- 1Hainan Academy of Ocean and Fisheries Sciences, Hainan, China
- 2Yazhou Bay Innovation Institute, College of Ecology and Environment, Hainan Tropical Ocean University, Sanya, China
Introduction: Seagrasses play a key role in biodiversity maintenance, sediment stabilization, and carbon reduction. Due to the significant decline of seagrass populations worldwide, many projects to restore seagrass by transplantation have been undertaken in recent decades. However, effective restoration technologies required to rapidly restore seagrass on Hainan Island are yet to be developed.
Methods: In this study, an experiment was carried out to investigate methods for alleviating continuous degradation of seagrass beds on Hainan Island. Seagrass were transplanted by “single-plant-fixed-distance transplantation” method, survival rate, shoot formation rate, coverage and shoot density of transplanting plants were monitored for 23 months.
Results: Over 67% of T. hemprichii and 90% of E. acoroides were still alive, shoot density were over 11 shoots/m2 and 15 shoots/m2 respectively after almost two years, E. acoroides was more likely to survive than T. hemprichii both in Gaolong Bay and Xincun lagoon. Because of the damage to below-ground tissues, the survival rate, shoot formation rate, coverage and shoot density decreased during the first one year after transplantation, then increased gradually.
Discussion: We considered transplantation established successfully of T. hemprichii and E. acoroides in the new site was about 12 months. In addition, T. hemprichii was suitable for transplanted in Xincun lagoon, and E. acoroides was suitable for transplanted in Gaolong bay. The results demonstrated that the growth of transplanted seagrass was greatly affected by suspend solids and DIN. The results of this study may provide data support and scientific basis for seagrass transplantation in South China.
1 Introduction
Seagrasses are flowering plants that grow predominantly in coastal areas, such as bays and lagoons. They play an important role in marine ecosystems (Duarte and Krause-Jensen, 2017). The seagrass can reduce water flow, stabilize sediment. Seagrass in coastal waters provide habitat and nursery for fish and invertebrates (Duarte and Chiscano, 1999), supply food for large herbivores (Klumpp et al., 1993). Also, seagrass play an important role in the nutrition transfer, nitrogen fixation and maintain the physical and chemical properties of marine organisms (Van de Koppel et al., 2015; Lee et al., 2016; Nordlund et al., 2016; Jahnke et al., 2019).
However, seagrass beds can be changed by both natural and anthropogenic influences (Tan et al., 2020). Physical disturbance, bitten by herbivore, intraspecific competition in seagrass, pollution of nutrients and flood waters with sediment may influence the biomass, distribution area and species composition of seagrass bed (Rasheed, 2004; Rasheed et al., 2014). Anthropogenic influences, such as coastal development, runoffs from coastal urban and agricultural, discharge of untreated sewage and industrial wastewater, have led to extensive losses of seagrasses shallows located near estuaries and coasts (Huang et al., 2006; Waycott et al., 2009; Arias-Ortiz et al., 2018). This reinforces the need for seagrass restoration that would lead to a reduction in the loss of seagrass and an enhancement of biodiversity in these important coastal ecosystems.
Various of seagrass habitat restoration methods have been investigated in the past years, dependent on species (Fonseca et al., 1983). The common restoration methods include habitat restoration (Tan et al., 2020), seed dispersal, and transplantation. Habitat restoration may be a very slow process, taking a long time. Since the high survival rate and growth rate, transplantation has been considered as an effective way to slow down the degradation of seagrass (Davis and Short, 1997; Orth et al., 1999; Fishman et al., 2004; Paling et al., 2007; Park and Lee, 2007; Bastyan and Cambridge, 2008). In the past few decades, transplantation have been developed and attempted in many countries (Li et al., 2010), and several transplantation have achieved successful, including transplantation of “sod”, “plug” and “peat pots” (all the plant materials and surrounding sediment were put into a peat pot, then planted into sediment with an artificial supporting medium or not), “staples” or “sprigs” (a length of rhizome including roots and shoots, fixed into the sediment by a wire staple) (Keulen et al., 2010). These seagrass transplantation methods have also been tried on Zostera marina (Short et al., 2002), Posidonia oceanica (Calvo et al., 2021; Mancini et al., 2021), Zostera noltii (Van Katwijk et al., 2009), Thalassia testudinum (Thorhaug, 1985), Halodule wrightii (Phillips, 1974), Syringodium filiforme (Thorhaug, 1985), Posidonia sinuosa (Paling et al., 2001), Amphibolis griffithii (Paling et al., 2001), Posidonia australis (Bastyan and Cambridge, 2008), Halophila ovalis (Kirkman, 1998) in the United States, Western Australia and North America. In Korea, Zostera marina become the most widely distributed seagrass species after transplantation (Park and Lee, 2007; Lee and Park, 2008). A few seagrass restorations have been trialed in China recent years. The restoration species include H. ovalis, Zostera japonica, Z. marina in Shandong province (Zhang et al., 2020). In Guangxi Zhuang Autonomous Region, the most suitable species for seagrass transplantation are Z. japonica, H. ovalis and Halophila beccarii, and the plug of transplantation method is more practical in the intertidal zone of Guangxi because of the higher survival rate (Qiu et al., 2014).
The total seagrass area of China (except Sansha city) is 11113.4 ha. The seagrass meadow in Hainan Province covers an area of 4457.0 ha, accounting for 40.0% of the total area (Li et al., 2022). It is mainly distributed in the eastern and southern coast of Hainan Province, such as Wenchang, Qionghai, Lingshui, and Sanya (Huang et al., 2006; Jiang et al., 2017). Due to human activities, such as artificial island construction, aquaculture and fishing, eutrophication of sea water (Ruiz et al., 2001; Moksnes et al., 2008; Fang et al., 2018; Cui et al., 2021), the meadows have been decling over the last year (Jiang et al., 2017). However, few studies focuse on the transplantation methods and continuous measurement of seagrass in Hainan province.
In this study, Thalassia hemprichii and Enhalus acoroides shoots were transplanted into Gaolong bay and Xincun Lagoon in May 2019 to establish seagrass transplantion in Hainan province. The physiological status of the transplanting were monitored every 4 months for a year. The survival rate, shoot formation rate, coverage and shoot density of transplants were monitored every 3 months for 23 months. The successful application of the transplants techniques not only provide practical guide for the meadow restoration in South China, but also provide valuable references for other seagrass species restoration.
2 Materials and methods
2.1 Study area
Gaolong bay (19°29′24.91”N, 110°48′53.66”E) is an open shallow water area with high salinity which located off the northeast coast of Hainan Island, China. The water exchanges well, and with a good self-purification ability. The bottom materials of Gaolong bay are predominantly coral debris, gravel, and sand (Zhou et al., 2012). The mainly human activities are fishing and building artificial islands.
Xincun lagoon (18°24′35.41”N, 110°0′38.90”E) is located on the southeast coast of Hainan Island. Relatively, the water of Xincun lagoon has low salinity and poor self-purification ability. The bottom material is sand-mud (Moksnes et al., 2008). Fishing, cage culture and ship anchoring are common in Xincun lagoon (Figure 1; Table 1).
2.2 Collection of transplanting shoots
The branching rate of rhizomes is an important indicator of seagrass cloning ability, a high branching rate indicating strong seagrass cloning ability (Sintes et al., 2005). The branching and rhizome elongation rates of T. hemprichii are higher than E. acoroides (Figure 2) (Marbà and Duarte, 1998). Although T. hemprichii and E. acoroides were not the only dominant seagrass species in Gaolong bay or Xincun lagoon, they possess strong adaptability and high survival rates. Therefore, they were selected for seagrass restoration in this study.
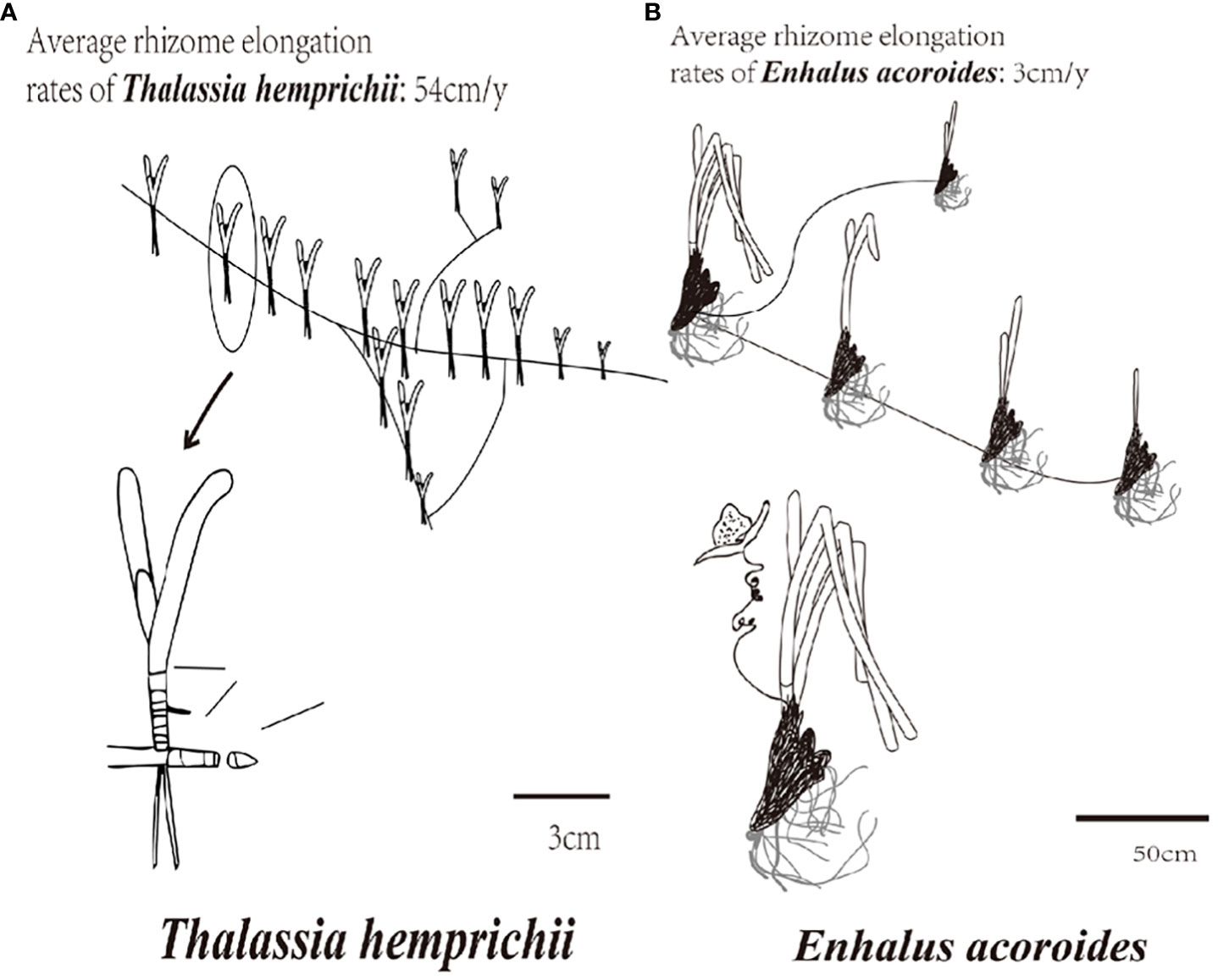
Figure 2 Branching rate and rhizome elongation rate of T. hemprichii and (E) acoroides. (A) T. hemprichii; (B) (E) acoroides.
In order to reduce the destruction of seagrass original habitat, meadows with areal extent more than 30 m2 and coverage greater than 80% were selected for donating shoots (Fang et al., 2018). All the donor shoots were collected from a healthy seagrass bed located in Lingshui Xincun lagoon seagrass special protected area (18°24′42′′N,109°57′58′′E), Hainan, China. The shoots were collected individually manually to reduce damage to the seagrass bed. Each transplanting shoot had three to five rhizome nodes and healthy leaves. The collected transplanting shoots were temporarily stored in large boxes with small quantity of cold seawater during transport to the transplanting location, preventing shoots desiccation. All the transplanting shoots were transplanted within 24 h.
2.3 Transplantation method
To compare the restoration performance of T. hemprichii and E. acoroides in Gaolong bay and Xincun lagoon, we selected the transplant sites with similar habitat conditions.
The transplanting shoots were planted manually by scuba divers through the frame method. We designed a fixing technique represented by an iron transplanting frame specifically. The iron transplanting frame was 1× 1 m, and divided internally into sixteen small squares evenly, one transplanting shoots in each square. A layer of fishing nets was attached on the surface of the transplanting frame by cable ties. Four 15 cm triangular irons legs were welded to the bottom of the transplanting frame at each of the corners. The transplant frame was inserted into bottom materials to make sure the transplanting shoots were anchored to the sediment (Figure 3).
Sixteen transplanting shoots were planted in each 1 × 1m transplanting frame, named transplanting unit. The transplanting unit was laid out in regular patterns, with the distance was 50 cm. We called this “single-plant-fixed-distance transplantation”, which was easy to conduct and less damage to surrounding habitats (Piazzi et al., 2021).
From March to April 2019, 90 transplanting units of T. hemprichii (including 1,440 transplanting shoots) and 10 transplanting units of E. acoroides (including 160 transplanting shoots) were transplanted in Gaolong bay. while 40 transplanting units of T. hemprichii and 60 transplanting units of E. acoroides were transplanted in Xincun lagoon, including 640 and 960 transplanting shoots respectively.
After transplantation, each transplanting unit was marked with serial numbers, transplantation time and other information were recorded. All the transplanting units placed were surrounded with plastic garden mesh (5 × 5 cm mesh size).
2.4 Environmental parameters
Water temperature was monitored by Pen Type Water Quality Meter (C-600, AZ Instrument Corp., Taiwan, China). To determine water salinity, suspended solids, dissolved inorganic nitrogen (DIN = nitrate + nitrite + ammonium) and dissolved inorganic phosphate (DIP), three replicate surface water samples were collected every 4-months by 200ml bottles. Water samples were stored on ice, then transport to the laboratory. Each water sample was filtered through Whatman GF/F filters (0.7 μm pore size) immediately. The content of suspended solids in the water was determined by weighing method. The DIN and DIP contents of the seawater samples were measured using standard colorimetric techniques by a spectrophotometer (TU-1900, Metash Instruments Co., Ltd, Shanghai, China). Nitrate, nitrite, ammonium and DIP were measured by zinc cadmium reduction method, hydrochloride naphthodiamide method, hypobromite oxidation method, and phosphorus molybdenum blue spectrophotometry, respectively (General Administration of Quality Supervision, 2008). Salinity was monitored using a Lab Salinometer (SYA2-2, National Ocean Technology Center, Tianjin, China).
2.5 Monitoring of transplantation
Statistical analyses were performed on monitoring the survival rate, shoot formation rate, coverage and shoot density of transplantation every 2 or 3 months for 23 months, from May 2019 to Mar 2021. Due to the Covid emergency, there was no monitoring in Dec 2020.
Survival rate (Renouard and Nisand, 2006): The percentage of living shoots after transplanting establishment. The survival rate of transplantation, which is the average of each transplanting unit, was calculated as follows:
In formula: L is seagrass survival rate (%), Ui is the number of surviving shoots in the ith transplanting unit, and U is the total number of transplanting shoots in each transplanting unit.
Shoot formation rate: The percentage of transplanting rhizomes with newly shoots. The shoot formation rate was estimated by counting the number of transplanting rhizomes with newly shoots or not respectively in each transplanting unit. The specific calculations were as follows:
where, B is the seagrass propagation rate (%), Ni is the number of transplanting rhizomes with newly shoots in the ith transplanting unit, and U is the total number of shoots in each transplanting unit. Coverage: The percentage of vertical projected area of vegetation (including stems, branches and leaves) on the ground against the total area of the transplanting unit. The coverage was estimate through a photogrammetry-based Chinese patent “A method for determining vegetation coverage based on grb and gridlines”. Firstly, suit images of each transplanting unit were acquired by scuba divers (Figure 4A). The color value of each pixel was changed into Visible-band Difference Vegetation Index (VDVI) (VDVI = (2 × G – R - B)/(2 × G + R + B)), which is designed to distinguish green vegetation (Wan et al., 2018; Ventura et al., 2022). Depending on threshold setting to 0.25, an image object was determined whether belonged to cover class, then this pixel was defined “vegetation point” (Figure 4B). The suit image was added grid lines of 20 × 20 pixels (Figure 4C). To distinguish the vegetation from sediment, we set two threshold values. When the “vegetation point” coverage was over 80% of a grid, the grid would be defined “vegetation grid”. If the “vegetation point” coverage was less than 20%, the grid would be defined “background grid”. Finally, the coverage was estimated by calculating the ratio of “vegetation grids” to total grids (Figure 4D).
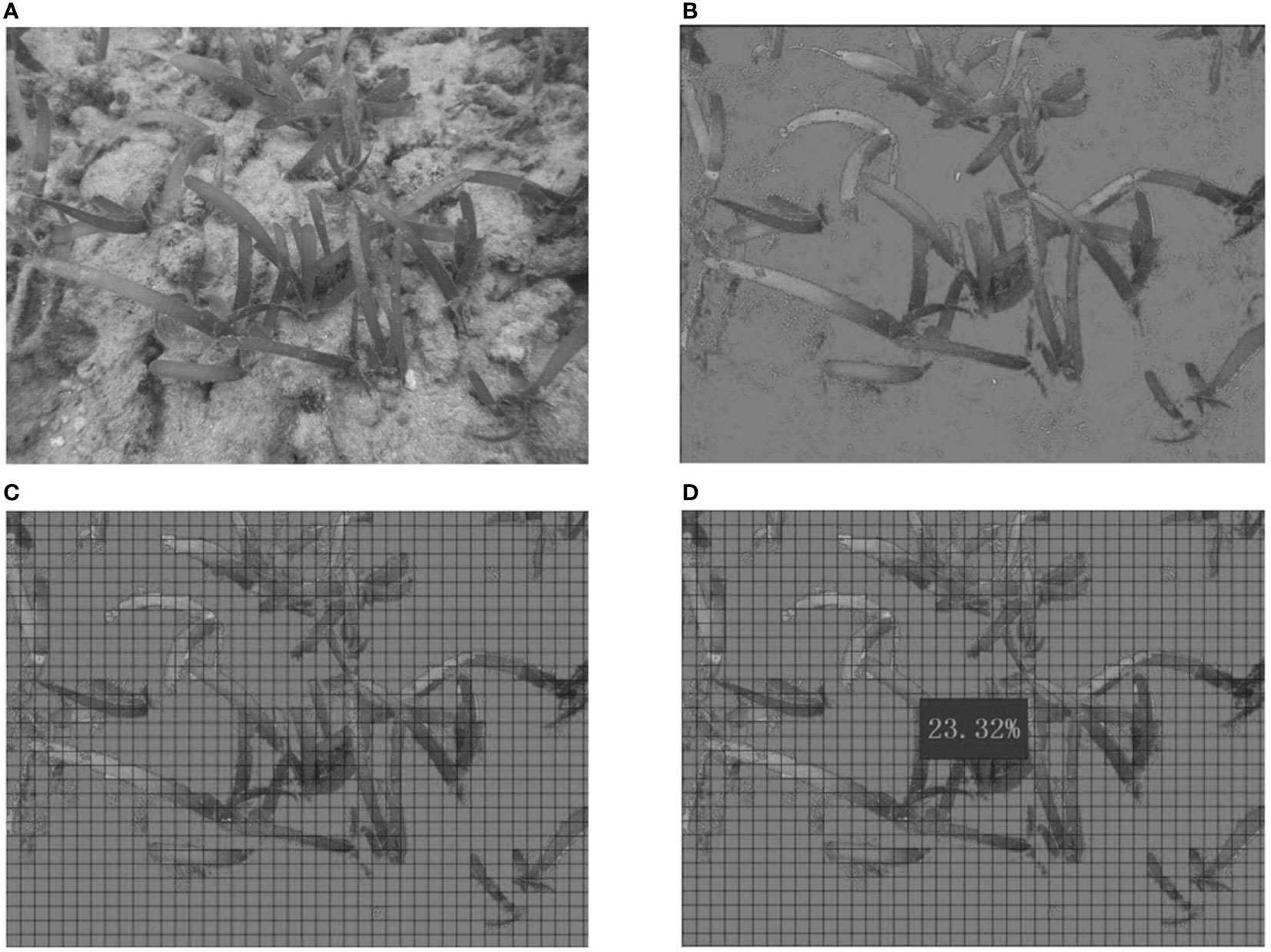
Figure 4 The method for determining vegetation coverage based on grb and gridlines. (A) the suit images of transplanting unit; (B) the color value of each pixel in the suit images was changed into VDVI; (C) the grid lines of 20 × 20 pixels were added on image; (D) the coverage was estimated.
Shoot density (Mancini et al., 2021): The number of shoots/m2. It was estimated by counting the number of surviving shoots in each transplanting unit (1 × 1 m).
2.6 Statistical analyses
All values were expressed as mean ± standard error. Microsoft office software was used to organize data and plot histograms. Statistical analyses were performed using SPSS 26.0 (SPSS Inc., Chicago, Illinois, USA). Data were tested for normality and homogeneity of variance to meet the assumptions of parametric statistics. Significant differences among sampling months were tested using a one-way analysis of variance (ANOVA) and least significant difference (LSD). Pearson correlation analysis was used to analyze the correlation of each parameter.
3 Results
3.1 Environmental parameters of transplanted site
The water temperature showed a clear temporal variation, it was high during summer and low during winter and spring. In Gaolong bay, the highest value was 30.12° in Aug 2019, and lowest value was 26.1° in Dec 2019. In Xincun lagoon, the highest value was 31.80° in Aug 2019, but the lowest value was 29.90° appeared in Apr 2020. The average of water temperature changed significantly (P<0.001) both in Gaolong bay and Xincun lagoon with sampling every 4-months (Figure 5A; Supplementary Table S1).

Figure 5 Seasonal changes in environmental parameters of transplanted site from Apr 2019 to Apr 2020. (A) water temperature; (B) salinity; (C) suspend solids; (D) DIN; (E) DIP.
The salinity was changed significantly (P<0.001) in Gaolong bay, the lowest value was 32.5723 in Apr 2019 while the highest was 33.9659 in Aug 2019. The salinity in Xincun lagoon showed the same variation pattern, with the highest value of 33.3534 in Aug 2019, but the lowest value 31.7700 appeared in Apr 2020 (Figure 5B; Supplementary Table S1). Overall, the salinity in summer was slightly higher than in other seasons, due to the rainfall and temperature.
The contents of suspend solids were increased from spring to winter both in Gaolong bay and Xincun lagoon. The highest value were 13.2733 mg/L and 8.5033 mg/L respectively in Gaolong bay and Xincun lagoon in Dec 2019, while the lowest values were 5.5000 mg/L in Gaolong bay in Apr 2019 and 4.7233 mg/L in Xincun lagoon in Apr 2020 (Figure 5C; Supplementary Table S1).
The water inorganic nutrients (DIN and DIP) concentrations were showed significantly (P<0.001) temporal variation in Gaolong bay. DIN concentration was highest (0.1077 mg/L) in Aug 2019 and lowest (0.0120 mg/L) in Apr 2020 (Figure 5D; Supplementary Table S1). DIP concentration was highest (0.0081 mg/L) in Aug 2019 and lowest (0.0012 mg/L) in Apr 2019 (Figure 5E; Supplementary Table S1). The water inorganic nutrients in Xincun lagoon were changed significantly (P<0.001) with sampling every 4 months, but did not show seasonal variation pattern (Figures 5D, E; Supplementary Table S1). In Gaolong bay, the higher water inorganic nutrients concentrations in summer may be related to the water pollution caused by over culture fisheries.
3.2 Comparison of transplantation performance of T. hemprichii
The survival rate of T. hemprichii transplanted in Gaolong bay and Xincun lagoon showed the same variation pattern. It was decline sharply at the first 13 months, then increased gradually. The survival rate of T. hemprichii transplanted in Gaolong bay was highest (90.42%) in May 2019 and lowest (56.18%) in Jun 2020, the highest and lowest value were 99.53% and 51.25% respectively in Xincun lagoon. The survival rate of T. hemprichii approached 70% by Mar 2021 both in Gaolong bay and Xincun (Figure 6A).
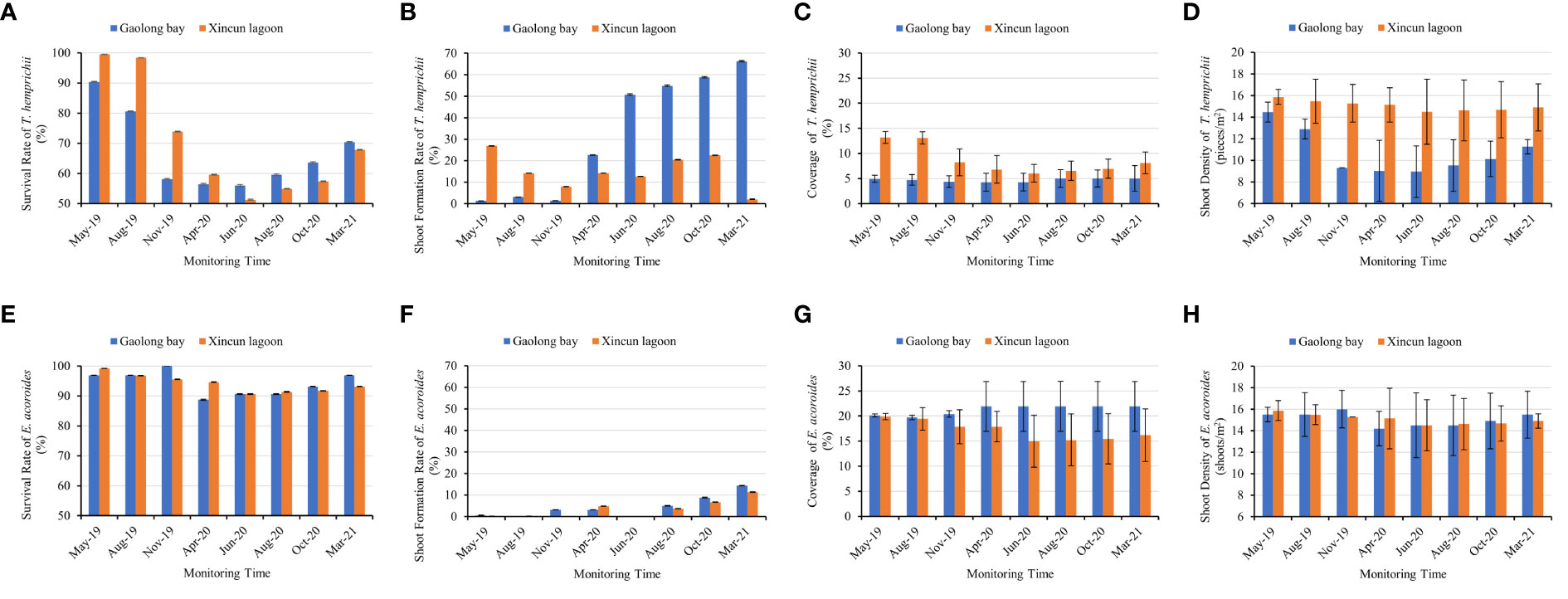
Figure 6 Comparison of the survival rate, shoot formation rate, coverage, and shoot density between T. hemprichii and (E) acoroides in Gaolong bay and Xincun lagoon. (A) the survival rate of T. hemprichii; (B) the shoot formation rate of T. hemprichii; (C) the coverage of T. hemprichii; (D) the shoot density of T. hemprichii; (E) the survival rate of (E) acoroides; (F) the shoot formation rate of E. acoroides; (G) the coverage of E. acoroides; (H) the shoot density of E. acoroides.
The shoot formation rate of T. hemprichii decreased firstly, the lowest value appears in Nov 2019 in Gaolong bay and from Nov 2019 to Jun 2020 in Xincun lagoon. Then the shoot formation rate of T. hemprichii increased rapidly, especially transplanted in Gaolong bay. After 18 months, the shoot formation rate of T. hemprichii transplanted in Xincun lagoon decreased sharply in Oct 2020, and it was only 2.03% in Mar 2021, while the shoot formation rate of T. hemprichii transplanted in Gaolong bay was as high as 66.25% (Figure 6B).
Coverage and shoot density are important indicators of seagrass community monitoring (Hashemi et al., 2005). The coverage and shoot density of T. hemprichii transplanted in Gaolong bay and Xincun lagoon were changed slightly, and with no obvious variation trend. After 23 months, the coverage of T. hemprichii transplanted in Gaolong bay and Xincun lagoon were 5% and 8.1%, the shoot density was 11.26 shoots/m2 and 14.9 shoots/m2, respectively (Figures 6C, D).
The survival rate, shoot formation rate, coverage and shoot density changed significantly (P<0.001) with sampling every 2 or 3 months (Supplementary Tables S2, S3).
3.3 Comparison of transplantation performance of E. acoroides
The survival rate of E. acoroides transplanted in Gaolong bay and Xincun lagoon showed a trend of slight decline and then rise. The survival rates were all above 90% except Apr 2020 in Gaolong bay. The survival rates were highest in Nov 2019 in Gaolong bay (100.00%) and in May 2019 in Xincun (99.17%), the lowest value appeared in Apr 2020 in Gaolong bay (88.75%) and in Jun 2020 in Xincun (90.63%) (Figure 6E).
The shoot formation rate was less than 5% until summer 2020 both in Gaolong bay and Xincun lagoon, then increased gradually. The highest value arose in Mar 2021, 14.38% and 11.35% respectively in Gaolong bay and Xincun lagoon (Figure 6F). The shoot formation rate changed significantly (P<0.001) with samplings.
The coverage of E. acoroides transplanted in Gaolong bay declined slightly before Aug 2019, the value was 19.70%. Then the coverage increased gradually, maintain the coverage at 21.90% from Apr 2020. In Xincun lagoon, the coverage declined from 19.88% to 14.97% until Jun 2020, then increased to 16.18% in Mar 2021 (Figure 6G).
The shoot density of E. acoroides declined first, and then risesd both in Gaolong bay and Xincun lagoon, and the mean shoot density remained around 15 shoots/m2. In Mar 2021, the shoot density was 15.50 shoots/m2 and 14.90 shoots/m2 respectively in Gaolong bay and Xincun lagoon (Figure 6H).
The difference of survival rate, coverage and shoot density among samplings was not significant (P>0.05) (Supplementary Tables S2, S3).
3.4 Analysis of significant differences between species in the same region
Independent sample t-test was used to analyze the survival rate, shoot formation rate, coverage and shoot density of T. hemprichii and E. acoroides after 23 months of transplantion.
Both in Gaolong Bay and Xincun lagoon, the survival rate, coverage and shoot density of E. acoroides were significantly higher than that of T. hemprichii (p<0.0001). The results indicating that E. acoroides was more likely to survive than T. hemprichii both in Gaolong bay and Xincun lagoon. Interestingly, T. hemprichii showed a stronger shoot formation ability, the shoot formation rate of T. hemprichii was significantly higher than that of E. acoroides (t=7.717, p<0.001).
3.5 Analysis of significant differences between regions of the same species
Independent sample t-test was used to analyze the survival rate, shoot formation rate, coverage and shoot density of in Gaolong bay and Xincun lagoon after 23 months of transplantion.
There was no significant difference in survival rate and shoot density of T. hemprichii between transplanted in Gaolong bay and Xincun lagoon (p>0.05). But the shoot formation rate in Gaolong bay was significantly higher than in Xincun lagoon (t=8.601, p<0.0001), the coverage showed the opposite result (t=-3.941, p<0.0001).
Similarly, there was no significant difference in survival rate, shoot formation rate and shoot density of E. acoroides between transplanted in Gaolong bay and Xincun lagoon (p>0.05). Only the coverage of Gaolong bay was significantly higher than that of Xincun lagoon (t=0.042, p=0.008).
Considering the final effectiveness of transplantion, we thought that T. hemprichii was suitable for transplanted in Xincun lagoon, and E. acoroides was suitable for transplanted in Gaolong bay (Figure 7).
3.6 Correlation analysis
The survival rate, shoot formation rate, coverage and shoot density of transplantion were taken into pertinence analysis with environmental parameters. The results showed that, there was no relationship of contents of suspend solids, DIN, DIN, and N:P ratio with survival rate, coverage and shoot density of transplantion in Gaolong bay (Figure 8A). While in Xincun lagoon, content of suspend solids and DIN had both significant negative correlations with shoot density (Figure 8B).
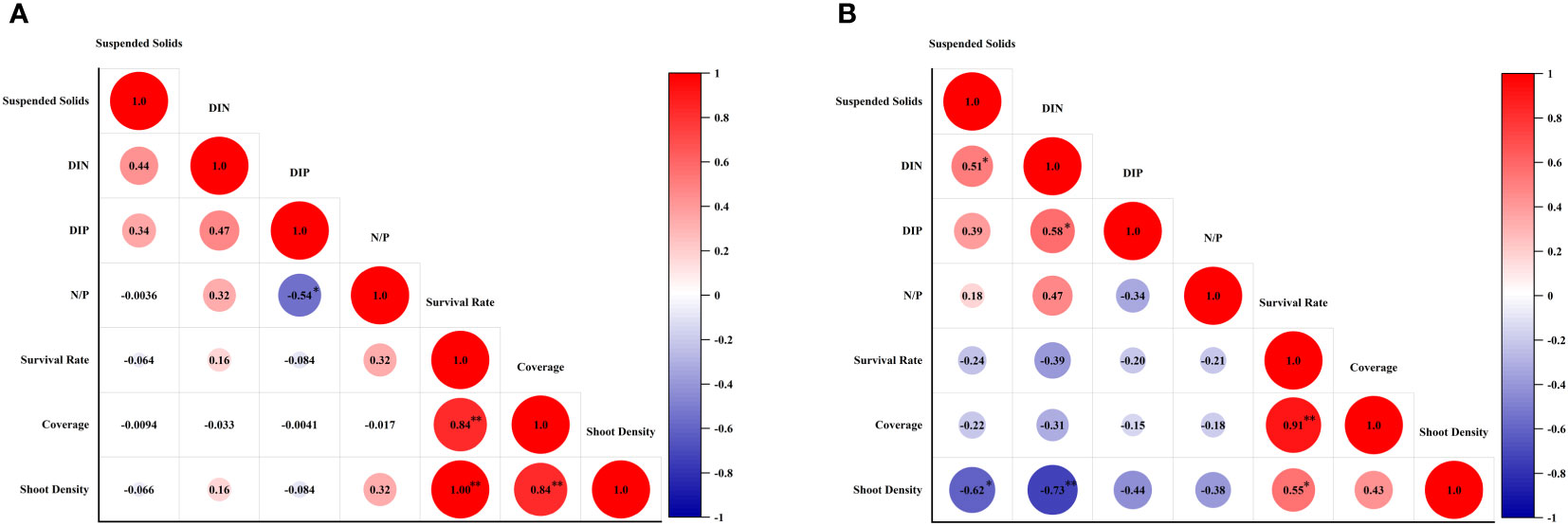
Figure 8 Correlation analysis between survival rate, shoot formation rate, coverage and shoot density of transplantion and environmental parameters. (A) the correlation analysis in Gaolong bay; (B) the correlation analysis in Xincun lagoon.
4 Discussion
4.1 The parameters in assessing success of seagrass transplantation
In order to promote effective restoration, there must be a clear definition of the success of seagrass transplantation. A number of criteria were used to evaluate the success of seagrass transplantation (Table 2). Usually, more than one parameter was considered to assess whether the ecological function of seagrass have been recovered after transplanted. Many parameters of habitat functions, such as abundance, composition, complexity of benthic organisms of the seagrass community and macroalgal were monitored in seagrass transplantation studies (Fonseca et al., 1996). We monitored the survival rate, shoot formation rate, coverage and shoot density to examine establishment of seagrass transplantation in this study.
The percentage of transplanting units surviving was the most common criteria (Fonseca et al., 1998), and was expressed as a percentage of the original number. When transplanting seagrasses, it is inevitable to sever the root and rhizome tissues of the donor shoots, especially in bare-root transplanting method, such as the staple method, TERFS method, and shell method (Fonseca et al., 1996; Calumpong and Fonseca, 2001; Short et al., 2002; Lee and Park, 2008; Cambridge and Kendrick, 2009). Sedimentassociated seagrass transplantiation methods, such as plug, sod, and core methods, have less impact on transplanting during the first 1-2 months. In this study, the survival rate of transplantation declined rapidly in first one year after transplantation, especially forT. hemprichii, which may be due to damage to underground tissues. The initial stress caused by root and rhizome tissues damage may lead to physiological and morphological changes of transplantation shoots (Struve et al., 2000). It was reported that, the photosynthetic efficiency of P. sinuosa (Horn et al., 2009), and the leaf length and width of T. testudinum decreased (Tussenbroek, 1996) after transplanting for 1-2 months. However, in this study, we did not observe significant changes in the morphology of transplantation shoots. After almost two years, more than 65% of T. hemprichii and 90% of E. acoroides were still alive, thanks to the seagrass shoots immobilized into the sediment.
Fonseca et al. (1998) thought that, random samples should be taken to measure the number of new shoots, because the addition of new shoots was a more accurate way to assess asexual reproductive activity of transplanting. Compared with sexual reproduction, clonal reproduction is the main way to maintain the regeneration and stability of seagrass population (Harwell and Rhode, 2007). In this study, we calculated shoot formation rate in place of shoots number. The shoot formation rates of transplants were less than 5% during first 5-6 months after transplantation. The low shoot formation rates may be due to transplanting damage in the initial months. From Nov 2020, the shoot formation rates increased greatly, indicated that, the transplanted plants had spread to where they lived and had been colonized successfully. However, for the seagrass plants with interlaced rhizomes, shoot formation rate may not be a good indicator.
The coverage usually measured by a 50 × 50 cm quadrat which divided into 5 × 5 cm grids. The quadrat randomly placed on the transplanting unit. The total number of seagrass shoots in a 5 × 5 cm grids was converted to the coverage of per square meter. In this study, we reduced the time of scuba diving spent underwater by measuring the coverage using a photogrammetry-based method.
Due to the damage of transplantation shoots, the shoot density usually decreases at the initial stage of transplantation, and then increases with the formation of lateral shoots (Orth et al., 1999; Park and Lee, 2007; Bastyan and Cambridge, 2008; Lee and Park, 2008). Thus, the increase of shoot density was an indicator of successful establishment of transplanting. However, in this study, the shoot density of T. hemprichii transplanted in Xincun lagoon and the shoot density of E. acoroides transplanted in Gaolong bay and Xincun lagoon did not decline during the early period of transplantation. Thus, we considered that, transplant establishment success could not be evaluated by just one indicator. Finally, we monitored the survival rate, shoot formation rate, coverage and shoot density, prominent change in morphology and growth of shoots to examine whether e seagrass transplantation was established successfully.
It was often thought that different species of seagrass transplantations took different times to establish successful transplantation in new locations (Campbell and Paling, 2003; Horn et al., 2009). Z. marina transplantation generally required a shorter establishment time compared to other seagrass species (Paling et al., 2007; Park and Lee, 2007). Different transplanting methods leaded to different establishment time of the same seagrass specie, even in the same location (Fonseca et al., 1996; Paling et al., 2001; Campbell and Paling, 2003; Horn et al., 2009). Park and Lee (2007) reported that, establishment time for Z. marina transplanting required 1.0-2.3 months by staple method, TERFS method took 2.2-2.6 months, and shell method took 3.2-3.7 months by. Based on the measurements of survival rate, shoot formation rate, coverage and shoot density, we concluded that transplantation established successfully of T. hemprichii and E. acoroides in the new site was about 12 months after transplantation. Because T. hemprichii and E. acoroides were hardly used in transplantation, we were unable to compare the results with other reports.
4.2 The influencing factors of seagrass transplantation
The survival and growth of transplanted seagrass plants were influenced by numerous factors, such as various environmental factors and human activities.
Water temperature is an important factor affecting the distribution and growth of seagrass (Robertson and Mann, 1984; Han et al., 2012). The heat tolerance of seagrass and the optimum temperatures of photosynthesis, respiration, and growth seagrass decided the direct effects of temperature changes. Under optimal growth temperature, water temperature was positive correlated to the shoot density, photosynthetic efficiency, and leaf growth rate of T. hemprichii strongly (Liu et al., 2020). With further rising temperature, the increase of leaf respiration rate was faster than the increase of photosynthetic rate, resulting in the continuous decline of photosynthetic respiration ratio, which destroyed the balance between photosynthetic rate and respiratory rate (Zimmerman et al., 1989). However, the correlation between seasonal water temperature and seagrass growth was weak in this study. We thought that, water temperatures during spring and autumn were within the optimum growth temperature range for T. hemprichii and E. acoroides, increased water temperatures may enhance seagrass productivities. However, the increased water temperature in summer exceeded the optimum temperature for seagrass growth, reduced the productivities of seagrass. Therefore, the seasonal variations between water temperature and seagrass productivity maybe cancel each other out. This conclusion is consistent with previous research (Barber and Behrens, 1985; Lee et al., 2005).
Salinity is a main environmental factor affecting the structure and function of seagrass community. Salinity change will have impact on seed germination, shoots formation, photosynthesis and respiration, growth and biomass distribution of seagrass, resulting distribution changes. At low salinities, although seagrass photosynthesis was maintained, productivity reduced by 20%. Higher than optimal salinity can also negatively affect seagrass. Under hypersaline conditions, production and biomass of A. antarctica decreasd (Walker, 1985). Because salt stress may limit growth by underexpanding cells and inhibiting the photosynthates and proteins. In our study, salinity of transplant sites was suitable for T. hemprichii and E. acoroides, no correlation between salinity and transplantation performance were observed.
The most immediate effect of an increase of suspend solids is a reduction in available light reaching the seagrass beds. Experimental studies had shown that, the decrease of light reduced plant size, leaves number of individual shoots, shoot density, leaf width and growth rate of seagrass, and increased in leaf length (Cambridge and Kendrick, 2009). In this study, when the content of suspend solids reached the highest value in Dec 2019, the survival rate, shoot formation rate and shoot density of T. hemprichii were at low level. Furthermore, we found a significant inverse correlation between the suspend solids and the shoot density in Xincun lagoon, meaning the higher suspend solids, the lower photosynthetic efficiency, ultimately leaded to lower shoot density.
The productivity of persistent seagrasses depends on the availability of nutrients, including water and sediment nutrients. It is usually considered that, sediment pore water is the main source of nutrients for seagrasses, because of the higher concentrations of nutrient compared to the surface water. However, studies have shown that seagrass can absorb nutrients through leaves and root tissue, seawater and sedimen nutrients contributed equally (Iizumi and Hattori, 1982; Lee and Dunton, 1999). To nutrient limited plants, the typical responses to N and/or P supplying were increases of shoot size, productivities, and biomass (Bulthuis et al., 1992; Lee and Dunton, 2000). But seagrass growth is not always limited by the concentration of nutrients in the surrounding environment, as increased nutrient supply has a limited effect on seagrass growth sometimes (Dennison et al., 1987; Lee and Dunton, 2000). Seagrass production may have little relationship with water nutrients (Lee and Dunton, 2000). Stimulated by phytoplanktonic, epiphytic and macroalgal growth, elevated water nutrient levels may have indirect negative effect on seagrasses. Due to water column nutrient enrichment, the primary producers throve, resulting in less light available to seagrasses. This conclusion was demonstrated by Burkholder et al. (1992) in Z. marina. For the same reason, DIN was significant negative correlations with shoot density in Xincun lagoon in this study.
Sediment types and grain-size characteristics have a great effect on the growth of seagrass (Eckrich and Holmquist, 2000). Park and Lee (2007) evaluated the feasibility and efficiency of Z. marina transplanting methods based on sediment type at different transplantation site. The results show that, the survival rate of shell method was higher in muddy (81.3%) and silty sediments (76.5%), and lower in sandy sediments (5.0%); While, both staple method and TERFS method had higher transplant survival rate. In this study, Gaolong bay was characterized by high coral debris, gravel and sand content in the sediment, whereas Xincun lagoon had mud and sand. Seagrass rhizomes transplanted by “singleplant-fixed-distance transplantation” method were inserted into the sediment firmly. There was no significant difference in survival rate and shoot density of seagrass between transplanted in Gaolong bay and Xincun lagoon after 23 months of transplantion. Our experiment shows that, an appropriate fixing technique is essential if transplantation is to be successful.
Over the last decade, seagrass beds are under a number of threats, particularly from human causes, such as shrimp and fish farming, shellfish harvesting, fishing through poisons, electricity and explosives, as well as trawling, pollution of aquaculture wastewater and dredging. Thankfully, this study was carried out without any human influence due to the plastic garden mesh set up around the transplanting area. The transplantation actions and protection measures for seagrass beds will play a positive role in reducing seagrass loss, optimizing biodiversity of seagrass bed, and enhancing ecosystem service (Possingham et al., 2015), especially for slow-growing species such as T. hemprichii and E. acoroides.
Data availability statement
The original contributions presented in this study are included in the article/Supplementary Material. Further inquiries can be directed at the corresponding authors.
Author contributions
JS: Data curation, Formal Analysis, Funding acquisition, Supervision, Writing – original draft, Writing – review & editing. LY: Writing – original draft. JZ: Investigation, Writing – review & editing. SJ: Writing – review & editing. YW: Writing – review & editing. DW: Writing – review & editing. ZC: Writing – original draft. CS: Funding acquisition, Writing – review & editing.
Funding
The author(s) declare financial support was received for the research, authorship, and/or publication of this article. This research was supported by National Natural Science Foundation of China (42166006, 42306169), Hainan Provincial Basic and Applied Basic Research Fund for High-Level Talents in Natural Science (421RC662, 323RC554), Fund of Department budget projects of Hainan Province in 2022 (KYL-2022-14), Fund projects of Zhilan (2022040321 B), Fund of Key Laboratory of Marine Ecological Conservation and Restoration, Ministry of Natural Resources, Fujian Provincial Key Laboratory of Marine Ecological Conservation and Restoration, (EPR2022005).
Conflict of interest
The authors declare that the research was conducted in the absence of any commercial or financial relationships that could be construed as a potential conflict of interest.
Publisher’s note
All claims expressed in this article are solely those of the authors and do not necessarily represent those of their affiliated organizations, or those of the publisher, the editors and the reviewers. Any product that may be evaluated in this article, or claim that may be made by its manufacturer, is not guaranteed or endorsed by the publisher.
Supplementary material
The Supplementary Material for this article can be found online at: https://www.frontiersin.org/articles/10.3389/fmars.2023.1294779/full#supplementary-material
Supplementary Table 1 | The environmental parameters monitoring results.
Supplementary Table 2 | The transplantation monitoring results in Gaolong bay.
Supplementary Table 3 | The transplantation monitoring results in Xincun lagoon.
References
Arias-Ortiz A., Serrano O., Masqué P., Lavery P. S., Mueller U., Kendrick G. A., et al. (2018). A marine heatwave drives massive losses from the world’s largest seagrass carbon stocks. Nat. Clim Change. 8, 338–344. doi: 10.1038/s41558018-0096-y
Barber B. J., Behrens P. J. (1985). Effects of elevSSated temperature on seasonal in situ leaf productivity of Thalassia testudinum Banks ex König and Syringodium filiforme Kützing. Aquat. Bot. 22, 61–69. doi: 10.1016/03043770(85)90029-4
Bastyan G. R., Cambridge M. L. (2008). Transplantation as a method for restoring the seagrass Posidonia australis. Estuarine Coast. Shelf Sci. 79 (2), 289–299. doi: 10.1016/j.ecss.2008.04.012
Bulthuis D. A., Axelrad D. M., Mickelson M. J. (1992). Growth of the seagrass Heterozostera tasmanica limited by nitrogen in Port Phillip Bay, Australia. Mar. Ecol. Prog. Ser. 89, 269–275. doi: 10.3354/meps089269
Burkholder J. M., Mason K. M., Glasgow H. B. Jr. (1992). Water column nitrate enrichment promotes decline of eelgrass Zostera marina: evidence from seasonal mesocosm experiments. Mar. Ecol. Prog. Ser. 81, 163–178. doi: 10.3354/meps081163
Calumpong H. P., Fonseca M. S. (2001). Seagrass transplantation and other seagrass restoration methods (Amsterdam: Global Seagrass Research Methods), 425–443. doi: 10.1016/B978-044450891-1/50023-2
Calvo S., Calvo R., Luzzu F., Raimondi V., Assenzo M., Cassetti F. P., et al. (2021). Performance assessment of Posidonia oceanica (L.) Delile restoration experiment on dead matte twelve years after planting-structural and functional meadow features. Water 13, 724. doi: 10.3390/w13050724
Cambridge M. L., Kendrick G. A. (2009). Contrasting responses of seagrass transplants (Posidonia australis) to nitrogen, phosphorus and iron addition in an estuary and a coastal embayment. J. Exp. Mar. Biol. Ecol. 371 (1), 34–41. doi: 10.1016/j.jembe.2009.01.001
Campbell M. L., Paling E. I. (2003). Evaluating vegetative transplant success in Posidonia Australis: a field trial with habitat enhancement. Mar. pollut. Bull. 46 (7), 828–834. doi: 10.1016/S0025-326X(03)00093-6
Cui L., Jiang Z., Huang X., Liu S. L., Wu Y. C., Fan M. L. (2021). Decade changes of the food web structure in tropical seagrass meadow: implication of eutrophication effects. Mar. pollut. Bull. 173, 113122. doi: 10.1016/j.marpolbul.2021.113122
Davis R. C., Short F. T. (1997). Restoring eelgrass, Zostera marina L., habitat using a new transplanting technique: the horizontal rhizome method. Aquat. Bot. 59 (1-2), 1–15. doi: 10.1016/S0304-3770(97)00034-X
Dennison W. C., Aller R. C., Alberte R. S. (1987). Sediment ammonium availability and eelgrass (Zostera marina) growth. Mar. Biol. 94, 469–477. doi: 10.1016/0198-0254(87)91016-8
Duarte C. M., Chiscano C. L. (1999). Seagrass biomass and production: a reassessment. Aquat. Bot. 65, 159–174. doi: 10.1016/S0304-3770(99)00038-8
Duarte C. M., Krause-Jensen D. (2017). Export from seagrass meadows contributes to marine carbon sequestration. Front. Mar. Sci. 4. doi: 10.3389/fmars.2017.00013
Eckrich C. E., Holmquist J. G. (2000). Trampling in a seagrass assemblage: direct effects, response of associated fauna, and the role of substrate characteristics. Mar. Ecol. Prog. Ser. 201, 199–209. doi: 10.3354/meps201199
Fang X., Hao C. L., Cheng C. G., Ni W., Wang J. C., Zhang Y. F. (2018). Pressure analysis and spatial quantitative assessment of impact of human activities on lagoon ecosystem: a case study in Xincun and Lian Lagoons, Hainan, China. Appl. Ecol. Environ. Res. 16, 6253–6266. doi: 10.15666/AEER/1605_62536266
Fishman J. R., Orth R. J., Marion S., Bieri J. (2004). A comparative test of mechanized and manual transplanting of eelgrass, Zostera marina, in Chesapeake Bay. Restor. Ecol. 12 (2), 214–219. doi: 10.1111/j.10612971.2004.00314.x
Fonseca M. S., Kenworthy W. J., Courtney F. X. (1996). Development of planted seagrass beds in Tampa Bay, Florida, USA. I. plant components. Mar. Ecol. Prog. 132 (1-3), 127–139. doi: 10.3354/meps132127
Fonseca M. S., Kenworthy W. J., Thayer G. W. (1998). Guidelines for the conservation and restoration of seagrasses in the United States and adjacent waters. US Dept. of Commerce, National Oceanic and Atmospheric Administration, Coastal Ocean Office. Silver Spring, MD. 111–138.
Fonseca M. S., Zieman J. C., Thayer G. W., Fisher J. S. (1983). The role of current velocity in structuring eelgrass (Zostera marina L.) meadows. Estuar. Coast. Shelf Sci. 17, 367–380. doi: 10.1016/0272-7714(83)90123-3
General Administration of Quality Supervision, I.a.Q.o.t.P.s.R.o.C., Standardization Aministration of the People’s Republic of China (2008). The Specification for Marine Monitoring, Part 4: Seawater analysis (in Chinese) (Beijing: the Standards Press of China), 4–2007.
Han T. S., Wan L., Xu X. B. (2012). Characteristics of the phytoplankton community in Xinchun Harbour, Lingshui, Hainan. J. Trop. Organ. 3, 180–185. doi: 10.15886/j.cnki.rdswxb.2012.02.004
Harwell M. C., Rhode J. M. (2007). Effects of edge/interior and patch structure on reproduction in Zostera marina L. @ in Chesapeake Bay, USA. Aquat. Bot. 87 (2), 147–154. doi: 10.1016/j.aquabot.2007.04.007
Hashemi A. M., Herbert S. J., Putnam D. H. (2005). Yield response of corn to crowding stress. American Society of Agronomy 97, 839–864. doi: 10.2134/agronj2003.0241
Horn L. E., Paling E. I., Keulen M. V. (2009). Photosynthetic recovery of transplanted Posidonia sinuosa, western Australia. Aquat. Bot. 90 (2), 149–156. doi: 10.1016/j.aquabot.2008.08.002
Huang X., Huang L., Li Y., Xu Z., Fong C. W., Huang D. (2006). Main seagrass beds and threats to their habitats in the coastal sea of South China. Chin. Sci. Bull. 51, 136–142. doi: 10.1007/s11434-006-9136-5
Iizumi H., Hattori A. (1982). Growth and organic production of eelgrass (Zostera marina L.) in temperate waters of the Pacific coast of Japan. III. The kinetics of nitrogen uptake. Aquat. Bot. 12, 245–256. doi: 10.1016/03043770(82)90020-1
Jahnke M., Gullstrom M., Larsson J., Asplund M. E., Mgeleka S., Silas M. O., et al. (2019). Population genetic structure and connectivity of the seagrass Thalassia hemprichii in the Western Indian Ocean is influenced by predominant ocean currents. Ecol. Evol. 9 (16), 8953–8964. doi: 10.1002/ece3.5420
Jiang Z., Liu S., Zhang J., Zhao C., Wu Y., Yu S., et al. (2017). Newly discovered seagrass beds and their potential for blue carbon in the coastal seas of Hainan Island, South China Sea. Mar. pollut. Bull. 125, 513–521. doi: 10.1016/j.marpolbul.2017.07.066
Keulen M. V., Paling E. I., Walker C. J. (2010). Effect of planting unit size and sediment stabilization on seagrass transplants in Western Australia. Restor. Ecol. 11 (1), 50–55. doi: 10.1046/j.1526-100X.2003.00036.x
Kirkman H. (1998). Pilot experiments on planting seedlings and small seagrass propagules in Western Australia. Mar. pollut. Bull. 37, 460–467. doi: 10.1016/S0025-326X(99)00146-0
Klumpp D. W., Salita-Espinosa J. T., Fortes M. D. (1993). Feeding ecology and trophic role of sea urchins in a tropical seagrass community. Aquat. Bot. 45, 205–229. doi: 10.1016/0304-3770(93)90022-O
Lee C. L., Huang Y. H., Chen C. H., Lin H. J. (2016). Remote underwater video reveals grazing preferences and drift export in multispecies seagrass beds. J. Exp. Mar. Biol. Ecol. 476, 1–7. doi: 10.1016/j.jembe.2015.12.004
Lee K. S., Dunton K. H. (1999). Inorganic nitrogen acquisition in the seagrass Thalassia testudinum: development of a whole-plant nitrogen budget. Limnol. Oceanogr. 44, 1204–1215. doi: 10.4319/lo.1999.44.5.1204
Lee K. S., Dunton K. H. (2000). Effects of nitrogen enrichment on biomass allocation, growth, and leaf morphology of the seagrass Thalassia testudinum. Mar. Ecol. Prog. Ser. 196, 39–48. doi: 10.3354/meps196039
Lee K. S., Park J. I. (2008). An effective transplanting technique using shells for restoration of Zostera marina habitats. Mar. pollut. Bull. 56 (5), 1015–1021. doi: 10.1016/j.marpolbul.2008.02.010
Lee K. S., Park S. R., Kim J. B. (2005). Production dynamics of the eelgrass, Zostera marina in two bay systems on the south coast of the Korean peninsula. Mar. Biol. 147, 1091–1108. doi: 10.1007/s00227-005-0011-8
Li Q., Jin R., Ye Z., Gu J., Dan L., He J., et al. (2022). Mapping seagrass meadows in coastal China using GEE. Geocarto Int. 37 (26), 12602–12617. doi: 10.1080/10106049.2022.2070672
Li W. T., Kim J. H., Park J. I., Lee K. S. (2010). Assessing establishment success of Zostera marina transplants through Measurements of shoot morphology and growth. Estuarine Coast. Shelf Sci. 88, 377–384. doi: 10.1016/j.ecss.2010.04.017
Liu J. P., Ang S. J., Mayfield A. B., Lin H. J. (2020). Influence of the seagrass Thalassia hemprichii on coral reef mesocosms exposed to ocean acidification and experimentally elevated temperatures. Sci. Total Environ. 700, 134464. doi: 10.1016/j.scitotenv.2019.134464
Mancini G., Casoli E., Ventura D., Jona Lasinio G. C., Belluscio A., Ardizzone G. D. (2021). An experimental investigation aimed at validating a seagrass restoration protocol based on transplantation. Biol. Conserv. 264, 109397. doi: 10.1016/j.biocon.2021.109397
Marbà N., Duarte C. M. (1998). Rhizome elongation and seagrass clonal growth. Mar. Ecol. Prog. Ser. 174, 269–280. doi: 10.3354/meps174269
Moksnes P. O., Gullström M., Tryman K., Baden S. (2008). Trophic cascades in a temperate seagrass community. Oikos 117, 763–777. doi: 10.1111/j.0030-1299.2008.16521.x
Nordlund L. M., Koch E. W., Barbier E. B., Creed J. C. (2016). Seagrass ecosystem services and their variability across genera and geographical regions. PloS One 11 (10), e0163091. doi: 10.1371/journal.pone.0163091
Orth R. J., Harwell M. C., Fishman J. R. (1999). A rapid and simple method for transplanting eelgrass using single, unanchored shoots. Aquat. Bot. 64 (1), 77–85. doi: 10.1016/S0304-3770(99)00007-8
Paling E. I., Van Keulen M., Tunbridge D. J. (2007). Seagrass transplanting in Cockburn Sound, Western Australia: a comparison of manual transplantation methodology using Posidonia sinuosa Cambridge et Kuo. Restor. Ecol. 15 (2), 240–249. doi: 10.1111/j.1526-100X.2007.00207.x
Paling E. I., van Keulen M., Wheeler K., Phillips J., Dyhrberg R. (2001). Mechanical seagrass transplantation in Western Australia. Ecol. Eng. 16 (3), 331–339. doi: 10.1016/S0925-8574(00)00119-1
Park J. I., Lee K. S. (2007). Site-specific success of three transplanting methods and the effect of planting time on the establishment of Zostera marina transplants. Mar. pollut. Bull. 54, 1238–1248. doi: 10.1016/j.marpolbul.2007.03.020
Phillips R. C. (1974). Transplantation of seagrasses, with special emphasis on eelgrass, Zostera marina L. Aquaculture 4, 161–176. doi: 10.1016/0044-8486(74)90031-3
Piazzi L., Acunto S., Frau F., Atzori F., Cinti M. F., Leone L., et al. (2021). Environmental engineering techniques to restore degraded Posidonia oceanica meadows. Water 13, 661. doi: 10.3390/w13050661
Possingham H. P., Bode M., Klein C. J. (2015). Optimal conservation outcomes require both restoration and protection. PloS Biol. 13, 1–16. doi: 10.1371/journal.pbio.1002052
Qiu G., Fan H., Zhou H., Li L., Li S., Pan L., et al. (2014). Transplantation techniques for restoring the intertidal seagrasses in Guangxi. Maine Sci. 1 (6), 24–30. doi: 10.11759/hykx20121104001
Rasheed M. A. (2004). Recovery and succession in a multi-species tropical seagrass meadow following experimental disturbance: the role of sexual and asexual reproduction. J. Exp. Mar. Biol. Ecol. 310, 13–45. doi: 10.1016/j.jembe.2004.03.022
Rasheed M. A., McKenna S. A., Carter A. B., Coles R. G. (2014). Contrasting recovery of shallow and deep water seagrass communities following climate associated losses in tropical north Queensland, Australia. Mar. pollut. Bull. 83, 491499. doi: 10.1016/j.marpolbul.2014.02.013
Renouard F., Nisand D. (2006). Impact of implant length and diameter on survival rates. Clin. Oral. Implants Res. 17 (S2), 35–51. doi: 10.1111/j.1600-0501.2006.01349.x
Robertson A. I., Mann K. H. (1984). Disturbance by ice and life-history adaptations of the seagrass Zostera marina. Mar. Biol. 80 (2), 131–141. doi: 10.1007/BF02180180
Ruiz J. M., Pérez M., Romero J. (2001). Effects of fish farm loadings on seagrass (Posidonia oceanica) distribution, growth and photosynthesis. Mar. pollut. Bull. 42, 749–760. doi: 10.1016/S0025-326X(00)00215-0
Short F. T., Davis R. C., Kopp B. S., Short C. A., Burdick D. M. (2002). Site-selection model for optimal transplantation of eelgrass Zostera marina in the northeastern us. Mar. Ecol. Progress. 227 (1), 253–267. doi: 10.3354/meps227253
Sintes T., Marbà N., Duarte C. M., Kendrick G. A. (2005). Nonlinear processes in seagrass colonisation explained by simple clonal growth rules. Oikos 108, 165–175. doi: 10.1111/j.0030-1299.2005.13331.x
Struve D. K., Burchfield L., Maupin C. (2000). Survival and growth of transplanted large- and small-caliper red oaks. J. Arboriculture. 26 (3), 162–169. doi: 10.48044/jauf.2000.019
Tan Y. M., Dalby O., Kendrick G. A., Statton J., Sinclair E. A., Fraser M. W., et al. (2020). Seagrass restoration is possible: insights and lessons from Australia and New Zealand. Front. Mar. Sci. 7. doi: 10.3389/fmars.2020.00617
Thorhaug A. (1985). Large-scale seagrass restoration in a damaged estuary. Mar. pollut. Bull. 16 (2), 55–62. doi: 10.1016/0025-326X(85)90124-9
Tussenbroek B. I. V. (1996). Leaf dimensions of transplants of Thalassia testudinum in a Mexican caribbean reef lagoon. Aquat. Bot. 55 (2), 133–138. doi: 10.1016/S0304-3770(96)01068-6
Van de Koppel J., van der Heide T., Altieri A. H., Eriksson B. K., Bouma T. J., Olff H., et al. (2015). Long-distance interactions regulate the structure and resilience of coastal ecosystems. Ann. Rev. Mar. Sci. 7, 139–158. doi: 10.1146/annurev-marine-010814-015805
Van Katwijk M. M., Bos A. R., De Jonge V. N., Hanssen L. S. A. M., Hermus D. C. R., de Jong D. J. (2009). Guidelines for seagrass restoration: importance of habitat selection and donor population, spreading of risks, and ecosystem engineering effects. Mar. pollut. Bull. 58, 179–188. doi: 10.1016/j.marpolbul.2008.09.028
Ventura D., Mancini G., Casoli E., Pace D. S., Lasinio G. J., Belluscio A., et al. (2022). Seagrass restoration monitoring and shallow-water benthic habitat mapping through a photogrammetry-based protocol. J. Environ. Management. 304, 114262. doi: 10.1016/j.jenvman.2021.114262
Walker D. I. (1985). Correlations between salinity and growth of the seagrass Amphibolis Antarctica (labill.) sonder & aschers., In Shark Bay, Western Australia, using a new method for measuring production rate. Aquat. Bot. 23 (1), 13–26. doi: 10.1016/0304-3770(85)90017-8
Wan L., Li Y., Cen H., Zhu J., Yin W., Wu W., et al. (2018). Combining UAV-based vegetation indices and image classification to estimate flower number in oilseed rape. Remote Sensing. 10 (9), 1484. doi: 10.3390/rs10091484
Waycott M., Duarte C. M., Carruthers T. J. B., Orth R. J., Dennison W. C., Olyarnik S., et al. (2009). Accelerating loss of seagrasses across the globe threatens coastal ecosystems. Proc. Natl. Acad. Sci. U. S. A. 106, 12377–12381. doi: 10.1073/pnas.0905620106
Zhang Y. H., Li C., Zhao J. S., Li W. T., Zhang P. D. (2020). Seagrass resilience: where and how to collect donor plants for the ecological restoration of eelgrass Zostera marina in Rongcheng Bay, Shandong Peninsula, China. Ecol. Eng. 158, 106029. doi: 10.1016/j.ecoleng.2020.106029
Zhou G., Huang H., Lian J., Zhang C., Li X. (2012). Habitat correlation of symbiodinium diversity in two reef-building coral species in an upwelling region, eastern Hainan Island, China. J. Mar. Biol. Assoc. UK. 92, 1309–1316. doi: 10.1017/S0025315411001548
Keywords: Thalassia hemprichii, Enhalus acoroides, Gaolong bay, Xincun lagoon, seagrass restoration
Citation: Shen J, Yin L, Zhang J, Jia S, Wang Y, Wang D, Cai Z and Chen S (2023) Restoration performance of Thalassia hemprichii and Enhalus acoroides at Gaolong bay and Xincun lagoon, Hainan Island, China. Front. Mar. Sci. 10:1294779. doi: 10.3389/fmars.2023.1294779
Received: 15 September 2023; Accepted: 24 November 2023;
Published: 12 December 2023.
Edited by:
Shaochun Xu, Chinese Academy of Sciences (CAS), ChinaReviewed by:
Daniele Ventura, Sapienza University of Rome, ItalyXia Zhang, Chinese Academy of Sciences (CAS), China
Copyright © 2023 Shen, Yin, Zhang, Jia, Wang, Wang, Cai and Chen. This is an open-access article distributed under the terms of the Creative Commons Attribution License (CC BY). The use, distribution or reproduction in other forums is permitted, provided the original author(s) and the copyright owner(s) are credited and that the original publication in this journal is cited, in accordance with accepted academic practice. No use, distribution or reproduction is permitted which does not comply with these terms.
*Correspondence: Zefu Cai, Y2FpemVmdUAxMjYuY29t; Shiquan Chen, Y2hlbnNxQGhuaGt5LmNu
†These authors have contributed equally to this work and share first authorship