- 1Department of Habitat Ecology, Wildlife Institute of India, Dehradun, Uttarakhand, India
- 2Environment Agency, Marine Threatened Species and Habitats, Terrestrial & Marine Biodiversity, Abu Dhabi, United Arab Emirates
The Andaman and Nicobar Islands, India, a geographically remote region, harbor a diverse island ecosystem. Limited exploration has hindered our understanding of marine floral biodiversity in this area. To address this gap, we investigated seagrass meadows in the Andaman and Nicobar Islands to understand their spatial distribution, species composition, and habitat characteristics. We assessed 66 seagrass meadows, including 32 newly discovered ones, filling data gaps in the region’s seagrass coldspots. Seagrasses were found across a wide range of depths, with the majority occurring in shallow subtidal waters (< 8 m). Large-sized species such as Thalassia hemprichii, Enhalus acoroides, Cymodocea rotundata, Cymodocea serrulata, and Syringodium isoetifolium dominated the littoral and shallow subtidal zones, while smaller species such as Halophila spp. and Halodule spp. exhibited broader depth distributions. H. beccarii and H. decipiens were strictly intertidal and subtidal species, respectively. Water depth significantly influenced seagrass occurrence (p < 0.0001), cover (β = -0.2759; SE = 0.02471; p < 0.0001), shoot densities (β = -0.3556; SE = 0.1231; p = 0.005), and biomass (β = -0.3526; SE = 0.1159; p = 0.003). Sand availability emerged as the second significant predictor of seagrass distribution, cover, and biomass (p values < 2e-16, < 2e-16, and 0.01, respectively). Habitat heterogeneity decreased with increasing water depth, and seagrass species exhibited strong preferences for specific substrata, resulting in spatial niche partitioning. Our study provides novel insights into the seagrass spatial diversity, habitat characteristics, and seagrass-environment relationship in the Andaman and Nicobar Islands. Further, it highlights the importance of water depth, habitat characteristics, and substratum heterogeneity in seagrass distribution and growth. Lastly, our findings imply that any change to the benthic profile of the meadows will influence the seagrass species distribution and growth. Understanding these factors is crucial for seagrass conservation and management in the region, aiding the development of targeted strategies to protect these valuable marine habitats and associated biodiversity.
1 Introduction
Seagrass meadows are critical marine ecosystems that provide numerous ecological and economic benefits. The diversity and distribution of seagrasses are influenced by various regulatory factors, including benthic light availability, sediment profile, wave exposure, water temperature, and nutrient dynamics (Dennison and Alberte, 1987; Duarte, 1991; Malmer and Grip, 1994; Greve and Binzer, 2004). These factors play a crucial role in the growth and persistence of seagrass populations, as seagrasses rely on optimal light for photosynthesis, suitable sediments for proliferation, and nutrients for growth (Duarte, 2002). Resultant declines in seagrass populations are reported due to light reduction (Short and Wyllie-Echeverria, 1996), altered nutrient dynamics (Lee and Dunton, 2000), and wave action (Japar Sidik et al., 2018). Impacts of wave exposure are more prominently seen in intertidal zones, which are at the transition of land and sea. Only species able to cope with the heavy tidal fluctuations and associated parameters can thrive in these littoral zones, thus restricting the upslope distribution of seagrasses (Hemminga and Duarte, 2000). Variations in water temperature, whether seasonal or due to climate change (marine heat waves), are also known to influence seagrass photosynthesis and productivity (Barber and Behrens, 1985; Seddon and Cheshire, 2001; Díaz-Almela et al., 2009; Garrabou et al., 2022). Nevertheless, seagrasses exhibit wide-ranging acclimation strategies as a response to changes in their natural environment (Vermaat et al., 1998; Lee et al., 2007). An experimental study in the Philippines demonstrated that seagrass species have a varied tolerance to enhanced siltation and altered light irradiance in the water column (Bach et al., 1998; Terrados et al., 1998). Besides, the shift in turbidity levels resulted in local variation in seagrass diversity, growth, and depth limits. Accordingly, global research provides substantial evidence that natural variability in the environment influences seagrass ecosystems at local and regional scales.
Additionally, unprecedented human-induced stressors catalyze the natural factors impacting seagrasses (Orth et al., 2006). The rise in anthropogenic footprints in the seagrass meadows is majorly attributed to dredging, marina construction, destructive fishing practices, land reclamation, nutrient and sediment loading, turbidity, and habitat alteration (Short et al., 2007). As a result, subsequent seagrass declines are reported worldwide (Waycott et al., 2009; Gunderson et al., 2016). Unfortunately, the degradation and loss of seagrass habitats have significant implications for the environment and society. With global recognition as a valuable marine ecosystem, seagrass shares a multifaceted relationship with its environment. Seagrass meadows provide essential services by maintaining the health of adjacent habitats such as coral reefs and mangroves (Green and Short, 2003; Short et al., 2007). They also serve as nursery grounds for commercially important fish and invertebrates, supporting fisheries and contributing to local economies (Hemminga and Duarte, 2000; Unsworth et al., 2019; Berkström et al., 2020; United Nations Environment Programme [UNEP], 2020). Furthermore, seagrasses are crucial for the survival of megaherbivores like dugongs, as they constitute their primary food source (Short et al., 2007). Under the socioeconomic context, seagrasses provide economical services higher than terrestrial habitats (Costanza et al., 2014) and support local communities’ livelihood and well-being (Mtwana Nordlund et al., 2016; Unsworth et al., 2019). Thus, seagrass losses or deterioration significantly impact these valuable services offered (Duarte, 2002; Orth et al., 2006). For instance, Unsworth (2007) reported a reduction in fish and invertebrate stocks in the Wakatobi Marine National Park, Indonesia, in response to seagrass degradation.
Similar seagrass trajectories are predicted to accelerate, particularly in developing countries, since these regions critically lack legislative measures to protect seagrasses (Duarte, 2002). Sadly, seagrass management has received little attention in the tropics than the adjacent coral reefs (Mumby et al., 2006). Although positive human interventions have reported an optimistic reversing of seagrass losses (Elliott et al., 2007; de los Santos et al., 2019; Tan et al., 2020), such initiatives, along with in situ seagrass management are yet substantially lacking in the tropics, including Indian waters. The widely accepted global seagrass distribution model classifies India into a diverse Indo-Pacific bioregion (Short et al., 2007). Of which the ecologically rich Andaman and Nicobar Islands (ANI) form the country’s second most varied seagrass habitats (Thangaradjou and Bhatt, 2018). Seagrass meadows in ANI are crucial to supporting the dugong’s remnant insular population (D’Souza et al., 2015). A recent study highlighted the importance of resource-rich seagrass beds for aggregating dugongs, possibly for calf protection (Gole et al., 2023). Despite such crucial ecological significance of seagrass meadows, there is a lack of information on their spatiotemporal changes, threats, and ecological and economic impacts in ANI. A report by Paulose et al. (2013) outlines the large-scale seagrass denudation in ANI (~1619 ha) caused by the Sumatra-Andaman earthquake and the 2004 Indian Ocean Tsunami. The severe deposition of marine debris from this disturbance has further altered the species distribution trends (Thangaradjou et al., 2010a). In addition, ANI is also a geologically vulnerable region, with reports of ~ 486 earthquakes (Richter scale >4; United States Geological Survey, 20231) and 25 cyclonic storms/depressions (Indian Meteorological Department, 20232) that have hit the islands’ coastline in the recent times (2018 to 2022). Unfortunately, the extent of seagrass loss to these threats remains uncertain due to sparse and discontinuous spatial seagrass assessments in the Islands.
For effective seagrass management, the accuracy of spatial studies and robust ecological knowledge is of utmost importance. Detailed information on species’ checklists, distribution ranges, depth limits, and, most importantly, regulatory factors governing distribution and growth is essential for translating seagrass science to management and conservation (Unsworth, 2007; International Seagrass Biology Workshop 13, 20133; McKenzie et al., 2020). A few significant challenges to studying seagrasses in ANI are the inaccessibility of sites to researchers, funds, and logistics required to perform marine surveys. Despite this, prior studies have given valuable baselines on species distribution, natural history, descriptive habitat preferences, and spatial extent of seagrasses in ANI (Jagtap, 1991; Jagtap, 1992; Das, 1996; Thangaradjou et al., 2010a; Thangaradjou et al., 2010b; Paulose et al., 2013; D’Souza et al., 2015; Ragavan et al., 2016; Savurirajan et al., 2018). However, a data gap is still reflected in many of the management crucial aspects of the region. Published literature largely lacks precise spatial information (locality) of meadows, hindering spatiotemporal comparisons like other global initiatives (Sudo et al., 2021). Despite three decades of seagrass exploration and research, only limited studies have a quantified seagrass-environment relationship (Savurirajan et al., 2018). Likewise, recent reports of new species’ local distribution and range (Savurirajan et al., 2015; Gole et al., 2022) indicate more seagrass exploration to upgrade species checklist and distribution trends. As most of the seagrass meadows in ANI are recovering from the impact of the 2004 tsunami, it is essential to update existing information on the species composition, distribution, and factors that determine the existing trends. To address these knowledge gaps, this study aimed to investigate the spatial diversity and distribution patterns of seagrass meadows along the accessible coastline of ANI. Contrary to prior spatial assessments, our work instead focused on studying the complex interactions of seagrass species with their natural environment. Thus, we also aimed to understand the environmental regulatory factors that influence seagrass occurrence and distribution locally. By conducting a comprehensive assessment of seagrass ecology, this study will complement the existing knowledge on seagrasses and collectively contribute to developing effective management and conservation strategies for seagrass habitats in ANI.
2 Materials and methods
2.1 Study area and site selection
India’s Andaman and Nicobar Islands support a tropical insular ecosystem as part of two global biodiversity hotspots (Myers et al., 2000). The ANI has a long and sheltered coastline spanning 1962 km (Andaman and Nicobar Administration, 20234), providing favorable conditions for the growth and diversification of shallow coastal habitats such as coral reefs, mangroves, and seagrasses (Tigga and Rao, 2004). We explored the coastal waters of ANI, ranging from a depth of 0.2 m (intertidal) to 37 m (deep waters), for seagrass presence between 2018 to 2022 (January to April). We identified seagrass meadows using three approaches: a) tapping local knowledge of fishers through community interactions, b) published literature and correspondences with prior seagrass researchers, and c) exploratory free dives or swims in potential sandy habitats. Based on the geographical proximity of the investigated regions, assuming these sites will have a shared environment for seagrasses, we broadly divided ANI into five island groups: 1) North and Middle Andaman (NMA), 2) South Andaman (SA), 3) Ritchie’s archipelago (RA), 4) Little Andaman (LA), and 5) Nicobar Islands (NIC) (Figure 1).
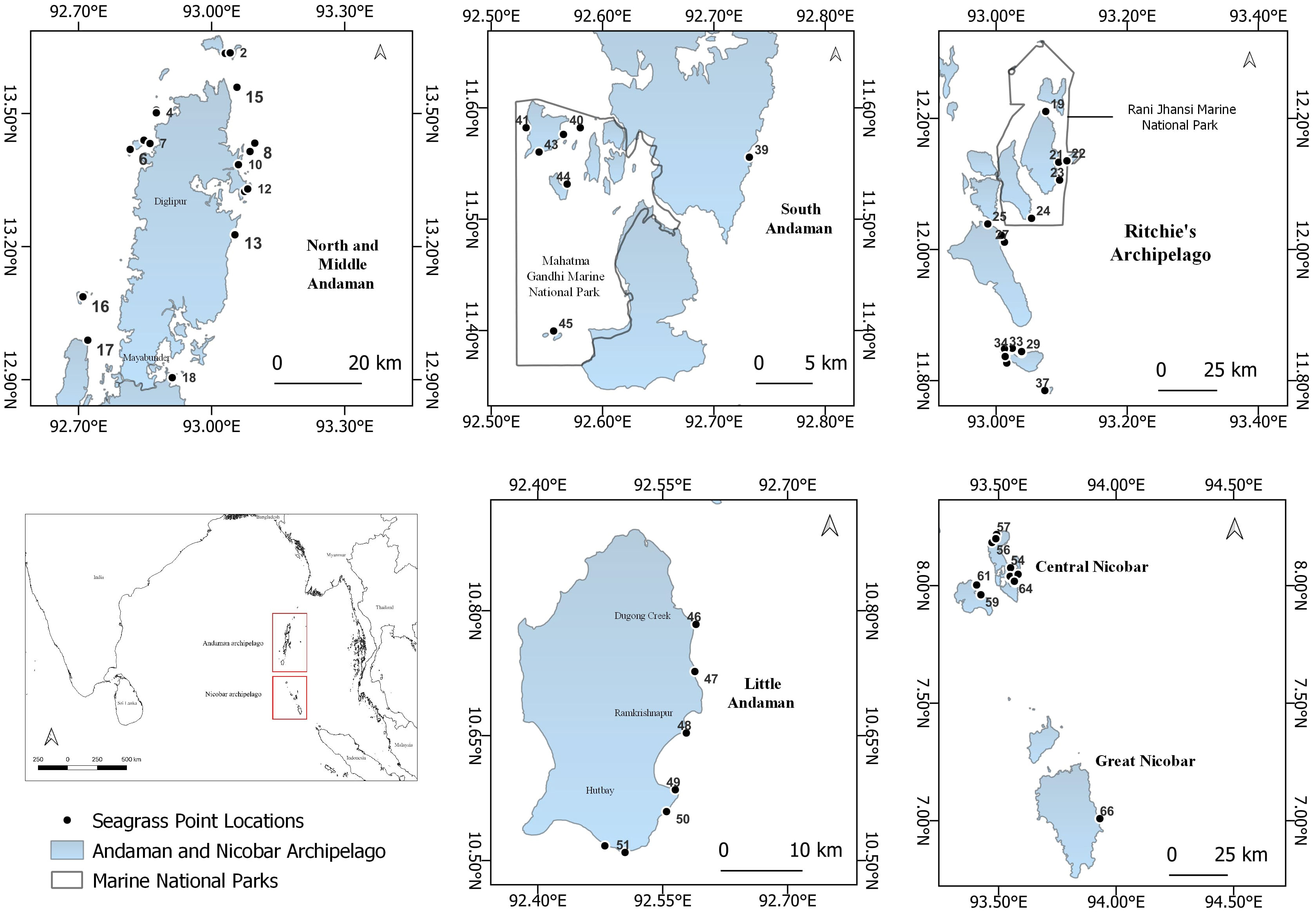
Figure 1 Seagrass distribution sites in the Andaman and Nicobar Islands (Sites codes and names in Supplementary Material Annexure 1).
2.2 Field surveys
We conducted intertidal surveys (0.2 to 0.5 m) during the spring tides when the region was maximally exposed, extending to the reef ward edges. We performed subtidal exploration using SCUBA diving-assisted surveys in both shallow and deep waters. However, due to saltwater crocodile hotspots in more than 80% of the sampled regions, deepwater investigations were limited to approximately 10 m, except for RA, which has a flourishing tourism industry and diving-friendly sites, allowing efforts up to 37 m. Coasts with strong wave action that hindered SCUBA exploration were excluded from the study. The line intercept transects (LIT; English et al., 1997) method was employed for systematically sampling seagrass meadows after locating them intertidally and sub-tidally. We surveyed 18 sites from NMA (25 LITs), 19 sites from RA (44 LITs), 8 from SA (11 LITs), 7 from LA (14 LITs), and 14 from NIC (20 LITs). At each site, the 50 m long LITs were placed perpendicular to the shore, and spatial replicates (3 to 4 LITs) were spaced 150-200 m apart. Within each transect, a 50 x 50 cm quadrat was used at every 5 meters to record seagrass meadow characteristics, including species composition, total and species-specific seagrass cover, shoot density, shoot length, total biomass (above and below ground, dry weight), and algal cover (epiphytic and non-epiphytic).
In addition, we recorded the following predictor variables at each sampling point: water depth (using a Dive Computer for subtidal meadows), pH and water temperature (measured using a handheld multi-parameter tester - Eutech Oaklon- PCS Testr 35), and salinity (measured using a handheld refractometer - LABART). We recorded major substrate types from the same quadrat for fine-scale habitat characterization, such as sand, dead coral with macroalgae, rock, rubble, and live coral. The habitat profile was calculated as the mean cover (%) from each sampling point, and it was further classified as either homogenous (100% sand) or heterogenous (approximately 50% sand along with other substrate types). The seagrass meadows were characterized based on the three criteria: a) observed depth gradient, b) seagrass cover, and c) species composition. We classified meadows’ depth profile as intertidal (0.2 to 0.5 m), shallow-subtidal (< 8 m), and deep waters (> 8 m; McKenzie et al., 2020). Based on the total seagrass cover, meadows were further profiled as a) sparse (0-25% cover), b) moderate (25-50% cover), c) dense (50-75% cover) and d) very dense (75-100% cover; Sabilah and Amran, 2020), and grouped as either mono-specific (single species) or mixed-species (2 or more co-occurring species).
2.3 Laboratory assessments
To estimate shoot density and biomass (above and below ground), we collected samples from a 20 X 20 cm smaller quadrat within each transect (n= 3/transect). The collected seagrass samples were stored in zip-lock bags and transported to the field base for further analysis. We rinsed the seagrass samples to remove sediment particles and algae and counted seagrass shoots (species-specific) to estimate shoot densities (shoots/m2). Lastly, we air-dried the seagrass samples and calculated total biomass (above and below ground - g/m2) on a micro-scale weighing balance (WENSAR PGB-220/0.001 to 200 g). Sediments were hand-scooped in triplicates from 0.4 m2 within the sampled seagrass beds and air-dried for texture analysis to assess the habitat suitability of the seagrass species.
2.4 Data analysis
2.4.1 Step 1-Data normalization and collinearity
We used a generalized linear model (GLM) framework to elucidate the influence of 11 potential explanatory variables on four response variables, namely seagrass occurrence, cover, biomass, and density. Since the normal distribution was not observed for three quantitative response variables (seagrass cover, density, and biomass), the data were transformed using Z-score normalization. Before GLM, collinearity among the 11 quantified explanatory variables (water depth, pH, water temperature, salinity, epiphytic macroalgae, non-epiphytic macroalgae, sand, rubble, dead-coral with macroalgae, live coral, and rock) was assessed using Variance Inflation Factor (VIF) values. The VIF value for sand, which is the primary substrate for seagrass occurrence and growth, was found to be considerably higher than the other independent variables. Therefore, we conducted a bivariate regression analysis to determine the strength and relationship of other independent variables with sand (Table 1). We observed that the least important variables for seagrass growth such as live coral and rock substantially correlated with sand (Table 1), so these two variables were removed from further analysis. The VIF values were rechecked, and it was found that all nine remaining explanatory variables exhibited low collinearity (VIF < 3), which is recommended for regression analysis (Bolker, 2008).
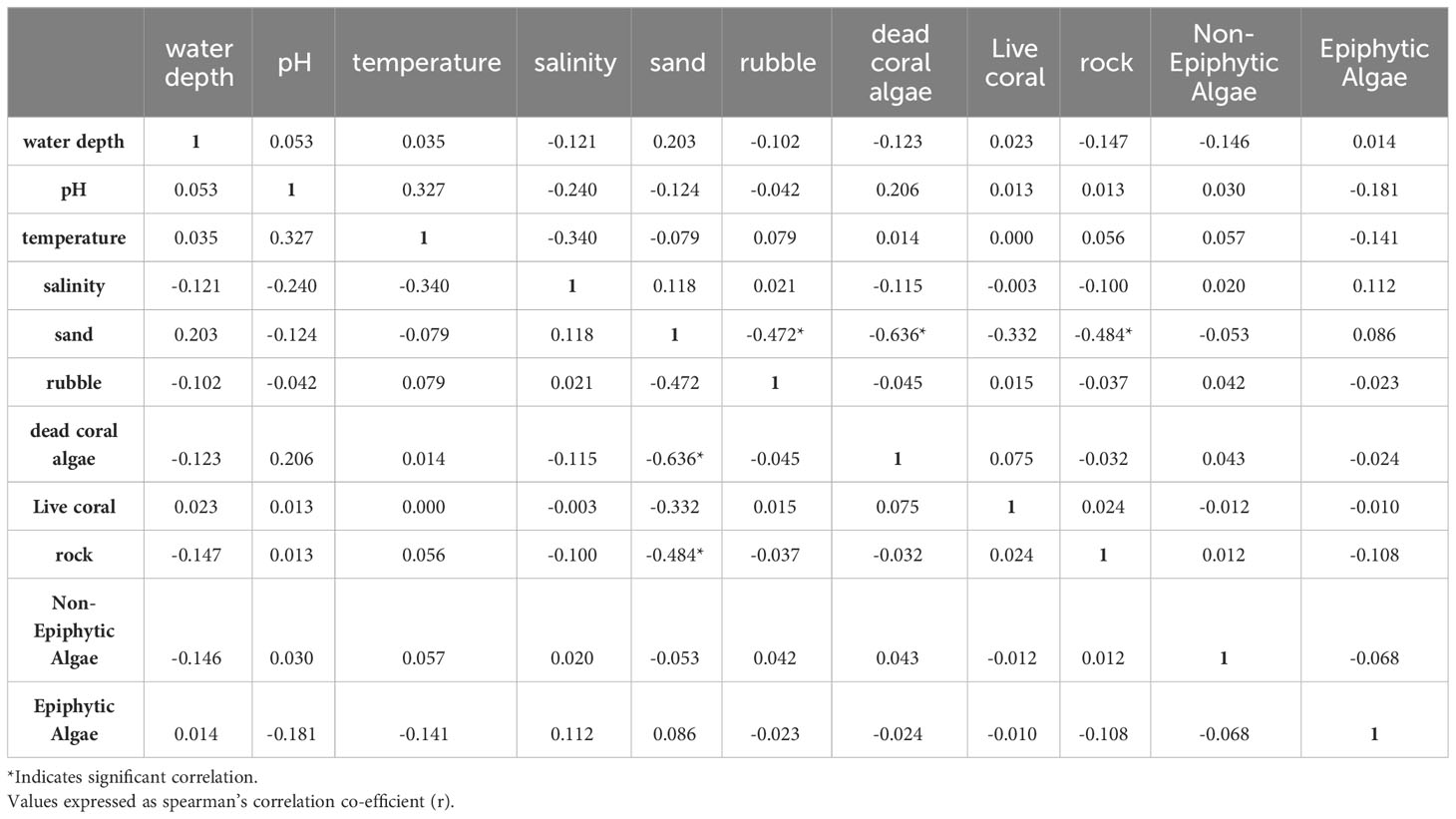
Table 1 Bivariate regression analysis carried out on 11 explanatory variables considered for the regression analysis.
2.4.2 Step 2-Regression analysis
We assumed the response variable of seagrass occurrence to follow a binomial distribution, and analysis was performed on the seagrass presence-absence matrix. An “Intercept-only” model was created with the response variable, and nine independent models were built for each predictor variable versus seagrass occurrence (Johnson and Omland, 2004). We excluded models with Akaike Information Criterion (AIC) values higher than the “Intercept-only” model from further analysis (Johnson and Omland, 2004; Arnold, 2010). We generated different combinations of informative models, and the model with the lowest AIC value and highest corresponding AIC weight was selected as the best-fit model. For quantitative variables, we assumed that the normalized data (Z-score) for seagrass cover (mean percentage), shoot densities (shoots/0.04 m2), and seagrass biomass (total dry weight/0.04 m2) followed a Gaussian distribution. Thus, GLM analyses were performed on their respective matrices. However, the AIC values of informative models did not differ significantly, making it challenging to select one best-fit model and potentially discarding relevant explanatory variables. To overcome this, we employed an information-theoretic model averaging approach. We created multiple combinations of models using the function “dredge” in the Mumin package in R (version 4.2.1). A total of 512 models were created for seagrass cover, density, and biomass. The models with the lowest AIC and AIC delta < 2 (indicating a small difference in AIC values) were averaged (Burnham and Anderson, 2002), providing a more robust understanding of the influence of potential explanatory variables on seagrass cover, density, and biomass.
2.4.3 Step 3-Species habitat and substratum preference
Canonical Correspondence Analysis allows for the exploration of relationships between species abundances and environmental variables. We performed Canonical Correspondence Analysis (CCA plots) on the species-densities matrix to assess the impact of habitat and sediment profile on seagrass species densities. All data analysis was conducted in R (version 4.2.1) using packages such as CAR, AICcmodavg, and ggplot2. Functions like glm, AIC, Loglik, and aictab were utilized to perform GLM analysis, calculate AIC values and likelihoods, and create plots (R core development team, 20195).
3 Results
3.1 Spatial distribution of seagrasses
The Andaman and Nicobar Islands (ANI) coastal waters host 12 species of seagrasses. Our study encompassed 66 meadows from 34 islands across five clusters, allowing us to identify 11 out of the 12 reported seagrass species in the region (Table 2). These seagrasses exhibited varying distribution patterns, ranging from dense and continuous beds to moderate to sparse stands. They were found in both homogeneous and heterogeneous habitats across different depth gradients. Among the identified species, Halophila ovalis and Halodule pinifolia demonstrated the widest distribution range, spanning from Landfall (Site 1), the northernmost limit, to Great Nicobar Islands (Site 66), the southernmost limit (Figure 1). These two species collectively occurred in over 50% of the investigated meadows. Following them, Halodule uninervis (34.8%), Thalassia hemprichii (31.8%), and Cymodocea rotundata (19.7%) were the next most frequently encountered species (Supplementary Material Annexure 1). However, the spatial spread of the remaining six species was relatively limited. An interesting finding was the rare occurrence of H. beccarii, observed only once at Pokkadera (Site 18) (Supplementary Material Annexure 1; Figure 1), indicating its scarcity in the study areas. Syringodium isoetifolium exhibited a restricted distribution, being confined to just five sites: Jua Tekdi (Site 21) and Vijay Nagar (Site 27) in the RA, and the shallow waters of Kardip (Site 53), Trinket (Site 63), and Al-Reak (Site 64) in the Nicobar Islands (Supplementary Material Annexure 1; Figure 1). Notably, C. serrulata was absent in the NMA sites, while H. minor and Enhalus acoroides were exclusively found in the RA and NIC regions (Supplementary Material Annexure 1). We observed highest seagrass coverage, and total plant biomass in shallow subtidal meadows of Safed Balu and Kardip respectively, in the Nicobar archipelago (Supplementary Material Annexure 1). Shoot densities however were the highest in the mixed intertidal meadow of Vijay Nagar (6392.5 ± 672.5 shoots/m2).
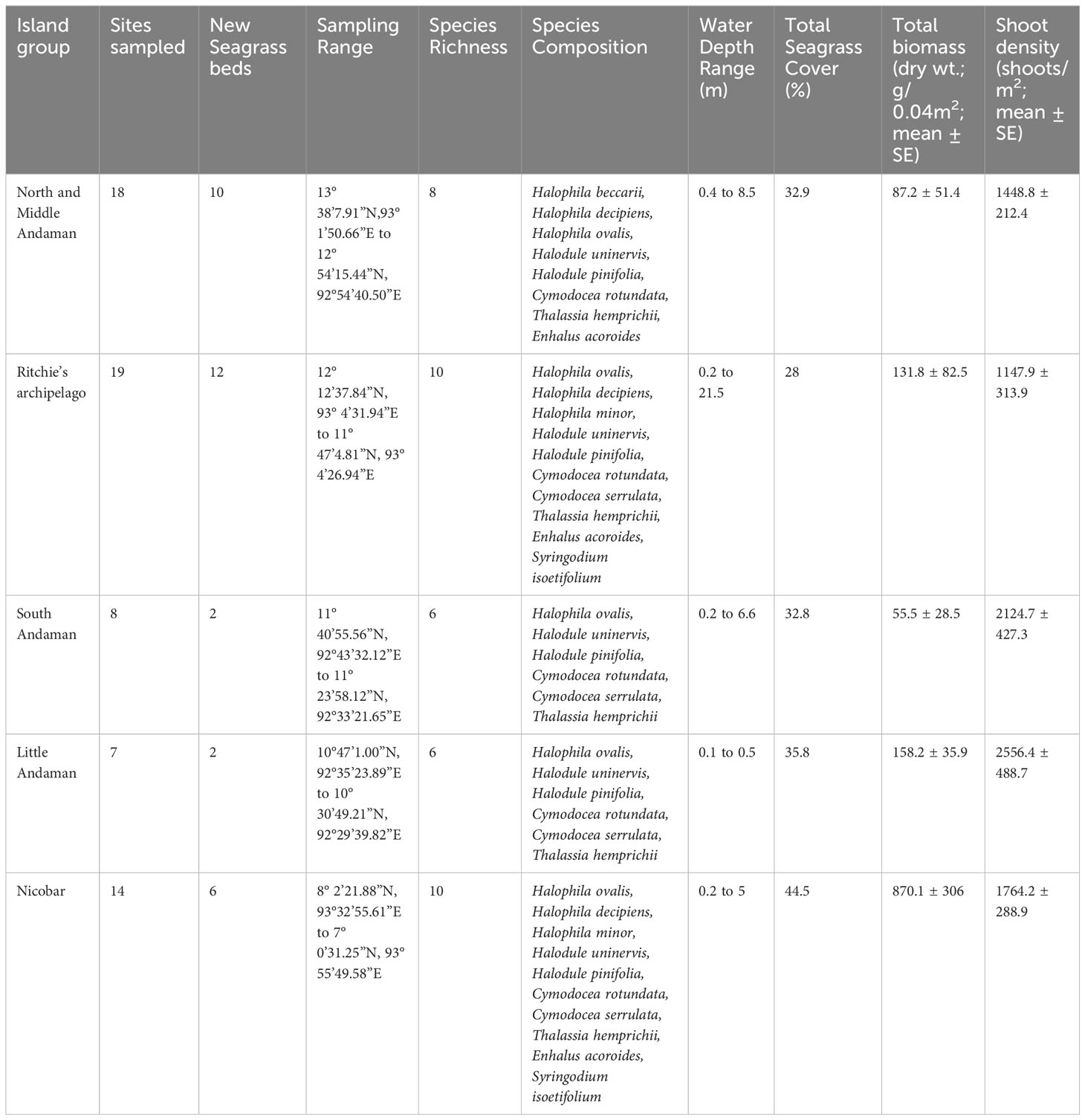
Table 2 Island wise summary of seagrass meadow characteristics in the Andaman and Nicobar Islands, India.
3.2 Species distribution across depth gradients
Seagrass meadows in the study area exhibited a range of depth distributions, occurring from the littoral zone (n=17) to subtidal regimes, shallow-subtidal (n=39), and deep waters (n=10; Supplementary Material Annexure 1). Most meadows in NMA, SA, and NIC were found in shallow-subtidal areas (Table 2). Deepwater seagrass beds were primarily restricted to RA, with the exception of Delgarno in the NMA (Table 2; Figure 1). In LA, seagrasses were exclusively intertidal and distributed along the island’s North-Eastern to Southern coast (Figure 1). No subtidal seagrasses were detected along Little Andaman’s West and North coasts. Intertidal and shallow-water seagrass meadows exhibited similar species richness, with ten species each and nine species overlapping between the two zones. The exceptions were H. beccarii, which was restricted to intertidal regions (0.4 m), and H. decipiens, which occurred exclusively in subtidal zones (3 to 21 m). T. hemprichii and C. rotundata were dominant species in the intertidal meadows (0 to 0.5 m), occurring with 88% and 59% frequency rates, respectively. However, T. hemprichii was observed colonizing down to a depth of 2.5 m. E. acoroides, Cymodocea spp., and S. isoetifolium had a down slope limit of 5 m, beyond which these species were not found. The Halophila spp. + Halodule spp. Complex, excluding H. beccarii, dominated the depth regimes beyond 5 m, accounting for approximately 96% of the observed occurrences, particularly within the range of 8-15 m (Supplementary Material Annexure 1; Figure 2). H. ovalis was the most frequent species (66% occurrence rate), followed by Halodule pinifolia (50%) and Halodule uninervis (39%) in the shallow-subtidal seagrass beds. H. ovalis also dominated the deepwater meadows, occurring in ~ 80% of the sites.
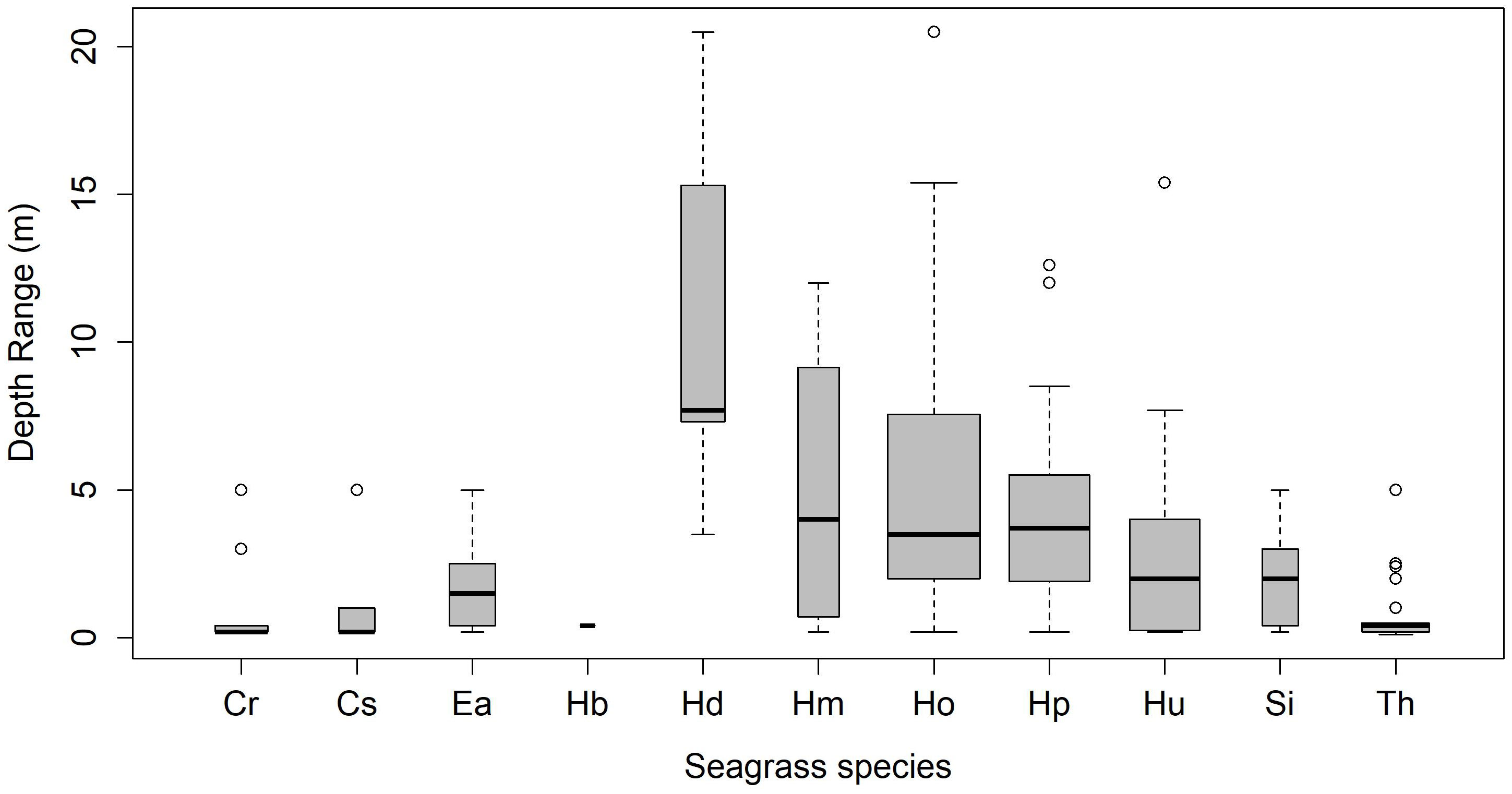
Figure 2 Depth wise distribution trends of seagrass species (Cr, Cymodocea rotundata; Cs, Cymodocea serrulata; Ea, Enhalus acoroides; Hb, Halophila beccarii; Hd, Halophila decipiens; Hm, Halophila minor; Ho, Halophila ovalis; Hp, Halodule pinifolia; Hu, Halodule uninervis; Si, Syringodium isoetifolium; Th, Thalassia hemprichii).
3.3 Meadow species composition and distribution trends
Based on depth profile and species composition, we classified the observed seagrass meadows into six categories; (1) Intertidal, mono-species meadows (n=4), (2) Intertidal, mixed-species meadows (n=13), (3) Shallow, mono-species meadows (n=15), (4) Shallow, mixed-species meadows (n=24), (5) Deep, mono-species meadows (n=3), (6) Deep, mixed-species meadows (n=7). Of the 66 meadows, 44 were mixed-species, while 22 were mono-specific (Supplementary Material Annexure 1). A clear trend of decreasing seagrass species richness and coverage with increasing water depth was observed. Mixed-species beds exhibited dense coverage and were limited to intertidal and shallow waters (Supplementary Material Annexure 1). In contrast, single species dominated all depth regimes but rarely formed dense meadows. In category 1, T. hemprichii was the dominant species in the sparse to moderate seagrass beds found in the heterogeneous habitats of RA and SA (Supplementary Material Annexure 1). In category 3, H. ovalis accounted for 40% of the sparse to moderate, mono-species meadows along with C. rotundata, H. decipiens, H. minor, H. ovalis, Halodule pinifolia, and Halodule uninervis. Similarly, H. ovalis, H. minor, and Halodule pinifolia formed deep water, mono-species stands in category 5 (Supplementary Material Annexure 1).
The species richness in mixed-seagrass meadows ranged from a minimum of two to eight species. Interestingly, we found that the probability of two co-occurring species was more frequently observed (>50% of meadows) than a greater number of species in a single mixed-species meadow. Six species exhibited the highest co-occurring tendencies; ‘Halophila spp. with Halodule spp.’ (in all-depth regimes), ‘T. hemprichii with C. rotundata’ (in intertidal habitats), and ‘S. isoetifolium with E. acoroides’ (in intertidal habitats). Three to eight co-occurring species characterized intertidal, mixed meadows (category 2) and showed a wide range of seagrass coverage ranging from sparse to very dense (Supplementary Material Annexure 1). Particularly within this category, we observed highly diverse (4 to 8 co-occurring species), dense, and contiguous seagrass meadows in sheltered bays such as Jua Tekdi (Site 21), Vijay Nagar (Site 27), Haddo (Site 38), Burmanallah (Site 39), and Light House-South Bay (Site 51) (Figure 1, Supplementary Material Annexure 1). Except for Haddo, all other intertidal seagrass-rich sites exhibited habitat heterogeneity (Supplementary Material Annexure 1). The mixed-species meadows of categories 4 and 6 were predominantly dominated by Halophila spp. and Halodule spp. Complex.
3.4 Habitat-sediment characterization and species’ affinities
Seagrasses were found predominantly in five habitat types; sand, rubble, dead coral with macroalgae, rock, and live coral (Supplementary Material Annexure 1; Figure 3). The sediment texture consisted of fine and coarse sand, clay, and silt. Homogeneous seagrass beds exhibited high siltation and turbidity. Sites in NMA and NIC were predominantly homogeneous, while LA and SA indicated habitat heterogeneity. RA displayed a more diverse habitat profile with a mix of homogeneous and heterogeneous benthic profiles (Supplementary Material Annexure 1). Seagrass patches in sites with fine sand were often found around dunes formed by crustacean burrows, such as Pokkadera, Dolphin, Temple, Nemo Reef, and Imli Dera (Figure 1). The presence of habitat heterogeneity supported highly diverse and extensive mixed-species meadows. The dominant non-epiphytic macroalgae were the Padina spp., followed by Halimeda spp. and Caulerpa spp. Epiphytic algal cover varied from thin or dense matt algal film over seagrass shoots. We observed the epiphyte Melobsia spp. on T. hemprichii shoots in Laxmanpur, Natural Bridge, and Burmanallah (Figure 1). 14 seagrass sites, mainly from NMA and RA, exhibited a high cover of mat-forming algae on shoots and the seabed (Supplementary Material Annexure 1).
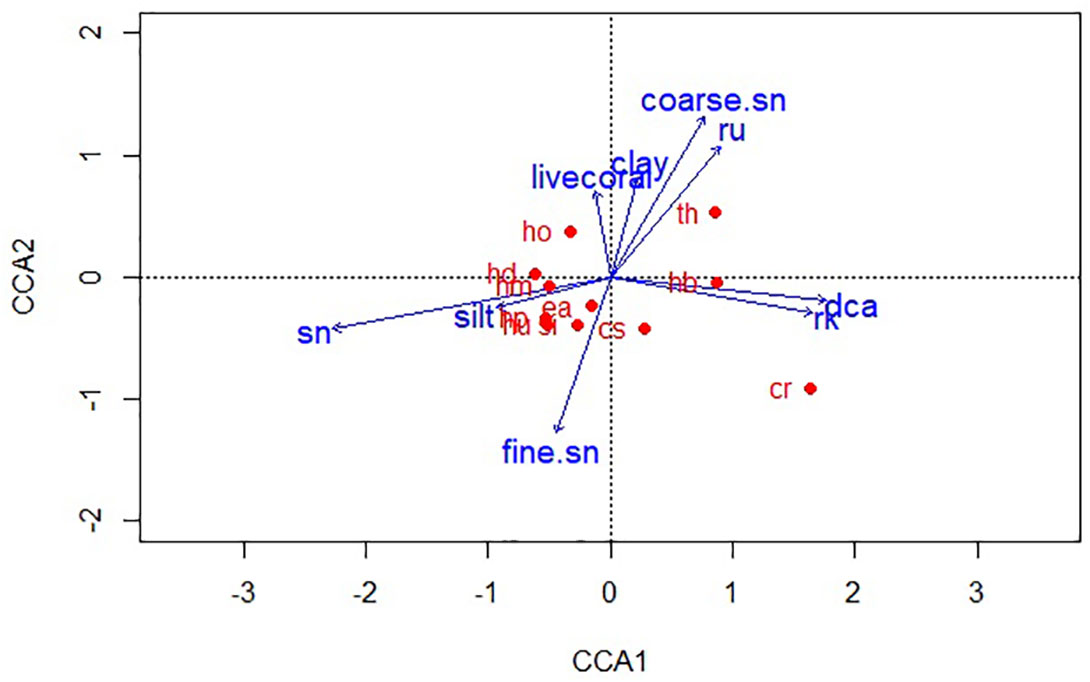
Figure 3 Canonical Correspondence Analysis ordination showing the influence of habitat profile and sediment texture on seagrass species’ shoot densities (sn, sand; fine.sn, fine sand; rk, rock; DCA, dead coral with macroalgae; coarse. sn, coarse sand; ru, rubble; cr, Cymodocea rotundata; cs, Cymodocea serrulata; ea, Enhalus acoroides; hb, Halophila beccarii; hd, Halophila decipiens; hm, Halophila minor; ho, Halophila ovalis; hp, Halodule pinifolia; hu, Halodule uninervis; si, Syringodium isoetifolium; th, Thalassia hemprichii).
Seagrass species’ densities clustered according to their habitat and substratum preferences, with Axes 1 and 2 of the CCA plots explaining a cumulative variance of 76. 32% (54.96% and 21.36%, respectively). While most species were dominant along a sediment gradient of sand, silt, and fine sand, distinct preferences for H. ovalis, T. hemprichii, and C. rotundata densities were observed. H. ovalis showed higher shoot densities in sandy substrata mixed with clay. It also occurred sparsely around the fringes of coral reefs in subtidal meadows (Figure 3). H. decipiens and H. minor displayed a strong preference for sandy habitats. H. beccarii was observed as a one-time occurrence in a muddy substratum, occupying fine sand mixed with mud near the high tide edges of Pokkadera (Site 18; Figure 1). E. acoroides and S. isoetifolium were positively associated with fine sand, while Halodule spp. was predominantly observed in silt. T. hemprichii, C. rotundata, and C. serrulata favored heterogeneous habitats consisting of sand, rock, dead coral with macroalgae, and rubble across all sites (Figure 3). However, there were differences in microhabitat preferences among these species. T. hemprichii densities correlated with coarse sand mixed with rubble, while C. rotundata was found in coarse sand as random sparse stands interspersed within the rubble, dead coral with macroalgae, and rocks. Additionally, C. rotundata, along with C. serrulata, also preferred fine sand in shallow waters or the upper edges of intertidal zones (Figure 3).
3.5 Factors influencing seagrass distribution and meadow characteristics
We used multiple regression models to examine factors influencing seagrass distribution and meadow characteristics (seagrass occurrence, cover, biomass, and shoot densities). Water depth and sand (except shoot densities) were the key environmental variables significantly predicting all response variables. Seagrass occurrence was found to decline with water depth (p=3.72e-15), as well as the presence of dead coral with macroalgae (p=<2e-16) and rubble (p=3.88e-10) (Figures 4A–C; Table 3). However, the presence of sand showed a positive association with seagrass occurrence (p- < 2e-16; Figure 4D, Table 3). Water depth exhibited a similar correlation with seagrass cover (β = -0.2759; SE= 0.02471; p= <2e-16), shoot densities (β = -0.3556; SE= 0.1231; p- 0.005) and biomass (β= -0.3526; SE= 0.1159; p=0.003). These variables decreased from littoral zones to deep waters (Figures 5A–C; Table 4). On the other hand, seagrass cover (β = 0.2924; SE= 0.03124; p- <2e-16) and biomass (β = 0.295; SE= 0.1171; p- 0.01) showed a positive relationship with the availability of sand (Figures 6A, B; Table 4). Furthermore, seagrass cover and biomass exhibited a positive correlation with the presence of epiphytic algae (β= 0.136, SE= 0.0244, p =<2e-16) and water temperature respectively (β= 0.4235, SE= 0.1103, p =0.0002) (Figures 6C, D; Table 4). Lastly, we observed a peak in seagrass biomass at approximately 2.5 m depth, with the highest coverage and shoot densities recorded up to 5 m depth (Figures 7A–C).
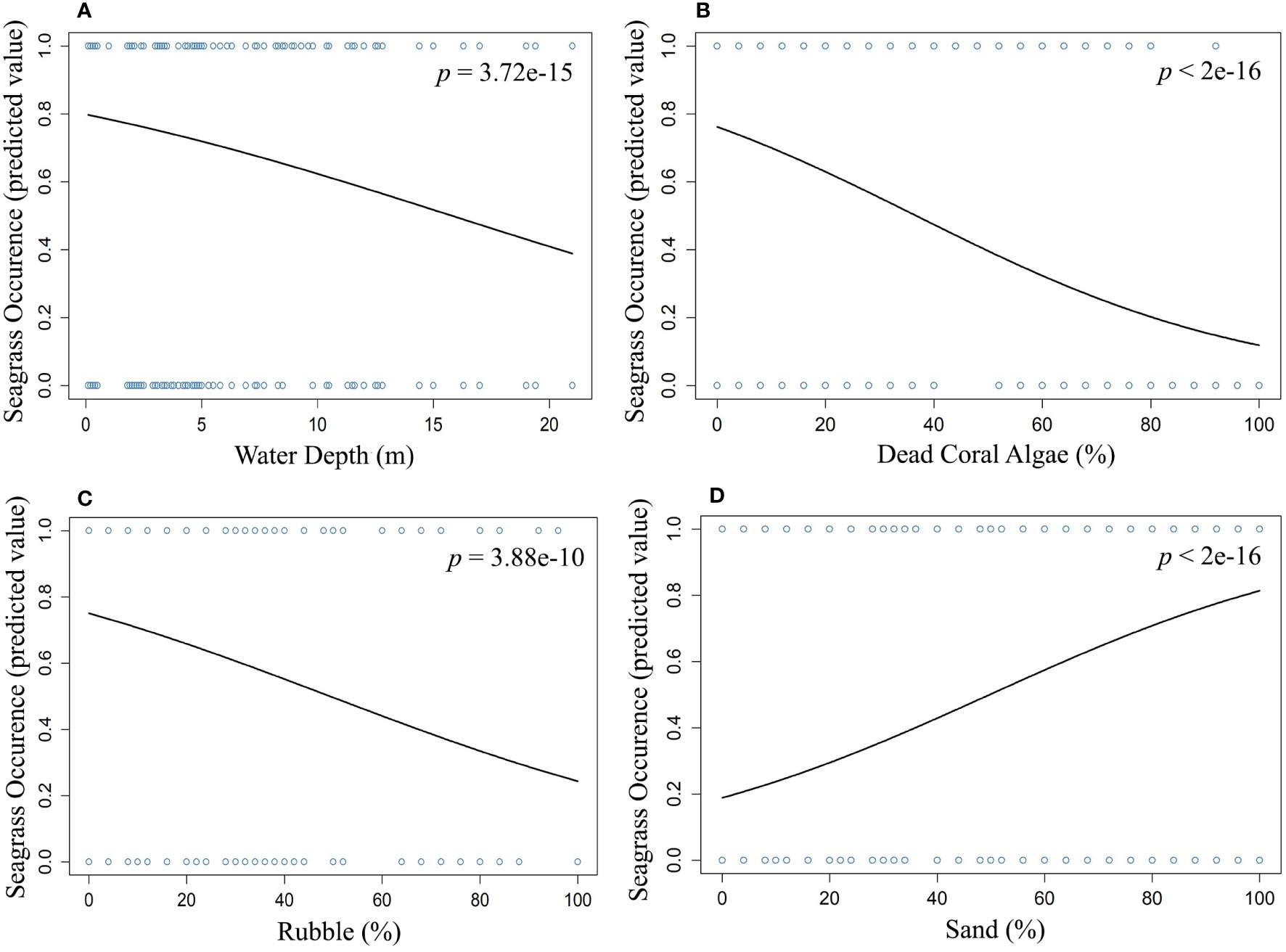
Figure 4 Influence and nature of the relationship between seagrass occurrence and (A) water depth, (B) dead coral with algae, (C) rubble, and (D) sand.
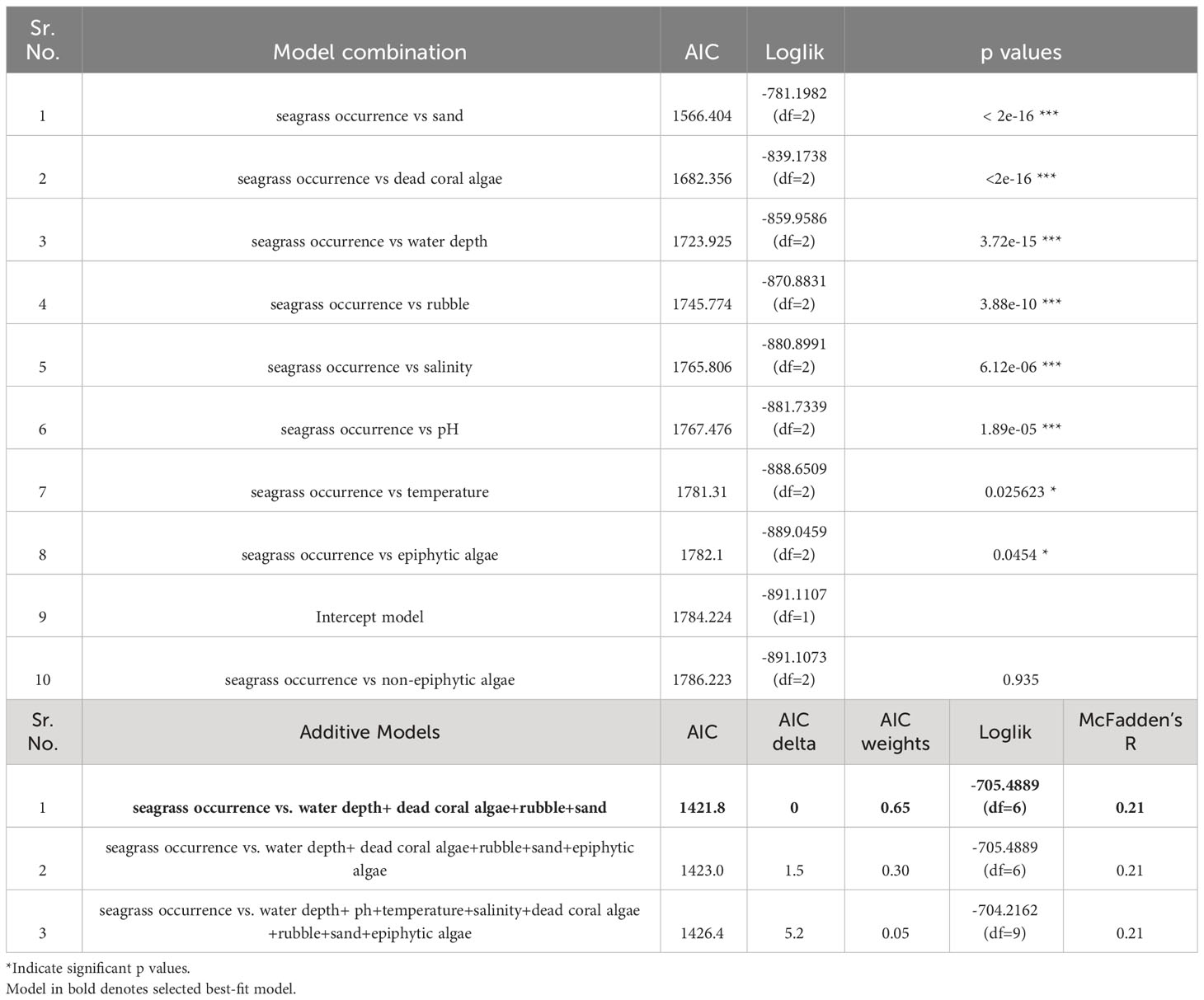
Table 3 Summary of logistic linear models used to identify key explanatory variables influencing seagrass occurrence in the Andaman and Nicobar Islands, India.
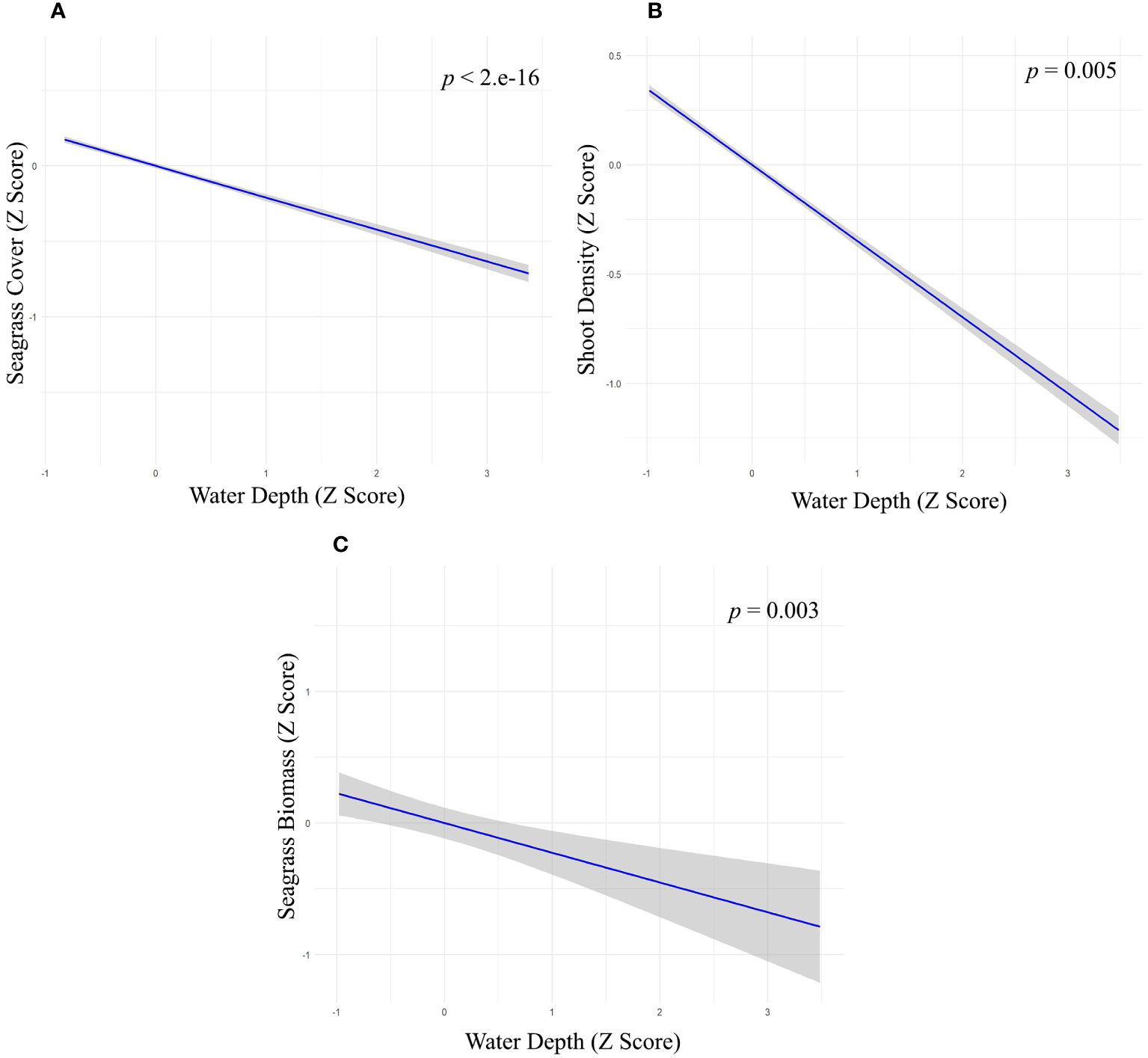
Figure 5 Relationship between water depth with (A) seagrass cover (Z-score values), (B) shoot density (Z-score values) and (C) seagrass biomass (Z-score values).
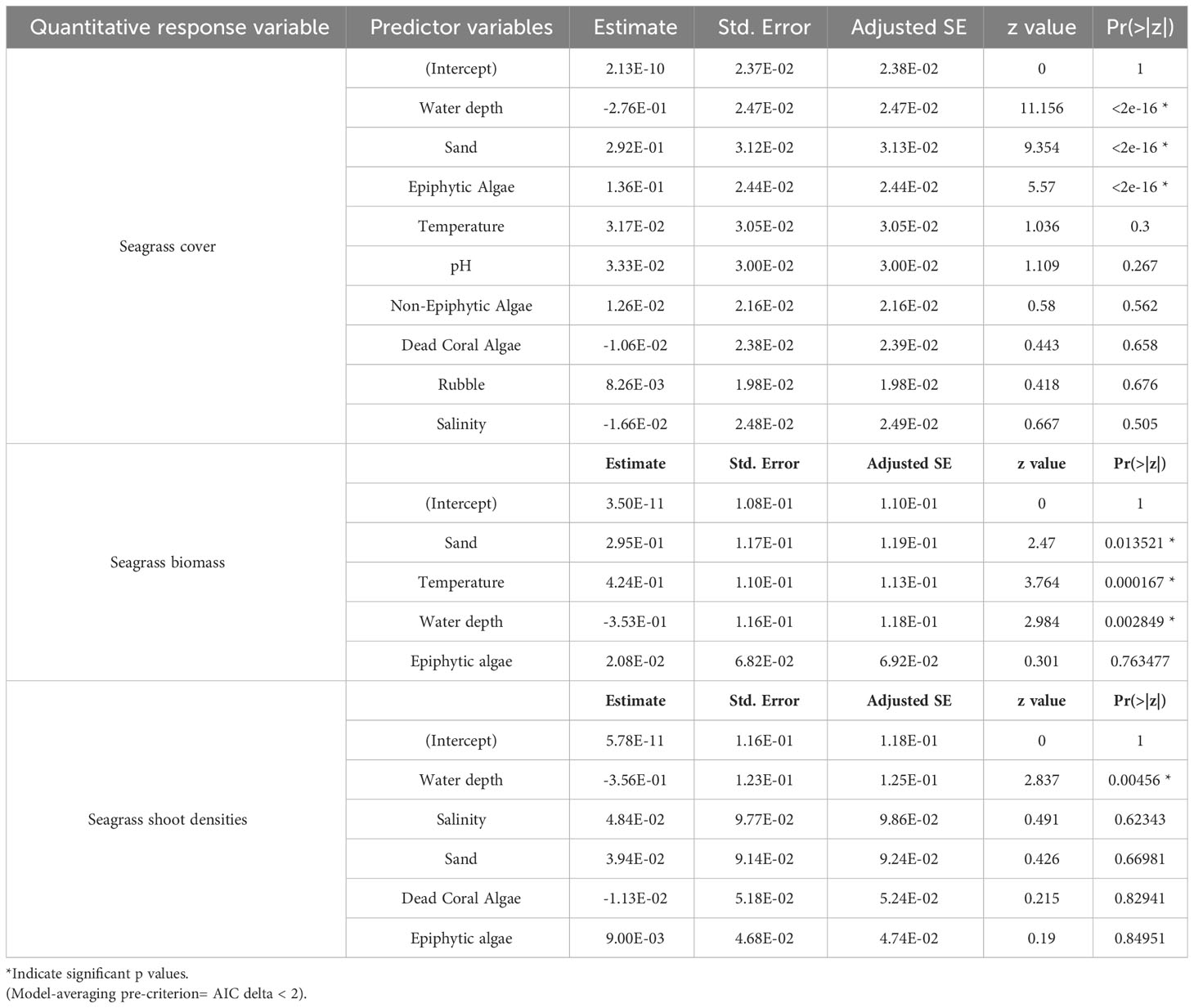
Table 4 Summary of gaussian model-averaged coefficients (full average) explaining influence of each explanatory variable on seagrass cover, biomass and shoot densities.
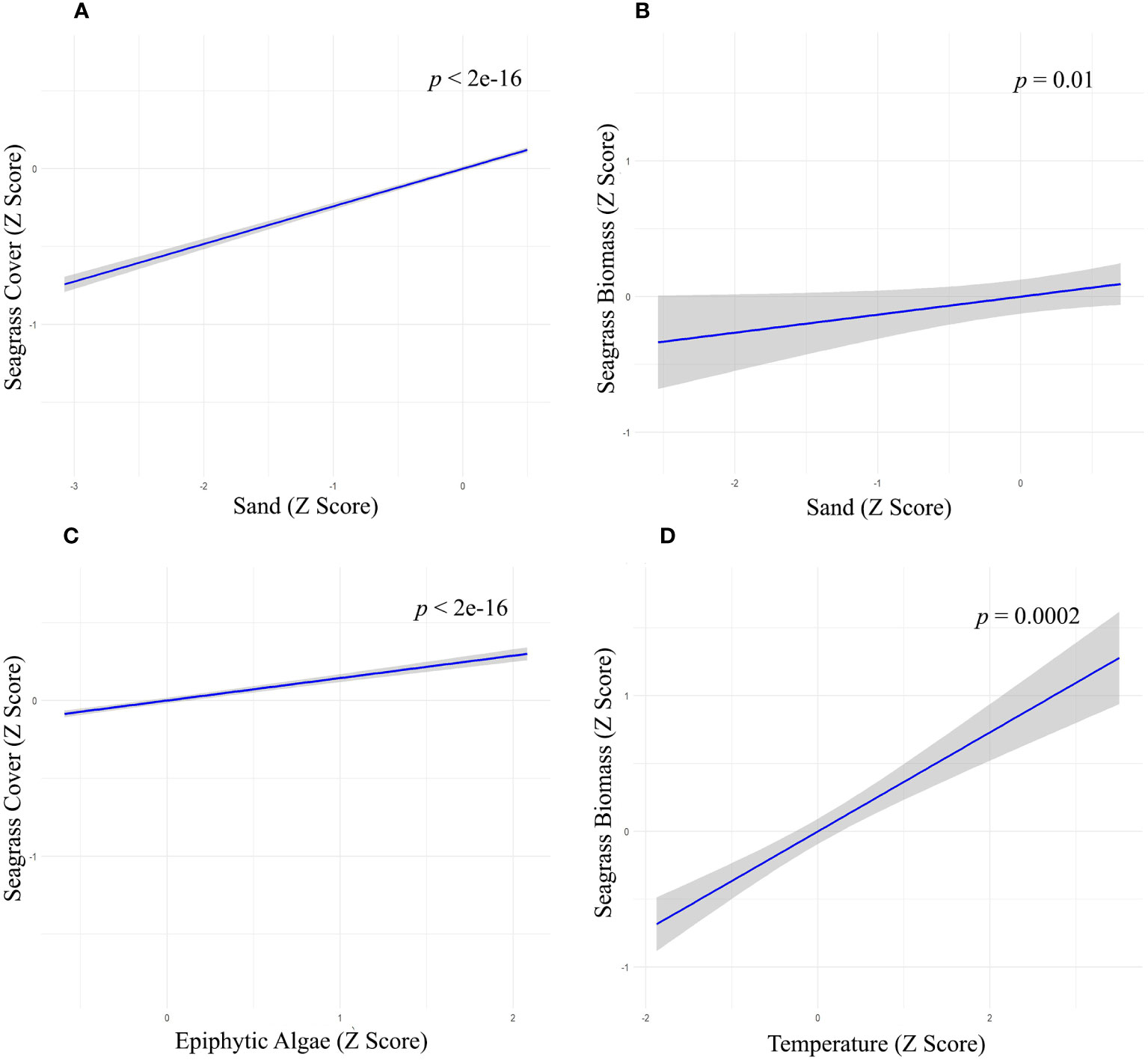
Figure 6 Relationship between Sand with (A) seagrass cover (Z-score values), (B) seagrass Biomass (Z-score values), (C) Epiphytic Algae on seagrass cover (Z-score values); (D) Water temperature on seagrass biomass (Z-score values).
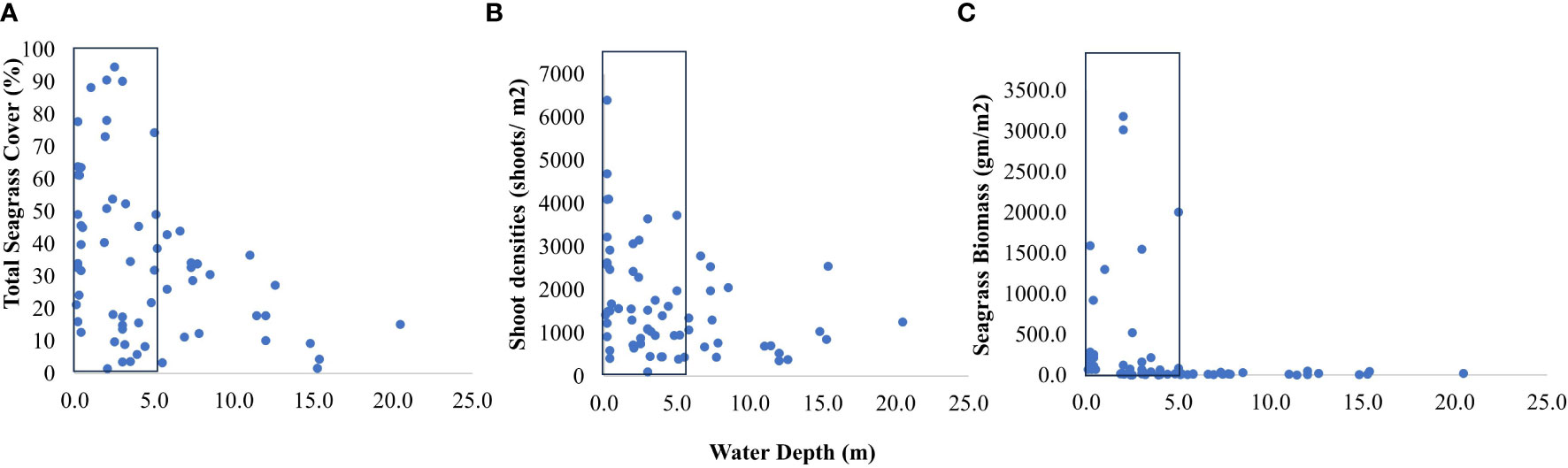
Figure 7 Response of seagrass growth; (A) seagrass cover, (B) shoot densities, and (C) biomass in relation to water depth; box indicates threshold depth limits with maximum values of response variables.
4 Discussion
The spatial diversity, distribution, and growth of seagrasses in the explored meadows are primarily determined by water depth, habitat heterogeneity, and substrate availability. Our findings reveal significant variations in species richness between intertidal/shallow and deepwater meadows, with the former habitats supporting higher species (10) than the latter (4). All 11 observed species were found up to a depth of 5 m, either in mono-species or mixed meadows. Beyond this depth, the species composition shifted toward exclusive dominance of Halophila spp. and Halodule spp. Complex, with Halophila ovalis and H. decipiens marking the deepest recorded seagrasses from our study area (ANI), reaching depths of 21 m. While the general depth limits for Indo-tropical seagrasses are commonly less than 10 m (Short et al., 2007), our findings, along with regional studies from Hervey Bay and Cape York (Queensland), the Red Sea, and the Mediterranean Sea, indicate region-specific deepwater trends (Den Hartog, 1971; Lee Long, 1996; Short et al., 2007). Halophila spp. has been recorded from depths of 50 m in the tropical Atlantic region, 70 m in the Red Sea (Short et al., 2007), and 90 m in the Coral Sea (Den Hartog, 1971) highlighting the species’ adaptability to deeper regimes. Additionally, H. decipiens has been reported from 58 m in the Great Barrier Reef (Lee Long, 1996), while H. ovalis is commonly observed beyond 35 m (Coles et al., 2000). The deepest recorded seagrass sample to date is a one-time dredged sample of H. stipulacea from 145 m (Lipkin et al., 2003; Short et al., 2007). Our findings are thus, consistent with global evidence, supporting the notion that Halophila spp. and Halodule spp. are adaptive to depths.
Although our observed depth-scaled distribution trends are consistent with previous local and regional assessments from ANI and mainland India (Das, 1996; Jagtap, 1996; Jagtap, 1998; D’Souza et al., 2015),we report some stark contrast for few species’ regional colonization depths. For instance, Jagtap et al. (2003) reported Thalassia hemprichii and Cymodocea serrulata from a depth range of 0-10 m in Southeast India, as opposed to restricted littoral distribution in our study (with one-time observations from 2.5 m and 5 m, respectively). Similarly, the compensation depth of Halodule uninervis, one of the deepest species from our sites (15 m), was 3 m from Tamil Nadu (Jagtap et al., 2003). We speculate these differences to be driven by local variation in the physical environment of the two regions. Palk Bay and the Gulf of Mannar in Tamil Nadu have a shallow continental shelf, a vast seagrass expanse (often 5 to 10 km from the shore), and a tidal amplitude of around 0.3 to 0.5 m (Geevarghese et al., 2018). In contrast, in ANI habitat discontinuity, narrow shelf and sharp depth slopes (a few meters from the shore), results in irregularity in seagrass distribution (Das, 1996; Geevarghese et al., 2018). Considering the limited physical variation in Tamil Nadu, water depth likely plays a lesser role as a regulatory variable in shaping seagrass species distribution. Nevertheless, in ANI, we propose that water depth favors certain adaptive species over others, thus influencing the colonization depths of seagrasses (Lee et al., 2007; Short et al., 2011).
In our study, furthermore, we suggest that water depth not only regulates the species distribution ranges, but also the plant growth. Overall, seagrass coverage, shoot densities, and community biomass declined in deep waters (critical limit between ~ 2 to 5 m) consistent with reports from other geographic regions such as the Southeast coast, insular Lakshadweep (Jagtap, 1996; Jagtap, 1998), north-western Cuba (Buesa, 1975), and from the Mediterranean Sea (Drew, 1978). As light attenuates strongly in deeper waters, the observed variations are a possible outcome of differential traits of seagrasses to adapt to depths (Lee et al., 2007; Short et al., 2011; Minguito-Frutos et al., 2023). Seagrass depth penetration and growth are directly influenced by light availability (Zieman and Wetzel, 1980; Duarte, 1991), owing to which conducive growth environment declines in the subtidal regions (Dennison and Alberte, 1987). Within similar environmental conditions, species-specific variation in colonization depths is a response to range of factors such as plant architecture, growth strategies, physiological responses, and acclimation potential (Dennison et al., 1993; Alcoverro et al., 2001; Greve and Binzer, 2004; Bité et al., 2007; Silva et al., 2013; Kilminster et al., 2015; Schubert et al., 2018; Tuya et al., 2019). A synthesis of the seagrass-water depth relationship indicates that seagrasses restrict their rhizome development in deeper regimes, with subsequent growth reduction (Duarte, 1991). This ability further is highly pronounced in pioneer, small-sized species with fast recolonization rates allowing them to cope up in deeper regimes. For instance, Halophila spp. and Halodule spp. can more efficiently regulate their respiratory demands in depths than large-sized seagrass species (Fourqurean et al., 1995). Therefore, deep water colonization of Halophila and Halodule species complex in our study could be accounted for higher acclimation potentials at greater depths (Minguito-Frutos et al., 2023). Since light attenuation is not the only predictor of depth distributions (Koch, 2001), in way forward, it would be critical to understand the fine-scale mechanisms which allow dominance of one species over the other across depth regimes.
Additionally, seagrass distribution is a product of habitat heterogeneity and substratum suitability from the sampled meadows. Heterogeneous habitats in the islands support a higher species richness (Das, 1996; Savurirajan et al., 2018). Our study reveals that variabilities in substratum types and habitat heterogeneity allow different species to dominate various spatial niches. Meadows with less substratum variability, such as those found in Little Andaman, Burmanallah, Laxmanpur, and Natural Bridge, had a vast extent but supported lower species richness. Our investigation of sediment texture affinities of seagrass species partially agrees with a previous study in the region by Savurirajan et al. (2018), which also reported high densities of H. ovalis in sand and clayey substrates. However, we observed T. hemprichii, E. acoroides, and Halodule pinifolia occupying coarse sand, fine sand, and silt, respectively, contrary to their preference for clayey sand in the previous study. It is important to note that the study by Savurirajan et al. (2018) focused on intertidal waters (< 2 m), while our study spanned depths up to 21 m. Therefore, the differences in spatial scale and sample sizes may contribute to the variations in results between the two studies. Additionally, we suggest that habitat and substratum profiles change from intertidal to deeper waters, limiting direct comparisons. In addition, the absence of H. decipiens from the intertidal regions is in line with the same report from South Andaman (Savurirajan et al., 2018). Yet, contrary to this study, we report C. serrulata and Syringodium isoetifolium from the littoral zones (0.2 to 0.4 m) of Ritchie’s archipelago and Little Andaman, updating the species’ distribution ranges.
Lastly, despite the vast spatial scale of our study, we did not observe H. ovata from the ANI’s seagrass checklist (12 species). Genus Halophila, with high taxonomic diversity, overlapping morphology, and phenotypic plasticity at local scales (Japar Sidik et al., 2010), has often led to species misidentification and systematic ambiguity (Fortes et al., 2018). The species was last reported in 2010 from ANI (Thangaradjou et al., 2010a; Thangaradjou et al., 2010b). A recent study (Ragavan et al., 2016) argued that H. ovata is a misidentified H. minor from all previous assessments in ANI. A similar report from Southeast Asia pointed to taxonomic discrepancies within Halophila spp., where the morphological resemblance between H. ovata and H. minor was “compounding” and has led to species misidentification (Fortes et al., 2018). Since all seagrass assessments in ANI have relied only on morphological traits for species identification, including our study, the possibility of misidentification cannot be ruled out. Thus, we suggest a more robust approach for species identification using molecular traits to resolve present inconsistencies.
4.1 Management recommendations
Based on our study findings, we propose the following recommendations for the effective management and conservation of seagrass ecosystems in the Andaman and Nicobar Islands (ANI):
4.1.1 Enhance legislative measures
The limited legislative measures safeguarding seagrass ecosystems in India include 1) recognition as ecologically sensitive habitats (Coastal Regulation Zone Category I; ICRZ Notification, 20216) and 2) protection under the Indian Wildlife (Protection) Act (WLPA), 1972 (Ramesh et al., 2018). Seagrass ecosystems in the ANI are protected as one of the environmentally critical habitats under the Category IA of the Island Coastal Regulation Zone (ICRZ, 20197). However, concerning are the revisions in the ICRZ 2019 of the Island Protection Zone Notification (2011) permitting eco-tourism and allied development in the ICRZ- IA (200 meters changed to 20-50 meters from the High tide line, ICRZ, 20198), where majority of seagrasses fall. We thus recommend a top-down revised approach to strengthen the existing legislative framework to provide stronger protection for seagrass ecosystems.
4.1.2 Expand the Marine Protected Area (MPA) Network
Less than 20% of the seagrass meadows we studied are protected as MPAs in ANI. On the contrary, a recent review of the Southeast Asian seagrasses (Sudo et al., 2021) suggests that the subtropical Ryukyuan archipelago in Japan, with a coastline of 650 km (one-third of ANI) and half the geographical expanse (~ 4600 km2) still protects more than 99% of their seagrass beds within its MPAs. The denoted figures shed light on the under-representation of seagrasses as valuable ecosystems that need protection in ANI. Within the remaining 80% of unprotected seagrasses in our study, which also includes the critical dugong habitats, the geographical remoteness of the islands (from human settlements) offers some level of natural protection.
However, these beds still fall on the geological fault lines of ANI, exposed to natural threats such as frequent cyclonic storms, earthquakes, and tsunamis, all known to impact seagrasses (Adulyanukosol and Poovachiranon, 2006; Sachithanandam et al., 2014). The rise in coastal development prospects in ANI is another matter of concern. It is likely to change seagrass ecosystems in the future, possibly with an aligning fate as reported from China (Jiang et al., 2020), Vietnam (Luong et al., 2012), Malaysia (Japar Sidik et al., 2018), and Europe (de los Santos et al., 2019), to name a few. Thus, there is a need to extend the network of MPAs to encompass a larger portion of seagrass beds, ensuring the conservation of these critical ecosystems. We suggest increasing the coverage of Marine Protected Areas (MPAs) and enforcement, following a potential NEOLI approach (Edgar et al., 2014) to include a more significant proportion of seagrass meadows, especially those identified as critical dugong habitats.
4.1.3 Assess socio-ecological-economic services
Need to conduct comprehensive research to evaluate the socio-ecological-economic services provided by seagrasses (Rahman and Yaakub, 2020). Under the ecological context, this assessment should focus on lesser-known fauna, such as marine invertebrates, which are currently understudied in the region since seagrass research is largely dugong-centrical. We further suggest investigating local communities’ perceptions and socioeconomic dependency on seagrass ecosystems. This should entail assessing the direct and indirect benefits of seagrasses, including their role in supporting fisheries, gleaning, shoreline protection, and tourism. Understanding the socioeconomic dynamics will inform policy and decision making (de la Torre-Castro et al., 2014; Campagne et al., 2015; Kilminster et al., 2015), along with developing sustainable management strategies that align with the needs and aspirations of local communities.
4.1.4 Quantify seagrass-centric threats
Though with a limited sample size, we observed increased sedimentation and subsequent mass die-off of coral reefs and enhanced turbidity in the seagrass beds from Shaheed Dweep and meadow-scarring in Swaraj Dweep, as a response to benthic dredging (for jetty construction) and boat anchorage respectively (personal observations). Nevertheless, these observations are mainly descriptive, lacking quantitative details of the damage or loss incurred. We, thus, suggest detailed studies to quantify the threats faced by seagrass meadows in ANI to inform policy-makers (Rahman, 2017). Assessment of the impacts of anthropogenic activities, such as dredging and boat anchorage, on seagrass health will help identify and develop targeted management strategies to minimize or mitigate these threats.
By implementing these recommendations, we can create a robust framework for the ‘habitat-level’ management and conservation of seagrass ecosystems in the Andaman and Nicobar Islands. Drawing from regional case studies (Fortes et al., 2018) and considering the unique context of ANI, these measures could be comprehensively put into a local-scale management perspective to create a roadmap for seagrass conservation for ANI.
5 Conclusion
Our study has provided updated information on the spatial distribution, habitat suitability, and depth ranges of seagrass meadows in the Andaman and Nicobar Islands (ANI). By conducting a comprehensive assessment across a broad spatial scale and depth gradients, we have filled significant data gaps in previously data-deficient regions of ANI. Our findings highlight key regulatory factors, such as water depth, influence seagrass growth, and species distribution. The observed differences in seagrass distribution along depth gradients can be attributed to various factors, including variations in covariates associated with water depth, species’ adaptability to different depth regimes, and habitat heterogeneity and substratum availability, which also change with depth. Any alterations in the benthic habitat profile of these meadows are likely to impact species distribution patterns and overall performance. Our study suggests that Halodule spp. and Halophila spp. are generalist species, capable of occupying suitable habitats across different depth regimes, while larger-sized species like Enhalus acoroides, Thalassia hemprichii, and Cymodocea spp. exhibit specialist characteristics. The presence of seagrasses in deepwater regions of Ritchie’s archipelago further confirms the availability of conducive growth environments in deeper regimes. Replicating similar investigations in other island groups would provide valuable insights to complement our findings. In summary, the outcomes of this study will have significant implications for the management and conservation of seagrass habitats in the region. The findings will provide valuable baseline information for policymakers, conservationists, and stakeholders and contribute to the broader scientific understanding of seagrass ecosystems and their responses to environmental changes.
Data availability statement
The raw data supporting the conclusions of this article will be made available by the authors, without undue reservation.
Author contributions
SG: conceptualization, data curation, sample processing, formal analysis, drafted the manuscript, SP: data curation, sample processing, NP: formal analysis, critical inputs in manuscript review, HD: methodology designing, supervision, manuscript review, SK and JJ: funding acquisition, methodology designing, resources, supervision, manuscript review. All authors contributed to the article and approved the submitted version.
Funding
The author(s) declare financial support was received for the research, authorship, and/or publication of this article. This work was funded by the CAMPA authority, Ministry of Environment, Forest, and Climate Change (MoEFCC), Government of India (Grant/Award Number: 13-28(01)/2015-CAMPA).
Acknowledgments
We acknowledge the support by the Chief Wildlife Warden, divisional forest officers and frontline staff (Department of Environment and Forests), administrative bodies, and the Indian Coastguard in the Islands for granting necessary work permits and logistical support. This work is a product of extensive field/ technical support given by the local communities, our boat crew, and Saw Tapori, Sohini Dudhat, Titiksh Kaka, Jyotish Kumar, Neeti Joshi, Ajay Kumar, Mohammed Hussain, Rammaya, Rajesh, Late Syed Hussain, Prasad Gaidhani, Esha Gokhale, Srabani Bose and Ankit Pacha. We lastly thank Ashish Jangid, Dr. N. Lakshminarayanan, Dr. Frank Sadrack, Chinmaya Ghanekar, and Mohit Mudaliar for their generous support in the statistical analysis of the data.
Conflict of interest
The authors declare that the research was conducted in the absence of any commercial or financial relationships that could be construed as a potential conflict of interest.
Publisher’s note
All claims expressed in this article are solely those of the authors and do not necessarily represent those of their affiliated organizations, or those of the publisher, the editors and the reviewers. Any product that may be evaluated in this article, or claim that may be made by its manufacturer, is not guaranteed or endorsed by the publisher.
Supplementary material
The Supplementary Material for this article can be found online at: https://www.frontiersin.org/articles/10.3389/fmars.2023.1251887/full#supplementary-material
Annexure 1 | Seagrass meadow characteristics of the 66 beds investigated from the Andaman and Nicobar Islands, India. Sites in bold indicate new meadow record. *Indicates new species distribution record for the region
Abbreviations
ANI, Andaman and Nicobar Islands.
Footnotes
- ^ https://earthquakes.usgs.gov/(accessed on March 2023).
- ^ https://mausam.imd.gov.in/(accessed on March 2023).
- ^ https://wsa.seagrassonline.org/(accessed on January 2023).
- ^ www.andaman.gov.in (accessed on February 2023).
- ^ https://www.R-project.org/(accessed on December 2022).
- ^ https://parivesh.nic.in/Notifications.aspx?id=CRZ/ (accessed on March 2023).
- ^ http://www.indiaenvironmentportal.org.in/content/461888/icrz-notification2019/.
- ^ https://environmentclearance.nic.in/writereaddata/SCZMADocument/ICRZ_Notification2019.pdf.
References
Adulyanukosol K., Poovachiranon S. (2006) in Proceedings of the 3rd International Symposium on SEASTAR2000 and Asian Bio-Logging Science, (The 7th SEASTAR2000 Workshop) Graduate School of Informatics. 41–50 (Kyoto: Kyoto University).
Alcoverro T., Manzanera M., Romero J. (2001). Annual metabolic carbon balance of the seagrass Posidonia oceanica: The importance of carbohydrate reserves. Mar. Ecol. Prog. Ser. 211, 105–116. doi: 10.3354/meps211105
Arnold T. W. (2010). Uninformative parameters and model selection using Akaike’s Information Criterion. J. Wildl. Manage. 74 (6), 1175–1178. doi: 10.2193/2009-367
Bach S. S., Borum J., Fortes M. D., Duarte C. M. (1998). Species composition and plant performance of mixed seagrass beds along a siltation gradient at Cape Bolinao, The Philippines. Mar. Ecol. Prog. Ser. 174, 247–256. doi: 10.3354/meps174247
Barber B. J., Behrens P. J. (1985). Effects of elevated temperature on seasonal in situ leaf productivity of Thalassia testudinum Banks ex König and Syringodium filiforme Kützing. Aquat. Bot. 22, 61–69. doi: 10.1016/0304-3770(85)90029-4
Berkström C., Eggertsen L., Goodell W., Cordeiro C. A. M. M., Lucena M. B., Gustafsson R., et al. (2020). Thresholds in seascape connectivity: the spatial arrangement of nursery habitats structure fish communities on nearby reefs. Ecography 43 (6), 882–896. doi: 10.1111/ecog.04868
Bité J. S., Campbell S. J., McKenzie L. J., Coles R. G. (2007). Chlorophyll fluorescence measures of seagrasses Halophila ovalis and Zostera capricorni reveal differences in response to experimental shading. Mar. Biol. 152, 405–414. doi: 10.1007/s00227-007-0700-6
Bolker B. M. (2008). Ecological models and data in R (Princeton: Princeton University Press). doi: 10.1515/9781400840908
Buesa R. (1975). Population and biological data on turtle grass (Thalassia testudinum Köni) on the north-western Cuban shelf. Aquac 4, 207–226. doi: 10.1016/0044-8486(74)90035-0
Burnham K. P., Anderson D. R. (2002). A practical information-theoretic approach. Model selection and multimodel inference. 2nd ed (New York: Springer). doi: 10.1007/b97636
Campagne C. S., Salles J., Boissery P., Deter J. (2015). The seagrass Posidonia oceanica: ecosystem services identification and economic evaluation of goods and benefits. Mar. pollut. Bull. 97, 391–400. doi: 10.1016/J.MARPOLBUL.2015.05.061
Coles R. G., Lee Long W. J., McKenzie L., Roelofs A., De’ath G. (2000). Stratification of seagrasses in the Great Barrier Reef world heritage area, north-eastern Australia, and the implications for management. Biol. Mar. Mediterr. 7, 345–348.
Costanza R., De Groot R., Sutton P., van der Ploeg S., Anderson S. J., Kubiszewski I., et al. (2014). Changes in the global value of ecosystem services. Glob. Environ. Change 26, 152–158. doi: 10.1016/j.gloenvcha.2014.04.002
Das H. S. (1996). Status of seagrass habitats of the Andaman and Nicobar coast (Coimbatore: Sálim Ali Centre for Ornithology and Natural History).
de la Torre-Castro M., Di Carlo G., Jiddawi N. S. (2014). Seagrass importance for a small-scale fishery in the tropics: the need for seascape management. Mar. pollut. Bull. 83, 398–407. doi: 10.1016/J.MARPOLBUL.2014.03.034
de los Santos C. B., Krause-Jensen D., Alcoverro T., Marbà N., Duarte C. M., Van Katwijk M. M., et al. (2019). Recent trend reversal for declining European seagrass meadows. Nat. Commun. 10, 3356. doi: 10.1038/s41467-019-11340-4
Den Hartog C. (1971). The dynamic aspect in the ecology of seagrass communities. Thalas. Jugoslav. 7, 101–112.
Dennison W. C., Alberte R. S. (1987). Photoadaptation and growth of Zostera marina L.(eelgrass) transplants along a depth gradient. J. Exp. Mar. Biol. Ecol. 98 (3), 265–282. doi: 10.1016/0022-0981(86)90217-0
Dennison W. C., Orth R. J., Moore K. A., Stevenson J. C., Carter V., Kollar S., et al. (1993). Assessing water quality with submersed aquatic vegetation. Bioscience 43, 86–94. doi: 10.2307/1311969
Díaz-Almela E., Marba N., Martínez R., Santiago R., Duarte C. M. (2009). Seasonal dynamics of Posidonia oceanica in Magalluf Bay (Mallorca, Spain): temperature effects on seagrass mortality. Limnol. Oceanogr 54 (6), 2170–2182. doi: 10.4319/lo.2009.54.6.2170
Drew E. A. (1978). Factors affecting photosynthesis and its seasonal variation in the seagrasses Cymodocea nodosa (Ucria) Aschers, and Posidonia oceanica (L.) Delile in the Mediterranean. J. Exp. Mar. Biol. Ecol. 31, 173–194. doi: 10.1016/0022-0981(78)90128-4
D’Souza E., Patankar V., Arthur R., Marbà N., Alcoverro T. (2015). Seagrass herbivory levels sustain site-fidelity in a remnant dugong population. PloS One 10 (10), e0141224. doi: 10.1371/journal.pone.0141224
Duarte C. M. (1991). Seagrass depth limits. Aquat. Bot. 40, 363–377. doi: 10.1016/0304-3770(91)90081-F
Duarte C. M. (2002). The future of seagrass meadows. Environ. Conserv. 29 (2), 192–206. doi: 10.1017/S0376892902000127
Edgar G. J., Stuart-Smith R. D., Willis T. J., Kininmonth S., Baker S. C., Banks S., et al. (2014). Global conservation outcomes depend on marine protected areas with five key features. Nature 506 (7487), 216–220. doi: 10.1038/nature13022
Elliott M., Burdon D., Hemingway K. L., Apitz S. E. (2007). Estuarine, coastal, and marine ecosystem restoration: confusing management and science–a revision of concepts. Estuar. Coast. Shelf Sci. 74 (3), 349–366. doi: 10.1016/j.ecss.2007.05.034
English S., Wilkinson C., Baker V. (1997). Survey manual for tropical marine resources. Australian Institute of Marine Science, Townsville, Australia.
Fortes M. D., Ooi J. L. S., Tan Y. M., Prathep A., Bujang J. S., Yaakub S. M. (2018). Seagrass in Southeast Asia: a review of status and knowledge gaps, and a road map for conservation. Bot. Mar. 61, 269–288. doi: 10.1515/bot-2018-0008
Fourqurean J. W., Powell G. V. N., Kenworthy W. J., Zieman J. C. (1995). The effects of long-term manipulation of nutrient supply on competition between the seagrasses Thalassia testudinum and Halodule wrightii in Florida Bay. Oikos 72, 349. doi: 10.2307/3546120
Garrabou J., Gómez-Gras D., Medrano A., Cerrano C., Ponti M. (2022). Marine heatwaves drive recurrent mass mortalities in the Mediterranean Sea. Glob. Change Biol. 28 (19), 5708–5725. doi: 10.1111/gcb.16301
Geevarghese G. A., Akhil B., Magesh G., Krishnan P., Purvaja R. (2018). A comprehensive geospatial assessment of seagrass distribution in India. Ocean Coast. Manage. 159, 16–25. doi: 10.1016/j.ocecoaman.2017.10.032
Gole S., Gaidhani P., Bose S., Pande A., Johnson J. A., Kuppusamy S. (2022). New distribution record of globally threatened Ocean Turf Grass Halophila beccarii Ascherso from the North Andaman Islands highlights the importance of seagrass exploratory surveys. JoTT 14 (1), 20406–20412. doi: 10.11609/jott.7719.14.1.20406-20412
Gole S., Prajapati S., Prabakaran N., Johnson J. A., Sivakumar K. (2023). Herd size dynamics and observations on the natural history of dugongs (Dugong dugon) in the andaman islands, India. Aquat. Mamm. 49 (1), 53–61. doi: 10.1578/AM.49.1.2023.53
Green E. P., Short F. T. (2003). World atlas of seagrasses (Berkeley: University of California Press).
Greve T. M., Binzer T. (2004). “Which factors regulate seagrass growth and distribution,” in European seagrasses: an introduction to monitoring and management. Eds. Borum J., Duarte C., Krause-Jensen D., Greve T. (Denmark: The M&MS project), 19–24.
Gunderson A. R., Armstrong E. J., Stillman J. H. (2016). Multiple stressors in a changing world: The need for an improved perspective on physiological responses to the dynamic marine environment. Ann. Rev. Mar. Sci. 8, 357–378. doi: 10.1146/annurev-marine-122414-033953
Hemminga M. A., Duarte C. M. (2000). Seagrass ecology (Cambridge: Cambridge University Press). doi: 10.1017/CBO9780511525551
Island Protection Zone Notification (IPZ) (2011). Ministry of Environment and Forests, Government of India, Notification in the Gazette of India, Extraordinary, Part-II, Section 3, Sub-section (ii) dated the 6th January 2011 (New Delhi, India: Ministry of Environment and Forests, Government of India).
Jagtap T. G. (1991). Distribution of seagrasses along the Indian coast. Aquat. Bot. 40, 379–386. doi: 10.1016/0304-3770(91)90082-G
Jagtap T. G. (1992). Marine flora of Nicobar group of islands in Andaman Sea. Indian J. Mar. Sci. 22, 56–58.
Jagtap T. G. (1996). Some quantitative aspects of structural components of seagrass meadows from the southeast coast of India. Bot. Mar. 39, 39–45. doi: 10.1515/botm.1996.39.1-6.39
Jagtap T. G. (1998). Structure of major seagrass beds from three coral reef atolls of Lakshadweep, Arabian Sea, India. Aquat. Bot. 60, 397–408. doi: 10.1016/S0304-3770(97)00092-2
Jagtap T. G., Komarpant D. S., Rodrigues R. S. (2003). Status of a seagrass ecosystem: an ecologically sensitive wetland habitat from India. Wetlands 23 (1), 161–170. doi: 10.1672/0277-5212(2003)023[0161:SOASEA]2.0.CO;2
Japar Sidik B., Muta Harah Z., Fakhrulddin M. L., Khairul Anwar M. S., Arshad A. (2010). Growth performance of Malaysian spoon grass, Halophila ovalis (R. Br.) Hooker f. under different substrate, salinity, and light regime. Coast. Mar. Sci. 34, 103–107.
Japar Sidik B., Muta Harah Z., Short F. T. (2018). “Seagrass in Malaysia: issues and challenges,” in The Wetland Book II: Distribution, Description and Conservation. Eds. Finlayson C.M., Milton G.R., Prentice R.C., Davidson N. C. (Berlin: Springer), 1875–18839. doi: 10.1007/s13280-010-0067-7
Jiang Z., Cui L., Liu S., Zhao C., Wu Y., Chen Q., et al. (2020). Historical changes in seagrass beds in a rapidly urbanizing area of Guangdong province: implications for conservation and management. Glob. Ecol. Conserv. 22, e01035. doi: 10.1016/j.gecco.2020.e01035
Johnson J. B., Omland K. S. (2004). Model selection in ecology and evolution. Trends Ecol. Evol. 19 (2), 101–108. doi: 10.1016/j.tree.2003.10.013
Kilminster K., McMahon K., Waycott M., Kendrick G. A., Scanes P., McKenzie L., et al. (2015). Unravelling complexity in seagrass systems for management: Australia as a microcosm. Sci. Total Environ. 534, 97–109. doi: 10.1016/J.SCITOTENV.2015.04.061
Koch E. W. (2001). Beyond light: Physical, geological, and geochemical parameters as possible submersed aquatic vegetation habitat requirements. Estuaries 24, 1–17. doi: 10.2307/1352808
Lee K. S., Dunton K. H. (2000). Effects of nitrogen enrichment on biomass allocation, growth, and leaf morphology of the seagrass Thalassia testudinum. Mar. Ecol. Prog. Ser. 196, 39–48. doi: 10.3354/meps196039
Lee K. S., Park S. R., Kim Y. K. (2007). Effects of irradiance, temperature, and nutrients on growth dynamics of seagrasses: a review. J. Exp. Mar. Biol. Ecol. 350 (1-2), 144–175. doi: 10.1016/j.jembe.2007.06.016
Lee Long W. J. (1996). Deepwater seagrasses in North-eastern Australia-How deep? How meaningful? (Sciences- University of Western Australia, Australia: Seagrass Biology-Proceedings of an International Workshop, Sciences UWA).
Lipkin Y., Beer S., Zakai D. (2003). “The seagrasses of the eastern Mediterranean and the Red Sea,” in World Atlas of Seagrasses. Eds. Green E. P., Short F. T. (London, England: University of California Press), 65–73.
Luong C. V., Thao N. V., Komatsu T., Ve N. D., Tien D. D. (2012). Status and threats on seagrass beds using GIS in Vietnam. In Proceedings SPIE – Int. Soc Opt. Eng. 8525, 12. doi: 10.1117/12.977277
Malmer A., Grip H. (1994). Converting tropical rainforest to forest plantation in Sabah, Malaysia. Part II. Effects on nutrient dynamics and net losses in stream water. Hydrol. Process. 8 (3), 195–209. doi: 10.1002/hyp.3360080303
McKenzie L., Nordlund L. M., Jones B. L., Cullen-Unsworth L. C., Roelfsema C. M., Unsworth R. K. (2020). The global distribution of seagrass meadows. Environ. Res. Lett. 15, 074041. doi: 10.1088/1748-9326/ab7d06
Minguito-Frutos M., Boada J., Pagès J. F., Marco-Méndez C., Arthur R., Adams M. P., et al. (2023). Species-specific acclimatization capacity of key traits explains global vertical distribution of seagrass species. Glob. Ecol. Biogeogr. 32 (6), 976–986. doi: 10.1111/geb.13673
Mtwana Nordlund L., Koch E. W., Barbier E. B., Creed J. C. (2016). Seagrass ecosystem services and their variability across genera and geographical regions. PloS One 11 (10), e0163091. doi: 10.1371/journal.pone.0163091
Mumby P. J., Dahlgren C. P., Harborne A. R., Kappel C. V., Micheli F., Brumbaugh D. R., et al. (2006). Fishing, trophic cascades, and the process of grazing on coral reefs. Science 311 (5757), 98–101. doi: 10.1126/science.1121129
Myers N., Mittermeier R. A., Mittermeier C. G., Da Fonseca G. A., Kent. J. (2000). Biodiversity hotspots for conservation priorities. Nat 403 (6772), 853–858. doi: 10.1038/35002501
Orth R. J., Carruthers T. J., Dennison W. C., Duarte C. M., Fourqurean J. W., Heck K. L., et al. (2006). A global crisis for seagrass ecosystems. Bioscience 56, 987–996. doi: 10.1641/0006-3568(2006)56[987:AGCFSE]2.0.CO;2
Paulose N. E., Dilipan E., Thangaradjou T. (2013). Integrating Indian remote sensing multi-spectral satellite and field data to estimate seagrass cover change in the Andaman and Nicobar Islands, India. Ocean Sci. J. 48, 173–181. doi: 10.1007/s12601-013-0014-1
Ragavan P., Jayaraj R. S., Muruganantham M., Jeeva C., Ubare V. V., Saxena A., et al. (2016). Species composition and distribution of seagrasses of the andaman and nicobar islands. Vegetos 29, 78–87.
Rahman S. A. (2017). “Johor’s Forest City faces critical challenges,” in Trends in Southeast Asia (Singapore: ISEAS-Yusof Ishak Institute).
Rahman S. A., Yaakub S. M. (2020). Socio-economic valuation of seagrass meadows in the Pulai River Estuary, Peninsular Malaysia, through a wellbeing lens. Mar. Freshw. Res. 71, 877–891. doi: 10.1071/MF19208
Ramesh R., Banerjee K., Selvam A. P., Lakshmi A., Krishnan P., Purvaja R. (2018). Legislation and policy options for conservation and management of seagrass ecosystems in India. Ocean Coast. Manage. 159, 46–50. doi: 10.1016/j.ocecoaman.2017.12.025
Sabilah A. A., Amran M. A. (2020). “Comparison of seagrass cover from multi-scale imagery,” in IOP Conference Series: Earth and Environmental Science, Vol. 564 (1), 012063. doi: 10.1088/1755-1315/564/1/012063
Sachithanandam V., Mageswaran T., Sridhar R., Purvaja R., Ramesh R. (2014). Assessment of Cyclone Lehar’s impact on seagrass meadows in Ross and Smith Islands, North Andaman. Nat. Hazards. 72, 1253–1258. doi: 10.1007/s11069-014-1040-8
Savurirajan M., Equbal J., Lakra R. K., Satyam K., Thiruchitrambalam G. (2018). Species diversity and distribution of seagrasses from the South Andaman, Andaman and Nicobar Islands, India. Bot. Mar. 61 (3), 225–234. doi: 10.1515/bot-2017-0109
Savurirajan M., Lakra R. K., Ganesh T. (2015). A new record of the seagrass Halophila beccarii Ascherson from the Port Blair coast, Andaman and Nicobar Islands, India. Bot. Mar. 58 (5), 409–413. doi: 10.1515/bot-2014-0076
Schubert N., Freitas C., Silva A., Costa M. M., Barrote I., Horta P. A., et al. (2018). Photo acclimation strategies in northeastern Atlantic seagrasses: Integrating responses across plant organizational levels. Sci. Rep. 8, 14825. doi: 10.1038/s41598-018-33259-4
Seddon S., Cheshire A. C. (2001). Photosynthetic response of Amphibolis Antarctica and Posidonia australis to temperature and desiccation using chlorophyll fluorescence. Mar. Ecol. Prog. Ser. 220, 119–130. doi: 10.3354/meps220119
Short F. T., Carruthers T., Dennison W., Waycott M. (2007). Global seagrass distribution and diversity: a bioregional model. J. Exp. Mar. Biol. Ecol. 350, 3–20. doi: 10.1016/j.jembe.2007.06.012
Short F. T., Polidoro B., Livingstone S. R., Carpenter K. E., Bandeira S., Bujang J. S., et al. (2011). Extinction risk assessment of the world’s seagrass species. Biol. Conserv. 144, 1961–1971. doi: 10.1016/j.biocon.2011.04.010
Short F. T., Wyllie-Echeverria S. (1996). Natural and human-induced disturbance of seagrasses. Environ. Conserv. 23, 17–27. doi: 10.1017/S0376892900038212
Silva J., Barrote I., Costa M. M., Albano S., Santos R. (2013). Physiological responses of Zostera marina and Cymodocea nodosa to light-limitation stress. PloS One 8, e81058. doi: 10.1371/journal.pone.0081058
Sudo K., Quiros T. A. L., Prathep A., Luong C. V., Lin H. J., Bujang J. S., et al. (2021). Distribution, temporal change, and conservation status of tropical seagrass beds in Southeast Asia: 2000–2020. Front. Mar. Sci. 8. doi: 10.3389/fmars.2021.637722
Tan Y. M., Dalby O., Kendrick G. A., Statton J., Sinclair E. A., Fraser M. W., et al. (2020). Seagrass restoration is possible: insights and lessons from Australia and New Zealand. Front. Mar. Sci. 7. doi: 10.3389/fmars.2020.00617
Terrados J., Duarte C. M., Fortes M. D., Borum J., Agawin N. S. R., Bach S., et al. (1998). Changes in community structure and biomass of seagrass communities along gradients of siltation in SE Asia. Estuar. Coast. Shelf Sci. 46 (5), 757–768. doi: 10.1006/ecss.1997.0304
Thangaradjou T., Bhatt J. R. (2018). Status of seagrass ecosystems in India. Ocean Coast. Manage. 159, 7–15. doi: 10.1016/j.ocecoaman.2017.11.025
Thangaradjou T., Sivakumar K., Nobi E. P., Dilipan E. (2010a). “Distribution of seagrasses along the Andaman and Nicobar Islands: a post tsunami survey,” in Recent trends in biodiversity of Andaman and Nicobar Islands. Eds. Raghunathan C., Sivaperuman C. (Kolkata, India: Zoological Survey of India), 157–160.
Thangaradjou T., Raviendran V. S., Sivakumar K., Kannan L., Khan S. A. (2010b). “Occurrence and distribution of seagrasses in great Nicobar Islands,” in Recent trends in biodiversity of Andaman and Nicobar Islands. Eds. Raghunathan C., Sivaperuman C. (Kolkata, India: Zoological Survey of India), 141–146.
Tigga M., Rao P. S. (2004). Marine flora of the Rani Jhansi Marine National Park, Andamans (India: Seaweed Res Utiln. Special), 23–25.
Tuya F., Fernández-Torquemada Y., Zarcero J., del Pilar-Ruso Y., Csenteri I., Espino F., et al. (2019). Biogeographical scenarios modulate seagrass resistance to small-scale perturbations. J. Ecol. 107, 1263–1275. doi: 10.1111/1365-2745.13114
United Nations Environment Programme [UNEP] (2020). Out of the Blue: The Value of Seagrasses to the Environment and to People (Nairobi: UNEP).
Unsworth R. K. (2007). Aspects of the ecology of Indo-Pacific seagrass systems (Doctoral dissertation, University of Essex).
Unsworth R. K., Nordlund L. M., Cullen-Unsworth L. C. (2019). Seagrass meadows support global fisheries production. Conserv. Lett. 12, e12566. doi: 10.1111/conl.12566
Vermaat J. E., Agawin N. S. R., Fortes M. D. (1998). The capacity of seagrasses to survive increased turbidity and siltation: The significance of growth form and light use. Oceanogr. Lit. Rev. 6 (45), 1002.
Waycott M., Duarte C. M., Carruthers T. J. B., Orth R. J., Dennison W. C., Olyarnik S., et al. (2009). Accelerating loss of seagrasses across the globe threatens coastal ecosystems. Proc. Natl. Acad. Sci. U.S.A. 106, 12377–12381. doi: 10.1073/pnas.0905620106
Keywords: intertidal, water depth, substrate availability, niche partitioning, seagrass distribution, dugongs, deepwater habitats
Citation: Gole S, Prajapati S, Prabakaran N, Das H, Kuppusamy S and Johnson JA (2023) Spatial diversity and habitat characteristics of seagrass meadows with management recommendations in the Andaman and Nicobar Islands, India. Front. Mar. Sci. 10:1251887. doi: 10.3389/fmars.2023.1251887
Received: 02 July 2023; Accepted: 06 November 2023;
Published: 07 December 2023.
Edited by:
Joao M. Neto, University of Coimbra, PortugalReviewed by:
Stuart James Kininmonth, The University of Queensland, AustraliaThadickal V. Joydas, King Fahd University of Petroleum and Minerals, Saudi Arabia
Copyright © 2023 Gole, Prajapati, Prabakaran, Das, Kuppusamy and Johnson. This is an open-access article distributed under the terms of the Creative Commons Attribution License (CC BY). The use, distribution or reproduction in other forums is permitted, provided the original author(s) and the copyright owner(s) are credited and that the original publication in this journal is cited, in accordance with accepted academic practice. No use, distribution or reproduction is permitted which does not comply with these terms.
*Correspondence: Jeyaraj Antony Johnson, amFqQHdpaS5nb3YuaW4=
†Present address: Sivakumar Kuppusamy, Department of Ecology, School of Life Sciences, Pondicherry University, Puducherry, India