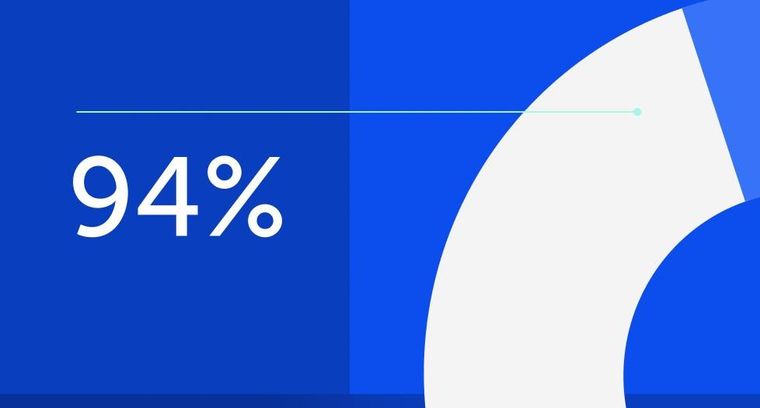
94% of researchers rate our articles as excellent or good
Learn more about the work of our research integrity team to safeguard the quality of each article we publish.
Find out more
ORIGINAL RESEARCH article
Front. Mar. Sci., 25 October 2023
Sec. Coral Reef Research
Volume 10 - 2023 | https://doi.org/10.3389/fmars.2023.1276400
This article is part of the Research TopicStony Coral Tissue Loss Disease in the CaribbeanView all 50 articles
Outbreaks of coral disease have been a dominant force shaping western Atlantic coral-reef assemblages since the late 1970s. Stony coral tissue loss disease (SCTLD) is nonetheless having an unprecedented impact in the region. Whereas numerous studies over the last decade have worked to characterize this novel pathogen and its impacts on coral populations, few have quantified its functional effects on reef ecosystems. Of particular importance is how SCTLD may be impacting the essential reef-accretion process and the myriad ecosystem services it supports. Here, we evaluated the impact of SCTLD on reef-accretion potential by estimating carbonate budgets and taxon-level carbonate production at 43 sites throughout the Florida Keys from 2016−2022. Average regional reef-accretion potential declined from an already low, but positive rate of 0.30 ± 0.16 mm y-1 (mean ± standard error) in 2016 before the disease was first observed, to a state of accretionary stasis (0.08 ± 0.12 mm y-1) by 2022. This 70% relative decline in reef-accretion potential was driven by the loss of reef-building corals, with significant decreases in carbonate production by massive taxa including Colpophyllia natans, Montastraea cavernosa, Pseudodiploria strigosa, Orbicella spp., and Siderastrea siderea, and increasing contributions from less susceptible, weedy taxa including Millepora spp., Agaricia spp., and Porites astreoides. In general, changes in taxon-level carbonate production following the SCTLD outbreak mirror long-term shifts in reef assemblages in response to previous stressors. One striking exception, however, is S. siderea, which had become increasingly dominant in recent decades, but declined significantly in response to SCTLD. Overall, by further decimating the already depauperate reef-building coral populations in the Florida Keys, SCTLD has caused a functionally significant shift in the composition of Florida’s coral-reef assemblages and accelerated the loss of regional reef-building capacity. The dire impacts of the disease in south Florida may serve as an early warning that the persistence of the invaluable ecological and socioeconomic functions coral reefs provide will be increasingly threatened throughout the western Atlantic in the aftermath of SCTLD.
Coral disease has played a central role in the decline of western Atlantic coral reefs over the last half a century. Beginning with the devastating impacts of white-band disease on acroporid coral populations in the late 1970s and early 1980s (Aronson and Precht, 2001), coral diseases have contributed to an unprecedented loss of foundational, reef-building species and the emergence of novel reef assemblages (Aronson and Precht, 2001; Aronson et al., 2004; Kuffner and Toth, 2016; Toth et al., 2019). The impact of coral diseases has accelerated in recent decades as rising ocean temperatures and other anthropogenic impacts (e.g., nutrients; Bruno et al., 2003) have driven increases in disease prevalence and virulence (Bruno et al., 2007; Brandt and McManus, 2009; Miller et al., 2009; Muller and van Woesik, 2012; Randall and van Woesik, 2015).
Despite the clear cumulative impact of disease as a driving force of western Atlantic reef ecology, significant disease outbreaks have historically affected only a handful of coral species and/or were limited to geographical or seasonal hotspots (Aronson and Precht, 2001; Muller and van Woesik, 2012; van Woesik and Randall, 2017). The novel epidemic known as stony coral tissue loss disease (SCTLD) has fundamentally overturned that paradigm. Because of its low host specificity (>20 susceptible species; DEP, 2018; Gintert et al., 2019; Muller et al., 2020), its rapid spread and high mortality rate, and its persistence across seasonal cycles, SCTLD is considered by some to be the most lethal coral disease on record (Precht et al., 2016; Walton et al., 2018; Alvarez-Filip et al., 2022).
SCLTD was first observed in southeast Florida in 2014 (Precht et al., 2016; Walton et al., 2018) and it has since spread to most countries/territories throughout the Caribbean (Kramer et al., 2019; Rosenau et al., 2021). In south Florida, its regional-scale expansion (Figure 1) has been linked to waterborne transport by bottom currents (Dobbelaere et al., 2020; Muller et al., 2020); however, more spatially random outbreaks of SCTLD throughout the Caribbean (Kramer et al., 2019), suggest that other vectors may also be important (e.g., ballast water, biofilms, etc.; Dahlgren et al., 2021; Rosenau et al., 2021; Evans et al., 2022; Studivan et al., 2022). Despite nearly a decade of research, the proximal causal agent(s) of SCLTD also remain unclear. The observation that antibiotics can slow progression of the disease (Aeby et al., 2019; Neely et al., 2020) and the relative enrichment of certain bacterial groups in affected tissue (Meyer et al., 2019; Landsberg et al., 2020) initially pointed to a bacterial cause; however, recent studies indicate that SCTLD primarily affects corals’ Symbiodiniaceae endosymbionts and may be viral in origin, with secondary bacterial infections of the coral host occurring opportunistically after initial infection (Landsberg et al., 2020; Work et al., 2021; Beavers et al., 2023).
Figure 1 Map showing the location of the Coral Reef Evaluation and Monitoring Program (CREMP) reef sites evaluated in this study. Colors indicate which CREMP survey year stony coral tissue loss disease (SCTLD) was first observed, demonstrating the clear temporal trends in the geographical spread of the disease. Map image is the intellectual property of Esri and is used herein under license. Copyright 2020 Esri and its licensors. All rights reserved.
Although important questions clearly remain about the origin, underlying etiology, and vectors spreading SCTLD, there is no question that it is having an unprecedented impact on western Atlantic coral assemblages. For example, the epidemic caused a ~30% decline in the density of coral colonies (individuals m-2) and a 60% decline in living coral tissue in southeast Florida from 2012–2016 (Walton et al., 2018). The effects on highly susceptible species have been especially severe, with corals in the families Meandrinidae (maze corals) and subfamily Faviinae (brain corals) frequently experiencing mortality rates of more than 50% (Walton et al., 2018; Gintert et al., 2019; Brandt et al., 2021; Alvarez-Filip et al., 2022). For the already rare coral Dendrogyra cylindrus (Modys et al., 2023), SCTLD has caused population declines upwards of 95% (Neely et al., 2021; Alvarez-Filip et al., 2022). As a result, D. cylindrus is now considered functionally extinct in south Florida (Neely et al., 2021).
Because of the disproportionate impact of SCTLD on massive, reef-building species, the disease is poised to accelerate the ongoing loss of key reef functions throughout the western Atlantic (Estrada-Saldívar et al., 2020). For example, in the Mexican Caribbean, SCTLD impacts from 2018–2020 had already caused a 30% decline in carbonate production (Alvarez-Filip et al., 2022): a change that has the potential to disrupt the dynamic balance between the processes of reef accretion and reef erosion (Perry et al., 2013). Reef accretion underpins the persistence of essential ecological and socioeconomic functions by creating complex habitat that supports reefs’ unparalleled marine biodiversity, fisheries production, shoreline protection, and tourism (Ferrario et al., 2014; Kuffner and Toth, 2016; Perry and Alvarez-Filip, 2019; Woodhead et al., 2019; Toth et al., 2023); however, coral disease and coral-bleaching events have caused significant losses of the most important western Atlantic reefs-building taxa, Acropora and Orbicella spp., which have driven regional-scale declines in carbonate production and reef-accretion capacity (Perry et al., 2013; Perry et al., 2015; Perry et al., 2018; Toth et al., 2019). As a result, reef erosion, rather than reef accretion was already the dominant process for an increasing number of western Atlantic reefs (Perry et al., 2013; Perry et al., 2018)—including ~30% of reefs in south Florida (Morris et al., 2022; Toth et al., 2022; also see Yates et al., 2017)—prior to the outbreak of SCTLD. The shift toward reef erosion is already degrading important habitat for fish and other reef-associated species (Alvarez-Filip et al., 2009; Pratchett et al., 2014) and has the potential to more than double coastal-flooding risk globally by the end of the century (Beck et al., 2018). With many western Atlantic reefs already balanced at a tipping point between accretion and erosion, the recent impacts of SCTLD portend grim consequences for the future of regional reef building and the critical ecosystem services it supports.
Since SCTLD was first observed in south Florida in 2014, it has gradually spread north and south along the Florida reef tract, through the Florida Keys (Muller et al., 2020), and reached the Dry Tortugas National Park in 2021 (Figure 1; Dobbelaere et al., 2022). Despite impacting Florida’s coral reefs for nearly a decade, however, the regional-scale effects of SCTLD on reef composition and function have yet to be comprehensively assessed. Given the already degraded state of Florida’s reefs (Ruzicka et al., 2013; Morris et al., 2022; Toth et al., 2022), the recent impacts of SCTLD could have significant implications for the ability of carbonate production to continue to support reef accretion and the essential ecosystem functions it supports. Indeed, Toth et al. (2022) showed that SCTLD had already caused a significant decline in net carbonate production in the Upper and Middle Keys between 2017 and 2019. In this study, we build upon those findings to quantify the functional impacts of SCTLD from 2016−2022 on overall and species-level contributions to regional carbonate production and reef-accretion potential across the entire Florida Keys reef tract.
The Coral Reef Evaluation and Monitoring Project (CREMP) has conducted annual benthic surveys of reefs throughout the Florida Keys since 1996 (Ruzicka et al., 2013). The sites are spread across three primary habitats (inshore-patch [1.8–12.8 m], offshore-shallow [1.8–7.3 m], and offshore-deep reefs [10.7–16.5 m]) and four geographic subregions (Upper, Middle, and Lower Keys and the Dry Tortugas) of the Florida Keys Reef Tract. Site descriptions and additional details about the CREMP surveys can be found at https://myfwc.com/research/habitat/coral/cremp/ and in Toth et al. (2022).
Carbonate-budget models provide a powerful means of translating reef-survey data on reef state, like those collected by CREMP, into quantitative estimates of the processes that determine the balance between reef accretion and erosion (Perry et al., 2012). Toth et al. (2022) used the CREMP data to calculate carbonate budgets for 46 reefs throughout the Florida Keys (n=38) and the Dry Tortugas National Park (n=8) from 1996−2019. In this study, we provide an updated carbonate-budget dataset that includes CREMP-survey data for those sites from 2020−2022. We use those data to quantify the impacts of SCTLD on gross and net carbonate production, bioerosion, and reef-accretion potential as well as species-level contributions to carbonate production throughout its endemic period in the region (2017–2022; Figure 1). The carbonate budgets were calculated in R Studio (R Core Team, 2023; Toth and Courtney, 2022) using the method proposed by Toth et al. (2022), which is an adaptation of the ReefBudget v2 methodology (Perry and Lange, 2019). All data, calculations, and necessary modifications to the ReefBudget v2 methodology are discussed in detail in Toth et al. (2022) and data and code for new analyses included in this study are provided in version 1.1 of the USGS software release by Toth and Courtney (2022).
Briefly, we calculated gross carbonate production (kg CaCO3 m-2 y-1) as the summed product of species-level coral and crustose coralline algae cover from CREMP and the area-normalized taxon-specific calcification rates proposed by Courtney et al. (2021). Site-level mean (± standard error [SE]) microbioerosion was estimated as the product of the recorded cover of consolidated substrate at each CREMP survey location across all years from 1996−2022 and the generalized western Atlantic microbioerosion rate of 0.24 kg CaCO3 m-2 y-1 suggested by Perry and Lange (2019). Site-level mean (± SE) bioerosion by the sponges Cliothosa delitrix, Cliona caribbaea, Cliona varians, and Pione lampa was calculated as the product of the mean (± SE) surface area of sponges at each site, which was recorded by CREMP from 2005–2009, and species-specific bioerosion rates (or, for P. lampa, the generalized sponge bioerosion rate) suggested by Perry and Lange (2019). Annual site-level bioerosion by the urchin Diadema antillarum was estimated as the product of their annual recorded densities (individuals m-2) by CREMP and a generalized D. antillarum bioerosion rate calculated based on the method suggested by Perry and Lange (2019) and an empirical distribution of D. antillarum test sizes in the Florida Keys from another study (Chiappone et al., 2008). No data were available from CREMP for the other western Atlantic bioeroding urchins (Echinometra lucunter, Echinometra viridis, and Eucidaris tribuloides) or the bioeroding sponge Siphonodictyon spp., so bioerosion by these taxa were not included in our carbonate budgets. CREMP also does not monitor parrotfish abundance, so we derived those data from sites surveyed by the National Oceanic and Atmospheric Administration’s Reef Visual Census program (https://grunt.sefsc.noaa.gov/rvc_analysis20/) that were within 10 km and in similar habitats as each CREMP site (see Toth and Courtney, 2022). We estimated parrotfish bioerosion for each CREMP site by combining parrotfish size-frequency data with size- and species-specific parrotfish bioerosion rates suggested by Perry and Lange (2019; see Toth et al. [2022] for details). Gross carbonate production and bioerosion were combined to estimate net carbonate production (kg CaCO3 m-2 y-1; mean ± SE) at each site for each year, which was converted to estimates of reef-accretion potential (cf. Perry et al., 2018) using the equation suggested by Kinsey (1985) and regional reef-framework porosity estimates from Toth et al. (2018a). We emphasize that reef-accretion potential likely represents a high-end estimate of realized accretion rates because it does not incorporate non-biological physical erosion or chemical dissolution, which Toth et al. (2022) estimated likely contributes at least an additional ~1 mm y-1 of erosion in the Florida Keys. Furthermore, the omission of some bioeroding taxa from our surveys, the possibility that sponge abundance has increased since they were last surveyed by CREMP in 2009, and the lack of co-located parrotfish data, suggest that our estimates of total bioerosion may be conservatively low and that reef-accretion potential is likely lower than our study indicates.
All statistical analyses for the present study were conducted in RStudio version 2023.03.0 (R Core Team, 2023). We first evaluated the overall, regional change in reef-accretion potential over time from one year before (2016) through each year of the SCTLD endemic period (2017−2022) using a linear-mixed effects model [LME; nlme package (Pinheiro et al., 2023)] with year as a fixed effect and random slopes and intercepts by site. Next, we used data on SCTLD occurrences from CREMP to identify the first year that the disease was detected at each site (Figure 1; Supplementary Figure S1) and create a categorical variable representing time since SCTLD detection (before, year of detection, and 1−5 years after detection; hereafter “SCTLD impact”). We note that the first detection of the disease in the CREMP surveys, which are conducted only once a year in the summer to early fall, likely does not represent the exact timing of the disease first impacting a particular site; however, it provides the best available approximation of the first year disease impact was recorded for the specific reefs assessed in our study. Three sites in the Dry Tortugas where SCTLD has not yet been observed (Loggerhead Patch and White Shoal) or was only observed in the most recent survey year (2022; Davis Rock), were excluded from the statistical analysis (but see Supplementary Figure S1). We used LMEs to evaluate how reef-accretion potential, gross carbonate production, and bioerosion changed based on SCTLD impact. SCTLD impact was treated as a fixed effect with random slopes and intercepts by site. We also used an LME to evaluate the interaction between the fixed effects of calcifier cover (coral cover + crustose coralline algae) and SCTLD impact to test whether SCTLD shifted the calcifying community towards taxa with faster (significant positive interaction) or slower (significant negative interaction) calcification rates.
Before running the LME models, we confirmed that there was no significant spatial autocorrelation in the carbonate-budget data by evaluating the correlation between distance matrices constructed from the latitude and longitude of the sites and the budget parameters using the ‘mantel’ function in the vegan package (Oksanen et al., 2020). We also evaluated changes in the fit of the models with six moving-average temporal autocorrelation structures (p and q = 0−2) using the ‘corARMA’ function in the nlme package. For the model evaluating reef-accretion potential over time, the autocorrelation structure of p=2 and q=0 provided the best fit based on Akaike’s Information Criterion (AIC); however, for all of the LMEs with SCTLD impact as a fixed effect, the models without a correlation structure had the lowest AIC. Evaluation of the models with the ‘acf’ function also did not show evidence of substantial temporal lags in the carbonate-budget data. Results of the autocorrelation analyses are available in Toth and Courtney (2022) (v.1.1).
To further evaluate the effects of SCTLD on carbonate production, we used LMEs to identify whether and how the absolute and relative (to total carbonate production at each site in each year) carbonate production by individual coral taxa changed following SCTLD detection. Based on the results of the main budget models, we made the simplifying assumption that the taxon-level data also did not have any significant spatial or temporal autocorrelation. The models included SCTLD impact as a fixed effect and site as a random intercept only, as inclusion of site as a random slope resulted in problems with model convergence due to overfitting. We also ran LME models evaluating the contributions of coral taxa to carbonate production within habitats and subregions, with SCTLD impact as a fixed effect and site as a random intercept. We summarized the fixed effects of the LMEs using the ‘anova’ function and evaluated pairwise differences among factor levels using the Tukey method in the package emmeans (Lenth, 2023). Finally, to investigate the impact of pre-SCTLD reef state on the response of reef assemblages, we ran linear regressions of the cover of coral taxa pre-SCTLD vs. the change in the cover of those taxa 5 years after SCTLD detection. We summarize the significant results of the statistical analysis below and the complete model outputs are available in Toth and Courtney (2022); (v.1.1).
Reef-accretion potential declined by >70% across the Florida Keys (N=43 sites) during the SCTLD endemic period, from 0.30 mm y-1 ± 0.16 (mean ± SE) in 2016, before SCTLD was first observed at the CREMP sites, to 0.08 mm y-1 ± 0.12 in 2022 (Figure 2A; Supplementary Table S1; LMEyear: F1,257 = 10.65, p=0.001). Because mean reef-accretion potential in 2022 cannot be statistically distinguished from zero (i.e., overlapping 95% confidence interval), the reefs of the Florida Keys can be considered to have entered accretionary stasis (or a net neutral carbonate budget). This loss of reef-accretion capacity was driven by a significant decline in gross carbonate production beginning one year after SCTLD detection at a given site (Figure 2B; LMESCTLD Impact=F6,195=4.56, p<0.001; Tukey test before SCTLD vs. 1−5 years after: p<0.05), with average gross carbonate production declining ~32% between 2016 and 2022, from 0.97 ( ± 0.16) kg CaCO3 m-2 y-1 to 0.66 ( ± 0.11) kg CaCO3 m-2 y-1, respectively. The temporal impact of SCTLD on reef-accretion potential was only statistically detectable one year after SCTLD was observed at the sites (Figure 2B; LMESCTLD Impact=F6,195=5.16, p<0.001; Tukey test before SCTLD vs. 1 year after: p=0.02), likely due to the significant decrease in total bioerosion 2, 3, and 4 years after SCTLD detection (Figure 2; LMESCTLD Impact=F6,195=1239, p<0.001).
Figure 2 The impacts of SCTLD on carbonate budgets across the Florida Keys Reef Tract. (A) Mean ( ± 95% confidence intervals [CIs]) changes in reef-accretion potential/net carbonate production, gross carbonate production, and bioerosion over the endemic period from 2016 to 2022. (B) Effects of SCTLD on reef-accretion potential, gross carbonate production, bioerosion, and the relationship between calcifier cover and gross carbonate production (“gross production~calcifier cover”) following SCTLD impact. Points represent estimated slope from the linear mixed-effect models and bars represent 95% CIs based standard errors of the models. Effects are significant when the 95% CIs do not overlap with zero (red vertical line). Significant negative effects for reef-accretion potential and gross carbonate production indicate that those processes have declined in response to SCLTD. The significant positive effects for bioerosion reflect a decline in bioerosion during some years following SCTLD. For gross production~calcifier cover, the significant negative interaction indicates that the calcifying assemblage shifted to taxa with slower calcification rates following the SCTLD outbreak.
As expected, coral cover was a significant driver of gross carbonate production (LMECoral Cover=F1,188=131.98, p<0.001); however, there was also a significant interaction effect between SCTLD impact and calcifier cover on gross carbonate production (LMESCTLD Impact*Calcifier Cover=F6,188=14.45, p<0.001), with the slope of the relationship between coral cover and gross production decreasing significantly following SCTLD detection (Figure 2B). This reflects a significant shift in calcifying assemblages towards more slowly calcifying taxa (i.e., Millepora alcicornis, Porites astreoides, and the Agaricia agaricites complex) following SCTLD, as described below (Figures 3, 4).
Figure 3 Linear relationships between pre-SCTLD percent (%) cover of coral taxa vs. the relative change in the % cover of those taxa 5 years after SCTLD was detected. Taxa are considered to have experienced a significant positive (negative) change in % cover if the 95% confidence interval (CI) of the % change in cover is above (below) zero. Taxa are categorized as “no change” if their 95% CI overlaps with zero. No linear relationship is shown for taxa that experienced no change. Only taxa with mean pre-SCTLD coral cover >0.1% are labelled.
Figure 4 Effects of SCTLD on the (A) absolute and (B) relative contribution of calcifying taxa to carbonate production in the years following SCTLD impact. Points represent estimated slope from the linear mixed-effect models and bars represent 95% confidence intervals based on standard errors of the models. Positive effects represent an increased contribution to carbonate production following SCTLD and are plotted to the right of the vertical red line (no effect) whereas negative effects represent a decreased contribution to carbonate production following SCTLD and are plotted to the left of the vertical red line. Only taxa whose carbonate production was significantly impacted by SCTLD are shown. Effects for taxa shown in gray were not significant for that response variable.
The most pronounced changes in coral cover following SCTLD impact were observed for taxa that had relatively high coral cover before the outbreak (Figure 3). Likewise, the sites that experienced the most severe declines in gross carbonate production and reef-accretion potential following SCTLD were generally those that had the highest pre-SCTLD carbonate production rates (Supplementary Table S1, Figure 1). The impact of SCTLD was particularly severe in patch-reef habitats (Supplementary Figure S2), where gross carbonate production declined 74%, from an average of 1.42 (± 0.26) kg CaCO3 m-2 y-1 before SCTLD to 0.37 (± 0.09) kg CaCO3 m-2 y-1 5-years after the disease was first detected, compared with the smaller absolute declines from 0.62 (± 0.19) to 0.38 (± 0.15) kg CaCO3 m-2 y-1 and 0.34 (± 0.08) to 0.18 (± 0.06) kg CaCO3 m-2 y-1, in offshore shallow and deep habitats, respectively. Larger effects of SCTLD were also observed in the Lower Keys where the disease has been present the longest (Supplementary Figure S3). One interesting exception to the generally declining carbonate budgets following the SCTLD outbreak is Jaap Reef, a patch reef in the Lower Keys where carbonate production and reef-accretion potential actually increased over the endemic period (Supplementary Figure S1A). SCTLD has never been observed at Jaap Reef by CREMP researchers, although its impact on surrounding reefs suggest that it likely has been present there.
Shifts in coral species composition resulted in significant changes in the absolute and relative contributions of numerous taxa to carbonate production following the SCLTD outbreak (Figure 4). The most substantial declines in absolute production were found for Colpophyllia natans, Montastraea cavernosa, Orbicella spp., Pseudodiploria strigosa, and Siderastrea siderea (Figure 4A); however, only C. natans, M. cavernosa, and Ps. strigosa also experienced significant declines in their relative contributions to carbonate production (Figure 4B). The largest declines in the most susceptible species, C. natans, M. cavernosa, and Ps. strigosa occurred in patch-reef habitats, where the populations of those taxa were the highest before the outbreak (Supplementary Figure S4). For Orbicella spp. and S. siderea, the most consistent declines in carbonate production occurred in the offshore habitats of the Upper Keys (Supplementary Figures S4, S5), where SCTLD has been affecting reefs the longest (Figure 1). In contrast to the declining trends in many coral species, the contributions of Millepora alcicornis, Porites astreoides, and the Agaricia agaricites complex increased significantly following SCTLD impact (Figure 4). The increase in M. alcicornis and P. astreoides was only significant in Lower Keys patch-reef habitats, whereas the increase in A. agaricites was fairly ubiquitous (Supplementary Figures S4, S5).
The unprecedented impacts of SCTLD on coral populations in the Florida Keys have exacerbated the already significant decline in regional carbonate production and reef-accretion potential that occurred over the last several decades (Toth et al., 2022). Previous studies suggested that by the beginning of the disease outbreak, reef-accretion potential in the Florida Keys had already declined by an order of magnitude from historic baselines and ~30% of Florida’s coral reefs were already eroding faster than they were growing (Morris et al., 2022; Toth et al., 2022). Our study indicates that SCTLD resulted in an additional >70% relative decline (0.22 mm y-1 absolute decline) in reef-accretion potential and a shift to accretionary stasis throughout the Florida Keys (Figure 2). Furthermore, by 2022, more than two-thirds of reefs in the Florida Keys were net erosional on average and, if the potential additional ~1 mm yr-1 contribution of non-biological erosion estimated by Toth et al. (2022) is considered, the actual percentage of eroding reefs could now be as high as 93%.
The decline in reef-accretion potential in the Florida Keys following the outbreak of SCTLD was driven by overall reductions in coral cover coupled with a significant shift in the relative contributions of coral taxa towards more slowly calcifying species (Figures 2−4). Like most of the western Atlantic, the reefs of the Florida Keys were geologically and historically dominated by just a handful of taxa: Acropora palmata in the shallowest depths and Orbicella spp. with moderate contributions from other long-lived massive coral taxa in deeper reef zones (Precht and Miller, 2007; Toth et al., 2019). Whereas populations of A. palmata have been severely diminished for decades due to the devastating impacts of white-band disease beginning in the late 1970s (Aronson and Precht, 2001; Precht and Miller, 2007), regional declines of massive reef-building corals began more recently. The percent cover of Orbicella spp. and its contribution to carbonate production declined significantly throughout the Florida Keys over the last two decades as a result of thermal stress and disease (Ruzicka et al., 2013; Toth et al., 2014; Courtney et al., 2020; Toth et al., 2022), and SCTLD reinforced that trend (Figures 3, 4). Regional populations of other massive coral taxa including C. natans, Ps. strigosa, and M. cavernosa had remained relatively stable in the decades prior to SCTLD, particularly in patch-reef habitats (Ruzicka et al., 2013; Toth et al., 2022; but see Courtney et al. (2020) who detected declines in carbonate production by M. cavernosa); however, those taxa experienced significant declines in the Florida Keys following the SCTLD outbreak, with the largest effects in the patch-reef habitats where they were historically most abundant (Figure 3; Supplementary Figure S4). This result underscores the high susceptibility of C. natans and Ps. strigosa to SCTLD (DEP, 2018) and supports previous observations of high disease prevalence for M. cavernosa in south Florida (Walton et al., 2018; Muller et al., 2020), despite its putatively lower suceptibility (DEP, 2018). Although these taxa were historically minor contributors to reef building relative to Orbicella spp., their loss nonetheless contributed to the regional decline in reef-accretion potential due to SCTLD (Figure 4).
By accelerating declines in massive, reef-building species, SCTLD has also reinforced the trend of increasing relative dominance by more weedy coral taxa (Darling et al., 2012) throughout the western Atlantic (Perry et al., 2015; Courtney et al., 2020; Estrada-Saldívar et al., 2020; Alvarez-Filip et al., 2022). In the Florida Keys, M. alcicornis, P. astreoides, and the A. agaricites complex all increased their contribution to carbonate production following the SCTLD outbreak (Figure 4). Because these corals are relatively unsusceptible to SCTLD (DEP, 2018; but see Brandt et al. (2021) for potential impacts on Agaricia spp.) they have maintained, or in the case of M. alcicornis, even increased percent cover in some locations, as more vulnerable taxa have declined (Figure 3). Increases in the relative contributions of both P. astreoides (Toth et al., 2019; Courtney et al., 2020; Gallery et al., 2021) and Millepora spp. (Toth et al., 2014; Courtney et al., 2020) have been previously documented in the Florida Keys, and all three taxa have become more common throughout the western Atlantic as thermal stress events and disease have decimated reef-building coral populations in recent decades (Aronson et al., 2004; Green et al., 2008; Alvarez-Filip et al., 2022). Although these weedy corals have been relatively successful in the short term, they were rare in the fossil record from the Florida Keys (Toth et al., 2019), and their relatively small size, short lifespans, and low rates of carbonate production (Courtney et al., 2021) make them a poor functional substitute for the reef-building species they have replaced (Alvarez-Filip et al., 2013). Their increasing contribution on Florida’s reefs is therefore unlikely to significantly mitigate the ongoing decline in regional reef-accretion potential.
Overall, SCTLD has accelerated decadal-scale shifts in the composition of western Atlantic reef assemblages in response to climate change and other anthropogenic stressors (Perry et al., 2015; Toth et al., 2019; Courtney et al., 2020; Alvarez-Filip et al., 2022). One striking exception to this pattern, however, is S. siderea. In recent decades, the relative abundance of this stress-tolerant coral (Darling et al., 2012) increased significantly throughout the Florida Keys (Ruzicka et al., 2013; Toth et al., 2014; Toth et al., 2019; Courtney et al., 2020). Although S. siderea was not an important regional contributor to reef accretion over geologic or historical timescales (Toth et al., 2019), in the years preceding the outbreak of SCTLD, its relative contribution to carbonate production in the Florida Keys had increased to a level similar to that of Orbicella spp. (Courtney et al., 2020; Toth et al., 2022). In a reversal of this long-term trend, however, the cover of S. siderea and its contribution to carbonate production declined significantly following the outbreak of SCTLD (Figures 3, 4). Although some researchers have raised questions about whether S. siderea is truly susceptible to SCTLD given differences in its disease epidemiology (DEP, 2018) and its low disease prevalence in some locations (e.g., Precht et al., 2016; Brandt et al., 2021), the unprecedented decline in S. siderea following the outbreak of SCTLD in the Florida Keys indicates that this species is being directly impacted by the disease (Walton et al., 2018; Estrada-Saldívar et al., 2020; Muller et al., 2020; Alvarez-Filip et al., 2022).
Despite declines in absolute carbonate production by both S. siderea and Orbicella spp., their relative contribution to carbonate production did not change following the SCTLD outbreak (Figure 4B) and they remain the dominant calcifiers in the region. This somewhat contradictory result reflects the fact that SCTLD reduced carbonate production by all major reef-building corals (Figure 3). In other words, S. siderea and Orbicella spp. were not disproportionately impacted by SCTLD relative to the other major reef-building taxa (Figure 4). Additionally, whereas highly susceptible taxa like C. natans experience rapid mortality from SCTLD (DEP, 2018), SCTLD impacts on S. siderea and Orbicella spp. are often more protracted because of their relatively large colony size and slower rates of disease progression (Meiling et al., 2020; Brandt et al., 2021). Indeed, when our results were parsed spatially, we found that SCTLD has only consistently affected carbonate production by S. siderea and Orbicella spp. on offshore reefs in the Upper Florida Keys (Supplementary Figures S4, 5), where the disease was observed earliest (Figure 1). This suggests that the regional impacts of SCTLD on S. siderea and Orbicella spp. populations are likely ongoing. The more gradual impacts of SCTLD on Orbicella spp. in particular may also help explain why Jaap Reef in the Lower Keys, which is composed almost entirely of Orbicella spp. corals (Ruzicka et al., 2013; Toth and Courtney, 2022), has apparently been unaffected by the disease so far (Supplementary Figure S1A). As the disease continues at endemic levels throughout the region, declines in carbonate production by S. siderea and Orbicella spp. will likely continue, which would further accelerate the decline in regional reef-accretion potential.
The ~30% relative change in gross carbonate production following the SCTLD outbreak in the Florida Keys was almost identical to that observed following the first few years of the outbreak in the Mexican Caribbean (Alvarez-Filip et al., 2022); however, because of the already degraded state of Florida’s reefs (Morris et al., 2022; Toth et al., 2022), the absolute impact of SCTLD on carbonate production in the Florida Keys was likely less severe than in many other western Atlantic locations (Estrada-Saldívar et al., 2020; Brandt et al., 2021; Alvarez-Filip et al., 2022), or those observed on less degraded reefs globally in response to recent coral bleaching events (e.g., Januchowski-Hartley et al., 2017; Lange and Perry, 2019; Courtney et al., 2022). Furthermore, whereas SCTLD resulted in a shift from marginally positive reef-accretion potential to accretionary stasis in the Florida Keys, the higher pre-SCTLD coral cover and the presence of remnant acroporid populations on many other western Atlantic reefs, may allow them to maintain or more rapidly recover positive reef-accretion potential despite significant SCTLD-driven declines of other functionally important taxa (Estrada-Saldívar et al., 2020; Alvarez-Filip et al., 2022). In south Florida, historic losses of key reef-building species (Toth et al., 2019; Toth et al., 2022), significant declines in coral cover (Ruzicka et al., 2013; Toth et al., 2014), and homogenization of remaining reef assemblages (Burman et al., 2012) left reefs more vulnerable to transitioning to net erosional states (Toth et al., 2018b).
Prior to the outbreak of SCTLD, the Florida Keys did still have a handful of relatively resilient reefs that had maintained higher coral cover and reef-accretion potential despite decades of decline elsewhere (i.e., patch-reef habitats in the Lower Keys; Supplementary Figure S1; Guest et al., 2018; Courtney et al., 2020; Elahi et al., 2022; Toth et al., 2022); however, these former “oases” (Guest et al., 2018) were far from immune to the impacts of SCTLD. In fact, the most profound effects of the disease on reef-accretion potential were observed at locations that had the highest pre-SCTLD coral cover and budgetary state (Figure 3; Supplementary Figures S1–S3). By decimating the remaining reef-building coral populations in the region, SCTLD is likely accelerating the ongoing erosion of functionally important reef structure (Yates et al., 2017; Kuffner et al., 2019). Indeed, whereas trends in gross carbonate production related to changes in coral cover were historically the most important drivers of reef-accretion potential in the Florida Keys (Toth et al., 2022), gross carbonate production is now negligible on most reefs (Figure 2; Supplementary Figure S1). As a result, changes in bioerosion, which in the Florida Keys is almost entirely driven by parrotfish and microbioeroders (Toth et al., 2022), are now having a larger proportional impact on the regional trajectories of reef-accretion potential (Figure 2A; Kuffner et al., 2019; Webb et al., 2023).
It’s important to note that other ongoing stressors were also likely impacting reefs in the Florida Keys during the SCTLD endemic period. In particular, although large-scale, regional coral bleaching did not occur in the region between 2017 and 2022, ecologically severe heat stress (>8 Degree Heating Weeks) was observed in 2019, 2020, and 2022 (NOAA Coral Reef Watch, 2023) and moderate bleaching was observed each summer (https://mote.org/research/program/coral-reef-science-monitoring/bleachwatch). Thermal stress and coral bleaching are known to make corals more vulnerable to disease and exacerbate coral-disease outbreaks (Bruno et al., 2007; Brandt and McManus, 2009; Miller et al., 2009; Muller and van Woesik, 2012; Randall and van Woesik, 2015). The coincidence of the initial SCTLD outbreak in southeast Florida with the onset of the most extreme thermal stress event (2014−2015) Florida’s reefs had experienced since the global mass bleaching event in 1997−1998 (Manzello, 2015; Precht et al., 2016), led some researchers to suggest that SCTLD is no exception to this principle (Precht et al., 2016); however, because SCTLD is now thought to primarily impact Symbiodiniaceae (Landsberg et al., 2020; Work et al., 2021; Beavers et al., 2023) and some researchers have observed reduced disease prevalence during the summer bleaching season (e.g., RRR & MAC [CREMP]), the exact relationship between SCLTD and thermal stress is somewhat unclear. We cannot eliminate the possibility that thermal stress played a role in the SCTLD-driven decline in coral populations in the Florida Keys since 2017, and changes in reef-accretion capacity will likely continue to be driven by the interaction of multiple disturbances into the future (Courtney et al., 2020; Cornwall et al., 2021; Alvarez-Filip et al., 2022; Toth et al., 2022). Nonetheless, the greatest absolute decline in carbonate production in our study consistently occurred in the first year after SCTLD detection (Figures 2, 4), suggesting our conclusions about the significant impacts of SCTLD are robust to other potential co-varying factors over the study period.
Reef accretion is essential to the ability of reefs to continue to provide coastal habitat supporting biodiversity, food production, and shoreline protection to communities around the world living on reef-lined coasts (Perry et al., 2018; Toth et al., 2018b; Toth et al., 2023). Ensuring these essential ecosystem services are maintained in a future increasingly characterized by the ongoing impacts of climate change is one of the most fundamental conservation challenges of our time, and it is one that will be particularly difficult in the western Atlantic following the devastating impacts of SCTLD. By accelerating losses of massive, reef-building corals, SCTLD is poised to exacerbate the trend of declining reef-building capacity potential to more than double coastal-flooding risk globally by the end of the century (Beck et al., 2018). With throughout the western Atlantic (Perry et al., 2015; Alvarez-Filip et al., 2022). In the Florida Keys, SCTLD has already driven a shift from net positive reef-accretion potential to accretionary stasis, and the effects on the dominant taxa responsible for carbonate production, Orbicella spp. and S. siderea, are likely ongoing in many parts of the region. This increasing shift to reef erosion is concerning both ecologically and economically as Florida’s reef habitats are home to a wide range of culturally and economically important fish species, contribute to a nearly $2 billion (USD) annual tourism industry, that supports ~20,000 jobs, and provide shoreline protection valued at >$600 million in coastal hazard risk reduction (Ault et al., 1998; Reguero et al., 2021; Gazal et al., 2022).
Local-scale coral restoration, in concert with global-scale action to mitigate climate change, has the potential to help revive reef accretion (Toth et al., 2022; Toth et al., 2023); however, our study underscores the importance of simultaneous efforts to manage the impacts of bioerosion (Kuffner and Toth, 2016), particularly on highly degraded reefs like those in south Florida. Whereas the efficacy of restoring important massive reef-building species could be limited in areas where the disease is still active, restoration of the non-suceptible historic reef-crest engineer, A. palmata, could have a substantial functional impact. Not only would restoration of this species have the greatest positive effect on reefs’ coastal protection function (Ferrario et al., 2014; Toth et al., 2023), but its capacity for rapid carbonate production could also allow A. palmata to return to its role as the dominant reef builder in the region (Alvarez-Filip et al., 2022; cf. Lange et al., 2023). Ultimately, however, the success of any restoration action will depend on whether the overwhelming effects of climate change can be mitigated (Toth et al., 2023), and this is likely particularly imperative for thermally sensitive taxa like A. palmata and Orbicella spp. (Precht and Miller, 2007). Perhaps one of the most important lessons that can be learned from the unprecedented impact of SCTLD on western Atlantic coral populations is that in a world increasingly characterized by environmental change, novelty is becoming the norm (Toth et al., 2019). Only by planning for the unexpected will reef management have the potential to ensure the persistence of reefs and the valuable ecological and socioeconomic functions they provide into the future.
Supporting data and the R script used to calculate and analyze the carbonate budgets in this study are provided in a U.S. Geological Survey software release available at https://doi.org/10.5066/P9APPZHJ.
The manuscript presents research on animals that do not require ethical approval for their study.
LT: Conceptualization, Formal Analysis, Methodology, Visualization, Writing – original draft. TC: Formal Analysis, Methodology, Writing – review & editing. MC: Data curation, Writing – review & editing. RR: Data curation, Project administration, Writing – review & editing.
The author(s) declare financial support was received for the research, authorship, and/or publication of this article. This research was funded by the U.S. Geological Survey, Coastal and Marine Hazards and Resources Program. CREMP is supported by the U.S. EPA Water Quality Protection Program award X7-00D39315-5 and the National Park Service.
We are grateful for the Coral Reef Evaluation and Monitoring Program and Rapid Visual Census (RVC) program for collecting the timeseries data used in this study. We thank Breanna Williams for extracting data from the RVC database for our sites in ArcGIS, Jeremiah Blondeau (NOAA) for helping with the interpretation of the RVC data, and Bryan McCloskey (USGS) for reviewing our R script. William F. Precht and the two journal reviewers provided valuable feedback on the manuscript. Any use of trade, firm, or product names is for descriptive purposes only and does not imply endorsement by the U.S. Government.
The authors declare that the research was conducted in the absence of any commercial or financial relationships that could be construed as a potential conflict of interest.
All claims expressed in this article are solely those of the authors and do not necessarily represent those of their affiliated organizations, or those of the publisher, the editors and the reviewers. Any product that may be evaluated in this article, or claim that may be made by its manufacturer, is not guaranteed or endorsed by the publisher.
The Supplementary Material for this article can be found online at: https://www.frontiersin.org/articles/10.3389/fmars.2023.1276400/full#supplementary-material
Aeby G. S., Ushijima B., Campbell J. E., Jones S., Williams G. J., Meyer J. L., et al. (2019). Pathogenesis of a tissue loss disease affecting multiple species of corals along the Florida reef tract. Front. Mar. Sci. 6. doi: 10.3389/fmars.2019.00678
Alvarez-Filip L., Carricart-Ganivet J. P., Horta-Puga G., Iglesias-Prieto R. (2013). Shifts in coral-assemblage composition do not ensure persistence of reef functionality. Sci. Rep. 3, srep03486. doi: 10.1038/srep03486
Alvarez-Filip L., Dulvy N. K., Gill J. A., Côté I. M., Watkinson A. R. (2009). Flattening of caribbean coral reefs: region-wide declines in architectural complexity. Proc. R. Soc. B: Biol. Sci. 276, 3019–3025. doi: 10.1098/rspb.2009.0339
Alvarez-Filip L., González-Barrios F. J., Pérez-Cervantes E., Molina-Hernández A., Estrada-Saldívar N. (2022). Stony coral tissue loss disease decimated Caribbean coral populations and reshaped reef functionality. Commun. Biol. 5, 440. doi: 10.1038/s42003-022-03398-6
Aronson R. B., Macintyre I. G., Wapnick C. M., O’Neill M. W. (2004). Phase shifts, alternative states, and the unprecedented convergence of two reef systems. Ecology 85, 1876–1891. doi: 10.1890/03-0108
Aronson R. B., Precht W. F. (2001). White band disease and the changing face of Caribbean coral reef. Hydrobiologia 406, 25–38. doi: 10.1023/A:1013103928980
Ault J. S., Bohnsack J. A., Meester G. A. (1998). A retrospective (1979-1996) multispecies assessment of coral reef fish stocks in the florida keys. Fishery Bull. 96, 395–414.
Beavers K. M., Van Buren E. W., Rossin A. M., Emery M. A., Veglia A. J., Karrick C. E., et al. (2023). Stony coral tissue loss disease induces transcriptional signatures of in situ degradation of dysfunctional Symbiodiniaceae. Nat. Commun. 14, 2915. doi: 10.1038/s41467-023-38612-4
Beck M. W., Losada I. J., Menéndez P., Reguero B. G., Díaz-Simal P., Fernández F. (2018). The global flood protection savings provided by coral reefs. Nat. Commun. 9, 2186. doi: 10.1038/s41467-018-04568-z
Brandt M. E., Ennis R. S., Meiling S. S., Townsend J., Cobleigh K., Glahn A., et al. (2021). The emergence and initial impact of stony coral tissue loss disease (SCTLD) in the United States virgin islands. Front. Mar. Sci. 8. doi: 10.3389/fmars.2021.715329
Brandt M. E., McManus J. W. (2009). Disease incidence is related to bleaching extent in reef-building corals. Ecology 90, 2859–2867. doi: 10.1890/08-0445.1
Bruno J. F., Petes L. E., Harvell C. D., Hettinger A. (2003). Nutrient enrichment can increase the severity of coral diseases. Ecol. Lett. 6, 1056–1061. doi: 10.1046/j.1461-0248.2003.00544.x
Bruno J. F., Selig E. R., Casey K. S., Page C. A., Willis B. L., Harvell C. D., et al. (2007). Thermal stress and coral cover as drivers of coral disease outbreaks. PloS Biol. 5, e124. doi: 10.1371/journal.pbio.0050124
Burman S. G., Aronson R. B., Van Woesik R. (2012). Biotic homogenization of coral assemblages along the Florida reef tract. Mar. Ecol. Prog. Ser. 467, 89–96. doi: 10.3354/meps09950
Chiappone M., Rutten L. M., Swanson D. W., Miller S. L. (2008). “Population status of the urchin Diadema antillarum in the Florida Keys 25 years after the Caribbean mass mortality,” in Proceedings of the 11th International Coral Reef Symposium. (Ft. Lauderdale), 706–710.
Cornwall C. E., Comeau S., Kornder N. A., Perry C. T., van Hooidonk R., DeCarlo T. M., et al. (2021). Global declines in coral reef calcium carbonate production under ocean acidification and warming. Proc. Natl. Acad. Sci. 118, e2015265118. doi: 10.1073/pnas.2015265118
Courtney T. A., Barkley H. C., Chan S., Couch C. S., Kindinger T. L., Oliver T. A., et al. (2022). Rapid assessments of Pacific Ocean net coral reef carbonate budgets and net calcification following the 2014–2017 global coral bleaching event. Limnology Oceanography 67, 1687–1700. doi: 10.1002/lno.12159
Courtney T. A., Barnes B. B., Chollett I., Elahi R., Gross K., Guest J. R., et al. (2020). Disturbances drive changes in coral community assemblages and coral calcification capacity. Ecosphere 11, e03066. doi: 10.1002/ecs2.3066
Courtney T. A., Chan S., Lange I. D., Perry C. T., Kriegman D. J., Andersson A. J. (2021). Area-normalized scaling of ReefBudget calcification, macrobioerosion, and microbioerosion rates for use with CoralNet Version 1.0. doi: 10.5281/zenodo.5140477
Dahlgren C., Pizarro V., Sherman K., Greene W., Oliver J. (2021). Spatial and temporal patterns of stony coral tissue loss disease outbreaks in the Bahamas. Front. Mar. Sci. 8. doi: 10.3389/fmars.2021.682114
Darling E. S., Alvarez-Filip L., Oliver T. A., McClanahan T. R., Côté I. M. (2012). Evaluating life-history strategies of reef corals from species traits. Ecol. Lett. 15, 1378–1386. doi: 10.1111/j.1461-0248.2012.01861.x
DEP. (2018). Case Definition: Stony Coral Tissue Loss Disease (SCTLD). Available at: https://floridadep.gov/rcp/coral/documents/stony-coral-tissue-loss-disease-sctld-case-definition.
Dobbelaere T., Holstein D. M., Muller E. M., Gramer L. J., McEachron L., Williams S. D., et al. (2022). Connecting the dots: transmission of stony coral tissue loss disease from the marquesas to the dry tortugas. Front. Mar. Sci. 9. doi: 10.3389/fmars.2022.778938
Dobbelaere T., Muller E. M., Gramer L. J., Holstein D. M., Hanert E. (2020). Coupled epidemio-hydrodynamic modeling to understand the spread of a deadly coral disease in Florida. Front. Mar. Sci. 7. doi: 10.3389/fmars.2020.591881
Elahi R., Edmunds P. J., Gates R. D., Kuffner I. B., Barnes B. B., Chollett I., et al. (2022). Scale dependence of coral reef oases and their environmental correlates. Ecol. Appl. 32, e2651. doi: 10.1002/eap.2651
Estrada-Saldívar N., Molina-Hernández A., Pérez-Cervantes E., Medellín-Maldonado F., González-Barrios F. J., Alvarez-Filip L. (2020). Reef-scale impacts of the stony coral tissue loss disease outbreak. Coral Reefs 39, 861–866. doi: 10.1007/s00338-020-01949-z
Evans J. S., Paul V. J., Kellogg C. A. (2022). Biofilms as potential reservoirs of stony coral tissue loss disease. Front. Mar. Sci. 9. doi: 10.3389/fmars.2022.1009407
Ferrario F., Beck M. W., Storlazzi C. D., Micheli F., Shepard C. C., Airoldi L. (2014). The effectiveness of coral reefs for coastal hazard risk reduction and adaptation. Nat. Commun. 5, 3794. doi: 10.1038/ncomms4794
Gallery D. N., Green M. L., Kuffner I. B., Lenz E. A., Toth L. T. (2021). Genetic structure and diversity of the mustard hill coral Porites astreoides along the Florida Keys reef tract. Mar. Biodiversity 51, 63. doi: 10.1007/s12526-021-01196-7
Gazal K., Andrew R., Burns R. (2022). Economic contributions of visitor spending in ocean recreation in the Florida keys national marine sanctuary. Water 14, 198. doi: 10.3390/w14020198
Gintert B. E., Precht W. F., Fura R., Rogers K., Rice M., Precht L. L., et al. (2019). Regional coral disease outbreak overwhelms impacts from a local dredge project. Environ. Monit. Assess. 191, 630. doi: 10.1007/s10661-019-7767-7
Green D. H., Edmunds P. J., Carpenter R. C. (2008). Increasing abundance of Porites astreoides on Caribbean reefs mediated by an overall decline in coral cover. Mar. Ecol. Prog. Ser. 359, 1–10. doi: 10.3354/meps07454
Guest J. R., Edmunds P. J., Gates R. D., Kuffner I. B., Andersson A. J., Barnes B. B., et al. (2018). A framework for identifying and characterising coral reef “oases“ against a backdrop of degradation. J. Appl. Ecol. 55, 2865–2875. doi: 10.1111/1365-2664.13179
Januchowski-Hartley F. A., Graham N. A. J., Wilson S. K., Jennings S., Perry C. T. (2017). Drivers and predictions of coral reef carbonate budget trajectories. Proc. R. Soc. B 284, 20162533. doi: 10.1098/rspb.2016.2533
Kinsey D. W. (1985). Metabolism, calcification and carbon production I: system level studies. Proc. Fifth Int. Coral Reef Congress Tahiti 4, 505–526.
Kramer P. R., Roth L., Lang J. (2019) Map of Stony Coral Tissue Loss Disease Outbreak in the Caribbean. Available at: www.agrra.org (Accessed May 2023).
Kuffner I. B., Toth L. T. (2016). A geological perspective on the degradation and conservation of western Atlantic coral reefs. Conserv. Biol. 30, 706–715. doi: 10.1111/cobi.12725
Kuffner I. B., Toth L. T., Hudson J. H., Goodwin W. B., Stathakopoulos A., Bartlett L. A., et al. (2019). Improving estimates of coral reef construction and erosion with in situ measurements. Limnology Oceanography 64, 2283–2294. doi: 10.1002/lno.11184
Landsberg J. H., Kiryu Y., Peters E. C., Wilson P. W., Perry N., Waters Y., et al. (2020). Stony coral tissue loss disease in Florida is associated with disruption of host–zooxanthellae physiology. Front. Mar. Sci. 7. doi: 10.3389/fmars.2020.576013
Lange I. D., Perry C. T. (2019). Bleaching impacts on carbonate production in the Chagos Archipelago: influence of functional coral groups on carbonate budget trajectories. Coral Reefs 38, 619–624. doi: 10.1007/s00338-019-01784-x
Lange I. D., Perry C. T., Stuhr M. (2023). Recovery trends of reef carbonate budgets at remote coral atolls 6 years post-bleaching. Limnology Oceanography 68, S8–S22. doi: 10.1002/lno.12066
Lenth R. V. (2023). emmeans: Estimated Marginal Means, aka Least-Squares Means. R package version 1.8.8. Available at: https://CRAN.R-project.org/package=emmeans.
Manzello D. P. (2015). Rapid recent warming of coral reefs in the Florida Keys. Nat. Sci. Rep. 5, srep16762. doi: 10.1038/srep16762
Meiling S., Muller E. M., Smith T. B., Brandt M. E. (2020). 3D photogrammetry reveals dynamics of stony coral tissue loss disease (SCTLD) lesion progression across a thermal stress event. Front. Mar. Sci. 7. doi: 10.3389/fmars.2020.597643
Meyer J. L., Castellanos-Gell J., Aeby G. S., Häse C. C., Ushijima B., Paul V. J. (2019). Microbial community shifts associated with the ongoing stony coral tissue loss disease outbreak on the Florida reef tract. Front. Microbiol. 10. doi: 10.3389/fmicb.2019.02244
Miller J., Muller E., Rogers C., Waara R., Atkinson A., Whelan K. R. T., et al. (2009). Coral disease following massive bleaching in 2005 causes 60% decline in coral cover on reefs in the US Virgin Islands. Coral Reefs 28, 925–937. doi: 10.1007/s00338-009-0531-7
Modys A. B., Toth L. T., Mortlock R. A., Oleinik A. E., Precht W. F. (2023). Discovery of a rare pillar coral (Dendrogyra cylindrus) death assemblage off southeast Florida reveals multi-century persistence during the late Holocene. Coral Reefs 42, 801–807. doi: 10.1007/s00338-023-02387-3
Morris J. T., Enochs I. C., Besemer N., Viehman T. S., Groves S. H., Blondeau J., et al. (2022). Low net carbonate accretion characterizes Florida’s coral reef. Sci. Rep. 12, 19582. doi: 10.1038/s41598-022-23394-4
Muller E. M., Sartor C., Alcaraz N. I., van Woesik R. (2020). Spatial epidemiology of the stony-coral-tissue-loss disease in Florida. Front. Mar. Sci. 7. doi: 10.3389/fmars.2020.00163
Muller E. M., van Woesik R. (2012). Caribbean coral diseases: primary transmission or secondary infection? Global Change Biol. 18, 3529–3535. doi: 10.1111/gcb.12019
Neely K. L., Lewis C. L., Lunz K. S., Kabay L. (2021). Rapid population decline of the pillar coral dendrogyra cylindrus along the Florida reef tract. Front. Mar. Sci. 8. doi: 10.3389/fmars.2021.656515
Neely K. L., Macaulay K. A., Hower E. K., Dobler M. A. (2020). Effectiveness of topical antibiotics in treating corals affected by Stony Coral Tissue Loss Disease. Peer J. 8, e9289. doi: 10.7717/peerj.9289
NOAA Coral Reef Watch. (2023). NOAA Coral Reef Watch Version 3.1 5 km Regional Bleaching Heat Stress Maps and Gauges (College Park, Maryland, USA). Available at: https://coralreefwatch.noaa.gov/product/vs/gauges/florida_keys.php. Data set accessed 2023-07-12.
Oksanen J., Simpson G., Blanchet F., Kindt R., Legendre P., Minchin P., et al. (2022). vegan: Community ecology package. R package version 2.6-4. Available at: https://CRAN.R-project.org/package=vegan.
Perry C. T., Alvarez-Filip L. (2019). Changing geo-ecological functions of coral reefs in the Anthropocene. Funct. Ecol. 33, 976–988. doi: 10.1111/1365-2435.13247
Perry C. T., Alvarez-Filip L., Graham N. A. J., Mumby P. J., Wilson S. K., Kench P. S., et al. (2018). Loss of coral reef growth capacity to track future increases in sea level. Nature 558, 396–400. doi: 10.1038/s41586-018-0194-z
Perry C. T., Edinger E. N., Kench P. S., Murphy G. N., Smithers S. G., Steneck R. S., et al. (2012). Estimating rates of biologically driven coral reef framework production and erosion: a new census-based carbonate budget methodology and applications to the reefs of Bonaire. Coral Reefs 31, 853–868. doi: 10.1007/s00338-012-0901-4
Perry C. T., Lange I. D. (2019). ReefBudget: Methodology Caribbean Version 2. Available at: https://geography.exeter.ac.uk/reefbudget/.
Perry C. T., Murphy G. N., Kench P. S., Smithers S. G., Edinger E. N., Steneck R. S., et al. (2013). Caribbean-wide decline in carbonate production threatens coral reef growth. Nat. Commun. 4. doi: 10.1038/ncomms2409
Perry C. T., Steneck R. S., Murphy G. N., Kench P. S., Edinger E. N., Smithers S. G., et al. (2015). Regional-scale dominance of non-framework building coral on Caribbean reefs affects carbonate production and future reef growth. Global Change Biol. 21, 1153–1164. doi: 10.1111/gcb.12792
Pinheiro J., Bates D. and, R Core Team. (2023). nlme: linear and nonlinear mixed effects models. R package version 3.1-153. Available at: https://CRAN.R-project.org/package=nlme.
Pratchett M. S., Hoey A. S., Wilson S. K. (2014). Reef degradation and the loss of critical ecosystem goods and services provided by coral reef fishes. Curr. Opin. Environ. Sustain. 7, 37–43. doi: 10.1016/j.cosust.2013.11.022
Precht W. F., Gintert B. E., Robbart M. L., Fura R., van Woesik R. (2016). Unprecedented disease-related coral mortality in southeastern Florida. Nat. Sci. Rep. 6, srep31374. doi: 10.1038/srep31374
Precht W. F., Miller S. L. (2007). “Ecological shifts along the Florida reef tract: the past as a key to the future,” in Geological Approaches to Coral Reef Ecology. Ed. Aronson R. B. (New York: Springer), 237–312.
Randall C. J., van Woesik R. (2015). Contemporary white-band disease in Caribbean coral driven by climate change. Nat. Climate Change 5, 375–379. doi: 10.1038/nclimate2530
R Core Team. (2023). R: A language and environment for statistical computing (Vienna, Austria: R Foundation for Statistical Computing). Available at: https://www.R-project.org/.
Reguero B. G., Storlazzi C. D., Gibbs A. E., Shope J. B., Cole A. D., Cumming K. A., et al. (2021). The value of US coral reefs for flood risk reduction. Nat. Sustain. 4, 688–698. doi: 10.1038/s41893-021-00706-6
Rosenau N. A., Gignoux-Wolfsohn S., Everett R. A., Miller A. W., Minton M. S., Ruiz G. M. (2021). Considering commercial vessels as potential vectors of stony coral tissue loss disease. Front. Mar. Sci. 8. doi: 10.3389/fmars.2021.709764
Ruzicka R. R., Colella M. A., Porter J. W., Morrison J. M., Kidney J. A., Brinkhuis V., et al. (2013). Temporal changes in benthic assemblages on Florida Keys reefs 11 years after the 1997/1998 El Niño. Mar. Ecol. Prog. Ser. 489, 125–141. doi: 10.3354/meps10427
Studivan M. S., Baptist M., Molina V., Riley S., First M., Soderberg N., et al. (2022). Transmission of stony coral tissue loss disease (SCTLD) in simulated ballast water confirms the potential for ship-born spread. Sci. Rep. 12, 19248. doi: 10.1038/s41598-022-21868-z
Toth L. T., Courtney T. A. (2022). Keys carbonate budget calculations and analysis. U.S. Geological Survey Software Release. doi: 10.5066/P9APPZHJ
Toth L. T., Courtney T. A., Colella M. A., Kupfner Johnson S. A., Ruzicka R. R. (2022). The past, present, and future of coral reef growth in the Florida Keys. Global Change Biol. 28, 5294–5309. doi: 10.1111/gcb.16295
Toth L. T., Kuffner I. B., Stathakopoulos A. (2018a). Descriptive core logs, core photographs, radiocarbon ages, and data on reef development for cores of holocene reef framework from the Florida keys reef tract. USGS Data Release. doi: 10.5066/F7NV9HJX
Toth L. T., Kuffner I. B., Stathakopoulos A., Shinn E. A. (2018b). A 3,000-year lag between the geological and ecological shutdown of Florida's coral reefs. Global Change Biol. 24, 5471–5483. doi: 10.1111/gcb.14389
Toth L. T., Stathakopoulos A., Kuffner I. B., Ruzicka R. R., Colella M. A., Shinn E. A. (2019). The unprecedented loss of Florida's reef-building corals and the emergence of a novel coral-reef assemblage. Ecology 100, e02781. doi: 10.1002/ecy.2781
Toth L. T., Storlazzi C. D., Whitcher E. M., Kuffner I. B., Quataert E., Reyns J., et al. (2023). The potential for coral reef restoration to mitigate coastal flooding as sea levels rise. Nat. Commun. 14, 2313. doi: 10.1038/s41467-023-37858-2
Toth L. T., van Woesik R., Murdoch T. J. T., Smith S. R., Ogden J. C., Precht W. F., et al. (2014). Do no-take reserves benefit Florida's corals? 14 years of change and stasis in the Florida Keys National Marine Sanctuary. Coral Reefs 33, 565–577. doi: 10.1007/s00338-014-1158-x
van Woesik R., Randall C. J. (2017). Coral disease hotspots in the Caribbean. Ecosphere 8, e01814. doi: 10.1002/ecs2.1814
Walton C. J., Hayes N. K., Gilliam D. S. (2018). Impacts of a regional, multi-year, multi-species coral disease outbreak in southeast Florida. Front. Mar. Sci. 5. doi: 10.3389/fmars.2018.00323
Webb A. E., Enochs I. C., van Hooidonk R., van Westen R. M., Besemer N., Kolodziej G., et al. (2023). Restoration and coral adaptation delay, but do not prevent, climate-driven reef framework erosion of an inshore site in the Florida Keys. Sci. Rep. 13, 258. doi: 10.1038/s41598-022-26930-4
Woodhead A. J., Hicks C. C., Norström A. V., Williams G. J., Graham N. A. J. (2019). Coral reef ecosystem services in the Anthropocene. Funct. Ecol. 33, 1023–1034. doi: 10.1111/1365-2435.13331
Work T. M., Weatherby T. M., Landsberg J. H., Kiryu Y., Cook S. M., Peters E. C. (2021). Viral-like particles are associated with endosymbiont pathology in Florida corals affected by stony coral tissue loss disease. Front. Mar. Sci. 8. doi: 10.3389/fmars.2021.750658
Keywords: Florida reef tract, coral disease, SCTLD, carbonate budget, reef erosion, reef growth, reef budget, reef function
Citation: Toth LT, Courtney TA, Colella MA and Ruzicka RR (2023) Stony coral tissue loss disease accelerated shifts in coral composition and declines in reef accretion potential in the Florida Keys. Front. Mar. Sci. 10:1276400. doi: 10.3389/fmars.2023.1276400
Received: 11 August 2023; Accepted: 09 October 2023;
Published: 25 October 2023.
Edited by:
Lorenzo Alvarez-Filip, National Autonomous University of Mexico, MexicoReviewed by:
Didier M. De Bakker, University of Exeter, United KingdomCopyright © 2023 Toth, Courtney, Colella and Ruzicka. This is an open-access article distributed under the terms of the Creative Commons Attribution License (CC BY). The use, distribution or reproduction in other forums is permitted, provided the original author(s) and the copyright owner(s) are credited and that the original publication in this journal is cited, in accordance with accepted academic practice. No use, distribution or reproduction is permitted which does not comply with these terms.
*Correspondence: Lauren T. Toth, bHRvdGhAdXNncy5nb3Y=
Disclaimer: All claims expressed in this article are solely those of the authors and do not necessarily represent those of their affiliated organizations, or those of the publisher, the editors and the reviewers. Any product that may be evaluated in this article or claim that may be made by its manufacturer is not guaranteed or endorsed by the publisher.
Research integrity at Frontiers
Learn more about the work of our research integrity team to safeguard the quality of each article we publish.