- School of Marine and Atmospheric Sciences, Stony Brook University, Southampton, NY, United States
Harmful algal blooms (HABs) such as those formed by the ichthyotoxic dinoflagellate, Margalefidinium (aka Cochlodinium) polykrikoides can have adverse effects on bivalves. While M. polykrikoides has caused significant die offs of bivalves and other marine organisms, the Northern quahog or hard clam, Mercenaria mercenaria, is comparatively more resistant to this HAB. This study quantified clearance rates of juvenile hard clams (10-20 mm) exposed to three different North American populations of M. polykrikoides (bloom, strain CP1, strain CPSB-1G) as well as the nonharmful cryptophyte, Rhodomonas salina and the nonharmful dinoflagellate, Gymnodinium aureolum, in single and mixed algal exposures. Multiple biovolume exposures with M. polykrikoides bloom water and R. salina (1,000, 1,500, 3,000 cells mL-1 M. polykrikoides biovolume equivalent) were completed to assess the effects of increasing biomass on hard clam clearance rates and selection. Hard clams opened and actively cleared algal mixtures at and below 1,000 M. polykrikoides cells mL-1. During single species exposures, strain CPSB-1G and R. salina were cleared significantly faster than wild M. polykrikoides populations and strain CP1. During mixed exposures, R. salina was cleared significantly faster than CPSB-1G but not other M. polykrikoides populations and there was no difference between hard clam clearance rates of G. aureolum and R. salina. Clearance rates of M. polykrikoides at ≥1,500 cells mL-1 M. polykrikoides/R. salina mixtures were not significantly different than zero unlike clearance of those at <1,000 cells mL-1 indicating a density dependent effect of blooms. Collectively, the results demonstrate that hard clams can actively clear M. polykrikoides cells at moderate (≤1,000 cells mL-1) but not elevated (> 1,000 cells mL-1) bloom densities. Given this, and the documented survival of hard clams during blooms, M. mercenaria may be candidate for aquaculture and restoration in regions prone to HABs caused by M. polykrikoides.
Introduction
Bivalve shellfish hold a key position in marine food webs (Officer et al., 1982; Abeels et al., 2012). As filter feeders, they are able to clear the water of phytoplankton and other particulates, thereby decreasing water turbidity (Coen et al., 2007; Wall et al., 2008). Important submerged aquatic vegetation, such as macroalgae and sea grasses, can benefit from the removal of phytoplankton and particulates by bivalves (Coen et al., 2007; Wall et al., 2008). Some bivalves, such as oysters, can also provide critical habitat by forming reefs composed of aggregations of live individuals (Newell, 2004; Coen et al., 2007). These aggregations provide habitat for many juvenile fish and invertebrates to hide from predators (Coen et al., 2007). Similarly, bivalve shells can provide habitat to other organisms that rely on hard substrate (Tolley and Volety, 2005) and promote survival and growth of early life stage bivalves and other calcifying organisms (Curtin et al., 2022). In addition to their ecological value, shellfish are commercially valuable (Lellis-Dibble et al., 2008). Coastal communities and countries around the world have a history of benefitting economically from shellfisheries along with having an integrated maritime culture based on harvesting and growing shellfish leading to millions of dollars in landings (Lellis-Dibble et al., 2008). Bivalve shellfish represent a $2B USD industry in the US (Ekstrom et al., 2015), a multi-million dollar industry on Long Island alone and are known to provide natural filtration for shallow embayed areas (Cerrato et al., 2004; Lellis-Dibble et al., 2008). Restoration efforts have been implemented to promote shellfish population recovery from anthropogenically-caused population declines, such as hard clam spawning sanctuaries in Great South Bay and Shinnecock Bay, NY (Doall et al., 2008; Gobler et al., 2022).
Harmful algal blooms (HABs) are an increasing threat to coastal areas causing environmental, ecological, and economic damage worldwide (Zingone and Enevoldsen, 2000; Haigh and Esenkulova, 2014). HAB species can range from toxin producers, species harmful to marine biota, and/or blooms that lead to hypoxia and/or coastal acidification (Zingone and Enevoldsen, 2000; Anderson et al., 2002; Levin et al., 2009; Griffith and Gobler, 2020). HABs are promoted by natural and anthropogenic conditions, with excessive nutrient loading and climate change being primary drivers (Heisler et al., 2008; Gobler et al., 2017). Margalefidinium Cochlodinium (a.k.a. Cochlodinium) polykrikoides is a HAB species that is globally distributed and has expanded during the past three decades (Kudela and Gobler, 2012; Griffith et al., 2019a). M. polykrikoides is a naked, chain-forming dinoflagellate which enhances its motility (Fraga, 1989; Smayda and Reynolds, 2003), helps to maintain surface positions in warmer waters (Fraga, 1989), and deters grazers (Jiang et al., 2009; Jiang et al., 2010). M. polykrikoides can outcompete other phytoplankton through allelopathy, specifically through the production of reactive oxygen species (ROS) that cause significant mortality in a wide range of marine organisms, including shellfish and finfish (Kim et al., 1999; Kim et al., 2000; Tang and Gobler, 2010). ROS on a cellular level can cause DNA damage, denature enzymes, and lead to the destruction of membrane integrity (Cunningham and Capone, 1992; Okamoto and Colepicolo, 1998). In addition to ROS production, M. polykrikoides may also have significant toxic effects through the production of extracellular polysaccharides (Kim et al., 2002). ROS production and extracellular mucus secretion lead to asphyxiation of fish through either direct damage to the gill lamellae by lipid peroxidation or by smothering through this extracellular polysaccharide secretion (Kim et al., 2000; Hallegraeff et al., 2017). These effects may be exacerbated during M. polykrikoides blooms due to their ability to vertically migrate within the water column (Gobler et al., 2008; Kim et al., 2010). By utilizing high light levels, congregating at high densities in surface waters during the day and migrating to the benthos at night (Cullen, 1985; Kim et al., 2010) M. polykrikoides can negatively impact benthic organisms including shellfish as well as pelagic finfish (Griffith et al., 2019c).
HABs, including M. polykrikoides, have led to the collapse of some bivalve fisheries (Bricelj and Kuenstner, 1989) and can affect bivalves in different ways depending on the bivalve species and life stage (Gobler et al., 2008; Tang and Gobler, 2009b; Griffith et al., 2019b; Griffith et al., 2019c). Some adult bivalves can experience mortality or possible gonadal depression when exposed to HABs (Bricelj et al., 2005) and accumulate high levels of toxins (Zingone and Enevoldsen, 2000). Compared to their adult counterparts, larval and juvenile bivalves are typically more susceptible to HABs (Gobler et al., 2008; Tang and Gobler, 2009b; Li et al., 2012; Griffith et al., 2019b). M. polykrikoides in particular can be highly toxic and lethal to early life stage bivalves and have been shown to cause mortality in larval and juvenile Crassostrea virginica (eastern oyster) and Argopecten irradians (bay scallop) (Gobler et al., 2008; Tang and Gobler, 2009b; Li et al., 2012; Griffith et al., 2019b). This HAB can cause gill tissue hyperplasia along with hemorrhaging that has led to mortality in juvenile bivalves (Gobler et al., 2008). In addition, larval and juvenile bivalve mortality increases when they are exposed to greater M. polykrikoides cell densities and are exposed for longer periods of time (Tang and Gobler, 2009b; Li et al., 2012; Griffith et al., 2019c). The specific growth phase of this dinoflagellate can also influence bivalve mortality with stationary phase being less toxic than exponential phase (Tang and Gobler, 2009b). Although gill tissue degradation in multiple bivalve species has been a major cause in mortality of juvenile bivalves (Gobler et al., 2008), species-specific responses to M. polykrikoides may also lead to greater stress on vulnerable populations (Griffith et al., 2019c). Hardier species such as Mercenaria mercenaria (hard clam) and Crassostrea virginica (eastern oyster) have displayed lower mortality compared to more sensitive species such as Argopecten irradians (bay scallop) (Griffith et al., 2019c).
Bivalves can selectively feed on certain species of phytoplankton based on nutritional value, size, biochemical cues, and toxicity (Newell and Jordan, 1983; Shumway et al., 1985; Espinosa et al., 2009; Espinosa et al., 2010). There are three areas where bivalves can selectively distinguish particles in their bodies; the ctenidia, the palps, and the gut (Shumway et al., 1985; Ward and Shumway, 2004). There can be preferential clearance upon the ctenidia, and gills, by the bivalve closing or retracting their incurrent siphon or selectively clearing certain species out of the water (Shumway et al., 1985; Ward et al., 1997; Baker, 1998; Ward and Shumway, 2004; Dunphy et al., 2006). Preferential clearance on the ctenidia will be addressed in this project as it has been observed in studies with several algal species present (Shumway et al., 1985; Ward et al., 1997; Baker, 1998; Ward et al., 1998; Ward and Shumway, 2004; Dunphy et al., 2006). The exact mechanism for selective clearance/particle sorting is still debated with some studies showing that it depends on the size of particles while others indicate it depends on particle composition (Levinton et al., 2002; Ward and Shumway, 2004; Dunphy et al., 2006). To date, no study has quantified the ability of juvenile bivalves to clear M. polykrikoides. Identifying the sensitivity of bivalve clearance to M. polykrikoides can improve the understanding of their threshold to major bloom events.
The objective of this study was to identify the effects of M. polykrikoides on clearance rates of juvenile hard clams, Mercenaria mercenaria. Wild bloom populations collected from Shinnecock Bay and Peconic Bay, both historical locations of M. polykrikoides blooms, along with cultured M. polykrikoides were used in these experiments. Juvenile hard clams (M. mercenaria) ranging from 10-20 mm in length were used in experimental trials to assess size class dependent clearance rates. Wild and cultured M. polykrikoides were used to identify how they each affect clearance rate of ecologically and economically important bivalve species. Cultured Gymnodinium aureolum, a nonharmful dinoflagellate, and Rhodomonas salina, a nonharmful cryptophyte, were used to compare juvenile bivalve clearance rate of non-toxic dinoflagellates and nanoplankton that mimicked the size and swimming behavior respectively, of M. polykrikoides in phytoplankton assemblages. These experiments were completed to address the following questions: (1) How does exposure to monospecific blooms and assemblages of M. polykrikoides affect clearance rates?, (2) How does exposure to M. polykrikoides within mixed assemblages of phytoplankton affect clearance rates?, and (3) How do changes in the total seston load/bloom density of M. polykrikoides in the water column affect juvenile hard clam clearance rates?
Methods
Algal cultures
Clonal cultures of M. polykrikoides were used for clearance rate experiments along with cultured R. salina and G. aureolum. M. polykrikoides cultures that were isolated from bloom water samples included strain CP1 from Flanders Bay, NY, in 2006 (Gobler et al., 2008) and strain CPSB-1G from Shinnecock Bay, NY, in 2010. Both strains have been shown to be harmful to bivalve and fish larvae (Tang and Gobler, 2009a; Tang and Gobler, 2009b; Griffith and Gobler, 2016), but have not yet been used to study their impact on bivalve clearance. G. aureolum (strain GymMHC-23) was isolated from Meetinghouse Creek, a tributary within Peconic Bay, NY and confirmed via sequencing of the 28S rRNA gene, and R. salina CCMP 1319 was provided by the National Center for Marine Algae (NCMA). Gymnodinium aureolum (Hulburt) G Hansen 2000, is different and distinct from the ichthyotoxic North European Gyrodinium aureolum which is now Karenia mikimotoi. While R. salina, G. aureolum, and M. polykrikoides differ in cellular diameter (15, 40, and 35 µm, respectively), hard clams are capable of clearing particles greater than 5 µm in diameter (Riisgård, 1988) up to at least 200 µm (Riisgård and Larsen, 2010).
All cultures were maintained in sterile GSe medium with a salinity of ~32, made with boiled and 0.2 μm filtered seawater (Doblin et al., 1999), and held at 21° C in an incubator that provided a light intensity of ~100 µmol m-2 s-2 with a 12 h light: 12 h dark photoperiod. Cultures were allowed to grow until they reached exponential growth phase to avoid any differences in toxicity amongst strains (Tang and Gobler, 2009a). Concentrations of all species and strains were quantified via flow cytometry using a Beckman Coulter CytoFLEX, imaging light microscopy using a Keyence BZ-X800, or hand counts through light microscopy.
Bloom water collection
Wild populations of M. polykrikoides from blooms were also used in this study to compare to laboratory grown cultures. Bloom water was obtained from regions that have a history of M. polykrikoides blooms (e.g. Peconic Bay and Shinnecock Bay). Sampling for bloom water took place opportunistically from July through September of 2020 and 2021. At each site, 20L of surface bloom water was collected in polyethylene carboys and physical parameters (water temperature, salinity, and dissolved oxygen) were measured with a YSI ProPlus probe. Although not monospecific, M. polykrikoides blooms constituted a majority of M. polykrikoides microscopically identified cells in the samples (>75%). Bloom water was used within a day of collection and diluted with UV-sterilized 1-μm filtered water from Old Fort Pond, Shinnecock Bay if too dense (>3,000 cells mL-1) for the purposes of these experiments. The strongly allelopathic nature of M. polykrikoides blooms reduces the presence of other, larger phytoplankton in bloom water (Tang and Gobler, 2010; Koch et al., 2014), minimizing their potential impact on experimental outcomes.
Juvenile hard clams
Juvenile clams (~10-20 mm) were hatchery spawned from wild brood stock. All individuals were held in a sea table with recirculating filtered (1 μm) Old Fort Pond seawater at experimental temperatures (~21°C) while being fed mixed live algae (M. muelleri, P. lutheri, R. salina, T. lutea) for at least one week before being used for clearance rate experiments (Helm et al., 2004). Bivalves were starved 24 h prior to conducting experiments to ensure some individuals opened during experiments (Shumway et al., 1985).
Clearance rate experiments
Juvenile bivalve clearance rates of M. polykrikoides, G. aureolum, and R. salina clearance rates were calculated using the clearance method (Riisgård, 2001). In the clearance method, clearance rate is measured as the volume of water cleared of suspended particles per unit of time. The reduction in the number of particles as a function of time is monitored by taking water samples at fixed time intervals and measuring the particle concentration. Clearance rate experiments were established using 250 mL glass beakers placed on multi-position magnetic stir plates (details below). For all experimental algal exposures (differing species and food levels), there were three control beakers with phytoplankton only and ten experimental beakers with bivalves of the same algal diet per treatment. Single species exposure experiments were completed to address question 1 where cultured and bloom M. polykrikoides concentrations represented bloom and sub-bloom concentrations: 1,000 cells mL-1, 750 cells mL-1, 500 cells mL-1, and 250 cells mL-1 (Tang and Gobler, 2009a; Tang and Gobler, 2009b). The highest concentration for experiments using cultured M. polykrikoides was 1,000 cells mL-1 which was limited by the density of scaled-up 4-liter cultures (<3,000 cells mL-1) that could be produced. Preliminary data (personal obs.) suggested that clams would cease to clear at 1,000 cells mL-1 although was not observed throughout this study. To address question 2, clonal culture CPSB-1G was used for M. polykrikoides, R. salina mixed culture experiments. Past studies have demonstrated that the clonal culture, CPSB-1G was of similar toxicity when compared to the clonal culture CP1 (Yang et al., 2022). Mixed culture experiments normalized at the 1,000 M. polykrikoides cell mL-1 biovolume level using five different proportions: (0 dinoflagellate, 0.25 dinoflagellate, 0.5 dinoflagellate, 0.75 dinoflagellate, or complete dinoflagellate) and R. salina was used to fulfill the remaining 0.75, 0.5, or 0.25 of cells present respectively. The experimental set up was identical for experiments with G. aureolum and R. salina with cell densities normalized to M. polykrikoides biovolume equivalent (i.e. μm3). To address question 3, several experiments using wild populations of M. polykrikoides at densities greater than 1,000 cells mL-1 with R. salina present were completed to observe behavior and clearance rates of juvenile clams when exposed to denser blooms.
Experimental beakers (250 mL) used for clearance rate experiments were gently homogenized using a 1 inch stir bar rotating at 60 rpm. One bivalve was placed on a plastic mesh platform in each beaker, keeping them ~2 cm above the stir bar. Bivalves were allowed to clear for one hour once they opened and/or extended their siphon. Duplicate samples were taken from each beaker once the bivalve opened and after the one-hour period. Samples were used to quantify concentrations of each algal species present, one preserved with 10% glutaraldehyde for flow cytometric quantification and the other with Lugol’s iodine for imaging or hand quantification using microscopy. Clearance rates were calculated using the equation below (Coughlan, 1969):
Where V is the volume of water in the container, n is the number of bivalves present (n = 1), t is time (1 hour), C0 is the initial concentration of algae, Ct is the concentration of algae after bivalves have been open for one hour, C0′ is the initial concentration of algae for the control, and Ct′ is the concentration after one hour. Clearance rate quantification was normalized to the dry weight of each bivalve individual allowing for a weight-based comparison between experiment types (Harke et al., 2011). Dry weights were obtained by removing all soft tissue from individual bivalves and keeping them in a drying oven for at least 48 hours before weighing them again on a balance. Changes in the cell densities of the control beakers were used to adjust the clearance rate of each replicate correcting for any loss of cells in the vessel.
Quantification of algal cells
Algal concentration was quantified via flow cytometry using a Beckman Coulter CytoFLEX, imaging light microscopy using a Keyence BZ-X800, or light microscopy. Flow cytometry employed the allophycocyanin (APC) channel with a 660/20 bandpass filter to obtain counts for live and preserved G. aureolum, and R. salina cells. Samples for both M. polykrikoides strains and bloom water were counted via light microscopy and imaging light microscopy to obtain total counts and distinguish between single cells and double/triple/quadruple chains.
Statistical analyses
Statistical analyses were performed using RStudio® statistical software (version 4.0.3). Outliers consisting of unrealistic clearance rates for juvenile clams (>50 L hr-1 g-1) were removed prior to analyses. To address unbalanced distributions and heterogenous variances bootstrap two-way and three-way ANOVAs were used to identify significant differences in clearance rates between varying densities, strains, and biovolumes of M. polykrikoides and species of algae. Beyond unbalanced distributions and heterogenous variances, bootstrapping requires minimal assumptions about normality and it allows statistical inferences to be drawn regarding whole population based on a limited data set (Efron, 1992). When significant differences were detected, a bootstrap Tukey’s honest significant difference test (Tukey’s HSD) was performed on the bootstrapped data to identify specific differences between groups. Additionally, bootstrap one-sample t-tests with a Bonferroni correction for type 1 errors were completed on clearance rate data for each group during all experiments to identify whether clearance rates were significantly different than zero. All assumptions were met for all statistical models. Bootstrap ANOVAs were performed using the lmboot package (Efron, 1992), bootstrap one-sample t-tests were completed using the MKinfer package (Efron, 1994), and Tukey’s HSD post-hoc comparison tests were completed using the boot package. Results for Tukey’s HSD tests are displayed in representative box plots via compact letter display where in groupings are based on significant differences (α = 0.05).
Results
Across all experiments, there was no detectable difference of hard clams altering opening and closing behavior when exposed to different strains or densities of M. polykrikoides with and without R. salina present. In contrast, clearance rates varied amongst different strains, species, and densities of algae.
Single species exposures
For the single algal species experiments, clearance rates were significantly different across the different phytoplankton populations (p<0.05; bootstrap two-way ANOVA, Figure 1). Hard clams cleared the clonal strain CPSB-1G faster than M. polykrikoides bloom water and the clonal CP1 strain (p<0.05; bootstrap Tukey’s HSD, Figure 1). Additionally, hard clams cleared R. salina faster than the CP1 strain (p<0.05; bootstrap Tukey’s HSD, Figure 1). Hard clam clearance rates of R. salina were not significantly different from clearance of CPSB-1G (p> 0.05; bootstrap two-way ANOVA, Figure 1) but were marginally faster than the clearance of M. polykrikoides bloom water (p=0.07; bootstrap Tukey’s HSD, Figure 1). Hard clam clearance rates of the clonal strain CP1 were not significantly different than the clearance of M. polykrikoides bloom water (p>0.05; bootstrap two-way ANOVA, Figure 1). Hard clam clearance rates of CP1(α′ = 0.0125, p=0.484; t-test) and bloom water were not significantly different than zero (α′ = 0.0125, p=0.186; t-test). Clearance rates for all single algal species exposures did not differ across cell densities examined (250 – 1,000 cells mL-1; p=0.163; bootstrap two-way ANOVA, Figure 1).
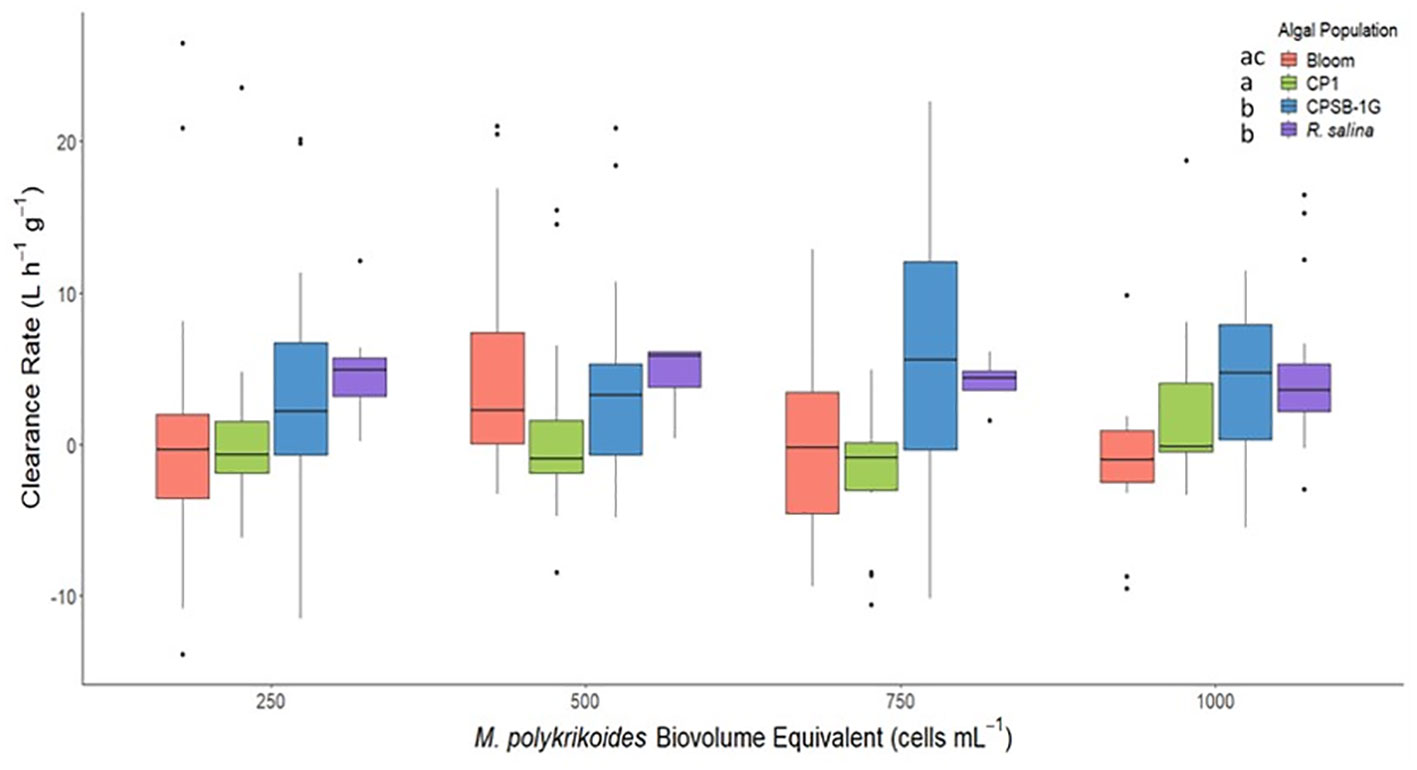
Figure 1 Clearance rates of M. mercenaria during single algal species exposures of M. polykrikoides bloom water, M. polykrikoides clonal isolates (CP1 & CPSB1-G) and R. salina at four densities (250, 500, 750 & 1000 cells mL-1 M. polykrikoides biovolume equivalent). Letters next to each algal population in figure legend indicates significantly different groups based on results of Tukey’s HSD tests. Boxes display the interquartile range (IQR) of each data set while bars within each box represent the median value. Whiskers represent the maximum and minimum for each data set if there are no possible outliers. Points past the whiskers display any possible outliers (true maximum or minimum) within the data.
Mixed culture/bloom exposures
Clearance rates were significantly different as a function of phytoplankton population present (p<0.05; bootstrap two-way ANOVA, Figure 2). During the M. polykrikoides bloom water/R. salina (Figure 2) mixture experiments, clearance of M. polykrikoides bloom water was not significantly different than clearance rates of R. salina (p>0.05; bootstrap Tukey’s HSD, Figure 2), and clearance of M. polykrikoides bloom water was significantly greater than zero (α′ = 0.0125, p<0.0125; t-test). In contrast, clearance rates of strain CPSB-1G were significantly slower than those of R. salina when the two algae were present together (p<0.05; bootstrap Tukey’s HSD, Figure 2). During the G. aureolum/R. salina (Figure 3) mixture experiments hard clam clearance of the two species was not significantly different (p>0.05; bootstrap two-way ANOVA, Figure 3). Similar to the single species exposures, there were no significant differences in clearance rates across the different proportions of dinoflagellates in the algal mixtures (25-75%; %; p>0.05; bootstrap two-way ANOVA, Figures 2, 3).
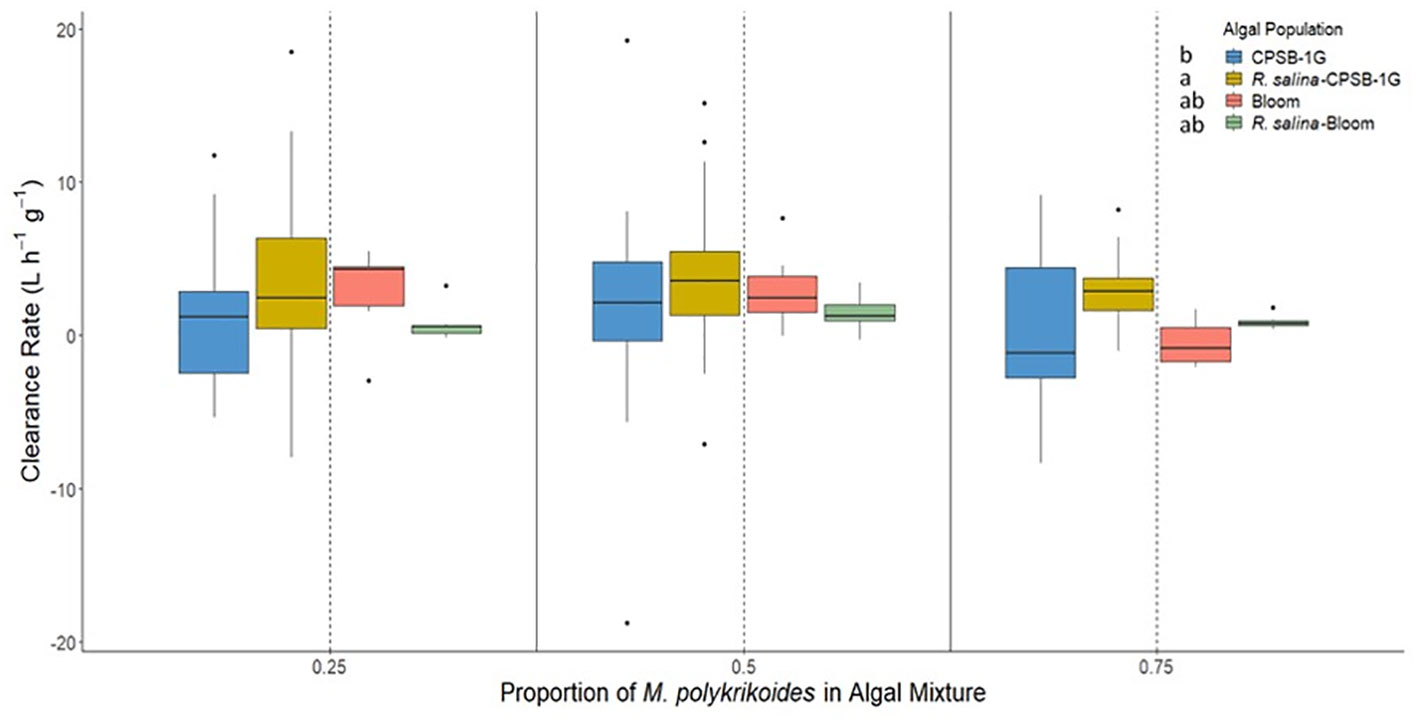
Figure 2 Clearance rates of M. mercenaria during mixed algal species exposures of M. polykrikoides bloom water with cultured R. salina and clonal isolate CPSB-1G with R. salina at different proportions of M. polykrikoides (0.25, 0.5, & 0.75) in the algal mixture. Each treatment was normalized to 1,000 cells mL-1 M. polykrikoides biovolume equivalent. Letters next to each algal population in figure legend indicates significantly different groups based on results of Tukey’s HSD tests. Boxes display the interquartile range (IQR) of each data set while bars within each box represent the median value. Whiskers represent the maximum and minimum for each data set if there are no possible outliers. Points past the whiskers display any possible outliers (true maximum or minimum) within the data.
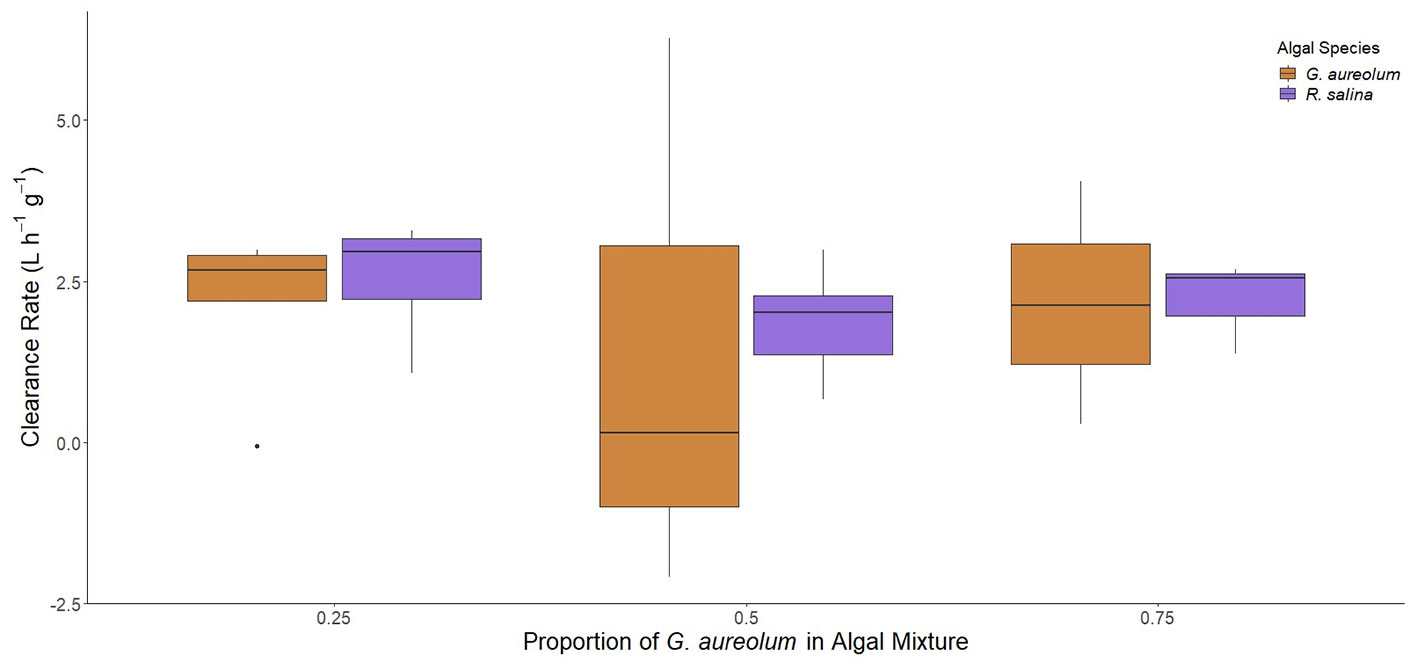
Figure 3 Clearance rates of M. mercenaria during mixed algal species exposures of cultured G. aureolum with R. salina at different proportions of G. aureolum (0.25, 0.5, 0.75) in the algal mixture. Each treatment was normalized to 1,000 cells mL-1 M. polykrikoides biovolume equivalent. Letters next to each algal population in figure legend indicates significantly different groups based on results of Tukey’s HSD tests. Boxes display the interquartile range (IQR) of each data set while bars within each box represent the median value. Whiskers represent the maximum and minimum for each data set if there are no possible outliers. Points past the whiskers display any possible outliers (true maximum or minimum) within the data.
Single versus mixed species comparisons
Hard clam clearance rates of M. polykrikoides within bloom water and CPSB-1G clonal cultures during single and mixed species exposures were compared. Clearance rates differed as a function of single versus mixed species exposures (p=0.08), but not across differing M. polykrikoides densities (p=0.196) and algal populations (p=0.275; bootstrap three-way ANOVA, Figure 4). More specifically, clearance rates of mixed species were marginally higher than single species exposures (p=0.08; bootstrap three-way ANOVA, Figure 4).
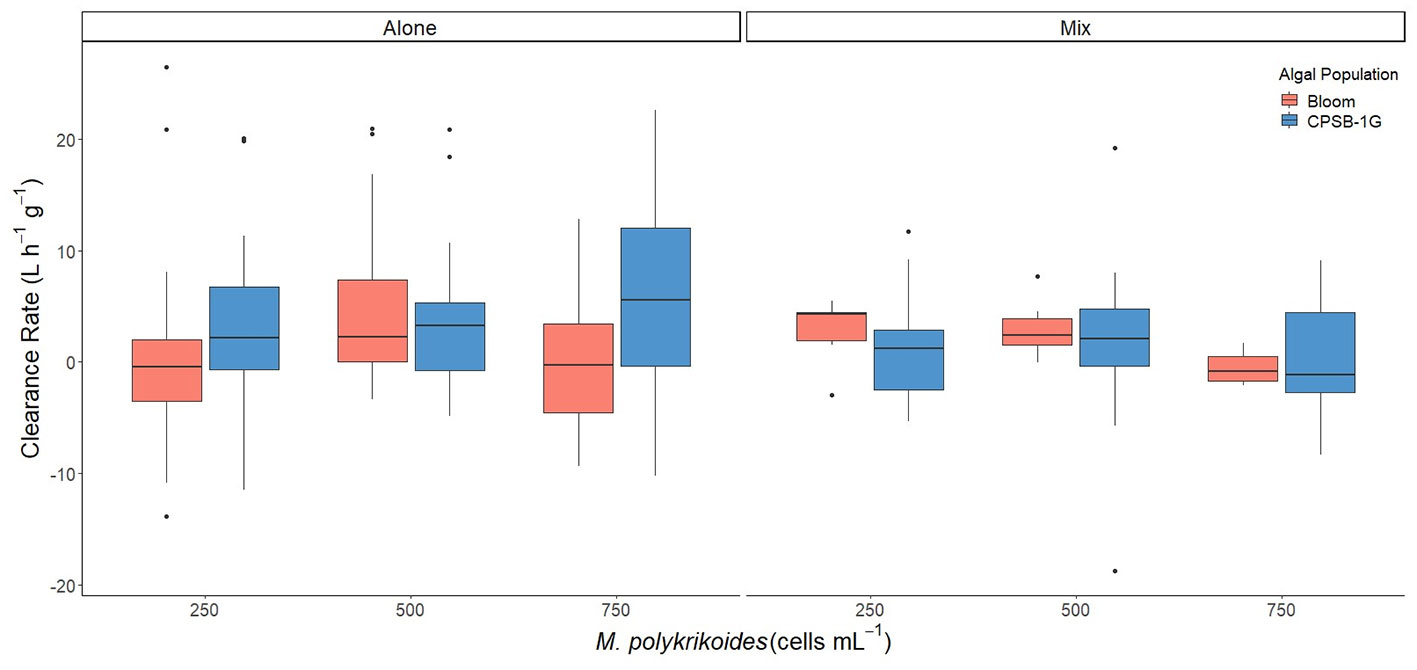
Figure 4 Clearance rates of M. mercenaria during single species exposures of M. polykrikoides bloom water and cultured clonal isolate CPSB-1G, and mixed algal species exposures of M. polykrikoides bloom water with cultured R. salina and CPSB-1G with R. salina. Boxes display the interquartile range (IQR) of each data set while bars within each box represent the median value. Whiskers represent the maximum and minimum for each data set if there are no possible outliers. Points past the whiskers display any possible outliers (true maximum or minimum) within the data.
Hard clam clearance rates of R. salina cells as a single species, mixed with CPSB-1G, and mixed within bloom water were compared. Clearance rates differed as a function of algal mixture (p<0.05; bootstrap two-way ANOVA, Figure 5) but not as a function of proportions of M. polykrikoides within the mixtures (p>0.05; bootstrap two-way ANOVA, Figure 5). Clearance rates of R. salina were significantly slower when it was mixed with bloom water compared to R. salina clearance rates when mixed with CPSB-1G or R. salina alone (p<0.05; bootstrap Tukey’s HSD, Figure 5). In contrast, clearance rates of R. salina as a uni-algal suspension or mixed with CPSB-1G were not significantly different (Figure 5).
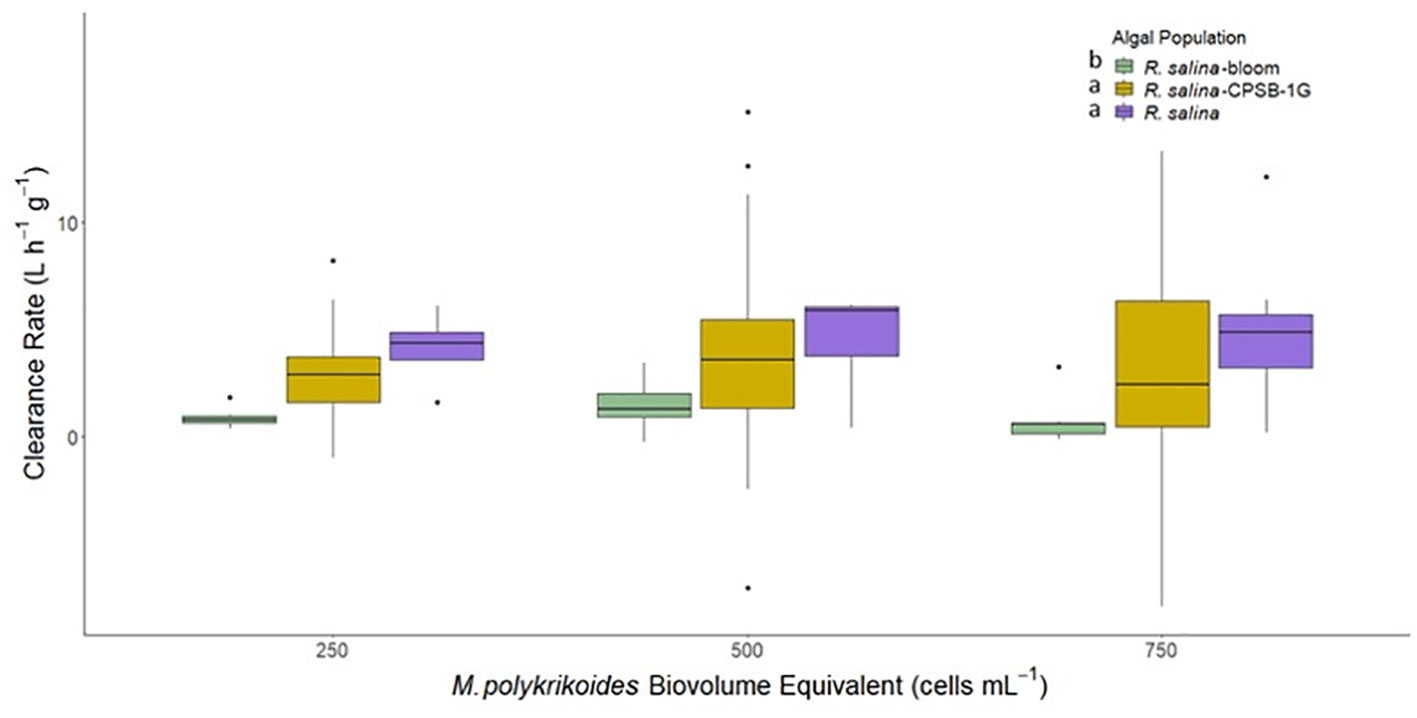
Figure 5 Clearance rates of M. mercenaria during single species exposures of R. salina and mixed algal exposures of R. salina with M. polykrikoides bloom water and cultured clonal isolate CPSB-1G. Letter next to each algal population in figure legend are results of Tukey’s HSD tests. Letters next to each algal population in figure legend indicates significantly different groups based on results of Tukey’s HSD tests. Boxes display the interquartile range (IQR) of each data set while bars within each box represent the median value. Whiskers represent the maximum and minimum for each data set if there are no possible outliers. Points past the whiskers display any possible outliers (true maximum or minimum) within the data.
Varying biovolume exposures
Clearance rates differed as a function of biovolume of phytoplankton (p<0.05; bootstrap three-way ANOVA, Figure 6) but not as a function of proportions of M. polykrikoides and differing algal species (p>0.05; bootstrap three-way ANOVA, Figure 6). Additionally, interactive effects between biovolume and proportions of M. polykrikoides led to significantly different clearance rates (p>0.05; bootstrap three-way ANOVA, Figure 6). Clearance rates at 1,000 cells mL-1 were not significantly different from the other two biovolume experiments (p>0.05; bootstrap Tukey’s HSD, Figure 6). M. polykrikoides clearance rates at 1,500 cells mL-1 and 3,000 cells mL-1 were not significantly different than zero (α′ = 0.0083, p>0.0083; t-test, Figure 6). R. salina clearance rates were not significantly different than zero at 3,000 cells mL-1(p>0.0083) but were significantly greater than zero at 1,500 cells mL-1 (α′ = 0.0083, p<0.0083; t-test, Figure 6).
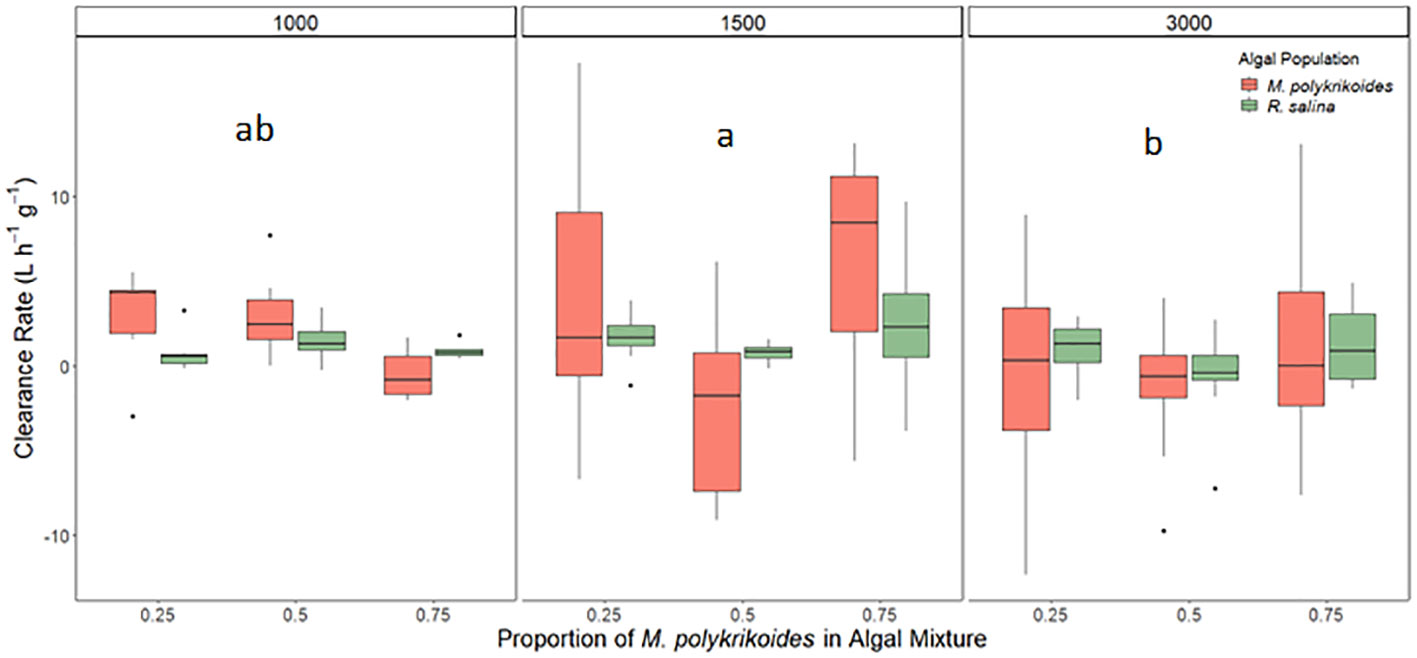
Figure 6 Clearance rates of M. mercenaria during mixed algal species exposures of M. polykrikoides bloom water with cultured R. salina at three different M. polykrikoides biovolume equivalent levels (1,000, 1,500, ∓ 3,000 cells mL-1). Letters within boxes for each biovolume level indicates significantly different groups based on results of Tukey’s HSD tests. Boxes display the interquartile range (IQR) of each data set while bars within each box represent the median value. Whiskers represent the maximum and minimum for each data set if there are no possible outliers. Points past the whiskers display any possible outliers (true maximum or minimum) within the data.
Discussion
During this study, hard clam clearance rate of M. polykrikoides varied as a function of algal populations, biomass level, and single versus mixed species exposures. There were substantial differences in hard clam clearance between some clonal strains of M. polykrikoides and bloom water suggesting clams and possibly other bivalves have strain-specific tolerances to this toxic alga. CPSB-1G strain was cleared at the highest rate amongst M. polykrikoides strains, being significantly higher than both CP1 and M. polykrikoides bloom water and matching the clearance of R. salina, a beneficial cryptophyte. Mixed algal assemblages slightly altered this relationship as the presence of CPSB-1G with R. salina yielded higher clearance of R. salina, yet the clearance of CPSB-1G in single species and mixed species exposures (with R. salina) were not different. Mixing R. salina into M. polykrikoides bloom water highlighted that R. salina clearance was significantly reduced in the presence of the harmful alga. In addition, clearance rates of M. polykrikoides bloom water at 1,500 cells mL-1 and higher were not significantly different than zero. Collectively, this variance in clearance rates across strains, single versus mixed species assemblages, and total biovolume exposure provide insight into hard clam behavior in the presence of M. polykrikoides and has implications for the resilience of M. mercenaria populations and aquaculture to this HAB.
M. polykrikoides toxicity and mechanisms affecting clearance rates
The dynamic nature of clearance rates of juvenile hard clams exposed to different strains of M. polykrikoides supports the need for multifaceted, unique approaches to understand the toxicity of this alga. M. polykrikoides is known to be toxic due to the production of ROS (superoxide anion, hydrogen peroxide, hydroxyl radical) and perhaps extracellular polysaccharides (Kim et al., 1999; Kim et al., 2002; Kim et al., 2009; Tang and Gobler, 2009a). Significant mortality events during HABs caused by M. polykrikoides have been observed across multiple taxa including finfish (Gobler et al., 2008; Dorantes-Aranda et al., 2009; Tang and Gobler, 2009a), shellfish (Kim et al., 2009; Tang and Gobler, 2009a; Tang and Gobler, 2009b; Li et al., 2012; Griffith et al., 2019c), reef building corals (Guzmán et al., 1990; Bauman et al., 2010; Richlen et al., 2010), copepods (Jiang et al., 2011), and shrimp (Pérez-Morales et al., 2017). Specifically, M. polykrikoides can also cause rapid mortality of larval stages of northwest Atlantic bivalves (Tang and Gobler, 2009b; Griffith and Gobler, 2016). In addition, it has been demonstrated that M. polykrikoides has species-specific effects on juvenile bivalves where a combination of bloom vertical migration, bloom duration, temperature, and inability for sessile fauna to evade blooms can lead to mortalities in Crassostrea virginica (eastern oyster; (Gobler et al., 2008; Li et al., 2012)), Argopecten irradians (bay scallop; (Gobler et al., 2008; Tang and Gobler, 2009a; Griffith et al., 2019c)), and Mytilus spp. (mussel species; (Curtiss et al., 2008)). In contrast, M. mercenaria (hard clam) has proven to be more resilient to this HAB, surviving dense blooms that have caused mortality or reduced growth rates in other bivalves such as M. virginica and A. irradians (Griffith et al., 2019c). Here, bivalve clearance rates were quantified to understand how M. polykrikoides might alter the behavior of these animals.
Bivalve clearance of HABs has been studied for a number of algal taxa with clearance rates varying between bivalve and algal species (Bricelj et al., 2001; Hegaret et al., 2007; Harke et al., 2011). Studies have been performed to address the effects of other toxins and harmful compounds, such as exposure to ROS produced by HABs on survival or clearance rate of bivalves (Jeong et al., 2004; Hegaret et al., 2007; Tang and Gobler, 2009b). Similar to the findings of this study, bivalves have been shown to clear M. polykrikoides at various rates (Jeong et al., 2004). Specifically, Jeong et al. (2004) demonstrated that mussel larvae can successfully clear M. polykrikoides cells from the water column after two weeks of development. Like all invertebrates, hard clams do not have acquired immunity and are limited to innate immunity through cellular response using hemocytes (Janeway, 1994). They also have an open circulatory system where particulates that are being taken in from the environment come into direct contact with clam hemolymph (Janeway, 1994; Hegaret, 2008). During a study by Hegaret (2008), the ROS-producing alga Heterosigma akashiwo displayed loss of shape and complexity and increased mortality as hard clam hemocytes engulfed cells through phagocytosis and use of an enzymatic chemical agent released by the hemocyte cells when hard clam hemolymph was exposed to H. akashiwo. Although H. akashiwo has cytotoxic properties that led to increased hard clam hemocyte death, suppressed phagocytosis, and increased apoptosis, hard clam hemocytes were the main drivers of harmful algal cell death in H. akashiwo and other HABs when compared to other bivalve hemocytes (Hegaret, 2008). M. polykrikoides cells may have a similar fate when cleared by juvenile hard clams.
The M. polykrikoides strain, CPSB-1G was cleared at rates similar to the nonharmful food source, R. salina, in single alga experiments while the CP1 strain and M. polykrikoides bloom populations were not cleared, suggesting possible hard clam resilience to a variety of M. polykrikoides strains and toxicities. Although hard clams have lower clearance rates than Eastern oysters and mussels (Bricelj and Malouf, 1984; Shumway et al., 1985; Leverone et al., 2007; Harke et al., 2011) they seem to display greater resistance to the effects of M. polykrikoides (Griffith et al., 2019c). Historically, hard clam populations have experienced mass die offs in response to some HABs, particularly those caused by Aureococcus anophagefferens (Gobler et al., 2005). In scenarios where hard clam populations have been restored to high densities or experimentally enhanced, this bivalve has been shown to clear the harmful brown tide alga at densities<105 cells mL-1 (Bricelj et al., 2001; Cerrato et al., 2004; Harke et al., 2011; Gobler et al., 2022).
While M. polykrikoides is a well-known toxic alga, there is variation in toxicity between strains of different origins (Kim et al., 2002; Tang and Gobler, 2009a; Wang et al., 2020; Yang et al., 2022), temperatures (Griffith and Gobler, 2016), and cellular growth stage (Tang and Gobler, 2009a; Griffith and Gobler, 2016). During this study, clams successfully cleared multiple strains of M. polykrikoides although the rates at which each strain was cleared varied, in some cases significantly. Clams cleared the clonal strain CPSB-1G faster than the CP1 strain and wild populations when exposed to each as monocultures. While in mixed assemblages with the non-harmful alga, R. salina, clearance of this non-harmful alga was faster than the clearance of CPSB-1G. Several possible scenarios regarding strain toxicity may be responsible for variation in clearance of M. polykrikoides strains and R. salina. The strain CPSB-1G has been shown to be similarly ichthyotoxic as strain CP1 (Yang et al., 2022). However, during this study, clams cleared CPSB-1G significantly faster than CP1, suggesting that clam clearance is not affected in the same manner as fish survival perhaps because the potentially toxic effects of this alga only manifest once a cell is physically in contact with the clam digestive tract (Rosa et al., 2018). We further note that there was large variance in the clearance rates of clams feeding on wild populations (e.g. Figure 6) and that this variation could have been driven by differing toxicity of bloom water collected from different sites on different dates (Table 1). It has been established that particle selection by bivalves is partly mediated by cell-surface lectins which differ across differing algal species and strains (Espinosa et al., 2009; Espinosa et al., 2010). While this was not addressed in this study, it is possible that the noxious nature of M. polykrikoides and its production of ROS result in the post-consumption rejection of cells as pseudo-feces (Ward et al., 1998; Ward and Shumway, 2004; Mafra et al., 2009) with the subsequent fate of the algal cells (reemergence, cysts formation, death) unknown.
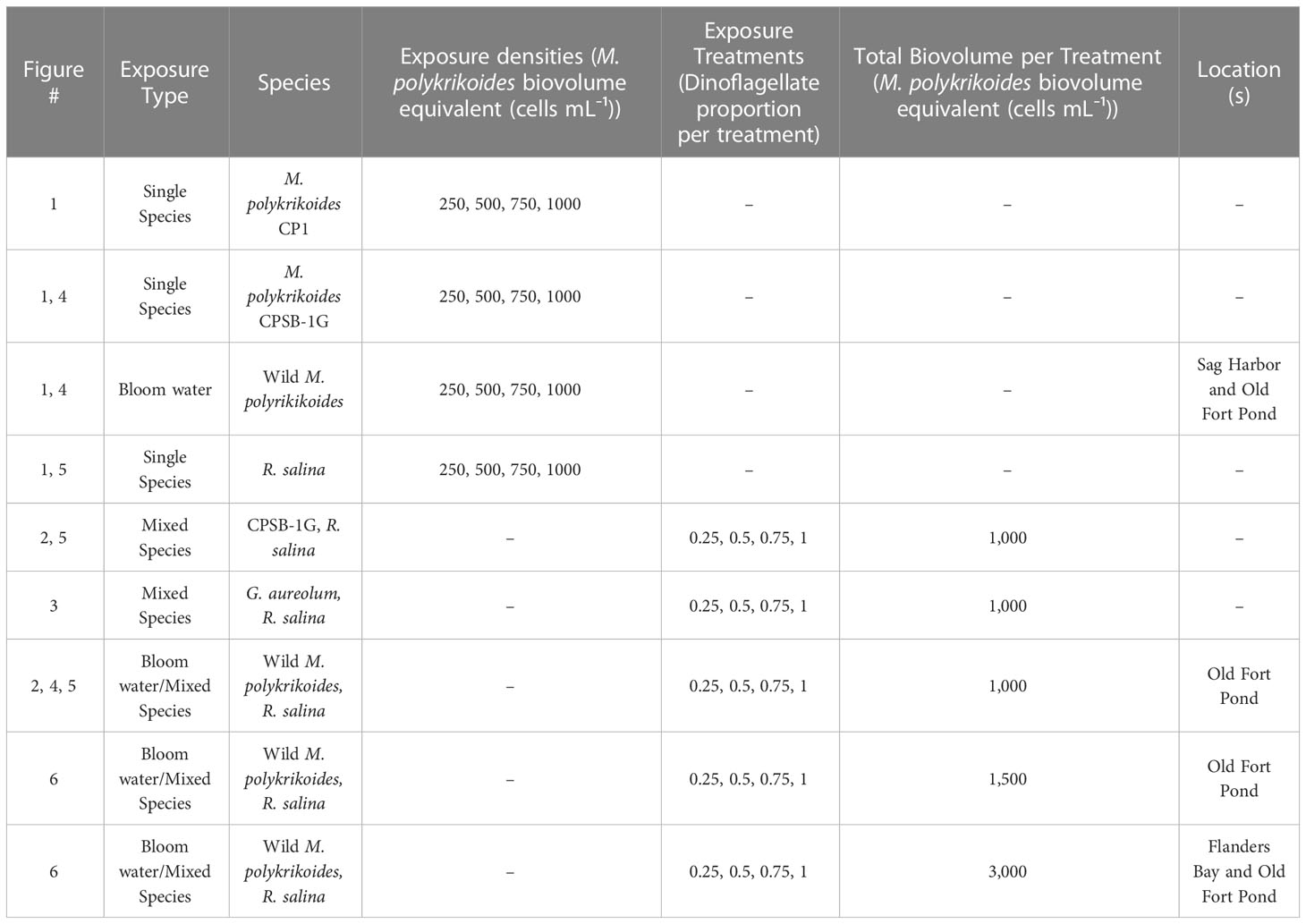
Table 1 Experimental design and details of algae used for clearance rate experiments using juvenile hard clams (M. mercenaria).
Beyond variation in toxicity due to genetic origin and biogeochemical parameters, the toxicity of clonal isolates of harmful algae can change over time (Villac et al., 1993; Bates, 1998; Bricelj et al., 2001; Furey et al., 2007). Although clonal strains are typically exposed to uniform conditions, toxin and or harmful compound concentrations can vary over time in culture as has been seen, for example, in the diatom Pseudo-nitzschia for which genetic changes, changes in the bacterial composition, and/or a lack of sexual reproduction (Villac et al., 1993; Bates, 1998) can cause a decrease in cellular toxin levels in as little as one year in culture (Villac et al., 1993). Aureococcus and Alexandrium are HABs that have also displayed decreases in toxicity in culture over time (Bricelj et al., 2004; Juhl et al., 2008; Harke et al., 2011). In contrast, regarding Karenia brevis, the “Wilson clone” has significantly higher brevetoxin production after six decades in of culture when compared to other more recently isolated strains (Lekan and Tomas, 2010). These toxicity changes in culture are consistent with the hypothesis that CPSB-1G and CP1 clones of M. polykrikoides may produce ROS at different rates and at rates that differ from prior studies (e.g. Tang and Gobler, 2009a; Yang et al., 2022)) and, therefore, were cleared at different rates by M. mercenaria (this study). This would be consistent with the more rapid clearance of strain CPSB-1G compared to wild populations and strain CP1, suggesting that overtime CPSB-1G had become less noxious compared to populations in the wild.
There were clear signs during this study that M. polykrikoides altered the feeding of M. mercenaria. While hard clam clearance of R. salina was not significantly different than CPSB-1G during single species exposure, clams cleared the cryptophyte significantly faster than M. polykrikoides during mixed algal treatments. This difference highlights the ability of hard clams to selectively clear non-harmful, beneficial algal species (Ward et al., 1998; Ward and Shumway, 2004; Mafra et al., 2009). In comparison, when the similar sized, nontoxic dinoflagellate G. aureolum was present with R. salina, hard clam clearance rates did not differ between the two species, emphasizing the noxious nature of M. polykrikoides relative to this non-harmful dinoflagellate. Similarly, the clearance of R. salina by the clams was significantly slower when mixed with M. polykrikoides bloom water compared to when the HAB was not present. The reduction in clearance rates of the cryptophyte may be due to higher exposure levels of ROS, extracellular polysaccharides, and other cytotoxic compounds mentioned previously (Kim et al., 2002; Hegaret, 2008; Yang et al., 2022). Finally, hard clams did not clear M. polykrikoides bloom water during single species exposures but when mixed with R. salina, they did successfully clear M. polykrikoides, highlighting possible facilitation of M. polykrikoides clearance by the nonharmful alga.
Allelopathy is the inhibition of one photosynthetic organism by another, and M. polykrikoides has been shown to be a strongly allelopathic HAB, likely due to the production of ROS (Tang and Gobler, 2010; Yang et al., 2022). Unlike toxicity amongst HABs that produce saxitoxin (Bricelj et al., 2005) and domoic acid (Bates, 1998), ROS are produced and exuded by actively growing M. polykrikoides cells and can have inhibitory effects on target algae in minutes-to-hours (Tang and Gobler, 2010). Prior studies on the allelopathic effects of M. polykrikoides have shown that R. salina is more resistant than other phytoplankton species but can still experience significant mortality after 24 h exposure to high densities of M. polykrikoides (2,400 cells mL-1; Tang and Gobler (2010)). During the present study, R. salina cells were alive and swimming in co-cultures with M. polykrikoides over the course of the 1-hour clearance rate experiments although signs of deformation and aggregation of some cells were observed. While such morphological changes may have altered clearance rates in mixed cultures, more work is needed to understand the quantitative impact of such changes. Culture experiments with ROS scavenging enzymes could provide additional insight on hard clam clearance in the presence of multiple algal species. In addition, quantification and examination of pseudofeces could indicate the extent to which hard clams ingested M. polykrikoides cells, and expelled live cells, expelled dead cells, or induced cyst formation.
A higher seston load can induce a variety of food selection responses in bivalves (Bricelj and Malouf, 1984). Some species adjust to larger particle loads by producing pseudo-feces while others, such as hard clams, control their ingestion by reducing clearance rates (Bricelj and Malouf, 1984; Shumway et al., 1985). During this study, higher biovolume experiments were completed using M. polykrikoides bloom water and R. salina to identify a possible threshold for juvenile hard clam clearance. There was no noticeable difference with regard to whether clams would open or close between total biovolume, species, or proportion of M. polykrikoides. In contrast, clams cleared M. polykrikoides at significantly lower rates during the higher biovolume treatments of 1,500 and 3,000 M. polykrikoides cells mL-1 compared to 1,000 M. polykrikoides cells mL-1, a finding consistent with prior studies showing that M. mercenaria reduces its feeding rate under higher particles loads (Bricelj and Malouf, 1984; Shumway et al., 1985). Additionally, clams still cleared R. salina during the 1,500 M. polykrikoides cells mL-1 biovolume experiments while not clearing M. polykrikoides highlighting possible selective clearance of the nonharmful algal as seen during the mixed culture experiments between CPSB-1G and R. salina. This finding also emphasizes that the impacts on clearance rates at high biovolume levels may be more a function of the toxicity of M. polykrikoides and/or total biovolume rather than total particle load given that the R. salina biovolume equivalent treatments had significantly higher cell densities. While M. mercenaria actively clears M. polykrikoides at densities of<1,000 cells mL-1, these findings suggest as blooms intensify, the filtration provided by this bivalve will subside.
Implications for shellfish aquaculture and restoration
Bivalve fisheries represent a multi-billion-dollar industry in the US but hard clam populations in the northeast US, including NY, have suffered significant reductions partly due to HABs (Cerrato et al., 2004; Gobler et al., 2005). In turn, these declines may permit for the intensification of some HABs (Cerrato et al., 2004; Gobler and Sunda, 2012). Although clearance rates of M. mercenaria exposed to bloom water were significantly lower compared to exposure to one of the clonal cultures, hard clams cleared M. polykrikoides from bloom water when mixed with the nonharmful alga R. salina. M. polykrikoides bloom densities can vary from 102 mL-1 to >104 mL-1 within dense bloom patches (Gobler et al., 2008; Kudela and Gobler, 2012). Such dense blooms are dynamic, migrating vertically daily and affecting surface and benthic fauna including bivalve shellfish (Park et al., 2001; Kim et al., 2010; Griffith and Gobler, 2016). Given this study explored the impacts of up to 3,000 cells mL-1 and found a cessation of feeding at ≥ 1,500 cells mL-1, it is likely extreme bloom densities would have been similarly impactful on M. mercenaria. Regardless, although M. polykrikoides is strongly toxic to many organisms including bivalves (Kudela and Gobler, 2012), hard clams are more resistant than other bivalve shellfish when exposed to blooms (Griffith et al., 2019c). Results herein demonstrate that at moderate M. polykrikoides densities (≤ 1,000 mL-1), hard clams can clear this HAB from the water column. Given that adult hard clams are generally more resilient to environmental stressors than juvenile stages (Kraeuter and Castagna, 2001), we hypothesize that clearance rates of adult hard clams would be less inhibited than the juveniles studied here.
Restoration is a major part of estuarine research and coastal environmental protection across the globe (Duarte et al., 2020). One avenue of such efforts includes shellfisheries restoration such as hard clam spawner sanctuaries aiming to restore hard clam populations in regions where they may be recruitment limited (Doall et al., 2008; Gobler et al., 2022). Along the US East Coast, hard clams can represent a significant proportion of the benthic community and, in some cases, may help mitigate HABs (Cerrato et al., 2004). For example, M. polykrikoides blooms occurred regularly in Shinnecock Bay, NY, USA, from 2006 to 2017 (Gobler et al., 2008; Hattenrath et al., 2016). An active restoration of the M. mercenaria population in this estuary increased densities of these bivalves by more than 17-fold and reduced the turnover time due to hard clam filtration of the western half of Shinnecock Bay from >21 days to< 10 days from 2012 to 2017 (Gobler et al., 2022). Consequently, there has not been a bloom of M. polykrikoides in western Shinnecock Bay since 2017.
Conclusions
While many bivalves can be inhibited by HABs, this study highlights the resilience of M. mercenaria exposed to moderately dense blooms (≤ 1,000 cells mL-1) of M. polykrikoides. Hard clams were capable of effectively clearing both M. polykrikoides bloom water and cultured clonal isolates when in mixed assemblages. While exposures to M. polykrikoides bloom water reduced the clearance of nonharmful algae and, the clearance rates of wild populations and some M. polykrikoides clones were lower than rates for nonharmful algae, clearance of this HAB persisted across densities below 1,500 cells mL-1 and across all ratios of HAB-to-non-HAB algae. Clearance rates differed between strains of M. polykrikoides in a manner that differed from their known ichthyotoxcity, highlighting the need for further studies to better understand factors that control bivalve clearance of this HAB. M. mercenaria has been shown to be more resistant to M. polykrikoides than other bivalves (Griffith et al., 2019c) and this study provides a mechanistic reason for this: the ability to clear this HAB, even at bloom densities. While the physiological means by which hard clams continue to clear when exposed to this ROS-producing alga are unknown, this study demonstrates that M. mercenaria may be an ideal bivalve to consider for restoration and aquaculture in regions prone to recurrent HABs caused by M. polykrikoides.
Data availability statement
The original contributions presented in the study are included in the article/supplementary material. Further inquiries can be directed to the corresponding author.
Ethics statement
The manuscript presents research on animals that do not require ethical approval for their study.
Author contributions
DD and CG contributed to conception and design of the study. DD performed experiments, organized the database., performed the statistical analysis and wrote the first draft of the manuscript. CG funded the study wrote sections of the manuscript. Both authors contributed to manuscript revision, read, and approved the submitted version. All authors contributed to the article and approved the submitted version.
Funding
This project was support by New York Sea Grant (award #R-FMB-38), the Laurie Landaeu Foundation, and the Chicago Community Trust. The authors acknowledge the support of Flynn DeLaney for statistical analyses.
Conflict of interest
The authors declare that the research was conducted in the absence of any commercial or financial relationships that could be construed as a potential conflict of interest.
Publisher’s note
All claims expressed in this article are solely those of the authors and do not necessarily represent those of their affiliated organizations, or those of the publisher, the editors and the reviewers. Any product that may be evaluated in this article, or claim that may be made by its manufacturer, is not guaranteed or endorsed by the publisher.
References
Abeels H. A., Loh A. N., Volety A. K. (2012). Trophic transfer and habitat use of oyster Crassostrea virginica reefs in southwest Florida, identified by stable isotope analysis. Mar. Ecol. Prog. Ser. 462, 125–142. doi: 10.3354/meps09824
Anderson D. M., Glibert P. M., Burkholder J. M. (2002). Harmful algal blooms and eutrophication: nutrient sources, composition, and consequences. Estuaries 25, 704–726. doi: 10.1007/BF02804901
Baker S. M. (1998). Selective feeding and biodeposition by zebra mussels and their relation to changes in phytoplankton composition and seston load. J. Shellfish Res. 17, 1207–1213.
Bates S. S. (1998). “Ecophysiology and metabolism of ASP toxin production,” in Physiological ecology of harmful algal blooms, (Heidelberg: Springer-Verlag) 405–426.
Bauman A. G., Burt J. A., Feary D. A., Marquis E., Usseglio P. (2010). Tropical harmful algal blooms: an emerging threat to coral reef communities? Mar. Pollut. Bull. 60, 2117–2122. doi: 10.1016/j.marpolbul.2010.08.015
Bricelj V. M., Connell L., Konoki K., MacQuarrie S. P., Scheuer T., Catterall W. A., et al. (2005). Sodium channel mutation leading to saxitoxin resistance in clams increases risk of PSP. Nature 434, 763–767. doi: 10.1038/nature03415
Bricelj V. M., Kuenstner S. H. (1989). “Effects of the “Brown tide” on the feeding physiology and growth of bay scallops and mussels,” in Novel phytoplankton blooms (Berlin, Heidelberg: Springer Berlin Heidelberg), 491–509.
Bricelj V. M., MacQuarrie S., Schaffner R. (2001). Differential effects of Aureococcus anophagefferens isolates (“brown tide”) in unialgal and mixed suspensions on bivalve feeding. Mar. Biol. 139, 605–616. doi: 10.1007/s002270100612
Bricelj V. M., MacQuarrie S. P., Smolowitz R. (2004). Concentration-dependent effects of toxic and non-toxic isolates of the brown tide alga Aureococcus anophagefferens on growth of juvenile bivalves. Mar. Ecol. Prog. Ser. 282, 101–114. doi: 10.3354/meps282101
Bricelj V. M., Malouf R. E. (1984). Influence of algal and suspended sediment concentrations on the feeding physiology of the hard clam Mercenaria mercenaria. Mar. Biol. 84, 155–165. doi: 10.1007/BF00393000
Cerrato R. M., Caron D. A., Lonsdale D. J., Rose J. M., Schaffner R. A. (2004). Effect of the northern quahog Mercenaria mercenaria on the development of blooms of the brown tide alga Aureococcus anophagefferens. Mar. Ecol. Prog. Ser. 281, 93–108. doi: 10.3354/meps281093
Coen L. D., Brumbaugh R. D., Bushek D., Grizzle R., Luckenbach M. W., Posey M. H., et al. (2007). Ecosystem services related to oyster restoration. Mar. Ecol. Prog. Ser. 341, 303–307. doi: 10.3354/meps341303
Coughlan J. (1969). The estimation of filtering rate from the clearance of suspensions. Mar. Biol. 2, 356–358. doi: 10.1007/BF00355716
Cullen J. J. (1985). Diel vertical migration by dinoflagellates: roles of carbohydrate metabolism and behavioral flexibility, Migration: Mechanisms and Adaptive Significance. Contr. Mar. Sci. 27, 135–152.
Cunningham K. A., Capone D. G. (1992). “Superoxide dismutase as a protective enzyme against oxygen toxicity: an overview and initial studies in Trichodesmium.,” in Marine pelagic cyanobacteria: Trichodesmium and other diazotrophs. Eds. Carpenter E. J., Capone D. G., Rueter J. G. (Dordrecht: Springer Netherlands), 331–341.
Curtin T. P., Volkenborn N., Dwyer I. P., Aller R. M., Zhu Q., Gobler C. J. (2022). Buffering muds with bivalve shell significantly increases the settlement, growth, survival, and burrowing of the early life stages of the Northern quahog, Mercenaria mercenaria, and other calcifying invertebrates. Estuar. Coast. Shelf Sci. 264, 107686. doi: 10.1016/j.ecss.2021.107686
Curtiss M. M., Langlois G. W., Busse L. B., Mazzillo F., Silver M. W. (2008). The emergence of Cochlodinium along the California Coast (USA). Harmful Algae 7, 337–346. doi: 10.1016/j.hal.2007.12.012
Doall M. H., Padilla D. K., Lobue C., Clapp C., Webb A. R., Hornstein J. (2008). Evaluating northern quahog (= hard clam, Mercenaria mercenaria L.) restoration: are transplanted clams spawning and reconditioning. J. Shellfish Res. 27, 1069–1080. doi: 10.2983/0730-8000-27.5.1069
Doblin M. A., Blackburn S. I., Hallegraeff G. M. (1999). Growth and biomass stimulation of the toxic dinoflagellate Gymnodinium catenatum (Graham) by dissolved organic substances. J. Exp. Mar. Biol. Ecol. 236, 33–47. doi: 10.1016/S0022-0981(98)00193-2
Dorantes-Aranda J. J., García-de laParra L. M., Alonso-Rodríguez R., Morquecho L. (2009). Hemolytic activity and fatty acids composition in the ichthyotoxic dinoflagellate Cochlodinium polykrikoides isolated from Bahía de la Paz, Gulf of California. Mar. pollut. Bull. 58, 1401–1405. doi: 10.1016/j.marpolbul.2009.06.007
Duarte C. M., Agusti S. E., Barbier S. E., Britten G. L., Castilla J. M., Gattuso J.-P. (2020). Rebuilding marine life. Nature 580, 39–51. doi: 10.1038/s41586-020-2146-7
Dunphy B. J., Hall J. A., Jeffs A. G., Wells R. M. G. (2006). Selective particle feeding by the Chilean oyster, Ostrea Chilensis; implications for nursery culture and broodstock conditioning. Aquaculture 261, 594–602. doi: 10.1016/j.aquaculture.2006.08.015
Efron B. (1992). “Bootstrap methods: another look at the jackknife,” in Breakthroughs in statistics: methodology and distribution. Eds. Kotz S., Johnson N. L. (New York, NY: Springer New York), 569–593.
Ekstrom J. A., Suatoni L., Cooley S. R., Pendleton L. H., Waldbusser G. G., Cinner J. E., et al. (2015). Vulnerability and adaptation of US shellfisheries to ocean acidification. Nat. Climate Change 5, 207–214. doi: 10.1038/nclimate2508
Espinosa E. P., Perrigault M., Ward J. E., Shumway S. E., Allam B. (2009). Lectins associated with the feeding organs of the oyster Crassostrea virginica can mediate particle selection. Biol. Bull. 217, 130–141. doi: 10.1086/BBLv217n2p130
Espinosa E. P., Perrigault M., Ward J. E., Shumway S. E., Allam B. (2010). Microalgal cell surface carbohydrates as recognition sites for particle sorting in suspension-feeding bivalves. Biol. Bull. 218, 75–86. doi: 10.1086/BBLv218n1p75
Fraga S. (1989). “Chainforming dinoflagellates: an adaptation to red tides,” in Red tides: biology, environmental science and toxicology, (Amsterdam, Netherlands: Elsevier) 281–284.
Furey A., García J., O’Callaghan K., Lehane M., Amandi M. F., James K. J. (2007). “Brevetoxins: structure, toxicology, and origin,” in Phycotoxins: chemistry and biochemistry., (Malden, Massachusetts, United States: Blackwell Publishing) 19–46.
Gobler C. J., Berry D. L., Anderson O. R., Burson A., Koch F., Rodgers B. S., et al. (2008). Characterization, dynamics, and ecological impacts of harmful Cochlodinium polykrikoides blooms on eastern Long Island, NY, USA. Harmful Algae 7, 293–307. doi: 10.1016/j.hal.2007.12.006
Gobler C. J., Doall M. H., Peterson B. J., Young C., DeLaney F., Wallace R. B. (2022). Rebuilding A collapsed bivalve population, restoring seagrass meadows, and eradicating harmful algal blooms in A temperate lagoon using spawner sanctuaries. Front. Mar. Sci. 9, 1258. doi: 10.3389/fmars.2022.911731
Gobler C. J., Doherty O. M., Hattenrath-Lehmann T. K., Griffith A. W., Kang Y., Litaker. R. W. (2017). Ocean warming since 1982 has expanded the niche of toxic algal blooms in the North Atlantic and North Pacific oceans. Proceed. Nat. Acad. Sci. 114, 4975–4980. doi: 10.1073/pnas.1619575114
Gobler C. J., Lonsdale D. J., Boyer G. L. (2005). A review of the causes, effects, and potential management of harmful brown tide blooms caused by Aureococcus anophagefferens (Hargraves et sieburth). Estuaries 28, 726–749. doi: 10.1007/BF02732911
Gobler C. J., Sunda W. G. (2012). Ecosystem disruptive algal blooms of the brown tide species, Aureococcus anophagefferens and Aureoumbra lagunensis. Harmful Algae 14, 36–45. doi: 10.1016/j.hal.2011.10.013
Griffith A. W., Doherty O. M., Gobler C. J. (2019a). Ocean warming along temperate western boundaries of the Northern Hemisphere promotes an expansion of Cochlodinium polykrikoides blooms. Proceed. R. Soc. B. 286, 20190340. doi: 10.1098/rspb.2019.0340
Griffith A. W., Gobler C. J. (2016). Temperature controls the toxicity of the ichthyotoxic dinoflagellate Cochlodinium polykrikoides. Mar. Ecol. Prog. Ser. 545, 63–76. doi: 10.3354/meps11590
Griffith A. W., Gobler C. J. (2020). Harmful algal blooms: A climate change co-stressor in marine and freshwater ecosystems. Harmful Algae 91, 101590. doi: 10.1016/j.hal.2019.03.008
Griffith A. W., Harke M. J., DePasquale E., Berry D. L., Gobler C. J. (2019b). The harmful algae, Cochlodinium polykrikoides and Aureococcus anophagefferens, elicit stronger transcriptomic and mortality response in larval bivalves (Argopecten irradians) than climate change stressors. Ecol. Evol. 9, 4931–4948. doi: 10.1002/ece3.5100
Griffith A. W., Shumway S. E., Gobler C. J. (2019c). Differential mortality of north atlantic bivalve molluscs during harmful algal blooms caused by the dinoflagellate, cochlodinium (a.k.a. Margalefidinium) polykrikoides. Estuaries Coasts 42, 190–203. doi: 10.1007/s12237-018-0445-0
Guzmán H. M., Cortés J., Glynn P. W., Richmond R. H. (1990). Coral mortality associated with dinoflagellate blooms in the eastern Pacific (Costa Rica and Panama). Mar. Ecol. Progr. Ser. 60, 299–303. doi: 10.3354/meps060299
Haigh N., Esenkulova S. (2014). Economic losses to the British Columbia salmon aquaculture industry due to harmful algal blooms 2009-2012. PICES Scientific Report. (British Columbia: PICES) 2.
Hallegraeff G., Dorantes Aranda J. J., Mardones J., Seger A. (2017). “Review of progress in our understanding of fish-killing microalgae: implications for management and mitigation,” in Proceedings of the 17th international conference on harmful algae 2016. International Society for the Study of Harmful Algae. (Paris: Intergovernmental Oceanographic Commission of UNESCO) 148–153.
Harke M. J., Gobler C. J., Shumway S. E. (2011). Suspension feeding by the Atlantic slipper limpet (Crepidula fornicata) and the northern quahog (Mercenaria mercenaria) in the presence of cultured and wild populations of the harmful brown tide alga, Aureococcus anophagefferens. Harmful Algae 10, 503–511. doi: 10.1016/j.hal.2011.03.005
Hattenrath-Lehmann T. K., Zhen Y., Wallace R. B., Tang Y. Z., Gobler C. J. (2016). Mapping the distribution of cysts from the toxic dinoflagellate Cochlodinium polykrikoides in bloom-prone estuaries by a novel fluorescence in situ hybridization assay. Applied and Environmental Microbiology 82 (4), 1114–1125.
Hegaret H. (2008). “Impacts of harmful algal blooms on physiological and cellular processes of bivalve molluscs,” (Storrs, Connecticut: University of Connecticut. PhD Dissertation).
Hegaret H., Wikfors G. H., Shumway S. E. (2007). Diverse feeding responses of five species of bivalve mollusc when exposed to three species of harmful algae. J. Shellfish Res. 26, 549–559. 511. doi: 10.2983/0730-8000(2007)26[549:DFROFS]2.0.CO;2
Heisler J., Glibert P. M., Burkholder J. M., Anderson D. M., Cochlan W., Dennison W. M., et al. (2008). Eutrophication and harmful algal blooms: A scientific consensus. Harmful Algae 8, 3–13. doi: 10.1016/j.hal.2008.08.006
Helm M. M., Bourne N., Lovatelli A. (2004). Hatchery culture of bivalves: a practical manual. (Rome, Italy: Food and Agriculture Organization).
Janeway M. (1994). “The role of microbial pattern recognition in self: nonself discrimination in innate and adaptive immunity,” in Phylogenetic perspectives in immunity, the insect host defence, (Boca Raton, FL: CRC Press) 15–122.
Jeong H. J., Song J. Y., Lee M. H., Kim S. T. (2004). Feeding by larvae of the mussel Mytilus galloprovincialis on red-tide dinoflagellates. J. Shellfish Res. 23, 185–196.
Jiang X., Lonsdale D. J., Gobler C. J. (2009). Deleterious consequences of a red tide dinoflagellate Cochlodinium polykrikoides for the calanoid copepod Acartia tonsa. Mar. Ecol. Prog. Ser. 390, 105–116. doi: 10.3354/meps08159
Jiang X., Lonsdale D. J., Gobler C. J. (2010). Grazers and vitamins shape chain formation in a bloom-forming dinoflagellate, Cochlodinium polykrikoides. Oecologia 164, 455–464. doi: 10.1007/s00442-010-1695-0
Jiang X., Lonsdale D. J., Gobler C. J. (2011). Rapid gain and loss of evolutionary resistance to the harmful dinoflagellate Cochlodinium polykrikoides in the copepod Acartia tonsa. Limnol. Oceanogr. 56, 947–954. doi: 10.4319/lo.2011.56.3.0947
Juhl A. R., Martins M. A., Anderson D. M. (2008). Toxicity of Alexandrium lusitanicum to gastropod larvae is not caused by paralytic-shellfish-poisoning toxins. Harmful Algae 7, 567–573. doi: 10.1016/j.hal.2007.12.019
Kim Y. S., Jeong M. S., Seong G. T., Han I. S., Lee Y. S. (2010). Diurnal vertical migration of Cochlodinium polykrikoides during the red tide in Korean coastal sea waters. J. Environ. Biol. 31, 687.
Kim M. S., Lee S. G., Kim H. G. (2000). Biochemical responses of fish exposed to a harmful dinoflagellate Cochlodinium polykrikoides. J. Exp. Mar. Biol. Ecol. 254, 131–141. doi: 10.1016/S0022-0981(00)00263-X
Kim M. S., Lee S. G., Lee M. K., Kim H. G., Jung J. (1999). Reactive oxygen species as causative agents in the ichthyotoxicity of the red tide dinoflagellate Cochlodinium polykrikoides. J. Plankton Res. 21, 2105–2115. doi: 10.1093/plankt/21.11.2105
Kim D., Oda T., Muramatsu T., Kim D., Matsuyama Y., Honjo T. (2002). Possible factors responsible for the toxicity of Cochlodinium polykrikoides, a red tide phytoplankton. Comp. Biochem. Physiol. C: Toxicol. Pharmacol. 132, 415–423. doi: 10.1016/S1532-0456(02)00093-5N/A
Kim D., Yamasaki Y., Yamatogi T., Yamaguchi K., Matsuyama Y., Kang Y.-S., et al. (2009). The possibility of reactive oxygen species (ROS)-independent toxic effects of Cochlodinium polykrikoides on damselfish (Chromis caerulea). Biosci. Biotechnol. Biochem. 73, 613–618. doi: 10.1271/bbb.80693
Koch F., Burson A., Tang Y. Z., Collier J. L., Fisher N. S., Sanudo-Wilhelmy S., et al. (2014). Alteration of plankton communities and biogeochemical cycles by harmful Cochlodinium polykrikoides (Dinophyceae) blooms. Harmful Algae 33, 41–54. doi: 10.1016/j.hal.2014.01.003
Kudela R. M., Gobler C. J. (2012). Harmful dinoflagellate blooms caused by Cochlodinium sp.: Global expansion and ecological strategies facilitating bloom formation. Harmful Algae 14, 71–86. doi: 10.1016/j.hal.2011.10.015
Lekan D. K., Tomas C. R. (2010). The brevetoxin and brevenal composition of three Karenia brevis clones at different salinities and nutrient conditions. Harmful Algae 9, 39–47. doi: 10.1016/j.hal.2009.07.004
Lellis-Dibble K. A., McGlynn K. E., Bigford T. E. (2008). Estuarine fish and shellfish species in U.S. commercial and recreational fisheries : economic value as an incentive to protect and restore estuarine habitat. United States: National Marine Fisheries Service., Office of Habitat Protection. Available at: https://repository.library.noaa.gov/view/noaa/3612.
Leverone J. R., Shumway S. E., Blake N. J. (2007). Comparative effects of the toxic dinoflagellate Karenia brevis on clearance rates in juveniles of four bivalve molluscs from Florida, USA. Toxicon 49, 634–645. doi: 10.1016/j.toxicon.2006.11.003
Levin L. A., Ekau W., Gooday A. J., Jorissen F., Middelburg J. J., Naqvi S. W. A., et al. (2009). Effects of natural and human-induced hypoxia on coastal benthos. Biogeosci. 6, 2063–2098. doi: 10.5194/bg-6-2063-2009
Levinton J., Ward J. E., Shumway S. E. (2002). Feeding responses of the bivalves Crassostrea gigas and Mytilus trossulus to chemical composition of fresh and aged kelp detritus. Mar. Biol. 141, 367–376. doi: 10.1007/s00227-002-0830-9
Li Y., Meseck S. L., Dixon M. S., Rivara K., Wikfors G. H. (2012). Temporal variability in phytoplankton removal by a commercial, suspended eastern oyster nursery and effects on local plankton dynamics. J. Shellfish Res. 31, 1077–1089. doi: 10.2983/035.031.0419
Mafra L. L., Bricelj V. M., Ouellette M., Léger M., Bates S. S. (2009). Mechanisms contributing to low domoic acid uptake by oysters feeding on Pseudo-nitzschia cells. I. Filtration and pseudofeces production. Aquat. Biol. 6, 201–212. doi: 10.3354/ab00121
Newell R. I. E. (2004). Ecosystem influences of natural and cultivated populations of suspension-feeding bivalve molluscs: a review. J. Shellfish Res. 23, 51+.
Newell R. I., Jordan S. J. (1983). Preferential ingestion of organic material by the American oyster Crassostrea virginica. Mar. Ecol. Prog. Ser. 13, 47–53. doi: 10.3354/meps013047
Officer M., Smayda T. J., Mann R. (1982). Benthic filter feeding: a natural eutrophication control. Mar. Ecol. Prog. Ser. 9, 203–210. doi: 10.3354/meps009203
Okamoto O. K., Colepicolo P. (1998). Response of superoxide dismutase to pollutant metal stress in the marine dinoflagellate gonyaulax polyedra. Comp. Biochem. Physiol. C: Pharmacol. Toxicol. Endocrinol. 119, 67–73. doi: 10.1016/S0742-8413(97)00192-8
Park J. G., Jeong M. K., Lee J. A., Cho K.-J., Kwon O. S. (2001). Diurnal vertical migration of a harmful dinoflagellate, Cochlodinium polykrikoides (Dinophyceae), during a red tide in coastal waters of Namhae Island, Korea. Phycologia 40, 292–297. doi: 10.2216/i0031-8884-40-3-292.1
Pérez-Morales A., Band-Schmidt M. J., Martínez-Díaz S. F. (2017). Mortality on zoea stage of the Pacific white shrimp Litopenaeus vannamei caused by Cochlodinium polykrikoides (Dinophyceae) and Chattonella spp. (Raphidophyceae). Mar. Biol. 164, 57. doi: 10.1007/s00227-017-3083-3
Richlen M. L., Morton S. L., JaMali E. A., Rajan A., Anderson D. M. (2010). The catastrophic 2008–2009 red tide in the Arabian gulf region, with observations on the identification and phylogeny of the fish-killing dinoflagellate Cochlodinium polykrikoides. Harmful Algae 9, 163–172. doi: 10.1016/j.hal.2009.08.013
Riisgård H. U. (1988). Efficiency of particle retention and filtration rate in 6 species of Northeast American bivalves. Mar. Ecol. Prog. Ser. 45, 217–223. doi: 10.3354/meps045217
Riisgård H. U. (2001). The stony road to reliable filtration rate measurements in bivalves: a reply. Mar. Ecol. Prog. Ser. 215, 307–310. doi: 10.3354/meps215307
Riisgård H. U., Larsen P. S. (2010). Particle capture mechanisms in suspension-feeding invertebrates. Mar. Ecol. Prog. Ser. 418, 255–293. doi: 10.3354/meps08755
Rosa M., Ward J. E., Shumway S. E. (2018). Selective capture and ingestion of particles by suspension-feeding bivalve molluscs: A review. J. Shellfish Res. 37, 727–746. doi: 10.2983/035.037.0405
Shumway S. E., Cucci T. L., Newell R. M., Yentsch M. M. (1985). Particle selection, ingestion, and absorption in filter-feeding bivalves. J. Exp. Mar. Biol. Ecol. 91, 77–92. doi: 10.1016/0022-0981(85)90222-9
Smayda T. J., Reynolds M. S. (2003). Strategies of marine dinoflagellate survival and some rules of assembly. J. Sea Res. 49, 95–106. doi: 10.1016/S1385-1101(02)00219-8
Tang Y. Z., Gobler C. J. (2009a). Characterization of the toxicity of Cochlodinium polykrikoides isolates from Northeast US estuaries to finfish and shellfish. Harmful Algae 8, 454–462. doi: 10.1016/j.hal.2008.10.001
Tang Y. Z., Gobler C. J. (2009b). Cochlodinium polykrikoides blooms and clonal isolates from the northwest Atlantic coast cause rapid mortality in larvae of multiple bivalve species. Mar. Biol. 156, 2601–2611. doi: 10.1007/s00227-009-1285-z
Tang Y. Z., Gobler C. J. (2010). Allelopathic effects of Cochlodinium polykrikoides isolates and blooms from the estuaries of Long Island, New York, on co-occurring phytoplankton. Mar. Ecol. Prog. Ser. 406, 19–31. doi: 10.3354/meps08537
Tolley S. G., Volety A. K. (2005). The role of oysters in habitat use of oyster reefs by resident fishes and decapod crustaceans. J. Shellfish Res. 24, 1007–1012. doi: 10.2983/0730-8000(2005)24[1007:TROOIH]2.0.CO;2
Villac M., Roelke D., Chavez F., Cifuentes L., Fryxell G. (1993). Pseudonitzschia australis Frenguelli and related species from the west coast of the U. S. A.: Occurrence and domoic acid production. J. Shellfish Res. 12, 457–465.
Wall C. C., Peterson B. J., Gobler M. J. (2008). Facilitation of seagrass Zostera marina productivity by suspension-feeding bivalves. Mar. Ecol. Prog. Ser. 357, 165–174. doi: 10.3354/meps07289
Wang H., Hu Z., Shang L., Leaw M. P., Lim P. T., Tang Y. Z. (2020). Toxicity comparison among four strains of Margalefidinium polykrikoides from China, Malaysia, and USA (belonging to two ribotypes) and possible implications. J. Exp. Mar. Biol. Ecol. 524, 151293. doi: 10.1016/j.jembe.2019.151293
Ward J. E., Levinton J. S., Shumway S. E., Cucci T. (1997). Site of particle selection in a bivalve mollusM. Nature 390, 131–132. doi: 10.1038/36481
Ward J. E., Levinton J. S., Shumway S. E., Cucci T. (1998). Particle sorting in bivalves: in vivo determination of the pallial organs of selection. Mar. Biol. 131, 283–292. doi: 10.1007/s002270050321
Ward J. E., Shumway S. E. (2004). Separating the grain from the chaff: particle selection in suspension-and deposit-feeding bivalves. J. Exp. Mar. Biol. Ecol. 300, 83–130. doi: 10.1016/j.jembe.2004.03.002
Yang H., Gobler C. J., Tang Y. Z. (2022). Consistency between the ichthyotoxicity and allelopathy among strains and ribotypes of Margalefidinium polykrikoides suggests that its toxins are allelochemicals. Front. Mar. Sci. 9, 941205. doi: 10.3389/fmars.2022.941205
Keywords: harmful algal blooms (HABs), Margalefidinium (Cochlodinium) polykrikoides, hard clam, Mercenaria mercenaria, bivalve, clearance rates
Citation: de Silva D and Gobler CJ (2023) Effects of the harmful alga Margalefidinium (aka Cochlodinium) polykrikoides on clearance rates of the hard clam, Mercenaria mercenaria. Front. Mar. Sci. 10:1252540. doi: 10.3389/fmars.2023.1252540
Received: 03 July 2023; Accepted: 03 August 2023;
Published: 28 August 2023.
Edited by:
Jose Luis Iriarte, Austral University of Chile, ChileReviewed by:
Gustaaf Marinus Hallegraeff, University of Tasmania, AustraliaYoonja Kang, Chonnam National University, Republic of Korea
Copyright © 2023 de Silva and Gobler. This is an open-access article distributed under the terms of the Creative Commons Attribution License (CC BY). The use, distribution or reproduction in other forums is permitted, provided the original author(s) and the copyright owner(s) are credited and that the original publication in this journal is cited, in accordance with accepted academic practice. No use, distribution or reproduction is permitted which does not comply with these terms.
*Correspondence: Christopher J. Gobler, Y2hyaXN0b3BoZXIuZ29ibGVyQHN0b255YnJvb2suZWR1