer presence on corals increases with nutrient input and promotes parrotfish bioerosion. Coral Reefs 39, 409–418. doi: 10.1007/s00338-020-01904-y
Rogers A., Blanchard J. L., Mumby P. J. (2014). Vulnerability of coral reef fisheries to a loss of structural complexity. Curr. Biol. 24, 1000–1005. doi: 10.1016/j.cub.2014.03.026
Rogers A., Blanchard J. L., Mumby P. J. (2018). Fisheries productivity under progressive coral reef degradation. J. Appl. Ecol. 55, 1041–1049. doi: 10.1111/1365-2664.13051
Santoso P., Setiawan F., Subhan B., Arafat D., Bengen D. G., Iqbal Sani L. M., et al. (2022). Influence of Coral Reef Rugosity on Fish Communities in Marine Reserves Around Lombok Island, Indonesia. Environ. Biol. Fishes 105, 105–117. doi: 10.1007/s10641-021-01198-1
Scoffin T. P. (1992). Taphonomy of coral reefs: a review. Coral Reefs 11, 57–77. doi: 10.1007/BF00357423
Scoffin T., Stearn C., Boucher D., Frydl P., Hawkins C., Hunter I., et al. (1980). Calcium carbonate budget of a fringing reef on the west coast of Barbados. II. Erosion sediments Internal Struct. Bull. Mar. Sci. 30, 475–508.
Shapiro S. S., Wilk M. B. (1965). An analysis of variance test for normality (complete samples). Biometrika 52, 591–611. doi: 10.1093/biomet/52.3-4.591
Shaver E. C., McLeod E., Hein M. Y., Palumbi S. R., Quigley K., Vardi T., et al. (2022). A roadmap to integrating resilience into the practice of coral reef restoration. Glob. Change Biol. 28, 4751–4764. doi: 10.1111/GCB.16212
Siebeck U. E., Marshall N. J., Klüter A., Hoegh-Guldberg O. (2006). Monitoring coral bleaching using a colour reference card. Coral Reefs 25, 453–460. doi: 10.1007/s00338-006-0123-8
Smith S. V., Key G. S. (1975). Carbon dioxide and metabolism in marine environments. Limnol. Oceanogr. 20, 493–495. doi: 10.4319/lo.1975.20.3.0493
Soong K. (1993). Colony size as a species character in massive reef corals. Coral Reefs 12, 77–83. doi: 10.1007/BF00302106/METRICS
Stearn C., Scoffin T., Martindale W. (1977). Calcium carbonate budget of a fringing reef on the west coast of Barbados. Part I - zonation and productivity. Bull. Mar. Sci. 27, 479–510.
Stella J. S., Wolfe K., Roff G., Rogers A., Priest M., Golbuu Y., et al. (2022). Functional and phylogenetic responses of motile cryptofauna to habitat degradation. J. Anim. Ecol. 91, 2203–2219. doi: 10.1111/1365-2656.13809
Storlazzi C. D., Gingerich S. B., Van Dongeren A., Cheriton O. M., Swarzenski P. W., Quataert E., et al. (2018). Most atolls will be uninhabitable by the mid-21st century because of sea-level rise exacerbating wave-driven flooding. Sci. Adv. 4, eaap9741. doi: 10.1126/sciadv.aap9741
Stuart-Smith R. D., Brown C. J., Ceccarelli D. M., Edgar G. J. (2018). Ecosystem restructuring along the Great Barrier Reef following mass coral bleaching. Nature 560, 92–96. doi: 10.1038/s41586-018-0359-9
Suggett D. J., Camp E. F., Edmondson J., Boström-Einarsson L., Ramler V., Lohr K., et al. (2019). Optimizing return-on-effort for coral nursery and outplanting practices to aid restoration of the Great Barrier Reef. Restor. Ecol. 27, 683–693. doi: 10.1111/rec.12916
Suggett D. J., van Oppen M. J. H. (2022). Horizon scan of rapidly advancing coral restoration approaches for 21st century reef management. Emerg. Top. Life Sci. 6, 125–136. doi: 10.1042/ETLS20210240
Sweatman H., Delean S., Syms C. (2011). Assessing loss of coral cover on Australia’s Great Barrier Reef over two decades, with implications for longer-term trends. Coral Reefs 30, 521–531. doi: 10.1007/s00338-010-0715-1
Tiddy I. C., Kaullysing D., Bailey D. M., Chumun P. K., Killen S. S., Le Vin A., et al. (2021). Outplanting of branching Acropora enhances recolonization of a fish species and protects massive corals from predation. Coral Reefs 40, 1549–1561. doi: 10.1007/s00338-021-02147-1
Tortolero-Langarica J. J. A., Rodríguez-Troncoso A. P., Cupul-Magaña A. L., Alarcón-Ortega L. C., Santiago-Valentín J. D. (2019). Accelerated recovery of calcium carbonate production in coral reefs using low-tech ecological restoration. Ecol. Eng. 128, 89–97. doi: 10.1016/J.ECOLENG.2019.01.002
Tribble G. W., Sansone F. J., Smith S. V. (1990). Stoichiometric modeling of carbon diagenesis within a coral reef framework. Geochim. Cosmochim. Acta 54, 2439–2449. doi: 10.1016/0016-7037(90)90231-9
Tribollet A., Decherf G., Hutchings P. A., Peyrot-Clausade M. (2002). Large-scale spatial variability in bioerosion of experimental coral substrates on the Great Barrier Reef (Australia): Importance of microborers. Coral Reefs 21, 424–432. doi: 10.1007/s00338-002-0267-0
Tribollet A., Golubic S. (2005). Cross-shelf differences in the pattern and pace of bioerosion of experimental carbonate substrates exposed for 3 years on the northern Great Barrier Reef, Australia. Coral Reefs 24, 422–434. doi: 10.1007/s00338-005-0003-7
UNEP-WCMC (2006). In the front line: shoreline protection and other ecosystem services from mangroves and coral reefs. (Cambridge, UK: UNEP-WCMC), 33.
Vander Voort G. F. (1999). Metallography, principles and practice. (Ohio: ASM international, Metals Park), 1–752.
Viehman T. S., Hench J. L., Griffin S. P., Malhotra A., Egan K., Halpin P. N. (2018). Understanding differential patterns in coral reef recovery: chronic hydrodynamic disturbance as a limiting mechanism for coral colonization. Mar. Ecol. Prog. Ser. 605, 135–150. doi: 10.3354/MEPS12714
Wild C., Huettel M., Klueter A., Kremb S. G., Rasheed M. Y. M., Jørgensen B. B. (2004). Coral mucus functions as an energy carrier and particle trap in the reef ecosystem. Nature 428, 66–70. doi: 10.1038/nature02344
Williams S. L., Sur C., Janetski N., Hollarsmith J. A., Rapi S., Barron L., et al. (2019). Large-scale coral reef rehabilitation after blast fishing in Indonesia. Restor. Ecol. 27, 447–456. doi: 10.1111/rec.12866
Wilson S. K., Graham N. A. J., Pratchett M. S., Jones G. P., Polunin N. V. C. (2006). Multiple disturbances and the global degradation of coral reefs: are reef fishes at risk or resilient? Glob. Change Biol. 12, 2220–2234. doi: 10.1111/J.1365-2486.2006.01252.X
Wolfe K., Kenyon T. M., Desbiens A., de la Motte K., Mumby P. J. (2023). Hierarchical drivers of cryptic biodiversity on coral reefs. Ecol. Monogr. 93, e1586. doi: 10.1002/ecm.1586
Xin L. H., Adzis K. A. A., Hyde J., Cob Z. C. (2016). Growth performance of acropora formosa in natural reefs and coral nurseries for reef restoration. AACL Bioflux 9, 1090–1100.
Yates K. K., Zawada D. G., Smiley N. A., Tiling-Range G. (2017). Divergence of seafloor elevation and sea level rise in coral reef ecosystems. Biogeosciences 14, 1739–1772. doi: 10.5194/bg-14-1739-2017
Young M. A. L., Bellwood D. R. (2011). Diel patterns in sea urchin activity and predation on sea urchins on the Great Barrier Reef. Coral Reefs 30, 729–736. doi: 10.1007/s00338-011-0754-2
Keywords: coral reef, coral restoration, coral calcification, reef accretion, skeletal traits, trade-offs, ecosystem services, carbonate budgets
Citation: Nuñez Lendo CI, Suggett DJ, Boote C, McArdle A, Nicholson F, Fisher EE, Smith D and Camp EF (2024) Carbonate budgets induced by coral restoration of a Great Barrier Reef site following cyclone damage. Front. Mar. Sci. 10:1298411. doi: 10.3389/fmars.2023.1298411
Received: 21 September 2023; Accepted: 04 December 2023;
Published: 26 January 2024.
Edited by:
Wei Jiang, Guangxi University, ChinaReviewed by:
Kimberly B. Ritchie, University of South Carolina Beaufort, United StatesKathleen Sullivan Sealey, University of Miami, United States
Copyright © 2024 Nuñez Lendo, Suggett, Boote, McArdle, Nicholson, Fisher, Smith and Camp. This is an open-access article distributed under the terms of the Creative Commons Attribution License (CC BY). The use, distribution or reproduction in other forums is permitted, provided the original author(s) and the copyright owner(s) are credited and that the original publication in this journal is cited, in accordance with accepted academic practice. No use, distribution or reproduction is permitted which does not comply with these terms.
*Correspondence: C. Isabel Nuñez Lendo, Y2FybWVsYS5udW5lekB1dHMuZWR1LmF1; aW5lemxlbmRvQGdtYWlsLmNvbQ==