- 1Instituto Universitario BIOTECMED, Universitat de València, Burjassot, Spain
- 2Departamento de Biología Vegetal, Área de Edafología y Química Agrícola, Facultad de Farmacia, Universitat de València, Burjassot, Spain
- 3AIMPLAS, Instituto Tecnológico del Plástico, Parque Tecnológico Valencia, Paterna, Spain
This study addresses one of the most common problems faced by the marine sector, namely the accumulation of organic matter and organisms on submerged surfaces. This biological phenomenon causes structural problems in aquatic systems and its mitigation implies a large economic outlay for marine aquaculture industry. Antifouling paints are being developed to help control this undesirable process; however, these treatments are problematic as they degrade and release biocides and heavy metals into the environment. In this context, our study focuses on developing more environmentally friendly antifouling strategies. For this purpose, we designed high-density polyethylene (HDPE) material functionalized with different copper compounds or silica, and subsequently tested their effects on biofilm formation by aquatic organisms at both laboratory and pilot scale. Bacterial species (Vibrio harveyi and Cellulophaga lytica) and diatoms (Nitzschia ovalis) known for producing biofilm were used. Our study revealed that material including copper pyrithione (CuPT) was highly effective in inhibiting bacterial and algal biofilm formation. Moreover, the ecotoxicological study covering three trophic levels (bacteria, algae and rotifers) indicated that none of the materials developed and tested herein was toxic. HDPE is easily moldable and suitable to produce built-in aquatic structures, and our results show that its functionalization with CuPT greatly improves its antifouling capacity. These findings represent a step forward in the fight against fouling in marine environments.
Introduction
In marine environments, any submerged surface is affected by biofouling. This phenomenon is described as the unwanted outcome of the accumulation of micro- and macro- organisms, including bacteria, seaweed and barnacles (Callow and Callow, 2011; Seo et al., 2021). It is a stepwise process encompassing three main stages: initially, different organic molecules adhere to the surface, creating a conditioning film that enables bacteria and diatoms to settle; the second step is reversible as bacteria and algae may detach from the surface; in the last stage, microorganisms have created an environment suitable for the attachment of protozoa and small invertebrates, which form macroscopic biofouling (Donlan, 2002; Salta et al., 2013; Ferrari et al., 2015).
The settlement of fouling organisms on the surface of submerged materials causes a series of biological, chemical and physical changes (levels of ions, oxygen, pH…), which promote coating deterioration due to the microbiologically influenced corrosion (MIC) (Ford et al., 1991; Gu, 2003). The adverse effects of marine biofouling are well documented, and are related to economic, environmental and/or safety issues (Endresen et al., 2003). Fouling degrades dock protections and mooring structures as well as causing surface roughness, leading to higher frictional resistance and thus increasing fuel consumption of ships (Endresen et al., 2003; Rosenhahn et al., 2010). Moreover, it is a major management issue in the aquaculture industry, since the settlement of organisms on nets and other structures may increase cage loads and reduce water exchange in cages, resulting in poor water quality and related adverse effects (Cardia and Lovatelli, 2016). Hence, it is fundamental to take measures to reduce the negative impact of biofouling on the marine industry.
In recent decades, high-density polyethylene (HDPE) has become one of the most widely used polymers due to its resistance to acids or solvents, impacts, traction, and to high or low temperatures (Geyer et al., 2017). Besides, HDPE is nontoxic, noncontaminating and fully recyclable. For all these reasons and given its resistance to marine organisms, abrasive seawater and UV rays, HDPE is used extensively in various industries such as the marine sector to build materials for boats or plumbing systems in ships, and for cages in aquaculture facilities (Saputra et al., 2021). Since these aquatic surfaces are susceptible to bacterial colonization, materials functionalized with antifouling agents could provide an excellent strategy to control the growth of microorganisms.
The antimicrobial properties of copper-containing composites have been recognized by numerous ancient civilizations and are, therefore, considered as one of the oldest fouling-degrading strategies (Woods Hole Oceanographic Institution, 1952). Although the precise antimicrobial mechanism of copper is not fully understood, two pathways have been proposed: i) membrane depolarization, which causes cell lysis due to a decrease in the difference in electric potential between the interior and exterior of the cell, and ii) reactive oxygen species (ROS) simultaneously oxidize diverse cellular substances (e.g., nucleic acids, proteins, and lipids) crucial for proper cell function (Mitra et al., 2019; Maan et al., 2020). The copper-based compounds most frequently used in antifouling paints included cuprous oxide (Cu2O), copper pyrithione (CuPT) and cuprous thiocyanate (CuSCN) (Paz-Villarraga et al., 2022).
Furthermore, some additives can act as surface modifiers, increasing efficiency and promoting anti-adhesion properties by modifying the wettability or hydrophobicity of surfaces and the surface-organism interactions (Yang et al., 2016; Eduok et al., 2017). The inclusion of silicon dioxide, also known as silica, could increase the hydrophobicity of the coating, which would result in reduced adhesion strength between the organism and the surface, thus facilitating removal (Hu et al., 2020).
In this study, HDPE has been functionalized with different copper compounds (Cu2O, CuSCN and CuPT) or silica, with subsequent testing of the antifouling properties of these materials. Laboratory and pilot scale assays were performed using bacterial species known for producing large amounts of biofilm and/or for their relevance in marine aquaculture environments (Vibrio harveyi and Cellulophaga lytica), as well as diatoms (Nitzschia ovalis) with high biofilm formation capacity. Moreover, to ensure that functionalized HDPE does not pose a hazard to marine environments, an ecotoxicological study was carried out using a battery of standardized bioassays covering three trophic levels: decomposers (bacteria), producers (algae) and primary consumers (rotifers).
Materials and methods
Bacterial and diatom culture conditions
Two marine bacterial strains, V. harveyi CECT (Spanish Collection of Type Cultures) 5156 and C. lytica CECT 5014 were selected for this study since both species are recognized as early colonizers in the fouling process and are natural inhabitants of the Mediterranean Sea (Karunasagar et al., 1996; Kientz et al., 2016). Bacteria were routinely grown in Marine Broth (MB), or Tripticase Soja Broth (TSB) supplemented with 2.5% of NaCl (TSB-3) at 28 °C for 24 h (V.harveyi), or 48 h (C. lytica). Bacteria were stored at −80°C in MB supplemented with 20% (v/v) glycerol.
The benthic microalgae Nitzschia ovalis BEA (Spanish Bank of Algae) 0423B, selected for this study was routinely grown in flasks containing 100 ml of natural seawater supplemented with Guillard f/2 medium (Guillard, 1975) inoculated with 1 x 105 cells/ml of the microalgae in pre-stationary phase and supplemented with sea water. Cultures were incubated for 7 days in climatic chambers at 21°C under a light intensity of 100 µmol photons m2/s.
Preparation of material (additives)
High Density Polyethylene (HDPE) was functionalized with Cu2O, CuPT, CuSCN and/or silica (biocides registered for use in the European Union). For this purpose, mixes were blended and extruded through a co-rotative twin-screw extruder. Once the compounds were obtained, sheets with a thickness of 300 µm were extruded using an L/D 30 single-screw extruder and a 100 mm wide flat head. These sheets were cut into 12 mm diameter discs or 10x22 cm sheets, as required for the assays. Sterilization of 12 mm discs was carried out by UV radiation in a laminar flow cabinet, with 2 hours of radiation total time (1 hour on each side). Rectangular sheets were washed with 96% ethanol before immersion in seawater tanks for biofilm formation assays.
Different sets of functionalized HDPE were prepared, with the compounds/additives separately/individually or combined at different concentrations (Table 1) to carry out the tests on a laboratory or pilot scale.
Characterization of material
The morphology of the functionalized HDPE was microscopically characterized by a scanning electron microscopy (SEM) (Hitachi FE-SEM S-4800) with an accelerated voltage of 10 kV. Samples were attached to a metal sample holder using double-sided adhesive tape with carbon and were coated with gold-palladium (Au-Pd) by sputtering to make them electrically conductive.
Bacterial biofilm formation assays
Biofilm formation assays were performed with selected bacteria at laboratory and pilot scale.
Laboratory scale. The biofilm formed onto the surface of the discs was quantified by a modified crystal violet (CV) staining method (Niu and Gilbert, 2004). Sterilized 12 mm discs were glued to the bottom of 24-wells cell culture plates. Briefly, overnight cultures in modified artificial seawater basal media [50 mM MgSO4, 10 mM CaCl2, 300 mM NaCl, 10 mM KCl, 0.0058% (wt/vol) K2HPO4, 10 μM FeSO4, 50 mM Tris-HCl (pH 7.5)] supplemented with 0.3% glycerol as carbon source (ASWgly) (Wang et al., 2010) were used to inoculate fresh ASWgly to a concentration of 1 x 106 cells/ml (V. harveyi) or 1 x 108 cells/ml (C. lytica). Then, cultures were placed into a 24-well cell culture plate (3 wells per strain and treatment) and left for static growth at 30°C 24 or 48 h for V.harveyi or C. lytica, respectively. After incubation, bacterial growth was measured at A595 before carefully removing the media by aspiration and staining the biofilm with CV (1 ml/well) for 30 min. Wells were rinsed with PBS (Phosphate Buffered Saline, pH 7) (1 ml/well) to remove the excess of CV stain until the non-disc control did not show visible traces of the dye and the violet stain was solubilized with 1 ml of 96% ethanol. All the experiments were performed in triplicate. The OD540 of the solubilized biofilm was measured and quantification of biofilm formation was calculated with the formula:
where AB is the OD540 of stained attached bacteria, CW is the OD540 or OD595 of stained control wells with no bacteria and CG is the OD595 of cells growth in suspended culture (Niu and Gilbert, 2004; Naves et al., 2008).
Pilot scale. The experiments were carried out in the aquarium facilities of the SCSIE (Central Support Service for Experimental Research) of the University of Valencia. The environmental conditions (temperature, humidity, etc.) were monitored daily. Assays were performed in 200 l tanks containing 100 l of natural seawater UV-sterilized. Seawater mesocosms were inoculated with an overnight washed culture in TSB-3 to a final concentration of 105 CFU/ml (dilution 1/1000). Sheets were submerged in the inoculated seawater and incubated for 14 days. Bacteria population was monitored periodically by plate counting onto Marine Agar (MA).
Biofilm formation was determined with the CV staining protocol similar to the one described before. Briefly, sheets with antifouling coatings were taken out of the tanks and placed in a vessel containing 0.1% CV solution for 30 min. Then, sheets were rinsed twice with PBS and air dried for 30 min. Afterwards, 6 cm2 strips were cut and placed in centrifuge plastic tubes containing 5 ml of absolute ethanol. The amount of biofilm was estimated through the CV resuspended in the ethanol. Three different experiments were performed and two strips for each treatment were recorded.
Diatom colonization assays
The algae biofilm assay was performed in 30 ml screw-tubes filled with 9 ml of sterile seawater supplemented with Guillard f/2 medium plus 1 ml of a culture of N. ovalis. Two discs were placed in each tube (one per treatment) and incubated 7 days at 22°C under a light intensity of 100 µmol photons m2/s.
Biomass adhered at the disc was indirectly measured by a colorimetric quantification of total carbohydrates using the phenol-sulfuric acid method, using glucose for the standard curve (Fournier, 2001). Basically, the two discs for treatment were washed with sterile seawater supplemented with Guillard f/2 medium and inserted into a new tube containing 500 µl of 4% phenol and the biomass was resuspended by agitation with the vortex. Later, 2.5 ml of sulfuric acid was added, and the mix was incubated for 5-10 min. The OD490 was measured, and ng of carbohydrates were calculated using the standard curve. Three different experiments were performed for every treatment studied.
Production of the leachates and ecotoxicological studies
Production of the leachate on functionalized HDPE sheets. For this purpose, glass tanks (30 cm height x 15 cm width x 15 cm depth) were filled with 5 l of synthetic marine water generated with Instant Ocean™ sea salts and dechlorinated tap water (final salinity 37 g/l). HDPE sheets (22 x 22 cm) were wrapped forming a cylinder, then a 36 Watt waterproof UV-C germicide lamp (JEBO®, 20 cm long x 10 cm in diameter) was placed inside (Figure 1). In order to avoid the water stratification and to assure a constant water movement, a 3.8-Watt (Sicce™) circulation pump generating a flow rate of 300 l/h was fixed in the tank bottom. The water temperature was set at 35°C. The total exposure time was 60 d. Samples were taken at 0, 30 and 60 days. Evaporation was checked twice a week and lost water replaced with deionized water.
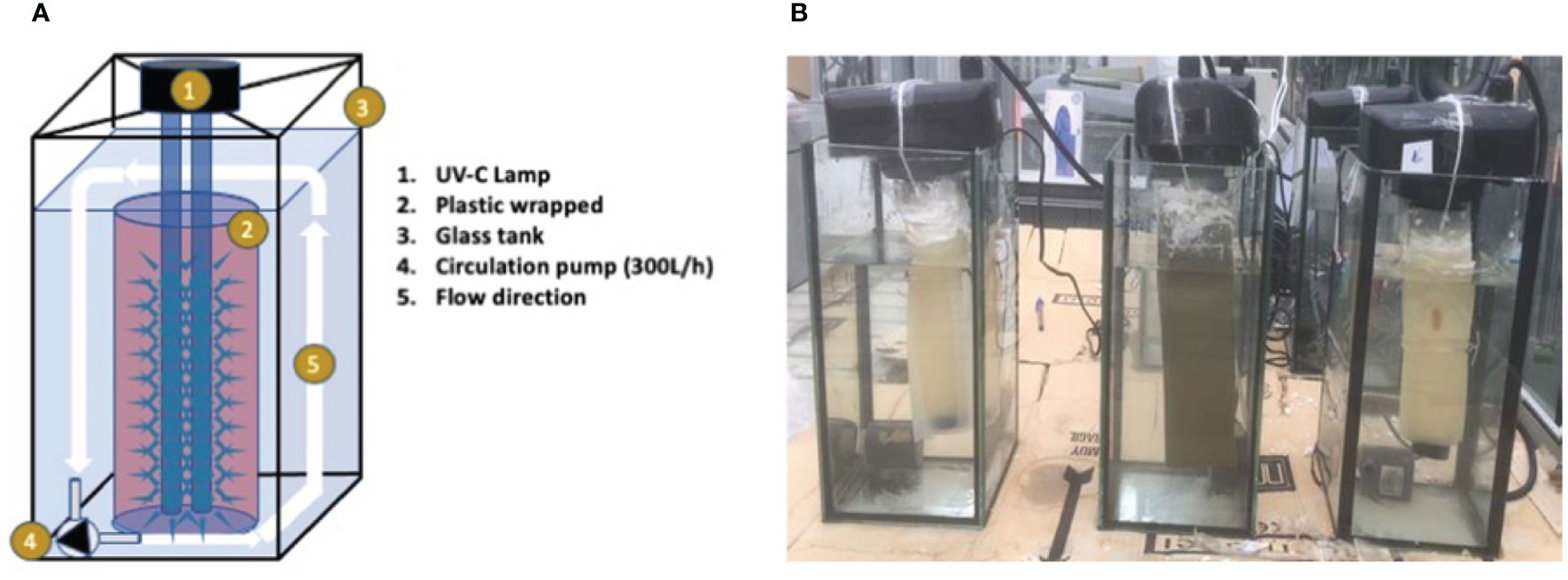
Figure 1 Leachate production setup. (A) Schematic diagram of the experimental setup to produce the leachates. (B) Experimental setup used for the environmental impact assessment of the leachates.
The ecotoxicological effect of the HDPE formulations was assessed using a battery of three bioassays based on standardized ISO guidelines.
Assays with the bacteria Aliivibrio fischeri. The ISO 11348-3 standard (ISO, 2022a) is based in the determination of the inhibitory effect of water samples on the light emission of A. fischeri (Luminescent bacteria) after exposure time of 15 min using freeze-dried bacteria (Zhang et al., 2020). The procedure was conducted according to the Biotox-STD® kit user´s manual of EPBI™ implemented in a handheld PD10 luminometer (Kikkoman™).
Acute toxicity test to the marine rotifer Brachionus plicatilis. The ISO 19820 specifies a method for the determination of the lethal effects of toxicants to B. plicatilis after 24 h or 48 h exposure (Jośko and Oleszczuk, 2012). The Rotoxkit M™ bioassay (MicroBioTests Inc.) was used in the study. Neonates of B. plicatilis were hatched from cysts 24 h before the assay. Mortality of organisms was checked after 24 h and 48 h of exposure to the leachates.
Marine algal growth inhibition test with Phaeodactylum tricornutum. The bioassay was conducted following the ISO 10253 guideline (ISO, 2022b), using the commercially available Algaltoxkit Marine™ (Microbiotests Inc.). The algal growth rate (r) was monitored during 24, 48 and 72 h in a spectrophotometric way at OD670 by means of 2021 Aurius™ spectrophotometer (CECIL Instruments™).
Bioassay data analysis. For the three bioassays, the 100%, 50%, 25%, 12.5% and 6.25% from the original leachate were assayed. According to ISO guidelines, all tests were conducted in triplicate. A probit regression (Litchfield and Wilcoxon, 1949) was used to determine the 50% Effective Concentration (EC50) in each bioassay: 72 h ECr50 in the algal bioassay, 15´ EC50 for the bacteria and 24-48 h EC50 in the rotifer test.
The 50% effect endpoint values (in % of dilution) were converted into toxic units (TU) according to the formula TU = 100/EC50, 100/ECr50 or 100/LC50 for bacteria, algae, and rotifers, respectively (Sprague and Ramsay, 2011).
Quality assurance. The quality of the results obtained in the bioassays was verified by means of positive controls. A. fischeri, P. tricornutum and B. plicatilis were exposed to a standard toxic in order to assure their sensitivity. For this purpose, potassium dichromate (K2Cr2O7) was used as toxic reference according to ISO guidelines.
Chemical analysis
The presence of metals in elutriates after the exposure to UV-C irradiation was determined by conducting chemical trace analysis. After each exposure time (30 d and 60 d) water aliquots were taken from each tank and copper (Cu) analyzed in a single quadrupole inductively coupled plasma mass spectrometry instrument (model 7900 ICP-MSAgilent Technologies™). Analyses were performed in the SCSIE.
Statistical analyses
Bacterial biofilm inhibition, algae colonization and ecotoxicological assays were statistically analyzed by ANOVA tests (p< 0.05). Significant differences between the control and tested groups were detected using Dunett’s test pairwise comparison method, with an α error of 0.05, obtained using RStudio version 2021.9.0.351 (RStudio Team, 2015).
Results
Surface morphology
The surface morphology of the different samples was observed with SEM. Micrographs of the HDPE discs are shown in Figure 2. No differences were observed between samples in the structure and morphology of their membranes.
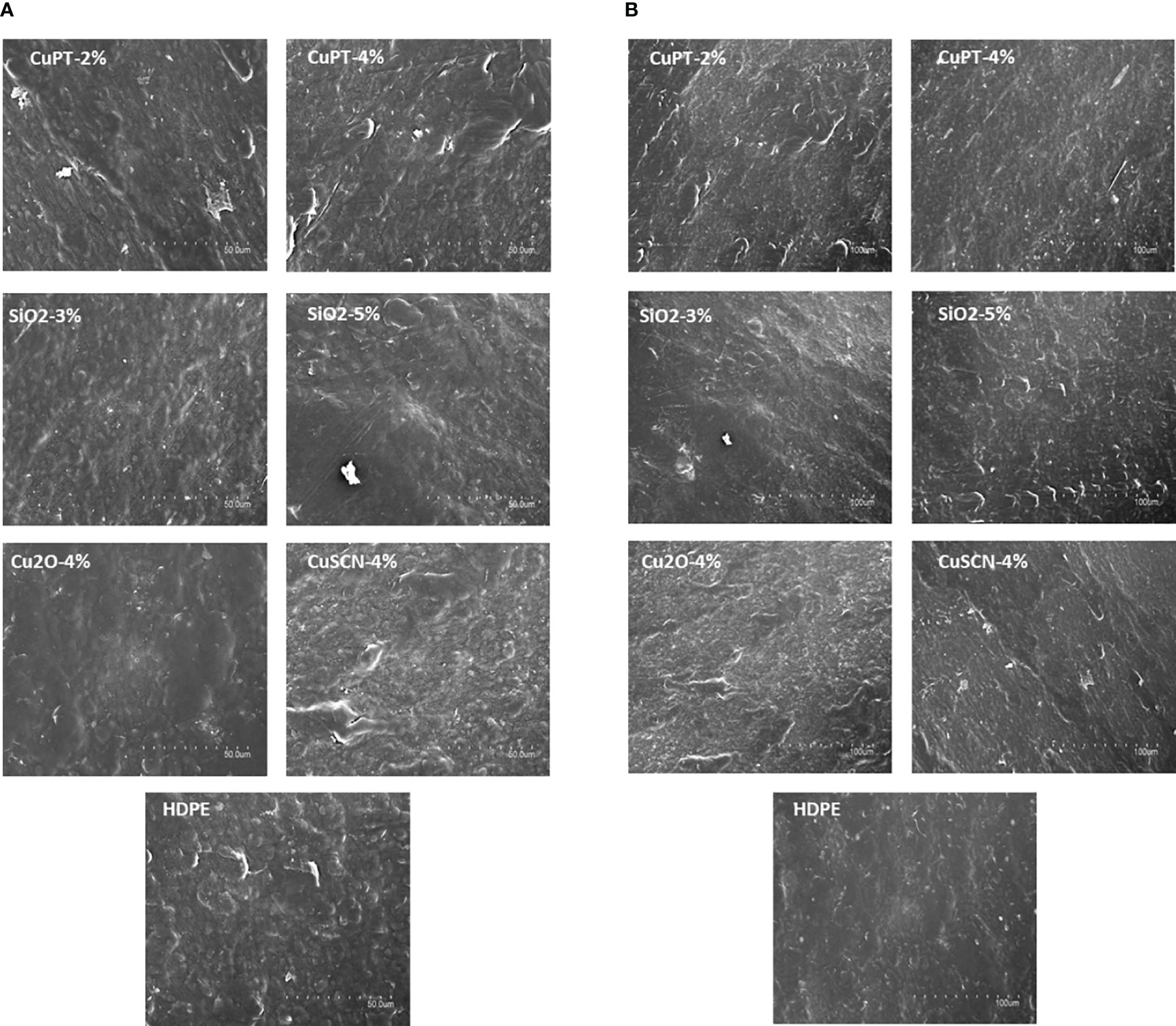
Figure 2 Surface SEM micrographs of high-density polyethylene (HDPE) materials. (A) SEM micrographs of HDPE discs at 1000x magnification. (B) Micrographs of the HDPE discs at 500x magnification.
Bacterial biofilm formation
In a first laboratory approach, the biofilm forming on the surface of the HDPE discs by the selected bacteria was investigated using a crystal violet assay and the BFI was calculated. In both bacterial species, CuPT-functionalized HDPE inhibited biofilm production (Figure 3), reducing it by between 12 and 40% with respect to the control (Table S1). By contrast, HDPE functionalization with CuSCN enhanced biofilm formation, mainly by V. harveyi, under test conditions, whereas no significant changes in biofilm formation were observed on discs treated with silica or Cu2O.
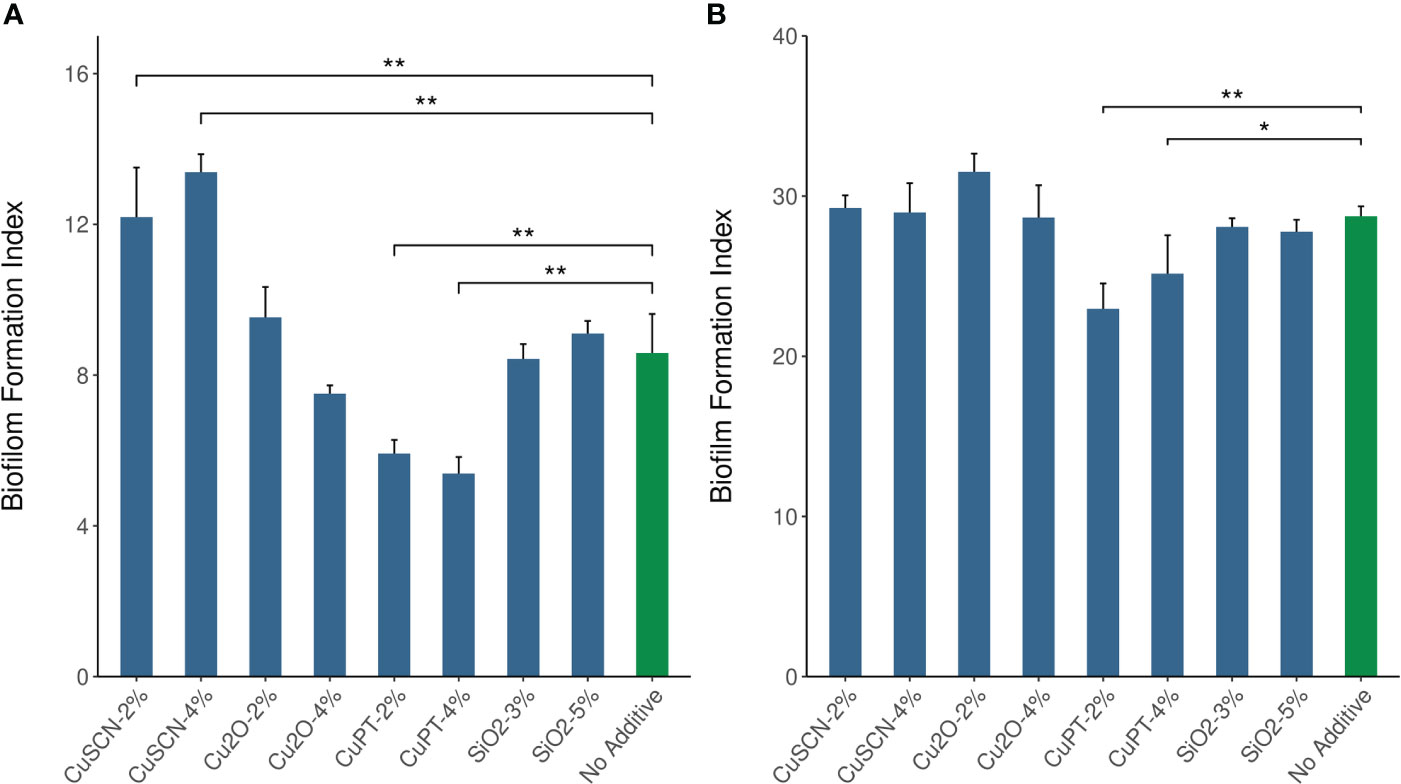
Figure 3 Quantification of biofilm. (A) Biofilm formed after 24h by Vibrio harveyi and (B) after 48h by Cellulophaga lytica on the surface of 12 mm HDPE discs functionalized with different additives and concentrations (%). Biofilm formation was determined by crystal violet staining (and represented as the Biofilm Formation Index (BFI) mean ± SD of three biological replicates. (**) and (*) indicate statistical significance in comparison with the control HDPE disc (p< 0.01 and p< 0.05, respectively).
The second approach was to assess biofilm formation at pilot scale, which also included combined treatments of HDPE. For this purpose, biofilm formation was investigated after 14 days in 100 l seawater mesocosms contaminated with bacterial populations, simulating natural environments (Pujalte et al., 1999). The number of bacteria in all these trials decreased slightly over time. For V. harveyi, the bacterial counts changed over the experimental period from 4.3x105 to 2x105 CFU/ml, and in the case of C. lytica from 7x105 to 9x104 CFU/ml).
We studied treatments with CuPT, (the most efficient additive in our laboratory assays) at a low concentration (2%), combined with Cu2O or silica, as well as a triple combination. We observed that CuPT was the only treatment that produced a notable reduction (at least 30%) in bacterial biofilm formation (Figure 4, Table S2), although this effect was not statistically significant. As occurred in the laboratory approach using CuSCN, the double combinations studied in mesocosms enhanced biofilm formation by both bacterial species under test conditions.
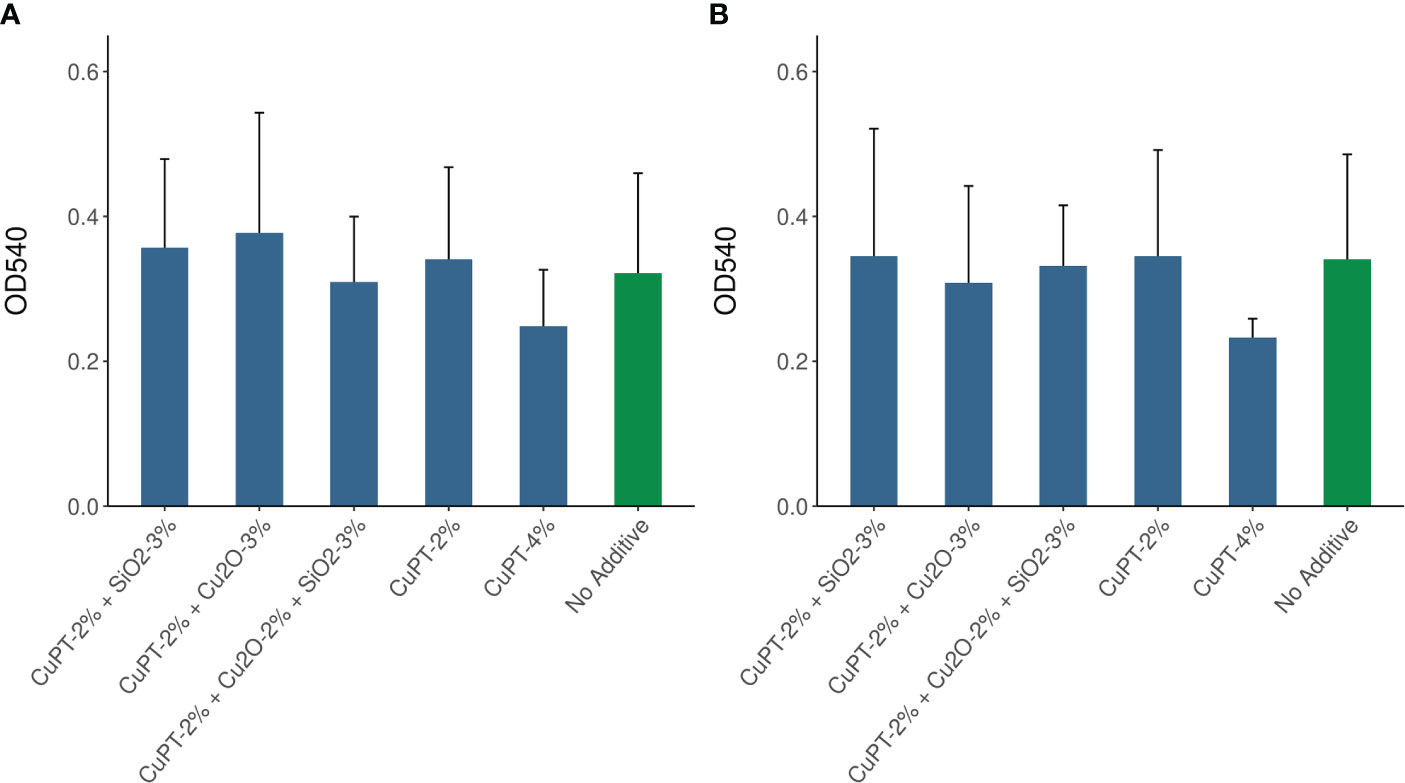
Figure 4 Quantification of biofilm. (A) Biofilm formed after 14 days of incubation for Vibrio harveyi and (B) Cellulophaga lytica on the surface of 10x22 cm sheets of HDPE functionalized with different additives and concentrations (%). Biofilm formation was determined with crystal violet staining and represented as OD540nm mean ± SD of three biological replicates.
Diatom colonization assays
Algae biomass formed by the selected diatomic algae was investigated after 7 days of incubation in suitable conditions. Total carbohydrates adhering to each type of functionalized HDPE were quantified and are represented in Figure 5. The results show that all the additives studied were effective in reducing biofilm formation by algae. The highest inhibition levels were observed for CuPT- or silica-treated HDPE (% reduction from 35 to 95 with respect to untreated HDPE) (Figure 5; Table S2).
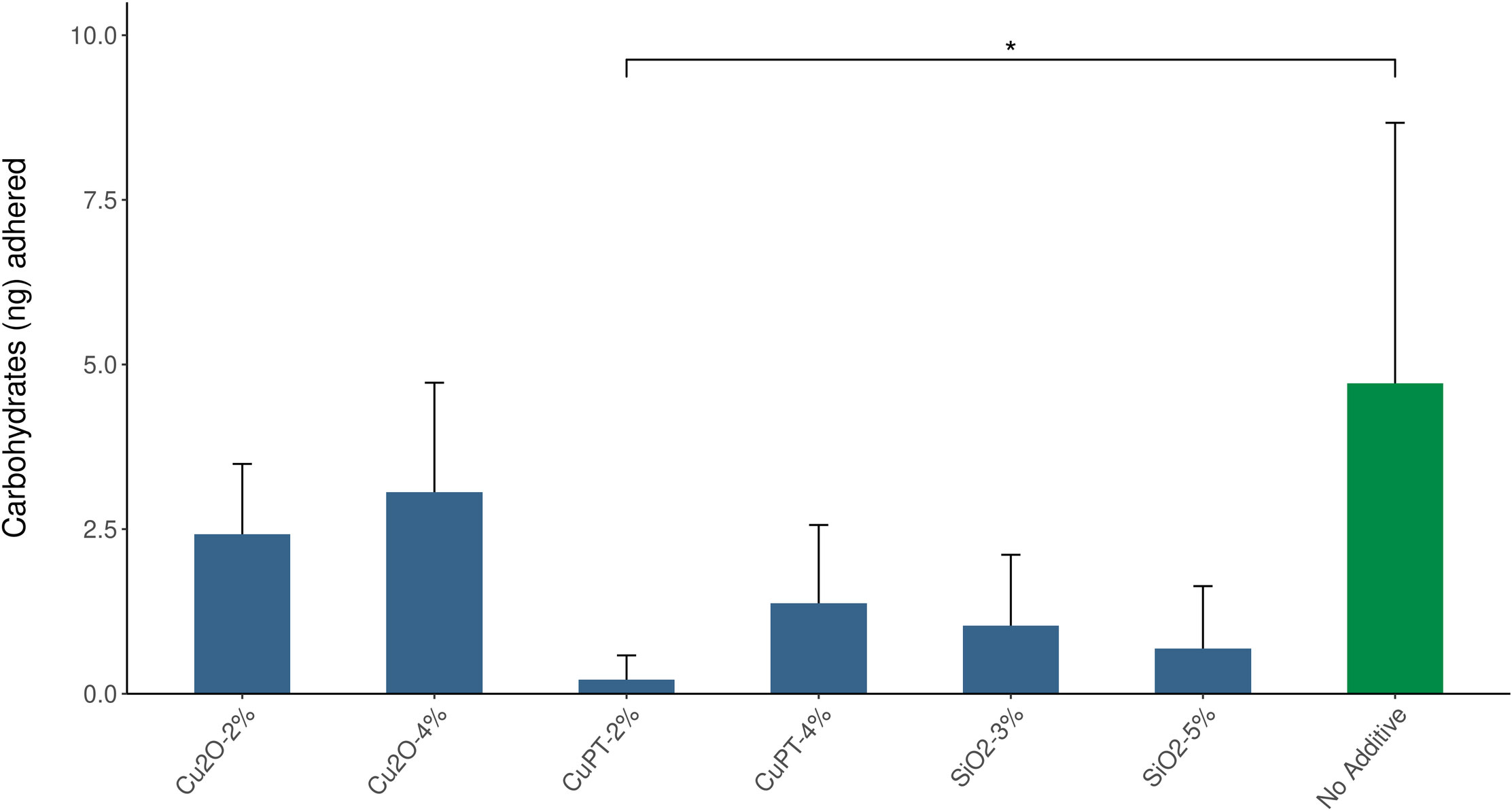
Figure 5 Quantification of algae colonization after 7 days for the algae Nitzschia ovalis on the surface of 12 mm discs of HDPE functionalized with different additives and concentrations (%). Level of colonization was determined by the phenol-sulfuric acid quantification method and represented as the mean amount of carbohydrates (ng) adhered ± SD to the surface for three biological replicates. (*) indicates statistical significance in comparison with control HDPE disc (p< 0.05).
Ecotoxicological studies
To evaluate the environmental impact, functionalized HDPE discs (Table 2) were subjected to a leaching test. When Cu2O or CuPT were added to HDPE, the amounts of copper released into the water registered low values of between 15 and > 130 µg/l of Cu (Table 2).
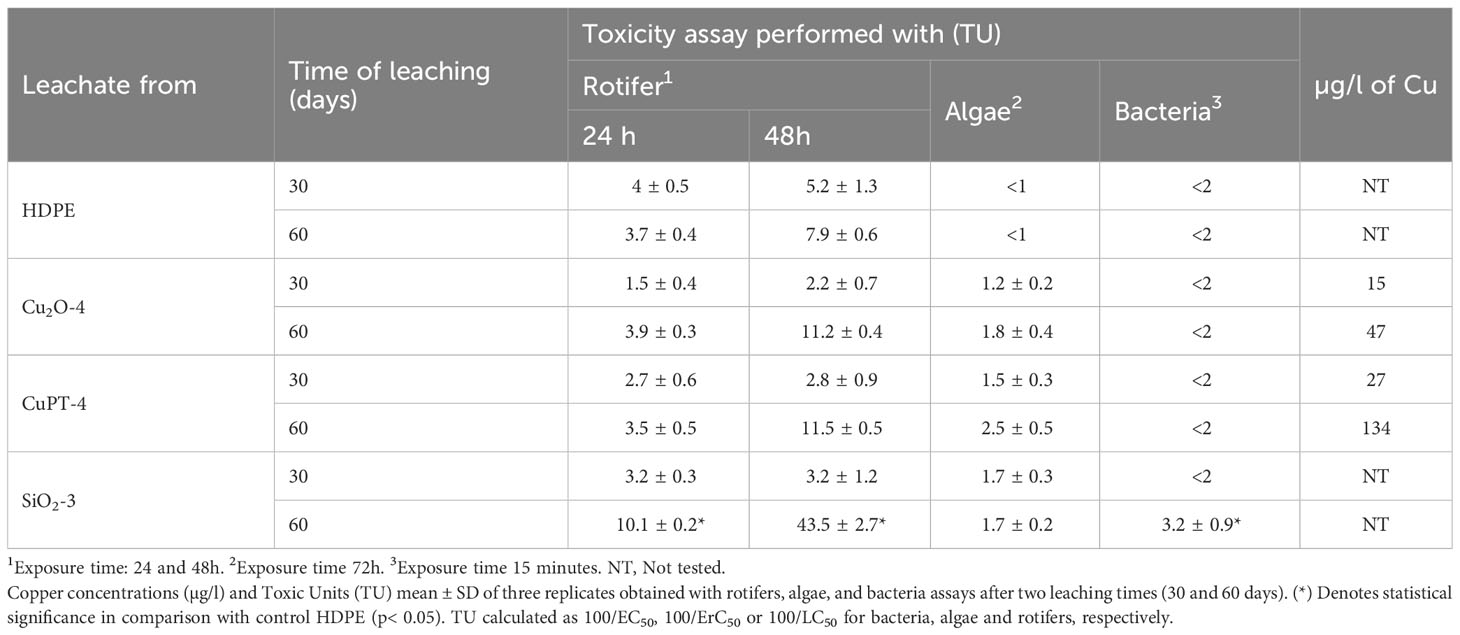
Table 2 Results of the ecotoxicological assays performed with the leachate from the different HDPE materials.
The leachates were assessed in a ecotoxicological study following a common methodology of evaluation at different levels of the trophic chain in the marine environment. Decomposer (bacteria), primary producer (algae) and consumer (rotifers) were selected. The toxicity of the leachates generated after 30 and 60 days was expressed in toxic units (TU) (Table 2). No toxic effects were found in any of the bioassays at the beginning of the experiment (0 day-leachate), i.e., in the freshly prepared synthetic seawater.
The ANOVA (p< 0.05) showed significant differences in toxicity values between the 30-day and 60-day leaching times for SiO2-3 with respect to B. plicatilis, as well as in the assays with A. fischeri. However, no statistically significant differences were observed in the P. tricornutum assays when comparing the control with the other functionalized material.
Except for HDPE-SiO2-3 in the 60-day leachate assayed with rotifers (TU: 43.5 ± 2.7), none of the generated leachates exhibited toxicity. Assays with the toxic standard (K2Cr2O7) showed that the sensitivity of the organisms was in agree with those values stablised by the ISO guidelines. The EC50 for the toxic standard for A. fischeri, P. tricornutum and B. plicatilis were 51.2 mg/l, 17.9 mg/l and 324.3 mg/l, respectively.
Discussion
Biofouling is an important and well-documented problem affecting the marine industry. An effective approach adopted to tackle this phenomenon is the use of antifouling paints, which include components able to inhibit or curb the settlement of marine organisms on submerged surfaces. These paints are designed to release biocides that prevent fouling organisms from becoming attached. In this context, frequently registered biocides are metal based, such as Cu2O, CuPT, zinc pyrithione, zineb and CuSCN. Cu2O is commonly used as the main biocide as it has a good cost, solubility and toxicity ratio (Brooks and Waldock, 2009).
Antifouling paints pose environmental risks as they are designed to be unstable and to dissolve in seawater during their service life. By contrast, the use of hard non-toxic coatings can reduce the input of paint components into the marine environment (Watermann and Eklund, 2019). HDPE is one of the polymers with the greatest number of industrial applications, including aquaculture. In the aquatic environment, the main advantages of HDPE include its resistance to corrosion and its 100% recyclability rates. In the present study, HDPE was functionalized with three different copper compounds to assess their efficacy in terms of inhibiting HDPE surface colonization by marine microorganisms. In addition, a surface modifying additive, silica, was also included in the experiments. Silica exerts a non-stick effect by modifying the wettability of the surface and, therefore, the interactions between the surface and the organisms present in seawater (Eduok et al., 2017). To our knowledge, this is the first report of research on functionalized HDPE implemented as an antifouling strategy in the marine environment.
For biofilm formation experiments, we selected two bacterial species; C. lytica, a microfouler commonly used in lab-based fouling assays (Briand, 2009; Leonardi and Ober, 2019) and V. harveyi, a natural inhabitant of seawater (Austin and Zhang, 2006). The diatom N. ovalis was also included in the tests as a representative of the benthic diatoms, reported as dominant contributors to marine biofilms (Abarzua and Jakubowski, 1995). Our results evidenced that the biofilm formed by algae on the functionalized HDPE surfaces was considerably reduced. Likewise, albeit to a lesser extent, CuPT functionalization was effective in reducing bacterial biofilm formation at both laboratory and pilot scales. Prior research describes that the first colonizers in biofilms are mainly bacteria whereas other more complex organisms, such as diatoms, adhere later on (Salta et al., 2013). This biological succession may explain the low algae biomass detected on the surface of the materials under study. With respect to additive combinations, CuPT concentration of around 4% seem necessary to gain benefits given that lower concentrations of this additive combined with Cu2O or silica did not give good results. None of these differences in colonization or biofilm formation would appear to be related to the surface morphology.
To assess the ecotoxicity of the new materials, except for the CuSCN-functionalized HDPE because of its poor results in laboratory-scale experiments with bacteria, they were exposed to the most unfavorable conditions, i.e., continuous UV-C light for 60 days in warm recirculating seawater (35°C). These experimental conditions are very harsh given the high material/seawater ratio in our artificial environment. By contrast, this ratio is practically negligible in the open sea, where the dilution factor under real conditions could be, at least, three orders of magnitude higher than in lab-based tests. The environmental impact study, following a common methodology of evaluation at different levels of the trophic chain, demonstrated that none of the additives were toxic.
Dissolved copper concentrations measured in aquatic habitats vary in time and space but often range from 0.069 to 16.0 μg/l, with the highest values found in marinas and harbors (Hall and Anderson, 1999). Schiff et al. (2004) estimated the copper emission rates from hard vinyl and modified epoxy coating with antifouling paints to be around 3.7 mg cm-2 day-1 and 4.3 mg cm-2 day-1, respectively. Despite the differences in the environmental conditions of their study and ours, the amount of copper released into seawater from functionalized HDPE in our assays was much lower than that reported for paints, indicating the low ecological impact of our additives. With reference to the higher copper emission rates from CuPT-functionalized HDPE, this finding may be due to the higher sensitivity of this compound to degradation by UV light (Thomas and Brooks, 2010).
In conclusion, our study revealed that CuPT was highly effective in inhibiting the formation of biofilm by both bacteria and algae. This reduction in biofilm formation would lead to a delay in the development of biofouling affecting installations in marine environments. Thus, CuPT-functionalized HDPE could be an environmentally friendly candidate to produce built-in structures or even for coating surfaces employed in the marine industry. Here we present the results of biofilm formation on HDPE-based materials submerged in controlled environments; however, further research should be done to confirm their antifouling effects in natural ecosystems.
Data availability statement
The original contributions presented in the study are included in the article/Supplementary Material. Further inquiries can be directed to the corresponding author.
Author contributions
ES: Conceptualization, Formal Analysis, Investigation, Methodology, Writing – original draft, Writing – review and editing. JB-C: Formal Analysis, Investigation, Methodology, Writing – original draft. OA-S: Formal Analysis, Investigation, Methodology, Writing – original draft. AG: Formal Analysis, Methodology, Resources, Writing – original draft. BF: Conceptualization, Data curation, Funding acquisition, Project administration, Resources, Supervision, Visualization, Writing – original draft, Writing – review and editing.
Funding
This study was supported by grants INNEST/2020/55 and INNEST/2020/66 from Agència Valenciana de la Innovació (Generalitat Valenciana, GV) and THINKINAZUL/2021/027 from MCIN (Ministerio de Ciencia e Innovación de España) with funding from European Union NextGeneration EU (PRTR-C17.I1) and GV.
Acknowledgments
We thank Dr. Antonia Rodrigo her help with the management of algae cultures.
Conflict of interest
The authors declare that the research was conducted in the absence of any commercial or financial relationships that could be construed as a potential conflict of interest.
Publisher’s note
All claims expressed in this article are solely those of the authors and do not necessarily represent those of their affiliated organizations, or those of the publisher, the editors and the reviewers. Any product that may be evaluated in this article, or claim that may be made by its manufacturer, is not guaranteed or endorsed by the publisher.
Supplementary material
The Supplementary Material for this article can be found online at: https://www.frontiersin.org/articles/10.3389/fmars.2023.1268695/full#supplementary-material
References
Abarzua S., Jakubowski S. (1995). Biotechnological investigation for the prevention of biofouling. I. Biological and biochemical principles for the prevention of biofouling. Mar. Ecol. Prog. Ser. 123, 301–312. doi: 10.3354/meps123301
Austin B., Zhang X. H. (2006). Vibrio harveyi: A significant pathogen of marine vertebrates and invertebrates. Lett. Appl. Microbiol. 43 (2), 119–124. doi: 10.1111/j.1472-765X.2006.01989.x
Briand J. F. (2009). Marine antifouling laboratory bioassays: An overview of their diversity. Biofouling. 25 (4), 297–311. doi: 10.1080/08927010902745316
Brooks S., Waldock M. (2009). “The use of copper as a biocide in marine antifouling paints,” in Advances in Marine Antifouling Coatings and Technologies (Sawston, Cambridge (UK): Woodhead Publishing Limited), 492–521. doi: 10.1533/9781845696313.3.492
Callow J. A., Callow M. E. (2011). Trends in the development of environmentally friendly fouling-resistant marine coatings. Nat. Commun. 2 (1), 244. doi: 10.1038/ncomms1251
Cardia F., Lovatelli A. (2016). “Aquaculture operations in floating HDPE cages,” in ,” in Fisheries and aquaculture (Rome: Food and Agriculture Organization of the United Nations (FAO) and Ministry of Agriculture of the Kingdom of Saudi Arabia), 176. Available at: http://www.fao.org/3/a-i4508e.pdf.
Donlan R. M. (2002). Biofilms: microbial life on surfaces, in Emerging Infect. Dis. 8 (9), 881–890. doi: 10.3201/eid0809.020063
Eduok U., Faye O., Szpunar J. (2017). Recent developments and applications of protective silicone coatings: A review of PDMS functional materials. Prog. Org Coatings 111 (August 2016), 124–163. doi: 10.1016/j.porgcoat.2017.05.012
Endresen Ø., Sørgård E., Sundet J. K., Dalsøren S. B., Isaksen I. S. A., Berglen T. F., et al. (2003). Emission from international sea transportation and environmental impact. J. Geophys Res. Atmos 108 (17), 4560. doi: 10.1029/2002JD002898
Ferrari M., Benedetti A., Santini E., Ravera F., Liggieri L., Guzman E., et al. (2015). Biofouling control by superhydrophobic surfaces in shallow euphotic seawater. Colloids Surfaces A Physicochem Eng. Asp 480, 369–375. doi: 10.1016/j.colsurfa.2014.11.009
Ford T., Sacco E., Black J., Kelley T., Goodacre R., Berkeley R. C. W., et al. (1991). Characterization of exopolymers of aquatic bacteria by pyrolysis-mass spectrometry. Appl. Environ. Microbiol. 57 (6), 1595–1601. doi: 10.1128/aem.57.6.1595-1601.1991
Fournier E. (2001). Colorimetric quantification of carbohydrates. Curr. Protoc. Food Anal. Chem. 00 (1), E1.1.1–E1.1.8. doi: 10.1002/0471142913.fae0101s00
Geyer R., Jambeck J. R., Law K. L. (2017). Production, use, and fate of all plastics ever made. Sci. Adv. [Internet] 3 (7), e1700782. doi: 10.1126/sciadv.1700782
Gu J. D. (2003). Microbiological deterioration and degradation of synthetic polymeric materials: Recent research advances. Int. Biodeterior. Biodegrad. 52 (2), 69–91. doi: 10.1016/S0964-8305(02)00177-4
Guillard R. R. L. (1975). “Culture of phytoplankton for feeding marine invertebrates” in Culture of marine invertebrate animals: proceedings—1st conference on culture of marine invertebrate animals greenport. Eds. Smith W. L., Chanley M. H. (Springer US), 29–60. doi: 10.1007/978-1-4615-8714-93
Hall L. W., Anderson R. D. (1999). A deterministic ecological risk assessment for copper in European saltwater environments. Mar. pollut. Bull. 38 (3), 207–218. doi: 10.1016/S0025-326X(98)00164-7
Hu P., Xie Q., Ma C., Zhang G. (2020). Silicone-based fouling-release coatings for marine antifouling. Langmuir 36 (9), 2170–2183. doi: 10.1021/acs.langmuir.9b03926
ISO (2022a). ISO 11348-3:2007 - Water quality — Determination of the inhibitory effect of water samples on the light emission of Vibrio fischeri (Luminescent bacteria test) — Part 3: Method using freeze-dried bacteria. Available at: https://www.iso.org/standard/40518.html.
ISO (2022b). ISO 10253:2016 - Water quality — Marine algal growth inhibition test with Skeletonema sp. and Phaeodactylum tricornutum. Available at: https://www.iso.org/standard/66657.html.
Jośko I., Oleszczuk P. (2012). The influence of ZnO and TiO2 nanoparticles on the toxicity of sewage sludges. Environ. Sci. Process Impacts 15 (1), 296–306. doi: 10.1039/C2EM30653K
Karunasagar I., Otta S. K., Karunasagar I. (1996). Biofilm formation by Vibrio harveyi on surfaces. Aquaculture. 140 (3), 241–245. doi: 10.1016/0044-8486(95)01180-3
Kientz B., Luke S., Vukusic P., Péteri R., Beaudry C., Renault T., et al. (2016). A unique self-organization of bacterial sub-communities creates iridescence in Cellulophaga lytica colony biofilms. Sci. Rep. 6 (July 2015), 1–11. doi: 10.1038/srep19906
Leonardi A. K., Ober C. K. (2019). Polymer-based marine antifouling and fouling release surfaces: Strategies for synthesis and modification. Annu. Rev. Chem. Biomol. Eng. 10, 241–264. doi: 10.1146/annurev-chembioeng-060718-030401
Litchfield J. T., Wilcoxon F. (1949). A simplified method of evaluating dose-effect experiments. J. Pharmacol. Exp. Ther. 96 (2), 99 LP–99 113.
Maan A. M. C., Hofman A. H., de Vos W. M., Kamperman M. (2020). Recent developments and practical feasibility of polymer-based antifouling coatings. Adv. Funct. Mater. 30 (33), 2000936. doi: 10.1002/adfm.202000936
Mitra D., Kang E.-T., Gee Neoh K. (2019). Antimicrobial copper-based materials and coatings: potential multifaceted biomedical applications. ACS Appl. Mater. Interfaces 12 (19), 21159–21182. doi: 10.1021/acsami.9b17815
Naves P., Del Prado G., Huelves L., Gracia M., Ruiz V., Blanco J., et al. (2008). Measurement of biofilm formation by clinical isolates of Escherichia coli is method-dependent. J. Appl. Microbiol. 105 (2), 585–590. doi: 10.1111/j.1365-2672.2008.03791.x
Niu C., Gilbert E. S. (2004). Colorimetric method for identifying plant essential oil components that affect biofilm formation and structure. Appl. Environ. Microbiol. 70 (12), 6951–6956. doi: 10.1128/AEM.70.12.6951-6956.2004
Paz-Villarraga C. A., Castro Í. B., Fillmann G. (2022). Biocides in antifouling paint formulations currently registered for use. Environ. Sci. pollut. Res. 29 (20), 30090–30101. doi: 10.1007/s11356-021-17662-5
Pujalte M. J., Ortigosa M., Macián M. C., Garay E. (1999). Aerobic and facultative anaerobic heterotrophic bacteria associated to Mediterranean oysters and seawater. Int. Microbiol. 2 (4), 259–266.
Rosenhahn A., Schilp S., Jürgen Kreuzer H., Grunze M., Egger D. A., Rissner F., et al. (2010). The role of “inert” surface chemistry in marine biofouling prevention. Phys. Chem. Chem. Phys. 12 (17), 4275–4286. doi: 10.1039/c001968m
RStudio Team (2015). RStudio: Integrated Development Environment for R (Boston, MA). Available at: http://www.rstudio.com/.
Salta M., Wharton J. A., Blache Y., Stokes K. R., Briand J. F. (2013). Marine biofilms on artificial surfaces: structure and dynamics. Environ. Microbiol. 15 (11), 2879–2893. doi: 10.1111/1462-2920.12186
Saputra N., Martana B., Noorohmah F., Rizal R. (2021). Study on the strength of a fishing boat made from plastic recycles. E3S Web Conf 328, 07002. doi: 10.1051/e3sconf/202132807002
Schiff K., Diehl D., Valkirs A. (2004). Copper emissions from antifouling paint on recreational vessels. Mar. Pollut. Bull. 48 (3–4), 371–377. doi: 10.1016/j.marpolbul.2003.08.016
Seo E., Lee J. W., Lee D., Seong M. R., Kim G. H., Hwang D. S., et al. (2021). Eco-friendly erucamide–polydimethylsiloxane coatings for marine anti-biofouling. Colloids Surfaces B Biointerfaces 207, 112003. doi: 10.1016/j.colsurfb.2021.112003
Sprague J. B., Ramsay B. A. (2011). Lethal Levels of Mixed Copper–Zinc Solutions for Juvenile Salmon. doi: 10.1139/f65-042
Thomas K. V., Brooks S. (2010). The environmental fate and effects of antifouling paint biocides. Biofouling. 26 (1), 73–88. doi: 10.1080/08927010903216564
Wang Y., Dufour Y. S., Carlson H. K., Donohue T. J., Marletta M. A., Ruby E. G. (2010). H-NOX-mediated nitric oxide sensing modulates symbiotic colonization by Vibrio fischeri. Proc. Natl. Acad. Sci. U.S.A. 107 (18), 8375–8380. doi: 10.1073/pnas.1003571107
Watermann B., Eklund B. (2019). Can the input of biocides and polymeric substances from antifouling paints into the sea be reduced by the use of non-toxic hard coatings? Mar. pollut. Bull. 144 (April), 146–151. doi: 10.1016/j.marpolbul.2019.04.059
Woods Hole Oceanographic Institution (1952). “The History of the Prevention of Fouling,” in Marine Fouling and its Prevention (Annapolis, Maryland (USA): Bureau of Ships, Navy Department, USA), 211–223. Available at: https://hdl.handle.net/1912/191.
Yang W., Zhao W., Liu Y., Hu H., Pei X., Wu Y., et al. (2016). The effect of wetting property on anti-fouling/foul-release performance under quasi-static/hydrodynamic conditions. Prog. Org Coat. 95, 64–71. doi: 10.1016/j.porgcoat.2016.02.018
Keywords: antifouling (AF), HDPE (high-density polyethylene), bacterial biofilm, algae colonization, aquaculture
Citation: Sanjuan E, Barriga-Cuartero J, Andreu-Sánchez O, González A and Fouz B (2023) Study of sustainable HDPE-based materials for aquaculture applications: effects on fouling. Front. Mar. Sci. 10:1268695. doi: 10.3389/fmars.2023.1268695
Received: 28 July 2023; Accepted: 13 September 2023;
Published: 03 October 2023.
Edited by:
S. S. S. Sarma, National Autonomous University of Mexico, MexicoReviewed by:
Matteo Oliva, Centro Interuniversitario di Biologia Marina ed Ecologia Applicata “G. Bacci” di Livorno (CIBM), ItalyAdrián Cervantes Martínez, University of Quintana Roo, Mexico
Copyright © 2023 Sanjuan, Barriga-Cuartero, Andreu-Sánchez, González and Fouz. This is an open-access article distributed under the terms of the Creative Commons Attribution License (CC BY). The use, distribution or reproduction in other forums is permitted, provided the original author(s) and the copyright owner(s) are credited and that the original publication in this journal is cited, in accordance with accepted academic practice. No use, distribution or reproduction is permitted which does not comply with these terms.
*Correspondence: Belén Fouz, YmVsZW4uZm91ekB1di5lcw==
†Present address: Eva Sanjuan, Dpto. Bioquímica, Microbiología, Biol. Celular y Genética, Facultad de Farmacia, Universidad de La Laguna, La Laguna, Spain
‡These authors have contributed equally to this work