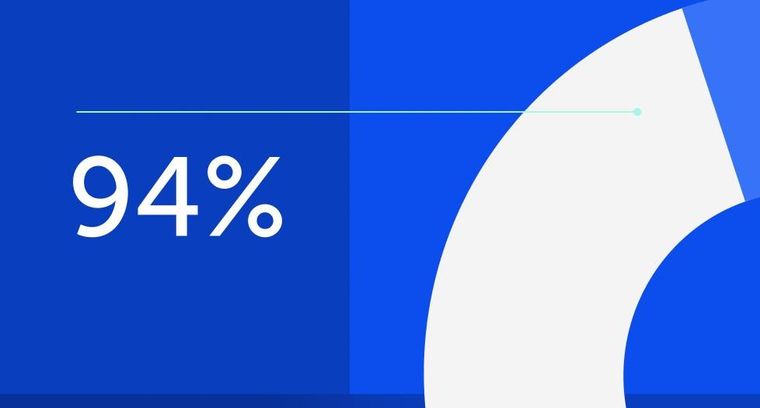
94% of researchers rate our articles as excellent or good
Learn more about the work of our research integrity team to safeguard the quality of each article we publish.
Find out more
ORIGINAL RESEARCH article
Front. Mar. Sci., 01 November 2023
Sec. Coral Reef Research
Volume 10 - 2023 | https://doi.org/10.3389/fmars.2023.1281691
This article is part of the Research TopicUsing Direct Microbiome Manipulation to Understand Causal Roles of Microbes in the Health and Stress Resistance of CoralsView all 6 articles
Introduction: Though bacterial pathogens and parasites can compromise coral health, coral microbiome research increasingly suggests a beneficial role for bacterial species living in coral tissue and mucus. Recent studies suggest the application of targeted antibiotic treatments, while inhibiting the growth of harmful bacteria, may have unintended and persistent impacts on coral health. Land-based coral nurseries use antiseptic treatments such as Lugol’s solution and KoralMD™ dip to reduce infectious agents as part of restoration best practices. These antiseptic treatments often halt tissue loss, but the short- or long-term effects of these treatments on the coral microbiome is unknown.
Methods: We conducted a controlled tank experiment to assess the effects of these broad-spectrum treatments on coral growth rates and microbial communities when used as a prophylactic measure on healthy corals. Sixty individuals from each of two genotypes of the coral species Acropora palmata and Orbicella faveolata were treated with either Lugol’s solution or KoralMD™. Coral tissue, mucus, and skeleton were sampled pre-treatment, during treatment, and 1 and 2 months after treatment to assess microbiome shifts and recovery. The impact of the two treatments on coral growth was assessed using surface area measurements from 3D imagery.
Results: Although we found that A. palmata treated with Lugol’s solution had significantly reduced growth rates compared with untreated controls, impacts of antiseptic treatment were otherwise limited and microbiomes were not significantly different by treatment either immediately after application or 2 months thereafter.
Discussion: Study of the effects of these widely-used interventions may have significant repercussions on management and propagation strategies for corals reared in land-based nurseries. Furthermore, our findings indicate that antiseptic treatments can be applied to mitigate coral health issues without long-term harmful effects or significant microbiome shifts.
Worldwide, corals are rapidly declining due to the loss of suitable habitat, anthropogenic disturbances at local and global scales, and disease outbreaks (Pandolfi et al., 2003; Bellwood et al., 2004; Carpenter et al., 2008; Walton et al., 2018). Coral reefs have degraded from a high level of stony coral coverage to an algae- and soft coral-dominated habitat (Hughes, 1994; Pandolfi et al., 2003; Bellwood et al., 2004; Arias-González et al., 2017). The loss of coral coverage and reef-building species leads to reef structure deterioration globally, with significant impacts on fisheries, tourism, and shoreline protection (Elliff and Silva, 2017). To aid in reef recovery, asexual coral propagation (fragmentation and recovery in a nursery setting) and outplanting (restoration of cultured corals to the reef) of coral has been implemented throughout the Caribbean (Schopmeyer et al., 2017; Boström-Einarsson et al., 2020). Land-based (ex situ) coral restoration facilities allow practitioners the flexibility to tend to corals without the limitations of field operations, the capacity for constant monitoring and care of corals during grow-out, and the ability to mitigate the effects of many stressors present in the natural environment (e.g., thermal stress). Innovations such as microfragmentation, the process of cutting large pieces of coral into several smaller pieces (Forsman et al., 2015; Page et al., 2018), have contributed to the success of land-based operations and facilitate significantly faster growth rates in certain coral species. Microfragmented pieces are often grown and cared for in ex situ systems. When fragments reach larger sizes, the process can be repeated or the corals may be outplanted to the reef (Forsman et al., 2015; Page et al., 2018).
While eliminating certain difficulties of in-water (in situ) aquaculture, ex situ restoration has its own set of challenges. Corals in ex situ aquaculture facilities are typically housed in large water tables or aquarium tanks that are supplied with real or artificial seawater in a flow-through or recirculating system. In flow-through systems, pathogens or parasites may be introduced from the local environment (Kent et al., 2009; Sheridan et al., 2013). In contrast, while recirculating systems that use artificial or filtered seawater are unlikely to be contaminated by pathogens entering via the water source, pathogens may nonetheless be introduced through human contact, newly-collected corals or live rock, or pathogen vectors such as snails (Antonius and Riegl, 1997; Williams and Miller, 2005; Williams and Miller, 2012). Further, the reuse of water in recirculating systems may compound issues of water quality and pathogen introduction if sufficient filtration is not used (Sheridan et al., 2013; Leal et al., 2016). A key challenge of housing corals in an ex situ facility is therefore consistent water quality and filtration. In order to maintain water quality, several filtration and sterilization methods are used, including sand filters, cartridge filters, bag filters and ultraviolet sterilization.
Despite these precautions, the high-density environment of coral aquaculture may allow pests and pathogenic microorganisms to spread at an accelerated rate (Sheridan et al., 2013). Corals can manifest symptoms of disease slowly in comparison to other marine species, allowing diseases or health issues to spread unnoticed (Kaczmarsky, 2006; Montano et al., 2015). Coral restoration practitioners often utilize hobbyist techniques from the aquarium trade and apply them to large-scale, land-based aquaculture systems. Frequently, aquarists may respond to health issues by fragmenting large impacted colonies (i.e., removing tissue with visible signs of active disease), conducting water changes, quarantining affected corals, or implementing antibiotic treatments. As each of these response types may significantly reduce production efficacy, practitioners may instead utilize antiseptic antimicrobial treatments to prevent issues before they arise. While antibiotics inhibit or kill specific microorganisms, antiseptics act more generally to inhibit growth of large classes of microorganisms and may be used as a prophylactic rather than reactive treatment (McDonnell and Russell, 1999). One such treatment is Lugol’s iodine solution (‘Lugol’s solution’): a mixture of deionized water, potassium iodide, and crystalized iodine. Due to the halogenic nature of iodine, this treatment acts as a bactericide, kills microorganisms and temporarily immobilizes pests (McDonnell and Russell, 1999; Lachapelle et al., 2013). In particular, treatment with Lugol’s solution effectively combats ciliates and flatworms (Carl, 2008). Approximately 70% of the Earth’s supply of natural iodine is found in the ocean (Carpenter et al., 2021), making iodine an essential minor element in seawater that may provide additional benefits when added directly to aquaria. Corals are placed into Lugol’s solution baths when they show signs of tissue recession or the presence of ciliates is suspected (Carl, 2008). Further, Lugol’s solution is commonly used prophylactically to prevent stress after microfragmentation, as fresh wounds may be particularly susceptible to infection. Another common aquarium dip is KoralMD (Brightwell Aquatics), which is used both as a prophylactic and to treat suspected parasites. KoralMD is a professional-strength coral dip which uses conifer and citrus oils along with proprietary cleaners to combat common pests. The plant-based essential oils present in KoralMD are designed to stun parasites and prevent them from being transferred across aquarium systems (Jack Kent, Brightwell Aquatics, personal comm.). Unlike Lugol’s solution, KoralMD is not recommended for direct application into aquarium water. While observationally these treatments are effective in halting tissue loss that may stem from bacterial infections, no publicly-accessible study has addressed the short- or long-term effects of Lugol’s solution or KoralMD on the endemic coral microbiome.
Corals host mutually favorable relationships with bacteria, viruses, fungi, archaea, and endolithic algae, which constitute the ‘coral holobiont’ (Rohwer et al., 2002; Ritchie, 2006; Thompson et al., 2015). The diverse array of bacterial species that the corals host is hypothesized to confer benefits to the coral including enhancement of larval settlement, production of antimicrobial compounds, and the provision of nutrients to both the coral and its algal symbiont (Thompson et al., 2015; van Oppen and Blackall, 2019). However, coral-bacterial symbiosis is fragile and easily disrupted by environmental stressors or the introduction of pathogens, leading to the reduction of host growth or to disease development (McDevitt-Irwin et al., 2017). Following disturbance, bacterial communities may remain in a disrupted or “dysbiotic” state for extended periods of time (Ainsworth et al., 2010; Egan and Gardiner, 2016). These shifts in microbial community composition in response to stress are often characterized by a decrease in beneficial bacterial species and an increase in opportunistic pathogens (Gilbert et al., 2016; West et al., 2019). Antibiotic treatment is widely employed in response to coral disease and may inhibit the growth of pathogenic or opportunistic species (Sweet et al., 2014; Aeby et al., 2019; Neely et al., 2020). The application of targeted antibiotic treatments, however, disrupts or depletes native coral microbiota and ultimately results in reduced photosynthetic efficiency (Gilbert et al., 2012), increased susceptibility to pathogenic infection (Mills et al., 2013), and increased mortality from bleaching (Glasl et al., 2016; Connelly et al., 2022). As the coral microbiome is believed to provide nutrients to the coral itself (Lema et al., 2014; Bourne et al., 2016), the application of antibiotics or antiseptic treatments may have long-term effects on coral growth rates. Broad-spectrum treatments employed by the aquarium and aquaculture trades to improve coral health may therefore have unanticipated, long-term effects on corals, with both direct impacts on coral health and indirect effects via the loss of essential beneficial microbial taxa.
To evaluate the impacts of prophylactic antiseptic treatments on coral growth rates and coral microbiomes in an ex situ restoration context, we applied Lugol’s solution and KoralMD to two species of coral (one branching and one massive species) in two distinct and complementary experiments conducted in controlled aquarium conditions. Coral treatment with Lugol’s solution and KoralMD followed standard schedules of treatment in aquaculture practice. Microbiomes were sampled and growth rates were measured throughout treatment and at one and two months of washout (60 days after last treatment). We aimed to assess whether coral growth rates were significantly impacted by the application of either treatment in the short- or long-term following treatment and whether microbiome community structure was significantly altered by the application of Lugol’s solution or KoralMD.
Application of Lugol’s solution and KoralMD treatment occurred in two sequential experiments with identical design at Mote Marine Laboratory’s Elizabeth Moore International Center for Coral Reef Research & Restoration (Summerland Key, FL, USA). In November 2021, corals of two genotypes each of Acropora palmata and Orbicella faveolata were exposed to a standard course of Lugol’s solution treatment as described below. In May 2022, corals of two genotypes each of A. palmata and O. faveolata were exposed to KoralMD (Brightwell Aquatics, Fort Payne, AL, USA) treatment. The genotypes used in this study had been held ex situ for several years prior to this experiment: the A. palmata used originated from larvae settled at Mote Marine Laboratory in 2013, produced from a batch cross of parent colonies collected from Elbow, Horseshoe and Sand Island reefs in the Florida Keys. O. faveolata were fragmented from wild colonies collected from near Key West Harbor (exact coordinates unknown) prior to 2010. The O. faveolata genotypes were determined to be distinct using 2bRAD sequencing in 2018. A. palmata genotypes were determined to be distinct using SNP analysis in 2020. Corals were fragmented 4 months prior to Lugol’s solution treatment and 3 months prior to treatment with KoralMD to an estimated size of 1 cm2.
For both experiments, each species was held in a separate tank with approximately 170 L of saltwater. Each tank was supplied with saltwater sourced from an adjacent seawater canal on a flowthrough system at a rate of 3.48 liters per minute. Temperature, pH, dissolved oxygen, and salinity were measured using a YSI (YSI Pro Plus, Xylem Inc, Washington, DC, USA) twice a day (8:00am and 12:00pm) three days a week (Monday, Wednesday, and Friday) for the duration of the experiment (approximately 2.5 months, Table 1). One powerhead pump (1000 GPH) was placed in each tank to provide sufficient flow. The racks holding the corals were set to be 18 cm below the surface of the water to keep light levels (photosynthetically active radiation, PAR) consistent with other ex situ nursery tanks onsite at roughly 300 micromoles per second. Tanks were covered to provide additional shade at 12:00pm every day to be consistent with other ex situ nursery tanks. Each tank contained 2 racks of corals: one rack with 50 untreated corals and one with 50 treated (Lugol’s solution or KoralMD) corals. Unrelated to this experiment, the water source for Mote Marine Laboratory’s ex situ nursery was changed from an 80 ft saltwater well to seawater sourced from a nearshore canal (10 ft below the surface) in late September 2021. Corals were acclimated to this new source of water for two months before initiation of the Lugol’s experiment. At the time of experiments using KoralMD, corals had acclimated to nearshore water for 8 months.
As all corals were ultimately destined for outplanting, standard coral restoration conditions for Mote Marine Laboratory were maintained. Untreated corals were housed in the same tank with treated corals to follow standard restoration practices for prophylactic treatment. KoralMD and Lugol’s solution treatments were applied following the standard schedule of treatment used by coral husbandry practitioners at Mote Marine Laboratory: corals were treated on Monday, Wednesday, and Friday for two weeks. Lugol’s solution baths were performed for ten minutes on treatment group corals at a concentration of 1 mL Lugol’s solution per 3 L of saltwater. KoralMD baths were performed on different coral individuals (i.e., corals not included in the previous experiment) in separate bins for 8 minutes at a concentration of 1 mL of KoralMD Pro (KoralMD professional-strength concentrated formulation) for every 2.5 L of saltwater following manufacturer recommendations. A powerhead was added to all treatment bins in both experiments to maintain flow during treatment and corals were lightly basted with a turkey baster to remove any potential biofouling organisms whilst applying the treatment. Following the bath with either solution, corals were rinsed with filtered, untreated seawater before being returned to their holding tank. To be consistent with standard facility-wide husbandry protocols, a broadcast feeding technique was used in each tank throughout the experiment. Three times a week, each tank was fed a total of 1 ounce of Golden Pearls aquarium food (Aquatic Foods & Blackworm Co.). The food was mixed in a one quart container of seawater, and dispersed throughout the tank. Corals were maintained following facility-wide cleaning protocols, including siphoning occasional detritus buildup and replacing racks when fouled by macroalgae and diatoms. Experimental execution (Figure 1) was identical between the two treatment regimes, save for the substitution of one genotype of A. palmata for a different genotype between experiments. All fragments of A. palmata genotype AP20 produced low DNA yields resulting from DNA isolation during the Lugol’s solution experiment, regardless of treatment, and as such were substituted during the KoralMD experiment with genotype AP5, a half-sibling of AP20 produced from the same batch cross performed in 2013.
Figure 1 Experimental Design. In two complementary but distinct experiments, corals of two species, A. palmata and O. faveolata, were exposed to the common coral prophylactics Lugol’s solution and KoralMD. Treated and untreated genotypes of the genets used in the study were housed within the same raceway to match standard restoration practices. Coral microbiome samples (n = 5 per genotype/timepoint/treatment) were collected prior to treatment (T0), 24 hours after first treatment (T1), after cessation of treatment (after 6 treatments; T2), 1 month post-treatment (T3), and 2 months post-treatment (T4). Sampled fragments were removed from experiment.
Samples of coral tissue, skeleton, and mucus were taken from five corals from each treatment (Lugol’s solution/KoralMD treated and control) at the following timepoints (Figure 1): prior to treatment (T0), 24 hours after the first treatment (T1), 24 hours after the final (6th) Lugol’s solution/KoralMD treatment (T2), 30 days after the final Lugol’s solution/KoralMD treatment (T3), and 60 days after the final treatment (T4). Using a flame sterilized razor blade, 2-3 polyps of O. faveolata and 6-8 polyps of A. palmata were excised from each individual and placed in a 1.5mL microcentrifuge tube containing 1mL of DNA/RNA shield (Zymo Research, R1100-250, Irvine, CA, USA). After sampling, fragments were removed from the study to heal over their wounds and were integrated into restoration pipelines and ultimat. Samples were transferred to a -80°C freezer for long-term storage. In preparation for DNA extractions, the samples were removed from the -80°C freezer and thawed on ice. With flame-sterilized tweezers, half of the biomass was transferred to a Disruptor Tube (Omega Bio-Tek, Norcross, GA, USA), the other half was kept as a bioarchive and returned to -80°C. DNA from each sample was isolated utilizing the E.Z.N.A.® Soil DNA Kit (Omega Bio-Tek, Norcross, GA, USA) with slight modifications to the manufacturer’s protocol to increase yield (Supp. Table 1). DNA isolates were stored at -80°C. DNA quantity and quality was assessed utilizing a NanoDrop spectrophotometer (Thermo Fisher Scientific™, Waltham, MA, USA); 67 (of 400) samples with lower DNA quality (A260/230 < 1.5) received additional cleanup with the DNeasy PowerClean Pro Cleanup Kit (Qiagen, Germantown, MD) upon submission for sequencing. Samples were submitted to MR DNA for 16S rRNA PCR amplification and sequencing (www.mrdnalab.com, Shallowater, TX, USA). Amplification of the 16S rRNA gene was conducted using the 515F-806R primer set, which targets the V4 region of the 16S rRNA, with barcodes on the forward primer (Apprill et al., 2015). The 16S rRNA gene V4 variable region was amplified via a 30-cycle PCR using the HotStarTaq Plus Master Mix Kit (Qiagen, Germantown, MD) under the following conditions: 95°C for 5 minutes, followed by 30 cycles of 95°C for 30 seconds, 53°C for 40 seconds and 72°C for 1 minute, after which a final elongation step at 72°C for 10 minutes was performed. After amplification, PCR products were checked in 2% agarose gel to determine the success of amplification and the relative intensity of bands. Samples were multiplexed using unique dual indices and are pooled together in equal proportions based on their molecular weight and DNA concentrations. Pooled samples were purified using calibrated Ampure XP beads (Beckman Coutler, CA, USA). Then the pooled and purified PCR product was used to prepare an Illumina DNA library. A PCR negative control was included in library preparation but did not produce a viable library. Paired-end sequencing was performed at MR DNA on an Illumina MiSeq following the manufacturer’s guidelines.
Upon receipt of raw sequence data from MR DNA, quality control was performed on sequenced reads to remove low-confidence reads as well as sequencing artifacts (Supp. Table 2). As samples from the two experiments were sequenced separately, quality control, sequence variant inference, and amplicon sequence variant (ASV) table construction was performed separately on each dataset. A total of 212,311,736 reads were processed across 400 samples (mean depth of 530,779.34 reads/sample) using DADA2 (Callahan et al., 2016) in R (version 4.0.3, R Core Team, 2021) as follows: based on quality plots, forward and reverse reads were truncated at their 3′ end at 220 and 200 base pairs, respectively. Sequences were also truncated at the first position having a quality score less than or equal to 2, and reads with a total expected error of >2 or with the presence of Ns were discarded, resulting in a total of 169,759,252 reads (mean depth of 424,398.13 reads/sample). A total of 138,596 sequence variants were assigned using the DADA2 denoising algorithm and taxonomy was assigned using the reference database SILVA (v138.1; Quast et al., 2012). Any sequencing reads identified as coral mitochondria, algal chloroplast, or other eukaryotic sequences were removed in the R package phyloseq (McMurdie and Holmes, 2013), which left a remaining 130,081 sequence variants in the dataset. In lieu of rarefaction, shown to artificially reduce diversity estimates in microbiome datasets (McMurdie and Holmes, 2014), sequencing artefacts (taxa with fewer than 15 read counts across all samples) were removed from the dataset for all analyses except alpha diversity, resulting in a new total of 102,028 taxa. After processing in phyloseq, ASVs classified only to a certain taxonomic level (e.g., annotated only to the family level) were renamed at lower taxonomic levels to reflect the lowest annotated level (e.g., ASV2, unknown genus in family Helicobacteraceae renamed at genus level to ‘Unclassified Helicobacteraceae’).
Alpha diversity analyses were performed on unpruned (no rare taxa removed) data rarefied to an even sampling depth of 2000 reads/sample. Alpha diversity was assessed using Simpson’s diversity index, and differences in diversity were tested with a Pairwise Wilcoxon Rank Sum Test with false discovery rate (FDR) correction for multiple testing. For beta diversity analyses, centered log-ratio (CLR) transformation was performed to capture ratio relationships between taxa using the tool clr from the microbiome package (Lahti and Shetty, 2012). Beta diversity analyses were performed on the pruned, CLR-transformed ASV table. Principal components analysis (PCA) was performed using Euclidean distances calculated from the CLR-transformed dataset (producing Aitchison distance (Gloor et al., 2017) with the phyloseq command ordinate (as RDA without constraints). Permutational Multivariate Analysis of Variance (PERMANOVA; Anderson, 2001) was performed on Euclidean distances to test for differences in beta diversity of bacterial community compositions among groups. PERMANOVA was performed using the function adonis from the package vegan (v2.5.5; Oksanen et al., 2019) and was followed by pairwise analysis of variance with pairwiseAdonis (v0.01; Martinez Arbizu, 2017) using Euclidean distance and 999 permutations. Permutational Analysis of Multivariate Dispersion analysis (PERMDISP; Anderson, 2006) was performed to examine shifts in multivariate dispersion over time and between groups using the function betadisper. Pairwise analysis was performed using the function permutest with FDR adjusted P-values (both in vegan v2.5.5; Oksanen et al., 2019). Metrics of alpha and beta diversity were compared across treatments through time to examine the effects on overall community composition and diversity. Analyses using relative abundance were performed on pruned data transformed to relative abundance. The R package ANCOM-II (v2.1, Kaul et al., 2017) was used to examine differential abundance of individual bacterial taxa in response to treatment with Lugol’s solution and KoralMD. ANCOM-II presents an analysis of changes in taxon relative abundance in comparison to the geometric mean of taxa in a given group, testing the null hypothesis that the average relative abundance of a given species in a group is equal to that in the other group. The pruned ASV table was first subset to contain only taxa with at least 20 counts in at least 10% of samples and summarized to the genus level with the tax_glom command in phyloseq. This resulted in a table with 35 genera within 400 samples with a median sequencing depth of 11,804 reads per sample.
Top-down 2D photos and 3D scans were taken of a subset of corals at each sampling time point to monitor growth rates throughout treatment and washout (Supp. Figure 1). During each of four time points (T0, T2, T3, T4; T1 was skipped as it was only one day after T0), the 3D surface area of a subset of 20 coral per species (5 coral per genotype per treatment; 40 total) was obtained utilizing a HDI Compact C210 3D light-structured scanner (Polyga, Burnaby, BC Canada) and following the methods described in Koch et al. (2021). In brief, at each timepoint, the scanner was calibrated with a 5 mm calibration grid prior to scan acquisition. The rotary table was set to turn and take an image of the coral every 30° to produce a total of 12 meshes that then were aligned using the FlexScan3D software (Polyga, Burnaby, BC Canada). The meshes were imported to the software and manually aligned. The functions “Fine Alignment” and “Smooth Merge” were used to finalize the scans, and any mesh editing (i.e., hole filling) was executed as necessary. Once the scan was finalized, the model was cropped to eliminate the plug on which each coral was mounted, and the surface area of the coral was calculated. Comparisons of surface area post-washout were performed for both coral species using a Pairwise Wilcoxon Rank Sum Test with false discovery rate (FDR) test correction for multiple comparisons. All reported variations are standard deviation.
Two distinct genotypes each of Acropora palmata and Orbicella faveolata were exposed to Lugol’s solution and KoralMD (Brightwell Aquatics) in two separate aquarium-based experiments to assess the effects of these broad-spectrum treatments on coral growth rates and microbiome composition. Coral tissue, mucus, and skeleton were sampled pre-treatment, during treatment, and 1- and 2-months post-treatment to assess shifts in bacterial community composition and structure. To assess growth rates, the surface area of the coral fragments was measured with 2D and 3D imagery. Across both experiments, no impacts of treatment were observed on coral mortality and all corals survived through 2 months post-washout.
Corals of both species and all genotypes increased in size over the course of both experiments (Figure 2, Supp. Table 3). When treatment with Lugol’s solution was initiated on 19 Nov 2021, A. palmata fragments averaged 688.33 ± 153.30 mm2, while O. faveolata fragments averaged 424.27 ± 63.36 mm2. All fragments (Lugol-treated and untreated) of A. palmata demonstrated signs of tissue recession as a result of pump failure between one and two months post-treatment and growth rates appeared to be stunted: in the 30 days between cessation of treatment and one month post-washout, corals grew an average of 136.70 ± 62.2 mm2, but between one and two months post-washout, corals only grew 95.5 ± 61.8 mm2. As a result, the two-month washout timepoint was excluded from further analysis. After 1 month of washout post-treatment, A. palmata fragments of both tested genotypes (AP20 and AP16) averaged 938.10 ± 226.09 mm2 in size, reflecting an average growth of 35.92 ± 12.05% from their original size (Supp. Table 3). Lugol-treated A. palmata, however, grew significantly slower than untreated conspecifics (p < 0.05, Supp. Table 4). Lugol-treated A. palmata grew by 27.15% ± 7.488%, while untreated controls grew by 44.69% ± 8.914% over the course of the experiment (Figure 2A, Supp. Table 3). Control corals of genotype AP20 had a significantly higher increase in size compared to AP16 (Supp. Table 4). After two months of washout post-treatment, O. faveolata fragments averaged 705.74 ± 110.42 mm2 in size, with an average growth of 34.14% ± 12.72% (Supp. Table 3). There were no significant differences in O. faveolata growth by treatment type (p > 0.05, Supp. Table 5), though untreated corals grew slightly more: Lugol-treated O. faveolata grew 30.18% ± 10.56%, while untreated controls grew 38.11% ± 13.97% over the course of the experiment (Figure 2A, Supp Table 3). There was no significant difference in growth rates between O. faveolata genotypes (Supp. Table 5).
Figure 2 Change in surface area of corals, A. palmata and O. faveolata, treated with Lugol’s solution (A) and KoralMD (B) compared to their respective control corals. Growth was significantly affected by Lugol’s solution in both genotypes of A. palmata. The error bars represent standard deviation. Boxes sharing a letter are not significantly different from each other (within genotype) using a significance level of p < 0.05.
Upon initiation of KoralMD treatment on 20 May 2022, A. palmata fragments averaged 196.49 ± 64.39 mm2, while O. faveolata fragments averaged 212.84 ± 50.15 mm2 (Figure 2B, Supp. Table 6). After 2 months of washout post-treatment, A. palmata fragments averaged 380.61 ± 95.43 mm2 in size, reflecting an average growth of 101.55% ± 38.50% (Supp. Table 6). There were no significant differences in growth rate by treatment (p > 0.05, Supp. Table 7), though untreated A. palmata grew slightly more than KoralMD-treated conspecifics (Figure 2B). KoralMD-treated A. palmata grew by 90.72% ± 28.82%, while untreated controls grew by 112.40% ± 45.14% over the course of the experiment (Supp. Table 6). There was no significant difference in growth rates between A. palmata genotypes in the KoralMD study (Supp. Table 7), though KoralMD-treated corals of genotype AP16 grew slower than all other groups, with an average growth of 72.91% ± 19.80%. In contrast, untreated AP5 had the highest growth at 150.63% ± 25.48%. Two months post-treatment, O. faveolata fragments averaged 357.53 ± 61.72 mm2 in size, with an average growth of 75.46% ± 44.46% (Supp. Table 6). There were no significant differences in O. faveolata growth by treatment type (Supp. Table 8), though treated corals appeared to grow more in comparison to original sizes. KoralMD-treated O. faveolata grew 87.19% ± 56.30%, while untreated controls grew 63.71% ± 26.43% over the course of the experiment (Figure 2B, Supp. Table 6). There were no significant differences in growth rates by genotype in O. faveolata (Supp. Table 8).
Microbial community composition of A. palmata and O. faveolata varied by coral species and genotype in both experiments (Supp. Figures 2, 3). Overall community composition did not appear to change significantly with time, with the same microbial taxa dominating at all time points in both species (Figure 3). Microbiomes of A. palmata were dominated by diverse rare taxa, with most taxa less than 2% relative abundance within each genotype and experiment (Figure 3A, Supp. Figure 2). The genus Candidatus Amoebophilus constituted an average of 24.37 ± 23.03% in samples from the Lugol experiment and 6.18 ± 15.17% in samples from the KoralMD experiment of both treatment types (Figure 3A). The genus Thalassotalea represented an average of 2.34 ± 5.25% and the genus MBIC10086 represented an average of 2.23 ± 6.44%. No other bacterial genus exceeded 2% relative abundance when examined across samples of both studies and both genotypes (Figure 3A). Across both studies, samples of O. faveolata from both genotypes (Figure 3B) were dominated by an unclassified species within the bacterial family Terasakiellaceae (mean relative abundance 55.64 ± 30.86%, max of 97.64%) and the genus Candidatus Amoebophilus (6.83 ± 7.74%, max of 40.12%). The genus-level clade Marine Methylotrophic Group 3 was also abundant, though variable (3.93 ± 7.49%, max of 36.15%, min of 0%). Minor taxa varied between genotypes and by study (Supp. Figure 3).
Figure 3 Relative abundance of the most abundant bacterial genera in samples of (A) Acropora palmata and (B) Orbicella faveolata exposed to Lugol’s solution (top panel) and KoralMD (Brightwell Aquatics, lower panel). Experimental treatment (control or treatment type) is listed on the x-axis. Microbial taxa are included in the plot if they had a relative abundance greater than 2% in more than 10% of samples across both experiments for each species. Each bar represents 10 corals, 5 of each of two genotypes. Samples taken after 2 months washout from treatment with Lugol’s solution were excluded from analysis due to unrelated tissue loss.
Microbial community composition was more strongly influenced by experimental timepoint than treatment type. Differences in microbial community composition (i.e., beta diversity) were found within each experiment in omnibus tests (Supp. Figure 4, F1,398 = 15.764, p = 0.001). However, the supporting R2 was only 0.038, indicating that experiment explained only 3.8% of the variation in microbiome composition. While significant differences were found in community composition between coral species, coral species also did not explain a large amount of variation in composition (R2 = 0.055, F1,398 = 23.33, p = 0.001). Within both experiments, the impact of treatment (treated vs. untreated) on community composition was insignificant (p > 0.05 for all comparisons), however, composition did vary significantly by experimental timepoint. For both species and treatment types and across both experiments, microbiomes after one day of treatment (T1) were not significantly different from initial samples taken pre-treatment (T0) (p > 0.05, pairwise PERMANOVA with FDR correction, Supp. Table 9, Supp. Table 10). In the Lugol’s solution experiment, microbiomes of A. palmata were indistinct from T0 through T2 (p > 0.05, Supp. Table 9), but by T3 (one month post-treatment), microbiomes of both Lugol-treated and untreated A. palmata were distinct in composition from initial samples (p < 0.01, Supp. Table 9). Both Lugol-treated and untreated A. palmata exhibited significant increases in microbial community dispersion (measured as distance-to-centroid) by one month post-washout (p < 0.05), but community dispersion was not significantly different between treated and untreated corals (p > 0.05, Supp. Figure 5). In the KoralMD experiment, microbial communities of both untreated and KoralMD-treated A. palmata were distinct from pre-treatment communities by T2 (cessation of treatment, ~2 weeks after initial sample) (p < 0.05, Supp. Table 10) and remained distinct from T0 at subsequent timepoints (p < 0.05, Supp. Table 10). A. palmata treated with KoralMD were significantly different in community composition from untreated conspecifics at T2 but became indistinguishable by treatment by one month post-treatment (Supp. Table 10). There were no significant differences in community dispersion through time in A. palmata in the KoralMD study (p > 0.05, Supp. Figure 5).
In the Lugol’s solution experiment, samples of both treated and untreated O. faveolata were distinct in microbial community composition from initial (T0) samples at exclusively T2 (immediately post-treatment, p < 0.01, Supp. Table 9) and T4 (2 months post-treatment, p < 0.01, Supp. Table 9). Microbial community dispersion was significantly higher in Lugol-treated O. faveolata at two months post-washout compared to before treatment (p < 0.05) and dispersion was also higher in Lugol-treated corals compared to untreated conspecifics at the culmination of the study (p < 0.05, Supp. Figure 5). Microbiomes of O. faveolata did not change significantly with time during the KoralMD experiment, whether treated with KoralMD or untreated conspecifics (p > 0.05, Supp. Table 10). There were no significant differences in community dispersion through time in O. faveolata of either treatment group in the KoralMD study (p > 0.05, Supp. Figure 5). In both studies, microbiomes of treated and untreated corals of either species were not significantly different, with few exceptions: in the Lugol’s solution experiment, microbiomes of Lugol-treated and untreated O. faveolata were distinct only at 2 months post-treatment (T4, p < 0.01, Supp. Table 9) and in the KoralMD experiment, microbiomes of KoralMD-treated and untreated A. palmata were distinct exclusively at the sampling point following cessation of treatment (T2, p < 0.01, Supp. Table 10).
Significant shifts did not occur in microbial community within-sample diversity (Shannon diversity index) of either species in response to either antiseptic treatment (Figure 4, Supp. Tables 11-14). While statistically insignificant, microbiome alpha diversity increased in samples of A. palmata between T0 and T3 of the Lugol’s solution experiment (Figure 4A, top panel, Supp. Table 11), but remained stable in the KoralMD experiment (Figure 4B, top panel, Supp. Table 13). Microbiome diversity in O. faveolata was more variable in response to Lugol’s solution, however diversity was not significantly different between T0 (pre-treatment), T2 (post-treatment) and T4 (2 months after treatment) within treated or untreated groups (Figure 4A, bottom panel, Supp. Table 12). At two months post-washout, Lugol-treated O. faveolata had significantly higher diversity than untreated conspecifics (p < 0.05, Pairwise Wilcoxon Rank Sum Test with FDR correction), though diversity was not significantly higher than pre-treatment within the Lugol-treated group. There were no significant differences in within-sample diversity between treatment groups or timepoints in O. faveolata during the KoralMD experiment (Figure 4B, bottom panel, Supp. Table 14).
Figure 4 Differences in Shannon diversity by treatment [Control vs Lugol (A) and Control vs KoralMD (B)] and coral species (Acropora palmata and Orbicella faveolata). Boxes sharing a letter are not significantly different from each other (within species and experiment, i.e. plot row) using an FDR corrected significance level of p < 0.05. No significant differences in Shannon diversity were found by treatment or time in the KoralMD experiment, nor in A. palmata from the Lugol’s solution experiment.
Shifts in individual taxa over the course of the experiment were examined as differential abundance using the tool ‘ANCOM-II’ (Figure 5, Supp. Figures 6, 7). There were no significant differences in relative abundance of individual taxa between A. palmata treated with Lugol’s solution and untreated conspecifics at one month post-washout (Figure 5A, left panel), Further, relative abundance of only one taxon, Litoribrevibacter, changed significantly over the course of the experiment, increasing slightly in treated corals while decreasing slightly in relative abundance in untreated corals (Supp. Figures 6A, B). Numerous genera, however, were significantly different between treated O. faveolata and untreated conspecifics, though magnitude of change was small (Figure 5B, left panel). While the relative abundance of many taxa changed over time in the Lugol’s experiment (Supp. Figures 6C, D), the magnitude of change and directionality was similar between both treatment groups for all taxa with few exceptions: Allofrancisella was significantly higher in relative abundance in both treated and untreated corals compared to T0, however, the magnitude of change was much greater between T0 and control corals at two months post-washout (Supp. Figures 6C, D). An unclassified genus from the family Terasakiellaceae was significantly higher in relative abundance in untreated controls compared to T0, however this genus was less abundant in Lugol-treated corals at two months post-washout compared to T0 (Supp. Figures 6C, D).
Figure 5 Volcano plot of results from differential abundance analysis with ANCOM-II on samples of Acropora palmata (A) and Orbicella faveolata (B) by treatment type at 2 months post-washout (1 month for Lugol-treated Acropora palmata, which experienced unrelated tissue loss at 2 months). Taxa with a total count of 10 in at least 10% of samples (‘N taxa’ in each panel) were included in the analysis. The W statistic represents the strength of the test for the taxa included. Taxa above the dashed line are significant with the null-hypothesis rejected 60% of the time (W = 0.6). Non-significant taxa are colored gray. The x-axis value presents the effect size as the CLR (centered log ratio)-transformed mean difference in abundance of a given species between the two groups being compared. A positive x-axis value indicates that a genus was more abundant in treated corals compared to untreated controls, and distance along x-axis indicates the magnitude of change.
No individual taxa were found to be differentially abundant in microbiomes of A. palmata or O. faveolata between fragments treated with KoralMD and untreated fragments at 2 months post-washout (Figures 5A, B, right panels). Numerous taxa differed, however, between samples taken at 2 months post-washout and initial samples (T0) for both treatment groups (Supp. Figure 7). The genus Vibrio exhibited a greater magnitude of change throughout the course of the experiment in untreated A. palmata fragments compared to KoralMD-treated A. palmata (Supp. Figures 7A, B). The genus Enterobacter and an unclassified taxon in the family Enterobacteraceae were found in reduced relative abundance in both groups at the end of the experiment compared to initial samples. When KoralMD-treated and untreated O. faveolata were compared to initial samples, the genera Pseudomonas and Vibrio were found in higher relative abundance in both groups compared to pre-treatment, and the fold change for Vibrio between initial samples and experiment end was higher for untreated O. faveolata samples than for KoralMD-treated samples (Supp. Figures 7C, D).
Productivity of coral aquaculture is strongly dependent on both the survival and growth rates of cultured corals. To maximize throughput, nursery corals are often kept at very high densities (5.5 corals [38.5cm² tissue] per gallon of saltwater in Mote’s ex situ nursery) which can expedite the proliferation of different pests and diseases. Broad-spectrum antibiotics may be applied in response to coral health issues that arise in culture, however, the success of antibiotic treatment is limited by the short half-life of many antibiotics (often requiring multiple rounds of administration) and by our limited knowledge of the causative agents of coral diseases (Sweet et al., 2012). Further, antibiotic treatment may exacerbate coral health issues by reducing or altering populations of beneficial bacteria within the coral holobiont and aquarium water (Glasl et al., 2016; Connelly et al., 2022). Indeed, broad-spectrum antibiotics have been shown to suppress the native coral microbiota, leading to negative effects on host health and the potential for proliferation of pathogens instead of the reduction thereof (Gilbert et al., 2012; Sweet and Bythell, 2015; Glasl et al., 2016; Connelly et al., 2022). Nonetheless, antibiotics have been successfully applied to arrest coral diseases including stony coral tissue loss disease (SCTLD) and white band disease (Sweet et al., 2014; Neely et al., 2020). Antibiotic use in marine environments has led to some apprehension due to the potential of inducing antibiotic resistance (Letchumanan et al., 2015; Tomova et al., 2015; Luis et al., 2019), as well as the need for repetitive administration to increase efficacy and to yield longer-lasting protection (Neely et al., 2020; Shilling et al., 2021).
These considerations have led to the development of alternative treatments for diseases in aquaculture, like the use of probiotics (beneficial microorganisms), that can complement the host microbiome without compromising it (Peixoto et al., 2017; Ushijima et al., 2023) as well as the widespread use of antiseptics and disinfectants in marine aquaculture (Chen et al., 2018; Luis et al., 2019). Antiseptics are administered as a preventative measure for pests and diseases when risk of infection is high, instead of once the individual has already been infected. Previous research suggests that the use of antiseptics may not strongly alter native microbiomes as antibiotics do; mice treated with 10% povidone-iodine (an antiseptic) exhibited no significant changes in skin microbial community structure compared to untreated conspecifics, while topical antibiotics applied singly or in combination induced an immediate shift in cutaneous microbial populations that persisted for multiple days after treatment (SanMiguel et al., 2017). Yet in this study, both topical antiseptics and antibiotics were sufficient to inhibit the growth of Staphylococcus spp. (SanMiguel et al., 2017), suggesting that antiseptics may be effective in preventing bacterial infection without causing long-term deleterious impacts on commensal microbiota. While antiseptics such as iodine have a broad spectrum of targets and mechanisms of action against bacteria (Gottardi, 1991; McDonnell and Russell, 1999), their impacts are more localized (application site-specific) rather than systemic, and their magnitude of impact is influenced by bacterial identity and load (SanMiguel et al., 2018).
The antimicrobial properties of iodine and of essential oils are well-determined in human and mammalian systems (Kalemba and Kunicka, 2003; Lachapelle et al., 2013). While Lugol’s solution is comprised of only potassium iodide and crystalized iodine, the ingredients of KoralMD are proprietary, but reported to consist of conifer and citrus oils. Volatile compounds found in conifer oils have mild antimicrobial properties against both gram-positive and -negative bacteria and fungi (Hong et al., 2004; Lee et al., 2009). Limonene, along with minor volatile compounds varying by species, can be derived from the peel of most citrus fruits and has strong inhibitory activity against gram-positive bacterial species (Dabbah et al., 1970; Li et al., 2019; Raspo et al., 2020). Iodine is bactericidal, fungicidal, and virucidal even at low concentrations, and impacts microbial proteins, nucleotides, and fatty acids, leading to rapid cell death (Gottardi, 1991; McDonnell and Russell, 1999). While iodine treatment is commonly implemented in large-scale aquaculture facilities (Chen et al., 2018), Mote Marine Laboratory has recently begun using KoralMD to stop tissue recession in cultured corals. With this study we aimed to characterize the impacts of these common antiseptic treatments on microbiome composition and growth rate of healthy corals. Evaluation of the long-term effects of these treatments on corals destined for restoration is critical to ensure that outplanted corals have the highest chance of survival and do not harbor dysbiotic microbiomes. The results from this study indicate that neither tested prophylactic treatment had significant or lasting effects on the microbiomes of visibly healthy corals. Our inability to detect a significant impact of treatment on coral microbiomes may have been influenced by our choice to house untreated corals in the same tank with treated corals following standard restoration practices for prophylactic treatment. While co-housing treated and untreated conspecifics in this manner may have allowed for exchange of microorganisms between treatment groups, this experimental design allowed us to evaluate whether these practices have a lasting impact on microbial community structure, necessitating the quarantine of untreated individuals from treated corals.
We found that bacterial communities of the elkhorn coral, Acropora palmata, were dominated by Candidatus Amoebophilus during exposure to Lugol’s solution, but communities were more diverse and more distinct by genotype during treatment with KoralMD (Figure 3). Candidatus Amoebophilus were originally identified as intracellular symbionts of amoebae but have since been identified in high abundance in healthy tissues of several Caribbean coral species (Apprill et al., 2016; Huggett and Apprill, 2019). Minor taxa that appeared to be consistently associated with A. palmata included Thalassotalea and Ascidiimonas, both of which are aerobic chemoheterotrophic species that have been identified in seawater and in other marine invertebrates (Sheu et al., 2016; Yoon et al., 2016). The genus P3OB-42, a member of Myxococcales that may play a commensal or beneficial role in acroporid microbiomes (Rosales et al., 2019; Klinges et al., 2022), was also found consistently in A. palmata during the KoralMD experiment. At one month post-washout from Lugol’s treatment, there were no significant differences in microbial community structure between untreated A. palmata and conspecifics treated with Lugol’s solution, and no taxa were found to be differentially abundant between the two groups (Figure 5). In contrast, many taxa changed with time over the course of the KoralMD experiment in both treated A. palmata and untreated conspecifics, though there were again no significant differences in relative abundance between treated and untreated corals at the end of the washout period (Figure 5). Interestingly, corals that were not treated showed an increase in the genus Vibrio, which includes many coral pathogens (Ben-Haim and Rosenberg, 2002; Ben-Haim, 2003; Luna et al., 2010). Although increases in this genus were found to be significant for both corals treated with KoralMD and untreated conspecifics, the magnitude of change was considerably greater for untreated A. palmata (>2 log-fold) than for corals treated with KoralMD.
In the present study, microbiomes of the mountainous star coral, Orbicella faveolata, were dominated by a single unclassified sequence variant from within the family Terasakiellaceae. Terasakiellaceae are known nitrogen cyclers from the order Rhodospirillales and are associated with healthy coral tissue (Moynihan et al., 2022; Pushpakumara et al., 2023). Microbial community richness and evenness, as measured by Shannon’s index of diversity, was significantly higher in O. faveolata treated with Lugol’s solution in comparison to untreated conspecifics by two months post-washout, however, diversity was not significantly higher than that at timepoint zero (start of experimental manipulation). This corresponded with a reduction in the family Terasakiellaceae in Lugol-treated O. faveolata compared to untreated conspecifics at two months post-washout. No taxa were significantly different in relative abundance between KoralMD-treated O. faveolata and untreated conspecifics and microbial community diversity was unchanged throughout the experiment in either treatment group. In both treatment groups, populations of the genera Vibrio and Pseudomonas increased in relative abundance from experiment start to two months post-washout. While these taxa have been found to be associated with coral stress (Luna et al., 2010; Kelly et al., 2014), both of these genera have demonstrated antagonistic activity against other pathogens when isolated from coral samples (Krediet et al., 2013; Vijay et al., 2021). As no visible signs of stress were observed in O. faveolata of either treatment group, the particular strains that increased in abundance in these corals may perform commensal roles in orbicellid microbiomes. Like A. palmata, microbiomes of O. faveolata had a significant proportion of the genus Candidatus Amoebophilus, suggesting that the shared Mote Marine Laboratory ex situ nursery environment may contribute to abundances of this taxon.
Coral microbiomes were relatively unreceptive to changes in community structure in response to antiseptic treatments, however, microbial communities appeared more variable with time in both treated and untreated corals in the Lugol’s solution experiment compared to the KoralMD experiment. There were no clear differences in water quality parameters between the two studies (Table 1), however, differences in seasonality between the two studies (November to January in Lugol’s solution study vs May to July in KoralMD study) could have contributed to a higher bacterial load in Mote’s source water canal as the KoralMD experiment extended into mid-summer. Nutrient (nitrate/nitrite/phosphate) levels were not measured in this study but were likely higher in July as Florida Keys watershed inputs are highest in the summer, especially in canals (Lapointe and Clark, 1992). A recent study found that seasonality did not contribute significantly to microbiome variation in Pacific Acropora spp. (Epstein et al., 2019), though a study on O. faveolata in the Caribbean indicated that there were greater differences in microbiome composition by season (September vs. March) than there were between yellow band-diseased and healthy colonies (Kimes et al., 2010). Nutrient enrichment has been previously found to contribute to microbiome dysbiosis as measured by increased community dispersion (Zaneveld et al., 2016; Maher et al., 2019). As no significant differences in dispersion occurred during the KoralMD experiment, it is unlikely that nutrient enrichment led to microbiome instability in this study.
In September 2021, Mote’s ex situ aquaculture water source was changed from well water collected 80 ft below the island to canal water collected 10 ft below the surface of the adjacent canal. While corals were allowed to acclimate to this new water source for two months prior to manipulation in the Lugol’s experiment, it is possible that corals of both species were still responding to this shift during the time of this experiment. Numerous microbial taxa were found to be differentially abundant in O. faveolata of both treatment groups between initiation and cessation of the Lugol’s experiment, and some of these taxa differed in relative abundance between the two treatment groups after two months of treatment washout. As O. faveolata are slow-growing and slow to exhibit signs of stress, as indicated by their high bleaching thresholds (Manzello et al., 2019; Parker et al., 2020), the two-month acclimation period allowed for these species to adapt to the new water source may not have been sufficient. The differences observed between the two treatment groups after two months of washout may therefore have been related to continued acclimation, with stochasticity between individuals contributing to greater community variability unrelated to treatment conditions (Zaneveld et al., 2017). In contrast, the microbiomes of A. palmata did not vary significantly throughout this study in either treatment group, and therefore may have been fully acclimated to the new water source before treatments were applied. Acroporid microbiomes have been previously described as ‘flexible,’ responding rapidly to environmental stressors through microbiome restructuring (Ziegler et al., 2019; Voolstra and Ziegler, 2020). Microbiome shifts in response to the change in water source may therefore have occurred in the two-month acclimation period before initial samples were collected. Only two genotypes per species were selected for use in this study to reduce microbiome variability between conspecifics within each treatment group, but this may have also limited our ability to detect microbiome response to these treatments that may occur in certain genotypes of A. palmata and O. faveolata.
Overall, these results support current restoration practices in that a 30-day washout period after treatment with Lugol’s solution or KoralMD is sufficient to ensure that microbiomes are stable before corals are reintroduced to the wild for restoration and research purposes. Further, our results indicate that these treatments do not have widespread impacts on native coral microbiota, however, administration of these treatments to corals showing signs of stress likely impacts opportunistic or pathogenic microbes based on the observed efficacy of both treatment types against tissue necrosis (Z. Craig, D. Merck, personal observation). Despite evidence that aseptic treatments likely have less systematic impacts on animal microbiomes than antibiotics do (SanMiguel et al., 2017; SanMiguel et al., 2018), these treatments should nonetheless be applied with caution, as some coral diseases progress slowly, taking days to weeks for signs of disease to manifest (Kaczmarsky, 2006; Montano et al., 2015). While prophylactic treatments may prevent some health issues from progressing, their efficacy against saprophytic bacteria and opportunistic microeukaryotes may prevent symptoms of disease from manifesting, therefore resulting in the unintended exposure of healthy corals to infected individuals (Kent et al., 2009; Sheridan et al., 2013). Further, the signs of stress exhibited by cultured corals may be indicative of abiotic issues associated with water quality or light stress, for example. Treatments such as Lugol’s solution or KoralMD may treat the symptoms of these issues (e.g. ciliates attracted to necrotic tissue) without addressing the underlying source.
Although neither KoralMD or Lugol’s solution significantly altered microbiomes, a slight reduction in the growth rate of Acropora palmata was observed after treatment with Lugol’s solution. In light of our observation that Lugol’s solution may repress growth rates of healthy A. palmata, future work is needed to evaluate the utility of this treatment on healthy corals. Additional observations using light microscopy were made separately from this study to understand the physical changes in A. cervicornis (related to A. palmata and a common restoration species) in response to treatment with KoralMD and Lugol’s solution. Lugol’s solution induced a more intense visible stress response than KoralMD: mesenterial filaments were observed in A. cervicornis after treatment with Lugol’s solution, but not after treatment with KoralMD, and sclerites were more exposed in individuals treated with Lugol’s solution (H. Koch, Mote Marine Laboratory, personal comm.). Corals returned to normal morphology 2 days after KoralMD treatment whereas those treated with Lugol’s solution returned to normal morphology 4 days after treatment (H. Koch, personal comm.). This observed retraction of coral tissue and mesenterial filament production may be indicators of stress and result in reduced acroporid growth rates. As growth rates of Orbicella faveolata were not significantly impacted by either treatment in this study, this species may be less susceptible to stress resulting from Lugol’s solution treatment. Nonetheless, we argue that treatment with Lugol’s solution is a valuable tool in the restoration practitioner’s toolkit as this treatment has been found to halt tissue loss. Corals exhibiting signs of recession have been found to recover (e.g., tissue loss halted, new tissue growing over dead skeleton, Z. Craig, personal observation) after being treated with Lugol’s solution, and treated corals continue to grow once outplanted onto the reef (Z. Craig, personal observation). Indeed, Lugol’s solution can be used by reef aquarists as a means to supplement aquarium invertebrates with iodine and iodate.
With this study, we assessed the impacts of two commonly-used antiseptics applied prophylactically on the coral microbiome and found minimal effect on community structure, diversity, or the relative abundance of individual taxa. By utilizing healthy corals for this study rather than actively diseased corals, we aimed to compare the long-term effects of these treatments on corals destined for restoration, rather than assess the efficacy of these treatments in mitigating coral health issues. The application of antiseptics on actively diseased corals may depend on the species of coral being affected: as growth rates were slightly reduced in A. palmata in response to Lugol’s Solution, KoralMD would be preferable for this species. Coral health issues are not uncommon when cultivating and rearing large populations of corals in high density, and treatment success is likely context-dependent, with outcomes varying by location and infrastructure. When rapid tissue loss is observed in a land-based aquaculture system, the root cause is not always apparent. All treatments should be discussed directly with a licensed aquaculture veterinarian. If simple solutions such as lighting or water quality adjustments do not resolve the problem, antiseptic solutions may be utilized. As many antiseptic treatments are commercially available for use in aquaculture, continued examination of the impacts of these treatments on native microbiota is essential. These results ultimately aid in optimizing coral production and treatment efficiency within a coral restoration facility by elucidating the benefits and any consequences of each treatment method, providing better use at the discretion of the coral practitioner.
Raw sequence data were deposited into the NCBI Sequence Read Archive (SRA) under BioProject PRJNA1022056. The datasets and scripts used for analysis in this study can be found in the following online repository: https://github.com/graceklinges/Lugol_KMD.
Ethical approval was not required for the study involving animals in accordance with the local legislation and institutional requirements because it is not required for corals. Appropriate collection permit information is included in manuscript text.
JK: Conceptualization, Data curation, Formal Analysis, Funding acquisition, Investigation, Methodology, Project administration, Supervision, Visualization, Writing – original draft, Writing – review & editing. ZC: Conceptualization, Data curation, Formal Analysis, Investigation, Methodology, Resources, Supervision, Visualization, Writing – original draft, Writing – review & editing, Funding acquisition. MD-M: Conceptualization, Data curation, Investigation, Methodology, Visualization, Writing – original draft, Writing – review & editing. DM: Investigation, Writing – original draft, Writing – review & editing. SB: Investigation, Writing – original draft, Writing – review & editing. AM: Investigation, Writing – original draft, Writing – review & editing. AC: Conceptualization, Funding acquisition, Project administration, Supervision, Validation, Writing – review & editing, Investigation.
The author(s) declare financial support was received for the research, authorship, and/or publication of this article. This work was funded by the Mote Marine Laboratory Protect Our Reefs Grant with award numbers POR_2020_24 and POR_2021_007.
We would like to acknowledge the assistance of multiple Mote Marine Laboratory interns for assisting with sample and image collection including Hailey Vaughan, Meghan Blaszynski, Mara Riese, Shara Sookhoo, Yuyang (Pike) Li, and Jacob Harding. We also thank staff at Mote Marine Laboratory’s Elizabeth Moore International Center for Coral Reef Research and Restoration for expertise and logistical help including Kyle Knoblock, Eleftherios Karabelas, and Erinn Muller. The use of nursery-grown corals was authorized by the Florida Keys National Marine Sanctuary under Special Activity License SAL-21-2048A and SAL-22-2406A-SCRP.
The authors declare that the research was conducted in the absence of any commercial or financial relationships that could be construed as a potential conflict of interest.
All claims expressed in this article are solely those of the authors and do not necessarily represent those of their affiliated organizations, or those of the publisher, the editors and the reviewers. Any product that may be evaluated in this article, or claim that may be made by its manufacturer, is not guaranteed or endorsed by the publisher.
The Supplementary Material for this article can be found online at: https://www.frontiersin.org/articles/10.3389/fmars.2023.1281691/full#supplementary-material
Aeby G. S., Ushijima B., Campbell J. E., Jones S., Williams G. J., Meyer J. L., et al. (2019). Pathogenesis of a tissue loss disease affecting multiple species of corals along the Florida Reef Tract. Front. Mar. Sci. 6. doi: 10.3389/fmars.2019.00678
Ainsworth T. D., Thurber R. V., Gates R. D. (2010). The future of coral reefs: a microbial perspective. Trends Ecol. Evol. 25, 233–240. doi: 10.1016/j.tree.2009.11.001
Anderson M. J. (2001). A new method for non-parametric multivariate analysis of variance. Austral Ecol. 26, 32–46. doi: 10.1111/j.1442-9993.2001.01070.pp.x
Anderson M. J. (2006). Distance-based tests for homogeneity of multivariate dispersions. Biometrics 62, 245–253. doi: 10.1111/j.1541-0420.2005.00440.x
Antonius A. A., Riegl B. (1997). A possible link between coral diseases and a corallivorous snail (Drupella cornus) outbreak in the Red Sea. Atoll Res. Bull. 447, 1–9. doi: 10.5479/si.00775630.447.1
Apprill A., McNally S., Parsons R., Weber L. (2015). Minor revision to V4 region SSU rRNA 806R gene primer greatly increases detection of SAR11 bacterioplankton. Aquat. Microb. Ecol. 75, 129–137. doi: 10.3354/ame01753
Apprill A., Weber L. G., Santoro A. E. (2016). Distinguishing between microbial habitats unravels ecological complexity in coral microbiomes. mSystems 1(5), e00143-16. doi: 10.1128/msystems.00143-16
Arias-González J. E., Fung T., Seymour R. M., Garza-Pérez J. R., Acosta-González G., Bozec Y.-M., et al. (2017). A coral-algal phase shift in Mesoamerica not driven by changes in herbivorous fish abundance. PloS One 12, e0174855. doi: 10.1371/journal.pone.0174855
Bellwood D. R., Hughes T. P., Folke C., Nyström M. (2004). Confronting the coral reef crisis. Nature 429, 827–833. doi: 10.1038/nature02691
Ben-Haim Y. (2003). Vibrio coralliilyticus sp. nov., a temperature-dependent pathogen of the coral Pocillopora damicornis. Int. J. Systematic Evolutionary Microbiol. 53, 309–315. doi: 10.1099/ijs.0.02402-0
Ben-Haim Y., Rosenberg E. (2002). A novel Vibrio sp. pathogen of the coral Pocillopora damicornis. Mar. Biol. 141, 47–55. doi: 10.1007/s00227-002-0797-6
Boström-Einarsson L., Babcock R. C., Bayraktarov E., Ceccarelli D., Cook N., Ferse S. C. A., et al. (2020). Coral restoration – A systematic review of current methods, successes, failures and future directions. PloS One 15, e0226631. doi: 10.1371/journal.pone.0226631
Bourne D. G., Morrow K. M., Webster N. S. (2016). Insights into the coral microbiome: underpinning the health and resilience of reef ecosystems. Annu. Rev. Microbiol. 70, 317–340. doi: 10.1146/annurev-micro-102215-095440
Callahan B. J., McMurdie P. J., Rosen M. J., Han A. W., Johnson A. J. A., Holmes S. P. (2016). DADA2: High-resolution sample inference from Illumina amplicon data. Nat. Methods 13, 581–583. doi: 10.1038/nmeth.3869
Carpenter K. E., Abrar M., Aeby G., Aronson R. B., Banks S., Bruckner A., et al. (2008). One-third of reef-building corals face elevated extinction risk from climate change and local impacts. Science 321, 560–563. doi: 10.1126/science.1159196
Carpenter L. J., Chance R. J., Sherwen T., Adams T. J., Ball S. M., Evans M. J., et al. (2021). Marine iodine emissions in a changing world. Proc. Math Phys. Eng. Sci. 477, 20200824. doi: 10.1098/rspa.2020.0824
Chen X., Lai C., Wang Y., Wei L., Zhong Q. (2018). Disinfection effect of povidone-iodine in aquaculture water of swamp eel (Monopterus albus). PeerJ 6, e5523. doi: 10.7717/peerj.5523
Connelly M. T., McRae C. J., Liu P.-J., Martin C. E., Traylor-Knowles N. (2022). Antibiotics alter Pocillopora coral-Symbiodiniaceae-bacteria interactions and cause microbial dysbiosis during heat stress. Front. Mar. Sci. 8. doi: 10.3389/fmars.2021.814124
Dabbah R., Edwards V. M., Moats W. A. (1970). Antimicrobial action of some citrus fruit oils on selected food-borne bacteria. Appl. Microbiol. 19, 27–31. doi: 10.1128/am.19.1.27-31.1970
Egan S., Gardiner M. (2016). Microbial Dysbiosis: Rethinking disease in marine ecosystems. Front. Microbiol. 0. doi: 10.3389/fmicb.2016.00991
Elliff C. I., Silva I. R. (2017). Coral reefs as the first line of defense: Shoreline protection in face of climate change. Mar. Environ. Res. 127, 148–154. doi: 10.1016/j.marenvres.2017.03.007
Epstein H. E., Smith H. A., Cantin N. E., Mocellin V. J. L., Torda G., van Oppen M. J. H. (2019). Temporal variation in the microbiome of Acropora coral species does not reflect seasonality. Front. Microbiol. 10. doi: 10.3389/fmicb.2019.01775
Forsman Z. H., Page C. A., Toonen R. J., Vaughan D. (2015). Growing coral larger and faster: micro-colony-fusion as a strategy for accelerating coral cover. PeerJ 3, e1313. doi: 10.7717/peerj.1313
Gilbert J. A., Hill R., Doblin M. A., Ralph P. J. (2012). Microbial consortia increase thermal tolerance of corals. Mar. Biol. 159, 1763–1771. doi: 10.1007/s00227-012-1967-9
Gilbert J. A., Quinn R. A., Debelius J., Xu Z. Z., Morton J., Garg N., et al. (2016). Microbiome-wide association studies link dynamic microbial consortia to disease. Nature 535, 94–103. doi: 10.1038/nature18850
Glasl B., Herndl G. J., Frade P. R. (2016). The microbiome of coral surface mucus has a key role in mediating holobiont health and survival upon disturbance. ISME J. 10, 2280–2292. doi: 10.1038/ismej.2016.9
Gloor G. B., Macklaim J. M., Pawlowsky-Glahn V., Egozcue J. J. (2017). Microbiome datasets are compositional: and this is not optional. Front. Microbiol. 8. doi: 10.3389/fmicb.2017.02224
Gottardi W. (1991). “Iodine and iodine compounds,” in Disinfection, sterilization, and preservation, 4th ed. Ed. Block S. S. (Philadelphia, Pa: Lea & Febiger), 152–166.
Hong E.-J., Na K.-J., Choi I.-G., Choi K.-C., Jeung E.-B. (2004). Antibacterial and antifungal effects of essential oils from coniferous trees. Biol. Pharm. Bull. 27, 863–866. doi: 10.1248/bpb.27.863
Huggett M. J., Apprill A. (2019). Coral microbiome database: Integration of sequences reveals high diversity and relatedness of coral-associated microbes. Environ. Microbiol. Rep. 11, 372–385. doi: 10.1111/1758-2229.12686
Hughes T. P. (1994). Catastrophes, phase shifts, and large-scale degradation of a Caribbean coral reef. Science 265, 1547–1551. doi: 10.1126/science.265.5178.1547
Kaczmarsky L. (2006). Coral disease dynamics in the central Philippines. Dis. Aquat. Org. 69, 9–21. doi: 10.3354/dao069009
Kalemba D., Kunicka A. (2003). Antibacterial and antifungal properties of essential oils. CMC 10, 813–829. doi: 10.2174/0929867033457719
Kaul A., Mandal S., Davidov O., Peddada S. D. (2017). Analysis of microbiome data in the presence of excess zeros. Front. Microbiol. 8. doi: 10.3389/fmicb.2017.02114
Kelly L. W., Williams G. J., Barott K. L., Carlson C. A., Dinsdale E. A., Edwards R. A., et al. (2014). Local genomic adaptation of coral reef-associated microbiomes to gradients of natural variability and anthropogenic stressors. Proc. Natl. Acad. Sci. 111, 10227–10232. doi: 10.1073/pnas.1403319111
Kent M. L., Feist S. W., Harper C., Hoogstraten-Miller S., Law J. M., Sánchez-Morgado J. M., et al. (2009). Recommendations for control of pathogens and infectious diseases in fish research facilities. Comp. Biochem. Physiol. Part C: Toxicol. Pharmacol. 149, 240–248. doi: 10.1016/j.cbpc.2008.08.001
Kimes N. E., Nostrand J. D. V., Weil E., Zhou J., Morris P. J. (2010). Microbial functional structure of Montastraea faveolata, an important Caribbean reef-building coral, differs between healthy and yellow-band diseased colonies. Environ. Microbiol. 12, 541–556. doi: 10.1111/j.1462-2920.2009.02113.x
Klinges J. G., Patel S. H., Duke W. C., Muller E. M., Vega Thurber R. L. (2022). Phosphate enrichment induces increased dominance of the parasite Aquarickettsia in the coral Acropora cervicornis. FEMS Microbiol. Ecol. 98, fiac013. doi: 10.1093/femsec/fiac013
Koch H. R., Wallace B., DeMerlis A., Clark A. S., Nowicki R. J. (2021). 3D Scanning as a tool to measure growth rates of live coral microfragments used for coral reef restoration. Front. Mar. Sci. 8. doi: 10.3389/fmars.2021.623645
Krediet C. J., Ritchie K. B., Alagely A., Teplitski M. (2013). Members of native coral microbiota inhibit glycosidases and thwart colonization of coral mucus by an opportunistic pathogen. ISME J. 7, 980–990. doi: 10.1038/ismej.2012.164
Lachapelle J.-M., Castel O., Casado A. F., Leroy B., Micali G., Tennstedt D., et al. (2013). Antiseptics in the era of bacterial resistance: a focus on povidone iodine. Clin. Pract. 10, 579–592. doi: 10.2217/cpr.13.50
Lahti L., Shetty S. (2012). microbiome R package. Available at: http://microbiome.github.com/microbiome.
Lapointe B. E., Clark M. W. (1992). Nutrient inputs from the watershed and coastal eutrophication in the Florida keys. Estuaries 15, 465–476. doi: 10.2307/1352391
Leal M. C., Ferrier-Pagès C., Petersen D., Osinga R. (2016). Coral aquaculture: applying scientific knowledge to ex situ production. Rev. Aquacult 8, 136–153. doi: 10.1111/raq.12087
Lee J.-H., Lee B.-K., Kim J.-H., Lee S. H., Hong S.-K. (2009). Comparison of chemical compositions and antimicrobial activities of essential oils from three conifer trees; Pinus densiflora, Cryptomeria japonica, and Chamaecyparis obtusa. J. Microbiol. Biotechnol. 19, 391–396. doi: 10.4014/jmb.0803.191
Lema K. A., Bourne D. G., Willis B. L. (2014). Onset and establishment of diazotrophs and other bacterial associates in the early life history stages of the coral Acropora millepora. Mol. Ecol. 23, 4682–4695. doi: 10.1111/mec.12899
Letchumanan V., Pusparajah P., Tan L. T.-H., Yin W.-F., Lee L.-H., Chan K.-G. (2015). Occurrence and antibiotic resistance of Vibrio parahaemolyticus from shellfish in Selangor, Malaysia. Front. Microbiol. 6. doi: 10.3389/fmicb.2015.01417
Li Z.-H., Cai M., Liu Y.-S., Sun P.-L., Luo S.-L. (2019). Antibacterial activity and mechanisms of essential oil from Citrus medica L. var. sarcodactylis. Molecules 24, 1577. doi: 10.3390/molecules24081577
Luis A. I. S., Campos E. V. R., De Oliveira J. L., Fraceto L. F. (2019). Trends in aquaculture sciences: from now to use of nanotechnology for disease control. Rev. Aquacult 11, 119–132. doi: 10.1111/raq.12229
Luna G. M., Bongiorni L., Gili C., Biavasco F., Danovaro R. (2010). Vibrio harveyi as a causative agent of the White Syndrome in tropical stony corals. Environ. Microbiol. Rep. 2, 120–127. doi: 10.1111/j.1758-2229.2009.00114.x
Maher R. L., Rice M. M., McMinds R., Burkepile D. E., Vega Thurber R. (2019). Multiple stressors interact primarily through antagonism to drive changes in the coral microbiome. Sci. Rep. 9, 1–12. doi: 10.1038/s41598-019-43274-8
Manzello D. P., Matz M. V., Enochs I. C., Valentino L., Carlton R. D., Kolodziej G., et al. (2019). Role of host genetics and heat-tolerant algal symbionts in sustaining populations of the endangered coral Orbicella faveolata in the Florida Keys with ocean warming. Global Change Biol. 25, 1016–1031. doi: 10.1111/gcb.14545
Martinez Arbizu P. (2017). pairwiseAdonis: Pairwise multilevel comparison using adonis. R Package version 0.0.1.
McDevitt-Irwin J. M., Baum J. K., Garren M., Thurber R. V. (2017). Responses of coral-associated bacterial communities to local and global stressors. Front. Mar. Sci. 4. doi: 10.3389/fmars.2017.00262
McDonnell G., Russell A. D. (1999). Antiseptics and disinfectants: activity, action, and resistance. Clin. Microbiol. Rev. 12, 147–179. doi: 10.1128/CMR.12.1.147
McMurdie P. J., Holmes S. (2013). phyloseq: An R package for reproducible interactive analysis and graphics of microbiome census data. PloS One 8, e61217. doi: 10.1371/journal.pone.0061217
McMurdie P. J., Holmes S. (2014). Waste not, want not: Why rarefying microbiome data is inadmissible. PloS Comput. Biol. 10, e1003531. doi: 10.1371/journal.pcbi.1003531
Mills E., Shechtman K., Loya Y., Rosenberg E. (2013). Bacteria appear to play important roles in both causing and preventing the bleaching of the coral Oculina patagonica. Mar. Ecol. Prog. Ser. 489, 155–162. doi: 10.3354/meps10391
Montano S., Strona G., Seveso D., Maggioni D., Galli P. (2015). Slow progression of black band disease in Goniopora cf. columna colonies may promote its persistence in a coral community. Mar. Biodiv 45, 857–860. doi: 10.1007/s12526-014-0273-9
Moynihan M. A., Goodkin N. F., Morgan K. M., Kho P. Y. Y., Lopes dos Santos A., Lauro F. M., et al. (2022). Coral-associated nitrogen fixation rates and diazotrophic diversity on a nutrient-replete equatorial reef. ISME J. 16, 233–246. doi: 10.1038/s41396-021-01054-1
Neely K. L., Macaulay K. A., Hower E. K., Dobler M. A. (2020). Effectiveness of topical antibiotics in treating corals affected by Stony Coral Tissue Loss Disease. PeerJ 8, e9289. doi: 10.7717/peerj.9289
Oksanen J., Blanchet F. G., Friendly M., Kindt R., Legendre P., McGlinn D., et al. (2019) vegan: Community Ecology Package. Available at: https://CRAN.R-project.org/package=vegan (Accessed May 15, 2020).
Page C. A., Muller E. M., Vaughan D. E. (2018). Microfragmenting for the successful restoration of slow growing massive corals. Ecol. Eng. 123, 86–94. doi: 10.1016/j.ecoleng.2018.08.017
Pandolfi J. M., Bradbury R. H., Sala E., Hughes T. P., Bjorndal K. A., Cooke R. G., et al. (2003). Global trajectories of the long-term decline of coral reef ecosystems. Science 301, 955–958. doi: 10.1126/science.1085706
Parker K. E., Ward J. O., Eggleston E. M., Fedorov E., Parkinson J. E., Dahlgren C. P., et al. (2020). Characterization of a thermally tolerant Orbicella faveolata reef in Abaco, The Bahamas. Coral Reefs 39, 675–685. doi: 10.1007/s00338-020-01948-0
Peixoto R. S., Rosado P. M., Leite D. C., de A., Rosado A. S., Bourne D. G. (2017). Beneficial microorganisms for corals (BMC): Proposed mechanisms for coral health and resilience. Front. Microbiol. 8. doi: 10.3389/fmicb.2017.00341
Pushpakumara B. L. D. U., Tandon K., Willis A., Verbruggen H. (2023). The Bacterial microbiome of the coral skeleton algal symbiont Ostreobium shows preferential associations and signatures of phylosymbiosis. Microb. Ecol. 86, 2032–2046. doi: 10.1007/s00248-023-02209-7
Quast C., Pruesse E., Yilmaz P., Gerken J., Schweer T., Yarza P., et al. (2012). The SILVA ribosomal RNA gene database project: improved data processing and web-based tools. Nucleic Acids Res. 41, D590–D596. doi: 10.1093/nar/gks1219
Raspo M. A., Vignola M. B., Andreatta A. E., Juliani H. R. (2020). Antioxidant and antimicrobial activities of citrus essential oils from Argentina and the United States. Food Bioscience 36, 100651. doi: 10.1016/j.fbio.2020.100651
R Core Team (2021). R: A language and environment for statistical computing. R Foundation for Statistical Computing, Vienna, Austria. Available at: https://www.R-project.org/.
Ritchie K. (2006). Regulation of microbial populations by coral surface mucus and mucus-associated bacteria. Mar. Ecol. Prog. Ser. 322, 1–14. doi: 10.3354/meps322001
Rohwer F., Seguritan V., Azam F., Knowlton N. (2002). Diversity and distribution of coral-associated bacteria. Mar. Ecol. Prog. Ser. 243, 1–10. doi: 10.3354/meps243001
Rosales S. M., Miller M. W., Williams D. E., Traylor-Knowles N., Young B., Serrano X. M. (2019). Microbiome differences in disease-resistant vs. susceptible Acropora corals subjected to disease challenge assays. Sci. Rep. 9, 18279. doi: 10.1038/s41598-019-54855-y
SanMiguel A. J., Meisel J. S., Horwinski J., Zheng Q., Bradley C. W., Grice E. A. (2018). Antiseptic agents elicit short-term, personalized, and body site–specific shifts in resident skin bacterial communities. J. Invest. Dermatol. 138, 2234–2243. doi: 10.1016/j.jid.2018.04.022
SanMiguel A. J., Meisel J. S., Horwinski J., Zheng Q., Grice E. A. (2017). Topical antimicrobial treatments can elicit shifts to resident skin bacterial communities and reduce colonization by Staphylococcus aureus competitors. Antimicrobial Agents Chemotherapy 61, e00774–17. doi: 10.1128/aac.00774-17
Schopmeyer S. A., Lirman D., Bartels E., Gilliam D. S., Goergen E. A., Griffin S. P., et al. (2017). Regional restoration benchmarks for Acropora cervicornis. Coral Reefs 36, 1047–1057. doi: 10.1007/s00338-017-1596-3
Sheridan C., Kramarsky-Winter E., Sweet M., Kushmaro A., Leal M. C. (2013). Diseases in coral aquaculture: causes, implications and preventions. Aquaculture 396–399, 124–135. doi: 10.1016/j.aquaculture.2013.02.037
Sheu S.-Y., Liu L.-P., Tang S.-L., Chen W.-M. (2016). Thalassotalea euphylliae sp. nov., isolated from the torch coral Euphyllia glabrescens. Int. J. Systematic Evolutionary Microbiol. 66, 5039–5045. doi: 10.1099/ijsem.0.001466
Shilling E. N., Combs I. R., Voss J. D. (2021). Assessing the effectiveness of two intervention methods for stony coral tissue loss disease on Montastraea cavernosa. Sci. Rep. 11, 8566. doi: 10.1038/s41598-021-86926-4
Sweet M., Bythell J. (2015). White Syndrome in Acropora muricata: Nonspecific bacterial infection and ciliate histophagy. Mol. Ecol. 24, 1150–1159. doi: 10.1111/mec.13097
Sweet M. J., Croquer A., Bythell J. C. (2014). Experimental antibiotic treatment identifies potential pathogens of white band disease in the endangered Caribbean coral Acropora cervicornis. Proc. R. Soc B 281, 20140094. doi: 10.1098/rspb.2014.0094
Sweet M., Jones R., Bythell J. (2012). Coral diseases in aquaria and in nature. J. Mar. Biol. Ass. 92, 791–801. doi: 10.1017/S0025315411001688
Thompson J. R., Rivera H. E., Closek C. J., Medina M. (2015). Microbes in the coral holobiont: partners through evolution, development, and ecological interactions. Front. Cell Infect. Microbiol. 4. doi: 10.3389/fcimb.2014.00176
Tomova A., Ivanova L., Buschmann A. H., Rioseco M. L., Kalsi R. K., Godfrey H. P., et al. (2015). Antimicrobial resistance genes in marine bacteria and human uropathogenic Escherichia coli from a region of intensive aquaculture. Environ. Microbiol. Rep. 7, 803–809. doi: 10.1111/1758-2229.12327
Ushijima B., Gunasekera S. P., Meyer J. L., Tittl J., Pitts K. A., Thompson S., et al. (2023). Chemical and genomic characterization of a potential probiotic treatment for stony coral tissue loss disease. Commun. Biol. 6, 1–13. doi: 10.1038/s42003-023-04590-y
van Oppen M. J. H., Blackall L. L. (2019). Coral microbiome dynamics, functions and design in a changing world. Nat. Rev. Microbiol. 17, 557–567. doi: 10.1038/s41579-019-0223-4
Vijay K., Kiran G. S., Divya S., Thangavel K., Thangavelu S., Dhandapani R., et al. (2021). Fatty acid methyl esters from the coral-associated bacterium Pseudomonas aeruginosa inhibit virulence and biofilm phenotypes in multidrug resistant Staphylococcus aureus: An in vitro approach. Front. Microbiol. 12. doi: 10.3389/fmicb.2021.631853
Voolstra C. R., Ziegler M. (2020). Adapting with microbial help: microbiome flexibility facilitates rapid responses to environmental change. BioEssays 42, 2000004. doi: 10.1002/bies.202000004
Walton C. J., Hayes N. K., Gilliam D. S. (2018). Impacts of a regional, multi-year, multi-species coral disease outbreak in Southeast Florida. Front. Mar. Sci. 0. doi: 10.3389/fmars.2018.00323
West A. G., Waite D. W., Deines P., Bourne D. G., Digby A., McKenzie V. J., et al. (2019). The microbiome in threatened species conservation. Biol. Conserv. 229, 85–98. doi: 10.1016/j.biocon.2018.11.016
Williams D., Miller M. (2005). Coral disease outbreak: Pattern, prevalence and transmission in Acropora cervicornis. Mar. Ecology-progress Ser. - Mar. ECOL-PROGR Ser. 301, 119–128. doi: 10.3354/meps301119
Williams D. E., Miller M. W. (2012). Attributing mortality among drivers of population decline in Acropora palmata in the Florida Keys (USA). Coral Reefs 31, 369–382. doi: 10.1007/s00338-011-0847-y
Yoon J., Oku N., Kasai H. (2016). Ascidiimonas aurantiaca gen. nov., sp. nov., a member of Flavobacteriaceae isolated from a sea squirt. Antonie van Leeuwenhoek 109, 501–508. doi: 10.1007/s10482-016-0655-z
Zaneveld J. R., Burkepile D. E., Shantz A. A., Pritchard C. E., McMinds R., Payet J. P., et al. (2016). Overfishing and nutrient pollution interact with temperature to disrupt coral reefs down to microbial scales. Nat. Commun. 7, 11833. doi: 10.1038/ncomms11833
Zaneveld J. R., McMinds R., Thurber R. V. (2017). Stress and stability: applying the Anna Karenina principle to animal microbiomes. Nat. Microbiol. 2, 17121. doi: 10.1038/nmicrobiol.2017.121
Keywords: aquaculture, coral, microbiome, antiseptic, coral disease, restoration
Citation: Klinges JG, Craig ZW, Villoch Diaz-Mauriño M, Merck DE, Brooks SN, Manfroy AA and Clark AS (2023) Common aquarium antiseptics do not cause long-term shifts in coral microbiota but may impact coral growth rates. Front. Mar. Sci. 10:1281691. doi: 10.3389/fmars.2023.1281691
Received: 22 August 2023; Accepted: 17 October 2023;
Published: 01 November 2023.
Edited by:
Kshitij Tandon, The University of Melbourne, AustraliaReviewed by:
Ashley M. Dungan, The University of Melbourne, AustraliaCopyright © 2023 Klinges, Craig, Villoch Diaz-Mauriño, Merck, Brooks, Manfroy and Clark. This is an open-access article distributed under the terms of the Creative Commons Attribution License (CC BY). The use, distribution or reproduction in other forums is permitted, provided the original author(s) and the copyright owner(s) are credited and that the original publication in this journal is cited, in accordance with accepted academic practice. No use, distribution or reproduction is permitted which does not comply with these terms.
*Correspondence: J. Grace Klinges, Z2tsaW5nZXNAbW90ZS5vcmc=
Disclaimer: All claims expressed in this article are solely those of the authors and do not necessarily represent those of their affiliated organizations, or those of the publisher, the editors and the reviewers. Any product that may be evaluated in this article or claim that may be made by its manufacturer is not guaranteed or endorsed by the publisher.
Research integrity at Frontiers
Learn more about the work of our research integrity team to safeguard the quality of each article we publish.