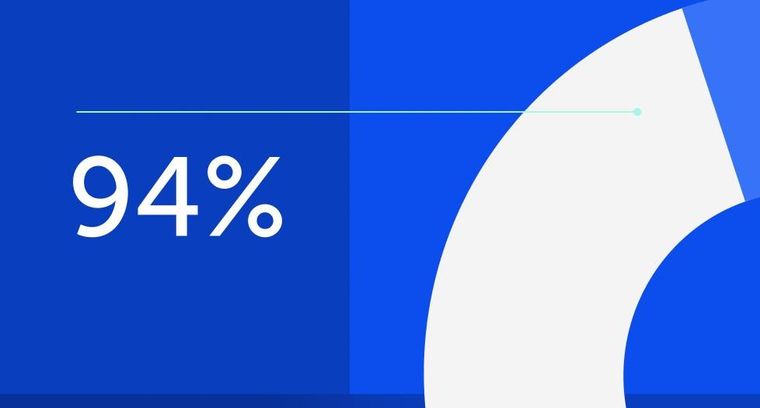
94% of researchers rate our articles as excellent or good
Learn more about the work of our research integrity team to safeguard the quality of each article we publish.
Find out more
ORIGINAL RESEARCH article
Front. Mar. Sci., 27 November 2023
Sec. Marine Ecosystem Ecology
Volume 10 - 2023 | https://doi.org/10.3389/fmars.2023.1254461
This article is part of the Research TopicSharing Technical Knowledge to Understand the Distribution Patterns and Migration History of Marine OrganismsView all 11 articles
Deep-sea squids are presumably vital components of largely undescribed marine ecosystems, yet limited access to specimens has hampered efforts to detail their ecological roles as predators and preys. Biochemical techniques such as stable isotope analyses, fatty acid analyses, and bomb calorimetry are increasingly recognized for their ability to infer trophic ecology and dietary information from small quantities of tissue. This study used five opportunistically collected Taningia danae specimens and one Chiroteuthis aff. veranii specimen retrieved from the Great Australian Bight, South Australia, to detail the trophic ecology of these poorly understood squids. Four body tissue types (i.e., arm, buccal mass, mantle, and digestive gland) were assessed for their utility in stable isotope (SI) and fatty acid (FA) analyses, and we found that the arm, buccal mass, and mantle tissues had similar SI and FA profiles, suggesting that they can be used interchangeably when the entire specimen is unavailable. δ13C, δ15N, and fatty acid data suggests that the T. danae and C. aff. veranii specimens lived in the Southern Ocean and were high-trophic-level predators, feeding on deep-sea fishes and small squids, while also taking advantage of the summer upwelling region of the Great Australian Bight. The fatty acid analysis and bomb calorimetry results indicate that these squids might be important reservoirs of essential FAs (EPA and DHA) for Southern Ocean predators and that the whole-body energy content of T. danae individuals can reach up to 362,250 kJ. Our findings indicate that these squids may be contributing greatly to the transport of nutrients and energy between the Southern Ocean deep-sea and the Great Australian Bight shelf–slope environments. In addition to building our understanding of the trophic ecology of two poorly understood deep-sea squids, these findings also highlight the utility of partial specimens and demonstrate the important ecological information that can be obtained from few samples that may be opportunistically collected.
Squids are vital components of marine ecosystems globally, occupying a broad range of ecological roles owing to their diverse morphology, habitat, behavior, and diet (Jereb and Roper, 2010; Coll et al., 2013). The 300 known species of squid range from low-trophic-level species in nearshore reefs to top predators in deep-sea and pelagic ecosystems (Navarro et al., 2013). Most squids are mesopredators feeding on small fishes and crustaceans and shunting key nutrients to large fishes, cetaceans, sharks, pinnipeds, and seabirds (De La Chesnais et al., 2019). Thus, squids are vital ecosystem members that facilitate energy and nutrient transfer in food webs (Merten et al., 2021) and can exert both top-down (i.e., predator influencing prey) and bottom-up (i.e., prey influencing predator) controls on a multitude of important marine species (De La Chesnais et al., 2019). The Southern Ocean alone is home to 42 different squid species, ranging in size from 5.7 cm (Abraliopsis gilchristi) to greater than 2 m (Mesonychoteuthis hamiltoni and Architeuthis dux) (Cherel, 2020). Furthermore, there is evidence to suggest that 12–24 million tonnes of cephalopods (primarily squids) might be consumed in the Southern Ocean annually (Santos et al., 2001) by at least 45 diverse predator species, showcasing the importance of squid as energy and nutrient resources in pelagic environments (Rodhouse, 2013). Additionally, global squid catches and abundances have both increased in recent decades (Doubleday et al., 2016), and their rapid growth and ability to adapt to environmental changes may make squid an especially ecologically and economically important taxa in changing ecosystems (Xavier et al., 2015).
Despite a global distribution and their increasingly important ecological roles, little is known about many squid species, with substantial knowledge gaps regarding diet, behavior, reproduction, distribution, physiology, and genetics (Albertin et al., 2012; Xavier et al., 2015; Xavier et al., 2018; Cherel, 2020; Ibáñez et al., 2021; Sato, 2021). The most researched are species that are commercially important, such as Humboldt squid (Dosidicus gigas) in the eastern Pacific Ocean or those that are easy to keep in aquaria (Roper and Shea, 2013). Comparatively little research effort has been applied to deep-sea and large-bodied squid species (Roper and Shea, 2013), owing to the myriad of difficulties associated with sampling animals occupying these mysterious habitats (Cherel et al., 2009b). Trawling often yields mixed and unreliable capture success (Roper and Shea, 2013), as large and fast squid are able to avoid trawl capture (Judkins et al., 2017), while trawls are known to damage delicate deep-sea specimens (Braid et al., 2014). Direct sightings of elusive species are becoming more common as camera technology becomes more affordable and accessible (O’Brien et al., 2018), but rarely do these provide information other than distribution, external morphology, and a snapshot of behavior (Kubodera et al., 2007; Gomes-Pereira and Tojeira, 2014; Hoving et al., 2014; Robinson et al., 2021). Much of the current genetic, physiological, and ecological data available on deep-sea and large squids has come from opportunistically retrieved carcasses, and beaks recovered from squid predators (Bolstad and O’Shea, 2004; Cherel and Hobson, 2005; Rosa et al., 2005). Research of large, deep-sea, or rarely sighted squids must be conducted whenever opportunities arise, and as they are abundant predators and preys, understanding their trophic interactions may be key for understanding food webs, nutrient dynamics, and ecosystems that are otherwise difficult to research and monitor.
Trophic ecology research aims to understand predator–prey interactions using methods such as direct observations, images or video recordings of predation events, gut content analyses, or molecular analyses of fatty acids (FAs) and stable isotopes (SIs) (Guerreiro et al., 2015; Da Silveira et al., 2020). Unfortunately, many of these methods are difficult to apply to inconspicuous marine species, especially large and deep-sea squids. Direct observation or image evidence of feeding events is difficult to attain due to the high cost and low success of these sampling methods (Robinson et al., 2021). Much of our understanding of large-bodied and deep-sea squids has instead come from investigations of deceased, opportunistically obtained specimens (see González et al., 2003; Rosa et al., 2005). In these cases, gut content analysis has varied success, typically requiring large sample sizes due to the frequency of empty stomachs, and the results are limited to identifiable prey items, which only represent the most recent feeding events (Braley et al., 2010; Rodhouse, 2013). Squids also have fast rates of digestion and a narrow esophagus that travels directly through the center of the brain, meaning that preys are thoroughly macerated with the beak and radula, reducing the incidence of identifiable stomach contents (Ibáñez et al., 2021).
Stable isotope analysis for trophic ecology primarily uses ratios of carbon-13 to carbon-12, and nitrogen-15 to nitrogen-14 isotopes in biological tissues (referred to as δ13C and δ15N) to infer foraging area and trophic level, respectively. δ13C varies predictably with latitude and is depleted in pelagic food webs vs. coastal and benthic zones (Cherel et al., 2000), while δ15N enriches as it travels up food webs with each successive link from prey to predator (Kelly, 2000). The use of these isotopes has been applied to studying the trophic ecology of squids and has also more recently been expanded into studies of ontogenic shifts in diet and species migrations (Merten et al., 2017; Golikov et al., 2019; Queirós et al., 2019). Stable isotope analysis is commonly used on cephalopod beaks, as these are undigestible hard parts readily found in the stomachs of their predators, including sperm whales (Cherel and Hobson, 2005). However, as SI analysis can be applied to any body tissue, it is an ideal tool for detailing the trophic ecology of opportunistically sampled animals, where only limited tissue types might be available (Jackson et al., 2007). Similar to δ13C, FAs differ at the base of the food web, as primary producers synthesize and retain unique FAs (Dalsgaard et al., 2003; Parrish, 2013). These FAs are assimilated in predators with minimal modification from prey items and thus can be used to infer a predator’s diet and trace nutrient pathways to basal food webs (e.g., pelagic zooplankton vs. diatoms vs. coastal macroalgae) (Desforges et al., 2022). Additionally, FAs can be used to explore the availability of key nutrients within an ecosystem and can indicate the potential role or importance of species as prey items (Parzanini et al., 2018). As with SIs, FAs can be measured from most body tissues, although the different physiological roles of varying tissues mean that they preferentially retain different FAs (Phillips et al., 2002), eliminating the possibility of cross-tissue comparisons. Regardless, FA analyses are an ideal tool for trophic ecologists working with rare and opportunistically collected samples, and multiple tissues should be collected to fully capitalize on these occasions.
The Great Australian Bight (GAB), located off the southern coast of Australia, is a site of high productivity, driven by the convergence of the warm eastward flowing Leeuwin current and the cold westward flowing Flinders current as well as annual summer upwelling events (Van Ruth et al., 2018). The GAB includes the shallow continental shelf area around the southern coast, including Kangaroo Island, as well as the continental slope and the abyssal plains, which exceed 5,000-m depth (Rogers et al., 2013). Over the past decade, efforts to improve our understanding of the deep-sea ecosystem of the GAB have increased substantially (e.g., the GAB Research Program and GAB Deepwater Survey by CSIRO). From 2013 to 2017, 304 survey operations were conducted from 200- to 5,000-m depths in the GAB. Of the 1,267 invertebrate species recorded, almost one quarter (401 species) of these were previously undescribed (MacIntosh et al., 2018), indicating how poorly understood the diversity of the benthic and abyssal depths are in this region.
The present study was developed from the opportunistic recovery of five large squid carcasses from the ocean surface in the eastern GAB in March of 2019. The largest of these specimens measured approximately 120 cm in mantle length, and all five of these specimens were identified as Taningia danae. Taningia danae is a globally distributed and abundant, but rarely seen or researched, species of deep-sea squid, with no tentacles (i.e., only has arms present) and two large photophores on the tips of two arms (Quetglas et al., 2006). In December of 2021, an additional smaller squid specimen, identified as Chiroteuthis aff. veranii, was retrieved from a similar location and included in the study due to its rarity and unknown diet and trophic role. The aim of this study was to use δ13C and δ15N SIs, FAs, and energy content to determine the ecological role of these elusive deep-sea squids in the GAB with reference to other cephalopod species encompassing global, southern temperate, and polar squid distributions. Two libraries of published SI and FA data were compiled for comparison with the data collected from the squids in this study. One dataset consisted of global squid data and was a source of comparison to other squid species that may have similar diets, trophic roles, and physiology. The second dataset included multi-taxa marine species (e.g., cetaceans, fishes, crustaceans, etc.) from the GAB and southeast Australia, the Southern Ocean, New Zealand, and the Antarctic (Supplementary Figure S1) and was used to assess potential trophic interactions between the squids and other local species. The specific objectives of this study were to (a) assess the utility of different body sections for trophic ecology by comparing SI, FA, and energy density data from four different body parts (arms, buccal mass, mantle, and digestive gland); (b) determine the foraging habitat and trophic role of Taningia danae and Chiroteuthis aff. veranii with comparison to published data from squid globally; and (c) describe the roles of these squids and potential trophic interactions within their identified food webs with comparison of published data collected from multi-taxa marine species across the southern hemisphere.
The six squid specimens analyzed in this study (“GAB squids”; five Taningia danae and one Chiroteuthis aff. veranii) were opportunistically collected from the eastern GAB, from 34-39°S and 135-139°E (Supplementary Figure S1) between 2019 and 2021 by Australian Fishing Enterprises. The deceased squids were initially spotted floating on the ocean surface by commercial tuna fishery spotter planes and subsequently collected by tuna fishing vessels. The squids were generally in good condition, with evidence of only minor post-mortem scavenging (eyes missing from seabird predation) and decomposition only on specimen Td3 (Supplementary Figure S2). The specimens were frozen onboard the vessels and transported to the Australian Fishing Enterprises facility in Port Lincoln, South Australia, where they were stored at –20°C until transport to Flinders University for subsampling. The specimens were thawed only sufficiently to be measured (e.g., mantle length, and arm length), described (i.e., noting distinguishing features such as arm and tentacle morphology and mantle and fin shape), and subsampled (Supplementary Table S1). Approximately 5 g of tissue from the mantle, arms, buccal mass, and digestive gland was sampled from each individual, cutting from the central portions of the tissues to avoid contamination, and immediately refrozen at –20°C for subsequent analyses. Approximately 3 g of each tissue type for each specimen was freeze-dried at –88°C for 72 h and then manually homogenized into a fine powder using a mortar and pestle.
Although squid mantle and arms have low lipid content (<2% wet weight, Phillips et al., 2002), making lipid removal not explicitly necessary for these tissues, the digestive gland has a high lipid content, which is variable both between and within squid species (6–55% wet weight; Phillips et al., 2002; Phillips et al., 2003; Pethybridge et al., 2012; Moovendhan et al., 2019). Therefore, lipid extraction was performed on all samples to ensure consistency across the study and to allow for comparisons with SI data available in the scientific literature, most of which have been chemically lipid extracted. Following a modified Bligh and Dyer (1959) protocol, lipids were removed by soaking the samples in 10 mL of 2:1 dichloromethane/methanol solution for 24 h. The delipidated samples were filtered and oven-dried at 60°C for 48 h to remove the remaining solvent. For each tissue type in each individual squid, one sample of approximately 1.50 mg ( ± 10%) of homogenized, delipidated tissue was weighed and placed into a tin capsule. The samples were analyzed in triplicate to confirm analytical precision.
Stable carbon and nitrogen isotope ratios were measured using an Isoprime GC5 continuous flow isotope ratio mass spectrometer with a vario ISOTOPE cube elemental analyzer (Elementar Australia Pty Ltd) at Flinders University Chemical Analysis Services. Blank tin capsules and standards were run throughout the analysis to measure analytical precision and correct for isotopic drift. The standards used were L-glutamic acid enriched in light C and N isotopes (12C and 14N) (USGS40) and heavy C and N isotopes (13C and 15N) (USGS41) (Sercon Ltd., UK). Stable isotope results were exported using the IonVantage software from Elementar and expressed in a standard notation as follows:
where X is 15N or 13C and R is the ratio of 15N to 14N or 13C to 12C in the sample, following the international standards of Pee Dee Belemnite for CO2, and atmospheric nitrogen for N2.
Three replicates of approximately 0.025 g per tissue type per individual squid specimen were weighed into 20-mL glass test tubes for FA extraction by direct transmethylation (Parrish et al., 2015). To separate the FAs from their glycerol backbones, tissue was soaked in 3 mL of 10:1:1 methanol/dichloromethane/hydrochloric acid solution at 80°C for 2 h. At the completion of the 2-h solvent soak, the samples were removed from heat and cooled to ambient temperature before the addition of 1 mL of milli-Q water and 1.8 mL of 2:1 hexane/dichloromethane solution, followed by vortex mixing and centrifuging at 2,000 rpm for 5 min. The upper layer containing the FA methyl esters (FAMEs) was removed and placed into 2-mL glass vials. The vials were placed under nitrogen gas streams to evaporate the solvent, leaving only pure FAMEs. The addition of 1.8 mL of hexane/dichloromethane solution and dehydration with nitrogen gas was repeated three times, maximizing the FA retention. The FAMEs were suspended in 1 mL of dichloromethane for subsequent quantification. Fatty acid methyl esters were identified and quantified using gas chromatography mass spectrophotometry (GCMS) analysis with Agilent Technologies 5975C Series GC/MSD (Agilent Technologies, Inc., USA) equipped with Triple-Axis HED-EM Detector, SGE Analytical Science (Ringwood, Victoria, Australia) BP21 capillary column (15 m length, 0.25 mm internal diameter, and 0.25 μm film thickness), Agilent 7683B Series Injector, and Agilent/HP 7683 automatic liquid sampler. Quality checks were performed throughout the analysis with a blank sample containing 1 mL dichloromethane at the start of each run, plus an internal FA standard (C4–C24) (Sigma-Aldrich, USA). The output was analyzed and processed using MassHunter Qualitative Analysis software and the National Institute of Standards and Technology (https://www.nist.gov) database of FA compounds. Individual FA values were reported as percentage of the total FAs per sample analyzed so that contributions to FA profiles could be established. Fatty acids contributing<0.1% across the 45 samples were removed, leaving 21 FAs for tissue and species comparisons within the GAB squids (Supplementary Table S2).
One sample of each T. danae tissue type was combusted in a bomb calorimeter (Parr 6200 Isoperibol with semi-micro bomb) to obtain the energy content of the tissues. Before measuring the samples, a standard of benzoic acid was run. For each T. danae sample, approximately 0.025 g of freeze-dried, homogenized tissue was pressed into a pellet, packed into a crucible, and ignited in the bomb calorimeter. The heat released was measured and converted to kilojoules per gram wet weight.
The isotopic compositions and FA profiles of the GAB squids alone were analyzed using PRIMER 7 + PERMANOVA (Anderson et al., 2008; Clarke and Gorley, 2015). To visually compare the isotopic composition across tissue types and species, a δ13C and δ15N biplot was created. Multivariate data visualization was used to assess if and how FA profiles differed across tissue type and species. Fatty acid data was square-root-transformed, and a resemblance matrix was generated using a Bray–Curtis measure of similarity. The data was visualized using a principal coordinates ordination (PCO) plot, and vectors were overlaid showing FAs with Pearson correlations >0.8 to indicate the direction and magnitude of the correlation with individual FAs. Similarity of Percentages (SIMPER) analyses were conducted using the previously generated resemblance matrices on factors of tissue type and species to determine the percentage dissimilarities between groups and the FAs contributing to the between-group differences.
The whole-body energy density of T. danae was roughly estimated using the energy density results for the mantle, arm, and digestive gland tissues. These were multiplied by the body weight proportions of T. danae given in Kelly (2019), wherein fins comprised 61% of body mass, mantle 14%, head and arms 23%, and viscera 4% (the digestive gland being 0.88%). Mantle energy density was multiplied by the fins + mantle proportions (0.75), arm energy density was multiplied by head and arms proportions (0.23), and digestive gland energy density was multiplied by the digestive gland proportion (0.0088). These were summed to provide an estimate of the whole animal energy density of T. danae tissues.
Additional SI and FA data were collected from published literature and legacy datasets (Nichols et al., 2023), enabling for the determination of the feeding ecology and trophic role of the GAB squids (available in Supplementary Tables S8–S12). Data were collected for (a) any squid species globally and (b) any marine species found in the southeast Australia, New Zealand, the Southern Ocean, and the Antarctica marine regions (henceforth referred to as southern marine species; Supplementary Figure S1).
Stable isotope data as mean δ13C and δ15N and associated metadata including species, location (sea or ocean sampled), and tissue type were collated from published literature. In total, 155 SI datapoints were used from the muscle and beak analyses of squid globally, and 293 data points were used from any tissue of multi-taxa southern marine species, including groups such as crustaceans, fishes, seabirds, and marine mammals. Beaks are commonly used for SI research of cephalopods but are comprised of chitin, which is known to be enriched in in 14-nitrogen, resulting in δ15N values lower than that of muscle tissues from the same animal. In previous works, the δ15N values of beaks have been adjusted for comparison to muscle samples by adding between 3.5‰ and 4.8‰ (Cherel and Hobson, 2005; Navarro et al., 2013; Guerreiro et al., 2015). Thus, we adjusted all beak δ15N data that were not already corrected in the source publication by + 3.5‰ to allow more reliable comparisons with our GAB squids. The triplicate SI ratios generated for each tissue type across the GAB squids were condensed to the mean muscle-specific isotope ratio per specimen. The isotope ratios in the digestive gland were depleted in both δ13C and δ15N and so were excluded from the muscle-specific mean. A δ13C and δ15N biplot was created to qualitatively compare the trophic ecology of the six specimens in this study with squid globally and to assess the role that these squids play within local food webs.
Fatty acid profiles were recorded as the percent contribution of each FA to the total FA quantity. For each profile, metadata including the species, taxon type (cephalopod, fish, chondrichthyan, etc.), location (sea or ocean sampled), and tissue type were recorded, as well as other information such as sex and maturity status, if available. If multiple FA profiles were reported within a single source for the same species, location, and tissue type, the mean FA profile across all samples was calculated for use. In total, 116 and 298 FA profiles were used from tissues of squids globally and tissues of multi-taxa southern marine species, respectively (metadata and citations can be found in the Supplementary Material).
Fatty acid data are reported differently throughout the literature. Up to 60 different FAs are often measurable on the GCMS; however, only those exceeding 2%, 1%, 0.2%, or 0.1% or simply those deemed “of interest” by the authors tend to be reported in the literature. Thus, depending on the author, the means of analysis, and tissue types, FA profiles may not be directly comparable. As this study combined FA data from a wide range of sources, many FAs needed to be grouped together to minimize the effects of differing sources. Firstly, FA profiles were standardized to sum up to 100%, eliminating the influence of minor FAs that were not measured or reported in many profiles. Secondly, FAs were filtered to further minimize any confounding effects of data source. For the global squid dataset, FAs reported in fewer than 50 samples and those with a mean of less than 0.5% across all samples were removed. For the southern marine species dataset, the mean percentage of each FA was calculated across each separate taxa group (cephalopods, fishes, chondrichthyes, etc.), and FAs with a mean of less than 2% within all taxa groups were removed. This ensured that any FAs important to only one taxon group were retained for analysis rather than be removed due to their lack of contribution in other taxon groups. This standardization process followed Meyer et al. (2019) and resulted in a 15 FA global squid dataset and a 12 FA southern marine, multi-taxon species dataset (Supplementary Table S2).
The triplicate FA profiles generated for each tissue type across the GAB squids were averaged to one profile per tissue type, per specimen, and included in the final FA datasets. The comparison of the six specimens within this study to squid globally was divided into two datasets: muscular tissue profiles (unspecified soft tissues, mantle, buccal mass, arm, and fin) and digestive tissue profiles (digestive gland, stomach, stomach fluid, cecum oil, and whole homogenized individuals). These datasets warranted a separate analysis, as the two tissue types have distinct FA profiles that are not directly comparable. Bray–Curtis similarity matrices were generated from the square-root-transformed data. The data were visualized using a PCO plot, and vectors were overlaid showing FAs with Pearson correlations >0.5. Similarity of Percentages (SIMPER) analyses were conducted using the previously generated resemblance matrices on factors of tissue type and location to determine the percentage dissimilarities between groups and the FAs contributing to the between-group differences.
Energy density data, as kilojoules per gram wet weight, were collected and analyzed for southern mid-trophic marine species (squids and fishes). In instances wherein one species had several differing energy densities available, the greatest kilojoules per gram value was retained for analysis. Additionally, body mass data were collected of all species included in the energy density analyses so that the approximate energy content per individual could be calculated. "As reported body masses can vary greatly; the greatest published body masses were used for analysis. This was so that the energy contents calculated per individual were more likely overestimations than underestimations, and thus comparisons made to T. danae energy content would be viable. Taningia danae whole-body energy density was multiplied by both the greatest individual body mass recorded, 161 kg, and a more conservative body mass, 60 kg (Roper and Jereb, 2010), to provide two points of comparison with the other southern marine species.
Stable isotope ratios differed between individual specimen, species, and tissue type (Table 1; Figure 1), with the highest δ15N found in the mantle and the lowest in the buccal mass. Digestive gland tissues were depleted in δ13C and δ15N versus the muscle tissues (mantle, arm, and buccal mass) (Figure 1). The carbon-to-nitrogen (C:N) ratio of all muscle tissues in all squid specimens ranged from 2.83 to 3.14, and the C:N ratio of the digestive gland was higher than that of the muscle tissues (Table 1).
Table 1 δ13C and δ15N (‰) composition and carbon-to-nitrogen ratio (C:N) of arm (A), buccal mass (B), mantle (M), and digestive gland (DG) from Taningia danae (Td) and Chiroteuthis aff. veranii (Cav) specimens collected from the eastern Great Australian Bight.
Figure 1 δ13C and δ15N (‰) composition of mantle, arm, buccal mass, and digestive gland from Taningia danae and Chiroteuthis aff. veranii collected from the eastern Great Australian Bight. Ellipses are drawn to illustrate the separation between muscle tissues and the digestive gland.
In T. danae and C. aff. veranii, saturated fatty acids (SFAs) and polyunsaturated fatty acids (PUFAs) were most abundant in the muscle tissues, while monounsaturated fatty acids (MUFAs) were most abundant in the digestive gland (Table 2). In muscles, the most abundant individual FAs were 16:0, 22:6ω3, and 20:5ω3, whereas in the digestive glands the most abundant were 16:0, 18:0, and 20:1ω9 (Table 2). The fatty acid profiles were differentiated primarily by tissue type (digestive gland or muscle tissue), followed by species (Figure 2), as the digestive gland FA profiles of T. danae were 19.09–20.10% dissimilar from the muscle FA profiles (Supplementary Table S3). This dissimilarity was influenced by higher proportions of 18:1ω9, 20:1ω9, and 24:1ω9 and lower proportions of 22:6ω3, 20:5ω3, and 16:0 in the digestive gland (Figure 2). The muscle tissues were all<10% dissimilar within each species (Supplementary Table S4). The muscle tissues of the T. danae specimens were 9.92–11.80% dissimilar to the muscles of the C. aff. veranii specimen (Supplementary Table S5). In total, 59% of this dissimilarity was influenced by the C. aff. veranii tissues having higher proportions of 16:1ω7t, 18:2ω6, and 20:5ω3 and lower proportions of 22:6ω3, 20:3ω3, and 24:0 versus the T. danae tissues.
Table 2 Percentage contribution of specific fatty acids to total FAs as mean ± standard deviation in the mantle, arm, buccal mass, and digestive gland tissues of Taningia danae and Chiroteuthis aff. veranii collected from the eastern Great Australian Bight.
Figure 2 Principal coordinates ordination plot of fatty acid (FA) profiles from Taningia danae and Chiroteuthis aff. veranii collected from the eastern Great Australian Bight. A vector showing Pearson correlations >0.8 is overlaid, indicating the FAs contributing to differences between samples, and polygons are drawn to show the grouping of muscle tissue profiles by specimen.
The digestive gland of T. danae had a greater energy content (5.94 kJ/g) than the muscular tissues which ranged from 2.53 kJ/g for the mantle tissue to only 0.50 kJ/g for the buccal mass (Table 3). Using the body part proportions given for T. danae in Kelly (2019), the whole-body energy density of T. danae is estimated at approximately 2.25 kJ/g.
Globally, squids had a δ13C range from -25.80‰ to -15.10‰ and a δ15N range from 6.30‰ to 17.90‰ (Figure 3). The GAB squids were comparatively depleted in δ13C, and most aligned with squid species collected from the Southern Ocean (Figure 3; Supplementary Figure S3). The GAB squids were also enriched in δ15N, with few squids from the Southern Ocean, southeast Australia, and New Zealand areas having higher δ15N in comparison (Figure 3; Supplementary Figure S4). Four specimens of T. danae (Td) from the Southern Ocean and southeast Australia had similarly enriched δ15N values compared to the GAB T. danae specimens (Figure 3). In contrast, one T. danae specimen from the Southern Ocean was considerably enriched in δ15N than the GAB specimens, at 15.30‰ (Figure 3). Chiroteuthis sp. and C. veranii specimens (two and three samples, respectively) had δ15N values ranging from 11.50‰ to 12.90‰ and δ13C values ranging from -20.00‰ to -16.50‰ (Figure 3).
Figure 3 Biplot of δ13C and δ15N (‰) from global squid studies. Great Australian Bight squid data used is mean muscle δ13C and δ15N. The data points referred to in the text are labeled with species abbreviation. GAB Td, Taningia danae from the eastern Great Australian Bight; GAB Cav, Chiroteuthis aff. veranii from the eastern Great Australian Bight; Mha, Mesonychoteuthis hamiltoni; Td, Taningia danae; Ln, Loliolus noctiluca; Cv, Chiroteuthis veranii; Cx, Chiroteuthis sp.
The SIMPER analysis revealed a 23.30% dissimilarity between the FA profiles of digestive gland and mantle muscle tissues from squids sampled globally. In total, 47% of this dissimilarity between tissue types was due to higher proportions of MUFAs (18:1, 20:1, 22:1, 16:1, and 24:1) in the digestive gland tissue, and 27.00% was attributed to higher proportions of 22:6ω3, 20:5ω3, and 16:0 in mantle tissue.
Globally, squid muscle tissue FA profiles were well separated by sampling area (Figure 4A), with GAB T. danae and GAB C. aff. veranii being least dissimilar to the squid samples taken from the northeast Atlantic (12.26% and 13.52% dissimilar, respectively) and southeast Australia (12.25% and 13.57% dissimilar, respectively; Supplementary Table S6). The GAB squid samples grouped closely with southeast Australian samples including Todarodes filippovae (Tf), Idioteuthis cordiformis (Ics), and Ommastrephes brevimanus (Omb) (Figure 4A). Two Architeuthis dux (Ad) samples were also similar in FAs, with one from eastern Australia and the other from the northeast Atlantic Ocean. The southeast Australia and Southern Ocean samples were differentiated from other areas by higher proportions of FAs 16:0, 20:1, 22:1, and 24:1. The Southern Ocean samples were differentiated from southeast Australia samples by higher proportions of FAs 16:1, 18:1, and 22:5ω3, while the southeast Australian samples, including the samples from this study, had higher 18:0. There were two samples taken from chiroteuthids, a C. veranii (Cv) sample from the northwestern Atlantic and a Chiroteuthis sp. (Cx) from southeast Australia. Both samples had lower proportions of FA 18:0 and higher proportions of 18:1 in the mantle versus the GAB C. aff. veranii. Zero Taningia spp. were found for comparison.
Figure 4 Principal coordinates ordination plots of fatty acid (FA) profiles of (A) squid muscle tissues (unspecified muscle, mantle, arms, fins, and buccal mass) and (B) squid digestive parts (digestive gland, whole squid, stomach, stomach fluid, and cecum oil) in studies conducted globally. Only FAs with a mean proportion of >0.5% across the global squid dataset and present in >50 of the samples were retained. A vector overlay showing Pearson correlations >0.5 indicates the individual FAs contributing to differences between samples. GAB, Great Australian Bight. Only samples referred to in the text are labeled with species abbreviation. Ad, Architeuthis dux; Omb, Ommastrephes bartramii; Tf, Todarodes filippovae; Cv, Chiroteuthis veranii; Cx, Chiroteuthis sp.; Ics, Idioteuthis cordiformis; Ome, Octopoteuthis megaptera; Ale, Ancistrocheirus lesueurii; Ha, Histioteuthis atlantica; Ll, Lycoteuthis lorigera; Max, Mastigoteuthis sp.; Ng, Nototodarus gouldi.
The fatty acid profiles from the digestive parts of squids (including whole squids, digestive glands, stomachs, stomach fluid, or cecum oil) showed less grouping by sampling area than was evident in the muscle tissues (Figure 4B). The Southern Ocean squid species remain characterized by MUFAs including 16:1, 18:1, and 24:1, as with the muscle FA profiles of Southern Ocean squid. The GAB T. danae was least dissimilar (19.30%) to the southeast Australian samples (Supplementary Table S7) and clustered close to several whole squid samples of Octopoteuthis megaptera (Ome), Lycoteuthis lorigera (Ll), Histioteuthis atlantica (Ha), Mastigoteuthis sp. (Max), Ancistrocheirus lesueurii (Ale), and O. bartramii (Omb), plus a digestive gland sample of Nototodarus gouldi (Ng). These samples were characterized by lower proportions of 14:0, 16:1, and 18:1 and higher proportions of 18:0 and 20:1. When filtering the dataset to include only the digestive gland samples and re-generating the PCO plot, the digestive gland samples of the GAB T. danae were distinct from all but one sample; Nototodarus gouldi (Ng) from southeast Australia (Supplementary Figure S5). No Taningia spp. FA samples were available for comparison.
The isotopic ratios of the marine species from southeast Australia, New Zealand, the Southern Ocean and Antarctica were well grouped by sample area along the δ13C axis, with samples with δ13C lower than -20‰ almost entirely Southern Ocean and Antarctic species (Figure 5). The GAB squids’ δ13C values (from -20.59‰ through -21.71‰) were most similar to taxa from the Southern Ocean. Overall, the southeast Australian samples were more enriched in δ15N over the Southern Ocean species but had a slightly smaller range, from 6.00‰ to 16.10‰ in the southeast Australian samples, versus 5.00‰ to 17.80‰ in Southern Ocean samples (Figure 5). The GAB squids had δ15N values similar to intermediate trophic level species such as fishes among the samples from southeast Australian taxa or similar to high-trophic-level species, such as seabirds, among the samples from Southern Ocean taxa.
Figure 5 Biplot of δ13C and δ15N (‰) from marine species sampled from southeast Australia, New Zealand, the Southern Ocean, and Antarctica. GAB squid data used is mean muscle δ13C and δ15N. GAB, Great Australian Bight. The data points referred to in the text are labeled with species abbreviation. Ec, Electrona carlsbergi; Ea, Electrona antarctica; Gyn, Gymnoscopelus nicholsi; Ml, Moroteuthopsis longimana; Mha, Mesonychoteuthis hamiltoni; Ln, Loliolus noctiluca.
The fatty acid profiles of the multi-taxon species sampled in this study were best grouped by taxon, with most variation occurring along the PCO1 axis (Figure 6). Squid FA profiles had higher proportions of 22:6ω3, 20:5ω3, 18:0, and 16:0 than other southeast Australia, New Zealand, Southern Ocean, and Antarctic taxa. The GAB squids were closely grouped and characterized by these same FAs, although they had relatively higher proportions of these FAs than most published squid samples (Supplementary Figure S6). The digestive gland profiles from GAB T. danae specimens Td1 and Td3 had higher proportions of MUFA and lower 22:6ω3 and 20:5ω3 than the other GAB squid muscle samples and were clustered closely to FA profiles from four fish species; Epigonus lenimen (Ele), E. carlsbergi (Ec), Lepidorhynchus denticulatus (Ld), and Nemichthys sp. (Nmx) (Figure 6).
Figure 6 Principal coordinates ordination plot of published fatty acid (FA) profiles of marine species collected in southeast Australia, New Zealand, the Southern Ocean, and Antarctica. Only FAs with a mean proportion >2% in all taxa groups were retained. A vector showing Pearson correlations >0.5 has been overlaid, indicating the individual FAs contributing to differences between samples. GAB: Great Australian Bight. The data points referred to in the text are labeled with species abbreviation. Nmx, Nemichthys sp.; Ele, Epigonus lenimen; Ec, Electrona carlsbergi; Ld, Lepidorhynchus denticulatus.
The whole-animal energy density per gram of T. danae is lower than all other southern marine squid and fish species that could be found in published literature (Figure 7; Supplementary Table S8). However, as T. danae is a large-bodied squid species, the whole-animal energy content of even the moderately sized individuals can surpass 130,000 kJ, which is higher than all species analyzed except for the toothfishes (Dissostichus spp.). The largest T. danae individual reliably measured, weighing 161 kg (Roper and Jereb, 2010), might contain an energy content of up to 362,250 kJ (Figure 7), greater than all other squids and fishes analyzed except for Antarctic toothfish, Dissostichus mawsoni.
Figure 7 Energy content of Southern Ocean fishes and squids as found in published literature. Whole-body energy densities, as kilojoules per gram wet weight, are shown with black columns. An estimate of the whole-animal energy content is shown with orange columns and was determined by multiplying the greatest recorded whole-body energy density by the greatest recorded whole-animal body mass.
Deep-sea squids are presumably vital components of these largely undescribed marine ecosystems, yet limited access to specimens has hampered efforts to detail their ecological roles as predators and prey. The opportunistic collection of five Taningia danae specimens and one Chiroteuthis aff. veranii specimen from the GAB enabled the comparison of FAs and SIs across four body tissue types showcasing that muscular tissues can be used interchangeably in the absence of entire specimens. It also suggested that the digestive gland offers insights into the foraging habitats of squids as predators while also being the key site of nutrient storage and indicating which nutrients are gained by the predators feeding on them. The SIs and FAs suggested that the T. danae and C. aff. veranii specimens probably lived in the Southern Ocean and were high-trophic-level predators likely consuming deep-sea fishes and small squids and are sources of essential FAs (EPA and DHA) and calories for Southern Ocean predators.
As this study was entirely opportunistic with regard to the GAB squid specimens, there are several limitations. These limitations may cause variation in both the stable isotope values recorded and the fatty acid results as well as affect the reliability of the results due to the small sample size. This should be considered when reading the hypotheses and conclusions presented here. In SI analysis, squid beaks are a commonly sampled tissue as they are retrieved frequently in predator stomachs (Hoving et al., 2014). Beaks contain chitin, which causes depletion in δ15N values, and there is tissue accumulation, rather than turnover, across an organism’s lifetime (Cherel and Hobson, 2005). Newly synthesized areas of the beak, such as the lower wing, have less chitin and can more reliably show recent dietary signatures, while the rostrum and whole homogenized beaks compress an entire lifetime of ecological information into a single isotopic ratio (Cherel and Hobson, 2005). There is some uncertainty when comparing beaks to muscle tissues, and therefore comparing the GAB squids data to published squid beak data may impact the comparison with squid globally. Correction values of +3.5‰ (Navarro et al., 2013) and +4.8‰ δ15N (Guerreiro et al., 2015) have been applied to beak isotopic ratios to make them more comparable to muscle tissues. However, different correction factors would be required for beak samples taken from the wing, rostrum, or whole beak, which has not, to the best of our knowledge, been developed yet. In this study, we applied a single, conservative correction factor of +3.5‰ to any beak samples taken from the literature that were not already corrected in the source publication. We did this so that these samples could be more reliably compared with the muscle samples taken from the GAB squids, but we are aware that applying a single-correction factor may have caused over- or under-corrections of particular samples, potentially impacting conclusions made here.
Additionally, Taningia danae is an ammoniacal squid, which means that their tissues contain elevated levels of ammonia, which helps the animal achieve neutral buoyancy and reduce energy costs (Clarke et al., 1979). Ammonia in the muscle tissues of elasmobranchs can have effects on SI values, and studies have suggested that rinsing samples in deionized water can remove the urea (Li et al., 2016). When comparing shark muscle samples with lipids extracted versus samples that were both lipid-extracted and deionized-water-rinsed, the urea extracted samples had δ15N values increased by just under 1.0‰ and δ13C values decreased by just under 1.0‰ (Martin and Jaquemet, 2019). To our knowledge, this method has not been tested in ammoniacal squid tissues and is not currently standard among SIA of squid muscle.
Comparisons among beak data, lipid extracted muscle data, and urea removed muscle data will likely require the development of a set of correction factors that could be applied to allow for better use of published data, which is a convenient way of utilizing opportunistic sampling events, regardless of how small sample sizes are.
In FA analysis, both decomposition and frozen storage are known to cause the degradation of PUFAs over time (Budge et al., 2006; Atayeter and Ercoşkun, 2011; Meyer et al., 2017). The GAB squid specimens were potentially decomposing in the environment for an unknown amount of time before retrieval and then were frozen for at least 3 years before samples were taken and processed for SI analysis and FA analysis. In another study, squid mantle frozen for 12 months at -20°C had proportions of PUFAs (especially 22:6ω3) decrease by approximately 10% and SFAs (especially 16:0 and 18:0) increase by approximately 10% versus fresh tissue (Atayeter and Ercoşkun, 2011). The GAB squids in this study were found to have relatively high 16:0 and 18:0 and low PUFAs in their tissues versus other squid samples, which may be an artefact of extended frozen storage or decomposition. Additionally, with the collection and use of published data, the storage period of many of those samples or levels of decomposition by the time of retrieval is unknown. Thus, we suggest that detailed tissue information is provided in future studies that use opportunistically collected specimens.
The clustering of the GAB squid muscle tissues (arm, buccal mass, and mantle) and the distinctiveness of the digestive glands suggest that the three muscle tissues could be used mostly equivalently, with similar SI and FA profiles. Often in opportunistic sampling of squid only a portion of the animal is retrieved, so being able to sample any of these muscle tissues and gain similar, useable results is vital for describing deep-sea and continental shelf food webs.
There are minor and variable isotopic differences among muscle tissue types, such as mantle being the most enriched in δ15N and buccal mass the least enriched, but these are likely due to the minor differences in protein content between the three tissue types (Shulman et al., 2002; Hobson and Cherel, 2006). Due to the limited number of squids with all three muscle tissue types available (three individuals across the two species), this could not be explored further within this study. However, these relatively minor isotopic differences should not preclude the interchangeable use of muscle tissues when equivalent tissues are unavailable across specimens, as the differences among these tissues do not exceed the differences among individuals of the same species. The digestive gland samples were relatively depleted in both δ13C and δ15N and increased in C:N ratio compared to the other tissues analyzed, which is likely due to the high oil content (Cherel et al., 2009a; Ruiz-Cooley et al., 2011; Svensson et al., 2016). Studies using SIs to examine the trophic ecology of squids will either need to limit comparisons to within digestive glands or develop mathematical correction factors as has been done with beaks and other tissues.
The digestive gland of squid has been the preferred source tissue for diet determination using FAs, as it is the primary lipid storage organ, incorporating FAs from the diet across the previous 10 days with minimal biomodification (Phillips et al., 2001; Stowasser et al., 2006). Additionally, the digestive gland has greater lipid content (13–54% wet weight) compared to muscle tissues (<2% wet weight) (Phillips et al., 2002; Shulman et al., 2002; Phillips et al., 2003) with phospholipids, which are involved in tissue structure, comprising much of this observed differences (20:5ω3, 22:6ω3, 16:0; Rosa et al., 2005). Squid digestive glands typically contain lower proportions of these phospholipid PUFAs and higher levels of key MUFAs (Phillips et al., 2001; Zhukova, 2019). The GAB squid results were consistent with data from previous Australian and Southern Ocean oegopsid studies, where the proportion of PUFA in the muscles was 42.40–58.10% versus 17.30–32.40% in the digestive gland, and the proportion of MUFA in the muscles was 13.60–21.80% versus 47.00–66.10% in the digestive gland (Phillips et al., 2001; Phillips et al., 2002; Pethybridge et al., 2013). However, the suite of FAs contributing to clustering of FA data by area appeared to be similar between muscle and digestive tissues—for example, the southeast Australian squid samples including the GAB squids were differentiated from the rest of the samples by higher proportions of 16:0, 18:0, and 22:1 in both plots, while the Southern Ocean samples were differentiated by higher proportions of 16:1, 18:1, and 24:1. Digestive gland tissue is not often available from opportunistically collected squid, but these results indicate that similar dietary information and ecological inferences may instead be attainable from muscle tissues.
In the future, multi-species comparisons of squid tissues through controlled feeding studies should aim to determine how SIs and dietary FAs incorporate into squid muscle and digestive gland tissues to understand the molecular processes unique to squids. The differences across tissue types observed in this study indicate that this is an important area of future work that should be undertaken on more easily accessible squid species, such as southern calamari Sepioteuthis australis.
δ13C from squid muscle tissues between -22.90‰ and -19.50‰ are classified as “subantarctic” (Guerreiro et al., 2015), a finding that fits well with both the global squids and southern multi-taxa SI data compiled here. The GAB squids, retrieved from latitudes of approximately 39-34°S, had δ13C ranging from -23.80‰ to -20.04‰, similar to SI ratios recorded in samples from the Kerguelen Plateau (49°S), Marion Island (47°S), Macquarie Island (55°S), and South Georgia (55°S). Therefore, the GAB squids having "subantarctic" carbon ratios might indicate that they lived further south than where they were collected and may have been moved to the GAB post-mortem, perhaps by a predator such as a sperm whale.
Alternatively, the GAB squids might have migrated from their subantarctic range north toward the GAB to feed in the high-productivity, cold-water upwelling food web that exists along the continental slope each austral summer (Van Ruth et al., 2018). The copepod FA biomarkers 20:1ω9 and 22:1ω9 (Parrish et al., 2005; Stowasser et al., 2009) are referenced frequently in FA studies of deep-sea and Southern Ocean squids, with several authors suggesting the presence of a copepod–myctophid–squid higher-order predator food chain within the Southern Ocean (Rodhouse et al., 1992; Phillips et al., 2001; Pethybridge et al., 2013). The GAB squids had high proportions of these FAs within their digestive glands and muscles, suggesting that the GAB squids likely feed within this food chain. The GAB T. danae and southeast Australian squids also had higher proportions of these two FAs in their digestive glands versus other Southern Ocean squids. Copepods are abundant in the eastern GAB (Van Ruth and Ward, 2009) and can significantly increase in population size in times of upwelling (Weikert, 1977; Jemi and Hatha, 2019), so it is possible that the GAB squids may have moved north to the continental slope to opportunistically forage upon fish schools that follow copepods around these upwelling regions. The rate of FA incorporation and isotopic turnover of squid muscle has yet to be determined, although studies on fishes have suggested a FA incorporation time of a few days or weeks, while the isotopes turnover was at a rate of several months (Young et al., 2010). This might further explain why the FA profiles of the GAB squids reflect more recent GAB feeding, while δ13C reflects a long-term subantarctic range.
If movements between the Southern Ocean and the GAB are regular for these squid species, whether for foraging or some other cause such as spawning, they may be previously unrecognized vectors of nutrient and energy transport between these environments. The role these squids play in linking the nutrient-rich shelf–slope environment of the GAB to the nutrient-poor Southern Ocean deep-sea may be important to the trophic functioning of both environments.
Squids in the Southern Ocean and southeast Australia occupy a broad range of trophic levels, with δ15N values ranging from 5.00‰ to 17.80‰—equating to four or five trophic levels (assuming an average trophic enrichment factor of approximately 2.75‰ δ15N per trophic level; Caut et al., 2009). The GAB squids with high δ15N values were at the second highest trophic level, just below the colossal squid Mesonychoteuthis hamiltoni from the Southern Ocean. Mesonychoteuthis hamiltoni is the heaviest known invertebrate (Rosa et al., 2017) and is thought to consume Antarctic toothfish (Dissostichus mawsoni, Remeslo et al., 2015), which, in turn, are high-trophic-level predators of mid- to large-sized cephalopods and fishes (Queirós et al., 2021). Additionally, the similarities between the GAB squid FAs and FA profiles from other large, actively predatory squid species including giant squid Architeuthis dux, Antarctic flying squid Todarodes filippovae, flying squid Ommastrephes bartramii, whiplash squid Idioteuthis cordiformis, and arrow squid Nototodarus gouldi suggest roles as active pelagic predators. When comparing the GAB squids’ δ15N to other taxa from the southern ecosystems, it appears that the GAB squids occupied a similar trophic level to a broader range of Southern Ocean top predators including seabirds, cetaceans, and other large squids. Thus, it is suggested that the GAB T. danae and C. aff. veranii were high-trophic-level predators within the Southern Ocean ecosystem.
Little is known of the diet of most deep-sea animals in general, and only a few opportunistic records of identifiable prey items have been recorded from the stomachs of large-bodied squids. Fortunately, some items have been identified from the stomach contents of two T. danae retrieved off the coast of Spain, consisting of a few blue whiting (Micromesistius poutassou) vertebrae, Gonatus sp. tentacle hooks, and integuments from crustaceans (González et al., 2003). These results are far from a comprehensive analysis of T. danae diet but do provide some evidence that T. danae is capable of feeding on active, pelagic fish and squid species as well as crustaceans. Chiroteuthis aff. veranii diet has not been described, although other chiroteuthids are known to feed on crustaceans, molluscs, and fish (Kubota et al., 1981). However, the C. aff. veranii specimen from this study is substantially larger than most previously studied Chiroteuthis veranii specimens [approximately 400 mm mantle length versus 50–297 mm in Rodhouse and Lu (1998); Mensch (2010), and Guerra et al. (2011)]. Therefore, the diet and general trophic ecology of the GAB C. aff. veranii specimen may be very different from what has previously been described for C. veranii globally.
Using the global mean trophic enrichment factors (2.75‰ for δ15N and 0.75‰ for δ13C; Caut et al., 2009), the predicted prey items of the GAB squids include Gymnoscopelus nicholsi, Electrona carlsbergi, and Electrona antarctica, three deep-sea myctophids abundant in the Southern Ocean (Kozlov, 1995), and Moroteuthopsis longimana, an Antarctic deep-sea squid. The maximum prey length suggested for squid are prey that measure between half to two thirds of the squid’s mantle length (Bidder, 1966), which would be 50–80 and 20–30 cm for T. danae and C aff. veranii, respectively. The myctophid species are small in length, with total length less than 20 cm (Collins et al., 2012), placing them within the GAB squids’ prey size range, but M. longimana can attain mantle lengths of up to 1 m (Laptikhovsky et al., 2013), which is out of this range.
The T. danae digestive gland FA profiles in this study further suggest deep-sea fish and squid predation. The T. danae digestive gland samples were similar in FA profile to whole homogenized fish samples and whole homogenized squid samples (Figure 6). The whole deep-sea fishes that were similar to the GAB T. danae digestive gland samples (and might represent potential prey species) were E. carlsbergi, Epigonus lenimen, Lepidorhynchus denticulatus, and Nemichthys sp. With the exception of Nemichthys sp., these species are within the size range of T. danae prey (<50 cm, Meynier et al., 2008; Pethybridge et al., 2013; Froese and Pauly, 2022) and are abundant in the deep-sea and continental slope communities of the Southern Ocean and southeast Australia (May and Blaber, 1989; Kozlov, 1995). The whole squid profiles and therefore possible squid prey species grouped closely to T. danae digestive glands were Octopoteuthis megaptera, Lycoteuthis lorigera, Histioteuthis atlantica, Mastigoteuthis sp., and Ancistrocheirus lesueurii. Comparatively less is known about the abundance of these species versus the potential fish prey, but they are all deep-sea and pelagic ones (SeaLifeBase 2022). In addition, apart from A. lesueurii which grows to 54 cm in mantle length (Hoving and Lipinski, 2015), these squid species are all classified as small with mantle lengths less than 25 cm (Lipiński, 1993; Hoving et al., 2007; Pethybridge et al., 2010; Kelly, 2019), again fitting within the suggested maximum prey size of T. danae.
The GAB squid had high proportions of EPA 20:5ω3 and DHA 22:6ω3 within their muscle tissues, even compared to other squids. This could be due to these FAs being taken in by the GAB squids through their diet and may be further evidence for a diet consisting of squid, as EPA and DHA are common in squid muscle tissues (Ozogul et al., 2008), and research has shown that fish that are fed squid diets have increased levels of these FAs in their tissues (Turner and Rooker, 2005; Sun et al., 2018). Additionally, dietary EPA and DHA have been linked to higher growth rates in fish (Lutfi et al., 2022), and therefore a diet rich in these two FAs may be necessary for the maintenance of muscle tissue synthesis in large squids such as the GAB squids in this study.
EPA and DHA are physiologically important for all omnivorous organisms, including humans (Gladyshev et al., 2013), and have been linked with a variety of important processes including anti-inflammatory processes, stress resistance, neural development, and behavior (Parrish, 2013; Zhukova, 2019). The high levels of these FAs in the tissues of the GAB squids may mean that these are important sources of dietary EPA and DHA for their known predator species including sperm whales (Physeter macrocephalus), northern elephant seals (Mirounga angustirostris), and seabirds (Cherel et al., 2009b; Guerreiro et al., 2015; Yoshino et al., 2020). Chiroteuthis aff. veranii could be especially important, as its similar proportions of EPA and DHA, but smaller body size, may make it an important source of these FAs for smaller predators such as swordfishes (e.g., Xiphias gladius), toothfishes (Dissostichus spp.) (Cherel and Hobson, 2005; Young et al., 2010), and deep-sea-foraging Southern Ocean cetaceans such as the pygmy sperm whale (Kogia breviceps) (Dos Santos and Haimovici, 2001).
Squids are generally less energy-dense than fishes, primarily due to lower levels of lipid and protein in their tissues (Croxall and Prince, 1982). This is reflected in the energy content of T. danae tissues, which was lower than the calorie content of all other deep-sea fishes and squids. The digestive gland, with a higher lipid content than the other tissues, also had a greater energy density. Many deep-sea squids, including T. danae, are considered ammoniacal. This results in a reduced energy density of muscle tissues, and a previous study estimates that ammoniacal squids have a whole-body energy density of 2.3 kJ/g (Clarke et al., 1985), which supports the T. danae whole-body energy density estimate made in this study.
Taningia danae has been noted in the diets of many marine predators globally, forming minor components of the diets of seabirds (Clarke et al., 1981; Guerreiro et al., 2015), marine mammals (Condit and Le Boeuf, 1984; MacLeod et al., 2003; Cherel et al., 2009b; Yoshino et al., 2020), sharks (Smale and Cliff, 1998; Mendonça, 2009; Ménard et al., 2013), and predatory fishes (Tsuchiya et al., 1998; Cherel et al., 2004; Potier et al., 2007; Ménard et al., 2013) and significant components of the diets of both sperm whales and sleeper sharks (identified in source as Somnoisus cf. microcephalus, likely Somniosus antarcticus) in the Southern Ocean (Cherel et al., 2004, Evans and Hindell, 2004). While T. danae is not densely calorific when compared to smaller Southern Ocean fish and squid species, their large body size means that, per individual, they are the second most calorie-rich mid-trophic species in the Southern Ocean. One T. danae individual might provide as many calories as several dozens of smaller prey items, with the added benefit of reducing the foraging costs for the predator (Evans and Hindell, 2004; Goldbogen et al., 2019). A previous study by Aoki et al. (2012) suggested that foraging on large squids may provide sperm whales with a high caloric intake for reduced energetic expenditure, especially given that the bursts of speed required to catch these squids can be highly costly (Aoki et al., 2012).
In the biodiverse and economically important areas of the Southern Ocean and the GAB, there is a need to fully understand the trophic interactions of higher-order predators such as large squids. The use of opportunistic sampling events is critical for studying elusive species in deep-sea areas, and this study has shown that even remnants of large squid muscle tissues floating on the sea surface can provide useful ecological information. Squid digestive glands are widely acknowledged to be effective in FA analysis to provide additional evidence of diet, but in this study, we found that muscle tissues can also be used. Researchers who intend to use opportunistic samples of squid in trophic ecology studies should collect any biological tissues that they have access to (e.g., arm, mantle, and buccal mass) for both SI analysis and FA analysis to gain information regarding potential diet, food chains, geographic origin, and trophic role. For the GAB squids, the comparison of multiple tissue types helped us determine that they were most likely living in the Southern Ocean and had a broad diet of deep-sea fishes and other squids. We also found that the GAB squids might play a role in providing important nutrients to other higher-order predators and could have an additional role in facilitating nutrient and energy transport between the Southern Ocean deep-sea and the GAB shelf–slope environments. Despite the small sample sizes, the lower quality of the GAB squids due to opportunistic sampling, and the other discussed limitations, this study has highlighted the types of ecological information that can be obtained from small and degraded biological samples, which can be important in building knowledge of trophic ecology in deep-sea squid research.
The raw data supporting the conclusions of this article will be made available by the authors without undue reservation.
Ethical approval was not required for the study involving animals in accordance with the local legislation and institutional requirements because the samples used were already deceased naturally upon collection.
BJ: Conceptualization, Data curation, Formal analysis, Visualization, Writing - original draft, Writing - review & editing. RB: Conceptualization, Funding acquisition, Supervision, Writing - original draft, Writing - review & editing. MD: Conceptualization, Funding acquisition, Supervision, Writing - review & editing. JH: Formal Analysis, Methodology, Supervision, Writing - review & editing. BM: Formal analysis, Investigation, Resources, Validation, Writing – review & editing. KR: Conceptualization, Resources, Writing – review & editing. LM: Conceptualization, Formal analysis, Funding acquisition, Methodology, Supervision, Writing - original draft, Writing - review & editing.
The author(s) declare financial support was received for the research, authorship, and/or publication of this article. Funding for this study was acquired by the Ecosystem Resilience Research Group (ERRG), the Southern Shark Ecology Group (SSEG), and Flinders Accelerator for Microbiome Exploration (FAME) at Flinders University, South Australia, as well as Australian Research Council DE220101409.
The authors would like to acknowledge that this study was made possible by samples obtained from the Australian Southern Bluefin Tuna Industry Association (ASBTIA), crew of Australian Fishing Enterprises, and Kim Mundy, who provided the squids. We would like to acknowledge the work of the Auckland University of Technology Lab for Cephalopod Ecology and Systematics (ALCES) in identifying the species of the squids sampled, the South Australian Museum (Shirley Sorokin, Andrea Crowther and Rachael King) for housing the squid specimens. We acknowledge funding for genetic identification from the Flinders Accelerator for Microbiome Exploration under project #010. The authors would like to acknowledge and thank those involved in dissections, lab work, and sample analysis including Zöe Doubleday, Erica Durante, Taryn-Lee Perrior, Chloe Roberts, Russel Fuller, the Marine Ecology class of 2021, and all others who attended the dissection at Flinders University.
Author KR is employed by Australian Southern Bluefin Tuna Industry Association.
The remaining authors declare that the research was conducted in the absence of any commercial or financial relationships that could be construed as a potential conflict of interest.
All claims expressed in this article are solely those of the authors and do not necessarily represent those of their affiliated organizations, or those of the publisher, the editors and the reviewers. Any product that may be evaluated in this article, or claim that may be made by its manufacturer, is not guaranteed or endorsed by the publisher.
The Supplementary Material for this article can be found online at: https://www.frontiersin.org/articles/10.3389/fmars.2023.1254461/full#supplementary-material
Albertin C. B., Bonnaud L., Brown C. T., Crookes-Goodson W. J., Da Fonseca R. R., Di Cristo C., et al. (2012). Cephalopod genomics: A plan of strategies and organization. Stand. Genomic Sci. 7, 175–188. doi: 10.4056/sigs.3136559
Anderson M. J., Gorley R. N., Clarke K. R. (2008). PERMANOVA+ for PRIMER: Guide to Software and Statistical Methods (Plymouth, UK: PRIMER-E).
Aoki K., Amano M., Mori K., Kourogi A., Kubodera T., Miyazaki N. (2012). Active hunting by deep-diving sperm whales: 3D dive profiles and maneuvers during bursts of speed. Mar. Ecol. Prog. Ser. 444, 289–301. doi: 10.3354/meps09371
Atayeter S., Ercoşkun H. (2011). Chemical composition of European squid and effects of different frozen storage temperatures on oxidative stability and fatty acid composition. J. Food Sci. Technol. 48, 83–89. doi: 10.1007/s13197-010-0139-5
Bidder A. M. (1966). “Feeding and digestion in cephalopods,” in Physiology of the mollusca, vol. 2 . Eds. Wilbur K., Yonge C. (New York, NY: Academic Press Inc), 97–124.
Bligh E. G., Dyer W. J. (1959). A rapid method of total lipid extraction and purification. N. Can. J. Biochem. Physiol. 37, 911–917. doi: 10.1139/o59-099
Bolstad K. S., O’Shea S. (2004). Gut contents of a giant squid Architeuthis dux (Cephalopoda: Oegopsida) from New Zealand waters. N.Z. J. Zool. 31, 15–21. doi: 10.1080/03014223.2004.9518354
Braid H. E., McBride P. D., Bolstad K. S. R. (2014). Molecular phylogenetic analysis of the squid family Mastigoteuthidae (Mollusca, Cephalopoda) based on three mitochondrial genes. Hydrobiologia 725, 145–164. doi: 10.3354/meps11008
Braley M., Goldsworthy S. D., Page B., Steer M., Austin J. J. (2010). Assessing morphological and DNA-based diet analysis techniques in a generalist predator, the arrow squid Nototodarus gouldi. Mol. Ecol. Resour. 10, 466–474. doi: 10.1111/j.1755-0998.2009.02767.x
Budge S. M., Iverson S. J., Koopman H. N. (2006). Studying trophic ecology in marine ecosystems using fatty acids: A primer on analysis and interpretation. Mar. Mamm. Sci. 22, 759–801. doi: 10.1111/j.1748-7692.2006.00079.x
Caut S., Angulo E., Courchamp F. (2009). Variation in discrimination factors (δ15N and δ13C): the effect of diet isotopic values and applications for diet reconstruction. J. Appl. Ecol. 46, 443–453. doi: 10.1111/j.1365-2664.2009.01620.x
Cherel Y. (2020). A review of Southern Ocean squids using nets and beaks. Mar. Biodivers. 50, 98. doi: 10.1007/s12526-020-01113-4
Cherel Y., Duhamel G., Gasco N. (2004). Cephalopod fauna of subantarctic islands: new information from predators. Mar. Ecol. Prog. Ser. 266, 143–156. doi: 10.3354/meps266143
Cherel Y., Fontaine C., Jackson G., Jackson C., Richard P. (2009a). Tissue, ontogenic and sex-related differences in δ13C and δ15N values of the oceanic squid Todarodes filippovae (Cephalopoda: Ommastrephidae). Mar. Biol. 156, 699–708. doi: 10.1007/s00227-008-1121-x
Cherel Y., Hobson K. A. (2005). Stable isotopes, beaks and predators: a new tool to study the trophic ecology of cephalopods, including giant and colossal squids. Proc. R. Soc B. 272, 1601–1607. doi: 10.1098/rspb.2005.3115
Cherel Y., Hobson K. A., Weimerskirch H. (2000). Using stable-isotope analysis of feathers to distinguish moulting and breeding origins of seabirds. Oecologia 122, 155–162. doi: 10.1007/pl00008843
Cherel Y., Ridoux V., Spitz J., Richard P. (2009b). Stable isotopes document the trophic structure of a deep-sea cephalopod assemblage including giant octopod and giant squid. Biol. Lett. 5, 364–367. doi: 10.1098/rsbl.2009.0024
Clarke A., Clarke M. R., Holmes L. J., Waters T. D. (1985). Calorific values and elemental analysis of eleven species of oceanic squids (Mollusca : Cephalopoda). J. Mar. Biolog. Assoc. U.K. 65, 983–986. doi: 10.1017/S0025315400019457
Clarke M. R., Croxall J. P., Prince P. A. (1981). Cephalopod remains in the regurgitations of the wandering albatross Diomedea exulans L. at South Georgia. Sci. Rep. Br. Antarct. Surv. 54, 9–21.
Clarke M. R., Denton E. J., Gilpin-Brown J. B. (1979). On the use of ammonium for buoyancy in squids. J. Mar. Biolog. Assoc. U.K. 59, 259–276. doi: 10.1017/S0025315400042570
Coll M., Navarro J., Olson R. J., Christensen V. (2013). Assessing the trophic position and ecological role of squids in marine ecosystems by means of food-web models. Deep-Sea Res. II: Top. Stud. Oceanogr. 95, 21–36. doi: 10.1016/j.dsr2.2012.08.020
Collins M. A., Stowasser G., Fielding S., Shreeve R., Xavier J. C., Venables H. J., et al. (2012). Latitudinal and bathymetric patterns in the distribution and abundance of mesopelagic fish in the Scotia Sea. Deep-Sea Res. II: Top. Stud. Oceanogr. 59-60, 189–198. doi: 10.1016/j.dsr2.2011.07.003
Condit R., Le Boeuf B. J. (1984). Feeding habits and feeding grounds of the northern elephant seal. J. Mammal. 65, 281–290. doi: 10.2307/1381167
Croxall J. P., Prince P. A. (1982). Calorific content of squid (Mollusca: Cephalopoda). Br. Antarct. Surv. Bull. 55, 27–31.
Dalsgaard J., St John M., Kattner G., Müller-Navarra D., Hagen W. (2003). Fatty acid trophic markers in the pelagic marine environment. Adv. Mar. Biol. 46, 225–340. doi: 10.1016/s0065-2881(03)46005-7
Da Silveira E. L., Semmar N., Cartes J. E., Tuset V. M., Lombarte A., Ballester E. L. C., et al. (2020). Methods for trophic ecology assessment in fishes: A critical review of stomach analyses. Rev. Fish. Sci. Aquac. 28, 71–106. doi: 10.1080/23308249.2019.1678013
De La Chesnais T., Fulton E. A., Tracey S. R., Pecl G. T. (2019). The ecological role of cephalopods and their representation in ecosystem models. Rev. Fish. Biol. Fish. 29, 313–334. doi: 10.1007/s11160-019-09554-2
Desforges J.-P., Kohlbach D., Carlyle C. G., Michel C., Loseto L. L., Rosenberg B., et al. (2022). Multi-dietary tracer approach reveals little overlap in foraging ecology between seasonally sympatric ringed and harp seals in the high Arctic. Front. Mar. Sci. 9. doi: 10.3389/fmars.2022.969327
Dos Santos R. A., Haimovici M. (2001). Cephalopods in the diet of marine mammals stranded or incidentally caught along southeastern and southern Brazil (21–34°S). Fish. Res. 52, 99–112. doi: 10.1016/S0165-7836(01)00234-X
Doubleday Z. A., Prowse T. A. A., Arkhipkin A., Pierce G. J., Semmens J., Steer M., et al. (2016). Global proliferation of cephalopods. Curr. Biol. 26, R406–R407. doi: 10.1016/j.cub.2016.04.002
Evans K., Hindell M. A. (2004). The diet of sperm whales (Physeter macrocephalus) in southern Australian waters. ICES J. Mar. Sci. 61, 1313–1329. doi: 10.1016/S1095-6433(03)00045-X
Froese R., Pauly D. (2022) FishBase. Available at: www.fishbase.org (Accessed 29 November, 2022).
Gladyshev M. I., Sushchik N. N., Makhutova O. N. (2013). Production of EPA and DHA in aquatic ecosystems and their transfer to the land. Prostaglandins Other Lipid Mediat. 107, 117–126. doi: 10.1016/j.prostaglandins.2013.03.002
Goldbogen J. A., Cade D. E., Wisniewska D. M., Potvin J., Segre P. S., Savoca M. S., et al. (2019). Why whales are big but not bigger: Physiological drivers and ecological limits in the age of ocean giants. Science 366, 1367–1372. doi: 10.1126/science.aax9044
Golikov A. V., Ceia F. R., Sabirov R. M., Ablett J. D., Gleadall I. G., Gudmundsson G., et al. (2019). The first global deep-sea stable isotope assessment reveals the unique trophic ecology of Vampire Squid Vampyroteuthis infernalis (Cephalopoda). Sci. Rep. 9, 19099. doi: 10.1038/s41598-019-55719-1
Gomes-Pereira J. N., Tojeira I. (2014). The cephalopod Taningia danae Joubin 1931 observed near bottom at over 2,000 m depth on Seine seamount. Mar. Biodivers. 44, 151–155. doi: 10.1007/s12526-013-0197-9
González Á., Guerra A., Rocha F. (2003). New data on the life history and ecology of the deep-sea hooked squid Taningia danae. Sarsia N. Atlantic Mar. Sci. 88, 297–301. doi: 10.1080/00364820310002524
Guerra Á., Portela J. M., Río J. L. D. (2011). Cephalopods caught in the outer Patagonian shelf and its upper and medium slope in relation to the main oceanographic features. Fish. Res. 109, 179–186. doi: 10.1016/j.fishres.2011.02.003
Guerreiro M., Phillips R., Cherel Y., Ceia F., Alvito P., Rosa R., et al. (2015). Habitat and trophic ecology of Southern Ocean cephalopods from stable isotope analyses. Mar. Ecol. Prog. Ser. 530, 119–134. doi: 10.3354/meps11266
Hobson K., Cherel Y. (2006). Isotopic reconstruction of marine food webs using cephalopod beaks: New insight from captively raised Sepia officinalis. Can. J. Zool. 84, 766–770. doi: 10.1139/Z06-049
Hoving H. J. T., Lipinski M. R. (2015). Observations on age and reproduction of the oceanic squid Ancistrocheirus lesueurii (d’Orbigny 1842) (Cephalopoda: ancistrocheiridae). J. Nat. Hist. 49, 1319–1325. doi: 10.1080/00222933.2013.840748
Hoving H. J. T., Lipinski M. R., Roeleveld M. A. C., Durholtz M. D. (2007). Growth and mating of southern African Lycoteuthis lorigera (Steenstrup 1875) (Cephalopoda; lycoteuthidae). Rev. Fish. Biol. Fish. 17, 259–270. doi: 10.1007/s11160-006-9031-9
Hoving H. J. T., Perez J. A. A., Bolstad K. S. R., Braid H. E., Evans A. B., Fuchs D., et al. (2014). The study of deep-sea cephalopods. Adv. Mar. Biol. 67, 235–359. doi: 10.1016/B978-0-12-800287-2.00003-2
Ibáñez C. M., Riera R., Leite T., Díaz-Santana-Iturrios M., Rosa R., Pardo-Gandarillas M. C. (2021). Stomach content analysis in cephalopods: past research, current challenges, and future directions. Rev. Fish. Biol. Fish. 31, 505–522. doi: 10.1007/s11160-021-09653-z
Jackson G. D., Bustamante P., Cherel Y., Fulton E. A., Grist E. P. M., Jackson C. H., et al. (2007). Applying new tools to cephalopod trophic dynamics and ecology: perspectives from the Southern Ocean Cephalopod Workshop, February 2–3, 2006. Rev. Fish. Biol. Fish. 17, 79–99. doi: 10.1007/s11160-007-9055-9
Jemi J. N., Hatha A. A. M. (2019). Copepod community structure during upwelling and non-upwelling seasons in coastal waters off Cochin, southwest coast of India. Acta Oceanol. Sin. 38, 111–117. doi: 10.1007/s13131-019-1491-6
Jereb P., Roper C. F. E. (2010). “Cephalopods of the world. An annotated and illustrated catalogue of cephalopod species known to date,” in Myopsid and Oegopsid Squids, vol. 2. (Italy: FAO).
Judkins H., Vecchione M., Cook A., Sutton T. (2017). Diversity of midwater cephalopods in the northern Gulf of Mexico: comparison of two collecting methods. Mar. Biodivers. 47, 647–657. doi: 10.1007/s12526-016-0597-8
Kelly J. (2000). Stable isotopes of carbon and nitrogen in the study of avian and mammalian trophic ecology. Can. J. Zool. 78, 1–27. doi: 10.1139/z99-165
Kelly J. T. (2019). Systematics of the Octopoteuthidae Berry 1912 (Cephalopoda: Oegopsida). [PhD dissertation] (Auckland, New Zealand: Auckland University of Technology).
Kozlov A. N. (1995). A review of the trophic role of mesopelagic fish of the Family Myctophidae in the Southern Ocean ecosystem. CCAMLR Sci. 2, 71–77.
Kubodera T., Koyama Y., Mori K. (2007). Observations of wild hunting behaviour and bioluminescence of a large deep-sea, eight-armed squid, Taningia danae. Proc. R. Soc B. 274, 1029–1034. doi: 10.1098/rspb.2006.0236
Kubota T., Koshiga M., Okutani T. (1981). Rare and interesting squid from Japan—VII. Some biological data on Chiroteuthis imperator from Suroga Bay, Japan (Cephalopoda: Chiroteuthidae). Venus Jap. J. Malacol. 40, 150–159.
Laptikhovsky V., Collins M. A., Arkhipkin A. (2013). First case of possible iteroparity among coleoid cephalopods: the giant warty squid Kondakovia longimana. J. Molluscan Stud. 79, 270–272. doi: 10.1093/mollus/eyt014
Li Y., Zhang Y., Hussey N. E., Dai X. (2016). Urea and lipid extraction treatment effects on δ15N and δ13C values in pelagic sharks. Rapid Commun. Mass Spectrom. 30, 1–8. doi: 10.1002/rcm.7396
Lipiński M. R. (1993). Description of mature males of the histioteuthid cephalopod Histioteuthis atlantica (Hoyle 1885) (Cephalopoda: oegopsida) from the South Atlantic Ocean. S. Afr. J. Mar. Sci. 13, 51–62. doi: 10.2989/025776193784287383
Lutfi E., Berge G., Baeverfjord G., Sigholt T., Bou M., Larsson T., et al. (2022). Increasing dietary levels of the omega-3 long-chain polyunsaturated fatty acids, EPA and DHA, improves the growth, welfare, robustness, and fillet quality of Atlantic salmon in sea cages. Br. J. Nutr. 129, 1–48. doi: 10.1017/s0007114522000642
MacIntosh H., Althaus F., Williams A., Tanner J. E., Alderslade P., Ahyong S. T., et al. (2018). Invertebrate diversity in the deep Great Australian Bight (200–5000 m). Mar. Biodivers. Rec. 11, 23. doi: 10.1186/s41200-018-0158-x
MacLeod C. D., Santos M. B., Pierce G. J. (2003). Review of data on diets of beaked whales: Evidence of niche separation and geographic segregation. J. Mar. Biolog. Assoc. U.K. 83, 651–665. doi: 10.1017/S0025315403007616h
Martin U., Jaquemet S. (2019). Effects of urea and lipid removal from Carcharhinus leucas and Galeocerdo cuvier white muscle on carbon and nitrogen stable isotope ratios. West. Indian Ocean J. Mar. Sci. 18, 47–56. doi: 10.4314/wiojms.v18i1.5
May J. L., Blaber S. J. M. (1989). Benthic and pelagic fish biomass of the upper continental slope off eastern Tasmania. Mar. Biol. 101, 11–25. doi: 10.1007/BF00393474
Ménard F., Potier M., Jaquemet S., Romanov E., Sabatié R., Cherel Y. (2013). Pelagic cephalopods in the western Indian Ocean: New information from diets of top predators. Deep-Sea Res. II: Top. Stud. Oceanogr. 95, 83–92. doi: 10.1016/j.dsr2.2012.08.022
Mendonça A. (2009). Diet of the blue shark, Prionace glauca, in the Northeast Atlantic. [master’s thesis] (Portugal: University of Porto).
Mensch R. (2010). A systematic review of the squid genus Chiroteuthis (Mollusca: Cephalopoda) in New Zealand waters. [master’s thesis] (Auckland, New Zealand: Auckland University of Technology).
Merten V., Bayer T., Reusch T. B. H., Puebla O., Fuss J., Stefanschitz J., et al. (2021). An integrative assessment combining deep-sea net sampling, in situ observations and environmental DNA analysis identifies Cabo Verde as a cephalopod biodiversity hotspot in the Atlantic Ocean. Front. Mar. Sci. 8, 760108. doi: 10.3389/fmars.2021.760108
Merten V., Christiansen B., Javidpour J., Piatkowski U., Puebla O., Gasca R., et al. (2017). Diet and stable isotope analyses reveal the feeding ecology of the orangeback squid Sthenoteuthis pteropus (Steenstrup 1855) (Mollusca, Ommastrephidae) in the eastern tropical Atlantic. PloS One 12, e0189691. doi: 10.1371/journal.pone.0189691
Meyer L., Pethybridge H., Nichols P. D., Beckmann C., Bruce B. D., Werry J. M., et al. (2017). Assessing the functional limitations of lipids and fatty acids for diet determination: the importance of tissue type, quantity, and quality. Front. Mar. Sci. 4, 369. doi: 10.3389/fmars.2017.00369
Meyer L., Pethybridge H., Nichols P. D., Beckmann C., Huveneers C. (2019). Abiotic and biotic drivers of fatty acid tracers in ecology: A global analysis of chondrichthyan profiles. Funct. Ecol. 33, 1243–1255. doi: 10.1111/1365-2435.13328
Meynier L., Morel P. C. H., MacKenzie D. D. S., MacGibbon A., Chilvers B. L., Duignan P. J. (2008). Proximate composition, energy content, and fatty acid composition of marine species from Campbell Plateau, New Zealand. N.Z. J. Mar. Freshw. Res. 42, 425–437. doi: 10.1080/00288330809509971
Moovendhan M., Shanmugam A., Shanmugam V. (2019). Nutritional composition of liver (Digestive gland) from thondi squid (Sepioteuthis lessoniana). Indian J. Mar. Sci. 48, 1398–1403.
Navarro J., Coll M., Somes C. J., Olson R. J. (2013). Trophic niche of squids: Insights from isotopic data in marine systems worldwide. Deep-Sea Res. II: Top. Stud. Oceanogr. 95, 93–102. doi: 10.1007/s00227-009-1281-3
Nichols P. D., Pethybridge H. R., Zhang B., Virtue P., Meyer L., Dhurmeea Z., et al. (2023). Fatty acid profiles of more than 470 marine species from the Southern Hemisphere. Ecology 104, e3888. doi: 10.1002/ecy.3888
O’Brien C. E., Roumbedakis K., Winkelmann I. E. (2018). The current state of cephalopod science and perspectives on the most critical challenges ahead from three early-career researchers. Front. Physiol. 9. doi: 10.3389/fphys.2018.00700
Ozogul Y., Duysak O., Ozogul F., Ozkutuk A. S., Tuereli C. (2008). Seasonal effects in the nutritional quality of the body structural tissue of cephalopods. Food Chem. 108, 847–852. doi: 10.1016/j.foodchem.2007.11.048
Parrish C. C. (2013). Lipids in marine ecosystems. ISRN Oceanography 2013, 604045. doi: 10.5402/2013/604045
Parrish C. C., Nichols P. D., Pethybridge H., Young J. W. (2015). Direct determination of fatty acids in fish tissues: quantifying top predator trophic connections. Oecologia 177, 85–95. doi: 10.1007/s00442-014-3131-3
Parrish C. C., Thompson R. J., Deibel D. (2005). Lipid classes and fatty acids in plankton and settling matter during the spring bloom in a cold ocean coastal environment. Mar. Ecol. Prog. Ser. 286, 57–68. doi: 10.3354/meps286057
Parzanini C., Parrish C. C., Hamel J. F., Mercier A. (2018). Functional diversity and nutritional content in a deep-sea faunal assemblage through total lipid, lipid class, and fatty acid analyses. PloS One 13, e0207395. doi: 10.1371/journal.pone.0207395
Pethybridge H., Daley R., Virtue P., Butler E. C. V., Cossa D., Nichols P. D. (2010). Lipid and mercury profiles of 61 midtrophic species collected off southeastern Australia. Mar. Freshw. Res. 61, 1092–1108. doi: 10.1071/MF09237
Pethybridge H. R., Nichols P. D., Virtue P., Jackson G. D. (2013). The foraging ecology of an oceanic squid, Todarodes filippovae: The use of signature lipid profiling to monitor ecosystem change. Deep-Sea Res. II: Top. Stud. Oceanogr. 95, 119–128. doi: 10.1016/j.dsr2.2012.07.025
Pethybridge H., Virtue P., Casper R., Yoshida T., Green C. P., Jackson G., et al. (2012). Seasonal variations in diet of arrow squid (Nototodarus gouldi): stomach content and signature fatty acid analysis. J. Mar. Biol. Assoc. U.K. 92, 187–196. doi: 10.1017/S0025315411000841
Phillips K. L., Nichols P. D., Jackson G. D. (2001). Predation on myctophids by the squid Moroteuthis ingens around Macquarie and Heard Islands: stomach contents and fatty acid analyses. Mar. Ecol. Prog. Ser. 215, 179–189. doi: 10.3354/meps215179
Phillips K. L., Nichols P. D., Jackson G. D. (2002). Lipid and fatty acid composition of the mantle and digestive gland of four Southern Ocean squid species: implications for food-web studies. Antarct. Sci. 14, 212–220. doi: 10.1017/s0954102002000044
Phillips K. L., Nichols P. D., Jackson G. D. (2003). Dietary variation of the squid Moroteuthis ingens at four sites in the Southern Ocean: stomach contents, lipid and fatty acid profiles. J. Mar. Biolog. Assoc. 83, 523–534. doi: 10.1017/S0025315403007446h
Potier M., Marsac F., Cherel Y., Lucas V., Sabatié R., Maury O., et al. (2007). Forage fauna in the diet of three large pelagic fishes (lancetfish, swordfish and yellowfin tuna) in the western equatorial Indian Ocean. Fish. Res. 83, 60–72. doi: 10.1016/j.fishres.2006.08.020
Queirós J. P., Phillips R. A., Baeta A., Abreu J., Xavier J. C. (2019). Habitat, trophic levels and migration patterns of the short-finned squid Illex argentinus from stable isotope analysis of beak regions. Polar Biol. 42, 2299–2304. doi: 10.1007/s00300-019-02598-x
Queirós J. P., Ramos J. A., Cherel Y., Franzitta M., Duarte B., Rosa R., et al. (2021). Cephalopod fauna of the Pacific Southern Ocean using Antarctic toothfish (Dissostichus mawsoni) as biological samplers and fisheries bycatch specimens. Deep-Sea Res. I: Oceanogr. Res. Papers 174, 103571. doi: 10.1016/j.dsr.2021.103571
Quetglas A., Fliti K., Massuti E., Refes W., Guijarro B., Zaghdoudi S. (2006). First record of Taningia danae (Cephalopoda: Octopoteuthidae) in the Mediterranean Sea. Sci. Mar. 70, 153–155. doi: 10.3989/scimar.2006.70n1153
Remeslo A. V., Yakushev M. R., Laptikhovsky V. (2015). Alien vs. Predator: interactions between the colossal squid (Mesonychoteuthis hamiltoni) and the Antarctic toothfish (Dissostichus mawsoni). J. Nat. Hist. 49, 2483–2491. doi: 10.1080/00222933.2015.1040477
Robinson N. J., Johnsen S., Brooks A., Frey L., Judkins H., Vecchione M., et al. (2021). Studying the swift, smart, and shy: Unobtrusive camera-platforms for observing large deep-sea squid. Deep-Sea Res. I: Oceanogr. Res. Papers 172, 103538. doi: 10.1016/j.dsr.2021.103538
Rodhouse P. G. K. (2013). Role of squid in the Southern Ocean pelagic ecosystem and the possible consequences of climate change. Deep-Sea Res. II: Top. Stud. Oceanogr. 95, 129–138. doi: 10.1016/j.dsr2.2012.07.001
Rodhouse P. G. K., Lu C. C. (1998). Chiroteuthis veranyi from the Atlantic sector of the Southern Ocean (Cephalopoda: Chiroteuthidae). S. Afr. J. Mar. Sci. 20, 311–322. doi: 10.2989/025776198784126593
Rodhouse P. G. K., White M. G., Jones M. R. R. (1992). Trophic relations of the cephalopod Martialia hyadesi (Teuthoidea: Ommastrephidae) at the Antarctic Polar Front, Scotia Sea. Mar. Biol. 114, 415–421. doi: 10.1007/BF0035003
Rogers P., Ward T., van Ruth P., Williams A., Bruce B. D., Connell S., et al. (2013). Physical processes, biodiversity and ecology of the Great Australian Bight region: a literature review (Australia: CSIRO).
Roper C. F. E., Jereb P. (2010). “Family octopoteuthidae,” in Cephalopods of the world. An annotated and illustrated catalogue of species known to date. Volume 2. Myopsid and Oegopsid Squids. FAO Species Catalogue for Fishery Purposes, vol. 4 . Eds. Jereb P., Roper C. F. E. (Rome: FAO), 262–268.
Roper C. F. E., Shea E. K. (2013). Unanswered questions about the giant squid Architeuthis (Architeuthidae) illustrate our incomplete knowledge of coleoid cephalopods. Am. Malacol. Bull. 31, 109–122. doi: 10.4003/006.031.0104
Rosa R., Lopes V. M., Guerreiro M., Bolstad K., Xavier J. C. (2017). Biology and ecology of the world’s largest invertebrate, the colossal squid (Mesonychoteuthis hamiltoni): a short review. Polar Biol. 40, 1871–1883. doi: 10.1007/s00300-017-2104-5
Rosa R., Pereira J., Nunes M. L. (2005). Biochemical composition of cephalopods with different life strategies, with special reference to a giant squid, Architeuthis sp. Mar. Biol. 146, 739–751. doi: 10.2307/3593118
Ruiz-Cooley R. I., Garcia K. Y., Hetherington E. D. (2011). Effects of lipid removal and preservatives on carbon and nitrogen stable isotope ratios of squid tissues: Implications for ecological studies. J. Exp. Mar. Biol. Ecol. 407, 101–107. doi: 10.1016/j.jembe.2011.07.002
Santos M. B., Clarke M. R., Pierce G. J. (2001). Assessing the importance of cephalopods in the diets of marine mammals and other top predators: problems and solutions. Fish. Res. 52, 121–139. doi: 10.1016/S0165-7836(01)00236-3
Sato N. (2021). A review of sperm storage methods and post-copulatory sexual selection in the Cephalopoda. Biol. J. Linn. Soc 134, 285–302. doi: 10.1093/biolinnean/blab096
Shulman G. E., Chesalin M. V., Abolmasova G. I., Yuneva T. V., Kideys A. (2002). Metabolic strategy in pelagic squid of genus Sthenoteuthis (Ommastrephidae) as the basis of high abundance and productivity. An overview of the Soviet investigations. Bull. Mar. Sci. 71, 815–836.
Smale M. J., Cliff G. (1998). Cephalopods in the diets of four shark species (Galeocerdo cuvier, Sphyrna lewini, S. zygaena and S. mokarran) from KwaZulu-Natal, South Africa. S. Afr. J. Mar. Sci. 20, 241–253. doi: 10.2989/025776198784126610
Stowasser G., McAllen R., Pierce G. J., Collins M. A., Moffat C. F., Priede I. G., et al. (2009). Trophic position of deep-sea fish—Assessment through fatty acid and stable isotope analyses. Deep-Sea Res. I: Oceanogr. Res. Papers 56, 812–826. doi: 10.1016/j.dsr.2008.12.016
Stowasser G., Pierce G. J., Moffat C. F., Collins M. A., Forsythe J. W. (2006). Experimental study on the effect of diet on fatty acid and stable isotope profiles of the squid Lolliguncula brevis. J. Exp. Mar. Biol. Ecol. 333, 97–114. doi: 10.1016/j.jembe.2005.12.008
Sun X., Guo H., Zhu K., Zhang N., Yu W., Wu N., et al. (2018). Feed type regulates the fatty acid profiles of golden pompano Trachinotus ovatus (Linnaeus 1758). J. Appl. Anim. Res. 46, 60–63. doi: 10.1080/09712119.2016.1259110
Svensson E., Schouten S., Hopmans E. C., Middelburg J. J., Sinninghe Damsté J. S. (2016). Factors controlling the stable nitrogen isotopic composition (δ15N) of lipids in marine animals. PloS One 11, e0146321. doi: 10.1371/journal.pone.0146321
Tsuchiya K., Okamoto H., Uozumi Y. (1998). Cephalopods eaten by pelagic fishes in the tropical East Pacific, with special reference to the feeding habitat of pelagic fish. La Mer 36, 57–66.
Turner J. P., Rooker J. R. (2005). Effect of diet on fatty acid compositions in Sciaenops ocellatus. J. Fish Biol. 67, 1119–1138. doi: 10.1111/j.0022-1112.2005.00816.x
Van Ruth P. D., Patten N. L., Doubell M. J., Chapman P., Rodriguez A. R., Middleton J. F. (2018). Seasonal- and event-scale variations in upwelling, enrichment and primary productivity in the eastern Great Australian Bight. Deep-Sea Res. II: Top. Stud. Oceanogr. 157-158, 36–45. doi: 10.1016/j.dsr2.2018.09.008
Van Ruth P. D., Ward T. M. (2009). Meso-zooplankton abundance, distribution and community composition in the Eastern Great Australian Bight. Trans. R. Soc S. Aust. 133, 274–283. doi: 10.1080/03721426.2009.10887124
Weikert H. (1977). Copepod carcasses in the upwelling region south of Cap Blanc, N.W. Africa. Mar. Biol. 42, 351–355. doi: 10.1007/BF00402197
Xavier J. C., Allcock A. L., Cherel Y., Lipinski M. R., Pierce G. J., Rodhouse P. G. K., et al. (2015). Future challenges in cephalopod research. J. Mar. Biol. Assoc. U.K. 95, 999–1015. doi: 10.1017/s0025315414000782
Xavier J. C., Cherel Y., Allcock L., Rosa R., Sabirov R. M., Blicher M. E., et al. (2018). A review on the biodiversity, distribution and trophic role of cephalopods in the Arctic and Antarctic marine ecosystems under a changing ocean. Mar. Biol. 165, 93. doi: 10.1007/s00227-018-3352-9
Yoshino K., Takahashi A., Adachi T., Costa D. P., Robinson P. W., Peterson S. H., et al. (2020). Acceleration-triggered animal-borne videos show a dominance of fish in the diet of female northern elephant seals. J. Exp. Biol. 223, 9. doi: 10.1242/jeb.212936
Young J. W., Guest M. A., Lansdell M., Phleger C. F., Nichols P. D. (2010). Discrimination of prey species of juvenile swordfish Xiphias gladius (Linnaeus 1758) using signature fatty acid analyses. Prog. Oceanogr. 86, 139–151. doi: 10.1016/j.pocean.2010.04.028
Keywords: deep-sea squid, trophic ecology, stable isotope analysis, fatty acid analysis, energy content, Taningia danae, Chiroteuthis aff. veranii
Citation: Jackel B, Baring R, Doane MP, Henkens J, Martin B, Rough K and Meyer L (2023) Towards unlocking the trophic roles of rarely encountered squid: Opportunistic samples of Taningia danae and a Chiroteuthis aff. veranii reveal that the Southern Ocean top predators are nutrient links connecting deep-sea and shelf-slope environments. Front. Mar. Sci. 10:1254461. doi: 10.3389/fmars.2023.1254461
Received: 07 July 2023; Accepted: 19 October 2023;
Published: 27 November 2023.
Edited by:
Tomihiko Higuchi, The University of Tokyo, Bunkyo, JapanReviewed by:
Kat Bolstad, Auckland University of Technology, Auckland, New ZealandCopyright © 2023 Jackel, Baring, Doane, Henkens, Martin, Rough and Meyer. This is an open-access article distributed under the terms of the Creative Commons Attribution License (CC BY). The use, distribution or reproduction in other forums is permitted, provided the original author(s) and the copyright owner(s) are credited and that the original publication in this journal is cited, in accordance with accepted academic practice. No use, distribution or reproduction is permitted which does not comply with these terms.
*Correspondence: Bethany Jackel, QmV0aGFueS5qYWNrZWxAZmxpbmRlcnMuZWR1LmF1
Disclaimer: All claims expressed in this article are solely those of the authors and do not necessarily represent those of their affiliated organizations, or those of the publisher, the editors and the reviewers. Any product that may be evaluated in this article or claim that may be made by its manufacturer is not guaranteed or endorsed by the publisher.
Research integrity at Frontiers
Learn more about the work of our research integrity team to safeguard the quality of each article we publish.