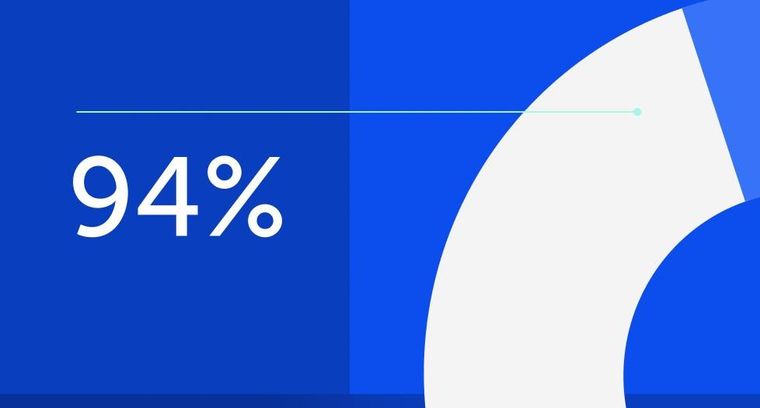
94% of researchers rate our articles as excellent or good
Learn more about the work of our research integrity team to safeguard the quality of each article we publish.
Find out more
REVIEW article
Front. Mar. Sci., 11 October 2023
Sec. Marine Ecosystem Ecology
Volume 10 - 2023 | https://doi.org/10.3389/fmars.2023.1271755
This article is part of the Research TopicUnderstanding the Response of Ecosystems to Increasing Human Pressures and Climate Change – Management OptionsView all 25 articles
Biological invasions, resulting from human activities, exert substantial impacts on ecosystems worldwide. This review focuses on marine invasive alien species (IAS) in Europe, examining the current state, proposing strategies to address the problem, and offering recommendations for enhanced management. Effective management of biological invasions relies on accessible, accurate data to inform decision-making. Information systems such as the European Alien Species Information Network (EASIN), Aquatic Non-Indigenous and Cryptogenic Species (AquaNIS), and World Register of Introduced Marine Species (WriMS) provide comprehensive databases on IAS, but their sustainability requires long-term maintenance, continuous updates, and support. Most countries lack specific monitoring programs for marine IAS, and standardization and improvement of monitoring methods are needed. Port monitoring plays a vital role in the early detection of new arrivals, and recent advancements in molecular techniques show promise for effective IAS monitoring. Risk screening tools are commonly employed to rank taxa based on their invasiveness potential in European regions, but variations in protocols can yield inconsistent results. European impact assessments highlight resource competition, novel habitat creation, and predation as primary mechanisms for negative impacts on biodiversity, while the creation of novel habitats represents a key mechanism for positive impacts. Preventing IAS introductions is critical, and measures such as ballast water treatment systems are implemented to reduce the likelihood of marine introductions. However, understanding introduction pathways remains uncertain for many IAS. Eradication and control efforts for marine IAS have limited success, emphasizing the need for enhanced biosecurity measures. Climate change, especially ocean warming, can intensify IAS impacts on native species and ecosystems. In climate change hotspots, some tropical aliens may, however, compensate for the loss of thermally sensitive natives with similar traits. Therefore, it is imperative to consider the interactions between climate change and IAS in developing effective management and conservation strategies. Enhancing IAS management in Europe entails i) securing adequate funding, ii) expanding the list of IAS of Union Concern to adequately cover marine invasions, iii) learning from countries with successful biosecurity practices, iv) sustaining information systems, v) improving monitoring and early warning systems with innovative technologies, vi) enhancing prediction models, vii) conducting integrated impact assessments and mapping cumulative IAS impacts, and vii) considering the potential benefits of IAS in ecosystem functioning and services.
Biological invasions or bioinvasions (Elton, 1958) are among the most influential human-driven processes impacting Earth’s terrestrial and aquatic ecosystems (Primack, 1995; Rilov and Crooks, 2009; Ehrenfeld, 2010; Vilà et al., 2011). Both native and non-native species have the potential to undergo exponential population growth and cause outbreaks, i.e., invasions. The dynamics of biological invasions arise from interspecific (direct or indirect) interactions, such as predation, competition, mutualism, or facilitation, often leading to the invader’s dominance over functionally similar species in the invaded community (Valéry et al., 2008; Valéry et al., 2009; Valeíry et al., 2013). The success and impact of a biological invasion depend on the interplay of ecological and biological characteristics of both the invader and the species in the invaded community, as well as the environmental conditions. Restricting the definition of biological invasions to a geographical phenomenon specific to non-indigenous species rather than an ecological one is not justified (Valéry et al., 2013). Therefore, invasive alien (=non-native, non-indigenous, exotic) species (IAS) should be regarded as a subset of invasive species, which can also include native or neonative (sensu Essl et al., 2019).
Non-indigenous species (NIS) are defined as species that have spread beyond their natural biogeographical range to new regions with the aid of human actions (Essl et al., 2018). IAS are defined by the European Union (EU) IAS Regulation as “alien species whose introduction or spread has been found to threaten or adversely impact upon biodiversity and related ecosystem services” (European Union (EU), 2014), giving the term “invasive” a negative connotation. IAS have rapidly increased worldwide (Seebens et al., 2017), resulting in significant economic costs (Diagne et al., 2021). IAS have the capacity to profoundly alter the structure and functioning of native communities, often leading to the loss of native biodiversity, disruption of ecosystem services, loss of socioeconomic values, and potential impacts on human health (Mazza et al., 2014; Tsirintanis et al., 2022). However, the impacts of IAS can have either (or both) “negative” (reducing the value of a specific property) or “positive” (increasing the value) consequences for specific ecological or socioeconomic attributes, and they can be highly context-dependent (Tsirintanis et al., 2022; Vimercati et al., 2022; Reise et al., 2023).
IAS are recognized in the Convention on Biological Diversity (CBD) as a cross-cutting issue with relevance across all thematic areas. Article 8(h) of the CBD explicitly states that “each contracting Party shall, as far as possible and as appropriate, prevent the introduction of, control or eradicate those alien species which threaten ecosystems, habitats or species”. Recently, the Kunming-Montreal Global Biodiversity Framework, under decision 15/4, has set the objective to “eliminate, minimize, reduce and or mitigate the impacts of invasive alien species on biodiversity and ecosystem services” through various approaches, with particular emphasis on eradicating or controlling IAS in priority sites, such as islands (Table 1). Multiple global and regional legislative instruments, policies, and guidelines have been established to contribute to the achievement of these global goals (see Table 1). Typically, species introduced before a specific cutoff date are not subject to biosecurity measures and are treated no differently than native species. In some cases, they may even become the focus of conservation efforts (Essl et al., 2018). Biosecurity efforts predominantly target neobiota, i.e., relatively recently introduced alien species or species that have not yet been introduced. However, there is no global consensus on this cutoff date, leading to the use of region-specific temporal thresholds in NIS databases. For example, in Europe and the Americas, the widely accepted cutoff date is 1492, which marks Christopher Columbus’s discovery of America and the related initiation of species introductions between the two continents. In the Mediterranean region, some databases have adopted the opening of the Suez Canal in 1869 as their temporal threshold, as it triggered a surge of Red Sea species into the Mediterranean Sea (Gatto et al., 2013; Essl et al., 2018).
Table 1 International policy context on biological invasions in coastal and marine environments with relevance for European Seas.
In the EU, the Biodiversity Strategy for 2030 has set the objective of effectively managing established IAS and reducing by 50% the number of Red List species they threaten by 2030. The Marine Strategy Framework Directive (MSFD) recognizes IAS as a significant pressure on marine ecosystems, negatively affecting environmental status. The MSFD indicates that achieving Good Environmental Status requires maintaining alien species at levels that do not cause adverse alterations to the marine ecosystems (Table 1).
In 2014, the EU implemented a comprehensive Regulation encompassing several key elements aimed at effectively managing invasive species (European Union (EU), 2014), hereafter called “the IAS Regulation”. The IAS Regulation is a vital biosecurity program that operates at a pan-European level. It mandates thorough risk assessments to assess the potential impact of invasive species and inform appropriate management strategies. It introduced the concept of an EU Black List, which comprises invasive species of Union Concern. The Black List serves as a basis for implementing specific rules and measures for the prevention of new introductions and further spread, early detection, rapid eradication, and management of IAS, thereby safeguarding the EU’s ecosystems. The Black List is dominated by terrestrial and freshwater species, with only two marine species currently included. The first marine species, namely, Plotosus lineatus, was introduced to the list in 2019 (European Union (EU), 2019), followed by Rugulopteryx okamurae in 2022 (European Union (EU), 2022).
This review aims to evaluate the current state of marine IAS in Europe and explore implemented or proposed strategies developed to date to mitigate IAS impacts. The review is structured to cover the existing knowledge base, information systems, methodologies for monitoring and predicting IAS distribution, pathway management, impact assessments, management options, and the combined effects of IAS and climate change. Drawing from this information, we offer recommendations on how to consider improving current practices for IAS management in Europe. Some of these lessons and approaches are centered in Europe but could be considered and adapted elsewhere.
Biological invasion management policies should rely on timely, accurate, publicly available data that are easily understood and usable for decision-making. For example, the effectiveness of IMO Ballast Water Management Convention (BWMC) measures for preventing the introduction of harmful aquatic organisms and pathogens can be assessed by estimating the reduction in the number of new arrivals through ballast water (Olenin et al., 2014). Similarly, the effectiveness of other conventions, directives, and agreements depends on reliable NIS monitoring data and targeted scientific research. Therefore, monitoring and research data should be collected, quality checked, harmonized, and presented through user-friendly and reliable information systems to be useful for management (Olenin et al., 2011; Lehtiniemi et al., 2015).
The utilization of NIS information systems for research is growing. These systems have been instrumental in compiling national and regional NIS inventories (e.g., Chainho et al., 2015; Ulman et al., 2017; Tsiamis et al., 2019), prioritizing the most impactful IAS, quantifying and summarizing ecological impacts of specific taxa (Katsanevakis et al., 2016), identifying major pathways and vectors of NIS introductions (Katsanevakis et al., 2013; Ojaveer et al., 2017; Pergl et al., 2020), and analyzing species traits and ecological preferences (Paavola et al., 2005; Cardeccia et al., 2018) (Table 2). The use of NIS information systems enhances the analytical and predictive nature of bioinvasion research, shifting from scientific curiosity (“nice to know”) to the “need to know” principle driven by management requirements (Olenin et al., 2011).
Table 2 Examples of currently active online information systems on marine, brackish, and coastal freshwater alien species relevant to Europe.
The European Commission launched the European Alien Species Information Network (EASIN) in 2012 to support European NIS management policies (Katsanevakis et al., 2012; Katsanevakis et al., 2015). EASIN provides easy and open access to harmonized data and information on alien and cryptogenic species, sourced from global, regional, and national databases and scientific literature (Trombetti et al., 2013), through online tools and web services (Figure 1). EASIN’s core component is the EASIN Catalogue, the most comprehensive European inventory of terrestrial, freshwater, and marine NIS. The Catalogue’s updating and quality assurance is managed by an international Editorial Board of taxonomic experts (Tsiamis et al., 2016). As of July 2023, EASIN included ~13,300 alien and cryptogenic (i.e., of unknown biogeographic status) species, of which ~1,700 were marine or oligohaline. Moreover, EASIN serves as the official information system for the European Commission to support the EU Regulation on IAS (European Union (EU), 2014). Specifically, EASIN features a Notification System that enables member states to promptly notify the Commission of new detections of IAS of EU concern and associated eradication measures.
Figure 1 European Alien Species Information Network (EASIN): schematic of its concept, main elements, and outputs (bottom left: marine alien species by country; bottom right: marine alien species by ecoregion).
Aquatic Non-Indigenous and Cryptogenic Species (AquaNIS), founded in 1997 as the “Baltic Sea Alien Species Database”, is likely the oldest international online database on aquatic NIS. Over time, it has expanded to cover all European regional seas and later incorporated datasets from other world regions. As of March 2023, AquaNIS contained data on nearly 5,500 NIS introduction events in 25 Large Marine Ecosystems (LMEs). The system features a flexible search engine with several criteria (taxonomy, geography, pathways, biological characteristics, etc.) and an analysis tool for comparing species lists in different LMEs, countries, regions, and time periods (Figure 2). AquaNIS data are regularly updated by the International Council for Exploration of the Seas Working Group on Introductions and Transfer of Marine Organisms (WGITMO). AquaNIS is increasingly used for assessing marine environmental status under the MSFD and supporting decision-making for the IMO Ballast Water Management Convention. Recently, it was equipped with an Early Warning System aimed at preventing the spread of harmful aquatic organisms and pathogens through ballast water.
Figure 2 Information system on Aquatic Non-Indigenous and Cryptogenic Species (AquaNIS) has a flexible search engine and a built-in comparative analysis tool, which makes it practical for management and useful for research.
The World Register of Introduced Marine Species (WRiMS) is a global database connected to the well-established World Register of Marine Species (WoRMS). WRiMS provides taxonomic information for marine species, utilizing the taxonomically authoritative classification and accepted names from WoRMS. It specifically focuses on introduced marine species, distinguishing their native and introduced geographic ranges (Costello et al., 2021). As of 2021, WRiMS included over 2,300 introduced species. The amount and quality of the information entered depend on the availability of experts to update its contents and are affected by regional biases in sampling and taxonomic effort. Despite some errors and outdated information, WRiMS is currently the most comprehensive standardized marine NIS database.
With the advent of Internet technologies and increasing demand from management and researchers, several NIS databases have emerged through short-term national or international projects. However, many of these databases prioritize their design using web technologies rather than focusing on data collection and creating ecologically meaningful output functionalities. At best, these databases prove useful toward the end of a project for generating reports and, occasionally, scholarly papers. However, the long-term utility of a database depends not only on the employed technologies and project deliverables but also on sustained user demand and post-project maintenance (Olenin et al., 2014). Unfortunately, securing funding for database collaboration, adaptation, improvement, and maintenance is often more challenging than developing new databases (Simpson et al., 2006). There are several examples of NIS databases that remained idle, with data not being updated for extended periods, or ceased to exist altogether, becoming inaccessible to users.
One notable example is the DAISIE information system, a product of the project DAISIE (Delivering Alien Invasive Species Inventories for Europe). The project, with a European Commission contribution of €2.4 million, spanned 3 years starting in February 2005 (DAISIE, 2009). Its goal was to create a comprehensive resource on biological invasions in Europe, through an international team of leading experts in biological invasions, cutting-edge database design and display technologies, and an extensive network of European collaborators and stakeholders (DAISIE, 2009). The system compiled and verified over 248 datasets from 98 European countries/regions, making it the world’s largest invasive species database.
However, “the DAISIE dataset is no longer maintained but can be used as a historical archive for researching and managing alien plants or compiling regional and national registries of alien species” (GBIF, 2023). While part of the data has been preserved and integrated into other databases, the European Alien Species Expertise Registry, the European Alien Species Database, and the European Invasive Alien Species Information System no longer exist. This is primarily because the project failed to establish mechanisms for long-term maintenance, continuous updates, and the transfer of technology to relevant European entities (e.g., EASIN) for storage, use, and future development.
Several key factors have been highlighted for sustainable database management and advancement (Olenin et al., 2002; Katsanevakis et al., 2012; Olenin et al., 2014; Katsanevakis et al., 2015; Costello et al., 2021):
• Determine the database’s intended purposes (e.g., research, management, environmental status assessment, and early warning). Ideally, a database should be multipurpose.
• Design a user-friendly technical system enabling easy searching, extraction, and basic data analysis.
• Ensure a constant flow of reliable data and engage a highly qualified editorial board.
• Obtain ongoing support from international, regional, or national environmental authorities.
• Due to the rapidly increasing volume of bioinvasion data, innovative approaches, e.g., utilizing artificial intelligence, are necessary for improved data collection, standardization, and analysis.
Monitoring recommendations, including sampling adequacy, coordination and coherence among programs, integration of existing monitoring, interoperability, adaptive monitoring, linkages to assessment needs, risk-based approaches, and the precautionary principle, are highlighted within the scope of implementing the MSFD (Zampoukas et al., 2014). Despite the high cost of inaction (Ahmed et al., 2022), challenges are evident in global efforts against biological invasions, with monitoring for timely detection of new NIS, their introduction pathways, spread, and impacts remaining costly and challenging. However, new technologies have the potential to revolutionize invasion monitoring by addressing some of the current difficulties. Here, we present an overview of the current monitoring focus and examples showcasing the potential of novel techniques to enhance the monitoring of marine biological invasions.
Regional Sea Conventions (RSCs) have set environmental objectives (Table 1) to tackle biological invasions. They have also implemented monitoring guidelines to aid NIS management across European Regional Seas Basins (Table 3). Collaborative efforts have been undertaken, such as initial port sampling guidelines developed jointly by OSPAR and HELCOM, and the continued activity of the joint task group on BWMC and Biofouling (JTG Ballast and Biofouling). Furthermore, OSPAR and HELCOM have formed an expert group on species invasions (JEG-NIS) to foster discussions on monitoring programs and facilitate the development of joint or coordinated monitoring initiatives wherever feasible.
Table 3 Brief overview of current efforts by Regional Sea Conventions (RSCs) toward improved monitoring and management of NIS in European Regional Seas Basins.
The MSFD’s requirements for assessing the impacts of marine NIS have had an important role in promoting common strategies to address NIS across RSCs. Many of the indicators and guidelines adopted by RSCs (Table 3) aim to align with EU requirements, facilitating reporting by contracting parties, which are also obliged to report under MSFD. The RSCs’ guidelines reflect synergistic top-down and bottom-up approaches to influence and align monitoring efforts at regional and national levels.
Most countries lack dedicated marine NIS monitoring programs (Lehtiniemi et al., 2015), relying instead on existing broad monitoring initiatives. However, NIS often receive limited attention in national monitoring programs (Ljungberg et al., 2011). This is noteworthy since monitoring of the arrival and spread of IAS are required by several international regulations (e.g., European Union (EU), 2008; European Union (EU), 2014), and information on the abundance/biomass of IAS and their impact is required by the MSFD for assessing good environmental status (GES) (Stæhr and Jakobsen, 2023). National inventories of marine NIS have been compiled and published for several EU countries, e.g., Greece (Zenetos et al., 2018; Zenetos et al., 2020), Italy (Occhipinti-Ambrogi et al., 2011; Servello et al., 2019), Portugal (Chainho et al., 2015), Malta (Evans et al., 2015), Norway (Sandvik et al., 2019), Denmark (Stæhr et al., 2020), and Belgium (Verleye et al., 2020), often prompted by international working groups on NIS such as those of ICES (ICES, 2022) in the Atlantic and CIESM in the Mediterranean.
In line with the MSFD, each EU Member State has established improved records of marine NIS in their seas. These baseline inventories were developed through the initial MSFD evaluation in 2012, updated information from EASIN, and an expert elicitation process (Tsiamis et al., 2019). The assessment revealed that Italy, France, Spain, and Greece have the highest NIS richness among member states, while Slovenia, Lithuania, Latvia, and Finland have the lowest. Among the EU ecoregions, the Levantine Sea has the highest NIS richness, followed by the western Mediterranean, North Sea, and Aegean Sea (Table 4).
Table 4 Numbers of alien and cryptogenic marine and oligohaline species reported in EASIN by ecoregion (sensu Spalding et al., 2007), ordered by species richness.
Ports are considered a key hub in the introduction of IAS (Miralles et al., 2021 and references therein) and are valuable sites for monitoring new NIS arrivals. One of the earliest port survey approaches is the CRIMP protocol, initially developed in 1995 to assess marine invasions and survey effectiveness in Australian ports (Hewitt and Martin, 1996). An updated version of the protocol was published in 2001 following 5 years of implementation in practice (Hewitt and Martin, 2001). The protocol was adopted by the IMO GloBallast program for port surveys. However, the CRIMP protocol relies heavily on scuba diving surveys, which are not feasible in all locations. In such cases, qualitative surveys, such as Rapid Assessment Surveys, can provide insights into the presence of alien species and changes in their spatial distribution (e.g., Cohen et al., 2005; Pederson et al., 2005; Ashton, 2006).
Baltic Sea Port Monitoring, based on established protocols (Hewitt and Martin, 2001; Power et al., 2006; Buschbaum et al., 2010; Andersen et al., 2023), was originally designed for granting exemptions from the BWMC. HELCOM’s port sampling protocol has been implemented in the Baltic Sea since 2012 (HELCOM, 2013; Outinen et al., 2021), though regular monitoring is lacking in most countries. Finland initiated a port monitoring program in 2022, and Denmark published a port monitoring report in 2022 that was expanded to compare environmental DNA from IAS across seasons (Knudsen et al., 2022). In the Mediterranean, a study compared environmental DNA (eDNA) levels inferred from metabarcoding with fishing fleet activity to detect IAS in harbors around Sicily and the northwestern Mediterranean (Aglieri et al., 2023). In the Bay of Biscay, eDNA metabarcoding was utilized on water samples from major ports for IAS monitoring (Borrell et al., 2017).
Recent years have witnessed an explosion in the application of molecular methods based on organismal or eDNA or RNA due to their rapid technological advancements (Fonseca et al., 2023). In the context of biodiversity monitoring, the most applied methods can be categorized into 1) methods targeted to specific species based on quantitative real-time PCR (qPCR) or digital PCR (dPCR; including digital droplet PCR) and 2) untargeted methods based on metabarcoding of amplified taxonomic marker sequences using “universal” primers with broad coverage. Both types of methods offer advantages over traditional monitoring, including enhanced sensitivity and the ability to identify sparse NIS populations, even when visually challenging to identify life stages or when local taxonomic expertise is lacking (Bowers et al., 2021). Sample collection and preservation are relatively straightforward, requiring smaller sediment volumes, while eDNA can be directly extracted from water filters. In recent years, numerous evaluation and proof-of-concept studies have demonstrated the utility of both approaches for NIS monitoring in the environment and transportation vectors such as ballast water (e.g., Zaiko et al., 2015; Borrell et al., 2017; Rey et al., 2018; Holman et al., 2019; Rey et al., 2020; Bowers et al., 2021; Duarte et al., 2021; Knudsen et al., 2022).
The obvious disadvantage of targeted methods is the requirement of species-specific assays for each NIS of interest, whereas metabarcoding can theoretically detect any species eDNA present in collected samples (Hablützel et al., 2023). When many species are of interest, metabarcoding therefore becomes more cost-efficient. Conversely, targeted approaches generally exhibit higher sensitivity and specificity, allowing for more accurate estimates of absolute abundance (McColl-Gausden et al., 2023; Sapkota et al., 2023). For successful NIS identification, both approaches rely on the availability of reference sequence data. The specificity of metabarcoding also depends on the phylogenetic resolution of the amplified taxonomic marker, which can be severely limited, e.g., when using partial sequences of the small subunit (18S) rRNA gene that may show little or no variation across metazoans, for which 12S or COI is commonly used. Insufficient database coverage can severely limit the utility of metabarcoding, especially in regions where baseline biodiversity is poorly characterized. For example, Pearman et al. (2021) found that only 31% of 18S and 4% of the unique COI metabarcoding sequence variants obtained from a diversity survey of marinas in Tahiti could be assigned to species. Metabarcoding of eDNA is also dependent on the genetic reference sequences deposited on genetic databases that originate from vouchered museum specimens, as this makes species identification from sequence reads more reliable (Pleijel et al., 2008; Buckner et al., 2021). It is important that the bioinformatic handling of eDNA metabarcode sequence data includes a validation step that allows for identification being based on vouchered sequence data, rather than the most prevalent sequences. It also underlines the continuous importance of having taxonomic expertise in museum collections and the value of natural history collections at museums (Rocha et al., 2014).
To estimate the database coverage of NIS in European waters, we cross-referenced species listed in the AquaNIS and EASIN databases with species in the sequence databases Midori v253 (Leray et al., 2022), PR2 v5.0.0 (Guillou et al., 2013), SILVA 138 SSURef and LSURef NR (Quast et al., 2013), MitoFish v2023-03-23 (Iwasaki et al., 2013), MetaZooGene (downloaded April 4, 2023; Bucklin et al., 2021), and a list of all rbcL gene entries from global data repositories (Omonhinmin and Onuselogu, 2022). Out of 2,197 NIS in European waters, sequence data for at least one taxonomic marker were available for 1,318 species (60%; see Table 5). For 854 species (39%), multiple marker sequences were available.
Table 5 Identified reference sequences per taxonomic marker for NIS encountered in European waters extracted from the EASIN and AquaNIS databases (in total 2,209 unique species) per marker and database (“*” denotes a marker from a mitochondria or chloroplast encoded gene).
Sampling design is critical for comprehensive biodiversity coverage, especially in heterogeneous habitats such as ports (Knudsen et al., 2022; Aglieri et al., 2023). Rey et al. (2020) demonstrated this in the Port of Bilbao and its upstream estuary, where 192 samples were taken from various locations using zooplankton nets, filtered water, sediment grabs, and settlement plates. Less than 1% of the species identified through COI and 7% through 18S rRNA metabarcoding were shared among all four sampling methods. Koziol et al. (2019) reported similar findings. This highlights the need for standardized eDNA monitoring protocols and further studies that compare eDNA and traditional monitoring methods.
Sampling design for eDNA monitoring must also consider variation in distribution across time and depth. Different depths harbor different NIS, and the eDNA they release to the water will vary (DiBattista et al., 2019; Canals et al., 2021; Merten et al., 2023). Organism distribution fluctuates throughout the year, resulting in seasonally dependent eDNA release (Sigsgaard et al., 2017; Agersnap et al., 2022; Knudsen et al., 2022; Baudry et al., 2023). Diurnal activity patterns impact eDNA levels (Jensen et al., 2022), necessitating nighttime sampling for monitoring nocturnal NIS.
Environmental RNA (eRNA), similar to eDNA, is shed by metazoans or exists in the form of whole live or dead individuals of smaller organisms, making it a potential monitoring target (Lejzerowicz et al., 2015; Keeley et al., 2018). eRNA has the disadvantage of lower stability in the environment (Kagzi et al., 2023) and requires stricter sample contamination and preservation protocols but is likely a better reflection of the presence of live organisms (Pochon et al., 2017).
Artificial intelligence (AI) applications for species recognition (Wäldchen and Mäder, 2018) can greatly facilitate marine NIS monitoring. AI has made significant advancements in various areas, including species identification. AI technology, powered by machine learning and neural networks, has revolutionized biodiversity monitoring and species identification, fostering NIS monitoring (Carvalho et al., 2023). Platforms like iNaturalist utilize AI to assign taxonomic names based on uploaded images, with expert verification and training for improved accuracy. Several organizations have developed AI systems for marine species detection, fish and plankton identification, benthic image annotation, and even stock assessment (e.g., Connolly et al., 2021). Tools like Linne Lens enable real-time identification of multiple species from photos and videos, providing instant species recognition using smartphones and internet connectivity. Automated species identification from images and videos has become widespread, offering a cost-efficient approach that archives valuable data for NIS monitoring.
Remote sensing using color infrared (IR) photos has been employed for NIS detection in shallow waters since the 1970s (e.g., water hyacinth, Rouse et al., 1975). Advances in imaging technologies and image processing algorithms have significantly enhanced the effectiveness of remote sensing. Remote sensing techniques are particularly valuable when target species form large homogenous patches, exhibit distinctive features (e.g., flowers), or possess unique chemical properties (He et al., 2015; Bolch et al., 2020). Roca et al. (2022) demonstrated the effective use of multispectral remote sensing data from drones and satellites to monitor the IAS of EU concern R. okamurae, providing crucial information for decision-making and species management. However, remote sensing in aquatic ecosystems has limitations due to various confounding factors. To overcome these limitations, high radiometric quality in images, thorough calibration processes, hyperspectral information, customized image timing, and radiative transfer modeling are often required for adequate detection and differentiation of submerged and water column IAS (Bolch et al., 2020).
Furthermore, data mining from social media, although with severe limitations, has been proposed as a promising source of NIS data (Caley and Cassey, 2023).
An increasingly relevant amount of data to support decision-making and reporting against international targets comes nowadays from citizen science (Pocock et al., 2019). Citizen science observations, especially for charismatic and visible IAS, complement regular monitoring (Giovos et al., 2019; Lehtiniemi et al., 2020). Although citizen-based observations of birds have been utilized for over a century, citizen science has gained wider popularity since the late 20th century (Tulloch et al., 2013). Online applications and global platforms have garnered immense participation and contribute daily to global biodiversity data (Seltzer, 2019). For example, iNaturalist has contributed over 58 million research-grade observations to the Global Biodiversity Information Facility (GBIF) as of March 2023, and these data have been integrated successfully with scientific research for various purposes, evident in over 3,403 publications citing the dataset (Nugent, 2018). Citizen science in environmental monitoring not only compensates for resource limitations in generating comprehensive and up-to-date species presence databases but also holds value beyond data provision, gradually being incorporated into solutions and mitigation actions (Pocock et al., 2019; Ferreira-Rodríguez et al., 2021).
A recent survey identified 103 citizen science initiatives related to biological invasions across 41 countries that contribute to research, policy, and management (Price-Jones et al., 2022). Among the 31 initiatives specifically focused on marine environments, nearly half (47%) aimed to collect species presence or abundance data to map their distribution and spread. NIS detection for early warning programs (16%) and compiling species lists (14%) were also common objectives. Interestingly, citizens are increasingly involved in gathering more complex information, such as evidence of NIS impacts on biodiversity (11%) and generating experimental data for scientific hypothesis testing (5%).
The potential for citizen science to contribute to biodiversity monitoring, including biological invasions, is indisputable (Pocock et al., 2018). However, uncertainties arise during sampling design, data collection, and statistical analyses of citizen science data, as well as linguistic uncertainties that affect information interpretation (Probert et al., 2022). Limitations of citizen science data include accuracy and uneven spatial distribution of observers (Wiggins and Crowston, 2011). Data quality decreases when species are difficult to identify or quantify (Lewandowski and Specht, 2015), especially in cases of low density (false negatives) or co-existence with morphologically similar species (false positives) (Fitzpatrick et al., 2009). Furthermore, citizen science often provides presence-only records, limiting data usefulness for range expansion calculations or species distribution models (Peron et al., 2016). Recognizing these challenges, efforts have been made to address uncertainties and enhance data reliability in citizen science (Probert et al., 2022).
The most successful instances of marine citizen science focused on the Mediterranean Sea are exemplified by the CIESM Jelly Watch Program initiatives related to jellyfish blooms. These stand out as the most impactful marine citizen science endeavors in the Mediterranean, achieving extensive time coverage, broad geographic reach, and significant citizen participation, resulting in a substantial number of reports (>24,000 jellyfish presence records, and a total of 115,367 presence+absence records) (Marambio et al., 2021). In Italy alone, data collected from 2009 to 2014 comprised >15,000 presence records contributed to the discovery of new NIS for Italy and the western Mediterranean (e.g., Phyllorhiza punctata and Mnemiopsis leiydi, in Boero et al., 2009) and even the finding of a jellyfish species new to science—undisputedly classified as cryptogenic in the northern Adriatic Sea (Piraino et al., 2014).
Coupling citizen science with eDNA monitoring is a promising approach in both marine (e.g., Tøttrup et al., 2021; Agersnap et al., 2022; Suzuki-Ohno et al., 2023) and freshwater habitats (Biggs et al., 2015). Citizen science involvement in eDNA monitoring allows for broader geographical sampling and public engagement in biodiversity research (Agersnap et al., 2022), including educational benefits (Tøttrup et al., 2021; Knudsen et al., 2023). However, careful consideration is needed to mitigate the increased risk of sample contamination from unwanted DNA due to the inexperience of participants in eDNA protocols. Incorporating negative and positive controls in sample analysis can improve the validity of citizen science-based eDNA monitoring (Tøttrup et al., 2021). Another advantage of citizen science is the potential cost reduction associated with eDNA monitoring, as demonstrated by studies in Denmark where volunteers collected and filtered water, eliminating the need for a field biologist. Leveraging citizen science and traditional approaches for eDNA monitoring can enhance understanding of biodiversity loss and the impacts of climate change, similar to approaches used for terrestrial organisms (Hudson et al., 2014; Newbold et al., 2015; Outhwaite et al., 2022). Furthermore, eDNA monitoring has shown superior performance compared to traditional surveys, leading to its implementation in national surveys (De Brauwer et al., 2023; Kelly et al., 2023).
As the costs of invasions are high, there is a global need to predict invasions before they occur and to adjust monitoring or management policies (Wylie and Janssen-May, 2017). Several attempts have been made, mainly using species distribution models (SDMs) to predict favorable areas for species (e.g., Kotta et al., 2016; Liversage et al., 2019; Poursanidis et al., 2022) and assess the vulnerability of marine protected areas (MPAs) to IAS (e.g., D’Amen and Azzurro, 2020a; Stæhr et al., 2023). Additionally, studies have explored factors contributing to successful invasions, such as life-history traits or global invasion history (Vilizzi et al., 2019; Vilizzi et al., 2021; D’Amen et al., 2022; D’Amen et al., 2023).
When modeling and projecting species invasions, several challenges arise, such as the need to extrapolate to novel conditions due to the lack of analogous conditions in the invaded region (Mesgaran et al., 2014), niche pioneering (part of a species’ fundamental ecological niche observed only in its invaded range) or niche expansion (Atwater et al., 2018), and niche unfilling (niche space that is occupied in the native but unoccupied in the invaded domain) (Strubbe et al., 2013). Biased predictions can result from excluding limiting variables from models, e.g., ignoring the minimum winter temperature for thermophilic Lessepsian species (Dimitriadis et al., 2020). Ignoring these challenges led to biased predictions of the lionfish distribution in the Mediterranean Sea (Poursanidis, 2015; D’Amen and Azzurro, 2020a). For example, predictions by Johnston and Purkis (2014), based on a biophysical model, incorrectly suggested that the lionfish would not successfully invade the Mediterranean, but the subsequent rapid expansion of the species proved these predictions false (Dimitriadis et al., 2020; Poursanidis et al., 2020; Poursanidis et al., 2022).
Over the past two decades, modeling the fundamental ecological niche (i.e., ecological niche models) and correlating the presence or absence of species with environmental factors (i.e., SDMs) have gained popularity for projecting the expansion of marine IAS (for thorough reviews, see Marcelino and Verbruggen, 2015; Robinson et al., 2017; Melo-Merino et al., 2020). To enhance predictive accuracy and overcome inherent limitations associated with correlative modeling tools, several advancements have been proposed. Hybrid distribution models, incorporating physiological performance estimates (called physiology SDMs), outperformed regular SDMs and provided more realistic range shift forecasts for marine invaders (Gamliel et al., 2020). Similarly, applying temperature constraints on the reproductive phenology of invaders improved the predictions by niche models (Chefaoui et al., 2019). To account for niche variations between native and invaded ranges, models coupled with univariate niche dynamics projected shifts under novel conditions (D’Amen and Azzurro, 2020b). The hypothesis of phylogenetic conservatism of ecological niches, which posits that closely related species share similar or identical niches, has been applied through supraspecific modeling units, i.e., combining occurrences of focal IAS and sister species in their native ranges. This approach has enhanced projections of invasion potential (Castaño-Quintero et al., 2020).
Monitoring marine NIS, whether using traditional or molecular methods, often suffers from imperfect detectability, which can lead to false predictions of occupancy (Issaris et al., 2012; Darling et al., 2017). Several methods have been developed to estimate occupancy based on presence–absence data, considering the imperfect detection of the target species (MacKenzie et al., 2006). These methods involve multiple visits to each site and have been widely applied in all environments. Cost-efficient protocols for data collection through scuba diving or snorkeling and modeling occupancy in the marine environment have been developed, involving multiple observers (Issaris et al., 2012). Such approaches have been used to document cascading effects due to native-invasive species interactions (Dimitriadis et al., 2021) for large-scale multi-species monitoring efforts (Gerovasileiou et al., 2017; Crocetta et al., 2021) or for explaining IAS spatial patterns (Salomidi et al., 2013). In monitoring programs coupling molecular and traditional methods, site occupancy–detection (SOD) modeling holds great promise for converting eDNA-positive detections into robust estimates of species distribution (Darling et al., 2017). Positive correlations have been observed, for example, between eDNA levels and tidewater in SOD for a marine endangered goby on the Californian coast (Schmelzle and Kinziger, 2016) and between oxygen levels and eDNA from an endangered crayfish threatened by the expansion of introduced crayfish (Baudry et al., 2023).
It is crucial to anticipate future invasions and their risks for effective strategy and policy development, risk management, and research prioritization (Ricciardi et al., 2017; Vaz et al., 2021). In the framework of the IAS Regulation, an important horizon scanning study was conducted at the European scale, bringing together international experts to identify potential IAS in terrestrial, freshwater, and marine environments (Roy et al., 2019). From an initial list of 329 species, 66 were identified as very high, high, or medium risk for the EU, including 16 marine species (P. lineatus, Codium parvulum, Crepidula onyx, Mytilopsis sallei, Acanthophora spicifera, Perna viridis, Potamocorbula amurensis, Symplegma reptans, Ascidia sydneiensis, Balanus glandula, Ciona savignyi, Dictyospaeria cavernosa, Didemnum perlucidum, Dorvillea similis, Rhodosoma turcicum, and Zostera japonica). Tsiamis et al. (2020) developed a scoring tool that aims at identifying the most likely invasive species in European waters. In the Baltic Sea, Jensen et al. (2023) conducted a horizon scanning study that identified 38 potential IAS, with 31 species meeting the invasiveness scoring criteria by Tsiamis et al. (2020). That horizon scan was combined with hydrodynamic models to predict the potential spread of these species after arrival in commercial harbors and marinas. Dobrzycka-Krahel and Medina-Villar (2023) developed a stepwise tool to identify potential IAS in the less saline parts of the Baltic. In Cyprus, horizon scanning using expert elicitation identified 45 marine species with potentially adverse impacts on biodiversity, economy, or human health, such as the venomous fish P. lineatus, a species of EU concern (Peyton et al., 2019; Peyton et al., 2020).
The first large-scale assessment of marine NIS pathways of introduction was conducted a decade ago (Katsanevakis et al., 2013), based on the Hulme et al. (2008) pathway classification. With the use of the EASIN Catalogue (version 2.3), the assessment identified 1,369 marine NIS in European seas, with 1,257 associated with likely pathways of introduction. The study revealed a rising trend in new introductions, with shipping as the primary pathway for over half of the species. The second-most common pathway was marine and inland corridors, mainly the Suez Canal, with aquaculture and aquarium trade following in terms of the numbers of introduced species. Interestingly, aquaculture showed a notable decrease in new introductions from 2001 to 2010, attributed to regulatory measures at national and European levels (e.g., ICES, 2005; European Union (EU), 2007). In contrast, introductions through other pathways, particularly aquarium trade, showed a consistent increase. The assessment underscored the ongoing expansion of the Suez Canal and the reduced barriers to the entry of Red Sea species as factors that are likely to facilitate the invasion of the Mediterranean Sea by additional Lessepsian species. These Lessepsian species have been greatly facilitated by climate change, and the increased temperatures of the eastern Mediterranean and currently dominate demersal communities (Box 1).
The CBD Pathway Classification Framework has become a global standard in recent years (Convention on Biological Diversity [CBD], 2014; Harrower et al., 2018). It consists of six broad categories: Release, Escape, Transport-contaminant, Transport-stowaway, Corridors, and Unaided. These are subdivided into several subcategories. EASIN has incorporated the CBD classification of pathways based on expert assessments that addressed implementation challenges (Pergl et al., 2020). According to the latest data in EASIN (March 2023), the main pathways of NIS introductions in Europe are “Transport-stowaway” and “Corridors”, followed by “Transport-contaminant”, “Escape from confinement”, and “Release in nature” (Figure 3A). However, when considering only high-impact NIS (as defined in EASIN), species introduced through “Transport-stowaway” and “Transport-contaminant” appear to have a greater impact compared to those introduced through “Corridors” (Figure 3B).
Figure 3 Number of marine NIS (A) and high-impact NIS (B) in European Seas known or likely to be introduced by each of the main pathways, according to the CBD classification. Percentages add to more than 100%, as some species are linked to more than one pathway. High-impact NIS are according to the EASIN classification. Data retrieved from EASIN (March 22, 2023). NIS, non-indigenous species; CBD, Convention on Biological Diversity; EASIN, European Alien Species Information Network.
Quantifying changes in pathways over time and space is crucial for understanding the dynamics of species introductions (Essl et al., 2015). These changes are influenced by complex interactions between environmental and socioeconomic factors, species traits, and the regions involved. Nunes et al. (2014) investigated the spatial distribution of initial introductions of marine NIS in European Seas, including all Mediterranean countries. They identified key entry points for invasions based on distinct geographic patterns related to different pathways (Figure 4). Aquaculture introductions were prominent in France and Italy, Lessepsian species were primarily found in Levantine Sea countries, shipping introductions were widespread near major ports, and species introduced through inland canals were primarily observed in the southern Baltic countries (Katsanevakis et al., 2014a; Nunes et al., 2014). In the Mediterranean, the Suez Canal was the most important pathway, responsible for over half of marine NIS introductions (Zenetos et al., 2012), whereas in all other European Seas, shipping was the dominant pathway (Nunes et al., 2014).
Figure 4 Pathways of introduction for first European records of marine NIS per recipient country (i.e., countries of initial introduction in Europe). For clarity, data are shown for countries with more than two recorded first introduction events (numbers shown next to the charts). Adapted from Nunes et al. (2014). NIS, non-indigenous species.
Pathway assessments for NIS entry and spread involve uncertainties, particularly when introductions are unintentional and poorly documented (Essl et al., 2015; Katsanevakis and Moustakas, 2018). Examples include species traveling as ship stowaways or using canals as corridors. Assigning specific pathways for these species often relies on assumptions or ecological inferences rather than concrete evidence. Overlooked or insufficiently studied pathways, such as aquarium trade (e.g., Padilla and Williams, 2004; Vranken et al., 2018) and marine litter (e.g., Barnes, 2002; Carlton and Fowler, 2018; Barry et al., 2023), may have greater significance than currently recognized. Transparently addressing these uncertainties and providing estimates of pathway assignment uncertainty would be valuable (Zenetos et al., 2012; Katsanevakis et al., 2013). Clear and consistent pathway definitions and guidelines are essential to ensure consistent application by different assessors, which can be facilitated through a pathway manual.
Secondary pathways of spread within Europe are important but poorly studied. Unaided dispersal by ocean currents is the most important secondary pathway, often surpassing primary pathways in importance. In the Aegean Sea, unaided dispersal from neighboring countries accounted for 56% of NIS introductions, followed by “Transport-stowaway” (35%) (Katsanevakis et al., 2020). In the Baltic Sea, shipping and natural NIS spread from the North Sea dominate among the pathways for established NIS (Ojaveer et al., 2017).
IAS impact and risk assessments are increasingly demanded by managers for informed decision-making. Risk screening can help identify species with invasive potential in the area of interest, requiring further analysis of their potential impacts (Ricciardi and Rasmussen, 1998; Copp et al., 2005). IAS often share life-history traits, such as frequent reproduction, large body size, long life span, high degree of omnivory, and a climate match with the area of interest (Statzner et al., 2008; Chan et al., 2021). Moreover, invasive species tend to have broad tolerance to abiotic conditions (Leuven et al., 2009) and a history of being invasive in other regions.
Several protocols exist for IAS impact and risk assessment, such as BPL/BINPAS (Olenin et al., 2007; Narščius et al., 2012), EICAT (Hawkins et al., 2015), SEICAT (Bacher et al., 2018), FISK and related tools (Copp et al., 2005; Copp, 2013), GABLIS (Essl et al., 2011), GB-NNRA (Baker et al., 2008), GISS (Nentwig et al., 2016), Harmonia+ (D’hondt et al., 2015), ISEIA (Branquart, 2009), and NGEIAAS (Sandvik et al., 2013). These tools rank taxa based on their threat level in the risk assessment area at a specified spatial scale. Until recently, there was no standardized and evidence-based system to classify the positive impacts of alien species; EICAT+ covered this gap by offering a protocol to categorize the magnitude of positive NIS impacts (Vimercati et al., 2022). The screening tools vary in objectives, taxonomic resolution, and target (e.g., specific habitats or pathways), as well as complexity, approaches to assess uncertainty, and scoring systems used. These variations may result in significant differences and inconsistencies in the assessment outcomes; selection of assessors, clear assessment guidelines, and adequate training are important in addition to arriving at final decisions collaboratively by consensus (González-Moreno et al., 2019).
A pan-European systematic review of NIS impacts (Katsanevakis et al., 2014b) identified 87 marine species in Europe with documented high impacts on biodiversity or ecosystem services. The study revealed that food provision was the most affected ecosystem service both positively and negatively. Other services negatively affected included ocean nourishment, recreation and tourism, and life cycle maintenance, while cognitive benefits, water purification, and climate regulation were among the services often positively impacted. Additionally, 49 assessed species were considered ecosystem engineers, altering habitats through physical or chemical modifications. The study acknowledged a potential bias against NIS, suggesting that positive impacts might be underestimated.
Tsirintanis et al. (2022) studied the impacts of biological invasions on biodiversity, ecosystem services, and human health in the Mediterranean Sea. They identified various biological mechanisms through which NIS affect Mediterranean ecosystems, resulting in both negative and positive impacts (Figures 5, S1; Table S1). Negative impacts on biodiversity were primarily due to competition for resources, followed by the creation of novel habitats and predation (Figure 5; Table S1). NIS structural ecosystem engineers can completely transform seascapes and substantially change community composition, leading to the loss of native species (García-Gómez et al., 2021; Mancuso et al., 2022). Alien predators and grazers cause significant negative impacts on Mediterranean ecosystems by consuming native biota (Sala et al., 2011; Kampouris et al., 2019). Predator–prey interactions in the marine environment are dynamic ecosystem processes influenced by local environmental factors and species’ ecological features, capable of affecting multiple food-web levels (Rilov, 2009).
Figure 5 Mechanisms (outer circle) of IAS impacts on biodiversity, ecosystem services, and human health (inner circle) in the Mediterranean Sea (circle compartment size corresponds to sample size). Based on Tsirintanis et al. (2022). IAS, invasive alien species.
Biofouling is the primary mechanism of negative impacts on provisioning services, with many IAS densely colonizing aquaculture facilities and reared species, leading to significant economic losses (Tsotsios et al., 2023). IAS also greatly impact cultural services through the degradation of highly valued habitats, algae massively washed ashore, and jellyfish blooms reaching coastal waters, negatively affecting tourism (e.g., Ghermandi et al., 2015; Ruitton et al., 2021). Habitat degradation is the primary mechanism through which IAS negatively impact regulating services. Regarding human health, IAS primarily cause negative impacts through stinging or poisonings/intoxications (Galil, 2018; Bédry et al., 2021) (Figure 5; Table S1).
Many positive NIS impacts have been reported in the European Seas (Katsanevakis et al., 2014b; Tsirintanis et al., 2022). In the Mediterranean, provisioning services benefit the most from NIS introductions through the provision of new commodities. Various fish, mollusks, and crustaceans have proven a boon for the fisheries and aquaculture sector, especially in the Levantine Sea (e.g., Katsanevakis et al., 2018). The creation of novel habitats is the most important mechanism of positive effects on biodiversity, as alien structural ecosystem engineers provide new habitats and shelter for various species through the formations they create (Katsanevakis et al., 2014b; Guy-Haim et al., 2018; Figure 5). Cultural services are positively affected by research conducted on NIS specimens for future potential exploitation of molecules for pharmaceutical or industrial applications (e.g., Genovese et al., 2012; Nekvapil et al., 2019). Regarding regulating services, the creation of novel habitats, carbon sequestration, and biofiltration are the most important mechanisms contributing to positive impacts (Figure 5; Table S1).
Evidence of reported impacts is mostly of medium strength (Figure 6; Table S1), predominantly from direct observations (e.g., novel habitat creation, competitive overgrowth of sessile organisms, or predation effects derived through stomach content analysis), followed by non-experimental-based correlations between a species presence/abundance and an impact, and modeling to project impact consequences (Figure 6; Table S1). Many reported impacts are only based on expert judgment. Only a small percentage of NIS impacts are supported by robust evidence from manipulative or natural experiments (Katsanevakis et al., 2014b; Tsirintanis et al., 2022; Figure 6).
Figure 6 Type of evidence of IAS impacts on biodiversity, ecosystem services, and human health in the Mediterranean Sea. Based on Tsirintanis et al. (2022). IAS, invasive alien species.
Several indices have been developed to assess NIS ecological impacts and ecological status considering NIS presence. ALEX (ALien biotic indEX; Çinar and Bakir, 2014) evaluates NIS impacts on benthic communities, aligning with the EU Water Framework Directive classification system; Piazzi et al. (2015) also recommended its application. ECOfast, an ecological evaluation index for shallow rocky reefs, was recently developed (Kytinou et al., 2023). ECOfast-NIS, a variant of this index, penalizes the presence of certain NIS that have negative impacts on local food webs. CIMPAL (Cumulative IMPacts of invasive ALien species) is a conservative additive model based on IAS and habitat distributions, reported magnitude of ecological impacts, and the strength of such evidence (Katsanevakis et al., 2016). CIMPAL has been implemented for the Mediterranean Sea (Katsanevakis et al., 2016), the European scale (Teixeira et al., 2019), and other marine regions like Maltese waters (Bartolo et al., 2021) and the Aegean Sea (Tsirintanis et al., 2023).
Despite extensive negative impacts, global documentation of marine IAS-related extinctions remains scarce. A recent global review on drivers of marine extinctions reported IAS as responsible for 27 out of 786 extinction cases (seven global and 20 local extinctions) (Nikolaou and Katsanevakis, 2023). Among the seven globally extinct species due to IAS, six were seabirds and one was a diadromous fish, while the invasive species causing the extinctions were not marine (e.g., invasive rats). In many reported extinctions, IAS were not the sole driver, and their contribution was often unknown, introducing uncertainty about their actual role as the cause of extinctions. The Mediterranean-endemic fan mussel Pinna nobilis is an example of IAS-related local extinctions in Europe. It experienced extensive local extinctions due to infection by the newly described protozoan Haplosporidium pinnae (likely introduced by shipping), putting the species at risk of global extinction (Katsanevakis et al., 2022). It is now critically endangered in the Red List (Kersting et al., 2019).
Although complete species extinction due to biological invasions is rare in the marine environment, dramatic declines in populations caused by predation or parasitism can lead to functional extinction (Boero et al., 2013). For instance, in the Baltic Sea, the invasion of the round goby Neogobius melanostomus resulted in a significant decline in the population of blue mussels (Mytilus edulis trossulus), leading to the disappearance of the mussel-created biotope, which served as a crucial habitat for wintering bird populations (Skabeikis et al., 2019).
The predatory impacts of IAS are often focused on, with most studies emphasizing the top-down predatory effects of invaders on native prey, although many species play both predator and prey roles in the ecosystem. The prey role is particularly interesting since nearly all NIS eventually become subject to predation by native predators, which can even lead to the control of IAS populations (e.g., Hunt and Yamada, 2003; Jensen et al., 2007), a process that often takes time (Santamaría et al., 2022). For example, in the Chesapeake Bay, USA, native blue crabs exert predation pressure on the invasive green crab to the point where there are no green crab populations left (DeRivera et al., 2005). In many cases, native predators may even benefit from the new prey (Crane et al., 2015; Pintor and Byers, 2015). Conversely, there are instances where the increased invasive resource leads to an increase in predator populations and results in increased predation on native species (Noonburg and Byers, 2005).
Prey naivety toward invasive predators has been extensively studied and documented (e.g., Sih et al., 2010; Anton et al., 2020). However, less focus has been given to the naivety of predators, although similar naivety may occur, especially toward novel prey (Reid et al., 2010; Santamaría et al., 2022). This can be particularly noticeable during the early stages of invasion, resulting in lower predation pressure on the novel species compared to native, more familiar prey (e.g., Carlsson et al., 2009; Santamaría et al., 2022).
Prevention of IAS introductions is the first line of defense (Olenin et al., 2011; Katsanevakis, 2022). According to Article 13 of the IAS Regulation, EU Member States need to “carry out a comprehensive analysis of the pathways of unintentional introduction and spread of invasive alien species of Union concern” in their marine waters and “establish and implement one single action plan or a set of action plans to address the priority pathways”.
To prevent introductions through shipping (Transport-stowaway), the most important pathway of marine introductions in the EU (Figure 3), a critical development was the entry into force of the IMO BWMC in 2017. The BWMC mandates all ships to adopt a ballast water management plan and, by September 2024, treat their ballast waters with an approved ballast water treatment system to diminish the survival probabilities of ballast water-transferred marine organisms. Although enforcement of the BWMC is challenging, it is expected to substantially reduce new introductions via ballast waters. In contrast, biofouling is currently regulated only voluntarily. The IMO’s Biofouling Guidelines (Resolution MEPC.207(62)2011) aim to establish a globally consistent approach to biofouling management. However, there is growing support for a new Biofouling IMO Convention, with intensive research focusing on efficient biofouling systems, including surveillance optimization (e.g., Abdo et al., 2018; Luoma et al., 2022) and hull cleaning (e.g., Morrisey and Woods, 2015; Zabin et al., 2016).
Corridors, particularly the Suez Canal, rank as the second-most significant introduction pathway in Europe (Figure 3). However, managing the Suez Canal to control invasions (e.g., implementing a salinity barrier or establishing locks to reduce current movement) falls beyond EU jurisdiction, and there is no political will from Egypt or the Barcelona Convention to undertake such measures (Galil et al., 2017). Nonetheless, there are arguments that consider climate change impacts in the eastern Mediterranean, Lessepsian species may not pose the primary threat to biodiversity and ecosystem services; instead, they could potentially play a role in securing ecosystem functions and services (see Box 1).
Regulation 708/2007 “concerning the use of alien and locally absent species in aquaculture” (European Union (EU), 2007) has been an important instrument for reducing aquaculture-introduced species. It was implemented well before the IAS Regulation, based on the ICES Code of Practice on the Introductions and Transfers of Marine Organisms (ICES, 2005), and resulted in a noticeable decline in new introductions (Katsanevakis et al., 2013). In contrast, the aquarium trade, a lesser but growing pathway (Zenetos and Galanidi, 2020), lacks EU-level regulation, leading to continued risks of new introductions. Numerous potentially invasive marine species are traded in EU markets (e.g., Mazza et al., 2015; Vranken et al., 2018).
In a recent systematic review of implemented species-specific eradication and control measures for marine IAS, only 31 studies covering 40 cases were found, of which 11 failed to achieve eradication or control targets (Table S2; Katsanevakis, 2022). These studies mainly focused on macroalgae (10), ascidians (7), and fish (seven; all related to lionfish). Physical methods were most commonly used (e.g., removal by divers, mechanical removal by trawling, dredging, or suction, jute matting, heat treatment, using traps, or promoting targeted fisheries), followed by chemical (using various chemicals such as bleach, herbicides, salt, acetic acid, copper sulfate, and sodium hypochlorite) and biological methods (using native predators or parasites).
Only six successful eradication cases have been reported in the global literature (Table S2): sodium hypochlorite used to eradicate the green alga Caulerpa taxifolia from California, USA (Anderson, 2005); physical removal by divers to eradicate the brown alga Ascophyllum nodosum from Redwood City, California, USA (Miller et al., 2004); a combination of physical removal by divers and heat treatment to eradicate the brown alga Undaria pinnatifida from a sunken trawler in Chatham Islands, New Zealand (Wotton et al., 2004); eradication of the sabellid polychaete Terebrasabella heterouncinata from an intertidal site in California, USA, by removing its main native host (Culver and Kuris, 2000); extensive chemical treatment with 187 tonnes of liquid sodium hypochlorite and 7.5 tonnes of copper sulfate to eradicate the mussel M. sallei from three sheltered marinas in the Darwin Harbour Estuary (Northern Territory, Australia) (Bax et al., 2002); and dredging to eradicate the invasive mussel Perna perna from a subtidal soft-sediment habitat in central New Zealand (Hopkins et al., 2011). Remarkably, successful eradication efforts have been reported only from the USA, New Zealand, and Australia; no successful eradication of a marine IAS from the EU has been reported.
In the EU, only four related studies appear in the literature (Uchimura et al., 2000; Žuljevic et al., 2001; Mancinelli et al., 2017; Kleitou et al., 2021). The first three are experimental investigations or proposals of control approaches, lacking large-scale implementation. Only the latter (Kleitou et al., 2021) made an effort to control lionfish populations in Cyprus, with partial success; lionfish removals significantly decreased its density and biomass (by >50%) in the short term, but long-term suppression requires repeated removals due to rapid population recovery. Another unpublished control effort in the EU (also from Cyprus) is the case of the silver-cheeked toad-fish Lagocephalus sceleratus, a toxic predatory fish with serious impacts on fisheries and human health. A targeted fishery by the small-scale fleet was promoted through fishers’ compensation based on the fished and incinerated biomass (Table S3). Although there has been no targeted monitoring to assess the measure’s effectiveness, empirical evidence from fishers supports its success in reducing the species’ biomass and mitigating its impacts; food web modeling indicates that L. sceleratus populations could have been higher without any measures, and continuous management is necessary to prevent the population’s rebound at high levels (Michailidis et al., 2023).
The only two species for which large-scale control efforts have been implemented in the EU (Pterois miles and L. sceleratus) have not been included in the IAS list of Union Concern of the IAS Regulation. Both species were proposed, but their inclusion in the latest (2022) update of the list was not approved. Conversely, there are no known successful control efforts for the only two marine species included in the Union List, i.e., the fish P. lineatus and the alga R. okamurae (Supplementary Text 1; Table S4). This highlights an inconsistency between the criteria for inclusion in the Union List (which may secure EU or national funding for management efforts) and existing applicable management options for specific marine IAS.
Reported successful eradication or control efforts globally (Katsanevakis, 2022) have highlighted several best practices (Table S2). The most critical factor for eradication success is a rapid response after detection (e.g., Bax et al., 2002; Miller et al., 2004; Anderson, 2005; Hopkins et al., 2011); delayed responses compromised many eradication efforts (e.g., Read et al., 2011; Sambrook et al., 2014). Developing rapid response mechanisms among EU member states (largely missing) is essential for successful eradication. Missing the critical time window for a rapid response makes eradication from the marine environment practically impossible. Once an IAS is established, alternative management strategies beyond eradication should be explored, essentially focusing on control (see Section 7.3) or considering the option of non-intervention (ignore).
Other best practices for successful eradication or control include flexibility in amending existing legislation (Bax et al., 2002); good coordination among local, regional, and national authorities and stakeholders (Anderson, 2005); effective communication with stakeholders and the local community to gain public support (Bax et al., 2002; Wotton et al., 2004); adequate and continuous funding (Wotton et al., 2004; Anderson, 2005; Hopkins et al., 2011; Sambrook et al., 2014); continuous monitoring (Culver and Kuris, 2000; Miller et al., 2004; Wotton et al., 2004; Anderson, 2005; Kleitou et al., 2021); and a good knowledge of biology and ecology of the IAS and underlying ecological theory to select appropriate eradication/control methods (Culver and Kuris, 2000; Wotton et al., 2004; Anderson, 2005; Hopkins et al., 2011; Green et al., 2014; Harris et al., 2020).
Managing marine IAS is more challenging than terrestrial and freshwater species due to the increased functional connectivity of the oceans (Kinlan and Gaines, 2003; Katsanevakis, 2022). Nevertheless, several management measures have been implemented (Section 7.2; Table S2), and potential additional options have been investigated (e.g., Thresher and Kuris, 2004; Giakoumi et al., 2019; Katsanevakis, 2022) (Table 6). The applicability of these measures depends on factors, such as effectiveness, technical feasibility, social acceptability, side impacts on native communities, and cost (Giakoumi et al., 2019). Some options, such as biological control using alien predators, parasites, or viral diseases, are strongly opposed by experts and stakeholders due to fears of irreversible detrimental side effects on native biodiversity. Despite their low expected effectiveness, soft measures like “education and awareness” or “environmental rehabilitation”, and inaction were ranked high by experts (Thresher and Kuris, 2004; Giakoumi et al., 2019). Commercial utilization of IAS has been widely suggested as a means of turning mitigation costs into profits for local populations (Mancinelli et al., 2017). Targeting and eating invaders, such as the lionfish (Kleitou et al., 2022), offers several supplementary advantages, such as raising public awareness about IAS and encouraging citizen participation in identifying new populations and engaging in other control measures (Nuñez et al., 2012).
A striking result reported by Thresher and Kuris (2004) was that the perceived likelihood of success of management options was negatively correlated with their acceptability. This suggests the need to enhance the effectiveness of existing techniques or increase the acceptability of potentially effective techniques (e.g., biological control and genetic technology to decrease pest viability) or develop new techniques that are both acceptable and effective (Thresher and Kuris, 2004).
Climate change, primarily ocean temperature increases, may facilitate the introduction and establishment of thermophilic NIS. It can also amplify the impacts associated with IAS, reducing the fitness of thermally sensitive species and thereby decreasing the resilience of native species, habitats, and ecosystems (Birchenough et al., 2015). The Mediterranean Sea, a semi-enclosed basin experiencing rapid warming compared to other marine regions (Schroeder et al., 2016), is a hotspot for bioinvasions by thermophilic Red Sea species (Costello et al., 2021; Box 1). In the Mediterranean, it was shown that an alien intertidal gastropod is much more resilient to warming than three native gastropod species, which may disappear in the future, leaving it the only large mollusk grazer in the region (Rilov et al., 2022).
Higher rates of between-continent dispersal events due to increasing international trade and human traveling are expected (Hewitt et al., 2018; Sardain et al., 2019; Roura-Pascual et al., 2021). For marine ecosystems, trade/transport and climate change are considered by invasion scientists as the primary drivers of IAS impacts until 2050 (Essl et al., 2020). The combined effects of climate and rapid transport could result in large-scale biotic homogenization, potentially exceeding the impact of either climate change or IAS acting alone due to context-dependent interactions (Gissi et al., 2021). Despite global climate change often facilitating IAS (Dukes and Mooney, 1999), these two issues are mostly treated independently (Pyke et al., 2008).
Climate change may affect IAS introduction pathways and vectors (Robinson et al., 2020). Melting Arctic ice caps have already facilitated new, faster shipping routes, connecting previously isolated ports and regions and increasing the chances of propagules surviving transit (Pyke et al., 2008; Miller and Ruiz, 2014; McCarthy et al., 2022). Climate change can also alter shipping connectivity by affecting trading patterns and tourism destinations, leading to increased propagule pressure in some locations and decreased pressure in others.
The effects of ocean climate change and acidification on NIS introductions and impacts are frequently discussed in the literature (Occhipinti-Ambrogi, 2021). However, causal effects are not well-documented. Studies that aimed to elucidate the influence of climate change on NIS tend to focus on the impact of increasing ocean temperature, with less attention to non-thermal factors associated with climate change (e.g., ocean acidification, salinity, dissolved oxygen, weather events, and hydrodynamic changes). Furthermore, limited research evaluates the effects of multiple factors and their interactions (Gissi et al., 2021), restricting our ability to robustly predict future IAS impacts.
Marine species are expected to undergo a general poleward expansion due to seawater warming (Pinsky et al., 2013; Poloczanska et al., 2016; Essl et al., 2019). Some evidence suggests that climate-related changes are increasing IAS abundances in marine systems (Sorte et al., 2010; García-Gómez et al., 2020; Stæhr et al., 2020). Among the different pathways of NIS introductions, the poleward expansion linked to ocean warming would be most relevant for secondary introductions. This is because southern seas, as hotspots of NIS introductions, could serve as source populations for further introductions to northerly regions as temperature conditions gradually become favorable there.
The rate of new NIS has significantly increased since the early 1980s (e.g., Zenetos et al., 2022; Jensen et al., 2023) possibly influenced by climate change-induced warming of the sea. Recent studies suggest a higher rate of new NIS arrivals in southern seas (Tsiamis et al., 2018; Tsiamis et al., 2019; Zenetos et al., 2022), with up to 76% of all NIS primary introductions in Europe originating from the Mediterranean Sea. This suggests the importance of secondary non-assisted dispersal for many observed NIS species in northern regions. In the OSPAR regions, secondary introductions accounted for only 5% of NIS introductions (Stæhr et al., 2022). The influence of climate-related warming on NIS introductions is not generally strongly supported by data and requires targeted species-specific analysis for the different European regions.
Climate change can alter the effectiveness of IAS management. Temperature dictates the life cycle of many IAS, influencing maturation, reproduction, establishment, and persistence (e.g., King et al., 2021; Teixeira Alves et al., 2021), with implications for eradication and population control under climate change. Some studies suggest that mechanical control of IAS becomes less efficient under climate change pressure (Hellmann et al., 2008; Pyke et al., 2008; Kernan, 2015), necessitating increased management efforts to achieve the same management goals (Teixeira Alves and Tidbury, 2022). Further research is needed to understand how both thermal and non-thermal factors of climate change influence IAS management.
In climate change hotspots, particularly in land-locked basins, such as the Mediterranean Sea, native biodiversity decline due to climate change may compromise ecosystem functioning and services (see Box 1). In such cases, thermophilic NIS could play a significant role in sustaining ecosystem functioning and services. As a result, a change in conservation goals has been proposed, moving from protecting native biodiversity to protecting functions and services (Rilov et al., 2019a; Rilov et al., 2020). Similarly, Reise et al. (2023) highlighted that some NIS in the Wadden Sea positively contributes to sediment stabilization, mud accretion, and diversification of lower food web levels, potentially benefiting foraging birds. They argued that these NIS have raised the tidal ecosystem’s capacity to adapt to environmental change rather than degrade it.
Global damage and management costs associated with biological invasions have exponentially increased in the last 50 years (Diagne et al., 2020). However, the allocation of economic resources toward invasive species prevention, control, research, long-term management, and eradication measures needs a substantial increase to offset the economic losses caused by direct and/or indirect impacts of invaders (Diagne et al., 2021).
The extensive economic impacts of invasions, reaching beyond administrative and national scales, highlight a clear discrepancy between the implementation of international agreements (Table 1) by local authorities and the achievement of broad policy objectives. Enhancing governance, encompassing the capacity to implement policies through expertise and resources, is crucial for preventing and managing biological invasions and their impacts. International initiatives and European Institutions play a critical role in supporting and expediting measures that require local implementation but rely on effective global and regional coordination. Strategic planning and securing adequate funding will be central to addressing many of the challenges raised in this review.
Currently, only two marine species (P. lineatus and R. okamurae) are included in the List of Invasive Alien Species of Union Concern, which does not reflect the status of marine biological invasions in the EU. Marine NIS thrive in the European seas, with EASIN listing 1,602 alien or cryptogenic species, while globally, WRiMS and AquaNIS currently report 2,781 and 2,028 species, respectively. The Mediterranean Sea, in particular, is a hotspot of biological invasions, harboring more NIS than any other sea globally (Costello et al., 2021). Many of these species are invasive, significantly impacting biodiversity, ecosystem services, and human health (Katsanevakis et al., 2014b; Tsirintanis et al., 2022). An EU horizon scanning exercise identified 18 marine species absent from or with a limited distribution in EU marine waters as potential candidates for inclusion in the list based on their impacts and management feasibility (Tsiamis et al., 2020). However, it seems that member states hesitate to include marine species in the list of IAS of Union Concern, assuming that management of marine IAS is impossible. As indicated in previous sections, managing marine IAS is more challenging than terrestrial or freshwater species, but it is not impossible. Hence, the current list of IAS of Union Concern does not fully acknowledge the threat marine IAS pose to the EU marine environment, and it needs to be supplemented based on current scientific advice and risk assessments (as per Article 5 of the IAS Regulation).
Ideally, a robust biosecurity system should encompass all three steps of the invasion process (pre-border, border, and post-border) and implement effective and timely interventions, drawing from countries with established cutting-edge biosecurity programs (Carvalho et al., 2023). New Zealand, Australia, and the USA have well-established biosecurity systems, as evidenced by their successful cases of marine IAS eradication or control (Table S2). For example, New Zealand’s Marine Biosecurity Team, operating under the Ministry of Fisheries since 1998, conducts various activities such as quarantine, surveillance, response to incidents, long-term control of established pests, and enforcement of legislation (Hewitt et al., 2004). Europe could benefit from the experience, learning from both successes and failures in managing IAS in these countries. Organizing workshops and meetings involving high-level policymakers, marine scientists, managers, and officials from various countries can promote collaborative knowledge sharing and mutual learning to enhance global marine IAS management.
Adequate EU funding is crucial to sustain key databases and online information systems. EASIN plays a central role in harmonizing and integrating information on NIS in Europe. It primarily acts as an aggregator that gathers data from various sources and provides efficient tools and services for access to harmonized datasets. However, the foundation of the European infrastructure relies on national institutions, local and regional networks, and online databases and initiatives. These entities are essential data suppliers to centralized systems like EASIN. Therefore, it is critical to financially support and sustain national institutions and scientific networks to ensure the continuous flow of information, knowledge, and expertise to EASIN and the scientific community.
NIS information systems should be multipurpose, following the principle of “gather data once, utilize it many times”. In addition to operational usage, these systems should continuously accumulate data for analysis and forecasting. Ideally, this should not only include information on species occurrences but also offer search functions for NIS biological traits, environmental tolerance limits, and their impacts on native biodiversity, ecosystem functioning, economy, and public health.
Continued efforts to increase the spatial and temporal coverage of marine IAS monitoring, as well as transboundary cooperation, are required. Aligned with the ethos of “take once, use many” and driven by the application of novel techniques such as eDNA, automated monitoring, and even citizen science, integration of NIS monitoring with other biodiversity monitoring programs, where applicable, is an opportunity to balance data collection against increasing costs/declining budgets. Automated eDNA monitoring (Hansen et al., 2020; Preston et al., 2023) and using citizen science for monitoring eDNA also have associated difficulties (Agersnap et al., 2022; Knudsen et al., 2023), and the eDNA metabarcoding itself (Fonseca, 2018) and species-specific eDNA detection are not without pitfalls and problems with interpretation (Klymus et al., 2019). The data analysis required when eDNA is to be interpreted is often complicated and is better off being aided by taxonomic experts who are familiar with the organisms known to inhabit the sampled area. Further, streamlining marine NIS data flow and reducing data time lags will enhance early warning systems and facilitate rapid response. Understanding introduction pathways is also crucial for implementing effective prevention measures and reducing new introductions.
Several studies have considered how man-made structures (offshore wind farms, wrecks, and oil and gas platforms) could act as de facto MPAs, facilitating colonization by both native and NIS (Birchenough and Degraer, 2020). It is important to highlight that the presence of these man-made structures will alter the species pool, with repercussions for trophic interactions (Mavraki et al., 2019) and secondary production, and may also serve as stepping stones for range-expanding (sometimes non-indigenous; Kerckhof et al., 2011) species altering population connectivity patterns (Henry et al., 2018; Coolen et al., 2020).
Accurately predicting the potential distribution of invasive species is crucial for global marine conservation. To improve predictions, it is imperative to consider the biological characteristics and distribution of the species, biotic interactions, and environmental conditions. Incorporating intrinsic traits in modeling can prove advantageous, as these traits can either facilitate higher adaptation rates or impose limitations on the invasion process (Gamliel et al., 2020). More data from both native and invaded ranges enhance prediction accuracy, allowing for a better assessment of the role of environmental factors in distribution and expansion potential. Removing noisy or uncertain predictors can further increase model accuracy. Integrating invasion dynamics like biotic interactions, dispersal limitations, and adaptation potential can inform potential niche conservatism violations (D’Amen and Azzurro, 2020b; Liu et al., 2020). As a final note, when selecting modeling approaches (e.g., correlative, mechanistic, and process-oriented), careful consideration of available input data accompanied by rigorous validation is essential (Melo-Merino et al., 2020).
Cumulative impact assessments of invasive species are valuable for several reasons. They offer a comprehensive understanding of the combined effects of multiple IAS on marine ecosystems, aiding policymakers and managers in understanding the extent and severity of ecological disturbances. This knowledge is crucial for devising effective strategies to prevent new invasions and mitigate existing impacts. As marine management shifts toward ecosystem-based spatial approaches, cumulative impact assessments become essential tools. They facilitate the integration of spatial information into environmental decisions and the setting of specific operational objectives. By identifying highly impacted areas, resources can be directed toward priority zones or targeted management actions for IAS.
Comprehensive large-scale analyses of the impacts of all alien marine species are urgently needed. Policymakers and managers, particularly in regions like the European Union, require a better understanding of invasive species’ impacts to meet environmental protection goals. Despite limitations and uncertainties in impact assessments, the adaptive management approach, involving monitoring, filling data gaps, and learning from management actions, offers a way to address and manage IAS impacts over time. In a limited-funding environment, decision-makers can efficiently allocate resources by focusing on sites, pathways, and species with high impacts and low uncertainty, increasing the chances of success in mitigating IAS effects. These initial successes can motivate further efforts to address biological invasions.
NIS have become a permanent component of contemporary ecosystems, and their potential benefits on ecosystem services, human wellbeing, and biodiversity should be thoroughly investigated (Schlaepfer et al., 2011; Vimercati et al., 2020; Vimercati et al., 2022). Many invasion studies are biased toward perceiving alien species as harmful due to their history of detrimental effects on ecosystems. Some reported negative impacts supported by limited strength of evidence may be influenced by this bias (Katsanevakis et al., 2014b; Tsirintanis et al., 2022), affecting impact assessments (e.g., compare Figures 5 and Figure S1). Scientists should adopt holistic approaches, considering both the negative and positive consequences of IAS on recipient ecosystems, relying on substantial evidence. The role of NIS in marine conservation, restoration, and securing ecosystem functioning and services, particularly in climate change hotspots, deserves serious consideration (see Box 1) (Mačić et al., 2018; Rilov et al., 2019a; Rilov et al., 2020).
In such regions heavily impacted by climate change, such as the eastern Mediterranean, IAS commercial exploitation becomes not merely a management choice but an essential measure to ensure the fishing industry’s viability and safeguard seafood supply from the ocean (Katsanevakis, 2022). However, in other regions, there are risks associated with promoting commercial utilization of IAS, and initiatives aimed at controlling IAS through human consumption should be carefully evaluated, as they could produce unintended outcomes contrary to their goals (Nuñez et al., 2012; Katsanevakis, 2022). This shift in perception could lead to illicit attempts to spread IAS to new areas, ultimately exacerbating their invasive potential (Mancinelli et al., 2017). Furthermore, it might create pressure to maintain and sustainably exploit these problematic species (Nuñez et al., 2012), as has happened in the cases of Rapana venosa in the Black Sea (Demirel et al., 2021) and the invasive red (Kamchatka) king crab (Paralithodes camtschaticus) fishery in the Barents Sea (Spiridonov, 2018).
Bioprospecting involves identifying and extracting new bioactive compounds with various potential applications, such as biomedicine, human health, food provision, nutraceuticals, cosmeceuticals, and the search for anti-fouling and antimicrobial agents. Managing IAS through prospecting can turn a threat into a resource, as demonstrated by growing research on invasive species, e.g., alien or native jellyfish (see Leone et al., 2015; Leone et al., 2019; De Domenico et al., 2023, and references therein), alien macroalgae (Misal and Sabale, 2016; Vitale et al., 2018; Cherry et al., 2019; Meinita et al., 2022), and even the poisonous L. sceleratus (Çavaş et al., 2020).
SK: Conceptualization, Funding acquisition, Investigation, Visualization, Writing – original draft, Writing – review & editing. SO: Conceptualization, Visualization, Writing – original draft, Writing – review & editing. RP-D: Conceptualization, Writing – original draft, Writing – review & editing. GR: Conceptualization, Writing – original draft, Writing – review & editing. PS: Conceptualization, Writing – original draft, Writing – review & editing. HTe: Conceptualization, Writing – original draft, Writing – review & editing. KT: Visualization, Writing – original draft, Writing – review & editing. SB: Conceptualization, Writing – original draft, Writing – review & editing. HJ: Conceptualization, Writing – original draft, Writing – review & editing. SWK: Writing – original draft, Writing – review & editing. AL: Data curation, Writing – original draft, Writing – review & editing. AM: Writing – original draft, Writing – review & editing. SP: Writing – original draft, Writing – review & editing. HTi: Writing – original draft, Writing – review & editing.
The author(s) declare financial support was received for the research, authorship, and/or publication of this article. This research was supported by the European Union’s Horizon 2020 research and innovation program HORIZON-CL6-2021-BIODIV-01-04 under grant agreement No. 101059877 “GES4SEAS - Achieving Good Environmental Status for maintaining ecosystem SErvices, by ASsessing integrated impacts of cumulative pressures”. GR and SP were supported by the European Union’s Horizon Europe research and innovation program HORIZON-CL6-2021-BIODIV-01-04 under grant agreement No. 101060072 “ACTNOW” - Advancing understanding of cumulative impacts on European marine biodiversity, ecosystem functions, and services for human wellbeing”. HTe was funded by FCT-Foundation for Science and Technology, I.P., under the project CEECIND/08095/2022 and CESAM FCT/MCTES (UIDP/50017/2020+UIDB/50017/2020+ LA/P/0094/2020). Funding for SB and HTe at Cefas was provided under the auspices of UKRI, Innovate UK through the Horizon Europe Guarantee scheme, grant number 10040226.
We would like to thank Dr. Irina Makarenko from the Permanent Secretariat of the Commission on the Protection of the Black Sea Against Pollution for providing information on current IAS-related policies in the Bucharest Convention and the EASIN team for providing their latest catalog of marine IAS (used for the analysis depicted in Table 5).
The authors declare that the research was conducted in the absence of any commercial or financial relationships that could be construed as a potential conflict of interest.
The author(s) declared that they were an editorial board member of Frontiers, at the time of submission. This had no impact on the peer review process and the final decision.
Box 1. The Levant bioinvasion and climate change hotspot: a look into the future of Mediterranean biodiversity
The southeastern Mediterranean, known as the Levantine basin or the Levant, is probably the most invaded region of the global ocean (Edelist et al., 2013; Costello et al., 2021). It is also one of the fastest-warming regions (Ozer et al., 2016; Rilov, 2016; Pastor et al., 2020) and a major global change hotspot, driven by fast tropicalization (Rilov et al., 2019b). Mollusca, for instance, is dominated by alien species due to the collapse of native populations (Rilov, 2016; Albano et al., 2021). The co-occurrence of intense warming and thermophilic bioinvasions makes it challenging to ascertain the primary cause of the native species decline (especially non-harvested ones). Experiments and correlative studies have indicated that warming is likely the main driver for species decline, such as in the case of the purple sea urchin Paracentrotus lividus (Yeruham et al., 2015; Yeruham et al., 2019), fish (Givan et al., 2018), and possibly mollusks (Albano et al., 2021). Recent experimental work further supported this, showing that tropical alien species are more resilient to warming than native species (Rilov et al., 2022).
Considering the formation of a new Levant ecosystem dominated by alien species, an important question arises: how does this process impact ecosystem functioning and services? To address this, indirect methods such as biological trait analysis can be used, using traits as proxies for functions. Recent research revealed distinct traits between native and alien assemblages, indicating that aliens cannot fully compensate for the loss of native species (Steger et al., 2022). Additionally, direct measurements of ecosystem functions through experiments have shown that alien macrophytes can restore lost biomass due to invasive rabbitfish grazing, unlike vulnerable native macroalgae (Peleg et al., 2020; Mulas et al., 2022), and therefore compensate for the reduction of blue carbon.
With ongoing warming and the influx of invaders to the Levant, the collapse of native species and the spread of alien domination are expected to rapidly extend westward and northward in the Mediterranean Sea. Thus, the current situation in the southeast corner of the Mediterranean Sea likely foreshadows the future of other parts of the basin, serving as a warning sign for the entire region (a “canary-in-the-coal-mine”). MPAs alone may not effectively combat NIS in such climate change and bioinvasion hotspots (Rilov et al., 2018; Frid et al., 2021). Given the native species collapse and proliferation of tropical aliens, regardless of protection from local human pressures, it is necessary to adapt and reconsider conservation objectives and indicators of success, adjusting criteria for good environmental status accordingly (Rilov et al., 2020).
The Red Sea alien lionfish Pterois miles and the alien urchin Diadema setosum meet again on the reefs of the Israeli coast (photo: G. Ra’anan).
All claims expressed in this article are solely those of the authors and do not necessarily represent those of their affiliated organizations, or those of the publisher, the editors and the reviewers. Any product that may be evaluated in this article, or claim that may be made by its manufacturer, is not guaranteed or endorsed by the publisher.
The Supplementary Material for this article can be found online at: https://www.frontiersin.org/articles/10.3389/fmars.2023.1271755/full#supplementary-material
Abdo D. A., Duggan R. L., McDonald J. I. (2018). Sounding out pests: the potential of hydroacoustics as a surveillance and compliance tool in aquatic biosecurity. Biol. Invasions 20, 3409–3416. doi: 10.1007/s10530-018-1792-2
Agersnap S., Sigsgaard E. E., Jensen M. R., Avila M. D. P., Carl H., Møller P. R., et al. (2022). A national scale “BioBlitz” using citizen science and eDNA metabarcoding for monitoring coastal marine fish. Front. Mar. Sci. 9. doi: 10.3389/fmars.2022.824100
Aglieri G., Quattrocchi F., Mariani S., Baillie C., Spatafora D., Di Franco A., et al. (2023). Fish eDNA detections in ports mirror fishing fleet activities and highlight the spread of non-indigenous species in the Mediterranean Sea. Mar. pollut. Bull. 189, 114792. doi: 10.1016/j.marpolbul.2023.114792
Ahmed D. A., Hudgins E. J., Cuthbert R. N., Kourantidou M., Diagne C., Haubrock P. J., et al. (2022). Managing biological invasions: the cost of inaction. Biol. Invasions 24, 1927–1946. doi: 10.1007/s10530-022-02755-0
Albano P. G., Steger J., Bošnjak M., Dunne B., Guifarro Z., Turapova E., et al. (2021). Native biodiversity collapse in the eastern Mediterranean. Proc. R. Soc B 288, 20202469. doi: 10.1098/rspb.2020.2469
Andersen N. R., Stæhr P. A. U., Andersen K. R., Buur H., Jakobsen H. H., Winding A., et al. (2023) Havneovervågning af ikke-hjemmehørende arter 2021 - Havstrategiens deskriptor 2. Aarhus University, Videnskabelig rapport fra DCE - Nationalt Center for Miljø og Energi nr. 534. Available at: https://dce.au.dk/udgivelser/vr/501-599/abstracts/no-534-harbor-monitoring-of-non-native-species-2021.
Anderson L. W. J. (2005). California’s reaction to caulerpa taxifolia: A model for invasive species rapid response. Biol. Invasions 7, 1003–1016. doi: 10.1007/s10530-004-3123-z
Anton A., Geraldi N. R., Ricciardi A., Dick J. T. A. (2020). Global determinants of prey naiveté to exotic predators. Proc. R. Soc. B. 287, 20192978. doi: 10.1098/rspb.2019.2978
AquaNIS (2023) Information system on Aquatic Non-Indigenous and Cryptogenic Species (World Wide Web electronic publication). Available at: www.corpi.ku.lt/databases/index.php/aquanis/ (Accessed February 27, 2023).
Ashton G. (2006). Rapid assessment of the distribution of marine non-native species in marinas in Scotland. Aquat. Invasions 1, 209–213. doi: 10.3391/ai.2006.1.4.3
Atwater D. Z., Ervine C., Barney J. N. (2018). Climatic niche shifts are common in introduced plants. Nat. Ecol. Evol. 2, 34–43. doi: 10.1038/s41559-017-0396-z
Bacher S., Blackburn T. M., Essl F., Genovesi P., Heikkilä J., Jeschke J. M., et al. (2018). Socio-economic impact classification of alien taxa (SEICAT). Methods Ecol. Evol. 9, 159–168. doi: 10.1111/2041-210X.12844
Baker R. H. A., Black R., Copp G. H., Haysom K. A., Hulme P. E., Thomas M. B., et al. (2008). “The UK risk assessment scheme for all non-native species,” in Biological invasions – from ecology to conservation, vol. 7 . Eds. Rabitsch W., Essl F., Klingenstein F. (Berlin: NEOBIOTA). 46–57.
Barnes D. K. A. (2002). Invasions by marine life on plastic debris. Nature 416, 808–809. doi: 10.1038/416808a
Barry P. J., Beraud C., Wood L. E., Tidbury H. J. (2023). Modelling of marine debris pathways into UK waters: Example of non-native crustaceans transported across the Atlantic Ocean on floating marine debris. Mar. pollut. Bull. 186, 114388. doi: 10.1016/j.marpolbul.2022.114388
Bartolo A. G., Tsiamis K., Küpper F. C. (2021). Identifying hotspots of non-indigenous species’ high impact in the Maltese islands (Central Mediterranean Sea). Mar. pollut. Bull. 164, 112016. doi: 10.1016/j.marpolbul.2021.112016
Baudry T., Laffitte M., Noizat C., Delaunay C., Ricou G., Vasselon V., et al. (2023). Influence of distance from source population and seasonality in eDNA detection of white-clawed crayfish, through qPCR and ddPCR assays. Environ. DNA 5, 733–749. doi: 10.1002/edn3.435
Bax N., Hayes K., Marshall A., Parry D., Thresher R. (2002). “Man-made marinas as sheltered islands for alien marine organisms: establishment and eradication of an alien invasive marine species,” in Turning the tide: the eradication of invasive species. Eds. Veitc C. R., Clout M. N. (Gland, Switzerland and Cambridge, UK: IUCN), 26–39.
Bédry R., de Haro L., Bentur Y., Senechal N., Galil B. S. (2021). Toxicological risks on the human health of populations living around the Mediterranean Sea linked to the invasion of nonindigenous marine species from the Red Sea: A review. Toxicon 191, 69–82. doi: 10.1016/j.toxicon.2020.12.012
Biggs J., Ewald N., Valentini A., Gaboriaud C., Dejean T., Griffiths R. A., et al. (2015). Using eDNA to develop a national citizen science-based monitoring programme for the great crested newt (Triturus cristatus). Biol. Conserv. 183, 19–28. doi: 10.1016/j.biocon.2014.11.029
Birchenough S. N. R., Degraer S. (2020). Science in support of ecologically sound decommissioning strategies for offshore man-made structures: taking stock of current knowledge and considering future challenges. ICES J. M. Sci. 77, 1075–1078. doi: 10.1093/icesjms/fsaa039
Birchenough S. N., Reiss H., Degraer S., Mieszkowska N., Borja Á., Buhl-Mortensen L., et al. (2015). Climate change and marine benthos: a review of existing research and future directions in the North Atlantic. Wires. Clim. Change 6, 203–223. doi: 10.1002/wcc.330
Boero F., Carlton J., Briand F., Kiessling W., Chenuil A., Voultsiadou E., et al. (2013). “Marine extinctions: patterns and processes – executive summary,” in CIESM Workshop Monograph, 45. Marine extinctions - patterns and processes. Ed. Briand F. (Monaco: CIESM Publisher), 5–19.
Boero F., Putti M., Trainito E., Prontera E., Piraino S., Shiganova T. A. (2009). First records of Mnemiopsis leidyi (Ctenophora) from the Ligurian, Thyrrhenian and Ionian Seas (Western Mediterranean) and first record of Phyllorhiza punctata (Cnidaria) from the Western Mediterranean. Aquat. Invasions 4, 675–680. doi: 10.3391/ai.2009.4.4.13
Bolch E. A., Santos M. J., Ante C., Khanna C., Basinger N. T., Reader M. O., et al. (2020). “Remote detection of invasive alien species,” in Remote sensing of plant biodiversity. Eds. Cavender-Bares J., Gamon J. A., Townsend P. A. (Cham, Switzerland: Springer). doi: 10.1007/978-3-030-33157-3_12
Borrell Y. J., Miralles L., Do Huu H., Mohammed-Geba K., Garcia-Vazquez E. (2017). DNA in a bottle - Rapid metabarcoding survey for early alerts of invasive species in ports. PloS One 12, e0183347. doi: 10.1371/journal.pone.0183347
Bowers H. A., Pochon X., von Ammon U., Gemmell N., Stanton J.-A. L., Jeunen G.-J., et al. (2021). Towards the optimization of eDNA/eRNA sampling technologies for marine biosecurity surveillance. Water 13, 1113. doi: 10.3390/w13081113
Branquart E. (2009). Guidelines for environmental impact assessment and list classification of non-native organisms in Belgium. Version 2.6.
Bucklin A., Peijnenburg K. T. C. A., Kosobokova K. N., O’Brien T. D., Blanco-Bercial L., Cornils A., et al. (2021). Toward a global reference database of COI barcodes for marine zooplankton. Mar. Biol. 168, 78. doi: 10.1007/s00227-021-03887-y
Buckner J. C., Sanders R. C., Faircloth B. C., Chakrabarty P. (2021). The critical importance of vouchers in genomics. eLife 10, e68264. doi: 10.7554/eLife.68264
Buschbaum C., Karez R., Lackschewitz D., Reise. K. (2010). Rapid assessment of neobiota in German coastal waters. HELCOM Indicator Fact Sheets and development of the HELCOM core set of indicators. HELCOM MONAS 13/2010, Document 6/4 (Russia: St. Petersburg).
Caley P., Cassey P. (2023). Do we need to mine social media data to detect exotic vertebrate-pest introductions? Wildlife Res. doi: 10.1071/WR22116
Canals O., Mendibil I., Santos M., Irigoien X., Rodríguez-Ezpeleta N. (2021). Vertical stratification of environmental DNA in the open ocean captures ecological patterns and behavior of deep-sea fishes. Limnol. Oceanogr. Lett. 6, 339–347. doi: 10.1002/lol2.10213
Cardeccia A., Marchini A., Occhipinti-Ambrogi A., Galil B., Gollasch S., Minchin D., et al. (2018). Assessing biological invasions in European Seas: Biological traits of the most widespread non-indigenous species. Estuar. Coast. Shelf Sci. 201, 17–28. doi: 10.1016/j.ecss.2016.02.014
Carlsson N. O., Sarnelle O., Strayer D. L. (2009). Native predators and exotic prey –an acquired taste? Front. Ecol. Environ. 7, 525–532. doi: 10.1890/080093
Carlton J. T., Fowler A. E. (2018). Ocean rafting and marine debris: A broader vector menu requires a greater appetite for invasion biology research support. Aquat. Invasions 13, 11–15. doi: 10.3391/ai.2018.13.1.02
Carvalho S., Shchepanik H., Aylagas E., Berumen M. L., Costa F. O., Costello M. J., et al. (2023). Hurdles and opportunities in implementing marine biosecurity systems in data-poor regions. BioScience 73, 494–512. doi: 10.1093/biosci/biad056
Castaño-Quintero S., Escobar-Luján J., Osorio-Olvera L., Peterson A. T., Chiappa-Carrara X., Martínez-Meyer E., et al. (2020). Supraspecific units in correlative niche modeling improves the prediction of geographic potential of biological invasions. PeerJ 8, e10454. doi: 10.7717/peerj.10454
Çavaş L., Bilgin Y., Yılmaz-Abesşka Y. (2020). Can bioactive peptides of Lagocephalus sceleratus be evaluated in the functional food industry? Biotech. Stud. 29, 77–84. doi: 10.38042/biost.2020.29.02.04
Chainho P., Fernandes A., Amorim A., Ávila S. P., Canning-Clode J., Castro J. J., et al. (2015). Non-indigenous species in Portuguese coastal areas, coastal lagoons, estuaries and islands. Estuar. Coast. Shelf Sci. 167, 199–211. doi: 10.1016/j.ecss.2015.06.019
Chan J., Zeng Y., Yeo D. C. J. (2021). Invasive species trait-based risk assessment for non-native freshwater fishes in a tropical city basin in Southeast Asia. PloS One 16, e0248480. doi: 10.1371/journal.pone.0248480
Chefaoui R. M., Serebryakova A., Engelen A. H., Viard F., Serrão E. A. (2019). Integrating reproductive phenology in ecological niche models changed the predicted future ranges of a marine invader. Divers. Distrib. 25, 688–700. doi: 10.1111/ddi.12910
Cherry P., O’Hara C., Magee P. J., McSorley E. M., Allsopp P. J. (2019). Risks and benefits of consuming edible seaweeds. Nutr. Rev. 77, 307–329. doi: 10.1093/nutrit/nuy066
Çinar M. E., Bakir K. (2014). ALien Biotic IndEX (ALEX)–A new index for assessing impacts of alien species on benthic communities. Mar. pollut. Bull. 87, 171–179. doi: 10.1016/j.marpolbul.2014.07.061
Cohen A. N., Harris L. H., Bingham B. L., Carlton J. T., Chapman J. W., Lambert C. C., et al. (2005). Rapid assessment survey for exotic organisms in southern california bays and harbors, and abundance in port and non-port areas. Biol. Invasions 7, 995–1002. doi: 10.1007/s10530-004-3121-1
Connolly R. M., Fairclough D. V., Jinks E. L., Ditria E. M., Jackson G., Lopez-Marcano S., et al. (2021). Improved accuracy for automated counting of a fish in baited underwater videos for stock assessment. Front. Mar. Sci. 8. doi: 10.3389/fmars.2021.658135
Convention on Biological Diversity [CBD] (2014). Pathways of introduction of invasive species, their prioritization and management (Montreal: CBD).
Coolen J. W. P., Boon A. R., Crooijmans R., van Pelt H., Kleissen F., Gerla D., et al. (2020). Marine stepping-stones: connectivity of Mytilus edulis populations between offshore energy installations. Mol. Ecol. 29, 686–703. doi: 10.1111/mec.15364
Copp G. H. (2013). The Fish Invasiveness Screening Kit (FISK) for non-native freshwater fishes: A summary of current applications. Risk Anal. 33, 1394–1396. doi: 10.1111/risa.12095
Copp G. H., Garthwaite R., Gozlan R. E. (2005). Risk identification and assessment of non-native freshwater fishes: a summary of concepts and perspectives on protocols for the UK. J. Appl. Ichthyol. 21, 371–373. doi: 10.1111/j.1439-0426.2005.00692.x
Costello M. J., Dekeyzer S., Galil B. S., Hutchings P., Katsanevakis S., Pagad S., et al. (2021). Introducing the world register of introduced marine species (WRiMS). Manage. Biol. Invasion 12, 792–811. doi: 10.3391/mbi.2021.12.4.02
Crane D. P., Farrell J. M., Einhouse D. W., Lantry J. R., Markham J. L. (2015). Trends in body condition of native piscivores following invasion of Lakes Erie and Ontario by the round goby. Freshw. Biol. 60, 111–124. doi: 10.1111/fwb.12473
Crocetta F., Al Mabruk S. A., Azzurro E., Bakiu R., Bariche M., Batjakas I. E., et al. (2021). New alien Mediterranean biodiversity records (November 2021). Mediterr. Mar. Sci. 22, 724–746. doi: 10.12681/mms.26668
Culver C. S., Kuris A. M. (2000). The apparent eradication of a locally established introduced marine pest. Biol. Invasions 2, 245–253. doi: 10.1023/A:1010082407254
D’Amen M., Azzurro E. (2020a). Lessepsian fish invasion in Mediterranean marine protected areas: a risk assessment under climate change scenarios. ICES J. Mar. Sci. 77, 388–397. doi: 10.1093/icesjms/fsz207
D’Amen M., Azzurro E. (2020b). Integrating univariate niche dynamics in species distribution models: A step forward for marine research on biological invasions. J. Biogeogr. 47, 686–697. doi: 10.1111/jbi.13761
D’Amen M., Smeraldo S., Azzurro E. (2023). Salinity, not only temperature, drives tropical fish invasions in the Mediterranean Sea, and surface-only variables explain it better. Coral Reefs 42, 467–472. doi: 10.1007/s00338-023-02357-9
D’Amen M., Smeraldo S., Di Franco A., Azzurro E. (2022). The spread of Lessepsian fish does not track native temperature conditions. ICES J. Mar. Sci. 79, 1864–1873. doi: 10.1093/icesjms/fsac121
Darling J. A., Galil B. S., Carvalho G. R., Rius M., Viard F., Piraino S. (2017). Recommendations for developing and applying genetic tools to assess and manage biological invasions in marine ecosystems. Mar. Policy 85, 54–64. doi: 10.1016/j.marpol.2017.08.014
De Brauwer M., Clarke L. J., Chariton A., Cooper M. K., de Bruyn M., Furlan E., et al. (2023). Best practice guidelines for environmental DNA biomonitoring in Australia and New Zealand. Environ. DNA 5, 417–423. doi: 10.1002/edn3.395
De Domenico S., De Rinaldis G., Mammone M., Bosch-Belmar M., Piraino S., Leone A. (2023). The zooxanthellate jellyfish holobiont cassiopea andromeda, a source of soluble bioactive compounds. Mar. Drugs 21, 272. doi: 10.3390/md21050272
Demirel N., Ulman A., Yıldız T., Ertör-Akyazi P. (2021). A moving target: Achieving good environmental status and social justice in the case of an alien species, Rapa whelk in the Black Sea. Mar. Policy 132, 104687. doi: 10.1016/j.marpol.2021.104687
DeRivera C. E., Ruiz G. M., Hines A., Jivoff P. (2005). Biotic resistance to invasion: native predator limits abundance and distribution of an introduced crab. Ecology 86, 3364–3376. doi: 10.1890/05-0479
D’hondt B., Vanderhoeven S., Roelandt S., Mayer F., Versteirt V., Adriaens T., et al. (2015). Harmonia + and Pandora +: risk screening tools for potentially invasive plants, animals and their pathogens. Biol. Invasions 17, 1869–1883. doi: 10.1007/s10530-015-0843-1
Diagne C., Leroy B., Gozlan R. E., Vaissière A. C., Assailly C., Nuninger. L., et al. (2020). InvaCost, a public database of the economic costs of biological invasions worldwide. Sci. Data. 7, 277. doi: 10.1038/s41597-020-00586-z
Diagne C., Leroy B., Vaissière A. C., Gozlan R. E., Roiz D., Jarić I., et al. (2021). High and rising economic costs of biological invasions worldwide. Nature 592, 571–576. doi: 10.1038/s41586-021-03405-6
DiBattista J. D., Reimer J. D., Stat M., Masucci G. D., Biondi P., De Brauwer M., et al. (2019). Digging for DNA at depth: rapid universal metabarcoding surveys (RUMS) as a tool to detect coral reef biodiversity across a depth gradient. PeerJ 7, e6379. doi: 10.7717/peerj.6379
Dimitriadis C., Fournari-Konstantinidou I., Sourbès L., Koutsoubas D., Katsanevakis S. (2021). Long term interactions of native and invasive species in a marine protected area suggest complex cascading effects challenging conservation outcomes. Diversity 13, 71. doi: 10.3390/d13020071
Dimitriadis C., Galanidi M., Zenetos A., Corsini-Foka M., Giovos I., Karachle P. K., et al. (2020). Updating the occurrences of Pterois miles in the Mediterranean Sea, with considerations on thermal boundaries and future range expansion. Mediterr. Mar. Sci. 21, 62–69. doi: 10.12681/mms.21845
Dobrzycka-Krahel A., Medina-Villar S. (2023). A new horizon-scanning tool to identify potential aquatic invasive alien species introduced into the Baltic Sea by shipping. Water 15, 531. doi: 10.3390/w15030531
Duarte S., Vieira P. E., Lavrador A. S., Costa F. O. (2021). Status and prospects of marine NIS detection and monitoring through (e)DNA metabarcoding. Sci. Total Environ. 751, 141729. doi: 10.1016/j.scitotenv.2020.141729
Dukes J. S., Mooney H. A. (1999). Does global change increase the success of biological invaders? Trends Ecol. Evol. 14, 135–139. doi: 10.1016/S0169-5347(98)01554-7
Edelist D., Rilov G., Golani D., Carlton J., Spanier E. (2013). Restructuring the Sea: profound shifts in the world’s most invaded marine ecosystem. Divers. Distrib. 19, 69–77. doi: 10.1111/ddi.12002
Ehrenfeld J. G. (2010). Ecosystem consequences of biological invasions. Annu. Rev. Ecol. Evol. Syst. 41, 59–80. doi: 10.1146/annurev-ecolsys-102209-144650
Essl F., Bacher S., Blackburn T., Booy O., Brundu G., Brunel S., et al. (2015). Crossing frontiers in tackling pathways of biological invasions. BioScience 65, 769–782. doi: 10.1093/biosci/biv082
Essl F., Bacher S., Genovesi P., Hulme P. E., Jeschke J. M., Katsanevakis S., et al. (2018). Which taxa are alien? Criteria, applications, and uncertainties. BioScience 68, 496–509. doi: 10.1093/biosci/biy057
Essl F., Dullinger S., Genovesi P., Hulme P. E., Jeschke J. M., Katsanevakis S., et al. (2019). A conceptual framework for range-expanding species that track human-induced environmental change. BioScience 69, 908–919. doi: 10.1093/biosci/biz101
Essl F., Lenzner B., Bacher S., Bailey S., Capinha C., Daehler C., et al. (2020). Drivers of future alien species impacts: an expert-based assessment. Glob. Change Biol. 26, 4880–4893. doi: 10.1111/gcb.15199
Essl F., Nehring S., Klingenstein F., Milasowszky N., Nowack C., Rabitsch W. (2011). Review of risk assessment systems of IAS in Europe and introducing the German-Austrian Black List Information System (GABLIS). J. Nat. Conserv. 19, 339–350. doi: 10.1016/j.jnc.2011.08.005
European Union (EU) (2007). Council Regulation 708/2007 concerning the use of alien and locally absent species in aquaculture. Off. J. Eur. Union L 168, 1–17.
European Union (EU) (2008). Directive 2008/56/EC of the European Parliament and of the Council of 17 June 2008 establishing a framework for community action in the field of marine environmental policy (Marine Strategy Framework Directive). Off. J. Eur. Union L 164, 19–40.
European Union (EU) (2014). Regulation (EU) No 1143/2014 of the European parliament and of the council of 22 October 2014 on the prevention and management of the introduction and spread of invasive alien species. Off. J. Eur. Union L 317, 35–55.
European Union (EU) (2019). Commission implementing Regulation (EU) 2019/1262 of 25 July 2019 amending Implementing Regulation (EU) 2016/1141 to update the list of invasive alien species of Union concern. Off. J. Eur. Union L 199, 1–4.
European Union (EU) (2022). Commission implementing Regulation (EU) 2022/1203 of 12 July 2022 amending Implementing Regulation (EU) 2016/1141 to update the list of invasive alien species of Union concern. Off. J. Eur. Union L 186, 10–13.
Evans J., Barbara J., Schembri P. J. (2015). Updated review of marine alien species and other ‘newcomers’ recorded from the Maltese Islands (Central Mediterranean). Mediterr. Mar. Sci. 16, 225–244. doi: 10.12681/mms.1064
Ferreira-Rodríguez N., Pave L. A. B., Cogălniceanu D. (2021). Integrating expert opinion and traditional ecological knowledge in invasive alien species management: Corbicula in Eastern Europe as a model. Biol. Invasions 4, 1087–1099. doi: 10.1007/s10530-020-02420-4
Fitzpatrick M. C., Preisser E. L., Ellison A. M., Elkinton J. S. (2009). Observer bias and the detection of low-density populations. Ecol. Appl. 19, 1673–1679. doi: 10.1890/09-0265.1
Fonseca V. G. (2018). Pitfalls in relative abundance estimation using eDNA metabarcoding. Mol. Ecol. Resour. 18, 923–926. doi: 10.1111/1755-0998.12902
Fonseca V. G., Davison P. I., Creach V., Stone D., Bass D., Tidbury H. J. (2023). The application of eDNA for monitoring aquatic non-indigenous species: practical and policy considerations. Diversity 15, 631. doi: 10.3390/d15050631
Frid O., Lazarus M., Malamud S., Belmaker J., Yahel R. (2021). Effects of marine protected areas on fish communities in a hotspot of climate change and invasion. Mediterr. Mar. Sci. 23, 157–190. doi: 10.12681/mms.26423
Galil B. (2018). “Poisonous and venomous: marine alien species in the Mediterranean Sea and human health,” in Invasive species and human health Ed. Mazza G., Tricarico E. (United Kingdom: CAB International), 1–15. doi: 10.1079/9781786390981.0001
Galil B., Marchini A., Occhipinti-Ambrogi A., Ojaveer H. (2017). The enlargement of the Suez Canal – Erythraean introductions and management challenges. Manage. Biol. Invasion 8, 141–152. doi: 10.3391/mbi.2017.8.2.02
Gamliel I., Buba Y., Guy-Haim T., Garval T., Willette D., Rilov G., et al. (2020). Incorporating physiology into species distribution models moderates the projected impact of warming on selected Mediterranean marine species. Ecography 43, 1090–1106. doi: 10.1111/ecog.04423
García-Gómez J. C., Florido M., Olaya-Ponzone L., Rey Díaz de Rada J., Donázar-Aramendía I., Chacón M., et al. (2021). Monitoring extreme impacts of rugulopteryx okamurae (Dictyotales, ochrophyta) in el estrecho natural park (Biosphere reserve). Showing radical changes in the underwater seascape. Front. Ecol. Evol. 9. doi: 10.3389/fevo.2021.639161
García-Gómez J. C., Sempere-Valverde J., González A. R., Martínez-Chacón M., Olaya-Ponzone L., Sánchez-Moyano E., et al. (2020). From exotic to invasive in record time: The extreme impact of Rugulopteryx okamurae (Dictyotales, Ochrophyta) in the strait of Gibraltar. Sci. Total Environ. 704, 135408. doi: 10.1016/j.scitotenv.2019.135408
Gatto F., Katsanevakis S., Vandekerkhove J., Zenetos A., Cardoso A. C. (2013). Evaluation of online information sources on alien species in Europe: the need of harmonization and integration. Environ. Manage. 51, 1137–1146. doi: 10.1007/s00267-013-0042-8
GBIF. (2023). The global biodiversity information facility: DAISIE - Inventory of alien invasive species in Europe. Available at: https://www.gbif.org/dataset/39f36f10-559b-427f-8c86-2d28afff68ca (Accessed March 24, 2023).
Genovese G., Faggio C., Gugliandolo C., Torre A., Spanò A., Morabito M., et al. (2012). In vitro evaluation of antibacterial activity of Asparagopsis taxiformis from the Straits of Messina against pathogens relevant in aquaculture. Mar. Environ. Res. 73, 1–6. doi: 10.1016/j.marenvres.2011.10.002
Gerovasileiou V., Akel E., Akyol O., Alongi G., Azevedo F., Babali N., et al. (2017). New mediterranean biodiversity records (July 2017). Mediterr. Mar. Sci. 18, 355–384. doi: 10.12681/mms.13771
Ghermandi A., Galil B., Gowdy J., Nunes P. A. L. D. (2015). Jellyfish outbreak impacts on recreation in the Mediterranean Sea: welfare estimates from a socioeconomic pilot survey in Israel. Ecosyst. Serv. 11, 140–147. doi: 10.1016/j.ecoser.2014.12.004
Giakoumi S., Katsanevakis S., Albano P. G., Azzurro E., Cardoso A. C., Cebrian E., et al. (2019). Management priorities for marine invasive species. Sci. Total Environ. 688, 976–982. doi: 10.1016/j.scitotenv.2019.06.282
Giovos I., Kleitou P., Poursanidis D., Batjakas I., Bernardi G., Crocetta F., et al. (2019). Citizen-science for monitoring marine invasions and stimulating public engagement: a case project from the eastern Mediterranean. Biol. Invasions 21, 3707–3721. doi: 10.1007/s10530-019-02083-w
Gissi E., Manea E., Mazaris A. D., Fraschetti S., Almpanidou V., Bevilacqua S., et al. (2021). A review of the combined effects of climate change and other human stressors on the marine environment. Sci. Total Environ. 755, 142564. doi: 10.1016/j.scitotenv.2020.142564
Givan O., Edelist D., Sonin O., Belmaker J. (2018). Thermal affinity as the dominant factor changing Mediterranean fish abundances. Glob. Change Biol. 24, e80–e89. doi: 10.1111/gcb.13835
González-Moreno P., Lazzaro L., Vilà M., Preda C., Adriaens T., Bacher S., et al. (2019). Consistency of impact assessment protocols for non-native species. NeoBiota 44, 1–25. doi: 10.3897/neobiota.44.31650
Green S. J., Dulvy N. K., Brooks A. M. L., Akins J. L., Cooper A. B., Miller S., et al. (2014). Linking removal targets to the ecological effects of invaders: a predictive model and field test. Ecol. Appl. 24, 1311–1322. doi: 10.1890/13-0979.1
Guillou L., Bachar D., Audic S., Bass D., Berney C., Bittner L., et al. (2013). The Protist Ribosomal Reference database (PR2): a catalog of unicellular eukaryote small sub-unit rRNA sequences with curated taxonomy. Nucleic Acids Res. 41 (Database issue), D597—D604. doi: 10.1093/nar/gks1160
Guy-Haim T., Lyons D. A., Kotta J., Ojaveer H., Queirós A. M., Chatzinikolaou E., et al. (2018). Diverse effects of invasive ecosystem engineers on marine biodiversity and ecosystem functions: A global review and meta-analysis. Glob. Change Biol. 24, 906–924. doi: 10.1111/gcb.14007
Hablützel P., Martínez. P. A., Bilsen A., Christodoulou M., Delacauw S., Deneudt K., et al. (2023) DNA-based monitoring of Non-Indigenous Species. GEANS– Genetic tools for Ecosystem health Assessment in the North Sea region. Available at: https://www.geans.eu/sites/geans.eu/files/managed/GEANS_Pilot%20NIS_copy.pdf (Accessed 10 July 2023).
Hansen B. K., Jacobsen M. W., Middelboe A. L., Preston C. M., Marin R., Bekkevold D., et al. (2020). Remote, autonomous real-time monitoring of environmental DNA from commercial fish. Sci. Rep. 10, 1. doi: 10.1038/s41598-020-70206-8
Harris H. E., Fogg A. Q., Gittings S. R., Ahrens R. N. M., Allen M. S., Patterson III W.F. (2020). Testing the efficacy of lionfish traps in the northern Gulf of Mexico. PloS One 15, e0230985. doi: 10.1371/journal.pone.0230985
Harrower C. A., Scalera R., Pagad S., Schonrogge K., Roy H. E. (2018). Guidance for interpretation of CBD categories on introduction pathways (Brussels: European Commission).
Hawkins C. L., Bacher S., Essl F., Hulme P. E., Jeschke J. M., Kühn I., et al. (2015). Framework and guidelines for implementing the proposed IUCN Environmental Impact Classification for Alien Taxa (EICAT). Divers. Distrib. 21, 1360–1363. doi: 10.1111/ddi.12379
He K. S., Bradley B. A., Cord A. F., Rocchini D., Tuanmu M.-N., Schmidtlein S., et al. (2015). Will remote sensing shape the next generation of species distribution models? Remote Sens. Ecol. Conserv. 1, 4–18. doi: 10.1002/rse2.7
HELCOM (2023) HELCOM Monitoring manual and guidelines. Available at: https://helcom.fi/action-areas/monitoring-and-assessment/monitoring-manual (Accessed 30 June 2023).
Hellmann J. J., Byers J. E., Bierwagen B. G., Dukes J. S. (2008). Five potential consequences of climate change for invasive species. Conserv. Biol. 22, 534–543. doi: 10.1111/j.1523-1739.2008.00951.x
Henry L.-A., Mayorga-Adame C. G., Fox A. D., Polton J. A., Ferris J. S., McLellan F., et al. (2018). Ocean sprawl facilitates dispersal and connectivity of protected species. Sci. Rep. 8, 11346. doi: 10.1038/s41598-018-29575-4
Hewitt M. J., Hourston M., McDonald J. I. (2018). A long way from home: Biosecurity lessons learnt from the impact of La Niña on the transportation and establishment of tropical portunid species. PloS One 13, e0202766. doi: 10.1371/journal.pone.0202766
Hewitt C. L., Martin R. B. (1996). Port surveys for introduced marine species – background considerations and sampling protocols. CRIMP technical report 4 (Hobart, TAS: Division of Fisheries, CSIRO).
Hewitt C. L., Martin R. B. (2001). Revised protocols for baseline port surveys for introduced marine species – design considerations, sampling protocols and taxonomic sufficiency. CRIMP technical report number 22 (Hobart,TAS: CSIRO Marine Research).
Hewitt C. L., Willing J., Bauckham A., Cassidy A. M., Cox C. M., Jones L., et al. (2004). New Zealand marine biosecurity: delivering outcomes in a fluid environment. New Zeal. J. Mar. Fresh. 38, 429–438. doi: 10.1080/00288330.2004.9517250
Holman L. E., de Bruyn M., Creer S., Carvalho G., Robidart J., Rius M. (2019). Detection of introduced and resident marine species using environmental DNA metabarcoding of sediment and water. Sci. Rep. 9, 11559. doi: 10.1038/s41598-019-47899-7
Hopkins G. A., Forrest B. M., Jiang W., Gardner J. P. A. (2011). Successful eradication of a non-indigenous marine bivalve from a subtidal soft-sediment environment. J. Appl. Ecol. 48, 424–431. doi: 10.1111/j.1365-2664.2010.01941.x
Hudson L. N., Newbold T., Contu S., Hill S. L., Lysenko I., De Palma A., et al. (2014). The PREDICTS database: a global database of how local terrestrial biodiversity responds to human impacts. Ecol. Evol. 4, 4701–4735. doi: 10.1002/ece3.1303
Hulme P. E., Bacher S., Kenis M., Klotz S., Kühn I., Minchin D., et al. (2008). Grasping at the routes of biological invasions: a framework for integrating pathways into policy. J. Appl. Ecol. 45, 403e414. doi: 10.1111/j.1365-2664.2007.01442.x
Hunt C. E., Yamada S. B. (2003). Biotic resistance experienced by an invasive crustacean in a temperate estuary. Biol. Invasions 5, 33–43. doi: 10.1023/A:1024011226799
ICES (2005). Code of practice on the introductions and transfers of marine organisms (Copenhagen: ICES).
ICES (2022). Working group on introductions and transfers of marine organisms (WGITMO). ICES scientific reports. (Copenhagen: ICES). 209. doi: 10.17895/ices.pub.21558855
Issaris Y., Katsanevakis S., Salomidi M., Tsiamis K., Katsiaras N., Verriopoulos G. (2012). Occupancy estimation of marine species: dealing with imperfect detectability. Mar. Ecol. Prog. Ser. 453, 95–106. doi: 10.3354/meps09668
Iwasaki W., Fukunaga T., Isagozawa R., Yamada K., Maeda Y., Satoh T. P., et al. (2013). MitoFish and MitoAnnotator: a mitochondrial genome database of fish with an accurate and automatic annotation pipeline. Mol. Biol. Evol. 30, 2531–2540. doi: 10.1093/molbev/mst141
Jensen K. R., Andersen P., Andersen N. R., Bruhn A., Buur A., Carl H., et al. (2023). Reviewing introduction histories, pathways, invasiveness, and impact of non-indigenous species in Danish marine waters. Diversity 15, 434. doi: 10.3390/d15030434
Jensen G. C., McDonald P. S., Armstrong D. A. (2007). Biotic resistance to green crab, Carcinus maenas, in California bays. Mar. Biol. 151, 2231–2243. doi: 10.1007/s00227-007-0658-4
Jensen M. R., Sigsgaard E. E., Ávila M. D. P., Agersnap S., Brenner-Larsen W., Sengupta M. E., et al. (2022). Short-term temporal variation of coastal marine eDNA. Environ. DNA 4, 747–762. doi: 10.1002/edn3.285
Johnston M. W., Purkis S. J. (2014). Are lionfish set for a Mediterranean invasion? Modelling explains why this is unlikely to occur. Mar. pollut. Bull. 88, 138–147. doi: 10.1016/j.marpolbul.2014.09.013
Kagzi K., Millette K. L., Littlefair J. E., Pochon X., Wood S. A., Fussmann G. F., et al. (2023). Assessing the degradation of environmental DNA and RNA based on genomic origin in a metabarcoding context. Environ. DNA 00, 1–16. doi: 10.1002/edn3.437
Kampouris T. E., Porter J. S., Sanderson W. G. (2019). Callinectes sapidus rathbun 1896 (Brachyura: Portunidae): An assessment on its diet and foraging behaviour, Thermaikos Gulf, NW Aegean Sea, Greece: Evidence for ecological and economic impacts. Crustacean Res. 48, 23–37. doi: 10.18353/crustacea.48.0_23
Katsanevakis S. (2022) Management Options for Marine IAS. Technical note prepared by IUCN for the European Commission. Available at: https://circabc.europa.eu/ui/group/4cd6cb36-b0f1-4db4-915e-65cd29067f49/library/367b19a5-e805-4ac0-97fc-6f86371ff683/details.
Katsanevakis S., Bogucarskis K., Gatto F., Vandekerkhove J., Deriu I., Cardoso A. C. (2012). Building the European Alien Species Information Network (EASIN): a novel approach for the exploration of distributed alien species data. BioInvasions Rec. 1, 235–245. doi: 10.3391/bir.2012.1.4.01
Katsanevakis S., Carella F., Çinar M. E., Čižmek H., Jimenez C., Kersting D. K., et al. (2022). “The fan mussel Pinna nobilis in the brink of extinction in the Mediterranean,” in Imperiled: the encyclopedia of conservation, vol. 2 . Eds. DellaSala D. A., Goldstein M. I. (The Netherlands: Elsevier), 700–709. doi: 10.1016/B978-0-12-821139-7.00070-2
Katsanevakis S., Coll M., Piroddi C., Steenbeek J., Ben Rais Lasram F., Zenetos A., et al. (2014a). Invading the Mediterranean Sea: biodiversity patterns shaped by human activities. Front. Mar. Sci. 1. doi: 10.3389/fmars.2014.00032
Katsanevakis S., Deriu I., D’Amico F., Nunes A. L., Pelaez Sanchez S., Crocetta F., et al. (2015). European Alien Species Information Network (EASIN): supporting European policies and scientific research. Manage. Biol. Invasion 6, 147–157. doi: 10.3391/mbi.2015.6.2.05
Katsanevakis S., Moustakas A. (2018). Uncertainty in marine invasion science. Front. Mar. Sci. 5. doi: 10.3389/fmars.2018.00038
Katsanevakis S., Rilov G., Edelist D. (2018). “Impacts of marine invasive alien species on European fisheries and aquaculture - plague or boon?,” in CIESM Monograph 50 - Engaging marine scientists and fishers to share knowledge and perceptions - early lessons. Ed. Briand F. (Monaco and Paris: CIESM Publisher) 125–132.
Katsanevakis S., Tempera F., Teixeira H. (2016). Mapping the impact of alien species on marine ecosystems: the Mediterranean Sea case study. Divers. Distrib. 22, 694–707. doi: 10.1111/ddi.12429
Katsanevakis S., Wallentinus I., Zenetos A., Leppäkoski E., Çinar M. E., Oztürk B., et al. (2014b). Impacts of invasive alien marine species on ecosystem services and biodiversity: a pan-European review. Aquat. Invasions 9, 391–423. doi: 10.3391/ai.2014.9.4.01
Katsanevakis S., Zenetos A., Belchior C., Cardoso A. C. (2013). Invading European Seas: assessing pathways of introduction of marine aliens. Ocean Coast. Manage. 76, 64–74. doi: 10.1016/j.ocecoaman.2013.02.024
Katsanevakis S., Zenetos A., Corsini-Foka M., Tsiamis K. (2020). “Biological invasions in the aegean sea: temporal trends, pathways, and impacts,” in The aegean sea environment: the natural system, handbook of environmental chemistry. Eds. Anagnostou C. L., Kostianoy A. G., Mariolakos I. D., Panayotidis P., Soilemezidou M., Tsaltas G. (Switzerland: Springer Nature). doi: 10.1007/698_2020_642
Keeley N., Wood S. A., Pochon X. (2018). Development and preliminary validation of a multi-trophic metabarcoding biotic index for monitoring benthic organic enrichment. Ecol. Indic. 85, 1044–1057. doi: 10.1016/j.ecolind.2017.11.014
Kelly R. P., Lodge D. M., Lee K. N., Theroux S., Sepulveda A. J., Scholin C. A., et al. (2023). Toward a national eDNA strategy for the United States. Environ. DNA. 00, 1–10. doi: 10.1002/edn3.432
Kerckhof F., Degraer S., Norro A., Rumes B. (2011). “Offshore intertidal hard substrata: a new habitat promoting non-indigenous species in the Southern North Sea: an exploratory study,” in Offshore wind farms in the belgian part of the north sea: selected findings from the baseline and targeted monitoring. Eds. Degraer S., Brabant R., Rumes B. (Brussels, Belgium: Royal Belgian Institute of Natural Sciences, Management Unit of the North Sea Mathematical Models, Marine Ecology and Management Section).
Kernan M. (2015). Climate change and the impact of invasive species on aquatic ecosystems. Aquat. Ecosyst. Health 18, 321–333. doi: 10.1080/14634988.2015.1027636
Kersting D., Benabdi M., Cižmek H., Grau A., Jimenez C., Katsanevakis S., et al. (2019). Pinna nobilis. The IUCN Red List of Threatened Species 2019: e.T160075998A160081499. (Gland: IUCN). doi: 10.2305/IUCN.UK.2019-3.RLTS.T160075998A160081499.en
King N. G., Wilmes S. B., Smyth D., Tinker J., Robins P. E., Thorpe J., et al. (2021). Climate change accelerates range expansion of the invasive non-native species, the Pacific oyster, Crassostrea gigas. ICES J. Mar. Sci. 78, 70–81. doi: 10.1093/icesjms/fsaa189
Kinlan B. P., Gaines S. D. (2003). Propagule dispersal in marine and terrestrial environments: a community perspective. Ecology 84, 2007–2020. doi: 10.1890/01-0622
Kleitou P., Hall-Spencer J. M., Rees S. E., Kletou D. (2022). Guide to lionfish management in the Mediterranean (Plymouth, UK: University of Plymouth), 62.
Kleitou P., Rees S., Cecconi F., Kletou D., Savva I., Cai L. L., et al. (2021). Regular monitoring and targeted removals can control lionfish in Mediterranean Marine Protected Areas. Aquat. Conserv. 31 2870–2882. doi: 10.1890/01-0622
Klymus K. E., Merkes C. M., Allison M. J., Goldberg C. S., Helbing C. C., Hunter M. E., et al. (2019). Reporting the limits of detection and quantification for environmental DNA assays. Environ. DNA 2 (3), 271–282. doi: 10.1002/edn3.29
Knudsen S. W., Hesselsøe M., Rytter M., Lillemark M. R., Tøttrup A. P., Rahbek C., et al. (2023). Detection of environmental DNA from amphibians in Northern Europe applied in citizen science. Environ. DNA 00, 1–20. doi: 10.1002/edn3.462
Knudsen S. W., Hesselsøe M., Thaulow J., Agersnap S., Hansen B. K., Jacobsen M. W., et al. (2022). Monitoring of environmental DNA from nonindigenous species of algae, dinoflagellates and animals in the North East Atlantic. Sci. Total Environ. 821, 153093. doi: 10.1016/j.scitotenv.2022.153093
Koziol A., Stat M., Simpson T., Jarman S., DiBattista J. D., Harvey E. S., et al. (2019). Environmental DNA metabarcoding studies are critically affected by substrate selection. Mol. Ecol. Resour. 19, 366–376. doi: 10.1111/1755-0998.12971
Kotta J., Nurkse K., Puntila R., Ojaveer H. (2016). Shipping and natural environmental conditions determine the distribution of the invasive non-indigenous round goby Neogobius melanostomus in a regional sea. Estuar. Coast. Shelf S. 169, 15–24. doi: 10.1016/j.ecss.2015.11.029
Kytinou E., Issaris Y., Sini M., Salomidi M., Katsanevakis S. (2023). ECOfast–An integrative ecological evaluation index for an ecosystem-based assessment of shallow rocky reefs. J. Environ. Manage. 344, 118323. doi: 10.1016/j.jenvman.2023.118323
Lehtiniemi M., Ojaveer H., David M., Galil B., Gollasch S., McKenzie C., et al. (2015). Dose of truth—monitoring marine non-indigenous species to serve legislative requirements. Mar. Policy 54, 26–35. doi: 10.1016/j.marpol.2014.12.015
Lehtiniemi M., Outinen O., Puntila-Dodd R. (2020). Citizen science provides added value in the monitoring for coastal non-indigenous species. J. Environ. Manage. 267, 110608. doi: 10.1016/j.jenvman.2020.110608
Lejzerowicz F., Esling P., Pillet L., Wilding T. A., Black K. D., Pawlowski J. (2015). High-throughput sequencing and morphology perform equally well for benthic monitoring of marine ecosystems. Sci. Rep. 5, 13932. doi: 10.1038/srep13932
Leone A., Lecci R. M., Durante M., Meli F., Piraino S. (2015). The bright side of gelatinous blooms: nutraceutical value and antioxidant properties of three mediterranean jellyfish (Scyphozoa). Mar. Drugs 13, 4654–4681. doi: 10.3390/md13084654
Leone A., Lecci R. M., Milisenda G., Piraino S. (2019). Mediterranean jellyfish as novel food: effects of thermal processing on antioxidant, phenolic, and protein contents. Eur. Food Res. Technol. 245, 1611–1627. doi: 10.1007/s00217-019-03248-6
Leray M., Knowlton N., Machida R. J. (2022). MIDORI2: A collection of quality controlled, preformatted, and regularly updated reference databases for taxonomic assignment of eukaryotic mitochondrial sequences. Environ. DNA 4, 894–907. doi: 10.1002/edn3.303
Leuven R. S. E. W., van der Velde G., Baijens I., Snijders J., van der Zwart C., Lenders H. J. R., et al. (2009). The river Rhine: a global highway for dispersal of aquatic invasive species. Biol. Invasions 11, 1989–2008. doi: 10.1007/s10530-009-9491-7
Lewandowski E., Specht H. (2015). Influence of volunteer and project characteristics on data quality of biological surveys. Conserv. Biol. 29, 713–723. doi: 10.1111/cobi.12481
Liu C., Wolter C., Xian W., Jeschke J. M. (2020). Species distribution models have limited spatial transferability for invasive species. Ecol. Lett. 23, 1682–1692. doi: 10.1111/ele.13577
Liversage K., Kotta J., Aps R., Fetissov M., Nurkse K., Orav-Kotta H., et al. (2019). Knowledge to decision in dynamic seas: Methods to incorporate non-indigenous species into cumulative impact assessments for maritime spatial planning. Sci. Total Environ. 658, 1452–1464. doi: 10.1016/j.scitotenv.2018.12.123
Ljungberg R., Pikkarainen A., Lehtiniemi M., Urho L. (2011). Detection of non-indigenous species in marine monitoring (in Finnish) (Helsinki: Suomen ympäristö).
Luoma E., Laurila-Pant M., Altarriba E., Nevalainen L., Helle I., Granhag L., et al. (2022). A multi-criteria decision analysis model for ship biofouling management in the Baltic Sea. Sci. Total Environ. 852, 158316. doi: 10.1016/j.scitotenv.2022.158316
Mačić V., Albano P. G., Almpanidou V., Claudet J., Corrales X., Essl F., et al. (2018). Biological invasions in conservation planning: A global systematic review. Front. Mar. Sci. 5. doi: 10.3389/fmars.2018.00178
MacKenzie D. I., Nichols J. D., Royle J. A., Pollock K. H., Bailey L. L., Hines J. E. (2006). Occupancy estimation and modeling: inferring patterns and dynamics of species occurrence (London: Elsevier Academic Press).
Mancinelli G., Chainho P., Cilenti L., Falco S., Kapiris K., Katselis G., et al. (2017). The Atlantic blue crab Callinectes sapidus in southern European coastal waters: Distribution, impact and prospective invasion management strategies. Mar. pollut. Bull. 119, 5–11. doi: 10.1016/j.marpolbul.2017.02.050
Mancuso F. P., D’Agostaro R., Milazzo M., Badalamenti F., Musco L., Mikac B., et al. (2022). The invasive seaweed Asparagopsis taxiformis erodes the habitat structure and biodiversity of native algal forests in the Mediterranean Sea. Mar. Environ. Res. 173, 105515. doi: 10.1016/j.marenvres.2021.105515
Marambio M., Canepa A., Lòpez L., Gauci A. A., Gueroun S. K. M., Zampardi S., et al. (2021). Unfolding jellyfish bloom dynamics along the mediterranean basin by transnational citizen science initiatives. Diversity 13, 274. doi: 10.3390/d13060274
Marcelino V. R., Verbruggen H. (2015). Ecological niche models of invasive seaweeds. J. Phycol. 51, 606–620. doi: 10.1111/jpy.12322
Mavraki N., Degraer S., Moens T., Vanaverbeke J. (2019). Functional differences in trophic structure of offshore wind farm communities: a stable isotope study. Mar. Environ. Res. 157, 104868. doi: 10.1016/j.marenvres.2019.104868
Mazza G., Aquiloni L., Inghilesi A. F., Giuliani C., Lazzaro L., Ferretti G., et al. (2015). Aliens just a click away: the online aquarium trade in Italy. Manage. Biol. Invasion 6, 253–261. doi: 10.3391/mbi.2015.6.3.04
Mazza G., Tricarico E., Genovesi P., Gherardi F. (2014). Biological invaders are threats to human health: an overview. Ethol. Ecol. Evol. 26, 112–129. doi: 10.1080/03949370.2013.863225
McCarthy A. H., Peck L. S., Aldridge D. C. (2022). Ship traffic connects Antarctica’s fragile coasts to worldwide ecosystems. Proc. Natl. Acad. Sci. U.S.A. 119, e2110303118. doi: 10.1073/pnas.2110303118
McColl-Gausden E. F., Weeks A. R., Coleman R., Song S., Tingley R. (2023). Using hierarchical models to compare the sensitivity of metabarcoding and qPCR for eDNA detection. Ecol. Inform. 75, 102072. doi: 10.1016/j.ecoinf.2023.102072
Meinita M. D. N., Harwanto D., Choi J. S. (2022). A concise review of the bioactivity and pharmacological properties of the genus Codium (Bryopsidales, Chlorophyta). J. Appl. Phycol. 34, 2827–2845. doi: 10.1007/s10811-022-02842-8
Melo-Merino S. M., Reyes-Bonilla H., Lira-Noriega A. (2020). Ecological niche models and species distribution models in marine environments: A literature review and spatial analysis of evidence. Ecol. Model. 415, 108837. doi: 10.1016/j.ecolmodel.2019.108837
Merten V., Puebla O., Bayer T., Reusch T. B. H., Fuss J., Stefanschitz J., et al. (2023). Arctic nekton uncovered by eDNA metabarcoding: Diversity, potential range expansions, and pelagic-benthic coupling. Environ. DNA 5, 503–518. doi: 10.1002/edn3.403
Mesgaran M. B., Cousens R. D., Webber B. L. (2014). Here be dragons: a tool for quantifying novelty due to covariate range and correlation change when projecting species distribution models. Divers. Distrib. 20, 1147–1159. doi: 10.1111/ddi.12209
Michailidis N., Chartosia N., Katsanevakis S. (2023). Exploring the role of fishing in a heavily bioinvaded shelf ecosystem. Fish. Res. 259, 106554. doi: 10.1016/j.fishres.2022.106554
Miller A. W., Chang A. L., Cosentino-Manning N., Ruiz G. M. (2004). A new record and eradication of the Northern Atlantic alga Ascophyllum nodosum (Phaeophyceae) from San Francisco Bay, California, USA. J. Phycol. 40, 1028–1031. doi: 10.1111/j.1529-8817.2004.04081.x
Miller A., Ruiz G. M. (2014). Arctic shipping and marine invaders. Nat. Climate Change 4, 413–416. doi: 10.1038/nclimate2244
Miralles L., Ibabe A., González M., García-Vázquez E., Borrell Y. J. (2021). “If you know the enemy and know yourself”: addressing the problem of biological invasions in ports through a new NIS invasion threat score, routine monitoring, and preventive action plans. Front. Mar. Sci. 8. doi: 10.3389/fmars.2021.633118
Misal B. N., Sabale A. B. (2016). Influence of seaweed liquid fertilizer (SLF) on seed germination and seedling growth of some crop plants. Indian J. Appl. Res. 6, 505–508.
Morrisey D., Woods C. (2015) In-water cleaning technologies: review of information. Available at: http://www.mpi.govt.nz/document-vault/10814.
Mulas M., Silverman J., Guy-Haim T., Noé S., Rilov G. (2022). High climate vulnerably of the Levantine endemic and endangered habitat-forming macroalga, Gongolaria rayssiae: implications for reef carbon budget. Front. Mari. Sci. 9. doi: 10.3389/fmars.2022.862332
Narščius A., Olenin S., Zaiko A., Minchin D. (2012). Biological invasion impact assessment system: From idea to implementation. Ecol. Inform. 7, 46–51. doi: 10.1016/j.ecoinf.2011.11.003
Nekvapil F., Aluas M., Barbu-Tudoran L., Suciu M., Bortnic R.-A., Glamuzina B., et al. (2019). From Blue Bioeconomy toward Circular Economy through High-Sensitivity Analytical Research on Waste Blue Crab Shells. ACS Sustain. Chem. Eng. 7, 16820–16827. doi: 10.1021/acssuschemeng.9b04362
Nentwig W., Bacher S., Pyšek P., Vilà M., Kumschick S. (2016). The generic impact scoring system (GISS): a standardized tool to quantify the impacts of alien species. Environ. Monit. Assess. 188, 315. doi: 10.1007/s10661-016-5321-4
Newbold T., Hudson L. N., Hill S. L., Contu S., Lysenko I., Senior R. A., et al. (2015). Global effects of land use on local terrestrial biodiversity. Nature 520, 45–50. doi: 10.1038/nature14324
Nikolaou A., Katsanevakis S. (2023). Marine extinctions and their drivers. Reg. Environ. Change 23, 88. doi: 10.1007/s10113-023-02081-8
Noonburg E. G., Byers J. E. (2005). More harm than good: when invader vulnerability to predators enhances impact on native species. Ecology 86, 2555–2560. doi: 10.1890/05-0143
Nugent J. (2018). iNaturalist: Citizen science for 21st-century naturalists Vol. 41 (Washington: ScienceScope), 12–15.
Nunes A. L., Katsanevakis S., Zenetos A., Cardoso A. C. (2014). Gateways to alien invasions in the European Seas. Aquat. Invasions 9, 133–144. doi: 10.3391/ai.2014.9.2.02
Nuñez M. A., Kuebbing S., Dimarco R. D., Simberloff D. (2012). Invasive Species: to eat or not to eat, that is the question. Conserv. Lett. 5, 334–341. doi: 10.1111/j.1755-263X.2012.00250.x
Occhipinti-Ambrogi A. (2021). Biopollution by invasive marine non-indigenous species: a review of potential adverse ecological effects in a changing climate. Int. J. Environ. Res. Public Health 18, 4268. doi: 10.3390/ijerph18084268
Occhipinti-Ambrogi A., Marchini A., Cantone G., Castelli A., Chimenz C., Cormaci M., et al. (2011). Alien species along the Italian coasts: an overview. Biol. invasions 13, 215–237. doi: 10.1007/s10530-010-9803-y
Ojaveer H., Olenin S., Narščius A., Florin A.-B., Ezhova E., Gollasch S., et al. (2017). Dynamics of biological invasions and pathways over time: a case study of a temperate coastal sea. Biol. Invasions 19, 799–813. doi: 10.1007/s10530-016-1316-x
Olenin S., Elliott M., Bysveen I., Culverhouse P., Daunys D., Dubelaar G. B. J., et al. (2011). Recommendations on methods for the detection and control of biological pollution in marine coastal waters. Mar. pollut. Bull. 62, 2598–2604. doi: 10.1016/j.marpolbul.2011.08.011
Olenin S., Leppäkoski E., Daunys D. (2002). “Internet database on alien species in the Baltic Sea,” in Invasive aquatic species of europe. Distribution, impacts and management. Eds. Leppäkoski E., Gollasch S., Olenin S. (Dordrecht: Springer), 525–528. doi: 10.1007/978-94-015-9956-6_52
Olenin S., Minchin D., Daunys D. (2007). Assessment of biopollution in aquatic ecosystems. Mar. pollut. Bull. 55, 379–394. doi: 10.1016/j.marpolbul.2007.01.010
Olenin S., Narščius A., Minchin D., David M., Galil B., Gollasch S., et al. (2014). Making non-indigenous species information systems practical for management and useful for research: an aquatic perspective. Biol. Conserv. 173, 98–107. doi: 10.1016/j.biocon.2013.07.040
Omonhinmin C., Onuselogu C. (2022). rbcL gene in global molecular data repository. Data Br. 42, 108090. doi: 10.1016/j.dib.2022.108090
OSPAR CEMP Guidelines (2022) Common Indicator: Changes to non-indigenous species communities (NIS3) (OSPAR Agreement 2018-04) updated 2022. Available at: https://www.ospar.org/documents?v=38992.
Outhwaite C. L., McCann P., Newbold T. (2022). Agriculture and climate change are reshaping insect biodiversity worldwide. Nature 605, 97–102. doi: 10.1038/s41586-022-04644-x
Outinen O., Puntila-Dodd R., Barda I., Brzana R., Hegele-Drywa J., Kalnina M., et al. (2021). The role of marinas in the establishment and spread of non-indigenous species in Baltic Sea fouling communities. Biofouling 37, 984–997. doi: 10.1080/08927014.2021.1996564
Ozer T., Gertman I., Kress N., Silverman J., Herut B. (2016). Interannual thermohaline, (1979–2014) and nutrient, (2002–2014) dynamics in the Levantine surface and intermediate water masses, SE Mediterranean Sea. Global Planet. Change 151, 60–67. doi: 10.1016/j.gloplacha.2016.04.001
Paavola M., Olenin S., Leppäkoski E. (2005). Are invasive species most successful in habitats of low native species richness across European brackish water seas? Estuar. Coast. Shelf S. 64, 738–750. doi: 10.1016/j.ecss.2005.03.021
Padilla D. K., Williams S. L. (2004). Beyond ballast water: aquarium and ornamental trades as sources of invasive species in aquatic ecosystems. Front. Ecol. Environ. 2, 131–138. doi: 10.1890/1540-9295(2004)002[0131:BBWAAO]2.0.CO;2
Pastor F., Valiente J. A., Khodayar S. (2020). A warming Mediterranean: 38 years of increasing sea surface temperature. Remote Sens. 12, 2687. doi: 10.3390/rs12172687
Pearman J. K., von Ammon U., Laroche O., Zaiko A., Wood S. A., Zubia M., et al. (2021). Metabarcoding as a tool to enhance marine surveillance of nonindigenous species in tropical harbors: A case study in Tahiti. Environ. DNA 3, 173–189. doi: 10.1002/edn3.154
Pederson J., Bullock R., Carlton J., Dijkstra J., Dobroski N., Dyrynda P., et al. (2005). Marine invaders in the northeast: rapid assessment survey of non-native and native marine species of floating dock communities, august 2003 (Cambridge, MA: MIT Sea Grant College Program).
Peleg O., Guy-Haim T., Yeruham E., Silverman J., Rilov G. (2020). Tropicalisation may invert trophic state and carbon budget of shallow temperate rocky reefs. J. Ecol. 108, 844–854. doi: 10.1111/1365-2745.13329
Pergl J., Brundu G., Harrower C. A., Cardoso A. C., Genovesi P., Katsanevakis S., et al. (2020). Applying the Convention on Biological Diversity Pathway Classification to alien species in Europe. NeoBiota 62, 333–363. doi: 10.3897/neobiota.62.53796
Peron G. R., Altwegg G. A., Jamie C. N., Spottiswoode C. N. (2016). Coupled range dynamics of brood parasites and their hosts responding to climate and vegetation changes. J. Anim. Ecol. 85, 1191–1199. doi: 10.1111/1365-2656.12546
Peyton J. M., Martinou A. F., Adriaens T., Chartosia N., Karachle P. K., Rabitsch W., et al. (2020). Horizon scanning to predict and prioritize invasive alien species with the potential to threaten human health and economies on Cyprus. Front. Ecol. Evol. 8. doi: 10.3389/fevo.2020.566281
Peyton J., Martinou A. F., Pescott O. L., Demetriou M., Adriaens T., Arianoutsou M., et al. (2019). Horizon scanning for invasive alien species with the potential to threaten biodiversity and human health on a Mediterranean island. Biol. Invasions 21, 2107–2125. doi: 10.1007/s10530-019-01961-7
Piazzi L., Gennaro P., Ceccherelli G. (2015). Suitability of the ALien Biotic IndEX (ALEX) for assessing invasion of macroalgae across different Mediterranean habitats. Mar. pollut. Bull. 97, 234–240. doi: 10.1016/j.marpolbul.2015.06.011
Pinsky M. L., Worm B., Fogarty M. J., Sarmiento J. L., Levin S. A. (2013). Marine taxa track local climate velocities. Science 341, 1239–1242. doi: 10.1126/science.1239352
Pintor L. M., Byers J. E. (2015). Do native predators benefit from non-native prey? Ecol. Lett. 18, 1174–1180. doi: 10.1111/ele.12496
Piraino S., Aglieri G., Martell L., Mazzoldi C., Melli V., Milisenda G., et al. (2014). Pelagia benovici sp. nov. (Cnidaria, Scyphozoa): a new jellyfish in the Mediterranean Sea. Zootaxa 3794, 455–468. doi: 10.11646/zootaxa.3794.3.7
Pleijel F., Jondelius U., Norlinder E., Nygren A., Oxelman B., Schander C., et al. (2008). Phylogenies without roots? A plea for the use of vouchers in molecular phylogenetic studies. Mol. Phylogenet. Evol. 48 (1), 369–371. doi: 10.1016/j.ympev.2008.03.024
Pochon X., Zaiko A., Fletcher L. M., Laroche O., Wood S. A. (2017). Wanted dead or alive? Using metabarcoding of environmental DNA and RNA to distinguish living assemblages for biosecurity applications. PloS One 12, e0187636. doi: 10.1371/journal.pone.0187636
Pocock M. J. O., Chandler M., Bonney R., Thornhill I., Albin A., August T., et al. (2018). “Chapter Six - A vision for global biodiversity monitoring with citizen science,” in Advances in ecological research. Eds. Bohan D. A., Dumbrell A. J., Woodward G., Jackson M. (Cambridge, MA: Academic Press), 169–223. doi: 10.1016/bs.aecr.2018.06.003
Pocock M. J. O., Roy H. E., August T., Kuria A., Barasa F., Bett J., et al. (2019). Developing the global potential of citizen science: Assessing opportunities that benefit people, society and the environment in East Africa. J. Appl. Ecol. 56, 274–281. doi: 10.1111/1365-2664.13279
Poloczanska E. S., Burrows M. T., Brown C. J., García Molinos J., Halpern B. S., Hoegh-Guldberg O., et al. (2016). Responses of marine organisms to climate change across oceans. Front. Mar. Sci. 3. doi: 10.3389/fmars.2016.00062
Poursanidis D. (2015). “Ecological niche modeling of the invasive lionfish Pterois miles (Bennett 1828) in the mediterranean sea,” in 11th Panhellenic Symposium on Oceanography and Fisheries, Mytilene, Greece. 621–624.
Poursanidis D., Kalogirou S., Azzurro E., Parravicini V., Bariche M., Zu Dohna H. (2020). Habitat suitability, niche unfilling and the potential spread of Pterois miles in the Mediterranean Sea. Mar. pollut. Bull. 154, 111054. doi: 10.1016/j.marpolbul.2020.111054
Poursanidis D., Kougioumoutzis K., Minasidis V., Chartosia N., Kletou D., Kalogirou S. (2022). Uncertainty in marine species distribution modelling: trying to locate invasion hotspots for pterois miles in the eastern mediterranean sea. J. Mar. Sci. Eng. 10, 729. doi: 10.3390/jmse10060729
Power A., Mitchell M., Walker R., Posey M., Alphin T., Belcher C. (2006). Baseline port surveys for introduced marine molluskan, crustacean and polychaete species in the south atlantic bight (Washington, DC: NOAA).
Preston C., Yamahara K., Pargett D., Weinstock C., Birch J., Roman B., et al. (2023). Autonomous eDNA collection using an uncrewed surface vessel over a 4200-km transect of the eastern Pacific Ocean. Environ. DNA. doi: 10.1002/edn3.468
Price-Jones V., Brown P. M. J., Adriaens T., Tricarico E., Farrow R. A., Cardoso A. C., et al. (2022). Eyes on the aliens: citizen science contributes to research, policy and management of biological invasions in Europe. NeoBiota 78, 1–24. doi: 10.3897/neobiota.78.81476
Probert A. F., Wegmann D., Volery L., Adriaens T., Bakiu R., Bertolino S., et al. (2022). Identifying, reducing, and communicating uncertainty in community science: a focus on alien species. Biol. Invasions 24, 3395–3421. doi: 10.1007/s10530-022-02858-8
Pyke C. R., Thomas R., Porter R. D., Hellmann J. J., Dukes J. S., Lodge D. M., et al. (2008). Current practices and future opportunities for policy on climate change and invasive species. Conserv. Biol. 22, 585–592. doi: 10.1111/j.1523-1739.2008.00956.x
Quast C., Pruesse E., Yilmaz P., Gerken J., Schweer T., Yarza P., et al. (2013). The SILVA ribosomal RNA gene database project: improved data processing and web-based tools. Nucleic Acids Res. 41 (Database issue), D590–D596. doi: 10.1093/nar/gks1219
Read G. B., Inglis G., Stratford P., Ahyong S. T. (2011). Arrival of the alien fanworm Sabella spallanzanii (Gmelin 1791) (Polychaeta: Sabellidae) in two New Zealand harbours. Aquat. Invasions 6, 273–279. doi: 10.3391/ai.2011.6.3.04
Reid A., Seebacher F., Ward A. (2010). Learning to hunt: the role of experience in predator success. Behaviour 147, 223–233. doi: 10.1163/000579509X12512871386137
Reise K., Buschbaum C., Lackschewitz D., Thieltges D. W., Waser A. M., Wegner K. M. (2023). Introduced species in a tidal ecosystem of mud and sand: curse or blessing? Mar. Biodivers. 53, 5. doi: 10.1007/s12526-022-01302-3
Rey A., Basurko O. C., Rodríguez-Ezpeleta N. (2018). The challenges and promises of genetic approaches for ballast water management. J. Sea Res. 133, 134–145. doi: 10.1016/j.seares.2017.06.001
Rey A., Basurko O. C., Rodriguez-Ezpeleta N. (2020). Considerations for metabarcoding-based port biological baseline surveys aimed at marine nonindigenous species monitoring and risk assessments. Ecol. Evol. 10, 2452–2465. doi: 10.1002/ece3.6071
Ricciardi A., Blackburn T. M., Carlton J. T., Dick J. T. A., Hulme P. E., Iacarella J. C., et al. (2017). Invasion science: a horizon scan of emerging challenges and opportunities. Trends Ecol. Evol. 32, 464–474. doi: 10.1016/j.tree.2017.03.007
Ricciardi A., Rasmussen J. B. (1998). Predicting the identity and impact of future biological invaders: a priority for aquatic resource management. Can. J. Fish. Aquat. Sci. 55, 1759–1765. doi: 10.1139/f98-066
Rilov G. (2009). “Predator-prey interactions of marine invaders,” in Biological invasions in marine ecosystems, vol. 204 . Eds. Rilov G., Crooks J. A. (Berlin, Germany: Springer). Ecological Studies. doi: 10.1007/978-3-540-79236-9_15
Rilov G. (2016). Multi-species collapses at the warm edge of a warming sea. Sci. Rep. 6, 36897. doi: 10.1038/srep36897
Rilov G., Crooks J. A. (2009). Biological invasions in marine ecosystems: ecological, management, and geographic perspectives (Berlin, Germany: Springer).
Rilov G., Fraschetti S., Gissi E., Pipitone C., Badalamenti F., Tamburello L., et al. (2020). A fast-moving target: achieving marine conservation goals under shifting climate and policies. Ecol. Appl. 30, e02009. doi: 10.1002/eap.2009
Rilov G., Klein L., Iluz D., Dubinsky Z., Guy-Haim T. (2022). Last snail standing? superior thermal resilience of an alien tropical intertidal gastropod over natives in an ocean-warming hotspot. Biol. Invasions 24, 3703–3719. doi: 10.1007/s10530-022-02871-x
Rilov G., Mazaris A., Stelzenmüller V., Helmuth B., Wahl M., Guy-Haim T., et al. (2019a). Adaptive marine conservation planning in the face of climate change: What can we learn from physiological, genetic and ecological studies? Global Ecol. Conserv. 17, e00566. doi: 10.1016/j.gecco.2019.e00566
Rilov G., Peleg O., Guy-Haim T. (2019b). “The restructuring of levant reefs by aliens, ocean warming and overfishing: implications to species interactions and ecosystem functions,” in Interactions in the marine benthos: global patterns and processes. Eds. Hawkins S. J., Bohn K., Firth L. B., Williams G. A. (Cambridge, UK: Cambridge University Press), 214–237. doi: 10.1017/9781108235792.010
Rilov G., Peleg O., Yeruham E., Garval T., Vichik A., Raveh O. (2018). Alien turf: overfishing, overgrazing and invader domination in southeastern Levant reef ecosystems. Aquat. Conserv. 28, 351–369. doi: 10.1002/aqc.2862
Robinson T. B., Martin N., Loureiro T. G., Matikinca P., Robertson M. P. (2020). Double trouble: The implications of climate change for biological invasions. NeoBiota 62, 463–487. doi: 10.3897/neobiota.62.55729
Robinson N. M., Nelson W. A., Costello M. J., Sutherland J. E., Lundquist C. J. (2017). A systematic review of marine-based species distribution models (SDMs) with recommendations for best practice. Front. Mar. Sci. 4. doi: 10.3389/fmars.2017.00421
Roca M., Dunbar M. B., Román A., Caballero I., Zoffoli M. L., Gernez P., et al. (2022). Monitoring the marine invasive alien species Rugulopteryx okamurae using unmanned aerial vehicles and satellites. Front. Mar. Sci. 9. doi: 10.3389/fmars.2022.1004012
Rocha L. A., Aleixo A., Allen G., Almeda F., Baldwin C. C., Barclay M. V., et al. (2014). Specimen collection: an essential tool. Science 344 (6186), 814–815. doi: 10.1126/science.344.6186.814
Roura-Pascual N., Leung B., Rabitsch W., Rutting L., Vervoort J., Bacher S., et al. (2021). Alternative futures for global biological invasions. Sustain. Sci. 16, 1637–1650. doi: 10.1007/s11625-021-00963-6
Rouse J. W., Benton A. R., Toler R. W., Haas R. H. (1975). Three examples of applied remote sensing of vegetation. In NASA earth resources survey symposium, vol 1-C (Houston, USA), 1797–1810.
Roy H. E., Bacher S., Essl F., Adriaens T., Aldridge D. C., Bishop J. D., et al. (2019). Developing a list of invasive alien species likely to threaten biodiversity and ecosystems in the European Union. Glob. Change Biol. 25, 1032–1048. doi: 10.1111/gcb.14527
Roy H. E., Preston C. D., Harrower C. A., Rorke S. L., Noble D., Sewell J., et al. (2014). GB Non-native Species Information Portal: documenting the arrival of non-native species in Britain. Biol. Invasions 16, 2495–2505. doi: 10.1007/s10530-014-0687-0
Ruitton S., Blanfuné A., Boudouresque C.-F., Guillemain D., Michotey V., Roblet S., et al. (2021). Rapid spread of the invasive brown alga rugulopteryx okamurae in a national park in provence (France, mediterranean sea). Water 13, 2306. doi: 10.3390/w13162306
Sala E., Kizilkaya Z., Yildirim D., Ballesteros E. (2011). Alien marine fishes deplete algal biomass in the eastern Mediterranean. PloS One 6, e17356. doi: 10.1371/journal.pone.0017356
Salomidi M., Katsanevakis S., Issaris Y., Tsiamis K., Katsiaras N. (2013). Anthropogenic disturbance of coastal habitats promotes the spread of the introduced scleractinian coral Oculina patagonica in the Mediterranean Sea. Biol. Invasions 15, 1961–1971. doi: 10.1007/s10530-013-0424-0
Sambrook K., Holt R. H. F., Sharp R., Griffith K., Roche R. C., Newstead R. G., et al. (2014). Capacity, capability and cross-border challenges associated with marine eradication programmes in Europe: The attempted eradication of an invasive non-native ascidian, Didemnum vexillum in Wales, United Kingdom. Mar. Policy 48, 51–58. doi: 10.1016/j.marpol.2014.03.018
Sandvik H., Dolmen D., Elven R., Falkenhaug T., Forsgren E., Hansen H., et al. (2019). Alien plants, animals, fungi and algae in Norway: an inventory of neobiota. Biol. Invasions 21, 2997–3012. doi: 10.1007/s10530-019-02058-x
Sandvik H., Sæther B.-E., Holmern T., Tufto J., Engen S., Roy H. E. (2013). Generic ecological impact assessments of alien species in Norway: a semi-quantitative set of criteria. Biodivers. Conserv. 22, 37–62. doi: 10.1007/s10531-012-0394-z
Santamaría J., Golo R., Verdura J., Tomas F., Ballesteros E., Alcoverro T., et al. (2022). Learning takes time: Biotic resistance by native herbivores increases through the invasion process. Ecol. Lett. 25, 2525–2539. doi: 10.1111/ele.14115
Sapkota R., Winding A., Stæhr P. A. U., Andersen N. R., Buur H., Hablutzel P. (2023). Use of metabarcoding to detect non-indigenous species in Danish harbours: Methods comparison (Denmark: Aarhus University, DCE – Danish Centre for Environment and Energy). Available at: https://dce2.au.dk/pub/TR267.pdf.
Sardain A., Sardain E., Leung B. (2019). Global forecasts of shipping traffic and biological invasions to 2050. Nat. Sustain. 2, 274–282. doi: 10.1038/s41893-019-0245-y
Schlaepfer M. A., Sax D. F., Olden J. D. (2011). The potential conservation value of non-native species. Conserv. Biol. 25, 428–437. doi: 10.1111/j.1523-1739.2010.01646.x
Schmelzle M. C., Kinziger A. P. (2016). Using occupancy modelling to compare environmental DNA to traditional field methods for regional-scale monitoring of an endangered aquatic species. Mol. Ecol. Resour. 16, 895–908. doi: 10.1111/1755-0998.12501
Schroeder K., Chiggiato J., Bryden H. L., Borghini M., Ben Ismail S. (2016). Abrupt climate shift in the Western Mediterranean Sea. Sci. Rep. 6, 23009. doi: 10.1038/srep23009
Seebens H., Blackburn T. M., Dyer E. E., Genovesi P., Hulme P. E., Jeschke J. M., et al. (2017). No saturation in the accumulation of alien species worldwide. Nat. Commun. 8, 14435. doi: 10.1038/ncomms14435
Seltzer C. (2019). Making Biodiversity Data Social, Shareable, and Scalable: Reflections on iNaturalist & citizen science. BISS 3, e46670. doi: 10.3897/biss.3.46670
Servello G., Andaloro F., Azzurro E., Castriota L., Catra M., Chiarore A., et al. (2019). Marine alien species in Italy: A contribution to the implementation of descriptor D2 of the marine strategy framework directive. Mediterr. Mar. Sci. 20, 1–48. doi: 10.12681/mms.18711
Sewell J., Bishop J. D. D., Seeley R. (2010). Through the portal – improving the flow of information on non-native species in Great Britain. Biol. Mar. Mediterr. 17, 76–77.
Sigsgaard E. E., Nielsen I. B., Carl H., Krag M. A., Knudsen S. W., Xing Y., et al. (2017). Seawater environmental DNA reflects seasonality of a coastal fish community. Mar. Biol. 164, 128. doi: 10.1007/s00227-017-3147-4
Sih A., Bolnick D. I., Luttbeg B., Orrock J. L., Peacor S. D., Pintor L. M., et al. (2010). Predator-prey naïveté, antipredator behavior, and the ecology of predator invasions. Oikos 119, 610–621. doi: 10.1111/j.1600-0706.2009.18039.x
Simpson A., Sellers E., Grosse A., Xie Y. (2006). Essential elements of online information networks on invasive alien species. Biol. Invasions 8, 1579–1587. doi: 10.1007/s10530-005-5850-1
Skabeikis A., Morkūnė R., Bacevičius E., Lesutienė J., Morkūnas J., Poškienė A., et al. (2019). Effect of round goby (Neogobius melanostomus) invasion on blue mussel (Mytilus edulis trossulus) population and winter diet of the long-tailed duck (Clangula hyemalis). Biol. Invasions 21, 911–992. doi: 10.1007/s10530-018-1869-y
Sorte C. J., Williams S. L., Zerebecki R. A. (2010). Ocean warming increases threat of invasive species in a marine fouling community. Ecology 91, 2198–2204. doi: 10.1890/10-0238.1
Spalding M. D., Fox H. E., Allen G. R., Davidson N., Ferdaña Z. A., Finlayson M. A. X., et al. (2007). Marine ecoregions of the world: a bioregionalization of coastal and shelf areas. BioScience 57, 573–583. doi: 10.1641/B570707
Spiridonov V. A. (2018). Editorial: Introduced species challenges and opportunities for marine conservation ecology and management practices: Notes inspired by a recent MSC certification. Aquat. Conserv. 28, 522–526. doi: 10.1002/aqc.2942
Stæhr P. A. U., Andersen N. R., Andersen K. R., Buur H., Jakobsen H. H., Larsen J., et al. (2023). Identification, dispersal, and possible mitigation responses for non-indigenous species in the Danish Wadden Sea area (Denmark: Aarhus University, DCE – Danish Centre for Environment and Energy). Available at: https://dce2.au.dk/pub/SR547.pdf. Scientific Report No. 547.
Stæhr P. A. U., Carbonell A., Guerin L., Kabuta S. H., Tidbury H., Viard F. (2022). Trends in new records of non-indigenous species (NIS) introduced by human activities in OSPAR 2023: the 2023 quality status report for the northeast atlantic (Denmark: London, UK: OSPAR Commission). Available at: https://oap.ospar.org/en/ospar-assessments/quality-status-reports/qsr-2023/indicator-assessments/trends-new-records-nis.
Stæhr P. A. U., Jakobsen H. H. (2023). Testing the D2C1 GES indicator for marine non-indigenous species with long-term data from Danish Seas (Denmark: Aarhus University, DCE – Danish Centre for Environment and Energy). Available at: http://dce2.au.dk/pub/SR546.pdf. Scientific Report No. 546.
Stæhr P. A., Jakobsen H. H., Hansen J. L., Andersen P., Christensen J., Göke C., et al. (2020). Trends in records and contribution of non-indigenous and cryptogenic species to marine communities in Danish waters: potential indicators for assessing impact. Aquat. Invasions 15, 217–244. doi: 10.3391/ai.2020.15.2.02
Statzner B., Bonada N., Dolédec S. (2008). Biological attributes discriminating invasive from native European stream macroinvertebrates. Biol. Invasions 10, 517–530. doi: 10.1007/s10530-007-9148-3
Steger J., Bošnjak M., Belmaker J., Galil B. S., Zuschin M., Albano P. G. (2022). Non-indigenous molluscs in the Eastern Mediterranean have distinct traits and cannot replace historic ecosystem functioning. Global Ecol. Biogeogr. 31, 89–102. doi: 10.1111/geb.13415
Strubbe D., Broennimann O., Chiron F., Matthysen E. (2013). Niche conservatism in non-native birds in Europe: niche unfilling rather than niche expansion. Glob. Ecol. Biogeogr. 22, 962–970. doi: 10.1111/geb.12050
Suzuki-Ohno Y., Tanabe A. S., Kasai A., Masuda R., Seino S., Dazai A., et al. (2023). Evaluation of community science monitoring with environmental DNA for marine fish species: “Fish survey project using environmental DNA”. Environ. DNA 5, 613–623. doi: 10.1002/edn3.425
Teixeira H., Zenetos A., Katsanevakis S., Korpinen S. (2019). Cumulative impact of marine alien species (CIMPAL) in european seas in Multiple pressures and their combined effects in Europe’s seas, ETC/ICM Technical Report 4/2019 (Magdeburg: European Topic Centre on Inland Coastal and Marine waters).
Teixeira Alves M., Taylor N. G., Tidbury H. J. (2021). Understanding drivers of wild oyster population persistence. Sci. Rep. 11, 7837. doi: 10.1038/s41598-021-87418-1
Teixeira Alves M., Tidbury H. J. (2022). Invasive non-native species management under climatic and anthropogenic pressure: application of a modelling framework. Manage. Biol. Invasion 13, 259. doi: 10.3391/mbi.2022.13.2.01
Thresher R. E., Kuris A. M. (2004). Options for managing invasive marine species. Biol. Invasions 6, 295–300. doi: 10.1023/B:BINV.0000034598.28718.2e
Tøttrup A. P., Svenningsen L., Rytter M., Lillemark M. R., Møller P., Knudsen S. W. (2021). Citizens in the lab: Performance and validation of eDNA results. Citizen Sci. Theory Pract. 6, 35. doi: 10.5334/cstp.382
Trombetti M., Katsanevakis S., Deriu I., Cardoso A. C. (2013). EASIN-Lit: a geo-database of published alien species records. Manage. Biol. Invasion 4, 261–264. doi: 10.3391/mbi.2013.4.3.08
Tsiamis K., Azzurro E., Bariche M., Çinar M. E., Crocetta F., De Clerck O., et al. (2020). Prioritizing marine invasive alien species in the European Union through horizon scanning. Aquat. Conserv. 30, 794–845. doi: 10.1002/aqc.3267
Tsiamis K., Gervasini E., D’Amico F., Deriu I., Katsanevakis S., Crocetta F., et al. (2016). The EASIN Editorial Board: quality assurance, exchange and sharing of alien species information in Europe. Manage. Biol. Invasion 7, 321–328. doi: 10.3391/mbi.2016.7.4.02
Tsiamis K., Palialexis A., Stefanova K., Gladan Ž.N., Skejić S., Despalatović M., et al. (2019). Non-indigenous species refined national baseline inventories: A synthesis in the context of the European Union’s Marine Strategy Framework Directive. Mar. pollut. Bull. 145, 429–435. doi: 10.1016/j.marpolbul.2019.06.012
Tsiamis K., Zenetos A., Deriu I., Gervasini E., Cardoso A. C. (2018). The native distribution range of the European marine non-indigenous species. Aquat. Invasions 13, 187–198. doi: 10.3391/ai.2018.13.2.01
Tsirintanis K., Azzurro E., Crocetta F., Dimiza M., Froglia C., Gerovasileiou V., et al. (2022). Bioinvasion impacts on biodiversity, ecosystem services, and human health in the Mediterranean Sea. Aquat. Invasions 17, 308–352. doi: 10.3391/ai.2022.17.3.01
Tsirintanis K., Sini M., Ragkousis M., Zenetos A., Katsanevakis S. (2023). Cumulative negative impacts of invasive alien species on marine ecosystems of the aegean sea. Biology 12, 933. doi: 10.3390/biology12070933
Tsotsios D., Moutopoulos D. K., Lattos A., Michaelidis B., Theodorou J. A. (2023). Impacts of the establishment of biofoulants on greek aquaculture: farmers’ Expert knowledge. J. Mar. Sci. Eng. 11, 1077. doi: 10.3390/jmse11051077
Tulloch A. I. T., Possingham H. P., Joseph L. N., Szabo J., Martin T. G. (2013). Realising the full potential of citizen science monitoring programs. Biol. Conserv. 165, 128–138. doi: 10.1016/j.biocon.2013.05.025
Uchimura M., Rival A., Nato A., Sandeaux R., Sandeaux J., Baccou J. C. (2000). Potential use of Cu2+, K+ and Na+ for the destruction of Caulerpa taxifolia: differential effects on photosynthetic parameters. J. Appl. Phycol. 12, 15–23. doi: 10.1023/A:1008108531280
Ulman A., Ferrario J., Occhpinti-Ambrogi A., Arvanitidis C., Bandi A., Bertolino M., et al. (2017). A massive update of non-indigenous species records in Mediterranean marinas. PeerJ 5, p.e3954. doi: 10.7717/peerj.3954
UNEP/MPA (2017). Action plan concerning species introductions and invasive species in the mediterranean sea (Greece: UN Environment/MAP Athens).
Valéry L., Fritz H., Lefeuvre J. C. (2013). Another call for the end of invasion biology. Oikos 122, 1143–1146. doi: 10.1111/j.1600-0706.2013.00445.x
Valéry L., Fritz H., Lefeuvre J. C., Simberloff D. (2008). In search of a real definition of the biological invasion phenomenon. Biol. Invasions 10, 1345–1351. doi: 10.1007/s10530-007-9209-7
Valéry L., Fritz H., Lefeuvre J. C., Simberloff D. (2009). Invasive species can also be native. Trends Ecol. Evol. 24, 585. doi: 10.1016/j.tree.2009.07.003
Vaz A. S., Novoa A., Vicente J. R., Honrado J. P., Shackleton R. T. (2021). Invaders on the horizon! Scanning the future of invasion science and management. Front. Ecol. Evol. 9. doi: 10.3389/fevo.2021.756339
Verleye T. J., De Raedemaecker F., Vandepitte L., Fockedey N., Lescrauwaet A.-K., De Pooter D., et al. (2020). Niet-inheemse soorten in het Belgisch deel van de Noordzee en aanpalende estuaria (Oostende: Vlaams Instituut voor de Zee (VLIZ).
Vilà M., Espinar J. L., Hejda M., Hulme P. E., Jarošík V., Maron J. L., et al. (2011). Ecological impacts of invasive alien plants: a meta-analysis of their effects on species, communities and ecosystems. Ecol. Lett. 14, 702–708. doi: 10.1111/j.1461-0248.2011.01628.x
Vilizzi L., Copp G. H., Adamovich B., Almeida D., Chan J., Davison P. I., et al. (2019). A global review and meta-analysis of applications of the freshwater Fish Invasiveness Screening Kit. Rev. Fish Biol. Fisher. 29, 529–568. doi: 10.1007/s11160-019-09562-2
Vilizzi L., Copp G. H., Hill J. E., Adamovich B., Aislabie L., Akin D., et al. (2021). A global-scale screening of non-native aquatic organisms to identify potentially invasive species under current and future climate conditions. Sci. Total Environ. 788, 147868. doi: 10.1016/j.scitotenv.2021.147868
Vimercati G., Kumschick S., Probert A. F., Volery L., Bacher S. (2020). The importance of assessing positive and beneficial impacts of alien species. NeoBiota 62, 525–545. doi: 10.3897/neobiota.62.52793
Vimercati G., Probert A. F., Volery L., Bernardo-Madrid R., Bertolino S., Céspedes V., et al. (2022). The EICAT+ framework enables classification of positive impacts of alien taxa on native biodiversity. PloS Biol. 20, e3001729. doi: 10.1371/journal.pbio.3001729
Vitale R. M., D’Aniello E., Gorbi S., Martella A., Silvestri C., Giuliani M. E., et al. (2018). Fishing for targets of alien metabolites: A novel peroxisome proliferator-activated receptor (PPAR) agonist from a marine pest. Mar. Drugs 16, 431. doi: 10.3390/md16110431
Vranken S., Bosch S., Peña V., Leliaert F., Mineur F., De Clerck O. (2018). A risk assessment of aquarium trade introductions of seaweed in European waters. Biol. Invasions 20, 1171–1187. doi: 10.1007/s10530-017-1618-7
Wäldchen J., Mäder P. (2018). Machine learning for image based species identification. Methods Ecol. Evol. 9, 2216–2225. doi: 10.1111/2041-210X.13075
Wiggins A., Crowston K. (2011). “From conservation to crowdsourcing: a typology of citizen science,” in Proceedings of the 44th Hawaii International Conference on System Sciences, Kauai, USA. doi: 10.1109/HICSS.2011.207
Wotton D. M., O’Brien C., Stuart M. D., Fergus D. J. (2004). Eradication success down under: heat treatment of a sunken trawler to kill the invasive seaweed Undaria pinnatifida. Mar. pollut. Bull. 49, 844–849. doi: 10.1016/j.marpolbul.2004.05.001
Wylie R., Janssen-May S. (2017). Red imported fire ant in Australia: What if we lose the war? Ecol. Manage. Restor. 18, 32–44. doi: 10.1111/emr.12238
Yeruham E., Rilov G., Shpigel M., Abelson A. (2015). Collapse of the echinoid Paracentrotus lividus populations in the Eastern Mediterranean - result of climate change? Sci. Rep. 5, 13479. doi: 10.1038/srep13479
Yeruham E., Shpigel M., Abelson A., Rilov G. (2019). Ocean warming and tropical invaders erode the performance of a key herbivore. Ecology 101, e02925. doi: 10.1002/ecy.2925
Zabin C., Davidson I., Ruiz G. (2016). In-water vessel cleaning: current and emerging technologies, associated risks, and management options for hawaii (Hawaii: Hawaii State Department of Land and Natural Resources, Division of Aquatic Resources).
Zaiko A., Samuiloviene A., Ardura A., Garcia-Vazquez E. (2015). Metabarcoding approach for nonindigenous species surveillance in marine coastal waters. Mar. pollut. Bull. 100, 53–59. doi: 10.1016/j.marpolbul.2015.09.030
Zampoukas N., Palialexis A., Duffek A., Graveland J., Giorgi G., Hagebro C., et al. (2014). Technical guidance on monitoring for the marine strategy framework directive in Joint research centre of the european commission. EUR 26499, Luxembourg (Luxembourg: Publications Office of the European Union). Available at: http://publications.jrc.ec.europa.eu/repository/bitstream/111111111/30508/1/lb-na-26499-en-n.pdf. JRC88073.
Zenetos A., Arianoutsou M., Bazos I., Balopoulou S., Corsini-Foka M., Dimiza M., et al. (2015). ELNAIS: A collaborative network on Aquatic Alien Species in Hellas (Greece). Manage. Biol. Invasion 6, 185–196. doi: 10.3391/mbi.2015.6.2.09
Zenetos A., Corsini-Foka M., Crocetta F., Gerovasileiou V., Karachle P. K., Simboura N., et al. (2018). Deep cleaning of alien and cryptogenic species records in the Greek Seas, (2018 update). Manage. Biol. Invasion 9, 209–226. doi: 10.3391/mbi.2018.9.3.04
Zenetos A., Galanidi M. (2020). Mediterranean non indigenous species at the start of the 2020s: recent changes. Mar. Biodivers. Rec. 13, 10. doi: 10.1186/s41200-020-00191-4
Zenetos A., Gofas S., Morri C., Rosso A., Violanti D., García Raso J. E., et al. (2012). Alien species in the Mediterranean Sea by 2012. A contribution to the application of European Union’s Marine Strategy Framework Directive (MSFD). Part 2. Introduction trends and pathways. Mediterr. Mar. Sci. 13, 328–352. doi: 10.12681/mms.327
Zenetos A., Karachle P. K., Corsini-Foka M., Gerovasileiou V., Simboura N., Xentidis N. J., et al. (2020). Is the trend in new introductions of marine non-indigenous species a reliable criterion for assessing good environmental status? The case study of Greece. Mediterr. Mar. Sci. 21, 75–793. doi: 10.12681/mms.25136
Zenetos A., Tsiamis K., Galanidi M., Carvalho N., Bartilotti C., Canning-Clode J., et al. (2022). Status and trends in the rate of introduction of marine non-indigenous species in european seas. Diversity 14, 1077. doi: 10.3390/d14121077
Keywords: alien species, biodiversity, biological invasions, ecosystem services, impacts, non-native, pathways, recommendations
Citation: Katsanevakis S, Olenin S, Puntila-Dodd R, Rilov G, Stæhr PAU, Teixeira H, Tsirintanis K, Birchenough SNR, Jakobsen HH, Knudsen SW, Lanzén A, Mazaris AD, Piraino S and Tidbury HJ (2023) Marine invasive alien species in Europe: 9 years after the IAS Regulation. Front. Mar. Sci. 10:1271755. doi: 10.3389/fmars.2023.1271755
Received: 02 August 2023; Accepted: 18 September 2023;
Published: 11 October 2023.
Edited by:
Chiara Piroddi, Joint Research Centre, ItalyReviewed by:
Ioannis A Giantsis, University of Western Macedonia, GreeceCopyright © 2023 Katsanevakis, Olenin, Puntila-Dodd, Rilov, Stæhr, Teixeira, Tsirintanis, Birchenough, Jakobsen, Knudsen, Lanzén, Mazaris, Piraino and Tidbury. This is an open-access article distributed under the terms of the Creative Commons Attribution License (CC BY). The use, distribution or reproduction in other forums is permitted, provided the original author(s) and the copyright owner(s) are credited and that the original publication in this journal is cited, in accordance with accepted academic practice. No use, distribution or reproduction is permitted which does not comply with these terms.
*Correspondence: Stelios Katsanevakis, c3RlbGlvc0BrYXRzYW5ldmFraXMuY29t
†Present address: Silvana N. R. Birchenough, Environmental Resource Management (ERM) Ltd, Norwich, United Kingdom
Disclaimer: All claims expressed in this article are solely those of the authors and do not necessarily represent those of their affiliated organizations, or those of the publisher, the editors and the reviewers. Any product that may be evaluated in this article or claim that may be made by its manufacturer is not guaranteed or endorsed by the publisher.
Research integrity at Frontiers
Learn more about the work of our research integrity team to safeguard the quality of each article we publish.